- 1School of Public Health, Qingdao University, Qingdao, China
- 2School of Life Science and Technology, ShanghaiTech University, Shanghai, China
- 3Linyi Center for Disease Control and Prevention, Linyi, China
Air pollution has become one of the most serious health risks as a result of industrialization, especially in developing countries. More attention has been drawn to the relationship between obesity/overweight and fine particulate matter (PM2.5). Especially for susceptible populations, the impact of air pollution on children and adolescents has attracted more public attentions. However, the detailed underlying mechanism influencing obesity or overweight under PM2.5 exposure is still unknown. Therefore, young mice were exposed to PM2.5 using the real-ambient exposure system that we previously established in Shijiazhuang city. Compared with the traditionally concentrated air particle (CAP) system, our real-ambient exposure system provides similar PM2.5 concentrations and characteristics as outdoor ambient air and minimizes the influence of external interfering factors. After 8 weeks of exposure to PM2.5, the weight of gonadal white adipose tissue (gWAT) and subcutaneous white adipose tissue (sWAT) was considerably increased, accompanied by a significantly enlarged size of adipocytes in sWAT. Importantly, multiomics analysis indicated altered metabolites involved in the lipid metabolism pathway, and transcriptomic analysis revealed notably changed signaling pathways related to fatty acid metabolism. Moreover, the mtDNA copy number, mitochondrial activity and fatty acid oxidation (FAO) were increased in the liver under PM2.5 exposure. Taken together, our research investigated the hypotrophy of adipose tissue in young mice, supported an imbalance in lipid metabolism based on multiomics analysis, and revealed disordered mitochondrial function under PM2.5 exposure. Our study provided new insight into the hazardous effects of air pollution, and extended our understanding on the underlying mechanism.
1 Introduction
Air pollution has become a global disease burden, contributing to 7.6% of the total annual mortality rate and 103.1 million deaths annually (Adar et al., 2014; Hassan Bhat et al., 2021). Recently, more and more studies revealed that air pollution contributed to the occurrence of obesity and overweight (Smith et al., 2020), especially in the pediatric population (Vrijheid et al., 2020). In fact, due to a faster rate of breathing, children can inhale more air pollutants than adults, which makes them one of the most susceptible populations to air pollution (Sly and Flack, 2008; Guo et al., 2019). A study showed that air pollution can cause disruptions of fatty acid metabolism in young people living near roads and may increase the risk of obesity-related metabolic diseases in adolescents (Chen et al., 2019). Accumulating epidemiological research has revealed that childhood exposure to air pollution contributes to overweight and a higher body mass index (BMI) in children (Tong et al., 2022). However, the underlying mechanism remains to be clarified. Therefore, in this study, young mice were used in our real-ambient exposure system to mimic the characteristics of childhood.
Overweight and obesity are characterized by the expansion of white adipose tissues (WATs), which occurs due to the storage of excessive lipid droplets, particularly in gonadal WAT (gWAT) and subcutaneous WAT (sWAT) (Scheja and Heeren, 2016). Obesity is usually accompanied by metabolic dysfunction, especially closely related to lipid metabolism. Additionally, the liver is a crucial organ in the regulation of lipid metabolism (Hellerstein, 1999; Wallace and Metallo, 2020). An increasing number of epidemiological studies have found that both short and long air pollution exposure contribute to an imbalance in lipid metabolism, which strongly facilitates the development of overweight and obesity (Kim et al., 2019; Furlong and Klimentidis, 2020; Simkova et al., 2020).
As the main site for the oxidation of fatty acids, mitochondria are crucial for maintaining the balance of lipid metabolism (Ipsen et al., 2018). Importantly, mitochondria are involved in the tricarboxylic acid cycle (TCA) and oxidative phosphorylation pathways. Accumulating studies have illustrated that air pollution contributes to mitochondrial dysfunction, mitochondrial metabolic abnormalities, and morphological abnormalities (Li et al., 2015a; Liu et al., 2020; Gao et al., 2022). It has been reported that the quantity and size of mitochondria are also changed under PM2.5 exposure (Mansouri et al., 2018).
The real-ambient exposure system that was previously established in Shijiazhuang city was applied in this study (Jiang et al., 2020; Ding et al., 2021). Compared to the concentrated ambient particle (CAP) exposure system, our system maintains the original chemical characteristics of PM2.5 and a concentration of PM2.5 similar to that of ambient PM2.5. In addition, our system maintains constant temperature, pressure, humidity, and low noise conditions without interrupting the normal activities and feeding of animals during the exposure period. Therefore, our real-ambient exposure system provides a perfect model for studying the harmful effects of PM2.5.
In our study, we examined the hypertrophy of adipose tissue in young mice, identified the disorder of lipid metabolism with multi-omics analysis and illustrated the increased mitochondrial activity in the real-ambient exposure system.
2 Materials and methods
2.1 Animals and whole-body inhaled PM exposure
Three-week-old female C57BL/6 mice were purchased in Beijing from Vital River Company and acclimated for 7 days after arrival. As per our previous protocol (Li et al., 2019; Jiang et al., 2020), the mice were randomly assigned to the filtered air (FA) and particulate matter (PM) groups in the real-ambient exposure system during winter in Shijiazhuang city (n = 10). Briefly, individually ventilated cages (IVCs) were linked directly to filtered air (FA group) or ambient outdoor air (PM group) after removing PM2.5 in ambient indoor air by three layers of high-efficiency particulate air (HEPA) filters. During the winter, the mice were exposed for 16 h per day (from 7:00 a.m. to 11:00 p.m.) for 4 or 8 weeks, and the mice were not restricted from eating or moving around. The experimental conditions were a 12-h daylight/12-h dark cycle. The room temperature was kept between 22 and 24°C. The experimental protocol was approved by the Animal Care and Use Committee of Qingdao University.
2.2 Tissue collection
After 4 or 8 weeks of PM2.5 exposure, inferior vena cava blood collection, and the serum was collected after centrifugation. The liver, gWAT and sWAT were collected and immediately fixed in 4% PFA or frozen at −80°C.
2.3 Pathological staining
The liver, gWAT and sWAT were fixed in 4% PFA, paraffin-embedded, sectioned, and stained with hematoxylin-eosin (H&E) to observe the histomorphology. Mitochondria-related genes, including CPT1 (catalog #ab128568; Abcam), CPT2 (catalog #ab181114; Abcam), PGC1α (catalog #2178s; Cell Signaling Technology, Danvers, MA) and COX4 (catalog #11967; Cell Signaling Technology, Danvers, MA), were examined by immunohistochemistry and immunofluorescence. Briefly, the paraffin-embedded tissues were sectioned, deparaffinized with xylene, dehydrated with ethanol, blocked with 10% FBS, incubated with primary and secondary antibodies, and stained with DAB substrate solution. Finally, under a microscope, the color of the antibody staining in the tissue sections was observed.
2.4 RNA extraction and qPCR
TRIzol reagent was utilized to extract total RNA from liver, gWAT, and sWAT tissues according to the user’s manual. Reverse transcription experiments were performed to obtain cDNA templates according to the instructions (Accurate Biology, Hunan), and further real-time quantitative PCR experiments were carried out to ascertain the expression of target genes using the QuantStudio Q7 RT–PCR system. The 2−ΔΔCT approach was used to calculate the target molecule’s expression level relative to β-actin. In Supplementary Table 1, the primer sequences applied in the studies are listed.
2.5 Lipid content
According to the user’s manual, the liver was homogenized in ethanol, and lipids were extracted from the supernatant after centrifugation. TG, TC and FFA levels in the liver and serum were measured using Nanjing Jiancheng kits, and VLDL levels in the liver and serum were measured using enzyme-linked immunosorbent assay (ELISA) kits (Fankew, Shanghai). After incubation for the indicated time, the absorbance was detected by a microplate spectrophotometer, and the concentration was calculated based on a standard curve.
2.6 Metabolomic analysis
The metabolites in serum and liver were analyzed by extraction, computer detection and statistical analysis. First, 25 mg liver was added to 500 μL of extract solution (methanol:acetonitrile:water = 2:2:1) and 100 μL of serum sample was added to 400 μL of extract solution (methanol:acetonitrile = 1:1), both of which contained the isotopically labeled internal standard mixture. Mix well and sonicate for 10 min under ice-water bath conditions, noting that the liver needs to be processed by grinding. The supernatant solution was obtained by centrifugation at 12,000 rpm for 15 min at 4°C after 1 hour at −40°C. The target compounds were chromatographically separated on a Waters ACQUITY UPLC BEH Amide (2.1 mm × 100 mm, 1.7 μm) column using a Vanquish (Thermo Fisher Scientific) ultra-performance liquid chromatograph. The A-phase of liquid chromatography was aqueous containing 25 mmol/L ammonium acetate and 25 mmol/L ammonia, and the B-phase was acetonitrile. The data collection was performed on a QE HFX mass spectrometer (Orbitrap MS, Thermo) under the control of acquisition software (Xcalibur, Thermo). The raw data were also processed using ProteoWizard software.
