- 1Biosystems & Integrative Sciences Institute, Faculty of Sciences, University of Lisbon, Lisbon, Portugal
- 2Laboratory of Pulmonary Investigation, Carlos Chagas Filho Institute of Biophysics, Federal University of Rio de Janeiro, Rio de Janeiro, Brazil
Respiratory diseases remain a major health concern worldwide because they subject patients to considerable financial and psychosocial burdens and result in a high rate of morbidity and mortality. Although significant progress has been made in understanding the underlying pathologic mechanisms of severe respiratory diseases, most therapies are supportive, aiming to mitigate symptoms and slow down their progressive course but cannot improve lung function or reverse tissue remodeling. Mesenchymal stromal cells (MSCs) are at the forefront of the regenerative medicine field due to their unique biomedical potential in promoting immunomodulation, anti-inflammatory, anti-apoptotic and antimicrobial activities, and tissue repair in various experimental models. However, despite several years of preclinical research on MSCs, therapeutic outcomes have fallen far short in early-stage clinical trials for respiratory diseases. This limited efficacy has been associated with several factors, such as reduced MSC homing, survival, and infusion in the late course of lung disease. Accordingly, genetic engineering and preconditioning methods have emerged as functional enhancement strategies to potentiate the therapeutic actions of MSCs and thus achieve better clinical outcomes. This narrative review describes various strategies that have been investigated in the experimental setting to functionally potentiate the therapeutic properties of MSCs for respiratory diseases. These include changes in culture conditions, exposure of MSCs to inflammatory environments, pharmacological agents or other substances, and genetic manipulation for enhanced and sustained expression of genes of interest. Future directions and challenges in efficiently translating MSC research into clinical practice are discussed.
1 Introduction
Respiratory diseases are primarily caused by genetic and environmental factors and/or social behaviors that lead to lung inflammation, injury, and remodeling, resulting in progressive deterioration of lung function and, ultimately, in respiratory failure (Aghasafari et al., 2019). These diseases are major health concerns worldwide because they result in a high rate of morbidity and disability, and subject patients to substantial financial and psychosocial burdens (Rehman et al., 2020; Soriano et al., 2020). Moreover, chronic obstructive pulmonary disease (COPD) and lower respiratory infections are two of the five leading causes of death worldwide, claiming over 6 million lives in 2019 according to data from the World Health Organization (World Health Organization, 2020). Despite remarkable progress in better understanding the pathologic mechanisms underlying severe respiratory diseases, the currently available therapies aim to alleviate symptoms and/or delay their natural progressive course but are unable to reverse morphologic and functional abnormalities already established in the lungs.
Mesenchymal stromal cells (MSCs) have been considered a versatile source for cell-based therapies and are at the forefront of the field of regenerative medicine. Based on the minimum criteria stablished by the International Society of Cellular Therapy (ISCT), human MSCs are multipotent progenitor cells that spontaneously adhere to plastic under standard culture conditions; express CD73, CD90, and CD105 cell-surface epitopes and lack CD14 (or CD11b), CD34, CD45, and human leukocytic antigen (HLA)-DR; and are able to differentiate in vitro into adipocytes, chondrocytes, and osteoblasts using differentiation protocols (Horwitz et al., 2005; Dominici et al., 2006). MSCs are present in virtually all tissues, but the most common sources used are bone marrow (BM), adipose tissue (AD), and umbilical cord (UC).
Over the past decade, an increasing body of literature has demonstrated the potential of MSCs in promoting therapeutic actions in various experimental models, such as acute respiratory distress syndrome (ARDS) (Németh et al., 2009; Krasnodembskaya et al., 2012; Silva et al., 2018; Lopes-Pacheco et al., 2020), allergic asthma (Goodwin et al., 2011; Lathrop et al., 2014; Malaquias et al., 2018; Castro et al., 2020), COPD/emphysema (Ingenito et al., 2012; Antunes et al., 2014; Poggio et al., 2018; Antunes et al., 2021), idiopathic pulmonary fibrosis (Cargnoni et al., 2012; Cahill et al., 2016; Moroncini et al., 2018), and silicosis (Lopes-Pacheco et al., 2013; Phinney et al., 2015; Lopes-Pacheco et al., 2016; Zhang et al., 2018a). Key therapeutic actions of MSCs include immunomodulation by inhibiting proliferation, maturation, and differentiation of immune cells (de Castro et al., 2019); anti-inflammatory, anti-apoptotic, and antimicrobial activities by secreting paracrine/endocrine mediators (chemokines, cytokines, growth factors, peptides, hormones, lipid mediators, mRNAs, and microRNAs) (Lopes-Pacheco et al., 2020; Lopes-Pacheco et al., 2016; da Silva et al., 2021) or by intercellular contact via ligand-receptor recognition and extracellular vesicles (EVs) (Abreu et al., 2021); and wound repair by secreting regenerative factors and transfer of organelles, such as mitochondria (Paliwal et al., 2018; de Carvalho et al., 2021).
The promising therapeutic actions of MSCs in experimental models of respiratory diseases have prompted their assessment in clinical investigations. Although MSC administration was well tolerated and demonstrated a good safety profile, the potential efficacy of MSCs was nevertheless limited (Weiss et al., 2013; Morales et al., 2015; McIntyre et al., 2018; Matthay et al., 2019; Aguiar et al., 2020). Such limited therapeutic effects have been attributed to several factors, including in vitro senescence, low number of MSCs infused, functional quiescence or low survival rate of MSCs after the infusion, poor engraftment, and MSC administration in the advanced stage of lung disease. Accordingly, various strategies have been proposed to preserve the stemness of MSCs as well as to functionally potentiate their therapeutic actions. These strategies consist of physical, chemical, and biological methods to condition cells before infusion, and they are based on the biological phenomenon of hormesis, i.e., brief exposure to low doses of an agent known to be harmful at higher doses may lead to beneficial effects (Calabrese et al., 2007). Alternatively, the therapeutic properties of MSCs can be genetically manipulated by overexpressing genes related to cell homing, survival, and immunomodulatory pathways, regardless of exogenous stimuli. In this narrative review, we describe several strategies that are under investigation in the experimental setting for the functional potentiation of the therapeutic properties of MSCs for respiratory diseases. Challenges and future directions are also discussed to efficiently translate MSC-based therapy into the clinical scenario.
2 Strategies to potentiate the therapeutic properties of MSCs
Various strategies have been investigated over the past decade to modulate the plasticity of MSCs and thus increase their survival, homing, and therapeutic properties according to the targeted diseases. These strategies include alterations in culture conditions (O2 or CO2 concentration, heat shock, nutrient deprivation, and others), exposure to inflammatory environments (cytokines/chemokines, growth factors, combinations thereof, or biologically relevant samples), pre-treatment with pharmacological or other chemical molecules, and genetic engineering to manipulate the expression levels of genes of interest (Figure 1).
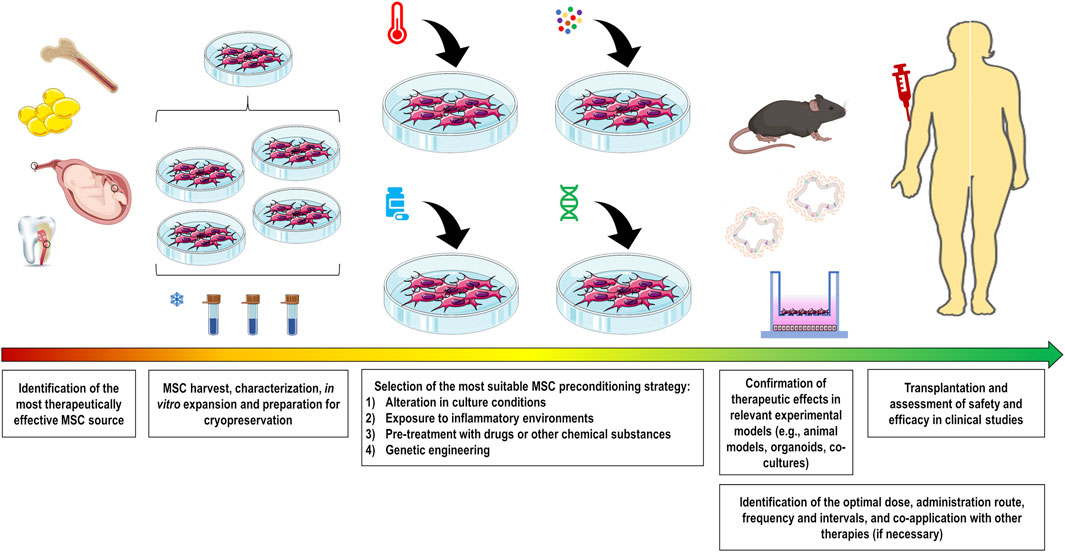
FIGURE 1. Comprehensive overview showing the production of preconditioned MSCs for pre-clinical and clinical use. Initially, the most therapeutically effective MSC source should be identified for the target disease. After harvesting, cells should be properly characterized and expanded to maximize the number of batches to be frozen. Preconditioning strategy should be selected to functionally potentiate MSC therapeutic properties. These strategies include alteration in culture conditions (O2 or CO2 concentration, heat shock, nutrient deprivation, and others), exposure to inflammatory conditions (cytokines/chemokines, growth factors, combinations thereof, or biologically relevant samples), pre-treatment with drugs or other substances, and genetic engineering to manipulate expression levels of the gene of interest. The therapeutic abilities of these cells should be validated in relevant experimental models for the subsequent assessment in clinical studies. To enhance the therapeutic outcomes, there are several MSC administration conditions that need to be optimized, including the identification of the optimal dose, administration route, frequency and intervals, and co-application with other therapies if necessary.
2.1 MSC preconditioning by altering culture conditions
The oxygen availability in MSC niches is significantly variable: 1%–7% in BM, 10%–15% in AD, and ≤5% in the female reproductive tract and birth-related tissues, such as placenta, UC, and Wharton’s jelly (WJ) (Amiri et al., 2015). However, MSCs are usually cultured at a normal atmospheric concentration of oxygen (21% O2), which may increase their susceptibility to oxidative stress-induced cellular damage (Boregowda et al., 2012; Amiri et al., 2015; Bétous et al., 2017). When cultured under hypoxia, MSCs exhibited a higher proliferation rate and formed a greater number of colonies compared with those cultured under normoxia (Lennon et al., 2001; Fehrer et al., 2007; Choi et al., 2014; Kwon et al., 2017). Furthermore, hypoxic conditions increased MSC stemness and migration (Das et al., 2012; Saller et al., 2012) and increased the expression of growth factors, such as vascular endothelial growth factor (VEGF), fibroblast growth factor (FGF)-2, and hepatocyte growth factor (HGF) (Choi et al., 2014; Lan et al., 2015; Gorin et al., 2016; Almeria et al., 2019), but reduced the expression of senescence-related β-galactosidase and pro-apoptotic markers (Fehrer et al., 2007; Kwon et al., 2017), as well as lower telomerase shortening rates (Lavrentieva et al., 2010).
Hypoxia-inducible factor (HIF)-1α, a master transcription factor, is stabilized in MSCs under hypoxic conditions (Hu et al., 2008; Li et al., 2017), which promotes a metabolic switch from oxidative phosphorylation to glycolysis and, consequently, decreases generation of reactive oxygen species (ROS) (Estrada et al., 2012; Sart et al., 2014a; Gonzalez-King et al., 2017; Contreras-Lopez et al., 2020). This alteration triggers activation of protein kinase C (PKC), which, in turn, activates nuclear factor kappa B (NF-κB) signaling and upregulates anti-oxidant and anti-apoptotic molecules, such as superoxide dismutase (SOD) and FGF-2 (Otani, 2004; Hu et al., 2008; Afzal et al., 2010). Hypoxia-preconditioned MSCs also demonstrated increased expression of chemokine receptors CXCR4, CXCR7, and CX3CR1, which are associated with the trafficking and homing of infused MSCs to the target organ (Haque et al., 2013). BM-, AD- and UC-MSCs under hypoxia demonstrated enhanced survival by upregulating Bag-1, Bcl-2, and Bcl-XL via phosphorylation of AKT (Hu et al., 2008; Bader et al., 2015) and by inhibiting oxidative stress-mediated apoptosis via inactivation of caspase-3/-7 activity and lactate dehydrogenase (LDH) release (Bader et al., 2015; Han et al., 2016a). Moreover, the composition of protein and RNA cargo packaged in MSC-derived EVs was substantially modified by hypoxic preconditioning (Gonzalez-King et al., 2017; Yuan et al., 2019; Koch et al., 2022). EVs from hypoxia-preconditioned BM-MSCs demonstrated increased concentration of miR-21, which can regulate cell survival by inhibiting apoptosis and stimulating proliferation (Cui et al., 2018).
Most respiratory diseases cause gas exchange impairment, therefore in vitro hypoxic preconditioning may adapt MSCs against stress due to an ischemic environment in vivo and increase their survival rate (Table 1). Compared with MSCs under normoxia, hypoxia-preconditioned BM-MSCs demonstrated increased expression of cytoprotective and regenerative factors, leading to greater reduction of interleukin (IL)-1β and IL-6 levels, airway constriction, lung edema, and fibrosis in experimental bleomycin-induced lung fibrosis (Lan et al., 2015). Hypoxia-preconditioned BM-MSCs were also effective at reducing ROS production, apoptosis of lung parenchymal cells and lung fibrosis in experimental radiation-induced lung injury (Li et al., 2017). Therapeutic effects of preconditioning with hypoxia were associated with increased survival of infused BM-MSCs in lung tissue, and upregulation of HIF-1α and AKT as well as SOD and HGF (Lan et al., 2015; Li et al., 2017). In experimental ischemia/reperfusion-induced lung injury, hypoxia-preconditioned BM-MSCs rapidly migrated toward injured lung tissue and reduced cell apoptosis and inflammatory responses related to ROS generation by upregulating glutathione, prostaglandin (PG)E2, IL-10 and Bcl-2, and downregulating p38/mitogen-associated protein kinase (MAPK) and the NF-κB signaling pathway (Liu et al., 2017). Moreover, BM-MSCs co-cultured with BM-derived lineage-positive blood cells under hypoxia have been shown to promote proliferation and polarization of macrophages to the M2 anti-inflammatory profile (Takizawa et al., 2017). BM-MSCs have also been shown to be resistant to a higher oxygen concentration (95% O2). Administration of conditioned media from hyperoxia-preconditioned BM-MSCs reduced pulmonary hypertension and lung structural abnormalities in a model of hyperoxia-induced neonatal lung injury (Waszak et al., 2012). Such effects were associated with an increase in stannoclacin-1 expression, which has anti-apoptotic actions (Waszak et al., 2012). On the other hand, hypercapnia is another pathologic condition that occurs frequently in chronic respiratory diseases. BM-MSCs cultured in 15% CO2 exhibited mitochondrial dysfunction and were unable to promote repair of human primary endothelial or small airway epithelial cells when co-cultured (Fergie et al., 2019), indicating that hypercapnic conditions may significantly hinder the therapeutic properties of MSCs.
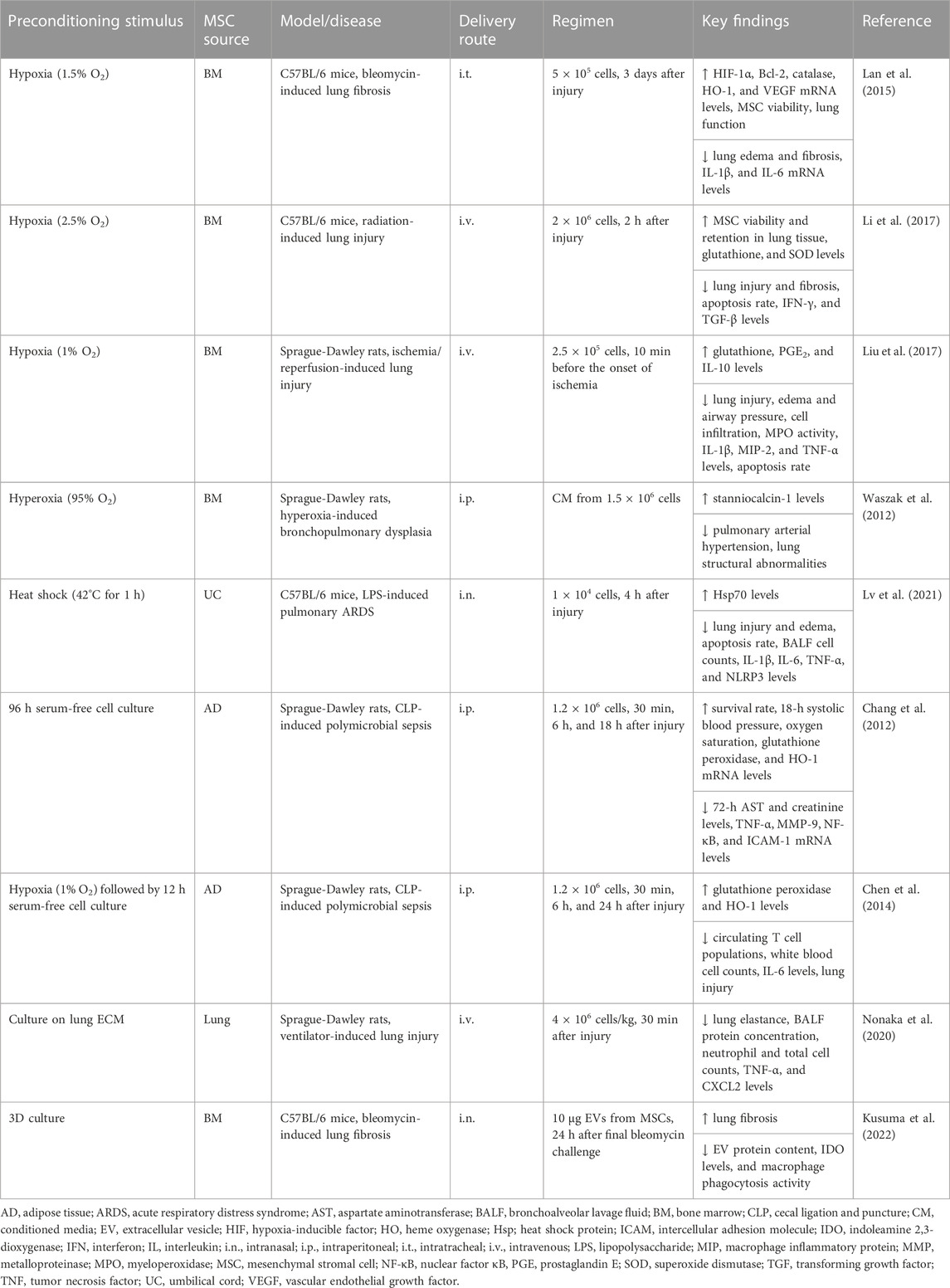
TABLE 1. In vivo preclinical studies assessing the efficacy of MSC preconditioning by altering the culture conditions.
Other strategies that have been investigated to precondition MSCs include heat shock and nutrient deprivation. Compared with normothermic conditions, exposure of BM-MSCs to a higher temperature (38.5°C) induced translocation of heat shock factor 1 (HSF-1) into the nucleus and increased cycloxygenase-2 (Cox-2) expression, which might enhance the immunogenic potential of MSCs (McClain-Caldwell et al., 2018). Heat shock-preconditioned BM-MSCs also induced greater production of IL-10 by co-cultured macrophages (McClain-Caldwell et al., 2018). Furthermore, BM-MSCs under hyperthermia (42°C for 60 min) demonstrated increased expression of heat shock protein (Hsp)27, Hsp70, and Hsp90 (Moloney et al., 2012). These Hsps contribute to cell survival by triggering the phosphoinositide 3-kinase (PI3K)/AKT, extracellular signal-regulated kinases (ERKs), and NF-κB signaling pathways, which upregulate expression of various anti-oxidants, anti-apoptotic and regenerative factors, such as VEGF, FGF-2, HGF, and insulin-like growth factor (IGF)-1 (Sart et al., 2014b). In experimental lipopolysaccharide (LPS)-induced ARDS, heat shock-preconditioned UC-MSCs reduced lung edema, inflammatory cell counts, and levels of inflammation-associated mediators (IL-1β, IL-6, tumor necrosis factor [TNF]-α) (Lv et al., 2021). Such effects were associated with increased Hsp70 levels and inhibition of NLRP3 inflammasome activation in alveolar macrophages (Lv et al., 2021).
