- 1Department of Neuro-Oncology, Chongqing University Cancer Hospital, Chongqing, China
- 2Department of Neurosurgery, The Affiliated Hospital of Kunming University of Science and Technology, Kunming, China
Acquired brain injury (ABI) is the most common disease of the nervous system, involving complex pathological processes, which often leads to a series of nervous system disorders. The structural destruction and dysfunction of the Neurovascular Unit (NVU) are prominent features of ABI. Therefore, understanding the molecular mechanism underlying NVU destruction and its reconstruction is the key to the treatment of ABI. SUMOylation is a protein post-translational modification (PTM), which can degrade and stabilize the substrate dynamically, thus playing an important role in regulating protein expression and biological signal transduction. Understanding the regulatory mechanism of SUMOylation can clarify the molecular mechanism of the occurrence and development of neurovascular dysfunction after ABI and is expected to provide a theoretical basis for the development of potential treatment strategies. This article reviews the role of SUMOylation in vascular events related to ABI, including NVU dysfunction and vascular remodeling, and puts forward therapeutic prospects.
1 Introduction
1.1 Neurovascular dysfunction after acquired brain injury
1.1.1 Pathology of ABI
Acquired Brain Injury (ABI) typically is classified into the two following types: traumatic brain injury (TBI) and non-TBI (non-TBI) (Bruns and Hauser, 2003), with the former having the highest incidence among neurological diseases (Maas et al., 2022). The causes of TBI include traffic accidents, violence, and other accidents1. The occurrence of non-TBI is related to stroke, neoplasm, infection, inflammation, anoxia, alcohol consumption, and drug use (Goldman et al., 2022). ABI leaves patients with physical and psychological sequelae, making it difficult for them to reintegrate into society, resulting in a great social burden (Menon and Bryant, 2019). ABI can cause vascular structure damage, dysfunction of the neurovascular unit (NVU), destruction of the blood-brain barrier (BBB), brain edema, activation of immunoreactive cells, the release of immune mediators, oxidative stress, mitochondrial and metabolic dysfunctions, and changes in cerebral blood flow (CBF) (Greve and Zink, 2009; Zibara et al., 2019). These eventually lead to headaches, cognitive changes, emotional anxiety, and a series of neurological disorders (Bedaso et al., 2018; Larsen et al., 2019; Chan and Woldeamanuel, 2020).
The primary injury of ABI is of the mechanical kind and includes the destruction of neurons, glia, and vascular structures. It is irreversible. However, secondary injury occurs over time and initiates multiple signaling cascades, which can change cell function and cause cell death, including oxidative stress, excitotoxicity, mitochondrial dysfunction, and inflammation (Lozano et al., 2015). Within minutes of ABI, astrocytes, and microglia begin to secrete pro-inflammatory factors (including interleukin-6, interleukin-1, tumor necrosis factor, etc.), causing local inflammation (Ghirnikar et al., 1998; Ekmark-Lewen et al., 2010; Homsi et al., 2010). These inflammatory factors actively recruit immune cells and glial cells, thus aggravating the occurrence of inflammation (Lossinsky and Shivers, 2004). Some studies have shown that microglia can maintain a state of enhanced inflammation for several months after ABI (Witcher et al., 2015). The inflammatory responses after ABI include the activation of immune cells in the central nervous system (CNS) and infiltration of peripheral immune cells through the BBB, which is mediated by a variety of inflammatory cytokines. The dysfunction of NVU and BBB caused by ABI allows activated white blood cells to migrate to the damaged brain parenchyma, which is also promoted by the upregulation of cell adhesion molecules. Activated white blood cells, microglia, and astrocytes produce ROS and inflammatory molecules, like cytokines and chemokines, leading to demyelination and destruction of the axonal cytoskeleton (Goldman et al., 2022). Over time, inflammatory events lead to brain edema and increased intracranial pressure (McGinn and Povlishock, 2016). ABI is characterized by the crossover and fusion of highly related neuropathological reactions, wherein severe and persistent inflammatory reactions may cause further deterioration. However, although neuroinflammation aggravates brain damage in the early stages of ABI, inflammation may also promote angiogenesis and neurogenesis at a later stage (Candelario-Jalil et al., 2022).
The vascular network of the brain originates from the internal carotid artery and goes deep into the brain. The maintenance of normal brain function requires the integrity of brain structure, normal synaptic activity, and smooth transmission of information. However, these require the coordination of different cells in the NVU and the structural integrity of BBB (Zhao et al., 2015) (Figure 1). In NVU, endothelial cells (ECs) are part of BBB. These ECs are related to several substrate-specific transport systems and can control the transport of nutrients, metabolites, and some essential molecules (Zhao et al., 2015). The concept of NVU regards the interaction among neurons, glial cells, and cerebral vessels as a whole, and promotes the previous treatment singly, such as neuron protection and vascular remodeling to the level of protection and repair of NVU, bringing new opportunities for research on the treatment of ABI.
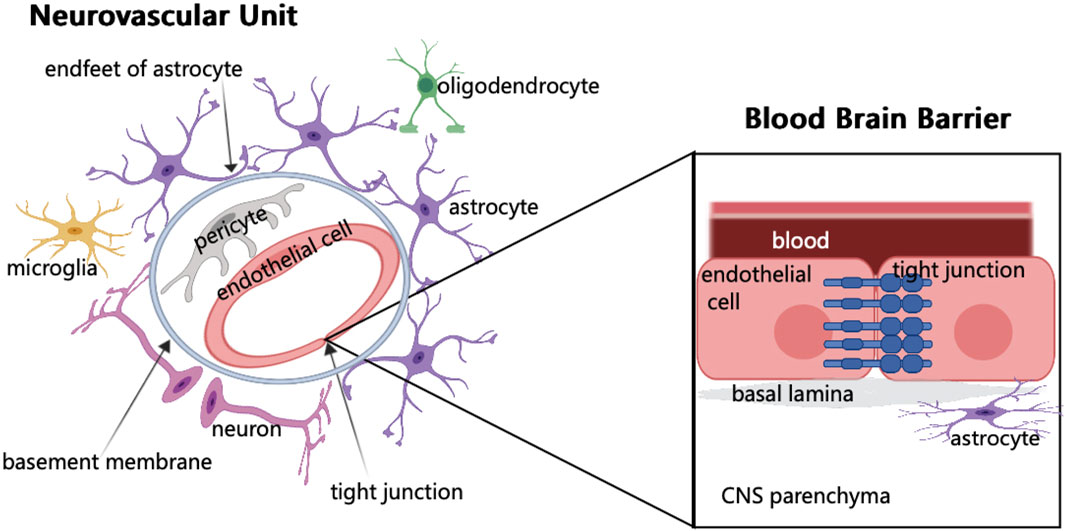
FIGURE 1. Schematic of neurovascular unit and blood brain barrier components. In NVU, endothelial cells are surrounded by pericytes, which are embedded in the basement membrane and surrounded by the endfeet of astrocytes. The synaptic connection between astrocytes and neurons regulates neurons. When brain tissue is injured, microglia respond first. In addition, NVU consists of few oligodendrocytes. The main component of BBB is endothelial cells. The junctions between endothelial cells are involved in regulating cell bypass permeability.
1.1.2 BBB damage and NVU dysfunction
BBB is formed by ECs of the capillary wall, astrocyte end-feet ensheathing the capillary, and pericytes embedded in the capillary basement membrane (Ballabh et al., 2004). The characteristics of BBB are mainly determined by the connection complex between brain ECs, including tight, adherens, and gap junctions. Although it has a strong similarity with the epithelium, the brain endothelial junction complex has a special structure and unique pattern of protein expression (Stamatovic et al., 2016). BBB is a highly selective physical barrier that strictly controls the microenvironment of brain cells. As the connection between ECs effectively limits the permeability of the adjacent cells, and these cells lack phagocytic vesicles, thus limiting the transport across the fine cytoplasm, the molecules are transported to CNS by active transport or passive diffusion (Daneman and Prat, 2015). Vascular ECs not only regulate the selectivity and permeability of BBB but also CBF, angiogenesis, and neuronal development (Iadecola, 2017). Although the barrier of the cerebrovascular system mainly comprises ECs, these are not functioning alone but as a part of the NVU, resulting in a complex molecular crosstalk network with different cellular elements in the NVU (Neuwelt et al., 2011). The formation and stability of cerebral vessels and BBB depend on the expression of growth factors, guiding molecules, and signal pathways (Andreone et al., 2015; Walchli et al., 2015). The function of BBB is to limit the circulation of harmful substances and blood cells in the blood in cerebral vessels which cannot enter the brain parenchyma (Winkler et al., 2011). Another important role of BBB is to reduce cross-cell permeability (Zhao et al., 2015). ABI can cause BBB damage, and various components in the blood will invade the brain tissue, resulting in cell infiltration and damage (Zhao et al., 2015; Montagne et al., 2017). BBB can cause an imbalance in CBF (Iadecola, 2017; Kisler et al., 2017), initially resulting in the disorder of the normal physiological function of the nervous system. Therefore, as a structure connecting the central nervous system and systemic circulation, BBB is the key to maintaining the homeostasis of the nervous system.
The concept of NVU emerged in 2001 to emphasize the relationship between the microvascular system and structural integrity and functional maintenance of brain cells, along with the coordinated response between the two in the event of a brain injury (Iadecola, 2017). NVU includes ECs, basement membranes, pericytes, astrocytes, microglia, and neurons, as well as oligodendrocytes (Sweeney et al., 2019). Among the many components, microglia can promote endothelial repair, thus maintaining endothelial integrity (Thurgur and Pinteaux, 2019). The function of neurons is to regulate the activity of enzymes to meet the metabolic needs of the brain (McConnell et al., 2017). In the vascular system of the brain, there is a three-dimensional vascular network comprising pial arteries, capillaries, ascending venules, and leptomeningeal veins. NVU exists in this vascular network (Blinder et al., 2013). This three-dimensional vascular network can enter the deep part of the brain, nourish the neurons, and transport the metabolites away (Kisler et al., 2017). The maintenance of normal brain function lies not only in the connection between neurons but also in the coordination between different components of the NVU (Lo and Rosenberg, 2009). NVU is indispensable for the normal maintenance of the function of the CNS. If NVU is destroyed, various cerebrovascular diseases can occur, eventually leading to nervous system dysfunction. However, NVU disorders are common in ABI, and the causal relationship between NVU dysfunction and diseases is not completely clear (McConnell et al., 2019).
1.1.3 New vessel formation after ABI
The main damages of ABI to cerebral vessels include hemorrhage, edema, abnormal CBF and destruction of BBB (Salehi et al., 2017). Several studies have shown that after cerebral vascular injury, it will try to repair it (Morgan et al., 2007; Park et al., 2009). Related studies have shown that the cerebrovascular system begins to repair within two to 3 weeks of damage, including not only larger blood vessels, but also smaller blood vessels, such as capillaries (Park et al., 2009; Siddiq et al., 2012). After ABI, the abnormal structure of vascular wall, swelling and apoptosis of endothelial cells and degradation of extracellular matrix could be seen under electron microscope (Danaila et al., 2013; Jullienne et al., 2014). The formation of new blood vessels after ABI is crucial to the recovery of nerve function. Existing literature shows that angiogenesis and vasculogenesis are two main mechanisms occurring after brain injury (Salehi et al., 2017). These complex but different processes play an important role in repairing the damaged vascular system after brain injury (Salehi et al., 2017). Angiogenesis refers to the formation of new blood vessels from the existing vascular system, while vasculogenesis is the occurrence of neovascularization.
Hypoxia caused by interruption of blood flow after ABI is the main cause of triggering angiogenesis (Gajavelli et al., 2015). This may be related to the expression regulation of hypoxia-related molecules, such as HIF-1 α (Anderson et al., 2009). Vascular endothelial cells are activated after ABI (Balabanov et al., 2001). Endothelial cells form vascular sprouts and migrate to hypoxia (Van Hove and Benoit, 2015). With the accumulation of pericytes, the damaged blood vessels were repaired (Hirota and Semenza, 2006). The occurrence of vasculogenesis mainly comes from endothelial progenitor cells, so this process is actually initiated in the bone marrow (Asahara et al., 1999). After ABI, endothelial progenitor cells peak within a week after a brief decrease, and play a role after maturation in the blood (Gong et al., 2011; Liu et al., 2011). In addition, endothelial progenitor cells also release some factors, which can promote the proliferation and migration of ECs and promote vasculogenesis (Ziegelhoeffer et al., 2004; Urbich et al., 2005).
After the occurrence of ABI, timely and effective intervention can restore the function of NVU to varying degrees. Angiogenesis and vascular remodeling can rebuild the BBB, protect brain tissue from further damage, provide necessary nutrition, and play a key role in nerve repair (Esquiva et al., 2018).
