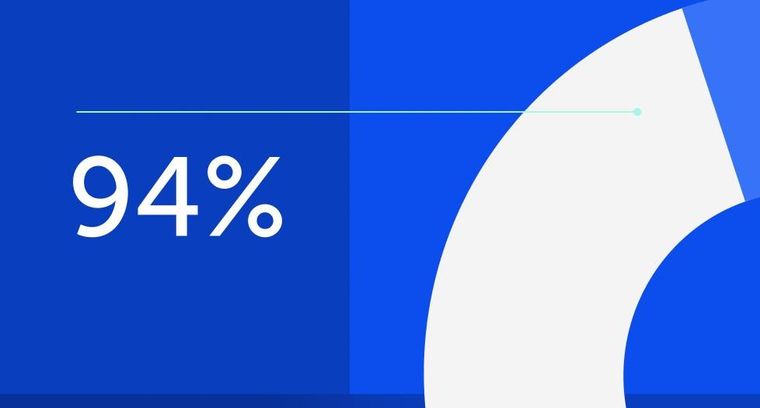
94% of researchers rate our articles as excellent or good
Learn more about the work of our research integrity team to safeguard the quality of each article we publish.
Find out more
REVIEW article
Front. Pharmacol., 26 October 2022
Sec. Experimental Pharmacology and Drug Discovery
Volume 13 - 2022 | https://doi.org/10.3389/fphar.2022.999300
This article is part of the Research TopicAntimalarial Chemotherapy in the XXIst Century Volume IIView all 5 articles
Malaria is caused by the protozoan Plasmodium sp and affects millions of people worldwide. Its clinical form ranges from asymptomatic to potentially fatal and severe. Current treatments include single drugs such as chloroquine, lumefantrine, primaquine, or in combination with artemisinin or its derivatives. Resistance to antimalarial drugs has increased; therefore, there is an urgent need to diversify therapeutic approaches. The disease cycle is influenced by biological, social, and anthropological factors. This longevity and complexity contributes to the records of drug resistance, where further studies and proposals for new therapeutic formulations are needed for successful treatment of malaria. Nanotechnology is promising for drug development. Preclinical formulations with antimalarial agents have shown positive results, but only a few have progressed to clinical phase. Therefore, studies focusing on the development and evaluation of antimalarial formulations should be encouraged because of their enormous therapeutic potential.
Malaria is a disease that affected 241 million people and led to 627,000 deaths worldwide in 2020. It is considered a significant public health problem that preferentially occurs in tropical and subtropical regions and is an endemic disease in 85 countries (World malaria report, 2021).
The incidence of malaria occurs in an environment conducive to the spread of the vector mosquito, geographically in developing and underdeveloped countries. Among the 85 countries reporting malaria cases in 2020, 29 accounted for 96% of malaria cases worldwide, and six countries in the African continent accounted for 55% of global malaria incidence. Malaria mortality rate (deaths per 100,000 inhabitants at risk) decreased from 30% in 2000 to 15% in 2015 and to 13% in 2019. However, in 2020, the mortality rate increased to 15%. The increased mortality rate of malaria in 2020 was associated with the interruption of medical services for malaria treatment due to COVID-19 pandemic (World malaria report, 2021).
The causative agent of malaria is the protozoan Plasmodium sp, which is inoculated into the human body by mosquitoes of the genus Anopheles during hematophagy. There is a complex mosquito-human-parasite cycle, and five species of the parasite can infect humans: Plasmodium falciparum, Plasmodium vivax, Plasmodium ovale, Plasmodium malariae, and Plasmodium knowlesi (Phillips et al., 2017; Ashley et al., 2018). Clinical manifestation of the disease in humans occurs due to the pre-programmed biology of the parasite in conjunction with the human pathophysiological response (Milner, 2018; Knackstedt et al., 2019). Two distinct stages occur in the life cycle of Plasmodium sp: sexual cycle in the vector mosquito and asexual cycle in the human host (Figure 1).
FIGURE 1. Life cycle of Plasmodium sp. The cycle can be divided into two stages: mosquito vector or sexual cycle and human host or asexual cycle. The mosquito ingests gametocytes while performing hematophagy. The zygote is formed from the union of gametocytes and generates oocyte. It crosses the intestinal wall and forms oocyst that releases sporozoites, which migrate to the mosquito’s salivary glands, completing the sexual cycle. The infected female Anopheles sp mosquito inoculates sporozoites, performs hematophagy, and begins the asexual cycle of Plasmodium sp in human. Sporozoites are transported to the liver through the blood, and asexual multiplication occurs in the hepatocytes, forming merozoites in the pre-erythrocytic cycle (Ashley et al., 2018). In P. vivax and P. ovale infections, some sporozoites differentiate in the liver to a latent form called hypnozoites. After rupture of the hepatocytes, merozoites are released into the bloodstream and penetrate the erythrocytes (erythrocyte phase), assuming a ring-shaped configuration (Coban et al., 2018). Proliferative schizogony occurs in infected erythrocytes, where merozoites multiply asexually, differentiating into schizonts and trophozoites. Erythrocytes rupture and release schizonts into the bloodstream, where one part differentiates into male and female gametocytes, and another part infects new erythrocytes (Phillips et al., 2017; Ashley et al., 2018). Image was created in BioRender.com.
The pathogenesis of malaria is related to the blood cycle. Symptoms usually appear approximately 10–15 days after infection, and the disease evolves with febrile response and potentially progresses to severe malaria, which is a consequence of parasite multiplication and invasion of red blood cells by parasites (Najer et al., 2018; Knackstedt et al., 2019). Malaria can be classified as asymptomatic, uncomplicated, and severe (World malaria report, 2020). Any Plasmodium sp can cause asymptomatic malaria (Brazier et al., 2017) or uncomplicated malaria, which is manifested as chills, sweating, headache, nausea, or vomiting without severe organ dysfunction (Phillips et al., 2017; Moxon et al., 2020). P. falciparum causes the most severe malaria disease, with severe organ damage, anemia, and hyperparasitemia (Brazier et al., 2017; Moxon et al., 2020).
Disruption of Plasmodium sp-infected erythrocytes leads to the release of malaria parasites and endotoxins, a complex of parasite DNA and hemozoin (Brazier et al., 2017; Ashley et al., 2018). Endotoxins are recognized by immune cells through Toll-like receptor 9 (TLR9), which increases the production of cytokines and chemokines (Karunaweera et al., 1992; Mubaraki et al., 2017). Oxidative stress increases the inflammatory response by releasing cytokines that cause organ damage (Dunst et al., 2017; Katsoulis et al., 2021). The membrane of erythrocytes infected by parasites hardens and loses its standard shape, contributing to the obstruction of capillaries and thrombus formation. Consequently, when vital organs are affected, severe malaria progresses to death (Coban et al., 2018; Klinkhamhom et al., 2020).
The first- and second-line of treatment recommended by the World Health Organization (WHO) for uncomplicated P. falciparum malaria and chloroquine-resistant P. vivax is artemisinin-based combination therapies (ACTs) (Report on antimalarial drug efficacy, 2020; World malaria report, 2020). This therapy combines an artemisinin derivative with a partner drug. Artemisinin compound plays an important role in reducing the number of parasites during the first 3 days of treatment. After this period, the partner drug eliminates the remaining parasites (Report on antimalarial drug efficacy, 2020).
Currently, several drugs that act during different stages of the parasite’s biological cycle are available for malaria prevention and cure (Figure 2). Most antimalarial agents target erythrocytic and asexual stages (Belete, 2020; Madhav and Hoda, 2021). Tafenoquine and primaquine are approved antimalarial agents against parasites and hypnozoites at the hepatic stage (Thriemer et al., 2017; Commons Id et al., 2019).
FIGURE 2. Current drug targets for malaria treatment: I—Asexual cycle; II—Liver cycle, III, IV, VI—Erythrocytic cycle, V—Asexual and sexual cycles. Source: Artemisinin-based combination therapies (ACTs) are recommended by the World Health Organization (World malaria report, 2020; Wicht et al., 2020; World malaria report, 2021).
The WHO currently recommends six ACTs as the first- and second-line of treatment for uncomplicated cases (Table 1). In regions where malaria transmission is moderate to high, the WHO recommends intermittent preventive treatment in pregnancy (IPTp) during consultation from the beginning of the second trimester of pregnancy (Table 2) (Report on antimalarial drug efficacy, 2020).
Over the years, several endemic areas worldwide have reported increased incidence of Plasmodium drug resistance. Increasing drug resistance of Plasmodium sp is one of the main factors responsible for treatment failure (Rai et al., 2017; L-QuraishAy et al., 2020). In addition, the use of drugs from the same chemical family or having a similar mode of action may have intensified cross-resistance to antimalarial drugs (Capela et al., 2019; Tse et al., 2019).
Several studies have reported that molecular mechanisms of resistance to antimalarial drugs occur in several species of parasites and include polymorphisms in proteins that alter the physiological regulation in the parasite (Menard and Dondorp, 2017; Wicht et al., 2020). The occurrence of polymorphisms makes it obvious that resistance to antimalarial drugs is associated with the genetic factors of parasites. Single, double, or quadruple mutations in different genes confer resistance in the parasite to antimalarial drugs. For example, mutations in the Pfmdr1, Pfcrt, Pfmrp, and Pfnhe1 genes confer drug resistance (Cubides et al., 2018; WWARN K13 Genotype-Phenotype Study Group, 2019).
Mutations in the Pfmdr1 membrane transporter found in Plasmodium sp digestive vacuoles may influence parasite susceptibility to chloroquine (Phillips et al., 2017; Bree and levy, 2018). The Pfcrt and Pfmdr1 are vital multidrug resistance proteins. The Pfmdr1 encodes a p-glycoprotein homolog that affects sensitivity to multiple antimalarial drugs (Ross et al., 2018; Xu et al., 2018) including artemisinin, mefloquine, lumefantrine, quinine, and chloroquine (Patel et al., 2017; Xu et al., 2018). Atovaquone is a synthetic hydroxynaphthoquinone with antiprotozoal activity. Atovaquone interferes with DNA synthesis by blocking mitochondrial transport of electrons from the protozoan respiratory chain, leading to cell death (Staines et al., 2018; Kathpalia et al., 2020). Unique mutations in the Pfcytb gene of P. falciparum caused resistance to atovaquone in in vitro/in vivo experiments (Staines et al., 2018; Kathpalia et al., 2020). The gene encoding P. falciparum Kelch 13 (PfKelch13) has been identified as a genetic determinant of resistance (Bree and levy, 2018; WWARN K13 Genotype-Phenotype Study Group, 2019). K13 mutations reduce protein function and cause artemisinin resistance by reducing its activation (Talman et al., 2019; Wicht et al., 2020).
