- 1Department of Pharmacology, Faculty of Pharmaceutical Sciences, Government College University Faisalabad, Faisalabad, Pakistan
- 2Riphah Institute of Pharmaceutical Sciences, Riphah International University, Lahore Campus, Lahore, Pakistan
Daphnetin (DAP), a coumarin derivative extracted from Daphne species, is biologically active phytochemical with copious bioactivities including anti-inflammatory, anti-oxidant, neuroprotective, analgesic, anti-pyretic, anti-malarial, anti-bacterial, anti-arthritic, neuroprotective, hepatoprotective, nephroprotective, and anti-cancer activities. A wide range of studies have been conducted exploring the significance and therapeutic potential of DAP. This study reviewed various databases such as NCBI, PubMed, Web of Science, Scopus and Google Scholar for published research articles regarding the sources, synthesis, and various bioactivities of DAP using different key words, including but not limited to “pharmacological activities,” “sources,” “neuroprotective effect,” “synthesis,” “cancer,” “anti-inflammatory effect” of “daphnetin.” Furthermore, this review encompasses both in-vivo and in-vitro studies on DAP for treating various diseases. A comprehensive review of the literature revealed that the DAP had a promising pharmacological and safety profile, and could be employed as a pharmaceutical moiety to treat a variety of illnesses including microbial infections, cancer, arthritis, hepatic damage, inflammation and neurological anomalies. The current review intends to provide an in-depth focus on all pharmacological activities and therapeutic approaches for the pharmaceutical and biomedical researchers.
1 Introduction
Phytochemicals are secondary metabolites that naturally exist in plants. These are categorized into various groups based on their chemical structure (Martinez et al., 2017). Coumarin is a naturally occurring secondary metabolite and benzopyrone derivative. This was one of the first metabolites to be identified in the 1930’s, and found in a variety of plant species (Archbold et al., 2011; Xu et al., 2011; Amin et al., 2014). A significant number of researches have been conducted to identify individual compounds, and develop procedures for their detection, synthesis, effectiveness and toxicity (Sovrlić and Manojlović, 2017). Since then, over 50 coumarins have been discovered in Daphne species. Depending on their structure, there are simple, dimeric or trimeric coumarins. Daphne contains various coumarins including: daphnetin, DAP-8-glucoside, daphnin, esculin, umbelliferone, and acetil-umbelliferon (Manojlović et al., 2012; Sovrlić et al., 2015). Rutarensin, daphnoretin, daphneretusin-A, dimethyl-daphnoretin-7-O-glucoside are categorized among dimeric coumarins while trimeric coumarin metabolites i.e., daphneretusin B, and triumbellin were also identified in Daphne species (Mansoor et al., 2013). This review elaborates sources, pharmacological activities as well as toxicity of daphnetin (DAP) so as to find and explore its therapeutic potential and promote the drug development.
DAP i.e., 7, 8-dihydroxycoumarin is generally an odorless and tasteless white or off-white powder that is freely soluble in ethanol, methanol and dimethyl-sulfoxide while slightly soluble in water (Shan et al., 2011). It has a molecular weight of 178.14 g/mole and melting point 262.0°C (Liao et al., 2013). It shows high solubility and permeability, and is metabolized by phase 1 reaction through CYP3A4 with a short half-life of 15 min. It exhibits poor bioavailability and absorbs through the intestine by passive diffusion. It is metabolized to methyl, glucuronide and sulfonate conjugated metabolites (Yang et al., 1999; Du et al., 2009; Shan, 2009; Liang et al., 2010; Chen, 2011; Zhang et al., 2014; Liang et al., 2015; Liang et al., 2017; Xia et al., 2018a). Some studies on DAP metabolism focused on glucuronidation, but other studies provided glimpse of other conjugated metabolites such as sulfonation, and methylation. In comparison to glucuronidation and sulfonation, the methylation pathway demonstrated a higher clearance rate (Liang et al., 2016).
The DAP is derived from different Daphne species. Daphne is a genus comprising 70 to 95 species of perennial and evergreen shrubs of Thymelaeaceae family that is indigenous to India, Europe, and North Africa. These plants are renowned for their fragrant flowers and brilliantly colored fruit (Riveiro et al., 2010). DAP-8-glucoside is derived from D. odora in which it is formed from DAP-7-glucoside (Ueno and Saito, 1976; Halda et al., 1998). Other sources of DAP include D. gnidium (isolated from the leaves and stems), D. mezereum (synthesized from shoots), D. giraldii, D. Koreana Nakai, D. tangutica and D. oleoides. Seventeen compounds including DAP were isolated from D. oleoides (Brown, 1986; Riaz et al., 2016; Han et al., 2020; Khouchlaa et al., 2021). D. pedunculata leaves and stems are also sources of DAP (Moshiashvili et al., 2020) as shown in Figure 1. E. lathyris Linnaeus, ethnically known as “Euphorbia semen” in East Asia, is also a source of coumarins including DAP. Previously, it was reported that simple coumarins including daphnetin, esculetin, esculin etc. Had been isolated and identified from the seeds of E. semen (Masamoto et al., 2003; Zhu et al., 2018). Different sources of DAP are shown in Figure 1.
This review focusses on the DAP-based treatment and prevention of diseases which are gradually receiving special attention due to underlying exceptional properties of DAP. In this context, an overview of DAP’s significance as an essential phytochemical and its intriguing uses have been presented and addressed. Relevant data were collected using various search engines such as Google scholar, PubMed, Web of Science, NCBI and Scopus by using the various search terms such as “daphnetin,” “Structure activity of daphnetin,” “sources of daphnetin,” “Synthesis of daphnetin,” “Traditional uses of Daphne species,” “isolation,” “physical properties,” “pharmacology of Daphentin” “hepatoprotective,” “neuroprotective,” “anti-inflammatory,” “anti-arthritic” and “anti-cancer” and “toxicity of daphnetin,” etc.
2 synthesis of Daphnetin
DAP, naturally occurring or synthesized, has oxygen-containing heterocycles with a characteristic benzo-α-pyrone framework (Xia et al., 2018b). It is biosynthesized from shikimate pathway from L-Tyrosine and L-Phenylalanine (Norma Francenia, 2019). Previously, it was synthesized when pyrogallol and malic acid were heated in concentrated H2SO4 under nitrogen presence. It is also synthesized from umbelliferone by hydroxylation as shown in Figure 2 (NDong et al., 2003; Pan et al., 2017; Wang et al., 2020a).
3 Pharmacological activities of Daphnetin
The DAP has been used to treat coagulation disorders, various skin diseases, rheumatoid arthritis (RA), cancer, lumbago, and fever (Tu et al., 2012; Wang et al., 2013). It exhibited numerous pharmacological activities, including analgesic, anti-pyretic (Singh et al., 2021a), anti-arthritic, anti-inflammatory, anti-oxidant (Qi et al., 2016; Lv et al., 2018), anti-proliferative (Fylaktakidou et al., 2004; Kostova et al., 2011), anti-bacterial (Cottigli et al., 2001), neuroprotective (Qi et al., 2016), cardio-protective, nephroprotective, stroke, coagulation disorders, ischemic brain injury, hepatoprotective and anti-cancer activities (Pinto and Silva, 2017; Zhang et al., 2018; Boulebd and Khodja, 2021) as mentioned in Figure 3. It has been used for treating RA and coagulation disorders for long duration without significant toxic effects (Du et al., 2014). Pharmacological actions of DAP are summarized in Table 1.
3.1 Neuroprotective action
Nerve cells interact with one another to carry out various physiological functions. Any communication breakdown in the brain, even in a solitary area, can impair the operation of other brain regions and is the main factor in catastrophic neurological illnesses or neurodegenerative disorders of the central and peripheral nervous systems. Progressive neuronal degeneration causes temporary or permanent sensory loss in a variety of neurodegenerative disorders. The reactive oxygen species (ROS) and inflammatory signaling molecules i.e., tumor necrosis factor alpha (TNF-α), and interleukin (IL)-6 are the key contributors to neurodegenerative disorders (Qi et al., 2016; Chitnis and Weiner, 2017; Boulebd and Khodja, 2021). For neuroprotective action, DAP reduces Toll-like receptor-4 (TLR-4), nuclear factor-ĸβ (NF-ĸβ), and other pro-inflammatory cytokines. It also inhibits JAK/STAT phosphorylation which is responsible for the increase of pro-inflammatory cytokines and enzymes, culminating in the reduction in COX-2 and inducible nitric oxide synthase (iNOS) levels. It significantly enhances the Nrf-2 expression. DAP is reported to enhance Heat shock protein (HSP)-70 by downregulating the expression of NF-ĸβ and mitogen-activated protein kinase (MAPK) at the molecular level, causing the enzymes to regulate neuronal apoptosis by increasing or decreasing the phosphorylation of pro-apoptotic proteins (Bax/Bad) and an anti-apoptotic protein (Bcl-2) (Singh et al., 2021a).
3.1.1 Cognition and memory
DAP has shown significant potential to prevent memory loss and cognition. It inhibited apoptosis and calcium overload induced by down-regulating NR2B-containing N-methyl-D-aspartate (NMDA) receptors as well as calcium accumulation which was activated by glutamate and caused excitation of neurons. DAP exhibited neuroprotective properties by preventing NMDA-induced neuronal cell loss and regulating the balance of Bcl-2 and Bax expression in cortisol neurons of mice (Yang et al., 2014).
The neuroprotective effect of DAP has been reported in the posterior cerebral artery occlusion (MCAO)/reperfusion mice model. The DAP (1 mg/kg) showed a substantial reduction in cerebral infarct volume (Du et al., 2014). It has a neuroprotective effect in stressed mice on microglial activation and its subsequent inflammatory response. The pre-incubation with DAP dramatically inhibited TNF-α and IL-1 production in lipopolysaccharide (LPS) or ß-amyloid activated BV2 cells.
The MAPK and protein kinase B (Akt) pathways play a negative role in the anti-inflammatory action of DAP. Furthermore, pre-treatment with Wortmannin, a PI-3 k/Akt inhibitor, resulted in a significant decrease in LPS-induced TNF-α and nitric oxide generation in BV2 cells, demonstrating an opposing role of the MAPK/Akt pathway in mediating anti-inflammatory effect of DAP. It also reduced the expression of NF-κB to promote neuroprotection (Yu et al., 2014a). The neuroprotective action of DAP is presented in Figure 4.