1.2 Protein post-translational modifications
Proteins play an important role in most biological processes. Their functions are regulated by several protein PTMs. PTMs refer to amino acid chain or terminal covalent enzyme modification, a reversible process that can affect the activity, localization, interaction, and function of target proteins (Wang et al., 2014). PTMs are the core of many cellular signaling events. Usually, PTMs can work alone or cooperatively. The crosstalk between PTMs often affects the physiological and pathological processes of cells through fine-tuning (Vu et al., 2018). PTMs usually occur in the C- or N-terminal of amino acid side chains of proteins, including the addition of chemical or functional groups, polypeptide chains, complex molecules, and modification of amino acids, depending on the type of PTMs (Xu et al., 2018). More than 450 kinds of PTMs have been found (Venne et al., 2014), including acetylation, ubiquitination, methylation, glycosylation, phosphorylation, and SUMOylation (Zheng and Shabek, 2017). Ubiquitin has lysine residues that act on the target protein and complete mono-ubiquitination or poly-ubiquitination under the action of ligase, binding enzyme, and activating enzyme. This process can be reversed by deubiquitinase (Deng et al., 2020). Phosphorylation mainly acts on serine, threonine, and tyrosine residues of target proteins (Olsen et al., 2006). Methylation mainly occurs on lysine and arginine residues. Lysine can be monomethylated, demethylated, or trimethylated by lysine methyltransferases; arginine can be monomethylated or dimethylated by arginine methyltransferases (Zhang et al., 2012; Yang and Bedford, 2013). Glycosylation can be linked to the side chain by oligosaccharide transferase by an amide bond (Moremen et al., 2012). As a PTM, SUMOylation has received more attention in recent years, because it is necessary to maintain genome integrity, molecular signal transduction, transcriptional regulation, and gene expression (Han et al., 2018). When PTMs in the cellular environment are dysfunctional, conformational changes of related proteins, imbalance of enzyme activity, abnormal protein folding, and production of toxic metabolites occur, all eventually leading to the disease state (Stram and Payne, 2016).
1.3 SUMOylation
SUMOylation is a common PTM of proteins. Small ubiquitin-like modifier (SUMO), similar to ubiquitin in structure, is an important protein discovered after ubiquitin (Yeh et al., 2000; Garcia-Rodriguez et al., 2016). Although the structure of SUMO is similar to that of ubiquitin, its cellular functions differ. The main function of ubiquitination is to degrade substrate proteins, while SUMOylation chiefly regulates cell localization, protein transcription, protein interaction, and DNA repair processes (Garcia-Rodriguez et al., 2016; Han et al., 2018). When SUMO binds to a protein, it can change the location, conformation, activity, and gene expression of the target protein, thus affecting many physiological and pathological processes (Chymkowitch et al., 2015).
Five SUMO homologous genes, named SUMO 1–5 are expressed in humans (Huang et al., 2004; Liang et al., 2016). SUMO 1–3 is widely expressed and participates in the modifications of thousands of proteins (Hendriks et al., 2017; Hendriks et al., 2018). The homology between SUMO2 and SUMO3 is very high, up to 97%. Therefore, the two cannot be completely distinguished and are usually termed SUMO2/3. They exist in various locations in the cell in a non-conjugated form and can be attached to the target protein after stimulating correspondingly (Enserink, 2015; Niskanen and Palvimo, 2017; Bernstock et al., 2018a). The expression of SUMO4–5 is limited, and it is not clear whether these can bind to the target protein (Celen and Sahin, 2020). In the SUMO family, SUMO2 has the highest abundance compared to the other members, so the lack of SUMO2 cannot be compensated by other analogs. In contrast, the functions of SUMO1 and SUMO3 can be compensated by the other analogs (Wang et al., 2014).
SUMOylation is a dynamic and reversible process (Figure 2), similar to the cascade reaction of ubiquitin enzymes (Gill, 2004). The SUMO subtype binds to the target protein through the three following steps: activation, heterodimer E1 enzyme (SAE1, SAE2), binding, E2 enzyme (UBE2I/UBC9), substrate modification, and E2 and E3 protein ligase interaction (Lv et al., 2018). The SUMOylation pathway is regulated by several enzymes, among which proteases and ligases are the most important regulatory modes. The most studied proteases and ligases are of the SENP and PIAS families, respectively (Lara-Urena et al., 2022). The proteases involved in SUMOylation modification are specific, and the most studied proteases are SENP1-3 and SENP5-7 of the SENP family (Kunz et al., 2018). SUMO-specific peptidases (SENP1, 2, 3, 5, 6, and 7) catalyze the deSUMOylation reaction, thus dissociating lysine residues of target proteins from SUMO (Rawlings et al., 2019). PIAS family is mainly involved in DNA binding and transcriptional regulation (Rabellino et al., 2017).
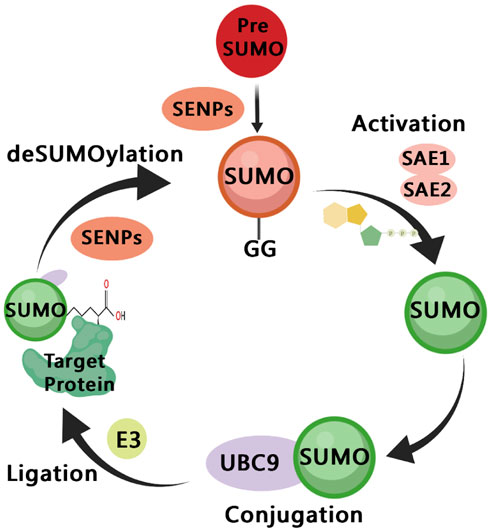
FIGURE 2. A diagram of the SUMOylation pathway. The precursor of small ubiquitin-like modifier (SUMO) are cleaved by SENPs, reveal the C-terminal diglycine motif (Maturation); It is then activated by the E1 enzyme SAE1/SAE2, which depends on ATP (Activation); The activated SUMO is transferred to UBC9 (Conjugation); SUMO is connected to the side chain of the specific lysine residue of the target protein, and this process involves E3 ligase (Ligation); SUMO is dissociated from the target protein, and the free SUMO can participate in the next catalytic cycle, which is regulated by SENPs (deSUMOylation).
Many studies have shown that abnormal regulation of SUMOylation is associated with a variety of diseases, including heart and neurodegenerative disorders (Da Silva-Ferrada et al., 2016; Mun et al., 2016). The balance of SUMOylation plays an important role in cardiac development, metabolism, and stress (Gupta et al., 2016; Gupta and Robbins, 2016; Liu et al., 2017). Ubc9-mediated SUMOylation can effectively reduce the incidence of proteotoxic heart diseases (Gupta et al., 2016). Moreover, therapeutic targets for cardiac fibrosis and heart failure are also closely related to Ubc9 (Liu et al., 2017). In neurodegenerative diseases, SUMOylation causes abnormal accumulation of HTT protein, leading to Huntington’s disease, which is related to abnormal degradation of the ubiquitin-proteasome pathway (Steffan et al., 2004). SUMOylation is also closely related to Alzheimer’s disease, which is related to the abnormal expression of amyloid- β and tau protein (Yang et al., 2017). Dysfunctional SUMOylation also plays an important role in cancer. The upregulation of SUMO binding enzyme increases SUMOylated proteins, leading to poor prognosis (Moschos et al., 2007; Chen et al., 2011; Zhang et al., 2013; Seeler and Dejean, 2017). In several cancers, the abnormal expression of SENPs may promote their progression (Ding et al., 2008; Bawa-Khalfe and Yeh, 2010; Sun et al., 2013). SUMOylation plays an important role in the study of drug resistance in hepatocellular carcinoma (Qin et al., 2014). Several therapeutic targets against cancer-related to SUMOylation are being studied. In short, the imbalance between SUMOylation and deSUMOylation is related to the occurrence and development of many diseases. Therefore, it is meaningful to study the relationship between SUMOylation and various diseases.
This review focuses on neurovascular dysfunction after ABI, with particular emphasis on the correlation between SUMOylation and neurovascular dysfunction. Actively examining potential molecular targets is needed and this review discusses the possibilities for the development of a new treatment for ABI.
2 Regulatory effects of SUMOylation on the components of NVU
2.1 The regulatory effects of SUMOylation on ECs
ECs in cerebral vessels are the core components of BBB, and their morphology and function are different from those of peripheral ECs (Daneman and Prat, 2015). The ECs in the cerebral vessels have more mitochondria than the peripheral ECs, consuming more energy to drive the ion gradient, which is important for enzyme activity (Daneman and Prat, 2015). ECs do not have small transcellular pores that allow free diffusion, so these can prevent rapid molecular exchange between blood and brain tissues (Pandit et al., 2020). The tight connection between ECs can completely close the gap between them to form continuous blood vessels (Tietz and Engelhardt, 2015). It also promotes the transmission of information between neurons and glial cells (Wang et al., 2015). Normal ECs also play an important role in regulating thrombosis and vasodilation (Findlay et al., 1989). ECs can secrete vasoactive factors to control vasodilation and contraction and vasoconstrictors, thus maintaining the balance in CBF (Sandoo et al., 2010). Endothelial cell dysfunction (ECD) destroys the integrity of BBB, leading to changes in CBF, eventually causing functional damage and degradation of the NVU (Iadecola, 2017), and mediating the occurrence and progression of ABI. The essence of ECD is a change in their functional phenotype, such as a deficiency in nitric oxide (NO), senescence of ECs, and increased expression of endothelial-leukocyte adhesion molecules, which are the basis for thrombosis and inflammation (Gimbrone and Garcia-Cardena, 2016; Anand et al., 2018; Harding et al., 2019). Given the importance of ECD in ABI, it remains the focus of future research.
2.1.1 EC senescence and apoptosis
Vascular aging can lead to many cardiovascular and cerebrovascular diseases, including stroke, hypertension, and coronary heart disease, and EC aging is an important characteristic of vascular aging (Ungvari et al., 2018). Reactive oxygen species (ROS) produced by mitochondrial dysfunction can activate microglia continuously (Faas and de Vos, 2020; Riley and Tait, 2020). The increase in ROS in microglia leads to cell death and neuroinflammation (Chiurazzi et al., 2020). Mature mitochondrial thioredoxin 2 (Trx2) is an important redox protein, which can eliminate ROS in time and slow down the senescence of VECs (Papaconstantinou, 2019). However, the precursor of Trx2 (PreTrx2) cannot perform the function of scavenging ROS. SUMOylation of Trx2 can reduce the accumulation of PreTrx2, promote the formation of Trx2, and effectively inhibit the senescence of ECs induced by ROS (Chen et al., 2019). Trx family of proteins reportedly helps reduce ischemic ABI (Otero-Losada et al., 2019). This may be related to the fact that cytoplasmic Trx1 and mitochondrial Trx2 can inhibit the production of free radicals, reduce oxidative stress, and mitochondria-dependent apoptosis (Chiueh et al., 2005). A previous study suggests that increased expression of Trx2 exerts a neuroprotective effect (Lee et al., 2017). A series of studies have obtained useful results, and the effect of Trx2’s SUMOylation on ABI is worthy of further study.
An animal study on pigs showed that increased expression of SUMO1 in the porcine arterial ECs could protect ECs, reduce EC apoptosis, and increase EC formation. Mechanistic studies have shown that SUMO1 promotes angiogenesis by regulating MMP13 expression and the JAK2/STAT5 signaling pathway, and similar results were obtained using mouse models (Yang et al., 2013). The effect of SUMOylation on endothelial cell apoptosis and senescence is not only that, we need to invest more research to prove it.
2.1.2 EC inflammation
GATA2, a transcription factor that regulates the expression of EC adhesion molecules, can be modified by SUMOylation, During inflammation, SENP1 promotes the deSUMOylation of GATA2 in ECs, increasing the stability of GATA2, thus finally aggravating endothelial inflammation (Qiu et al., 2017). NLRP3 inflammatory bodies can activate IL-1 β and increase the intensity of inflammation (Wang et al., 2017). Several studies have shown that the initiation and activation of NLRP3 are significantly upregulated after ABI (O'Brien et al., 2020). SUMOylation deletion of NLRP3 can increase the expression of IL-1 β, while the deletion of SENP7 downregulates the expression of IL-1 β, indicating that NLRP3 can inhibit the progression of inflammation through SUMOylation (Barry et al., 2018). Here, SUMOylation seems to show anti-inflammatory effects.
Angiotensin II (Ang II) is a key molecule in the renin-angiotensin system that regulates blood pressure, which can promote the production of ROS, apoptosis, and inflammation in ECs (Benigni et al., 2010). The activation of transcription factor 3 (ATF3), an adaptive reactive protein, and its abnormal expression lead to atherosclerosis (Forrester et al., 2018). Silencing ATF3 can inhibit the lipotoxicity of cerebral microvascular ECs (Nyunt et al., 2019). Another study also supports this view (Aung et al., 2016). Interestingly, however, another study reports opposite results, whereby after ATF3 gene knockout, the infarct size increased significantly in the mouse model of cerebral ischemia (Wang et al., 2012). ATF3 also inhibits the apoptosis of neurons and activation of microglia in the rat model of cerebral ischemia and alleviates ABI in rats (Ma et al., 2022). The study showed that compared to the SUMOylation mutant group, the SUMOylation degree and SUMO1-mediated expression of wild-type ATF3 increased significantly, thus accelerating the process of ECD induced by Ang II (Zhang et al., 2016). Simultaneously, ATF3 is a stress-induced adaptive response protein, which is associated with immune responses, tumors, inflammation, and other diseases (Zhou et al., 2011). ATF3 or SUMO1 gene knockout can inhibit the expression of inflammatory molecules induced by Ang II, while wild-type ATF3 can reduce the production of NO. Thus, SUMOylation of ATF3 can increase the stability of ATF3 and promote ECD mediated by angiotensin-converting enzyme II (Zhang et al., 2016).
