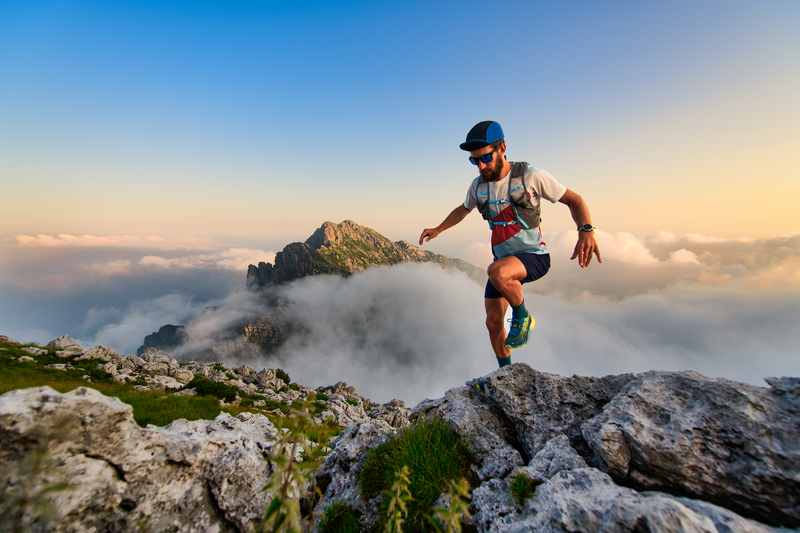
94% of researchers rate our articles as excellent or good
Learn more about the work of our research integrity team to safeguard the quality of each article we publish.
Find out more
REVIEW article
Front. Pharmacol. , 25 January 2023
Sec. Neuropharmacology
Volume 14 - 2023 | https://doi.org/10.3389/fphar.2023.1097284
This article is part of the Research Topic Gut Microbiota as a Therapeutic Target in Neuropsychiatric Disorders: Current status and future directions View all 13 articles
Second-generation antipsychotics (SGAs) are the mainstay of treatment for schizophrenia and other neuropsychiatric diseases but cause a high risk of disruption to lipid metabolism, which is an intractable therapeutic challenge worldwide. Although the exact mechanisms underlying this lipid disturbance are complex, an increasing body of evidence has suggested the involvement of the gut microbiota in SGA-induced lipid dysregulation since SGA treatment may alter the abundance and composition of the intestinal microflora. The subsequent effects involve the generation of different categories of signaling molecules by gut microbes such as endogenous cannabinoids, cholesterol, short-chain fatty acids (SCFAs), bile acids (BAs), and gut hormones that regulate lipid metabolism. On the one hand, these signaling molecules can directly activate the vagus nerve or be transported into the brain to influence appetite via the gut–brain axis. On the other hand, these molecules can also regulate related lipid metabolism via peripheral signaling pathways. Interestingly, therapeutic strategies directly targeting the gut microbiota and related metabolites seem to have promising efficacy in the treatment of SGA-induced lipid disturbances. Thus, this review provides a comprehensive understanding of how SGAs can induce disturbances in lipid metabolism by altering the gut microbiota.
The use of antipsychotic medications as a treatment for patients with schizophrenia is surging, and the incidence of schizophrenia is also rising dramatically worldwide (Hert et al., 2011; Gonçalves et al., 2015). However, long-term use of these drugs can cause numerous adverse effects on patients, especially the disruption of lipid levels, including high-density lipoprotein (HDL), low-density lipoprotein (LDL), triglyceride (TG), and total cholesterol (TC) (Jaberi et al., 2020). Although individuals with schizophrenia may exhibit dyslipidemia before the initiation of treatment, mounting evidence has shown that antipsychotics can independently induce further abnormalities. It has been noted that patients with first-episode schizophrenia have abnormal lipid profiles, and those with multiple-episode schizophrenia are more likely to have dyslipidemia (Mackin et al., 2007; Vancampfort et al., 2015; Mhalla et al., 2018; Pillinger et al., 2019; Yang et al., 2022). Notably, second-generation antipsychotics (SGAs) have stronger associations with lipid abnormalities than first-generation antipsychotics (FGAs) have (Buhagiar and Jabbar, 2019). Metabolic abnormalities especially lipid metabolism disorders, are major risk factors contributing to cardiovascular events (Fan et al., 2013). They also play a role in the pathophysiological process of systemic organ damage and are causative factors in the development and progression of atherosclerotic cardiovascular disease. According to previous reports, patients with schizophrenia have a life expectancy that could be 15 years shorter than that of the general population. Additionally, more than two-thirds of patients with schizophrenia die from coronary heart disease, which is significantly higher than the mortality rate in the general population (Hennekens et al., 2005). A growing body of research indicated that SGA-induced disturbances of lipid metabolism and other metabolic abnormalities are the key factor linking to an increased risk of cardiovascular disease in patients with schizophrenia, in addition to some confounding risk factors such as smoking, physical inactivity, unhealthy lifestyle, and poor dietary habits (Arias et al., 2018).
Although the particular processes by which SGAs cause dysfunctional lipid metabolism are complex, a growing body of evidence suggests that the gut microbiota is involved in SGA-induced defects in lipid metabolism. From birth, humans have microbes in their digestive tract (Yatsunenko et al., 2012). Hundreds of millions of microorganisms, including bacteria, fungi, and viruses, exist in the healthy human gastrointestinal system, forming a microbial community that has a major impact on the body (Mirzaei and Maurice, 2017). A large number of these bacteria make up the collective intestinal flora. The intestinal flora contains 1000 to 1500 species of bacteria, which outnumber the body’s cells by more than 10 times (Kim and Jazwinski, 2018) and have more than 100 times the total number of genes as humans (Cox et al., 2019). These bacteria play important roles in host metabolism, digestion, the immune system, and the central nervous system (John and Mullin, 2016; Rogers et al., 2016; Dinan and Cryan, 2017; Ipci et al., 2017; Kanji et al., 2018). The theory that the gut microbiota affects lipid metabolism has been extensively studied in mice. For example, germ-free (GF) mice on a chow diet showed lower fasting systemic TG, TC, HDL cholesterol, and portal vein TG (Martinez-Guryn et al., 2018), as well as higher liver cholesterol and lower TG levels, than conventionally raised (Conv-R) mice (Rabot et al., 2010). Rabot et al. found that Conv-R mice had increased blood TG, HDL, and TC levels after consuming a high-fat diet (Rabot et al., 2010). To maintain the same weight as Conv-R mice, GF mice had to increase their caloric intake by at least 30% (Hsiao et al., 2008). Further evidence that the intestinal flora affects lipid metabolism has been observed in fecal transplantation experiments. Peter et al. showed for the 1 time that the ability of the gut microbiota to harvest energy from the diet was a transmissible trait. GF mice colonized with an ‟obesity microbiota” had a much higher increase in total fat than GF mice colonized with a ‟lean microbiota” (Turnbaugh et al., 2006). Similarly, obese patients with reduced microbial gene abundance (40%) showed more pronounced metabolic disturbances and had increased total serum cholesterol and serum TG levels (Cotillard et al., 2013).
The sex and age of the host as well as the site in the gastrointestinal tract influence the makeup and variety of the intestinal flora (Kim and Jazwinski, 2018; Cox et al., 2019). Independent of host variables, diet, lifestyle, and medicine can alter the composition of the gut flora (Kanji et al., 2018). Studies in recent years have shown that SGAs have some antibacterial activity and can alter the gut microbiota of patients with psychosis (Nehme et al., 2018; Ait Chait et al., 2020). Olanzapine can have direct antibacterial in vitro effects against the mammalian gut bacteria Escherichia coli and Enterococcus faecalis, which are the two most common species in the intestine (E. coli: Proteobacteria; E. faecalis: Firmicutes) (Morgan et al., 2014). Similarly, chlorpromazine (Kristiansen, 1979) has shown antibacterial effects against Mycobacterium tuberculosis in vitro, and thioridazine (Thorsing et al., 2013) acts against methicillin-resistant Staphylococcus aureus. These medications targeted a more comparable pattern of species than their degree of chemical similarity would suggest (Maier et al., 2018). This raises the possibility that direct bacterial inhibition by SGAs is not merely a side effect but also a part of their molecular mechanism.
The effect of gut microbes on lipid metabolism has been supported by many in vivo and in vitro studies, and evidence of the effect of SGAs on gut microbes is gradually emerging with the advancement of microbiological research techniques. Furthermore, positive results have been achieved with therapeutic strategies that directly target the gut microbiota and related metabolites, thereby ameliorating antipsychotic-induced disorders of lipid metabolism. This certainly identifies the gut microbiome as a potential target and establishes that the potential mechanism underlying lipid metabolism disturbances associated with antipsychotics is worthy of further investigation. However, the role of the gut microbiome in antipsychotic-induced disorders of lipid metabolism has not been systematically explained. This review aims to provide a comprehensive understanding of the potential for SGAs to alter the gut microbiota and promote adverse lipid metabolism events. Keyword search on PubMed is detailed in Figure 1, based on the Preferred Reporting Item Guidelines for Systematic Reviews and Meta-Analyses.
Research on the microbiota in schizophrenia patients treated with SGAs is very scarce within the general microbiota literature. Several studies have investigated the effect of the gut microbiome on animal and human models (Table 1; Table 2). Mice treated with risperidone (Ridaura et al., 2013; Bahr et al., 2015b; Riedl et al., 2021) and olanzapine (Morgan et al., 2014) have increased ratios of Firmicutes to Bacteroidetes, which is one of the distinguishing features of the microbiota of obese individuals (Turnbaugh et al., 2006; Schwiertz et al., 2010; Ferrer et al., 2013). Firmicutes and Proteobacteria are the two major phyla associated with the human intestinal microbiome, constituting the majority of intestinal bacteria (approximately 90%) (Human Microbiome Project Consortium, 2012). These studies have been well replicated in humans (Bahr et al., 2015a; Yuan et al., 2018; Ma et al., 2020). Exceptions to this rule are Kao et al. (Kao et al., 2018) and Pelka et al. (Pełka-Wysiecka et al., 2019). These studies showed no significant effects of olanzapine on the gut microbiota in female rats or in women with schizophrenia. The results of studies on changes in the phylum Actinomycetes are also inconsistent. Bahr et al. (Bahr et al., 2015b) found an increase in the relative abundance of the actinomycete clade in the feces of mice treated with risperidone, whereas Davey et al. (Davey et al., 2012) showed that the relative abundance of the actinomycete clade in mice administered olanzapine was decreased. During olanzapine treatment, the relative abundance of Erysipelotrichi and Gammaproteobacteria increased, while the relative abundance of Bacteroidia decreased (Morgan et al., 2014). Both Erysipelotrichi and Gammaproteobacteria are associated with non-alcoholic fatty liver disease (NAFLD) independent of weight gain (Spencer et al., 2011; Henao-Mejia et al., 2012). Risperidone treatment increased the relative abundance of Allobaculum spp. Bacteroides spp. Bifidobacterium spp. and E. coli and decreased the relative abundance of Lactobacillus spp. Alistipes spp. Akkermansia spp, and Clostridium coccoides groups (Ridaura et al., 2013; Bahr et al., 2015b; Yuan et al., 2018). It should be noted that the results of studies on the change in relative abundance of Bacteroides spp. Are inconsistent: one study showed an increase, while another showed no significant change (Bahr et al., 2015b; Yuan et al., 2018). Furthermore, the abundance of Bifidobacterium spp. In the feces of mice treated with risperidone was negatively correlated with serum LDL levels; E. coli was negatively correlated with serum TG levels (Yuan et al., 2018). However, there is little evidence regarding the relationship between changes in lipid metabolism and alterations in the gut microbiota in humans following SGA treatment. Of particular interest is the search for potential probiotic bacteria such as Akkermansia muciniphila, which is a previously reported ‘lean gut microbiota’ species. A. muciniphila, a member of the phylum Verrucomicrobia, is the only species of the genus Akkermansia. A. muciniphila is a mucin degrader in the intestine and is significantly and negatively associated with altered fat metabolism and obesity (Henao-Mejia et al., 2012; Schneeberger et al., 2015). A significantly reduced abundance of fecal A. muciniphila was found in patients with bipolar disorder who were treated with a range of SGAs, such as clozapine, olanzapine, and risperidone, compared to controls (Flowers et al., 2017).
To describe the relationship between intestinal flora and SGA-induced lipid metabolism, Davey et al. investigated the effect of antibiotic-induced alterations in the gut microbiota on the metabolism of female rats treated with olanzapine (Davey et al., 2013). They found that clinically relevant doses of olanzapine accelerated metabolic disturbances and weight gain in C57BL/6J mice fed a high-fat diet. When the rats were treated with both olanzapine and a cocktail of broad-spectrum antibiotics, including oral neomycin, metronidazole, and polymyxin, the increases in the proportions of Firmicutes and Bacteroidetes bacteria were reversed, and this treatment reversed the olanzapine-induced metabolic disturbances and weight gain induced by high-fat diets in C57BL/6J mice. Thus, Morgan et al. conducted a further study and found that this phenomenon was consistent with a previously described study conducted under sterile conditions but that olanzapine-induced metabolic disturbances and weight gain occurred soon after gut microbial colonization (Morgan et al., 2014). Further experimental work has been conducted on mice treated with prebiotics in combination with SGAs. Coadministration of olanzapine and the prebiotic B-GOS led to a significant increase in circulating levels of TNFα in mice, which has been reported to affect lipid metabolism, elevate fecal Bifidobacterium spp. And reduce body weight, and these effects were not seen in response to olanzapine or B-GOS treatment alone (Kao et al., 2018). Similarly, the probiotic A. muciniphila was observed to have a similar effect (Huang et al., 2021). These studies suggest that intestinal microbes are necessary and sufficient for SGA-induced disruption of lipid metabolism. It is worth noting that none of these experiments were replicated in humans.
Accumulating evidence shows that female patients who take SGAs seem to have poorer lipid profiles than those of male patients, as well as a higher prevalence of metabolic syndrome and cardiovascular risk factors, including weight gain and dyslipidemia (Chen et al., 2020). It is noteworthy that sex-dependent differences in the host’s metabolism may be associated with gut microbiota (Wu et al., 2007; Lange et al., 2017). For example, women usually have considerably higher Firmicutes:Bacteroidetes ratios as compared to men in a population-based cross-sectional investigation (Koliada et al., 2021). Given that SGA-induced lipid disturbances are frequently associated with an increased ratio of Firmicutes to Bacteroidetes, this finding raises the possibility that women are more susceptible than men to abnormal lipid metabolism (Morgan et al., 2014). Another substantial indication that men and women have different microbes is the fact that sex hormones can affect the composition of the host microbiome. Significant changes in the host gut microbiota, such as a drop in the abundance of butyrate-producing bacteria and a decline in alpha diversity, are linked to elevated levels of estrogen in pregnant women (Koren et al., 2012). These differentiations can result in a significant impact on SAG-induced changes in lipid metabolism between genders. Unfortunately, available studies are not enough to systematically explain the link between sex differences in gut microbes and sex differences in disorders of lipid metabolism caused by antipsychotics. However, this phenomenon might offer some guidance for future studies on sex differences regarding the side effects of SGAs.
