- 1Department of Respiratory and Critical Care Medicine, Aerospace Center Hospital, Peking University Aerospace School of Clinical Medicine, Beijing, China
- 2Department of Neurology, Zhongnan Hospital of Wuhan University, Wuhan, China
- 3Department of Neurology, The Affiliated Hospital of Chifeng University, Chifeng, China
- 4Department of Psychological Medicine, The Affiliated Hospital of Chifeng University, Chifeng, China
- 5Department of Anatomy, College of Basic Medicine, Chifeng University Health Science Center, Chifeng, China
- 6Department of Neurology,Aerospace Center Hospital, Peking University Aerospace School of Clinical Medicine, Beijing, China
- 7Tianjin Medical University Cancer Institute and Hospital, National Clinical Research Center for Cancer, Tianjin’s Clinical Research Center for Cancer, Key Laboratory of Cancer Prevention and Therapy, Tianjin, China
Epigallocatechin 3-gallate (EGCG), an abundant polyphenolic component derived from green tea extract, possesses versatile bioactivities that can combat many diseases. During the last decade, EGCG was shown to be effective in experimental models of Parkinson’s disease (PD). Several experimental studies have suggested that it has pleiotropic neuroprotective effects, which has enhanced the appeal of EGCG as a therapeutic strategy in PD. In this review, we compiled recent updates and knowledge of the molecular mechanisms underlying the neuroprotective effects of EGCG in PD. We focused on the effects of EGCG on apoptosis, oxidative stress, inflammation, ferroptosis, modulation of dopamine production, and the aggregation of α-synuclein. The review highlights the pharmacological features of EGCG and its therapeutic implications in PD. Taken together, the accumulated data indicate that EGCG is a promising neuroprotective compound for the treatment of PD.
Introduction
Parkinson’s disease (PD) is the second most common neurodegenerative disease. It is characterized by motor and non-motor symptom (Postuma et al., 2015). The degeneration of dopaminergic neurons located in the substantia nigra pars compacta (SNpc) of the brainstem (Wang et al., 2021), which leads to the depletion of striatal dopamine levels (Meder et al., 2019), is the major pathological feature of PD, along with the presence of Lewy bodies (LBs), which mainly consist of misfolded α-synuclein, ubiquitin, PTEN-induced kinase-1 (PINK1), parkin, and other proteins, in the surviving neurons (Cookson, 2005; Abeliovich and Flint, 2006). PD affects more than 2% of the population older than 65 years old (Aarsland et al., 2017), and is becoming a major age-related health problem (Zou et al., 2015; Hirsch et al., 2016; Savica et al., 2016).
Despite intensive research, the molecular mechanisms involved in the degeneration of dopaminergic neurons remains poorly understood (Dos Santos et al., 2022). Oxidative stress (Fahn and Cohen, 1992; Dionísio et al., 2021), mitochondrial dysfunction (Park et al., 2018), neuroinflammation (Kip and Parr-Brownlie, 2022), iron dysregulation (Vuuren et al., 2020), ferroptosis (Ko et al., 2021; Mahoney-Sánchez et al., 2021; Wu et al., 2021), protein misfolding and degradation dysfunction (Haque et al., 2022), and environmental and genetic factors (Kline et al., 2021) probably play an important role in the pathogenesis of PD. The available therapeutic options for PD are limited, and only provide symptomatic relief, rather than halting the progression of the disease, in addition to having serious side effects (Wang et al., 2021). Increasing numbers of studies have been performed to identify neuroprotective compounds that can prevent dopaminergic neuron injury, and thereby retard disease progression and add further benefits to current therapy (Kujawska and Jodynis-Liebert, 2018; Dos Santos et al., 2022).
In this context, nutraceuticals have gained tremendous interest in recent decades, due to their long history of use (Payne et al., 2022). Various nutraceuticals exhibit antioxidative, anti-inflammatory, and anti-aging properties, and have been studied in the treatment of PD. Phytochemicals are biologically active nutraceutical plant chemicals that are typically secondary metabolites present in plants, such as green tea polyphenols, anthocyanidins, carotenoids, phytoestrogens, and terpenoids (Balakrishnan et al., 2021). Many phytochemicals have emerged as potential multi-target agents for the treatment of PD, due to their diverse actions (Peluso and Serafini, 2017).
Several dietary phytochemicals have been investigated in PD due to their potential beneficial and neuroprotective effects, including green tea catechins, such as epigallocatechin 3-gallate (EGCG) (Gonçalves et al., 2021). EGCG is an abundant polyphenolic component of green tea extract, and has exhibited versatile bioactivities in combating several diseases (Zhang et al., 2020; Fernandes et al., 2021). During the last decade, EGCG has been shown to be effective in experimental models of PD (Payne et al., 2022). Mounting evidence from experimental studies has suggested that EGCG exerts pleiotropic neuroprotective effects, which has led to emergence of EGCG as a therapeutic strategy for PD.
We here compiled recent updates on the use, and reports on the cellular and molecular mechanisms of neuroprotection of EGCG in PD. In this review, we focused on the effects of EGCG apoptosis, oxidation, inflammation, dopamine production, and the aggregation of α-synuclein. By highlighting the pharmacological features of EGCG and its therapeutic implications in PD, this review suggests that EGCG may be a promising neuroprotective compound for the treatment of PD.
Source, biochemistry, and bioavailability of EGCG
Green tea contains six main catechin compounds, i.e., gallocatechin, catechin, epicatechin (EC), epicatechin gallate (ECG), epigallocatechin (EGC), and EGCG. EGCG is the most active component and best-studied polyphenol in green tea. Each two hundred and fifty milliliters (1.25% w/v) of green tea contains around 177 mg of EGCG (Alam et al., 2022). EGCG (C22H18O11) is a flavanol catechin, and is an ortho-benzoyl benzopyran byproduct, comprised of three hydroxyphenyl and hydroxybenzoate moieties marked A, B, C, and D (Payne et al., 2022) (Figure 1). The benzopyran ring, which has a phenyl group at C2 and a gallate group at C3, is made up of ring A and C. The B ring has positional 3,4,5-trihydroxyl groups, and the D ring gallate group (a galloyl moiety) is configured as an ester at C3. EGCG has reactive oxygen species (ROS)-deactivating properties due to the contribution of the B and D rings. The D ring has been shown to have anticancer and anti-inflammation characteristics (Payne et al., 2022). EGCG has seven hydroxyl radicals distributed among three aromatic rings, which confers water solubility, causing EGCG to have high blood-brain barrier (BBB) permeability (Payne et al., 2022). It has been reported that EGCG permeates the BBB within 0.5 h (Unno et al., 2017). The BBB permeability of EGCG were decreased by 57.54% (Unno et al., 2017). Although EGCG has good pharmacological and biological activity, the bioavailability of oral EGCG is relatively poor. A previous study showed that the highest plasma concentration of EGCG was only 0.15 µM after a human ingested two cups of green tea (Zhang et al., 2013). Oral EGCG was not stable in intestinal and blood environment, most of EGCG was not absorbed, and its bioavailability was reduced. The bioavailability of oral EGCG could be signifificantly improved through structure modification or nano-materials dependant protection and delivery (Dai et al., 2019).
The medicinal properties of green tea are derived from EGC esterification with gallic acid (i.e., galloylation). Thus, green tea has antioxidative mechanisms provided by EGCG (Payne et al., 2022). The unique chemical structure and makeup of EGCG confer its highly antioxidative and anti-inflammatory properties. EGCG is a peroxynitrite scavenger that reduces the nitration of tyrosine, and scavenges hydrogen peroxide and superoxide anions, thereby blocking ROS-induced DNA damage. EGCG have exhibited many disease-alleviating properties particularly regarding neuroprotective effects (Figure 2).
