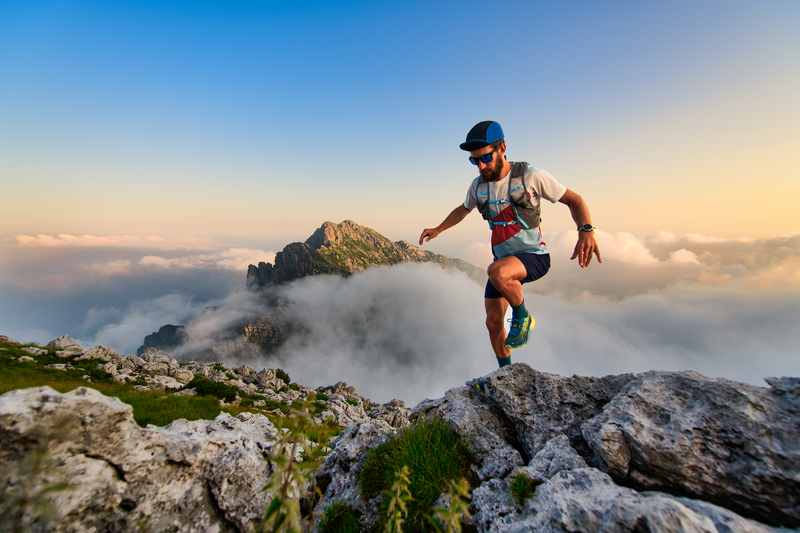
94% of researchers rate our articles as excellent or good
Learn more about the work of our research integrity team to safeguard the quality of each article we publish.
Find out more
REVIEW article
Front. Pharmacol. , 10 May 2023
Sec. Ethnopharmacology
Volume 14 - 2023 | https://doi.org/10.3389/fphar.2023.1117337
This article is part of the Research Topic Natural Products in Regulating Mitochondrial Dysfunction View all 7 articles
Parkinson’s disease (PD), the second most common neurodegenerative disease worldwide, often occurs in middle-aged and elderly individuals. The pathogenesis of PD is complex and includes mitochondrial dysfunction, and oxidative stress. Recently, natural products with multiple structures and their bioactive components have become one of the most important resources for small molecule PD drug research targeting mitochondrial dysfunction. Multiple lines of studies have proven that natural products display ameliorative benefits in PD treatment by regulating mitochondrial dysfunction. Therefore, a comprehensive search of recent published articles between 2012 and 2022 in PubMed, Web of Science, Elesvier, Wliey and Springer was carried out, focusing on original publications related to natural products against PD by restoring mitochondrial dysfunction. This paper presented the mechanisms of various kinds of natural products on PD-related mitochondrial dysfunction regulation and provided evidence that natural products are promising to be developed as drugs for PD therapeutics.
Parkinson’s disease (PD) is a progressive debilitating neurodegenerative disease worldwide that affects approximately 0.1%–0.2% of the general population but approximately 1% of the population over the age of 60 years in modern countries (Wright Willis et al., 2010; Zou et al., 2014). As an age-related neurodegenerative disease, PD onset has shown a serious increase in prevalence rates in the past 60 years (Muhammad et al., 2022). Due to global population aging, the prevalence of PD is expected to increase, and therapies for this disease may face large challenges in the future. The main clinical manifestations of PD are bradykinesia, tremor, postural instability, cognitive impairment and other nonmotor impairments (Blauwendraat et al., 2020; Mohammadipour et al., 2020), and the main pathological features are progressive loss of dopaminergic neurons in the substantia nigra striatum, deposition of neuronal α-synaptic nucleoproteins and formation of Lewy bodies with complex pathogenesis, including mitochondrial dysfunction, oxidative stress, neuroinflammation and so on (Kalia and Lang, 2015). Various treatment strategies have been put into effect, for example, clinical treatment with pharmacotherapy such as levodopa, dopamine agonists and anticholinergics, as well as nondrug interventions such as surgery and exercise (Voon et al., 2017). However, long-term use of these treatments can lead to serious side effects but fail to completely halt disease progression. Therefore, it is of great significance to develop novel drugs that are safe and effective for PD prevention and treatment.
Mitochondria are vital organelles that provide energy to cells and play a particularly important role as the cellular “powerhouse” of dopaminergic neurons (Schapira and Patel, 2014; Gao et al., 2022). Increasing evidence supports the critical role of mitochondrial dysfunction, such as adenosine triphosphate (ATP) depletion, oxidative stress elevation, aberrant mitochondria-dependent apoptosis and other effects, in the development of PD (Cong et al., 2016; Shahba et al., 2021), suggesting that targeting mitochondrial dysfunction is a promising therapeutic target for PD treatments. Natural products originated from plants, animals, or other natural sources have been applied as substances with therapeutic potential to treat different diseases. As reported in previous studies, many kinds of natural products, such as flavonoids and polyphenols, have manifested the strong effects of PD treatment by targeting mitochondrial dysfunction (Kim et al., 2017; van der Merwe et al., 2017). Therefore, this paper provides a brief review of the roles that various natural products play by targeting mitochondrial dysfunction in PD, highlighting the mechanisms of effects to shed light on developing novel therapeutics for PD.
Mitochondria are the energy suppliers of the cells. Mitochondrial respiration can support a vast amount of energy in the ATP form by the oxidative phosphorylation cycle and regulate energy-dependent cell functions, including intermediary metabolism, protein folding, and cell motility (Wallace et al., 1999; Jin et al., 2021). In addition, mitochondria are dynamic organelles that undergo constant fusion and fission (Ashrafi and Schwarz, 2013). Mitophagy is the selective clearance of damaged mitochondria via autophagy. Mitochondria strive to maintain a balance between biogenesis and mitophagy to maintain mitochondrial quality (Ventura-Clapier et al., 2008; Voigt et al., 2016; Wang et al., 2021). In addition, reactive oxygen species (ROS), which are intimately related to oxidative stress, can be generated through the electron carriers of the respiratory chain (Wang et al., 2015). ROS cause mitochondrial membrane lipid peroxidation and protein nitrification; damage mitochondrial DNA, mitochondrial membrane structure and electron transport chain enzyme complexes; weaken or prevent oxidative phosphorylation; reduce ATP production; release caspase-3; and trigger further mitochondrial damage, which leads to mitochondrial dysfunction (Ali et al., 2015; Badshah et al., 2016).
A number of studies have shown that dysfunction of related mitochondrial respiration is of great importance in PD progression (Figure 1). Neurons have a higher energy demand to maintain their function. ATP deficiency induced by mitochondrial dysfunction leads to a decrease in vesicular dopamine uptake, resulting in increased dopaminergic degradation in PD neurons (Wu et al., 2021). ROS, which are a prime culprit for causing mitochondrial dysfunction, play a pivotal role in the pathogenesis of PD (Bhattacharjee and Borah, 2016; Lu et al., 2016). In previous studies, it was also reported that impaired mitochondrial biogenesis led to depletion of mitochondria and that the imbalance of biogenesis and mitophagy is a mechanism mediating PD (Zhu et al., 2012; Liu et al., 2021a). Mitochondrial fission is a process of mitochondrial dynamics. When equilibrium is disrupted, excessive mitochondrial fragmentation leads to mitochondrial dysfunction and neuronal death in PD (Wang et al., 2022). In addition, mitochondrial apoptosis has also been reported in many PD studies (Khan et al., 2018; Balakrishnan et al., 2021). Thus, it is obvious that neurons are highly sensitive to mitochondrial dysfunction in PD and that mitochondria may be a target with great potential.
FIGURE 1. Primary mitochondrial dysfunction associated pathways and disease progression in Parkinson’s disease. Mechanisms of mitochondrial dysfunction in Parkinson’s disease include dysregulation of mitochondrial respiration, apoptosis, dynamics, biogenesis and mitophagy related to a variety of molecules. Mitochondrial dysfunction resulted in progressive loss of dopaminergic neurons in the substantia nigra striatum and ultimately cause severe motor and nonmotor disorders in Parkinson’s disease.
Natural products have been shown to have therapeutic potential for various diseases. Many kinds of natural products, such as flavonoids, polyphenols, terpenoids, and glycosides, have strong effects on PD by targeting mitochondrial dysfunction (Figure 2). Then, we reviewed the natural compounds and their molecular mechanisms by targeting mitochondrial dysfunction from different mechanisms of action (Table 1).
Mitochondria are double-layer organelles where aerobic respiration occurs. It is the major site that generates ATP via oxidative phosphorylation. The electron transfer chain, which is composed of approximately 80 polypeptides and located in the inner membrane of mitochondria, plays a vital role in ATP production. The inner membrane of mitochondria contains different transmembrane protein complexes (I-V) (Yadav et al., 2022). Along with the circulation of electrons through the entire electron transfer chain, different protein complexes set up mitochondrial membrane potential (MMP) across the inner mitochondria membrane and maintain mitochondrial integrity and perform its normal function (Papa et al., 2012). Electrons are prone to leak out of complex I and complex III in the electron transfer chain and transfer to O2 to produce superoxide radicals and hydrogen peroxide, which are referred as ROS (Sies et al., 2017). A balanced amount of mitochondrial ROS is involved in various beneficial processes such as various signaling pathways (Bhat et al., 2015). However, aberrant production of ROS causes severe oxidative stress and triggers PD pathogenesis via mtDNA damage, and lipid peroxidation associated with mitochondrial dysfunction (Ali et al., 2015; Badshah et al., 2016). In the respiratory chain, damage to any of the complexes also causes severe cell and mitochondrial dysfunction such as loss of MMP in PD (Qi et al., 2019). Mitochondrial respiration is an important process through which mitochondria can provide energy that supports physiological activity and body function. Many natural compounds and plant extracts can beneficially function in mitochondrial dysregulation. Most of them are flavonoids and polyphenols, in addition to glycosides, terpenoids, etc.