2.7 Lipidomics analysis
The adipose tissue was ground, and water and methanol solution were added to the homogenate at a ratio of 2:1:4 to extract lipid components. To enable subsequent measurement, 250 mL of methyl tert-butyl ether (MTBE) comprising lipid standards was added after vortex mixing. The samples were bathed in 10°C water for 30 min (ultrasonic frequency 40 kHz, power 100 W). The organic phase was then separated by centrifugation at 10°C (1,400 × g) for 10 min. LC-MS analysis was performed using ultra-high pressure liquid chromatography combined with a Sciex Triple TOF 6600 liquid chromatograph. The Thermo Hypersil Gold C18 column (100 mm × 2.1 mm, 1.9 μm) was used. The mobile phase A was water-acetonitrile (6:4 v/v, containing 0.1% formic acid and 10 mmol ammonium formate), and the mobile phase B was acetonitrile-isopropanol (1:9 v/v, containing 0.1% formic acid and 1 mmol ammonium formate). Data analysis was performed using Peak view software to analyze lipid composition.
2.8 Transcriptome analysis
Total RNA was extracted from the liver, and the quality of the RNA was examined for concentration and degradation. mRNA was enriched with oligo-(dT) beads, and subsequently, cDNA was generated with poly (A) added to the 3′ end. After purification, sequencing adapters were added to the QiaQuick PCR kit, agarose gel electrophoresis was used to assess the size of the fragments, and PCR was performed to amplify the cDNA. After library construction, the quality inspection of the library was qualified, and PE150 sequencing was performed using the Illumina NovaSeq platform. KOBAS software was used to perform a KEGG enrichment analysis.
2.9 Statistical analysis
GraphPad Prism 8.3.0 software was used for the statistical analysis of the experimental data, and the unpaired two-tailed t-test was run. Error bars represent ± SEM, and statistical significance was considered when the p value was less than 0.05. *, p < 0.05, **, p < 0.01, and ***, p < 0.001. Regarding metabolomics analysis, after preprocessing the data, a set of observed potentially correlated variables was transformed into linearly uncorrelated variables by orthogonal transformation using principal component analysis (PCA). Using SIMCA software (V16.0.2, Sartorius Stedim Data Analytics AB, Umea, Sweden), the data were formatted by logarithmic (LOG) transformation plus centralization (CTR), and then automatic modeling analysis was performed to obtain scores indicating the first and second ranked principal components, respectively [PC (1) and PC (2)]. The orthogonal partial least squares-discriminant analysis (OPLS-DA) statistical method was also used to filter out orthogonal variables in metabolites that were not correlated with categorical variables, and non-orthogonal and orthogonal variables were analyzed separately. Thus, a more reliable letter of correlation between group differences of metabolites and experimental groups was obtained. Finally, SIMCA software was used to obtain the Variable Importance in the Projection (VIP) by logarithmic (LOG) transformation plus UV formatting of the data. VIP is the importance of the variables to the model, which describes the overall contribution of each variable to the model, and is usually set at a threshold value of VIP > 1, p value < 0.05.
3 Results
3.1 Chronic real-ambient PM2.5 exposure facilitated the hypertrophy of white adipose tissue
To examine the adverse effect of PM2.5 on adipose tissue, young mice were exposed to the real-ambient exposure system in Shijiazhuang during the winter of 2019. During the exposure period, the daily average ambient PM2.5 concentration reached 140.8 μg/m3 (Ji et al., 2022). After exposure for 4 or 8 weeks, the sWAT and gWAT were isolated and weighed. Interestingly, after 8 weeks of exposure, the gWAT and sWAT mass increased significantly in the PM group compared with the FA group (Figures 1A, B). Considering the close crosstalk between liver and adipose tissue (Scheja and Heeren, 2016), the liver weight was evaluated as well. Conversely, the liver weight was markedly reduced in the PM group compared with the FA group (Figure 1C). Because of the contribution of hypertrophic adipocytes to the growth of adipose tissue (Ghaben and Scherer, 2019), hematoxylin-eosin (H&E) staining was used to determine the adipocyte size in sWAT and gWAT. Consistently, after exposure for 8 weeks, the adipocytes of the sWAT in the PM group were considerably larger than those in the FA group, accompanied by a slightly increased adipocyte size in the gWAT in the PM groups (Figure 1D). Furthermore, the histomorphology of the liver was detected with H&E staining. However, the morphology was similar in the FA and PM groups (Figure 1D). Considering the pivotal role of fatty acids during the crosstalk of adipose tissue and liver (Fuchs et al., 2022), the levels of free fatty acids (FFAs) and very-low-density lipoproteins (VLDLs) in the liver and serum were measured by ELISA. We found a trend of higher levels of FFAs in the liver and lower levels in the serum in the PM group (Figure 1E, G). Importantly, the level of serum VLDL was strikingly increased in the PM group, accompanied by a decreasing trend in the liver, indicating the influx of VLDLs from hepatocytes to serum (Figure 1F, H). Taken together, our findings showed that chronic exposure to PM2.5 exacerbated the expansion of fat tissue.
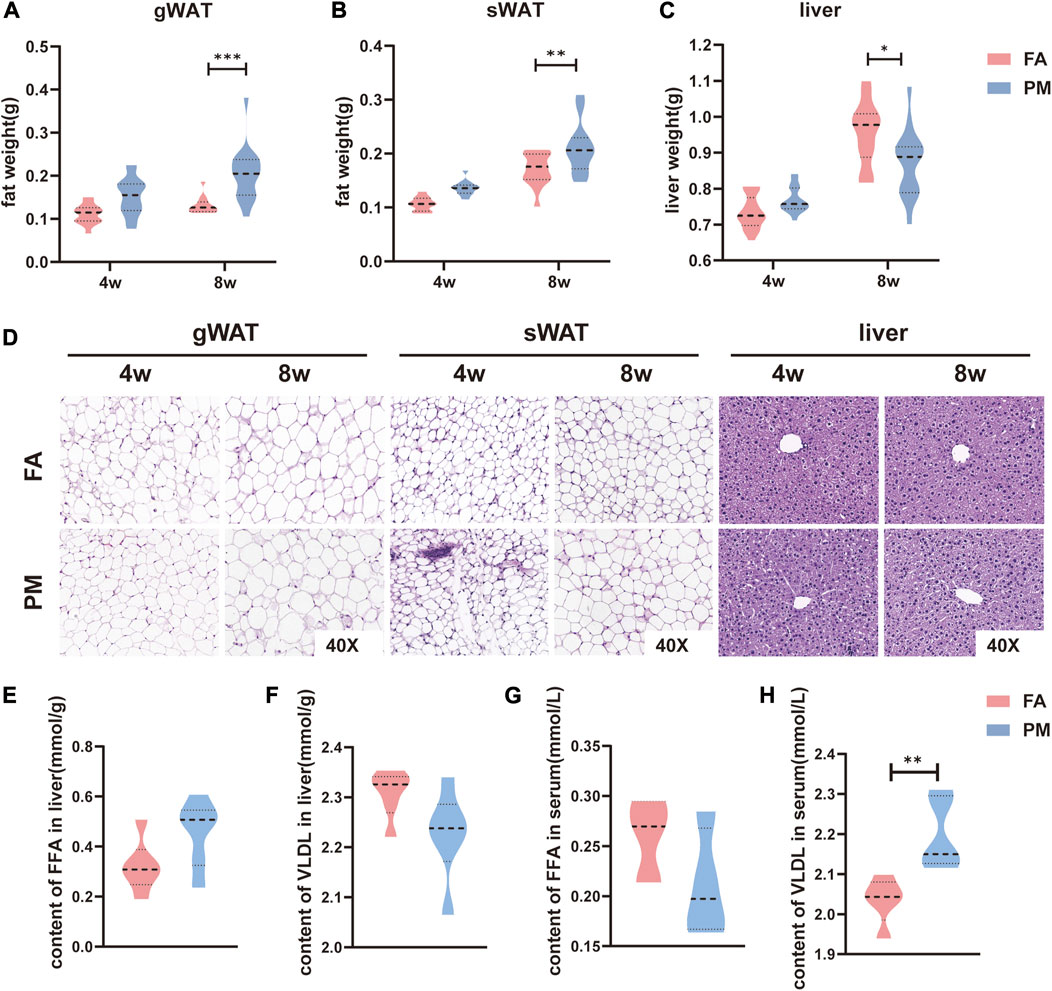
FIGURE 1. Hypertrophy of adipose tissue overgrowth in young mice after PM2.5 exposure (A-C) The mass of gWAT, sWAT and liver tissue after exposure to PM2.5 for 4 and 8 weeks (n = 17–20). (D) Hematoxylin and eosin (H&E) staining was used to observe the morphology of adipose tissue and liver tissue (n = 4). (E-F) The contents of FFAs and VLDL in the liver after exposure for 8 weeks (n = 5). (G-H) Serum FFA and VLDL contents after exposure for 8 weeks (n = 5). *, p < 0.05; **, p < 0.01; ***, p < 0.001.
