- 1First College of Clinical Medicine, Shandong University of Traditional Chinese Medicine, Jinan, China
- 2College of Integrated Traditional Chinese and Western Medicine, Hebei University of Chinese Medicine, Shijiazhuang, China
- 3Department of Orthopedics, Affiliated Hospital of Shandong University of Traditional Chinese Medicine, Jinan, China
Bone homeostasis depends on a precise dynamic balance between bone resorption and bone formation, involving a series of complex and highly regulated steps. Any imbalance in this process can cause disturbances in bone metabolism and lead to the development of many associated bone diseases. Autophagy, one of the fundamental pathways for the degradation and recycling of proteins and organelles, is a fundamental process that regulates cellular and organismal homeostasis. Importantly, basic levels of autophagy are present in all types of bone-associated cells. Due to the cyclic nature of autophagy and the ongoing bone metabolism processes, autophagy is considered a new participant in bone maintenance. Novel therapeutic targets have emerged as a result of new mechanisms, and bone metabolism can be controlled by interfering with autophagy by focusing on certain regulatory molecules in autophagy. In parallel, several studies have reported that various natural products exhibit a good potential to mediate autophagy for the treatment of metabolic bone diseases. Therefore, we briefly described the process of autophagy, emphasizing its function in different cell types involved in bone development and metabolism (including bone marrow mesenchymal stem cells, osteoblasts, osteocytes, chondrocytes, and osteoclasts), and also summarized research advances in natural product-mediated autophagy for the treatment of metabolic bone disease caused by dysfunction of these cells (including osteoporosis, rheumatoid joints, osteoarthritis, fracture nonunion/delayed union). The objective of the study was to identify the function that autophagy serves in metabolic bone disease and the effects, potential, and challenges of natural products for the treatment of these diseases by targeting autophagy.
Introduction
The bone acts as the primary structural component of the human body, which serves the functions of providing structural support, protection and movement, mineral storage, hematopoiesis, hormone secretion, and cognitive regulation. The supposedly robust and static bone tissue is dynamically remodeled continuously and regularly to carry out these intricate duties (Hadjidakis and Androulakis, 2006). This is necessary for microfracture recovery, the maintenance of calcium homeostasis, and the adaptation of the bone to different stresses (Sims and Gooi, 2008). Any imbalance in this process can cause disturbances in bone metabolism, leading to the development of many bone diseases such as osteoporosis (OP), osteoarthritis (OA), rheumatoid arthritis (RA), and fracture nonunion/delayed union, which can seriously impair patients’ quality of life and possibly put their lives in danger (Wang S. et al., 2020). Although various drugs have helped to reduce pain, restore bone strength, prevent bone deformities, and maintain daily activities to some extent, they have always had less satisfactory drawbacks, such as the inability to reverse the disease, high costs, restrictions on long-term drug use, and side effects (Ruderman, 2012; Sugiyama et al., 2015; Hunter and Bierma-Zeinstra, 2019). As a result, the current treatment strategy for these common metabolic bone diseases is largely conservative. Researchers have never stopped looking for novel medications, but the creation of new medications must be supported by a thorough comprehension of the disease mechanisms.
Autophagy provides a new perspective for understanding metabolic bone disease, which is an intracellular survival mechanism critical for cellular function (Kuma and Mizushima, 2010). Autophagy is constitutive as well as adaptive; under normal physiological conditions, basal levels of autophagy help remove damaged and malfunctioning cellular components and maintain basic energy homeostasis, whereas, under various stress conditions (especially nutritional deficiencies), upregulation of autophagy generates energy to maintain metabolism by providing additional nutrients from the recovered cellular components (Kim and Lee, 2014; Li et al., 2020b). The role of this mechanism transcends a single cell type or tissue and extends to the entire organism. Autophagy is the fundamental process that maintains cellular and organismal homeostasis (Dikic and Elazar, 2018). Novel therapeutic targets have emerged as a result of new mechanisms, and altering autophagy by focusing on certain regulatory molecules in autophagy can affect a number of disease processes (Al-Bari et al., 2021). Thus, autophagy is a crucial pharmacological target for drug development and therapeutic intervention in a variety of diseases, including bone metabolic disorders. More intriguingly, natural compounds derived from plants, animals, and microorganisms have been demonstrated to have the ability to influence autophagy through a variety of mechanisms, and as a result, may be crucial in the prevention or treatment of metabolic bone disease, implying the emergence of alternative drugs with lower costs, fewer side effects, and longer-term applications (Luk et al., 2020; Zheng H. et al., 2020; Mueller et al., 2021).
In this review, we provided a review of the current knowledge on the role of autophagy in bone metabolism disorders, to explore the potential relationship between autophagy and bone metabolism disorders. To serve as a resource for future studies, we also presented an overview of the functions and mechanisms of natural compounds that have been suggested to control autophagy in the treatment of prevalent metabolic bone disease in recent years.
Initiation and regulation of autophagy
Mammals have so far been classified as having three different types of autophagy: chaperone-mediated autophagy (CMA), micro-autophagy, and macro-autophagy (Mizushima and Komatsu, 2011). This paper focuses on macro-autophagy, henceforth referred to as autophagy. Autophagy is influenced by multiple signaling pathways, of which the most widely studied are the phosphoinositide3 kinase (PI3K)/protein kinase B (Akt) and 5ʹ AMP-activated protein kinase pathways. Together, these pathways converge on the mammalian target of rapamycin (mTOR). mTOR recruits various proteins to form two distinct complexes, mTORC1, and mTORC2. mTORC1 is involved in the regulation of autophagy and is a recognized negative regulator of autophagy (Jung et al., 2010; Chen X. D. et al., 2020). Autophagy is a highly conserved process that typically consists of several phases, including initiation, nucleation, elongation, maturation, and degradation (Yin et al., 2016; Markaki and Tavernarakis, 2020). The UNC-51-like kinase (ULK1) complex (which is composed of ULK1, ATG101, ATG13 and FAK family kinase-interacting protein of 200 kDa (FIP200), etc.) is activated and recruits the class 3 PI3K complex (which is composed of vacuolar protein sorting (Vps) 15, Vps34, ATG14 and Beclin1, etc.) to the autophagy initiation site to form an isolated membrane. autophagy-related protein (Atg) seven then binds the Atg12-Atg5-Atg16 complex and microtubule-associated protein one light chain 3 (LC3) I with phosphatidylethanolamine (PE) to produce LC3 II (Rabinowitz and White., 2010). LC3 II modifies the expanding phagophore to participate in cargo recognition and recruitment (Li et al., 2020a). The phagophore eventually enlarges and closes to form an autophagosome, a double membrane structure (Ravikumar et al., 2010). After maturing into the autophagolysosome, which is made up of the autophagosome and lysosome, acidic proteases break down the autophagolysosome and its contents into amino acids, lipids, nucleotides, and energy for cellular recycling (Ravikumar et al., 2010) (Figure 1).
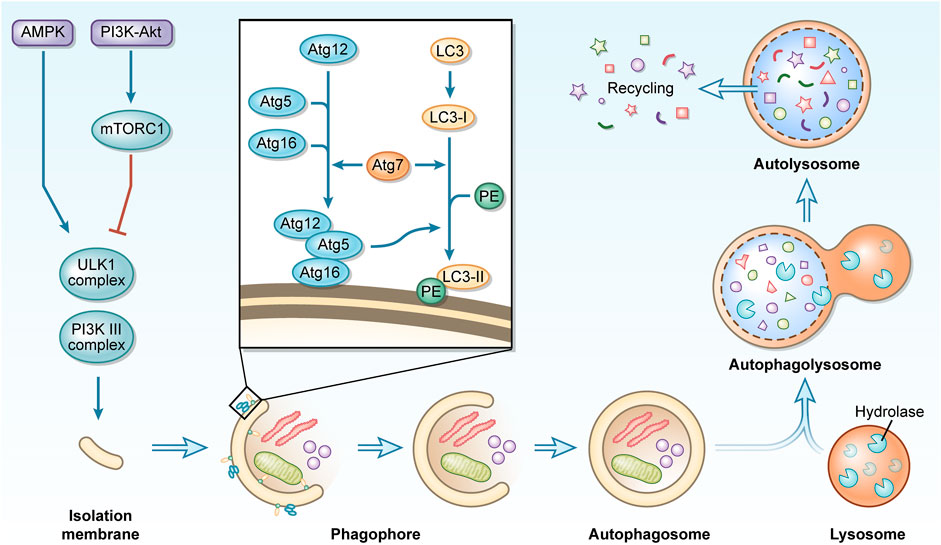
FIGURE 1. A concise description of the macro-autophagy process. During macro-autophagy, cellular components are sequestered in autophagosomes and transported to lysosomes for degradation to achieve recycling use.
Autophagy in bone metabolism
As an active metabolic tissue, bone undergoes a continuous remodeling cycle. Cells of different lineages perform specific skeletal functions, with osteoblasts and chondrocytes from bone marrow mesenchymal stem cells (BMSCs) shaping bone for maximum adaptability and osteoclasts of the monocyte/macrophage lineage resorbing large surfaces of predominantly cancellous bone to maintain mineral homeostasis (Zaidi, 2007). This complex mechanism of simultaneous activation couples bone formation and bone resorption, carefully balancing the development and maintenance of bone size, shape, and integrity (Boyle et al., 2003; Zaidi, 2007). Osteocytes, which are descended from osteoblasts, operate as sensors embedded in the matrix and may translate mechanical stimuli into biochemical signals (Palumbo and Ferretti, 2021). With the cyclic nature of autophagy and the continuous remodeling of bone tissue, and the levels of basal autophagy found to be present in all bone-related cells, it is reasonable to assume that autophagy plays an important role in bone homeostasis.
Autophagy in BMSCs
Autophagy participates in fundamental processes such as stem cell quiescence, self-renewal, differentiation, and pluripotency by regulating cell remodeling, and metabolism, and as an important mechanism of quality control (Boya et al., 2018). Adult stem cells known as BMSCs are pluripotent and may differentiate into a variety of cell types, including osteoblasts, adipocytes, chondrocytes, and neurons, etc (Chen et al., 2018). Autophagy in BMSCs is activated in response to environmental induction and hormones, which are essential for the survival, anti-aging, and differentiation of BMSCs. Early activation of autophagy can effectively inhibit apoptosis in BMSCs under conditions of hypoxia (28), serum deprivation (Zhang et al., 2012), oxidative stress (Song et al., 2014; Fan et al., 2019), inflammatory environments (Yang et al., 2016), highly saturated fatty acid environments (Liu et al., 2018), radiation (Alessio et al., 2015), and glucocorticoids administration (Wang et al., 2015). When exposed for an excessively long or severe period, this defense mechanism causes a switch from protective to destructive autophagy, which appears to depend on the intensity and duration of the stressful environment (Hu et al., 2019).
We concentrate on the function of autophagy in the osteogenic differentiation of BMSCs since specialized differentiation of BMSCs, such as differentiation to neurons (Li et al., 2016) or adipocytes (Song et al., 2015), needs the participation of autophagy. Undifferentiated BMSCs tend to accumulate large amounts of undegraded autophagic vacuoles with limited autophagic conversion, which continues to increase when osteogenic differentiation is initiated (Nuschke et al., 2014), which may be driven by elevated bioenergetic needs. Autophagy provides the morphology and structure required to support osteogenic differentiation, the energy required for metabolic remodeling and anabolic precursors (Ceccariglia et al., 2020), which is also reflected in the dependence of autophagy on 5’ AMP-activated protein kinase (AMPK)/Akt/mTOR signaling early in osteogenic differentiation (Pantovic et al., 2013), and inhibition of autophagy would significantly inhibit the osteogenic differentiation capacity of BMSCs. Furthermore, the efficiency of the autophagic process is decreased with age (Wu et al., 2009), which for BMSCs is frequently reflected in an imbalance of osteogenic and lipogenic differentiation, leading to bone loss and fat accumulation (Moerman et al., 2004). Inhibition of autophagy can put young BMSCs into a relatively senescent state, predisposing them to lipogenic differentiation. In contrast, the autophagy activator rapamycin reverses this property, which may be due to the correlation between the regulation of autophagy on reactive oxygen species (ROS) levels and the expression of P53 (a regulator of senescence) (Ma et al., 2018), suggesting that reasonable maintenance of autophagy levels would be beneficial for the prevention of aging and rejuvenation of BMSCs (Yang et al., 2018; Kondrikov et al., 2020) (Figures 2A,B).
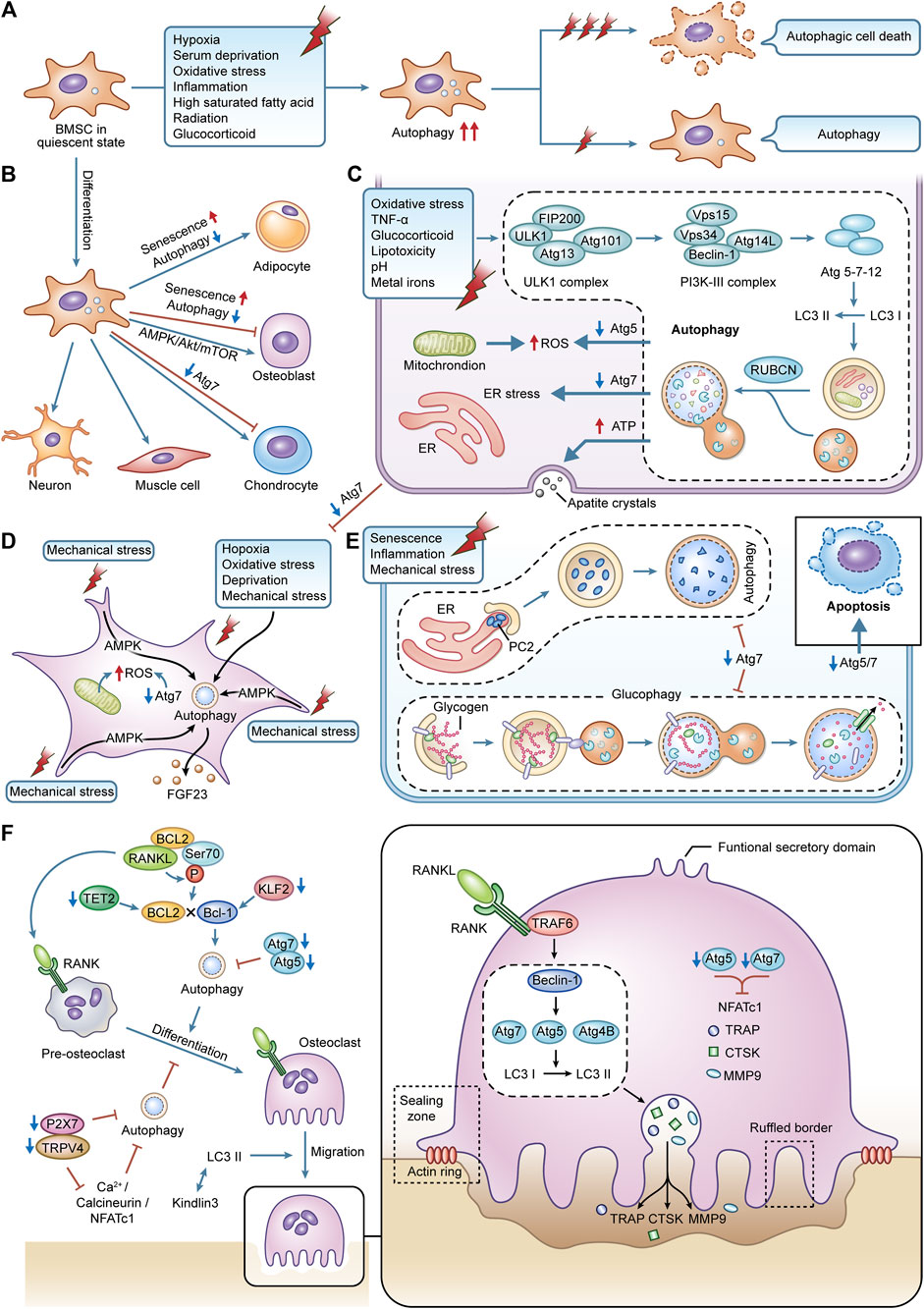
FIGURE 2. The effects of autophagy in various bone cell. (A) autophagy in BMSCs is activated during cellular differentiation, responding to external stress. (B) the specialized differentiation process in BMSCs is modified by autophagy. (C) autophagy in osteoblasts is activated caused by the stimulation of external stress. It can maintain redox balance within osteoblasts and affect the cellular mineralization and differentiation process. (D) terminally differentiated osteocytes can maintain lifespan and adapt to harsh environments via autophagy. The interaction between autophagy and mechanical stress is critical for the functions of osteocytes. (E) autophagy is an essential part of BMSCs differentiating into chondrocytes. Autophagy can regulate the secretion of type II collagen. Glycophagy can supply energy for chondrocytes. (F) the differentiation, migration, and bone resorption functions of osteoclasts are supported by autophagy.