The activation of focal adhesion kinase (FAK) can promote the progression of EC inflammation, and disturbed flow (D-flow) can induce EC senescence. D-flow can induce ROS production, further activating FAK through SUMOylation of FAK K152, forming a positive feedback loop, which induces EC activation and senescence. The site-directed mutation of FAK affecting SUMOylation reverses this phenomenon (Velatooru et al., 2021). Moreover, FAK mediates the expression of IL-6 in the brain and promotes inflammation (Keasey et al., 2018). Another study also showed that the mouse model of stroke had significant anti-inflammatory and neuroprotective effects after treatment with FAK inhibitors (Jia et al., 2022).
Extracellular signal-regulated kinase 5 (ERK5) is an anti-atherosclerotic factor (Heo et al., 2014), and its activation leads to the upregulation of PPAR, resulting in anti-inflammatory and anti-atherosclerotic effects (Woo et al., 2008). ERK5 can reduce its expression through the SUMOylation of PIAS1, thus accelerating the progression of inflammation (Paez-Mayorga et al., 2018). Moreover, in the case of hemodynamic abnormality, the expression of p53 and ERK5 is regulated by SENP2. In the mouse model carrying a SENP2 deletion, the degree of SUMOylation increased, and increased apoptosis and expression of ECs were observed, while in the SUMOylation mutation group, the apoptosis and expression of ECs were inhibited after overexpression of p53 and ERK5 (Heo et al., 2013). However, another study reports opposite conclusions. Ponatinib, a tyrosine kinase inhibitor (TKI), can upregulate SUMOylation of ERK5, promote the inflammatory progression of ECs, and disrupt the normal state of blood vessels (Paez-Mayorga et al., 2018). Protein kinase C ζ (PKC ζ) can induce SUMOylation of p53 and accelerate apoptosis of ECs (Heo et al., 2011; Dehnavi et al., 2019). A previous study suggests that increased expression of PKC ζ may play a role in preventing cognitive impairment in mice (Wu et al., 2012).
The regulatory effects of SUMOylation on endothelial cell inflammation show two completely opposite effects. Proinflammatory or anti-inflammatory effects are related to different molecules SUMOylation. However, it is uncertain whether such a role is beneficial or harmful. Considering the core role of ECs in NVU and BBB, the influence of SUMOylation on ECs may be an interesting research direction.
2.2 The regulatory effects of SUMOylation on neurons
The role of SUMOylation in neuroprotection has been studied for a long time now. After transient cerebral ischemia, the expression of SUMO2/3 in the hippocampus and cerebral cortex increases significantly, and the activation of the SUMOylation pathway can block the activation of oxidative stress responses and protect neurons (Yang et al., 2008). In the transgenic mouse model, increased expressions of SUMO1–3 were detected in the brain of mice after cerebral ischemia (Yang et al., 2014), however, SUMO1–3 gene knockouts showed limited neurological recovery after cerebral ischemia (Zhang et al., 2017). These studies show that overexpression of SUMO1/2 or Ubc9 can protect neurons while silencing SUMO2/3 is not conducive to neuronal survival (Datwyler et al., 2011; Lee et al., 2011; Zhang et al., 2019). The specific deletion of pericytes in SENP1 can enlarge the range of focal cerebral ischemia, aggravate motor dysfunction, and significantly increase neuronal damage after cerebral ischemia in mice. In the in vitro model, knockout of SENP1 from pericytes could activate the apoptosis process and destroy the integrity of the BBB (Sun et al., 2020). This is contrary to the conclusions of previous studies. A recent study has shown that the knockout of the SUMO2 gene in mouse neurons shows significant impairment in cognitive functions in mice. This process does not involve changes in gene structure and neuron morphology but is related to the impairment in synaptic SUMOylation (Yu et al., 2020). Another study using neural stem cells showed that mice with middle cerebral artery occlusion had strong hypoxia resistance after overexpression of SUMO E2 ligase (Ubc9), along with an enhanced differentiation ability of neurons in vitro (Bernstock et al., 2019). The expression of SUMOylation proteins increases significantly in the brain during coma. Subsequent in vivo and in vitro experiments have shown that brain SUMOylation can induce ischemic tolerance (Bernstock et al., 2018a), which can enhance the neuroprotective effect of SUMOylation by inhibiting the expression of SENP2 (Bernstock et al., 2018b).
Ion homeostasis involves almost all physiological and pathological processes, and sodium/calcium exchanger-3 (NCX3) plays a protective role after cerebral ischemia. A study on the model of cerebral infarction in rats shows that SUMO1 silencing aggravated brain injury after cerebral ischemia, and the combination of SUMO1 and NCX3 could enhance the neuroprotective effect after cerebral ischemia (Cuomo et al., 2016). In one study, the authors explored the therapeutic targets of neuronal apoptosis from the perspectives of biology and chemistry and found that SUMOylation played a neuroprotective role through drug activation (Krajnak and Dahl, 2018).
Hypothermic brain protection strategies have long been used clinically but their underlying molecular mechanism warrant investigation. After permanent occlusion of the middle cerebral artery in mice, hypothermic brain protection can greatly promote the level of SUMOylated proteins in the brain, thus protecting mice from ischemic injury (Lee et al., 2014). Hypoxia and hypothermia can promote SUMOylation of neural stem cells and resist hypoxia injury, while Ubc9 gene knockout can reverse this ability. SUMOylation is an important mechanism in neural stem cells for protection against hypoxia injury and can improve the survival rate and neural repair ability of neural stem cells after transplantation (Cai et al., 2022). Targeted temperature management (TTM) cools the whole body or target organs by lowering the temperature. TTM is of great significance in ischemia and reperfusion injury after ABI. TTM can induce SUMOylation, promote the regulation of unfolded protein responses, inhibit apoptosis and neuroinflammation, and plays a protective role in the brain (Talma et al., 2016).
With regard to the effects of SUMOylation on neurons, studies have shown that SUMOylation is more beneficial, but more work is needed to determine the exact effect of SUMOylation on neurons. In addition, the related mechanism is worthy of further study.
2.3 The regulatory effects of SUMOylation on microglia
Annexin-A1 SUMOylation regulates microglial polarization after cerebral ischemia by modulating the IκB kinase stability via selective autophagy and significantly improves the neurological functions in a mouse model of ischemic (Li et al., 2021). The expression of SENP6 in microglia increases significantly after cerebral ischemia, and after downregulation of SENP6 in microglia, positive results were obtained, whereby not only did the area of cerebral infarction reduce significantly but the motor and cognitive functions of mice with cerebral ischemia also improved substantially (Mao et al., 2022). However, opposite results have also been reported. Alcohol causes inflammation in the hippocampus of rats, which is caused by an increase in the number of microglia and is mediated by p-NF-κB-p65. The overexpression of SENP6 can inhibit this process, prevent the progression of neuroinflammation, and play a neuroprotective role (Li et al., 2019). Intermittent hypoxia can cause neuronal inflammation and neuronal apoptosis in mice, likely due to the downregulation of the expression of PPAR γ due to increased SUMOylation, and further downregulation of SENP1, together aggravating neuroinflammation and neuronal apoptosis (Wang et al., 2021b). Another study reported similar results. SENP1 overexpression inhibits inflammation caused by intermittent hypoxia, while SENP1 knockout leads to cognitive decline in mice (Wang et al., 2021c). Moreover, by regulating SUMOylation of NF- κB essential modulator (NEMO), overexpression of SENP1 can reduce the activation of NF- κB, thus inhibiting the inflammatory responses induced by microglia (Yang et al., 2020).
The effect of SUMOylation on microglia showed different results. SUMOylation may improve cognitive function through microglia, but it may also aggravate neuroinflammation. The difference in results may be due to different research methods, but it also suggests the diversity of SUMOylation in regulating the function of microglia. In view of the long-term pro-inflammatory effect of microglia on neuroinflammation, we should make more efforts in this research direction.
2.4 The regulatory effects of SUMOylation on astrocytes
Guanosine plays an active role in many diseases, especially neuroprotection (Lanznaster et al., 2016; Tasca et al., 2018). For example, guanosine has an anti-inflammatory effect during astrocyte senescence (Souza et al., 2016). Guanosine also has a neuroprotective effect on cerebral ischemia, which may be related to its ability to reduce the production of ROS (Dal-Cim et al., 2019). Guanosine can stimulate the proliferation of neural stem cells through the activation of CREB (Su et al., 2013). A recent study has shown that the neuroprotective effect of guanosine is related to the SUMOylation of neurons and astrocytes, and guanosine receptor antagonists can reverse this effect, suggesting that guanosine can play a role as a SUMOylation enhancer for neuroprotection (Zanella et al., 2020) (Table 1).
3 Regulatory effects of SUMOylation on vascular remodeling
3.1 Effects of SUMOylation on vascular smooth muscle cells
VSMCs are the main components of the vascular wall, which play a key role in the stability of vascular structure and the maintenance of normal vascular pulsation (Lacolley et al., 2017). VSMCs are characterized by the expression of contractile proteins, including actin and myosin (Poittevin et al., 2014). VSMCs regulate CBF by contracting and relaxing blood vessels, changing the diameter of blood vessels, and regulating the supply of oxygen and nutrients (Hartmann et al., 2022). VSMCs are regulated by several molecular mechanisms, affecting the expression of related genes, thus changing vascular tension (Liu and Lin, 2022). The renin-angiotensin system (RAS) is the key medium for VSMCs to perform their physiological functions (Griendling et al., 1997), and the Notch signaling pathway regulates the migration and adhesion of VSMCs (Sorrentino et al., 2022). VSMCs mainly include two phenotypes. Contractile VSMCs function mostly when blood vessels are healthy, and switch to synthetic VSMC phenotype after vascular damage (Rensen et al., 2007; Touyz et al., 2018). The changes in VSMCs are related to cell signaling proteins, injury stimulation, and cell-to-cell contact. After a neurovascular injury, VSMCs dedifferentiate and promote vascular repair (Davis-Dusenbery et al., 2011). Generally, normal VSMC function is very important for the maintenance and repair of neurovascular function, and VSMC dysfunction leads to the progression of vascular disease.
In the biological process underlying VSMC proliferation, Kruppel-like factor 4 (KLF4) acts as a switch (Nie et al., 2016). When the reaction between KLF4 and the SUMO-binding enzyme, Ubc9 is inhibited, the proliferation of VSMCs decreases. Another study reports a similar conclusion (Li et al., 2018). In addition, peroxisome proliferator-activated receptors (PPARs), an important regulator of lipid metabolism, also play an important role in the proliferation of VSMCs. SUMOylation of PPAR γ one promotes the proliferation and migration of VSMCs (Lim et al., 2009; Wadosky and Willis, 2012). The Rho-specific guanine nucleotide dissociation inhibitor (RhoGDI) can regulate the proliferation of VSMCs and the stability of the thrombus. When Ang II receptor one is activated, Ang II can increase the stability of RhoGDI and promote the proliferation of VSMCs through SUMOylation (Dai et al., 2019). Myocardin is a factor regulating the differentiation of smooth muscle cells (SMCs), which can be modified by SUMO1 to increase protein stability, and this process is reversibly regulated by SENP2, which is known to promote the phenotypic switching of VSMCs (Liang et al., 2022).
Autophagy is a research hotspot, and its activation is related to the proliferation of VSMCs under hypoxic stress (Wen et al., 2019). In the mouse model of hypoxic pulmonary hypertension, the overexpression of SUMO1 activates the autophagy pathway, resulting in the dedifferentiation of VSMCs from the pulmonary artery and enhanced VSMC proliferation (Yao et al., 2019). In hypoxic mice, the expression of SUMO1 is upregulated, and a significant increase in VSMC proliferation, migration, dedifferentiation, and autophagy is observed. Downregulation of SUMO1 expression can reverse these phenotypes (Yao et al., 2019). On the contrary, another study concluded that HIF-1 α could increase the expression of the downstream gene, VEGF, and enhance the proliferation of pulmonary artery smooth muscles in rats through SENP1-mediated deSUMOylation (Zhou et al., 2016). These two studies on pulmonary artery smooth muscles have shown opposite results. Thus, evaluating the role of SUMOylation in cerebral VSMCs is a potential research direction.
As a SUMOylation proteolytic enzyme, SENP3 plays an important role in the occurrence and development of many tumors (Han et al., 2010). Studies have shown that a high expression of SENP3 is closely related to arterial remodeling. It can significantly promote the proliferation and migration of VSMCs while silencing SENP3 reverses this effect and slows down vascular remodeling in mice. In addition, increased SENP3 expression can also enlarge the area of the remodeled arterial intima (Cai et al., 2021). Another study showed that SUMOylation had a positive effect on VSMCs, increasing vascular intimal thickness and muscle fiber production in mice, all promoting vascular remodeling (Dai et al., 2019).
