- Department of Nephrology, Shanghai Municipal Hospital of Traditional Chinese Medicine, Shanghai University of Traditional Chinese Medicine, Shanghai, China
Background: Renal repair is closely related to the prognosis of acute kidney injury (AKI) and has attracted increasing attention in the research field. However, there is a lack of a comprehensive bibliometric analysis in this research area. This study aims at exploring the current status and hotspots of renal repair research in AKI from the perspective of bibliometrics.
Methods: Studies published between 2002 and 2022 related to kidney repair after AKI were collected from Web of Science core collection (WoSCC) database. Bibliometric measurement and knowledge graph analysis to predict the latest research trends in the field were performed using bibliometrics software CiteSpace and VOSviewer.
Results: The number of documents related to kidney repair after AKI has steadily increased over 20 years. The United States and China contribute more than 60% of documents and are the main drivers of research in this field. Harvard University is the most active academic institution that contributes the most documents. Humphreys BD and Bonventre JV are the most prolific authors and co-cited authors in the field. The American Journal of Physiology-Renal Physiology and Journal of the American Society of Nephrology are the most popular journals in the field with the greatest number of documents. “exosome”, “macrophage polarization”, “fibroblast”, and” aki-ckd transition” are high-frequency keywords in this field in recent years. Extracellular vesicles (including exosomes), macrophage polarization, cell cycle arrest, hippo pathway, and sox9 are current research hotspots and potential targets in this field.
Conclusion: This is the first comprehensive bibliometric study on the knowledge structure and development trend of AKI-related renal repair research in recent years. The results of the study comprehensively summarize and identify research frontiers in AKI-related renal repair.
1 Introduction
Acute kidney injury (AKI) is a critical illness characterized by a sudden deterioration of kidney function, initial mortality, high morbidity, and high burden on the health system (Bonventre and Yang, 2011; Susantitaphong et al., 2013). Research on the chronic sequelae of AKI has increased dramatically in the last decade. Recent clinical data suggest that AKI is an important risk influence for progression to chronic kidney disease (CKD) (Coca et al., 2012). Due to the complexity of the structure of the kidney and the incomplete understanding of the pathophysiology of AKI, regenerative medicine for kidney disease is unfeasible. Recovery in surviving patients with AKI depends primarily on the reversal of hemodynamic damage, removal of the cause of kidney injury, and successful repair of the renal parenchyma (Basile et al., 2016). Therefore, developing effective treatment strategies to promote the repair of damaged renal tissue is critical for improving the short- and long-term prognosis of AKI. In the past few decades, much research has revolved around the endogenous repair and regeneration mechanisms of the kidney, including stem cell therapy and exosomes (Fais et al., 2016; Squillaro et al., 2016). In addition, the cellular and molecular mechanisms behind maladaptive repair and renal fibrosis, including epithelial-mesenchymal transformation (EMT), cellular senescence, G2/M cell cycle arrest, fibroblast, and immune cell activation, are constantly updated (Rayego-Mateos et al., 2022). As a result, kidney repair is becoming an important area of AKI research.
At present, bibliometric analysis is mainly used to analyze the development trend, academic exchange and core influence of literature in specific scientific fields (Chen and Song, 2019). Moreover, bibliometric analysis can also be used to explore leading journals in the field, core author teams, and current research frontiers and trends (Merigó and Núñez, 2016; Wu et al., 2021). However, to our knowledge, there is no systematic evaluation exploring kidney repair in AKI. In the present study, we conducted a bibliometric analysis of research related to kidney repair in AKI and explored emerging trends in this field.
2 Materials and methods
2.1 Data source and search strategy
Web of Science is one of the most authoritative and comprehensive database platforms in the world, with comprehensive content and high quality of academic journals, and is currently the most usually used database for bibliometric analysis (Luo et al., 2022; Zhou et al., 2022). Therefore, Web of Science core collection (WoSCC) was used as the data basis for our article. At the same time, so as to ensure the comprehensiveness and accuracy of the retrieved data, the citation index is selected as SCI-Expanded. The search keyword was “((((TS=(acute kidney injury)) OR TS=(acute kidney failure)) OR TS=(acute renal failure)) OR TS=(acute renal injury)) AND TS=(kidney repair) AND Document types = (ARTICLE OR REVIEW) AND Language = (English)”, and the time span was selected from 1 January 2002 to 31 December 2022. In addition, all valid bibliographic data, including year of publication, title, author name, nationality, affiliation, abstract, keywords, journal name, etc., are saved in plain text format file format in the WoSCC database.
2.2 Bibliometric analysis and visualization
Bibliometrics is an independent discipline and provides quantitative methods for reviewing and investigating existing literature in a particular field (Gureyev and Mazov, 2022). During the analysis, detailed information such as authors, keywords, journals, countries, institutions, references, etc., Can be obtained. Visualization helps to uncover the intrinsic connections between this information, such as different authors having the same research topic, research priorities from different institutions, new theories from existing institutions, and so on. For data analysis and visualization, such as country, region co-occurrence, journal dual-maps, high-frequency keyword tendency, co-cited references, and reference bursts, networks between countries, institutional researchers, and co-occurrence analysis, etc., we used both VOSviewer and CiteSpace (Synnestvedt et al., 2005; van Eck and Waltman, 2010). In addition, in the analysis, Taiwan is classified as the People’s Republic of China; England, Scotland, Northern Ireland and Wales are classified as the United Kingdom.
3 Results
3.1 Trend of global publications and citations
Between 2002 and 2022, 1,600 articles (Figure 1) were collected from WoSCC, including 1,160 articles and 440 reviews, and all publications were published in English. The 1,160 papers used in this study were written by 7,893 authors from 1,612 institutions in 56 countries, published in 481 journals, and cited 56,264 reference form 4,528 journals. Over the past 20 years, research on kidney repair in AKI has continuously increased (Figure 2A). These papers were cited an average of 36.57 times per paper, for a total of 73,190 citations.
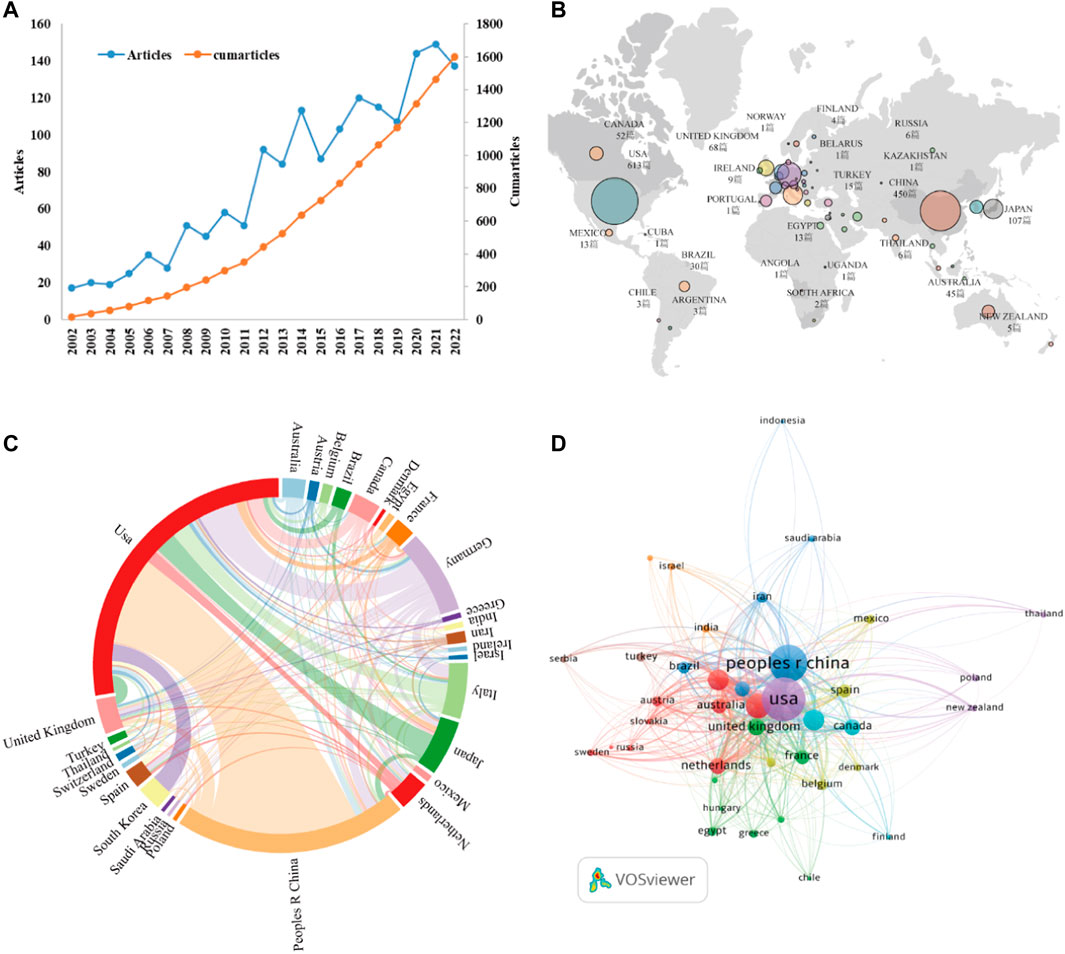
FIGURE 2. (A) The annual number and cumulative publications related to this topic. (B) An overview of the number of articles published by country in the world. (C) Academic cooperation between countries/regions on this topic. The thickness of segment indicated the frequency of cooperation. (D) The citation network of countries/regions mapped through VOSviewer.
