- 1Department of Traditional Chinese Medicine, Shengjing Hospital of China Medical University, Shenyang, China
- 2Liaoning Key Laboratory of Research and Application of Animal Models for Environmental and Metabolic Diseases, Medical Research Center, Shengjing Hospital of China Medical University, Shenyang, China
Gastrointestinal cancer (GIC) poses a serious threat to human health globally. Curcumin (CUR), a hydrophobic polyphenol extracted from the rhizome of Curcuma longa, has shown reliable anticancer function and low toxicity, thereby offering broad research prospects. Numerous studies have demonstrated the pharmacological mechanisms underlying the effectiveness of CUR against GIC, including the induction of apoptosis and autophagy, arrest of the cell cycle, inhibition of the epithelial–mesenchymal transition (EMT) processes, inhibition of cell invasion and migration, regulation of multiple signaling pathways, sensitization to chemotherapy and reversal of resistance to such treatments, and regulation of the tumor survival environment. It has been confirmed that CUR exerts its antitumor effects on GIC through these mechanisms in vitro and in vivo. Moreover, treatment with CUR is safe and tolerable. Newly discovered types of regulated cell death (RCD), such as pyroptosis, necroptosis, and ferroptosis, may provide a new direction for research on the efficacy of CUR against GIC. In this review, we discuss the recently found pharmacological mechanisms underlying the effects of CUR against GIC (gastric and colorectal cancers). The objective is to provide a reference for further research on treatments against GIC.
1 Introduction
Gastrointestinal cancer (GIC) is characterized by high incidence and mortality rates, posing a serious threat to human health globally. According to the Global Cancer Statistics 2020 estimates (Sung et al., 2021), gastric cancer (GC) ranked fifth and fourth among cancers in terms of incidence (>1 million new cases) and mortality (769,000 deaths), respectively. Similarly, colorectal cancer (CRC) accounts for >1.9 million new cases and 935,000 deaths, ranking third and second among cancers, respectively.
Surgical treatment, radiotherapy, and chemotherapy have traditionally been the main strategies for the treatment of GIC. However, owing to the low rate of early detection and high rate of postoperative recurrence associated with GIC, the effectiveness of radiotherapy and chemotherapy may be compromised by the occurrence of severe undesirable side effects. In the search for effective treatments with fewer side effects, an increasing number of research studies are focused on traditional herbal medicines and their monomer compounds.
Curcumin (CUR) was first discovered by Vogel and Pelletier (Prasad et al., 2014), and is the most important component of the rhizomes of turmeric (Curcuma longa) (Waly et al., 2018). CUR is a hydrophobic polyphenol that has been approved by the US Food and Drug Administration based on its bio-safety (Mashayekhi-Sardoo et al., 2021). Moreover, it has demonstrated a wide range of pharmacological activities, such as antibacterial (Ibarra-Martinez et al., 2022), anti-inflammatory (Yan et al., 2021), antioxidant (Xu et al., 2021), and antitumor (Zhang et al., 2020). CUR has been widely reported to inhibit the proliferation of tumor cells in a concentration-and time-dependent manner in vitro (Li et al., 2017a; Fan et al., 2020; Mao et al., 2021). According to relevant clinical trials on safety and toxicity, the acceptable dose of CUR for maximum efficacy is 4–8 g per day. It has been reported that humans can tolerate treatment with CUR at a dose up to 12 g per day (Barati et al., 2019).
In this review article, we discuss the pharmacological mechanisms underlying the effects of CUR against GIC based on recent evidence derived from in vitro and in vivo experiments, as well as clinical trials (Figure 1). The objective of this review is to provide a reference for further research on the treatment of GIC using CUR.
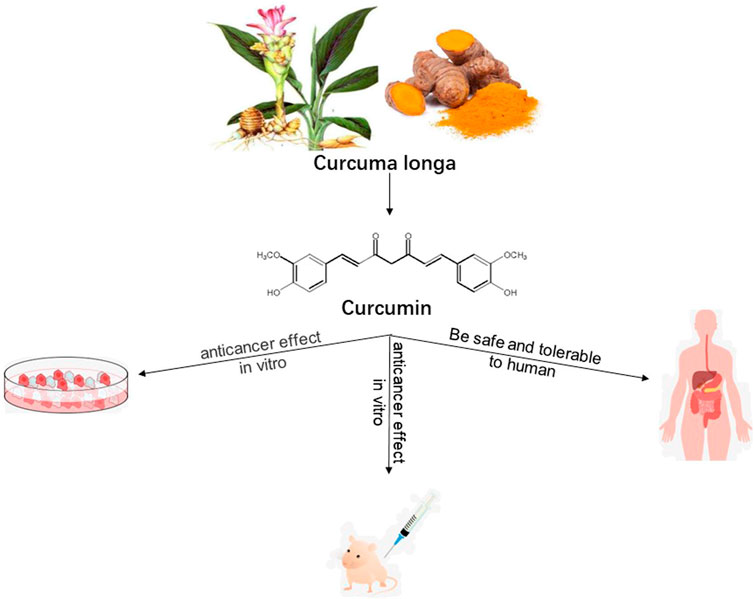
FIGURE 1. Research method utilized to summarize the pharmacological action of curcumin against gastrointestinal cancers.
2 Curcumin in gastrointestinal cancer (gastric cancer and colorectal cancer)
2.1 Inhibition of gastrointestinal cancer cell proliferation and blockage of cell cycle
It is widely recognized that CUR inhibits the proliferation and cell cycle of GIC cells. Cyclin D1 (CCND1) and cyclin-dependent kinase 4 (CDK4), a serine/threonine kinase which binds to CCND, can regulate cell transition from G1 to S phase (Yin et al., 2018). Sun et al. (Sun et al., 2019) found that CUR significantly inhibited cell cycle progression of SGC7901 cells in the G0/G1-S phase, increased the cell number in the G0/G1 phase, and downregulated the expression of B-cell lymphoma 2 (Bcl-2), CDK4, and CCND1 proteins in both cells and tissues.
Herrero et al. (De La Parte et al., 2021) found that CUR inhibited the proliferation of CRC cells CC531 in vitro and reduced the tumor volume in liver implants in vivo. Mao et al. reported that CUR inhibited tumorsphere formation and reduced cell viability in a concentration-dependent manner in LGR5 (+) colorectal cancer stem cells (CSCs). Inhibition of the proliferation of HCT-116 and LoVo CRC cell lines by CUR has also been reported (Mao et al., 2021).
Blakemore et al. (Blakemore et al., 2013) elucidated the stage of arrest induced by CUR in eight CRC cell lines (i.e., Caco-2, DLD-1, HCA-7, HCT116p53+/+,HCT116p53−/−, HCT116p21−/−, HT-29, and SW480). The results showed that the majority of these eight cell lines were arrested at the G2/M transition, with some cells arrested in mitosis. Notably, HCT116 and Caco-2 cells presented the highest levels of mitotic arrest.
2.2 Induction of cell apoptosis
Apoptosis is an indispensable mechanism for maintaining normal and healthy bodily functions. Any change in apoptotic patterns can enhance the survival and progression of cancer cells (Li et al., 2017b; Liang et al., 2018). CUR can promote the apoptosis of tumor cells by regulating multiple signaling pathways, upregulating proapoptotic genes, or downregulating antiapoptotic genes, thus inhibiting cancer progression (Liang et al., 2018).
2.2.1 Activation of the p53 signaling pathway
The tumor suppressor gene p53 plays an important role in the pathology of human cancer. The protein p53 inhibits the growth of cancer cells by regulating cell cycle arrest, cell apoptosis, and DNA repair (Hafner et al., 2019). Upregulation of p53 expression inhibits the proliferation and induces apoptosis of cancer cells (Su et al., 2017).
It has been shown that treatment with CUR for 24 h inhibited the proliferation and apoptosis of GC cells SGC-7901 and BGC-823 in a dose-dependent manner. Further studies found that this effect was related to the activation of the p53 signaling pathway through the upregulation of p53 and p21 (Fu et al., 2018).
By taking advantage of the waiting period before surgery, He et al. (2011) treated a group of CRC patients with CUR. The results showed that weight loss was improved in patients treated with CUR. In addition, by analyzing in human CRC tissue specimens obtained at the time of diagnosis (biopsy via colonoscopy) and surgery (surgical removal of CRC tissue), they demonstrated that CUR reduced the serum levels of TNF-α, induced apoptosis of CRC cells, upregulated p53 expression, and regulated the expression of apoptosis-related Bax and Bcl-2.
2.2.2 Inhibition of the phosphoinositide-3-kinase/Akt (PI3K/Akt) signaling pathway
The PI3K/Akt/mechanistic target of rapamycin kinase (PI3K/Akt/mTOR) signaling pathway is a well-studied antiapoptotic and pro-proliferative signaling pathway (Braglia et al., 2020). It has been revealed that this pathway is activated in approximately one-third of human cancers (Khorasani et al., 2021). Abnormal activation and overexpression of key signal molecules in the PI3K/Akt signaling pathway may promote the occurrence and development of GIC.
By treating SGC-7901, BGC-823, and MKN28 cell lines with increasing amounts of CUR for 24, 48, and 72 h, Li et al. (2017a) found that CUR could inhibit the proliferation and induce apoptosis of GC cells in a time- and dose-dependent manner. The results of western blotting assays showed that treatment with CUR downregulated the expression of phospho-Akt together with that of downstream phospho-mTOR and phospho-p70s6K in a dose-dependent manner. These findings indicated that the activation of the PI3K/Akt/mTOR signaling pathway was suppressed in GC cells following treatment with CUR. Liu et al. (2018) reported that CUR could inhibit the PI3K/Akt signaling pathway and activate the activity of p21 protein, thus promoting apoptosis in GC cell line AGS.
It has been reported that CUR downregulated PI3K and Akt expression while promoting apoptosis in the early stages of CRC induced by 1,2-dimethylhydrazine dihydrochloride in rats (Rana et al., 2015). Also, the results of western blotting assays showed that CUR upregulated the expression of pro-apoptotic Bcl-2 family members (Bcl-2 associated agonist of cell death [Bad] and Bax) and cysteine protease family apoptosis executioner (CASP3/9), whereas it downregulated that of antiapoptotic Bcl-2 protein.
2.2.3 Inhibition of the Wnt/β-catenin signaling pathway
β-catenin can be activated by Wnt signaling and is involved in different types of signal transduction. Studies have confirmed that it is essential for tumor growth (Zheng et al., 2017). The regulation of the Wnt/β-catenin signaling pathway is highly relevant to the metabolism of cancer cells (Cantor & Sabatini, 2012), and plays an important role in the occurrence, progression, and prognosis of various types of cancer in humans. Activation of the Wnt/β-catenin signaling pathway results in the development of resistance to chemotherapy in several types of cancer (Sun et al., 2012; Nagaraj et al., 2015). Therefore, it is hypothesized that it could effectively inhibit tumor growth by targeting the Wnt/β-catenin signaling pathway (Zheng et al., 2017).
Zheng et al. (2017) found that CUR significantly attenuated cell viability and induced tumor cell apoptosis in vitro in GC cell lines SNU-1, SNU-5, and AGS. CUR significantly suppressed the levels of Wnt3a, LDL receptor related protein 6 (LRP6), phospho-LRP6, β-catenin, phospho-β-catenin, c-Myc, and survivin, demonstrating that Wnt/β-catenin signaling was downregulated. In an in vivo experiment, AGS cells were used to establish a xenograft tumor model in male BALB/c nude mice. The CUR treatment group received CUR (1 mg/kg body weight) through gavage once daily, and the results showed that xenograft growth was inhibited by treatment with CUR.
