- 1Department of Pharmacology, Weill Cornell Medicine- Qatar, Doha, Qatar
- 2National Heart and Lung Institute, Imperial College London, London, United Kingdom
Stent thrombosis remains one of the main causes that lead to vascular stent failure in patients undergoing percutaneous coronary intervention (PCI). Type 2 diabetes mellitus is accompanied by endothelial dysfunction and platelet hyperactivity and is associated with suboptimal outcomes following PCI, and an increase in the incidence of late stent thrombosis. Evidence suggests that late stent thrombosis is caused by the delayed and impaired endothelialization of the lumen of the stent. The endothelium has a key role in modulating inflammation and thrombosis and maintaining homeostasis, thus restoring a functional endothelial cell layer is an important target for the prevention of stent thrombosis. Modifications using specific molecules to induce endothelial cell adhesion, proliferation and function can improve stents endothelialization and prevent thrombosis. Blood endothelial progenitor cells (EPCs) represent a potential cell source for the in situ-endothelialization of vascular conduits and stents. We aim in this review to summarize the main biofunctionalization strategies to induce the in-situ endothelialization of coronary artery stents using circulating endothelial stem cells.
Introduction
Cardiovascular diseases are the most prevalent non-communicable diseases worldwide, accounting for 31% of all deaths (WHO, 2017). Coronary artery disease (CAD) is the most common type of cardiovascular disease, causing the majority of cardiovascular-related deaths worldwide (Okrainec et al., 2004). The main cause of CAD is the accumulation of fatty and fibrous materials in the wall of the coronary artery forming an atherosclerotic lesion, which eventually leads to arterial occlusion (Ross, 1993). The growing size of the formed lesion can be sufficient to block the blood flow, however most clinical complications result from thrombus formation. A thrombus obstructs blood flow to the heart muscle leading to myocardial ischemia and infarction (Lusis, 2000).
Percutaneous coronary intervention (PCI) is a non-surgical revascularization technique used to treat obstructive coronary arteries. PCI has become the treatment of choice for CAD. The implantation of intracoronary stents is one of the major PCI techniques used to relive the narrowing of coronary arteries (Smith et al., 2001). Although stenting have improved the acute outcomes of PCI, the long-term outcomes are still hindered by other factors such as age and other comorbidities (Smith et al., 2001). Stent thrombosis and restenosis remain the main causes that lead to vascular stent failure in patients undergoing PCI (Seabra-Gomes, 2006; Chaabane et al., 2013). Stent implantation causes mechanical vascular injury characterized by endothelial denudation and platelet activation, leading to thrombosis and stenosis (Kipshidze et al., 2004; Otsuka et al., 2012; Chaabane et al., 2013).
Evidence suggests that type 2 diabetes mellitus is associated with suboptimal outcomes following PCI or revascularization (Seabra-Gomes, 2006; Bittl, 2015; Koskinas et al., 2016). Type 2 diabetes mellitus is characterized by hyperglycemia and insulin resistance leading to endothelial dysfunction. The abnormal reactivity of diabetic endothelial cells is associated with an increased rate of cardiovascular events (Seabra-Gomes, 2006). In addition, patients with diabetes are at higher risk to develop coronary lesions in stented vessels and are presented with higher rates of completely occlusive restenosis following PCI (Seabra-Gomes, 2006). Diabetes also results in platelet dysfunction and hypo-responsiveness to antiplatelet treatments, increasing the risk of stent thrombosis (Gum et al., 2003; Watala et al., 2004; Angiolillo et al., 2005).
It has been suggested that inducing the rapid endothelialization of stents might improve the outcomes of PCI (Finn et al., 2007). Rapid endothelialization of blood-contacting devices and surfaces is desired due to the anti-thrombotic and anti-adhesive properties of endothelial cells, thus preventing the recruitment and adhesion of platelets and leukocytes to the stented area (Sousa et al., 2003). Establishing a functional endothelial cell layer rapidly after stent implantation might prevent stent thrombosis (Kong et al., 2004). Thus, in this review, we highlight the role of endothelial cells in protecting from stent thrombosis in the context of diabetes, and summarize the main studies that investigated biofunctionalization strategies to induce the in-situ endothelialization of coronary artery stents using circulating endothelial stem cells.
Pathogenesis of stent thrombosis: Role of endothelial cells
Coronary stents are prosthetic cylindrical meshes inserted into the coronary artery using a catheter to relieve the narrowing of the artery and improve blood flow to the heart muscle (Meads et al., 2000). Stents provide a permanent scaffolding for the vessel wall, thus inhibiting the arterial recoil and restenosis associated with plain old balloon angioplasty (Meads et al., 2000; Garcia-Garcia et al., 2006; Seabra-Gomes, 2006). To improve the outcomes of PCI, stents have evolved in terms of design and composition, from bare metal stents (BMS), to drug eluting stents (DES) and bioresorbable vascular scaffolds (BRS). We refer the reader to these reviews on the evolution of stents types, designs and materials (O’Brien and Carroll, 2009; Borhani et al., 2018; Torii et al., 2020; Scafa Udriște et al., 2021).
Stent thrombosis is the occlusion of a coronary artery stent by a thrombus. Standard definitions and classifications of stent thrombosis has been proposed by the Academic Research Consortium (ARC) (Garcia-Garcia et al., 2018). Stent thrombosis is classified into early, late or very late thrombosis according to the elapsed time from stent implantation, and could also be defined according to the degree of certainty as definitive, probable, or silent occlusion (Garcia-Garcia et al., 2018). The reported incidence of stent thrombosis was< 1% for early stent thrombosis (D'Ascenzo et al., 2013), 0.5–1% for late stent thrombosis (D'Ascenzo et al., 2013) and 0.2–0.4% per year for very late stent thrombosis with second generation DES while 2% was reported with 1st generation DES (Biondi-Zoccai et al., 2006). Although stent thrombosis incidence remains low, it constitutes a significant public health issue due to the high number of implanted stents worldwide and the major consequences of thrombotic events (Gori et al., 2019). The mortality caused by stent thrombosis has been reported to be as high as 45% (Biondi-Zoccai et al., 2006). Additionally, stent thrombosis was shown to be accountable for 20% of all myocardial infarction cases following PCI (Gori et al., 2019). Four factors have been identified to influence stent thrombosis including the used device, implantation procedure, patient status, and type of lesion.
The pathophysiological response to stent implantation involves wound healing processes including thrombosis, inflammation, and remodeling (Chaabane et al., 2013). The stenting process leads to a partial or complete denudation of the endothelial cell layer, stretching of the artery, and mechanical vascular injury. This induces platelet activation and adhesion, and the deposition of fibrin on the site of injury. The activated platelets express adhesion molecules, such as P-selectin, which leads to the recruitment of inflammatory cells (Costa and Simon, 2005). The recruited platelets and leukocytes respond by releasing growth factors and cytokines that induce smooth muscle cell proliferation, migration, and deposition of extracellular matrix proteins in the intima of the artery, leading to in-stent restenosis (Chaabane et al., 2013).
Endothelial cells play an important role in protecting from thrombosis and inflammation and maintaining blood fluidity. The release of vasoprotective and thromboresistant agents such as Nitric oxide (NO) and prostacyclin prevents platelet activation and thrombus formation. Von Willebrand factor secretion is also an important factor that modulates platelets adhesion and aggregation under shear conditions (van Hinsbergh, 2012). Additionally, the normal endothelium activates fibrinolysis through the secretion of tissue plasminogen activator; an important mechanism for the resolution of thrombi (Oliver James et al., 2005). Endothelial injury leads to a disturbed production of these protective molecules, and an increase in the expression of adhesion molecules leading to thrombosis, leukocyte recruitment and smooth muscle cell dysregulation (van Hinsbergh, 2012).
The vascular endothelium is also an important interface between the vascular wall and the blood components, and its absence leads to the exposure of the subendothelial elements. The direct interaction of the blood with the subendothelial elements might trigger platelet adhesion leading to thrombosis (Palmaz, 1992). Additionally, implanted stent strut or coating material may induce stent thrombosis (Palmaz, 1992; Jaffer et al., 2015; Georgiadou and Voudris, 2017). It has been determined that the degree of stent coverage with endothelial cells is “the most powerful histological predictor” of stent thrombosis (Finn et al., 2007; Georgiadou and Voudris, 2017). Additionally, the degree of neointima formation following mechanical injury was found to be correlated with the rate of re-endothelialization (Douglas et al., 2013). The delayed stent coverage with endothelial cells in addition to the constant fibrin deposition and inflammation are associated with late and very late stent thrombosis, and the risk is greatly increased in stents with more than 30% uncovered struts (Finn et al., 2007; Claessen et al., 2014).
