- 1Department of Orthopaedics, The Second Affiliated Hospital and Yuying Children's Hospital of Wenzhou Medical University, Wenzhou, Zhejiang, China
- 2School of Biomedical Sciences, The University of Western Australia, Perth, WA, Australia
- 3Department of Endocrinology, The First Affiliated Hospital of Guangdong Pharmaceutical University, Guangzhou, Guangdong, China
- 4The State Key Laboratory of Pharmaceutical Biotechnology, Institute of Functional Biomolecules, Nanjing University, Nanjing, China
- 5Research Center for Drug Discovery, School of Pharmaceutical Sciences, Sun Yat-sen University, Guangzhou, China
- 6UWA Dental School, The University of Western Australia, Perth, WA, Australia
Parthenolide (PTL or PAR) was first isolated from Magnolia grandiflora and identified as a small molecule cancer inhibitor. PTL has the chemical structure of C15H20O3 with characteristics of sesquiterpene lactones and exhibits the biological property of inhibiting DNA biosynthesis of cancer cells. In this review, we summarise the recent research progress of medicinal PTL, including the therapeutic effects on skeletal diseases, cancers, and inflammation-induced cytokine storm. Mechanistic investigations reveal that PTL predominantly inhibits NF-κB activation and other signalling pathways, such as reactive oxygen species. As an inhibitor of NF-κB, PTL appears to inhibit several cytokines, including RANKL, TNF-α, IL-1β, together with LPS induced activation of NF-κB and NF-κB -mediated specific gene expression such as IL-1β, TNF-α, COX-2, iNOS, IL-8, MCP-1, RANTES, ICAM-1, VCAM-1. It is also proposed that PTL could inhibit cytokine storms or hypercytokinemia triggered by COVID-19 via blocking the activation of NF-κB signalling. Understanding the pharmacologic properties of PTL will assist us in developing its therapeutic application for medical conditions, including arthritis, osteolysis, periodontal disease, cancers, and COVID-19-related disease.
Introduction
Parthenolide (PTL or PAR) has been widely used as a herbal medicine for various health conditions (Pareek et al., 2011). It was originally isolated from plants of the Asteraceae family during the 1970s, is known to contain sesquiterpene lactones (SLs), and has been used for the treatment of migraine, inflammation, arthritis, and tumors (Hall et al., 1980; Heinrich et al., 1998; Schinella et al., 1998). It has been well established that sesquiterpene, sharing a similar structural feature with lignans, diterpenes, triterpenes, and polyphenols and a biological property on an inhibitory effect of nuclear factor kappa B (NF-κB) activity (Nam, 2006).
NF-κB proteins exist in the cytoplasm in a complex with inhibitors of NF-κB (IκB), which are in an inactivated state, but become phosphorylated upon activation. They are subsequently ubiquitinated and degraded by proteasome-mediated pathways (May and Ghosh, 1997). This permits NF-κB proteins to be released from the complex and translocates to the nucleus (May and Ghosh, 1997), and regulate the transcription of a large number of genes required for inflammatory response, chemokine activation, cell adhesion and immune regulations (Nam, 2006).
PTL shares structural similarities with PTL analogues such as germacrane-type sesquiterpenoids, which bares the same carbon skeleton (7-isopropyl-4,10-dimethylcyclodecane) (Figure 1). They all are highly oxygenated with ester functionalities. In addition, they display structural differences, including the varied configurations of carbon-carbon double bond and diverse types and substitutional positions of ester functionalities (Figure 1). However, the relationship of these diverse chemical structures and their biological functions remains to be investigated.
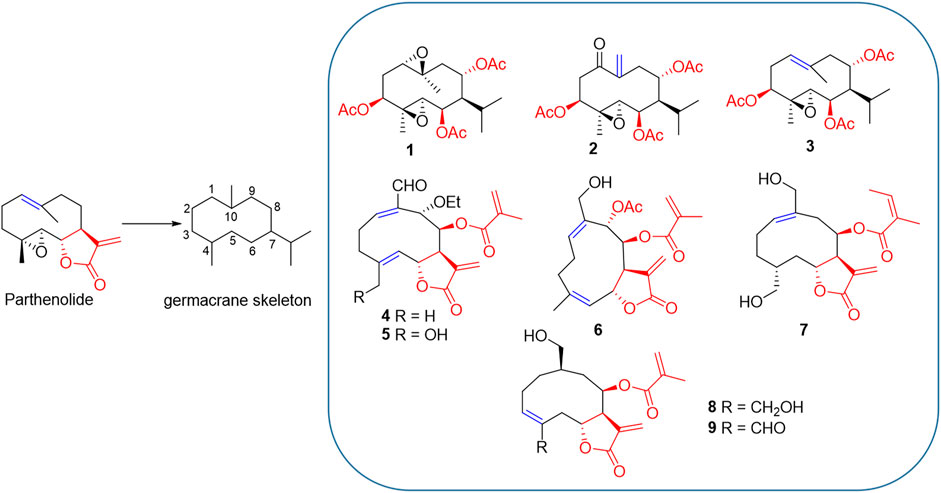
FIGURE 1. PTL and its analogues 1–9. Structural similarity: 1) they are all germacrane-type sesquiterpenoids sharing the same carbon skeleton (7-isopropyl-4,10-dimethylcyclodecane); 2) they all are highly oxygenated and have ester functionalities. Structural differences: 1) the configuration of carbon-carbon double bond (blue) is varied; 2) the types and substitutional positions of ester functionalities (red) are diverse.
Recent studies have shown the pharmacological effect of PTL on cancers, and structural modification of PTL could improve anticancer activity (Liu and Wang, 2022). In this review, we summarize the recent progress of the role of PTL and analogues in various conditions with emphasis on osteolytic diseases, primary and metastasis bone cancers, and COVID-19, which helps to fill the gap of knowledge through our investigation and discussion of the role of PTL and its derivatives in skeletal disease and cytokine storm. Understanding the pharmacological mechanisms of sesquiterpene lactone in the pathogenesis of various diseases will be important in developing an practical approach to prevent and treat these medical conditions.
The role of PTL in skeletal disorders
Previous studies have shown that sesquiterpene lactone has anti-inflammatory effects (Schinella et al., 1998). It was subsequently revealed that it could block lipopolysaccharide (LPS)-induced osteolysis via the inhibition of osteoclast formation and bone resorption (Yip et al., 2004). Mechanically, PTL was found to inhibit NF-κB activity induced by pro-inflammatory cytokines such as tumour necrosis factor (TNF)-α, interleukin (IL)-1β, and receptor activator of nuclear factor kappa-Β ligand (RANKL), all of which have an inductive effect on osteoclast formation and activation (Yip et al., 2004). Further, PTL was found to attenuate polyethylene particles induced peri-implant osteolysis in a mouse model (Li et al., 2014; Zawawi et al., 2015). Consistently, PTL also was found to reduce empty lacunae and osteoclastic bone surface resorption induced by polyethylene particles in a murine calvarial model of peri-implant osteolysis for inflammation related orthopaedic aseptic implant loosening (Zawawi et al., 2015). Further, it prevents ovariectomy-induced bone loss in vivo by inhibiting osteoclast activity (Idris et al., 2010). The inhibition of osteoclast formation and bone resorption is vital to the therapeutic inhibition and prevention of osteolysis.
