- 1UMR152 PHARMADEV, IRD, UPS, Université de Toulouse, Toulouse, France
- 2Université Paris-Saclay, CNRS, BioCIS, Orsay, France
- 3CNR Du Paludisme, AP-HP, Hôpital Bichat–Claude Bernard, Paris, France
- 4Joint Research Unit 1158 BioEcoAgro, University Lille, JUNIA, INRAE, University Liège, UPJV, University Artois, ULCO, VilleneuveD’Ascq, France
- 5Laboratoire de Recherche en Sciences Végétales (UMR 5546), CNRS, Université de Toulouse, Toulouse, France
- 6MetaboHUB, National Infrastructure of Metabolomics and Fluxomics, Toulouse, France
- 7Facultad de Farmacia y Bioquímica, Universidad Nacional de la Amazonía Peruana (UNAP), Iquitos, Peru
- 8Centro de Investigación de Recursos Naturales, Universidad Nacional de la Amazonía Peruana (UNAP), Iquitos, Peru
- 9Facultad de Ingeniería Química, Universidad Nacional de la Amazonía Peruana (UNAP), Iquitos, Peru
- 10International Joint Laboratory of Molecular Anthropological Oncology (LOAM), National Cancer Institute, Lima, Perú
High prevalence of parasitic or bacterial infectious diseases in some world areas is due to multiple reasons, including a lack of an appropriate health policy, challenging logistics and poverty. The support to research and development of new medicines to fight infectious diseases is one of the sustainable development goals promoted by World Health Organization (WHO). In this sense, the traditional medicinal knowledge substantiated by ethnopharmacology is a valuable starting point for drug discovery. This work aims at the scientific validation of the traditional use of Piper species (“Cordoncillos”) as firsthand anti-infectious medicines. For this purpose, we adapted a computational statistical model to correlate the LCMS chemical profiles of 54 extracts from 19 Piper species to their corresponding anti-infectious assay results based on 37 microbial or parasites strains. We mainly identified two groups of bioactive compounds (called features as they are considered at the analytical level and are not formally isolated). Group 1 is composed of 11 features being highly correlated to an inhibiting activity on 21 bacteria (principally Gram-positive strains), one fungus (C. albicans), and one parasite (Trypanosoma brucei gambiense). The group 2 is composed of 9 features having a clear selectivity on Leishmania (all strains, both axenic and intramacrophagic). Bioactive features in group 1 were identified principally in the extracts of Piper strigosum and P. xanthostachyum. In group 2, bioactive features were distributed in the extracts of 14 Piper species. This multiplexed approach provided a broad picture of the metabolome as well as a map of compounds putatively associated to bioactivity. To our knowledge, the implementation of this type of metabolomics tools aimed at identifying bioactive compounds has not been used so far.
Introduction
In Amazonia, tropical diseases have a high prevalence due to multiple reasons, including a lack of an appropriate health policy, a climate conducive to diseases, challenging logistics and poverty. Neglected tropical diseases (NTDs) are a group of 20 conditions (caused by viruses, protozoa, helminths and bacteria) prioritized by the World Health Organization (WHO). The NTDs are responsible for approximately 200,000 deaths and the loss of 19 million disability-adjusted life years (DALYs) annually. In 2020, new infections by Plasmodium spp. and Leishmania spp. worldwide were estimated at 241 and 1 million, respectively. Even if Plasmodium is not strictly classified as a NTD, it caused around 627,000 deaths in 2020, with two-thirds of these deaths (470,000) being due to treatment disruptions during the COVID-19 pandemic (WHO, 2021). Leishmania spp. and Trypanosoma spp. are protozoan parasites responsible for diseases labeled as NTDs stricto sensu. The ambitious 10-year WHO plan to defeat NTDs is based on three pillars: control, elimination and eradication (WHO, 2022a). The support of research and development of new medicines to fight infectious diseases is one of the sustainable development goals promoted by WHO (WHO, 2022b). Drug resistance is a widespread concern in medical care, and the increase of drug-resistant infections is faster than the pace of the development of new drugs approved for use in humans. Therefore, every input into the search for new antimicrobial agents is welcome (Yan et al., 2021). Ethnopharmacology is the interdisciplinary study of the knowledge or practices of traditional cultures related to plants, animals, or mineral used for therapeutic purposes (SFE, 2022). Such knowledge can be valued as the starting point for drug discovery, especially in the case of infectious diseases which are prevalent among such populations.
Piperaceae is a pantropical family composed of eight genera. Two genera are the most representative in this family: Piper and Peperomia (Vásquez-Ocmín et al., 2017; Salehi et al., 2019). Among these, Piper is the most diverse and representative genus, encompassing ca. 2600 species (Trujillo et al., 2022). Besides pungent compounds, many Piper species produce essential oils and are hence highly aromatic, explaining their use for cooking and medicinal purposes (Ruiz-Vásquez et al., 2022). In Peru, Piper spp. (called “Cordoncillos”) have been used for a very long time in traditional medicine as a “first-hand treatment”, especially in the villages far away from major cities and medical care. The necessary scientific validation of traditional uses implies the isolation of the main bioactive compounds by successive fractionations using chromatographic techniques and biological activity testing (bio-guided isolation). Such an approach has evidenced interesting activities for Piper spp. as anti-inflammatory, antiparasitic, antibacterial, etc. (Mgbeahuruike et al., 2017; Durant-Archibold et al., 2018). Even if bio-guided fractionation is still used with some success in the natural product chemistry field, current trends involve streamlining the cost, effort, and time (Vásquez-Ocmín et al., 2022). Metabolomics is a holistic approach allowing rapid detection and putative identification (i.e., annotation) of numerous metabolites, along with data mining on multiple datasets. Metabolomics aims to comprehensively map all biochemical reactions in a given system and has become a key to deciphering their biological roles, hence becoming mainstream in natural product chemistry and drug discovery. This approach can be divided into targeted and untargeted analyses (Alarcon-Barrera et al., 2022). Two spectral techniques are regularly employed for metabolomics analysis: mass spectrometry (MS) and nuclear magnetic resonance (NMR) spectroscopy, both being assisted by bioinformatics and statistical analysis. Our team has solid expertise in implementing straightforward and effective workflows to decipher anti-infectious compounds using untargeted metabolomics (Vásquez-Ocmín et al., 2021a).
This work aims at the scientific validation of the use of Piper species as firsthand anti-infectious medicines. We adapted a statistical model to correlate the LCMS chemical profiles of 54 extracts from 19 Piper species to their corresponding anti-infectious assay results based on 37 microbial or parasites strains. This multiplexed approach led to the annotation of compounds bearing these activities.
Materials and methods
Ethnopharmacology and plant material
Based on the encouraging previous results of our research group on the anti-infectious activities of Piper species (Vásquez-Ocmín et al., 2021a), ethnopharmacological surveys were realized as part of the project “Compuestos bioactivos in vitro a partir de especies vegetales Amazónicas”. The surveys were undertaken between July and December 2020 in communities of three Amazonian regions of Peru: Cusco, Loreto and San Martin. People of these communities were interrogated about the main use of medicinal Piper species (“Cordoncillos”), including their use against malaria, “uta” (local name for leishmaniasis) and bacteria. According to ethnopharmacological studies, we collected 8 species in Cusco, 10 species in Loreto, and 1 species in San Martin.
Thus, different parts of these nineteen plants were collected, then identified and deposited in the Herbarium Amazonense (AMAZ), Iquitos, Peru. This project was realized in accordance with the guidelines pertaining to ethnopharmacological studies and edited by the Laboratorio de Investigacion de Productos Naturales Antiparasitarios de la Amazonia (LIPNAA) of the Universidad Nacional de la Amazonia Peruana (UNAP) (Resolucion Rectoral Nº 1312-2020-UNAP).
Preparation of plant extracts
One hundred grams of each air-dried and ground plant (leaves, leaves and stems, aerial parts) were soaked in 1 L of each of the following solvents successively: hexane, methylene chloride, methanol, ethanol/water (7:3, v/v), and aqueous. These macerations were performed during 21 days each for hexane, methylene chloride and ethanol/water, and for 15 days in methanol and aqueous extracts, with solvents being changed every 3 days. The extracts were then filtered through a paper filter and evaporated under reduced pressure below 40 °C. Dry extracts were stored at −4 °C until use. Extracts were solubilized in DMSO at a concentration of 10 mg/mL for in vitro bioassays and HPLC-MS analysis.
Cell lines and microorganism culture
HUVEC cells: Human umbilical vein endothelial cells (HUVECs) were maintained in culture in RPMI 1640 medium (Invitrogen, Life Technologies) supplemented with 10% heat-inactivated fetal bovine serum (Invitrogen Life Technologies) and 1 mM glutamine (Invitrogen Life Technologies) (Vásquez-Ocmín et al., 2018).
RAW264.7: The mouse monocyte/macrophage cell line RAW264.7 was maintained in culture in DMEM (Invitrogen, Life Technologies) supplemented with 10% heat-inactivated fetal bovine serum (Vásquez-Ocmín et al., 2018).
Plasmodium: The P. falciparum chloroquine-sensitive strain 3D7 was obtained from the Malaria French National Reference Center (CNR Paludisme, Hôpital Bichat Claude Bernard, Paris) and was maintained in O+ human erythrocytes in RPMI 1640 medium (Invitrogen, Life Technologies) supplemented with 25 mM HEPES (Sigma), 25 mM NaHCO3 (Sigma) and 0.5% Albumax II (Invitrogen, Life Technologies) at 37 °C in a candle-jar method following the Trager and Jensen conditions (Trager and Jensen, 1976; Lambros and Vanderberg, 1979; Vásquez-Ocmín et al., 2018).
Leishmania: The Leishmania donovani MHOM/ET/67/HU3, L. amazonensis MHOM/BR/73/M2269, and L. braziliensis MHOM/BR/75/M2903 b strains were maintained routinely in vitro culture. Passages in macrophages RAW 264.7 were carried out regularly to preserve the virulence of the strain, then they were recovered for culture maintenance in the promastigote form. For assays, parasites were maintained as promastigote forms in M-199 medium (Sigma) supplemented with 40 mM HEPES, 100 mM adenosine, 0.5 mg/L hemin and 10% fetal bovine serum (FBS) at 25 °C in a dark environment (Balaraman et al., 2015; Vásquez-Ocmín et al., 2018).
Trypanosomes: Trypomastigotes of T b. gambiense (FéoITMAP/1893 strain) were grown in HMI9 medium constituted of prepacked Iscove’s modified Dulbecco’s medium (Thermo-Fisher, Les Ulis, France) supplemented with 36 mM NaHCO3, 1 mM hypoxanthine, 0.05 mM bathocuproine, 0.16 mM thymidine, 0.2 mM 2-mercapthoethanol, 1.5 mM L-cysteine, 10% heat-inactivated foetal bovine serum, 100 IU penicillin and 100 μg mL-1 streptomycin. Parasites were incubated in a Series 8,000 direct-heat CO2 incubator (Thermo-Fisher, Les Ulis, France) at 37 °C in a water-saturated atmosphere containing 5% CO2 (Pomel et al., 2015; Vásquez-Ocmín et al., 2018).
Bacteria and yeast: Most microbial strains were diluted in BH medium (Brain Heart), MH medium (Mueller Hinton) for Candida sp. and Mycobacterium sp., or WW medium (Wilkins-West) for Streptococcus sp. and stored at −20 °C. Then strains were subcultured at 20 °C on RC (Ringer Cysteine) medium for 24 h before tests (Bocquet et al., 2019).
In vitro antiprotozoal activity
In vitro antiplasmodial activity on P. falciparum
Assays were realized with a suspension of erythrocytes at 1% parasitemia containing more than 85% ring stage obtained by repeated sorbitol treatment and incubated with the compounds at concentrations ranging between 0.49 and 100 µM or µg/mL, obtained by serial dilution, in duplicates. Two controls were used, parasites without drug and parasites with chloroquine at concentrations ranging between 0.49 and 1000 nM. Plates were incubated for 44 h at 37 °C in a candle jar (Vásquez-Ocmín et al., 2018; 2021a).