The proliferation of VSMCs is very important for the stability of normal vascular structure. Related studies have shown that SUMOylation can promote the proliferation and migration of VSMCs, but there are different research conclusions. Generally speaking, the role of SUMOylation on VSMCs can not be ignored and needs to be further explored.
3.2 Effects of SUMOylation on angiogenesis
Angiogenesis plays a key role in various physiological and pathological processes, including inflammation, diabetes, infection, and cancer. Angiogenesis means to regenerate and maintain the structure of blood vessels based on the original blood vessels, which is a dynamic and complex process (Carmeliet and Jain, 2011). Blood vessels originate from endothelial progenitor cells through a process called vasculogenesis, and further collateral circulation formation occurs, referred to as angiogenesis (Carmeliet, 2003). After ABI, angiogenesis helps restore the supply of oxygen and nutrients to the damaged brain, stabilize blood perfusion in the brain, maintain the survival of neurons, and promote the recovery of the nervous system. Research on VEGF1–5 and their receptors, placental growth factors (PLGFs), fibroblast growth factors (FGFF1, FGFF2) and their receptors, transforming growth factors, and tumor necrosis factor has been conducted. They are angiogenic factors and anti-angiogenic factors (Huang and Bao, 2004; Ucuzian et al., 2010). There are many sources of angiogenic factors, including ECs, fibroblasts, platelets, and cancer cells (Ucuzian et al., 2010).
VEGFs mediate physiological processes mainly by activating VEGFR2, which participates in cell migration, regulation of endothelial connections, and angiogenesis (Simons et al., 2016). During the process of pathological angiogenesis in VEGFR2, the loss of SENP1 hinders pathological angiogenesis and tissue repair and reduces VEGF-induced angiogenesis (Zhou et al., 2018). SUMOylation not only regulates PTMs of proteins but also regulates VEGFR at the gene level. Related studies have shown that PROX1, which regulates lymphangiogenesis, is also regulated by SUMOylation when it induces VEGFR expression (Pan et al., 2009). Basic FGF (FGF2) is a pro-angiogenic factor, which can activate the FGF receptor-1 (FGFR1) of ECs to promote angiogenesis. Under hypoxia, FGFR is SUMOylated, which promotes VEGF2 aggregation but limits VEGF1 aggregation, thus activating the VEGFR2 signal and enhancing angiogenesis (Zhu et al., 2022). However, some studies have shown that VEGFR-2 can specifically bind to SUMO1, inhibit the angiogenic signal pathway in non-small cell lung cancer cells, and prevent the malignant progression of tumor cells (Wang and Jiang, 2020).
The notch signaling pathway plays an important role in many physiological and pathological processes, as well as vascular diseases. The activation of the Notch1 pathway in ECs can inhibit the expression of VEGFR, restrict the transmission of the VEGF signaling axis, and reduce angiogenesis (Benedito et al., 2009). The absence of SENP1 in ECs leads to long-term SUMOylation of the Notch1 signaling pathway and slows down the speed of retinal vascularization. Notch1 can reversibly regulate signal transduction for endothelial angiogenesis through SUMOylation (Zhu et al., 2017). In neurovascular diseases, the regulatory role of SUMOylation on Notch pathway deserves further exploration.
In a study on cerebral ischemic stroke, inhibition of the AKT/mTOR pathway was found to promote angiogenesis and neurogenesis and improve CBF and glucose metabolism (Zhao et al., 2019). However, the opposite results have been obtained in a study on cardiovascular disease. In vitro and in vivo experimental studies have shown that SENP2 gene deficiency can improve cardiac function after myocardial infarction in mice owing to the increase in the SUMOylation activity of targeting protein kinase B (AKT), which promotes cardiomyocyte proliferation and angiogenesis (Chen et al., 2021). In another study, similar results were obtained. A decrease in the level of SUMOylation reduced AKT activation and cell proliferation. Interestingly, the activation of AKT is not regulated by the classical PI3K pathway (de la Cruz-Herrera et al., 2015). A study on cardiac ischemia-reperfusion injury showed that after the injury, the activity of the deSUMOylation enzyme decreased, while some genes related to angiogenesis showed SUMOylation changes, indicating its important role in the process of cardiac angiogenesis (Hotz et al., 2020). The difference in the regulation of AKT in cardio-cerebral angiogenesis may be the potential research direction of assessing the role of SUMOylation on the regulation of neuroangiogenesis.
A recent study showed that Astragaloside IV (AS-IV) could stabilize HIF-1 α protein by activating the SUMOylation pathway, and could promote the proliferation and migration of VECs, thus improving angiogenesis under hypoxic conditions and accelerating wound healing (Wang B. et al., 2021). SUMOylation is also closely related to angiogenesis in tumor-related research. For example, angiogenesis under hypoxia is promoted in prostate cancer cells, which is regulated by androgen receptor-dependent SUMOylation (Vlachostergios and Papandreou, 2012).
4 Conclusion and perspective
ABI remains a major health problem, posing a heavy burden on these patients and their families. Many studies have focused on the treatment of ABI, including targets for molecular therapy and drug development, and some promising research directions have been applied in clinical settings. However, considering the complexity of ABI and individual differences among patients, the effectiveness of monotherapy cannot be exaggerated, because a single treatment cannot solve pathological progression.
In this review, we briefly report the composition and normal physiological functioning of the neurovasculature. This paper focuses on the role of SUMOylation in phenotypes related to neurovascular pathological progress, including EC disturbance, VSMC proliferation, angiogenesis, etc. We also discussed the neuroprotective effects of SUMOylation. For easier elaboration, we wrote the above sections separately but in reality, physiological and pathological processes are not strictly independent but occur almost simultaneously and involve mutual integration. For example, the improvement in EC function also has a positive effect on angiogenesis; SMC proliferation is also a part of vascular remodeling. ECs are not only the basis of VSMC proliferation, angiogenesis, and vascular remodeling but also many scholars of several fields, resulting in the accumulation of research results, so we focused on this aspect in the review. However, its limitation is that we only reviewed the correlation between SUMOylation and ABI, and did not detail its internal relationship and causal relationship.
Remarkable progress has been made in the study of SUMOylation and ABI worldwide. However, given the complexity of the pathological mechanism of ABI, the development of treatment is still challenging. The purpose of this review is to understand how neurovascular disorders associated with ABI occur; how SUMOylation is involved in these injuries, and how SUMOylation improves ABI. It is important to understand the role of SUMOylation in ABI as it may be the potential molecular mechanism underlying ABI or brain protection. We hope that this review will provide theoretical references for future research on SUMOylation and ABI.
Author contributions
PL, LL, and JH prepared the manuscript. DM, SL, JR, JC, RT, and YS edited and revised the manuscript. HY and SZ supervised the manuscript’s preparation.
Funding
This work was funded by the 2022 Decision Consulting and Management Innovation Guidance Planning Project of Chongqing Shapingba District Science and Technology Bureau (Grant Number: Jcd202277), the 2022 Technology Foresight and Institutional Innovation Project of Chongqing Science and Technology Bureau (Grant Number: CSTB2022TFII-OFX0006) and the Special Project for Improving the Scientific Research Capacity of Chongqing University Cancer Hospital.
Conflict of interest
The authors declare that the research was conducted in the absence of any commercial or financial relationships that could be construed as a potential conflict of interest.
Publisher’s note
All claims expressed in this article are solely those of the authors and do not necessarily represent those of their affiliated organizations, or those of the publisher, the editors and the reviewers. Any product that may be evaluated in this article, or claim that may be made by its manufacturer, is not guaranteed or endorsed by the publisher.
References
Anand, A. R., Rachel, G., and Parthasarathy, D. (2018). HIV proteins and endothelial dysfunction: Implications in cardiovascular disease. Front. Cardiovasc Med. 5, 185. doi:10.3389/fcvm.2018.00185
Anderson, J., Sandhir, R., Hamilton, E. S., and Berman, N. E. (2009). Impaired expression of neuroprotective molecules in the HIF-1alpha pathway following traumatic brain injury in aged mice. J. Neurotrauma 26 (9), 1557–1566. doi:10.1089/neu.2008.0765
Andreone, B. J., Lacoste, B., and Gu, C. (2015). Neuronal and vascular interactions. Annu. Rev. Neurosci. 38, 25–46. doi:10.1146/annurev-neuro-071714-033835
Asahara, T., Masuda, H., Takahashi, T., Kalka, C., Pastore, C., Silver, M., et al. (1999). Bone marrow origin of endothelial progenitor cells responsible for postnatal vasculogenesis in physiological and pathological neovascularization. Circ. Res. 85 (3), 221–228. doi:10.1161/01.res.85.3.221
Aung, H. H., Altman, R., Nyunt, T., Kim, J., Nuthikattu, S., Budamagunta, M., et al. (2016). Lipotoxic brain microvascular injury is mediated by activating transcription factor 3-dependent inflammatory and oxidative stress pathways. J. Lipid Res. 57 (6), 955–968. doi:10.1194/jlr.M061853
Balabanov, R., Goldman, H., Murphy, S., Pellizon, G., Owen, C., Rafols, J., et al. (2001). Endothelial cell activation following moderate traumatic brain injury. Neurol. Res. 23 (2-3), 175–182. doi:10.1179/016164101101198514
Ballabh, P., Braun, A., and Nedergaard, M. (2004). The blood-brain barrier: An overview: Structure, regulation, and clinical implications. Neurobiol. Dis. 16 (1), 1–13. doi:10.1016/j.nbd.2003.12.016
Barry, R., John, S. W., Liccardi, G., Tenev, T., Jaco, I., Chen, C. H., et al. (2018). SUMO-mediated regulation of NLRP3 modulates inflammasome activity. Nat. Commun. 9 (1), 3001. doi:10.1038/s41467-018-05321-2
Bawa-Khalfe, T., and Yeh, E. T. (2010). SUMO losing balance: SUMO proteases disrupt SUMO homeostasis to facilitate cancer development and progression. Genes. Cancer 1 (7), 748–752. doi:10.1177/1947601910382555
Bedaso, A., Geja, E., Ayalew, M., Oltaye, Z., and Duko, B. (2018). Post-concussion syndrome among patients experiencing head injury attending emergency department of Hawassa University Comprehensive specialized hospital, Hawassa, southern Ethiopia. J. Headache Pain 19 (1), 112. doi:10.1186/s10194-018-0945-0
Benedito, R., Roca, C., Sorensen, I., Adams, S., Gossler, A., Fruttiger, M., et al. (2009). The notch ligands Dll4 and Jagged1 have opposing effects on angiogenesis. Cell. 137 (6), 1124–1135. doi:10.1016/j.cell.2009.03.025
Benigni, A., Cassis, P., and Remuzzi, G. (2010). Angiotensin II revisited: New roles in inflammation, immunology and aging. EMBO Mol. Med. 2 (7), 247–257. doi:10.1002/emmm.201000080
Bernstock, J. D., Peruzzotti-Jametti, L., Leonardi, T., Vicario, N., Ye, D., Lee, Y. J., et al. (2019). SUMOylation promotes survival and integration of neural stem cell grafts in ischemic stroke. EBioMedicine 42, 214–224. doi:10.1016/j.ebiom.2019.03.035