PTL consistently inhibits pro-inflammatory cytokine production and exhibits protective effects against the progression of collagen-induced arthritis in a rat model (Liu et al., 2015). PTL could inhibit ADAMTS and MMP—mediated degeneration of cartilage and osteoarthritis via its potential effect on chondrocytes (Legendre et al., 2005; El Mabrouk et al., 2007). At the cellular level, PTL can suppress LPS and TNF-α-induced increases in matrix metalloproteinase (MMP)-1, MMP-3, inducible nitric oxide synthase (iNOS), and IL-1β mRNA in chondrocytes (Liu et al., 2015), and inhibits TNF-α induced catabolism of aggrecan in cultured human chondrocytes (Zhou et al., 2008). During chemotherapy, it could also inhibit methotrexate (MTX)-induced osteoclastogenesis via attenuating TNF-α levels and the activation of NF-κB (King et al., 2012).
PTL and analogues have a protective effect on arthritis (Nam, 2006; Parada-Turska et al., 2008; Xu et al., 2009; Li et al., 2010; Liu et al., 2015). PTL inhibited the proliferation of rheumatoid arthritis fibroblast-like synoviocytes (RA-FLS) (Parada-Turska et al., 2008), as well as the expression of RANKL mRNA and protein in RA-FLS (Li et al., 2010). Interestingly, PTL has been found to attenuate neuropathy pains partly via the inhibition of intracellular signalling pathways NF-κB and MEK1/2 (Popiolek-Barczyk et al., 2014; Popiolek-Barczyk et al., 2015), which would be beneficial for arthritis related pain.
More recently, PTL was found to enhance alkaline phosphatase activity and mineralized nodule formation of osteoblasts in human periodontal ligament-derived cells (Zhang et al., 2017). These stimulated effect on osteoblasts were further evident by the increased expression of osteogenesis-related gene/protein expression of osteoblasts in the presence of TNF-α. Further, PTL inhibited the NF-κB/p50 pathway and resisted the inhibition of Wnt/beta-catenin signalling induced by TNF-α (Zhang et al., 2017). Interestingly, PTL increased cell viability and inhibited H2O2-induced apoptosis, indicating its role in inhibiting oxidative stress and cellular apoptosis in osteoblasts (Mao and Zhu, 2018). However, more recently, PTL was found to reduce the activity of ALP, alizarin red-positive mineralization, and the expression of ALP and osteocalcin mRNA using human periosteum-derived cells (hPDCs) (Park et al., 2020). In addition, PTL also attenuated the increased osteoblastic differentiation of TNF-α -treated hPDCs via the suppression of JNK signalling (Park et al., 2020). Consistently, PTL was previously found to have no inhibitory effects on osteoblast function using primary calvarial osteoblasts (Idris et al., 2010). The discrepancy observed in these studies might be due to the variation of cell types and culture conditions used, indicating the need for further research.
At the molecular level, PTL displays inhibitory effects on NF-κB, both at its transcriptional level and by direct inhibition of associated kinases, IKK-beta (IKK-β) (Mathema et al., 2012). Inhibition of NF-κB will influence the gene expression, including IL-1β, TNF-α, COX-2, iNOS, IL-8, MCP-1, RANTES, ICAM-1, and VCAM-1 (Nam, 2006). These molecules are critical for pro-inflammatory response, chemokine activation, cell adhesion and immune regulations (Nam, 2006).
NF-κB signaling is key to osteoclastogenesis. Previous studies have found that NF-κB subunits p50−/− and p52−/− null mice display osteopetrosis owing to lack of osteoclast formation (Franzoso et al., 1997; Iotsova et al., 1997). Conversely, activation of NF-κB resulted in excessive osteoclasts and osteolysis (Boyce et al., 1999; Xu et al., 2009). PTL appears to inhibit osteoclastogenesis and NF-κB activity induced by RANKL and LPS. For example, research suggests PTL is able to inhibit NF-κB activation by the attenuation of TNF-α induced IκB kinase complex activation (Hehner et al., 1999). Additionally, PTL and analogues appear to suppress NF-κB activity and RANKL-induced IκBα degradation (Yip et al., 2004; Qin et al., 2015).
In addition to its inhibitory role in the regulation of NF-κB, PTL was found to exert a biological effect as a phosphorylation inhibitor for signal transducer and activator of transcription (STAT1 and STAT3), and to prevent STAT1 and STAT3 DNA binding activity. Through this property, PTL can inhibit STAT-mediated transcriptional suppression of pro-apoptotic genes (Mathema et al., 2012).
More recently, PTL was found to inhibit inflammasome activity via the ATPase activity of nucleotide-binding oligomerization domain (NACHT), leucine rich repeat (LRR) and pyrin domain containing (PYD) 3 (NLRP3) (Juliana et al., 2010). NLRP3 is a member of the NACHT, LRR and PYD domains-containing protein 3 (NALP3) inflammasome complex, an upstream activator of NF-κB signalling. Through this action, PTL regulates the inflammatory response, immune regulation, and apoptosis. Collectively, PTL and sesquiterpene lactone compounds were able to affect NF-κB and other signalling molecules that results in osteoclast formation, bone resorption and osteolysis (Table 1).
The role of PTL in cancers
NF-κB signalling pathway is constitutively activated in many types of cancer cells. Under physiological condition, inactive form of NF-κB is mainly bound to its inhibitor IκB and present in the cytoplasm. Upon activation by signalling molecules, IκBα is phosphorylated and degraded and releases NF-κB, which will transfer from the cytoplasm to the nucleus (Karin, 1999). Targeting NF-κB to reduce overexpression or activation of NF-κB, and its anti-apoptotic effect hint a role of PTL as a potential therapeutic target for the treatment of cancers (Fuchs, 2010), including gastric cancer (Li et al., 2018; Liu et al., 2020), pancreatic cancer (Yip-Schneider et al., 2005; Liu et al., 2010; Liu et al., 2017), hepatic cancer (Carlisi et al., 2011; Kim et al., 2012; Liu et al., 2013), lung cancer (Li et al., 2020; Sun et al., 2020; Wu et al., 2020), ovarian cancer (Lee et al., 2012; Takai et al., 2013; Kwak et al., 2014), glioblastoma (Anderson and Bejcek, 2008; Hexum et al., 2015; Ding et al., 2020), and oral cancer (Yu et al., 2015; Baskaran et al., 2017), just to name a few examples. In this review, we will focus on our discussion in more detail regarding the role of PTL in cancers related to the skeletal system (Table 1).
Research suggests that PTL could be a therapeutic agent for the treatment of osteosarcoma. PTL was found to sensitize radioresistant osteosarcoma cells and greatly reduce the prevalence of relapse and metastatic progression (Sugiyasu et al., 2011; Zuch et al., 2012). PTL also induced cell death in human osteosarcoma cells via reactive oxygen species (ROS)-mediated autophagy (Yang et al., 2016), and through caspase-independent and AIF-mediated signalling molecules (D'Anneo et al., 2013). In a murine animal model, PTL was able to inhibit lung colonization of osteosarcoma cells (Kishida et al., 2007). Taken together, these findings suggested that PTL through its inhibitory effect on NF-κB might serve as an antimetastatic drug.