All molecular targets of antimalarial drugs have not been defined. Drug resistance can occur via several pathways, such as processes that reduce drug toxicity, some catalytic processes that promote changes in enzyme reactions, or amplification of the gene encoding the target enzyme or transporter that pumps the drug out of the parasite (Ross et al., 2018; Deda et al., 2020). Surveillance of resistance to antimalarials is performed using three complementary approaches: 1) in vivo studies to evaluate the efficacy of medications in patients, 2) in vitro studies to assess the parasite susceptibility to medicinal products, and 3) molecular studies to detect genetic mutations and/or gene copy number alterations associated with drug resistance (Xu et al., 2018; Nsanzabana, 2019; Report on antimalarial drug efficacy, 2020). Many studies have described factors that indicate resistance to most drugs used to treat malaria and reveal possible targets for new drugs. With the advancement of molecular biology, metabolomics and proteomics details of the parasite support the development of new pharmacological agents such as nanopharmaceuticals. The work between academic research and pharmaceutical industry is essential and positive for treating malaria cases in endemic regions with efficient and technically targeted approaches.
The most evident disadvantage of conventional antimalarial drugs is Plasmodium sp resistance due to genetic polymorphisms (Bree and levy, 2018; Wicht et al., 2020). However, other disadvantages that influence malaria treatment with conventional antimalarial drugs include low water solubility, low bioavailability, side effects, and relatively short half-life (Alven and Aderibigbe, 2020; Rashidzadeh et al., 2021; Souza Botelho et al., 2021). Side effects frequently related to conventional antimalarial drugs include abdominal pain and gastrointestinal symptoms such as vomiting, jaundice, itching, hypoglycemia, anemia, dizziness, coma, and altered consciousness (Novitt-Moreno et al., 2021; Rashidzadeh et al., 2021). In addition, during prolonged use, there is a risk of hemolysis (tafenoquine and primaquine), retinopathy, mental confusion, cardiac complications (tafenoquine and chloroquine) (Novitt-Moreno et al., 2021), and gastric irritation (primaquine) (da Silva de Barros et al., 2021). Skin hypersensitivity reactions to sulfadoxine-pyrimethamine (Stevens-Johnson syndrome), severe hepatoxicity, and neuropsychiatric reactions to mefloquine have also been reported (Frey et al., 2010; Ashley and Phyo, 2018). In many cases, serious side effects resulted in treatment discontinuation (Brito-Sousa et al., 2019; Who, 2019).
Metabolic pathways, such as nucleic acid synthesis, heme detoxification, oxidative fatty acid synthesis, and stress, are the primary targets for development of new drugs (Baruah et al., 2017; Oyelade et al., 2019). In the search for new treatments, pharmaceutical companies have studied a variety of drug candidates for malaria control and elimination (Belete, 2020). New agents such as arterolane, cipargamin, and KAF156 have the potential to replace ACTs that fail to treat malaria infection. Therefore, there is an urgent need to reassess the current combination therapy for malaria treatment (Ashley and Phyo, 2018; Moyo et al., 2020).
To develop antiparasitic molecules, phenotypic screening studies of the parasite are essential (Cowell and Winzeler, 2019; Yahiya et al., 2019). In addition, phenotypic screening for the entire biological cycle of Plasmodium sp is needed to gather data in chemical libraries and enable discovery of multiple substances that have potential as antimalarials (Cowell and Winzeler, 2019; Yahiya et al., 2019).
Kae609, KAF156, DSM265, and MMV048 are the four most advanced antimalarials have emerged from multidisciplinary collaboration and are currently in phase II trials. The main objective of malaria treatment and elimination strategies is to target multiple stages of the parasite cycle (Summers et al., 2021). Combined antimalarial treatments that do not present artemisinin (ART) are recommended by the WHO when unavailable or suitable for treatment (Who, 2015). An open randomized phase III clinical study compared the efficacy of quinine/clindamycin with artemether/lumefantrine in treating uncomplicated malaria in children below 5 years of age and did not find evidence for the use of quinine/clindamycin when artemether/lumefantrine is still effective (Obonyo et al., 2022). A single monthly prophylactic antimalarial drug composed of a combination of naphthoquinone-azithromycin (NQAZ) was used in a randomized, placebo-controlled, double-blind study to evaluate its protective effect against Plasmodium infection. Treatment with NQAZ showed 93.62% protective efficacy with a 95% confidence interval [CI] of 91.72–95.52 (Yang et al., 2021). A two-group, multicenter, and randomized comparative study compared the efficacy of a dispersible tablet composed of a combination of a fixed dose of arterolane maleate (AM) 37.5 mg and piperaquine phosphate (PQP) 187.5 mg and that of artemether-lumefantrine (AL) in pediatric patients with P. falciparum infection. Both treatments were considered safe with good tolerance, and the efficacy of the AM-PQP combination was compared to that of AL (Toure et al., 2016). There is an urgent need to develop rapid action antimalarials that act during the asexual stage in the blood to reduce the propensity to generate resistance. The four most successful antimalarials primarily target multiple stages of the malaria parasite’s life cycle (Ashton et al., 2019).
Recent studies have demonstrated the potential of nanotechnology for the treatment of different diseases through multiple techniques (Figure 3). Nanostructured drug delivery systems have clinical applications in the treatment of immunological disorders such as allergy, cancer, arteriosclerosis, diabetes, and malaria (Calderó et al., 2017; Charlie-Silva et al., 2018). FDA have already approved nanotherapies for a variety of applications, but at the best of our knowledge, none yet for malaria treatment (Mitchell et al., 2021).
FIGURE 3. Classes of Nanoparticles. Nanoparticles can be divided into organic (lipid-based and polymeric) and inorganic. Each class embodies several NPs with the most relevant highlighted in the figure.
A considerable improvement in the pharmacokinetics and efficiency of the encapsulated nano drug was observed compared with that of the encapsulated free drug (Abdolmaleki et al., 2021; Biosca et al., 2021). Critical studies based on nanotechnology for the development of antimalarial drugs are aimed to solve key problem in malaria treatment, such as disease severity, and are focused on reduced level of drug toxicity, interruption of transmission of Plasmodium sp, increased efficacy of drugs, and mainly, combating multidrug resistance (Manconi et al., 2019; Nnamani et al., 2021). The use of nanostructured drug delivery systems can potentially solve the critical issue of Plasmodium sp multidrug resistance to drugs used for a long time in endemic regions, offering a new possibility of using the same drugs at manometric concentrations with reduced side effects (Marwah et al., 2020; Elmi et al., 2021).
Targeting a drug to unveil its precise mechanism of action is a crucial strategy for treating malaria. Several barriers must be eliminated to allow the drug to reach the intracellular parasite. Bioavailability, concentration, and elimination of drugs are important factors that need to be considered for successful treatment. Nanopharmaceuticals have a promising prospect (Panzarini et al., 2018; Akpa et al., 2020; Pestehchian et al., 2020; Zhang et al., 2020; Guo et al., 2021; Wang et al., 2021). The application of nano-based delivery systems as carriers of antimalarial drugs has resolved some essential issues, such as increased therapeutic effect of conventional antimalarials with decreased resistance of Plasmodium sp and selective distribution of drugs (Abazari et al., 2020; Rashidzadeh et al., 2021) (Figure 4).
FIGURE 4. Nanocarriers. (A) Nanocarriers targeting. A schematic diagram represents the active and passive targeting of NPs. In passive targeting, NPs are carried by red blood cells and in the bloodstream to all tissues. During active targeting, NPs are conjugated with affinity ligands on their surface to enhance their uptake by the target site and cells. Different targeting moieties, such as antibodies, integrins, folate, glucose, or transferrin, can interact with molecules on the target cell surface. (B) Nanocarrier uptake mechanism. In the blood, nanocarriers can be targeted to recognize only parasitized red blood cells. These reduce the chances of resistant pathogen strains and side effects for the patient since the intake is considerably low compared to traditional treatments. In addition, Plasmodium induces new permeation pathways (NPPs) that confer increased permeability to the pRBC by changing the osmotic stability and enabling nanocarriers to enter the pRBC. In other tissues, intracellular uptake of nanocarriers follows different endocytosis pathways. When nanocarriers reach the cell surface, they are taken up by endocytosis depending on their shape, charge, size, or surface. Endocytosis can occur by macropinocytosis, driven by membrane ruffling and actin protrusions. After engulfment, they fuse with lysosomal compartments to content degradation. Clathrin-mediated endocytosis is based on clathrin-coated pits on the cytosolic side of the membrane forming clathrin-coated vesicles that undergo vesicular trafficking. Caveolae-mediated endocytosis undergoes the same dynamics. However, they fuse to caveosomes, avoiding lysosomal degradation.
Cerebral malaria is considered to be extremely severe, which is manifested by intense inflammatory conditions and severe organ damage. The drugs used for the treatment of cerebral malaria are generally administered intravenously; therefore, drug concentration and side effects are the major issues that hinder the treatment of patients with cerebral malaria. Nanostructured delivery systems can potentially treat malaria with less harm to patient (Golenser et al., 2020; Agbo et al., 2021). Different polymer-based nanoparticle structures, such as drug delivery systems, have been studied and improved in multiple studies (Nosrati et al., 2018; Ramazani et al., 2018; Abazari et al., 2020; Dias et al., 2020).
In vitro and in vivo studies used the Pfs25 sex stage gene from P. falciparum, harmonized by codon in Escherichia coli (CHrPfs25), as antigen conjugated to the gold nanoparticle (GN) in different sizes and shapes. GNs can act as a vaccine delivery vehicle because they strongly induce antibodies that block P. falciparum transmission. Authors found IgG from mice immunized with different GN-particles with highly potent blocking activity regardless of IgG isotype differences (Kumar et al., 2015).
