- 1Institute of Forensic Sciences, Suzhou Medical College, Soochow University, Suzhou, China
- 2Department of Orthopedics, Dongtai People’s Hospital, Dongtai, China
- 3Department of Obstetrics and Gynecology, The Affiliated Suzhou Hospital of Nanjing Medical University, Suzhou, China
Neurological diseases such as traumatic brain injury, cerebral ischemia, Parkinson’s, and Alzheimer’s disease usually occur in the central and peripheral nervous system and result in nervous dysfunction, such as cognitive impairment and motor dysfunction. Long-term clinical intervention is necessary for neurological diseases where neural stem cell transplantation has made substantial progress. However, many risks remain for cell therapy, such as puncture bleeding, postoperative infection, low transplantation success rate, and tumor formation. Sustained drug delivery, which aims to maintain the desired steady-state drug concentrations in plasma or local injection sites, is considered as a feasible option to help overcome side effects and improve the therapeutic efficiency of drugs on neurological diseases. Natural polymers such as silk fibroin have excellent biocompatibility, which can be prepared for various end-use material formats, such as microsphere, gel, coating/film, scaffold/conduit, microneedle, and enables the dynamic release of loaded drugs to achieve a desired therapeutic response. Sustained-release drug delivery systems are based on the mechanism of diffusion and degradation by altering the structures of silk fibroin and drugs, factors, and cells, which can induce nerve recovery and restore the function of the nervous system in a slow and persistent manner. Based on these desirable properties of silk fibroin as a carrier with sustained-release capacity, this paper discusses the role of various forms of silk fibroin-based drug delivery materials in treating neurological diseases in recent years.
1 Introduction
Neurological diseases, both acute and chronic neurological diseases, result in a loss of neuronal function due to the damage of neurons and neuronal networks, which may cause permanent disability such as cognitive impairment (Balcom et al., 2021). Therefore, appropriate treatment is critical for neurological diseases. Innovative and effective therapeutic strategies are urgently required to repair injured neural tissue. Stem cell-based therapy is becoming increasingly popular and has been used in a variety of neurological diseases, such as traumatic brain injury (Mahmood et al., 2004), Parkinson’s disease (Bouchez et al., 2008), spinal cord injury (Abrams et al., 2009), amyotrophic lateral sclerosis (Forostyak et al., 2011), and multiple sclerosis diseases (Yan et al., 2018). However, the low efficiency and high cost of the delivery system limited its practical application. Gene therapy shows promising superiority in rare genetic diseases but often suffers from irreversible adverse reactions (Morris and Schorge, 2022). A recent study also has found that regeneration and repair of nerve tissue can be stimulated by biomaterials (Lim and Spector, 2017; Shan et al., 2019; Yonesi et al., 2021). Moreover, nano-and-micron technologies also offer the possibility of biomaterial-cell interactions (Orive et al., 2009). Biomaterials can not only help transplanted cells survive and incorporate into damaged regions but also enable sustained drug delivery on a site-by-site basis. Therefore, biomaterials can improve drug delivery to the brain and promote neuronal regeneration and repair in combination with cell therapies (Elliott Donaghue et al., 2014). Biomaterials can be classified into natural or synthetic according to the origin and the components, such as extracellular matrix (ECM) (Song et al., 2018). In contrast to natural biomaterials, synthetic materials lack cell binding domains, resulting in weak bioactivity toward cells in vivo (Troy et al., 2021). The natural biomaterials commonly used in nerve regeneration are polyesters, proteins, and polysaccharides, such as silk fibroin (SF), alginate, hyaluronic acid, collagen, chitosan, and gelatin (Fornasari et al., 2020). SF has a long history of application as a drug carrier in different systems. The capability of purification, biocompatibility, and excellent binding capacity for a wide range of drugs of SF-based natural biomaterials makes it attractive for use as drug delivery carriers (Farokhi et al., 2020). But the use of SF as a drug carrier in neurological diseases has not been reported. Based on the advantages mentioned above, SF is expected to become a drug carrier for central nervous system diseases. However, it is different in the characteristics of central nervous system diseases, and different diseases require the application of different forms of SF to achieve the delivery of drugs and achieve the purpose of treating diseases. In our group’s research, SF hydrogels are effective in regenerating brain tissue, repairing damaged brain tissue, and improving neurologic recovery (Chen et al., 2022a; Zhang et al., 2022; Yang et al., 2022). Particular attention is being paid to the use of hydrogels in drug delivery due to their unique physical properties. As a result of their porosity, drugs can be loaded into the hydrogel matrix and released at a rate dependent on the diffusion coefficient between the small molecules or macromolecules through the gel matrix (Hoare and Kohane, 2008). There are also porous 3D structures made out of SF, including films, sponges, foams, and scaffolds that are useful for biomedical applications such as tissue engineering and implantable medical devices. Methods of use include filling, application, and injection. SF films were well tolerated in the brain without acute neuroinflammation, cell death, or altered brain function (Tang-Schomer et al., 2019). As a drug carrier and cell culture substrate, SF films were used to simulate the in vivo interface between drug reservoirs and brain cells for drug delivery in the brain (Tang-Schomer et al., 2019). Small molecules released from the SF membrane can penetrate the hippocampal region and remain in the ipsilateral hemisphere (Tang-Schomer et al., 2019). A variety of processing methods are available to tune the morphological and molecular mechanisms for functionalized SF materials as drug carriers to treat brain disease as follows (Figure 1). Dhyani et al. developed a UV-based strategy to modify SF nanogels and encapsulate bioactive molecules, which could be released at different rates. The bioactive molecules exhibited various delivery behaviors at specific regions to achieve the desired multiple biological effects (Dhyani and Singh, 2017). The carriers derived from the regenerated SF show high universality for small molecule drugs (adenosine (Wilz et al., 2008)), proteins (enzymes (Wang et al., 2007a), antibodies (Guziewicz et al., 2011)), antitumor drugs (Yucel et al., 2014; Montalbán et al., 2018), antibacterial drugs (Pritchard et al., 2013; Zhong et al., 2015), neurotrophic factors and cells (mesenchymal stem cells (Martín-Martín et al., 2019), sciatic nerve Schwann cells (Yang et al., 2007; Gu et al., 2014), neurons, etc.). Therefore, SF as a drug carrier is a suitable platform to achieve the treatment of neurological diseases. The timeline of SF as the drug carrier in neurological disease as follows (Figure 2). However, the research on SF as a drug delivery system for neurological diseases is still limited, such as how to strengthen the biological characteristics of SF itself, change the morphology of SF, and the effect of drug-loading to meet the needs of specific neurological diseases. In this review, we will focus on the advantages of different forms and applications (filling, application, and injection) of SF, and drugs delivered by SF for the treatment of neurological diseases, as well as the latest applications (including treatment and detection) in a few neurological diseases (including hemorrhagic and ischemic stroke, Alzheimer’s disease and Parkinson’s, traumatic brain trauma, glioblastoma and neuroblastoma, epilepsy, etc.).
When the nervous system suffered from injured, the body experiences several changes, including a higher level of reactive oxygen species (ROS), an increase in inflammation, ruptured blood vessels, nerve damage or rupture, and neuron death. Cells, growth factors, and chemical drugs can be carried into the body to repair damage by various forms of SF. A) The hemostatic ability of SF can be stimulated by the cross-linking of Tyr under the action of the inducer. B) The RGD sequence of SF (Arg-Gly-Asp) is conducive to cell adhesion, so SF can ensure the transport and adhesion of neural stem cells to the site of injury, further proliferation, and differentiation to repair the injured nerve. C) Although SF itself has a little anti-inflammatory effect, it can be combined with other antibacterial materials to form new biomaterials, which can inhibit or kill microorganisms and reduce cell death.
2 Different forms of silk fibroin carrier for drug delivery
A major aim of advanced drug carrier is to deliver the drug to the desired area and thereby reduce the systemic dose to decrease side effects (Farokhi et al., 2020). SF carrier was first reported in 1989 and a series of studies revealed the feasibility of SF carriers to load cells, enzymes, and small molecules. The material parameters of SF and the drug release behavior of SF hydrogels can be thoroughly investigated by experimental rheological methods, coupled models of affinity-based diffusion, degradation, and mechanical behavior. The result showed that SF had good mechanical properties and the ability to slow release and control release. Based on these properties, the drug release characteristics could be predicted, and the drug dosage could be adjusted in time (Gharehnazifam et al., 2021). The mechanical properties of the electrospinning SF mats were improved after wetting, which was more suitable to be used as drug carrier (Phamornnak et al., 2022). The electrospinning SF mat has good physical properties and can be used as neural substrates to promote the growth of neurons (Nune et al., 2019). In conclusion, various forms of SF scaffolds have different rheological and mechanical characteristics and are suitable for the treatment of different neurological diseases (Li et al., 2016; Montalbán et al., 2018; Cui et al., 2021). Continuous research indicated that various drugs could be regulated for sustained release by changing the state, conformations, and nanostructures of SF and blending with other materials, which implies the advantages of SF as an ideal smart carrier to better simulate the microenvironment in vivo (Mathur and Gupta, 2010; Wang et al., 2016a; Kapoor and Kundu, 2016; Farokhi et al., 2018; Gou et al., 2019; Yavuz et al., 2019; Wang et al., 2020; Horo et al., 2021). SF has multiple features that are beneficial to nerve regeneration. SF microspheres, gels, scaffolds, catheters, and other forms (Rockwood et al., 2011) were prepared to encapsulate stem cells (Ciocci et al., 2018), neurons (Zhang et al., 2012; White et al., 2015), and growth factors (Uebersax et al., 2007) to clarify the role and advantages of SF in the recovery of central and peripheral nervous systems.
2.1 SF microspheres
Polymer microspheres with the size of 1–500 μm have been used widely to encapsulate drugs. SF microspheres of different sizes have changeable penetrability, which can deliver drugs and active factors to different lesion sites in the human body. Compared with synthetic polymeric microspheres, biodegradable SF microspheres have several unique advantages in the delivery of various drugs, including proteins, nucleic acids, polypeptides, and other bio-macromolecules. Wang et al. evaluated the delivery of growth factors from polylactic co-glycolic acid (PLGA) and SF microspheres on human bone marrow-derived mesenchymal stem cells (hMSCs)-derived osteochondral differentiation. The result showed better osteogenic differentiation of hMSCs in the SF microsphere system because SF microspheres showed quicker sustained release of growth factors than PLGA microspheres. However, the fabrication process of SF microspheres is complicated (Wang et al., 2009). Gong et al. developed a polyethylene glycol (PEG) assisted emulsification method to encapsulate octreotide acetate with SF microspheres and octreotide could release slowly from SF microspheres for more than 28 days in rats after intramuscular injection (Gong et al., 2019). Shen et al. prepared BMP-2 encapsulated with SF microspheres in SF/nHAp porous scaffolds, which showed a sustained release of BMP-2 for 3 weeks (Shen et al., 2016). In another study, the composite alginate hydrogels combined with SF microspheres could provide a more sustained release of IGF-1 than microspheres without SF (Feng et al., 2020). The SF microspheres have a promising future as long-acting controlled release delivery. Hino et al. reported that the secondary structure of SF microspheres obtained by spray-drying changed from a β-sheet to a random structure. Due to water absorption and subsequent recrystallization, its conformation changes into β-sheet again. The β-folded structure is the most stable structure in the secondary structure of SF, which may affect its sustained release performance. The deficiency of the spray drying method is that the conformations conversion to the β-folded structure in SF microspheres may be obstructed if the particles are dried too fast. Lammel et al. produced SF particles by salting SF solution with potassium phosphate. Compared with emulsification, salt leaching voids the use of organic solvents and is friendly and safe. However, the whole experiment condition is in the environment of PH = 8, and the particles will gather into non-dispersible aggregates with poor pelletizing if PH = 5, suggesting that the change of PH value will affect the aggregation of SF microspheres (Lammel et al., 2010). Therefore, the specific production process for SF microspheres limited its practical application in biomedicine to a certain extent.
