- 1Key Laboratory of Liver and Kidney Diseases (Ministry of Education), Institute of Liver Diseases, Shanghai Key Laboratory of Traditional Chinese Clinical Medicine, Shuguang Hospital Affiliated to Shanghai University of Traditional Chinese Medicine, Shanghai, China
- 2Institute of Clinical Pharmacology, Shuguang Hospital Affiliated to Shanghai University of Traditional Chinese Medicine, Shanghai, China
The incidence of obesity and associated metabolic diseases is increasing globally, adversely affecting human health. Dietary fats, especially triglycerides, are an important source of energy for the body, and the intestine absorbs lipids through a series of orderly and complex steps. A long-term high-fat diet leads to intestinal dysfunction, inducing obesity and metabolic disorders. Therefore, regulating dietary triglycerides absorption is a promising therapeutic strategy. In this review, we will discuss diverse aspects of the dietary triglycerides hydrolysis, fatty acid uptake, triglycerides resynthesis, chylomicron assembly, trafficking, and secretion processes in intestinal epithelial cells, as well as potential targets in this process that may influence dietary fat-induced obesity and metabolic diseases. We also mention the possible shortcomings and deficiencies in modulating dietary lipid absorption targets to provide a better understanding of their administrability as drugs in obesity and related metabolic disorders.
1 Introduction
Obesity is a growing global health crisis. Obesity leads to a number of comorbidities such as non-alcoholic fatty liver disease (NAFLD), diabetes, hyperlipidemia, cardiovascular disease, and cancer, causing an enormous health burden and harm life quality (Chooi et al., 2019). To curb the obesity epidemic, lifestyle intervention becomes a lifelong process. However, obesity is difficult to control and remains a serious public health problem worldwide (Seidell and Halberstadt, 2015).
Increased food energy consumption is the leading cause of obesity and metabolic diseases (Vandevijvere et al., 2015). Triglyceride (TG) is the main dietary lipid, and nearly 90%–95% of the energy produced by fat is derived from TG (Iqbal and Hussain, 2009). Clinical and animal studies have demonstrated the importance of a high-fat diet (HFD) in developing obesity and metabolic disorders. In animal studies, HFD has been widely used to induce obesity, NAFLD, type 2 diabetes mellitus, hyperinsulinemia, and hyperlipidemia in mouse models (Heydemann, 2016; Recena Aydos et al., 2019; Li et al., 2020). Increased fat absorption is one of the primary impetuses of obesity and metabolic diseases (Luo et al., 2018). In contrast, restricting TG absorption improves the obesity phenotype.
The intestine, which absorbs nutrients, is of principal importance in regulating dietary fat absorption. Nearly 95% of dietary TG can be absorbed and undergo digestion, uptake, resynthesis, and secretion into the circulation in chylomicron (CM) (Mansbach and Siddiqi, 2016; Zembroski et al., 2021). Dietary TG is essential for the maintenance of systemic lipid homeostasis. Excessive intake of TG causes systemic metabolic disorders and intestinal lipid metabolism disorders. In humans, as little as 3 days of HFD can significantly decrease gastrointestinal transit time (Clegg et al., 2011). Moreover, a chronic HFD markedly affects intestinal physiology and even influences the absorption of other macronutrients. The regulation of essential genes involved in this process may disrupt the entry of dietary TG into the circulatory system. In this review, we have insight into the role of dietary TG absorption in obesity and metabolic diseases. We will overview the dietary TG absorption process in the intestine, especially focusing on the interaction between TG absorption, intestinal function, and metabolic diseases, as well as the key proteins that regulate intestinal lipid mobilization and metabolism. We hope to provide a novel perspective for the preventing and treating of obesity and metabolic diseases.
2 Overview of dietary triglyceride absorption process in intestine
2.1 Dietary triglyceride digestion
TG digestion begins upon contacting the lingual lipase secreted by the lingual gland. Digestion continues under the action of gastric enzymes in the stomach. The coarse fat emulsion enters the duodenum as lipid droplets and is solubilized by the action of bile acid. TG is further digested and hydrolyzed in the lumen by several pancreatic lipases (PL) to yield sn-2-monoacylglycerol (2-MAG) and fatty acids (FAs). 2-MAG and long-chain FA (LCFA) are taken up by intestinal epithelial cells effectively (Figure 1).
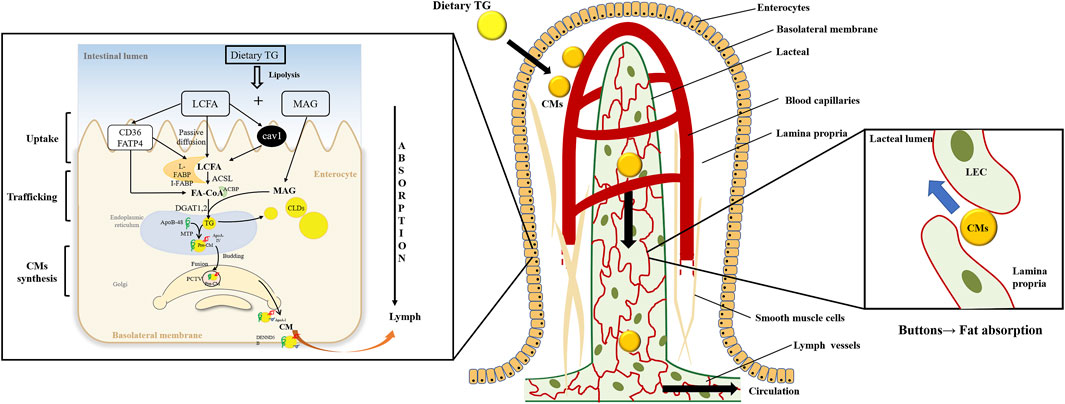
FIGURE 1. Dietary triglyceride transport from the intestinal lumen, enterocytes, and lacteals. Dietary triglyceride (TG) is hydrolyzed into long-chain fatty acids (LCFAs) and monoacylglycerols (MAGs) in the intestinal lumen. The enterocytes uptake the digestion products and traffic them into the endoplasmic reticulum (ER). And TG is resynthesized in the ER, which may bud off the ER membrane to form cytoplasmic lipid droplets (CLDs) or be assembled to form pre-chylomicrons (pre-CMs). The pre-CMs are transported to Golgi in pre-chylomicron transport vesicle (PCTV) for further mature. Then mature chylomicrons (CMs) exit the basolateral membrane to lamina propria. CMs are transported into the lacteals through the buttons junction of lymphatic endothelial cells (LECs) and secreted into the lymphatic vessels before entering circulations.
2.2 Long-chain fatty acid uptake and traffic
Transport of LCFA and MAG in enterocytes occurs by passive diffusion. In addition, transporters promote LCFA take-up, especially when the concentrations of LCFA are low in the lumen. Various LCFA transporters, including the cluster of differentiation 36 (CD36), fatty acid transport protein 4 (FATP4), and the plasma membrane-associated fatty acid-binding protein, are involved in the transportation process (Stahl et al., 1999; Drover et al., 2005). Moreover, Caveolae-mediated endocytosis also contributes to FAs uptake, and caveolin-1 plays a vital role in forming caveolae (Parton and del Pozo, 2013). Although specific 2-MAG transporter has not been reported, some in vitro experiments suggest that FAs transporters are partially involved in this process (Murota et al., 2001; Murota K, 2005).
LCFAs and 2-MAG traverse across the cytoplasm to reach the endoplasmic reticulum (ER) membrane in enterocytes. Cytoplasmic fatty acid-binding proteins such as intestinal FABP (I-FABP) and liver FABP (L-FABP) are involved in intracellular LCFAs transportation (Storch and Corsico, 2008).
2.3 Intracellular triglyceride resynthesis
The first step of TG resynthesis is adding the coenzyme A (CoA) group to the LCFAs, which is catalyzed by long-chain acyl-CoA synthetases (ACSL) (Ellis et al., 2010). FATP4 has acyl CoA synthetase activity and is also involved in FAs acylation (Milger et al., 2006). The newly generated FA-CoA is bound to an acyl-CoA binding protein, and this process can regulate cellular FA-CoA disposal (Niot et al., 2009).
In the ER membrane, two multi-enzymatic systems catalyze FA-CoA re-esterified into TG: the glycerol-3-phosphate pathway and the monoacylglycerol pathway. In enterocytes, TG resynthesis is mainly along the monoacylglycerol pathway, and nearly 80% of the resynthesized TG is produced from this pathway (Kern and Borgstrom, 1965). Monoacylglycerol acyltransferase (MGAT) is a key enzyme that transports FA-acyl-CoA to 2-MAG to generate DAG. DAG is further esterified by diacylglycerol acyltransferase (DGAT) with an FA-CoA to yield TG (Cases et al., 1998).
2.4 Enterocyte cytoplasmic lipid droplets storage
The re-esterified TG in intestinal epithelial cells is either transported to the ER to synthesize lipoproteins or stored in cytoplasmic lipid droplets (CLDs) in a highly dynamic state (Demignot et al., 2014). In enterocytes, the biogenesis mechanisms of CLD remains unclear. This process may consist of re-esterified TG accumulation and lens formation in the ER bilayer, and then the CLD enters the cytoplasm by budding (Zembroski et al., 2021). Intestinal CLDs are divided into different dynamic lipid pools due to three different origin sources: 1) dietary lipids taken up on the apical side of enterocytes; 2) circulation lipids absorbed at the basolateral side of enterocytes; 3) de novo FAs synthesis. CLDs synthesized from different sources may undergo different metabolic fate. FAs delivered from the apical side are preferably used for TG synthesis, while the FAs delivered from the basolateral side are used for phospholipid synthesis and preferential oxidation (Storch et al., 2008).
2.5 Chylomicron assembly, trafficking, and secretion
The resynthesized TG is transported to the ER to synthesize CMs. In the inner leaflet of ER, microsomal triglyceride transfer protein (MTP) facilitates lipidation of apolipoprotein B-48 (ApoB-48) to form the pre-CM. The pre-CMs is then secreted from the ER and transported to the Golgi via pre-CM transport vesicles (PCTVs) for further maturation. In Golgi, pre-CM is further modified by addition of ApoA-I and glycosylating ApoB-48 to form mature CM [reviewed in (D'Aquila et al., 2016)]. Mature CM is excreted from the Golgi in large vesicles and then releases from the basolateral side of the intestinal epithelial cells into the lamina propria by exocytosis. Lacteal drains CMs into the lymphatic system and finally enters into circulation (D'Aquila et al., 2016).
3 Impacts of dietary triglyceride on obesity and metabolic diseases
3.1 Influence of triglyceride consumption
The amount of dietary TG intake is a decisive factor in obesity and metabolic diseases. The human body has a good absorption and clearance capacity of dietary TG. A single intake of 15 g of dietary TG did not affect postprandial lipids and lipoprotein in healthy adults (Dubois et al., 1998). Although postprandial hyperlipemia is a normal physiological response to acute dietary TG intake, increased TG intake may break the balance, leading to stepwise severe lipidaemia (Dubois et al., 1998). An intake of over 40 g of fat per meal in healthy adults can lead to lipidaemia, which can be exacerbated by successive lipid-containing meals (Dubois et al., 1998). Another study in obese individuals also confirmed that ingesting 40 g of fat can increase the postprandial plasma TG concentration (Vors et al., 2015). Postprandial states are repeated during waking hours, and pathological postprandial hyperlipidemia persists for a long time, which leads to the accumulation of postprandial TG-rich lipoproteins. Their remnants in the circulation may have a profound effect on cardiovascular disease, obesity, and metabolic diseases. In patients with insulin resistance (IR), elevated serum TG is often found in non-fasting state (Moreno-Perez et al., 2021).
3.2 Impacts of the composition of fatty acids
The progression of metabolic diseases is not only related to dietary TG intake but also to its composition. Dietary TG is a group of compounds with various chemical properties, and the FA and TG compositions of all lipids vary significantly (Ye et al., 2019). From the perspective of intestinal TG absorption, the rate order of dietary TG digestion is commonly reported as long-chain length TG < medium-chain length TG (MCT) < short-chain length TG (Ye et al., 2019). PL cleaves the sn-1 and sn-3 sites of TG to form free FAs and 2-MAG (Figure 2A). LCFAs and 2-MAG are re-esterified to form resynthesized TG and CM, which are then secreted into the lymphatic system. In contrast, SCFAs (2–4C) and MCFAs (6–12C) are soluble in the aqueous phase of intestinal contents, absorbed in combination with albumin, and delivered to the liver through the portal vein or directly absorbed in the stomach (Thomson et al., 1989). Due to the lack of FAs for 2-MAG re-esterification, the amount of CM secretions in lymph is significantly decreased. MCT administration does not induce an apparent maximum number of CM particles in lymph. Diets containing MCFA are less likely to predispose to obesity than those containing LCFA, and MCT may be a treatment for postprandial hypertriglyceridemia (Papamandjaris et al., 1998; Folwaczny et al., 2021). Patients with metabolic disorders have recently paid wide attention to DAG. DAG does not follow the monoacylglycerol pathway for TG resynthesis. DAGs, in particular sn-1,3-DAG, are digested in the small intestine by lipase to synthesize sn-1-MAG or sn-3 MAG (Lee et al., 2020). Compared to 2-MAG, the re-esterification ability of sn-1-MAG and sn-3-MAG is poor. This unique metabolic pattern hinders the release of CM, producing a lipid-lowering effect (Lee et al., 2020).
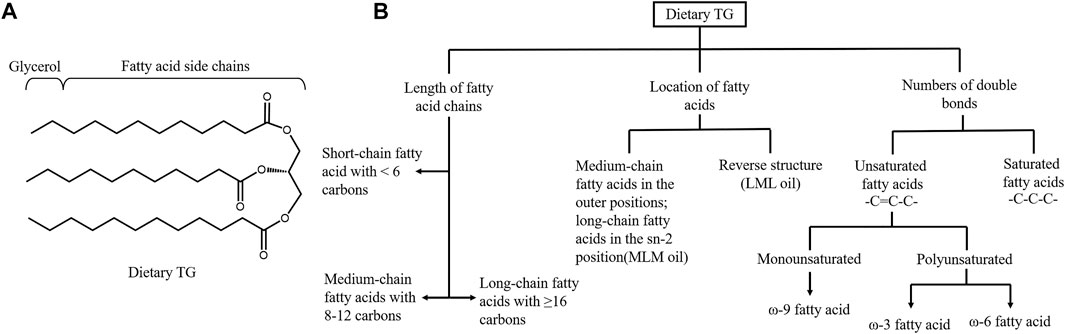
FIGURE 2. Structure and classification of dietary triglyceride. (A) Structure of triglyceride (TG). Pancreatic lipase hydrolyzes the sn-1 and sn-3 positions of TGs to generate sn-2 MGs and free fatty acids. (B) Dietary triglyceride can be classified based on the length of fatty acid (FA) chains, the location of different length of FAs in TG and the numbers of double bonds in the hydrocarbon chains. Basing on the numbers of carbons, FAs can be subcategorized into short, medium and long-chain FAs. Three FAs in the TG contribute to the different structured oil, including medium-chain FA in the outer positions of the TG and long-chain FA in the sn-2 position and the reverse structure. Unsaturated FAs contains double bonds while saturated FAs only have single bonds. Unsaturated FAs are further categorized in to monounsaturated FAs and polyunsaturated according to the number of the double bonds.
The location of FAs in TG can also affect lipids absorption efficiency and metabolic syndrome (Figure 2B). Ye et al. demonstrated that short-chain saturated FAs at −1,3 position could favor lipid digestion. Lipids with faster digestion may affect serum lipid profiles more easily and persistently (Ye et al., 2019). In infants, the amount of double bonds (unsaturated FAs) was positively related to fat absorption (Hageman et al., 2019). However, the lipid absorption efficiency cannot be used alone to determine the effect on metabolic syndrome. Although the MCFA and MCT are easily absorbed, they are systematically associated with higher energy expenditure and are also recommended to control obesity phenotypes (Rial et al., 2016). MCFAs can significantly reduce late apoptotic and necrotic cells, decrease the levels of inflammatory markers and exert benefits in the treatment of NAFLD (Wang et al., 2016). Polyunsaturated fats are more easily absorbed than LCFAs and are inversely associated with insulin sensitivity (Bray et al., 2002).