The stent design and composition are of the main factors that influence stent endothelialization and endothelial cell recovery following PCI (Cornelissen and Vogt, 2019). The surface topography of the stent affects cell adhesion and alignment. It has been shown that a topography resulting in elongated and aligned cells could accelerate the development of a healthy endothelium layer (Claessen et al., 2014). Additionally, the non-physiological nature of the stent material could affect the migration and adhesion of endothelial cells and thus biocompatibility is a key factor in improving endothelialization (Van der Heiden et al., 2013). Endothelialization is also influenced by the thickness of the strut and was shown to be improved in stents with thinner struts (Cornelissen and Vogt, 2019). Additionally, the types of drugs and polymers used in the stent affect cell adhesion and proliferation. While the antiproliferative drugs used in DES reduce neointima formation and in-stent restenosis, they also delay the endothelization of the stent leading to late stent thrombosis (Finn et al., 2007). The incidence of thrombosis in BMS and DES was not shown to be different, and the polymers used in BRS were shown to induce thrombosis (Buchanan et al., 2012). To reduce the occurrence of thrombotic events, dual anti-platelet therapy (aspirin and a P2Y12 inhibitor) is given to patients following PCI (Seabra-Gomes, 2006).
Stent thrombosis and diabetes
In diabetes mellitus, patients usually present with platelet dysfunction, hyperactivity or hypo-responsiveness, increasing their risk of stent thrombosis (Yuan and Xu, 2018). Additionally, the vascular endothelium is dysfunctional in response to hyperglycemia, and the proliferation and wound healing responses are impaired in this subgroup of patients (Triggle et al., 2020). Hyperglycemia results in the impairment of endothelial cells, reducing the generation of the vasodilator NO, thus favoring a vasoconstrictive state through the increase in vasoconstrictors and pro-thrombotic mediators, endothelin-1 (ET-1) and thromboxane A2 (TXA2). This imbalance disturbs the vascular tone and results in an increase in smooth muscle proliferation and migration, accompanied by an increased secretion of inflammatory cytokines and prothrombotic factors. The reduction in NO, and the increase of ET-1 and TXA2 induces platelet activation and thrombosis with the potential contribution of an elevated generation of prostacyclin that activates TXA2 receptors (Beckman et al., 2002; Seabra-Gomes, 2006; Vanhoutte and Tang, 2008). These conditions promote thrombus formation (Figure 1A). The incidence of stent thrombosis in patients with diabetes was found to be double that for patients without diabetes (Wiviott et al., 2008). Additionally, insulin was found to play a major role in influencing thrombosis. The chronic activation of endothelial cells by insulin might affect the production of vasoprotective and antithrombotic factors, activating a prothrombotic and proinflammatory status (Wu and Thiagarajan, 1996; Angiolillo et al., 2005). The prothrombotic status in these patients decreases their response to anti-platelet agents. The dysfunctional platelets in patients with diabetes are less sensitive to aspirin increasing their risk of ischemic events (Gum et al., 2003; Watala et al., 2004; Angiolillo et al., 2005). There is also evidence of the negative effect of the common anti-diabetes drug, metformin, on endothelial proliferation on stents releasing mTOR inhibitors, as was shown in vitro and in rabbit model (Habib et al., 2013a; Habib et al., 2013b). In terms of the time of occurrence, a meta-analysis of stent thrombosis in patients with and without diabetes have shown that both subgroups had a similar rate of early stent thrombosis following PCI with DES, however, diabetes was associated with an increase in the incidence of late stent thrombosis (Yuan and Xu, 2018).
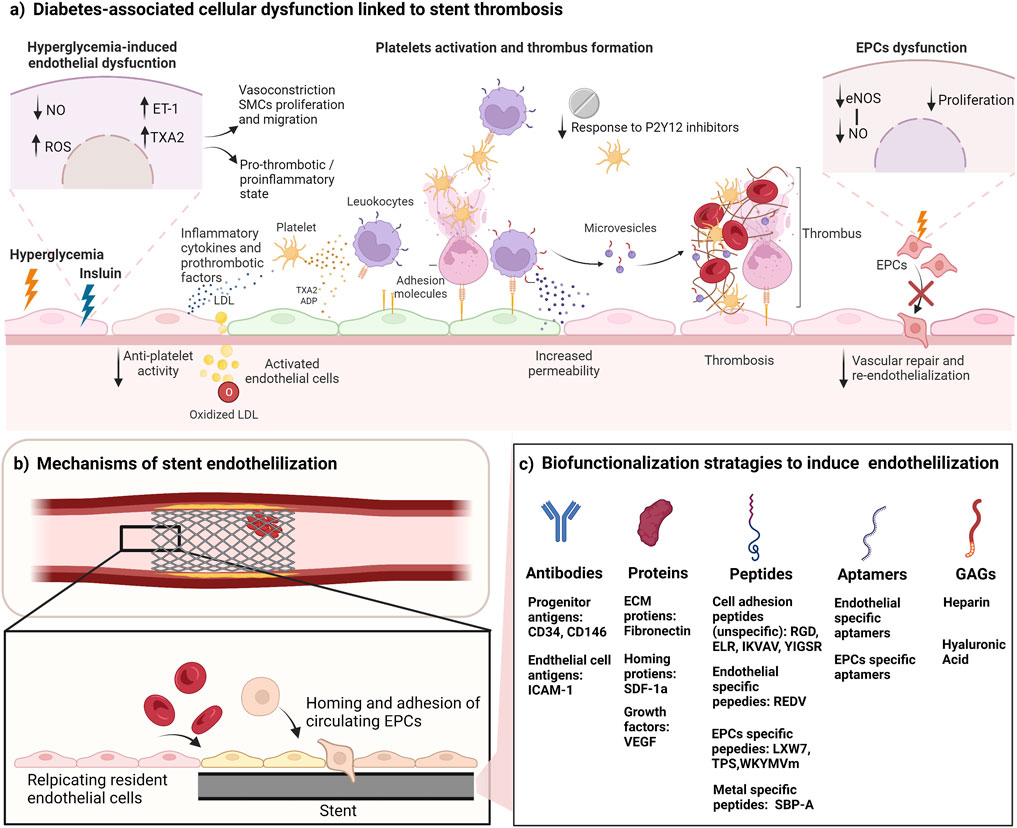
FIGURE 1. Biofunctionalization of stents to improve endothelialization and reduce thrombosis. (A) Cellular dysfunction in diabetes leads to high risk of stent thrombosis. Hyperglycemia results in vascular dysfunction characterized by reduced generation of NO, and induced synthesis of ET-1 and TXA2, resulting in a proinflammatory, pro-thrombotic and vasoconstrictive state. In addition, the chronic activation of endothelial cells by insulin affects the production of vasoprotective and antithrombotic factors. Diabetes also causes platelet hyperactivity, and hypo-responsiveness to anti-platelets drugs. Activated platelets bind to the vascular endothelium directly through adhesion molecules and stimulate an inflammatory response. Platelets also deposit chemokines into the surface of endothelial cells leading to leukocyte recruitment, and platelets can bind to leukocytes that adhere to the endothelial layer. Platelets also can influence endothelial cells by their secretion of vasoactive molecules (such as ADP, serotonin and TXA2) from their granules. The regenerative mechanisms by EPCs are also impaired due to EPCs dysfunction characterized by reduced EPC proliferation, and impaired eNOS and NO production. (B) Endothelialization of stents can reduce stent thrombosis. Stent endothelialization happens through 2 mechanisms: resident cell replication and EPC recruitment. Both mechanisms are impaired in diabetes. Targeting these mechanisms can enhance the endothelialization rate. (C) Biofunctionalization strategies to promote stent endothelialization. Surface biofunctionalization with mimicry factors aims to induce EPCs mobilization, capture, adhesion, and proliferation. Some of the listed factors also induce the proliferation of resident endothelial cells. NO, nitric oxide; eNOS, endothelial nitric oxide synthase; ROS, reactive oxygen species; TAX2, thromboxane A2; ET-1, endothelin-1; ADP, Adenosine diphosphate; LDL, low density lipoprotein; SMCs, smooth muscle cells; EPCs, endothelial progenitor cells; ICAM-1, intercellular adhesion molecule 1; ECM, extracellular matrix; SDF-1a, stromal cell-derived factor 1; VEGF, vascular endothelial growth factor; GAGs, glycosaminoglycans. Figure was created by BioRender.com.
Given the important role of the endothelium in the protection from thrombosis, re-endothelialization is a key therapeutic target to improve the outcomes of stent implantation in patients with diabetes, and to maintain an antithrombotic and anti-inflammatory status at the site of implantation (Douglas et al., 2013). The gradual endothelialization of stents protects from the thrombotic events, however, this process is slow in BMS, and the drugs used in DES inhibit endothelial cell proliferation and complete coverage. Thus, there is a need for a modulation in the composition of the stents to induce rapid endothelial cell adhesion and proliferation and full stent coverage soon after implantation.