Neuroprotective properties of EGCG in PD
During the last two decades, studies have increasingly focused on the neuroprotective properties of EGCG in PD. In the early 2000s, the potent neuroprotective effects of EGCG were studied in 6-hydroxydopamine (6-OHDA)-induced PC12 cells. These studies suggested that EGCG has neuroprotective effects against 6-OHDA-induced neuronal apoptosis (Jin et al., 2001). Since these first reports on the neuroprotective effects of EGCG in PD, EGCG has received significant attention as a therapeutic agent, due to its multiple molecular mechanisms of action in PD. The potential neuroprotective effects in the context of PD have been thoroughly studied in both in vitro and in vivo models, allowing a deeper understanding of the molecular cascades through which EGCG exerts its neuroprotective actions on PD (Table 1).
Protection against apoptosis
Apoptosis is activated via the intrinsic or extrinsic pathways, and has been extensively documented in PD (Stern, 1996; Dionísio et al., 2021). Apoptosis has been implicated as the main mechanisms of neuronal death in the SNpc in PD. Apoptotic cell death has been observed in cell culture and animal models of PD, and also in nigrostriatal regions of the brains of patients with PD at postmortem (Lev et al., 2003). Targeting apoptosis is regarded as one strategy for preventing dopaminergic neuron death (Vila et al., 2001; Ma et al., 2016).
Jin et al.’s pioneering study showed that preincubation with EGCG inhibited 6-OHDA-induced apoptosis in PC12 cells (Jin et al., 2001), which was further corroborated by the same group’s later studies (Nie et al., 2002a; 2002b). After these studies, evidence suggesting that EGCG exerts neuroprotective effects against apoptosis in PD has accumulated. Levites and others have shown that EGCG prevented both 6-OHDA-induced expression of several mRNAs, such as Bad, Bax, and Mdm2, and resulted in a decrease in Bcl-w, Bcl-2, and Bcl-x(L). EGCG exerted neuroprotective effects against 6-OHDA caused SH-SY5Y cells toxicity through increasing phosphorylated protein kinase C (PKC), suggesting that EGCG exert neuroprotective effects against oxidative stress-induced cell death through activation of PKC and modulation of apoptosis (Levites et al., 2002). Chan and others have shown that pretreatment of SH-SY5Y cells with EGCG at 0.1–10 μM significantly attenuated cell death induced by 6-OHDA. EGCG (1 μM) prevented 6-OHDA-induced activity decline of STAT3. These data clearly demonstrated that EGCG inhibited 6-OHDA-induced oxidative stress-dependent cell death through re-stimulation of the STAT3 signaling pathway (Wang et al., 2009). EGCG inhibited 6-OHDA-induced neurotoxicity in SH-SY5Y cells expressing A53T-mutated α-synuclein, by which sensitivity to 6-OHDA was increased, causing oxidative stress (Ma et al., 2010). EGCG protected against 6-OHDA-induced neurotoxicity in N27 cells. Pretreatment with EGCG prevented the 6-OHDA-induced activation of caspase-3 activity (Chen et al., 2015). In the 6-OHDA-treated SK-N-AS cell PD model, EGCG inhibited the upregulation of α-synuclein, and significantly reduced caspase-3 immunoreactivity (Özduran et al., 2022). A recent study has suggested that leptin-conjugated phosphatidic acid liposomes containing EGCG and resveratrol reduced 1-methyl-4-phenylpyridinium (MPP+)-induced apoptosis in SH-SY5Y cells (Kuo et al., 2021). EGCG and resveratrol, encapsulated in liposomes, could reduce expression of Bax and α-synuclein, and increase levels of Bcl-2, tyrosine hydroxylase (TH), and the dopamine transporter (Kuo et al., 2021). EGCG also inhibited apoptosis induced by paraquat (PQ) in PC12 cells (Hou et al., 2008), by inhibiting the loss of mitochondrial membrane potential (MMP) as well as reducing caspase-3 activity, and by downregulating levels of the pro-apoptotic protein Smac in the cytosol (Hou et al., 2008). Furthermore, EGCG inhibited apoptosis induced by rotenone in vivo (Tseng et al., 2020). In rotenone-challenged rat PD models, EGCG treatment prevented most of the rotenone-induced motor dysfunctions. EGCG reduced the levels of the apoptotic marker caspase-3 in the striatum of these rats (Tseng et al., 2020). Taken together, EGCG shows potential in inhibiting apoptosis in both in vivo and in vitro PD models.
Protection against oxidative stress
Oxidative stress is one of the main factors in the pathogenesis of PD (Percário et al., 2020; Dorszewska et al., 2021). The oxidative stress hypothesis of PD was proposed in 1992 (Fahn and Cohen, 1992), and holds that oxidative stress leads to the neurodegeneration of dopaminergic neurons, resulting in the pathogenesis of PD (Subramaniam and Chesselet, 2013). Accumulating evidence has suggested a number of sources and mechanisms for oxidative stress in PD, which include nicotinamide adenine dinucleotide phosphate oxidase (NOX) activation, mitochondrial dysfunction, the catabolism of dopamine by auto-oxidation, iron (Fe2+) accumulation (Wang et al., 2021). Oxidative stress causes injury to macromolecular components (i.e., DNA, proteins, and lipids) (Pires et al., 2019a; 2019b; 2019c; 2019d, 2020), resulting in cellular dysfunction and, eventually, dopaminergic neuron death (Wang et al., 2021). Given the important role of oxidative stress in PD, antioxidant supplements could be a reasonable therapeutic approach to halting PD progression (Chang and Chen, 2020), as it could mitigate oxidative stress-dependent neuronal injury (Buendia et al., 2016).
Ye et al. (2012) and Lee et al. (2013) highlighted the EGCG-mediated decrease in PD-related neurotoxin-induced ROS production in their in vitro experiments. Ye et al. showed that EGCG inhibits MPP+-induced oxidative stress in PC12 cells via the SIRT1/PGC-1α signaling pathway (Ye et al., 2012). Specifically, EGCG significantly increased cell viability and decreased MPP+-induced ROS production, and potentiated MPP+-induced upregulation of Sirtuin 1 (SIRT1), peroxisome proliferator-activated receptor gamma (PPARgamma) coactivator-1α(PGC-1α), glutathione peroxidase (GPX1), and superoxide dismutase 1 (SOD1) (Ye et al., 2012). Lee et al. demonstrated that EGCG could inhibit L-3,4-dihydroxyphenylalanine-induced oxidative stress-dependent PC12 cell death, which was reflected by a reduction in ROS generation and production of thiobarbituric acid reactive substances, and by an increased intracellular level of glutathione (GSH) (Lee et al., 2013).
EGCG also plays a neuroprotective role in PD through antioxidant mechanisms in vivo PD animal models. In a 1-methyl-4-phenyl-1,2,3,6 -tetrahydropyridine (MPTP)-induced PD model, EGCG rescued MPTP-induced neurotoxicity by decreasing serum protein carbonyls, implying that EGCG reduced oxidative stress in mice (Xu et al., 2017). In agreement with these findings, Pinto and others revealed that EGCG reverted behavioral changes in 6-OHDA-induced male Wistar rats, which were reflected by increased locomotor activity, decreased rotational behavior, antidepressive effects, and improvement of cognitive dysfunction. EGCG reversed the striatal oxidative stress and inhibited immunohistochemistry changes, indicating that EGCG likely exerts neuroprotective effects by its powerful antioxidant and anti-inflammatory properties (Bitu Pinto et al., 2015). This observation was corroborated by other studies, which reported that EGCG protects and prevents PQ-induced oxidative stress-dependent neurodegeneration in Drosophila melanogaster (Bonilla-Ramirez et al., 2013; Martinez-Perez et al., 2018). Recent evidence has indicated that EGCG reversed rotenone-induced neurochemical and motor dysfunctions in rats by reducing lipid peroxidation (LPO) and nitric oxide (NO) levels (Tseng et al., 2020). This study substantiated previous indications that EGCG had neuroprotective effects in PD by anti-oxidant, anti-neuroinflammation, and anti-apoptosis activities (Tseng et al., 2020). Taken together, EGCG shows potential in inhibiting neurotoxin-induced oxidative stress injury in both in vitro and in vivo PD models.