Dietary TG can be classified into saturated, monounsaturated, and polyunsaturated fats based on the double bonds in the hydrocarbon chain. Although there are few reports on the difference in lipid absorption between saturated FAs and unsaturated FAs, both can significantly increase postprandial plasma TG (Folwaczny et al., 2021). Unsaturated FAs enriched diets such as the Mediterranean diet have been reported to have a potential anti-inflammation and anti-obesity effect. Conversely, western diets and diets rich in saturated fats accelerate the synthesis of pro-inflammatory chemokines and cytokines, contributing to the development of metabolic diseases (Ravaut et al., 2020).
4 Impacts of high-fat diet-induced obesity and metabolic diseases on intestinal function
4.1 Changes in intestinal morphology
Intestinal epithelial cells exhibit significant cell replacement and are well-adapted to different nutritional states of the body. Dietary lipids strongly stimulate mucosal regeneration (Buts et al., 1990). Short-term (3 weeks) HFD (containing 40% lipids) significantly increased the mitosis index of jejunum in B6D2F1 mice, increasing villous size and absorption area. The expression of genes related to LCFA uptake, transport, and lipoprotein synthesis is also induced during HFD feeding (Petit et al., 2007). However, after returning to the chow diet, the changes described above also returned to normal, demonstrating that the intestine has a powerful adaptation to cope with dietary fat. With the prolongation of HFD (59% kcal from fat) intake, Swiss mice fed with a HFD for 8 weeks showed a decreased length of the small intestine and decreased circumference of the ileum. In the small intestine of mouse, morphometric parameters, including the villi, crypts, muscular layer, and total wall were decreased in the distal part but increased in the proximal part (Soares et al., 2015). When the C57/BL6 mice were fed a HFD (60% kcal from fat) for 14 weeks, the length of villi in the small intestine and the depth of crypts in the colon were significantly shorter than those in control. The 14-week HFD led to increased intestinal stem cell counts, apoptosis rate, and decreased goblet cell numbers and mucin levels (Xie et al., 2020).
The intestinal epithelial basement membrane prevents CMs from randomly entering the lamina propria. During acute HFD, CM leaves the intestinal cells and accumulates in the intercellular space, putting pressure on the tight connections between cells. The basement membrane may rupture due to stretching, resulting in increased mucosal permeability and facilitating the entry of CMs and other harmful substances into the lamina propria (Zhou et al., 2020). The exact mechanism of CM transport from basolateral membrane requires further investigation. Nevertheless, the leaky gut and impaired tight connections between cells provide a better understanding of HFD-induced intestinal barrier damage and metabolic disorders caused by intestinal dysfunction.
Intestinal neuron loss was found in the duodenum of mice fed a HFD (72% kcal from fat) for 8 weeks (Stenkamp-Strahm et al., 2013). According to statistics, the proportion of obese people in patients with gastroparesis can reach 29% (Parkman et al., 2021). The hospitalization rate of obese gastroparesis patients accounted for 13.75% of the total gastroparesis patients (Dahiya et al., 2022). Neuron loss-induced changes in gastrointestinal motility also contribute to the development and maintenance of obesity. Moreover, intestinal blood flow can be up to twice as high after dietary lipid ingestion than after fasting, a phenomenon called postprandial congestion. LCFAs are more effective than other nutrients in inducing hyperemia in the distal small intestine (Eduardo, 2005). Increased intestinal blood flow enhances free FA extraction from circulation into enterocytes, providing mitochondrial oxidation substrates and influencing intestinal metabolism. Recently, Koffert et al. reported that obesity could not be normalized by bariatric surgery. Although postoperative patients lost 24% of their weight, they still exhibited increased FA uptake and unchanged duodenal blood flow (Koffert et al., 2018).
Intestinal epithelial cells respond to lumen stimulation by producing chemokines and cytokines that induce the accumulation of lamina propria immune cells in areas of inflammation. Dietary nutrients, especially lipids, can have side effects that trigger immune cell activation. More free FAs derived from a HFD are cytotoxic and can disrupt the intestinal immune system [reviewed in (Basson et al., 2020)]. Tamala et al. demonstrated that reduced numbers of small intestinal intraepithelial lymphocytes and lamina propria lymphocytes could be found in mice fed HFD for only 1-day. HFD feeding for 3 weeks induced atrophy of gut-associated lymphoid tissue (Tanaka et al., 2020). In DSS-induced colitis mice, HFD aggravates experimental colitis by decreasing the number of goblet cells and mucin levels (Lee et al., 2017). In clinical patients, a large amount of animal lipid intake plays a role in the pathogenesis of inflammatory bowel disease (Ji et al., 2011). In addition, dietary fat-activated mast cells release mucosal mast cell protease II (RMCPII), which directly increases the permeability of epithelial cells by reducing the expression of tight junction-related proteins (Scudamore et al., 1998). RMCPII can also selectively attack type IV collagen, a vital component of the basal membrane (Scudamore et al., 1998). The activation of mucosal mast cells may be involved in intestinal permeability and insular membrane incompleteness, facilitating fat transport and uptake (Ji et al., 2012).
4.2 Changes in dietary nutrient absorption
Dietary TG is a potent suppressor of further energy intake, but chronic HFD reduces the satiating effect of enteral oleic acid infusion (Covasa and Ritter, 1999). In obese rats, chronic HFD feeding leads to increased gastric emptying, partly due to impaired cholecystokinin signaling (Covasa and Ritter, 2000). In human studies, obesity is also closely associated with rapid gastric emptying and fasting gastric volume (Acosta et al., 2015). Although obese patients obtain the overload energy intake from the diet, increased gastric emptying causing decreased fullness could contribute to greater energy intake (Paulino et al., 2008).
HFD consumption can directly or indirectly affect each step of TG absorption. Chronic HFD could increase the synthesis and release of pancreatic lipase, facilitating TG digestion (Sabb et al., 1986). Unlike the genes related to TG absorption in the duodenum mucosa that are decreased by a single HFD, chronic HFD can increase the expression of these genes, with the middle part of the small intestine obtaining the most pronounced effect (de Wit et al., 2008; Yoshioka et al., 2008). C57BL/6 mice fed HFD for up to 12 weeks exhibited a dramatic upregulation of LCFA uptake/trafficking (Fatp-4, I-Fabp, L-Fabp, Mtp) and lipoprotein synthesis (ApoA-IV) gene expression (Torelli Hijo et al., 2019).
HFD can modulate nutrient transporters that may further influence overall nutrient uptake. High-fat consumption decreases the expression of cholesterol transporter niemann-pick C1-like 1, influencing cholesterol absorption (de Vogel-van den Bosch et al., 2008). The peptide cotransporter-1 solute carrier transporter 15A1 protein, which transports dipeptide and tripeptide products of protein digestion in the small intestine, was also reduced in HFD-fed mice, suggesting that HFD-fed mice suffer from impaired peptide absorption (Do et al., 2014). Moreover, carbohydrates transporter GLUT2 content also decreased significantly (Torelli Hijo et al., 2019). Surprisingly, the downregulated expression of nutrient transporters in mice was detected in the third week of HFD, though the obesity phenotype occurred after 6 weeks (Torelli Hijo et al., 2019). Moreover, HFD also enhances sodium transporter Na (+)-H (+) exchanger 3 content, partly contributing to the hypertension progression in obesity (Torelli Hijo et al., 2019). Mariana et al. demonstrated that HFD feeding affects the expression of intestinal nutrient transporters in hyperthyroidism or hypothyroidism mice through an independent mechanism of PPAR-α (Losacco et al., 2018). High levels of fat intake can have a negative effect on nutritional status, introducing an obesity-related malnutrition concept.
4.3 Changed the cytoplasmic lipid droplets storage in enterocytes
Enterocytes can not only transport dietary TG but also act as storage organs to store the resynthesed TG in CLDs. In obesity, fat is deposited in the liver and adipose tissues. Similarly, ectopic fat deposition has also been observed in the intestine. Dietary fat intake can lead to a first increase and then decrease in the number and size of CLDs (Uchida et al., 2012). In HFD-induced obese mice and ob/ob mice, mucosa accumulated larger CLDs in the fed state (Uchida et al., 2012). In vitro, Caco-2/15 enterocytes, with prolonged exposure to high lipid concentrations, also exhibited CLD growth (Auclair et al., 2019). D'Aquila et al. found that diet-induced obese mice altered the CLD proteome, with increased or unique subgroup of lipid-related proteins involved in steroid synthesis, TG synthesis, and lipolysis (D'Aquila et al., 2019). Some candidate protein species and locations, such as perilipin family members, are also differed in the HFD model. Lee et al. found that perilipin family members act differently in the dietary fat challenge. Perilipin2 and perilipin3 proteins were present in the lean and dietary fat-challenged mice. Meanwhile, in acute and chronic HFD-fed mice, only perilipin2 coated CLDs in enterocytes and stabilized stored TG (Lee et al., 2009). Chronic HFD-induced obesity influences the expression of genes involved in CLD metabolism. Compared with lean mice, DIO mice had lower levels of genes related to lipolysis and fatty acid oxidation (FAO), and genes associated with TG synthesis, CM synthesis, TG storage, and lipolysis were not induced by acute dietary fat challenge (Uchida et al., 2012; D'Aquila et al., 2019). However, during the acute dietary fat challenge, DIO mice did not show increased expression of these genes. Further, the levels of genes mediating CLD hydrolysis were markedly lower 2 h after the challenge than baseline (Uchida et al., 2012).
4.4 Changed chylomicron secretion
An increased dietary TG load may lead to three different models of CM secretion: i) expansion of the size of CMs, ii) an increase in the number of CMs, and iii) a combination of these processes. In insulin-deficient rat model, the secretion rate of CMs is decreased, whereas the size of CMs is increased (Martins et al., 1994). To adapt to these changes in CM secretion, plasma TG clearance is enhanced in HFD mice. In HFD induced obese mice, the expression of intestinal ApoC-III gene, a potent inhibitor of blood CM clearance lipoprotein lipase, was decreased. In contrast, the expression of ApoC-II, a strong activator of lipoprotein lipase, was increased. High ApoC-II/III ratio contributes to increased clearance of CMs from the blood (Petit et al., 2007). Moreover, FAO is activated to metabolize lipids in enterocytes, preventing excess dietary TG from being transported into circulation (Kondo et al., 2006; de Wit et al., 2008). The decreased CM secretion rate and enhanced lipid oxidation and clearance function may be among the reasons for the decrease in TG levels in mice fed a TG-rich diet. However, plasma TG level changes after lipid challenge are inversely correlated with the body weight increase (Ji and Friedman, 2008). Kondo et al. also demonstrated that fat-mediated lipid metabolic adaptation was related to susceptibility to obesity (Kondo et al., 2006). The increasing number of CMs in response to dietary TG load may lead to increased CM remnants, resulting in an increased risk of diabetes and atherosclerosis (Tomkin and Owens, 2001). CMs and very low-density lipoproteins are the primary TG sources for peripheral cells and tissues. In the postprandial state, the number and size of CMs in the circulation increase markedly. Compared to very low-density lipoprotein, CMs are the first lipoprotein lipase substrates, allowing very low-density lipoprotein to remain in circulation for a prolonged period of time, increasing the risk of atherosclerosis (Potts et al., 1991).
5 Effects of the genes involved in regulating dietary triglyceride absorption on obesity and related metabolic disorders
5.1 Focusing on dietary triglyceride digestion
Bile acids (BAs) emulsify dietary lipids and facilitate the hydrolysis and absorption of fats in the proximal intestine. The composition of BAs can determine the absorption efficiency of dietary lipids. Mice lacking 12α-hydroxylase cytochrome P450 family 8 subfamily B member 1gene (Cyp8b1−/−) have altered BA composition, impaired absorption of dietary TG, increased fecal TG levels, decreased body weight and improved glucose tolerance (Bertaggia et al., 2017; Higuchi et al., 2020). The 12α hydroxylated BAs, such as cholic acid, taurocholate acid and glycocholic acid, have been reported to be associated with IR and high TG levels (Haeusler et al., 2013). Cyp8b1−/− mice treated with 12α-hydroxylated BA taurocholic acid or CA restored the absorption of lipids (Bertaggia et al., 2017; Higuchi et al., 2020). However, using a bile acid sequestrant to modulate the bile acid pool is also accompanied by persistent gastrointestinal adverse reactions, such as constipation, bloating, and hypertriglyceridemia (Goldfine et al., 2010) (Table 1).
PL is responsible for the hydrolysis of 50%–70% of dietary lipids (Armand, 2007; Birari and Bhutani, 2007). PL inhibitors influence the root cause of obesity (Kumar and Chauhan, 2021). Orlistat, a well-known PL inhibitor, is the only authorized anti-obesity drug (McClendon et al., 2009). However, the use of orlistat is associated with gastrointestinal adverse effects, such as oily stools and diarrhea (Filippatos et al., 2008). Apart from Orlistat, plenty of phytoconstituents, including flavonoids, saponins, alkaloids, and terpenoids, also have profound inhibitory effects on PL (Rajan et al., 2020; Kumar and Chauhan, 2021). Though blocking fat digestion is an exciting opportunity for treating obesity, the unabsorbed lipid transport into the lower intestine can activate GPR119 to slow gastric emptying (Higuchi et al., 2020).
5.2 Focus on long-chain fatty acid uptake and traffic in enterocytes
5.2.1 Long-chain fatty acid uptake
Intestinal absorption of LCFA requires CD36. Cd36 gene deficiency (Cd36−/−) mice showed a 50% reduction in FA uptake in proximal enterocytes (Nassir et al., 2007). However, based on fecal lipid content and blood appearance, Cd36−/− mice showed no evidence of reduced lipid absorption. They only had a reduced rate of TG entry into lymph and serum (Goudriaan et al., 2002). The unaltered lipid uptake in Cd36−/− mice may be due in part to ultimate compensation by the transfer of the lipid absorption sites to the distal parts of small intestine (Nassir et al., 2007). However, CD36 also influences the catabolism of TG-rich CMs in plasma. In Cd36−/−mice, lipoprotein lipase activity was normal, but the clearance of intestine-derived lipoproteins was slow (Drover et al., 2005). In addition, people with CD36 deficiency develop postprandial hypertriglyceridemia, IR, and cardiovascular disease through enhanced lipoprotein remnant levels in plasma (Masuda et al., 2009). Hajri et al. demonstrated that global Cd36−/− mice were protected against obesity, mainly caused by impaired FA sensing by adipocytes and elevated leptin levels (Hajri et al., 2007). However, Cd36 deletion in endothelial cells result in a leaky epithelial barrier and inflammation (Cifarelli et al., 2017).
In the FATP family, FATP4 is mainly expressed in jejunum (Buttet et al., 2014). In vivo, isolated enterocytes with a 48% reduction in FATP4 protein showed a 40% reduction in FA uptake (Gimeno et al., 2003). Nevertheless, Fatp4 deletion (Fatp4−/−) mice exhibited no modified fat absorption and had no protection against obesity during HFD feeding (Shim et al., 2009). However, FATP4 is not ineffective in regulating metabolic disorders. For example, Fatp4 deletion plays a crucial role in oleic acid-induced GLP-1 secretion, enhancing glucose-dependent insulin secretion and inhibiting gastric emptying (Poreba et al., 2012).