Microorganisms and their metabolites are crucial in understanding how the gut microbiome is implicated in SGA-induced systemic lipid disorders (Skonieczna-Żydecka et al., 2019). Short-chain fatty acids (SCFAs), bile acids (BAs), and neurotransmitters are among the metabolites that the intestinal microbiota can create. Bacteroidetes and Firmicutes can create butyric acid, which accounts for approximately 20% and 60% of the total intestinal flora, respectively, while Proteobacteria and Actinobacteria produce very small amounts of SCFAs (5%–10% and 3%, respectively). Sulfate-reducing bacteria may use lactic acid to make acetic acid and hydrogen sulfide, while Veillonellaceae can convert it to propionic acid. Bacteroidetes is a phylum that can convert succinic acid to propionic acid, and its population density is related to the amount of propionic acid in the intestine (Karlsson et al., 2013). The dominant genera for BA production are Lactobacillus, Bifidobacterium, Enterobacter, Anaplasma, and Clostridium (Krautkramer et al., 2021). In addition, Candida, Streptococcus, and Escherichia can produce 5-hydroxytryptamine (5-HT; serotonin) (Krautkramer et al., 2021). Approximately 36% of the small molecules in human blood are produced or modified by microbial metabolism. The total SCFA concentration in the colons of GF mice is 100 times higher than that of ordinary animals. Acetic acid is the most concentrated SCFA in organisms and is central to carbohydrate and lipid metabolic pathways (Kimura et al., 2020). Miller et al. used radioisotope analysis and showed that the main pathway for bacterial production of acetate is the Wood–Ljungdahl pathway (Miller and Wolin, 1996). Moreover, olanzapine treatment of patients with schizophrenia significantly increased plasma acetate concentrations (Kao et al., 2018). Increased levels of the Kyoto Encyclopedia of Genes and Genomes (KEGG) metabolic pathways of butyric acid and propionic acid were found in a group of schizophrenia patients treated with risperidone (Bahr et al., 2015a). Hepatocytes create primary BAs, which are then 7-dehydroxylated by intestinal bacteria to produce secondary BAs. The gut microbiome affects the composition of the BA pool, such as the primary BA/secondary BA ratio, and thus affects the function of BAs, especially the metabolism of lipids (Wahlström et al., 2016). SGAs can cause an increase in total serum BAs. Specific SGAs, including chlorpromazine (BREUER, 1965), olanzapine (Lui et al., 2009), haloperidol (Fuller et al., 1977), risperidone (Wright and Vandenberg, 2007), and quetiapine (Shpaner et al., 2008), have been reported to cause cholestasis in a small number of patients taking the medication and are unpredictable with no significant correlation between dose and the duration of administration.
The intestinal microbiota signals to enteroendocrine (EE) cells through metabolites in multiple ways, resulting in the secretion of a range of intestinal hormones, such as glucagon-like peptide 1 (GLP-1), 5-HT, gastrin, leptin, cholecystokinin (CCK) and peptide tyrosine-tyrosine (PYY) (Martin et al., 2019). First, the microbiota produces SCFAs, which signal to EE cells through free fatty acid receptors 2 or 3 (FFAR2/3) (Offermanns, 2014) or by activating nuclear histone deacetylase (HDAC) (Waldecker et al., 2008; Fellows et al., 2018; Larraufie et al., 2018). Second, secondary BAs signal to EE cells via the Takeda G-protein-coupled BA receptor TGR5 or the nuclear receptor known as farnesoid X receptor (FXR) (Wahlström et al., 2016). Numerous human and animal studies have demonstrated that leptin, ghrelin (Sentissi et al., 2008), and 5-HT levels (Bahr et al., 2015a) have a substantial positive link to aberrant lipid profiles and body mass index before and after SGA treatment in schizophrenia patients. This supports the idea that the intestinal flora and its metabolites play an important role in SGA-induced metabolic abnormalities.
There appear to be distinct bacteria that are more or less related to specific classes of lipids. Gut commensal microorganisms (Bacteroides, Prevotella and Porphyromonas) are significantly altered by SGAs, and they can produce sphingolipids, including ceramide phospholipids and deoxy sphingolipids (Brown et al., 2019). Acute SGA treatment dramatically altered the homeostasis of central and peripheral sphingolipids (Castillo et al., 2016; Weston-Green et al., 2018). Notably, sphingolipids from bacteria were incorporated into the mammalian sphingolipid pathway (Johnson et al., 2020). The probiotic Bacteroides has also been shown to produce the endothelin-like molecule N-acyl-3-hydroxypalmitoyl-glycine (commendamide) (Cohen et al., 2015; Lynch et al., 2017). Furthermore, olanzapine-induced metabolic effects have been shown to be dependent on the endogenous cannabinoid system (Abolghasemi et al., 2021). Everard et al. showed that treating obese mice with A. muciniphila increased intestinal 2-oleoylglycerol (2-OG), 2-arachidonoylglycerol (2-AG) and 2-palmitoylglycerol (2-PG) levels (Everard et al., 2013). However, a recent study reported that A. muciniphila exerted its beneficial effects on metabolism independent of general changes in plasma endocannabinoidome mediators (Depommier et al., 2021). The gut microbiota–endocannabinoid axis is a key topic in the studies listed above, and it is likely to be a new target for SGA-induced lipid metabolism disorders.
Current evidence suggests that hyperphagic effects are responsible for a large percentage of the observed aberrations in lipid profiles, and there is a lack of satiety in both human and animal models in the presence of SGAs (Hartfield et al., 2003; Huang et al., 2020). The diversity of the gut flora is vital for appetite and metabolism regulation. Different gut bacteria and metabolites influence the gut’s ability to perceive nutrients, influencing the host’s appetite and energy metabolism (Oliphant and Allen-Vercoe, 2019). This is where the gut–brain axis becomes active. The gut–brain axis is a fundamental mechanism that links biochemical signals from the gastrointestinal tract to brain function (Carabotti et al., 2015). A sophisticated network of neurons regulates energy homeostasis in the host. Two types of neurons are particularly important for appetite control: neurons that express the neuropeptide proopiomelanocortin (POMC) (Baldini and Phelan, 2019) and those that express neuropeptide Y/agouti-related peptide (NPY/AgRP) (Han et al., 2018). These neurons interact with each other to form a switch that instantly adjusts appetite (Quarta et al., 2021). Among them, POMC neurons promote satiety, while AgRP neurons increase appetite.
On the one hand, gastrointestinal hormones affect the balance of the POMC/AgRP system, which controls appetite. Leptin (Endomba et al., 2020), CCK (Fan et al., 2004), PYY (Loh et al., 2015), GLP-1 (Teff et al., 2013), and 5-HT (Sohn et al., 2011; Bonn et al., 2013) activate POMC neuronal activity via receptors in the hypothalamus and inhibit NPY in AgRP neurons, sending appetite suppressant signals and regulating energy homeostasis and metabolism. Ghrelin is the only known gut hormone that promotes appetite by directly activating AgRP neurons and increasing the inhibitory effect of AgRP neurons on POMC neurons (Lage et al., 2010; Varela et al., 2011). On the other hand, the intestinal flora metabolites SCFAs and BAs can also influence appetite via the gut–brain axis. An increase in acetate production activates the parasympathetic nervous system, leading to an increase in gastrin secretion, which promotes host appetite (Perry et al., 2016). SCFAs also have the potential to enter the circulation, cross the blood‒brain barrier, and directly affect the central nervous system (Morrison and Preston, 2016). In addition, BAs can reach the hypothalamus and are highly correlated with circulating BA levels, which can reach the hypothalamus via passive diffusion, causing a brief increase in hypothalamic BA concentrations and triggering the expression of the AgRP/NPY neuronal membrane receptor TGR5, which in turn regulates appetite (Perino et al., 2021). It is worth noting that an imbalance in the amount of proinflammatory pathogenic bacteria can compromise intestinal wall integrity, affecting brain–gut axis transmission (Küme et al., 2017; Tilg et al., 2020). A study showed that the mRNA levels of NPY and AgRP were significantly increased in the hypothalamus of olanzapine-administered rats and were considerably lower than those in normal animals (Zhu Z et al., 2022). Some notable causes include several of these mechanisms affecting neuronal function through the gut–brain axis, which leads to hyperphagia and results in abnormal lipid profiles. Interestingly, this study showed that olanzapine-induced increases in weight gain percentage (WG%) occurred only when the vagus nerve was intact, while the negative effects of olanzapine-induced increases in white adipose tissue percentage (WAT%) and decreases in brown adipose tissue percentage (BAT%) were reversed by the disruption of the gut microbiota–brain axis (vagotomy), suggesting that an intact gut microbiota–brain axis may be necessary for olanzapine-induced disruption of lipid metabolism.
Lipopolysaccharide (LPS), a component of the outer membrane of most Gram-negative bacteria, is released upon bacterial cell death and enters the circulation through a ‟leaky gut”, resulting in increased levels of LPS in the blood (known as endotoxemia, which is a leading cause of metabolic diseases, such as insulin resistance, and is promoted by increased IL-6 and tumor necrosis factor (TNF) (Tilg et al., 2020)), which acts as a powerful stimulator of host immunity (Park and Lee, 2013). LPS is detected by Toll-like receptor 4 (TLR4) on the immune cell surface, resulting in the release of numerous cytokines and chemokines (Rhee, 2014). LPS can also interact directly with lipid molecules. All lipoproteins can bind to LPS and neutralize its toxicity in vitro and in vivo (Barcia and Harris, 2005).
SCFAs are used as a carbon source for the production of important endogenous host metabolites, such as fat and cholesterol (Besten et al., 2013). SCFAs produced by the intestinal flora are rapidly absorbed by colonic cells, due in part to monocarboxylate transporters, including the proton-coupled monocarboxylate transporter 1 (MCT1) and sodium-coupled monocarboxylate transporter 1 (SMCT1) (Dalile et al., 2019). The principal substrates for lipid synthesis in rat colonic epithelial cells, which convert SCFAs to acetyl coenzyme A (CoA), are acetate and butyrate (Zambell et al., 2003). CoA generates energy through the tricarboxylic acid cycle and produces palmitic acid under the action of the cytoplasmic enzyme system, which can be transferred to mitochondria to lengthen the carbon chain and form triglycerides with other substances stored in adipose tissue. In contrast, SCFAs that are not digested in colon cells enter the portal circulation of the liver through the basolateral membrane and provide substrates for hepatocyte energy metabolism. Carbohydrate-responsive element-binding protein (ChREBP) plays a key role in this process (Iizuka et al., 2020). A member of the acetyl-CoA synthetase short-chain family, encoded by Acss2, is induced by ChREBP and converts acetate to acetyl-CoA, which is used as a substrate for lipogenesis (BERG, 1956). Thus, regulating lipogenic gene expression and hepatic acetyl-CoA production from gut microbial acetate by inhibiting hepatic ChREBP is expected to prevent SGA-induced TG accumulation by inhibiting lipogenic gene expression and hepatic acetyl-CoA production. SCFAs are also involved in the biosynthesis of cholesterol and fatty acids in hepatocytes (Dalile et al., 2019). Chen et al. performed radiolabeling studies and showed that acetate was involved in the increase in de novo fat synthesis. Furthermore, antibiotic-treated mice showed reduced de novo fat synthesis (Kindt et al., 2018).
In addition, SCFAs are signaling molecules that regulate host-related functions mainly through two signaling pathways: the HDAC and G protein-coupled receptor signaling pathways. SCFAs have been shown to bind to the G protein-coupled receptors GPR43/FFAR2 and Gpr41/FFAR3 (Kimura et al., 2020), leading to further activation of downstream signaling cascades, including the phospholipase C (PLC), mitogen-activated protein kinase (MAPK), phospholipase A2 (PLA2) and nuclear factor-κB (NF-κB) pathways. Acetate inhibits insulin-mediated fat accumulation and improves lipid and glucose metabolism via GPR43. Mice lacking GPR43 were obese on a normal diet, whereas mice specifically overexpressing GPR43 in adipose tissue remained lean even when fed a high-fat diet. Both types of mice recovered under sterile conditions or after being treated with antibiotics (Kimura et al., 2013). GPR41 has been shown to regulate host energy homeostasis in a gut microbiota–dependent manner. Mice with knockout of the GPR41 gene exhibited a leaner body weight, but this difference was not observed in GF mice (Samuel et al., 2008). SCFAs also activate AMP-activated protein kinase (AMPK), a downstream signal of the G-protein-coupled receptor signaling pathway, and AMPK activation increases peroxisome proliferator-activated receptor-γ coactivator 1α (PGC-1α) expression in adipose tissue and skeletal muscle (Taylor et al., 2005; Wan et al., 2014; Yan et al., 2016). In addition, PGC-1α regulates the transcriptional activity of peroxisome proliferator-activated receptor α (PPARα) and peroxisome proliferator-activated receptor γ (PPARγ) (Muoio et al., 2002; Lin et al., 2005). Butyrate and propionate can activate PPARγ (Alex et al., 2013). Activation of liver and adipose tissue PPARγ by SCFAs regulates lipid metabolism by increasing energy expenditure, reducing inflammation in adipose tissue, improving insulin sensitivity, reducing body weight, and decreasing hepatic TG accumulation (Besten et al., 2015). A study in fish showed that the effects of olanzapine on lipid metabolism may be related to the regulation of the gut microbiota–SCFA–PPAR signaling pathway (Chang et al., 2022). The gut microbiome was significantly altered in carp that were administered olanzapine, as evidenced by an increase in the abundance of SCFA-producing bacteria, which led to an increase in the production of SCFAs. In addition, many genes that are components of the PPAR signaling pathway were significantly altered; specifically, the mRNA levels of genes related to lipid synthesis (including PPARγ, fatty acid synthase (FAS), and SREBP1) were significantly increased, and lipolysis-related genes (such as hormone-sensitive lipase (HSL) and PPARα) were significantly decreased. The activated AMPK signaling pathway can also promote the expression of HSL and adipose triglyceride lipase (ATGL), which promote lipolysis (Cantó and Auwerx, 2010; Deng et al., 2020; Guo et al., 2020; Tang et al., 2020). Jocken et al. performed in vitro experiments with a human white adipocyte model (human multipotent adipose tissue-derived stem (hMADS) cells). Acetate was found to be the main driver of the antilipolytic effect of SCFAs and attenuated HSL phosphorylation in hMADS adipocytes in a Gi-coupled manner (Jocken et al., 2017). This is reminiscent of the fact that the effect of SGAs on AMPK may also be an indirect consequence of the activation of AMPK by SCFAs in peripheral tissues. Indeed, olanzapine can reduce AMPK phosphorylation and activation in hepatocytes and 3T3-L1 cells, accompanied by a concomitant increase in SREBP-dependent lipid synthesis (Oh et al., 2011; Li et al., 2016). Interestingly, acetate supplementation did not attenuate olanzapine-induced weight gain in mice but appeared to increase it (Kao et al., 2019a). This concept of SCFA-induced weight gain appears to be consistent with the olanzapine-induced increase in plasma acetate (Kao et al., 2018).
