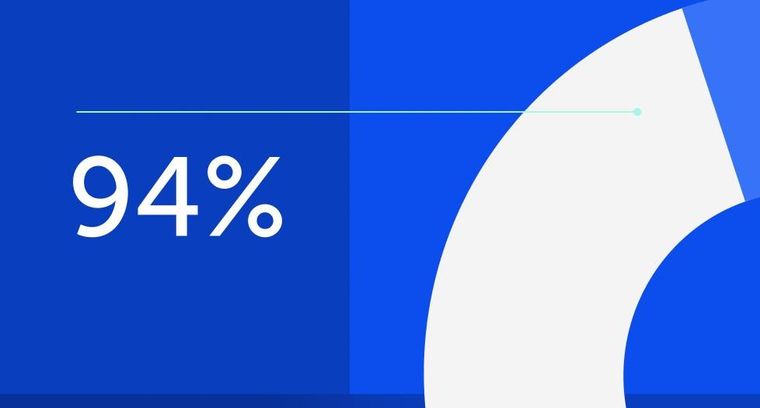
94% of researchers rate our articles as excellent or good
Learn more about the work of our research integrity team to safeguard the quality of each article we publish.
Find out more
REVIEW article
Front. Pharmacol., 15 March 2023
Sec. Pharmacology of Anti-Cancer Drugs
Volume 14 - 2023 | https://doi.org/10.3389/fphar.2023.1101320
This article is part of the Research TopicStimuli responsive nanocarriers: New insights and future perspectives in cancer managementView all 5 articles
Nanoconstructs are made up of nanoparticles and ligands, which can deliver the loaded cargo at the desired site of action. Various nanoparticulate platforms have been utilized for the preparation of nanoconstructs, which may serve both diagnostic as well as therapeutic purposes. Nanoconstructs are mostly used to overcome the limitations of cancer therapies, such as toxicity, nonspecific distribution of the drug, and uncontrolled release rate. The strategies employed during the design of nanoconstructs help improve the efficiency and specificity of loaded theranostic agents and make them a successful approach for cancer therapy. Nanoconstructs are designed with a sole purpose of targeting the requisite site, overcoming the barriers which hinders its right placement for desired benefit. Therefore, instead of classifying modes for delivery of nanoconstructs as actively or passively targeted systems, they are suitably classified as autonomous and nonautonomous types. At large, nanoconstructs offer numerous benefits, however they suffer from multiple challenges, too. Hence, to overcome such challenges computational modelling methods and artificial intelligence/machine learning processes are being explored. The current review provides an overview on attributes and applications offered by nanoconstructs as theranostic agent in cancer.
Cancer is one of the major causes of mortality globally, and if a unique and adaptable anticancer platform is not created, it may account for 70% of all deaths over the next 2 decades (Dai et al., 2017). As per CA: A Cancer Journal for Clinicians, approximately 19.3 million cancer patients were diagnosed, and approximately 10 million deaths were reported in 2020 (Sung et al., 2021). It is estimated that cancer incidences will worsen in the upcoming years by increasing the number of cancer-related deaths to 13.1 million by 2030 (Chaturvedi et al., 2019).
Nanotechnology contributed enormously in cancer therapy and opens unique paradigm to address issues with existing chemotherapeutic agents (Bae et al., 2011). Nanomedicines have vast applicability in the diagnosis, and treatment of disease. It is a painless therapy that improves human health and can be used as a molecular tool for specialized medical interventions at the molecular level. Nanosystems have been used in the last 10 years for a variety of purposes, including drug supply (Goldberg et al., 2007; Ferrari, 2010), tissue regeneration (Goldberg et al., 2007), detection at the molecular level, molecular imaging (Godin et al., 2011; Hu et al., 2011), and direct therapy of various cancers, such as melanoma, lung cancer, and breast cancer (Gazeau et al., 2008; Mamo et al., 2010).
Theranosis is an important tool in efforts to apply precision medicine in clinical practice, which uses imaging agents to target diseases at the molecular level while combining therapy and diagnostics. Cancer theranosis is proven to be incredibly helpful for both doctors and patients. The basic objective of nanotechnology is to enable nanoparticle-based agents to efficiently and selectively distribute payload, while avoiding toxic manifestation, as well as to reliably track noninvasively delivered therapeutic efficacy over time. The designed theranostic agents may be stimuli-responsive, targeted, or nontargeted. Targeted theranostic carriers incorporate a targeting ligand that can adhere to the receptors overexpressed in tumors. Currently, nanoconstructs are a promising approach to cancer treatment, and they are generated by combining nanoparticles (NPs) with ligands. They have a simple design, geometry, and stability, that can be delivered either actively or passively. Nanoconstructs overcome the drawbacks of conventional cancer therapies, such as toxicity and untoward biodistribution. However, they suffer from some known limitations, such as biocompatibility, uneven distribution, toxicity and lack of precision. To address these issues, the concept of autonomous and nonautonomous drug delivery was introduced in which the blood flow parameters and tumor microenvironment (TME)-related factors were taken into consideration. This review covers the fundamental aspects of the importance, effect, and potential of nanoconstructs while focusing on the most recent advancements of nanoconstructs in cancer diagnosis and therapy.
Cancer is a state in which a few cells of the body multiply uncontrollably and spread to other parts of the body. DNA damage, the major contributory factor for cancer, dysregulates various mechanisms that turn into specific malignant diseases. An unhealthy lifestyle and age has a significant influence on cancer occurrence (Wu et al., 2018; Quazi, 2022) Chikara et al. have reported that 19.3 million new cases of the cancer and more than 10 million deaths were reported in 2020, in worldwide (Chhikara and Parang, 2022). In the US, 1.9 million new cases and 609,360 deaths were reported in 2023 (Siegel et al., 2022).
Defective genetic changes or mutations in any of the listed genes, such as cytochrome P450 (CYP19, CYP2D6, CYP1A1), S-transferase (e.g., GSTM1, GSTP1), BRCA1/2, ATM, NBS1, PTEN, checkpoint kinase 2 (CHEK2), BRCA1 interacting protein (BRIP1), Nibrin (NBN), partner and localizer of BRCA2 (PALB2), RAD51C, RAD51D, malignant ovarian epithelial (MRE11A), Fanconi anemia, complementation group M (FANCM), tumor protein (p53), RAD50, BRCA1-associated ring domain (BARD1), alcohol, one-carbon metabolism genes (e.g., ADH1C, MTHFR), genes associated with DNA repair (XRCC1, XRCC3) and cell signaling receptors such as ER(Estrogen receptor), PR(Progesterone receptor), TNF-α(Tumor necrosis factor – α), may also contribute to the development of cancer (Easton et al., 2015; Walsh, 2015; Neidhardt et al., 2017). Furthermore, tumor heterogeneity, the TME, CSCs(Cancer stem cells), and epigenetics are factors responsible for the development of cancer, which may be accompanied by the development of chemotherapeutic resistance and recurrence associated with cancer (Jain et al., 2020).
The TME plays an important role in the development and poor prognosis of cancer (Baghban et al., 2020). The TME is highly heterogeneous in nature, and diverse cell populations make it more complex. The TME is highly associated with alteration or remodeling of the extracellular matrix (ECM), immune cells and other cellular processes in the development of drug resistance and relapse (Deepak et al., 2020). Tumor-associated markers such as tumor-infiltrating lymphocytes (TILs), tumor-associated macrophages (TAMs), cancer-associated fibroblasts (CAFs) and cancer-associated adipocytes (CAAs) are related to immune/tumor interactions, which contribute to the development of chemoresistance. Consistent tumor growth creates a hypoxic zone, acidic pH, and an anaerobic environment in the tumor mass (Lee and Griffiths, 2020). At the metastatic stage of cancer, chemotherapy is often not a promising therapy that will give a selective and broader response to patients (Jain et al., 2020).
Immunotherapeutic drugs have shown excellent growth by not only being the treatment for primary level cancer but also in avoiding metastasis and decreasing recurrence rates (Mahapatro and Singh, 2011). However, autoimmune problems are a main adverse effect of immune therapy. Furthermore, studies show that immune therapy is not as efficient for tumor cells as lymphoma (Kroemer and Zitvogel, 2018). A unique ECM is created by cancers that immune cells find difficult to infiltrate (Rosenberg et al., 2008). (Rosenberg et al., 2008). There are different types of immunotherapy for cancer, such as immune checkpoint inhibitors, T-cell transfer therapy, monoclonal antibodies, and vaccines. Immune checkpoints such as CLTA-4 and PD-L1 bind with other proteins of tumor cells and communicate with the immune system to allow for cancer development. Hence, various checkpoint inhibitors are used, such as PLGA-ICG-R837 for anti-CTLA4, and chimeric antigen receptor-mediated nanomedicines, which targets T cells (Cremolini et al., 2021).
‘Nanoconstructs’ are two-component structures made of a ‘hard’ nanoparticle core and a ‘soft’ shell of biomolecular ligands that are often used for targeted applications drug delivery (Dam et al., 2014). Nanosystems contain the active cargo and carrier for the delivery of drugs, which are preserved, transported over biological barriers, and then released as payloads (Allen and Cullis, 2004). The primary benefits of nanoparticle-based chemotherapy are the rise in treatment specificity, increasing drug deposition at the targeted region, and lowering peripheral toxicity (Schmidt, 1990). The nanoconstructs should be designed in such a way that they can retain a variety of agents on the surface or inside its core to form an ideal tool for combination therapy. After the administration of nanoconstructs, their ultimate fate depends not only on the surface chemistry but also on the whole vasculature, which includes pressure, velocities, and heterogeneities in tissues. Hence, to determine whether the nanoconstructs reached the walls of vessels or persisted in blood, a computational modeling technique was used based on 4S parameters (size, shape, surface and stiffness) (Başağaoğlu et al., 2013; Coclite et al., 2017). Therefore, computational methods are used for complex phenomena, and designing optimal nanoconstructs for biomedical applications. (Cervadoro et al., 2018).
Computational models can be used to understand the complex processes associated with the TME, which play a crucial role in designing nanoconstructs. It helps in reducing the time and cost of production and helps in deciding the optimized dose. These models can demonstrate the distribution of drugs or theranostic agents inside the tumor, rate of flow, unbinding and binding of drugs to cancer cells, permeability, etc (Hubbard et al., 2017). These models determine the heterogenicity of cancer, interactions between cells, and cell behavior. They convert the hypotheses into mathematical rules and help in running the simulation experiments that discover the behavior of these converted hypotheses. There are various computational models used for cancer, such as lattice-based methods, off lattice methods, and boundary tracking models (Metzcar et al., 2019).
Cell-based computational models, also known as individual-based models or discrete models, are currently very popular for cancer; they simulate the cells that interact with virtual tissues and observe their single-cell behaviour and role in controlling cancer. Mathematical modeling helps provide valuable information about the tumor growth mechanisms and angiogenic process (Zangooei et al., 2021). Hence, these models can be employed to understand the interaction between cancer and the therapeutic modality, which in turn will ease the design of optimal nanoconstructs for biomedical applications where experimental measurements are important (Cervadoro et al., 2018).
Nanoparticles (NPs) are known as particles with a diameter less than 1,000 nm and special characteristics that are often absent from major samples of similar types of material (Boisseau and Loubaton, 2011). All these can be categorized as 0D, 1D, 2D, or 3D depending on how the nanoparticle is shaped overall (Laurent et al., 2008). The layers of surface, shell, and core make up the primary components of NPs (Tiwari et al., 2012). They have become very popular in diverse disciplines because of their high surface to volume ratio, dissimilarity, submicron size, and better targeting abilities (Shin et al., 2016; Gavas et al., 2021).
