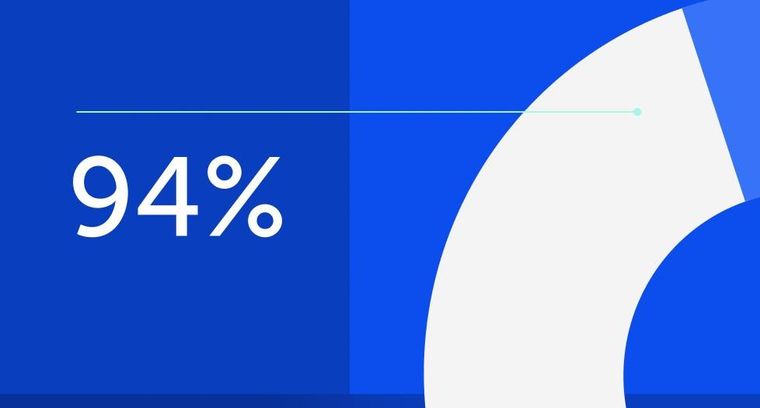
94% of researchers rate our articles as excellent or good
Learn more about the work of our research integrity team to safeguard the quality of each article we publish.
Find out more
SYSTEMATIC REVIEW article
Front. Pharmacol., 03 March 2023
Sec. Ethnopharmacology
Volume 14 - 2023 | https://doi.org/10.3389/fphar.2023.1053680
This article is part of the Research TopicNatural Products for Neuroprotection and NeuroregenerationView all 9 articles
Objective: Over the last decade, researchers have sought to develop novel medications against dementia. One potential agent under investigation is cannabinoids. This review systematically appraised and meta-analyzed published pre-clinical research on the mechanism of endocannabinoid system modulation in glial cells and their effects on cognitive function in animal models of Alzheimer’s disease (AD).
Methods: A systematic review complying with PRISMA guidelines was conducted. Six databases were searched: EBSCOHost, Scopus, PubMed, CINAHL, Cochrane, and Web of Science, using the keywords AD, cannabinoid, glial cells, and cognition. The methodological quality of each selected pre-clinical study was evaluated using the SYRCLE risk of bias tool. A random-effects model was applied to analyze the data and calculate the effect size, while I2 and p-values were used to assess heterogeneity.
Results: The analysis included 26 original articles describing (1050 rodents) with AD-like symptoms. Rodents treated with cannabinoid agonists showed significant reductions in escape latency (standard mean difference [SMD] = −1.26; 95% confidence interval [CI]: −1.77 to −0.76, p < 0.00001) and ability to discriminate novel objects (SMD = 1.40; 95% CI: 1.04 to 1.76, p < 0.00001) compared to the control group. Furthermore, a significant decrease in Aβ plaques (SMD = −0.91; 95% CI: −1.55 to −0.27, p = 0.006) was observed in the endocannabinoid-treated group compared to the control group. Trends were observed toward neuroprotection, as represented by decreased levels of glial cell markers including glial fibrillary acid protein (SMD = −1.47; 95% CI: −2.56 to −0.38, p = 0.008) and Iba1 (SMD = −1.67; 95% CI: −2.56 to −0.79, p = 0.0002). Studies on the wild-type mice demonstrated significantly decreased levels of pro-inflammatory markers TNF-α, IL-1, and IL-6 (SMD = −2.28; 95% CI: −3.15 to −1.41, p = 0.00001). Despite the non-significant decrease in pro-inflammatory marker levels in transgenic mice (SMD = −0.47; 95% CI: −1.03 to 0.08, p = 0.09), the result favored the endocannabinoid-treated group over the control group.
Conclusion: The revised data suggested that endocannabinoid stimulation promotes cognitive function via modulation of glial cells by decreasing pro-inflammatory markers in AD-like rodent models. Thus, cannabinoid agents may be required to modulate the downstream chain of effect to enhance cognitive stability against concurrent neuroinflammation in AD. Population-based studies and well-designed clinical trials are required to characterize the acceptability and real-world effectiveness of cannabinoid agents.
Systematic Review Registration: [https://inplasy.com/inplasy-2022-8-0094/], identifier [Inplasy Protocol 3770].
Alzheimer’s disease (AD) is a progressive neurological illness most commonly linked to memory loss and cognitive impairment (Liu & Li, 2019). However, other clinical manifestations are becoming more widely recognized. The presence of amyloid plaques and neurofibrillary tangles (NFTs) is currently essential for a pathological diagnosis (Mattson & Arumugam, 2018). AD is the major cause of dementia worldwide. Apart from a small percentage of cases attributed to familial genetic mutations, most AD cases do not have a clear underlying cause (Alzheimer’s Association Report, 2020). Proteinopathy (amyloid and tau) is a common feature in patients with AD and is typically associated with other age-related disorders, including cerebrovascular and Lewy body diseases (Deture & Dickson, 2019). Although cannabinoids have been rigorously studied in animal and clinical contexts in the field of AD, the cellular and biomolecular mechanisms and targets remain to be elucidated, challenging efforts to develop effective diagnostic tools and disease-modifying therapeutics (Deture & Dickson, 2019).
Since ancient times, Indian hemp, also known as cannabis sativa L. (Cannabis sativa), has been grown mostly in Central Asia (China and India) (Russo et al., 2008). It contains chemically active substances, including alkaloids, flavonoids, terpenoids, and cannabinoids (Andre et al., 2016). The most potent substances are terpenophenolic compounds, known as cannabinoids, which are primarily deposited in the female flowers' trichome cavity (Taura et al., 2007; Hartsel et al., 2016; Degenhardt et al., 2017). Numerous traditional medical applications of cannabis have already been established and are now accepted practices in medicine (Balant et al., 2021).
Phytocannabinoids, a naturally occurring, distinctive family of secondary metabolites, were believed to be responsible for the medicinal properties of cannabis. In 1964, Mechoulam and others first isolated and structurally elucidated the most abundant phytocannabinoid, a psychoactive ()-trans-9-tetrahydrocannabinol (THC) (Gaoni & Mechoulam, 1964). A non-psychoactive cannabidiol (CBD) was initially extracted in 1940 (Adams et al., 1939) and its full chemical structure was elucidated in 1963 (Mechoulam & Shvo, 1963). The finding of endogenous cannabinoids (endocannabinoids) in vertebrates as a result of the separation of phytocannabinoids from the cannabis plant has accelerated the growth of cannabis-related research (Turner et al., 2017).
Followers of Tantric Buddhism and Hinduism have utilized C. sativa flowers and resins in India and Tibet to aid in meditation and spirit communication (Schultes et al., 1992). The vegetal oils and proteins in C. sativa seeds were primarily employed by Chinese healers. According to Anwar et al. (2006), C. sativa seeds are high in linoleic acid, which doctors suggested using topically for inflammatory illnesses like eczema and psoriasis (Jeong et al., 2014). Historically, C. sativa was reportedly utilized by ancient Egyptian ladies to ease pain and elevate their mood, according to Diodorus Siculus (about 60 B.C.) (Bonini et al., 2018). Pliny the Elder, a Roman historian, remarked on the use of C. sativa roots for pain relief (Ryz et al., 2017). C. sativa was used in nineteenth-century English medicine as an analgesic, anti-inflammatory, anti-emetic, and anti-convulsant (Bonini et al., 2018; Balant et al., 2021).
The endocannabinoid system (ECS) consists of peptide (hemopressin derivatives) and lipid endocannabinoid (eCB) mediators, cannabinoid receptors, membrane transporters, and metabolic enzymes (Augusto et al., 2021). Within the central nervous system (CNS), the ECS regulates synaptic transmission, synaptic plasticity, and cytokine release, in addition to playing a neuroprotective role against neuronal injury (Cristino et al., 2019). The two primary receptors that make up the ECS are cannabinoid receptor type 1 (CB1R) and cannabinoid receptor type 2 (CB2R). As the most abundant G protein-coupled receptor (GPCR) in the brain, CB1R is found in the anterior cingulate cortex, prefrontal cortex, striatum, and hippocampus (Morena & Campolongo, 2014; Di Marzo et al., 2015). Another GPCR, CB2R is expressed mostly in the cellular immune system (natural killer cells, B cells, macrophages, and activated microglia) (Zou & Kumar, 2018) and recent immunostaining and western blotting studies have identified its weak expression in healthy neural tissue of rats, mice, ferrets, and human (Stempel et al., 2016; Zou & Kumar, 2018; Komorowska-Müller & Schmöle, 2021). AEA and 2-AG are the two most studied endocannabinoids, with the former a partial agonist at CB1R and CB2R and the latter a full agonist at these two sites when expressed at concentrations 200 times greater than those found in the CNS (Bajaj et al., 2021). Besides CB1R and CB2R, the ECS has several other receptors, including nuclear receptors and transient receptor potential channel ionotropic receptors (Marcu & Schechter, 2016). Derived from lipid membrane components that go through on-demand synthesis, endocannabinoids inhibit the retrograde of neurotransmitter release from adjacent neurons via CB1R-mediated signaling. The degradative enzymes also have a significant role in regulating endocannabinoid activity efficiently, with 2-AG predominantly degraded by monoacylglycerol lipase (MAGL) and fatty acid amide hydrolase (FAAH) playing the same role for AEA (Tanaka et al., 2020).
Accumulating evidence suggests the benefits of endocannabinoids on emotion and cognitive abilities (Morena & Campolongo, 2014; Kruk-Slomka et al., 2017). Infusions of AM251 and rimonabant, CB1R antagonists/inverse agonists, into the hippocampus region dramatically diminished memory updating, limited behavioral flexibility, and promoted the forgetting of fearful memories in Wistar rats (Lunardi et al., 2020). Conversely, the microinjection of arachidonylcyclopropylamide (1–4 ng/rat), a CB1R agonist, into the rat’s basolateral amygdala ameliorated a scopolamine-induced memory consolidation deficit (Nedaei et al., 2016). Additionally, endocannabinoid signaling engaged dorsal striatum glucocorticoids by promoting memory consolidation in Wistar rats, as evidenced by enhanced retention avoidance following inhibitory avoidance training (Siller-Pérez et al., 2019). In the Morris water maze test, the CB agonist WIN55,212–2 affected spatial memory acquisition but not consolidation. Moreover, endocannabinoid signaling also facilitated reward-based motor sequence learning, which was disrupted in CB1 and diacylglycerol lipase-a (DGLa) knockout mice (Tanigami et al., 2019).
Major advancements in the understanding of microglia and astrocytes have been made in recent years. Glial cells are activated or reactivated in a variety of pathogenic states, including stroke, trauma, tumor growth, and neurodegenerative disorders. Neuroinflammation can cause two distinct forms of reactive astrocytes, A1 and A2, which correspond to the M1/M2 phenotypes of microglia and macrophage classification (Liddelow & Barres, 2017).
Microglia play essential roles in CNS development, immunological surveillance, and maintenance of neuronal function (Maurya et al., 2021). Microglia are activated and acquire different transcriptome profiles in neurological diseases, including disease-associated microglia (DAM), which have been linked to neurodegenerative disorders (Deczkowska et al., 2018). Microglia also have a detrimental role in learning and memory capabilities under inflammatory and disease conditions. Minett et al. reported that the presence of dementia was positively associated with microglial activation markers (CD68 and CD64), with an inverse relationship with Iba1, a pan microglial marker (Minett et al., 2016), as indicated by a loss of microglial motility necessary to support neurons (Franco-Bocanegra et al., 2019). Persistent inflammatory pain elevated microglial activity in the dentate gyrus (DG) and cornu ammonis (CA1 subfield) regions, as evidenced by cellular changes that impaired spatial learning and memory abilities (Mohammadi et al., 2020). Transformation of the microglial phenotype from an active to an alternate state is important to maintain neuronal stability. CD200R, a receptor expressed by microglial cells, interacts with CD200 molecules released by neurons, astrocytes, and oligodendrocytes to enhance phagocytosis, providing a potential neuroprotective effect. CD200 suppression by hippocampus induction via AAV injection revealed severe impairment of synaptic and cognitive function (Feng et al., 2019).
Astrocytes are the most abundant and largest type of glial cells in the CNS, where they play a critical role in synaptic transmission and plasticity, neuroprotection, and maintenance of CNS homeostasis (Kasatkina et al., 2021). Astrocytes are also essential contributors to information processing and cognitive behavior. In the context of AD, astrocytes have received less attention than microglia or neurons. Fortunately, recent technologically advanced tools such as optogenetics, scRNAseq, in vivo imaging, and the growing use of animal and cell models (iPSCs), have illuminated the functions of astrocytes in normal and pathological conditions (Escartin et al., 2021; Sanchez-Varo et al., 2022). In in vitro and in vivo experiments, Lee et al. (2014)demonstrated that astrocytes are necessary for novel object recognition behavior and functional gamma oscillations maintenance. Moreover, evidence supports the role of astrocytes in the regulation of neuronal oscillations and cognitive flexibility via the Ca2+ binding protein S100β at the medial prefrontal cortex (mPFC) of rats (Brockett et al., 2018). Regarding the entorhinal cortex-dentate gyrus circuit, a review by Di Castro and Volterra showed that increased TNFα levels during infection/inflammation processes led to uncontrolled astrocyte glutamate release, altering perforant path (PP) excitatory projections onto dentate granule cells (GC). The disruption of this PP-GC synapse processing ultimately impaired contextual memory performance (Di Castro & Volterra, 2022). Zhang et al. (2020) demonstrated that IL-10, an anti-inflammatory cytokine, is essential in the modulation of A1 astrocyte activation, learning, memory dysfunction, and depressive-like behavior.
Microglial cell exposure to cannabinoids may result in the beneficial promotion of neurotrophic capabilities (Saijo & Glass, 2011). This is observed particularly when homeostatic microglia synthesize endogenous cannabinoids (AEA and 2-AG) along with the low expression of cannabinoid receptors (CB1R and CB2R). Microglia produce more endocannabinoids, which upregulate CB2R expression when activated, promoting a protective microglial phenotype by increasing the production of the neuroprotective molecules while decreasing the production of pro-inflammatory components (Komorowska-Müller & Schmöle, 2021). Additionally, microglial cells play a critical role in synaptic stripping, which occurs when synapses are removed from damaged neurons (Kettenmann et al., 2013). As the constant sensors of microenvironment changes in the CNS and restorer of tissue homeostasis, microglia not only serve as the primary immune cells of the CNS but also regulate the innate immune functions of astrocytes. The modulation of astrocyte function by endocannabinoid signaling is thought to be regulated by astrocyte Ca2+ mobilization by CB1R, which occurs throughout the rodent brain as well as in cortical and hippocampal human tissue (Bernal-Chico et al., 2022). Both microglia and astrocytes establish autocrine feedback and bidirectional communication for a tight reciprocal modulation upon CNS insult or injury by releasing a range of signaling molecules (Jha et al., 2019). Hence, this review critically discusses and summarizes the glial-endocannabinoid system effects on AD in animal models.
The research question was generated based on the PICO model of research question as follows. In AD-like animal models, P) would modulation of the endocannabinoid system influence glial cells I) compared to control C) to improve cognitive function O)? As there is growing data regarding the effects of endocannabinoid and glial cells on cognition, the present study aimed to provide a detailed systematic literature review of existing animal research examining the effects of endocannabinoid modulation of glial cells and its mechanism on the cognitive domains relevant to AD. The research papers were selected in this review to meet the following objectives: 1) to evaluate the potential mechanisms of endocannabinoid mediated by glial cells on cognitive measurement in AD-like animal models; 2) to determine the amelioration of neuro-inflammatory and other relevant pathological markers based on the effects in endocannabinoid-based pre-clinical models of cognitive impairment; and 3) to compare the effects of cannabinoid administration on cognitive function and make recommendations for future research.
This systematic review conducted a meta-analysis of studies performed on rodent models of AD to provide a comprehensive evaluation and understanding of the efficacy of the endocannabinoid system in modulating glial cells in the context of cognitive function.