Caveolar vesicle structures are formed by the caveolin proteins, which are present in a single caveola and can directly bind to FAs (Trigatti et al., 1999; Parton and Simons, 2007). Global Caveolin-1 deletion (Cav1−/−) mice showed protection against diet-induced obesity, partly due to the higher presence of steatorrhea (Razani et al., 2002; Siddiqi et al., 2013). However, serum TG, cholesterol, and free FA levels were severely elevated in Cav1−/−mice, especially in the postprandial state (Razani et al., 2002). Intestinal specific-Caveolin-1 deletion (Cav1iEC−KO) mice decreased circulating free FAs and low-density lipoproteins cholesterol levels, preventing HFD-induced obesity and rapid increase in low-density lipoproteins cholesterol (Otis et al., 2017). Moreover, global Cav1−/− mice exhibited elevated very low-density lipoproteins and low-density lipoproteins cholesterol levels, mainly resulting from reduced lipid storage by adipocytes and hepatic lipoprotein cholesterol uptake (Frank et al., 2008). The increased circulating free FA levels in Cav1iEC−KO mice may be due to decreased hepatic FAs instead of altered intestinal processing (Otis et al., 2017).
5.2.2 Long-chain fatty acid traffic
FAs are transported to specific metabolic sites within enterocytes by FABPs. In vitro, both L-FABP and I-FABP are involved in FA uptake from the intestinal lumen and circulating blood into enterocytes (Alpers et al., 2000). The function of the two FABPs is independent because eliminating one gene does not cause compensatory upregulation of the other (Storch and Thumser, 2010). I-Fabp deletion (I-Fabp−/−) mice exhibited a leaner phenotype when fed a HFD than wild-type mice, whereas L-Fabp deletion (L-Fabp−/−) mice displayed higher body weight and body fat mass (Gajda et al., 2013; Lackey et al., 2020). There are also some contrasting results. Newberry et al. found that L-Fabp−/− mice were protective against western diet-induced obesity and hepatic steatosis (Newberry et al., 2006). During HFD feeding, male I-Fabp−/− mice had greater liver mass and gained more weight than wild-type mice. However, female I-Fabp−/− mice exhibited smaller livers and lower weight gain (Agellon et al., 2007). These different phenotypes may result from various factors such as lipid species in the HFD, sex, residual background genes, and even living environment (Newberry et al., 2015). Although L-Fabp−/− mice exhibited different changes in body weight, lower liver TG content and decreased FAO were consistent (Newberry et al., 2006). L-Fabp−/− obese mice had normal blood glucose and lipid levels, lower intestinal TG secretion rate, and greater exercise tolerance (Gajda et al., 2013).
The leaner phenotype of I-Fabp−/− mice is not dependent on fat malabsorption. Instead, the shortened movement time of the small intestine and blunt villi lead to changes in nutrient absorption, thus affecting systemic energy metabolism (Lackey et al., 2020). Notably, the intestinal tissue in I-Fabp−/− mice was more fragile, with thinner muscular layer and higher permeability in the proximal small intestine. I-Fabp−/− mice exhibited intestinal dysbiosis and even pathophysiological changes under HFD feeding (Lau et al., 2016; Lackey et al., 2020). In humans, L-FABP is more abundant than I-FABP. The I-FABP mutation can increase the affinity to FA, which is associated with hypertriglyceridemia and IR in Pima Indians (Baier et al., 1995).
5.3 Focus on the intestinal triglyceride resynthesis
5.3.1 Long-chain fatty acid-coenzyme A synthesis
ACSL catalyzes the first step of TG resynthesis. ACSL5 and ACSL3 are the main synthetases in the intestine and ACSL5 contributes 60%–80% of the total activity of intestinal ACSL activity (Meller N et al., 2013). ACSL5 transports FAs for lipid biosynthesis. During the neonatal period, Acsl5 variant is associated with recurrent vomiting, diarrhea, and failure to thrive (Al-Thihli et al., 2021). The administration of MCT that is absorbed directly through the portal vein or stomach can attenuate the aforementioned adverse effects, reflecting the importance of ACSL5 in promoting long-chain length TG absorption (Al-Thihli et al., 2021). Global Acsl5 deletion (Acsl5−/−) mice exhibited decreased TG absorption, lower fat mass, lower blood TG and glucose levels, and increased insulin sensitivity without acute postnatal complications (Bowman et al., 2016). Meanwhile, dietary LCFA absorption and body weight gain remained unaffected in Acsl5−/− mice, possibly due to the residual ACSL activity that can maintain normal LCFA absorption (Meller N et al., 2013). However, Acsl5 deficiency may impair intestinal epithelium. Reduced Acsl5 levels can lead to high concentrations of ceramide-mediated apoptosis and oxidative stress of unesterified FAs in intestinal epithelial cells (Al-Thihli et al., 2021). Acsl3 gene expression is induced by the liver X receptor, and liver X receptor agonists promote Acsl3 expression and delay lipid secretion in zebrafish (Cruz-Garcia and Schlegel, 2014).
5.3.2 DAG synthesis
In the MGAT family, MGAT2 is highly expressed in the intestine of human and mouse, and MGAT3 is only highly expressed in the distal small intestine of human. Mice without global Mgat2 genes (Mgat2−/−) exhibited resistance to HFD-induced obesity, although they consumed and absorbed normal amounts of dietary fat (Yen et al., 2009). In global Mgat2−/− mice, intestine-specific overexpression of Mgat2 restored fat absorption rate and metabolic efficiency and promoted weight gain during HFD (Gao et al., 2013). In addition, mice with intestinal-specific Mgat2 deficiency exhibited similar delayed fat absorption and increased energy expenditure. They were equally resistant to HFD-induced NAFLD, hypercholesterolemia, and glucose intolerance (Nelson et al., 2014). In addition, global Mgat2−/− mice showed increased GLP-1 and PYY secretion, which contributed to appetite reduction and glycemic control (Yen et al., 2009). However, global Mgat2−/− mice fed a fat-free diet also showed increased energy expenditure, which suggested that MGAT2 modulates energy expenditure and may be independent of dietary fat absorption (Nelson et al., 2011).
Recently, some small-molecule MGAT2 inhibitors with significant structural diversity may be used to treat obesity and metabolic diseases further (Devasthale and Cheng, 2018). However, MGAT2 inhibitors result in minor gastrointestinal adverse reactions that may be related to the unique presence of MGAT3 in the human intestine. Although the role of MGAT3 in lipid absorption has been recognized for a long time, this gene is lacking in the intestinal tract of mice and other lower animals. The construction of an appropriate animal model can help further understand the physiological functions of MGAT3.
5.3.3 Triglyceride resynthesis
DGAT enzyme is encoded by two non-homologous genes, DGAT1 and DGAT2 (Yen et al., 2008). Deletion of the Dgat1 gene (Dgat1−/−) increased energy expenditure in mice, improved leptin and insulin sensitivity, and improved resistance to diet-induced obesity and NAFLD (Chen et al., 2002). In addition, in lipid stress testing and HFD feeding studies, gut-specific deletion of Dgat1 gene or DGAT1 inhibitors led to increased accumulation of total neutral lipid droplets in proximal intestinal epithelial cells and the delayed release of CMs into the blood (Ables et al., 2012; Maciejewski et al., 2013; Hung and Buhman, 2019). Notably, after restoring the function of intestinal DGAT1 in Dgat1−/−mice, the resistance to HFD-induced hepatic steatosis and obesity disappeared (Lee et al., 2010).
Studies have also suggested that the effect of DGAT2 on intestinal fat absorption is greater than that of DGAT1 (Buhman et al., 2002). DGAT2 is necessary for the growth and development of mice, as Dgat2 gene deletion mice die after birth due to insufficient fat and severely impaired skin permeability barrier function (Stone et al., 2004). In DGAT1-deficient human intestinal stem cell-like organoids, DGAT2 overexpression partially compensated for CLD formation and protected against oleic acid-induced toxicity (van Rijn et al., 2019). Furthermore, Dgat2 overexpressed mice exhibited higher CM secretion rates, contributing to hypertriglyceridemia and hepatic steatosis (Uchida et al., 2013). Since only DGAT1 is highly expressed in the human small intestine, and DGAT2 is expressed only at very low levels, DGAT2 is defined as non-functional. DGAT1 mutation is associated with congenital diarrheal disorders, intestinal failure, and aberrant lipid metabolism (van Rijn et al., 2018). DGAT1 inhibitors can lead to severe gastrointestinal reactions, such as diarrhea and nausea. The intestinal cytotoxic FA produced by DGAT1 inhibitors in humans is similar to the inhibition of DGAT1 and DGAT2 simultaneously in mice (Denison et al., 2014; van Rijn et al., 2018).
5.4 Focus on lipid pools
5.4.1 Formation of cytoplasmic lipid droplets
The number of CLDs in enterocytes synthesizes a dynamic lipid pool (Figure 3). Diverse proteomics have novel roles in regulating the balance of TG storage in CLDs or secretion in CMs (D'Aquila et al., 2019). More than 180 proteins are associated with the CLD fraction, among which the perilipin family has been widely reported (D'Aquila et al., 2015). In the perilipin family, perilipin2 is highly expressed in the small intestine. A global absence of the perilipin2 protein (Plin2-null) prevented HFD-induced obesity, IR, and liver steatosis, partly by limiting energy intake (McManaman et al., 2013). Plin2-null mice had increased fecal TG levels and decreased enterocyte CLD content in a 4-day HFD feeding experiment (Frank et al., 2015). Moreover, the metabolic benefits of perilipin2 deletion may also be due to increased energy expenditure or altered intestinal structure and function (Frank et al., 2015; Xiong et al., 2017).
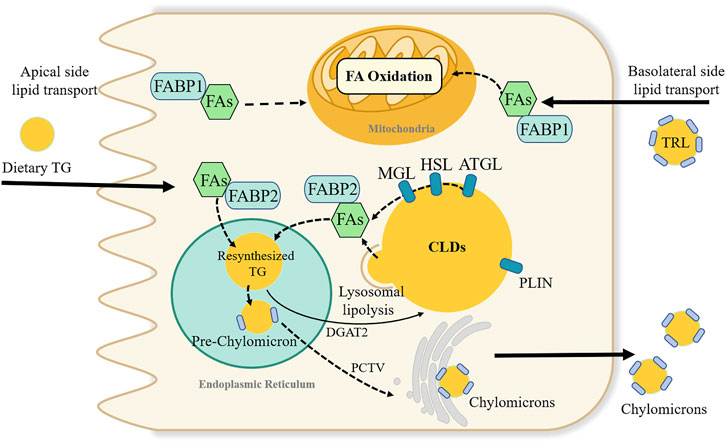
FIGURE 3. Dynamic lipids pool and proposed fatty acids metabolic fate in intestinal enterocyte. Fatty acids (FAs) in enterocytes can source from the apical side of enterocytes or the basolateral side. FAs absorbed from the intestinal lumen preferentially direct the triglyceride (TG) synthesis and undergoes secretion into lymph in chylomicrons. While, FAs from the circulatory system, i.e., basolateral side source of FAs are pre-utilized for phospholipid synthesis and FA oxidation. FAs binding to fatty acid-binding protein 1 is suggested to transported FAs towards the FA oxidation pathway. Many proteins like perilipin family involve in the stabilizing and swelling of cytoplasmic lipid droplets (CLDs) TG stored in CLDs can be break down by a series of lipases, or through lysosomal lipolysis pathway. Adipocyte triglyceride lipase (ATGL), hormone sensitive lipase (HSL) and monoacylglycerol lipase (MGL); diacylglycerol acyltransferase (DGAT2); PCTV; triglyceride-rich lipoproteins (TRL); perilipin (PLIN).
CLD membrane is another candidate that can influence the size of CLDs. Phosphatidylcholine (PC) is a prominent phospholipid found in CLD membranes. Lysophosphatidylcholine acyltransferases3 (LPCAT3) are involved in the re-acylation of PC in the intestine (Zhang et al., 2021). Intestine-specific Lpcat3 deficient mice exhibited enterocyte lipid accumulation and significantly reduced lipid absorption and circulating lipids and lipoproteins, which may be due to decreased protein levels of CD36 and FATP4 protein (Li et al., 2015; Kabir et al., 2016). Although whole Lpcat3 deficiency can cause neonatal lethality, which can be saved by PC/olive oil supplementation, some survivors showed larger small intestines with shorter and wider villi. Moreover, acute liver-specific Lpcat3 deletion exacerbates lipid-induced ER stress and hepatic steatosis (Rong et al., 2015).
Lipid fusion between CLDs facilitates their growth. Cell death-inducing DNA fragmentation factor alpha-like effector (CIDE) family proteins, which are enriched at CLD-CLD contact site, mediate the transfer of lipids from small CLDs to large CLDs (Chen et al., 2020). In the CIDE family, CIDEB is low expressed in the intestine but high expressed in the liver. Mice with global deletion of Cideb (Cideb−/−) had lower levels of plasma TG, improved IR and protection against HFD-induced obesity and fatty liver (Li et al., 2007). In the intestine, Cideb−/− mice exhibited impaired CM secretion and increased CLD accumulation in enterocytes. Moreover, overexpression of Cideb can increase TG secretion, indicating that CIDEB may also be involved in intestinal CM lipidation (Zhang et al., 2014).
5.4.2 Cytoplasmic lipid droplets breakdown
Cytoplasmic hydrolysis involves three sequential enzymes: ATGL and its coactivator comparative gene identification-58 (Cgi-58), hormone-sensitive lipase, and monoacylglycerol lipase (MGL). First, ATGL hydrolyzes TG to FA and DAG. Intestinal-specific deletion of Atgl (AtgliKO) increases CLDs in enterocytes but does not influence TG absorption, suggesting that CM secretion might be ATGL-independent (Obrowsky et al., 2013). During HFD feeding, AtgliKO mice had similar plasma lipid parameters and body weights to those of wild-type mice. Small intestinal-specific overexpression of Atgl mice showed a slight elevated enzymatic activity and unchanged CM secretion (Korbelius et al., 2022). In comparison, mice deprived of Cgi-58 in the intestine exhibited inefficient CM secretion, reduced plasma TG concentration, and increased CLD accumulation in the first proximal segment of 5 equal segments of the small intestinal, even during fasting conditions (Xie et al., 2014). Intestine-specific Cgi-58/Atgl double knockout mice exhibited increased lipid accumulation, but luminal lipid absorption and TG secretion rates were unaffected (Korbelius et al., 2019). The synthesis of CMs seems to bypass ATGL/Cgi-58 mediated catabolism. CLDs accumulated in enterocytes 2 h post-gavage of lipids, which is more likely to be caused by circulating lipid uptake, leading to decreased plasma TG (Korbelius et al., 2019). Consistently, both Cgi-58 single-knockout mice and Cgi-58/Atgl double-knockout mice exhibited attenuated hepatic steatosis (Xie et al., 2014; Korbelius et al., 2019). ATGL plays a vital role in the lipolysis to provide energy, so Atgl-deficient mice are prone to hypoglycemia. During prolonged fasting, Atgl-deficient mice stay in a torpor-like state (Schoiswohl et al., 2010).
Hormone-sensitive lipase catalyzes the conversion of DAG to FA and MAG. Interestingly, mice with intestine-specific hormone-sensitive lipase deletion did not display impaired TG metabolism but had increased plasma cholesterol concentrations and cholesteryl ester accumulation in the small intestine, suggesting that hormone-sensitive lipase may play a more important role in maintaining cholesterol homeostasis (Obrowsky et al., 2012).
MGL is the enzyme involved in the final step of TG lipolysis, which can hydrolyze MAG. Chon et al. demonstrated that the primary function of MGL was to convert dietary MGs to TG instead of CLD degradation in the intestine (Chon et al., 2012). Intestinal-specific Mgl overexpression (iMgl) mice exhibited unaltered dietary fat absorption. Body weight, hepatic and serum TG levels of iMgl mice were higher than those of wild-type mice after only 3 weeks of HFD feeding. This may be due to the decreased levels of 2-arachidonyl glycerol caused by Mgl overexpression, which led to the hyperphagic behavior and decreased energy expenditure (Chon et al., 2012). Global Mgl deletion mice showed delayed intestinal fat absorption, prolonged TG retention in the ileum, decreased body weight gain, better IR and prevented hepatic steatosis (Tardelli et al., 2019; Yoshida et al., 2019). Global deletion of Mgl affected entire energy homeostasis, which was partly due to higher levels of 2-arachidonyl glycerol-induced central orexigenic stimuli (Taschler et al., 2011).