Polymeric material-based nanoplatforms represent a class of widely used tuneable targeted delivery systems that can specifically deliver drug cargo to the desired site in a tumor. Nanoconstructs containing polymers such as poly(ε-caprolactone), (poly-l-lysine (PLL), poly(lactic-co-glycolic acid) (PLGA), and poly(propylene oxide) (PPO) are used for anticancer therapy. There are several advantages of using polymers, such as improved drug solubility, drug release, bioavailability, biodegradation, and reduced toxicity. Polymers for nanoconstructs are selected on the basis of target cancer cells, TME and toxicity status. Several lipid-based nanoplatforms are also being explored for the development of nanoconstructs (Alven and Aderibigbe, 2020).
In addition to organic material-based nanoconstructs, inorganic nanoconstructs based on iron oxide, gold, silver and other nonmetallic materials have been explored for their potential therapeutic benefits. Among them, black phosphorus(BP) has become very popular in two-dimensional nanomaterials because of its special characteristics and structure. Its special features, such as biocompatibility and thermal, optical, electrical, and drug-loading characteristics, have increased its demand compared to graphene-containing 2D nanomaterials.
Due to the puckered honeycomb structure of BP nanomaterials, where each phosphorus atom is sp3 hybridized with a tetrahedral configuration, they exhibit exceptional optoelectronic, thermal, and mechanical capabilities. This gives them the ability to act as photothermal agents, which cause the photothermal ablation of tumors by converting NIR irradiation to heat. Additionally, the energy produced by the excitation of BP NPs can be transmitted to ambient oxygen and result in the formation of reactive oxygen species (ROS), which are crucial for the photodynamic therapy of tumors. Apart from the aforementioned materials used in the development of nanoconstructs, other organic NPs, inorganic NPs, and hybrid NPs are often utilized in the preparation of nanoconstructs. Representative examples of NPs are shown in Figure 1, and the approved marketed formulations used in cancer therapy are compiled in Table 1.
FIGURE. 1. Nanotheranostics used in cancer therapy and imaging. Adapted from (Silva et al., 2019). © 2019 by the authors distributed under the terms and conditions of the Creative Commons Attribution (CC BY) license.
The development or engineering of a gene or drug release system with outstanding loading capacity to target tumors without affecting healthy cells is essential for effective cancer therapy. It is important to understand the targeting mechanisms and the cycle of interaction between cancer cells, NPs and tumors. Passive, active targeting, stimuli-responsive, and magnetic targeting are the few representative categories into which the targeting systems are divided.
The diffusion-mediated drug delivery method known as passive targeting includes the development of a complex of drug carriers. The complex of drug and carrier is transported to the target site via the bloodstream. Passive targeting nanoconstructs passively use the unique properties of solid tumors, such as a vasculature that is leaky and has reduced lymphatic clearance, to allow nanoconstructs to escape from the vasculature of the tumor and concentrate inside the tissues of the tumor via EPR (Black et al., 2014). For effective passive drug targeting, several characteristics, including molecular weight, surface charge, the surface’s nature, and its size, are important. For example, stealth liposomes coated with polyethylene glycol (PEG) will flow in the blood, and the surface charge on these liposomes has a major role in their longevity in blood circulation. PLGA-based NPs have the potential to load a high amount of drug and provide high specificity, stability, controlled drug release and a lower degradation rate (Rezvantalab et al., 2018). Polyvinyl alcohol (PVA) is also used for passive targeting, which helps in encapsulating hydrophobic drugs and their controlled release at specific tumor sites (Wei et al., 2020). The most often used method for the delivery of drugs in malignant cells is passive targeting, as shown in Figure 2.
FIGURE. 2. Passive and active targeting methods in drug delivery. Adapted from (Jain et al., 2020). © 2020 Elsevier B. V. All rights reserved.
Active targeting is dependent on particular ligands or molecules, which bind to receptors or molecules that are particularly overexpressed on the target cells (Goldberg et al., 2013). This method of targeting is also known as ligand-mediated targeting (Ko et al., 2013). Here, NPs that contain ligands with specific properties, such as uptake and retention, must be in the target’s proximity to increase affinity. In active targeting, the nanoconstructs are delivered into the TME, which results in minimal damage to healthy cells. In this approach, strong binding ligands were used that bind with target receptors to initiate ligand‒receptor complex formation (Kue et al., 2016). In Figure 2, receptor-mediated endocytosis is explained, in which receptors such as folate and transferrin are present on the surface of the cell and capture specific target molecules or ligands such as proteins, peptides, and sugars. In this process, macromolecules are transported from the extracellular fluid. This approach helps enhance changes in the binding of NPs to cancer cells, improving drug penetration (Chaturvedi et al., 2019).
Cancer is characterized by the unique TME and abnormal cells and their complex mechanism. As discussed, conventional therapies for cancer have limitations because of nonspecific targeting and biodistribution. The performance of nanoconstructs can be affected by external stimuli (light, magnetic field, etc.) and internal stimuli (pH, enzymes, oxidative stress, etc.). During internal stimuli-responsive targeting, compounds such as calcium carbonate and glutathione are used with nanocarriers for pH-responsiveness. The responsiveness to pH/H2O2 was imparted to human serum albumin (HSA)-coated manganese dioxide (MnO2) NPs through albumin-based biomineralization of Mn2+. Generally, transducers convert external stimuli such as magnetic fields or phototherapy to physical quantities such as light radiation, which can be further converted into heat for effective cell killing (Fang et al., 2021).
In one of the published reports, magnetic field was utilized for the targeted delivery of the drug (Alexiou et al., 2011). In this system, superparamagnetic NPs were coated with chemotherapeutic agents and administered under the influence of a strong magnetic field to target the tumor. As a result, targeted delivery of the drug occurs in the tumor region. This technique is currently a promising approach because of fewer side effects and high specificity (Alexiou et al., 2011).
Conventional chemotherapy without target selectivity usually produces significant side effects, reducing the effectiveness of a particular therapy. As a result, drug delivery methods need to ensure that they release drugs selectively and effectively up to the intracellular level at particular sites and result in low toxicity to maintain the quality of life of the patient. The appropriate choice of the ligands that may get adhered with particular receptors in cancer cells is one of the successful techniques for tumor-specific drug release. To design tumor-selective drug targeting systems, several ligands, such as hyaluronic acid, folic acid, peptides, and antibodies, have been widely utilized. To improve trafficking at the intracellular level for anticancer drugs, peptides or ligands that penetrate cells for tight junction valves are also used. In order to enhance the target selectivity and cellular uptake, different types of ligands are utilized in combination (Nguyen et al., 2021).
Delivering the therapeutic moiety with a specific ligand that has greater affinity to the pathological site is one of the best ways to enhance the effectiveness and safety of the drug. These types of systems offer advantages such as preventing toxicity to healthy cells and afford flexibility for optimizing the drug. Various ligands ranging from small molecules to aptamers and antibody fragments have been developed for this purpose. The application of ligand-targeted delivery systems extends to diagnosis as well. There are several factors that decide the selection of a targeting ligand. The ligand size that plays a role in pharmacokinetics of the drug and the ability of the ligand to bind to various receptor surfaces are crucial parameters. The binding affinity of the ligand influences the amount of the drug at the targeted site, while its chemistry can affect its binding capability with receptors. Immunogenicity, as in the cases of antibody‒drug conjugates, may affect the drug’s concentration and its half-life. Apart from this, selectivity of the target and other challenges, such as production cost and time, are the key criteria for ligand selection. Although there are techniques to overcome some challenges associated with ligands, for example, the stability can be increased by decorating the surfaces with PEG, these points should be keenly considered in the selection process of a ligand for achieving receptor-targeted drug delivery (Srinivasarao and Low, 2017).
Cancer therapy is a novel intervention that offers potential benefits to both physicians and patients. This system incorporates diagnostic capabilities aligned with targeted delivery for real-time monitoring of cancer therapy (Ryu et al., 2012). Cancer theranosis depends on the depiction of different phenotypes at the cellular level detected by theranosis agent at the targeted tumor location, allowing for cancer therapy and observation of the theranostic compounds (Table 2).
Nanotechnology has been identified as a possible option in cancer diagnosis and therapy. The majority of organic (polymer/lipids/dendrimers/liposomes, etc.) as well as inorganic NPs (metallic NPs/carbon nanotubes/quantum dots, etc.) imparted with imaging, treatment, and targeting abilities. The application of theranostic NPs in chemotherapy, photothermal therapy, siRNA/miRNA delivery, and other cancer treatments is currently the subject of substantial research.
Black Phosphorus became very popular in two-dimensional nanomaterials because of its special characteristics and structure. To enhance the stability of these types of nanoconstructs, heterogeneous doping is used. BP can be conjugated with various types of metals, polymers, folic acid, albumin, etc., and give theranostic effects in the biomedical field. Stimuli-responsive nanoconstructs can be developed to offer pH-mediated activation followed by NIR irradiation to offer stimuli-responsive BP-based anticancer therapy (Pandey et al., 2021). In one such application, the BP system has demonstrated its potential use in gene delivery. Mcl-1 belongs to Bcl-2 group and is a possible target for cancer therapy. Breast cancer cells also showed Mcl-1 amplification. BP nanomaterials conjugated with PLL for Cas13a/crRNA delivery were created to target Mcl-1 transcription. In vitro experiments on AGS cells revealed a 58.64% decrease in Mcl-1 expression as well as a suppression of cell activity (Schacter et al., 2014).
In addition to magnetic resonance imaging (MRI), optical imaging methods have been used for these nanoparticulate systems. Because of their bright fluorescence and great photostability, quantum dots have been frequently utilized in nanoparticulate systems to examine particle transport in vitro and in vivo (Bae et al., 2011).
Among the stimuli-responsive systems, the pH-responsive system carries a great advantage of modifying the delivery system as per the internal environment. One example is mesoporous silica nanoparticles (MSNs), which are novel pH-responsive drug delivery systems where the drug is attached to the surface via covalent bonds, which are pH-responsive linkages. These are a class of upconversion NPs that are a special type of optical nanomaterial doped with lanthanide ions and exhibit a wide range of electronic transitions in the 4f electron shell. These NPs have the ability to upconvert two or more photons of lower energy into one photon of higher energy. The nanoconstruct is prepared using copper ions and metal-phenolic networks of tannic acid on the surface of mesoporous silica-coated upconversion NPs. Real-time monitoring is possible using these systems with simultaneous drug release at the target site. Anticancer drugs have been incorporated into these systems; thus, this nanoparticulate form helps in carrying out efficient cancer therapy (Hu et al., 2019).
Moreover, for better cancer imaging and therapy, nanoconstructs are prepared with copper sulphide (CuS) NPs on the surface of mesoporous silica nanoshells containing porphyrin molecules and labelled with [89Zr]. These hybrid nanoconstructs were biocompatible, enhanced tumor deposition, and increased blood retention time. It can help in tetramodal imaging during cancer therapy with complete tumor elimination without any side effects. It is an efficient approach to combine imaging and therapeutic benefits in a single nanoconstruct (Goel et al., 2018).
Hyaluronic acid, as a targeting moiety for CD44 receptor-overexpressing cancer cells, works very well for controlling tumor growth and alterations. The trio-responsive chemo-phototherapy nanoconstruct includes various agents such as chemotherapeutic drug (DOX), duo-photothermal agents such as CuS and graphene oxide, and hyaluronic acid as the targeting moiety. The nanoconstruct shows proper drug release and increased photothermal properties with efficient ROS generation as the three major responses. High deposition and retention of nanoconstructs is seen in tumors, which is reflected by the photothermal and biodistribution profile. These types of nanoconstructs inhibit tumor growth by analyzing and identifying the tumor volume, apoptosis, and proliferation speed. Thus, these nanoconstructs show great potential in the translational cancer nanomedicine field (Poudel et al., 2020).