In vitro antileishmanial activity on L. donovani, L. amazonensis, and L. braziliensis axenic amastigotes
A suspension of promastigotes in growth plateau-phase was incubated at 37 °C in 5% CO2 for 3 days to obtain the amastigote form in promastigote medium supplemented with 2 mM CaCl2, 2 mM MgCl2, and a pH adjusted to 5.5. The axenic amastigote suspension containing 1.106 parasites/mL was incubated for 72 h with the compounds at 37 °C in 5% CO2 in the dark. Tested compounds or extracts were obtained by serial dilution and ranged between 0.49 and 100 µM or µg/mL. There were two controls: parasites without drug and parasites treated with miltefosine at the same concentrations as the compounds tested (Vásquez-Ocmín et al., 2018; 2021a).
In vitro antileishmanial activity on L. donovani, L. amazonenesis and L. braziliensis intramacrophage amastigote form
Macrophages were seeded into a 96 well microtitration plate at a density of 100,000 cells/well in 100 μL and incubated in a 5% CO2 at 37 °C for 24 h. After removing the medium, cells were incubated with 100 μL of fresh DMEM containing a suspension of promastigotes in the growth plateau phase at a rate of 1 cell per 10 parasites. After incubation under a 5% CO2 atmosphere at 37 °C for 24 h (the time needed by the parasite to infect the macrophage), the culture medium was replaced with 100 μL of fresh DMEM with different concentrations of compounds as previously for a new incubation period of 48 h. Controls were parasites alone in DMEM medium, axenic amastigotes, macrophages alone, infected macrophages and infected macrophages with different concentrations of miltefosine (Balaraman et al., 2015; Vásquez-Ocmín et al., 2018; 2021a).
Determination of IC50, CC50 and selectivity index for Plasmodium, Leishmania, and Trypanosoma
After incubation, the plates were subjected to 3 freeze/thaw cycles to achieve complete cell lysis. The cell lysis suspension was diluted 1:1 except for Plasmodium plates that have been diluted 1:10 in lysis buffer (10 mM NaCl, 1 mM Tris HCl pH 8, 2.5 mM EDTA pH 8, 0.05% SDS, 0.01 mg/mL proteinase K and 1X SYBR Green). SYBR Green incorporation in cell DNA amplification was determined using the Master epRealplex cycler® (Eppendorf, France) and the following program to increase SYBR Green incorporation: 90 °C for 1 min, decrease in temperature from 90 °C to 10 °C for 5 min with fluorescence reading at 10 °C for 1 min and a new reading at 10 °C for 2 min. Molecules were tested in duplicate. Compounds with different SYBR Green fluorescence values from duplicates were retested. The cytotoxicity of the compounds was expressed as IC50 (concentration of drug inhibiting the parasite growth by 50%, comparatively to the controls), CC50 (Cytotoxic Concentration 50%: concentration inhibiting macrophages growth by 50%). IC50 and CC50 were calculated by nonlinear regression using ICEstimator 1.2 version (http://www.antimalarial-icestimator.net/MethodIntro.htm). Selectivity index (SI) for antiplasmodial and anti-Trypanosoma activities were calculated as the HUVEC’s CC50 divided to IC50 of Plasmodium 3D7 and Trypanosoma, and for Leishmania assays as the ratio of RAW 364.7 CC50 to intramacrophage amastigote IC50 value (Vásquez-Ocmín et al., 2018; 2021a).
In vitro evaluation on bloodstream form of Trypanosoma brucei gambiense
For each extract, twelve two-fold serial dilutions from 100 μg/mL to 0.049 μg/mL were performed in 96-well microplates in 100 µL HMI9 medium. Parasites were then added to each well to reach a final density of 4.104 cells/mL in 200 µL. Following 3 days of incubation at 37 °C with 5% CO2 in a water-saturated atmosphere, 20 µL of resazurin at 450 µM in water was added to each well for a final concentration of 40.9 nM to evaluate cell viability. Plates were then incubated for 6 h at 37 °C with 5% CO2 in water-saturated atmosphere and the conversion of resazurin to resorufin was quantified by measuring the absorbance at 570 nm (resofurin) and 600 nm (resazurin) using the microplate reader Spark® (Lyon, France) Pentamidine di-isethionate was used as the reference compound (Pomel et al., 2015; Vásquez-Ocmín et al., 2018; 2021a).
Antimicrobial assay
Minimal Inhibitory Concentration (MIC) determinations of crude extracts were carried out using the agar dilution method stipulated by the Clinical and Laboratory Standards Institute (CLSI, 2006). Antimicrobial activity was evaluated for the first time against a panel of 36 pathogenic and multi-drug-resistant bacteria, which, in most cases, have been recently isolated from human infections. For comparison, reference strains from the American Type Culture Collection (ATCC) were included.
The inhibitory concentrations ranged between 0.075 and 1.2 mg/mL in five dilutions (1.2, 0.6, 0.3, 0.15 and 0.075 mg/mL); 0.075 mg/mL was considered a low enough concentration for a preliminary screening. Petri dishes were inoculated with strains (104 CFU, obtained by dilution in brain heart medium, BH) using a Steer’s replicator and were incubated at 37°C for 24 h. MIC was defined as the lowest concentration of extract without bacterial growth after incubation. The extracts with MIC ≤1.2 mg/mL were tested in triplicate at lower concentrations (mean absolute deviation is done for values: 1.2 ± 0.4; 0.6 ± 0.2; 0.3 ± 0.1; 0.15 ± 0.05; 0.075 ± 0.03). The standards (gentamicin, vancomycin, amoxicillin, amphotericin B, fluconazole, and sertaconazole) were tested in triplicate in 12 concentrations ranging from 0.03 to 64 mg/mL.
Cytotoxic assay
Cytotoxicity was evaluated on RAW 264.7 macrophages for Leishmania assays and HUVECs for Plasmodium and Trypanosoma. The cells were seeded at a density of 50,000 cells per well in 100 µl of DMEM in a 96-well microtiter plate. After incubation in a 5% CO2 at 37 °C for 24h, the culture medium was replaced with 100 µl of fresh DMEM containing serial dilutions of the compounds tested. The concentrations of the compounds are the same as for intramacrophage Leishmania or Plasmodium assays. The plates were incubated for 48 h at 37 °C with 5% CO2. Antiprotozoal assays have been performed only with compounds not demonstrating cytotoxicity (Vásquez-Ocmín et al., 2021a).
Liquid chromatography and mass spectrometry data mining
HPLC-MS analyses
Extracts were analyzed by liquid chromatography performed on an Agilent 1260 series HPLC coupled to a 6530 QToF (Agilent Technologies). Chromatography separations were performed on a XSelect CSH C18 column, 130Å, 2.5 µm, 2.1 × 75 mm –(Waters). The mobile phase comprised water (0.1% formic acid) A) and acetonitrile (ACN) B). A stepwise gradient method at a constant flow rate of 0.35 mL/min was applied as follows: 5%–100% B (0–9.5 min), 100% B (4.5 min) and 4 min equilibration at 5% B. The mass spectrometer settings were: positive ESI mode, 50-3200 mass range calibration, and 2 GHz acquisition rate. Ionization source conditions were drying gas temperature 325 °C, drying gas flow rate 10 L/min, nebulizer 35 psig, fragmentor 150 V, and skimmer 65 V. Range of m/z was 200–1700. Purine C5H4N4 [M + H]+ ion (m/z 121.050873) and the hexakis-(1H,1H, 3H-tetrafluoropropoxy)-phosphazene C18H18F24N3O6P3 [M + H]+ ion (m/z 922.009798) were used as internal lock masses. Full scans were acquired at a resolution of 11,000 (at m/z 922). MS-MS acquisitions were performed using three collision energies: 10, 20, and 40 eV. Three of the most intense ions (top 3) per cycle were selected. MS-MS acquisition parameters were defined as follows: m/z range 100–1200, default charge of 1, minimum intensity of 5,000 counts, rate/time = 3 spectra/s, isolation width: Narrow (1.3 u).
Data processing
For untargeted metabolomics, the LC-MS data were processed according to the MSCleanR workflow (Fraisier-Vannier et al., 2020; Vásquez-Ocmín et al., 2021b). Briefly, a batch in positive ionization (PI) was processed with MS-DIAL version 4.90 (Tsugawa et al., 2015). MS1 and MS2 tolerances were set to 0.01 and 0.05 Da, respectively, in centroid mode for each dataset. Peaks were aligned on a QC reference with an RT tolerance of 0.2 min, a mass tolerance of 0.015 Da, and a minimum peak height detection at 1 × 105. MS-DIAL data was deconvoluted together with MS-CleanR by selecting all filters with a minimum blank ratio set to 0.8 and a maximum relative standard deviation (RSD) set to 40. The maximum mass difference for feature relationship detection was set to 0.005 Da, and the maximum RT difference was set to 0.025 min. Pearson correlation links were used with a correlation ≥0.8 and a p-value significance threshold of 0.05. Two peaks were kept in each cluster for further database requests and the kept features were annotated with MS-FINDER version 3.52 (Tsugawa et al., 2016). The MS1 and MS2 tolerances were set to 5 and 15 ppm, respectively. The formula finder was exclusively based on C, H, O, and N atoms. Three levels of compounds annotation from several sets of data were carried out. Metabolite annotation at level 1 using MS-DIAL: i) the experimental LC-MS/MS data of 500 compounds (retention time, exact mass and fragmentation) were used as references; ii) the Mass spectral records from MS-DIAL, MONA (MassBank of North America), and GNPS (Global Natural Product Social Molecular Networking) databases were used for spectral match applying a dot product score cut-off of 800. Metabolite annotation at level 2 was prioritized according to: i) a search would be made using MSFinder for a match with compounds identified in the literature for the Piper genus (genus level) and Piperaceae family (family level) (Dictionary of Natural Products version 28.2, CRC Press) based on exact mass and in silico fragmentation. For metabolite annotation level 3, a search was made using MS-FINDER for a match with natural compounds included in their databases embedded (PlantCyc, ChEBI, NANPDB, COCONUT, and KNApSAcK) (generic level) (Vásquez-Ocmín et al., 2023).
Mass spectra similarity networking was carried out from PI mode using MetGem (Olivon et al., 2018) on the final annotated. msp file and metadata file for PI obtained with MSCleanR. Values used were MS2 m/z tolerance = 0.02 Da, minimum matched peaks = 4 and minimal cosine score value = 0.7. The visualization of the molecular network (MN) was performed on Cytoscape version 3.9.1 (Shannon et al., 2003). The list of compound mass, retention time, row ID and peak height was exported in CSV format. Then, this format was used for the statistical data analysis.
Statistical analyses
The final annotated untargeted metabolome feature matrix and biological assay results datasets were analyzed using the R package MixOmics (http://mixomics.org/), which is dedicated to the integrative analysis of ‘omics’ data (Le Cao et al., 2016). Biological assays results expressed in IC50 were transformed in pIC50 (-log10 [IC50]) for further statistical correlation analysis. MIC values were log transformed. The LC-MS dataset (m/z × RT × peak area) was normalized to total ion chromatogram and scaled to unit variance. A vertical integration approach has been afforded to leverage on multiplexed measurements for the same extract (González et al., 2008). To highlight the overall correlation between chemical fingerprints and biological assays. A regularized Canonical Correlation Analysis (rCCA) was done using a cross validation approach for tuning regularization parameters. The correlation structure of the two-block data matrices was displayed on clustered image map (CIM) and relevance network using 0.3 as cutoff correlation value.