BAs bind to FXR and TGR5 in the host and regulate lipid and energy metabolism (Chiang and Ferrell, 2019). FXR is a transcription factor that binds to the promoter region and induces the expression of multiple target genes and is expressed in the liver, ileum, kidney, and other tissues (Lefebvre et al., 2009; Teodoro et al., 2011). The most potent ligand for FXR is chenodeoxycholic acid (CDCA), followed by cholic acid (CA), deoxycholic acid (DCA), and lithocholic acid (LCA), all of which are FXR agonists. CDCA is converted to ursodeoxycholic acid in humans through a sequence of processes, and it does not activate FXR but rather inhibits FXR activity (Wang et al., 1999; Mueller et al., 2015). In addition, Sayin et al. identified two natural FXR antagonists: the taurine-conjugated murine BAs tauro-α-muricholic acid (TαMCA) and tauro-β-muricholic acid (TβMCA) (Sayin et al., 2013). TGR5 is a binding G-protein-coupled receptor expressed in tissues such as the intestine, liver, and brown‒white adipose tissue. TGR5 is mainly activated by the secondary BAs LCA and DCA (Maruyama et al., 2002; Kawamata et al., 2003).
FXR-deficient animals had increased hepatic and serum TG and cholesterol levels (Sinal et al., 2000). This finding indicates that FXR is required for lipid metabolism and energy homeostasis (Trauner et al., 2010). Reduced sterol-response element-binding protein-1c (SREBP-1c) expression caused by natural or synthetic FXR agonists via the FXR-SHP (small heterodimer partner) pathway could explain the inhibitory effect of BAs on TG production (Watanabe et al., 2004). In addition, Caron et al. used immortalized human hepatocyte (IHH) and HepaRG cell lines, which are glucose-responsive human hepatocyte lines, to show that the activation of FXR inhibits the transcriptional activity of ChREBP in human hepatocytes (Caron et al., 2013). BAs can also induce the expression of the human PPARα gene, which is a nuclear receptor that controls lipid and glucose metabolism and exerts anti-inflammatory effects via FXR (Pineda Torra et al., 2003). The activation of FXR has been shown to induce a decrease in serum apolipoprotein (Apo) CIII concentrations, leading to the amelioration of TG-rich remnant lipoprotein metabolism to reduce serum TG levels and cardiovascular risk profiles (Claudel et al., 2003). The FXR signaling pathway in mice and humans is significantly affected by SGAs. At present, pharmacological therapies that target FXR in combination with SGAs are still needed to translate the positive findings of these studies into practical outcomes. Exposure of a mouse precision-cut liver slice (PCLS) model to chlorpromazine significantly altered cholesterol and BA cellular transport regulated by FXR and BA regulation of glucose and lipid metabolism via FXR (Szalowska et al., 2013). In addition, a study also showed the downregulation of FXR targets such as Bsep, Mdr3, Ntcp, and Cyp8b1. This finding was consistent with that observed in chlorpromazine-treated HepaRG cells (Anthérieu et al., 2013). As a next step, more experiments on the effect of SGAs on FXR are needed to further understand the beneficial effects of chlorpromazine.
TGR5 has also been shown to be a BA-responsive receptor involved in host lipid metabolism. In muscle and brown adipose tissue, TGR5 may play a role in energy homeostasis by promoting intracellular thyroid hormone activity and thereby increasing energy expenditure (Watanabe et al., 2006). In addition, TGR5 has been shown to activate PPARα and PGC-1α to increase mitochondrial oxidative phosphorylation and energy metabolism (Chiang and Ferrell, 2020). However, there are limited data on changes in TGR5 receptor activity in schizophrenia patients during the use of SGAs.
Ishøy et al. published the first clinical data supporting the use of the GLP-1 agonist liraglutide to treat clozapine-induced lipid profile disturbances and weight gain in schizophrenia (Ishøy et al., 2013). Consistent with this study, Larsen et al. and Siskind et al. demonstrated that GLP-1 agonists could be effective in reducing clozapine- or olanzapine-induced lipid metabolism disorders (Kouidrat and Amad, 2019). GLP-1, a glucose-dependent incretin, plays a crucial role in lipid metabolism and body weight maintenance by binding to the GLP-1 receptor (GLP-1R). Many human tissues, including the pancreas, liver, muscle, fat, gastrointestinal tract, heart, and brain, express GLP-1R (Campbell and Drucker, 2013). When bound to GLP-1, GLP-1R acts through its coupled G protein (Gαs) in pancreatic β-cells, activating adenylyl cyclase and increasing the intracellular levels of cyclic adenosine monophosphate (cAMP) (Doyle and Egan, 2007); this increase in cAMP exerts a series of effects. The activation of factors (protein kinase A (PKA) (Béguin et al., 1999) and exchange protein directly activated by cAMP (EPAC) (Kang et al., 2008)) leads to calcium influx, increased transcription of the proinsulin gene, and the stimulation of insulin secretion. In addition, GLP-1R may regulate pancreatic β-cell metabolism by activating the phosphoinositide 3-kinase (PI3K)/AKT (protein kinase B)/mTOR (mammalian target of rapamycin) and MAPK signaling pathways (Rowlands et al., 2018). The binding of GLP to GLP-1R in adipocytes activates the adenylyl cyclase (AC)/cAMP signaling pathway, regulates the apoptosis and proliferation of preadipocytes through various cellular signaling pathways, such as extracellular signal–regulated kinase (ERK), protein kinase C (PKC), and AKT, and alters the expression of PPARγ and its target genes (Challa et al., 2012; Chen et al., 2017). Furthermore, by reducing macrophage infiltration in adipose tissue, GLP-1 can directly block the inflammatory signaling pathway, improving insulin resistance, lowering liver fat levels, and considerably alleviating NAFLD (Blaslov et al., 2014). This explains how GLP-1R might decrease hepatic substrate supply (e.g., glucose and non-esterified fatty acids (NEFAs)) by affecting adipose tissue, which may be partially responsible for the overall effect. GLP-1R-based treatment of metabolic diseases has been reported to act on hepatocyte lipid metabolism through PI3K, type 1 protein phosphatase (PP-1), and PKC (Redondo et al., 2003). Interestingly, a study showed that liraglutide ameliorated hepatocyte steatosis by inducing autophagy through the AMPK/mTOR pathway (He et al., 2016). Additionally, GLP-1 may promote hepatocyte survival by downregulating microRNA-23, resulting in increased expression of PGC-1α and uncoupling protein 2 (UCP2) (Wang C et al., 2015). Recent studies have shown that GLP1/GLP-1R signaling is involved in the effect of brexpiprazole, a new multitarget antipsychotic drug (APD) approved by the US FDA in 2015 that induces disorders of glucose and lipid metabolism (Li et al., 2021). Brexpiprazole administration significantly reduced the protein and mRNA levels of GLP1 in the pancreas and small intestine by inhibiting Ca2+/calmodulin-dependent kinase IIα (CaMKIIα), AMPK, and β-catenin. Brexpiprazole administration also caused islet dysfunction and decreased GLP-1R, PI3K, and IRβ expression in the pancreas. Cotreatment with liraglutide and brexpiprazole is an effective strategy for certain aberrant metabolisms.
Leptin was found to be involved in lipid metabolism and energy balance by mediating certain signaling pathways. Leptin inhibits acetyl-CoA carboxylase (ACC) activity by activating AMPK in skeletal muscle, thereby stimulating the oxidation of fatty acids (Minokoshi et al., 2002). Consistently, another study revealed that the activation of AMPK can have a therapeutic effect on metabolic syndrome only if leptin is present and active (Stockebrand et al., 2013). In addition, p38 MAPK may also contribute to the effect of leptin on fatty acid oxidation (Dardeno et al., 2010). In non-adipose tissues, leptin may promote fatty acid oxidation by activating PPARα-induced CoA expression via signal transducer and activator of transcription 3 (STAT3) (Unger et al., 1999). Maya et al. found that leptin could regulate lipid metabolism and inflammation by modulating the PI3K/Akt/mTOR pathway (Maya-Monteiro and Bozza, 2008). Consistent with this report, Schmidt et al. found that olanzapine simultaneously upregulated the mTOR pathway and downstream signaling cascades, including the activation of mTORC1, in mice (Schmidt et al., 2013). mTORC1 activation interferes with lipid and energy metabolism, leading to the upregulation of lipid biosynthesis and the accumulation of TGs. Furthermore, activation of the mTOR pathway inhibits autophagy, thereby increasing intracellular lipid accumulation (Zhuo et al., 2022). Enhanced mTOR activity disrupts hepatic lipid homeostasis by regulating the expression of the transcription factor SREBP-1c (Takashima et al., 2009).
To date, the mechanisms of SGA-induced metabolic changes have not been thoroughly investigated. However, in clinical treatment, side effects of SGAs on lipid metabolism can usually be suppressed by other drugs, and some interventions have yielded significant results. Researchers found that metformin, a biguanide antihyperglycemic agent, had a positive effect on the lipid profile, insulin resistance, and body weight in patients with schizophrenia, which has been supported by animal (Zhu W et al., 2022) and human models (Wu et al., 2016; Vancampfort et al., 2019; Jiang et al., 2020). Interestingly, the intestinal flora plays a vital role in the positive effects of metformin. Luo et al. (Luo et al., 2021) and Wang et al. (Wang et al., 2021) found that metformin not only prevented olanzapine-induced disruption of the lipid profile and hepatic histopathological changes but also partially reversed olanzapine-induced alterations in the gut microbiota and helped correct peripheral and central satiety-related neuropeptide disorders. This finding demonstrated that the gut–brain axis is a mediator by which metformin ameliorates SGA-induced metabolic dysfunction. Statins are also considered a potential preventive and therapeutic approach to reduce SGA-induced weight gain and dyslipidemia in patients with schizophrenia. It has been reported that pravastatin (Vincenzi et al., 2014), atorvastatin (Ojala et al., 2008), lovastatin (Ghanizadeh et al., 2014), rosuvastatin (Hert et al., 2006), or simvastatin (Tajik-Esmaeeli et al., 2017) in combination with SGAs can reduce TC, LDL cholesterol, and TG levels in patients with schizophrenia. Animal studies have shown that statins improve SGA-induced metabolic disturbances partly due to statin-mediated modulation of BAT activity (Liu et al., 2020) and inhibition of the hepatic mTOR signaling pathway (Liu et al., 2019). Interestingly, statins were also recently shown to improve the gut microbiota, which seems to partially explain the associated clinical improvements (Kim et al., 2019; Vieira-Silva et al., 2020).
New biological therapeutic strategies, including probiotics, prebiotics, gut hormone, and fecal microbiota transplantation (FMT), are being explored to directly target the gut microbiota and its metabolite products to improve SGA-induced dyslipidemia. Probiotics have been shown to play a vital role in lipid homeostasis in the host (Table 3). However, there have been few studies on the effects of probiotics and prebiotics on SGA-induced changes in lipid metabolism and energy. Tomasik et al. discovered that probiotics and prebiotics could alleviate SGA-induced gastrointestinal distress (Tomasik et al., 2015). However, the effects of probiotics and prebiotics on SGA-induced changes in lipid metabolism are unclear and controversial because the effects of these factors on lipid metabolism are strain and population specific. For example, the probiotic A. muciniphila (Huang et al., 2021) or prebiotic B-GOS (Kao et al., 2018) can partially reverse olanzapine-induced disturbances in the gut microbiota and lipid metabolism in rats. The probiotic mixture VSL#3, a mixture of eight different bacterial probiotic species, was shown to attenuate olanzapine-induced body weight gain, uterine fat deposition, and dyslipidemia (Dhaliwal et al., 2019). Importantly, while their effectiveness has been relatively well documented in animal studies, translation to humans has sometimes shown controversy. Kao et al. found that B-GOS supplementation did not affect SGA-induced weight gain or changes in circulating metabolic markers, contrary to their observations in rats (Kao et al., 2019b). Yang et al. reported that the addition of probiotics, including Bifidobacterium and Lactobacillus, was not sufficient to reduce weight gain in patients with schizophrenia, nor did it significantly improve lipid profiles (Yang et al., 2021). In comparison, the combined use of probiotics and dietary fiber was effective in reducing olanzapine-induced weight gain without any apparent adverse effects while maintaining the desired psychopathological effect (Liu et al., 2021; Huang et al., 2022a; Huang et al., 2022b). Therefore, more randomized controlled trials in humans are needed to translate beneficial findings in animals. Indeed, A. muciniphila has been shown to be safe and effective in human trials, and pasteurized A. muciniphila is more effective than live A. muciniphila (Depommier et al., 2019). In addition, the gut hormone GLP-1 has demonstrated the potential to improve SGA-induced disorders of lipid metabolism. The combination of liraglutide, a GLP-1 receptor agonist, and SGAs has potential benefits on body weight and lipid metabolism in patients with schizophrenia, but patients must receive daily subcutaneous injections and have a relatively high rate of adverse events (Whicher et al., 2019). In contrast, FMT, which is being researched as an alternative to SGAs (Settanni et al., 2021), lacks experimental data to demonstrate its potential in SGA-induced metabolic disorders.
Long-term use of SGAs can cause weight gain and increase lipids, which can lead to an increased chance of patients suffering from metabolic syndrome, thereby increasing the risk that they will develop hypertension and cardiovascular and cerebrovascular diseases. During this process, the microbiome is both essential and sufficient, and several pathways involved in lipid metabolism have been postulated (Figure 2). First, SGAs directly inhibit the growth of microbial species that produce specific lipids (e.g., endogenous cannabinoids, and cholesterol). Second, SCFAs and BAs produced by the gut microbiota can regulate gut hormones such as CCK, PYY, GLP-1, and 5-HT. On the one hand, these signaling molecules can stimulate the vagus nerve or be carried into the brain to affect appetite via the gut–brain axis; on the other hand, they can regulate lipid metabolism via peripheral signaling pathways (Figure 3).