3.2 Countries/regions and institutions analysis
As stated by the world map (Figure 2B), the publications on this topic came from researchers in 56 countries. The specific number of posts and citations of each country is shown in Table 1, among which the United States (613), China (450), Germany (162), Italy (108), and Japan (107) contributed the most. In addition, Italy, the United States, and Germany have higher citation rates than China and Japan. An interactive collaboration map depicts partnerships between countries (Figure 2C). According to Figure 2D, citation relationships between the 30 countries/regions which published no less than nine publications were revealed. Despite publishing more documents, China does not cooperate with other countries as commonly as the United States and Germany.
On the word of CiteSpace, 1,600 papers were provided by 1,612 different institutions. Table 2 lists the top 10 institutions with the most articles. It can be found that these 10 institutions are all from the United States and China, which is also in line with the country’s ranking of the number of documents. In institutional cooperation map with VOSviewer (Figure 3A), there is closer cooperation between various U.S. institutions, centered on Harvard University, than Chinese institutions. In terms of citation analysis of institutions, the top three institutions with the most citations are Harvard University (8,885), Brigham and womens hospital (3,424), and Univ Pittsburgh (2,801) (Figure 3B). In addition, the above chart shows that Harvard’s work in this area of research started earlier, while the impact of the research from Brigham and womens hospital was stronger.
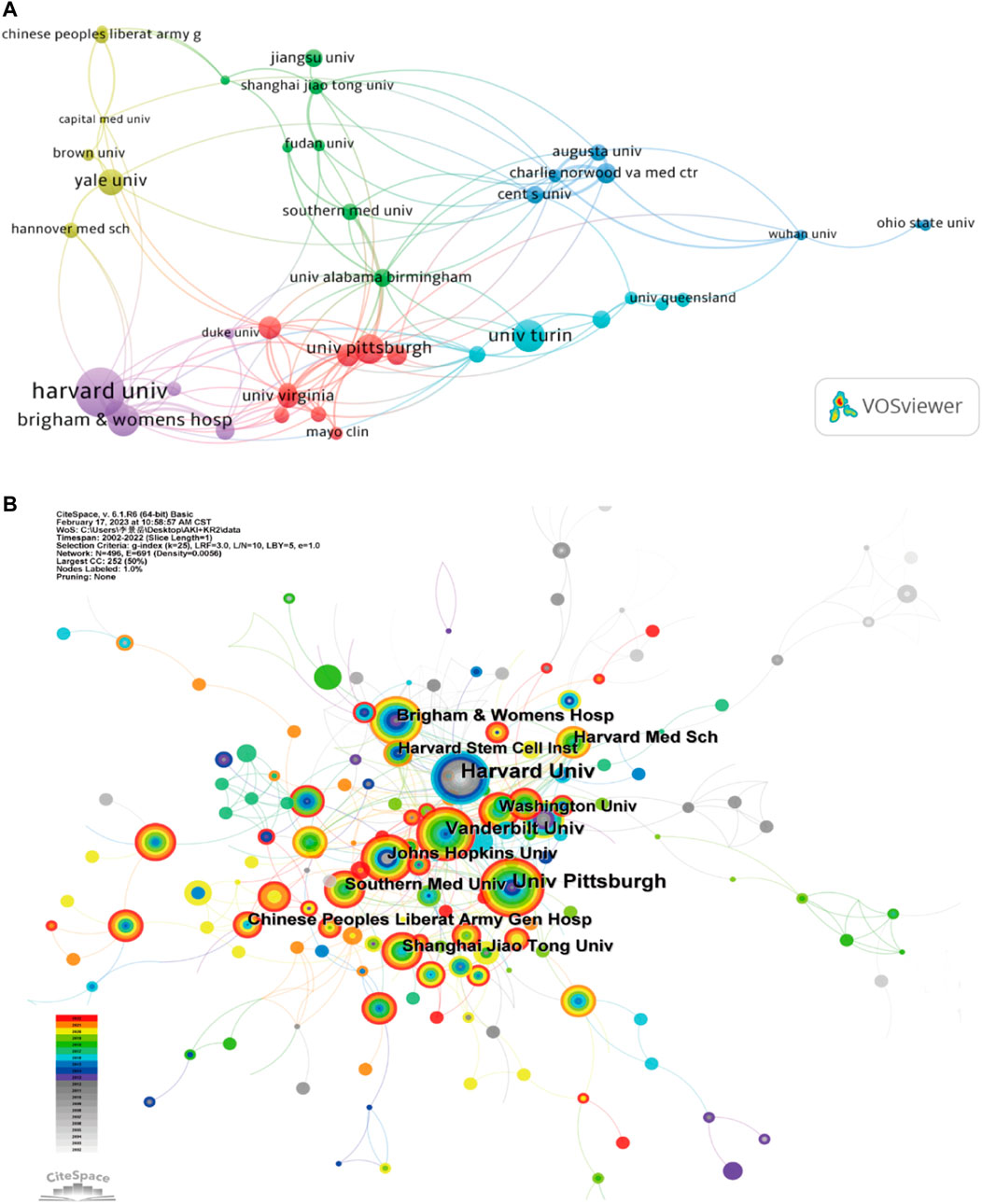
FIGURE 3. (A) Institutional cooperation diagram based on VOSviewer. (B) Institutional citation network generated by CiteSpace.
3.3 Authors and co-cited authors
A total of 7,893 authors participated in the study of kidney repair at AKI. The top 10 most productive authors and co-cited authors were provided in Table 3. Humphreys BD, Dong Z and Bonventre JV were the top three contributing authors with 30, 27 and 25 publications, respectively. As shown in Figure 4B, the 50 authors with the highest total link strength (TLS) were divided into three main clusters through co-citation analysis. The TLS of Bonventre JV, Humphreys BD and Togel F were 16,837, 18,201, and 17,045, respectively.
3.4 Journal analysis
A total of 481 journals published papers on this topic, of which 99 published more than five. In Table 4, the top 10 journals covered 27.63% of the publications, with a total of 442 articles. American Journal of Physiology-Renal Physiology contributed the most articles (n = 97). According to the 2021 Journal Citation Report (JCR), 5 of all top 10 journals are in Q1 in the academic rankings. Two had impact factor (IF) greater than 10, and Kidney International was the journal with maximum IF (18.998).
As shown in Figure 5, double-graph overlapping journals were mainly used to visualize the citation path between the cited journal and the cited journal. The citation path started with the cited journal in the left half and ended with the cited journal in the right half, while the topics covered by the journal are marked. It could be found that publications on the themes of “Health, Nursing and Medicine” were most often quoted, establishing two main citation paths that started with “Molecular, Biology and Immunology” and “Medicine, Medical and Clinical”.
3.5 Analysis of co-cited references
In total, 1,600 articles under this topic cited 56,264 references. In Table 5, the article entitled “Intrinsic epithelial cells repair the kidney after injury” by Humphreys BD had the most co-citations (n = 233) (Humphreys et al., 2008). Tögel F’ paper “Administered mesenchymal stem cells protect against ischemic acute renal failure through differentiation-independent mechanisms” ranked the second with 215 co-citations (Tögel et al., 2005).
In Figure 6A, the co-citation network of references was visualized via CiteSpace, and a total of 12 clusters containing keywords were identified. Multiple cell types and cell components are clustered together, including mesenchymal stem cells, renal tubule, extracellular vesicle, macrophage phenotype. In addition, renal fibrosis and age-associated change are also being found in clustering. The timeline view of co-cited references was publicized in Figure 6B, which is important for observing how research hotspots have changed over time. Nine clusters used title words as label source, then displayed different positions and colors on the timeline according to differences in publication time. The recent clusters on the timeline were “#4 renal fibrosis”, “#5 extracellular vesicle”, and “#2 macrophage phenotype”.
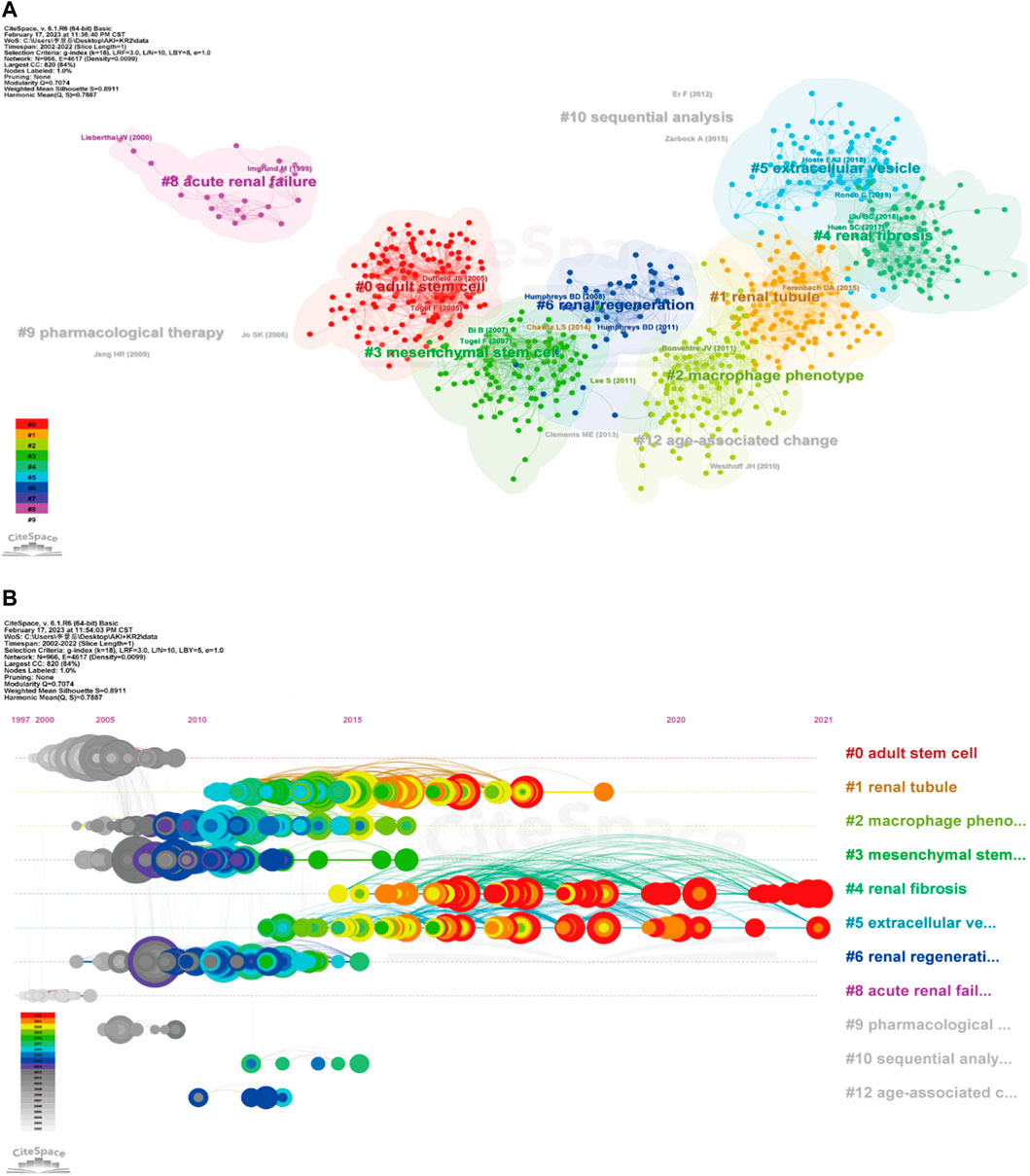
FIGURE 6. Cluster diagram of co-cited references using keywords as label source (A), and timeline view of co-cited references via title words (B) were visualized through CiteSpace.