Results
Ethnopharmacological survey
Our set of 19 samples was collected in three Amazonian regions of Peru, where the use of these species of “Cordoncillos” in traditional medicine is widespread. All information about the collected samples are presented in Supplementary Table S1. Among the plants studied in this work, 10 (52.6%) had not been subject to chemical analysis so far. In our previous work (Vásquez-Ocmín et al., 2021a), Piper casapiense, P. strigosum and P. pseudoarboreum showed promising antiprotozoal activity. Thus, these plants were re-collected in other areas of the Loreto region.
Anti-infectious activity
Antiprotozoal activity
The IC50 values for antiplasmodial, antileishmanial and antitrypanosomal activities of each extract are shown in Table 1. We considered an extract active and worthy to be further studied when its IC50 value was below 10 μg/ml. Four extracts were active in this case regarding the 3D7 P. falciparum chloroquine-sensitive strain assay: the hexane and methylene chloride extracts of P. crassinervium, the methanol extract of P. stellipilum, and the methylene chloride extract of P. xanthostachyum. Only the methylene chloride extracts of P. crassinervium, and P. oblongum were active on three strains of Leishmania (both axenic and intra-macrophagic amastigotes). For the other active extracts, six were not active on intra-macrophagic forms: hexane and methylene chloride of P. heterophyllum, hexane, methylene chloride and water extracts of P. sancti-felicis (L. amazonensis) and a hexane extract of P. crassinervium (L. donovani). Hexane extract of P. “cordatomentosa” was active on all the strains, both axenic and intramacrophagic amastigotes, except on axenic amastigote forms of L. donovani. Five extracts were active only on L. amazonenesis, and L. braziliensis (both axenic and intramacrophagic amastigotes): methylene chloride extracts of P. calvescentinerve, Piper crassinervium, and P. oblongum, and the methylene chloride and hexane extracts of Piper “cordatomentosa”, and P. crassinervium. Five extracts were active only on L. donovani, and L. braziliensis (both axenic and intramacrophagic amastigotes), the methylene chloride extract of P. crassinervium, and the hexane and methylene chloride extracts of P. heterophyllum, and P. oblongum. Hexane extract of P. glabribaccum was not active on axenic amastigote forms of L. donovani. Two extracts were active on L. donovani, and L. amazonenesis, both forms, methylene chloride extracts of P. crassinervium, and P. oblongum. Selective activity on L. braziliensis were observed for twelve extracts, the hexane and methylene chloride extracts Piper sancti-felicis, Piper stellipilum, and P. xanthostachyum; hexane and methylene chloride extracts of P. stellipilum, and hexane and methylene chloride extracts of P. calvescentinerve and of P. divaricatum. None of the extracts showed selective activity on L. donovani or L. amazonensis.
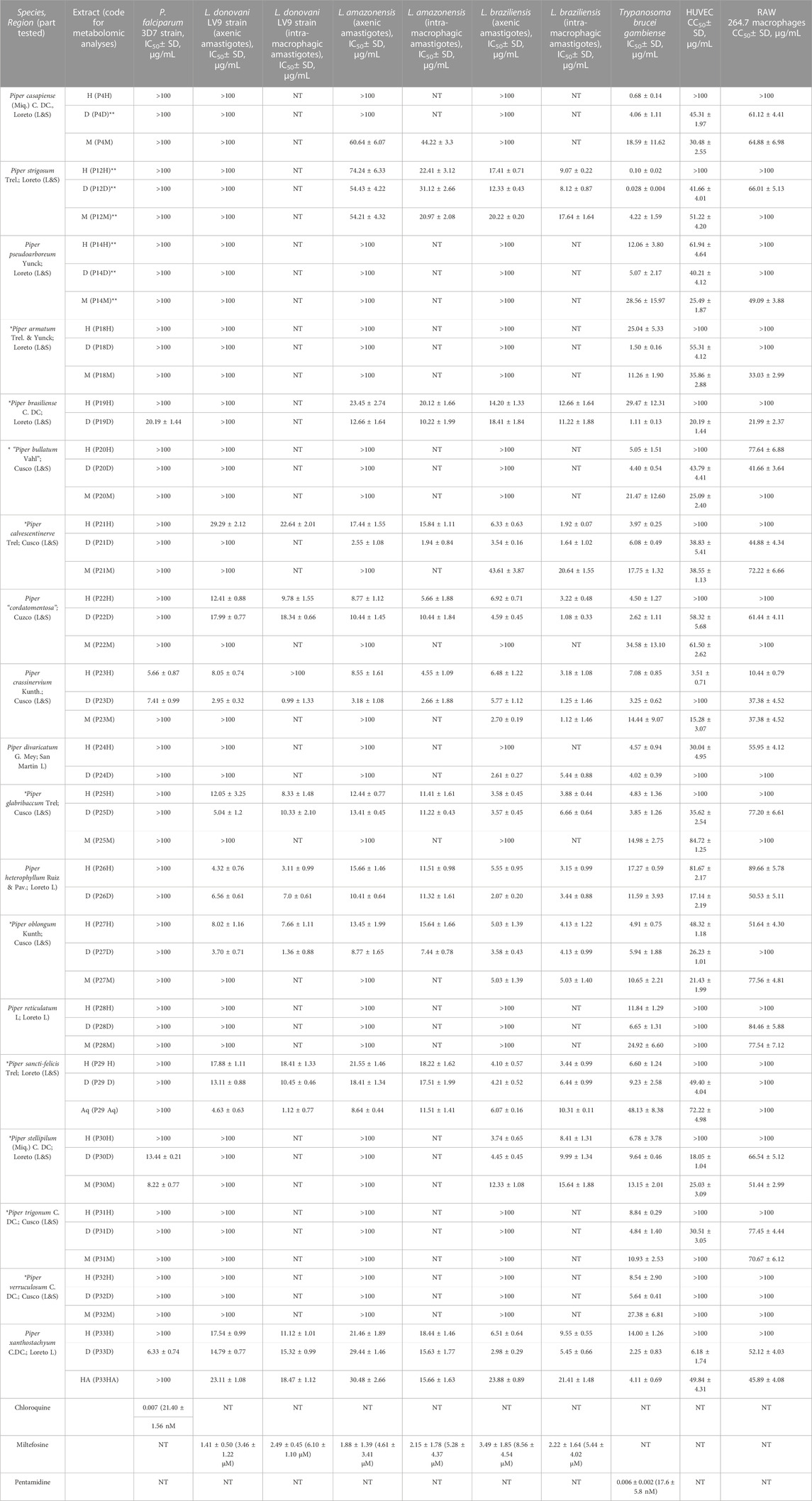
TABLE 1. In vitro antiprotozoal and cytotoxicity activities of Piper extracts. M = methanol; H = hexane; D = methylene chloride (D stands for dichloromethane); HA = hydroalcoholic; Aq = aqueous; NT = not tested. *Species showing no reference in phytochemical literature on Web of Science and PubMed. **Extracts previously tested (Vásquez-Ocmín et al., 2021a) (the plants in this work come from different collection 331 site). L = leaves; L&S = Leaves and stems; AP = aerial parts; “” = species with temporary botanical name (these species need more taxonomical 332 studies).
Selectivity index (SI) values for antiprotozoal activities (Table 2) were ranked as low >10 < 50, high >51 < 99, and very high >100. For antimalarial activity, the only active extract displaying a low SI (13.50) was the methylene chloride extract of P. crassinervium. For the antileishmanial activity, methylene chloride extract of P. crassinervium and hexane extracts of Piper glabribaccum and P. heterophyllum showed a low SI on Leishmania donovani with 37.76, 12.0, and 28.82 respectively; methylene chloride and aqueous extracts of P. oblongum and P. sancti-felicis showed high SI with 73.53 and 89.29 respectively. For L. amazonenesis, three extracts presented a low SI, methylene chloride extracts of P. “cordatomentosa”, P. crassinervium, and P. oblongum with 13.39, 14.05, and 13.44 respectively. For L. braziliensis, 17 extracts displayed low SI, the hexane extracts of P. strigosum, P. “cordatomentosa”, P. glabribaccum, P. heterophyllum, P. oblongum, P. sancti-felicis, P. stellipilum, and P. xanthostachyum (11.02, 31.06, 25.77, 28.46, 12.50, 29.07, 11.89, 10.47), methylene chloride extracts of Piper calvescentinerve, P. crassinervium, P. divaricatum, P. glabribaccum, P. heterophyllum, P. oblongum, and P. sancti-felicis (27.37, 29.90, 18.38, 11.59, 14.69, 24.21, 15.53, respectively); methanol extracts of Piper crassinervium, and P. oblongum (33.36 and 15.42). Only the hexane and methylene chloride extracts of P. calvescentinerve and P. “cordatomentosa” presented a high SI with 52.08 and 56.89, respectively.
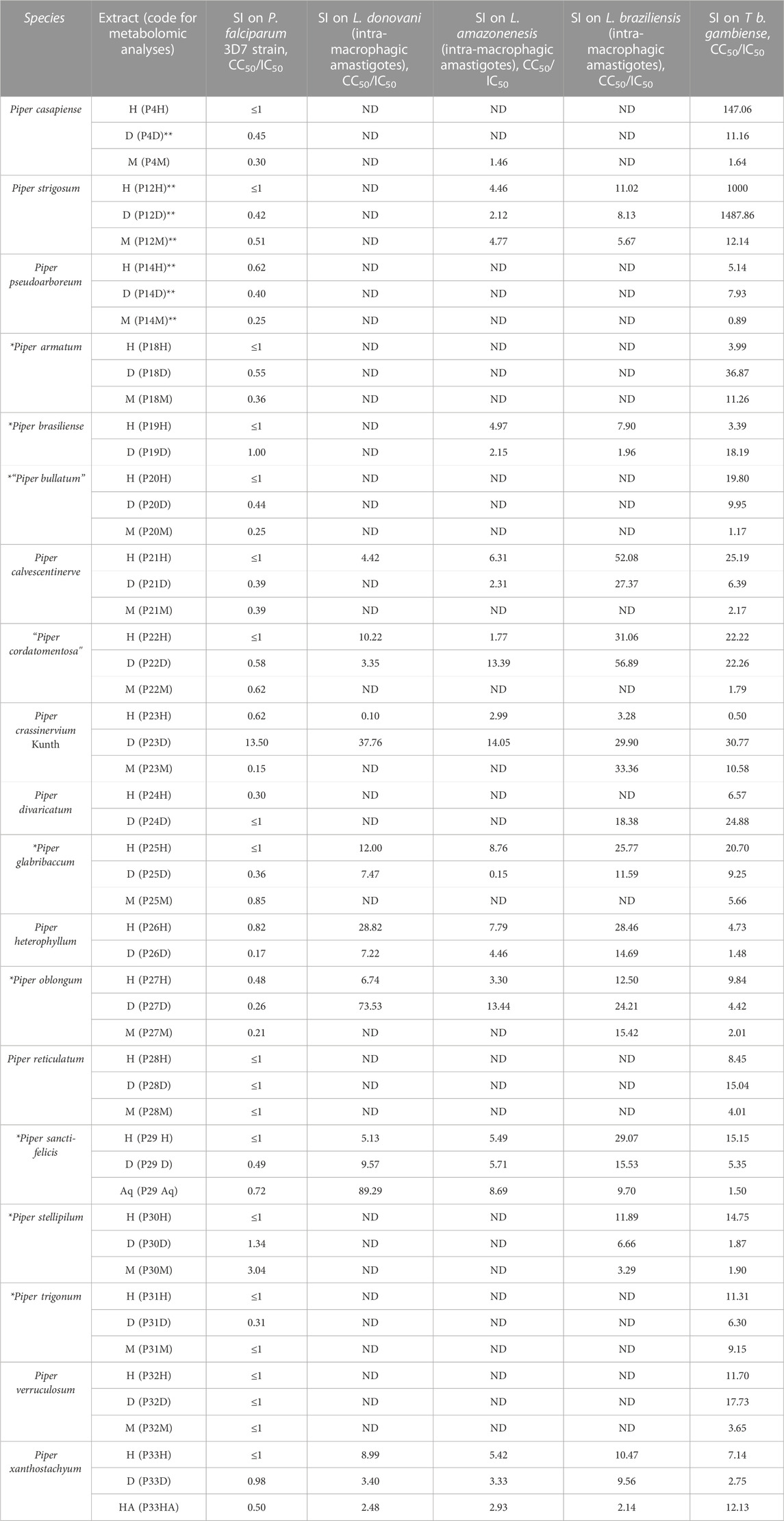
TABLE 2. Selectivity index of Piper extracts calculated with CC50 on HUVEC cells for P. falciparum and T. b. gambiense, and CC50 on RAW 264.7 cells for Leishmania strains. M = methanol; H = hexane; D = methylene chloride (D stands for dichloromethane); Aq = aqueous; ND = not determined. *Species with no phytochemical reference in Web of Science and PubMed. **Extracts previously tested (Vásquez-Ocmín et al., 2021a) (the plants come from different collection site). L = leaves; L&S = Leaves and stems. “” = species with a temporary botanical name (these species need further taxonomic study).
