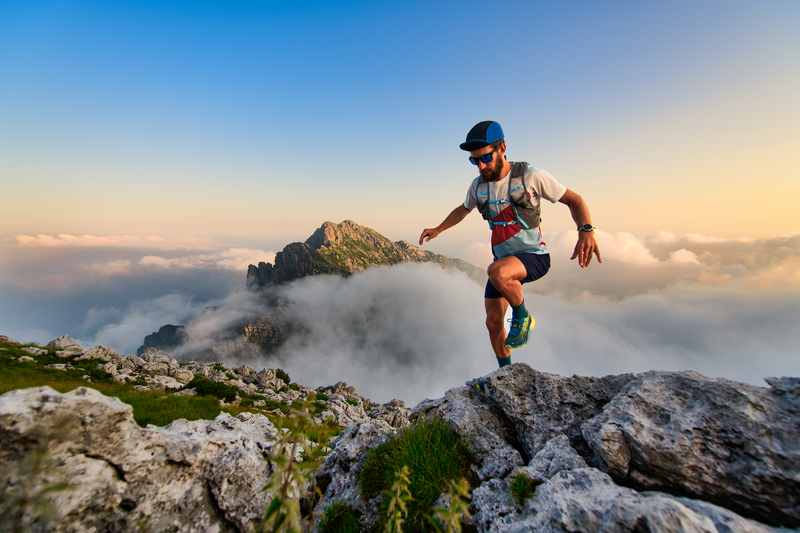
95% of researchers rate our articles as excellent or good
Learn more about the work of our research integrity team to safeguard the quality of each article we publish.
Find out more
ORIGINAL RESEARCH article
Front. Pharmacol. , 03 February 2023
Sec. Cardiovascular and Smooth Muscle Pharmacology
Volume 14 - 2023 | https://doi.org/10.3389/fphar.2023.1103971
This article is part of the Research Topic Novel and current therapeutic approaches for treatment of cardiovascular disorders View all 9 articles
Introduction: The protective effects of astaxanthin against myocardial ischemia-reperfusion injuries are well documented, although the mechanisms are not defined.
Methods: The anoxia-reoxygenation injury model was established after astaxanthin treated H9c2 cells for 24 h. Cell viability, lactate dehydrogenase, oxidative stress level and western blot were tested. Secondly, measured the effects of astaxanthin pretreatment on microRNA expression in a rat myocardial cell anoxia-reoxygenation injury model.
Results: After anoxia-reoxygenation injury, in a dose dependent manner, astaxanthin increased cell viability, superoxide dismutase and glutathione peroxidase activity, decreased lactate dehydrogenase and malondialdehyde levels, downregulated protein expression of caspase-3, caspase-8, nuclear factor erythroid-2-related factor 2 and heme oxygenase-1, and upregulated the Bcl-2/Bax ratio. High-throughput sequencing and qPCR showed that microRNAs rno-miR-125b-5p and rno-let-7c-1-3p were differentially expressed (|log2| ≥ 0.585, q < 0.1) between the normal, anoxia-reoxygenation, and astaxanthin (1.25 μM) groups. Kyoto Encyclopedia of Genes and Genomes and GO Gene ontology pathway enrichment analyses showed that TNF signaling, axon guidance, NF-κB signaling pathway, and other pathways displayed differentially expressed microRNA target genes associated with myocardial injuries.
Discussion: These results suggested that thetarget genes of rno-miR-125b-5p were enriched in inflammation and apoptosis-related signaling pathways. Also, the results imply that simultaneous targeting of these related signaling pathways could significantly prevent myocardial anoxia-reoxygenation injury in the presence of astaxanthin.
Astaxanthin, 3,3′-dihydroxy-β,β-carotene-4,4′-dione, occurs in shrimp and crab shells, red hair fruit yeast, rainbow-sounding red algae, fish, and the showy feathers of birds (Fassett and Coombes, 2011). Astaxanthin is composed of a six-node ring structure of four isoprene units linked by a conjugated double bond with one isoprene unit at each end. The conjugated polyene structure of the astaxanthin molecule is susceptible to oxidation and extremely unstable; thus, astaxanthin possesses high antioxidant activity as a quencher of singlet oxygen. Astaxanthin is reported to have anti-aging, anti-cancer, immune system regulation, and cardiovascular disease prevention properties (Zhang and Wang, 2015; Eren et al., 2019; Sztretye et al., 2019; Yin et al., 2021). Notably, Chen et al. (2020) found that astaxanthin had significant protective and damage mitigation effects in prevention and treatment of cardiovascular disease.
Myocardial ischemia-reperfusion (I/R) injury is a pathological process in which the myocardium is damaged after partial or complete acute blockage of the coronary arteries followed by restoration of normal perfusion (Wei et al., 2014). The mechanism of I/R injury appears to be related to a massive production of intracellular oxygen free radicals, calcium ion overload, and the inflammatory effect of leukocytes. Several basic studies have validated the ability of astaxanthin pretreatment to alleviate oxidative stress, apoptosis, and inflammatory responses induced by myocardial anoxia-reoxygenation (A/R) injury (Fassett and Coombes, 2009; Gai et al., 2020; Kohandel et al., 2021).
MicroRNAs are small, endogenous, non-coding RNAs composed of 19–25 nucleotides. MicroRNAs post-transcriptionally regulate gene expression, and their regulatory networks are involved in many biological processes (Chen et al., 2019), such as embryogenesis (Wójcik et al., 2017), cell proliferation and differentiation (Miao et al., 2017; Wu et al., 2018), apoptosis (Koo and Kwon, 2018), and tumorigenesis (Honardoost and Rad, 2018). In the cardiovascular system, microRNAs regulate growth and contraction of cardiomyocytes (Alikunju et al., 2022), cardiac rhythm (Briasoulis et al., 2019; Cao et al., 2019), plaque formation (Meng et al., 2020), lipid metabolism, and angiogenesis (Barwari et al., 2016; Du et al., 2021). By affecting microRNA-mRNA networks, astaxanthin can alter signaling pathways and cell death in cardiovascular disease (Chaboksafar et al., 2022), type 2 diabetes (Shokri-Mashhadi et al., 2021), Parkinson’s disease (Shen et al., 2021), and tumors (Faraone et al., 2020). These effects suggest that astaxanthin-induced expression of microRNAs can be used to assess therapeutic effects. However, it is not known whether the protective effects of astaxanthin in myocardial A/R are related to microRNA expression.