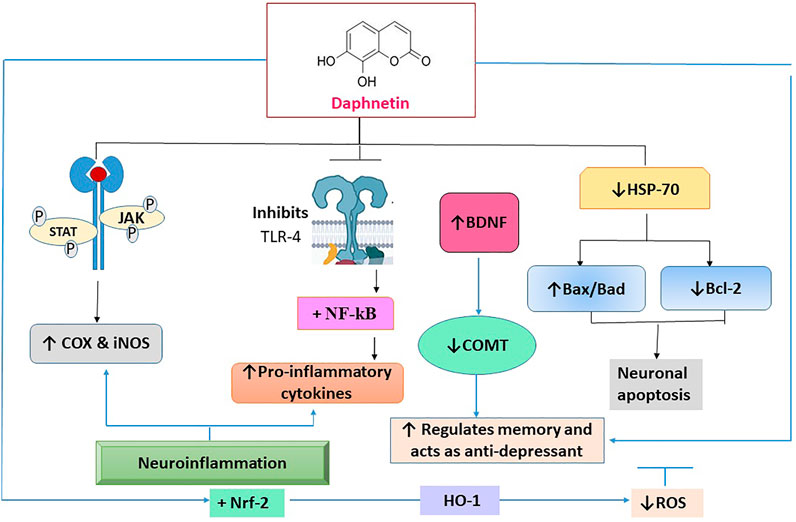
FIGURE 4. The molecular interaction of multiple mediators implicated in the neuroprotective effect of daphnetin. TLR-4: toll-like receptor -4; NF-ĸβ: nuclear factor-ĸβ; Janus kinase: JAK/STAT; COX-2: cyclooxygenase -2; iNOS: inducible nitric oxide; Nrf-2: Nuclear factor erythroid 2-related factor; MAPK: mitogen activated protein kinase; Bcl-2: pro-apoptotic proteins; Bax/Bad; anti-apoptotic protein; ROS: reactive oxygen species.
In another study, the neuroprotective effect in mice was studied by utilizing the middle cerebral artery occlusion (MCAO)/R model. It reduced the extent of the MCAO/R-induced cerebral infarct, neuronal apoptosis, and brain IL-1β, IL-6, TLR-4/NF-kB, and TNF-α levels in the cerebral cortex (Vivier et al., 2016). Lei Shen and his coworkers showed that DAP had reduced endotoxin lethality in a mouse model of LPS-induced endotoxemia and suppressed the inflammatory response to LPS in Raw264.7 cells by inhibiting ROS generation, JAK1 and JAK2, and enhancing suppression of STAT1 and STAT3 phosphorylation, and ultimately prevented the STAT1 and STAT3 transport in the nucleus (Shen et al., 2017).
Several preclinical researches have revealed that DAP had improved spatial memory and depressed behavior. The chronic unexpected stress mice model was used to assess the effect of DAP. It improved the Chronic Unpredictable Stress (CUS) impaired spatial memory as indicated by mouse performance in the Morris water maze test. DAP (2 and 8 mg/kg) injection reduced the immobilization time in a forced swim test compared to the CUS-treated group, confirming the involvement of DAP in improving spatial memory and restoring depressive behavior in mice (Liao et al., 2013).
3.1.2 Stroke
Cerebral ischemia is caused by impaired blood flow and is accompanied by an inflammatory reaction, release of cytokines, and inflammatory mediators that play a pivotal role in the development of stroke (Iadecola and Alexander, 2001). The TLR4 is highly induced after reperfusion injury. In an earlier study, DAP was used for treating cerebral ischemia/reperfusion injury and it was found to exhibit neuroprotective and anti-inflammatory effect by inhibiting TLR4/NF-kβ pathway, alleviating the production of inflammatory cytokines and neural cell apoptosis (Liu et al., 2016a). DAP (at 5, 10, 25, 50, 75, and 100 μM/L) displayed dose-dependent neuroprotective action in glutamate-induced toxicity in hippocampal HT22 cells and ischemic brain injury by restoring reduced glutathione (GSH) and superoxide dismutase (SOD) (Du et al., 2014).
It was further found that DAP suppressed oxidative stress and cell apoptosis in hippocampal neurons. It increased nuclear translocation of nuclear factor erythroid 2 (Nrf2) and HO-1 expression in neurons exposed to reoxygenation-induced cell injury at 10, 20, and 40 µM doses. DAP inhibited oxidative stress and neuronal apoptosis by activating Nrf2/HO-1 signaling pathway (Zhi et al., 2019).
3.2 Hepatoprotective action
Excessive generation of ROS causes significant damage to cell membrane phospholipids, proteins and DNA leading to a variety of disorders (Blair, 2008; Mendes-Braz and Martins, 2018; Zhao et al., 2018). DAP reduced hepatotoxicity caused by tert-butyl hydroperoxide (t-BHP) and acetaminophen through the modulating Nrf2/Trx-1 pathway. It effectively prevented t-BHP-induced hepatotoxicity by regulating Nrf2/Trx-1 pathway in HepG2 cells. Moreover, it inhibited ASK1/JNK activation and decreased the acute liver failure (ALF), cytochrome C, and Bax mitochondrial translocation, all of which concomitantly restored the mitochondrial function. It is also found that DAP inhibited inflammatory reactions in the liver by inactivating the thioredoxin-interacting protein (Txnip)/NLRP3 inflammasome. It also improved the Nrf2 nuclear translocation and Trx-1 expression (Lv et al., 2020). It inhibited MAPK, NF-kβ, nucleotide binding domain like receptor protein 3 (NLRP3) and decreased the pro-inflammatory cytokines in acute liver failure (ALF) (Lv et al., 2018). The hepatoprotective mechanism of DAP is given in Figure 4.
A study reported that the DAP had reduced the lipid accumulation within the hepatocytes by regulating PI3K expression and pAKT/AKT levels. Moreover, it decreased the insulin resistance by promoting the hepatocellular glucose uptake through upregulating the expression of Nrf2. In addition, DAP reduced the level of ROS in hepatocytes by downregulating the expression of CYP2E1 and CYP4A (Liu et al., 2019). In an earlier study, DAP improved carbon tetrachloride (CCL4)-induced biochemical changes. It decreased the CCL4 induced-lipid peroxidation and boosted the antioxidant defense system. DAP induced nuclear translocation of Nrf2 related factor 2 to induce the expression of hydroxyl ion. Thus, DAP prevented the hepatotoxicity induced by oxidative stress by activating Nrf2-mediated hydroxyl ion expression (Mohamed et al., 2014).
3.3 Effect on heavy metal and endotoxin induced lung injury
It is found that DAP exhibited a phenomenal anti-oxidant in arsenic-induced cytotoxicity in human lung epithelial cells. DAP (at 2.5, 5, 10 g/ml) progressively shielded Beas-2B cells from NaAsO2-induced apoptosis as well as arsenic cytotoxicity via Nrf2-dependent pathway and increased GSH level (Lv et al., 2019).
Endotoxin is an important toxin precipitating lung injury and is responsible for increased serum concentration of all cytokines and growth factors (Rojas et al., 2005). DAP at 5 and 10 mg/kg provided considerable protection from endotoxin-induced acute lung injury in mice by inhibiting the activation of macrophages and human alveolar epithelial cells through reducing the production of inflammatory mediators, induction of TNF-α induced protein 3 (TNFAIP3) and decreasing the expression of iNOS and NF-κB to attenuate inflammation. It also downregulated the phosphorylation of MAPKs including p38, extracellular signal regulated kinase (ERK), and JNK kinases (Yu et al., 2014b).
3.5 Anti-bacterial action
DAP was investigated for anti-bacterial activity against Helicobacter pylori; a Gram-negative bacterium that usually colonizes stomach causing gastritis and peptic ulcers (Zali, 2011). DAP showed virtuous activity against multidrug resistant (MDR) H. pylori via enhancing DNA damage, phosphatidylserine (PS) translocation, and recA expression while downregulating blood group antigen binding adhesion (babA) and urease l (urel) with the decrease in the attachment of H. pylori to GES-1 cells with minimal inhibitory concentration (MIC) from 25 to 100 μg/ml (Wang et al., 2019); (Walker and Crabtree, 1998). It also showed antibacterial activity by destroying cell wall and preventing membrane coherence of Pseudomonas fluorescens and Shewanella putrefaciens with MIC of 0.16 and 0.08 mg/ml respectively (Liu et al., 2020). In another study, the effect of DAP on the Ralstonia solanacearum was investigated in which it was found that DAP had exhibited strongest anti-bacterial effect due to the presence of hydroxylation at C6, C7, or C8 which increased its anti-bacterial effect by destructing the bio-membrane against R. solanacearum with the MIC at 64 mg/L (Yang et al., 2016).
DAP (at 10 mg/kg i.p.) was used to treat bacterial pneumonia caused by methicillin-resistant Staphylococcus aureus (MRSA) in C57BL/6 mice. It protected against inflammation, tissue damage, and stimulated the mTOR-dependent autophagy pathway, which resulted in the increased bactericidal activity of macrophages by suppressing ROS production (Zhang et al., 2019).
3.6 Anti-malarial action
Malaria is one of the major fatal diseases, affecting around 1 million people worldwide and leading to death (Hu et al., 2018). DAP and its two derivatives, DAP78 and DAP79, have demonstrated anti-malarial activity against Plasmodium falciparum; nevertheless, DAP functions as an iron chelator, and its anti-malarial potency decreased significantly with time, leading its chelating action to be abolished (Huang et al., 2006). In another investigation, DAP was found to have a high iron chelating activity when compared to the potent iron-chelator desferroxamine B at different dosages (Mu et al., 2002). It caused 50% inhibition of 3H-hypoxanthine incorporation by P. falciparum at 25 and 40 µM. DAP did not immediately generate superoxide under in-vitro conditions, therefore it is not considered an oxidant. However, during in-vivo studies, it significantly prolonged the survival of mice infected with P. yoelli (Yang et al., 1992). Wang et al. (2000) reported the schizontocidal activity of DAP by using P. falciparum FCC1 strain in-vitro. The in-vivo activity was evaluated against P. berghei in Anka mice at the dosage of 10–100 mg/kg/day which demonstrated positive outcome.
3.7 Anti-inflammatory and anti-arthritic actions
The excess endogenous production of ROS leads to oxidative stress due to decreased concentration of GSH, SOD, and increased level of malondialdehyde (MDA). Oxidative stress also causes activation of the NF-κB pathway. This pathway controls the release of different cytokines by directing the expression of number of pro-inflammatory cytokines, inhibiting the apoptosis proteins (IAPS) and COX-2 which leads to inflammation. For anti-inflammatory action, DAP inhibits these pathways.
Adjuvant-induced arthritis is an autoimmune disorder characterized by chronic inflammation of joints that exhibits the same pathological response as that of RA (Connor et al., 1995). Various pro-inflammatory mediators play a significant role in the pathogenesis of this disorder (Barsante et al., 2005). DAP significantly attenuated the poly-arthritis by suppressing the production of pro-inflammatory cytokines (IL-1 and TNF-α) (Gao et al., 2008).
In another study, DAP alleviated the inflammation and pathological changes in the joint tissue, synovial hyperplasia, and chondrocyte degeneration in collagen-induced arthritis (CIA) in female rats at 1 and 4 mg/kg by restoring the expression of Th1/Th2/Th17 type cytokines, Foxp3, IL-17, IL-6, TGF- β, IL-4, and IFN- γ (Tu et al., 2012). In another study, it inhibited the proliferation of fibroblast-like synoviocytes (FLS) in rats with CIA and induced apoptosis by suppressing PI3k/AKT/MTOR signaling pathway at 0-60 μg/ml (Deng et al., 2020). In another study, DAP was combined with B cell lymphoma 2 targeted small interfering RNA (si-Bcl2) on fibroblast-like synoviocytes (FLS) in rats with CIA by downregulation of Bcl2. When si-Bcl2 was combined with DAP (40 μg/ml), it increased the effect via promoting apoptosis on RAFLS and by reducing the expression of Bcl2 and STAT3 (Chen et al., 2018).