Targeted alpha therapy is another useful radiotherapeutic technique that can be used as a promising treatment against cancer. The main limitations of this technique are the conjugation of radionuclides with vectors and confinement of the dose. In this study, silica NPs were conjugated with transferrin and a chelator and loaded with 225Ac. The resultant nanoconstructs were highly and selectively cytotoxic and facilitated better excretion and minimal deposition in bones (Pallares et al., 2020).
Dam et al. (2014) reported an improvement in the in vitro efficiency of nanoconstructs containing gold by increasing the loading of G-quadruplex aptamers, which shows how citrate-type buffers at low pH can be used to load the outer surface of gold nanostars (AuNSs) with oligonucleotides and DNA aptamers (Dam et al., 2014).
Currently, polymeric nanoconstructs are being employed for cancer theranosis because of the flexibility associated with their surface modification, stimuli responsiveness and ability to incorporate hydrophilic as well as lipophilic bioactives or diagnostic agents. One such example is deformable discoidal nanoconstructs, which are used as a novel delivery approach for imaging and therapeutic purposes. These are made by the polymerization of PEG and PLGA in a discoidal shape. These polymer matrices include hydrophobic and hydrophilic microdomains that act as pockets for various imaging and therapeutic compounds. These particles reduce rapid sequestration from the Mononuclear phagocyte system (MPS) by circulating in the bloodstream for a longer duration. Additionally, these polymeric matrices can easily integrate contrast agents, lipid-drug conjugates, and polymer-drug conjugates, leading to the creation of true theranosis agents (Palange et al., 2017).
Currently, fluorescence resonance energy transfer (FRET) is a novel optical imaging modality that may be used to monitor drug release from NPs at the targeted tumor location (Caldorera-Moore et al., 2011). In this regard, doxorubicin(DOX)-loaded polyethylene glycol-block-peptide (FFKY)-block-tetraphenylethylene (PEG-Pep-TPE/DOX) NPs were developed and monitored for changes in FRET signals in A549 cells. Synergism was observed between DOX and the self-assembled peptide with real-time observation of drug release from the developed system (Wang et al., 2020).
In addition, PLGA-based nanoconstructs were explored to offer radiodynamic therapy. This anticancer therapy is based on the production of ROS at the tumor site. It mainly works on the hypoxia caused by the tumor, due to which a reduction in oxygen levels results in the production of ROS. There is one novel approach in which nanoconstructs are made of PLGA NPs that are loaded with verteporfin and perfluorooctylbromide. These nanoconstructs, when placed under normoxic and hypoxic conditions, show a sudden increase in ROS production. This therapy has killed ∼60% of pancreatic cancer cells in humans and suppressed the growth of tumors within 2 weeks. These success rates show that nanoconstructs based on radiodynamic therapy provide better and non-invasive treatment for deeply located hypoxic tumor (Clement et al., 2021).
Other polymeric nanoconstructs, such as polyurethanes (PU) nanoconstructs, are widely used for biomedical applications because they are part of the stimuli-responsive and biodegradable material class. PU nanoconstructs are simple delivery systems for drug and cancer treatment. These types of nanoconstructs have various types of properties, such as rapid drug release, the solubility of hydrophobic-type chemo drugs, targeting, improving efficiency, and stimuli sensitivity. They can be conjugated with ligands for active targeting. They are sensitive to pH, temperature, stimuli, and various external factors; hence, all these factors make them perfect nanocarriers (Gajbhiye et al., 2020).
Among lipid-based nanoconstructs, PEGylated squalene (SQ-PEG)-based nanoconstructs were also used for cancer therapy. They assemble with lipophilic pyropheophorbide-a (Ppa) and form nanoconstructs, with an average size of 200 nm and drug loading capacity of 18% (w/w). These nanoconstructs show 99.99% fluorescence quenching. Its bioavailability and phototoxicity can be identified through light eradication in vivo. These nanoconstructs show good diagnostic potential when tested in the chick embryo model implanted with U87MG glioblastoma microtumors (Adriouach et al., 2019).
Apart from polymer- and lipid-based nanoconstructs, dendrimer-based nanoconstructs offer multiple benefits as theranostic agents. Dendrimers made up of poly(amidoamine) (PAMAM) have been widely used for cancer nanomedicine applications and are a family of synthetic macromolecules that contain various types of functional groups and well-organized interior structures. First-generation dendrimers have one main limitation of their small size, which causes limited capacity for drug loading, and resist passive targeting for a tumor because of increased retention time and permeability. They are also not stimuli-responsive nanoconstructs, so to overcome all these limitations, superstructured dendrimeric nanoconstructs (SDNs) were generated.
PAMAM dendrimers are spherical, highly branching macromolecules that can stabilize metal NPs such as gold NPs while encapsulating active compounds. A study explored the theranosis potential of curcumin-loaded dendrimer-gold hybrid structures. By combining generation five poly(amidoamine) dendrimers with PEGylated amine-terminated AuCl4− ions, a dendrimer-gold hybrid structure was developed. The final hybrid system was loaded with curcumin attached to the MUC-1 aptamer. The results showed increased cellular cytotoxicity in HT29 and C26 cells in comparison to the nontargeted system and established potential in both cancer therapy and CT scan-based tumor imaging (Alibolandi et al., 2018).
Another study demonstrated the chemical production of unimolecular micelle-based hyperbranched PAMAM dendrimers conjugated with F3 peptide to target cellular nucleolin overexpressed in MDA-MB-231 cells. PAMAM micelles with F3 attachment (PAMAM-DOX-F3) demonstrated better uptake in MDA-MB-231 cells. For PET imaging, the 64Cu was chelated to micelles to monitor their pharmacokinetic behaviour. Serial PET imaging revealed that 64Cu-PAMAM-DOX-F3 accumulated in MDA-MB-231 tumors quickly, effectively, and persistently compared to 64Cu-PAMAM-DOX. The distribution characteristics in other organs and tissues were remarkably comparable (Yang et al., 2018).
The number of approved therapies that use the simultaneous intake of two or more pharmacological therapeutic compounds or a combination of different treatment approaches has progressively expanded (Gane et al., 2017). Often, even the most potent drug molecule may not be enough to completely cure the problem. Because of this, the modern administration of two or more therapeutic agents may aid in synergistically achieving more intracellular targets and destroying the targets more efficiently (Gee et al., 2005). One such example is the drug fumagillin, which was administered as a single injection of αVβ3 integrin-targeted paramagnetic NPs when given in combination with oral atorvastatin in experimental rabbits, where antiangiogenic activity was observed with a prolonged effect (Winter et al., 2008). In another study, protein-based hybrid NPs encapsulating docetaxel (DTX) and poly (sodium-4-styrenesulfonate)/DOX -modified gold nanorods were developed for combined plasmonic-based photothermal therapy (PPTT) and dual-chemotherapy. The release of DOX was controlled by NIR radiation, while DTX was released following diffusion. The cytotoxicity results in MDA-MB-231 cells demonstrated synergism between the two drugs followed by NIR irradiation (Villar-Alvarez et al., 2019). In one of the reports, a polyvalent theranostic nanocarrier was developed with a core made of superparamagnetic iron oxide nanoparticles (SPIONs) and a surface made of folic acid-polyamidoamine dendrimers (FA-PAMAM). To increase its solubility and evaluate its therapeutic potential, a very effective hydrophobic anticancer drug called 3,4-difluorobenzylidene-curcumin (CDF) was also coloaded in the FA-PAMAM dendrimer. Targeted NPs that were produced as a result (SPIONs@FA-PAMAM-CDF) have strong MR contrast and better anticancer activity on SKOV3 and HeLa cancer cells (Luong et al., 2017).
The Enhanced Permeability and Retention Effect (EPR) effect is a process that is explained by the hyperpermeation and extended retention of biomolecules encapsulated in nanocarriers, such as lipoproteins, hormones, and albumins, retained in solid tumors which contains leaky vasculature, elevated endothelial macromolecule transcytosis, and a lack of functional lymphatic drainage inside its interstitium (Figure 3).
FIGURE. 3. Challenges in the delivery of nanoconstructs. Adapted from (Saw et al., 2021) © 2021 Wiley-VCH GmbH distributed under the terms and conditions of the Creative Commons Attribution (CC BY) license.
To obtain EPR effect-based selective tumor deposition, low extravasation of normal-type tissue and renal clearance are needed. The nanoconstructs’ reduced particle size, which is lower than tumour vascular permeability, allowed them to easily pass through and produce the EPR effect (600–800 nm). However, the nanoconstructs have the bigger particle size than the pore of blood capillary (6–12 nm) and renal filtration (5–6 nm), influences the targeting of NPs (Choi et al., 2009).
Multiple tumors may develop tumor interstitium with different ECM (e.g., fibrin, fibronectin, hyaluronan collagen, and proteoglycans), tumor parenchyma and stroma cell compositions due to variations in the tumor type and stage. The variations in physical rigidity and pressure ranges may affect the distribution and transport of nanoconstructs in the tumor, which may further affect their therapeutic efficacy (Swartz and Lund, 2012; Perry et al., 2017). Hepatocellular carcinoma, Kaposi sarcoma, renal cell carcinoma, and cancer of the neck and head (Regev et al., 2005) are known to be high-level EPR tumors, whereas pancreatic ductal carcinoma and prostate cancer (Maeda, 2015) are referred to as low-EPR tumors.
There are other factors that hinder nanoconstructs distribution and deposition in tumors other than drawbacks of the EPR effect, such as biological and physical hurdles in delivering an adequate dosage of a therapeutic drug to the targeted site of the tumor. (Bae and Park, 2011). After intravenous delivery, nanoconstructs undergo multiple events, such as adsorption of proteins, diffusion of particles, aggregation, shear force destruction and hydrolysis. (Florence, 2012; Lazarovits et al., 2015). These types of events influence the number of nanoconstructs that eventually reach the tumor. The degree to which these events impact nanoconstructs tumor accumulation may vary depending on the physicochemical characteristics of the nanoconstructs (Gould et al., 2015). Another challenge is incorrect perceptions derived from the selection of in vivo models at the preclinical stage. In vivo studies performed on animal-derived cancer models do not show a resemblance to human cancers. The ratio of a person’s body weight to their tumor’s size is comparatively lower than those in animal models. As a result, it is not a surprise that the amount of nanoconstructs reaching the tumor sites in humans is probably less than the requisite therapeutic range.
Despite the issues outlined above, several ways to address these problems may facilitate nanoconstructs penetration and deposition into tumors. A generic classification adapted to facilitate drug release and enhance the effectiveness of nanoconstructs comprises autonomous and nonautonomous systems of drug delivery.
Nonautonomous cancer treatment was classified as actively and passively aimed nanoconstructs. The modification of TME with the help of NPs can be considered a promising approach for the management of cancer (Jia et al., 2021). It is an established fact that cancer cells survive in an oxygen-deficient environment known as a hypoxic environment. The integral switch to switch off mitochondrial energy production is independent of ATP and oxygen supply and switches on the alternate pathways to produce energy in oxygen-deficient environments. Thus, by stopping the cells from switching on the alternate pathway, cancer growth can be controlled. To make this possible, many NPs are coming into play. For example, the mitochondrial chaperone TRAP-1 is structurally and functionally similar to the Hsp90 family of proteins, which have the potential to switch on alternate pathways for energy production in tumor cells. Hence, nanocarriers to deliver TRAP-1 inhibitors were developed in which iron oxide nanoparticles (IONs) were conjugated to the Hsp90 inhibitor geldanamycin (GA) and the mitochondria localization signal (MLS) peptide to enable selective tumor targeting (Amash et al., 2020). One of the recent studies examined the impact of the surface curvature of nanoconstructs on endosomal pathways using CpG (cytosine-phosphate-guanine)-conjugated spiky and spherical gold NPs. Administration of spherical NPs followed by spiky NPs prompted the production of larger late-stage endosomes. The outcomes from this research demonstrate a possible impact of nanoconstructs design on intracellular fate (Lee et al., 2022).
