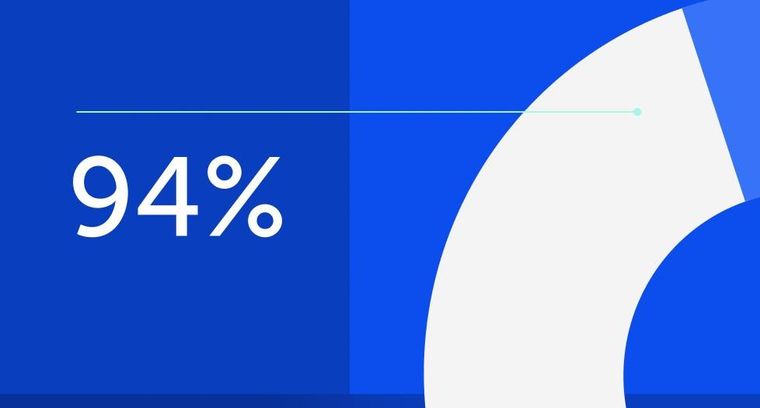
94% of researchers rate our articles as excellent or good
Learn more about the work of our research integrity team to safeguard the quality of each article we publish.
Find out more
REVIEW article
Front. Pharmacol., 27 September 2022
Sec. Inflammation Pharmacology
Volume 13 - 2022 | https://doi.org/10.3389/fphar.2022.997403
This article is part of the Research TopicStreaming Inflammation: From Damage to Healing and Resilience - Volume IIView all 11 articles
Polyunsaturated fatty acids (PUFAs) are structural components of membrane phospholipids in cells. PUFAs regulate cellular function through the formation of derived lipid mediators termed eicosanoids. The oxygenation of 20-carbon PUFAs via the oxygenases cyclooxygenases, lipoxygenases, or cytochrome P450, generates a class of classical eicosanoids including prostaglandins, thromboxanes and leukotrienes, and also the more recently identified hydroxy-, hydroperoxy-, epoxy- and oxo-eicosanoids, and the specialized pro-resolving (lipid) mediators. These eicosanoids play a critical role in the regulation of inflammation in the blood and the vessel. While arachidonic acid-derived eicosanoids are extensively studied due to their pro-inflammatory effects and therefore involvement in the pathogenesis of inflammatory diseases such as atherosclerosis, diabetes mellitus, hypertension, and the coronavirus disease 2019; in recent years, several eicosanoids have been reported to attenuate exacerbated inflammatory responses and participate in the resolution of inflammation. This review focused on elucidating the biosynthesis and the mechanistic signaling of eicosanoids in inflammation, as well as the pro-inflammatory and anti-inflammatory effects of these eicosanoids in the blood and the vascular wall.
Eicosanoids are a family of fatty acid metabolites generated from 20-carbon polyunsaturated fatty acids (PUFAs) synthesized by enzymatic oxygenation pathways involving a distinct family of enzymes, the oxygenases (Khanapure et al., 2007). Eicosanoids are not stored, but promptly synthesized de novo after cell activation (Bozza et al., 2011) through a highly regulated event, primarily involving three oxygenases: cyclooxygenases (COXs), P450 cytochrome epoxygenases (CYP450), and lipoxygenases (LOXs) (Alvarez and Lorenzetti, 2021). The formed eicosanoids function to regulate a physiological response, including tissue homeostasis, pain, host defense, and inflammation (Esser-von Bieren, 2019). Due to the observed critical role of eicosanoids in physiological and pathological inflammation, they have been implicated in the pathogenesis of major diseases including cardiovascular disease, diabetes mellitus, hypertension, and more recently, the coronavirus disease 2019 (COVID-19) (Wang and Dubois, 2010; Fava and Bonafini, 2018; Hammock et al., 2020; Bosma et al., 2022). Although eicosanoids are usually associated with pro-inflammatory responses (Aoki and Narumiya, 2012), they are also known to play a key role in reducing inflammation by promoting the resolution of inflammation (Pan et al., 2022), limiting immune cell infiltration, and initiating tissue repair mechanisms (Serhan and Levy, 2018; Díaz Del Campo et al., 2022). This review focuses on the role of eicosanoids on inflammation in the blood and the vascular wall and discusses key discoveries related to the regulatory mechanism of these lipid mediators in inflammation in the blood vessel.
The superclass of eicosanoids expressed in the blood includes classical eicosanoids, prostaglandins (PGs), leukotrienes (LTs), and thromboxanes (Txs), and the more recently discovered, specialized pro-resolving (lipid) mediators (SPMs), as well as hydroxy-, hydroperoxy-, epoxy-, and oxoeicosanoids (Fahy et al., 2009). The SPMs include lipoxins (LXs), resolvins, protectins (PD), their aspirin-triggered (AT) isomers, and maresins (MaR) (Chiang and Serhan, 2020). One of the best-studied classes of these lipid mediators are the eicosanoids derived from the 20-carbon PUFAs such as eicosapentaenoic acid (EPA; 20:5 ω-3), dihomo-γ-linolenic acid (DGLA; 20:3 ω-6), and arachidonic acid (AA; 20:4 ω-6) (Astarita et al., 2015) (Figure 1), with the last being the most abundant PUFA in the phospholipid of human cell membranes (Sonnweber et al., 2018). Regarding the newly discovered SPMs, LXs are generated from AA, E-series resolvins (RvEs) are synthesized from EPA, and D-series resolvins (RvDs), PDs, and MaRs are formed from docosahexaenoic acid (DHA; 22:6 ω-3) (Calder, 2020a; Chiang and Serhan, 2020) (Figure 2). The initial event of eicosanoid biosynthesis consists of cellular activation which leads to an increased influx of calcium; and subsequently, the translocation of cytoplasmic phospholipase A2 (cPLA2) to the membrane resulting in the cleavage of the PUFA from the sn-2 position of the glycerophospholipid (Dennis et al., 2011) to be further oxygenated by respective enzymes. Eicosanoid biosynthesis differs between the type of PUFA being oxidized and the enzymes metabolizing those PUFAs. The freed PUFAs can be oxygenated by several enzymes including COXs, LOXs and CYP450s (Hajeyah et al., 2020) (Figure 1). An alternative biosynthesis pathway forming the AT-isomers can be triggered by aspirin. For example, in the presence of aspirin, AA and EPA form 15(R)-HpETE and 18(R)-HpEPE, respectively (Calder, 2020a) (Figure 2).
FIGURE 1. Eicosanoid biosynthesis in the blood and the vessel upon an inflammatory stimulus. AA: arachidonic acid; COX-1: cyclooxygenase-1; COX-2: cyclooxygenase-2; cPLA2: cytoplasmic phospholipase A2; CYP450: P450 cytochrome epoxygenase; CysLTs: cysteinyl leukotrienes; DGLA: dihomo-γ-linolenic acid; EPA: eicosapentaenoic acid; LTA4: leukotriene A4; LTB4: leukotriene B4; LTC4: leukotriene C4; LTD4: leukotriene D4; LTE4: leukotriene E4; PGD1: prostaglandin D1; PGD2: prostaglandin D2; PGD3: prostaglandin D3; PGE1: prostaglandin E1; PGE2: prostaglandin E2; PGE3: prostaglandin E3; PGI2: prostaglandin I2 or prostacyclin; PGI3: prostaglandin I3; TxA1: thromboxane A1; TxA2: thromboxane A2; TxA3: thromboxane A3; 5(S)-HEPE: 5(S)-hydroxyeicosapentaenoic acid; 5(S)-HpEPE: 5(S)-hydroperoxyeicosapentaenoic acid; 5(S)-HETE: 5(S)-hydroxyeicosatetraenoic acid; 5(S)-HpETE: 5(S)-hydroperoxyeicosatetraenoic acid; 5-LOX: 5-lipoxygenase; 5,6-EET: 5,6-epoxyeicosatrienoic acid; 8,9-EET: 8,9-epoxyeicosatrienoic acid; 8,9-EETeTr: 8,9-epoxyeicosatetraenoic acid; 11,12-EET: 11,12-epoxyeicosatrienoic acid; 11,12-EETeTr: 11,12-epoxyeicosatetraenoic acid; 12(S)-HETE: 12(S)-hydroxyeicosatetraenoic acid; 12(S)-HpETE: 12(S)-hydroperoxyeicosatetraenoic acid; 12(S)-HETE: 12(S)-hydroxyeicosatetraenoic acid; 12(S)-HpETE: 12(S)-hydroperoxyeicosatetraenoic acid; 12(S)-HETrE: 12(S)-hydroxyeicosatrienoic acid; 12(S)-HpETrE: 12(S)-hydroperoxyeicosatrienoic acid; 12-LOX: 12-lipoxygenase; 14,15-EET: 14,15-epoxyeicosatrienoic acid; 14,15-EETeTr: 14,15-epoxyeicosatetraenoic acid; 15-LOX: 15-lipoxygenase; 15(S)-HETE: 15(S)-hydroxyeicosatetraenoic acid; 15(S)-HpETE: 15(S)-hydroperoxyeicosatetraenoic acid; 15(S)-HETE: 15(S)-hydroxyeicosatetraenoic acid; 15(S)-HpETE: 15(S)-hydroperoxyeicosatetraenoic acid; 15(S)-HETrE: 15(S)-hydroxyeicosatrienoic acid; 15(S)-HpETrE: 15(S)-hydroperoxyeicosatrienoic acid; 17,18-EETeTr: 17,18-epoxyeicosatetraenoic acid; 18(R)HpEPE: 18(R)-hydroperoxyeicosapentaenoic acid; 18(R)-HEPE: 18(R)-hydroxyeicosapentaenoic acid; 19-HEPE: 19-hydroxyeicosapentaenoic acid; 20-HEPE: 20-hydroxyeicosapentaenoic acid; 20-HETE: 20-hydroxyeicosatetraenoic acid.
FIGURE 2. Transcellular biosynthesis of eicosanoids in the blood and the vascular wall. AA: arachidonic acid; COX-2: cyclooxygenase-2; CYP450: P450 cytochrome epoxygenase; DHA: docosahexaenoic acid; EPA: eicosapentaenoic acid; LTA4: leukotriene A4; LXs: lipoxins; LXA4: lipoxin A4; LXB4: lipoxin B4; MaR1: maresin 1; PD1/NPD1: protectin D1/neuroprotectin D1; RvD1-2: resolvin D1-2; RvD3-4: resolvin D3-4; RvD5: resolvin D5; RvD6: resolvin D6; RvE1: resolvin E1; RvE2: resolvin E2; RvE3: resolvin E3; RvE4: resolvin E4; 5-LOX: 5-lipoxygenase; 12(S)-LOX: 12(S)-lipoxygenase; 14(S)-HpDHA: 14(S)-hydroperoxydocosahexaenoic acid; 15-LOX: 15-lipoxygenase; 15(S)-HEPE: 15(S)-hydroxyeicosapentaenoic acid; 15(S)-HpEPE: 15(S)-hydroperoxyeicosapentaenoic acid; 15(S)-HETE: 15(S)-hydroxyeicosatetraenoic acid; 15(S)-HpETE: 15(S)-hydroperoxyeicosatetraenoic acid; 15-epi-LXs: 15-epi-lipoxins; 17(S)-HDHA: 17(S)-hydroxydocosahexaenoic acid; 17(S)-HpDHA: 17(S)-hydroperoxydocosahexaenoic acid; 18(R)-HEPE: 18(R)-hydroxyeicosapentaenoic acid; 18(R)-HpEPE: 18(R)-hydroperoxyeicosapentaenoic acid.
Cyclooxygenases are a widely distributed enzyme in mammalian tissues and exist in two isoforms, COX-1 and COX-2 (Vane et al., 1998). The activation of COX leads to the generation of PGs and Txs, which are collectively named as prostanoids. COX oxygenates AA into series 2 PGs (PGD2, PGE2, PGI2, and TxA2) (Figure 1). Series 1 PGs (PGD1, PGE1, and TxA1) and series 3 PGs (PGD3, PGE3, PGI3, and TxA3,) are produced from the oxygenation of DGLA and EPA, respectively (Lagarde et al., 2013; Sergeant et al., 2016) (Figure 1).
In patients taking aspirin, COX-2 is involved in the formation of LXs and RvEs through transcellular biosynthesis (Figure 2). In endothelial cells, aspirin causes an irreversible acetylation of COX-2, which oxygenates AA to form 15(R)-hydroxyeicosatetraenoic acid (15(R)-HETE) and EPA to form 18(R)-hydroxyeicosapentaenoic acid (18(R)-HEPE). While 15(R)-HETE is further used by adherent leukocyte and other endothelial cells to form the 15-epimeric-LXs (15-epi-LXs) (Fu et al., 2020), 18(R)-HEPE is further metabolized into RvE1 and RvE2. It is important to mention that 18(R)-HEPE can also be formed through oxygenation of EPA by CYP450s (Serhan and Petasis, 2011).
Lipoxygenases are a family of nonheme iron-containing enzymes (Kuhn et al., 2015) which are categorized accordingly to their positional specificity of AA oxygenation: 5-LOX, 12-LOX, and 15-LOX. LOX isozymes are further characterized by tissue expression and stereospecificity (S or R), such as the platelet-type 12-(S)-LOX and the epithelial 12-(R)-LOX (Brash, 1999), as an example. Regarding the expression of LOXs in the blood, 12(S)-LOX is only expressed in platelets and 15-LOX-1 is expressed in eosinophils, monocytes, macrophages, and reticulocytes (Jiang et al., 2006). The expression of 5-LOX is found in myeloid cells including neutrophils, macrophages, monocytes and basophils (Yeung et al., 2017).