In a previous study, DAP reduced the serum level of Th17, Th2, and Th1 type cells and upregulated the levels of Tregs in arthritis rats at 1 and 4 mg/kg. It also decreased RORγt, NF-kB, and CD77 in joint tissue while increased the expression of Foxp3 and IL-10. Thus it modulated the balance of Tregs and Th17 cells and is considered to be an effective agent in the treatment of CIA in rats (Yao et al., 2011).
Zhang et al. (2020) reported the chondroprotective effect of DAP against osteoarthritis. DAP (at 12, 24 and 48 ng/ml) profoundly protected chondrocytes of rabbits by averting IL-1β, -6,-12, MMP3,-9, and -13 and decreasing the caspase-3 and BAX while increasing BCL-2. In another study, DAP exhibited anti-arthritic action by demethylation of pro-apoptotic genes in synovial cells (FasL and P53). For this purpose, MTT analysis was performed on CIA-treated rat synovial cells to determine the inhibitory effect of DAP and DNA methyltransferase inhibitor drug (5-aza-dc) in the range of 1.25-40 μg/ml. It inhibited cell growth in synovial cells in a dose and time dependent manner (Shu et al., 2014). Zheng et al. reported anti-arthritic action of DAP using collagen induced FLS. It escalated caspase 3, 8, and 9, Bax, FasL, and cytochrome c (Cyt-c) with the reduction in Bcl-2 and enhanced the Cyt-c discharge from mitochondria to the cytosol (Zheng et al., 2020). In another study, DAP at 4 and 8 mg/kg inhibited spinal glial activation in murine mice provoked by CFA. It also decreased the expression of pro-inflammatory cytokines. It inhibited the NF-κβ pathway and activated the Nrf2/HO-1 signaling pathway (Yang et al., 2021a).
3.8 Osteoporosis
Glucocorticoids are effective agents in treating inflammatory and autoimmune diseases. While its long-term usage results in osteoporosis. DAP at 1 and 4 mg/kg exhibited the therapeutic action against dexamethasone induced osteoporosis in male rats by restoring bone mineral content, microstructure parameters, and bone turnover. In-vitro, it promoted osteoblast proliferation, differentiation, and mineralization in pre-osteoblasts by activating Wnt/GSK-3β/β catenin signaling pathway (Wang et al., 2020b).
3.9 Multiple sclerosis
Multiple sclerosis (MS) is an inflammatory and neurodegenerative illness that is identified by projected inflammation, axonal injury, and demyelination. DAP (8 mg/kg) has exhibited an immune-regulatory role in autoimmune encephalomyelitis in the murine model used for MS (Leung et al., 2011). Autoimmune encephalomyelitis is a demyelinating inflammatory illness of the central nervous system caused in experimental animals by an immune response to myelin epitopes (Fletcher et al., 2010). T cells attract macrophages, microglia, and astrocytes which release inflammatory mediators such as nitric oxide (NO), and ROS. DAP therapy lowered the level of pro-inflammatory cytokines, induced heme oxygenase-1 (HO-1), decreased the level of MDA, and displayed anti-inflammatory and neuroprotective effects in mice at 8 mg/kg (Wang et al., 2020c). In a previous study, DAP administered for 28 days mitigated the encephalomyelitis in mice via suppressing the activation, maturation, and antigen-presenting capability of Dendritic cells, and regulated NF-κB signaling (Wang et al., 2016).
3.10 Systemic lupus erythematosus
Li et al. reported the anti-inflammatory potential of DAP in the NZB/WF1 systemic lupus erythematosus (SLE) murine model. In the SLE-prone NZB/W F1 mice, DAP (at 5 mg/kg) treatment enhanced the survival rates, reduced renal damage and blood urea nitrogen levels, and lowered the serum autoantibody production. Furthermore, its therapy significantly reduced the serum levels of TNF-α and IL-6, inhibited NF-kB activity, lowered the nuclear factor of activated T-cell protein production, and increased the A20 protein expression in SLE-prone NZB/W F1 mice. Finally, DAP reduced the inflammation in the NZB/WF1 murine SLE model via NF-κB suppression mediated by A20 overexpression (Li et al., 2017).
3.11 Anti-psoriasis action
Psoriasis is a chronic inflammatory disease of the skin characterized by excessive proliferation, abnormal differentiation of keratinocytes, and infiltration of inflammatory cells into the epidermis and dermis. Hyperproliferation of keratinocytes and extreme inflammatory response play a pivotal role in its pathogenesis. Cytokines secreted by immune cells cause keratinocytes’ hyperproliferation which produces pro-inflammatory cytokines to potentiate inflammatory response. A previous study showed the anti-psoriatic activity of DAP in HaCaT keratinocytes mouse which occurred through the downregulation of inflammatory cytokines and suppression of NF-κB signaling pathway (Gao et al., 2020). DAP also decreased the epidermal hyperplasia and infiltration of inflammatory cells in imiquimod induced skin lesions in mice. In another research, DAP above 40 μM caused a decrease in cell viability in human HaCaT keratinocytes by upregulation of IL-1, -6, -8, TNF-α, and IL-23A while inhibiting P65 phosphorylation and nuclear translocation. Additionally, it improved the inflammation, erythema, scaling, and epidermal thickness of psoriatic mice (Gao et al., 2020).
3.12 Anticancer action
DAP is known for anticancer potential against leukemia, ovary, kidney, colon, and liver cancers. Uncontrolled proliferation and suppression of apoptosis lead to cancer. Mitogen pathways are responsible for regulating apoptosis. DAP is a protein kinase inhibitor; therefore, it significantly suppresses this pathway and acts as an anti-proliferative agent. It also acts at different phases of the cell cycle. DAP inactivates Akt/NF-κB (an anti-apoptotic pathway), JNK, MAPK, and ERK pathways that are responsible for causing cancer. DAP activated Keap1-Nrf2 pathway that protected the cell against oxidative stress by activating transcription of several cytoprotective genes thus helping to combat cancer (Figure 4) (Jiménez-Orozco et al., 2020). In a previous study, effect of DAP (at 2.5, 5, and 10 μg/ml) on tert-butyl hydroperoxide (t-BHP) induced mitochondrial dysfunction and cell death in C57B1/6 mice and RAW 264.7 cells revealed that the DAP suppressed the production of ROS by stimulating various anti-oxidant genes and activating Nrf2 pathway that protected the body against oxidative damage. Activation of Nrf2 pathway suppressed NLRP3 activation, thus inhibiting the activation of caspases and release of pro-inflammatory cytokines. In this way, DAP protected the body from cell death and mitochondrial dysfunction (Lv et al., 2017).
In another study, anti-proliferative properties of DAP in cancer cells were reported. DAP inhibited migration and invasion of highly metastatic murine osteosarcoma LM8 cells. It reduced the intracellular stress fibers and filopodia. It also decreased the expressions of RhoA and Cdc42 (Fukuda et al., 2016).
3.12.1 Kidney cancer
The human renal cell carcinoma (RCC) accounts for up to 90% of kidney cancers due to the alterations of the genes responsible for controlling cell division (Motzer et al., 1996; Hsieh et al., 2017). MAPKs pathway causes activation of transcription factors which in turn regulate gene expression, thus controlling the cell growth, differentiation, and proliferation. ERK pathway also controls the proliferation and differentiation and survival of cells. DAP prevented the RCC proliferation by inhibiting ERK/MAPK pathway and upregulated the differentiation mediated by p38 MAP kinase. It also suppressed the G1 to S phase transition by inhibiting DNA synthesis at 10 and 50 µM in AQ-498 cells (Finn et al., 2004). The anticancer mechanism of DAP is shown in Figure 4. p38 MAP kinase is intrinsically involved in mediating the effect of DAP in A-498 cells. Moreover, DAP is involved in promoting the cellular maturation and is considered to be a new less toxic approach for treating poorly differentiated RCC (Finn et al., 2004).
3.12.2 Ovarian cancer
Ovarian cancer is the sixth most common cancer among European women (Colombo et al., 2006). Autophagy, apoptosis, and ROS production can trigger cell death and help to treat cancer. AMPK/Akt/mTOR pathway is associated with autophagy and apoptosis. In an earlier study, DAP exhibited the anticancer potential in A2780 xenograft tumor model against ovarian cancer at 0, 5, 10, 20, and 40 μg/ml in-vitro and 30 mg/kg in-vivo by inducing cell death, increasing ROS production, inducing autophagy, and inhibiting the cell proliferation (Fan et al., 2021a).
3.12.3 Leukemia
Benzene is a chemical present in the atmosphere that can cause different types of leukemia. Exposure to the vapors of benzene leads to oxidative damage, inflammatory responses, changes in cell cycle progression, and DNA damage (Huff, 2007). In a benzene-induced leukemia study, treatment of rats with DAP at 12.5, 25, and 50 mg/kg caused an increased blood count, and hemoglobin concentration, reduced the level of inflammatory mediators, and inhibited ROS production to retard cancer progression (Pei et al., 2021).
3.12.4 Liver cancer
A previous study reported the therapeutic potential of DAP against liver cancer. Hepatocellular carcinoma (HCC) was induced in Wistar rats by diethyl nitrosamine (DEN) (200 mg/kg) and its effect was enhanced by phenobarbital for 4 weeks. DAP (at 10, 20, and 30 mg/kg) repressed the biochemical parameters with enhanced levels of GSH, glutathione S-transferase (GST), SOD and CAT while decreasing the level of MDA. It also reduced the inflammatory markers such as COX-2, NF-κB, prostaglandin (PGE2), IL-1β, IL-6, and TNF-α in treated rats (Li et al., 2022).
In another study, DAP inhibited the progression of hepatocellular carcinoma in Huh7 and SK-HEP-1 cell lines. DAP suppressed the cell viability and tumorigenesis, promoted the apoptosis of cells, and induced the arrest the cells in G1 phase dose-dependently which were rescued by SKL 2001, an activator of Wnt/β-catenin signaling. Thus, DAP exerted an antitumor role through the inactivation of Wnt/β-catenin signaling (Liu et al., 2022).
3.12.5 Breast cancer
Cell proliferation and estrogenicity lead to the tumor development in breast. DAP acts at different phases of cell cycle thus controlling cell proliferation and tumor development. Cyclin D1 is a major protein for the initiation of cell cycle and proliferation of cells. In a previous study, DAP suppressed cyclin D1, thereby preventing the proliferation in MCF-7 cells. It is also a protein kinase inhibitor which leads to the inhibition of proliferation. It did not possess estrogenic activity (Jiménez-Orozco et al., 2011).
In another study. DAP inhibited p-AKT which reduced NF- κB in mammary cancer. It was considered to be an effective agent in the treatment of mammary cancer in rats by suppressing the Nrf-2-Keap1 pathway and NF- κB expression (Kumar et al., 2016a).