Stent biofunctionalization to induce endothelialization with circulating endothelial progenitor cells
Endothelialization of stents happens through two main mechanisms: (I) the proliferation and migration of the resident cells at the site of injury, and (II) the homing and adhesion of circulating endothelial progenitor cells (EPCs) (Ong et al., 2005) (Figure 1B). Mature endothelial cells have a low proliferation and replication capacity, thus their participation in the endothelialization process is slow and limited. It is hypothesized that EPCs play a major role in the endothelialization process. EPCs are progenitors that circulate in the blood and have the ability to differentiate to mature endothelial cells and to participate in angiogenesis and neovascularization processes (Medina et al., 2017). Since their discovery by Asahara et al. (1997) in 1997, many attempts have been made to isolate EPCs using varying methods, which resulted in the identification of multiple cell populations that have been categorized under the EPC terminology (Medina et al., 2017). The main identified sub-populations are early EPCs (expressing CD31, CD45 and CD14, and lack expression of CD133) and late EPCs (expressing CD34, CD31 and CD133 and lack expression of the hematopoietic markers CD45, CD14, and CD115) (Tura et al., 2013; O'Neill TJtWamhoff et al., 2005; Zentilin et al., 2006). The late EPCs have been recently recognized to be the “true EPCs” due to their ability to differentiate into a stable mature endothelial phenotype, and to participate directly in the neovascularization process by incorporating into the vasculature (Yoder et al., 2007; Medina et al., 2010a; Keighron et al., 2018). A recent study used single-cell RNA-sequencing analysis (scRNA-seq) to identify specific markers in late EPCs, and found that this subpopulation expressed high levels of bone morphogenetic protein 2 and 4 (BMP 2 and 4) and ephrin B2 (EFNB2) when compared to other types of endothelial cells (Abdelgawad et al., 2021). BMP 2 and 4 were also found to be selectively expressed by late, but not, early EPCs, and to regulate EPC commitment and angiogenic potential (Smadja et al., 2008). Late EPCs and HUVECs share high expression of neuropilin 1 (NRP1) and Vascular endothelial growth factor (VEGF-C) (Abdelgawad et al., 2021), both important factors for the differentiation of endothelial precursors (Cimato et al., 2009; Zhang et al., 2019; Abdelgawad et al., 2021). This expression pattern could be used for the identification and differentiation between subpopulations of EPCs. We refer the reader to these reviews on the detailed differences between these subtypes and their therapeutic potential in many settings including diabetes (Medina et al., 2010b; Yoder, 2012; Pelliccia et al., 2022a; Triggle et al., 2022a).
Biofunctionalization of blood contacting implants and stents using attracting molecules (such as antibodies, proteins, glycosaminoglycan (GAGs), peptides and aptamers) have been proposed to induce endothelialization (Figure 1C). Other delivery approaches have been investigated such as nanoparticles and magnetic molecules. These modifications provide mimicry factors that aim to induce cell capture, adhesion, and proliferation of endothelial progenitors and/or influence their mobilization, taking advantage of their ability to migrate to the site of injury during vascular repair processes. Table 1 summarized some of the recent studies investigating the use of these factors to induce stent endothelialization. We also refer the reader to a comprehensive review on the chemistry aspect of biofunctionalization to incorporate these molecules into the surfaces of medical devices (Spicer et al., 2018).
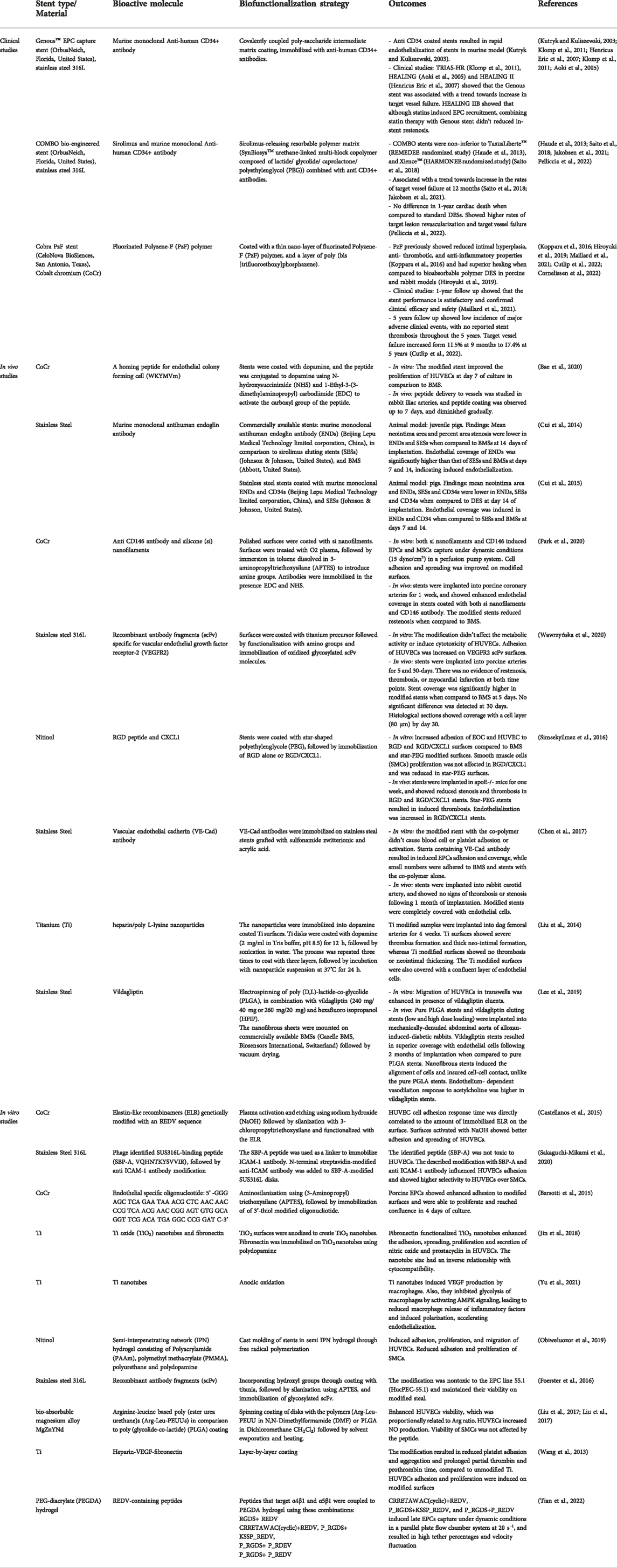
TABLE 1. Summary of the recent studies investigating the use of biomolecules to induce stent endothelialization.
To this date, the main clinically applied biofunctionalization strategy to induce EPCs capture and stent endothelization is the use of monoclonal antibodies against CD34, represented by the Genous™ EPC capture stent and the COMBO bio-engineered stent (OrbusNeich, Florida, United States) (Klomp et al., 2009; Tomasevic et al., 2019) (Table 1). CD34 biofunctionalized stents showed a great promise in early in vivo models, as they resulted in the rapid endothelialization of stents in a murine model (Kutryk and Kuliszewski, 2003). Also, early ex-vivo and clinical studies showed the rapid endothelialization of BMS (Larsen et al., 2012) and DES (Granada et al., 2010; Nakazawa et al., 2010), and for that it was hypothesized that these stents will protect from stent thrombosis. Despite their initial promise, recent clinical studies comparing the performance of the Genous™ EPC capture stent with DES didn’t show superior results in terms of their protection from lumen loss and restenosis. Studies including the TRIAS-HR (71), HEALING and HEALING II (Duckers et al., 2007) showed that the Genous stent was associated with a trend towards increase in target vessel failure. In light of these findings, it was thought that combining the CD34 capture antibody with an anti-proliferative drug will improve these outcomes, thus the novel COMBO bio-engineered stent was developed.
The COMBO bio-engineered stent (OrbusNeich, Florida, United States), is a new generation DES which contains a sirolimus-releasing resorbable polymer matrix to reduce restenosis, in addition to the CD34 coating to induce endothelization. Although comparative clinical trials have shown that COMBO stents were non-inferior to other DESs including TaxusLiberte™ (REMEDEE randomized study) (Haude et al., 2013), and Xience™ (HARMONEE randomized study) (Saito et al., 2018), the COMBO stents were associated with a trend towards increase in the rates of target vessel failure at 12 months (Saito et al., 2018; Jakobsen et al., 2021). Additionally, a recent systematic review including a total of 3961 patients and comparing the COMBO EPC-capturing DES against standard DES from 4 randomized controlled trials, showed no difference in 1-year cardiac death when compared to standard DESs. However, COMBO stent was associated with higher rates of target lesion revascularization and target vessel failure (Pelliccia et al., 2022b). Thus the benefit of these stents in inducing rapid endothelization should be weighed against the possible risk of induced hyperplastic reactions and their consequences (Pelliccia et al., 2022b).