The autonomous delivery systems are described as ‘therapeutic missiles’, as they can transport the nanoconstructs to the target regardless of the flow of blood and its direction. Recent advancements in autonomous-type delivery systems indicate that biomimetic-type delivery and biohybrid bacteria have the desired therapeutic potential (Yu et al., 2020). In one report, a nanomedicine-loaded bacterium that can propel itself was used to cure cancer. This autonomous swimmer destructs itself and delivers the anticancer medication at the targeted site (Figure 4). Incubation and electroporation were two methods that were utilized for introducing NPs into the bacteria, where incubation was found to be less effective than electroporation. More precisely, DOX-containing 100-nm liposomes were injected into motile bacteria (Salmonella) (Zoaby et al., 2017). When the bacteria enter cancer cells, the drug is internally released to kill the cancer cell. Salmonella was chosen over E. coli to build the nanoswimmer platform due to its higher velocity in the TME and its capacity to target tumors and penetrate triple-negative cancer cells. In general, the TME favours bacterial motility, which is dependent on glucose and pH (Pontier-Bres et al., 2012).
FIGURE. 4. Autonomous delivery systems. Adapted from (Saw et al., 2021) © 2021 Wiley-VCH GmbH distributed under the terms and conditions of the Creative Commons Attribution (CC BY) license.
The sciences of nanomedicine and artificial intelligence are two highly powerful tools for advancing the cause of personalized medicine. Drug synergy is a constant problem for cancer patients receiving any type of drug administration because it depends on time, dose, and patient at any point in the therapeutic process. Furthermore, due to significant intratumor and interpatient heterogeneities, it is difficult to rationally design diagnostic and therapeutic delivery systems and study their outcomes. Closing these gaps and improving the accuracy of diagnosis, drug delivery, and therapy requires the integration of artificial intelligence technologies (especially data mining, neural networks, and machine learning) (Soltani et al., 2021).
An innovative statistical method for developing controlled release drug delivery systems(CRDDS) is the artificial neural network (ANN). When there is no obvious functional dependence between the inputs and outputs, it is one of the best methods to be relied upon. ANNs can also be used to model complex biological data and nonlinear systems (Cheung and Rubin, 2021). Other uses for ANNs include cancer classification, predicting protein secondary structures, and solving multiresponse and multivariate system problems (Bi et al., 2019). The relationship between process variables, formulation, and CRDDS drug release profiles is not implicit or linear. As a result, related networks can be used in conjunction with other ANN model types. These ANN models can be used to depict the relationship between process variables, formulation, and response, such as in vitro drug release patterns (Patel and Patel, 2016). In comparison to conventional therapies, the application of AI (Artificial Intelligence) in the formulation of nanotheranosis can be a great benefit for better positioning of diagnostic and therapeutic substances into the body. If imaging agents and medications need to be loaded into a certain carrier, predictive AI algorithms can be utilized to forecast encapsulation efficiency (EE%) (Soltani et al., 2021). For instance, a QSPR model was used to predict, with greater than 90% accuracy, whether molecules may be loaded into the carrier based on the circumstances of the encapsulation process and their chemical makeup. A similar method can be employed to evaluate the cytotoxicity of other NPs and to assess the impact of surface modification on biocompatibility. When talking about medical imaging, it is important to consider how AI can contribute to image analysis. By using the aforementioned methods in imaging from nanos, one can better comprehend the therapeutic efficacies and particle biological dispersion patterns (Xu et al., 2019).
Currently, Nanorobotics is the emerging technology of constructing robots in the nanometric scale, which are creating a niche in the biomedical field (Raaja et al., 2016). In similar line, DNA based nanomaterials controlled by aptamer encoded logic gate can be utilized as autonomous delivery systems (Douglas et al., 2012). Since DNA is a natural substrate for computing, it has benefitted a diverse set of logic circuits and robotics (Katsnelson, 2012). In this context, DNA origami can be utilized to develop nanorobots which can intercommunicate and can be activated to release the drug cargo at the targeted site. This will allow the real time monitoring, faster delivery and computer assisted drug delivery (Khulbe, 2014; Devasena Umai et al., 2018).
There are multiple other examples in which DNA nanorobots can target HER2-positive breast cancer cells to induce apoptosis (Ma et al., 2019), deliver drug cargo to the cancer tissue mediated by nucleolin targeted therapy (Li et al., 2018), identification of cancer biomarkers (Mirzaiebadizi et al., 2022) and act as biosensors to allow the detection of target oligonucleotides and miRNAs (Domljanovic et al., 2022).
Conventional drug delivery systems for anticancer therapy frequently lack the ability to target medications to certain organs or tissues of interest (tumors), as well as the ability to track or image the in vivo fate and assess the effectiveness of drug administration. Imaging tumor regression in patients after targeted therapy X-ray, CT or radiography, will be considered a separate intervention altogether. Multiple objectives can be accomplished with a single dose by co-administering the image guided molecules incorporated in the delivery system. It may facilitate evaluation of extent of drug targeting, sites of localization, excretion, and imaging (Iyer et al., 2012).
In one of the reports, PSMA aptamer-conjugated DOX-loaded iron oxide NPs were developed that could be used as theranostic agents in prostate cancer. These systems detect prostate cancer sites (by MRI) and even deliver the drug DOX to the target tissue (Yu et al., 2011).
Treatments that comprise biocompatible NPs could provide precise optical/MR imaging and treatment to cells that overexpress folate receptors. The co-encapsulation of DTX and NIR dyes into NPs, which are novel additions to nano delivery systems for the detection, diagnosis, and treatment of malignancies, was also produced using a modified solvent diffusion approach (Santra et al., 2009).
In one such approach, light-activated theranostic NPs made up of PEG-modified polyacrylamide combined with iron oxide NPs and photofrin, a strong photosensitizer. were used for the imaging and photodynamic treatment of brain malignancies., F3 peptide was used as a ligand to specifically target nucleolin receptors present on endothelial cells and tumor cells. This system showed antitumor effects in a rat brain tumor model for brain targeting (Reddy et al., 2006).
Another method relies on bifluorescence resonance energy transfer (Bi-FRET) technology, which was applied to quantum dot (QD)-aptamer conjugates for simultaneous tumor imaging and DOX delivery. A functional RNA aptamer was attached to the surface of quantum dots and DOX was embedded in the aptamer., By triggering the fluorescence of QDs, this nanosystem was able to visualize drug delivery as well as to target prostate cancer cells (Bagalkot et al., 2007).
In continuation, pH-responsive polymeric micelles were developed with an aim of in vivo imaging and photodynamic therapy of tumors (Zhong et al., 2019), where, methoxy PEG was conjugated with a pH-sensitive polymer, poly (b-amino ester). Self-assembly of the block copolymer with protoporphyrin IX, a radiosensitizer, led to the formation of nanomicelles. pH-responsive release of protoporphyrin IX was observed in the acidic environment of tumors. Clear tumor accumulation and complete tumor ablation were observed by fluorescence imaging using tumor-bearing mouse models. Thus, these systems show great potential as photo dynamic theranosis (Koo et al., 2010).
Nanoconstructs designed for theranostic applications in cancer offer improved detection, precise delivery of drug cargo to tumors and fewer devastating effects on healthy organs. Additionally, imaging approaches can evaluate the effectiveness of medications in real time by using customized probes. Understanding the TME and cancer-associated events with the help of computational modeling will enable the development of nanoconstructs with desired attributes. A plethora of nanotechnology-based platforms have been explored for theranostic applications, including nanomaterials from organic and inorganic origins. Exogenous and endogenous stimuli play vital roles in controlling drug release and augmenting the autonomous and nonautonomous mechanisms of targetability. The full potential of DNA origami, nanorobots, artificial intelligence and machine learning has yet to be explored for the development of nanoconstructs for cancer theranosis. Although enormous progress has been made in the development of nanoconstructs for theranostic application, very few of them could advance to the clinical phase of investigation. However, the solution always lies in advanced nanomaterials, which will open up a new vista for the development of multimodal NPs equipped with better diagnostic and therapeutic capabilities. The desired outcomes through the advent of nanotechnology can only be facilitated by conducting multidisciplinary studies in a large cohort of patients.
SM: Writing; TB: Writing; HK: Writing, Re-writing and Editing; RJ: Re-writing and Editing; SS: Re-writing and Editing, VJ: Re-writing and Supervision.
The authors declare that the research was conducted in the absence of any commercial or financial relationships that could be construed as a potential conflict of interest.
All claims expressed in this article are solely those of the authors and do not necessarily represent those of their affiliated organizations, or those of the publisher, the editors and the reviewers. Any product that may be evaluated in this article, or claim that may be made by its manufacturer, is not guaranteed or endorsed by the publisher.