The Preferred Reporting Items for Systematic Review and Meta-Analyses (PRISMA) criteria were used to conduct this systematic review. The protocol for this systematic review was registered in the INPLASY database (registration no INPLASY202280094). Only studies on glial cell alterations regulated by endocannabinoids were included in this review, along with behavioral evaluations following any quantity of cannabinoid agonist treatment or cannabinoid receptor knockout in mice or rats. For the meta-analysis, data that reported similar or related outcomes under similar or related experimental circumstances were pooled to calculate the final effect size. Other studies were qualitatively synthesized in this systematic review but were eliminated from the meta-analysis because they described different measurements or distinct sets of experiments.
Studies comparing the effects of endocannabinoid modulation on glial cells and related to behavior evaluation in rodents (mice and rats of either sex) were considered. The search was restricted to English-language articles that provided proof of experimental work in rodents. No consideration was given to the number of animals used in the experiment, transgenic or wild type, or the length of time the animals were exposed to the treatment. Studies with insufficient data were excluded from the meta-analysis. Results that allowed for the creation of pooled data were selected for inclusion in the meta-analysis.
The articles were initially screened based on their titles and abstracts, and any irrelevant studies were excluded. Two reviewers separately examined the abstracts to identify articles that fulfilled the inclusion criteria. Any disagreement was resolved by a discussion with a third reviewer. The remaining items were reviewed by reading the entire texts. Articles that were unrelated or that did not have the entire text available were excluded. The eligibility criteria were established based on the defined criteria for study inclusion and exclusion.
The criteria for the selection of articles were based on the Population, Intervention, Comparison, and Outcomes (PICO) model as a framework to establish the inclusion criteria. The four main identification components were: 1) AD animal model; 2) endocannabinoid system (ligand, receptors, enzymes, exogenous cannabinoid, etc.); 3) glial cells (microglia, astrocytes, oligodendrocytes); and 4) cognitive outcome (maze/NOR, etc.). The inclusion and exclusion criteria are summarized in Table 1.
To identify studies examining endocannabinoid neuroprotective properties in AD models of rodents, a thorough literature search was conducted. The pertinent publications were gathered from six credible databases: five from the EBSCOhost platform (Academic Search Complete, CINAHL Plus with Full Text, Cochrane Central Register of Controlled Trials, MEDLINE Complete, and the Psychology and Behavioral Sciences Collection). Additionally, Scopus was also searched. The keywords used for the literature search were as follows.
i) “Alzheimer* disease” OR “dementia” OR “mental disorder” OR “mental deterioration” OR “neurodegenerati*” OR “neuroinflammat*”
ii) “endocannabinoid*” OR “endocannabinoid system*” OR “cannabinoid receptor*” OR “cannabinoid agonist*” OR “cannabinoid ligand*” OR “cannabinoid*” OR “cannabis” OR “cannabis sativa” OR “cannabidiol” OR “cbd” OR “tetrahydrocannabinol” OR “thc”
iii) “microglia*” OR “autophag*” OR “microglia* activation” OR “microglia* stimulation” OR “microglia* function” OR “microglia* polarization” OR “microglia* propert*” OR “glia*” OR “gli*” OR “immune cell*” OR “immunomodulat*” OR “neuroprotect*” OR “astrocyte*” OR “astrogli*” OR “oligodendrocyte*” OR “ependymal cell*”
iv) “cogniti*” OR “intelligence*” OR “intellectual*” OR “executive function*” OR “think*” OR “learn*” OR “memor*” OR “judge*” OR “knowledge” OR “mind” OR “thought” OR “behavio*”
Only studies concerning animal models were selected for further consideration. All the articles identified during the search were exported to Mendeley (Mendeley Ltd. © 2008—2020 version 1.19.8) and duplicate records were removed.
Bias is a divergence from the truth in outcomes or inferences that can lead to systematic errors. The risks of bias among the included articles were assessed using a checklist developed by the Systematic Review Centre for Laboratory Animal Experimentation (SYRCLE) and based on the Cochrane Collaboration RoB Tool (Hooijmans et al., 2014). The checklist contains ten items within ten main domains: sequence generation, allocation concealment, baseline characteristics, random outcome assessment, random housing, blinding of the investigator, blinding of outcome evaluators, selective outcome reporting, incomplete outcome data, and other sources of bias. For the judgment of bias, the answer was either “Yes” to indicate a low risk of bias, “No” to indicate a high risk of bias, or “NC” to indicate an unclear level of bias due to inadequate information.
Evidence across studies was assessed for quality according to the Grades of Recommendation, Assessment, Development, and Evaluation (GRADE) Working Group (Schünemann et al., 2008). This evaluation considered the risk of bias within individual studies, the precision of effect estimates, heterogeneity, the directness of the evidence, and the risk of publication bias (Higgins et al., 2011).
The primary outcomes obtained in the selected articles described the presentation of general cognitive function (memory, learning, orientation, and attention) and the modifications observed as a result of endocannabinoid modulation methods in AD animal models. Moreover, secondary outcomes representing the animal’s biochemical characteristics and histological analysis were also observed. These included glial cell and inflammatory markers, amyloid burden, oxidative stress markers, synaptic plasticity, enzymatic levels, and cellular apoptosis.
The meta-analysis was performed using RevMan version 5.4 (The Nordic Cochrane Centre, The Cochrane Collaboration, Copenhagen, Denmark). The results consisted of several outcome variables including the number of right quadrant/range crossings during Morris water maze tests, levels of glial cell and inflammatory markers, and brain amyloid beta (Aβ), which were determined using a random-effects model to account for the possibility of heterogeneity because identical outcome variables were used to analyze various AD animal models in each study. The standard mean difference (SMD) and 95 percent confidence intervals (CIs) were calculated using a random-effects model for each study. To determine heterogeneity, the Q-statistic test was utilized. P <0.05 indicated heterogeneity among the studies. I2 values were used to measure the heterogeneity. When p < 0.05, heterogeneity was considered to be present. I2 = 0%, 0 < I2 ≤ 25%, 25% < I2 ≤ 75%, and I2 > 75% indicated no, mild, moderate, and high heterogeneity, respectively. By removing the included data one at a time, a sensitivity analysis was carried out to determine if these modifications altered the estimated cumulative result effect size when there was substantial heterogeneity in the data (Bown and Sutton, 2010). Data were extracted from plots/images using WebPlotDigitizer version 4.5 (Cramond et al., 2019). A meta-analysis was then performed on the extracted data using RevMan 5.4. The presence of publication bias was investigated using a graphical funnel plot (Figure 1).
FIGURE 1. Funnel plots of publication bias. (A) CB treatment versus model for escape latency. (B) CB treatment versus model for recognition index. (C) CB treatment versus model for amyloid plaque. (D) CB treatment versus model for GFAP. (E) CB treatment versus model for Iba1. (F) CB treatment versus model for pro-inflammatory cytokines in transgenic mice. (G) CB treatment versus model for pro-inflammatory cytokines of non-transgenic mice.
The databases were searched from January to July 2022, and a total of 1,498 records were identified. Subsequently, 1,347 irrelevant articles were excluded (most did not use AD animal models or did not report the association between endocannabinoid-glial cells-behavior and cognition), leaving 151 records. Further assessment revealed that 87 articles were duplicates, which were removed. A further 17 records were excluded due to either absence or inability to access the full texts (Supplementary Table S1). After reviewing the full texts of the remaining 47 records, 26 studies met the inclusion criteria. Additionally, more than half of the articles included were published within the last 10 years (i.e., 2012–2022). The relevance of the inclusion criteria for this review exists since 2005, when the first included article was published. Thereafter, articles on the involvement of the endocannabinoid system in modulating glial cells in AD-like rodent models indicate an ongoing research interest. Figure 2 illustrates the PRISMA flow diagram of the search results.
The systematic literature search found no human clinical evidence of the effects of cannabinoid treatment on cognition in AD based on the observation of glial endocannabinoid signaling. This may be because the symptoms of AD typically appear in humans at the moderate to advanced stages of disease. Moreover, the pathological evidence can only be observed in post-mortem harvesting of brain tissue. Mammalian rodent models such as mice and rats that are commonly used for AD are more receptive to transgenic procedures, easier to maintain, less expensive, and have shorter lifespans than invertebrates and non-mammalian vertebrates (NHP). As such, utilizing mouse models with human neurodegeneration may be more appropriate and realistic (Leung & Jia, 2016). Furthermore, the cognitive capacities in rodent models have been extensively studied and faithfully mimic the neurodegeneration and accompanying cognitive and behavioral abnormalities as those seen in humans (Webster et al., 2014). Rats and mice are generally models that best suit this research area. The identified rat/mouse studies in the present review fulfilled the criteria for the association of endocannabinoid-glial cell-cognitive function.
Exploring the pathophysiology and determining the therapeutic efficacy of AD requires a reliable experimental model. Of the 26 included articles, most toxin-induced AD models did not accurately reflect acute toxicity-induced neurodegeneration. Some did not create NFTs or Aβ deposition linked to AD. The transgenic mouse models with specific transgenes to replicate AD pathology appeared to be a more suitable tool for therapeutic discovery. To provide objective support and unbiased evidence for the continued application of cannabinoid-based agents as candidate drugs in the clinical treatment of AD, this study thoroughly examined and systematically analyzed the neuroprotective effects of endocannabinoid-mediated glial cells in various rodent models of AD. A summary of the key characteristics of the included studies is provided in Table 2.
The selected studies used two kinds of animals: rats (8 studies) and mice (18 studies; 16 transgenic and two wild-type or non-transgenic). The number of animals used in each group of the included studies ranged between 5 and 15 animals per group. However, no study described the method for determining the sample size. Eighteen studies used male animals, four used female animals, and two used both male and female animals. The 26 included studies involved six types of induction leading to AD rodent models, including i) stereotaxic injection of Aβ or streptozotocin (STZ); ii) transgenic mice such as familial AD (FAD)/TgAPP/APP/PS1/tau-transgenic; iii) transgenic crossed with CB2−/− mice, iv) aging-related animal models; v) D-galactose alone or combined with aluminum chloride (AlCl3)-induced AD; and vi) D-galactose combined with ovariectomy.
Amyloid peptide AD induction via direct intracerebral injection into distinct brain regions causes deficits in learning and memory abilities, which trigger behavioral changes comparable to those seen in AD. However, this treatment does not mimic the slow neurodegenerative process because it is an acute toxicity model. The intracerebroventricular (i.c.v) microinjection of Aβ (1–42) into the hippocampus was performed as a single injection on the first day (Martín-Moreno et al., 2011), daily for 7 days (Ramírez et al., 2005), repeated every other day on days 3, 5, 7 (Fakhfouri et al., 2012; Patricio-Martínez et al., 2019), and daily for 14 days (Wu et al., 2013). Meanwhile, streptozotocin (STZ) is another neurotoxin agent administered through i.c.v injections to establish the AD model (Xiang et al., 2022). Following the tau or Aβ-dependent increase in CB2R expression, four studies used genetic mouse models to determine if deletion of CB2 encoding cnr2 genes also modulated disease vulnerability (Schmöle et al., 2015; 2018) and provided neuroprotection (Galán-Ganga et al., 2021; J; Zhang & Chen, 2018). Conversely, APP23 mice with CB1 deficiency showed cognitive deficits despite a reduction in APP processing accompanied by lowered plaque load (Stumm et al., 2013). D-galactose, a neurotoxic agent that induced AD (Chen et al., 2017) resulted in cognitive impairments and neurodegeneration in rats, but lacked AD-related pathological hallmarks. The combination of D-galactose + AlCl3 resulted in obvious alterations in the CA1 sub-field of the model group, with disruption of the pyramidal cell layer and cellular degeneration (Mahdi et al., 2021). Evaluation of the restoration of cognitive impairment by CB2R activation was reported in bilaterally ovariectomized female rats administered D-galactose injections (Abd El-Rahman & Fayed, 2022). The anti-inflammatory and memory-enhancing effects of CB1/2 receptor stimulation in normal-aged rats were also investigated since natural aging is associated with increased microglial activation (Marchalant et al., 2008).
Transgenic (Tg) mouse-based models have become mainstream in pre-clinical research on AD. FAD, TgAPP, APP, PS1, and tau-transgenic models are best suited for quantifying amyloid plaque burden and were used in most of the selected studies. Among 16 transgenic mice studies, APP/PS1 was the most used animal model (9 studies), followed by 5xFAD (4 studies). APP23, TgAPP, and hTau models were used in one study each. APP-based animals were the first approach to reproduce and analyze the histopathological progression of cerebral Aβ (Sanchez-Varo et al., 2022). APP/PS1 was reported in Swedish mutant mice with L166P mutation in the presenilin 1 gene (Li et al., 2019), whereas a new generation of double Tg mice (5xFAD) co-expressed human APP and PS1 or PS2 mutations (Aparicio et al., 2018). A TgAPP mice model expressed the human APP containing a double mutation (Lys 670-Asn/Met 671-Leu) (Martín-Moreno et al., 2012), while an APP23 mice model showed hemizygous expression of the AD-linked KM670/671NL double mutation (Swedish mutation) of human APP (Stumm et al., 2013). Only one tauopathy model was included, in which the six isoforms of wild-type (WT) human tau were expressed in a mouse model with hTau (Galán-Ganga et al., 2021).
The cannabinoids agents used in the studies included WIN55,212-2, a non-selective CBR agonist (Marchalant et al., 2008; Fakhfouri et al., 2012; Mahdi et al., 2021); selective CB2R agonists such as AM1241 (Abd El-Rahman & Fayed, 2022); JWH-015 (Li et al., 2019), MDA7 (Wu et al., 2013; 2017); β-caryophyllene (Cheng et al., 2014); JWH-133 (Aso et al., 2013); ACEA a CB1R agonist (Patricio-Martínez et al., 2019); MAGL inhibitors like JZL184 (R. Chen et al., 2012); and FAAH inhibitors like URB (Vázquez et al., 2015). Other agents included tetrahydrocannabinol (THC) as a psychoactive, cannabidiol (CBD) as a non-psychoactive, or a combination of THC and CBD (Aso et al., 2016). Some studies used atypical CBRs like O-1602 (Xiang et al., 2022) or C. sativa leaf extract (Chen et al., 2017).
(1) Cognitive assessment
All the included studies performed behavioral tests to assess animal learning and memory function. These tests included a two-object recognition test alone and with an active avoidance test (4 of 26), a novel object recognition test (NOR) (5 of 26), Morris water maze (MWM) tests (16 of 26), and eight-arm radial tests (1 of 26). We separated the data into three categories according to different animal model species such as “wild-type” (Martín-Moreno et al., 2011; Xiang et al., 2022), “transgenic mice” (Aparicio et al., 2018; Aso et al., 2012; Aso et al., 2013; Aso et al., 2015; Aso et al., 2016; R; Chen et al., 2012; Cheng et al., 2014; Galán-Ganga et al., 2021; C; Li et al., 2019; Martín-moreno et al., 2012; Schmöle et al., 2015; Schmöle et al., 2018; Stumm et al., 2013; Vázquez et al., 2015; Wu et al., 2017; J; Zhang and Chen, 2018) and “rats” (Abd El-Rahman and Fayed, 2022; N. Y; Chen et al., 2017; Fakhfouri et al., 2012; Mahdi et al., 2021; Marchalant et al., 2008; Patricio-Martínez et al., 2019; Ramírez et al., 2005; Wu et al., 2013) to reduce variability across groups for analysis. We used ImageJ (Rawak Software Inc., Stuttgart, Germany) and RevMan 5.4 to quantify and analyze the data from four included studies. To investigate the protective impact of endocannabinoid-mediated glial cells on escape latency in MVM, novel object recognition, pathological determination, glial cell activation, and anti-neuroinflammation action, we performed a meta-analysis of the outcome and displayed the data via a forest plot.