The LOXs are able to oxygenate AA to form hydroperoxyeicosatetraenoic acids (HpETEs) (Figure 1), which are rapidly converted to hydroxy derivative HETEs in the blood (Tourdot and Holinstat, 2017). In a similar manner, the LOX-derived eicosanoids from DGLA and EPA are converted to hydroperoxyeicosatrienoic acids (HpETrEs) and hydroperoxyeicosapentaenoic acids (HpEPEs), which are further hydrolyzed to hydroxyeicosatrienoic acids (HETrEs) and hydroxyeicosapentaenoic acids (HEPEs), respectively (Yeung et al., 2017). 5-LOX is best known for its ability to produce LTs (Figure 1). The oxygenation of AA by 5-LOX generates 5(S)-HpETE, which is further converted to the unstable leukotriene A4 (LTA4). This intermediate eicosanoid is either converted to the leukotriene B4 (LTB4) or leukotriene C4 (LTC4) in cells that possess LTC4 synthase activity, such as platelets and endothelial cells, and sequential degradation of the LTC4 by peptidases forms LTD4 and LTE4 (Figure 1). These three products, LTC4, LTD4, and LTE4, are collectively named cysteinyl LTs (cysLTs). The production of cysLTs appears to be restricted to leukocytes, including eosinophils, basophils, and macrophages. However, under inflammatory stimulus, transcellular activity can result in cysLTs formation in endothelial cells (Feinmark and Cannon, 1986). This mechanism favors cells unable to produce LTA4, such as vascular endothelial cells, platelets and blood peripheral monocytes, to use LTA4 generated from surrounding cells (such as leukocytes) to produce LTC4 and the other cysLTs (Colazzo et al., 2017). LTA4 can also be used by other cells in the blood to form the LXs. Lipoxins A (LXA4) and B (LXB4) are formed through a transcellular mechanism between polymorphonuclear leukocytes (PMNs) (5-LOX) and platelets (12(S)-LOX) (Recchiuti and Serhan, 2012). In addition to the transcellular mechanism, lipoxins are synthesized from AA via 15-LOX in neutrophils and monocytes. In these cells, AA is converted to 15(S)-hydroperoxyeicosatetraenoic acid (15(S)-HpETE), which is subsequently converted to lipoxins A and B (Chandrasekharan and Sharma-Walia, 2015) (Figure 2).
The LOXs are also involved in the biosynthesis of others SPMs derived from DHA and EPA. The D-series resolvins 1–6 (RvD1-RvD6) are SPMs derived from the DHA-derived 17(S)-hydroperoxydocosahexaenoic acid (17(S)-HpDHA), which is synthesized through the oxygenation of DHA by 5-LOX in PMNs and macrophages (Serhan and Levy, 2018) (Figure 2). DHA is also a precursor for the maresins (MaR) and protectins. Maresin 1 (MaR1) is generated from the precursor DHA-derived 14(S)-hydroperoxydocosahexaenoic acid (14(S)-HpDHA) and its biosynthesis was first described in human macrophages via 12-LOX-mediated biosynthesis (Serhan et al., 2009). MaR1 is also synthesized during platelet-PMN interactions (Serhan and Levy, 2018). In leukocytes, the biosynthesis of the protectin D1/neuroprotectin D1 (PD1/NPD1) has the DHA-derived 17(S)-HpDHA as the intermediate precursor and it occurs via a 15-LOX-mediated pathway (Serhan et al., 2015; Chiang and Serhan, 2020) (Figure 2). Also in leukocytes, aspirin triggers the biosynthesis of the DHA-derived aspirin-triggered neuroprotection D1/protectin D1 [AT-(NPD1/PD1)] (Serhan et al., 2011a; Serhan et al., 2015). The E-series resolvins 1–4 (RvE1-4) are generated from a common precursor, the EPA-derived 18(R)-hydroxyeicosapentaenoic acid (18(R)-HEPE) (Figure 2). RvE1 and RvE2 are synthesized by PMNs via the 5-LOX pathway, whereas RvE3 is synthesized by eosinophils via the 12/15-LOX pathways (Serhan and Petasis, 2011; Isobe et al., 2012). Currently, the synthesis of RvE4 has only been shown in in vitro studies using purified recombinant human 5-LOX and 15-LOX (Serhan and Petasis, 2011; Libreros et al., 2020).
CYP450s belong to a family of heme-containing monooxygenases (Cook et al., 2016) that are known for their role in the metabolism of eicosanoids from PUFAs (Zhao et al., 2021). CYP epoxygenase metabolizes AA into epoxyeicosatrienoic acids (EETs) (Figure 1). Four regioisomeric cis-epoxyeicosatrienoic acids have been described: 5.6-, 8.9-, 11.12-, and 14,15-EET. Upon hydration by soluble epoxide hydrolase (sEH), EETs are rapidly converted to more stable and less biologically active metabolites, dihydroxyeicosatrienoic acids (DHETs) (Spiecker and Liao, 2005). Additionally, members of the CYP4A and CYP4F subfamilies also oxygenate AA to produce 20-hydroxyeicosatetraenoic acid (20-HETE) (Arnold et al., 2010) (Figure 1), which undergoes additional oxidation to 20-hydroxy-prostaglandin G2 and H2 (Schwartzman et al., 1989; Kaduce et al., 2004; Hoxha and Zappacosta, 2020). EPA can also be a substrate for CYP450 catalysis. The major CYP450-dependent metabolites derived from EPA include epoxyeicosatetraenoic acids (EETeTrs, 5.6-, 8.9-, 11.12-, and 14,15-EETeTrs), 19- and 20-hydroxyeicosapentaenoic acids (19- and 20-HEPE) (Figure 1).
PGs exert their biological effects in the blood in an autocrine and paracrine manner by activating their respective cell surface G protein-coupled receptors (GPCRs) (Ricciotti and FitzGerald, 2011; Biringer, 2021). There are at least eight known prostanoid receptor subfamilies in the blood and the vascular wall (Funk, 2001) (Table 1). Four of the receptor subtypes bind PGE2, E prostanoid receptor (EP) 1, EP2, EP3, and EP4 in platelets and vascular smooth muscle cells (VSMCs) and two bind PGD2 (DP1 and DP2) (Aoki and Narumiya, 2012). While PGF2α binds to FP, the PGI2 and TxA2 receptors are known as IP and TP, respectively (Wang and Dubois, 2010). The IP is expressed in the endothelium, VSMCs and platelets. There are two isoforms of human TP (TPα, TPβ) in platelets, vascular smooth muscle cells, and macrophages, and FP (FPA, FPB) in VSMCs (Ricciotti and FitzGerald, 2011; Gilroy and Bishop-Bailey, 2019). DP2 is also known as a chemoattractant receptor-homologous molecule (CRTH/DP2) expressed in T helper 2 cells, that responds to PGD2 but belongs to the family of chemokine receptors (Ricciotti and FitzGerald, 2011; Aoki and Narumiya, 2012).
The prostanoid receptors couple to a range of intracellular signaling pathways that mediate the effects of receptor activation in the cell (Table 1). While EP2, EP4, IP, and DP1 receptors activate adenylyl cyclase (AC) via Gαs, increasing intracellular cyclic adenosine monophosphate (cAMP) and protein kinase A (PKA) activity, EP1, FP, and TP activate phosphatidylinositol metabolism via Gαq, leading to the formation of inositol triphosphate (IP3) via the mobilization of intracellular free calcium (Ca2+) (Huang et al., 2004a). In addition to signaling through Gαq, the FP and TP receptors couples to the small G-protein Rho via a Gα12/13-dependent mechanism (Ricciotti and FitzGerald, 2011). EP3 isoforms can couple via Gαi or Gα12 to elevate intracellular Ca2+, inhibit cAMP generation, and activate Rho (Ricciotti and FitzGerald, 2011). The DP2 couples to a Gαi to inhibit cAMP synthesis and increase intracellular Ca2+ (Schuligoi et al., 2010).
There are four known LT receptors subfamilies (Table 1). Two GPCRs are known to be associated with LTB4, leukotriene B4 receptor (BLT) BLT1 and BLT2. While BLT1 is known to be expressed on a number of blood cells including leukocytes (Yokomizo et al., 1997), eosinophils (Tager et al., 2000), cluster of differentiation (CD) 4+ and CD8+ effector T cells (Goodarzi et al., 2003; Tager et al., 2003), dendritic cells (Toda et al., 2010) and macrophages (Serezani et al., 2011), BLT2 is expressed ubiquitously in leukocytes, with high expression in mononuclear cells, such as CD8+ and CD4+ T-cells, and CD14+ monocytes (Toda et al., 2002). In leukocytes, BLT1 is coupled to the pertussis toxin-sensitive G protein (Gαi) and its activation by LTB4 promotes Ca2+ mobilization, leukocyte chemotactic migration and lysosomal release (Goldman et al., 1985). In monocytes, both BLT1 and BLT2 have been reported to couple to Gαi to induce phosphorylation of mitogen-activated protein kinases (MAPKs) and PI3K/Akt (phosphatidylinositol 3-kinase/protein kinase B, Akt is also known as protein kinase B (PKB)), and nuclear factor-κB (NF-κB) activation (Sánchez-Galán et al., 2009). However, in human umbilical vein endothelial cells (HUVECs) LTB4 increases HUVEC adhesiveness for polymorphonuclear neutrophils (PMNs) through the increase of intracellular Ca2+, but it does not depend on pertussis toxin-sensitive G proteins (Palmblad et al., 1994). The BLT2 receptor is considered to be a receptor for several oxidized fatty acids, including 12-hydroxyheptadecatrienoic acid (12-HHT) and hydroxyeicosatetraenoic acids (HETEs) (Yokomizo et al., 2000) and in the blood vessel, BLT2 is expressed in endothelial cells (Yokomizo et al., 2018).
CysLTs regulate cell function through the cysteinyl leukotriene receptors CysLT1 and CysLT2 (Funk, 2001) (Table 1). CysLT1 is known as a high-affinity receptor for LTD4, whereas CysLT2 has similar affinity to LTC4 and LTD4 (Woszczek et al., 2007). Duah et al. (Duah et al., 2013) has demonstrated that while CysLT1 activation elicits proliferation of endothelial cells via extracellular signal-regulated kinase (ERK) phosphorylation, activation of CysLT2 increases intracellular Ca2+ and leads to endothelial cell contraction and barrier disruption via the Rho kinase pathway. Moreover, the in vitro activation of CysLT1 by LTD4 in monocyte/macrophage U937 cells produces second intracellular messengers through phospholipase Cβ (Crooke et al., 1989). LTD4 induces Ca2+ response via the pertussis toxin-sensitive G protein (Gαi/o) in these cells (Pollock and Creba, 1990; Capra, 2004). In addition, LTC4 has been shown to activate CysLT2 in mouse platelets ex vivo to induce α-granule and TxA2 secretion (Capra et al., 2003; Cummings et al., 2013). In endothelial cells, CysLT2 couples to Gαq/11 to activates PLCβ and IP3 signaling, and increase intracellular Ca2+ release, in response to interferon-γ (IFN-γ) stimulation in vitro (Woszczek et al., 2007).
The CYP-derived epoxyeicosatrienoic acids (EETs) activates peroxisome proliferator-activated receptor γ (PPARγ) in endothelial cells in the presence of an epoxide hydrolase-specific inhibitor (Liu et al., 2005). Additionally, EETs inhibit the NF-κB activation and attenuate the NF-κB-dependent inflammatory responses by reducing cytokine-induced leukocyte adhesion to the vasculature (Node et al., 1999). Some vascular-related actions of the EETs include the activation of the signal transducer and activator of transcription 3 (STAT3) (Table 1). Specifically, 14,15-EET stimulates the tyrosine phosphorylation of STAT3 and its translocation from the cytoplasm to the nucleus to bind to vascular endothelial growth factor (VEGF) promoter in a Src-STAT3 activation signaling-dependent manner, which leads to VEGF expression and angiogenesis (Cheranov et al., 2008).
Hydroxyeicosanoids are known to activate cells through a number of mechanisms including activation of GPCRs. The ω-6-derived 12(S)-HETrE inhibits platelet function through selectively binding to the Gαs-coupled prostacyclin receptor and activates a PKA-dependent signaling pathway (Tourdot et al., 2017) (Table 1). More recently, Cebo et al. has suggested that 12(S)-HETrE promotes C-X-C chemokine receptor type 7 (ACKR3, also known as CXCR7) ligation coordinated with IP to trigger the cAMP-PKA signaling pathway. Enhanced platelet expression of the chemokine receptor ACKR3/CXCR7 has been reported in coronary artery disease patients with reduced platelet aggregation (Cebo et al., 2022).
The eicosanoid 12(S)-HETE acts through binding to the G-coupled protein receptor 31 (GPR31) in platelets and human umbilical vein endothelial cells (HUVECs) (Van Doren et al., 2021) (Table 1). In platelets, 12(S)-HETE-GPR31 signals through Gαi to induce platelet activation and thrombosis. Activation of the GPR31 inhibits AC activity and results in Ras-related protein 1 (Rap1) and p38 activation (Van Doren et al., 2019). 20-HETE affects vascular function by binding to G-protein coupled receptor 75 (GPR75) coupled to Gαq/11 in endothelial cells which results in PLC-IP3-mediated increases in intracellular Ca2+ (Garcia et al., 2017), activation of the Rho kinase (Randriamboavonjy et al., 2003) and the mitogen activated protein (MAP) kinase pathways (Muthalif et al., 2000) (Table 1). Additionally, studies have demonstrated that 20-HETE stimulates the production of inflammatory cytokines, including interleukin-8 (IL-8), IL-13, IL-4, and PGE2, in endothelial cells via activation of NF-kB and MAPK/ERK signaling pathways (Ishizuka et al., 2008), resulting in endothelial cell activation and endothelial dysfunction (Singh et al., 2007). In addition to regulation of the cells through activation of GPCRs, hydroxyeicosanoids, such as 11(S)-HpDPAω-6 and 14(S)-HpDPAω-6 selectively activate PPARα in platelets ex vivo which results in inhibition of PKC activity and reduction in Ca2+ mobilization (Yeung et al., 2020) (Table 1).
