- 1Department of Nutrition and Dietetics, Gazi University, Emek, Ankara, Turkey
- 2MERCK S.P.A., Rome, Italy
- 3Department of Pharmacy, University of Naples “Federico II”, Naples, Italy
- 4Medical Oncology, Department of Precision Medicine, Università degli Studi della Campania “Luigi Vanvitelli”, Naples, Italy
- 5Department of Experimental Therapeutics, The University of Texas MD Anderson Cancer Center, Houston, TX, United States
- 6Department of Histology and Embryology, Kütahya Health Sciences University, Kütahya, Turkey
- 7Department of Translational Medical Science and ImmunoNutritionLab at CEINGE Biotechnologies Research Center and Task Force for Microbiome Studies, University of Naples Federico II, Naples, Italy
- 8Department of Agricultural Sciences, University of Naples Federico II, Portici, Italy
Gastrointestinal cancer represents one of the most diagnosed types of cancer. Cancer is a genetic and multifactorial disease, influenced by the host and environmental factors. It has been stated that 20% of cancer is caused by microorganisms such as Helicobacter pylori, hepatitis B and C virus, and human papillomavirus. In addition to these well-known microorganisms associated with cancer, it has been shown differences in the composition of the microbiota between healthy individuals and cancer patients. Some studies have suggested the existence of the selected microorganisms and their metabolites that can promote or inhibit tumorigenesis via some mechanisms. Recent findings have shown that gut microbiome and their metabolites can act as cancer promotors or inhibitors. It has been shown that gastrointestinal cancer can be caused by a dysregulation of the expression of non-coding RNA (ncRNA) through the gut microbiome. This review will summarize the latest reports regarding the relationship among gut microbiome, ncRNAs, and gastrointestinal cancer. The potential applications of diagnosing and cancer treatments will be discussed.
1 Introduction
“Microbiome” and “cancer” are the most popular topics of today’s modern age. All human surfaces and all the cavities that communicate with the outside are populated by an ecosystem of microorganisms, such as bacteria, viruses, protozoa, fungi, and archaea. This population of microbes has a complex, individual, and variable nature; its composition is influenced by the genetics of the host, dietary habits, lifestyle, and microbial exposure at birth (Zitvogel et al., 2016). An average of ten times more bacteria has been calculated than the number of cells in the human body (Guarner and Malagelada, 2003), between 500 and 1,000 different species of microorganisms. The scientific community has agreed on defining “microbiota” as the totality of microbial organisms present in certain environments, while the term “microbiome” the genetic information inherent in the microbiota itself. The microbiome presents a great variability in its composition both between different subjects and within the same subject, in different anatomical sites and tissues (Guarner et al., 2003). Individual and inter-individual differences are thought to be the underlying cause of many diseases and health problems, in gastrointestinal (GI) cancers; therefore, they play a key role in both diagnosis and treatment.
Although cancer is generally accepted as a disease caused by genetic characteristics and environmental factors, it has been reported that the effect of microorganisms on cancer could be approximately 20% of cancers human beings suffer from (De Martel et al., 2012). Digestive system cancers account for approximately one-third of cancers that result in death. At least 15%–20% of cancers are triggered by infectious agents; 20%–30% are associated with tobacco products, 30%–35% with diet, lack of physical activity, and/or energy balance disorder (obesity) (Anand et al., 2008). Targeted therapies are effective in the treatment of certain types of cancer. Many cancer types are treated by traditional chemotherapy treatments with varying efficacy and side effects. Cancer prevention is the main target, and it is reported that a better understanding of the interactions between gut microbiota, barrier function, and inflammatory responses is significant in identifying new targets in cancer therapy (Bultman, 2016; Sun and Kato, 2016).
This review aims to examine the role of the gut microbiota in the development of digestive system cancers, point out the potential factors-metabolites that can modulate this relationship, and comprehensively evaluate their potential role in diagnosis and treatment.
2 GI microbiome
Despite the heterogeneity found in the microbiome (Arumugam et al., 2011), based on the analysis of deoxyribonucleic acid (DNA) sequences from 39 samples belonging to subjects of six different nationalities, three main bacterial strains, which are Bacteroides, Prevotella, and Ruminococcus have been identified which are independent of age, sex, geographical area or diet, and based on the prevalence of one of them, each individual is cataloged in one of three enterotypes. This enterotype is linked to specific functions of the subject, such as the production of some nutrients like vitamins and the predisposition to some non-communicable diseases (NCDs) (Kau et al., 2011).
The number of microorganisms is very high in the oral cavity, where it consists mainly of Firmicutes and Proteobacteria. At the level of the esophagus, the Firmicutes increase even more. In the stomach involved in the secretion of hydrochloric acid in which the pH is strongly acidic (pH ≈ 2), there are a few bacteria among which the most abundant are the Firmicutes, Proteobacteria, and Actinobacteria. The duodenum also has a low microbial population due to the rapid transit time and the secretion of bile and pancreatic fluids that kill most of the ingested bacteria, and because of propulsive motor activity that prevents stable colonization of the lumen.
There is a progressive increase in the number of species from mouth to ileum: first, the Gram (−) and then the obligate anaerobes increase. At the level of mouth and ileum, in which the digestion and absorption of monosaccharides, amino acids, and fatty acids take place, there are Enterococci and Lactobacilli, while in the ileum and the colon (at the level of which the bile acid is absorbed) there is a myriad of bacteria, including Ruminococcus, Staphylococcus, Streptococcus, Peptococcus, Escherichia, Eubacterium, Clostridium, and many others are less abundant such as Proteobacteria, Actinobacteria and Fusobacteria (Hold et al., 2002; Hayashi et al., 2003; Eckburg et al., 2005; Hayashi et al., 2005; Wang et al., 2005; Delgado et al., 2006; Chung et al., 2016; Lu et al., 2016). The gut microbiome is the set of symbiotic microorganisms, which are bacteria, viruses, fungi, and protozoa, found in the digestive tract of mankind, about 1010-1012cells/Gram or milliliter of luminal content, 500–1,000 different species, mostly anaerobic non-sporogenous, and species that cannot be identified by conventional methods (Backhed et al., 2005; Qin et al., 2010).
Most of the species that make up the gut microbiome of the gastrointestinal tract (GIT) are included in the five main phyla in the taxonomic classification. They are listed as Firmicutes (60%–80%), Bacteroidetes (15%–25%), Actinobacteria (2.5%–5%), Proteobacteria (1%–10%) and Verrucomicrobia (0.1%–2.2%). The main bacterial phyla and species in the human digestive tract microbiota are shown in Table 1 (Tözün, 2019).
There is fast-growing literature examining and reporting the relationship between the bacterial composition, structure, and functional capacity of the microbiome in health and diseases. In the gut microbiome, a decrease in beneficial bacteria populations and an increase in pathogenic bacteria populations, changes in bacterial content, and deterioration of balance are defined as dysbiosis. Dysbiosis affects health negatively by preventing the regular functioning of the immune system and may play a role in the pathogenesis of some diseases (von Martels et al., 2017). Dysbiosis has been correlated with inflammatory diseases (Frank et al., 2007; Qin et al., 2010; Jostins et al., 2012), metabolic disorders (Ley et al., 2005), and tumors (Lertpiriyapong et al., 2014; Lam et al., 2017; Zitvogel et al., 2017).
3 Drivers of the gut microbiome composition
The lack of adequate laboratory techniques has for a long time conditioned the study of the intestinal microbiome, being the classical inadequate cultivation method since most of the flora is made up of anaerobic germs. Fortunately, the development of techniques based on the sequencing of the 16S ribosomal RNA (16S rRNA) has facilitated the identification and classification of bacteria.
The colonization of the gastrointestinal tract begins at birth with Bacteroides and Bifidobacteria in the event of a vaginal delivery. On the other hand, it mostly begins with feeding in the cesarean delivery.
In addition to the type of birth, the type of feeding is also a significant determinant of the intestinal microbiota of infants. While Bifidobacterium and Lactobacillus provide colonization in the intestinal microbiota of breastfed infants; Enterococcus, Enterobacteria, Bacteroides, Clostridium, and other anaerobic Streptococcus bacteria provide colonization in the intestinal microbiota of formula-fed infants (Groer et al., 2014). Microbiome composition and diversity may vary depending on age. The first year of life is fundamental for the establishment of the microbiome, which then variably evolves into adulthood from person to person (Gronlund et al., 1999; Harmsen et al., 2000; Palmer et al., 2007). In children between 1 and 7 years of age, there is a greater number of Enterobacteria than in adults with a greater abundance of general Bifidobacterium and Clostridium (Enck et al., 2009; Agans et al., 2011). The composition of the microbiota is very dynamic in the first 3 years of life and then becomes more stable and complex in adulthood (Turroni et al., 2009). In geriatric age, there is a significant variation in its composition with an increase in Bacteroides, Escherichia coli, Streptococcus, Clostridia, Lactobacilli, and Bifidobacterium decrease (Woodmansey, 2007).
The microbiome is particularly abundant in the cecum and the right colon due to the large availability of substrates and the favorable environment for bacterial growth (low transit time, ready availability of nutrients, favorable pH, etc.) (Savage, 1977; Bengmark, 2001). The stomach has a bacterial concentration of about 107 bacteria, therefore much lower than the distal tracts of the gastrointestinal system. The duodenum bacteria have been little studied, except for their involvement in some diseases, such as irritable bowel syndrome (IBS), in which both bacterial growth and a reduction in microbial diversity have been found (Giamarellos-Bourboulis et al., 2015). In any case, tumors of the duodenum are rare, and the relevance of bacterial involvement has not yet been investigated extensively. Besides, although the jejunum and ileum are the longest part of the gastrointestinal system and with the widest contact surface, the recovery of samples in this region remains a challenge (Wang and Yang, 2013). Molecular analyses of the 16S rRNA gene have shown that jejunum hosts a bacterial community less complex than the distal ileum and the large intestine (Wang et al., 2005); nevertheless, the knowledge on the microbial community of the small intestine is still limited both in healthy individuals and patients. On the other hand, the colon hosts one of the most populous and diverse bacterial communities that are distributed between the lumen and the mucosa (Wang et al., 2003; Eckburg et al., 2005).
Dietary patterns play a significant role in the modulation of the gut microbiome. Accumulated literature investigating the roles of nutrition in the modulation of the microbiome. Nutrition has the potential to affect gut microbiota composition and function by influencing microbial diversity, microbial taxonomy, gene expressions, and enzyme activities. Macronutrient and phytochemical content of the diet; some specific foods (probiotics, fermented foods, whole grains, oilseeds, and fruits, etc.) and the overall diet have the potential to modulate the gut microbiome in a short time (Beslenme and Besinler, 2017). In one of the first intervention studies examining the effects of short- and long-term dietary habits on the microbiome, it was shown that long-term dietary habits were associated with three enterotypes (Bacteroides, Prevotella, and Ruminoccous) determined through dominant groups. Bacteroides enterotypes were defined in diets rich in animal protein and fat, and Prevotella enterotypes in diets rich in complex carbohydrates. At the same time, in this study, it was shown that the change in gut microbiome started within 24 h of short-term dietary intervention but did not cause significant changes to affect enterotypes (Wu et al., 2011). In another dietary intervention study, butyrate production increased when the fiber content of the diet was increased; the fat content decreased for 2 weeks; and secondary bile acid synthesis, colonic mucosal proliferation, and inflammation have been shown to decrease (O’Keefe et al., 2015). For example, the gut microbiome of individuals who are carnivores or vegetarians differs. Changes in diet cause changes in the number and content of microorganisms in the microbiome. It was found that the ratios of Prevotella, Lactobacillus, and Bifidobacterium bacteria and fecal short-chain fatty acid (SCFA) levels were high in the microbiota of individuals with high adherence to the Mediterranean diet (MedDiet), and Clostridium species are seen to be lower (Del Chierico et al., 2014; De Filippis et al., 2016).
Interestingly, the diet influences the composition of the gut microbiome especially if the new eating habits last over time (David et al., 2014; Xu and Knight, 2015). A study suggests that low-fiber diet cause some reversible microbiota changes in mice (Sonnenburg et al., 2016), but the diversity of the microbiota is decreased if the diet continues for several generations. It is also noted that a modern diet low in fiber contributes to the loss of taxa over generations and may be responsible for the lower diversity microbiota observed in the industrialized world (Sonnenburg et al., 2016).
There is also a high interindividual and geographical diversity (De Filippo et al., 2010; Arumugam et al., 2011; Fallani et al., 2011). Nutritional habits are also reported to play a significant role in this diversity. The study of African children showed a greater presence of Prevotella, Xylanibacter, and Treponema, which possess enzymes to maximize the extraction of energy from xylans and cellulose, present in large quantities in the low-calorie but a high-fiber diet of these children (De Filippo et al., 2010). In studies conducted in adult groups, the diets of those living in rural areas were based on plant-based foods, the diversity in microbial composition was high and Prevotella was dominant; on the other hand, it has been found that microbial diversity is less and Bacteroides are dominant in those living in developed societies that consume foods rich in animal foods and processed products (Yatsunenko et al., 2012; Ou et al., 2013). All these pieces of evidence shed light on the need for well-designed studies that will evaluate the nutritional microbiota relationship from different aspects to develop correct nutritional recommendations for the regulation of microbiota.
As well as diet, even antibiotic treatments, invasive pathogens, drug-taking, or stress can disturb the balance of organisms and cause dysbiosis. It shows that antibiotic therapy in the first years of life increases the risk of inflammatory bowel disease (Shaw et al., 2010). A study showed that the microbiota is modified by the administration of antibiotic therapy (Dethlefsen et al., 2008). It has been known for a long time that excessive use of antibiotics has some negative effects on increasing antibiotic-resistant pathogens (Dethlefsen et al., 2008).
All these factors, individually or in combination, affect the intestinal microbiome, and alteration of the intestinal microbiome is related to various diseases including obesity, type 2 diabetes, atherosclerosis, cancer neurological and psychiatric diseases, inflammatory bowel diseases (IBD), and autoimmune diseases (Raoult, 2008; Sekirov et al., 2010; Holmes et al., 2011). Hence, the human microbiome including the GI tract represents a real organ adapted to protect our life and our wellbeing but is also willing to be altered in response to any factors.
4 Functions and metabolic clusters of the GI microbiome
The intestinal flora has a metabolic, structural, and protective role; carries out functions such as metabolizing the indigestible components of the diet, producing vitamins, contributing to the fortification of the mucosal barrier, and preventing the increase of pathogenic microorganisms. Therefore, the microbiota plays an important role in the development and regulation of the immune system. There is evidence in favor of the role of the microbiota in the development of B lymphocytes and the regulation of T helper lymphocytes. Several studies in the mouse have shown that tolerogenic dendritic cells and regulatory T cells (Tregs) are absent in germ-free mice and that both commensal bacteria and their metabolites, such as butyric acid, are necessary for the development of Tregs (Atarashi et al., 2011; Arpaia et al., 2013; Furusawa et al., 2013).