MSCs can be induced into a quiescent state by serum depletion, which reduces nucleotide and protein synthesis and other ATP-consuming functions, thus facilitating their survival in ischemic environments (Moya et al., 2017; Ferro et al., 2019). BM-MSC quiescence has been shown to maintain cell viability and function for up 14 days in vitro as well as enhance their survival after infusion in mice for up 7 days (Moya et al., 2017). BM-MSC preconditioning with serum depletion inhibits the mammalian target of rapamycin (mTOR), a master regulator of protein translation and proliferation, and stimulates autophagy to protect MSCs against deleterious ischemic conditions. When the autophagic process was pharmacologically inhibited, there was a significant decrease in the survival rate of BM-MSCs under ischemic conditions in vitro (Moya et al., 2017). In another study, BM-MSCs were maintained under serum-depleted culture for up 75 days. MSCs transiently altered their morphology and behavior during serum depletion, but maintained their stemness and survived in a quiescent state (Ferro et al., 2019). Preconditioning with serum depletion was found to induce Janus kinase/signal transducer and activator of transcription (Jak/STAT) anti-apoptotic activity, and BM-MSCs used lipid β-oxidation as an alternative energy source (Ferro et al., 2019). On the other hand, AD-MSCs underwent apoptosis by serum depletion, but were shown to increase levels of anti-oxidant factors (glutathione peroxidase and HO-1) and reduce levels of inflammation-related mediators (IL-1β, MIP-1α, and TNF-α), yielding attenuated lung and heart injury and an improved survival rate in experimental cecal ligation and puncture (CLP)-induced sepsis (Chang et al., 2012; Chen et al., 2014).
Although MSCs are often maintained in two-dimensional (2D) monolayer cultures, three-dimensional (3D) culture systems have emerged as an interesting approach, because they may more closely replicate the original physiologic properties of cells and thus enhance their stemness (McKee and Chaudhry, 2017; Deng et al., 2020). The differentiation potential and immunomodulatory properties of MSCs can be influenced not only by soluble bioactive factors and intercellular contact but also by the presence of extracellular matrix (ECM) components, because these elements can control cellular behavior during homeostasis and disease progression (Deng et al., 2020; Raman et al., 2022). Among the supporting materials for 3D cultures (also known as spheroids), hydrogels have been the most commonly used in recent investigations (Murua et al., 2008; McKee and Chaudhry, 2017). Hydrogel encapsulation was able to enhance the viability and proliferation rate of MSCs (Ansari et al., 2017), and chondrogenic (Dvořáková et al., 2014) and osteogenic (Steinmetz et al., 2015) differentiation, as well as promote neovascularization (Chen et al., 2015a) and wound repair (Rustad et al., 2012). AD-MSC spheroids have also been found to have greater levels of pluripotency markers (i.e., CXCR4, Nanog, Sox2, and Oct4), suggesting an increase in MSC stemness in the 3D configuration (Cheng et al., 2013).
Compared with 2D monolayer cultures, MSC spheroids further produced anti-inflammatory and regenerative mediators, including PGE2, VEGF, and FGF-2 (Bhang et al., 2011; Ceccaldi et al., 2012; Follin et al., 2016). MSC spheroids also stabilized HIF-1α and upregulated levels of anti-apoptotic markers (e.g., Bcl-XL) (Bartosh et al., 2010; Bhang et al., 2011). By using dynamic methods with spinner flasks and a rotating bioreactor, BM-MSC spheroids upregulated IL-24 levels, and their conditioned medium was able to impair the viability of prostate cancer cells (Frith et al., 2010). BM-MSCs were cultured in alginate-based hydrogels with different stiffnesses and then exposed to TNF-α. BM-MSCs preconditioned in the softer hydrogel demonstrated increased clustering of TNF receptors, which led to greater NF-κB activation and downstream responses by exposure to TNF-α (Wong et al., 2020). In a recent study, lung derived MSCs were cultured on lung ECM to biophysically precondition cells in a microenvironment that resembles lung tissue. Administration of preconditioned MSCs led to improved lung elastance and reduced levels of TNF-α and CXCL2 in the bronchoalveolar lavage fluid (BALF) of experimental ventilator-induced lung injury (Nonaka et al., 2020). However, another study reported that 3D culture conditions may hinder certain therapeutic properties of MSCs (Kusuma et al., 2022). Compared with EVs obtained from 2D MSC cultures, BM-MSC spheroid-derived EVs demonstrated reduced anti-inflammatory and anti-fibrotic actions in experimental bleomycin-induced lung fibrosis. Reduced macrophage phagocytosis activity was also observed after in vitro incubation with BM-MSC spheroid-derived EVs. Proteomic profiling revealed a significant reduction in the protein content of MSC spheroid-derived EVs and in their global ontology using functional enrichment analysis (Kusuma et al., 2022).
These studies demonstrate that changes in culture conditions potentially have an impact on the therapeutic properties of MSCs. Further studies should be performed to elucidate the potential effects of these preconditioned methods for different respiratory diseases. Moreover, the utility of 3D culture using different supporting materials as a preconditioning method for MSC-based therapies should be further investigated.
2.2 MSC preconditioning in an inflammatory environment
There has been increasing interest in preconditioning MSCs in an inflammatory environment by using cytokines/chemokines, growth factors released under pro-inflammatory conditions, or biologically relevant samples from patients, such as BALF and serum (Table 2). Experimental evidence has indicated that MSCs are able to detect the environmental inflammatory signals through their damage- and pathogen-associated surface receptors and tailor their responses according to these stimuli (Waterman et al., 2010; de Castro et al., 2019; Islam et al., 2019). For instance, stimulation of Toll-like receptor (TLR)3 and TLR4 induced generation of regulatory T cells (Treg) in a cell contact-dependent manner (Rashedi et al., 2017). Nevertheless, stimulation of TLR3 and TLR4 led to subsequent activation of different downstream signaling pathways, and MSCs were thus polarized into two different profiles with significantly distinct responses (Waterman et al., 2010). Activation of TLR3 with polyinosinic:polycytidylic acid (poly I:C) led to an anti-inflammatory MSC profile with activation of the Notch signaling pathway and inhibition of TH1/TH17 cell expansion (Rashedi et al., 2017) as well as production of indoleamine 2,3-dioxygenase (IDO), IL-1RN, IL-4, and PGE2 (Waterman et al., 2010; Zhao et al., 2014a; Kim et al., 2018). Poly I:C-preconditioned UC-MSCs also inhibited miR-143 expression and increased production of Cox-2 and macrophage anti-inflammatory activity in experimental CLP-induced sepsis (Zhao et al., 2014a). On the other hand, exposure of MSCs to LPS (a TLR4 agonist) induced a pro-inflammatory MSC profile with upregulation of IL-6, IL-8, and transforming growth factor (TGF)-β (Waterman et al., 2010). Under inflammatory conditions, preconditioning with LPS was found to improve BM-MSC survival (Gupta et al., 2018; Saeedi et al., 2019). Furthermore, when BM-MSCs were harvested from TLR4-deficient mice, they demonstrated impaired survival under in vitro inflammatory conditions and were therapeutically inefficient after infusion in an animal model of Escherichia coli-induced ARDS (Gupta et al., 2018).
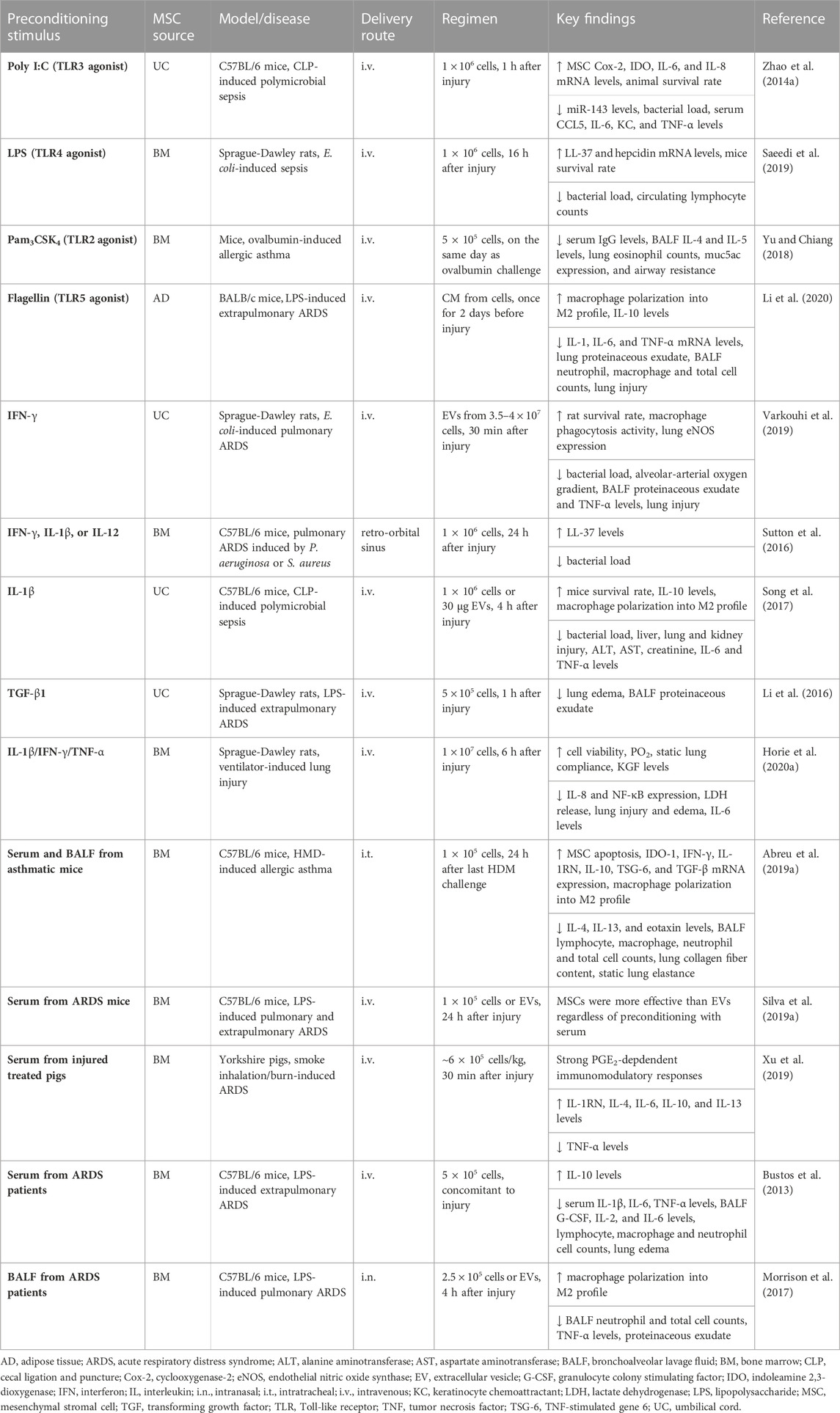
TABLE 2. In vivo preclinical studies assessing the efficacy of MSC preconditioning in inflammatory conditions.
The microenvironmental conditions of a disease may have a different impact on MSC TLR activation in vivo, and MSCs express various TLRs in addition to TLR3 and TLR4 (de Castro et al., 2019). Activation of TLR2 inhibited chemotaxis of BM-MSCs and mitigated MSC-mediated expansion of the Treg population in vitro (Lei et al., 2011). In experimental ovalbumin-induced allergic asthma, activation of BM-MSC TLR2 by the agonist Pam3CSK4 led to a reduction in levels of BALF TH2 cytokines (IL-4 and IL-5) and eosinophil counts in lungs, resulting in attenuation of airway resistance in response to incremental doses of methacholine (Yu and Chiang, 2018). Furthermore, activation of UC-MSC TLR5 by flagellin promoted increased expression of CCL24, IL-10, and TGF-β, whereas expression of CCL5 and IP-10 was reduced (Li et al., 2015). Preconditioning with flagellin enhanced the therapeutic actions of AD-MSCs, resulting in a decrease in lung exudate, cell infiltration, and levels of inflammation-associated mediators (IL-1, IL-6, monocyte chemoattractant protein [MCP]-1 and TNF-α) in experimental LPS-induced ARDS (Li et al., 2020). Conditioned media from flagellin-preconditioned MSCs also induced macrophages to polarize into an M2 anti-inflammatory profile (Li et al., 2020).
Under normal physiologic conditions, MSCs exhibit very low expression of major histocompatibility complex (MHC) type I, whereas type II is present intracellularly but absent on the cell surface (Le Blanc et al., 2003). Upon interferon (IFN)-γ stimulation, MHC class II and costimulatory factor (CD40, CD80, and CD86) can traffic to the cell surface, but MSCs are still able to escape recognition by alloreactive T cells and displayed enhanced immunomodulatory activities (Le Blanc et al., 2003; Ankrum et al., 2014; De Witte et al., 2016). Proteomic analysis revealed that preconditioning with IFN-γ altered BM-MSC expression of over 200 proteins, of which 169 were upregulated and 41 were downregulated (Guan et al., 2017). Exposure of MSCs to IFN-γ led to upregulation of IDO (De Witte et al., 2016; Wang et al., 2016; Guan et al., 2017), several immunomodulatory mediators (CCL2, HGF, PGE2, and TFG-β) (De Witte et al., 2016), adhesion molecules (ICAM-1 and VCAM-1) (Wang et al., 2016; Guan et al., 2017) and chemokines (CXCL9, CXCL10, CXCL11) (Wang et al., 2016), and promoted inhibition of NK cell activation (Noone et al., 2013) and immunosuppressive effects on T lymphocytes (Krampera et al., 2006). When IFN-γ blocking antibody was used, BM-MSC-induced immunosuppression was abrogated (Krampera et al., 2006). The protective effect of BM-MSCs against ovalbumin-induced allergic asthma was also abrogated when cells were infused in IFN-γ null mice (Goodwin et al., 2011). In another study, preconditioning with IFN-γ inhibited mTOR signaling by early phosphorylation of STAT1 and STAT3, increasing the ability of BM-MSCs to suppress T cell proliferation (Vigo et al., 2017). IFN-γ-preconditioned BM-MSCs were also effective at inhibiting T cell proliferation and subsequent secretion of TH1 cytokines by upregulating programmed cell death-1 ligands (PDL-1), regardless of IDO upregulation (Chinnadurai et al., 2014). Furthermore, when co-cultured with activated lymphocytes, IFN-γ-preconditioned MSCs reduced TH17 cell counts and production of IFN-γ and TNF-α (Wang et al., 2016). Although preconditioning with IFN-γ also led to increased expression of MHC type I, it significantly reduced the susceptibility of UC-MSCs to NK cytotoxicity by reducing the cell surface expression of NKG2D (Noone et al., 2013). In experimental E. coli-induced ARDS, EVs from both naive and IFN-γ-preconditioned UC-MSCs were similarly able to reduce mortality and improve bacterial killing and phagocytosis (Varkouhi et al., 2019). However, EVs from IFN-γ-preconditioned UC-MSCs were more effective at reducing alveolar protein leak, TNF-α levels, and alveolar-arterial oxygen gradient but enhanced production of endothelial nitric oxide synthase (eNOS) (Varkouhi et al., 2019).
In addition to IFN-γ, TNF-α, IL-1β, and IL-17 have been identified as key inflammatory cytokines to enhance the therapeutic properties of MSCs before infusion. Preconditioning with TNF-α promoted upregulation of several immunomodulatory factors in MSCs, including IDO, HGF, PGE2, and TNF-α-stimulated gene 6 (TSG-6), although to a lesser extent in comparison with MSC preconditioning with IFN-γ (English et al., 2007; Prasanna et al., 2010; De Witte et al., 2016). Nevertheless, preconditioning with TNF-α had superior effects on migration of BM- and UC-MSCs and secretion of IL-8 in comparison with preconditioning with IFN-γ (Hemeda et al., 2010). In experimental CLP-induced sepsis, infusion of BM-MSCs exposed to TNF-α neutralizing antibody or harvested from TNF-R1 knockout mice was unable to protect against the deleterious effects of sepsis (Németh et al., 2009). Conditioned media from TNF-α-preconditioned BM-MSCs induced Cox-2/PGE2 signaling activation and led to inhibition of B-cell IgE production and histamine release, thus alleviating allergic symptoms (Su et al., 2015). On the other hand, global transcriptome profiling of IL-1β-preconditioned BM-MSCs revealed upregulation of several genes related to NF-κB signaling and its downstream responses, namely, cell survival, migration, cytokine production, angiogenesis, and immune responses (Carrero et al., 2012). IL-1β-preconditioned UC-MSCs demonstrated higher migration to inflammatory foci and led to increased percentages of Treg and TH2 cells and decreased TH1 and TH17 cell counts in mesenteric lymph nodes and spleen (Fan et al., 2012). BM-MSCs preconditioned with IL-1β, IL-12, or IFN-γ also demonstrated increased secretion of the antimicrobial peptide LL-37, thus significantly reducing the rate of growth of Pseudomonas aeruginosa, Staphylococcus aureus, and Streptococcus pneumonia (Sutton et al., 2016). Furthermore, preconditioning with IL-1β was reported to potentiate gingival derived-MSC immunomodulatory and wound repair properties by upregulating expression of TGF-β and matrix metalloproteinases (MMPs) (Magne et al., 2020). In another study, IL-1β-preconditioned UC-MSCs produced EVs with high levels of miR-146a, which resulted in M2 polarization when transferred to BM-derived macrophages in vitro (Song et al., 2017). Administration of IL-1β-preconditioned UC-MSCs led to decreased serum levels of IL-6, TNF-α, alanine aminotransferase and aspartate aminotransferase, and liver, lung, and kidney injury and increased bacterial clearance and survival in experimental CLP-induced sepsis (Song et al., 2017).
Differentiation and proliferation of MSCs have been modulated by preconditioning with IL-17 in a dose-dependent manner (Huang et al., 2009; Sivanathan et al., 2015). These effects were associated with the generation of ROS from stimulation of TNF receptor-associated factor 6 (TRAF-6) and the Act-1 adaptor that activated the MEK/ERK signaling pathway (Huang et al., 2009). MSC preconditioning with IL-17 also promoted greater osteogenic differentiation and MSC migration by increasing expression of CXCL6, MMP1, and MMP13 (Huang et al., 2009; Noh, 2012; Sivanathan et al., 2015) and inhibited adipogenic differentiation by upregulating IL-6 and IL-8 upon differentiation (Shin et al., 2009). The immunosuppressive potential of BM-MSCs was enhanced by preconditioning with IL-17, leading to inhibition of effector T cell proliferation and decreased secretion of TH1 cytokines (IFN-γ, IL-2, and TNF-α) and promoted Treg cell expansion (Sivanathan et al., 2015; Sivanathan et al., 2017). Furthermore, IL-17-preconditioned BM-MSCs demonstrated expression of MHC class I, II and costimulatory molecules comparable with that of naive MSCs, indicating they maintained a hypoimmunogenic profile (Sivanathan et al., 2017).
Growth factors have also been considered as preconditioning factors to enhance the therapeutic properties of MSCs. In this context, preconditioning with TGF-β promoted BM-MSC mobilization and migration during bone remodeling and facilitated peripheral tissue healing via the non-canonical Smad-independent signaling pathway (Dubon et al., 2018). TGF-β-preconditioned UC-MSCs demonstrated an extended period of survival in the lungs of experimental LPS-induced ARDS, and reduced lung edema and BALF neutrophil counts (Li et al., 2016). Furthermore, preconditioning with FGF-2 enhanced the angiogenic properties of dental pulp-derived MSCs by increasing expression of HGF and VEGF more efficiently than hypoxia preconditioning (Gorin et al., 2016). In addition to a single cytokine or growth factor, preconditioning with a cocktail of inflammatory mediators has been investigated. For instance, IFN-γ and TNF-α were found to work synergistically in enhancing the immunomodulatory properties of MSCs. MSC preconditioning with both cytokines led to inhibition of complement activation by increasing the production of factor H in a time- and dose-dependent manner (Tu et al., 2010). IFN-γ and TNF-α promoted chromatin remodeling closer to the transcriptional start site for IDO1, which remained altered even during cryopreservation (Gonzalez et al., 2016). After thawing, previously preconditioned BM-MSCs demonstrated quick accumulation of high levels of IDO1 mRNA upon re-exposure to these cytokines (Gonzalez et al., 2016). BM-MSC preconditioning with IFN-γ/TNF-α increased IDO activity, which promoted monocyte differentiation into M2 anti-inflammatory macrophages, which, in turn, induced suppression of T cell proliferation (François et al., 2012a). Exposure of BM-MSCs to IFN-γ/TNF-α led to secretion of PD-1 ligands (PD-L1 and PD-L2), which downregulated IL-2 and suppressed CD4+ T cell activation, inducing immunosuppression by irreversible hyporesponsiveness and subsequent apoptosis (Davies et al., 2017). In another study, inhibition of T cell proliferation by IFN-γ/TNF-α-preconditioned BM-MSCs was associated with upregulation of NOS2 and subsequent increase in NO generation as well as attenuation of delayed hypersensitivity reactions (Szabó et al., 2015).