The Wnt/β-catenin signaling pathway has been considered one of the most frequently dysregulated pathways in CRC (McCubrey et al., 2016). Its abnormal activation is related to cell proliferation, invasiveness, and resistance to therapy, indicating its potential value as a target in the treatment of CRC (Bahrami et al., 2017). Li et al. (2018) found that high levels of paternally expressed gene 10 (PEG10) increased the expression of Wnt1 and β-catenin in colon tissues, and this effect may be regulated by miR-491. By upregulating miR-491 and inhibiting the Wnt/β-catenin signaling pathway, CUR inhibited the expression of PEG10, thereby inducing apoptosis and impairing the proliferation of HCT-116 cells.
Axin2, a downstream gene of the Wnt/β-catenin signaling pathway, has been associated with cell proliferation, mutation, migration, and apoptosis (Gunes et al., 2010; Aristizabal-Pachon et al., 2015). Hao et al. (Hao et al., 2021) found that the Wnt/β-catenin pathway was activated in human CRC samples, accompanied by an increased mRNA expression of Axin2. Furthermore, they demonstrated that CUR could reduce Axin2 in the Wnt/β-catenin pathway, thus inhibiting CRC in vivo.
2.2.4 Induction of reactive oxygen species production
Reactive oxygen species (ROS), a general term describing oxygen or oxygen-containing highly reactive molecules, acts as a double-edged sword for cancer cells; these molecules act differently at different stages of cancer (Prasad et al., 2017). ROS can promote the proliferation and growth of cancer cells by activating a variety of cell signaling pathways, while the excessive accumulation of ROS can lead to cell damage and apoptosis (Liang et al., 2021).
Tong et al. (Tong et al., 2020) found that high concentrations of CUR (≥20 μmol/L) increased the levels of ROS in human GC cells, leading to mitochondrial damage, DNA damage, and apoptosis. These findings indicated that the anticancer effect of CUR in GC may be closely related to its pro-oxidative effect at high concentrations and elevation in the levels of ROS in cancer cells. In addition, the investigators demonstrated that CUR induced DNA demethylation in human GC cells through the damaged DNA repair-p53-p21/growth arrest and DNA damage inducible alpha-cyclin (GADD45A-cyclin)/CDK-retinoblastoma/E2F-DNA methyltransferase1 (E2F-DNMT1) axis.
Liang et al. (2014) found that CUR induced apoptosis combined with the production of ROS in BGC-823 cells. The apoptosis induced by CUR was reduced by adding an antioxidant (N-acetyl cysteine [NAC] or trion) to inhibit ROS production. Additional experiments showed that the apoptotic signal regulating the kinase 1-mitogen-activated protein kinase 4-JUN N-terminal kinase (ASK1-MKK4-JNK) signaling pathway was activated by oxidative stress induced by CUR, further leading to apoptosis.
Sritharan & Sivalingam (2021) found that treatment with CUR induced apoptosis, ROS generation, and a decrease in MMP in HT-29 and HCT-116 cell lines. Pre-treatment with NAC effectively reduced the levels of ROS and prevented MMP loss in HT-29 cells; however, it was less effective in HCT-116 cells. Pre-treatment of HCT-116 cells with NAC for 24 h upregulated the protein expression of total p53. This evidence demonstrated that CUR induced ROS-mediated apoptosis in CRC cell lines, and this effect may be mediated by p53.
2.2.5 Activation of the mitochondrial pathway
Liu et al. (2014) treated SGC7901 cells with co-administration of CUR at serial concentrations and diazoxide–an adenosine triphosphate-sensitive (ATP-sensitive) potassium (KATP) channel opener. They found that CUR induced the opening of KATP channels, leading to loss of the mitochondrial membrane potential (MMP) in a dose-dependent manner; moreover, it induced apoptosis of tumor cells.
Inhibition of glycolysis has been a therapeutic target in cancer (Hamanaka & Chandel, 2012). The regulation of key glycolytic enzymes, such as hexokinase 2 (HK2), is an effective approach to the inhibition of glycolysis (Patra et al., 2013). Wang K. et al (2015) found that the uptake of glucose, as well as the production of ATP and lactic acid, were inhibited by CUR in a dose-dependent manner in human CRC cells HCT116 and HT29. Further study showed that CUR induced the dissociation of HK2 from the mitochondria and triggered mitochondria-mediated apoptosis. Collectively, the evidence demonstrated that CUR inhibited glycolysis and promoted mitochondria-mediated apoptosis by regulating HK2 in CRC cells.
Similarly, it has been reported that CUR induced apoptosis through the mitochondrial cell death pathway in LoVo cells (Guo et al., 2013). Guo et al. found that CUR induced apoptosis in LoVo cells, accompanied by the release of lactate dehydrogenase, collapse of MMP, arrest of the cell cycle, and activation of CASP3/9. Moreover, the results of western blotting analysis showed that CUR upregulated the expression of cytochrome c, Bax, and p53, whereas it downregulated that of Bcl-2 and survivin in LoVo cells.
2.3 Induction of autophagy
Autophagy is a catabolic degradation process in which cellular proteins or organelles are degraded in the lysosome and recycled. This process plays an important and complicated role in the occurrence and development of cancer, as well as the development of resistance to therapy (Li et al., 2017a). Studies utilizing transmission electron microscopy revealed the presence of autophagosomes in GC cells (SGC-7901 and BGC-823) treated with CUR (Fu et al., 2018). Li et al. (2017a) found that CUR induced the formation of acidic vesicular organelles, conversion of LC3-I to LC3-II, and upregulation of the expression of autophagy-related proteins, such as beclin 1 (BECN1), autophagy related 7 (ATG7), and ATG5–12 conjugate in BGC-823, SGC-7901, and MKN-28 cells. These results demonstrated that CUR induced autophagy in GC cells. Interestingly, CUR and autophagy inhibitor 3-methyladenine co-treatment attenuated the viability of GC cells and increased apoptosis. In conclusion, they suggested that CUR induced a protective autophagy, which could be antagonistic apoptotic cell death in GC cells.
Mao et al. (2021) found that CUR induced autophagy in colorectal CSCs in a concentration-dependent manner. The results obtained from transmission electron micrographs confirmed this finding. In addition, co-treatment with hydroxychloroquine (an autophagy inhibitor) significantly decreased the cell proliferation induced by CUR.
2.4 Inhibition of epithelial–mesenchymal transition processes
By converting the epithelial characteristics of cells into mesenchymal attributes, EMT stimulates the progression of cancer. This is achieved by enhancing the invasive, migratory, and metastatic abilities of cancer cells. In recent years, studies have focused on elucidating the mechanism involved in the inhibition of EMT by treatment with CUR in GIC cells (Suarez-Carmona et al., 2017).
2.4.1 Inhibition of the Wnt/β-catenin pathway
The Wnt/β-catenin signaling pathway, an important pathway in EMT, plays an important role in embryogenesis and human diseases, including various types of cancer.
Zhang et al. (2020) reported that CUR inhibited EMT processes, cell migration, invasion, and cytoskeletal remodeling, and induced apoptosis in SGC-7901 cells. The results of reverse transcription-quantitative polymerase chain reaction, western blotting, and co-immunoprecipitation analyses showed downregulation of the mRNA and protein expression of sonic hedgehog signaling molecule (SHH), GLI family zinc finger 1 (GLI1), and forkhead box M1 (FOXM1) in the SHH signaling pathway, and β-catenin in the Wnt signaling pathway. These findings indicated that CUR inhibits the SHH and Wnt signaling pathways. This inhibition of the aforementioned signaling pathway, as well as the addition of CUR, also suppressed the EMT process. In addition, the evidence demonstrated an interaction between GLI1 and β-catenin, which could be inhibited by CUR.
Zhang et al. (2016) found that CUR inhibited EMT in CRC cells via the naked cuticle homolog 2-Wnt-C-X-C motif chemokine receptor 4 (NKD2-Wnt-CXCR4) signaling pathway. The results showed that CUR significantly inhibited the proliferation of CRC cells and upregulated the expression of NKD2 in SW620 CRC cells. These changes resulted in the regulation of key markers (downregulation of β-catenin and transcription factor 4 [TCF4] expression, upregulation of axin) of Wnt signaling. In addition, the progression of EMT was inhibited through the overexpression of E-cadherin and downregulation of vimentin. CUR also inhibited tumor metastasis by significantly reducing the expression of CXCR4.
Chen et al. (2020) reported that CUR downregulated the expression of transcription factors to promote EMT in CRC cells by reducing methylation in the promoter of caudal type homeobox 2 (CDX2) and inhibiting the CDX2/Wnt3a/β-catenin signaling pathway. In human SW480 CRC cells, CUR inhibited EMT, accompanied by the downregulation of DNMT1 and DNMT3a expression, and reduction of the methylation levels in the CDX2 promoter in a concentration-dependent manner. Treatment with CUR reduced the expression levels of N-cadherin, vimentin, Wnt3a, Snail1, and Twist, as well as the nuclear translocation levels of β-catenin in a concentration-dependent manner. In contrast, the expression levels of E-cadherin were increased.
2.4.2 Regulation of miR-200c/EPM5
The tumor suppressor miR-200c is upregulated by CUR in CRC cells (SW620 and HT29) (Wang et al., 2020). As a consequence of EMT repression, cell migration and invasion were also inhibited by CUR. Treatment with CUR also downregulated the expression of EPM5 (also termed PRICKLE2), a direct target of miR-200c. Further investigation indicated that EPM5 acted as a downstream of the CUR/miR-200c cascade, and miR-200c mediated the CUR-repressed EMT by direct repression of EPM5.
2.5 Inhibition of invasion and migration
Metastasis of a malignant tumor is often the main reason for failure of therapy. Therefore, an important part of the research on anticancer drugs is focused on the inhibition of tumor metastasis.
Numerous studies (Liu et al., 2018; Sun et al., 2019; Tong et al., 2020; Zhang et al., 2020) have demonstrated that CUR could inhibit the invasion and migration of GC cells. Liu et al. reported that CUR inhibited the expression of miRNA-21 and decreased that of matrix metalloproteinase 2 (MMP2) and MMP9, thus inhibiting the invasion and migration of AGS cells (Liu et al., 2018). Zhang et al. (Zhang et al., 2020) found that CUR affected the migration, invasion, and cytoskeletal remodeling of SGC-7901 cells by regulating the GLI1-β-catenin pathway.
In vitro and in vivo studies have shown that CUR inhibited the invasion, metastasis, and angiogenesis of CRC cells (Chen et al., 2013; Calibasi-Kocal et al., 2019; De La Parte et al., 2021). According to Chen et al. (Chen et al., 2013), the antimetastatic effect of CUR on CRC cells is related to the downregulation of Sp-1 (transcription factor), FAK (cell adhesion component), and CD24, as well as the upregulation of E-cadherin expression.
Herrero et al. (De La Parte et al., 2021) reported the antitumor effect of CUR against liver implants from CRC both in vitro and in vivo. Importantly, the investigators quantified the total and individual liver lobe tumor volume in untreated and CUR-treated WAG/RijHsd tumor-bearing rats. They found that CUR reduced the tumor volume in liver implants. Similar findings (Chen et al., 2013) in a mouse model have also been reported.
2.6 Sensitization to chemotherapy and reversal of resistance to chemotherapy
2.6.1 Gastric cancer
Chemotherapy, one of the most important treatment options for GC, greatly improves the prognosis and survival of patients with GC. However, the occurrence of chemotherapy-resistant cancer cells has resulted in treatment failure or disease recurrence. Furthermore, the severe adverse effects caused by chemotherapy drugs negatively impact patient quality of life. CUR is a natural plant extract with a good safety profile. Hence, its role in sensitizing cancer cells to chemotherapy and reversing resistance to treatment has become an important topic of research in the GC setting. Drugs commonly used in chemotherapy against GC include 5-fluorouracil (5-FU), cisplatin (DDP), doxorubicin (DOX), etc.