Recent studies have shown that the SPMs also exert their effects in the blood to regulate inflammation through GPCRs (Table 1). These receptors are typically able to interact with more than one SPM and conversely some SPMs are able to interact with several receptors, leading to some overlapping downstream signals and pathways. RvE1 binds to BLT1 on neutrophils (Arita et al., 2007), to the chemokine-like receptor 1 (ChemR23) and to the resolvin E1 receptor (ERV1) on monocyte/macrophages (Freire et al., 2017), platelets (Fredman et al., 2010), neutrophils (Chiang and Serhan, 2020), and VSMCs (Ho et al., 2010). The activation of BLT1 by RvE1 induces phosphorylation of the ribosomal protein S6 (rS6) in neutrophils (Freire et al., 2017), as well as RvE1 activation of ERV1/ChemR23, which results in phosphorylation of Akt and rS6 to enhance phagocytosis by human macrophages (Ohira et al., 2010). Additionally, treatment of HEK-ChemR23 cells with pertussis toxin inhibited RvE1-dependent ERK activation (Serhan et al., 2011b). Although it was shown in HEK cells, the pertussis toxin-sensitive G protein (Gαi/o)-dependent pathway has already been shown to be activated by LTD4 in macrophages, suggesting that ChemR23 might couple to a Gαi/o to activate intracellular signaling in cells in the blood.
Regarding the D-series resolvins, while in human VSMCs RvD1 binds to the formyl peptide receptor 2 (ALX/FPR2) (also known as LXA4 receptor) (Ho et al., 2010), studies have suggested that RvD1 may interact with two GPCRs the ALX/FPR2 and the G-protein coupled receptor 32 (GPR32) in leukocytes and platelets (Krishnamoorthy et al., 2010; Lannan et al., 2017). Notably, lipoxins have been found to interact with the same receptors as RvD1, the ALX/FPR2 and GPR32 receptors (Chandrasekharan and Sharma-Walia, 2015). Recently, RvD2 was shown to bind to the G protein-coupled receptor 18 (GRP18) in leukocytes, including PMN, monocytes, and macrophages (Chiang et al., 2015). In macrophages, activation of GRP18 by RvD2 leads to cAMP release and phosphorylation of select kinases and transcription factors, such as cAMP-response element binding protein (CREB) and STAT3 (Chiang and Serhan, 2020). The RvD5 was described to activate the RvD1 receptor GPR32 in leukocytes and macrophages to reduce the expression of NF-κB (Chiang et al., 2012) (Table 1).
The MaR1 activates the leucine-rich repeat-containing G protein-coupled receptor 6 (LGR6) in neutrophils and macrophages/monocytes to increase the phosphorylation of CREB and ERK (Chiang et al., 2019; Chiang and Serhan, 2020). Moreover, studies have shown that MaR1 suppresses NF-κB activation in VSMC and vascular endothelial cells in vitro (Chatterjee et al., 2014; Akagi et al., 2015). PD1/NPD1 binds to the G protein-coupled receptor 37 (GPR37) to increase intracellular Ca2+ in macrophages (Chiang and Serhan, 2020) (Table 1).
PGs play a key role in the generation of the inflammatory response (Ricciotti and FitzGerald, 2011). They are ubiquitously produced and act as autocrine and paracrine lipid mediators to maintain local hemostasis in the body (Funk, 2001). While PG production is generally very low in uninflamed tissues, it increases immediately in acute inflammation before the recruitment of leukocytes and the infiltration of immune cells (Ricciotti and FitzGerald, 2011). In the blood vessel, one member of the PG family, PGE2, is synthesized mainly by platelets and macrophages (Cook, 2005). PGE2 has vasodilation effects and increases the permeability of postcapillary venules, early events in the inflammatory response (Funk, 2001) (Figure 3). Furthermore, PGs may synergize in the blood vessel with other pro-inflammatory mediators, such as histamine or bradykinin, to increase vascular permeability and promote edema (Funk, 2001; Khanapure et al., 2007).
FIGURE 3. Pro-inflammatory effects of eicosanoids in the blood and the vascular wall. AA: arachidonic acid; COX: cyclooxygenase; CYP450: P450 cytochrome epoxygenase; CysLTs: cysteinyl leukotrienes; LTB4: leukotriene B4; MCP-1: monocyte chemoattractant protein-1; PGE2: prostaglandin E2; TxA2: thromboxane A2; VSMC: vascular smooth muscle cell; 5-LOX: 5-lipoxygenase; 12(S)-LOX: 12(S)-lipoxygenase; 12(S)-HETE: 12(S)-hydroxyeicosatetraenoic acid; 15-LOX: 15-lipoxygenase; 15(S)-HETE: 15(S)-hydroxyeicosatetraenoic acid; 20-HETE: 20-hydroxyeicosatetraenoic acid.
TxA2 is synthesized by macrophages and monocytes on the blood, and in large quantities by platelets (Cook, 2005; Ricciotti and FitzGerald, 2011; Yeung and Holinstat, 2011). Although TxA2 is an unstable compound with a half-life of 20–30 s (Cook, 2005), it has a wide range of effects on the blood vessel (Figure 3). TxA2 is a potent vasoconstrictor and VSMC mitogen. It is produced by aggregating platelets and acts as a direct platelet activator in addition to amplifying the platelet response to other platelet agonists (Praticò and Dogné, 2009).
CysLTs are potent pro-inflammatory mediators produced during vascular injury (Colazzo et al., 2017). The cysLTs induce eosinophil and monocyte chemotaxis and activation (Funk, 2001), potentiate platelet activation (Yeung et al., 2017), promote vascular smooth muscle constriction and increase vascular permeability in post-capillary venules (Poeckel and Funk, 2010) (Figure 3). Duah et al. (Duah et al., 2013) demonstrated that LTC4 and LTD4 regulate endothelial cell function in vitro through the increase of endothelial contraction and induction of barrier disruption in the endothelial cell monolayer. In the same study, they also demonstrated that the cysLTs are able to promote attachment of leukocytes to the endothelial monolayer.
Although most attention has been focused on the COX-dependent pathway of the prostanoids’ biosynthesis, the 5-LOX-catalyzed oxygenation of AA play a role in inflammation through the formation of LTs (Poeckel and Funk, 2010). The 5-LOX pathway has long been recognized as a pro-inflammatory cascade and LTs are lipid mediators involved in inflammation and chemotaxis (Funk, 2001). Expression of 5-LOX is usually absent under normal physiologic conditions, but is induced by pro-inflammatory stimuli. Leukotriene B4 (LTB4) is a potent chemotactic effect on leukocytes (Yokomizo et al., 2018) and has been implicated in atherosclerosis (Bäck et al., 2005; Ketelhuth et al., 2015). In vivo and in vitro studies have shown that LTB4 promotes neutrophil chemotaxis, traffic and adhesion of monocytes to vascular endothelial cells (Friedrich et al., 2003), and increases the formation of monocyte chemoattractant protein-1 (MCP-1) (Huang et al., 2004b) (Figure 3).
The early studies with 12(S)-hydroxyeicosatetraenoic acid (12(S)-HETE) had described anti-inflammatory, antiplatelet and anti-thrombotic effects (Fonlupt et al., 1991; Lagarde et al., 2018). However, most recent studies have shown that 12(S)-HETE potentiates platelet activation, thrombin generation, and calcium mobilization in the platelet (Yeung and Holinstat, 2011) (Figure 3). Furthermore, in vitro treatment of human aortic endothelial cells with 12(S)-HETE increased monocyte binding to endothelial cells (Patricia et al., 1999).
The role of 20-hydroxyeicosatetraenoic acid (20-HETE) in the regulation of vascular tone and homeostasis promoting a prohypertensive response is due to its potent vasoactive effect (Miyata and Roman, 2005). 20-HETE causes vasoconstriction through its regulation of intracellular signaling (Muthalif et al., 2000) and membrane depolarization (Obara et al., 2002) in smooth muscle cells. Furthermore, studies have demonstrated that 20-HETE stimulates the production of inflammatory cytokines, including IL-8, IL-13, IL-4, and PGE2, in endothelial cells (Ishizuka et al., 2008) resulting in endothelial cell activation and endothelial dysfunction (Singh et al., 2007) (Figure 3).
Prostacyclin (PGI2) has been characterized to inhibit platelet aggregation and exerts vasodilator functions, as well as counterbalancing the actions of TxA2 (Schmid and Brüne, 2021) (Figure 4). It is produced primarily by vascular endothelial and VSMCs, but other cells such as fibroblasts and dendritic cells also synthesize PGI2 (Dorris and Peebles, 2012). PGI2 inhibits LPS-induced expression of pro-inflammatory cytokines in macrophages, dendritic cells, T cells and endothelial cells (Luttmann et al., 1996; Zhou et al., 2007a; Zhou et al., 2007b; Di Francesco et al., 2009). PGI2 can synergize with the anti-inflammatory cytokines IL-4 and IL-13 to selectively inhibit the release of pro-inflammatory cytokines from human peripheral mononuclear blood cells (Luttmann et al., 1999). Under inflammatory conditions including atherosclerosis, the production of PGI2 may increase, and this has been commonly considered a protective mechanism. Nonetheless, due to PGI2 acting mainly on TP receptors in vessels with limited IP receptor expression, an increase of its synthesis may lead to increased endothelium-derived vasoconstrictor activity (Luo et al., 2016).
FIGURE 4. Anti-inflammatory effects of eicosanoids in the blood and the vessel wall. AA: arachidonic acid; DGLA: dihomo-γ-linolenic acid; DHA: docosahexaenoic acid; EETs: epoxyeicosatrienoic acids; EPA: eicosapentaenoic acid; COX: cyclooxygenase; COX-1: cyclooxygenase-1; COX-2: cyclooxygenase-2; CYP450: cytochrome epoxygenase; ICAM-1: intercellular adhesion molecule 1; IFN-γ: interferon-γ; LTs: leukotrienes; LTB4: leukotriene B4; LXs: lipoxins; MaR1: maresin 1; Nrf2: nuclear factor-erythroid factor 2-related factor 2; PD1/NPD1: protectin D1/neuroprotectin D1; PGD2: prostaglandin D2; PGD3: prostaglandin D3; PGE1: prostaglandin E1; PGI2: prostaglandin I2 or prostacyclin; PGI3: prostaglandin I3; PMN: polymorphonuclear leukocytes; RvDs: D-series resolvins; RvEs: E-series resolvins; ROS: reactive oxygen species; TNFα: tumor necrosis factor α; VCAM-1: vascular cell adhesion molecule 1; 12-HEPE: 12-hydroxyeicosapentaenoic acid; 15-HEPE: 15-hydroxyeicosapentaenoic acid; 5-HETE: 5-hydroxyeicosatetraenoic acid; 5-LOX: 5-lipoxygenase; 12-LOX: 12-lipoxygenase; 12(S)-HETrE: 12(S)-hydroxyeicosatrienoic acid; 15-LOX: 15-lipoxygenase; 15-HEPE: 15(S)-hydroxyeicosapentaenoic acid; 15-HETE: 15(S)-hydroxyeicosatetraenoic acid; 15(S)-HETrE: 15(S)-hydroxyeicosatrienoic acid.
Series 1 and series 3 PGs are well-known to inhibit platelet activity both in vivo and in vitro (Lagarde et al., 2013; Sergeant et al., 2016). PGE1 has been considered of biological interest as strong inhibitor of platelet function (Minno et al., 1979; Colman and Figures, 1984), whereas PGD3 effectively oppose the transmigration of neutrophils on endothelial cells promoted by PGD2 (Tull et al., 2009). Thus, PGD3 and PGI3 have been exhibited potent anti-aggregatory effect in vitro in human platelet experiments (Whitaker et al., 1979; Fischer and Weber, 1985) (Figure 4).
The lipoxins A (LXA4) and B (LXB4) were two of the first SPMs to be identified and play a critical role in the down-regulation of acute inflammation and enhancement of resolution (Serhan, 2005). LXs increase monocyte chemotaxis and adherence, without causing degranulation or elevation of reactive oxygen species (ROS) (Scalia et al., 1997). It has been established that LXs regulate anti-inflammatory signaling in vascular homeostasis through the stimulation of PGI2 secretion by human endothelial cells (Brezinski et al., 1989) (Figure 4).
Upon vascular injury, the resolvins D1 (RvD1) and D2 (RvD2) have been shown to regulate VSMC phenotypic response, including inhibition of proliferation, migration, monocyte adhesion, ROS production, and inflammatory cytokine expression (Miyahara et al., 2013) (Figure 4). Using mass spectrometry approaches, Cherpokova et al. (Cherpokova et al., 2019) has identified the kinetics of the formation of SPMs in the clot using a deep vein thrombosis animal model. In the same study, administration of RvD4 reduced thrombus burden, with less neutrophil infiltration and more pro-resolving monocytes in the clot. RvD5 promotes pro-resolving effects through enhancement of phagocytosis and reduction of expression of tumor necrosis factor α (TNFα) in neutrophils and macrophages (Chiang et al., 2012; Werz et al., 2018).