Flavanoids isolated from various sources induced mitochondrial protection and improved mitochondrial function in PD by upregulating the production of ATP, and ROS, and maintaining the mitochondrial membrane potential and activity of mitochondrial complexes. The dicarbonyl compound methylglyoxal (MG) has been shown to be linked to PD development by inducing mitochondrial dysfunction (Bakala et al., 2012). Exposure of SH-SY5Y cells to MG caused decreases in cell viability, intracellular ATP, and mitochondrial membrane potential. The flavonoid naringenin is extracted from the pericarp of Citrus reticulata Blanco, known as 5,7-dihydroxy-2-(4-hydroxyphenyl)-2,3-dihydrochromen-4-one. It is one of the most important polyphenolic flavanones and has been reported to have neuroprotective effects in PD possessing potent free radical scavenging properties (Lou et al., 2014). SH-SY5Y cells were pretreated for 2 h with naringenin (at 10–80 μM) and then challenged with MG at 500 μM for 24 h. Naringenin significantly increased MMP, ATP, and complex I and V activity, and attenuated the effects on mitochondrial function and the redox environment (de Oliveira et al., 2019). In another study, 80 μM Naringenin treatment in paraquat-induced SH-SY5Y cells resulted in increased cell viability, reduced oxidative stress, elevated MMP, and higher cellular ATP levels. In paraquat-induced rats, 40 mg/kg naringenin treatment resulted in significant neuroprotection against paraquat -induced behavioral deficits, oxidative stress, mitochondrial dysfunction, and astrocytosis (Ahmad et al., 2021). Calycosin, an isoflavonoid extracted from Astragalus membranaceus. , has been revealed to exhibit neuroprotective functions against cerebral ischemia and reperfusion-induced neurological injury, high glucose-induced oxidative stress and neuroinflammation, as well as neuronal apoptosis in earlier studies (Wang and Zhao, 2016; Hsu et al., 2020). Chaouhan et al. (2022) reported that calycosin administration increased ATP and MMP. Flies feed with 100 μM calycosin exhibited significant resistance against paraquat-induced mortality and locomotor deficits in terms of reduced oxidative stress, loss of DA neurons, depletion of dopamine content. Nobiletin is a natural polymethoxylated flavone. The disruption of mitochondrial respiratory complexes I-IV and the enhancement of ROS induced by lipopolysaccharide were ameliorated by nobiletin pretreatment, which also enhanced MMP in BV-2 microglial cells (Qi et al., 2019). Silibinin is a flavonoid extracted and isolated from the fruit of Silybum marianum (L.) Gaertn and has been widely used to exploit drugs for the treatment of diseases. Previous studies showed that silibinin could potentially exert protective effects against neuronal diseases including PD (Kujawska and Jodynis-Liebert, 2018). Silibinin restored MMP decline in mitochondrial respiration (Liu et al., 2021b). Baicalein, 5, 6, 7-trihydroxyflavone, is a flavonoid and is mainly derived from the root of the herb Scutellaria baicalensis Georgi, which is a well-known traditional Chinese medicine (Kuang et al., 2017). Baicalein has neuroprotective effects in PD models by exerting anti-inflammatory, anti-apoptosis, and antioxidative effects. In previous studies, it has been manifested that baicalein protected PC12 and SH-SY5Y cells against neurotoxicity induced by several toxic substances and ameliorated the neurotoxicity in rats (Mu et al., 2009; Zhang et al., 2012). It has been reported that 10 μM baicalein increased cell viability and restored mitochondrial function in SH-SY5Y cells. Baicalein administration (5 mg/kg) prevented rotenone-induced behavioral deficits, dopaminergic neuronal loss, and mitochondrial dysfunction in mice by restoring mitochondrial disorders of MMP decline. Hyperoside, which is a flavonoid glycoside, maintained MMP and ROS and improved mitochondrial function (Kwon et al., 2019). Quercetin (3,5,7,30,40-pentahydroxyflavone), found in Bupleurum chinense or Crataegus pinnatifida, has been proven to have neuroprotective and cognitive enhancing effects in different brain injury models (Selvakumar et al., 2012). A recent study reported that administration of quercetin (10 mg/kg) reduced aluminum-induced oxidative stress including decreasing ROS production and increasing mitochondrial superoxide dismutase (Sharma et al., 2016).
In addition, advanced glycation end products (AGEs) ligate to the receptor of AGEs (RAGE), improving activation of the transcription factor NF-κB, which may be involved in the development of neurodegenerative diseases (Qin et al., 2009). Myricitrin, a flavanoid contained in the root bark of Myrica cerifera, alleviated MG-induced dysfunction of mitochondrial bioenergetics, including an increase in ATP and MMP and a decrease in ROS at concentrations of 1 and 10 μm, and the possible mechanism is through inhibiting the AGE/RAGE/NF-κB pathway (Wang et al., 2014). Icaritin, a flavonoid extracted from Epimedium sagittatum maxim, reversed the decline in the levels of ATP, ADP, inosine, and citric acid in the substantia nigra of PD mice induced by MPTP. Icaritin improved the levels of SDHA, VDAC and ATP5B, which are closely related to mitochondrial respiration and energy supply (Wu et al., 2021).
In previous studies, various phenols and polyphenols exerted potent mitochondrial protection effects, such as resveratrol, mangiferin, curcumin, tea polyphenols, etc. Resveratrol is a natural polyphenolic compound sustained in a variety of plant species, such as Veratrum grandiflorum and Polygonum cuspidatum. It has been reported that resveratrol has potent potential for PD therapy via multiple mitochondria-related pathways (Yadav et al., 2022). The functional impacts of resveratrol on mitochondrial bioenergetics included a decrease in ROS, an increase in complex I and citrate synthase activities, basal oxygen consumption, mitochondrial ATP production, and an attenuated loss of MMP (Ferretta et al., 2014; Lin et al., 2018; Wang et al., 2018). Mangiferin is the primary polyphenol component of Mangifera indica L., possessing neuroprotective effects in PD. Mangiferin (20 μg/mL) pretreatment of rotenone- or MPTP-treated cells resulted in a higher MMP and significantly enhanced ATP levels in SK-N-SH neuroblastoma cells and a PD mouse model (Kavitha et al., 2014; Wang et al., 2022). Demethoxycurcumin (a natural derivative of curcumin), paeonolum and α-Arbutin, which are extracted from Curcuma longa, Ericaceae species, and Moutan cortex, respectively, have been shown to prevent MMP loss and ROS production (Lu et al., 2015; Ramkumar et al., 2017; Ding et al., 2020). In addition, Sativex® (a mixture of 9-tetrahydrocannabinol and cannabidiol) significantly increased mitochondrial complex II activity and complex IV protein levels in mice (Khatri and Juvekar, 2016). Oleuropein, isolated from Fraxinus rhynchophylla, plays a role as a protective molecule against glutamate-induced mitochondrial dysfunction by regulating ATP and ROS levels (Kim et al., 2018). Additionally, Parkin deficiency is able to trigger mitochondrial dysfunction and dopaminergic neuronal loss (Lim and Ng, 2009). Polydatin, a nonglycosylated derivative of resveratrol extracted from grapes, protected mitochondrial function by increasing MMP and decreasing ROS in cells exposed to rotenone or knocked down Parkin (Bai et al., 2020). Putative kinase 1 (PINK1), linked to familial Parkinson’s disease, is known to affect mitochondrial function (Kim et al., 2012). Curcumin is an active natural polyphenolic compound extracted from rhizomes of C. longa, which is widely used. It has been reported that curcumin has multiple pharmacological effects, including the ability to inhibit the key characteristic features of PD such as ROS production, apoptosis, and cognitive deficits in cell cultures and animal models (Yang et al., 2005). In a recent study, it was shown that curcumin at different doses (50, 100, and 200 mg/kg) could significantly rescue complex II activity in mice that were given chronic administration of rotenone for 3 weeks and had significant alterations in mitochondrial enzyme complex activity (Khatri and Juvekar, 2016). The PINK1 gene plays a vital role in the maintenance and regulation of healthy mitochondria, and mutations in the PINK1 gene result in an autosomal recessive form of early-onset PD (Schapira AH et al., 1998). Subsequently, it was also reported that curcumin at a concentration of 2 μM increased MMP and spare respiratory capacity in paraquat-treated PINK1 siRNA and control SH-SY5Y cells (van der Merwe et al., 2017).
Several triterpenoids are capable of repairing the function of mitochondrial bioenergetics, including ginsenoside Re, ursolic, celastrol and mogroside V. Ginsenoside Re is one of the primary biologically active components of ginseng. Ginsenoside Re enhanced NO production and was capable of reversing the deficit in complex IV activity in PINK1 null cells by increasing LRPPRC, Hsp90, and Hsp60 levels, which are mitochondria-related complex IV assembly factors (Kim et al., 2012). Ursolic acid, a pentacyclic triterpenoid carboxylic acid, is found in many plant species. In rotenone-induced damage rats, treatment with ursolic acid at 5 and 10 mg/kg prevented inhibition of mitochondrial complex I activity in the mid-brain (Peshattiwar et al., 2020). Celastrol, a natural triterpene, protected SH-SY5Y cells from rotenone-induced MMP loss and ROS production (Choi et al., 2014). SIRT3 is present in the mitochondria and participates in multiple mitochondrial functions, including maintaining ATP levels (Ahn et al., 2008). Mogroside V, as a bioactive triterpene, recovered ROS and increased MMP, ATP production and the oxygen consumption rate in a dose-dependent manner, which may be associated with SIRT3 upregulation (Luo et al., 2022).
Treatment with the phenylethanoid glycoside echinacoside significantly attenuated the MMP decrease induced by 6-hydroxydopamine (6-OHDA) in PC12 cells (Wang et al., 2015). Astragalus polysaccharide is one of the main active ingredients in astragalus. Astragalus polysaccharide maintained MMP and ROS and improved mitochondrial function (Liu H. et al., 2018). Additionally, cordycepin (3′-deoxyadenosine) is the main bioactive ingredient isolated from Cordyceps militaris. It effectively preserves mitochondrial function by increasing MMP, ATP content, and complex I activity and decreasing ROS levels (Zhang et al., 2021).
Pretreatment of cells with 50 nM anthraquinone, extracted from edible fungi Pleurotus ostreatus, reversed the decrease in MMP induced by 6-OHDA (Bindhu et al., 2020). Treatment with 5 μM embelin resulted in a time-dependent enhancement of the basal oxygen consumption rate and ATP production in rat N27 cells (Rao et al., 2020).
Many plant extracts restored MMP and decreased mitochondrial depolarization, including the extracts of Calyptranthes grandifolia leaves (Kich et al., 2016) and the seaweeds Sargassum muticum, Codium tomentosum, and Ulva compressa (Silva et al., 2018). The extracts of Eucommia ulmoides, Zizyphus spinachristi fruits and Citrus bergamia fruits restored both MMP and ROS (Kwon et al., 2014; Singh et al., 2018; Ferlazzo et al., 2020). Extracts of Acanthopanax senticosus and Ganoderma lucidum reversed mitochondrial membrane potential collapse and ATP depletion caused by MPTP (Liu S. M. et al., 2018; Ren et al., 2019). Bacopa monnieri L. has been proven to have neuroprotective effects, and the standardized extract also plays a role by targeting mitochondrial respiration. Pretreatment with a standardized extract of Bacopa monnieria maintained complex I activity in SK-N-SH cells and complexes II-III activity in the mouse striatal region and prevented MMP loss and ROS production (Singh et al., 2012, 2013; Hosamani et al., 2016). Tomato seed extracts efficiently restored ROT-induced activity loss of complexes I-II (in the hippocampus) and complexes II-III (in the striatum) (Gokul and Muralidhara, 2014). Rao et al. (2016) studied the neuroprotective efficacy of saffron methanolic extract and its bioactive constituent crocin. They both increased the activity of complexes I-III (Rao et al., 2016). Supplementation of the ethyl acetate extract of Morinda citrifolia significantly augmented the activity of complex I by 22% and complex IV by 23% compared to only ROT-treated rats (Kishore Kumar et al., 2017). The standardized extract of Centella asiatica ECa233 (30 mg/kg) protected against the inhibition of complex I and an increase in ROS (Teerapattarakan et al., 2018). Selaginella delicatula extract restored rotenone-induced perturbations in the activity levels of complexes I-II, MMP and activity of ATPases to normalcy among mice (Chandran and Muralidhara, 2013). Piper longum L. extract, containing two active alkaloids, reversed the reduction in MMP in MN9D cells caused by rotenone and protected mitochondrial complex I activity (Wang et al., 2016). Pretreatment with red clover (Trifolium pratense) extract and the individual isoflavone daidzein both decreased ROS levels and enhanced the oxygen consumption rate (de Rus Jacquet et al., 2021).
