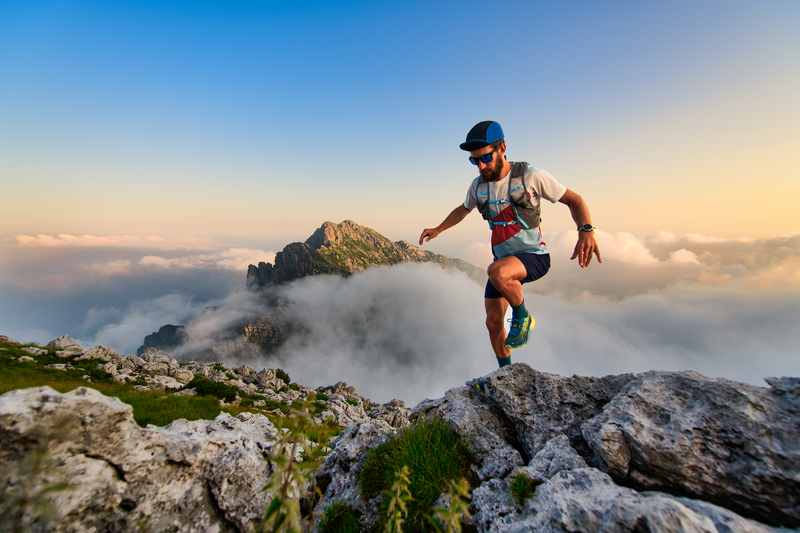
95% of researchers rate our articles as excellent or good
Learn more about the work of our research integrity team to safeguard the quality of each article we publish.
Find out more
ORIGINAL RESEARCH article
Front. Pharmacol. , 16 March 2023
Sec. Neuropharmacology
Volume 14 - 2023 | https://doi.org/10.3389/fphar.2023.1135929
Synthetic cannabinoids have exhibited unpredictable abuse liabilities, especially self-administration (SA) responses in normal rodent models, despite seemingly inducing addiction-like effects in humans. Thus, an efficient pre-clinical model must be developed to determine cannabinoid abuse potential in animals and describe the mechanism that may mediate cannabinoid sensitivity. The Cryab knockout (KO) mice were recently discovered to be potentially sensitive to the addictive effects of psychoactive drugs. Herein, we examined the responses of Cryab KO mice to JWH-018 using SA, conditioned place preference, and electroencephalography. Additionally, the effects of repeated JWH-018 exposure on endocannabinoid- and dopamine-related genes in various addiction-associated brain regions were examined, along with protein expressions involving neuroinflammation and synaptic plasticity. Cryab KO mice exhibited greater cannabinoid-induced SA responses and place preference, along with divergent gamma wave alterations, compared to wild-type (WT) mice, implying their higher sensitivity to cannabinoids. Endocannabinoid- or dopamine-related mRNA expressions and accumbal dopamine concentrations after repeated JWH-018 exposure were not significantly different between the WT and Cryab KO mice. Further analyses revealed that repeated JWH-018 administration led to possibly greater neuroinflammation in Cryab KO mice, which may arise from upregulated NF-κB, accompanied by higher expressions of synaptic plasticity markers, which might have contributed to the development of cannabinoid addiction-related behavior in Cryab KO mice. These findings signify that increased neuroinflammation via NF-κB may mediate the enhanced addiction-like responses of Cryab KO mice to cannabinoids. Altogether, Cryab KO mice may be a potential model for cannabinoid abuse susceptibility.
The development of synthetic cannabinoids was initially aimed at understanding the endocannabinoid system and developing potential pharmacotherapies for cannabinoid-induced disorders (de Luca and Fattore, 2018; Diao and Huestis, 2019). However, the growing number of novel synthetic cannabinoids has become a major public health concern as the identification of specific synthetic cannabinoids consumed by abusers became difficult. Additionally, novel cannabinoids are classified under the highest drug schedule due to their adverse effects and lack of medical use (Drug Enforcement Administration, 2015). Prevalence of their abuse might be due to their stronger effects compared to trans-Δ⁹-tetrahydrocannabinol (THC), the main psychoactive component of marijuana (Weinstein et al., 2017), and being “legally” available online. Despite inducing adverse effects (MacDonald and Pappas, 2016; Schmitz and Richert, 2020; National Institute on Drug Abuse, 2021), synthetic cannabinoids have persistently circumvented legal regulations and initial detection owing to their varying chemical composition, resulting from the constant modification of functional groups. Thus, evaluating the potential dangers of these substances has piqued the interest of the scientific community.
The pharmacological properties of synthetic cannabinoids are similar to those of exogenous phytocannabinoids, with synthetic cannabinoids being more potent due to their higher affinity for cannabinoid receptors (Tai and Fantegrossi, 2014). Recent studies have suggested that CB1 receptors are ubiquitously expressed throughout the central nervous system, forming part of the endocannabinoid system (Fagundo et al., 2013), which is interconnected with the mesolimbic dopaminergic pathway, a signaling network implicated in mediating drug addiction (Adinoff, 2004; Vlachou and Panagis, 2014). The endocannabinoid system may mediate addiction-like behaviors by regulating γ-Aminobutyric acid (GABA)/glutamate neurotransmission via retrograde signaling of CB1 receptors expressed on GABAergic/glutamatergic neurons, thus influencing dopaminergic neuronal activity (Parsons and Hurd, 2015). This may provide the basis for cannabinoid-induced addiction in humans, leading to cannabinoid use disorders. However, some studies have demonstrated that not all synthetic cannabinoids can induce significant addiction-like behaviors in animal models (Polissidis et al., 2009; Hyatt and Fantegrossi, 2014; Tampus et al., 2015; Rodríguez-Arias et al., 2016; Bilel et al., 2019), especially in self-administration (SA) paradigms, contradictory to clinical reports and personal accounts (Le Boisselier et al., 2017; de Luca and Fattore, 2018; Leung et al., 2020). This presents several challenges in screening the abuse potential of novel cannabinoids and the involvement of complex neural mechanisms in the pathophysiology of cannabinoid abuse. Thus, there is a need to develop an efficient tool for evaluating the abuse potential of synthetic cannabinoids.
Accumulating evidence suggest the involvement of neuroinflammation in cannabinoid addiction, potentially entailing both pro- and anti-inflammatory activities (Kozela et al., 2010; Kinsey et al., 2011; Javed et al., 2016; Bayazit et al., 2017). Some cannabinoids also seem to mitigate the addictive effects of abused drugs (Xi et al., 2011; Delis et al., 2017; Lin et al., 2018). Increased neuroinflammation induced by drugs of abuse may modulate glutamate synapses via increased astroglia glutamate reuptake (Boycott et al., 2008; Ramos et al., 2010), thus affecting glutamate-dependent synaptic plasticity (Park et al., 2015), which may reinforce drug-seeking behavior (Kauer and Malenka, 2007). Since some cannabinoids may reduce cytokine production (Xu et al., 2007) and deactivate glial cells (Rodrigues et al., 2014), their potential effects on neuroinflammation may provide another possible mechanism that may also mediate cannabinoid addiction development. This further suggests their inconsistent addiction-inducing effects in rodent models to probably involve inflammatory processes.
Transgenic mice models have been widely used to elucidate the pathophysiology of substance abuse disorder (Spanagel and Sanchis-Segura, 2003; Sora et al., 2010). These models are commonly developed based on genes that are differentially expressed in response to drugs of abuse. These genes are then modified in mice to generate transgenic rodent models (Yazdani et al., 2015; Szumlinski et al., 2017). Preliminary findings have suggested that treatment with methamphetamine or cocaine modulates the expression of Cryab in mice (Ministry of Food and Drug Safety, 2021). Cryab codes a small heat shock protein (CRYAB) with anti-inflammatory functions, such as reducing apoptosis through enhancing PI3K and activating AKT signaling (Ren et al., 2018), and inhibiting inflammatory responses in glial cells by downregulating pro-inflammatory mediators (Kuipers et al., 2017; Guo et al., 2019). Although initially, CRYAB functions as a molecular chaperone and aggregates misfolded proteins (Dai et al., 2022), thus preventing detrimental protein accumulation during stress (Calderwood et al., 2019). Furthermore, it may also participate in various types of cancer (Zhang et al., 2019), providing it a potential anti-inflammatory and anti-apoptotic role. Although the administration of addictive drugs may alter the expression of Cryab, the role of Cryab in cannabinoid-induced addiction has not yet been investigated. Therefore, a Cryab transgenic mouse model may be possibly used to determine the abuse potential of novel substances and identify susceptibility to potentially addictive drugs, such as synthetic cannabinoids.
Thus, this study aimed to provide an animal model that would be susceptible to cannabinoid abuse by determining the responses of Cryab knockout (KO) mice to 1-naphthalenyl(1-pentyl-1H-indol-3-yl)-methanone (JWH-018; a representative cannabinoid) in addiction-associated behavioral paradigms. Given the effect of Cryab on inflammation and the involvement of inflammation in addiction development, Cryab KO mice might exhibit increased sensitivity to JWH-018 abuse. As neural electrical activity indicates physiological responses to drugs of abuse, the electroencephalogram of Cryab KO mice may perhaps also differ from those of wild-type (WT) mice after repeated JWH-018 exposure. Since cannabinoid addiction may also involve endocannabinoid, dopaminergic, and neuroinflammatory pathways, repeated exposure to JWH-018 might differentially modulate endocannabinoid-related, dopamine-related, and neuroinflammation-related genes and/or proteins in Cryab KO mice, contributing to their divergent cannabinoid-induced behaviors when compared with WT mice.
The Department of Pharmacy, Chungbuk National University (Cheongju City, Korea) provided two male and two female Cryab KO mice (aged 8 weeks). Transgenic mice were bred with C57BL/6N mice (aged 8 weeks, 22–25 g) obtained from Hanlim Animal Laboratory Co. (Hwasung, Korea), to obtain heterozygous Cryab (Cryab Het) mice. All procured mice were acclimatized in the animal room for 1 week prior to being used. Male and female Cryab Het mice (aged 8 weeks, F1) were subsequently bred to obtain male Cryab KO mice (F2), which were used for all experiments in this study (aged 8–12 weeks, 25–30 g). Newborn pups were genotyped at 3–4 weeks old using DNA from the tail. Only male mice were used for behavioral and molecular experiments. Mice for experiments were housed together in cages (4–6 mice per cage). To obtain next generation mice (F3), male and female Cryab Het mice (F2) were bred. All mice were housed in a room under controlled conditions (circadian cycle, 12-h light/dark cycle (7 AM–7 PM); temperature, 22°C ± 2°C) and had access to food ad libitum. The schedule of experiments is presented in Figure 1. Animal treatment and maintenance procedures were performed according to the Principles of Laboratory Animal Care (NIH Publication No. 85–23, revised 1985) and the Animal Care and Use Guidelines of Sahmyook University, South Korea (SYUIACUC 2021-020 and SYUIACUC 2022-001).
FIGURE 1. Schedule of behavioral experiments. Mice are genotyped between 3 and 5 weeks of age using tail samples. Mice are then segregated according to genotype. Starting at 8 weeks of age, only male mice are housed according to the experiment. Brains may be collected at the end of each behavioral experiment.
JWH-018 was synthesized according to reported procedures (Huffman et al., 2005). In brief, indole was reacted with pentyl bromide and potassium hydroxide in DMF. The obtained N-pentylindole was acylated with 1-naphthoyl chloride in the presence of Me2AlCl in dichloromethane to produce JWH-018. The structure was confirmed by the following spectroscopic analyses: 1H-NMR (500 MHz, CDCl3) δ 8.52-8.48 (m, 1H), 8.20 (d, J = 8.6 Hz, 1H), 7.95 (t, J = 8.3 Hz, 1H), 7.90 (d, J = 8.0 Hz, 1H), 7.65 (dd, J = 6.9, 1.1 Hz, 1H), 7.54-7.45 (m, 3H), 7.40-7.35 (m, 4H), 4.05 (t, J = 7.4 Hz, 2H), 1.82-1.76 (m, 2H), 1.32-1.22 (m, 4H), 0.85 (t, J = 7.2 Hz, 3H); 13C-NMR (125 MHz, CDCl3) δ 192.1, 139.2, 138.1, 137.1, 133.8, 130.9, 130.1, 128.3, 127.1, 126.8, 126.4, 126.1, 125.9, 124.7, 123.7, 123.0, 122.9, 117.6, 110.1, 47.3, 29.6, 29.0, 22.3, 14.0; HR-MS calculated for C24H23NO [M + H]+ 342.1852, found 342.1852; HPLC purity 99.46%. In all experiments, JWH-018 was dissolved in a solution comprising Tween 80, absolute ethanol, and 0.9% (w/v) saline (SAL) in the ratio 1:1:48 to obtain the desired dosage (0.3 mg/kg body weight). JWH-018 was selected as it has been used in several early cannabinoid-based studies, and the dose was selected based on reports that demonstrated significant addiction-related effects of JWH-018 and other cannabinoids in rodents (de Luca et al., 2015; Ossato et al., 2017).