Autophagy in osteoblasts
Osteoblasts are the primary constructors of bone, and these cells deposit bone matrix through continuous synthesis and resection activities (Yin et al., 2019). Deletion of autophagy-related genes impairs autophagy and negatively affects osteoblast activity and function. Increased susceptibility to oxidative stress damage and suppression of osteoblast proliferation and differentiation result from the deletion of Atg5 (Weng et al., 2018). Specific knockdown of Atg7 induces endoplasmic reticulum (ER) stress during bone development and remodeling stages thereby promoting apoptosis in osteoblasts (Li H. et al., 2018), and this deletion, if present early in the differentiation of the osteoblast lineage, can even affect the transition of osteoblasts to osteocytes and disrupt the formation and maintenance of the osteocyte network, so severely that more than 50% of mice with Atg7 knockdown will fracture as a result (Piemontese et al., 2016).
At the same time, autophagy facilitates the survival of osteoblasts under various stressful environments. Through the ER stress pathway, early activation of autophagy in osteoblasts can successfully reduce intracellular ROS levels and remove damaged mitochondria, counteracting the damage brought on by oxidative stress (Li D. Y. et al., 2017). This protective effect is equally beneficial in alleviating osteoblast apoptosis induced by toxic stimuli such as tumor necrosis factor-alpha (TNF-α) (Zheng L. W. et al., 2020), glucocorticoids (Wang et al., 2019), lipotoxicity (Al Saedi et al., 2020), acidity (Zhang et al., 2017), and metal ions (Xu G. et al., 2021; Liu et al., 2021).
Osteoblasts are dedicated mineralized cells, and the presence of double-membrane autophagosomes containing apatitic pinpoint-like structures was found in primary osteoblast cell lines, suggesting that intracellular mineralization, one of the mechanisms of mineralization, may be mediated by autophagy, with autophagosomes acting as carriers of the secretion of mineralization (Nollet et al., 2014). This shift from a low steady state to a high autophagic flux may be designed to meet the high-energy demands of active osteoblasts during mineralization, thus promoting a high synthesis of secreted proteins and the removal of misfolded bone matrix proteins. In contrast, the deletion of Atg7 and Beclin1 significantly reduces the mineralization efficiency of osteoblast cell lines (Nollet et al., 2014). Osteoblast proliferation to mineralization is inhibited when FIP200 (a component of the complex that initiates autophagosome formation) is specifically deleted (Liu et al., 2013). In contrast, deletion of RUBCN (a negative regulator gene of autophagosome-lysosome fusion) leads to enhanced osteoblast differentiation and mineralization and elevated expression of key transcription factors related to osteoblast function such as Runx2 and Bglap/Osteocalcin, which may be achieved through accelerated autophagic degradation of the intracellular structural domain of NOTCH (Yoshida et al., 2022) (Figure 2C).
Autophagy in osteocytes
Organelle recycling is required to give nourishment and adapt to the new environment as a result of the major changes in the spatial location and morphology of cells during the transition of osteoblasts to osteocytes (Dallas and Bonewald, 2010). Considering that those mature osteocytes are terminally differentiated cells that do not actively divide, intracellular degradation mechanisms are required to protect them from undergoing cell death (Stroikin et al., 2005). However, because of their lengthy longevity, they must be able to endure conditions with low oxygen, high levels of oxidative stress, nutrient deprivation, and constant mechanical stress. This biological evidence highlight autophagy as a potential mechanism to ensure the survival of osteocytes in their unique microenvironment. Osteocyte resistance to oxidative stress and hypoxia-induced cell death is naturally facilitated by osteoocytes higher autophagic activity than osteoblasts (Zahm et al., 2011; Kurihara et al., 2021). The oxidative stress in the bones of young mice climbs to elderly levels when Atg7 is lacking, and this causes skeletal abnormalities that are comparable to those brought on by aging (Onal et al., 2013).
The osteocytes are mechanoreceptive cells, which mediate the adaptive response to bone loading, perceive mechanical forces and translate them into graded structures as well as ensure metabolic changes (Qin et al., 2020). Mechanical loading is a specific and potent stimulus for osteocytes, which improves bone strength and inhibits defects in bone aging (Klein-Nulend et al., 2012). Autophagy is highly sensitive to the changes in mechanical stress in slime mold and mammalian cells (King et al., 2011). Periodic mechanical stretching alters the size and shape of osteocytes and promotes the network development of osteocytes, which is associated with upregulated autophagy (Inaba et al., 2017). Fluid shear stress-induced autophagy in osteocytes in vitro promotes osteocyte survival by preserving adenosine triphosphate (ATP), which is an adaptive response of osteocytes to mechanical stress (Zhang et al., 2018). Furthermore, the interaction between autophagy and mechanical tension is essential for regulating the secretory capacity of osteocytes, and mechanical tension can activate autophagy and enhance the secretion of fibroblast growth factor-23 (FGF23) (a homeostatic regulator within mineralization and phosphate) in osteocytes via AMPK signaling, thereby promoting the development and formation of osteoblasts (Xu H. et al., 2021) (Figure 2D).
Autophagy in chondrocytes
Most bones develop by endochondral ossification. Apoptosis of chondrocytes, calcification of the mesenchyme, new bone deposition, and longitudinal development of the diaphysis are all ongoing processes that occur in the cartilage growth plate at the confluence of the epiphysis and the diaphysis. The cartilage growth plate is mainly composed of chondrocytes and extracellular matrix (ECM). Chondrocyte proliferation, differentiation, hypertrophy, and ECM formation are essential for skeletal development and linear growth (Berendsen and Olsen, 2015). Autophagy is required for the conversion of mesenchymal stem cells to proliferating chondrocytes, as reflected by the completion of a multi-step differentiation process from mesenchymal condensation to calcification during normal ATDC5 cell culture, whereas COL2A1 expression is completely blocked during the culture of Atg7-deficient ATDC5 cells (Wang X. J. et al., 2021). Even if the transformation can be completed, deletion of Atg5 or Atg7 in the chondrocyte will also result in decreased chondrocyte proliferation and higher apoptosis, as well as related growth retardation and shorter bone length in mice (Vuppalapati et al., 2015; Wang X. J. et al., 2021). At the same time, due to the vascularization of the growth plate, chondrocytes grow in a hypoxic and nutrient-deficient environment. It is also necessary to rely on autophagosomes to selectively wrap glycogen in the chondrocytes so that the glycogen is catabolized to glucose to provide energy, which is known as glycophagy (Vuppalapati et al., 2015; Ueno and Komatsu, 2017).
On the other hand, functional cartilage requires the homeostasis of chondrocytes and the integrity of cartilage ECM (Luo et al., 2019). Autophagy regulates the secretion of type II collagen, which is a major component of cartilage ECM, and deletion of Atg7 would result in type II procollagen not being transported and remaining within the ER (Cinque et al., 2015). Under the stresses of aging, inflammation and mechanical stimulation, increased apoptosis, decreased ECM production and excessive activation of proteases in chondrocytes contribute to the degeneration of cartilage and the destruction of the joint microarchitecture, leading to the development of diseases such as OA and RA. Contrarily, increased autophagy in chondrocytes can reduce the progression of these disorders by influencing intracellular metabolic processes, demonstrating the benefits of autophagy on chondrocyte survival and prevention of cartilage deterioration (Tian et al., 2021) (Figure 2E).
Autophagy in osteoclasts
Hematopoietic cell-derived mononuclear osteoclast precursors are drawn to resorption sites where they combine to become terminally differentiated multinucleated osteoclasts (Montaseri et al., 2020). The alternating between migration and phases of bone resorption as well as considerable phenotypic alterations show that they are extremely mobile (Roy and Roux, 2020). After adhering to the area of bone resorption, osteoclasts undergo cytoskeletal reorganization and polarization, leading to the formation of a series of membrane domains such as the sealing zone, ruffled border, basolateral domain and functional secretory domain, which work together to complete the osteolysis process when osteoclasts lyse bone tissue (Georgess et al., 2014). The sealing zone, formed by a dense arrangement of actin-rich podosomes, forms a unique microenvironment between the cells and the bone surface. The transient reabsorption complex, which consists of actin rings and a ruffled border, is sealed off from the extracellular fluid in this region to form an absorption lacuna (Saltel et al., 2008). The ruffled border is formed by the fusion of acid-donating vesicles, which release hydrolases such as cathepsin K (CTSK), matrix metalloproteinase9 (MMP9), and tartrate-resistant acidic phosphatase (TRAP), and is the site truly responsible for bone resorption (Saltel et al., 2008). This fusion process takes place in the sealing zone, where several intracellular membranes are moved to create lengthy folds resembling fingers. Furthermore, in the non-resorption/migration state of osteoclasts, the relaxed osteoclasts undergo depolarization as they switch from the sealing zone to the podosome belts (Ory et al., 2008).
Autophagy is essential for osteoclast differentiation, migration and maintenance of bone resorption function. The receptor activator of nuclear factor-κB (NF-κB) (RANK)/receptor activator of NF-κB ligand (RANKL)/osteoprotegerin system mediates the process of osteoclast differentiation involving a series of signaling molecules, and the calcium signaling pathway Ca2+/calcineurin/nuclear factor of activated T-cell c1 (NFATc1), as one of the key pathways, can be mediated by Ca2+-permeable channels such as transient receptor potential vanilloid 4 (TRPV4) or P2X7 receptor (P2X7R). On the other hand, by inhibiting autophagy and Ca2+/calcineurin/NFATc1 signaling, suppression of either TRPV4 or P2X7R prevents osteoclast differentiation (Cao et al., 2019; Ma et al., 2022). The expression of Atg5, Atg7, Atg4B, and Beclin1 is increased in RANKL-stimulated bone marrow macrophages, and inhibition of Beclin1 will significantly reduce RANKL-mediated Atg activation and osteoclast differentiation (Arai et al., 2019). Meanwhile, after RANKL stimulation, the ubiquitin ligase tumor necrosis factor receptor-associated factor 6 (TRAF6) is recruited to RANK, thereby initiating Atg and subsequent osteoclasts differentiation at an early stage by mediating Beclin1 ubiquitination (Arai et al., 2019). In addition, RANKL can promote osteoclastogenesis via the B-cell lymphoma 2 (BCL2)/Beclin1 pathway, and the mechanism may be related to the fact that RANKL induces Beclin1-dependent protective autophagy by promoting BCL2 phosphorylation at the Ser70 site in osteoclast precursors (Ke et al., 2019; Ke et al., 2022). The tet methylcytosine dioxygenase 2 (TET2)/Beclin1 or kruppel-like factor 2 (KLF2)/Beclin1 autophagy-related pathways have also been shown to promote osteoclastogenesis (Laha et al., 2019; Yang et al., 2022). Deletion of Atg5 or Atg7 would also impede autophagy, which would result in a reduction in osteoclast differentiation and the expression of osteoclast markers such as NFATc1, TRAP, CTSK, and MMP9 (Lin et al., 2016; Chen W. et al., 2021).
One of the key components of the osteoclast’s exercise of bone resorption is migration over the bone matrix. Podosome rings undergo continuous and rapid assembly disassembly and drive osteoclast migration by exerting traction on the bone surface (Georgess et al., 2014). kindlin3 is an important bridging protein in the podosome, and downregulation of autophagy due to the deletion of LC3 II would enhance the interaction between kindlin3 and integrins, thereby inhibiting the breakdown of the abandoned podosome rings and leading to the disassembly of the actin cytoskeleton and impaired migration of osteoclasts (Zhang Y. et al., 2020). For optimal resorption of the bone matrix, osteoclasts require lysosomal transport and fusion to produce fold-edge boundaries and gaps for acidic resorption (Na et al., 2020). The fusion of lysosomes with the border of the fold is similar to the fusion of lysosomes with autophagosomes, suggesting that autophagy proteins are involved in regulating lysosomal localization and the release of reabsorbed molecules (Dawodu et al., 2018). Atg5, Atg7, and Atg4B, together with LC3, which is necessary for the formation of actin rings and the release of tissue proteinase K, are required for the construction of the edge of the fold (DeSelm et al., 2011; Chung et al., 2012) (Figure 2F).
CMA and micro-autophagy in bone
As with macro-autophagy, CMA similarly responds to nutrient deficiency (Cuervo et al., 1995), oxidative stress (Kaushik and Cuervo, 2006), hypoxia (Hubbi et al., 2013), genotoxic (Park et al., 2015) and other stimuli. Unlike macro-autophagy, CMA does not utilize autophagosomes, chaperone HSC70 and cochaperones deliver protein cargoes containing specific KFERQ-like sequences directly to the lysosome, where they are then transported via lysosome-associated membrane protein type 2A (LAMP2A) translocation system is transferred into the lysosome (Sahu et al., 2011; Akel et al., 2022). Compared to the wild type, vertebral cancellous bone mass was significantly lower in LAMP2A and LAMP2C global knockout mice, which was associated with increased osteoclastogenesis due to increased RANKL expression (Akel et al., 2022). BMSCs also exhibited higher CMA activity during osteogenic differentiation, this trend promotes the transition of BMSCs to OBs while inhibiting the potential of BMSCs to differentiate into lipogenic cells and chondrocytes (Gong et al., 2021). Downregulation of LAMP2A in BMSCs is also closely associated with impaired osteogenic differentiation during aging (Gong et al., 2021). Furthermore, leptin affects CMA-mediated expression of megalin (lipoprotein-related protein 2, a key receptor for 25(OH)D3 entry into BMSCs) by inhibiting the levels of LAMP2A and HSC70, thereby increasing the utilization of 25(OH)D3 by BMSCs and making 25(OH)D3-induced enhanced osteogenic differentiation potential of BMSCs, which also suggests that CMA is essential for the role of vitamin D in bone health (He et al., 2021).
During microautophagy, lysosomes and late endosomes capture a small amount of surrounding cytoplasm through membrane protrusion and invagination and degrade it in the endolysosomal lumen (Sahu et al., 2011). In mammalian cells, the exact mechanisms of microautophagy regulation remain largely elusive, with only studies exploring the association of dysfunctional microautophagy with various neurodegenerative diseases (e.g., Alzheimer’s disease, Parkinson’s disease, and amyotrophic lateral sclerosis) and cancer, the knowledge that may open a new window for the use of microautophagy in the skeletal domain (Wang L. et al., 2022).
Mitophgay in bone
Lemaster first defined “mitochondrial autophagy” to emphasize the non-random nature of the mitochondrial autophagic process (Lemasters, 2005). This selective autophagy process mediates mitochondrial quality control by removing damaged mitochondria and coordinating the dynamic balance between mitochondrial and cellular energy requirements (Liang et al., 2020). Similarly, this activity plays an important role in bone cells to mediate the homeostasis of bone metabolism. An increase in mitochondrial mass implies an accumulation of damaged mitochondria and requires a corresponding mitochondrial autophagic activity to maintain a good mitochondrial mass. The induction of mitochondrial autophagy eliminates damaged and unnecessary mitochondria from dental pulp stem cells and preserves healthy mitochondria, which promotes their differentiation into osteoblasts (Maity et al., 2022). During TNF-α-induced osteoblast senescence, the impaired bone anabolic activity can be ameliorated by restoring mitochondrial dysfunction and promoting mitochondrial autophagy (Lu et al., 2022). Furthermore, in vitro estrogen administration also promoted mitochondrial autophagy to some extent, which effectively increased osteoblast activity and promoted their proliferation (Sun et al., 2018). Interestingly, just like autophagy, the role played by mitochondrial autophagy seems to depend on the spatiotemporal location of the cell. In high-glucose-treated osteoblasts, mitochondrial autophagy accelerates their osteogenic dysfunction, and pharmacological and genetic inhibition of mitochondrial autophagy can effectively rescue osteoblast differentiation and mineralization (Zhao et al., 2020). the PTEN-induced putative kinase 1 (PINK1)/Parkin pathway is a key player in regulating mitochondrial homeostasis and the most important player in mitochondrial autophagy (Ploumi et al., 2017). For osteoclasts, the mitochondrial deacetylase sirtuin three promotes mitochondrial metabolism and mitochondrial autophagy in osteoclasts by deacetylating PINK1, which in turn promotes osteoclast differentiation. Thus, although osteoclast progenitors from sirtuin 3-deficient aged mice are able to differentiate into osteoclasts, however, these differentiated cells exhibit impaired polykaryon formation and resorption activity, further emphasizing the importance of mitochondrial autophagy regulation in bone cells and its contribution to skeletal disease (Ling et al., 2021).