In a study, AGS cells were treated with CUR, DOX, and their combination (DOX-CUR). The results showed that all these treatments significantly inhibited the viability, tumor spheroid formation, migration, and invasion in AGS cells. Notably, the DOX-CUR combination exhibited a more significant anticancer activity than monotherapy with CUR or DOX (Amoodizaj et al., 2020). Yu et al. (2011) found that chemotherapeutics (etoposide and DOX) induced apoptosis and activated NF-κB in SGC-7901 cells. The combination of CUR and chemotherapeutics induced apoptosis, while the activation of NF-κB was attenuated and the expression of the downstream antiapoptotic genes Bcl-2 and Bcl-xL was downregulated.
By establishing a 5-FU-resistant GC cell line (SGCR/5-FU), Kang et al. (2016) found that cytosolic IκBα degradation and NF-κB nuclear translocation were increased in these cells. Treatment with CUR reversed 5-FU resistance and inhibited the proliferation of GC cells by inhibiting the NF-κB signaling pathway. Moreover, downregulation of tumor necrosis factor-α (TNF-α) mRNA was induced by CUR in GC cells.
A regimen combining 5-FU with DDP (FP) is typically used in chemotherapy against GC. He et al. (2017) found that CUR enhanced the effects of treatment with FP on the viability, colony formation, and migratory abilities of MGC-803 cells. This effect may be associated with the apoptosis induced by CUR via the CASP3/8, Bcl-2, and Bax signaling pathways.
The chemotherapeutic regimen combining 5-FU, folinic acid, plus OXA (FOLFOX) plays an important role in the treatment of GC. Nevertheless, it is associated with extensive side effects and the development of resistance in some patients (Kim et al., 2011). Zhou et al. (2016) explored the synergistic antitumor effect of CUR and FOLFOX against GC in vitro and in vivo. Using cell proliferation assays and assessment of apoptosis, they demonstrated the synergistic antitumor effect of CUR in combination with 5-FU and OXA (5-FU/OXA). This effect may be associated with the downregulation of Bcl-2 expression and upregulation of Bax and CASP3/8/9 expression caused by CUR. In addition, the in vivo data showed that the combination of CUR and 5-FU/OXA significantly inhibited the growth of BGC-823 xenograft tumors.
2.6.2 Colorectal cancer
Chemotherapy is an important therapeutic modality in the treatment of CRC. However, toxicity and the development of resistance limit its efficacy. CUR could synergize with DDP to inhibit CRC cells and effectively suppress the occurrence of resistance to DDP in those cells (Zheng Z.-h. et al, 2021; Fan et al., 2022). Fan et al. (2022) reported that this effect of CUR was achieved by targeting the miRNA-137-glutaminase axis. They found that DDP-resistant CRC cells (HT-29) displayed an increased glutamine metabolism, and this elevation could be attenuated by CUR. Further study showed that miR-137, which directly targets glutaminase, was induced by treatment with CUR, leading to sensitization of CRC cells to DDP. According to a study conducted by Zheng et al., the reversal effect of CUR on resistance to DDP in CRC cells was based on the downregulation of lncRNA KCNQ1 opposite strand/antisense transcript 1 (KCNQ1OT1) expression. KCNQ1OT1 is upregulated in HCT8/DDP cells and regulates their proliferation and apoptosis by blocking the suppressive effect of miR-497 on Bcl-2 expression. In vitro and vivo experiments showed that CUR reversed the development of resistance to DDP in CRC by downregulating KCNQ1OT1 expression, thereby regulating the miR-497/Bcl-2 axis.
It was reported that >15% of patients are resistant to treatment with 5-FU-based chemotherapeutic regimens (Shakibaei et al., 2014). Thus, the resistance to 5-FU is also a research focus in the CRC setting. Studies (Shakibaei et al., 2014; Zhang et al., 2018; Lu et al., 2020; Zheng X. et al, 2021) confirmed that CUR could enhance the sensitivity of CRC cells to 5-FU and reverse the occurrence of resistance. Nicotinamide N-methyltransferase (NNMT) is highly expressed in a wide variety of cancers. It has been reported that NNMT could enhance resistance to 5-FU in human CRC cells (Xie et al., 2016; Li G. et al, 2021). Li et al. (Li G. et al, 2021) found that the effect of CUR on reversing NNMT-induced resistance to 5-FU was related to ROS production and cell cycle arrest. They demonstrated that CUR inhibited the expression of NNMT, though the precise mechanism involved in this process is unclear. Zheng X. et al (2021) showed synergistic inhibitory effects of CUR and 5-FU both in vivo and in vitro in CRC SW620 cells. These effects may be attributed to inhibition of STAT1 activation to reduce L1 expression and induce a higher level of cell apoptosis. By establishing the 5-FU resistant HCT-116 cell line, Lu et al. (2020) found that CUR reversed resistance to 5-FU in CRC cells through inhibition of the EMT process. This effect may be achieved by regulation of the TET1-NKD-Wnt pathway. Zhang et al. (2018) reported the important role of nuclear factor erythroid 2-related factor (Nrf2) in the effect of CUR on the reversal of resistance to 5-FU in CRC. CUR suppressed the mRNA and protein expression of Nrf2, which further reduced the expression levels of Bcl-2. These effects promoted apoptosis in HCT-8/5-FU cells and reversed MDR.
OXA is a platinum-based chemotherapeutic agent that plays an important role in the treatment of CRC (Yin et al., 2019); nevertheless, the development of resistance limits its efficacy. Han et al. (2020) established the OXA-resistant CRC cell line HCT116/OXA, and found that CUR could reverse resistance to its OXA. Furthermore, they suggested that the mechanism underlying this effect may be the inhibition of excision repair cross-complementation 1 (ERCC1) through upregulation of miRNA-409-3p. These alterations result in inhibition of the expression of drug resistance-related proteins [Bcl-2, glutathione S-transferase-π (GST-π), mitochondrial ribosomal protein (MRP), P-gp, and survivin]. Yin et al. (2019) also established an OXA-resistant cell line (HCT116/OXA) to investigate the effect of CUR on resistance to OXA in CRC. According to the results, CUR could reverse resistance to OXA in CRC through inhibition of EMT by suppressing the transforming growth factor-β/Smads (TGF-β/Smads) pathway both in vitro and in vivo. TGF-β, regulated by Smad and non-Smad signaling pathways, is an important inducer of EMT (Katsuno et al., 2013). EMT has been gradually recognized as an important mechanism in the development of drug resistance in tumors (Yin et al., 2019). Ruiz et al. (Ruiz de Porras et al., 2016) found that NF-κB was overactivated in OXA-resistant CRC cells, and this overactivation could be attenuated by treatment with CUR. Meanwhile, they found that treatment with CUR plus OXA could revert resistance to OXA, potentially through inhibition of the NF-κB signaling cascade. In addition, the investigators reported that C-X-C motif chemokine ligand 1 (CXCL1) was regulated by NF-κB; its expression was upregulated in resistant cells, but downregulated after treatment with CUR plus OXA. Thus, they speculated that CXCL1 could be used as a predictive marker in patients with CRC. The majority of studies on CRC cells are conducted in vitro. Guo et al. (Guo L.-d. et al, 2015) evaluated the therapeutic effect of CUR and OXA in vivo by establishing xenografts through subcutaneous implantation of LoVo CRC cells in nude mice. The results showed that the combinatorial administration of CUR and OXA inhibited the growth of CRC in nude mice, caused cell cycle arrest, and induced apoptosis. In addition, some important signaling molecules were tested; the results showed that the expression of Bax, CASP3, and PARP was significantly increased, whereas that of Bcl-2, survivin, HSP70, pro-CASP3, and pro-PARP was markedly suppressed in tumor cells.
Su et al. (2018) also reported that CUR could reduce resistance to irinotecan (CPT-11). LoVo/CPT-11, an established CPT-11-resistant CRC cell line, exhibited markedly higher expression levels (mRNA and protein) of colon CSC markers (CD44, CD133, epithelial cell adhesion molecule [EpCAM], and CD24) compared with parental cells. Furthermore, treatment with CUR attenuated resistance to CPT-11, accompanied by reduction in the expression levels of CSC identification markers. In addition, they found that CUR induced cell apoptosis in sphere-forming cells, and the combination of CUR and CPT-11 could increase the apoptosis-inducing effect.
FOLFOX is the most commonly used combination chemotherapeutic regimen for CRC (Al Sabbagh et al., 2021). In recent years, studies (including clinical trials) on CUR and FOLFOX have been conducted. James et al. (James et al., 2015) established ex vivo CSC models from patient-derived colorectal liver metastases. They found that the combination of CUR and 5-FU/OXA could significantly reduce the number of spheroids, while the levels of CSC markers (ALDH and CD133) were also decreased. A phase I clinical trial (James et al., 2015) performed in the United Kingdom in patients with liver metastases from CRC showed that addition of daily oral CUR (0.5–2 g) to FOLFOX chemotherapy is safe and tolerable. A phase IIa (Howells et al., 2019), two-armed, randomized, and controlled clinical trial further demonstrated the safety and tolerability of this combination in patients with metastatic CRC who were randomly assigned to receive either FOLFOX or CUFOX (FOLFOX +2 g oral CUR per day). A significant difference in overall survival was observed between patients receiving FOLFOX and CUFOX. The concentration of plasma CXCL1 [growth-regulated oncogene-α (GRO-α)], which is associated with metastatic spread in CRC, was 1.7-fold higher in the FOLFOX group versus the CUFOX group; however, the difference was not statistically significant. Hence, it is suggested that the combination treatment has the potential to provide benefit to patients, though the currently available evidence is insufficient. Both phase I and II trials involved a small number of patients (12 and 28, respectively) and lacked a placebo control group. Thus, the clinical efficacy of CUR as an adjunct of FOLFOX chemotherapy is uncertain, and phase III trials are warranted.
3 Curcumin in gastric cancer
The unique anti-GC mechanism of CUR, which is described in detail below, combined with the above common anti-GIC mechanism of CUR is illustrated in Figure 2 and summarized in Table 1.
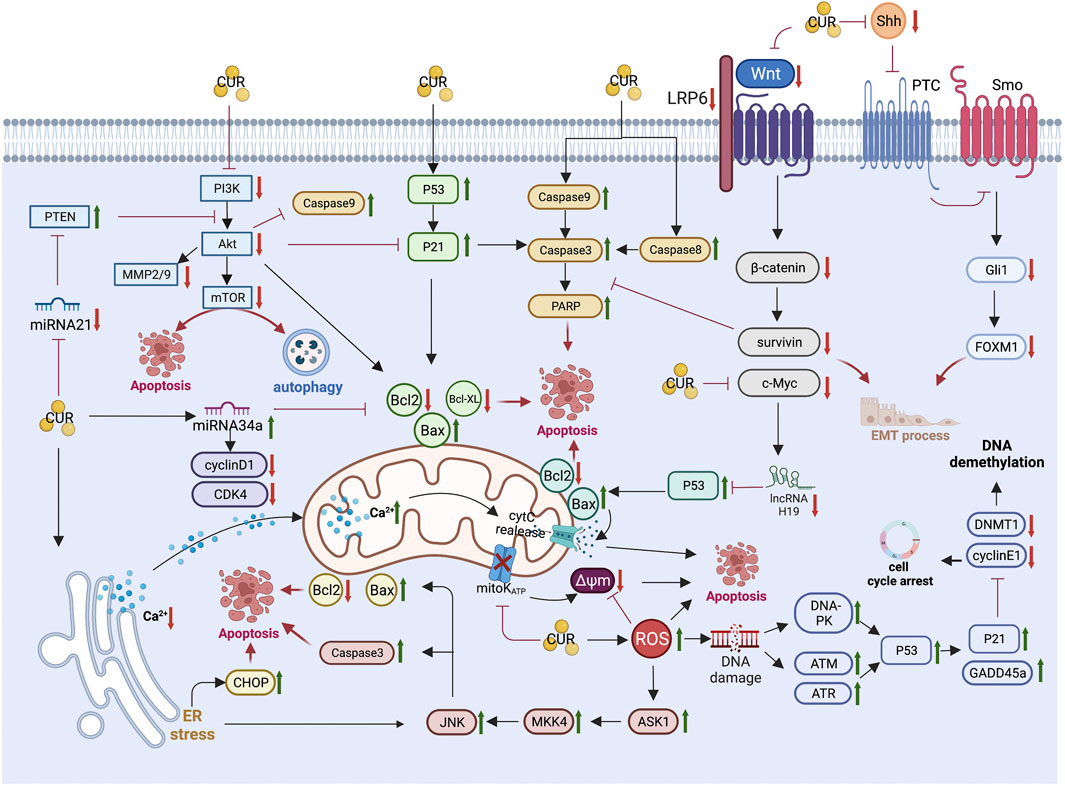
FIGURE 2. Pharmacological mechanism underlying the effects of curcumin on gastric cancer (Created with Biorender.com). PI3K, Phosphatidylinositol 3-kinase; Akt, Protein kinase B; mTOR, mammalian target of rapamycin; MMP2/9, matrix metalloproteinase 2/9; PTEN, phosphatase and tensin homolog; CDK4, cyclin-dependent kinase 4; Bcl2,B-cell lymphoma 2; Bax, B-cell lymphoma-2 (Bcl-2)-associated X protein; PARP, Poly (ADP-ribose) polymerase; LRP6, lipoprotein receptor-related protein 6; Shh, sonic hedgehog; Gli1, Glioma-associated oncogene homologue 1; FoxM1, forkhead box transcription factor M1; EMT, epithelial-mesenchymal transition; cytC, cytochrome C; Δψm, mitochondrial membrane potential; ER, endoplasmic reticulum; CHOP, CCAAT/enhancer binding protein homologous protein; JNK, c-Jun N-terminal kinases; MKK4, mitogen-activated protein kinase kinase 4; ASK1, apoptosis signal-regulated kinase 1; ROS, reactive oxygen species; DNA-PK, DNA-dependent protein kinase; ATM, Ataxia-telangiectasia mutated; ATR, ataxia telangiectasia and Rad3-related; GADD45a, growth arrest and DNA damage-inducible protein GADD45 alpha; DNMT1, DNA methyltransferase 1.