Rat cardiomyocyte H9c2 cells are immortalized cells with a cardiac phenotype; H9c2 cells are the most commonly used cell type for isolated primary cardiomyocyte characteristics, they are easily accessible and cultured (Chou et al., 2010; Kuznetsov et al., 2015; Upadhyay et al., 2020; Hu et al., 2022), and they have been used as models in ischemia and reperfusion studies (Han et al., 2004; Wang et al., 2016; Shengm et al., 2019; Luo et al., 2020; Ma et al., 2020). Thus, we analyzed the expression of microRNAs following astaxanthin treatment in an anoxia-reoxygenation system for H9c2 cardiomyocytes to uncover targets of astaxanthin action and guide strategies for myocardial A/R protection.
Astaxanthin (purity ≥98%, A114383, Aladdin), and Trolox (T137260-1g, Aladdin) were dissolved in DMSO, ensuring that the DMSO content in the cell culture medium was less than 1%. Anoxic solution was composed of NaH2PO4 0.9 mM, NaHCO3 6 mM, CaCl2 1.8 mM, MgSO4 1.2 mM, sodium lactate 40 mM, HEPES 20 mM, NaCl 98.5 mM, and KCl 10 mM, pH 6.8. Reoxygenation solution (Chen et al., 2020) was NaCl 129.5 mM, KCl 5 mM NaH2PO4 0.9 mM, NaHCO3 20 mM, CaCl2 1.8 mM, MgSO4 1.2 mM, glucose 5.5 mM, and HEPES 20 mM, pH 7.4.
H9c2 cells (GNR-5, Stem Cell Bank, Chinese Academy of Sciences, Shanghai, China) were cultured in DMEM (11995073, Gibco, United States) with 10% (v/v) fetal bovine serum (F8192, Sigma) and 1% (v/v) penicillin-streptomycin solution (Gibco) at 37°C with 5% CO2 and 95% air. Cells were pretreated for 24 h with astaxanthin (0.312, 0.625, 1.25, 2.5, and 5 μM); Trolox (1.25 μM) was used as a positive control. To simulate myocardial ischemia-reperfusion in vitro, a cardiomyocyte anoxia-reoxygenation model was constructed. The anoxic solution was pre-warmed in a 37°C water bath for 30 min and ventilated with a mixture of 95% N2 and 5% CO2 for 50 min. The cells were washed twice with pre-warmed saturated anoxic solution, an appropriate amount of pre-saturated anoxic solution was added, the cells were placed in an anoxic device and 95% N2-5% CO2 was introduced for 30 min. Then the device was clamped shut and incubated for 2.5 h in a 37°C incubator (without gas introduction). The reoxygenation solution was similarly pre-warmed and passed through a mixture of 95% O2-5% CO2 for 50 min. After establishing acute anoxia, the cells were washed twice with the reoxygenation solution, an appropriate amount of pre-saturated reoxygenation solution was added, the cells were placed in a reoxygenation device and 95% O2-5% CO2 was introduced for 30 min. Then the device was clamped shut and placed in a 37°C incubator (without gas introduction) for 1.5 h before indicators were measured, show as Figure 1.
FIGURE 1. Astaxanthin pretreatment scheme. Cardiomyocytes were pretreated with astaxanthin (AST, 0.313 –5 μM) for 24 h before A/R injury.
Cell viability assays were performed using the CCK-8 kit (BS350A, Biosharp) on 96-well plates according to the manufacturer’s instructions. CCK-8 reagent (10 μL) was added to each well and incubated for 1–2 h at 37°C in the dark. The absorbance was measured at 450 nm using an enzyme marker (PerkinElmer Victor 1420, United States).
Cultures from each treatment subgroup were collected and the lactate dehydrogenase (LDH) content in the supernatant was measured (kit A020-2-2, built in Nanjing, China) to assess cell damage according to the kit instructions.
Antioxidant enzymes are crucial for cells to dispel oxygen radicals and maintain redox homeostasis. Cells from each treatment group were homogenized; protein content was measured by BCA. Superoxide dismutase (SOD), glutathione peroxidase (GSH-Px), and malondialdehyde (MDA) kits (A001-3-2, A005-1-2, A003-4-1 built in Nanjing, China) were used to assess whether astaxanthin upregulated the antioxidant capacity of H9c2 cardiomyocytes after A/R injury.
Cells were collected after 24 h of astaxanthin pretreatment and anoxic reoxygenation. Cells were lysed for 20 min in RIPA buffer (HYK1001, MedChem Express, NJ, United States) containing protease inhibitors and then centrifuged for 15 min at 4°C, 5,550 ×g. A BCA kit was used to measure the protein content of the supernatants. The primary antibodies used in the experiment were specific for nuclear factor erythroid-2-related factor 2 (Nrf2), heme oxygenase 1 (HO-1), Bax, Bcl-2, caspase-3, caspase-8, GAPDH, and LaminB. The secondary antibodies were anti-rabbit immunoglobulin and anti-mouse immunoglobulin.
The cells were diluted with 1×105/DMEM and then seeded on 10 cm diameter plates, pretreated with astaxanthin for 24 h, and collected after anoxic-reoxygenation. Total RNA was extracted. Nanophotometer spectrophotometer was used for assessment of RNA purity (OD260/280 and OD260/230 ratio).
We used high-throughput sequencing to measure the abundance of microRNAs of the control, A/R, and A/R+astaxanthin groups. The experiment was performed at The Beijing Genomics Institute. Differentially expressed microRNAs were defined by log2 values, |log2 change values| ≥ 0.585, p < 0.05, or q < 0.01.
Extracted RNA was converted to cDNA with reverse transcriptase. The primers were selected for the tailing method and real-time PCR was performed using the SYBR-Green polymerase chain reaction kit (Shanghai Bioengineering Technology Co., Ltd.). On the LightCycler® 96 Real-Time Polymerase Chain Reaction System, operations were conducted according to the manufacturer’s instructions. Table 1 contains the PCR primer sequences. The expression levels of microRNAs were normalized to U6 snRNA. Statistical treatment was performed using a t-test with a threshold of p < 0.05.
The KEGG and GO databases were used to identify the functions of microRNAs and the signaling pathways in which they are involved, and to assess the microRNA-mRNA regulatory interactions in astaxanthin-treated H9c2 cardiomyocytes.
All experiments were repeated three times and statistical analysis was performed using Graphpad 8.0, SPSS statistical software. p-values were analyzed using one-way ANOVA and Tukey’s post-hoc test; p < 0.05 was considered statistically significant.
We found that the cardiomyocyte survival of the A/R group was 65%, which was significantly reduced compared with the control group. The lactate dehydrogenase (LDH) content in the cell supernatant was about 95 U/L, and twice as high as that of the control group (p < 0.01; Figures 2A,B); these results were similar to findings of Liu et al. (2021). Compared with the A/R group, the astaxanthin group showed a dose-dependent increase in cell survival and decreasing supernatant lactate dehydrogenase level (p < 0.05 and p < 0.01). In addition, the Yaghooti et al. found that astaxanthin significantly improved cell survival of mesenchymal stem cells after palmitate injury (Yaghooti et al., 2019). On the basis of these results, we selected astaxanthin concentrations of 0.625, 1.25, and 2.5 μM for subsequent experiments.