Baseline locomotor activity was evaluated using a square Plexiglas open-field arena (42 cm × 42 cm × 42 cm), as per the methodology described in previous studies (Custodio et al., 2018; dela Peña et al., 2019; Kim et al., 2019). Mice were placed at the center of the arena and allowed to explore for 12 min for three consecutive days. The recorded data of the first 2 min were excluded from the analysis, as this period was considered a habituation period. The distance moved (cm) and the movement duration (s) of each mouse was measured using an automated system (EthoVision, Noldus, Netherlands).
Basic cognition in mice was assessed using a “Y”-shaped maze comprising three identical arms (each arm spaced at an angle of 120°) with each arm measuring 5 cm × 35 cm × 10 cm. Each mouse was placed at the end of an arm and allowed to explore the maze freely for 8 min. Spontaneous alternating behavior and total entry in each arm were obtained following a previous study (Custodio et al., 2018). The alternation behavior (actual alternation) was described as the consecutive entry into three arms (continuous exploration of three different arms (e.g., ABC, BCA, or CAB) with stepwise sequence combinations. The maximum number of alternations was defined as the total number of arms entered minus two. The percentage spontaneous alternation behavior was calculated as follows: spontaneous alternation behavior (%) = (actual alternations/maximum alternations) × 100.
General motor balance and coordination of mice were measured using a rotating rod (Ugo Basile, Varese, Italy) at a fixed speed of 36 rpm, as per the methodology described in previous studies (Custodio et al., 2018; dela Peña et al., 2019). Two consecutive days before the experiment, the mice were allowed to habituate and were trained to run on the rotating rod for 3 min (T1 and T2). On the day of the experiment, “challenge day” (Ch), the mice were placed on the rotating rod for 10 min. Mice that fell during the experiment were gently placed back onto the rota-rod. The falling latency and frequency were recorded.
The impulsive behavior of the mice was assessed using a 50 cm long cylindrical Plexiglass rod support with a round Plexiglass platform (diameter = 20 cm; thickness = 1 cm) on both ends. Each mouse was placed at the center of the platform, and its behavior was observed for 10 min. The first fall latency and falling frequency were recorded.
Inherent anxiety-like behavior in mice was determined using a plus-maze comprising four arms, two open and two closed arms measuring 30 cm × 6 cm (closed arms were enclosed by 20 cm high walls). The center of the maze contained a 6 cm × 6 cm delimited area. At the start of each test, individual mice were placed at the center, facing one of the open arms. The percentage of entries (100 × open/total entries) and time spent in the open arms were calculated after each 5 min test, similar to a previous study (dela Peña et al., 2019). The EthoVision system was used for automated recording and analysis.
The mice were anesthetized with 0.02 mL Zoletil® (50 mg/mL) and Rompun® (xylazine 23.32 mg/mL) prepared in SAL. A three-channel tethered head mount (8200K3-iS/iSE) was placed and fixed with stainless screws positioned in the frontal region (A/P: −1.0 mm, M/L −1.5 mm) and posterior brain (A/P: −1.0 mm, M/L ±1.5 mm), conductive epoxy, and dental cement. Standard operating procedures were performed to minimize animal discomfort. The mice were allowed to recover for 5 days before recording. Data acquisition and analyses were performed according to the methodology described in previous studies (Botanas et al., 2021; Custodio et al., 2021) but with some modifications. On the last recovery day, mice were allowed to acclimatize to the EEG apparatus for 2 h (unrecorded). On the next day, a baseline recording for drug-naïve mice was performed for 2 h with an initial 10 min of habituation using a computer-based software (Pinnacle Technology, Inc., Lawrence, KS, United States). One group of mice was treated with JWH-018 (0.3 mg/kg body weight) or vehicle (VEH) for 7 days. After the initial baseline recording (Day 0), EEG was recorded on the first and last day of treatment. EEG wave changes relative to the baseline recording were calculated.
The SA test was performed as reported previously (Custodio et al., 2022). Lever pressing training, which was based on food pellet reward under continuous reinforcement, was performed for three consecutive days [30 min per session (AM and PM sessions)]. In this study, lever pressing behavior was determined when mice earned 15 reinforcers at the end of the session on the third day and 75% of responses were on the active lever (Thomsen and Caine, 2011). After training, surgery was performed to insert a 0.28 mm inner diameter catheter into the jugular vein and mid-scapular region of mice anesthetized with Zoletil® (50 mg/mL) and Rompun® (xylazine 23.32 mg/mL) prepared in SAL. The catheterized mice were allowed to recover for 5 days. During recovery, the catheters were infused daily with 0.02 mL of 0.9% SAL containing heparin (20 IU/mL) and gentamicin (0.08 mg/mL). Catheter patency was determined by flushing catheters with a 0.02 mL of the Zoletil®/Rompun® mixture, and the loss of muscle tone was observed in mice within 10 s of infusion. During the SA experiment, catheters were flushed with SAL containing gentamicin or heparin immediately before and after each SA session. Mice were assessed daily in 2-h SA sessions under a fixed-ratio (FR) 1 schedule for 7 days, followed by assessment with an FR2 schedule for 3 days (Graphic State Notation 4; Coulbourn Instruments, Whitehall, PA, United States). Mice were fed a pellet diet (2 g) daily. In each session, both left and right levers were available. Left (active) lever pressing was followed by infusion of 0.05 mL JWH-018 (0.03 mg/kg body weight/infusion) or SAL over 10 s. During the infusion, the stimulus light over the lever was illuminated for 20 s. Lever presses during “time-out” periods were recorded but had no effect. Right (inactive) lever presses were also recorded, but not reinforced. Active and inactive lever presses and the number of infusions for each daily session were recorded for evaluating SA behavior.
The apparatus comprised two compartments (dimensions = 17.4 cm × 12.7 cm × 12.7 cm) with a removable guillotine door that separated the compartments. One compartment had smooth black walls and white flooring, whereas the other had white-dotted black walls and textured white flooring. An illumination of 12 lux was maintained throughout the experiment. A computer system (EthoVision) was used for recording animal movements and stay durations in the compartments. The protocol was performed according to previous studies with some modifications (Kim et al., 2019; Custodio et al., 2020; Sayson et al., 2020). The test comprised the following three phases: (A) habituation (days 1–3) and pre-conditioning (day 4; 15 min), (B) conditioning (days 5–12; 30 min), and (C) post-conditioning (day 13; 15 min). During habituation, mice were allowed to freely explore the entire apparatus. An initial trial (pre-conditioning) was performed to determine the stay duration of each mouse in each of the compartments. Mice were assigned to groups based on the pre-conditioning phase such that their non-preferred side was designated as the drug-paired compartment. In the conditioning phase, mice were administered JWH-018 (0.3 mg/kg body weight) or VEH and placed in the drug-paired compartment. On alternate days, the mice received VEH and were confined to the VEH-paired compartment. During the post-conditioning phase, the mice were not treated and were allowed to explore both compartments (similar to the pre-conditioning phase). The CPP score was calculated as the difference in the time spent by the mice in their respective drug-paired compartments between the post-conditioning and pre-conditioning phases.
Drug-naïve or JWH-018-treated mice (7 days) were euthanized and decapitated to excise the brain 30 min after the last treatment. The ventral tegmental area (VTA) and nucleus accumbens (NAC) were isolated using a mouse brain matrix on ice. The samples were rapidly frozen at −80°C before further processing. The subsequent steps followed previous methods (Custodio et al., 2022) and were according to MIQE guidelines. Total RNA was isolated with TRIzol® (Invitrogen, Carlsbad, CA, United States), following the manufacturer’s protocol, and purified using the Hybrid-RTM kit (Geneall Biotechnology, Seoul, Korea). RNA concentrations were determined using Colibri Microvolume Spectrometer (Titertek-Berthold, Pforzheim, Germany). Total RNA (1 μg) was reverse-transcribed into complementary DNA (cDNA) using AccuPower® CycleScript RT PreMix (Bioneer, Seoul, Korea), following the manufacturer’s instructions. Aliquots of cDNA were stored at −20°C. Target genes were amplified using custom sequence-specific primers (Cosmogenetech, Seoul, Korea) and detected using SYBR® Green (Solgent, Daejeon, Korea). The primer sequences are shown in Supplementary Table S1. The input cDNA concentration was 2.5 μg/μL. The PCR conditions were as follows: 94°C for 1 min (denaturing step), followed by annealing at primer-specific temperature for 1 min, and 72°C for 45 s. qRT-PCR was performed using samples in triplicate. The expression of target genes was normalized to that of Gapdh. The results are expressed as relative expression calculated using the 2−ΔΔCT method (VanGuilder et al., 2008).
Brains of a separate cohort of VEH or JWH-018-exposed (7-day treatment) mice were collected and isolated similarly as described in the previous section. Protein extraction was done according to previous methods with slight modifications (Sayson et al., 2020). Brain tissues were lysed in 400 μL homogenization buffer [radioimmunoprecipitation assay buffer (Biosesang Inc., Seongnam, Korea) supplemented with cOmplete™ ULTRA protease inhibitor cocktail tablets (05892791001, Sigma-Aldrich) and PhosSTOP™ phosphatase inhibitor cocktail tablets (04906845001, Sigma-Aldrich)]. The tissue extracts were centrifuged at 16,000 g at 4°C for 20 min. Dopamine concentration was determined using the dopamine ELISA kit (KA1887, Abnova, Taiwan), following the manufacturer’s instructions. Briefly, standards, controls, and samples were acylated and incubated with dopamine antiserum for 2 h at room temperature (20°C–25°C) on a shaker (approximately 600 rpm). Next, the samples in the wells were washed and incubated with the enzyme conjugate for 30 min. After washing the samples, the substrate was pipetted into individual wells, and the samples were incubated for 25 min. The stop solution was then added to each well. The absorbance of the reaction mixture was measured at 450 nm using an EMax Plus Microplate Reader (Molecular Devices; San Jose, CA, United States). All measurements were performed in duplicates.
The striatum (STR) of VEH or JWH-018-exposed (7-day treatment) mice was isolated. Procedure was done according to previous studies (Sayson et al., 2020; Custodio et al., 2021). The samples were then heated at 95°C for 5 min. Protein lysates (20 μg) were subjected to sodium dodecyl sulfate-polyacrylamide gel electrophoresis on a 12% gel. The resolved proteins were transferred onto nitrocellulose membranes. The membrane was blocked with 5% bovine serum albumin (BSA) prepared in Tris-buffered saline containing 0.1% Tween-20 (TBST) for 1 h and then incubated overnight with specific primary antibodies at 4°C. Then, the membrane was washed with TBST and incubated with horseradish peroxidase-conjugated anti-rabbit (1:3,000) or anti-mouse secondary antibodies (1:5,000) for 1 h. Protein bands were visualized based on enhanced chemiluminescence (Clarity Western ECL; Bio-Rad Laboratories, Hercules, CA, USA) using the ChemiDoc Imaging System (Image Lab software, version 6.0; Bio-Rad). The levels of phosphorylation-independent proteins were normalized to those of β-Actin. The levels of the phosphorylated form of proteins were normalized to those of the total form of proteins. Fold change was determined by normalizing the values of the test groups to those of the WT VEH group. The antibodies used for the Western blot analysis are listed in Supplementary Table S2.
Standard protocols were used, following previously described methods (Custodio et al., 2022). After the last SA session, the mice were intracardially perfused with perfusion solution [0.05 M phosphate-buffered saline (PBS)] and perfusate [4% paraformaldehyde (PFA) in 0.1 M phosphate buffer]. The brain tissues were stored in PFA solution at 4°C overnight, followed by incubation with 30% sucrose solution at 4°C. The brain samples were sectioned to a thickness of 35 μm using a Leica CM1850 cryostat (Wetzlar, Germany), following mouse brain stereotaxic coordinates (Paxinos and Franklin, 2001). For this experiment, the STR was selected for analysis as this region is commonly implicated in inflammation-associated behavioral alterations, such as addiction (Krasnova et al., 2016; Vonder Haar et al., 2019). The brain sections were carefully washed with 1× PBS and subsequently incubated in a protein-blocking solution (5% BSA and 0.3% Triton X-100 in 1× PBS) for 1 h at room temperature. Next, the sections were incubated with primary antibodies diluted in a protein-blocking solution for 3 days at 4°C. The primary antibody used in this analysis is listed in Supplementary Table S2. After washing, the samples were incubated overnight with Alexa fluor-555-conjugated goat anti-mouse (Thermo Fisher Scientific A32727, RRID: AB_2633276) antibody at 4°C. The samples were washed, incubated with Hoechst for 10 min, and mounted on 76 mm × 26 mm × 1 mm clean positively charged microscope slides (Walter Products Inc., Canada). Mounted sections were cured with Permount® Mounting Medium UN1294 (Fisher Chemical, NJ, United States), covered with 24 mm × 50 mm microscope cover glasses (Marienfeld Laboratory Glassware, Germany), and allowed to dry for 24 h at room temperature. The corrected total cell fluorescence (CTCF) level was measured in each sample using ImageJ 1.53k (NIH, Maryland, United States) as reported previously (Hammond, 2014).