The MWM test is a popular method used to assess spatial learning and memory. It is utilized in AD models to test cognitive function, memory, and medication efficacy. After training days, the MWM probe test is performed by removing the platform and allowing the tested animals to swim freely for 60 s. The amount of time spent in the target quadrant (where the platform is located on the training days) and the number of times the animal passes through it are assumed to signify the degree of memory consolidation during training. Six of 16 studies used rats as models, while the remaining 10 studies used mice. A total of 142 animals were treated with various cannabinoids at different doses and durations ranging from acute administration up to 5 months of chronic treatment (Wu et al., 2017). Another 142 vehicle-treated animals were added as controls. Univariate statistical analysis in all three subgroups (Figure 3) using a random-effects model showed that cannabinoid treatment significantly reduced the time to escape from the platform according to the overall effect size (SMD = −1.26; 95% CI: −1.77 to −0.76, p < 0.00001). The highest heterogeneity belonged to the transgenic mice group (77%, p = 0.0002). No heterogeneity was observed in the non-transgenic mice (wild-type) subgroup (I2 = 0%, p = 0.88), while moderate heterogeneity was seen in the rat subgroup (I2 = 68%, p = 0.008).
The novel object recognition (NOR) test is used to assess recognition memory. An investigator blinded to the treatment groups conducts the exam over 3 days. On day one, the mice are acclimatized for 20 min to the empty arena. On day 2, mice are returned to the arena for 6 minutes with two identical objects. On the third day, one of the two familiar objects is replaced with a new object of a different material, color, and shape. Six minutes are given for mice to explore the arena and the total time spent exploring each object is recorded. Exploration is defined as nodding and sniffing at an object from a distance of <2 cm. The details are presented in the form of a recognition index (RI). Rodents administered cannabinoid agonists better discriminated the novel object compared to the vehicle-treated group according to the overall effect size (SMD = 1.40; 95% CI: 1.04 to 1.76, p < 0.00001). Interestingly, no heterogeneity was observed (I2 = 0, p = 0.76) (Figure 4).
(2) Pathological determination
The amyloid hypothesis proposes that regardless of hereditary or sporadic condition, senile plaques develop in the brain due to amyloid peptide accumulation and aggregation. Fourteen of the 26 studies described the pathological assessments of Aβ burden in mice, in which all Aβ plaques formed in transgenic mice. Two studies involved FAAH inactivation (FAAH−/−) while another two studies featured cannabinoid receptor deficiency (CB1−/− and CB2−/−, respectively). The remaining studies used transgenic mice to produce Aβ plaques. The amount of plaques was determined from immunohistochemical analysis and was analyzed using RevMan 5.4. With moderate heterogeneity (I2 = 71 percent, p < 0.0001), univariate statistical analysis revealed a significant decrease in Aβ plaques according to the overall effect size (SMD = −0.91; 95% CI: −1.55 to −0.27, p = 0.006) toward the endocannabinoid-treated group over the vehicle-control group (Figure 5). The results of subgroup analysis indicated that endocannabinoid treatment significantly decreased Aβ plaques in the cortex (p < 0.04) compared to the hippocampus (p = 0.07).
The two most common Aβ species end at residues 40 and 42; the latter exhibits a stronger propensity for aggregation and is markedly more neurotoxic due to the presence of two additional hydrophobic amino acids. Three of the 26 studies reported Aβ oligomeric assessments such as Aβ1–42 pathology; another three studies reported on soluble Aβ40 and Aβ42.
(1) Glial cell activation
Twenty of the 26 selected studies ran immunoreaction tests with either Iba1 as a marker for microglia, GFAP as a marker for astrocytes, or both immunoreaction markers. Of these 20 studies, six conducted assessments of both GFAP and Iba1, eight assessed GFAP only, and six assessed Iba1 only. The remaining six studies conducted other microglial investigations such as microglial phenotyping, cell numbers, and macrophage infiltration instead of markers of glial cell immunoreaction. Besides the Iba1 marker, other microglial markers (CD68 and CD11b) were also applied. Furthermore, the CD68 and CD11b microglial markers were co-assessed with GFAP and they simultaneously reduced with cannabinoid treatment. One study reported that CD68 microglia expression was reduced following AM1241 administration (Abd El-Rahman and Fayed, 2022). Genetically CB2-deleted transgenic mice showed decreased microglial CD40 expression compared with normal transgenic (Schmöle et al., 2015). Decreased CD11b microglial marker was observed following MDA7 treatment in Aβ1–40 fibrils injected rats (Wu et al., 2013) and JZL184 treatment in 5XFAD transgenic mice (R. Chen et al., 2012). There was one study that indirectly used active caspase 3 to represent the presence of glial cells marked by positive-immunoreactivity paralleled with enhanced active caspase 3 immunostaining (Fakhfouri et al., 2012).
Iba and GFAP expression levels were increased in the AD model compared with normal vehicle administration animals. With cannabinoid stimulation, our analysis found that nine of 11 overall studies involving Iba1 reported its reduced expression compared to the control, while two studies showed non-significant changes. Similarly, following cannabinoid treatment, 11 of 14 studies reported reduced GFAP expression compared to the control; two other studies showed a non-significant result, and one reported increased GFAP expression. The non-significant difference in glial cell activity between the cannabinoid and control groups appeared when AβPP/PS1 mice were treated at advanced stages (Aso et al., 2016). All six studies combining Iba1 and GFAP observed decreased levels for both markers following cannabinoid administration. The results of univariate statistical analysis revealed a significant decrease in GFAP in the endocannabinoid-treated group compared to the vehicle-controlled group with an overall estimate (SMD = −1.47; 95% CI: −2.56 to −0.38, p = 0.008) −1.28 and substantial heterogeneity (I2 = 82%, p = 0.00001) (Figure 6). Similarly, Iba1 expression was significantly decreased in the endocannabinoid-treated group compared to the vehicle-controlled group according to the overall effect (SMD = −1.67; 95% CI: −2.56 to −0.79, p = 0.0002) with moderate heterogeneity (I2 = 70%, p = 0.0004) (Figure 7). Finally, the remaining six of 25 studies that performed microglia investigations reported decreased microglial number (Ramírez et al., 2005) (Aparicio et al., 2018), activity (Fakhfouri et al., 2012) and infiltration (Schmöle et al., 2015), as well as a stable morphology (Schmöle et al., 2018; Galán-Ganga et al., 2021). Although not included in the statistical analysis in this review due to their distinctive evaluation of glial cells, the decrease in microglial activity was observed as a response to endocannabinoid modulation.
(2) Anti-neuroinflammatory action
Fourteen of 26 studies were conducted on neuro-inflammatory markers, including eight studies using transgenic mice and six studies using wild-type mice or rats. A total of 76 animals received different cannabinoid agents at different doses, while 74 vehicle-treated animals were added as controls. Two studies each used CB2R and FAAH knockout mice, respectively. Most studies assessing inflammatory parameters showed a significant reduction in pro-inflammatory cytokine levels following cannabinoid administration, indicating the anti-inflammatory effect of cannabinoids by repressing activated microgliosis and astrocytosis. The evaluated variables included IL1β (7 studies), IL6 (7 studies), tumor necrosis factor-alpha (TNF-α) (10 studies), IFN-γ (2 studies), NFκB p65 (3 studies), and COX2 (2 studies). Out of 14 studies that assessed inflammatory cytokines, 11 reported on more than one cytokine, with the remaining three studies reporting only one cytokine. Of the 14 studies reporting neuroinflammation measurement, 11 were involved with cannabinoid agonist administration, one study concerned CB2R deletion while the remaining two studies utilized FAAH deletion animal models. Microglia and astrocytes are essential modulators of neuroinflammation in the central nervous system, responding quickly to infections, stress, and injury. Microglia and astrocyte activation leads to neuroinflammation-mediated neurodegeneration in the pathological development of AD, which will be elaborated on later.
Among 14 studies reporting neuroinflammatory markers in the endocannabinoid modulation of glial cells, 11 described a reduction in neuroinflammation in the treatment group compared to the control group. However, three other studies reported the opposite in transgenic (male) mice overexpressing hTAUP301S with concurrent CB2R deletion (Galán-Ganga et al., 2021), and FAAH knockout mice (Vázquez et al., 2015; Aparicio et al., 2018). These controversial findings are, at present, hard to translate, but could be due to animals with CB2R or FAAH deletions. Therefore, sensitivity analysis was performed in the present study by excluding the most outliers to control for heterogeneity and overall effect. The inflammatory mediators extracted for data analysis were TNF-α, IL-1β, and IL-6. Overall, 21 measurements of cytokines were reported, among which only five described increased neuroinflammation.
The univariate analysis of transgenic mice included six studies. The results revealed a non-significant decrease in all three cytokines (p = 0.09) in the endocannabinoid-treated group compared to the vehicle-controlled group according to the overall effect (SMD = −0.47; 95% CI: −1.03 to 0.08, p = 0.09) with nearly-substantial heterogeneity (I2 = 74%, p = 0.00001) (Figure 8). Three studies of wild-type mice included in univariate statistical analysis showed significantly reduced levels of all three cytokine types (SMD = −2.28; 95% CI: −3.15 to −1.41, p = 0.00001) in the endocannabinoid-treated group compared to the vehicle-controlled group with moderate heterogeneity (I2 = 37%, p = 0.14) (Figure 9).
(3) Other endocannabinoid mechanisms of action
FIGURE 8. Forest plot for comparison: Cannabinoid versus AD model. Outcome: pro-inflammatory cytokines in transgenic mice.
FIGURE 9. Forest plot for comparison: Cannabinoid versus AD model. Outcome: pro-inflammatory cytokines in wild type mice.
Endocannabinoid-mediated glial cells orchestrate alterations in biochemical expression toward neuroprotection, representing cognitive amelioration. Parameters relevant to neuroinflammatory and other mechanisms of AD involving several tests were recorded. Evaluations of oxidative stress, including those for nestin (Mahdi et al., 2021), NO • and nitric oxide synthase (iNOS) (Li et al., 2019; Patricio-Martínez et al., 2019; Vázquez et al., 2015), superoxide dismutase (SOD) (Aso et al., 2013; N. Y; Chen et al., 2017; Mahdi et al., 2021), and malondialdehyde (MDA) (N. Y. Chen et al., 2017; Xiang et al., 2022) expression were observed as these play pivotal synergistic roles in the pathogenesis of neurodegenerative processes, particularly in AD. Other mechanisms involve the expression of cAMP response element-binding protein (CREB) (Abd El-Rahman and Fayed, 2022), brain-derived neurotrophic factor (BDNF) (Galán-Ganga et al., 2021), PSD-95 (Aso et al., 2016; J; Zhang and Chen, 2018) (Stumm et al., 2013), and long-term potentiation (LTP) (R. Chen et al., 2012; Wu et al., 2013; Wu et al., 2017) for the assessment of neurogenesis and synaptic plasticity.
Caspase 3 (Fakhfouri et al., 2012) and Fluoro-jade C (J. Zhang and Chen, 2018) (R. Chen et al., 2012) as markers of apoptosis and degeneration of neurons were also evaluated. The expression of sex-determining region Y-box 2 (Sox2) (Wu et al., 2017), mitogen-activated protein kinase 3 (MAPK3), proteasome subunit, beta type, 2 (PSMB2), thioredoxin 2 (Txn2), and wingless-related integration site 16 (Wnt16) genes (Aso et al., 2015) were maintained following the administration of cannabinoid agents. The expression of membrane-tethered disintegrin and metalloproteases (ADAM17) responsible for the release of soluble TNF-α (Schmöle et al., 2018) and p-Ser9-GSK3b, which mediates tau’s hyper-phosphorylation (Aso et al., 2012; Martín-Moreno et al., 2012) were also investigated. However, those parameters were not used for univariate statistical analysis since each of them was not a component in the outcome criteria of this review, nor did all studies provide the same parameters.
Based on the SYRCLE risk of bias tool to evaluate the methodological quality of the 26 animal studies, the number of items with low risk of bias divided by the total number of items was used to determine each study’s quality score (Table 3). The quality scores were in the range of 0%–60%. Only one of the 26 included studies showed low bias risks in six domains (60%), while three studies scored 50%. According to the bias type, only the baseline characteristic domain achieved a score >50%. The scores were rated based on ‘yes’ answers.
TABLE 3. Methodological quality of the included studies. A, sequence generation; B, baseline characteristics; C, allocation concealment; D, random housing; E, blinded intervention; F, random outcome assessment; G, blinded outcome assessment; H, incomplete outcome data; I, selective outcome reporting; J, other sources of bias. Y: yes; N: no; NC: not clear.
The low-risk scores were generally low in most studies, which could be attributed to the large number of uncertain scores due to a lack of information as most of the studies did not describe in detail each component of bias in their methodology, even though the authors might have performed some of the bias surveillance checklists on the animals during their laboratory work. This is the reason why the uncertain answer covers most of the domains. Overall, the high risk of bias showed the lowest score among the three bias indicators in all domains (Figure 10).
This literature review presents the first systematic analysis of pre-clinical data on the modulation of glial cells by cannabinoids that affects cognitive performance. Evidence of the impact of glial cell endocannabinoid signaling on cognitive deficits in AD was demonstrated by the attenuation of memory impairment (Dominicis et al., 2022). The summary of the glial cell endocannabinoid mechanisms in cognition extracted from each article is presented in Supplementary Table S2.
Studies applying AD animal models have demonstrated the great potential of the endocannabinoid system to control neurodegeneration. Interestingly, the endocannabinoid system’s interaction with glial cells fundamentally determines its role. The neurochemical and biomolecular reaction of glial cells modulated by the endocannabinoid system maintained the stability of the brain/neural microenvironment and ensured neural cell resilience and ultrastructure. This neuro-immunomodulation of glial cells by endocannabinoids eventually displayed the behavioral and cognitive responses that can then be observed and translated into the improvement of the symptoms of dementia, which impair learning and memory in AD.
Clinical practice typically focuses on symptomatic treatment by prescribing cholinesterase inhibitors like donepezil and partial NMDA receptor antagonist-like memantine. Most of these AD treatments target a single pathogenic route; however, some treatments have undesirable side effects (Alzheimer’s Association Report, 2020). In contrast, natural products are characterized by their ability to effectively target numerous pathological disease pathways while having fewer negative effects (Paes-Colli et al., 2022). Overall, the administration of cannabis plants containing cannabinoid agonists improves cognitive deficits in several domains (Amanullah et al., 2017). Although cognitive neurobiology is not fully understood, a large body of data suggests the involvement of several brain networks, with intricate interactions between distinct signaling systems (Rapaka et al., 2021). Therefore, it is unlikely that the endocannabinoid-mediated glial mechanism of action can be wholly attributed to a single specific signaling pathway. Indeed, the pre-clinical studies discussed in this review demonstrate that exogenous cannabinoid treatment for cognitive deficits affects biochemical parameters such as inflammatory and oxidative stress markers, receptor subunit proteins, and signaling proteins. The roles of these systems in the potential mechanisms underlying the effect of cannabinoids on cognitive function are discussed below.
In animal studies, the restoration of cognitive/behavioral function from endocannabinoid modulation involves several strategies ranging from observation of endocannabinoid changes or cannabinoid receptor deficiency to the administration of exogenous cannabinoid (cannabis extract/cannabinoid constituent/cannabinoid agonist/antagonist). In this review, the effects of glial cell endocannabinoid signaling on cognition were impactful, in which the rodent models of AD showed preserved learning and memory. Cognitive recovery occurs following the administration of cannabinoids agonist. The endocannabinoid system, comprised of cannabinoid (CB: CB1 and CB2) receptors and their endogenous ligands, is engaged in various physiological tasks, most notably memory and learning. Numerous investigations have revealed that the endocannabinoid system strictly regulates cognition-related functions (Kruk-Slomka et al., 2017). The result from the analysis in the present review demonstrated that endocannabinoid-mediated glial cells exhibited considerable efficacy in shortening escape latency in pre-clinical AD models (p < 0.00001) and retaining recognition memory (p = 0.002). As the results of the recognition index and MWM tests were improved, experimental evidence indicates that this mechanism explains the beneficial effects of cannabinoids to stimulate endocannabinoid-mediated glial cells (Duffy et al., 2021).