Adriouach, S., Vorobiev, V., Trefalt, G., Allémann, E., Lange, N., and Babič, A. (2019). Squalene-PEG: Pyropheophorbide-a nanoconstructs for tumor theranostics. Nanomed. Nanotechnol. Biol. Med. 15, 243–251. doi:10.1016/j.nano.2018.09.013
Akanda, M., Getti, G., Nandi, U., Mithu, M. S., and Douroumis, D. (2021). Bioconjugated solid lipid nanoparticles (SLNs) for targeted prostate cancer therapy. Int. J. Pharm. 599, 120416. doi:10.1016/j.ijpharm.2021.120416
Alexiou, C., Tietze, R., Schreiber, E., Jurgons, R., Richter, H., Trahms, L., et al. (2011). Cancer therapy with drug loaded magnetic nanoparticlesmagnetic drug targeting. J. Magn. Magn. Mater. 323, 1404–1407. doi:10.1016/j.jmmm.2010.11.059
Alibolandi, M., Hoseini, F., Mohammadi, M., Ramezani, P., Einafshar, E., Taghdisi, S. M., et al. (2018). Curcumin-entrapped MUC-1 aptamer targeted dendrimer-gold hybrid nanostructure as a theranostic system for colon adenocarcinoma. Int. J. Pharm. 549, 67–75. doi:10.1016/j.ijpharm.2018.07.052
Allen, T. M., and Cullis, P. R. (2004). Drug delivery systems: Entering the mainstream. Science 303, 1818–1822. doi:10.1126/science.1095833
Alven, S., and Aderibigbe, B. A. (2020). Efficacy of polymer-based nanocarriers for co-delivery of curcumin and selected anticancer drugs. Nanomaterials 10, 1556–1628. doi:10.3390/nano10081556
Amash, V., Paithankar, K., Dharaskar, S. P., Arunachalam, A., and Amere Subbarao, S. (2020). Development of nanocarrier-based mitochondrial chaperone, TRAP-1 inhibitor to combat cancer metabolism. ACS Appl. Bio Mater. 3, 4188–4197. doi:10.1021/acsabm.0c00268
Bae, Y. H., and Park, K. (2011). Targeted drug delivery to tumors: Myths, reality and possibility. J. Control. Release 153, 198–205. doi:10.1016/j.jconrel.2011.06.001
Bae, K. H., Chung, H. J., and Park, T. G. (2011). Nanomaterials for cancer therapy and imaging. Mol. Cells 31, 295–302. doi:10.1007/s10059-011-0051-5
Bagalkot, V., Zhang, L., Levy-Nissenbaum, E., Jon, S., Kantoff, P. W., Langery, R., et al. (2007). Quantum dot-aptamer conjugates for synchronous cancer imaging, therapy, and sensing of drug delivery based on Bi-fluorescence resonance energy transfer. Nano Lett. 7, 3065–3070. doi:10.1021/nl071546n
Baghban, R., Roshangar, L., Jahanban-Esfahlan, R., Seidi, K., Ebrahimi-Kalan, A., Jaymand, M., et al. (2020). Tumor microenvironment complexity and therapeutic implications at a glance. Cell Commun. Signal. 18, 59. doi:10.1186/s12964-020-0530-4
Başağaoğlu, H., Allwein, S., Succi, S., Dixon, H., Carrola, J. T., and Stothoff, S. (2013). Two- and three-dimensional lattice Boltzmann simulations of particle migration in microchannels. Microfluid. Nanofluid. 15, 785–796. doi:10.1007/s10404-013-1191-z
Basu, A., Upadhyay, P., Ghosh, A., Bose, A., Gupta, P., Chattopadhyay, S., et al. (2021). Hyaluronic acid engrafted metformin loaded graphene oxide nanoparticle as CD44 targeted anti-cancer therapy for triple negative breast cancer. Biochim. Biophys. Acta - Gen. Subj. 1865, 129841. doi:10.1016/j.bbagen.2020.129841
Bhagwat, G. S., Athawale, R. B., Gude, R. P., Md, S., Alhakamy, N. A., Fahmy, U. A., et al. (2020). Formulation and development of transferrin targeted solid lipid nanoparticles for breast cancer therapy. Front. Pharmacol. 11, 614290. doi:10.3389/fphar.2020.614290
Bi, W. L., Hosny, A., Schabath, M. B., Giger, M. L., Birkbak, N. J., Mehrtash, A., et al. (2019). Artificial intelligence in cancer imaging: Clinical challenges and applications. Ca. Cancer J. Clin. 69, 21552. doi:10.3322/caac.21552
Black, K. C. L., Wang, Y., Luehmann, H. P., Cai, X., Xing, W., Pang, B., et al. (2014). Radioactive 198Au-doped nanostructures with different shapes for in vivo analyses of their biodistribution, tumor uptake, and intratumoral distribution. ACS Nano 8, 4385–4394. doi:10.1021/nn406258m
Boisseau, P., and Loubaton, B. (2011). Nanomedicine, nanotechnology in medicine. Comptes Rendus Phys. 12, 620–636. doi:10.1016/j.crhy.2011.06.001
Caldorera-Moore, M. E., Liechty, W. B., and Peppas, N. A. (2011). Responsive theranostic systems: Integration of diagnostic imaging agents and responsive controlled release drug delivery carriers. Acc. Chem. Res. 44, 1061–1070. doi:10.1021/ar2001777
Cervadoro, A., Coclite, A., Di Mascolo, D., Ferreira, M., Palange, A. L., Palomba, R., et al. (2018). “Smart nanoconstructs for theranostics in cancer and cardiovascular diseases,” in Core-shell nanostructures drug delivery and theranostics challenges, strategies prospects for novel carrier. Systems, 297–321. doi:10.1016/C2016-0-03458-7
Charbe, N. B., Lagos, C. F., Ortiz, C. A. V., Tambuwala, M., Palakurthi, S. S., and Zacconi, F. C. (2022). PCSK9 conjugated liposomes for targeted delivery of paclitaxel to the cancer cell: A proof-of-concept study. Biomed. Pharmacother. 153, 113428. doi:10.1016/j.biopha.2022.113428
Chaturvedi, V. K., Singh, A., Singh, V. K., and Singh, M. P. (2019). Cancer nanotechnology: A new revolution for cancer diagnosis and therapy. Curr. Drug Metab. 20, 416–429. doi:10.2174/1389200219666180918111528
Cheung, H. M. C., and Rubin, D. (2021). Challenges and opportunities for artificial intelligence in oncological imaging. Clin. Radiol. 76, 728–736. doi:10.1016/j.crad.2021.03.009
Chhikara, B. S., and Parang, K. (2022). Global cancer statistics 2022: The trends projection analysis. Chem. Biol. Lett. 2023, 451. Available at: https://pubs.thesciencein.org/cbl.
Choi, H. S., Ipe, B. I., Misra, P., Lee, J. H., Bawendi, M. G., and Frangioni, J. V. (2009). Tissue- and organ-selective biodistribution of NIR fluorescent quantum dots. Nano Lett. 9, 2354–2359. doi:10.1021/nl900872r
Clement, S., Guller, A., Mahbub, S. B., and Goldys, E. M. (2021). Oxygen-carrying polymer nanoconstructs for radiodynamic therapy of deep hypoxic malignant tumors. Biomedicines 9, 322. doi:10.3390/biomedicines9030322
Coclite, A., Mollica, H., Ranaldo, S., Pascazio, G., de Tullio, M. D., and Decuzzi, P. (2017). Predicting different adhesive regimens of circulating particles at blood capillary walls. Microfluid. Nanofluid. 21, 168. doi:10.1007/s10404-017-2003-7
Cordeiro, R., Carvalho, A., Durães, L., and Faneca, H. (2022). Triantennary GalNAc-functionalized multi-responsive mesoporous silica nanoparticles for drug delivery targeted at asialoglycoprotein receptor. Int. J. Mol. Sci. 23, 6243. doi:10.3390/ijms23116243
Cremolini, C., Vitale, E., Rastaldo, R., and Giachino, C. (2021). Advanced nanotechnology for enhancing immune checkpoint blockade therapy. Nanomaterials 11, 661–726. doi:10.3390/nano11030661
Cycle, M.-C., Jose, S., Cinu, T. A., Sebastian, R., Shoja, M. H., Aleykutty, N. A., et al. (2019). Transferrin-conjugated docetaxel–PLGA nanoparticles for tumor targeting: Influence on MCF-7 cell cycle. Polym. (Basel). 11, 1–20. doi:10.3390/polym11111905
d’Avanzo, N., Torrieri, G., Figueiredo, P., Celia, C., Paolino, D., Correia, A., et al. (2021). LinTT1 peptide-functionalized liposomes for targeted breast cancer therapy. Int. J. Pharm. 597, 120346. doi:10.1016/j.ijpharm.2021.120346
Dai, W., Wang, X., Song, G., Liu, T., He, B., Zhang, H., et al. (2017). Combination antitumor therapy with targeted dual-nanomedicines. Adv. Drug Deliv. Rev. 115, 23–45. doi:10.1016/j.addr.2017.03.001
Dam, D. H. M., Lee, R. C., and Odom, T. W. (2014). Improved in vitro efficacy of gold nanoconstructs by increased loading of G-quadruplex aptamer. Nano Lett. 14, 2843–2848. doi:10.1021/nl500844m
Dash, B. S., Lu, Y. J., Chen, H. A., Chuang, C. C., and Chen, J. P. (2021). Magnetic and GRPR-targeted reduced graphene oxide/doxorubicin nanocomposite for dual-targeted chemo-photothermal cancer therapy. Mater. Sci. Eng. C 128, 112311. doi:10.1016/j.msec.2021.112311
De, K. (2021). Decapeptide modified doxorubicin loaded solid lipid nanoparticles as targeted drug delivery system against prostate cancer. Langmuir 37, 13194–13207. doi:10.1021/acs.langmuir.1c01370
Deepak, K. G. K., Vempati, R., Nagaraju, G. P., Dasari, V. R., Nagini, S., Rao, D. N., et al. (2020). Tumor microenvironment: Challenges and opportunities in targeting metastasis of triple negative breast cancer. Pharmacol. Res. 153, 104683. doi:10.1016/j.phrs.2020.104683
Deng, L., Xu, Y., Sun, C., Yun, B., Sun, Q., Zhao, C., et al. (2018). Functionalization of small black phosphorus nanoparticles for targeted imaging and photothermal therapy of cancer. Sci. Bull. 63, 917–924. doi:10.1016/j.scib.2018.05.022
Devasena Umai, R., Brindha Devi, P., and Thiruchelvi, R. (2018). A review on dna nanobots – a new technique for cancer treatment. Asian J. Pharm. Clin. Res. 11, 61–64. doi:10.22159/ajpcr.2018.v11i6.25015
Domljanovic, I., Loretan, M., Kempter, S., Acuna, G. P., Kocabey, S., and Ruegg, C. (2022). DNA origami book biosensor for multiplex detection of cancer-associated nucleic acids. Nanoscale 14, 15432–15441. doi:10.1039/d2nr03985k
Dong, Y., Fu, R., Yang, J., Ma, P., Liang, L., Mi, Y., et al. (2019). Folic acid-modified ginsenoside Rg5-loaded bovine serum albumin nanoparticles for targeted cancer therapy in vitro and in vivo. Int. J. Nanomed. 14, 6971–6988. doi:10.2147/IJN.S210882
Douglas, S. M., Bachelet, I., and Church, G. M. (2012). A logic-gated nanorobot for targeted transport of molecular payloads. Sci. (80- 335, 831–834. doi:10.1126/science.1214081
Duwa, R., Banstola, A., Emami, F., Jeong, J. H., Lee, S., and Yook, S. (2020). Cetuximab conjugated temozolomide-loaded poly (lactic-co-glycolic acid) nanoparticles for targeted nanomedicine in EGFR overexpressing cancer cells. J. Drug Deliv. Sci. Technol. 60, 101928. doi:10.1016/j.jddst.2020.101928
Easton, D. F., Pharoah, P. D. P., Antoniou, A. C., Tischkowitz, M., Tavtigian, S. V., Nathanson, K. L., et al. (2015). Gene-panel sequencing and the prediction of breast-cancer risk. N. Engl. J. Med. 372, 2243–2257. doi:10.1056/NEJMsr1501341
Edelman, R., Assaraf, Y. G., Levitzky, I., Shahar, T., and Livney, Y. D. (2017). Hyaluronic acid-serum albumin conjugate-based nanoparticles for targeted cancer therapy. Oncotarget 8, 24337–24353. doi:10.18632/oncotarget.15363