In addition, PTL has potential effects on multiple myeloma (MM) (Suvannasankha et al., 2008; Gunn et al., 2011; Kong et al., 2015). It was found that PTL has anti-cancer stem cell activity (Gunn et al., 2011), as well as direct effects on MM cells and the bone marrow microenvironment in myeloma (Suvannasankha et al., 2008). The inhibitory effects of PTL in MM via targeting tumor necrosis factor receptor-associated factor 6 (TRAF6) and NF-κB pathways (Kong et al., 2015). PTL-induced apoptosis in MM cells involves ROS generation and cell sensitivity depends on catalase activity (Wang et al., 2006). In addition, PTL has inhibitory effects on angiogenesis induced by human MM cells (Kong et al., 2008).
PTL also has effects on cancers that are accompanied by bone osteolytic conditions such as breast cancer (Chen et al., 2009; Idris et al., 2009; Marino et al., 2017). It is therefore suggested that targeting NF-κB may be of value in the treatment of breast cancer related osteolysis (Marino et al., 2017), and the administration of PTL might be effective in preventing breast cancer mediated osteolysis (Chen et al., 2009; Idris et al., 2009).
Leukemia is often accompanied with severe bone loss due to the dysregulation in leukemia cells and bone microenvironment during leukemogenesis (Cheung et al., 2018; Anderson et al., 2020). PTL as a natural product has been suggested to target the leukemia stem cells (LSCs) in acute myeloid leukemia (AML) (Siveen et al., 2017) via regulating ROS levels and the anti-proliferative activity of cancer cells (Kempema et al., 2015). A panel of novel modified PTL analogues were found to possess significantly improved anti-leukemic potency against primary AML cells, with low toxicity against normal mature and progenitor hematopoietic cells via P450-mediated pathways (Kolev et al., 2014). PTL was found to have a radiosensitization effect in prostate cancer cells. Further, PTL inhibits NF-κB activity and the expression of phosphatase and tensin homologue deleted on chromosome 10 (PTEN) that is involved in the radiosensitization effect (Sun et al., 2007; Watson et al., 2009; Mendonca et al., 2017).
More recently, PTL was found to inhibit the growth of prostate cancer cells dose-dependently and reduce prostate cancer cell-osteoclast co-cultures mediated osteoclast formation, suggesting that PTL could reduce prostate cancer related osteolysis (Marino et al., 2019).
The role of PTL in cytokine storm
Cytokines play a vital role in the homeostasis of the immune system. A cytokine storm could occur when a large number of cytokines are released in the body instantaneously, which may be life threatening and lead to multiple organ failure. A cytokine storm is also called hypercytokinemia, which is usually induced by an infection, autoimmune disorder, or other inflammatory disease.
A cytokine storm triggered by COVID-19 has been associated with respiratory failure and lung fibrosis (Cao, 2020; Yang et al., 2020; Lowery et al., 2021). It is evident that multiple inflammatory cytokines, including TNF-α, IL-1β, IL-6, and IL-10 play a significant role in the pathogenesis of COVID-19 induced morbidity and mortality (Peddapalli et al., 2021). For instance, increased IL-6 levels in circulating were tested in patients with cardiovascular conditions with a poor prognosis of COVID-19 (Clerkin et al., 2020; Guo et al., 2020). NF-κB signalling pathways are common to COVID-19 induced cytokine responses (Kircheis et al., 2020; Kandasamy, 2021; Peddapalli et al., 2021). The inhibition of NF-κB has therefore been proposed to be a target for the treatment of COVID-19 (Kircheis et al., 2020; Kandasamy, 2021; Kircheis et al., 2021). For example, propolis and digitoxin have been found to suppress levels of the cytokines and have benefits for the treatment of the comorbidities in COVID-19 patients (Berretta et al., 2020; Pollard et al., 2020).
In line with this, PTL, as a previously identified NF-κB inhibitor has been proposed to treat COVID-19 (Bahrami et al., 2020; Soleymani et al., 2022). Interestingly, using molecular docking, PTL analogues were found to binds with high affinity to the selected target of SARSCoV-2, which might serve as a potential candidate for anti-SARS-CoV-2 therapy (Ouled Aitouna et al., 2021; Lakhera et al., 2022). Consistently, feverfew which contains the major ingredient of PTL has long been used as a traditional medicine for the treatment of fever, migraine headache, and inflammatory conditions (De Weerdt et al., 1996; Koprowska and Czyz, 2010). More recently, PTL was found covalently bind to Cys-191 or Cys-194 of the coronavirus papain-like protease and inhibit its deISGylation and activity by allosteric regulation (Zou et al., 2022). Collectively, PTL is thought to be beneficial for the treatment of COVID-19 via the inhibition of cytokine storm with NF-κB signalling pathways activation (Bahrami et al., 2020; Ouled Aitouna et al., 2021; Soleymani et al., 2022) (Table 1).
The role of PTL in periodontal disease
Periodontal disease is one the most common non-communicable diseases in humans, where nearly 1 billion people are affected (GBD, 2016 Disease and Injury Incidence and Prevalence Collaborators, 2017). The disease involves gingivitis and periodontitis and is caused by oral infection (Li et al., 2000). The current understanding of periodontal disease pathogenesis demonstrates host-related inflammatory responses triggered by bacterial pathogens whereby an influx of immune-host mediators such as prostaglandins, leukotrienes, complement activation products, chemokines, and cytokines are released to form a sophisticated network of interactions between elements of innate and adaptive immune systems (Hajishengallis et al., 2020). These complex interactions lead to inflammation-induced bone loss, primarily mediated by a triad of RANKL and NF-κB signalling pathway (Hajishengallis et al., 2020).
Recent research has explored the potential therapeutic effects of PTL for periodontal disease in both an in vitro model and on human alveolar bone tissue (Zhang et al., 2014; Zhang et al., 2017). PTL appears to inhibit the activation of major inflammatory pathways involved in periodontal disease via NF-κB and ERK signalling pathways, in addition to the expression of inflammatory and osteoclastogenic genes in lipopolysaccharide-stimulated human periodontal ligament cells (Zhang et al., 2014). Whilst PTL could also promote osteoblast differentiation via the Wnt/β-catenin signaling pathway and might be a pivotal target for periodontal bone regeneration (Zhang et al., 2017). Periodontitis would be a significant health burden if it was left without treatment. It will not only lead to teeth loss, but it can cause severe consequences to general health. The systemic-oral link is well established, and poorly controlled periodontitis can complicate the management of systemic conditions such as diabetes and cardiovascular diseases (Kane, 2017). PTL offers an excellent opportunity to enhance the current regimens in the management of periodontal disease, and further studies are required.