Numerous memory processes, including consolidation, destabilization, and extinction, are highly influenced by the endocannabinoid system (Lunardi et al., 2020). Memory phase, brain site, and task-dependent manners may be affected by endocannabinoid-induced signaling pathways. Various experiments and clinical studies have shown that CB1R ligands affect memory and learning. Although CB1R ligands can both enhance and impair memory, each does so differently. The activation of cannabinoid CB1R in the basolateral amygdala (BLA) alleviated the memory impairment caused by scopolamine in adult male Wistar rats (Nedaei et al., 2016). The immediate footshock (context pre-exposure facilitation effect) and reversal learning, however, were inhibited by the hippocampal injection of CB1 antagonist (Lunardi et al., 2020). Such contradictory results could be attributed to the variations in behavioral tasks performed, handling techniques, the timing of drug delivery or the type of medication therapy, or other experimental settings, as well as doses and CB compounds chosen (Kruk-Slomka et al., 2017).
CB2R activation increases microglial cell migration and proliferation while reducing the release of inflammatory factors like TNF-α and free radicals, suggesting that anti-inflammatory effects occur secondary to activated CB2R in microglia (Rapaka et al., 2021). These effects also restore dendritic complexity in the cortex and improve memory for novel objects while not affecting plaque deposition or spatial memory (C. Li et al., 2019). CB2 agonists like MDA7 suppress microglial activation by Aβ fibril and facilitate Aβ disposal (Wu et al., 2013). CB2 knockout mice also exhibited better spatial memory in the Y-maze test (Y. Li and Kim, 2016). Memory and learning can be both facilitated and attenuated by CB2R ligands. These various memory effects may be primarily related to the pharmacokinetic properties of the CB2R ligands that have been tested and antioxidant properties that are indicated by both agonists and antagonists of these receptors (Kruk-Slomka et al., 2017). Overall, memory processes are modulated by CB1R and CB2R and they can be specifically targeted for pharmacological therapeutics to elicit the desired effects and prevent the undesirable ones once the roles of each kind of receptor are thoroughly characterized.
CB2R manipulation has been proposed to affect cognitive impairment because of its anti-inflammatory function and is commonly upregulated via microglial activity during neuroinflammation (Komorowska-Müller and Schmöle, 2021). As shown in our results, the implications of the pharmacological activation and genetic modification of CB2R in the AD mouse model are different in terms of how they affect the microglial activity and AD-induced neuroinflammation. While the pharmacological treatment requires CB2Rs to bind with molecules or ligands for activation to reduce the secretion of pro-inflammatory cytokines and improve cognitive behavior (Aso et al., 2013; Cheng et al., 2014; C; Li et al., 2019; Wu et al., 2013), CB2R deletion caused adverse effects (Galán-Ganga et al., 2021; Schmöle et al., 2015; Schmöle et al., 2018; J; Zhang and Chen, 2018). Moreover, differences in experimental designs may also have influenced the cognitive outcomes. We hypothesize that these could be attributed to the nature of CB2R itself, whether it is deficient, activated, or inactivated. When CB2R is deleted or antagonized, it mitigates inflammatory response, making it a neuroprotective factor. The same effect occurs when there is a presence of cannabinoid agonists. However, in progressing AD conditions, the inactivation or unoccupied of CB2R may lead to CB2 overexpression. This excess of CB2 during microglial activation would promote inflammatory cytokine release leading to neurodegeneration. Still, additional experiments are required to validate this hypothesis. Therefore, selective or non-selective cannabinoid agonists are crucial for microglial CB2R activation, with anti-inflammatory cytokine release to reverse the devastating ongoing inflammatory process in AD and overcome cognitive deterioration.
In synaptic plasticity, the downstream pathways of phosphoinositide 3-kinase (PI3K), phospholipase C-γ (PLCγ), and MAPK are activated as a result of BDNF’s high affinity binding to tropomyosin receptor kinase B (TrkB) (Guo et al., 2018). Depending on the route that is activated, the downstream physiological consequence of BDNF/Trk B activation followed by phosphorylation of CREB may involve modulation and enhancement of synaptic plasticity (De Vincenti et al., 2019), greater dendritic growth and branching (González-Gutiérrez et al., 2020), upregulation of diacylglycerol (DAG) synthesis, and promoted growth of neuronal fibers (Kowiański et al., 2018; Bergen, 2020). In the downstream regulation of synaptic signaling, endocannabinoid signaling appears to interact with BDNF activities. In neuroplasticity, the strength of synaptic signaling plays a significant role. The mechanisms of long-term depression (LTD) and LTP are particularly crucial to the neurobiology of memory and learning. Endocannabinoids play significant roles in controlling glutamatergic and GABAergic synaptic transmission as many retrograde messengers throughout the CNS (Dow-Edwards et al., 2017; Bergen, 2020).
In exploring endocannabinoid-mediated neurogenesis and neuroplasticity, Ferreira et al. discovered that CB1R selective activation and CB1R/CB2R non-selective activation increased cell proliferation and increased DG and subventricular zone (SVZ) cell proliferation, respectively. Regarding neuronal differentiation, both subtypes of cannabinoid receptors also enhanced neuronal differentiation in the DG and SVZ neurogenic niches (Ferreira et al., 2018). In the same study, Ferreira demonstrated that CREB is a key regulator of BDNF-induced gene expression and might also be the common linking element. In addition, De Chiara et al. uncovered a novel mechanism by which BDNF influences the function of striatal CB1R (De Chiara et al., 2010). In another study, Abd El-Rahman and Fayed (2022) reported improved cognition in D-galactose-injected ovariectomized rats via CB2R activation modulating the CREB/BDNF signaling pathway. This promoted CREB phosphorylation for the expression of various pro-survival genes such as BDNF and Bcl-2 which later enhanced the expression of both antioxidant enzymes and the antiapoptotic protein and reduced delayed neuronal death. Throughout neurogenesis, BDNF may be necessary for cannabinoid-induced effects on cellular proliferation and neuronal differentiation.
Glial cells play roles in several physiological processes, including programmed cell death, cell surveillance, the removal of newborn apoptotic neurons, neural plasticity, and synaptic pruning, among many others, that are essential for brain development and the maintenance of homeostasis in the adult brain (Sanchez-Varo et al., 2022). Neuroinflammation in AD driven by Aβ-induced neurotoxicity is alleviated by enhancing microglial endocannabinoid signaling. In the CA1 neurons of APP/PS1 transgenic mice, the LTP elicited by high-frequency electric stimulation was markedly impaired but restored with MDA7 treatment along with recovery of Sox (a neural stem cell marker) and decreased Iba1 marker and CB2 expression (Wu et al., 2017). Aso et al. (2016)reported that the administration of THC and CBD advanced stages altered the imbalance between excitatory and inhibitory neuronal activity in the somatosensory cortex of aged APP/PS1 mice, as evidenced by decreased GluR2/3 expression levels and increased GABA-A Rα1 expression. Via CB1R activation, THC and CBD both reduced the deleterious impact of Aβ on GABAergic function, which in turn improved cognitive function, facilitating inhibitory GABAergic activity in the somatosensory cortex despite the lack of changes in Iba1 and GFAP. A MAGL inhibitor instead of a cannabinoid agonist showed a decreased cognitive deficit with a reduction in astrocytic marker and CB2 expression in TG-CB2-KO mice treated with JZL184. The restoration of downregulated PSD95 and glutamate receptor subunit expression is likely linked to the improvement in cognitive function (J. Zhang and Chen, 2018).
The results of the analysis in the present review demonstrated that endocannabinoid-modulating glial cells decreased amyloid plaques in the brain by enhancing amyloid clearance. Only one study in this review did not observe a decrease in beta-amyloid levels (Aso et al., 2012). Similarly, transgenic mice carrying a particular mutation in Beclin 1 (F121A) showed constitutively activated autophagy in the brain (among other tissues) and dramatically reduced amyloid accumulation (Rocchi et al., 2017). This may suggest that the agent promoting autophagy plays a pivotal role in plaque clearance and, thus, amelioration of cognition (Tamagno et al., 2018). Aβ may precipitate in brain tissues and trigger neuroinflammation by glial cells. Glial cells may also respond to endocannabinoid stimulation by activating upstream and downstream pathway cascades. These actions, which are required to ascertain the underlying neuroinflammatory mechanism, are regulated toward a safer mode without harming the surrounding neuron area.
Nevertheless, correlation analysis of the association between Aβ pathology and cognitive function showed that Aβ-42 was not significantly related to cognitive changes (El-Bakly et al., 2019). Instead of Aβ precipitation, glial cells directly affect behavioral function. The included studies showed that a decrease in glial cell activity was associated with the restoration of cognitive impairment in animals, as evidenced in the early and pre-symptomatic stages of AD when CB2R glial cell activation in APP/PS1 mice attenuated AD-dependent neuroinflammation (Aso et al., 2013). Although treatment with JWH-133 (Aso et al., 2013), THC/CBD (Aso et al., 2016), and JWH-015 (C. Li et al., 2019) decreased the release of pro-inflammatory cytokines, the Aβ plaque burden was unaffected. However, these treatments ameliorated cognitive performance in mice. Intriguingly, compared to the early symptomatic state, this impact was greater when the treatment was administered during the pre-symptomatic phase (Aso et al., 2013). Despite the association of CB2R overexpression with tauopathy in transgenic mice and patients with AD (Galán-Ganga et al., 2021), the amyloid burden causing CB2R overexpression was not correlated with cognitive status in humans (Solas et al., 2013). Consistent with a human study, Ma et al. (2021) reported that early Aβ accumulation had an independent effect on cognitive decline when mediated by either tau-related pathology, neurodegeneration, and neuroinflammation in subsequent cognitive decline in patients with mild cognitive impairment. Similarly, a cross-sectional study of 598 amyloid-positive participants found that participants with normal cognition and hippocampal volume were associated with preservation of high levels of soluble Aβ-42 despite increasing brain amyloidosis compared to those with mild cognitive impairment (MCI) and AD (Sturchio et al., 2021). Thus, the evidence showed that accompanying factors along with Aβ are required to induce cognitive deficits.
The findings from the current analysis suggest that the cognitive symptoms of AD may begin to appear upon the activation of neuro-immunological processes within the brain. Plaque accumulation does not immediately impair cognition but eventually precipitates the pathological process, symptom severity, and disease progression. Chronic treatment may be beneficial for attenuating the inflammatory state to augment cognitive function, which, in turn, promotes the clearance of protein precipitates. Thus, future studies should focus on tau pathology since brain hyperphosphorylated tau accumulation correlates more closely with cognitive decline than Aβ deposits (Johnson et al., 2016; Sanchez-Varo et al., 2022).
Through toll-like receptor (TLR) activation, lipopolysaccharides (LPS) induce systemic inflammatory reactions. LPS binding triggers the activation of NF-κB to TLR4 on the microglia surface, which activates many signal transduction pathways, including MAPK, PI3K/AKT, and mTOR. Microglial activation mediates neuroinflammation following aberrant stimulation; tissue damage; and the presence of pathogens, infection or injury, and neurotoxins. Microglia will then aggregate, migrate, proliferate, phagocytose, present the antigen to T-cells, release various oxidants, and activate a variety of proteins and genes. Through NF-κB activation, microglia enhance the release of proinflammatory cytokines such as IL-1β, TNF-α, iNOS, reactive oxygen species (ROS), cyclooxygenase (COX)-1 and COX-2, and several neurotoxic agents, resulting in neuronal dysfunction and cell death. In chronic neuroinflammation, those cytokines and neurotoxic chemicals are released over a longer period, contributing to prolonged neurodegeneration.
CB1R/CB2R activation in the microglia recruits a variety of intracellular protein kinases that are implicated in the expression of various anti-inflammatory protein genes. Endocannabinoids inhibit MAPK pathway activity in activated microglia, leading to decreased IL-1β, IL-6, and TNF-α levels. In most of the selected studies, the endogenous cannabinoids AEA and 2-AG broadly modulated the immune system by increasing the production of anti-inflammatory cytokines such as IL-10 while decreasing the production of pro-inflammatory molecules via CB1R and CB2R activation in microglia cells (Krishnan and Chatterjee, 2012; Rapaka et al., 2021). CB2R is expressed by activated microglia in the brains of patients with AD and also in a similar model of dementia. The magnitude of reaction to partial and full cannabinoid agonists may be significantly influenced by receptor density. Cannabinoids elicit CB2R activation in microglia, which is responsible for their immunological effects. For example, CB2R activation inhibits the release of pro-inflammatory mediators, including TNF- α and free radicals, while promoting microglial cell proliferation and migration (Carrier et al., 2004; Dominicis et al., 2022). Therefore, activating CB2R in microglia might favorably affect inflammation. Additionally, the involvement of other functioning ‘endocannabinoidome’ receptors may contribute to the enhancement of the glial endocannabinoid signaling effects in AD (Cristino et al., 2019). Before initiating anti-inflammatory actions, CB2R activation by cannabinoid agonists establishing receptor-ligand interaction is assumed to further decrease CB2R expression, marked by the concurrent reduction of CB2 protein and microglial marker. Following this, the initial low CB1R expression during progressive and advanced AD may, in turn, become upregulated once the inflammation is resolved. However, additional experiments are required to validate this hypothesis. Our present work highlighted the reversal of microglia-mediated neuroinflammation following cannabinoids treatment (Marchalant et al., 2008; Martín-Moreno et al., 2012; Stumm et al., 2013; Aso et al., 2016; Wu et al., 2017) (Xiang et al., 2022) (C. Li et al., 2019) (Vázquez et al., 2015) (Schmöle et al., 2015) (Cheng et al., 2014) (Table 2).
Our work revealed reduced immunoreactivity in glial cells, as evidenced by lower levels of GFAP (astrocytes) and Iba1 (microglia) in the cannabinoid-exposed animal model compared to the AD animal model. These reductions indicated that glial cells undergo polarization from A1/M1 to A2/M2 states. M2 microglia are an alternate microglia activation thought to be a phenotype of anti-inflammatory response, with neuroprotective effects and a role in tissue repair (Figure 11). Stimulation of interleukin-4/13 (IL-4)/IL-13) promoted M2 microglia predominance (Kwon and Koh, 2020; Tang and Le, 2016; Colonna and Butovsky, 2017). Markers of inflammation were dramatically upregulated in M1 microglia, while anti-inflammation factors were upregulated in M2 microglia of mice and rats (Lam et al., 2017).
The most evident phenotype of AD is cognitive and memory decline; thus, mitigating this dysfunction is the most crucial factor in determining how well potential medications are modeled and their treatment effectiveness. The attenuation of cognitive deficits in the transgenic AD model may occur due to the clearance of amyloid plaques, depending on the state of microglial polarization, which plays a critical role in this condition. The Aβ plaques in the alternative microglial states are believed to undergo either degradation by Aβ-degrading proteases (Saido and Leissring, 2012) or through autophagosomes for autophagy (Daily and Amer, 2020). In the brains of patients with AD, the latter process is more prevalent (Nilsson et al., 2013). As novel stimulation of memory and to augment the impact of systemic aspect on cognitive fitness, autophagy induction is not restricted to microglia but also occurs in hippocampal neurons to enhance activity-dependent functional and structural synaptic plasticity (Glatigny et al., 2019), astrocyte pruning of axons (Song et al., 2008), and increased myelination by oligodendrocytes (Smith et al., 2013).