Natural product-targeted autophagy for the treatment of metabolic bone disease
The growing body of research connecting autophagy and bone metabolic hints at the possibility of treating metabolic bone disease by inhibiting autophagy. Natural materials derived from natural plants and animals, minerals and their processed products are characterized by novel and diverse structures, better activity and less toxic side effects, and are a “treasure trove” for the development of drugs and nutrients (Li et al., 2021). With the increasing use of natural products, a variety of natural compounds have been screened as effective modulators of autophagy. Importantly, several of these natural compounds can cure metabolic bone disease by targeting autophagy through a number of distinct modes of action. The discovery and research of natural compounds that control autophagy now focus mostly on autophagy inducers and inhibitors because of the dual nature of autophagy during bone metabolism. The regulatory function of these natural products in OP, RA, OA, and fracture nonunion/delayed union is highlighted in this section through activation or inhibition of autophagy.
Osteoporosis
The majority of patients with OP are unaware that they have this sneaky illness, which is defined by decreased bone density, degeneration of the microarchitecture of bone tissue, increased skeletal fragility, and an increased risk of fractures, including hip, spine, and wrist fractures (Sànchez-Riera et al., 2010). The pathogenesis of this most common skeletal disease is based on an imbalance in the activity of osteoblasts and osteoclasts during bone metabolism (Zaidi, 2007). OP is classified as primary or secondary OP, and the former includes postmenopausal OP and senile OP. Due to diverse underlying causes and rates of development, autophagy has distinct functions in the various OP. In the early stage of the postmenopausal OP, estrogen decreases and bone metabolism has a high-conversion pattern, at this time osteoclastic bone resorption is enhanced and exceeds bone formation, and autophagy plays a more important role in bone resorption (Li et al., 2020b). Progressively lower levels of autophagy in osteocytes with aging are thought to be the underlying cause of bone loss (Chen et al., 2014), and defective autophagy in BMSCs due to aging will also lead to an imbalance in osteogenic and lipogenic differentiation. Bone conversion is delayed in the advanced stages of senile OP or postmenopausal OP (Ma et al., 2018). The predominant pathogenesis of secondary OP, such as the most prevalent glucocorticoid-induced OP, is characterized by impaired osteogenic differentiation, increased osteoblast and osteocyte apoptosis, and prolonged osteoclast lifespan, which results in reduced bone formation and early massive bone loss (Jia et al., 2006; Wang T. et al., 2020). While autophagy plays a role in promoting osteoclastic bone resorption and maintaining the survival of osteoblasts as well as osteocytes, this is usually dependent on glucocorticoid dose and duration of treatment and is regulated by systemic metabolism (Chen et al., 2014). Furthermore, in the disturbed bone microenvironment caused by oxidative stress or inflammation, damaging apoptosis of bone cells and enhanced osteoclast activity are the main causes of bone loss, and the level of autophagy often depends on the changes in the microenvironment and the severity of the stress.
Based on our current review, the mechanisms of action of natural products that target autophagy for the treatment of OP can be divided into four groups: promoting autophagy to encourage osteogenic differentiation of BMSCs and osteoblast mineralization, inhibiting autophagy to prevent the differentiation of osteoclasts, inhibiting autophagy to prevent apoptosis in bone cells, and raising the level of protective autophagy in stressful environments. Leonurine, the active ingredient of Leonurus japonicus, promotes the proliferation of BMSCs in SD rats, upregulates the gene and protein levels of Atg5, Atg7, and LC3, and promotes the differentiation of BMSCs toward osteoblasts through activation of autophagy that depends on the PI3K/Akt/mTOR pathway (Zhao et al., 2021). Icariin is an important active ingredient in Epimedium brevicornum and is mainly metabolized to Icaritin after ingestion. Icariin reduces ovariectomy (OVX)-induced osteoclast formation in OP mice and promotes osteogenic differentiation by enhancing autophagy in BMSCs (Liang et al., 2019). Similar to osthole, a key component of Cnidium monnieri and Angelica pubescens, osthole is a coumarin derivative that alleviates OP symptoms in OVX mice by preserving autophagy and encouraging osteogenic differentiation of BMSCs (Zheng et al., 2019). Arbutin, a natural hydroquinone glycoside abundant in plants such as Vaccinium, Asteraceae and Ericaceae, promotes bone formation by activating autophagy to promote osteoblast differentiation and mineralization and attenuate dexamethasone-induced bone mass and loss of trabecular bone structure (Zhang et al., 2021a). In addition, Ginsenoside Rg3, an extract of Panax ginseng, significantly attenuated OVX-induced weight gain, decreased bone mineral density and histological alterations in femoral tissue in rats. And in vitro, it significantly enhances AMPK signaling, autophagy, osteogenic differentiation and mineralization, inhibits mTOR signaling, and attenuates OVX-induced osteoporosis (Zhang X. et al., 2020).
Kaempferol, a natural flavonol, exists in large quantities as a dietary substance in fruits and vegetables such as tea, citrus fruits and cauliflower, and is also widely distributed in medicinal herbs such as Bauhinia microstachya, Chromolaena odorata and Ardisia japonica (Bangar et al., 2022). Concentrations of Kaempferol above 50 μM inhibit RANKL-induced osteoclast differentiation and formation of resorption pits in RAW 264.7 cells, with the mechanism being related to degradation of p62/SQSTM1 (autophagy-related scaffold protein) to inhibit autophagy and activate apoptosis (Kim et al., 2018). Ursolic acid, a pentacyclic triterpene found mainly in the Lamiaceae family, ameliorates OVX-induced osteoporosis in rats by blocking the autophagic process, reducing the expression of the major transcription factor and NFATc1 affecting osteoclast formation and the activity of the inhibitor of NF-κB kinase/NF-κB of its upstream pathway (Zheng H. et al., 2020).
Autophagy may be a major self-protective mechanism for osteocytes in response to excess glucocorticoids (Li et al., 2020b). Enhanced autophagy in osteocytes treated with low doses of glucocorticoids acts as anti-apoptotic self-protection (Wang T. et al., 2020), therefore, activation of appropriate levels of autophagy to protect osteocytes from apoptosis is a promising strategy to combat glucocorticoid-induced OP and glucocorticoid-associated osteonecrosis of the femoral head. Pinocembrin, a natural flavonoid compound isolated from compositae and propolis, attenuates dexamethasone-induced active damage and apoptosis in mice with long bone cell Y4 by inhibiting PI3K/Akt/mTOR signaling to activate autophagy (Wang X. Y. et al., 2020). It is also important to activate autophagy in osteoblasts to maintain cellular activity and prevent apoptosis. The bisbenzylisoquinoline alkaloid fangchinoline, which is obtained from the roots of Stephania tetrandra, shares the same structural features as tetrandrine (Zhang et al., 2021b). Fangchinoline administration dramatically lowers osteoblast apoptosis in prednisolone-induced osteoporosis rats by triggering autophagy, improves altered microstructural parameters in rat vertebrae, and avoids bone loss (Zhu W. et al., 2019). Similarly, iridoid glycoside Aucubin, which is abundant in Eucommia ulmoides, and monoterpene glucoside Paeoniflorin, which is abundant in the roots of Paeonia lactiflora, can enhance autophagy via AMPK and Akt/mTOR signaling pathways, respectively, to prevent glucocorticoid-induced apoptosis in osteoblasts, thereby effectively reducing OP symptoms (Yang et al., 2021; Yue et al., 2021). Another mechanism strongly linked to OP is the NF-κB signaling, which when activated promotes osteoclast activation and creation whereas when inactivated encourages osteoblast differentiation in vitro and bone formation in vivo (Nandy et al., 2018). In contrast, Timosaponin B-II, a component of the major steroidal saponin of Anemarrhena asphodeloides, attenuates high glucose-induced oxidative stress and osteoblast apoptosis by inhibiting the mTOR/NF-κB pathway to activate autophagy (Wang N. et al., 2021).
Oxidative stress is a key mechanism leading to the uncoupling of osteoclast and osteoblast functions in OP (Domazetovic et al., 2017), which may be brought on by excessive ROS production and then brings on OP (Baek et al., 2010). In age-induced oxidative stress, the excessive production of ROS impairs the proliferation and osteogenic differentiation of BMSCs, blocks the maturation of osteoblast precursors and induces apoptosis by inhibiting osteoblast mineralization (Coipeau et al., 2009; Cervellati et al., 2014). Contrarily, it is now generally accepted that the degree of autophagy is inversely connected with oxidative stress and that activating autophagy lowers oxidative stress damage and apoptosis while inhibiting autophagy would increase oxidative stress in osteoblasts (Li D. Y. et al., 2017). Other studies have shown that the damage caused by oxidative stress to osteoblasts can be mitigated by the early initiation of autophagy (Yin et al., 2019). Moreover, activation of autophagic activity also reduces apoptosis in osteocytes under high oxidative stress (Manolagas and Parfitt, 2010). The phenolic glycoside Curculigoside, which is abundant in Curculigo orchioides, inhibits phosphorylation of Forkhead box O1 (FOXO1), an upstream protein of antioxidant enzymes, and increases the expression of FOXO1 in osteoblasts with iron overload, thereby increasing the levels of antioxidant enzymes and LC3, promoting osteoblast autophagy and mineralization, and inhibiting oxidative damage caused by iron overload (Zhang et al., 2019). Similarly, monotropein, an iridoid glycoside extracted from the roots of Morind officinalis, similarly inhibits H2O2-induced reactive oxygen species production in osteoblasts, enhances autophagy-mediated antioxidant effects via the Akt/mTOR pathway, and guards against oxidative stress in osteoblasts (Shi et al., 2020).
The upregulation of systemic inflammation is an important mechanism in the aging process, often referred to as inflammatory aging (Flynn et al., 2019). The ongoing stimulation of inflammatory pathways in bone tissue, such as NF-κB signaling, throughout this process has a number of detrimental impacts on the preservation of bone mass, including the suppression of osteoblast development and mineralization and aberrant activation of osteoclast activity (Park et al., 2007; Chang et al., 2009). The current study suggests that autophagy is closely related to inflammation in the development of OP. For example, the inflammatory factor TNF-α induces increased expression of ATG7 and Beclin1 in arthritis models (Lin et al., 2013), and low concentrations of interleukin (IL) 17A activates autophagy via the c-Jun amino-terminal kinase (JNK) pathway, thereby promoting osteoclast differentiation and bone resorption activity (Ke et al., 2018). Therefore, reconciling inflammation with autophagy levels would be beneficial to inhibit osteoclast activity and bone resorption. For instance, autophagy is in charge of osteoclast development and enhanced activity in lipopolysaccharide-induced inflammatory bone loss (Chen L. et al., 2020). Extracts of Glycyrrhiza root isoliquiritigenin can inhibit RANKL-induced NF-κB expression and nuclear translocation, and suppress the expression of LC3 II and Beclin1 in vitro. And by drastically decreasing NF-κB-dependent autophagy of osteoclast precursors and consequently limiting osteoclast development, it may be able to treat lipopolysaccharide-induced inflammatory bone deterioration (Liu et al., 2016) (Table 1; and Figure 3).
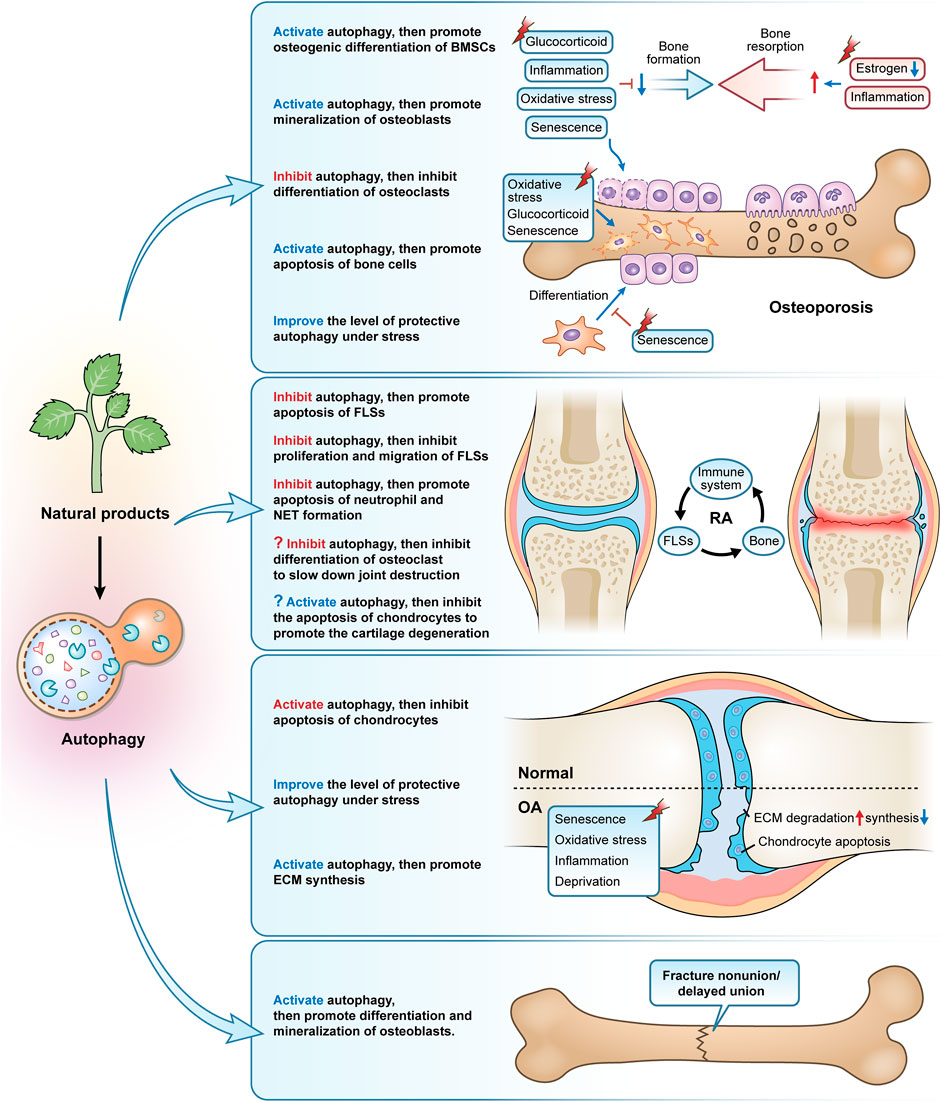
FIGURE 3. The primary modes and mechanisms of natural products targeting autophagy to regulate bone metabolism disorders. Natural products, used as autophagy activators or inhibitors, can effectively control osteoporosis, rheumatoid arthritis, osteoarthritis, and fracture nonunion/delayed union.
Rheumatoid arthritis
RA is characterized by progressive inflammation and destruction of bone and cartilage in the affected joint, which essentially depends on the interaction between the immune system, fibroblast-like synoviocytes (FLSs) and bone (Komatsu and Takayanagi, 2022). The immune system promotes the tissue-destroying properties of FLSs and influences the function of bone cells, such as increased bone resorption by osteoclasts (Komatsu and Takayanagi, 2022).