Protection against neuroinflammation
Since McGeer and others observed activated microglial infiltration in the SN of the postmortem PD brain, in the early 1980s (McGeer et al., 1988), numerous studies have focused on the role played by neuroinflammation in the pathogenesis of PD. These studies have revealed that cytokine-induced inflammatory responses play an important role in this disease (Liu et al., 2022). Activation of astrocytes/microglia and peripheral immune cell infiltration, a process called neuroinflammation, are observed in PD (Kip and Parr-Brownlie, 2022). Chronic inflammation and neuroinflammation triggers neuronal damage and plays a vital role in PD pathology (Tansey and Romero-Ramos, 2019; Pajares et al., 2020; Yang et al., 2020; Badanjak et al., 2021; Hirsch and Standaert, 2021; Kip and Parr-Brownlie, 2022). Mounting evidence has indicated that targeting chronic inflammation may be a potential therapeutic target for PD, and pharmacologically reducing neuroinflammation via therapeutic compounds maybe prevent or delay progression of PD (Wang et al., 2015; Hassanzadeh and Rahimmi, 2018; Lee et al., 2019, 2021; Kip and Parr-Brownlie, 2022).
Remarkably, EGCG exhibits anti-inflammatory activities in vitro. Le and others have shown that EGCG potently down-regulates inducible NO synthase (iNOS) and tumor necrosis factor-α (TNF-α) expression, thereby inhibiting lipopolysaccharide (LPS)-activated microglial secretion of nitric oxide (NO) and TNF-α. In addition, EGCG inhibited neuronal injury in SH-SY5Y and in primary rat mesencephalic cultures through microglial activation, which suggested that EGCG functions as a potent inhibitor of microglial activation, thereby alleviating microglia-mediated dopaminergic neuron injury in PD (Li et al., 2004). Additionally, EGCG suppresses 6-OHDA-induced expression of TNF-α and IL-1β in SK-N-AS cells, thereby inhibiting apoptotic pathways and enhancing survival (Özduran et al., 2022).
Recently, several in vivo findings have provided evidence for possible anti-inflammatory effects of EGCG in PD. Al-Amri et al. reported that pretreatment with EGCG decreased TNF-α and NO, and markedly increased the number and density of TH-immunoreactive neurons in the midbrain of PD model rats (Al-Amri et al., 2013). Likewise, EGCG reduced the rotenone-induced increase in NO levels in the striatum and reduced the levels of neuroinflammatory markers of model rats (Tseng et al., 2020). Interestingly, recent data have demonstrated that EGCG-loaded liposomes decreased the production of NO and TNF-α in LPS-induced BV-2 microglia, attenuated LPS-induced pro-inflammatory cytokine levels, and restored motor impairment in vivo in a PD rat model, suggesting that EGCG exerts a neuroprotective effect by modulating microglial activation (Cheng et al., 2021).
Collectively, these data indicate that EGCG maybe play a neuroprotective role by inhibiting neuroinflammation in both in vivo and in vitro PD models.
Protection against ferroptosis
Recent studies have suggested that EGCG may regulate ferroptosis, which is an iron-dependent regulated cell death pathway involving a lethal accumulation of lipid peroxides that is triggered by a combination of iron toxicity, LPO, and plasma membrane damage (Zheng and Conrad, 2020; Chen et al., 2021; Stockwell, 2022) (Figure 3). Ferroptosis, characterised by iron-dependent LPO, shares several features with PD pathophysiology. Interestingly, several major pathological hallmarks of PD are known key features and/or triggers in the ferroptosis pathway (Mahoney-Sánchez et al., 2021). These include iron overload (Dexter et al., 1987), increased LPO (Dexter et al., 1986; de Farias et al., 2016), SLC7A11 downregulation (Vallerga et al., 2020), DJ-1 depletion (Cao et al., 2020), GSH level reduction (Sofic et al., 1992; Sian et al., 1994), and CoQ10 level reduction (Battino et al., 1996; Mischley et al., 2012). Increasingly, studies have revealed that α-synuclein regulates both iron and lipid metabolism, suggesting a possible interplay between ferroptosis and dysregulated α-synuclein (Angelova et al., 2020). Taken together, these studies strongly implicate ferroptosis in the neurodegeneration observed in PD.
Reddy and coworkers have shown that EGCG can affect brain iron homeostasis in 6-OHDA-induced N27 cells (Chen et al., 2015). EGCG pretreatment counteracted 6-OHDA-induced increased expression of divalent metal transporter-1 (DMT1) and hepcidin and decreased expression of the iron-export protein ferroportin 1 (Fpn1), leading to a 28% reduction in Fe2+ uptake. Pretreatment with EGCG prevented the 6-OHDA-induced activation of caspase-3 activity, indicating that EGCG inhibits 6-OHDA-induced neurotoxicity by regulating iron homeostasis (Chen et al., 2015). This observation was corroborated by other studies, which showed that EGCG upregulated Fpn1 in the SN and reduced oxidative stress, thereby exerting a neuroprotective effect against MPTP-induced neurotoxicity in mice (Xu et al., 2017). However, the study by Lee et al. (2013) demonstrated that EGCG increased intracellular levels of GSH in a PD model. Recent evidence indicated that EGCG reversed rotenone-induced lipid peroxidation (LPO) production (Tseng et al., 2020), which substantiate previous indications showing that EGCG treatment provided protection and prevention from the PQ-induced increase in LPO and neurodegeneration in dj-1-β-knockdown Drosophila melanogaster (Martinez-Perez et al., 2018).
In summary, these studies suggested that EGCG inhibits iron overload, decreased LPO, and increased GSH levels in PD models, which are the three major hallmarks of ferroptosis. However, further research is needed to strengthen this hypothesis and provide more detailed mechanisms underlining EGCG inhibition of ferroptosis, such as whether EGCG regulates the ferroptosis signaling pathway and ferroptosis regulators.
Modulation of dopamine production
PD is a neurodegenerative disease caused by the death of dopaminergic neurons located in the SNpc of the brainstem, resulting in the depletion of striatal dopamine, an important neurotransmitter in the brain (Meder et al., 2019). Loss of more than 80% of the dopaminergic neurons in the SNpc affects the nigrostriatal circuits in the midbrain, leading to typical PD motor symptoms, which include tremor at rest, rigidity, slowness or absence of voluntary movement, postural instability, and freezing (Masato et al., 2019; Latif et al., 2021). The recovery of striatal DA content is an important target in PD treatment. Therefore, dopamine replacement therapy, compensating for the lack of dopamine, is the classic treatment for motor symptoms of PD (Ferrazzoli et al., 2016).
A previous study revealed that EGCG (400 mg/kg) protected against MPTP-induced functional and neurochemical deficits, resulting in increased striatal dopamine concentrations in an MPTP-induced PD model in male C57 black mice (Xu et al., 2017). A single intraperitoneal injection of LPS (15 mg/kg) resulted in a decrease in dopamine levels and reduced the number and the density of TH-positive neurons in the midbrain in male Sprague–Dawley rats. Pretreatment with EGCG (10 mg/kg) preserved the number of TH-positive neurons and increased dopamine levels, indicating that EGCG protected against LPS-induced neurotoxicity by reducing inflammatory mediators and preserving dopamine levels in the midbrain (Al-Amri et al., 2013).