3.1 Activation of caspase 3 signaling pathway and regulation of Bcl-2-associated X, Bcl-2
Activation of the cysteine protease (caspase) family plays a critical role in apoptosis and is considered a direct effector of apoptosis. Caspase 3 (CASP3) is the main effector molecule of apoptosis; it can lyse DNA repair-related molecules, apoptosis inhibitory proteins, extracellular matrix proteins, and skeleton proteins. The activation of CASP3 signals an reliable marker for apoptosis (Crowley & Waterhouse, 2016). Members of the Bcl-2 family play a central role in the regulation of apoptosis and influence the cell cycle, particularly the transition from quiescence to proliferation (Xu et al., 2011). He et al. (He et al., 2017) demonstrated that CUR inhibited the proliferation and promoted apoptosis of MGC803 cells via the activation of CASP3/8, downregulation of Bcl-2, and upregulation of Bcl-2-associated X (Bax).
3.2 Downregulation of miRNA-21
It has been found that miRNA-21 is highly expressed in GC tissues (Liu et al., 2018). CUR induced the activity of phosphatase and tensin homolog (PTEN) and inhibited that of the PI3K/Akt signaling pathway by inhibiting miRNA-21 expression in AGS cells. These effects resulted in the inhibition of biological activity and induced apoptosis of AGS cells. Similar findings were also obtained in MGC803 cells (Liu et al., 2018). Qiang et al. (Qiang et al., 2019) found that CUR induced apoptosis, inhibited the expression of phospho-Akt protein, upregulated PTEN expression, and downregulated the levels of miRNA-21 in MGC803 cells. These results suggested that CUR negatively modulated the miR-21/PTEN/Akt pathway in MGC803 cells.
3.3 Downregulation of long non-coding RNA H19
It is gradually accepted that long non-coding RNA (lncRNAs) play multiple roles in the progression of cancer. Previous studies (Song et al., 2013; Wang J. et al, 2015) have reported that H19 is abnormally upregulated in GC. This upregulation directly inhibits p53 activation, thereby promoting GC progression (Yang et al., 2012). Liu et al. (Liu et al., 2016) found that CUR inhibited the expression of H19 and upregulated that of p53 in a concentration-dependent manner in SGC7901 cells. Further investigation showed that CUR downregulated the expression of the c-Myc oncogene. In addition, the downregulation of H19 expression induced by CUR could be reversed by exogenous c-Myc protein. This observation suggested that CUR inhibited the proliferation and induced apoptosis of GC cells by downregulating the c-Myc/H19 pathway.
3.4 Upregulation of miR-34a
It has been revealed that miR-34a plays a critical role in GC (Wong et al., 2011). Sun et al. (Sun et al., 2019) found that CUR could upregulate miR-34a expression, inhibit proliferation, and induce apoptosis in SGC7901 cells. Administration of CUR or miR-34a in GC cells or tissues decreased the expression of Bcl-2, CDK4, and CCND1. It is suggested that CUR may downregulate Bcl-2, CDK4, and CCND1 expression by upregulating miR-34a, thus inducing apoptosis in GC.
3.5 Activation of the endoplasmic reticulum stress pathway
Cao et al. (2013) indicated that CUR could function through endoplasmic reticulum (ER) stress and mitochondria functional pathways in GC cells AGS, based on the upregulation of CCAAT/enhancer binding protein homologous protein (CHOP), phosphorylation of JNK and downregulation of SERCA2ATPase, release of cytochrome c, reduction of Bcl-2, and loss of MMP.
3.6 Suppression of gastric acid secretion
The development of GC is often accompanied by excessive gastric acid secretion (Deng et al., 2012). It is widely thought that the acidic environment in the stomach contributes to the development of GC (Sheng et al., 2018). Therefore, hyperacidity may be related to the progression of GC. Gastrin, an inducer of gastric acid secretion, is a valuable marker for GC screening (Waldum et al., 2017). Zhou et al. (2017) evaluated the mechanism through which CUR inhibits GC by affecting gastric acid secretion both in vitro and in vivo. The results showed that CUR significantly inhibited GC progression through an increase in gastric pH and suppression of gastrin-mediated acid secretion.
3.7 Inhibition of angiogenesis and lymphangiogenesis
Vascular endothelial growth factor (VEGF) can promote angiogenesis and growth of cancer cells, and is considered the most important and specific angiogenic factor. Signal transduction and transcription activator 3 (STAT3) is highly expressed in GC, and is closely related to cancer cell stage, invasion depth, lymph node metastasis, and cancer grade. Overexpression of STAT3 and VEGF in GC cells induced an increase in microvascular density and disease progression. Using BALB/C mice grafted with a mouse gastric adenocarcinoma cell line MFC as an experimental model, Wang et al. (2017) investigated the antitumor activity of CUR. The results showed that CUR inhibited the proliferation of GC by downregulating the differentiated embryonic chondrocyte-expressed gene 1-hypoxia inducible factor 1 subunit alpha-STAT3-VEGF (DEC1-HIF1A-STAT3-VEGF) signal transduction pathway.
Huang et al. (2017) reported that tumor-derived mesenchymal stem cells (MSC) are important for tumor angiogenesis, and investigated the mechanism through which CUR mediates angiogenesis to regulate GC cell-derived MSC (GC-MSC). The results showed that CUR reduced GC-MSC-derived angiogenesis by inhibiting the NF-κB/VEGF signaling pathway, which plays a critical role in tube formation, migration, and colony formation induced by GC-MSC.
High mobility group box 1 (HMGB1) and vascular endothelial growth factor D (VEGFD) are associated with lymphangiogenesis and tumor metastasis in GC. Da et al. (2019) found that CUR may inhibit lymphangiogenesis in GC cells (AGS and SGC-7901) through the inhibition of HMGB1/VEGFD signaling. In addition, they investigated the effect of CUR on lymphatic vessel density in a nude mouse model using SGC7901 cells. The results indicated that CUR inhibited lymph node metastasis of GC, as evidenced by the reduced tumor volume, decreased protein expression of prospero homeobox 1 (PROX1), podoplanin (PDPN), and vascular endothelial growth factor receptor 3 (VEGFR3), and downregulated mRNA expression of lymphatic vessel endothelial receptor 1 (LYVE1), PROX1, PDPN, and VEGFR3 (Da et al., 2015).
4 Curcumin in colorectal cancer
The anti-CRC mechanism of CUR, which is described in detail below, combined with the above common anti-GIC mechanism of CUR is illustrated in Figure 3 and summarized in Table 2.
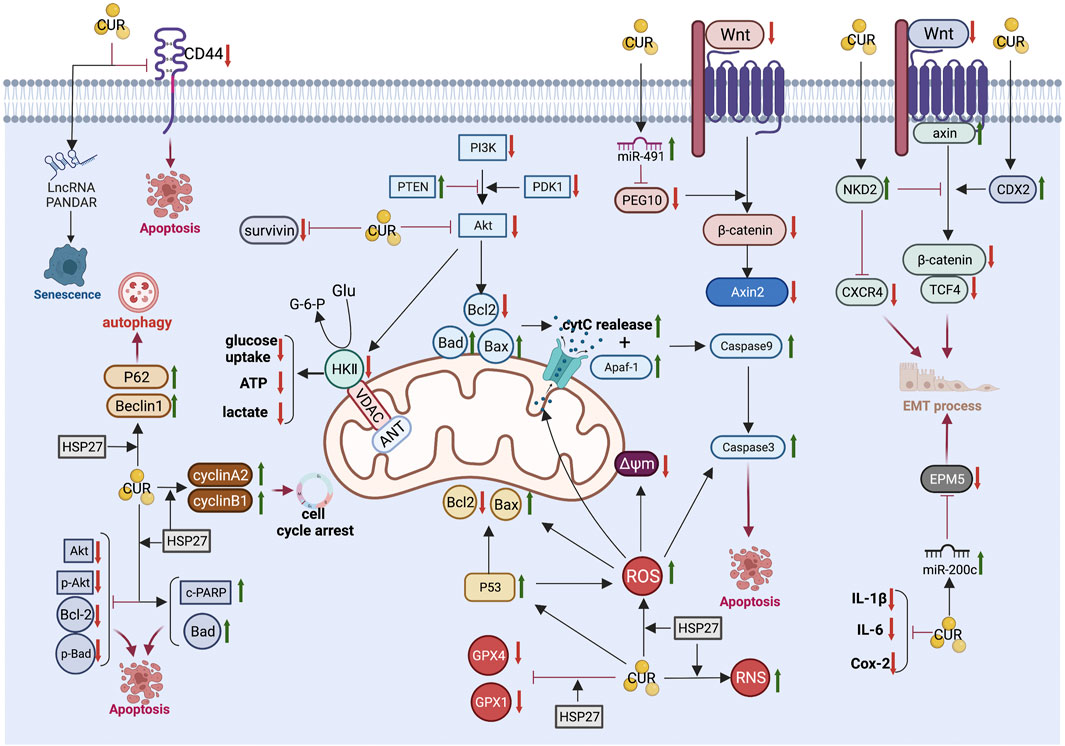
FIGURE 3. Pharmacological mechanism underlying the effects of curcumin on colorectal cancer (Created with Biorender.com). LncRNA, long non-coding RNA; PI3K, Phosphatidylinositol 3-kinase; Akt, Protein kinase B; PTEN, phosphatase and tensin homolog; PDK1, phosphoinositide-dependent protein kinase-1; Bcl2, B-cell lymphoma 2; Bax, B-cell lymphoma-2 (Bcl-2)-associated X protein; Bad, BCL2 associated agonist of cell death; cytC, cytochrome C; Δψm, mitochondrial membrane potential; Apaf-1, Apoptotic protease activating factor 1; PEG10, paternally expressed gene 10; Axin2, axis inhibition protein 2; NKD2, Naked cuticle homolog 2; CXCR4, chemokine receptor 4; CDX2, caudal type homeobox 2; TCF4, transcription factor 4; EMT, epithelial-mesenchymal transition; IL-6, interleukin-6; IL-1β, interleukin-1β; Cox-2,cyclooxygenase-2; ROS, reactive oxygen species; RNS, reactive nitrogen species; HSP27, Heat shock protein 27; GPX4, Glutathione peroxidase 4; GPX1, Glutathione peroxidase-1; c-PARP, cleaved poly (ADP-ribose) polymerase; GluGlucose.