3.13 Nephroprotective action
The DAP showed nephroprotective effect against cisplatin-induced nephrotoxicity by suppressing the NF-κB signaling pathway and activating the Nrf2 pathway when C57BL/6 mice were treated with DAP at 2.5–10 mg/kg. It decreased the blood urea nitrogen and creatinine levels along with the reduction of ROS (Zhang et al., 2018). Another study stated that the DAP (at 40 mg/kg) restored the weight loss, blood urea, kidney index, and creatinine levels in cisplatin-induced acute nephrotoxicity. It remarkably increased sirtuins (SIRT1, SIRT6) and Nrf2 with an increased SOD and GSH levels, and the reduction in MDA and MPO levels in wild-type mice (Fan et al., 2020). The nephroprotective mechanism of DAP is shown in Figure 4. A study also reported the preventive potential of DAP in diabetic nephropathy in mesangial cells at 10-40 µM by preventing cell proliferation, protection against oxidative stress and inflammation by targeting Nrf2/keap1, and Akt/NF-kB inflammatory pathways (Shen et al., 2017; Xu et al., 2019).
DAP protected the mice from gentamicin-induced nephrotoxicity at 40 mg/kg by preventing renal injury and decreasing cell damage. It upregulated the expression of Nrf2, and antioxidant enzymes such as HO-1, NQ01, GCLC and GCLM (Fan et al., 2021b).
3.14 Other actions of Daphnetin
Song et al. investigated the potential effect of DAP as immunosuppressive agent in BALB/c mice using a 100 μl emulsion comprising of 100 μg OVA as prototype antigen. DAP (at 5, 10, and 20 mg/kg i.p.) downregulated the OVA-specific antibody IgG subclasses IgG1 and IgG2b and reduced the growth of Th1 and Th2 cytokines as well as restrained in-vivo splenocytes proliferation (Song et al., 2021).
It was reported that the pretreatment with DAP (at 1, 10, 20, and 40 µM) improved the cell viability in rat insulinoma (INS-1) cells that were previously exposed to streptozotocin (STZ) as compared to INS-1 cells (Negative control). It also improved the insulin secretion in the INS-1 cells. Thus, the antidiabetic effect of DAP relied on insulin stimulating, and antiapoptotic actions (Vinayagam and Xu, 2017).
DAP (at 4–16 mg/kg) considerably improved the experimental colitis by suppressing the colonic inflammation, improving colonic integrity, and restoring the immune and metabolic homeostasis. It increased the abundance of short-chain fatty acid producing microbiota of gut that were responsible for the increased development of T reg cells and the reduced pro-inflammatory Th 17 cell differentiation (Ji et al., 2019).
Previously, it was found that the DAP inhibited melanin biosynthesis by suppressing the expression of microphthalmia associated transcription factor responsible for melanogenesis, and also inhibited melanogenic enzymes such as tyrosine and tyrosine-related proteins in B16F10 cells. DAP downregulated the phosphorylation of kinases such as PKA, ERK, mitogen and stress activated protein kinase (MSK)-1 and cAMP response element binding protein (CREB). It inhibited the melanin synthesis, and exhibited the anti-pigmentation activity by modulating PKA/CREB, and ERK/MSK1/CREB pathways (Nam et al., 2022).
DAP possesses analgesic action. In a previous study, DAP at 10 mg/kg averted reserpine induced fibromyalgia (chronic pain syndrome along with depression) in mice. DAP effectively averted fibromyalgia by downregulating monoamine oxidase-A (MAO-A), glutamate level, IL-1β, and TNF-α while elevating the GSH, dopamine, serotonin and norepinephrine levels (Singh et al., 2021b).
A previous study reported that DAP reduced Toll-like receptor-4 (TLR4) expression and suppressed the activation of the NF-κB signaling pathway in acute pancreatitis showing its potential to avert pancreatitis (Liu et al., 2016b). DAP as an emulsion [locus bean gum (0.5%) and sodium alginate (1.5%)] is used in the food industry as an additive, preservative and packaging material. It is used as a preservative because of antimicrobial and antioxidant properties (Liu et al., 2021; Cheng, 2022).
Wen et al. investigated the percutaneous absorption of DAP in rat abdominal skin using various chemical enhancers. The experiment was performed using isopropyl myristate as a vehicle while other enhances of O-acylmenthol derivatives were synthesized from which only M-LA was explored to improve the DAP permeation. Its effects were also pronounced when DAP was used with span 80 (Wen et al., 2009).
Yang and his coworkers investigated the effect of DAP on ischemia repurfusion (I/R) injury. DAP (at 2.5, 5,10, and 20 mg/kg) decreased the myocardial I/R injury with improved cardiac function in treated cells. It reduced the apoptosis, oxidative stress, and inflammation both under in-vitro and in-vivo experiments. It also reduced the risk of ventricular arrhythmias by downregulation of TLR4, MyD88, and NF-κβ in I/R mice (Yang et al., 2021b).
In another study, DAP exhibited anti-angiogenic properties through inhibition of different stages of angiogenesis such as migration, invasion, and tube formation. It suppressed the NF-κB pathway, TNF-α induced IκBα degradation and translocation of the NF- κB-p65 protein. It significantly decreased the expression of c-Src, FAK, ERK1/2, Akt, VEGFR2, Inos, and MMP2 as well as induced apoptosis (Kumar et al., 2016b).
DAP also exhibited the protection against LPS induced inflammatory bone destruction in murine osteolysis model. It inhibited RANKL-induced osteoclast differentiation, fusion and bone resorption. It inhibited the activation of ERK and NFATc1 signaling cascade, so it has the potential to be used for the treatment of inflammatory osteolysis (Wu et al., 2019). Zhou and Zhang et al. investigated the in-vitro integration of DAP-Cu (II) complex with calf thymus DNA (ctDNA). The findings exhibited that the DAP and Cu2+ exerted synergistic pharmacological actions (Zhou et al., 2016).
4 Toxicity studies
A previous study reported the Minimum inhibitory concentration (MIC of DAP (25 μg/ml) against highly resistant H. pylori isolated from human gastric antrum. Using the Cell Counting Kit-8 (CCK-8), the sub-minimum inhibitory concentration (MIC) of DAP was studied in GES-1 cells. DAP was well tolerated by GES-1 cells, and there was insignificant difference on cytotoxic effect of DAP by culture media conditions (Wang et al., 2019). Furthermore, the acute toxicity of DAP was evaluated using the Bacterial reverse mutation assay (Ames test), which revealed no genetic toxicity. Bone marrow micronucleus test revealed that DAP) had no effect on mouse bone marrow cells at various concentrations (0.75, 1.5, and 3 mg/kg). DAP was prepared for an acute skin allergy test at a final concentration of 4 mg/ml, indicating that it is non-allergenic. A local mucosa stimulation test was done on the oral mucosa of rabbits which revealed no oral mucosa ulceration, erosion, erythema and irritation caused by DAP.
In mice, the maximal oral toxic dose of DAP was greater than 100 mg/kg (Nanzhen et al., 2018). Hippocampal HT-22 cell line was used with 5 mM glutamate and different concentrations of DAP. After 12 h of incubation, 100 mΜ DAP protected HT-22 cells in concentration dependent manner against glutamate toxicity (Du et al., 2014). BV2 microglia were used and treated with 0-160 μM concentrations of DAP which showed insignificant change in cell survival rate (Yu et al., 2014a). Indeed, the in-vitro and in-vivo studies confirmed that DAP was devoid of any significant toxicity at pharmacologically relevant concentrations.
5 Structure activity relationship
There is a series of DAP derivatives ranging from 1-22 that showed moderate inhibitory or activating action on GPCRs resultantly responsible for copious pharmacological activities. The activity of GPCRs depends on the chemical alteration of hydroxyl groups at the C-7, C-8, and C-3/C-4 positions of DAP (Satô and Hasegawa, 1969; Wang et al., 2020a). The C-7 and C-8 substituents were generated through phenolic O-acylation/O-alkylation (nucleophilic acyl/alkyl substitution), whereas the C-3 and C-4 substituents were formed using Pechmann condensation. DAP 2, 3, 4, 5, 15, 16, 18, 19, and 20 cause moderate activation on GPCRs while 3-5, and 19 exhibit profound activation with EC50 of 1.18–1.91 nM (Wang et al., 2020a).
Substitution at C-3 or C-4 of DAP produces different derivatives. The anti-oxidant activities of different DAP derivatives were evaluated. The catechol group was considered a key pharmacophore for the anti-oxidant activity. The introduction of electron-withdrawing hydrophilic group at the C-4 position increased the anti-oxidant potential but it was not observed with C-3 substitution. Introduction of the hydrophobic phenyl group produced negative effect on the anti-oxidant activity at C-3 and C-4. The 4-carboxymethyl DAP exerted the most powerful anti-oxidant activity. It also displayed strong metabolic stability (Dar et al., 2015; Xia et al., 2018b).
6 Discussion
The inclusion of multiple research investigations on pharmacological mechanisms of DAP in treating numerous chronic conditions was a main emphasis of the current study. Consequently, the review offers a comprehensive outline of the prospective medicinal uses of this phytochemical. The current study uncovered the pharmacological and therapeutic benefits of DAP for human health. Several medicinal plants are rich source of bioactive compounds which exhibit numerous pharmacological activities, minimal side effects, and the potential source of novel drugs for the treatment of diseases. Many drugs currently available in the market have either been directly or indirectly derived from the traditional plants. By reviewing the available information, it was determined that DAP was a bioactive constituent with a variety of effects against bacterial microorganisms, inflammation, malarial parasite, viral infections, cardiovascular diseases, rheumatoid arthritis, kidney disorders, cerebral disorders, various cancers, lung infections, melanogenesis, bowel diseases, oxidative stress, diabetes mellitus, and others. However, its impact against different cancer types are particularly important in the therapy of numerous malignant diseases.
DAP is a simple coumarin derivative with a variety of therapeutic benefits in preclinical research and mostly isolated from the Daphne genus. Thus, there are areas to work on the isolation from other genus and synthesis in laboratory. Furthermore, identification of various intermediate metabolites may broaden the range of biologically active compounds to be tested for various ailments.
In numerous preclinical researches, DAP’s neuroprotective effects have been thoroughly documented. Studies have demonstrated the protective effect of DAP against ischemic/reperfusion injury, and spatial memory impairment caused by CUS, NMDA-induced excitotoxicity, glutamate-excited HT-22 cells, as well as cerebral ischemia (Liao et al., 2013; Du et al., 2014; Yang et al., 2014; Liu et al., 2016a; Berman and Bayati, 2018). The neuroprotective effect can be significantly achieved by the modification of the TLR-4/NF-κB, HSP70, JAK/STAT, and Nrf-2/HO−1 downstream pathways (Figure 4). DAP’s potential as a neuroprotective compound could further be supported in preclinical research by examining its impact on the Aβ amyloid, tau, Parkinson’s, and Huntingtin proteins.