3.2 Lipidomic remodeling of WAT and metabolomic reprogramming of serum and liver to PM2.5 exposure
It is generally known that lipid metabolism regulates the growth of adipose tissue (Samuel and Shulman, 2016). After exposure for 8 weeks, lipidomic analysis was performed in gWAT and sWAT. Based on PLS-DA, the lipid signature of gWAT and sWAT in the PM group was considerably distinct from that of the FA group (Figures 2A, D). According to volcano plots, some notably changed lipid species were observed in gWAT and sWAT (Figures 2B, E). To further identify the specific lipid species that were affected by PM2.5, all significantly altered lipid species were visualized by heatmaps. A total of 18 lipid species markedly changed in gWAT (Figure 2C), while nine species considerably changed in sWAT (Figure 2E). The lipid classes included diacylglycerol (DG), triacylglycerol (TG), acyl-carnitine coenzyme Q (CoQ), phosphatidylcholine (PC), phosphatidylethanolamine (PE), phosphatidylglycerol (PG), phosphatidylserine (PS), sphingomyelin (SM), phosphatidylethanol (PET), lyso-phosphatidylethanolamine (LPE), lyso-phosphatidylcholine (LPC) and lyso-phosphatidylglycerol (LPG) (Figure 2F).
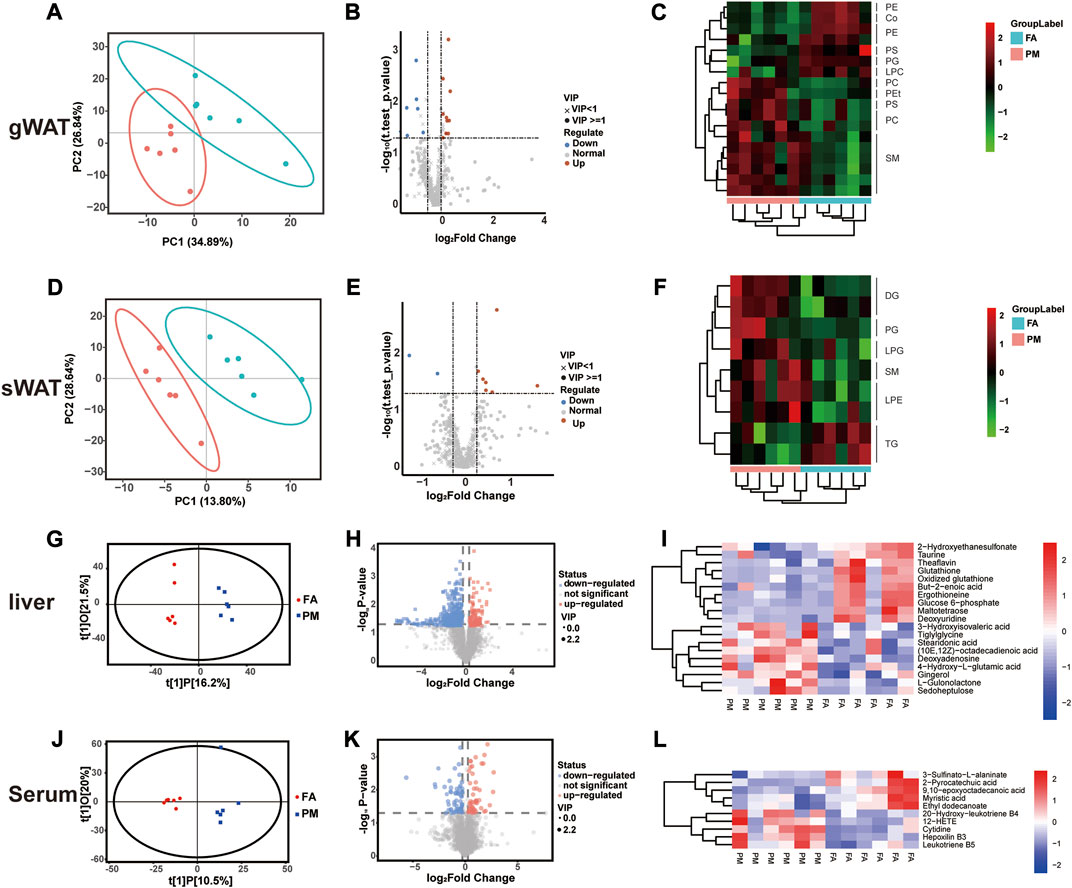
FIGURE 2. Metabolic reprogramming in gWAT, sWAT, liver and serum based on multiomics analysis (A–F) The lipidomic analysis results of gWAT and sWAT (n = 6), including PLS-DA plots (A, D), volcano plots (B, E), and cluster heatmaps (C, F). (G–H) Liver and serum metabolomic analysis results (n = 6), including OPLS-DA plots (G, J), volcano plots (H, K), and cluster heatmaps (I, L). PC [1] and PC [2] in partial least squares discriminant analysis (PLS-DA) denote the scores of the first and second ranked principal components, respectively. t[1]P in orthogonal partial least squares discriminant analysis (OPLS-DA) denotes the prediction score of the first principal component, indicating the difference between sample groups; t[1]O denotes the score of the orthogonal principal component, indicating the difference within sample groups.
The liver plays a crucial role in the expansion of adipose tissue by regulating lipid influx, and metabolites usually circulate in the bloodstream to realize crosstalk among tissues (Scheja and Heeren, 2016). Therefore, the metabolites in the liver and serum were examined with metabolomics analysis. Consistently, based on OPLS-DA, the metabolomic profiling of liver and serum in the PM group was notably distinct from that in the FA group (R2X = 0.36, R2Y = 0.989, Q2 = 0.211 in Figure 2G, R2X = 0.407, R2Y = 0.882, Q2 = 0.0422 in Figure 2J) (Figures 2G, J). Based on volcano and clustering heatmaps, when compared to the FA group, various metabolites in the liver and serum displayed significant differences in the PM group (Figures 2H, K). Consistently, the level of TG in the liver was increased after exposure to PM2.5 (Figure 2I). Glutathione is believed to be a key regulator in fat burning and storage (Rom et al., 2020). Glutathione was altered in the liver during PM2.5 exposure, accompanied by significant downregulation of the unsaturated fatty acid but-2-enoic acid (Figure 2I). At the same time, we also found that PM2.5 exposure had an effect on glucose metabolism, and its key metabolite glucose 6-phosphate was significantly reduced at the end of the exposure (Figure 2I). 12-Hydroxyeicosatetraenoic acid (12-HETE) and leukotriene B5 are considered inflammation markers (Maaløe et al., 2011; Cheng et al., 2019). Both of these metabolites notably increased in serum after PM exposure (Figure 2L). Taken together, these results revealed glycolipid metabolic remodeling in adipose tissue and liver when exposed to PM2.5.
3.3 Altered metabolism-related signaling pathways based on transcriptome analysis during PM2.5 exposure
To investigate the underlying molecular mechanism, the liver was subjected to transcriptomic analysis. KEGG enrichment analysis revealed the top 20 upregulated and downregulated signaling pathways in the liver. Intriguingly, the notably changed signaling pathways included metabolic pathways, fatty acid metabolism, fatty acid degradation, fatty acid elongation, biosynthesis of unsaturated fatty acids, oxidative phosphorylation (Figure 3A). These pathways are well known to be involved in regulating lipid metabolic homeostasis. Besides, in agreement with the results of the metabolomic analysis of this study, glycolysis/gluconeogenesis, and pentose and glucuronate interconversion pathways were remarkably altered (Figure 3A).