FIGURE 2. Schematic presentation of the potential mechanism of lipid metabolism disorders secondary to SGA treatment based on the gut microbiota. Treatment with SGAs may increase the relative ratio of Firmicutes to Bacteroidetes bacteria, As well as decrease the relative abundance of Bifidobacterium and Akkermansia muciniphila. The products of the gut microbiota (lipids, LPS, SCFAs, and BAs) change as a result of this transformation. SCFAs activate FFAR2/3 or HDAC. BAs send signals to EE cells through TGR5 or nuclear FXR, allowing EE cells to synthesize and secrete various gut hormones. LPS, SCFAs, BAs, and gut hormones are important players in interorgan crosstalk by affecting appetite, regulating gut integrity, and improving liver, pancreas, and adipose tissue function and lipid metabolism. POMC, Proopiomelanocortin; CART, Cocaine- and amphetamine-regulated transcript; NPY, Neuropeptide Y; AgRP, Agouti-related peptide; FXR, Farnesoid X receptor; HDAC, Histone deacetylase; ATP, Adenosine triphosphate; TGR5, Takeda G protein-coupled receptor 5; FFAR2/3, Free fatty acid receptors 2/3; MCT1, Proton-coupled monocarboxylate transporters 1; SMCT1, Sodium-coupled monocarboxylate transporters 1; BAs, Bile acids; SCFAs, Short-chain fatty acids; LPS, Lipopolysaccharide; SGAs, Second-generation antipsychotics.
FIGURE 3. Regulation of lipid metabolism by SCFAs, BAs, leptin and GLP-1. The major signaling pathways including AMPK, MAPK, PI3K/Akt/mTOR and cAMP/PKA/CREB-P work systematically in concert to regulate fatty acid oxidation, fatty acid synthesis, protein synthesis and mitochondrial oxidative phosphorylation and energy metabolism. SCFAs: Short-chain fatty acids; GLP-1: Glucagon-like peptide 1; BAs, Bile acids; FFAR2/3, Free fatty acid receptors 2/3; GLP-1R, GLP-1 receptor; NTCP, Na+-taurocholate cotransporting polypeptide; OATP, Organic anion transporting polypeptide; TGR5, Takeda G protein-coupled receptor 5; AMPK, AMP-activated protein kinase; PGC-1α, Peroxisome proliferator-activated receptor-γ coactivator 1α; PPARα, Peroxisome proliferator-activated receptor α; PPARγ, Peroxisome proliferator-activated receptor γ; HSL, Hormone-sensitive lipase; ATGL, Adipose triglyceride lipase; ACC, Acetyl coenzyme A carboxylase; SREBP-1c, Sterol response element-binding protein-1c; JAK2, Janus kinase-2; MAPK, Mitogen-activated protein kinase; IRS, Insulin receptor substrate; PDK, Phosphoinositide-dependent protein kinase; PKC, Protein kinase C; PI3K, Phosphoinositide 3-kinase; AKT, Protein kinase B (PKB); mTOR, Mammalian target of rapamycin; GTP, Guanosine triphosphate; AC, Adenyl cyclase; cAMP, Cyclic adenosine monophosphate; PKA, Protein kinase A; CREB-P, Phosphorylated CREB (cAMP-response element-binding protein); FXR, Farnesoid X receptor; RXR, Retinoid X receptor; CRE, cAMP response element; SHP, Small heterodimer partner; FAS, Fatty acid synthase.
However, many unanswered questions remain. Which components of the gut microbiota and host metabolism are chiefly associated with schizophrenia? Are the microbiota changes observed in schizophrenia treated with SGAs secondary to SGA treatment? What are the metabolic side effects of SGAs and their impact on the microbiota? Do changes in the gut microbiota affect the efficacy of SGAs? To answer these questions, further experimental data are needed. This will lead to improved schizophrenia treatment options, individualized therapy, and the prediction and mitigation of side effects.
Current strategies to modulate the gut microbiome to improve SGA-induced lipid metabolism disturbances are particularly promising. Prebiotics, probiotics, and FMT have achieved certain curative effects in animal experiments. As a next step, more randomized controlled trials into humans are needed to translate the beneficial findings in animals. It is important to observe changes in host lipid metabolism after concurrent administration of SGAs and the abovementioned treatments. Compared with prebiotic therapy and other drug interventions, probiotic treatment offers superior specificity and safety. To develop this specific microbial therapeutic approach, a better understanding of the precise role of microbes in SGA-related lipid metabolism and elucidation of the linkages between specific microbiota and lipid profiles of the gastrointestinal tract will be needed. Furthermore, proper exercise and diet must not be overlooked.
HC collected the literature and wrote the article. TC conducted the preliminary research. BZ, HC supervised the study. All authors reviewed and approved the manuscript.
This work was supported in part by the grants from Hunan Provincial Natural Science Foundation of China (2021JJ30922), Hunan Provincial Health Commission Research Project (202113010595), Talent Project established by Chinese Pharmaceutical Association Hospital Pharmacy Department (No. CPA-Z05-ZC-2021-003), the Fundamental Research Funds for the Central Universities of Central South University (2021zzts1073) and New Clinical Medical Technology Project of the Second Xiangya Hospital of Central South University (202194).
We thank AJE (https://www.aje.cn) for editing this manuscript.
The authors declare that the research was conducted in the absence of any commercial or financial relationships that could be construed as a potential conflict of interest.
All claims expressed in this article are solely those of the authors and do not necessarily represent those of their affiliated organizations, or those of the publisher, the editors and the reviewers. Any product that may be evaluated in this article, or claim that may be made by its manufacturer, is not guaranteed or endorsed by the publisher.
Abolghasemi, A., Manca, C., Iannotti, F. A., Shen, M., Leblanc, N., Lacroix, S., et al. (2021). Assessment of the effects of dietary vitamin D levels on olanzapine-induced metabolic side effects: Focus on the endocannabinoidome-gut microbiome Axis. Int. J. Mol. Sci. 22, 12361. doi:10.3390/ijms222212361
Ait Chait, Y., Mottawea, W., Tompkins, T. A., and Hammami, R. (2020). Unravelling the antimicrobial action of antidepressants on gut commensal microbes. Sci. Rep. 10, 17878. doi:10.1038/s41598-020-74934-9
Alex, S., Lange, K., Amolo, T., Grinstead, J. S., Haakonsson, A. K., Szalowska, E., et al. (2013). Short-chain fatty acids stimulate angiopoietin-like 4 synthesis in human colon adenocarcinoma cells by activating peroxisome proliferator-activated receptor γ. Mol. Cell. Biol. 33, 1303–1316. doi:10.1128/MCB.00858-12
An, H. M., Park, S. Y., Lee, D. K., Kim, J. R., Cha, M. K., Lee, S. W., et al. (2011). Antiobesity and lipid-lowering effects of Bifidobacterium spp. in high fat diet-induced obese rats. Lipids health Dis. 10, 116. doi:10.1186/1476-511X-10-116
Anthérieu, S., Bachour-El Azzi, P., Dumont, J., Abdel-Razzak, Z., Guguen-Guillouzo, C., Fromenty, B., et al. (2013). Oxidative stress plays a major role in chlorpromazine-induced cholestasis in human HepaRG cells. Hepatol. Baltim. Md 57, 1518–1529. doi:10.1002/hep.26160
Arias, L. H. M., Fadrique, R. S., García, S. P., Gil, M. S., Lobato, C. T., and Ortega, P. G. (2018). Antipsychotics and cardiovascular risk: A case/non-case study. Psychiatry Res. 270, 341–347. doi:10.1016/j.psychres.2018.09.014
Bahr, S. M., Tyler, B. C., Wooldridge, N., Butcher, B. D., Burns, T. L., Teesch, L. M., et al. (2015a). Use of the second-generation antipsychotic, risperidone, and secondary weight gain are associated with an altered gut microbiota in children. Transl. psychiatry 5, e652. doi:10.1038/tp.2015.135
Bahr, S. M., Weidemann, B. J., Castro, A. N., Walsh, J. W., deLeon, O., Burnett, C. M. L., et al. (2015b). Risperidone-induced weight gain is mediated through shifts in the gut microbiome and suppression of energy expenditure. EBioMedicine 2, 1725–1734. doi:10.1016/j.ebiom.2015.10.018
Baldini, G., and Phelan, K. D. (2019). The melanocortin pathway and control of appetite-progress and therapeutic implications. J. Endocrinol. 241, R1-R33–R33. doi:10.1530/JOE-18-0596
Barcia, A. M., and Harris, H. W. (2005). Triglyceride-rich lipoproteins as agents of innate immunity. Clin. Infect. Dis. official Publ. Infect. Dis. Soc. Am. 41 (7), S498–S503. doi:10.1086/432005
Béguin, P., Nagashima, K., Nishimura, M., Gonoi, T., and Seino, S. (1999). PKA-Mediated phosphorylation of the human K(ATP) channel: Separate roles of Kir6.2 and SUR1 subunit phosphorylation. EMBO J. 18, 4722–4732. doi:10.1093/emboj/18.17.4722
Berg, P., and With the technical assistance of Georgia Newton, (1956). Acyl adenylates; an enzymatic mechanism of acetate activation. J. Biol. Chem. 222, 991–1013. doi:10.1016/s0021-9258(20)89957-8
Besten, G. d.n, Bleeker, A., Gerding, A., van Eunen, K., Havinga, R., van Dijk, T. H., et al. (2015). Short-chain fatty acids protect against high-fat diet-induced obesity via a pparγ-dependent switch from lipogenesis to fat oxidation. Diabetes 64, 2398–2408. doi:10.2337/db14-1213
Besten, G. den, Lange, K., Havinga, R., van Dijk, T. H., Gerding, A., van Eunen, K., et al. (2013). Gut-derived short-chain fatty acids are vividly assimilated into host carbohydrates and lipids. Am. J. physiology. Gastrointest. liver physiology 305, G900–G910. doi:10.1152/ajpgi.00265.2013
Blaslov, K., Bulum, T., Zibar, K., and Duvnjak, L. (2014). Incretin based therapies: A novel treatment approach for non-alcoholic fatty liver disease. World J. gastroenterology 20, 7356–7365. doi:10.3748/wjg.v20.i23.7356
Bonn, M., Schmitt, A., Lesch, K.-P., van Bockstaele, E. J., and Asan, E. (2013). Serotonergic innervation and serotonin receptor expression of NPY-producing neurons in the rat lateral and basolateral amygdaloid nuclei. Brain Struct. Funct. 218, 421–435. doi:10.1007/s00429-012-0406-5
Breuer, R. I. (1965). Chlorpromazine hepatotoxicity manifested by A selective and sustained rise of serum alkaline phosphatase activity; report of A case. Am. J. Dig. Dis. 10, 727–731. doi:10.1007/BF02236073
Brown, E. M., Ke, X., Hitchcock, D., Jeanfavre, S., Avila-Pacheco, J., Nakata, T., et al. (2019). Bacteroides-derived sphingolipids are critical for maintaining intestinal homeostasis and symbiosis. Cell. host microbe 25, 668–680. e7. doi:10.1016/j.chom.2019.04.002
Buhagiar, K., and Jabbar, F. (2019). Association of first-vs. Second-generation antipsychotics with lipid abnormalities in individuals with severe mental illness: A systematic review and meta-analysis. Clin. drug Investig. 39, 253–273. doi:10.1007/s40261-019-00751-2
Campbell, J. E., and Drucker, D. J. (2013). Pharmacology, physiology, and mechanisms of incretin hormone action. Cell. metab. 17, 819–837. doi:10.1016/j.cmet.2013.04.008
Cantó, C., and Auwerx, J. (2010). AMP-activated protein kinase and its downstream transcriptional pathways. Cell. Mol. life Sci. CMLS 67, 3407–3423. doi:10.1007/s00018-010-0454-z
Carabotti, M., Scirocco, A., Maselli, M. A., and Severi, C. (2015). The gut-brain axis: Interactions between enteric microbiota, central and enteric nervous systems. Ann. gastroenterology 28, 203–209.
