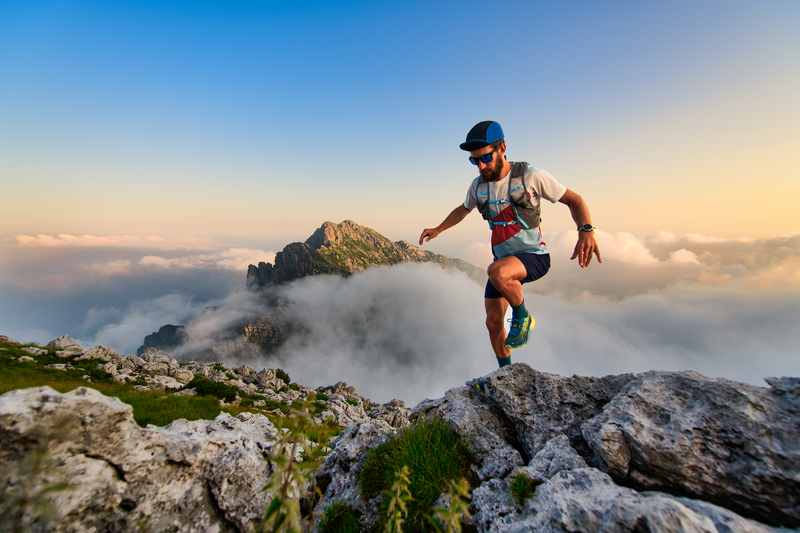
94% of researchers rate our articles as excellent or good
Learn more about the work of our research integrity team to safeguard the quality of each article we publish.
Find out more
REVIEW article
Front. Pharmacol. , 17 March 2023
Sec. Integrative and Regenerative Pharmacology
Volume 14 - 2023 | https://doi.org/10.3389/fphar.2023.1131001
This article is part of the Research Topic Comprehensive Biomaterial Strategies for Musculoskeletal Tissue Regeneration View all 9 articles
Exosomes are extracellular vesicles (EVs) containing various ingredients such as DNA, RNA, lipids and proteins, which play a significant role in intercellular communication. Numerous studies have demonstrated the important role of exosomes in bone regeneration through promoting the expression of osteogenic-related genes and proteins in mesenchymal stem cells. However, the low targeting ability and short circulating half-life of exosomes limited their clinical application. In order to solve those problems, different delivery systems and biological scaffolds have been developed. Hydrogel is a kind of absorbable biological scaffold composed of three-dimensional hydrophilic polymers. It not only has excellent biocompatibility and superior mechanical strength but can also provide a suitable nutrient environment for the growth of the endogenous cells. Thus, the combination between exosomes and hydrogels can improve the stability and maintain the biological activity of exosomes while achieving the sustained release of exosomes in the bone defect sites. As an important component of the extracellular matrix (ECM), hyaluronic acid (HA) plays a critical role in various physiological and pathological processes such as cell differentiation, proliferation, migration, inflammation, angiogenesis, tissue regeneration, wound healing and cancer. In recent years, hyaluronic acid-based hydrogels have been used as an exosome delivery system for bone regeneration and have displayed positive effects. This review mainly summarized the potential mechanism of HA and exosomes in promoting bone regeneration and the application prospects and challenges of hyaluronic acid-based hydrogels as exosome delivery devices in bone regeneration.
Bone regeneration is a complex, multi-stage physiological process involving a variety of cells, cytokines, chemokines, growth factors, and intracellular and extracellular signaling pathways (Dimitriou et al., 2011; Majidinia et al., 2018; Salhotra et al., 2020). Fracture healing is the most common form of bone regeneration in clinical settings. It consists of two main mechanisms: direct and indirect remodeling, with the latter predominating (Ferguson et al., 1999; Dimitriou et al., 2011; St-Arnaud and Naja, 2011). Direct remodeling usually requires anatomical reduction and strict stability. Once these conditions are met, the lamellar bone and the Haversian systems are able to regenerate with fewer calluses (Marsell and Einhorn, 2011). Indirect remodeling, on the other hand, is not required to meet the conditions of anatomical reduction and strict stability. It is mainly formed calluses through both intramembranous and endochondral ossification (Marsell and Einhorn, 2011; St-Arnaud and Naja, 2011). Because of the limited ability of bones to self-heal after injury, it is urgent for clinicians to repair large bone defects caused by various reasons such as fractures, traumatic injuries, tumor removal, and infection, and restore their function (Bai et al., 2018; Wang et al., 2019; Yu et al., 2020). It has been demonstrated that once the length of the bone defect exceeds 2 to 2.5 times the diameter of the damaged bone, the self-healing ability of bone tissue alone is not enough (Wiese and Pape, 2010). Hence, some additional clinical treatments are needed to keep the bone defect site stable and create a suitable microenvironment for bone regeneration, thus achieving better bone regeneration and functional reconstruction of the defect site (Elliott et al., 2016; Zhang et al., 2022). Currently, autografts and allografts remain the main methods for repairing bone defects (Dimitriou et al., 2011; Chen and Lv, 2018). Nevertheless, the limited graft supply, donor area complications, immune rejection, and spread of infectious diseases associated with bone grafting have prompted the search for new bone substitutes to achieve the regeneration and functional reconstruction of bone tissue (Polo-Corrales et al., 2014; Amirazad et al., 2022).
Extracellular vesicles (EVs), including exosomes, endosomes, microparticles, apoptotic bodies and other different subtypes, are nanoscale biological vesicles released by different cells (Lotvall et al., 2014; Teng and Fussenegger, 2020). Exosomes are EVs with an average diameter of 100 nm, which contain different components such as nucleic acids, proteins, lipids, and metabolites, depending on the various sources of cells (Kalluri and LeBleu, 2020). Recently, exosomes derived from different kinds of cells have shown enormous potential in bone regeneration and repair as a key element of cell-free therapy (Zhai et al., 2020). This cell-free therapy strategy based on exosomes not only avoids the immune risks relevant to cell therapy and improves the low homing efficiency of transplanted cells, but it also can achieve the repair and regeneration of the defective bone tissue by regulating the inflammatory response and promoting angiogenesis and osteogenesis (Eggenhofer et al., 2014; Sun et al., 2022; Zhang et al., 2022). And since exosomes do not have the ability to self-replicate, the risk of iatrogenic tumor formation is also greatly reduced (Sun et al., 2022). Additionally, exosomes have high stability, can better maintain biological activity, and also have an intrinsic homing effect that can target organs (Lou et al., 2017; Yang et al., 2020). Unfortunately, exosomes have a short half-life in the body and are quickly cleared through body fluids without encapsulation (Riau et al., 2019). Therefore, it is very necessary to find a delivery vehicle that can achieve sustained release of exosomes at the site of injury while maintaining the bioactivity of exosomes.
In recent years, hyaluronic acid-based hydrogels have received extensive attention for the delivery of exosomes from different cell sources (Xin et al., 2022). Hyaluronic acid (HA) is a naturally occurring unbranched glycosaminoglycan (GAG) that is composed of repeating units of the disaccharide β-1,4-D-glucuronic acid-β-1,3-N-acetyl-D-glucosamine (Burdick and Prestwich, 2011; Abatangelo et al., 2020). It is widely present in mammalian tissues and plays a critical role in cell differentiation, proliferation, migration, inflammation, angiogenesis, wound healing, cancer, diabetes and many other physiological and pathological processes (Passi and Vigetti, 2019; Marinho et al., 2021). Besides, hyaluronic acid-based hydrogels prepared by different physical or chemical methods as delivery vehicles for exosomes, mesenchymal stem cells (MSCs), drugs and growth factors, have been extensively studied in various disease models such as osteoarthritis, bone defects and cardiac repair (Figure 1) (Park et al., 2017; Jin Y. et al., 2020; Zhang et al., 2021b; Zou et al., 2021; Wang L. et al., 2022; Sang et al., 2022).
In this review, we described the synthesis and degradation of HA, the application of modified hyaluronic acid-based hydrogels in bone regeneration, the potential mechanism of hyaluronic acid in promoting bone regeneration, and the role of exosomes in bone regeneration. Finally, the applications, prospects and challenges of hyaluronic acid-based hydrogels as exosome delivery systems in bone regeneration were summarized.
HA is a linear non-sulfated GAG formed by repeating disaccharides units (D-glucuronic acid and N-acetyl-D-glucosamine) linked alternately by β-1,3 and β-1,4 glycosidic bonds (Passi and Vigetti, 2019; Fang et al., 2021). Most cells in the body are capable of synthesizing HA (Marinho et al., 2021). Specially, the synthesis of HA differs from that of other types of GAGs, which are produced in the Golgi apparatus, whereas HA is produced by the hyaluronic acid synthases (HASes) in the inner leaflet of the cell membrane (Weigel et al., 1997; Passi and Vigetti, 2019; Abatangelo et al., 2020). The total content of HA in the human body is about 15 g, which is mainly in the skin and musculoskeletal tissues (Knopf-Marques et al., 2016). In mammals, HA is mainly synthesized by three types of HAS: HAS1, HAS2 and HAS3(Passi and Vigetti, 2019; Marinho et al., 2021). These three types of HAS use UDP-glucuronic acid and UDP-N-acetylglucosamine in the cytoplasm as substrates to catalyze repetitive sugar polymerization and extrude HA into the extracellular matrix (ECM) through the pore-like structures in the cell membrane (Vigetti et al., 2014; Kobayashi et al., 2020; Marinho et al., 2021). Due to the difference in the catalytic activities of these three types of enzymes, the molecular weight (MW) of the synthesized HA molecules ranges from 20,000 up to millions of Daltons (Vigetti et al., 2014; Trombino et al., 2019). For example, HAS1 and HAS2 can synthesize polymers with a high MW (larger than 2 × 106 Da), while HAS3 synthesizes polymers with a low MW (1 × 105 to 1 × 106 Da) (Itano et al., 1999; Abatangelo et al., 2020). Moreover, the turnover of HA in the body is quick, the tissue half-lives range from several hours to a few days, and the daily turnover is about one-third of the total (Monslow et al., 2015; Trombino et al., 2019; Marinho et al., 2021). The HA in the blood is mainly degraded by the lymphatic system and liver, while HA in tissues is mainly degraded by hyaluronidases (HYALs), reactive oxygen species, superoxide dismutase, and nitric oxide produced by inflammation or damaged tissues (Collins and Birkinshaw, 2013; Garantziotis and Savani, 2019; Heldin et al., 2019; Abatangelo et al., 2020). In mammals, various HYALs such as HYAL 1-4, HYALP1 and PH-20 can degrade HA by catalyzing the hydrolysis of the β-1,4 glycosidic bonds between hexosamine and D-glucuronic acid residues (Stern and Jedrzejas, 2006; Stern et al., 2007; Knopf-Marques et al., 2016; Abatangelo et al., 2020). HYAL-1 and HYAL-2 are the main HYALs in mammals and widely exist in various somatic tissues (Kobayashi et al., 2020). PH-20 is the most active HYAL in mammals; it is mainly present on the surface of the sperm head and is essential for the fertilization of sperm (Weber et al., 2019; Kobayashi et al., 2020). These HYALs are capable of degrading HA into many small fragments. For example, HYAL-2 can break down HA into fragments of about 20 kDa, which are normally internalized into cells and subsequently further cleaved into smaller oligomeric HA by HYAL-1 in lysosomes (Tammi et al., 2001; Lee-Sayer et al., 2015; Abatangelo et al., 2020; Marinho et al., 2021). However, when the number of small fragments of HA in the ECM exceeds the clearance capacity of cells, these fragments can play an important role in biological processes such as angiogenesis and inflammatory responses (Bohaumilitzky et al., 2017; Passi and Vigetti, 2019). The balance between the synthesis and degradation of HA determines its MW and content, while the MW of HA determines its different biological functions (Abatangelo et al., 2020; Kobayashi et al., 2020; Marinho et al., 2021). For instance, the HA with high MW has angiogenesis-inhibiting, anti-inflammatory, immune-suppressive and cell proliferation-inhibiting effects, while the HA with low MW can induce extracellular matrix-associated cytokine activity, angiogenesis, tissue inflammation, and cell death (West and Kumar, 1989; McKee et al., 1996; Passi and Vigetti, 2019; Marinho et al., 2021; Ding et al., 2022).