Several other cocktails of inflammatory mediators have been used to precondition MSCs. Compared with preconditioning with IFN-γ/TNFα, exposure of nasal mucosa-derived MSCs to IL-1β/IFN-γ/TNFα enhanced neutrophilia by further increasing IL-8 secretion in a mechanism mediated by activation of STAT5 and p38/MAPK signaling (Hackel et al., 2021). On the other hand, IL-1β/IFN-γ/TNFα-preconditioned BM-MSCs reduced structural abnormalities and inflammation in lungs and restored oxygenation and improved lung compliance in experimental ventilator-induced lung injury (Horie et al., 2020a). The enhanced epithelial wound repair was mediated in part by keratinocyte growth factor (KGF) secretion (Horie et al., 2020a). In another study, IL-1β/TNFα-preconditioned BM-MSCs prolonged graft survival by stimulating lung-derived myeloid cells to inhibit lymphocyte proliferation and promote Treg cell expansion (Murphy et al., 2019). Immunosuppressive actions of BM-MSCs were also remarkably increased by preconditioning with IL-17/IFN-γ/TNF-α in an inducible NO synthase (iNOS)-induced mechanism (Han et al., 2014). LPS and TNF-α acted synergistically and increased arginase-1 and PGE2 secretion by preconditioned MSCs. LPS/TNF-α-preconditioned BM-MSCs also induced macrophage polarization in vitro to an M2 profile and improved osteogenesis (Croes et al., 2015; Lin et al., 2017). IL-1β/IL-6/IL-23-preconditioned AD- and BM-MSCs displayed no changes in morphology, immunophenotype, and costimulatory factors, with an exception for upregulation of CD45. Furthermore, MSC immunomodulatory activity was well preserved with increased IL-10 secretion and decreased IL-4 after preconditioning with IL-1β/IL-6/IL-23 (Pourgholaminejad et al., 2016).
To more closely replicate lung inflammatory milieu, BALF and serum from animal models or patients with inflammatory lung conditions have been used as surrogates in MSC preconditioning. Exposure of MSCs to BALF from patients with cystic fibrosis (CF) infected with Aspergillus sp. induced rapid MSC apoptosis partly related to the presence of fungal-produced gliotoxin, which led to mitochondrial dysfunction (Abreu et al., 2020). RNA analysis revealed differential expression of transcripts involved in IFN signaling, antimicrobial activity, and cell death by MSCs exposed to CF BALF positive versus negative to Aspergillus infection (Abreu et al., 2020). BM-MSCs were also induced to undergo apoptosis by exposure to either BALF or serum from experimental house dust mite (HDM)-induced allergic asthma, and this process increased expression of immunomodulatory mediators (IDO-1, IFN-γ, IL-1RN, IL-10, TSG-6, and TGF-β) (Abreu et al., 2019a). Serum was more effective than BALF in preconditioning BM-MSCs and led to a significant reduction in lung inflammatory cell counts and levels of TH2 cytokines (IL-4 and IL-13) and eotaxin, and lung function improved in a murine model of HDM-induced allergic asthma (Abreu et al., 2019a).
BM-MSCs were preconditioned with serum from experimental LPS-induced ARDS and their EVs were harvested. Regardless of preconditioning with serum, MSCs and EVs were able to reduce levels of inflammation-related mediators (IL-6, keratinocyte chemoattractant [KC], TGF-β, TNF-α), alveolar collapse, and BALF inflammatory cell counts (Silva et al., 2019a). Nevertheless, MSCs were more effective than EVs in reducing lung edema and fibrosis, resulting in improved lung function in both experimental pulmonary and extrapulmonary ARDS (Silva et al., 2019a). A significant immunomodulatory response in a PGE2-dependent mechanism was observed when BM-MSCs were reconditioned with serum from porcine smoke inhalation/burn-induced ARDS treated with MSCs (Xu et al., 2019). BM-MSCs were also preconditioned with serum from patients with moderate to severe ARDS, which contained high levels of IL-6, IL-8, and IL-10 (Bustos et al., 2013). After preconditioning with serum, BM-MSCs demonstrated enhanced expression of IL-1RN and IL-10 in vitro and significantly reduced BALF inflammatory cell counts, lung injury, and vascular permeability in experimental LPS-induced ARDS (Bustos et al., 2013).
BALF from either patients with ARDS or health controls (HC) demonstrated no cytotoxicity to MSCs, but they clearly modulated MSC expression of various pro- and anti-inflammatory mediators (Enes et al., 2021). IL-1β in HC and ARDS BALF was predictive of MSC production of certain pro-inflammatory mediators, such as IL-6 and IL-8. MSCs exposed to HC BALF, but not to ARDS BALF, demonstrated increased expression of HLA class II factors, suggesting that MSCs can be stimulated by normal lung milieu to cooperate in immune surveillance (Enes et al., 2021). Another study demonstrated that preconditioning with ARDS BALF promoted anti-inflammatory actions of BM-MSCs and in vitro macrophage phagocytosis activity (Morrison et al., 2017). Nevertheless, conditioned media from ARDS BALF-preconditioned BM-MSCs were less effective in promoting monocyte polarization to an anti-inflammatory profile than conditioned media from CF BALF-preconditioned MSCs (Abreu et al., 2019b).
These studies demonstrate that disease environmental conditions have a major impact on MSC survival and immunomodulatory activities, and the microenvironments in certain diseases (or sub-phenotypes) may be more suited to facilitating MSC activation and promoting optimal MSC therapeutic actions.
2.3 MSC preconditioning with pharmacological agents or other substances
In preclinical research, MSCs have been preconditioned with a range of drugs or other chemical agents not only to enhance their therapeutic properties and homing but also to improve their survival rate and resistance against any harmful stimuli they may encounter (Table 3). For instance, exposure of MSCs to sub-lethal doses of H2O2 (≤50 µM) was shown to increase their resistance against a lethal concentration of this chemical agent (Sart et al., 2014b). BM-MSCs exposed to low doses of H2O2 exhibited increased expression of CXCR4 and migration-mediated stromal cell-derived factor (SDF)-1α (Li et al., 2009). H2O2-preconditioned BM-MSCs were also protected against apoptosis induced by a higher concentration of H2O2 (500 µM) due to activation of the ERK pathway, which upregulated anti-apoptotic factors such as Bcl-2 and Bcl-XL (Li et al., 2009; Sart et al., 2014b). In AD-MSCs, long-term exposure to a low dose (10 µM) of H2O2 led to increased survival by upregulating nuclear factor-erythroid 2-related factor 2 (Nrf2) and several anti-oxidant mediators (catalase, glutathione peroxidase-1, heme oxygenase (HO)-1 and SOD) but reduced intracellular ROS levels and expression of Cox-2 and IL-1β (Garrido-Pascual et al., 2020). Administration of H2O2-preconditioned UC-MSCs reduced lung remodeling by attenuating expression of α-smooth muscle actin (α-SMA) and TGF-β as well as myeloperoxidase (MPO) activity in experimental bleomycin-induced lung fibrosis (Mahmoudi et al., 2020). Preconditioning with pyrogallol was also able to enhance UC-MSC therapeutic properties via activation of the Nrf2/HO-1 signaling pathway, leading to a reduction of lung injury, epithelial cell apoptosis, MPO activity, and levels of inflammation-associated mediators (IL-6, IL-8, MCP-1, and TNF-α) in experimental LPS-induced ARDS (Zhang et al., 2022).
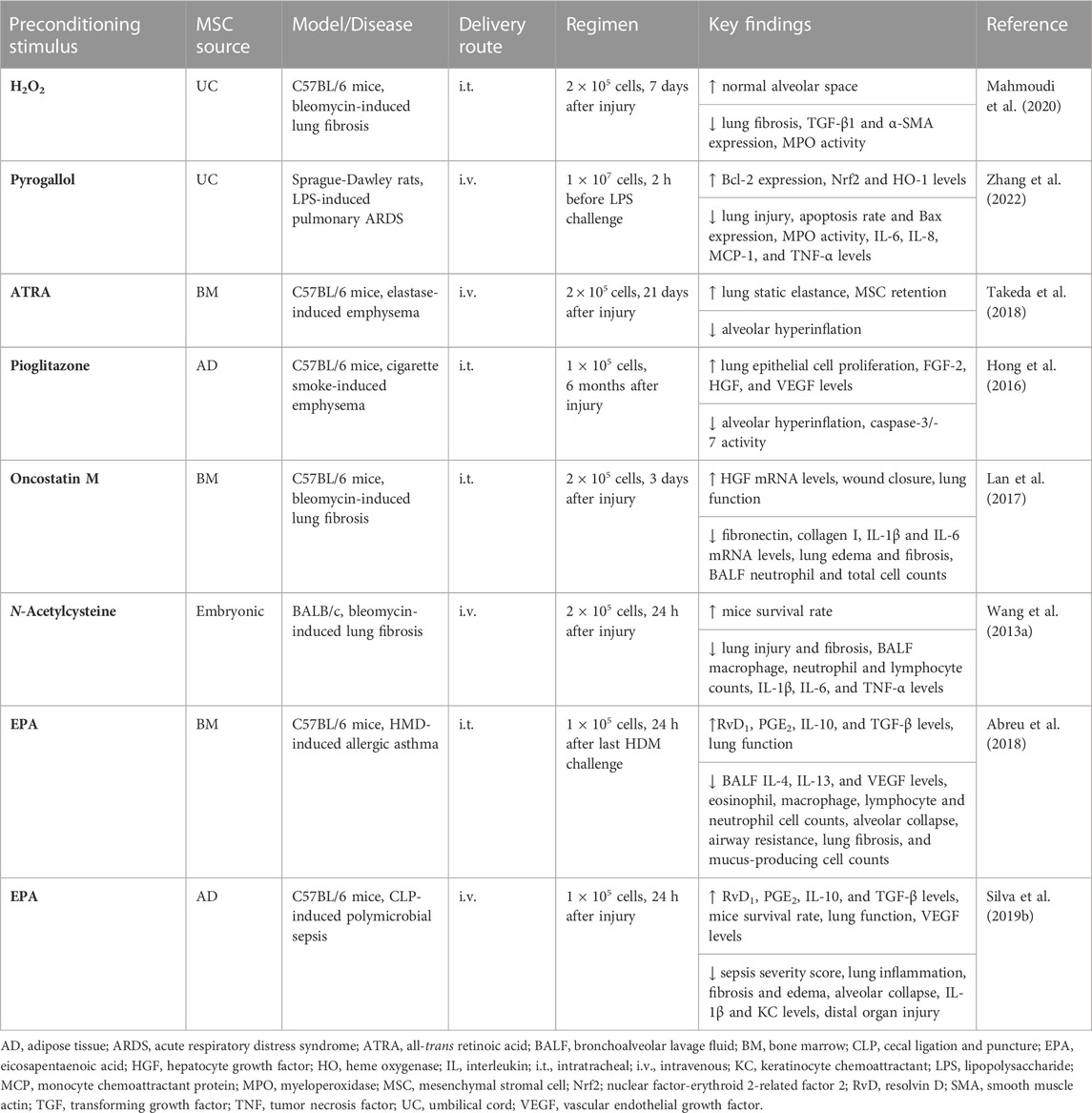
TABLE 3. In vivo preclinical studies assessing the efficacy of MSC preconditioning with pharmacological agents or other substances.
Although MSCs are subjected to the lung first-pass effect and are thus easily trapped in pulmonary capillaries after systemic infusion (Fischer et al., 2009), their retention in lung tissue rarely exceeds a few days (Leblond et al., 2009; Wang et al., 2009; De Oliveira et al., 2017; de Witte et al., 2018). As MSC engraftment is primarily mediated by the interaction between CXCR4 and SDF-1 (Jin et al., 2018), pharmacological modulation of these may enable improved MSC homing to injured tissues. UC-MSC preconditioning with low doses of valproic acid, a histone deacetylase inhibitor, enhanced MSC recruitment to injury sites by upregulating CXCR4 and increased MSC anti-inflammatory responses (Marquez-Curtis et al., 2014; Lim et al., 2017). Preconditioning with rapamycin, ethionamide, or the DNA methyltransferase inhibitor, 5-azacytidine, was able to increase MSC CXCR4 expression, thus improving the migration ability of UC- and WJ-MSCs (Lee et al., 2015; Zheng et al., 2019; Lee et al., 2020). When co-cultured with activated T cells, rapamycin- or 5-azacytidine-preconditioned MSCs demonstrated immunosuppressive properties by upregulating Cox-2 and PGE2 (Lee et al., 2015; Wang et al., 2017). BM-MSC homing and CXCR4 expression were also increased by resveratrol treatment before preconditioning with SDF-1α (Hajinejad et al., 2018). In addition to these compounds, screening of ∼9000 signal-transduction modulators was performed to identify novel compounds able to enhance MSC surface expression of homing ligands (Levy et al., 2015). In this context, preconditioning with Ro-31-8425 enabled the in vivo delivery BM-MSCs to inflammatory sites in a CD11a-dependent mechanism (Levy et al., 2015).
Desferrioxamine and 2,4-dinitrophenol, two hypoxia-mimetic agents, were able to enhance MSC homing and immunomodulatory actions (Najafi and Sharifi, 2013; Khan et al., 2016). BM-MSCs preconditioned with 2,4-dinitrophenol exhibited increased expression of genes related to cell adhesion and angiogenesis (Khan et al., 2016); preconditioning of BM-MSCs with low doses of desferrioxamine led to stabilization of HIF-1α by preventing its hydroxylation, decreased mitochondrial activity and apoptosis, and upregulation of glycolysis-related genes (Fujisawa et al., 2018). Short-term exposure of BM-MSCs to the volatile anesthetic isoflurane also increased expression of HIF-1α, CXCR4, and SDF-1 (Sun et al., 2015). On the other hand, exposure of BM-MSCs to curcumin, a natural dietary product, and subsequent hypoxia improved cell survival and promoted mitochondria fusion (Wang et al., 2020). Preconditioning with curcumin/hypoxia suppressed BM-MSC apoptosis by mitigating cytochrome c release from the mitochondria and caspase-3 cleavage (Wang et al., 2020). Moreover, curcumin/hypoxia-preconditioned BM-MSCs accelerated wound repair in vivo (Wang et al., 2020). Although these preconditioning strategies led to more MSCs moving into and remaining in certain injured tissues longer, their efficacy for respiratory diseases has yet to be assessed.
All-trans retinoic acid (ATRA) regulates transcription of genes related to apoptosis, differentiation, and immune responses. When BM-MSCs were preconditioned with ATRA, there was increased expression of Cox-2, CXCR4, CCR2, HIF-1, angiopoietin (Ang)-2, and Ang-4, which was abrogated by Cox-2 inhibition (Pourgholaminejad et al., 2016). BM-MSCs preconditioned with ATRA also demonstrated enhanced potential of wound repair in vivo (Pourgholaminejad et al., 2016). In this context, preconditioning with ATRA promoted in vitro activation of BM-MSC p70S6 kinase-1 and led to significant improvements in lung structure (mean linear intercepts and alveolar surface area) and function (static lung compliance) after infusion of ATRA-preconditioned MSCs in experimental elastase-induced emphysema (Takeda et al., 2018). AD-MSCs preconditioned with pioglitazone, an antidiabetic drug that binds to peroxisome proliferator-activated receptor (PPAR)-γ, also enhanced lung tissue repair and upregulated VEGF in vitro (Hong et al., 2016). In experimental emphysema induced by cigarette smoke, administration of pioglitazone-preconditioned AD-MSCs led to mitigation of lung structure abnormalities measured by mean linear intercepts and increased expression of several growth factors (FGF-2, HGF, and VEGF) (Hong et al., 2016).
Screening of a library containing over 1400 bioactive compounds approved by the US Food and Drug Administration identified tetrandrine, a calcium channel inhibitor, as a potential hit for MSC preconditioning. In vitro exposure of BM-MSCs to tetrandrine increased expression of PGE2 by the NF-κB/Cox-2 signaling pathway and attenuated TNF-α secretion by LPS-activated macrophages (Yang et al., 2016). Furthermore, preconditioning with oncostatin M was effective in promoting BM-MSC migration and increasing HGF expression (Lan et al., 2017). BM-MSCs preconditioned with oncostatin M improved lung function and reduced lung edema, inflammatory cell counts, and fibrosis in bleomycin-induced lung fibrosis and reduced mRNA expression of fibronectin and type I collagen in co-cultured fibroblasts (Lan et al., 2017). The anti-oxidant ability of MSCs preconditioned with the mucolytic agent N-acetylcysteine improved in vitro, leading to increased cellular glutathione levels and attenuated ROS generation (Wang et al., 2013a). In experimental bleomycin-induced lung fibrosis, MSCs preconditioned with N-acetylcysteine reduced lung inflammation and fibrosis, BALF inflammatory cell counts, and levels of inflammation-associated mediators (IL-1β, IL-6, and TNF-α), which resulted in a significant improvement in the survival rate (Wang et al., 2013a).
The omega-3 fatty acid eicosapentaenoic acid (EPA) is another agent that can improve the therapeutic properties of MSCs. Preconditioning with EPA increased the formation of lipid bodies in vitro and secretion of resolvin-D1, PGE2, IL-10, and TGF-β by AD- and BM-MSCs without affecting cell viability (Abreu et al., 2018; Silva et al., 2019b). BM-MSCs preconditioned with EPA decreased BALF inflammatory cell counts, levels of TH2 cytokines (IL-4 and IL-13), lung fibrosis, and the presence of mucus-producing cells, and improved lung function in experimental HDM-induced allergic asthma (Abreu et al., 2018). Administration of MSCs preconditioned with EPA also promoted polarization of lung macrophages to an M2 anti-inflammatory profile (Abreu et al., 2018). In experimental CLP-induced sepsis, preconditioning of AD-MSCs with EPA led to a reduction of lung inflammation, edema and alveolar collapse, and levels of IL-1β, KC, and TGF-β, and increased VEGF levels and improved lung function (Silva et al., 2019b). Moreover, administration of AD-MSCs preconditioned with EPA reduced tissue injury not only in lungs but also in distal organs, thus resulting in a decrease in sepsis severity score and improvement in the survival rate (Silva et al., 2019b).
Overall, MSC preconditioning with drugs and other substances is an interesting approach and has been demonstrated to enhance MSC homing and immunomodulatory actions. However, there are still limited data on MSC preconditioning with each drug for respiratory diseases, and further studies are warranted.
2.4 Potentiation of MSC therapeutic properties by genetic engineering
Genetic engineering has been used extensively in experimental research not only to enhance the beneficial effects of therapies but also to unravel their mechanism of action by overexpressing or knocking down genes of interest. In MSC-based therapy, several genes related to survival, engraftment, and immunomodulatory actions have been targeted for enhanced and sustained expression to potentiate the therapeutic properties of MSCs (Table 4).
Although CXCR4 and SDF-1 play a fundamental role in driving migration of MSCs to injured sites, the surface expression of the CXCR4 can decline due to successive replications, which can limit MSC homing. Overexpression of CXCR4 in BM-MSCs using a lentiviral vector was shown to enhance MSC migration in vitro (Yang et al., 2015). In experimental LPS-induced ARDS, administration of BM-MSCs overexpressing CXCR4 reduced lung injury, inflammation and edema, and TNF-α levels and increased IL-10 levels. BM-MSC homing in injured lung tissue was also facilitated by overexpressing CXCR4 (Yang et al., 2015). Alternatively, PGE2 can facilitate MSC migration by activating E-prostanoid 2 (EP2) receptor, which in turn stimulates focal adhesion kinase (FAK) and the ERK1/2 signaling pathway. When FAK and ERK1/2 were inhibited, PGE2-mediated BM-MSC migration was also mitigated (Lu et al., 2017). Overexpression of EP2 enhanced retention of BM-MSCs in lungs and increased IL-10 levels, but reduced IL-1β and TNF-α levels, lung injury, edema and endothelial permeability in experimental LPS-induced ARDS (Han et al., 2016b). Overexpression of the receptor tyrosine kinase-like orphan receptor 2 (ROR2) was also able to increase retention of BM-MSCs in lung tissue after LPS challenge, leading to further reduction in lung injury, edema and fibrosis, and IL-1β and IL-6 levels, compared with unmodified MSCs, in experimental LPS-induced ARDS (Cai et al., 2016).
Because MSCs can promote protection of lung tissue against oxidative injury, overexpression of antioxidant mediators, such as HO-1 and SOD, have been assessed to further enhance the therapeutic properties of MSCs. BM-MSCs expressing human HO-1 under the control of surfactant protein C promoter were harvested from transgenic mice (Liang et al., 2011). Administration of these MSCs in an experimental mouse model of hypoxia-induced pulmonary arterial hypertension led to a reduction of right ventricular hypertrophy and systolic pressure (Liang et al., 2011). Such effects were associated with inhibition of smooth muscle cell proliferation and decrease levels of inflammation-related mediators (Liang et al., 2011). When LPS-injured pulmonary endothelial cells were co-cultured with BM-MSCs overexpressing HO-1, they showed increased Nrf2 activation and attenuated NF-κB activation, leading to more effective recovery of SOD and glutathione peroxidase activity, but suppressed production of lipid peroxide, malondialdehyde (MDA), and levels of inflammation-related mediators (IL-1β, IL-6, and TNF-α) (Chen et al., 2018). In this co-culture system, levels of HGF and IL-10 produced by BM-MSCs overexpressing HO-1 were also higher after pulmonary endothelial cells were exposed to LPS (Chen et al., 2018). Compared with unmodified MSCs, administration of BM-MSCs overexpressing HO-1 further reduced lung injury, edema, neutrophilia, and levels of IL-1β and TNF-α, but increased levels of HGF, KGF, and IL-10 in serum and lung tissue, and improved the 7-day survival rate in experimental LPS-induced ARDS (Chen et al., 2019). Nrf2-overexpressing MSCs derived from amniotic membrane were also more effective than unmodified MSCs at reducing lung epithelial cell apoptosis, edema, injury, and fibrosis in experimental LPS-induced ARDS (Zhang et al., 2018b). In another study, SOD-overexpressing BM-MSCs attenuated lung epithelial cell apoptosis, inflammatory and fibrosis, and increased IL-10 levels and 30-day survival rate in radiation-induced lung injury (Chen et al., 2017a).