CB2R activation induces phagocytosis (Mecha et al., 2015) and switches the M1 phenotype of microglial cells to the M2 phenotype (Orihuela et al., 2016). As M2 microglia upregulate endocannabinoid receptor expression, increased endocannabinoid release may, in turn, activate CB1R or CB2R and distinct endocannabinoids signaling cascades, intensifying the M2 profile (Mecha et al., 2016; Dominicis et al., 2022). The exposure of rat or human microglia to low concentrations of 2-AG and AEA increases the expression of the M2 marker Arg-1, Ym1/2, along with other markers of alternative phenotype such as suppressor of cytokine signaling 3 (SOCS 3) (Mecha et al., 2015). A range of endogenous, photogenic, and synthetic cannabinoids have been explored for their dual targeting of CB2R/PPARs to provide neuroprotective activities, mainly in rodent models of many neurodegenerative diseases (Lago-Fernandez et al., 2021). Ji et al. (2018) reported that antagonizing PPAR gamma promoted polarization from the M1 to the M2 phenotype in primary microglia, as indicated by increased M2 marker levels to enhance autophagy via LKB1–AMPK signaling and inhibited NF-κB–IKKβ activation. Toll-like receptor 2 (TLR2)-mediated autophagy may also regulate the M1/M2 phenotypic switch in BV2 microglial cells (Ma et al., 2020). The anti-inflammatory cytokine IL-4 causes M2 polarization of microglia and activates autophagic flux (Tang et al., 2019). The stimulation of autophagy may also cause microglial polarization into the M2 phenotype and suppress the inflammatory process (Zubova et al., 2022). Therefore, the selection of cannabinoid base medicine is pivotal for regulating microglia toward M2 polarization, enhancing the upstream signaling cascade of autophagy, and downregulating neuroinflammation.
The antioxidant and anti-inflammatory effects of phytocannabinoids are principally responsible for improving patient health, even though there remains much to learn about cannabis-based medicine (CBM)-mediated modulation of the ECS (Maayah et al., 2020). The so-called endocannabinoidome, which is an ECS expansion to incorporate the receptors, enzymes, and second messengers under cannabinoids regulation, has diverse targets for these phytocannabinoids (Ligresti et al., 2016). In addition to CB1 and CB2, other receptors respond to these molecules, including the transient receptor potential cation channel subfamily M member 8 (TRPM8) and transient receptor potential channels of vanilloid type-1 (TRPV1) (De Petrocellis et al., 2007); the orphan G-protein-coupled receptors (GPCR) 55, 18, and 119 (GPR55, GPR18, and GPR119); and peroxisome proliferator-activated receptor gamma (PPAR-α) (Cristino et al., 2019).
Emerging evidence suggests that ECS can modify the expression and/or activity of enzymes implicated in the formation of these small reactive molecules such as NADPH oxidase enzymes 2 and 4 (NOX2 and NOX4) and also control cellular ROS/RNS generation by regulating mitochondrial-derived ROS/RNS (Lipina & Hundal, 2016). CB1 activation increases redox imbalance, whereas CB2 stimulation reduces ROS/RNS production (Han et al., 2009). By increasing intracellular SOD and GSH levels, lowering the oxidized glutathione (GSSG) level, raising the GSH/GSSG ratio, and reducing NOX2 expression, AEA protected a mouse hippocampal neuron cell line against H2O2-induced redox imbalance. These effects were nullified by the administration of a CB1 antagonist or CB1-siRNA, indicating the ability of AEA to reduce oxidative stress in hippocampal neurons that might be mediated by CB1 activation (Jia et al., 2014). Moreover, arachidonyl-2-chloroethylamide activates mitochondrial CB1, reducing oxidative stress and exerting neuroprotective effects on I/R damage (Paloczi et al., 2018). CB2 activation seems to play a role in mitigating I/R damage by reducing ROS/RNS generation and lipid peroxidation (Paloczi et al., 2018). Palmitoylethanolamide (PEA), dependent on PPAR-α, protects glia from oxidative stress by decreasing MDA production (Scuderi et al., 2018). TRPV1 is involved in vascular dementia once activated by AEA, which reduces oxidative stress, improves learning and memory, and enhances neuroprotection (Gallelli et al., 2018). Additionally, N-arachidonoyldopamine (NADA), an endogenous ligand of CB1, TRPV1, and PPAR-γ, belongs to the endovanilloid class of endocannabinoids and has anti-inflammatory and antioxidant effects on glial cells.
Our findings indicated that cannabinoids play roles as antioxidants by activating GPR55, where acute administration of O-1602 elevated SOD and depressed MDA with concomitant reduced microglial activation (Xiang et al., 2022). Chronic daily cannabinoid treatment with JWH-133 also ameliorated cognitive symptoms in an experimental model of AD by reducing inflammation, oxidative stress, and microgliosis via CB2R activation (Aso et al., 2013). Similarly, reduced astrocyte activation and lipid peroxidation were observed in chronic EFC treatment (Chen et al., 2017). The decreased MDA levels and increased SOD activities in the rat brains following WIN administration could be attributed to the positive effects of WIN on maintaining redox balance as well as their interactions with various signaling pathways that orchestrate neuronal survival, differentiation, and death (Mahdi et al., 2021).
The different mechanisms by which astrocytes interact with other cell types are essentially controlled by intracellular Ca2+ concentrations. Astrocytic CB1 responds to endocannabinoids generated during neuronal activity by eliciting increased Ca2+ levels, as evidenced by astrocytic-neuron communication in synaptic physiology (Bernal-Chico et al., 2022). As a general and fundamental mode of endocannabinoid signaling in the modulation of astrocyte activity, astrocyte Ca2+ mobilization via CB1R is thought to occur in the cortex and hippocampal human tissue and throughout the rat brain. Astrocytic CB1 activation causes increased cytosolic Ca2+ levels, which helps to release gliotransmitters like glutamate and D-serine and indirectly facilitate excitatory transmission (Han et al., 2012; Mahmoud et al., 2019; Robin et al., 2018). This discovery underscores the importance of CB1R in the modulation of synaptic activity by astrocytic cells while also emphasizing the unique role of astroglial CB1 in the effects of cannabis-based medications in humans. Despite a few studies that expressed CB2R in astrocytic cells, there is currently a lack of strong evidence for the existence of astroglial CB2R under physiological settings. Surprisingly, one of the studies included in this review reported reduced GFAP in APP transgenic mice lacking CB2R following 3 weeks of JZL184 administration (Zhang & Chen, 2018). In that study, GFAP was used instead of Iba1 to assess its association with CB2R. The CB2R deficiency might have resulted in reduced microglia activation, which may also indirectly influence astrocyte action, represented by decreased GFAP, which is required for the close relationship between CB2R and astrocyte compared to microglia.
Glial cells regulate not only aggregated protein clearance but also the anti-neuroinflammatory, immunomodulation, redox stability, and eventually neuroplasticity causing amelioration of learning and memory, which are key mechanisms occurring with endocannabinoid modulation. As with microglia, the astrocyte react to brain damage or neurodegeneration as an adaptive mechanism in response to injury or illness. Astrocytes undergo reactive astrogliosis in pathological conditions such as the accumulation of Aβ and pathogenic tau. The re-expression of nestin and the upregulation of glial fibrillary acid protein (GFAP) and vimentin in the above conditions are considered markers of astrocyte reactivity (Sanchez-Varo et al., 2022). Mahdi et al. reported that increased nestin levels might affect stem cell migration and differentiation, as evidenced by improved cellular activity in the brain, GFAP levels, and cognitive status following treatment with WIN or donepezil in AlCl3 and D-galactose-induced AD rats (Mahdi et al., 2021).
Overall, the present works showed that cannabinoid agonists decreased astrocyte activation. In MAGL-deficient mice, Chen et al. (2012) reported that decreased GFAP with MAGL inhibition was connected to greater hippocampus LTP and enhanced learning and memory via a CB1 receptor-dependent mechanism. Reducing astrocyte activation reduced oxidative stress after daily EFC treatment for 14 weeks in D-galactose-induced AD rats (Chen et al., 2017). Wu et al. (2013)reported that chronic MDA7 administration promoted Aβ clearance, ameliorated Aβ-induced glia activation and production of IL-1β, and restored CB2 expression with subsequent synaptic plasticity, memory, and cognition in Aβ1–40 fibrils injected in the hippocampus. Later, Wu et al. (2017)showed that using the same agents with longer duration showed the same results in APP/PS1 mice. Sox expression was recovered, suggesting that MDA7-mediated microglia CB2R activation rescued neurogenesis and improved cognition in the AD mouse model. A low extent of astroglial activation should be aligned with CB1 activation. However, the corresponding cognitive deficits in APP23/CB1−/− mice showed decreased astroglial marker and also reduced sAPPa, its C-terminal a and b fragments, and Aβ1-40 peptide in the brain (Stumm et al., 2013), which might reflect a lower amyloid plaque load.
Our work has accumulated evidence on the mutual response of microglia and astrocytes. Most of the articles evaluating both microglial and astrocytes reported increased activity of both in neuroinflammation and decreased reactivity in neuroprotection. Compelling evidence suggests synchronization and communication between microglia and astrocytes in healthy and diseased brains (Vainchtein & Molofsky, 2020). Microglia, in particular via nuclear factor-B (NF-κB) signaling, may potentiate the inflammatory activation of astrocytes by elevating cytokine and chemokine expression levels (Kirkley et al., 2017). Cytokines (TNF-α, IL-1, and C1q) released by microglia may alter the supportive function of astroglia (Liddelow et al., 2017). In an in vitro cell culture study, Kim et al. (2021) showed that astrocyte-microglia cross-talk is advantageous for microglia proliferation with M2 type acquisition and A2 type polarization of astrocytes. In AD mouse models where the complement system was engaged, the interaction between the complement factor C3 produced by astrocytes and the microglial C3a receptor (C3aR) controlled the dynamic regulation of microglial phagocytosis (Lian et al., 2016).
Microglia and astrocyte interactions are also observed. Aso et al. conducted two stages of initiation of cannabinoid treatment in APP/PS1, in which the presymptomatic stage showed restoration of the long-term memory decline. However, they reported no appreciable changes in aversive avoidance learning capacity when therapy was initiated at the symptomatic stage. Despite no significant decrease in Aβ plaques, the GSK3, p38, and SAPK/JNK kinase activity may have decreased, which would explain the decline in tau hyperphosphorylation level, establishing a role of CB2R in GSKβ modulation (Aso et al., 2013). The combination of THC and CBD reduced soluble Aβ42, but not Aβ40 protein levels, thus lowering the levels of the most hazardous soluble Aβ form in APP/PS1 animals to provide a protective effect and increase the expression of thioredoxin 2 and Wnt16 to contribute to axonal integrity (Aso et al., 2015). Through CB1R activation, the above combination also supports inhibitory GABAergic activity in the somatosensory cortex by reducing the negative impact of A on GABAergic function and, ultimately, on cognitive performance. In addition, chronic stimulation of CB1R significantly suppresses glutamatergic activity, which helps to enhance cognitive performance by reversing the alterations in neuronal excitability observed in APP/PS1 animals (Aso et al., 2016). The ubiquitous distribution of the endocannabinoid system and its multifunctionality suggest that the favorable cognitive effects reported in APP/PS1 following chronic treatment with natural cannabinoids may result from parallel mechanisms.
Cannabinoids have recently been studied in several fields, including neuroscience. Although pre-clinical research has produced compelling results, some clinical trials have also reported potentially positive outcomes. A systematic review and meta-analysis found strong evidence of the effectiveness of cannabinoids for the treatment of dementia-related neuropsychiatric symptoms (Bahji et al., 2020). No randomized controlled trials (RCTs) on the use of cannabinoids to treat cognitive deficits in dementia have yet been conducted, but a systematic review of RCTs on the effectiveness of cannabinoids for treating dementia showed improvement in the behavioral and psychological symptoms of dementia (BPSD) for nabilone over THC (Charernboon et al., 2021). A systematic review of human studies reported mixed findings on the effects of ECS on cognition in AD in investigations range from epigenetic to imaging and blood and cerebrospinal fluid studies (Berry et al., 2020). The major challenges of human studies compared to animal studies are the lack of an ability to sample the brain tissues of humans. Thus, glial cells cannot be sampled in living human brains except when there is a strong indication for surgical intervention. Therefore, the current practical method of obtaining human brain samples through post-mortem examinations, in which data from a comprehensive review demonstrated the association of endocannabinoids and glial cells in AD (Berry et al., 2020). Although complete tissue findings have been reported, the lack of simultaneous cognitive function is a notable shortcoming of post-mortem samples. Thus, animal studies should reflect complementary work in humans. The exploitation of appropriate investigative modalities such as AD-specific plasma biomarkers for screening (Cullen et al., 2021) and performing positron emission tomography (PET) scans may provide molecular imaging to potentially unveil diagnostic CB receptors (Ahmad et al., 2014; Ahmad et al., 2016) and glial cell changes (Edison et al., 2018; Cavaliere et al., 2020) to identify subtle findings in AD research in humans.
This review has several limitations. Since only a limited number of studies were included, only an evidence-backed basis for subsequent experiments through the systematic review of some of these potential mechanisms was provided. Moreover, the meta-analysis showed high heterogeneity in certain parameters, which frequently occurs in animal studies due to differences in experimental methods. The high proportion of unclear risk of bias (Figure 10) resulted from inadequate information regarding the assessment of certain types of bias domains reported by the authors in a primary study without knowing whether they had assessed a particular type of bias. Therefore, the risk of bias analysis in primary studies should be improved by encouraging researchers conducting animal studies to comply with the recently revised ARRIVE guidelines 2.0 (du Sert et al., 2020). These guidelines ensure a standard of reporting by prompting authors and journals to identify the minimal information required to report in publications describing animal experiments to allow accurate and transparent reporting.
Based on the data of the included studies analyzing the pharmacological properties of selective CB1R, CB2R, or non-selective cannabinoid agonists, we speculate that non-selective cannabinoid agonists might offer a better outcome due to their flexibility on either CB1 or CB2 receptors, which exert different mechanisms. CB1R provides cognitive improvement against synaptic dysfunction while CB2R provides neuroprotection against inflammatory responses. However, additional research is required to validate this speculation. The classification of CB1R and CB2R agonists and their respective pharmacodynamic properties according to the abovementioned mechanism may provide information to allow more precise targeting in therapeutic strategies for AD. The communication between microglia and astrocytes is also complex in the context of achieving the treatment aim of preserving cognitive functions. The analysis results indicated that pharmacological manipulation of endocannabinoid system-mediated glial cells through the intake of cannabinoid agonists may be a candidate for the clinical treatment of AD. The present study summarized and discussed the possible pharmacological mechanisms involved and also addressed the importance of the effects of endocannabinoids on symptomatology-dependent immunomodulation as a primary treatment target in AD combined with anti-amyloidogenic agents as secondary and complementary therapies.
To our knowledge, this is the first systematic review to integrate the results of ECS-linked glial cell changes in AD from all known animal research studies published in the last 17 years. ECS signaling that directly shifts microglial morphology into the neuroprotective (M2) and homeostatic (M0) phenotype would be the main events toward a major outcome of cognitive improvement, in which synaptic plasticity modulation, synaptic pruning, and neural trophic support are considered the crucial physiological roles of microglia. We postulate that the ECS effects from the administration of cannabinoid agonists lead to endocannabinoid modulation of glial cells, particularly CB2R activation, to influence the mechanistic sequence as follows: 1) glial cell phenotype transformation toward the alternative state, 2) increased anti-inflammatory cytokine and reduced pro-inflammatory cytokine levels, 3) promotion of glial cell autophagy for clearance of protein aggregation, 4) decreased ROS/RNS generation and lipid peroxidation with increased antioxidant levels, and 5) amelioration and maintenance of synaptic plasticity. The findings support the view that changes in CB1R, CB2R, central AEA concentration, FAAH, and MAGL activity occur in AD animals despite some methodologically heterogeneous data. In general, the findings in this review provide knowledge to establish ECS biomarkers in AD and may offer opportunities for the development of novel drugs.
In conclusion, CB2R in glial cell activation is the rate-limiting step before endocannabinoids exert their neuroprotective effects. Additionally, the synergistic involvement of CB1R is required to amplify the positive neurocognitive impact. As the endocannabinoid system is a near-ubiquitous regulator of neuronal communication throughout the brain, studies involving transcriptional and epigenetic mechanisms are needed to elucidate endocannabinoid-related synaptic plasticity.