Lysosomal acid lipase is a key enzyme in the lysosomal TG lipolysis pathway. In mice, lysosomal acid lipase deficiency leads to a large accumulation of TG in the small intestine, severe fat malabsorption, and steatorrhea (Du et al., 2001). Lipophagy is a form of macroautophagy initiated by phagocytosis of CLDs by autophagosomes, but the large size of CLDs impairs its recruitment, leading to partial lipophagy of CLDs. The underlying recruitment mechanisms for CLD fragmentation and specific anchoring targets on CLD remain undefined. However, inhibition of the fusion of autophagosomes with lysosomes or lysosomal acid lipase can accumulate CLDs in Caco-2 cells (Khaldoun et al., 2014). In an in vivo study, DGAT1−/− mice were resistant to diet-induced obesity, in part due to disruption of lysosomal function, which in turn induced abnormal CLD accumulation in enterocytes. Although there were no fat absorption disorders in DGAT1−/− mice, TG secretion was slow (Hung and Buhman, 2019).
5.4.3 Cytoplasmic lipid droplets pool
Synthesis of CLDs can temporarily store dietary lipids in epithelial cells, attenuate postprandial lipid fluctuations, and protect against the cellular lipotoxicity caused by the large influx of dietary FAs (Schaffer, 2003). Lipids of basolateral origin can also play a role in adjusting lipidemia. Enhanced basolateral lipid substrate transport in the gut can reduce circulating lipids and promote intestinal FAO, thus playing a protective role against diet-induced obesity (Li D et al., 2019). However, basolateral lipids contribute to FAO in intestinal cells and effectively maintain diet-derived CMs secretion.
5.5 Focus on chylomicron assembly and trafficking
5.5.1 Chylomicron assembly
In ER lumen, MTP mediates lipidation of ApoB-48 to form pre-CM particles. Mice with conditional Mtp knockout in villus enterocytes (MtpiKO) showed impaired CM assembly, increased accumulation of TG in enterocytes, and no CM particles in the secretory pathway. However, although MtpiKO mice exhibited decreased plasma and hepatic TG content, hepatic lipogenesis and low-density lipoproteins secretion increased, suggesting an unexpected interaction between intestinal and hepatic lipid metabolism (Xie et al., 2006). Xie et al. also found that MtpiKO mice fed a high-fat-fructose-cholesterol diet for 10 weeks exhibited a sustained reduction in hepatic TG (Xie et al., 2021). MTP inhibitor lomitapide, has also shown beneficial effects in improving obesity, blood glucose, lipids, and blood vessels (Hooper et al., 2015; Munkhsaikhan et al., 2022). Lomitapide, however, targets both intestinal and hepatic MTP. Therefore, it can lead to lipid accumulation in the liver and contribute to the progression of NAFLD and even fibrosis (Liu et al., 2017). Intestine-specific MTP inhibitor JTT-130 avoids lipid deposition in the liver and plays in a diet-dependent manner to reduce food intake, reduce body weight, and increase insulin sensitivity (Hata et al., 2011). In hepatic steatosis and fibrosis, blockage of CM assembly by MtpiKO can increase intestinal permeability, increase hepatic lipogenesis, and delay the remission of liver inflammation and fibrogenic signals (Xie et al., 2021). Patients with MTP gene mutations are characterized by an inability to produce CMs in the intestine, resulting in malnutrition and abetalipoproteinemia (Berriot-Varoqueaux N, 2000).
ApoB-48 is encoded by the ApoB gene and edited by Apobec-1 after transcription to generate a 48% of the length of ApoB-100 protein. Given that ApoB exists as ApoB-100 in the liver, defective translation of full-length ApoB leads to defective assembly of ApoB-containing lipoproteins from both enterocytes and hepatocytes, which is associated with familial hypobetalipoproteinemia. Patients with familial hypobetalipoproteinemia are at higher risk for obesity and NAFLD (Di Filippo et al., 2014). Other factors, such as hepatic high mobility group box-1 in intestinal epithelial cells, can also influence ApoB-48 expression, leading to lipid accumulation in enterocytes and decreased CM formation, thereby protecting mice from obesity and fatty liver (Gaskell et al., 2020).
Exchangeable apolipoproteins can also influence metabolism. ApoA-IV is the most responsive lipoprotein to dietary lipids (Qu et al., 2019). ApoA-IV can directly interact with ApoB, and when ApoA-IV is intracellularly retained in the ER, pre-CM trafficking from the ER to the Golgi is significantly reduced, resulting in delayed ApoB secretion and decreased lipid absorption efficiency under HFD (Weinberg et al., 2012). Moreover, ApoA-IV can facilitate pre-CM expansion by stabilizing pre-CM and ApoB-48, further influencing the size of CMs (Weinberg et al., 2012). Overexpression of ApoA-IV can enhance lipid transport in intestinal enterocytes by increasing the size of the secreted lipoproteins (Lu et al., 2006). ApoA-IV knockout mice exhibited reduction in plasma TG and cholesterol levels, without lipid malabsorption (Kohan et al., 2012). ApoA-I deficient mice showed enhanced dietary TG absorption but accelerated clearance of postprandial TG, which could protect mice from HFD-induced hepatic lipid deposition (Karavia et al., 2012). Though apoC-III overexpression inhibited CM secretion, transgenic mice exhibited fewer and smaller CLDs in enterocytes and secreted smaller and less density CMs (Li D et al., 2019). Moreover, excessive apoC-III can inhibit intestinal uptake of TG-rich lipoproteins from the basolateral surface in a dose-dependent manner, increasing the risk of atherogenesis.
5.5.2 Chylomicron trafficking
The PCTV budding complex contains ApoB-48, L-FABP, CD36, COPII and vesicle-associated membrane protein7 (VAMP7) proteins. The binding of L-FABP to the ER initiates PCTV budding. L-Fabp deletion mice have only 21% PCTV budding activity (Siddiqi et al., 2010). SAR1B is a GTPase required for ER movement to the Golgi apparatus of pre-CM transport vesicles. In humans, SAR1B gene mutations are related to chylomicron retention disease (Levy et al., 2014). Mice with a human SAR1B genetic defect (Sar1b−/−) reproduce some chylomicron retention disease features. Sar1b−/− mice exhibited decreased ApoB and Mtp expression, contributing to the malabsorption of fat and decreased secretion of CM (Auclair et al., 2021). Correspondingly, Sar1b overexpression (Sar1b+/+) can enhance intestinal fat absorption under HFD condition. Moreover, Sar1b +/+ mice showed phenotypes of dyslipidemia, obesity, and liver steatosis and were susceptible to IR (Levy et al., 2014). In Caco-2/15 cells, the deletion of SAR1B decreased CM output but did not completely eliminate CM secretion because SAR1A expression can be compensatory elevated (Sane et al., 2017).
Transmembrane proteins are also involved in PCTV formation. Anti-ApoB-48, anti-VAMP7 antibodies, and gene deletion of L-FABP and CD36 can all inhibit PCTV formation (Siddiqi et al., 2010). Anti-VAMP7 antibodies eliminated in 85% of PCTV transfer from ER to Golgi apparatus (Siddiqi et al., 2006). HFD can inhibit the expression of VAMP7, and ginger-derived nanoparticles can induce the expression of VAMP7. Sorting miR-375 into exosomes via VAMP7 can improve insulin sensitivity without affecting dietary fat absorption (Kumar et al., 2021). Moreover, soluble N-ethylmaleimide-sensitive factor attachment protein receptor complex participates in the process of PCTV-Golgi membrane fusion. Syntaxin-binding protein 5 is a negative regulator of soluble N-ethylmaleimide-sensitive factor attachment protein receptor, and its downregulation may be involved in the glucose-mediated mobilization of TG (Xiao et al., 2019).
5.6 Focus on chylomicron passaging through the lamina propria
Mature CMs are transported to the basolateral membrane via budding CM secretory vesicles, secreted into the intercellular space by exocytosis, and further into the lamina propria. In DENN domain-containing protein 5b knockout (Dennd5b−/−) mice, CM transport out of enterocytes was impaired, and massive CM secretory vesicles were accumulated in the enterocytes, as CM secretory vesicles from the Golgi failed to fuse with the basolateral membrane. Dennd5b−/− mice were resistant to western diet-induced obesity, exhibited improved plasma and hepatic lipid concentrations and reduced aortic atherosclerosis (Gordon et al., 2019). However, Mobilia et al. also demonstrated that the significant improvement of metabolic disorder in global Dennd5b−/− mice were also mediated by downregulated hepatic lipid metabolism genes (Mobilia et al., 2022). Therefore, future studies in cell-specific knockout mice are needed to distinguish the beneficial metabolic effects of Denn5b in different tissues. Furthermore, pleomorphic adenoma gene-like 2 (Plagl2) regulates lipid entry into the lacteals. Plagl2 deletion mice develop severe emaciation due to the blockage of CMs from the lamina propria into the lacteals, and exhibit growth arrest and even die of starvation shortly after birth. Plagl2 null enterocytes can assemble and secrete CMs, but cannot exit the gut interstitium. PLAGL2 is involved in CM modification and is necessary for subsequent CM uptake (Van Dyck et al., 2007).
5.7 Focus on lymphatic lipid transport
5.7.1 Lacteal survival
Lacteals are in permanent regeneration and slow proliferation to replace damaged cells (Norden and Kume, 2020). Defective growth of lymphatic vessels in the lacteals can lead to dietary lipid malabsorption and prevent obesity. Vascular endothelial growth factor (VEGF) receptor 3 (VEGFR3) is a vital mediator in lacteal survival (Figure 4A). Chy mice, with Vegfr3 inactivated mutation, exhibited defective lymphatic vessels. Chy mice exhibited significantly increased TG accumulation in enterocytes and increased fecal lipid content, contributing to the reduced postprandial plasma TG levels and protecting against obesity. The mechanism is partly related to the mutant of Vegfr3 significantly reducing nitric oxide production, which is required for CM to be released into circulation (Shew et al., 2018). Moreover, VEGF-C is an indispensable molecular regulator of intestinal lymph angiogenesis, although VEGF-D is also a ligand of VEGFR3 (Nurmi et al., 2015). Gut microbiota can influence VEGF-C production by villus macrophages and further affect the lacteal integrity. Because the recognition of microbial components via the toll-like receptors/myeloid differentiation factor 88 complex is a vital upstream signal for VEGF-C production, depletion of gut microbiota can break down this process, causing decreased VEGF-C expression and resulting in lacteal regression, specifically in jejunum and ileum (Suh et al., 2019). Genetical deletion of Vegfc (Vegfc−/−) can lead to lacteal regression without affecting other lymphatic beds, thereby influencing lipid absorption and enhancing excretion of FAs and cholesterol in faces. Vegfc−/− mice exhibited improved glucose metabolism and were protected from HFD-induced obesity (Nurmi et al., 2015). Bernier-Latmani et al. found that Notch signaling also contributes to the continuous regeneration of lacteals (Bernier-Latmani et al., 2015). Notch ligand delta-like 4 (Dll4) is the ligand of the Notch receptor. Mice with genetic ablation of Dll4 (Dll4−/−) in LECs exhibited shorter lacteals and the survival and migration of IEC were unable to maintain (Bernier-Latmani et al., 2015). VEGFR3 and VEGF2 activate Dll4 expression in lacteals. The altered VEGFC/D-VEGFR2/3 signaling may induce lipid absorption defects partly through Notch signaling. Moreover, Calcitonin receptor-like receptor (Calcrl) has also been reported as an essential upstream regulator of the Notch pathway (Figure 4A). Compared with control mice, mice with temporal and spatial deletion of Calcrl (Calcrlfl/fL/Prox1-CreERT2) developed intestinal lymphangiectasia and lacteals uptake lipids defecits and exhibited reduced weight gain (Davis et al., 2017).
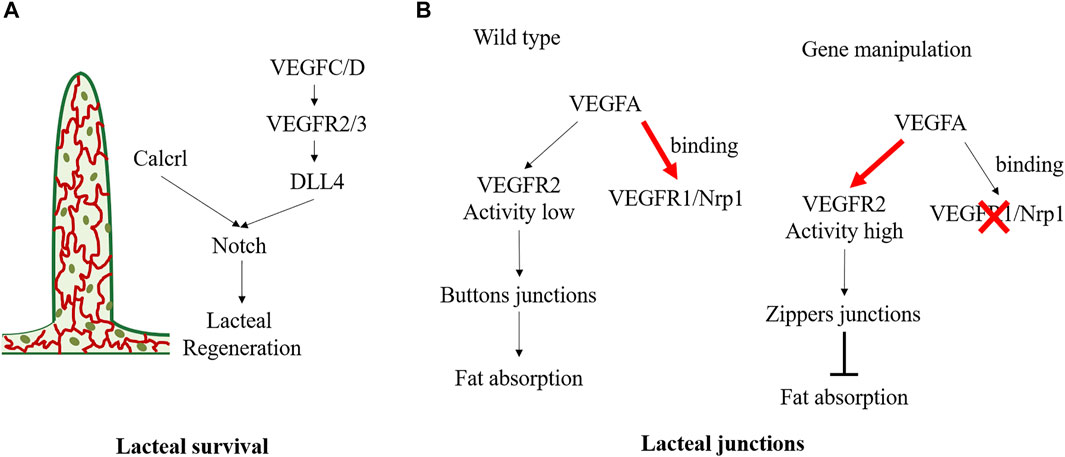
FIGURE 4. Molecular mechanisms regulating the status of lacteal survival and lacteal junctions. (A) VEGFC/D signals activate VEGFR2/3 and further promote lacteal DLL4 expression and Notch signaling. And Calcrl is also the upstream of Notch/DLL4 signaling in maintaining lacteal regeneration. (B) In physiological state, VEGFA binding to VEGFR1and Nrp1 on blood endothelial cells resulting in low VEGFR2 activity in lymphatic endothelial cell, which maintains the buttons junctions and facilitates fat absorption. Deletion of endothelial Nrp1 and VEGFR-1 results in high VEGFR2 signaling activity, leading to the formation of zippers junction and impairs fat absorption.
5.7.2 Lacteal junctions
There are button junctions and zipper junctions between endothelial cells in lacteal. The button junction has open and closed regions, which allows CMs transport, but the zipper junction only has the closed region, preventing CM uptake (Figure 4B). VEGF-A-VEGFR2 interaction can regulate endothelial cell junction, influence CMs production, and exert an obesity protection effect. Normally, VEGF-A binds VEGFR1 and Neuropilin1 (Nrp1) on blood endothelial cells. However, in Nrp1/Vegfr1 deletion mice, the bioavailability of VEGF-A significantly increased, and signaling through VEGFR2 leads to lacteal junction zippering and CM uptake defection (Zhang et al., 2018). Compared with HFD wild-type mice, Nrp1/Vegfr1 deletion mice showed decreased fat mass, improved glucose tolerance, and reduced plasma TG, total cholesterol, and HDL concentrations, and no hepatic steatosis (Zhang et al., 2018). Lymphatic Dll4 mutation also contributes to a shift of button junction to continuous zipper junction, resulting in inefficient CM uptake. Moreover, Dll4−/− mice exhibited a significantly blunted increase in plasma TG and free FA (Bernier-Latmani et al., 2015). In contrast, dexamethasone, a drug that induces the transition of the tracheal lymphatic zipper to button-like junctions, can reduce the number of zipper connections and increase CM uptake (Zhang et al., 2018).