The use of CD34 antibody to capture EPCs has also been proposed for other medical devices, vascular grafts and tissue engineering scaffolds. Nevertheless, because CD34 is not specific to EPCs, it has been suggested that other CD34 positive cells in the blood will compete with EPCs to adhere to the immobilized antibody (Sidney et al., 2014). This could be a contributing factor to the hyperplasia observed in the CD34 biofunctionalized stents. The use of other antibodies against CD133, and VE-Cadherin, amongst other antigens, have been reported to influence EPCs capture, however these stents have met mixed success in vivo (Sedaghat et al., 2013; Van der Heiden et al., 2013). Therefore, there is a need to incorporate other specific bioactive moieties to induce the specific recruitment of EPCs without inducing hyperplasia and restenosis.
Other EPC capturing strategies have been investigated in vitro and in vivo, however these approaches are yet to be validated and translated to clinical use (Table 1 respectively). Growth factors such as vascular endothelial growth factor (VEGF) have been used to induce EPCs adhesion and growth (Van der Heiden et al., 2013). Mobilization of stem cells using chemokines such as stromal cell derived factor 1a (SDF-1a) have also been investigated (Zhang et al., 2011). However, these factors are not specific to EPCs and might result in similar outcomes to what has been observed in CD34 coated stents.
A more specific approach to capture EPCs is the use of specific short peptide ligands and aptamers. These ligands provide an advantage over large biomolecules, because controlling the configuration and folding of large biomolecules is challenging during the biofunctionalization process. The literature describes the use of peptides with different specificities: (i) metal-binding peptides, (ii) non-specific cell adhesion peptides, (iii) endothelial cell-specific peptides and (iv) EPC-specific peptides. Metal-binding peptides are used as linkers to allow further modifications of the stent surface. Examples include the stainless-steel specific peptide SBP-A (Sakaguchi-Mikami et al., 2020).
One of the commonly used peptides that has shown enhanced cell biocompatibility is RGD (Arginyl-glycyl-aspartic acid) peptide, which is the principle ligand responsible for cell binding to the ECM (Bellis, 2011). Other peptides have been investigated such as the laminin derived sequences IKVAV (isoleucine-lysine-valine-alanine-valine) and YIGSR (Tyrosine-Isoleucine-Glycine-Serine-Arginine) (Massia and Hubbell, 1991; Grant et al., 1992). These peptides enhance the non-specific adhesion of cells to biofunctionalized surfaces. Peptides targeting endothelial cells have also been investigated, including REDV (Arginine-Glutamate-Aspartate-Valine) (Hubbell et al., 1991). Specific peptides to EPCs have been identified such as the disulfide cyclic octa-peptide (cGRGDdvc, also known as LXW7) (Hao et al., 2017), TPS (Threonine-Proline-Serine-Leucine-Glutamate-Glutamine-Arginine-Threonine-Valine-Tyrosine-Alanine-Lysine) (Veleva et al., 2007), and WKYMVm (Trp-Lys-Tyr-Met-Val-D-Met) (Bae et al., 2020a). These peptides interact with the integrins -which are adhesion receptors on the cells - and activate them, resulting in enhanced cell adhesion and binding. EPCs specific aptamers or oligonucleotides have been also tested (Barsotti et al., 2015). These bioactive molecules hold a great promise for the biofunctionalization of stents due to their specificity and ease of incorporation.
Challenges facing stent endothelialization with EPCs
One of the main challenges facing the in situ endothelialization with circulating EPCs is their low numbers in the blood (Yoder, 2012). These numbers were also shown to be reduced in disease states such as diabetes. Thus, strategies to boost the numbers of EPCs might be required. One example is the use of pharmacological induction using agents with known effects on EPCs such as statins. It was observed during the HEALING IIB study that statin therapy has increased the numbers of EPCs by 5.6-fold, and that the combination of statin therapy with EPC capturing stents resulted in optimal coverage of the stents (den Dekker et al., 2011). EPCs numbers could be boosted by other strategies such as combining more than one capturing molecule or incorporating chemokine or growth-factor-releasing nanoparticles within the coating of the stent. Additionally, local or systematic injection of autologous EPCs could help to boost the endothelialization of the stent.
Another limitation of stent endothelialization with EPCs is the variability in the intrinsic regenerative potential between patients, which might be affected by diabetes, cardiovascular diseases or other comorbidities (Emmert and Hoerstrup, 2016). This is important to consider particularly because the whole concept of in situ endothelialization depends on the intrinsic regenerative potential, and any impairment of this potential will affect the rate of endothelialization (Stassen et al., 2017). It was shown that EPCs function and regenerative ability is impaired in diabetes (Triggle et al., 2022b). This, in addition to vascular endothelial dysfunction, reduces the potential of stent coverage. Thus, enhancing endothelial and EPC function in these patients should be a target to improve endothelialization, in combination with stent biofunctionalization. Antidiabetic drugs with endothelial and cardioprotective effects (such as vildagliptin) (Lee et al., 2019) could be investigated in combination with the biofunctionalized stents.
In conclusion, stent endothelialization represents a potential target to reduce in-stent thrombosis following PCI. Specific biofunctionalization of stents is required to induce endothelialization without evoking restenosis. Targeting EPC and endothelial dysfunction in diabetes are key strategies to aid in the endothelialization process.
Author contributions
IM and CT developed the theoretical framework of the manuscript. IM wrote the manuscript. IM, CT, and BA-S reviewed and edited the manuscript.
Funding
This work was made possible by an Early Career Researcher Award ECRA02-007-3-006 from the Qatar National Research Fund (a member of The Qatar Foundation). IM is supported by the L’Oréal-UNESCO For Women in Science Young Talents Program/Middle East, 2020. The statements made herein are solely the responsibility of the authors. BA-S is funded by the British Heart Foundation Imperial Centre of Research Excellence Award—RE/18/4/34215.
Acknowledgments
Figure 1 was created using BioRender.com.
Conflict of interest
The authors declare that the research was conducted in the absence of any commercial or financial relationships that could be construed as a potential conflict of interest.
Publisher’s note
All claims expressed in this article are solely those of the authors and do not necessarily represent those of their affiliated organizations, or those of the publisher, the editors and the reviewers. Any product that may be evaluated in this article, or claim that may be made by its manufacturer, is not guaranteed or endorsed by the publisher.