FIGURE 2. Astaxanthin alleviates A/R damage to H9c2 cardiomyocytes by maintaining cellular activity (A), increasing expression of antioxidant enzymes (C,D) and reducing LDH and MDA levels (B, E). *, vs. A/R group (*p < 0.05; **p < 0.01); #, astaxanthin vs. Trolox group (#p < 0.05; ##p < 0.01). A/R, anoxia reoxygenation; AST, astaxanthin.
As shown in Figures 2C–E, superoxide dismutase (SOD) and glutathione peroxidase (GSH-Px) activities were significantly decreased by 11.3 U/mgprot and 8 U/mgprot (p < 0.01), and the level of malondialdehyde (MDA) was significantly increased by 12 nmol/mgprot (p < 0.01) in the A/R group compared with the control group. The activities of SOD and GSH-Px were significantly increased and MDA level was significantly lower in the astaxanthin group compared with the A/R group (p < 0.05 or p < 0.01). At an astaxanthin concentration of 1.25 μM, the activity of SOD and GSH-Px increased to 78 U/mgprot and 15.2 U/mgprot, which was higher than 11 U/mgprot and 10 U/mgprot in A/R group. The MDA level was significantly reduced to 14.7 nmol/mgprot, approximately 10 nmol/mgprot lower than the A/R group. Compared with the Trolox positive control group, the SOD and GSH-Px activities were slightly lower in the 0.625 μM astaxanthin concentration group (p < 0.05 or p < 0.01), and the MDA content was slightly higher in the Trolox group (p < 0.05). At a concentration of 1.25 μM astaxanthin, compared with the Trolox group, SOD activity was slightly higher, GSH-Px activity was the same, and MDA content was significantly decreased by 6 nmol/mgprot (p < 0.05). At an astaxanthin concentration of 2.5 μM, the SOD activity was on par with the Trolox group, GSH-Px activity was significantly increased by 8 U/mgprot (p < 0.01), and MDA content was significantly lower (p < 0.05) than the Trolox group. Su et al. (2022) studied the trend of various antioxidant enzymes in kidney I/R injury. As a powerful natural antioxidant, astaxanthin protects against damage and maintains redox balance by activating antioxidant enzymes. Astaxanthin protects against hypertensive vascular remodeling injury by increasing the level of the SOD that prevents oxidative injury (Chen et al., 2020).
We found that Bcl-2/Bax ratio were reduced and expression of caspase-8 and caspase-3 increased in each group with A/R injury compared with the normal group (p < 0.01; Figures 4A–C). Bcl-2/Bax ratio were significantly higher in the astaxanthin groups compared with the A/R group, and the expression of caspase-3 and caspase-8 was also significantly lower (p < 0.05 or p < 0.01). Bcl-2/Bax ratio were higher in the astaxanthin group compared to the Trolox positive control group (p < 0.01). Many studies have shown that astaxanthin can inhibit apoptosis (Cui et al., 2020; Zhang et al., 2021; Abbaszadeh et al., 2022).
The Nrf2/HO-1 pathway is an important antioxidant mechanism. We investigated the effect of astaxanthin treatment on the Nrf2/HO-1 pathway in A/R-injured H9c2 cardiomyocytes (Figure 3). Oxidative stress caused by A/R injury increased Nrf2 nuclear translocation and decreased HO-1 content (p < 0.05). Astaxanthin increased the nuclear translocation of Nrf2 and the amount of HO-1 compared with the A/R group (p < 0.05 and p < 0.01, Figures 4D, E). In addition, the expression level of HO-1 protein in the control group was higher than that in the A/R group. This finding suggested that the function of cells and mitochondria would be affected, and cell homeostasis would be unbalanced due to the abnormal production of reactive oxygen species and energy metabolism during anoxia-reoxygenation injury, thus resulting in the decrease of HO-1 protein synthesis. Second, inhibitors of Nrf2, such as Bach1, also inhibit the synthesis of HO-1 (Lignitto et al., 2019); Researchers indicated that inhibition of PI3K-Akt pathway can significantly reduce the expression of HO-1 protein, thus weakening the protective effect of HO-1 on cardiomyocytes (Sun et al., 2012). Astaxanthin alleviates anoxia-reoxygenation injury in cardiomyocytes and maintains cellular vitality. Therefore, in the presence of astaxanthin, the increase of Nrf2 can promote an increase of HO-1.
FIGURE 4. Astaxanthin pretreatment reduced apoptosis-related protein content (A–C) and enhanced nuclear Nrf2 and HO-1 protein content (D,E) in cardiomyocytes after A/R injury. *, vs. A/R group (*p < 0.05; **p < 0.01); #, astaxanthin vs. Trolox group (#p < 0.05; ##p < 0.01). A/R, anoxia reoxygenation; AST, astaxanthin.
To investigate a link between the protective effect of astaxanthin against A/R damage of cardiomyocytes and microRNA regulation, we constructed a control group, an A/R group, and an astaxanthin+A/R group. After filtering for poor-quality tags, splice contaminants, and sequences with less than 18 nucleotides, >90.8% clean tags were obtained from each group (Supplementary Table SA1; Supplementary Datasheet S1). These tags were between 20–24 nt, with a peak at 23 nt (Supplementary Figures SA1–SA3), and they were comparable in size to animal microRNAs. Taking AR2 as an example, based on the miRBase database, the intergenic, mature sequences, and unmap tags accounted for 28.57%, 22.34%, and 15.44%, respectively (Table 2).
The screening criteria for differentially expressed microRNAs were q < 0.05 and |log2 change value| ≥ 0.585. Tables 3, 4 and Figure 5 show that the number of differential microRNAs identified in the AR/C group was 47 (14 upregulated and 33 downregulated) and the number of differential microRNAs in the AR/astaxanthin group was 16 (7 upregulated and 9 downregulated). To identify the key microRNAs whereby astaxanthin exerted a protective effect and selecting microRNAs that astaxanthin was capable of back-regulating abnormal changes in AR, three eligible microRNAs were found, of which rno-miR-125b-5p, rno-miR-146a-5p were upregulated and rno-let-7c-1-3p was downregulated.
FIGURE 5. RNA-Seq-based differential microRNA heat maps were plotted against the expression level of each sample.
As verified by qPCR (e.g., Figures 6A–C), we found that the expression of rno-miR-125b-5p and rno-let-7c-1-3p in each treatment group was consistent with the sequencing results, and the qPCR results were generally in good agreement with the RNA-seq results.
FIGURE 6. RT-qPCR analysis of the expression of rno-miR125b-5p (A), Let-7c-1-3p (B). (C) Comparison of RNA-seq and RT-qPCR results for selected differentially expressed microRNAs (*p < 0.05; **p < 0.01). A/R, anoxia reoxygenation; AST, astaxanthin.