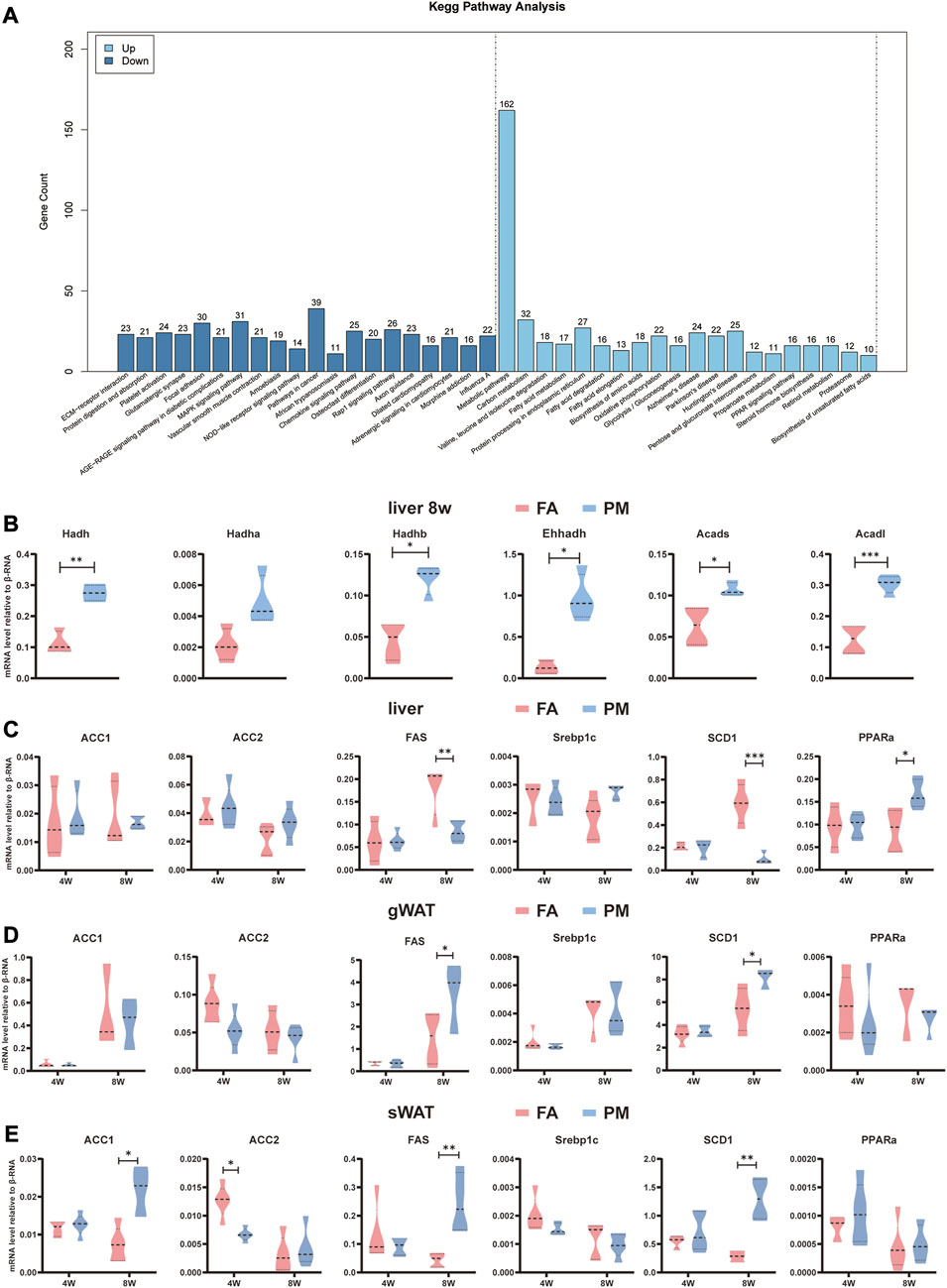
FIGURE 3. Altered metabolism-related signaling pathways based on transcriptome analysis (A) KEGG enrichment analysis according to transcriptome analysis in the liver after exposure for 8 weeks. Differential genes were screened according to p value < 0.05 and |log2FC|>12. The vertical axis indicates the number of differentially expressed genes, while the horizontal axis reflects the enrichment of the top 20 pathways that were significantly upregulated or downregulated (n = 4). (B–D) Relative mRNA levels of genes related to fatty acid synthesis and fatty acid oxidation in the liver, sWAT, and gWAT (n = 5). (E) Relative mRNA levels of β-oxidation-related genes in the liver (n = 5). *, p < 0.05; **, p < 0.01; ***, p < 0.001.
Acetyl-CoA carboxylase 1 (ACC1), acetyl-CoA carboxylase 2 (ACC2), fatty acid synthetase (FAS), sterol regulatory element-binding transcription factor 1 (Srebp1c), and stearoyl-coenzyme A desaturase 1 (SCD1) participate in the de novo lipogenesis (DNL) pathway. Peroxisome proliferator-activated receptor alpha (PPARα) plays a key role in lipid oxidation. Compared to the FA group, the mRNA levels of FAS and SCD1 were dramatically reduced (Figure 3B), whereas the mRNA levels of PPARα significantly increased in PM group after exposed for 8w (Figure 3C). Hydroxy acyl-CoA dehydrogenase (Hadh), acyl-CoA dehydrogenase (Acad), enoyl-CoA hydratase and 3-hydroxy acyl CoA dehydrogenase (Ehhadh) and cytochrome P450, family 4, subfamily a (Cyp4a) are involved in lipid oxidation (Hoek-van den Hil et al., 2013; Ipsen et al., 2018). These genes were found altered by transcriptome analysis, and were further validated with qPCR. Consistently, Hadh, Hadhb, Ehhadh, Acad long chain (Acadl) and Acad short chain (Acads) were greatly enhanced in the liver in the PM group (Figure 3C). In addition, the expression of metabolism-related genes was examined in gWAT and sWAT. The findings revealed that after exposure to PM2.5, the levels of FAS and SCD1 were considerably increased in gWAT and sWAT, accompanied by higher levels of ACC1 in sWAT (Figures 3D, E). These results suggested that PM2.5 exposure disturbed signaling pathways involved in metabolism in the liver and adipose tissue.
3.4 Increased mitochondrial activity under PM2.5 exposure
The primary function of mitochondria is maintaining energy homeostasis by breaking down lipids and other molecules (Thorp, 2019). Therefore, mitochondrial activity was evaluated after PM2.5 exposure. After 8 weeks of exposure, the mtDNA copy number were comparative in FA and PM groups in sWAT, gWAT and liver based on qPCR assays (Figure 4A). Carnitine palmitoyltransferase (CPT1, CPT2), as a mitochondrial enzyme, is critical in fatty acid oxidation (FAO) (Nassir and Ibdah, 2014). Peroxisome proliferator-activated receptor-gamma coactivator 1α (PGC1α) is considered a primary regulator of mitochondrial biosynthesis (Li et al., 2016; Jamwal et al., 2021). The levels of CPT1, CPT2, and PGC1α were investigated using qPCR and IHC staining in liver, gWAT, and sWAT. The levels of CPT2 and PGC1α were considerably increased in sWAT, and the mRNA expression of CPT1, CPT2 and PGC1α was considerably increased in the liver (Figures 4B–D). Consistently, the protein levels of these genes markedly increased in the gWAT, sWAT and liver after exposure for 8 weeks (Figure 4E). As a marker of mitochondria, cytochrome c oxidase subunit 4 (Cox4) was determined by immunofluorescence labeling. We found increased expression of Cox4 in gWAT and sWAT after exposure to PM2.5 (Figure 4E). In addition, the mRNA expression of Cox4 was significantly elevated in the liver after exposed to PM2.5 for 8 weeks (Figure 4D). These findings revealed that the number and activity of mitochondria increased during PM2.5 exposure.