The gut microbiome also has many roles in the digestion and metabolism of nutrients. The intestinal flora, influencing the metabolism with specific enzymes, increases the extraction of energy and therefore influences the control of body weight. Resident bacteria are a fundamental line of resistance to colonization by exogenous microbes, reducing the availability of nutrients to potential pathogens (Bernet et al., 1994; Hooper et al., 1999), competing for sites of attack on the brush border of intestinal epithelial cells, and producing some antimicrobial substances (Brook, 1999; Lievin et al., 2000). Besides, among the components of the human microbiota are listed those that cause fermentation (80%) such as Lactobacillus and Bifidobacteria, and those that cause putrefaction of the remains (20%) such as Escherichia, Bacteroides, Eubacteria, and Clostridium. The fermentation of non-digestible food residues and endogenous mucus produced by the epithelium is the main source of energy in the colon. The fibers are resistant to digestion in the small intestine due to the conformation of the glycosidic bond: the enzymes of the human small intestine can only split α-type bonds, but not those of the β type. This explains why amylose, a glucose polymer with α-1,4 glycosidic bond, is digestible, while cellulose, a glucose polymer with β-1,4 glycosidic bond, is indigestible due to the lack of the specific enzyme. The distinctive feature of the carbohydrate type is the difference in hydrolysis by the enzymes of the human digestive system. Digestible carbohydrates are broken down into their monomers and absorbed from the small intestine, while non-digestible carbohydrates reach the colon. Another distinguishing feature is the use of carbohydrates reaching the colon by the colon microbiota. Carbohydrates that can be used by the microbiota can shape microbiota composition and function (Beslenme and Besinler, 2017).
In the cecum and right colon, fermentation is very intense with a high production of SCFAs (acetate, propionate, and butyrate), which generate an acid pH (range 5–6) and rapid bacterial growth. By contrast, the substrate in the left or distal colon is less available; the pH is almost neutral; the putrefactive processes become quantitatively more important, and the activity of the bacterial population is lower. Putrefaction is another anaerobic metabolism of peptides and proteins (elastin and collagen from food sources, pancreatic enzymes, exfoliated epithelial cells, and lysed bacteria, etc.); it also produces SCFAs but, at the same time, generates potentially toxic substances including ammonia, amines, phenols, thiols, and indoles (Macfarlane et al., 1986).
SCFAs (i.e. acetic, propionic, and butyric acids) are released because of the fermentation of some dietary components, such as dietary fiber by the colon microbiota (Smith et al., 2013). Current evidence shows that with low dietary fiber, microbial diversity is significantly reduced, and there are significant changes in microbiota composition. On the other hand, increasing dietary fiber intake increases intestinal microbial richness and/or diversity. It has also been reported to increase bacterial gene expressions for butyrate production and decrease genes required for secondary bile acid synthesis (Cotillard et al., 2013). It has been shown that long-term high fiber intake in the diet can shape the enterotype of the microbiota, especially prevotella colonization (Ou et al., 2013).
SCFAs have important functions in the physiology of the host. To illustrate, butyrate is the main source of energy for the epithelium of the colon and appears to have a protective effect against colon cancer; acetate and propionate are metabolized by the liver (propionate) or by peripheral tissues, in particular by muscles (acetate), and can play a role as modulators of glucose and cholesterol metabolism; butyrate and propionate favor the aboral transition of feces (Chen et al., 1984; Berggren et al., 1996; Wong et al., 2006). SCFAs have broad effects on many cell types, including myeloid cells with anti-inflammatory effects and regulatory T cells in the colon (Smith et al., 2013).
SCFAs can exert powerful immunomodulatory effects by suppressing the activation of the nuclear factor-kB and/or by acting on the G protein-coupled receptors as demonstrated by the acetate and increasing the expression of the Treg gene (Furusawa et al., 2013). This effect translates into some studies of an increase in immunological tolerance and protection from inflammatory or allergic diseases (Honda and Littman, 2012). On the other hand, there is also very limited evidence that this fermentation may negatively impact colon cancer (O’Keefe et al., 2015).
Unlike the other cells of the human body, which use glucose as primary energy, the colonocytes, rapidly proliferating cells, exploit butyrate for 60%–70% of their energy (Fung et al., 2012; Bode et al., 2013; Chang et al., 2014). As a fatty acid, butyrate is beta-oxidized in mitochondria (Chang et al., 2014). Interestingly, butyrate has been shown to have a higher antimutagenic effect compared to organic acids (acetic acid, lactic acid, and pyruvic acid) produced by Lactobacillus and Bifidobacteria (Chong, 2014).
According to Warburg’s hypothesis, cancer cells consume glucose (Goncalves and Martel, 2013), so the butyrate in these cells is not metabolized, accumulates in the nucleus where it inhibits histone deacetylases (HDAC) (Larrosa et al., 2006; Smith et al., 2013; Singh et al., 2014), and therefore the cell cycle progression. Moreover, the bacteria that produce SCFAs seem to affect the enterocyte cycle in the colon; in particular, butyrate inhibits cell proliferation, stimulates differentiation in epithelial neoplastic cell lines in vitro, and promotes the return from neoplastic to non-neoplastic phenotype (Gibson et al., 1992; Siavoshian et al., 2000). The mechanisms underlying the cancer prevention of fiber are of course not related to butyrate alone. Insoluble fiber types such as cellulose are not fermented by intestinal bacteria and accelerate intestinal transit. Decreased intestinal transit rate is thought to be effective in preventing cancer in that they reduce exposure of colon cells and exogenous carcinogens (such as heterocyclic amines from meats) (Bultman, 2016).
Finally, the colon microorganisms assisted by the presence of SCFAs also play a role in the synthesis of some vitamins (B1, B2, B3, B6, B12, pantothenic, biotin, and folic acid) and the absorption of some minerals such as calcium, magnesium, and iron (Pierro et al., 1996).
5 GI cancers and microbiome
5.1 Esophagus cancer
Esophageal cancers are the sixth leading cause of death (Mayer RJ, 2003). Normally, the number of microorganisms in the esophagus is low. It is reported that Streptococcus viridans is the most common in the esophageal microbiota (Yang et al., 2009). The transition from Gram (+) bacteria to Gram (−) bacteria predominance in the esophageal flora induces an important inflammatory response that triggers dysbiosis. Loss of bacterial diversity and atrophic gastritis are increasingly associated with distal esophageal cancer. Higher levels of Campylobacter concisus and Campylobacter rectus have been found in patients with premalignant Barrett’s esophagus and are associated with pathogenesis (WHO, 2020). In the scans performed on adenocarcinomas, it has been shown that the microbiota includes Campylobacter and some Escherichia coli species (Zaidi et al., 2016).
5.2 Gastric tumor
The involvement of Helicobacter pylori in gastric cancer is the best-known example of a microbial infection related to cancer in the human gastrointestinal tract. In the early 1990s, these flagellated Gram (−) bacteria were classified in group I of carcinogens by the International Agency for Cancer Research (IARC, 1994).
Several studies have shown that Helicobacter pylori are implicated in the onset of gastric adenocarcinoma; specifically, the deficiency of the aforementioned bacterium coincides with the increase in the incidence of this tumor form due to the consequent alterations in the physiology of the gastrointestinal tract (secretion of gastric acid, hormones, and immunocytes) and changes the composition of the microbiota since it alters the proportion of other microbial species present (Peek and Blaser, 2002). Studies have shown that changes in microbiota diversity occur during the sequence of chronic gastritis-atrophic gastritis-intestinal metaplasia-dysplasia (Aviles-Jimenez et al., 2014; Eun et al., 2014), with an increase of 21 groups, including Peptostreptococcus stomatis, Parvimonas micra, Fusobacterium nucleatum, and the depletion of 10 bacterial groups (Coker et al., 2018).
Helicobacter pylori play a role in oncogenesis at the gastric level through three mechanisms (Polk and Peek, 2010). First, helicobacter pylori inject two cytotoxins, VacA and CagA into the host cell, which activates oncogenic signal transduction pathways (Fuhler et al., 2009; Queiroz et al., 2012; Hoekstra et al., 2016). Secondly, it induces the production of reactive oxygen species (ROS) that activate inflammatory pathways. Finally, causing atrophic gastritis is characterized by the destruction of the parietal cells that produce acid so that there is a compensatory upregulation of gastrin that stimulates the cells to produce more acid, but also activates oncogenic signals. It is shown that Helicobacter pylori are responsible for chronic atrophic gastritis while it is the consequent change in the microbial flora responsible for the progression to gastric cancer. Following the reduction of gastric acidity, the carcinogenic power of some bacterial strains may increase. In addition to these, the microbiota is also populated with bacteria that reduce nitrates, it follows the formation of nitrites and carcinogenic N-nitroso compounds (Lundberg et al., 1994; Naylor and Axon, 2003; Jakszyn et al., 2006; Brawner et al., 2014).
5.3 Colorectal cancer
Colorectal cancer is one of the most common cancers in the world, and it is a partly hereditary tumor, partly influenced by external factors and lifestyle. The colon has the most populous bacterial community in the human body with a prevalence of Firmicutes and Bacteroidetes (Eckburg et al., 2005; Arumugam et al., 2011) and many more immune cells than other mucous membranes and lymphoid tissues. Moreover, the association between inflammation and cancer is particularly strong in colorectal cancer and indeed patients with chronic intestinal inflammation increase the risk of cancer by 2–10 times (Itzkowitz and Harpaz, 2004). Recent evidence shows that even some bacteria, such as Bacteroides fragilis, Escherichia coli, and Peptostreptococcus anaerobius could promote colorectal cancer by the activation of Th17 cells (Wu et al., 2009), direct DNA damage (Cuevas-Ramos et al., 2010; Arthur et al., 2012), and induction of cholesterol synthesis (Tsoi et al., 2017).
It has not yet been defined what triggers the start of the mutations responsible for the transformation of adenocarcinoma (Sears and Pardoll, 2011), but from metagenomic studies on fecal microbiota (Sobhani et al., 2011; Wang et al., 2012a; Zackular et al., 2014; Zeller et al., 2014; Feng et al., 2015; Yu et al., 2017a) and mucosal (Marchesi et al., 2011; Chen et al., 2012; Allali et al., 2015; Nakatsu et al., 2015; Flemer et al., 2017) of the patient with colorectal cancer, a dysbiosis and an abundance of Fusobacterium, especially Fusobacterium nucleatum (Castellarin et al., 2012), Peptostreptococcus, Parvimonas, and Porphyromonas (Drewes et al., 2017; Dai et al., 2018) which are the components of the oral microbiota have been noted. This suggests that carcinogenesis is associated with bacterial translocation from the mouth to the intestine (Atarashi et al., 2017).
Fusobacterium, in Fusobacterium nucleatum, is a commensal of the oral cavity, but if present in abundance in the colon-rectum, it is associated with metastasis of regional lymph nodes (Warnakulasuriya, 2009) and tumor localization in the colorectal colon (Mima et al., 2016). Fusobacterium nucleatum promotes colon cancer through multiple mechanisms. Fusobacterium nucleatum has a direct interaction with epithelial cells; it attacks and invades epithelial cells with FadA adhesion molecules that bind E-cadherin on the cell surface and activates Wnt. It is internalized and activates other inflammatory genes (Rubinstein et al., 2013). This mechanism is confirmed by the presence of high expression of the FadA gene in adenomas and colorectal tumors (Zeller et al., 2014). With the Fap2 protein, Fusobacterium nucleatum also binds a receptor on T lymphocytes and natural killer (NK) cells and blocks the cytotoxic activity on tumor cells (Mima et al., 2015). Moreover, the bacterium could also modify the tumor microenvironment (Kostic et al., 2013) and induce the expression of microRNA-21 (Yang et al., 2017). It is interesting to point out that the increase in Fusobacterium nucleatum is linked to different clinical and histological parameters, such as lower T cell infiltration (Mima et al., 2015), advanced disease status, and low survival (Flanagan et al., 2014; Mima et al., 2016). However, the difficulty is to understand the causal link: is the bacterium the cause of carcinoma or is it only the end-user of an environment altered by the tumor? There is no study confirming the cause and evidence that a transplant of specific bacteria can cause a tumor in the recipient as seen in obesity. However, there is quite convincing evidence of how extensive alterations of the bacterial flora led to the creation of a pro-oncogenic environment that favors the neoplastic transformation of the cells. This is the so-called hypothesis of the alpha bug, that is a small number of bacteria, acting as alpha subjects, would be the promoters of the carcinogenesis damaging the DNA and would favor the transformation of the remaining bacterial population of the organ concerned (Tjalsma et al., 2012).
The microbiota can also act at a distance because it can alter the intestinal barrier. The intestinal epithelium consists of a single layer of cells that separates the microbiota in the lumen from the intraepithelial lymphocytes and the cells of the innate and adaptive immune system present in the lamina propria. A thin layer of mucus produced by the goblet cells covers the epithelium of the colon and prevents direct contact of the microbes with the epithelium (Takiishi et al., 2017). A diet low in fiber can cause a condition of dysbiosis in which the butyrate-producing bacteria decrease and increase Akkermansiamuciniphilia and Bacteroides caccae which degrade the mucus (DeGruttola et al., 2016). All this leads to a condition of a “leaky gut”, and therefore increased permeability of the intestinal barrier. Clinical and experimental data suggest the importance of intestinal hyperpermeability in the inflammatory changes of various diseases including GI cancers (Fukui, 2016).
On the other hand, several metagenomic studies have identified significant enrichment of butyrate-producing bacteria in healthy controls compared to those affected by colorectal cancer (Itzkowitz and Harpaz, 2004) and in patients suffering from a reduction of commensal bacteria (Bifidobacteria, lactobacillus, Ruminococcus) and producing butyrate (Lachnospiraceae and Faecalibacterium) (Chen et al., 2012; Borges-Canha et al., 2015; Baxter et al., 2016; Hold, 2016). SCFAs enhance barrier functions and exert anti-inflammatory and tolerogenic effects on immune cells through mechanisms such as G-protein-coupled receptor (Gpr)-mediated sensitization of IEC inflammations and reduction of IEC oxygen concentrations and induction of hypoxia-induced factor (HIF) (Tilg et al., 2018). In this way, SCFAs have potent anti-inflammatory and anticancer effects (Bultman, 2014). Butyrate suppresses carcinogenesis through multiple mechanisms; as a histone deacetylase inhibitor, regulates cell proliferation and apoptosis (Donohoe et al., 2014), binds G proteins implicated in tumor suppression (Singh et al., 2014) and induces Treg cells, maintains the barrier function epithelial increasing the expression of tight junctions (Ploger et al., 2012) which are important for preventing inflammation. It has been found that Africans have an abundance of butyrate-producing bacteria and a reduced risk of colorectal cancer when compared to African Americans with a high risk. Faecalibacterium prausnitzii is the major producer of butyrate together with Clostridium IV and XIVa (Ou et al., 2013). Lachnospiraceae, another butyrate producer, is reduced in the feces of colorectal cancer patients (Baxter et al., 2016). One could imagine that the reduction of butyrate-producing bacteria and other short-chain fatty acids may contribute to the progression of colorectal cancer. It is interesting to note that Fusobacterium also produces butyrate but uses it as a substrate, amino acids, for example, lysine, which develop ammonia which is harmful to the intestine.
Except for all this information, the use of the microbiome in diagnosis is another question that needs to be answered. Given the spread of rectal colon cancer, biomarkers are being researched to allow the screening. Case-control cohort studies conducted in America (Zackular et al., 2014; Baxter et al., 2016; Vogtmann et al., 2016), Europe (Zeller et al., 2014; Feng et al., 2015), and China (Yu et al., 2017a) give hope that markers can be identified in the fecal microbiota as 20 genes closely associated with colorectal cancer have been found. One of these genes is that linked to the Fusobacterium nucleatum which also gives information on the prognosis, unfortunately negative (Flanagan et al., 2014; Mima et al., 2016; Yamaoka et al., 2018). The fecal microbiome does not correspond perfectly to the mucosal one (Flemer et al., 2017) and is more useful for identifying risk factors associated with colorectal cancer. From the diagnostic point of view, the combination of 4 markers to beat us (Fusobacterium nucleatum, Clostridium Hathaway, Bacteriodes clarus, and an indefinite species “m7”) turns out to be more accurate than Fusobacterium nucleatum alone (Liang et al., 2017). Besides, comparative studies between healthy people and patients on the oral microbiota have also identified differences that could be used as markers to diagnose esophagus cancer (Chen et al., 2015; Yu et al., 2017a; Peters et al., 2017) and pancreas (Farrell et al., 2012; Torres et al., 2015).