The resolvin E1 (RvE1) was the first isolated and studied E-series resolvin and possesses anti-inflammatory and pro-resolving actions (Serhan and Petasis, 2011). In the blood, RvE1 has been shown to negatively regulate leukocytes in vivo and platelets ex vivo (Figure 4), by reducing U46619-, a TP receptor agonist, and ADP-stimulated platelet aggregation, and TxA2 generation (Dona et al., 2008), suggesting that RvE1 might inhibit P2Y12 receptor in platelets. Currently, P2Y12 receptor antagonists are used in association with aspirin as the most widely used antiplatelet therapy in cardiovascular diseases (Jackson and Schoenwaelder, 2003). In addition, RvE1 initiates resolution of inflammation through repolarization of human M1 macrophages toward resolution-type macrophages (Herová et al., 2015). Since RvE1 activates the BLT1 receptor in neutrophils, LTB4 action is inhibited which reduces LTB4-pro-inflammatory responses (Arita et al., 2007) (Figure 4).
RvE2 has also been reported to promote anti-inflammatory and pro-resolving effects through ex vivo inhibition of PMN chemotaxis and enhancement of nonphlogistic phagocytosis by macrophages (Oh et al., 2012). Recently, the inhibitory effect of RvE3 on neutrophil chemotaxis in vitro has been demonstrated (Isobe et al., 2012) and a new member of the EPA-derived resolvins E has been identified and termed resolvin E4 (RvE4). RvE4 is produced under physiologic hypoxia and has a resolving function. It stimulates human M2 macrophage efferocytosis of senescent erythrocytes and apoptotic neutrophils in vitro (Libreros et al., 2020) (Figure 4).
Maresin 1 (MaR1) plays a role in the resolution of inflammation by reducing platelet aggregation ex vivo (Freedman et al., 2020) and stimulating phagocytosis and efferocytosis in human and mouse phagocytes (Chiang and Serhan, 2020) (Figure 4).
Studies have demonstrated that protectin D1/Neuroprotection D1 (PD1/NPD1) increases phagocytosis in macrophages, regulates TNFα and IFNγ secretion by activated T cells in vitro (Ariel et al., 2005), and limits transendothelial migration of leukocytes to prevent the infiltration of leukocytes into sites of inflammation (Serhan et al., 2015; Calder, 2020a; Chiang and Serhan, 2020) (Figure 4). Moreover, synthetic PD1/NPD1 attenuated human PMN transmigration in vivo and in vitro in response to LTB4 and T cells (Serhan et al., 2015).
Human leukocytes can form AT-(NPD1/PD1) via aspirin-acetylated COX-2 (Serhan et al., 2015). Studies have demonstrated that AT-(NPD1/PD1) has potent protective actions comparable to NPD1/PD1 in vitro and in vivo, reducing transendothelial PMN migration and enhancing efferocytosis of apoptotic human PMN by macrophages (Serhan et al., 2011a).
In neutrophils, EPA and AA are metabolized by 5-LOX to form 5-hydroxyeicosatetraenoic acid (5-HEPE) and 5-hydroxyeicosatetraenoic acid (5-HETE), respectively. Both 5-HEPE and 5-HETE have been shown to induce antioxidative enzymes in vascular endothelial cells through activation of a nuclear factor-erythroid factor 2-related factor 2 (Nrf2)-dependent mechanism through their metabolites, 5-oxo-EPE and 5-oxo-HETE (Nagahora et al., 2017) (Figure 4).
The 12(S)-hydroxyeicosapentaenoic acid (12(S)-HEPE) is the eicosanoid formed through oxygenation of EPA by 12(S)-LOX. Pre-treatment of whole blood with EPA prolonged the time of clot retraction ex vivo (Figure 4), suggesting that EPA-derived eicosanoids, such as 12(S)-HEPE, might regulate blood clotting and play a role in clot resolution (Ikei et al., 2012).
DGLA is an ω-6 fatty acid that is oxidized in the platelet by 12(S)-LOX to form 12(S)-HETrE. This metabolite has been shown to attenuate platelet activity and thrombosis (Ikei et al., 2012). The antiplatelet role of 12-HETrE was determined by demonstrating its ability to inhibit platelet aggregation in vitro and attenuate clot formation in vivo (Figure 4) through selective activation of the prostacyclin receptor in platelets (Yeung et al., 2016; Tourdot et al., 2017; Yeung and Holinstat, 2017).
The 15-LOX-derived eicosanoids from AA, DGLA, and EPA are 15(S)-hydroxyeicosatetraenoic acid (15(S)-HETE), 15-hydroxyeicosatrienoic acid (15(S)-HETrE), and 15(S)-hydroxyeicosapentaenoic acid (15(S)-HEPE), respectively. While 15(S)-HETrE, 15(S)-HETE and 15(S)-HEPE have been shown to inhibit platelet reactivity (Guichardant et al., 1988; Vanderhoek et al., 1991) (Figure 4), other studies have observed a pro-aggregatory effect of 15(S)-HETE on platelet function (Setty and Stuart, 1986; Vijil et al., 2014) and an increase of clot formation in human whole blood pre-treated ex vivo with 15(S)-HETE (Lundqvist et al., 2016) (Figure 3).
Moreover, studies have suggested that DGLA inhibits the synthesis in vitro of LTB4 in neutrophils through the formation of 15(S)-HETrE (Iversen et al., 1991; Chilton-Lopez et al., 1996) (Figure 4). Additionally, 15(S)-HETE has been shown to inhibit LTB4-induced chemotaxis of PMNs in vitro (Ternowitz et al., 1988; Takata et al., 1994).
Epoxyeicosatrienoic acids (EETs) are generated from AA by CYP450 enzymes and promote the active termination of inflammation by a broad array of anti-inflammatory and pro-resolving actions. EETs were found to have direct effects on the large-conductance Ca2+-activated potassium (K+) channels in vascular smooth muscle cells (Campbell and Harder, 1999). This mechanism contributes to the effect of EETs as endothelium-derived hyperpolarizing factor to hyperpolarize and relax arterial smooth muscle (Li and Campbell, 1997). EETs present functional relevance in vascular inflammation primarily due to their role in the reduction of vascular cell adhesion molecule 1 (VCAM-1), E-selectin and intercellular adhesion molecule 1 (ICAM-1) expression, and prevention of leukocyte adhesion to the vascular wall (Node et al., 1999) (Figure 4).
Polyunsaturated fatty acids and their bioactive eicosanoids play a critical role in human health and diseases through regulating inflammation in the blood and the vessel. The role of the ω-6 PUFA AA in inflammation through formation of eicosanoids is well established. While the AA-derived eicosanoids, including PGE2, TxA2 and LTs, are well-known as pro-inflammatory mediators in the blood, the COX-derived PGs PGI2 from AA, and the PGs series 1 and 3 from EPA and DGLA, respectively, have a critical role in counterbalancing pro-inflammatory states to attenuate inflammation in the blood and the vascular wall. More recently, studies in the eicosanoid-inflammation field have additionally identified a wide class of bioactive metabolites and SPMs derived from AA, DGLA, EPA, and DHA. As the classic eicosanoids (PGs, LTs and Txs), the bioactive metabolites have pro- or anti-inflammatory effects, whereas the SPMs are currently extensively studied due to their effects on the attenuation of pro-inflammatory eicosanoid actions and active contribution to the resolution of inflammatory tissue. This review has outlined the function of COX-, LOX- and CYP 450-derived eicosanoids from PUFAs and elucidated their mechanistic regulation of the inflammation process in the blood and the vessel.
As a result of their widespread expression in the blood and the vascular wall, eicosanoids and their metabolites are involved in the pathogenesis and the development of inflammatory diseases such as atherosclerosis, hypertension, diabetes mellitus and more recently, COVID-19. As an example, alterations in the formation of bioactive metabolites, such as 20-HETE, have been reported in inflammatory diseases such as hypertension, diabetes (Miyata and Roman, 2005) and cardiovascular disease (Zu et al., 2016). Due to their critical involvement in eicosanoid biosynthesis, alterations in the expression of oxygenases also play a role in the pathogenesis of inflammatory diseases such as atherosclerosis and diabetes. While the upregulation of 5-LOX expression, leading to production of Cys-LTs and LTB4, has been reported at the site of atherosclerotic plaques (Whatling et al., 2007; Riccioni et al., 2010), increased 12-LOX activity or expression has been implicated in the functional loss of insulin secretion or production in beta-cells of the pancreatic islets, which may impair blood glucose regulation leading to the development of diabetes (Ma et al., 2010). Synthesis of PGE2 has also been suggested to be up-regulated in atherosclerosis. Using an animal model of atherosclerosis, Gross et al. have shown that PGE2 is produced in the arterial wall in response to inflammation and is detected in atherosclerotic plaques. In addition, the authors have demonstrated that PGE2 enhances atherothrombosis in vivo (Gross et al., 2007). Increased production of PGE2 was recently identified in the blood of COVID-19 patients. These patients were found to have higher PGE2 levels which were correlated positively with the severity of the disease (Ricke-Hoch et al., 2021). Coronavirus infection activates endoplasmic reticulum stress signaling, which, in turn, can induce the biosynthesis of PGE2 (Chopra et al., 2019). Thus, an increased level of PGE2 may be involved in the hyperinflammatory response in COVID 19 infection (Hammock et al., 2020).
Due to their effects on promoting inflammation, the eicosanoids are potential targets for the treatment of these diseases, as well as the enzymes and receptors implicated in their formation. For example, the inhibition of 12-LOX in platelets, using the pharmacological inhibitor ML355, reduces platelet aggregation ex vivo and impairs clot formation in vivo (Adili et al., 2017). The prostacyclin analogs, such as iloprost and selexipag, are used to treat pulmonary arterial hypertension due to their vasodilatory and anti-platelet effects through activation of the prostacyclin receptor (Sitbon et al., 2015; Mandras et al., 2021). The inhibition of pro-aggregatory effects of TxA2 through acetylation of COX-1 in platelets is the pharmacological basis for aspirin, used in association with a P2Y12 receptor antagonist in dual antiplatelet therapy to treat cardiovascular diseases and prevent the recurrence of major cardiovascular events due to thrombosis (Schrör and Rauch, 2015). The role of TxA2 in the impairing endothelial function is highly associated with pathogenesis of atherosclerosis. Studies have shown that mice deficient in TP and IP demonstrated an accelerated atherogenesis in the blood vessel (Kobayashi et al., 2004). Notably, the acetylation of COX by aspirin can trigger alternative biosynthesis pathways forming bioactive metabolites and SPMs (Figure 2) which might provide additional anti-inflammatory effects promoted by aspirin treatment.
Studies have shown that increased intake of ω-3 PUFAs (EPA and DHA) results in increased amounts of these fatty acids in blood lipids, leukocytes and platelets (Browning et al., 2012). The increased level of ω-3 PUFAs in leukocytes and platelets has been demonstrated to result in a reduction of the capacity of these cells to produce pro-inflammatory eicosanoids from AA, such as PGs and LTs (Calder, 2020b), and to regulate the function of these cells by attenuating platelet reactivity and increasing leukocyte response to inflammation (Faber et al., 2011; Yamaguchi et al., 2022). Notably, the concentration of several bioactive metabolites, including hydroxy- and epoxyeicosanoids derived from AA, EPA and DHA, were increased in the plasma of normo- and hyperlipidemic patients following supplementation with EPA and DHA (Schuchardt et al., 2014; Schmöcker et al., 2018). Moreover, studies have detected higher levels of the SPMs, such as RvD1 and RvD2, in the plasma and serum of individuals with an increased intake of EPA and DHA (Calder, 2020a). Thus, given the evidence of diverse supplementary studies, modulating the levels of PUFAs mediated by ingestion or supplementation might provide beneficial effects in attenuating the inflammation process in the blood and the vessel.
The SPMs have been recently described as positive modulators on resolution and termination of inflammation. Studies have indicated that RvE1 might control vascular inflammation in atherosclerosis. RvE1 has been shown to protect against atherogenesis in an animal model of atherosclerosis (Hasturk et al., 2015) and Laguna-Fernandez et al. (Laguna-Fernandez et al., 2018) have demonstrated that targeted deletion of the RvE1 receptor ERV1/Chem23 in a hyperlipidemic animal model was associated with proatherogenic signaling in macrophages, increased oxidized low-density lipoprotein uptake, reduced phagocytosis, and increased atherosclerotic plaque size and necrotic core formation, suggesting that RvE1 might have protective effects during atheroprogression (Salic et al., 2016). Additionally, the administration of the D-series resolvin RvD4 to mice of a deep vein thrombosis model has been shown to reduce thrombus formation and improve clot resolution (Cherpokova et al., 2019), suggesting that the delivery of SPMs might help to regulate thrombosis and inflammation in cardiovascular diseases. Thus, SPMs may be considered as potential therapeutic approaches for prevention or resolution of inflammation or insult in the vessel.
The discovery of SPMs was first reported in exudates (Serhan et al., 2011b) and the investigation of the effects of SPMs on the blood and the vessel is currently in early stages. Studies using in vitro assays and animal models have described the SPMs’ ability to contribute to resolution of inflammation through regulation of cell function in the blood and the vessel (see review (Chiang and Serhan, 2020)), but the physiological relevance of these effects depends on the endogenous concentration of SPM in vivo. The biosynthesis of SPMs has been characterized using in vitro studies (Isobe et al., 2012; Libreros et al., 2020; Perry et al., 2020) and other studies have demonstrated the ability of blood cells such as neutrophils and macrophages to form SPMs in vitro (Werz et al., 2018; Mainka et al., 2022). In addition, despite several studies having detected SPMs in human samples including plasma and serum (see review (Calder, 2020a)), the concentration of SPMs was at low levels (picogram/picomolar to nanogram/nanomolar range) (Mainka et al., 2022; Schebb et al., 2022) and the analysis of low concentrations of low SPMs can be an analytical challenge and it may affect the detection and quantification process of these metabolites in the sample. Indeed, there is a current controversy in the field based on differences in the methodology and analytical instrumentation used to detect the SPMs in biological samples (Schebb et al., 2022), which demonstrates that a deeper investigation is warranted to provide a better understanding of the concentration range of SPMs circulating in the human bloodstream and whether SPMs at these concentrations are able to regulate resolution of inflammation in the blood and the vessel.