Bernstock, J. D., Yang, W., Ye, D. G., Shen, Y., Pluchino, S., Lee, Y. J., et al. (2018a). SUMOylation in brain ischemia: Patterns, targets, and translational implications. J. Cereb. Blood Flow. Metab. 38 (1), 5–16. doi:10.1177/0271678X17742260
Bernstock, J. D., Ye, D., Smith, J. A., Lee, Y. J., Gessler, F. A., Yasgar, A., et al. (2018b). Quantitative high-throughput screening identifies cytoprotective molecules that enhance SUMO conjugation via the inhibition of SUMO-specific protease (SENP)2. FASEB J. 32 (3), 1677–1691. doi:10.1096/fj.201700711R
Blinder, P., Tsai, P. S., Kaufhold, J. P., Knutsen, P. M., Suhl, H., and Kleinfeld, D. (2013). The cortical angiome: An interconnected vascular network with noncolumnar patterns of blood flow. Nat. Neurosci. 16 (7), 889–897. doi:10.1038/nn.3426
Bruns, J., and Hauser, W. A. (2003). The epidemiology of traumatic brain injury: A review. Epilepsia 44 (10), 2–10. doi:10.1046/j.1528-1157.44.s10.3.x
Cai, H., Ma, X., Lu, D., Chen, L., Bian, X., Zhang, N., et al. (2022). Mild hypothermia promotes ischemic tolerance and survival of neural stem cell grafts by enhancing global SUMOylation. Oxid. Med. Cell. Longev. 2022, 6503504. doi:10.1155/2022/6503504
Cai, Z., Wang, Z., Yuan, R., Cui, M., Lao, Y., Wang, Y., et al. (2021). Redox-sensitive enzyme SENP3 mediates vascular remodeling via de-SUMOylation of beta-catenin and regulation of its stability. EBioMedicine 67, 103386. doi:10.1016/j.ebiom.2021.103386
Candelario-Jalil, E., Dijkhuizen, R. M., and Magnus, T. (2022). Neuroinflammation, stroke, blood-brain barrier dysfunction, and imaging modalities. Stroke 53 (5), 1473–1486. doi:10.1161/STROKEAHA.122.036946
Carmeliet, P. (2003). Angiogenesis in health and disease. Nat. Med. 9 (6), 653–660. doi:10.1038/nm0603-653
Carmeliet, P., and Jain, R. K. (2011). Molecular mechanisms and clinical applications of angiogenesis. Nature 473 (7347), 298–307. doi:10.1038/nature10144
Celen, A. B., and Sahin, U. (2020). Sumoylation on its 25th anniversary: Mechanisms, pathology, and emerging concepts. FEBS J. 287 (15), 3110–3140. doi:10.1111/febs.15319
Chan, T. L. H., and Woldeamanuel, Y. W. (2020). Exploring naturally occurring clinical subgroups of post-traumatic headache. J. Headache Pain 21 (1), 12. doi:10.1186/s10194-020-1080-2
Chen, C., Wang, K., Zhang, H., Zhou, H. J., Chen, Y., and Min, W. (2019). A unique SUMO-interacting motif of Trx2 is critical for its mitochondrial presequence processing and anti-oxidant activity. Front. Physiol. 10, 1089. doi:10.3389/fphys.2019.01089
Chen, S. F., Gong, C., Luo, M., Yao, H. R., Zeng, Y. J., and Su, F. X. (2011). Ubc9 expression predicts chemoresistance in breast cancer. Chin. J. Cancer 30 (9), 638–644. doi:10.5732/cjc.011.10084
Chen, Y., Xu, T., Li, M., Li, C., Ma, Y., Chen, G., et al. (2021). Inhibition of SENP2-mediated Akt deSUMOylation promotes cardiac regeneration via activating Akt pathway. Clin. Sci. (Lond) 135 (6), 811–828. doi:10.1042/CS20201408
Chiueh, C. C., Andoh, T., and Chock, P. B. (2005). Induction of thioredoxin and mitochondrial survival proteins mediates preconditioning-induced cardioprotection and neuroprotection. Ann. N. Y. Acad. Sci. 1042, 403–418. doi:10.1196/annals.1338.034
Chiurazzi, M., Di Maro, M., Cozzolino, M., and Colantuoni, A. (2020). Mitochondrial dynamics and microglia as new targets in metabolism regulation. Int. J. Mol. Sci. 21 (10), 3450. doi:10.3390/ijms21103450
Chymkowitch, P., Nguea, P. A., and Enserink, J. M. (2015). SUMO-Regulated transcription: Challenging the dogma. Bioessays 37 (10), 1095–1105. doi:10.1002/bies.201500065
Cuomo, O., Pignataro, G., Sirabella, R., Molinaro, P., Anzilotti, S., Scorziello, A., et al. (2016). Sumoylation of LYS590 of NCX3 f-loop by SUMO1 participates in brain neuroprotection induced by ischemic preconditioning. Stroke 47 (4), 1085–1093. doi:10.1161/STROKEAHA.115.012514
Da Silva-Ferrada, E., Ribeiro-Rodrigues, T. M., Rodriguez, M. S., and Girao, H. (2016). Proteostasis and SUMO in the heart. Int. J. Biochem. Cell. Biol. 79, 443–450. doi:10.1016/j.biocel.2016.09.015
Dai, F., Qi, Y., Guan, W., Meng, G., Liu, Z., Zhang, T., et al. (2019). RhoGDI stability is regulated by SUMOylation and ubiquitination via the AT1 receptor and participates in Ang II-induced smooth muscle proliferation and vascular remodeling. Atherosclerosis 288, 124–136. doi:10.1016/j.atherosclerosis.2019.07.010
Dal-Cim, T., Poluceno, G. G., Lanznaster, D., de Oliveira, K. A., Nedel, C. B., and Tasca, C. I. (2019). Guanosine prevents oxidative damage and glutamate uptake impairment induced by oxygen/glucose deprivation in cortical astrocyte cultures: Involvement of A(1) and A(2A) adenosine receptors and PI3K, MEK, and PKC pathways. Purinergic Signal 15 (4), 465–476. doi:10.1007/s11302-019-09679-w
Danaila, L., Popescu, I., Pais, V., Riga, D., Riga, S., and Pais, E. (2013). Apoptosis, paraptosis, necrosis, and cell regeneration in posttraumatic cerebral arteries. Chir. (Bucur) 108 (3), 319–324.
Daneman, R., and Prat, A. (2015). The blood-brain barrier. Cold Spring Harb. Perspect. Biol. 7 (1), a020412. doi:10.1101/cshperspect.a020412
Datwyler, A. L., Lattig-Tunnemann, G., Yang, W., Paschen, W., Lee, S. L., Dirnagl, U., et al. (2011). SUMO2/3 conjugation is an endogenous neuroprotective mechanism. J. Cereb. Blood Flow. Metab. 31 (11), 2152–2159. doi:10.1038/jcbfm.2011.112
Davis-Dusenbery, B. N., Wu, C., and Hata, A. (2011). Micromanaging vascular smooth muscle cell differentiation and phenotypic modulation. Arterioscler. Thromb. Vasc. Biol. 31 (11), 2370–2377. doi:10.1161/ATVBAHA.111.226670
de la Cruz-Herrera, C. F., Campagna, M., Lang, V., del Carmen Gonzalez-Santamaria, J., Marcos-Villar, L., Rodriguez, M. S., et al. (2015). SUMOylation regulates AKT1 activity. Oncogene 34 (11), 1442–1450. doi:10.1038/onc.2014.48
Dehnavi, S., Sadeghi, M., Penson, P. E., Banach, M., Jamialahmadi, T., and Sahebkar, A. (2019). The role of protein SUMOylation in the pathogenesis of atherosclerosis. J. Clin. Med. 8 (11), 1856. doi:10.3390/jcm8111856
Deng, L., Meng, T., Chen, L., Wei, W., and Wang, P. (2020). The role of ubiquitination in tumorigenesis and targeted drug discovery. Signal Transduct. Target Ther. 5 (1), 11. doi:10.1038/s41392-020-0107-0
Ding, X., Sun, J., Wang, L., Li, G., Shen, Y., Zhou, X., et al. (2008). Overexpression of SENP5 in oral squamous cell carcinoma and its association with differentiation. Oncol. Rep. 20 (5), 1041–1045.
Ekmark-Lewen, S., Lewen, A., Israelsson, C., Li, G. L., Farooque, M., Olsson, Y., et al. (2010). Vimentin and GFAP responses in astrocytes after contusion trauma to the murine brain. Restor. Neurol. Neurosci. 28 (3), 311–321. doi:10.3233/RNN-2010-0529
Enserink, J. M. (2015). Sumo and the cellular stress response. Cell. Div. 10, 4. doi:10.1186/s13008-015-0010-1
Esquiva, G., Grayston, A., and Rosell, A. (2018). Revascularization and endothelial progenitor cells in stroke. Am. J. Physiol. Cell. Physiol. 315 (5), C664-C674–C674. doi:10.1152/ajpcell.00200.2018
Faas, M. M., and de Vos, P. (2020). Mitochondrial function in immune cells in health and disease. Biochim. Biophys. Acta Mol. Basis Dis. 1866 (10), 165845. doi:10.1016/j.bbadis.2020.165845
Findlay, J. M., Weir, B. K., Kanamaru, K., and Espinosa, F. (1989). Arterial wall changes in cerebral vasospasm. Neurosurgery 25 (5), 736–745. discussion 745-736. doi:10.1097/00006123-198911000-00008
Forrester, S. J., Booz, G. W., Sigmund, C. D., Coffman, T. M., Kawai, T., Rizzo, V., et al. (2018). Angiotensin II signal transduction: An update on mechanisms of physiology and pathophysiology. Physiol. Rev. 98 (3), 1627–1738. doi:10.1152/physrev.00038.2017
Gajavelli, S., Kentaro, S., Diaz, J., Yokobori, S., Spurlock, M., Diaz, D., et al. (2015). Glucose and oxygen metabolism after penetrating ballistic-like brain injury. J. Cereb. Blood Flow. Metab. 35 (5), 773–780. doi:10.1038/jcbfm.2014.243
Garcia-Rodriguez, N., Wong, R. P., and Ulrich, H. D. (2016). Functions of ubiquitin and SUMO in DNA replication and replication stress. Front. Genet. 7, 87. doi:10.3389/fgene.2016.00087
Ghirnikar, R. S., Lee, Y. L., and Eng, L. F. (1998). Inflammation in traumatic brain injury: Role of cytokines and chemokines. Neurochem. Res. 23 (3), 329–340. doi:10.1023/a:1022453332560
Gill, G. (2004). SUMO and ubiquitin in the nucleus: Different functions, similar mechanisms? Genes. Dev. 18 (17), 2046–2059. doi:10.1101/gad.1214604
Gimbrone, M. A., and Garcia-Cardena, G. (2016). Endothelial cell dysfunction and the pathobiology of atherosclerosis. Circ. Res. 118 (4), 620–636. doi:10.1161/CIRCRESAHA.115.306301
Goldman, L., Siddiqui, E. M., Khan, A., Jahan, S., Rehman, M. U., Mehan, S., et al. (2022). Understanding acquired brain injury: A review. Biomedicines 10 (9), 2167. doi:10.3390/biomedicines10092167
Gong, D., Zhang, S., Liu, L., Dong, J., Guo, X., Hao, M., et al. (2011). Dynamic changes of vascular endothelial growth factor and angiopoietin-1 in association with circulating endothelial progenitor cells after severe traumatic brain injury. J. Trauma 70 (6), 1480–1484. doi:10.1097/TA.0b013e31821ac9e1
Greve, M. W., and Zink, B. J. (2009). Pathophysiology of traumatic brain injury. Mt. Sinai J. Med. 76 (2), 97–104. doi:10.1002/msj.20104
Griendling, K. K., Ushio-Fukai, M., Lassegue, B., and Alexander, R. W. (1997). Angiotensin II signaling in vascular smooth muscle. New concepts. Hypertension 29 (1), 366–373. doi:10.1161/01.hyp.29.1.366
Gupta, M. K., McLendon, P. M., Gulick, J., James, J., Khalili, K., and Robbins, J. (2016). UBC9-Mediated sumoylation favorably impacts cardiac function in compromised hearts. Circ. Res. 118 (12), 1894–1905. doi:10.1161/CIRCRESAHA.115.308268
Gupta, M. K., and Robbins, J. (2016). Making the connections: Autophagy and post-translational modifications in cardiomyocytes. Autophagy 12 (11), 2252–2253. doi:10.1080/15548627.2016.1215384
Han, Y., Huang, C., Sun, X., Xiang, B., Wang, M., Yeh, E. T., et al. (2010). SENP3-mediated de-conjugation of SUMO2/3 from promyelocytic leukemia is correlated with accelerated cell proliferation under mild oxidative stress. J. Biol. Chem. 285 (17), 12906–12915. doi:10.1074/jbc.M109.071431
Han, Z. J., Feng, Y. H., Gu, B. H., Li, Y. M., and Chen, H. (2018). The post-translational modification, SUMOylation, and cancer (Review). Int. J. Oncol. 52 (4), 1081–1094. doi:10.3892/ijo.2018.4280
Harding, I. C., Mitra, R., Mensah, S. A., Nersesyan, A., Bal, N. N., and Ebong, E. E. (2019). Endothelial barrier reinforcement relies on flow-regulated glycocalyx, a potential therapeutic target. Biorheology 56 (2-3), 131–149. doi:10.3233/BIR-180205
Hartmann, D. A., Coelho-Santos, V., and Shih, A. Y. (2022). Pericyte control of blood flow across microvascular zones in the central nervous system. Annu. Rev. Physiol. 84, 331–354. doi:10.1146/annurev-physiol-061121-040127
Hendriks, I. A., Lyon, D., Su, D., Skotte, N. H., Daniel, J. A., Jensen, L. J., et al. (2018). Site-specific characterization of endogenous SUMOylation across species and organs. Nat. Commun. 9 (1), 2456. doi:10.1038/s41467-018-04957-4
Hendriks, I. A., Lyon, D., Young, C., Jensen, L. J., Vertegaal, A. C., and Nielsen, M. L. (2017). Site-specific mapping of the human SUMO proteome reveals co-modification with phosphorylation. Nat. Struct. Mol. Biol. 24 (3), 325–336. doi:10.1038/nsmb.3366
Heo, K. S., Chang, E., Le, N. T., Cushman, H., Yeh, E. T., Fujiwara, K., et al. (2013). De-SUMOylation enzyme of sentrin/SUMO-specific protease 2 regulates disturbed flow-induced SUMOylation of ERK5 and p53 that leads to endothelial dysfunction and atherosclerosis. Circ. Res. 112 (6), 911–923. doi:10.1161/CIRCRESAHA.111.300179
Heo, K. S., Cushman, H. J., Akaike, M., Woo, C. H., Wang, X., Qiu, X., et al. (2014). ERK5 activation in macrophages promotes efferocytosis and inhibits atherosclerosis. Circulation 130 (2), 180–191. doi:10.1161/CIRCULATIONAHA.113.005991