It is worth noting that lymphatics are vital in maintaining intestinal homeostasis, impaired lacteals exacerbate intestinal mucosal inflammation and contribute to increased risk of obesity and cardiovascular disease (Jiang et al., 2019; Cifarelli et al., 2021). Mice heterozygous for Prospero-related homeobox1(Prox1+/−) had defective lymphatic vascular integrity, and the accumulation of lymph can lead to adipocyte proliferation and development of spontaneous adult-onset obesity (Harvey et al., 2005). In addition, CD36 is not only expressed in enterocytes but also highly expressed in lacteals. Mice with inducible Cd36 deletion in LECs had increased discontinuous button junctions in lacteals and submucosal lymphatic vessels, resulting in lymph leakage (Cifarelli et al., 2021). Immune cells from lymph can traffic to adipose tissue causing proinflammatory changes, which are closely linked to systemic glucose intolerance. FAs from the lymph can expand mesenteric and visceral adipose depots, similar to previously mentioned Prox1+/− mice (Cifarelli et al., 2021). In addition, lymphatic vessels also contribute to maintaining gastrointestinal homeostasis. Davis et al. reported that Calcrlfl/fl/Prox1-CreERT2 mice with lymphatic insufficiency impaired the resolution of intestinal inflammation from drug-induced acute mucosal injury and exhibited dilated lacteals and protein-losing enteropathy (Davis et al., 2017).
6 Conclusion and perspectives
Obesity poses a severe threat to human health. The disorder of dietary TG absorption dramatically affects the occurrence and development of metabolic diseases. Prolonged HFD feeding can change intestinal morphology, induce altered morphometric parameters and gastrointestinal motility, and impair the intestinal mechanical and immune barrier. In contrast, restricting TG absorption ameliorates the obesity phenotype, indicating a promising strategy for anti-obesity.
Dietary TG absorption involves various regulatory genes and proteins that play a vital role in regulating the related processes. Besides, the length of the carbon chains, the number of unsaturated bonds, and the location of FAs in dietary TG affect absorption efficiency and metabolic phenotypes. The undergoing studies are limited in: i) the expected efficacy is unsatisfied by intervening in certain essential genes or proteins (e.g., CD36, FABPs, DGAT, SAR1A, etc.); ii) lacking clinical safety and efficacy of the dietary TG absorption-related synthetic small biomolecules; iii) unexpected side effects by inhibiting certain key targets, such as severe gastrointestinal reactions (e.g., CD36, DGAT1 etc.), systemic dyslipidemia (CD36, caveolin-1, etc.), and other adverse reactions that interrupt the long-term treatment. Furthermore, differences in sex, living environment, circadian rhythms, and dietary nutrient structure also hunt the therapeutic benefits. Therefore, regulating dietary TG absorption to control HFD-induced obesity is still challenging.
We note that recently published high-quality studies have indicated some key targets of the intestinal lipid absorption process are promising in therapeutic prospects, such as MGAT2 inhibitors and intestinal-specific MTP inhibitors. TG absorption is an efficient and delicate process. Further research is needed to balance the metabolic benefits and side effects. Combination therapy that regulates multiple targets of intestinal lipid absorption may be an alternative and safe therapeutic approach.
Author contributions
XL and QL contributed equally to this work. They conceived and designed the study, and drafted the manuscript. YP and SC were responsible for the collection of articles and resolved problems through discussion. All authors reviewed the manuscript, which was revised in response to comments from YZ and YH. YH monitored the project and had overall responsibility for the paper.
Funding
This work was supported by the National Natural Science Foundation of China (No. 81830119, 82174249 and 82204840), Yangfan Project of Shanghai Science and Technology Commission (No. 22YF1449400), Siming Youth Fund of Shuguang Hospital (No. SGKJ-202113).
Conflict of interest
The authors declare that the research was conducted in the absence of any commercial or financial relationships that could be construed as a potential conflict of interest.
Publisher’s note
All claims expressed in this article are solely those of the authors and do not necessarily represent those of their affiliated organizations, or those of the publisher, the editors and the reviewers. Any product that may be evaluated in this article, or claim that may be made by its manufacturer, is not guaranteed or endorsed by the publisher.
References
Ables, G. P., Yang, K. J., Vogel, S., Hernandez-Ono, A., Yu, S., Yuen, J. J., et al. (2012). Intestinal DGAT1 deficiency reduces postprandial triglyceride and retinyl ester excursions by inhibiting chylomicron secretion and delaying gastric emptying. J. Lipid Res. 53 (11), 2364–2379. doi:10.1194/jlr.M029041
Acosta, A., Camilleri, M., Shin, A., Vazquez-Roque, M. I., Iturrino, J., Burton, D., et al. (2015). Quantitative gastrointestinal and psychological traits associated with obesity and response to weight-loss therapy. Gastroenterology 148 (3), 537–546. doi:10.1053/j.gastro.2014.11.020
Agellon, L. B., Drozdowski, L., Li, L., Iordache, C., Luong, L., Clandinin, M. T., et al. (2007). Loss of intestinal fatty acid binding protein increases the susceptibility of male mice to high fat diet-induced fatty liver. Biochim. Biophys. Acta 1771 (10), 1283–1288. doi:10.1016/j.bbalip.2007.08.004
Al-Thihli, K., Afting, C., Al-Hashmi, N., Mohammed, M., Sliwinski, S., Al Shibli, N., et al. (2021). Deficiency of acyl-CoA synthetase 5 is associated with a severe and treatable failure to thrive of neonatal onset. Clin. Genet. 99 (3), 376–383. doi:10.1111/cge.13883
Alpers, D. H., Bass, N. M., Engle, M. J., and DeSchryver-Kecskemeti, K. (2000). Intestinal fatty acid binding protein may favor differential apical fatty acid binding in the intestine. Biochim. Biophys. Acta 1483 (3), 352–362. doi:10.1016/s1388-1981(99)00200-0
Armand, M. (2007). Lipases and lipolysis in the human digestive tract: Where do we stand? Curr. Opin. Clin. Nutr. Metab. Care 10 (2), 156–164. doi:10.1097/MCO.0b013e3280177687
Auclair, N., Patey, N., Melbouci, L., Ou, Y., Magri-Tomaz, L., Sane, A., et al. (2019). Acylated ghrelin and the regulation of lipid metabolism in the intestine. Sci. Rep. 9 (1), 17975. doi:10.1038/s41598-019-54265-0
Auclair, N., Sane, A. T., Ahmarani, L., Patey, N., Beaulieu, J. F., Peretti, N., et al. (2021). Sar1b mutant mice recapitulate gastrointestinal abnormalities associated with chylomicron retention disease. J. Lipid Res. 62, 100085. doi:10.1016/j.jlr.2021.100085
Baier, L. J., Sacchettini, J. C., Knowler, W. C., Eads, J., Paolisso, G., Tataranni, P. A., et al. (1995). An amino acid substitution in the human intestinal fatty acid binding protein is associated with increased fatty acid binding, increased fat oxidation, and insulin resistance. J. Clin. Invest. 95 (3), 1281–1287. doi:10.1172/JCI117778
Basson, A. R., Chen, C., Sagl, F., Trotter, A., Bederman, I., Gomez-Nguyen, A., et al. (2020). Regulation of intestinal inflammation by dietary fats. Front. Immunol. 11, 604989. doi:10.3389/fimmu.2020.604989
Bernier-Latmani, J., Cisarovsky, C., Demir, C. S., Bruand, M., Jaquet, M., Davanture, S., et al. (2015). DLL4 promotes continuous adult intestinal lacteal regeneration and dietary fat transport. J. Clin. Invest. 125 (12), 4572–4586. doi:10.1172/JCI82045
Berriot-Varoqueaux N, A. L., Samson-Bouma, M., and Wetterau, J. R. (2000). The role of the microsomal triglygeride transfer protein in abetalipoproteinemia. Annu. Rev. Nutr. 20, 663–697. doi:10.1146/annurev.nutr.20.1.663
Bertaggia, E., Jensen, K. K., Castro-Perez, J., Xu, Y., Di Paolo, G., Chan, R. B., et al. (2017). Cyp8b1 ablation prevents Western diet-induced weight gain and hepatic steatosis because of impaired fat absorption. Am. J. Physiol. Endocrinol. Metab. 313 (2), E121–E133. doi:10.1152/ajpendo.00409.2016
Birari, R. B., and Bhutani, K. K. (2007). Pancreatic lipase inhibitors from natural sources: Unexplored potential. Drug Discov. Today 12 (19-20), 879–889. doi:10.1016/j.drudis.2007.07.024
Bowman, T. A., O'Keeffe, K. R., D'Aquila, T., Yan, Q. W., Griffin, J. D., Killion, E. A., et al. (2016). Acyl CoA synthetase 5 (ACSL5) ablation in mice increases energy expenditure and insulin sensitivity and delays fat absorption. Mol. Metab. 5 (3), 210–220. doi:10.1016/j.molmet.2016.01.001
Bray, G. A., Lovejoy, J. C., Smith, S. R., DeLany, J. P., Lefevre, M., Hwang, D., et al. (2002). The influence of different fats and fatty acids on obesity, insulin resistance and inflammation. J. Nutr. 132 (9), 2488–2491. doi:10.1093/jn/132.9.2488
Buhman, K. K., Smith, S. J., Stone, S. J., Repa, J. J., Wong, J. S., Knapp, F. F., et al. (2002). DGAT1 is not essential for intestinal triacylglycerol absorption or chylomicron synthesis. J. Biol. Chem. 277 (28), 25474–25479. doi:10.1074/jbc.M202013200
Buts, J. P., Vijverman, V., Barudi, C., De Keyser, N., Maldague, P., and Dive, C. (1990). Refeeding after starvation in the rat: Comparative effects of lipids, proteins and carbohydrates on jejunal and ileal mucosal adaptation. Eur. J. Clin. Invest. 20 (4), 441–452. doi:10.1111/j.1365-2362.1990.tb01882.x
Buttet, M., Traynard, V., Tran, T. T., Besnard, P., Poirier, H., and Niot, I. (2014). From fatty-acid sensing to chylomicron synthesis: Role of intestinal lipid-binding proteins. Biochimie 96, 37–47. doi:10.1016/j.biochi.2013.08.011
Cases, S., Smith, S. J., Zheng, Y. W., Myers, H. M., Lear, S. R., Sande, E., et al. (1998). Identification of a gene encoding an acyl CoA:diacylglycerol acyltransferase, a key enzyme in triacylglycerol synthesis. Proc. Natl. Acad. Sci. U. S. A. 95 (22), 13018–13023. doi:10.1073/pnas.95.22.13018
Chen, F. J., Yin, Y., Chua, B. T., and Li, P. (2020). CIDE family proteins control lipid homeostasis and the development of metabolic diseases. Traffic 21 (1), 94–105. doi:10.1111/tra.12717
Chen, H. C., Smith, S. J., Ladha, Z., Jensen, D. R., Ferreira, L. D., Pulawa, L. K., et al. (2002). Increased insulin and leptin sensitivity in mice lacking acyl CoA:diacylglycerol acyltransferase 1. J. Clin. Investigation 109 (8), 1049–1055. doi:10.1172/JCI14672
Chon, S. H., Douglass, J. D., Zhou, Y. X., Malik, N., Dixon, J. L., Brinker, A., et al. (2012). Over-expression of monoacylglycerol lipase (MGL) in small intestine alters endocannabinoid levels and whole body energy balance, resulting in obesity. PLoS One 7 (8), e43962. doi:10.1371/journal.pone.0043962
Chooi, Y. C., Ding, C., and Magkos, F. (2019). The epidemiology of obesity. Metabolism 92, 6–10. doi:10.1016/j.metabol.2018.09.005
Cifarelli, V., Appak-Baskoy, S., Peche, V. S., Kluzak, A., Shew, T., Narendran, R., et al. (2021). Visceral obesity and insulin resistance associate with CD36 deletion in lymphatic endothelial cells. Nat. Commun. 12 (1), 3350. doi:10.1038/s41467-021-23808-3
Cifarelli, V., Ivanov, S., Xie, Y., Son, N. H., Saunders, B. T., Pietka, T. A., et al. (2017). CD36 deficiency impairs the small intestinal barrier and induces subclinical inflammation in mice. Cell Mol. Gastroenterol. Hepatol. 3 (1), 82–98. doi:10.1016/j.jcmgh.2016.09.001
Clegg, M. E., McKenna, P., McClean, C., Davison, G. W., Trinick, T., Duly, E., et al. (2011). Gastrointestinal transit, post-prandial lipaemia and satiety following 3 days high-fat diet in men. Eur. J. Clin. Nutr. 65 (2), 240–246. doi:10.1038/ejcn.2010.235
Covasa, M., and Ritter, R. C. (2000). Adaptation to high-fat diet reduces inhibition of gastric emptying by CCK and intestinal oleate. Am. J. Physiol. Regul. Integr. Comp. Physiol. 278 (1), R166–R170. doi:10.1152/ajpregu.2000.278.1.R166
Covasa, M., and Ritter, R. C. (1999). Reduced sensitivity to the satiation effect of intestinal oleate in rats adapted to high-fat diet. Am. J. Physiol. 277 (1), R279–R285. doi:10.1152/ajpregu.1999.277.1.R279
Cruz-Garcia, L., and Schlegel, A. (2014). Lxr-driven enterocyte lipid droplet formation delays transport of ingested lipids. J. Lipid Res. 55 (9), 1944–1958. doi:10.1194/jlr.M052845
D'Aquila, T., Hung, Y. H., Carreiro, A., and Buhman, K. K. (2016). Recent discoveries on absorption of dietary fat: Presence, synthesis, and metabolism of cytoplasmic lipid droplets within enterocytes. Biochim. Biophys. Acta 1861, 730–747. doi:10.1016/j.bbalip.2016.04.012
D'Aquila, T., Sirohi, D., Grabowski, J. M., Hedrick, V. E., Paul, L. N., Greenberg, A. S., et al. (2015). Characterization of the proteome of cytoplasmic lipid droplets in mouse enterocytes after a dietary fat challenge. PLoS One 10 (5), e0126823. doi:10.1371/journal.pone.0126823
D'Aquila, T., Zembroski, A. S., and Buhman, K. K. (2019). Diet induced obesity alters intestinal cytoplasmic lipid droplet morphology and proteome in the postprandial response to dietary fat. Front. Physiol. 10, 180. doi:10.3389/fphys.2019.00180