At least one extract of all nineteen plants, except P. heterophyllum, was active on T b. gambiense at IC50 ≤ 10 μg/mL (Table 1). The only two plants that displayed activity in all extracts were P. strigosum and P. divaricatum. Among all active extracts, five had very good activity with a IC50 ≤ 1 μg/mL, hexane and methylene chloride extracts of P. strigosum, hexane extract of P. casapiense, and methylene chloride extracts of P. armatum, and P. brasiliense. Especially, the methylene chloride extract from Piper strigosum was very active with an IC50 at 0.028 μg/mL. Three extracts presented a high SI, hexane extract of P. casapiense with 147.06 and the hexane and methylene chloride extracts of P. strigosum with 1000 and 1487.86, respectively. Twenty extracts displayed a lower yet interesting SI between 10.58 and 36.87.
Antimicrobial activity
Antimicrobial assay results are presented in (Supplementary Table S2) (17 Gram-positive bacteria, 13 Gram-negative bacteria, 2 yeasts). Extracts were more active on Gram-positive bacteria and yeast. The IC50 cut-off values were set at ≤ 0.5 mg/mL for good activity and ≤0.09 mg/mL for very good activity. Among the very active extracts, methylene chloride extract of P. strigosum is the most representative, being very active on all Gram-positive strains [(excepted: Staphylococcus warneri (T26A1)], only on two Gram-negative strains [(Stenotrophomonas maltophilia (21170) and Burkholderia cepacia (13003)], and on the two Candida albicans strains. This extract was also quite active on Streptococcus pyogenes (16135). Other extracts with good activity on Gram-positive bacteria can be pointed out: methylene chloride extracts of P. xantochyma and P. brasiliense, and hydroalcoholic extract of P. xantochyma. Extracts with very good activity on Gram-negative bacteria are: methylene chloride extracts of P. xantochyma and P. divaricatum on B. cepacia (13003) and Pseudomonas aeruginosa (ATCC 27583), respectively. Extracts with very good activity on Candida strains were methylene chloride extracts of P. pseudoarboreum, P. divaricatum, and P. heterophyllum and hexane extract of P. sancti-felicis. Extracts with good activity on Gram-positive bacteria and Candida were principally methylene chloride of P. brasiliense and a hydroalcoholic extract of P. xantochyma.
LCMS data mining and statistical correlation analysis
After the application MS-CleanR workflow to the LCMS data, we obtained 123 unique metabolite features (m/z × RT × Peak area). Among these, 56 compounds were annotated at the genus level (45.53%), 6 compounds at the family level (4.88%), and 55 compounds at the generic level (44.72%), leaving 6 compounds unannotated (4.88%) (Table 3). The MSMS fragmentation pathway of these compounds was used to establish a molecular network (Figure 1). Using NPClassifire and ClassyFire (Djoumbou Feunang et al., 2016; Kim et al., 2021), major phytochemical classes and subclasses of compounds were identified. The main classes of compounds identified were alkaloids, amino acids, fatty acids, polyketides, shikimates, phenylpropanoids, and terpenoids (metadata in Supplementary Table S3).
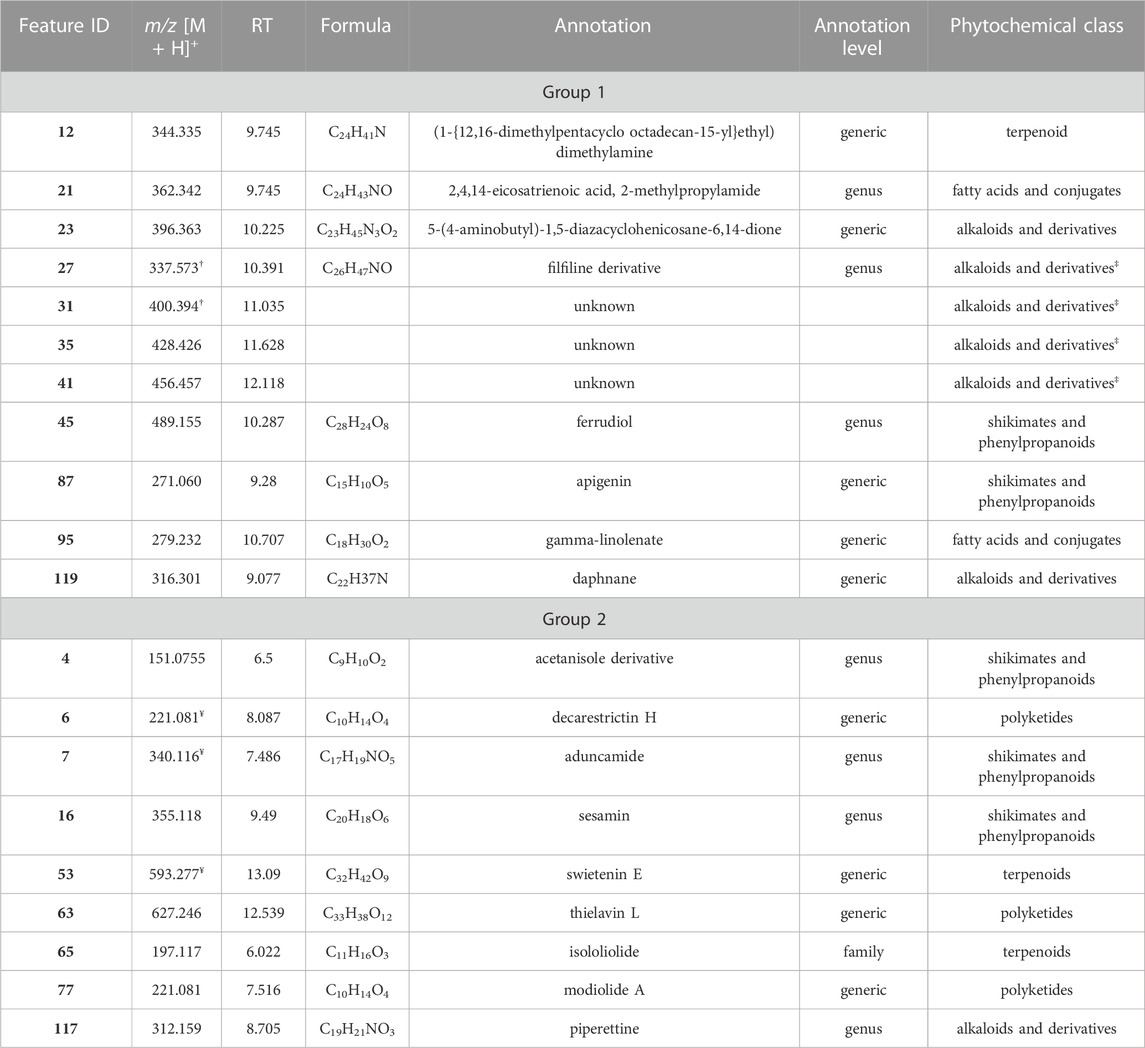
TABLE 3. Features identified as responsible for bioactivity. † = [M + H-H2O]+; ¥ = [M + Na]+; ‡ = based on molecular networking.
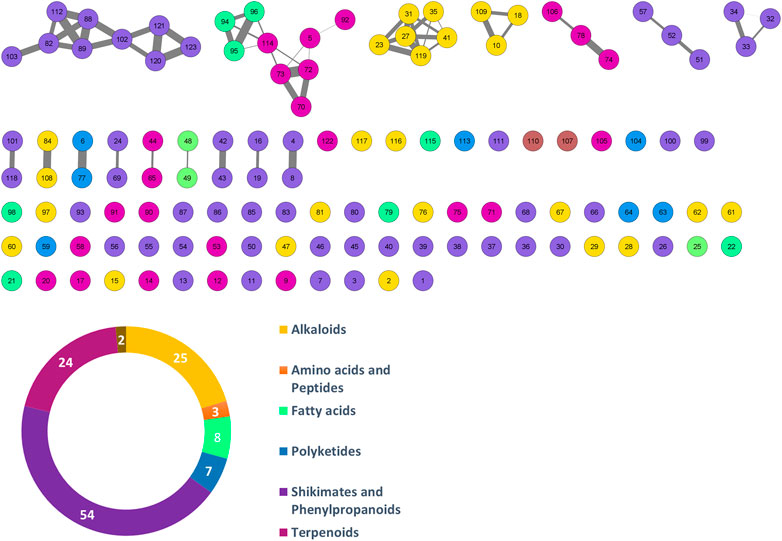
FIGURE 1. Molecular networking of putative compounds. A feature ID is allocated in each node. The colors in the network and pie chart relate to the phytochemical classes. The number of features for each class is also shown in the pie chart.
A heatmap of correlation analysis (Clustered Image Map, CIM) is shown in Figures 2–A. For this analysis, we compiled all in vitro assay results (30 bacterial strains, 2 fungal strains, 4 parasitic strains and 2 cell lines; Table 1, Supplementary Table S2) versus the LCMS features. This figure results from a setting of the threshold of correlation at 0.3 (the heatmap is shown in extenso in Supplemenytary Datasheet S1). Two groups of features responsible for activities can be distinguished. Group 1 is composed of 11 features (12, 21, 23, 27, 31, 35, 41, 45, 87, 95, and 119) having activity on 21 bacteria, one fungus (C. albicans ATCC 10231), and one parasite (T. b. gambiense). Activity on bacteria was mostly concentrated on Gram-positive strains [(except for Burkholderia cepacia (13003), Stenotrophomonas maltophilia (21170), and the three strains of Escherichia coli)]. Group 2 is composed of 9 features (4, 6, 7, 16, 53, 63, 65, 77, and 117). This group was selective on Leishmania (all strains, both axenic and intramacrophagic). We also performed a relevance network (RN) analysis on the same datasets to verify and complete the CIM results (Figures 2–B). RN showed two main clusters of features linked to the activity, the same as in the CIM. The LC-MSMS molecular network (Figure 1) allowed to determine that six features identified in the Group 1 belong to the alkaloids class, while others are mostly self-loops (i.e., compounds not having structurally-related analogs in the extract) belonging to varied phytochemical classes. In the case of Leishmania-specific compounds (Group 2), they mostly belong to the polyketides or shikimates and phenylpropanoids classes, and are found only as self-loops or only related to one compound and not correlated to any activity (i.e., features 16 and 4).