2.2 Silk fibroin hydrogels
With unique biocompatibility and biodegradability, SF hydrogels are one of the effective candidate materials for biomedical engineering, with many properties such as capacity to hold water, stability of shape and modifications to the functionality possibility. Consequently, a three-dimensional microenvironment that mimics extracellular matrix can be created, allowing cellular behavior and tissue function to be regulated. In addition to physical and chemical hydrogels, the SF hydrogels make promising biopolymeric matrices that are capable of controlling drug release (Tomeh et al., 2019). Wu et al. incubated the diluted silk solution at 60°C for 24 h to make it self-assemble to form a physical hydrogel and then dissolved doxorubicin (DOX) directly into the hydrogel to form hydrogel-loaded DOX. DOX can be released in and out of the body for more than 4 weeks and tumors treated with DOX-loaded filamentous hydrogels showed a larger necrotic area than those treated with free DOX (Wu et al., 2016). Lovett et al. mixed SF with bevacizumab solution to induce gelation by ultrasound and the SF hydrogels achieve drug-release for more than 3 months (Lovett et al., 2015). Attention should be paid that the influences, such as an increase in local temperatures, an engineering shear, and gas-liquid interface increase, affected the gelation of SF during the ultrasound process.
Chen et al. mixed SF hydrogels with carboxymethyl chitosan (CMCS) into SF/CMCS hydrogels, which can be easily controlled in terms of thickness, quality, and shape by electrodeposition on graphite (Chen et al., 2021). Guziewicz et al. developed a lyophilized SF hydrogel, which makes SF hydrogels suitable for long-term sustained release applications in antibody release (Guziewicz et al., 2011). In terms of physical strength and stability, hydrogels made by chemicals are highly effective compared with physical hydrogels. The common preparation methods for chemical hydrogels include photopolymerization, chemical crosslinking agent, and irradiation. He et al. found that tannic acid-reinforced methacrylated chitosan/methacrylated SF hydrogels (TA-CSMA/SFMA) had a significant effect on wound healing in a full-thickness skin defect model (He et al., 2020). It should be noted that the addition of a photoinducer may cause adverse reactions, such as surface oxidation. Kim et al. optimized bone tissue engineering using SF composite hydrogels with HAP nanoparticles (NPs) by irradiating SF composite hydrogels with HAP nanoparticles (NPs) and these hydrogels enhanced the osteogenic differentiation of hMSCs in vitro (Kim et al., 2017). Zhou et al. prepared porous polyvinyl alcohol/SF/nano-hydroxyapatite (PVA/SF/N-HA) composite hydrogels by genipin (GP) crosslinking and the composite hydrogels could be a novel approach to corneal tissue engineering (Zhou et al., 2019). Therefore, both physical and chemical cross-linking can induce gelation of SF solution, and the corresponding SF gels loaded with drugs can be prepared with the conditions optimized using improved methodology according to the performance of loaded drugs and experimental purposes. In biomedical applications, SF hydrogels are limited by their poor mechanical properties and swelling behavior (Yang et al., 2022). The biological safety of SF hydrogels and their composite hydrogel materials, hydrogels’ drug loading efficiency, and the excellent injection performance of hydrogels is important challenges in the field of regenerative medicine (Liu et al., 2022a).
2.3 Silk fibroin coating/film
SF coating can be obtained by dipping coating and layer-by-layer assembly. The application of SF coating can improve the “ initial burst release” of drugs and prolong the release time of drugs. Meanwhile, the behavior of drug-release can be precisely controlled by adjusting the thickness and crystallinity of SF coating. Pritchard et al. coated adenosine tablets with SF solution and put them into phosphate buffer solution (PBS) after methanol treatment for in vitro release. They found that the increase of the crystal content or the thickness of SF coating could deliver adenosine for several weeks and then degrade in the body, requiring no surgical removal (Pritchard et al., 2010). Wang et al. prepared SF coating by a step deposition process and used it as a carrier to incorporate drugs and the release duration of these adulterated molecules can be prolonged by controlling the coating structure and suppressing the initial burst (Wang et al., 2007b).
SF membrane can be obtained by solution pouring and freeze-drying. Lee et al. investigated antithrombogenicity and surface properties of SF and S-carboxymethyl keratin (SCMK) polymers by solvent cast, which show better antithrombotic properties than SF or SCMK alone (Lee et al., 1998). Seib et al. poured the SF solution onto the substrate and dried it to generate a SF membrane with different crystallinity. After different steam temperatures, dried SF membranes were immersed in doxorubicin solution and then were implanted in the corresponding tumor sites in breast tumor mice. The result showed that the different crystallinity of SF membrane produced at different temperatures provided a controlled release of doxorubicin and limited adverse reactions in the treatment of human breast cancer (Seib and Kaplan, 2012). The result suggests these systems as a good candidate material for SF coating or film to control drug release, when considering the all-aqueous process involved, coatings with conformal properties, and the degradability and biocompatibility of SF coating or film. A number of drawbacks, however, include expensive coatings, which together reduce affordability and commercial viability, and prevention of direct contact between loaded drugs and cells by coatings to the observation that insufficient manifestation of their effects may occur (Kwon et al., 2021).
2.4 Silk fibroin scaffold/conduit
SF porous scaffolds have well-interconnected pores and can well simulate the three-dimensional network environment in organisms (Moisenovich et al., 2019), to facilitate cell adhesion, proliferation, and growth (Abdel-Naby et al., 2017). The preparation methods of SF porous scaffold include freeze-drying, salt leaching, and porogen leaching. A frozen-dried SF/hyaluronan scaffold was prepared, and 3 weeks of mesenchymal stem cell culture were performed on the scaffolds by Garcia-Fuentes et al. In comparison to plain SF scaffolds, stem cell cultures on SF/hyaluronan scaffolds produced more effective tissue formation (Garcia-Fuentes et al., 2009). A salt leaching method was used to prepare demineralized bone matrix-SF (DBM/SF) scaffolds. The scaffolds can promote cell attachment and growth, and it may be a suitable candidate material for bone tissue engineering (Ding et al., 2015). However, traditional porous scaffolds generated by porogen can hardly control the distribution of scaffolds’ pores. Ze et al. combined supercritical carbon dioxide (SC-CO2) technology with porogen leaching using ammonium bicarbonate (AB) particles as a pore-forming agent, which could overcome the disadvantages of traditional porogen leaching and prepared scaffold was loaded with Schwann cells to demonstrate its good cytocompatibility (Li et al., 2019). Zhang et al. prepared bioactive glass (MBG)/SF scaffolds with aspirin to repair calvarial defects in the mice model. The MBG/SF scaffolds have been shown to enhance drug loading in vitro, increase drug release rate, and stimulate bone regeneration in vivo (Zhang et al., 2014).
SF conduits are more widely used in the preparation of nerve catheters (NC). The use of artificial nerve conduits (NCs) can be useful in the treatment of damaged peripheral nerves in certain cases, however, their therapeutic effect is often not enough effective (Madduri and Gander, 2012). Therefore, it is necessary to manufacture NCs to achieve a better therapeutic effect. Studies have shown that SF NCs promote axon regeneration in the short nerve gap thanks to the slow degradation of SFs (Wang et al., 2008; Cao and Wang, 2009). Schwann cells, in particular, can survive, adhere, and migrate along silk fibers due to their affinity for them (Yang et al., 2007). SF conduits are produced in three major ways: mold casting, staining, and electrospinning. Yang et al. used stainless steel casting molds by lyophilized demoulding to provide SF-NC and SF vessels that are implanted into the back of adult rabbits could degrade rapidly and meet the requirements of peripheral nerve regeneration compared with SF fibers (Yang et al., 2009). Ghaznavi et al. used catheterization immersed in SF/polyethylene oxide to examine the cellular inflammatory response and functional recovery in models of sciatic nerve defects and the catheter had physical and biological properties of promoting nerve repair from proximal to distal nerve stump (Ghaznavi et al., 2011). Wang et al. combined SF with PLGA to prepare nerve guidance conduits (NGCs), which showed the strength of good biocompatibility, stiffness, and high porosity (Wang et al., 2015). In addition to the traditional methods mentioned above, Carvalho et al. developed an SF-based cross-linking catheter for the delivery of neurotrophic factors. Studies have shown that this design has significantly improved retrograde transport, neuronal protection, and motor nerve reinnervation, and to some extent solved the defect of incomplete nerve regeneration caused by a lack of neurotrophic factors in the proximal region (Carvalho et al., 2021). You et al. fabricated a multi-channel bioactive SF nanofiber catheter, which can promote neuron-like development and directional neurite extension in vitro and in vivo spinal cord injury models (You et al., 2020). Despite its advantages, the scaffold can also be disadvantageous. It is difficult to distribute cells evenly and remain underpopulated in the central or core regions (Luetchford et al., 2020). The scaffold design relies on a bottom-up approach that may spread cells evenly and shape them into the final 3D structure by layering different cells or drugs (Chen et al., 2011). Likewise, simple hollow SF NGCs cannot enhance functional and sensory recovery after peripheral nerve injuries (PNIs). It has been shown that including neurotrophic factors (NTFs) in NGCs can significantly improve nerve regeneration (Carvalho et al., 2021). It is important, therefore, to find optimal delivery methods for drugs such as neurotrophic factors (NTFs) that are extracted from SF conduits.
2.5 Silk fibroin microparticles/nanoparticles
It has been reported that nanoparticles are effective carriers for drugs that promote efficacy. It shows unique characteristics due to the “size effect”. The common methods for preparing SF nanoparticles include desolvation, salt leaching, and supercritical fluid technologies. Kundu et al. prepared SF nanoparticles loaded with VEGF using a desolvation technique using dimethyl sulphoxide (DMSO) as the desolvation solvent. In vitro release experiments showed that there was no sudden release of VEGF in the drug delivery system, which could be used as an efficient release system of VEGF (Kundu et al., 2010). Lammel et al. produced SF particles by salting the SF solution with potassium phosphate. Compared with emulsification, salt leaching avoids the use of organic solvents and is friendly and safe. The whole experimental condition is in the environment of PH = 8. Increasing SF concentration will lead to larger particles. When PH = 5, particles will gather into non-dispersible aggregates with poor spheronization (Lammel et al., 2010). The preparation of SF nanoparticles was carried out by solution-enhanced dispersion by supercritical CO2 (SEDS). The SEDS process was used to encapsulate the non-steroidal anti-inflammatory drug Indomethacin (IDMC) in SF nanoparticles. The experimental results showed that SF nanoparticles prepared by the SEDS process were suitable for drug delivery as a biocompatible carrier and feasible for drug delivery control (Zhao et al., 2012). There is a great potential for SF microparticles/nanoparticles to be used as delivery systems for a wide variety of therapeutic agents, such as small molecules, protein drugs, genes, and vaccines, which enhances the biological stability and improves the drug delivery (Pham and Tiyaboonchai, 2020). The unspecific targeting of SF microparticles and nanoparticles may result in low therapeutic efficiency and systemic toxicity, due to their inefficiency as drug delivery systems.
2.6 Silk fibroin microneedle
In order to deliver localized treatment, microneedles (MNs) were used to penetrate the layers of the cuticle, which can achieve subcutaneous injections and continuously releases the drug directly into interstitial fluid or blood vessels by adjusting the swelling properties of MNs. The production method of SF microneedles is relatively simple and usually cast by mold. Qi et al. poured proline/melatonin/SF mixture into a polydimethylsiloxane microneedle mold to obtain drug-loaded SF microneedles and microneedles incorporated the drug into the body through the skin and maintained a high concentration of melatonin in the blood of rat insomnia model (Qi et al., 2021). Yavuz et al. reported that levonorgestrel was loaded into microparticles and then injected into silk-based microneedles, which could achieve continuous release for up to a year for unwanted pregnancy (Yavuz et al., 2020). Microneedle devices can also provide highly reproducible results and therapeutic benefits by penetrating through the cuticle into the interstitial fluid. However, there are some limitations to microneedles in biomedical applications, such as skin irritation or allergy to sensitive skin and broken microneedle tips left in the skin (Waghule et al., 2019).