In cancer, cells divide uncontrollably and metastasize other tissues. The findings of current review indicated that the DAP was pharmacologically effective against different type of cancers including cancers of kidney, liver, ovary and leukemia via inhibiting the proliferation and promotion of apoptosis making it a viable adjuvant in the treatment of cancer.
The inflammation and oxidative stress are increased in severe joint inflammatory conditions such as osteoarthritis and RA. The upsurge of pro-inflammatory cytokines, NF-κB, myeloperoxidase, iNOS, NOS, COX-2, and other mediators worsen the disease (Prasad et al., 2021). Thus, the analysis of previous studies indicated that the DAP was effective in retarding the progression of RA and other inflammatory diseases even at low doses via inhibiting the pro-inflammatory cytokines, NF-κB and MMP levels, and restored the protein expressions.
The DAP and derivatives were effective against several bacterial infections as well as different malarial parasites via suppressing the metabolic functions of these microbes as demonstrated by diverse in-vitro and in-vivo studies. Such studies are need to be extended to antibiotic resistant microbes so as to treat and prevent drug resistant infections. The hepatoxicity could be due to infections, malignant diseases and drug therapy. Previous investigations indicated that the DAP had antioxidant potential and inhibited lipid and protein oxidation, decreased ROS, and pro-inflammatory cytokines to prevent and treat hepatotoxicity (Khan et al., 2019).
Several intriguing pharmacological investigations on DAP against different diseases have outlined the mechanisms supporting the use of DAP as supplementary therapy. Further research is needed on long-term toxicity, information on potential medication interactions and its effect as adjuvant therapy against chronic diseases. To further support the clinical value in medical practice, supplementary clinical trials are also required. Previous studies show that DAP is a suitable candidate for the drug development.
7 Conclusion and future perspectives
This review provides pertinent information regarding the pharmacological aspects of DAP to explore its hidden potential as it targets various molecular and cellular pathways to combat numerous inflammatory disorders, infectious diseases, neurological disorders, hepatotoxicity, nephrotoxicity, psoriasis, diabetic nephropathy, leukemia, and other cancers. DAP exhibited no mutagenic effect, allergenic action, sensitization, mucosal irritation, erythema, and mortality in toxicity studies. The information summarized above will be used for the development of an effective formulation for the treatment of various ailments without significant adverse/toxic effects.
On the basis of literature reviewed, it has been found that DAP exhibited remarkable pharmacological profile and it could be used as a treatment or adjuvant for the treatment of different disorders. Thus, still its biosynthesis and structure activity relationship should be critically analyzed. The effect of DAP on H. pylori was investigated but other microorganisms causing gastrointestinal infections should be investigated to reduce their impact on human and animal health. The development of nanoformulations of DAP need attention to enhance its therapeutic effect and half-life. The synergistic effect of DAP with commercially available drugs should be studied to enhancing their effects in treating various diseases. As DAP attenuates the activation of microglia that plays a crucial role in the pathogenesis of multiple neurodegenerative diseases, this evidence suggests the possibility of DAP as treatment option for Huntington and Parkinson’s disease. Further research should be implicated to explore its various bioactivities and mechanisms. Toxicity study of DAP and its derivatives should be conducted in detail to assure their safety in human and animals (Lu et al., 2021).
Author contributions
AS and MA: Conceptualization, visualization, review, supervision, analysis, review, and editing. MJ, AX: Literature search, collection of data, writing original draft.
Conflict of interest
The authors declare that the research was conducted in the absence of any commercial or financial relationships that could be construed as a potential conflict of interest.
Publisher’s note
All claims expressed in this article are solely those of the authors and do not necessarily represent those of their affiliated organizations, or those of the publisher, the editors and the reviewers. Any product that may be evaluated in this article, or claim that may be made by its manufacturer, is not guaranteed or endorsed by the publisher.
References
Amin, K. M., Abdel Gawad, N. M., Abdel Rahman, D. E., and El Ashry, M. K. M. (2014). New series of 6-substituted coumarin derivatives as effective factor Xa inhibitors: Synthesis, in vivo antithrombotic evaluation and molecular docking. Bioorg. Chem. 52, 31–43. doi:10.1016/j.bioorg.2013.11.002
Archbold, J. K., Flanagan, J. U., Watkins, H. A., Gingell, J. J., and Hay, D. L. (2011). Structural insights into RAMP modification of secretin family G protein-coupled receptors: implications for drug development. Trends Pharmacol. Sci. 32 (10), 591–600. doi:10.1016/j.tips.2011.05.007
Barsante, M. M., Roffe, E., Yokoro, C. M., Tafuri, W. L., Souza, D. G., Pinho, V., et al. (2005). Anti-inflammatory and analgesic effects of atorvastatin in a rat model of adjuvant-induced arthritis. Eur. J. Pharmacol. 516 (3), 282–289. doi:10.1016/J.EJPHAR.2005.05.005
Berman, T., and Bayati, A. (2018). What are neurodegenerative diseases and how do they affect the brain? Front. Young Minds 6. doi:10.3389/frym.2018.00070
Blair, I. A. (2008). DNA adducts with lipid peroxidation products. J. Biol. Chem. 283 (23), 15545–15549. doi:10.1074/jbc.R700051200
Boulebd, H., and Khodja, I. A. (2021). A detailed DFT-based study of the free radical scavenging activity and mechanism of daphnetin in physiological environments. Phytochemistry 189, 112831. doi:10.1016/j.phytochem.2021.112831
Brown, S. A., Biosynthesis of daphnetin in Daphne mezereum L. Z. für Naturforsch. C, 1986. 41(3), 247–252. doi:10.1515/znc-1986-0301
Chen, X., Kuang, N., Zeng, X., Zhang, Z., Li, Y., Liu, W., et al. (2018). Effects of daphnetin combined with Bcl2-siRNA on antiapoptotic genes in synovial fibroblasts of rats with collagen-induced arthritis. Mol. Med. Rep. 17 (1), 884–890. doi:10.3892/mmr.2017.8008
Chen, L. (2011). Effects of Glycyrrhiza uralensis on rat intestinal absorption and metabolism of daphnetin. Nanjing: Nanjing University of Chinese Medicine.
Cheng, H. (2022). Characterization of sodium alginate—locust bean gum films Reinforced with daphnetin Emulsions for the Development of active packaging. Polymers 14, 731. doi:10.3390/polym14040731
Chitnis, T., and Weiner, H. L. (2017). CNS inflammation and neurodegeneration. J. Clin. Invest. 127 (10), 3577–3587. doi:10.1172/JCI90609
Colombo, N., Van Gorp, T., Parma, G., Amant, F., Gatta, G., Sessa, C., et al. (2006). Ovarian cancer. Crit. Rev. Oncol. Hematol. 60 (2), 159–179. doi:10.1016/j.critrevonc.2006.03.004
Connor, J. R., Manning, P. T., Settle, S. L., Moore, W. M., Jerome, G. M., Webber, R. K., et al. (1995). Suppression of adjuvant-induced arthritis by selective inhibition of inducible nitric oxide synthase. Eur. J. Pharmacol. 273 (1), 15–24. doi:10.1016/0014-2999(94)00672-T
Cottigli, F., Loy, G., Garau, D., Floris, C., CasuM., , Pompei, R., et al. (2001). Antimicrobial evaluation of coumarins and flavonoids from the stems of Daphne gnidium L. Phytomedicine 8 (4), 302–305. doi:10.1078/0944-7113-00036
Dar, M. Y., Ara, T., and Akbar, S. (2015). Isolation of daphnetin 8-methyl ether from Daphne oleoides and its anti-bacterial activity. J. Phytopharm. 4 (4), 224–226. doi:10.31254/phyto.2015.4407
Deng, H., Zheng, M., Hu, Z., Zeng, X., Kuang, N., and Fu, Y. (2020). Effects of daphnetin on the autophagy signaling pathway of fibroblast-like synoviocytes in rats with collagen-induced arthritis (CIA) induced by TNF-α. Cytokine 127, 154952. doi:10.1016/j.cyto.2019.154952
Du, Q., Di, L. Q., Shan, J. J., Liu, T. S., and Zhang, X. Z. (2009). Intestinal absorption of daphnetin by rats single pass perfusion in situ. Yao xue xue bao = Acta Pharm. Sin. 44 (8), 922–926. PMID: 20055163.
Du, G., Tu, H., Li, X., Pei, A., Chen, J., Miao, Z., et al. (2014). Daphnetin, a natural coumarin derivative, provides the neuroprotection against glutamate-induced toxicity in HT22 cells and ischemic brain injury. Neurochem. Res. 39 (2), 269–275. doi:10.1007/s11064-013-1218-6
Fan, X., Wei, W., Huang, J., Peng, L., and Ci, X. (2020). Daphnetin attenuated cisplatin-induced acute nephrotoxicity with enhancing antitumor activity of cisplatin by upregulating SIRT1/SIRT6-nrf2 pathway. Front. Pharmacol. 11, 579178. doi:10.3389/fphar.2020.579178
Fan, X., Xie, M., Zhao, F., Li, J., Fan, C., Zheng, H., et al. (2021a). Daphnetin triggers ROS-induced cell death and induces cytoprotective autophagy by modulating the AMPK/Akt/mTOR pathway in ovarian cancer. Phytomedicine. 82, 153465. doi:10.1016/j.phymed.2021.153465
Fan, X., Gu, W., Gao, Y., Ma, N., Fan, C., and Ci, X. (2021b). Daphnetin ameliorated GM-induced renal injury through the suppression of oxidative stress and apoptosis in mice. Int. Immunopharmacol. 96, 107601. doi:10.1016/j.intimp.2021.107601
Finn, G. J., Creaven, B. S., and Egan, D. A. (2004). Daphnetin induced differentiation of human renal carcinoma cells and its mediation by p38 mitogen-activated protein kinase. Biochem. Pharmacol. 67 (9), 1779–1788. doi:10.1016/j.bcp.2004.01.014
Fletcher, J. M., Lalor, S. J., Sweeney, C. M., TubridyN., , and Mills, K. H. G. (2010). T cells in multiple sclerosis and experimental autoimmune encephalomyelitis. Clin. Exp. Immunol. 162 (1), 1–11. doi:10.1111/j.1365-2249.2010.04143.x
Fukuda, H., Nakamura, S., Chisaki, Y., Takada, T., Toda, Y., Murata, H., et al. (2016). Daphnetin inhibits invasion and migration of LM8 murine osteosarcoma cells by decreasing RhoA and Cdc42 expression. Biochem. Biophys. Res. Commun. 471 (1), 63–67. doi:10.1016/j.bbrc.2016.01.179
Fylaktakidou, K. C., Hadjipavlou-Litina, D. J., Litinas, K. E., and Nicolaides, D. N. (2004). Natural and synthetic coumarin derivatives with anti-inflammatory/antioxidant activities. Curr. Pharm. Des. 10 (30), 3813–3833. doi:10.2174/1381612043382710
Gao, Q., Shan, J., Di, L., Jiang, L., and Xu, H. (2008). Therapeutic effects of daphnetin on adjuvant-induced arthritic rats. J. Ethnopharmacol. 120 (2), 259–263. doi:10.1016/j.jep.2008.08.031
Gao, J., Chen, F., Fang, H., Mi, J., Qi, Q., and Yang, M. (2020). Daphnetin inhibits proliferation and inflammatory response in human HaCaT keratinocytes and ameliorates imiquimod-induced psoriasis-like skin lesion in mice. Biol. Res. 53, 48. doi:10.1186/s40659-020-00316-0
Halda, J. J., Horáček, L., and Panarotto, P. (1998). Some taxonomic problems in the genus Daphne L. Czechia: Okresní muzeum Orlických hor.