4.1 Downregulation of CD44 expression
CD44, a family of transmembrane glycoproteins, is an important cancer stem cell marker in CRC. It is involved in multiple cellular biological functions, such as proliferation, migration, and survival (Thapa & Wilson, 2016; Chen et al., 2018). Fan et al. (Fan et al., 2020) examined the relationship between the anticancer effect of CUR and CD44 in CRC. Following treatment with CUR, the expression of CD44 was downregulated in HCT-116 and HCT-8 cell lines in a dose-dependent manner. In addition, CUR specifically inhibited CD44 + CRC cells. Knockdown of CD44 expression using siRNA led to a significant decrease in the inhibitory effect of CUR against CD44 + CRC cells. Further investigation indicated that the apoptosis of CD44 + CRC cells induced by CUR may be associated with the binding of CUR and the CD44 molecule, which could result in a higher uptake of CUR.
4.2 Regulation of heat shock protein 27
Heat shock protein 27 (HSP27), a small heat shock protein, was found to be lowly expressed in normal cells; however, its levels increase following exposure to survival stress (Hou et al., 2016). High expression of HSP27 has been associated with growth, progression, and metastasis of cancer cells (Hung et al., 2017).
Liang et al. (2018) demonstrated that silencing of HSP27 inhibited apoptosis and autophagy, consequently inducing resistance to treatment with CUR in CRC cells. They found that DLD-1 cells (with high HSP27 expression) are more sensitive to treatment with CUR than HT-29 cells (with low HSP27 expression). HSP27 knockdown (HSP27-KD) cells presented resistance to CUR, accompanied by decreased levels of antiapoptotic proteins (phospho-Akt, Akt, Bcl-2, and phospho-Bad) and increased levels of apoptotic proteins (Bad and cleaved PARP). A series of CUR-induced effects were abolished in HSP27-KD cells, including apoptosis, G2/M cell cycle arrest, oxidative stress, autophagy, blockage of Akt signaling, and mitochondria-mediated apoptosis. In addition, HSP27-KD cells had decreased levels of antiapoptotic proteins (phospho-Akt, Akt, Bcl-2, and phospho-Bad) and increased levels of apoptotic proteins (Bad and cleaved PARP). These data indicated that HSP27-KD induced resistance to CUR in CRC cells.
4.3 Induction of senescence
Chen et al. (2017) demonstrated that treatment with low-dose CUR induced senescence and promoted CDKN1A antisense DNA damage activated RNA (PANDAR) expression in CRC DLD-1 cells. Notably, silencing of lncRNA PANDAR in these cells may switch senescence to apoptosis partly by regulating the expression of p53-upregulated modulator of apoptosis (PUMA). They did not find a difference in the expression of PANDAR between CRC tissues and corresponding normal tissues. Knockdown of PANDAR by si-RNA did not significantly change the proliferation of CRC DLD-1 cells. Moreover, low-dose CUR (5 μM) induced senescence but not apoptosis in DLD-1 cells, along with upregulation of PANDAR expression. Interestingly, silencing of PANDAR in DLD-1 cells treated with CUR was linked to enhanced apoptosis and a significant reduction in senescent cells. They also found that silencing of PANDAR stimulated the mRNA expression of the pro-apoptotic gene PUMA in DLD-1 cells treated with CUR.
4.4 Binding to sirtuin 1 and reduction of its stability
Sirtuin 1 (SIRT1) is a NAD+-dependent histone/protein deacetylase with several physiological functions, such as metabolic regulation, differentiation, and stress response (Lee et al., 2018). It is involved in the progression of CRC (Song et al., 2018) and highly expressed in human CRC tissues compared with adjacent normal tissues (Song & Surh, 2012). Lee et al. (Lee et al., 2018) found that CUR downregulated the expression of SIRT1 protein, but not mRNA, in human CRC cells (HCT-116, DLD-1, and HCT-15). These findings suggested that the regulation of SIRT1 by treatment with CUR is post-translational. Results obtained through nano-liquid chromatography tandem mass spectrometry indicated that CUR directly bound to cysteine 67 of SIRT1 and resulted in structural modification. Consequently, it promoted the ubiquitin-dependent proteasomal degradation of SIRT1, which is overexpressed in CRC cells. In conclusion, by binding to SIRT1 and decreasing its stability, CUR suppressed its oncogenicity in human CRC cells and inhibited disease progression both in vitro and in vivo.
4.5 Inhibition of anchorage-independent growth of colorectal cancer cells
As an in vitro characteristic of tumorigenic cells, anchorage-independent growth (colony-forming capacity in semisolid medium) has been considered a marker of transformed cells which differentiates them from normal cells (Borowicz et al., 2014). In tumor cells, the anchorage-independent growth is related to their tumorigenic and metastatic potential in vivo (Choi et al., 2021). The aberrant epigenetic landscape (i.e., heritable alterations in gene expression without changes in DNA sequence) may increase the complexity of CRC initiation and progression.
Deleted in lung and esophageal cancer 1 (DLEC1), a tumor suppressor gene, could reduce transcriptional activity and promote hypermethylation in several types of cancer, including CRC (Guo Y. et al, 2015). Guo et al. (Guo Y. et al, 2015) investigated the inhibitory role of DLEC1 in anchorage-independent growth of human colorectal adenocarcinoma HT29 cells and epigenetic regulation by CUR. The results revealed the tumor inhibitory role of DLEC1, and indicated its association with the inhibition of anchorage-independent growth of HT29 cells by treatment with CUR. In addition, the investigators demonstrated that CUR suppressed the anchorage-independent growth of HT29 cells by upregulating DLEC1 and reducing CpG methylation in HT29 cells. This activity may be involved in lowering the protein expression of DNMTs and HDACs.
4.6 Modulation of colonic microbial ecology
It has been well established that the inflammatory microenvironment and intestinal microbiota influence the progression of colitis-associated CRC (Schwabe & Jobin, 2013; McFadden et al., 2015). McFadden et al. (McFadden et al., 2015) used interleukin-10 (IL-10) -/- mice on 129/SvEv background as a model of colitis-associated CRC to evaluate the role of CUR in modulating colonic microbial ecology and preventing the progression of chronic colitis to CRC. Wild-type or IL-10 -/- mice (age: 10 weeks) received six weekly intraperitoneal injections of azoxymethane or phosphate-buffered saline, along with supplementation with a control or CUR initiated at the same time. Stools were collected every 4 weeks for microbial community analysis. The results showed that dietary CUR reduced or entirely prevented the development of CRC in the azoxymethane-induced CRC model in a dose-dependent manner. The chemoprophylactic effects appeared to be related to the ecological regulation of colonic microorganisms by CUR, rather than the reduction of inflammation. These findings suggested that the role of CUR in CRC prevention is associated with the maintenance of a more diverse colon microbial ecology.
5 Conclusion
GIC are associated with high morbidity and mortality rates, thus posing a serious threat to human life and health. Currently, surgery and chemotherapy are the main treatment options for GIC; nevertheless, their efficacy is limited. In numerous studies, CUR, a plant extract with a good safety profile, has exhibited pharmacological effects on GIC both in vivo and in vitro. As demonstrated in the present review, CUR can effectively inhibit GlC through multiple targets, mechanisms, and pathways. Despite the potential of CUR in drug development and application to the treatment of GIC, there remain some deficiencies in the current studies that warrant further investigation.
Regulated cell death (RCD) mainly includes apoptosis, autophagy, pyroptosis, necroptosis, and ferroptosis. Studies investigating RCD induced by CUR in GIC mainly focus on apoptosis and autophagy. It remains unclear whether CUR could induce pyroptosis, necroptosis, and ferroptosis in GIC. These three modes of RCD were recently proposed, and have become hot topics in antitumor drug research, particularly with regard to apoptosis-resistant cells. It has been reported that CUR promoted pyroptosis in liver cancer cells (Li W.-f. et al, 2021), induced necroptosis in prostate and lung cancer cells (Lee et al., 2021), and induced ferroptosis in breast (Li et al., 2020) and lung cancer cells (Tang et al., 2021). Further research on the potential induction of pyroptosis, necroptosis, and ferroptosis by CUR in GIC, as well as the mechanisms involved in these processes, is required.
CUR has exhibited good antitumor activity and low toxicity. Nevertheless, its poor solubility in water (456 μg/L) and poor stability in aqueous solution result in an insufficient concentration in serum and tissues, leading to low bioavailability and clinical efficacy (Jakubek et al., 2019). To resolve this problem, researchers have turned their attention to CUR analogs and nanopreparations. This attempt is focused on overcoming its deficiencies in terms of water solubility, bioavailability, and stability, while retaining its excellent antitumor effect. CUR analogs (e.g., WZ35 (He et al., 2019), M37 (Liang et al., 2017), and EF24 (He et al., 2016)) and CUR nanoparticles (e.g., CUR micelles (Lin et al., 2020), CUR-carrying nanoliposomes (Angeline et al., 2020), and solid lipid nanoparticle (Mohamed et al., 2021)) have exerted excellent antitumor effects on GIC. This evidence suggests a breakthrough in the application of CUR to the treatment of GIC and provides broad research prospects.
Author contributions
Conceptualization, YF and JL; writing—original draft preparation,YF; writing—review and editing, YF and XZ; visualization, YT, SC, and JL; funding acquisition, YT. All authors have read and agreed to the published version of the manuscript.
Funding
This work was supported by the National Natural Science Foundation of China (Nos. 81902694) and 345 Talent Project of Shengjing Hospital.
Acknowledgments
We apologize to colleagues whose important work could not be cited due to space constraints.
Conflict of interest
The authors declare that the research was conducted in the absence of any commercial or financial relationships that could be construed as a potential conflict of interest.
Publisher’s note
All claims expressed in this article are solely those of the authors and do not necessarily represent those of their affiliated organizations, or those of the publisher, the editors and the reviewers. Any product that may be evaluated in this article, or claim that may be made by its manufacturer, is not guaranteed or endorsed by the publisher.
References
Al Sabbagh, C., Agapova, E., Boudy, V., and Mignet, N. (2021). Stability of calcium levofolinate, 5-fluorouracil and oxaliplatin (FOLFOX) mixture. J. Oncol. Pharm. Pract. 1, 1. doi:10.1177/10781552211020808
Amoodizaj, F. F., Baghaeifar, S., Taheri, E., Jadid, M. F. S., Safi, M., Sani, N. S., et al. (2020). Enhanced anticancer potency of doxorubicin in combination with curcumin in gastric adenocarcinoma. J. Biochem. Mol. Toxicol. 34 (6), e22486. doi:10.1002/jbt.22486
Angeline, N., Suhito, I. R., Kim, C.-H., Hong, G.-P., Park, C. G., Bhang, S. H., et al. (2020). A fibronectin-coated gold nanostructure composite for electrochemical detection of effects of curcumin-carrying nanoliposomes on human stomach cancer cells. ANALYST 145 (2), 675–684. doi:10.1039/c9an01553a
Aristizabal-Pachon, A. F., Carvalho, T. I., Carrara, H. H., Andrade, J., and Takahashi, C. S. (2015). AXIN2 polymorphisms, the beta-catenin destruction complex expression profile and breast cancer susceptibility. Asian pac. J. Cancer Prev. 16 (16), 7277–7284. doi:10.7314/apjcp.2015.16.16.7277
Bahrami, A., Amerizadeh, F., ShahidSales, S., Khazaei, M., Ghayour-Mobarhan, M., Sadeghnia, H. R., et al. (2017). Therapeutic potential of targeting wnt/-catenin pathway in treatment of colorectal cancer: Rational and progress. J. Cell. Biochem. 118 (8), 1979–1983. doi:10.1002/jcb.25903
Barati, N., Momtazi-Borojeni, A. A., Majeed, M., and Sahebkar, A. (2019). Potential therapeutic effects of curcumin in gastric cancer. J. Cell. Physiol. 234 (3), 2317–2328. doi:10.1002/jcp.27229
Blakemore, L. M., Boes, C., Cordell, R., and Manson, M. M. (2013). Curcumin-induced mitotic arrest is characterized by spindle abnormalities, defects in chromosomal congression and DNA damage. Carcinogenesis 34 (2), 351–360. doi:10.1093/carcin/bgs345
Borowicz, S., Van Scoyk, M., Avasarala, S., Rathinam, M. K. K., Tauler, J., Bikkavilli, R. K., et al. (2014). The soft agar colony formation assay. J. Vis. Exp. 1 (92), e51998. doi:10.3791/51998
Braglia, L., Zavatti, M., Vinceti, M., Martelli, A. M., and Marmiroli, S. (2020). Deregulated PTEN/PI3K/AKT/mTOR signaling in prostate cancer: Still a potential druggable target? Biochim. Biophys. Acta. Mol. Cell Res. 1867 (9), 118731. doi:10.1016/j.bbamcr.2020.118731
Calibasi-Kocal, G., Pakdemirli, A., Bayrak, S., Ozupek, N. M., Sever, T., Basbinar, Y., et al. (2019). Curcumin effects on cell proliferation, angiogenesis and metastasis in colorectal cancer. J. Buon 24 (4), 1482–1487.