In addition to the above-mentioned forms of SF carriers for drug delivery, Hu et al. used the electrostatic spinning method of preparation of the regenerated SF nanofibers and cultivated Schwann cells on SF nanofibers. They found that the regeneration of electrostatic spinning nanofibers simulates the structure and function of extracellular matrix form and the adhesion, growth, and proliferation of Schwann cells, showing excellent biocompatibility (Hu et al., 2012). In addition, studies have explored that straining flow spinning (SFS) can be used to regenerate high-performance silk fibers as scaffolds for axonal growth induction and guidance and spontaneous cellular connection of dissociated neurons (Mercado et al., 2020). Zhu et al. prepared a porous scaffold called SF sponge to grow primary cortical cells (with or without microglia removal). The results showed that neuronal maturation and synaptic development were reduced in fibroin sponges depleted by microglia (Zhu et al., 2020). Overall, a variety of SF forms, as biomaterial carriers, can be generated in a variety of applications using the method described. We summarized the advantages and disadvantages of different forms of SF carriers for drug delivery (Table 1).
3 The application of SF-based drug delivery materials in neurological diseases
There are significant socioeconomic implications for sufferers and society as a whole from neurological diseases. The penetration of drugs into the brain has been achieved using a variety of strategies (Islam et al., 2020). A variety of drug delivery systems and drug delivery vehicles have been reported to be feasible to SF (Wani and Veerabhadrappa, 2018). Previous studies have confirmed that SF showed good biocompatibility with nerve tissues and cells, which may be used to prepare nerve conduits or drug delivery vectors to treat neurological diseases (Yang et al., 2007; Tang et al., 2009). Therefore, we have summarized the applications of SF-based drug delivery materials (Figure 3) and the possible molecular mechanism (Figure 4) in neurological diseases.
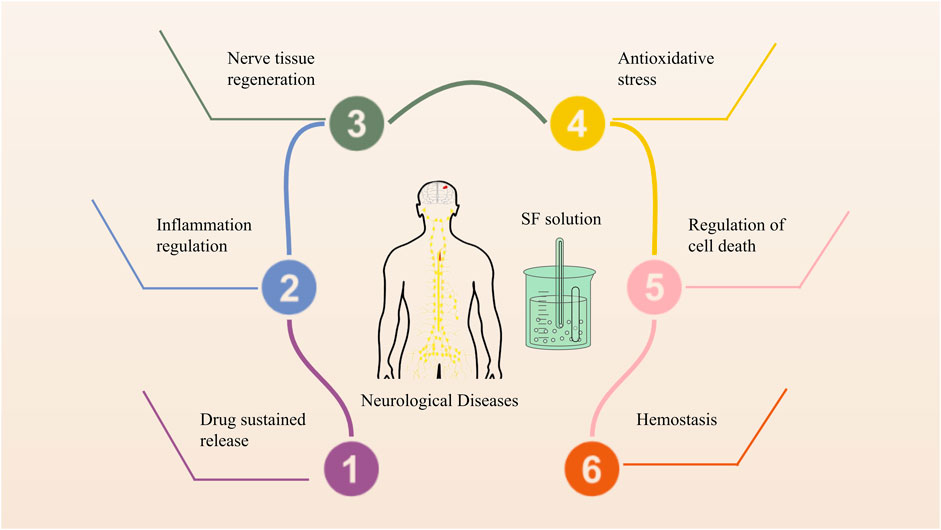
FIGURE 4. The possible molecular mechanism of SF-based drug delivery materials in neurological diseases.
In terms of sustained release systems, SF is a new option. As the core material, it is possible to design SF into many forms, including microspheres, hydrogels, films, scaffolds, nanoparticles, and microneedles and widely used in drugs or cells delivery. This process that has resulted in SF being used as a carrier for neurological diseases by laying the basic principles, such as traumatic brain injury, intracerebral hemorrhage, ischemic stroke, Alzheimer’s disease, Parkinson’s disease, glioblastoma, neuroblastoma, and epilepsy. The functions of SF as a carrier in neurological diseases include controlled and sustained drug release, filling the damaged cavity, avoiding volatilization of gaseous drugs, and providing a microenvironment for recovery.
SF has been widely used as a drug-carrier matrix and provides different protection mechanisms according to the characteristics of silk fibroin and the contained drug. SF has been widely used for the preparation of sustained drug delivery systems. SF-based drug delivery materials also regulate cellular homeostasis, such as hemostasis and nerve regeneration, and act as prime modulators of cellular dysfunction, such as inflammation, oxidative stress, cell death, and nerve regeneration, contributing to treat neurological diseases.
3.1 Vascular diseases
SF hydrogels bring drugs into the site of neuronal damage in the brain (targeted administration, and provides the microenvironment for stroke recovery to realize treatment. Stroke, including ischemic and hemorrhagic strokes, is one of the causes of neuron damage in the human body, and the regeneration capacity of neurons is very limited. Current strategies aimed at enhancing brain tissue’s limited capacity for spontaneous regeneration, include using biologics, small molecules, and cells to treat diseases and SF has been used as an effective delivery vector. Noh et al. reported that SF loaded with Brain Factor-7 peptide reduces brain damage and improves neurological function after stroke by reducing the generation of ROS (Noh et al., 2020a). Gorenkova et al. reported that self-assembled SF hydrogels filled the stroke cavity conducive to stroke recovery and there was no significant microglia/macrophage reaction (Gorenkova et al., 2019). Sun et al. reported that a 3D scaffold made of IKVAV-modified SF hydrogels may be useful for the differentiation and maturity of neuronal stem cells (Sun et al., 2017). Fernández-García et al. found that SF hydrogel-loaded with bone marrow mesenchymal stem cells (MSCs-SF) was better to implant into an infarcted hemisphere than SF hydrogels without MSCs, suggesting that SF is an effective delivery vector for stem cells in stroke (Fernández-García et al., 2018). Our group has recently prepared injectable SF hydrogels loaded with hydrogen sulfide (H2S@SF) for the treatment of severe intracerebral hemorrhage. The result showed that the neuroprotective effect of H2S@SF hydrogels on intracerebral hemorrhage was superior to that of SF hydrogels alone because of sustained H2S release and localized targeted delivery from H2S@SF hydrogels (Zhang et al., 2022). In general, it is possible to use SF-based drugs for the development of brain tissue engineering in stroke patients.
3.2 Neurodegenerative diseases
At present, the application of SF in AD is the detection and diagnosis of the disease. SF can also be used for the treatment of Parkinson’s disease, and using SF to deliver drugs is simpler and more effective than the traditional treatment of Parkinson’s disease. Alzheimer’s disease (AD) is pathologically defined by the presence of neurofibrillary tangles and the accumulation of amyloid-beta (Aβ) in the brain (Hardy and Higgins, 1992). Goncalves et al. developed a new prototype of an electrochemical immunosensor with aminoacid-long amyloid-β peptides fixed in layer-by-layer (LbL) films containing SF to make a preliminary diagnosis of AD (Gonçalves et al., 2016). Liu et al. developed an immune electrochemical interface of conductive SF-based immunoparticles (CSIPs) by electrophoretic/electropolymerization strategy that can be easily integrated into point-of-care testing (PoCT) devices. The interface comprises electropolymerized Poly 3,4-ethylene dioxythiophene (PEDOT) bridged conductive SF-based immune particles (CSIPs) to achieve the Aβ detection in the blood of patients with AD (Liu et al., 2018). There is no valid data to support the application of SF-loaded drugs in the treatment of AD, and as a result, SF-based materials can be used in treating AD in a much broader range of applications.
Parkinson’s disease (PD) is characterized by the progressive loss of dopaminergic neurons of the substantia nigra pars compacta that project to the striatum, which translates into reduced dopamine levels. Levodopa, a precursor of dopamine, is the most common method for increasing dopamine concentration in PD patients (Hayes et al., 2019). Acharya et al. devised a new method for converting tyrosine to L-DOPA that is cheaper and more efficient. By fixing tyrosinase to SF, they found the optimal conditions for L-DOPA production and also showed greater stability, allowing mass production (Acharya et al., 2008). However, it is urgent to fill the gaps in the application of SF-loaded drugs in the treatment of PD.
3.3 Traumatic brain injury
After SF hydrogels and porous scaffolds are injected or implanted into the site of traumatic brain injury, it can play its anti-inflammatory effect and the role of related drugs to promote the regeneration and differentiation of damaged neurons to achieve the purpose of treatment. Sultan et al. showed that human MSCs with brain-derived neurotrophic factor (BDNF) can be encapsulated with SF-based hydrogels and BDNF-hMSC was transplanted into rat brain to treat traumatic brain injury (TBI), which represents a critical setup towards brain injury treatment for clinical application. Encapsulated BDNF-HMSC induces neurogenesis by secreting BDNF in vitro cell culture and contributes to the growth and differentiation of neurons at the injured site by eliminating oxidative stress in vivo TBI model (Sultan et al., 2021). Jiang et al. fabricated porous scaffolds using cross-linked collagen and SF and co-cultured them with human umbilical cord MSCs (hUCMSCs) to form implantable complexes. The SF implant complex is injected directly into the site of injury in a TBI canine model. The result showed that the porous SF scaffolds loaded with hUCMSCs could suppress inflammation, inhibit glial proliferation, promote vascular regeneration, induce neuronal cytoskeleton and axon regeneration, and enhance synapses and myelination (Jiang et al., 2021). Our group has recently prepared injectable SF hydrogels loaded with hydrogen sulfide (H2S@SF) to treat TBI and surfaces filled with H2S@SF hydrogels can achieve local administration and sustained-release H2S, avoiding volatile and toxic side effects. Moreover, surfaces filled with H2S@SF hydrogels are capable of penetrating the blood-brain barrier, enabling non-invasive local delivery of H2S after brain injury (Chen et al., 2022b). SF can be formulated into various material formats and different forms of SF carriers, as promising drug delivery systems, should be further studied in the future.
3.4 Brain tumors
Using SF microneedles or hydrogels can achieve targeted drug delivery, control drug release, and improve drug utilization rate (compared with traditional drug delivery), to achieve the purpose of treating nervous system tumors. Ribeiro et al. reported that SF hydrogels were able to suppress the growth of human neuronal glioblastoma (U251) in response to conformational transitions from the unordered coil to the β-sheet structure, suggesting that SF is a very useful tool in specific drug delivery to target glioblastoma (Ribeiro et al., 2018). Wang et al. prepared SF microneedle (SMN) patches to inhibit neovascularization, anti-angiogenesis, and induction of tumor cell apoptosis by remoting activation and controlling the release of Bevacizumab, thrombin, and temozolomide (Wang et al., 2022). SF microneedles provide a vehicle for drug release in the desired manner. Pandey et al. constructed SF nanoparticles loaded with DOX and the DOX-SF nanoparticles induced the exogenous apoptosis pathway of glioblastoma cell lines, C-6 and LN-229 (Pandey et al., 2020). Thermal tumor ablation can be achieved through photothermal therapy using a light absorber under near-infrared (NIR) irradiation. Nevertheless, due to leakage of light absorbent, near-infrared irradiation may damage adjacent tissues. To reduce this damage, Yao et al. developed a bioinspired green hydrogel (BVSF) integrating bioproduct biliverdin to stimulate healing wounds and produce angiogenesis by controllable NIR irradiation Yao et al., 2020. An indocyanine green-SF nanoparticle platform (ICG-SFNPs) for photo-thermal treatment of glioblastoma has been developed by Xu et al. It was effective at accumulating inside tumors in mice bearing nude C6 gliomas when ICG-SFNPs were injected into their veins (Xu et al., 2018; ZhuGe et al., 2019). Coburn et al. found that treated with SF hydrogels containing a continuous release of vincristine and doxorubicin significantly reduced the growth of neuroblastoma tumors. Vincristine delivery significantly slows tumor growth and increases the availability of oncology drugs. In addition, intratumor drug concentration was significantly higher when the hydrogel was injected into the tumor site than when intravenous (Coburn et al., 2017). Therefore, these results provide a powerful tool for the investigation of different forms of SF carriers on the programmed cell death in tumors.