All mice were randomized for treatment. The researchers were blinded to the treatment of the animals while conducting the tests and analyzing the data. Statistical analyses were performed using GraphPad Prism v7 (GraphPad Software Inc., San Diego, CA, United States). Data are presented as the mean ± standard error of the mean (S.E.M.), unless specified otherwise. The means were analyzed using one-way or two-way analysis of variance (ANOVA) with or without repeated measures (RM), followed by Tukey’s or Bonferroni’s multiple comparison test, when appropriate. Differences were considered statistically significant at p < 0.05. The p-value and detailed statistical analysis (e.g., genotype, treatment, time, days, etc.) are indicated.
Although controversial, inherent aberrant behaviors were previously correlated with substance abuse co-morbidity (Herrero et al., 2008; Volkow, 2009; Kabir et al., 2016), therefore we screened the general behavior of Cryab KO mice in various behavioral assays. OFT revealed that Cryab KO mice innately possessed lower spontaneous locomotor activity than WT mice, as evidenced by their lower total distance moved (Figure 2A; genotype: F1,18 = 29.6; p < 0.001; test day: F2,36 = 27.0; p < 0.001) and movement duration (Figure 2B; genotype: F1,18 = 37.3; p < 0.001; test day: F2,36 = 35.1; p < 0.001), even across multiple testing sessions. Spontaneous alternation (Figure 2C) in the Y-maze were similar between mice genotypes, while total entry (Figure 2D; t = 5.14, df = 17) also suggested lower locomotor activity in Cryab KO mice. Other behaviors in rota-rod (Figures 2E, F), cliff avoidance (Figures 2G, H), and EPM (Figures 2I, J) exhibited no differences between WT and Cryab KO mice. Drugs of abuse also modulate neural electrical activity (Newton et al., 2003; Malyshevskaya et al., 2017; Zanettini et al., 2019; Nukitram et al., 2021), suggesting that EEG alterations may co-manifest alongside the addiction-inducing effects of some psychoactive drugs. Aberrant EEG patterns may also indicate potentially modified neuropsychological responses to addictive drugs. Accordingly, 2-h baseline EEG recording data of mice (Figure 2K) showed that the delta-, theta-, alpha-, beta-, and gamma-wave levels of Cryab KO mice were lower than WT mice [absolute power (μV2) of WT mice was set to 100%]. Sample EEG traces of mice (Figure 2L) showed comparable patterns between genotype.
FIGURE 2. General behavior of wild-type (WT) and Cryab knockout (KO) mice. At 5–7 weeks old, pups were tested for their baseline behaviors. The open-field test shows the locomotor activity indicated by (A) distance moved (cm) and (B) movement duration (s) recorded for 10 min over three consecutive days. Y-maze indicates short-term memory in terms of (C) spontaneous alternating behavior (%) and (D) total entries. Latency of first fall (s) and falling frequency (n) in the (E,F) rota-rod and (G,H) cliff avoidance tests determine motor balance/coordination and impulsive tendencies, respectively. Anxiety is measured by the (I) percentage entry (%) and (J) percentage time spent (%) in the open arms in the elevated maze plus test. (K) Baseline electroencephalogram (EEG) results reveals the power of delta, theta, alpha, beta, and gamma in mice, recorded for 2 h (drug-free). (L) Sample EEG traces shows a 2-s activity during a 2-h recording. Data expressed as mean ± S.E.M., except (K) and (L). n = 9–10. ***p < 0.001 (vs. WT; Bonferroni post hoc analysis or t-test).
To evaluate the responses of mice to the reinforcing effect of JWH-018, we exposed WT and Cryab KO mice to JWH-018 SA. Cryab KO obtained higher active lever responses (Figure 3A) in FR1 (F3,38 = 3.94; p < 0.05) and FR2 [treatment (F3,38 = 2.99; p < 0.05); SA days (F2,76 = 4.38; p < 0.05)], along with greater number of infusions (Figure 3C) and FR1 (F3,38 = 4.9; p < 0.01) and FR2 (F3,38 = 3.49; p < 0.05), compared to WT mice. Average obtained infusions (Figure 3D) all throughout the SA sessions were also significantly higher (F3,24 = 51.3; p < 0.001) in Cryab KO mice compared to their WT and VEH counterparts. The varying behavior of mice during SA indicate the manifestation of JWH-018-induced reinforcing effects in Cryab KO mice. Aside from that, the rewarding property of JWH-018 was also evaluated in WT and Cryab KO mice through the CPP paradigm. JWH-018 appeared to induce rewarding effects in Cryab KO mice (Figure 3E), as evidenced by their higher CPP score (F3,24 = 5.59; p < 0.01) compared to their VEH counterpart, but not in WT mice.
FIGURE 3. Response of wild-type (WT) and Cryab knockout (KO) mice to JWH-018 addiction-related behavioral paradigms. Self-administration (SA) responses of mice are indicated by (A) active and (B) inactive lever responses and the (C) number of infusions, along with (D) mean number of infusions, which were recorded each day for a 2-h session. n = 9–11. Place preference is indicated by (E) Conditioned place preference (CPP) score of mice, which is obtained by the difference between the stay duration of mice in the drug-paired compartment between post- and pre-conditioning. n = 7. Changes in gamma-wave power of mice after (F) acute or (G) repeated treatments over 30-min bins were determined by 2-h electroencephalography (EEG) recordings, and the 2-h averages (H) were compared. n = 6–9. Data expressed as mean ± S.E.M. *p < 0.05, **p < 0.01, and ***p < 0.001 (vs. VEH; Tukey post hoc analysis). #p < 0.05, ##p < 0.01, and ###p < 0.001 (vs. WT; Tukey post hoc analysis).
Gamma-wave generation is also altered in chronic cannabis users owing to their continuous intake of cannabinoids (Skosnik et al., 2012; Liu et al., 2022), as evidenced by in vivo and in vitro studies (Sales-Carbonell et al., 2013). Thus, gamma wave alterations in mice following repeated JWH-018 administration were also determined through EEG. Gamma-wave power after acute JWH-018 treatment (Figure 3F) was significantly different among mice with a treatment-time interaction (F3,42 = 5.71; p < 0.01), specifically the change in gamma-wave power in Cryab KO mice was lesser than WT mice only after 60 min post-treatment. Repeated JWH-018 administration also induced significant differences in change in gamma-wave power (Figure 3G) among mice (F3,42 = 5.71; p < 0.01), showing that gamma-wave power in Cryab KO mice increased significantly from baseline, unlike WT mice, all throughout the recording period. The change in gamma-wave power in mice during the 2-h recording (Figure 3H) was significantly different among groups (F3,27 = 3.21; p < 0.05). The change in gamma-wave power of Cryab KO mice was significantly higher than WT mice after repeated JWH-018 treatment, but not after acute exposure.
The abuse liabilities of THC and synthetic cannabinoids are usually attributed to their influence on CB1 receptors expressed in the VTA and NAC, which are major brain regions associated with the development of addiction-related behaviors (Nestler, 2001). Cannabinoids may also affect the expression of CB2 receptors (Sun et al., 2017), along with enzymes that degrade endocannabinoids (Li et al., 2019). Thus, we measured the mRNA levels of endocannabinoid-related genes in the VTA and NAC of mice repeatedly treated with JWH-018. Cnr1 (Figure 4A; F1,20 = 38.8; p < 0.001), Cnr2 (Figure 4B; F1,16 = 26.2; p < 0.001), and Mgll (Figure 4D; F1,16 = 41; p < 0.001) mRNA levels were downregulated in the VTA of WT and Cryab KO mice following repeated JWH-018 treatment. However, Cnr1, Cnr2, Faah, and Mgll mRNA levels in the VTA were not significantly different between WT and Cryab KO mice. In the NAC, Cnr2 (Figure 4F; F1,17 = 10.9; p < 0.01) and Mgll (Figure 4H; F1,18 = 16.4; p < 0.001) mRNA levels were also downregulated only in WT mice following repeated JWH-018 exposure. Similar to the VTA, Cnr1, Cnr2, Faah, and Mgll mRNA levels in the NAC were also not significantly different between genotypes. Faah expressions in both VTA and NAC (Figures 4C, G) were unaltered, although Cnr1 expression in NAC (Figure 4E) exhibited treatment differences between groups (F1,20 = 6.65; p < 0.05).
FIGURE 4. Expression levels of endocannabinoid system-related and dopamine-related genes and dopamine concentration in wild-type (WT) and Cryab knockout (KO) mice after repeated JWH-018 treatment. Expressions of Cnr1, Cnr2, Faah, and Mgll in the (A–D) ventral tegmental area (VTA) and (E–H) nucleus accumbens (NAC) were determined through quantitative real-time polymerase chain reaction (qRT-PCR) following repeated JWH-018 treatment. The expression levels of (I) Dat, (J) Drd1, (K) Drd2, and (L) Vmat2 in the NAC of mice following repeated JWH-018 treatment were also identified through qRT-PCR. Enzyme-linked immunosorbent assay determined the dopamine concentrations in mouse NAC after (M) acute or (N) repeated JWH-018 treatment. Data expressed as mean ± S.E.M. n = 4–6. *p < 0.05, **p < 0.01, ***p < 0.001 (vs. drug-naïve; Tukey post hoc analysis).
Since endocannabinoid signaling within the VTA may influence dopaminergic neurotransmission, divergent addiction responses in mice genotypes might be reflected by differential cannabinoid-mediated regulation of the VTA and distinct changes in dopamine-related markers in the NAC. Accordingly, we determined the mRNA levels of dopamine-related genes in the NAC of mice following repeated JWH-018 treatment. No significant alterations were detected in Drd1 (Figure 4J) and Drd2 (Figure 4K) mRNA levels, whereas the expression levels of Dat (Figure 4I; F1,16 = 8.23; p < 0.05) and Vmat2 (Figure 4L; F1,20 = 8.63; p < 0.01) in WT mice were downregulated. We also determined the accumbal dopamine concentration in mice following acute and repeated JWH-018 administration to verify the potential effect of cannabinoid treatment on dopamine-mediated responses. Accumbal dopamine concentrations in mice showed no significant differences between the genotypes following repeated JWH-018 treatment (Figure 4N), although significant differences (treatment: F1,16 = 10.4; p < 0.01; genotype: F1,16 = 6.04; p < 0.05) were observed among groups following acute JWH-018 treatment (Figure 4M).
Striatal neuroinflammatory processes may possibly potentiate addiction-related behavior (Rodrigues et al., 2014; Kohno et al., 2019). Studies have mentioned that CRYAB may produce anti-inflammatory effects by inhibiting cytokine expression through various pathways (Shao et al., 2013; Qiu et al., 2016; Somade et al., 2019; Xu et al., 2019). Thus, CRYAB may influence addiction development through its impact on neuroinflammation. In here, we first identified the effect of CRYAB expression on the inflammation-related PI3K-AKT-GSK3 pathway (Xu et al., 2013), which may also modulate NF-κB expression (Xie and Wang, 2022), following repeated JWH-018 administration. Representative blots are shown in Figure 5A. JWH-018 exposure increased CRYAB expression (Figure 5B; genotype: F1,15 = 156; p < 0.001; treatment: F1,15 = 7.51; p < 0.05) in WT mice. PI3K (Figure 5C) and p-AKT levels (Figure 5D) were not significantly different between groups. p-GSK-3β levels (Figure 5E; treatment: F1,16 = 4.85; p < 0.05) were decreased in Cryab KO mice following repeated JWH-018 administration, while GSK-3β expression (Figure 5F; genotype: F1,16 = 15; p < 0.01, treatment: F1,16 = 11.5; p < 0.01) was significantly higher in Cryab KO mice than their WT and VEH counterpart. Interestingly, repeated JWH-018 administration in the absence of functional CRYAB resulted in a higher NF-κB expression (Figure 5G) in Cryab KO mice than WT mice (F1,15 = 17.2; p < 0.001).
FIGURE 5. Expression of Protein expressions along the PI3K-AKT-GSK3 pathway and inflammation-related proteins in wild-type (WT) and Cryab knockout (KO) mice following JWH-018 exposure. (A) Representative blots showing the expression of target proteins in mice STR. Western blotting revealed the expression levels of (B) CRYAB, (C) PI3K, (D) p-AKT, (E) GSK-3β, (F) p-GSK-3β, (G) NF-κB, (H) GFAP, (I) TNF-α, (J) IL-1β, and (K) IL-6 in the STR of JWH-018-treated mice. n = 4–5. Data expressed as mean ± S.E.M. *p < 0.05, **p < 0.01, and ***p < 0.001 (vs. VEH; Tukey’s post hoc analysis). #p < 0.05 and ###p < 0.001 (vs. WT; Tukey’s post hoc analysis).
NF-κB may also influence neuroinflammation by mediating inflammatory cytokine gene transcription (Liu et al., 2017). Accordingly, we verified the expression of glial cell activity and neuroinflammatory markers in mice STR. While TNF-α (Figure 5I) and IL-1β (Figure 5J) were unaltered in mice after JWH-018 exposure, GFAP (Figure 5H; F1,16 = 13.1; p < 0.001) and IL-6 (Figure 5K; F1,15 = 12.7; p < 0.01) expressions were higher in Cryab KO mice than WT mice.