CiteSpace’s citation burst analysis can uncover studies that have received widespread attention (Synnestvedt, Chen et al., 2005). The 25 references had strongest citation bursts and their most cited time periods were shown in Figure 7. The references that maintain the citation peak until 2020 to 2022 were: “Ferenbach DA, 2015, NAT REV NEPHROL, V11, P264, DOI 10.1038/nrneph.2015.3” (Ferenbach and Bonventre, 2015), “Venkatachalam MA, 2015, J AM SOC NEPHROL, V26, P1765, DOI 10.1681/ASN.2015010006” (Venkatachalam et al., 2015) and “Zuk A, 2016, ANNU REV MED, V67, P293, DOI 10.1146/annurev-med-050214-013407” (Zuk and Bonventre, 2016). Importantly, six of the top 10 co-cited publications are from Prof. Bonventre’s research team, demonstrating the impact of the lab’s research in this field.
3.6 Analysis of keywords
The co-occurrence network map and overlay map of the author keywords can explore the cluster distribution characteristics and time variation rules of the keywords. According to Figure 8A, VOSviewer shown the 10 main author keyword clusters. The purple cluster mainly contains several pathological processes related to tubular epithelial cell injury and repair, such as “apoptosis”, “stem cell”, and “mesenchymal stromal cell”. The blue cluster focuses on “Inflammation”, “cytokine”, “oxidative stress and “autophagy”. In the green cluster, cellular senescence is the focus of research attention. In Figure 8B, author keywords appeared in different colors depending on the average year of occurrence. In recent years, “exosome”, “macrophage polarization”, “fibroblast”, “metabolism”, “sox9”, and “aki-ckd transition” appeared frequently, denoting the conversion of AKI to CKD is a current research focus to intervene through multiple pathways.
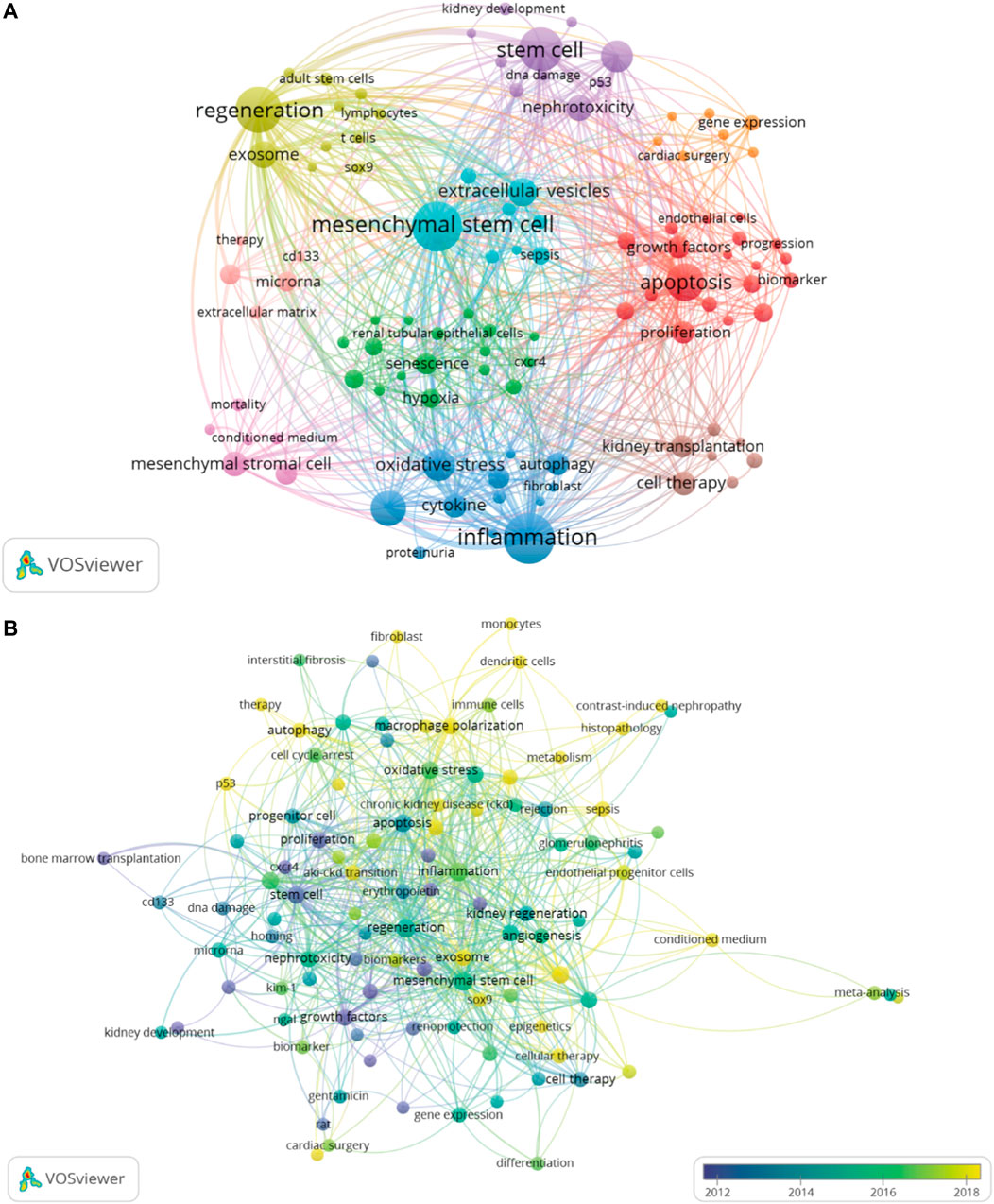
FIGURE 8. The author keywords co-occurrence network map, which showed the distribution of clusters of major keywords (A). The overlay map displayed that author keywords are colored based on average occurrence time (B). The overlay visualization map showed the color change of the author keywords based on average occurrence time.
In Figure 9A, we also identified research hotspots by keywords with strong citation outbursts. The citation bursts for keywords like “extracellular vesicles”, “fibrosis”, “aki-ckd transition”, and “inflammation” are still occurring, demonstrating that these words may convert to new hot spots. Alluvial flow diagram can also observe how concepts change over time. In Figure 9B, each of these “blocks” is named after the most critical keyword in the cluster, while the lines connect the same keywords in different years. In addition to showing the flow dynamics of some concepts such as stem cells over time, it can also explore some new research hotspots.