3.5 Epilepsy
Adenosine-carrying SF microspheres, nanofilm coatings, microneedles, and hydrogels can not only treat epilepsy but also monitor the delivery of drugs. Continual monitoring for symptoms and immediate in situ treatment for seizures are crucial for patients with epilepsy, chronic and non-communicable brain diseases affecting people of all ages. Wilz et al. developed a novel adenosine-release system based on SF by insetting adenosine-containing microspheres into a nanomembrane-coated SF scaffolds and were prepared. In this study, it was found that kindling acquisition occurs in a dose-dependent manner when treated with adenosine-releasing brain implants with the desired target release dose, suggesting that implant-derived adenosine might prevent epileptogenesis (Wilz et al., 2008). Zhang et al. developed sensor-equipped devices using a conductive SF hydrogel (CSFH) combination with microneedles array to treat epilepsy for real-time monitoring of the drug delivery (Zhang et al., 2020). These results, based on different SF designs, offer a rapid and sustained treatment to stop or prevent epilepsy.
Neurological diseases can also be classified as chronic or acute according to the speed of onset. Chronic injury can use various forms of SF to achieve the purpose of sustained and controlled release. Acute injury can lead to structural changes and defects. In addition to the sustained release and controlled release of the drug, it also needs to fill the damage site. Therefore, it is suitable for in situ administration with SF hydrogels to fill the defect site and provide a microenvironment suitable for nerve recovery after injury. The application of different forms of SF depends on the pathophysiological mechanisms of various neurological diseases (existing research) as follows (Table 2).
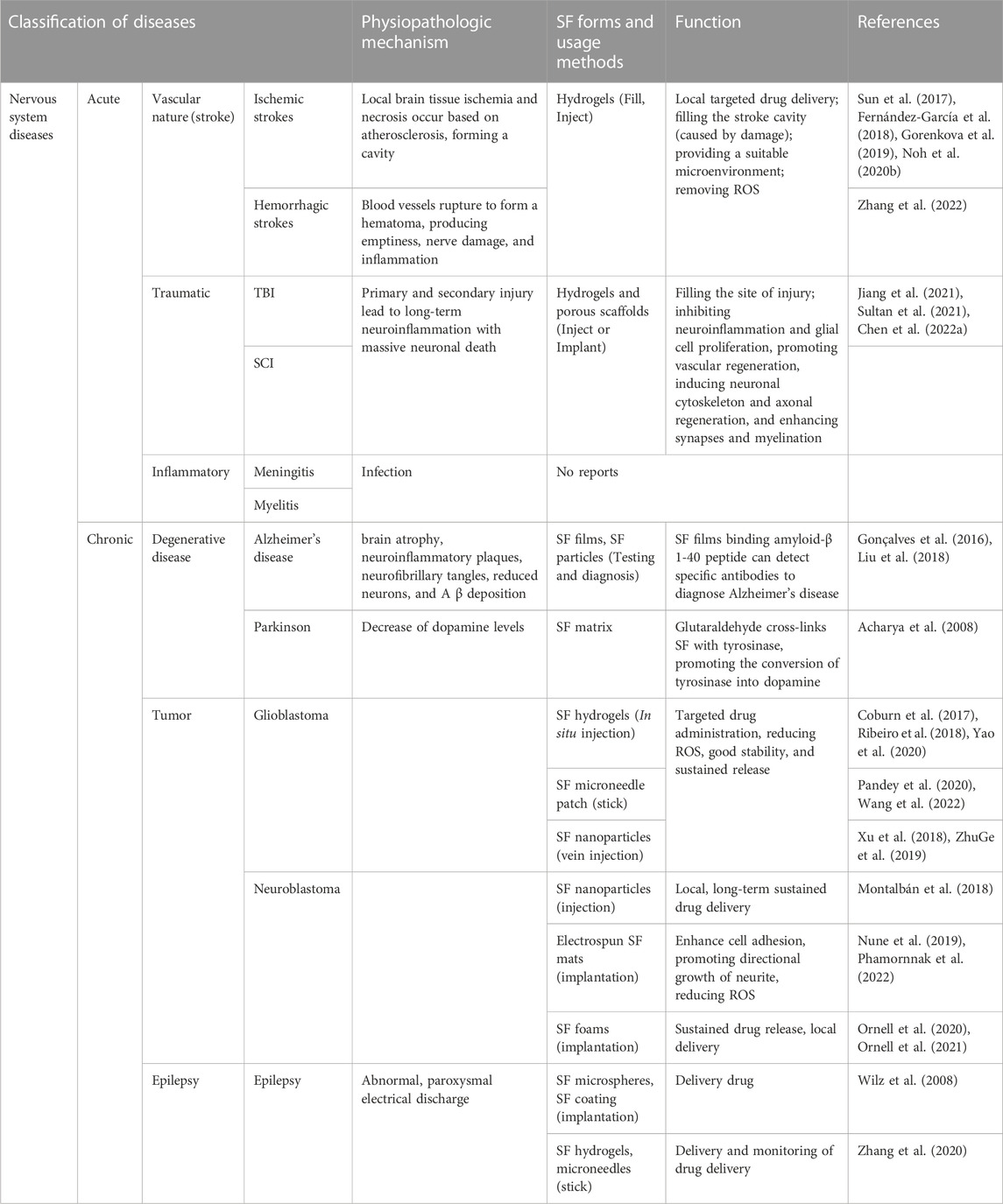
TABLE 2. The application of different SF forms depends on the pathophysiological mechanisms of various neurological diseases.
4 Conclusion
In conclusion, the safety and tolerability of SF as a drug carrier implanted or injected into the human body have been confirmed, indicating the feasibility of SF in the treatment of brain diseases and spinal cord injury (Chung et al., 2014; Wang et al., 2016b; Fernández-García et al., 2016; Li et al., 2016; Jiao et al., 2017; Jiang et al., 2020; Jiang et al., 2021; Wang et al., 2021; Liu et al., 2022b). Due to its biodegradable, biocompatible, mechanically strong characteristic, and better cell adhesion ability (Shen et al., 2022), SF shows great potential for sustained delivery applications of drugs and genes. SF is a versatile material that can be made into a wide range of forms, like microspheres, hydrogels, films, scaffolds, nanoparticles, and microneedles for drug delivery, which have triggered and expanded the use of SF as a carrier of neurological disorders. Biocompatible and biodegradable silk-derived materials can be used to treat neurological diseases if we can improve silk morphologies and the effects of loaded drugs to meet the needs of specific diseases. SF alleviates ischemic injury, protects nerve cells, and improves memory deficits by reducing ROS production (Noh et al., 2020b). The SF hydrogel was injected into the site of ischemic brain injury to fill the site, showing good biocompatibility and providing a good microenvironment for nerve cell regeneration (Gorenkova et al., 2019). Silk fibroin protein has good cell adhesion (Shen et al., 2022). Nevertheless, the treatment effect of a single SF on stroke is limited (Zhang et al., 2022). Therefore, more and more people use SF as a drug delivery system and obtain various forms of modified SF through various methods, or composite materials prepared with SF and other materials to improve the therapeutic effect of nervous system diseases (Sun et al., 2017). In addition, it has been found in the treatment of traumatic brain injury that SF hydrogels carry drugs that can penetrate the blood-brain barrier (Chen et al., 2022a) and inhibit glial proliferation and promote vascular regeneration (Jiang et al., 2021). SF has shown excellent drug targeting and controlled release in the treatment of nervous system tumors (Coburn et al., 2017; Yao et al., 2020). The drug delivery system and sensing device formed by the preparation of SF can control drug release and monitor drug delivery, effectively preventing and treating epilepsy (Wilz et al., 2008; Zhang et al., 2020). SF can also be used as an immune sensing system to detect Aβ levels in the blood to diagnose Alzheimer’s disease (Hardy and Higgins, 1992; Gonçalves et al., 2016; Liu et al., 2018). In addition to its applications in brain diseases, SF has also played a role in the treatment of spinal cord injuries. Liu et al. prepared a hydrogel that can be formed in situ for the treatment of spinal cord injury. The hydrogel is biocompatible, stretchable, mechanically matched, and adhesive to the primary spinal cord. At the same time, the hydrogel fills in the damaged area, promoting nerve regeneration and, ultimately, functional recovery (Liu et al., 2022b). As with brain diseases, the treatment of spinal cord injury uses SF to deliver NSCs (Jiang et al., 2020) and neurotrophic factors (Li et al., 2018) to promote nerve regeneration after spinal cord injury. Now, it has been found that human olfactory ecto-mesenchymal stem cells (hOE-MSCs) can differentiate into motor neurons and promote the recovery of motor function after spinal cord injury, which provides a new promising direction for clinical stem cell therapy (Hamidabadi et al., 2021). However, SF-based materials are still in their infancy, and many aspects require further development and improvement before they can be used to treat most neurological diseases. Alginate-magnetic short nanofibers 3D composite hydrogel enhances the biological activity of hOE-MSCs (Karimi et al., 2021). Therefore, we considered whether we could make a composite of SF together with alginate, magnetic short nanofibers or polycaprolactone (PCL), and poly lactide-co-glycolic acid (PLGA) (Zare et al., 2021). It can not only make the material have good biocompatibility, and optimize the mechanical properties of the material, but also enhance the activity of the cells transported by the material, to promote nerve regeneration after injury. In a word, fabricating various forms of SF-based biomaterials promising approaches to achieve sustained release of drugs and genes in neurological diseases.
Author contributions
Conceptualization, XH, YA, and CC; investigation, XH, YA, and CC; writing—original draft preparation, XH, HS, and MZ; writing—review and editing, XH, HS, and MZ; supervision, HS and MZ; funding acquisition, HS and MZ All authors have read and agreed to the published version of the manuscript.
Funding
This work was supported by the National Natural Science Foundation of China (No. 82071382, No. 81601306); The Priority Academic Program Development of Jiangsu Higher Education Institutions (PAPD); Jiangsu 333 High Level Talent Training Project (2022); The Jiangsu Maternal and Child Health Research Key Project (F202013); Jiangsu Talent Youth Medical Program (QNRC2016245); Shanghai Key Lab of Forensic Medicine (KF2102); Suzhou Science and Technology Development Project (SYS2020089); The Fifth Batch of Gusu District Health Talent Training Project (GSWS2019060); Undergraduate Extracurricular Academic Research Project (KY20220012Z).
Acknowledgments
We are grateful to Professor Qiang Lu for constructive discussion and critical reading.
Conflict of interest
The authors declare that the research was conducted in the absence of any commercial or financial relationships that could be construed as a potential conflict of interest.
Publisher’s note
All claims expressed in this article are solely those of the authors and do not necessarily represent those of their affiliated organizations, or those of the publisher, the editors and the reviewers. Any product that may be evaluated in this article, or claim that may be made by its manufacturer, is not guaranteed or endorsed by the publisher.