Mitochondrial biogenesis is a pivotal biological process that plays a critical role in maintaining mitochondrial homeostasis, and ultimately adapts to the cellular physiological demand for energy supply. Mitochondrial biogenesis is the process in which existing mitochondria divide directly to produce new mitochondria. The original mtDNA is indispensable for the mitochondrial biogenesis process due to its obligation to encode essential mitochondrial tRNAs and RNAs (Richter-Dennerlein et al., 2015). Peroxisome proliferator-activated receptor gamma coactivator (PGC)-1α is a member of a family of transcription coactivators that play a central role in the regulation of mitochondrial biogenesis and cellular energy metabolism (Finck and Kelly, 2006; Handschin and Spiegelman, 2006). PGC-1α activation is attributed to adenosine monophosphate-activated protein kinase (AMPK) induced by SIRT1 (Fernandez-Marcos and Auwerx, 2011). PGC-1α can function to activate nuclear respiratory factor (Nrf) 1/2 as a transcriptional modulator, and then bind to the mitochondrial transcription factor A (TFAM) promoter to activate TFAM and complete and promote the replication and transcription of mtDNA. Cytochrome c oxidase (MtCO-1/COX1) is a mtDNA-encoded polypeptide and is a downstream target of PGC-1α. Upregulation of COX1 transcripts provides evidence for the activation of mitochondrial biogenesis and respiratory activity (Peshattiwar et al., 2020). Therefore, the biogenesis of new mitochondria depends on the activation of SIRT1/AMPK/PGC-1α-Nrf-TFAM pathway. Plenty of studies have shown that anomalous expression of SIRT1/AMPK and the decreased expression of PGC-1α, Nrf and TFAM leaded to mitochondrial dysfunction in PD (Li et al., 2017; Mohammadipour et al., 2020).
Mitophagy is a type of selective autophagy that controls the quantity and quality of mitochondria and maintains the normal function of the mitochondrial network. Abnormal mitophagy can cause many pathological changes that can lead to Alzheimer’s disease and Parkinson’s disease (Fang X et al., 2022). Phosphatase and tensin homolog (PTEN)-induced PINK1 and Parkin, the two PD-associated genes, are involved in the selective removal of damaged mitochondria (Geisler et al., 2010). PINK1 acts upstream of Parkin in the mitochondrial quality control pathway, and the two synergistically mediate the polyubiquitination process of damaged mitochondrial surface structures or functional proteins, and play a key role in depolarizing mitophagy degradation (Matsuda et al., 2010). LC3 is the most widely used autophagosome marker to evaluate autophagic flux. During autophagy, LC3I, the cytoplasmic form, is conjugated with phosphatidylethanolamine to form LC3II, which is recruited to autophagosome membranes (Klionsky et al., 2021). During the late stages of autophagy, p62 and p62-bound polyubiquitinated proteins that are incorporated into the autophagosome are degraded in autolysosomes. Accordingly, the level of p62 indicates damaged mitochondria accumulation instead of mitophagy (Lin et al., 2014). Studies have revealed that PINK1/Parkin-dependent mitophagy augmentation occurred in treated PD models and exerted neuroprotective effects along with decreased p62 protein and increased Parkin and LC3-II levels (Kuang et al., 2017; Palikaras and Tavernarakis, 2020; Chaouhan et al., 2022).
Balance maintenance of mitochondrial biogenesis and autophagy plays a crucial role in controlling mitochondrial physiology and function (Narendra et al., 2008). Mitochondrial dysfunction of impaired mitochondrial biogenesis has been observed in neurodegenerative diseases, including Parkinson’s disease (Zhu et al., 2012). Various natural products have shown significant effects on regulating mitochondrial biogenesis and mitophagy.
The flavonoid baicalein could also increase autophagic flux in rotenone-treated mice by increasing the LC3B-II protein level (Kuang et al., 2017). PTEN-induced PINK1 and Parkin, the two PD-associated genes, are involved in the selective removal of damaged mitophagy (Geisler et al., 2010). Silibinin promoted clearance of the toxic effects of damaged mitochondria. MPTP-injected mice were protected against dopaminergic neuronal loss by oral administration of silibinin (280 mg/kg), which increased the expression of PTEN-PINK1 and Parkin, suggesting mitophagy activation (Liu et al., 2021a). The level of p62, an essential mitophagy regulator that indicates the accumulation of damaged mitochondria, was observed to be dramatically increased in paraquat-exposed flies. However, calycosin treatment inhibited this effect. It also decreased the phosphorylation levels of S6K and 4EBP1, indicating mitophagy stimulation (Chaouhan et al., 2022).
Resveratrol regulated mitochondrial biogenesis and mitophagy in PD by regulating the PGC-1α, LC3-II, p62 protein-related pathways, etc. Resveratrol also promoted LC3-II accumulation, inhibited p62 expression and augmented autophagic flux, which was inhibited by rotenone in SH-SY5Y cells (Lin et al., 2014; Lin et al., 2018). A significant resveratrol (25 μM) -mediated increase in the PGC-1α transcriptional activity of downstream genes TFAM and COX 1, the mtDNA/nDNA ratio, and enhanced macroautophagic flux through upregulating LC3-II levels was observed in skin fibroblasts from PD patients (Ferretta et al., 2014). Resveratrol at a concentration of 20 μM promoted LC3-II accumulation, inhibited p62 expression and augmented autophagic flux, which was inhibited by rotenone in SH-SY5Y cells (Lin et al., 2014; Lin et al., 2018). Vanillic acid is a phenolic compound found in various plants and fruits. Its treatment resulted in significant increases in the mRNA expression of PGC-1α and TFAM, and treatment with 300 µM for 24 h significantly elevated the mtDNA copy number and mitochondrial mass of SH-SY5Y cells (Ay, 2022). Urolithin A, a natural compound produced by gut bacteria from ingested ellagitannins and ellagic acid, decreased p62 protein levels and increased LC3-II and other related protein levels of Parkin and PINK1, regulating mitophagy (Qiu et al., 2022). Polydatin increased the expression of LC3-II, indicating that autophagic flux was augmented by polydatin (Bai et al., 2020).
Ursolic acid caused a prominent upregulation of COX1, the downstream gene of PGC-1α, in treated rats at a dose of 10 mg/kg, manifesting the promotion of mitochondrial biogenesis (Peshattiwar et al., 2020).
Pretreatment with the alkaloids piperine and piperlonguminine upregulated LC3-I/II in neurons compared to those without pretreatment, implying an increase in mitophagy (Wang et al., 2016). Nuclear factor erythroid 2-related factor 2-keap1 signaling pathways function to promote mitochondrial biogenesis and cell proliferation (Barone et al., 2011). The alkaloids nicotine and caffeine played a role in the activation of the nuclear factor erythroid 2-related factor 2-Keap1 and PGC-1α signaling pathways, thus regulating mitochondrial biogenesis (Zhou et al., 2019).
Embelin, a natural benzoquinone compound, increased mtDNA levels in a dose- and time-dependent manner in N27 cells. It also caused a substantial increase in the mitochondrial biogenesis regulators of pAMPK, SIRT1, PGC1α and mRNA levels of its downstream targets (Nrf 1/2 and TFAM) (Rao et al., 2020).
Incubation of PC12 cells with alkaloid component extracts from Huperzia selago and Diphasiastrum complanatum for 24 h evoked significant upregulation of the expression of the gene for DNA polymerase γ (Polg), which is responsible for replication of mtDNA and its repair processes (Lenkiewicz et al., 2016). Ginseng total protein administration led to an increase in mtDNA levels and supported mitochondrial biogenesis in PINK1B9-mutated Drosophila (Liu et al., 2020). Theobroma cocoa extract at a concentration of 10 μg/mL produced significant upregulation of PPARγ, PGC1α, Nrf2, TFAM, and COX4 proteins in 1-methyl-4-phenyl-1,2,3,6- pyridine (MPP+)-treated SH-SY5Y cells, mediating mitochondrial biogenesis (Chidambaram et al., 2020). Supplementation with Artemisia argyi extract increased LC3B expression in a dose-dependent manner in comparison with cells treated with MPP+ alone (Wu et al., 2022).
Mitochondria are dynamic organelles that undergo constant fusion and fission and control mitochondrial morphology, providing energy for cells, which is vital for retaining the usual mitochondrial functions as well as cell endurance (Suarez-Rivero et al., 2016). Mitochondrial fusion supplements enzymes and mitochondrial gene products in partially damaged mitochondria to optimize mitochondrial function and reduce the accumulation of age-related mutations in the mitochondrial genome (Westermann, 2012). Fission events create multiple small mitochondria and contribute to separating the fragments of damaged mitochondria from healthy mitochondria, further facilitating their clearance by mitophagy or apoptosis when under high levels of cellular stress (Twig et al., 2008; Lin et al., 2018). Several GTPases mediate mitochondrial dynamics, such as mitochondrial fission 1 (Fis1) and dynamin-related protein-1 (Drp1) for fission in the cytoplasm and mitofusions (Mfn1 and Mfn2) and Optic Atrophy-1 (Opa1) for fusion located on the mitochondrial membrane (Zhang et al., 2021). In addition, the balance between mitochondrial fusion and fission events has been reported to be regulated by PGC-1α and 1β (Scarpulla, 2011). Unbalanced fusion and fission occur in PD with increased fragmented mitochondria or aberrant expression of related genes, and ultimately increased oxidative stress (Kim et al., 2018; Lin et al., 2018) (Chandra et al., 2019).
Silibinin administration to MPTP-treated mice restored mitochondrial dynamic disorder by decreasing Drp1 expression and increasing Mfn1 expression in the hippocampus (Liu et al., 2021b). Quercetin attenuated aluminum-induced mitochondrial swelling, loss of cristae and chromatin condensation and restored the size of mitochondria to normality in the aluminum-treated rat hippocampus, improving mitochondrial integrity and function (Sharma et al., 2016).
Resveratrol pretreatment reversed high percentages of short and fragmented mitochondria in rotenone-exposed SH-SY5Y cells (Lin et al., 2018). Oleuropein, a polyphenolic compound extracted from F. rhynchophylla, amended a glutamate-induced mitochondrial dynamic imbalance and reduced the number of cells with fragmented mitochondria, regulating the phosphorylation of Drp1 at amino acid residue serine 637 (Kim et al., 2018). MPTP promoted Drp1 translocation to mitochondria. However, mangiferin (10 and 40 mg/kg) markedly inhibited this effect to prevent MPTP-induced excessive mitochondrial fission with reversed expression of mitophagic proteins, including PINK1, Parkin, NIX, BNIP3, FUNDC1 and p62, and mitigated mitochondria with a disrupted and swollen structure, vague mitochondrial cristae, and condensate matrix (Wang et al., 2022).
Treatment with 0.1% standardized methanolic extract of Mucuna pruriens seeds significantly reduced the number of damaged, swollen and clearly fragmented mitochondria in presynaptic boutons of antennal lobes of PINK1B9 mutant flies compared with untreated mutants (Poddighe et al., 2014). 4-Hydroxyisophthalic acid is a bioactive component extracted from the roots of Decalepis hamiltonii. Fragmented mitochondrial cristae caused by paraquat exposure could be markedly reduced in the brains of flies fed 4-hydroxyisophthalic acid (Niveditha and Shivanandappa, 2018). Pretreatment with cocoa (10 μg/mL) extract downregulated the expression of mitochondrial Fis1 and upregulated the expression of Mfn2 proteins to balance mitochondrial dynamics in SH-SY5Y cells (Chidambaram et al., 2020). Artemisia leaf extract downregulated mitochondrial fission proteins (Drp1 and p-Drp1) and protected the mitochondria (Wu et al., 2022).