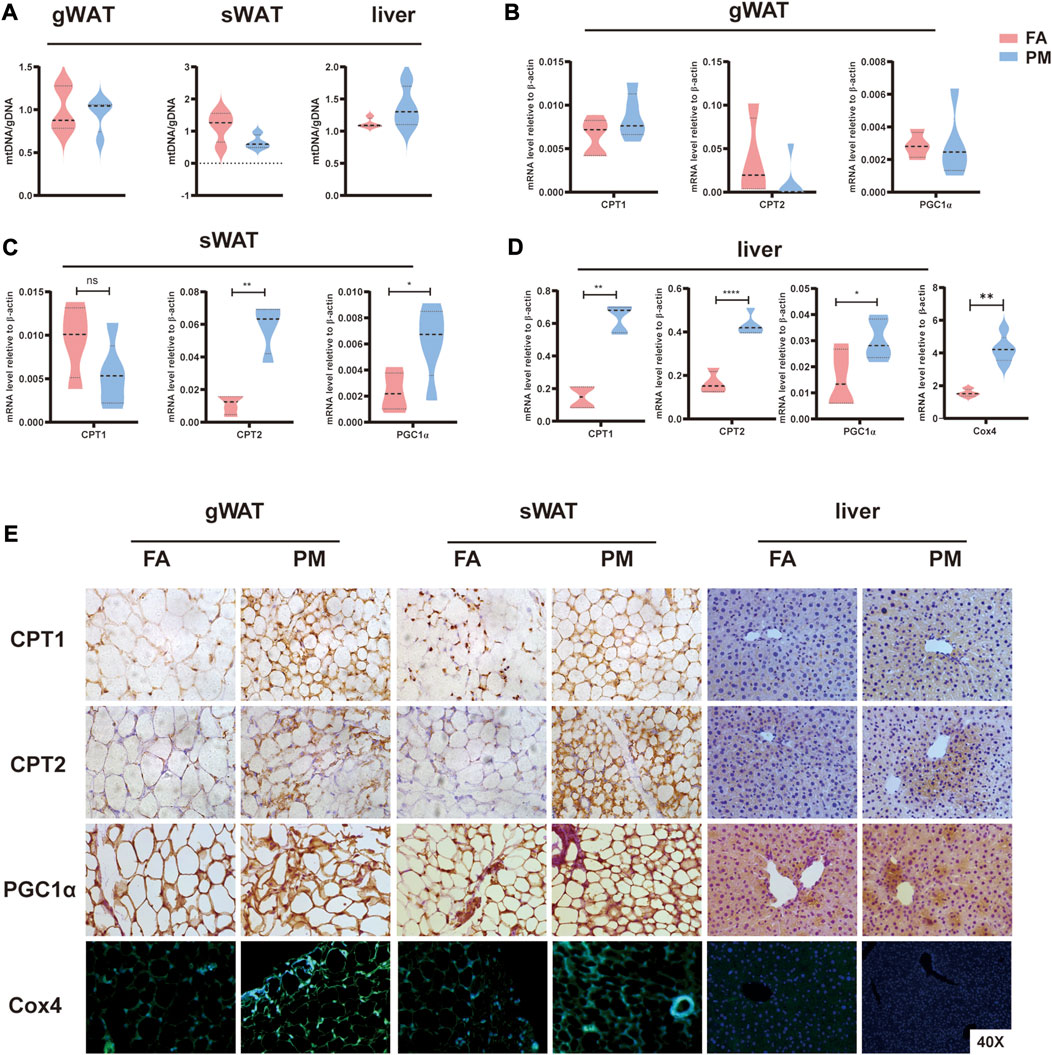
FIGURE 4. Abnormal mitochondrial activity in liver and adipose tissue under PM2.5 exposure (A) mtDNA copy number levels in gWAT, sWAT and liver tissue (n = 5). (B–D) The mRNA levels of the CPT1, CPT2, and PGC1α enzymes in gWAT, sWAT and liver tissue, respectively (n = 5). (E) Immunohistochemistry and immunofluorescence techniques were used to detect CPT1, CPT2, and PGC1α protein levels and mitochondrial numbers (Cox4 labeling) in gWAT, sWAT and liver tissue (n = 3). *, p < 0.05; **, p < 0.01; ***, p < 0.001.
4 Discussion
Air pollution has been considered as a worldwide public health concern and has drawn more attention recently (Ostro et al., 2018). As a primary component in air pollution, PM2.5 directly enters the blood and contributes to multiorgan toxicities (Goldberg and Villeneuve, 2008; Xu et al., 2016; Xu et al., 2018). Recently, accumulating research has shown that exposure to PM2.5 can act as an independent factor to disrupt the balance of lipid metabolism (McGuinn et al., 2019; Zhang et al., 2021). Air pollution can increase the BMI of children and can increase the probability of developing obesity, as reported by most epidemiological studies (Tong et al., 2022). The underlying molecular mechanisms, however, are not completely understood. Consistently, in our research, PM2.5 inhalation exposure caused remarkable hypertrophy of adipose tissue, accompanied by disturbances in lipid metabolism in young mice.
The PM2.5 concentration in Shijiazhuang is extremely high, with annual average PM2.5 concentrations as high as 138.8 μg/m3 in 2014–2016 (Xie et al., 2019). Therefore, our real-ambient exposure system was built in Shijiazhuang city, as described in our previous reports (Li et al., 2019). Simulating real environmental exposure is one of the innovative points of this paper. Our real-ambient exposure system built in Shijiazhuang provided flawless control with filtered air in which the PM was undetectable and air that maintained a similar and changeable PM concentration in the exposure chamber coinciding with the outdoor air environment. Moreover, in the real-ambient system, the characteristics of PM were comparable with those of outdoor air pollution. The composition of PM2.5 in our system was already measured in our previous study, with PAHs, PCBs and polychlorinated dibenzo-p-dioxins (PCDD) in organic extracts and metals and trace elements (Zn, Mn, As, Pb, Li, Cr, NO3− and SO42−) in the water-soluble fraction (Li et al., 2020). In addition, our system also maintains constant temperature, pressure, humidity and low noise levels, minimizing the effects on animal activity. Our system provides a perfect model for studying whole-body inhalation exposure to air pollution. In fact, we have already reported the toxicities of PM2.5 on the lung and cardiovascular system by applying the real-ambient exposure system (Cui et al., 2020; Su et al., 2020; Zhang et al., 2022).
According to a World Health Organization survey in 2020, 38.9 million children under the age of five were overweight worldwide, and the number of adolescents and adults population suffering from obesity reached 340 million and 650 million, respectively (Rajagopalan et al., 2018). A nationwide survey of obesity and overweight prevalence based on Chinese standards found overweight rates of 6.8% and 11.1% in children (<6 years) and adolescents (6–17 years), respectively, and obesity rates of 3.6% and 7.9% (Pan et al., 2021). Obesity in early childhood not only substantially increases the chances of obesity in adulthood but also causes sequelae, such as premature death, type 2 diabetes and cardiovascular disease. Recently accumulating epidemiological studies have supported the link between air pollution and obesity (Dong et al., 2014; Li et al., 2015b; Rohde and Muller, 2015). For instance, prenatal exposure to air pollution or childhood exposure to traffic pollution increases BMI and contributes to the development of obesity (Fioravanti et al., 2018; Tong et al., 2022). Consistently, our results show that whole-body PM2.5 exposure leads to significant hypertrophy of white adipose tissue in young mice, including sWAT and gWAT.
It is well known that the development of overweight and obesity is accompanied by excessive storage of lipids in adipose tissue (Park et al., 2021). Lipid metabolism was reported to be influenced by PM2.5 exposure, thereby affecting the expansion of adipose tissue (Zou, 2010; Chen et al., 2012; Pei et al., 2013). Lipidomics enables the systematic analysis of overall lipids, which in turn can accurately identify key lipid biomarkers in metabolic regulation and ultimately uncover the mechanisms of lipid action in a variety of life activities (Brügger, 2014). Based on lipidomics, the lipid metabolism was found disturbed in white adipose tissue after exposed to PM2.5 for 8 weeks. FFAs in tissues are stored and transported in the form of DG and TG and provide energy to the body (Alves-Bezerra and Cohen, 2017). Through lipidomics, our study found that TG was significantly reduced in sWAT after 8 weeks of PM2.5 exposure, but DG, a precursor of TG, was significantly increased. In addition, PC (a precursor for cholesterol synthesis, which turns excess lipids into smaller droplets for excretion) (Cole et al., 2011) and SM (which regulates cell membrane fluidity and cell transduction) (Hori et al., 2021) were significantly increased in gWAT.
The crosstalk between the liver and adipose tissue is pivotal for regulating lipid metabolism. For example, FFAs are released into the blood by WAT, enter and provide rapid mobilization fuel for the liver and ATP for gluconeogenesis and are esterified into more complex molecules, such as VLDL, in the liver (Scheja and Heeren, 2016). In addition to lipid metabolism, the liver plays a crucial role in the metabolism of carbohydrates, proteins, and amino acids, as well as drugs (Han et al., 2016; Piccinin et al., 2019). Metabolomics can help us to comprehensively analyze the alterations of key metabolites in disease and physiological processes, predict biomarkers of disease, and find active drivers of biological processes (Rinschen et al., 2019). In our study, the results of hepatic metabolomics showed a considerable drop in glutathione and glucose 6-phosphate levels, after 8 weeks of PM2.5 exposure. Among them, glutathione plays a significant role in the TCA cycle and can promote lipid metabolism (Gao et al., 2021). In addition, there was a significant increase in stearidonic acid, which is an omega-3 polyunsaturated fatty acid that affects steroid synthesis and alters the lipid profile (Shahidi and Ambigaipalan, 2018).
Transcriptome analysis in the liver was performed to investigate the altered signaling pathways in order to further study the underlying molecular mechanism. Overall, the metabolic pathways were found significant upregulated after exposed to PM2.5, which was consistent with the metabolomic results. Our results showed that fatty acid metabolism, fatty acid degradation, fatty acid elongation, biosynthesis of unsaturated fatty acids, oxidative phosphorylation pathways are significantly altered in the liver after 8 weeks of PM2.5 exposure. These signaling pathway are involved in regulating the balance of lipid and fatty acid, including Tiglylglycine, Stearidonic acid, (10E,12Z)−octadecadienoic acid and so on. Moreover, according to transcriptome analysis the Glycolysis/Gluconeogenesis pathway was upregulated in PM group, consistent with the alteration of Glucose-6-phosphate in metabolomics analysis. Subsequently, the genes related to fatty acid synthesis and oxidation were validated by qPCR. After 8 weeks of exposure, the expression level of PPARα, a key factor controlling fatty acid oxidation, was enhanced, and the expression levels of the DNL-related genes FAS and SCD1 were decreased. Plasma levels of VLDL increased significantly after 8 weeks of exposure. Similarly, the mRNA levels of FAS and SCD1 in gWAT and sWAT increased markedly, and ACC1 also increased markedly in sWAT.