The studies using in vitro and in vivo approaches in cellular and animal models, and the analysis of samples collected from humans, have significantly contributed to the current understanding of the mechanistic regulation of eicosanoids in inflammation. It resulted in a large body of evidence about the role of the classical pro- and anti-inflammatory eicosanoids derived from the 20-carbon PUFAs AA, DGLA and EPA, in inflammation in the blood. However, a better understanding of the mechanistic regulatory effects of the most recently discovered eicosanoids, including SPMs and bioactive metabolites, in the regulation of inflammatory states and their contribution to the resolution of inflammation in the blood and the vascular wall is warranted. Furthermore, it is important to highlight that, although there is evidence of the synthesis of SPMs by cells in the blood, whether the biosynthesis of some SPMs occurs in the blood and the biological relevance of this process still need to be further elucidate. Hence, the role of eicosanoids in inflammation in the blood and the vessel is currently a focus of much research in the inflammation field which might help to position the anti-inflammatory bioactive eicosanoids as a novel therapeutic approach to treat inflammatory diseases that affects the blood and the vascular wall.
AY and EB performed literature search, wrote the manuscript and created figures. MH wrote and edited the manuscript. MH, AY, and EB proofed the manuscript.
This study was supported by NIH grants R35 GM131835 (MH), T32 HL007853 (AY), and UL1 TR002240 (MH and AY).
MH is a consultant and equity holder for Veralox therapeutics and Cereno Scientific.
The remaining authors declare that the research was conducted in the absence of any commercial or financial relationships that could be construed as a potential conflict of interest.
All claims expressed in this article are solely those of the authors and do not necessarily represent those of their affiliated organizations, or those of the publisher, the editors and the reviewers. Any product that may be evaluated in this article, or claim that may be made by its manufacturer, is not guaranteed or endorsed by the publisher.
Adili, R., Tourdot, B. E., Mast, K., Yeung, J., Freedman, J. C., Green, A., et al. (2017). First selective 12-LOX inhibitor, ML355, impairs thrombus formation and vessel occlusion in vivo with minimal effects on hemostasis. Arterioscler. Thromb. Vasc. Biol. 37 (10), 1828–1839. doi:10.1161/ATVBAHA.117.309868
Akagi, D., Chen, M., Toy, R., Chatterjee, A., and Conte, M. S. (2015). Systemic delivery of proresolving lipid mediators resolvin D2 and maresin 1 attenuates intimal hyperplasia in mice. Faseb J. 29 (6), 2504–2513. doi:10.1096/fj.14-265363
Alvarez, M. L., and Lorenzetti, F. (2021). Role of eicosanoids in liver repair, regeneration and cancer. Biochem. Pharmacol. 192, 114732. doi:10.1016/j.bcp.2021.114732
Aoki, T., and Narumiya, S. (2012). Prostaglandins and chronic inflammation. Trends Pharmacol. Sci. 33 (6), 304–311. doi:10.1016/j.tips.2012.02.004
Ariel, A., Li, P. L., Wang, W., Tang, W. X., Fredman, G., Hong, S., et al. (2005). The docosatriene protectin D1 is produced by TH2 skewing and promotes human T cell apoptosis via lipid raft clustering. J. Biol. Chem. 280 (52), 43079–43086. doi:10.1074/jbc.M509796200
Arita, M., Ohira, T., Sun, Y-P., Elangovan, S., Chiang, N., and Serhan, C. N. (2007). Resolvin E1 selectively interacts with leukotriene B4 receptor BLT1 and ChemR23 to regulate inflammation. J. Immunol. 178 (6), 3912–3917. doi:10.4049/jimmunol.178.6.3912
Arnold, C., Konkel, A., Fischer, R., and Schunck, W-H. (2010). Cytochrome P450-dependent metabolism of ω-6 and ω-3 long-chain polyunsaturated fatty acids. Pharmacol. Rep. 62 (3), 536–547. doi:10.1016/s1734-1140(10)70311-x
Astarita, G., Kendall, A. C., Dennis, E. A., and Nicolaou, A. (2015). Targeted lipidomic strategies for oxygenated metabolites of polyunsaturated fatty acids. Biochim. Biophys. Acta 1851 (4), 456–468. doi:10.1016/j.bbalip.2014.11.012
Bäck, M., Bu, D-X., Bränström, R., Sheikine, Y., Yan, Z-Q., and Hansson, G. K. (2005). Leukotriene B4 signaling through NF-κB-dependent BLT1 receptors on vascular smooth muscle cells in atherosclerosis and intimal hyperplasia. Proc. Natl. Acad. Sci. 102 (48), 17501–17506.
Biringer, R. G. (2021). A review of prostanoid receptors: Expression, characterization, regulation, and mechanism of action. J. Cell Commun. Signal 15 (2), 155–184. doi:10.1007/s12079-020-00585-0
Bosma, K. J., Kaiser, C. E., Kimple, M. E., and Gannon, M. (2022). Effects of arachidonic acid and its metabolites on functional beta-cell mass. Metabolites 12 (4), 342. doi:10.3390/metabo12040342
Bozza, P. T., Bakker-Abreu, I., Navarro-Xavier, R. A., and Bandeira-Melo, C. (2011). Lipid body function in eicosanoid synthesis: An update. Prostagl. Leukot. Essent. Fat. Acids 85 (5), 205–213. doi:10.1016/j.plefa.2011.04.020
Brash, A. R. (1999). Lipoxygenases: Occurrence, functions, catalysis, and acquisition of substrate. J. Biol. Chem. 274 (34), 23679–23682. doi:10.1074/jbc.274.34.23679
Brezinski, M. E., Gimbrone, M. A., Nicolaou, K., and Serhan, C. N. (1989). Lipoxins stimulate prostacyclin generation by human endothelial cells. FEBS Lett. 245 (1-2), 167–172. doi:10.1016/0014-5793(89)80214-5
Browning, L. M., Walker, C. G., Mander, A. P., West, A. L., Madden, J., Gambell, J. M., et al. (2012). Incorporation of eicosapentaenoic and docosahexaenoic acids into lipid pools when given as supplements providing doses equivalent to typical intakes of oily fish. Am. J. Clin. Nutr. 96 (4), 748–758. doi:10.3945/ajcn.112.041343
Calder, P. C. (2020). n-3 PUFA and inflammation: from membrane to nucleus and from bench to bedside. Proc. Nutr. Soc. 79 (4), 1–13. doi:10.1017/S0029665120007077
Calder, P. C. (2020). Eicosapentaenoic and docosahexaenoic acid derived specialised pro-resolving mediators: Concentrations in humans and the effects of age, sex, disease and increased omega-3 fatty acid intake. Biochimie 178, 105–123. doi:10.1016/j.biochi.2020.08.015
Campbell, W. B., and Harder, D. R. (1999). Endothelium-derived hyperpolarizing factors and vascular cytochrome P450 metabolites of arachidonic acid in the regulation of tone. Circ. Res. 84 (4), 484–488. doi:10.1161/01.res.84.4.484
Capra, V., Accomazzo, M. R., Ravasi, S., Parenti, M., Macchia, M., Nicosia, S., et al. (2003). Involvement of prenylated proteins in calcium signaling induced by LTD4 in differentiated U937 cells. Prostagl. Other Lipid Mediat 71 (3-4), 235–251. doi:10.1016/s1098-8823(03)00045-5
Capra, V. (2004). Molecular and functional aspects of human cysteinyl leukotriene receptors. Pharmacol. Res. 50 (1), 1–11. doi:10.1016/j.phrs.2003.12.012
Cebo, M., Dittrich, K., Fu, X., Manke, M. C., Emschermann, F., Rheinlaender, J., et al. (2022). Platelet ACKR3/CXCR7 favors antiplatelet lipids over an atherothrombotic lipidome and regulates thromboinflammation. J. Am. Soc. Hematol. 139 (11), 1722–1742. doi:10.1182/blood.2021013097
Chandrasekharan, J. A., and Sharma-Walia, N. (2015). Lipoxins: nature's way to resolve inflammation. J. Inflamm. Res. 8, 181–192. doi:10.2147/JIR.S90380
Chatterjee, A., Sharma, A., Chen, M., Toy, R., Mottola, G., and Conte, M. S. (2014). The pro-resolving lipid mediator maresin 1 (MaR1) attenuates inflammatory signaling pathways in vascular smooth muscle and endothelial cells. PLoS One 9 (11), e113480. doi:10.1371/journal.pone.0113480
Cheranov, S. Y., Karpurapu, M., Wang, D., Zhang, B., Venema, R. C., and Rao, G. N. (2008). An essential role for SRC-activated STAT-3 in 14,15-EET-induced VEGF expression and angiogenesis. Blood 111 (12), 5581–5591. doi:10.1182/blood-2007-11-126680
Cherpokova, D., Jouvene, C. C., Libreros, S., DeRoo, E. P., Chu, L., de la Rosa, X., et al. (2019). Resolvin D4 attenuates the severity of pathological thrombosis in mice. Blood 134 (17), 1458–1468. doi:10.1182/blood.2018886317
Chiang, N., Dalli, J., Colas, R. A., and Serhan, C. N. (2015). Identification of resolvin D2 receptor mediating resolution of infections and organ protection. J. Exp. Med. 212 (8), 1203–1217. doi:10.1084/jem.20150225
Chiang, N., Fredman, G., Bäckhed, F., Oh, S. F., Vickery, T., Schmidt, B. A., et al. (2012). Infection regulates pro-resolving mediators that lower antibiotic requirements. Nature 484 (7395), 524–528. doi:10.1038/nature11042
Chiang, N., Libreros, S., Norris, P. C., de la Rosa, X., and Serhan, C. N. (2019). Maresin 1 activates LGR6 receptor promoting phagocyte immunoresolvent functions. J. Clin. Invest 129 (12), 5294–5311. doi:10.1172/JCI129448
Chiang, N., and Serhan, C. N. (2020). Specialized pro-resolving mediator network: An update on production and actions. Essays Biochem. 64 (3), 443–462. doi:10.1042/EBC20200018
Chilton-Lopez, L., Surette, M. E., Swan, D. D., Fonteh, A. N., Johnson, M. M., and Chilton, F. H. (1996). Metabolism of gammalinolenic acid in human neutrophils. J. Immunol. 156 (8), 2941–2947.
Chopra, S., Giovanelli, P., Alvarado-Vazquez, P. A., Alonso, S., Song, M., Sandoval, T. A., et al. (2019). IRE1α-XBP1 signaling in leukocytes controls prostaglandin biosynthesis and pain. Science 365 (6450), eaau6499. doi:10.1126/science.aau6499
Colazzo, F., Gelosa, P., Tremoli, E., Sironi, L., and Castiglioni, L. (2017). Role of the cysteinyl leukotrienes in the pathogenesis and progression of cardiovascular diseases. Mediat. Inflamm. 2017, 2432958. doi:10.1155/2017/2432958
Colman, R. W., and Figures, W. R. (1984). Characteristics of an ADP receptor mediating platelet activation. Mol. Cell Biochem. 59 (1), 101–111. doi:10.1007/BF00231307
Cook, D., Finnigan, J., Cook, K., Black, G., and Charnock, S. (2016). Cytochromes P450: History, classes, catalytic mechanism, and industrial application. Adv. Protein Chem. Struct. Biol. 105, 105–126. doi:10.1016/bs.apcsb.2016.07.003
Cook, J. A. (2005). Eicosanoids. Crit. Care Med. 33 (1), S488–S491. doi:10.1097/01.ccm.0000196028.19746.42
Crooke, S. T., Mattern, M., Sarau, H. M., Winkler, J. D., Balcarek, J., Wong, A., et al. (1989). The signal transduction system of the leukotriene D4 receptor. Trends Pharmacol. Sci. 10 (3), 103–107. doi:10.1016/0165-6147(89)90206-x
Cummings, H. E., Liu, T., Feng, C., Laidlaw, T. M., Conley, P. B., Kanaoka, Y., et al. (2013). Cutting edge: Leukotriene C4 activates mouse platelets in plasma exclusively through the type 2 cysteinyl leukotriene receptor. J. Immunol. 191 (12), 5807–5810. doi:10.4049/jimmunol.1302187
Dennis, E. A., Cao, J., Hsu, Y-H., Magrioti, V., and Kokotos, G. (2011). Phospholipase A2 enzymes: Physical structure, biological function, disease implication, chemical inhibition, and therapeutic intervention. Chem. Rev. 111 (10), 6130–6185. doi:10.1021/cr200085w
Di Francesco, L., Totani, L., Dovizio, M., Piccoli, A., Di Francesco, A., Salvatore, T., et al. (2009). Induction of prostacyclin by steady laminar shear stress suppresses tumor necrosis factor-alpha biosynthesis via heme oxygenase-1 in human endothelial cells. Circ. Res. 104 (4), 506–513. doi:10.1161/CIRCRESAHA.108.191114
Díaz Del Campo, L. S., Rodrigues-Díez, R., Salaices, M., Briones, A. M., and García-Redondo, A. B. (2022). Specialized pro-resolving lipid mediators: New therapeutic approaches for vascular remodeling. Int. J. Mol. Sci. 23 (7).