Dahiya, D. S., Perisetti, A., Al-Haddad, M., Kichloo, A., Sharma, R., Cheng, C. I., et al. (2022). Obese gastroparesis inpatient admissions: Trends and outcomes from 2007-2017 in the United States. Ann. Gastroenterol. 35 (3), 249–259. doi:10.20524/aog.2022.0702
Davis, R. B., Kechele, D. O., Blakeney, E. S., Pawlak, J. B., and Caron, K. M. (2017). Lymphatic deletion of calcitonin receptor-like receptor exacerbates intestinal inflammation. JCI Insight 2 (6), e92465. doi:10.1172/jci.insight.92465
de Vogel-van den Bosch, H. M., de Wit, N. J. W., Hooiveld, G. J. E. J., Vermeulen, H., van der Veen, J. N., Houten, S. M., et al. (2008). A cholesterol-free, high-fat diet suppresses gene expression of cholesterol transporters in murine small intestine. Am. J. Physiology-Gastrointestinal Liver Physiology 294 (5), G1171–G1180. doi:10.1152/ajpgi.00360.2007
de Wit, N. J., Bosch-Vermeulen, H., de Groot, P. J., Hooiveld, G. J., Bromhaar, M. M., Jansen, J., et al. (2008). The role of the small intestine in the development of dietary fat-induced obesity and insulin resistance in C57BL/6J mice. BMC Med. Genomics 1, 14. doi:10.1186/1755-8794-1-14
Demignot, S., Beilstein, F., and Morel, E. (2014). Triglyceride-rich lipoproteins and cytosolic lipid droplets in enterocytes: Key players in intestinal physiology and metabolic disorders. Biochimie 96, 48–55. doi:10.1016/j.biochi.2013.07.009
Denison, H., Nilsson, C., Lofgren, L., Himmelmann, A., Martensson, G., Knutsson, M., et al. (2014). Diacylglycerol acyltransferase 1 inhibition with AZD7687 alters lipid handling and hormone secretion in the gut with intolerable side effects: A randomized clinical trial. Diabetes Obes. Metab. 16 (4), 334–343. doi:10.1111/dom.12221
Devasthale, P., and Cheng, D. (2018). Monoacylglycerol acyltransferase 2 (MGAT2) inhibitors for the treatment of metabolic diseases and nonalcoholic steatohepatitis (NASH). J. Med. Chem. 61 (22), 9879–9888. doi:10.1021/acs.jmedchem.8b00864
Di Filippo, M., Moulin, P., Roy, P., Samson-Bouma, M. E., Collardeau-Frachon, S., Chebel-Dumont, S., et al. (2014). Homozygous MTTP and APOB mutations may lead to hepatic steatosis and fibrosis despite metabolic differences in congenital hypocholesterolemia. J. Hepatol. 61 (4), 891–902. doi:10.1016/j.jhep.2014.05.023
Do, T. T., Hindlet, P., Waligora-Dupriet, A. J., Kapel, N., Neveux, N., Mignon, V., et al. (2014). Disturbed intestinal nitrogen homeostasis in a mouse model of high-fat diet-induced obesity and glucose intolerance. Am. J. Physiol. Endocrinol. Metab. 306 (6), E668–E680. doi:10.1152/ajpendo.00437.2013
Drover, V. A., Ajmal, M., Nassir, F., Davidson, N. O., Nauli, A. M., Sahoo, D., et al. (2005). CD36 deficiency impairs intestinal lipid secretion and clearance of chylomicrons from the blood. J. Clin. Investigation 115 (5), 1290–1297. doi:10.1172/jci21514
Du, H., Heur, M., Duanmu, M., Grabowski, G. A., Hui, D. Y., Witte, D. P., et al. (2001). Lysosomal acid lipase-deficient mice: Depletion of white and Brown fat, severe hepatosplenomegaly, and shortened life span. J. Lipid Res. 42 (4), 489–500. doi:10.1016/s0022-2275(20)31157-3
Dubois, C., Beaumier, G., Juhel, C., Armand, M., Portugal, H., Pauli, A. M., et al. (1998). Effects of graded amounts (0-50 g) of dietary fat on postprandial lipemia and lipoproteins in normolipidemic adults. Am. J. Clin. Nutr. 67 (1), 31–38. doi:10.1093/ajcn/67.1.31
Eduardo, d. A.-N. J. (2005). The role of macronutrients in gastrointestinal blood flow. Curr. Opin. Clin. Nutr. Metab. Care 8, 552–556. doi:10.1097/01.mco.0000170755.32996.1d
Ellis, J. M., Frahm, J. L., Li, L. O., and Coleman, R. A. (2010). Acyl-coenzyme A synthetases in metabolic control. Curr. Opin. Lipidol. 21 (3), 212–217. doi:10.1097/mol.0b013e32833884bb
Filippatos, T. D., Derdemezis, C. S., Gazi, I. F., Nakou, E. S., Mikhailidis, D. P., and Elisaf, M. S. (2008). Orlistat-associated adverse effects and drug interactions: A critical review. Drug Saf. 31 (1), 53–65. doi:10.2165/00002018-200831010-00005
Folwaczny, A., Waldmann, E., Altenhofer, J., Henze, K., and Parhofer, K. G. (2021). Postprandial lipid metabolism in normolipidemic subjects and patients with mild to moderate hypertriglyceridemia: Effects of test meals containing saturated fatty acids, mono-unsaturated fatty acids, or medium-chain fatty acids. Nutrients 13 (5), 1737. doi:10.3390/nu13051737
Frank, D. N., Bales, E. S., Monks, J., Jackman, M. J., MacLean, P. S., Ir, D., et al. (2015). Perilipin-2 modulates lipid absorption and microbiome responses in the mouse intestine. PLoS One 10 (7), e0131944. doi:10.1371/journal.pone.0131944
Frank, P. G., Pavlides, S., Cheung, M. W., Daumer, K., and Lisanti, M. P. (2008). Role of caveolin-1 in the regulation of lipoprotein metabolism. Am. J. Physiol. Cell Physiol. 295 (1), C242–C248. doi:10.1152/ajpcell.00185.2008
Gajda, A. M., Zhou, Y. X., Agellon, L. B., Fried, S. K., Kodukula, S., Fortson, W., et al. (2013). Direct comparison of mice null for liver or intestinal fatty acid-binding proteins reveals highly divergent phenotypic responses to high fat feeding. J. Biol. Chem. 288 (42), 30330–30344. doi:10.1074/jbc.M113.501676
Gao, Y., Nelson, D. W., Banh, T., Yen, M. I., and Yen, C. E. (2013). Intestine-specific expression of MOGAT2 partially restores metabolic efficiency in Mogat2-deficient mice. J. Lipid Res. 54 (6), 1644–1652. doi:10.1194/jlr.M035493
Gaskell, H., Ge, X., Desert, R., Das, S., Han, H., Lantvit, D., et al. (2020). Ablation of Hmgb1 in intestinal epithelial cells causes intestinal lipid accumulation and reduces NASH in mice. Hepatol. Commun. 4 (1), 92–108. doi:10.1002/hep4.1448
Gimeno, R. E., Hirsch, D. J., Punreddy, S., Sun, Y., Ortegon, A. M., Wu, H., et al. (2003). Targeted deletion of fatty acid transport protein-4 results in early embryonic lethality. J. Biol. Chem. 278 (49), 49512–49516. doi:10.1074/jbc.M309759200
Goldfine, A. B., Fonseca, V. A., Jones, M. R., Wang, A. C., Ford, D. M., and Truitt, K. E. (2010). Long-term safety and tolerability of colesevelam HCl in subjects with type 2 diabetes. Horm. Metab. Res. 42 (1), 23–30. doi:10.1055/s-0029-1241195
Gordon, S. M., Neufeld, E. B., Yang, Z., Pryor, M., Freeman, L. A., Fan, X., et al. (2019). DENND5B regulates intestinal triglyceride absorption and body mass. Sci. Rep. 9 (1), 3597. doi:10.1038/s41598-019-40296-0
Goudriaan, J. R., Dahlmans, V. E., Febbraio, M., Teusink, B., Romijn, J. A., Havekes, L. M., et al. (2002). Intestinal lipid absorption is not affected in CD36 deficient mice. Mol. Cell Biochem. 239 (1-2), 199–202. doi:10.1023/a:1020575412789
Haeusler, R. A., Astiarraga, B., Camastra, S., Accili, D., and Ferrannini, E. (2013). Human insulin resistance is associated with increased plasma levels of 12α-hydroxylated bile acids. Diabetes 62 (12), 4184–4191. doi:10.2337/db13-0639
Hageman, J. H. J., Keijer, J., Dalsgaard, T. K., Zeper, L. W., Carriere, F., Feitsma, A. L., et al. (2019). Free fatty acid release from vegetable and bovine milk fat-based infant formulas and human milk during two-phase in vitro digestion. Food Funct. 10 (4), 2102–2113. doi:10.1039/c8fo01940a
Hajri, T., Hall, A. M., Jensen, D. R., Pietka, T. A., Drover, V. A., Tao, H., et al. (2007). CD36-facilitated fatty acid uptake inhibits leptin production and signaling in adipose tissue. Diabetes 56 (7), 1872–1880. doi:10.2337/db06-1699
Harvey, N. L., Srinivasan, R. S., Dillard, M. E., Johnson, N. C., Witte, M. H., Boyd, K., et al. (2005). Lymphatic vascular defects promoted by Prox1 haploinsufficiency cause adult-onset obesity. Nat. Genet. 37 (10), 1072–1081. doi:10.1038/ng1642
Hata, T., Mera, Y., Ishii, Y., Tadaki, H., Tomimoto, D., Kuroki, Y., et al. (2011). JTT-130, a novel intestine-specific inhibitor of microsomal triglyceride transfer protein, suppresses food intake and gastric emptying with the elevation of plasma peptide YY and glucagon-like peptide-1 in a dietary fat-dependent manner. J. Pharmacol. Exp. Ther. 336 (3), 850–856. doi:10.1124/jpet.110.176560
Heydemann, A. (2016). An overview of murine high fat diet as a model for type 2 diabetes mellitus. J. Diabetes Res. 2016, 2902351. doi:10.1155/2016/2902351
Higuchi, S., Ahmad, T. R., Argueta, D. A., Perez, P. A., Zhao, C., Schwartz, G. J., et al. (2020). Bile acid composition regulates GPR119-dependent intestinal lipid sensing and food intake regulation in mice. Gut 69 (9), 1620–1628. doi:10.1136/gutjnl-2019-319693
Hooper, A. J., Burnett, J. R., and Watts, G. F. (2015). Contemporary aspects of the biology and therapeutic regulation of the microsomal triglyceride transfer protein. Circ. Res. 116 (1), 193–205. doi:10.1161/CIRCRESAHA.116.304637
Hung, Y. H., and Buhman, K. K. (2019). DGAT1 deficiency disrupts lysosome function in enterocytes during dietary fat absorption. Biochim. Biophys. Acta Mol. Cell Biol. Lipids 1864 (4), 587–595. doi:10.1016/j.bbalip.2018.10.007
Iqbal, J., and Hussain, M. M. (2009). Intestinal lipid absorption. Am. J. Physiol. Endocrinol. Metab. 296 (6), E1183–E1194. doi:10.1152/ajpendo.90899.2008
Ji, H., and Friedman, M. I. (2008). Reduced hepatocyte fatty acid oxidation in outbred rats prescreened for susceptibility to diet-induced obesity. Int. J. Obes. (Lond) 32 (8), 1331–1334. doi:10.1038/ijo.2008.71
Ji, Y., Sakata, Y., and Tso, P. (2011). Nutrient-induced inflammation in the intestine. Curr. Opin. Clin. Nutr. Metab. Care 14 (4), 315–321. doi:10.1097/MCO.0b013e3283476e74
Ji, Y., Sakata, Y., Yang, Q., Li, X., Xu, M., Yoder, S., et al. (2012). Activation of rat intestinal mucosal mast cells by fat absorption. Am. J. Physiol. Gastrointest. Liver Physiol. 302 (11), G1292–G1300. doi:10.1152/ajpgi.00011.2012
Jiang, X., Tian, W., Nicolls, M. R., and Rockson, S. G. (2019). The lymphatic system in obesity, insulin resistance, and cardiovascular diseases. Front. Physiol. 10, 1402. doi:10.3389/fphys.2019.01402
Kabir, I., Li, Z., Bui, H. H., Kuo, M. S., Gao, G., and Jiang, X. C. (2016). Small intestine but not liver lysophosphatidylcholine acyltransferase 3 (Lpcat3) deficiency has a dominant effect on plasma lipid metabolism. J. Biol. Chem. 291 (14), 7651–7660. doi:10.1074/jbc.M115.697011
Karavia, E. A., Papachristou, D. J., Liopeta, K., Triantaphyllidou, I. E., Dimitrakopoulos, O., and Kypreos, K. E. (2012). Apolipoprotein A-I modulates processes associated with diet-induced nonalcoholic fatty liver disease in mice. Mol. Med. 18, 901–912. doi:10.2119/molmed.2012.00113
Kern, F., and Borgstrom, B. (1965). Quantitative study of the pathways of triglyceride synthesis by hamster intestinal mucosa. Biochim. Biophys. Acta 98 (3), 520–531. doi:10.1016/0005-2760(65)90148-7
Khaldoun, S. A., Emond-Boisjoly, M. A., Chateau, D., Carriere, V., Lacasa, M., Rousset, M., et al. (2014). Autophagosomes contribute to intracellular lipid distribution in enterocytes. Mol. Biol. Cell 25 (1), 118–132. doi:10.1091/mbc.E13-06-0324
Koffert, J., Stahle, M., Karlsson, H., Iozzo, P., Salminen, P., Roivainen, A., et al. (2018). Morbid obesity and type 2 diabetes alter intestinal fatty acid uptake and blood flow. Diabetes Obes. Metab. 20 (6), 1384–1390. doi:10.1111/dom.13228
Kohan, A. B., Wang, F., Li, X., Bradshaw, S., Yang, Q., Caldwell, J. L., et al. (2012). Apolipoprotein A-IV regulates chylomicron metabolism-mechanism and function. Am. J. Physiol. Gastrointest. Liver Physiol. 302 (6), G628–G636. doi:10.1152/ajpgi.00225.2011
Kondo, H., Minegishi, Y., Komine, Y., Mori, T., Matsumoto, I., Abe, K., et al. (2006). Differential regulation of intestinal lipid metabolism-related genes in obesity-resistant A/J vs. obesity-prone C57BL/6J mice. Am. J. Physiol. Endocrinol. Metab. 291 (5), E1092–E1099. doi:10.1152/ajpendo.00583.2005
Korbelius, M., Vujic, N., Kuentzel, K. B., Obrowsky, S., Rainer, S., Haemmerle, G., et al. (2022). Enterocyte-specific ATGL overexpression affects intestinal and systemic cholesterol homeostasis. Biochim. Biophys. Acta Mol. Cell Biol. Lipids 1867 (4), 159121. doi:10.1016/j.bbalip.2022.159121
Korbelius, M., Vujic, N., Sachdev, V., Obrowsky, S., Rainer, S., Gottschalk, B., et al. (2019). ATGL/CGI-58-Dependent hydrolysis of a lipid storage pool in murine enterocytes. Cell Rep. 28 (7), 1923–1934. doi:10.1016/j.celrep.2019.07.030
Kumar, A., and Chauhan, S. (2021). Pancreatic lipase inhibitors: The road voyaged and successes. Life Sci. 271, 119115. doi:10.1016/j.lfs.2021.119115
Kumar, A., Ren, Y., Sundaram, K., Mu, J., Sriwastva, M. K., Dryden, G. W., et al. (2021). miR-375 prevents high-fat diet-induced insulin resistance and obesity by targeting the aryl hydrocarbon receptor and bacterial tryptophanase (tnaA) gene. Theranostics 11 (9), 4061–4077. doi:10.7150/thno.52558
Lackey, A. I., Chen, T., Zhou, Y. X., Bottasso Arias, N. M., Doran, J. M., Zacharisen, S. M., et al. (2020). Mechanisms underlying reduced weight gain in intestinal fatty acid-binding protein (IFABP) null mice. Am. J. Physiol. Gastrointest. Liver Physiol. 318 (3), G518–G530. doi:10.1152/ajpgi.00120.2019
Lau, E., Marques, C., Pestana, D., Santoalha, M., Carvalho, D., Freitas, P., et al. (2016). The role of I-FABP as a biomarker of intestinal barrier dysfunction driven by gut microbiota changes in obesity. Nutr. Metab. (Lond) 13, 31. doi:10.1186/s12986-016-0089-7