Glutamate reuptake in synapses is said to decrease, via GLT-1 downregulation, following an increase in cytokine expression and astrocyte activation (Boycott et al., 2008; Ramos et al., 2010), possibly altering glutamate concentration. Thus, we determined the mRNA expressions of glutamate transporter subtypes in JWH-018 exposed mice. Expression level of Eaat2 mRNA (Figure 6A) was significantly decreased in Cryab KO mice and was lower than WT mice (genotype: F1,16 = 10.4; p < 0.01; treatment: F1,16 = 6.51; p < 0.05) after repeated treatment of JWH-018. Eaat3 (Figure 6B) and Eaat4 (Figure 6C) mRNA levels were both increased in WT mice (Eaat3: F1,20 = 12.5; p < 0.01, Eaat4: F1,20 = 32.5; p < 0.001), while only Eaat4 mRNA was upregulated in Cryab KO mice after repeated JWH-018 administration.
FIGURE 6. Glutamate transporter mRNA levels and plasticity-related protein expressions in wild-type (WT) and Cryab knockout (KO) mice following JWH-018 exposure. mRNA expression levels of (A) Eaat2 (B) Eaat3, and (C) Eaat4 in JWH-018-exposed mice was determined by quantitative real-time polymerase chain reaction. n = 5–6. (D) Representative blots showing the expression of target proteins in mice STR. Western blotting revealed the expression levels of (E) GluA1, (F) GluA2, (G) p-CREB/CREB, (H) ΔFosB, (I) p-mTOR/mTOR, and (J) BDNF in the STR of JWH-018-exposed mice. n = 4–5. Data expressed as mean ± S.E.M. *p < 0.05 and **p < 0.01 (vs. VEH; Tukey’s post hoc analysis). #p < 0.05 (vs. WT; Tukey’s post hoc analysis).
Alterations in glutamate neurotransmission have been previously associated with the development of drug addiction, including those induced by psychostimulants (Kalivas, 2007) and cannabinoids (Cohen et al., 2019). These are generally characterized by changes in glutamate receptors and markers of synaptic plasticity in brain areas mediating addiction-related behaviors (Kauer and Malenka, 2007; Park et al., 2015). In here, we determined the expressions of glutamate receptors and synaptic plasticity markers in the STR (Figure 6D). GluA1 (Figure 6E; F1,16 = 5.92; p < 0.05), but not GluA2 (Figure 6F), expression showed significant differences among treatment groups, but only an increased trend in JWH-018-exposed Cryab KO mice can be observed. While p-CREB expressions of mice (Figure 6G) exhibited no significant variations among groups, ΔFosB expression (Figure 6H; genotype: F1,16 = 7.69; p < 0.01; treatment: F1,16 = 11.4; p < 0.05) was significantly higher in JWH-018-exposed Cryab KO mice than WT. p-mTOR (Figure 6I; treatment: F1,16 = 10.6; p < 0.01) and BDNF (Figure 6J genotype/treatment interaction: F1,15 = 5.21; p < 0.05) levels were increased in Cryab KO mice after repeated JWH-018 administration.
To verify the potential involvement of increased NF-κB expression in the manifestation of cannabinoid-induced addiction-related behaviors, we determined the striatal NF-κB expression of mice that underwent JWH-018 SA. NF-κB CTCF (Figure 7B) was significantly greater in Cryab KO mice (genotype: F1,8 = 12.2; p < 0.01; treatment: F1,8 = 18.8; p < 0.01) compared to their WT and VEH counterparts after JWH-018 SA.
FIGURE 7. NF-κB immunoreactivity in mice from JWH-018 self-administration. (A) Immunofluorescence analysis of NF-κB and Hoechst staining in VEH- or JWH-018-exposed WT and Cryab KO mice at ×150 magnification (merged images are shown). (B) Analysis through ImageJ determined the corrected total cell fluorescence of NF-κB in mice following JWH-018 self-administration. n = 3. Data expressed as mean ± S.E.M. *p < 0.05 (vs. VEH; Tukey’s post hoc analysis). #p < 0.05 (vs. WT; Tukey’s post hoc analysis).
Cryab KO mice displayed generally similar baseline behavior with WT mice (Figures 2C, E–J), other than the marked hypoactivity in OFT (Figures 2A, B). This kind of phenotype was previously observed to occur alongside other aberrant behaviors, such as anxiety (Wood et al., 1987), depression (Ota et al., 2018), or autism spectrum disorder (Wang et al., 2018; Angelakos et al., 2019). Animal models for depression, which were also observed to possess inherently low spontaneous locomotor activity (Skalisz et al., 2002; Rygula et al., 2005; Ota et al., 2018), demonstrated increased sensitivity to cocaine (Lepsch et al., 2005; Ribeiro Do Couto et al., 2009; Xu et al., 2020) and nicotine (Cruz et al., 2008). The hypoactive behavior of Cryab KO mice might then indicate a possibility for them to likewise exhibit aberrant responses to psychoactive drugs. Furthermore, Cryab KO and WT mice might differentially respond to addictive drugs also due to their divergent baseline EEG recordings (Figure 2K). Human EEG data demonstrated persistent changes in the delta, alpha, and gamma waves of subjects repeatedly exposed to addictive drugs, such as cocaine (Reid et al., 2006) and marijuana (Herning et al., 2008), indicating the involvement of EEG waves in the neuropsychological effects of addictive drugs. Together, the hypoactivity and baseline EEG of Cryab KO mice might render them potentially sensitive to psychoactive substances, probably including synthetic cannabinoids. We then subsequently investigated the behavioral responses of Cryab KO mice to the effects of the synthetic cannabinoid JWH-018 using paradigms that generally indicate the rewarding and reinforcing effects of drugs.
Cryab KO mice exhibited potentially higher sensitivity to the addiction-related effects of JWH-018, as evidenced by their increased responses in intravenous SA (Figures 3A–C) and CPP (Figure 3E) tests compared to WT mice. While several studies have reported the addiction-like effects of synthetic cannabinoids, including JWH-018 (Hur et al., 2021), in healthy rodent subjects (Cha et al., 2014; de Luca et al., 2015), some cannabinoids still do not exhibit significant abuse potential in animal behavioral paradigms (Tampus et al., 2015; Bilel et al., 2019). This suggests the involvement of complex neurological mechanisms (that mediate the addictive effects of cannabinoids) other than the typical mesolimbic dopaminergic pathway. The results of the SA and CPP tests were consistent with this hypothesis, as WT mice did not exhibit significant preference or tendency for self-administering JWH-018 at 0.03 mg/kg/infusion or 0.3 mg/kg, unlike with previous studies. This discrepancy among studies investigating intravenous JWH-018 SA in WT mice might be attributed primarily to methodological differences, given that some studies required long-term exposure of mice in JWH-018 SA (de Luca et al., 2015), with some using adolescent mice with potentially different drug sensitivity (Margiani et al., 2022). However, the fact that Cryab KO mice exhibited modest SA responses and place preference for JWH-018 indicated cannabinoid-induced addiction-like behaviors, suggesting cannabinoid abuse susceptibility.
EEG recordings revealed significantly different gamma-wave power changes in WT and Cryab KO mice following repeated exposures to JWH-018 (Figures 3F–H). Gamma oscillations are involved in both cognition and perceptive functions and may be mediated by CB1 receptors via GABA interneurons (Skosnik et al., 2012), indicating the potential involvement of gamma-wave alterations in cannabinoid-mediated effects, which may include addiction. Previous studies have also reported that repeated exposure to various addictive drugs increases gamma-wave production (Abiero et al., 2020; Custodio et al., 2020), which was also observed in Cryab KO mice after repeated JWH-018 exposure. However, gamma-wave production is generally associated with attention and perception (Müller et al., 2000; Tallon-Baudry et al., 2005), which may implicate the JWH-018-induced increase in Cryab KO mice gamma-wave power as an improvement in receptivity and cognition. While this may not entirely indicate an addiction response or vulnerability, the divergent changes in gamma-wave power between WT and Cryab KO mice might still indicate different neurophysiological modifications in brain substrates that may contribute to the observed disparity in the cannabinoid-induced, addiction-like responses between genotypes. Thus far, behavioral and EEG data suggest the Cryab KO mice to be a potential animal model of cannabinoid abuse susceptibility.
As previously mentioned, psychoactive cannabinoids generally activate CB1 receptors on GABA/glutamatergic neurons located in the VTA and NAC, thus modulating the release of GABA/glutamate (Cristino et al., 2020; Spanagel, 2020) and influencing dopamine-mediated activities, such as addiction responses. Thus, a dysregulation in these pathways may influence cannabinoid-induced responses. Endocannabinoid-related mRNA expression levels (Figures 4A–H) suggest a possible downregulation of endocannabinoid signaling in WT and Cryab KO mice VTA, and perhaps also in WT mice NAC, after repeated JWH-018 treatment. While frequent exposure to cannabinoids potentially downregulates cannabinoid receptors in some brain regions (Scherma et al., 2016; van de Giessen et al., 2017; Kesner and Lovinger, 2021), similar to our qRT-PCR data, comparable JWH-018-induced mRNA alterations were detected between Cryab KO and WT mice, indicating possibly similar effects on dopaminergic signaling. Since alterations in cannabinoid receptor-encoding gene expressions may indicate GABA/glutamate signaling adaptations and dopaminergic activity dysregulation (Maldonado et al., 2011; Colizzi et al., 2016; Zehra et al., 2018; Pintori et al., 2021), comparable modifications in both Cryab KO and WT mice might suggest similarities in the expression of cannabinoid-induced behaviors. As this was not the case, given the SA and CPP results, the disparity between the JWH-018-induced behavioral responses of WT and Cryab KO mice might involve divergent modifications in dopaminergic signaling.
Drug addiction is frequently attributed to alterations in dopamine neurotransmission, along with upregulated accumbal dopamine levels (Fleckenstein et al., 2007; MacNicol, 2017; Jiménez-González et al., 2022). Expressions of dopamine-related mRNA in the NAC of JWH-018-exposed mice (Figures 4I–L) suggest a possible dampening of dopaminergic activity. The same phenomenon was also observed in previous studies showing the blunting of dopaminergic activity following chronic cannabinoid exposure (van de Giessen et al., 2017; Kesner and Lovinger, 2021). It was previously suggested that genes influencing dopamine availability tend to also downregulate following chronic cannabinoid treatments (Perdikaris et al., 2018). The Dat and Vmat2 downregulation in WT mice may suggest a compensatory response to promote dopamine availability, which is a phenomenon also found during drug tolerance and in chronic methamphetamine users (Wilson et al., 1996; Graves et al., 2021). Although there are only limited studies describing the effect of cannabinoids on Vmat2 mRNA, its downregulation may denote possible dopamine release dysregulation (German et al., 2015) in WT mice in response to cannabinoids. One study suggested that reduced Vmat2 mRNA expression may lead to inhibition of vesicular dopamine and potentially increase cytosolic dopamine levels (Sun et al., 2014). The lack of such changes in Cryab KO mice may imply their resilience to cannabinoid-induced dopaminergic alterations, further suggesting dopamine-independent mechanisms mediating their higher cannabinoid sensitivity. Nevertheless, downregulation of endocannabinoid signaling may have contributed to possible dopaminergic desensitization following repeated JWH-018 administration, which is a potential theory behind cannabinoid addiction pathophysiology (Zehra et al., 2018). On the other hand, the involvement of dopamine mediation might still not be ruled out completely. Studies demonstrating dopamine level upregulation after acute cannabinoid administration (de Luca et al., 2015; Ossato et al., 2017; Pintori et al., 2021), along with our ELISA results following acute JWH-018 treatment (Figure 4M), may still implicate transient dopamine increments in addiction-related brain regions to the addictive properties of cannabinoids. However, probably due to the absence of sustained dopaminergic activation via endocannabinoid signaling downregulation, some cannabinoids may have appeared to lack robust abuse potential following prolonged exposure (Cha et al., 2014; Tampus et al., 2015), as opposed to recognized abused drugs that show persistent increases in dopamine concentration and activity even after repeated exposures (Nestby et al., 1997; Cadoni et al., 2000), resulting in evident abuse liability. Therefore, the differential responses between Cryab KO and WT mice to the addiction-inducing effects of JWH-018 may implicate the involvement of other neurological mechanisms beyond the endocannabinoid or dopaminergic systems.