These data, which are likely to be used in the clinic in the future, need to be investigated with further studies. Fecal microbiota analysis showed that there is an association between colorectal cancer and increased lipopolysaccharide metabolism (LPS) which increases the activation of inflammatory pathways by TLRs whose substrates are derived from various bacterial products (LPS, PAMPs) (Zeller et al., 2014).
6 Potential underlying mechanisms of microbiome in GI cancers
Possible mechanisms regarding the role of the gut microbiome in cancer development or prevention are schematized in Figure 1. There are many hypotheses about these mechanisms, and there are many factors that modulate this positive or negative effect. The relationship between cancer and microbiota has intrigued the biomedical community since the late 19th century when William Coley began treating sarcomas by injecting bacteria, the so-called “Coley’s toxin”. Subsequently, other microbial agents and their products were isolated to try to cure malignant tumors, such as bladder cancer via Mycobacterium bovis (Kiselyov et al., 2015) or melanoma via Herpes virus (Greig, 2015), or pancreatic cancer with Listeria monocytogenes (Le et al., 2015). However, little has yet been proven regarding the role of microbiota in the development of gastrointestinal cancers. The current evidence is carcinogenesis of certain specific bacteria found in the gut shows that it can stimulate the immune system by showing modulating effects, stimulating inflammation, and increasing cell proliferation (Abreu and Peek, 2014; Lynch and Pedersen, 2016).
Gut microbiome could modulate many systemic immune response elements such as the regulation of hematopoiesis, development of intestinal macrophages, and T cell response. The immune system participates in the initial phase of the suppression of malignant transformation, destroying the cells already infected with oncogenic viruses and recognizing the cells that express the tumor-associated antigens (TAAs) (Vesely et al., 2011). The immunogenicity of a tumor cell, and therefore its susceptibility to immunosurveillance, requires the combination of antigens, such as TAAs, and an immune system capable of responding to specific molecular patterns (DAMPs) (Kroemer et al., 2015). The intestinal mucosa signals a threat to the innate immune system, through toll-like receptors that recognize and bind specific microbial macromolecules, and this triggers a protective response by commensal bacteria, an inflammatory response to pathogenic organisms, or the trigger of apoptosis. Therefore, the commensal bacteria of the gastrointestinal tract play an active role in the development and homeostasis of the immune system.
The change in the diversity and distribution of bacteria is the most important factor that causes chronic inflammation and the onset of cancer progression. In the specific case of IBD, the mucus layer is thinner and less continuous, the tight junctions are destroyed, and the space between epithelial cells is increased. Therefore, the translocation capacity of the bacteria increases, and all this leads to the triggering of the recurrent chronic inflammatory process with the release of pro-inflammatory cytokines, Tumor Necrosis Factor (TNF), and Interferon-gamma (IFN-γ). Patients with IBD are more susceptible than others to develop colorectal cancer since chronic persistent inflammation determines an abnormal proliferation of tissue that first becomes metaplastic and then neoplastic. A few direct pieces of evidence in favor of the ability of commensal bacteria to promote tumor immunosurveillance are provided by the correlation of the growth and infiltration of major melanomas in mice with less expression in the microbiota of Bifidobacterium species (Sivan et al., 2015).
The increase in some tumors seems to be related to the reduction of certain microbial species, caused by modern lifestyles, which introduce ultra-processed foods (Oikonomopoulou et al., 2013) and dysbiosis by frequent antibiotic therapies. Consistent with this, an epidemiological study conducted on 125441 people demonstrated the correlation between repeated use of penicillin, cephalosporins, or macrolides with lung, prostate, and bladder cancer, and tetracycline with breast cancer (Boursi et al., 2015). Antibiotics likely favor the development of tumors by modifying the composition of the microbiota. Furthermore, the use of antibiotics during cancer therapies reduces their effectiveness. The mucosal barrier in this case has a double offense, on the part of chemotherapy and antibiotics, which can lead to the proliferation of opportunistic pathogenic bacteria that can move through the compromised epithelium and cause infection (Taur and Pamer, 2016). There is also evidence on patients confirming that treatment with Gram (+) active antibiotics is associated with shorter progression-free survival and overall survival in patients with chronic lymphatic leukemia treated with cyclophosphamide and cisplatin (Pflug et al., 2016).
Identifying microbial agents that have a causal link with the process of carcinogenesis is an important issue since it was estimated that 15.0% of new cancer cases were due to infectious agents (Plummer et al., 2016). The oncogenic mechanisms of these complex bacterial communities have not yet been fully clarified. However, there is also some evidence that some bacteria can produce toxins and metabolites that are involved in oncogenesis. Examples are CagA and VacA, the toxins produced by Helicobacter pylori, or deoxycholic acid, a metabolite of the genus Clostridium that predisposes to cancer of the colon and liver. In addition to some bacteria, there are also oncogenic viruses, such as the Papillomavirus, which are positively correlated with cancer of the cervix, head-neck, bladder, and uterus (Rooney et al., 2015). Screenings of oncogenic bacteria and viruses in GI cancers may open areas of research for composing cancer control strategies.
7 Microbiome, diet, and GI cancers: Emerging positive and negative issues
Nutrition can prevent or increase GI cancers by modulating the microbiota. Considering the positive restoration of gut modulation, the first thing that comes to mind is undoubtedly the MedDiet. The MedDiet is among the most healthy and sustainable diet models, which is a diet model mainly based on vegetables, fruits, legumes, nuts, beans, cereals, grains, fish, and unsaturated fats such as olive oil. It has been reported that compliance with the MedDiet model positively affects the gut microbiome ecosystem and has positive roles in ensuring the immune system and metabolic balance of the human organism (Tsigalou et al., 2021). The MedDiet is based on regular consumption of monounsaturated fatty acids (MUFAs) and polyunsaturated fatty acids (PUFAs), polyphenols, and other antioxidants, high intake of prebiotic fiber and low-glycemic carbohydrates, and consumption of more plant proteins than animal proteins. Garcia-Mantrana et al. (2018) noted that a higher Firmicutes-Bacteroidetes ratio was associated with lower adherence to the MedDiet, whereas the greater presence of Bacteroidetes was associated with lower animal protein intake. Also, higher Bifidobacteria counts and higher total SCFAs were associated with greater consumption of plant-based nutrients such as plant proteins and polysaccharides (Garcia-Mantrana et al., 2018). Consistent with all these, it has been reported in the literature that as adherence to the MedDiet increases, mortality is lower and the frequency of gastrointestinal system diseases such as NCDs, low-grade inflammation, and Crohn’s disease decrease (Khalili et al., 2020; Tsigalou et al., 2021). Studies investigating the effects of MedDiet on GI cancers, especially colon cancer, are intense. In this context, in the European Prospective Investigation into Cancer and Nutrition (EPIC) cohort, it was found that adherence to the MedDiet delayed the onset of colorectal cancer. Although the mechanisms associated with this are not clear, it has been reported that DNA methylation of cg20674490-RUNX3 may play a mediator role in this relationship (Fasanelli et al., 2019). In another study, no negative relationship was found between MedDiet compliance and the incidence of colon, proximal colon, distal colon, and rectum cancers in the Dutch population, and this study shed light on the fact that many genomic and environmental factors can modulate this relationship (Schulpen and van den Brandt, 2020). Contrary to MedDiet, the negative effects of Western diets on the gut microbiome ecosystem have been known for a long time. The low fiber but high fat and carbohydrate content of the Western diet is the basis of dysbiosis and related GI problems (Tomasello et al., 2016). Russell et al. administered a high-protein diet for 4 weeks, which resulted in a decrease in the total number of bacteria and microorganisms that produce butyrate, which is known as a protective agent associated with lowering cancer risk, such as Roseburia/Eubacterium rectale (Russell et al., 2011).
Besides, the bacterial products that come from protein metabolism are thought to promote cancer and include N-nitroso compounds, ammonia, hydrogen sulfide, and polyamines (Louis et al., 2014). Roasted meat is of particular concern due to the formation of heterocyclic amines (HCAs) that are metabolized by colon bacteria in compounds that damage DNA (Huycke and Gaskins, 2004). High-fat diets significantly reduced fecal SCFAs concentration compared to low-fat diets; it is also well known to stimulate secondary bile acid secretion and increase the fecal concentration of secondary bile acids such as deoxycholic acid (DCA). Changes in the microbiota with the increase in secondary bile acids increase both intestinal permeability and endotoxin production. This situation plays an important role in the physiopathology of many chronic diseases, including cancer (Brinkworth et al., 2009). In addition, in the Western diet model, which includes frequently consumed processed foods, the intestinal barrier function may be further impaired by certain food additives, including dietary emulsifiers, and may cause significant health problems (Tilg et al., 2018).
There are also some foods and food components that are converted into onco-suppressive metabolites in GI. In the first place are the fibers and/or polysaccharides such as starch, cellulose, pectins, and gums, which reduce the risk of colorectal cancer through some mechanisms. The first mechanism is that insoluble fibers in food such as whole grains, green leafy vegetables, and dried fruits may assist in accelerating intestinal transit, therefore, reducing exposure to carcinogens that may be present. The second mechanism is that soluble fiber, contained in legumes, fresh fruit, and potatoes, sees the action of the microbiota in forming butyrate. Therefore, only soluble fibers generate butyrate, and only a long time dietary intake can bring important changes in the species of the microbiota that produce SCFAs (Sonnenburg et al., 2016; Wu et al., 2016). In addition to fibers, soy-based products such as tofu, miso, and soy milk, which contain daidzein (an isoflavone) in a form require a certain metabolism by the flora to be activated as an antioxidant. Interestingly, only 30%–40% of Westerners can metabolize daidzein in equol, while that percentage doubles in Asians (Bultman, 2016). Cruciferous vegetables are another example of bioactive compounds such as glucosinolates and their hydrolysis products including indoles and isothiocyanates (ITCs), and accumulated literature has reported that high intake of cruciferous vegetables has been associated with a lower risk of certain types of cancer including colorectal cancer (Higdon et al., 2007; Miękus et al., 2020). When these raw vegetables are cut, the glucosinolates are converted by the myrosinase (thioglucosidase) into sulforaphane (SFN) which acts as an anti-inflammatory, as an HDAC inhibitor activity (Clarke et al., 2011). However, the cruciferous are generally cooked and the heat denatures the myrosinases since that is temperature sensitive and can be inactivated upon exposure to temperatures over 60°C. However, human gut microbiota can secrete their myrosinase and can be a mediator to transform glucosinolate precursors in cruciferous vegetables to active isothiocyanates (Tian et al., 2018) and in the colon, the bacterial thioglucosidases convert the glucosinolates into isothiocyanates which exert their beneficial effects (Tian et al., 2018; Parchem et al., 2020). Finally, berries and berry constituents containing ellagic acid are metabolized to urolithin by microflora with an inhibitory function of ROS; cyclooxygenase-2 (COX-2), c-Jun (a component of activator protein-1 (AP-1)), inducible nitric oxide synthase (iNOS) expression; NF-κB and TNFα in preneoplastic tissues especially in the esophagus (Stoner et al., 2008).
The first strategies that come to mind in the prevention and treatment of GI cancers by modulation of gut microbiota or correction of dysbiosis are also biotics (Fong et al., 2020). Probiotics are identified as “live microorganisms that when administered in adequate amounts, confer a health benefit on the host” (Hill et al., 2014). The microorganisms most used as probiotics are the species from the genera Lactobacillus, Bifidobacterium, and other genera Bacillus, Propionibacterium, Streptococcus, Escherichia, and also Saccharomyces are also employed (O’sullivan et al., 1992; Rabah et al., 2017). They can be found and added to numerous types of products including foods and dietary supplements. Probiotic strains must be i) sufficiently characterized; ii) safe; iii) supported by at least one positive human clinical trial with accepted scientific standards and iv) alive in the product at an acceptable dose throughout shelf life (Binda et al., 2020). In the literature, it was shown that the involvement of probiotics in enhancing intestinal barrier functions, maintaining the integrity of narrow junctions, maturation of Tregs, activation of NK cells with direct elimination of bacteria, increasing mucin production from Globet cells, defensins from Paneth cells, secretion of IgA and anti-inflammatory cytokines from immune cells (Gallo et al., 2016).
Prebiotics are recently defined as “the substrates that are selectively utilized by host microorganisms conferring health benefits” (Gibson et al., 2017). Plenty of fermentable carbohydrates have been suggested to include a prebiotic effect, but the dietary prebiotics frequently reported to promote health especially intestinal benefits are the fructans (fructooligosaccharides and inulin) and galactans (galactooligosaccharides) as non-digestible oligosaccharides (Rastall and Gibson, 2015; Gibson et al., 2017). However, by the current definition, the term prebiotic not only covers oligosaccharides, but also includes other food components and metabolites (such as CLA, PUFAs, SCFAs, and dietary polyphenols), making them candidate prebiotics, additionally considering the benefits to regions outside the GI and includes various categories other than food (Gibson et al., 2017). Ingestion of prebiotics is associated with anticarcinogenic effects. Prebiotics reduce pH via forming SCFAs-organic acids in the colon as they are fermented and/or degraded by enzymes (β-fructanosidase β-galactosidase), which are prevalent in Bifidobacteria (Scott et al., 2013), thus exerting anti-inflammatory activity, stimulating Treg cells, and reducing IFN-γ (Gibson et al., 1999). Prebiotics are also able to inhibit the adhesion of pathogens to the intestinal epithelium, preventing translocation (Wang et al., 2012b; de Jesus Raposo et al., 2016; Mendis et al., 2016). Moreover, it has been shown that prebiotics increases the height of the villi, the depth of the crypts, the number of epithelial cells, and the thickness of the mucosal layer of the jejunum and the colon (Kleessen et al., 2003; de Jesus Raposo et al., 2016). Finally, another mechanism of anticancer activity is the detoxification of genotoxins such as nitrosamides and hydrogen peroxide in the gut (Wollowski et al., 2001). It plays a role in all these effects together with probiotics.
In addition to probiotics, postbiotics have recently come to the forefront due to their lower risk compared to probiotics and their modulating effect on the microbiome (Żółkiewicz et al., 2020). The International Scientific Association of Probiotics and Prebiotics (ISAPP) defined a postbiotic as a “preparation of inanimate microorganisms and/or their components that confers a health benefit on the host”. ISAPP has noted that effective postbiotics must contain inactivated microbial cells (micro and macromolecules) or cell fractions, with or without metabolites, that contribute to observed health benefits when administered in adequate amounts (Salminen et al., 2021). In addition to its health benefits, there is evidence in the literature that it supports the treatment of colorectal cancer and reduces side effects by supporting and modulating immune system function. It is reported that it does this by showing antioxidant, antiproliferative, and anti-inflammatory effects (Rad et al., 2021). However, as it is still a new emerging topic, there is a need for longer-term, large-scale randomized-controlled studies investigating the effects of postbiotics on GI cancers.
8 Impact of gut microbiome on anticancer therapy
It is clear in recent years that the long-term response of anticancer drugs depends on the immune response, and the microbiome can play an important role in this (Galluzzi et al., 2015). Therefore, in addition to the mechanism of carcinogenesis, the intestinal microbiota plays a role in mediating the results of anticancer therapies, giving hope for a potential improvement in treatment and reduction of toxicity through the manipulation of the microbiota. In preclinical studies, it was found that the composition of the intestinal microbiota correlates with antitumor immunity and therapeutic efficacy (Iida et al., 2013; Viaud et al., 2013; Sivan et al., 2015; Vetizou et al., 2015). For example, Bifidobacterium breve improves the efficacy of anti-programmed death 1 (PD-1), The cytotoxic T-lymphocyte–associated antigen 4 (CTLA-4) blockade depends on some Bacteroides species (Vetizou et al., 2015), and platinum-based chemotherapy requires an intact intestinal microbiota (Iida et al., 2013). Indeed, it has been shown that a massive presence of Bifidobacterium improves the efficacy of anti-PD-1 in reducing melanoma growth through dendritic cells that become more active in presenting melanoma antigen to T lymphocytes. In a study, microbial correlations were found in response to anti-PD-1 therapy in patients with metastatic melanoma (Peek and Crabtree, 2006). Patients who respond to therapy have an abundance of Faelibacterium while those who do not respond will have an abundance of other Bacteroidales species (Gopalakrishnan et al., 2018).