With its capacity to produce inflammatory mediators such as matrix-degrading enzymes, cytokines, and chemokines, FLSs are thought to play a significant role in the pathogenesis of RA, ultimately leading to the destruction of bone and cartilage (Karami et al., 2020). By preventing excessive immune cell activation and cytokine generation, the proper operation of the apoptotic program can reduce inflammation (Vomero et al., 2018). However, studies have found a reduced rate of apoptosis and apoptotic mediators of FLSs in RA, suggesting that these cells are resistant to apoptosis (Firestein et al., 1995). Activation of autophagy was shown to be an important pathway of the anti-apoptosis of FLSs in RA, this can be explained by the fact that autophagy is a cellular pro-survival mechanism and high levels of autophagy in FLSs, although the detailed mechanism between autophagy and apoptosis remains to be elucidated (Shin et al., 2010). Baicalin and Silibinin are two different flavonoid compounds from the dried roots of Scutellaria baicalensis and Silybum marianum, respectively, both of which can induce apoptosis and reduce inflammation by inhibiting autophagy in RA FLSs (Tong et al., 2018; Chen X. et al., 2022). Similarly, Oridonin, a kaurene-type diterpenoid isolated from Rabdosia rubescens, and Daphnetin, a coumarin derivative widely distributed in the thymelaeaceae family, also have the ability to inhibit the autophagy of RA FLSs and thus inhibit proliferation and induce apoptosis (Deng et al., 2020; He et al., 2020). Triptolide, a significant epoxy diterpene lactone derived from Tripterygium wilfordii, prevents cell migration and preserves the redox status of RA FLSs by preventing autophagy (Xie et al., 2019). The cytokine IL 21, a key immunomodulator, invokes a variety of immunomodulatory functions in RA including FLSs proliferation, the differentiation of T-cell subsets, and B-cell activation, and induces autophagy in adjuvant arthritis (AA)-FLSs through a PI3K/Akt-dependent manner (Niu et al., 2010; Dinesh and Rasool, 2019). In contrast, Berberine, an isoquinoline alkaloid (Zhu et al., 2022) widely distributed in the Berberidaceae, Papaveraceae, Menispermaceae, Ranunculaceae, and other botanical families, inhibits IL 21/IL 21R-mediated autophagy of AA-FLSs, inhibits the proliferation of a subpopulation of CD4+ T cells (T helper lymphocyte 17 (Th17)), and induces cellular differentiation of another subpopulation of CD4+ T cells (regulatory T lymphocyte (Treg)) that are suppressive of RA, thereby restoring an immune imbalance of Th17/Treg (Dinesh and Rasool, 2019). Additionally, excessive levels of pro-inflammatory cytokines cause neutrophils and activated macrophages to secrete ROS, which is then created by mitochondria to the extent of 90% (Zhang et al., 2016). Resveratrol, a non-flavonoid polyphenolic compound from Vitis, can lead to the accumulation of ROS by inhibiting autophagy, which in turn induces mitochondrial dysfunction and leads to apoptosis in FLSs (Cao et al., 2018).
Immune cells of various subtypes have a role in the development and progression of RA. As well as having a phenotype of delayed apoptosis and releasing high amounts of degradative enzymes and reactive oxygen species, activated neutrophils can also induce autoimmunity and worsen tissue damage by forming neutrophil extracellular traps (NETs) (Cascão et al., 2010; Khandpur et al., 2013). Several natural products, including Andrographolide, a diterpene compound derived from Andrographis paniculata, quercetin, a flavonoid compound derived from various vegetables and fruits, and Emodin, an anthraquinone derivative widely found in herbs such as Rheum palmatum, Polygonum cuspidatum, and Cassiae semen, exhibit effects of inhibiting the autophagy of neutrophils, promoting apoptosis and inhibiting NETs formation (Li X. et al., 2019; Zhu M. et al., 2019; Yuan et al., 2020) (Table 2; Figure 3).
We concentrate further on how autophagy contributes to RA joint degeneration. First, the secretion of pro-inflammatory factors and RANKL in FLSs allow osteoclast differentiation to be induced and bone resorption to be activated. The activation of autophagy is demonstrated by the high expression of Beclin1 and Atg7 in osteoclasts of human RA, while overexpression of Beclin1 induces osteoclastogenesis and significantly enhances their resorptive capacity (Lin et al., 2013). In the Atg7-deficient RA mice model, inhibition of autophagy reduces the number of osteoclasts and resists TNF-α-induced bone erosion (Lin et al., 2013). Similar to this, in bone marrow mononuclear cells from RA mice caused by K/BxN serum, there is a dramatically increased expression of autophagy-related genes such as Beclin1, Atg7, and LC3 II, and the production of autophagic vesicles is greatly enhanced during osteoclast differentiation (Laha et al., 2019).
Secondly, one of the primary factors contributing to the degeneration and loss of articular cartilage in RA is thought to be the apoptosis of articular chondrocytes. The autophagy inhibitor 3-MA increases joint inflammation and cartilage damage and induces chondrocyte apoptosis in rats with AA, whereas the autophagy activator Rapa reduces joint inflammation and chondrocyte apoptosis, suggesting that autophagy activation ameliorates damage to chondrocytes in AA rats by inhibiting apoptosis (Zhou et al., 2019). The accumulation of large amounts of acid in the synovial fluid is one of the important pathological features of RA, and elevated acid has been found in synovial biopsies of patients with early RA (Chang et al., 2005). Extracellular acidification causes chondrocytes to undergo apoptosis through the action of acid-sensitive ion channel 1a (ASIC1a), which is a crucial factor in the destruction of articular cartilage in RA (Xie et al., 2018). Contrarily, several studies have shown that estrogen protects articular cartilage from acidosis-induced damage by encouraging the breakdown of the ASIC1a protein. This is related to the fact that estrogen increases autophagy in chondrocytes to some extent, which in turn encourages the breakdown of the ASIC1a protein, which is reliant on the autophagy-lysosome pathway (Xie et al., 2018; Song et al., 2020; Su et al., 2021). However, we would like to ask, in the treatment of RA, should we choose to inhibit autophagy to attenuate osteoclast differentiation and bone resorption, or should we choose to promote autophagy to protect chondrocyte apoptosis and cartilage metabolism and repair? Does this rely on various RA triggers, phases, or the activation of autophagy brought on by various substances and signals? Nevertheless, RA can be treated by regulating autophagy in a cell-specific manner. But despite the fact that we have not yet discovered any studies on the pertinent natural products that interfere in the treatment of RA by focusing on autophagy in osteoclasts or chondrocytes, we do think that this may be an intriguing area for future investigation (Figure 3).
Osteoarthritis
OA is the most common degenerative joint disease, manifested by articular cartilage erosion, synovial inflammation, osteoid formation, and subchondral osteosclerosis (Hunter and Bierma-Zeinstra, 2019). The most severe degenerative alterations among them are found in articular cartilage, which is intimately connected to the metabolic imbalance and abnormal apoptosis of chondrocytes due to aging or overuse of cartilage (Fujii et al., 2022). Therefore, modulating chondrocyte behavior and thus restoring homeostasis of articular cartilage is a central theme in the study of OA (van der Kraan, 2012). It is debatable whether OA causes an increase or decrease in the amount of autophagy. An earlier study has demonstrated that ULK1, Beclin1, and LC3 proteins are expressed in articular chondrocytes of both humans and mice and are reduced in aging or surgery-induced OA as well as increased apoptosis of chondrocytes (Caramés et al., 2010). Another study from the same time period, however, found increased expression of LC3 and Beclin1 in OA chondrocytes, particularly when the chondrocytes were experiencing nutritional stress and catabolism, and this study attributed this differential result to the different locations of the collected samples of OA cartilage (Sasaki et al., 2012). A recent study explained this issue in a targeted manner by observing changes in autophagy in different weight-bearing states and at different stages of OA, and it was observed that autophagy was stronger in weight-bearing areas than in the non-weight-bearing areas and was stronger in the 4-week group than in the 10-week group, in other words, autophagy was differentially expressed in different stages of OA, with stronger expression in the early stages of OA and diminishing expression as the disease progressed (Zhang et al., 2022). In any case, however, these results demonstrate that autophagy is a protective process for maintaining homeostasis in the cartilage. Therefore, starting with chondrocyte autophagy and activating the autophagy activity of chondrocytes can effectively reverse the state of autophagic failure and provide an effective way to prevent and treat OA degeneration.
Currently, most studies based on natural products for the treatment of OA have been conducted by inducing autophagy in cartilage, and these products include the saponin Astragaloside IV from Astragalus membranaceus (Liu et al., 2017), polyphenol curcumin from Curcuma longa (Li X. et al., 2017; Chen T. et al., 2021; Yao et al., 2021; Jin et al., 2022), coumarins isopsoralen from Psoralea corylifolia seeds (Chen Z. et al., 2020), Icariin (Mi et al., 2018; Tang et al., 2021a), furocoumarin Columbianetin from the root of Radix Angelicae Pubescentis (Chen W. et al., 2022), glucoxilxanthone mangiferin from in various parts of Mangifera indica (Li Y. et al., 2019), flavonoid baicalin from the root of Scutellaria baicalensis (Li Z. et al., 2020), polyphenolic compound chlorogenic acid from coffee and plants such as Lonicera japonica (Zada et al., 2021), polyphenol anthocyanidin delphinidin from various brightly colored fruits and vegetables (Lee et al., 2020), polyphenol tannin punicalagin from the peel of Punica granatum (Kong et al., 2020), natural carotenoid compound Lycopene from bright red-orange fruits and vegetables such as Lycopersicon esculentum (Wu et al., 2021), polymethoxylated flavonoid Sinensetin from citrus fruits (Zhou et al., 2021), isoflavonoid glabridin from the root of Glycyrrhiza glabra (Dai et al., 2021), triterpenoid saponin compound saikosaponin D from Bupleurum falcatum (Jiang et al., 2020), polyphenols (-)-Epigallocatechin 3-gallate from green tea (Huang et al., 2020), flavonoid rhoifolin from Rhus succedanea (Yan et al., 2021), quercetin (Lv et al., 2022), and naphthoquinone compound Shikonin from the root of Lithospermum erythrorhizon (Wang A. et al., 2022). Because of the large number, we have summarized in the table the specific mechanisms involved in these studies. Inhibiting chondrocyte apoptosis, regulating the metabolic balance of ECM synthesis and degradation, and re-establishing the disturbed microenvironment within the cartilage are all ways that these medications affect the autophagic process of chondrocytes in OA caused by various triggers (aging, inflammatory factors, oxidative stress, oxygen-glucose deprivation, and serum deprivation) (Table 3; Figure 3).
Fracture nonunion/delayed union
Despite the strong self-healing ability of bone tissue, about 5–10% of patients still experience problems with fracture healing (Nelson et al., 2003). LC3 II is upregulated in bone tissue after internal fixation in a rat femoral fracture model and positively correlates with the number of cells positive for Proliferating cell nuclear antigen (a key protein for osteoblast proliferation) (Zhou et al., 2015). Systemic administration of rapamycin-induced autophagy in a rat femur fracture model, significantly promoting mineralization, formation, and remodeling of bone scabs and the expression of proliferating cell nuclear antigen and vascular endothelial growth factor (Yang et al., 2015). It has also been demonstrated that AMPK activation accelerates the healing of fractures by increasing autophagy, which improves osteoblast differentiation and mineralization (Li G. et al., 2018). Autophagy, therefore, presents a possible therapeutic target for the clinical treatment of fracture nonunion/delayed union given the significant role it plays in bone development and bone mineralization. However, there are focused research on medications, particularly those involving natural compounds, that monitor and target autophagy using fracture nonunion or delayed union models (only two items). β-Ecdysterone, a polyhydroxylated steroid hormone found mainly in Achyranthes bidentata and Cyanotis arachnoidea, activates autophagy by inhibiting PI3K/Akt/mTOR signaling pathway in femoral tissue of rats with femoral fractures, promoting osteoblast differentiation and mineralization, inhibiting apoptosis, and accelerating fracture healing (Tang et al., 2021b). Similarly, curcumin-treated rat femur fracture osteoblasts exhibited rapid fracture healing and autophagy activation, whereas the rate of fracture healing was noticeably slowed in the presence of curcumin plus 3-MA (Li Y. et al., 2018) (Figure 3).
Conclusion and prospects for the future
The orderly conduct of bone metabolic processes and the maintenance of homeostasis in bone depend on the coordinated cooperation of multiple cell types, which requires the proper functioning of BMSCs, osteoblasts, osteocytes, osteoclasts and chondrocytes. It has been established through investigation of certain autophagy-related gene knockdowns that autophagy is crucial to the maintenance of these cell types’ functional integrity. Consequently, increasing autophagy may be a key therapeutic focus for treating metabolic bone disease. Meanwhile, recent studies have reported the therapeutic role of natural products as inducers or inhibitors of autophagy in various diseases, including diseases caused by various disorders of bone metabolism, such as OP, RA, OA, and fracture nonunion/delayed union.
Despite the surprising results, there are still some challenges that have not been overcome and are inevitable to be addressed. On the one hand, both bone metabolism and autophagy are dynamic processes, with different metabolic bone diseases and affected bone cells, various disease stages, varying trigger intensities and durations, and different degrees of inhibition or activation of autophagy in different individuals. This undoubtedly increases the demand for precise regulation of autophagy. We must be cautious when identifying autophagic “defects” and determining the “positive or negative” functions performed by autophagy before we seek to restore cellular viability by the elimination of cellular damage via autophagy. For example, excessive activation may result in increased secondary mineral deposition and bone fragility even if impaired autophagic activity in osteocytes is linked to decreased bone mass and slowed bone turnover (Li et al., 2020b). For many skeletal tissues with low cell numbers and extended lifespans, excessive induction of autophagy may accelerate apoptosis and senescence thereby exacerbating the disease state (Shapiro et al., 2014). Another illustration is the transformation of early activated protective autophagy into destructive autophagy with ongoing or increased stress, which necessitates an accurate measurement of the spatiotemporal location and autophagic state of the afflicted cells. Therefore, there is no consensus on the therapeutic potential of autophagy. The stage of autophagy should be included in follow-up research, and it is necessary to build a dynamic observation system of autophagy under various illness phases in order to standardize and unify follow-up investigations. The close association between bone cells in particular calls for increased specific targeting of autophagy, which requires us to identify the level of autophagy in the main affected bone cells in different metabolic bone diseases; for example, bone resorption by osteoclasts and bone formation by osteoblasts both require autophagic activity, which requires us to choose between inhibition or activation of autophagy.
On the other hand, to cope with the need for precise autophagic regulation, it is necessary to continuously figure out the effective dose of natural products, early and late administration, specific targeting of different cells, precise regulation and range maintenance of autophagic levels, and differential responses of different individuals. The bioavailability of many natural compounds is constrained by features including poor absorption, quick metabolism, and rapid elimination (Rahman et al., 2020). As a result, it may be advantageous to structurally alter natural products based on the control of autophagy to enhance their targeting, efficacy, and safety. Also, the development and utilization of nanocarriers may be effective in improving the stability, solubility, and sustainability of natural products, as demonstrated by excellent precedents such as the treatment of ischemia-reperfusion with curcumin and the promotion of nerve recovery with resveratrol (Kalani et al., 2016; Fan et al., 2020). Furthermore, translation to clinical application is a difficult threshold to cross in the short term, and although some natural products have shown promising results in clinical trials for the treatment of skeletal disorders, it has not been elucidated whether their effects are associated with autophagy. A recent randomized, placebo-controlled trial in middle-aged adults investigated the role of Urolithin A, a natural compound produced by intestinal flora following ingestion of pomegranate, berries and nuts, in improving muscle performance by promoting mitochondrial autophagy, which may inform subsequent clinical studies of natural products modulating autophagy in the treatment of skeletal disorders (Singh et al., 2022).
Finally, although we have reviewed studies on the regulation of autophagy by natural products to treat common metabolic bone diseases, more metabolic bone diseases should be included, such as osteolysis, Paget disease of bone, and osteogenesis imperfecta, and there is still a paucity of studies on natural products in the treatment of these diseases, and the role of autophagy in these diseases is obscure. What’s more, it is anticipated that more pertinent mechanisms will be discovered, leading to an increase in the validity of the theoretical underpinnings and options for natural product-targeted autophagy in the treatment of various metabolic bone diseases. In any case, the regulation of autophagy is thought to be an exciting strategy for drug development.
Author contributions
ZL was responsible for the collection, collation of data, and writing of the original manuscript. DL, HS, and HX were accountable for collection of data. GT and ZX were responsible for concept development and manuscript revision. All authors reviewed and accepted the final version of the manuscript.
Funding
This work was supported by the National Natural Science Foundation of China (No. 82174410), Natural Science Foundation of Shandong Province (ZR202011050051), Natural Science Foundation of Shandong Province (ZR2020MH362) and the Construction Project of Inheritance Studio of National Famous Old Chinese Medicine Experts [Chinese Medicine Education Letter (2022) grant No. 75].