Two important enzymes, monoamine oxidase (MAO) and catechol-O-methyl transferase (COMT), are needed for the catabolism of dopamine, through which dopamine is changed to its inactive metabolites (Latif et al., 2021). MAO first converts dopamine to 3,4-dihydroxyphenylacetaldehyde (DOPAL). Aldehyde dehydrogenase then converts DOPAL to 3,4-dihydroxyphenylacetic acid (DOPAC). In the COMT pathway, dopamine is converted to 3-methoxytyramine, which is further reduced to homovanillic acid (HVA), which is subsequently eliminated via the urine (Latif et al., 2021). In the MPTP-induced PD murine model, EGCG inhibits the loss of TH-positive cells located in the SN and the reduction of TH activity in the striatum. At the same time, EGCG preserves dopamine and its metabolites, DOPAC and HVA, in the striatum (Choi et al., 2002).
Modulation of α-Synuclein
The pathological hallmarks of PD are the presence of LBs in different brain regions, which are primarily composed of misfolded and aggregated α-synuclein (Singh et al., 2017). Increasing evidence has indicated that α-synuclein plays a pivotal role in PD pathogenesis. It has been reported that α-synuclein aggregation is one of the leading causes for dopaminergic neuron dysfunction and death (Srinivasan et al., 2021). The multifactorial events involved in this process includes increased oxidative stress, inflammation, mitochondrial dysfunction, and ubiquitin-proteasome system (UPS) dysfunction, which lead to the accumulation of abnormal or misfolded α-synuclein (Javed et al., 2018). These aggregates undergo several key stages of oligomerization, fibrillation, and aggregation. Recent studies have proposed that α-synuclein aggregates can disrupt synaptic regulation, impair neuronal signaling, and eventually lead to neuronal death (Volpicelli-Daley et al., 2011; Zoey et al., 2021). The α-synuclein oligomers induce mitochondrial dysfunction and cause neuroinflammation, oxidative stress, endoplasmic reticulum stress, and inhibition of proteasomal activity and autophagy (Ghiglieri et al., 2018; Javed et al., 2018). An imbalance in the homeostasis of α-synuclein might result in accumulation of α-synuclein and aggregation. The α-synuclein oligomer hypothesis of PD for dopaminergic neuron cell death holds that α-synuclein forms transiently unstable oligomers, which exert cytotoxic effects and are eventually converted to thermodynamically more stable amyloid fibrils (Gadhe et al., 2022).
EGCG inhibits α-synuclein fibrillogenesis in cell-free assays (Bieschke et al., 2010). After this was published, many studies investigated whether EGCG has the ability to remodel α-synuclein aggregates in cell-based models, and found that EGCG could reduce α-synuclein fibril-induced cytotoxicity by remodeling the α-synuclein structure (Bieschke et al., 2010). EGCG binds to α-synuclein amyloid fibrils and oligomers, thereby directly altering their morphology. It as shown that EGCG directly binds to β-sheet-rich aggregates, mediating a conformational change without disassembling them into small diffusible oligomers or monomers (Bieschke et al., 2010). Subsequently, it was shown that EGCG can robustly disaggregate pre-formed oligomers and dose-dependently inhibit α-synuclein aggregation (Caruana et al., 2011). Another study revealed that EGCG can reduce the ability of oligomers to bind to membranes, in addition to affecting oligomer size distribution or secondary structure, to prevent cytotoxicity (Lorenzen et al., 2014).
Jha and coworkers have shown that high dose EGCG decreased fibrillization kinetics, and concentration-dependently reduced the toxicity of α-synuclein aggregates. EGCG induced nontoxic aggregates to form smaller sized fibrils, indicating that EGCG may decrease α-synuclein aggregate-induced cytotoxicity by its ability to reduce the exposure of a hydrophobic surface (Jha et al., 2017).
Taken together, these studies suggested that EGCG have the poteential to protect against α-synuclein-induced cytotoxicity by modulating the α-synuclein aggregation pathway toward formation of nontoxic aggregates. Moreover, EGCG ameliorates cytotoxicity induced by α-synuclein oligomers, possibly by reducing the extent of toxic aggregate-induced cell membrane permeabilization.
Future prospective and challenges
There are still some challenges on EGCG new drug development regarding to PD. The first challenge is that the stability of EGCG is poor, the absorption rate is low, the bioavailability of oral EGCG was relatively poor needs to be improved (Dai et al., 2019). Another challenge is BBB penetration property of EGCG (Zhang et al., 2020).
However, some authors have suggested new techniques to improve the bioavailability of EGCG, such as nanoparticle-based delivery systems, structurally modified molecules of catechins, or co-administration with other drugs or bioactive compounds (Cai et al., 2018; Gonçalves et al., 2021). Simultaneously, the precise molecular mechanism underlying the action of EGCG is not fully understood. The detailed cell signaling pathway through which EGCG exerts its neuroprotective effects require further investigation. Mechanistic research that can help to define the function of EGCG could provide further benefits for human health. To date, reliable clinical data describing the neuroprotective effects of EGCG for the treatment of PD are lacking. However, the beneficial effect of EGCG in PD still needs to be confirmed in larger animals or even in humans before they are applied in clinical settings. Hence, these aspects of EGCG need to be studied in future, and clinical trials on its efficacy and safety should be performed. EGCG remains a potential and promising therapeutic strategy in the battle against PD.
Conclusion
In conclusion, we here summarized the neuroprotective roles of EGCG shown in both in vitro and in vivo PD models. The studies summarized in this review clearly revealed that EGCG may have the potential to be a novel drug for the treatment of PD, to prevent neurodegeneration due to its multi-targeted actions. The published research suggests that the molecular mechanisms by which EGCG exerts neuroprotective benefits include inhibition of apoptosis, oxidative stress, inflammation, and ferroptosis, modulation of dopamine production, and the aggregation of α-synuclein (Figure 4).
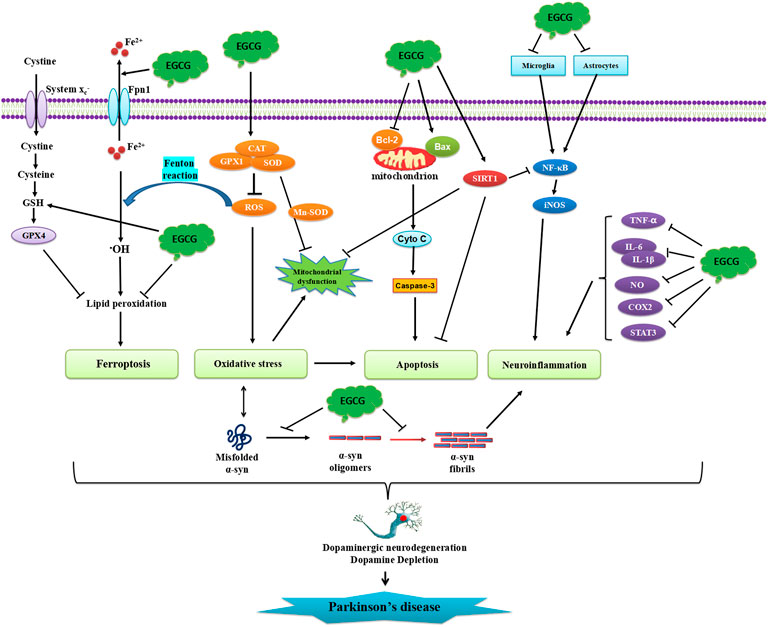
FIGURE 4. Schematic illustration of neuroprotective effects of EGCG in PD. EGCG can attenuate α-synuclein aggregation, oligomerization, and fibrillation. EGCG can also inhibit protein misfolding, oxidative stress, neuronal apoptosis, and neuroinflammatory responses.
Author contributions
YW, SW and HW conceived of and designed the study. YW and HW provided administrative support. All authors analysed and interpreted the data. YW, SW, and HW wrote the manuscript. All authors read and approved the final manuscript.