Mitochondria are crucial to the regulation of intrinsic apoptosis. In the mitochondrial apoptotic pathway, the antiapoptotic Bcl-2 and proapoptotic Bax/Bak markers have a primary role. Bax and Bak can be activated and accumulate at the outer mitochondrial membrane (Debatin et al., 2002). Then, they oligomerize and create multimeric pore complexes that alter the permeability of the outer mitochondrial membrane sufficiently, and induce leakage and overactivation of Cytochrome C (Cyt-c) (Jin et al., 2021). Cyt-c is an important mediator in the mitochondrial-associated pathway, which ultimately leads to activation of caspases (Badshah et al., 2015). Inappropriate mitochondrial apoptosis is a crucial process causing neurotoxicity in PD. Thus, a variety of natural products inhibit mitochondrial apoptosis to exert neuroprotective effects by regulating related molecules.
Baicalein inhibited the rotenone-induced increase in caspase-3 activity and significantly prevented rotenone-induced cleaved caspase-3 protein expression in mice (Kuang et al., 2017). Quercetin significantly reduced the Bax/Bcl-2 ratio and prevented the release of Cyt-c and subsequent activation of caspase-3, protecting the hippocampal region of the rat brain (Sharma et al., 2016). Pretreatment with naringenin increased the antiapoptotic protein Bcl-2 while decreasing the proapoptotic protein Bax and inhibited the MG-triggered release of Cyt-c from the mitochondria to the cytosol in SH-SY5Y cells. Meanwhile, it also significantly attenuated proapoptotic enzyme caspase-3/9 activation (de Oliveira et al., 2019). In addition, calycosin supplements alleviate paraquat -induced neurodegeneration by suppressing JNK phosphorylation and caspase-3 activation, which is responsible for DA neuronal cell death in exposed organisms (Chaouhan et al., 2022).
Glutamate treatment induced a decrease in Bcl-2 and an increase in Bax compared to control HT-22 cells. However, pretreatment with oleuropein maintained Bax/Bcl-2 expression levels in HT-22 cells exposed to glutamate treatment (Kim et al., 2018). Mangiferin prevented the increase in the expression of cytosolic Cyt-c and caspase-3/9 observed in rotenone -treated SK-N-SH cells (Kavitha et al., 2014). Green tea polyphenols increased the dysfunction of the mitochondrial apoptosis-related protein Bcl-2 and decreased Bax and caspase-3. When neurons were incubated with siBcl-2, the neuroprotective effect was abrogated (Cong et al., 2016). In rotenone-treated SH-SY5Y cells, the expression of Bax, Bad, caspase-3, caspase-6, caspase-8, and caspase-9 in mitochondria and Cyt-c in the cytosol was increased, whereas the distribution of Bcl-2, Bcl-xL and Cyt-c in mitochondria was significantly decreased. Pretreatment of cells with demethoxycurcumin gradually restored the excessive expression of these proteins (Ramkumar et al., 2017).
Caspase 3 activity and Cyt-c release into the cytosol were found to be decreased following dose-dependent treatment with mogroside V, indicating that mogroside V inhibited mitochondrial apoptosis (Luo et al., 2022).
Astragalus polysaccharide played a role in MPTP-induced PD mice by increasing Bcl-2 and decreasing the expression of Bax, Cyt-c, pro-caspase-3, and caspase-3 protein significantly compared with the control group (Liu H. et al., 2018).
MN9D cells exposed to the alkaloids piperine and piperlonguminine prior to rotenone application had lower cytosolic Cyt-c levels than cells treated with rotenone only, which indicated that the apoptosis induced by rotenone was inhibited (Wang et al., 2016).
Isolongifolene is a novel tricyclic sesquiterpene compound isolated from the herb Murraya koenigii. The biochemical effects of mitochondrial apoptosis in rotenone-treated rats were mitigated by isolongifolene (10 mg/kg), which increased the expression of anti-apoptotic Bcl-2, reduced the expression of pro-apoptotic Bax, inhibited Cyt-c release from mitochondria, and reduced the activation of caspases (Balakrishnan et al., 2021).
Plant extracts also have outstanding effects on hindering mitochondrial apoptosis. Many natural product extracts regulated apoptosis by inhibiting the activity of caspase-3/9, including the water extract of the Edible bird’s nest (Yew et al., 2014), ethanol and hexane extracts of C. grandifolia leaves (Kich et al., 2016), extracts of seaweeds (S. muticum, C. tomentosum, Padina pavonica, U. compressa) (Silva et al., 2018), and extracts of Echium amoenum petals (Sadeghi et al., 2018). Ganoderma lucidum extract and Z. spinachristi fruit extract suppressed the activation of caspase-3/9 and Cyt-c release from mitochondria into the cytoplasm (Singh et al., 2018; Ren et al., 2019). In addition, many plant extracts significantly upregulated the level of anti-apoptotic Bcl-2, downregulated the level of pro-apoptotic Bax and caspase-3/9 and blocked Cyt-c release into the cytosol, thereby alleviating neuronal loss, including the root bark extract of Paeonia suffruticosa, stem bark extract of E. ulmoides, and fruit extract of M. citrifolia (Kim et al., 2014; Kwon et al., 2014; Kishore Kumar et al., 2017). Theobroma cocoa extract increased the expression of the antiapoptotic protein Bcl-2 (Chidambaram et al., 2020). Additionally, pretreatment with the methanol extract of Humulus japonicus (100 and 200 μg/mL) significantly decreased the expression of cleaved PARP, cleaved caspase-9 and cleaved caspase-3. Meanwhile, Cyt-c release from the mitochondria to the cytosol was also significantly suppressed (Ryu et al., 2017). The incubation of SH-SY5Y cells with 6-OHDA significantly upregulated Bax and p53 proteins as well as downregulated Bcl-2 protein and the activity of caspase-3, which were significantly counteracted by preexposure to bergamot juice at both 0.5% and 1% concentrations (Ferlazzo et al., 2020).
As the study demonstrated, G. lucidum extract treatment could regulate mitochondrial mobility by increasing and decreasing the speed in the anterograde and retrograde directions, respectively (Ren et al., 2019). Pretreatment of SK-N-SH cells with the alkaloids piperine and piperlonguminine blocked the opening of the mitochondrial permeability transition pore (Wang et al., 2016).
Previous in vitro and clinical studies have shown that most natural compounds have no significant toxic effects within the concentration range of administration in PD (Kuang et al., 2017; Ding et al., 2020; Ahmad et al., 2021). Baicalein is not mutagenic or genotoxic and showed safety profile in preclinical and clinical toxicity studies. In a Phase I, randomized, double-blind, single-dose trial, baicalein was safe with no signs of toxicity at oral doses of 100–2,800 mg in healthy humans (Yarla et al., 2016). Silybin, silydianin, and silychristin were not cytotoxic or genotoxic at a concentration of 100 μM. Silymarin is safe in humans at therapeutic doses and is well tolerated even at a high dose of 700 mg three times a day for 24 weeks (Soleimani et al., 2019).
Natural components have high safety and serve neuroprotective roles through multiple molecular pathways. However, for this reason, when they are pharmacologically active, they exert nonspecific off-target effects on normal tissues. The toxic effects of natural products vary with the biological species and route of administration. Additionally, several natural compounds may transform into toxic substances in the process of metabolism. Therefore, side effects cannot be neglected while achieving therapeutic efficacy. Quercetin is a flavonoid that can form a semiquinone in the metabolic process, thus causing cytotoxicity (Metodiewa D et al., 1999). When several flavonoids such as naringin, and quercetin were detected, quercetin showed mutagenicity, and mutagenic activity could be detected (Bjeldanes and Chang., 1977). 945 mg/m2 was a safe dose of quercetin, while some patients exhibited emesis, hypertension, nephrotoxicity, and decreased serum potassium with its higher dose (Ferry DR et al., 1996; Bai et al., 2023). It has been reported that high dose curcumin induced apoptosis of normal human lymphocytes and noncancer cell lines (Li W et al., 2017; Eguchi et al., 2022). Nephrotoxic toxicity and gastrointestinal problems were detected after administration of resveratrol (Talib et al., 2022). Ursolic acid may cause liver injury at higher doses of 74, 98, and 130 mg/m2 with some patients exhibiting diarrhoea and elevated serum activity of aspartate aminotransferase, alanine aminotransferase and c-glu-tamyltransferase (Wang XH et al., 2013). In particular, piperine was related to decreased serum protein and increased levels of aspartate aminotransferase and alkaline phosphatase in rats, suggestive of the hepatotoxicity of piperine (Bai et al., 2023). Oral administration of anthraquinones can cause different side effects. It has been reported that a short-term toxicity of 6 weeks of 120 mg/kg oral administration was observed in female rats including disintegration, necrotic changes, and perinuclear vacuolation in the liver and kidney, which were relieved after embelin discontinuance (Poojari, 2014). When the animals received 5.44 mg/kg body weight or more of anthraquinone, acute and subchronic oral toxicity of anthraquinones including anemia and hypothyroidism, was observed in both the male and female rats (Qu et al., 2022).
Here, we presented studies of the neuroprotective effects of multiple natural products by targeting PD mitochondrial dysfunction. Targeting mitochondrial dysfunction is quite important for PD therapeutics, in which various kinds of natural products can play a part. Not only can pure natural products that cover diversiform structures ameliorate mitochondrial damage, but plant extracts sustaining variant components can also come into play; thus, the treatment and improvement of PD disease by natural products can be realized. As summarized above, the pharmacological mechanisms of natural products mainly include regulating mitochondrial respiration, dynamics, apoptosis, biogenesis and mitophagy. Most natural products (such as varieties of flavonoids, polyphenols, terpenoids, etc.) can regulate mitochondrial respiration through the production of ATP, maintenance of MMP and so on. Furthermore, in apoptosis, natural products also exert significant effects by regulating related apoptotic proteins. However, on the sides of dynamics, biogenesis and mitophagy, there are relatively few reports and in-depth investigations. In addition, it is essential to emphasize that it is difficult to illustrate the explicit structure-bioactivity relationship between natural products with structural diversity and PD established on different experimental models.
While many natural products have shown potential to treat PD, there are currently some limitations and challenges. First, many natural products can only be studied in vitro or in animal trials, and more clinical trials are needed to demonstrate their efficacy and safety in humans with Parkinson’s disease. In addition, the efficacy and dosage of natural products are not stable, which brings some risks to the treatment of PD. In order to overcome these limitations and challenges, future research requires more basic experimental studies to gain insight into the mechanism of action of natural products on mitochondrial dysfunction. In addition, more clinical studies are needed to evaluate the safety of natural products and their efficacy in treating human PD. Some of the best natural products can be synthesized and yield more stable, safe and controllable therapeutic doses, which will be a priority for future research.
In conclusion, natural products have great potential to be developed into new drugs for PD with exact effects on mitochondrial dysfunction. Substances of natural origins seem to be accepted more easily by patients since they are considered healthier than fully synthetic drugs. It is necessary to conduct further studies on the related mitochondrial dysfunction mechanisms of PD and other preclinical and clinical studies.
WW and TH guided the scope and research background of the research, and wrote the manuscript. TH and XL wrote the original version of this manuscript. AS and YZ performed the figures. ZX, LM, TW, and ZL critically revised the manuscript. All authors listed have made a substantial, direct, and intellectual contribution to the work and approved it for publication. All authors contributed to the article and approved the submitted version.