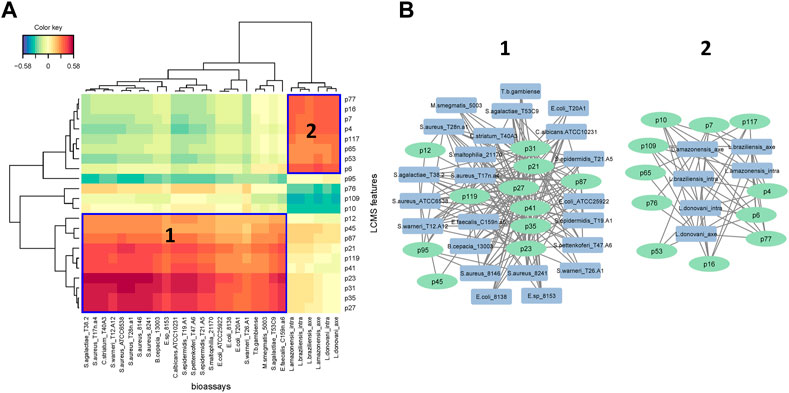
FIGURE 2. (A) Clustered Image Map (CIM) performed on the two-blocks of data sets. The plot depicts the correlation between X = LCMS features (p = peak) data and Y = bioassays. Variables were selected across two dimensions and clustered with a complete Euclidean distance method. Blue squares represent the two main groups of features identified by activities (B) The two main clusters of interaction represented by the relevance network (RN). The two analyses were tuned to a threshold of interaction of 0.3.
Figure 3 shows a heatmap depicting how the features are distributed among the extracts. Features of Group 1 are mainly distributed in all extracts of P. strigosum (features 12, 21, 23, 31, 41, 27 and 35) and P. xanthostachyum (features 45 and 87). Features of Group 2 are found in the hexane and methylene chloride extracts of P. sancti-felicis and hexane extracts of P. calvescentinerve, P. cordatomentosa and P. crassinervium (features 6, 7, 16 and 77), methylene chloride extracts of P. pseudoarboreum, P. calvescentinerve, P. divaricatum, P. glabribaccum, P. bullatum, P. heterophyllum, P. reticulatum, P. oblongum, P. trigonum, P. crassinervium and P. cordatomentosa, and the aqueous extract of P. sancti-felicis (features 4, 53, 63, 65 and 117). Feature 95 is found in the methanol and hexane extracts of P. reticulatum and the hexane extracts of P. pseudoarboreum, P armatum, P. glabribaccum, P. oblongum and P. verruculosum.
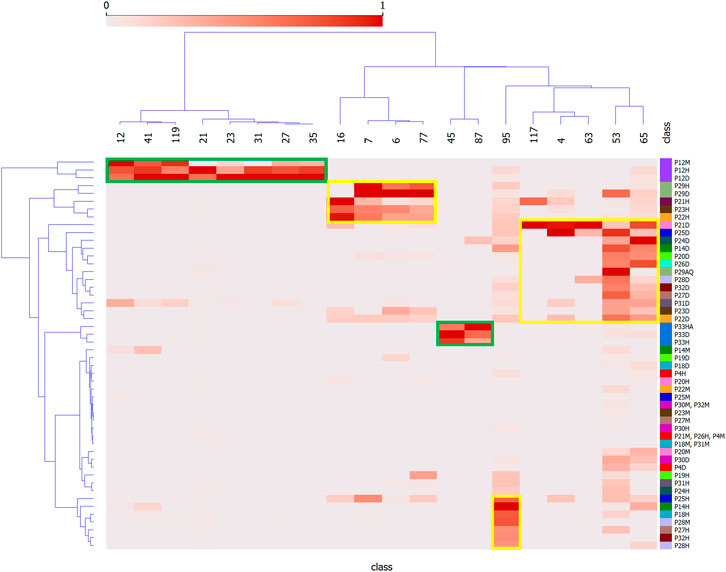
FIGURE 3. Heatmap of correlation between plant extracts and presumed bioactive peak features. Y = Piper extracts, and X = features of LC-MSMS analysis as shown in Figures 2A,B. Green and yellow squares represent the distribution of the bioactive features identified for the Groups 1 and 2 in the Piper extracts.
Discussion
Plants from the Piper genus are currently used by the Amerindian communities in Peru in different preparations (infusion of different parts, juice directly ingested, cataplasms made of crushed leaves or stems, etc.) to treat different diseases (Odonne et al., 2009, 2011; Vásquez-Ocmín et al., 2021a). For the present study, we conducted ethnopharmacological surveys in Loreto and Cusco, Peruvian Amazonian departments heavily impacted by protozoal diseases. Indeed, Loreto has always been the department with the greatest number of malaria cases (principally P. vivax), mostly affecting children from birth to age 11 (84.5% in 2020) (Ministerio de Salud del Perú, 2021). Leishmaniasis occurrence in Peru is principally due to cutaneous (L. amazonesis, 17,892 cases over the last 5 years) and mucosal forms (L. braziliensis, L. peruviana, L. lainsoni, L. guyanensis, 1,642 cases in the last 5 years). To date, in 2002, 70% of the cases of both forms were concentrated in 7 departments: Madre de Dios, Cusco (Echarate and Kosñipata districts), Piura, Junín, Loreto, Cajamarca and San Martín (Ministerio de Salud del Perú, 2022). In Peru, plant-based treatments are very common and even recommended by the health personnel. It is estimated that approximately 5,000 species are used in the traditional medicine (Vásquez-Ocmín et al., 2018) among which the Piper species studied in this work represent 0.34%. We targeted the ethnopharmacological survey on “Cordoncillos” (little cords), a word used to describe pepper vines in Amazonia because the presence of nodes on the stems reminds us of a nudo de soga (node of cord). While this terminology would initially direct the sample collection, we took great care of properly identifying the species upon collection. In two cases, we could not fully ascertain the species and further taxonomic examination is underway.
Chemical profiling of natural extracts can be readily obtained, but for metabolomic purposes, data need to be ‘preprocessed’ (peak picking, alignment, clustering, integration and normalization) to obtain a clean dataset able to provide meaningful information upon statistical analysis. The large abundance and complexity of variables are also challenging and require to apply an appropriate statistical method to answer a specific biological question (Hervé et al., 2018). Few metabolomic studies on Piper species using NMR (Yamaguchi et al., 2011; Uckele et al., 2021) or LCMS have been reported so far (Vásquez-Ocmín et al., 2021a). The Piper genus is a good model to apply our framework for two reasons: i) Piper species are particularly abundant in Peru (∼260 according to www.tropicos.org), many of them used in traditional medicine; ii) many studies, including numerous phytochemical ones, were carried out on this genus (∼9,000 results found in www.webofscience.com for “Piper”, consulted on 1 July 2022), allowing a good metabolome coverage.
We propose in this work a computational statistical analysis to integrate two heterodimensional datasets: the features (compounds) obtained by LC-MSMS analysis of Piper extracts (Supplementary Table S3), and the in vitro biological activity of these extracts on parasites, bacteria and mammalian cells (Table 1; Supplementary Table S2), with the aim to outline most active compounds. To leverage on this multiplexed approach, we applied a regularized Canonical Correlation Analysis (rCCA). This method aims to extract correlated information by maximizing their correlation via canonical variates between two datasets acquired on the same samples (vertical integration). The results of rCCA model were displayed using Clustered Image Maps (CIM) to highlight the correlation level of variables from all datasets, ordered through unsupervised clustering on both samples and compounds simultaneously (González et al., 2012). Another approach for displaying net-like correlation structures between two data sets is to use Relevance Networks (RN). This method generates a graph where nodes represent variables, and edges represent variable associations (Butte et al., 2000). Given an appropriate threshold, the RN highlights main correlation between both datasets (Figures 2–B). To our knowledge, the implementation of this type of metabolomics framework aimed at identifying bioactive compounds has not been used so far. However, a study has been recently published using rCCA to combine multiparametric analysis of adjuvanticity in vivo with immunological profiles in vitro (cytokines, chemokines, and growth factor secretion analyzed by flow cytometry) of a library of compounds derived from hot-water extracts of herbal medicines (Hioki et al., 2022).
Figure 2 strikingly shows that the antibacterial and antileishmanial activities are correlated with two distinct groups of compounds. This is by no means surprising as these organisms have significantly different physiological organization, leading to different drugs currently used against these infections. The species showing the most significant antibacterial activities are P. strigosum and P. xanthostachyum, and the species showing the most significant antileishmanial activities are P. pseudoarboreum, P. calvescentinerve, P. glabribaccum, P. reticulatum and P. sancti-felicis. The compounds correlated with these activities within these extracts are rather specific to these species (Figure 3). An overall weak activity of these Piper extracts on P. falciparum (Table 1) is an unexpected result, as Piper species are usually reputed for their antimalarial potential.
Features from Group 1 were active not only on bacteria but also on one fungus and one parasite. Although the high cross-activity observed for this group of compounds (Figure 2 and Supplementary Datasheet S1) could foretell of a lack of selectivity, these compounds did not cause cytotoxicity in mammalian cells. Six of the features of Group 1 annotated as alkaloid derivatives were grouped in a same cluster in the molecular networking, suggesting a similar biosynthesis pathway. Only three of them were annotated in our workflow, suggesting that the three unannotated ones may have an original structure. The reliability of the annotation or classification (NPClassifire and ClassyFire) part of the workflow may nevertheless be tempered, as shown by the case of feature 27, classified by the workflow as an alkaloid, but annotated as filfiline, a fatty amide previously isolated from the roots of Piper retrofractum (Banerji et al., 2002). The broad bioactivity spectrum of alkaloids is well known, i.e., antibacterial, antiparasitic, anticancer, but also their cytotoxicity (Newman and Cragg, 2007; 2020; Daley and Cordell, 2021; Yan et al., 2021). The mechanism of action of antibacterial alkaloids is mainly described as disrupting the bacterial membrane, affecting DNA function and inhibiting protein synthesis (Ananthan et al., 2009; Pan et al., 2014; Kelley et al., 2013; Li et al., 2014; Larghi et al., 2015). Antibacterial alkaloids (MIC <10 μM/mL) are as diverse as isoquinolines, aporphines, phenanthrenes, quinolines and indoles and their activity is mostly oriented against Gram-positive bacteria (Porras et al., 2021). Quinoleines like 8-hydroxyquinoline and its derivatives and evocarpine are active on bacteria associated with respiratory system infections: Mycobacterium tuberculosis, S. aureus, and MRSA (Methicillin-resistant Staphylococcus aureus) (MIC ≤10 μM/mL). Aporphine alkaloid derivatives exhibit high broad-spectrum activity against Gram-positive bacteria: S. agalactiae, S. aureus, S. epidermidis, E. faecalis, and as in our study E. coli (Hamoud et al., 2015; Tan et al., 2015). Even if the chemical array within Piper spp. Is impressively diverse, nitrogen-containing compounds are limited, i.e., alkaloids like piperines or amides like piplartine, phenethyls, and diaminodiamides. Amides are known to have fungicidal, cytotoxic, or antiprotozoal activities. With regard to fungicidal activity, dehydropipernonaline and nigramide R previously isolated from P. retrofractum displayed potent growth inhibition of Cladosporium cladosporioides and cytotoxicity against the L5178Y mouse lymphoma cell line (IC50 values of 8.9 µM and 9.3 µM, respectively) (Muharini et al., 2015). Amide piplartine isolated from a methanol extract of P. retrofractum showed good activity on Leishmania donovani with an IC50 value at 7.5 µM (Bodiwala et al., 2007). In Group 1, features correlated with a fungicidal activity would only target C. albicans. Other active compounds identified in Group 1 were one terpenoid, two fatty acids, one shikimate and phenylpropanoid derivative and one polyketide.
Compounds from the Group 2, characterized as being rather specific to Leishmania, are labeled as polyketides 3), shikimates and phenylpropanoids 3), terpenoids 2) and alkaloids 1). Their lack of activity on Trypanosoma is not surprising (Figure 2), as even though both parasites belong to the same Trypanosomatidae order, the current treatments are not similar. These two parasites cause different pathologies, in distinct geographical areas. They evolved differently and show evolutionary discrepancies in their mechanisms that may explain variations in sensitivity to treatments (Fernandes et al., 2020; Van den Broeck et al., 2020). A cross-reading of Figures 2, 3, suggests that extracts of P. calvescentinerve and P. glabribaccum and features from Group 2 have not only a selectivity for Leishmania but also a good selectivity index. Selectivity is a key parameter for antileishmanial drugs: antimonials, amphotericin B, paromomycin sulfate and miltefosine have variable efficacy against the Leishmania species but have significant adverse effects (Rao et al., 2019). Tremendous efforts have been put into the understanding of the Leishmania biology, leading to the identification of numerous putative targets: ergosterol and its biosynthetic pathway [i.e., amphotericine B and enzymes like squalene synthase (SQS) or sterol methyltransferase (SMT)], the glycolytic pathway necessary to provide glucose as an energy source, DNA topoisomerases, enzymes of the polyamine biosynthetic pathway (i.e., arginase, ornithine decarboxylase, s-adenosylmethionine decarboxylase, and spermidine synthase), redox metabolism pathway (Nagle et al., 2014; Raj et al., 2020).