Several anti-inflammatory mediators targeted for genetic manipulation are produced endogenously by MSCs or stimulated by MSCs to be produced by other cells during resolution of inflammation. For instance, IL-10 upregulation has been documented extensively after MSC administration in experimental models. Interestingly, serum IL-10 levels were sustainably greater in mice infused with BM-MSCs overexpressing IL-10 compared with direct injection of IL-10 (Wang et al., 2018a). In experimental LPS-induced ARDS, BM-MSCs overexpressing IL-10 increased the presence of IL-10-producing T cells and B cells in both spleen and lung, which may protect mice against the deleterious effects of LPS challenge, thus improving the survival rate (Wang et al., 2018a). Both unmodified MSCs and UC-MSCs overexpressing IL-10 were able to improve lung static compliance in experimental E. coli-induced ARDS. However, UC-MSCs overexpressing IL-10 were more effective than unmodified MSCs at reducing the alveolar-arterial gradient and neutrophil infiltration in lungs, and increased the percentage of lung macrophages and their phagocytic activity (Jerkic et al., 2019). In another study, mice were subjected to lung injury by instillation of HCl, and administration of unmodified MSCs further aggravated the deleterious effects (Islam et al., 2019). Administration of MSCs overexpressing IL-10 otherwise reduced structural lung injury, fibrosis, and inflammation in experimental HCl-induced lung injury (Islam et al., 2019).
IL-1 receptor-like 1 (IL-1RL1), developmental endothelial locus-1 (Del-1), and angiotensin-converting enzyme (ACE)2 are other anti-inflammatory mediators genetically manipulated in MSCs. Overexpression of IL-1RL1, an antagonist receptor for IL-33, enhanced the therapeutic properties of AD-MSCs, leading to decreased structural lung abnormalities, IFN-γ, IL-1β, IL-33, and TLR4 mRNA levels, and increased IL-10 levels after MSCs were infused in experimental LPS-induced ARDS (Martínez-González et al., 2013). BM-MSCs overexpressing Del-1, an anti-inflammatory mediator that inhibits endothelial adhesion of leukocytes, also promoted further therapeutic benefits in experimental LPS-induced ARDS (Zhao et al., 2014b). Compared with unmodified MSCs, BM-MSCs overexpressing Del-1 were more effective at mitigating lung structural abnormalities, edema, neutrophil counts, TNF-α levels, and MPO activity (Zhao et al., 2014b). Although human MSCs do not express endogenous ACE2, a mediator that protects lung tissue against injury by counteracting the effects of angiotensin II, its introduction into UC-MSCs by lentiviral vectors further mitigated symptoms in experimental bleomycin-induced lung fibrosis (Min et al., 2015). UC-MSCs overexpressing ACE2 led to recovery of SOD and glutathione levels, reduction in MDA levels as well as lung injury and fibrosis (Min et al., 2015). In experimental LPS-induced ARDS, BM-MSCs overexpressing ACE2 were also more effective than unmodified MSCs, resulting in a greater reduction in lung injury, vascular permeability, BALF neutrophil counts, IL-1β and IL-6 levels, but increased levels of lung IL-10 and eNOS (He et al., 2015).
Other strategies that have been investigated include the overexpression of mediators that were mechanistically identified as key factors in epithelial and endothelial repair. MSC overexpression of angiopoietin (Ang)-1 or VEGF, two pro-angiogenic factors, improved disease symptoms in animal models (Mei et al., 2007; Xu et al., 2008; Chen et al., 2015b). Compared with unmodified MSCs, BM-MSCs overexpressing Ang-1 reduced BALF neutrophil and total cell counts, levels of inflammation-related mediators (IFN-γ, IL-1β, IL-6, and TNF-α), MPO activity, and alveolar proteinaceous exudate in experimental LPS-induced ARDS (Mei et al., 2007; Xu et al., 2008). In experimental elastase-induced emphysema, BM-MSCs with cis-resveratrol-induced Hsp70 promoter-regulated VEGFA expression were infused and demonstrated to enhance lung function and levels of VEGF and anti-oxidant mediators (HO-1, Nrf2 and SOD), and reduced lung structural abnormalities (Chen et al., 2015b). A subset of placenta-derived MSCs expressing platelet-derived growth factor (PDGF) receptor-β also demonstrated greater expression of pro-angiogenic factors (Ang-1, Ang-2, FGF, PDGF, and VEGF) as well as an increased proliferation rate and wound repair ability in comparison with MSCs not expressing PDGR receptor-β (Wang et al., 2018b). Furthermore, dental pulp-derived MSCs overexpressing HIF-1α demonstrated enhanced angiogenic ability by increasing the amount of the Notch ligand Jagged1 loaded into their EVs (Gonzalez-King et al., 2017).
Compared with unmodified MSCs, administration of BM-MSCs overexpressing FGF-2 was more effective at reducing lung injury, edema, neutrophil counts, MPO activity, and TNF-α levels in experimental LPS-induced ARDS (Zhao et al., 2015). Overexpression of KGF also enhanced the therapeutic actions of BM-MSCs in experimental LPS-induced ARDS, leading not only to improved pulmonary vascular permeability but also mitigated pro-inflammatory responses, which resulted in a reduction in the severity score and mortality rate (Chen et al., 2013). Furthermore, the therapeutic effects of MSCs were enhanced by overexpressing HGF. When co-cultured with MSCs, dendritic cells were converted into the regulatory profile in a mechanism dependent on MSC-secreted HGF (Lu et al., 2019). MSCs overexpressing HGF induced immune tolerance, thus reducing dendritic cell aggregation in the lung tissue of experimental LPS-induced ARDS (Lu et al., 2019). In experimental radiation-induced lung injury, BM-MSCs overexpressing HGF decreased lung histopathologic abnormalities, epithelial cell apoptosis, and mRNA levels of inflammatory (ICAM, IFN-γ, IL-6, and TNF-α) and fibrotic factors (col1α1, col3α1, and TGF-β) (Wang et al., 2013b). Overexpression of HGF in UC-MSCs reduced tracheal occlusion and apoptosis, IFN-γ and TGF-β mRNA levels in allograft trachea, and the percentage of Treg cells and the ratio TH1/TH2 in the spleen of experimental bronchiolitis obliterans (Cao et al., 2016). HGF-overexpressing MSCs were also able to protect lung tissue against injury by HCl instillation (Islam et al., 2019).
Engineered MSCs that overexpress any of the aforementioned factors have been shown to efficiently potentiate therapeutic actions by simultaneously increasing the expression levels of the cited protein and secreting other paracrine immunomodulatory mediators in lung tissue. Furthermore, genetically engineered cells were used in patients with pulmonary arterial hypertension and some positive effects were observed in an early-stage clinical trial (Granton et al., 2015). Nevertheless, genetic modification is usually performed with a viral vector to achieve high transduction efficacy. For such an approach, it can take a few months to acquire genetically modified MSCs for harvesting in sufficient number for infusion, which might limit their use for acute respiratory diseases.
3 Insights from early-stage clinical investigations of MSC-based therapy
MSCs are able to avoid host immune surveillance (Le Blanc et al., 2003; Ankrum et al., 2014; De Witte et al., 2016) and have a long-established safety record. To the best of our knowledge, assessments of MSC preconditioning strategies are still under investigation in pre-clinical studies and there is no clinical trial using preconditioned MSCs in patients with respiratory diseases. Several clinical trials are currently investigating non-preconditioned MSC-based therapy for respiratory diseases, in particular for COVID-19 (da Silva et al., 2021). However, despite some positive effects, clinical benefits were not clearly demonstrated in early-stage clinical studies of MSCs in ARDS and COPD (Weiss et al., 2013; Matthay et al., 2019).
Sixty-two patients with moderate to severe COPD were enrolled in a placebo-controlled, randomized phase 2 clinical trial (Weiss et al., 2013). Although systemic infusion of BM-MSCs was well tolerated and safe, no differences in lung function assessments and the 6-min walking test were observed among the cohorts. However, patients who had increased circulating C-reactive protein (CRP) levels at study entry demonstrated a reduction in CRP levels after treatment with BM-MSCs (Weiss et al., 2013). In a post hoc analysis of clinical trial data, patients were stratified according to the baseline CRP levels (Weiss et al., 2021). Surprisingly, patients with COPD with higher baseline CRP levels (≥4 mg/L) treated with BM-MSCs demonstrated significant improvements in forced vital capacity, forced expiratory volume in 1 s, and the 6-min walking test at 120 days (Weiss et al., 2021). Although variable, these improvements persisted over the 2-year observation period (Weiss et al., 2021).
Sixty patients with moderate to severe ARDS were enrolled in a double-blind, randomized phase 2a clinical trial (Matthay et al., 2019). Intravenous infusion of allogenic BM-MSCs did not induce hemodynamic or respiratory side effects, and thus proved to be safe. Although MSC efficacy could not be properly supported and this was attributed to reduced MSC viability after thawing, patients treated with BM-MSCs with greater viability after thawing demonstrated a reduction in plasma Ang-2 levels after 6 h (Matthay et al., 2019). A subsequent analysis of these data identified a nested cohort of patients with ARDS treated with BM-MSCs who presented a significant reduction of airspace total protein, Ang-2, IL-6, and TNF-R1 levels within 48 h after infusion of BM-MSCs (Wick et al., 2021).
Similar findings have been observed in clinical trials using MSCs to treat graft-versus-host disease (GvHD). Fifty-five patients with GvHD were enrolled in a multicenter phase 2 clinical trial. No side effects during or immediately after MSC infusion were reported. A sub-population demonstrated a complete response and had lower transplantation-associated mortality 1 year after MSC infusion compared with patients who had only partial or no response (Le Blanc et al., 2008). A retrospective study analyzed a cohort of 37 children with GvHD treated with BM-MSCs, and a significant increase in survival rate was reported in complete responders compared with partial and non-responders (Ball et al., 2013).
These findings support the hypothesis of stratifying patients according to disease sub-phenotypes (Matthay et al., 2020; Vasquez et al., 2021) to identify those who are most likely to benefit from MSC therapy. In this context, some studies have applied integrated genomic approaches or cluster analysis to define sub-phenotypes in patients with sepsis (Davenport et al., 2016; Scicluna et al., 2017) and ARDS (Bos et al., 2017). Patient stratification and treatment in a personalized fashion have also been successfully performed in other diseases, such as CF (Lopes-Pacheco, 2020; Lopes-Pacheco et al., 2021). Moreover, determination of a panel of biomarkers that more precisely differentiate disease sub-phenotypes may facilitate the identification of patients who might best respond to MSC therapy.
4 Translational challenges and future directions
Despite the significant progress in assessing MSC-based therapy for respiratory diseases, there is a lack of consistency among the different experimental studies, which might also play a role in the limited success in clinical investigations. For instance, increasing evidence has shown that MSCs from different sources retain certain organ-specific functions and properties, including gene expression and stability, cell-surface proteins, differentiation patterns, secreted cytokine profile, and immunomodulatory ability (Ostanin et al., 2011; Viero Nora et al., 2012; Elahi et al., 2016; Heo et al., 2016; Sacchetti et al., 2016). Accordingly, such differences may have a major impact on MSC survival and therapeutic properties (Wagner et al., 2007; Antunes et al., 2014; Chao et al., 2014; Silva et al., 2018), and further experimental studies should be carried out to comparatively assess the effects of MSCs from different sources and clarify whether cells from one source may promote greater therapeutic responses than others for respiratory diseases.
The influence of donor intrinsic variability on the properties of MSCs has been highlighted in recent studies. MSCs from different donors preconditioned or not with cytokines and hypoxia exhibited a variable degree of immunomodulatory actions (François et al., 2012a; Amati et al., 2017; Kang et al., 2018). MSCs harvested from donors of different ages also demonstrated distinct in vitro differentiation potential and proliferation rates (Zaim et al., 2012; Mehrian et al., 2020). The infusion of aged MSCs or their EVs led to impaired immunomodulatory actions (Yu et al., 2014; Huang et al., 2019) or even deleterious effects in disease models (Lee and Yu, 2020). Moreover, certain diseases can alter MSC niche or cell metabolism, thus promoting a negative impact on their therapeutic ability when they are harvested for either autologous or allogeneic infusion (Silva et al., 2014; Antebi et al., 2018; Kim et al., 2020; Antunes et al., 2021). To identify MSCs of potential clinical use, a number of reference biomarkers should be validated for donor selection and thus the number of batches of good donors should be maximized. Nevertheless, scalability remains limited because successive replications of MSCs and long-term culture to acquire large quantities of cells may affect their properties (Lee et al., 2009; Zaim et al., 2012). The development of scalable platforms, such as bioreactors, is urgently needed to generate a higher number of good-quality MSCs for clinical use.
Some conflicting data exist on whether the freezing/thawing process may be detrimental to the cells. Although some studies have indicated that both freshly thawed and continuously cultured MSCs have similar anti-inflammatory activities (Cruz et al., 2015; Tan et al., 2019; Horie et al., 2020b), others have suggested that freshly thawed MSCs are less effective than continuously cultured MSCs (François et al., 2012b; Chinnadurai et al., 2016). One report also argued that cryopreserved MSCs may require a recovery period before they may be infused (François et al., 2012b). Further studies should investigate the effects of continuously cultured and freshly thawed MSCs in disease-specific models. Meanwhile, MSC cryopreservation and storage conditions should be optimized to prevent reduction in their viability (Matthay et al., 2019). This is of particular relevance for acute respiratory diseases, which occur with rapid onset and progression and there is insufficient time for expanding MSCs. Overall, there is a lack of standardization in MSC manufacturing practices among different facilities and academic centers in the United States and Europe (Trento et al., 2018; Phinney et al., 2019). Accordingly, well-standardized and regulated manufacturing practices need to be successfully implemented among MSC distributors (Fernández-Santos et al., 2022). Specific legislation should be also established for the commercialization if cell therapy products are to become a ready-to-use therapy.
Although most clinical investigations have been using systemic infusions of MSCs, there is no consensus on the optimal delivery route. Differences in the early biodistribution of MSCs were observed after their infusion via different routes (Cardenes et al., 2019). Systemic infusion (e.g., intravenous) ensures a broad distribution of cells throughout the body, and local infusion (e.g., intratracheal or endobronchial) can deliver MSCs directly into the pulmonary compartments. Both delivery routes might provide specific advantages for each respiratory disease, but only a few experimental studies have comparatively assessed different delivery routes and therapeutic responses were equivalent (Curley et al., 2013; Antunes et al., 2014; Alcayaga-Miranda et al., 2015; Devaney et al., 2015). The therapeutic window and index of MSC infusion have been also investigated. In experimental models, further therapeutic responses were observed when MSCs were infused soon after the initial injury (Chang et al., 2012; Hayes et al., 2015; Horie et al., 2020b), which suggests that MSCs might be more effective in acute inflammatory conditions. On the other hand, MSCs were less effective in promoting repair when tissue remodeling was already established (Lopes-Pacheco et al., 2014; Mariñas-Pardo et al., 2014; Sabry et al., 2014; Kitoko et al., 2018). One potential explanation for this limited tissue repair is the short period of permanence of cells in the injured tissue, which might be overcome, at least partially, by preconditioning methods that enhance MSC retention (as described in previous sections) or repeated infusions of MSCs. In this context, multiple infusions of cells were demonstrated to maintain therapeutic benefits for a longer period and promoted a more efficient tissue repair in various experimental models (Lopes-Pacheco et al., 2013; Poggio et al., 2018; Horie et al., 2020b; Castro et al., 2020).
Another important fact to be considered is the co-application of other therapies with MSCs. Many respiratory diseases can be caused by or predispose to infections, therefore MSCs have been used concomitantly with antibiotics in experimental models, and therapeutic responses were superior with combined therapy (Mei et al., 2010; Alcayaga-Miranda et al., 2015; Sung et al., 2016; Horie et al., 2020b). Synergistic effects were also observed with the combination of MSCs with N-acetylcysteine (Shin et al., 2019) or pre-activated disaggregated platelets (Chen et al., 2017b). On the other hand, extracorporeal membrane oxygenation (ECMO) is a therapeutic modality frequently used in critical care illness. In an ex vivo model, increased pressure and reduced flow through the apparatus were observed after MSC infusion because cells were adhering to membrane oxygenation fibers (Millar et al., 2019; von Bahr et al., 2019). Further studies should be performed to determine potential synergistic and interference effects of MSCs with other therapies commonly used in patients with respiratory diseases.
5 Outlook and conclusion
MSCs have been investigated extensively over the last decade as a potential therapy for numerous diseases. However, despite the promising therapeutic effects observed in experimental models, MSC efficacy has fallen far short in most clinical trials for respiratory diseases. Several functional enhancement strategies have been investigated to potentiate the therapeutic actions of MSCs and overcome the limitations associated with reduced MSC efficacy in clinical trials. Genetic manipulation and preconditioning methods can target different properties of MSCs, such as homing, survival, and immunomodulatory activities, and they may be used individually or in combination to further enhance therapeutic outcomes. Patients may not respond equally to MSC therapy, and their stratification based on disease sub-phenotypes may facilitate identification of those who might best benefit from MSC therapy. The environmental conditions of disease can have a major impact on the therapeutic actions of MSCs, therefore different MSC preconditioning strategies can be used to treat disease sub-phenotypes in a personalized medicine approach. Although MSCs still hold immense promise due to their multifaceted mechanisms of action and therapeutic abilities, it has become evident that further experimental research is required to better understand the optimal way to use MSCs and how to translate this information into clinical trials to achieve the greatest clinical outcomes.
Author contributions
ML-P wrote the first version of the manuscript and prepared Figure and Tables 1–4. PR critically reviewed and edited the manuscript. Both authors approved the final version of the manuscript.
Funding
This study was supported by the Brazilian Council for Scientific and Technological Development (CNPq) 443824/2018-5, 403485/2020-7, 408124/2021-0, the Rio de Janeiro State Research Foundation (FAPERJ) E-26/010.001488/2019, and National Institute of Science and Technology for Regenerative Medicine 465656/2014-5.
Acknowledgments
The authors acknowledge Mrs. Moira Elizabeth Schottler and Ms. Lorna O’Brien (authorserv.com) for their assistance in editing the manuscript.
Conflict of interest
The authors declare that the research was conducted in the absence of any commercial or financial relationships that could be construed as a potential conflict of interest.
The handling editor CA declared a past collaboration with the author PR.
Publisher’s note
All claims expressed in this article are solely those of the authors and do not necessarily represent those of their affiliated organizations, or those of the publisher, the editors and the reviewers. Any product that may be evaluated in this article, or claim that may be made by its manufacturer, is not guaranteed or endorsed by the publisher.