Cantor, J. R., and Sabatini, D. M. (2012). Cancer cell metabolism: One hallmark, many faces. Cancer Discov. 2 (10), 881–898. doi:10.1158/2159-8290.CD-12-0345
Cao, A. L., Li, Q., Yin, P. H., Dong, Y., Shi, H. L., Wang, L., et al. (2013). Curcumin induces apoptosis in human gastric carcinoma AGS cells and colon carcinoma HT-29 cells through mitochondrial dysfunction and endoplasmic reticulum stress. APOPTOSIS 18 (11), 1391–1402. doi:10.1007/s10495-013-0871-1
Chen, C.-C., Sureshbabul, M., Chen, H.-W., Lin, Y.-S., Lee, J.-Y., Hong, Q.-S., et al. (2013). Curcumin suppresses metastasis via sp-1, FAK inhibition, and E-cadherin upregulation in colorectal cancer. Evid. Based. Complement. Altern. Med. 2013, 541695. doi:10.1155/2013/541695
Chen, C., Zhao, S., Karnad, A., and Freeman, J. W. (2018). The biology and role of CD44 in cancer progression: Therapeutic implications. J. Hematol. Oncol. 11, 64. doi:10.1186/s13045-018-0605-5
Chen, T., Yang, C., Xi, Z., Chen, F., and Li, H. (2020). Reduced caudal type homeobox 2 (CDX2) promoter methylation is associated with curcumin's suppressive effects on epithelial-mesenchymal transition in colorectal cancer cells. Med. Sci. Monit. 26, e926443. doi:10.12659/MSM.926443
Chen, T., Yang, P., Wang, H., and He, Z.-Y. (2017). Silence of long noncoding RNA PANDAR switches low-dose curcumin-induced senescence to apoptosis in colorectal cancer cells. Onco. Targets. Ther. 10, 483–491. doi:10.2147/OTT.S127547
Choi, J. Y., Lee, Y. S., Shim, D. M., and Seo, S. W. (2021). PTCH1 regulates anchorage-independent growth and bone invasion of non-small cell lung cancer cells. BONE 144, 115829. doi:10.1016/j.bone.2020.115829
Crowley, L. C., and Waterhouse, N. J. (2016). Detecting cleaved caspase-3 in apoptotic cells by flow cytometry. Cold Spring Harb. Protoc. 2016 (11), 1. doi:10.1101/pdb.prot087312
Da, W., Zhang, J., Zhang, R., and Zhu, J. (2019). Curcumin inhibits the lymphangiogenesis of gastric cancer cells by inhibiton of HMGB1/VEGF-D signaling. Int. J. Immunopathol. Pharmacol. 33, 1. doi:10.1177/2058738419861600
Da, W., Zhu, J., Wang, L., and Sun, Q. (2015). Curcumin suppresses lymphatic vessel density in an in vivo human gastric cancer model. Tumour Biol. 36 (7), 5215–5223. doi:10.1007/s13277-015-3178-8
De La Parte, B. H., Rodeno-Casado, M., Correcher, S. I., Medina, C. M., and Garcia-Alonso, I. (2021). Curcumin reduces colorectal cancer cell proliferation and migration and slows in vivo growth of liver metastases in rats. BIOMEDICINES 9 (9), 1183. doi:10.3390/biomedicines9091183
Deng, K., Lin, S., Zhou, L., Li, Y., Chen, M., Wang, Y., et al. (2012). High levels of aromatic amino acids in gastric juice during the early stages of gastric cancer progression. PLOS ONE 7 (11), e49434. doi:10.1371/journal.pone.0049434
Fan, W.-h., Wang, F.-c., Jin, Z., Zhu, L., and Zhang, J.-x. (2022). Curcumin synergizes with cisplatin to inhibit colon cancer through targeting the MicroRNA-137-glutaminase Axis. Curr. Med. Sci. 42 (1), 108–117. doi:10.1007/s11596-021-2469-0
Fan, X., Zhu, M., Qiu, F., Li, W., Wang, M., Guo, Y., et al. (2020). Curcumin may be a potential adjuvant treatment drug for colon cancer by targeting CD44. Int. Immunopharmacol. 88, 106991. doi:10.1016/j.intimp.2020.106991
Fu, H. B., Wang, C. M., Yang, D. J., Wei, Z. R., Xu, J. P., Hu, Z. Q., et al. (2018). Curcumin regulates proliferation, autophagy, and apoptosis in gastric cancer cells by affecting PI3K and P53 signaling. J. Cell. Physiol. 233 (6), 4634–4642. doi:10.1002/jcp.26190
Gunes, E. G., Pinarbasi, E., and Pinarbasi, H. (2010). AXIN2 polymorphism and its association with astrocytoma in a Turkish population. Mol. Med. Rep. 3 (4), 705–709. doi:10.3892/mmr_00000321
Guo, L.-d., Chen, X.-j., Hu, Y.-h., Yu, Z.-j., Wang, D., and Liu, J.-z. (2013). Curcumin inhibits proliferation and induces apoptosis of human colorectal cancer cells by activating the mitochondria apoptotic pathway. Phytother. Res. 27 (3), 422–430. doi:10.1002/ptr.4731
Guo, L.-d., Shen, Y.-q., Zhao, X.-h., Guo, L.-j., Yu, Z.-j., Wang, D., et al. (2015a). Curcumin combined with oxaliplatin effectively suppress colorectal carcinoma in vivo through inducing apoptosis. Phytother. Res. 29 (3), 357–365. doi:10.1002/ptr.5257
Guo, Y., Shu, L., Zhang, C., Su, Z.-Y., and Kong, A.-N. T. (2015b). Curcumin inhibits anchorage-independent growth of HT29 human colon cancer cells by targeting epigenetic restoration of the tumor suppressor gene DLEC1. Biochem. Pharmacol. 94 (2), 69–78. doi:10.1016/j.bcp.2015.01.009
Hafner, A., Bulyk, M. L., Jambhekar, A., and Lahav, G. (2019). The multiple mechanisms that regulate p53 activity and cell fate. Nat. Rev. Mol. Cell Biol. 20 (4), 199–210. doi:10.1038/s41580-019-0110-x
Hamanaka, R. B., and Chandel, N. S. (2012). Targeting glucose metabolism for cancer therapy. J. Exp. Med. 209 (2), 211–215. doi:10.1084/jem.20120162
Han, W., Yin, H., Ma, H., Wang, Y., Kong, D., and Fan, Z. (2020). Curcumin regulates ERCC1 expression and enhances oxaliplatin sensitivity in resistant colorectal cancer cells through its effects on miR-409-3p. Evid. Based. Complement. Altern. Med. 2020, 8394574. doi:10.1155/2020/8394574
Hao, J., Dai, X., Gao, J., Li, Y., Hou, Z., Chang, Z., et al. (2021). Curcumin suppresses colorectal tumorigenesis via the Wnt/β-catenin signaling pathway by downregulating Axin2. Oncol. Lett. 21 (3), 186. doi:10.3892/ol.2021.12447
He, B., Wei, W., Liu, J., Xu, Y., and Zhao, G. (2017). Synergistic anticancer effect of curcumin and chemotherapy regimen FP in human gastric cancer MGC-803 cells. Oncol. Lett. 14 (3), 3387–3394. doi:10.3892/ol.2017.6627
He, G., Feng, C., Vinothkumar, R., Chen, W., Dai, X., Chen, X., et al. (2016). Curcumin analog EF24 induces apoptosis via ROS-dependent mitochondrial dysfunction in human colorectal cancer cells. Cancer Chemother. Pharmacol. 78 (6), 1151–1161. doi:10.1007/s00280-016-3172-x
He, W., Xia, Y. Q., Cao, P. H., Hong, L., Zhang, T. T., Shen, X., et al. (2019). Curcuminoid WZ35 synergize with cisplatin by inducing ROS production and inhibiting TrxR1 activity in gastric cancer cells. J. Exp. Clin. Cancer Res. 38, 207. doi:10.1186/s13046-019-1215-y
He, Z.-Y., Shi, C.-B., Wen, H., Li, F.-L., Wang, B.-L., and Wang, J. (2011). Upregulation of p53 expression in patients with colorectal cancer by administration of curcumin. Cancer Invest. 29 (3), 208–213. doi:10.3109/07357907.2010.550592
Hou, A., Zhao, L., Zhao, F., Wang, W., Niu, J., Li, B., et al. (2016). Expression of MECOM is associated with unfavorable prognosis in glioblastoma multiforme. Onco. Targets. Ther. 9, 315–320. doi:10.2147/OTT.S95831
Howells, L. M., Iwuji, C. O. O., Irving, G. R. B., Barber, S., Walter, H., Sidat, Z., et al. (2019). Curcumin combined with FOLFOX chemotherapy is safe and tolerable in patients with metastatic colorectal cancer in a randomized phase IIa trial. J. Nutr. 149 (7), 1133–1139. doi:10.1093/jn/nxz029
Huang, F., Yao, Y., Wu, J., Liu, Q., Zhang, J., Pu, X., et al. (2017). Curcumin inhibits gastric cancer-derived mesenchymal stem cells mediated angiogenesis by regulating NF-kappa B/VEGF signaling. Am. J. Transl. Res. 9 (12), 5538–5547.