References
Abdelgawad, M. E., Desterke, C., Uzan, G., and Naserian, S. (2021). Single-cell transcriptomic profiling and characterization of endothelial progenitor cells: new approach for finding novel markers. Stem Cell Res. Ther. 12 (1), 145. doi:10.1186/s13287-021-02185-0
Angiolillo, D. J., Fernandez-Ortiz, A., Bernardo, E., Ramirez, C., Sabate, M., Jimenez-Quevedo, P., et al. (2005). Platelet function profiles in patients with type 2 diabetes and coronary artery disease on combined aspirin and clopidogrel treatment. Diabetes 54 (8), 2430–2435. doi:10.2337/diabetes.54.8.2430
Aoki, J., Serruys, P. W., van Beusekom, H., Ong, A. T., McFadden, E. P., Sianos, G., et al. (2005). Endothelial progenitor cell capture by stents coated with antibody against CD34: The HEALING-FIM (healthy endothelial accelerated lining inhibits neointimal growth-first in man) registry. J. Am. Coll. Cardiol. 45 (10), 1574–1579. doi:10.1016/j.jacc.2005.01.048
Asahara, T., Murohara, T., Sullivan, A., Silver, M., van der Zee, R., Li, T., et al. (1997). Isolation of putative progenitor endothelial cells for angiogenesis. Sci. (New York, NY) 275 (5302), 964–967. doi:10.1126/science.275.5302.964
Bae, I-H., Jeong, M. H., Park, D. S., Lim, K. S., Shim, J. W., Kim, M. K., et al. (2020). Mechanical and physio-biological properties of peptide-coated stent for re-endothelialization. Biomater. Res. 24, 4. doi:10.1186/s40824-020-0182-x
Bae, I-H., Jeong, M. H., Park, D. S., Lim, K. S., Shim, J. W., Kim, M. K., et al. (2020). Mechanical and physio-biological properties of peptide-coated stent for re-endothelialization. Biomater. Res. 24 (1), 4–9. doi:10.1186/s40824-020-0182-x
Barsotti, M. C., Al Kayal, T., Tedeschi, L., Dinucci, D., Losi, P., Sbrana, S., et al. (2015). Oligonucleotide biofunctionalization enhances endothelial progenitor cell adhesion on cobalt/chromium stents. J. Biomed. Mat. Res. A 103 (10), 3284–3292. doi:10.1002/jbm.a.35461
Beckman, J. A., Creager, M. A., and Libby, P. (2002). Diabetes and atherosclerosis: epidemiology, pathophysiology, and management. JAMA 287 (19), 2570–2581. doi:10.1001/jama.287.19.2570
Bellis, S. L. (2011). Advantages of RGD peptides for directing cell association with biomaterials. Biomaterials 32 (18), 4205–4210. doi:10.1016/j.biomaterials.2011.02.029
Biondi-Zoccai, G. G., Agostoni, P., Sangiorgi, G. M., Airoldi, F., Cosgrave, J., Chieffo, A., et al. (2006). Incidence, predictors, and outcomes of coronary dissections left untreated after drug-eluting stent implantation. Eur. Heart J. 27 (5), 540–546. doi:10.1093/eurheartj/ehi618
Bittl, J. A. (2015). Percutaneous coronary interventions in the diabetic patient: Where do we stand? Circ. Cardiovasc. Interv. 8 (4), e001944. doi:10.1161/CIRCINTERVENTIONS.114.001944
Borhani, S., Hassanajili, S., Ahmadi Tafti, S. H., and Rabbani, S. (2018). Cardiovascular stents: overview, evolution, and next generation. Prog. Biomater. 7 (3), 175–205. doi:10.1007/s40204-018-0097-y
Buchanan, G. L., Basavarajaiah, S., and Chieffo, A. (2012). Stent thrombosis: Incidence, predictors and new technologies. Thrombosis 2012, 956962. doi:10.1155/2012/956962
Castellanos, M. I., Zenses, A-S., Grau, A., Rodríguez-Cabello, J. C., Gil, F. J., Manero, J. M., et al. (2015). Biofunctionalization of REDV elastin-like recombinamers improves endothelialization on CoCr alloy surfaces for cardiovascular applications. Colloids Surf. B Biointerfaces 127, 22–32. doi:10.1016/j.colsurfb.2014.12.056
Chaabane, C., Otsuka, F., Virmani, R., and Bochaton-Piallat, M. L. (2013). Biological responses in stented arteries. Cardiovasc. Res. 99 (2), 353–363. doi:10.1093/cvr/cvt115
Chen, H., Wang, X., Zhou, Q., Xu, P., Liu, Y., Wan, M., et al. (2017). Preparation of vascular endothelial cadherin loaded-amphoteric copolymer decorated coronary stents for Anticoagulation and endothelialization. Langmuir 33 (46), 13430–13437. doi:10.1021/acs.langmuir.7b03064
Cimato, T., Beers, J., Ding, S., Ma, M., McCoy, J. P., Boehm, M., et al. (2009). Neuropilin-1 Identifies endothelial precursors in human and murine embryonic stem cells before CD34 expression. Circulation 119 (16), 2170–2178. doi:10.1161/CIRCULATIONAHA.109.849596
Claessen, B. E., Henriques, J. P., Jaffer, F. A., Mehran, R., Piek, J. J., and Dangas, G. D. (2014). Stent thrombosis: A clinical perspective. JACC. Cardiovasc. Interv. 7 (10), 1081–1092. doi:10.1016/j.jcin.2014.05.016
Cornelissen, A., Sakamoto, A., Sato, Y., Kawakami, R., Mori, M., Kawai, K., et al. (2022). COBRA PzF™ coronary stent in clinical and preclinical studies: setting the stage for new antithrombotic strategies? Future Cardiol. 18 (3), 207–217. doi:10.2217/fca-2021-0057
Cornelissen, A., and Vogt, F. J. (2019). The effects of stenting on coronary endothelium from a molecular biological view: time for improvement? J. Cell. Mol. Med. 23 (1), 39–46. doi:10.1111/jcmm.13936
Costa, M. A., and Simon, D. I. (2005). Molecular basis of restenosis and drug-eluting stents. Circulation 111 (17), 2257–2273. doi:10.1161/01.CIR.0000163587.36485.A7
Cui, S., Liu, J-H., Song, X-T., Ma, G-L., Du, B-J., Lv, S-Z., et al. (2014). A novel stent coated with antibodies to endoglin inhibits neointimal formation of porcine coronary arteries. Biomed. Res. Int. 2014, 428619. doi:10.1155/2014/428619
Cui, S., Song, X-T., Ding, C., Meng, L-J., Lv, S-Z., and Li, K. (2015). Comparison of reendothelialization and neointimal formation with stents coated with antibodies against endoglin and CD34 in a porcine model. Drug Des. devel. Ther. 9, 2249–2256. doi:10.2147/DDDT.S81257
Cutlip, D. E., Jauhar, R., Meraj, P., Garratt, K. N., Novack, V., Novack, L., et al. (2022). Five-year clinical outcomes of the cobra polyzene f nanocoated coronary stent system. Cardiovasc. Revasc. Med. 41, 76–80. doi:10.1016/j.carrev.2021.12.030
D'Ascenzo, F., Bollati, M., Clementi, F., Castagno, D., Lagerqvist, B., de la Torre Hernandez, J. M., et al. (2013). Incidence and predictors of coronary stent thrombosis: evidence from an international collaborative meta-analysis including 30 studies, 221, 066 patients, and 4276 thromboses. Int. J. Cardiol. 167 (2), 575–584. doi:10.1016/j.ijcard.2012.01.080
den Dekker, W. K., Houtgraaf, J. H., Onuma, Y., Benit, E., de Winter, R. J., Wijns, W., et al. (2011). Final results of the HEALING IIB trial to evaluate a bio-engineered CD34 antibody coated stent (Genous™Stent) designed to promote vascular healing by capture of circulating endothelial progenitor cells in CAD patients. Atherosclerosis 219 (1), 245–252. doi:10.1016/j.atherosclerosis.2011.06.032
Douglas, G., Van Kampen, E., Hale, A. B., McNeill, E., Patel, J., Crabtree, M. J., et al. (2013). Endothelial cell repopulation after stenting determines in-stent neointima formation: Effects of bare-metal vs. drug-eluting stents and genetic endothelial cell modification. Eur. Heart J. 34 (43), 3378–3388. doi:10.1093/eurheartj/ehs240
Duckers, H. J., Silber, S., de Winter, R., den Heijer, P., Rensing, B., Rau, M., et al. (2007). Circulating endothelial progenitor cells predict angiographic and intravascular ultrasound outcome following percutaneous coronary interventions in the HEALING-II trial: evaluation of an endothelial progenitor cell capturing stent. EuroIntervention 3 (1), 67–75.
Emmert, M. Y., and Hoerstrup, S. P. (2016). Tissue engineered heart valves: moving towards clinical translation. Expert Rev. Med. Devices 13 (5), 417–419. doi:10.1586/17434440.2016.1171709
Finn, A. V., Joner, M., Nakazawa, G., Kolodgie, F., Newell, J., John, M. C., et al. (2007). Pathological correlates of late drug-eluting stent thrombosis: Strut coverage as a marker of endothelialization. Circulation 115 (18), 2435–2441. doi:10.1161/CIRCULATIONAHA.107.693739
Foerster, A., Hołowacz, I., Sunil Kumar, G., Anandakumar, S., Wall, J., Wawrzyńska, M., et al. (2016). Stainless steel surface functionalization for immobilization of antibody fragments for cardiovascular applications. J. Biomed. Mat. Res. A 104 (4), 821–832. doi:10.1002/jbm.a.35616
Garcia-Garcia, H. M., McFadden, E. P., Farb, A., Mehran, R., Stone, G. W., Spertus, J., et al. (2018). Standardized end point definitions for coronary intervention trials: the academic research consortium-2 consensus document. Circulation 137 (24), 2635–2650. doi:10.1161/CIRCULATIONAHA.117.029289
Garcia-Garcia, H. M., Vaina, S., Tsuchida, K., and Serruys, P. W. (2006). Drug-eluting stents. Arch. Cardiol. Mex. 76 (3), 297–319.