The oxaliplatin and cyclophosphamide chemotherapeutics are both less effective in tumor reduction in immunodeficient mice, in germ-free mice, or treated with broad-spectrum antibiotics (Zitvogel et al., 2016). In a recent study of patients with non-small cell renal and urothelial lung cancer, the authors found a negative correlation between antibiotic use and response to anti-PD-1 therapy (Routy et al., 2018). These results corroborate the importance of intestinal microbiota in the response to immunotherapy and that microbial destruction by antibiotics may hinder the effectiveness of immune checkpoint blockade (Jobin, 2018).
The role of intestinal microbiota has also been investigated in chemoresistance (Yu et al., 2017b), and it has been found that in the presence of Fusobacterium nucleatum autophagy mechanisms that confer resistance are activated. Furthermore, intestinal microbiota can predict whether a patient receiving ipilimumab will develop autoimmune colitis (Dubin et al., 2016). For instance, the toxicity of immunotherapies can be mitigated by Bifidobacterium (Wang et al., 2018).
9 GI cancer hallmarks and potential clinical applications
9.1 Role of non-coding RNAs and gut microbiome in GI cancers
In the last decade, the function of non-coding RNAs (ncRNAs) in cancer development has been clarified. They have a pivotal role in gene expression, cancer progression, and cell-cell communication through the involvement of extracellular vesicles (EV). ncRNAs have been classified into diverse classes based on their length, structure, and location. The important ncRNA types with well-defined roles in cancers are microRNAs (miRNA), circular RNAs (circRNA), PIWI-interacting RNAs (piRNA), and long-ncRNAs (lncRNA) (Yan and Bu, 2021). Small RNAs known as miRNAs typically have a length of 20–22 nucleotides (nt). Targeted mRNA is degraded by the RNA-induced silencing complex (RISC) when miRNAs bind to the complementary region in the target mRNA (Chang et al., 2015). piRNA, which is 24–30 nt in length, was first discovered in the Drosophila. It is mostly found in germline cells and interacts with members of the PIWI family of proteins to help regulate chromatin’s epigenetic state. (Tsai et al., 2020). LncRNAs and circRNAs are both longer than 200 nt, although circRNAs are ring-like whereas lncRNAs are linear. LncRNAs and circRNAs both fold into complicated second structures that allow them to interact with DNA, RNA, and proteins. They can both be created from exon, intron, intergenic region, or 5/3-untranslated sequences (Yang et al., 2018). ncRNAs can act as tumor suppressors and oncogenes in cancers and they are considered promising diagnostic and therapeutic markers. As of late numerous studies have appeared that the microbiota can influence the event and improvement of cancer by influencing the expression of ncRNA (Table 2).
miRNAs play a crucial role in the relationship between the host and the microbiota in cancer. Numerous studies suggest that Helicobacter pylori infection may influence the host miRNA expression. Chang et al. showed that Helicobacter pylori-positive gastric cancer patients had considerably greater levels of miR99b-3p, miR-564, and miR-638 compared to Helicobacter pylori-negative patients, despite exhibiting significantly less of miR-204-5p, miR-338-5p, miR-375, and miR-548c-3p (Chang et al., 2015). MiR-18a-3p and miR-4286 levels were substantially larger in gastric cancer associated with Helicobacter pylori, according to research by Tsai et al. In a group of gastric cancer patients, serum expression of miR-18a-3p and miR-4286 was positively and significantly associated with Helicobacter pylori. Additionally, invasion, tumor stage, tumor size, and lymph node metastasis were all strongly linked with miR-18a-3p and miR-4286 (Tsai et al., 2020). Another study showed that Helicobacter pylori infection enhanced miR-223-3p expression, which in turn activated the NF-kB pathway and contributed to the development of gastric cancer (Yang et al., 2018). Interestingly, the Ebstein-Barr virus can affect cell signaling pathways, influence gene expression, cause methylation of the host genome, cause infected gastric epithelial cells to generate a tumor microenvironment, and initiate and progress gastric cancer. Ebstein-Barr virus-associated gastric carcinoma is a subtype of gastric cancer that has morphologic traits that are like those of cells going through the epithelial-to-mesenchymal transition (Sun et al., 2020). In one study, miR-200a and miR-200b, which are connected to the epithelial-mesenchymal transition and were assessed in gastric carcinoma, were expressed at lower levels in Ebstein-Barr virus-associated gastric carcinoma than in Ebstein-Barr virus-negative carcinoma (Shinozaki et al., 2010).
Numerous studies have demonstrated that the gut microbiome affects the expression of miRNAs and their target genes in colorectal cancer (Cougnoux et al., 2014; Yu et al., 2017b; Yang et al., 2017). According to one study, Fusobacterium nucleatum enhanced tumor cell miR-21 levels by turning on the TLR4-MyD88 signaling cascade, which in turn increased CRC cell growth and tumor development in mice (Yang et al., 2017). Other research has demonstrated that patients with high miR-21 and Fusobacterium nucleatum DNA levels consistently had a higher risk of negative outcomes. It has also been noted that Fusobacterium nucleatum enhances chemoresistance to CRC via changing autophagy in a way that is miRNA-dependent (Yu et al., 2017b).
Like Fusobacterium nucleatum, it has been shown that different Escherichia coli bacteria contribute favorably to CRC carcinogenesis in a way that is reliant on miRNA (Cougnoux et al., 2014). Tan et al. demonstrated that probiotics could inhibit the expression of the oncogenes hsa-mir-153 and hsa-mir-429 and increase the expression of the tumor suppressors hsa-mir-140 and hsa-mir-132, whereas pathobionts could increase the expression of the oncogenes hsa-mir-223, hsa-mir-96, and hsa-mir-106a (Tan et al., 2021). The research revealed that the development of colorectal cancer and microbiota-mediated colorectal carcinogenesis depend on these miRNAs (Figure 2A, B).
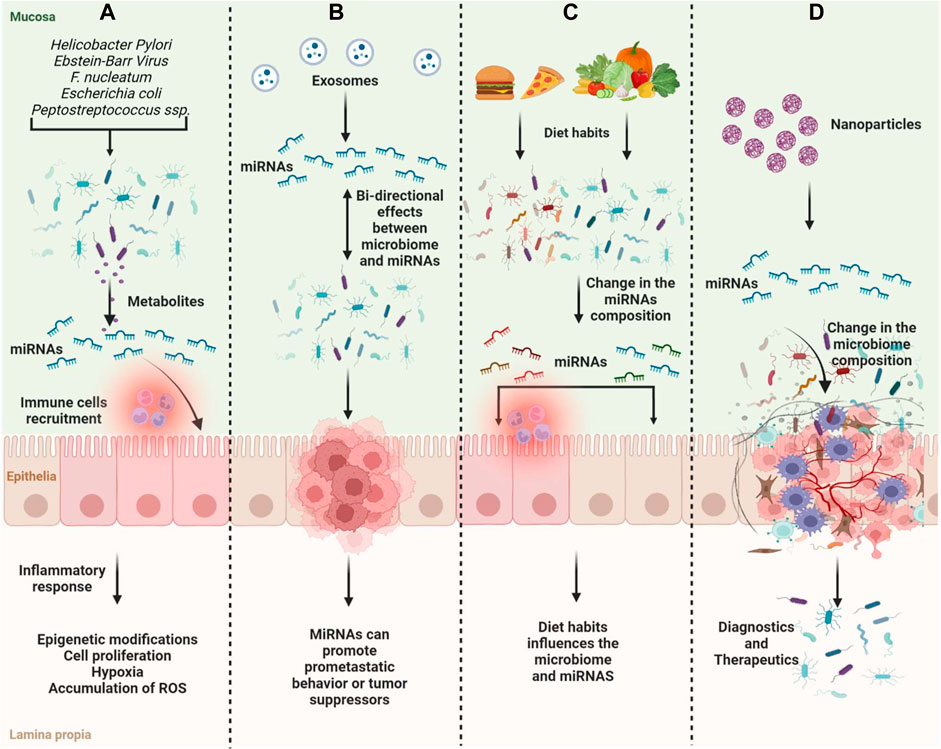
FIGURE 2. Scheme of the gut microbiome and miRNAs on tumor suppression and oncogenesis. (A) Microbiome compositions can induce changes in miRNA expression and induce inflammation, immune recruitment, and epigenetic modifications associated with cancer progression; (B) MiRNAs and microbiota have bi-directional effects on cancer development; (C) Diet habits can influence the microbiome composition along with miRNA expression; (D) ncRNAs can be used as therapeutics influencing the microbiome composition or as diagnostics.
LncRNA is a subclass of non-coding RNA that has been linked to intestinal microbiota, tumor progression, and colorectal carcinogenesis (Hayashi et al., 2003; Delgado et al., 2006). Four lncRNAs, including LINC00355, KCNQ1OT1, LINC00491, and HOTAIR, were found in the probiotic and pathobiont environments while two lncRNAs, including LINC00355 and KCNQ1OT1, were found in the pathogenic environment, according to Tan et al. Patients with CRC who express these lncRNAs strongly have poor prognostic value (Tsai et al., 2020).
Diet and dietary habits influence the microbiome, which is important in triggering the association between cancer and diet (Guz et al., 2021). On the other hand, tumor-associated-miRNAs can regulate the growth and composition of the gut microbiota along with the change in the metabolism (Yuan et al., 2019). Metabolites produced by the microbiome can change gene expression including miRNAs (Figure 2C).
The miRNA expression is regulated by gut microbiota, and aberrations in their expression can lead to pathological processes involved in cancer development and progression. MiR-17-92, miR-21, and miR-503 result to be more expressed in tumoral tissues compared with normal (Yuan et al., 2018). MiR-21 reduces the expression of RAS p21 and programmed cell death-4. In CRC, infection of Fusobacterium nucleatum increases the expression of miR-21 and down-modulation of miR-18a and miR-4802 (Wang et al., 2021). Crosstalk between microRNA-microbiota in the gut plays a pivotal role in gut homeostasis. microRNAs secreted by intestinal epithelial cells or diet-derived can influence the microbiota composition. There is a bi-directional regulation of miRNAs released and microbiota composition (Bi et al., 2020). Delivery of miRNAs may be explored as a therapeutic strategy due to the potential therapeutic interaction between miRNAs and bacteria (Figure 2D). Despite many studies, the gut microbiome and ncRNAs’ relationship and pathway mechanisms are still not fully known. Clarifying this process is very important for understanding the biology of cancer and developing treatment options.
10 Conclusion
It has been shown that changes in the microbiota that cause dysbiosis are associated with various diseases, especially cancer. In recent years, it has been suggested that the gut microbiota, especially dysbiosis, or some specific microorganism species play an important role in the development of cancer, especially GI cancers. In this context, it is not easy to come to a definitive general judgment. Because many factors modulate this relationship, both host and microorganism, as well as the environment.
The research has made important progress in the study of microbiota to facilitate the diagnosis and treatment of human diseases and to prevent them. Targeted microbiota interventions with natural foods and nutraceuticals including biotics may be used to prevent cancer, particularly in patients at risk and specific manipulations of the microbiota could be introduced into the clinic as an adjuvant regimen to increase efficacy and ideally to reduce the side effects of anti-cancer treatments. Of course, the most important issue in this application is to do this in expert monitoring and to consider the possible profit/loss ratio.
The accumulation of scientific evidence offers opportunities for the development of new applications in cancer diagnosis and management. The microorganisms involved in the pathogenesis can offer valid biomarkers to get more information about the disease, the response to treatment, and its handling. Recent studies report that ncRNAs play an important role in cancer development by regulating several mechanisms involved in cell proliferation, differentiation, and apoptosis, including gut microbiome composition. ncRNAs represent promising approaches as diagnostics and therapeutics to explore in the future. However, further investigations are needed to deeply understand the role of ncRNAs in GI cancer.
While waiting for further evidence to introduce the microbiota among the tools of precision medicine and nutrition, the most immediate approach is to dietary intervention enriching with fibers, and foods rich in bioactive substances and through pro-prebiotic foods, etc., limiting the consumption of processed foods and some certain type of animal foods. Along the same line, there is important evidence that the MedDiet is has a protective effect on GI cancers by modulating the microbiota. In addition to healthy nutrition practices, rational drug use strategies should be developed, and especially antibiotic treatments should be applied rationally to prevent all health problems related to dysbiosis, including GI cancers. Considering all these issues, it will be more realistic to develop microbiota-oriented strategies, especially in the prevention and treatment of GI cancers.
Author contributions
DA, RBC, and PA: Conceptualization, Writing—Review and Editing; EC, OC, ADB, MFN, IC, FM, AB, and SKS: Writing—Original Draft Preparation; RC: Conceptualization, Writing—Review and Editing, Supervision, Funding Acquisition.
Funding
The paper was funded under the National Recovery and Resilience Plan (NRRP), Mission 4 Component 2 Investment 1.3-Call for tender No. 341 of 15 March 2022 of Italian Ministry of University and Research funded by the European Union—NextGenerationEU; Award Number: Project code PE00000003, Concession Decree No. 1550 of 11 October 2022 adopted by the Italian Ministry of University and Research, CUP D93C22000890001, Project title “ON Foods-Research and innovation network on food and nutrition Sustainability, Safety and Security—Working ON Foods.
Acknowledgments
Figures were created with BioRender.com.
Conflict of interest
EC was employed by the company MERCK S.P.A.
The remaining authors declare that the research was conducted in the absence of any commercial or financial relationships that could be construed as a potential conflict of interest.
Publisher’s note
All claims expressed in this article are solely those of the authors and do not necessarily represent those of their affiliated organizations, or those of the publisher, the editors and the reviewers. Any product that may be evaluated in this article, or claim that may be made by its manufacturer, is not guaranteed or endorsed by the publisher.