A DNA vaccine study was carried out using magnetic vectors composed of superparamagnetic iron oxide nanoparticles (SPIONs), hyaluronic acid (HA), and polyethyleneimine (PEI) added to the DNA encoding PyMSP1 19 of Plasmodium yoelii. The complex induced cellular and humoral immunity against the antigen PyMSP1 19 with increased production of antibodies by an external magnetic stimulus. The immunization with the complexes induced dendritic cell maturation through the upregulation of CD86 expression in the spleen. The presence of secondary effector T cell-mediated immune responses was noted as CD4 helper T cells (Th). The complexes induced antigen-specific Th1 and Th17 cells (Al-Deen et al., 2017). In addition, a non-inflammatory delivery system based on polystyrene nanoparticles (PSNPs) complexed with antigen MSP4/5 (surface proteins of P. falciparum/P. vivax merozoites) and Freund’s adjuvants and alum was tested in a mice model. The non-inflammatory adjuvants associated with PSNPs induced a Th1 immune response acting similarly to a vaccine. The PSNPs-MSP4/5 conjugates induced immune responses by Th1 and Th2, and antibody subclasses IgG1, IgG2a, and IgG2b. The response using adjuvants was even higher. IL-4-associated with Th2 responses induced IgG1 antibodies, and IgG2 antibodies were related to Th1 responses and dependent on IFN-γ. Immunization protection against malaria blood-stage infection may depend on IFN-γ production (Wilson et al., 2019).
Herein, we aimed to discuss the potential application of nanotechnology in developing new antimalarial drugs and the gap between preclinical and clinical studies based on nanotechnology. Table 3 presents the potential and limitations of nanotechnology.
The number of publications on nanoparticles in treating malaria over time is increasing. For instance, a search on the National Library of Medicine USA databases found only one publication from 1990 to 2000 and 8 from 2001 to 2010. Herein, a total of 103 publications that address the study of nanoparticles in malaria treatment were analyzed. They are divided yearly into the following numbers: 2017-5 articles, 2018-9 articles, 2019-13 articles, 2020-24 articles, and 2021-21 articles (Figure 5). In 2022, a growing number of publications that address studies related to nanoparticles in treating malaria will be noticeable. In 2022, 31 publications related to this field are available for consultation.
FIGURE 5. Currently published articles on nano-formulated therapies against malaria. The illustration shows that data recorded from 2017 to 2022 were evaluated and retrieved from the National Library of Medicine USA databases (MEDLINE∕PUBMED—NLM), Scientific Electronic Library Online (SciELO), and Google Scholar (Google Scholar).
From 2019 to 2022, multiple studies based on nanotechnology application in malaria treatment reaffirmed positive conclusions, suggesting the potential of nanosystems. Conversely, only a few ongoing clinical trials based on drug delivery for malaria treatment have been reported. In the last decade, dendrimers have caught interest for several biological applications, such as drug and gene delivery and diagnostic[S1] imaging agents (Araujo et al., 2018; Dias et al., 2020). Dendrimers are quasi-spherical, nanometer-sized, tree-like polymeric macromolecules containing many reactive functional subgroups, branches, and protected interior spaces (Chauhan, 2018; Sherje et al., 2018). The ramifications of the nanostructure generate layers, or “generations”, which characterize the size of the cavity, being the most reported in the first, second, or third generations (one, two, or three layers). These nanosystems can carry hydrophilic and hydrophobic drugs, as they have many functional groups in the periphery (Chis et al., 2020). Furthermore, they target drugs to specific sites and favor cellular uptake, mainly by endocytosis and passive diffusion (Russier et al., 2015; da Silva Santos et al., 2016; Akbarzadeh et al., 2018).
Preclinical studies using nanotechnology to treat malaria are summarized in Table 4. For instance, ultrasmall gold nanoparticles based on glucose or nanogold clusters (Glc-NCs) were developed for use in the intraerythrocytic stage of P. falciparum (in vitro) in both phases of parasitic development (asexual and sexual) without nonspecific connections or red blood cell lysis. Glc-NCs loaded with ciprofloxacin showed 50% higher antibiotic effect than that of free drug, demonstrating its therapeutic potential (Varela-Aramburu et al., 2020). Silver nanoparticles (AgNPs) synthesized using Artemisia sp leaf extract showed high antimalarial activity in P. falciparum cultures (Avitabile et al., 2020). AgNPs from Salvia officinalis leaf extract showed hepatoprotective and antiplasma effects, reducing parasitemia and hepatic oxidative stress markers in an experimental malaria model (Metwally et al., 2021). Moreover, AgNPs from Indigofera oblongifolia leaf extract decreased parasitemia and showed anti-inflammatory, antioxidant, and anti-apoptotic effects in mice infected by Plasmodium chabaudi (Dkhil et al., 2019).
Hollow nanoparticles of mesoporous ferrite (HMFNs) with ferromagnetic properties were prepared using artemisinin and targeted to hemozoin produced by P. falciparum in infected erythrocytes. It acted as a targeted delivery system, increasing the local concentration of ART through its association with heparin, suggesting its potential in antimalarial therapy (Wang et al., 2021). Surface-loaded nanoparticles containing ART in two types of formulations (nanospheres and nanocapsules) had longer elimination half-life than that of an ART solution in ethanol, suggesting their potential as intravenous antimalarial agents (Gérard Yaméogo et al., 2020). Furthermore, a stable ART-based injectable nanocomplex, composed of dimorphic artesunate-choline (dACC) micelles coated with hyaluronic acid (HA), demonstrated safety and antimalarial activity in in vitro and in vivo experiments compared to those of free artemisinin/artesunate, suggesting it as a promising injectable alternative to the traditional artesunate (ATS) used in malaria treatment (He et al., 2021).
In vitro and in vivo studies have shown that lipid nanoemulsions with modified surface are ideal carriers of dihydroartemisinin (Umeyor et al., 2019). Ester-linked dihydroartemisinin trimer (DHA 3) prepared as self-assembled nanoparticles (DHA 3 NPs) demonstrated superior antimalarial effects compared to that of control in a murine experimental model, with improved cure rate and survival time and reduced recurrence rate in experimental animals (Guo et al., 2021). ATS fortified with iron oxide-coated nanoparticles showed increased cytotoxicity and selective damage to P. falciparum in a murine experimental model, suggesting a potent antimalarial agent against artemisinin-resistant malaria (Kannan et al., 2019). Nanostructured lipid carriers (NLCs) loaded with ATS for intranasal administration (ATS-NLCs) showed great potential as an alternative to parenteral administration in hard-to-reach regions, simplifying the treatment of severe and cerebral malaria in adults and children (Agbo et al., 2021). The nanocapsules based on artesunate-heparin conjugate (ATS-HEP-NCPs) were used as drug delivery vehicle for intracellular release; in vitro and in vivo experiments showed increased stability and improved pharmacokinetic properties (Ismail et al., 2019). In a murine experimental model, the triple combination containing atovaquone in a lyophilized suspension of proguanil-artesunate demonstrated prophylactic efficacy.
In addition, the use of double (atovaquone/proguanil) and triple (atovaquone/proguanil/artesunate) combination formulations resulted in a complete cure (Kathpalia et al., 2020). The injectable pasty polymer, formulated for controlled release of artemisinin, reduced parasitemia and severe symptoms in cerebral malaria and increased survival of animals, with an increase in the half-life of the drug compared to that of free drug in an experimental murine model (Golenser et al., 2020). The nanovector immunoliposomal encapsulating the antimalarials pyronaridine and atovaquone, which block the development of gametocytes through targeting glycophorin in vitro, presented significantly higher efficacy than that of the free forms (Biosca et al., 2019).
The improved solubility of lumefantrine (LUM) in an aqueous medium by using lumefantrine phospholipid complex (LMF-PC) enhanced antimalarial activity in a murine model (Kaur et al., 2021). The matrices of microneedle (MN) containing ART (MN-ART) and LUM (MN-LUM) in nanosuspensions, applied as intradermal devices, showed increased solubility of drugs and potential as an alternative treatment for malaria in endemic regions with scarce resources (Volpe-Zanutto et al., 2021). Transdermal artemether nanogel (TMT) as NLCs have demonstrated good antimalarial properties in in vitro and ex vivo skin permeation experiments. They offered 100% cure and negligible side effects when two adhesives were applied per week at a lower dose than that of free TMT (Nnamani et al., 2021). Zein nanoparticles loaded with TMT showed good encapsulation efficiency, reduced hemolysis, and prolonged therapeutic effect compared to that of free TMT (Boateng-Marfo et al., 2021). Caprol-based NLCs were prepared to improve the bioavailability of artemether/lumefantrine antimalarials; they showed improved oral bioavailability, antimalarial activity, and potential compared to those of free antimalarials (Akpa et al., 2020). In addition, nanocapsules (NC-ATM) showed decreased neurotoxic and cardiotoxic effects in mice infected with Plasmodium berghei, a safe alternative to TMT for the treatment of malaria (Moreira Souza et al., 2020). Flash nanoprecipitation (FNP) is a technique that enables the production of nanoparticles from laboratory-scale to industrial pilot-scale. Lumefantrine was processed by FNP to obtain 200 nm nanoparticles with increased bioavailability and dissolution kinetics suitable for industrial-scale production (Ristroph et al., 2019). The technique (FNP) was employed for formulation of the substance OZ439 (artefenomel), which is active against drug-resistant malaria, and the formulation showed potential as a single-dose cure. Powder formulations using spray dryer is appropriate for industrial-scale production. In vitro tests have shown that the formulation of the antimalarial OZ439 in a single-dose oral form had good stability against humidity and temperature (Ristroph et al., 2019). The oral bioavailability of OZ439 and the adjustment of interactions by selecting alternative systems such as milk-like lipid nanoparticles or powder systems can provide different possibilities for enhanced solubilization and absorption for this drug (Salim et al., 2019).
Neutral zwitterionic amphiphiles forming nanoparticles (PBMA-MESBMA) using curcumin and targeted to infected erythrocytes showed effective and faster antimalarial activity than that of free curcumin in an animal model (Biosca et al., 2021). Nutrisomes (phospholipid vesicles + Nutriose® FM06) were modified to obtain new systems with increased efficacy of curcumin as an antimalarial agent after oral administration. Eudragit® L100 (EUD) was added to these vesicles to improve their in vitro and in vivo performance, which showed an increased ability to neutralize oxidative stress in intestinal cells and increased survival of infected mice compared to controls treated with free curcumin (Manconi et al., 2019). The nanocapsules (NCP80) and nanospheres (NSP80) of polysorbate 80 and nanocapsules (NCEUD) and nanospheres (NSEUD) of Eudragit RS 100 containing quinine were evaluated for their effects on the surface characteristics and antimalarial efficacy in vitro and in vivo. Cationic and anionic nanocapsules have been developed to deliver quinine to erythrocytes using Eudragit RS 100. An improvement in antiplasmodial efficacy was observed along with altered characteristics of the cationic nanocapsules with quinine such as protection against light and improvement of quinine partition coefficient in vitro. Improvement in biodistribution of quinine by NCEUD and increase in the half-life of elimination of quinine in vivo suggest it as a potential alternative for the treatment of malaria (Michels et al., 2019). The nanoformulation of curcumin in combination with the compound benzothiophene 6 (3-bromine-N-(4-fluorobenzyl)-benzo[b]thiophene-2-carboxamide) showed sustained release of curcumin, increased stability and solubility in aqueous medium, and antimalarial activity in in vivo and in vitro experiments (Ghosh and Banerjee, 2020).