Han, S., Li, L.-z., and Song, S.-j. (2020). Daphne giraldii Nitsche (Thymelaeaceae): Phytochemistry, pharmacology and medicinal uses. Phytochemistry 171, 112231. doi:10.1016/j.phytochem.2019.112231
Hsieh, J. J., Purdue, M. P., Signoretti, S., Swanton, C., Albiges, L., Schmidinger, M., et al. (2017). Renal cell carcinoma. Nat. Rev. Dis. Prim. 3 (1), 17009–17019. doi:10.1038/nrdp.2017.9
Hu, X.-L., Gao, C., Xu, Z., Liu, M. L., Feng, L. S., and Zhang, G. D. (2018). Recent development of coumarin derivatives as potential antiplasmodial and antimalarial agents. Curr. Top. Med. Chem. 18 (2), 114–123. doi:10.2174/1568026618666171215101158
Huang, F., Tang, L. H., Yu, L. Q., Ni, Y. C., Wang, Q. M., and Nan, F. J. (2006). In vitro potentiation of antimalarial activities by daphnetin derivatives against Plasmodium falciparum. Biomed. Environ. Sci. 19 (5), 367–370. PMID: 17190189.
Huff, J. (2007). Benzene-induced cancers: Abridged history and occupational health impact. Int. J. Occup. Environ. Health 13 (2), 213–221. doi:10.1179/oeh.2007.13.2.213
Iadecola, C., and Alexander, M. J. (2001). Cerebral ischemia and inflammation. Curr. Opin. Neurol. 14 (1), 89–94. doi:10.1097/00019052-200102000-00014
Ji, J., Ge, X., Chen, Y., Zhu, B., Wu, Q., Zhang, J., et al. (2019). Daphnetin ameliorates experimental colitis by modulating microbiota composition and Treg/Th17 balance. FASEB 33(8), 9308–9322. doi:10.1096/fj.201802659RR
Jiménez-Orozco, F. A., Rosales, A. A. R., Vega-Lopez, A., Dominguez-Lopez, M. L., Garcia-Mondragon, M. J., Maldonado-Espinoza, A., et al. (2011). Differential effects of esculetin and daphnetin on in vitro cell proliferation and in vivo estrogenicity. Eur. J. Pharmacol. 668 (1), 35–41. doi:10.1016/j.ejphar.2011.06.024
Jiménez-Orozco, F. A., Ranđelović, I., Hegedüs, Z., Vega-Lopez, A., and Martínez-Flores, F. (2020). In vitro anti-proliferative effect and in vivo antitumor action of daphnetin in different tumor cells. Cir. Cir. 88 (6), 765–771. doi:10.24875/ciru.20000197
Khan, H., Ullah, H., and Nabavi, S. M. (2019). Mechanistic insights of hepatoprotective effects of curcumin: Therapeutic updates and future prospects. Food Chem. Toxicol. 124, 182–191. doi:10.1016/j.fct.2018.12.002
Khouchlaa, A., El Menyiy, N., Guaouguaou, F. E., El Baaboua, A., Charfi, S., Lakhdar, F., et al. (2021). Ethnomedicinal use, phytochemistry, pharmacology, and toxicology of Daphne gnidium: A review. J. Ethnopharmacol. 275, 114124. doi:10.1016/j.jep.2021.114124
Kostova, I., Bhatia, S., Grigorov, P., BalkanSky, S., Parmar, V. S., Prasad, A. K., et al. (2011). Coumarins as antioxidants. Curr. Med. Chem. 18 (25), 3929–3951. doi:10.2174/092986711803414395
Kumar, A., Jha, S., and Pattanayak, S. P. (2016a). Daphnetin ameliorates 7, 12-dimethylbenz[a]anthracene-induced mammary carcinogenesis through Nrf-2-Keap1 and NF-κB pathways. Biomed. Pharmacother. 82, 439–448. doi:10.1016/j.biopha.2016.05.028
Kumar, A., Sunita, P., Jha, S., and Pattanayak, S. P. (2016b). Daphnetin inhibits TNF-α and VEGF-induced angiogenesis through inhibition of the IKK s/IκBα/NF-κB, Src/FAK/ERK 1/2 and Akt signalling pathways. Clin. Exp. Pharmacol. Physiol. 43 (10), 939–950. doi:10.1111/1440-1681.12608
Leung, G., Sun, W., Zheng, L., BrookeS, S., TullyM., , and Shi, R. (2011). Anti-acrolein treatment improves behavioral outcome and alleviates myelin damage in experimental autoimmune encephalomyelitis mouse. Neuroscience 173, 150–155. doi:10.1016/j.neuroscience.2010.11.018
Li, M., Shi, X., Chen, F., and Hao, F. (2017). Daphnetin inhibits inflammation in the NZB/W F1 systemic lupus erythematosus murine model via inhibition of NF-κB activity. Exp. Ther. Med. 13 (2), 455–460. doi:10.3892/etm.2016.3971
Li, T., Yang, G., Hao, Q., Zhang, X., and Zhang, X. (2022). Daphnetin ameliorates the expansion of chemically induced hepatocellular carcinoma via reduction of inflammation and oxidative stress. J. Oleo Sci. 71 (4), 575–585. doi:10.5650/jos.ess21415
Liang, S.-C., Ge, G. B., Liu, H. X., Zhang, Y. Y., Wang, L. M., Zhang, J. W., et al. (2010). Identification and characterization of human UDP-glucuronosyltransferases responsible for the in vitro glucuronidation of daphnetin. Drug Metab. Dispos. 38 (6), 973–980. doi:10.1124/dmd.109.030734
Liang, S.-C., Ge, G. B., Xia, Y. L., Zhang, J. W., Qi, X. Y., Tu, C. X., et al. (2015). In vitro evaluation of the effect of 7-methyl substitution on glucuronidation of daphnetin: metabolic stability, isoform selectivity, and bioactivity analysis. J. Pharm. Sci. 104 (10), 3557–3564. doi:10.1002/jps.24538
Liang, S.-C., Xia, Y. L., Hou, J., Ge, G. B., Zhang, J. W., He, Y. Q., et al. (2016). Methylation, glucuronidation, and sulfonation of daphnetin in human hepatic preparations in vitro: Metabolic profiling, pathway comparison, and bioactivity analysis. J. Pharm. Sci. 105 (2), 808–816. doi:10.1016/j.xphs.2015.10.010
Liang, S., Ge, G. b., Xia, Y. l., Qi, X. y., Wang, A. x., Tu, C. x., et al. (2017). In vitro metabolism of daphnetin in rat liver S9 fractions. Yao Xue Xue Bao 52(2), 291–295. PMID: 29979523
Liao, M. J., Lin, L. F., Zhou, X., Zhou, X. W., Xu, X., Cheng, X., et al. (2013). Daphnetin prevents chronic unpredictable stress-induced cognitive deficits. Fundam. Clin. Pharmacol. 27 (5), 510–516. doi:10.1111/j.1472-8206.2012.01049.x
Liu, J., Chen, Q., Jian, Z., Shao, L., Jin, T., Zhu, X., et al. (2016a). Daphnetin protects against cerebral ischemia/reperfusion injury in mice via inhibition of TLR4/NF-κB signaling pathway. Biomed. Res. Int. 2016, 2816056. doi:10.1155/2016/2816056
Liu, Z., Liu, J., Zhao, K., Shi, Q., Zuo, T., Wang, G., et al. (2016b). Role of daphnetin in rat severe acute pancreatitis through the regulation of TLR4/NF-[Formula: see text]B signaling pathway activation. Am. J. Chin. Med. 44 (01), 149–163. doi:10.1142/S0192415X16500105
Liu, Y., Liao, L., Chen, Y., and Han, F. (2019). Effects of daphnetin on lipid metabolism, insulin resistance and oxidative stress in OA-treated HepG2 cells. Mol. Med. Rep. 19 (6), 4673–4684. doi:10.3892/mmr.2019.10139
Liu, W., Mei, J., and Xie, J. (2020). Elucidating Antibacterial activity and mechanism of daphnetin against Pseudomonas fluorescens and Shewanella putrefaciens. J. Food Qual. 2011, 1–10. doi:10.1155/2020/6622355
Liu, W., Mei, J., and Xie, J. (2021). Effect of locust bean gum-sodium alginate coatings incorporated with daphnetin emulsions on the quality of Scophthalmus maximus at refrigerated condition. Int. J. Biol. Macromol. 170, 129–139. doi:10.1016/j.ijbiomac.2020.12.089
Liu, C., Liu, H., Pan, J., Lin, R., Chen, Y., and Zhang, C. (2022). Daphnetin inhibits the survival of hepatocellular carcinoma cells through regulating Wnt/β-catenin signaling pathway. Drug Dev. Res. 83, 952. doi:10.1002/ddr.21920
Lu, Y., Pang, J., Wang, G., Hu, X., Li, X., Li, G., et al. (2021). Quantitative proteomics approach to investigate the antibacterial response of Helicobacter pylori to daphnetin, a traditional Chinese medicine monomer. RSC Adv. 11 (4), 2185–2193. doi:10.1039/D0RA06677J
Lv, H., Liu, Q., Zhou, J., Tan, G., Deng, X., and Ci, X. (2017). Daphnetin-mediated Nrf2 antioxidant signaling pathways ameliorate tert-butyl hydroperoxide (t-BHP)-induced mitochondrial dysfunction and cell death. Free Radic. Biol. Med. 106, 38–52. doi:10.1016/j.freeradbiomed.2017.02.016
Lv, H., Fan, X., Wang, L., Feng, H., and Ci, X. (2018). Daphnetin alleviates lipopolysaccharide/d-galactosamine-induced acute liver failure via the inhibition of NLRP3, MAPK and NF-κB, and the induction of autophagy. Int. J. Biol. Macromol. 119, 240–248. doi:10.1016/j.ijbiomac.2018.07.101
Lv, X., Li, Y., Xiao, Q., and Li, D. (2019). Daphnetin activates the Nrf2-dependent antioxidant response to prevent arsenic-induced oxidative insult in human lung epithelial cells. Chem. Biol. Interact. 302, 93–100. doi:10.1016/j.cbi.2019.02.001
Lv, H., Zhu, C., Wei, W., Lv, X., Yu, Q., Deng, X., et al. (2020). Enhanced Keap1-Nrf2/Trx-1 axis by daphnetin protects against oxidative stress-driven hepatotoxicity via inhibiting ASK1/JNK and Txnip/NLRP3 inflammasome activation. Phytomedicine 71, 153241. doi:10.1016/j.phymed.2020.153241
Manojlović, N. T., Maskovic, P., Vasiljevic, P., Jelic, R., Juskovic, M., Sovrlic, M., et al. (2012). HPLC Analysis, antimicrobial and antioxidant activities of Daphne cneorum L. Hem. Ind. 66 (5), 709–716. doi:10.2298/HEMIND120114029M
Mansoor, F., Anis, I., Ali, S., Choudhary, M. I., and Shah, M. R. (2013). New dimeric and trimeric coumarin glucosides from Daphne retusa Hemsl. Fitoterapia 88, 19–24. doi:10.1016/j.fitote.2013.03.029
Martinez, K. B., Mackert, J. D., and McIntosh, M. K. (2017). “Chapter 18 - polyphenols and intestinal health,” in Nutrition and functional foods for healthy aging. Editor R. R. Watson (Cambridge: Academic Press), 191–210. doi:10.1016/B978-0-12-805376-8.00018-6
Masamoto, Y., Ando, H., Murata, Y., Shimoishi, Y., Tada, M., and Takahata, K. (2003). Mushroom tyrosinase inhibitory activity of esculetin isolated from seeds of Euphorbia lathyris L. Biosci. Biotechnol. Biochem. 67 (3), 631–634. doi:10.1271/bbb.67.631
Mendes-Braz, M., and Martins, J. O. (2018). Diabetes mellitus and liver surgery: the effect of diabetes on oxidative stress and inflammation. Mediat. Inflamm. 2018, 2456579. doi:10.1155/2018/2456579
Mohamed, M. R., Emam, M. A., Hassan, N. S., and Mogadem, A. I. (2014). Umbelliferone and daphnetin ameliorate carbon tetrachloride-induced hepatotoxicity in rats via nuclear factor erythroid 2-related factor 2-mediated heme oxygenase-1 expression. Environ. Toxicol. Pharmacol. 38 (2), 531–541. doi:10.1016/j.etap.2014.08.004
Moshiashvili, G., Tabatadze, N., and Mshvildadze, V. (2020). The genus Daphne: A review of its traditional uses, phytochemistry and pharmacology. Fitoterapia 143, 104540. doi:10.1016/j.fitote.2020.104540
Motzer, R. J., Bander, N. H., and Nanus, D. M. J. N. E. J. o. M. (1996). Renal-cell carcinoma. N. Engl. J. Med. 335 (12), 865–875. doi:10.1056/NEJM199609193351207
Mu, L., Wang, Q., and Ni, Y. (2002). In vitro antimalarial effect of daphnetin relating to its iron-chelating activity. Zhongguo ji Sheng Chong xue yu ji Sheng Chong Bing za zhi= Chin. J. Parasitol. Parasit. Dis. 20 (2), 83–85. PMID: 12567565.