Mitochondria are considered the main sites of fatty acid oxidation and serve as the “powerhouse” for energy production. PM2.5 in ambient air induces mitochondrial damage in the mouse organism, including liver, heart and other tissues (Qiu et al., 2019; Jiang et al., 2021). Mitochondrial damage and lipid metabolism disorders promote each other. In detail, when mitochondrial dysfunction affects the homeostasis of lipid metabolism, increased tissue lipid supply and incomplete lipid oxidation will act back on the mitochondria (Gonzalez-Franquesa and Patti, 2017; Sangwung et al., 2020). Considering the central role in metabolism, mitochondrial activity was examined in our study (Xu et al., 2011; Mansouri et al., 2018). The main pathway of fat degradation is mitochondrial FAO (Houten et al., 2016). CPT1 and CPT2 are located in the inner and outer mitochondrial membranes, respectively. CPT1 and CPT2 transfer fatty acids into the mitochondria for further processing and play an integral role in the mitochondrial FAO process (Nassir and Ibdah, 2014). PGC1α, an important regulator of mitochondrial proliferation, was reported to expand the mitochondrial number and mitochondrial oxidative activity by boosting CPT1 activity (Villena, 2015; Jamwal et al., 2021). The mRNA and protein expression levels of CPT1, CPT2, and PGC1α were substantially higher in the liver and adipose tissue after PM2.5 exposure.
However, our study was conducted in winter time to ensure exposure to high PM2.5 concentration, resulting in relative short-term exposure. Moreover, we applied young female mice, which could not observe the gender difference under air pollution. In addition, we only examined the external exposure dose, but the internal exposure dose was not evaluated in the study.
5 Conclusion
In summary, our study reported the hypertrophy of adipose tissue and revealed a comprehensive analysis of lipidomic and metabolic profiles combined with transcriptomics analysis in young mice under real-ambient exposure to PM2.5. The real-ambient exposure system provided a perfect model to study the hazardous effects of air pollution due to similar concentrations and chemical characteristics of PM2.5 between the exposure system and outdoor air. The differences in metabolic compositions reflected the imbalance in lipid metabolism. Taken together, our research investigated the hypotrophy of adipose tissue in young mice, supported an imbalance in lipid metabolism based on multi-omics analysis, and revealed disordered mitochondrial function under PM2.5 exposure. The present study further extends our understanding about the health effects of exposure to PM2.5 at an early age and provide evidence-based support for epidemiological studies on air pollution increasing the prevalence of overweight or obesity in children and adolescents.
Data availability statement
Original datasets are available in a publicly accessible repository: The original contributions presented in the study are publicly available. This data can be found here: www.ebi.ac.uk/metabolights/MTBLS7396, www.ebi.ac.uk/metabolights/MTBLS7397.
Ethics statement
The animal study was reviewed and approved by Animal Care and Use Committee of Qingdao University.
Author contributions
HS and TG: Conceptualization, methodology, responsible for data management, investigation, and paper writing; JaY, JZ, YH, CL, JW, and JL: Data collection and investigation; YaZ and LC: Data Curation, Investigation; YuZ: Conceptualization, methodology; MJ: Conceptualization, methodology, thesis revision, supervision, project management, and funding acquisition.
Funding
This work was supported by grants from the Program of the National Natural Science Foundation of China (82073597 and 91943301).
Conflict of interest
The authors declare that the research was conducted in the absence of any commercial or financial relationships that could be construed as a potential conflict of interest.
Publisher’s note
All claims expressed in this article are solely those of the authors and do not necessarily represent those of their affiliated organizations, or those of the publisher, the editors and the reviewers. Any product that may be evaluated in this article, or claim that may be made by its manufacturer, is not guaranteed or endorsed by the publisher.
Supplementary material
The Supplementary Material for this article can be found online at: https://www.frontiersin.org/articles/10.3389/fphar.2023.1122615/full#supplementary-material
References
Adar, S. D., Filigrana, P. A., Clements, N., and Peel, J. L. (2014). Ambient coarse particulate matter and human health: A systematic review and meta-analysis. Curr. Environ. Health Rep. 1, 258–274. doi:10.1007/s40572-014-0022-z
Alves-Bezerra, M., and Cohen, D. E. (2017). Triglyceride metabolism in the liver. Compr. Physiol. 8 (1), 1–8. doi:10.1002/cphy.c170012
Brügger, B. (2014). Lipidomics: Analysis of the lipid composition of cells and subcellular organelles by electrospray ionization mass spectrometry. Annu Rev. Biochem 83, 79–98. doi:10.1146/annurev-biochem-060713-035324
Chen, Y-C., Chen, P-C., Hsieh, W-S., Portnov, B. A., Chen, Y-A., and Lee, Y. L. (2012). Environmental factors associated with overweight and obesity in Taiwanese children. Paediatr. Perinat. Epidemiol. 26 (6), 561–571. doi:10.1111/ppe.12001
Chen, Z., Newgard, C. B., Kim, J. S., Iikayeva, O., Alderete, T. L., Thomas, D. C., et al. (2019). Near-roadway air pollution exposure and altered fatty acid oxidation among adolescents and young adults - the interplay with obesity. Environ. Int. 130, 104935. doi:10.1016/j.envint.2019.104935
Cheng, Q., Tian, L., Liang, H., and Luo, Y. (2019). Research progress of 12-HETE in the inflammation and oxidative stress. Zhonghua Wei Zhong Bing Ji Jiu Yi Xue 31 (12), 1555–1558. doi:10.3760/cma.j.issn.2095-4352.2019.12.027
Cole, L. K., Dolinsky, V. W., Dyck, J. R. B., and Vance, D. E. (2011). Impaired phosphatidylcholine biosynthesis reduces atherosclerosis and prevents lipotoxic cardiac dysfunction in ApoE-/- Mice. Circ. Res. 108 (6), 686–694. doi:10.1161/CIRCRESAHA.110.238691
Cui, L., Shi, L., Li, D., Li, X., Su, X., Chen, L., et al. (2020). Real-ambient particulate matter exposure-induced cardiotoxicity in C57/B6 mice. Front. Pharmacol. 11, 199. doi:10.3389/fphar.2020.00199
Ding, H., Jiang, M., Li, D., Zhao, Y., Yu, D., Zhang, R., et al. (2021). Effects of real-ambient PM2.5 exposure on lung damage modulated by Nrf2-/. Front. Pharmacol. 12, 662664. doi:10.3389/fphar.2021.662664
Dong, G. H., Qian, Z., Liu, M. M., Wang, D., Ren, W. H., Flick, L. H., et al. (2014). Ambient air pollution and the prevalence of obesity in Chinese children: The seven northeastern cities study. Obesity 22 (3), 795–800. doi:10.1002/oby.20198
Fioravanti, S., Cesaroni, G., Badaloni, C., Michelozzi, P., Forastiere, F., and Porta, D. (2018). Traffic-related air pollution and childhood obesity in an Italian birth cohort. Environ. Res. 160, 479–486. doi:10.1016/j.envres.2017.10.003
Fuchs, C. D., Radun, R., Dixon, E. D., Mlitz, V., Timelthaler, G., Halilbasic, E., et al. (2022). Hepatocyte-specific deletion of adipose triglyceride lipase (adipose triglyceride lipase/patatin-like phospholipase domain containing 2) ameliorates dietary induced steatohepatitis in mice. Hepatology 75 (1), 125–139. doi:10.1002/hep.32112
Furlong, M. A., and Klimentidis, Y. C. (2020). Associations of air pollution with obesity and body fat percentage, and modification by polygenic risk score for BMI in the UK Biobank. Environ. Res. 185, 109364. doi:10.1016/j.envres.2020.109364
Gao, G., Xie, Z., Li, E-W., Yuan, Y., Fu, Y., Wang, P., et al. (2021). Dehydroabietic acid improves nonalcoholic fatty liver disease through activating the Keap1/Nrf2-ARE signaling pathway to reduce ferroptosis. J. Nat. Med. 75 (3), 540–552. doi:10.1007/s11418-021-01491-4
Gao, M., Liang, C., Hong, W., Yu, X., Zhou, Y., Sun, R., et al. (2022). Biomass-related PM2.5 induces mitochondrial fragmentation and dysfunction in human airway epithelial cells. Environ. Pollut. 292, 118464. doi:10.1016/j.envpol.2021.118464
Ghaben, A. L., and Scherer, P. E. (2019). Adipogenesis and metabolic health. Nat. Rev. Mol. Cell Biol. 20 (4), 242–258. doi:10.1038/s41580-018-0093-z