Funding
This work was supported in part by the National Natural Science Foundation of China (61971011, 81260196, 81450036), Natural Science Foundation of Inner Mongolia Autonomous Region (IMAR) (2021MS08131, 2020MS08175, 2021LHMS08024, 2022MS08046), Science Foundation of AMHT (2020YK02, 2021YK05), Science Foundation of CASIC (2020-LCYL-009), Science Foundation of ASCH (YN202104), Hygiene and Health Development Scientific Research Fostering Plan of Haidian District Beijing (HP 2021-19-50701), and Science Foundation of Universities of IMAR (NJZY19218).
Conflict of interest
The authors declare that the research was conducted in the absence of any commercial or financial relationships that could be construed as a potential conflict of interest.
Publisher’s note
All claims expressed in this article are solely those of the authors and do not necessarily represent those of their affiliated organizations, or those of the publisher, the editors and the reviewers. Any product that may be evaluated in this article, or claim that may be made by its manufacturer, is not guaranteed or endorsed by the publisher.
References
Aarsland, D., Creese, B., Politis, M., Chaudhuri, K. R., Ffytche, D. H., Weintraub, D., et al. (2017). Cognitive decline in Parkinson disease. Nat. Rev. Neurol. 13 (4), 217–231. doi:10.1038/nrneurol.2017.27
Abeliovich, A., and Flint, B. M. (2006). Parkinsonism genes: Culprits and clues. J. Neurochem. 99 (4), 1062–1072. doi:10.1111/j.1471-4159.2006.04102.x
Al-Amri, J. S., Hagras, M. M., and Mohamed, I. M. (2013). Effect of epigallocatechin-3-gallate on inflammatory mediators release in LPS-induced Parkinson's disease in rats. Indian J. Exp. Biol. 51 (5), 357–362.
Alam, M., Ali, S., Ashraf, G. M., Bilgrami, A. L., Yadav, D. K., and Hassan, M. I. (2022). Epigallocatechin 3-gallate: From green tea to cancer therapeutics. Food Chem. 379, 132135. doi:10.1016/j.foodchem.2022.132135
Angelova, P. R., Choi, M. L., Berezhnov, A. V., Horrocks, M. H., Hughes, C. D., De, S., et al. (2020). Alpha synuclein aggregation drives ferroptosis: An interplay of iron, calcium and lipid peroxidation. Cell Death Differ. 27 (10), 2781–2796. doi:10.1038/s41418-020-0542-z
Badanjak, K., Fixemer, S., Smajić, S., Skupin, A., and Grünewald, A. (2021). The contribution of microglia to neuroinflammation in Parkinson's disease. Int. J. Mol. Sci. 22 (9), 4676. doi:10.3390/ijms22094676
Balakrishnan, R., Azam, S., Cho, D. Y., Su-Kim, I., and Choi, D. K. (2021). Natural phytochemicals as novel therapeutic strategies to prevent and treat Parkinson's disease: Current knowledge and future perspectives. Oxid. Med. Cell. Longev. 2021, 6680935. doi:10.1155/2021/6680935
Battino, M., Littarru, G. P., Gorini, A., and Villa, R. F. (1996). Coenzyme Q, peroxidation and cytochrome oxidase features after Parkinson's-like disease by MPTP toxicity in intra-synaptic and non-synaptic mitochondria from Macaca fascicularis cerebral cortex and hippocampus: Action of dihydroergocriptine. Neurochem. Res. 21 (12), 1505–1514. doi:10.1007/BF02533098
Bieschke, J., Russ, J., Friedrich, R. P., Ehrnhoefer, D. E., Wobst, H., Neugebauer, K., et al. (2010). EGCG remodels mature alpha-synuclein and amyloid-beta fibrils and reduces cellular toxicity. Proc. Natl. Acad. Sci. U. S. A. 107 (17), 7710–7715. doi:10.1073/pnas.0910723107
Bitu Pinto, N., da Silva Alexandre, B., Neves, K. R., Silva, A. H., Leal, L. K., and Viana, G. S. (2015). Neuroprotective properties of the standardized extract from camellia sinensis (green tea) and its main bioactive components, epicatechin and epigallocatechin gallate, in the 6-OHDA model of Parkinson's disease. Evid. Based. Complement. Altern. Med. 2015, 161092. doi:10.1155/2015/161092
Bonilla-Ramirez, L., Jimenez-Del-Rio, M., and Velez-Pardo, C. (2013). Low doses of paraquat and polyphenols prolong life span and locomotor activity in knock-down parkin Drosophila melanogaster exposed to oxidative stress stimuli: Implication in autosomal recessive juvenile parkinsonism. Gene 512 (2), 355–363. doi:10.1016/j.gene.2012.09.120
Buendia, I., Michalska, P., Navarro, E., Gameiro, I., Egea, J., and León, R. (2016). Nrf2-ARE pathway: An emerging target against oxidative stress and neuroinflammation in neurodegenerative diseases. Pharmacol. Ther. 157, 84–104. doi:10.1016/j.pharmthera.2015.11.003
Cai, Z. Y., Li, X. M., Liang, J. P., Xiang, L. P., Wang, K. R., Shi, Y. L., et al. (2018). Bioavailability of tea catechins and its improvement. Molecules 23, E2346. doi:10.3390/molecules23092346
Cao, J., Chen, X., Jiang, L., Lu, B., Yuan, M., Zhu, D., et al. (2020). DJ-1 suppresses ferroptosis through preserving the activity of S-adenosyl homocysteine hydrolase. Nat. Commun. 11 (1), 1251. doi:10.1038/s41467-020-15109-y
Caruana, M., Högen, T., Levin, J., Hillmer, A., Giese, A., and Vassallo, N. (2011). Inhibition and disaggregation of α-synuclein oligomers by natural polyphenolic compounds. FEBS Lett. 585 (8), 1113–1120. doi:10.1016/j.febslet.2011.03.046
Chang, K. H., and Chen, C. M. (2020). The role of oxidative stress in Parkinson's disease. Antioxidants (Basel) 9 (7), E597. doi:10.3390/antiox9070597
Chao, J., Lau, W. K., Huie, M. J., Ho, Y. S., Yu, M. S., Lai, C. S. W., et al. (2010). A pro-drug of the green tea polyphenol (-)-epigallocatechin-3-gallate (EGCG) prevents differentiated SH-SY5Y cells from toxicity induced by 6-hydroxydopamine. Neurosci. Lett. 469 (3), 360–364. doi:10.1016/j.neulet.2009.12.028
Chen, D., Kanthasamy, A. G., and Reddy, M. B. (2015). EGCG protects against 6-OHDA-induced neurotoxicity in a cell culture model. Park. Dis. 2015, 843906. doi:10.1155/2015/843906
Chen, X., Kang, R., Kroemer, G., and Tang, D. (2021). Ferroptosis in infection, inflammation, and immunity. J. Exp. Med. 218 (6), e20210518. doi:10.1084/jem.20210518
Cheng, C. Y., Barro, L., Tsai, S. T., Feng, T. W., Wu, X. Y., Chao, C. W., et al. (2021). Epigallocatechin-3-Gallate-Loaded liposomes favor anti-inflammation of microglia cells and promote neuroprotection. Int. J. Mol. Sci. 22 (6), 3037. doi:10.3390/ijms22063037
Choi, J. Y., Park, C. S., Kim, D. J., Cho, M. H., Jin, B. K., Pie, J. E., et al. (2002). Prevention of nitric oxide-mediated 1-methyl-4-phenyl-1, 2, 3, 6-tetrahydropyridine-induced Parkinson's disease in mice by tea phenolic epigallocatechin 3-gallate. Neurotoxicology 23 (3), 367–374. doi:10.1016/s0161-813x(02)00079-7