Conclusion
In short, PTL has therapeutic effects on skeletal diseases, primary and metastasis bone cancers, and inflammation-induced cytokine storm. As summarised in Figure 2, PTL displays inhibitory effects on cytokine-mediated NF-κB by direct inhibition of IKK-β (Mathema et al., 2012), or indirect inhibitory effects on inflammasome activity via NLRP3 (Juliana et al., 2010) and ROS (Kempema et al., 2015) (Figure 2). PTL also act as a phosphorylation inhibitor for STAT1 and STAT3 (Mathema et al., 2012) (Figure 2). Further understanding the mechanistic insights into the role of PTL in a disease specific manner will enable us to develop therapeutic applications of PTL for arthritis, osteoporosis, periodontal disease, cancer, and COVID-19. In addition, there are limitations regarding the clinical use of PTL, including pharmacological doses, routes of drug delivery and double-blind clinical trial studies, and future studies in addressing these issues will enhance the clinical applications of PTL in various diseases. Previous studies have focused on the efficacy of PTL in various conditions with a lack of reports on toxicity assessments. As a general rule, since PTL is an NF-κB inhibitor, it might cause liver toxicity when used to overdose. Thus, further systemic evaluation of liver toxicity will help to better understand the druggability of PTL.
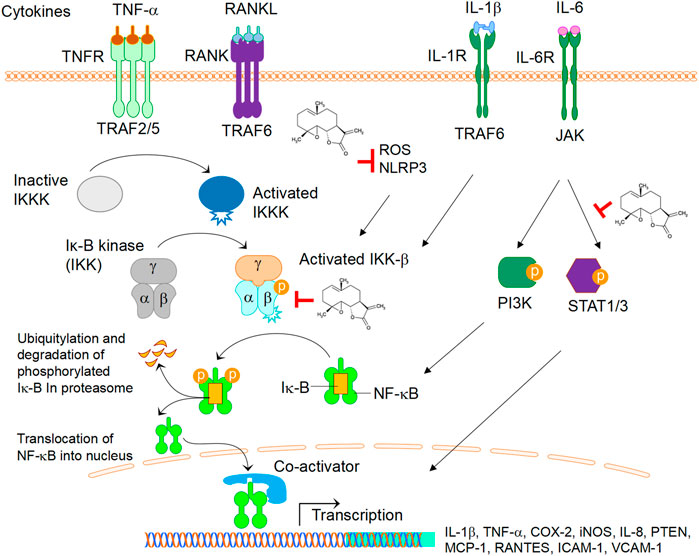
FIGURE 2. The role of PTL in cytokines-mediated signalling activation. Cytokines TNF-α, RANKL, IL-1β, IL-6, upon binding to their respective receptors, activate several signalling cascades leading to down-stream the transcriptions of genes such as IL-1β, TNF-α, COX-2, iNOS, IL-8, PTEN, MCP1, RANTES, ICAM, VCAM. Note that PTL displays inhibitory effects on NF-κB by direct inhibition of IKK-β, inflammasome activity via NLRP3, or ROS. PTL also act as a phosphorylation inhibitor for STAT1 and STAT3. Abbreviations: TNF-α, tumor necrosis factor alpha; RANKL, receptor activator of nuclear factor kappa-Β ligand; IL-1β, interleukin 1 beta; IL-6, interleukin 6; COX-2, cyclooxygenase-2; iNOS, inducible nitric oxide synthase; IL-8, interleukin 8; PTEN, phosphatase and tensin Homolog; MCP-1, monocyte chemoattractant protein-1; RANTES, regulated upon activation, normal T cell expressed and secreted; ICAM-1, intercellular adhesion molecule 1; VCAM-1, vascular cell adhesion molecule 1.
Author contributions
SZ and JX drafted the manuscript. PS, SB, OC, XP, and QG contributed to figure formulation and literature collection. SB and JX revised the manuscript. RT, OK, and JX contributed to evaluation and assistance in the process of manuscript preparation and supervised the study.
Funding
This work was partly supported by a research grant from the National Natural Science Funding of China (81802235), Zhejiang Experimental Animal Science and Technology Project of China (2018C37112), and Wenzhou basic science research plan project (Y20180033). This work was also partly supported by a collaborative research award (RCA, 2020) from The University of Western Australia (UWA). SZ and PS made overseas collaborative visits to UWA. OC was visiting student at JX’s lab at UWA.
Conflict of interest
The authors declare that the research was conducted in the absence of any commercial or financial relationships that could be construed as a potential conflict of interest.
Publisher’s note
All claims expressed in this article are solely those of the authors and do not necessarily represent those of their affiliated organizations, or those of the publisher, the editors and the reviewers. Any product that may be evaluated in this article, or claim that may be made by its manufacturer, is not guaranteed or endorsed by the publisher.
References
Anderson, D., Skut, P., Hughes, A. M., Ferrari, E., Tickner, J., Xu, J., et al. (2020). The bone marrow microenvironment of pre-B acute lymphoblastic leukemia at single-cell resolution. Sci. Rep. 10 (1), 19173. doi:10.1038/s41598-020-76157-4
Anderson, K. N., and Bejcek, B. E. (2008). Parthenolide induces apoptosis in glioblastomas without affecting NF-kappaB. J. Pharmacol. Sci. 106 (2), 318–320. doi:10.1254/jphs.sc0060164
Bahrami, M., Kamalinejad, M., Latifi, S. A., Seif, F., and Dadmehr, M. (2020). Cytokine storm in COVID-19 and parthenolide: Preclinical evidence. Phytother. Res. 34 (10), 2429–2430. doi:10.1002/ptr.6776
Baskaran, N., Selvam, G. S., Yuvaraj, S., and Abhishek, A. (2017). Parthenolide attenuates 7,12-dimethylbenz[a]anthracene induced hamster buccal pouch carcinogenesis. Mol. Cell Biochem. 440, 11–22. doi:10.1007/s11010-017-3151-5
Berretta, A. A., Silveira, M. A. D., Condor Capcha, J. M., and De Jong, D. (2020). Propolis and its potential against SARS-CoV-2 infection mechanisms and COVID-19 disease: Running title: Propolis against SARS-CoV-2 infection and COVID-19. Biomed. Pharmacother. 131, 110622. doi:10.1016/j.biopha.2020.110622
Boyce, B. F., Xing, L., Franzoso, G., and Siebenlist, U. (1999). Required and nonessential functions of nuclear factor-kappa B in bone cells. Bone 25 (1), 137–139. doi:10.1016/s8756-3282(99)00105-2
Cao, X. (2020). COVID-19: Immunopathology and its implications for therapy. Nat. Rev. Immunol. 20 (5), 269–270. doi:10.1038/s41577-020-0308-3
Carlisi, D., D'Anneo, A., Angileri, L., Lauricella, M., Emanuele, S., Santulli, A., et al. (2011). Parthenolide sensitizes hepatocellular carcinoma cells to TRAIL by inducing the expression of death receptors through inhibition of STAT3 activation. J. Cell Physiol. 226 (6), 1632–1641. doi:10.1002/jcp.22494