Goldberg, M. J. Roeh., and Villeneuve, P. J. (2008). A systematic review of the relation between long-term exposure to ambient air pollution and chronic diseases. Rev. Environ. Health 23 (4), 243–297. doi:10.1515/reveh.2008.23.4.243
Gonzalez-Franquesa, A., and Patti, M-E. (2017). Insulin resistance and mitochondrial dysfunction. Adv. Exp. Med. Biol. 982, 465–520. doi:10.1007/978-3-319-55330-6_25
Guo, C., Hoek, G., Chang, L-Y., Bo, Y., Lin, C., Huang, B., et al. (2019). Long-term exposure to ambient fine particulate matter (PM2.5) and lung function in children, adolescents, and young adults: A longitudinal cohort study. Environ. Health Perspect. 127 (12), 127008. doi:10.1289/EHP5220
Han, H-S., Kang, G., Kim, J. S., Choi, B. H., and Koo, S-H. (2016). Regulation of glucose metabolism from a liver-centric perspective. Exp. Mol. Med. 48, e218. doi:10.1038/emm.2015.122
Hassan Bhat, T., Jiawen, G., and Farzaneh, H. (2021). Air pollution health risk assessment (AP-HRA), principles and applications. Int. J. Environ. Res. Public Health 18 (4), 1935. doi:10.3390/ijerph18041935
Hellerstein, M. K. (1999). De novo lipogenesis in humans: Metabolic and regulatory aspects. Eur. J. Clin. Nutr. 53 (1), S53–S65. doi:10.1038/sj.ejcn.1600744
Hoek-van den Hil, E. F., Keijer, J., Bunschoten, A., Vervoort, J. J. M., Stankova, B., Bekkenkamp, M., et al. (2013). Quercetin induces hepatic lipid omega-oxidation and lowers serum lipid levels in mice. PLoS One 8 (1), e51588. doi:10.1371/journal.pone.0051588
Hori, A., Ishida, F., Nakazawa, H., Yamaura, M., Morita, S., Uehara, T., et al. (2021). Serum sphingomyelin species profile is altered in hematologic malignancies. Clin. Chim. Acta 514, 29–33. doi:10.1016/j.cca.2020.11.024
Houten, S. M., Violante, S., Ventura, F. V., and Wanders, R. J. A. (2016). The biochemistry and physiology of mitochondrial fatty acid β-oxidation and its genetic disorders. Annu Rev. Physiol. 78, 23–44. doi:10.1146/annurev-physiol-021115-105045
Ipsen, D. H., Lykkesfeldt, J., and Tveden-Nyborg, P. (2018). Molecular mechanisms of hepatic lipid accumulation in non-alcoholic fatty liver disease. Cell Mol. Life Sci. 75 (18), 3313–3327. doi:10.1007/s00018-018-2860-6
Jamwal, S., Blackburn, J. K., and Elsworth, J. D. (2021). PPARγ/PGC1α signaling as a potential therapeutic target for mitochondrial biogenesis in neurodegenerative disorders. Pharmacol. Ther. 219, 107705. doi:10.1016/j.pharmthera.2020.107705
Ji, X., Liu, R., Guo, J., Li, Y., Cheng, W., Pang, Y., et al. (2022). Olfactory bulb microglia activation mediated neuronal death in real-ambient particulate matter exposure mice with depression-like behaviors. Sci. total Environ. 821, 153456. doi:10.1016/j.scitotenv.2022.153456
Jiang, J., Liang, S., Zhang, J., Du, Z., Xu, Q., Duan, J., et al. (2021). Melatonin ameliorates PM2.5 -induced cardiac perivascular fibrosis through regulating mitochondrial redox homeostasis. J. Pineal Res. 70 (1), e12686. doi:10.1111/jpi.12686
Jiang, M., Li, D., Piao, J., Li, J., Sun, H., Chen, L., et al. (2020). Real-ambient exposure to air pollution exaggerates excessive growth of adipose tissue modulated by Nrf2 signal. Sci. total Environ. 730, 138652. doi:10.1016/j.scitotenv.2020.138652
Kim, J. S., Chen, Z., Alderete, T. L., Toledo-Corral, C., Lurmann, F., Berhane, K., et al. (2019). Associations of air pollution, obesity and cardiometabolic health in young adults: The Meta-AIR study. Environ. Int. 133, 105180. doi:10.1016/j.envint.2019.105180
Li, D., Chen, S., Li, Q., Chen, L., Zhang, H., Li, H., et al. (2020). Caloric restriction attenuates C57BL/6 J mouse lung injury and extra-pulmonary toxicity induced by real ambient particulate matter exposure. Part. Fibre Toxicol. 17, 22–17. doi:10.1186/s12989-020-00354-2
Li, D., Zhang, R., Cui, L., Chu, C., Zhang, H., Sun, H., et al. (2019). Multiple organ injury in male C57BL/6J mice exposed to ambient particulate matter in a real-ambient PM exposure system in Shijiazhuang, China. Environ. Pollut. 248, 874–887. doi:10.1016/j.envpol.2019.02.097
Li, M., Qian, Z., Vaughn, M., Boutwell, B., Ward, P., Lu, T., et al. (2015). Sex-specific difference of the association between ambient air pollution and the prevalence of obesity in Chinese adults from a high pollution range area: 33 communities Chinese health study. Atmos. Environ. X. 117, 227–233. doi:10.1016/j.atmosenv.2015.07.029
Li, R., Kou, X., Geng, H., Xie, J., Tian, J., Cai, Z., et al. (2015). Mitochondrial damage: An important mechanism of ambient PM2.5 exposure-induced acute heart injury in rats. J. Hazard Mater 287, 392–401. doi:10.1016/j.jhazmat.2015.02.006
Li, Y., Xu, S., Li, J., Zheng, L., Feng, M., Wang, X., et al. (2016). SIRT1 facilitates hepatocellular carcinoma metastasis by promoting PGC-1α-mediated mitochondrial biogenesis. Oncotarget 7 (20), 29255–29274. doi:10.18632/oncotarget.8711
Liu, X., Zhao, X., Li, X., Lv, S., Ma, R., Qi, Y., et al. (2020). PM2.5 triggered apoptosis in lung epithelial cells through the mitochondrial apoptotic way mediated by a ROS-DRP1-mitochondrial fission axis. J. Hazard Mater 397, 122608. doi:10.1016/j.jhazmat.2020.122608
Maaløe, T., Schmidt, E. B., Svensson, M., Aardestrup, I. V., and Christensen, J. H. (2011). The effect of n-3 polyunsaturated fatty acids on leukotriene B and leukotriene B production from stimulated neutrophil granulocytes in patients with chronic kidney disease. Prostagl. Leukot. Essent. Fat. Acids 85 (1), 37–41. doi:10.1016/j.plefa.2011.04.004
Mansouri, A., Gattolliat, C-H., and Asselah, T. (2018). Mitochondrial dysfunction and signaling in chronic liver diseases. Gastroenterology 155 (3), 629–647. doi:10.1053/j.gastro.2018.06.083
McGuinn, L. A., Schneider, A., McGarrah, R. W., Ward-Caviness, C., Neas, L. M., Di, Q., et al. (2019). Association of long-term PM2. 5 exposure with traditional and novel lipid measures related to cardiovascular disease risk. Environ. Int. 122, 193–200. doi:10.1016/j.envint.2018.11.001
Nassir, F., and Ibdah, J. A. (2014). Role of mitochondria in nonalcoholic fatty liver disease. Int. J. Mol. Sci. 15 (5), 8713–8742. doi:10.3390/ijms15058713
Ostro, B., Spadaro, J. V., Gumy, S., Mudu, P., Awe, Y., Forastiere, F., et al. (2018). Assessing the recent estimates of the global burden of disease for ambient air pollution: Methodological changes and implications for low- and middle-income countries. Environ. Res. 166, 713–725. doi:10.1016/j.envres.2018.03.001
Pan, X-F., Wang, L., and Pan, A. (2021). Epidemiology and determinants of obesity in China. Lancet Diabetes Endocrinol. 9 (6), 373–392. doi:10.1016/S2213-8587(21)00045-0
Park, Y. J., Lee, S., Lim, S., Nahmgoong, H., Ji, Y., Huh, J. Y., et al. (2021). DNMT1 maintains metabolic fitness of adipocytes through acting as an epigenetic safeguard of mitochondrial dynamics. Proc. Natl. Acad. Sci. U S A 118 (11), e2021073118. doi:10.1073/pnas.2021073118
Pei, Z., Flexeder, C., Fuertes, E., Thiering, E., Koletzko, B., Cramer, C., et al. (2013). Early life risk factors of being overweight at 10 years of age: Results of the German birth cohorts GINIplus and LISAplus. Eur. J. Clin. Nutr. 67 (8), 855–862. doi:10.1038/ejcn.2013.80
Piccinin, E., Villani, G., and Moschetta, A. (2019). Metabolic aspects in NAFLD, NASH and hepatocellular carcinoma: The role of PGC1 coactivators. Nat. Rev. Gastroenterol. Hepatol. 16 (3), 160–174. doi:10.1038/s41575-018-0089-3
Qiu, Y-N., Wang, G-H., Zhou, F., Hao, J-J., Tian, L., Guan, L-F., et al. (2019). PM2.5 induces liver fibrosis via triggering ROS-mediated mitophagy. Ecotoxicol. Environ. Saf. 167, 178–187. doi:10.1016/j.ecoenv.2018.08.050
Rajagopalan, S., Al-Kindi, S. G., and Brook, R. D. (2018). Air pollution and cardiovascular disease: JACC state-of-the-art review. J. Am. Coll. Cardiol. 72 (17), 2054–2070. doi:10.1016/j.jacc.2018.07.099
Rinschen, M. M., Ivanisevic, J., Giera, M., and Siuzdak, G. (2019). Identification of bioactive metabolites using activity metabolomics. Nat. Rev. Mol. Cell Biol. 20 (6), 353–367. doi:10.1038/s41580-019-0108-4
Rohde, R. A., and Muller, R. A. (2015). Air pollution in China: Mapping of concentrations and sources. PLoS One 10 (8), e0135749. doi:10.1371/journal.pone.0135749
Rom, O., Liu, Y., Liu, Z., Zhao, Y., Wu, J., Ghrayeb, A., et al. Glycine-based treatment ameliorates NAFLD by modulating fatty acid oxidation, glutathione synthesis, and the gut microbiome. Sci. Transl. Med. 2020;12, eaaz2841, doi:10.1126/scitranslmed.aaz2841572).