References
Abreu, S. C., Lopes-Pacheco, M., Silva, A. L. da, Xisto, D. G., Oliveira, T. B. de, Kitoko, J. Z., et al. (2018). Eicosapentaenoic acid enhances the effects of mesenchymal Stromal cell therapy in experimental allergic asthma. Front. Immunol. 9, 1147. doi:10.3389/fimmu.2018.01147
Abreu, S. C., Xisto, D. G., de Oliveira, T. B., Blanco, N. G., de Castro, L. L., Kitoko, J. Z., et al. (2019). Serum from asthmatic mice potentiates the therapeutic effects of mesenchymal stromal cells in experimental allergic asthma. Stem Cells Transl. Med. 8, 301–312. doi:10.1002/sctm.18-0056
Abreu, S. C., Enes, S. R., Dearborn, J., Goodwin, M., Coffey, A., Borg, Z. D., et al. (2019). Lung inflammatory environments differentially alter mesenchymal stromal cell behavior. Am. J. Physiol. - Lung Cell Mol. Physiol. 317, L823–L831. doi:10.1152/ajplung.00263.2019
Abreu, S. C., Hampton, T. H., Hoffman, E., Dearborn, J., Ashare, A., Sidhu, K. S., et al. (2020). Differential effects of the cystic fibrosis lung inflammatory environment on mesenchymal stromal cells. Am. J. Physiol. - Lung Cell Mol. Physiol. 319, L908–L925. doi:10.1152/AJPLUNG.00218.2020
Abreu, S. C., Lopes-Pacheco, M., Weiss, D. J., and Rocco, P. R. M. (2021). Mesenchymal stromal cell-derived extracellular vesicles in lung diseases: Current status and perspectives. Front. Cell Dev. Biol. 9, 600711. doi:10.3389/fcell.2021.600711
Afzal, M. R., Haider, H. K., Idris, N. M., Jiang, S., Ahmed, R. P. H., and Ashraf, M. (2010). Preconditioning promotes survival and angiomyogenic potential of mesenchymal stem cells in the infarcted heart via NF-kappaB signaling. Antioxidants Redox Signal 12, 693–702. doi:10.1089/ars.2009.2755
Aghasafari, P., George, U., and Pidaparti, R. (2019). A review of inflammatory mechanism in airway diseases. Inflamm. Res. 68, 59–74. doi:10.1007/s00011-018-1191-2
Aguiar, F. S., Melo, A. S., Araújo, A. M. S., Cardoso, A. P., De Souza, S. A. L., Lopes-Pacheco, M., et al. (2020). Autologous bone marrow-derived mononuclear cell therapy in three patients with severe asthma. Stem Cell Res. Ther. 11, 167. doi:10.1186/s13287-020-01675-x
Alcayaga-Miranda, F., Cuenca, J., Martin, A., Contreras, L., Figueroa, F. E., and Khoury, M. (2015). Combination therapy of menstrual derived mesenchymal stem cells and antibiotics ameliorates survival in sepsis. Stem Cell Res. Ther. 6, 199. doi:10.1186/s13287-015-0192-0
Almeria, C., Weiss, R., Roy, M., Tripisciano, C., Kasper, C., Weber, V., et al. (2019). Hypoxia conditioned mesenchymal stem cell-derived extracellular vesicles induce increased vascular tube formation in vitro. Front. Bioeng. Biotechnol. 7, 292. doi:10.3389/fbioe.2019.00292
Amati, E., Sella, S., Perbellini, O., Alghisi, A., Bernardi, M., Chieregato, K., et al. (2017). Generation of mesenchymal stromal cells from cord blood: Evaluation of in vitro quality parameters prior to clinical use. Stem Cell Res. Ther. 8, 14. doi:10.1186/s13287-016-0465-2
Amiri, F., Jahanian-Najafabadi, A., and Roudkenar, M. H. (2015). In vitro augmentation of mesenchymal stem cells viability in stressful microenvironments: In vitro augmentation of mesenchymal stem cells viability. Cell Stress Chaperones 20, 237–251. doi:10.1007/s12192-014-0560-1
Ankrum, J. A., Ong, J. F., and Karp, J. M. (2014). Mesenchymal stem cells: Immune evasive, not immune privileged. Nat. Biotechnol. 32, 252–260. doi:10.1038/nbt.2816
Ansari, S., Chen, C., Hasani-Sadrabadi, M. M., Yu, B., Zadeh, H. H., Wu, B. M., et al. (2017). Hydrogel elasticity and microarchitecture regulate dental-derived mesenchymal stem cell-host immune system cross-talk. Acta Biomater. 60, 181–189. doi:10.1016/j.actbio.2017.07.017
Antebi, B., Walker, K. P., Mohammadipoor, A., Rodriguez, L. A., Montgomery, R. K., Batchinsky, A. I., et al. (2018). The effect of acute respiratory distress syndrome on bone marrow-derived mesenchymal stem cells. Stem Cell Res. Ther. 9, 251. doi:10.1186/s13287-018-0981-3
Antunes, M. A., Abreu, S. C., Cruz, F. F., Teixeira, A. C., Lopes-Pacheco, M., Bandeira, E., et al. (2014). Effects of different mesenchymal stromal cell sources and delivery routes in experimental emphysema. Respir. Res. 15, 118. doi:10.1186/s12931-014-0118-x
Antunes, M. A., Braga, C. L., Oliveira, T. B., Kitoko, J. Z., Castro, L. L., Xisto, D. G., et al. (2021). Mesenchymal stromal cells from emphysematous donors and their extracellular vesicles are unable to reverse cardiorespiratory dysfunction in experimental severe emphysema. Front. Cell Dev. Biol. 9, 661385. doi:10.3389/fcell.2021.661385
Bader, A. M., Klose, K., Bieback, K., Korinth, D., Schneider, M., Seifert, M., et al. (2015). Hypoxic preconditioning increases survival and pro-Angiogenic capacity of human cord blood mesenchymal stromal cells in vitro. PLoS One 10, e0138477. doi:10.1371/journal.pone.0138477
Ball, L. M., Bernardo, M. E., Roelofs, H., van Tol, M. J. D., Contoli, B., Zwaginga, J. J., et al. (2013). Multiple infusions of mesenchymal stromal cells induce sustained remission in children with steroid-refractory, grade III-IV acute graft-versus-host disease. Br. J. Haematol. 163, 501–509. doi:10.1111/bjh.12545
Bartosh, T. J., Ylöstalo, J. H., Mohammadipoor, A., Bazhanov, N., Coble, K., Claypool, K., et al. (2010). Aggregation of human mesenchymal stromal cells (MSCs) into 3D spheroids enhances their antiinflammatory properties. Proc. Natl. Acad. Sci. U S A 107, 13724–13729. doi:10.1073/pnas.1008117107
Bétous, R., Renoud, M-L., Hoede, C., Gonzalez, I., Jones, N., Longy, M., et al. (2017). Human adipose-derived stem cells expanded under ambient oxygen concentration accumulate oxidative DNA lesions and experience procarcinogenic DNA replication stress. Stem Cells Transl. Med. 6, 68–76. doi:10.5966/sctm.2015-0401
Bhang, S. H., Cho, S. W., La, W. G., Lee, T. J., Yang, H. S., Sun, A. Y., et al. (2011). Angiogenesis in ischemic tissue produced by spheroid grafting of human adipose-derived stromal cells. Biomaterials 32, 2734–2747. doi:10.1016/j.biomaterials.2010.12.035
Boregowda, S. V., Krishnappa, V., Chambers, J. W., Lograsso, P. V., Lai, W. T., Ortiz, L. A., et al. (2012). Atmospheric oxygen inhibits growth and differentiation of marrow-derived mouse mesenchymal stem cells via a p53-dependent mechanism: Implications for long-term culture expansion. Stem Cells 30, 975–987. doi:10.1002/stem.1069
Bos, L. D., Schouten, L. R., van Vught, L. A., Wiewel, M. A., Ong, D. S. Y., Cremer, O., et al. (2017). Identification and validation of distinct biological phenotypes in patients with acute respiratory distress syndrome by cluster analysis. Thorax 72, 876–883. doi:10.1136/thoraxjnl-2016-209719
Bustos, M. L., Huleihel, L., Meyer, E. M., Donnenberg, A. D., Donnenberg, V. S., Sciurba, J. D., et al. (2013). Activation of human mesenchymal stem cells impacts their therapeutic abilities in lung injury by increasing interleukin (IL)-10 and IL-1RN levels. Stem Cells Transl. Med. 2, 884–895. doi:10.5966/sctm.2013-0033
Cahill, E. F., Kennelly, H., Carty, F., Mahon, B. P., and English, K. (2016). Hepatocyte growth factor is required for mesenchymal stromal cell protection against bleomycin-induced pulmonary fibrosis. Stem Cells Transl. Med. 5, 1307–1318. doi:10.5966/sctm.2015-0337
Cai, S-X., Liu, A-R., Chen, S., He, H-L., Chen, Q-H., Xu, J-Y., et al. (2016). The orphan receptor tyrosine kinase ROR2 facilitates MSCs to repair lung injury in ARDS animal model. Cell Transpl. 25, 1561–1574. doi:10.3727/096368915X689776
Calabrese, E. J., Bachmann, K. A., Bailer, A. J., Bolger, P. M., Borak, J., Cai, L., et al. (2007). Biological stress response terminology: Integrating the concepts of adaptive response and preconditioning stress within a hormetic dose-response framework. Toxicol. Appl. Pharmacol. 222, 122–128. doi:10.1016/j.taap.2007.02.015
Cao, X-P., Han, D-M., Zhao, L., Guo, Z-K., Xiao, F-J., Zhang, Y-K., et al. (2016). Hepatocyte growth factor enhances the inflammation-alleviating effect of umbilical cord-derived mesenchymal stromal cells in a bronchiolitis obliterans model. Cytotherapy 18, 402–412. doi:10.1016/j.jcyt.2015.12.006
Cardenes, N., Aranda-Valderrama, P., Carney, J. P., Sellares Torres, J., Alvarez, D., Kocyildirim, E., et al. (2019). Cell therapy for ARDS: Efficacy of endobronchial versus intravenous administration and biodistribution of MAPCs in a large animal model. BMJ Open Respir. Res. 6, e000308. doi:10.1136/bmjresp-2018-000308
Cargnoni, A., Ressel, L., Rossi, D., Poli, A., Arienti, D., Lombardi, G., et al. (2012). Conditioned medium from amniotic mesenchymal tissue cells reduces progression of bleomycin-induced lung fibrosis. Cytotherapy 14, 153–161. doi:10.3109/14653249.2011.613930
Carrero, R., Cerrada, I., Lledó, E., Dopazo, J., García-García, F., Rubio, M. P., et al. (2012). IL1β induces mesenchymal stem cells migration and leucocyte chemotaxis through NF-κB. Stem Cell Rev. Rep. 8, 905–916. doi:10.1007/s12015-012-9364-9
Castro, L. L., Kitoko, J. Z., Xisto, D. G., Olsen, P. C., Guedes, H. L. M., Morales, M. M., et al. (2020). Multiple doses of adipose tissue-derived mesenchymal stromal cells induce immunosuppression in experimental asthma. Stem Cells Transl. Med. 9, 250–260. doi:10.1002/sctm.19-0120
Ceccaldi, C., Fullana, S. G., Alfarano, C., Lairez, O., Calise, D., Cussac, D., et al. (2012). Alginate scaffolds for mesenchymal stem cell cardiac therapy: Influence of alginate composition. Cell Transpl. 21, 1969–1984. doi:10.3727/096368912X647252
Chang, C-L., Leu, S., Sung, H-C., Zhen, Y-Y., Cho, C-L., Chen, A., et al. (2012). Impact of apoptotic adipose-derived mesenchymal stem cells on attenuating organ damage and reducing mortality in rat sepsis syndrome induced by cecal puncture and ligation. J. Transl. Med. 10, 244. doi:10.1186/1479-5876-10-244
Chao, Y. H., Wu, H. P., Wu, K. H., Tsai, Y. G., Peng, C. T., Lin, K. C., et al. (2014). An increase in CD3+CD4+CD25+ regulatory T cells after administration of umbilical cord-derived mesenchymal stem cells during sepsis. PLoS One 9, e110338. doi:10.1371/journal.pone.0110338
Chen, J., Li, C., Gao, X., Li, C., Liang, Z., Yu, L., et al. (2013). Keratinocyte growth factor gene delivery via mesenchymal stem cells protects against lipopolysaccharide-induced acute lung injury in mice. PLoS One 8, e83303. doi:10.1371/journal.pone.0083303
Chen, H-H., Chang, C-L., Lin, K-C., Sung, P-H., Chai, H-T., Zhen, Y-Y., et al. (2014). Melatonin augments apoptotic adipose-derived mesenchymal stem cell treatment against sepsis-induced acute lung injury. Am. J. Transl. Res. 6, 439–458.
Chen, S., Shi, J., Zhang, M., Chen, Y., Wang, X., Zhang, L., et al. (2015). Mesenchymal stem cell-laden anti-inflammatory hydrogel enhances diabetic wound healing. Sci. Rep. 5, 18104. doi:10.1038/srep18104
Chen, Y-B., Lan, Y-W., Chen, L-G., Huang, T-T., Choo, K-B., Cheng, W. T. K., et al. (2015). Mesenchymal stem cell-based HSP70 promoter-driven VEGFA induction by resveratrol alleviates elastase-induced emphysema in a mouse model. Cell Stress Chaperones 20, 979–989. doi:10.1007/s12192-015-0627-7
Chen, H-X., Xiang, H., Xu, W-H., Li, M., Yuan, J., Liu, J., et al. (2017). Manganese superoxide dismutase gene-modified mesenchymal stem cells attenuate acute radiation-induced lung injury. Hum. Gene Ther. 28, 523–532. doi:10.1089/hum.2016.106
Chen, C-H., Chen, Y-L., Sung, P-H., Sun, C-K., Chen, K-H., Chen, Y-L., et al. (2017). Effective protection against acute respiratory distress syndrome/sepsis injury by combined adipose-derived mesenchymal stem cells and preactivated disaggregated platelets. Oncotarget 8, 82415–82429. doi:10.18632/oncotarget.19312
Chen, X., Zhang, Y., Wang, W., Liu, Z., Meng, J., and Han, Z. (2018). Mesenchymal stem cells modified with heme oxygenase-1 have enhanced paracrine function and attenuate lipopolysaccharide-induced inflammatory and oxidative damage in pulmonary microvascular endothelial cells. Cell Physiol. Biochem 49, 101–122. doi:10.1159/000492847
Chen, X., Wu, S., Tang, L., Ma, L., Wang, F., Feng, H., et al. (2019). Mesenchymal stem cells overexpressing heme oxygenase-1 ameliorate lipopolysaccharide-induced acute lung injury in rats. J. Cell Physiol. 234, 7301–7319. doi:10.1002/jcp.27488
Cheng, N-C., Chen, S-Y., Li, J-R., and Young, T-H. (2013). Short-term spheroid formation enhances the regenerative capacity of adipose-derived stem cells by promoting stemness, angiogenesis, and chemotaxis. Stem Cells Transl. Med. 2, 584–594. doi:10.5966/sctm.2013-0007
Chinnadurai, R., Copland, I. B., Patel, S. R., and Galipeau, J. (2014). Ido-independent suppression of T cell effector function by IFN-γ-licensed human mesenchymal stromal cells. J. Immunol. 192, 1491–1501. doi:10.4049/jimmunol.1301828
Chinnadurai, R., Copland, I. B., Garcia, M. A., Petersen, C. T., Lewis, C. N., Waller, E. K., et al. (2016). Cryopreserved mesenchymal stromal cells are susceptible to T-cell mediated apoptosis which is partly rescued by IFNγ licensing. Stem Cells 34, 2429–2442. doi:10.1002/stem.2415
Choi, J. R., Pingguan-Murphy, B., Wan Abas, W. A. B., Noor Azmi, M. A., Omar, S. Z., Chua, K. H., et al. (2014). Impact of low oxygen tension on stemness, proliferation and differentiation potential of human adipose-derived stem cells. Biochem Biophys. Res. Commun. 448, 218–224. doi:10.1016/j.bbrc.2014.04.096
Contreras-Lopez, R., Elizondo-Vega, R., Paredes, M. J., Luque-Campos, N., Torres, M. J., Tejedor, G., et al. (2020). HIF1α-dependent metabolic reprogramming governs mesenchymal stem/stromal cell immunoregulatory functions. FASEB J. 34, 8250–8264. doi:10.1096/fj.201902232R
Croes, M., Oner, F. C., Kruyt, M. C., Blokhuis, T. J., Bastian, O., Dhert, W. J. A., et al. (2015). Proinflammatory mediators enhance the osteogenesis of human mesenchymal stem cells after lineage commitment. PLoS One 10, e0132781. doi:10.1371/journal.pone.0132781
Cruz, F. F., Borg, Z. D., Goodwin, M., Sokocevic, D., Wagner, D., McKenna, D. H., et al. (2015). Freshly thawed and continuously cultured human bone marrow-derived mesenchymal stromal cells comparably ameliorate allergic airways inflammation in immunocompetent mice. Stem Cells Transl. Med. 4, 615–624. doi:10.5966/sctm.2014-0268
Cui, G-H., Wu, J., Mou, F-F., Xie, W-H., Wang, F-B., Wang, Q-L., et al. (2018). Exosomes derived from hypoxia-preconditioned mesenchymal stromal cells ameliorate cognitive decline by rescuing synaptic dysfunction and regulating inflammatory responses in APP/PS1 mice. FASEB J. 32, 654–668. doi:10.1096/fj.201700600R
Curley, G. F., Ansari, B., Hayes, M., Devaney, J., Masterson, C., Ryan, A., et al. (2013). Effects of intratracheal mesenchymal stromal cell therapy during recovery and resolution after ventilator-induced lung injury. Anesthesiology 118, 924–932. doi:10.1097/ALN.0b013e318287ba08
da Silva, K. N., Gobatto, A. L. N., Costa-Ferro, Z. S. M., Cavalcante, B. R. R., Caria, A. C. I., de Aragão França, L. S., et al. (2021). Is there a place for mesenchymal stromal cell-based therapies in the therapeutic armamentarium against COVID-19? Stem Cell Res. Ther. 12, 425. doi:10.1186/s13287-021-02502-7
Das, B., Bayat-Mokhtari, R., Tsui, M., Lotfi, S., Tsuchida, R., Felsher, D. W., et al. (2012). HIF-2α suppresses p53 to enhance the stemness and regenerative potential of human embryonic stem cells. Stem Cells 30, 1685–1695. doi:10.1002/stem.1142
Davenport, E. E., Burnham, K. L., Radhakrishnan, J., Humburg, P., Hutton, P., Mills, T. C., et al. (2016). Genomic landscape of the individual host response and outcomes in sepsis: A prospective cohort study. Lancet Respir. Med. 4, 259–271. doi:10.1016/S2213-2600(16)00046-1
Davies, L. C., Heldring, N., Kadri, N., and Le Blanc, K. (2017). Mesenchymal stromal cell secretion of programmed death-1 ligands regulates T cell mediated immunosuppression. Stem Cells 35, 766–776. doi:10.1002/stem.2509
de Carvalho, L. R. P., Abreu, S. C., de Castro, L. L., Andrade da Silva, L. H., Silva, P. M., Vieira, J. B., et al. (2021). Mitochondria-rich fraction isolated from mesenchymal stromal cells reduces lung and distal organ injury in experimental sepsis. Crit. Care Med. 49, e880–e890. doi:10.1097/ccm.0000000000005056
de Castro, L. L., Lopes-Pacheco, M., Weiss, D. J., Cruz, F. F., and Rocco, P. R. M. (2019). Current understanding of the immunosuppressive properties of mesenchymal stromal cells. J. Mol. Med. 97, 605–618. doi:10.1007/s00109-019-01776-y
De Oliveira, H. D., De Melo, E. B. B., Silva, J. D., Kitoko, J. Z., Gutfilen, B., Barboza, T., et al. (2017). Therapeutic effects of bone marrow-derived mononuclear cells from healthy or silicotic donors on recipient silicosis mice. Stem Cell Res. Ther. 8, 259. doi:10.1186/s13287-017-0699-7
De Witte, S. F. H., Franquesa, M., Baan, C. C., and Hoogduijn, M. J. (2016). Toward development of imesenchymal stem cells for immunomodulatory therapy. Front. Immunol. 6, 648. doi:10.3389/fimmu.2015.00648
de Witte, S. F. H., Luk, F., Sierra Parraga, J. M., Gargesha, M., Merino, A., Korevaar, S. S., et al. (2018). Immunomodulation by therapeutic mesenchymal stromal cells (MSC) is triggered through phagocytosis of MSC by monocytic cells. Stem Cells 36, 602–615. doi:10.1002/stem.2779
Deng, Z., Fear, M. W., Suk Choi, Y., Wood, F. M., Allahham, A., Mutsaers, S. E., et al. (2020). The extracellular matrix and mechanotransduction in pulmonary fibrosis. Int. J. Biochem Cell Biol. 126, 105802. doi:10.1016/j.biocel.2020.105802
Devaney, J., Horie, S., Masterson, C., Elliman, S., Barry, F., O’Brien, T., et al. (2015). Human mesenchymal stromal cells decrease the severity of acute lung injury induced by E. coli in the rat. Thorax 70, 625–635. doi:10.1136/thoraxjnl-2015-206813