Cookson, M. R. (2005). The biochemistry of Parkinson's disease. Annu. Rev. Biochem. 74, 29–52. doi:10.1146/annurev.biochem.74.082803.133400
Dai, W., Ruan, C., Zhang, Y., Wang, J., Han, J., Shao, Z., et al. (2019). Bioavailability enhancement of EGCG by structural modification and nano-delivery: A review. J. Funct. FOODS 65 (C), 103732. doi:10.1016/j.jff.2019.103732
de Farias, C. C., Maes, M., Bonifácio, K. L., Bortolasci, C. C., de Souza Nogueira, A., Brinholi, F. F., et al. (2016). Highly specific changes in antioxidant levels and lipid peroxidation in Parkinson's disease and its progression: Disease and staging biomarkers and new drug targets. Neurosci. Lett. 617, 66–71. doi:10.1016/j.neulet.2016.02.011
Dexter, D., Carter, C., Agid, F., Agid, Y., Lees, A. J., Jenner, P., et al. (1986). Lipid peroxidation as cause of nigral cell death in Parkinson's disease. Lancet 2 (8507), 639–640. doi:10.1016/s0140-6736(86)92471-2
Dexter, D. T., Wells, F. R., Agid, F., Agid, Y., Lees, A. J., Jenner, P., et al. (1987). Increased nigral iron content in postmortem parkinsonian brain. Lancet 2 (8569), 1219–1220. doi:10.1016/s0140-6736(87)91361-4
Dionísio, P. A., Amaral, J. D., and Rodrigues, C. (2021). Oxidative stress and regulated cell death in Parkinson's disease. Ageing Res. Rev. 67, 101263. doi:10.1016/j.arr.2021.101263
Dorszewska, J., Kowalska, M., Prendecki, M., Piekut, T., Kozłowska, J., and Kozubski, W. (2021). Oxidative stress factors in Parkinson's disease. Neural Regen. Res. 16 (7), 1383–1391. doi:10.4103/1673-5374.300980
Dos Santos, M. G., Schimith, L. E., André-Miral, C., Muccillo-Baisch, A. L., Arbo, B. D., and Hort, M. A. (2022). Neuroprotective effects of resveratrol in in vivo and in vitro experimental models of Parkinson's disease: A systematic review. Neurotox. Res. 40 (1), 319–345. doi:10.1007/s12640-021-00450-x
Fahn, S., and Cohen, G. (1992). The oxidant stress hypothesis in Parkinson's disease: Evidence supporting it. Ann. Neurol. 32 (6), 804–812. doi:10.1002/ana.410320616
Fernandes, L., Cardim-Pires, T. R., Foguel, D., and Palhano, F. L. (2021). Green tea polyphenol epigallocatechin-gallate in amyloid aggregation and neurodegenerative diseases. Front. Neurosci. 15, 718188. doi:10.3389/fnins.2021.718188
Fernandes, L., Messias, B., Pereira-Neves, A., Azevedo, E. P., Araujo, J., Foguel, D., et al. (2020). Green tea polyphenol microparticles based on the oxidative coupling of EGCG inhibit amyloid aggregation/cytotoxicity and serve as a platform for drug delivery. ACS Biomater. Sci. Eng. 6 (8), 4414–4423. doi:10.1021/acsbiomaterials.0c00188
Ferrazzoli, D., Carter, A., Ustun, F. S., Palamara, G., Ortelli, P., Maestri, R., et al. (2016). Dopamine replacement therapy, learning and reward prediction in Parkinson's disease: Implications for rehabilitation. Front. Behav. Neurosci. 10, 121. doi:10.3389/fnbeh.2016.00121
Gadhe, L., Sakunthala, A., Mukherjee, S., Gahlot, N., Bera, R., Sawner, A. S., et al. (2022). Intermediates of α-synuclein aggregation: Implications in Parkinson's disease pathogenesis. Biophys. Chem. 281, 106736. doi:10.1016/j.bpc.2021.106736
Ghiglieri, V., Calabrese, V., and Calabresi, P. (2018). Alpha-Synuclein: From early synaptic dysfunction to neurodegeneration. Front. Neurol. 9, 295. doi:10.3389/fneur.2018.00295
Gonçalves, P. B., Sodero, A., and Cordeiro, Y. (2021). Green tea epigallocatechin-3-gallate (EGCG) targeting protein misfolding in drug discovery for neurodegenerative diseases. Biomolecules 11 (5), 767. doi:10.3390/biom11050767
Haque, M. E., Akther, M., Azam, S., Kim, I. S., Lin, Y., Lee, Y. H., et al. (2022). Targeting α-synuclein aggregation and its role in mitochondrial dysfunction in Parkinson's disease. Br. J. Pharmacol. 179 (1), 23–45. doi:10.1111/bph.15684
Hassanzadeh, K., and Rahimmi, A. (2018). Oxidative stress and neuroinflammation in the story of Parkinson's disease: Could targeting these pathways write a good ending? J. Cell. Physiol. 234 (1), 23–32. doi:10.1002/jcp.26865
Hirsch, E. C., and Standaert, D. G. (2021). Ten unsolved questions about neuroinflammation in Parkinson's disease. Mov. Disord. 36 (1), 16–24. doi:10.1002/mds.28075
Hirsch, L., Jette, N., Frolkis, A., Steeves, T., and Pringsheim, T. (2016). The incidence of Parkinson's disease: A systematic review and meta-analysis. Neuroepidemiology 46 (4), 292–300. doi:10.1159/000445751
Hou, R. R., Chen, J. Z., Chen, H., Kang, X. G., Li, M. G., and Wang, B. R. (2008). Neuroprotective effects of (-)-epigallocatechin-3-gallate (EGCG) on paraquat-induced apoptosis in PC12 cells. Cell Biol. Int. 32 (1), 22–30. doi:10.1016/j.cellbi.2007.08.007
Javed, H., Nagoor Meeran, M. F., Azimullah, S., Adem, A., Sadek, B., and Ojha, S. K. (2018). Plant extracts and phytochemicals targeting α-synuclein aggregation in Parkinson's disease models. Front. Pharmacol. 9, 1555. doi:10.3389/fphar.2018.01555
Jha, N. N., Kumar, R., Panigrahi, R., Navalkar, A., Ghosh, D., Sahay, S., et al. (2017). Comparison of α-synuclein fibril inhibition by four different amyloid inhibitors. ACS Chem. Neurosci. 8 (12), 2722–2733. doi:10.1021/acschemneuro.7b00261
Jin, C. F., Shen, S. R., and Zhao, B. L. (2001). Different effects of five catechins on 6-hydroxydopamine-induced apoptosis in PC12 cells. J. Agric. Food Chem. 49 (12), 6033–6038. doi:10.1021/jf010903r
Kang, K. S., Wen, Y., Yamabe, N., Fukui, M., Bishop, S. C., and Zhu, B. T. (2010). Dual beneficial effects of (-)-epigallocatechin-3-gallate on levodopa methylation and hippocampal neurodegeneration: In vitro and in vivo studies. PLoS One 5 (8), e11951. doi:10.1371/journal.pone.0011951
Kim, J. S., Kim, J. M., Jeong-Ja, O., and Jeon, B. S. (2010). Inhibition of inducible nitric oxide synthase expression and cell death by (-)-epigallocatechin-3-gallate, a green tea catechin, in the 1-methyl-4-phenyl-1, 2, 3, 6-tetrahydropyridine mouse model of Parkinson's disease. J. Clin. Neurosci. 17 (9), 1165–1168. doi:10.1016/j.jocn.2010.01.042
Kip, E., and Parr-Brownlie, L. C. (2022). Reducing neuroinflammation via therapeutic compounds and lifestyle to prevent or delay progression of Parkinson's disease. Ageing Res. Rev. 78, 101618. doi:10.1016/j.arr.2022.101618