References
Abdel-Naby, W., Cole, B., Liu, A., Liu, J., Wan, P., Guaiquil, V. H., et al. (2017). Silk-derived protein enhances corneal epithelial migration, adhesion, and proliferation. Investigative Ophthalmol. Vis. Sci. 58 (3), 1425–1433. doi:10.1167/iovs.16-19957
Abrams, M. B., Dominguez, C., Pernold, K., Reger, R., Wiesenfeld-Hallin, Z., Olson, L., et al. (2009). Multipotent mesenchymal stromal cells attenuate chronic inflammation and injury-induced sensitivity to mechanical stimuli in experimental spinal cord injury. Restor. neurology Neurosci. 27 (4), 307–321. doi:10.3233/RNN-2009-0480
Acharya, C., Kumar, V., Sen, R., and Kundu, S. C. (2008). Performance evaluation of a silk protein-based matrix for the enzymatic conversion of tyrosine to L-DOPA. Biotechnol. J. 3 (2), 226–233. doi:10.1002/biot.200700120
Ayutsede, J., Gandhi, M., Sukigara, S., Ye, H., Hsu, C. M., Gogotsi, Y., et al. (2006). Carbon nanotube reinforced Bombyx mori silk nanofibers by the electrospinning process. Biomacromolecules 7 (1), 208–214. doi:10.1021/bm0505888
Balcom, E. F., Nath, A., and Power, C. (2021). Acute and chronic neurological disorders in COVID-19: Potential mechanisms of disease. Brain 144 (12), 3576–3588. doi:10.1093/brain/awab302
Bouchez, G., Sensebé, L., Vourc'h, P., Garreau, L., Bodard, S., Rico, A., et al. (2008). Partial recovery of dopaminergic pathway after graft of adult mesenchymal stem cells in a rat model of Parkinson's disease. Neurochem. Int. 52 (7), 1332–1342. doi:10.1016/j.neuint.2008.02.003
Cao, Y., and Wang, B. (2009). Biodegradation of silk biomaterials. Int. J. Mol. Sci. 10 (4), 1514–1524. doi:10.3390/ijms10041514
Carvalho, C. R., Chang, W., Silva-Correia, J., Reis, R. L., Oliveira, J. M., and Kohn, J. (2021). Engineering silk fibroin-based nerve conduit with neurotrophic factors for proximal protection after peripheral nerve injury. Adv. Healthc. Mater. 10 (2), e2000753. doi:10.1002/adhm.202000753
Chen, M., Wang, X., Ye, Z., Zhang, Y., Zhou, Y., and Tan, W. S. (2011). A modular approach to the engineering of a centimeter-sized bone tissue construct with human amniotic mesenchymal stem cells-laden microcarriers. Biomaterials 32 (30), 7532–7542. doi:10.1016/j.biomaterials.2011.06.054
Chen, X., Huang, X., Liu, C., Li, S., Yang, Z., Zhang, F., et al. (2022). Surface-fill H(2)S-releasing silk fibroin hydrogel for brain repair through the repression of neuronal pyroptosis. Acta biomater. 154, 259–274. doi:10.1016/j.actbio.2022.11.021
Chen, X., Huang, X., Liu, C., Li, S., Yang, Z., Zhang, F., et al. (2022). Surface-fill H(2)S-releasing silk fibroin hydrogel for brain repair through the repression of neuronal pyroptosis. Acta biomater. 154, 259–274. doi:10.1016/j.actbio.2022.11.021
Chen, Y., Wu, T., Huang, S., Suen, C. W., Cheng, X., Li, J., et al. (2019). Sustained release SDF-1α/TGF-β1-loaded silk fibroin-porous gelatin scaffold promotes cartilage repair. ACS Appl. Mater. interfaces 11 (16), 14608–14618. doi:10.1021/acsami.9b01532
Chen, Z., Zhang, X., Liang, J., Ji, Y., Zhou, Y., and Fang, H. (2021). Preparation of silk fibroin/carboxymethyl chitosan hydrogel under low voltage as a wound dressing. Int. J. Mol. Sci. 22 (14), 7610. doi:10.3390/ijms22147610
Chung, Y. G., Algarrahi, K., Franck, D., Tu, D. D., Adam, R. M., Kaplan, D. L., et al. (2014). The use of bi-layer silk fibroin scaffolds and small intestinal submucosa matrices to support bladder tissue regeneration in a rat model of spinal cord injury. Biomaterials 35 (26), 7452–7459. doi:10.1016/j.biomaterials.2014.05.044
Ciocci, M., Cacciotti, I., Seliktar, D., and Melino, S. (2018). Injectable silk fibroin hydrogels functionalized with microspheres as adult stem cells-carrier systems. Int. J. Biol. Macromol. 108, 960–971. doi:10.1016/j.ijbiomac.2017.11.013
Coburn, J., Harris, J., Zakharov, A. D., Poirier, J., Ikegaki, N., Kajdacsy-Balla, A., et al. (2017). Implantable chemotherapy-loaded silk protein materials for neuroblastoma treatment. Int. J. Cancer 140 (3), 726–735. doi:10.1002/ijc.30479
Cui, Y., Zhang, F., Chen, G., Yao, L., Zhang, N., Liu, Z., et al. (2021). A stretchable and transparent electrode based on PEGylated silk fibroin for in vivo dual-modal neural-vascular activity probing. Adv. Mat. 33 (34), e2100221. doi:10.1002/adma.202100221
Demura, M., and Asakura, T. (1989). Immobilization of glucose oxidase with Bombyx mori silk fibroin by only stretching treatment and its application to glucose sensor. Biotechnol. Bioeng. 33 (5), 598–603. doi:10.1002/bit.260330513
Dhyani, V., and Singh, N. (2017). Modifications of silk film for dual delivery. Biomed. Mater. (Bristol, Engl. 12 (4), 045017. doi:10.1088/1748-605X/aa71bb
Ding, X., Wei, X., Huang, Y., Guan, C., Zou, T., Wang, S., et al. (2015). Delivery of demineralized bone matrix powder using a salt-leached silk fibroin carrier for bone regeneration. J. Mater. Chem. B 3 (16), 3177–3188. doi:10.1039/c5tb00046g
Elliott Donaghue, I., Tam, R., Sefton, M. V., and Shoichet, M. S. (2014). Cell and biomolecule delivery for tissue repair and regeneration in the central nervous system. J. Control Release 190, 219–227. doi:10.1016/j.jconrel.2014.05.040
Farokhi, M., Mottaghitalab, F., Reis, R. L., Ramakrishna, S., and Kundu, S. C. (2020). Functionalized silk fibroin nanofibers as drug carriers: Advantages and challenges. J. Control Release 321, 324–347. doi:10.1016/j.jconrel.2020.02.022
Farokhi, M., Mottaghitalab, F., Samani, S., Shokrgozar, M. A., Kundu, S. C., Reis, R. L., et al. (2018). Silk fibroin/hydroxyapatite composites for bone tissue engineering. Biotechnol. Adv. 36 (1), 68–91. doi:10.1016/j.biotechadv.2017.10.001
Feng, J., Wu, Y., Chen, W., Li, J., Wang, X., Chen, Y., et al. (2020). Sustained release of bioactive IGF-1 from a silk fibroin microsphere-based injectable alginate hydrogel for the treatment of myocardial infarction. J. Mater. Chem. B 8 (2), 308–315. doi:10.1039/c9tb01971e
Fernández-García, L., Marí-Buyé, N., Barios, J. A., Madurga, R., Elices, M., Pérez-Rigueiro, J., et al. (2016). Safety and tolerability of silk fibroin hydrogels implanted into the mouse brain. Acta biomater. 45, 262–275. doi:10.1016/j.actbio.2016.09.003
Fernández-García, L., Pérez-Rigueiro, J., Martinez-Murillo, R., Panetsos, F., Ramos, M., Guinea, G. V., et al. (2018). Cortical reshaping and functional recovery induced by silk fibroin hydrogels-encapsulated stem cells implanted in stroke animals. Front. Cell. Neurosci. 12, 296. doi:10.3389/fncel.2018.00296
Fornasari, B. E., Carta, G., Gambarotta, G., and Raimondo, S. (2020). Natural-based biomaterials for peripheral nerve injury repair. Front. Bioeng. Biotechnol. 8, 554257. doi:10.3389/fbioe.2020.554257
Forostyak, S., Jendelova, P., Kapcalova, M., Arboleda, D., and Sykova, E. (2011). Mesenchymal stromal cells prolong the lifespan in a rat model of amyotrophic lateral sclerosis. Cytotherapy 13 (9), 1036–1046. doi:10.3109/14653249.2011.592521
Garcia-Fuentes, M., Meinel, A. J., Hilbe, M., Meinel, L., and Merkle, H. P. (2009). Silk fibroin/hyaluronan scaffolds for human mesenchymal stem cell culture in tissue engineering. Biomaterials 30 (28), 5068–5076. doi:10.1016/j.biomaterials.2009.06.008
Gharehnazifam, Z., Dolatabadi, R., Baniassadi, M., Shahsavari, H., Kajbafzadeh, A. M., Abrinia, K., et al. (2021). Computational analysis of vincristine loaded silk fibroin hydrogel for sustained drug delivery applications: Multiphysics modeling and experiments. Int. J. Pharm. 609, 121184. doi:10.1016/j.ijpharm.2021.121184
Ghaznavi, A. M., Kokai, L. E., Lovett, M. L., Kaplan, D. L., and Marra, K. G. (2011). Silk fibroin conduits: A cellular and functional assessment of peripheral nerve repair. Ann. plastic Surg. 66 (3), 273–279. doi:10.1097/SAP.0b013e3181e6cff7
Gonçalves, J. M., Lima, L. R., Moraes, M. L., and Ribeiro, S. J. L. (2016). Immunosensor for diagnosis of Alzheimer disease using amyloid-β 1-40 peptide and silk fibroin thin films. C, Mater. Biol. Appl. 68, 338–342. doi:10.1016/j.msec.2016.05.101
Gong, H., Wang, J., Zhang, J., Wu, J., Zheng, Z., Xie, X., et al. (2019). Control of octreotide release from silk fibroin microspheres. C, Mater. Biol. Appl. 102, 820–828. doi:10.1016/j.msec.2019.05.004
Gorenkova, N., Osama, I., Seib, F. P., and Carswell, H. V. O. (2019). In vivo evaluation of engineered self-assembling silk fibroin hydrogels after intracerebral injection in a rat stroke model. ACS biomaterials Sci. Eng. 5 (2), 859–869. doi:10.1021/acsbiomaterials.8b01024