The nanoemulsion delivery system of azacarbazoles, derived from carbazole (9 H-carbazole) with a concentrated form of ethyl esters of polyunsaturated fatty acids n-3 and n-6, provided evidence of increased antiplasmodial activities in vitro, without cytotoxic effects against mammalian cells, showing rapid absorption after intragastric administration (Jaromin et al., 2021). In addition, thiazoline nanoemulsion (10-(4,5-dihydrothiazol-2-yl)thio)decan-1-ol), a synthetic compound similar to 3-alkylidene marine alkaloid being reported as an antimalarial substance, reduced in vivo parasitemia and increased antimalarial activity in vitro (Silva and cordoso., 2020). The 407 poloxamer nanomicelles loaded with pyrimethamine showed potent antimalarial activity and lower liver damage in a murine experiment than that of the free compound, indicating potential for adaptation as an antimalarial formulation (Pestehchian et al., 2020). In vitro and in vivo experiments have shown that hybrid dendritic-linear-dendritic block copolymer mycelial transporters based on Pluronic® F127 (HDLDBC-bGMPA) are promising for the development of future antimalarial drugs aimed at penetrating erythrocytes (<30 nm) infected by Plasmodium sp (Martí Coma-Cros et al., 2019). In vitro and in vivo experiments of artelinic acid (AD) derivatives formulated as liposomes (ADLs) showed superior antimalarial efficacy compared with that of the control groups. Pharmacokinetic results of ADLs demonstrated the slowest elimination and highest total concentration in plasma, showing potential for the treatment of malaria (Duan et al., 2020).
Furthermore, primaquine polymeric microparticles (PPM) in in vivo experiments using a murine model showed partial efficacy and protection against parasite development compared with that of free primaquine, suggesting the potential of this drug delivery system for the treatment of malaria (da Silva de Barros et al., 2021). Three drug carriers based on lipid formulations loaded with primaquine, solid lipid nanoparticles, nanoemulsions and nanostructured lipid carriers were developed and evaluated; all lipid formulations could successfully protect erythrocytes from cell lysis caused by free primaquine (Wu et al., 2021). Phospholipid-free unilamellar vesicles (PFSUVs) composed of Tween 80 and cholesterol were evaluated in experimental models in vitro and in vivo. They effectively delivered primaquine to the liver, selectively targeted hepatocytes, and reduced erythrocyte uptake compared to that of free primaquine, leading to reduced erythrocyte toxicity (Al Fayes et al., 2021). In vivo experiments with PEGylated liposomes containing primaquine and chloroquine for the treatment of malaria in the hepatic stage demonstrated that liposomal membrane fluidity was greatly affected by the double burden of primaquine and chloroquine drugs, and additional studies related to stabilization of these liposomes are needed (Miatmoko et al., 2021). Decoquinate (DQN) is a molecule that can potentially function as a substitute for active primaquine against malaria in the hepatic stage was directed to hepatocytes infected by the parasite using a liposomal transporter system in vitro and in vivo. The study concluded that glycyrrhizic acid receptors participated in the targeted delivery of DQN liposomes to the hepatocytes (Marwah et al., 2020). Multilamellar nanoliposomes stabilized by hydrogen bonds containing chloroquine targeted to infected erythrocytes showed antiparasitic effect superior to that of free chloroquine in in vitro and in vivo studies, being permissive for smaller and larger molecules (Fotoran et al., 2019). The loading of chloroquine in G2 nanodendrimers showed antiplasmodial activity and decreased toxicity of structured nano chloroquine compared to that of free chloroquine, indicating that this compound is an effective antiplasmodic agent in vivo in a murine model (Elmi et al., 2021). 1H NMR spectroscopy was used to study the effect of anionic linear globular dimerized nanocomplexes based on curcumin loaded with chloroquine G2 and antiplasmodial effect against P. falciparum in vitro (Elmi et al., 2021). In addition, dextran NPs loaded with chloroquine diphosphate (CHQ-DEX-NPs) were developed to overcome resistance of P. falciparum to chloroquine in vitro. CHQ-DEX-NPs could trigger the parasite’s apoptotic pathway by accumulating in food vacuoles and were found safe for parenteral administration (Kashyap et al., 2018). The biocompatible and biodegradable nanoparticles of chitosan-tripolyphosphate-chloroquine triggered elimination of multidrug-resistant parasites through redox action, modulating pro- and anti-inflammatory responses, suggesting a new approach to treat multidrug-resistant malaria. The CS-TPP CQ nanoparticles killed the parasite and diminished the production of the pro-inflammatory cytokines TNF-α and IFN-γ, and increased the anti-inflammatory cytokines IL-10 and TGF-β (Das et al., 2021). The incorporation of proteins or peptides of interest can occur both during the preparation of the NP (e.g., antigens), and through their complexation/conjugation on the nanocarrier surface (e.g., plasmids or antibodies). For example, Cherif et al. (2011) developed polymeric NPs formed by pDNA complexed with PEI (cationic polymer) and PLA (biocompatible polymer), which were tested in vivo against P. yoelii in different administration routes (IV, SC, and IP) in mice. They observed an increase in the immune response regarding the levels of IgG, T cells (CD4+ and CD8+), IFN-γ and IL-12. Another study carried out by Collins et al. (2017) developed NPs conjugated to the surface antigen of the circumsporozoite protein CSP-hepatitis B, acting in the pre-erythrocyte stage of infection by P. falciparum. Moderate levels of protection were induced through the immune response mediated by CSP-specific antibodies. In addition, this vaccine was immunogenic (cellular and humoral immune response) at low doses. Also, when administered with Abisco-100 and Matrix-M adjuvants, it induced protection against transgenic sporozoites (Collins, 2017).
Articles have discussed the multi-component vaccination to challenge both humoral and cellular immunity and adjuvants as the best strategy to reach all stages of malaria.
The association of polymeric and lipid NPs is also described, as reported by Kumar et al. (2015). Nanoemulsion containing squalene, chitosan/PLGA NPs and CHrPfs25 were developed and evaluated against P. falciparum. Results regarding the functional immunogenicity generated by CHrPfs25 are promising. Authors found IgG from mice immunized with different GN-particles with highly potent blocking activity regardless of IgG isotype differences. Most studies in this article suggest nanoformulations as drug delivery systems, indicating their potential for the treatment of malaria (Table 4).
Nanotechnology has enormous potential for the development of new drugs against malaria, although there are limitations. Despite significant advantages of nano-pharmaceutical release systems, factors such as high preparation cost, interaction with biological components, difficulty in production scale up depending on the development method, failure to define the appropriate route of administration, and failure in therapeutic reproducibility demotivated researchers to lead preclinical research for clinical application (Neves Borgheti-Cardoso et al., 2020; Rashidzadeh et al., 2021).
The cost of producing nanosystems is a primary concern for their use in treating diseases such as malaria. The large-scale production of nano drugs to provide access in malaria predominant regions is difficult due to financial, logistical, and political issues, among other limiting factors (Feng et al., 2019; Ristroph et al., 2019). In addition, majority of malaria-affected population is concentrated in developing countries, which have low resources invested in health and medical care. This panorama of resource scarcity failed to foster industry interest in the development of new antimalarial medicines on a large scale because of low financial returns (Ristroph et al., 2019; Volpe-Zanutto et al., 2021).
Despite considerable volume of studies based on nanotechnology application for the treatment of malaria, it is possible to perceive a difference between the number of preclinical and clinical studies, which are lower. Preclinical studies that employ nanoparticles in other diseases, such as cancer, tumors, and Alzheimer’s disease, present more signifying results and often involve theranostic nanomedicine (Gandhi et al., 2020; Abdolmaleki et al., 2021). High cost of preparation, drug administration routes, bioaccumulation, toxicity, and interaction with biological components are some critical issues to be resolved to contemplate the success of new nanotechnological strategies for the treatment of malaria (Apolinário et al., 2020; Rashidzadeh et al., 2021).
To overcome the clinical failures of antimalarial therapy, developing new medicines is urgent and necessary. However, developing a new drug is expensive, complex, and time-consuming. In this case, strategies that increase the therapeutic efficacy of current conventional antimalarial drugs and reduce their toxicity are promising alternatives. For that, nanotechnology can be considered an approach to solving these inherent limitations, such as the low water solubility, biodegradability, bioavailability, and parasite resistance (Jawahar et al., 2019; Puttappa et al., 2019; Rashidzadeh et al., 2019; Abazari et al., 2020; Bagheri et al., 2020). Several organic, inorganic, and hybrid nanometric systems were discussed in this review and offer many alternatives as drug delivery systems for antimalarial drugs (Table 3). Therefore, these efforts must be continued to accelerate the clinical application of these systems to treat malaria.
Some companies have explored the potential of dendrimers. For instance, Starpharma developed the DEP® platform for drug delivery applied to antitumor drugs. Or diagnostic kits component as 3DNA® from Gemisphere, or transfection agents such as Polyfect® and Superfect® from Qiagen (Dias et al., 2020). In addition, according to information from the American repository ClinicalTrial.gov, some clinical trials evaluate drugs associated with dendrimers for treating cancer, bacterial infection, or COVID-19 (Caminade, 2022). However, up to date, there are no dendrimer-based medicines to treat malaria, either under clinical evaluation or commercialization.
Malaria is a disease affecting millions of people worldwide. Despite the current treatments, resistance to antimalarial drugs has increased. Advances in nanotechnology for the development of new drug delivery systems are promising and being increasingly studied in preclinical tests, with significant and instigating results. However, no nano-formulated antimalarial drug is currently available for clinical use.
New approaches to malaria treatment are urgently needed owing to the complexity of the biological cycle of the parasite, spread of the disease in conditions where sanitary and social conditions are precarious, and reports of resistance of the parasite to conventional therapies. The interaction between academic research, pharmaceutical industry, and political leaders is the most promising path for the development of new drugs and nanostructured drug delivery systems.
All authors listed have made a substantial, direct, and intellectual contribution to the work and approved it for publication.