Chen, Y. C., Sosnoski, D. M., Gandhi, U. H., Novinger, L. J., Prabhu, K. S., and Mastro, A. M. (2009). Selenium modifies the osteoblast inflammatory stress response to bone metastatic breast cancer. Carcinogenesis 30 (11), 1941–1948. doi:10.1093/carcin/bgp227
Cheung, L. C., Tickner, J., Hughes, A. M., Skut, P., Howlett, M., Foley, B., et al. (2018). New therapeutic opportunities from dissecting the pre-B leukemia bone marrow microenvironment. Leukemia 32 (11), 2326–2338. doi:10.1038/s41375-018-0144-7
Clerkin, K. J., Fried, J. A., Raikhelkar, J., Sayer, G., Griffin, J. M., Masoumi, A., et al. (2020). COVID-19 and cardiovascular disease. Circulation 141 (20), 1648–1655. doi:10.1161/CIRCULATIONAHA.120.046941
D'Anneo, A., Carlisi, D., Lauricella, M., Emanuele, S., Di Fiore, R., Vento, R., et al. (2013). Parthenolide induces caspase-independent and AIF-mediated cell death in human osteosarcoma and melanoma cells. J. Cell Physiol. 228 (5), 952–967. doi:10.1002/jcp.24131
De Weerdt, C. J., Bootsma, H. P., and Hendriks, H. (1996). Herbal medicines in migraine prevention Randomized double-blind placebo-controlled crossover trial of a feverfew preparation. Phytomedicine 3 (3), 225–230. doi:10.1016/S0944-7113(96)80057-2
Ding, Y., Xue, Q., Liu, S., Hu, K., Wang, D., Wang, T., et al. (2020). Identification of parthenolide dimers as activators of pyruvate kinase M2 in xenografts of glioblastoma multiforme in vivo. J. Med. Chem. 63 (4), 1597–1611. doi:10.1021/acs.jmedchem.9b01328
El Mabrouk, M., Sylvester, J., and Zafarullah, M. (2007). Signaling pathways implicated in oncostatin M-induced aggrecanase-1 and matrix metalloproteinase-13 expression in human articular chondrocytes. Biochim. Biophys. Acta 1773 (3), 309–320. doi:10.1016/j.bbamcr.2006.11.018
Franzoso, G., Carlson, L., Xing, L., Poljak, L., Shores, E. W., Brown, K. D., et al. (1997). Requirement for NF-kappaB in osteoclast and B-cell development. Genes Dev. 11 (24), 3482–3496. doi:10.1101/gad.11.24.3482
Fuchs, O. (2010). Transcription factor NF-κB inhibitors as single therapeutic agents or in combination with classical chemotherapeutic agents for the treatment of hematologic malignancies. Curr. Mol. Pharmacol. 3 (3), 98–122. doi:10.2174/1874467211003030098
GBD 2016 Disease and Injury Incidence and Prevalence Collaborators (2017). Global, regional, and national incidence, prevalence, and years lived with disability for 328 diseases and injuries for 195 countries, 1990-2016: A systematic analysis for the global burden of disease study 2016. Lancet 390 (10100), 1211–1259. doi:10.1016/S0140-6736(17)32154-2
Gunn, E. J., Williams, J. T., Huynh, D. T., Iannotti, M. J., Han, C., Barrios, F. J., et al. (2011). The natural products parthenolide and andrographolide exhibit anti-cancer stem cell activity in multiple myeloma. Leuk. Lymphoma 52 (6), 1085–1097. doi:10.3109/10428194.2011.555891
Guo, W., Li, M., Dong, Y., Zhou, H., Zhang, Z., Tian, C., et al. (2020). Diabetes is a risk factor for the progression and prognosis of COVID-19. Diabetes Metab. Res. Rev. 36, e3319. doi:10.1002/dmrr.3319
Hajishengallis, G., Chavakis, T., and Lambris, J. D. (2020). Current understanding of periodontal disease pathogenesis and targets for host-modulation therapy. Periodontol 84 (1), 14–34. doi:10.1111/prd.12331
Hall, I. H., Starnes, C. O., Lee, K. H., and Waddell, T. G. (1980). Mode of action of sesquiterpene lactones as anti-inflammatory agents. J. Pharm. Sci. 69 (5), 537–543. doi:10.1002/jps.2600690516
Hehner, S. P., Hofmann, T. G., Droge, W., and Schmitz, M. L. (1999). The antiinflammatory sesquiterpene lactone parthenolide inhibits NF-kappa B by targeting the I kappa B kinase complex. J. Immunol. 163 (10), 5617–5623. doi:10.4049/jimmunol.163.10.5617
Heinrich, M., Robles, M., West, J. E., Ortiz de Montellano, B. R., and Rodriguez, E. (1998). Ethnopharmacology of Mexican asteraceae (compositae). Annu. Rev. Pharmacol. Toxicol. 38, 539–565. doi:10.1146/annurev.pharmtox.38.1.539
Hexum, J. K., Becker, C. M., Kempema, A. M., Ohlfest, J. R., Largaespada, D. A., and Harki, D. A. (2015). Parthenolide prodrug LC-1 slows growth of intracranial glioma. Bioorg Med. Chem. Lett. 25 (12), 2493–2495. doi:10.1016/j.bmcl.2015.04.058
Idris, A. I., Krishnan, M., Simic, P., Landao-Bassonga, E., Mollat, P., Vukicevic, S., et al. (2010). Small molecule inhibitors of IkappaB kinase signaling inhibit osteoclast formation in vitro and prevent ovariectomy-induced bone loss in vivo. FASEB J. 24 (11), 4545–4555. doi:10.1096/fj.10-164095
Idris, A. I., Libouban, H., Nyangoga, H., Landao-Bassonga, E., Chappard, D., and Ralston, S. H. (2009). Pharmacologic inhibitors of IkappaB kinase suppress growth and migration of mammary carcinosarcoma cells in vitro and prevent osteolytic bone metastasis in vivo. Mol. Cancer Ther. 8 (8), 2339–2347. doi:10.1158/1535-7163.MCT-09-0133
Iotsova, V., Caamano, J., Loy, J., Yang, Y., Lewin, A., and Bravo, R. (1997). Osteopetrosis in mice lacking NF-kappaB1 and NF-kappaB2. Nat. Med. 3 (11), 1285–1289. doi:10.1038/nm1197-1285
Juliana, C., Fernandes-Alnemri, T., Wu, J., Datta, P., Solorzano, L., Yu, J. W., et al. (2010). Anti-inflammatory compounds parthenolide and Bay 11-7082 are direct inhibitors of the inflammasome. J. Biol. Chem. 285 (13), 9792–9802. doi:10.1074/jbc.M109.082305
Kandasamy, M. (2021). NF-κB signalling as a pharmacological target in COVID-19: Potential roles for IKKβ inhibitors. Naunyn Schmiedeb. Arch. Pharmacol. 394 (3), 561–567. doi:10.1007/s00210-020-02035-5
Karin, M. (1999). How NF-kappaB is activated: The role of the IkappaB kinase (IKK) complex. Oncogene 18 (49), 6867–6874. doi:10.1038/sj.onc.1203219
Kempema, A. M., Widen, J. C., Hexum, J. K., Andrews, T. E., Wang, D., Rathe, S. K., et al. (2015). Synthesis and antileukemic activities of C1-C10-modified parthenolide analogues. Bioorg Med. Chem. 23 (15), 4737–4745. doi:10.1016/j.bmc.2015.05.037