This work was financially supported by the Support Program for Science and Technology Department of Sichuan Province (No. 2021YFS0230) and the Clinical Research Incubation Program of West China Hospital (No. 19HXFH010).
The authors declare that the research was conducted in the absence of any commercial or financial relationships that could be construed as a potential conflict of interest.
All claims expressed in this article are solely those of the authors and do not necessarily represent those of their affiliated organizations, or those of the publisher, the editors and the reviewers. Any product that may be evaluated in this article, or claim that may be made by its manufacturer, is not guaranteed or endorsed by the publisher.
Ahmad, M. H., Fatima, M., Ali, M., Rizvi, M. A., and Mondal, A. C. (2021). Naringenin alleviates paraquat-induced dopaminergic neuronal loss in SH-SY5Y cells and a rat model of Parkinson's disease. Neuropharmacology 201, 108831. doi:10.1016/j.neuropharm.2021.108831
Ahn, B. H., Kim, H. S., Song, S., Lee, I. H., Liu, J., Vassilopoulos, A., et al. (2008). A role for the mitochondrial deacetylase Sirt3 in regulating energy homeostasis. Proc. Natl. Acad. Sci. U. S. A. 105 (38), 14447–14452. doi:10.1073/pnas.0803790105
Ali, T., Badshah, H., Kim, T. H., and Kim, M. O. (2015). Melatonin attenuates D-galactose-induced memory impairment, neuroinflammation and neurodegeneration via RAGE/NF-K B/JNK signaling pathway in aging mouse model. J. Pineal Res. 58 (1), 71–85. doi:10.1111/jpi.12194
Ashrafi, G., and Schwarz, T. L. (2013). The pathways of mitophagy for quality control and clearance of mitochondria. Cell Death Differ. 20 (1), 31–42. doi:10.1038/cdd.2012.81
Ay, M. (2022). Vanillic acid induces mitochondrial biogenesis in SH-SY5Y cells. Mol. Biol. Rep. 49 (6), 4443–4449. doi:10.1007/s11033-022-07284-6
Badshah, H., Ali, T., Kim, M. J., Kim, M., Abid, N. B., Shah, S. A., et al. (2015). Co-treatment with anthocyanins and vitamin C ameliorates ethanol- induced neurodegeneration via modulation of GABAB receptor signaling in the adult rat brain. CNS neurological Disord. drug targets 14 (6), 791–803. doi:10.2174/1871527314666150225142919
Badshah, H., Ali, T., and Kim, M. O. (2016). Osmotin attenuates LPS-induced neuroinflammation and memory impairments via the TLR4/NFκB signaling pathway. Sci. Rep. 6, 24493. doi:10.1038/srep24493
Bai, H., Ding, Y., Li, X., Kong, D., Xin, C., Yang, X., et al. (2020). Polydatin protects SH-SY5Y in models of Parkinson's disease by promoting Atg5-mediated but parkin-independent autophagy. Neurochem. Int. 134, 104671. doi:10.1016/j.neuint.2020.104671
Bai, X., Bian, Z., and Zhang, M. (2023). Targeting the Nrf2 signaling pathway using phytochemical ingredients: A novel therapeutic road map to combat neurodegenerative diseases. Phytomedicine 109, 154582. doi:10.1016/j.phymed.2022.154582
Bakala, H., Hamelin, M., Mary, J., Borot-Laloi, C., and Friguet, B. (2012). Catalase, a target of glycation damage in rat liver mitochondria with aging. Biochim. Biophys. Acta 1822 (10), 1527–1534. doi:10.1016/j.bbadis.2012.05.016
Balakrishnan, R., Vijayraja, D., Mohankumar, T., Manimaran, D., Ganesan, P., Choi, D. K., et al. (2021). Isolongifolene mitigates rotenone-induced dopamine depletion and motor deficits through anti-oxidative and anti-apoptotic effects in a rat model of Parkinson's disease. J. Chem. Neuroanat. 112, 101890. doi:10.1016/j.jchemneu.2020.101890
Barone, M. C., Sykiotis, G. P., and Bohmann, D. (2011). Genetic activation of Nrf2 signaling is sufficient to ameliorate neurodegenerative phenotypes in a Drosophila model of Parkinson's disease. Dis. Model Mech. 4 (5), 701–707. doi:10.1242/dmm.007575
Bhat, A. H., Dar, K. B., Anees, S., Zargar, M. A., Masood, A., Sofi, M. A., et al. (2015). Oxidative stress, mitochondrial dysfunction and neurodegenerative diseases; a mechanistic insight. Biomed. Pharmacother. 74, 101–110. doi:10.1016/j.biopha.2015.07.025
Bhattacharjee, N., and Borah, A. (2016). Oxidative stress and mitochondrial dysfunction are the underlying events of dopaminergic neurodegeneration in homocysteine rat model of Parkinson's disease. Neurochem. Int. 101, 48–55. doi:10.1016/j.neuint.2016.10.001
Bindhu, J., Das, A., and Sakthivel, K. M. (2020). Anthraquinone from edible fungi Pleurotus ostreatus protects human SH-SY5Y neuroblastoma cells against 6-hydroxydopamine-induced cell death-preclinical validation of gene knockout possibilities of PARK7, PINK1, and SNCA1 using CRISPR SpCas9. Appl. Biochem. Biotechnol. 191 (2), 555–566. doi:10.1007/s12010-019-03188-7
Bjeldanes, L. .F., and Chang, G. .W. (1977). Mutagenic activity of quercetin and related compounds. Science 197 (4303), 577–578. doi:10.1126/science.327550
Blauwendraat, C., Nalls, M. A., and Singleton, A. B. (2020). The genetic architecture of Parkinson's disease. Lancet Neurol. 19 (2), 170–178. doi:10.1016/S1474-4422(19)30287-X
Casarejos, M. J., Perucho, J., Gomez, A., Munoz, M. P., Fernandez-Estevez, M., Sagredo, O., et al. (2013). Natural cannabinoids improve dopamine neurotransmission and tau and amyloid pathology in a mouse model of tauopathy. J. Alzheimers Dis. 35 (3), 525–539. doi:10.3233/JAD-130050
Chandra, G., Shenoi, R. A., Anand, R., Rajamma, U., and Mohanakumar, K. P. (2019). Reinforcing mitochondrial functions in aging brain: An insight into Parkinson's disease therapeutics. J. Chem. Neuroanat. 95, 29–42. doi:10.1016/j.jchemneu.2017.12.004
Chandran, G., and Muralidhara, (2013). Neuroprotective effect of aqueous extract of Selaginella delicatula as evidenced by abrogation of rotenone-induced motor deficits, oxidative dysfunctions, and neurotoxicity in mice. Cell Mol. Neurobiol. 33 (7), 929–942. doi:10.1007/s10571-013-9959-y
Chaouhan, H. S., Li, X., Sun, K. T., Wang, I. K., Yu, T. M., Yu, S. H., et al. (2022). Calycosin alleviates paraquat-induced neurodegeneration by improving mitochondrial functions and regulating autophagy in a Drosophila model of Parkinson's disease. Antioxidants (Basel) 11 (2), 222. doi:10.3390/antiox11020222
Chidambaram, S. B., Bhat, A., Ray, B., Sugumar, M., Muthukumar, S. P., Manivasagam, T., et al. (2020). Cocoa beans improve mitochondrial biogenesis via PPARγ/PGC1α dependent signalling pathway in MPP+ intoxicated human neuroblastoma cells (SH-SY5Y). Nutr. Neurosci. 23 (6), 471–480. doi:10.1080/1028415X.2018.1521088
Choi, B. S., Kim, H., Lee, H. J., Sapkota, K., Park, S. E., Kim, S., et al. (2014). Celastrol from 'Thunder God Vine' protects SH-SY5Y cells through the preservation of mitochondrial function and inhibition of p38 MAPK in a rotenone model of Parkinson's disease. Neurochem. Res. 39 (1), 84–96. doi:10.1007/s11064-013-1193-y
Cong, L., Cao, C., Cheng, Y., and Qin, X. Y. (2016). Green tea polyphenols attenuated glutamate excitotoxicity via antioxidative and antiapoptotic pathway in the primary cultured cortical neurons. Oxid. Med. Cell Longev. 2016, 2050435. doi:10.1155/2016/2050435
de Oliveira, M. R., Custodio de Souza, I. C., and Furstenau, C. R. (2019). Promotion of mitochondrial protection by naringenin in methylglyoxal-treated SH-SY5Y cells: Involvement of the Nrf2/GSH axis. Chem. Biol. Interact. 310, 108728. doi:10.1016/j.cbi.2019.108728
de Rus Jacquet, A., Ambaw, A., Tambe, M. A., Ma, S. Y., Timmers, M., Grace, M. H., et al. (2021). Neuroprotective mechanisms of red clover and soy isoflavones in Parkinson's disease models. Food Funct. 12 (23), 11987–12007. doi:10.1039/d1fo00007a
Debatin, K. M., Poncet, D., and Kroemer, G. (2002). Chemotherapy: Targeting the mitochondrial cell death pathway. Oncogene 21 (57), 8786–8803. doi:10.1038/sj.onc.1206039
Ding, Y., Kong, D., Zhou, T., Yang, N. D., Xin, C., Xu, J., et al. (2020). α-Arbutin protects against Parkinson's disease-associated mitochondrial dysfunction in vitro and in vivo. Neuromolecular Med. 22 (1), 56–67. doi:10.1007/s12017-019-08562-6
Eguchi, N., Damyar, K., Alexander, M., Dafoe, D., Lakey, J. R. T., and Ichii, H. (2022). Anti-oxidative therapy in islet cell transplantation. Antioxidants (Basel) 11 (6), 1038. doi:10.3390/antiox11061038
Fang, X., Wu, H., Wei, J., Miao, R., and Zhang, Y. (2022). Research progress on the pharmacological effects of berberine targeting mitochondria. Front. Endocrinol. (Lausanne) 13, 982145. doi:10.3389/fendo.2022.982145
Ferlazzo, N., Cirmi, S., Maugeri, A., Russo, C., Lombardo, G. E., Gangemi, S., et al. (2020). Neuroprotective effect of bergamot juice in 6-OHDA-induced SH-SY5Y cell death, an in vitro model of Parkinson's disease. Pharmaceutics 12 (4), 326. doi:10.3390/pharmaceutics12040326
Fernandez-Marcos, P. J., and Auwerx, J. (2011). Regulation of PGC-1α, a nodal regulator of mitochondrial biogenesis. Am. J. Clin. Nutr. 93 (4), 884S–890S. doi:10.3945/ajcn.110.001917
Ferretta, A., Gaballo, A., Tanzarella, P., Piccoli, C., Capitanio, N., Nico, B., et al. (2014). Effect of resveratrol on mitochondrial function: Implications in parkin-associated familiar Parkinson's disease. Biochim. Biophys. Acta 1842 (7), 902–915. doi:10.1016/j.bbadis.2014.02.010
Ferry, D. .R., Smith, A., Malkhandi, J., Fyfe, D. .W., deTakats, P. .G., Anderson, D., et al. (1996). Phase I clinical trial of the flavonoid quercetin: Pharmacokinetics and evidence for in vivo tyrosine kinase inhibition. Clin. Cancer Res. 2 (4), 659–668.