References
Abreu, M. T., and Peek, R. M. (2014). Gastrointestinal malignancy and the microbiome. Gastroenterology 146 (6), 1534–1546. e3. doi:10.1053/j.gastro.2014.01.001
Agans, R., Rigsbee, L., Kenche, H., Michail, S., Khamis, H. J., and Paliy, O. (2011). Distal gut microbiota of adolescent children is different from that of adults. FEMS Microbiol. Ecol. 77 (2), 404–412. doi:10.1111/j.1574-6941.2011.01120.x
Allali, I., Delgado, S., Marron, P. I., Astudillo, A., Yeh, J. J., Ghazal, H., et al. (2015). Gut microbiome compositional and functional differences between tumor and non-tumor adjacent tissues from cohorts from the US and Spain. Gut microbes 6 (3), 161–172. doi:10.1080/19490976.2015.1039223
Anand, P., Kunnumakara, A. B., Sundaram, C., Harikumar, K. B., Tharakan, S. T., Lai, O. S., et al. (2008). Cancer is a preventable disease that requires major lifestyle changes. Pharm. Res. 25 (9), 2097–2116. doi:10.1007/s11095-008-9661-9
Arpaia, N., Campbell, C., Fan, X., Dikiy, S., van der Veeken, J., deRoos, P., et al. (2013). Metabolites produced by commensal bacteria promote peripheral regulatory T-cell generation. Nature 504 (7480), 451–455. doi:10.1038/nature12726
Arthur, J. C., Perez-Chanona, E., Muhlbauer, M., Tomkovich, S., Uronis, J. M., Fan, T. J., et al. (2012). Intestinal inflammation targets cancer-inducing activity of the microbiota. Science 338 (6103), 120–123. doi:10.1126/science.1224820
Arumugam, M., Raes, J., Pelletier, E., Le Paslier, D., Yamada, T., Mende, D. R., et al. (2011). Enterotypes of the human gut microbiome. Nature 473 (7346), 174–180. doi:10.1038/nature09944
Atarashi, K., Suda, W., Luo, C., Kawaguchi, T., Motoo, I., Narushima, S., et al. (2017). Ectopic colonization of oral bacteria in the intestine drives TH1 cell induction and inflammation. Science 358 (6361), 359–365. doi:10.1126/science.aan4526
Atarashi, K., Tanoue, T., Shima, T., Imaoka, A., Kuwahara, T., Momose, Y., et al. (2011). Induction of colonic regulatory T cells by indigenous Clostridium species. Science 331 (6015), 337–341. doi:10.1126/science.1198469
Aviles-Jimenez, F., Vazquez-Jimenez, F., Medrano-Guzman, R., Mantilla, A., and Torres, J. (2014). Stomach microbiota composition varies between patients with non-atrophic gastritis and patients with intestinal type of gastric cancer. Sci. Rep. 4, 4202. doi:10.1038/srep04202
Backhed, F., Ley, R. E., Sonnenburg, J. L., Peterson, D. A., and Gordon, J. I. (2005). Host-bacterial mutualism in the human intestine. Science 307 (5717), 1915–1920. doi:10.1126/science.1104816
Baxter, N. T., Mtt, R., Rogers, M. A., and Schloss, P. D. (2016). Microbiota-based model improves the sensitivity of fecal immunochemical test for detecting colonic lesions. Genome Med. 8 (1), 37. doi:10.1186/s13073-016-0290-3
Bengmark, S. (2001). Pre-pro- and synbiotics. Curr. Opin. Clin. Nutr. metabolic care 4 (6), 571–579. doi:10.1097/00075197-200111000-00019
Berggren, A. M., Nyman, E. M., Lundquist, I., and Bjorck, I. M. (1996). Influence of orally and rectally administered propionate on cholesterol and glucose metabolism in obese rats. Br. J. Nutr. 76 (2), 287–294. doi:10.1079/bjn19960032
Bernet, M. F., Brassart, D., Neeser, J. R., and Servin, A. L. (1994). Lactobacillus acidophilus LA 1 binds to cultured human intestinal cell lines and inhibits cell attachment and cell invasion by enterovirulent bacteria. Gut 35 (4), 483–489. doi:10.1136/gut.35.4.483
Beslenme, B. D. Z., and Besinler, F. (2017). Mikrobiyota. Tüba-mikrobiyota ve i?nsan sağlığı sempozyumu raporu. Ankara, Turkey: Türkiye Bilimler Akademisi.
Bi, K., Zhang, X., Chen, W., and Diao, H. (2020). MicroRNAs regulate intestinal immunity and gut microbiota for gastrointestinal health: A comprehensive review. Genes (Basel) 11 (9), 1075. doi:10.3390/genes11091075
Binda, S., Hill, C., Johansen, E., Obis, D., Pot, B., Sanders, M. E., et al. (2020). Criteria to qualify microorganisms as “probiotic” in foods and dietary supplements. Front. Microbiol. 11 (1662), 1662. doi:10.3389/fmicb.2020.01662
Bode, L. M., Bunzel, D., Huch, M., Cho, G. S., Ruhland, D., Bunzel, M., et al. (2013). In vivo and in vitro metabolism of trans-resveratrol by human gut microbiota. Am. J. Clin. Nutr. 97 (2), 295–309. doi:10.3945/ajcn.112.049379
Borges-Canha, M., Portela-Cidade, J. P., Dinis-Ribeiro, M., Leite-Moreira, A. F., and Pimentel-Nunes, P. (2015). Role of colonic microbiota in colorectal carcinogenesis: A systematic review. Rev. espanola enfermedades Dig. organo Of. Soc. Espanola Patol. Dig. 107 (11), 659–671. doi:10.17235/reed.2015.3830/2015
Boursi, B., Mamtani, R., Haynes, K., and Yang, Y. X. (2015). Recurrent antibiotic exposure may promote cancer formation-Another step in understanding the role of the human microbiota? Eur. J. cancer 51 (17), 2655–2664. doi:10.1016/j.ejca.2015.08.015
Brawner, K. M., Morrow, C. D., and Smith, P. D. (2014). Gastric microbiome and gastric cancer. Cancer J. 20 (3), 211–216. doi:10.1097/PPO.0000000000000043
Brinkworth, G. D., Noakes, M., Clifton, P. M., and Bird, A. R. (2009). Comparative effects of very low-carbohydrate, high-fat and high-carbohydrate, low-fat weight-loss diets on bowel habit and faecal short-chain fatty acids and bacterial populations. Br. J. Nutr. 101 (10), 1493–1502. doi:10.1017/S0007114508094658
Brook, I. (1999). Bacterial interference. Crit. Rev. Microbiol. 25 (3), 155–172. doi:10.1080/10408419991299211
Bultman, S. J. (2014). Emerging roles of the microbiome in cancer. Carcinogenesis 35 (2), 249–255. doi:10.1093/carcin/bgt392
Bultman, S. J. (2016). The microbiome and its potential as a cancer preventive intervention. Seminars Oncol. 43, 97–106. doi:10.1053/j.seminoncol.2015.09.001
Castellarin, M., Warren, R. L., Freeman, J. D., Dreolini, L., Krzywinski, M., Strauss, J., et al. (2012). Fusobacterium nucleatum infection is prevalent in human colorectal carcinoma. Genome Res. 22 (2), 299–306. doi:10.1101/gr.126516.111
Chang, H., Kim, N., Park, J. H., Nam, R. H., Choi, Y. J., Lee, H. S., et al. (2015). Different microRNA expression levels in gastric cancer depending on Helicobacter pylori infection. Gut Liver 9 (2), 188–196. doi:10.5009/gnl13371
Chang, P. V., Hao, L., Offermanns, S., and Medzhitov, R. (2014). The microbial metabolite butyrate regulates intestinal macrophage function via histone deacetylase inhibition. Proc. Natl. Acad. Sci. U. S. A. 111 (6), 2247–2252. doi:10.1073/pnas.1322269111
Chen, W., Liu, F., Ling, Z., Tong, X., and Xiang, C. (2012). Human intestinal lumen and mucosa-associated microbiota in patients with colorectal cancer. PloS one 7 (6), e39743. doi:10.1371/journal.pone.0039743
Chen, W. J., Anderson, J. W., and Jennings, D. (1984). Propionate may mediate the hypocholesterolemic effects of certain soluble plant fibers in cholesterol-fed rats. Proc. Soc. Exp. Biol. Med. Soc. Exp. Biol. Med. 175 (2), 215–218. doi:10.3181/00379727-175-41791
Chen, X., Winckler, B., Lu, M., Cheng, H., Yuan, Z., Yang, Y., et al. (2015). Oral microbiota and risk for esophageal squamous cell carcinoma in a high-risk area of China. PloS one 10 (12), e0143603. doi:10.1371/journal.pone.0143603
Chong, E. S. L. (2014). A potential role of probiotics in colorectal cancer prevention: Review of possible mechanisms of action. World J. Microbiol. Biotechnol. 30 (2), 351–374. doi:10.1007/s11274-013-1499-6
Chung, C. S., Chang, P. F., Liao, C. H., Lee, T. H., Chen, Y., Lee, Y. C., et al. (2016). Differences of microbiota in small bowel and faeces between irritable bowel syndrome patients and healthy subjects. Scand. J. gastroenterology 51 (4), 410–419. doi:10.3109/00365521.2015.1116107
Clarke, J. D., Riedl, K., Bella, D., Schwartz, S. J., Stevens, J. F., and Ho, E. (2011). Comparison of isothiocyanate metabolite levels and histone deacetylase activity in human subjects consuming broccoli sprouts or broccoli supplement. J. Agric. food Chem. 59 (20), 10955–10963. doi:10.1021/jf202887c
Coker, O. O., Dai, Z., Nie, Y., Zhao, G., Cao, L., Nakatsu, G., et al. (2018). Mucosal microbiome dysbiosis in gastric carcinogenesis. Gut 67 (6), 1024–1032. doi:10.1136/gutjnl-2017-314281
Cotillard, A., Kennedy, S. P., Kong, L. C., Prifti, E., Pons, N., Le Chatelier, E., et al. (2013). Dietary intervention impact on gut microbial gene richness. Nature 500 (7464), 585–588. doi:10.1038/nature12480
Cougnoux, A., Dalmasso, G., Martinez, R., Buc, E., Delmas, J., Gibold, L., et al. (2014). Bacterial genotoxin colibactin promotes colon tumour growth by inducing a senescence-associated secretory phenotype. Gut 63 (12), 1932–1942. doi:10.1136/gutjnl-2013-305257
Cuevas-Ramos, G., Petit, C. R., Marcq, I., Boury, M., Oswald, E., and Nougayrede, J. P. (2010). Escherichia coli induces DNA damage in vivo and triggers genomic instability in mammalian cells. Proc. Natl. Acad. Sci. U. S. A. 107 (25), 11537–11542. doi:10.1073/pnas.1001261107
Dai, Z., Coker, O. O., Nakatsu, G., Wu, W. K. K., Zhao, L., Chen, Z., et al. (2018). Multi-cohort analysis of colorectal cancer metagenome identified altered bacteria across populations and universal bacterial markers. Microbiome 6 (1), 70. doi:10.1186/s40168-018-0451-2
David, L. A., Maurice, C. F., Carmody, R. N., Gootenberg, D. B., Button, J. E., Wolfe, B. E., et al. (2014). Diet rapidly and reproducibly alters the human gut microbiome. Nature 505 (7484), 559–563. doi:10.1038/nature12820
De Filippis, F., Pellegrini, N., Vannini, L., Jeffery, I. B., La Storia, A., Laghi, L., et al. (2016). High-level adherence to a Mediterranean diet beneficially impacts the gut microbiota and associated metabolome. Gut 65 (11), 1812–1821. doi:10.1136/gutjnl-2015-309957
De Filippo, C., Cavalieri, D., Di Paola, M., Ramazzotti, M., Poullet, J. B., Massart, S., et al. (2010). Impact of diet in shaping gut microbiota revealed by a comparative study in children from Europe and rural Africa. Proc. Natl. Acad. Sci. U. S. A. 107 (33), 14691–14696. doi:10.1073/pnas.1005963107
de Jesus Raposo, M. F., de Morais, A. M., and de Morais, R. M. (2016). Emergent sources of prebiotics: Seaweeds and microalgae. Mar. drugs 14 (2), 27. doi:10.3390/md14020027
De Martel, C., Ferlay, J., Franceschi, S., Vignat, J., Bray, F., Forman, D., et al. (2012). Global burden of cancers attributable to infections in 2008: A review and synthetic analysis. lancet Oncol. 13 (6), 607–615. doi:10.1016/S1470-2045(12)70137-7
DeGruttola, A. K., Low, D., Mizoguchi, A., and Mizoguchi, E. (2016). Current understanding of dysbiosis in disease in human and animal models. Inflamm. Bowel Dis. 22 (5), 1137–1150. doi:10.1097/MIB.0000000000000750
Del Chierico, F., Vernocchi, P., Dallapiccola, B., and Putignani, L. (2014). Mediterranean diet and health: Food effects on gut microbiota and disease control. Int. J. Mol. Sci. 15 (7), 11678–11699. doi:10.3390/ijms150711678
Delgado, S., Suarez, A., and Mayo, B. (2006). Identification of dominant bacteria in feces and colonic mucosa from healthy Spanish adults by culturing and by 16S rDNA sequence analysis. Dig. Dis. Sci. 51 (4), 744–751. doi:10.1007/s10620-006-3201-4
Dethlefsen, L., Huse, S., Sogin, M. L., and Relman, D. A. (2008). The pervasive effects of an antibiotic on the human gut microbiota, as revealed by deep 16S rRNA sequencing. PLoS Biol. 6 (11), e280. doi:10.1371/journal.pbio.0060280
Donohoe, D. R., Holley, D., Collins, L. B., Montgomery, S. A., Whitmore, A. C., Hillhouse, A., et al. (2014). A gnotobiotic mouse model demonstrates that dietary fiber protects against colorectal tumorigenesis in a microbiota- and butyrate-dependent manner. Cancer Discov. 4 (12), 1387–1397. doi:10.1158/2159-8290.CD-14-0501
Drewes, J. L., White, J. R., Dejea, C. M., Fathi, P., Iyadorai, T., Vadivelu, J., et al. (2017). High-resolution bacterial 16S rRNA gene profile meta-analysis and biofilm status reveal common colorectal cancer consortia. NPJ biofilms microbiomes 3, 34. doi:10.1038/s41522-017-0040-3
Dubin, K., Callahan, M. K., Ren, B., Khanin, R., Viale, A., Ling, L., et al. (2016). Intestinal microbiome analyses identify melanoma patients at risk for checkpoint-blockade-induced colitis. Nat. Commun. 7, 10391. doi:10.1038/ncomms10391
Eckburg, P. B., Bik, E. M., Bernstein, C. N., Purdom, E., Dethlefsen, L., Sargent, M., et al. (2005). Diversity of the human intestinal microbial flora. Science 308 (5728), 1635–1638. doi:10.1126/science.1110591
Enck, P., Zimmermann, K., Rusch, K., Schwiertz, A., Klosterhalfen, S., and Frick, J. S. (2009). The effects of maturation on the colonic microflora in infancy and childhood. Gastroenterology Res. Pract. 2009, 752401. doi:10.1155/2009/752401
Eun, C. S., Kim, B. K., Han, D. S., Kim, S. Y., Kim, K. M., et al. (2014). Differences in gastric mucosal microbiota profiling in patients with chronic gastritis, intestinal metaplasia, and gastric cancer using pyrosequencing methods. Helicobacter 19 (6), 407–416. doi:10.1111/hel.12145
Fallani, M., Amarri, S., Uusijarvi, A., Adam, R., Khanna, S., Aguilera, M., et al. (2011). Determinants of the human infant intestinal microbiota after the introduction of first complementary foods in infant samples from five European centres. Microbiology 157, 1385–1392. doi:10.1099/mic.0.042143-0
Farrell, J. J., Zhang, L., Zhou, H., Chia, D., Elashoff, D., Akin, D., et al. (2012). Variations of oral microbiota are associated with pancreatic diseases including pancreatic cancer. Gut 61 (4), 582–588. doi:10.1136/gutjnl-2011-300784
Fasanelli, F., Giraudo, M. T., Vineis, P., Fiano, V., Fiorito, G., Grasso, C., et al. (2019). DNA methylation, colon cancer and mediterranean diet: Results from the EPIC-Italy cohort. Epigenetics 14 (10), 977–988. doi:10.1080/15592294.2019.1629230
Feng, Q., Liang, S., Jia, H., Stadlmayr, A., Tang, L., Lan, Z., et al. (2015). Gut microbiome development along the colorectal adenoma-carcinoma sequence. Nat. Commun. 6, 6528. doi:10.1038/ncomms7528
Flanagan, L., Schmid, J., Ebert, M., Soucek, P., Kunicka, T., Liska, V., et al. (2014). Fusobacterium nucleatum associates with stages of colorectal neoplasia development, colorectal cancer and disease outcome. Eur. J. Clin. Microbiol. Infect. Dis. 33 (8), 1381–1390. doi:10.1007/s10096-014-2081-3
Flemer, B., Lynch, D. B., Brown, J. M., Jeffery, I. B., Ryan, F. J., Claesson, M. J., et al. (2017). Tumour-associated and non-tumour-associated microbiota in colorectal cancer. Gut 66 (4), 633–643. doi:10.1136/gutjnl-2015-309595
Fong, W., Li, Q., and Yu, J. (2020). Gut microbiota modulation: A novel strategy for prevention and treatment of colorectal cancer. Oncogene 39 (26), 4925–4943. doi:10.1038/s41388-020-1341-1
Frank, D. N., St Amand, A. L., Feldman, R. A., Boedeker, E. C., Harpaz, N., and Pace, N. R. (2007). Molecular-phylogenetic characterization of microbial community imbalances in human inflammatory bowel diseases. Proc. Natl. Acad. Sci. U. S. A. 104 (34), 13780–13785. doi:10.1073/pnas.0706625104
Fuhler, G. M., Tyl, M. R., Olthof, S. G., Lyndsay Drayer, A., Blom, N., and Vellenga, E. (2009). Distinct roles of the mTOR components Rictor and Raptor in MO7e megakaryocytic cells. Eur. J. Haematol. 83 (3), 235–245. doi:10.1111/j.1600-0609.2009.01263.x
Fukui, H. (2016). Increased intestinal permeability and decreased barrier function: Does it really influence the risk of inflammation? Inflamm. Intest. Dis. 1 (3), 135–145. doi:10.1159/000447252