In order to exert its biological properties, HA needs to interact with receptors on the surface of the target cell to activate relevant signaling pathways within the cell. Presently, the interaction effects of a variety of receptors such as cluster of differentiation-44 (CD44), receptor for hyaluronic acid-mediated motility (RHAMM), cell migration inducing hyaluronidase 1 (CEMIP), hyaluronic acid-receptor for endocytosis (HARE), lymphatic vessel endothelial receptor-1 (LYVE-1), Toll-like receptors (TLR) and other receptors with HA have been widely researched (Garantziotis and Savani, 2019). CD44 and RHAMM are major HA receptors (Abatangelo et al., 2020). Previous research has established that the binding of HA and CD44 can affect various processes such as cell adhesion, bone metabolism, migration, inflammation, tumor growth and metastasis (Lesley et al., 1993; Ishida et al., 1997). Additionally, the study of Leng et al. (2019) suggested that CD44 and RHAMM have overlapping functions in regulating cell behavior and that HA binding to both receptors can regulate the motility and proliferation of myoblasts and connective tissue cells. Another study found that HA activates protein tyrosine kinases and ERK1, 2-MAP kinase cascades by interacting with CD44, RHAMM, and growth factors, thereby increasing the random movement ability of cells (Tolg et al., 2014; Knopf-Marques et al., 2016). Furthermore, it was shown that CEMIP, an HA-binding protein engaged in HA depolymerization, controls endochondral ossification through the metabolism of HA (Shimoda et al., 2017). Besides, high-MW HA can disrupt macrophage colony-stimulating factor signal transduction in osteoclast precursors and the TLR4, inhibit osteoclast differentiation, and thus prevent bone resorption in vivo (Chang et al., 2007).
Although the mechanism of interaction between HA and various receptors has been elucidated in many studies, the specific mechanism of HA in promoting osteogenesis still remains unclarified. Despite this, there are still studies constantly showing that HA is useful for the osteogenesis process. Previous studies have demonstrated that HA can limit osteoblast-mediated osteoclast genesis by interacting with CD44, thus playing an important role in bone metabolism and the communication between osteocytes and osteocytes or osteoclasts (Fujii et al., 2003; Kawano et al., 2011). It has also been shown that HA can bind to CD44 on the surface of human dental pulp stem cells (hDPSCs) and promote the expression of bone-related markers and osteogenic differentiation by inducing the YAP/TAZ pathway (La Noce et al., 2021). Moreover, the study by Huang et al. (2003) revealed that HA can increase the proliferation and differentiation of osteoprogenitor cells into the osteoblastic phenotype, as well as enhance the osteoinductive and osteogenic properties of bone graft materials and substitutes. This effect was dose-specific and molecular weight-specific; specifically, the HA with a high MW can increase the activity of alkaline phosphatase (ALP) and promote cell mineralization in a dose-dependent manner, while the HA with a low MW works well in increasing cell proliferation and the expression of osteocalcin mRNA (Huang et al., 2003). Recently, Lu et al. (2022) constructed an instantly fixable and self-adaptive hybrid cross-linked scaffold (HCLS) using dopamine-modified HA, micron hydroxyapatite, type I collagen and other materials to mimic the natural bone ECM. This scaffold not only has excellent deformation and mechanical matching ability but also provides an appropriate immune microenvironment to regulate the M2 phenotype polarization of macrophages. It has been demonstrated that the M2 macrophages play an active role in bone regeneration by secreting BMP-2, IL-10 and TGF-β to stimulate osteoblast differentiation and bone formation (Xiong et al., 2020; Li et al., 2021; Schlundt et al., 2021). Hence, when this scaffold is applied to cranial defect models in rabbits and beagle dogs, it can recruit endogenous stem cells in situ, rapidly initiate angiogenesis and osteogenesis, and accelerate osteogenic differentiation to promote cranial bone regeneration (Lu et al., 2022).
Additionally, HA also has an effect on angiogenesis, which is also essential for bone regeneration. Park et al. (2016) prepared a catechol-functionalized hyaluronic acid hydrogel loaded with human adipose-derived stem cells and applied it in the mouse hindlimb ischemia and critical-sized calvarial bone defects models, respectively. Their study illustrated that this hydrogel has excellent biocompatibility and tissue adhesiveness and can significantly promote angiogenesis and bone reconstruction at the defect site. Precious studies have shown that the Notch signaling pathway plays an important role in both bone development and remodeling (Zanotti and Canalis, 2016). It has also been demonstrated that Jagged-1 is the most highly expressed Notch ligand in fracture repair, and when it interacts with a membrane-bound receptor (Notch1-4) on the receiving cell, Notch signaling will be activated, thus promoting osteogenesis (Dishowitz et al., 2014). Consequently, Deng et al. (2021) conjugate the porous HA hydrogels with Jagged-1 ligand to enhance osteogenesis and bone regeneration by upregulating the Notch intracellular domain (NICD) and downstream msh homeobox 2 (MSX2, one of the Notch-signaling-related genes) to active Notch signaling. Furthermore, this functionalized Jagged-1 ligand can indirectly promote bone regeneration by regulating macrophage recruitment and promoting angiogenesis. Regrettably, although many previous studies have reported the ability of HA in promoting osteogenesis, the pathways and specific mechanisms involved in this process remain to be further investigated.
Although HA has great biocompatibility, biodegradability, and hydrophilicity, its mechanical properties are poorer than those of normal bone tissue, and the unmodified HA is susceptible to being degraded by HASes (Zhao et al., 2016; Fang et al., 2021; Pérez et al., 2021; Ding et al., 2022). Hence, in order to overcome these problems, researchers always modify HA and prepare it into hydrogel through various physical or chemical approaches to improve its mechanical properties, stability and half-life (Ahmadian et al., 2020; Marinho et al., 2021; Pérez et al., 2021). There are three types of sites in each disaccharide unit of HA that can be chemically modified, including carboxyl, hydroxyl and N-acetyl groups (Fang et al., 2021; Pérez et al., 2021). HA is mainly modified through its carboxyl groups, and the modification of its carboxyl groups using carbodiimide derivatives is one of the most widely used methods (Schanté et al., 2011; Pérez et al., 2021). Specifically, the carboxyl groups of HA can be amidated by 1- ethyl-3-[3-(dimethylamino)-propyl]-carbodiimide (EDC), 2-chloro-1-methylpyridinium iodide (CMPI), 2-chloro-dimethoxy-1,3,5-triazine (CDMT), 1,1′-carbonyldiimidazole, hexamethylenediamine (HMDA) and react with different crosslinking agents to generate hyaluronic acid-based hydrogels (Magnani et al., 2000; Park et al., 2002; Schanté et al., 2011; D'Este et al., 2012; Yan et al., 2012; Liang et al., 2015; Wang L. et al., 2018; Fang et al., 2021). Furthermore, it also can be esterified by alkyl halides, tosylate, diazomethane and epoxides, for tissue engineering and drug delivery (Burdick and Prestwich, 2011; Schanté et al., 2011; Collins and Birkinshaw, 2013). The hydroxyl groups of HA can form ether bonds under the modification of epoxides, divinyl sulfone, and ethylene sulfide, but they can also form ester bonds under the modification of octenyl succinic anhydride (OSA), activated compounds, and methacrylic anhydride (Schanté et al., 2011). The N-acetyl groups of HA can be deacetylated to form amino groups, which can react with the carboxyl group of HA to form an auto-crosslinked hydrogel or react with the active acid to form amide bonds (Schanté et al., 2011; Collins and Birkinshaw, 2013). For instance, the study of Zhang et al. (2020b) revealed that the HA modified by methacrylic anhydride can form lighted-cured hydrogels with good biocompatibility and mechanical properties in the presence of other crosslinking agents. In addition, Poldervaart et al. (2017) modified the naturally occurring extracellular matrix HA and prepared a photo-crosslinked methacrylated hyaluronic acid (MeHA) hydrogel with better mechanical strength and long-term stability using 3D bioprinting technology. In in vitro experiments, hydrogels with high concentrations of MeHA polymers can promote osteogenic differentiation of MSCs without additional osteogenic stimulation. Another study indicated that the combination of glycidyl methacrylate-modified hyaluronic acid-based hydrogels with appropriate osteoinductive molecules [such as morphogenetic protein 2 (BMP-2) and vascular endothelial-derived growth factor (VEGF)] can induce the formation of mineralized bone tissue and promote bone growth in vivo (Patterson et al., 2010). Moreover, the temporal progression of bone formation and remodeling can be regulated by changing the degradation rate of the hydrogel scaffolds (Patterson et al., 2010).
Currently, various crosslinking strategies for HA have been developed, such as click chemistry, Schiff base reactions, enzymatic crosslinking, disulfide crosslinking, radical polymerization crosslinking, and condensation reactions (Trombino et al., 2019; Ding et al., 2022). These hyaluronic acid-based hydrogels are widely used in tissue engineering, regenerative medicine, cell delivery, drug delivery and exosome delivery (Trombino et al., 2019; Bao et al., 2021; Flegeau et al., 2021; Hamilton et al., 2021; Yang et al., 2021; Cheng et al., 2022; Flegeau et al., 2022; Mi et al., 2022). Because of their high reactivity, selectivity, and yield, click chemistry strategies such as Diels–Alder reaction, Azide-Alkyn cycloaddition reaction, Thiol-ene reaction, Aldehyde-Hydrazide coupling and others have emerged as the most promising strategy for preparing hydrogels under mild conditions (Pérez et al., 2021). Since various modification and cross-linking strategies of HA have been described in detail in previous literature, this article will not introduce them further (Schanté et al., 2011; Collins and Birkinshaw, 2013; Khunmanee et al., 2017; Pérez et al., 2021; Di Mola et al., 2022). Table 1 lists various crosslinking strategies and applications of hyaluronic acid-based hydrogels.