Fang, Z., Shen, Y., and Gao, D. (2021). Stimulus-responsive nanocarriers for targeted drug delivery. New J. Chem. 45, 4534–4544. doi:10.1039/d0nj05169a
Ferrari, M. (2010). Frontiers in cancer nanomedicine: Directing mass transport through biological barriers. Trends Biotechnol. 28, 181–188. doi:10.1016/j.tibtech.2009.12.007
Florence, A. T. (2012). Targeting” nanoparticles: The constraints of physical laws and physical barriers. J. Control. Release 164, 115–124. doi:10.1016/j.jconrel.2012.03.022
Gajbhiye, K. R., Chaudhari, B. P., Pokharkar, V. B., Pawar, A., and Gajbhiye, V. (2020). Stimuli-responsive biodegradable polyurethane nano-constructs as a potential triggered drug delivery vehicle for cancer therapy. Int. J. Pharm. 588, 119781. doi:10.1016/j.ijpharm.2020.119781
Gane, E. J., Hyland, R. H., Yang, Y., Svarovskaia, E., Stamm, L. M., Brainard, D. M., et al. (2017). Efficacy of ledipasvir plus sofosbuvir for 8 or 12 Weeks in patients with hepatitis C virus genotype 2 infection. Gastroenterology 152, 1366–1371. doi:10.1053/j.gastro.2017.01.017
Gavas, S., Quazi, S., and Karpiński, T. M. (2021). Nanoparticles for cancer therapy: Current progress and challenges. Nanoscale Res. Lett. 16, 173. doi:10.1186/s11671-021-03628-6
Gazeau, F., Lévy, M., and Wilhelm, C. (2008). Optimizing magnetic nanoparticle design for nanothermotherapy. Nanomedicine 3, 831–844. doi:10.2217/17435889.3.6.831
Gee, J. M. W., Howell, A., Gullick, W. J., Benz, C. C., Sutherland, R. L., Santen, R. J., et al. (2005). Consensus statement. Workshop on therapeutic resistance in breast cancer: Impact of growth factor signalling pathways and implications for future treatment. Endocr. Relat. Cancer 12, S1–S7. doi:10.1677/erc.1.01054
Godin, B., Tasciotti, E., Liu, X., Serda, R. E., and Ferrari, M. (2011). Multistage nanovectors: From concept to novel imaging contrast agents and therapeutics. Acc. Chem. Res. 44, 979–989. doi:10.1021/ar200077p
Goel, S., Ferreira, C. A., Chen, F., Ellison, P. A., Siamof, C. M., Barnhart, T. E., et al. (2018). Activatable hybrid nanotheranostics for tetramodal imaging and synergistic photothermal/photodynamic therapy. Adv. Mater. 30, 1704367. doi:10.1002/adma.201704367
Goldberg, M., Langer, R., and Jia, X. (2007). Nanostructured materials for applications in drug delivery and tissue engineering. J. Biomater. Sci. Polym. Ed. 18, 241–268. doi:10.1163/156856207779996931
Goldberg, M. S., Hook, S. S., Wang, A. Z., Bulte, J. W. M., Patri, A. K., Uckun, F. M., et al. (2013). Biotargeted nanomedicines for cancer: Six tenets before you begin. Nanomedicine 8, 299–308. doi:10.2217/nnm.13.3
Gould, S. E., Junttila, M. R., and de Sauvage, F. J. (2015). Translational value of mouse models in oncology drug development. Nat. Med. 21, 431–439. doi:10.1038/nm.3853
Handali, S., Moghimipour, E., Kouchak, M., Ramezani, Z., Amini, M., Angali, K. A., et al. (2019). New folate receptor targeted nano liposomes for delivery of 5-fluorouracil to cancer cells: Strong implication for enhanced potency and safety. Life Sci. 227, 39–50. doi:10.1016/j.lfs.2019.04.030
Hasannia, M., Lamei, K., Abnous, K., Taghdisi, S. M., Nekooei, S., Nekooei, N., et al. (2023). Targeted poly(L-glutamic acid)-based hybrid peptosomes co-loaded with doxorubicin and USPIONs as a theranostic platform for metastatic breast cancer. Nanomed. Nanotechnol. Biol. Med. 48, 102645. doi:10.1016/j.nano.2022.102645
Hu, Y., Fine, D. H., Tasciotti, E., Bouamrani, A., and Ferrari, M. (2011). Nanodevices in diagnostics. Wiley Interdiscip. Rev. Nanomed. Nanobiotechnol. 3, 11–32. doi:10.1002/wnan.82
Hu, C., Fan, F., Qin, Y., Huang, C., Zhang, Z., Guo, Q., et al. (2018). Redox-sensitive folate-conjugated polymeric nanoparticles for combined chemotherapy and photothermal therapy against breast cancer. J. Biomed. Nanotechnol. 14, 2018–2030. doi:10.1166/jbn.2018.2647
Hu, F., Liu, B., Chu, H., Liu, C., Li, Z., Chen, D., et al. (2019). Real-time monitoring of pH-responsive drug release using a metal-phenolic network-functionalized upconversion nanoconstruct. Nanoscale 11, 9201–9206. doi:10.1039/c9nr01892a
Hubbard, M. E., Jove, M., Loadman, P. M., Phillips, R. M., Twelves, C. J., and Smye, S. W. (2017). Drug delivery in a tumour cord model: A computational simulation. R. Soc. Open Sci. 4, 170014. doi:10.1098/rsos.170014
Iyer, A. K., He, J., and Amiji, M. M. (2012). Image-guided nanosystems for targeted delivery in cancer therapy. Curr. Med. Chem. 19, 3230–3240. doi:10.2174/092986712800784685
Jain, V., Kumar, H., Anod, H. V., Chand, P., Gupta, N. V., Dey, S., et al. (2020). A review of nanotechnology-based approaches for breast cancer and triple-negative breast cancer. J. Control. Release 326, 628–647. doi:10.1016/j.jconrel.2020.07.003
Jain, P., Pandey, V., and Soni, V. (2022). Bioconjugate-loaded solid lipid nanoparticles for enhanced anticancer drug delivery to brain cancer cells: An in vitro evaluation. Indian J. Med. Res. 0, 139–148. doi:10.4103/ijmr.ijmr_514_19
Jani, P., Suman, S., Subramanian, S., Korde, A., Gohel, D., Singh, R., et al. (2021). Development of mitochondrial targeted theranostic nanocarriers for treatment of gliomas. J. Drug Deliv. Sci. Technol. 64, 102648. doi:10.1016/j.jddst.2021.102648
Jia, M., Zhang, D., Zhang, C., and Li, C. (2021). Nanoparticle-based delivery systems modulate the tumor microenvironment in pancreatic cancer for enhanced therapy. J. Nanobiotechnol. 19, 384. doi:10.1186/s12951-021-01134-6
Kang, S. J., Jeong, H. Y., Kim, M. W., Jeong, I. H., Choi, M. J., You, Y. M., et al. (2018). Anti-EGFR lipid micellar nanoparticles co-encapsulating quantum dots and paclitaxel for tumor-targeted theranosis. Nanoscale 10, 19338–19350. doi:10.1039/c8nr05099f
Khulbe, P. (2014). Nanorobots: A review. Int. J. Pharm. Sci. Res. 5, 2164. Available at:. doi:10.13040/IJPSR.0975-8232.5
Kim, M. W., Jeong, H. Y., Kang, S. J., Choi, M. J., You, Y. M., Im, C. S., et al. (2017). Cancer-targeted nucleic acid delivery and quantum dot imaging using EGF receptor aptamer-conjugated lipid nanoparticles. Sci. Rep. 7, 9474. doi:10.1038/s41598-017-09555-w
Ko, A. H., Tempero, M. A., Shan, Y. S., Su, W. C., Lin, Y. L., Dito, E., et al. (2013). A multinational phase 2 study of nanoliposomal irinotecan sucrosofate (PEP02, MM-398) for patients with gemcitabine-refractory metastatic pancreatic cancer. Br. J. Cancer 109, 920–925. doi:10.1038/bjc.2013.408
Koo, H., Lee, H., Lee, S., Min, K. H., Kim, M. S., Lee, D. S., et al. (2010). In vivo tumor diagnosis and photodynamic therapy via tumoral pH-responsive polymeric micelles. Chem. Commun. 46, 5668–5670. doi:10.1039/c0cc01413c
Kroemer, G., and Zitvogel, L. (2018). Cancer immunotherapy in 2017: The breakthrough of the microbiota. Nat. Rev. Immunol. 18, 87–88. doi:10.1038/nri.2018.4
Kue, C. S., Kamkaew, A., Burgess, K., Kiew, L. V., Chung, L. Y., and Lee, H. B. (2016). Small molecules for active targeting in cancer. Med. Res. Rev. 36, 494–575. doi:10.1002/med.21387
Kundu, B. K., PragtiCarlton Ranjith, W. A., Shankar, U., Kannan, R. R., Mobin, S. M., Bandyopadhyay, A., et al. (2022). Cancer-targeted chitosan-biotin-conjugated mesoporous silica nanoparticles as carriers of zinc complexes to achieve enhanced chemotherapy in vitro and in vivo. ACS Appl. Bio Mater. 5, 190–204. doi:10.1021/acsabm.1c01041
Kuo, Y.-C., and Chen, Y.-C. (2015). Targeting delivery of etoposide to inhibit the growth of human glioblastoma multiforme using lactoferrin-and folic acid-grafted poly (lactide-co-glycolide) nanoparticles. Int. J. Pharm. 479, 138–149. doi:10.1016/j.ijpharm.2014.12.070
Laurent, S., Forge, D., Port, M., Roch, A., Robic, C., Vander Elst, L., et al. (2008). Magnetic iron oxide nanoparticles: Synthesis, stabilization, vectorization, physicochemical characterizations and biological applications. Chem. Rev. 108, 2064–2110. doi:10.1021/cr068445e
Lazarovits, J., Chen, Y. Y., Sykes, E. A., and Chan, W. C. W. (2015). Nanoparticle–blood interactions: The implications on solid tumour targeting. Chem. Commun. 51, 2756–2767. doi:10.1039/C4CC07644C
Lee, S. H., and Griffiths, J. R. (2020). How and why are cancers acidic? Carbonic anhydrase ix and the homeostatic control of tumour extracellular ph. Cancers (Basel) 12, 1616–1623. doi:10.3390/cancers12061616
Lee, K., Jung, I., and Odom, T. W. (2022). Delivery order of nanoconstructs affects intracellular trafficking by endosomes. J. Am. Chem. Soc. 144, 5274–5279. doi:10.1021/jacs.2c02276
Li, J., Yang, X., Yang, P., and Gao, F. (2017). Hyaluronic acid–conjugated silica nanoparticles for breast cancer therapy. Inorg. Nano-Metal Chem. 47, 777–782. doi:10.1080/15533174.2016.1218509
Li, S., Jiang, Q., Liu, S., Zhang, Y., Tian, Y., Song, C., et al. (2018). A DNA nanorobot functions as a cancer therapeutic in response to a molecular trigger in vivo. Nat. Biotechnol. 36, 258–264. doi:10.1038/nbt.4071
Li, L., He, S., Yu, L., Elshazly, E. H., Wang, H., Chen, K., et al. (2019). Codelivery of DOX and siRNA by folate-biotin-quaternized starch nanoparticles for promoting synergistic suppression of human lung cancer cells. Drug Deliv. 26, 499–508. doi:10.1080/10717544.2019.1606363
Li, R., Gao, R., Wang, Y., Liu, Z., Xu, H., Duan, A., et al. (2020). Gastrin releasing peptide receptor targeted nano-graphene oxide for near-infrared fluorescence imaging of oral squamous cell carcinoma. Sci. Rep. 10, 11434. doi:10.1038/s41598-020-68203-y
Li, G., Zhang, X., Fei, X., Li, J., Liu, H., Liu, W., et al. (2022). Chiral FA conjugated CdTe/CdS quantum dots for selective cancer ablation. ACS Nano 16, 12991–13001. doi:10.1021/acsnano.2c05517
Luong, D., Sau, S., Kesharwani, P., and Iyer, A. K. (2017). Polyvalent folate-dendrimer-coated iron oxide theranostic nanoparticles for simultaneous magnetic resonance imaging and precise cancer cell targeting. Biomacromolecules 18, 1197–1209. doi:10.1021/acs.biomac.6b01885
Ma, W., Zhan, Y., Zhang, Y., Shao, X., Xie, X., Mao, C., et al. (2019). An Intelligent DNA Nanorobot with in vitro enhanced protein lysosomal degradation of HER2. Nano Lett. 19, 4505–4517. doi:10.1021/acs.nanolett.9b01320