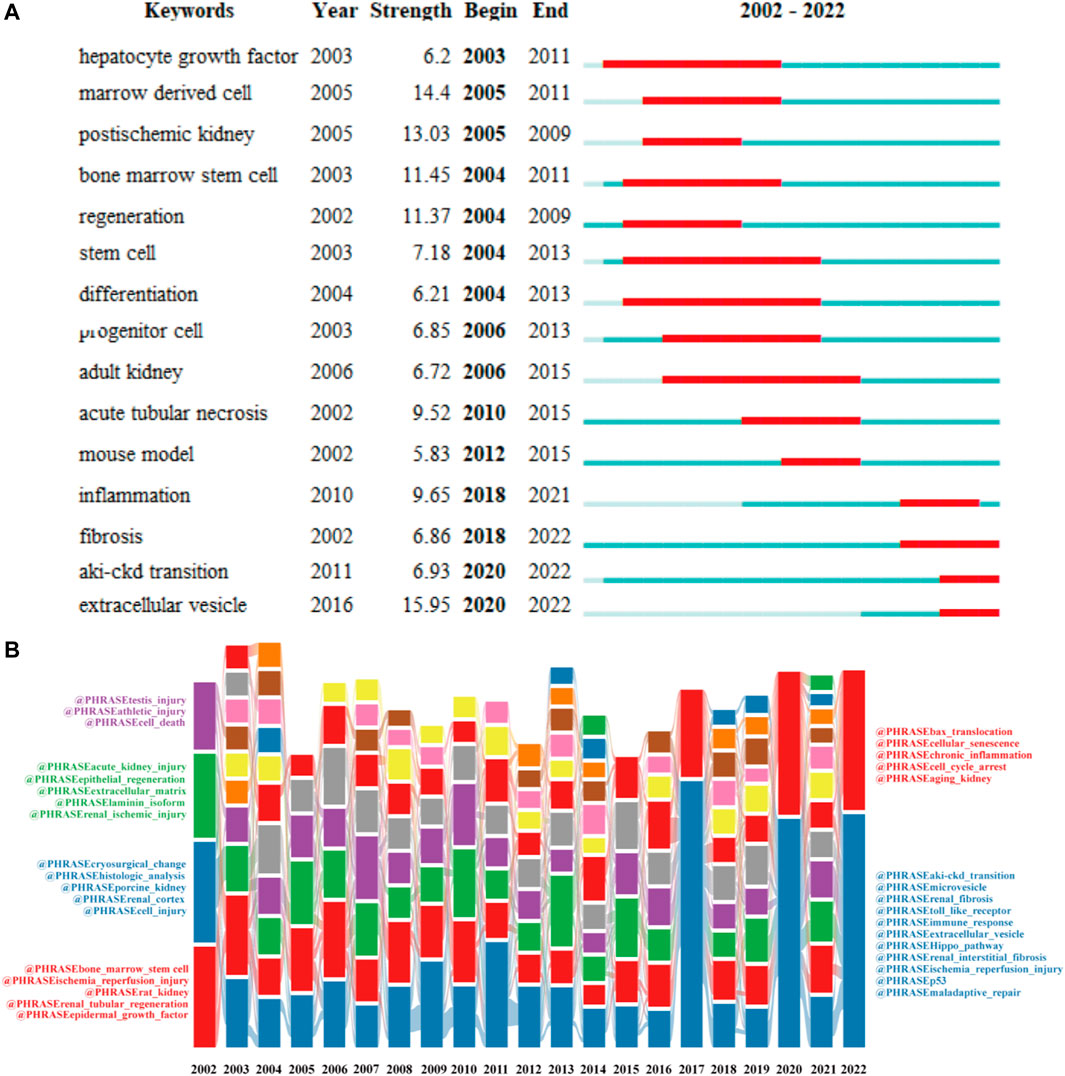
FIGURE 9. Top 20 keywords with the strong citation bursts (A). Alluvial flow diagrams reflect the flow of concepts to see how concepts change over time (B).
4 Discussion
From 2002 to 2022, there has been a rapid increase in the number of annual publications on kidney repair in AKI, indicating that kidney repair will remain a research hotspot in the future. In this study, we provide a comprehensive overview of the development of AKI-related renal repair research through bibliometric analysis, with a focus on possible research hotspots. Globally, the leading countries in kidney repair research are the United States, China, and Germany. This is also supported by an analysis of institutional publications, with 7 of the top 10 productive institutions in the United States and the rest in China (Table 2).
Upon author analysis, Humphreys BD and Bonventre JV were among the top 10 most productive authors and the top 10 most co-cited authors (Table 3), and there is a close collaboration between these two authors (Figure 4A). The most cited study, “Intrinsic epithelial cells repair the kidney after injury,” written by Humphreys BD, Valerius MT, et al., focuses on the main mechanisms of post-ischemic tubular injury in adult mammalian kidneys (Humphreys, Valerius et al., 2008). The work of Humphreys BD’s team also includes interventions in renal fibrosis, cell cycle arrest, and stem cell therapy to facilitate the repair of damaged kidneys after AKI (Liu et al., 2014; Humphreys et al., 2016; Kefaloyianni et al., 2016). The second most cited study, “Administered mesenchymal stem cells protect against ischemic acute renal failure through differentiation-independent mechanism,” written by Tögel F, Hu Z, et al., is on the prevention of ischemic AKI by administering mesenchymal stem cells (Tögel, et al., 2005). The third most cited study, “Cellular pathophysiology of ischemic acute kidney injury,” is a review written by Bonventre JV, Yang L, et al., which elaborates on the cytopathophysiological processes of ischemic AKI and repair (Bonventre and Yang, 2011). Moreover, Bonventre JV’s team studied the molecular markers related to AKI (Han et al., 2002). Additionally, Humphreys BD and Bonventre JV are in the Renal Division, Department of Medicine, Brigham and Women’s Hospital, Harvard Institutes of Medicine. This also shows the dominance of Brigham and Women’s Hospital in this field.
On the basis of the top journals, the American Journal of Physiology-Renal Physiology, Journal of the American Society of Nephrology, and Kidney International had the greatest number of publications on kidney repair in AKI, and these had guidelines for manuscript proposal (Table 4). This study also illustrated that the top 10 journals on the topic included professional journals in the field of nephrology and journals in other fields like stem cell research, and molecular science, indicating that there is a multidisciplinary development trend in this field.
Citation burst, a phenomenon whereby terms such as references and keywords receive a lot of attention and reflect dynamic changes over a certain period of time, was seen. In Figure 7, the 25 references with the strongest citation bursts had a relatively short active period of up to 5 years. The following 3 articles were the most recent of the 25 citation bursts: “Zuk and Bonventre, 2016, ANNU REV MED, V67, P293, DOI 10.1146/annurev-med-050214–013407” describes the complex pathophysiological mechanisms of AKI, biomarkers and therapeutic strategies to prevent the progression of AKI to CKD (Zuk and Bonventre, 2016); “Venkatachalam MA, 2015, J AM SOC NEPHROL, V26, P1765, DOI 10.1681/ASN.2015010006” reviews the progression mechanism of further deterioration of renal structure following AKI transformation to CKD (Venkatachalam, et al., 2015); “Ferenbach and Boventre, 2015, NAT REV NEPHROL, V11, P264, DOI 10.1038/nrneph. 2015.3” explores the archetypal mechanisms by which severely damaged kidneys regenerate and how adaptive repair processes can become maladaptive (Ferenbach and Bonventre, 2015).
Typically, keywords are used in bibliometrics to get an overview of the development of a field. Figure 8B (which colors keywords according to the average year they appear) and Figure 9 show how kidney repair research has evolved over time. The above data shows that the study on kidney repair after AKI can be mainly divided into two important development directions. The first research direction focused on the restoration and regeneration of epithelial cell function. The acute phase of AKI is characterized by cell death, followed by a recovery phase, in which there is an activation of protective and regenerative mechanisms in the surviving cells to restore the properties and functions of epithelial cells (Ruiz-Ortega et al., 2020). A range of cells, such as resident renal progenitor cells and stem cells, are thought to contribute to kidney repair and regeneration (Rayego-Mateos, et al., 2022). Renal injury leads to local microenvironment recruitment and activation of progenitor cells and cell-dependent tissue repair (Huang et al., 2021). Progenitor cells of the proximal tubule are more resistant to death and replace injured cells by differentiating into tubular epithelial cells (Gupta et al., 2006; Lazzeri et al., 2018). In addition to renal progenitor cells, subsequent studies have found that circulating bone marrow stem cells and kidney-resident stem cells also contribute to kidney repair and regeneration (Li et al., 2010). Therefore, the regenerative potential of pluripotent embryonic stem cells (ESCs), induced pluripotent stem cells (iPSCs), and mesenchymal stem cells (MSCs) have been gradually emphasized in AKI research. Recent studies have found that the nephroprotective effects of stem cell transplantation may depend on its paracrine effects, including the release of growth factors, chemokines, cytokines, and extracellular vesicles to induce proximal tubular cell proliferation, dedifferentiation, and angiogenesis (Ranghino et al., 2017; Liu et al., 2020). At present, extracellular vesicles and exosomes are still potential research hotspots. The second research direction focuses on the exploration of renal repair mechanisms dominated by renal fibrosis. After kidney injury, various intrinsic repair processes are rapidly activated, and some pathophysiological processes constitute maladaptive repair, which may promote fibrosis and lead to a progressive decline in renal function (Rayego-Mateos et al., 2021). The major role of oxidative stress in the progression of AKI to CKD initially attracted attention in research. Defense mechanisms against hypoxia and oxidative stress in cells, such as hypoxia-inducible factor (HIF) and nuclear factor E2-related factor 2, are considered likely to be suitable therapeutic targets (Kim et al., 2009; Kapitsinou et al., 2012). Subsequently, other signaling pathways activated due to maladaptive repair like mitochondrial dysfunction and epigenetic changes have been found as potential therapeutic targets for intervention in renal fibrosis after AKI (Cianciolo Cosentino et al., 2013; Stallons et al., 2014). In recent studies, a variety of pathological mechanisms are closely related to maladaptive kidney repair, including macrophage polarization, cell cycle arrest, and cell senescence. In addition, new molecules and pathways, such as the Hippo signaling pathway, Sox9, and p53, have also begun to receive attention in this field. In addition, activation of the senescence mechanism in renal tubular cells and the accumulation of senescent cells will lead to the failure of regeneration after AKI to CKD conversion (Gire and Dulic, 2015; Kim et al., 2019). Overall, CiteSpace’s keyword exploration also helps reveal future trends in space. We concluded that extracellular vesicles (including exosomes), macrophage polarization, and Hippo pathway may be current research hotspots, which will be described in detail below. Additionally, cell cycle arrest, cellular senescence, and Sox9 are also potential research directions and targets.