Kline, E. M., Houser, M. C., Herrick, M. K., Seibler, P., Klein, C., West, A., et al. (2021). Genetic and environmental factors in Parkinson's disease converge on immune function and inflammation. Mov. Disord. 36 (1), 25–36. doi:10.1002/mds.28411
Ko, C. J., Gao, S. L., Lin, T. K., Chu, P. Y., and Lin, H. Y. (2021). Ferroptosis as a major factor and therapeutic target for neuroinflammation in Parkinson's disease. Biomedicines 9 (11), 1679. doi:10.3390/biomedicines9111679
Kujawska, M., and Jodynis-Liebert, J. (2018). Polyphenols in Parkinson's disease: A systematic review of in vivo studies. Nutrients 10 (5), E642. doi:10.3390/nu10050642
Kuo, Y. C., Wang, I. H., and Rajesh, R. (2021). Use of leptin-conjugated phosphatidic acid liposomes with resveratrol and epigallocatechin gallate to protect dopaminergic neurons against apoptosis for Parkinson's disease therapy. Acta Biomater. 119, 360–374. doi:10.1016/j.actbio.2020.11.015
Latif, S., Jahangeer, M., Maknoon Razia, D., Ashiq, M., Ghaffar, A., Akram, M., et al. (2021). Dopamine in Parkinson's disease. Clin. Chim. Acta. 522, 114–126. doi:10.1016/j.cca.2021.08.009
Lee, J. H., Kim, H. J., Kim, J. U., Yook, T. H., Kim, K. H., Lee, J. Y., et al. (2021). A novel treatment strategy by natural products in NLRP3 inflammasome-mediated neuroinflammation in alzheimer's and Parkinson's disease. Int. J. Mol. Sci. 22 (3), 1324. doi:10.3390/ijms22031324
Lee, M. Y., Choi, E. J., Lee, M. K., and Lee, J. J. (2013). Epigallocatechin gallate attenuates L-DOPA-induced apoptosis in rat PC12 cells. Nutr. Res. Pract. 7 (4), 249–255. doi:10.4162/nrp.2013.7.4.249
Lee, Y., Lee, S., Chang, S. C., and Lee, J. (2019). Significant roles of neuroinflammation in Parkinson's disease: Therapeutic targets for PD prevention. Arch. Pharm. Res. 42 (5), 416–425. doi:10.1007/s12272-019-01133-0
Lev, N., Melamed, E., and Offen, D. (2003). Apoptosis and Parkinson's disease. Prog. Neuropsychopharmacol. Biol. Psychiatry 27, 245–250. doi:10.1016/S0278-5846(03)00019-8
Levites, Y., Amit, T., Youdim, M. B., and Mandel, S. (2002). Involvement of protein kinase C activation and cell survival/ cell cycle genes in green tea polyphenol (-)-epigallocatechin 3-gallate neuroprotective action. J. Biol. Chem. 277 (34), 30574–30580. doi:10.1074/jbc.M202832200
Li, R., Huang, Y. G., Fang, D., and Le, W. D. (2004). (-)-Epigallocatechin gallate inhibits lipopolysaccharide-induced microglial activation and protects against inflammation-mediated dopaminergic neuronal injury. J. Neurosci. Res. 78 (5), 723–731. doi:10.1002/jnr.20315
Liu, T. W., Chen, C. M., and Chang, K. H. (2022). Biomarker of neuroinflammation in Parkinson's disease. Int. J. Mol. Sci. 23 (8), 4148. doi:10.3390/ijms23084148
Lorenzen, N., Nielsen, S. B., Yoshimura, Y., Vad, B. S., Andersen, C. B., Betzer, C., et al. (2014). How epigallocatechin gallate can inhibit α-synuclein oligomer toxicity in vitro. J. Biol. Chem. 289 (31), 21299–21310. doi:10.1074/jbc.M114.554667
Ma, C., Pan, Y., Yang, Z., Meng, Z., Sun, R., Wang, T., et al. (2016). Pre-administration of BAX-inhibiting peptides decrease the loss of the nigral dopaminergic neurons in rats. Life Sci. 144, 113–120. doi:10.1016/j.lfs.2015.11.019
Ma, L., Cao, T. T., Kandpal, G., Warren, L., Fred Hess, J., Seabrook, G. R., et al. (2010). Genome-wide microarray analysis of the differential neuroprotective effects of antioxidants in neuroblastoma cells overexpressing the familial Parkinson's disease alpha-synuclein A53T mutation. Neurochem. Res. 35 (1), 130–142. doi:10.1007/s11064-009-0038-1
Mahoney-Sánchez, L., Bouchaoui, H., Ayton, S., Devos, D., Duce, J. A., and Devedjian, J. C. (2021). Ferroptosis and its potential role in the physiopathology of Parkinson's Disease. Prog. Neurobiol. 196, 101890. doi:10.1016/j.pneurobio.2020.101890
Martinez-Perez, D. A., Jimenez-Del-Rio, M., and Velez-Pardo, C. (2018). Epigallocatechin-3-Gallate protects and prevents paraquat-induced oxidative stress and neurodegeneration in knockdown dj-1-β Drosophila melanogaster. Neurotox. Res. 34 (3), 401–416. doi:10.1007/s12640-018-9899-x
Masato, A., Plotegher, N., Boassa, D., and Bubacco, L. (2019). Impaired dopamine metabolism in Parkinson's disease pathogenesis. Mol. Neurodegener. 14 (1), 35. doi:10.1186/s13024-019-0332-6
McGeer, P. L., Itagaki, S., Boyes, B. E., and McGeer, E. G. (1988). Reactive microglia are positive for HLA-DR in the substantia nigra of Parkinson's and Alzheimer's disease brains. Neurology 38 (8), 1285–1291. doi:10.1212/wnl.38.8.1285
Meder, D., Herz, D. M., Rowe, J. B., Lehéricy, S., and Siebner, H. R. (2019). The role of dopamine in the brain - lessons learned from Parkinson's disease. Neuroimage 190, 79–93. doi:10.1016/j.neuroimage.2018.11.021
Mischley, L. K., Allen, J., and Bradley, R. (2012). Coenzyme Q10 deficiency in patients with Parkinson's disease. J. Neurol. Sci. 318 (1-2), 72–75. doi:10.1016/j.jns.2012.03.023
Ng, C. H., Guan, M. S., Koh, C., Ouyang, X., Yu, F., Tan, E. K., et al. (2012). AMP kinase activation mitigates dopaminergic dysfunction and mitochondrial abnormalities in Drosophila models of Parkinson's disease. J. Neurosci. 32 (41), 14311–14317. doi:10.1523/JNEUROSCI.0499-12.2012
Nie, G., Cao, Y., and Zhao, B. (2002a). Protective effects of green tea polyphenols and their major component, (-)-epigallocatechin-3-gallate (EGCG), on 6-hydroxydopamine-induced apoptosis in PC12 cells. Redox Rep. 7 (3), 171–177. doi:10.1179/135100002125000424
Nie, G., Jin, C., Cao, Y., Shen, S., and Zhao, B. (2002b). Distinct effects of tea catechins on 6-hydroxydopamine-induced apoptosis in PC12 cells. Arch. Biochem. Biophys. 397 (1), 84–90. doi:10.1006/abbi.2001.2636
Özduran, G., Becer, E., Vatansever, H. S., and Yücecan, S. (2022). Neuroprotective effects of catechins in an experimental Parkinson's disease model and SK-N-as cells: Evaluation of cell viability, anti-inflammatory and anti-apoptotic effects. Neurol. Res. 44 (6), 511–523. doi:10.1080/01616412.2021.2024715
Pajares, M., I Rojo, A., Manda, G., Boscá, L., and Cuadrado, A. (2020). Inflammation in Parkinson's disease: Mechanisms and therapeutic implications. Cells 9 (7), E1687. doi:10.3390/cells9071687
Park, J. S., Davis, R. L., and Sue, C. M. (2018). Mitochondrial dysfunction in Parkinson's disease: New mechanistic insights and therapeutic perspectives. Curr. Neurol. Neurosci. Rep. 18 (5), 21. doi:10.1007/s11910-018-0829-3