Hung, C.-S., Huang, C.-Y., Lee, C.-H., Chen, W.-Y., Huang, M.-T., Wei, P.-L., et al. (2017). IGFBP2 plays an important role in heat shock protein 27-mediated cancer progression and metastasis. Oncotarget 8 (33), 54978–54992. doi:10.18632/oncotarget.18989
Ibarra-Martinez, D., Munoz-Ortega, M. H., Quintanar-Stephano, A., Martinez-Hernandez, S. L., Avila-Blanco, M. E., and Ventura-Juarez, J. (2022). Antibacterial activity of supernatants of Lactoccocus lactis, Lactobacillus rhamnosus, Pediococcus pentosaceus and curcumin against Aeromonas hydrophila. in vitro study. Vet. Res. Commun. 46, 459–470. doi:10.1007/s11259-021-09871-7
Jakubek, M., Kejik, Z., Kaplanek, R., Hromadka, R., Sandrikova, V., Sykora, D., et al. (2019). Strategy for improved therapeutic efficiency of curcumin in the treatment of gastric cancer. Biomed. Pharmacother. 118, 109278. doi:10.1016/j.biopha.2019.109278
James, M. I., Iwuji, C., Irving, G., Karmokar, A., Higgins, J. A., Griffin-Teal, N., et al. (2015). Curcumin inhibits cancer stem cell phenotypes in ex vivo models of colorectal liver metastases, and is clinically safe and tolerable in combination with FOLFOX chemotherapy. Cancer Lett. 364 (2), 135–141. doi:10.1016/j.canlet.2015.05.005
Kang, Y., Hu, W., Bai, E., Zheng, H., Liu, Z., Wu, J., et al. (2016). Curcumin sensitizes human gastric cancer cells to 5-fluorouracil through inhibition of the NF kappa B survival-signaling pathway. Onco. Targets. Ther. 9, 7373–7384. doi:10.2147/OTT.S118272
Katsuno, Y., Lamouille, S., and Derynck, R. (2013). TGF-beta signaling and epithelial-mesenchymal transition in cancer progression. Curr. Opin. Oncol. 25 (1), 76–84. doi:10.1097/CCO.0b013e32835b6371
Khorasani, A. B. S., Pourbagheri-Sigaroodi, A., Pirsalehi, A., Safaroghli-azar, A., Zali, M. R., and Bashash, D. (2021). The PI3K/Akt/mTOR signaling pathway in gastric cancer; from oncogenic variations to the possibilities for pharmacologic interventions. Eur. J. Of Pharmacol. 898, 173983. doi:10.1016/j.ejphar.2021.173983
Kim, H. K., Choi, I. J., Kim, C. G., Kim, H. S., Oshima, A., Michalowski, A., et al. (2011). A gene expression signature of acquired chemoresistance to cisplatin and fluorouracil combination chemotherapy in gastric cancer patients. PLOS ONE 6 (2), e16694. doi:10.1371/journal.pone.0016694
Lee, Y.-H., Song, N.-Y., Suh, J., Kim, D.-H., Kim, W., Ann, J., et al. (2018). Curcumin suppresses oncogenicity of human colon cancer cells by covalently modifying the cysteine 67 residue of SIRT1. Cancer Lett. 431, 219–229. doi:10.1016/j.canlet.2018.05.036
Lee, Y.-J., Park, K.-S., and Lee, S.-H. (2021). Curcumin targets both apoptosis and necroptosis in acidity-tolerant prostate carcinoma cells. Biomed. Res. Int. 2021, 8859181. doi:10.1155/2021/8859181
Li, B., Shi, C., Li, B., Zhao, J.-M., and Wang, L. (2018). The effects of Curcumin on HCT-116 cells proliferation and apoptosis via the miR-491/PEG10 pathway. J. Cell. Biochem. 119 (4), 3091–3098. doi:10.1002/jcb.26449
Li, G., Fang, S., Shao, X., Li, Y., Tong, Q., Kong, B., et al. (2021a). Curcumin reverses NNMT-induced 5-fluorouracil resistance via increasing ROS and cell cycle arrest in colorectal cancer cells. BIOMOLECULES 11 (9), 1295. doi:10.3390/biom11091295
Li, R., Zhang, J., Zhou, Y., Gao, Q., Wang, R., Fu, Y., et al. (2020). Transcriptome investigation and in vitro verification of curcumin-induced HO-1 as a feature of ferroptosis in breast cancer cells. Oxidative Med. And Cell. Longev. 2020, 3469840. doi:10.1155/2020/3469840
Li, W.-f., Gong, Y.-x., Li, H.-f., Sun, F.-l., Li, W.-l., Chen, D.-q., et al. (2021b). Curcumin activates ROS signaling to promote pyroptosis in hepatocellular carcinoma HepG2 cells. Vivo 35 (1), 249–257. doi:10.21873/invivo.12253
Li, W., Zhou, Y., Yang, J., Li, H. N., Zhang, H. H., and Zheng, P. (2017a). Curcumin induces apoptotic cell death and protective autophagy in human gastric cancer cells. Oncol. Rep. 37 (6), 3459–3466. doi:10.3892/or.2017.5637
Li, W., Zhou, Y., Yang, J., Zhang, H. H., Zhao, S. L., Zhang, T., et al. (2017b). Curcumin induces apoptosis and protective autophagy in human gastric cancer cells with different degree of differentiation. Zhonghua Zhong Liu Za Zhi 39 (7), 490–496. doi:10.3760/cma.j.issn.0253-3766.2017.07.003
Liang, B., Liu, Z., Cao, Y., Zhu, C., Zuo, Y., Huang, L., et al. (2017). MC37, a new mono-carbonyl curcumin analog, induces G2/M cell cycle arrest and mitochondria-mediated apoptosis in human colorectal cancer cells. Eur. J. Pharmacol. 796, 139–148. doi:10.1016/j.ejphar.2016.12.030
Liang, H. H., Huang, C. Y., Chou, C. W., Makondi, P. T., Huang, M. T., Wei, P. L., et al. (2018). Heat shock protein 27 influences the anti-cancer effect of curcumin in colon cancer cells through ROS production and autophagy activation. Life Sci. 209, 43–51. doi:10.1016/j.lfs.2018.07.047
Liang, T., Zhang, X., Xue, W., Zhao, S., Zhang, X., and Pei, J. (2014). Curcumin induced human gastric cancer BGC-823 cells apoptosis by ROS-mediated ASK1-MKK4-JNK stress signaling pathway. Int. J. Mol. Sci. 15 (9), 15754–15765. doi:10.3390/ijms150915754
Liang, X., Wang, P., Yang, C., Huang, F., Wu, H., Shi, H., et al. (2021). Galangin inhibits gastric cancer growth through enhancing STAT3 mediated ROS production. Front. Pharmacol. 12, 646628. doi:10.3389/fphar.2021.646628
Lin, X., Wang, L., Zhao, L., Zhu, Z., Chen, T., Chen, S., et al. (2020). Curcumin micelles suppress gastric tumor cell growth by upregulating ROS generation, disrupting redox equilibrium and affecting mitochondrial bioenergetics. Food Funct. 11 (5), 4146–4159. doi:10.1039/d0fo00260g
Liu, G., Xiang, T., Wu, Q.-F., and Wang, W.-X. (2016). Curcumin suppresses the proliferation of gastric cancer cells by downregulating H19. Oncol. Lett. 12 (6), 5156–5162. doi:10.3892/ol.2016.5354
Liu, W. W., Huang, M. X., Zou, Q. Q., and Lin, W. Y. (2018). Curcumin suppresses gastric cancer biological activity by regulation of miRNA-21: An in vitro study. Int. J. Clin. Exp. Pathol. 11 (12), 5820–5829.
Liu, X., Sun, K., Chen, H., Song, A., Zhang, X., Zhang, X., et al. (2014). Curcumin inhibits proliferation of gastric cancer cells by impairing ATP-sensitive potassium channel opening. World J. Surg. Oncol. 12, 389. doi:10.1186/1477-7819-12-389
Lu, Y., Zhang, R., Zhang, X., Zhang, B., and Yao, Q. (2020). Curcumin may reverse 5-fluorouracil resistance on colonic cancer cells by regulating TET1-NKD-Wnt signal pathway to inhibit the EMT progress. Biomed. Pharmacother. 129, 110381. doi:10.1016/j.biopha.2020.110381
Mao, X., Zhang, X., Zheng, X., Chen, Y., Xuan, Z., and Huang, P. (2021). Curcumin suppresses LGR5(+) colorectal cancer stem cells by inducing autophagy and via repressing TFAP2A-mediated ECM pathway. J. Nat. Med. 75 (3), 590–601. doi:10.1007/s11418-021-01505-1
Mashayekhi-Sardoo, H., Mashayekhi-Sardoo, A., Roufogalis, B. D., Jamialahmadi, T., and Sahebkar, A. (2021). Impact of curcumin on microsomal enzyme activities: Drug interaction and chemopreventive studies. Curr. Med. Chem. 28 (34), 7122–7140. doi:10.2174/0929867328666210329123449
McCubrey, J. A., Rakus, D., Gizak, A., Steelman, L. S., Abrams, S. L., Lertpiriyapong, K., et al. (2016). Effects of mutations in Wnt/β-catenin, hedgehog, Notch and PI3K pathways on GSK-3 activity-Diverse effects on cell growth, metabolism and cancer. Biochim. Biophys. Acta 1863 (12), 2942–2976. doi:10.1016/j.bbamcr.2016.09.004
McFadden, R. M. T., Larmonier, C. B., Shehab, K. W., Midura-Kiela, M., Ramalingam, R., Harrison, C. A., et al. (2015). The role of curcumin in modulating colonic microbiota during colitis and colon cancer prevention. Inflamm. Bowel Dis. 21 (11), 2483–2494. doi:10.1097/MIB.0000000000000522
Mohamed, J. M., Alqahtani, A., Ahmad, F., Krishnaraju, V., and Kalpana, K. (2021). Pectin co-functionalized dual layered solid lipid nanoparticle made by soluble curcumin for the targeted potential treatment of colorectal cancer. Carbohydr. Polym. 252, 117180. doi:10.1016/j.carbpol.2020.117180
Nagaraj, A. B., Joseph, P., Kovalenko, O., Singh, S., Armstrong, A., Redline, R., et al. (2015). Critical role of Wnt/β-catenin signaling in driving epithelial ovarian cancer platinum resistance. Oncotarget 6 (27), 23720–23734. doi:10.18632/oncotarget.4690
Patra, K. C., Wang, Q., Bhaskar, P. T., Miller, L., Wang, Z. B., Wheaton, W., et al. (2013). Hexokinase 2 is required for tumor initiation and maintenance and its systemic deletion is therapeutic in mouse models of cancer. Cancer cell 24 (2), 213–228. doi:10.1016/j.ccr.2013.06.014
Prasad, S., Gupta, S. C., and Tyagi, A. K. (2017). Reactive oxygen species (ROS) and cancer: Role of antioxidative nutraceuticals. Cancer Lett. 387, 95–105. doi:10.1016/j.canlet.2016.03.042
Prasad, S., Tyagi, A. K., and Aggarwal, B. B. (2014). Recent developments in delivery, bioavailability, absorption and metabolism of curcumin: The golden pigment from golden spice. Cancer Res. Treat. 46 (1), 2–18. doi:10.4143/crt.2014.46.1.2
Qiang, Z. R., Meng, L. Y., Yi, C. X., Yu, L. Y., Chen, W. X., and Sha, W. H. (2019). Curcumin regulates the miR-21/PTEN/Akt pathway and acts in synergy with PD98059 to induce apoptosis of human gastric cancer MGC-803 cells. J. Int. Med. Res. 47 (3), 1288–1297. doi:10.1177/0300060518822213
Rana, C., Piplani, H., Vaish, V., Nehru, B., and Sanyal, S. N. (2015). Downregulation of PI3-K/Akt/PTEN pathway and activation of mitochondrial intrinsic apoptosis by Diclofenac and Curcumin in colon cancer. Mol. Cell. Biochem. 402 (1-2), 225–241. doi:10.1007/s11010-015-2330-5
Ruiz de Porras, V., Bystrup, S., Martinez-Cardus, A., Pluvinet, R., Sumoy, L., Howells, L., et al. (2016). Curcumin mediates oxaliplatin-acquired resistance reversion in colorectal cancer cell lines through modulation of CXC-Chemokine/NF-κB signalling pathway. Sci. Rep. 6, 24675. doi:10.1038/srep24675
Schwabe, R. F., and Jobin, C. (2013). The microbiome and cancer. Nat. Rev. Cancer 13 (11), 800–812. doi:10.1038/nrc3610
Shakibaei, M., Buhrmann, C., Kraehe, P., Shayan, P., Lueders, C., and Goel, A. (2014). Curcumin chemosensitizes 5-fluorouracil resistant MMR-deficient human colon cancer cells in high density cultures. PLOS ONE 9 (1), e85397. doi:10.1371/journal.pone.0085397
Sheng, J., Sun, L.-B., Zhao, S.-F., Qi, W.-W., Lv, J., Zhang, Z.-G., et al. (2018). Acidic stress induces protective autophagy in SGC7901 cells. J. Int. Med. Res. 46 (8), 3285–3295. doi:10.1177/0300060518768167
Song, H. J., Sun, W. L., Ye, G. L., Ding, X. Y., Liu, Z., Zhang, S. J., et al. (2013). Long non-coding RNA expression profile in human gastric cancer and its clinical significances. J. Transl. Med. 11, 225. doi:10.1186/1479-5876-11-225
Song, N.-Y., Lee, Y.-H., Na, H.-K., Baek, J.-H., and Surh, Y.-J. (2018). Leptin induces SIRT1 expression through activation of NF-E2-related factor 2: Implications for obesity-associated colon carcinogenesis. Biochem. Pharmacol. 153, 282–291. doi:10.1016/j.bcp.2018.02.001
Song, N.-Y., and Surh, Y.-J. (2012). “Janus-faced role of SIRT1 in tumorigenesis,” in Nutrition and physical activity in aging, obesity, and cancer. Editors Y. J. Surh, Y. S. Song, J. Y. Han, T. W. Jun, and H. K. Na, 10–19.