Samuel, V. T., and Shulman, G. I. (2016). The pathogenesis of insulin resistance: Integrating signaling pathways and substrate flux. J. Clin. Invest 126 (1), 12–22. doi:10.1172/JCI77812
Sangwung, P., Petersen, K. F., Shulman, G. I., and Knowles, J. W. (2020). Mitochondrial dysfunction, insulin resistance, and potential genetic implications. Endocrinology 161 (4), bqaa017. doi:10.1210/endocr/bqaa017
Scheja, L., and Heeren, J. (2016). Metabolic interplay between white, beige, Brown adipocytes and the liver. J. Hepatol. 64 (5), 1176–1186. doi:10.1016/j.jhep.2016.01.025
Shahidi, F., and Ambigaipalan, P. (2018). Omega-3 polyunsaturated fatty acids and their health benefits. Annu Rev. Food Sci. Technol. 9, 345–381. doi:10.1146/annurev-food-111317-095850
Simkova, S., Veleminsky, M., and Sram, R. J. (2020). The impact of air pollution to obesity. Neuro Endocrinol. Lett. 41 (3), 146–153.
Sly, P. D., and Flack, F. (2008). Susceptibility of children to environmental pollutants. Ann. N. Y. Acad. Sci. 1140 (1), 163–183. doi:10.1196/annals.1454.017
Smith, J. D., Fu, E., and Kobayashi, M. A. (2020). Prevention and management of childhood obesity and its psychological and health comorbidities. Annu Rev. Clin. Psychol. 16, 351–378. doi:10.1146/annurev-clinpsy-100219-060201
Su, X., Tian, J., Li, B., Zhou, L., Kang, H., Pei, Z., et al. (2020). Ambient PM2.5 caused cardiac dysfunction through FoxO1-targeted cardiac hypertrophy and macrophage-activated fibrosis in mice. Chemosphere 247, 125881. doi:10.1016/j.chemosphere.2020.125881
Thorp, E. B. (2019). Mitochondrial indigestion after lipid scavenging. Circ. Res. 125 (12), 1103–1105. doi:10.1161/CIRCRESAHA.119.316200
Tong, J., Ren, Y., Liu, F., Liang, F., Tang, X., Huang, D., et al. (2022). The impact of PM2.5 on the growth curves of children's obesity indexes: A prospective cohort study. Front. Public Health 10, 843622. doi:10.3389/fpubh.2022.843622
Villena, J. A. (2015). New insights into PGC-1 coactivators: Redefining their role in the regulation of mitochondrial function and beyond. FEBS J. 282 (4), 647–672. doi:10.1111/febs.13175
Vrijheid, M., Fossati, S., Maitre, L., Márquez, S., Roumeliotaki, T., Agier, L., et al. (2020). Early-life environmental exposures and childhood obesity: An exposome-wide approach. Environ. Health Perspect. 128 (6), 67009. doi:10.1289/EHP5975
Wallace, M., and Metallo, C. M. (2020). Tracing insights into de novo lipogenesis in liver and adipose tissues. Semin. Cell Dev. Biol. 108, 65–71. doi:10.1016/j.semcdb.2020.02.012
Xie, Y., Liu, Z., Wen, T., Huang, X., Liu, J., Tang, G., et al. (2019). Characteristics of chemical composition and seasonal variations of PM2. 5 in Shijiazhuang, China: Impact of primary emissions and secondary formation. Sci. Total Environ. 677, 215–229. doi:10.1016/j.scitotenv.2019.04.300
Xu, M-X., Qin, Y-T., Ge, C-X., Gu, T-T., Lou, D-S., Li, Q., et al. (2018). Activated iRhom2 drives prolonged PM2. 5 exposure-triggered renal injury in Nrf2-defective mice. Nanotoxicology 12 (9), 1045–1067. doi:10.1080/17435390.2018.1513093
Xu, M-X., Zhu, Y-F., Chang, H-F., and Liang, Y. (2016). Nanoceria restrains PM2. 5-induced metabolic disorder and hypothalamus inflammation by inhibition of astrocytes activation related NF-κB pathway in Nrf2 deficient mice. Free Radic. Biol. Med. 99, 259–272. doi:10.1016/j.freeradbiomed.2016.08.021
Xu, X., Liu, C., Xu, Z., Tzan, K., Zhong, M., Wang, A., et al. (2011). Long-term exposure to ambient fine particulate pollution induces insulin resistance and mitochondrial alteration in adipose tissue. Toxicol. Sci. 124 (1), 88–98. doi:10.1093/toxsci/kfr211
Zhang, R., Chen, S., Chen, L., Ye, L., Jiang, Y., Peng, H., et al. (2022). Single-cell transcriptomics reveals immune dysregulation mediated by IL-17A in initiation of chronic lung injuries upon real-ambient particulate matter exposure. Part Fibre Toxicol. 19 (1), 42. doi:10.1186/s12989-022-00483-w
Zhang, X., Zhang, J., Wu, Y., Nan, B., Huang, Q., Du, X., et al. (2021). Dynamic recovery after acute single fine particulate matter exposure in male mice: Effect on lipid deregulation and cardiovascular alterations. J. Hazard Mater 414, 125504. doi:10.1016/j.jhazmat.2021.125504
Keywords: PM2.5, young mice, adipose tissue, lipid metabolism, mitochondria, multiomics
Citation: Si H, Gao T, Yang J, Zhu J, Han Y, Li C, Wang J, Li J, Zhao Y, Chen L, Zheng Y and Jiang M (2023) Multi-omics reveals hypertrophy of adipose tissue and lipid metabolism disorder via mitochondria in young mice under real-ambient exposure to air pollution. Front. Pharmacol. 14:1122615. doi: 10.3389/fphar.2023.1122615
Received: 13 December 2022; Accepted: 02 March 2023;
Published: 23 March 2023.
Edited by:
Zhiyuan Meng, Yangzhou University, ChinaReviewed by:
Saba Imdad, Cheongju University, Republic of KoreaSen Yan, China Agricultural University, China
Copyright © 2023 Si, Gao, Yang, Zhu, Han, Li, Wang, Li, Zhao, Chen, Zheng and Jiang. This is an open-access article distributed under the terms of the Creative Commons Attribution License (CC BY). The use, distribution or reproduction in other forums is permitted, provided the original author(s) and the copyright owner(s) are credited and that the original publication in this journal is cited, in accordance with accepted academic practice. No use, distribution or reproduction is permitted which does not comply with these terms.
*Correspondence: Menghui Jiang, jiangmenghui@qdu.edu.cn
†These authors have contributed equally to this work and share first authorship