We used the TargetScan (8.0), microRNAda (v3.3a), and RNAhybrid databases to predict target genes for rno-miR-125b-5p and rno-let-7c-1-3p.
We used the GO database (http://www.geneontology.org) to identify enrichment of the target genes. The GO database is a comprehensive overview of the gene functions of an organism divided into three ontologies, namely Biological process, Cellular component, and Molecular function (Wang et al., 2021). Because rno-let-7c-1-3p had no more than ten target genes, we analyzed rno-miR-125b-5p. Figure 7 is the GO enrichment analysis of rno-miR-125b-5p target genes (q ≤ 0.01). The target genes were associated with branching involved in biological processes such as histone lysine methylation, histone methylation, and protein phosphorylation. The GO cellular components were the nucleus and the synaptic precursor, and the GO molecular functions were histone methyltransferase activity (H3-K9 specific), histone-lysine N-methyltransferase activity, and protein kinase activity.
The KEGG Pathway enrichment analysis (Figure 8) showed that the target genes of the differentially expressed microRNAs across treatments were significantly enriched in the TNF signaling pathway, axon guidance, and the NF-κB pathway. The target genes of the TNF signaling pathway enriched by miR125b-5p were Tnfrsf1b, Nod2, Rela, Map2k7, Lif, and Tnfaip3; the NF-κB signaling pathway contains target genes that Rela, Map2k7, Syk, and Cxcl12. The Ras signaling pathway contains the target genes Syngap1, Ntrk2, Ralgds, Rela, Rac1, and Sos2; the MAPK signaling pathway contains the target genes Ntrk2, Rela, Map2k7, Rac1, Dusp3, and Sos2; the PI3K-AKT signaling pathway contains the target genes Ntrk2, Syk, Rela, Rac1, Itga7 and Sos2; the FC epsilon RI signaling pathway contains the target genes Syk, Map2k7, Rac1, and Sos2; the Toll-like receptor signaling pathway contains the target genes Rela, Map2k7, Rac1 (Figure 9). Rela, Rac1, and Map2k7 target genes participate in multiple signaling pathways and may be associated with protection against myocardial anoxia-reoxygenation damage; thus, these genes are value for follow-up studies.
Astaxanthin efficiently scavenges superoxide anions, hydroxyl radicals, and lipid-free radicals (Zuluaga et al., 2018). Many studies on the cardioprotective effects of astaxanthin demonstrate its powerful antioxidant activity in prevention and treatment of ischemia-reperfusion lesions in vivo (Xue et al., 2019; Wang et al., 2021). And astaxanthin has been reported to protect the myocardium from ischemia/reperfusion injury by decreasing oxidative stress and apoptosis (Adluri et al., 2013). Moreover, the beneficial effects of astaxanthin on IRI-induced increase in plasma and cardiac levels of oxidative stress (Pongkan et al., 2017). Similar effects have been observed in an in vitro study, wherein administration of astaxanthin protected myocardial cells from anoxia/reoxygenation injury (Gai et al., 2020). However, the mechanism of astaxanthin protection against myocardial ischemia-reperfusion injury was unknown. We studied the mechanism of myocardial ischemia-reperfusion injury in vitro.
When myocardium undergoes ischemia-reperfusion injury, myocardial cell permeability increases, and a large amount of lactate dehydrogenase (LDH) is released; thus, extracellular LDH activity can reflect the degree of myocardial cell damage (Gai et al., 2020). Our LDH measurements showed that, compared to the anoxia reoxygenation group, astaxanthin improved the survival of H9c2 cardiomyocytes that would have had significant A/R injury and LDH leakage (Figures 2A,B). Excess reactive oxygen species is a major contributor to myocardial ischemia-reperfusion injury because it leads to oxidative stress that, in turn, causes apoptosis of cardiomyocytes. Antioxidant enzyme activity decreases when cells are damaged. SOD can remove superoxide anions to produce H2O2. GSH-Px catalyzes the conversion of lipid hydroperoxide (LOOH) produced during lipid peroxidation to the corresponding alcohol lipid hydroxide (LOH), blocking the side chain cycle reaction of lipid peroxidation. We found that SOD and GSH-Px levels were reduced and MDA level was increased in the A/R group compared with the normal group. In the astaxanthin group, intracellular SOD and GSH-Px levels were increased, and malondialdehyde level was decreased in cardiac myocytes (Figures 2C–E). The results suggested that astaxanthin can scavenge free radicals, increase the activity of antioxidant enzymes, and reduce oxidative damage during A/R.
Anoxia reoxygenation promotes cardiomyocyte expression of apoptosis-related proteins such as caspase-8 and caspase-3, initiates apoptotic signaling pathways, and induces apoptosis in cardiomyocytes. The astaxanthin group had significantly reduced A/R damage in cardiomyocytes and increased expression of Bcl-2, activated caspase-8/caspase-3, and delayed onset of apoptosis (Figures 4A–C). All these results confirmed that astaxanthin can alleviate oxidative damage during myocardial ischemia-reperfusion.
Heme oxygenase-1 (HO-1) is involved in preventing oxidative stress and in anti-apoptotic processes; HO-1 has an important protective function in A/R injury. Nuclear factor erythroid 2-related factor 2 (Nrf2) is present in oxygen-depleted organs and is an important regulator of oxidative stress (Cui et al., 2020). The Nrf2/HO-1 signaling pathway is an important intracellular antioxidant pathway. In response to oxidative stress, Nrf2 dissociates from kelch-like ECH-associated protein 1 and translocates from the cytoplasm to the nucleus to induce antioxidant and detoxification genes (Kohandel et al., 2021). Astaxanthin activated the Nrf2/HO-1 pathway to suppress oxidative stress, alleviating myocardial injury and ischemia-reperfusion injury of femur and liver. Astaxanthin alleviates cardiomyocyte apoptosis after coronary microembolization by inhibiting oxidative stress via Nrf2/HO-1 pathway in rats (Zuluaga et al., 2019; Cui et al., 2020). We found that the Nrf2/HO-1 pathway was adaptively activated to counteract A/R damage of cardiomyocytes. Astaxanthin pretreatment enhanced the activation of the Nrf2/HO-1 pathway, which promoted the antioxidant stress response (Figures 4D,E). Although there have been numerous studies on the protective effects of astaxanthin in a variety of diseases, to the best of our knowledge, our study is the first to demonstrate the contribution of the Nrf2/HO-1 pathway to the protective effects of astaxanthin in myocardial ischemia-reperfusion.