Studies have suggested the involvement of CRYAB and cannabinoids in the anti-inflammatory action of the PI3K-AKT-GSK3 pathway (Ozaita et al., 2007; Xu et al., 2013; Zhu et al., 2015), which may also modulate the expression of NF-κB (Bathina and Das, 2018), a transcription factor broadly associated with immune system-related and inflammation-related gene regulation (Beurel et al., 2015; Liu et al., 2017). Several factors (Beurel et al., 2015; Liu et al., 2017; Feng and Lu, 2021), including some heat shock proteins (Schell et al., 2005), were reported to potentially influence neuroinflammation by regulating NF-κB expression/activity, and when modulated, altered complex behaviors such as addiction (Rodrigues et al., 2014; Nennig and Schank, 2017; Morcuende et al., 2021). More recently, CRYAB was described to potentially regulate NF-κB nuclear translocation (Shao et al., 2013; Qiu et al., 2016), which is essential for inflammatory cytokine and chemokine transcription (Somade et al., 2019). Based on the possible correlation between cannabinoid addiction and neuroinflammation, CRYAB, via NF-κB, may potentially influence addiction-related behaviors. Increased CRYAB (Figure 5B) and negligible changes along the PI3K-AKT-GSK3 pathway (Figures 5C–F) in JWH-018-exposed WT mice may imply the occurrence of only minor neuroinflammation. In contrast, JWH-018-exposed Cryab KO mice seemed to suggest potential downstream neuroinflammatory alterations, given by increased GSK-3β (Figure 5F) and NF-κB (Figure 5G) expressions. From this observation, functional CRYAB expression might have a role in the anti-inflammatory effect of cannabinoids, as the presence or absence of CRYAB resulted in significant genotype differences in the expression of NF-κB following JWH-018 exposure. Furthermore, NF-κB expression and translocation may also influence neuroinflammation by mediating inflammatory cytokine gene transcription and astroglia activation (Young et al., 2011; Liu et al., 2017). While JWH-018 exposure induced no changes in WT mice, the higher expression of glial fibrillary acidic protein (GFAP; Figure 5H) and IL-6 (Figure 5K) in Cryab KO mice may indicate a higher state of neuroinflammation compared to WT mice, which may have been a consequence of increased NF-κB expression following repeated JWH-018 administration. Intriguingly, this had no effect on TNF-α and IL-1β expressions, unlike in previous reports (Nennig and Schank, 2017), which could suggest that the alteration of CRYAB expression may have specificity to IL-6 transcription. Our results contradict some previous studies, given that cannabinoids are understood to be anti-inflammatory and JWH-018 seemed to potentially induce inflammation in Cryab KO mice. However, according to previous studies (Pintori et al., 2021; Margiani et al., 2022), JWH-018 may potentially promote neuroinflammation in mice following previous repeated exposures. Thus, the absence of CRYAB might have exacerbated the neuroinflammatory effect of JWH-018 in Cryab KO mice, resulting in the observed alterations in NF-κB, GFAP, and IL-6 expressions, given also that CRYAB, possessing an anti-inflammatory function, might have rendered the Cryab KO mice susceptible to inflammatory insults.
Increased neuroinflammation, specifically due to increased cytokine and astrocyte activation (Boycott et al., 2008), may result to the downregulation of glutamate transporter (GLT-1) (Ramos et al., 2010), potentially increasing synaptic glutamate concentration. Glutamate reuptake was also reported to be reduced in the STR of cocaine-exposed rodents (Parikh et al., 2014; Smaga et al., 2020), potentially increasing the availability of glutamate in the synapse. Taking together the alterations in glutamate transporter mRNA (Figures 6A–C), there might be an overall decrease in glutamate reuptake in Cryab KO mice, suggesting potentially higher glutamate levels in the STR of Cryab KO mice, compared to that in WT after JWH-018 exposure, which may have implications in glutamate-mediated long-term potentiation. While glutamate-mediated cannabinoid addiction may entail their regulation of glutamate activation of medium spiny neurons in the NAC (Cohen et al., 2019), other drugs of abuse elicit glutamate-mediated addiction through long-term potentiation of the glutamatergic synapse (LaLumiere and Kalivas, 2008; Gipson et al., 2014; Griffin et al., 2015) via potential neuroinflammatory mechanisms (Hutchinson et al., 2012; Zhu et al., 2018). Similarly, the expressions of synaptic plasticity markers in Cryab KO mice (Figures 6G–J) may suggest the occurrence of neuronal adaptations following JWH-018 exposure, which may have contributed to the development and maintenance of drug-induced addiction (Tsai, 2007; Post and Kalivas, 2013; Zhang et al., 2014; Sutton and Caron, 2015; Ucha et al., 2020; García-García et al., 2021) in Cryab KO mice. While it is still uncertain whether these changes were indeed a result of increased glutamate receptor activation, given the lack of genotype variations in GluA1 and GluA2 expressions, although with marked differences among groups (Figures 6E, F), the lack of CRYAB during JWH-018 administration might still ultimately induce positive effects on drug cue reinforcement. Since glutamate receptor activation may reinforce addiction-related behaviors through postsynaptic alterations (Gass and Olive, 2008; Joffe et al., 2014; Nennig and Schank, 2017), the greater striatal synaptic adaptations in drug-exposed Cryab KO mice may have induced stronger improved habit-associated learning from neuroinflammation-mediated long-term potentiation. This can be supported by NF-κB fluorescent signaling in Cryab KO mice from JWH-018 SA (Figures 7A, B), implying that upregulated NF-κB expression might indeed be involved in the manifestation of cannabinoid-induced addiction-like behaviors in Cryab KO mice, even through various means of JWH-018 exposure (daily repeated treatment or self-administered). Therefore, functional CRYAB deficiency may have enhanced the occurrence of increased striatal synaptic plasticity via NF-κB-modulated neuroinflammation following JWH-018 exposure, potentially corroborating Cryab in neuroinflammation-mediated cannabinoid addiction.
In conclusion, Cryab KO mice exhibit higher cannabinoid-induced addiction than WT mice. Their divergent responses to cannabinoids compared to WT may involve neuroinflammation-mediated synaptic plasticity, due to functional CRYAB deletion (Figure 8). Such behavior might not also involve genotype differences in cannabinoid-induced alterations in the endocannabinoid or dopaminergic systems. Given these findings, future studies are imperative to investigate the behavioral responses of Cryab KO mice to other types of cannabinoids to further verify the involvement of Cryab in general cannabinoid addiction. Taken together, the Cryab KO mice might represent one of the potential mechanisms for enhanced cannabinoid abuse susceptibility and be an efficient tool for screening the abuse potential of novel synthetic cannabinoids, contributing to more reliable and accurate evaluation regarding the potential dangers of these substances in humans.
FIGURE 8. Schematic diagram for a proposed mechanism underlying the enhanced JWH-018 abuse susceptibility of Cryab knockout (KO) mice. Repeated administration of cannabinoids, such JWH-018, may result to a reduction in cannabinoid receptor (CBR) expression, leading to a desensitized dopamine (DA) system in both Cryab KO and wild-type (WT) mice. Due to CRYAB deficiency, repeated JWH-018 administration resulted in higher striatal neuroinflammation, leading to higher neuroadaptations. These changes may potentially contribute to the abuse susceptibility of Cryab KO mice to cannabinoids.
The original contributions presented in the study are included in the article/Supplementary Material, further inquiries can be directed to the corresponding authors.
The animal study was reviewed and approved by Animal Care and Use Guidelines of Sahmyook University.
LS, JC, and HK were responsible for the study design. LS, DO, HL, MK, RC, JY, CL, YL, and HC contributed to data acquisition, analysis, and interpretation of data. LS drafted the manuscript. All authors contributed to and approved the final version of the manuscript.
This work was supported by the Ministry of Food and Drug Safety (19182MFDS410 and 22214MFDS252) and the National Research Foundation of Korea (2020R1F1A1075633).
The authors declare that the research was conducted in the absence of any commercial or financial relationships that could be construed as a potential conflict of interest.
All claims expressed in this article are solely those of the authors and do not necessarily represent those of their affiliated organizations, or those of the publisher, the editors and the reviewers. Any product that may be evaluated in this article, or claim that may be made by its manufacturer, is not guaranteed or endorsed by the publisher.
The Supplementary Material for this article can be found online at: https://www.frontiersin.org/articles/10.3389/fphar.2023.1135929/full#supplementary-material
Abiero, A., Botanas, C. J., Custodio, R. J., Sayson, L. V., Kim, M., Lee, H. J., et al. (2020). 4-MeO-PCP and 3-MeO-PCMo, new dissociative drugs, produce rewarding and reinforcing effects through activation of mesolimbic dopamine pathway and alteration of accumbal CREB, deltaFosB, and BDNF levels. Psychopharmacol. Berl. 237, 757–772. doi:10.1007/s00213-019-05412-y
Adinoff, B. (2004). Neurobiologic processes in drug reward and addiction. Harv Rev. Psychiatry 12, 305–320. doi:10.1080/10673220490910844
Angelakos, C. C., Tudor, J. C., Ferri, S. L., Jongens, T. A., and Abel, T. (2019). Home-cage hypoactivity in mouse genetic models of autism spectrum disorder. Neurobiol. Learn Mem. 165, 107000. doi:10.1016/J.NLM.2019.02.010
Bathina, S., and Das, U. N. (2018). Dysregulation of PI3K-Akt-mTOR pathway in brain of streptozotocin-induced type 2 diabetes mellitus in Wistar rats. Lipids Health Dis. 17, 168. doi:10.1186/s12944-018-0809-2
Bayazit, H., Selek, S., Karababa, I. F., Cicek, E., and Aksoy, N. (2017). Evaluation of oxidant/antioxidant status and cytokine levels in patients with cannabis use disorder. Clin. Psychopharmacol. Neurosci. 15, 237–242. doi:10.9758/cpn.2017.15.3.237
Beurel, E., Grieco, S. F., and Jope, R. S. (2015). Glycogen synthase kinase-3 (GSK3): Regulation, actions, and diseases. Pharmacol. Ther. 148, 114–131. doi:10.1016/j.pharmthera.2014.11.016
Bilel, S., Tirri, M., Arfè, R., Stopponi, S., Soverchia, L., Ciccocioppo, R., et al. (2019). Pharmacological and behavioral effects of the synthetic cannabinoid AKB48 in rats. Front. Neurosci. 13, 1163. doi:10.3389/fnins.2019.01163
Botanas, C. J., Perez Custodio, R. J., Kim, H. J., de la Pena, J. B., Sayson, L. V., Ortiz, D. M., et al. (2021). R (−)-methoxetamine exerts rapid and sustained antidepressant effects and fewer behavioral side effects relative to S (+)-methoxetamine. Neuropharmacology 193, 108619. doi:10.1016/j.neuropharm.2021.108619
Boycott, H. E., Wilkinson, J. A., Boyle, J. P., Pearson, H. A., and Peers, C. (2008). Differential involvement of TNFα in hypoxic suppression of astrocyte glutamate transporters. Glia 56, 998–1004. doi:10.1002/glia.20673
Cadoni, C., Solinas, M., and di Chiara, G. (2000). Psychostimulant sensitization: Differential changes in accumbal shell and core dopamine. Eur. J. Pharmacol. 388, 69–76. doi:10.1016/S0014-2999(99)00824-9
Calderwood, S. K., Repasky, E. A., Neckers, L., and Hightower, L. E. (2019). The IXth CSSI international symposium on heat shock proteins in biology and medicine: Stress responses in health and disease: Alexandria old town, alexandria, Virginia, november 10–13, 2018. Cell. Stress Chaperones 24, 1–6. doi:10.1007/S12192-018-00966-W
Cha, H. J., Lee, K. W., Song, M. J., Hyeon, Y. J., Hwang, J. Y., Jang, C. G., et al. (2014). Dependence potential of the synthetic cannabinoids JWH-073, JWH-081, and JWH-210: In vivo and in vitro approaches. Biomol. Ther. Seoul. 22, 363–369. doi:10.4062/biomolther.2014.039
Cohen, K., Weizman, A., and Weinstein, A. (2019). Modulatory effects of cannabinoids on brain neurotransmission. Eur. J. Neurosci. 50, 2322–2345. doi:10.1111/EJN.14407
Colizzi, M., McGuire, P., Pertwee, R. G., and Bhattacharyya, S. (2016). Effect of cannabis on glutamate signalling in the brain: A systematic review of human and animal evidence. Neurosci. Biobehav Rev. 64, 359–381. doi:10.1016/j.neubiorev.2016.03.010
Cristino, L., Bisogno, T., and di Marzo, V. (2020). Cannabinoids and the expanded endocannabinoid system in neurological disorders. Nat. Rev. Neurol. 16, 9–29. doi:10.1038/s41582-019-0284-z
Cruz, F. C., DeLucia, R., and Planeta, C. S. (2008). Effects of chronic stress on nicotine-induced locomotor activity and corticosterone release in adult and adolescent rats. Addict. Biol. 13, 63–69. doi:10.1111/j.1369-1600.2007.00080.x
Custodio, R. J. P., Botanas, C. J., de la Peña, J. B., dela Peña, I. J., Kim, M., Sayson, L. V., et al. (2018). Overexpression of the thyroid hormone-responsive (THRSP) gene in the striatum leads to the development of inattentive-like phenotype in mice. Neuroscience 390, 141–150. doi:10.1016/j.neuroscience.2018.08.008
Custodio, R. J. P., Kim, M., Sayson, L. V., Lee, H. J., Ortiz, D. M., Kim, B. N., et al. (2021). Low striatal T3 is implicated in inattention and memory impairment in an ADHD mouse model overexpressing thyroid hormone-responsive protein. Commun. Biol. 4, 1101–1114. doi:10.1038/s42003-021-02633-w
Custodio, R. J. P., Kim, M., Sayson, L. V., Ortiz, D. M., Buctot, D., Lee, H. J., et al. (2022). Regulation of clock and clock-controlled genes during morphine reward and reinforcement: Involvement of the period 2 circadian clock. J. Psychopharmacol. 36, 875–891. doi:10.1177/02698811221089040
Custodio, R. J. P., Sayson, L. V., Botanas, C. J., Abiero, A., You, K. Y., Kim, M., et al. (2020). 25B-NBOMe, a novel N-2-methoxybenzyl-phenethylamine (NBOMe) derivative, may induce rewarding and reinforcing effects via a dopaminergic mechanism: Evidence of abuse potential. Addict. Biol. 25, e12850. doi:10.1111/adb.12850
Dai, A., Guo, X., Yang, X., Li, M., Fu, Y., and Sun, Q. (2022). Effects of the CRYAB gene on stem cell-like properties of colorectal cancer and its mechanism. J. Cancer Res. Ther. 18, 1328–1337. doi:10.4103/JCRT.JCRT_212_22
de Luca, M. A., Bimpisidis, Z., Melis, M., Marti, M., Caboni, P., Valentini, V., et al. (2015). Stimulation of in vivo dopamine transmission and intravenous self-administration in rats and mice by JWH-018, a Spice cannabinoid. Neuropharmacology 99, 705–714. doi:10.1016/j.neuropharm.2015.08.041
de Luca, M. A., and Fattore, L. (2018). Therapeutic use of synthetic cannabinoids: Still an open issue? Clin. Ther. 40, 1457–1466. doi:10.1016/j.clinthera.2018.08.002
dela Peña, I. J. I., Botanas, C. J., de la Peña, J. B., Custodio, R. J., dela Peña, I., Ryoo, Z. Y., et al. (2019). The atxn7-overexpressing mice showed hyperactivity and impulsivity which were ameliorated by atomoxetine treatment: A possible animal model of the hyperactive-impulsive phenotype of adhd. Prog. Neuropsychopharmacol. Biol. Psychiatry 88, 311–319. doi:10.1016/j.pnpbp.2018.08.012
Delis, F., Polissidis, A., Poulia, N., Justinova, Z., Nomikos, G. G., Goldberg, S. R., et al. (2017). Attenuation of cocaine-induced conditioned place preference and motor activity via cannabinoid CB2 receptor agonism and cb1 receptor antagonism in rats. Int. J. Neuropsychopharmacol. 20, 269–278. doi:10.1093/ijnp/pyw102
Diao, X., and Huestis, M. A. (2019). New synthetic cannabinoids metabolism and strategies to best identify optimal marker metabolites. Front. Chem. 7, 109. doi:10.3389/fchem.2019.00109
Drug Enforcement Administration (2015). Drugs of abuse 2015 edition: A dea resource guide. Springfield: Drug Enforcement Administration.