Finck, B. N., and Kelly, D. P. (2006). PGC-1 coactivators: Inducible regulators of energy metabolism in health and disease. J. Clin. Invest. 116 (3), 615–622. doi:10.1172/JCI27794
Gao, X. Y., Yang, T., Gu, Y., and Sun, X. H. (2022). Mitochondrial dysfunction in Parkinson's disease: From mechanistic insights to therapy. Front. Aging Neurosci. 14, 885500. doi:10.3389/fnagi.2022.885500
Geisler, S., Holmstrom, K. M., Skujat, D., Fiesel, F. C., Rothfuss, O. C., Kahle, P. J., et al. (2010). PINK1/Parkin-mediated mitophagy is dependent on VDAC1 and p62/SQSTM1. Nat. Cell Biol. 12 (2), 119–131. doi:10.1038/ncb2012
Gokul, K., and Muralidhara, (2014). Oral supplements of aqueous extract of tomato seeds alleviate motor abnormality, oxidative impairments and neurotoxicity induced by rotenone in mice: Relevance to Parkinson's disease. Neurochem. Res. 39 (7), 1382–1394. doi:10.1007/s11064-014-1323-1
Handschin, C., and Spiegelman, B. M. (2006). Peroxisome proliferator-activated receptor gamma coactivator 1 coactivators, energy homeostasis, and metabolism. Endocr. Rev. 27 (7), 728–735. doi:10.1210/er.2006-0037
Hosamani, R., Krishna, G., and Muralidhara, (2016). Standardized Bacopa monnieri extract ameliorates acute paraquat-induced oxidative stress, and neurotoxicity in prepubertal mice brain. Nutr. Neurosci. 19 (10), 434–446. doi:10.1179/1476830514Y.0000000149
Hsu, C. C., Kuo, T. W., Liu, W. P., Chang, C. P., and Lin, H. J. (2020). Calycosin preserves BDNF/TrkB signaling and reduces post-stroke neurological injury after cerebral ischemia by reducing accumulation of hypertrophic and TNF-alpha-containing microglia in rats. J. Neuroimmune Pharmacol. 15 (2), 326–339. doi:10.1007/s11481-019-09903-9
Jin, X., Guo, J. L., Wang, L., Zhong, X., Yao, W. F., Gao, H., et al. (2021). Natural products as pharmacological modulators of mitochondrial dysfunctions for the treatments of alzheimer's disease: A comprehensive review. Eur. J. Med. Chem. 218, 113401. doi:10.1016/j.ejmech.2021.113401
Kalia, L. V., and Lang, A. E. (2015). Parkinson's disease. Lancet 386 (9996), 896–912. doi:10.1016/S0140-6736(14)61393-3
Kavitha, M., Manivasagam, T., Essa, M. M., Tamilselvam, K., Selvakumar, G. P., Karthikeyan, S., et al. (2014). Mangiferin antagonizes rotenone: Induced apoptosis through attenuating mitochondrial dysfunction and oxidative stress in SK-N-sh neuroblastoma cells. Neurochem. Res. 39 (4), 668–676. doi:10.1007/s11064-014-1249-7
Khan, A., Ali, T., Rehman, S. U., Khan, M. S., Alam, S. I., Ikram, M., et al. (2018). Neuroprotective effect of quercetin against the detrimental effects of LPS in the adult mouse brain. Front. Pharmacol. 9, 1383. doi:10.3389/fphar.2018.01383
Khatri, D. K., and Juvekar, A. R. (2016). Neuroprotective effect of curcumin as evinced by abrogation of rotenone-induced motor deficits, oxidative and mitochondrial dysfunctions in mouse model of Parkinson's disease. Pharmacol. Biochem. Behav. 150, 39–47. doi:10.1016/j.pbb.2016.09.002
Kich, D. M., Bitencourt, S., Alves, C., Silva, J., Pinteus, S., Pedrosa, R., et al. (2016). Neuromodulatory effects of Calyptranthes grandifolia extracts against 6-hydroxydopamine-induced neurotoxicity in SH-SY5Y cells. Biomed. Pharmacother. 84, 382–386. doi:10.1016/j.biopha.2016.09.063
Kim, H. G., Park, G., Piao, Y., Kang, M. S., Pak, Y. K., Hong, S. P., et al. (2014). Effects of the root bark of Paeonia suffruticosa on mitochondria-mediated neuroprotection in an MPTP-induced model of Parkinson's disease. Food Chem. Toxicol. 65, 293–300. doi:10.1016/j.fct.2013.12.037
Kim, K. H., Song, K., Yoon, S. H., Shehzad, O., Kim, Y. S., and Son, J. H. (2012). Rescue of PINK1 protein null-specific mitochondrial complex IV deficits by ginsenoside Re activation of nitric oxide signaling. J. Biol. Chem. 287 (53), 44109–44120. doi:10.1074/jbc.M112.408146
Kim, M. H., Min, J. S., Lee, J. Y., Chae, U., Yang, E. J., Song, K. S., et al. (2018). Oleuropein isolated from Fraxinus rhynchophylla inhibits glutamate-induced neuronal cell death by attenuating mitochondrial dysfunction. Nutr. Neurosci. 21 (7), 520–528. doi:10.1080/1028415X.2017.1317449
Kim, S. M., Park, Y. J., Shin, M. S., Kim, H. R., Kim, M. J., Lee, S. H., et al. (2017). Acacetin inhibits neuronal cell death induced by 6-hydroxydopamine in cellular Parkinson's disease model. Bioorg Med. Chem. Lett. 27 (23), 5207–5212. doi:10.1016/j.bmcl.2017.10.048
Kishore Kumar, S. N., Deepthy, J., Saraswathi, U., Thangarajeswari, M., Yogesh Kanna, S., Ezhil, P., et al. (2017). Morinda citrifolia mitigates rotenone-induced striatal neuronal loss in male Sprague-Dawley rats by preventing mitochondrial pathway of intrinsic apoptosis. Redox Rep. 22 (6), 418–429. doi:10.1080/13510002.2016.1253449
Klionsky, D. J., Abdel-Aziz, A. K., Abdelfatah, S., Abdellatif, M., Abdoli, A., Abel, S., et al. (2021). Guidelines for the use and interpretation of assays for monitoring autophagy (4th edition)1. Autophagy 17 (1), 1–382. doi:10.1080/15548627.2020.1797280
Kuang, L., Cao, X., and Lu, Z. (2017). Baicalein protects against rotenone-induced neurotoxicity through induction of autophagy. Biol. Pharm. Bull. 40 (9), 1537–1543. doi:10.1248/bpb.b17-00392
Kujawska, M., and Jodynis-Liebert, J. (2018). Polyphenols in Parkinson's disease: A systematic review of in vivo studies. Nutrients 10 (5), 642. doi:10.3390/nu10050642
Kwon, S. H., Lee, S. R., Park, Y. J., Ra, M., Lee, Y., Pang, C., et al. (2019). Suppression of 6-hydroxydopamine-induced oxidative stress by hyperoside via activation of Nrf2/HO-1 signaling in dopaminergic neurons. Int. J. Mol. Sci. 20 (23), 5832. doi:10.3390/ijms20235832
Kwon, S. H., Ma, S. X., Hong, S. I., Kim, S. Y., Lee, S. Y., and Jang, C. G. (2014). Eucommia ulmoides Oliv. bark. attenuates 6-hydroxydopamine-induced neuronal cell death through inhibition of oxidative stress in SH-SY5Y cells. J. Ethnopharmacol. 152 (1), 173–182. doi:10.1016/j.jep.2013.12.048
Lenkiewicz, A. M., Czapski, G. A., Jesko, H., Wilkaniec, A., Szypula, W., Pietrosiuk, A., et al. (2016). Potent effects of alkaloid-rich extract from Huperzia selago against sodium nitroprusside-evoked PC12 cells damage via attenuation of oxidative stress and apoptosis. Folia Neuropathol. 54 (2), 156–166. doi:10.5114/fn.2016.60361
Li, P. A., Hou, X., and Hao, S. (2017). Mitochondrial biogenesis in neurodegeneration. J. Neurosci. Res. 95 (10), 2025–2029. doi:10.1002/jnr.24042
Li, W., Zhou, Y., Yang, J., Zhang, H. H., Zhao, S. L., Zhang, T., et al. (2017). Curcumin induces apoptosis and protective autophagy in human gastric cancer cells with different degree of differentiation. Zhonghua Zhong Liu Za Zhi 39 (7), 490–496. doi:10.3760/cma.j.issn.0253-3766.2017.07.003
Lim, K. L., and Ng, C. H. (2009). Genetic models of Parkinson disease. Biochim. Biophys. Acta 1792 (7), 604–615. doi:10.1016/j.bbadis.2008.10.005
Lin, K. L., Lin, K. J., Wang, P. W., Chuang, J. H., Lin, H. Y., Chen, S. D., et al. (2018). Resveratrol provides neuroprotective effects through modulation of mitochondrial dynamics and ERK1/2 regulated autophagy. Free Radic. Res. 52 (11-12), 1371–1386. doi:10.1080/10715762.2018.1489128
Lin, T. K., Chen, S. D., Chuang, Y. C., Lin, H. Y., Huang, C. R., Chuang, J. H., et al. (2014). Resveratrol partially prevents rotenone-induced neurotoxicity in dopaminergic SH-SY5Y cells through induction of heme oxygenase-1 dependent autophagy. Int. J. Mol. Sci. 15 (1), 1625–1646. doi:10.3390/ijms15011625
Liu, H., Chen, S., Guo, C., Tang, W., Liu, W., and Liu, Y. (2018a). Astragalus polysaccharide protects neurons and stabilizes mitochondrial in a mouse model of Parkinson disease. Med. Sci. Monit. 24, 5192–5199. doi:10.12659/MSM.908021
Liu, M., Yu, S., Wang, J., Qiao, J., Liu, Y., Wang, S., et al. (2020). Ginseng protein protects against mitochondrial dysfunction and neurodegeneration by inducing mitochondrial unfolded protein response in Drosophila melanogaster PINK1 model of Parkinson's disease. J. Ethnopharmacol. 247, 112213. doi:10.1016/j.jep.2019.112213
Liu, S. M., Li, X. Z., Zhang, S. N., Yang, Z. M., Wang, K. X., Lu, F., et al. (2018b). Acanthopanax senticosus protects structure and function of mesencephalic mitochondria in A mouse model of Parkinson's disease. Chin. J. Integr. Med. 24 (11), 835–843. doi:10.1007/s11655-018-2935-5
Liu, X., Liu, W., Wang, C., Chen, Y., Liu, P., Hayashi, T., et al. (2021a). Silibinin attenuates motor dysfunction in a mouse model of Parkinson's disease by suppression of oxidative stress and neuroinflammation along with promotion of mitophagy. Physiol. Behav. 239, 113510. doi:10.1016/j.physbeh.2021.113510
Liu, X., Wang, C., Liu, W., Song, S., Fu, J., Hayashi, T., et al. (2021b). Oral administration of silibinin ameliorates cognitive deficits of Parkinson's disease mouse model by restoring mitochondrial disorders in Hippocampus. Neurochem. Res. 46 (9), 2317–2332. doi:10.1007/s11064-021-03363-5
Lou, H., Jing, X., Wei, X., Shi, H., Ren, D., and Zhang, X. (2014). Naringenin protects against 6-OHDA-induced neurotoxicity via activation of the Nrf2/ARE signaling pathway. Neuropharmacology 79, 380–388. doi:10.1016/j.neuropharm.2013.11.026