Caron, S., Huaman Samanez, C., Dehondt, H., Ploton, M., Briand, O., Lien, F., et al. (2013). Farnesoid X receptor inhibits the transcriptional activity of carbohydrate response element binding protein in human hepatocytes. Mol. Cell. Biol. 33, 2202–2211. doi:10.1128/MCB.01004-12
Castillo, R. I., Rojo, L. E., Henriquez-Henriquez, M., Silva, H., Maturana, A., Villar, M. J., et al. (2016). From molecules to the clinic: Linking schizophrenia and metabolic syndrome through sphingolipids metabolism. Front. Neurosci. 10, 488. doi:10.3389/fnins.2016.00488
Challa, T. D., Beaton, N., Arnold, M., Rudofsky, G., Langhans, W., and Wolfrum, C. (2012). Regulation of adipocyte formation by GLP-1/GLP-1R signaling. J. Biol. Chem. 287, 6421–6430. doi:10.1074/jbc.M111.310342
Chang, X., Shen, Y., Yun, L., Wang, X., Feng, J., Yang, G., et al. (2022). The antipsychotic drug olanzapine altered lipid metabolism in the common carp (Cyprinus carpio L.): Insight from the gut microbiota-SCFAs-liver axis. Sci. total Environ. 856, 159054. doi:10.1016/j.scitotenv.2022.159054
Chen, A., Park, T. Y., Li, K. J., and DeLisi, L. E. (2020). Antipsychotics and the microbiota. Curr. Opin. psychiatry 33, 225–230. doi:10.1097/YCO.0000000000000594
Chen, J., Zhao, H., Ma, X., Zhang, Y., Lu, S., Wang, Y., et al. (2017). GLP-1/GLP-1R signaling in regulation of adipocyte differentiation and lipogenesis. Cell. physiology Biochem. Int. J. Exp. Cell. physiology, Biochem. Pharmacol. 42, 1165–1176. doi:10.1159/000478872
Chiang, J. Y. L., and Ferrell, J. M. (2020). Bile acid receptors FXR and TGR5 signaling in fatty liver diseases and therapy. Am. J. physiology. Gastrointest. liver physiology 318, G554-G573–G573. doi:10.1152/ajpgi.00223.2019
Chiang, J. Y. L., and Ferrell, J. M. (2019). Bile acids as metabolic regulators and nutrient sensors. Annu. Rev. Nutr. 39, 175–200. doi:10.1146/annurev-nutr-082018-124344
Claudel, T., Inoue, Y., Barbier, O., Duran-Sandoval, D., Kosykh, V., Fruchart, J., et al. (2003). Farnesoid X receptor agonists suppress hepatic apolipoprotein CIII expression. Gastroenterology 125, 544–555. doi:10.1016/s0016-5085(03)00896-5
Cohen, L. J., Kang, H.-S., Chu, J., Huang, Y.-H., Gordon, E. A., Reddy, B. V. B., et al. (2015). Functional metagenomic discovery of bacterial effectors in the human microbiome and isolation of commendamide, a GPCR G2A/132 agonist. Proc. Natl. Acad. Sci. U. S. A. 112, E4825–E4834. doi:10.1073/pnas.1508737112
Cotillard, A., Kennedy, S. P., Kong, L. C., Prifti, E., Pons, N., Le Chatelier, E., et al. (2013). Dietary intervention impact on gut microbial gene richness. Nature 500, 585–588. doi:10.1038/nature12480
Cox, L. M., Abou-El-Hassan, H., Maghzi, A. H., Vincentini, J., and Weiner, H. L. (2019). The sex-specific interaction of the microbiome in neurodegenerative diseases. Brain Res. 1724, 146385. doi:10.1016/j.brainres.2019.146385
Crovesy, L., El-Bacha, T., and Rosado, E. L. (2021). Modulation of the gut microbiota by probiotics and symbiotics is associated with changes in serum metabolite profile related to a decrease in inflammation and overall benefits to metabolic health: A double-blind randomized controlled clinical trial in women with obesity. Food. Funct. 12, 2161–2170. doi:10.1039/d0fo02748k
Cussotto, S., Strain, C. R., Fouhy, F., Strain, R. G., Peterson, V. L., Clarke, G., et al. (2019). Differential effects of psychotropic drugs on microbiome composition and gastrointestinal function. Psychopharmacology 236, 1671–1685. doi:10.1007/s00213-018-5006-5
Dalile, B., van Oudenhove, L., Vervliet, B., and Verbeke, K. (2019). The role of short-chain fatty acids in microbiota-gut-brain communication. Nat. Rev. Gastroenterology hepatology 16, 461–478. doi:10.1038/s41575-019-0157-3
Dardeno, T. A., Chou, S. H., Moon, H.-S., Chamberland, J. P., Fiorenza, C. G., and Mantzoros, C. S. (2010). Leptin in human physiology and therapeutics. Front. Neuroendocrinol. 31, 377–393. doi:10.1016/j.yfrne.2010.06.002
Davey, K. J., Cotter, P. D., O'Sullivan, O., Crispie, F., Dinan, T. G., Cryan, J. F., et al. (2013). Antipsychotics and the gut microbiome: Olanzapine-induced metabolic dysfunction is attenuated by antibiotic administration in the rat. Transl. psychiatry 3, e309. doi:10.1038/tp.2013.83
Davey, K. J., O'Mahony, S. M., Schellekens, H., O'Sullivan, O., Bienenstock, J., Cotter, P. D., et al. (2012). Gender-dependent consequences of chronic olanzapine in the rat: Effects on body weight, inflammatory, metabolic and microbiota parameters. Psychopharmacology 221, 155–169. doi:10.1007/s00213-011-2555-2
Deng, M., Qu, F., Chen, L., Liu, C., Zhang, M., Ren, F., et al. (2020). SCFAs alleviated steatosis and inflammation in mice with NASH induced by MCD. J. Endocrinol. 245, 425–437. doi:10.1530/JOE-20-0018
Depommier, C., Everard, A., Druart, C., Plovier, H., van Hul, M., Vieira-Silva, S., et al. (2019). Supplementation with Akkermansia muciniphila in overweight and obese human volunteers: A proof-of-concept exploratory study. Nat. Med. 25, 1096–1103. doi:10.1038/s41591-019-0495-2
Depommier, C., Vitale, R. M., Iannotti, F. A., Silvestri, C., Flamand, N., Druart, C., et al. (2021). Beneficial effects of Akkermansia muciniphila are not associated with major changes in the circulating endocannabinoidome but linked to higher mono-palmitoyl-glycerol levels as new PPARα agonists. Cells 10, 185. doi:10.3390/cells10010185
Dhaliwal, N., Dhaliwal, J., Singh, D. P., Kondepudi, K. K., Bishnoi, M., and Chopra, K. (2019). The probiotic mixture VSL#3 reverses olanzapine-induced metabolic dysfunction in mice. Methods Mol. Biol. Clift. N.J.) 2011, 531–544. doi:10.1007/978-1-4939-9554-7_31
Dinan, T. G., and Cryan, J. F. (2017). Microbes, immunity, and behavior: Psychoneuroimmunology meets the microbiome. Neuropsychopharmacology, 42. official publication of the American College of Neuropsychopharmacology, 178–192. doi:10.1038/npp.2016.103
Ding, W., Shi, C., Chen, M., Zhou, J., Long, R., and Guo, X. (2017). Screening for lactic acid bacteria in traditional fermented Tibetan yak milk and evaluating their probiotic and cholesterol-lowering potentials in rats fed a high-cholesterol diet. J. Funct. Foods 32, 324–332. doi:10.1016/j.jff.2017.03.021
Doyle, M. E., and Egan, J. M. (2007). Mechanisms of action of glucagon-like peptide 1 in the pancreas. Pharmacol. Ther. 113, 546–593. doi:10.1016/j.pharmthera.2006.11.007
Endomba, F. T., Tankeu, A. T., Nkeck, J. R., and Tochie, J. N. (2020). Leptin and psychiatric illnesses: Does leptin play a role in antipsychotic-induced weight gain? Lipids health Dis. 19, 22. doi:10.1186/s12944-020-01203-z
Everard, A., Belzer, C., Geurts, L., Ouwerkerk, J. P., Druart, C., Bindels, L. B., et al. (2013). Cross-talk between Akkermansia muciniphila and intestinal epithelium controls diet-induced obesity. Proc. Natl. Acad. Sci. U. S. A. 110, 9066–9071. doi:10.1073/pnas.1219451110
Fan, W., Ellacott, K. L. J., Halatchev, I. G., Takahashi, K., Yu, P., and Cone, R. D. (2004). Cholecystokinin-mediated suppression of feeding involves the brainstem melanocortin system. Nat. Neurosci. 7, 335–336. doi:10.1038/nn1214
Fan, Z., Wu, Y., Shen, J., Ji, T., and Zhan, R. (2013). Schizophrenia and the risk of cardiovascular diseases: A meta-analysis of thirteen cohort studies. J. psychiatric Res. 47, 1549–1556. doi:10.1016/j.jpsychires.2013.07.011
Fellows, R., Denizot, J., Stellato, C., Cuomo, A., Jain, P., Stoyanova, E., et al. (2018). Microbiota derived short chain fatty acids promote histone crotonylation in the colon through histone deacetylases. Nat. Commun. 9, 105. doi:10.1038/s41467-017-02651-5
Ferrer, M., Ruiz, A., Lanza, F., Haange, S.-B., Oberbach, A., Till, H., et al. (2013). Microbiota from the distal guts of lean and obese adolescents exhibit partial functional redundancy besides clear differences in community structure. Environ. Microbiol. 15, 211–226. doi:10.1111/j.1462-2920.2012.02845.x
Flowers, S. A., Baxter, N. T., Ward, K. M., Kraal, A. Z., McInnis, M. G., Schmidt, T. M., et al. (2019). Effects of atypical antipsychotic treatment and resistant starch supplementation on gut microbiome composition in a cohort of patients with bipolar disorder or schizophrenia. Pharmacotherapy 39, 161–170. doi:10.1002/phar.2214
Flowers, S. A., Evans, S. J., Ward, K. M., McInnis, M. G., and Ellingrod, V. L. (2017). Interaction between atypical antipsychotics and the gut microbiome in a bipolar disease cohort. Pharmacotherapy 37, 261–267. doi:10.1002/phar.1890
Fuller, C. M., Yassinger, S., Donlon, P., Imperato, T. J., and Ruebner, B. (1977). Haloperidol-induced liver disease. West. J. Med. 127, 515.
Ghanizadeh, A., Rezaee, Z., Dehbozorgi, S., Berk, M., and Akhondzadeh, S. (2014). Lovastatin for the adjunctive treatment of schizophrenia: A preliminary randomized double-blind placebo-controlled trial. Psychiatry Res. 219, 431–435. doi:10.1016/j.psychres.2014.06.039
Gonçalves, P., Araújo, J. R., and Martel, F. (2015). Antipsychotics-induced metabolic alterations: Focus on adipose tissue and molecular mechanisms. Eur. Neuropsychopharmacol. J. Eur. Coll. Neuropsychopharmacol. 25, 1–16. doi:10.1016/j.euroneuro.2014.11.008
Guerrero-Bonmatty, R., Gil-Fernández, G., Rodríguez-Velasco, F. J., and Espadaler-Mazo, J. (2021). A combination of lactoplantibacillus plantarum strains CECT7527, CECT7528, and CECT7529 plus monacolin K reduces blood cholesterol: Results from a randomized, double-blind, placebo-controlled study. Nutrients 13, 1206. doi:10.3390/nu13041206
Guo, L., Kang, J. S., Park, Y. H., Je, B. I., Lee, Y. J., Kang, N. J., et al. (2020). S-petasin inhibits lipid accumulation in oleic acid-induced HepG2 cells through activation of the AMPK signaling pathway. Food & Funct. 11, 5664–5673. doi:10.1039/d0fo00594k
Han, Y., Xia, G., and Wu, Q. (2018). Functional interrogation of the AgRP neural circuits in control of appetite, body weight, and behaviors. Adv. Exp. Med. Biol. 1090, 1–16. doi:10.1007/978-981-13-1286-1_1
Hartfield, A. W., Moore, N. A., and Clifton, P. G. (2003). Effects of clozapine, olanzapine and haloperidol on the microstructure of ingestive behaviour in the rat. Psychopharmacology 167, 115–122. doi:10.1007/s00213-002-1368-8
He, Q., Sha, S., Sun, L., Zhang, J., and Dong, M. (2016). GLP-1 analogue improves hepatic lipid accumulation by inducing autophagy via AMPK/mTOR pathway. Biochem. biophysical Res. Commun. 476, 196–203. doi:10.1016/j.bbrc.2016.05.086
Henao-Mejia, J., Elinav, E., Jin, C., Hao, L., Mehal, W. Z., Strowig, T., et al. (2012). Inflammasome-mediated dysbiosis regulates progression of NAFLD and obesity. Nature 482, 179–185. doi:10.1038/nature10809
Hennekens, C. H., Hennekens, A. R., Hollar, D., and Casey, D. E. (2005). Schizophrenia and increased risks of cardiovascular disease. Am. heart J. 150, 1115–1121. doi:10.1016/j.ahj.2005.02.007
Hert, M. de, Detraux, J., van Winkel, R., Yu, W., and Correll, C. U. (2011). Metabolic and cardiovascular adverse effects associated with antipsychotic drugs. Nat. Rev. Endocrinol. 8, 114–126. doi:10.1038/nrendo.2011.156
Hert, M. de, Kalnicka, D., van Winkel, R., Wampers, M., Hanssens, L., van Eyck, D., et al. (2006). Treatment with rosuvastatin for severe dyslipidemia in patients with schizophrenia and schizoaffective disorder. J. Clin. psychiatry 67, 1889–1896. doi:10.4088/jcp.v67n1208
Hijova, E., Strojný, L., Bertková, I., Bomba, A., and Štofilová, J. (2020). Dietary Lactobacillus plantarum LS/07 and inulin in the management of chronic disease risk factors. Acta biochim. Pol. 67, 447–451. doi:10.18388/abp.2020_5404
Hsiao, W. W. L., Metz, C., Singh, D. P., and Roth, J. (2008). The microbes of the intestine: An introduction to their metabolic and signaling capabilities. Endocrinol. metabolism Clin. N. Am. 37, 857–871. doi:10.1016/j.ecl.2008.08.006
Hu, X., Wang, T., Li, W., Jin, F., and Wang, L. (2013). Effects of NS Lactobacillus strains on lipid metabolism of rats fed a high-cholesterol diet. Lipids health Dis. 12, 67. doi:10.1186/1476-511X-12-67
Huang, D., Gao, J., Li, C., Nong, C., Huang, W., Zheng, X., et al. (2021). A potential probiotic bacterium for antipsychotic-induced metabolic syndrome: Mechanisms underpinning how Akkermansia muciniphila subtype improves olanzapine-induced glucose homeostasis in mice. Psychopharmacology 238, 2543–2553. doi:10.1007/s00213-021-05878-9
Huang, J., Hei, G.-R., Yang, Y., Liu, C.-C., Xiao, J.-M., Long, Y.-J., et al. (2020). Increased appetite plays a key role in olanzapine-induced weight gain in first-episode schizophrenia patients. Front. Pharmacol. 11, 739. doi:10.3389/fphar.2020.00739
Huang, J., Kang, D., Zhang, F., Yang, Y., Liu, C., Xiao, J., et al. (2022a). Probiotics plus dietary fiber supplements attenuate olanzapine-induced weight gain in drug-naïve first-episode schizophrenia patients: Two randomized clinical trials. Schizophr. Bull. 48, 850–859. doi:10.1093/schbul/sbac044