Fagundo, A. B., de la Torre, R., Jiménez-Murcia, S., Agüera, Z., Pastor, A., Casanueva, F. F., et al. (2013). Modulation of the endocannabinoids N-arachidonoylethanolamine (AEA) and 2-arachidonoylglycerol (2-AG) on executive functions in humans. PLoS One 8, e66387. doi:10.1371/journal.pone.0066387
Feng, Y., and Lu, Y. (2021). Immunomodulatory effects of dopamine in inflammatory diseases. Front. Immunol. 12, 663102. doi:10.3389/fimmu.2021.663102
Fleckenstein, A. E., Volz, T. J., Riddle, E. L., Gibb, J. W., and Hanson, G. R. (2007). New insights into the mechanism of action of amphetamines. Annu. Rev. Pharmacol. Toxicol. 47, 681–698. doi:10.1146/annurev.pharmtox.47.120505.105140
García-García, F., Priego-Fernández, S., López-Muciño, L. A., Acosta-Hernández, M. E., and Peña-Escudero, C. (2021). Increased alcohol consumption in sleep-restricted rats is mediated by delta FosB induction. Alcohol 93, 63–70. doi:10.1016/J.ALCOHOL.2021.02.004
Gass, J. T., and Olive, M. F. (2008). Glutamatergic substrates of drug addiction and alcoholism. Biochem. Pharmacol. 75, 218–265. doi:10.1016/J.BCP.2007.06.039
German, C. L., Baladi, M. G., McFadden, L. M., Hanson, G. R., and Fleckenstein, A. E. (2015). Regulation of the dopamine and vesicular monoamine transporters: Pharmacological targets and implications for disease. Pharmacol. Rev. 67, 1005–1024. doi:10.1124/pr.114.010397
Gipson, C. D., Kupchik, Y. M., and Kalivas, P. W. (2014). Rapid, transient synaptic plasticity in addiction. Neuropharmacology 76, 276–286. doi:10.1016/j.neuropharm.2013.04.032
Graves, S. M., Schwarzschild, S. E., Tai, R. A., Chen, Y., and Surmeier, D. J. (2021). Mitochondrial oxidant stress mediates methamphetamine neurotoxicity in substantia nigra dopaminergic neurons. Neurobiol. Dis. 156, 105409. doi:10.1016/j.nbd.2021.105409
Griffin, W. C., Ramachandra, V. S., Knackstedt, L. A., and Becker, H. C. (2015). Repeated cycles of chronic intermittent ethanol exposure increases basal glutamate in the nucleus accumbens of mice without affecting glutamate transport. Front. Pharmacol. 6, 27. doi:10.3389/fphar.2015.00027
Guo, Y. S., Liang, P., Lu, S. Z., Chen, R., Yin, Y., and Zhou, J. (2019). Extracellular αB-crystallin modulates the inflammatory responses. Biochem. Biophys. Res. Commun. 508, 282–288. doi:10.1016/j.bbrc.2018.11.024
Hammond, L. (2014). Measuring cell fluorescence using ImageJ — the open Lab book v1.0. The open Lab book. Available at: https://theolb.readthedocs.io/en/latest/imaging/measuring-cell-fluorescence-using-imagej.html (Accessed October 4, 2022).
Herning, R. I., Better, W., and Cadet, J. L. (2008). EEG of chronic marijuana users during abstinence: Relationship to years of marijuana use, cerebral blood flow and thyroid function. Clin. Neurophysiol. 119, 321–331. doi:10.1016/j.clinph.2007.09.140
Herrero, M. J., Domingo-Salvany, A., Torrens, M., Brugal, M. T., de Hoz, L. D. L. F., Gómez, R. B., et al. (2008). Psychiatric comorbidity in young cocaine users: Induced versus independent disorders. Addiction 103, 284–293. doi:10.1111/j.1360-0443.2007.02076.x
Huffman, J. W., Zengin, G., Wu, M. J., Lu, J., Hynd, G., Bushell, K., et al. (2005). Structure-activity relationships for 1-alkyl-3-(1-naphthoyl)indoles at the cannabinoid CB 1 and CB 2 receptors: Steric and electronic effects of naphthoyl substituents. New highly selective CB 2 receptor agonists. Bioorg Med. Chem. 13, 89–112. doi:10.1016/j.bmc.2004.09.050
Hur, K. H., Ma, S. X., Lee, B. R., Ko, Y. H., Seo, J. Y., Ryu, H. W., et al. (2021). Abuse potential of synthetic cannabinoids: Am-1248, cb-13, and pb-22. Biomol. Ther. Seoul. 29, 384–391. doi:10.4062/biomolther.2020.212
Hutchinson, M. R., Northcutt, A. L., Hiranita, T., Wang, X., Lewis, S. S., Thomas, J., et al. (2012). Opioid activation of toll-like receptor 4 contributes to drug reinforcement. Soc. Neurosci. 32, 11187–11200. doi:10.1523/JNEUROSCI.0684-12.2012
Hyatt, W. S., and Fantegrossi, W. E. (2014). Δ9-THC exposure attenuates aversive effects and reveals appetitive effects of K2/’Spice’ constituent JWH-018 in mice. Behav. Pharmacol. 25, 253–257. doi:10.1097/FBP.0000000000000034
Javed, H., Azimullah, S., Haque, M. E., and Ojha, S. K. (2016). Cannabinoid type 2 (CB2) receptors activation protects against oxidative stress and neuroinflammation associated dopaminergic neurodegeneration in rotenone model of Parkinson’s disease. Front. Neurosci. 10, 321. doi:10.3389/fnins.2016.00321
Jiménez-González, A., Gómez-Acevedo, C., Ochoa-Aguilar, A., and Chavarría, A. (2022). The role of glia in addiction: Dopamine as a modulator of glial responses in addiction. Cell. Mol. Neurobiol. 42, 2109–2120. doi:10.1007/s10571-021-01105-3
Joffe, M., Grueter, C. A., and Grueter, B. A. (2014). Biological substrates of addiction. Wiley Interdiscip. Rev. Cogn. Sci. 5, 151–171. doi:10.1002/wcs.1273
Kabir, Z. D., Lee, A. S., and Rajadhyaksha, A. M. (2016). L-Type Ca2+ channels in mood, cognition and addiction: Integrating human and rodent studies with a focus on behavioural endophenotypes. J. Physiology 594, 5823–5837. doi:10.1113/JP270673
Kalivas, P. W. (2007). Cocaine and amphetamine-like psychostimulants: Neuro circuitry and glutamate neuroplasticity. Dialogues Clin. Neurosci. 9, 389–397. doi:10.31887/DCNS.2007.9.4/PKALIVAS
Kauer, J. A., and Malenka, R. C. (2007). Synaptic plasticity and addiction. Nat. Rev. Neurosci. 8;(11), 844–858. doi:10.1038/nrn2234
Kesner, A. J., and Lovinger, D. M. (2021). Cannabis use, abuse, and withdrawal: Cannabinergic mechanisms, clinical, and preclinical findings. J. Neurochem. 157, 1674–1696. doi:10.1111/jnc.15369
Kim, M., Custodio, R. J., Botanas, C. J., de la Peña, J. B., Sayson, L. V., Abiero, A., et al. (2019). The circadian gene, Per2, influences methamphetamine sensitization and reward through the dopaminergic system in the striatum of mice. Addict. Biol. 24, 946–957. doi:10.1111/adb.12663
Kinsey, S. G., Mahadevan, A., Zhao, B., Sun, H., Naidu, P. S., Razdan, R. K., et al. (2011). The CB2 cannabinoid receptor-selective agonist O-3223 reduces pain and inflammation without apparent cannabinoid behavioral effects. Neuropharmacology 60, 244–251. doi:10.1016/j.neuropharm.2010.09.004
Kohno, M., Link, J., Dennis, L. E., McCready, H., Huckans, M., Hoffman, W. F., et al. (2019). Neuroinflammation in addiction: A review of neuroimaging studies and potential immunotherapies. Pharmacol. Biochem. Behav. 179, 34–42. doi:10.1016/j.pbb.2019.01.007
Kozela, E., Pietr, M., Juknat, A., Rimmerman, N., Levy, R., and Vogel, Z. (2010). Cannabinoids Delta(9)-tetrahydrocannabinol and cannabidiol differentially inhibit the lipopolysaccharide-activated NF-kappaB and interferon-beta/STAT proinflammatory pathways in BV-2 microglial cells. J. Biol. Chem. 285, 1616–1626. doi:10.1074/jbc.M109.069294
Krasnova, I. N., Justinova, Z., and Cadet, J. L. (2016). Methamphetamine addiction: Involvement of CREB and neuroinflammatory signaling pathways. Psychopharmacol. Berl. 233, 1945–1962. doi:10.1007/s00213-016-4235-8
Kuipers, H. F., Yoon, J., van Horssen, J., Han, M. H., Bollyky, P. L., Palmer, T. D., et al. (2017). Phosphorylation of αB-crystallin supports reactive astrogliosis in demyelination. Proc. Natl. Acad. Sci. U. S. A. 114, E1745–E1754. doi:10.1073/PNAS.1621314114/SUPPL_FILE/PNAS.1621314114.SD01.XLSX
LaLumiere, R. T., and Kalivas, P. W. (2008). Glutamate release in the nucleus accumbens core is necessary for heroin seeking. J. Neurosci. 28, 3170–3177. doi:10.1523/JNEUROSCI.5129-07.2008
Le Boisselier, R., Alexandre, J., Lelong-Boulouard, V., and Debruyne, D. (2017). Focus on cannabinoids and synthetic cannabinoids. Clin. Pharmacol. Ther. 101, 220–229. doi:10.1002/cpt.563
Lepsch, L. B., Gonzalo, L. A., Magro, F. J. B., Delucia, R., Scavone, C., and Planeta, C. S. (2005). Exposure to chronic stress increases the locomotor response to cocaine and the basal levels of corticosterone in adolescent rats. Addict. Biol. 10, 251–256. doi:10.1080/13556210500269366
Leung, J., Chan, G. C. K., Hides, L., and Hall, W. D. (2020). What is the prevalence and risk of cannabis use disorders among people who use cannabis? A systematic review and meta-analysis. Addict. Behav. 109, 106479. doi:10.1016/j.addbeh.2020.106479
Li, R., Fukumori, R., Takeda, T., Song, Y., Morimoto, S., Kikura-Hanajiri, R., et al. (2019). Elevation of endocannabinoids in the brain by synthetic cannabinoid JWH-018: Mechanism and effect on learning and memory. Sci. Rep. 9, 9621. doi:10.1038/S41598-019-45969-4
Lin, X., Dhopeshwarkar, A. S., Huibregtse, M., MacKie, K., and Hohmann, A. G. (2018). Slowly signaling G protein-biased CB2 cannabinoid receptor agonist LY2828360 suppresses neuropathic pain with sustained efficacy and attenuates morphine tolerance and dependence. Mol. Pharmacol. 93, 49–62. doi:10.1124/mol.117.109355
Liu, T., Zhang, L., Joo, D., and Sun, S. C. (2017). NF-κB signaling in inflammation. Signal Transduct. Target Ther. 2, 17023. doi:10.1038/sigtrans.2017.23
Liu, Y., Chen, Y., Fraga-González, G., Szpak, V., Laverman, J., Wiers, R. W., et al. (2022). Resting-state EEG, substance use and abstinence after chronic use: A systematic review. Clin. EEG Neurosci. 53, 344–366. doi:10.1177/15500594221076347
MacDonald, K., and Pappas, K. (2016). WHY not pot?: A review of the brain-based risks of cannabis. Innov. Clin. Neurosci. 13, 13–22. Available at: https://pubmed.ncbi.nlm.nih.gov/27354924/ (Accessed October 17, 2022).