Maeda, H. (2015). Toward a full understanding of the EPR effect in primary and metastatic tumors as well as issues related to its heterogeneity. Adv. Drug Deliv. Rev. 91, 3–6. doi:10.1016/j.addr.2015.01.002
Mahapatro, A., and Singh, D. K. (2011). Biodegradable nanoparticles are excellent vehicle for site directed in-vivo delivery of drugs and vaccines. J. Nanobiotechnol. 9, 55. doi:10.1186/1477-3155-9-55
Mamo, T., Moseman, E. A., Kolishetti, N., Salvador-Morales, C., Shi, J., Kuritzkes, D. R., et al. (2010). Emerging nanotechnology approaches for HIV/AIDS treatment and prevention. Nanomedicine 5, 269–285. doi:10.2217/nnm.10.1
Marshall, S., Panrak, Y., Makchuchit, N., Jaroenpakdee, P., Saelim, B., Taweesap, M., et al. (2022). Anti-EpCAM and anti-EGFR targeted theranostic functionalized I-131 labeled poly(lactic-co-glycolic acid) (PLGA) radio-nanotherapeutic for cancer treatment. J. Med. Imaging Radiat. Sci. 53, S33. doi:10.1016/j.jmir.2022.10.108
Mbatha, L. S., Maiyo, F. C., and Singh, M. (2019). Dendrimer functionalized folate-targeted gold nanoparticles for luciferase gene silencing in vitro: A proof of principle study. Acta Pharm. 69, 49–61. doi:10.2478/acph-2019-0008
Mbatha, L. S., Maiyo, F., Daniels, A., and Singh, M. (2021). Dendrimer-coated gold nanoparticles for efficient folate-targeted mRNA delivery in vitro. Pharmaceutics 13, 900. doi:10.3390/pharmaceutics13060900
Metzcar, J., Wang, Y., Heiland, R., and Macklin, P. (2019). A review of cell-based computational modeling in cancer biology. JCO Clin. cancer Inf. 3, 1–13. doi:10.1200/CCI.18.00069
Mirzaiebadizi, A., Ravan, H., Dabiri, S., Mohammadi, P., Shahba, A., Ziasistani, M., et al. (2022). An intelligent DNA nanorobot for detection of MiRNAs cancer biomarkers using molecular programming to fabricate a logic-responsive hybrid nanostructure. Bioprocess Biosyst. Eng. 45, 1781–1797. doi:10.1007/s00449-022-02785-x
Mosafer, J., Abnous, K., Tafaghodi, M., Mokhtarzadeh, A., and Ramezani, M. (2017). In vitro and in vivo evaluation of anti-nucleolin-targeted magnetic PLGA nanoparticles loaded with doxorubicin as a theranostic agent for enhanced targeted cancer imaging and therapy. Eur. J. Pharm. Biopharm. 113, 60–74. doi:10.1016/j.ejpb.2016.12.009
Neidhardt, G., Hauke, J., Ramser, J., Groß, E., Gehrig, A., Müller, C. R., et al. (2017). Association between loss-of-function mutations within the FANCM gene and early-onset familial breast cancer. JAMA Oncol. 3, 1245–1248. doi:10.1001/jamaoncol.2016.5592
Nguyen, P. V., Allard-Vannier, E., Chourpa, I., and Hervé-Aubert, K. (2021). Nanomedicines functionalized with anti-EGFR ligands for active targeting in cancer therapy: Biological strategy, design and quality control. Int. J. Pharm. 605, 120795. doi:10.1016/j.ijpharm.2021.120795
Omurtag Ozgen, P. S., Atasoy, S., Zengin Kurt, B., Durmus, Z., Yigit, G., and Dag, A. (2020). Glycopolymer decorated multiwalled carbon nanotubes for dual targeted breast cancer therapy. J. Mater. Chem. B 8, 3123–3137. doi:10.1039/c9tb02711d
Palange, A. L., Palomba, R., Rizzuti, I. F., Ferreira, M., and Decuzzi, P. (2017). Deformable discoidal polymeric nanoconstructs for the precise delivery of therapeutic and imaging agents. Mol. Ther. 25, 1514–1521. doi:10.1016/j.ymthe.2017.02.012
Palanikumar, L., Kim, J., Oh, J. Y., Choi, H., Park, M. H., Kim, C., et al. (2018). Hyaluronic acid-modified polymeric gatekeepers on biodegradable mesoporous silica nanoparticles for targeted cancer therapy. ACS Biomater. Sci. Eng. 4, 1716–1722. doi:10.1021/acsbiomaterials.8b00218
Pallares, R. M., Agbo, P., Liu, X., An, D. D., Gauny, S. S., Zeltmann, S. E., et al. (2020). Engineering mesoporous silica nanoparticles for targeted alpha therapy against breast cancer. ACS Appl. Mater. Interfaces 12, 40078–40084. doi:10.1021/acsami.0c11051
Palombarini, F., Masciarelli, S., Incocciati, A., Liccardo, F., Di Fabio, E., Iazzetti, A., et al. (2021). Self-assembling ferritin-dendrimer nanoparticles for targeted delivery of nucleic acids to myeloid leukemia cells. J. Nanobiotechnol. 19, 172. doi:10.1186/s12951-021-00921-5
Pandey, A., Nikam, A. N., Padya, B. S., Kulkarni, S., Fernandes, G., Shreya, A. B., et al. (2021). Surface architectured black phosphorous nanoconstructs based smart and versatile platform for cancer theranostics. Coord. Chem. Rev. 435, 213826. doi:10.1016/j.ccr.2021.213826
Patel, J., and Patel, A. (2016). “Artificial neural networking in controlled drug delivery,” in Artificial neural network for drug design, delivery and disposition (Elsevier), 195–218. doi:10.1016/B978-0-12-801559-9.00010-7
Patel, J., Amrutiya, J., Bhatt, P., Javia, A., Jain, M., and Misra, A. (2018a). Targeted delivery of monoclonal antibody conjugated docetaxel loaded PLGA nanoparticles into EGFR overexpressed lung tumour cells. J. Microencapsul. 35, 204–217. doi:10.1080/02652048.2018.1453560
Patel, N. R., Piroyan, A., Ganta, S., Morse, A. B., Candiloro, K. M., Solon, A. L., et al. (2018b). In vitro and in Vivo evaluation of a novel folate-targeted theranostic nanoemulsion of docetaxel for imaging and improved anticancer activity against ovarian cancers. Cancer Biol. Ther. 19, 554–564. doi:10.1080/15384047.2017.1395118
Peng, F., Zhao, F., Shan, L., Li, R., Jiang, S., and Zhang, P. (2021). Black phosphorus nanosheets-based platform for targeted chemo-photothermal synergistic cancer therapy. Colloids Surfaces B Biointerfaces 198, 111467. doi:10.1016/j.colsurfb.2020.111467
Perry, J. L., Reuter, K. G., Luft, J. C., Pecot, C. V., Zamboni, W., and DeSimone, J. M. (2017). Mediating passive tumor accumulation through particle size, tumor type, and location. Nano Lett. 17, 2879–2886. doi:10.1021/acs.nanolett.7b00021
Pillay, N. S., Daniels, A., and Singh, M. (2020). Folate-targeted transgenic activity of dendrimer functionalized selenium nanoparticles in vitro. Int. J. Mol. Sci. 21, 7177–7217. doi:10.3390/ijms21197177
Pontier-Bres, R., Prodon, F., Munro, P., Rampal, P., Lemichez, E., Peyron, J. F., et al. (2012). Modification of Salmonella typhimurium motility by the probiotic yeast strain Saccharomyces boulardii. PLoS One 7, e33796. doi:10.1371/journal.pone.0033796
Poudel, K., Banstola, A., Tran, T. H., Thapa, R. K., Gautam, M., Ou, W., et al. (2020). Hyaluronic acid wreathed, trio-stimuli receptive and on-demand triggerable nanoconstruct for anchored combinatorial cancer therapy. Carbohydr. Polym. 249, 116815. doi:10.1016/j.carbpol.2020.116815
Prajapati, S. K., Jain, A., Shrivastava, C., and Jain, A. K. (2019). Hyaluronic acid conjugated multi-walled carbon nanotubes for colon cancer targeting. Int. J. Biol. Macromol. 123, 691–703. doi:10.1016/j.ijbiomac.2018.11.116
Pramanik, A., Xu, Z., Ingram, N., Coletta, P. L., Millner, P. A., Tyler, A. I. I., et al. (2022). Hyaluronic-acid-tagged cubosomes deliver cytotoxics specifically to CD44-positive cancer cells. Mol. Pharm. 19, 4601–4611. doi:10.1021/acs.molpharmaceut.2c00439
Quazi, S. (2022). Telomerase gene therapy: A remission toward cancer. Med. Oncol. 39, 105. doi:10.1007/s12032-022-01702-2
Raaja, D. K., Ajay, V., Jayadev, S. G., Kumar, M., Karthikeyan, N. S., Ravichandran, C., et al. (2016). A mini review on nanobots in human surgery and cancer therapy. 222–226.
Reddy, G. R., Bhojani, M. S., McConville, P., Moody, J., Moffat, B. A., Hall, D. E., et al. (2006). Vascular targeted nanoparticles for imaging and treatment of brain tumors. Clin. Cancer Res. 12, 6677–6686. doi:10.1158/1078-0432.CCR-06-0946
Regev, R., Yeheskely-hayon, D., Katzir, H., and Eytan, G. D. (2005). Transport of anthracyclines and mitoxantrone across membranes by a flip-flop mechanism. Biochem. Pharmacol. 70, 161–169. doi:10.1016/j.bcp.2005.03.032
Rezvantalab, S., Drude, N. I., Moraveji, M. K., Güvener, N., Koons, E. K., Shi, Y., et al. (2018). PLGA-based nanoparticles in cancer treatment. Front. Pharmacol. 9, 1260. doi:10.3389/fphar.2018.01260
Rosenberg, S. A., Restifo, N. P., Yang, J. C., Morgan, R. A., and Dudley, M. E. (2008). Adoptive cell transfer: A clinical path to effective cancer immunotherapy. Nat. Rev. Cancer 8, 299–308. doi:10.1038/nrc2355
Ryu, J. H., Koo, H., Sun, I. C., Yuk, S. H., Choi, K., Kim, K., et al. (2012). Tumor-targeting multi-functional nanoparticles for theragnosis: New paradigm for cancer therapy. Adv. Drug Deliv. Rev. 64, 1447–1458. doi:10.1016/j.addr.2012.06.012
Santra, S., Kaittanis, C., Grimm, J., and Perez, J. M. (2009). Drug/dye-loaded, multifunctional iron oxide nanoparticles for combined targeted cancer therapy and dual optical/magnetic resonance imaging. Small 5, 1862–1868. doi:10.1002/smll.200900389
Saw, W. S., Anasamy, T., Foo, Y. Y., Kwa, Y. C., Kue, C. S., Yeong, C. H., et al. (2021). Delivery of nanoconstructs in cancer therapy: Challenges and therapeutic opportunities. Adv. Ther. 4, 2000206. doi:10.1002/adtp.202000206
Schacter, J. L., Henson, E. S., and Gibson, S. B. (2014). Estrogen regulation of anti-apoptotic Bcl-2 family member Mcl-1 expression in breast cancer cells. PLoS One 9, e100364. doi:10.1371/journal.pone.0100364
Schmidt, P. C. (1990). Encyclopedia of pharmaceutical technology. Editors J. Swarbrick, J. C. Boylan, and M. Dekker (New York: Inc.), 1, 494. hardcov. Price: see review text. Pharm. Unserer Zeit. doi:10.1002/pauz.19900190309
Scialla, S., Hanafy, M. S., Wang, J.-L., Genicio, N., Costa Da Silva, M., Costa, M., et al. (2023). Targeted treatment of triple-negative-breast cancer through pH-triggered tumour associated macrophages using smart theranostic nanoformulations. Int. J. Pharm. 632, 122575. doi:10.1016/j.ijpharm.2022.122575
Shin, W.-K., Cho, J., Kannan, A. G., Lee, Y.-S., and Kim, D.-W. (2016). Cross-linked composite gel polymer electrolyte using mesoporous methacrylate-functionalized SiO2 nanoparticles for lithium-ion polymer batteries. Sci. Rep. 6, 26332. doi:10.1038/srep26332
Siegel, R. L., Miller, K. D., Fuchs, H. E., and Jemal, A. (2022). Cancer statistics, 2022. Ca. Cancer J. Clin. 72, 7–33. doi:10.3322/caac.21708
Silva, C. O., Pinho, J. O., Lopes, J. M., Almeida, A. J., Gaspar, M. M., and Reis, C. (2019). Current trends in cancer nanotheranostics: Metallic, polymeric, and lipid-based systems. Pharmaceutics 11, 22. doi:10.3390/pharmaceutics11010022