Gou, S., Huang, Y., Wan, Y., Ma, Y., Zhou, X., Tong, X., et al. (2019). Multi-bioresponsive silk fibroin-based nanoparticles with on-demand cytoplasmic drug release capacity for CD44-targeted alleviation of ulcerative colitis. Biomaterials 212, 39–54. doi:10.1016/j.biomaterials.2019.05.012
Gu, Y., Zhu, J., Xue, C., Li, Z., Ding, F., Yang, Y., et al. (2014). Chitosan/silk fibroin-based, Schwann cell-derived extracellular matrix-modified scaffolds for bridging rat sciatic nerve gaps. Biomaterials 35 (7), 2253–2263. doi:10.1016/j.biomaterials.2013.11.087
Guziewicz, N., Best, A., Perez-Ramirez, B., and Kaplan, D. L. (2011). Lyophilized silk fibroin hydrogels for the sustained local delivery of therapeutic monoclonal antibodies. Biomaterials 32 (10), 2642–2650. doi:10.1016/j.biomaterials.2010.12.023
Hamidabadi, H. G., Simorgh, S., Kamrava, S. K., Namjoo, Z., Bagher, Z., Bojnordi, M. N., et al. (2021). Promoting motor functions in a spinal cord injury model of rats using transplantation of differentiated human olfactory stem cells: A step towards future therapy. Behav. Brain Res. 405, 113205. doi:10.1016/j.bbr.2021.113205
Hardy, J. A., and Higgins, G. A. (1992). Alzheimer's disease: The amyloid cascade hypothesis. Sci. (New York, N.Y.) 256 (5054), 184–185. doi:10.1126/science.1566067
Hayes, M. T., Brown, V., Tan, E. J., Chevalier, A., D'Souza, M., Rissel, C., et al. (2019). Patterns and costs of health-care utilisation in Australian children: The first 5 years. Am. J. Med. 132 (7), 802–808. doi:10.1111/jpc.14292
He, X., Liu, X., Yang, J., Du, H., Chai, N., Sha, Z., et al. (2020). Tannic acid-reinforced methacrylated chitosan/methacrylated silk fibroin hydrogels with multifunctionality for accelerating wound healing. Carbohydr. Polym. 247, 116689. doi:10.1016/j.carbpol.2020.116689
Hino, T., Tanimoto, M., and Shimabayashi, S. (2003). Change in secondary structure of silk fibroin during preparation of its microspheres by spray-drying and exposure to humid atmosphere. J. colloid interface Sci. 266 (1), 68–73. doi:10.1016/s0021-9797(03)00584-8
Hoare, T. R., and Kohane, D. S. (2008). Hydrogels in drug delivery: Progress and challenges. Polymer 49 (8), 1993–2007. doi:10.1016/j.polymer.2008.01.027
Horo, H., Bhattacharyya, S., Mandal, B., and Kundu, L. M. (2021). Synthesis of functionalized silk-coated chitosan-gold nanoparticles and microparticles for target-directed delivery of antitumor agents. Carbohydr. Polym. 258, 117659. doi:10.1016/j.carbpol.2021.117659
Hu, A., Zuo, B., Zhang, F., Lan, Q., and Zhang, H. (2012). Electrospun silk fibroin nanofibers promote Schwann cell adhesion, growth and proliferation. Neural Regen. Res. 7 (15), 1171–1178. doi:10.3969/j.issn.1673-5374.2012.15.008
Islam, Y., Leach, A. G., Smith, J., Pluchino, S., Coxonl, C. R., Sivakumaran, M., et al. (2020). Peptide based drug delivery systems to the brain. Nano Express 1 (1), 012002. doi:10.1088/2632-959x/ab9008
Jiang, J., Dai, C., Liu, X., Dai, L., Li, R., Ma, K., et al. (2021). Implantation of regenerative complexes in traumatic brain injury canine models enhances the reconstruction of neural networks and motor function recovery. Theranostics 11 (2), 768–788. doi:10.7150/thno.50540
Jiang, J. P., Liu, X. Y., Zhao, F., Zhu, X., Li, X. Y., Niu, X. G., et al. (2020). Three-dimensional bioprinting collagen/silk fibroin scaffold combined with neural stem cells promotes nerve regeneration after spinal cord injury. Neural Regen. Res. 15 (5), 959–968. doi:10.4103/1673-5374.268974
Jiao, G., Lou, G., Mo, Y., Pan, Y., Zhang, Z., Guo, R., et al. (2017). A combination of GDNF and hUCMSC transplantation loaded on SF/AGs composite scaffolds for spinal cord injury repair. C, Mater. Biol. Appl. 74, 230–237. doi:10.1016/j.msec.2016.12.017
Kapoor, S., and Kundu, S. C. (2016). Silk protein-based hydrogels: Promising advanced materials for biomedical applications. Acta biomater. 31, 17–32. doi:10.1016/j.actbio.2015.11.034
Karimi, S., Bagher, Z., Najmoddin, N., Simorgh, S., and Pezeshki-Modaress, M. (2021). Alginate-magnetic short nanofibers 3D composite hydrogel enhances the encapsulated human olfactory mucosa stem cells bioactivity for potential nerve regeneration application. Int. J. Biol. Macromol. 167, 796–806. doi:10.1016/j.ijbiomac.2020.11.199
Katayama, H., Issiki, M., and Yoshitomi, H. (2000). Application of fibroin in controlled release tablets containing theophylline. Biol. Pharm. Bull. 23 (10), 1229–1234. doi:10.1248/bpb.23.1229
Kim, D. H., Viventi, J., Amsden, J. J., Xiao, J., Vigeland, L., Kim, Y. S., et al. (2010). Dissolvable films of silk fibroin for ultrathin conformal bio-integrated electronics. Nat. Mater. 9 (6), 511–517. doi:10.1038/nmat2745
Kim, M. H., Kim, B. S., Lee, J., Cho, D., Kwon, O. H., and Park, W. H. (2017). Silk fibroin/hydroxyapatite composite hydrogel induced by gamma-ray irradiation for bone tissue engineering. Biomaterials Res. 21, 12. doi:10.1186/s40824-017-0098-2
Kim, U. J., Park, J., Kim, H. J., Wada, M., and Kaplan, D. L. (2005). Three-dimensional aqueous-derived biomaterial scaffolds from silk fibroin. Biomaterials 26 (15), 2775–2785. doi:10.1016/j.biomaterials.2004.07.044
Kundu, J., Chung, Y. I., Kim, Y. H., Tae, G., and Kundu, S. C. (2010). Silk fibroin nanoparticles for cellular uptake and control release. Int. J. Pharm. 388 (1-2), 242–250. doi:10.1016/j.ijpharm.2009.12.052
Kwon, G., Heo, B., Kwon, M. J., Kim, I., Chu, J., Kim, B. Y., et al. (2021). Effect of silk fibroin biomaterial coating on cell viability and intestinal adhesion of probiotic bacteria. J. Microbiol. Biotechnol. 31 (4), 592–600. doi:10.4014/jmb.2103.03031
Lammel, A. S., Hu, X., Park, S. H., Kaplan, D. L., and Scheibel, T. R. (2010). Controlling silk fibroin particle features for drug delivery. Biomaterials 31 (16), 4583–4591. doi:10.1016/j.biomaterials.2010.02.024
Lee, K. Y., Kong, S. J., Park, W. H., Ha, W. S., and Kwon, I. C. (1998). Effect of surface properties on the antithrombogenicity of silk fibroin/S-carboxymethyl kerateine blend films. Polym. Ed. 9 (9), 905–914. doi:10.1163/156856298x00235
Li, G., Che, M. T., Zeng, X., Qiu, X. C., Feng, B., Lai, B. Q., et al. (2018). Neurotrophin-3 released from implant of tissue-engineered fibroin scaffolds inhibits inflammation, enhances nerve fiber regeneration, and improves motor function in canine spinal cord injury. J. Biomed. Mater. Res. Part A 106 (8), 2158–2170. doi:10.1002/jbm.a.36414
Li, G., Che, M. T., Zhang, K., Qin, L. N., Zhang, Y. T., Chen, R. Q., et al. (2016). Graft of the NT-3 persistent delivery gelatin sponge scaffold promotes axon regeneration, attenuates inflammation, and induces cell migration in rat and canine with spinal cord injury. Biomaterials 83, 233–248. doi:10.1016/j.biomaterials.2015.11.059
Li, M., Ogiso, M., and Minoura, N. (2003). Enzymatic degradation behavior of porous silk fibroin sheets. Biomaterials 24 (2), 357–365. doi:10.1016/s0142-9612(02)00326-5
Li, Z.-H., Wang, L., Dai, H.-L., Wang, X.-Y., Li, J.-S., and Zhao, Z. (2019). Fabrication, characterization, and in vitro evaluation of biomimetic silk fibroin porous scaffolds via supercritical CO2 technology. J. Supercrit. Fluids 150, 86–93. doi:10.1016/j.supflu.2019.04.006
Li, Z., Yang, Y., Yao, J., Shao, Z., and Chen, X. (2017). A facile fabrication of silk/MoS2 hybrids for Photothermal therapy. C, Mater. Biol. Appl. 79, 123–129. doi:10.1016/j.msec.2017.05.010
Lim, T. C., and Spector, M. (2017). Biomaterials for enhancing CNS repair. Transl. Stroke Res. 8 (1), 57–64. doi:10.1007/s12975-016-0470-x
Liu, J., Ge, X., Liu, L., Xu, W., and Shao, R. (2022). Challenges and opportunities of silk protein hydrogels in biomedical applications. Mater. Adv. 3 (5), 2291–2308. doi:10.1039/d1ma00960e
Liu, T. C., Lee, Y. C., Ko, C. Y., Liu, R. S., Ke, C. C., Lo, Y. C., et al. (2018). Highly sensitive/selective 3D nanostructured immunoparticle-based interface on a multichannel sensor array for detecting amyloid-beta in Alzheimer's disease. Theranostics 8 (15), 4210–4225. doi:10.7150/thno.25625
Liu, Y., Zhang, Z., Zhang, Y., Luo, B., Liu, X., Cao, Y., et al. (2022). Construction of adhesive and bioactive silk fibroin hydrogel for treatment of spinal cord injury. Acta biomater.