MacNicol, B. (2017). The biology of addiction. Can. J. Anesth. 64, 141–148. doi:10.1007/s12630-016-0771-2
Maldonado, R., Berrendero, F., Ozaita, A., and Robledo, P. (2011). Neurochemical basis of cannabis addiction. Neuroscience 181, 1–17. doi:10.1016/j.neuroscience.2011.02.035
Malyshevskaya, O., Aritake, K., Kaushik, M. K., Uchiyama, N., Cherasse, Y., Kikura-Hanajiri, R., et al. (2017). Natural (Δ9-THC) and synthetic (JWH-018) cannabinoids induce seizures by acting through the cannabinoid CB1 receptor. Sci. Rep. 7, 10516. doi:10.1038/s41598-017-10447-2
Margiani, G., Castelli, M. P., Pintori, N., Frau, R., Ennas, M. G., Orrù, V., et al. (2022). Adolescent self-administration of the synthetic cannabinoid receptor agonist JWH-018 induces neurobiological and behavioral alterations in adult male mice. Psychopharmacology 239:(10), 3083–3102. doi:10.1007/S00213-022-06191-9
Ministry of Food and Drug Safety (2021). Development of sensitized screening method for addiction/dependence of abuse drugs. MFDS. Available at: https://scienceon.kisti.re.kr/commons/util/originalView.do?cn=TRKO202200005149&dbt=TRKO&rn= (Accessed February 20, 2023).
Morcuende, A., Navarrete, F., Nieto, E., Manzanares, J., and Femenía, T. (2021). Inflammatory biomarkers in addictive disorders. Biomolecules 11, 1824. doi:10.3390/biom11121824
Müller, M. M., Gruber, T., and Keil, A. (2000). Modulation of induced gamma band activity in the human EEG by attention and visual information processing. Int. J. Psychophysiol. 38, 283–299. doi:10.1016/S0167-8760(00)00171-9
National Institute on Drug Abuse (2021). Is marijuana safe and effective as medicine? National Institute on drug abuse (NIDA). National institutes of health. Available at: https://nida.nih.gov/publications/research-reports/marijuana/marijuana-safe-effective-medicine (Accessed October 17, 2022).
Nennig, S. E., and Schank, J. R. (2017). The role of NFkB in drug addiction: Beyond inflammation. Alcohol Alcohol. 52, 172–179. doi:10.1093/alcalc/agw098
Nestby, P., Vanderschuren, L. J. M. J., de Vries, T. J., Hogenboom, F., Wardeh, G., Mulder, A. H., et al. (1997). Ethanol, like psychostimulants and morphine, causes long-lasting hyperreactivity of dopamine and acetylcholine neurons of rat nucleus accumbens: Possible role in behavioural sensitization. Psychopharmacol. Berl. 133, 69–76. doi:10.1007/s002130050373
Nestler, E. J. (2001). Molecular basis of long-term plasticity underlying addiction. Nat. Rev. Neurosci. 2, 119–128. doi:10.1038/35053570
Newton, T. F., Cook, I. A., Kalechstein, A. D., Duran, S., Monroy, F., Ling, W., et al. (2003). Quantitative EEG abnormalities in recently abstinent methamphetamine dependent individuals. Clin. Neurophysiol. 114, 410–415. doi:10.1016/S1388-2457(02)00409-1
Nukitram, J., Cheaha, D., and Kumarnsit, E. (2021). Spectral power and theta-gamma coupling in the basolateral amygdala related with methamphetamine conditioned place preference in mice. Neurosci. Lett. 756, 135939. doi:10.1016/j.neulet.2021.135939
Ossato, A., Uccelli, L., Bilel, S., Canazza, I., di Domenico, G., Pasquali, M., et al. (2017). Psychostimulant effect of the synthetic cannabinoid JWH-018 and AKB48: Behavioral, neurochemical, and dopamine transporter scan imaging studies in mice. Front. Psychiatry 8, 130. doi:10.3389/fpsyt.2017.00130
Ota, S. M., Suchecki, D., and Meerlo, P. (2018). Chronic social defeat stress suppresses locomotor activity but does not affect the free-running circadian period of the activity rhythm in mice. Neurobiol. Sleep. Circadian Rhythms 5, 1–7. doi:10.1016/J.NBSCR.2018.03.002
Ozaita, A., Puighermanal, E., and Maldonado, R. (2007). Regulation of PI3K/Akt/GSK-3 pathway by cannabinoids in the brain. J. Neurochem. 102, 1105–1114. doi:10.1111/j.1471-4159.2007.04642.x
Parikh, V., Naughton, S. X., Shi, X., Kelley, L. K., Yegla, B., Tallarida, C. S., et al. (2014). Cocaine-induced neuroadaptations in the dorsal striatum: Glutamate dynamics and behavioral sensitization. Neurochem. Int. 75, 54–65. doi:10.1016/J.NEUINT.2014.05.016
Park, H., Han, K.-S., Seo, J., Lee, J., Dravid, S. M., Woo, J., et al. (2015). Channel-mediated astrocytic glutamate modulates hippocampal synaptic plasticity by activating postsynaptic NMDA receptors. Mol. Brain 8, 7. doi:10.1186/s13041-015-0097-y
Parsons, L. H., and Hurd, Y. L. (2015). Endocannabinoid signalling in reward and addiction. Nat. Rev. Neurosci. 16, 579–594. doi:10.1038/nrn4004
Paxinos, G., and Franklin, K. B. J. (2001). Paxinos and franklin’s the mouse brain in stereotaxic coordinates. Available at: https://books.google.com/books?hl=en&lr=&id=x3aQDwAAQBAJ&oi=fnd&pg=PP1&dq=related:2wOjGlM4MNoJ:scholar.google.com/&ots=mAGLt_LNcu&sig=LmcHDk5xWmonT8ydLdNV2Lue5J0 (Accessed October 10, 2022).
Perdikaris, P., Tsarouchi, M., Fanarioti, E., Natsaridis, E., Mitsacos, A., and Giompres, P. (2018). Long lasting effects of chronic WIN55,212-2 treatment on mesostriatal dopaminergic and cannabinoid systems in the rat brain. Neuropharmacology 129, 1–15. doi:10.1016/j.neuropharm.2017.11.005
Pintori, N., Castelli, M. P., Miliano, C., Simola, N., Fadda, P., Fattore, L., et al. (2021). Repeated exposure to JWH-018 induces adaptive changes in the mesolimbic and mesocortical dopaminergic pathways, glial cells alterations, and behavioural correlates. Br. J. Pharmacol. 178, 3476–3497. doi:10.1111/bph.15494
Polissidis, A., Chouliara, O., Galanopoulos, A., Marselos, M., Papadopoulou-Daifoti, Z., and Antoniou, K. (2009). Behavioural and dopaminergic alterations induced by a low dose of WIN 55,212-2 in a conditioned place preference procedure. Life Sci. 85, 248–254. doi:10.1016/j.lfs.2009.05.015
Post, R. M., and Kalivas, P. (2013). Bipolar disorder and substance misuse: Pathological and therapeutic implications of their comorbidity and cross-sensitisation. Br. J. Psychiatry 202, 172–176. doi:10.1192/BJP.BP.112.116855
Qiu, J., Yan, Z., Tao, K., Li, Y., Li, Y., Li, J., et al. (2016). Sinomenine activates astrocytic dopamine D2 receptors and alleviates neuroinflammatory injury via the CRYAB/STAT3 pathway after ischemic stroke in mice. J. Neuroinflammation 13, 263. doi:10.1186/s12974-016-0739-8
Ramos, K. M., Lewis, M. T., Morgan, K. N., Crysdale, N. Y., Kroll, J. L., Taylor, F. R., et al. (2010). Spinal upregulation of glutamate transporter GLT-1 by ceftriaxone: Therapeutic efficacy in a range of experimental nervous system disorders. Neuroscience 169, 1888–1900. doi:10.1016/J.NEUROSCIENCE.2010.06.014
Reid, M. S., Flammino, F., Howard, B., Nilsen, D., and Prichep, L. S. (2006). Topographic imaging of quantitative EEG in response to smoked cocaine self-administration in humans. Neuropsychopharmacology 31, 872–884. doi:10.1038/sj.npp.1300888
Ren, Z., Yang, M., Guan, Z., and Yu, W. (2018). Astrocytic α7 nicotinic receptor activation inhibits amyloid-β aggregation by upregulating endogenous αB-crystallin through the PI3K/akt signaling pathway. Curr. Alzheimer Res. 16, 39–48. doi:10.2174/1567205015666181022093359
Ribeiro Do Couto, B., Aguilar, M. A., Lluch, J., Rodríguez-Arias, M., and Miñarro, J. (2009). Social experiences affect reinstatement of cocaine-induced place preference in mice. Psychopharmacol. Berl. 207, 485–498. doi:10.1007/s00213-009-1678-1
Rodrigues, L. C. M., Gobira, P. H., de Oliveira, A. C., Pelição, R., Teixeira, A. L., Moreira, F. A., et al. (2014). Neuroinflammation as a possible link between cannabinoids and addiction. Acta Neuropsychiatr. 26, 334–346. doi:10.1017/neu.2014.24
Rodríguez-Arias, M., Roger-Sánchez, C., Vilanova, I., Revert, N., Manzanedo, C., Miñarro, J., et al. (2016). Effects of cannabinoid exposure during adolescence on the conditioned rewarding effects of WIN 55212-2 and cocaine in mice: Influence of the novelty-seeking trait. Neural Plast. 2016, 6481862. doi:10.1155/2016/6481862
Rygula, R., Abumaria, N., Flügge, G., Fuchs, E., Rüther, E., and Havemann-Reinecke, U. (2005). Anhedonia and motivational deficits in rats: Impact of chronic social stress. Behav. Brain Res. 162, 127–134. doi:10.1016/j.bbr.2005.03.009
Sales-Carbonell, C., Rueda-Orozco, P. E., Soria-Gómez, E., Buzsáki, G., Marsicano, G., and Robbe, D. (2013). Striatal GABAergic and cortical glutamatergic neurons mediate contrasting effects of cannabinoids on cortical network synchrony. Proc. Natl. Acad. Sci. U. S. A. 110, 719–724. doi:10.1073/pnas.1217144110
Sayson, L. V., Custodio, R. J. P., Ortiz, D. M., Lee, H. J., Kim, M., Jeong, Y., et al. (2020). The potential rewarding and reinforcing effects of the substituted benzofurans 2-EAPB and 5-EAPB in rodents. Eur. J. Pharmacol. 885, 173527. doi:10.1016/j.ejphar.2020.173527
Schell, M. T., Spitzer, A. L., Johnson, J. A., Lee, D., and Harris, H. W. (2005). Heat shock inhibits NF-kB activation in a dose- and time-dependent manner. J. Surg. Res. 129, 90–93. doi:10.1016/j.jss.2005.05.025
Scherma, M., Dessì, C., Muntoni, A. L., Lecca, S., Satta, V., Luchicchi, A., et al. (2016). Adolescent Δ 9-tetrahydrocannabinol exposure alters WIN55,212-2 self-administration in adult rats. Neuropsychopharmacology 41, 1416–1426. doi:10.1038/npp.2015.295
Schmitz, N., and Richert, L. (2020). Pharmacists and the future of cannabis medicine. J. Am. Pharm. Assoc. 60, 207–211. doi:10.1016/J.JAPH.2019.11.007
Shao, W., Zhang, S. Z., Tang, M., Zhang, X. H., Zhou, Z., Yin, Y. Q., et al. (2013). Suppression of neuroinflammation by astrocytic dopamine D2 receptors via αb-crystallin. Nature 494, 90–94. doi:10.1038/nature11748
Skalisz, L. L., Beijamini, V., Joca, S. L., Vital, M. A. B. F., da Cunha, C., and Andreatini, R. (2002). Evaluation of the face validity of reserpine administration as an animal model of depression-Parkinson’s disease association. Prog. Neuropsychopharmacol. Biol. Psychiatry 26, 879–883. doi:10.1016/S0278-5846(01)00333-5
Skosnik, P. D., D’Souza, D. C., Steinmetz, A. B., Edwards, C. R., Vollmer, J. M., Hetrick, W. P., et al. (2012). The effect of chronic cannabinoids on broadband eeg neural oscillations in humans. Neuropsychopharmacology 37, 2184–2193. doi:10.1038/npp.2012.65
Smaga, I., Gawlińska, K., Frankowska, M., Wydra, K., Sadakierska-Chudy, A., Suder, A., et al. (2020). Extinction training after cocaine self-administration influences the epigenetic and genetic machinery responsible for glutamatergic transporter gene expression in male rat brain. Neuroscience 451, 99–110. doi:10.1016/J.NEUROSCIENCE.2020.10.001