Lee, B., Fast, A. M., Zhu, J., Cheng, J. X., and Buhman, K. K. (2010). Intestine-specific expression of acyl CoA:diacylglycerol acyltransferase 1 reverses resistance to diet-induced hepatic steatosis and obesity in Dgat1-/- mice. J. Lipid Res. 51 (7), 1770–1780. doi:10.1194/jlr.M002311
Lee, B., Zhu, J., Wolins, N. E., Cheng, J. X., and Buhman, K. K. (2009). Differential association of adipophilin and TIP47 proteins with cytoplasmic lipid droplets in mouse enterocytes during dietary fat absorption. Biochim. Biophys. Acta 1791 (12), 1173–1180. doi:10.1016/j.bbalip.2009.08.002
Lee, J. C., Lee, H. Y., Kim, T. K., Kim, M. S., Park, Y. M., Kim, J., et al. (2017). Obesogenic diet-induced gut barrier dysfunction and pathobiont expansion aggravate experimental colitis. PLoS One 12 (11), e0187515. doi:10.1371/journal.pone.0187515
Lee, Y. Y., Tang, T. K., Phuah, E. T., Tan, C. P., Wang, Y., Li, Y., et al. (2020). Production, safety, health effects and applications of diacylglycerol functional oil in food systems: A review. Crit. Rev. Food Sci. Nutr. 60 (15), 2509–2525. doi:10.1080/10408398.2019.1650001
Levy, E., Spahis, S., Garofalo, C., Marcil, V., Montoudis, A., Sinnet, D., et al. (2014). Sar1b transgenic male mice are more susceptible to high-fat diet-induced obesity, insulin insensitivity and intestinal chylomicron overproduction. J. Nutr. Biochem. 25 (5), 540–548. doi:10.1016/j.jnutbio.2014.01.004
Li D, R. C., Johnson, Z. K., Bae, M., Muter, A., Heussinger, A. E., Tambini, N., et al. (2019). Intestinal basolateral lipid substrate transport is linked to chylomicron secretion and is regulated by apoC-III. J. Lipid Res. 60 (9), 1503–1515. doi:10.1194/jlr.M092460
Li, J., Wu, H., Liu, Y., and Yang, L. (2020). High fat diet induced obesity model using four strainsof mice: Kunming, C57BL/6, BALB/c and ICR. Exp. Anim. 69 (3), 326–335. doi:10.1538/expanim.19-0148
Li, J. Z., Ye, J., Xue, B., Qi, J., Zhang, J., Zhou, Z., et al. (2007). Cideb regulates diet-induced obesity, liver steatosis, and insulin sensitivity by controlling lipogenesis and fatty acid oxidation. Diabetes 56 (10), 2523–2532. doi:10.2337/db07-0040
Li, Z., Jiang, H., Ding, T., Lou, C., Bui, H. H., Kuo, M. S., et al. (2015). Deficiency in lysophosphatidylcholine acyltransferase 3 reduces plasma levels of lipids by reducing lipid absorption in mice. Gastroenterology 149 (6), 1519–1529. doi:10.1053/j.gastro.2015.07.012
Liu, X., Men, P., Wang, Y., Zhai, S., Zhao, Z., and Liu, G. (2017). Efficacy and safety of lomitapide in hypercholesterolemia. Am. J. Cardiovasc Drugs 17 (4), 299–309. doi:10.1007/s40256-017-0214-7
Losacco, M. C., de Almeida, C. F. T., Hijo, A. H. T., Bargi-Souza, P., Gama, P., Nunes, M. T., et al. (2018). High-fat diet affects gut nutrients transporters in hypo and hyperthyroid mice by PPAR-a independent mechanism. Life Sci. 202, 35–43. doi:10.1016/j.lfs.2018.03.053
Lu, S., Yao, Y., Cheng, X., Mitchell, S., Leng, S., Meng, S., et al. (2006). Overexpression of apolipoprotein A-IV enhances lipid secretion in IPEC-1 cells by increasing chylomicron size. J. Biol. Chem. 281 (6), 3473–3483. doi:10.1074/jbc.M502501200
Luo, H., Jiang, M., Lian, G., Liu, Q., Shi, M., Li, T. Y., et al. (2018). AIDA selectively mediates downregulation of fat synthesis enzymes by ERAD to retard intestinal fat absorption and prevent obesity. Cell Metab. 27 (4), 843–853. doi:10.1016/j.cmet.2018.02.021
Maciejewski, B. S., LaPerle, J. L., Chen, D., Ghosh, A., Zavadoski, W. J., McDonald, T. S., et al. (2013). Pharmacological inhibition to examine the role of DGAT1 in dietary lipid absorption in rodents and humans. Am. J. Physiol. Gastrointest. Liver Physiol. 304 (11), G958–G969. doi:10.1152/ajpgi.00384.2012
Mansbach, C. M., and Siddiqi, S. (2016). Control of chylomicron export from the intestine. Am. J. Physiol. Gastrointest. Liver Physiol. 310 (9), G659–G668. doi:10.1152/ajpgi.00228.2015
Martins, I. J., Sainsbury, A. J., Mamo, J. C., and Redgrave, T. G. (1994). Lipid and apolipoprotein B48 transport in mesenteric lymph and the effect of hyperphagia on the clearance of chylomicron-like emulsions in insulin-deficient rats. Diabetologia 37 (3), 238–246. doi:10.1007/BF00398049
Masuda, D., Hirano, K., Oku, H., Sandoval, J. C., Kawase, R., Yuasa-Kawase, M., et al. (2009). Chylomicron remnants are increased in the postprandial state in CD36 deficiency. J. Lipid Res. 50 (5), 999–1011. doi:10.1194/jlr.P700032-JLR200
McClendon, K. S., Riche, D. M., and Uwaifo, G. I. (2009). Orlistat: Current status in clinical therapeutics. Expert Opin. Drug Saf. 8 (6), 727–744. doi:10.1517/14740330903321485
McManaman, J. L., Bales, E. S., Orlicky, D. J., Jackman, M., MacLean, P. S., Cain, S., et al. (2013). Perilipin-2-null mice are protected against diet-induced obesity, adipose inflammation, and fatty liver disease. J. Lipid Res. 54 (5), 1346–1359. doi:10.1194/jlr.M035063
Meller N, M. M., Wong, W. P., Altemus, J. B., and Sehayek, E. (2013). Targeting of Acyl-CoA synthetase 5 decreases jejunal fatty acid activation with no effect on dietary long-chain fatty acid absorption. Lipids Health Dis. 12, 88. doi:10.1186/1476-511X-12-88
Milger, K., Herrmann, T., Becker, C., Gotthardt, D., Zickwolf, J., Ehehalt, R., et al. (2006). Cellular uptake of fatty acids driven by the ER-localized acyl-CoA synthetase FATP4. J. Cell Sci. 119, 4678–4688. doi:10.1242/jcs.03280
Mobilia, M., Whitus, C., Karakashian, A., Lu, H. S., Daugherty, A., and Gordon, S. M. (2022). Dennd5b-Deficient mice are resistant to PCSK9-induced hypercholesterolemia and diet-induced hepatic steatosis. J. Lipid Res. 63 (12), 100296. doi:10.1016/j.jlr.2022.100296
Moreno-Perez, B., Benito, E., Civera, M., Alabadi, B., Martinez-Hervas, S., Peiro, M., et al. (2021). Postprandial triglyceridaemia is modulated by insulin resistance but not by grade of obesity in abdominal and morbid obese subjects. Int. J. Clin. Pract. 75 (4), e13776. doi:10.1111/ijcp.13776
Munkhsaikhan, U., Kwon, Y., Sahyoun, A. M., Ait-Aissa, K., Kassan, A., and Kassan, M. (2022). The microsomal triglyceride transfer protein inhibitor lomitapide improves vascular function in mice with obesity. Obes. (Silver Spring) 30 (4), 893–901. doi:10.1002/oby.23389
Murota, K., Matsui, N., Kawada, T., Takahashi, N., and Fushuki, T. (2001). Inhibitory effect of monoacylglycerol on fatty acid uptake into rat intestinal epithelial cells. Biosci. Biotechnol. Biochem. 65 (6), 1441–1443. doi:10.1271/bbb.65.1441
Murota, K. S. J., and Storch, J. (2005). Uptake of micellar long-chain fatty acid and sn-2-monoacylglycerol into human intestinal Caco-2 cells exhibits characteristics of protein-mediated transport. J. Nutr. 135 (7), 1626–1630. doi:10.1093/jn/135.7.1626
Nassir, F., Wilson, B., Han, X., Gross, R. W., and Abumrad, N. A. (2007). CD36 is important for fatty acid and cholesterol uptake by the proximal but not distal intestine. J. Biol. Chem. 282 (27), 19493–19501. doi:10.1074/jbc.M703330200
Nelson, D. W., Gao, Y., Spencer, N. M., Banh, T., and Yen, C. L. (2011). Deficiency of MGAT2 increases energy expenditure without high-fat feeding and protects genetically obese mice from excessive weight gain. J. Lipid Res. 52 (9), 1723–1732. doi:10.1194/jlr.M016840
Nelson, D. W., Gao, Y., Yen, M. I., and Yen, C. L. (2014). Intestine-specific deletion of acyl-CoA:monoacylglycerol acyltransferase (MGAT) 2 protects mice from diet-induced obesity and glucose intolerance. J. Biol. Chem. 289 (25), 17338–17349. doi:10.1074/jbc.M114.555961
Newberry, E. P., Kennedy, S., Xie, Y., Luo, J., Jiang, H., Ory, D. S., et al. (2015). Phenotypic divergence in two lines ofL-Fabp−/−mice reflects substrain differences and environmental modifiers. Am. J. Physiology-Gastrointestinal Liver Physiology 309 (8), G648–G661. doi:10.1152/ajpgi.00170.2015
Newberry, E. P., Xie, Y., Kennedy, S. M., Luo, J., and Davidson, N. O. (2006). Protection against Western diet-induced obesity and hepatic steatosis in liver fatty acid-binding protein knockout mice. Hepatology 44 (5), 1191–1205. doi:10.1002/hep.21369
Niot, I., Poirier, H., Tran, T. T., and Besnard, P. (2009). Intestinal absorption of long-chain fatty acids: Evidence and uncertainties. Prog. Lipid Res. 48 (2), 101–115. doi:10.1016/j.plipres.2009.01.001
Norden, P. R., and Kume, T. (2020). Molecular mechanisms controlling lymphatic endothelial junction integrity. Front. Cell Dev. Biol. 8, 627647. doi:10.3389/fcell.2020.627647
Nurmi, H., Saharinen, P., Zarkada, G., Zheng, W., Robciuc, M. R., and Alitalo, K. (2015). VEGF-C is required for intestinal lymphatic vessel maintenance and lipid absorption. EMBO Mol. Med. 7 (11), 1418–1425. doi:10.15252/emmm.201505731
Obrowsky, S., Chandak, P. G., Patankar, J. V., Pfeifer, T., Povoden, S., Schreiber, R., et al. (2012). Cholesteryl ester accumulation and accelerated cholesterol absorption in intestine-specific hormone sensitive lipase-null mice. Biochim. Biophys. Acta 1821 (11), 1406–1414. doi:10.1016/j.bbalip.2012.07.013
Obrowsky, S., Chandak, P. G., Patankar, J. V., Povoden, S., Schlager, S., Kershaw, E. E., et al. (2013). Adipose triglyceride lipase is a TG hydrolase of the small intestine and regulates intestinal PPARα signaling. J. Lipid Res. 54 (2), 425–435. doi:10.1194/jlr.M031716
Otis, J. P., Shen, M. C., Quinlivan, V., Anderson, J. L., and Farber, S. A. (2017). Intestinal epithelial cell caveolin 1 regulates fatty acid and lipoprotein cholesterol plasma levels. Dis. Model Mech. 10 (3), 283–295. doi:10.1242/dmm.027300
Papamandjaris, A. A., MacDougall, D. E., and Jones, P. J. (1998). Medium chain fatty acid metabolism and energy expenditure: Obesity treatment implications. Life Sci. 62 (14), 1203–1215. doi:10.1016/s0024-3205(97)01143-0
Parkman, H. P., Van Natta, M., Yamada, G., Grover, M., McCallum, R. W., Sarosiek, I., et al. (2021). Body weight in patients with idiopathic gastroparesis. Neurogastroenterol. Motil. 33 (2), e13974. doi:10.1111/nmo.13974
Parton, R. G., and del Pozo, M. A. (2013). Caveolae as plasma membrane sensors, protectors and organizers. Nat. Rev. Mol. Cell Biol. 14 (2), 98–112. doi:10.1038/nrm3512
Parton, R. G., and Simons, K. (2007). The multiple faces of caveolae. Nat. Rev. Mol. Cell Biol. 8 (3), 185–194. doi:10.1038/nrm2122
Paulino, G., Darcel, N., Tome, D., and Raybould, H. (2008). Adaptation of lipid-induced satiation is not dependent on caloric density in rats. Physiol. Behav. 93 (4-5), 930–936. doi:10.1016/j.physbeh.2007.12.015
Petit, V., Arnould, L., Martin, P., Monnot, M. C., Pineau, T., Besnard, P., et al. (2007). Chronic high-fat diet affects intestinal fat absorption and postprandial triglyceride levels in the mouse. J. Lipid Res. 48 (2), 278–287. doi:10.1194/jlr.M600283-JLR200
Poreba, M. A., Dong, C. X., Li, S. K., Stahl, A., Miner, J. H., and Brubaker, P. L. (2012). Role of fatty acid transport protein 4 in oleic acid-induced glucagon-like peptide-1 secretion from murine intestinal L cells. Am. J. Physiol. Endocrinol. Metab. 303 (7), E899–E907. doi:10.1152/ajpendo.00116.2012
Potts, J. L., Fisher, R. M., Humphreys, S. M., Coppack, S. W., Gibbons, G. F., and Frayn, K. N. (1991). Chylomicrons are the preferred substrate for lipoprotein lipase in vivo. Biochem. Soc. Trans. 19 (3), 314S. doi:10.1042/bst019314s
Qu, J., Ko, C. W., Tso, P., and Bhargava, A. (2019). Apolipoprotein A-IV: A multifunctional protein involved in protection against atherosclerosis and diabetes. Cells 8 (4), 319. doi:10.3390/cells8040319
Rajan, L., Palaniswamy, D., and Mohankumar, S. K. (2020). Targeting obesity with plant-derived pancreatic lipase inhibitors: A comprehensive review. Pharmacol. Res. 155, 104681. doi:10.1016/j.phrs.2020.104681
Ravaut, G., Legiot, A., Bergeron, K. F., and Mounier, C. (2020). Monounsaturated fatty acids in obesity-related inflammation. Int. J. Mol. Sci. 22 (1), 330. doi:10.3390/ijms22010330
Razani, B., Combs, T. P., Wang, X. B., Frank, P. G., Park, D. S., Russell, R. G., et al. (2002). Caveolin-1-deficient mice are lean, resistant to diet-induced obesity, and show hypertriglyceridemia with adipocyte abnormalities. J. Biol. Chem. 277 (10), 8635–8647. doi:10.1074/jbc.M110970200
Recena Aydos, L., Aparecida do Amaral, L., Serafim de Souza, R., Jacobowski, A. C., Freitas Dos Santos, E., and Rodrigues Macedo, M. L. (2019). Nonalcoholic fatty liver disease induced by high-fat diet in C57bl/6 models. Nutrients 11 (12), 3067. doi:10.3390/nu11123067
Rial, S. A., Karelis, A. D., Bergeron, K. F., and Mounier, C. (2016). Gut microbiota and metabolic health: The potential beneficial effects of a medium chain triglyceride diet in obese individuals. Nutrients 8 (5), 281. doi:10.3390/nu8050281
Rong, X., Wang, B., Dunham, M. M., Hedde, P. N., Wong, J. S., Gratton, E., et al. (2015). Lpcat3-dependent production of arachidonoyl phospholipids is a key determinant of triglyceride secretion. Elife 4, e06557. doi:10.7554/eLife.06557
Sabb, J. E., Godfrey, P. M., and Brannon, P. M. (1986). Adaptive response of rat pancreatic lipase to dietary fat: Effects of amount and type of fat. J. Nutr. 116 (5), 892–899. doi:10.1093/jn/116.5.892