Exosomes are EVs that are released by various cell types and are widely found in the interstitial spaces and body fluids (Zhang et al., 2019b; Brigstock, 2021). Exosomes from various origins can influence gene expression and signaling pathways regulation in recipient cells by delivering biomolecules such as mRNA, miRNA, and proteins (Akbari et al., 2020; Kalluri and LeBleu, 2020; Hu et al., 2021; Sun et al., 2022). It has been demonstrated that tetraspanins, integrins, immunoglobulins, proteoglycans and lectins all play a role in the binding of EVs to recipient cells (Mulcahy et al., 2014; Hoshino et al., 2015; Mathieu et al., 2019). Exosomes can enter target cells through various forms of endocytosis, such as clathrin-mediated endocytosis, caveolin-dependent endocytosis, macropinocytosis and phagocytosis, and lipid raft-mediated endocytosis (Figure 2), and then release their contents (Mulcahy et al., 2014; Pegtel and Gould, 2019; Ginini et al., 2022; van Niel et al., 2022). And exosomes follow the endosome pathway after being internalized by the recipient cells, from early endosomes to late endosomes to MVBs (Gurung et al., 2021; Khan and Steeg, 2021). Eventually, some of them are degraded after fusion with lysosomes, while others escape the lysosomal pathway and transfer their contents into the cytoplasm of recipient cells, mediating the changes of recipient cells (Gurung et al., 2021; Khan and Steeg, 2021; van Niel et al., 2022). Moreover, exosomes can also fuse directly with the plasma membrane of recipient cells (Mulcahy et al., 2014; Mathieu et al., 2019; Teng and Fussenegger, 2020; Gurung et al., 2021; Kimiz-Gebologlu and Oncel, 2022). On the one hand, exosomes release their contents into the cytoplasm of recipient cells, and on the other hand, the direct interaction between exosomes and the receptors on the surface of recipient cells induces downstream signaling cascades (Gurung et al., 2021). Recently, exosomes have been widely used for bone regeneration due to their biocompatibility, biostability and low immunogenicity (Tan et al., 2020; Wang et al., 2020). In the bone defects microenvironment consisting of various cells, such as osteoblasts, osteoclasts, endothelial cells, immune cells, and MSCs, exosomes can participate in the regulation of angiogenesis, osteogenesis and inflammation by being phagocytosed by these target cells and interacting with them (Safari et al., 2021; Zhu et al., 2021; Zhang et al., 2022). For instance, Kang et al. (2022) designed an exosome-functionalized cell-free PLGA/Exo-Mg-GA MOF composite scaffold that simultaneously enhances angiogenesis, osteogenesis, and anti-inflammatory capacity. The human adipose stem cell-derived exosomes (hADSCs-Exos) released from this scaffold can enter the human bone marrow-derived mesenchymal stem cells (hBMSCs) and human umbilical vein endothelial cells (HUVECs) co-cultured with the scaffold through phagocytosis to promote osteogenic differentiation and accelerate bone reconstruction.
FIGURE 2. Exosomes enter target cells through various forms of endocytosis, including clathrin-mediated endocytosis, caveolin-dependent endocytosis, macropinocytosis and phagocytosis, and lipid raft-mediated endocytosis.
It was reported that exosomes secreted by MSCs derived from human induced pluripotent stem cells (hiPSCs) and the exosomes derived from human adipose stem cells, bone marrow mesenchymal stem cells (BMSCs), and other types of cells all have the potential to promote osteogenesis (Qi et al., 2016; Li et al., 2018; Chen et al., 2019; Zhang et al., 2020a). They can regulate the expression of osteogenic factors and osteogenesis-related proteins in the MSCs through the bioactive molecules they contain (Narayanan et al., 2016). Exosomes secreted by MSCs derived from hiPSCs (hiPSC-MSC-Exos), for example, not only affect in vitro proliferation and osteogenic differentiation of BMSCs by enhancing osteogenic gene and protein expression [such as Collagen type I (COL 1), Runt-related transcription factor 2 (RUNX-2) and ALP], but also stimulate bone regeneration of critical-sized calvarial defects in ovariectomized rats (Qi et al., 2016). And there are also studies demonstrating that exosomes secreted by MSCs derived from hiPSCs also can be internalized by BMSCs to enhance the osteoinductivity of beta-tricalcium phosphate (β-TCP) and promote the repair of calvarial bone defects in rats by regulating the PI3K/Akt signaling pathway (Zhang et al., 2016). Another study illustrated that optimized bone marrow mesenchymal stem cells-derived osteoinductive exosomes (BMSCs-OI-Exos) can enhance bone regeneration by targeting Acvr2b/Acvr1 via multiple miRNAs (such as let-7a-5p, let-7c-5p, miR-328a-5p, and miR-31a-5p) and activating Smad1/5/9 phosphorylation through Bmpr2/Acvr2b competitive receptors (Liu et al., 2021a). Moreover, there are also studies revealing that miR-5,106 in M2 macrophage-derived exosomes (M2D-Exos) can induce the osteogenic differentiation of BMSCs and promote femoral fracture healing in mice by targeting the salt-inducible kinases 2 and 3 (SIK2 and SIK3) (Xiong et al., 2020). And the study of Zhang et al. (2021a) indicated that bone marrow mesenchymal stem cells-derived exosomes (BMSCs-Exos) can also reduce the osteoporosis in rats by delivering miR-935 to osteoblasts and targeting signal transducer and activator of transcription 1 (STAT1), inhibiting its expression, and promoting the proliferation and differentiation of osteoblasts.
As is known to us, the lack of a vascular network at the bone defect will prevent the formation of new bone, and adequate blood supply is an important basis for bone generation (Wu et al., 2021; Zhang et al., 2022). Angiogenesis and osteogenesis are closely related, and only by improving both at the same time can the remodeling of new bone be better improved (Behera et al., 2021; Wu et al., 2021). For instance, Wang et al. (2022b) manifested that macrophages-derived exosomes (MФs-derived exosomes) can promote the migration of endothelial cells and enhance the expression of angiogenic-related genes, thus promoting angiogenesis, while increasing ALP activity and the expression of osteogenic-related genes in BMSCs. Besides, the study of Zhang et al. (2019a) showed that human umbilical cord mesenchymal stem cells-derived exosomes (huMSCs-Exos) can indirectly promote fracture healing by enhancing the angiogenic capacity of HUVECs through increasing the expression of VEGF and hypoxia inducible factor-1α (HIF-1α). And another study revealed that BMSCs preconditioned with a low dose of dimethyloxaloylglycine (DMOG), DMOG-MSC-Exos can activate the AKT/mTOR signaling pathway and enhance the bone regeneration at the site of calvarial defects in rats by promoting angiogenesis (Liang et al., 2019). There are also studies reporting that BMSCs-derived exosomes can activate the lnc-H19/Tie2-NO signaling pathway in the MSCs and endothelial cells (ECs) by regulating the expression of the angiogenic factor angiopoietin 1 (Angpt1), thus significantly promoting endothelial angiogenesis and osteogenesis (Behera et al., 2021). Moreover, Zhang et al. (2020a) also illustrated that BMSCs-derived exosomes can promote the osteogenesis and angiogenesis of a femoral non-union rat model through activating the BMP-2/Smad1/RUNX2, and HIF-1α/VEGF signaling pathways. Table 2 is a summary of the studies on the applications of exosomes in promoting osteogenesis and angiogenesis mentioned above.
TABLE 2. Summary of relevant studies on the application of exosomes in promoting osteogenesis and angiogenesis.
Additionally, exosomes can also serve as delivery vehicles for therapeutic drugs, targeting delivery small molecules or nucleic acid drugs to specific cells or tissues, thus increasing the local concentration of therapeutic drugs and reducing side effects (Liao et al., 2019; Chinnappan et al., 2020; Elsharkasy et al., 2020; Liang et al., 2020; Herrmann et al., 2021; Liang et al., 2021; Wang et al., 2021). For example, a new engineered exosome was constructed through encapsulating a plasmid carrying the VEGF gene into a chondrogenic progenitor cells-derived exosomes. Following by combining it with polycaprolactone 3D-printed porous bone scaffolds and applying it to rat radial defect model. This engineered exosome exhibited favorable osteogenesis and angiogenesis effects in vivo (Zha et al., 2021). Moreover, the role of exosomes as drug carriers has also been studied in other pathological conditions, such as malignant gliomas and colorectal cancer (Liang et al., 2020; Wang et al., 2021).
All in all, exosomes derived from various cells that have osteogenic potential not only can directly promote the bone regeneration by enhancing osteogenic or the expression of osteogenic-related genes but also can indirectly promote bone regeneration by promoting the angiogenesis in bone defect sites. In addition, exosomes can also be used to carry small molecules or nucleic acid drugs to the target cells, which can promote osteogenesis and angiogenesis.
Hydrogel is a new type of biological scaffold composed of three-dimensional hydrophilic polymer chains with excellent biocompatibility, absorbability, and controlled mechanical properties that can mimic the natural ECM and provide a suitable growth environment for endogenous cells, and has been widely used in bone regeneration (Pishavar et al., 2021; Sun et al., 2022). Recently, various hyaluronic acid-based hydrogels have become a promising biomaterial and have been widely used in the biomedical field due to their biocompatibility, biodegradability, non-toxicity, high hydrophilicity, viscoelasticity, and other properties (Ahmadian et al., 2020; Ding et al., 2022). Although hyaluronic acid-based hydrogels have displayed promising effects in bone regeneration, it is not enough to implant a HA scaffold into the damaged area for tissue repair alone (Zhou et al., 2020; Deng et al., 2021; Arcos et al., 2022). Therefore, various cell-derived exosomes have been successfully introduced into hyaluronic acid-based hydrogels in order to better achieve bone regeneration at the defect areas. Figure 3 displayed the schematic diagram of hyaluronic acid-based hydrogel loaded with exosomes for bone regeneration.
FIGURE 3. Schematic diagram of hyaluronic acid-based hydrogel loaded with exosomes for bone regeneration.
It was reported that hyaluronic acid-based hydrogels loaded with exosomes can exert a variety of effects, such as promoting osteogenesis, angiogenesis and regulating inflammation response. For example, Yang et al. (2020) used an injectable hyaluronic acid-alginate (HA-ALG) hydrogel system with huMSCs-Exos and hydroxyapatite (HAP) encapsulated in situ to repair the cranial defects in rats. The in vitro experiments indicated that this HA-ALG hydrogel system with huMSCs-Exos and HAP encapsulated in situ can promote the proliferation, migration and osteogenic differentiation of the murine calvariae preosteoblast cell line (MC3T3-E1) by upregulating the expression of osteogenic-related genes and proteins such as ALP, osteocalcin (OCN) and Collagen type I alpha1(COL1A1). And in the rat cranial defect model, this HA-ALG hydrogel system encapsulating huMSCs-Exos and HAP can not only promote osteogenesis, but also effectively promote angiogenesis.
Additionally, vasculatures, as the major source of oxygen, nutrients, growth factors, immune cells, hormones and more, are indispensable in the repair and regeneration of various tissues (Liu et al., 2017; Chen et al., 2018; Mu et al., 2022). Zhang et al. (2021b) used hyaluronic acid-based hydrogels encapsulated with umbilical mesenchymal stem cells-derived exosomes (uMSCs-Exos) in combination with nanohydroxyapatite/poly-ε-caprolactone (nHP) scaffolds for the repair of cranial defects in rats. It has been shown that miR-21, the most abundant miRNA in uMSCs-Exos, can act as an intercellular messenger to promote angiogenesis by inhibiting the NOTCH1/DLL4 pathway. Furthermore, in vitro experiments have indicated that the uMSCs-Exos have no significant effect on the osteogenic differentiation of BMSCs, but they can indirectly promote bone formation by promoting angiogenesis and the proliferation and migration of the endothelial progenitor cells (EPCs).