This work was supported by grants from Universidade Federal Fluminense (PROPPI/UFF), Coordenação de Aperfeiçoamento de Pessoal de Nível Superior (CAPES) Grant [001], Programa de Biotecnologia da Universidade Federal Fluminense (UFF), Programa de Pós Graduação em Biologia Molecular Celular (UNIRIO), Universidade Federal do Estado do Rio de Janeiro (UNIRIO), Fundação Carlos Chagas Filho de Amparo à Pesquisa do Estado do Rio de Janeiro (FAPERJ) Grants (E-26/010.000983/2019, E-26/203.290/2017, and E-26/2010.592/2019, E-26/201.448/2021), Conselho Nacional de Desenvolvimento Científico e Tecnológico (CNPq), and Instituto Oswaldo Cruz, FIOCRUZ.
We also acknowledge financial support by the European Community’s Seventh Framework Programme (FP7-2007-2013) under grant agreement (HEALTH-F4-2011-282095) (TARKINAID).
Author FN was employed by Empresa Brasileira de Pesquisa Agropecuária, EMBRAPA.
The remaining authors declare that the research was conducted in the absence of any commercial or financial relationships that could be construed as a potential conflict of interest.
All claims expressed in this article are solely those of the authors and do not necessarily represent those of their affiliated organizations, or those of the publisher, the editors and the reviewers. Any product that may be evaluated in this article, or claim that may be made by its manufacturer, is not guaranteed or endorsed by the publisher.
Abazari, M., Ghaffari, A., Rashidzadeh, H., Momeni Badeleh, S., and Maleki, Y. (2020). Current status and future outlook of nano-based systems for burn wound management. J. Biomed. Mat. Res. B Appl. Biomater. 108, 1934–1952. doi:10.1002/jbm.b.34535
Abdolmaleki, A., Asadi, A., Gurushankar, K., Shayan, T. K., and Sarvestani, F. A. (2021). Importance of nano medicine and new drug therapies for cancer. Adv. Pharm. Bull. 11, 450–457. doi:10.34172/apb.2021.052
Agbo, C. P., Ugwuanyi, T. C., Ugwuoke, W. I., Mcconville, C., Attama, A. A., and Ofokansi, K. C. (2021). Intranasal artesunate-loaded nanostructured lipid carriers: A convenient alternative to parenteral formulations for the treatment of severe and cerebral malaria. J. Control. Release 334, 224–236. doi:10.1016/j.jconrel.2021.04.020
Akbarzadeh, A., Khalilov, R., Mostafavi, E., Annabi, N., Abasi, E., Kafshdooz, T., et al. (2018). Role of dendrimers in advanced drug delivery and biomedical applications: A review. Exp. Oncol. 40, 178–183. doi:10.31768/2312-8852.2018.40(3):178-183
Akpa, P. A., Ugwuoke, J. A., Attama, A. A., Nnenna Ugwu, C., Nneoma Ezeibe, E., Momoh, M. A., et al. (2020). Improved antimalarial activity of caprol-based nanostructured lipid carriers encapsulating artemether-lumefantrine for oral administration. Afr. Health Sci. 20 (4), 1679–1697. doi:10.4314/ahs.v20i4.20
Al Fayes, N., Böttger, R., Rouhollahi, E., Cullis, P. R., Witzigmann, D., and Li, S. D. (2021). Improved liver delivery of primaquine by phospholipid-free small unilamellar vesicles with reduced hemolytic ToxicityNo title. Mol. Pharm. 19 (6), 1778–1785. doi:10.1021/acs.molpharmaceut.1c00520
Al-Deen, F. M., Xiang, S. D., Ma, C., Wilson, K., Coppel, R. L., Selomulya, C., et al. (2017).Magnetic nanovectors for the development of DNA blood-stage malaria vaccines, Nanomaterials (Basel), 7. , E30. doi:10.3390/nano7020030
Alven, S., and Aderibigbe, B. A. (2020). Nanoparticles formulations of artemisinin and derivatives as potential therapeutics for the treatment of cancer, leishmaniasis and Malaria. Pharmaceutics 12 (8), 748. doi:10.3390/pharmaceutics12080748
Apolinário, A. C., Salata, G. C., Bianco, A. F. R., Fukumori, C., and Lopes, L. B. (2020). Opening the pandora's box of nanomedicine: There is needed plenty of room at the bottom. Quimica Nova 43, 212–225. doi:10.21577/0100-4042.20170481
Araujo, R. V., Santos, S. D. S., Igne Ferreira, E., and Giarolla, J. (2018). New advances in general biomedical applications of PAMAM dendrimers. Molecules 23, E2849. doi:10.3390/molecules23112849
Ashley, E. A., and Phyo, A. P. (2018). Drugs in development for malaria. Drugs 78, 861–879. doi:10.1007/s40265-018-0911-9
Ashley, E. A., Pyae Phyo, A., and Woodrow, C. J. (2018). Malaria. Lancet 391, 1608–1621. doi:10.1016/S0140-6736(18)30324-6
Ashton, T. D., Devine, S. M., Möhrle, J. J., Laleu, B., Burrows, J. N., Charman, S. A., et al. (2019). The development process for discovery and clinical advancement of modern antimalarials. J. Med. Chem. 62, 10526–10562. doi:10.1021/acs.jmedchem.9b00761
Avitabile, E., Senes, N., D’avino, C., Tsamesidis, I., Pinna, A., Medici, S., et al. (2020). The potential antimalarial efficacy of hemocompatible silver nanoparticles from Artemisia species against P. falciparum parasite. PLoS ONE 15, 02385322–e238617. doi:10.1371/journal.pone.0238532
Bagheri, A. G., Golenser, J., and Greiner, A. (2020). Controlled and manageable release of antimalarial Artemisone by encapsulation in biodegradable carriers. Eur. Polym. J. 129, 109625. doi:10.1016/j.eurpolymj.2020.109625
Baruah, U. K., Gowthamarajan, K., Vanka, R., Karri, V. V. S. R., Selvaraj, K., and Jojo, G. M. (2017). Malaria treatment using novel nano-based drug delivery systems. J. Drug Target. 25, 567–581. doi:10.1080/1061186X.2017.1291645
Belete, T. M. (2020). Recent progress in the development of new antimalarial drugs with novel targets. Drug Des. devel. Ther. 14, 3875–3889. doi:10.2147/DDDT.S265602
Biosca, A., Cabanach, P., Abdulkarim, M., Gumbleton, M., Gómez-Canela, C., Ramírez, M., et al. (2021). Zwitterionic self-assembled nanoparticles as carriers for Plasmodium targeting in malaria oral treatment. J. Control. Release 331, 364–375. doi:10.1016/j.jconrel.2021.01.028
Biosca, A., Dirscherl, L., Moles, E., Imperial, S., and Fernàndez-Busquets, X. (2019). An immunopegliposome for targeted antimalarial combination therapy at the nanoscale. Pharmaceutics 11, 3411–E419. doi:10.3390/pharmaceutics11070341
Boateng-Marfo, Y., Dong, Y., Kiong Ng, W., and Lin, H.-S. (2021). Artemether-loaded zein nanoparticles: An innovative intravenous dosage form for the management of severe malaria. Int. J. Mol. Sci. 22 (3), 1141. doi:10.3390/ijms22031
Brazier, A. J., Avril, M., Bernabeu, M., Benjamin, M., Smith, J. D., and Dzikowski, R. (2017). Pathogenicity determinants of the human malaria parasite plasmodium falciparum have ancient origins. ASM Journals 2. doi:10.1128/mSphere.00348-16
Brito-Sousa, J. D., Santos, T. C., Avalos, S., Fontecha, G., Melo, G. C., Val, F., et al. (2019). Clinical spectrum of primaquine-induced hemolysis in glucose-6-phosphate dehydrogenase deficiency: A 9-year hospitalization-based study from the Brazilian amazon. Clin. Infect. Dis. 69, 1440–1442. doi:10.1093/cid/ciz122
Calderó, G., Fornaguera, C., Zadoina, L., Dols-Perez, A., and Solans, C. (2017). Design of parenteral MNP-loaded PLGA nanoparticles by a low-energy emulsification approach as theragnostic platforms for intravenous or intratumoral administration. Colloids Surf. B Biointerfaces 160, 535–542. doi:10.1016/j.colsurfb.2017.09.060
Caminade, A. M. (2022). Dendrimers, an emerging opportunity in personalized medicine? J. Pers. Med. 12, 1334. doi:10.3390/jpm12081334
Capela, R., Moreira, R., and Lopes, F. (2019). An overview of drug resistance in protozoal diseases. Int. J. Mol. Sci. 20, E5748. doi:10.3390/ijms20225748
Charlie-Silva, I., Fraceto, L. F., and De Melo, N. F. S. (2018). Progress in nano-drug delivery of artemisinin and its derivatives: Towards to use in immunomodulatory approaches. Artif. Cells Nanomed. Biotechnol. 46, S611–S620. doi:10.1080/21691401.2018.1505739
Chauhan, A. S. (2018). Dendrimers for drug delivery. Molecules 23, E938. doi:10.3390/molecules23040938
Cherif, M. S., Shuaibu, M. N., Kurosaki, T., Helegbe, G. K., Kikuchi, M., Yanagi, T., et al. (2011). Immunogenicity of novel nanoparticle-coated MSP-1 C-terminus malaria DNA vaccine using different routes of administration. Vaccine 29 (48), 9038–9050. doi:10.1016/j.vaccine.2011.09.031
Chis, A. A., Dobrea, C., Morgovan, C., Arseniu, A. M., Rus, L. L., Butuca, A., et al. (2020). Applications and limitations of dendrimers in biomedicine. Molecules 25, E3982. doi:10.3390/molecules25173982
Coban, C., Lee, M. S. J., and Ishii, K. J. (2018). Tissue-specific immunopathology during malaria infection. Nat. Rev. Immunol. 18 (4), 266–278. doi:10.1038/nri.2017.138
Collins, K. A., Snaith, R., Cottingham, M. G., Gilbert, S. C., and Hill, A. V. s. (2017). Enhancing protective immunity to malaria with a highly immunogenic virus-like particle vaccine. Scientific Reports 7 (1). doi:10.1038/srep46621
Commons, , Robert, J., Simpson, , Julie, A., Thriemer, , Kamala, , et al. (2019). The efficacy of dihydroartemisinin-piperaquine and artemether-lumefantrine with and without primaquine on plasmodium vivax recurrence: A systematic review and individual patient data meta-analysis. PLOS MEDICINE 16, 10. doi:10.1371/journal.pmed.1002928
Cowell, A. N., and Winzeler, E. A. (2019). Advances in omics-based methods to identify novel targets for malaria and other parasitic protozoan infections. London, United Kingdom: BioMed Central Ltd.