Nam, G., An, S. K., Park, I. C., Bae, S., and Lee, J. H. (2022). Daphnetin inhibits α-MSH-induced melanogenesis via PKA and ERK signaling pathways in B16F10 melanoma cells. Biosci. Biotechnol. Biochem. 86 (5), 596–609. doi:10.1093/bbb/zbac016
Nanzhen, K., Jieying, W., Wenwei, Z., and Xiaoping, Z. (2018). Toxicological studies of daphnetin. Pharmacogn. Mag. 14 (58), 561. doi:10.4103/pm.pm_523_17
NDong, C., Anzellotti, D., Ibrahim, R. K., Huner, N. P. A., and Sarhan, F. (2003). Daphnetin methylation by a novel O-methyltransferase is associated with cold acclimation and photosystem II excitation pressure in rye. J. Biol. Chem. 278 (9), 6854–6861. doi:10.1074/jbc.M209439200
Norma Francenia, S.-S. (2019). Shikimic acid pathway in biosynthesis of phenolic compounds. Vienna, Austria: IntechOpen. doi:10.5772/intechopen.83815
Pan, L., Li, X., Jin, H., Yang, X., and Qin, B. (2017). Antifungal activity of umbelliferone derivatives: Synthesis and structure-activity relationships. Microb. Pathog. 104, 110–115. doi:10.1016/j.micpath.2017.01.024
Pei, Q., Hu, P., Zhang, H., Li, H., Yang, T., and Liu, R. (2021). Daphnetin exerts an anticancer effect by attenuating the pro‐inflammatory cytokines. J. Biochem. Mol. Toxicol. 35 (6), 1–8. doi:10.1002/jbt.22759
Pinto, D. C., and Silva, A. (2017). Anticancer natural coumarins as lead compounds for the discovery of new drugs. Curr. Top. Med. Chem. 17 (29), 3190–3198. doi:10.2174/1568026618666171215095750
Prasad, S., Kulshreshtha, A., Lall, R., and Gupta, S. C. (2021). Inflammation and ROS in arthritis: management by ayurvedic medicinal plants. Food Funct. 12 (18), 8227–8247. doi:10.1039/D1FO01078F
Qi, Z., Qi, S., Gui, L., Shen, L., and Feng, Z. (2016). Daphnetin protects oxidative stress-induced neuronal apoptosis via regulation of MAPK signaling and HSP70 expression. Oncol. Lett. 12 (3), 1959–1964. doi:10.3892/ol.2016.4849
Riaz, M., Saleem, A., Siddique, S., Khan, B. A., Nur-e-Alam, M., Shahzad-ul-Hussan, S., et al. (2016). Phytochemistry of Daphne oleoides. Nat. Prod. Res. 30 (8), 880–897. doi:10.1080/14786419.2015.1092146
Riveiro, M. E., De KimpeN., , Moglioni, A., Vazquez, R., MonczorF., , Shayo, C., et al. (2010). Coumarins: old compounds with novel promising therapeutic perspectives. Curr. Med. Chem. 17 (13), 1325–1338. doi:10.2174/092986710790936284
Rojas, M., Woods, C. R., Mora, A. L., Xu, J., and Brigham, K. L. (2005). Endotoxin-induced lung injury in mice: structural, functional, and biochemical responses. Am. J. Physiol. Lung Cell. Mol. Physiol. 288 (2), L333–L341. doi:10.1152/ajplung.00334.2004
Satô, M., and Hasegawa, M. (1969). Conversion of daphnin to daphnetin-8-glucoside in Daphne odora. Phytochemistry 8 (7), 1211–1214. doi:10.1016/S0031-9422(00)85559-4
Shan, J. (2009). Studies on oral absorption and metabolism of the main active constituents of Zushima (D). Nanjing: Nanjing University of Chinese Medicine.
Shan, J., Zhu, H., Geng, T., Zhang, L., and Ding, A. (2011). Determination of equilibrium solubility and apparent oil/water partition coefficient of Daphnetin. J. Nanjing Univ. Traditional Chin. Med. 35, 3144–3146.
Shen, L., Zhou, T., Wang, J., Sang, X., Lan, L., Luo, L., et al. (2017). Daphnetin reduces endotoxin lethality in mice and decreases LPS-induced inflammation in Raw264.7 cells via suppressing JAK/STATs activation and ROS production. Inflamm. Res. 66 (7), 579–589. doi:10.1007/s00011-017-1039-1
Shu, K., Kuang, N., Zhang, Z., Hu, Z., Zhang, Y., Fu, Y., et al. (2014). Therapeutic effect of daphnetin on the autoimmune arthritis through demethylation of proapoptotic genes in synovial cells. J. Transl. Med. 12 (1), 287. doi:10.1186/s12967-014-0287-x
Singh, L., Singh, A. P., and Bhatti, R. (2021a). Mechanistic interplay of various mediators involved in mediating the neuroprotective effect of daphnetin. Pharmacol. Rep. 73, 1220–1229. doi:10.1007/s43440-021-00261-z
Singh, L., Kaur, A., Singh, A. P., and Bhatti, R. (2021b). Daphnetin, a natural coumarin averts reserpine-induced fibromyalgia in mice: modulation of MAO-A. Exp. Brain Res. 239 (5), 1451–1463. doi:10.1007/s00221-021-06064-1
Song, B.-C., Jiang, M. M., Zhang, S., Ma, H., Liu, M., Fu, Z. R., et al. (2021). Immunosuppressive activity of daphnetin on the humoral immune responses in ovalbumin-sensitized BALB/c mice. Immunopharmacol. Immunotoxicol. 43 (2), 171–175. doi:10.1080/08923973.2021.1872618
Sovrlić, M. M., and Manojlović, N. T. (2017). Plants from the genus Daphne: A review of its traditional uses, phytochemistry, biological and pharmacological activity. Serbian J. Exp. Clin. Res. 18 (1), 69–80. doi:10.1515/sjecr-2016-0024
Sovrlić, M., Vasiljevic, P., Juskovic, M., Maskovic, P., and Manojlovic, N. (2015). Phytochemical, antioxidant and antimicrobial profiles of extracts of Daphne alpina (Thymelaeaceae) L leaf and twig from Mt Kopaonik (Serbia). Trop. J. Pharm. Res. 14 (7), 1239–1248. doi:10.4314/TJPR.V14I7.17
Tu, L., Li, S., Fu, Y., Yao, R., Zhang, Z., Yang, S., et al. (2012). The therapeutic effects of daphnetin in collagen-induced arthritis involve its regulation of Th17 cells. Int. Immunopharmacol. 13 (4), 417–423. doi:10.1016/j.intimp.2012.04.001
Ueno, K., and Saito, N. (1976). Daphnetin, isolated from Daphne odora. Acta Crystallogr. Sect. B 32 (3), 946–948. doi:10.1107/S0567740876004275
Vinayagam, R., and Xu, B. (2017). 7, 8-Dihydroxycoumarin (daphnetin) protects INS-1 pancreatic β-cells against streptozotocin-induced apoptosis. Phytomedicine 24, 119–126. doi:10.1016/j.phymed.2016.11.023
Vivier, D., Chen, Q., Jian, Z., Xiong, X., Shao, L., Jin, T., et al. (2016). Daphnetin protects against cerebral ischemia/reperfusion injury in mice via inhibition of TLR4/NF-κB signaling pathway. Biomed. Res. Int. 59, 2816056–2816335. doi:10.1155/2016/2816056
Walker, M., and Crabtree, J. E. (1998). Helicobacter pylori infection and the pathogenesis of duodenal ulceration. Ann. N. Y. Acad. Sci. 859 (1), 96–111. doi:10.1111/j.1749-6632.1998.tb11114.x
Wang, Q. M., Ni, Y. C., Xu, Y. Q., Ha, S. H., and Cai, Y. (2000). The schizontocidal activity of daphnetin against malaria parasites in vitro and in vivo. Zhongguo ji sheng chong xue yu ji sheng chong bing za zhi = Chin. J. Parasitol. Parasit. Dis. 18 (4), 204–206. PMID: 12567659.