Conflict of interest
The authors declare that the research was conducted in the absence of any commercial or financial relationships that could be construed as a potential conflict of interest.
Publisher’s note
All claims expressed in this article are solely those of the authors and do not necessarily represent those of their affiliated organizations, or those of the publisher, the editors and the reviewers. Any product that may be evaluated in this article, or claim that may be made by its manufacturer, is not guaranteed or endorsed by the publisher.
References
Akel, N., MacLeod, R. S., Berryhill, S. B., Laster, D. J., Dimori, M., Crawford, J. A., et al. (2022). Loss of chaperone-mediated autophagy is associated with low vertebral cancellous bone mass. Sci. Rep. 12 (1), 3134. doi:10.1038/s41598-022-07157-9
Al Saedi, A., Myers, D. E., Stupka, N., and Duque, G. (2020). 1, 25(OH)(2)D(3) ameliorates palmitate-induced lipotoxicity in human primary osteoblasts leading to improved viability and function. Bone 141, 115672. doi:10.1016/j.bone.2020.115672
Al-Bari, M., Ito, Y., Ahmed, S., Radwan, N., Ahmed, H. S., and Eid, N. (2021). Targeting autophagy with natural products as a potential therapeutic approach for cancer. Int. J. Mol. Sci. 22 (18), 9807. doi:10.3390/ijms22189807
Alessio, N., Del, G. S., Capasso, S., Di Bernardo, G., Cappabianca, S., Cipollaro, M., et al. (2015). Low dose radiation induced senescence of human mesenchymal stromal cells and impaired the autophagy process. Oncotarget 6 (10), 8155–8166. doi:10.18632/oncotarget.2692
Arai, A., Kim, S., Goldshteyn, V., Kim, T., Park, N. H., Wang, C. Y., et al. (2019). Beclin1 modulates bone homeostasis by regulating osteoclast and chondrocyte differentiation. J. Bone Min. Res. 34 (9), 1753–1766. doi:10.1002/jbmr.3756
Baek, K. H., Oh, K. W., Lee, W. Y., Lee, S. S., Kim, M. K., Kwon, H. S., et al. (2010). Association of oxidative stress with postmenopausal osteoporosis and the effects of hydrogen peroxide on osteoclast formation in human bone marrow cell cultures. Calcif. Tissue Int. 87 (3), 226–235. doi:10.1007/s00223-010-9393-9
Bangar, S. P., Chaudhary, V., Sharma, N., Bansal, V., Ozogul, F., and Lorenzo, J. M. (2022). Kaempferol: A flavonoid with wider biological activities and its applications. Crit. Rev. Food Sci. Nutr., 1–25. doi:10.1080/10408398.2022.2067121
Berendsen, A. D., and Olsen, B. R. (2015). Bone development. Bone. 80, 14–18. doi:10.1016/j.bone.2015.04.035
Boya, P., Codogno, P., and Rodriguez-Muela, N. (2018). Autophagy in stem cells: Repair, remodelling and metabolic reprogramming. Development 145 (4), dev146506. doi:10.1242/dev.146506
Boyle, W. J., Simonet, W. S., and Lacey, D. L. (2003). Osteoclast differentiation and activation. Nature 423 (6937), 337–342. doi:10.1038/nature01658
Cao, B., Dai, X., and Wang, W. (2019). Knockdown of TRPV4 suppresses osteoclast differentiation and osteoporosis by inhibiting autophagy through Ca(2+) -calcineurin-NFATc1 pathway. J. Cell. Physiol. 234 (5), 6831–6841. doi:10.1002/jcp.27432
Cao, W., Zhang, J., Wang, G., Lu, J., Wang, T., and Chen, X. (2018). Reducing-autophagy derived mitochondrial dysfunction during resveratrol promotes fibroblast-like synovial cell apoptosis. Anat. Rec. 301 (7), 1179–1188. doi:10.1002/ar.23798
Caramés, B., Taniguchi, N., Otsuki, S., Blanco, F. J., and Lotz, M. (2010). Autophagy is a protective mechanism in normal cartilage, and its aging-related loss is linked with cell death and osteoarthritis. Arthritis Rheum. 62 (3), 791–801. doi:10.1002/art.27305
Cascão, R., Rosário, H. S., Souto-Carneiro, M. M., and Fonseca, J. E. (2010). Neutrophils in rheumatoid arthritis: More than simple final effectors. Autoimmun. Rev. 9 (8), 531–535. doi:10.1016/j.autrev.2009.12.013
Ceccariglia, S., Cargnoni, A., Silini, A. R., and Parolini, O. (2020). Autophagy: A potential key contributor to the therapeutic action of mesenchymal stem cells. Autophagy 16 (1), 28–37. doi:10.1080/15548627.2019.1630223
Cervellati, C., Bonaccorsi, G., Cremonini, E., Romani, A., Fila, E., Castaldini, M. C., et al. (2014). Oxidative stress and bone resorption interplay as a possible trigger for postmenopausal osteoporosis. Biomed. Res. Int. 2014, 569563. doi:10.1155/2014/569563
Chang, J., Wang, Z., Tang, E., Fan, Z., McCauley, L., Franceschi, R., et al. (2009). Inhibition of osteoblastic bone formation by nuclear factor-kappaB. Nat. Med. 15 (6), 682–689. doi:10.1038/nm.1954
Chang, X., Yamada, R., and Yamamoto, K. (2005). Inhibition of antithrombin by hyaluronic acid may be involved in the pathogenesis of rheumatoid arthritis. Arthritis Res. Ther. 7 (2), R268–R273. doi:10.1186/ar1487
Chen, K., Yang, Y. H., Jiang, S. D., and Jiang, L. S. (2014). Decreased activity of osteocyte autophagy with aging may contribute to the bone loss in senile population. Histochem. Cell Biol. 142 (3), 285–295. doi:10.1007/s00418-014-1194-1
Chen, X., He, Y., and Lu, F. (2018). Autophagy in stem cell biology: A perspective on stem cell self-renewal and differentiation. Stem Cells Int. 2018, 9131397. doi:10.1155/2018/9131397
Chen, L., Yang, Y., Bao, J., Wang, Z., Xia, M., Dai, A., et al. (2020a). Autophagy negative-regulating wnt signaling enhanced inflammatory osteoclastogenesis from pre-OCs in vitro. Biomed. Pharmacother. 126, 110093. doi:10.1016/j.biopha.2020.110093
Chen, X. D., Tan, J. L., Feng, Y., Huang, L. J., Zhang, M., and Cheng, B. (2020b). Autophagy in fate determination of mesenchymal stem cells and bone remodeling. World J. Stem Cells 12 (8), 776–786. doi:10.4252/wjsc.v12.i8.776
Chen, T., Zhou, R., Chen, Y., Fu, W., Wei, X., Ma, G., et al. (2021). Curcumin ameliorates IL-1β-induced apoptosis by activating autophagy and inhibiting the NF-κB signaling pathway in rat primary articular chondrocytes. Cell Biol. Int. 45 (5), 976–988. doi:10.1002/cbin.11541
Chen, W., Xian, G., Gu, M., Pan, B., Wu, X., Ye, Y., et al. (2021). Autophagy inhibitors 3-MA and LY294002 repress osteoclastogenesis and titanium particle-stimulated osteolysis. Biomater. Sci. 9 (14), 4922–4935. doi:10.1039/d1bm00691f
Chen, W., Zheng, H., Zhang, X., Xu, Y., Fu, Z., Ji, X., et al. (2022a). Columbianetin alleviates lipopolysaccharides (LPS)-induced inflammation and apoptosis in chondrocyte through activation of autophagy by inhibiting serum and glucocorticoid-induced protein kinase 1 (SGK1) expression. Bioengineered 13 (2), 4051–4062. doi:10.1080/21655979.2022.2032970
Chen, X., Wang, Y., Cai, J., Wang, S., Cheng, Z., Zhang, Z., et al. (2022b). Anti-inflammatory effect of baicalin in rats with adjuvant arthritis and its autophagy- related mechanism. Technol. Health Care. 30 (S1), 191–200. doi:10.3233/THC-228018
Chen, Z., Li, C., Qian, Y. H., Fu, Y., and Feng, Z. M. (2020c). Enhancement of autophagy flux by isopsoralen ameliorates interleukin-1β-stimulated apoptosis in rat chondrocytes. J. Asian Nat. Prod. Res. 22 (2), 179–192. doi:10.1080/10286020.2018.1537265
Chung, Y. H., Yoon, S. Y., Choi, B., Sohn, D. H., Yoon, K. H., Kim, W. J., et al. (2012). Microtubule-associated protein light chain 3 regulates cdc42-dependent actin ring formation in osteoclast. Int. J. Biochem. Cell Biol. 44 (6), 989–997. doi:10.1016/j.biocel.2012.03.007
Cinque, L., Forrester, A., Bartolomeo, R., Svelto, M., Venditti, R., Montefusco, S., et al. (2015). FGF signalling regulates bone growth through autophagy. Nature 528 (7581), 272–275. doi:10.1038/nature16063
Coipeau, P., Rosset, P., Langonne, A., Gaillard, J., Delorme, B., Rico, A., et al. (2009). Impaired differentiation potential of human trabecular bone mesenchymal stromal cells from elderly patients. Cytotherapy 11 (5), 584–594. doi:10.1080/14653240903079385
Cuervo, A. M., Knecht, E., Terlecky, S. R., and Dice, J. F. (1995). Activation of a selective pathway of lysosomal proteolysis in rat liver by prolonged starvation. Am. J. Physiol. 269, C1200–C1208. doi:10.1152/ajpcell.1995.269.5.C1200
Dai, J., Zhang, Y., Chen, D., Chen, D., Li, X., Wang, J., et al. (2021). Glabridin inhibits osteoarthritis development by protecting chondrocytes against oxidative stress, apoptosis and promoting mTOR mediated autophagy. Life Sci. 268, 118992. doi:10.1016/j.lfs.2020.118992
Dallas, S. L., and Bonewald, L. F. (2010). Dynamics of the transition from osteoblast to osteocyte. Ann. N. Y. Acad. Sci. 1192, 437–443. doi:10.1111/j.1749-6632.2009.05246.x
Dawodu, D., Patecki, M., Hegermann, J., Dumler, I., Haller, H., and Kiyan, Y. (2018). OxLDL inhibits differentiation and functional activity of osteoclasts via scavenger receptor-A mediated autophagy and cathepsin K secretion. Sci. Rep. 8 (1), 11604. doi:10.1038/s41598-018-29963-w
Deng, H., Zheng, M., Hu, Z., Zeng, X., Kuang, N., and Fu, Y. (2020). Effects of Daphnetin on the autophagy signaling pathway of fibroblast-like synoviocytes in rats with collagen-induced arthritis (CIA) induced by TNF-α. Cytokine 127, 154952. doi:10.1016/j.cyto.2019.154952
DeSelm, C. J., Miller, B. C., Zou, W., Beatty, W. L., van Meel, E., Takahata, Y., et al. (2011). Autophagy proteins regulate the secretory component of osteoclastic bone resorption. Dev. Cell 21 (5), 966–974. doi:10.1016/j.devcel.2011.08.016
Dikic, I., and Elazar, Z. (2018). Mechanism and medical implications of mammalian autophagy. Nat. Rev. Mol. Cell Biol. 19 (6), 349–364. doi:10.1038/s41580-018-0003-4
Dinesh, P., and Rasool, M. (2019). Berberine mitigates IL-21/IL-21r mediated autophagic influx in fibroblast-like synoviocytes and regulates Th17/treg imbalance in rheumatoid arthritis. Apoptosis 24 (7-8), 644–661. doi:10.1007/s10495-019-01548-6
Domazetovic, V., Marcucci, G., Iantomasi, T., Brandi, M. L., and Vincenzini, M. T. (2017). Oxidative stress in bone remodeling: Role of antioxidants. Clin. Cases Min. Bone Metab. 14 (2), 209–216. doi:10.11138/ccmbm/2017.14.1.209
Fan, P., Yu, X. Y., Xie, X. H., Chen, C. H., Zhang, P., Yang, C., et al. (2019). Mitophagy is a protective response against oxidative damage in bone marrow mesenchymal stem cells. Life Sci. 229, 36–45. doi:10.1016/j.lfs.2019.05.027
Fan, Y., Li, Y., Huang, S., Xu, H., Li, H., and Liu, B. (2020). Resveratrol-primed exosomes strongly promote the recovery of motor function in SCI rats by activating autophagy and inhibiting apoptosis via the PI3K signaling pathway. Neurosci. Lett. 736, 135262. doi:10.1016/j.neulet.2020.135262
Firestein, G. S., Yeo, M., and Zvaifler, N. J. (1995). Apoptosis in rheumatoid arthritis synovium. J. Clin. Invest. 96 (3), 1631–1638. doi:10.1172/JCI118202
Flynn, M. G., Markofski, M. M., and Carrillo, A. E. (2019). Elevated inflammatory status and increased risk of chronic disease in chronological aging: Inflamm-aging or inflamm-inactivity. Aging Dis. 10 (1), 147–156. doi:10.14336/AD.2018.0326
Fujii, Y., Liu, L., Yagasaki, L., Inotsume, M., Chiba, T., and Asahara, H. (2022). Cartilage homeostasis and osteoarthritis. Int. J. Mol. Sci. 23 (11), 6316. doi:10.3390/ijms23116316
Georgess, D., Machuca-Gayet, I., Blangy, A., and Jurdic, P. (2014). Podosome organization drives osteoclast-mediated bone resorption. Cell adh. Migr. 8 (3), 191–204. doi:10.4161/cam.27840
Gong, Y., Li, Z., Zou, S., Deng, D., Lai, P., Hu, H., et al. (2021). Vangl2 limits chaperone-mediated autophagy to balance osteogenic differentiation in mesenchymal stem cells. Dev. Cell 56 (14), 2103–2120.e9. doi:10.1016/j.devcel.2021.06.011
Hadjidakis, D. J., and Androulakis, I. I. (2006). Bone remodeling. Ann. N. Y. Acad. Sci. 1092, 385–396. doi:10.1196/annals.1365.035
He, Q., Qin, R., Glowacki, J., Zhou, S., Shi, J., Wang, S., et al. (2021). Synergistic stimulation of osteoblast differentiation of rat mesenchymal stem cells by leptin and 25(oh)D(3) is mediated by inhibition of chaperone-mediated autophagy. Stem Cell Res. Ther. 12 (1), 557. doi:10.1186/s13287-021-02623-z
He, S. D., Huang, S. G., Zhu, H. J., Luo, X. G., Liao, K. H., Zhang, J. Y., et al. (2020). Oridonin suppresses autophagy and survival in rheumatoid arthritis fibroblast-like synoviocytes. Pharm. Biol. 58 (1), 146–151. doi:10.1080/13880209.2020.1711783
Hu, C., Zhao, L., Wu, D., and Li, L. (2019). Modulating autophagy in mesenchymal stem cells effectively protects against hypoxia- or ischemia-induced injury. Stem Cell Res. Ther. 10 (1), 120. doi:10.1186/s13287-019-1225-x
Huang, H. T., Cheng, T. L., Ho, C. J., Huang, H. H., Lu, C. C., Chuang, S. C., et al. (2020). Intra-articular injection of (-)-Epigallocatechin 3-gallate to attenuate articular cartilage degeneration by enhancing autophagy in a post-traumatic osteoarthritis rat model. Antioxidants (Basel) 10 (1), E8. doi:10.3390/antiox10010008
Hubbi, M. E., Hu, H., KshitizAhmed, I., Levchenko, A., and Semenza, G. L. (2013). Chaperone-mediated autophagy targets hypoxia-inducible factor-1Α (Hif-1Α) for lysosomal degradation. J. Biol. Chem. 288 (15), 10703–10714. doi:10.1074/jbc.M112.414771
Hunter, D. J., and Bierma-Zeinstra, S. (2019). Osteoarthritis. Lancet 393 (10182), 1745–1759. doi:10.1016/S0140-6736(19)30417-9