The compounds labeled as shikimates or phenylpropanoids (acetanisole, aduncamide, and sesamin) or alkaloid (piperettine) were annotated at the genus level because they had previously been isolated from Piper tuberculatum, Piper nigrum, P. longum, Piper aduncum, Piper puberulum, P. austrosinense, Piper brachystachyum, P. mullesua, P. retrofractum, P. sarmentosum and P. sylvaticum (Spring and Stark, 1950; Orjala et al., 1993; De Araujo-Junior et al., 1997; Puri et al., 1998; Umezawa, 2003; Tuntiwachwuttikul et al., 2006). Among these compounds, only the lignan sesamin was reported to show activity on L. amazonensis with an IC50 of 44.6 μM/mL and was not cytotoxic for mouse macrophage cells (CC50 > 100 μg/mL, SI > 6) (Pulivarthi et al., 2015). A study based on computational methods suggests that sesamin could be a promising inhibitor of the L. donovani CRK12 receptor (binding affinity of −8.5 kcal/mol) (Broni et al., 2021). Nevertheless, sesamin, isolated from a hexane extract of P. retrofractum (IC50 of the extract = 5 μg/mL), was inactive on L. donovani (Bodiwala et al., 2007) (Bodiwala et al., 2007). This compound was also inactive on Plasmodium falciparum K1 multidrug resistant strain, M. tuberculosis H37Ra, and Candida albicans (EC50 > 20 μg/mL, MIC >200 μg/mL and IC50 > 50 μg/mL, respectively). Cubein, another lignan isolated from Piper cubeba, was shown to be active on L. donovani (IC50 = 28.0 µM). Interestingly, sesamin is connected in the MN with an unknown compound (RT = 6.577 [M + H]+ = 360.145). Piperettine has been previously isolated from P. nigrum and P. aurantiacum and was shown to be active on epimastigotes and amastigotes of Trypanosoma cruzi (IC50 = 10.67 and 7.40 µM, respectively) (Ribeiro et al., 2004). In our work, the compound annotated as piperettine was only detected in P. calvescentinerve extracts (Figure 3) but was not labeled as being correlated with any activity on T b. gambiense (Supplementary Datasheet S1). The use of this Piper species as a medicinal plant with various indications can partly be validated by the fact that it is an inhibitor of 5-lipoxygenase (76.02 µM) (Muthuraman et al., 2019), a key enzyme involved in the biosynthesis of pro-inflammatory leukotrienes, provided its concentration is sufficient in the traditional preparations. Aduncamide was shown to present a moderate antineuroinflammatory activity (IC50 at 26 ± 8.3 µM) by the Griess method on LPS-stimulated BV-2 cells (Zheng et al., 2021), to be cytotoxic for KB nasopharyngeal carcinoma cells (ED50 = 5.7 μg/mL) and to inhibit growth Gram-positive Burkholderia subtilis and Mycobacterium luteus, while being less active towards Gram-negative E. coli (Orjala et al., 1993). In our work, the compound annotated as aduncamide was identified in extracts of P. glabribaccum, P. calvescentinerve and P. cordatomentosa, whose extracts are among the most active on Leishmania. Two compounds were labeled as 10-membered lactone macrolides and annotated as decarestrictin H and modiolide A, previously isolated from the fungi Penicillium simplicissimum and Stagonospora cirsii, respectively (Grabley et al., 1992; Evidente et al., 2008). These two last annotations, although resulting from similar fragmentation patterns, may be subject to caution. Indeed, these compounds were annotated at the generic level and no macrolide has been identified in Piper spp. so far (only a macrolactam, laevicarpin, previously isolated from P. laevicarpus) (da Silva A. Maciel et al., 2016). Furthermore, macrolides are well-known antibacterial compounds and thus should logically rather be clustered in Group 1, if ever present in the extracts. A compound annotated as apigenin was detected only in P. xanthostachyum and was identified as being correlated to the antibacterial activity (Figure 2A). This may appear surprising given that apigenin is a common and ubiquitous flavonoid with no antibacterial activity. The number of possible flavonoid isomers being quite large, this annotation may also be subject to caution, notwithstanding the fact that the actual compound (p87) being present in the extract remains of interest from the antibacterial perspective. Other compounds were annotated as flavonoids in the extracts (most of the compounds in Group 3, see Supplementary Datasheet S1), characterized by a moderate activity of the extracts containing them, a moderate correlation with all the activities without any clear selectivity. These compounds were annotated at the genus level, as they were previously isolated from Piper species (Matsui and Munakata, 1976; Lago et al., 2004; González et al., 2022). Antiprotozoal or antimicrobial activity of flavonoids is well documented (Graf et al., 2005; Ortiz et al., 2017; 2020). Two compounds were annotated as pinocembrine and pinostrobin, both belonging to the flavanones subclass. Pinocembrine displays antifungal (C. cladosporioides and C. sphaerospermum) or antibacterial (Enterococcus faecalis, M. tuberculosis) potential (Lago et al., 2004; Jeong et al., 2009; Gröblacher et al., 2012), while showing either no cytotoxicity on healthy and cancerous cell lines (RAW 264.7, epidermoid carcinoma of the oral cavity (KB), human small cell lung cancer (NCI-H187), metastatic murine colon 26-L5 carcinoma, PANC-1 human pancreatic cancer, metastatic human HT-1080 fibrosarcoma), or high cytotoxicity (Awale et al., 2009; Yenjai and Wanich, 2010; Lee et al., 2013). Pinostrobin showed weak antimalaria activity on P. falciparum (Kaur et al., 2009) but significant potential as a cancer chemopreventive agent (Gu et al., 2002).
Some of these Piper compounds are therefore of interest in an isolation and factual testing perspective. Compounds annotated as aduncamide, sesamin, and apigenin, along with the unknown compounds correlated to the activity, could be subjected to mass-targeted isolation (Vásquez-Ocmín et al., 2022), in order to confirm their annotation or identify their structure, as well as confirm their biological potential.
Conclusion
Hyphenated analytical techniques are very useful to provide highly informative chemical profiling of complex metabolomes. Nevertheless, deciphering such profiles to determine the compounds responsible for the biological activity remains a challenge. By correlating these chemical profiles with biological assay results, we propose a workflow of integrated metabolomics statistical tools to provide a broad picture of the metabolome as well as a map of compounds putatively associated to the bioactivity. The structure of these compounds can either be annotated from previous works or remain unknown at this stage, but in any case, they require a formal isolation step to confirm their structure and activity. Nevertheless, an initial data mining on analytical-scale data proves very effective for prioritizing the compounds to target. The relevance of our approach has been validated on a set of Piper species tested for their anti-infectious diseases at the extract level. Such an approach can be extended to any type of natural extract, particularly when prior phytochemical data is available in the literature.
Data availability statement
The original contributions presented in the study are included in the article/Supplementary Material, further inquiries can be directed to the corresponding authors.
Author contributions
Conceptualization: PV-O, AM, GM, and LRM; Methodology: PV-O, AM, GM, and LRM; Chemical data acquisition/curation: PV-O, KL, GM, AG, and AM; Statistical analysis: PV-O and GM; Biological data curation: SC, VR, SB, and SP; Investigation: PV-O, SC, VR, GM, SP, AG, KL, ID, LR-V, HR, WR, SB, LRM, and AM. PV-O wrote the original draft and all the authors contributed to the final manuscript.
Funding
Authors acknowledge the funding support of the Universidad Nacional de la Amazonia Peruana (UNAP) (Resolución Rectoral N°1312-2020-UNAP, 9/12/2020) and Financial support from the French National Infrastructure for Metabolomics and Fluxomics, Grant MetaboHUB-ANR-11-INBS-0010.
Acknowledgments
Authors are indebted to the communities cited in this work for their essential contribution in order to spread their ancestral knowledge. The authors thank IRD the French National Research Institute for Sustainable Development (IRD), UMR 152 PHARMADEV, IRD-UPS (Toulouse, France) for the post-doctoral fellowship to PV-O (contract number 04077858). The authors thank MINEDU (Ministerio de Educación), Lima, Peru, convenio MINEDU-UNAP (Decreto Supremo 146-2020, EF/Resolución Rectoral N°1312-2020-UNAP, 9/12/2020). We also appreciate the important collaboration of Juan Celedonio Ruiz (Herbarium Amazonense (AMAZ) de la Universidad Nacional de la Amazonía Peruana (UNAP), Iquitos, Perú). The authors are also grateful to Amélie Perez (MetaboHUB-MetaToul-Agromix, LRSV, Auzeville-Tolosane), Guillaume Cabanac (IRIT, Toulouse), and Rizwana Zaffaroullah (CNR du Paludisme, AP-HP, Hôpital Bichat - Claude Bernard, F-75018 Paris, France).
Conflict of interest
The authors declare that the research was conducted in the absence of any commercial or financial relationships that could be construed as a potential conflict of interest.
Publisher’s note
All claims expressed in this article are solely those of the authors and do not necessarily represent those of their affiliated organizations, or those of the publisher, the editors and the reviewers. Any product that may be evaluated in this article, or claim that may be made by its manufacturer, is not guaranteed or endorsed by the publisher.
Supplementary material
The Supplementary Material for this article can be found online at: https://www.frontiersin.org/articles/10.3389/fphar.2023.1100542/full#supplementary-material
References
Alarcon-Barrera, J. C., Kostidis, S., Ondo-Mendez, A., and Giera, M. (2022). Recent advances in metabolomics analysis for early drug development. Drug Discov. Today 27, 1763–1773. doi:10.1016/j.drudis.2022.02.018
Awale, S., Miyamoto, T., Linn, T. Z., Li, F., Win, N. N., Tezuka, Y., et al. (2009). Cytotoxic constituents of Soymida febrifuga from Myanmar. J. Nat. Prod. 72, 1631–1636. doi:10.1021/np9003323
Balaraman, K., Vieira, N. C., Moussa, F., Vacus, J., Cojean, S., Pomel, S., et al. (2015). In vitro and in vivo antileishmanial properties of a 2-n-propylquinoline hydroxypropyl β-cyclodextrin formulation and pharmacokinetics via intravenous route. Biomed. Pharmacother. 76, 127–133. doi:10.1016/j.biopha.2015.10.028
Banerji, A., Sarkar, M., Datta, R., Sengupta, P., and Abraham, K. (2002). Amides from Piper brachystachyum and Piper retrofractum. Phytochemistry 59, 897–901. doi:10.1016/S0031-9422(01)00364-8
Bocquet, L., Sahpaz, S., Bonneau, N., Beaufay, C., Mahieux, S., Samaillie, J., et al. (2019). Phenolic compounds from Humulus lupulus as natural antimicrobial products: New weapons in the fight against methicillin resistant Staphylococcus aureus, Leishmania mexicana and Trypanosoma brucei Strains. Molecules 24, E1024. doi:10.3390/molecules24061024
Bodiwala, H. S., Singh, G., Singh, R., Dey, C. S., Sharma, S. S., Bhutani, K. K., et al. (2007). Antileishmanial amides and lignans from Piper cubeba and Piper retrofractum. J. Nat. Med. 61, 418–421. doi:10.1007/s11418-007-0159-2
Broni, E., Kwofie, S. K., Asiedu, S. O., Miller, W. A., and Wilson, M. D. (2021). A molecular modeling approach to identify potential antileishmanial compounds against the cell division cycle (cdc)-2-related kinase 12 (CRK12) receptor of Leishmania donovani. Biomolecules 11, 458. doi:10.3390/biom11030458
Butte, A. J., Tamayo, P., Slonim, D., Golub, T. R., and Kohane, I. S. (2000). Discovering functional relationships between RNA expression and chemotherapeutic susceptibility using relevance networks. Proc. Natl. Acad. Sci. 97, 12182–12186. doi:10.1073/pnas.220392197
CLSI (2006). Methods for dilution antimicrobial susceptibility test for bacteria that grow aerobically, aproved standard. Available at: https://infostore.saiglobal.com/en-us/standards/CLSI-M7-A7-7ED-2006-357843_SAIG_CLSI_CLSI_815015/ (Accessed September 2, 2022).