Fung, K. Y., Cosgrove, L., Lockett, T., Head, R., and Topping, D. L. (2012). A review of the potential mechanisms for the lowering of colorectal oncogenesis by butyrate. Br. J. Nutr. 108 (5), 820–831. doi:10.1017/S0007114512001948
Furusawa, Y., Obata, Y., Fukuda, S., Endo, T. A., Nakato, G., Takahashi, D., et al. (2013). Commensal microbe-derived butyrate induces the differentiation of colonic regulatory T cells. Nature 504 (7480), 446–450. doi:10.1038/nature12721
Gallo, A., Passaro, G., Gasbarrini, A., Landolfi, R., and Montalto, M. (2016). Modulation of microbiota as treatment for intestinal inflammatory disorders: An uptodate. World J. gastroenterology 22 (32), 7186–7202. doi:10.3748/wjg.v22.i32.7186
Galluzzi, L., Buque, A., Kepp, O., Zitvogel, L., and Kroemer, G. (2015). Immunological effects of conventional chemotherapy and targeted anticancer agents. Cancer Cell 28 (6), 690–714. doi:10.1016/j.ccell.2015.10.012
Garcia-Mantrana, I., Selma-Royo, M., Alcantara, C., and Collado, M. C. (2018). Shifts on gut microbiota associated to mediterranean diet adherence and specific dietary intakes on general adult population. Front. Microbiol. 9, 890. doi:10.3389/fmicb.2018.00890
Giamarellos-Bourboulis, E., Tang, J., Pyleris, E., Pistiki, A., Barbatzas, C., Brown, J., et al. (2015). Molecular assessment of differences in the duodenal microbiome in subjects with irritable bowel syndrome. Scand. J. gastroenterology 50 (9), 1076–1087. doi:10.3109/00365521.2015.1027261
Gibson, G. R., Hutkins, R., Sanders, M. E., Prescott, S. L., Reimer, R. A., Salminen, S. J., et al. (2017). Expert consensus document: The International Scientific Association for Probiotics and Prebiotics (ISAPP) consensus statement on the definition and scope of prebiotics. Nat. Rev. Gastroenterology Hepatology 14 (8), 491–502. doi:10.1038/nrgastro.2017.75
Gibson, P. R., Moeller, I., Kagelari, O., Folino, M., and Young, G. P. (1992). Contrasting effects of butyrate on the expression of phenotypic markers of differentiation in neoplastic and non-neoplastic colonic epithelial cells in vitro. J. gastroenterology hepatology 7 (2), 165–172. doi:10.1111/j.1440-1746.1992.tb00956.x
Gibson, P. R., Rosella, O., Wilson, A. J., Mariadason, J. M., Rickard, K., Byron, K., et al. (1999). Colonic epithelial cell activation and the paradoxical effects of butyrate. Carcinogenesis 20 (4), 539–544. doi:10.1093/carcin/20.4.539
Goncalves, P., and Martel, F. (2013). Butyrate and colorectal cancer: The role of butyrate transport. Curr. drug Metab. 14 (9), 994–1008. doi:10.2174/1389200211314090006
Gopalakrishnan, V., Spencer, C. N., Nezi, L., Reuben, A., Andrews, M. C., Karpinets, T. V., et al. (2018). Gut microbiome modulates response to anti-PD1 immunotherapy in melanoma patients. Science 359 (6371), 97–103. doi:10.1126/science.aan4236
Greig, S. L. (2015). Brexpiprazole: First global approval. Drugs 75 (14), 1687–1697. doi:10.1007/s40265-015-0462-2
Groer, M. W., Luciano, A. A., Dishaw, L. J., Ashmeade, T. L., Miller, E., and Gilbert, J. A. (2014). Development of the preterm infant gut microbiome: A research priority. Microbiome 2 (1), 38–8. doi:10.1186/2049-2618-2-38
Gronlund, M. M., Lehtonen, O. P., Eerola, E., and Kero, P. (1999). Fecal microflora in healthy infants born by different methods of delivery: Permanent changes in intestinal flora after cesarean delivery. J. Pediatr. gastroenterology Nutr. 28 (1), 19–25. doi:10.1097/00005176-199901000-00007
Guarner, F., Casellas, F., Borruel, N., Antolìn, M., Videla, S., and VilasecaMalagelada, J. (2003). Gut flora in health and disease. Gut flora health Dis. Lancet 361, 512–519. doi:10.1016/S0140-6736(03)12489-0
Guarner, F., and Malagelada, J. R. (2003). Gut flora in health and disease. Lancet 361 (9356), 512–519. doi:10.1016/S0140-6736(03)12489-0
Guz, M., Jeleniewicz, W., Malm, A., and Korona-Glowniak, I. (2021). A crosstalk between diet, microbiome and microRNA in epigenetic regulation of colorectal cancer. Nutrients 13 (7), 2428. doi:10.3390/nu13072428
Harmsen, H. J., Wildeboer-Veloo, A. C., Raangs, G. C., Wagendorp, A. A., Klijn, N., Bindels, J. G., et al. (2000). Analysis of intestinal flora development in breast-fed and formula-fed infants by using molecular identification and detection methods. J. Pediatr. gastroenterology Nutr. 30 (1), 61–67. doi:10.1097/00005176-200001000-00019
Hayashi, H., Sakamoto, M., Kitahara, M., and Benno, Y. (2003). Molecular analysis of fecal microbiota in elderly individuals using 16S rDNA library and T-RFLP. Microbiol. Immunol. 47 (8), 557–570. doi:10.1111/j.1348-0421.2003.tb03418.x
Hayashi, H., Takahashi, R., Nishi, T., Sakamoto, M., and Benno, Y. (2005). Molecular analysis of jejunal, ileal, caecal and recto-sigmoidal human colonic microbiota using 16S rRNA gene libraries and terminal restriction fragment length polymorphism. J. Med. Microbiol. 54, 1093–1101. doi:10.1099/jmm.0.45935-0
Higdon, J. V., Delage, B., Williams, D. E., and Dashwood, R. H. (2007). Cruciferous vegetables and human cancer risk: Epidemiologic evidence and mechanistic basis. Pharmacol. Res. 55 (3), 224–236. doi:10.1016/j.phrs.2007.01.009
Hill, C., Guarner, F., Reid, G., Gibson, G. R., Merenstein, D. J., Pot, B., et al. (2014). Expert consensus document: The International Scientific Association for Probiotics and Prebiotics consensus statement on the scope and appropriate use of the term probiotic. Nat. Rev. Gastroenterology hepatology 11, 506–514. doi:10.1038/nrgastro.2014.66
Hoekstra, E., Das, A. M., Swets, M., Cao, W., van der Woude, C. J., Bruno, M. J., et al. (2016). Increased PTP1B expression and phosphatase activity in colorectal cancer results in a more invasive phenotype and worse patient outcome. Oncotarget 7 (16), 21922–21938. doi:10.18632/oncotarget.7829
Hold, G. L. (2016). Gastrointestinal microbiota and colon cancer. Dig. Dis. 34 (3), 244–250. doi:10.1159/000443358
Hold, G. L., Pryde, S. E., Russell, V. J., Furrie, E., and Flint, H. J. (2002). Assessment of microbial diversity in human colonic samples by 16S rDNA sequence analysis. FEMS Microbiol. Ecol. 39 (1), 33–39. doi:10.1111/j.1574-6941.2002.tb00904.x
Holmes, E., Li, J. V., Athanasiou, T., Ashrafian, H., and Nicholson, J. K. (2011). Understanding the role of gut microbiome-host metabolic signal disruption in health and disease. Trends Microbiol. 19 (7), 349–359. doi:10.1016/j.tim.2011.05.006
Honda, K., and Littman, D. R. (2012). The microbiome in infectious disease and inflammation. Annu. Rev. Immunol. 30, 759–795. doi:10.1146/annurev-immunol-020711-074937
Hooper, L. V., Xu, J., Falk, P. G., Midtvedt, T., and Gordon, J. I. (1999). A molecular sensor that allows a gut commensal to control its nutrient foundation in a competitive ecosystem. Proc. Natl. Acad. Sci. U. S. A. 96 (17), 9833–9838. doi:10.1073/pnas.96.17.9833
Huycke, M. M., and Gaskins, H. R. (2004). Commensal bacteria, redox stress, and colorectal cancer: Mechanisms and models. Exp. Biol. Med. 229 (7), 586–597. doi:10.1177/153537020422900702
IARC (1994). Schistosomes, liver flukes and Helicobacter pylori. IARC Monogr. Eval. Carcinog. Risks Hum. 61, 1–241.
Iida, N., Dzutsev, A., Stewart, C. A., Smith, L., Bouladoux, N., Weingarten, R. A., et al. (2013). Commensal bacteria control cancer response to therapy by modulating the tumor microenvironment. Science 342 (6161), 967–970. doi:10.1126/science.1240527
Itzkowitz, S. H., and Harpaz, N. (2004). Diagnosis and management of dysplasia in patients with inflammatory bowel diseases. Gastroenterology 126 (6), 1634–1648. doi:10.1053/j.gastro.2004.03.025
Jakszyn, P., Bingham, S., Pera, G., Agudo, A., Luben, R., Welch, A., et al. (2006). Endogenous versus exogenous exposure to N-nitroso compounds and gastric cancer risk in the European Prospective Investigation into Cancer and Nutrition (EPIC-EURGAST) study. Carcinogenesis 27 (7), 1497–1501. doi:10.1093/carcin/bgl019
Jobin, C. (2018). Precision medicine using microbiota. Science 359 (6371), 32–34. doi:10.1126/science.aar2946
Jostins, L., Ripke, S., Weersma, R. K., Duerr, R. H., McGovern, D. P., Hui, K. Y., et al. (2012). Host-microbe interactions have shaped the genetic architecture of inflammatory bowel disease. Nature 491 (7422), 119–124. doi:10.1038/nature11582
Kau, A. L., Ahern, P. P., Griffin, N. W., Goodman, A. L., and Gordon, J. I. (2011). Human nutrition, the gut microbiome and the immune system. Nature 474 (7351), 327–336. doi:10.1038/nature10213
Khalili, H., Håkansson, N., Chan, S. S., Chen, Y., Lochhead, P., Ludvigsson, J. F., et al. (2020). Adherence to a mediterranean diet is associated with a lower risk of later-onset Crohn's disease: Results from two large prospective cohort studies. Gut 69 (9), 1637–1644. doi:10.1136/gutjnl-2019-319505
Kiselyov, A., Bunimovich-Mendrazitsky, S., and Startsev, V. (2015). Treatment of non-muscle invasive bladder cancer with Bacillus Calmette-Guerin (BCG): Biological markers and simulation studies. BBA Clin. 4, 27–34. doi:10.1016/j.bbacli.2015.06.002
Kleessen, B., Hartmann, L., and Blaut, M. (2003). Fructans in the diet cause alterations of intestinal mucosal architecture, released mucins and mucosa-associated bifidobacteria in gnotobiotic rats. Br. J. Nutr. 89 (5), 597–606. doi:10.1079/BJN2002827
Kostic, A. D., Chun, E., Robertson, L., Glickman, J. N., Gallini, C. A., Michaud, M., et al. (2013). Fusobacterium nucleatum potentiates intestinal tumorigenesis and modulates the tumor-immune microenvironment. Cell host microbe 14 (2), 207–215. doi:10.1016/j.chom.2013.07.007
Kroemer, G., Senovilla, L., Galluzzi, L., Andre, F., and Zitvogel, L. (2015). Natural and therapy-induced immunosurveillance in breast cancer. Nat. Med. 21 (10), 1128–1138. doi:10.1038/nm.3944
Lam, S. Y., Yu, J., Wong, S. H., Peppelenbosch, M. P., and Fuhler, G. M. (2017). The gastrointestinal microbiota and its role in oncogenesis. Best Pract. Res. Clin. gastroenterology 31 (6), 607–618. doi:10.1016/j.bpg.2017.09.010
Larrosa, M., Gonzalez-Sarrias, A., Garcia-Conesa, M. T., Tomas-Barberan, F. A., and Espin, J. C. (2006). Urolithins, ellagic acid-derived metabolites produced by human colonic microflora, exhibit estrogenic and antiestrogenic activities. J. Agric. food Chem. 54 (5), 1611–1620. doi:10.1021/jf0527403
Le, D. T., Wang-Gillam, A., Picozzi, V., Greten, T. F., Crocenzi, T., Springett, G., et al. (2015). Safety and survival with GVAX pancreas prime and Listeria Monocytogenes-expressing mesothelin (CRS-207) boost vaccines for metastatic pancreatic cancer. J. Clin. Oncol. official J. Am. Soc. Clin. Oncol. 33 (12), 1325–1333. doi:10.1200/JCO.2014.57.4244
Lertpiriyapong, K., Whary, M. T., Muthupalani, S., Lofgren, J. L., Gamazon, E. R., Feng, Y., et al. (2014). Gastric colonisation with a restricted commensal microbiota replicates the promotion of neoplastic lesions by diverse intestinal microbiota in the Helicobacter pylori INS-GAS mouse model of gastric carcinogenesis. Gut 63 (1), 54–63. doi:10.1136/gutjnl-2013-305178
Ley, R. E., Backhed, F., Turnbaugh, P., Lozupone, C. A., Knight, R. D., and Gordon, J. I. (2005). Obesity alters gut microbial ecology. Proc. Natl. Acad. Sci. U. S. A. 102 (31), 11070–11075. doi:10.1073/pnas.0504978102
Liang, Q., Chiu, J., Chen, Y., Huang, Y., Higashimori, A., Fang, J., et al. (2017). Fecal bacteria act as novel biomarkers for noninvasive diagnosis of colorectal cancer. Clin. cancer Res. official J. Am. Assoc. Cancer Res. 23 (8), 2061–2070. doi:10.1158/1078-0432.CCR-16-1599
Lievin, V., Peiffer, I., Hudault, S., Rochat, F., Brassart, D., Neeser, J. R., et al. (2000). Bifidobacterium strains from resident infant human gastrointestinal microflora exert antimicrobial activity. Gut 47 (5), 646–652. doi:10.1136/gut.47.5.646
Louis, P., Hold, G. L., and Flint, H. J. (2014). The gut microbiota, bacterial metabolites and colorectal cancer. Nat. Rev. Microbiol. 12 (10), 661–672. doi:10.1038/nrmicro3344
Lu, Y., Chen, J., Zheng, J., Hu, G., Wang, J., Huang, C., et al. (2016). Mucosal adherent bacterial dysbiosis in patients with colorectal adenomas. Sci. Rep. 6, 26337. doi:10.1038/srep26337
Lundberg, J. O., Weitzberg, E., Lundberg, J. M., and Alving, K. (1994). Intragastric nitric oxide production in humans: Measurements in expelled air. Gut 35 (11), 1543–1546. doi:10.1136/gut.35.11.1543
Lynch, S. V., and Pedersen, O. (2016). The human intestinal microbiome in health and disease. N. Engl. J. Med. 375 (24), 2369–2379. doi:10.1056/NEJMra1600266
Macfarlane, G. T., Cummings, J. H., and Allison, C. (1986). Protein degradation by human intestinal bacteria. J. general Microbiol. 132 (6), 1647–1656. doi:10.1099/00221287-132-6-1647
Marchesi, J. R., Dutilh, B. E., Hall, N., Peters, W. H., Roelofs, R., Boleij, A., et al. (2011). Towards the human colorectal cancer microbiome. PloS one 6 (5), e20447. doi:10.1371/journal.pone.0020447
Mayer Rj, P. C. E. (2003). Esophageal cancer. Esophageal cancer NEngl JMed 349, 2241–2252. doi:10.1056/NEJMra035010
Mendis, M., Leclerc, E., and Simsek, S. (2016). Arabinoxylans, gut microbiota and immunity. Carbohydr. Polym. 139, 159–166. doi:10.1016/j.carbpol.2015.11.068
Miękus, N., Marszałek, K., Podlacha, M., Iqbal, A., Puchalski, C., and Świergiel, A. H. (2020). Health benefits of plant-derived sulfur compounds, glucosinolates, and organosulfur compounds. Molecules 25 (17), 3804. doi:10.3390/molecules25173804
Mima, K., Nishihara, R., Qian, Z. R., Cao, Y., Sukawa, Y., Nowak, J. A., et al. (2016). Fusobacterium nucleatum in colorectal carcinoma tissue and patient prognosis. Gut 65 (12), 1973–1980. doi:10.1136/gutjnl-2015-310101
Mima, K., Sukawa, Y., Nishihara, R., Qian, Z. R., Yamauchi, M., Inamura, K., et al. (2015). Fusobacterium nucleatum and T cells in colorectal carcinoma. JAMA Oncol. 1 (5), 653–661. doi:10.1001/jamaoncol.2015.1377
Nakatsu, G., Li, X., Zhou, H., Sheng, J., Wong, S. H., Wu, W. K., et al. (2015). Gut mucosal microbiome across stages of colorectal carcinogenesis. Nat. Commun. 6, 8727. doi:10.1038/ncomms9727
Naylor, G., and Axon, A. (2003). Role of bacterial overgrowth in the stomach as an additional risk factor for gastritis. Can. J. gastroenterology = J. Can. de gastroenterologie 17, 13B–17B. doi:10.1155/2003/350347
O'sullivan, M., Thornton, G., O'sullivan, G., and Collins, J. (1992). Probiotic bacteria: Myth or reality? Trends food Sci. Technol. 3, 309–314. doi:10.1016/s0924-2244(10)80018-4
Oikonomopoulou, K., Brinc, D., Kyriacou, K., and Diamandis, E. P. (2013). Infection and cancer: Revaluation of the hygiene hypothesis. Clin. cancer Res. official J. Am. Assoc. Cancer Res. 19 (11), 2834–2841. doi:10.1158/1078-0432.CCR-12-3661
O’Keefe, S., Li, J., Lahti, L., Ou, J., Carbonero, F., Mohammed, K., et al. (2015). Fat, fibre and cancer risk in African Americans and rural Africans. Nat. Commun. 6, 6342–6355. doi:10.1038/ncomms7342
Ou, J., Carbonero, F., Zoetendal, E. G., DeLany, J. P., Wang, M., Newton, K., et al. (2013). Diet, microbiota, and microbial metabolites in colon cancer risk in rural Africans and African Americans. Am. J. Clin. Nutr. 98 (1), 111–120. doi:10.3945/ajcn.112.056689
Palmer, C., Bik, E. M., DiGiulio, D. B., Relman, D. A., and Brown, P. O. (2007). Development of the human infant intestinal microbiota. PLoS Biol. 5 (7), e177. doi:10.1371/journal.pbio.0050177
Parchem, K., Piekarska, A., and Bartoszek, A. (2020). “Chapter 3 - enzymatic activities behind degradation of glucosinolates,” in Glucosinolates: Properties, recovery, and applications. Editor C. M. Galanakis (Cambridge: Academic Press).