Kim, I. H., Kim, S. W., Kim, S. H., Lee, S. O., Lee, S. T., Kim, D. G., et al. (2012). Parthenolide-induced apoptosis of hepatic stellate cells and anti-fibrotic effects in an in vivo rat model. Exp. Mol. Med. 44 (7), 448–456. doi:10.3858/emm.2012.44.7.051
King, T. J., Georgiou, K. R., Cool, J. C., Scherer, M. A., Ang, E. S., Foster, B. K., et al. (2012). Methotrexate chemotherapy promotes osteoclast formation in the long bone of rats via increased pro-inflammatory cytokines and enhanced NF-κB activation. Am. J. Pathol. 181 (1), 121–129. doi:10.1016/j.ajpath.2012.03.037
Kircheis, R., Haasbach, E., Lueftenegger, D., Heyken, W. T., Ocker, M., and Planz, O. (2020). NF-κB pathway as a potential target for treatment of critical stage COVID-19 patients. Front. Immunol. 11, 598444. doi:10.3389/fimmu.2020.598444
Kircheis, R., Schuster, M., and Planz, O. (2021). COVID-19: Mechanistic model of the african paradox supports the central role of the NF-κB pathway. Viruses 13 (9), 1887. doi:10.3390/v13091887
Kishida, Y., Yoshikawa, H., and Myoui, A. (2007). Parthenolide, a natural inhibitor of Nuclear Factor-kappaB, inhibits lung colonization of murine osteosarcoma cells. Clin. Cancer Res. 13 (1), 59–67. doi:10.1158/1078-0432.CCR-06-1559
Kolev, J. N., O'Dwyer, K. M., Jordan, C. T., and Fasan, R. (2014). Discovery of potent parthenolide-based antileukemic agents enabled by late-stage P450-mediated C-H functionalization. ACS Chem. Biol. 9 (1), 164–173. doi:10.1021/cb400626w
Kong, F., Chen, Z., Li, Q., Tian, X., Zhao, J., Yu, K., et al. (2008). Inhibitory effects of parthenolide on the angiogenesis induced by human multiple myeloma cells and the mechanism. J. Huazhong Univ. Sci. Technol. Med. Sci. 28 (5), 525–530. doi:10.1007/s11596-008-0508-8
Kong, F. C., Zhang, J. Q., Zeng, C., Chen, W. L., Ren, W. X., Yan, G. X., et al. (2015). Inhibitory effects of parthenolide on the activity of NF-κB in multiple myeloma via targeting TRAF6. J. Huazhong Univ. Sci. Technol. Med. Sci. 35 (3), 343–349. doi:10.1007/s11596-015-1435-0
Koprowska, K., and Czyz, M. (2010). Molecular mechanisms of parthenolide's action: Old drug with a new face. Postepy Hig. Med. Dosw (Online) 64, 100–114.
Kwak, S. W., Park, E. S., and Lee, C. S. (2014). Parthenolide induces apoptosis by activating the mitochondrial and death receptor pathways and inhibits FAK-mediated cell invasion. Mol. Cell Biochem. 385 (1-2), 133–144. doi:10.1007/s11010-013-1822-4
Lakhera, S., Devlal, K., Ghosh, A., Chowdhury, P., and Rana, M. (2022). Modelling the DFT structural and reactivity study of feverfew and evaluation of its potential antiviral activity against COVID-19 using molecular docking and MD simulations. Chem. Zvesti 76 (5), 2759–2776. doi:10.1007/s11696-022-02067-6
Lee, C. S., Kim, Y. J., Lee, S. A., Myung, S. C., and Kim, W. (2012). Combined effect of Hsp90 inhibitor geldanamycin and parthenolide via reactive oxygen species-mediated apoptotic process on epithelial ovarian cancer cells. Basic Clin. Pharmacol. Toxicol. 111 (3), 173–181. doi:10.1111/j.1742-7843.2012.00883.x
Legendre, F., Bogdanowicz, P., Boumediene, K., and Pujol, J. P. (2005). Role of interleukin 6 (IL-6)/IL-6R-induced signal tranducers and activators of transcription and mitogen-activated protein kinase/extracellular. J. Rheumatol. 32 (7), 1307–1316.
Li, H., Lu, H., Lv, M., Wang, Q., and Sun, Y. (2018). Parthenolide facilitates apoptosis and reverses drug-resistance of human gastric carcinoma cells by inhibiting the STAT3 signaling pathway. Oncol. Lett. 15 (3), 3572–3579. doi:10.3892/ol.2018.7739
Li, X., Huang, R., Li, M., Zhu, Z., Chen, Z., Cui, L., et al. (2020). Parthenolide inhibits the growth of non-small cell lung cancer by targeting epidermal growth factor receptor. Cancer Cell Int. 20 (1), 561. doi:10.1186/s12935-020-01658-1
Li, X., Kim, K. W., Cho, M. L., Ju, J. H., Kang, C. M., Oh, H. J., et al. (2010). IL-23 induces receptor activator of NF-kappaB ligand expression in fibroblast-like synoviocytes via STAT3 and NF-kappaB signal pathways. Immunol. Lett. 127 (2), 100–107. doi:10.1016/j.imlet.2009.10.012
Li, X., Kolltveit, K. M., Tronstad, L., and Olsen, I. (2000). Systemic diseases caused by oral infection. Clin. Microbiol. Rev. 13 (4), 547–558. doi:10.1128/CMR.13.4.547
Li, Y., Zhang, C., Zhou, X., Wang, H., Mao, Y., and Wang, X. (2014). Parthenolide inhibits polyethylene particle-induced mouse calvarial osteolysis in vivo. J. Surg. Res. 187 (1), 176–181. doi:10.1016/j.jss.2013.10.027
Liu, D., Liu, Y., Liu, M., Ran, L., and Li, Y. (2013). Reversing resistance of multidrug-resistant hepatic carcinoma cells with parthenolide. Future Oncol. 9 (4), 595–604. doi:10.2217/fon.13.15
Liu, J. W., Cai, M. X., Xin, Y., Wu, Q. S., Ma, J., Yang, P., et al. (2010). Parthenolide induces proliferation inhibition and apoptosis of pancreatic cancer cells in vitro. J. Exp. Clin. Cancer Res. 29, 108. doi:10.1186/1756-9966-29-108
Liu, M., Yang, Y., Liu, D., Cao, Y., and Li, Y. (2020). Parthenolide increases the sensitivity of gastric cancer cells to chemotherapy. J. Tradit. Chin. Med. 40 (6), 908–916. doi:10.19852/j.cnki.jtcm.2020.06.002
Liu, Q., Zhao, J., Tan, R., Zhou, H., Lin, Z., Zheng, M., et al. (2015). Parthenolide inhibits pro-inflammatory cytokine production and exhibits protective effects on progression of collagen-induced arthritis in a rat model. Scand. J. Rheumatol. 44 (3), 182–191. doi:10.3109/03009742.2014.938113
Liu, W., Wang, X., Sun, J., Yang, Y., Li, W., and Song, J. (2017). Parthenolide suppresses pancreatic cell growth by autophagy-mediated apoptosis. Onco Targets Ther. 10, 453–461. doi:10.2147/OTT.S117250