Cubides, J. R., Camargo-Ayala, P. A., Niño, C. H., Garzón-Ospina, D., Ortega-Ortegón, A., Ospina-Cantillo, E., et al. (2018). Simultaneous detection of Plasmodium vivax dhfr, dhps, mdr1 and crt-o resistance-associated mutations in the Colombian Amazonian region. Malar. J. 17, 130. doi:10.1186/s12936-018-2286-5
Da Silva De Barros, A. O., Portilho, F. L., Dos Santos Matos, A. P., Ricci-Junior, E., Alencar, L. M. R., Dos Santos, C. C., et al. (2021). Preliminary studies on drug delivery of polymeric primaquine microparticles using the liver high uptake effect based on size of particles to improve malaria treatment. Mat. Sci. Eng. C Mat. Biol. Appl. 128, 112275. doi:10.1016/j.msec.2021.112275
Da Silva Santos, S., Igne Ferreira, E., and Giarolla, J. (2016). Dendrimer prodrugs. Molecules 21, 686. doi:10.3390/molecules21060686
Das, S., Tripathy, S., Pramanik, P., Saha, B., and Roy, S. (2021). A novel nano-anti-malarial induces redox damage and elicits cytokine response to the parasite. Cytokine 144, 155555. doi:10.1016/j.cyto.2021.155555
Dawre, S. (2018). Enhanced antimalalarial activity of a prolonged release in situ gel of arteetherlumefantrine in a murine model. European Journal of Pharmaceutics and Biopharmaceutics 123, 95107. doi:10.1016/j.ejpb.2017.11.002
Deda, D. K., Iglesias, B. A., Alves, E., Araki, K., and Garcia, C. R. S. (2020). Porphyrin derivative nanoformulations for therapy and antiparasitic agents. Molecules 25, 20800–E2131. doi:10.3390/molecules25092080
Dias, A. P., Da Silva Santos, S., Da Silva, J. V., Parise-Filho, R., Igne Ferreira, E., Seoud, O. E., et al. (2020). Dendrimers in the context of nanomedicine. Int. J. Pharm. 573, 118814. doi:10.1016/j.ijpharm.2019.118814
Dkhil, M. A., Al-Shaebi, E. M., and Al-Quraishy, S. (2019). Effect of indigofera oblongifolia on the hepatic oxidative status and expression of inflammatory and apoptotic genes during blood-stage murine malaria. Oxid. Med. Cell. Longev. 2019, 8264861. doi:10.1155/2019/8264861
Duan, S., Wang, R., Wang, R., Tang, J., Xiao, X., Li, N., et al. (2020). In vivo antimalarial activity and pharmacokinetics of artelinic acid-choline derivative liposomes in rodents. Parasitology 147, 58–64. doi:10.1017/S0031182019001306
Dunst, J., Kamena, F., and Matuschewski, K. (2017). Cytokines and chemokines in cerebral malaria pathogenesis. Front. Cell. Infect. Microbiol. 7, 324. doi:10.3389/fcimb.2017.00324
Elmi, T., Ardestani, M. S., Motevalian, M., Hesari, A. K., Hamzeh, M. S., Zamani, Z., et al. (2021). Antiplasmodial effect of nano dendrimer G2 loaded with chloroquine in mice infected with plasmodium berghei. Acta Parasitol. 1, 298–308. doi:10.1007/s11686-021-00459-4
Feng, J., Markwalter, C. E., Tian, C., Armstrong, M., and Prud'homme, R. K. (2019). Translational formulation of nanoparticle therapeutics from laboratory discovery to clinical scale. J. Transl. Med. 17, 200. doi:10.1186/s12967-019-1945-9
Fotoran, W. L., Müntefering, T., Kleiber, N., Miranda, B. N. M., Liebau, E., Irvine, D. J., et al. (2019). A multilamellar nanoliposome stabilized by interlayer hydrogen bonds increases antimalarial drug efficacy. Nanomedicine 22, 102099. doi:10.1016/j.nano.2019.102099
Frey, S. G., Chelo, D., Kinkela, M. N., Djoukoue, F., Tietche, F., Hatz, C., et al. (2010). Artesunate-mefloquine combination therapy in acute plasmodium falciparum malaria in young children: A field study regarding neurological and neuropsychiatric safety. Malar. J. 9, 291. doi:10.1186/1475-2875-9-291
Gandhi, H., Sharma, A. K., Mahant, S., and Kapoor, D. N. (2020). Recent advancements in brain tumor targeting using magnetic nanoparticles. Ther. Deliv. 11, 97–112. doi:10.4155/tde-2019-0077
Gérard Yaméogo, J. B., Mazet, R., Wouessidjewe, D., Choisnard, L., Godin-Ribuot, D., Putaux, J. L., et al. (2020). Pharmacokinetic study of intravenously administered artemisinin-loaded surface-decorated amphiphilic γ-cyclodextrin nanoparticles. Mat. Sci. Eng. C Mat. Biol. Appl. 106, 110281. doi:10.1016/j.msec.2019.110281
Ghosh, A., and Banerjee, T. (2020). Nanotized curcumin-benzothiophene conjugate: A potential combination for treatment of cerebral malaria. IUBMB Life 72, 2637–2650. doi:10.1002/iub.2394
Golenser, J., Salaymeh, N., Higazi, A. A., Alyan, M., Daif, M., Dzikowski, R., et al. (2020). Treatment of experimental cerebral malaria by slow release of artemisone from injectable pasty formulation. Front. Pharmacol. 11, 846–849. doi:10.3389/fphar.2020.00846
Guo, W., Li, N., Ren, G., Wang, R. R., Chai, L., Li, Y., et al. (2021). Murine pharmacokinetics and antimalarial pharmacodynamics of dihydroartemisinin trimer self-assembled nanoparticles. Parasitol. Res. 120, 2827–2837. doi:10.1007/s00436-021-07208-6
He, W., Du, Y., Li, C., Wang, J., Wang, Y., Dogovski, Y., et al. (2021). Dimeric artesunate-choline conjugate micelles coated with hyaluronic acid as a stable, safe and potent alternative anti-malarial injection of artesunate. Int. J. Pharm. 609, 121138. doi:10.1016/j.ijpharm.2021.121138
Hu, Q. (2020). Transferrin conjugated ph-and redox-responsive poly(Amidoamine) dendrimer conjugate as an efficient drug delivery carrier for cancer therapy. International Journal of Nanomedicine 15, 27512764. doi:10.2147/IJN.S238536
Ismail, M., Du, Y., Ling, L., and Li, X. (2019). Artesunate-heparin conjugate based nanocapsules with improved pharmacokinetics to combat malaria. Int. J. Pharm. 562, 162–171. doi:10.1016/j.ijpharm.2019.03.031
Jaromin, A., Parapini, S., Basilico, N., Zaremba-Czogalla, M., Lewińska, A., Zagórska, A., et al. (2021). Azacarbazole n-3 and n-6 polyunsaturated fatty acids ethyl esters nanoemulsion with enhanced efficacy against Plasmodium falciparum. Bioact. Mat. 6, 1163–1174. doi:10.1016/j.bioactmat.2020.10.004
Jawahar, N., Baruah, U. K., and Singh, V. (2019). Co-delivery of chloroquine phosphate and azithromycin nanoparticles to overcome drug resistance in malaria through intracellular targeting. J. Pharm. Sci. Res. 11, 33–40.
Kannan, D., Yadav, N., Ahmad, S., Namdev, P., Bhattacharjee, S., Lochab, B., et al. (2019). Pre-clinical study of iron oxide nanoparticles fortified artesunate for efficient targeting of malarial parasite. EBioMedicine 45, 261–277. doi:10.1016/j.ebiom.2019.06.026
Karunaweera, N. D., Grau, G. E., Gamage, P., Carter, R., and Mendis, K. N. (1992). Dynamics of fever and serum levels of tumor necrosis factor are closely associated during clinical paroxysms in Plasmodium vivax malaria. Proc. Natl. Acad. Sci. U. S. A. 89, 3200–3203. doi:10.1073/pnas.89.8.3200
Kashyap, A., Kaur, R., Baldi, A., Jain, U. K., Chandra, R., and Madan, J. (2018). Chloroquine diphosphate bearing dextran nanoparticles augmented drug delivery and overwhelmed drug resistance in Plasmodium falciparum parasites. Int. J. Biol. Macromol. 114, 161–168. doi:10.1016/j.ijbiomac.2018.03.102
Kathpalia, H., Juvekar, S., Mohanraj, K., Apsingekar, M., and Shidhaye, S. (2020). Investigation of pre-clinical pharmacokinetic parameters of atovaquone nanosuspension prepared using a pH-based precipitation method and its pharmacodynamic properties in a novel artemisinin combination. J. Glob. Antimicrob. Resist. 22, 248–256. doi:10.1016/j.jgar.2020.02.018
Katsoulis, O., Georgiadou, A., and Cunnington, A. J. (2021). Immunopathology of acute kidney injury in severe malaria. Lausanne, Switzerland: Frontiers Media SA.