Dona, M., Fredman, G., Schwab, J. M., Chiang, N., Arita, M., Goodarzi, A., et al. (2008). Resolvin E1, an EPA-derived mediator in whole blood, selectively counterregulates leukocytes and platelets. Blood 112 (3), 848–855. doi:10.1182/blood-2007-11-122598
Dorris, S. L., and Peebles, R. S. (2012). PGI2 as a regulator of inflammatory diseases. Mediat. Inflamm. 2012, 926968. doi:10.1155/2012/926968
Duah, E., Adapala, R. K., Al-Azzam, N., Kondeti, V., Gombedza, F., Thodeti, C. K., et al. (2013). Cysteinyl leukotrienes regulate endothelial cell inflammatory and proliferative signals through CysLT₂ and CysLT₁ receptors. Sci. Rep. 3, 3274. doi:10.1038/srep03274
Esser-von Bieren, J. (2019). Eicosanoids in tissue repair. Immunol. Cell Biol. 97 (3), 279–288. doi:10.1111/imcb.12226
Faber, J., Berkhout, M., Vos, A. P., Sijben, J. W., Calder, P. C., Garssen, J., et al. (2011). Supplementation with a fish oil-enriched, high-protein medical food leads to rapid incorporation of EPA into white blood cells and modulates immune responses within one week in healthy men and women. J. Nutr. 141 (5), 964–970. doi:10.3945/jn.110.132985
Fahy, E., Subramaniam, S., Murphy, R. C., Nishijima, M., Raetz, C. R., Shimizu, T., et al. (2009). Update of the LIPID MAPS comprehensive classification system for lipids. J. Lipid Res. 50 Suppl, S9–S14. doi:10.1194/jlr.R800095-JLR200
Fava, C., and Bonafini, S. (2018). Eicosanoids via CYP450 and cardiovascular disease: Hints from genetic and nutrition studies. Prostagl. Other Lipid Mediat 139, 41–47. doi:10.1016/j.prostaglandins.2018.10.001
Feinmark, S. J., and Cannon, P. J. (1986). Endothelial cell leukotriene C4 synthesis results from intercellular transfer of leukotriene A4 synthesized by polymorphonuclear leukocytes. J. Biol. Chem. 261 (35), 16466–16472. doi:10.1016/s0021-9258(18)66589-5
Fischer, S., and Weber, P. C. (1985). Thromboxane (TX)A3 and prostaglandin (PG)I3 are formed in man after dietary eicosapentaenoic acid: Identification and quantification by capillary gas chromatography-electron impact mass spectrometry. Biomed. Mass Spectrom. 12 (9), 470–476. doi:10.1002/bms.1200120905
Fonlupt, P., Croset, M., and Lagarde, M. (1991). 12-HETE inhibits the binding of PGH2/TXA2 receptor ligands in human platelets. Thromb. Res. 63 (2), 239–248. doi:10.1016/0049-3848(91)90287-7
Fredman, G., Van Dyke, T. E., and Serhan, C. N. (2010). Resolvin E1 regulates adenosine diphosphate activation of human platelets. Arterioscler. Thromb. Vasc. Biol. 30 (10), 2005–2013. doi:10.1161/ATVBAHA.110.209908
Freedman, C., Tran, A., Tourdot, B. E., Kalyanaraman, C., Perry, S., Holinstat, M., et al. (2020). Biosynthesis of the maresin intermediate, 13S,14S-Epoxy-DHA, by human 15-lipoxygenase and 12-lipoxygenase and its regulation through negative allosteric modulators. Biochemistry 59 (19), 1832–1844. doi:10.1021/acs.biochem.0c00233
Freire, M. O., Dalli, J., Serhan, C. N., and Van Dyke, T. E. (2017). Neutrophil resolvin E1 receptor expression and function in type 2 diabetes. J. Immunol. 198 (2), 718–728. doi:10.4049/jimmunol.1601543
Friedrich, E. B., Tager, A. M., Liu, E., Pettersson, A., Owman, C., Munn, L., et al. (2003). Mechanisms of leukotriene B4--triggered monocyte adhesion. Arterioscler. Thromb. Vasc. Biol. 23 (10), 1761–1767. doi:10.1161/01.ATV.0000092941.77774.3C
Fu, T., Mohan, M., Brennan, E. P., Woodman, O. L., Godson, C., Kantharidis, P., et al. (2020). Therapeutic potential of lipoxin A4 in chronic inflammation: Focus on cardiometabolic disease. ACS Pharmacol. Transl. Sci. 3 (1), 43–55. doi:10.1021/acsptsci.9b00097
Funk, C. D. (2001). Prostaglandins and leukotrienes: Advances in eicosanoid biology. Science 294 (5548), 1871–1875. doi:10.1126/science.294.5548.1871
Garcia, V., Gilani, A., Shkolnik, B., Pandey, V., Zhang, F. F., Dakarapu, R., et al. (2017). 20-HETE signals through G-protein-coupled receptor GPR75 (gq) to affect vascular function and trigger hypertension. Circ. Res. 120 (11), 1776–1788. doi:10.1161/CIRCRESAHA.116.310525
Gilroy, D. W., and Bishop-Bailey, D. (2019). Lipid mediators in immune regulation and resolution. Br. J. Pharmacol. 176 (8), 1009–1023. doi:10.1111/bph.14587
Goldman, D. W., Chang, F. H., Gifford, L. A., Goetzl, E. J., and Bourne, H. R. (1985). Pertussis toxin inhibition of chemotactic factor-induced calcium mobilization and function in human polymorphonuclear leukocytes. J. Exp. Med. 162 (1), 145–156. doi:10.1084/jem.162.1.145
Goodarzi, K., Goodarzi, M., Tager, A. M., Luster, A. D., and von Andrian, U. H. (2003). Leukotriene B4 and BLT1 control cytotoxic effector T cell recruitment to inflamed tissues. Nat. Immunol. 4 (10), 965–973. doi:10.1038/ni972
Gross, S., Tilly, P., Hentsch, D., Vonesch, J-L., and Fabre, J-E. (2007). Vascular wall-produced prostaglandin E2 exacerbates arterial thrombosis and atherothrombosis through platelet EP3 receptors. J. Exp. Med. 204 (2), 311–320. doi:10.1084/jem.20061617
Guichardant, M., Naltachayan-Durbin, S., and Lagarde, M. (1988). Occurrence of the 15-hydroxy derivative of dihomogammalinolenic acid in human platelets and its biological effect. Biochim. Biophys. Acta 962 (1), 149–154. doi:10.1016/0005-2760(88)90106-3
Hajeyah, A. A., Griffiths, W. J., Wang, Y., Finch, A. J., and O’Donnell, V. B. (2020). The biosynthesis of enzymatically oxidized lipids. Front. Endocrinol. 11, 910. doi:10.3389/fendo.2020.591819
Hammock, B. D., Wang, W., Gilligan, M. M., and Panigrahy, D. (2020). Eicosanoids: The overlooked storm in coronavirus disease 2019 (COVID-19)? Am. J. Pathol. 190 (9), 1782–1788. doi:10.1016/j.ajpath.2020.06.010
Hasturk, H., Abdallah, R., Kantarci, A., Nguyen, D., Giordano, N., Hamilton, J., et al. (2015). Resolvin E1 (RvE1) attenuates atherosclerotic plaque formation in diet and inflammation-induced atherogenesis. Arterioscler. Thromb. Vasc. Biol. 35 (5), 1123–1133. doi:10.1161/ATVBAHA.115.305324
Herová, M., Schmid, M., Gemperle, C., and Hersberger, M. (2015). ChemR23, the receptor for chemerin and resolvin E1, is expressed and functional on M1 but not on M2 macrophages. J. Immunol. 194 (5), 2330–2337. doi:10.4049/jimmunol.1402166
Ho, K. J., Spite, M., Owens, C. D., Lancero, H., Kroemer, A. H., Pande, R., et al. (2010). Aspirin-triggered lipoxin and resolvin E1 modulate vascular smooth muscle phenotype and correlate with peripheral atherosclerosis. Am. J. Pathol. 177 (4), 2116–2123. doi:10.2353/ajpath.2010.091082
Hoxha, M., and Zappacosta, B. (2020). CYP-Derived eicosanoids: Implications for rheumatoid arthritis. Prostagl. Other Lipid Mediat 146, 106405. doi:10.1016/j.prostaglandins.2019.106405
Huang, J. S., Ramamurthy, S. K., Lin, X., and Le Breton, G. C. (2004). Cell signalling through thromboxane A2 receptors. Cell Signal 16 (5), 521–533. doi:10.1016/j.cellsig.2003.10.008
Huang, L., Zhao, A., Wong, F., Ayala, J. M., Struthers, M., Ujjainwalla, F., et al. (2004). Leukotriene B4 strongly increases monocyte chemoattractant protein-1 in human monocytes. Arterioscler. Thromb. Vasc. Biol. 24 (10), 1783–1788. doi:10.1161/01.ATV.0000140063.06341.09
Ikei, K. N., Yeung, J., Apopa, P. L., Ceja, J., Vesci, J., Holman, T. R., et al. (2012). Investigations of human platelet-type 12-lipoxygenase: Role of lipoxygenase products in platelet activation. J. Lipid Res. 53 (12), 2546–2559. doi:10.1194/jlr.M026385
Ishizuka, T., Cheng, J., Singh, H., Vitto, M. D., Manthati, V. L., Falck, J. R., et al. (2008). 20-Hydroxyeicosatetraenoic acid stimulates nuclear factor-kappaB activation and the production of inflammatory cytokines in human endothelial cells. J. Pharmacol. Exp. Ther. 324 (1), 103–110. doi:10.1124/jpet.107.130336
Isobe, Y., Arita, M., Matsueda, S., Iwamoto, R., Fujihara, T., Nakanishi, H., et al. (2012). Identification and structure determination of novel anti-inflammatory mediator resolvin E3, 17,18-dihydroxyeicosapentaenoic acid. J. Biol. Chem. 287 (13), 10525–10534. doi:10.1074/jbc.M112.340612
Iversen, L., Fogh, K., Bojesen, G., and Kragballe, K. (1991). Linoleic acid and dihomogammalinolenic acid inhibit leukotriene B4 formation and stimulate the formation of their 15-lipoxygenase products by human neutrophils in vitro. Evidence of formation of antiinflammatory compounds. Agents Actions 33 (3-4), 286–291. doi:10.1007/BF01986575
Jackson, S. P., and Schoenwaelder, S. M. (2003). Antiplatelet therapy: In search of the 'magic bullet'. Nat. Rev. Drug Discov. 2 (10), 775–789. doi:10.1038/nrd1198
Jiang, W. G., Watkins, G., Douglas-Jones, A., and Mansel, R. E. (2006). Reduction of isoforms of 15-lipoxygenase (15-LOX)-1 and 15-LOX-2 in human breast cancer. Prostagl. Leukot. Essent. Fat. Acids 74 (4), 235–245. doi:10.1016/j.plefa.2006.01.009
Kaduce, T. L., Fang, X., Harmon, S. D., Oltman, C. L., Dellsperger, K. C., Teesch, L. M., et al. (2004). 20-hydroxyeicosatetraenoic acid (20-HETE) metabolism in coronary endothelial cells. J. Biol. Chem. 279 (4), 2648–2656. doi:10.1074/jbc.M306849200
Ketelhuth, D. F., Hermansson, A., Hlawaty, H., Letourneur, D., Yan, Z-Q., and Bäck, M. (2015). The leukotriene B4 receptor (BLT) antagonist BIIL284 decreases atherosclerosis in ApoE-/- mice. Prostagl. Other Lipid Mediat 121, 105–109. doi:10.1016/j.prostaglandins.2015.05.007
Khanapure, S. P., Garvey, D. S., Janero, D. R., and Letts, L. G. (2007). Eicosanoids in inflammation: Biosynthesis, pharmacology, and therapeutic frontiers. Curr. Top. Med. Chem. 7 (3), 311–340. doi:10.2174/156802607779941314
Kobayashi, T., Tahara, Y., Matsumoto, M., Iguchi, M., Sano, H., Murayama, T., et al. (2004). Roles of thromboxane A(2) and prostacyclin in the development of atherosclerosis in apoE-deficient mice. J. Clin. Invest 114 (6), 784–794. doi:10.1172/JCI21446
Krishnamoorthy, S., Recchiuti, A., Chiang, N., Yacoubian, S., Lee, C. H., Yang, R., et al. (2010). Resolvin D1 binds human phagocytes with evidence for proresolving receptors. Proc. Natl. Acad. Sci. U. S. A. 107 (4), 1660–1665. doi:10.1073/pnas.0907342107
Kuhn, H., Banthiya, S., and van Leyen, K. (2015). Mammalian lipoxygenases and their biological relevance. Biochim. Biophys. Acta 1851 (4), 308–330. doi:10.1016/j.bbalip.2014.10.002