Inaba, N., Kuroshima, S., Uto, Y., Sasaki, M., and Sawase, T. (2017). Cyclic mechanical stretch contributes to network development of osteocyte-like cells with morphological change and autophagy promotion but without preferential cell alignment in rat. Biochem. Biophys. Rep. 11, 191–197. doi:10.1016/j.bbrep.2017.04.018
Jia, D., O'Brien, C. A., Stewart, S. A., Manolagas, S. C., and Weinstein, R. S. (2006). Glucocorticoids act directly on osteoclasts to increase their life span and reduce bone density. Endocrinology 147 (12), 5592–5599. doi:10.1210/en.2006-0459
Jiang, J., Meng, Y., Hu, S., Botchway, B., Zhang, Y., and Liu, X. (2020). Saikosaponin D: A potential therapeutic drug for osteoarthritis. J. Tissue Eng. Regen. Med. 14 (8), 1175–1184. doi:10.1002/term.3090
Jin, Z., Chang, B., Wei, Y., Yang, Y., Zhang, H., Liu, J., et al. (2022). Curcumin exerts chondroprotective effects against osteoarthritis by promoting AMPK/PINK1/Parkin-mediated mitophagy. Biomed. Pharmacother. 151, 113092. doi:10.1016/j.biopha.2022.113092
Jung, C. H., Ro, S. H., Cao, J., Otto, N. M., and Kim, D. H. (2010). MTOR regulation of autophagy. FEBS Lett. 584 (7), 1287–1295. doi:10.1016/j.febslet.2010.01.017
Kalani, A., Chaturvedi, P., Kamat, P. K., Maldonado, C., Bauer, P., Joshua, I. G., et al. (2016). Curcumin-loaded embryonic stem cell exosomes restored neurovascular unit following ischemia-reperfusion injury. Int. J. Biochem. Cell Biol. 79, 360–369. doi:10.1016/j.biocel.2016.09.002
Karami, J., Masoumi, M., Khorramdelazad, H., Bashiri, H., Darvishi, P., Sereshki, H. A., et al. (2020). Role of autophagy in the pathogenesis of rheumatoid arthritis: Latest evidence and therapeutic approaches. Life Sci. 254, 117734. doi:10.1016/j.lfs.2020.117734
Kaushik, S., and Cuervo, A. M. (2006). Autophagy as a cell-repair mechanism: Activation of chaperone-mediated autophagy during oxidative stress. Mol. Asp. Med. 27 (5-6), 444–454. doi:10.1016/j.mam.2006.08.007
Ke, D., Fu, X., Xue, Y., Wu, H., Zhang, Y., Chen, X., et al. (2018). IL-17A regulates the autophagic activity of osteoclast precursors through RANKL-JNK1 signaling during osteoclastogenesis in vitro. Biochem. Biophys. Res. Commun. 497 (3), 890–896. doi:10.1016/j.bbrc.2018.02.164
Ke, D., Ji, L., Wang, Y., Fu, X., Chen, J., Wang, F., et al. (2019). JNK1 regulates RANKL-induced osteoclastogenesis via activation of a novel bcl-2-beclin1-autophagy pathway. Faseb J. 33 (10), 11082–11095. doi:10.1096/fj.201802597RR
Ke, D., Yu, Y., Li, C., Han, J., and Xu, J. (2022). Phosphorylation of BCL2 at the Ser70 site mediates RANKL-induced osteoclast precursor autophagy and osteoclastogenesis. Mol. Med. 28 (1), 22. doi:10.1186/s10020-022-00449-w
Khandpur, R., Carmona-Rivera, C., Vivekanandan-Giri, A., Gizinski, A., Yalavarthi, S., Knight, J. S., et al. (2013). NETs are a source of citrullinated autoantigens and stimulate inflammatory responses in rheumatoid arthritis. Sci. Transl. Med. 5 (178), 178ra40–178r. doi:10.1126/scitranslmed.3005580
Kim, C. J., Shin, S. H., Kim, B. J., Kim, C. H., Kim, J. H., Kang, H. M., et al. (2018). The effects of kaempferol-inhibited autophagy on osteoclast formation. Int. J. Mol. Sci. 19 (1), E125. doi:10.3390/ijms19010125
Kim, K. H., and Lee, M. S. (2014). Autophagy--A key player in cellular and body metabolism. Nat. Rev. Endocrinol. 10 (6), 322–337. doi:10.1038/nrendo.2014.35
King, J. S., Veltman, D. M., and Insall, R. H. (2011). The induction of autophagy by mechanical stress. Autophagy 7 (12), 1490–1499. doi:10.4161/auto.7.12.17924
Klein-Nulend, J., Bacabac, R. G., and Bakker, A. D. (2012). Mechanical loading and how it affects bone cells: The role of the osteocyte cytoskeleton in maintaining our skeleton. Eur. Cell. Mater. 24, 278–291. doi:10.22203/ecm.v024a20
Komatsu, N., and Takayanagi, H. (2022). Mechanisms of joint destruction in rheumatoid arthritis - immune cell-fibroblast-bone interactions. Nat. Rev. Rheumatol. 18 (7), 415–429. doi:10.1038/s41584-022-00793-5
Kondrikov, D., Elmansi, A., Bragg, R. T., Mobley, T., Barrett, T., Eisa, N., et al. (2020). Kynurenine inhibits autophagy and promotes senescence in aged bone marrow mesenchymal stem cells through the aryl hydrocarbon receptor pathway. Exp. Gerontol. 130, 110805. doi:10.1016/j.exger.2019.110805
Kong, J., Wang, J., Gong, X., Zheng, X., and Chen, T. (2020). Punicalagin inhibits tert-butyl hydroperoxide-induced apoptosis and extracellular matrix degradation in chondrocytes by activating autophagy and ameliorates murine osteoarthritis. Drug Des. devel. Ther. 14, 5521–5533. doi:10.2147/DDDT.S282932
Kuma, A., and Mizushima, N. (2010). Physiological role of autophagy as an intracellular recycling system: With an emphasis on nutrient metabolism. Semin. Cell Dev. Biol. 21 (7), 683–690. doi:10.1016/j.semcdb.2010.03.002
Kurihara, M., Mukudai, Y., Watanabe, H., Asakura, M., Abe, Y., Houri, A., et al. (2021). Autophagy prevents osteocyte cell death under hypoxic conditions. Cells Tissues Organs 210 (5-6), 326–338. doi:10.1159/000519086
Laha, D., Deb, M., and Das, H. (2019). KLF2 (Kruppel-Like factor 2 [lung]) regulates osteoclastogenesis by modulating autophagy. Autophagy 15 (12), 2063–2075. doi:10.1080/15548627.2019.1596491
Lee, D. Y., Park, Y. J., Song, M. G., Kim, D. R., Zada, S., and Kim, D. H. (2020). Cytoprotective effects of delphinidin for human chondrocytes against oxidative stress through activation of autophagy. Antioxidants (Basel) 9 (1), E83. doi:10.3390/antiox9010083
Lemasters, J. J. (2005). Selective mitochondrial autophagy, or mitophagy, as a targeted defense against oxidative stress, mitochondrial dysfunction, and aging. Rejuvenation Res. 8 (1), 3–5. doi:10.1089/rej.2005.8.3
Li, B., Duan, P., Li, C., Jing, Y., Han, X., Yan, W., et al. (2016). Role of autophagy on bone marrow mesenchymal stem-cell proliferation and differentiation into neurons. Mol. Med. Rep. 13 (2), 1413–1419. doi:10.3892/mmr.2015.4673
Li, D. Y., Yu, J. C., Xiao, L., Miao, W., Ji, K., Wang, S. C., et al. (2017a). Autophagy attenuates the oxidative stress-induced apoptosis of Mc3T3-E1 osteoblasts. Eur. Rev. Med. Pharmacol. Sci. 21 (24), 5548–5556. doi:10.26355/eurrev_201712_13991
Li, X., Feng, K., Li, J., Yu, D., Fan, Q., Tang, T., et al. (2017b). Curcumin inhibits apoptosis of chondrocytes through activation ERK1/2 signaling pathways induced autophagy. Nutrients 9 (4), E414. doi:10.3390/nu9040414
Li, G., Chen, L., and Chen, K. (2018a). Curcumin promotes femoral fracture healing in a rat model by activation of autophagy. Med. Sci. Monit. 24, 4064–4072. doi:10.12659/MSM.908311
Li, H., Li, D., Ma, Z., Qian, Z., Kang, X., Jin, X., et al. (2018b). Defective autophagy in osteoblasts induces endoplasmic reticulum stress and causes remarkable bone loss. Autophagy 14 (10), 1726–1741. doi:10.1080/15548627.2018.1483807
Li, Y., Su, J., Sun, W., Cai, L., and Deng, Z. (2018c). AMP-activated protein kinase stimulates osteoblast differentiation and mineralization through autophagy induction. Int. J. Mol. Med. 41 (5), 2535–2544. doi:10.3892/ijmm.2018.3498
Li, X., Yuan, K., Zhu, Q., Lu, Q., Jiang, H., Zhu, M., et al. (2019a). Andrographolide ameliorates rheumatoid arthritis by regulating the apoptosis-NETosis balance of neutrophils. Int. J. Mol. Sci. 20 (20), E5035. doi:10.3390/ijms20205035
Li, Y., Wu, Y., Jiang, K., Han, W., Zhang, J., Xie, L., et al. (2019b). Mangiferin prevents TBHP-induced apoptosis and ECM degradation in mouse osteoarthritic chondrocytes via restoring autophagy and ameliorates murine osteoarthritis. Oxid. Med. Cell. Longev. 2019, 8783197. doi:10.1155/2019/8783197
Li, X., He, S., and Ma, B. (2020a). Autophagy and autophagy-related proteins in cancer. Mol. Cancer 19 (1), 12. doi:10.1186/s12943-020-1138-4
Li, X., Xu, J., Dai, B., Wang, X., Guo, Q., and Qin, L. (2020b). Targeting autophagy in osteoporosis: From pathophysiology to potential therapy. Ageing Res. Rev. 62, 101098. doi:10.1016/j.arr.2020.101098
Li, Z., Cheng, J., and Liu, J. (2020). Baicalin protects human OA chondrocytes against IL-1β-induced apoptosis and ECM degradation by activating autophagy via MiR-766-3p/AIFM1 Axis. Drug Des. devel. Ther. 14, 2645–2655. doi:10.2147/DDDT.S255823
Li, Y. Q., Zhang, F., Yu, L. P., Mu, J. K., Yang, Y. Q., Yu, J., et al. (2021). Targeting PINK1 using natural products for the treatment of human diseases. Biomed. Res. Int. 2021, 4045819. doi:10.1155/2021/4045819
Liang, K. X., Kristiansen, C. K., Mostafavi, S., Vatne, G. H., Zantingh, G. A., Kianian, A., et al. (2020). Disease-specific phenotypes in ipsc-derived neural stem cells with polg mutations. EMBO Mol. Med. 12 (10), e12146. doi:10.15252/emmm.202012146
Liang, X., Hou, Z., Xie, Y., Yan, F., Li, S., Zhu, X., et al. (2019). Icariin promotes osteogenic differentiation of bone marrow stromal cells and prevents bone loss in OVX mice via activating autophagy. J. Cell. Biochem. 120 (8), 13121–13132. doi:10.1002/jcb.28585
Lin, N. Y., Beyer, C., Giessl, A., Kireva, T., Scholtysek, C., Uderhardt, S., et al. (2013). Autophagy regulates TNFα-mediated joint destruction in experimental arthritis. Ann. Rheum. Dis. 72 (5), 761–768. doi:10.1136/annrheumdis-2012-201671
Lin, N. Y., Chen, C. W., Kagwiria, R., Liang, R., Beyer, C., Distler, A., et al. (2016). Inactivation of autophagy ameliorates glucocorticoid-induced and ovariectomy-induced bone loss. Ann. Rheum. Dis. 75 (6), 1203–1210. doi:10.1136/annrheumdis-2015-207240
Ling, W., Krager, K., Richardson, K. K., Warren, A. D., Ponte, F., Aykin-Burns, N., et al. (2021). Mitochondrial Sirt3 contributes to the bone loss caused by aging or estrogen deficiency. Jci Insight 6 (10), 146728. doi:10.1172/jci.insight.146728
Liu, F., Fang, F., Yuan, H., Yang, D., Chen, Y., Williams, L., et al. (2013). Suppression of autophagy by FIP200 deletion leads to osteopenia in mice through the inhibition of osteoblast terminal differentiation. J. Bone Min. Res. 28 (11), 2414–2430. doi:10.1002/jbmr.1971
Liu, S., Zhu, L., Zhang, J., Yu, J., Cheng, X., and Peng, B. (2016). Anti-osteoclastogenic activity of isoliquiritigenin via inhibition of NF-κB-dependent autophagic pathway. Biochem. Pharmacol. 106, 82–93. doi:10.1016/j.bcp.2016.03.002
Liu, J., Meng, Q., Jing, H., and Zhou, S. (2017). Astragaloside IV protects against apoptosis in human degenerative chondrocytes through autophagy activation. Mol. Med. Rep. 16 (3), 3269–3275. doi:10.3892/mmr.2017.6980
Liu, Y., Wang, N., Zhang, S., and Liang, Q. (2018). Autophagy protects bone marrow mesenchymal stem cells from palmitate-induced apoptosis through the ROS-JNK/p38 MAPK signaling pathways. Mol. Med. Rep. 18 (2), 1485–1494. doi:10.3892/mmr.2018.9100
Liu, P., Cui, Y., Liu, M., Xiao, B., Zhang, J., Huang, W., et al. (2021). Protective effect of mitophagy against aluminum-induced MC3T3-E1 cells dysfunction. Chemosphere 282, 131086. doi:10.1016/j.chemosphere.2021.131086
Lu, Z. F., Jiang, L., Lesani, P., Zhang, W. J., Li, N., Luo, D., et al. (2022). Nicotinamide mononucleotide alleviates osteoblast senescence induction and promotes bone healing in osteoporotic mice. J. Gerontol. A Biol. Sci. Med. Sci., glac175. doi:10.1093/gerona/glac175
Luk, H. Y., Appell, C., Chyu, M. C., Chen, C. H., Wang, C. Y., Yang, R. S., et al. (2020). Impacts of green tea on joint and skeletal muscle health: Prospects of translational nutrition. Antioxidants (Basel) 9 (11), E1050. doi:10.3390/antiox9111050
Luo, P., Gao, F., Niu, D., Sun, X., Song, Q., Guo, C., et al. (2019). The role of autophagy in chondrocyte metabolism and osteoarthritis: A comprehensive research review. Biomed. Res. Int. 2019, 5171602. doi:10.1155/2019/5171602
Lv, S., Wang, X., Jin, S., Shen, S., Wang, R., and Tong, P. (2022). Quercetin mediates TSC2-RHEB-mTOR pathway to regulate chondrocytes autophagy in knee osteoarthritis. Gene 820, 146209. doi:10.1016/j.gene.2022.146209
Ma, Y., Qi, M., An, Y., Zhang, L., Yang, R., Doro, D. H., et al. (2018). Autophagy controls mesenchymal stem cell properties and senescence during bone aging. Aging Cell 17 (1), e12709. doi:10.1111/acel.12709
Ma, Y., Di, R., Zhao, H., Song, R., Zou, H., and Liu, Z. (2022). P2X7 receptor knockdown suppresses osteoclast differentiation by inhibiting autophagy and Ca(2+)/calcineurin signaling. Mol. Med. Rep. 25 (5), 160. doi:10.3892/mmr.2022.12677
Maity, J., Barthels, D., Sarkar, J., Prateeksha, P., Deb, M., Rolph, D., et al. (2022). Ferutinin induces osteoblast differentiation of dpscs via induction of Klf2 and autophagy/mitophagy. Cell Death Dis. 13 (5), 452. doi:10.1038/s41419-022-04903-9
Manolagas, S. C., and Parfitt, A. M. (2010). What Old means to bone. Trends Endocrinol. Metab. 21 (6), 369–374. doi:10.1016/j.tem.2010.01.010
Markaki, M., and Tavernarakis, N. (2020). Autophagy mechanisms and roles: Recent advances and implications. Febs J. 287 (23), 5024–5026. doi:10.1111/febs.15573
Mi, B., Wang, J., Liu, Y., Liu, J., Hu, L., Panayi, A. C., et al. (2018). Icariin activates autophagy via down-regulation of the NF-κB signaling-mediated apoptosis in chondrocytes. Front. Pharmacol. 9, 605. doi:10.3389/fphar.2018.00605