MicroRNAs are a class of small, non-coding RNAs that regulate more than 30% of genes by inhibiting the translation of their mRNAs or promoting mRNA degradation (Poller et al., 2018). Recent studies have identified novel mechanisms of regulatory networks between microRNAs and mRNAs that control the pathophysiology of cardiovascular disease (Gong et al., 2020; Liu et al., 2021). Astaxanthin can protect cardiomyocytes from anoxia/reoxygenation injury by regulating the miR-138/HIF-1α axis (Gai et al., 2020). Therefore, we systematically analyzed the microRNAs that responded to astaxanthin and protected cardiomyocytes from A/R injury.
We used RNA-seq and RT-qPCR validation to identify differentially expressed microRNAs associated with astaxanthin protection against myocardial A/R injury. In our study, rno-miR-125b-5p expression in the three treatment groups was control group >astaxanthin+A/R group >A/R group. Therefore, A/R damage decreased rno-miR-125b-5p expression. MiR-125b-5p has low abundance in patients with acute ischemic stroke and ischemic cardiomyopathy, and miR-125b-5p can be used as a diagnostic biomarker of heart failure (Tiedt et al., 2017; Bayoumi et al., 2018). We found that predicted target genes of rno-miR-125b-5p were enriched in signaling pathways related to oxidative stress, inflammation, and apoptosis. Many studies have shown that microRNAs can treat diseases associated with oxidative stress (Wang et al., 2019). Rno-miR-125b-5p is closely associated with oxidative stress-induced diseases and protects endothelial cells from hydrogen peroxide-induced oxidative stress (Wei et al., 2017). In our data, the rno-miR-125b-5p target gene Rac1 (small Rac family GTPase 1) can activate MAPK and PI3K-AKT signaling pathways. Because the mechanism of anoxia-reoxygenation-induced myocardial injury is crucial, myocardial oxidative stress will generate a large amount of reactive oxygen species, and astaxanthin is a natural antioxidant. Our results also show that rno-miR-125b-5p was a important target in astaxanthin antioxidant effect on myocardial anoxia-reoxygenation injury, and astaxanthin can increase the expression of rno-miR-125-b-5p after anoxia-reoxygenation injury. Therefore, we speculate that rno-miR-125b-5p is a target for astaxanthin’s involvement in protection from myocardial injury.
In addition, miR-125b-5p acts as a protective agent for ischemic cardiomyocytes by inhibiting the pro-apoptotic genes p53, Bak1, and Klf13 (Bayoumi et al., 2018; Zhu et al., 2018). Apoptosis has multiple pathways, such as oxidative stress, inflammation, and DNA damage. We found that the target genes of rno-miR-125b-5p were significantly enriched in the TNF signaling pathway, in which TNF-α could activate three downstream signaling pathways: caspase protease, JNK signaling pathway, and NF-κB signaling pathway. TNF-α dissociates SODD bound to TNFR1 on the cell membrane, exposing the dead zone of TNFR1, and FADD binds to TNFR1 to activate downstream caspase-8 and caspase-3, leading to apoptosis. Astaxanthin reduced the levels of caspase-8 and caspase-3, which suggested that astaxanthin inhibits apoptosis by enhancing the expression of rno-miR-125b-5p. TNFR1 also binds to TRADD, RIPI, and TRAF2/5, activates the IKK kinase complex, phosphorylates and degrades IKBα, separates it from NF-κB, and activates the inflammatory response. MiR-125b-5p suppresses excessive inflammation in human arthritic chondrocytes by downregulating Rela target genes (Rasheed et al., 2019). LIF, a proinflammatory factor regulated by miR-125b-5p, is a protective factor against acutely stressed myocardium during ischemia-reperfusion (Zouein et al., 2013). Further study is needed to determine whether astaxanthin can inhibit the inflammatory response of rno-miR-125b-5p to anoxia-reoxygenation injury.
Although the regulatory function of rno-miR-125b-5p is not fully understood, current data suggest that upregulation of rno-miR-125b-5p is beneficial for protecting against myocardial anoxia-reoxygenation injury. In a follow-up study, we used qPCR for different treatment groups to verify differential expression of Rac1, the target gene of miR125b-5p. Some studies showed that Rac1 activation can aggravate myocardial injury (Wang et al., 2022). Rac1 can activate ERK/P38MAPK signaling pathway (Zhu et al., 2009). In the future, we will investigate whether astaxanthin can inhibit apoptosis by modulating the miR125b-5p/Rac1/ERK/P38MAPK signaling pathway and protect the heart muscle from anoxia and reoxygenation damage by modulating the miR125b-5p/Rac1/ERK/P38MAPK signaling pathway. In addition, several studies have evaluated the antioxidant effects of orally and intravenously administered astaxanthin in I/R models (Gross and Lockwood, 2004; Dilli et al., 2022). We demonstrated that astaxanthin improved cell survival and inhibited oxidative stress and apoptosis after myocardial anoxic-reoxygenation injury. Therefore, our results can serve as a reference for in vivo model studies.
Astaxanthin pretreatment reduced myocardial injury and increased cardiomyocyte survival, and inhibited apoptosis and oxidative stress damage. We identified differentially expressed microRNAs and related target genes that, with astaxanthin pretreatment, protect cardiomyocytes from A/R injury. These target genes can be used to further investigate their regulatory relationships.
The datasets presented in this study can be found in online repositories. The NCBI serial number: PRJNA908639. https://www.ncbi.nlm.nih.gov/sra/PRJNA908639.
XZ and MX conducted the experiments and drafted the manuscript. SC and BC analyzed the microRNAs expression profiles. HL provided critical revision of the manuscript. ZL oversaw the study, and provided the funding. All authors reviewed the manuscript.
This research was funded by the National Key Research and Development Program of China (grant number 2018YFD0901102) (grant number 2021R1013003) and the Marine Economic Development Special Project of Fujian Province (grant number FJHJF-L-2022-9).
The authors thank AiMi Academic Services (www.aimieditor.com) for English language editing and review services.
The authors declare that the research was conducted in the absence of any commercial or financial relationships that could be construed as a potential conflict of interest.
All claims expressed in this article are solely those of the authors and do not necessarily represent those of their affiliated organizations, or those of the publisher, the editors and the reviewers. Any product that may be evaluated in this article, or claim that may be made by its manufacturer, is not guaranteed or endorsed by the publisher.