Heo, K. S., Lee, H., Nigro, P., Thomas, T., Le, N. T., Chang, E., et al. (2011). PKCζ mediates disturbed flow-induced endothelial apoptosis via p53 SUMOylation. J. Cell. Biol. 193 (5), 867–884. doi:10.1083/jcb.201010051
Hirota, K., and Semenza, G. L. (2006). Regulation of angiogenesis by hypoxia-inducible factor 1. Crit. Rev. Oncol. Hematol. 59 (1), 15–26. doi:10.1016/j.critrevonc.2005.12.003
Homsi, S., Piaggio, T., Croci, N., Noble, F., Plotkine, M., Marchand-Leroux, C., et al. (2010). Blockade of acute microglial activation by minocycline promotes neuroprotection and reduces locomotor hyperactivity after closed head injury in mice: A twelve-week follow-up study. J. Neurotrauma 27 (5), 911–921. doi:10.1089/neu.2009.1223
Hotz, P. W., Wiesnet, M., Tascher, G., Braun, T., Muller, S., and Mendler, L. (2020). Profiling the murine SUMO proteome in response to cardiac ischemia and reperfusion injury. Molecules 25 (23), 5571. doi:10.3390/molecules25235571
Huang, W. C., Ko, T. P., Li, S. S., and Wang, A. H. (2004). Crystal structures of the human SUMO-2 protein at 1.6 A and 1.2 A resolution: Implication on the functional differences of SUMO proteins. Eur. J. Biochem. 271 (20), 4114–4122. doi:10.1111/j.1432-1033.2004.04349.x
Huang, Z., and Bao, S. D. (2004). Roles of main pro- and anti-angiogenic factors in tumor angiogenesis. World J. Gastroenterol. 10 (4), 463–470. doi:10.3748/wjg.v10.i4.463
Iadecola, C. (2017). The neurovascular unit coming of age: A journey through neurovascular coupling in health and disease. Neuron 96 (1), 17–42. doi:10.1016/j.neuron.2017.07.030
Jia, C., Lovins, C., Malone, H. M., Keasey, M. P., and Hagg, T. (2022). Female-specific neuroprotection after ischemic stroke by vitronectin-focal adhesion kinase inhibition. J. Cereb. Blood Flow. Metab. 42 (10), 1961–1974. doi:10.1177/0271678X221107871
Jullienne, A., Roberts, J. M., Pop, V., Paul Murphy, M., Head, E., Bix, G. J., et al. (2014). Juvenile traumatic brain injury induces long-term perivascular matrix changes alongside amyloid-beta accumulation. J. Cereb. Blood Flow. Metab. 34 (10), 1637–1645. doi:10.1038/jcbfm.2014.124
Keasey, M. P., Jia, C., Pimentel, L. F., Sante, R. R., Lovins, C., and Hagg, T. (2018). Blood vitronectin is a major activator of LIF and IL-6 in the brain through integrin-FAK and uPAR signaling. J. Cell. Sci. 131 (3), jcs202580. doi:10.1242/jcs.202580
Kisler, K., Nelson, A. R., Montagne, A., and Zlokovic, B. V. (2017). Cerebral blood flow regulation and neurovascular dysfunction in Alzheimer disease. Nat. Rev. Neurosci. 18 (7), 419–434. doi:10.1038/nrn.2017.48
Krajnak, K., and Dahl, R. (2018). Small molecule SUMOylation activators are novel neuroprotective agents. Bioorg Med. Chem. Lett. 28 (3), 405–409. doi:10.1016/j.bmcl.2017.12.028
Kunz, K., Piller, T., and Muller, S. (2018). SUMO-specific proteases and isopeptidases of the SENP family at a glance. J. Cell. Sci. 131 (6), jcs211904. doi:10.1242/jcs.211904
Lacolley, P., Regnault, V., Segers, P., and Laurent, S. (2017). Vascular smooth muscle cells and arterial stiffening: Relevance in development, aging, and disease. Physiol. Rev. 97 (4), 1555–1617. doi:10.1152/physrev.00003.2017
Lanznaster, D., Dal-Cim, T., Piermartiri, T. C., and Tasca, C. I. (2016). Guanosine: A neuromodulator with therapeutic potential in brain disorders. Aging Dis. 7 (5), 657–679. doi:10.14336/AD.2016.0208
Lara-Urena, N., Jafari, V., and Garcia-Dominguez, M. (2022). Cancer-associated dysregulation of sumo regulators: Proteases and ligases. Int. J. Mol. Sci. 23 (14), 8012. doi:10.3390/ijms23148012
Larsen, E. L., Ashina, H., Iljazi, A., Al-Khazali, H. M., Seem, K., Ashina, M., et al. (2019). Acute and preventive pharmacological treatment of post-traumatic headache: A systematic review. J. Headache Pain 20 (1), 98. doi:10.1186/s10194-019-1051-7
Lee, J. C., Park, J. H., Kim, I. H., Cho, G. S., Ahn, J. H., Tae, H. J., et al. (2017). Neuroprotection of ischemic preconditioning is mediated by thioredoxin 2 in the hippocampal CA1 region following a subsequent transient cerebral ischemia. Brain Pathol. 27 (3), 276–291. doi:10.1111/bpa.12389
Lee, Y. J., Mou, Y., Klimanis, D., Bernstock, J. D., and Hallenbeck, J. M. (2014). Global SUMOylation is a molecular mechanism underlying hypothermia-induced ischemic tolerance. Front. Cell. Neurosci. 8, 416. doi:10.3389/fncel.2014.00416
Lee, Y. J., Mou, Y., Maric, D., Klimanis, D., Auh, S., and Hallenbeck, J. M. (2011). Elevated global SUMOylation in Ubc9 transgenic mice protects their brains against focal cerebral ischemic damage. PLoS One 6 (10), e25852. doi:10.1371/journal.pone.0025852
Li, J. Y., Liu, C. P., Shiao, W. C., Jayakumar, T., Li, Y. S., Chang, N. C., et al. (2018). Inhibitory effect of PDGF-BB and serum-stimulated responses in vascular smooth muscle cell proliferation by hinokitiol via up-regulation of p21 and p53. Arch. Med. Sci. 14 (3), 579–587. doi:10.5114/aoms.2018.75085
Li, Q., Liu, D., Pan, F., Ho, C. S. H., and Ho, R. C. M. (2019). Ethanol exposure induces microglia activation and neuroinflammation through TLR4 activation and SENP6 modulation in the adolescent rat Hippocampus. Neural Plast. 2019, 1648736. doi:10.1155/2019/1648736
Li, X., Xia, Q., Mao, M., Zhou, H., Zheng, L., Wang, Y., et al. (2021). Annexin-A1 SUMOylation regulates microglial polarization after cerebral ischemia by modulating IKKα stability via selective autophagy. Sci. Adv. 7 (4), eabc5539. doi:10.1126/sciadv.abc5539
Liang, M., Cai, Z., Jiang, Y., Huo, H., Shen, L., and He, B. (2022). SENP2 promotes VSMC phenotypic switching via myocardin de-SUMOylation. Int. J. Mol. Sci. 23 (20), 12637. doi:10.3390/ijms232012637
Liang, Y. C., Lee, C. C., Yao, Y. L., Lai, C. C., Schmitz, M. L., and Yang, W. M. (2016). SUMO5, a novel poly-SUMO isoform, regulates PML nuclear bodies. Sci. Rep. 6, 26509. doi:10.1038/srep26509
Lim, S., Ahn, B. Y., Chung, S. S., Park, H. S., Cho, B. J., Kim, M., et al. (2009). Effect of a peroxisome proliferator-activated receptor gamma sumoylation mutant on neointimal formation after balloon injury in rats. Atherosclerosis 206 (2), 411–417. doi:10.1016/j.atherosclerosis.2009.02.031
Liu, L., Wei, H., Chen, F., Wang, J., Dong, J. F., and Zhang, J. (2011). Endothelial progenitor cells correlate with clinical outcome of traumatic brain injury. Crit. Care Med. 39 (7), 1760–1765. doi:10.1097/CCM.0b013e3182186cee
Liu, S., and Lin, Z. (2022). Vascular smooth muscle cells mechanosensitive regulators and vascular remodeling. J. Vasc. Res. 59 (2), 90–113. doi:10.1159/000519845
Liu, Y., Zhao, D., Qiu, F., Zhang, L. L., Liu, S. K., Li, Y. Y., et al. (2017). Manipulating PML SUMOylation via silencing UBC9 and RNF4 regulates cardiac fibrosis. Mol. Ther. 25 (3), 666–678. doi:10.1016/j.ymthe.2016.12.021
Lo, E. H., and Rosenberg, G. A. (2009). The neurovascular unit in health and disease: Introduction. Stroke 40 (3), S2–S3. doi:10.1161/STROKEAHA.108.534404
Lossinsky, A. S., and Shivers, R. R. (2004). Structural pathways for macromolecular and cellular transport across the blood-brain barrier during inflammatory conditions. Review. Histol. Histopathol. 19 (2), 535–564. doi:10.14670/HH-19.535
Lozano, D., Gonzales-Portillo, G. S., Acosta, S., de la Pena, I., Tajiri, N., Kaneko, Y., et al. (2015). Neuroinflammatory responses to traumatic brain injury: Etiology, clinical consequences, and therapeutic opportunities. Neuropsychiatr. Dis. Treat. 11, 97–106. doi:10.2147/NDT.S65815
Lv, Z., Yuan, L., Atkison, J. H., Williams, K. M., Vega, R., Sessions, E. H., et al. (2018). Molecular mechanism of a covalent allosteric inhibitor of SUMO E1 activating enzyme. Nat. Commun. 9 (1), 5145. doi:10.1038/s41467-018-07015-1
Ma, N., Li, G., and Fu, X. (2022). Protective role of activating transcription factor 3 against neuronal damage in rats with cerebral ischemia. Brain Behav. 12 (4), e2522. doi:10.1002/brb3.2522
Maas, A. I. R., Menon, D. K., Manley, G. T., Abrams, M., Akerlund, C., Andelic, N., et al. (2022). Traumatic brain injury: Progress and challenges in prevention, clinical care, and research. Lancet Neurol. 21, 1004–1060. doi:10.1016/S1474-4422(22)00309-X
Mao, M., Xia, Q., Zhan, G. F., Chu, Q. J., Li, X., and Lian, H. K. (2022). SENP6 induces microglial polarization and neuroinflammation through de-SUMOylation of Annexin-A1 after cerebral ischaemia-reperfusion injury. Cell. Biosci. 12 (1), 113. doi:10.1186/s13578-022-00850-2
McConnell, H. L., Kersch, C. N., Woltjer, R. L., and Neuwelt, E. A. (2017). The translational significance of the neurovascular unit. J. Biol. Chem. 292 (3), 762–770. doi:10.1074/jbc.R116.760215
McConnell, H. L., Li, Z., Woltjer, R. L., and Mishra, A. (2019). Astrocyte dysfunction and neurovascular impairment in neurological disorders: Correlation or causation? Neurochem. Int. 128, 70–84. doi:10.1016/j.neuint.2019.04.005
McGinn, M. J., and Povlishock, J. T. (2016). Pathophysiology of traumatic brain injury. Neurosurg. Clin. N. Am. 27 (4), 397–407. doi:10.1016/j.nec.2016.06.002
Menon, D. K., and Bryant, C. (2019). Time for change in acquired brain injury. Lancet Neurol. 18 (1), 28. doi:10.1016/S1474-4422(18)30463-0
Montagne, A., Zhao, Z., and Zlokovic, B. V. (2017). Alzheimer's disease: A matter of blood-brain barrier dysfunction? J. Exp. Med. 214 (11), 3151–3169. doi:10.1084/jem.20171406
Moremen, K. W., Tiemeyer, M., and Nairn, A. V. (2012). Vertebrate protein glycosylation: Diversity, synthesis and function. Nat. Rev. Mol. Cell. Biol. 13 (7), 448–462. doi:10.1038/nrm3383
Morgan, R., Kreipke, C. W., Roberts, G., Bagchi, M., and Rafols, J. A. (2007). Neovascularization following traumatic brain injury: Possible evidence for both angiogenesis and vasculogenesis. Neurol. Res. 29 (4), 375–381. doi:10.1179/016164107X204693
Moschos, S. J., Smith, A. P., Mandic, M., Athanassiou, C., Watson-Hurst, K., Jukic, D. M., et al. (2007). SAGE and antibody array analysis of melanoma-infiltrated lymph nodes: Identification of Ubc9 as an important molecule in advanced-stage melanomas. Oncogene 26 (29), 4216–4225. doi:10.1038/sj.onc.1210216
Mun, M. J., Kim, J. H., Choi, J. Y., Kim, M. S., Jang, W. C., Lee, J. J., et al. (2016). Polymorphisms of small ubiquitin-related modifier genes are associated with risk of Alzheimer's disease in Korean: A case-control study. J. Neurol. Sci. 364, 122–127. doi:10.1016/j.jns.2016.03.023
Neuwelt, E. A., Bauer, B., Fahlke, C., Fricker, G., Iadecola, C., Janigro, D., et al. (2011). Engaging neuroscience to advance translational research in brain barrier biology. Nat. Rev. Neurosci. 12 (3), 169–182. doi:10.1038/nrn2995
Nie, C. J., Li, Y. H., Zhang, X. H., Wang, Z. P., Jiang, W., Zhang, Y., et al. (2016). SUMOylation of KLF4 acts as a switch in transcriptional programs that control VSMC proliferation. Exp. Cell. Res. 342 (1), 20–31. doi:10.1016/j.yexcr.2016.03.001
Niskanen, E. A., and Palvimo, J. J. (2017). Chromatin SUMOylation in heat stress: To protect, pause and organise? SUMO stress response on chromatin. Bioessays 39 (6), 1600263. doi:10.1002/bies.201600263
Nyunt, T., Britton, M., Wanichthanarak, K., Budamagunta, M., Voss, J. C., Wilson, D. W., et al. (2019). Mitochondrial oxidative stress-induced transcript variants of ATF3 mediate lipotoxic brain microvascular injury. Free Radic. Biol. Med. 143, 25–46. doi:10.1016/j.freeradbiomed.2019.07.024
O'Brien, W. T., Pham, L., Symons, G. F., Monif, M., Shultz, S. R., and McDonald, S. J. (2020). The NLRP3 inflammasome in traumatic brain injury: Potential as a biomarker and therapeutic target. J. Neuroinflammation 17 (1), 104. doi:10.1186/s12974-020-01778-5