4.1 Extracellular vesicles (EVs)
Besides soluble cytokines, EVs such as exosomes (30–100 nm in diameter) and microvesicles (100–1,000 nm in diameter) have been described as a different form of cell-to-cell communication through the horizontal transfer of microRNAs (miRNAs), mRNAs, and proteins-to-target cells (Lu et al., 2022). Apart from the stem cells mentioned above, all regions of the nephron can release EVs. Since the study by Camussi et al. found that microvesicles from mesenchymal stem cells (MSCs) may activate proliferation in renal tubular cells surviving injury through horizontal transfer of mRNA, there is increasing evidence supporting the involvement of EVs in pathophysiology and repair process of AKI (Bruno et al., 2009; Camussi et al., 2010). Research have found that renal proximal tubular epithelial cells (RPTECs) of injured kidneys can release EVs carrying miR-216a and stimulate nearby RPTECs for epithelial-mesenchymal conversion via the PTEN/Akt pathway, followed by renal interstitial fibrosis (Qu et al., 2019). Tubular cells can also communicate bidirectionally with other cell types involved in kidney injury through EVs. For example, damaged PTECs release EVs, which stimulate macrophage activation and infiltration and amplify the inflammatory response (Lv et al., 2018). Specifically, EVs can influence processes such as inflammation, apoptosis, autophagy, oxidative stress, and cell proliferation, thereby contributing to the development of AKI (Han and Lee, 2019).
Over the past few years, EVs have received a lot of attention as a promising pro-regenerative entity and possible alternative to cell therapy due to their involvement in cell differentiation, proliferation, and angiogenesis, as well as the regulation of extracellular matrix turnover during regeneration. There is considerable evidence that EVs have a nephroprotective effect on improving kidney injury in different experimental AKI models. Various AKI models have shown the protective effects of EVs derived from bone marrow MSCs (BM-MSCs). MiR-199a-3p derived from BM-MSC-EVs prevents renal I/R injury and apoptosis by modulating the Akt and Erk1/2 signaling pathways (Zhu et al., 2019). The important role of miRNAs transferred by BM-MSC-EVs in post-AKI recovery and regeneration was also revealed, while miRNA depletion significantly reduced its intrinsic regeneration potential in AKI (Collino et al., 2015). In different AKI models, umbilical cord MSC (UC-MSC)-derived EVs have also been shown to be beneficial. UC-MSC-derived EVs can enhance proliferation and angiogenesis in a HIF-1α-independent manner to improve renal function after unilateral I/R (Zou et al., 2016). Furthermore, UC-MSC-EV can also promote recovery from kidney injury by increasing HGF expression, and its mechanism may be related to the transfer of RNA to injured renal tubular cells and activation of Erk1/2 signaling to accelerate the dedifferentiation and growth of renal tubular cells (Ju et al., 2015). In addition to the above sources, the effect of EVs derived from human placental MSCs, adipose MSCs, liver MSCs, and macrophages and endothelial progenitor cells have also been studied in post-AKI regeneration and recovery, and some new evidence has been obtained (Gao et al., 2020; Grange et al., 2020; Zhang et al., 2020). Several promising approaches are also being explored, including modifying the EVs of MSCs to enhance their targeting of the kidneys, or artificially altering the active molecules delivered in the EVs. Although the mechanisms of current EVs-based studies remain murky in most cases, many examples of beneficial effects dominated by MSC-derived EVs suggest that they have great potential to be used as a basis for the development of novel post-AKI renal repair and regenerative therapies.
4.2 Macrophage polarization
Macrophages are the main immune cells in the normal kidneys and are thought to be core members in the pathogenesis of AKI. Macrophages can change their phenotype according to the surrounding microenvironment. Macrophages that infiltrate the kidneys have a profound effect on kidney damage, repair, and fibrosis due to different polarization states (Lee et al., 2011). Typically, macrophages accumulate in the kidneys after injury and undergo a transition from a pro-inflammatory M1 phenotype to an alternatively activated M2 phenotype (Engel and Chade, 2019). Therefore, M2 macrophage polarization is essential for inflammation inhibition, remodeling, and AKI recovery. Macrophage depletion in the later stages of the Ischemia-reperfusion injury (IRI) model reduces PTECs proliferation and delays renal repair, while IL-4 polarizes metastasis-induced repair of M2 macrophages (Vinuesa et al., 2008; Meng et al., 2022). Interestingly, PTECs can also modulate the phenotype of macrophages. In sepsis-induced AKI, CSF2 secreted by injured TECs can facilitate the transition from M1 to M2 macrophages in a dose- and time-dependent mode (Li et al., 2020). The mechanism by which macrophages promote tubular repair is complex. Macrophage-derived Wnt7b, BRP-39, and IL-22 have been identified as factors that directly promote tubular repair after ischemic injury (Lin et al., 2010; Kulkarni et al., 2014). Vascular-resident CD169+ macrophages limit neutrophil infiltration after ischemic damage by down-regulating the expression of intercellular adhesion molecule-1 on vascular endothelial cells, and they indirectly promote tubular repair (Karasawa et al., 2015). Therefore, macrophage phenotype plays a pivotal role in kidney repair. However, it is difficult to maintain a macrophage phenotype for a successful switch in vivo. Lipoclysin-2 (Lcn-2) is an effective regulator of macrophage polarization that stabilizes the M2 macrophage phenotype (Mertens et al., 2020). Additionally, when kidney injury is unresolved and progressive, M2 macrophages can become profibrotic through mesothelial-to-mesenchymal transition (Huen and Cantley, 2015). Overall, targeting the regulation of macrophage phenotypes is a promising treatment to promote renal repair after AKI. Although some details remain to be clarified, such as the precise molecular characteristics and cell fate plasticity of macrophage subsets in vivo after AKI, the direct extrapolation of relative M1/M2 expression profiles defined in vitro and its biological effects on in vivo repair responses remain to be confirmed.
4.3 Cell cycle arrest and cellular senescence
The mitotic cell cycle consists of four stages, including G0-G1, S, G2 and M, and is controlled by four checkpoints, including G1/S, S, G2/M and M (Hartwell and Weinert, 1989). However, failure to pass one of these checkpoints can induce cell cycle arrest or cell death at a specific stage. Under physiological conditions, the rate of renal cell turnover is very low, and RPTECs are mostly maintained in the G0-G1 phase (Canaud and Bonventre, 2015). After kidney injury, surviving RPTECs enter the cell cycle and divide to replace the damaged cells. Early research suggests that cell cycle arrest may be a protective mechanism for post-AKI RPTECs, promoting DNA damage repair or preventing cell division when DNA damage cannot be repaired (De Chiara et al., 2021). Studies have found that p21 is induced in the kidneys after several types of AKI, including cisplatin administration, ischemia-reperfusion, and ureteral obstruction, and causes cell cycle arrest. However, the deletion of the p21 gene leads to more severe kidney injury (Megyesi et al., 1998; Megyesi et al., 2001). The Bonventre laboratory study confirms that G2/M cycle arrest of epithelial cells exacerbates the renal fibrotic process in ischemic, toxic and obstructive AKI models; however, JNK or p53 inhibitors can rescue the subprocess (Yang et al., 2010). Additionally, prolonged blockade of the G1 or G2/M stages after AKI leads to senescence-associated secretory phenotype (SASP), which includes the secretion of profibrotic and pro-inflammatory factors, exacerbating kidney damage and forming a vicious cycle (Yu and Bonventre, 2020). Targeting cell cycle arrest is a potential innovative strategy to improve kidney repair and prevent CKD, as studies targeting p21, p53, Cyclin G1 (CG1) and HDAC inhibitors have progressed in animal experiments (Skrypnyk et al., 2016; Fu et al., 2017; Canaud et al., 2019). With the further recognition of the importance of cell cycle abnormalities in renal repair after AKI, therapeutic strategies that target this process deserve the attention of researchers.
4.4 Hippo signaling pathway
The Hippo pathway is highly evolutionarily conserved in mammals and consists of a three-step kinase cascade of MST1/2 (also known as STK4/3), LATS1/2, and YAP/TAZ (also known as WWTR1) (Callus et al., 2006). This pathway regulates cell growth and fate decisions, organ size, and regeneration through phosphorylation and inactivation of its downstream effector YAP/TAZ (Ma et al., 2019). In recent years, the association between the Hippo pathway and renal repair and fibrosis after AKI has attracted attention. Studies have found that YAP expression and nuclear distribution are enhanced in renal RPTC in AKI patients and I/R mice, while histological recovery is delayed in YAP/TAZ double-knockout mice (Chen et al., 2018). The EGFR-PI3K-Akt pathway and RacGAP1 can help RTECs recover from acute injury by activating YAP (Zhou et al., 2020). There is considerable evidence that YAP may promote proliferation and redifferentiation of reconstituted epithelium after acute I/R-induced AKI (Xu et al., 2016). However, the function of the Hippo pathway in kidney repair after AKI may be bidirectional. Sustained activation of YAP/TAZ in severe AKI impedes redifferentiation of dedifferentiated tubular cells and promotes renal fibrosis, leading to maladaptive repair and CKD (Xu et al., 2021). Epithelial-mesenchymal transformation (EMT), G2/M cell cycle arrest, and macrophage M2 polarization have been associated to Hippo signaling-mediated renal fibrosis (Seo et al., 2016; Feng et al., 2018; Zheng et al., 2021). In summary, proper regulation of the Hippo signaling pathway, especially YAP intervention in repair, may be an effective prevention target for AKI-CKD conversion after AKI. Although the above evidence is convincing, the correlation between the hippo pathway and AKI needs to be further studied, and the underlying mechanisms and pathways need to be further elucidated.