Lovett, M. L., Wang, X., Yucel, T., York, L., Keirstead, M., Haggerty, L., et al. (2015). Silk hydrogels for sustained ocular delivery of anti-vascular endothelial growth factor (anti-VEGF) therapeutics. Eur. J. Pharm. Biopharm. official J. Arbeitsgemeinschaft fur Pharmazeutische Verfahrenstechnik e.V 95 (1), 271–278. doi:10.1016/j.ejpb.2014.12.029
Luetchford, K. A., Chaudhuri, J. B., and De Bank, P. A. (2020). Silk fibroin/gelatin microcarriers as scaffolds for bone tissue engineering. Mater Sci. Eng. C Mater Biol. Appl. 106, 110116. doi:10.1016/j.msec.2019.110116
Madduri, S., and Gander, B. (2012). Growth factor delivery systems and repair strategies for damaged peripheral nerves. J. Control. release official J. Control. Release Soc. 161 (2), 274–282. doi:10.1016/j.jconrel.2011.11.036
Mahmood, A., Lu, D., and Chopp, M. (2004). Intravenous administration of marrow stromal cells (MSCs) increases the expression of growth factors in rat brain after traumatic brain injury. J. neurotrauma 21 (1), 33–39. doi:10.1089/089771504772695922
Martín-Martín, Y., Fernández-García, L., Sanchez-Rebato, M. H., Marí-Buyé, N., Rojo, F. J., Pérez-Rigueiro, J., et al. (2019). Evaluation of neurosecretome from mesenchymal stem cells encapsulated in silk fibroin hydrogels. Sci. Rep. 9 (1), 8801. doi:10.1038/s41598-019-45238-4
Mathur, A. B., and Gupta, V. (2010). Silk fibroin-derived nanoparticles for biomedical applications. Nanomedicine Lond. Engl. 5 (5), 807–820. doi:10.2217/nnm.10.51
Mercado, J., Pérez-Rigueiro, J., González-Nieto, D., Lozano-Picazo, P., López, P., Panetsos, F., et al. (2020). Regenerated silk fibers obtained by straining flow spinning for guiding axonal elongation in primary cortical neurons. ACS biomaterials Sci. Eng. 6 (12), 6842–6852. doi:10.1021/acsbiomaterials.0c00985
Minoura, N., Aiba, S., Higuchi, M., Gotoh, Y., Tsukada, M., and Imai, Y. (1995). Attachment and growth of fibroblast cells on silk fibroin. Biochem. biophysical Res. Commun. 208 (2), 511–516. doi:10.1006/bbrc.1995.1368
Moisenovich, M. M., Plotnikov, E. Y., Moysenovich, A. M., Silachev, D. N., Danilina, T. I., Savchenko, E. S., et al. (2019). Effect of silk fibroin on neuroregeneration after traumatic brain injury. Neurochem. Res. 44 (10), 2261–2272. doi:10.1007/s11064-018-2691-8
Montalbán, M. G., Coburn, J. M., Lozano-Pérez, A. A., Cenis, J. L., Víllora, G., and Kaplan, D. L. (2018). Production of curcumin-loaded silk fibroin nanoparticles for cancer therapy. Nanomater. (Basel, Switz. 8 (2), 126. doi:10.3390/nano8020126
Morris, G., and Schorge, S. (2022). Gene therapy for neurological disease: State of the art and opportunities for next-generation approaches. Neuroscience 490, 309–314. doi:10.1016/j.neuroscience.2022.03.010
Napavichayanun, S., Bonani, W., Yang, Y., Motta, A., and Aramwit, P. (2019). Fibroin and polyvinyl alcohol hydrogel wound dressing containing silk sericin prepared using high-pressure carbon dioxide. Adv. wound care 8 (9), 452–462. doi:10.1089/wound.2018.0856
Noh, Y., Ahn, J. H., Lee, J. W., Hong, J., Lee, T. K., Kim, B., et al. (2020). Brain Factor-7® improves learning and memory deficits and attenuates ischemic brain damage by reduction of ROS generation in stroke in vivo and in vitro. Lab. Anim. Res. 36, 24. doi:10.1186/s42826-020-00057-x
Noh, Y., Ahn, J. H., Lee, J. W., Hong, J., Lee, T. K., Kim, B., et al. (2020). Brain Factor-7® improves learning and memory deficits and attenuates ischemic brain damage by reduction of ROS generation in stroke in vivo and in vitro. Laboratory animal Res. 36, 24. doi:10.1186/s42826-020-00057-x
Nune, M., Manchineella, S., T, G. S. N. K., and K S, N. (2019). Melanin incorporated electroactive and antioxidant silk fibroin nanofibrous scaffolds for nerve tissue engineering. C, Mater. Biol. Appl. 94, 17–25. doi:10.1016/j.msec.2018.09.014
Oral, C. B., Yetiskin, B., and Okay, O. (2020). Stretchable silk fibroin hydrogels. Int. J. Biol. Macromol. 161, 1371–1380. doi:10.1016/j.ijbiomac.2020.08.040
Orive, G., Anitua, E., Pedraz, J. L., and Emerich, D. F. (2009). Biomaterials for promoting brain protection, repair and regeneration. Neuroscience 10 (9), 682–692. doi:10.1038/nrn2685
Ornell, K. J., Chiu, B., and Coburn, J. M. (2021). Development of a dinutuximab delivery system using silk foams for GD2 targeted neuroblastoma cell death. J. Biomed. Mater. Res. Part A 109 (8), 1393–1405. doi:10.1002/jbm.a.37131
Ornell, K. J., Taylor, J. S., Zeki, J., Ikegaki, N., Shimada, H., Coburn, J. M., et al. (2020). Local delivery of dinutuximab from lyophilized silk fibroin foams for treatment of an orthotopic neuroblastoma model. Cancer Med. 9 (8), 2891–2903. doi:10.1002/cam4.2936
Pandey, V., Haider, T., Chandak, A. R., Chakraborty, A., Banerjee, S., and Soni, V. (2020). Surface modified silk fibroin nanoparticles for improved delivery of doxorubicin: Development, characterization, in-vitro studies. Int. J. Biol. Macromol. 164, 2018–2027. doi:10.1016/j.ijbiomac.2020.07.326
Pham, D. T., and Tiyaboonchai, W. (2020). Fibroin nanoparticles: A promising drug delivery system. Drug Deliv. 27 (1), 431–448. doi:10.1080/10717544.2020.1736208
Phamornnak, C., Han, B., Spencer, B. F., Ashton, M. D., Blanford, C. F., Hardy, J. G., et al. (2022). Instructive electroactive electrospun silk fibroin-based biomaterials for peripheral nerve tissue engineering. Biomater. Adv. 141, 213094. doi:10.1016/j.bioadv.2022.213094
Pritchard, E. M., Szybala, C., Boison, D., and Kaplan, D. L. (2010). Silk fibroin encapsulated powder reservoirs for sustained release of adenosine. J. Control. release official J. Control. Release Soc. 144 (2), 159–167. doi:10.1016/j.jconrel.2010.01.035
Pritchard, E. M., Valentin, T., Panilaitis, B., Omenetto, F., and Kaplan, D. L. (2013). Antibiotic-releasing silk biomaterials for infection prevention and treatment. Adv. Funct. Mater. 23 (7), 854–861. doi:10.1002/adfm.201201636
Qi, Z., Cao, J., Tao, X., Wu, X., Kundu, S. C., and Lu, S. (2021). Silk fibroin microneedle patches for the treatment of insomnia. Pharmaceutics 13 (12), 2198. doi:10.3390/pharmaceutics13122198
Ribeiro, M., de Moraes, M. A., Beppu, M. M., Garcia, M. P., Fernandes, M. H., Monteiro, F. J., et al. (2015). Development of silk fibroin/nanohydroxyapatite composite hydrogels for bone tissue engineering. Eur. Polym. J. 67, 66–77. doi:10.1016/j.eurpolymj.2015.03.056
Ribeiro, V. P., Silva-Correia, J., Gonçalves, C., Pina, S., Radhouani, H., Montonen, T., et al. (2018). Rapidly responsive silk fibroin hydrogels as an artificial matrix for the programmed tumor cells death. PloS one 13 (4), e0194441. doi:10.1371/journal.pone.0194441
Rockwood, D. N., Preda, R. C., Yücel, T., Wang, X., Lovett, M. L., and Kaplan, D. L. (2011). Materials fabrication from Bombyx mori silk fibroin. Nat. Protoc. 6 (10), 1612–1631. doi:10.1038/nprot.2011.379
Seib, F. P., and Kaplan, D. L. (2012). Doxorubicin-loaded silk films: Drug-silk interactions and in vivo performance in human orthotopic breast cancer. Biomaterials 33 (33), 8442–8450. doi:10.1016/j.biomaterials.2012.08.004
Shan, D., Ma, C., and Yang, J. (2019). Enabling biodegradable functional biomaterials for the management of neurological disorders. Adv. Drug Deliv. Rev. 148, 219–238. doi:10.1016/j.addr.2019.06.004
Shen, X., Zhang, Y., Gu, Y., Xu, Y., Liu, Y., Li, B., et al. (2016). Sequential and sustained release of SDF-1 and BMP-2 from silk fibroin-nanohydroxyapatite scaffold for the enhancement of bone regeneration. Biomaterials 106, 205–216. doi:10.1016/j.biomaterials.2016.08.023
Shen, Y., Wang, X., Li, B., Guo, Y., and Dong, K. (2022). Development of silk fibroin-sodium alginate scaffold loaded silk fibroin nanoparticles for hemostasis and cell adhesion. Int. J. Biol. Macromol. 211, 514–523. doi:10.1016/j.ijbiomac.2022.05.064
Sommer, M. R., Vetsch, J. R., Leemann, J., Müller, R., Studart, A. R., and Hofmann, S. (2017). Silk fibroin scaffolds with inverse opal structure for bone tissue engineering. J. Biomed. Mater. Res. Part B, Appl. biomaterials 105 (7), 2074–2084. doi:10.1002/jbm.b.33737
Song, R., Murphy, M., Li, C., Ting, K., Soo, C., and Zheng, Z. (2018). Current development of biodegradable polymeric materials for biomedical applications. Drug Des. Devel Ther. 12, 3117–3145. doi:10.2147/DDDT.S165440
Sultan, M. T., Choi, B. Y., Ajiteru, O., Hong, D. K., Lee, S. M., Kim, H. J., et al. (2021). Reinforced-hydrogel encapsulated hMSCs towards brain injury treatment by trans-septal approach. Biomaterials 266, 120413. doi:10.1016/j.biomaterials.2020.120413
Sun, W., Incitti, T., Migliaresi, C., Quattrone, A., Casarosa, S., and Motta, A. (2017). Viability and neuronal differentiation of neural stem cells encapsulated in silk fibroin hydrogel functionalized with an IKVAV peptide. J. tissue Eng. Regen. Med. 11 (5), 1532–1541. doi:10.1002/term.2053
Tamada, Y. (2005). New process to form a silk fibroin porous 3-D structure. Biomacromolecules 6 (6), 3100–3106. doi:10.1021/bm050431f
Tang, S., Liao, X., Shi, B., Qu, Y., Huang, Z., Lin, Q., et al. (2014). The effects of controlled release of neurotrophin-3 from PCLA scaffolds on the survival and neuronal differentiation of transplanted neural stem cells in a rat spinal cord injury model. PloS one 9 (9), e107517. doi:10.1371/journal.pone.0107517
Tang, X., Ding, F., Yang, Y., Hu, N., Wu, H., and Gu, X. (2009). Evaluation on in vitro biocompatibility of silk fibroin-based biomaterials with primarily cultured hippocampal neurons. J. Biomed. Mater. Res. Part A 91 (1), 166–174. doi:10.1002/jbm.a.32212
Tang, Y., Cao, C., Ma, X., Chen, C., and Zhu, H. (2006). Study on the preparation of collagen-modified silk fibroin films and their properties. Biomed. Mater. (Bristol, Engl. 1 (4), 242–246. doi:10.1088/1748-6041/1/4/010
Tang-Schomer, M. D., Kaplan, D. L., and Whalen, M. J. (2019). Film interface for drug testing for delivery to cells in culture and in the brain. Acta biomater. 94, 306–319. doi:10.1016/j.actbio.2019.02.052
Tomeh, M. A., Hadianamrei, R., and Zhao, X. (2019). Silk fibroin as a functional biomaterial for drug and gene delivery. Pharmaceutics 11 (10), 494. doi:10.3390/pharmaceutics11100494
Troy, E., Tilbury, M. A., Power, A. M., and Wall, J. G. (2021). Nature-based biomaterials and their application in biomedicine. Polym. (Basel) 13 (19), 3321. doi:10.3390/polym13193321
Uebersax, L., Mattotti, M., Papaloïzos, M., Merkle, H. P., Gander, B., and Meinel, L. (2007). Silk fibroin matrices for the controlled release of nerve growth factor (NGF). Biomaterials 28 (30), 4449–4460. doi:10.1016/j.biomaterials.2007.06.034
Unger, R. E., Wolf, M., Peters, K., Motta, A., Migliaresi, C., and James Kirkpatrick, C. (2004). Growth of human cells on a non-woven silk fibroin net: A potential for use in tissue engineering. Biomaterials 25 (6), 1069–1075. doi:10.1016/s0142-9612(03)00619-7