Lu, M., Su, C., Qiao, C., Bian, Y., Ding, J., and Hu, G. (2016). Metformin prevents dopaminergic neuron death in MPTP/P-induced mouse model of Parkinson's disease via autophagy and mitochondrial ROS clearance. Int. J. Neuropsychopharmacol. 19 (9), pyw047. doi:10.1093/ijnp/pyw047
Lu, X. L., Lin, Y. H., Wu, Q., Su, F. J., Ye, C. H., Shi, L., et al. (2015). Paeonolum protects against MPP(+)-induced neurotoxicity in zebrafish and PC12 cells. BMC Complement. Altern. Med. 15, 137. doi:10.1186/s12906-015-0661-0
Luo, H., Peng, C., Xu, X., Peng, Y., Shi, F., Li, Q., et al. (2022). The protective effects of mogroside V against neuronal damages by attenuating mitochondrial dysfunction via upregulating Sirtuin3. Mol. Neurobiol. 59 (4), 2068–2084. doi:10.1007/s12035-021-02689-z
Matsuda, N., Sato, S., Shiba, K., Okatsu, K., Saisho, K., Gautier, C. A., et al. (2010). PINK1 stabilized by mitochondrial depolarization recruits Parkin to damaged mitochondria and activates latent Parkin for mitophagy. J. Cell Biol. 189 (2), 211–221. doi:10.1083/jcb.200910140
Metodiewa, D., Jaiswal, A. .K., Cenas, N., and Dickancaité, E. (1999). Quercetin may act as a cytotoxic prooxidant after its metabolic activation to semiquinone and quinoidal product. Free Radic. Biol. Med. 26 (1-2), 107–116. doi:10.1016/s0891-5849(98)00167-1
Mohammadipour, A., Haghir, H., and Ebrahimzadeh Bideskan, A. (2020). A link between nanoparticles and Parkinson's disease. Which nanoparticles are most harmful? Rev. Environ. Health 35 (4), 545–556. doi:10.1515/reveh-2020-0043
Mu, X., He, G., Cheng, Y., Li, X., Xu, B., and Du, G. (2009). Baicalein exerts neuroprotective effects in 6-hydroxydopamine-induced experimental parkinsonism in vivo and in vitro. Pharmacol. Biochem. Behav. 92 (4), 642–648. doi:10.1016/j.pbb.2009.03.008
Muhammad, F., Liu, Y., Zhou, Y., Yang, H., and Li, H. (2022). Antioxidative role of traditional Chinese medicine in Parkinson's disease. J. Ethnopharmacol. 285, 114821. doi:10.1016/j.jep.2021.114821
Narendra, D., Tanaka, A., Suen, D. F., and Youle, R. J. (2008). Parkin is recruited selectively to impaired mitochondria and promotes their autophagy. J. Cell Biol. 183 (5), 795–803. doi:10.1083/jcb.200809125
Niveditha, S., and Shivanandappa, T. (2018). Neuroprotective action of 4-Hydroxyisophthalic acid against paraquat-induced motor impairment involves amelioration of mitochondrial damage and neurodegeneration in Drosophila. Neurotoxicology 66, 160–169. doi:10.1016/j.neuro.2018.04.006
Palikaras, K., and Tavernarakis, N. (2020). Regulation and roles of mitophagy at synapses. Mech. Ageing Dev. 187, 111216. doi:10.1016/j.mad.2020.111216
Papa, S., Martino, P. L., Capitanio, G., Gaballo, A., De Rasmo, D., Signorile, A., et al. (2012). The oxidative phosphorylation system in mammalian mitochondria. Adv. Exp. Med. Biol. 942, 3–37. doi:10.1007/978-94-007-2869-1_1
Peshattiwar, V., Muke, S., Kaikini, A., Bagle, S., Dighe, V., and Sathaye, S. (2020). Mechanistic evaluation of Ursolic acid against rotenone induced Parkinson's disease-emphasizing the role of mitochondrial biogenesis. Brain Res. Bull. 160, 150–161. doi:10.1016/j.brainresbull.2020.03.003
Poddighe, S., De Rose, F., Marotta, R., Ruffilli, R., Fanti, M., Secci, P. P., et al. (2014). Mucuna pruriens (Velvet bean) rescues motor, olfactory, mitochondrial and synaptic impairment in PINK1B9 Drosophila melanogaster genetic model of Parkinson's disease. PLoS One 9 (10), e110802. doi:10.1371/journal.pone.0110802
Poojari, R. (2014). Embelin - a drug of antiquity: Shifting the paradigm towards modern medicine. Expert Opin. Investig. Drugs 23 (3), 427–444. doi:10.1517/13543784.2014.867016
Qi, G., Mi, Y., Fan, R., Li, R., Liu, Z., and Liu, X. (2019). Nobiletin protects against systemic inflammation-stimulated memory impairment via MAPK and NF-κB signaling pathways. J. Agric. Food Chem. 67 (18), 5122–5134. doi:10.1021/acs.jafc.9b00133
Qin, Y. H., Dai, S. M., Tang, G. S., Zhang, J., Ren, D., Wang, Z. W., et al. (2009). HMGB1 enhances the proinflammatory activity of lipopolysaccharide by promoting the phosphorylation of MAPK p38 through receptor for advanced glycation end products. J. Immunol. 183 (10), 6244–6250. doi:10.4049/jimmunol.0900390
Qiu, J., Chen, Y., Zhuo, J., Zhang, L., Liu, J., Wang, B., et al. (2022). Urolithin A promotes mitophagy and suppresses NLRP3 inflammasome activation in lipopolysaccharide-induced BV2 microglial cells and MPTP-induced Parkinson's disease model. Neuropharmacology 207, 108963. doi:10.1016/j.neuropharm.2022.108963
Qu, J., Pei, L., Wang, X., Fu, S., Yong, L., Xiao, X., et al. (2022). Acute and subchronic oral toxicity of anthraquinone in sprague dawley rats. Int. J. Environ. Res. Public Health 19 (16), 10413. doi:10.3390/ijerph191610413
Ramkumar, M., Rajasankar, S., Gobi, V. V., Dhanalakshmi, C., Manivasagam, T., Justin Thenmozhi, A., et al. (2017). Neuroprotective effect of Demethoxycurcumin, a natural derivative of Curcumin on rotenone induced neurotoxicity in SH-SY 5Y Neuroblastoma cells. BMC Complement. Altern. Med. 17 (1), 217. doi:10.1186/s12906-017-1720-5
Rao, S. P., Sharma, N., and Kalivendi, S. V. (2020). Embelin averts MPTP-induced dysfunction in mitochondrial bioenergetics and biogenesis via activation of SIRT1. Biochim. Biophys. Acta Bioenerg. 1861 (3), 148157. doi:10.1016/j.bbabio.2020.148157
Rao, S. V., MuralidharaYenisetti, S. C., and Rajini, P. S. (2016). Evidence of neuroprotective effects of saffron and crocin in a Drosophila model of parkinsonism. Neurotoxicology 52, 230–242. doi:10.1016/j.neuro.2015.12.010
Ren, Z. L., Wang, C. D., Wang, T., Ding, H., Zhou, M., Yang, N., et al. (2019). Ganoderma lucidum extract ameliorates MPTP-induced parkinsonism and protects dopaminergic neurons from oxidative stress via regulating mitochondrial function, autophagy, and apoptosis. Acta Pharmacol. Sin. 40 (4), 441–450. doi:10.1038/s41401-018-0077-8
Richter-Dennerlein, R., Dennerlein, S., and Rehling, P. (2015). Integrating mitochondrial translation into the cellular context. Nat. Rev. Mol. Cell Biol. 16 (10), 586–592. doi:10.1038/nrm4051
Ryu, Y. K., Kang, Y., Go, J., Park, H. Y., Noh, J. R., Kim, Y. H., et al. (2017). Humulus japonicus prevents dopaminergic neuron death in 6-hydroxydopamine-induced models of Parkinson's disease. J. Med. Food 20 (2), 116–123. doi:10.1089/jmf.2016.3851
Sadeghi, L., Tanwir, F., and Yousefi Babadi, V. (2018). Physiological and biochemical effects of Echium amoenum extract on Mn(2+)-imposed Parkinson like disorder in rats. Adv. Pharm. Bull. 8 (4), 705–713. doi:10.15171/apb.2018.079
Scarpulla, R. C. (2011). Metabolic control of mitochondrial biogenesis through the PGC-1 family regulatory network. Biochimica Biophysica Acta (BBA) - Mol. Cell Res. 1813 (7), 1269–1278. doi:10.1016/j.bbamcr.2010.09.019
Schapira, A. H., Gu, M., Taanman, J. W., Tabrizi, S. J., Seaton, T., Cleeter, M., et al. (1998). Mitochondria in the etiology and pathogenesis of Parkinson's disease. Ann. Neurol. 448 (3), S89–S98. doi:10.1002/ana.410440714
Schapira, A. H., and Patel, S. (2014). Targeting mitochondria for neuroprotection in Parkinson disease. JAMA Neurol. 71 (5), 537–538. doi:10.1001/jamaneurol.2014.64
Selvakumar, K., Bavithra, S., Suganthi, M., Benson, C. S., Elumalai, P., Arunkumar, R., et al. (2012). Protective role of quercetin on PCBs-induced oxidative stress and apoptosis in hippocampus of adult rats. Neurochem. Res. 37 (4), 708–721. doi:10.1007/s11064-011-0661-5
Shahba, S., Mehrzad, J., and Malvandi, A. M. (2021). Neuroimmune disruptions from naturally occurring levels of mycotoxins. Environ. Sci. Pollut. Res. Int. 28, 32156–32176. doi:10.1007/s11356-021-14146-4
Sharma, D. R., Wani, W. Y., Sunkaria, A., Kandimalla, R. J., Sharma, R. K., Verma, D., et al. (2016). Quercetin attenuates neuronal death against aluminum-induced neurodegeneration in the rat hippocampus. Neuroscience 324, 163–176. doi:10.1016/j.neuroscience.2016.02.055
Sies, H., Berndt, C., and Jones, D. P. (2017). Oxidative stress. Annu. Rev. Biochem. 86, 715–748. doi:10.1146/annurev-biochem-061516-045037
Silva, J., Alves, C., Pinteus, S., Mendes, S., and Pedrosa, R. (2018). Neuroprotective effects of seaweeds against 6-hydroxidopamine-induced cell death on an in vitro human neuroblastoma model. BMC Complement. Altern. Med. 18 (1), 58. doi:10.1186/s12906-018-2103-2
Singh, M., Murthy, V., and Ramassamy, C. (2013). Neuroprotective mechanisms of the standardized extract of Bacopa monniera in a paraquat/diquat-mediated acute toxicity. Neurochem. Int. 62 (5), 530–539. doi:10.1016/j.neuint.2013.01.030
Singh, M., Murthy, V., and Ramassamy, C. (2012). Standardized extracts of Bacopa monniera protect against MPP+- and paraquat-induced toxicity by modulating mitochondrial activities, proteasomal functions, and redox pathways. Toxicol. Sci. 125 (1), 219–232. doi:10.1093/toxsci/kfr255
Singh, V., Essa, M. M., Guizani, N., Balakrishnan, R., Hemalatha, T., Manivasagam, T., et al. (2018). Protective effect of Zizyphus spinachristi on MPP+-induced oxidative stress. Front. Biosci. (Scholar Ed. 10 (2), 285–299. doi:10.2741/s516
Soleimani, V., Delghandi, P. S., Moallem, S. A., and Karimi, G. (2019). Safety and toxicity of silymarin, the major constituent of milk thistle extract: An updated review. Phytother. Res. 33 (6), 1627–1638. doi:10.1002/ptr.6361