Georgiadou, P., and Voudris, V. (2017). Platelet activation and stent thrombosis. Hell. J. Cardiol. 58 (1), 49–50. doi:10.1016/j.hjc.2017.03.013
Gori, T., Polimeni, A., Indolfi, C., Räber, L., Adriaenssens, T., and Münzel, T. (2019). Predictors of stent thrombosis and their implications for clinical practice. Nat. Rev. Cardiol. 16 (4), 243–256. doi:10.1038/s41569-018-0118-5
Granada, J. F., Inami, S., Aboodi, M. S., Tellez, A., Milewski, K., Wallace-Bradley, D., et al. (2010). Development of a novel prohealing stent designed to deliver sirolimus from a biodegradable abluminal matrix. Circ. Cardiovasc. Interv. 3 (3), 257–266. doi:10.1161/CIRCINTERVENTIONS.109.919936
Grant, D. S., Kinsella, J. L., Fridman, R., Auerbach, R., Piasecki, B. A., Yamada, Y., et al. (1992). Interaction of endothelial cells with a laminin A chain peptide (SIKVAV) in vitro and induction of angiogenic behavior in vivo. J. Cell. Physiol. 153 (3), 614–625. doi:10.1002/jcp.1041530324
Gum, P. A., Kottke-Marchant, K., Welsh, P. A., White, J., and Topol, E. J. (2003). A prospective, blinded determination of the natural history of aspirin resistance among stable patients with cardiovascular disease. J. Am. Coll. Cardiol. 41 (6), 961–965. doi:10.1016/s0735-1097(02)03014-0
Habib, A., Karmali, V., Polavarapu, R., Akahori, H., Nakano, M., Yazdani, S., et al. (2013). Metformin impairs vascular endothelial recovery after stent placement in the setting of locally eluted mammalian target of rapamycin inhibitors via S6 kinase-dependent inhibition of cell proliferation. J. Am. Coll. Cardiol. 61 (9), 971–980. doi:10.1016/j.jacc.2012.12.018
Habib, A., Karmali, V., Polavarapu, R., Akahori, H., Pachura, K., and Finn, A. V. (2013). Metformin impairs endothelialization after placement of newer generation drug eluting stents. Atherosclerosis 229 (2), 385–387. doi:10.1016/j.atherosclerosis.2013.06.001
Hao, D., Xiao, W., Liu, R., Kumar, P., Li, Y., Zhou, P., et al. (2017). Discovery and characterization of a potent and specific peptide ligand targeting endothelial progenitor cells and endothelial cells for tissue regeneration. ACS Chem. Biol. 12 (4), 1075–1086. doi:10.1021/acschembio.7b00118
Haude, M., Lee, S. W., Worthley, S. G., Silber, S., Verheye, S., Erbs, S., et al. (2013). The REMEDEE trial: A randomized comparison of a combination sirolimus-eluting endothelial progenitor cell capture stent with a paclitaxel-eluting stent. JACC. Cardiovasc. Interv. 6 (4), 334–343. doi:10.1016/j.jcin.2012.10.018
Hiroyuki, J., Hiroyoshi, M., Qi, C., Matthew, K., Sho, T., Atsushi, S., et al. (2019). Thromboresistance and functional healing in the COBRA PzF stent versus competitor DES: Implications for dual antiplatelet therapy. EuroIntervention 15 (4), e342–e353. doi:10.4244/EIJ-D-18-00740
Hubbell, J. A., Massia, S. P., Desai, N. P., and Drumheller, P. D. (1991). Endothelial cell-selective materials for tissue engineering in the vascular graft via a new receptor. Biotechnology. 9 (6), 568–572. doi:10.1038/nbt0691-568
Jaffer, I. H., Fredenburgh, J. C., Hirsh, J., and Weitz, J. I. (2015). Medical device-induced thrombosis: what causes it and how can we prevent it? J. Thromb. Haemost. 1, s72–81. doi:10.1111/jth.12961
Jakobsen, L., Christiansen, E. H., Freeman, P., Kahlert, J., Veien, K., Maeng, M., et al. (2021). randomized clinical comparison of the dual-therapy cd34 antibody-covered sirolimus-eluting combo stent with the sirolimus-eluting orsiro stent in patients treated with percutaneous coronary intervention: the sort out x trial. Circulation 143 (22), 2155–2165. doi:10.1161/CIRCULATIONAHA.120.052766
Jin, Z., Yan, X., Liu, G., and Lai, M. (2018). Fibronectin modified TiO2 nanotubes modulate endothelial cell behavior. J. Biomater. Appl. 33 (1), 44–51. doi:10.1177/0885328218774512
Keighron, C., Lyons, C. J., Creane, M., O'Brien, T., and Liew, A. (2018). Recent advances in endothelial progenitor cells toward their use in clinical translation. Front. Med. 5, 354. doi:10.3389/fmed.2018.00354
Kipshidze, N., Dangas, G., Tsapenko, M., Moses, J., Leon, M. B., Kutryk, M., et al. (2004). Role of the endothelium in modulating neointimal formation: vasculoprotective approaches to attenuate restenosis after percutaneous coronary interventions. J. Am. Coll. Cardiol. 44 (4), 733–739. doi:10.1016/j.jacc.2004.04.048
Klomp, M., Beijk, M. A., Varma, C., Koolen, J. J., Teiger, E., Richardt, G., et al. (2011). 1-year outcome of TRIAS HR (TRI-stent adjudication study-high risk of restenosis) a multicenter, randomized trial comparing genous endothelial progenitor cell capturing stents with drug-eluting stents. JACC. Cardiovasc. Interv. 4 (8), 896–904. doi:10.1016/j.jcin.2011.05.011
Klomp, M., Beijk, M. A., Varma, C., Koolen, J. J., Teiger, E., Richardt, G., et al. (2011). 1-Year outcome of trias hr (tri-stent adjudication study–high risk of restenosis): a multicenter, randomized trial comparing genous endothelial progenitor cell capturing stents with drug-eluting stents. JACC. Cardiovasc. Interv. 4 (8), 896–904. doi:10.1016/j.jcin.2011.05.011
Klomp, M., Beijk, M. A. M., and de Winter, R. J. (2009). Genous endothelial progenitor cell-capturing stent system: A novel stent technology. Expert Rev. Med. Devices 6 (4), 365–375. doi:10.1586/erd.09.16
Kong, D., Melo, L. G., Mangi, A. A., Zhang, L., Lopez-Ilasaca, M., Perrella, M. A., et al. (2004). Enhanced inhibition of neointimal hyperplasia by genetically engineered endothelial progenitor cells. Circulation 109 (14), 1769–1775. doi:10.1161/01.CIR.0000121732.85572.6F
Koppara, T., Sakakura, K., Pacheco, E., Cheng, Q., Zhao, X., Acampado, E., et al. (2016). Preclinical evaluation of a novel polyphosphazene surface modified stent. Int. J. Cardiol. 222, 217–225. doi:10.1016/j.ijcard.2016.07.181
Koskinas, K. C., Siontis, G. C., Piccolo, R., Franzone, A., Haynes, A., Rat-Wirtzler, J., et al. (2016). Impact of diabetic status on outcomes after revascularization with drug-eluting stents in relation to coronary artery disease complexity: patient-level pooled analysis of 6081 patients. Circ. Cardiovasc. Interv. 9 (2), e003255. doi:10.1161/CIRCINTERVENTIONS.115.003255
Kutryk, .M. J., and Kuliszewski, M. (2003). In vivo endothelial progenitor cell seeding for the accelerated endothelialization of endovascular devices. NEW YORK, NY 10011 USA: American Journal of Cardiology; 2003: EXCERPTA MEDICA INC 650 AVENUE OF THE AMERICAS.