Huang, J., Liu, C., Yang, Y., Kang, D., Xiao, J., Long, Y., et al. (2022b). The effects of probiotics plus dietary fiber on antipsychotic-induced weight gain: A randomized clinical trial. Transl. psychiatry 12, 185. doi:10.1038/s41398-022-01958-2
Huang, Y., Wang, J., Cheng, Y., and Zheng, Y. (2010). The hypocholesterolaemic effects of Lactobacillus acidophilus American type culture collection 4356 in rats are mediated by the down-regulation of Niemann-Pick C1-like 1. Br. J. Nutr. 104, 807–812. doi:10.1017/S0007114510001285
Human Microbiome Project Consortium (2012). Structure, function and diversity of the healthy human microbiome. Nature 486, 207–214. doi:10.1038/nature11234
Iizuka, K., Takao, K., and Yabe, D. (2020). ChREBP-mediated regulation of lipid metabolism: Involvement of the gut microbiota, liver, and adipose tissue. Front. Endocrinol. 11, 587189. doi:10.3389/fendo.2020.587189
Ipci, K., Altıntoprak, N., Muluk, N. B., Senturk, M., and Cingi, C. (2017). The possible mechanisms of the human microbiome in allergic diseases. Eur. archives oto-rhino-laryngology official J. Eur. Fed. Oto-Rhino-Laryngological Soc. (EUFOS) Affil. Ger. Soc. Oto-Rhino-Laryngology - Head Neck Surg. 274, 617–626. doi:10.1007/s00405-016-4058-6
Ishøy, P. L., Knop, F. K., Vilsbøll, T., Glenthøj, B. Y., and Ebdrup, B. H. (2013). Sustained weight loss after treatment with a glucagon-like peptide-1 receptor agonist in an obese patient with schizophrenia and type 2 diabetes. Am. J. psychiatry 170, 681–682. doi:10.1176/appi.ajp.2013.12101344
Jaberi, N., Faramarzi, E., Farahbakhsh, M., Ostadarahimi, A., Asghari Jafarabadi, M., and Fakhari, A. (2020). Prevalence of metabolic syndrome in schizophrenia patients treated with antipsychotic medications. Casp. J. Intern. Med. 11, 310–314. doi:10.22088/cjim.11.3.310
Jiang, W.-L., Cai, D.-B., Yin, F., Zhang, L., Zhao, X.-W., He, J., et al. (2020). Adjunctive metformin for antipsychotic-induced dyslipidemia: A meta-analysis of randomized, double-blind, placebo-controlled trials. Transl. psychiatry 10, 117. doi:10.1038/s41398-020-0785-y
Jocken, J. W. E., González Hernández, M. A., Hoebers, N. T. H., van der Beek, , Christina, M., Essers, Y. P. G., et al. (2017). Short-chain fatty acids differentially affect intracellular lipolysis in a human white adipocyte model. Front. Endocrinol. 8, 372. doi:10.3389/fendo.2017.00372
John, G. K., and Mullin, G. E. (2016). The gut microbiome and obesity. Curr. Oncol. Rep. 18, 45. doi:10.1007/s11912-016-0528-7
Johnson, E. L., Heaver, S. L., Waters, J. L., Kim, B. I., Bretin, A., Goodman, A. L., et al. (2020). Sphingolipids produced by gut bacteria enter host metabolic pathways impacting ceramide levels. Nat. Commun. 11, 2471. doi:10.1038/s41467-020-16274-w
Jung, S., Lee, Y. J., Kim, M., Kim, M., Kwak, J. H., Lee, J.-W., et al. (2015). Supplementation with two probiotic strains, Lactobacillus curvatus HY7601 and Lactobacillus plantarum KY1032, reduced body adiposity and Lp-PLA (2) activity in overweight subjects. J. Funct. FOODS 19, 744–752. doi:10.1016/j.jff.2015.10.006
Kang, G., Leech, C. A., Chepurny, O. G., Coetzee, W. A., and Holz, G. G. (2008). Role of the cAMP sensor Epac as a determinant of KATP channel ATP sensitivity in human pancreatic beta-cells and rat INS-1 cells. J. physiology 586, 1307–1319. doi:10.1113/jphysiol.2007.143818
Kanji, S., Fonseka, T. M., Marshe, V. S., Sriretnakumar, V., Hahn, M. K., and Müller, D. J. (2018). The microbiome-gut-brain axis: Implications for schizophrenia and antipsychotic induced weight gain. Eur. archives psychiatry Clin. Neurosci. 268, 3–15. doi:10.1007/s00406-017-0820-z
Kao, A. C.-C., Chan, K. W., Anthony, D. C., Lennox, B. R., and Burnet, P. W. (2019a). Prebiotic reduction of brain histone deacetylase (HDAC) activity and olanzapine-mediated weight gain in rats, are acetate independent. Neuropharmacology 150, 184–191. doi:10.1016/j.neuropharm.2019.02.014
Kao, A. C.-C., Safarikova, J., Marquardt, T., Mullins, B., Lennox, B. R., and Burnet, P. W. J. (2019b). Pro-cognitive effect of a prebiotic in psychosis: A double blind placebo controlled cross-over study. Schizophrenia Res. 208, 460–461. doi:10.1016/j.schres.2019.03.003
Kao, A. C.-C., Spitzer, S., Anthony, D. C., Lennox, B., and Burnet, P. W. J. (2018). Prebiotic attenuation of olanzapine-induced weight gain in rats: Analysis of central and peripheral biomarkers and gut microbiota. Transl. psychiatry 8, 66. doi:10.1038/s41398-018-0116-8
Karlsson, F. H., Tremaroli, V., Nookaew, I., Bergström, G., Behre, C. J., Fagerberg, B., et al. (2013). Gut metagenome in European women with normal, impaired and diabetic glucose control. Nature 498, 99–103. doi:10.1038/nature12198
Kawamata, Y., Fujii, R., Hosoya, M., Harada, M., Yoshida, H., Miwa, M., et al. (2003). A G protein-coupled receptor responsive to bile acids. J. Biol. Chem. 278, 9435–9440. doi:10.1074/jbc.M209706200
Kim, J., Lee, H., An, J., Song, Y., Lee, C.-K., Kim, K., et al. (2019). Alterations in gut microbiota by statin therapy and possible intermediate effects on hyperglycemia and hyperlipidemia. Front. Microbiol. 10, 1947. doi:10.3389/fmicb.2019.01947
Kim, S.-W., Park, K.-Y., Kim, B., Kim, E., and Hyun, C.-K. (2013). Lactobacillus rhamnosus GG improves insulin sensitivity and reduces adiposity in high-fat diet-fed mice through enhancement of adiponectin production. Biochem. biophysical Res. Commun. 431, 258–263. doi:10.1016/j.bbrc.2012.12.121
Kim, S., and Jazwinski, S. M. (2018). The gut microbiota and healthy aging: A mini-review. Gerontology 64, 513–520. doi:10.1159/000490615
Kimura, I., Ichimura, A., Ohue-Kitano, R., and Igarashi, M. (2020). Free fatty acid receptors in health and disease. Physiol. Rev. 100, 171–210. doi:10.1152/physrev.00041.2018
Kimura, I., Ozawa, K., Inoue, D., Imamura, T., Kimura, K., Maeda, T., et al. (2013). The gut microbiota suppresses insulin-mediated fat accumulation via the short-chain fatty acid receptor GPR43. Nat. Commun. 4, 1829. doi:10.1038/ncomms2852
Kindt, A., Liebisch, G., Clavel, T., Haller, D., Hörmannsperger, G., Yoon, H., et al. (2018). The gut microbiota promotes hepatic fatty acid desaturation and elongation in mice. Nat. Commun. 9, 3760. doi:10.1038/s41467-018-05767-4
Koliada, A., Moseiko, V., Romanenko, M., Lushchak, O., Kryzhanovska, N., Guryanov, V., et al. (2021). Sex differences in the phylum-level human gut microbiota composition. BMC Microbiol. 21, 131. doi:10.1186/s12866-021-02198-y
Koren, O., Goodrich, J. K., Cullender, T. C., Spor, A., Laitinen, K., Bäckhed, H. K., et al. (2012). Host remodeling of the gut microbiome and metabolic changes during pregnancy. Cell. 150, 470–480. doi:10.1016/j.cell.2012.07.008
Kouidrat, Y., and Amad, A. (2019). GLP-1 agonists for metabolic disorders in schizophrenia. Schizophrenia Res. 204, 448–449. doi:10.1016/j.schres.2018.09.019
Krautkramer, K. A., Fan, J., and Bäckhed, F. (2021). Gut microbial metabolites as multi-kingdom intermediates. Nat. Rev. Microbiol. 19, 77–94. doi:10.1038/s41579-020-0438-4
Kristiansen, J. E. (1979). Experiments to illustrate the effect of chlorpromazine on the permeability of the bacterial cell wall. Acta pathologica Microbiol. Scand. Sect. B, Microbiol. 87, 317–319. doi:10.1111/j.1699-0463.1979.tb02445.x
Küme, T., Acar, S., Tuhan, H., Çatlı, G., Anık, A., Gürsoy Çalan, Ö., et al. (2017). The relationship between serum zonulin level and clinical and laboratory parameters of childhood obesity. J. Clin. Res. Pediatr. Endocrinol. 9, 31–38. doi:10.4274/jcrpe.3682
Lage, R., Vázquez, M. J., Varela, L., Saha, A. K., Vidal-Puig, A., Nogueiras, R., et al. (2010). Ghrelin effects on neuropeptides in the rat hypothalamus depend on fatty acid metabolism actions on BSX but not on gender. FASEB J. official Publ. Fed. Am. Soc. Exp. Biol. 24, 2670–2679. doi:10.1096/fj.09-150672
Lange, B., Mueller, J. K., Leweke, F. M., and Bumb, J. M. (2017). How gender affects the pharmacotherapeutic approach to treating psychosis - a systematic review. Expert Opin. Pharmacother. 18, 351–362. doi:10.1080/14656566.2017.1288722
Larraufie, P., Martin-Gallausiaux, C., Lapaque, N., Dore, J., Gribble, F. M., Reimann, F., et al. (2018). SCFAs strongly stimulate PYY production in human enteroendocrine cells. Sci. Rep. 8, 74. doi:10.1038/s41598-017-18259-0
Lefebvre, P., Cariou, B., Lien, F., Kuipers, F., and Staels, B. (2009). Role of bile acids and bile acid receptors in metabolic regulation. Physiol. Rev. 89, 147–191. doi:10.1152/physrev.00010.2008
Li, D.-J., Yue, Q., Liu, L., Che, K.-K., Liu, X.-M., and Hu, C.-H. (2021). Brexpiprazole caused glycolipid metabolic disorder by inhibiting GLP1/GLP1R signaling in rats. Acta Pharmacol. Sin. 42, 1267–1279. doi:10.1038/s41401-021-00680-x
Li, Y., Zhao, X., Feng, X., Liu, X., Deng, C., and Hu, C.-H. (2016). Berberine alleviates olanzapine-induced adipogenesis via the ampkα-SREBP pathway in 3T3-L1 cells. Int. J. Mol. Sci. 17, 1865. doi:10.3390/ijms17111865
Lin, J., Handschin, C., and Spiegelman, B. M. (2005). Metabolic control through the PGC-1 family of transcription coactivators. Cell. metab. 1, 361–370. doi:10.1016/j.cmet.2005.05.004
Liu, C., Kang, D., Xiao, J., Huang, Y., Peng, X., Wang, W., et al. (2021). Dietary fiber and probiotics for the treatment of atypical antipsychotic-induced metabolic side effects: Study protocol for a randomized, double-blind, placebo-controlled trial. Trials 22, 159. doi:10.1186/s13063-021-05123-w
Liu, X.-M., Zhao, X.-M., Deng, C., Zeng, Y.-P., and Hu, C.-H. (2019). Simvastatin improves olanzapine-induced dyslipidemia in rats through inhibiting hepatic mTOR signaling pathway. Acta Pharmacol. Sin. 40, 1049–1057. doi:10.1038/s41401-019-0212-1
Liu, X., Feng, X., Deng, C., Liu, L., Zeng, Y., and Hu, C.-H. (2020). Brown adipose tissue activity is modulated in olanzapine-treated young rats by simvastatin. BMC Pharmacol. Toxicol. 21, 48. doi:10.1186/s40360-020-00427-0
Loh, K., Herzog, H., and Shi, Y.-C. (2015). Regulation of energy homeostasis by the NPY system. Trends Endocrinol. metabolism TEM 26, 125–135. doi:10.1016/j.tem.2015.01.003
Lui, S. Y., Tso, S., Lam, M., and Cheung, E. F. C. (2009). Possible olanzapine-induced hepatotoxicity in a young Chinese patient. Hong Kong Med. J. = Xianggang yi xue za zhi 15, 394.
Luo, C., Wang, X., Huang, H.-X., Mao, X.-Y., Zhou, H.-H., and Liu, Z.-Q. (2021). Coadministration of metformin prevents olanzapine-induced metabolic dysfunction and regulates the gut-liver axis in rats. Psychopharmacology 238, 239–248. doi:10.1007/s00213-020-05677-8
Lynch, A., Crowley, E., Casey, E., Cano, R., Shanahan, R., McGlacken, G., et al. (2017). The Bacteroidales produce an N-acylated derivative of glycine with both cholesterol-solubilising and hemolytic activity. Sci. Rep. 7, 13270. doi:10.1038/s41598-017-13774-6
Ma, X., Asif, H., Dai, L., He, Y., Zheng, W., Wang, D., et al. (2020). Alteration of the gut microbiome in first-episode drug-naïve and chronic medicated schizophrenia correlate with regional brain volumes. J. psychiatric Res. 123, 136–144. doi:10.1016/j.jpsychires.2020.02.005
Mackin, P., Bishop, D., Watkinson, H., Gallagher, P., and Ferrier, I. N. (2007). Metabolic disease and cardiovascular risk in people treated with antipsychotics in the community. Br. J. psychiatry J. Ment. Sci. 191, 23–29. doi:10.1192/bjp.bp.106.031716
Maier, L., Pruteanu, M., Kuhn, M., Zeller, G., Telzerow, A., Anderson, E. E., et al. (2018). Extensive impact of non-antibiotic drugs on human gut bacteria. Nature 555, 623–628. doi:10.1038/nature25979
Martin, A. M., Sun, E. W., Rogers, G. B., and Keating, D. J. (2019). The influence of the gut microbiome on host metabolism through the regulation of gut hormone release. Front. physiology 10, 428. doi:10.3389/fphys.2019.00428
Martinez-Guryn, K., Hubert, N., Frazier, K., Urlass, S., Musch, M. W., Ojeda, P., et al. (2018). Small intestine microbiota regulate host digestive and absorptive adaptive responses to dietary lipids. Cell. host microbe 23, 458–469. e5. doi:10.1016/j.chom.2018.03.011
Maruyama, T., Miyamoto, Y., Nakamura, T., Tamai, Y., Okada, H., Sugiyama, E., et al. (2002). Identification of membrane-type receptor for bile acids (M-BAR). Biochem. biophysical Res. Commun. 298, 714–719. doi:10.1016/s0006-291x(02)02550-0
Maya-Monteiro, C. M., and Bozza, P. T. (2008). Leptin and mTOR: Partners in metabolism and inflammation. Cell. cycleGeorget. Tex.) 7, 1713–1717. doi:10.4161/cc.7.12.6157
Mhalla, A., Bel Hadj Salah, W., Mensi, R., Amamou, B., Messaoud, A., Gassab, L., et al. (2018). Lipid profile in schizophrenia: Case control study. La Tunis. medicale 96, 22.