da Silva, A., Maciel, D., Freitas, V. P., Conserva, G. A. A., Alexandre, T. R., Purisco, S. U., et al. (2016). Bioactivity-guided isolation of laevicarpin, an antitrypanosomal and anticryptococcal lactam from Piper laevicarpu (Piperaceae). Fitoterapia 111, 24–28. doi:10.1016/j.fitote.2016.04.005
Daley, S., and Cordell, G. A. (2021). Alkaloids in contemporary drug discovery to meet global disease needs. Molecules 26, 3800. doi:10.3390/molecules26133800
De Araujo-Junior, J. X., Da-Cunha, E. V. L., Chaves, M. C. D. O., and Gray, A. I. (1997). Piperdardine, a piperidine alkaloid from Piper tuberculatum. Phytochemistry 44, 559–561. doi:10.1016/S0031-9422(96)00503-1
de Salud del Perú, M. (2022). Boletín Epidemiológico del Perú-Situación epidemiológica de la Leishmaniasis en el Perú SE 12-2022. Available at: https://www.dge.gob.pe/epipublic/uploads/boletin/boletin_202212_22_181950_2.pdf (Accessed October 2, 2021).
Djoumbou Feunang, Y., Eisner, R., Knox, C., Chepelev, L., Hastings, J., Owen, G., et al. (2016). ClassyFire: Automated chemical classification with a comprehensive, computable taxonomy. J. Cheminformatics 8, 61. doi:10.1186/s13321-016-0174-y
Durant-Archibold, A. A., Santana, A. I., and Gupta, M. P. (2018). Ethnomedical uses and pharmacological activities of most prevalent species of genus piper in Panama: A review. J. Ethnopharmacol. 217, 63–82. doi:10.1016/j.jep.2018.02.008
Evidente, A., Cimmino, A., Berestetskiy, A., Andolfi, A., and Motta, A. (2008). Stagonolides G−I and Modiolide A, nonenolides produced by Stagonospora cirsii, a potential mycoherbicide for Cirsium arvense. J. Nat. Prod. 71, 1897–1901. doi:10.1021/np800415w
Fernandes, P. M., Kinkead, J., McNae, I. W., Vásquez-Valdivieso, M., Wear, M. A., Michels, P. A. M., et al. (2020). Kinetic and structural studies of Trypanosoma and Leishmania phosphofructokinases show evolutionary divergence and identify AMP as a switch regulating glycolysis versus gluconeogenesis. FEBS J. 287, 2847–2861. doi:10.1111/febs.15177
Fraisier-Vannier, O., Chervin, J., Cabanac, G., Puech, V., Fournier, S., Durand, V., et al. (2020). MS-CleanR: A feature-filtering workflow for untargeted LC–MS based metabolomics. Anal. Chem. 92, 9971–9981. doi:10.1021/acs.analchem.0c01594
González, A. S., Tellini, V. H. S., and Gutiérrez, D. M. B. (2022). Study of the dermal anti-inflammatory, antioxidant, and analgesic activity of pinostrobin. Heliyon 8, e10413. doi:10.1016/j.heliyon.2022.e10413
González, I., Cao, K.-A. L., Davis, M. J., and Déjean, S. (2012). Visualising associations between paired ‘omics’ data sets. BioData Min. 5, 19. doi:10.1186/1756-0381-5-19
González, I., Déjean, S., Martin, P. G. P., and Baccini, A. (2008). Cca: An R package to extend canonical correlation analysis. J. Stat. Softw. 23, 1–14. doi:10.18637/jss.v023.i12
Grabley, S., Hammann, P., Hütter, K., Kirsch, R., Kluge, H., Thiericke, R., et al. (1992). Secondary metabolites by chemical screening 20 decarestrictines, a new family of inhibitors of cholesterol biosynthesis from Penicillium III. Decarestrictines E M. J. Antibiot. 45, 1176–1181. doi:10.7164/antibiotics.45.1176
Graf, B. A., Milbury, P. E., and Blumberg, J. B. (2005). Flavonols, flavones, flavanones, and human health: Epidemiological evidence. J. Med. Food 8, 281–290. doi:10.1089/jmf.2005.8.281
Gröblacher, B., Kunert, O., and Bucar, F. (2012). Compounds of Alpinia katsumadai as potential efflux inhibitors in Mycobacterium smegmatis. Bioorg. Med. Chem. 20, 2701–2706. doi:10.1016/j.bmc.2012.02.039
Gu, J.-Q., Park, E. J., Vigo, J. S., Graham, J. G., Fong, H. H. S., Pezzuto, J. M., et al. (2002). Activity-guided isolation of constituents of Renealmia nicolaioides with the potential to induce the hhase II enzyme quinone reductase. J. Nat. Prod. 65, 1616–1620. doi:10.1021/np020249p
Hamoud, R., Reichling, J., and Wink, M. (2015). Synergistic antibacterial activity of the combination of the alkaloid sanguinarine with EDTA and the antibiotic streptomycin against multidrug resistant bacteria. J. Pharm. Pharmacol. 67, 264–273. doi:10.1111/jphp.12326
Hervé, M. R., Nicolè, F., and Lê Cao, K.-A. (2018). Multivariate analysis of multiple datasets: A practical guide for chemical ecology. J. Chem. Ecol. 44, 215–234. doi:10.1007/s10886-018-0932-6
Hioki, K., Hayashi, T., Natsume-Kitatani, Y., Kobiyama, K., Temizoz, B., Negishi, H., et al. (2022). Machine learning-assisted screening of herbal medicine extracts as vaccine adjuvants. Front. Immunol. 13. Available at: https://www.frontiersin.org/articles/10.3389/fimmu.2022.847616 (Accessed September 7, 2022).
Jeong, K.-W., Lee, J.-Y., Kang, D.-I., Lee, J.-U., Shin, S. Y., and Kim, Y. (2009). Screening of flavonoids as candidate antibiotics against Enterococcus faecalis. J. Nat. Prod. 72, 719–724. doi:10.1021/np800698d
Kaur, K., Jain, M., Kaur, T., and Jain, R. (2009). Antimalarials from nature. Bioorg. Med. Chem. 17, 3229–3256. doi:10.1016/j.bmc.2009.02.050
Kelley, C., Lu, S., Parhi, A., Kaul, M., Pilch, D. S., and LaVoie, E. J. (2013). Antimicrobial activity of various 4- and 5-substituted 1-phenylnaphthalenes. Eur. J. Med. Chem. 60, 395–409. doi:10.1016/j.ejmech.2012.12.027
Kim, H. W., Wang, M., Leber, C. A., Nothias, L.-F., Reher, R., Kang, K. B., et al. (2021). NPClassifier: A deep neural network-based structural classification tool for natural products. J. Nat. Prod. 84, 2795–2807. doi:10.1021/acs.jnatprod.1c00399
Lago, J. H. G., Ramos, C. S., Casanova, D. C. C., Morandim, A. de A., Bergamo, D. C. B., Cavalheiro, A. J., et al. (2004). Benzoic acid derivatives from Piper species and their fungitoxic activity against Cladosporium cladosporioides and C. sphaerospermum. J. Nat. Prod. 67, 1783–1788. doi:10.1021/np030530j
Lambros, C., and Vanderberg, J. P. (1979). Synchronization of Plasmodium falciparum erythrocytic stages in culture. J. Parasitol. 65, 418–420. doi:10.2307/3280287
Larghi, E. L., Bracca, A. B. J., Aguilar, A. A. A., Heredia, D. A., Pergomet, J. L., Simonetti, S. O., et al. (2015). Neocryptolepine: A promising indoloisoquinoline alkaloid with interesting biological activity. Evaluation of the drug and its most relevant analogs. Curr. Top. Med. Chem. 15, 1683–1707. doi:10.2174/1568026615666150427113937
Le Cao, K.-A., Rohart, F., Gonzalez, I., Dejean, S., Gautier, B., Bartolo, F., et al. (2016). mixOmics: Omics data integration project. R package version 6.1.1. Available at: https://CRAN.R-project.org/package=mixOmics.
Lee, C., Lee, J. W., Jin, Q., Jang, D. S., Lee, S.-J., Lee, D., et al. (2013). Inhibitory constituents of the heartwood of Dalbergia odorifera on nitric oxide production in RAW 264.7 macrophages. Bioorg. Med. Chem. Lett. 23, 4263–4266. doi:10.1016/j.bmcl.2013.04.032
Li, N., Tan, S., Cui, J., Guo, N., Wang, W., Zu, Y., et al. (2014). PA-1, a novel synthesized pyrrolizidine alkaloid, inhibits the growth of Escherichia coli and Staphylococcus aureus by damaging the cell membrane. J. Antibiot. 67, 689–696. doi:10.1038/ja.2014.49
Matsui, K., and Munakata, K. (1976). Four new neolignans from Piper futokadzura. Tetrahedron Lett. 17, 4371–4374. doi:10.1016/0040-4039(76)80118-9
Mgbeahuruike, E. E., Yrjönen, T., Vuorela, H., and Holm, Y. (2017). Bioactive compounds from medicinal plants: Focus on Piper species. South Afr. J. Bot. 112, 54–69. doi:10.1016/j.sajb.2017.05.007
Ministerio de Salud del Perú (2021). Boletín Epidemiológico del Perú SE 02-2021. Available at: https://cdn.www.gob.pe/uploads/document/file/1865086/Bolet%C3%ADn%20epidemiol%C3%B3gico%20del%20Per%C3%BA%202021.pdf?v=1629922432 (Accessed October 2, 2021).