Suarez-Rivero, J. M., Villanueva-Paz, M., de la Cruz-Ojeda, P., de la Mata, M., Cotan, D., Oropesa-Avila, M., et al. (2016). Mitochondrial dynamics in mitochondrial diseases. Diseases 5 (1), 1. doi:10.3390/diseases5010001
Talib, W. H., Awajan, D., Hamed, R. A., Azzam, A. O., Mahmod, A. I., and Al-Yasari, I. H. (2022). Combination anticancer therapies using selected phytochemicals. Molecules 27 (17), 5452. doi:10.3390/molecules27175452
Teerapattarakan, N., Benya-Aphikul, H., Tansawat, R., Wanakhachornkrai, O., Tantisira, M. H., and Rodsiri, R. (2018). Neuroprotective effect of a standardized extract of Centella asiatica ECa233 in rotenone-induced parkinsonism rats. Phytomedicine 44, 65–73. doi:10.1016/j.phymed.2018.04.028
Twig, G., Elorza, A., Molina, A. J., Mohamed, H., Wikstrom, J. D., Walzer, G., et al. (2008). Fission and selective fusion govern mitochondrial segregation and elimination by autophagy. EMBO J. 27 (2), 433–446. doi:10.1038/sj.emboj.7601963
van der Merwe, C., van Dyk, H. C., Engelbrecht, L., van der Westhuizen, F. H., Kinnear, C., Loos, B., et al. (2017). Curcumin rescues a PINK1 knock down SH-SY5Y cellular model of Parkinson's disease from mitochondrial dysfunction and cell death. Mol. Neurobiol. 54 (4), 2752–2762. doi:10.1007/s12035-016-9843-0
Ventura-Clapier, R., Garnier, A., and Veksler, V. (2008). Transcriptional control of mitochondrial biogenesis: The central role of PGC-1alpha. Cardiovasc Res. 79 (2), 208–217. doi:10.1093/cvr/cvn098
Voigt, A., Berlemann, L. A., and Winklhofer, K. F. (2016). The mitochondrial kinase PINK1: Functions beyond mitophagy. J. Neurochem. 139 (1), 232–239. doi:10.1111/jnc.13655
Voon, V., Napier, T. C., Frank, M. J., Sgambato-Faure, V., Grace, A. A., Rodriguez-Oroz, M., et al. (2017). Impulse control disorders and levodopa-induced dyskinesias in Parkinson's disease: An update. Lancet Neurol. 16 (3), 238–250. doi:10.1016/S1474-4422(17)30004-2
Wallace, D. C., Brown, M. D., and Lott, M. T. (1999). Mitochondrial DNA variation in human evolution and disease. Gene 238 (1), 211–230. doi:10.1016/s0378-1119(99)00295-4
Wang, H., Dong, X., Liu, Z., Zhu, S., Liu, H., Fan, W., et al. (2018). Resveratrol suppresses rotenone-induced neurotoxicity through activation of SIRT1/akt1 signaling pathway. Anat. Rec. Hob. 301 (6), 1115–1125. doi:10.1002/ar.23781
Wang, H., Liu, J., Gao, G., Wu, X., Wang, X., and Yang, H. (2016). Protection effect of piperine and piperlonguminine from Piper longum L. alkaloids against rotenone-induced neuronal injury. Brain Res. 1639, 214–227. doi:10.1016/j.brainres.2015.07.029
Wang, X. H., Zhou, S. Y., Qian, Z. Z., Zhang, H. L., Qiu, L. H., Song, Z., et al. (2013). Evaluation of toxicity and single-dose pharmacokinetics of intravenous ursolic acid liposomes in healthy adult volunteers and patients with advanced solid tumors. Expert Opin. Drug Metab. Toxicol. 9, 117–125. doi:10.1517/17425255.2013.738667
Wang, X. L., Feng, S. T., Wang, Y. T., Zhang, N. N., Guo, Z. Y., Yan, X., et al. (2022). Mangiferin, a natural glucoxilxanthone, inhibits mitochondrial dynamin-related protein 1 and relieves aberrant mitophagic proteins in mice model of Parkinson's disease. Phytomedicine 104, 154281. doi:10.1016/j.phymed.2022.154281
Wang, X. L., Feng, S. T., Wang, Z. Z., Chen, N. H., and Zhang, Y. (2021). Role of mitophagy in mitochondrial quality control: Mechanisms and potential implications for neurodegenerative diseases. Pharmacol. Res. 165, 105433. doi:10.1016/j.phrs.2021.105433
Wang, X., and Zhao, L. (2016). Calycosin ameliorates diabetes-induced cognitive impairments in rats by reducing oxidative stress via the PI3K/Akt/GSK-3beta signaling pathway. Biochem. Biophys. Res. Commun. 473 (2), 428–434. doi:10.1016/j.bbrc.2016.03.024
Wang, Y. H., Xuan, Z. H., Tian, S., and Du, G. H. (2015). Echinacoside protects against 6-hydroxydopamine-induced mitochondrial dysfunction and inflammatory responses in PC12 cells via reducing ROS production. Evid. Based Complement. Altern. Med. 2015, 189239. doi:10.1155/2015/189239
Wang, Y. H., Yu, H. T., Pu, X. P., and Du, G. H. (2014). Myricitrin alleviates methylglyoxal-induced mitochondrial dysfunction and AGEs/RAGE/NF-κB pathway activation in SH-SY5Y cells. J. Mol. Neurosci. 53 (4), 562–570. doi:10.1007/s12031-013-0222-2
Westermann, B. (2012). Bioenergetic role of mitochondrial fusion and fission. Biochim. Biophys. Acta 1817 (10), 1833–1838. doi:10.1016/j.bbabio.2012.02.033
Wright Willis, A., Evanoff, B. A., Lian, M., Criswell, S. R., and Racette, B. A. (2010). Geographic and ethnic variation in Parkinson disease: A population-based study of US medicare beneficiaries. Neuroepidemiology 34 (3), 143–151. doi:10.1159/000275491
Wu, H., Liu, X., Gao, Z. Y., Lin, M., Zhao, X., Sun, Y., et al. (2021). Icaritin provides neuroprotection in Parkinson's disease by attenuating neuroinflammation, oxidative stress, and energy deficiency. Antioxidants (Basel) 10 (4), 529. doi:10.3390/antiox10040529
Wu, L. K., Agarwal, S., Kuo, C. H., Kung, Y. L., Day, C. H., Lin, P. Y., et al. (2022). Artemisia Leaf Extract protects against neuron toxicity by TRPML1 activation and promoting autophagy/mitophagy clearance in both in vitro and in vivo models of MPP+/MPTP-induced Parkinson's disease. Phytomedicine 104, 154250. doi:10.1016/j.phymed.2022.154250
Yadav, E., Yadav, P., Khan, M. M. U., Singh, H., and Verma, A. (2022). Resveratrol: A potential therapeutic natural polyphenol for neurodegenerative diseases associated with mitochondrial dysfunction. Front. Pharmacol. 13, 922232. doi:10.3389/fphar.2022.922232
Yang, F., Lim, G. P., Begum, A. N., Ubeda, O. J., Simmons, M. R., Ambegaokar, S. S., et al. (2005). Curcumin inhibits formation of amyloid beta oligomers and fibrils, binds plaques, and reduces amyloid in vivo. J. Biol. Chem. 280 (7), 5892–5901. doi:10.1074/jbc.M404751200
Yarla, N. S., Bishayee, A., Sethi, G., Reddanna, P., Kalle, A. M., Dhananjaya, B. L., et al. (2016). Targeting arachidonic acid pathway by natural products for cancer prevention and therapy. Semin. Cancer Biol. 40-41, 48–81. doi:10.1016/j.semcancer.2016.02.001
Yew, M. Y., Koh, R. Y., Chye, S. M., Othman, I., and Ng, K. Y. (2014). Edible bird's nest ameliorates oxidative stress-induced apoptosis in SH-SY5Y human neuroblastoma cells. BMC complementary Altern. Med. 14, 391. doi:10.1186/1472-6882-14-391
Zhang, X. L., Huang, W. M., Tang, P. C., Sun, Y., Zhang, X., Qiu, L., et al. (2021). Anti-inflammatory and neuroprotective effects of natural cordycepin in rotenone-induced PD models through inhibiting Drp1-mediated mitochondrial fission. Neurotoxicology 84, 1–13. doi:10.1016/j.neuro.2021.02.002
Zhang, Z., Cui, W., Li, G., Yuan, S., Xu, D., Hoi, M. P., et al. (2012). Baicalein protects against 6-OHDA-induced neurotoxicity through activation of Keap1/Nrf2/HO-1 and involving PKCα and PI3K/AKT signaling pathways. J. Agric. Food Chem. 60 (33), 8171–8182. doi:10.1021/jf301511m
Zhou, Z. D., Xie, S. P., Saw, W. T., Ho, P. G. H., Wang, H., Lei, Z., et al. (2019). The therapeutic implications of tea polyphenols against dopamine (DA) neuron degeneration in Parkinson's disease (PD). Cells 8 (8), 911. doi:10.3390/cells8080911
Zhu, J. H., Gusdon, A. M., Cimen, H., Van Houten, B., Koc, E., and Chu, C. T. (2012). Impaired mitochondrial biogenesis contributes to depletion of functional mitochondria in chronic MPP+ toxicity: Dual roles for ERK1/2. Cell Death Dis. 3 (5), e312. doi:10.1038/cddis.2012.46
Keywords: Parkinson’s disease, natural products, mitochondrial dysfunction, pharmacological mechanisms, flavanoids, phenols
Citation: He T, Lin X, Su A, Zhang Y, Xing Z, Mi L, Wei T, Li Z and Wu W (2023) Mitochondrial dysfunction-targeting therapeutics of natural products in Parkinson’s disease. Front. Pharmacol. 14:1117337. doi: 10.3389/fphar.2023.1117337
Received: 06 December 2022; Accepted: 02 May 2023;
Published: 10 May 2023.
Edited by:
Xin Wang, Hokkaido University, JapanCopyright © 2023 He, Lin, Su, Zhang, Xing, Mi, Wei, Li and Wu. This is an open-access article distributed under the terms of the Creative Commons Attribution License (CC BY). The use, distribution or reproduction in other forums is permitted, provided the original author(s) and the copyright owner(s) are credited and that the original publication in this journal is cited, in accordance with accepted academic practice. No use, distribution or reproduction is permitted which does not comply with these terms.
*Correspondence: Wenshuang Wu, d2Vuc2h1YW5nX3d1QDE2My5jb20=
†These authors have contributed equally to this work and share first authorship
Disclaimer: All claims expressed in this article are solely those of the authors and do not necessarily represent those of their affiliated organizations, or those of the publisher, the editors and the reviewers. Any product that may be evaluated in this article or claim that may be made by its manufacturer is not guaranteed or endorsed by the publisher.
Research integrity at Frontiers
Learn more about the work of our research integrity team to safeguard the quality of each article we publish.