Waghule, T., Singhvi, G., Dubey, S. K., Pandey, M. M., Gupta, G., Singh, M., et al. (2019). Microneedles: A smart approach and increasing potential for transdermal drug delivery system. Biomed. Pharmacother. 109, 1249–1258. doi:10.1016/j.biopha.2018.10.078
Wang, J., Yang, Q., Cheng, N., Tao, X., Zhang, Z., Sun, X., et al. (2016). Collagen/silk fibroin composite scaffold incorporated with PLGA microsphere for cartilage repair. C, Mater. Biol. Appl. 61, 705–711. doi:10.1016/j.msec.2015.12.097
Wang, T. G., Xu, J., Zhu, A. H., Lu, H., Miao, Z. N., Zhao, P., et al. (2016). Human amniotic epithelial cells combined with silk fibroin scaffold in the repair of spinal cord injury. Neural Regen. Res. 11 (10), 1670–1677. doi:10.4103/1673-5374.193249
Wang, X. H., Tang, X. C., Li, X., Qin, J. Z., Zhong, W. T., Wu, P., et al. (2021). Implantation of nanofibrous silk scaffolds seeded with bone marrow stromal cells promotes spinal cord regeneration (6686 words). Artif. cells, nanomedicine, Biotechnol. 49 (1), 699–708. doi:10.1080/21691401.2021.2013250
Wang, X., Hu, X., Daley, A., Rabotyagova, O., Cebe, P., and Kaplan, D. L. (2007). Nanolayer biomaterial coatings of silk fibroin for controlled release. J. Control. release official J. Control. Release Soc. 121 (3), 190–199. doi:10.1016/j.jconrel.2007.06.006
Wang, X., Kim, H. J., Xu, P., Matsumoto, A., and Kaplan, D. L. (2005). Biomaterial coatings by stepwise deposition of silk fibroin. Langmuir ACS J. surfaces colloids 21 (24), 11335–11341. doi:10.1021/la051862m
Wang, X., Wenk, E., Matsumoto, A., Meinel, L., Li, C., and Kaplan, D. L. (2007). Silk microspheres for encapsulation and controlled release. J. Control. release official J. Control. Release Soc. 117 (3), 360–370. doi:10.1016/j.jconrel.2006.11.021
Wang, X., Wenk, E., Zhang, X., Meinel, L., Vunjak-Novakovic, G., and Kaplan, D. L. (2009). Growth factor gradients via microsphere delivery in biopolymer scaffolds for osteochondral tissue engineering. J. Control. release official J. Control. Release Soc. 134 (2), 81–90. doi:10.1016/j.jconrel.2008.10.021
Wang, Y., Fan, S., Li, Y., Niu, C., Li, X., Guo, Y., et al. (2020). Silk fibroin/sodium alginate composite porous materials with controllable degradation. Int. J. Biol. Macromol. 150, 1314–1322. doi:10.1016/j.ijbiomac.2019.10.141
Wang, Y. L., Gu, X. M., Kong, Y., Feng, Q. L., and Yang, Y. M. (2015). Electrospun and woven silk fibroin/poly(lactic-co-glycolic acid) nerve guidance conduits for repairing peripheral nerve injury. Neural Regen. Res. 10 (10), 1635–1642. doi:10.4103/1673-5374.167763
Wang, Y., Rudym, D. D., Walsh, A., Abrahamsen, L., Kim, H. J., Kim, H. S., et al. (2008). In vivo degradation of three-dimensional silk fibroin scaffolds. Biomaterials 29 (24-25), 3415–3428. doi:10.1016/j.biomaterials.2008.05.002
Wang, Z., Yang, Z., Jiang, J., Shi, Z., Mao, Y., Qin, N., et al. (2022). Silk microneedle patch capable of on-demand multidrug delivery to the brain for glioblastoma treatment. Adv. Mat. 34 (1), e2106606. doi:10.1002/adma.202106606
Wani, S. U. D., and Veerabhadrappa, G. H. (2018). Silk fibroin based drug delivery applications: Promises and challenges. Curr. Drug Targets 19 (10), 1177–1190. doi:10.2174/1389450119666171227205525
White, J. D., Wang, S., Weiss, A. S., and Kaplan, D. L. (2015). Silk-tropoelastin protein films for nerve guidance. Acta biomater. 14, 1–10. doi:10.1016/j.actbio.2014.11.045
Wilz, A., Pritchard, E. M., Li, T., Lan, J. Q., Kaplan, D. L., and Boison, D. (2008). Silk polymer-based adenosine release: Therapeutic potential for epilepsy. Biomaterials 29 (26), 3609–3616. doi:10.1016/j.biomaterials.2008.05.010
Wu, H., Liu, S., Xiao, L., Dong, X., Lu, Q., and Kaplan, D. L. (2016). Injectable and pH-responsive silk nanofiber hydrogels for sustained anticancer drug delivery. ACS Appl. Mater. interfaces 8 (27), 17118–17126. doi:10.1021/acsami.6b04424
Xu, H. L., ZhuGe, D. L., Chen, P. P., Tong, M. Q., Lin, M. T., Jiang, X., et al. (2018). Silk fibroin nanoparticles dyeing indocyanine green for imaging-guided photo-thermal therapy of glioblastoma. Drug Deliv. 25 (1), 364–375. doi:10.1080/10717544.2018.1428244
Yan, L., Jiang, B., Niu, Y., Wang, H., Li, E., Yan, Y., et al. (2018). Intrathecal delivery of human ESC-derived mesenchymal stem cell spheres promotes recovery of a primate multiple sclerosis model. Cell. death Discov. 4, 28. doi:10.1038/s41420-018-0091-0
Yang, C., Li, S., Huang, X., Chen, X., Shan, H., Chen, X., et al. (2022). Silk fibroin hydrogels could Be therapeutic biomaterials for neurological diseases. Oxid. Med. Cell. Longev. 2022, 2076680. doi:10.1155/2022/2076680
Yang, Y., Chen, X., Ding, F., Zhang, P., Liu, J., and Gu, X. (2007). Biocompatibility evaluation of silk fibroin with peripheral nerve tissues and cells in vitro. Biomaterials 28 (9), 1643–1652. doi:10.1016/j.biomaterials.2006.12.004
Yang, Y., Zhao, Y., Gu, Y., Yan, X., Liu, J., Ding, F., et al. (2009). Degradation behaviors of nerve guidance conduits made up of silk fibroin in vitro and in vivo. Polym. Degrad. Stab. 94 (12), 2213–2220. doi:10.1016/j.polymdegradstab.2009.09.002
Yao, Q., Lan, Q. H., Jiang, X., Du, C. C., Zhai, Y. Y., Shen, X., et al. (2020). Bioinspired biliverdin/silk fibroin hydrogel for antiglioma photothermal therapy and wound healing. Theranostics 10 (25), 11719–11736. doi:10.7150/thno.47682
Yavuz, B., Chambre, L., Harrington, K., Kluge, J., Valenti, L., and Kaplan, D. L. (2020). Silk fibroin microneedle patches for the sustained release of levonorgestrel. ACS Appl. bio Mater. 3 (8), 5375–5382. doi:10.1021/acsabm.0c00671
Yavuz, B., Chambre, L., and Kaplan, D. L. (2019). Extended release formulations using silk proteins for controlled delivery of therapeutics. Expert Opin. drug Deliv. 16 (7), 741–756. doi:10.1080/17425247.2019.1635116
Yonesi, M., Garcia-Nieto, M., Guinea, G. V., Panetsos, F., Pérez-Rigueiro, J., and González-Nieto, D. (2021). Silk fibroin: An ancient material for repairing the injured nervous system. Pharmaceutics 13 (3), 429. doi:10.3390/pharmaceutics13030429
You, R., Zhang, Q., Li, X., Yan, S., Luo, Z., Qu, J., et al. (2020). Multichannel bioactive silk nanofiber conduits direct and enhance axonal regeneration after spinal cord injury. ACS biomaterials Sci. Eng. 6 (8), 4677–4686. doi:10.1021/acsbiomaterials.0c00698
Yucel, T., Lovett, M. L., Giangregorio, R., Coonahan, E., and Kaplan, D. L. (2014). Silk fibroin rods for sustained delivery of breast cancer therapeutics. Biomaterials 35 (30), 8613–8620. doi:10.1016/j.biomaterials.2014.06.030
Zare, P., Pezeshki-Modaress, M., Davachi, S. M., Zare, P., Yazdian, F., Simorgh, S., et al. (2021). Alginate sulfate-based hydrogel/nanofiber composite scaffold with controlled Kartogenin delivery for tissue engineering. Carbohydr. Polym. 266, 118123. doi:10.1016/j.carbpol.2021.118123
Zhang, J., Li, S., Yang, Z., Liu, C., Chen, X., Zhang, Y., et al. (2022). Implantation of injectable SF hydrogel with sustained hydrogen sulfide delivery reduces neuronal pyroptosis and enhances functional recovery after severe intracerebral hemorrhage. Biomater. Adv. 135, 212743. doi:10.1016/j.bioadv.2022.212743
Zhang, Q., Zhao, Y., Yan, S., Yang, Y., Zhao, H., Li, M., et al. (2012). Preparation of uniaxial multichannel silk fibroin scaffolds for guiding primary neurons. Acta biomater. 8 (7), 2628–2638. doi:10.1016/j.actbio.2012.03.033
Zhang, S., Zhou, Z., Zhong, J., Shi, Z., Mao, Y., and Tao, T. H. (2020). Body-integrated, enzyme-triggered degradable, silk-based mechanical sensors for customized health/fitness monitoring and in situ treatment. Adv. Sci. (Weinheim, Baden-Wurttemberg, Ger. 7 (13), 1903802. doi:10.1002/advs.201903802
Zhang, X., Zhang, J., and Shi, B. (2014). Mesoporous bioglass/silk fibroin scaffolds as a drug delivery system: Fabrication, drug loading and release in vitro and repair calvarial defects in vivo. J. Wuhan Univ. Technology-Mater. Sci. Ed. 29 (2), 401–406. doi:10.1007/s11595-014-0929-0
Zhao, Y., Gong, J., Niu, C., Wei, Z., Shi, J., Li, G., et al. (2017). A new electrospun graphene-silk fibroin composite scaffolds for guiding Schwann cells. Polym. Ed. 28 (18), 2171–2185. doi:10.1080/09205063.2017.1386835
Zhao, Z., Chen, A., Li, Y., Hu, J., Liu, X., Li, J., et al. (2012). Fabrication of silk fibroin nanoparticles for controlled drug delivery. J. Nanoparticle Res. 14 (4), 736. doi:10.1007/s11051-012-0736-5
Zhong, T., Jiang, Z., Wang, P., Bie, S., Zhang, F., and Zuo, B. (2015). Silk fibroin/copolymer composite hydrogels for the controlled and sustained release of hydrophobic/hydrophilic drugs. Int. J. Pharm. 494 (1), 264–270. doi:10.1016/j.ijpharm.2015.08.035
Zhou, H., Wang, Z., Cao, H., Hu, H., Luo, Z., Yang, X., et al. (2019). Genipin-crosslinked polyvinyl alcohol/silk fibroin/nano-hydroxyapatite hydrogel for fabrication of artificial cornea scaffolds-a novel approach to corneal tissue engineering. Polym. Ed. 30 (17), 1604–1619. doi:10.1080/09205063.2019.1652418
Zhu, H., Qiao, X., Liu, W., Wang, C., and Zhao, Y. (2020). Microglia play an essential role in synapse development and neuron maturation in tissue-engineered neural tissues. Front. Neurosci. 14, 586452. doi:10.3389/fnins.2020.586452
ZhuGe, D. L., Wang, L. F., Chen, R., Li, X. Z., Huang, Z. W., Yao, Q., et al. (2019). Cross-linked nanoparticles of silk fibroin with proanthocyanidins as a promising vehicle of indocyanine green for photo-thermal therapy of glioma. Artif. cells, nanomedicine, Biotechnol. 47 (1), 4293–4304. doi:10.1080/21691401.2019.1699819
Glossary
Keywords: silk fibroin, sustained release, neurological diseases, TBI, traumatic brain injury, drug delivery
Citation: Huang X, An Y, Yuan S, Chen C, Shan H and Zhang M (2023) Silk fibroin carriers with sustained release capacity for treating neurological diseases. Front. Pharmacol. 14:1117542. doi: 10.3389/fphar.2023.1117542
Received: 06 December 2022; Accepted: 21 April 2023;
Published: 05 May 2023.
Edited by:
Rebeca Alvariño, Universidad de Santiago de Compostela, SpainReviewed by:
Masoumeh Haghbin Nazarpak, Amirkabir University of Technology, IranSara Simorgh, Iran University of Medical Sciences, Iran
Copyright © 2023 Huang, An, Yuan, Chen, Shan and Zhang. This is an open-access article distributed under the terms of the Creative Commons Attribution License (CC BY). The use, distribution or reproduction in other forums is permitted, provided the original author(s) and the copyright owner(s) are credited and that the original publication in this journal is cited, in accordance with accepted academic practice. No use, distribution or reproduction is permitted which does not comply with these terms.
*Correspondence: Haiyan Shan, Z2hvc3RxdGhAMTYzLmNvbQ==; Mingyang Zhang, bWluZ3lhbmd6aGFuZ0BzdWRhLmVkdS5jbg==
†These authors have contributed equally to this work