Peek, R. M., and Blaser, M. J. (2002). Helicobacter pylori and gastrointestinal tract adenocarcinomas. Nat. Rev. Cancer 2 (1), 28–37. doi:10.1038/nrc703
Peek, R. M., and Crabtree, J. E. (2006). Helicobacter infection and gastric neoplasia. J. pathology 208 (2), 233–248. doi:10.1002/path.1868
Peters, B. A., Wu, J., Pei, Z., Yang, L., Purdue, M. P., Freedman, N. D., et al. (2017). Oral microbiome composition reflects prospective risk for esophageal cancers. Cancer Res. 77 (23), 6777–6787. doi:10.1158/0008-5472.CAN-17-1296
Pflug, N., Kluth, S., Vehreschild, J. J., Bahlo, J., Tacke, D., Biehl, L., et al. (2016). Efficacy of antineoplastic treatment is associated with the use of antibiotics that modulate intestinal microbiota. Oncoimmunology 5 (6), e1150399. doi:10.1080/2162402X.2016.1150399
Pickard, J. M., Zeng, M. Y., Caruso, R., and Núñez, G. (2017). Gut microbiota: Role in pathogen colonization, immune responses, and inflammatory disease. Immunol. Rev. 279 (1), 70–89. doi:10.1111/imr.12567
Pierro, A., van Saene, H. K., Donnell, S. C., Hughes, J., Ewan, C., Nunn, A. J., et al. (1996). Microbial translocation in neonates and infants receiving long-term parenteral nutrition. Archives Surg. 131 (2), 176–179. doi:10.1001/archsurg.1996.01430140066018
Ploger, S., Stumpff, F., Penner, G. B., Schulzke, J. D., Gabel, G., Martens, H., et al. (2012). Microbial butyrate and its role for barrier function in the gastrointestinal tract. Ann. N. Y. Acad. Sci. 1258, 52–59. doi:10.1111/j.1749-6632.2012.06553.x
Plummer, M., de Martel, C., Vignat, J., Ferlay, J., Bray, F., and Franceschi, S. (2016). Global burden of cancers attributable to infections in 2012: A synthetic analysis. Lancet Glob. health 4 (9), e609–e616. doi:10.1016/S2214-109X(16)30143-7
Polk, D. B., and Peek, R. M. (2010). Helicobacter pylori: Gastric cancer and beyond. Nat. Rev. Cancer 10 (6), 403–414. doi:10.1038/nrc2857
Qin, J., Li, R., Raes, J., Arumugam, M., Burgdorf, K. S., Manichanh, C., et al. (2010). A human gut microbial gene catalogue established by metagenomic sequencing. Nature 464 (7285), 59–65. doi:10.1038/nature08821
Queiroz, K. C., Milani, R., Ruela-de-Sousa, R. R., Fuhler, G. M., Justo, G. Z., Zambuzzi, W. F., et al. (2012). Violacein induces death of resistant leukaemia cells via kinome reprogramming, endoplasmic reticulum stress and Golgi apparatus collapse. PloS one 7 (10), e45362. doi:10.1371/journal.pone.0045362
Rabah, H., Rosa do Carmo, F. L., and Jan, G. (2017). Dairy propionibacteria: Versatile probiotics. Microorganisms 5 (2), 24. doi:10.3390/microorganisms5020024
Rad, A. H., Aghebati-Maleki, L., Kafil, H. S., and Abbasi, A. (2021). Molecular mechanisms of postbiotics in colorectal cancer prevention and treatment. Crit. Rev. food Sci. Nutr. 61 (11), 1787–1803. doi:10.1080/10408398.2020.1765310
Raoult, D. (2008). Obesity pandemics and the modification of digestive bacterial flora. Eur. J. Clin. Microbiol. Infect. Dis. official Publ. Eur. Soc. Clin. Microbiol. 27 (8), 631–634. doi:10.1007/s10096-008-0490-x
Rastall, R. A., and Gibson, G. R. (2015). Recent developments in prebiotics to selectively impact beneficial microbes and promote intestinal health. Curr. Opin. Biotechnol. 32, 42–46. doi:10.1016/j.copbio.2014.11.002
Rooney, M. S., Shukla, S. A., Wu, C. J., Getz, G., and Hacohen, N. (2015). Molecular and genetic properties of tumors associated with local immune cytolytic activity. Cell 160 (1-2), 48–61. doi:10.1016/j.cell.2014.12.033
Routy, B., Le Chatelier, E., Derosa, L., Duong, C. P. M., Alou, M. T., Daillere, R., et al. (2018). Gut microbiome influences efficacy of PD-1-based immunotherapy against epithelial tumors. Science 359 (6371), 91–97. doi:10.1126/science.aan3706
Rubinstein, M. R., Wang, X., Liu, W., Hao, Y., Cai, G., and Han, Y. W. (2013). Fusobacterium nucleatum promotes colorectal carcinogenesis by modulating E-cadherin/β-catenin signaling via its FadA adhesin. Cell host microbe 14 (2), 195–206. doi:10.1016/j.chom.2013.07.012
Russell, W. R., Gratz, S. W., Duncan, S. H., Holtrop, G., Ince, J., Scobbie, L., et al. (2011). High-protein, reduced-carbohydrate weight-loss diets promote metabolite profiles likely to be detrimental to colonic health. Am. J. Clin. Nutr. 93 (5), 1062–1072. doi:10.3945/ajcn.110.002188
Salminen, S., Collado, M. C., Endo, A., Hill, C., Lebeer, S., Quigley, E. M. M., et al. (2021). The International Scientific Association of Probiotics and Prebiotics (ISAPP) consensus statement on the definition and scope of postbiotics. Nat. Rev. Gastroenterology hepatology 18 (9), 649–667. doi:10.1038/s41575-021-00440-6
Savage, D. C. (1977). Microbial ecology of the gastrointestinal tract. Annu. Rev. Microbiol. 31, 107–133. doi:10.1146/annurev.mi.31.100177.000543
Schulpen, M., and van den Brandt, P. A. (2020). Mediterranean diet adherence and risk of colorectal cancer: The prospective Netherlands cohort study. Eur. J. Epidemiol. 35 (1), 25–35. doi:10.1007/s10654-019-00549-8
Scott, K. P., Gratz, S. W., Sheridan, P. O., Flint, H. J., and Duncan, S. H. (2013). The influence of diet on the gut microbiota. Pharmacol. Res. 69 (1), 52–60. doi:10.1016/j.phrs.2012.10.020
Sears, C. L., and Pardoll, D. M. (2011). Perspective: Alpha-bugs, their microbial partners, and the link to colon cancer. J. Infect. Dis. 203 (3), 306–311. doi:10.1093/jinfdis/jiq061
Sekirov, I., Russell, S. L., Antunes, L. C., and Finlay, B. B. (2010). Gut microbiota in health and disease. Physiol. Rev. 90 (3), 859–904. doi:10.1152/physrev.00045.2009
Shaw, S. Y., Blanchard, J. F., and Bernstein, C. N. (2010). Association between the use of antibiotics in the first year of life and pediatric inflammatory bowel disease. Official J. Am. Coll. Gastroenterology| ACG 105 (12), 2687–2692. doi:10.1038/ajg.2010.398
Shinozaki, A., Sakatani, T., Ushiku, T., Hino, R., Isogai, M., Ishikawa, S., et al. (2010). Downregulation of microRNA-200 in EBV-associated gastric carcinoma. Cancer Res. 70 (11), 4719–4727. doi:10.1158/0008-5472.CAN-09-4620
Siavoshian, S., Segain, J. P., Kornprobst, M., Bonnet, C., Cherbut, C., Galmiche, J. P., et al. (2000). Butyrate and trichostatin A effects on the proliferation/differentiation of human intestinal epithelial cells: Induction of cyclin D3 and p21 expression. Gut 46 (4), 507–514. doi:10.1136/gut.46.4.507
Singh, N., Gurav, A., Sivaprakasam, S., Brady, E., Padia, R., Shi, H., et al. (2014). Activation of Gpr109a, receptor for niacin and the commensal metabolite butyrate, suppresses colonic inflammation and carcinogenesis. Immunity 40 (1), 128–139. doi:10.1016/j.immuni.2013.12.007
Sivan, A., Corrales, L., Hubert, N., Williams, J. B., Aquino-Michaels, K., Earley, Z. M., et al. (2015). Commensal Bifidobacterium promotes antitumor immunity and facilitates anti-PD-L1 efficacy. Science 350 (6264), 1084–1089. doi:10.1126/science.aac4255
Smith, P. M., Howitt, M. R., Panikov, N., Michaud, M., Gallini, C. A., Bohlooly-y, M., et al. (2013). The microbial metabolites, short-chain fatty acids, regulate colonic Treg cell homeostasis. Science 341 (6145), 569–573. doi:10.1126/science.1241165
Sobhani, I., Tap, J., Roudot-Thoraval, F., Roperch, J. P., Letulle, S., Langella, P., et al. (2011). Microbial dysbiosis in colorectal cancer (CRC) patients. PloS one 6 (1), e16393. doi:10.1371/journal.pone.0016393
Sommer, F., and Bäckhed, F. (2016). Know your neighbor: Microbiota and host epithelial cells interact locally to control intestinal function and physiology. BioEssays 38 (5), 455–464. doi:10.1002/bies.201500151
Sonnenburg, E. D., Smits, S. A., Tikhonov, M., Higginbottom, S. K., Wingreen, N. S., and Sonnenburg, J. L. (2016). Diet-induced extinctions in the gut microbiota compound over generations. Nature 529 (7585), 212–215. doi:10.1038/nature16504
Stoner, G. D., Wang, L-S., and Casto, B. C. (2008). Laboratory and clinical studies of cancer chemoprevention by antioxidants in berries. Carcinogenesis 29 (9), 1665–1674. doi:10.1093/carcin/bgn142
Sun, J., and Kato, I. (2016). Gut microbiota, inflammation and colorectal cancer. Genes and Dis. 3 (2), 130–143. doi:10.1016/j.gendis.2016.03.004
Sun, K., Jia, K., Lv, H., Wang, S. Q., Wu, Y., Lei, H., et al. (2020). EBV-Positive gastric cancer: Current knowledge and future perspectives. Front. Oncol. 10, 583463. doi:10.3389/fonc.2020.583463
Takiishi, T., Fenero, C. I. M., and Câmara, N. O. S. (2017). Intestinal barrier and gut microbiota: Shaping our immune responses throughout life. Tissue Barriers 5 (4), e1373208–e. doi:10.1080/21688370.2017.1373208
Tan, X., Mao, L., Huang, C., Yang, W., Guo, J., Chen, Z., et al. (2021). Comprehensive analysis of lncRNA-miRNA-mRNA regulatory networks for microbiota-mediated colorectal cancer associated with immune cell infiltration. Bioengineered 1, 3410–3425. doi:10.1080/21655979.2021.1940614
Taur, Y., and Pamer, E. G. (2016). Microbiome mediation of infections in the cancer setting. Genome Med. 8 (1), 40. doi:10.1186/s13073-016-0306-z
Tian, S., Liu, X., Lei, P., Zhang, X., and Shan, Y. (2018). Microbiota: A mediator to transform glucosinolate precursors in cruciferous vegetables to the active isothiocyanates. J. Sci. food Agric. 98 (4), 1255–1260. doi:10.1002/jsfa.8654
Tilg, H., Adolph, T. E., Gerner, R. R., and Moschen, A. R. (2018). The intestinal microbiota in colorectal cancer. Cancer Cell 33 (6), 954–964. doi:10.1016/j.ccell.2018.03.004
Tjalsma, H., Boleij, A., Marchesi, J. R., and Dutilh, B. E. (2012). A bacterial driver-passenger model for colorectal cancer: Beyond the usual suspects. Nat. Rev. Microbiol. 10 (8), 575–582. doi:10.1038/nrmicro2819
Tomasello, G., Mazzola, M., Leone, A., Sinagra, E., Zummo, G., Farina, F., et al. (2016). Nutrition, oxidative stress and intestinal dysbiosis: Influence of diet on gut microbiota in inflammatory bowel diseases. Biomed. Pap. Med. Fac. Univ. Palacky, Olomouc, Czechoslov. 160 (4), 461–466. doi:10.5507/bp.2016.052
Torres, P. J., Fletcher, E. M., Gibbons, S. M., Bouvet, M., Doran, K. S., and Kelley, S. T. (2015). Characterization of the salivary microbiome in patients with pancreatic cancer. PeerJ 3, e1373. doi:10.7717/peerj.1373
Tözün, A. (2019). Bağırsak mikrobiyatası gastrointestinal kanserler. İSTANBUL: Acibadem Mehmet Ali Aydinlar University Research Information System.