Lagarde, M., Bernoud-Hubac, N., Calzada, C., Véricel, E., and Guichardant, M. (2013). Lipidomics of essential fatty acids and oxygenated metabolites. Mol. Nutr. Food Res. 57 (8), 1347–1358. doi:10.1002/mnfr.201200828
Lagarde, M., Guichardant, M., Bernoud-Hubac, N., Calzada, C., and Véricel, E. (2018). Oxygenation of polyunsaturated fatty acids and oxidative stress within blood platelets. Biochim. Biophys. Acta Mol. Cell Biol. Lipids 1863 (6), 651–656. doi:10.1016/j.bbalip.2018.03.005
Laguna-Fernandez, A., Checa, A., Carracedo, M., Artiach, G., Petri, M. H., Baumgartner, R., et al. (2018). ERV1/ChemR23 signaling protects against atherosclerosis by modifying oxidized low-density lipoprotein uptake and phagocytosis in macrophages. Circulation 138 (16), 1693–1705. doi:10.1161/CIRCULATIONAHA.117.032801
Lannan, K. L., Spinelli, S. L., Blumberg, N., and Phipps, R. P. (2017). Maresin 1 induces a novel pro-resolving phenotype in human platelets. J. Thromb. Haemost. 15 (4), 802–813. doi:10.1111/jth.13620
Li, P-L., and Campbell, W. B. (1997). Epoxyeicosatrienoic acids activate K+ channels in coronary smooth muscle through a guanine nucleotide binding protein. Circ. Res. 80 (6), 877–884. doi:10.1161/01.res.80.6.877
Libreros, S., Shay, A. E., Nshimiyimana, R., Fichtner, D., Martin, M. J., Wourms, N., et al. (2020). A new E-series resolvin: RvE4 stereochemistry and function in efferocytosis of inflammation-resolution. Front. Immunol. 11, 631319. doi:10.3389/fimmu.2020.631319
Liu, Y., Zhang, Y., Schmelzer, K., Lee, T. S., Fang, X., Zhu, Y., et al. (2005). The antiinflammatory effect of laminar flow: The role of PPARgamma, epoxyeicosatrienoic acids, and soluble epoxide hydrolase. Proc. Natl. Acad. Sci. U. S. A. 102 (46), 16747–16752. doi:10.1073/pnas.0508081102
Lundqvist, A., Sandstedt, M., Sandstedt, J., Wickelgren, R., Hansson, G. I., Jeppsson, A., et al. (2016). The arachidonate 15-lipoxygenase enzyme product 15-HETE is present in Heart tissue from patients with ischemic Heart disease and enhances clot formation. PLoS One 11 (8), e0161629. doi:10.1371/journal.pone.0161629
Luo, W., Liu, B., and Zhou, Y. (2016). The endothelial cyclooxygenase pathway: Insights from mouse arteries. Eur. J. Pharmacol. 780, 148–158. doi:10.1016/j.ejphar.2016.03.043
Luttmann, W., Herzog, V., Matthys, H., Thierauch, K-H., Virchow, J. C., and Kroegel, C. (1999). Modulation of cytokine release from mononuclear cells by prostacyclin, IL-4 and IL-13. Cytokine 11 (2), 127–133. doi:10.1006/cyto.1998.0410
Luttmann, W., Herzog, V., Virchow, J-C., Matthys, H., Thierauch, K-H., and Kroegel, C. (1996). Prostacyclin modulates granulocyte/macrophage colony-stimulating factor release by human blood mononuclear cells. Pulm. Pharmacol. 9 (1), 43–48. doi:10.1006/pulp.1996.0005
Ma, K., Nunemaker, C. S., Wu, R., Chakrabarti, S. K., Taylor-Fishwick, D. A., and Nadler, J. L. (2010). 12-Lipoxygenase products reduce insulin secretion and {beta}-Cell viability in human islets. J. Clin. Endocrinol. Metab. 95 (2), 887–893. doi:10.1210/jc.2009-1102
Mainka, M., George, S., Angioni, C., Ebert, R., Goebel, T., Kampschulte, N., et al. (2022). On the biosynthesis of specialized pro-resolving mediators in human neutrophils and the influence of cell integrity. Biochim. Biophys. Acta Mol. Cell Biol. Lipids 1867 (3), 159093. doi:10.1016/j.bbalip.2021.159093
Mandras, S., Kovacs, G., Olschewski, H., Broderick, M., Nelsen, A., Shen, E., et al. (2021). Combination therapy in pulmonary arterial hypertension-targeting the nitric oxide and prostacyclin pathways. J. Cardiovasc Pharmacol. Ther. 26 (5), 453–462. doi:10.1177/10742484211006531
Minno, G. D., Silver, M., and Gaetano, G. D. (1979). Prostaglandins as inhibitors of human platelet aggregation. Br. J. Haematol. 43 (4), 637–647. doi:10.1111/j.1365-2141.1979.tb03797.x
Miyahara, T., Runge, S., Chatterjee, A., Chen, M., Mottola, G., Fitzgerald, J. M., et al. (2013). D-series resolvin attenuates vascular smooth muscle cell activation and neointimal hyperplasia following vascular injury. Faseb J. 27 (6), 2220–2232. doi:10.1096/fj.12-225615
Miyata, N., and Roman, R. J. (2005). Role of 20-hydroxyeicosatetraenoic acid (20-HETE) in vascular system. J. Smooth Muscle Res. 41 (4), 175–193. doi:10.1540/jsmr.41.175
Muthalif, M. M., Benter, I. F., Khandekar, Z., Gaber, L., Estes, A., Malik, S., et al. (2000). Contribution of Ras GTPase/MAP kinase and cytochrome P450 metabolites to deoxycorticosterone-salt-induced hypertension. Hypertension 35 (1), 457–463. doi:10.1161/01.hyp.35.1.457
Nagahora, N., Yamada, H., Kikuchi, S., Hakozaki, M., and Yano, A. (2017). Nrf2 activation by 5-lipoxygenase metabolites in human umbilical vascular endothelial cells. Nutrients 9 (9), 1001. doi:10.3390/nu9091001
Node, K., Huo, Y., Ruan, X., Yang, B., Spiecker, M., Ley, K., et al. (1999). Anti-inflammatory properties of cytochrome P450 epoxygenase-derived eicosanoids. Science 285 (5431), 1276–1279. doi:10.1126/science.285.5431.1276
Obara, K., Koide, M., and Nakayama, K. (2002). 20-Hydroxyeicosatetraenoic acid potentiates stretch-induced contraction of canine basilar artery via PKC alpha-mediated inhibition of KCa channel. Br. J. Pharmacol. 137 (8), 1362–1370. doi:10.1038/sj.bjp.0704960
Oh, S. F., Dona, M., Fredman, G., Krishnamoorthy, S., Irimia, D., and Serhan, C. N. (2012). Resolvin E2 formation and impact in inflammation resolution. J. Immunol. 188 (9), 4527–4534. doi:10.4049/jimmunol.1103652
Ohira, T., Arita, M., Omori, K., Recchiuti, A., Van Dyke, T. E., and Serhan, C. N. (2010). Resolvin E1 receptor activation signals phosphorylation and phagocytosis. J. Biol. Chem. 285 (5), 3451–3461. doi:10.1074/jbc.M109.044131
Palmblad, J., Lerner, R., and Larsson, S. H. (1994). Signal transduction mechanisms for leukotriene B4 induced hyperadhesiveness of endothelial cells for neutrophils. J. Immunol. 152 (1), 262–269.
Pan, W. H., Hu, X., Chen, B., Xu, Q. C., and Mei, H. X. (2022). The effect and mechanism of lipoxin A4 on neutrophil function in LPS-induced Lung injury. Inflammation 1, 1. doi:10.1007/s10753-022-01666-5
Patricia, M. K., Kim, J. A., Harper, C. M., Shih, P. T., Berliner, J. A., Natarajan, R., et al. (1999). Lipoxygenase products increase monocyte adhesion to human aortic endothelial cells. Arterioscler. Thromb. Vasc. Biol. 19 (11), 2615–2622. doi:10.1161/01.atv.19.11.2615
Perry, S. C., Kalyanaraman, C., Tourdot, B. E., Conrad, W. S., Akinkugbe, O., Freedman, J. C., et al. (2020). 15-Lipoxygenase-1 biosynthesis of 7S,14S-diHDHA implicates 15-lipoxygenase-2 in biosynthesis of resolvin D5. J. Lipid Res. 61 (7), 1087–1103. doi:10.1194/jlr.RA120000777
Poeckel, D., and Funk, C. D. (2010). The 5-lipoxygenase/leukotriene pathway in preclinical models of cardiovascular disease. Cardiovasc Res. 86 (2), 243–253. doi:10.1093/cvr/cvq016
Pollock, K., and Creba, J. (1990). Leukotriene D4 induced calcium changes in U937 cells may utilize mechanisms additional to inositol phosphate production that are pertussis toxin insensitive but are blocked by phorbol myristate acetate. Cell Signal 2 (6), 563–568. doi:10.1016/0898-6568(90)90078-o
Praticò, D., and Dogné, J-M. (2009). Vascular biology of eicosanoids and atherogenesis. Expert Rev. Cardiovasc Ther. 7 (9), 1079–1089. doi:10.1586/erc.09.91
Randriamboavonjy, V., Busse, R., and Fleming, I. (2003). 20-HETE-induced contraction of small coronary arteries depends on the activation of Rho-kinase. Hypertension 41 (1), 801–806. doi:10.1161/01.HYP.0000047240.33861.6B
Recchiuti, A., and Serhan, C. N. (2012). Pro-resolving lipid mediators (SPMs) and their actions in regulating miRNA in novel resolution circuits in inflammation. Front. Immunol. 3, 298. doi:10.3389/fimmu.2012.00298
Riccioni, G., Bäck, M., and Capra, V. (2010). Leukotrienes and atherosclerosis. Curr. Drug Targets 11 (7), 882–887. doi:10.2174/138945010791320881
Ricciotti, E., and FitzGerald, G. A. (2011). Prostaglandins and inflammation. Arterioscler. Thromb. Vasc. Biol. 31 (5), 986–1000. doi:10.1161/ATVBAHA.110.207449
Ricke-Hoch, M., Stelling, E., Lasswitz, L., Gunesch, A. P., Kasten, M., Zapatero-Belinchón, F. J., et al. (2021). Impaired immune response mediated by prostaglandin E2 promotes severe COVID-19 disease. PloS one 16 (8), e0255335. doi:10.1371/journal.pone.0255335
Salic, K., Morrison, M. C., Verschuren, L., Wielinga, P. Y., Wu, L., Kleemann, R., et al. (2016). Resolvin E1 attenuates atherosclerosis in absence of cholesterol-lowering effects and on top of atorvastatin. Atherosclerosis 250, 158–165. doi:10.1016/j.atherosclerosis.2016.05.001
Sánchez-Galán, E., Gómez-Hernández, A., Vidal, C., Martín-Ventura, J. L., Blanco-Colio, L. M., Muñoz-García, B., et al. (2009). Leukotriene B4 enhances the activity of nuclear factor-kappaB pathway through BLT1 and BLT2 receptors in atherosclerosis. Cardiovasc Res. 81 (1), 216–225.
Scalia, R., Gefen, J., Petasis, N. A., Serhan, C. N., and Lefer, A. M. (1997). Lipoxin A4 stable analogs inhibit leukocyte rolling and adherence in the rat mesenteric microvasculature: Role of P-selectin. Proc. Natl. Acad. Sci. U. S. A. 94 (18), 9967–9972. doi:10.1073/pnas.94.18.9967
Schebb, N. H., Kühn, H., Kahnt, A. S., Rund, K. M., O'Donnell, V. B., Flamand, N., et al. (2022). Formation, signaling and occurrence of specialized pro-resolving lipid mediators-what is the evidence so far? Front. Pharmacol. 13, 838782. doi:10.3389/fphar.2022.838782
Schmid, T., and Brüne, B. (2021). Prostanoids and resolution of inflammation–beyond the lipid-mediator class switch. Front. Immunol. 12, 2838. doi:10.3389/fimmu.2021.714042
Schmöcker, C., Zhang, I. W., Kiesler, S., Kassner, U., Ostermann, A. I., Steinhagen-Thiessen, E., et al. (2018). Effect of omega-3 fatty acid supplementation on oxylipins in a routine clinical setting. Int. J. Mol. Sci. 19 (1), 180. doi:10.3390/ijms19010180
Schrör, K., and Rauch, B. H. (2015). Aspirin and lipid mediators in the cardiovascular system. Prostagl. Other Lipid Mediat 121 (1), 17–23.