Bone homeostasis is achieved by keeping the balance between osteoblasts and osteoclasts, which is strictly regulated by immune cells, endocrine system and osteocytes (Kong et al., 2019). And macrophages play a crucial role in bone homeostasis as a subpopulation of cells of the innate immune system (Kong et al., 2019; Li et al., 2019). When local stimulated by microenvironmental cytokines, macrophages can be divided into two phenotypes: M1 (secreting pro-inflammatory cytokines) and M2 (producing anti-inflammatory cytokines). And in the process of bone healing, only when the ratio of M1 and M2 macrophages reach a proper balance, can bone regeneration be better promoted (Li et al., 2019; Liu et al., 2021b). Previous studies have shown that M2 macrophages can upregulate ALP and increase bone mineralization, while M1 macrophages can inhibit osteoblasts and bone formation (Kong et al., 2019). Mi et al. (2022) designed a cocktail therapy to prepare an injectable hyaluronic acid-based hydrogel with anti-inflammatory, antibacterial, self-healing and tissue adhesion properties using aldehyde quaternary ammonium modified HA and hydrazine group modified HA. Subsequently, engineered ECs-derived exosomes (ECs-Exos miR-26a−5p) and the inositol-requiring enzyme 1alpha (IRE-1α) inhibitor APY29 were loaded into the prepared HA hydrogels and injected in situ into the fracture site of a mouse model. In this way, the ECs-ExosmiR-26a−5p and APY29 loaded in the HA hydrogel can achieve sustained release and function at the injury site. Additionally, it can inhibit inflammatory cytokines and osteoclast differentiation and promote osteogenic differentiation and macrophage M2 polarization, showing an excellent effect in fracture repair (Mi et al., 2022). Table 3 is a summary of relevant studies on the application of hyaluronic acid-based hydrogels as exosomes delivery system in bone regeneration.
TABLE 3. Summary of relevant studies on the application of hyaluronic acid-based hydrogels as exosomes delivery system in bone regeneration.
With the development of new crosslinking strategies and the improvement of existing ones, more and more effective hyaluronic acid-based hydrogels for exosome delivery will emerge. By changing the concentration and molecular weight of HA polymer, hyaluronic acid-based hydrogels with different viscoelasticity and mechanical properties can be obtained to better meet the needs of different tissue repair (Passi and Vigetti, 2019; Fang et al., 2021). Additionally, the research on the bone regeneration microenvironment continues to deepen, and more bone regeneration strategies will appear in the future. For example, by adding different therapeutic components to the hydrogels, the hydrogels can have different effects on various processes such as inflammation, osteogenic differentiation, angiogenesis, and immune regulation in the bone regeneration process, thus promoting bone regeneration more efficiently. In this review, we summarized the use of hyaluronic acid-based hydrogels as exosome delivery systems for bone regeneration. Hyaluronic acid-based hydrogels have good biocompatibility, non-toxicity, controlled degradation, injectability, and adjusted mechanical properties. Besides, the hyaluronic acid-based hydrogels loaded with growth factors, exosomes or other therapeutic components can also achieve sustained release and action of exosomes and other therapeutic components at the site of the injury, which has good prospects for bone regeneration. However, natural HA cannot form hydrogel alone due to its high hydrophilicity and susceptibility to degradation by HASes. Therefore, it is often necessary to modify and crosslink natural HA to obtain hyaluronic acid-based hydrogels that can be used in tissue repair and regeneration. The development of different modification and crosslinking strategies has greatly improved the stability and mechanical properties of hyaluronic acid-based hydrogels, but there are still many challenges in their biomedical application and clinical translation. Firstly, the current modification or crosslinking strategies of HA may involve toxic catalysts or initiators or produce toxic by-products. Consequently, it is essential to develop new and better HA modification and crosslinking strategies. Secondly, the interactions and influence between the obtained hyaluronic acid-based hydrogels and the exosomes they loaded as well as the surrounding tissues need to be further explored. In addition, more studies are needed to clarify the role and mechanism of exosomes from different call sources in bone regeneration.
HD searched the literature and wrote this manuscript. JW and RA reviewed this manuscript. HD, JW, and RA conceived and revised this manuscript. RA provided funding support for this manuscript. All authors contributed to the manuscript and approved the submitted version.
The National Natural Science Foundation of China (grant 81901977 to RA) provides support for this work.
The authors declare that the research was conducted in the absence of any commercial or financial relationships that could be construed as a potential conflict of interest.
All claims expressed in this article are solely those of the authors and do not necessarily represent those of their affiliated organizations, or those of the publisher, the editors and the reviewers. Any product that may be evaluated in this article, or claim that may be made by its manufacturer, is not guaranteed or endorsed by the publisher.
Abatangelo, G., Vindigni, V., Avruscio, G., Pandis, L., and Brun, P. (2020). Hyaluronic acid: Redefining its role. Cells 9 (7), 1743. doi:10.3390/cells9071743
Ahmadian, E., Dizaj, S. M., Eftekhari, A., Dalir, E., Vahedi, P., Hasanzadeh, A., et al. (2020). The potential applications of hyaluronic acid hydrogels in biomedicine. Drug Res. (Stuttg) 70 (1), 6–11. doi:10.1055/a-0991-7585
Akbari, A., Jabbari, N., Sharifi, R., Ahmadi, M., Vahhabi, A., Seyedzadeh, S. J., et al. (2020). Free and hydrogel encapsulated exosome-based therapies in regenerative medicine. Life Sci. 249, 117447. doi:10.1016/j.lfs.2020.117447
Amirazad, H., Dadashpour, M., and Zarghami, N. (2022). Application of decellularized bone matrix as a bioscaffold in bone tissue engineering. J. Biol. Eng. 16 (1), 1. doi:10.1186/s13036-021-00282-5
Arcos, D., Gomez-Cerezo, N., Saiz-Pardo, M., de Pablo, D., Ortega, L., Enciso, S., et al. (2022). Injectable mesoporous bioactive nanoparticles regenerate bone tissue under osteoporosis conditions. Acta Biomater. 151, 501–511. doi:10.1016/j.actbio.2022.07.067
Bai, X., Gao, M., Syed, S., Zhuang, J., Xu, X., and Zhang, X. Q. (2018). Bioactive hydrogels for bone regeneration. Bioact. Mater 3 (4), 401–417. doi:10.1016/j.bioactmat.2018.05.006
Bao, Z., Yu, A., Shi, H., Hu, Y., Jin, B., Lin, D., et al. (2021). Glycol chitosan/oxidized hyaluronic acid hydrogel film for topical ocular delivery of dexamethasone and levofloxacin. Int. J. Biol. Macromol. 167, 659–666. doi:10.1016/j.ijbiomac.2020.11.214
Behera, J., Kumar, A., Voor, M. J., and Tyagi, N. (2021). Exosomal lncRNA-H19 promotes osteogenesis and angiogenesis through mediating Angpt1/Tie2-NO signaling in CBS-heterozygous mice. Theranostics 11 (16), 7715–7734. doi:10.7150/thno.58410
Bencherif, S. A., Siegwart, D. J., Srinivasan, A., Horkay, F., Hollinger, J. O., Washburn, N. R., et al. (2009). Nanostructured hybrid hydrogels prepared by a combination of atom transfer radical polymerization and free radical polymerization. Biomaterials 30 (29), 5270–5278. doi:10.1016/j.biomaterials.2009.06.011
Bohaumilitzky, L., Huber, A. K., Stork, E. M., Wengert, S., Woelfl, F., and Boehm, H. (2017). A trickster in disguise: Hyaluronan's ambivalent roles in the matrix. Front. Oncol. 7, 242. doi:10.3389/fonc.2017.00242
Brigstock, D. R. (2021). Extracellular vesicles in organ fibrosis: Mechanisms, therapies, and diagnostics. Cells 10 (7), 1596. doi:10.3390/cells10071596
Burdick, J. A., and Prestwich, G. D. (2011). Hyaluronic acid hydrogels for biomedical applications. Adv. Mater 23 (12), H41–H56. doi:10.1002/adma.201003963
Cadamuro, F., Russo, L., and Nicotra, F. (2020). Biomedical hydrogels fabricated using diels–alder crosslinking. Eur. J. Org. Chem. 2021 (3), 374–382. doi:10.1002/ejoc.202001042
Chang, E. J., Kim, H. J., Ha, J., Kim, H. J., Ryu, J., Park, K. H., et al. (2007). Hyaluronan inhibits osteoclast differentiation via Toll-like receptor 4. J. Cell Sci. 120 (1), 166–176. doi:10.1242/jcs.03310
Chen, C. W., Wang, L. L., Zaman, S., Gordon, J., Arisi, M. F., Venkataraman, C. M., et al. (2018). Sustained release of endothelial progenitor cell-derived extracellular vesicles from shear-thinning hydrogels improves angiogenesis and promotes function after myocardial infarction. Cardiovasc Res. 114 (7), 1029–1040. doi:10.1093/cvr/cvy067
Chen, F., Le, P., Lai, K., Fernandes-Cunha, G. M., and Myung, D. (2020). Simultaneous interpenetrating polymer network of collagen and hyaluronic acid as an in situ-forming corneal defect filler. Chem. Mater 32 (12), 5208–5216. doi:10.1021/acs.chemmater.0c01307
Chen, F., Ni, Y., Liu, B., Zhou, T., Yu, C., Su, Y., et al. (2017). Self-crosslinking and injectable hyaluronic acid/RGD-functionalized pectin hydrogel for cartilage tissue engineering. Carbohydr. Polym. 166, 31–44. doi:10.1016/j.carbpol.2017.02.059
Chen, G., and Lv, Y. (2018). Decellularized bone matrix scaffold for bone regeneration. Methods Mol. Biol. 1577, 239–254. doi:10.1007/7651_2017_50
Chen, S., Tang, Y., Liu, Y., Zhang, P., Lv, L., Zhang, X., et al. (2019). Exosomes derived from miR-375-overexpressing human adipose mesenchymal stem cells promote bone regeneration. Cell Prolif. 52 (5), e12669. doi:10.1111/cpr.12669