Miller, T. L., and Wolin, M. J. (1996). Pathways of acetate, propionate, and butyrate formation by the human fecal microbial flora. Appl. Environ. Microbiol. 62, 1589–1592. doi:10.1128/aem.62.5.1589-1592.1996
Minokoshi, Y., Kim, Y.-B., Peroni, O. D., Fryer, L. G. D., Müller, C., Carling, D., et al. (2002). Leptin stimulates fatty-acid oxidation by activating AMP-activated protein kinase. Nature 415, 339–343. doi:10.1038/415339a
Mirzaei, M. K., and Maurice, C. F. (2017). Ménage à trois in the human gut: Interactions between host, bacteria and phages. Nat. Rev. Microbiol. 15, 397–408. doi:10.1038/nrmicro.2017.30
Morgan, A. P., Crowley, J. J., Nonneman, R. J., Quackenbush, C. R., Miller, C. N., Ryan, A. K., et al. (2014). The antipsychotic olanzapine interacts with the gut microbiome to cause weight gain in mouse. PloS one 9, e115225. doi:10.1371/journal.pone.0115225
Morrison, D. J., and Preston, T. (2016). Formation of short chain fatty acids by the gut microbiota and their impact on human metabolism. Gut microbes 7, 189–200. doi:10.1080/19490976.2015.1134082
Mueller, M., Thorell, A., Claudel, T., Jha, P., Koefeler, H., Lackner, C., et al. (2015). Ursodeoxycholic acid exerts farnesoid X receptor-antagonistic effects on bile acid and lipid metabolism in morbid obesity. J. hepatology 62, 1398–1404. doi:10.1016/j.jhep.2014.12.034
Muoio, D. M., Way, J. M., Tanner, C. J., Winegar, D. A., Kliewer, S. A., Houmard, J. A., et al. (2002). Peroxisome proliferator-activated receptor-alpha regulates fatty acid utilization in primary human skeletal muscle cells. Diabetes 51, 901–909. doi:10.2337/diabetes.51.4.901
Nami, Y., Bakhshayesh, R. V., Manafi, M., and Hejazi, M. A. (2019). Hypocholesterolaemic activity of a novel autochthonous potential probiotic Lactobacillus plantarum YS5 isolated from yogurt. LWT-FOOD Sci. Technol. 111, 876–882. doi:10.1016/j.lwt.2019.05.057
Nehme, H., Saulnier, P., Ramadan, A. A., Cassisa, V., Guillet, C., Eveillard, M., et al. (2018). Antibacterial activity of antipsychotic agents, their association with lipid nanocapsules and its impact on the properties of the nanocarriers and on antibacterial activity. PloS one 13, e0189950. doi:10.1371/journal.pone.0189950
Offermanns, S. (2014). Free fatty acid (FFA) and hydroxy carboxylic acid (HCA) receptors. Annu. Rev. Pharmacol. Toxicol. 54, 407–434. doi:10.1146/annurev-pharmtox-011613-135945
Oh, K.-J., Park, J., Lee, S. Y., Hwang, I., Kim, J. B., Park, T.-S., et al. (2011). Atypical antipsychotic drugs perturb AMPK-dependent regulation of hepatic lipid metabolism. Am. J. physiology. Endocrinol. metabolism 300, E624–E632. doi:10.1152/ajpendo.00502.2010
Ojala, K., Repo-Tiihonen, E., Tiihonen, J., and Niskanen, L. (2008). Statins are effective in treating dyslipidemia among psychiatric patients using second-generation antipsychotic agents. J. Psychopharmacol. Oxf. Engl. 22, 33–38. doi:10.1177/0269881107077815
Oliphant, K., and Allen-Vercoe, E. (2019). Macronutrient metabolism by the human gut microbiome: Major fermentation by-products and their impact on host health. Microbiome 7, 91. doi:10.1186/s40168-019-0704-8
Park, B. S., and Lee, J.-O. (2013). Recognition of lipopolysaccharide pattern by TLR4 complexes. Exp. Mol. Med. 45, e66. doi:10.1038/emm.2013.97
Park, D.-Y., Ahn, Y.-T., Park, S.-H., Huh, C.-S., Yoo, S.-R., Yu, R., et al. (2013). Supplementation of Lactobacillus curvatus HY7601 and Lactobacillus plantarum KY1032 in diet-induced obese mice is associated with gut microbial changes and reduction in obesity. PloS one 8, e59470. doi:10.1371/journal.pone.0059470
Pełka-Wysiecka, J., Kaczmarczyk, M., Bąba-Kubiś, A., Liśkiewicz, P., Wroński, M., Skonieczna-Żydecka, K., et al. (2019). Analysis of gut microbiota and their metabolic potential in patients with schizophrenia treated with olanzapine: Results from a six-week observational prospective cohort study. J. Clin. Med. 8, 1605. doi:10.3390/jcm8101605
Perino, A., Velázquez-Villegas, L. A., Bresciani, N., Sun, Y., Huang, Q., Fénelon, V. S., et al. (2021). Central anorexigenic actions of bile acids are mediated by TGR5. Nat. Metab. 3, 595–603. doi:10.1038/s42255-021-00398-4
Perry, R. J., Peng, L., Barry, N. A., Cline, G. W., Zhang, D., Cardone, R. L., et al. (2016). Acetate mediates a microbiome-brain-β-cell axis to promote metabolic syndrome. Nature 534, 213–217. doi:10.1038/nature18309
Pillinger, T., D'Ambrosio, E., McCutcheon, R., and Howes, O. D. (2019). Correction to: Is psychosis a multisystem disorder? A meta-review of central nervous system, immune, cardiometabolic, and endocrine alterations in first-episode psychosis and perspective on potential models. Mol. psychiatry 24, 928. doi:10.1038/s41380-018-0275-2
Pineda Torra, I., Claudel, T., Duval, C., Kosykh, V., Fruchart, J.-C., and Staels, B. (2003). Bile acids induce the expression of the human peroxisome proliferator-activated receptor alpha gene via activation of the farnesoid X receptor. Mol. Endocrinol. Baltim. Md 17, 259–272. doi:10.1210/me.2002-0120
Quarta, C., Claret, M., Zeltser, L. M., Williams, K. W., Yeo, G. S. H., Tschöp, M. H., et al. (2021). POMC neuronal heterogeneity in energy balance and beyond: An integrated view. Nat. Metab. 3, 299–308. doi:10.1038/s42255-021-00345-3
Rabot, S., Membrez, M., Bruneau, A., Gérard, P., Harach, T., Moser, M., et al. (2010). Germ-free C57BL/6J mice are resistant to high-fat-diet-induced insulin resistance and have altered cholesterol metabolism. FASEB J. official Publ. Fed. Am. Soc. Exp. Biol. 24, 4948–4959. doi:10.1096/fj.10-164921
Rather, S. A., Pothuraju, R., Sharma, R. K., De, S., Mir, N. A., and Jangra, S. (2014). Anti-obesity effect of feeding probiotic dahi containing Lactobacillus casei NCDC 19 in high fat diet-induced obese mice. Int. J. DAIRY Technol. 67, 504–509. doi:10.1111/1471-0307.12154
Redondo, A., Trigo, M. V., Acitores, A., Valverde, I., Villanueva-Peñacarrillo, M. L., and Acitores, A. (2003). Cell signalling of the GLP-1 action in rat liver. Mol. Cell. Endocrinol. 204, 43–50. doi:10.1016/s0303-7207(03)00146-1
Rhee, S. H. (2014). Lipopolysaccharide: Basic biochemistry, intracellular signaling, and physiological impacts in the gut. Intestinal Res. 12, 90–95. doi:10.5217/ir.2014.12.2.90
Ridaura, V. K., Faith, J. J., Rey, F. E., Cheng, J., Duncan, A. E., Kau, A. L., et al. (2013). Gut microbiota from twins discordant for obesity modulate metabolism in mice. Sci. (New York, N.Y.) 341, 1241214. doi:10.1126/science.1241214
Riedl, R. A., Burnett, C. M. L., Pearson, N. A., Reho, J. J., Mokadem, M., Edwards, R. A., et al. (2021). Gut microbiota represent a major thermogenic biomass. Funct. Oxf. Engl. 2, zqab019. doi:10.1093/function/zqab019
Rogers, G. B., Keating, D. J., Young, R. L., Wong, M.-L., Licinio, J., and Wesselingh, S. (2016). From gut dysbiosis to altered brain function and mental illness: Mechanisms and pathways. Mol. psychiatry 21, 738–748. doi:10.1038/mp.2016.50
Rowlands, J., Heng, J., Newsholme, P., and Carlessi, R. (2018). Pleiotropic effects of GLP-1 and analogs on cell signaling, metabolism, and function. Front. Endocrinol. 9, 672. doi:10.3389/fendo.2018.00672
Safavi, M., Farajian, S., Kelishadi, R., Mirlohi, M., and Hashemipour, M. (2013). The effects of synbiotic supplementation on some cardio-metabolic risk factors in overweight and obese children: A randomized triple-masked controlled trial. Int. J. food Sci. Nutr. 64, 687–693. doi:10.3109/09637486.2013.775224
Salaj, R., Stofilová, J., Soltesová, A., Hertelyová, Z., Hijová, E., Bertková, I., et al. (2013). The effects of two Lactobacillus plantarum strains on rat lipid metabolism receiving a high fat diet. TheScientificWorldJournal 2013, 135142. doi:10.1155/2013/135142
Samuel, B. S., Shaito, A., Motoike, T., Rey, F. E., Backhed, F., Manchester, J. K., et al. (2008). Effects of the gut microbiota on host adiposity are modulated by the short-chain fatty-acid binding G protein-coupled receptor, Gpr41. Gpr41. Proc. Natl. Acad. Sci. U. S. A. 105, 16767–16772. doi:10.1073/pnas.0808567105
Sayin, S. I., Wahlström, A., Felin, J., Jäntti, S., Marschall, H.-U., Bamberg, K., et al. (2013). Gut microbiota regulates bile acid metabolism by reducing the levels of tauro-beta-muricholic acid, a naturally occurring FXR antagonist. Cell. metab. 17, 225–235. doi:10.1016/j.cmet.2013.01.003
Schmidt, R. H., Jokinen, J. D., Massey, V. L., Falkner, K. C., Shi, X., Yin, X., et al. (2013). Olanzapine activates hepatic mammalian target of rapamycin: New mechanistic insight into metabolic dysregulation with atypical antipsychotic drugs. J. Pharmacol. Exp. Ther. 347, 126–135. doi:10.1124/jpet.113.207621
Schneeberger, M., Everard, A., Gómez-Valadés, A. G., Matamoros, S., Ramírez, S., Delzenne, N. M., et al. (2015). Akkermansia muciniphila inversely correlates with the onset of inflammation, altered adipose tissue metabolism and metabolic disorders during obesity in mice. Sci. Rep. 5, 16643. doi:10.1038/srep16643
Schwiertz, A., Taras, D., Schäfer, K., Beijer, S., Bos, N. A., Donus, C., et al. (2010). Microbiota and SCFA in lean and overweight healthy subjects. Obes. (Silver Spring, Md 18, 190–195. doi:10.1038/oby.2009.167
Sentissi, O., Epelbaum, J., Olié, J.-P., and Poirier, M.-F. (2008). Leptin and ghrelin levels in patients with schizophrenia during different antipsychotics treatment: A review. Schizophr. Bull. 34, 1189–1199. doi:10.1093/schbul/sbm141
Settanni, C. R., Ianiro, G., Bibbò, S., Cammarota, G., and Gasbarrini, A. (2021). Gut microbiota alteration and modulation in psychiatric disorders: Current evidence on fecal microbiota transplantation. Prog. neuro-psychopharmacology Biol. psychiatry 109, 110258. doi:10.1016/j.pnpbp.2021.110258
Shpaner, A., Li, W., Ankoma-Sey, V., and Botero, R. C. (2008). Drug-induced liver injury: Hepatotoxicity of quetiapine revisited. Eur. J. gastroenterology hepatology 20, 1106–1109. doi:10.1097/MEG.0b013e3282f8e3a0
Sinal, C. J., Tohkin, M., Miyata, M., Ward, J. M., Lambert, G., and Gonzalez, F. J. (2000). Targeted disruption of the nuclear receptor FXR/BAR impairs bile acid and lipid homeostasis. Cell. 102, 731–744. doi:10.1016/s0092-8674(00)00062-3
Singh, T. P., Malik, R. K., Katkamwar, S. G., and Kaur, G. (2015). Hypocholesterolemic effects of Lactobacillus reuteri LR6 in rats fed on high-cholesterol diet. Int. J. food Sci. Nutr. 66, 71–75. doi:10.3109/09637486.2014.953450
Skonieczna-Żydecka, K., Łoniewski, I., Misera, A., Stachowska, E., Maciejewska, D., Marlicz, W., et al. (2019). Second-generation antipsychotics and metabolism alterations: A systematic review of the role of the gut microbiome. Psychopharmacology 236, 1491–1512. doi:10.1007/s00213-018-5102-6
Sohn, J.-W., Xu, Y., Jones, J. E., Wickman, K., Williams, K. W., and Elmquist, J. K. (2011). Serotonin 2C receptor activates a distinct population of arcuate pro-opiomelanocortin neurons via TRPC channels. Neuron 71, 488–497. doi:10.1016/j.neuron.2011.06.012
Sohn, M., Na, G. Y., Chu, J., Joung, H., Kim, B.-K., and Lim, S. (2021). Efficacy and safety of Lactobacillus plantarum K50 on lipids in Koreans with obesity: A randomized, double-blind controlled clinical trial. Front. Endocrinol. 12, 790046. doi:10.3389/fendo.2021.790046
Spencer, M. D., Hamp, T. J., Reid, R. W., Fischer, L. M., Zeisel, S. H., and Fodor, A. A. (2011). Association between composition of the human gastrointestinal microbiome and development of fatty liver with choline deficiency. Gastroenterology 140, 976–986. doi:10.1053/j.gastro.2010.11.049
Stockebrand, M., Sauter, K., Neu, A., Isbrandt, D., and Choe, C. (2013). Differential regulation of AMPK activation in leptin- and creatine-deficient mice. FASEB J. official Publ. Fed. Am. Soc. Exp. Biol. 27, 4147–4156. doi:10.1096/fj.12-225136
Szalowska, E., Stoopen, G., Groot, M. J., Hendriksen, P. J. M., and Peijnenburg, Ad A. C. M. (2013). Treatment of mouse liver slices with cholestatic hepatotoxicants results in down-regulation of Fxr and its target genes. BMC Med. genomics 6, 39. doi:10.1186/1755-8794-6-39
Tajik-Esmaeeli, S., Moazen-Zadeh, E., Abbasi, N., Shariat, S. V., Rezaei, F., Salehi, B., et al. (2017). Simvastatin adjunct therapy for negative symptoms of schizophrenia: A randomized double-blind placebo-controlled trial. Int. Clin. Psychopharmacol. 32, 87–94. doi:10.1097/YIC.0000000000000159
Takashima, M., Ogawa, W., Emi, A., and Kasuga, M. (2009). Regulation of SREBP1c expression by mTOR signaling in hepatocytes. Kobe J. Med. Sci. 55, E45.