The original contributions presented in the study are included in the article/Supplementary Material. Further inquiries can be directed to the corresponding authors.
MK devised the conceptual ideas and developed the protocol for the systematic review. MR provided coaching and advice on implementing the systematic searches. RA and MH organized the table of literature matrix from the included studies. SV revised and provided guidance on appropriate language and wording. MN and SS assessed the included studies based on the ‘SYRCLE’ risk of bias tool. VT drew a figure diagram. KW revised the appropriate and validated references. MuAA provided the computational framework and analyzed the data. NM helped in interpreting the results. MoAA guided data extraction and the appropriate method of systematic review based on PRISMA. MM was involved in supervising and planning the work.
This work was supported by Universiti Putra Malaysia (UPM) who sponsored the research grants from the Ministry of Higher Education of Malaysia, namely, the Fundamental Research Grant Scheme (FRGS) (FRGS/1/2019/SKK08/UPM/02/16).
All the involved colleagues and the supervisory committee for their encouragement and full support until this manuscript was ready for publication.
The authors declare that the research was conducted in the absence of any commercial or financial relationships that could be construed as a potential conflict of interest.
All claims expressed in this article are solely those of the authors and do not necessarily represent those of their affiliated organizations, or those of the publisher, the editors, and the reviewers. Any product that may be evaluated in this article, or claim that may be made by its manufacturer, is not guaranteed or endorsed by the publisher.
The Supplementary Material for this article can be found online at: https://www.frontiersin.org/articles/10.3389/fphar.2023.1053680/full#supplementary-material
Abd El-Rahman, S. S., and Fayed, H. M. (2022). Improved cognition impairment by activating cannabinoid receptor type 2: Modulating CREB/BDNF expression and impeding TLR-4/NFκBp65/M1 microglia signaling pathway in D-galactose-injected ovariectomized rats. Plos One 17 (3), e0265961. doi:10.1371/journal.pone.0265961
Adams, R., Hunt, M., Clark, J. H., 62, V., Clark^, J. H., and Washington, D. C. (1939). Structure of cannabidiol, a product isolated from the marihuana extract of Minnesota wild hemp. I (IN collaboration with the treasury department. NARCOTICS Lab. 123 (4), 196–200.
Ahmad, R., Goffin, K., Van den Stock, J., De Winter, F. L., Cleeren, E., Bormans, G., et al. (2014). In vivo type 1 cannabinoid receptor availability in Alzheimer’s disease. Eur. Neuropsychopharmacol. 24 (2), 242–250. doi:10.1016/j.euroneuro.2013.10.002
Ahmad, R., Postnov, A., Bormans, G., Versijpt, J., Vandenbulcke, M., and Van Laere, K. (2016). Decreased in vivo availability of the cannabinoid type 2 receptor in Alzheimer’s disease. Eur. J. Nucl. Med. Mol. Imaging 43 (12), 2219–2227. doi:10.1007/s00259-016-3457-7
Alzheimer’s Association Report (2020). 2020 Alzheimer’s disease facts and figures. Alzheimer’s Dementia 16 (3), 391–460. doi:10.1002/alz.12068
Amanullah, S., Shivakumar, K., Hassan, S., Canfield, A., and Cole, J. (2017). “Chapter 86 - synthetic cannabinoids in dementia,” in Handbook of cannabis and related pathologies (Elsevier). doi:10.1016/B978-0-12-800756-3/00100-9
Andre, C. M., Hausman, J. F., and Guerriero, G. (2016). Cannabis sativa: The plant of the thousand and one molecules. Front. Plant Sci. 7 (FEB2016), 19–17. doi:10.3389/fpls.2016.00019
Anwar, F., Latif, S., and Ashraf, M. (2006). Analytical characterization of hemp (Cannabis sativa) seed oil from different agro-ecological zones of Pakistan. JAOCS, J. Am. Oil Chemists’ Soc. 83 (4), 323–329. doi:10.1007/s11746-006-1207-x
Aparicio, N., Grande, M. T., Ruiz de Martín Esteban, S., López, A., Ruiz-Pérez, G., Amores, M., et al. (2018). Role of interleukin 1-beta in the inflammatory response in a fatty acid amide hydrolase-knockout mouse model of Alzheimer’s disease. Biochem. Pharmacol. 157 (9), 202–209. doi:10.1016/j.bcp.2018.09.009
Aso, E., Andrés-Benito, P., and Ferrer, I. (2016). Delineating the efficacy of a cannabis-based medicine at advanced stages of dementia in a murine model. J. Alzheimer’s Dis. 54 (3), 903–912. doi:10.3233/JAD-160533
Aso, E., Juvés, S., Maldonado, R., and Ferrer, I. (2013). CB2 cannabinoid receptor agonist ameliorates alzheimer-like phenotype in AβPP/PS1 mice. J. Alzheimer’s Dis. 35 (4), 847–858. doi:10.3233/JAD-130137
Aso, E., Palomer, E., Juvés, S., Maldonado, R., Muoz, F. J., and Ferrer, I. (2012). CB1 agonist ACEA protects neurons and reduces the cognitive impairment of AβPP/PS1 mice. J. Alzheimer’s Dis. 30 (2), 439–459. doi:10.3233/JAD-2012-111862
Aso, E., Sánchez-Pla, A., Vegas-Lozano, E., Maldonado, R., and Ferrer, I. (2015). Cannabis-based medicine reduces multiple pathological processes in AβPP/PS1 mice. J. Alzheimer’s Dis. 43 (3), 977–991. doi:10.3233/JAD-141014
Augusto, R., Reis, D. M., Isaac, A. R., Freitas, H. R., Almeida, M. M., Schuck, P. F., et al. (2021). Quality of life and a surveillant endocannabinoid system. Front. Neurosci. 15 (10), 747229. doi:10.3389/fnins.2021.747229
Bahji, A., Meyyappan, A. C., and Hawken, E. R. (2020). Cannabinoids for the neuropsychiatric symptoms of dementia: A systematic review and meta-analysis. Can. J. Psychiatry 65 (6), 365–376. doi:10.1177/0706743719892717
Bajaj, S., Jain, S., Vyas, P., Bawa, S., and Vohora, D. (2021). The role of endocannabinoid pathway in the neuropathology of Alzheimer ’ s disease: Can the inhibitors of MAGL and FAAH prove to be potential therapeutic targets against the cognitive impairment associated with Alzheimer ’ s disease. Brain Res. Bull. 174 (4), 305–322. doi:10.1016/j.brainresbull.2021.06.022
Balant, M., Gras, A., Ruz, M., Vallès, J., Vitales, D., and Garnatje, T. (2021). Traditional uses of Cannabis: An analysis of the CANNUSE database. J. Ethnopharmacol. 279, 114362. doi:10.1016/j.jep.2021.114362
Bergen, M. (2020). The changing brain: The interactive role of brain-derived neurotrophic factor, cannabinoids, and the endocannabinoid system in neurogenic and neuroplastic processes of the brain. Univ. Sask. Undergrad. Res. J. 6 (2). doi:10.32396/usurj.v6i2.460
Bernal-Chico, A., Tepavcevic, V., Manterola, A., Utrilla, C., Matute, C., and Mato, S. (2022). Endocannabinoid signaling in brain diseases: Emerging relevance of glial cells. Glia, Febr. 71, 103–126. doi:10.1002/glia.24172
Berry, A. J., Zubko, O., Reeves, S. J., and Howard, R. J. (2020). Endocannabinoid system alterations in alzheimer’s disease: A systematic review of human studies. Brain Res. 1749 (8), 147135. doi:10.1016/j.brainres.2020.147135
Bonini, S. A., Premoli, M., Tambaro, S., Kumar, A., Maccarinelli, G., Memo, M., et al. (2018). Cannabis sativa: A comprehensive ethnopharmacological review of a medicinal plant with a long history. J. Ethnopharmacol. 227 (5), 300–315. doi:10.1016/j.jep.2018.09.004
Brockett, A. T., Kane, G. A., Monari, P. K., Briones, B. A., Vigneron, P. A., Barber, G. A., et al. (2018). Evidence supporting a role for astrocytes in the regulation of cognitive flexibility and neuronal oscillations through the Ca2+ binding protein S100β. PLoS ONE 13 (4), 1–19. doi:10.1371/journal.pone.0195726
Carrier, E. J., Kearn, C. S., Barkmeier, A. J., Breese, N. M., Yang, W., Nithipatikom, K., et al. (2004). Cultured rat microglial cells synthesize the endocannabinoid 2-arachidonylglycerol, which increases proliferation via a CB2 receptor-dependent mechanism. Mol. Pharmacol. 65 (4), 999–1007. doi:10.1124/mol.65.4.999
Cavaliere, C., Tramontano, L., Fiorenza, D., Alfano, V., Aiello, M., and Salvatore, M. (2020). Gliosis and neurodegenerative diseases: The role of PET and MR imaging. Front. Cell. Neurosci. 14 (4), 75–13. doi:10.3389/fncel.2020.00075
Charernboon, T., Lerthattasilp, T., and Supasitthumrong, T. (2021). Effectiveness of cannabinoids for treatment of dementia: A systematic review of randomized controlled trials. Clin. Gerontol. 44 (1), 16–24. doi:10.1080/07317115.2020.1742832
Chen, N. Y., Liu, C. W., Lin, W., Ding, Y., Bian, Z. Y., Huang, L., et al. (2017). Extract of fructus cannabis ameliorates learning and memory impairment induced by D-galactose in an aging rats model. Evidence-Based Complementary Altern. Med. 2017, 4757520. doi:10.1155/2017/4757520
Chen, R., Zhang, J., Wu, Y., Wang, D., Feng, G., Tang, Y. P., et al. (2012). Monoacylglycerol lipase is a therapeutic target for alzheimer’s disease. Cell Rep. 2 (5), 1329–1339. doi:10.1016/j.celrep.2012.09.030
Cheng, Y., Dong, Z., and Liu, S. (2014). β-caryophyllene ameliorates the Alzheimer-like phenotype in APP/PS1 mice through CB2 receptor activation and the PPARγ pathway. Pharmacology 94 (1–2), 1–12. doi:10.1159/000362689
Colonna, M., and Butovsky, O. (2017). Microglia function in the central nervous system during health and neurodegeneration. Annu. Rev. Immunol. 35 (1), 441–468. doi:10.1146/annurev-immunol-051116-052358
Cramond, F., O’mara-Eves, A., Doran-Constant, L., Rice, A. S. C., Macleod, M., and Thomas, J. (2019). The development and evaluation of an online application to assist in the extraction of data from graphs for use in systematic reviews [version 2; referees: 3 approved]. Wellcome Open Res. 3, 1–19. doi:10.12688/wellcomeopenres.14738.2
Cristino, L., Bisogno, T., and Marzo, V. Di. (2019). Cannabinoids and the expanded endocannabinoid system in neurological disorders. Nat. Rev. Neurol. 16, 9–29. doi:10.1038/s41582-019-0284-z
Cullen, N. C., Leuzy, A., Janelidze, S., Palmqvist, S., Svenningsson, A. L., Stomrud, E., et al. (2021). Plasma biomarkers of Alzheimer’s disease improve prediction of cognitive decline in cognitively unimpaired elderly populations. Nat. Commun. 12 (1), 3555–3559. doi:10.1038/s41467-021-23746-0
Daily, K. P., and Amer, A. (2020). Microglial autophagy-mediated clearance of amyloid-beta plaques is dysfunctional in Alzheimer’s disease mice. Alzheimer’s Dementia 16 (S3), 44120. doi:10.1002/alz.044120
De Chiara, V., Angelucci, F., Rossi, S., Musella, A., Cavasinni, F., Cantarella, C., et al. (2010). Brain-derived neurotrophic factor controls cannabinoid CB1 receptor function in the striatum. J. Neurosci. 30 (24), 8127–8137. doi:10.1523/JNEUROSCI.1683-10.2010
De Petrocellis, L., Starowicz, K., Moriello, A. S., Vivese, M., Orlando, P., and Di Marzo, V. (2007). Regulation of transient receptor potential channels of melastatin type 8 (TRPM8): Effect of cAMP, cannabinoid CB1 receptors and endovanilloids. Exp. Cell Res. 313 (9), 1911–1920. doi:10.1016/j.yexcr.2007.01.008
De Vincenti, A. P., Ríos, A. S., Paratcha, G., and Ledda, F. (2019). Mechanisms that modulate and diversify BDNF functions: Implications for hippocampal synaptic plasticity. Front. Cell. Neurosci. 13, 1–7. doi:10.3389/fncel.2019.00135
Deczkowska, A., Keren-shaul, H., Weiner, A., Colonna, M., Schwartz, M., and Amit, I. (2018). Disease-associated microglia: A universal immune sensor of neurodegeneration. Cell 173 (5), 1073–1081. doi:10.1016/j.cell.2018.05.003
Degenhardt, F., Stehle, F., and Kayser, O. (2017). “The biosynthesis of cannabinoids,” in Handbook of cannabis and related pathologies: Biology, Pharmacology, diagnosis, and treatment (Elsevier). doi:10.1016/B978-0-12-800756-3.00002-8
Deture, M. A., and Dickson, D. W. (2019). The neuropathological diagnosis of Alzheimer ’ s disease. Mol. Neurodegener. BMC, 5, 32–18. doi:10.1186/s13024-019-0333-5
Di Castro, M. A., and Volterra, A. (2022). Astrocyte control of the entorhinal cortex-dentate gyrus circuit: Relevance to cognitive processing and impairment in pathology. Glia 70 (8), 1536–1553. doi:10.1002/glia.24128
Di Marzo, V., Stella, N., and Zimmer, A. (2015). Endocannabinoid signalling and the deteriorating brain. Nat. Rev. Neurosci. 16 (1), 30–42. doi:10.1038/nrn3876
Dominicis, N. D., Oddi, S., and Maccarrone, M. (2022). Microglial endocannabinoid signalling in AD. cell 22, 1–22.