Cheng, G., Zhu, D., Huang, K., and Caranasos, T. G. (2022). Minimally invasive delivery of a hydrogel-based exosome patch to prevent heart failure. J. Mol. Cell Cardiol. 169, 113–121. doi:10.1016/j.yjmcc.2022.04.020
Chinnappan, M., Srivastava, A., Amreddy, N., Razaq, M., Pareek, V., Ahmed, R., et al. (2020). Exosomes as drug delivery vehicle and contributor of resistance to anticancer drugs. Cancer Lett. 486, 18–28. doi:10.1016/j.canlet.2020.05.004
Collins, M. N., and Birkinshaw, C. (2013). Hyaluronic acid based scaffolds for tissue engineering--a review. Carbohydr. Polym. 92 (2), 1262–1279. doi:10.1016/j.carbpol.2012.10.028
Crescenzi, V., Francescangeli, A., Renier, D., and Bellini, D. (2002). New cross-linked and sulfated derivatives of partially deacetylated hyaluronan: Synthesis and preliminary characterization. Biopolymers 64 (2), 86–94. doi:10.1002/bip.10131
D'Este, M., Alini, M., and Eglin, D. (2012). Single step synthesis and characterization of thermoresponsive hyaluronan hydrogels. Carbohydr. Polym. 90 (3), 1378–1385. doi:10.1016/j.carbpol.2012.07.007
Dahlmann, J., Krause, A., Moller, L., Kensah, G., Mowes, M., Diekmann, A., et al. (2013). Fully defined in situ cross-linkable alginate and hyaluronic acid hydrogels for myocardial tissue engineering. Biomaterials 34 (4), 940–951. doi:10.1016/j.biomaterials.2012.10.008
Das, D., Pham, T. T. H., and Noh, I. (2018). Characterizations of hyaluronate-based terpolymeric hydrogel synthesized via free radical polymerization mechanism for biomedical applications. Colloids Surf. B Biointerfaces 170, 64–75. doi:10.1016/j.colsurfb.2018.05.059
Deng, Y., Li, R., Wang, H., Yang, B., Shi, P., Zhang, Y., et al. (2021). Biomaterial-mediated presentation of jagged-1 mimetic ligand enhances cellular activation of Notch signaling and bone regeneration. ACS Nano 16, 1051–1062. doi:10.1021/acsnano.1c08728
Di Mola, A., Landi, M. R., Massa, A., D'Amora, U., and Guarino, V. (2022). Hyaluronic acid in biomedical fields: New trends from chemistry to biomaterial applications. Int. J. Mol. Sci. 23 (22), 14372. doi:10.3390/ijms232214372
Dimitriou, R., Jones, E., McGonagle, D., and Giannoudis, P. V. (2011). Bone regeneration: Current concepts and future directions. BMC Med. 9, 66. doi:10.1186/1741-7015-9-66
Ding, Y. W., Wang, Z. Y., Ren, Z. W., Zhang, X. W., and Wei, D. X. (2022). Advances in modified hyaluronic acid-based hydrogels for skin wound healing. Biomater. Sci. 10, 3393–3409. doi:10.1039/d2bm00397j
Dishowitz, M. I., Zhu, F., Sundararaghavan, H. G., Ifkovits, J. L., Burdick, J. A., and Hankenson, K. D. (2014). Jagged1 immobilization to an osteoconductive polymer activates the Notch signaling pathway and induces osteogenesis. J. Biomed. Mater Res. A 102 (5), 1558–1567. doi:10.1002/jbm.a.34825
Eggenhofer, E., Luk, F., Dahlke, M. H., and Hoogduijn, M. J. (2014). The life and fate of mesenchymal stem cells. Front. Immunol. 5, 148. doi:10.3389/fimmu.2014.00148
Elliott, D. S., Newman, K. J. H., Forward, D. P., Hahn, D. M., Ollivere, B., Kojima, K., et al. (2016). A unified theory of bone healing and nonunion: BHN theory. Bone & Jt. J. 98-B (7), 884–891. doi:10.1302/0301-620X.98B7.36061
Elsharkasy, O. M., Nordin, J. Z., Hagey, D. W., de Jong, O. G., Schiffelers, R. M., Andaloussi, S. E., et al. (2020). Extracellular vesicles as drug delivery systems: Why and how? Adv. Drug Deliv. Rev. 159, 332–343. doi:10.1016/j.addr.2020.04.004
Fang, Y., Shi, L., Duan, Z., and Rohani, S. (2021). Hyaluronic acid hydrogels, as a biological macromolecule-based platform for stem cells delivery and their fate control: A review. Int. J. Biol. Macromol. 189, 554–566. doi:10.1016/j.ijbiomac.2021.08.140
Ferguson, C., Alpern, E., Miclau, T., and Helms, J. A. (1999). Does adult fracture repair recapitulate embryonic skeletal formation? Mech. Dev. 87 (1-2), 57–66. doi:10.1016/s0925-4773(99)00142-2
Fisher, S. A., Anandakumaran, P. N., Owen, S. C., and Shoichet, M. S. (2015). Tuning the microenvironment: Click-crosslinked hyaluronic acid-based hydrogels provide a platform for studying breast cancer cell invasion. Adv. Funct. Mater. 25 (46), 7163–7172. doi:10.1002/adfm.201502778
Flegeau, K., Gauthier, O., Rethore, G., Autrusseau, F., Schaefer, A., Lesoeur, J., et al. (2021). Injectable silanized hyaluronic acid hydrogel/biphasic calcium phosphate granule composites with improved handling and biodegradability promote bone regeneration in rabbits. Biomater. Sci. 9 (16), 5640–5651. doi:10.1039/d1bm00403d
Flegeau, K., Puiggali-Jou, A., and Zenobi-Wong, M. (2022). Cartilage tissue engineering by extrusion bioprinting utilizing porous hyaluronic acid microgel bioinks. Biofabrication 14 (3), 034105. doi:10.1088/1758-5090/ac6b58
Fu, S., Dong, H., Deng, X., Zhuo, R., and Zhong, Z. (2017). Injectable hyaluronic acid/poly(ethylene glycol) hydrogels crosslinked via strain-promoted azide-alkyne cycloaddition click reaction. Carbohydr. Polym. 169, 332–340. doi:10.1016/j.carbpol.2017.04.028
Fujii, Y., Fujii, K., Nakano, K., and Tanaka, Y. (2003). Crosslinking of CD44 on human osteoblastic cells upregulates ICAM-1 and VCAM-1. FEBS Lett. 539 (1-3), 45–50. doi:10.1016/s0014-5793(03)00182-0
Garantziotis, S., and Savani, R. C. (2019). Hyaluronan biology: A complex balancing act of structure, function, location and context. Matrix Biol. 78-79, 1–10. doi:10.1016/j.matbio.2019.02.002
Ginini, L., Billan, S., Fridman, E., and Gil, Z. (2022). Insight into extracellular vesicle-cell communication: From cell recognition to intracellular fate. Cells 11 (9), 1375. doi:10.3390/cells11091375
Gurung, S., Perocheau, D., Touramanidou, L., and Baruteau, J. (2021). The exosome journey: From biogenesis to uptake and intracellular signalling. Cell Commun. Signal 19 (1), 47. doi:10.1186/s12964-021-00730-1
Gwon, K., Kim, E., and Tae, G. (2017). Heparin-hyaluronic acid hydrogel in support of cellular activities of 3D encapsulated adipose derived stem cells. Acta Biomater. 49, 284–295. doi:10.1016/j.actbio.2016.12.001
Hamilton, M., Harrington, S., Dhar, P., and Stehno-Bittel, L. (2021). Hyaluronic acid hydrogel microspheres for slow release stem cell delivery. ACS Biomater. Sci. Eng. 7 (8), 3754–3763. doi:10.1021/acsbiomaterials.1c00658
Heldin, P., Lin, C.-Y., Kolliopoulos, C., Chen, Y.-H., and Skandalis, S. S. (2019). Regulation of hyaluronan biosynthesis and clinical impact of excessive hyaluronan production. Matrix Biol. : J. Int. Soc. For Matrix Biol. 78-79, 100–117. doi:10.1016/j.matbio.2018.01.017
Herrmann, I. K., Wood, M. J. A., and Fuhrmann, G. (2021). Extracellular vesicles as a next-generation drug delivery platform. Nat. Nanotechnol. 16 (7), 748–759. doi:10.1038/s41565-021-00931-2
Hoshino, A., Costa-Silva, B., Shen, T. L., Rodrigues, G., Hashimoto, A., Tesic Mark, M., et al. (2015). Tumour exosome integrins determine organotropic metastasis. Nature 527 (7578), 329–335. doi:10.1038/nature15756
Hu, Y., Li, X., Zhang, Q., Gu, Z., Luo, Y., Guo, J., et al. (2021). Exosome-guided bone targeted delivery of Antagomir-188 as an anabolic therapy for bone loss. Bioact. Mater 6 (9), 2905–2913. doi:10.1016/j.bioactmat.2021.02.014
Huang, L., Cheng, Y. Y., Koo, P. L., Lee, K. M., Qin, L., Cheng, J. C. Y., et al. (2003). The effect of hyaluronan on osteoblast proliferation and differentiation in rat calvarial-derived cell cultures. J. Biomed. Mater. Res. Part A 66 (4), 880–884. doi:10.1002/jbm.a.10535
Ilochonwu, B. C., Mihajlovic, M., Maas-Bakker, R. F., Rousou, C., Tang, M., Chen, M., et al. (2022). Hyaluronic acid-PEG-based diels-alder in situ forming hydrogels for sustained intraocular delivery of bevacizumab. Biomacromolecules 23 (7), 2914–2929. doi:10.1021/acs.biomac.2c00383
Ishida, O., Tanaka, Y., Morimoto, I., Takigawa, M., and Eto, S. (1997). Chondrocytes are regulated by cellular adhesion through CD44 and hyaluronic acid pathway. J. Bone Mineral Res. : Official J. Am. Soc. For Bone Mineral Res. 12 (10), 1657–1663. doi:10.1359/jbmr.1997.12.10.1657
Itano, N., Sawai, T., Yoshida, M., Lenas, P., Yamada, Y., Imagawa, M., et al. (1999). Three isoforms of mammalian hyaluronan synthases have distinct enzymatic properties. J. Biol. Chem. 274 (35), 25085–25092. doi:10.1074/jbc.274.35.25085
Jin, X., Shang, Y., Zou, Y., Xiao, M., Huang, H., Zhu, S., et al. (2020a). Injectable hypoxia-induced conductive hydrogel to promote diabetic wound healing. ACS Appl. Mater Interfaces 12 (51), 56681–56691. doi:10.1021/acsami.0c13197
Jin, Y., Koh, R. H., Kim, S.-H., Kim, K. M., Park, G. K., and Hwang, N. S. (2020b). Injectable anti-inflammatory hyaluronic acid hydrogel for osteoarthritic cartilage repair. Mater. Sci. Eng. C, Mater. For Biol. Appl. 115, 111096. doi:10.1016/j.msec.2020.111096
Jo, Y. J., Gulfam, M., Jo, S. H., Gal, Y. S., Oh, C. W., Park, S. H., et al. (2022). Multi-stimuli responsive hydrogels derived from hyaluronic acid for cancer therapy application. Carbohydr. Polym. 286, 119303. doi:10.1016/j.carbpol.2022.119303
Kalluri, R., and LeBleu, V. S. (2020). The biology, function, and biomedical applications of exosomes. Science 367 (6478), eaau6977. doi:10.1126/science.aau6977
Kang, Y., Xu, C., Meng, L., Dong, X., Qi, M., and Jiang, D. (2022). Exosome-functionalized magnesium-organic framework-based scaffolds with osteogenic, angiogenic and anti-inflammatory properties for accelerated bone regeneration. Bioact. Mater 18, 26–41. doi:10.1016/j.bioactmat.2022.02.012
Kawano, M., Ariyoshi, W., Iwanaga, K., Okinaga, T., Habu, M., Yoshioka, I., et al. (2011). Mechanism involved in enhancement of osteoblast differentiation by hyaluronic acid. Biochem Biophys. Res. Commun. 405 (4), 575–580. doi:10.1016/j.bbrc.2011.01.071