Sane, A. T., Seidman, E., Peretti, N., Kleme, M. L., Delvin, E., Deslandres, C., et al. (2017). Understanding chylomicron retention disease through Sar1b gtpase gene disruption: Insight from cell culture. Arterioscler. Thromb. Vasc. Biol. 37 (12), 2243–2251. doi:10.1161/ATVBAHA.117.310121
Schaffer, J. E. (2003). Lipotoxicity: When tissues overeat. Curr. Opin. Lipidol. 14 (3), 281–287. doi:10.1097/00041433-200306000-00008
Schoiswohl, G., Schweiger, M., Schreiber, R., Gorkiewicz, G., Preiss-Landl, K., Taschler, U., et al. (2010). Adipose triglyceride lipase plays a key role in the supply of the working muscle with fatty acids. J. Lipid Res. 51 (3), 490–499. doi:10.1194/jlr.M001073
Scudamore, C. L., Jepson, M. A., Hirst, B. H., Hugh, R., and Miller, P. (1998). The rat mucosal mast cell chymase, RMCP-II, alters epithelial cell monolayer permeability in association with altered distribution of the tight junction proteins ZO-1 and occludin. Eur. J. Cell Biol. 75 (4), 321–330. doi:10.1016/s0171-9335(98)80065-4
Seidell, J. C., and Halberstadt, J. (2015). The global burden of obesity and the challenges of prevention. Ann. Nutr. Metab. 66, 7–12. doi:10.1159/000375143
Shew, T., Wolins, N. E., and Cifarelli, V. (2018). VEGFR-3 signaling regulates triglyceride retention and absorption in the intestine. Front. Physiol. 9, 1783. doi:10.3389/fphys.2018.01783
Shim, J., Moulson, C. L., Newberry, E. P., Lin, M. H., Xie, Y., Kennedy, S. M., et al. (2009). Fatty acid transport protein 4 is dispensable for intestinal lipid absorption in mice. J. Lipid Res. 50 (3), 491–500. doi:10.1194/jlr.M800400-JLR200
Siddiqi, S. A., Mahan, J., Siddiqi, S., Gorelick, F. S., and Mansbach, C. M. (2006). Vesicle-associated membrane protein 7 is expressed in intestinal ER. J. Cell Sci. 119, 943–950. doi:10.1242/jcs.02803
Siddiqi, S., Saleem, U., Abumrad, N. A., Davidson, N. O., Storch, J., Siddiqi, S. A., et al. (2010). A novel multiprotein complex is required to generate the prechylomicron transport vesicle from intestinal ER. J. Lipid Res. 51 (7), 1918–1928. doi:10.1194/jlr.M005611
Siddiqi, S., Sheth, A., Patel, F., Barnes, M., and Mansbach, C. M. (2013). Intestinal caveolin-1 is important for dietary fatty acid absorption. Biochim. Biophys. Acta 1831 (8), 1311–1321. doi:10.1016/j.bbalip.2013.05.001
Soares, A., Beraldi, E. J., Ferreira, P. E., Bazotte, R. B., and Buttow, N. C. (2015). Intestinal and neuronal myenteric adaptations in the small intestine induced by a high-fat diet in mice. BMC Gastroenterol. 15, 3. doi:10.1186/s12876-015-0228-z
Stahl, A., Hirsch, D. J., Gimeno, R. E., Punreddy, S., Ge, P., Watson, N., et al. (1999). Identification of the major intestinal fatty acid transport protein. Mol. Cell 4 (3), 299–308. doi:10.1016/s1097-2765(00)80332-9
Stenkamp-Strahm, C. M., Kappmeyer, A. J., Schmalz, J. T., Gericke, M., and Balemba, O. (2013). High-fat diet ingestion correlates with neuropathy in the duodenum myenteric plexus of obese mice with symptoms of type 2 diabetes. Cell Tissue Res. 354 (2), 381–394. doi:10.1007/s00441-013-1681-z
Stone, S. J., Myers, H. M., Watkins, S. M., Brown, B. E., Feingold, K. R., Elias, P. M., et al. (2004). Lipopenia and skin barrier abnormalities in DGAT2-deficient mice. J. Biol. Chem. 279 (12), 11767–11776. doi:10.1074/jbc.M311000200
Storch, J., and Corsico, B. (2008). The emerging functions and mechanisms of mammalian fatty acid-binding proteins. Annu. Rev. Nutr. 28, 73–95. doi:10.1146/annurev.nutr.27.061406.093710
Storch, J., and Thumser, A. E. (2010). Tissue-specific functions in the fatty acid-binding protein family. J. Biol. Chem. 285 (43), 32679–32683. doi:10.1074/jbc.R110.135210
Storch, J., Zhou, Y. X., and Lagakos, W. S. (2008). Metabolism of apical versus basolateral sn-2-monoacylglycerol and fatty acids in rodent small intestine. J. Lipid Res. 49 (8), 1762–1769. doi:10.1194/jlr.M800116-JLR200
Suh, S. H., Choe, K., Hong, S. P., Jeong, S. H., Makinen, T., Kim, K. S., et al. (2019). Gut microbiota regulates lacteal integrity by inducing VEGF-C in intestinal villus macrophages. EMBO Rep. 20 (4), e46927. doi:10.15252/embr.201846927
Tanaka, S., Nemoto, Y., Takei, Y., Morikawa, R., Oshima, S., Nagaishi, T., et al. (2020). High-fat diet-derived free fatty acids impair the intestinal immune system and increase sensitivity to intestinal epithelial damage. Biochem. Biophys. Res. Commun. 522 (4), 971–977. doi:10.1016/j.bbrc.2019.11.158
Tardelli, M., Bruschi, F. V., Claudel, T., Fuchs, C. D., Auer, N., Kunczer, V., et al. (2019). Lack of monoacylglycerol lipase prevents hepatic steatosis by favoring lipid storage in adipose tissue and intestinal malabsorption. J. Lipid Res. 60 (7), 1284–1292. doi:10.1194/jlr.M093369
Taschler, U., Radner, F. P., Heier, C., Schreiber, R., Schweiger, M., Schoiswohl, G., et al. (2011). Monoglyceride lipase deficiency in mice impairs lipolysis and attenuates diet-induced insulin resistance. J. Biol. Chem. 286 (20), 17467–17477. doi:10.1074/jbc.M110.215434
Thomson, A. B., Keelan, M., Garg, M. L., and Clandinin, M. T. (1989). Intestinal aspects of lipid absorption: In review. Can. J. Physiol. Pharmacol. 67 (3), 179–191. doi:10.1139/y89-031
Tomkin, G. H., and Owens, D. (2001). Abnormalities in apo B-containing lipoproteins in diabetes and atherosclerosis. Diabetes Metab. Res. Rev. 17 (1), 27–43. doi:10.1002/dmrr.179
Torelli Hijo, A. H., Coutinho, C. P., Alba-Loureiro, T. C., Moreira Leite, J. S., Bargi-Souza, P., and Goulart-Silva, F. (2019). High fat diet modulates the protein content of nutrient transporters in the small intestine of mice: Possible involvement of PKA and PKC activity. Heliyon 5 (10), e02611. doi:10.1016/j.heliyon.2019.e02611
Trigatti, B. L., Anderson, R. G., and Gerber, G. E. (1999). Identification of caveolin-1 as a fatty acid binding protein. Biochem. Biophys. Res. Commun. 255 (1), 34–39. doi:10.1006/bbrc.1998.0123
Uchida, A., Slipchenko, M. N., Eustaquio, T., Leary, J. F., Cheng, J. X., and Buhman, K. K. (2013). Intestinal acyl-CoA:diacylglycerol acyltransferase 2 overexpression enhances postprandial triglyceridemic response and exacerbates high fat diet-induced hepatic triacylglycerol storage. Biochim. Biophys. Acta 1831 (8), 1377–1385. doi:10.1016/j.bbalip.2013.04.013
Uchida, A., Whitsitt, M. C., Eustaquio, T., Slipchenko, M. N., Leary, J. F., Cheng, J. X., et al. (2012). Reduced triglyceride secretion in response to an acute dietary fat challenge in obese compared to lean mice. Front. Physiol. 3, 26. doi:10.3389/fphys.2012.00026
Van Dyck, F., Braem, C. V., Chen, Z., Declercq, J., Deckers, R., Kim, B. M., et al. (2007). Loss of the PlagL2 transcription factor affects lacteal uptake of chylomicrons. Cell Metab. 6 (5), 406–413. doi:10.1016/j.cmet.2007.09.010
van Rijn, J. M., Ardy, R. C., Kuloğlu, Z., Härter, B., van Haaften-Visser, D. Y., van der Doef, H. P. J., et al. (2018). Intestinal failure and aberrant lipid metabolism in patients with DGAT1 deficiency. Gastroenterology 155 (1), 130–143. doi:10.1053/j.gastro.2018.03.040
van Rijn, J. M., van Hoesel, M., de Heus, C., van Vugt, A. H. M., Klumperman, J., Nieuwenhuis, E. E. S., et al. (2019). DGAT2 partially compensates for lipid-induced ER stress in human DGAT1-deficient intestinal stem cells. J. Lipid Res. 60 (10), 1787–1800. doi:10.1194/jlr.M094201
Vandevijvere, S., Chow, C. C., Hall, K. D., Umali, E., and Swinburn, B. A. (2015). Increased food energy supply as a major driver of the obesity epidemic: A global analysis. Bull. World Health Organ 93 (7), 446–456. doi:10.2471/BLT.14.150565
Vors, C., Pineau, G., Drai, J., Meugnier, E., Pesenti, S., Laville, M., et al. (2015). Postprandial endotoxemia linked with chylomicrons and lipopolysaccharides handling in obese versus lean men: A lipid dose-effect trial. J. Clin. Endocrinol. Metab. 100 (9), 3427–3435. doi:10.1210/JC.2015-2518
Wang, B., Li, L., Fu, J., Yu, P., Gong, D., Zeng, C., et al. (2016). Effects of long-chain and medium-chain fatty acids on apoptosis and oxidative stress in human liver cells with steatosis. J. Food Sci. 81 (3), H794–H800. doi:10.1111/1750-3841.13210
Weinberg, R. B., Gallagher, J. W., Fabritius, M. A., and Shelness, G. S. (2012). ApoA-IV modulates the secretory trafficking of apoB and the size of triglyceride-rich lipoproteins. J. Lipid Res. 53 (4), 736–743. doi:10.1194/jlr.M019992
Xiao, C., Stahel, P., Carreiro, A. L., Hung, Y. H., Dash, S., Bookman, I., et al. (2019). Oral glucose mobilizes triglyceride stores from the human intestine. Cell Mol. Gastroenterol. Hepatol. 7 (2), 313–337. doi:10.1016/j.jcmgh.2018.10.002
Xie, P., Guo, F., Ma, Y., Zhu, H., Wang, F., Xue, B., et al. (2014). Intestinal Cgi-58 deficiency reduces postprandial lipid absorption. PLoS One 9 (3), e91652. doi:10.1371/journal.pone.0091652
Xie, Y., Ding, F., Di, W., Lv, Y., Xia, F., Sheng, Y., et al. (2020). Impact of a highfat diet on intestinal stem cells and epithelial barrier function in middleaged female mice. Mol. Med. Rep. 21 (3), 1133–1144. doi:10.3892/mmr.2020.10932
Xie, Y., Newberry, E. P., Brunt, E. M., Ballentine, S. J., Soleymanjahi, S., Molitor, E. A., et al. (2021). Inhibition of chylomicron assembly leads to dissociation of hepatic steatosis from inflammation and fibrosis. J. Lipid Res. 62, 100123. doi:10.1016/j.jlr.2021.100123
Xie, Y., Newberry, E. P., Young, S. G., Robine, S., Hamilton, R. L., Wong, J. S., et al. (2006). Compensatory increase in hepatic lipogenesis in mice with conditional intestine-specific Mttp deficiency. J. Biol. Chem. 281 (7), 4075–4086. doi:10.1074/jbc.M510622200
Xiong, X., Bales, E. S., Ir, D., Robertson, C. E., McManaman, J. L., Frank, D. N., et al. (2017). Perilipin-2 modulates dietary fat-induced microbial global gene expression profiles in the mouse intestine. Microbiome 5 (1), 117. doi:10.1186/s40168-017-0327-x
Ye, Z., Cao, C., Li, R., Cao, P., Li, Q., and Liu, Y. (2019). Lipid composition modulates the intestine digestion rate and serum lipid status of different edible oils: A combination of in vitro and in vivo studies. Food Funct. 10 (3), 1490–1503. doi:10.1039/c8fo01290c
Yen, C. L., Cheong, M. L., Grueter, C., Zhou, P., Moriwaki, J., Wong, J. S., et al. (2009). Deficiency of the intestinal enzyme acyl CoA:monoacylglycerol acyltransferase-2 protects mice from metabolic disorders induced by high-fat feeding. Nat. Med. 15 (4), 442–446. doi:10.1038/nm.1937
Yen, C. L., Stone, S. J., Koliwad, S., Harris, C., and Farese, R. V. (2008). Thematic review series: Glycerolipids. DGAT enzymes and triacylglycerol biosynthesis. J. Lipid Res. 49 (11), 2283–2301. doi:10.1194/jlr.R800018-JLR200
Yoshida, K., Kita, Y., Tokuoka, S. M., Hamano, F., Yamazaki, M., Sakimura, K., et al. (2019). Monoacylglycerol lipase deficiency affects diet-induced obesity, fat absorption, and feeding behavior in CB1 cannabinoid receptor-deficient mice. FASEB J. 33 (2), 2484–2497. doi:10.1096/fj.201801203R
Yoshioka, M., Bolduc, C., Raymond, V., and St-Amand, J. (2008). High-fat meal-induced changes in the duodenum mucosa transcriptome. Obes. (Silver Spring) 16 (10), 2302–2307. doi:10.1038/oby.2008.352
Zembroski, A. S., Xiao, C., and Buhman, K. K. (2021). The roles of cytoplasmic lipid droplets in modulating intestinal uptake of dietary fat. Annu. Rev. Nutr. 41, 79–104. doi:10.1146/annurev-nutr-110320-013657
Zhang, F., Zarkada, G., Han, J., Li, J., Dubrac, A., Ola, R., et al. (2018). Lacteal junction zippering protects against diet-induced obesity. Science 361 (6402), 599–603. doi:10.1126/science.aap9331
Zhang, L. J., Wang, C., Yuan, Y., Wang, H., Wu, J., Liu, F., et al. (2014). Cideb facilitates the lipidation of chylomicrons in the small intestine. J. Lipid Res. 55 (7), 1279–1287. doi:10.1194/jlr.M046482
Zhang, Q., Yao, D., Rao, B., Jian, L., Chen, Y., Hu, K., et al. (2021). The structural basis for the phospholipid remodeling by lysophosphatidylcholine acyltransferase 3. Nat. Commun. 12 (1), 6869. doi:10.1038/s41467-021-27244-1
Keywords: chylomicron, dietary fat absorption, enterocyte, obesity, triglycerides
Citation: Li X, Liu Q, Pan Y, Chen S, Zhao Y and Hu Y (2023) New insights into the role of dietary triglyceride absorption in obesity and metabolic diseases. Front. Pharmacol. 14:1097835. doi: 10.3389/fphar.2023.1097835
Received: 14 November 2022; Accepted: 20 January 2023;
Published: 02 February 2023.
Edited by:
Yanwen Wang, National Research Council Canada (NRC), CanadaReviewed by:
Hong-Ping Guan, Rezubio Pharmaceuticals Co. Ltd., ChinaChangting Xiao, University of Saskatchewan, Canada
Copyright © 2023 Li, Liu, Pan, Chen, Zhao and Hu. This is an open-access article distributed under the terms of the Creative Commons Attribution License (CC BY). The use, distribution or reproduction in other forums is permitted, provided the original author(s) and the copyright owner(s) are credited and that the original publication in this journal is cited, in accordance with accepted academic practice. No use, distribution or reproduction is permitted which does not comply with these terms.
*Correspondence: Yu Zhao, Y2F0aHkxNTBAMTM5LmNvbQ==; Yiyang Hu, eXlodWxpdmVyQDE2My5jb20=
†These authors have contributed equally to this work and share first authorship