Mizushima, N., and Komatsu, M. (2011). Autophagy: Renovation of cells and tissues. Cell 147 (4), 728–741. doi:10.1016/j.cell.2011.10.026
Moerman, E. J., Teng, K., Lipschitz, D. A., and Lecka-Czernik, B. (2004). Aging activates adipogenic and suppresses osteogenic programs in mesenchymal marrow stroma/stem cells: The role of PPAR-gamma2 transcription factor and TGF-beta/BMP signaling pathways. Aging Cell 3 (6), 379–389. doi:10.1111/j.1474-9728.2004.00127.x
Montaseri, A., Giampietri, C., Rossi, M., Riccioli, A., Del, F. A., and Filippini, A. (2020). The role of autophagy in osteoclast differentiation and bone resorption function. Biomolecules 10 (10), E1398. doi:10.3390/biom10101398
Mueller, A. L., Payandeh, Z., Mohammadkhani, N., Mubarak, S., Zakeri, A., Alagheband, B. A., et al. (2021). Recent advances in understanding the pathogenesis of rheumatoid arthritis: New treatment strategies. Cells 10 (11), 3017. doi:10.3390/cells10113017
Na, W., Lee, E. J., Kang, M. K., Kim, Y. H., Kim, D. Y., Oh, H., et al. (2020). Aesculetin inhibits osteoclastic bone resorption through blocking ruffled border formation and lysosomal trafficking. Int. J. Mol. Sci. 21 (22), E8581. doi:10.3390/ijms21228581
Nandy, A., Lin, L., Velentzas, P. D., Wu, L. P., Baehrecke, E. H., and Silverman, N. (2018). The NF-κB factor relish regulates Atg1 expression and controls autophagy. Cell Rep. 25 (8), 2110–2120. doi:10.1016/j.celrep.2018.10.076
Nelson, F. R., Brighton, C. T., Ryaby, J., Simon, B. J., Nielson, J. H., Lorich, D. G., et al. (2003). Use of physical forces in bone healing. J. Am. Acad. Orthop. Surg. 11 (5), 344–354. doi:10.5435/00124635-200309000-00007
Niu, X., He, D., Zhang, X., Yue, T., Li, N., Zhang, J. Z., et al. (2010). IL-21 regulates Th17 cells in rheumatoid arthritis. Hum. Immunol. 71 (4), 334–341. doi:10.1016/j.humimm.2010.01.010
Nollet, M., Santucci-Darmanin, S., Breuil, V., Al-Sahlanee, R., Cros, C., Topi, M., et al. (2014). Autophagy in osteoblasts is involved in mineralization and bone homeostasis. Autophagy 10 (11), 1965–1977. doi:10.4161/auto.36182
Nuschke, A., Rodrigues, M., Stolz, D. B., Chu, C. T., Griffith, L., and Wells, A. (2014). Human mesenchymal stem cells/multipotent stromal cells consume accumulated autophagosomes early in differentiation. Stem Cell Res. Ther. 5 (6), 140. doi:10.1186/scrt530
Onal, M., Piemontese, M., Xiong, J., Wang, Y., Han, L., Ye, S., et al. (2013). Suppression of autophagy in osteocytes mimics skeletal aging. J. Biol. Chem. 288 (24), 17432–17440. doi:10.1074/jbc.M112.444190
Ory, S., Brazier, H., Pawlak, G., and Blangy, A. (2008). Rho GTPases in osteoclasts: Orchestrators of podosome arrangement. Eur. J. Cell Biol. 87 (8-9), 469–477. doi:10.1016/j.ejcb.2008.03.002
Palumbo, C., and Ferretti, M. (2021). The osteocyte: From "prisoner" to "orchestrator. J. Funct. Morphol. Kinesiol. 6 (1), 28. doi:10.3390/jfmk6010028
Pantovic, A., Krstic, A., Janjetovic, K., Kocic, J., Harhaji-Trajkovic, L., Bugarski, D., et al. (2013). Coordinated time-dependent modulation of AMPK/Akt/mTOR signaling and autophagy controls osteogenic differentiation of human mesenchymal stem cells. Bone 52 (1), 524–531. doi:10.1016/j.bone.2012.10.024
Park, B. K., Zhang, H., Zeng, Q., Dai, J., Keller, E. T., Giordano, T., et al. (2007). NF-kappaB in breast cancer cells promotes osteolytic bone metastasis by inducing osteoclastogenesis via GM-CSF. Nat. Med. 13 (1), 62–69. doi:10.1038/nm1519
Park, C., Suh, Y., and Cuervo, A. M. (2015). Regulated degradation of chk1 by chaperone-mediated autophagy in response to dna damage. Nat. Commun. 6, 6823. doi:10.1038/ncomms7823
Piemontese, M., Onal, M., Xiong, J., Han, L., Thostenson, J. D., Almeida, M., et al. (2016). Low bone mass and changes in the osteocyte network in mice lacking autophagy in the osteoblast lineage. Sci. Rep. 6, 24262. doi:10.1038/srep24262
Ploumi, C., Daskalaki, I., and Tavernarakis, N. (2017). Mitochondrial biogenesis and clearance: A balancing act. Febs J. 284 (2), 183–195. doi:10.1111/febs.13820
Qin, L., Liu, W., Cao, H., and Xiao, G. (2020). Molecular mechanosensors in osteocytes. Bone Res. 8, 23. doi:10.1038/s41413-020-0099-y
Rabinowitz, J. D., and White, E. (2010). Autophagy and metabolism. Science 330 (6009), 1344–1348. doi:10.1126/science.1193497
Rahman, H. S., Othman, H. H., Hammadi, N. I., Yeap, S. K., Amin, K. M., Abdul, S. N., et al. (2020). Novel drug delivery systems for loading of natural plant extracts and their biomedical applications. Int. J. Nanomed. 15, 2439–2483. doi:10.2147/IJN.S227805
Ravikumar, B., Sarkar, S., Davies, J. E., Futter, M., Garcia-Arencibia, M., Green-Thompson, Z. W., et al. (2010). Regulation of mammalian autophagy in physiology and pathophysiology. Physiol. Rev. 90 (4), 1383–1435. doi:10.1152/physrev.00030.2009
Roy, M., and Roux, S. (2020). Rab GTPases in osteoclastic bone resorption and autophagy. Int. J. Mol. Sci. 21 (20), E7655. doi:10.3390/ijms21207655
Ruderman, E. M. (2012). Overview of safety of non-biologic and biologic DMARDs. Rheumatol. Oxf. 51, i37–i43. doi:10.1093/rheumatology/kes283
Sahu, R., Kaushik, S., Clement, C. C., Cannizzo, E. S., Scharf, B., Follenzi, A., et al. (2011). Microautophagy of cytosolic proteins by late endosomes. Dev. Cell 20 (1), 131–139. doi:10.1016/j.devcel.2010.12.003
Saltel, F., Chabadel, A., Bonnelye, E., and Jurdic, P. (2008). Actin cytoskeletal organisation in osteoclasts: A model to decipher transmigration and matrix degradation. Eur. J. Cell Biol. 87 (8-9), 459–468. doi:10.1016/j.ejcb.2008.01.001
Sànchez-Riera, L., Wilson, N., Kamalaraj, N., Nolla, J. M., Kok, C., Li, Y., et al. (2010). Osteoporosis and fragility fractures. Best. Pract. Res. Clin. Rheumatol. 24 (6), 793–810. doi:10.1016/j.berh.2010.10.003
Sasaki, H., Takayama, K., Matsushita, T., Ishida, K., Kubo, S., Matsumoto, T., et al. (2012). Autophagy modulates osteoarthritis-related gene expression in human chondrocytes. Arthritis Rheum. 64 (6), 1920–1928. doi:10.1002/art.34323
Shapiro, I. M., Layfield, R., Lotz, M., Settembre, C., and Whitehouse, C. (2014). Boning up on autophagy: The role of autophagy in skeletal biology. Autophagy 10 (1), 7–19. doi:10.4161/auto.26679
Shi, Y., Liu, X. Y., Jiang, Y. P., Zhang, J. B., Zhang, Q. Y., Wang, N. N., et al. (2020). Monotropein attenuates oxidative stress via akt/mTOR-mediated autophagy in osteoblast cells. Biomed. Pharmacother. 121, 109566. doi:10.1016/j.biopha.2019.109566
Shin, Y. J., Han, S. H., Kim, D. S., Lee, G. H., Yoo, W. H., Kang, Y. M., et al. (2010). Autophagy induction and CHOP under-expression promotes survival of fibroblasts from rheumatoid arthritis patients under endoplasmic reticulum stress. Arthritis Res. Ther. 12 (1), R19. doi:10.1186/ar2921
Sims, N. A., and Gooi, J. H. (2008). Bone remodeling: Multiple cellular interactions required for coupling of bone formation and resorption. Semin. Cell Dev. Biol. 19 (5), 444–451. doi:10.1016/j.semcdb.2008.07.016
Singh, A., D'Amico, D., Andreux, P. A., Fouassier, A. M., Blanco-Bose, W., Evans, M., et al. (2022). Urolithin a improves muscle strength, exercise performance, and biomarkers of mitochondrial health in a randomized trial in middle-aged adults. Cell Rep. Med. 3 (5), 100633. doi:10.1016/j.xcrm.2022.100633
Song, C., Song, C., and Tong, F. (2014). Autophagy induction is a survival response against oxidative stress in bone marrow-derived mesenchymal stromal cells. Cytotherapy 16 (10), 1361–1370. doi:10.1016/j.jcyt.2014.04.006
Song, B. Q., Chi, Y., Li, X., Du, W. J., Han, Z. B., Tian, J. J., et al. (2015). Inhibition of notch signaling promotes the adipogenic differentiation of mesenchymal stem cells through autophagy activation and PTEN-PI3K/AKT/mTOR pathway. Cell. Physiol. biochem. 36 (5), 1991–2002. doi:10.1159/000430167
Song, S. J., Tao, J. J., Li, S. F., Qian, X. W., Niu, R. W., Wang, C., et al. (2020). 17Β-Estradiol attenuates rat articular chondrocyte injury by targeting ASIC1a-mediated apoptosis. Mol. Cell. Endocrinol. 505, 110742. doi:10.1016/j.mce.2020.110742
Stroikin, Y., Dalen, H., Brunk, U. T., and Terman, A. (2005). Testing the "garbage" accumulation theory of ageing: Mitotic activity protects cells from death induced by inhibition of autophagy. Biogerontology 6 (1), 39–47. doi:10.1007/s10522-004-7382-y
Su, J. W., Li, S. F., Tao, J. J., Xu, Y. Y., Wang, K., Qian, X. W., et al. (2021). Estrogen protects against acidosis-mediated articular chondrocyte injury by promoting ASIC1a protein degradation. Eur. J. Pharmacol. 908, 174381. doi:10.1016/j.ejphar.2021.174381
Sugiyama, T., Kim, Y. T., and Oda, H. (2015). Osteoporosis therapy: A novel insight from natural homeostatic system in the skeleton. Osteoporos. Int. 26 (2), 443–447. doi:10.1007/s00198-014-2923-y
Sun, X., Yang, X., Zhao, Y., Li, Y., and Guo, L. (2018). Effects of 17ß-estradiol on mitophagy in the murine Mc3T3-E1 osteoblast cell line is mediated via G protein-coupled estrogen receptor and the erk1/2 signaling pathway. Med. Sci. Monit. 24, 903–911. doi:10.12659/msm.908705
Tang, Y., Li, Y., Xin, D., Chen, L., Xiong, Z., and Yu, X. (2021a). Icariin alleviates osteoarthritis by regulating autophagy of chondrocytes by mediating PI3K/AKT/mTOR signaling. Bioengineered 12 (1), 2984–2999. doi:10.1080/21655979.2021.1943602
Tang, Y., Mo, Y., Xin, D., Xiong, Z., Zeng, L., Luo, G., et al. (2021b). Regulation of osteoblast autophagy based on PI3K/AKT/mTOR signaling pathway study on the effect of Β-ecdysterone on fracture healing. J. Orthop. Surg. Res. 16 (1), 719. doi:10.1186/s13018-021-02862-z
Tian, Z., Zhang, X., and Sun, M. (2021). Phytochemicals mediate autophagy against osteoarthritis by maintaining cartilage homeostasis. Front. Pharmacol. 12, 795058. doi:10.3389/fphar.2021.795058
Tong, W. W., Zhang, C., Hong, T., Liu, D. H., Wang, C., Li, J., et al. (2018). Silibinin alleviates inflammation and induces apoptosis in human rheumatoid arthritis fibroblast-like synoviocytes and has a therapeutic effect on arthritis in rats. Sci. Rep. 8 (1), 3241. doi:10.1038/s41598-018-21674-6
Ueno, T., and Komatsu, M. (2017). Autophagy in the liver: Functions in health and disease. Nat. Rev. Gastroenterol. Hepatol. 14 (3), 170–184. doi:10.1038/nrgastro.2016.185
van der Kraan, P. M. (2012). Osteoarthritis year 2012 in review: Biology. Osteoarthr. Cartil. 20 (12), 1447–1450. doi:10.1016/j.joca.2012.07.010
Vomero, M., Barbati, C., Colasanti, T., Perricone, C., Novelli, L., Ceccarelli, F., et al. (2018). Autophagy and rheumatoid arthritis: Current knowledges and future perspectives. Front. Immunol. 9, 1577. doi:10.3389/fimmu.2018.01577
Vuppalapati, K. K., Bouderlique, T., Newton, P. T., Kaminskyy, V. O., Wehtje, H., Ohlsson, C., et al. (2015). Targeted deletion of autophagy genes Atg5 or Atg7 in the chondrocytes promotes caspase-dependent cell death and leads to mild growth retardation. J. Bone Min. Res. 30 (12), 2249–2261. doi:10.1002/jbmr.2575
Wang, L., Fan, J., Lin, Y. S., Guo, Y. S., Gao, B., Shi, Q. Y., et al. (2015). Glucocorticoids induce autophagy in rat bone marrow mesenchymal stem cells. Mol. Med. Rep. 11 (4), 2711–2716. doi:10.3892/mmr.2014.3099
Wang, L., Heckmann, B. L., Yang, X., and Long, H. (2019). Osteoblast autophagy in glucocorticoid-induced osteoporosis. J. Cell. Physiol. 234 (4), 3207–3215. doi:10.1002/jcp.27335
Wang, S., Deng, Z., Ma, Y., Jin, J., Qi, F., Li, S., et al. (2020a). The role of autophagy and mitophagy in bone metabolic disorders. Int. J. Biol. Sci. 16 (14), 2675–2691. doi:10.7150/ijbs.46627
Wang, T., Liu, X., and He, C. (2020b). Glucocorticoid-induced autophagy and apoptosis in bone. Apoptosis 25 (3-4), 157–168. doi:10.1007/s10495-020-01599-0
Wang, X. Y., Gong, L. J., Huang, J. M., Jiang, C., and Yan, Z. Q. (2020c). Pinocembrin alleviates glucocorticoid-induced apoptosis by activating autophagy via suppressing the PI3K/Akt/mTOR pathway in osteocytes. Eur. J. Pharmacol. 880, 173212. doi:10.1016/j.ejphar.2020.173212
Wang, L., Klionsky, D. J., and Shen, H. M. (2022a). The emerging mechanisms and functions of microautophagy. Nat. Rev. Mol. Cell Biol. doi:10.1038/s41580-022-00529-z
Wang, A., Fang, S., Zhong, L., Lu, M., Zhou, H., Huang, W., et al. (2022b). Shikonin, a promising therapeutic drug for osteoarthritis that acts via autophagy activation. Int. Immunopharmacol. 106, 108563. doi:10.1016/j.intimp.2022.108563
Wang, N., Xu, P., Wu, R., Wang, X., Wang, Y., Shou, D., et al. (2021). Timosaponin BII improved osteoporosis caused by hyperglycemia through promoting autophagy of osteoblasts via suppressing the mTOR/NFκB signaling pathway. Free Radic. Biol. Med. 171, 112–123. doi:10.1016/j.freeradbiomed.2021.05.014
Wang, X. J., Tian, W., Xu, W. W., Lu, X., Zhang, Y. M., Li, L. J., et al. (2021). Loss of autophagy causes increased apoptosis of tibial plateau chondrocytes in Guinea pigs with spontaneous osteoarthritis. Cartilage 13, 796S–807S. doi:10.1177/19476035211044820