4.5 Sox9
The kidneys can regenerate after injury; however, the intrinsic molecular mechanisms remain unknown. The transcription factor Sox9 is important in organogenesis during mammalian embryonic development. Simultaneously, Sox9 is a transcription factor essential for normal kidney development, and recent studies have highlighted the importance of its expression in the repair process of renal tubular epithelial cells (Kumar et al., 2015; Kang et al., 2016). In the early stages of kidney injury, Sox9 expression is significantly upregulated within damaged tubular epithelial cells, and approximately 40% of Sox9-positive cells proliferate and expand after kidney injury. Despite the recovery of kidney function, Sox9 activation was found to persist on day 28. Furthermore, multiple upstream and downstream molecules or mechanisms are involved in the renal repair process via Sox9 in AKI. Sox9 drives the upregulation of VGF nerve growth factor in multiple AKI models and acts as a stress-responsive protective gene in TECs (Kim et al., 2020). In IRI and folic acid (FA)-induced AKI models, early growth response 1 (EGR1) can increase Sox9 expression in kidney TECs by directly binding to the promoter of the Sox9 gene, consequently promoting Sox9 cell proliferation by activating the Wnt/β-catenin pathway (Chen et al., 2022). A recent study found that kidney-resident Sox9+ TECs can be activated and secrete various factors to promote tissue repair, of which S100A9 may be a key factor associated with the renal repair pathway (Nie et al., 2023). Although the upregulation of Sox9 was identified as an early event after AKI in these studies, the primary player in kidney repair as tubular epithelial cells or resident tubular progenitor cells remains controversial. In addition to being involved in the intrinsic repair of the kidney, Sox9 plays a role in maladaptive repair and chronic fibrosis in the AKI-CKD transition. SOX9 has been associated with human renal fibrosis and is required for experimentally-induced renal fibrosis in mice (Raza et al., 2021). Both embryonic stem cell-derived extracellular vesicles (ESC-EVs) and human amnion-derived mesenchymal stem cells (hAD-MSCs)-derived exosomes activate Sox9 in TECs to promote physiological repair after AKI and inhibit the progression of fibrotic processes (Zhu et al., 2017; Yu et al., 2021). In conclusion, although Sox9 has been associated with kidney repair after AKI, a further in-depth research is still necessary.
5 Limitation
First, not all studies from the databases were included and data were taken from the WoSCC database only. Second, we only selected studies and review articles written in English, articles published in non-English or non-research/review articles were not included in this study, leading to some omissions. Third, data were obtained through bibliometric software based on machine learning, which may lead to bias in bibliometric research and discussion.
Data availability statement
The original contributions presented in the study are included in the article/Supplementary Material, further inquiries can be directed to the corresponding author.
Author contributions
JL and XG designed the work for this article. JL reviewed the literature and wrote this paper. XG won the research fundings supporting this manuscript. All authors approved the paper for publication.
Funding
This project is supported by National Natural Science Foundation of China (No.82074387 and No.81873280).
Conflict of interest
The authors declare that the research was conducted in the absence of any commercial or financial relationships that could be construed as a potential conflict of interest.
Publisher’s note
All claims expressed in this article are solely those of the authors and do not necessarily represent those of their affiliated organizations, or those of the publisher, the editors and the reviewers. Any product that may be evaluated in this article, or claim that may be made by its manufacturer, is not guaranteed or endorsed by the publisher.
References
Basile, D. P., Bonventre, J. V., Mehta, R., Nangaku, M., Unwin, R., Rosner, M. H., et al. (2016). Progression after AKI: Understanding maladaptive repair processes to predict and identify therapeutic treatments. J. Am. Soc. Nephrol. 27 (3), 687–697. doi:10.1681/asn.2015030309
Bonventre, J. V., and Yang, L. (2011). Cellular pathophysiology of ischemic acute kidney injury. J. Clin. Invest. 121 (11), 4210–4221. doi:10.1172/jci45161
Bruno, S., Grange, C., Deregibus, M. C., Calogero, R. A., Saviozzi, S., Collino, F., et al. (2009). Mesenchymal stem cell-derived microvesicles protect against acute tubular injury. J. Am. Soc. Nephrol. 20 (5), 1053–1067. doi:10.1681/asn.2008070798
Callus, B. A., Verhagen, A. M., and Vaux, D. L. (2006). Association of mammalian sterile twenty kinases, Mst1 and Mst2, with hSalvador via C-terminal coiled-coil domains, leads to its stabilization and phosphorylation. Febs J. 273 (18), 4264–4276. doi:10.1111/j.1742-4658.2006.05427.x
Camussi, G., Deregibus, M. C., Bruno, S., Cantaluppi, V., and Biancone, L. (2010). Exosomes/microvesicles as a mechanism of cell-to-cell communication. Kidney Int. 78 (9), 838–848. doi:10.1038/ki.2010.278
Canaud, G., and Bonventre, J. V. (2015). Cell cycle arrest and the evolution of chronic kidney disease from acute kidney injury. Nephrol. Dial. Transpl. 30 (4), 575–583. doi:10.1093/ndt/gfu230
Canaud, G., Brooks, C. R., Kishi, S., Taguchi, K., Nishimura, K., Magassa, S., et al. (2019). Cyclin G1 and TASCC regulate kidney epithelial cell G(2)-M arrest and fibrotic maladaptive repair. Sci. Transl. Med. 11 (476), eaav4754. doi:10.1126/scitranslmed.aav4754
Chen, C., and Song, M. (2019). Visualizing a field of research: A methodology of systematic scientometric reviews. PLoS One 14 (10), e0223994. doi:10.1371/journal.pone.0223994
Chen, J. W., Huang, M. J., Chen, X. N., Wu, L. L., Li, Q. G., Hong, Q., et al. (2022). Transient upregulation of EGR1 signaling enhances kidney repair by activating SOX9(+) renal tubular cells. Theranostics 12 (12), 5434–5450. doi:10.7150/thno.73426
Chen, J., You, H., Li, Y., Xu, Y., He, Q., and Harris, R. C. (2018). EGF receptor-dependent YAP activation is important for renal recovery from AKI. J. Am. Soc. Nephrol. 29 (9), 2372–2385. doi:10.1681/asn.2017121272
Cianciolo Cosentino, C., Skrypnyk, N. I., Brilli, L. L., Chiba, T., Novitskaya, T., Woods, C., et al. (2013). Histone deacetylase inhibitor enhances recovery after AKI. J. Am. Soc. Nephrol. 24 (6), 943–953. doi:10.1681/asn.2012111055
Coca, S. G., Singanamala, S., and Parikh, C. R. (2012). Chronic kidney disease after acute kidney injury: A systematic review and meta-analysis. Kidney Int. 81 (5), 442–448. doi:10.1038/ki.2011.379
Collino, F., Bruno, S., Incarnato, D., Dettori, D., Neri, F., Provero, P., et al. (2015). AKI recovery induced by mesenchymal stromal cell-derived extracellular vesicles carrying MicroRNAs. J. Am. Soc. Nephrol. 26 (10), 2349–2360. doi:10.1681/asn.2014070710
De Chiara, L., Conte, C., Antonelli, G., and Lazzeri, E. (2021). Tubular cell cycle response upon AKI: Revising old and new paradigms to identify novel targets for CKD prevention. Int. J. Mol. Sci. 22 (20), 11093. doi:10.3390/ijms222011093
Engel, J. E., and Chade, A. R. (2019). Macrophage polarization in chronic kidney disease: A balancing act between renal recovery and decline? Am. J. Physiol. Ren. Physiol. 317 (6), F1409–f1413. doi:10.1152/ajprenal.00380.2019
Fais, S., O'Driscoll, L., Borras, F. E., Buzas, E., Camussi, G., Cappello, F., et al. (2016). Evidence-based clinical use of nanoscale extracellular vesicles in nanomedicine. ACS Nano 10 (4), 3886–3899. doi:10.1021/acsnano.5b08015
Feng, Y., Liang, Y., Zhu, X., Wang, M., Gui, Y., Lu, Q., et al. (2018). The signaling protein Wnt5a promotes TGFβ1-mediated macrophage polarization and kidney fibrosis by inducing the transcriptional regulators Yap/Taz. J. Biol. Chem. 293 (50), 19290–19302. doi:10.1074/jbc.RA118.005457
Ferenbach, D. A., and Bonventre, J. V. (2015). Mechanisms of maladaptive repair after AKI leading to accelerated kidney ageing and CKD. Nat. Rev. Nephrol. 11 (5), 264–276. doi:10.1038/nrneph.2015.3
Fu, S., Tang, Y., Huang, X. R., Feng, M., Xu, A. P., and Lan, H. Y. (2017). Smad7 protects against acute kidney injury by rescuing tubular epithelial cells from the G1 cell cycle arrest. Clin. Sci. (Lond) 131 (15), 1955–1969. doi:10.1042/cs20170127