Kaur, R., Gorki, V., Katare, O. P., Dhingra, N., Chauhan, M., Kaur, R., et al. (2021). Improved biopharmaceutical attributes of lumefantrine using choline mimicking drug delivery system: Preclinical investigation on NK-65 P.berghei murine model. Expert Opin. Drug Deliv. 18, 1533–1552. doi:10.1080/17425247.2021.1946512
Klinkhamhom, A., Glaharn, S., Srisook, C., Ampawong, S., Krudsood, S., Ward, S. A., et al. (2020). M1 macrophage features in severe Plasmodium falciparum malaria patients with pulmonary oedema. Malar. J. 19, 182. doi:10.1186/s12936-020-03254-0
Knackstedt, S. L., Georgiadou, A., Apel, F., Abu-Abed, U., Moxon, C. A., Cunnington, A. J., et al. (2019). Neutrophil extracellular traps drive inflammatory pathogenesis in malaria. Sci. Immunol. 4, eaaw0336–336. doi:10.1126/sciimmunol.aaw0336
Kumar, R., Ray, P. C., Datta, D., Bansal, G. P., Angov, E., and Kumar, N. (2015). Nanovaccines for malaria using Plasmodium falciparum antigen Pfs25 attached gold nanoparticles. Vaccine 33, 5064–5071. doi:10.1016/j.vaccine.2015.08.025
L-Quraishay, S., Murshed, M., Delic, D., Al-Shaebi, E. M., Qasem, M. a. A., Mares, M. M., et al. (2020). Plasmodium chabaudi-infected mice spleen response to synthesized silver nanoparticles from Indigofera oblongifolia extract. Lett. Appl. Microbiol. 71, 542–549. doi:10.1111/lam.13366
Madhav, H., and Hoda, N. (2021). An insight into the recent development of the clinical candidates for the treatment of malaria and their target proteins. Eur. J. Med. Chem. 210, 112955. doi:10.1016/j.ejmech.2020.112955
Manconi, M., Manca, M. L., Escribano-Ferrer, E., Coma-Cros, E. M., Biosca, A., Lantero, E., et al. (2019). Nanoformulation of curcumin-loaded eudragit-nutriosomes to counteract malaria infection by a dual strategy: Improving antioxidant intestinal activity and systemic efficacy. Int. J. Pharm. 556, 82–88. doi:10.1016/j.ijpharm.2018.11.073
Martí Coma-Cros, E., Lancelot, A., San Anselmo, M., Neves Borgheti-Cardoso, L., Valle-Delgado, J. J., Serrano, J. L., et al. (2019). Micelle carriers based on dendritic macromolecules containing bis-MPA and glycine for antimalarial drug delivery. Biomater. Sci. 7, 1661–1674. doi:10.1039/c8bm01600c
Marwah, M., Narain Srivastava, P., Mishra, S., and Nagarsenker, M. (2020). Functionally engineered ‘hepato-liposomes’: Combating liver-stage malaria in a single prophylactic dose. Int. J. Pharm. 587, 119710. doi:10.1016/j.ijpharm.2020.119710
Menard, D., and Dondorp, A. (2017). Antimalarial drug resistance: A threat to malaria elimination. Cold Spring Harb. Perspect. Med. 7, a025619–a025624. doi:10.1101/cshperspect.a025619
Metwally, D. M., Alajmi, R. A., El-Khadragy, M. F., and Al-Quraishy, S. (2021). Silver nanoparticles biosynthesized with Salvia officinalis leaf exert protective effect on hepatic tissue injury induced by plasmodium chabaudi. Front. Vet. Sci. 7, 620665. doi:10.3389/fvets.2020.620665
Miatmoko, A., Nurjannah, I., Nehru, N. F., Rosita, N., Hendradi, E., Sari, R., et al. (2021). Interactions of primaquine and chloroquine with PEGylated phosphatidylcholine liposomes. Sci. Rep. 11, 12420. doi:10.1038/s41598-021-91866-0
Michels, L. R., Maciel, T. R., Nakama, K. A., Teixeira, F. E. G., De Carvalho, F. B., Gundel, A., et al. (2019). Effects of surface characteristics of polymeric nanocapsules on the pharmacokinetics and efficacy of antimalarial quinine. Int. J. Nanomedicine 14, 10165–10178. doi:10.2147/IJN.S227914
Milner, D. A. (2018). Malaria pathogenesis. Cold Spring Harb. Perspect. Med. 8, a025569. doi:10.1101/cshperspect.a025569
Mitchell, M. J., Billingsley, M. M., Haley, R. M., Wechsler, M. E., Peppas, N. A., and Langer, R. (2021). Engineering precision nanoparticles for drug delivery. Nat. Rev. Drug Discov. 20, 101–124. doi:10.1038/s41573-020-0090-8
Moreira Souza, A. C., Grabe-Guimarães, A., Cruz, J. D. S., Santos-Miranda, A., Farah, C., Teixeira Oliveira, L., et al. (2020). Mechanisms of artemether toxicity on single cardiomyocytes and protective effect of nanoencapsulation. Br. J. Pharmacol. 177, 4448–4463. doi:10.1111/bph.15186
Moxon, C. A., Gibbins, M. P., Mcguinness, D., Milner, D. A., and Marti, M. (2020). New insights into malaria pathogenesis. Annu. Rev. Pathol. 15, 315–343. doi:10.1146/annurev-pathmechdis-012419-032640
Moyo, P., Mugumbate, G., Eloff, J. N., Louw, A. I., Maharaj, V. J., and Birkholtz, L. M. (2020). Natural products: A potential source of malaria transmission blocking drugs? Pharmaceuticals (Basel) 13(9 ), 251. doi:10.3390/ph13090251
Mubaraki, M. A., Hafiz, T. A., Al-Quraishy, S., and Dkhil, M. A. (2017). Oxidative stress and genes regulation of cerebral malaria upon Zizyphus spina-christi treatment in a murine model. Microb. Pathog. 107, 69–74. doi:10.1016/j.micpath.2017.03.017
Najer, A., Palivan, C. G., Beck, H. P., and Meier, W. (2018). Challenges in malaria management and a glimpse at some nanotechnological approaches. Singapore: Springer Singapore.
Nawwab al-deen, F. M. (2017). Magnetic nanovectors for the development of DNA blood-stage malaria vaccines 7 (2), 10. doi:10.3390/nano7020030
Neves Borgheti-Cardoso, L., San Anselmo, M., Lantero, E., Lancelot, A., Serrano, J. L., Hernández-Ainsa, S., et al. (2020). Promising nanomaterials in the fight against malaria. J. Mat. Chem. B 8, 9428–9448. doi:10.1039/d0tb01398f
Nnamani, P. O., Ugwu, A. A., Nnadi, O. H., Kenechukwu, F. C., KennethOfokansi, C., Attama, A. A., et al. (2021). Formulation and evaluation of transdermal nanogel for delivery of artemether. Drug Deliv. Transl. Res. 1, 1655–1674. doi:10.1007/s13346-021-00951-4
Nosrati, H., Salehiabar, M., Bagheri, Z., Rashidzadeh, H., Davaran, S., and Danafar, H. (2018). Preparation, characterization, and evaluation of amino acid modified magnetic nanoparticles: Drug delivery and MRI contrast agent applications. Pharm. Dev. Technol. 23, 1156–1167. doi:10.1080/10837450.2018.1536995
Novitt-Moreno, A., Martidis, A., Gonzalez, V., Ransom, J., Scott, C. B., Dow, G., et al. (2021). Long-term safety of the tafenoquine antimalarial chemoprophylaxis regimen: A 12-month, randomized, double-blind, placebo-controlled trial. Travel Med. Infect. Dis. 45, 102211. doi:10.1016/j.tmaid.2021.102211
Nsanzabana, C. (2019). Tropical medicine and infectious disease resistance to artemisinin combination therapies (ACTs): Do not forget the partner drug!. Trop. Med. Infect. Dis. 4 (1), 26. doi:10.3390/tropicalmed4010026
Obonyo, C. O., Juma, E. A., Were, V. O., and Ogutu, B. R. (2022). Efficacy of 3-day low dose quinine plus clindamycin versus artemether-lumefantrine for the treatment of uncomplicated plasmodium falciparum malaria in Kenyan children (CLINDAQUINE): An open-label randomized trial. Malar. J. 21, 30–11. doi:10.1186/s12936-022-04050-8
Owonubi, S. J. (2018). Characterization and in vitro release kinetics of antimalarials from whey protein-based hydrogel biocomposites. International Journal of Industrial Chemistry 9 (1), 3952. doi:10.1007/s40090-018-0139-2
Oyelade, J., Isewon, I., Aromolaran, O., Uwoghiren, E., Dokunmu, T., Rotimi, S., et al. (2019). Computational identification of metabolic pathways of plasmodium falciparum using the k-shortest path algorithm. Int. J. Genomics 2019, 1750291. doi:10.1155/2019/1750291
Panzarini, E., Mariano, S., Carata, E., Mura, F., Rossi, M., and Dini, L. (2018). Intracellular transport of silver and gold nanoparticles and biological responses: An update. Int. J. Mol. Sci. 19 (5), 1305. doi:10.3390/ijms19051305
Patel, P., Bharti, P. K., Bansal, D., Ali, N. A., Raman, R. K., Mohapatra, P. K., et al. (2017). Prevalence of mutations linked to antimalarial resistance in plasmodium falciparum from Chhattisgarh, central India: A malaria elimination point of view. Sci. Rep. 7, 16690. doi:10.1038/s41598-017-16866-5
Pestehchian, N., Vafaei, M. R., Nematolahy, P., Varshosaz, J., Yousefi, H. A., Bide, V. Z., et al. (2020). A new effective antiplasmodial compound: Nanoformulated pyrimethamine. J. Glob. Antimicrob. Resist. 20, 309–315. doi:10.1016/j.jgar.2019.08.002
Phillips, M. A., Burrows, J. N., Manyando, C., Van Huijsduijnen, R. H., Van Voorhis, W. C., and Wells, T. N. C. (2017). Malaria. Nat. Rev. Dis. Prim. 3, 17050. doi:10.1038/nrdp.2017.50
Powles, L., Wilson, K. L., Xiang, S. D., Coppel, R. L., Ma, C., Selomulya, C., et al. (2020). Pullulan-Coated Iron Oxide Nanoparticles for Blood-Stage Malaria Vaccine Delivery. Vaccines 8 (4), 651. doi:10.3390/vaccines8040651
Puttappa, N., Kumar, R. S., Kuppusamy, G., and Radhakrishnan, A. (2019). Nano-facilitated drug delivery strategies in the treatment of plasmodium infection. Acta Trop. 195, 103–114. doi:10.1016/j.actatropica.2019.04.020
Rai, M., Paralikar, P., Jogee, P., Agarkar, G., Ingle, A. P., Derita, M., et al. (2017). Synergistic antimicrobial potential of essential oils in combination with nanoparticles: Emerging trends and future perspectives. Amsterdam, Netherlands: Elsevier B.V.
Ramazani, A., Keramati, M., Malvandi, H., Danafar, H., and Kheiri Manjili, H. (2018). Preparation and in vivo evaluation of anti-plasmodial properties of artemisinin-loaded PCL–PEG–PCL nanoparticles. Pharm. Dev. Technol. 23, 911–920. doi:10.1080/10837450.2017.1372781
Rashidzadeh, H., Salimi, M., Sadighian, S., Rostamizadeh, K., and Ramazani, A. (2019). In vivo antiplasmodial activity of curcumin-loaded nanostructured lipid carriers. Curr. Drug Deliv. 16, 923–930. doi:10.2174/1567201816666191029121036
Rashidzadeh, H., Tabatabaei Rezaei, S. J., Adyani, S. M., Abazari, M., Rahamooz Haghighi, S., Abdollahi, H., et al. (2021). Recent advances in targeting malaria with nanotechnology-based drug carriers. Pharm. Dev. Technol. 26, 807–823. doi:10.1080/10837450.2021.1948568
Report on antimalarial drug efficacy (2020). Report on antimalarial drug efficacy, resistance and response: 10 years of surveillance (2010-2019). Geneva, Switzerland: WHO.