Weng, Y. M., Ke, C. R., Kong, J. Z., Chen, H., Hong, J. J., and Zhou, D. S. (2018). The significant role of ATG5 in the maintenance of normal functions of Mc3T3-E1 osteoblast. Eur. Rev. Med. Pharmacol. Sci. 22 (5), 1224–1232. doi:10.26355/eurrev_201803_14462
Wu, J. J., Quijano, C., Chen, E., Liu, H., Cao, L., Fergusson, M. M., et al. (2009). Mitochondrial dysfunction and oxidative stress mediate the physiological impairment induced by the disruption of autophagy. Aging (Albany NY) 1 (4), 425–437. doi:10.18632/aging.100038
Wu, Z., Zhang, X., Li, Z., Wen, Z., and Lin, Y. (2021). Activation of autophagy contributes to the protective effects of Lycopene against oxidative stress-induced apoptosis in rat chondrocytes. Phytother. Res. 35 (7), 4032–4045. doi:10.1002/ptr.7127
Xie, Y. Y., Li, Y., Zhou, R. P., Dai, B. B., Qian, Y. J., Wu, X. S., et al. (2018). Effects of autophagy on acid-sensing ion channel 1A-mediated apoptosis in rat articular chondrocytes. Mol. Cell. Biochem. 443 (1-2), 181–191. doi:10.1007/s11010-017-3223-6
Xie, C., Jiang, J., Liu, J., Yuan, G., and Zhao, Z. (2019). Triptolide suppresses human synoviocyte MH7A cells mobility and maintains redox balance by inhibiting autophagy. Biomed. Pharmacother. 115, 108911. doi:10.1016/j.biopha.2019.108911
Xu, G., Li, X., Zhu, Z., Wang, H., and Bai, X. (2021a). Iron overload induces apoptosis and cytoprotective autophagy regulated by ROS generation in mc3t3-E1 cells. Biol. Trace Elem. Res. 199 (10), 3781–3792. doi:10.1007/s12011-020-02508-x
Xu, H., Xia, M., Sun, L., Wang, H., and Zhang, W. B. (2021b). Osteocytes enhance osteogenesis by autophagy-mediated FGF23 secretion under mechanical tension. Front. Cell Dev. Biol. 9, 782736. doi:10.3389/fcell.2021.782736
Yan, J., Ni, B., Sheng, G., Zhang, Y., Xiao, Y., Ma, Y., et al. (2021). Rhoifolin ameliorates osteoarthritis via regulating autophagy. Front. Pharmacol. 12, 661072. doi:10.3389/fphar.2021.661072
Yang, G. E., Duan, X., Lin, D., Li, T., Luo, D., Wang, L., et al. (2015). Rapamycin-induced autophagy activity promotes bone fracture healing in rats. Exp. Ther. Med. 10 (4), 1327–1333. doi:10.3892/etm.2015.2660
Yang, R., Ouyang, Y., Li, W., Wang, P., Deng, H., Song, B., et al. (2016). Autophagy plays a protective role in tumor necrosis factor-α-induced apoptosis of bone marrow-derived mesenchymal stem cells. Stem Cells Dev. 25 (10), 788–797. doi:10.1089/scd.2015.0387
Yang, M., Wen, T., Chen, H., Deng, J., Yang, C., and Zhang, Z. (2018). Knockdown of insulin-like growth factor 1 exerts a protective effect on hypoxic injury of aged BM-MSCs: Role of autophagy. Stem Cell Res. Ther. 9 (1), 284. doi:10.1186/s13287-018-1028-5
Yang, L., Liu, S., Mu, S., Guo, R., Zhou, L., and Fu, Q. (2021). Paeoniflorin attenuates dexamethasone-induced apoptosis of osteoblast cells and promotes bone formation via regulating AKT/mTOR/Autophagy signaling pathway. Evid. Based. Complement. Altern. Med. 2021, 6623464. doi:10.1155/2021/6623464
Yang, C., Tao, H., Zhang, H., Xia, Y., Bai, J., Ge, G., et al. (2022). TET2 regulates osteoclastogenesis by modulating autophagy in OVX-induced bone loss. Autophagy, 1–13. doi:10.1080/15548627.2022.2048432
Yao, J., Liu, X., Sun, Y., Dong, X., Liu, L., and Gu, H. (2021). Curcumin-alleviated osteoarthritic progression in rats fed a high-fat diet by inhibiting apoptosis and activating autophagy via modulation of MicroRNA-34a. J. Inflamm. Res. 14, 2317–2331. doi:10.2147/JIR.S312139
Yin, Z., Pascual, C., and Klionsky, D. J. (2016). Autophagy: Machinery and regulation. Microb. Cell 3 (12), 588–596. doi:10.15698/mic2016.12.546
Yin, X., Zhou, C., Li, J., Liu, R., Shi, B., Yuan, Q., et al. (2019). Autophagy in bone homeostasis and the onset of osteoporosis. Bone Res. 7, 28. doi:10.1038/s41413-019-0058-7
Yoshida, G., Kawabata, T., Takamatsu, H., Saita, S., Nakamura, S., Nishikawa, K., et al. (2022). Degradation of the NOTCH intracellular domain by elevated autophagy in osteoblasts promotes osteoblast differentiation and alleviates osteoporosis. Autophagy 18, 2323–2332. doi:10.1080/15548627.2021.2017587
Yuan, K., Zhu, Q., Lu, Q., Jiang, H., Zhu, M., Li, X., et al. (2020). Quercetin alleviates rheumatoid arthritis by inhibiting neutrophil inflammatory activities. J. Nutr. Biochem. 84, 108454. doi:10.1016/j.jnutbio.2020.108454
Yue, C., Jin, H., Zhang, X., Li, W., Wang, D., Tong, P., et al. (2021). Aucubin prevents steroid-induced osteoblast apoptosis by enhancing autophagy via AMPK activation. J. Cell. Mol. Med. 25 (21), 10175–10184. doi:10.1111/jcmm.16954
Zada, S., Pham, T. M., Hwang, J. S., Ahmed, M., Lai, T. H., Elashkar, O., et al. (2021). Chlorogenic acid protects human chondrocyte C28/I2 cells from oxidative stress-induced cell death through activation of autophagy. Life Sci. 285, 119968. doi:10.1016/j.lfs.2021.119968
Zahm, A. M., Bohensky, J., Adams, C. S., Shapiro, I. M., and Srinivas, V. (2011). Bone cell autophagy is regulated by environmental factors. Cells Tissues Organs 194 (2-4), 274–278. doi:10.1159/000324647
Zaidi, M. (2007). Skeletal remodeling in health and disease. Nat. Med. 13 (7), 791–801. doi:10.1038/nm1593
Zhang, Q., Yang, Y. J., Wang, H., Dong, Q. T., Wang, T. J., Qian, H. Y., et al. (2012). Autophagy activation: A novel mechanism of atorvastatin to protect mesenchymal stem cells from hypoxia and serum deprivation via AMP-activated protein kinase/mammalian target of rapamycin pathway. Stem Cells Dev. 21 (8), 1321–1332. doi:10.1089/scd.2011.0684
Zhang, J., Song, X., Cao, W., Lu, J., Wang, X., Wang, G., et al. (2016). Autophagy and mitochondrial dysfunction in adjuvant-arthritis rats treatment with resveratrol. Sci. Rep. 6, 32928. doi:10.1038/srep32928
Zhang, Z., Lai, Q., Li, Y., Xu, C., Tang, X., Ci, J., et al. (2017). Acidic pH environment induces autophagy in osteoblasts. Sci. Rep. 7, 46161. doi:10.1038/srep46161
Zhang, B., Hou, R., Zou, Z., Luo, T., Zhang, Y., Wang, L., et al. (2018). Mechanically induced autophagy is associated with ATP metabolism and cellular viability in osteocytes in vitro. Redox Biol. 14, 492–498. doi:10.1016/j.redox.2017.10.021
Zhang, Q., Zhao, L., Shen, Y., He, Y., Cheng, G., Yin, M., et al. (2019). Curculigoside protects against excess-iron-induced bone loss by attenuating akt-FoxO1-dependent oxidative damage to mice and osteoblastic MC3T3-E1 cells. Oxid. Med. Cell. Longev. 2019, 9281481. doi:10.1155/2019/9281481
Zhang, X., Huang, F., Chen, X., Wu, X., and Zhu, J. (2020a). Ginsenoside Rg3 attenuates ovariectomy-induced osteoporosis via AMPK/mTOR signaling pathway. Drug Dev. Res. 81 (7), 875–884. doi:10.1002/ddr.21705
Zhang, Y., Cui, Y., Wang, L., and Han, J. (2020b). Autophagy promotes osteoclast podosome disassembly and cell motility athrough the interaction of Kindlin3 with LC3. Cell. Signal. 67, 109505. doi:10.1016/j.cellsig.2019.109505
Zhang, Y., Li, M., Liu, Z., and Fu, Q. (2021a). Arbutin ameliorates glucocorticoid-induced osteoporosis through activating autophagy in osteoblasts. Exp. Biol. Med. 246 (14), 1650–1659. doi:10.1177/15353702211002136
Zhang, Y., Wang, S., Chen, Y., Zhang, J., Yang, J., Xian, J., et al. (2021b). Fangchinoline inhibits human esophageal cancer by transactivating ATF4 to trigger both noxa-dependent intrinsic and DR5-dependent extrinsic apoptosis. Front. Oncol. 11, 666549. doi:10.3389/fonc.2021.666549
Zhang, S. L., Zhang, K. S., Wang, J. F., Wang, Y. C., Zhang, H., Tang, C., et al. (2022). Corresponding changes of autophagy-related genes and proteins in different stages of knee osteoarthritis: An animal model study. Orthop. Surg. 14 (3), 595–604. doi:10.1111/os.13057
Zhao, W., Zhang, W., Ma, H., and Yang, M. (2020). Nipa2 regulates osteoblast function by modulating mitophagy in type 2 diabetes osteoporosis. Sci. Rep. 10 (1), 3078. doi:10.1038/s41598-020-59743-4
Zhao, B., Peng, Q., Poon, E., Chen, F., Zhou, R., Shang, G., et al. (2021). Leonurine promotes the osteoblast differentiation of rat BMSCs by activation of autophagy via the PI3K/Akt/mTOR pathway. Front. Bioeng. Biotechnol. 9, 615191. doi:10.3389/fbioe.2021.615191
Zheng, X., Yu, Y., Shao, B., Gan, N., Chen, L., and Yang, D. (2019). Osthole improves therapy for osteoporosis through increasing autophagy of mesenchymal stem cells. Exp. Anim. 68 (4), 453–463. doi:10.1538/expanim.18-0178
Zheng, H., Feng, H., Zhang, W., Han, Y., and Zhao, W. (2020a). Targeting autophagy by natural product ursolic acid for prevention and treatment of osteoporosis. Toxicol. Appl. Pharmacol. 409, 115271. doi:10.1016/j.taap.2020.115271
Zheng, L. W., Wang, W. C., Mao, X. Z., Luo, Y. H., Tong, Z. Y., and Li, D. (2020b). TNF-α regulates the early development of avascular necrosis of the femoral head by mediating osteoblast autophagy and apoptosis via the P38 MAPK/NF-κB signaling pathway. Cell Biol. Int. 44 (9), 1881–1889. doi:10.1002/cbin.11394
Zhou, Q., Luo, D., Li, T., Liu, Z., Zou, W., Wang, L., et al. (2015). Bone fracture in a rat femoral fracture model is associated with the activation of autophagy. Exp. Ther. Med. 10 (5), 1675–1680. doi:10.3892/etm.2015.2752
Zhou, R., Zhu, F., Wu, X., Song, S., Chen, Y., Zhu, C., et al. (2019). Effects of autophagy on apoptosis of articular chondrocytes in adjuvant arthritis rats. J. Cell. Mol. Med. 23 (11), 7879–7884. doi:10.1111/jcmm.14629
Zhou, W., Shi, Y., Wang, H., Yu, C., Zhu, H., and Wu, A. (2021). Sinensetin reduces osteoarthritis pathology in the tert-butyl hydroperoxide-treated chondrocytes and the destabilization of the medial meniscus model mice via the AMPK/mTOR signaling pathway. Front. Pharmacol. 12, 713491. doi:10.3389/fphar.2021.713491
Zhu, M., Yuan, K., Lu, Q., Zhu, Q., Zhang, S., Li, X., et al. (2019a). Emodin ameliorates rheumatoid arthritis by promoting neutrophil apoptosis and inhibiting neutrophil extracellular trap formation. Mol. Immunol. 112, 188–197. doi:10.1016/j.molimm.2019.05.010
Zhu, W., Ding, W., Shang, X., Zhu, D., and Dai, X. (2019b). Fangchinoline promotes autophagy and inhibits apoptosis in osteoporotic rats. Med. Sci. Monit. 25, 324–332. doi:10.12659/MSM.912624
Zhu, W. Q., Wu, H. Y., Sun, Z. H., Guo, Y., Ge, T. T., Li, B. J., et al. (2022). Current evidence and future directions of berberine intervention in depression. Front. Pharmacol. 13, 824420. doi:10.3389/fphar.2022.824420
Glossary
OP osteoporosis
OA osteoarthritis
RA rheumatoid arthritis
CMA chaperone-mediated autophagy
PI3K phosphoinositide3 kinase
Akt protein kinase B
AMPK 5ʹ AMP-activated protein kinase
mTOR mammalian target of rapamycin
mTORC mammalian target of rapamycin complex
ULK1 UNC-51-like kinase
FIP200 FAK family kinase-interacting protein of 200 kDa
Vps vacuolar protein sorting
Atg autophagy-related protein
LC3 microtubule-associated protein one light chain three
PE phosphatidylethanolamine
BMSCs bone marrow mesenchymal stem cells
ROS oxygen species
ER endoplasmic reticulum
TNF-α tumor necrosis factor-alpha
ATP adenosine triphosphate
FGF23 fibroblast growth factor-23
ECM extracellular matrix
PC2 type II procollagen
CTSK cathepsin K
MMM9 matrix metalloproteinase nine
TRAP tartrate-resistant acidic phosphatase
NF-κB nuclear factor-κB
RANK receptor activator of NF-κB
RANKL receptor activator of NF-κB ligand
NFATc1 nuclear factor of activated T-cell c1
TRPV4 transient receptor potential vanilloid four
P2X7R P2X7 receptor
TRAF6 tumor necrosis factor receptor-associated factor 6
BCL2 B-cell lymphoma two
TET2 tet methylcytosine dioxygenase 2
KLF2 kruppel-like factor 2
LAMP2A lysosome-associated membrane protein type 2A
PINK1 PTEN-induced putative kinase one
OVX ovariectomy
FOXO1 Forkhead box O1
IL interleukin
JNK c-Jun amino-terminal kinase
FLSs fibroblast-like synoviocytes
AA adjuvant arthritis
Th17 T helper lymphocyte 17
Treg regulatory T lymphocyte
NETs neutrophil extracellular traps
ERK extracellular signal-regulated kinases
PINK1 PTEN-induced putative kinase one
LAMP lysosomal associated membrane protein
Keywords: autophagy, natural products, bone metabolism, osteoporosis, rheumatoid arthritis, osteoarthritis, fracture nonunion/delayed union
Citation: Li Z, Li D, Su H, Xue H, Tan G and Xu Z (2022) Autophagy: An important target for natural products in the treatment of bone metabolic diseases. Front. Pharmacol. 13:999017. doi: 10.3389/fphar.2022.999017
Received: 20 July 2022; Accepted: 08 November 2022;
Published: 18 November 2022.
Edited by:
Xiaofeng Zhu, Jinan University, ChinaReviewed by:
Dipranjan Laha, National Institutes of Health (NIH), United StatesManjinder Singh, Chitkara University, India
Takuma Matsubara, Kyushu Dental University, Japan
Copyright © 2022 Li, Li, Su, Xue, Tan and Xu. This is an open-access article distributed under the terms of the Creative Commons Attribution License (CC BY). The use, distribution or reproduction in other forums is permitted, provided the original author(s) and the copyright owner(s) are credited and that the original publication in this journal is cited, in accordance with accepted academic practice. No use, distribution or reproduction is permitted which does not comply with these terms.
*Correspondence: Guoqing Tan, eXhrbXRAaG90bWFpbC5jb20=; Zhanwang Xu, eHp3NjAwMUAxNjMuY29t