Dominici, M., Le Blanc, K., Mueller, I., Slaper-Cortenbach, I., Marini, F. C., Krause, D. S., et al. (2006). Minimal criteria for defining multipotent mesenchymal stromal cells. The International Society for Cellular Therapy position statement. Cytotherapy 8, 315–317. doi:10.1080/14653240600855905
Dubon, M. J., Yu, J., Choi, S., and Park, K. (2018). Transforming growth factor β induces bone marrow mesenchymal stem cell migration via noncanonical signals and N-cadherin. J. Cell Physiol. 233, 201–213. doi:10.1002/jcp.25863
Dvořáková, J., Kučera, L., Kučera, J., Švík, K., Foglarová, M., Muthný, T., et al. (2014). Chondrogenic differentiation of mesenchymal stem cells in a hydrogel system based on an enzymatically crosslinked tyramine derivative of hyaluronan. J. Biomed. Mater Res. - Part A 102, 3523–3530. doi:10.1002/jbm.a.35033
Elahi, K. C., Klein, G., Avci-Adali, M., Sievert, K. D., Macneil, S., and Aicher, W. K. (2016). Human mesenchymal stromal cells from different sources diverge in their expression of cell surface proteins and display distinct differentiation patterns. Stem Cells Int. 2016, 5646384. doi:10.1155/2016/5646384
Enes, S. R., Hampton, T. H., Barua, J., McKenna, D. H., Dos Santos, C. C., Amiel, E., et al. (2021). Healthy versus inflamed lung environments differentially affect mesenchymal stromal cells. Eur. Respir. J. 58, 2004149. doi:10.1183/13993003.04149-2020
English, K., Barry, F. P., Field-Corbett, C. P., and Mahon, B. P. (2007). IFN-γ and TNF-α differentially regulate immunomodulation by murine mesenchymal stem cells. Immunol. Lett. 110, 91–100. doi:10.1016/j.imlet.2007.04.001
Estrada, J. C., Albo, C., Benguría, A., Dopazo, A., López-Romero, P., Carrera-Quintanar, L., et al. (2012). Culture of human mesenchymal stem cells at low oxygen tension improves growth and genetic stability by activating glycolysis. Cell Death Differ. 19, 743–755. doi:10.1038/cdd.2011.172
Fan, H., Zhao, G., Liu, L., Liu, F., Gong, W., Liu, X., et al. (2012). Pre-treatment with IL-1β enhances the efficacy of MSC transplantation in DSS-induced colitis. Cell Mol. Immunol. 9, 473–481. doi:10.1038/cmi.2012.40
Fehrer, C., Brunauer, R., Laschober, G., Unterluggauer, H., Reitinger, S., Kloss, F., et al. (2007). Reduced oxygen tension attenuates differentiation capacity of human mesenchymal stem cells and prolongs their lifespan. Aging Cell 6, 745–757. doi:10.1111/j.1474-9726.2007.00336.x
Fergie, N., Todd, N., McClements, L., McAuley, D., O’Kane, C., and Krasnodembskaya, A. (2019). Hypercapnic acidosis induces mitochondrial dysfunction and impairs the ability of mesenchymal stem cells to promote distal lung epithelial repair. FASEB J. 33, 5585–5598. doi:10.1096/fj.201802056R
Fernández-Santos, M. E., Garcia-Arranz, M., Andreu, E. J., García-Hernández, A. M., López-Parra, M., Villarón, E., et al. (2022). Optimization of mesenchymal stromal cell (MSC) manufacturing processes for a better therapeutic outcome. Front. Immunol. 13, 918565. doi:10.3389/fimmu.2022.918565
Ferro, F., Spelat, R., Shaw, G., Duffy, N., Islam, M. N., O’Shea, P. M., et al. (2019). Survival/Adaptation of bone marrow-derived mesenchymal stem cells after long-term starvation through selective processes. Stem Cells 37, 813–827. doi:10.1002/stem.2998
Fischer, U. M., Harting, M. T., Jimenez, F., Monzon-Posadas, W. O., Xue, H., Savitz, S. I., et al. (2009). Pulmonary passage is a major obstacle for intravenous stem cell delivery: The pulmonary first-pass effect. Stem Cells Dev. 18, 683–692. doi:10.1089/scd.2008.0253
Follin, B., Juhl, M., Cohen, S., Pedersen, A. E., Kastrup, J., and Ekblond, A. (2016). Increased paracrine immunomodulatory potential of mesenchymal stromal cells in three-dimensional culture. Tissue Eng. Part B Rev. 22, 322–329. doi:10.1089/ten.TEB.2015.0532
François, M., Romieu-Mourez, R., Li, M., and Galipeau, J. (2012). Human MSC suppression correlates with cytokine induction of indoleamine 2,3-dioxygenase and bystander M2 macrophage differentiation. Mol. Ther. 20, 187–195. doi:10.1038/mt.2011.189
François, M., Copland, I. B., Yuan, S., Romieu-Mourez, R., Waller, E. K., and Galipeau, J. (2012). Cryopreserved mesenchymal stromal cells display impaired immunosuppressive properties as a result of heat-shock response and impaired interferon-γ licensing. Cytotherapy 14, 147–152. doi:10.3109/14653249.2011.623691
Frith, J. E., Thomson, B., and Genever, P. G. (2010). Dynamic three-dimensional culture methods enhance mesenchymal stem cell properties and increase therapeutic potential. Tissue Eng. - Part C Methods 16, 735–749. doi:10.1089/ten.tec.2009.0432
Fujisawa, K., Takami, T., Okada, S., Hara, K., Matsumoto, T., Yamamoto, N., et al. (2018). Analysis of metabolomic changes in mesenchymal stem cells on treatment with desferrioxamine as a hypoxia mimetic compared with hypoxic conditions. Stem Cells 36, 1226–1236. doi:10.1002/stem.2826
Garrido-Pascual, P., Alonso-Varona, A., Castro, B., Burón, M., and Palomares, T. (2020). H2O2-preconditioned human adipose-derived stem cells (HC016) increase their resistance to oxidative stress by overexpressing Nrf2 and bioenergetic adaptation. Stem Cell Res. Ther. 11, 335. doi:10.1186/s13287-020-01851-z
Gonzalez, RoviraY. I., Lynch, P. J., Thompson, E. E., Stultz, B. G., and Hursh, D. A. (2016). In vitro cytokine licensing induces persistent permissive chromatin at the Indoleamine 2,3-dioxygenase promoter. Cytotherapy 18, 1114–1128. doi:10.1016/j.jcyt.2016.05.017
Gonzalez-King, H., García, N. A., Ontoria-Oviedo, I., Ciria, M., Montero, J. A., and Sepúlveda, P. (2017). Hypoxia inducible factor-1α potentiates jagged 1-mediated angiogenesis by mesenchymal stem cell-derived exosomes. Stem Cells 35, 1747–1759. doi:10.1002/stem.2618
Goodwin, M., Sueblinvong, V., Eisenhauer, P., Ziats, N. P., LeClair, L., Poynter, M. E., et al. (2011). Bone marrow-derived mesenchymal stromal cells inhibit Th2-mediated allergic airways inflammation in mice. Stem Cells 29, 1137–1148. doi:10.1002/stem.656
Gorin, C., Rochefort, G. Y., Bascetin, R., Ying, H., Lesieur, J., Sadoine, J., et al. (2016). Priming dental pulp stem cells with fibroblast growth factor-2 increases angiogenesis of implanted tissue-engineered constructs through hepatocyte growth factor and vascular endothelial growth factor secretion. Stem Cells Transl. Med. 5, 392–404. doi:10.5966/sctm.2015-0166
Granton, J., Langleben, D., Kutryk, M. B., Camack, N., Galipeau, J., Courtman, D. W., et al. (2015). Endothelial NO-synthase gene-enhanced progenitor cell therapy for pulmonary arterial hypertension: The PHACeT trial. Circ. Res. 117, 645–654. doi:10.1161/CIRCRESAHA.114.305951
Guan, Q., Ezzati, P., Spicer, V., Krokhin, O., Wall, D., and Wilkins, J. A. (2017). Interferon γ induced compositional changes in human bone marrow derived mesenchymal stem/stromal cells. Clin. Proteomics 14, 26. doi:10.1186/s12014-017-9161-1
Gupta, N., Sinha, R., Krasnodembskaya, A., Xu, X., Nizet, V., Matthay, M. A., et al. (2018). The TLR4-PAR1 Axis regulates bone marrow mesenchymal stromal cell survival and therapeutic capacity in experimental bacterial pneumonia. Stem Cells 36, 796–806. doi:10.1002/stem.2796
Hackel, A., Aksamit, A., Bruderek, K., Lang, S., and Brandau, S. (2021). TNF-α and IL-1β sensitize human MSC for IFN-γ signaling and enhance neutrophil recruitment. Eur. J. Immunol. 51, 319–330. doi:10.1002/eji.201948336
Hajinejad, M., Pasbakhsh, P., Omidi, A., Mortezaee, K., Nekoonam, S., Mahmoudi, R., et al. (2018). Resveratrol pretreatment enhanced homing of SDF-1α-preconditioned bone marrow-derived mesenchymal stem cells in a rat model of liver cirrhosis. J. Cell Biochem 119, 2939–2950. doi:10.1002/jcb.26500
Han, X., Yang, Q., Lin, L., Xu, C., Zheng, C., Chen, X., et al. (2014). Interleukin-17 enhances immunosuppression by mesenchymal stem cells. Cell Death Differ. 21, 1758–1768. doi:10.1038/cdd.2014.85
Han, Y. S., Lee, J. H., Yoon, Y. M., Yun, C. W., Noh, H., and Lee, S. H. (2016). Hypoxia-induced expression of cellular prion protein improves the therapeutic potential of mesenchymal stem cells. Cell Death Dis. 7, e2395. doi:10.1038/cddis.2016.310
Han, J., Lu, X., Zou, L., Xu, X., and Qiu, H. (2016). E-prostanoid 2 receptor overexpression promotes mesenchymal stem cell attenuated lung injury. Hum. Gene Ther. 27, 621–630. doi:10.1089/hum.2016.003
Haque, N., Rahman, M. T., Abu Kasim, N. H., and Alabsi, A. M. (2013). Hypoxic culture conditions as a solution for mesenchymal stem cell based regenerative therapy. Sci. World J. 27, 632972. doi:10.1155/2013/632972
Hayes, M., Masterson, C., Devaney, J., Barry, F., Elliman, S., O’Brien, T., et al. (2015). Therapeutic efficacy of human mesenchymal stromal cells in the repair of established ventilator-induced lung injury in the rat. Anesthesiology 122, 363–373. doi:10.1097/ALN.0000000000000545
He, H., Liu, L., Chen, Q., Liu, A., Cai, S., Yang, Y., et al. (2015). Mesenchymal stem cells overexpressing angiotensin-converting enzyme 2 rescue lipopolysaccharide-induced lung injury. Cell Transpl. 24, 1699–1715. doi:10.3727/096368914X685087
Hemeda, H., Jakob, M., Ludwig, A. K., Giebel, B., Lang, S., and Brandau, S. (2010). Interferon-γ and tumor necrosis factor-α differentially affect cytokine expression and migration properties of mesenchymal stem cells. Stem Cells Dev. 19, 693–706. doi:10.1089/scd.2009.0365
Heo, J. S., Choi, Y., Kim, H. S., and Kim, H. O. (2016). Comparison of molecular profiles of human mesenchymal stem cells derived from bone marrow, umbilical cord blood, placenta and adipose tissue. Int. J. Mol. Med. 37, 115–125. doi:10.3892/ijmm.2015.2413
Hong, Y., Kim, Y-S., Hong, S-H., and Oh, Y-M. (2016). Therapeutic effects of adipose-derived stem cells pretreated with pioglitazone in an emphysema mouse model. Exp. Mol. Med. 48, e266. doi:10.1038/emm.2016.93
Horie, S., Gaynard, S., Murphy, M., Barry, F., Scully, M., O’Toole, D., et al. (2020). Cytokine pre-activation of cryopreserved xenogeneic-free human mesenchymal stromal cells enhances resolution and repair following ventilator-induced lung injury potentially via a KGF-dependent mechanism. Intensive Care Med. Exp. 8, 8. doi:10.1186/s40635-020-0295-5
Horie, S., Masterson, C., Brady, J., Loftus, P., Horan, E., O’Flynn, L., et al. (2020). Umbilical cord-derived CD362+ mesenchymal stromal cells for E. coli pneumonia: Impact of dose regimen, passage, cryopreservation, and antibiotic therapy. Stem Cell Res. Ther. 11, 116. doi:10.1186/s13287-020-01624-8
Horwitz, E. M., Le Blanc, K., Dominici, M., Mueller, I., Slaper-Cortenbach, I., Marini, F. C., et al. (2005). Clarification of the nomenclature for MSC: The international society for cellular therapy position statement. Cytotherapy 7, 393–395. doi:10.1080/14653240500319234
Hu, X., Yu, S. P., Fraser, J. L., Lu, Z., Ogle, M. E., Wang, J. A., et al. (2008). Transplantation of hypoxia-preconditioned mesenchymal stem cells improves infarcted heart function via enhanced survival of implanted cells and angiogenesis. J. Thorac. Cardiovasc Surg. 135, 799–808. doi:10.1016/j.jtcvs.2007.07.071
Huang, H., Kim, H. J., Chang, E. J., Lee, Z. H., Hwang, S. J., Kim, H. M., et al. (2009). IL-17 stimulates the proliferation and differentiation of human mesenchymal stem cells: Implications for bone remodeling. Cell Death Differ. 16, 1332–1343. doi:10.1038/cdd.2009.74
Huang, R., Qin, C., Wang, J., Hu, Y., Zheng, G., Qiu, G., et al. (2019). Differential effects of extracellular vesicles from aging and young mesenchymal stem cells in acute lung injury. Aging (Albany NY) 11, 7996–8014. doi:10.18632/aging.102314
Ingenito, E. P., Tsai, L., Murthy, S., Tyagi, S., Mazan, M., and Hoffman, A. (2012). Autologous lung-derived mesenchymal stem cell transplantation in experimental emphysema. Cell Transpl. 21, 175–189. doi:10.3727/096368910X550233
Islam, D., Huang, Y., Fanelli, V., Delsedime, L., Wu, S., Khang, J., et al. (2019). Identification and modulation of microenvironment is crucial for effective mesenchymal stromal cell therapy in acute lung injury. Am. J. Respir. Crit. Care Med. 199, 1214–1224. doi:10.1164/rccm.201802-0356OC
Jerkic, M., Masterson, C., Ormesher, L., Gagnon, S., Goyal, S., Rabani, R., et al. (2019). Overexpression of IL-10 enhances the efficacy of human umbilical-cord-derived mesenchymal stromal cells in E. Coli pneumosepsis. J. Clin. Med. 8, 847. doi:10.3390/jcm8060847
Jin, W., Liang, X., Brooks, A., Futrega, K., Liu, X., Doran, M. R., et al. (2018). Modelling of the SDF-1/CXCR4 regulated in vivo homing of therapeutic mesenchymal stem/stromal cells in mice. PeerJ 6, e6072. doi:10.7717/peerj.6072
Kang, I., Lee, B-C., Choi, S. W., Lee, J. Y., Kim, J-J., Kim, B-E., et al. (2018). Donor-dependent variation of human umbilical cord blood mesenchymal stem cells in response to hypoxic preconditioning and amelioration of limb ischemia. Exp. Mol. Med. 50, 35–15. doi:10.1038/s12276-017-0014-9
Khan, I., Ali, A., Akhter, M. A., Naeem, N., Chotani, M. A., Mustafa, T., et al. (2016). Preconditioning of mesenchymal stem cells with 2,4-dinitrophenol improves cardiac function in infarcted rats. Life Sci. 162, 60–69. doi:10.1016/j.lfs.2016.08.014
Kim, D. S., Lee, W. H., Lee, M. W., Park, H. J., Jang, I. K., Lee, J. W., et al. (2018). Involvement of TLR3-dependent PGES expression in immunosuppression by human bone marrow mesenchymal stem cells. Stem Cell Rev. Rep. 14, 286–293. doi:10.1007/s12015-017-9793-6
Kim, J-H., Han, J., Seo, D., Yoon, J. H., Yoon, D., Hong, J., et al. (2020). Characterization of mesenchymal stem cells derived from patients with cerebellar ataxia: Downregulation of the anti-inflammatory secretome profile. Cells 9, 212. doi:10.3390/cells9010212
Kitoko, J. Z., de Castro, L. L., Nascimento, A. P., Abreu, S. C., Cruz, F. F., Arantes, A. C., et al. (2018). Therapeutic administration of bone marrow-derived mesenchymal stromal cells reduces airway inflammation without up-regulating Tregs in experimental asthma. Clin. Exp. Allergy 48, 205–216. doi:10.1111/cea.13048
Koch, B., Geßner, A., Farmand, S., Fuhrmann, D. C., Chiocchetti, A. G., Schubert, R., et al. (2022). Effects of hypoxia on RNA cargo in extracellular vesicles from human adipose-derived stromal/stem cells. Int. J. Mol. Sci. 23, 7384. doi:10.3390/ijms23137384
Krampera, M., Cosmi, L., Angeli, R., Pasini, A., Liotta, F., Andreini, A., et al. (2006). Role for interferon-γ in the immunomodulatory activity of human bone marrow mesenchymal stem cells. Stem Cells 24, 386–398. doi:10.1634/stemcells.2005-0008
Krasnodembskaya, A., Samarani, G., Song, Y., Zhuo, H., Su, X., Lee, J. W., et al. (2012). Human mesenchymal stem cells reduce mortality and bacteremia in gram-negative sepsis in mice in part by enhancing the phagocytic activity of blood monocytes. Am. J. Physiol. - Lung Cell Mol. Physiol. 302, 1003–1013. doi:10.1152/ajplung.00180.2011
Kusuma, G. D., Li, A., Zhu, D., McDonald, H., Inocencio, I. M., Chambers, D. C., et al. (2022). Effect of 2D and 3D culture microenvironments on mesenchymal stem cell-derived extracellular vesicles potencies. Front. Cell Dev. Biol. 10, 819726. doi:10.3389/fcell.2022.819726
Kwon, S. Y., Chun, S. Y., Ha, Y. S., Kim, D. H., Kim, J., Song, P. H., et al. (2017). Hypoxia enhances cell properties of human mesenchymal stem cells. Tissue Eng. Regen. Med. 14, 595–604. doi:10.1007/s13770-017-0068-8
Lan, Y. W., Choo, K. B., Chen, C. M., Hung, T. H., Chen, Y. B., Hsieh, C. H., et al. (2015). Hypoxia-preconditioned mesenchymal stem cells attenuate bleomycin-induced pulmonary fibrosis. Stem Cell Res. Ther. 6, 97. doi:10.1186/s13287-015-0081-6
Lan, Y-W., Theng, S-M., Huang, T-T., Choo, K-B., Chen, C-M., Kuo, H-P., et al. (2017). Oncostatin M-preconditioned mesenchymal stem cells alleviate bleomycin-induced pulmonary fibrosis through paracrine effects of the hepatocyte growth factor. Stem Cells Transl. Med. 6, 1006–1017. doi:10.5966/sctm.2016-0054
Lathrop, M. J., Brooks, E. M., Bonenfant, N. R., Sokocevic, D., Borg, Z. D., Goodwin, M., et al. (2014). Mesenchymal stromal cells mediate Aspergillus hyphal extract-induced allergic airway inflammation by inhibition of the Th17 signaling pathway. Stem Cells Transl. Med. 3, 194–205. doi:10.5966/sctm.2013-0061
Lavrentieva, A., Majore, I., Kasper, C., and Hass, R. (2010). Effects of hypoxic culture conditions on umbilical cord-derived human mesenchymal stem cells. Cell Commun. Signal 8, 18. doi:10.1186/1478-811X-8-18
Le Blanc, K., Tammik, C., Rosendahl, K., Zetterberg, E., and Ringdén, O. (2003). HLA expression and immunologic properties of differentiated and undifferentiated mesenchymal stem cells. Exp. Hematol. 31, 890–896. doi:10.1016/S0301-472X(03)00110-3
Le Blanc, K., Frassoni, F., Ball, L., Locatelli, F., Roelofs, H., Lewis, I., et al. (2008). Mesenchymal stem cells for treatment of steroid-resistant, severe, acute graft-versus-host disease: A phase II study. Lancet (London, Engl. 371, 1579–1586. doi:10.1016/S0140-6736(08)60690-X
Leblond, A-L., Naud, P., Forest, V., Gourden, C., Sagan, C., Romefort, B., et al. (2009). Developing cell therapy techniques for respiratory disease: Intratracheal delivery of genetically engineered stem cells in a murine model of airway injury. Hum. Gene Ther. 20, 1329–1343. doi:10.1089/hum.2009.035
Lee, B-C., and Yu, K-R. (2020). Impact of mesenchymal stem cell senescence on inflammaging. BMB Rep. 53, 65–73. doi:10.5483/BMBRep.2020.53.2.291
Lee, K. A., Shim, W., Paik, M. J., Lee, S. C., Shin, J. Y., Ahn, Y. H., et al. (2009). Analysis of changes in the viability and gene expression profiles of human mesenchymal stromal cells over time. Cytotherapy 11, 688–697. doi:10.3109/14653240902974032