Somade, O. T., Ajayi, B. O., Tajudeen, N. O., Atunlute, E. M., James, A. S., and Kehinde, S. A. (2019). Camphor elicits up-regulation of hepatic and pulmonary pro-inflammatory cytokines and chemokines via activation of NF-kB in rats. Pathophysiology 26, 305–313. doi:10.1016/j.pathophys.2019.07.005
Sora, I., Li, B., Igari, M., Hall, F. S., and Ikeda, K. (2010). Transgenic mice in the study of drug addiction and the effects of psychostimulant drugs. Ann. N. Y. Acad. Sci. 1187, 218–246. doi:10.1111/J.1749-6632.2009.05276.X
Spanagel, R. (2020). Cannabinoids and the endocannabinoid system in reward processing and addiction: From mechanisms to interventions. Dialogues Clin. Neurosci. 22, 241–250. doi:10.31887/DCNS.2020.22.3/RSPANAGEL
Spanagel, R., and Sanchis-Segura, C. (2003). The use of transgenic mice to study addictive behavior. Clin. Neurosci. Res. 3, 325–331. doi:10.1016/S1566-2772(03)00094-X
Sun, L., Dong, R., Xu, X., Yang, X., and Peng, M. (2017). Activation of cannabinoid receptor type 2 attenuates surgery-induced cognitive impairment in mice through anti-inflammatory activity. J. Neuroinflammation 14, 138. doi:10.1186/s12974-017-0913-7
Sun, Y., Li, Y.-S., Yang, J.-W., Yu, J., Wu, Y.-P., and Li, B.-X. (2014). Exposure to atrazine during gestation and lactation periods: Toxicity effects on dopaminergic neurons in offspring by downregulation of Nurr1 and VMAT2. Int. J. Mol. Sci. 15, 2811–2825. doi:10.3390/ijms15022811
Sutton, L. P., and Caron, M. G. (2015). Essential role of D1R in the regulation of mTOR complex1 signaling induced by cocaine. Neuropharmacology 99, 610–619. doi:10.1016/J.NEUROPHARM.2015.08.024
Szumlinski, K. K., Lominac, K. D., Campbell, R. R., Cohen, M., Fultz, E. K., Brown, C. N., et al. (2017). Methamphetamine addiction vulnerability: The glutamate, the bad, and the ugly. Biol. Psychiatry 81, 959–970. doi:10.1016/j.biopsych.2016.10.005
Tai, S., and Fantegrossi, W. E. (2014). Synthetic cannabinoids: Pharmacology, behavioral effects, and abuse potential. Curr. Addict. Rep. 1, 129–136. doi:10.1007/s40429-014-0014-y
Tallon-Baudry, C., Bertrand, O., Hénaff, M.-A., Isnard, J., and Fischer, C. (2005). Attention modulates gamma-band oscillations differently in the human lateral occipital cortex and fusiform gyrus. Cereb. Cortex 15, 654–662. doi:10.1093/cercor/bhh167
Tampus, R., Yoon, S. S., de la Peña, J. B., Botanas, C. J., Kim, H. J., Seo, J. W., et al. (2015). Assessment of the abuse liability of synthetic cannabinoid agonists JWH-030, JWH-175, and JWH-176. Biomol. Ther. Seoul. 23, 590–596. doi:10.4062/biomolther.2015.120
Thomsen, M., and Caine, S. B. (2011). False positive in the intravenous drug self-administration test in C57BL/6J mice. Behav. Pharmacol. 22, 239–247. doi:10.1097/FBP.0b013e328345f8f2
Tsai, S. J. (2007). Increased central brain-derived neurotrophic factor activity could be a risk factor for substance abuse: Implications for treatment. Med. Hypotheses 68, 410–414. doi:10.1016/J.MEHY.2006.05.035
Ucha, M., Roura-Martínez, D., Ambrosio, E., and Higuera-Matas, A. (2020). The role of the mTOR pathway in models of drug-induced reward and the behavioural constituents of addiction. J. Psychopharmacol. 34, 1176–1199. doi:10.1177/0269881120944159
van de Giessen, E., Weinstein, J. J., Cassidy, C. M., Haney, M., Dong, Z., Ghazzaoui, R., et al. (2017). Deficits in striatal dopamine release in cannabis dependence. Mol. Psychiatry 22, 68–75. doi:10.1038/mp.2016.21
VanGuilder, H. D., Vrana, K. E., and Freeman, W. M. (2008). Twenty-five years of quantitative PCR for gene expression analysis. Biotechniques 44, 619–626. doi:10.2144/000112776
Vlachou, S., and Panagis, G. (2014). Regulation of brain reward by the endocannabinoid system: A critical review of behavioral studies in animals. Curr. Pharm. Des. 20, 2072–2088. doi:10.2174/13816128113199990433
Volkow, N. D. (2009). Substance use disorders in Schizophrenia - clinical implications of comorbidity. Schizophr. Bull. 35, 469–472. doi:10.1093/schbul/sbp016
Vonder Haar, C., Ferland, J. M. N., Kaur, S., Riparip, L. K., Rosi, S., and Winstanley, C. A. (2019). Cocaine self-administration is increased after frontal traumatic brain injury and associated with neuroinflammation. Eur. J. Neurosci. 50, 2134–2145. doi:10.1111/ejn.14123
Wang, S.-H., Hsiao, P.-C., Yeh, L.-L., Liu, C.-M., Liu, C.-C., Hwang, T.-J., et al. (2018). Polygenic risk for schizophrenia and neurocognitive performance in patients with schizophrenia. Genes. Brain Behav. 17, 49–55. doi:10.1111/gbb.12401
Weinstein, A. M., Rosca, P., Fattore, L., and London, E. D. (2017). Synthetic cathinone and cannabinoid designer drugs pose a major risk for public health. Front. Psychiatry 8, 156. doi:10.3389/fpsyt.2017.00156
Wilson, J. M., Kalasinsky, K. S., Levey, A. I., Bergeron, C., Reiber, G., Anthony, R. M., et al. (1996). Striatal dopamine nerve terminal markers in human, chronic methamphetamine users. Nat. Med. 2 (6), 699–703. doi:10.1038/nm0696-699
Wood, N. C., Hamilton, I., Axon, A. T. R., Khan, S. A., Quirke, P., Mindham, R. H. S., et al. (1987). Abnormal intestinal permeability. Br. J. Psychiatry 150, 853–856. doi:10.1192/bjp.150.6.853
Xi, Z. X., Peng, X. Q., Li, X., Song, R., Zhang, H. Y., Liu, Q. R., et al. (2011). Brain cannabinoid CB- receptors modulate cocaine's actions in mice. Nat. Neurosci. 14, 1160–1166. doi:10.1038/nn.2874
Xie, S., and Wang, X. (2022). CRYAB reduces cigarette smoke-induced inflammation, apoptosis, and oxidative stress by retarding PI3K/Akt and NF-κB signaling pathways in human bronchial epithelial cells. Allergol. Immunopathol. Madr. 50, 23–29. doi:10.15586/AEI.V50I5.645
Xu, F., Yu, H., Liu, J., and Cheng, L. (2013). αb-crystallin regulates oxidative stress-induced apoptosis in cardiac H9c2 cells via the PI3K/AKT pathway. Mol. Biol. Rep. 40, 2517–2526. doi:10.1007/s11033-012-2332-2
Xu, H., Cheng, C. L., Chen, M., Manivannan, A., Cabay, L., Pertwee, R. G., et al. (2007). Anti-inflammatory property of the cannabinoid receptor-2-selective agonist JWH-133 in a rodent model of autoimmune uveoretinitis. J. Leukoc. Biol. 82, 532–541. doi:10.1189/JLB.0307159
Xu, L., Nan, J., and Lan, Y. (2020). The nucleus accumbens: A common target in the comorbidity of depression and addiction. Front. Neural Circuits 14, 37. doi:10.3389/fncir.2020.00037
Xu, W., Guo, Y., Huang, Z., Zhao, H., Zhou, M., Huang, Y., et al. (2019). Small heat shock protein CRYAB inhibits intestinal mucosal inflammatory responses and protects barrier integrity through suppressing IKKβ activity. Mucosal Immunol. 12, 1291–1303. doi:10.1038/s41385-019-0198-5
Yazdani, N., Parker, C. C., Shen, Y., Reed, E. R., Guido, M. A., Kole, L. A., et al. (2015). Hnrnph1 is A quantitative trait gene for methamphetamine sensitivity. PLoS Genet. 11, e1005713. doi:10.1371/journal.pgen.1005713
Young, A. M. H., Campbell, E., Lynch, S., Suckling, J., and Powis, S. J. (2011). Aberrant NF-kappaB expression in autism spectrum condition: A mechanism for neuroinflammation. Front. Psychiatry 2, 27. doi:10.3389/fpsyt.2011.00027
Zanettini, C., Scaglione, A., Keighron, J. D., Giancola, J. B., Lin, S.-C., Newman, A. H., et al. (2019). Pharmacological classification of centrally acting drugs using EEG in freely moving rats: An old tool to identify new atypical dopamine uptake inhibitors. Neuropharmacology 161, 107446. doi:10.1016/j.neuropharm.2018.11.034
Zehra, A., Burns, J., Liu, C. K., Manza, P., Wiers, C. E., Volkow, N. D., et al. (2018). Cannabis addiction and the brain: A review. J. Neuroimmune Pharmacol. 13, 438–452. doi:10.1007/s11481-018-9782-9
Zhang, J. F., Liu, J., Wu, J. L., Li, W. F., Chen, Z. W., and Yang, L. S. (2019). Progression of the role of CRYAB in signaling pathways and cancers. Onco Targets Ther. 12, 4129–4139. doi:10.2147/OTT.S201799
Zhang, Y., Crofton, E. J., Li, D., Lobo, M. K., Fan, X., Nestler, E. J., et al. (2014). Overexpression of DeltaFosB in nucleus accumbens mimics the protective addiction phenotype, but not the protective depression phenotype of environmental enrichment. Front. Behav. Neurosci. 8, 297. doi:10.3389/fnbeh.2014.00297
Zhu, R., Bu, Q., Fu, D., Shao, X., Jiang, L., Guo, W., et al. (2018). Toll-like receptor 3 modulates the behavioral effects of cocaine in mice. J. Neuroinflammation 15, 93–11. doi:10.1186/s12974-018-1130-8
Keywords: cannabinoid, Cryab, JWH-018, neuroinflammation, NF-κB, synaptic plasticity, addiction susceptibility, drug abuse
Citation: Sayson LV, Ortiz DM, Lee HJ, Kim M, Custodio RJP, Yun J, Lee CH, Lee YS, Cha HJ, Cheong JH and Kim HJ (2023) Deletion of Cryab increases the vulnerability of mice to the addiction-like effects of the cannabinoid JWH-018 via upregulation of striatal NF-κB expression. Front. Pharmacol. 14:1135929. doi: 10.3389/fphar.2023.1135929
Received: 02 January 2023; Accepted: 07 March 2023;
Published: 16 March 2023.
Edited by:
Francisco Navarrete Rueda, Miguel Hernández University of Elche, SpainReviewed by:
Elena Martín-García, Pompeu Fabra University, SpainCopyright © 2023 Sayson, Ortiz, Lee, Kim, Custodio, Yun, Lee, Lee, Cha, Cheong and Kim. This is an open-access article distributed under the terms of the Creative Commons Attribution License (CC BY). The use, distribution or reproduction in other forums is permitted, provided the original author(s) and the copyright owner(s) are credited and that the original publication in this journal is cited, in accordance with accepted academic practice. No use, distribution or reproduction is permitted which does not comply with these terms.
*Correspondence: Jae Hoon Cheong, Y2hlb25namhAamJudS5hYy5rcg==; Hee Jin Kim, aGpraW1Ac3l1LmFjLmty
Disclaimer: All claims expressed in this article are solely those of the authors and do not necessarily represent those of their affiliated organizations, or those of the publisher, the editors and the reviewers. Any product that may be evaluated in this article or claim that may be made by its manufacturer is not guaranteed or endorsed by the publisher.
Research integrity at Frontiers
Learn more about the work of our research integrity team to safeguard the quality of each article we publish.