Wang, Y., Li, C. F., Pan, L. M., and Gao, Z. L. (2013). 7, 8-Dihydroxycoumarin inhibits A549 human lung adenocarcinoma cell proliferation by inducing apoptosis via suppression of Akt/NF-κB signaling. Exp. Ther. Med. 5 (6), 1770–1774. doi:10.3892/etm.2013.1054
Wang, D., Lu, Z., Zhang, H., Jin, S. F., Yang, H., Li, Y. M., et al. (2016). Daphnetin alleviates experimental autoimmune encephalomyelitis via regulating dendritic cell activity. CNS Neurosci. Ther. 22 (7), 558–567. doi:10.1111/cns.12537
Wang, G., Pang, J., Hu, X., Nie, T., Lu, X., Li, X., et al. (2019). Daphnetin: A novel anti-Helicobacter pylori agent. Int. J. Mol. Sci. 20 (4), 850. doi:10.3390/ijms20040850
Wang, Y., Wang, J., Fu, Z., Sheng, R., Wu, W., Fan, J., et al. (2020a). Syntheses and evaluation of daphnetin derivatives as novel G protein-coupled receptor inhibitors and activators. Bioorg. Chem. 104, 104342. doi:10.1016/j.bioorg.2020.104342
Wang, Y., Chen, J., Chen, J., Dong, C., Yan, X., Zhu, Z., et al. (2020b). Daphnetin ameliorates glucocorticoid-induced osteoporosis via activation of Wnt/GSK-3β/β-catenin signaling. Toxicol. Appl. Pharmacol. 409, 115333. doi:10.1016/j.taap.2020.115333
Wang, D., Zhu, B., Liu, X., Han, Q., Ge, W., Zhang, W., et al. (2020c). Daphnetin ameliorates experimental autoimmune encephalomyelitis through regulating heme oxygenase-1. Neurochem. Res. 45 (4), 872–881. doi:10.1007/s11064-020-02960-0
Wen, Z., Fang, L., and He, Z. (2009). Effect of chemical enhancers on percutaneous absorption of daphnetin in isopropyl myristate vehicle across rat skin in vitro. Drug Deliv. 16 (4), 214–223. doi:10.1080/10717540902836715
Wu, Z., Wu, H., Li, C., Fu, F., Ding, J., Shao, S., et al. (2019). Daphnetin attenuates LPS-induced osteolysis and RANKL mediated osteoclastogenesis through suppression of ERK and NFATc1 pathways. J. Cell. Physiol. 234 (10), 17812–17823. doi:10.1002/jcp.28408
Xia, Y.-L., Dou, T. Y., Liu, Y., Wang, P., Ge, G. B., and Yang, L. (2018a). In vitro evaluation of the effect of C-4 substitution on methylation of 7, 8-dihydroxycoumarin: metabolic profile and catalytic kinetics. R. Soc. Open Sci. 5 (1), 171271. doi:10.1098/rsos.171271
Xia, Y., Chen, C., Liu, Y., Ge, G., Dou, T., and Wang, P. (2018b). Synthesis and structure-activity relationship of daphnetin derivatives as potent antioxidant agents. Mol. (Basel, Switz. 23 (10), 2476. doi:10.3390/molecules23102476
Xu, W. C., Shen, J. G., and Jiang, J. Q. (2011). Phytochemical and biological studies of the plants from the genus Daphne. Chem. Biodivers. 8 (7), 1215–1233. doi:10.1002/cbdv.201000117
Xu, K., Guo, L., Bu, H., and Wang, H. (2019). Daphnetin inhibits high glucose-induced extracellular matrix accumulation, oxidative stress and inflammation in human glomerular mesangial cells. J. Pharmacol. Sci. 139 (2), 91–97. doi:10.1016/j.jphs.2018.11.013
Yang, Y.-Z., Ranz, A., Pan, H. Z., Zhang, Z. N., Lin, X. B., and Meshnick, S. R. (1992). Daphnetin: a novel antimalarial agent with in vitro and in vivo activity. Am. J. Trop. Med. Hyg. 46 (1), 15–20. doi:10.4269/ajtmh.1992.46.15
Yang, E. B., Zhao, Y. N., Zhang, K., and Mack, P. (1999). Daphnetin, one of coumarin derivatives, is a protein kinase inhibitor. Biochem. Biophys. Res. Commun. 260 (3), 682–685. doi:10.1006/bbrc.1999.0958
Yang, L., Yang, Q., Zhang, K., Li, Y. J., Wu, Y. M., Liu, S. B., et al. (2014). Neuroprotective effects of daphnetin against NMDA receptor-mediated excitotoxicity. Molecules 19 (9), 14542–14555. doi:10.3390/molecules190914542
Yang, L., Ding, W., Xu, Y., Wu, D., Li, S., Chen, J., et al. (2016). New insights into the antibacterial activity of hydroxycoumarins against Ralstonia solanacearum. Molecules 21 (4), 468. doi:10.3390/molecules21040468
Yang, Y., Sheng, Q., Nie, Z., Liu, L., Zhang, W., Chen, G., et al. (2021a). Daphnetin inhibits spinal glial activation via Nrf2/HO-1/NF-κB signaling pathway and attenuates CFA-induced inflammatory pain. Int. Immunopharmacol. 98, 107882. doi:10.1016/j.intimp.2021.107882
Yang, F., Jiang, X., Cao, H., Shuai, W., Zhang, L., Wang, G., et al. (2021b). Daphnetin preconditioning decreases cardiac injury and susceptibility to ventricular arrhythmia following ischaemia-reperfusion through the TLR4/MyD88/NF-?b signalling pathway. Pharmacology 106 (7-8), 369–383. doi:10.1159/000513631
Yang, S., Song, Y., Wang, Q., Liu, Y., Wu, Z., Duan, X., et al. (2021c). Daphnetin ameliorates acute lung injury in mice with severe acute pancreatitis by inhibiting the JAK2–STAT3 pathway. Sci. Rep. 11 (1), 11491. doi:10.1038/s41598-021-91008-6
Yang, T., Wang, X., Guo, L., Zheng, F., Meng, C., Zheng, Y., et al. (2022). Daphnetin inhibits corneal inflammation and neovascularization on a mouse model of corneal alkali burn. Int. Immunopharmacol. 103, 108434. doi:10.1016/j.intimp.2021.108434
Yao, R., Fu, Y., Li, S., Tu, L., Zeng, X., and Kuang, N. (2011). Regulatory effect of daphnetin, a coumarin extracted from Daphne odora, on the balance of Treg and Th17 in collagen-induced arthritis. Eur. J. Pharmacol. 670 (1), 286–294. doi:10.1016/j.ejphar.2011.08.019
Yu, W., Wang, H., Ying, H., Yu, Y., Chen, D., Ge, W., et al. (2014a). Daphnetin attenuates microglial activation and proinflammatory factor production via multiple signaling pathways. Int. Immunopharmacol. 21 (1), 1–9. doi:10.1016/j.intimp.2014.04.005
Yu, W.-w., Lu, Z., Zhang, H., Kang, Y. h., Mao, Y., Wang, H. h., et al. (2014b). Anti-inflammatory and protective properties of daphnetin in endotoxin-induced lung injury. J. Agric. Food Chem. 62 (51), 12315–12325. doi:10.1021/jf503667v
Zali, M. R. (2011). Facing resistance of H. pylori infection. Gastroenterol. Hepatol. Bed Bench 4 (1), 3–11.
Zhang, W., Di, L. q., Li, J. S., Shan, J. j., Kang, A., Qian, S., et al. (2014). The effects of Glycyrrhizae uralenis and its major bioactive components on pharmacokinetics of daphnetin in Cortex daphnes in rats. J. Ethnopharmacol. 154 (3), 584–592. doi:10.1016/j.jep.2014.03.047
Zhang, L., Gu, Y., Li, H., Cao, H., Liu, B., Zhang, H., et al. (2018). Daphnetin protects against cisplatin-induced nephrotoxicity by inhibiting inflammatory and oxidative response. Int. Immunopharmacol. 65, 402–407. doi:10.1016/j.intimp.2018.10.018
Zhang, W., Zhuo, S., He, L., Cheng, C., Zhu, B., Lu, Y., et al. (2019). Daphnetin prevents methicillin-resistant Staphylococcus aureus infection by inducing autophagic response. Int. Immunopharmacol. 72, 195–203. doi:10.1016/j.intimp.2019.04.007
Zhang, X., Yao, J., Wu, Z., Zou, K., Yang, Z., Huang, X., et al. (2020). Chondroprotective and antiarthritic effects of Daphnetin used in vitro and in vivo osteoarthritis models. Life Sci. 240, 116857. doi:10.1016/j.lfs.2019.116857
Zhao, H., Jiang, Z., Chang, X., Xue, H., Yahefu, W., and Zhang, X. (2018). 4-Hydroxyphenylacetic acid prevents acute APAP-induced liver injury by increasing phase II and antioxidant enzymes in mice. Front. Pharmacol. 9, 653. doi:10.3389/fphar.2018.00653
Zheng, M., Kuang, N., Zeng, X., Wang, J., Zou, Y., and Fu, Y. (2020). Daphnetin induces apoptosis in fibroblast-like synoviocytes from collagen-induced arthritic rats mainly via the mitochondrial pathway. Cytokine 133, 155146. doi:10.1016/j.cyto.2020.155146
Zhi, J., Duan, B., Pei, J., Wu, S., and Wei, J. (2019). Daphnetin protects hippocampal neurons from oxygen-glucose deprivation–induced injury. J. Cell. Biochem. 120 (3), 4132–4139. doi:10.1002/jcb.27698
Zhou, X., Zhang, C., Zhang, G., and Liao, Y. (2016). Intercalation of the daphnetin–Cu (II) complex with calf thymus DNA. RSC Adv. 6 (7), 5408–5418. doi:10.1039/C5RA22274E
Keywords: daphnetin, neuroprotective, anti-inflammatory, anti-bacterial, psoriasis
Citation: Javed M, Saleem A, Xaveria A and Akhtar MF (2022) Daphnetin: A bioactive natural coumarin with diverse therapeutic potentials. Front. Pharmacol. 13:993562. doi: 10.3389/fphar.2022.993562
Received: 13 July 2022; Accepted: 09 September 2022;
Published: 29 September 2022.
Edited by:
Mansour Sobeh, Mohammed VI Polytechnic University, MoroccoReviewed by:
Gabin Bitchagno, Mohammed VI Polytechnic University, MoroccoLatifa Bouissane, Université Sultan Moulay Slimane, Morocco
Copyright © 2022 Javed, Saleem, Xaveria and Akhtar. This is an open-access article distributed under the terms of the Creative Commons Attribution License (CC BY). The use, distribution or reproduction in other forums is permitted, provided the original author(s) and the copyright owner(s) are credited and that the original publication in this journal is cited, in accordance with accepted academic practice. No use, distribution or reproduction is permitted which does not comply with these terms.
*Correspondence: Ammara Saleem, YW1tYXJhc2FsZWVtQGdjdWYuZWR1LnBr, YW1hcmFmdXJxYW43ODZAaG90bWFpbC5jb20=; Muhammad Furqan Akhtar, ZnVycWFuLnBoYXJtYWNpc3RAZ21haWwuY29t