The Supplementary Material for this article can be found online at: https://www.frontiersin.org/articles/10.3389/fphar.2023.1103971/full#supplementary-material
Abbaszadeh, F., Jorjani, M., Joghataei, M, T., and Mehrabi, S. (2022). Astaxanthin modulates autophagy, apoptosis, and neuronal oxidative stress in a rat model of compression spinal cord injury. Neurochem. Res. 47 (7), 2043–2051. doi:10.1007/s11064-022-03593-1
Adluri, R. S., Thirunavukkarasu, M., Zhan, L., Maulik, N., Svennevig, K., Bagchi, M., et al. (2013). Cardioprotective efficacy of a novel antioxidant mix VitaePro against ex vivo myocardial ischemia-reperfusion injury. Cell Biochem. Biophys. 67, 281–286. doi:10.1007/s12013-011-9300-7
Alikunju, S., Niranjan, N., Mohsin, M., Sayed, N., and Sayed, D. (2022). G3bp1-microRNA-1 axis regulates cardiomyocyte hypertrophy. Cell. Signal. 91, 110245. doi:10.1016/j.cellsig.2022.110245
Barwari, T., Joshi, A., and Mayr, M. (2016). MicroRNAs in cardiovascular disease. J. Am. Coll. Cardiol. 68, 2577–2584. doi:10.1016/j.jacc.2016.09.945
Bayoumi, A. S., Park, K. M., Wang, Y., Teoh, J. P., Aonuma, T., Tang, Y., et al. (2018). A carvedilol-responsive microRNA, miR-125b-5p protects the heart from acute myocardial infarction by repressing pro-apoptotic bak1 and klf13 in cardiomyocytes. J. Mol. Cell. Cardiol. 114, 72–82. doi:10.1016/j.yjmcc.2017.11.003
Briasoulis, A., Sharma, S., Telila, T., Mallikethi-Reddy, S., Papageorgiou, N., Oikonomou, E., et al. (2019). MicroRNAs in atrial fibrillation. Curr. Med. Chem. 26, 855–863. doi:10.2174/0929867324666170920151024
Cao, F., Li, Z., Ding, W. M., Yan, L., and Zhao, Q. Y. (2019). LncRNA PVT1 regulates atrial fibrosis via miR-128-3p-SP1-TGF-β1-Smad axis in atrial fibrillation. Mol. Med. 25, 7. doi:10.1186/s10020-019-0074-5
Chaboksafar, M., Fakhr, L., Kheirouri, S., and Alizadeh, M. (2022). The effects of astaxanthin supplementation on expression of microRNAs involved in cardiovascular diseases: A systematic review of current evidence. Int. J. Food. Sci. Nutr. 1, 1019–1029. doi:10.1080/09637486.2022.2123909
Chen, L., Heikkinen, L., Wang, C., Yang, Y., Sun, H., and Wong, G. (2019). Trends in the development of miRNA bioinformatics tools. Brief. Bioinform. 20, 1836–1852. doi:10.1093/bib/bby054
Chen, Y., Li, S., Guo, Y., Yu, H., Bao, Y., Xin, X., et al. (2020). Astaxanthin attenuates hypertensive vascular remodeling by protecting vascular Smooth muscle cells from oxidative stress-induced mitochondrial dysfunction. Oxid. Med. Cell. Longev. 2020, 4629189. doi:10.1155/2020/4629189
Chou, H, C., Chen, Y, W., Lee, T, R., Wu, F. S., Chan, H. T., Lyu, P. C., et al. (2010). Proteomics study of oxidative stress and src kinase inhibition in H9C2 cardiomyocytes: A cell model of heart ischemia-reperfusion injury and treatment. Free Radic. Biol. Med. 49 (1), 96–108. doi:10.1016/j.freeradbiomed.2010.04.001
Cui, G., Li, L., Xu, W., Wang, M., Jiao, D., Yao, B., et al. (2020). Astaxanthin protects ochratoxin A-induced oxidative stress and apoptosis in the heart via the Nrf2 pathway. Oxid. Med. Cell. Longev. 7639109, 7639109. doi:10.1155/2020/7639109
Dilli, D., Tasoglu, İ., Sarı, E., Akduman, H., Yumuşak, N., Tumer, N. B., et al. (2022). Therapeutic role of astaxanthin and resveratrol in an experimental rat model of supraceliac aortic ischemia-reperfusion. Am. J. Perinatol. doi:10.1055/s-0042-1748324
Du, H., Zhao, Y., Li, H., Wang, D. W., and Chen, C. (2021). Roles of MicroRNAs in glucose and lipid metabolism in the heart. Front. Cardiovasc. Med. 8, 716213. doi:10.3389/fcvm.2021.716213
Eren, B., Tuncay Tanrıverdi, S., Aydın Köse, F., and Özer, Ö. (2019). Antioxidant properties evaluation of topical astaxanthin formulations as anti-aging products. J. Cosmet. Dermatol. 18, 242–250. doi:10.1111/jocd.12665
Faraone, I., Sinisgalli, C., Ostuni, A., Armentano, M. F., Carmosino, M., Milella, L., et al. (2020). Astaxanthin anticancer effects are mediated through multiple molecular mechanisms: A systematic review. Pharmacol. Res. 155, 104689. doi:10.1016/j.phrs.2020.104689
Fassett, R. G., and Coombes, J. S. (2009). Astaxanthin, oxidative stress, inflammation and cardiovascular disease. Future Cardiol. 5, 333–342. doi:10.2217/fca.09.19
Fassett, R. G., and Coombes, J. S. (2011). Astaxanthin: A potential therapeutic agent in cardiovascular disease. Mar. Drugs. 9, 447–465. doi:10.3390/md9030447
Gai, Y. S., Ren, Y. H., Gao, Y., and Liu, H. N. (2020). Astaxanthin protecting myocardial cells from hypoxia/reoxygenation injury by regulating miR-138/HIF-1α axis. Eur. Rev. Med. Pharmacol. Sci. 24, 7722–7731. doi:10.26355/eurrev_202007_22276
Gong, C., Zhou, X., Lai, S., Wang, L., and Liu, J. (2020). Long noncoding RNA/circular RNA-miRNA-mRNA axes in ischemia-reperfusion injury. Biomed. Res. Int. 2020, 8838524. doi:10.1155/2020/8838524
Gross, G, J., and Lockwood, S, F. (2004). Cardioprotection and myocardial salvage by a disodium disuccinate astaxanthin derivative (Cardax). Life Sci. 75 (2), 215–224. doi:10.1016/j.lfs.2003.12.006
Han, H., Long, H., Wang, H., Wang, J., Zhang, Y., and Wang, Z. (2004). Progressive apoptotic cell death triggered by transient oxidative insult in H9c2 rat ventricular cells: A novel pattern of apoptosis and the mechanisms. Am. Physiol. Heart Circ. Physiol. 286, H2169–H2182. doi:10.1152/ajpheart.00199.2003
Honardoost, M., and Rad, S. (2018). Triangle of AKT2, miRNA, and tumorigenesis in different cancers. Appl. Biochem. Biotechnol. 185, 524–540. doi:10.1007/s12010-017-2657-3