Muharini, R., Liu, Z., Lin, W., and Proksch, P. (2015). New amides from the fruits of Piper retrofractum. Tetrahedron Lett. 56, 2521–2525. doi:10.1016/j.tetlet.2015.03.116
Muthuraman, S., Sinha, S., Vasavi, C. S., Waidha, K. M., Basu, B., Munussami, P., et al. (2019). Design, synthesis and identification of novel coumaperine derivatives for inhibition of human 5-LOX: Antioxidant, pseudoperoxidase and docking studies. Bioorg. Med. Chem. 27, 604–619. doi:10.1016/j.bmc.2018.12.043
Nagle, A. S., Khare, S., Kumar, A. B., Supek, F., Buchynskyy, A., Mathison, C. J. N., et al. (2014). Recent developments in drug discovery for leishmaniasis and human african trypanosomiasis. Chem. Rev. 114, 11305–11347. doi:10.1021/cr500365f
Newman, D. J., and Cragg, G. M. (2007). Natural products as sources of new drugs over the last 25 years. J. Nat. Prod. 70, 461–477. doi:10.1021/np068054v
Newman, D. J., and Cragg, G. M. (2020). Natural products as sources of new drugs over the nearly four decades from 01/1981 to 09/2019. J. Nat. Prod. 83, 770–803. doi:10.1021/acs.jnatprod.9b01285
Olivon, F., Elie, N., Grelier, G., Roussi, F., Litaudon, M., and Touboul, D. (2018). MetGem software for the generation of molecular networks based on the t-SNE algorithm. Anal. Chem. 90, 13900–13908. doi:10.1021/acs.analchem.8b03099
Orjala, J., Wright, A. D., Rali, T., and Sticher, O. (1993). Aduncamide, a cytotoxic and antibacterial b-phenylethylamine-derived amide from Piper aduncum. Nat. Product. Lett. 2, 231–236. doi:10.1080/10575639308043814
Ortiz, S., Dali-Yahia, K., Vásquez-Ocmín, P., Grougnet, R., Grellier, P., Michel, S., et al. (2017). Heme-binding activity of methoxyflavones from Pentzia monodiana maire (asteraceae). Fitoterapia 118, 1–5. doi:10.1016/j.fitote.2017.01.012
Ortiz, S., Vásquez-Ocmín, P. G., Cojean, S., Bouzidi, C., Michel, S., Figadère, B., et al. (2020). Correlation study on methoxylation pattern of flavonoids and their heme-targeted antiplasmodial activity. Bioorg. Chem. 104, 104243. doi:10.1016/j.bioorg.2020.104243
Pomel, S., Dubar, F., Forge, D., Loiseau, P. M., and Biot, C. (2015). New heterocyclic compounds: Synthesis and antitrypanosomal properties. Bioorg. Med. Chem. 23, 5168–5174. doi:10.1016/j.bmc.2015.03.029
Porras, G., Chassagne, F., Lyles, J. T., Marquez, L., Dettweiler, M., Salam, A. M., et al. (2021). Ethnobotany and the role of plant natural products in antibiotic drug discovery. Chem. Rev. 121, 3495–3560. doi:10.1021/acs.chemrev.0c00922
Pulivarthi, D., Steinberg, K. M., Monzote, L., Piñón, A., and Setzer, W. N. (2015). Antileishmanial activity of compounds isolated from Sassafras albidum. Nat. Prod. Commun. 10, 1934578X1501000–1230. doi:10.1177/1934578x1501000723
Puri, B., Hall, A., Harborne, J. B., Baxter, H., and Moss, G. P. (1998). “Lignans,” in Phytochemical dictionary (London: CRC Press).
Raj, S., Sasidharan, S., Balaji, S. N., and Saudagar, P. (2020). An overview of biochemically characterized drug targets in metabolic pathways of Leishmania parasite. Parasitol. Res. 119, 2025–2037. doi:10.1007/s00436-020-06736-x
Rao, S. P. S., Barrett, M. P., Dranoff, G., Faraday, C. J., Gimpelewicz, C. R., Hailu, A., et al. (2019). Drug discovery for Kinetoplastid diseases: Future directions. ACS Infect. Dis. 5, 152–157. doi:10.1021/acsinfecdis.8b00298
Ribeiro, T. S., Freire-de-Lima, L., Previato, J. O., Mendonça-Previato, L., Heise, N., and Freire de Lima, M. E. (2004). Toxic effects of natural piperine and its derivatives on epimastigotes and amastigotes of Trypanosoma cruzi. Bioorg. Med. Chem. Lett. 14, 3555–3558. doi:10.1016/j.bmcl.2004.04.019
Ruiz-Vásquez, L., Ruiz Mesia, L., Caballero Ceferino, H. D., Ruiz Mesia, W., Andrés, M. F., Díaz, C. E., et al. (2022). Antifungal and herbicidal potential of Piper essential oils from the Peruvian Amazonia. Plants 11, 1793. doi:10.3390/plants11141793
Salehi, B., Zakaria, Z. A., Gyawali, R., Ibrahim, S. A., Rajkovic, J., Shinwari, Z. K., et al. (2019). Piper species: A comprehensive review on their phytochemistry, biological activities and applications. Molecules 24, 1364. doi:10.3390/molecules24071364
SFE (2022). Ethnopharmacologie, plantes médicinales, médecine traditionnelle. Société Française d’Ethnopharmacologie. Available at: http://www.ethnopharmacologia.org/definition/ (Accessed July 26, 2022).
Shannon, P., Markiel, A., Ozier, O., Baliga, N. S., Wang, J. T., Ramage, D., et al. (2003). Cytoscape: A software environment for integrated models of biomolecular interaction networks. Genome Res. 13, 2498–2504. doi:10.1101/gr.1239303
Spring, F. S., and Stark, J. (1950). Piperettine from Piper nigrum; its isolation, identification, and synthesis. J. Chem. Soc., 1177–1180. doi:10.1039/JR9500001177
Tan, K. K., Khoo, T. J., Rajagopal, M., and Wiart, C. (2015). Antibacterial alkaloids from Artabotrys crassifolius Hook.f. & thomson. Nat. Prod. Res. 29, 2346–2349. doi:10.1080/14786419.2015.1013954
Trager, W., and Jensen, J. B. (1976). Human malaria parasites in continuous culture. Science 193, 673–675. doi:10.1126/science.781840
Trujillo, W., Trujillo, E. T., Ortiz-Morea, F. A., Toro, D. A., and Jaramillo, M. A. (2022). New Piper species from the eastern slopes of the Andes in northern South America. PhytoKeys 206, 25–48. doi:10.3897/phytokeys.206.75971
Tsugawa, H., Cajka, T., Kind, T., Ma, Y., Higgins, B., Ikeda, K., et al. (2015). MS-DIAL: Data-independent MS/MS deconvolution for comprehensive metabolome analysis. Nat. Methods 12, 523–526. doi:10.1038/nmeth.3393
Tsugawa, H., Kind, T., Nakabayashi, R., Yukihira, D., Tanaka, W., Cajka, T., et al. (2016). Hydrogen rearrangement rules: Computational MS/MS fragmentation and structure elucidation using MS-FINDER software. Anal. Chem. 88, 7946–7958. doi:10.1021/acs.analchem.6b00770
Tuntiwachwuttikul, P., Phansa, P., Pootaeng-on, Y., and Taylor, W. C. (2006). Chemical constituents of the roots of piper sarmentosum. Chem. Pharm. Bull. 54, 149–151. doi:10.1248/cpb.54.149
Uckele, K. A., Jahner, J. P., Tepe, E. J., Richards, L. A., Dyer, L. A., Ochsenrider, K. M., et al. (2021). Phytochemistry reflects different evolutionary history in traditional classes versus specialized structural motifs. Sci. Rep. 11, 17247. doi:10.1038/s41598-021-96431-3
Umezawa, T. (2003). Diversity in lignan biosynthesis. Phytochem. Rev. 2, 371–390. doi:10.1023/B:PHYT.0000045487.02836.32
Van den Broeck, F., Savill, N. J., Imamura, H., Sanders, M., Maes, I., Cooper, S., et al. (2020). Ecological divergence and hybridization of Neotropical Leishmania parasites. Proc. Natl. Acad. Sci. U. S. A. 117, 25159–25168. doi:10.1073/pnas.1920136117
Vásquez-Ocmín, P., Cojean, S., Rengifo, E., Suyyagh-Albouz, S., Amasifuen Guerra, C. A., Pomel, S., et al. (2018). Antiprotozoal activity of medicinal plants used by Iquitos-Nauta road communities in Loreto (Peru). J. Ethnopharmacol. 210, 372–385. doi:10.1016/j.jep.2017.08.039
Vásquez-Ocmín, P. G., Gadea, A., Cojean, S., Marti, G., Pomel, S., Van Baelen, A.-C., et al. (2021a). Metabolomic approach of the antiprotozoal activity of medicinal Piper species used in Peruvian Amazon. J. Ethnopharmacol. 264, 113262. doi:10.1016/j.jep.2020.113262
Vásquez-Ocmín, P. G., Gallard, J.-F., Van Baelen, A.-C., Leblanc, K., Cojean, S., Mouray, E., et al. (2022). Biodereplication of antiplasmodial extracts: Application of the amazonian medicinal plant piper coruscans kunth. Molecules 27, 7638. doi:10.3390/molecules27217638
Vásquez-Ocmín, P. G., Marti, G., Bonhomme, M., Mathis, F., Fournier, S., Bertani, S., et al. (2021b). Cannabinoids vs. whole metabolome: Relevance of cannabinomics in analyzing Cannabis varieties. Anal. Chim. Acta 1184, 339020. doi:10.1016/j.aca.2021.339020
Vásquez-Ocmín, P. G., Marti, G., Gadea, A., Cabanac, G., Vásquez-Briones, J. A., Casavilca-Zambrano, S., et al. (2023). Metabotyping of Andean pseudocereals and characterization of emerging mycotoxins. Food Chem. 407, 135134. doi:10.1016/j.foodchem.2022.135134
Vásquez-Ocmín, P., Haddad, M., Gadea, A., Jullian, V., Castillo, D., Paloque, L., et al. (2017). A new phthalide derivative from Peperomia nivalis. Nat. Prod. Res. 31, 138–142. doi:10.1080/14786419.2016.1219857
WHO (2022a). Neglected tropical diseases. Available at: https://www.who.int/health-topics/neglected-tropical-diseases (Accessed July 25, 2022).
WHO (2022b). The sustainable development goals. Available at: https://www.un.org/sustainabledevelopment/ (Accessed October 3, 2022).
WHO (2021). World malaria report 2021. Available at: https://www.who.int/teams/global-malaria-programme/reports/world-malaria-report-2021 (Accessed July 26, 2022).
Yamaguchi, L. F., Freitas, G. C., Yoshida, N. C., Silva, R. A., Gaia, A. M., Silva, A. M., et al. (2011). Chemometric analysis of ESIMS and NMR data from Piper species. J. Braz. Chem. Soc. 22, 2371–2382. doi:10.1590/S0103-50532011001200019
Yan, Y., Li, X., Zhang, C., Lv, L., Gao, B., and Li, M. (2021). Research progress on antibacterial activities and mechanisms of natural alkaloids: A review. Antibiotics 10, 318. doi:10.3390/antibiotics10030318
Yenjai, C., and Wanich, S. (2010). Cytotoxicity against KB and NCI-H187 cell lines of modified flavonoids from Kaempferia parviflora. Bioorg. Med. Chem. Lett. 20, 2821–2823. doi:10.1016/j.bmcl.2010.03.054
Keywords: anti-infectious diseases, metabolomics, Peruvian amazonia, Piper, Cordoncillos
Citation: Vásquez-Ocmín PG, Cojean S, Roumy V, Marti G, Pomel S, Gadea A, Leblanc K, Dennemont I, Ruiz-Vásquez L, Ricopa Cotrina H, Ruiz Mesia W, Bertani S, Ruiz Mesia L and Maciuk A (2023) Deciphering anti-infectious compounds from Peruvian medicinal Cordoncillos extract library through multiplexed assays and chemical profiling. Front. Pharmacol. 14:1100542. doi: 10.3389/fphar.2023.1100542
Received: 16 November 2022; Accepted: 04 May 2023;
Published: 05 June 2023.
Edited by:
Caio Pinho Fernandes, Universidade Federal do Amapá, BrazilReviewed by:
Edward Emmanuel Kweku Baidoo, Berkeley Lab (DOE), United StatesWen-bin Zhang, Renmin Hospital of Wuhan University, China
Copyright © 2023 Vásquez-Ocmín, Cojean, Roumy, Marti, Pomel, Gadea, Leblanc, Dennemont, Ruiz-Vásquez, Ricopa Cotrina, Ruiz Mesia, Bertani, Ruiz Mesia and Maciuk. This is an open-access article distributed under the terms of the Creative Commons Attribution License (CC BY). The use, distribution or reproduction in other forums is permitted, provided the original author(s) and the copyright owner(s) are credited and that the original publication in this journal is cited, in accordance with accepted academic practice. No use, distribution or reproduction is permitted which does not comply with these terms.
*Correspondence: Pedro G. Vásquez-Ocmín, dmFzY28yMjI0QGdtYWlsLmNvbQ==; Alexandre Maciuk, YWxleGFuZHJlLm1hY2l1a0B1bml2ZXJzaXRlLXBhcmlzLXNhY2xheS5mcg==
†These authors have contributed equally to this work