Dow-Edwards, D., and Silva, L. (2017). Endocannabinoids in brain plasticity: Cortical maturation, HPA axis function and behavior. Brain Res. 1654, 157–164. doi:10.1016/j.brainres.2016.08.037
du Sert, N. P., Ahluwalia, A., Alam, S., Avey, M. T., Baker, M., Browne, W. J., et al. (2020). Reporting animal research: Explanation and elaboration for the arrive guidelines 2.0. PLoS Biol. 18 (7). doi:10.1371/journal.pbio.3000411
Duffy, S. S., Hayes, J. P., Fiore, N. T., and Moalem-Taylor, G. (2021). The cannabinoid system and microglia in health and disease. Neuropharmacology 190 (12), 108555. doi:10.1016/j.neuropharm.2021.108555
Edison, P., Donat, C. K., and Sastre, M. (2018). In vivo imaging of glial activation in alzheimer’s disease. Front. Neurology 9 (AUG), 1–10. doi:10.3389/fneur.2018.00625
El-Bakly, W., Wagdy, O., Sobhy, A., abo elenain, O., Riad, M. S., El Sayed, M., et al. (2019). The efficacy and underlying mechanism of phosphodiesterase- 5 inhibitors in preventing cognitive impairment and alzheimer pathology: A systematic review of animal studies. Behav. Brain Res. 372 (6), 112004. doi:10.1016/j.bbr.2019.112004
Escartin, C., Galea, E., Lakatos, A., O’Callaghan, J. P., Petzold, G. C., Serrano-Pozo, A., et al. (2021). Reactive astrocyte nomenclature, definitions, and future directions. Nat. Neurosci. 24 (3), 312–325. doi:10.1038/s41593-020-00783-4
Fakhfouri, G., Ahmadiani, A., Rahimian, R., Grolla, A. A., Moradi, F., and Haeri, A. (2012). WIN55212-2 attenuates amyloid-beta-induced neuroinflammation in rats through activation of cannabinoid receptors and PPAR-γ pathway. Neuropharmacology 63 (4), 653–666. doi:10.1016/j.neuropharm.2012.05.013
Feng, D., Huang, A., Yan, W., and Chen, D. (2019). CD200 dysfunction in neuron contributes to synaptic deficits and cognitive impairment. Biochem. Biophysical Res. Commun. 516 (4), 1053–1059. doi:10.1016/j.bbrc.2019.06.134
Ferreira, F. F., Ribeiro, F. F., Rodrigues, R. S., Sebastião, A. M., and Xapelli, S. (2018). Brain-derived neurotrophic factor (BDNF) role in cannabinoid-mediated neurogenesis. Front. Cell. Neurosci. 12 (11), 1–16. doi:10.3389/fncel.2018.00441
Franco-Bocanegra, D. K., George, B., Lau, L. C., Holmes, C., Nicoll, J. A. R., and Boche, D. (2019). Microglial motility in alzheimer’s disease and after Aβ42 immunotherapy: A human post-mortem study. Acta Neuropathol. Commun. 7 (1), 174. doi:10.1186/s40478-019-0828-x
Galán-Ganga, M., Rodríguez-Cueto, C., Merchán-Rubira, J., Hernández, F., Ávila, J., Posada-Ayala, M., et al. (2021). Cannabinoid receptor CB2 ablation protects against TAU induced neurodegeneration. Acta Neuropathol. Commun. 9 (1), 90–18. doi:10.1186/s40478-021-01196-5
Gallelli, C. A., Calcagnini, S., Romano, A., Koczwara, J. B., de Ceglia, M., Dante, D., et al. (2018). Modulation of the oxidative stress and lipid peroxidation by endocannabinoids and their lipid analogues. Antioxidants 7 (7), 93. doi:10.3390/antiox7070093
Gaoni, Y., and Mechoulam, R. (1964). Isolation, structure, and partial synthesis of an active constituent of hashish. J. Am. Chem. Soc. 86 (8), 1646–1647. doi:10.1021/ja01062a046
Glatigny, M., Moriceau, S., Rivagorda, M., Ramos-Brossier, M., Nascimbeni, A. C., Lante, F., et al. (2019). Autophagy is required for memory formation and reverses age-related memory decline. Curr. Biol. 29 (3), 435–448.e8. doi:10.1016/j.cub.2018.12.021
González-Gutiérrez, A., Lazo, O. M., and Bronfman, F. C. (2020). The Rab5-rab11 endosomal pathway is required for bdnf-induced CREB transcriptional regulation in hippocampal neurons. J. Neurosci. Res. 40 (42), 8042–8054. doi:10.1523/JNEUROSCI.2063-19.2020
Guo, W., Nagappan, G., and Lu, B. (2018). Differential effects of transient and sustained activation of BDNF-TrkB signaling. Dev. Neurobiol. 78 (7), 647–659. doi:10.1002/dneu.22592
Han, J., Kesner, P., Metna-Laurent, M., Duan, T., Xu, L., Georges, F., et al. (2012). Acute cannabinoids impair working memory through astroglial CB1 receptor modulation of hippocampal LTD. Cell 148 (5), 1039–1050. doi:10.1016/j.cell.2012.01.037
Han, K. H., Lim, S., Ryu, J., Lee, C. W., Kim, Y., Kang, J. H., et al. (2009). CB1 and CB2 cannabinoid receptors differentially regulate the production of reactive oxygen species by macrophages. Cardiovasc. Res. 84 (3), 378–386. doi:10.1093/cvr/cvp240
Hartsel, J. A., Eades, J., Hickory, B., and Makriyannis, A. (2016). “Cannabis sativa and hemp,” in Nutraceuticals: Efficacy, safety and toxicity (Elsevier). doi:10.1016/B978-0-12-802147-7.00053-X
Hooijmans, C. R., Rovers, M. M., De Vries, R. B. M., Leenaars, M., Ritskes-Hoitinga, M., and Langendam, M. W. (2014). SYRCLE’s risk of bias tool for animal studies. BMC Med. Res. Methodol. 14 (1), 43–49. doi:10.1186/1471-2288-14-43
Jeong, M., Cho, J., Shin, J. I., Jeon, Y. J., Kim, J. H., Lee, S. J., et al. (2014). Hempseed oil induces reactive oxygen species- and C/EBP homologous protein-mediated apoptosis in MH7A human rheumatoid arthritis fibroblast-like synovial cells. J. Ethnopharmacol. 154 (3), 745–752. doi:10.1016/j.jep.2014.04.052
Jha, M. K., Jo, M., Kim, J. H., and Suk, K. (2019). Microglia-astrocyte crosstalk: An intimate molecular conversation. Neuroscientist 25 (3), 227–240. doi:10.1177/1073858418783959
Ji, J., Xue, T. F., Guo, X. D., Yang, J., Guo, R. B., Wang, J., et al. (2018). Antagonizing peroxisome proliferator-activated receptor γ facilitates M1-to-M2 shift of microglia by enhancing autophagy via the LKB1–AMPK signaling pathway. Aging Cell 17 (4), 1–16. doi:10.1111/acel.12774
Jia, J., Ma, L., Wu, M., Zhang, L., Zhang, X., Zhai, Q., et al. (2014). Anandamide protects HT22 cells exposed to hydrogen peroxide by inhibiting CB1 receptor-mediated type 2 NADPH oxidase. Oxidative Med. Cell. Longev. 2014, 893516. doi:10.1155/2014/893516
Johnson, K. A., Schultz, A., Betensky, R. A., Becker, J. A., Sepulcre, J., Rentz, D., et al. (2016). Tau positron emission tomographic imaging in aging and early Alzheimer disease. Ann. Neurology 79 (1), 110–119. doi:10.1002/ana.24546
Kasatkina, L. A., Rittchen, S., and Sturm, E. M. (2021). Neuroprotective and immunomodulatory action of the endocannabinoid system under neuroinflammation. Int. J. Mol. Sci. 22 (11), 5431. doi:10.3390/ijms22115431
Kettenmann, H., Kirchhoff, F., and Verkhratsky, A. (2013). Microglia: New roles for the synaptic stripper. Neuron 77 (1), 10–18. doi:10.1016/j.neuron.2012.12.023
Kim, S., and Son, Y. (2021). Astrocytes stimulate microglial proliferation and m2 polarization in vitro through crosstalk between astrocytes and microglia. Int. J. Mol. Sci. 22 (16), 8800. doi:10.3390/ijms22168800
Kirkley, K. S., Popichak, K. A., Afzali, M. F., Legare, M. E., and Tjalkens, R. B. (2017). Microglia amplify inflammatory activation of astrocytes in manganese neurotoxicity. J. Neuroinflammation 14 (1), 99–17. doi:10.1186/s12974-017-0871-0
Komorowska-Müller, J. A., and Schmöle, A. C. (2021). CB2 receptor in microglia: The guardian of self-control. Int. J. Mol. Sci. 22 (1), 19–27. doi:10.3390/ijms22010019
Kowiański, P., Lietzau, G., Czuba, E., Waśkow, M., Steliga, A., and Moryś, J. (2018). Bdnf: A key factor with multipotent impact on brain signaling and synaptic plasticity. Cell. Mol. Neurobiol. 38 (3), 579–593. doi:10.1007/s10571-017-0510-4
Krishnan, G., and Chatterjee, N. (2012). Endocannabinoids alleviate proinflammatory conditions by modulating innate immune response in muller glia during inflammation. Glia 60 (11), 1629–1645. doi:10.1002/glia.22380
Kruk-Slomka, M., Dzik, A., Budzynska, B., and Biala, G. (2017). Endocannabinoid system: The direct and indirect involvement in the memory and learning processes—a short review. Mol. Neurobiol. 54 (10), 8332–8347. doi:10.1007/s12035-016-0313-5
Kwon, H. S., and Koh, S. H. (2020). Neuroinflammation in neurodegenerative disorders: The roles of microglia and astrocytes. Transl. Neurodegener. 9 (1), 42–12. doi:10.1186/s40035-020-00221-2
Lago-Fernandez, A., Zarzo-Arias, S., Jagerovic, N., and Morales, P. (2021). Relevance of peroxisome proliferator activated receptors in multitarget paradigm associated with the endocannabinoid system. Int. J. Mol. Sci. 22 (3), 1001–1028. doi:10.3390/ijms22031001
Lam, D., Lively, S., and Schlichter, L. C. (2017). Responses of rat and mouse primary microglia to pro- and anti-inflammatory stimuli: Molecular profiles, K+ channels and migration. J. Neuroinflammation 14 (1), 1–30. doi:10.1186/s12974-017-0941-3
Lee, H. S., Ghetti, A., Pinto-Duarte, A., Wang, X., Dziewczapolski, G., Galimi, F., et al. (2014). Astrocytes contribute to gamma oscillations and recognition memory. Proc. Natl. Acad. Sci. U. S. A. 111 (32), E3343–E3352. doi:10.1073/pnas.1410893111
Leung, C., and Jia, Z. (2016). Mouse genetic models of human brain disorders. Front. Genet. 7 (MAR), 40–20. doi:10.3389/fgene.2016.00040
Li, C., Shi, J., Wang, B., Li, J., and Jia, H. (2019). CB2 cannabinoid receptor agonist ameliorates novel object recognition but not spatial memory in transgenic APP/PS1 mice. Neurosci. Lett. 707 (11), 134286. doi:10.1016/j.neulet.2019.134286
Li, Y., and Kim, J. (2016). CB2 cannabinoid receptor knockout in mice impairs contextual long-term memory and enhances spatial working memory. Neural Plast. 2016, 9817089. doi:10.1155/2016/9817089
Lian, H., Litvinchuk, A., Chiang, A. C. A., Aithmitti, N., Jankowsky, J. L., and Zheng, H. (2016). Astrocyte-microglia cross talk through complement activation modulates amyloid pathology in mouse models of alzheimer’s disease. J. Neurosci. 36 (2), 577–589. doi:10.1523/JNEUROSCI.2117-15.2016
Liddelow, S. A., and Barres, B. A. (2017). Reactive astrocytes: Production, function, and therapeutic potential. Immunity 46 (6), 957–967. doi:10.1016/j.immuni.2017.06.006
Liddelow, S. A., Guttenplan, K. A., Clarke, L. E., Bennett, F. C., Bohlen, C. J., Schirmer, L., et al. (2017). Neurotoxic reactive astrocytes are induced by activated microglia. Nature 541 (7638), 481–487. doi:10.1038/nature21029
Ligresti, A., De Petrocellis, L., and Di Marzo, V. (2016). From phytocannabinoids to cannabinoid receptors and endocannabinoids: Pleiotropic physiological and pathological roles through complex pharmacology. Physiol. Rev. 96 (4), 1593–1659. doi:10.1152/physrev.00002.2016
Lipina, C., and Hundal, H. S. (2016). Modulation of cellular redox homeostasis by the endocannabinoid system. Open Biol. 6 (4), 150276. doi:10.1098/rsob.150276
Liu, J., and Li, L. (2019). Targeting autophagy for the treatment of alzheimer’s disease: Challenges and opportunities. Front. Mol. Neurosci. 12 (8), 203–209. doi:10.3389/fnmol.2019.00203
Lunardi, P., de Souza, L. W., dos Santos, B., Popik, B., and de Oliveira Alvares, L. (2020). Effect of the endocannabinoid system in memory updating and forgetting. Neuroscience 444, 33–42. doi:10.1016/j.neuroscience.2020.07.045
Ma, K., Guo, J., Wang, G., Ni, Q., and Liu, X. (2020). Toll-like receptor 2–mediated autophagy promotes microglial cell death by modulating the microglial M1/M2 phenotype. Inflammation 43 (2), 701–711. doi:10.1007/s10753-019-01152-5
Ma, L. Z., Hu, H., Wang, Z. T., Ou, Y. N., Dong, Q., Tan, L., et al. (2021). P-tau and neurodegeneration mediate the effect of β-amyloid on cognition in non-demented elders. Alzheimer’s Res. Ther. 13 (1), 200–211. doi:10.1186/s13195-021-00943-z
Maayah, Z. H., Takahara, S., Ferdaoussi, M., and Dyck, J. R. B. (2020). The molecular mechanisms that underpin the biological benefits of full-spectrum cannabis extract in the treatment of neuropathic pain and inflammation. Biochimica Biophysica Acta - Mol. Basis Dis. 1866 (7), 165771. doi:10.1016/j.bbadis.2020.165771
Mahdi, O., Chiroma, S. M., Baharuldin, M. T. H., Nor, N. H. M., Taib, C. N. M., Jagadeesan, S., et al. (2021). Win55,212-2 attenuates cognitive impairments in alcl3 + d-galactose-induced alzheimer’s disease rats by enhancing neurogenesis and reversing oxidative stress. Biomedicines 9 (9), 1–22. doi:10.3390/biomedicines9091270
Mahmoud, S., Gharagozloo, M., Simard, C., and Gris, D. (2019). Astrocytes maintain glutamate homeostasis in the cns by controlling the balance between glutamate uptake and release. Cells 8 (2), 1–27. doi:10.3390/cells8020184
Marchalant, Y., Cerbai, F., Brothers, H. M., and Wenk, G. L. (2008). Cannabinoid receptor stimulation is anti-inflammatory and improves memory in old rats. Neurobiol. Aging 29 (12), 1894–1901. doi:10.1016/j.neurobiolaging.2007.04.028
Marcu, J. P., and Schechter, J. B. (2016). Molecular pharmacology of CB1 and CB2 cannabinoid receptors neuropathology of drug addictions and substance misuse. Elsevier Inc. 1 (2). doi:10.1016/B978-0-12-800213-1.00066-3
Martín-moreno, A. M., Brera, B., Spuch, C., Carro, E., García-garcía, L., Delgado, M., et al. (2012). Prolonged oral cannabinoid administration prevents neuroinflammation, lowers β-amyloid levels and improves cognitive performance in Tg APP 2576 mice. J. Neuroinflammation 16, 8–15. doi:10.1186/1742-2094-9-8
Martín-Moreno, A. M., Reigada, D., Ramírez, B. G., Mechoulam, R., Innamorato, N., Cuadrado, A., et al. (2011). Cannabidiol and other cannabinoids reduce microglial activation in vitro and in vivo: Relevance to alzheimer’s disease. Mol. Pharmacol. 79 (6), 964–973. doi:10.1124/mol.111.071290
Mattson, M. P., and Arumugam, T. V. (2018). Hallmarks of brain aging: Adaptive and pathological modification by metabolic states. Cell Metab. 27 (6), 1176–1199. doi:10.1016/j.cmet.2018.05.011
Maurya, S. K., Bhattacharya, N., Mishra, S., Bhattacharya, A., Banerjee, P., Senapati, S., et al. (2021). Microglia speci fi c drug targeting using natural products for the regulation of redox imbalance in neurodegeneration. Front. Pharmacol. 12 (4), 1–18. doi:10.3389/fphar.2021.654489