Liu, X., and Wang, X. (2022). Recent advances on the structural modification of parthenolide and its derivatives as anticancer agents. Chin. J. Nat. Med. 20 (11), 814–829. doi:10.1016/S1875-5364(22)60238-3
Lowery, S. A., Sariol, A., and Perlman, S. (2021). Innate immune and inflammatory responses to SARS-CoV-2: Implications for COVID-19. Cell Host Microbe 29 (7), 1052–1062. doi:10.1016/j.chom.2021.05.004
Mao, W., and Zhu, Z. (2018). Parthenolide inhibits hydrogen peroxideinduced osteoblast apoptosis. Mol. Med. Rep. 17 (6), 8369–8376. doi:10.3892/mmr.2018.8908
Marino, S., Bishop, R. T., Carrasco, G., Logan, J. G., Li, B., and Idris, A. I. (2019). Pharmacological inhibition of NFκB reduces prostate cancer related osteoclastogenesis in vitro and osteolysis ex vivo. Calcif. Tissue Int. 105 (2), 193–204. doi:10.1007/s00223-019-00538-9
Marino, S., Bishop, R. T., Logan, J. G., Mollat, P., and Idris, A. I. (2017). Pharmacological evidence for the bone-autonomous contribution of the NFκB/β-catenin axis to breast cancer related osteolysis. Cancer Lett. 410, 180–190. doi:10.1016/j.canlet.2017.09.034
Mathema, V. B., Koh, Y. S., Thakuri, B. C., and Sillanpaa, M. (2012). Parthenolide, a sesquiterpene lactone, expresses multiple anti-cancer and anti-inflammatory activities. Inflammation 35 (2), 560–565. doi:10.1007/s10753-011-9346-0
May, M. J., and Ghosh, S. (1997). Rel/NF-kappa B and I kappa B proteins: An overview. Semin. Cancer Biol. 8 (2), 63–73. doi:10.1006/scbi.1997.0057
Mendonca, M. S., Turchan, W. T., Alpuche, M. E., Watson, C. N., Estabrook, N. C., Chin-Sinex, H., et al. (2017). DMAPT inhibits NF-κB activity and increases sensitivity of prostate cancer cells to X-rays in vitro and in tumor xenografts in vivo. Free Radic. Biol. Med. 112, 318–326. doi:10.1016/j.freeradbiomed.2017.08.001
Nam, N. H. (2006). Naturally occurring NF-kappaB inhibitors. Mini Rev. Med. Chem. 6 (8), 945–951. doi:10.2174/138955706777934937
Ouled Aitouna, A., Belghiti, M. E., Esme, A., Anouar, E., Ouled Aitouna, A., Zeroual, A., et al. (2021). Chemical reactivities and molecular docking studies of parthenolide with the main protease of HEP-G2 and SARS-CoV-2. J. Mol. Struct. 1243, 130705. doi:10.1016/j.molstruc.2021.130705
Parada-Turska, J., Mitura, A., Brzana, W., Jablonski, M., Majdan, M., and Rzeski, W. (2008). Parthenolide inhibits proliferation of fibroblast-like synoviocytes in vitro. Inflammation 31 (4), 281–285. doi:10.1007/s10753-008-9076-0
Pareek, A., Suthar, M., Rathore, G. S., and Bansal, V. (2011). Feverfew (tanacetum parthenium L.): A systematic review. Pharmacogn. Rev. 5 (9), 103–110. doi:10.4103/0973-7847.79105
Park, J. H., Kang, Y. H., Hwang, S. C., Oh, S. H., and Byun, J. H. (2020). Parthenolide has negative effects on in vitro enhanced osteogenic phenotypes by inflammatory cytokine TNF-alpha via inhibiting JNK signaling. Int. J. Mol. Sci. 21 (15), 5433. doi:10.3390/ijms21155433
Peddapalli, A., Gehani, M., Kalle, A. M., Peddapalli, S. R., Peter, A. E., and Sharad, S. (2021). Demystifying excess immune response in COVID-19 to reposition an orphan drug for down-regulation of NF-κB: A systematic review. Viruses 13 (3), 378. doi:10.3390/v13030378
Pollard, B. S., Jc, B. L., and Pollard, J. R. (2020). Classical drug digitoxin inhibits influenza cytokine storm, with implications for covid-19 therapy. Vivo 34 (6), 3723–3730. doi:10.21873/invivo.12221
Popiolek-Barczyk, K., Kolosowska, N., Piotrowska, A., Makuch, W., Rojewska, E., Jurga, A. M., et al. (2015). Parthenolide relieves pain and promotes M2 microglia/macrophage polarization in rat model of neuropathy. Neural Plast. 2015, 676473. doi:10.1155/2015/676473
Popiolek-Barczyk, K., Makuch, W., Rojewska, E., Pilat, D., and Mika, J. (2014). Inhibition of intracellular signaling pathways NF-κB and MEK1/2 attenuates neuropathic pain development and enhances morphine analgesia. Pharmacol. Rep. 66 (5), 845–851. doi:10.1016/j.pharep.2014.05.001
Qin, S., Ang, E., Dai, L., Yang, X., Ye, D., Chen, H., et al. (2015). Natural germacrane sesquiterpenes inhibit osteoclast formation, bone resorption, RANKL-induced NF-κB activation, and IκBα degradation. Int. J. Mol. Sci. 16 (11), 26599–26607. doi:10.3390/ijms161125972
Schinella, G. R., Giner, R. M., Recio, M. C., Mordujovich de Buschiazzo, P., Rios, J. L., and Manez, S. (1998). Anti-inflammatory effects of South American tanacetum vulgare. J. Pharm. Pharmacol. 50 (9), 1069–1074. doi:10.1111/j.2042-7158.1998.tb06924.x
Siveen, K. S., Uddin, S., and Mohammad, R. M. (2017). Targeting acute myeloid leukemia stem cell signaling by natural products. Mol. Cancer 16 (1), 13. doi:10.1186/s12943-016-0571-x
Soleymani, S., Naghizadeh, A., Karimi, M., Zarei, A., Mardi, R., Kordafshari, G., et al. (2022). COVID-19: General strategies for herbal therapies. J. Evid. Based Integr. Med. 27, 2515690X211053641. doi:10.1177/2515690X211053641
Sugiyasu, K., Nanno, K., Tamai, N., Hashimoto, N., Kishida, Y., Yoshikawa, H., et al. (2011). Radio-sensitization of the murine osteosarcoma cell line LM8 with parthenolide, a natural inhibitor of NF-κB. Oncol. Lett. 2 (3), 407–412. doi:10.3892/ol.2011.277
Sun, L., Yuan, W., Wen, G., Yu, B., Xu, F., Gan, X., et al. (2020). Parthenolide inhibits human lung cancer cell growth by modulating the IGF1R/PI3K/Akt signaling pathway. Oncol. Rep. 44 (3), 1184–1193. doi:10.3892/or.2020.7649
Sun, Y., St Clair, D. K., Fang, F., Warren, G. W., Rangnekar, V. M., Crooks, P. A., et al. (2007). The radiosensitization effect of parthenolide in prostate cancer cells is mediated by nuclear factor-kappaB inhibition and enhanced by the presence of PTEN. Mol. Cancer Ther. 6 (9), 2477–2486. doi:10.1158/1535-7163.MCT-07-0186