Larsen, K., Cheng, C., Tempel, D., Parker, S., Yazdani, S., den Dekker, W. K., et al. (2012). Capture of circulatory endothelial progenitor cells and accelerated re-endothelialization of a bio-engineered stent in human ex vivo shunt and rabbit denudation model. Eur. Heart J. 33 (1), 120–128. doi:10.1093/eurheartj/ehr196
Lee, C. H., Hsieh, M. J., Chang, S. H., Hung, K. C., Wang, C. J., Hsu, M. Y., et al. (2019). Nanofibrous vildagliptin-eluting stents enhance re-endothelialization and reduce neointimal formation in diabetes: In vitro and in vivo. Int. J. Nanomedicine 14, 7503–7513. doi:10.2147/IJN.S211898
Liu, J., Wang, P., Chu, C-C., and Xi, T. (2017). A novel biodegradable and biologically functional arginine-based poly (ester urea urethane) coating for Mg–Zn–Y–Nd alloy: enhancement in corrosion resistance and biocompatibility. J. Mat. Chem. B 5 (9), 1787–1802. doi:10.1039/c6tb03147a
Liu, J., Wang, P., Chu, C-C., and Xi, T. (2017). Arginine-leucine based poly (ester urea urethane) coating for Mg-Zn-Y-Nd alloy in cardiovascular stent applications. Colloids Surf. B Biointerfaces 159, 78–88. doi:10.1016/j.colsurfb.2017.07.031
Liu, T., Zeng, Z., Liu, Y., Wang, J., Maitz, M. F., Wang, Y., et al. (2014). Surface modification with dopamine and heparin/poly-l-lysine nanoparticles provides a favorable release behavior for the healing of vascular stent lesions. ACS Appl. Mat. Interfaces 6 (11), 8729–8743. doi:10.1021/am5015309
Maillard, L., de Labriolle, A., Brasselet, C., Faurie, B., Durel, N., de Poli, F., et al. (2021). Evaluation of the safety and efficacy of the cobra pzf nanocoated coronary stent in routine, consecutive, prospective, and high-risk patients: the e-cobra study. Catheter. Cardiovasc. Interv. 98 (1), 45–54. doi:10.1002/ccd.29065
Massia, S. P., and Hubbell, J. A. (1991). Human endothelial cell interactions with surface-coupled adhesion peptides on a nonadhesive glass substrate and two polymeric biomaterials. J. Biomed. Mat. Res. 25 (2), 223–242. doi:10.1002/jbm.820250209
Meads, C., Cummins, C., Jolly, K., Stevens, A., Burls, A., and Hyde, C. (2000). Coronary artery stents in the treatment of ischaemic heart disease: A rapid and systematic review. Health Technol. Assess. 4 (23), 1–153. doi:10.3310/hta4230
Medina, R. J., Barber, C. L., Sabatier, F., Dignat-George, F., Melero-Martin, J. M., Khosrotehrani, K., et al. (2017). Endothelial progenitors: A consensus statement on nomenclature. Stem Cells Transl. Med. 6 (5), 1316–1320. doi:10.1002/sctm.16-0360
Medina, R. J., O'Neill, C. L., Sweeney, M., Guduric-Fuchs, J., Gardiner, T. A., Simpson, D. A., et al. (2010). Molecular analysis of endothelial progenitor cell (EPC) subtypes reveals two distinct cell populations with different identities. BMC Med. Genomics 3, 18. doi:10.1186/1755-8794-3-18
Medina, R. J., O'Neill, C. L., Sweeney, M., Guduric-Fuchs, J., Gardiner, T. A., Simpson, D. A., et al. (2010). Molecular analysis of endothelial progenitor cell (EPC) subtypes reveals two distinct cell populations with different identities. BMC Med. Genomics 3, 18. doi:10.1186/1755-8794-3-18
Nakazawa, G., Granada, J. F., Alviar, C. L., Tellez, A., Kaluza, G. L., Guilhermier, M. Y., et al. (2010). Anti-CD34 antibodies immobilized on the surface of sirolimus-eluting stents enhance stent endothelialization. JACC. Cardiovasc. Interv. 3 (1), 68–75. doi:10.1016/j.jcin.2009.09.015
O'Neill TJt, , Wamhoff, B. R., Owens, G. K., and Skalak, T. C. (2005). Mobilization of bone marrow-derived cells enhances the angiogenic response to hypoxia without transdifferentiation into endothelial cells. Circ. Res. 97 (10), 1027–1035. doi:10.1161/01.RES.0000189259.69645.25
Obiweluozor, F. O., Tiwari, A. P., Lee, J. H., Batgerel, T., Kim, J. Y., Lee, D., et al. (2019). Thromboresistant semi-IPN hydrogel coating: towards improvement of the hemocompatibility/biocompatibility of metallic stent implants. Mat. Sci. Eng. C Mat. Biol. Appl. 99, 1274–1288. doi:10.1016/j.msec.2019.02.054
O’Brien, B., and Carroll, W. (2009). The evolution of cardiovascular stent materials and surfaces in response to clinical drivers: A review. Acta Biomater. 5 (4), 945–958. doi:10.1016/j.actbio.2008.11.012
Okrainec, K., Banerjee, D. K., and Eisenberg, M. J. (2004). Coronary artery disease in the developing world. Am. Heart J. 148 (1), 7–15. doi:10.1016/j.ahj.2003.11.027
Oliver James, J., Webb David, J., and Newby David, E. (2005). Stimulated tissue plasminogen activator release as a marker of endothelial function in humans. Arterioscler. Thromb. Vasc. Biol. 25 (12), 2470–2479. doi:10.1161/01.ATV.0000189309.05924.88
Ong, A. T., Aoki, J., Kutryk, M. J., and Serruys, P. W. (2005). How to accelerate the endothelialization of stents. Arch. Mal. Coeur Vaiss. 98 (2), 123–126.
Otsuka, F., Finn, A. V., Yazdani, S. K., Nakano, M., Kolodgie, F. D., and Virmani, R. (2012). The importance of the endothelium in atherothrombosis and coronary stenting. Nat. Rev. Cardiol. 9 (8), 439–453. doi:10.1038/nrcardio.2012.64
Palmaz, J. C. (1992). Intravascular stenting: From basic research to clinical application. Cardiovasc. Interv. Radiol. 15 (5), 279–284. doi:10.1007/BF02733951
Park, K-S., Kang, S. N., Kim, D. H., Kim, H-B., Im, K. S., Park, W., et al. (2020). Late endothelial progenitor cell-capture stents with CD146 antibody and nanostructure reduce in-stent restenosis and thrombosis. Acta Biomater. 111, 91–101. doi:10.1016/j.actbio.2020.05.011
Pelliccia, F., Pasceri, V., Zimarino, M., De Luca, G., De Caterina, R., Mehran, R., et al. (2022). Endothelial progenitor cells in coronary atherosclerosis and percutaneous coronary intervention: A systematic review and meta-analysis. Cardiovasc. Revascularization Med. 42, 94–99. doi:10.1016/j.carrev.2022.02.025
Pelliccia, F., Zimarino, M., De Luca, G., Viceconte, N., Tanzilli, G., and De Caterina, R. (2022). Endothelial progenitor cells in coronary artery disease: from bench to bedside. Stem Cells Transl. Med. 11 (5), 451–460. doi:10.1093/stcltm/szac010
Ross, R. (1993). The pathogenesis of atherosclerosis: A perspective for the 1990s. Nature 362 (6423), 801–809. doi:10.1038/362801a0
Saito, S., Krucoff, M. W., Nakamura, S., Mehran, R., Maehara, A., Al-Khalidi, H. R., et al. (2018). Japan-united states of america harmonized assessment by randomized multicentre study of orbusneich’s combo stent (japan-usa harmonee) study: primary results of the pivotal registration study of combined endothelial progenitor cell capture and drug-eluting stent in patients with ischaemic coronary disease and non-st-elevation acute coronary syndrome. Eur. Heart J. 39 (26), 2460–2468. doi:10.1093/eurheartj/ehy275
Sakaguchi-Mikami, A., Fujimoto, K., Taguchi, T., Isao, K., and Yamazaki, T. (2020). A novel biofunctionalizing peptide for metallic alloy. Biotechnol. Lett. 42 (5), 747–756. doi:10.1007/s10529-020-02832-1
Scafa Udriște, A., Niculescu, A-G., Grumezescu, A. M., and Bădilă, E. (2021). Cardiovascular stents: a review of past, current, and emerging devices. Materials 14 (10), 2498. doi:10.3390/ma14102498
Seabra-Gomes, R. (2006). Percutaneous coronary interventions with drug eluting stents for diabetic patients. Heart 92 (3), 410–419. doi:10.1136/hrt.2005.062992
Sedaghat, A., Sinning, J-M., Paul, K., Kirfel, G., Nickenig, G., and Werner, N. (2013). First in vitro and in vivo results of an anti-human CD133-antibody coated coronary stent in the porcine model. Clin. Res. Cardiol. 102 (6), 413–425. doi:10.1007/s00392-013-0547-4
Sidney, L. E., Branch, M. J., Dunphy, S. E., Dua, H. S., and Hopkinson, A. (2014). Concise review: evidence for CD34 as a common marker for diverse progenitors. Stem Cells 32 (6), 1380–1389. doi:10.1002/stem.1661
Simsekyilmaz, S., Liehn, E. A., Weinandy, S., Schreiber, F., Megens, R. T., Theelen, W., et al. (2016). Targeting in-stent-stenosis with RGD- and CXCL1-coated mini-stents in mice. PLoS One 11 (5), e0155829. doi:10.1371/journal.pone.0155829
Smadja, D. M., Bièche, I., Silvestre, J-S., Germain, S., Cornet, A., Laurendeau, I., et al. (2008). Bone morphogenetic proteins 2 and 4 are selectively expressed by late outgrowth endothelial progenitor cells and promote neoangiogenesis. Arterioscler. Thromb. Vasc. Biol. 28 (12), 2137–2143. doi:10.1161/ATVBAHA.108.168815