Mecha, M., Carrillo-Salinas, F. J., Feliú, A., Mestre, L., and Guaza, C. (2016). Microglia activation states and cannabinoid system: Therapeutic implications. Pharmacol. Ther. 166, 40–55. doi:10.1016/j.pharmthera.2016.06.011
Mecha, M., Feliú, A., Carrillo-Salinas, F. J., Rueda-Zubiaurre, A., Ortega-Gutiérrez, S., de Sola, R. G., et al. (2015). Endocannabinoids drive the acquisition of an alternative phenotype in microglia. Brain, Behav. Immun. 49, 233–245. doi:10.1016/j.bbi.2015.06.002
Mechoulam, R., and Shvo, Y. (1963). Hashish-I. The structure of cannabidiol. Tetrahedron 19 (12), 2073–2078. doi:10.1016/0040-4020(63)85022-X
Minett, T., Classey, J., Matthews, F. E., Fahrenhold, M., Taga, M., Brayne, C., et al. (2016). Microglial immunophenotype in dementia with Alzheimer’s pathology. J. Neuroinflammation 13 (1), 1–10. doi:10.1186/s12974-016-0601-z
Mohammadi, M., Manaheji, H., Maghsoudi, N., Danyali, S., Baniasadi, M., and Zaringhalam, J. (2020). Microglia dependent BDNF and proBDNF can impair spatial memory performance during persistent inflammatory pain. Behav. Brain Res. 390 (3), 112683. doi:10.1016/j.bbr.2020.112683
Morena, M., and Campolongo, P. (2014). The endocannabinoid system: An emotional buffer in the modulation of memory function. Neurobiol. Learn. Mem. 112, 30–43. doi:10.1016/j.nlm.2013.12.010
Nedaei, S. E., Rezayof, A., Pourmotabbed, A., Nasehi, M., and Zarrindast, M. R. (2016). Activation of endocannabinoid system in the rat basolateral amygdala improved scopolamine-induced memory consolidation impairment. Behav. Brain Res. 311, 183–191. doi:10.1016/j.bbr.2016.05.043
Nilsson, P., Loganathan, K., Sekiguchi, M., Matsuba, Y., Hui, K., Tsubuki, S., et al. (2013). Aβ secretion and plaque formation depend on autophagy. Cell Rep. 5 (1), 61–69. doi:10.1016/j.celrep.2013.08.042
Orihuela, R., McPherson, C. A., and Harry, G. J. (2016). Microglial M1/M2 polarization and metabolic states. Br. J. Pharmacol. 173 (4), 649–665. doi:10.1111/bph.13139
Paes-Colli, Y., Aguiar, A. F. L., Isaac, A. R., Ferreira, B. K., Campos, R. M. P., Trindade, P. M. P., et al. (2022). Phytocannabinoids and cannabis-based products as alternative pharmacotherapy in neurodegenerative diseases: From hypothesis to clinical practice. Front. Cell. Neurosci. 16 (5), 1–18. doi:10.3389/fncel.2022.917164
Paloczi, J., Varga, Z. V., Hasko, G., and Pacher, P. (2018). Neuroprotection in oxidative stress-related neurodegenerative diseases: Role of endocannabinoid system modulation. Antioxidants Redox Signal. 29 (1), 75–108. doi:10.1089/ars.2017.7144
Patricio-Martínez, A., Sánchez-Zavaleta, R., Angulo-Cruz, I., Gutierrez-Praxedis, L., Ramírez, E., Martínez-García, I., et al. (2019). The acute activation of the CB1 receptor in the Hippocampus decreases neurotoxicity and prevents spatial memory impairment in rats lesioned with β-amyloid 25–35. Neuroscience 416, 239–254. doi:10.1016/j.neuroscience.2019.08.001
Ramírez, B. G., Blázquez, C., Gómez Del Pulgar, T., Guzmán, M., and De Ceballos, M. L. (2005). Prevention of Alzheimer’s disease pathology by cannabinoids: Neuroprotection mediated by blockade of microglial activation. J. Neurosci. 25 (8), 1904–1913. doi:10.1523/JNEUROSCI.4540-04.2005
Rapaka, D., Bitra, V. R., Challa, S. R., and Adiukwu, P. C. (2021). Potentiation of microglial endocannabinoid signaling alleviates neuroinflammation in Alzheimer’s disease. Neuropeptides 90 (6), 102196. doi:10.1016/j.npep.2021.102196
Robin, L. M., Oliveira da Cruz, J. F., Langlais, V. C., Martin-Fernandez, M., Metna-Laurent, M., Busquets-Garcia, A., et al. (2018). Astroglial CB1 receptors determine synaptic D-serine availability to enable recognition memory. Neuron 98 (5), 935–944.e5. doi:10.1016/j.neuron.2018.04.034
Rocchi, A., Yamamoto, S., Ting, T., Fan, Y., Sadleir, K., Wang, Y., et al. (2017). A Becn1 mutation mediates hyperactive autophagic sequestration of amyloid oligomers and improved cognition in Alzheimer’s disease. PLoS Genet. 13 (8), 1–26. doi:10.1371/journal.pgen.1006962
Russo, E. B., Jiang, H. E., Li, X., Sutton, A., Carboni, A., Del Bianco, F., et al. (2008). Phytochemical and genetic analyses of ancient cannabis from Central Asia. J. Exp. Bot. 59 (15), 4171–4182. doi:10.1093/jxb/ern260
Ryz, N. R., Remillard, D. J., and Russo, E. B. (2017). Cannabis roots: A traditional therapy with future potential for treating inflammation and pain. Cannabis Cannabinoid Res. 2 (1), 210–216. doi:10.1089/can.2017.0028
Saido, T., and Leissring, M. A. (2012). Proteolytic degradation of amyloid β-protein. Cold Spring Harb. Perspect. Med. 2 (6), a006379. doi:10.1101/cshperspect.a006379
Saijo, K., and Glass, C. K. (2011). Microglial cell origin and phenotypes in health and disease. Nat. Rev. Immunol. 11 (11), 775–787. doi:10.1038/nri3086
Sanchez-Varo, R., Mejias-Ortega, M., Fernandez-Valenzuela, J. J., Nuñez-Diaz, C., Caceres-Palomo, L., Vegas-Gomez, L., et al. (2022). Transgenic mouse models of alzheimer’s disease: An integrative analysis. Int. J. Mol. Sci. 23 (10), 5404–5446. doi:10.3390/ijms23105404
Schmöle, A. C., Lundt, R., Ternes, S., Albayram, Ö., Ulas, T., Schultze, J. L., et al. (2015). Cannabinoid receptor 2 deficiency results in reduced neuroinflammation in an Alzheimer’s disease mouse model. Neurobiol. Aging 36 (2), 710–719. doi:10.1016/j.neurobiolaging.2014.09.019
Schmöle, A. C., Lundt, R., Toporowski, G., Hansen, J. N., Beins, E., Halle, A., et al. (2018). Cannabinoid receptor 2-deficiency ameliorates disease symptoms in a mouse model with alzheimer’s disease-like pathology. J. Alzheimer’s Dis. 64 (2), 379–392. doi:10.3233/JAD-180230
Schünemann, H. J., Oxman, A. D., Brozek, J., Glasziou, P., Bossuyt, P., Chang, S., et al. (2008). Grade: Assessing the quality of evidence for diagnostic recommendations. ACP J. Club 149 (6), JC6. doi:10.7326/0003-4819-149-12-200812160-02002
Scuderi, C., Bronzuoli, M. R., Facchinetti, R., Pace, L., Ferraro, L., Broad, K. D., et al. (2018). Ultramicronized palmitoylethanolamide rescues learning and memory impairments in a triple transgenic mouse model of Alzheimer’s disease by exerting anti-inflammatory and neuroprotective effects. Transl. Psychiatry 8 (1), 32. doi:10.1038/s41398-017-0076-4
Siller-Pérez, C., Fuentes-Ibañez, A., Sotelo-Barrera, E. L., Serafín, N., Prado-Alcalá, R. A., Campolongo, P., et al. (2019). Glucocorticoid interactions with the dorsal striatal endocannabinoid system in regulating inhibitory avoidance memory. Psychoneuroendocrinology 99 (3), 97–103. doi:10.1016/j.psyneuen.2018.08.021
Smith, C. M., Mayer, J. A., and Duncan, I. D. (2013). Autophagy promotes oligodendrocyte survival and function following dysmyelination in a long-lived myelin mutant. J. Neurosci. 33 (18), 8088–8100. doi:10.1523/JNEUROSCI.0233-13.2013
Solas, M., Francis, P. T., Franco, R., and Ramirez, M. J. (2013). CB2 receptor and amyloid pathology in frontal cortex of Alzheimer’s disease patients. Neurobiol. Aging 34 (3), 805–808. doi:10.1016/j.neurobiolaging.2012.06.005
Song, J. W., Misgeld, T., Kang, H., Knecht, S., Lu, J., Cao, Y., et al. (2008). Lysosomal activity associated with developmental axon pruning. J. Neurosci. 28 (36), 8993–9001. doi:10.1523/JNEUROSCI.0720-08.2008
Stempel, A. V., Stumpf, A., Zhang, H., Xi, Z., Zimmer, A., Schmitz, D., et al. (2016). Cannabinoid type 2 receptors mediate a cell type-specific plasticity in the Hippocampus. Neuron 90, 795–809. doi:10.1016/j.neuron.2016.03.034
Stumm, C., Hiebel, C., Hanstein, R., Purrio, M., Nagel, H., Conrad, A., et al. (2013). Cannabinoid receptor 1 deficiency in a mouse model of Alzheimer’s disease leads to enhanced cognitive impairment despite of a reduction in amyloid deposition. Neurobiol. Aging 34 (11), 2574–2584. doi:10.1016/j.neurobiolaging.2013.05.027
Sturchio, A., Dwivedi, A. K., Young, C. B., Malm, T., Marsili, L., Sharma, J. S., et al. (2021). High cerebrospinal amyloid-β 42 is associated with normal cognition in individuals with brain amyloidosis. EClinicalMedicine 38, 100988. doi:10.1016/j.eclinm.2021.100988
Tamagno, E., Guglielmotto, M., Monteleone, D., Manassero, G., Vasciaveo, V., and Tabaton, M. (2018). The unexpected role of Aβ 1-42 monomers in the pathogenesis of alzheimer’s disease. J. Alzheimer’s Dis. 62 (3), 1241–1245. doi:10.3233/JAD-170581
Tanaka, M., Sackett, S., and Zhang, Y. (2020). Endocannabinoid modulation of microglial phenotypes in neuropathology. Front. Neurology 11 (2), 87. doi:10.3389/fneur.2020.00087
Tang, R. H., Qi, R. Q., and Liu, H. Y. (2019). Interleukin-4 affects microglial autophagic flux. Neural Regen. Res. 14 (9), 1594–1602. doi:10.4103/1673-5374.255975
Tang, Y., and Le, W. (2016). Differential roles of M1 and M2 microglia in neurodegenerative diseases. Mol. Neurobiol. 53 (2), 1181–1194. doi:10.1007/s12035-014-9070-5
Tanigami, H., Yoneda, M., Tabata, Y., Echigo, R., Kikuchi, Y., Yamazaki, M., et al. (2019). Endocannabinoid signaling from 2-arachidonoylglycerol to CB1 cannabinoid receptor facilitates reward-based learning of motor sequence. Neuroscience 421, 1–16. doi:10.1016/j.neuroscience.2019.09.040
Taura, F., Sirikantaramas, S., Shoyama, Y., Shoyama, Y., and Morimoto, S. (2007). Phytocannabinoids in cannabis sativa: Recent studies on biosynthetic enzymes. Chem. Biodivers. 4 (8), 1649–1663. doi:10.1002/cbdv.200790145
Turner, S. E., Williams, C. M., Iversen, L., and Whalley, B. J. (2017). “Molecular pharmacology of phytocannabinoids,” in Progress in the chemistry of organic natural product, 103. doi:10.1007/978-3-319-45541-9_3s
Vainchtein, I. D., and Molofsky, A. V. (2020). Astrocytes and microglia: In sickness and in health. Trends Neurosci. 43 (3), 144–154. doi:10.1016/j.tins.2020.01.003
Vázquez, C., Tolón, R. M., Grande, M. T., Caraza, M., Moreno, M., Koester, E. C., et al. (2015). Endocannabinoid regulation of amyloid-induced neuroinflammation. Elsevier Ltd. 36 (11), 3008–3019. doi:10.1016/j.neurobiolaging.2015.08.003
Webster, S. J., Bachstetter, A. D., Nelson, P. T., Schmitt, F. A., and Van Eldik, L. J. (2014). Using mice to model Alzheimer’s dementia: An overview of the clinical disease and the preclinical behavioral changes in 10 mouse models. Front. Genet. 5 (APR), 88–23. doi:10.3389/fgene.2014.00088
Wu, J., Bie, B., Yang, H., Xu, J. J., Brown, D. L., and Naguib, M. (2013). Activation of the CB2 receptor system reverses amyloid-induced memory deficiency. Neurobiol. Aging 34 (3), 791–804. doi:10.1016/j.neurobiolaging.2012.06.011
Wu, J., Hocevar, M., Foss, J. F., Bie, B., and Naguib, M. (2017). Activation of CB2 receptor system restores cognitive capacity and hippocampal Sox2 expression in a transgenic mouse model of Alzheimer’s disease. Eur. J. Pharmacol. 811, 12–20. doi:10.1016/j.ejphar.2017.05.044
Xiang, X. T., Wang, X., Wu, Y. M., Hu, J., Li, Y. Y., Jin, S. Y., et al. (2022). Activation of GPR55 attenuates cognitive impairment, oxidative stress, neuroinflammation, and synaptic dysfunction in a streptozotocin-induced Alzheimer’s mouse model. Pharmacol. Biochem. Behav. 214 (2021), 173340. doi:10.1016/j.pbb.2022.173340
Zhang, H. Y., Wang, Y., He, Y., Wang, T., Huang, X. H., Zhao, C. M., et al. (2020). A1 astrocytes contribute to murine depression-like behavior and cognitive dysfunction, which can be alleviated by IL-10 or fluorocitrate treatment. J. Neuroinflammation 17 (1), 200–213. doi:10.1186/s12974-020-01871-9
Zhang, J., and Chen, C. (2018). Alleviation of neuropathology by inhibition of monoacylglycerol lipase in APP transgenic mice lacking CB2 receptors. Mol. Neurobiol. 55 (6), 4802–4810. doi:10.1007/s12035-017-0689-x
Zou, S., and Kumar, U. (2018). Cannabinoid receptors and the endocannabinoid system: Signaling and function in the central nervous system. Int. J. Mol. Sci. 19 (3), 833. doi:10.3390/ijms19030833
Keywords: Alzheimer’s disease, cognition, dementia, endocannabinoid, glial cell, microglia, astrocyte, systematic review
Citation: Kamaruzzaman MA, Romli MH, Abas R, Vidyadaran S, Hidayat Baharuldin MT, Nasaruddin ML, Thirupathirao V, Sura S, Warsito K, Mohd Nor NH, Azwaruddin MA, Alshawsh MA and Mohd Moklas MA (2023) Regulatory role of the endocannabinoid system on glial cells toward cognitive function in Alzheimer’s disease: A systematic review and meta-analysis of animal studies. Front. Pharmacol. 14:1053680. doi: 10.3389/fphar.2023.1053680
Received: 26 September 2022; Accepted: 08 February 2023;
Published: 03 March 2023.
Edited by:
Lee Wei Lim, The University of Hong Kong, Hong Kong SAR, ChinaReviewed by:
Haolong Liu, Health Science Centre, Peking University, ChinaCopyright © 2023 Kamaruzzaman, Romli, Abas, Vidyadaran, Hidayat Baharuldin, Nasaruddin, Thirupathirao, Sura, Warsito, Mohd Nor, Azwaruddin, Alshawsh and Mohd Moklas. This is an open-access article distributed under the terms of the Creative Commons Attribution License (CC BY). The use, distribution or reproduction in other forums is permitted, provided the original author(s) and the copyright owner(s) are credited and that the original publication in this journal is cited, in accordance with accepted academic practice. No use, distribution or reproduction is permitted which does not comply with these terms.
*Correspondence: Mohamad Aris Mohd Moklas, YXJpc0B1cG0uZWR1Lm15; Mohammed Abdullah Alshawsh, YWxzaGF3ZXNobWFtQHVtLmVkdS5teQ==
Disclaimer: All claims expressed in this article are solely those of the authors and do not necessarily represent those of their affiliated organizations, or those of the publisher, the editors and the reviewers. Any product that may be evaluated in this article or claim that may be made by its manufacturer is not guaranteed or endorsed by the publisher.
Research integrity at Frontiers
Learn more about the work of our research integrity team to safeguard the quality of each article we publish.