Schuchardt, J. P., Schmidt, S., Kressel, G., Willenberg, I., Hammock, B. D., Hahn, A., et al. (2014). Modulation of blood oxylipin levels by long-chain omega-3 fatty acid supplementation in hyper- and normolipidemic men. Prostagl. Leukot. Essent. Fat. Acids 90 (2-3), 27–37. doi:10.1016/j.plefa.2013.12.008
Schuligoi, R., Sturm, E., Luschnig, P., Konya, V., Philipose, S., Sedej, M., et al. (2010). CRTH2 and D-type prostanoid receptor antagonists as novel therapeutic agents for inflammatory diseases. Pharmacology 85 (6), 372–382. doi:10.1159/000313836
Schwartzman, M. L., Falck, J., Yadagiri, P., and Escalante, B. (1989). Metabolism of 20-hydroxyeicosatetraenoic acid by cyclooxygenase. Formation and identification of novel endothelium-dependent vasoconstrictor metabolites. J. Biol. Chem. 264 (20), 11658–11662. doi:10.1016/s0021-9258(18)80115-6
Serezani, C. H., Lewis, C., Jancar, S., and Peters-Golden, M. (2011). Leukotriene B4 amplifies NF-κB activation in mouse macrophages by reducing SOCS1 inhibition of MyD88 expression. J. Clin. Invest 121 (2), 671–682. doi:10.1172/JCI43302
Sergeant, S., Rahbar, E., and Chilton, F. H. (2016). Gamma-linolenic acid, dihommo-gamma linolenic, eicosanoids and inflammatory processes. Eur. J. Pharmacol. 785, 77–86. doi:10.1016/j.ejphar.2016.04.020
Serhan, C. N., Dalli, J., Colas, R. A., Winkler, J. W., and Chiang, N. (2015). Protectins and maresins: New pro-resolving families of mediators in acute inflammation and resolution bioactive metabolome. Biochim. Biophys. Acta 1851 (4), 397–413. doi:10.1016/j.bbalip.2014.08.006
Serhan, C. N., Fredman, G., Yang, R., Karamnov, S., Belayev, L. S., Bazan, N. G., et al. (2011). Novel proresolving aspirin-triggered DHA pathway. Chem. Biol. 18 (8), 976–987. doi:10.1016/j.chembiol.2011.06.008
Serhan, C. N., Krishnamoorthy, S., Recchiuti, A., and Chiang, N. (2011). Novel anti-inflammatory--pro-resolving mediators and their receptors. Curr. Top. Med. Chem. 11 (6), 629–647. doi:10.2174/1568026611109060629
Serhan, C. N., and Levy, B. D. (2018). Resolvins in inflammation: Emergence of the pro-resolving superfamily of mediators. J. Clin. Invest 128 (7), 2657–2669. doi:10.1172/JCI97943
Serhan, C. N. (2005). Lipoxins and aspirin-triggered 15-epi-lipoxins are the first lipid mediators of endogenous anti-inflammation and resolution. Prostagl. Leukot. Essent. Fat. Acids 73 (3-4), 141–162. doi:10.1016/j.plefa.2005.05.002
Serhan, C. N., and Petasis, N. A. (2011). Resolvins and protectins in inflammation resolution. Chem. Rev. 111 (10), 5922–5943. doi:10.1021/cr100396c
Serhan, C. N., Yang, R., Martinod, K., Kasuga, K., Pillai, P. S., Porter, T. F., et al. (2009). Maresins: Novel macrophage mediators with potent antiinflammatory and proresolving actions. J. Exp. Med. 206 (1), 15–23. doi:10.1084/jem.20081880
Setty, B. N., and Stuart, M. J. (1986). 15-Hydroxy-5,8,11,13-eicosatetraenoic acid inhibits human vascular cyclooxygenase. Potential role in diabetic vascular disease. J. Clin. Invest 77 (1), 202–211. doi:10.1172/JCI112277
Singh, H., Cheng, J., Deng, H., Kemp, R., Ishizuka, T., Nasjletti, A., et al. (2007). Vascular cytochrome P450 4A expression and 20-hydroxyeicosatetraenoic acid synthesis contribute to endothelial dysfunction in androgen-induced hypertension. Hypertension 50 (1), 123–129. doi:10.1161/HYPERTENSIONAHA.107.089599
Sitbon, O., Channick, R., Chin, K. M., Frey, A., Gaine, S., Galiè, N., et al. (2015). Selexipag for the treatment of pulmonary arterial hypertension. N. Engl. J. Med. 373 (26), 2522–2533. doi:10.1056/NEJMoa1503184
Sonnweber, T., Pizzini, A., Nairz, M., Weiss, G., and Tancevski, I. (2018). Arachidonic acid metabolites in cardiovascular and metabolic diseases. Int. J. Mol. Sci. 19 (11), 3285. doi:10.3390/ijms19113285
Spiecker, M., and Liao, J. K. (2005). Vascular protective effects of cytochrome p450 epoxygenase-derived eicosanoids. Arch. Biochem. Biophys. 433 (2), 413–420. doi:10.1016/j.abb.2004.10.009
Tager, A. M., Bromley, S. K., Medoff, B. D., Islam, S. A., Bercury, S. D., Friedrich, E. B., et al. (2003). Leukotriene B4 receptor BLT1 mediates early effector T cell recruitment. Nat. Immunol. 4 (10), 982–990. doi:10.1038/ni970
Tager, A. M., Dufour, J. H., Goodarzi, K., Bercury, S. D., von Andrian, U. H., and Luster, A. D. (2000). BLTR mediates leukotriene B(4)-induced chemotaxis and adhesion and plays a dominant role in eosinophil accumulation in a murine model of peritonitis. J. Exp. Med. 192 (3), 439–446. doi:10.1084/jem.192.3.439
Takata, S., Matsubara, M., Allen, P. G., Janmey, P. A., Serhan, C. N., and Brady, H. R. (1994). Remodeling of neutrophil phospholipids with 15(S)-hydroxyeicosatetraenoic acid inhibits leukotriene B4-induced neutrophil migration across endothelium. J. Clin. Invest 93 (2), 499–508. doi:10.1172/JCI116999
Ternowitz, T., Fogh, K., and Kragballe, K. (1988). 15-Hydroxyeicosatetraenoic acid (15-HETE) specifically inhibits LTB4-induced chemotaxis of human neutrophils. Skin. Pharmacol. 1 (2), 93–99. doi:10.1159/000210754
Toda, A., Terawaki, K., Yamazaki, S., Saeki, K., Shimizu, T., and Yokomizo, T. (2010). Attenuated Th1 induction by dendritic cells from mice deficient in the leukotriene B4 receptor 1. Biochimie 92 (6), 682–691. doi:10.1016/j.biochi.2009.12.002
Toda, A., Yokomizo, T., and Shimizu, T. (2002). Leukotriene B4 receptors. Prostagl. Other Lipid Mediat 68-69, 575–585. doi:10.1016/s0090-6980(02)00056-4
Tourdot, B. E., Adili, R., Isingizwe, Z. R., Ebrahem, M., Freedman, J. C., Holman, T. R., et al. (2017). 12-HETrE inhibits platelet reactivity and thrombosis in part through the prostacyclin receptor. Blood Adv. 1 (15), 1124–1131. doi:10.1182/bloodadvances.2017006155
Tourdot, B. E., and Holinstat, M. (2017). Targeting 12-lipoxygenase as a potential novel antiplatelet therapy. Trends Pharmacol. Sci. 38 (11), 1006–1015. doi:10.1016/j.tips.2017.08.001
Tull, S. P., Yates, C. M., Maskrey, B. H., O'Donnell, V. B., Madden, J., Grimble, R. F., et al. (2009). Omega-3 fatty acids and inflammation: Novel interactions reveal a new step in neutrophil recruitment. PLoS Biol. 7 (8), e1000177. doi:10.1371/journal.pbio.1000177
Van Doren, L., Nguyen, N., Garzia, C., Fletcher, E., Stevenson, R., Jaramillo, D., et al. (2019). Blockade of lipid receptor GPR31 suppresses platelet reactivity and thrombosis with minimal effect on hemostasis. Blood 134, 1064. doi:10.1182/blood-2019-126111
Van Doren, L., Nguyen, N., Garzia, C., Fletcher, E. K., Stevenson, R., Jaramillo, D., et al. (2021). Lipid receptor GPR31 (G-Protein-Coupled receptor 31) regulates platelet reactivity and thrombosis without affecting hemostasis. Arterioscler. Thromb. Vasc. Biol. 41 (1), e33–e45. doi:10.1161/ATVBAHA.120.315154
Vanderhoek, J. Y., Schoene, N. W., and Pham, P. P. (1991). Inhibitory potencies of fish oil hydroxy fatty acids on cellular lipoxygenases and platelet aggregation. Biochem. Pharmacol. 42 (4), 959–962. doi:10.1016/0006-2952(91)90062-a
Vane, J., Bakhle, Y., and Botting, R. (1998). Cyclooxygenases 1 and 2. Annu. Rev. Pharmacol. Toxicol. 38 (1), 97–120. doi:10.1146/annurev.pharmtox.38.1.97
Vijil, C., Hermansson, C., Jeppsson, A., Bergström, G., and Hultén, L. M. (2014). Arachidonate 15-lipoxygenase enzyme products increase platelet aggregation and thrombin generation. PLoS One 9 (2), e88546. doi:10.1371/journal.pone.0088546
Wang, D., and Dubois, R. N. (2010). Eicosanoids and cancer. Nat. Rev. Cancer 10 (3), 181–193. doi:10.1038/nrc2809
Werz, O., Gerstmeier, J., Libreros, S., De la Rosa, X., Werner, M., Norris, P. C., et al. (2018). Human macrophages differentially produce specific resolvin or leukotriene signals that depend on bacterial pathogenicity. Nat. Commun. 9 (1), 59. doi:10.1038/s41467-017-02538-5
Whatling, C., McPheat, W., and Herslöf, M. (2007). The potential link between atherosclerosis and the 5-lipoxygenase pathway: Investigational agents with new implications for the cardiovascular field. Expert Opin. Investig. Drugs 16 (12), 1879–1893. doi:10.1517/13543784.16.12.1879
Whitaker, M. O., Wyche, A., Fitzpatrick, F., Sprecher, H., and Needleman, P. (1979). Triene prostaglandins: Prostaglandin D3 and icosapentaenoic acid as potential antithrombotic substances. Proc. Natl. Acad. Sci. U. S. A. 76 (11), 5919–5923. doi:10.1073/pnas.76.11.5919
Woszczek, G., Chen, L-Y., Nagineni, S., Alsaaty, S., Harry, A., Logun, C., et al. (2007). IFN-gamma induces cysteinyl leukotriene receptor 2 expression and enhances the responsiveness of human endothelial cells to cysteinyl leukotrienes. J. Immunol. 178 (8), 5262–5270. doi:10.4049/jimmunol.178.8.5262
Yamaguchi, A., Stanger, L., Freedman, C., Prieur, A., Thav, R., Tena, J., et al. (2022). Supplementation with omega-3 or omega-6 fatty acids attenuates platelet reactivity in postmenopausal women. Clin. Transl. Sci. 1, 1.
Yeung, J., Adili, R., Yamaguchi, A., Freedman, C. J., Chen, A., Shami, R., et al. (2020). Omega-6 DPA and its 12-lipoxygenase-oxidized lipids regulate platelet reactivity in a nongenomic PPARα-dependent manner. Blood Adv. 4 (18), 4522–4537. doi:10.1182/bloodadvances.2020002493
Yeung, J., Hawley, M., and Holinstat, M. (2017). The expansive role of oxylipins on platelet biology. J. Mol. Med. Berl. 95 (6), 575–588. doi:10.1007/s00109-017-1542-4
Yeung, J., and Holinstat, M. (2011). 12-lipoxygenase: A potential target for novel anti-platelet therapeutics. Cardiovasc Hematol. Agents Med. Chem. 9 (3), 154–164. doi:10.2174/187152511797037619
Yeung, J., and Holinstat, M. (2017). Who is the real 12-HETrE? Prostagl. Other Lipid Mediat 132, 25–30. doi:10.1016/j.prostaglandins.2017.02.005
Yeung, J., Tourdot, B. E., Adili, R., Green, A. R., Freedman, C. J., Fernandez-Perez, P., et al. (2016). 12(S)-HETrE, a 12-lipoxygenase oxylipin of dihomo-γ-linolenic acid, inhibits thrombosis via Gαs signaling in platelets. Arterioscler. Thromb. Vasc. Biol. 36 (10), 2068–2077. doi:10.1161/ATVBAHA.116.308050
Yokomizo, T., Izumi, T., Chang, K., Takuwa, Y., and Shimizu, T. (1997). A G-protein-coupled receptor for leukotriene B4 that mediates chemotaxis. Nature 387 (6633), 620–624. doi:10.1038/42506
Yokomizo, T., Kato, K., Terawaki, K., Izumi, T., and Shimizu, T. (2000). A second leukotriene B(4) receptor, BLT2. A new therapeutic target in inflammation and immunological disorders. J. Exp. Med. 192 (3), 421–432. doi:10.1084/jem.192.3.421
Yokomizo, T., Nakamura, M., and Shimizu, T. (2018). Leukotriene receptors as potential therapeutic targets. J. Clin. Invest 128 (7), 2691–2701. doi:10.1172/JCI97946
Zhao, M., Ma, J., Li, M., Zhang, Y., Jiang, B., Zhao, X., et al. (2021). Cytochrome P450 enzymes and drug metabolism in humans. Int. J. Mol. Sci. 22 (23), 12808. doi:10.3390/ijms222312808
Zhou, W., Blackwell, T. S., Goleniewska, K., O'Neal, J. F., Fitzgerald, G. A., Lucitt, M., et al. (2007). Prostaglandin I2 analogs inhibit Th1 and Th2 effector cytokine production by CD4 T cells. J. Leukoc. Biol. 81 (3), 809–817. doi:10.1189/jlb.0606375
Zhou, W., Hashimoto, K., Goleniewska, K., O'Neal, J. F., Ji, S., Blackwell, T. S., et al. (2007). Prostaglandin I2 analogs inhibit proinflammatory cytokine production and T cell stimulatory function of dendritic cells. J. Immunol. 178 (2), 702–710. doi:10.4049/jimmunol.178.2.702
Keywords: eicosanoids, inflammation, oxygenases, blood, blood vessel
Citation: Yamaguchi A, Botta E and Holinstat M (2022) Eicosanoids in inflammation in the blood and the vessel. Front. Pharmacol. 13:997403. doi: 10.3389/fphar.2022.997403
Received: 18 July 2022; Accepted: 05 September 2022;
Published: 27 September 2022.
Edited by:
Pallavi R. Devchand, University of Calgary, CanadaReviewed by:
Hong Yong Peh, Brigham and Women’s Hospital and Harvard Medical School, United StatesCopyright © 2022 Yamaguchi, Botta and Holinstat. This is an open-access article distributed under the terms of the Creative Commons Attribution License (CC BY). The use, distribution or reproduction in other forums is permitted, provided the original author(s) and the copyright owner(s) are credited and that the original publication in this journal is cited, in accordance with accepted academic practice. No use, distribution or reproduction is permitted which does not comply with these terms.
*Correspondence: Michael Holinstat, bWhvbGluc3RAdW1pY2guZWR1
Disclaimer: All claims expressed in this article are solely those of the authors and do not necessarily represent those of their affiliated organizations, or those of the publisher, the editors and the reviewers. Any product that may be evaluated in this article or claim that may be made by its manufacturer is not guaranteed or endorsed by the publisher.
Research integrity at Frontiers
Learn more about the work of our research integrity team to safeguard the quality of each article we publish.