Smith, S. C., Dove, J. T., Jacobs, A. K., Kennedy, J. W., Kereiakes, D., Kern, M. J., et al. (2001). ACC/AHA guidelines for percutaneous coronary intervention (revision of the 1993 ptca guidelines)-executive summary: a report of the american college of cardiology/american heart association task force on practice guidelines (committee to revise the 1993 guidelines for percutaneous transluminal coronary angioplasty) endorsed by the society for cardiac angiography and interventions. Circulation 103 (24), 3019–3041. doi:10.1161/01.cir.103.24.3019
Sousa, J. E., Serruys, P. W., and Costa, M. A. (2003). New frontiers in cardiology: drug-eluting stents: Part I. Circulation 107 (17), 2274–2279. doi:10.1161/01.CIR.0000069330.41022.90
Spicer, C. D., Pashuck, E. T., and Stevens, M. M. (2018). Achieving controlled biomolecule–biomaterial conjugation. Chem. Rev. 118 (16), 7702–7743. doi:10.1021/acs.chemrev.8b00253
Stassen, O., Muylaert, D. E. P., Bouten, C. V. C., and Hjortnaes, J. (2017). Current challenges in translating tissue-engineered heart valves. Curr. Treat. Options Cardiovasc. Med. 19 (9), 71. doi:10.1007/s11936-017-0566-y
Tian, Y., Seeto, W. J., Páez-Arias, M. A., Hahn, M. S., and Lipke, E. A. (2022). Endothelial colony forming cell rolling and adhesion supported by peptide-grafted hydrogels. Acta Biomater. S1742 7061. 00523–532. doi:10.1016/j.actbio.2022.08.047
Tomasevic, M., Rakocevic, J., Dobric, M., Aleksandric, S., and Labudovic, M. (2019). Capturing endothelial cells by coronary stents - from histology to clinical outcomes. Serbian J. Exp. Clin. Res. 0 (0). doi:10.2478/sjecr-2019-0018
Torii, S., Jinnouchi, H., Sakamoto, A., Kutyna, M., Cornelissen, A., Kuntz, S., et al. (2020). Drug-eluting coronary stents: Insights from preclinical and pathology studies. Nat. Rev. Cardiol. 17 (1), 37–51. doi:10.1038/s41569-019-0234-x
Triggle, C. R., Ding, H., Marei, I., Anderson, T. J., and Hollenberg, M. D. (2020). Why the endothelium? The endothelium as a target to reduce diabetes-associated vascular disease. Can. J. Physiol. Pharmacol. 98 (7), 415–430. doi:10.1139/cjpp-2019-0677
Triggle, C. R., Marei, I., Ye, K., Ding, H., Anderson, T. J., Hollenberg, M. D., et al. (2022). Repurposing metformin for vascular disease. Curr. Med. Chem. 29. doi:10.2174/0929867329666220729154615
Triggle, C. R., Mohammed, I., Bshesh, K., Marei, I., Ye, K., Ding, H., et al. (2022). Metformin: Is it a drug for all reasons and diseases? Metabolism. 133, 155223. doi:10.1016/j.metabol.2022.155223
Tura, O., (2013) Skinner Em Fau - Barclay, G. R., Barclay Gr Fau - Samuel, K., Samuel, K., Fau - Gallagher, R. C. J., Gallagher Rc Fau - Brittan, M., et al. . Late outgrowth endothelial cells resemble mature endothelial cells and are not derived from bone marrow. Stem Cells. 338–348.doi:10.1002/stem.1280
Van der Heiden, K., Gijsen, F. J., Narracott, A., Hsiao, S., Halliday, I., Gunn, J., et al. (2013). The effects of stenting on shear stress: relevance to endothelial injury and repair. Cardiovasc. Res. 99 (2), 269–275. doi:10.1093/cvr/cvt090
van Hinsbergh, V. W. M. (2012). Endothelium--role in regulation of coagulation and inflammation. Semin. Immunopathol. 34 (1), 93–106. doi:10.1007/s00281-011-0285-5
Vanhoutte, P. M., and Tang, E. H. (2008). Endothelium-dependent contractions: when a good guy turns bad. J. Physiol. 586 (22), 5295–5304. doi:10.1113/jphysiol.2008.161430
Veleva, A. N., Cooper, S. L., and Patterson, C. (2007). Selection and initial characterization of novel peptide ligands that bind specifically to human blood outgrowth endothelial cells. Biotechnol. Bioeng. 98 (1), 306–312. doi:10.1002/bit.21420
Wang, H., Yin, T., Ge, S., Zhang, Q., Dong, Q., Lei, D., et al. (2013). Biofunctionalization of titanium surface with multilayer films modified by heparin‐VEGF‐fibronectin complex to improve endothelial cell proliferation and blood compatibility. J. Biomed. Mat. Res. A 101 (2), 413–420. doi:10.1002/jbm.a.34339
Watala, C., Golanski, J., Pluta, J., Boncler, M., Rozalski, M., Luzak, B., et al. (2004). Reduced sensitivity of platelets from type 2 diabetic patients to acetylsalicylic acid (aspirin)-its relation to metabolic control. Thromb. Res. 113 (2), 101–113. doi:10.1016/j.thromres.2003.12.016
Wawrzyńska, M., Kraskiewicz, H., Paprocka, M., Krawczenko, A., Bielawska-Pohl, A., Biały, D., et al. (2020). Functionalization with a VEGFR2-binding antibody fragment leads to enhanced endothelialization of a cardiovascular stent in vitro and in vivo. J. Biomed. Mat. Res. B Appl. Biomater. 108 (1), 213–224. doi:10.1002/jbm.b.34380
Wiviott, S. D., Braunwald, E., Angiolillo, D. J., Meisel, S., Dalby, A. J., Verheugt, F. W., et al. (2008). Greater clinical benefit of more intensive oral antiplatelet therapy with prasugrel in patients with diabetes mellitus in the trial to assess improvement in therapeutic outcomes by optimizing platelet inhibition with prasugrel-thrombolysis in myocardial Infarction 38. Circulation 118 (16), 1626–1636. doi:10.1161/CIRCULATIONAHA.108.791061
Wu, K. K., and Thiagarajan, P. (1996). Role of endothelium in thrombosis and hemostasis. Annu. Rev. Med. 47 (0066-4219), 315–331. doi:10.1146/annurev.med.47.1.315
Yoder, M. C. (2012). Human endothelial progenitor cells. Cold Spring Harb. Perspect. Med. 2 (7), a006692. doi:10.1101/cshperspect.a006692
Yoder, M. C., Mead, L. E., Prater, D., Krier, T. R., Mroueh, K. N., Li, F., et al. (2007). Redefining endothelial progenitor cells via clonal analysis and hematopoietic stem/progenitor cell principals. Blood 109 (5), 1801–1809. doi:10.1182/blood-2006-08-043471
Yu, W. P., Ding, J. L., Liu, X. L., Zhu, G. D., Lin, F., Xu, J. J., et al. (2021). Titanium dioxide nanotubes promote M2 polarization by inhibiting macrophage glycolysis and ultimately accelerate endothelialization. Immun. Inflamm. Dis. 9 (3), 746–757. doi:10.1002/iid3.429
Yuan, J., and Xu, G. M. (2018). Early and late stent thrombosis in patients with versus without diabetes mellitus following percutaneous coronary intervention with drug-eluting stents: A systematic review and meta-analysis. Am. J. Cardiovasc. Drugs 18 (6), 483–492. doi:10.1007/s40256-018-0295-y
Zentilin, L., Tafuro, S., Zacchigna, S., Arsic, N., Pattarini, L., Sinigaglia, M., et al. (2006). Bone marrow mononuclear cells are recruited to the sites of VEGF-induced neovascularization but are not incorporated into the newly formed vessels. Blood 107 (9), 3546–3554. doi:10.1182/blood-2005-08-3215
Zhang, H-f., Wang, Y-l., Tan, Y-z., Wang, H-j., Tao, P., and Zhou, P. (2019). Enhancement of cardiac lymphangiogenesis by transplantation of CD34+VEGFR-3+ endothelial progenitor cells and sustained release of VEGF-C. Basic Res. Cardiol. 114 (6), 43. doi:10.1007/s00395-019-0752-z
Keywords: in-stent thrombosis, diabetes, cellular dysfunction, endothelialization, endothelial progenitor cells, biofunctionalization, cell capture
Citation: Marei I, Ahmetaj-Shala B and Triggle CR (2022) Biofunctionalization of cardiovascular stents to induce endothelialization: Implications for in- stent thrombosis in diabetes. Front. Pharmacol. 13:982185. doi: 10.3389/fphar.2022.982185
Received: 30 June 2022; Accepted: 31 August 2022;
Published: 10 October 2022.
Edited by:
Ali H. Eid, Qatar University, QatarReviewed by:
Tim Murphy, University of New South Wales, AustraliaMohamed Noureldein, Michigan Medicine, University of Michigan, United States
Copyright © 2022 Marei, Ahmetaj-Shala and Triggle. This is an open-access article distributed under the terms of the Creative Commons Attribution License (CC BY). The use, distribution or reproduction in other forums is permitted, provided the original author(s) and the copyright owner(s) are credited and that the original publication in this journal is cited, in accordance with accepted academic practice. No use, distribution or reproduction is permitted which does not comply with these terms.
*Correspondence: Isra Marei, aXltMjAwMUBxYXRhci1tZWQuY29ybmVsbC5lZHU=; Chris R. Triggle, Y2h0MjAxMUBxYXRhci1tZWQuY29ybmVsbC5lZHU=