Hu, B., Zhen, D., Bai, M., Xuan, T., Wang, Y., Liu, M., et al. (2022). Ethanol extracts of Rhaponticum uniflorum (L.) DC flowers attenuate doxorubicin-induced cardiotoxicity via alleviating apoptosis and regulating mitochondrial dynamics in H9c2 cells. J. Ethnopharmacol. 2022, 288. doi:10.1016/j.jep.2021.114936
Kohandel, Z., Farkhondeh, T., Aschner, M., and Samarghandian, S. (2021). Nrf2 a molecular therapeutic target for Astaxanthin. Biomed. Pharmacother. 137, 111374. doi:10.1016/j.biopha.2021.111374
Koo, K. H., and Kwon, H. (2018). MicroRNA miR-4779 suppresses tumor growth by inducing apoptosis and cell cycle arrest through direct targeting of PAK2 and CCND3. Cell. death. Dis. 9, 77. doi:10.1038/s41419-017-0100-x
Kuznetsov, A, V., Javadov, S., Sickinger, S., Frotschnig, S., and Grimm, M. (2015). H9c2 and HL-1 cells demonstrate distinct features of energy metabolism, mitochondrial function and sensitivity to hypoxia-reoxygenation. Biochim. Biophys. Acta 1853 (2), 276–284. doi:10.1016/j.bbamcr.2014.11.015
Lignitto, L., Leboeuf, S, E., Homer, H., Jiang, S., Askenazi, M., Karakousi, T. R., et al. (2019). Nrf2 activation promotes lung cancer metastasis by inhibiting the degradation of Bach1. Cell 178 (2), 316–329. doi:10.1016/j.cell.2019.06.003
Liu, X, J., Lv, Y, F., Cui, W, Z., Li, Y., Liu, Y., Xue, Y. T., et al. (2021). Icariin inhibits hypoxia/reoxygenation-induced ferroptosis of cardiomyocytes via regulation of the Nrf2/HO-1 signaling pathway. FEBS Open Bio 11 (11), 2966–2976. doi:10.1002/2211-5463.13276
Luo, C., Zhang, Y., Guo, H., Han, X., Ren, J., and Liu, J. (2020). Ferulic acid attenuates hypoxia/reoxygenation injury by suppressing mitophagy through the PINK1/parkin signaling pathway in H9c2 cells. Front. Pharmacol. 11 (103), 103. doi:10.3389/fphar.2020.00103
Ma, Z., Lan, Y, H., Liu, Z, W., Yang, M. X., Zhang, H., and Ren, J. Y. (2020). mir-19a suppress apoptosis of myocardial cells inratswithmyocardial ischemia/reperfusion through PTEN/AKT/P-AKT signaling pathway. Eur. Rev. Med. Pharmacol. Sci. 24 (6), 3322–3330. doi:10.26355/eurrev_202003_20700
Meng, X., Yin, J., Yu, X., and Guo, Y. (2020). MicroRNA-205-5p promotes unstable atherosclerotic plaque formation in vivo. Cardiovasc. Drugs. Ther. 34, 25–39. doi:10.1007/s10557-020-06935-9
Miao, X., Wu, X., and Shi, W. (2017). MicroRNA-346 regulates neural stem cell proliferation and differentiation by targeting KLF4. Am. J. Transl. Res. 9, 5400–5410.
Poller, W., Dimmeler, S., Heymans, S., Zeller, T., Haas, J., Karakas, M., et al. (2018). Non-coding RNAs in cardiovascular diseases: Diagnostic and therapeutic perspectives. Eur. Heart. J. 39, 2704–2716. doi:10.1093/eurheartj/ehx165
Pongkan, W., Takatori, O., Ni, Y., Xu, L., Nagata, N., Chattipakorn, S. C., et al. (2017). β-Cryptoxanthin exerts greater cardioprotective effects on cardiac ischemia-reperfusion injury than astaxanthin by attenuating mitochondrial dysfunction in mice. Mol. Nutr. Food Res. 61, 1601077. doi:10.1002/mnfr.201601077
Rasheed, Z., Rasheed, N., Abdulmonem, W. A., and Khan, M. I. (2019). Author correction: MicroRNA-125b-5p regulates IL-1β induced inflammatory genes via targeting TRAF6-mediated MAPKs and NF-κB signaling in human osteoarthritic chondrocytes. Sci. Rep. 9, 14729. doi:10.1038/s41598-019-50844-3
Shen, D. F., Qi, H. P., Ma, C., Chang, M. X., Zhang, W. N., and Song, R. R. (2021). Astaxanthin suppresses endoplasmic reticulum stress and protects against neuron damage in Parkinson's disease by regulating miR-7/SNCA axis. Neurosci. Res. 165, 51–60. doi:10.1016/j.neures.2020.04.003
Shengm, Z., Lu, W., Zuo, Z., Wang, D., Zuo, P., Yao, Y., et al. (2019). MicroRNA-7b attenuates ischemia/reperfusion-induced H9C2 cardiomyocyte apoptosis via the hypoxia inducible factor-1/p-p38 pathway. Cell Biochem. 120 (6), 9947–9955. doi:10.1002/jcb.28277
Shokri-Mashhadi, N., Tahmasebi, M., Mohammadi-Asl, J., Zakerkish, M., and Mohammadshahi, M. (2021). The antioxidant and anti-inflammatory effects of astaxanthin supplementation on the expression of miR-146a and miR-126 in patients with type 2 diabetes mellitus: A randomised, double-blind, placebo-controlled clinical trial. Int. J. Clin. Pract. 75, e14022. doi:10.1111/ijcp.14022
Sun, G. B., Sun, X., Wang, M., Ye, J. X., Si, J. Y., Xu, H. B., et al. (2012). Oxidative stress suppression by luteolin-induced heme oxygenase-1 expression. Toxicol. Appl. Pharmacol. 265 (2), 229–240. doi:10.1016/j.taap.2012.10.002
Sztretye, M., Dienes, B., Gönczi, M., Czirják, T., Csernoch, L., Dux, L., et al. (2019). Astaxanthin: A potential mitochondrial-targeted antioxidant treatment in diseases and with aging. Oxid. Med. Cell. Longev. 2019, 3849692. doi:10.1155/2019/3849692
Tiedt, S., Prestel, M., Malik, R., Schieferdecker, N., Duering, M., Kautzky, V., et al. (2017). RNA-seq identifies circulating miR-125a-5p, miR-125b-5p, and miR-143-3p as potential biomarkers for acute ischemic stroke. Circ. Res. 121, 970–980. doi:10.1161/CIRCRESAHA.117.311572
Upadhyay, S., Mantha, A, K., and Dhiman, M. (2020). Glycyrrhiza glabra (Licorice) root extract attenuates doxorubicin-induced cardiotoxicity via alleviating oxidative stress and stabilising the cardiac health in H9c2 cardiomyocytes. J. Ethnopharmacol. 258 (112690), 112690. doi:10.1016/j.jep.2020.112690
Wang, J. K., Wang, Z., and Li, G. (2019). MicroRNA-125 in immunity and cancer. Cancer. Lett. 454, 134–145. doi:10.1016/j.canlet.2019.04.015