Sritharan, S., and Sivalingam, N. (2021). Curcumin induced apoptosis is mediated through oxidative stress in mutated p53 and wild type p53 colon adenocarcinoma cell lines. J. Biochem. Mol. Toxicol. 35 (1), e22616. doi:10.1002/jbt.22616
Su, P. X., Wang, F. Q., Qi, B., Wang, T., and Zhang, S. B. (2017). P53 regulation-association long non-coding RNA (LncRNA PRAL) inhibits cell proliferation by regulation of P53 in human lung cancer. Med. Sci. Monit. 23, 1751–1758. doi:10.12659/MSM.900205
Su, P., Yang, Y., Wang, G., Chen, X., and Ju, Y. (2018). Curcumin attenuates resistance to irinotecan via induction of apoptosis of cancer stem cells in chemoresistant colon cancer cells. Int. J. Oncol. 53 (3), 1343–1353. doi:10.3892/ijo.2018.4461
Suarez-Carmona, M., Lesage, J., Cataldo, D., and Gilles, C. (2017). EMT and inflammation: Inseparable actors of cancer progression. Mol. Oncol. 11 (7), 805–823. doi:10.1002/1878-0261.12095
Sun, C., Zhang, S., Liu, C., and Liu, X. (2019). Curcumin promoted miR-34a expression and suppressed proliferation of gastric cancer cells. Cancer biother. Radiopharm. 34 (10), 634–641. doi:10.1089/cbr.2019.2874
Sun, Y., Campisi, J., Higano, C., Beer, T. M., Porter, P., Coleman, I., et al. (2012). Treatment-induced damage to the tumor microenvironment promotes prostate cancer therapy resistance through WNT16B. Nat. Med. 18 (9), 1359–1368. doi:10.1038/nm.2890
Sung, H., Ferlay, J., Siegel, R. L., Laversanne, M., Soerjomataram, I., Jemal, A., et al. (2021). Global cancer statistics 2020: GLOBOCAN estimates of incidence and mortality worldwide for 36 cancers in 185 countries. Ca. Cancer J. Clin. 71 (3), 209–249. doi:10.3322/caac.21660
Tang, X., Ding, H., Liang, M., Chen, X., Yan, Y., Wan, N., et al. (2021). Curcumin induces ferroptosis in non-small-cell lung cancer via activating autophagy. Thorac. Cancer 12 (8), 1219–1230. doi:10.1111/1759-7714.13904
Thapa, R., and Wilson, G. D. (2016). The importance of CD44 as a stem cell biomarker and therapeutic target in cancer. Stem Cells Int. 2016, 2087204. doi:10.1155/2016/2087204
Tong, R., Wu, X., Liu, Y., Liu, Y., Zhou, J., Jiang, X., et al. (2020). Curcumin-induced DNA demethylation in human gastric cancer cells is mediated by the DNA-damage response pathway. Oxidative Med. And Cell. Longev. 2020, 2543504. doi:10.1155/2020/2543504
Waldum, H. L., Sagatun, L., and Mjones, P. (2017). Gastrin and gastric cancer. Front. Endocrinol. 8, 1. doi:10.3389/fendo.2017.00001
Waly, M. I., Al-Hinai, S., Al-Bulushi, I., and Rahman, M. S. (2018). Protective effect of curcumin against nitrosamine-induced gastric cancer in rats. FASEB J. 23, 288–293. doi:10.3746/pnf.2018.23.4.288
Wang, H., Cai, X., and Ma, L. (2020). Curcumin modifies epithelial-mesenchymal transition in colorectal cancer through regulation of miR-200c/EPM5. Cancer Manag. Res. 12, 9405–9415. doi:10.2147/CMAR.S260129
Wang, J., Song, Y. X., and Wang, Z. N. (2015a). Non-coding RNAs in gastric cancer. Gene 560 (1), 1–8. doi:10.1016/j.gene.2015.02.004
Wang, K., Fan, H., Chen, Q., Ma, G., Zhu, M., Zhang, X., et al. (2015b). Curcumin inhibits aerobic glycolysis and induces mitochondrial-mediated apoptosis through hexokinase II in human colorectal cancer cells in vitro. Anticancer. Drugs 26 (1), 15–24. doi:10.1097/CAD.0000000000000132
Wang, X.-P., Wang, Q.-X., Lin, H.-P., and Chang, N. (2017). Anti-tumor bioactivities of curcumin on mice loaded with gastric carcinoma. Food Funct. 8 (9), 3319–3326. doi:10.1039/c7fo00555e
Wong, M. Y. W., Yu, Y., Walsh, W. R., and Yang, J. L. (2011). microRNA-34 family and treatment of cancers with mutant or wild-type p53 (Review). Int. J. Oncol. 38 (5), 1189–1195. doi:10.3892/ijo.2011.970
Xie, X., Liu, H., Wang, Y., Zhou, Y., Yu, H., Li, G., et al. (2016). Nicotinamide N-methyltransferase enhances resistance to 5-fluorouracil in colorectal cancer cells through inhibition of the ASK1-p38 MAPK pathway. Oncotarget 7 (29), 45837–45848. doi:10.18632/oncotarget.9962
Xu, G. W., Gu, Y. Q., Yan, N., Li, Y. F., Sun, L., and Li, B. (2021). Curcumin functions as an anti-inflammatory and antioxidant agent on arsenic-induced hepatic and kidney injury by inhibiting MAPKs/NF-kappa B and activating Nrf2 pathways. Environ. Toxicol. 36 (11), 2161–2173. doi:10.1002/tox.23330
Xu, J. D., Cao, X. X., Long, Z. W., Liu, X. P., Furuya, T., Xu, J. W., et al. (2011). BCL2L10 protein regulates apoptosis/proliferation through differential pathways in gastric cancer cells. J. Pathol. 223 (3), 400–409. doi:10.1002/path.2811
Yan, S. X., Zhou, M., Zheng, X. Y., Xing, Y. Y., Dong, J., Yan, M. W., et al. (2021). Anti-inflammatory effect of curcumin on the mouse model of myocardial infarction through regulating macrophage polarization. Mediat. Of Inflamm. 2021, 9976912. doi:10.1155/2021/9976912
Yang, F., Bi, J., Xue, X., Zheng, L., Zhi, K., Hua, J., et al. (2012). Up-regulated long non-coding RNA H19 contributes to proliferation of gastric cancer cells. FEBS J. 279 (17), 3159–3165. doi:10.1111/j.1742-4658.2012.08694.x
Yin, J. H., Wang, L., Wang, Y., Shen, H. L., Wang, X. J., and Wu, L. (2019). Curcumin reverses oxaliplatin resistance in human colorectal cancer via regulation of TGF-β/Smad2/3 signaling pathway. Onco. Targets. Ther. 12, 3893–3903. doi:10.2147/OTT.S199601
Yin, S., Du, W. Z., Wang, F., Han, B., Cui, Y. Q., Yang, D. B., et al. (2018). MicroRNA-326 sensitizes human glioblastoma cells to curcumin via the SHH/GLI1 signaling pathway. Cancer Biol. Ther. 19 (4), 260–270. doi:10.1080/15384047.2016.1250981
Yu, L.-L., Wu, J.-G., Dai, N., Yu, H.-G., and Si, J.-M. (2011). Curcumin reverses chemoresistance of human gastric cancer cells by downregulating the NF-kappa B transcription factor. Oncol. Rep. 26 (5), 1197–1203. doi:10.3892/or.2011.1410
Zhang, C., He, L.-J., Ye, H.-Z., Liu, D.-F., Zhu, Y.-B., Miao, D.-D., et al. (2018). Nrf2 is a key factor in the reversal effect of curcumin on multidrug resistance in the HCT-8/5-Fu human colorectal cancer cell line. Mol. Med. Rep. 18 (6), 5409–5416. doi:10.3892/mmr.2018.9589
Zhang, X., Zhang, C. L., Ren, Z. H., Zhang, F. F., Xu, J. Y., Zhang, X., et al. (2020). Curcumin affects gastric cancer cell migration, invasion and cytoskeletal remodeling through gli1-beta-catenin. Cancer Manag. Res. 12, 3795–3806. doi:10.2147/CMAR.S244384
Zhang, Z., Chen, H., Xu, C., Song, L., Huang, L., Lai, Y., et al. (2016). Curcumin inhibits tumor epithelial-mesenchymal transition by downregulating the Wnt signaling pathway and upregulating NKD2 expression in colon cancer cells. Oncol. Rep. 35 (5), 2615–2623. doi:10.3892/or.2016.4669
Zheng, R. Z., Deng, Q. H., Liu, Y. H., and Zhao, P. J. (2017). Curcumin inhibits gastric carcinoma cell growth and induces apoptosis by suppressing the wnt/β-catenin signaling pathway. Med. Sci. Monit. 23, 163–171. doi:10.12659/MSM.902711
Zheng, X., Yang, X., Lin, J., Song, F., and Shao, Y. (2021a). Low curcumin concentration enhances the anticancer effect of 5-fluorouracil against colorectal cancer. Phytomedicine. 85, 153547. doi:10.1016/j.phymed.2021.153547
Zheng, Z.-h., You, H.-y., Feng, Y.-j., and Zhang, Z.-t. (2021b). LncRNA KCNQ1OT1 is a key factor in the reversal effect of curcumin on cisplatin resistance in the colorectal cancer cells. Mol. Cell. Biochem. 476 (7), 2575–2585. doi:10.1007/s11010-020-03856-x
Zhou, S., Yao, D., Guo, L., and Teng, L. (2017). Curcumin suppresses gastric cancer by inhibiting gastrin-mediated acid secretion. Febs Open Bio 7 (8), 1078–1084. doi:10.1002/2211-5463.12237
Keywords: gastrointestinal cancer, gastric cancer, colorectal cancer, curcumin, pharmacological mechanism
Citation: Fan Y, Zhang X, Tong Y, Chen S and Liang J (2022) Curcumin against gastrointestinal cancer: A review of the pharmacological mechanisms underlying its antitumor activity. Front. Pharmacol. 13:990475. doi: 10.3389/fphar.2022.990475
Received: 10 July 2022; Accepted: 09 August 2022;
Published: 02 September 2022.
Edited by:
Yi-Ming Zhou, Fudan University, ChinaReviewed by:
Samson Mathews Samuel, Weill Cornell Medicine- Qatar, QatarLaiba Arshad, Forman Christian College, Pakistan
Copyright © 2022 Fan, Zhang, Tong, Chen and Liang. This is an open-access article distributed under the terms of the Creative Commons Attribution License (CC BY). The use, distribution or reproduction in other forums is permitted, provided the original author(s) and the copyright owner(s) are credited and that the original publication in this journal is cited, in accordance with accepted academic practice. No use, distribution or reproduction is permitted which does not comply with these terms.
*Correspondence: Jingjing Liang, bGlhbmdqakBzai1ob3NwaXRhbC5vcmc=