Lee, S., Kim, H-S., Roh, K-H., Lee, B-C., Shin, T-H., Yoo, J-M., et al. (2015). DNA methyltransferase inhibition accelerates the immunomodulation and migration of human mesenchymal stem cells. Sci. Rep. 5, 8020. doi:10.1038/srep08020
Lee, N-H., Myeong, S. H., Son, H. J., Hwang, J. W., Lee, N. K., Chang, J. W., et al. (2020). Ethionamide preconditioning enhances the proliferation and migration of human Wharton’s jelly-derived mesenchymal stem cells. Int. J. Mol. Sci. 21, 7013. doi:10.3390/ijms21197013
Lei, J., Wang, Z., Hui, D., Yu, W., Zhou, D., Xia, W., et al. (2011). Ligation of TLR2 and TLR4 on murine bone marrow-derived mesenchymal stem cells triggers differential effects on their immunosuppressive activity. Cell Immunol. 271, 147–156. doi:10.1016/j.cellimm.2011.06.014
Lennon, D. P., Edmison, J. M., and Caplan, A. I. (2001). Cultivation of rat marrow-derived mesenchymal stem cells in reduced oxygen tension: Effects on in vitro and in vivo osteochondrogenesis. J. Cell Physiol. 187, 345–355. doi:10.1002/jcp.1081
Levy, O., Mortensen, L. J., Boquet, G., Tong, Z., Perrault, C., Benhamou, B., et al. (2015). A small-molecule screen for enhanced homing of systemically infused cells. Cell Rep. 10, 1261–1268. doi:10.1016/j.celrep.2015.01.057
Li, S., Deng, Y., Feng, J., and Ye, W. (2009). Oxidative preconditioning promotes bone marrow mesenchymal stem cells migration and prevents apoptosis. Cell Biol. Int. 33, 411–418. doi:10.1016/j.cellbi.2009.01.012
Li, Q., Ma, Y., Li, L., Bao, J., and Zhang, L. (2015). Flagellin influences the expression of a variety of important cytokines and chemokines without affecting the immune status of umbilical cord mesenchymal stem cells. Mol. Med. Rep. 12, 6955–6961. doi:10.3892/mmr.2015.4276
Li, D., Liu, Q., Qi, L., Dai, X., Liu, H., and Wang, Y. (2016). Low levels of TGF-β1 enhance human umbilical cord-derived mesenchymal stem cell fibronectin production and extend survival time in a rat model of lipopolysaccharide-induced acute lung injury. Mol. Med. Rep. 14, 1681–1692. doi:10.3892/mmr.2016.5416
Li, B., Li, C., Zhu, M., Zhang, Y., Du, J., Xu, Y., et al. (2017). Hypoxia-induced mesenchymal stromal cells exhibit an enhanced therapeutic effect on radiation-induced lung injury in mice due to an increased proliferation potential and enhanced antioxidant ability. Cell Physiol. Biochem 44, 1295–1310. doi:10.1159/000485490
Li, R., Li, Y., and Dong, X. (2020). Preconditioning mesenchymal stromal cells with flagellin enhances the anti-inflammatory ability of their secretome against lipopolysaccharide-induced acute lung injury. Mol. Med. Rep. 22, 2753–2766. doi:10.3892/mmr.2020.11380
Liang, O. D., Mitsialis, S. A., Chang, M. S., Vergadi, E., Lee, C., Aslam, M., et al. (2011). Mesenchymal stromal cells expressing heme oxygenase-1 reverse pulmonary hypertension. Stem Cells 29, 99–107. doi:10.1002/stem.548
Lim, J., Lee, S., Ju, H., Kim, Y., Heo, J., Lee, H-Y., et al. (2017). Valproic acid enforces the priming effect of sphingosine-1 phosphate on human mesenchymal stem cells. Int. J. Mol. Med. 40, 739–747. doi:10.3892/ijmm.2017.3053
Lin, T., Pajarinen, J., Nabeshima, A., Lu, L., Nathan, K., Jämsen, E., et al. (2017). Preconditioning of murine mesenchymal stem cells synergistically enhanced immunomodulation and osteogenesis. Stem Cell Res. Ther. 8, 277. doi:10.1186/s13287-017-0730-z
Liu, Y-Y., Chiang, C-H., Hung, S-C., Chian, C-F., Tsai, C-L., Chen, W-C., et al. (2017). Hypoxia-preconditioned mesenchymal stem cells ameliorate ischemia/reperfusion-induced lung injury. PLoS One 12, e0187637. doi:10.1371/journal.pone.0187637
Lopes-Pacheco, M., Xisto, D. G., Ornellas, F. M., Antunes, M. A., Abreu, S. C., Rocco, P. R. M., et al. (2013). Repeated administration of bone marrow-derived cells prevents disease progression in experimental silicosis. Cell Physiol. Biochem 32, 1681–1694. doi:10.1159/000356603
Lopes-Pacheco, M., Ventura, T. G., Oliveira, H. D. de, Monção-Ribeiro, L. C., Gutfilen, B., Souza, S. A. L. de, et al. (2014). Infusion of bone marrow mononuclear cells reduces lung fibrosis but not inflammation in the late stages of murine silicosis. PLoS One 9, e109982. doi:10.1371/journal.pone.0109982
Lopes-Pacheco, M., Bandeira, E., and Morales, M. M. (2016). Cell-based therapy for silicosis. Stem Cells Int. 2016, 5091838. doi:10.1155/2016/5091838
Lopes-Pacheco, M., Robba, C., Rocco, P. R. M., and Pelosi, P. (2020). Current understanding of the therapeutic benefits of mesenchymal stem cells in acute respiratory distress syndrome. Cell Biol. Toxicol. 36, 83–102. doi:10.1007/s10565-019-09493-5
Lopes-Pacheco, M., Pedemonte, N., and Veit, G. (2021). Discovery of CFTR modulators for the treatment of cystic fibrosis. Expert Opin. Drug Discov. 16, 897–913. doi:10.1080/17460441.2021.1912732
Lopes-Pacheco, M. (2020). CFTR modulators: The changing face of cystic fibrosis in the era of precision medicine. Front. Pharmacol. 10, 1662. doi:10.3389/fphar.2019.01662
Lu, X., Han, J., Xu, X., Xu, J., Liu, L., Huang, Y., et al. (2017). PGE2 promotes the migration of mesenchymal stem cells through the activation of FAK and ERK1/2 pathway. Stem Cells Int. 2017, 8178643. doi:10.1155/2017/8178643
Lu, Z., Chang, W., Meng, S., Xu, X., Xie, J., Guo, F., et al. (2019). Mesenchymal stem cells induce dendritic cell immune tolerance via paracrine hepatocyte growth factor to alleviate acute lung injury. Stem Cell Res. Ther. 10, 372. doi:10.1186/s13287-019-1488-2
Lv, H., Yuan, X., Zhang, J., Lu, T., Yao, J., Zheng, J., et al. (2021). Heat shock preconditioning mesenchymal stem cells attenuate acute lung injury via reducing NLRP3 inflammasome activation in macrophages. Stem Cell Res. Ther. 12, 290. doi:10.1186/s13287-021-02328-3
Magne, B., Dedier, M., Nivet, M., Coulomb, B., Banzet, S., Lataillade, J-J., et al. (2020). IL-1β-Primed mesenchymal stromal cells improve epidermal substitute engraftment and wound healing via matrix metalloproteinases and transforming growth factor-β1. J. Invest Dermatol 140, 688–698. doi:10.1016/j.jid.2019.07.721
Mahmoudi, T., Abdolmohammadi, K., Bashiri, H., Mohammadi, M., Rezaie, M. J., Fathi, F., et al. (2020). Hydrogen peroxide preconditioning promotes protective effects of umbilical cord vein mesenchymal stem cells in experimental pulmonary fibrosis. Adv. Pharm. Bull. 10, 72–80. doi:10.15171/apb.2020.009
Malaquias, M. A. S., Oyama, L. A., Jericó, P. C., Costa, I., Padilha, G., Nagashima, S., et al. (2018). Effects of mesenchymal stromal cells play a role the oxidant/antioxidant balance in a murine model of asthma. Allergol. Immunopathol. Madr. 46, 136–143. doi:10.1016/j.aller.2017.06.003
Mariñas-Pardo, L., Mirones, I., Amor-Carro, O., Fraga-Iriso, R., Lema-Costa, B., Cubillo, I., et al. (2014). Mesenchymal stem cells regulate airway contractile tissue remodeling in murine experimental asthma. Allergy 69, 730–740. doi:10.1111/all.12392
Marquez-Curtis, L. A., Qiu, Y., Xu, A., and Janowska-Wieczorek, A. (2014). Migration, proliferation, and differentiation of cord blood mesenchymal stromal cells treated with histone deacetylase inhibitor valproic Acid. Stem Cells Int. 2014, 610495. doi:10.1155/2014/610495
Martínez-González, I., Roca, O., Masclans, J. R., Moreno, R., Salcedo, M. T., Baekelandt, V., et al. (2013). Human mesenchymal stem cells overexpressing the IL-33 antagonist soluble IL-1 receptor-like-1 attenuate endotoxin-induced acute lung injury. Am. J. Respir. Cell Mol. Biol. 49, 552–562. doi:10.1165/rcmb.2012-0406OC
Matthay, M. A., Calfee, C. S., Zhuo, H., Thompson, B. T., Wilson, J. G., Levitt, J. E., et al. (2019). Treatment with allogeneic mesenchymal stromal cells for moderate to severe acute respiratory distress syndrome (START study): A randomised phase 2a safety trial. Lancet Respir. Med. 7, 154–162. doi:10.1016/S2213-2600(18)30418-1
Matthay, M. A., Arabi, Y. M., Siegel, E. R., Ware, L. B., Bos, L. D. J., Sinha, P., et al. (2020). Phenotypes and personalized medicine in the acute respiratory distress syndrome. Intensive Care Med. 46, 2136–2152. doi:10.1007/s00134-020-06296-9
McClain-Caldwell, I. A. N., Vitale-Cross, L. Y. N. N., Mayer, B., Krepuska, M., Boyajian, M., Myneni, V., et al. (2018). Immunogenic potential of human bone marrow mesenchymal stromal cells is enhanced by hyperthermia. Cytotherapy 20, 1437–1444. doi:10.1016/j.jcyt.2018.10.002
McIntyre, L. A., Stewart, D. J., Mei, S. H. J., Courtman, D., Watpool, I., Granton, J., et al. (2018). Cellular immunotherapy for septic shock: A phase I clinical trial. Am. J. Respir. Crit. Care Med. 197, 337–347. doi:10.1164/rccm.201705-1006OC
McKee, C., and Chaudhry, G. R. (2017). Advances and challenges in stem cell culture. Colloids Surfaces B Biointerfaces 159, 62–77. doi:10.1016/j.colsurfb.2017.07.051
Mehrian, M., Lambrechts, T., Marechal, M., Luyten, F. P., Papantoniou, I., and Geris, L. (2020). Predicting in vitro human mesenchymal stromal cell expansion based on individual donor characteristics using machine learning. Cytotherapy 22, 82–90. doi:10.1016/j.jcyt.2019.12.006
Mei, S. H. J., McCarter, S. D., Deng, Y., Parker, C. H., Liles, W. C., and Stewart, D. J. (2007). Prevention of LPS-induced acute lung injury in mice by mesenchymal stem cells overexpressing angiopoietin. PLoS Med. 4, e269–e1537. doi:10.1371/journal.pmed.0040269
Mei, S. H. J., Haitsma, J. J., Dos Santos, C. C., Deng, Y., Lai, P. F. H., Slutsky, A. S., et al. (2010). Mesenchymal stem cells reduce inflammation while enhancing bacterial clearance and improving survival in sepsis. Am. J. Respir. Crit. Care Med. 182, 1047–1057. doi:10.1164/rccm.201001-0010OC
Millar, J. E., Von Bahr, V., Malfertheiner, M. V., Ki, K. K., Redd, M. A., Bartnikowski, N., et al. (2019). Administration of mesenchymal stem cells during ECMO results in a rapid decline in oxygenator performance. Thorax 74, 194–196. doi:10.1136/thoraxjnl-2017-211439
Min, F., Gao, F., Li, Q., and Liu, Z. (2015). Therapeutic effect of human umbilical cord mesenchymal stem cells modified by angiotensin-converting enzyme 2 gene on bleomycin-induced lung fibrosis injury. Mol. Med. Rep. 11, 2387–2396. doi:10.3892/mmr.2014.3025
Moloney, T. C., Hoban, D. B., Barry, F. P., Howard, L., and Dowd, E. (2012). Kinetics of thermally induced heat shock protein 27 and 70 expression by bone marrow-derived mesenchymal stem cells. Protein Sci. 21, 904–909. doi:10.1002/pro.2077
Morales, M. M., Souza, S. A. L. L., Loivos, L. P., Lima, M. A., Szklo, A., Vairo, L., et al. (2015). Pilot safety study of intrabronchial instillation of bone marrow-derived mononuclear cells in patients with silicosis. BMC Pulm. Med. 15, 66. doi:10.1186/s12890-015-0061-8
Moroncini, G., Paolini, C., Orlando, F., Capelli, C., Grieco, A., Tonnini, C., et al. (2018). Mesenchymal stromal cells from human umbilical cord prevent the development of lung fibrosis in immunocompetent mice. PLoS One 13, e0196048. doi:10.1371/journal.pone.0196048
Morrison, T. J., Jackson, M. V., Cunningham, E. K., Kissenpfennig, A., McAuley, D. F., O’Kane, C. M., et al. (2017). Mesenchymal stromal cells modulate macrophages in clinically relevant lung injury models by extracellular vesicle mitochondrial transfer. Am. J. Respir. Crit. Care Med. 196, 1275–1286. doi:10.1164/rccm.201701-0170OC
Moya, A., Larochette, N., Paquet, J., Deschepper, M., Bensidhoum, M., Izzo, V., et al. (2017). Quiescence preconditioned human multipotent stromal cells adopt a metabolic profile favorable for enhanced survival under ischemia. Stem Cells 35, 181–196. doi:10.1002/stem.2493
Murphy, N., Treacy, O., Lynch, K., Morcos, M., Lohan, P., Howard, L., et al. (2019). TNF-α/IL-1β-licensed mesenchymal stromal cells promote corneal allograft survival via myeloid cell-mediated induction of Foxp3+ regulatory T cells in the lung. FASEB J. 33, 9404–9421. doi:10.1096/fj.201900047R
Murua, A., Portero, A., Orive, G., Hernández, R. M., de Castro, M., and Pedraz, J. L. (2008). Cell microencapsulation technology: Towards clinical application. J. Control Release 132, 76–83. doi:10.1016/j.jconrel.2008.08.010
Najafi, R., and Sharifi, A. M. (2013). Deferoxamine preconditioning potentiates mesenchymal stem cell homing in vitro and in streptozotocin-diabetic rats. Expert Opin. Biol. Ther. 13, 959–972. doi:10.1517/14712598.2013.782390
Németh, K., Leelahavanichkul, A., Yuen, P. S. T., Mayer, B., Parmelee, A., Doi, K., et al. (2009). Bone marrow stromal cells attenuate sepsis via prostaglandin E 2-dependent reprogramming of host macrophages to increase their interleukin-10 production. Nat. Med. 15, 42–49. doi:10.1038/nm.1905
Noh, M. (2012). Interleukin-17A increases leptin production in human bone marrow mesenchymal stem cells. Biochem Pharmacol. 83, 661–670. doi:10.1016/j.bcp.2011.12.010
Nonaka, P. N., Falcones, B., Farre, R., Artigas, A., Almendros, I., and Navajas, D. (2020). Biophysically preconditioning mesenchymal stem cells improves treatment of ventilator-induced lung injury. Arch. Bronconeumol 56, 179–181. doi:10.1016/j.arbres.2019.08.014
Noone, C., Kihm, A., English, K., O’Dea, S., and Mahon, B. P. (2013). IFN-γ stimulated human umbilical-tissue-derived cells potently suppress NK activation and resist NK-mediated cytotoxicity in vitro. Stem Cells Dev. 22, 3003–3014. doi:10.1089/scd.2013.0028
Ostanin, A. A., Petrovskii, Y. L., Shevela, E. Y., and Chernykh, E. R. (2011). Multiplex analysis of cytokines, chemokines, growth factors, MMP-9 and TIMP-1 produced by human bone marrow, adipose tissue, and placental mesenchymal stromal cells. Bull. Exp. Biol. Med. 151, 133–141. doi:10.1007/s10517-011-1275-2
Otani, H. (2004). Reactive oxygen species as mediators of signal transduction in ischemic preconditioning. Antioxidants Redox Signal 6, 449–469. doi:10.1089/152308604322899521
Paliwal, S., Chaudhuri, R., Agrawal, A., and Mohanty, S. (2018). Regenerative abilities of mesenchymal stem cells through mitochondrial transfer. J. Biomed. Sci. 25, 31. doi:10.1186/s12929-018-0429-1
Phinney, D. G., Di Giuseppe, M., Njah, J., Sala, E., Shiva, S., St Croix, C. M., et al. (2015). Mesenchymal stem cells use extracellular vesicles to outsource mitophagy and shuttle microRNAs. Nat. Commun. 6, 8472. doi:10.1038/ncomms9472
Phinney, D. G., and Galipeau, J.MSC COMMITTEE OF THE INTERNATIONAL SOCIETY OF CELL AND GENE THERAPY (2019). Manufacturing mesenchymal stromal cells for clinical applications: A survey of good manufacturing practices at U.S. Academic centers. Cytotherapy 21, 782–792. doi:10.1016/j.jcyt.2019.04.003
Poggio, H. A., Antunes, M. A., Rocha, N. N., Kitoko, J. Z., Morales, M. M., Olsen, P. C., et al. (2018). Impact of one versus two doses of mesenchymal stromal cells on lung and cardiovascular repair in experimental emphysema. Stem Cell Res. Ther. 9, 296. doi:10.1186/s13287-018-1043-6
Pourgholaminejad, A., Aghdami, N., Baharvand, H., and Moazzeni, S. M. (2016). The effect of pro-inflammatory cytokines on immunophenotype, differentiation capacity and immunomodulatory functions of human mesenchymal stem cells. Cytokine 85, 51–60. doi:10.1016/j.cyto.2016.06.003
Prasanna, S. J., Gopalakrishnan, D., Shankar, S. R., and Vasandan, A. B. (2010). Pro-inflammatory cytokines, IFNgamma and TNFalpha, influence immune properties of human bone marrow and Wharton jelly mesenchymal stem cells differentially. PLoS One 5, e9016. doi:10.1371/journal.pone.0009016
Raman, N., Imran, S. A. M., Noordin, K. B. A. A., Zaman, W. S. W. K., and Nordin, F. (2022). Mechanotransduction in mesenchymal stem cells (MSCs) differentiation: A review. Int. J. Mol. Sci. 23, 4580. doi:10.3390/ijms23094580
Rashedi, I., Gómez-Aristizábal, A., Wang, X. H., Viswanathan, S., and Keating, A. (2017). TLR3 or TLR4 activation enhances mesenchymal stromal cell-mediated Treg induction via Notch signaling. Stem Cells 35, 265–275. doi:10.1002/stem.2485
Rehman, A. U., Ahmad Hassali, M. A., Muhammad, S. A., Shah, S., Abbas, S., Hyder Ali, I. A. B., et al. (2020). The economic burden of chronic obstructive pulmonary disease (COPD) in the USA, Europe, and asia: Results from a systematic review of the literature. Expert Rev. Pharmacoecon Outcomes Res. 20, 661–672. doi:10.1080/14737167.2020.1678385
Rustad, K. C., Wong, V. W., Sorkin, M., Glotzbach, J. P., Major, M. R., Rajadas, J., et al. (2012). Enhancement of mesenchymal stem cell angiogenic capacity and stemness by a biomimetic hydrogel scaffold. Biomaterials 33, 80–90. doi:10.1016/j.biomaterials.2011.09.041
Sabry, M. M., Elkalawy, S. A-E., Abo-Elnour, R. K. E-D., and Abd-El-Maksod, D. F. (2014). Histolgical and immunohistochemical study on the effect of stem cell therapy on bleomycin induced pulmonary fibrosis in albino rat. Int. J. Stem Cells 7, 33–42. doi:10.15283/ijsc.2014.7.1.33
Sacchetti, B., Funari, A., Remoli, C., Giannicola, G., Kogler, G., Liedtke, S., et al. (2016). No identical “mesenchymal stem cells” at different times and sites: Human committed progenitors of distinct origin and differentiation potential are incorporated as adventitial cells in microvessels. Stem Cell Rep. 6, 897–913. doi:10.1016/j.stemcr.2016.05.011
Saeedi, P., Halabian, R., and Fooladi, A. A. I. (2019). Antimicrobial effects of mesenchymal stem cells primed by modified LPS on bacterial clearance in sepsis. J. Cell Physiol. 234, 4970–4986. doi:10.1002/jcp.27298
Saller, M. M., Prall, W. C., Docheva, D., Schönitzer, V., Popov, T., Anz, D., et al. (2012). Increased stemness and migration of human mesenchymal stem cells in hypoxia is associated with altered integrin expression. Biochem Biophys. Res. Commun. 423, 379–385. doi:10.1016/j.bbrc.2012.05.134