Suvannasankha, A., Crean, C. D., Shanmugam, R., Farag, S. S., Abonour, R., Boswell, H. S., et al. (2008). Antimyeloma effects of a sesquiterpene lactone parthenolide. Clin. Cancer Res. 14 (6), 1814–1822. doi:10.1158/1078-0432.CCR-07-1359
Takai, E., Taniguchi, F., Nakamura, K., Uegaki, T., Iwabe, T., and Harada, T. (2013). Parthenolide reduces cell proliferation and prostaglandin E2 [corrected] in human endometriotic stromal cells and inhibits development of endometriosis in the murine model. Fertil. Steril. 100 (4), 1170–1178. doi:10.1016/j.fertnstert.2013.06.028
Wang, W., Adachi, M., Kawamura, R., Sakamoto, H., Hayashi, T., Ishida, T., et al. (2006). Parthenolide-induced apoptosis in multiple myeloma cells involves reactive oxygen species generation and cell sensitivity depends on catalase activity. Apoptosis 11 (12), 2225–2235. doi:10.1007/s10495-006-0287-2
Watson, C., Miller, D. A., Chin-Sinex, H., Losch, A., Hughes, W., Sweeney, C., et al. (2009). Suppression of NF-kappaB activity by parthenolide induces X-ray sensitivity through inhibition of split-dose repair in TP53 null prostate cancer cells. Radiat. Res. 171 (4), 389–396. doi:10.1667/RR1394.1
Wu, L. M., Liao, X. Z., Zhang, Y., He, Z. R., Nie, S. Q., Ke, B., et al. (2020). Parthenolide augments the chemosensitivity of non-small-cell lung cancer to cisplatin via the PI3K/AKT signaling pathway. Front. Cell Dev. Biol. 8, 610097. doi:10.3389/fcell.2020.610097
Xu, J., Wu, H. F., Ang, E. S., Yip, K., Woloszyn, M., Zheng, M. H., et al. (2009). NF-kappaB modulators in osteolytic bone diseases. Cytokine Growth Factor Rev. 20 (1), 7–17. doi:10.1016/j.cytogfr.2008.11.007
Yang, C., Yang, Q. O., Kong, Q. J., Yuan, W., and Ou Yang, Y. P. (2016). Parthenolide induces reactive oxygen species-mediated autophagic cell death in human osteosarcoma cells. Cell Physiol. Biochem. 40 (1-2), 146–154. doi:10.1159/000452532
Yang, L., Liu, S., Liu, J., Zhang, Z., Wan, X., Huang, B., et al. (2020). COVID-19: Immunopathogenesis and immunotherapeutics. Signal Transduct. Target Ther. 5 (1), 128. doi:10.1038/s41392-020-00243-2
Yip, K. H., Zheng, M. H., Feng, H. T., Steer, J. H., Joyce, D. A., and Xu, J. (2004). Sesquiterpene lactone parthenolide blocks lipopolysaccharide-induced osteolysis through the suppression of NF-kappaB activity. J. Bone Min. Res. 19 (11), 1905–1916. doi:10.1359/JBMR.040919
Yip-Schneider, M. T., Nakshatri, H., Sweeney, C. J., Marshall, M. S., Wiebke, E. A., and Schmidt, C. M. (2005). Parthenolide and sulindac cooperate to mediate growth suppression and inhibit the nuclear factor-kappa B pathway in pancreatic carcinoma cells. Mol. Cancer Ther. 4 (4), 587–594. doi:10.1158/1535-7163.MCT-04-0215
Yu, H. J., Jung, J. Y., Jeong, J. H., Cho, S. D., and Lee, J. S. (2015). Induction of apoptosis by parthenolide in human oral cancer cell lines and tumor xenografts. Oral Oncol. 51 (6), 602–609. doi:10.1016/j.oraloncology.2015.03.003
Zawawi, M. S., Marino, V., Perilli, E., Cantley, M. D., Xu, J., Purdue, P. E., et al. (2015). Parthenolide reduces empty lacunae and osteoclastic bone surface resorption induced by polyethylene particles in a murine calvarial model of peri-implant osteolysis. J. Biomed. Mater Res. A 103 (11), 3572–3579. doi:10.1002/jbm.a.35484
Zhang, X., Chen, Q., Liu, J., Fan, C., Wei, Q., Chen, Z., et al. (2017). Parthenolide promotes differentiation of osteoblasts through the wnt/β-catenin signaling pathway in inflammatory environments. J. Interferon Cytokine Res. 37 (9), 406–414. doi:10.1089/jir.2017.0023
Zhang, X., Fan, C., Xiao, Y., and Mao, X. (2014). Anti-inflammatory and antiosteoclastogenic activities of parthenolide on human periodontal ligament cells in vitro. Evid. Based Complement. Altern. Med. 2014, 546097. doi:10.1155/2014/546097
Zhou, H. Q., Zhang, F. C., and Rk, W. (2008). Parthenolide inhibits tumor necrosis factor-alpha induced catabolism of aggrecan in chondrocytes in osteoarthritis: In vitro experiment with cultured human chondrocytes. Zhonghua Yi Xue Za Zhi 88 (11), 764–768.
Zou, Z., Shan, H., Sun, D., Xia, L., Shi, Y., Wan, J., et al. (2022). Parthenolide reveals an allosteric mode to inhibit the deISGylation activity of SARS-CoV-2 papain-like protease. Acta Biochim. Biophys. Sin. (Shanghai) 54 (8), 1133–1139. doi:10.3724/abbs.2022092
Keywords: parthenolide, skeletal diseases, cancers, inflammation, osteolysis
Citation: Zhu S, Sun P, Bennett S, Charlesworth O, Tan R, Peng X, Gu Q, Kujan O and Xu J (2023) The therapeutic effect and mechanism of parthenolide in skeletal disease, cancers, and cytokine storm. Front. Pharmacol. 14:1111218. doi: 10.3389/fphar.2023.1111218
Received: 29 November 2022; Accepted: 17 February 2023;
Published: 09 March 2023.
Edited by:
Per-Johan Jakobsson, Karolinska Institutet (KI), SwedenReviewed by:
Laiba Arshad, Forman Christian College, PakistanQiang Guo, Third Xiangya Hospital, Central South University, China
Copyright © 2023 Zhu, Sun, Bennett, Charlesworth, Tan, Peng, Gu, Kujan and Xu. This is an open-access article distributed under the terms of the Creative Commons Attribution License (CC BY). The use, distribution or reproduction in other forums is permitted, provided the original author(s) and the copyright owner(s) are credited and that the original publication in this journal is cited, in accordance with accepted academic practice. No use, distribution or reproduction is permitted which does not comply with these terms.
*Correspondence: Sipin Zhu, c2lwaW56aHVAMTYzLmNvbQ==; Jiake Xu, amlha2UueHVAdXdhLmVkdS5hdQ==
†These authors have contributed equally to this work