Olsen, J. V., Blagoev, B., Gnad, F., Macek, B., Kumar, C., Mortensen, P., et al. (2006). Global, in vivo, and site-specific phosphorylation dynamics in signaling networks. Cell. 127 (3), 635–648. doi:10.1016/j.cell.2006.09.026
Otero-Losada, M., Udovin, L., Kobiec, T., and Toro-Urrego, N. (2019). Long-term effects of hypoxia-reoxygenation on thioredoxins in rat central nervous system. Curr. Pharm. Des. 25 (45), 4791–4798. doi:10.2174/1381612825666191211111926
Paez-Mayorga, J., Chen, A. L., Kotla, S., Tao, Y., Abe, R. J., He, E. D., et al. (2018). Ponatinib activates an inflammatory response in endothelial cells via ERK5 SUMOylation. Front. Cardiovasc Med. 5, 125. doi:10.3389/fcvm.2018.00125
Pan, M. R., Chang, T. M., Chang, H. C., Su, J. L., Wang, H. W., and Hung, W. C. (2009). Sumoylation of Prox1 controls its ability to induce VEGFR3 expression and lymphatic phenotypes in endothelial cells. J. Cell. Sci. 122 (18), 3358–3364. doi:10.1242/jcs.050005
Pandit, R., Chen, L., and Gotz, J. (2020). The blood-brain barrier: Physiology and strategies for drug delivery. Adv. Drug Deliv. Rev. 165-166, 1–14. doi:10.1016/j.addr.2019.11.009
Papaconstantinou, J. (2019). The role of signaling pathways of inflammation and oxidative stress in development of senescence and aging phenotypes in cardiovascular disease. Cells 8 (11), 1383. doi:10.3390/cells8111383
Park, E., Bell, J. D., Siddiq, I. P., and Baker, A. J. (2009). An analysis of regional microvascular loss and recovery following two grades of fluid percussion trauma: A role for hypoxia-inducible factors in traumatic brain injury. J. Cereb. Blood Flow. Metab. 29 (3), 575–584. doi:10.1038/jcbfm.2008.151
Poittevin, M., Lozeron, P., Hilal, R., Levy, B. I., Merkulova-Rainon, T., and Kubis, N. (2014). Smooth muscle cell phenotypic switching in stroke. Transl. Stroke Res. 5 (3), 377–384. doi:10.1007/s12975-013-0306-x
Qin, Y., Bao, H., Pan, Y., Yin, M., Liu, Y., Wu, S., et al. (2014). SUMOylation alterations are associated with multidrug resistance in hepatocellular carcinoma. Mol. Med. Rep. 9 (3), 877–881. doi:10.3892/mmr.2014.1882
Qiu, C., Wang, Y., Zhao, H., Qin, L., Shi, Y., Zhu, X., et al. (2017). The critical role of SENP1-mediated GATA2 deSUMOylation in promoting endothelial activation in graft arteriosclerosis. Nat. Commun. 8, 15426. doi:10.1038/ncomms15426
Rabellino, A., Andreani, C., and Scaglioni, P. P. (2017). The role of PIAS SUMO E3-ligases in cancer. Cancer Res. 77 (7), 1542–1547. doi:10.1158/0008-5472.CAN-16-2958
Rawlings, N., Lee, L., Nakamura, Y., Wilkinson, K. A., and Henley, J. M. (2019). Protective role of the deSUMOylating enzyme SENP3 in myocardial ischemia-reperfusion injury. PLoS One 14 (4), e0213331. doi:10.1371/journal.pone.0213331
Rensen, S. S., Doevendans, P. A., and van Eys, G. J. (2007). Regulation and characteristics of vascular smooth muscle cell phenotypic diversity. Neth Heart J. 15 (3), 100–108. doi:10.1007/BF03085963
Riley, J. S., and Tait, S. W. (2020). Mitochondrial DNA in inflammation and immunity. EMBO Rep. 21 (4), e49799. doi:10.15252/embr.201949799
Salehi, A., Zhang, J. H., and Obenaus, A. (2017). Response of the cerebral vasculature following traumatic brain injury. J. Cereb. Blood Flow. Metab. 37 (7), 2320–2339. doi:10.1177/0271678X17701460
Sandoo, A., van Zanten, J. J., Metsios, G. S., Carroll, D., and Kitas, G. D. (2010). The endothelium and its role in regulating vascular tone. Open Cardiovasc Med. J. 4, 302–312. doi:10.2174/1874192401004010302
Seeler, J. S., and Dejean, A. (2017). SUMO and the robustness of cancer. Nat. Rev. Cancer 17 (3), 184–197. doi:10.1038/nrc.2016.143
Siddiq, I., Park, E., Liu, E., Spratt, S. K., Surosky, R., Lee, G., et al. (2012). Treatment of traumatic brain injury using zinc-finger protein gene therapy targeting VEGF-A. J. Neurotrauma 29 (17), 2647–2659. doi:10.1089/neu.2012.2444
Simons, M., Gordon, E., and Claesson-Welsh, L. (2016). Mechanisms and regulation of endothelial VEGF receptor signalling. Nat. Rev. Mol. Cell. Biol. 17 (10), 611–625. doi:10.1038/nrm.2016.87
Sorrentino, S., Polini, A., Arima, V., Romano, A., Quattrini, A., Gigli, G., et al. (2022). Neurovascular signals in amyotrophic lateral sclerosis. Curr. Opin. Biotechnol. 74, 75–83. doi:10.1016/j.copbio.2021.10.021
Souza, D. G., Bellaver, B., Bobermin, L. D., Souza, D. O., and Quincozes-Santos, A. (2016). Anti-aging effects of guanosine in glial cells. Purinergic Signal 12 (4), 697–706. doi:10.1007/s11302-016-9533-4
Stamatovic, S. M., Johnson, A. M., Keep, R. F., and Andjelkovic, A. V. (2016). Junctional proteins of the blood-brain barrier: New insights into function and dysfunction. Tissue Barriers 4 (1), e1154641. doi:10.1080/21688370.2016.1154641
Steffan, J. S., Agrawal, N., Pallos, J., Rockabrand, E., Trotman, L. C., Slepko, N., et al. (2004). SUMO modification of Huntingtin and Huntington's disease pathology. Science 304 (5667), 100–104. doi:10.1126/science.1092194
Stram, A. R., and Payne, R. M. (2016). Post-translational modifications in mitochondria: Protein signaling in the powerhouse. Cell. Mol. Life Sci. 73 (21), 4063–4073. doi:10.1007/s00018-016-2280-4
Su, C., Wang, P., Jiang, C., Ballerini, P., Caciagli, F., Rathbone, M. P., et al. (2013). Guanosine promotes proliferation of neural stem cells through cAMP-CREB pathway. J. Biol. Regul. Homeost. Agents 27 (3), 673–680.
Sun, M., Chen, X., Yin, Y. X., Gao, Y., Zhang, L., Chen, B., et al. (2020). Role of pericyte-derived SENP1 in neuronal injury after brain ischemia. CNS Neurosci. Ther. 26 (8), 815–828. doi:10.1111/cns.13398
Sun, Z., Hu, S., Luo, Q., Ye, D., Hu, D., and Chen, F. (2013). Overexpression of SENP3 in oral squamous cell carcinoma and its association with differentiation. Oncol. Rep. 29 (5), 1701–1706. doi:10.3892/or.2013.2318
Sweeney, M. D., Zhao, Z., Montagne, A., Nelson, A. R., and Zlokovic, B. V. (2019). Blood-brain barrier: From physiology to disease and back. Physiol. Rev. 99 (1), 21–78. doi:10.1152/physrev.00050.2017
Talma, N., Kok, W. F., de Veij Mestdagh, C. F., Shanbhag, N. C., Bouma, H. R., and Henning, R. H. (2016). Neuroprotective hypothermia - why keep your head cool during ischemia and reperfusion. Biochim. Biophys. Acta 1860 (11), 2521–2528. doi:10.1016/j.bbagen.2016.07.024
Tasca, C. I., Lanznaster, D., Oliveira, K. A., Fernandez-Duenas, V., and Ciruela, F. (2018). Neuromodulatory effects of guanine-based purines in health and disease. Front. Cell. Neurosci. 12, 376. doi:10.3389/fncel.2018.00376
Thurgur, H., and Pinteaux, E. (2019). Microglia in the neurovascular unit: Blood-brain barrier-microglia interactions after central nervous system disorders. Neuroscience 405, 55–67. doi:10.1016/j.neuroscience.2018.06.046
Tietz, S., and Engelhardt, B. (2015). Brain barriers: Crosstalk between complex tight junctions and adherens junctions. J. Cell. Biol. 209 (4), 493–506. doi:10.1083/jcb.201412147
Touyz, R. M., Alves-Lopes, R., Rios, F. J., Camargo, L. L., Anagnostopoulou, A., Arner, A., et al. (2018). Vascular smooth muscle contraction in hypertension. Cardiovasc Res. 114 (4), 529–539. doi:10.1093/cvr/cvy023
Ucuzian, A. A., Gassman, A. A., East, A. T., and Greisler, H. P. (2010). Molecular mediators of angiogenesis. J. Burn Care Res. 31 (1), 158–175. doi:10.1097/BCR.0b013e3181c7ed82
Ungvari, Z., Tarantini, S., Donato, A. J., Galvan, V., and Csiszar, A. (2018). Mechanisms of vascular aging. Circ. Res. 123 (7), 849–867. doi:10.1161/CIRCRESAHA.118.311378
Urbich, C., Aicher, A., Heeschen, C., Dernbach, E., Hofmann, W. K., Zeiher, A. M., et al. (2005). Soluble factors released by endothelial progenitor cells promote migration of endothelial cells and cardiac resident progenitor cells. J. Mol. Cell. Cardiol. 39 (5), 733–742. doi:10.1016/j.yjmcc.2005.07.003
Van Hove, A. H., and Benoit, D. S. (2015). Depot-based delivery systems for pro-angiogenic peptides: A review. Front. Bioeng. Biotechnol. 3, 102. doi:10.3389/fbioe.2015.00102
Velatooru, L. R., Abe, R. J., Imanishi, M., Gi, Y. J., Ko, K. A., Heo, K. S., et al. (2021). Disturbed flow-induced FAK K152 SUMOylation initiates the formation of pro-inflammation positive feedback loop by inducing reactive oxygen species production in endothelial cells. Free Radic. Biol. Med. 177, 404–418. doi:10.1016/j.freeradbiomed.2021.09.023
Venne, A. S., Kollipara, L., and Zahedi, R. P. (2014). The next level of complexity: Crosstalk of posttranslational modifications. Proteomics 14 (4-5), 513–524. doi:10.1002/pmic.201300344
Vlachostergios, P. J., and Papandreou, C. N. (2012). The role of the small ubiquitin-related modifier (SUMO) pathway in prostate cancer. Biomolecules 2 (2), 240–255. doi:10.3390/biom2020240
Vu, L. D., Gevaert, K., and De Smet, I. (2018). Protein Language: Post-translational modifications talking to each other. Trends Plant Sci. 23 (12), 1068–1080. doi:10.1016/j.tplants.2018.09.004
Wadosky, K. M., and Willis, M. S. (2012). The story so far: Post-translational regulation of peroxisome proliferator-activated receptors by ubiquitination and SUMOylation. Am. J. Physiol. Heart Circ. Physiol. 302 (3), H515–H526. doi:10.1152/ajpheart.00703.2011
Walchli, T., Wacker, A., Frei, K., Regli, L., Schwab, M. E., Hoerstrup, S. P., et al. (2015). Wiring the vascular network with neural cues: A CNS perspective. Neuron 87 (2), 271–296. doi:10.1016/j.neuron.2015.06.038
Wang, B., Zhang, C., Chu, D., Ma, X., Yu, T., Liu, X., et al. (2021a). Astragaloside IV improves angiogenesis under hypoxic conditions by enhancing hypoxia‑inducible factor‑1α SUMOylation. Mol. Med. Rep. 23 (4), 244. doi:10.3892/mmr.2021.11883
Wang, H., Xiong, W., Hang, S., Wang, Y., Zhang, S., and Liu, S. (2021b). Depletion of SENP1-mediated PPARγ SUMOylation exaggerates intermittent hypoxia-induced cognitive decline by aggravating microglia-mediated neuroinflammation. Aging (Albany NY) 13 (11), 15240–15254. doi:10.18632/aging.203084
Wang, H., Yang, T., Sun, J., Zhang, S., and Liu, S. (2021c). SENP1 modulates microglia-mediated neuroinflammation toward intermittent hypoxia-induced cognitive decline through the de-SUMOylation of NEMO. J. Cell. Mol. Med. 25 (14), 6841–6854. doi:10.1111/jcmm.16689
Wang, L., Deng, S., Lu, Y., Zhang, Y., Yang, L., Guan, Y., et al. (2012). Increased inflammation and brain injury after transient focal cerebral ischemia in activating transcription factor 3 knockout mice. Neuroscience 220, 100–108. doi:10.1016/j.neuroscience.2012.06.010
Wang, L., Wansleeben, C., Zhao, S., Miao, P., Paschen, W., and Yang, W. (2014a). SUMO2 is essential while SUMO3 is dispensable for mouse embryonic development. EMBO Rep. 15 (8), 878–885. doi:10.15252/embr.201438534
Wang, M., and Jiang, X. (2020). SUMOylation of vascular endothelial growth factor receptor 2 inhibits the proliferation, migration, and angiogenesis signaling pathway in non-small cell lung cancer. Anticancer Drugs 31 (5), 492–499. doi:10.1097/CAD.0000000000000896
Wang, R., Wang, Y., Mu, N., Lou, X., Li, W., Chen, Y., et al. (2017). Activation of NLRP3 inflammasomes contributes to hyperhomocysteinemia-aggravated inflammation and atherosclerosis in apoE-deficient mice. Lab. Investig. 97 (8), 922–934. doi:10.1038/labinvest.2017.30
Wang, Y. C., Peterson, S. E., and Loring, J. F. (2014b). Protein post-translational modifications and regulation of pluripotency in human stem cells. Cell. Res. 24 (2), 143–160. doi:10.1038/cr.2013.151
Wang, Y., Wang, N., Cai, B., Wang, G. Y., Li, J., and Piao, X. X. (2015). In vitro model of the blood-brain barrier established by co-culture of primary cerebral microvascular endothelial and astrocyte cells. Neural Regen. Res. 10 (12), 2011–2017. doi:10.4103/1673-5374.172320
Wen, J., Wang, J., Guo, L., Cai, W., Wu, Y., Chen, W., et al. (2019). Chemerin stimulates aortic smooth muscle cell proliferation and migration via activation of autophagy in VSMCs of metabolic hypertension rats. Am. J. Transl. Res. 11 (3), 1327–1342.