Ristroph, K. D., Feng, J., Mcmanus, S. A., Zhang, Y., Gong, K., Ramachandruni, H., et al. (2019). Spray drying OZ439 nanoparticles to form stable, water-dispersible powders for oral malaria therapy. J. Transl. Med. 17, 97. doi:10.1186/s12967-019-1849-8
Ross, L. S., Dhingra, S. K., Mok, S., Yeo, T., Wicht, K. J., Kümpornsin, K., et al. (2018). Emerging Southeast Asian PfCRT mutations confer Plasmodium falciparum resistance to the first-line antimalarial piperaquine. Nat. Commun. 9, 3314. doi:10.1038/s41467-018-05652-0
Russier, J., Grillaud, M., and Bianco, A. (2015). Elucidation of the cellular uptake mechanisms of polycationic HYDRAmers. Bioconjug. Chem. 26, 1484–1493. doi:10.1021/acs.bioconjchem.5b00270
Sahoo, R. K., and Khan, J. (2020). PEGylated Dendrimer Mediated Delivery of Bortezomib: Drug Conjugation versus Encapsulation. International Journal of Pharmaceutics 584, 119389. doi:10.1016/j.ijpharm.2020.119389
Salim, M., Khan, J., Ramirez, G., Murshed, M., Clulow, A. J., Hawley, A., et al. (2019). Impact of ferroquine on the solubilization of artefenomel (OZ439) during in vitro lipolysis in milk and implications for oral combination therapy for malaria. Mol. Pharm. 16, 1658–1668. doi:10.1021/acs.molpharmaceut.8b01333
Sherje, A. P., Jadhav, M., Dravyakar, B. R., and Kadam, D. (2018). Dendrimers: A versatile nanocarrier for drug delivery and targeting. Int. J. Pharm. 548, 707–720. doi:10.1016/j.ijpharm.2018.07.030
Silva, M. G. D., Cardoso, J. F., Perasoli, F. B., Branquinho, R. T., Mourao, R. S., Tavares, H. D. S., et al. (2020). Nanoemulsion composed of 10-(4, 5-dihydrothiazol-2-yl)thio)decan-1-ol), a synthetic analog of 3-alkylpiridine marine alkaloid: Development, characterization, and antimalarial activity. Eur. J. Pharm. Sci. 151, 105382. doi:10.1016/j.ejps.2020.105382
Souza Botelho, M., Bolfi, F., Leite, R. G. O. F., Leite, M. S. F., Banzato, L. R., Soares, L. T., et al. (2021). Systematic review and meta-analysis of the safety of chloroquine and hydroxychloroquine from randomized controlled trials on malarial and non-malarial conditions. Syst. Rev. 10, 294–319. doi:10.1186/s13643-021-01835-x
Staines, H. M., Burrow, R., Teo, B. H. Y., Ster, I. C., Kremsner, P. G., and Krishna, S. (2018). Clinical implications of plasmodium resistance to atovaquone/proguanil: A systematic review and meta-analysis. J. Antimicrob. Chemother. 73 (3), 581–595. doi:10.1093/jac/dkx431
Summers, R. L., Pasaje, C. F. A., Pisco, J. P., Striepen, J., Luth, M. R., Kumpornsin, K., et al. (2021). Chemogenomics identifies acetyl-coenzyme A synthetase as a target for malaria treatment and prevention. Cell Chem. Biol. 29, 191–201. doi:10.1016/j.chembiol.2021.07.010
Talman, A. M., Clain, J., Duval, R., Ménard, R., and Ariey, F. (2019). Artemisinin bioactivity and resistance in malaria parasites. Trends Parasitol. 35, 953–963. doi:10.1016/j.pt.2019.09.005
Thriemer, K., Ley, B., Bobogare, A., Dysoley, L., Alam, M. S., Pasaribu, A. P., et al. (2017). Challenges for achieving safe and effective radical cure of plasmodium vivax: A round table discussion of the APMEN vivax working group. Malar. J. 16, 141. doi:10.1186/s12936-017-1784-1
Toure, O. A., Valecha, N., Tshefu, A. K., Thompson, R., Krudsood, S., Gaye, O., et al. (2016). A phase 3, double-blind, randomized study of arterolane maleate-piperaquine phosphate vs artemether-lumefantrine for falciparum malaria in adolescent and adult patients in asia and africa. Clin. Infect. Dis. 62, 964–971. doi:10.1093/cid/ciw029
Tse, E. G., Korsik, M., and Todd, M. H. (2019). The past, present and future of anti-malarial medicines. Malar. J. 18 (1), 93. doi:10.1186/s12936-019-2724-z
Umeyor, C. E., Obachie, O., Chukwuka, R., and Attama, A. (2019). Development insights of surface modified lipid nanoemulsions of dihydroartemisinin for malaria chemotherapy: Characterization, and in vivo antimalarial evaluation. Recent Pat. Biotechnol. 13, 149–165. doi:10.2174/1872208313666181204095314
Varela-Aramburu, S., Ghosh, C., Goerdeler, F., Priegue, P., Moscovitz, O., and Seeberger, P. H. (2020). Targeting and inhibiting plasmodium falciparum using ultra-small gold nanoparticles. ACS Appl. Mat. Interfaces 12, 43380–43387. doi:10.1021/acsami.0c09075
Volpe-Zanutto, F., Ferreira, L. T., Permana, A. D., Kirkby, M., Paredes, A. J., Vora, L. K., et al. (2021). Artemether and lumefantrine dissolving microneedle patches with improved pharmacokinetic performance and antimalarial efficacy in mice infected with Plasmodium yoelii. J. Control. Release 333, 298–315. doi:10.1016/j.jconrel.2021.03.036
Wang, X., Xie, Y., Jiang, N., Wang, J., Liang, H., Liu, D., et al. (2021). Enhanced antimalarial efficacy obtained by targeted delivery of artemisinin in heparin-coated magnetic hollow mesoporous nanoparticles. ACS Appl. Mat. Interfaces 13, 287–297. doi:10.1021/acsami.0c20070
Who, (2019). World malaria report 2019. Avaiable at:https://apps.who.int/iris/handle/10665/330011.Accessed 4 December 2019.
World malaria report, (2021). World malaria report 2021. Geneva, Switzerland: World Health Organization.
Who, (2015). Guidelines for the treatment of malaria. 3rd edition. Geneva, Switzerland: World Health Organization, 299.
World malaria report, (2020). World malaria report 2020: 20 years of global progress and challenges. Geneva, Switzerland: World Health Organization.
Wicht, K. J., Mok, S., and Fidock, D. A. (2020). Molecular mechanisms of drug resistance in plasmodium falciparum malaria. Annu. Rev. Microbiol. 74, 431–454. doi:10.1146/annurev-micro-020518-115546
Wilson, K. L., Pouniotis, D., Hanley, J., Xiang, S. D., Ma, C., Coppel, R. L., et al. (2019). A synthetic nanoparticle based vaccine approach targeting MSP4/5 is immunogenic and induces moderate protection against murine blood-stage malaria. Front. Immunol. 10, 331. doi:10.3389/fimmu.2019.00331
Wu, K. W., Sweeney, C., Dudhipala, N., Lakhani, P., Chaurasiya, N. D., Tekwani, B. L., et al. (2021). Primaquine loaded solid lipid nanoparticles (SLN), nanostructured lipid carriers (NLC), and nanoemulsion (NE): Effect of lipid Matrix and surfactant on drug entrapment, in vitro release, and ex vivo hemolysis. AAPS PharmSciTech 22, 240. doi:10.1208/s12249-021-02108-5
WWARN K13 Genotype-Phenotype Study Group (2021). Association of mutations in the Plasmodium falciparum Kelch13 gene (Pf3D7_1343700) with parasite clearance rates after artemisinin-based treatments-a WWARN individual patient data meta-analysis. BMC medicine 17, 11. doi:10.1186/s12916-018-1207-3
Xu, C., Wei, Q., Yin, K., Sun, H., Li, J., Xiao, T., et al. (2018). Surveillance of antimalarial resistance Pfcrt, Pfmdr1, and Pfkelch13 polymorphisms in african plasmodium falciparum imported to shandong province, China. Sci. Rep. 8 (1), 1. doi:10.1038/s41598-018-31207-w
Yahiya, S., Rueda-Zubiaurre, A., Delves, M. J., Fuchter, M. J., and Baum, J. (2019). The antimalarial screening landscape—Looking beyond the asexual blood stage. Curr. Opin. Chem. Biol. 50, 1–9. doi:10.1016/j.cbpa.2019.01.029
Yang, H., Wang, J., Liu, H., Zhao, Y., Lakshmi, S., Li, X., et al. (2021). Efficacy and safety of a naphthoquine-azithromycin coformulation for malaria prophylaxis in southeast asia: A phase 3, double-blind, randomized, placebo-controlled trial. Clin. Infect. Dis. 73, e2470–e2476. doi:10.1093/cid/ciaa1018
Keywords: malaria, treatment, pre-clinical study, nanotechnology, infectious disease
Citation: Chaves JB, Portugal Tavares de Moraes B, Regina Ferrarini S, Noé da Fonseca F, Silva AR and Gonçalves-de-Albuquerque CF (2022) Potential of nanoformulations in malaria treatment. Front. Pharmacol. 13:999300. doi: 10.3389/fphar.2022.999300
Received: 20 July 2022; Accepted: 03 October 2022;
Published: 26 October 2022.
Edited by:
Rafael V. C. Guido, University of São Paulo, BrazilReviewed by:
Raj Kumar, University of Nebraska Medical Center, United StatesCopyright © 2022 Chaves, Portugal Tavares de Moraes, Regina Ferrarini, Noé da Fonseca, Silva and Gonçalves-de-Albuquerque. This is an open-access article distributed under the terms of the Creative Commons Attribution License (CC BY). The use, distribution or reproduction in other forums is permitted, provided the original author(s) and the copyright owner(s) are credited and that the original publication in this journal is cited, in accordance with accepted academic practice. No use, distribution or reproduction is permitted which does not comply with these terms.
*Correspondence: Cassiano Felippe Gonçalves-de-Albuquerque, Y2Fzc2lhbm8uYWxidXF1ZXJxdWVAdW5pcmlvLmJy
Disclaimer: All claims expressed in this article are solely those of the authors and do not necessarily represent those of their affiliated organizations, or those of the publisher, the editors and the reviewers. Any product that may be evaluated in this article or claim that may be made by its manufacturer is not guaranteed or endorsed by the publisher.
Research integrity at Frontiers
Learn more about the work of our research integrity team to safeguard the quality of each article we publish.