Khan, I., and Steeg, P. S. (2021). Endocytosis: A pivotal pathway for regulating metastasis. Br. J. Cancer 124 (1), 66–75. doi:10.1038/s41416-020-01179-8
Khunmanee, S., Jeong, Y., and Park, H. (2017). Crosslinking method of hyaluronic-based hydrogel for biomedical applications. J. Tissue Eng. 8, 2041731417726464. doi:10.1177/2041731417726464
Kimiz-Gebologlu, I., and Oncel, S. S. (2022). Exosomes: Large-scale production, isolation, drug loading efficiency, and biodistribution and uptake. J. Control Release 347, 533–543. doi:10.1016/j.jconrel.2022.05.027
Knopf-Marques, H., Pravda, M., Wolfova, L., Velebny, V., Schaaf, P., Vrana, N. E., et al. (2016). Hyaluronic acid and its derivatives in coating and delivery systems: Applications in tissue engineering, regenerative medicine and immunomodulation. Adv. Healthc. Mater 5 (22), 2841–2855. doi:10.1002/adhm.201600316
Kobayashi, T., Chanmee, T., and Itano, N. (2020). Hyaluronan: Metabolism and function. Biomolecules 10 (11), 1525. doi:10.3390/biom10111525
Kong, L., Wang, Y., Smith, W., and Hao, D. (2019). Macrophages in bone homeostasis. Curr. Stem Cell Res. Ther. 14 (6), 474–481. doi:10.2174/1574888X14666190214163815
La Noce, M., Stellavato, A., Vassallo, V., Cammarota, M., Laino, L., Desiderio, V., et al. (2021). Hyaluronan-based gel promotes human dental pulp stem cells bone differentiation by activating YAP/TAZ pathway. Cells 10 (11), 2899. doi:10.3390/cells10112899
Lee-Sayer, S. S., Dong, Y., Arif, A. A., Olsson, M., Brown, K. L., and Johnson, P. (2015). The where, when, how, and why of hyaluronan binding by immune cells. Front. Immunol. 6, 150. doi:10.3389/fimmu.2015.00150
Lei, M., Chen, G., Zhang, M., Lei, J., Li, T., Li, D., et al. (2021). A pH-sensitive drug delivery system based on hyaluronic acid co-deliver doxorubicin and aminoferrocene for the combined application of chemotherapy and chemodynamic therapy. Colloids Surf. B Biointerfaces 203, 111750. doi:10.1016/j.colsurfb.2021.111750
Leng, Y., Abdullah, A., Wendt, M. K., and Calve, S. (2019). Hyaluronic acid, CD44 and RHAMM regulate myoblast behavior during embryogenesis. Matrix Biol. 78-79, 236–254. doi:10.1016/j.matbio.2018.08.008
Lesley, J., Hyman, R., and Kincade, P. W. (1993). CD44 and its interaction with extracellular matrix. Adv. Immunol. 54, 271–335. doi:10.1016/s0065-2776(08)60537-4
Li, S., Pei, M., Wan, T., Yang, H., Gu, S., Tao, Y., et al. (2020). Self-healing hyaluronic acid hydrogels based on dynamic Schiff base linkages as biomaterials. Carbohydr. Polym. 250, 116922. doi:10.1016/j.carbpol.2020.116922
Li, W., Liu, Y., Zhang, P., Tang, Y., Zhou, M., Jiang, W., et al. (2018). Tissue-engineered bone immobilized with human adipose stem cells-derived exosomes promotes bone regeneration. ACS Appl. Mater Interfaces 10 (6), 5240–5254. doi:10.1021/acsami.7b17620
Li, Y., Kong, N., Li, Z., Tian, R., Liu, X., Liu, G., et al. (2019). Bone marrow macrophage M2 polarization and adipose-derived stem cells osteogenic differentiation synergistically promote rehabilitation of bone damage. J. Cell Biochem 120 (12), 19891–19901. doi:10.1002/jcb.29297
Li, Z., Wang, Y., Li, S., and Li, Y. (2021). Exosomes derived from M2 macrophages facilitate osteogenesis and reduce adipogenesis of BMSCs. Front. Endocrinol. (Lausanne) 12, 680328. doi:10.3389/fendo.2021.680328
Liang, B., Liang, J. M., Ding, J. N., Xu, J., Xu, J. G., and Chai, Y. M. (2019). Dimethyloxaloylglycine-stimulated human bone marrow mesenchymal stem cell-derived exosomes enhance bone regeneration through angiogenesis by targeting the AKT/mTOR pathway. Stem Cell Res. Ther. 10 (1), 335. doi:10.1186/s13287-019-1410-y
Liang, G., Zhu, Y., Ali, D. J., Tian, T., Xu, H., Si, K., et al. (2020). Engineered exosomes for targeted co-delivery of miR-21 inhibitor and chemotherapeutics to reverse drug resistance in colon cancer. J. Nanobiotechnology 18 (1), 10. doi:10.1186/s12951-019-0563-2
Liang, J., Cheng, L., Struckhoff, J. J., and Ravi, N. (2015). Investigating triazine-based modification of hyaluronan using statistical designs. Carbohydr. Polym. 132, 472–480. doi:10.1016/j.carbpol.2015.06.067
Liang, Y., Duan, L., Lu, J., and Xia, J. (2021). Engineering exosomes for targeted drug delivery. Theranostics 11 (7), 3183–3195. doi:10.7150/thno.52570
Liao, W., Du, Y., Zhang, C., Pan, F., Yao, Y., Zhang, T., et al. (2019). Exosomes: The next generation of endogenous nanomaterials for advanced drug delivery and therapy. Acta Biomater. 86, 1–14. doi:10.1016/j.actbio.2018.12.045
Liu, A., Lin, D., Zhao, H., Chen, L., Cai, B., Lin, K., et al. (2021a). Optimized BMSC-derived osteoinductive exosomes immobilized in hierarchical scaffold via lyophilization for bone repair through Bmpr2/Acvr2b competitive receptor-activated Smad pathway. Biomaterials 272, 120718. doi:10.1016/j.biomaterials.2021.120718
Liu, K., Luo, X., Lv, Z.-Y., Zhang, Y.-J., Meng, Z., Li, J., et al. (2021b). Macrophage-derived exosomes promote bone mesenchymal stem cells towards osteoblastic fate through microRNA-21a-5p. Front. Bioeng. Biotechnol. 9, 801432. doi:10.3389/fbioe.2021.801432
Liu, L., You, Z., Yu, H., Zhou, L., Zhao, H., Yan, X., et al. (2017). Mechanotransduction-modulated fibrotic microniches reveal the contribution of angiogenesis in liver fibrosis. Nat. Mater 16 (12), 1252–1261. doi:10.1038/nmat5024
Lotvall, J., Hill, A. F., Hochberg, F., Buzas, E. I., Di Vizio, D., Gardiner, C., et al. (2014). Minimal experimental requirements for definition of extracellular vesicles and their functions: A position statement from the international society for extracellular vesicles. J. Extracell. Vesicles 3, 26913. doi:10.3402/jev.v3.26913
Lou, G., Chen, Z., Zheng, M., and Liu, Y. (2017). Mesenchymal stem cell-derived exosomes as a new therapeutic strategy for liver diseases. Exp. Mol. Med. 49 (6), e346. doi:10.1038/emm.2017.63
Lu, G., Xu, Y., Liu, Q., Chen, M., Sun, H., Wang, P., et al. (2022). An instantly fixable and self-adaptive scaffold for skull regeneration by autologous stem cell recruitment and angiogenesis. Nat. Commun. 13 (1), 2499. doi:10.1038/s41467-022-30243-5
Magnani, A., Rappuoli, R., Lamponi, S., and Barbucci, R. (2000). Novel polysaccharide hydrogels: Characterization and properties. Polym. Adv. Technol. 11 (8-12), 488–495. doi:10.1002/1099-1581(200008/12)11:8/12<488::aid-pat995>3.0.co;2-5
Majidinia, M., Sadeghpour, A., and Yousefi, B. (2018). The roles of signaling pathways in bone repair and regeneration. J. Cell Physiol. 233 (4), 2937–2948. doi:10.1002/jcp.26042
Manzi, G., Zoratto, N., Matano, S., Sabia, R., Villani, C., Coviello, T., et al. (2017). Click" hyaluronan based nanohydrogels as multifunctionalizable carriers for hydrophobic drugs. Carbohydr. Polym. 174, 706–715. doi:10.1016/j.carbpol.2017.07.003
Marinho, A., Nunes, C., and Reis, S. (2021). Hyaluronic acid: A key ingredient in the therapy of inflammation. Biomolecules 11 (10), 1518. doi:10.3390/biom11101518
Marsell, R., and Einhorn, T. A. (2011). The biology of fracture healing. Injury 42 (6), 551–555. doi:10.1016/j.injury.2011.03.031
Mathieu, M., Martin-Jaular, L., Lavieu, G., and Thery, C. (2019). Specificities of secretion and uptake of exosomes and other extracellular vesicles for cell-to-cell communication. Nat. Cell Biol. 21 (1), 9–17. doi:10.1038/s41556-018-0250-9
McKee, C. M., Penno, M. B., Cowman, M., Burdick, M. D., Strieter, R. M., Bao, C., et al. (1996). Hyaluronan (HA) fragments induce chemokine gene expression in alveolar macrophages. The role of HA size and CD44. J. Clin. Investigation 98 (10), 2403–2413. doi:10.1172/JCI119054
Mi, B., Chen, L., Xiong, Y., Yang, Y., Panayi, A. C., Xue, H., et al. (2022). Osteoblast/osteoclast and immune cocktail therapy of an exosome/drug delivery multifunctional hydrogel accelerates fracture repair. ACS Nano 16, 771–782. doi:10.1021/acsnano.1c08284
Monslow, J., Govindaraju, P., and Pure, E. (2015). Hyaluronan - a functional and structural sweet spot in the tissue microenvironment. Front. Immunol. 6, 231. doi:10.3389/fimmu.2015.00231
Mu, J., Li, L., Wu, J., Huang, T., Zhang, Y., Cao, J., et al. (2022). Hypoxia-stimulated mesenchymal stem cell-derived exosomes loaded by adhesive hydrogel for effective angiogenic treatment of spinal cord injury. Biomater. Sci. 10 (7), 1803–1811. doi:10.1039/d1bm01722e
Mulcahy, L. A., Pink, R. C., and Carter, D. R. (2014). Routes and mechanisms of extracellular vesicle uptake. J. Extracell. Vesicles 3, 24641. doi:10.3402/jev.v3.24641
Narayanan, R., Huang, C. C., and Ravindran, S. (2016). Hijacking the cellular mail: Exosome mediated differentiation of mesenchymal stem cells. Stem Cells Int. 2016, 3808674. doi:10.1155/2016/3808674
Park, H. J., Jin, Y., Shin, J., Yang, K., Lee, C., Yang, H. S., et al. (2016). Catechol-functionalized hyaluronic acid hydrogels enhance angiogenesis and osteogenesis of human adipose-derived stem cells in critical tissue defects. Biomacromolecules 17 (6), 1939–1948. doi:10.1021/acs.biomac.5b01670
Park, S.-N., Park, J.-C., Kim, H. O., Song, M. J., and Suh, H. (2002). Characterization of porous collagen/hyaluronic acid scaffold modified by 1-ethyl-3-(3-dimethylaminopropyl)carbodiimide cross-linking. Biomaterials 23 (4), 1205–1212. doi:10.1016/s0142-9612(01)00235-6
Park, S. K., Ha, M., Kim, E. J., Seo, Y. A., Lee, H. J., Myung, D., et al. (2022). Hyaluronic acid hydrogels crosslinked via blue light-induced thiol-ene reaction for the treatment of rat corneal alkali burn. Regen. Ther. 20, 51–60. doi:10.1016/j.reth.2022.03.005
Park, Y.-B., Ha, C.-W., Lee, C.-H., Yoon, Y. C., and Park, Y.-G. (2017). Cartilage regeneration in osteoarthritic patients by a composite of allogeneic umbilical cord blood-derived mesenchymal stem cells and hyaluronate hydrogel: Results from a clinical trial for safety and proof-of-concept with 7 Years of extended follow-up. Stem Cells Transl. Med. 6 (2), 613–621. doi:10.5966/sctm.2016-0157