Gao, F., Zuo, B., Wang, Y., Li, S., Yang, J., and Sun, D. (2020). Protective function of exosomes from adipose tissue-derived mesenchymal stem cells in acute kidney injury through SIRT1 pathway. Life Sci. 255, 117719. doi:10.1016/j.lfs.2020.117719
Gire, V., and Dulic, V. (2015). Senescence from G2 arrest, revisited. Cell Cycle 14 (3), 297–304. doi:10.1080/15384101.2014.1000134
Grange, C., Papadimitriou, E., Dimuccio, V., Pastorino, C., Molina, J., O'Kelly, R., et al. (2020). Urinary extracellular vesicles carrying klotho improve the recovery of renal function in an acute tubular injury model. Mol. Ther. 28 (2), 490–502. doi:10.1016/j.ymthe.2019.11.013
Gupta, S., Verfaillie, C., Chmielewski, D., Kren, S., Eidman, K., Connaire, J., et al. (2006). Isolation and characterization of kidney-derived stem cells. J. Am. Soc. Nephrol. 17 (11), 3028–3040. doi:10.1681/asn.2006030275
Gureyev, V. N., and Mazov, N. A. (2022). Bibliometrics as a promising tool for solving publication ethics issues. Heliyon 8 (3), e09123. doi:10.1016/j.heliyon.2022.e09123
Han, S. J., and Lee, H. T. (2019). Mechanisms and therapeutic targets of ischemic acute kidney injury. Kidney Res. Clin. Pract. 38 (4), 427–440. doi:10.23876/j.krcp.19.062
Han, W. K., Bailly, V., Abichandani, R., Thadhani, R., and Bonventre, J. V. (2002). Kidney injury molecule-1 (KIM-1): A novel biomarker for human renal proximal tubule injury. Kidney Int. 62 (1), 237–244. doi:10.1046/j.1523-1755.2002.00433.x
Hartwell, L. H., and Weinert, T. A. (1989). Checkpoints: Controls that ensure the order of cell cycle events. Science 246 (4930), 629–634. doi:10.1126/science.2683079
Huang, J., Kong, Y., Xie, C., and Zhou, L. (2021). Stem/progenitor cell in kidney: Characteristics, homing, coordination, and maintenance. Stem Cell Res. Ther. 12 (1), 197. doi:10.1186/s13287-021-02266-0
Huen, S. C., and Cantley, L. G. (2015). Macrophage-mediated injury and repair after ischemic kidney injury. Pediatr. Nephrol. 30 (2), 199–209. doi:10.1007/s00467-013-2726-y
Humphreys, B. D., Cantaluppi, V., Portilla, D., Singbartl, K., Yang, L., Rosner, M. H., et al. (2016). Targeting endogenous repair pathways after AKI. J. Am. Soc. Nephrol. 27 (4), 990–998. doi:10.1681/asn.2015030286
Humphreys, B. D., Valerius, M. T., Kobayashi, A., Mugford, J. W., Soeung, S., Duffield, J. S., et al. (2008). Intrinsic epithelial cells repair the kidney after injury. Cell Stem Cell 2 (3), 284–291. doi:10.1016/j.stem.2008.01.014
Ju, G. Q., Cheng, J., Zhong, L., Wu, S., Zou, X. Y., Zhang, G. Y., et al. (2015). Microvesicles derived from human umbilical cord mesenchymal stem cells facilitate tubular epithelial cell dedifferentiation and growth via hepatocyte growth factor induction. PLoS One 10 (3), e0121534. doi:10.1371/journal.pone.0121534
Kang, H. M., Huang, S., Reidy, K., Han, S. H., Chinga, F., and Susztak, K. (2016). Sox9-Positive progenitor cells play a key role in renal tubule epithelial regeneration in mice. Cell Rep. 14 (4), 861–871. doi:10.1016/j.celrep.2015.12.071
Kapitsinou, P. P., Jaffe, J., Michael, M., Swan, C. E., Duffy, K. J., Erickson-Miller, C. L., et al. (2012). Preischemic targeting of HIF prolyl hydroxylation inhibits fibrosis associated with acute kidney injury. Am. J. Physiol. Ren. Physiol. 302 (9), F1172–F1179. doi:10.1152/ajprenal.00667.2011
Karasawa, K., Asano, K., Moriyama, S., Ushiki, M., Monya, M., Iida, M., et al. (2015). Vascular-resident CD169-positive monocytes and macrophages control neutrophil accumulation in the kidney with ischemia-reperfusion injury. J. Am. Soc. Nephrol. 26 (4), 896–906. doi:10.1681/asn.2014020195
Kefaloyianni, E., Muthu, M. L., Kaeppler, J., Sun, X., Sabbisetti, V., Chalaris, A., et al. (2016). ADAM17 substrate release in proximal tubule drives kidney fibrosis. JCI Insight 1 (13), e87023. doi:10.1172/jci.insight.87023
Kim, J., Seok, Y. M., Jung, K. J., and Park, K. M. (2009). Reactive oxygen species/oxidative stress contributes to progression of kidney fibrosis following transient ischemic injury in mice. Am. J. Physiol. Ren. Physiol. 297 (2), F461–F470. doi:10.1152/ajprenal.90735.2008
Kim, J. Y., Bai, Y., Jayne, L. A., Abdulkader, F., Gandhi, M., Perreau, T., et al. (2020). SOX9 promotes stress-responsive transcription of VGF nerve growth factor inducible gene in renal tubular epithelial cells. J. Biol. Chem. 295 (48), 16328–16341. doi:10.1074/jbc.RA120.015110
Kim, M. G., Yang, J., Ko, Y. S., Lee, H. Y., Oh, S. W., Cho, W. Y., et al. (2019). Impact of aging on transition of acute kidney injury to chronic kidney disease. Sci. Rep. 9 (1), 18445. doi:10.1038/s41598-019-54585-1
Kulkarni, O. P., Hartter, I., Mulay, S. R., Hagemann, J., Darisipudi, M. N., Kumar Vr, S., et al. (2014). Toll-like receptor 4-induced IL-22 accelerates kidney regeneration. J. Am. Soc. Nephrol. 25 (5), 978–989. doi:10.1681/asn.2013050528
Kumar, S., Liu, J., Pang, P., Krautzberger, A. M., Reginensi, A., Akiyama, H., et al. (2015). Sox9 activation highlights a cellular pathway of renal repair in the acutely injured mammalian kidney. Cell Rep. 12 (8), 1325–1338. doi:10.1016/j.celrep.2015.07.034
Lazzeri, E., Angelotti, M. L., Peired, A., Conte, C., Marschner, J. A., Maggi, L., et al. (2018). Endocycle-related tubular cell hypertrophy and progenitor proliferation recover renal function after acute kidney injury. Nat. Commun. 9 (1), 1344. doi:10.1038/s41467-018-03753-4
Lee, S., Huen, S., Nishio, H., Nishio, S., Lee, H. K., Choi, B. S., et al. (2011). Distinct macrophage phenotypes contribute to kidney injury and repair. J. Am. Soc. Nephrol. 22 (2), 317–326. doi:10.1681/asn.2009060615
Li, Y., Zhai, P., Zheng, Y., Zhang, J., Kellum, J. A., and Peng, Z. (2020). Csf2 attenuated sepsis-induced acute kidney injury by promoting alternative macrophage transition. Front. Immunol. 11, 1415. doi:10.3389/fimmu.2020.01415
Lin, S. L., Li, B., Rao, S., Yeo, E. J., Hudson, T. E., Nowlin, B. T., et al. (2010). Macrophage Wnt7b is critical for kidney repair and regeneration. Proc. Natl. Acad. Sci. U. S. A. 107 (9), 4194–4199. doi:10.1073/pnas.0912228107
Liu, D., Cheng, F., Pan, S., and Liu, Z. (2020). Stem cells: A potential treatment option for kidney diseases. Stem Cell Res. Ther. 11 (1), 249. doi:10.1186/s13287-020-01751-2
Liu, J., Krautzberger, A. M., Sui, S. H., Hofmann, O. M., Chen, Y., Baetscher, M., et al. (2014). Cell-specific translational profiling in acute kidney injury. J. Clin. Invest. 124 (3), 1242–1254. doi:10.1172/jci72126
Lu, Y., Wang, L., Zhang, M., and Chen, Z. (2022). Mesenchymal stem cell-derived small extracellular vesicles: A novel approach for kidney disease treatment. Int. J. Nanomedicine 17, 3603–3618. doi:10.2147/ijn.s372254
Luo, F., Li, Y., Zhang, Y., Song, Y., and Diao, J. (2022). Bibliometric analysis of IgA vasculitis nephritis in children from 2000 to 2022. Front. Public Health 10, 1020231. doi:10.3389/fpubh.2022.1020231
Lv, L. L., Feng, Y., Wen, Y., Wu, W. J., Ni, H. F., Li, Z. L., et al. (2018). Exosomal CCL2 from tubular epithelial cells is critical for albumin-induced tubulointerstitial inflammation. J. Am. Soc. Nephrol. 29 (3), 919–935. doi:10.1681/asn.2017050523
Ma, S., Meng, Z., Chen, R., and Guan, K. L. (2019). The hippo pathway: Biology and pathophysiology. Annu. Rev. Biochem. 88, 577–604. doi:10.1146/annurev-biochem-013118-111829
Megyesi, J., Andrade, L., Vieira, J. M., Safirstein, R. L., and Price, P. M. (2001). Positive effect of the induction of p21WAF1/CIP1 on the course of ischemic acute renal failure. Kidney Int. 60 (6), 2164–2172. doi:10.1046/j.1523-1755.2001.00044.x
Megyesi, J., Safirstein, R. L., and Price, P. M. (1998). Induction of p21WAF1/CIP1/SDI1 in kidney tubule cells affects the course of cisplatin-induced acute renal failure. J. Clin. Invest. 101 (4), 777–782. doi:10.1172/jci1497
Meng, X., Jin, J., and Lan, H. Y. (2022). Driving role of macrophages in transition from acute kidney injury to chronic kidney disease. Chin. Med. J. Engl. 135 (7), 757–766. doi:10.1097/cm9.0000000000002100
Merigó, J. M., and Núñez, A. (2016). Influential journals in health research: A bibliometric study. Glob. Health 12 (1), 46. doi:10.1186/s12992-016-0186-4
Mertens, C., Kuchler, L., Sola, A., Guiteras, R., Grein, S., Brüne, B., et al. (2020). Macrophage-derived iron-bound lipocalin-2 correlates with renal recovery markers following sepsis-induced kidney damage. Int. J. Mol. Sci. 21 (20), 7527. doi:10.3390/ijms21207527