Soltani, M., Moradi Kashkooli, F., Souri, M., Zare Harofte, S., Harati, T., Khadem, A., et al. (2021). Enhancing clinical translation of cancer using nanoinformatics. Cancers (Basel) 13, 2481. doi:10.3390/cancers13102481
Srinivasarao, M., and Low, P. S. (2017). Ligand-targeted drug delivery. Chem. Rev. 117, 12133–12164. doi:10.1021/acs.chemrev.7b00013
Sung, H., Ferlay, J., Siegel, R. L., Laversanne, M., Soerjomataram, I., Jemal, A., et al. (2021). Global cancer statistics 2020: GLOBOCAN estimates of incidence and mortality worldwide for 36 cancers in 185 countries. Ca. Cancer J. Clin. 71, 209–249. doi:10.3322/caac.21660
Suo, X., Eldridge, B. N., Zhang, H., Mao, C., Min, Y., Sun, Y., et al. (2018). P-Glycoprotein-Targeted photothermal therapy of drug-resistant cancer cells using antibody-conjugated carbon nanotubes. ACS Appl. Mater. Interfaces 10, 33464–33473. doi:10.1021/acsami.8b11974
Swartz, M. A., and Lund, A. W. (2012). Lymphatic and interstitial flow in the tumour microenvironment: Linking mechanobiology with immunity. Nat. Rev. Cancer 12, 210–219. doi:10.1038/nrc3186
Thakur, C. K., Neupane, R., Karthikeyan, C., Ashby, C. R., Babu, R. J., Boddu, S. H. S., et al. (2022). Lysinated multiwalled carbon nanotubes with carbohydrate ligands as an effective nanocarrier for targeted doxorubicin delivery to breast cancer cells. Molecules 27, 7461. doi:10.3390/molecules27217461
Thitichai, N., Thanapongpibul, C., Theerasilp, M., Sungkarat, W., and Nasongkla, N. (2019). Study of biodistribution and systemic toxicity of glucose functionalized SPIO/DOX micelles. Pharm. Dev. Technol. 24, 935–946. doi:10.1080/10837450.2019.1569679
Tiwari, J. N., Tiwari, R. N., and Kim, K. S. (2012). Zero-dimensional, one-dimensional, two-dimensional and three-dimensional nanostructured materials for advanced electrochemical energy devices. Prog. Mater. Sci. 57, 724–803. doi:10.1016/j.pmatsci.2011.08.003
Uzonwanne, V. O., Navabi, A., Obayemi, J. D., Hu, J., Salifu, A. A., Ghahremani, S., et al. (2022). Triptorelin-functionalized PEG-coated biosynthesized gold nanoparticles: Effects of receptor-ligand interactions on adhesion to triple negative breast cancer cells. Biomater. Adv. 136, 212801. doi:10.1016/j.bioadv.2022.212801
Vahedi, N., Tabandeh, F., and Mahmoudifard, M. (2022). Hyaluronic acid–graphene quantum dot nanocomposite: Potential target drug delivery and cancer cell imaging. Biotechnol. Appl. Biochem. 69, 1068–1079. doi:10.1002/bab.2178
Villar-Alvarez, E., Cambón, A., Pardo, A., Arellano, L., Marcos, A. V., Pelaz, B., et al. (2019). Combination of light-driven co-delivery of chemodrugs and plasmonic-induced heat for cancer therapeutics using hybrid protein nanocapsules. J. Nanobiotechnol. 17, 106. doi:10.1186/s12951-019-0538-3
Walsh, C. S. (2015). Two decades beyond BRCA1/2: Homologous recombination, hereditary cancer risk and a target for ovarian cancer therapy? Gynecol. Oncol. 137, 343–350. doi:10.1016/j.ygyno.2015.02.017
Wang, T. T., Wei, Q. C., Zhang, Z. T., Lin, M. T., Chen, J. J., Zhou, Y., et al. (2020). AIE/FRET-based versatile PEG-Pep-TPE/DOX nanoparticles for cancer therapy and real-time drug release monitoring. Biomater. Sci. 8, 118–124. doi:10.1039/c9bm01546a
Wang, K., Shang, F., Chen, D., Cao, T., Wang, X., Jiao, J., et al. (2021). Protein liposomes-mediated targeted acetylcholinesterase gene delivery for effective liver cancer therapy. J. Nanobiotechnol. 19, 31. doi:10.1186/s12951-021-00777-9
Wei, Y., Xia, H., Zhang, F., Wang, K., Luo, P., Wu, Y., et al. (2019). Theranostic nanoprobe mediated simultaneous monitoring and inhibition of P-glycoprotein potentiating multidrug-resistant cancer therapy. Anal. Chem. 91, 11200–11208. doi:10.1021/acs.analchem.9b02118
Wei, Q. Y., Xu, Y. M., and Lau, A. T. Y. (2020). Recent progress of nanocarrier-based therapy for solid malignancies. Cancers (Basel) 12, 2783–2837. doi:10.3390/cancers12102783
Winter, P. M., Caruthers, S. D., Zhang, H., Williams, T. A., Wickline, S. A., and Lanza, G. M. (2008). Antiangiogenic synergism of integrin-targeted fumagillin nanoparticles and atorvastatin in atherosclerosis. JACC Cardiovasc. Imaging 1, 624–634. doi:10.1016/j.jcmg.2008.06.003
Wu, S., Zhu, W., Thompson, P., and Hannun, Y. A. (2018). Evaluating intrinsic and non-intrinsic cancer risk factors. Nat. Commun. 9, 3490. doi:10.1038/s41467-018-05467-z
Xu, Y., Hosny, A., Zeleznik, R., Parmar, C., Coroller, T., Franco, I., et al. (2019). Deep learning predicts lung cancer treatment response from serial medical imaging. Clin. Cancer Res. 25, 3266–3275. doi:10.1158/1078-0432.CCR-18-2495
Yang, J., Lu, W., Xiao, J., Zong, Q., Xu, H., Yin, Y., et al. (2018). A positron emission tomography image-guidable unimolecular micelle nanoplatform for cancer theranostic applications. Acta Biomater. 79, 306–316. doi:10.1016/j.actbio.2018.08.036
Yu, M. K., Kim, D., Lee, I. H., So, J. S., Jeong, Y. Y., and Jon, S. (2011). Image-guided prostate cancer therapy using aptamer-functionalized thermally cross-linked superparamagnetic iron oxide nanoparticles. Small 7, 2241–2249. doi:10.1002/smll.201100472
Yu, H., Yang, Z., Li, F., Xu, L., and Sun, Y. (2020). Cell-mediated targeting drugs delivery systems. Drug Deliv. 27, 1425–1437. doi:10.1080/10717544.2020.1831103
Zangooei, M. H., Margolis, R., and Hoyt, K. (2021). Multiscale computational modeling of cancer growth using features derived from microCT images. Sci. Rep. 11, 18524. doi:10.1038/s41598-021-97966-1
Zhai, J., Luwor, R. B., Ahmed, N., Escalona, R., Tan, F. H., Fong, C., et al. (2018). Paclitaxel-loaded self-assembled lipid nanoparticles as targeted drug delivery systems for the treatment of aggressive ovarian cancer. ACS Appl. Mater. Interfaces 10, 25174–25185. doi:10.1021/acsami.8b08125
Zhang, F., Peng, F., Qin, L., Yang, D., Li, R., Jiang, S., et al. (2019). pH/near infrared dual-triggered drug delivery system based black phosphorus nanosheets for targeted cancer chemo-photothermal therapy. Colloids Surfaces B Biointerfaces 180, 353–361. doi:10.1016/j.colsurfb.2019.04.021
Zheng, M., Jiang, T., Yang, W., Zou, Y., Wu, H., Liu, X., et al. (2019). The siRNAsome: A cation-free and versatile nanostructure for siRNA and drug Co-delivery. Angew. Chem. 131, 4992–4996. doi:10.1002/ange.201814289
Zhong, S., Chen, C., Yang, G., Zhu, Y., Cao, H., Xu, B., et al. (2019). Acid-Triggered nanoexpansion polymeric micelles for enhanced photodynamic therapy. ACS Appl. Mater. Interfaces 11, 33697–33705. doi:10.1021/acsami.9b12620
Zoaby, N., Shainsky-Roitman, J., Badarneh, S., Abumanhal, H., Leshansky, A., Yaron, S., et al. (2017). Autonomous bacterial nanoswimmers target cancer. J. Control. Release 257, 68–75. doi:10.1016/j.jconrel.2016.10.006
AChE Acetylcholinesterase
AuNSs Gold nanostars
ANN Artificial neural network
AI Artificial intelligence
BP Black phosphorus
CAAs Cancer-associated adipocytes
CAFs Cancer-associated fibroblasts
CDF 3,4-difluorobenzylidene-curcumin
CSCs Cancer stem cells
CuS Copper sulfide
DOX Doxorubicin
DTX Docetaxel
ECM Extracellular matrix
EPR Enhanced permeability and retention
ER Estrogen receptor
FA Folic acid
FRET Fluorescence resonance energy transfer
GA Geldanamycin
HSA Human serum albumin
IONs Iron oxide nanoparticles
MLS Mitochondria localization signal
MnO2 Manganese dioxide
MPS Mononuclear phagocyte system
MSNs Mesoporous silica nanoparticles
NPs Nanoparticles
PAMAM Poly(amidoamine)
PEG Polyethylene glycol
PLGA Poly(lactic-co-glycolic acid)
PLL Poly-l-lysine
Ppa Pyropheophorbide-a
PPO Poly (propylene oxide)
PPTT Plasmonic-based photothermal therapy
PR Progesterone receptor
PU Polyurethanes
PVA Polyvinyl alcohol
ROS Reactive oxygen species
SDNs Structured dendrimeric nanoconstructs
SPIONs Superparamagnetic iron oxide nanoparticles
SQ-PEG PEGylated squalene
TAMs Tumor-associated macrophages
TILs Tumor-infiltrating lymphocytes
TME Tumor microenvironment
TNF-α Tumor necrosis factor-alpha
TPE Tetraphenylethylene
CRDDS Controlled release drug delivery system
QD Quantum dot
ICG Indocyanine green
EGFR Epidermal growth factor receptor
Rg5 Ginsenoside Rg5
BSA Bovine serum albumin
HA Hyaluronic acid
LF Lactoferrin
SLN Solid lipid nanoparticle
MWCNT Multiwalled Carbon Nanotubes
GEM Gemcitabine
SFB Sorafenib
GalNAc Triantennary N-acetylgalactosamine
GRPR Gastrin-releasing peptide receptor
BPNS Black phosphorus nanosheets;
BPNPs Black phosphorus nanoparticles
DMKE O,O′- dimyristyl-N-lysyl glutamate
Keywords: nanoconstructs, drug delivery, nanoparticles, theranostic application, cancer, autonomous delivery
Citation: Mishra S, Bhatt T, Kumar H, Jain R, Shilpi S and Jain V (2023) Nanoconstructs for theranostic application in cancer: Challenges and strategies to enhance the delivery. Front. Pharmacol. 14:1101320. doi: 10.3389/fphar.2023.1101320
Received: 17 November 2022; Accepted: 06 March 2023;
Published: 15 March 2023.
Edited by:
Ashwni Verma, University at Buffalo, United StatesReviewed by:
Sanjoy Das, Dibrugarh University, IndiaCopyright © 2023 Mishra, Bhatt, Kumar, Jain, Shilpi and Jain. This is an open-access article distributed under the terms of the Creative Commons Attribution License (CC BY). The use, distribution or reproduction in other forums is permitted, provided the original author(s) and the copyright owner(s) are credited and that the original publication in this journal is cited, in accordance with accepted academic practice. No use, distribution or reproduction is permitted which does not comply with these terms.
*Correspondence: Vikas Jain, dmlrYXNqYWluQGpzc3VuaS5lZHUuaW4=
Disclaimer: All claims expressed in this article are solely those of the authors and do not necessarily represent those of their affiliated organizations, or those of the publisher, the editors and the reviewers. Any product that may be evaluated in this article or claim that may be made by its manufacturer is not guaranteed or endorsed by the publisher.
Research integrity at Frontiers
Learn more about the work of our research integrity team to safeguard the quality of each article we publish.