Tang, T., Song, J., Li, J., Wang, H., Zhang, Y., and Suo, H. (2020). A synbiotic consisting of Lactobacillus plantarum S58 and hull-less barley β-glucan ameliorates lipid accumulation in mice fed with a high-fat diet by activating AMPK signaling and modulating the gut microbiota. Carbohydr. Polym. 243, 116398. doi:10.1016/j.carbpol.2020.116398
Taylor, E. B., Lamb, J. D., Hurst, R. W., Chesser, D. G., Ellingson, W. J., Greenwood, L. J., et al. (2005). Endurance training increases skeletal muscle LKB1 and PGC-1alpha protein abundance: Effects of time and intensity. Am. J. physiology Endocrinol. metabolism 289, E960–E968. doi:10.1152/ajpendo.00237.2005
Teff, K. L., Rickels, M. R., Grudziak, J., Fuller, C., Nguyen, H.-L., and Rickels, K. (2013). Antipsychotic-induced insulin resistance and postprandial hormonal dysregulation independent of weight gain or psychiatric disease. Diabetes 62, 3232–3240. doi:10.2337/db13-0430
Teodoro, J. S., Rolo, A. P., and Palmeira, C. M. (2011). Hepatic FXR: Key regulator of whole-body energy metabolism. Trends Endocrinol. metabolism TEM 22, 458–466. doi:10.1016/j.tem.2011.07.002
Thakkar, P. N., Patel, A., Modi, H. A., and Prajapati, J. B. (2020). Hypocholesterolemic effect of potential probiotic Lactobacillus fermentum strains isolated from traditional fermented foods in wistar rats. Probiotics Antimicrob. proteins 12, 1002–1011. doi:10.1007/s12602-019-09622-w
Thorsing, M., Klitgaard, J. K., Atilano, M. L., Skov, M. N., Kolmos, H. J., Filipe, S. R., et al. (2013). Thioridazine induces major changes in global gene expression and cell wall composition in methicillin-resistant Staphylococcus aureus USA300. PloS one 8, e64518. doi:10.1371/journal.pone.0064518
Ticinesi, A., Milani, C., Lauretani, F., Nouvenne, A., Mancabelli, L., Lugli, G. A., et al. (2017). Gut microbiota composition is associated with polypharmacy in elderly hospitalized patients. Sci. Rep. 7, 11102. doi:10.1038/s41598-017-10734-y
Tilg, H., Zmora, N., Adolph, T. E., and Elinav, E. (2020). The intestinal microbiota fuelling metabolic inflammation. Nat. Rev. Immunol. 20, 40–54. doi:10.1038/s41577-019-0198-4
Tomasik, J., Yolken, R. H., Bahn, S., and Dickerson, F. B. (2015). Immunomodulatory effects of probiotic supplementation in schizophrenia patients: A randomized, placebo-controlled trial. Biomark. insights 10, 47–54. doi:10.4137/BMI.S22007
Trauner, M., Claudel, T., Fickert, P., Moustafa, T., and Wagner, M. (2010). Bile acids as regulators of hepatic lipid and glucose metabolism. Dig. Dis. (Basel, Switz. 28, 220–224. doi:10.1159/000282091
Turnbaugh, P. J., Ley, R. E., Mahowald, M. A., Magrini, V., Mardis, E. R., and Gordon, J. I. (2006). An obesity-associated gut microbiome with increased capacity for energy harvest. Nature 444, 1027–1031. doi:10.1038/nature05414
Unger, R. H., Zhou, Y. T., and Orci, L. (1999). Regulation of fatty acid homeostasis in cells: Novel role of leptin. Proc. Natl. Acad. Sci. U. S. A. 96, 2327–2332. doi:10.1073/pnas.96.5.2327
Vancampfort, D., Firth, J., Correll, C. U., Solmi, M., Siskind, D., Hert, M., et al. (2019). The impact of pharmacological and non-pharmacological interventions to improve physical health outcomes in people with schizophrenia: A meta-review of meta-analyses of randomized controlled trials. World psychiatry official J. World Psychiatric Assoc. (WPA) 18, 53–66. doi:10.1002/wps.20614
Vancampfort, D., Stubbs, B., Mitchell, A. J., Hert, M. de, Wampers, M., Ward, P. B., et al. (2015). Risk of metabolic syndrome and its components in people with schizophrenia and related psychotic disorders, bipolar disorder and major depressive disorder: A systematic review and meta-analysis. World psychiatry official J. World Psychiatric Assoc. (WPA) 14, 339–347. doi:10.1002/wps.20252
Varela, L., Vázquez, M. J., Cordido, F., Nogueiras, R., Vidal-Puig, A., Diéguez, C., et al. (2011). Ghrelin and lipid metabolism: Key partners in energy balance. J. Mol. Endocrinol. 46, R43–R63. doi:10.1677/JME-10-0068
Vieira-Silva, S., Falony, G., Belda, E., Nielsen, T., Aron-Wisnewsky, J., Chakaroun, R., et al. (2020). Statin therapy is associated with lower prevalence of gut microbiota dysbiosis. Nature 581, 310–315. doi:10.1038/s41586-020-2269-x
Vincenzi, B., Stock, S., Borba, C. P. C., Cleary, S. M., Oppenheim, C. E., Petruzzi, L. J., et al. (2014). A randomized placebo-controlled pilot study of pravastatin as an adjunctive therapy in schizophrenia patients: Effect on inflammation, psychopathology, cognition and lipid metabolism. Schizophrenia Res. 159, 395–403. doi:10.1016/j.schres.2014.08.021
Wahlström, A., Sayin, S. I., Marschall, H.-U., and Bäckhed, F. (2016). Intestinal crosstalk between bile acids and microbiota and its impact on host metabolism. Cell. metab. 24, 41–50. doi:10.1016/j.cmet.2016.05.005
Waldecker, M., Kautenburger, T., Daumann, H., Busch, C., and Schrenk, D. (2008). Inhibition of histone-deacetylase activity by short-chain fatty acids and some polyphenol metabolites formed in the colon. J. Nutr. Biochem. 19, 587–593. doi:10.1016/j.jnutbio.2007.08.002
Wan, Z., Root-McCaig, J., Castellani, L., Kemp, B. E., Steinberg, G. R., and Wright, D. C. (2014). Evidence for the role of AMPK in regulating PGC-1 alpha expression and mitochondrial proteins in mouse epididymal adipose tissue. Obes. (Silver Spring, Md.) 22, 730–738. doi:10.1002/oby.20605
Wang, H., Chen, J., Hollister, K., Sowers, L. C., and Forman, B. M. (1999). Endogenous bile acids are ligands for the nuclear receptor FXR/BAR. Mol. Cell. 3, 543–553. doi:10.1016/s1097-2765(00)80348-2
Wang, J., Zhang, H., Chen, X., Chen, Y., Menghebilige, , and Bao, Q. (2012). Selection of potential probiotic lactobacilli for cholesterol-lowering properties and their effect on cholesterol metabolism in rats fed a high-lipid diet. J. dairy Sci. 95, 1645–1654. doi:10.3168/jds.2011-4768
Wang, X., Huang, H., Zhu, Y., Li, S., Zhang, P., Jiang, J., et al. (2021). Metformin acts on the gut-brain axis to ameliorate antipsychotic-induced metabolic dysfunction. Biosci. trends 15, 321–329. doi:10.5582/bst.2021.01317
Wang, Y., Xu, N., Xi, A., Ahmed, Z., Zhang, B., and Bai, X. (2009). Effects of Lactobacillus plantarum MA2 isolated from Tibet kefir on lipid metabolism and intestinal microflora of rats fed on high-cholesterol diet. Appl. Microbiol. Biotechnol. 84, 341–347. doi:10.1007/s00253-009-2012-x
Wang, C, C., Li, Q., Wang, W., Guo, L., Guo, C., Sun, Y., et al. (2015). GLP-1 contributes to increases in PGC-1α expression by downregulating miR-23a to reduce apoptosis. Biochem. biophysical Res. Commun. 466, 33–39. doi:10.1016/j.bbrc.2015.08.092
Wang, J, J., Tang, H., Zhang, C., Zhao, Y., Derrien, M., Rochervan-Hylckama Vlieg, E., et al. (2015). Modulation of gut microbiota during probiotic-mediated attenuation of metabolic syndrome in high fat diet-fed mice. ISME J. 9, 1–15. doi:10.1038/ismej.2014.99
Watanabe, M., Houten, S. M., Mataki, C., Christoffolete, M. A., Kim, B. W., Sato, H., et al. (2006). Bile acids induce energy expenditure by promoting intracellular thyroid hormone activation. Nature 439, 484–489. doi:10.1038/nature04330
Watanabe, M., Houten, S. M., Wang, L., Moschetta, A., Mangelsdorf, D. J., Heyman, R. A., et al. (2004). Bile acids lower triglyceride levels via a pathway involving FXR, SHP, and SREBP-1c. J. Clin. investigation 113, 1408–1418. doi:10.1172/JCI21025
Weston-Green, K., Babic, I., Santis, M. de, Pan, B., Montgomery, M. K., Mitchell, T., et al. (2018). Disrupted sphingolipid metabolism following acute clozapine and olanzapine administration. J. Biomed. Sci. 25, 40. doi:10.1186/s12929-018-0437-1
Whicher, C. A., Price, H. C., Phiri, P., Rathod, S., Barnard-Kelly, K., Reidy, C., et al. (2019). Liraglutide and the management of overweight and obesity in people with schizophrenia, schizoaffective disorder and first-episode psychosis: Protocol for a pilot trial. Trials 20, 633. doi:10.1186/s13063-019-3689-5
Wright, T. M., and Vandenberg, A. M. (2007). Risperidone- and quetiapine-induced cholestasis. Ann. Pharmacother. 41, 1518–1523. doi:10.1345/aph.1K145
Wu, R.-R., Zhang, F.-Y., Gao, K.-M., Ou, J.-J., Shao, P., Jin, H., et al. (2016). Metformin treatment of antipsychotic-induced dyslipidemia: An analysis of two randomized, placebo-controlled trials. Mol. psychiatry 21, 1537–1544. doi:10.1038/mp.2015.221
Wu, R.-R., Zhao, J.-P., Zhai, J.-G., Guo, X.-F., and Guo, W.-B. (2007). Sex difference in effects of typical and atypical antipsychotics on glucose-insulin homeostasis and lipid metabolism in first-episode schizophrenia. J. Clin. Psychopharmacol. 27, 374–379. doi:10.1097/JCP.0b013e3180cac8db
Xie, N., Cui, Y., Yin, Y.-N., Zhao, X., Yang, J.-W., Wang, Z.-G., et al. (2011). Effects of two Lactobacillus strains on lipid metabolism and intestinal microflora in rats fed a high-cholesterol diet. BMC complementary Altern. Med. 11, 53. doi:10.1186/1472-6882-11-53
Xie, Y., Zhang, H., Liu, H., Xiong, L., Gao, X., Jia, H., et al. (2015). Hypocholesterolemic effects of Kluyveromyces marxianus M3 isolated from Tibetan mushrooms on diet-induced hypercholesterolemia in rat. Braz. J. Microbiol. Publ. Braz. Soc. Microbiol. 46, 389–395. doi:10.1590/S1517-838246220131278
Yan, M., Audet-Walsh, É., Manteghi, S., Dufour, C. R., Walker, B., Baba, M., et al. (2016). Chronic AMPK activation via loss of FLCN induces functional beige adipose tissue through PGC-1α/ERRα. Genes. Dev. 30, 1034–1046. doi:10.1101/gad.281410.116
Yang, Y., Long, Y., Kang, D., Liu, C., Xiao, J., Wu, R., et al. (2021). Effect of Bifidobacterium on olanzapine-induced body weight and appetite changes in patients with psychosis. Psychopharmacology 238, 2449–2457. doi:10.1007/s00213-021-05866-z
Yang, Y., Xie, P., Long, Y., Huang, J., Xiao, J., Zhao, J., et al. (2022). Previous exposure to antipsychotic drug treatment is an effective predictor of metabolic disturbances experienced with current antipsychotic drug treatments. BMC psychiatry 22, 210. doi:10.1186/s12888-022-03853-y
Yatsunenko, T., Rey, F. E., Manary, M. J., Trehan, I., Dominguez-Bello, M. G., Contreras, M., et al. (2012). Human gut microbiome viewed across age and geography. Nature 486, 222–227. doi:10.1038/nature11053
Yin, Y.-N., Yu, Q.-F., Fu, N., Liu, X.-W., and Lu, F.-G. (2010). Effects of four Bifidobacteria on obesity in high-fat diet induced rats. World J. gastroenterology 16, 3394–3401. doi:10.3748/wjg.v16.i27.3394
Yoo, S.-R., Kim, Y.-J., Park, D.-Y., Jung, U.-J., Jeon, S.-M., Ahn, Y.-T., et al. (2013). Probiotics L. plantarum and L. curvatus in combination alter hepatic lipid metabolism and suppress diet-induced obesity. Obes. (Silver Spring, Md.) 21, 2571–2578. doi:10.1002/oby.20428
Yuan, X., Wang, Y., Li, X., Jiang, J., Kang, Y., Pang, L., et al. (2021). Gut microbial biomarkers for the treatment response in first-episode, drug-naïve schizophrenia: A 24-week follow-up study. Transl. psychiatry 11, 422. doi:10.1038/s41398-021-01531-3
Yuan, X., Zhang, P., Wang, Y., Liu, Y., Li, X., Kumar, B. U., et al. (2018). Changes in metabolism and microbiota after 24-week risperidone treatment in drug naïve, normal weight patients with first episode schizophrenia. Schizophrenia Res. 201, 299–306. doi:10.1016/j.schres.2018.05.017
Zambell, K. L., Fitch, M. D., and Fleming, S. E. (2003). Acetate and butyrate are the major substrates for de novo lipogenesis in rat colonic epithelial cells. J. Nutr. 133, 3509–3515. doi:10.1093/jn/133.11.3509
Zheng, J., Lin, Z., Ko, C.-Y., Xu, J.-H., Lin, Y., and Wang, J. (2022). Analysis of gut microbiota in patients with exacerbated symptoms of schizophrenia following therapy with amisulpride: A pilot study. Behav. Neurol. 2022, 4262094. doi:10.1155/2022/4262094
Zhu, W, W., Ding, C., Huang, P., Ran, J., Lian, P., Tang, Y., et al. (2022). Metformin Ameliorates Hepatic Steatosis induced by olanzapine through inhibiting LXRα/PCSK9 pathway. Sci. Rep. 12, 5639. doi:10.1038/s41598-022-09610-1
Zhu, Z, Z., Gu, Y., Zeng, C., Yang, M., Yu, H., Chen, H., et al. (2022). Olanzapine-induced lipid disturbances: A potential mechanism through the gut microbiota-brain axis. Front. Pharmacol. 13, 897926. doi:10.3389/fphar.2022.897926
Keywords: gut microbiome, SGAs, lipid disturbances, gut microbiota-brain axis, SCFAs, BAs
Citation: Chen H, Cao T, Zhang B and Cai H (2023) The regulatory effects of second-generation antipsychotics on lipid metabolism: Potential mechanisms mediated by the gut microbiota and therapeutic implications. Front. Pharmacol. 14:1097284. doi: 10.3389/fphar.2023.1097284
Received: 13 November 2022; Accepted: 12 January 2023;
Published: 25 January 2023.
Edited by:
Laura Orio, Complutense University of Madrid, SpainReviewed by:
Mary V. Seeman, University of Toronto, CanadaCopyright © 2023 Chen, Cao, Zhang and Cai. This is an open-access article distributed under the terms of the Creative Commons Attribution License (CC BY). The use, distribution or reproduction in other forums is permitted, provided the original author(s) and the copyright owner(s) are credited and that the original publication in this journal is cited, in accordance with accepted academic practice. No use, distribution or reproduction is permitted which does not comply with these terms.
*Correspondence: Bikui Zhang, NTA1OTk1QGNzdS5lZHUuY24=; Hualin Cai, aHVhbGluY2FpQGNzdS5lZHUuY24=
Disclaimer: All claims expressed in this article are solely those of the authors and do not necessarily represent those of their affiliated organizations, or those of the publisher, the editors and the reviewers. Any product that may be evaluated in this article or claim that may be made by its manufacturer is not guaranteed or endorsed by the publisher.
Research integrity at Frontiers
Learn more about the work of our research integrity team to safeguard the quality of each article we publish.