Winkler, E. A., Bell, R. D., and Zlokovic, B. V. (2011). Central nervous system pericytes in health and disease. Nat. Neurosci. 14 (11), 1398–1405. doi:10.1038/nn.2946
Witcher, K. G., Eiferman, D. S., and Godbout, J. P. (2015). Priming the inflammatory pump of the CNS after traumatic brain injury. Trends Neurosci. 38 (10), 609–620. doi:10.1016/j.tins.2015.08.002
Woo, C. H., Shishido, T., McClain, C., Lim, J. H., Li, J. D., Yang, J., et al. (2008). Extracellular signal-regulated kinase 5 SUMOylation antagonizes shear stress-induced antiinflammatory response and endothelial nitric oxide synthase expression in endothelial cells. Circ. Res. 102 (5), 538–545. doi:10.1161/CIRCRESAHA.107.156877
Wu, D. M., Lu, J., Zheng, Y. L., Zhang, Y. Q., Hu, B., Cheng, W., et al. (2012). Small interfering RNA-mediated knockdown of protein kinase C zeta attenuates domoic acid-induced cognitive deficits in mice. Toxicol. Sci. 128 (1), 209–222. doi:10.1093/toxsci/kfs124
Xu, H., Wang, Y., Lin, S., Deng, W., Peng, D., Cui, Q., et al. (2018). Ptmd: A database of human disease-associated post-translational modifications. Genomics Proteomics Bioinforma. 16 (4), 244–251. doi:10.1016/j.gpb.2018.06.004
Yang, P., Zhang, Y., Xu, J., Zhang, S., Yu, Q., Pang, J., et al. (2013). SUMO1 regulates endothelial function by modulating the overall signals in favor of angiogenesis and homeostatic responses. Am. J. Transl. Res. 5 (4), 427–440.
Yang, T., Sun, J., Wei, B., and Liu, S. (2020). SENP1-mediated NEMO de-SUMOylation inhibits intermittent hypoxia induced inflammatory response of microglia in vitro. J. Cell. Physiol. 235 (4), 3529–3538. doi:10.1002/jcp.29241
Yang, W., Sheng, H., Thompson, J. W., Zhao, S., Wang, L., Miao, P., et al. (2014). Small ubiquitin-like modifier 3-modified proteome regulated by brain ischemia in novel small ubiquitin-like modifier transgenic mice: Putative protective proteins/pathways. Stroke 45 (4), 1115–1122. doi:10.1161/STROKEAHA.113.004315
Yang, W., Sheng, H., Warner, D. S., and Paschen, W. (2008). Transient global cerebral ischemia induces a massive increase in protein sumoylation. J. Cereb. Blood Flow. Metab. 28 (2), 269–279. doi:10.1038/sj.jcbfm.9600523
Yang, Y., and Bedford, M. T. (2013). Protein arginine methyltransferases and cancer. Nat. Rev. Cancer 13 (1), 37–50. doi:10.1038/nrc3409
Yang, Y., He, Y., Wang, X., Liang, Z., He, G., Zhang, P., et al. (2017). Protein SUMOylation modification and its associations with disease. Open Biol. 7 (10), 170167. doi:10.1098/rsob.170167
Yao, Y., Li, H., Da, X., He, Z., Tang, B., Li, Y., et al. (2019). SUMOylation of Vps34 by SUMO1 promotes phenotypic switching of vascular smooth muscle cells by activating autophagy in pulmonary arterial hypertension. Pulm. Pharmacol. Ther. 55, 38–49. doi:10.1016/j.pupt.2019.01.007
Yeh, E. T., Gong, L., and Kamitani, T. (2000). Ubiquitin-like proteins: New wines in new bottles. Gene 248 (1-2), 1–14. doi:10.1016/s0378-1119(00)00139-6
Yu, S., Galeffi, F., Rodriguiz, R. M., Wang, Z., Shen, Y., Lyu, J., et al. (2020). Small ubiquitin-like modifier 2 (SUMO2) is critical for memory processes in mice. FASEB J. 34 (11), 14750–14767. doi:10.1096/fj.202000850RR
Zanella, C. A., Tasca, C. I., Henley, J. M., Wilkinson, K. A., and Cimarosti, H. I. (2020). Guanosine modulates SUMO2/3-ylation in neurons and astrocytes via adenosine receptors. Purinergic Signal 16 (3), 439–450. doi:10.1007/s11302-020-09723-0
Zhang, H., Huang, D., Zhou, J., Yue, Y., and Wang, X. (2019). SUMOylation participates in induction of ischemic tolerance in mice. Brain Res. Bull. 147, 159–164. doi:10.1016/j.brainresbull.2019.02.012
Zhang, H., Kuai, X., Ji, Z., Li, Z., and Shi, R. (2013). Over-expression of small ubiquitin-related modifier-1 and sumoylated p53 in colon cancer. Cell. Biochem. Biophys. 67 (3), 1081–1087. doi:10.1007/s12013-013-9612-x
Zhang, L., Liu, X., Sheng, H., Liu, S., Li, Y., Zhao, J. Q., et al. (2017). Neuron-specific SUMO knockdown suppresses global gene expression response and worsens functional outcome after transient forebrain ischemia in mice. Neuroscience 343, 190–212. doi:10.1016/j.neuroscience.2016.11.036
Zhang, X., Wen, H., and Shi, X. (2012). Lysine methylation: Beyond histones. Acta Biochim. Biophys. Sin. (Shanghai) 44 (1), 14–27. doi:10.1093/abbs/gmr100
Zhang, Z. B., Ruan, C. C., Chen, D. R., Zhang, K., Yan, C., and Gao, P. J. (2016). Activating transcription factor 3 SUMOylation is involved in angiotensin II-induced endothelial cell inflammation and dysfunction. J. Mol. Cell. Cardiol. 92, 149–157. doi:10.1016/j.yjmcc.2016.02.001
Zhao, Y. H., Liu, N. W., Ke, C. C., Liu, B. W., Chen, Y. A., Luo, C., et al. (2019). Combined treatment of sodium ferulate, n-butylidenephthalide, and ADSCs rehabilitates neurovascular unit in rats after photothrombotic stroke. J. Cell. Mol. Med. 23 (1), 126–142. doi:10.1111/jcmm.13894
Zhao, Z., Nelson, A. R., Betsholtz, C., and Zlokovic, B. V. (2015). Establishment and dysfunction of the blood-brain barrier. Cell. 163 (5), 1064–1078. doi:10.1016/j.cell.2015.10.067
Zheng, N., and Shabek, N. (2017). Ubiquitin ligases: Structure, function, and regulation. Annu. Rev. Biochem. 86, 129–157. doi:10.1146/annurev-biochem-060815-014922
Zhou, F., Dai, A., Jiang, Y., Tan, X., and Zhang, X. (2016). SENP‑1 enhances hypoxia‑induced proliferation of rat pulmonary artery smooth muscle cells by regulating hypoxia‑inducible factor‑1α. Mol. Med. Rep. 13 (4), 3482–3490. doi:10.3892/mmr.2016.4969
Zhou, H. J., Xu, Z., Wang, Z., Zhang, H., Zhuang, Z. W., Simons, M., et al. (2018). SUMOylation of VEGFR2 regulates its intracellular trafficking and pathological angiogenesis. Nat. Commun. 9 (1), 3303. doi:10.1038/s41467-018-05812-2
Zhou, H., Shen, D. F., Bian, Z. Y., Zong, J., Deng, W., Zhang, Y., et al. (2011). Activating transcription factor 3 deficiency promotes cardiac hypertrophy, dysfunction, and fibrosis induced by pressure overload. PLoS One 6 (10), e26744. doi:10.1371/journal.pone.0026744
Zhu, X., Ding, S., Qiu, C., Shi, Y., Song, L., Wang, Y., et al. (2017). SUMOylation negatively regulates angiogenesis by targeting endothelial NOTCH signaling. Circ. Res. 121 (6), 636–649. doi:10.1161/CIRCRESAHA.117.310696
Zhu, X., Qiu, C., Wang, Y., Jiang, Y., Chen, Y., Fan, L., et al. (2022). FGFR1 SUMOylation coordinates endothelial angiogenic signaling in angiogenesis. Proc. Natl. Acad. Sci. U. S. A. 119 (26), e2202631119. doi:10.1073/pnas.2202631119
Zibara, K., Ballout, N., Mondello, S., Karnib, N., Ramadan, N., Omais, S., et al. (2019). Combination of drug and stem cells neurotherapy: Potential interventions in neurotrauma and traumatic brain injury. Neuropharmacology 145 (1), 177–198. doi:10.1016/j.neuropharm.2018.09.032
Keywords: acquired brain injury, neurovascular dysfunction, small ubiquitin-like modifier, SUMO, post-translational modifications
Citation: Luo P, Li L, Huang J, Mao D, Lou S, Ruan J, Chen J, Tang R, Shi Y, Zhou S and Yang H (2023) The role of SUMOylation in the neurovascular dysfunction after acquired brain injury. Front. Pharmacol. 14:1125662. doi: 10.3389/fphar.2023.1125662
Received: 16 December 2022; Accepted: 10 March 2023;
Published: 22 March 2023.
Edited by:
Chongjie Cheng, Chongqing Medical University, ChinaReviewed by:
Cheng Yin, Sichuan Academy of Medical Sciences and Sichuan Provincial People’s Hospital, ChinaZhaohua Tang, First Affiliated Hospital of Chongqing Medical University, China
Copyright © 2023 Luo, Li, Huang, Mao, Lou, Ruan, Chen, Tang, Shi, Zhou and Yang. This is an open-access article distributed under the terms of the Creative Commons Attribution License (CC BY). The use, distribution or reproduction in other forums is permitted, provided the original author(s) and the copyright owner(s) are credited and that the original publication in this journal is cited, in accordance with accepted academic practice. No use, distribution or reproduction is permitted which does not comply with these terms.
*Correspondence: Shuai Zhou, enNodWFpQGt1c3QuZWR1LmNu; Haifeng Yang, NDI5ODEwNTYwQHFxLmNvbQ==
†These authors have contributed equally to this work