Sart, S., Agathos, S. N., and Li, Y. (2014). Process engineering of stem cell metabolism for large scale expansion and differentiation in bioreactors. Biochem Eng. J. 84, 74–82. doi:10.1016/j.bej.2014.01.005
Sart, S., Ma, T., and Li, Y. (2014). Preconditioning stem cells for in vivo delivery. Biores Open Access 3, 137–149. doi:10.1089/biores.2014.0012
Scicluna, B. P., van Vught, L. A., Zwinderman, A. H., Wiewel, M. A., Davenport, E. E., Burnham, K. L., et al. (2017). Classification of patients with sepsis according to blood genomic endotype: A prospective cohort study. Lancet Respir. Med. 5, 816–826. doi:10.1016/S2213-2600(17)30294-1
Shin, J. H., Shin, D. W., and Noh, M. (2009). Interleukin-17A inhibits adipocyte differentiation in human mesenchymal stem cells and regulates pro-inflammatory responses in adipocytes. Biochem Pharmacol. 77, 1835–1844. doi:10.1016/j.bcp.2009.03.008
Shin, J. H., Ryu, C-M., Ju, H., Yu, H. Y., Song, S., Shin, D-M., et al. (2019). Synergistic effects of N-acetylcysteine and mesenchymal stem cell in a lipopolysaccharide-induced interstitial cystitis rat model. Cells 9, 86. doi:10.3390/cells9010086
Silva, J. D., Paredes, B. D., Araújo, I. M., Lopes-Pacheco, M., Oliveira, M. V., Suhett, G. D., et al. (2014). Effects of bone marrow-derived mononuclear cells from healthy or acute respiratory distress syndrome donors on recipient lung-injured Mice. Crit. Care Med. 42, e510–e524. doi:10.1097/CCM.0000000000000296
Silva, J. D., Lopes-Pacheco, M., Paz, A. H. R., Cruz, F. F., Melo, E. B., de Oliveira, M. V., et al. (2018). Mesenchymal stem cells from bone marrow, adipose tissue, and lung tissue differentially mitigate lung and distal organ damage in experimental acute respiratory distress syndrome. Crit. Care Med. 46, e132–e140. doi:10.1097/CCM.0000000000002833
Silva, J. D., de Castro, L. L., Braga, C. L., Oliveira, G. P., Trivelin, S. A., Barbosa-Junior, C. M., et al. (2019). Mesenchymal stromal cells are more effective than their extracellular vesicles at reducing lung injury regardless of acute respiratory distress syndrome etiology. Stem Cells Int. 2019, 8262849. doi:10.1155/2019/8262849
Silva, J. D., Lopes-Pacheco, M., de Castro, L. L., Kitoko, J. Z., Trivelin, S. A., Amorim, N. R., et al. (2019). Eicosapentaenoic acid potentiates the therapeutic effects of adipose tissue-derived mesenchymal stromal cells on lung and distal organ injury in experimental sepsis. Stem Cell Res. Ther. 10, 264. doi:10.1186/s13287-019-1365-z
Sivanathan, K. N., Rojas-Canales, D. M., Hope, C. M., Krishnan, R., Carroll, R. P., Gronthos, S., et al. (2015). Interleukin-17A-Induced human mesenchymal stem cells are superior modulators of immunological function. Stem Cells 33, 2850–2863. doi:10.1002/stem.2075
Sivanathan, K. N., Rojas-Canales, D., Grey, S. T., Gronthos, S., and Coates, P. T. (2017). Transcriptome profiling of IL-17a preactivated mesenchymal stem cells: A comparative study to unmodified and IFN-γ modified mesenchymal stem cells. Stem Cells Int. 2017, 1025820. doi:10.1155/2017/1025820
Song, Y., Dou, H., Li, X., Zhao, X., Li, Y., Liu, D., et al. (2017). Exosomal miR-146a contributes to the enhanced therapeutic efficacy of interleukin-1β-primed mesenchymal stem cells against sepsis. Stem Cells 35, 1208–1221. doi:10.1002/stem.2564
Soriano, J. B., Kendrick, P. J., Paulson, K. R., Gupta, V., Abrams, E. M., Adedoyin, R. A., et al. (2020). Prevalence and attributable health burden of chronic respiratory diseases, 1990–2017: A systematic analysis for the global burden of disease study 2017. Lancet Respir. Med. 8, 585–596. doi:10.1016/S2213-2600(20)30105-3
Steinmetz, N. J., Aisenbrey, E. A., Westbrook, K. K., Qi, H. J., and Bryant, S. J. (2015). Mechanical loading regulates human MSC differentiation in a multi-layer hydrogel for osteochondral tissue engineering. Acta Biomater. 21, 142–153. doi:10.1016/j.actbio.2015.04.015
Su, W., Wan, Q., Huang, J., Han, L., Chen, X., Chen, G., et al. (2015). Culture medium from TNF-α-stimulated mesenchymal stem cells attenuates allergic conjunctivitis through multiple antiallergic mechanisms. J. Allergy Clin. Immunol. 136, 423–432. doi:10.1016/j.jaci.2014.12.1926
Sun, Y., Li, Q., Yan, J., Hu, R., and Jiang, H. (2015). Isoflurane preconditioning promotes the survival and migration of bone marrow stromal cells. Cell Physiol. Biochem. 36, 1331–1345. doi:10.1159/000430300
Sung, P-H., Chiang, H-J., Chen, C-H., Chen, Y-L., Huang, T-H., Zhen, Y-Y., et al. (2016). Combined therapy with adipose-derived mesenchymal stem cells and ciprofloxacin against acute urogenital organ damage in rat sepsis syndrome induced by intrapelvic injection of cecal bacteria. Stem Cells Transl. Med. 5, 782–792. doi:10.5966/sctm.2015-0116
Sutton, M. T., Fletcher, D., Ghosh, S. K., Weinberg, A., van Heeckeren, R., Kaur, S., et al. (2016). Antimicrobial properties of mesenchymal stem cells: Therapeutic potential for cystic fibrosis infection, and treatment. Stem Cells Int. 2016, 5303048. doi:10.1155/2016/5303048
Szabó, E., Fajka-Boja, R., Kriston-Pál, É., Hornung, Á., Makra, I., Kudlik, G., et al. (2015). Licensing by inflammatory cytokines abolishes heterogeneity of immunosuppressive function of mesenchymal stem cell population. Stem Cells Dev. 24, 2171–2180. doi:10.1089/scd.2014.0581
Takeda, K., Ning, F., Domenico, J., Okamoto, M., Ashino, S., Kim, S-H., et al. (2018). Activation of p70S6 kinase-1 in mesenchymal stem cells is essential to lung tissue repair. Stem Cells Transl. Med. 7, 551–558. doi:10.1002/sctm.17-0200
Takizawa, N., Okubo, N., Kamo, M., Chosa, N., Mikami, T., Suzuki, K., et al. (2017). Bone marrow-derived mesenchymal stem cells propagate immunosuppressive/anti-inflammatory macrophages in cell-to-cell contact-independent and -dependent manners under hypoxic culture. Exp. Cell Res. 358, 411–420. doi:10.1016/j.yexcr.2017.07.014
Tan, Y., Salkhordeh, M., Wang, J-P., McRae, A., Souza-Moreira, L., McIntyre, L., et al. (2019). Thawed mesenchymal stem cell product shows comparable immunomodulatory potency to cultured cells in vitro and in polymicrobial septic animals. Sci. Rep. 9, 18078. doi:10.1038/s41598-019-54462-x
Trento, C., Bernardo, M. E., Nagler, A., Kuçi, S., Bornhäuser, M., Köhl, U., et al. (2018). Manufacturing mesenchymal stromal cells for the treatment of graft-versus-host disease: A survey among centers affiliated with the European society for blood and marrow transplantation. Biol. Blood Marrow Transpl. 24, 2365–2370. doi:10.1016/j.bbmt.2018.07.015
Tu, Z., Li, Q., Bu, H., and Lin, F. (2010). Mesenchymal stem cells inhibit complement activation by secreting factor H. Stem Cells Dev. 19, 1803–1809. doi:10.1089/scd.2009.0418
Varkouhi, A. K., Jerkic, M., Ormesher, L., Gagnon, S., Goyal, S., Rabani, R., et al. (2019). Extracellular vesicles from interferon-γ-primed human umbilical cord mesenchymal stromal cells reduce Escherichia coli-induced acute lung injury in rats. Anesthesiology 130, 778–790. doi:10.1097/ALN.0000000000002655
Vasquez, C. R., Gupta, S., Miano, T. A., Roche, M., Hsu, J., Yang, W., et al. (2021). Identification of distinct clinical subphenotypes in critically ill patients with COVID-19. Chest 160, 929–943. doi:10.1016/j.chest.2021.04.062
Viero Nora, C. C., Camassola, M., Bellagamba, B., Ikuta, N., Christoff, A. P., Da Silva Meirelles, L., et al. (2012). Molecular analysis of the differentiation potential of murine mesenchymal stem cells from tissues of endodermal or mesodermal origin. Stem Cells Dev. 21, 1761–1768. doi:10.1089/scd.2011.0030
Vigo, T., Procaccini, C., Ferrara, G., Baranzini, S., Oksenberg, J. R., Matarese, G., et al. (2017). IFN-γ orchestrates mesenchymal stem cell plasticity through the signal transducer and activator of transcription 1 and 3 and mammalian target of rapamycin pathways. J. Allergy Clin. Immunol. 139, 1667–1676. doi:10.1016/j.jaci.2016.09.004
von Bahr, V., Millar, J. E., Malfertheiner, M. V., Ki, K. K., Passmore, M. R., Bartnikowski, N., et al. (2019). Mesenchymal stem cells may ameliorate inflammation in an ex vivo model of extracorporeal membrane oxygenation. Perfusion 34, 15–21. doi:10.1177/0267659119830857
Wagner, W., Roderburg, C., Wein, F., Diehlmann, A., Frankhauser, M., Schubert, R., et al. (2007). Molecular and secretory profiles of human mesenchymal stromal cells and their abilities to maintain primitive hematopoietic progenitors. Stem Cells 25, 2638–2647. doi:10.1634/stemcells.2007-0280
Wang, H., Cao, F., De, A., Cao, Y., Contag, C., Gambhir, S. S., et al. (2009). Trafficking mesenchymal stem cell engraftment and differentiation in tumor-bearing mice by bioluminescence imaging. Stem Cells 27, 1548–1558. doi:10.1002/stem.81
Wang, Q., Zhu, H., Zhou, W-G., Guo, X-C., Wu, M-J., Xu, Z-Y., et al. (2013). N-acetylcysteine-pretreated human embryonic mesenchymal stem cell administration protects against bleomycin-induced lung injury. Am. J. Med. Sci. 346, 113–122. doi:10.1097/MAJ.0b013e318266e8d8
Wang, H., Yang, Y-F., Zhao, L., Xiao, F-J., Zhang, Q-W., Wen, M-L., et al. (2013). Hepatocyte growth factor gene-modified mesenchymal stem cells reduce radiation-induced lung injury. Hum. Gene Ther. 24, 343–353. doi:10.1089/hum.2012.177
Wang, Q., Yang, Q., Wang, Z., Tong, H., Ma, L., Zhang, Y., et al. (2016). Comparative analysis of human mesenchymal stem cells from fetal-bone marrow, adipose tissue, and Warton’s jelly as sources of cell immunomodulatory therapy. Hum. Vaccines Immunother. 12, 85–96. doi:10.1080/21645515.2015.1030549
Wang, B., Lin, Y., Hu, Y., Shan, W., Liu, S., Xu, Y., et al. (2017). mTOR inhibition improves the immunomodulatory properties of human bone marrow mesenchymal stem cells by inducing COX-2 and PGE2. Stem Cell Res. Ther. 8, 292. doi:10.1186/s13287-017-0744-6
Wang, C., Lv, D., Zhang, X., Ni, Z-A., Sun, X., and Zhu, C. (2018). Interleukin-10-Overexpressing mesenchymal stromal cells induce a series of regulatory effects in the inflammatory system and promote the survival of endotoxin-induced acute lung injury in mice model. DNA Cell Biol. 37, 53–61. doi:10.1089/dna.2017.3735
Wang, S., Mo, M., Wang, J., Sadia, S., Shi, B., Fu, X., et al. (2018). Platelet-derived growth factor receptor beta identifies mesenchymal stem cells with enhanced engraftment to tissue injury and pro-angiogenic property. Cell Mol. Life Sci. 75, 547–561. doi:10.1007/s00018-017-2641-7
Wang, X., Shen, K., Wang, J., Liu, K., Wu, G., Li, Y., et al. (2020). Hypoxic preconditioning combined with curcumin promotes cell survival and mitochondrial quality of bone marrow mesenchymal stem cells, and accelerates cutaneous wound healing via PGC-1α/SIRT3/HIF-1α signaling. Free Radic. Biol. Med. 159, 164–176. doi:10.1016/j.freeradbiomed.2020.07.023
Waszak, P., Alphonse, R., Vadivel, A., Ionescu, L., Eaton, F., and Thébaud, B. (2012). Preconditioning enhances the paracrine effect of mesenchymal stem cells in preventing oxygen-induced neonatal lung injury in rats. Stem Cells Dev. 21, 2789–2797. doi:10.1089/scd.2010.0566
Waterman, R. S., Tomchuck, S. L., Henkle, S. L., and Betancourt, A. M. (2010). A new mesenchymal stem cell (MSC) paradigm: Polarization into a pro-inflammatory MSC1 or an immunosuppressive MSC2 phenotype. PLoS One 5, e10088. doi:10.1371/journal.pone.0010088
Weiss, D. J., Casaburi, R., Flannery, R., LeRoux-Williams, M., and Tashkin, D. P. (2013). A placebo-controlled, randomized trial of mesenchymal stem cells in COPD. Chest 143, 1590–1598. doi:10.1378/chest.12-2094
Weiss, D. J., Segal, K., Casaburi, R., Hayes, J., and Tashkin, D. (2021). Effect of mesenchymal stromal cell infusions on lung function in COPD patients with high CRP levels. Respir. Res. 22, 142. doi:10.1186/s12931-021-01734-8
Wick, K. D., Leligdowicz, A., Zhuo, H., Ware, L. B., and Matthay, M. A. (2021). Mesenchymal stromal cells reduce evidence of lung injury in patients with ARDS. JCI Insight 6, e148983. doi:10.1172/jci.insight.148983
Wong, S. W., Lenzini, S., Cooper, M. H., Mooney, D. J., and Shin, J-W. (2020). Soft extracellular matrix enhances inflammatory activation of mesenchymal stromal cells to induce monocyte production and trafficking. Sci. Adv. 6, eaaw0158. doi:10.1126/sciadv.aaw0158
World Health Organization (2020) The top 10 causes of death. Available at: https://www.who.int/news-room/fact-sheets/detail/the-top-10-causes-of-death [Accessed August 14, 2022].
Xu, J., Qu, J., Cao, L., Sai, Y., Chen, C., He, L., et al. (2008). Mesenchymal stem cell-based angiopoietin-1 gene therapy for acute lung injury induced by lipopolysaccharide in mice. J. Pathol. 214, 472–481. doi:10.1002/path.2302
Xu, A. L., Rodriguez, L. A., Walker, K. P., Mohammadipoor, A., Kamucheka, R. M., Cancio, L. C., et al. (2019). Mesenchymal stem cells reconditioned in their own serum exhibit augmented therapeutic properties in the setting of acute respiratory distress syndrome. Stem Cells Transl. Med. 8, 1092–1106. doi:10.1002/sctm.18-0236
Yang, J-X., Zhang, N., Wang, H-W., Gao, P., Yang, Q-P., and Wen, Q-P. (2015). CXCR4 receptor overexpression in mesenchymal stem cells facilitates treatment of acute lung injury in rats. J. Biol. Chem. 290, 1994–2006. doi:10.1074/jbc.M114.605063
Yang, Z., Concannon, J., Ng, K. S., Seyb, K., Mortensen, L. J., Ranganath, S., et al. (2016). Tetrandrine identified in a small molecule screen to activate mesenchymal stem cells for enhanced immunomodulation. Sci. Rep. 6, 30263. doi:10.1038/srep30263
Yu, H. C., and Chiang, B. L. (2018). Toll-like receptor 2 ligation of mesenchymal stem cells alleviates asthmatic airway inflammation. J. Allergy Clin. Immunol. 142, 284–287. doi:10.1016/j.jaci.2017.12.996
Yu, K-R., Lee, J. Y., Kim, H-S., Hong, I-S., Choi, S. W., Seo, Y., et al. (2014). A p38 MAPK-mediated alteration of COX-2/PGE2 regulates immunomodulatory properties in human mesenchymal stem cell aging. PLoS One 9, e102426. doi:10.1371/journal.pone.0102426
Yuan, O., Lin, C., Wagner, J., Archard, J. A., Deng, P., Halmai, J., et al. (2019). Exosomes derived from human primed mesenchymal stem cells induce mitosis and potentiate growth factor secretion. Stem Cells Dev. 28, 398–409. doi:10.1089/scd.2018.0200
Zaim, M., Karaman, S., Cetin, G., and Isik, S. (2012). Donor age and long-term culture affect differentiation and proliferation of human bone marrow mesenchymal stem cells. Ann. Hematol. 91, 1175–1186. doi:10.1007/s00277-012-1438-x
Zhang, E., Yang, Y., Chen, S., Peng, C., Lavin, M. F., Yeo, A. J., et al. (2018). Bone marrow mesenchymal stromal cells attenuate silica-induced pulmonary fibrosis potentially by attenuating Wnt/β-catenin signaling in rats. Stem Cell Res. Ther. 9, 311. doi:10.1186/s13287-018-1045-4
Zhang, S., Jiang, W., Ma, L., Liu, Y., Zhang, X., and Wang, S. (2018). Nrf2 transfection enhances the efficacy of human amniotic mesenchymal stem cells to repair lung injury induced by lipopolysaccharide. J. Cell Biochem 119, 1627–1636. doi:10.1002/jcb.26322
Zhang, Y., Yang, S., Qiu, Z., Huang, L., Huang, L., Liang, Y., et al. (2022). Pyrogallol enhances therapeutic effect of human umbilical cord mesenchymal stem cells against LPS-mediated inflammation and lung injury via activation of Nrf2/HO-1 signaling. Free Radic. Biol. Med. 191, 66–81. doi:10.1016/j.freeradbiomed.2022.08.030
Zhao, X., Liu, D., Gong, W., Zhao, G., Liu, L., Yang, L., et al. (2014). The toll-like receptor 3 Ligand, Poly(I:C), improves immunosuppressive function and therapeutic effect of mesenchymal stem cells on sepsis via inhibiting MiR-143. Stem Cells 32, 521–533. doi:10.1002/stem.1543
Zhao, Y-F., Xiong, W., and Wu, X-L. (2014). Mesenchymal stem cell-based developmental endothelial locus-1 gene therapy for acute lung injury induced by lipopolysaccharide in mice. Mol. Med. Rep. 9, 1583–1589. doi:10.3892/mmr.2014.1988
Zhao, Y-F., Luo, Y-M., Xiong, W., Ding, W., Li, Y-R., Zhao, W., et al. (2015). Mesenchymal stem cell-based FGF2 gene therapy for acute lung injury induced by lipopolysaccharide in mice. Eur. Rev. Med. Pharmacol. Sci. 19, 857–865.
Zheng, J., Li, H., He, L., Huang, Y., Cai, J., Chen, L., et al. (2019). Preconditioning of umbilical cord-derived mesenchymal stem cells by rapamycin increases cell migration and ameliorates liver ischaemia/reperfusion injury in mice via the CXCR4/CXCL12 axis. Cell Prolif. 52, e12546. doi:10.1111/cpr.12546
Keywords: cell therapy, genetic engineering, immunomodulation, inflammation, lung, preconditioning, remodeling
Citation: Lopes-Pacheco M and Rocco PRM (2023) Functional enhancement strategies to potentiate the therapeutic properties of mesenchymal stromal cells for respiratory diseases. Front. Pharmacol. 14:1067422. doi: 10.3389/fphar.2023.1067422
Received: 20 October 2022; Accepted: 06 March 2023;
Published: 16 March 2023.
Edited by:
Cassiano Felippe Gonçalves-de-Albuquerque, Rio de Janeiro State Federal University, BrazilReviewed by:
Dean Philip John Kavanagh, University of Birmingham, United KingdomRenata Szydlak, Jagiellonian University Medical College, Poland
Copyright © 2023 Lopes-Pacheco and Rocco. This is an open-access article distributed under the terms of the Creative Commons Attribution License (CC BY). The use, distribution or reproduction in other forums is permitted, provided the original author(s) and the copyright owner(s) are credited and that the original publication in this journal is cited, in accordance with accepted academic practice. No use, distribution or reproduction is permitted which does not comply with these terms.
*Correspondence: Miquéias Lopes-Pacheco, bWxvcGVzMDgxMUBnbWFpbC5jb20=; Patricia R. M. Rocco, cHJtcm9jY29AZ21haWwuY29t