Tsai, C. C., Chen, T. Y., Tsai, K. J., Lin, M. W., Hsu, C. Y., Wu, D. C., et al. (2020). NF-κB/miR-18a-3p and miR-4286/BZRAP1 axis may mediate carcinogenesis in Helicobacter pylori-associated gastric cancer. Biomed. Pharmacother. 132, 110869. doi:10.1016/j.biopha.2020.110869
Tsigalou, C., Paraschaki, A., Karvelas, A., Kantartzi, K., Gagali, K., Tsairidis, D., et al. (2021). Gut microbiome and Mediterranean diet in the context of obesity. Current knowledge, perspectives and potential therapeutic targets. Metab. Open 9, 100081. doi:10.1016/j.metop.2021.100081
Tsoi, H., Chu, E. S. H., Zhang, X., Sheng, J., Nakatsu, G., Ng, S. C., et al. (2017). Peptostreptococcus anaerobius induces intracellular cholesterol biosynthesis in colon cells to induce proliferation and causes dysplasia in mice. Gastroenterology 152 (6), 1419–1433. doi:10.1053/j.gastro.2017.01.009
Turroni, F., Foroni, E., Pizzetti, P., Giubellini, V., Ribbera, A., Merusi, P., et al. (2009). Exploring the diversity of the bifidobacterial population in the human intestinal tract. Appl. Environ. Microbiol. 75 (6), 1534–1545. doi:10.1128/AEM.02216-08
Vesely, M. D., Kershaw, M. H., Schreiber, R. D., and Smyth, M. J. (2011). Natural innate and adaptive immunity to cancer. Annu. Rev. Immunol. 29, 235–271. doi:10.1146/annurev-immunol-031210-101324
Vetizou, M., Pitt, J. M., Daillere, R., Lepage, P., Waldschmitt, N., Flament, C., et al. (2015). Anticancer immunotherapy by CTLA-4 blockade relies on the gut microbiota. Science 350 (6264), 1079–1084. doi:10.1126/science.aad1329
Viaud, S., Saccheri, F., Mignot, G., Yamazaki, T., Daillere, R., Hannani, D., et al. (2013). The intestinal microbiota modulates the anticancer immune effects of cyclophosphamide. Science 342 (6161), 971–976. doi:10.1126/science.1240537
Vogtmann, E., Hua, X., Zeller, G., Sunagawa, S., Voigt, A. Y., Hercog, R., et al. (2016). Colorectal cancer and the human gut microbiome: Reproducibility with whole-genome shotgun sequencing. PloS one 11 (5), e0155362. doi:10.1371/journal.pone.0155362
von Martels, J. Z., Sadabad, M. S., Bourgonje, A. R., Blokzijl, T., Dijkstra, G., Faber, K. N., et al. (2017). The role of gut microbiota in health and disease: In vitro modeling of host-microbe interactions at the aerobe-anaerobe interphase of the human gut. Anaerobe 44, 3–12. doi:10.1016/j.anaerobe.2017.01.001
Wang, F., Yin, Q., Chen, L., and Davis, M. M. (2018). Bifidobacterium can mitigate intestinal immunopathology in the context of CTLA-4 blockade. Proc. Natl. Acad. Sci. U. S. A. 115 (1), 157–161. doi:10.1073/pnas.1712901115
Wang, H. B., Wang, P. Y., Wang, X., Wan, Y. L., and Liu, Y. C. (2012). Butyrate enhances intestinal epithelial barrier function via up-regulation of tight junction protein Claudin-1 transcription. Dig. Dis. Sci. 57 (12), 3126–3135. doi:10.1007/s10620-012-2259-4
Wang, M., Ahrne, S., Jeppsson, B., and Molin, G. (2005). Comparison of bacterial diversity along the human intestinal tract by direct cloning and sequencing of 16S rRNA genes. FEMS Microbiol. Ecol. 54 (2), 219–231. doi:10.1016/j.femsec.2005.03.012
Wang, Q., Ding, H., Dong, G., Xu, L., Jiang, F., and Mao, Q. (2021). Bi-direction effects between microbiome and MiRNAs in carcinogenesis. J. Cancer Res. Clin. Oncol. 147 (5), 1299–1305. doi:10.1007/s00432-021-03567-w
Wang, T., Cai, G., Qiu, Y., Fei, N., Zhang, M., Pang, X., et al. (2012). Structural segregation of gut microbiota between colorectal cancer patients and healthy volunteers. ISME J. 6 (2), 320–329. doi:10.1038/ismej.2011.109
Wang, X., Heazlewood, S. P., Krause, D. O., and Florin, T. H. (2003). Molecular characterization of the microbial species that colonize human ileal and colonic mucosa by using 16S rDNA sequence analysis. J. Appl. Microbiol. 95 (3), 508–520. doi:10.1046/j.1365-2672.2003.02005.x
Wang, Z. K., and Yang, Y. S. (2013). Upper gastrointestinal microbiota and digestive diseases. World J. gastroenterology 19 (10), 1541–1550. doi:10.3748/wjg.v19.i10.1541
Warnakulasuriya, S. (2009). Global epidemiology of oral and oropharyngeal cancer. Oral Oncol. 45 (4-5), 309–316. doi:10.1016/j.oraloncology.2008.06.002
Wollowski, I., Rechkemmer, G., and Pool-Zobel, B. L. (2001). Protective role of probiotics and prebiotics in colon cancer. Am. J. Clin. Nutr. 73 (2), 451S–5s. doi:10.1093/ajcn/73.2.451s
Wong, J. M., de Souza, R., Kendall, C. W., Emam, A., and Jenkins, D. J. (2006). Colonic health: Fermentation and short chain fatty acids. J. Clin. gastroenterology 40 (3), 235–243. doi:10.1097/00004836-200603000-00015
Woodmansey, E. J. (2007). Intestinal bacteria and ageing. J. Appl. Microbiol. 102 (5), 1178–1186. doi:10.1111/j.1365-2672.2007.03400.x
Wu, G. D., Chen, J., Hoffmann, C., Bittinger, K., Chen, Y-Y., Keilbaugh, S. A., et al. (2011). Linking long-term dietary patterns with gut microbial enterotypes. Science 334 (6052), 105–108. doi:10.1126/science.1208344
Wu, G. D., Compher, C., Chen, E. Z., Smith, S. A., Shah, R. D., Bittinger, K., et al. (2016). Comparative metabolomics in vegans and omnivores reveal constraints on diet-dependent gut microbiota metabolite production. Gut 65 (1), 63–72. doi:10.1136/gutjnl-2014-308209
Wu, S., Rhee, K. J., Albesiano, E., Rabizadeh, S., Wu, X., Yen, H. R., et al. (2009). A human colonic commensal promotes colon tumorigenesis via activation of T helper type 17 T cell responses. Nat. Med. 15 (9), 1016–1022. doi:10.1038/nm.2015
Xu, Z., and Knight, R. (2015). Dietary effects on human gut microbiome diversity. Br. J. Nutr. 113, S1–S5. doi:10.1017/S0007114514004127
Yamaoka, Y., Suehiro, Y., Hashimoto, S., Hoshida, T., Fujimoto, M., Watanabe, M., et al. (2018). Fusobacterium nucleatum as a prognostic marker of colorectal cancer in a Japanese population. J. gastroenterology 53 (4), 517–524. doi:10.1007/s00535-017-1382-6
Yan, H., and Bu, P. (2021). Non-coding RNA in cancer. Essays Biochem. 65 (4), 625–639. doi:10.1042/EBC20200032
Yang, F., Xu, Y., Liu, C., Ma, C., Zou, S., Xu, X., et al. (2018). NF-κB/miR-223-3p/ARID1A axis is involved in Helicobacter pylori CagA-induced gastric carcinogenesis and progression. Cell Death Dis. 9 (1), 12. doi:10.1038/s41419-017-0020-9
Yang, L., Lu, X., Nossa, C. W., Francois, F., Peek, R. M., and Pei, Z. (2009). Inflammation and intestinal metaplasia of the distal esophagus are associated with alterations in the microbiome. Gastroenterology 137 (2), 588–597. doi:10.1053/j.gastro.2009.04.046
Yang, Y., Weng, W., Peng, J., Hong, L., Yang, L., Toiyama, Y., et al. (2017). Fusobacterium nucleatum increases proliferation of colorectal cancer cells and tumor development in mice by activating toll-like receptor 4 signaling to nuclear factor-κb, and up-regulating expression of MicroRNA-21. Gastroenterology 152 (4), 851–866. doi:10.1053/j.gastro.2016.11.018
Yatsunenko, T., Rey, F. E., Manary, M. J., Trehan, I., Dominguez-Bello, M. G., Contreras, M., et al. (2012). Human gut microbiome viewed across age and geography. Nature 486 (7402), 222–227. doi:10.1038/nature11053
Yu, J., Feng, Q., Wong, S. H., Zhang, D., Liang, Q. Y., Qin, Y., et al. (2017). Metagenomic analysis of faecal microbiome as a tool towards targeted non-invasive biomarkers for colorectal cancer. Gut 66 (1), 70–78. doi:10.1136/gutjnl-2015-309800
Yu, T., Guo, F., Yu, Y., Sun, T., Ma, D., Han, J., et al. (2017). Fusobacterium nucleatum promotes chemoresistance to colorectal cancer by modulating autophagy. Cell 170 (3), 548–563. e16. doi:10.1016/j.cell.2017.07.008
Yuan, C., Burns, M. B., Subramanian, S., and Blekhman, R. (2018). Interaction between host MicroRNAs and the gut microbiota in colorectal cancer. mSystems 3 (3), e00205–17. doi:10.1128/mSystems.00205-17
Yuan, C., Steer, C. J., and Subramanian, S. (2019). Host–MicroRNA–microbiota interactions in colorectal cancer. Genes 10, 270. doi:10.3390/genes10040270
Zackular, J. P., Rogers, M. A., Mtt, R., and Schloss, P. D. (2014). The human gut microbiome as a screening tool for colorectal cancer. Cancer Prev. Res. 7 (11), 1112–1121. doi:10.1158/1940-6207.CAPR-14-0129
Zaidi, A. H., Kelly, L. A., Kreft, R. E., Barlek, M., Omstead, A. N., Matsui, D., et al. (2016). Associations of microbiota and toll-like receptor signaling pathway in esophageal adenocarcinoma. BMC cancer 16 (1), 52. doi:10.1186/s12885-016-2093-8
Zeller, G., Tap, J., Voigt, A. Y., Sunagawa, S., Kultima, J. R., Costea, P. I., et al. (2014). Potential of fecal microbiota for early-stage detection of colorectal cancer. Mol. Syst. Biol. 10, 766. doi:10.15252/msb.20145645
Zitvogel, L., Ayyoub, M., Routy, B., and Kroemer, G. (2016). Microbiome and anticancer immunosurveillance. Cell 165 (2), 276–287. doi:10.1016/j.cell.2016.03.001
Zitvogel, L., Daillere, R., Roberti, M. P., Routy, B., and Kroemer, G. (2017). Anticancer effects of the microbiome and its products. Nat. Rev. Microbiol. 15 (8), 465–478. doi:10.1038/nrmicro.2017.44
Żółkiewicz, J., Marzec, A., Ruszczyński, M., and Feleszko, W. (2020). Postbiotics-A step beyond pre- and probiotics. Nutrients 12 (8), 2189. doi:10.3390/nu12082189
Glossary
NCDs Non-communicable diseases
GI Gastrointestinal
DNA Deoxyribonucleic acid
nc-RNAs Non-coding RNAs
16S rRNA 16S ribosomal RNA
SCFAs Short-chain fatty acids
IBD Inflammatory bowel disease
Tregs Regulatory T cells
NK Natural killer
HIF Hypoxia-induced factor
LPS Lipopolysaccharide metabolism
TAAs Tumor-associated antigens
TNF Tumor Necrosis Factor
IFN-γ Interferon-gamma
MedDiet Mediterranean diet
MUFAs Monounsaturated fatty acids
PUFAs Polyunsaturated fatty acids
EPIC European Prospective Investigation into Cancer and Nutrition
DCA Deoxycholic acid
ITCs Isothiocyanates
COX-2 Cyclooxygenase-2
AP-1 Activator protein-1
iNOS Inducible nitric oxide synthase
ISAPP International Scientific Association of Probiotics and Prebiotics
PD-1 Anti-programmed death 1
CTLA4 Cytotoxic T-lymphocyte–associated antigen 4
TLRs Toll-Like Receptors
PAMPs Pathogen-associated molecular patterns
HDAC Histone deacetylases
ROS Reactive oxygen specie
SFN Sulforaphane
GIT Gastrointestinal tract
ncRNA non-coding RNA
NT Nucleotides
Keywords: microbiome, gastrointestinal cancer, non-coding RNAs, therapeutics, diagnosis
Citation: Ağagündüz D, Cocozza E, Cemali Ö, Bayazıt AD, Nanì MF, Cerqua I, Morgillo F, Saygılı SK, Berni Canani R, Amero P and Capasso R (2023) Understanding the role of the gut microbiome in gastrointestinal cancer: A review. Front. Pharmacol. 14:1130562. doi: 10.3389/fphar.2023.1130562
Received: 23 December 2022; Accepted: 10 January 2023;
Published: 24 January 2023.
Edited by:
Ludovico Abenavoli, Magna Græcia University, ItalyReviewed by:
Natasa Milic, University of Novi Sad, SerbiaRaffaele Addeo, Aslnapoli2nord Oncologia, Italy
Copyright © 2023 Ağagündüz, Cocozza, Cemali, Bayazıt, Nanì, Cerqua, Morgillo, Saygılı, Berni Canani, Amero and Capasso. This is an open-access article distributed under the terms of the Creative Commons Attribution License (CC BY). The use, distribution or reproduction in other forums is permitted, provided the original author(s) and the copyright owner(s) are credited and that the original publication in this journal is cited, in accordance with accepted academic practice. No use, distribution or reproduction is permitted which does not comply with these terms.
*Correspondence: Raffaele Capasso, cmFmY2FwYXNAdW5pbmEuaXQ=; Paola Amero, UEFtZXJvQG1kYW5kZXJzb24ub3Jn