Passi, A., and Vigetti, D. (2019). Hyaluronan as tunable drug delivery system. Adv. Drug Deliv. Rev. 146, 83–96. doi:10.1016/j.addr.2019.08.006
Patterson, J., Siew, R., Herring, S. W., Lin, A. S., Guldberg, R., and Stayton, P. S. (2010). Hyaluronic acid hydrogels with controlled degradation properties for oriented bone regeneration. Biomaterials 31 (26), 6772–6781. doi:10.1016/j.biomaterials.2010.05.047
Pegtel, D. M., and Gould, S. J. (2019). Exosomes. Annu Rev. Biochem 88, 487–514. doi:10.1146/annurev-biochem-013118-111902
Pérez, L. A., Hernández, R., Alonso, J. M., Pérez-González, R., and Sáez-Martínez, V. (2021). Hyaluronic acid hydrogels crosslinked in physiological conditions: Synthesis and biomedical applications. Biomedicines 9 (9), 1113. doi:10.3390/biomedicines9091113
Pishavar, E., Luo, H., Naserifar, M., Hashemi, M., Toosi, S., Atala, A., et al. (2021). Advanced hydrogels as exosome delivery systems for osteogenic differentiation of MSCs: Application in bone regeneration. Int. J. Mol. Sci. 22 (12), 6203. doi:10.3390/ijms22126203
Poldervaart, M. T., Goversen, B., de Ruijter, M., Abbadessa, A., Melchels, F. P. W., Oner, F. C., et al. (2017). 3D bioprinting of methacrylated hyaluronic acid (MeHA) hydrogel with intrinsic osteogenicity. PLoS One 12 (6), e0177628. doi:10.1371/journal.pone.0177628
Polo-Corrales, L., Latorre-Esteves, M., and Ramirez-Vick, J. E. (2014). Scaffold design for bone regeneration. J. Nanosci. Nanotechnol. 14 (1), 15–56. doi:10.1166/jnn.2014.9127
Qi, X., Zhang, J., Yuan, H., Xu, Z., Li, Q., Niu, X., et al. (2016). Exosomes secreted by human-induced pluripotent stem cell-derived mesenchymal stem cells repair critical-sized bone defects through enhanced angiogenesis and osteogenesis in osteoporotic rats. Int. J. Biol. Sci. 12 (7), 836–849. doi:10.7150/ijbs.14809
Raia, N. R., Jia, D., Ghezzi, C. E., Muthukumar, M., and Kaplan, D. L. (2020). Characterization of silk-hyaluronic acid composite hydrogels towards vitreous humor substitutes. Biomaterials 233, 119729. doi:10.1016/j.biomaterials.2019.119729
Riau, A. K., Ong, H. S., Yam, G. H. F., and Mehta, J. S. (2019). Sustained delivery system for stem cell-derived exosomes. Front. Pharmacol. 10, 1368. doi:10.3389/fphar.2019.01368
Safari, B., Davaran, S., and Aghanejad, A. (2021). Osteogenic potential of the growth factors and bioactive molecules in bone regeneration. Int. J. Biol. Macromol. 175, 544–557. doi:10.1016/j.ijbiomac.2021.02.052
Salhotra, A., Shah, H. N., Levi, B., and Longaker, M. T. (2020). Mechanisms of bone development and repair. Nat. Rev. Mol. Cell Biol. 21 (11), 696–711. doi:10.1038/s41580-020-00279-w
Sang, X., Zhao, X., Yan, L., Jin, X., Wang, X., Wang, J., et al. (2022). Thermosensitive hydrogel loaded with primary chondrocyte-derived exosomes promotes cartilage repair by regulating macrophage polarization in osteoarthritis. Tissue Eng. Regen. Med. 19 (3), 629–642. doi:10.1007/s13770-022-00437-5
Schanté, C. E., Zuber, G., Herlin, C., and Vandamme, T. F. (2011). Chemical modifications of hyaluronic acid for the synthesis of derivatives for a broad range of biomedical applications. Carbohydr. Polym. 85 (3), 469–489. doi:10.1016/j.carbpol.2011.03.019
Schlundt, C., Fischer, H., Bucher, C. H., Rendenbach, C., Duda, G. N., and Schmidt-Bleek, K. (2021). The multifaceted roles of macrophages in bone regeneration: A story of polarization, activation and time. Acta Biomater. 133, 46–57. doi:10.1016/j.actbio.2021.04.052
Shimoda, M., Yoshida, H., Mizuno, S., Hirozane, T., Horiuchi, K., Yoshino, Y., et al. (2017). Hyaluronan-binding protein involved in hyaluronan depolymerization controls endochondral ossification through hyaluronan metabolism. Am. J. Pathol. 187 (5), 1162–1176. doi:10.1016/j.ajpath.2017.01.005
St-Arnaud, R., and Naja, R. P. (2011). Vitamin D metabolism, cartilage and bone fracture repair. Mol. Cell Endocrinol. 347 (1-2), 48–54. doi:10.1016/j.mce.2011.05.018
Stern, R., and Jedrzejas, M. J. (2006). Hyaluronidases: Their genomics, structures, and mechanisms of action. Chem. Rev. 106 (3), 818–839. doi:10.1021/cr050247k
Stern, R., Kogan, G., Jedrzejas, M. J., and Soltes, L. (2007). The many ways to cleave hyaluronan. Biotechnol. Adv. 25 (6), 537–557. doi:10.1016/j.biotechadv.2007.07.001
Sun, J., Yin, Z., Wang, X., and Su, J. (2022). Exosome-laden hydrogels: A novel cell-free strategy for in-situ bone tissue regeneration. Front. Bioeng. Biotechnol. 10, 866208. doi:10.3389/fbioe.2022.866208
Tammi, R., Rilla, K., Pienimaki, J. P., MacCallum, D. K., Hogg, M., Luukkonen, M., et al. (2001). Hyaluronan enters keratinocytes by a novel endocytic route for catabolism. J. Biol. Chem. 276 (37), 35111–35122. doi:10.1074/jbc.M103481200
Tan, S. H. S., Wong, J. R. Y., Sim, S. J. Y., Tjio, C. K. E., Wong, K. L., Chew, J. R. J., et al. (2020). Mesenchymal stem cell exosomes in bone regenerative strategies-a systematic review of preclinical studies. Mater. Today. Bio 7, 100067. doi:10.1016/j.mtbio.2020.100067
Teng, F., and Fussenegger, M. (2020). Shedding light on extracellular vesicle biogenesis and bioengineering. Adv. Sci. (Weinh) 8 (1), 2003505. doi:10.1002/advs.202003505
Tolg, C., McCarthy, J. B., Yazdani, A., and Turley, E. A. (2014). Hyaluronan and RHAMM in wound repair and the “cancerization” of stromal tissues. BioMed Res. Int. 2014, 103923–104018. doi:10.1155/2014/103923
Trombino, S., Servidio, C., Curcio, F., and Cassano, R. (2019). Strategies for hyaluronic acid-based hydrogel design in drug delivery. Pharmaceutics 11 (8), 407. doi:10.3390/pharmaceutics11080407
van Niel, G., Carter, D. R. F., Clayton, A., Lambert, D. W., Raposo, G., and Vader, P. (2022). Challenges and directions in studying cell-cell communication by extracellular vesicles. Nat. Rev. Mol. Cell Biol. 23 (5), 369–382. doi:10.1038/s41580-022-00460-3
Vigetti, D., Viola, M., Karousou, E., De Luca, G., and Passi, A. (2014). Metabolic control of hyaluronan synthases. Matrix Biol. 35, 8–13. doi:10.1016/j.matbio.2013.10.002
Wang, G., Cao, X., Dong, H., Zeng, L., Yu, C., and Chen, X. (2018a). A hyaluronic acid based injectable hydrogel formed via photo-crosslinking reaction and thermal-induced diels-alder reaction for cartilage tissue engineering. Polym. (Basel) 10 (9), 949. doi:10.3390/polym10090949
Wang, J., Liu, S., Li, J., Zhao, S., and Yi, Z. (2019). Roles for miRNAs in osteogenic differentiation of bone marrow mesenchymal stem cells. Stem Cell Res. Ther. 10 (1), 197. doi:10.1186/s13287-019-1309-7
Wang, J., Tang, W., Yang, M., Yin, Y., Li, H., Hu, F., et al. (2021). Inflammatory tumor microenvironment responsive neutrophil exosomes-based drug delivery system for targeted glioma therapy. Biomaterials 273, 120784. doi:10.1016/j.biomaterials.2021.120784
Wang, L., Wang, J., Zhou, X., Sun, J., Zhu, B., Duan, C., et al. (2020). A new self-healing hydrogel containing hucMSC-derived exosomes promotes bone regeneration. Front. Bioeng. Biotechnol. 8, 564731. doi:10.3389/fbioe.2020.564731
Wang, L., Zhang, D., Ren, Y., Guo, S., Li, J., Ma, S., et al. (2022a). Injectable hyaluronic acid hydrogel loaded with BMSC and NGF for traumatic brain injury treatment. Mater. Today Bio 13, 100201. doi:10.1016/j.mtbio.2021.100201
Wang, L., Zhou, Y., Dai, J., Jiang, S., Lu, Y., Sun, M., et al. (2018b). 2-Thiophene ethylamine modified hyaluronic acid with its application on hepatocytes culture. Mater Sci. Eng. C Mater Biol. Appl. 88, 157–165. doi:10.1016/j.msec.2018.03.009
Wang, R., Huang, X., Zoetebier, B., Dijkstra, P. J., and Karperien, M. (2023). Enzymatic co-crosslinking of star-shaped poly(ethylene glycol) tyramine and hyaluronic acid tyramine conjugates provides elastic biocompatible and biodegradable hydrogels. Bioact. Mater 20, 53–63. doi:10.1016/j.bioactmat.2022.05.020
Wang, Z., Zhao, F., Zhao, Y., Bai, L., and Hang, R. (2022b). Simultaneously enhanced osteogenesis and angiogenesis via macrophage-derived exosomes upon stimulation with titania nanotubes. Biomater. Adv. 134, 112708. doi:10.1016/j.msec.2022.112708
Weber, G. C., Buhren, B. A., Schrumpf, H., Wohlrab, J., and Gerber, P. A. (2019). Clinical applications of hyaluronidase. Adv. Exp. Med. Biol. 1148, 255–277. doi:10.1007/978-981-13-7709-9_12
Weigel, P. H., Hascall, V. C., and Tammi, M. (1997). Hyaluronan synthases. J. Biol. Chem. 272 (22), 13997–14000. doi:10.1074/jbc.272.22.13997
Wiese, A., and Pape, H. C. (2010). Bone defects caused by high-energy injuries, bone loss, infected nonunions, and nonunions. Orthop. Clin. North Am. 41 (1), 1–4. doi:10.1016/j.ocl.2009.07.003
Wu, D., Chang, X., Tian, J., Kang, L., Wu, Y., Liu, J., et al. (2021). Bone mesenchymal stem cells stimulation by magnetic nanoparticles and a static magnetic field: Release of exosomal miR-1260a improves osteogenesis and angiogenesis. J. Nanobiotechnology 19 (1), 209. doi:10.1186/s12951-021-00958-6
Wu, L., Di Cio, S., Azevedo, H. S., and Gautrot, J. E. (2020). Photoconfigurable, cell-remodelable disulfide cross-linked hyaluronic acid hydrogels. Biomacromolecules 21 (12), 4663–4672. doi:10.1021/acs.biomac.0c00603
Xin, L., Wei, C., Tong, X., Dai, Y., Huang, D., Chen, J., et al. (2022). In situ delivery of apoptotic bodies derived from mesenchymal stem cells via a hyaluronic acid hydrogel: A therapy for intrauterine adhesions. Bioact. Mater 12, 107–119. doi:10.1016/j.bioactmat.2021.10.025
Xiong, Y., Chen, L., Yan, C., Zhou, W., Yu, T., Sun, Y., et al. (2020). M2 Macrophagy-derived exosomal miRNA-5106 induces bone mesenchymal stem cells towards osteoblastic fate by targeting salt-inducible kinase 2 and 3. J. Nanobiotechnology 18 (1), 66. doi:10.1186/s12951-020-00622-5