Wang, W., Liu, T., Liu, Y., Yu, L., Yan, X., Weng, W., et al. (2021). Astaxanthin attenuates alcoholic cardiomyopathy via inhibition of endoplasmic reticulum stress-mediated cardiac apoptosis. Toxicol. Appl. Pharmacol. 412, 115378. doi:10.1016/j.taap.2020.115378
Wang, Y., Jian, Y., Zhang, X., Ni, B., Wang, M., and Pan, C. (2022). Melatonin protects H9c2 cardiomyoblasts from oxygen-glucose deprivation and reperfusion-induced injury by inhibiting Rac1/JNK/Foxo3a/Bim signaling pathway. Cell Biol. Int. 46 (3), 415–426. doi:10.1002/cbin.11739
Wang, Z., Yu, J., Wu, J., Qi, F., and Wang, H., (2016). Scutellarin protects cardiomyocyte ischemia-reperfusion injury by reducing apoptosis and oxidative stress. Life Sci. 157 (200-7), 200–207. doi:10.1016/j.lfs.2016.01.018
Wei, B., Li, W. W., Ji, J., Hu, Q. H., and Ji, H. (2014). The cardioprotective effect of sodium tanshinone IIA sulfonate and the optimizing of therapeutic time window in myocardial ischemia/reperfusion injury in rats. Atherosclerosis 235, 318–327. doi:10.1016/j.atherosclerosis.2014.05.924
Wei, M., Gan, L., Liu, Z., Kong, L. H., Chang, J. R., Chen, L. H., et al. (2017). MiR125b-5p protects endothelial cells from apoptosis under oxidative stress. Biomed. Pharmacother. 95, 453–460. doi:10.1016/j.biopha.2017.08.072
Wójcik, A. M., Nodine, M. D., and Gaj, M. D. (2017). miR160 and miR166/165 contribute to the LEC2-mediated auxin response involved in the somatic embryogenesis induction in arabidopsis. Front. Plant. Sci. 8, 2024. doi:10.3389/fpls.2017.02024
Wu, X., Zhao, X., and Miao, X. (2018). MicroRNA-374b promotes the proliferation and differentiation of neural stem cells through targeting Hes1. Biochem. Biophys. Res. Commun. 503, 593–599. doi:10.1016/j.bbrc.2018.06.044
Xue, Y., Sun, C., Hao, Q., and Cheng, J. (2019). Astaxanthin ameliorates cardiomyocyte apoptosis after coronary microembolization by inhibiting oxidative stress via Nrf2/HO-1 pathway in rats. Naunyn. Schmiedeb. Arch. Pharmacol. 392, 341–348. doi:10.1007/s00210-018-1595-0
Yaghooti, H., Mohammadtaghvaei, N., and Mahboobnia, K. (2019). Effects of palmitate and astaxanthin on cell viability and proinflammatory characteristics of mesenchymal stem cells. Int. Immunopharmacol. 68, 164–170. doi:10.1016/j.intimp.2018.12.063
Yin, Y., Xu, N., Shi, Y., Zhou, B., Sun, D., Ma, B., et al. (2021). Astaxanthin protects dendritic cells from lipopolysaccharide-induced immune dysfunction. Mar. Drugs. 19, 346. doi:10.3390/md19060346
Zhang, L., and Wang, H. (2015). Multiple mechanisms of anti-cancer effects exerted by astaxanthin. Mar. Drugs. 13, 4310–4330. doi:10.3390/md13074310
Zhang, X, S., Lu, Y., Li, W., Tao, T., Peng, L., Wang, W. H., et al. (2021). Astaxanthin ameliorates oxidative stress and neuronal apoptosis via SIRT1/NRF2/Prx2/ASK1/p38 after traumatic brain injury in mice. Br. J. Pharmacol. 178 (5), 1114–1132. doi:10.1111/bph.15346
Zhu, H., Shan, L., and Peng, T. (2009). Rac1 mediates sex difference in cardiac tumor necrosis factor-alpha expression via NADPH oxidase-ERK1/2/p38 MAPK pathway in endotoxemia. J. Mol. Cell Cardiol. 47 (2), 264–274. doi:10.1016/j.yjmcc.2009.05.002
Zhu, L. P., Tian, T., Wang, J. Y., He, J. N., Chen, T., Pan, M., et al. (2018). Hypoxia-elicited mesenchymal stem cell-derived exosomes facilitates cardiac repair through miR-125b-mediated prevention of cell death in myocardial infarction. Theranostics 8, 6163–6177. doi:10.7150/thno.28021
Zouein, F. A., Kurdi, M., and Booz, G. W. (2013). LIF and the heart: Just another brick in the wall? Eur. Cytokine. Netw. 24, 11–19. doi:10.1684/ecn.2013.0335
Zuluaga, M., Choudat, L., Aid-launais, R., Thibaudeau, O., Louedec, L., Letourneur, D., et al. (2019). Astaxanthin complexes to attenuate muscle damage after in vivo femoral ischemia-reperfusion. Mar. Drugs 17 (6), 354. doi:10.3390/md17060354
Keywords: astaxanthin, ischemia-reperfusion injury, microRNA, target genes, antioxidant activity
Citation: Zhang X, Xu M, Cai S, Chen B, Lin H and Liu Z (2023) Effects of astaxanthin on microRNA expression in a rat cardiomyocyte anoxia-reoxygenation model. Front. Pharmacol. 14:1103971. doi: 10.3389/fphar.2023.1103971
Received: 21 November 2022; Accepted: 23 January 2023;
Published: 03 February 2023.
Edited by:
Vittoria Di Mauro, Humanitas Research Hospital, ItalyReviewed by:
Chen Fleischmann, Hebrew University of Jerusalem, IsraelCopyright © 2023 Zhang, Xu, Cai, Chen, Lin and Liu. This is an open-access article distributed under the terms of the Creative Commons Attribution License (CC BY). The use, distribution or reproduction in other forums is permitted, provided the original author(s) and the copyright owner(s) are credited and that the original publication in this journal is cited, in accordance with accepted academic practice. No use, distribution or reproduction is permitted which does not comply with these terms.
*Correspondence: Zhiyu Liu, TGl1enlAZmFmdS5lZHUuY24=
†These authors have contributed equally to this work
Disclaimer: All claims expressed in this article are solely those of the authors and do not necessarily represent those of their affiliated organizations, or those of the publisher, the editors and the reviewers. Any product that may be evaluated in this article or claim that may be made by its manufacturer is not guaranteed or endorsed by the publisher.
Research integrity at Frontiers
Learn more about the work of our research integrity team to safeguard the quality of each article we publish.