Nie, H., Zhao, Z., Zhou, D., Li, D., Wang, Y., Ma, Y., et al. (2023). Activated SOX9+ renal epithelial cells promote kidney repair through secreting factors. Cell Prolif. 56, e13394. doi:10.1111/cpr.13394
Qu, N. Y., Zhang, Z. H., Zhang, X. X., Xie, W. W., and Niu, X. Q. (2019). Microvesicles containing microRNA-216a secreted by tubular epithelial cells participate in renal interstitial fibrosis through activating PTEN/AKT pathway. Eur. Rev. Med. Pharmacol. Sci. 23 (15), 6629–6636. doi:10.26355/eurrev_201908_18552
Ranghino, A., Bruno, S., Bussolati, B., Moggio, A., Dimuccio, V., Tapparo, M., et al. (2017). The effects of glomerular and tubular renal progenitors and derived extracellular vesicles on recovery from acute kidney injury. Stem Cell Res. Ther. 8 (1), 24. doi:10.1186/s13287-017-0478-5
Rayego-Mateos, S., Campillo, S., Rodrigues-Diez, R. R., Tejera-Muñoz, A., Marquez-Exposito, L., Goldschmeding, R., et al. (2021). Interplay between extracellular matrix components and cellular and molecular mechanisms in kidney fibrosis. Clin. Sci. (Lond). 135 (16), 1999–2029. doi:10.1042/cs20201016
Rayego-Mateos, S., Marquez-Expósito, L., Rodrigues-Diez, R., Sanz, A. B., Guiteras, R., Doladé, N., et al. (2022). Molecular mechanisms of kidney injury and repair. Int. J. Mol. Sci. 23 (3), 1542. doi:10.3390/ijms23031542
Raza, S., Jokl, E., Pritchett, J., Martin, K., Su, K., Simpson, K., et al. (2021). SOX9 is required for kidney fibrosis and activates NAV3 to drive renal myofibroblast function. Sci. Signal 14 (672), eabb4282. doi:10.1126/scisignal.abb4282
Ruiz-Ortega, M., Rayego-Mateos, S., Lamas, S., Ortiz, A., and Rodrigues-Diez, R. R. (2020). Targeting the progression of chronic kidney disease. Nat. Rev. Nephrol. 16 (5), 269–288. doi:10.1038/s41581-019-0248-y
Seo, E., Kim, W. Y., Hur, J., Kim, H., Nam, S. A., Choi, A., et al. (2016). The Hippo-Salvador signaling pathway regulates renal tubulointerstitial fibrosis. Sci. Rep. 6, 31931. doi:10.1038/srep31931
Skrypnyk, N. I., Sanker, S., Skvarca, L. B., Novitskaya, T., Woods, C., Chiba, T., et al. (2016). Delayed treatment with PTBA analogs reduces postinjury renal fibrosis after kidney injury. Am. J. Physiol. Ren. Physiol. 310 (8), F705–f716. doi:10.1152/ajprenal.00503.2015
Squillaro, T., Peluso, G., and Galderisi, U. (2016). Clinical trials with mesenchymal stem cells: An update. Cell Transpl. 25 (5), 829–848. doi:10.3727/096368915x689622
Stallons, L. J., Whitaker, R. M., and Schnellmann, R. G. (2014). Suppressed mitochondrial biogenesis in folic acid-induced acute kidney injury and early fibrosis. Toxicol. Lett. 224 (3), 326–332. doi:10.1016/j.toxlet.2013.11.014
Susantitaphong, P., Cruz, D. N., Cerda, J., Abulfaraj, M., Alqahtani, F., Koulouridis, I., et al. (2013). World incidence of AKI: A meta-analysis. Clin. J. Am. Soc. Nephrol. 8 (9), 1482–1493. doi:10.2215/cjn.00710113
Synnestvedt, M. B., Chen, C., and Holmes, J. H. (2005). CiteSpace II: Visualization and knowledge discovery in bibliographic databases. AMIA Annu. Symp. Proc. 2005, 724–728.
Tögel, F., Hu, Z., Weiss, K., Isaac, J., Lange, C., and Westenfelder, C. (2005). Administered mesenchymal stem cells protect against ischemic acute renal failure through differentiation-independent mechanisms. Am. J. Physiol. Ren. Physiol. 289 (1), F31–F42. doi:10.1152/ajprenal.00007.2005
van Eck, N. J., and Waltman, L. (2010). Software survey: VOSviewer, a computer program for bibliometric mapping. Scientometrics 84 (2), 523–538. doi:10.1007/s11192-009-0146-3
Venkatachalam, M. A., Weinberg, J. M., Kriz, W., and Bidani, A. K. (2015). Failed tubule recovery, AKI-CKD transition, and kidney disease progression. J. Am. Soc. Nephrol. 26 (8), 1765–1776. doi:10.1681/asn.2015010006
Vinuesa, E., Hotter, G., Jung, M., Herrero-Fresneda, I., Torras, J., and Sola, A. (2008). Macrophage involvement in the kidney repair phase after ischaemia/reperfusion injury. J. Pathol. 214 (1), 104–113. doi:10.1002/path.2259
Wu, H., Zhou, Y., Xu, L., Tong, L., Wang, Y., Liu, B., et al. (2021). Mapping knowledge structure and research frontiers of ultrasound-induced blood-brain barrier opening: A scientometric study. Front. Neurosci. 15, 706105. doi:10.3389/fnins.2021.706105
Xu, D., Chen, P. P., Zheng, P. Q., Yin, F., Cheng, Q., Zhou, Z. L., et al. (2021). KLF4 initiates sustained YAP activation to promote renal fibrosis in mice after ischemia-reperfusion kidney injury. Acta Pharmacol. Sin. 42 (3), 436–450. doi:10.1038/s41401-020-0463-x
Xu, J., Li, P. X., Wu, J., Gao, Y. J., Yin, M. X., Lin, Y., et al. (2016). Involvement of the hippo pathway in regeneration and fibrogenesis after ischaemic acute kidney injury: YAP is the key effector. Clin. Sci. (Lond). 130 (5), 349–363. doi:10.1042/cs20150385
Yang, L., Besschetnova, T. Y., Brooks, C. R., Shah, J. V., and Bonventre, J. V. (2010). Epithelial cell cycle arrest in G2/M mediates kidney fibrosis after injury. Nat. Med. 16 (5), 535–543. doi:10.1038/nm.2144
Yu, L., Liu, S., Wang, C., Zhang, C., Wen, Y., Zhang, K., et al. (2021). Embryonic stem cell-derived extracellular vesicles promote the recovery of kidney injury. Stem Cell Res. Ther. 12 (1), 379. doi:10.1186/s13287-021-02460-0
Yu, S. M., and Bonventre, J. V. (2020). Acute kidney injury and maladaptive tubular repair leading to renal fibrosis. Curr. Opin. Nephrol. Hypertens. 29 (3), 310–318. doi:10.1097/mnh.0000000000000605
Zhang, C., Shang, Y., Chen, X., Midgley, A. C., Wang, Z., Zhu, D., et al. (2020). Supramolecular nanofibers containing arginine-glycine-aspartate (RGD) peptides boost therapeutic efficacy of extracellular vesicles in kidney repair. ACS Nano 14 (9), 12133–12147. doi:10.1021/acsnano.0c05681
Zheng, Z., Li, C., Shao, G., Li, J., Xu, K., Zhao, Z., et al. (2021). Hippo-YAP/MCP-1 mediated tubular maladaptive repair promote inflammation in renal failed recovery after ischemic AKI. Cell Death Dis. 12 (8), 754. doi:10.1038/s41419-021-04041-8
Zhou, W., Zhao, S., Xu, S., Sun, Z., Liang, Y., and Ding, X. (2020). RacGAP1 ameliorates acute kidney injury by promoting proliferation and suppressing apoptosis of renal tubular cells. Biochem. Biophys. Res. Commun. 527 (3), 624–630. doi:10.1016/j.bbrc.2020.04.140
Zhou, Y., Hu, F., Cui, Y., Wu, H., Hu, S., and Wei, W. (2022). Bibliometric analysis of research on immunogenic cell death in cancer. Front. Pharmacol. 13, 1029020. doi:10.3389/fphar.2022.1029020
Zhu, F., Chong Lee Shin, O. L. S., Pei, G., Hu, Z., Yang, J., Zhu, H., et al. (2017). Adipose-derived mesenchymal stem cells employed exosomes to attenuate AKI-CKD transition through tubular epithelial cell dependent Sox9 activation. Oncotarget 8 (41), 70707–70726. doi:10.18632/oncotarget.19979
Zhu, G., Pei, L., Lin, F., Yin, H., Li, X., He, W., et al. (2019). Exosomes from human-bone-marrow-derived mesenchymal stem cells protect against renal ischemia/reperfusion injury via transferring miR-199a-3p. J. Cell Physiol. 234 (12), 23736–23749. doi:10.1002/jcp.28941
Zou, X., Gu, D., Xing, X., Cheng, Z., Gong, D., Zhang, G., et al. (2016). Human mesenchymal stromal cell-derived extracellular vesicles alleviate renal ischemic reperfusion injury and enhance angiogenesis in rats. Am. J. Transl. Res. 8 (10), 4289–4299.
Keywords: bibliometrics, acute kidney injury, kidney repair, research hotspots, visualization
Citation: Li J and Gong X (2023) Bibliometric and visualization analysis of kidney repair associated with acute kidney injury from 2002 to 2022. Front. Pharmacol. 14:1101036. doi: 10.3389/fphar.2023.1101036
Received: 17 November 2022; Accepted: 12 April 2023;
Published: 20 April 2023.
Edited by:
Joseph Bonventre, Brigham and Women’s Hospital and Harvard Medical School, United StatesReviewed by:
Qingjun Pan, Affiliated Hospital of Guangdong Medical University, ChinaShan Mou, Shanghai Jiao Tong University, China
Copyright © 2023 Li and Gong. This is an open-access article distributed under the terms of the Creative Commons Attribution License (CC BY). The use, distribution or reproduction in other forums is permitted, provided the original author(s) and the copyright owner(s) are credited and that the original publication in this journal is cited, in accordance with accepted academic practice. No use, distribution or reproduction is permitted which does not comply with these terms.
*Correspondence: Xuezhong Gong, c2huYW5zaGFuQHllYWgubmV0