Yan, X. M., Seo, M. S., Hwang, E. J., Cho, I. H., Hahn, S. K., and Sohn, U. D. (2012). Improved synthesis of hyaluronic acid hydrogel and its effect on tissue augmentation. J. Biomater. Appl. 27 (2), 179–186. doi:10.1177/0885328211398508
Yang, R., Liu, X., Ren, Y., Xue, W., Liu, S., Wang, P., et al. (2021). Injectable adaptive self-healing hyaluronic acid/poly (gamma-glutamic acid) hydrogel for cutaneous wound healing. Acta Biomater. 127, 102–115. doi:10.1016/j.actbio.2021.03.057
Yang, S., Zhu, B., Yin, P., Zhao, L., Wang, Y., Fu, Z., et al. (2020). Integration of human umbilical cord mesenchymal stem cells-derived exosomes with hydroxyapatite-embedded hyaluronic acid-alginate hydrogel for bone regeneration. ACS Biomater. Sci. Eng. 6 (3), 1590–1602. doi:10.1021/acsbiomaterials.9b01363
Yu, T., Wang, H., Zhang, Y., Wang, X., and Han, B. (2020). The delivery of RNA-interference therapies based on engineered hydrogels for bone tissue regeneration. Front. Bioeng. Biotechnol. 8, 445. doi:10.3389/fbioe.2020.00445
Zanotti, S., and Canalis, E. (2016). Notch signaling and the skeleton. Endocr. Rev. 37 (3), 223–253. doi:10.1210/er.2016-1002
Zha, Y., Li, Y., Lin, T., Chen, J., Zhang, S., and Wang, J. (2021). Progenitor cell-derived exosomes endowed with VEGF plasmids enhance osteogenic induction and vascular remodeling in large segmental bone defects. Theranostics 11 (1), 397–409. doi:10.7150/thno.50741
Zhai, M., Zhu, Y., Yang, M., and Mao, C. (2020). Human mesenchymal stem cell derived exosomes enhance cell-free bone regeneration by altering their miRNAs profiles. Adv. Sci. (Weinh) 7 (19), 2001334. doi:10.1002/advs.202001334
Zhang, J., Liu, X., Li, H., Chen, C., Hu, B., Niu, X., et al. (2016). Exosomes/tricalcium phosphate combination scaffolds can enhance bone regeneration by activating the PI3K/Akt signaling pathway. Stem Cell Res. Ther. 7 (1), 136. doi:10.1186/s13287-016-0391-3
Zhang, L., Jiao, G., Ren, S., Zhang, X., Li, C., Wu, W., et al. (2020a). Exosomes from bone marrow mesenchymal stem cells enhance fracture healing through the promotion of osteogenesis and angiogenesis in a rat model of nonunion. Stem Cell Res. Ther. 11 (1), 38. doi:10.1186/s13287-020-1562-9
Zhang, M., Li, Y., Feng, T., Li, R., Wang, Z., Zhang, L., et al. (2022). Bone engineering scaffolds with exosomes: A promising strategy for bone defects repair. Front. Bioeng. Biotechnol. 10, 920378. doi:10.3389/fbioe.2022.920378
Zhang, Q., Wei, X., Ji, Y., Yin, L., Dong, Z., Chen, F., et al. (2020b). Adjustable and ultrafast light-cured hyaluronic acid hydrogel: Promoting biocompatibility and cell growth. J. Mater Chem. B 8 (25), 5441–5450. doi:10.1039/c9tb02796c
Zhang, Y., Cao, X., Li, P., Fan, Y., Zhang, L., Ma, X., et al. (2021a). microRNA-935-modified bone marrow mesenchymal stem cells-derived exosomes enhance osteoblast proliferation and differentiation in osteoporotic rats. Life Sci. 272, 119204. doi:10.1016/j.lfs.2021.119204
Zhang, Y., Hao, Z., Wang, P., Xia, Y., Wu, J., Xia, D., et al. (2019a). Exosomes from human umbilical cord mesenchymal stem cells enhance fracture healing through HIF-1α-mediated promotion of angiogenesis in a rat model of stabilized fracture. Cell Prolif. 52 (2), e12570. doi:10.1111/cpr.12570
Zhang, Y., Liu, S., Li, T., Zhang, L., Azhar, U., Ma, J., et al. (2020c). Cytocompatible and non-fouling zwitterionic hyaluronic acid-based hydrogels using thiol-ene "click" chemistry for cell encapsulation. Carbohydr. Polym. 236, 116021. doi:10.1016/j.carbpol.2020.116021
Zhang, Y., Liu, Y., Liu, H., and Tang, W. H. (2019b). Exosomes: Biogenesis, biologic function and clinical potential. Cell Biosci. 9, 19. doi:10.1186/s13578-019-0282-2
Zhang, Y., Xie, Y., Hao, Z., Zhou, P., Wang, P., Fang, S., et al. (2021b). Umbilical mesenchymal stem cell-derived exosome-encapsulated hydrogels accelerate bone repair by enhancing angiogenesis. ACS Appl. Mater Interfaces 13 (16), 18472–18487. doi:10.1021/acsami.0c22671
Zhao, N., Wang, X., Qin, L., Zhai, M., Yuan, J., Chen, J., et al. (2016). Effect of hyaluronic acid in bone formation and its applications in dentistry. J. Biomed. Mater Res. A 104 (6), 1560–1569. doi:10.1002/jbm.a.35681
Zhou, Y., Gu, Z., Liu, J., Huang, K., Liu, G., and Wu, J. (2020). Arginine based poly (ester amide)/hyaluronic acid hybrid hydrogels for bone tissue Engineering. Carbohydr. Polym. 230, 115640. doi:10.1016/j.carbpol.2019.115640
Zhu, G., Zhang, T., Chen, M., Yao, K., Huang, X., Zhang, B., et al. (2021). Bone physiological microenvironment and healing mechanism: Basis for future bone-tissue engineering scaffolds. Bioact. Mater 6 (11), 4110–4140. doi:10.1016/j.bioactmat.2021.03.043
Ziadlou, R., Rotman, S., Teuschl, A., Salzer, E., Barbero, A., Martin, I., et al. (2021). Optimization of hyaluronic acid-tyramine/silk-fibroin composite hydrogels for cartilage tissue engineering and delivery of anti-inflammatory and anabolic drugs. Mater Sci. Eng. C Mater Biol. Appl. 120, 111701. doi:10.1016/j.msec.2020.111701
Zou, Y., Li, L., Li, Y., Chen, S., Xie, X., Jin, X., et al. (2021). Restoring cardiac functions after myocardial infarction-ischemia/reperfusion via an exosome anchoring conductive hydrogel. ACS Appl. Mater. Interfaces 13 (48), 56892–56908. doi:10.1021/acsami.1c16481
EV extracellular vesicle
ECM extracellular matrix
HA hyaluronic acid
GAG glycosaminoglycan
MSC mesenchymal stem cell
HASes hyaluronic acid synthases
MW molecular weight
HYALs hyaluronidases
CD44 cluster of differentiation-44
RHAMM receptor for hyaluronic acid-mediated motility
CEMIP cell migration inducing hyaluronidase 1
HARE hyaluronic acid-receptor for endocytosis
LYVE-1:Lymphatic Vessel Endothelial Receptor-1
TLR Toll-like receptors
hDPSCs human dental pulp stem cells
ALP alkaline phosphatase
HCLS hybrid cross-linked scaffold
NCID Notch intracellular domain
MSX2 msh homeobox 2
EDC 1- ethyl-3-[3-(dimethylamino)-propyl]-carbodiimide
CMPI 2-chloro-1-methylpyridinium iodide
CDMT 2-chloro-dimethoxy-1,3,5-triazine
HMDA hexamethylenediamine
OSA octenyl succinic anhydride
MeHA methacrylated hyaluronic acid
BMP-2 morphogenetic protein 2
VEGF vascular endothelial-derived growth factor
PLGA Poly (lactic acid-co-glycolic acid)
GA gallic acid
MOF metal-organic framework
hADSCs-Exos human adipose stem cell-derived exosomes
hBMSCs human bone marrow-derived mesenchymal stem cells
HUVECs human umbilical vein endothelial cells
hiPSCs human induced pluripotent stem cells
BMSCs bone marrow mesenchymal stem cells
hiPSC-MSC-Exos exosomes secreted by mesenchymal stem cells derived from human induced pluripotent stem cells
COL 1 Collagen type I
RUNX-2 Runt-related transcription factor 2
β-TCP beta-tricalcium phosphate
BMSCs-OI-Exos BMSCs-derived osteoinductive exosomes
Acvr2b activin A receptor, type IIb
Acvr1 activin A receptor type 1
Bmpr2 bone morphogenetic protein receptor 2
M2D-Exos M2 macrophage-derived exosomes
SIK2 salt-inducible kinases 2
SIK3 salt-inducible kinases 3
BMSCs-Exos BMSCs-derived exosomes
STAT1 signal transducer and activator of transcription 1
MФs-derived exosomes macrophages-derived exosomes
huMSCs-Exos human umbilical cord mesenchymal stem cells-derived exosomes
HIF-1α hypoxia inducible factor-1α
DMOG dimethyloxaloylglycine
DMOG-MSC-Exos BMSCs preconditioned with a low dose of DMOG
AKT protein kinase B
mTOR Mechanistic target of rapamycin
Tie2 Tyrosine-protein kinase receptor 2
NO nitric oxide
lnc-H19 long non-coding RNA-H19
ECs endothelial cells
Smad1 SMAD family member 1
Angp1 angiogenic factor angiopoietin 1
MBG mesoporous bioactive glass
PI3K Phosphoinositide 3-kinase
Smad5 SMAD family member 5
Smad9 SMAD family member 9
BSP bone sialoprotein; Bmpr1a
ATF4 activating transcription factor 4
p-AKT phosphorylated Akt
p-mTOR phosphorylated-mTOR
OCN osteocalcin
OGN Osteoglycin
OPN osteopontin
BBB blood brain barrier
5-FU 5-fluorouracil
HA-ALG hyaluronic acid-alginate
HAP hydroxyapatite
COL1A1 Collagen type I alpha1
uMSCs-Exos umbilical mesenchymal stem cell-derived exosomes
nHP nanohydroxyapatite/poly-ε-caprolactone
DLL4 Delta-like ligand 4
EPCs endothelial progenitor cells
IRE-1α inositol-requiring enzyme 1alpha
VEGFA vascular endothelial growth factor A
Keywords: hyaluronic acid, hydrogel, extracellular vesicles, exosomes, bone regeneration
Citation: Deng H, Wang J and An R (2023) Hyaluronic acid-based hydrogels: As an exosome delivery system in bone regeneration. Front. Pharmacol. 14:1131001. doi: 10.3389/fphar.2023.1131001
Received: 24 December 2022; Accepted: 03 March 2023;
Published: 17 March 2023.
Edited by:
Silvia Fare’, Polytechnic University of Milan, ItalyReviewed by:
Diego Velasco, Universidad Carlos III de Madrid de Madrid, SpainCopyright © 2023 Deng, Wang and An. This is an open-access article distributed under the terms of the Creative Commons Attribution License (CC BY). The use, distribution or reproduction in other forums is permitted, provided the original author(s) and the copyright owner(s) are credited and that the original publication in this journal is cited, in accordance with accepted academic practice. No use, distribution or reproduction is permitted which does not comply with these terms.
*Correspondence: Jiecong Wang, d2FuZ2ppZWNvbmcxOTgyQHNpbmEuY29t; Ran An, YW5fcmFuMjAxOEAxNjMuY29t
Disclaimer: All claims expressed in this article are solely those of the authors and do not necessarily represent those of their affiliated organizations, or those of the publisher, the editors and the reviewers. Any product that may be evaluated in this article or claim that may be made by its manufacturer is not guaranteed or endorsed by the publisher.
Research integrity at Frontiers
Learn more about the work of our research integrity team to safeguard the quality of each article we publish.