Payne, A., Nahashon, S., Taka, E., Adinew, G. M., and Soliman, K. (2022). Epigallocatechin-3-Gallate (EGCG): New therapeutic perspectives for neuroprotection, aging, and neuroinflammation for the modern age. Biomolecules 12 (3), 371. doi:10.3390/biom12030371
Peluso, I., and Serafini, M. (2017). Antioxidants from black and green tea: From dietary modulation of oxidative stress to pharmacological mechanisms. Br. J. Pharmacol. 174 (11), 1195–1208. doi:10.1111/bph.13649
Percário, S., da Silva Barbosa, A., Varela, E., Gomes, A. R. Q., Ferreira, M. E. S., de Nazare Araujo Moreira, T., et al. (2020). Oxidative stress in Parkinson's disease: Potential benefits of antioxidant supplementation. Oxid. Med. Cell. Longev. 2020, 2360872. doi:10.1155/2020/2360872
Pires, F., Geraldo, V., Antunes, A., Marletta, A., Oliveira, O. N., and Raposo, M. (2019a). Effect of blue light irradiation on the stability of phospholipid molecules in the presence of epigallocatechin-3-gallate. Colloids Surf. B Biointerfaces 177, 50–57. doi:10.1016/j.colsurfb.2019.01.042
Pires, F., Geraldo, V., Antunes, A., Marletta, A., Oliveira, O. N., and Raposo, M. (2019b). On the role of epigallocatechin-3-gallate in protecting phospholipid molecules against UV irradiation. Colloids Surf. B Biointerfaces 173, 312–319. doi:10.1016/j.colsurfb.2018.09.065
Pires, F., Geraldo, V., Rodrigues, B., Granada-Flor, A., de Almeida, R., Oliveira, O. N., et al. (2019c). Evaluation of EGCG loading capacity in DMPC membranes. LANGMUIR 35, 6771–6781. doi:10.1021/acs.langmuir.9b00372
Pires, F., Magalhães-Mota, G., Geraldo, V., Ribeiro, P. A., Oliveira, O. N., and Raposo, M. (2020). The impact of blue light in monolayers representing tumorigenic and nontumorigenic cell membranes containing epigallocatechin-3-gallate. Colloids Surf. B Biointerfaces 193, 111129. doi:10.1016/j.colsurfb.2020.111129
Pires, F., Santos, J. F., Bitoque, D., Silva, G. A., Marletta, A., Nunes, V. A., et al. (2019d). Polycaprolactone/gelatin nanofiber membranes containing EGCG-loaded liposomes and their potential use for skin regeneration. ACS Appl. Bio Mat. 2, 4790–4800. doi:10.1021/acsabm.9b00524
Postuma, R. B., Berg, D., Stern, M., Poewe, W., Olanow, C. W., Oertel, W., et al. (2015). MDS clinical diagnostic criteria for Parkinson's disease. Mov. Disord. 30, 1591–1601. doi:10.1002/mds.26424
Roy, S., and Bhat, R. (2019). Suppression, disaggregation, and modulation of γ-Synuclein fibrillation pathway by green tea polyphenol EGCG. Protein Sci. 28 (2), 382–402. doi:10.1002/pro.3549
Savica, R., Grossardt, B. R., Bower, J. H., Ahlskog, J. E., and Rocca, W. A. (2016). Time trends in the incidence of Parkinson disease. JAMA Neurol. 73 (8), 981–989. doi:10.1001/jamaneurol.2016.0947
Sian, J., Dexter, D. T., Lees, A. J., Daniel, S., Agid, Y., Javoy-AgidF., , et al. (1994). Alterations in glutathione levels in Parkinson's disease and other neurodegenerative disorders affecting basal ganglia. Ann. Neurol. 36 (3), 348–355. doi:10.1002/ana.410360305
Singh, S. K., Dutta, A., and Modi, G. (2017). α-Synuclein aggregation modulation: An emerging approach for the treatment of Parkinson's disease. Future Med. Chem. 9 (10), 1039–1053. doi:10.4155/fmc-2017-0016
Sofic, E., Lange, K. W., Jellinger, K., and Riederer, P. (1992). Reduced and oxidized glutathione in the substantia nigra of patients with Parkinson's disease. Neurosci. Lett. 142 (2), 128–130. doi:10.1016/0304-3940(92)90355-b
Srinivasan, E., Chandrasekhar, G., Chandrasekar, P., Anbarasu, K., Vickram, A. S., Karunakaran, R., et al. (2021). Alpha-synuclein aggregation in Parkinson's disease. Front. Med. 8, 736978. doi:10.3389/fmed.2021.736978
Stockwell, B. R. (2022). Ferroptosis turns 10: Emerging mechanisms, physiological functions, and therapeutic applications. CELL 185, 2401–2421. doi:10.1016/j.cell.2022.06.003
Subramaniam, S. R., and Chesselet, M. F. (2013). Mitochondrial dysfunction and oxidative stress in Parkinson's disease. Prog. Neurobiol. 106-107, 17–32. doi:10.1016/j.pneurobio.2013.04.004
Tai, K. K., and Truong, D. D. (2010). (-)-Epigallocatechin-3-gallate (EGCG), a green tea polyphenol, reduces dichlorodiphenyl-trichloroethane (DDT)-induced cell death in dopaminergic SHSY-5Y cells. Neurosci. Lett. 482 (3), 183–187. doi:10.1016/j.neulet.2010.06.018
Tansey, M. G., and Romero-Ramos, M. (2019). Immune system responses in Parkinson's disease: Early and dynamic. Eur. J. Neurosci. 49 (3), 364–383. doi:10.1111/ejn.14290
Teng, Y., Zhao, J., Ding, L., Ding, Y., and Zhou, P. (2019). Complex of EGCG with Cu(II) suppresses amyloid aggregation and Cu(II)-Induced cytotoxicity of α-synuclein. Molecules 24 (16), E2940. doi:10.3390/molecules24162940
Tseng, H. C., Wang, M. H., Chang, K. C., Soung, H. S., Fang, C. H., Lin, Y. W., et al. (2020). Protective effect of (-)Epigallocatechin-3-gallate on rotenone-induced parkinsonism-like symptoms in rats. Neurotox. Res. 37 (3), 669–682. doi:10.1007/s12640-019-00143-6
Unno, K., Pervin, M., Nakagawa, A., Iguchi, K., Hara, A., Takagaki, A., et al. (2017). Blood-brain barrier permeability of green tea catechin metabolites and their neuritogenic activity in human neuroblastoma SH-SY5Y cells. Mol. Nutr. Food Res. 61 (12), 1700294. doi:10.1002/mnfr.201700294
Vallerga, C. L., Zhang, F., Fowdar, J., McRae, A. F., Qi, T., Nabais, M. F., et al. (2020). Analysis of DNA methylation associates the cystine-glutamate antiporter SLC7A11 with risk of Parkinson's disease. Nat. Commun. 11 (1), 1238. doi:10.1038/s41467-020-15065-7
Vila, M., Jackson-Lewis, V., Vukosavic, S., Djaldetti, R., Liberatore, G., Offen, D., et al. (2001). Bax ablation prevents dopaminergic neurodegeneration in the 1-methyl- 4-phenyl-1, 2, 3, 6-tetrahydropyridine mouse model of Parkinson's disease. Proc. Natl. Acad. Sci. U. S. A. 98 (5), 2837–2842. doi:10.1073/pnas.051633998
Volpicelli-Daley, L. A., Luk, K. C., Patel, T. P., Tanik, S. A., Riddle, D. M., Stieber, A., et al. (2011). Exogenous α-synuclein fibrils induce Lewy body pathology leading to synaptic dysfunction and neuron death. Neuron 72 (1), 57–71. doi:10.1016/j.neuron.2011.08.033
Vuuren, M., Nell, T. A., Carr, J. A., Kell, D. B., and Pretorius, E. (2020). Iron dysregulation and inflammagens related to oral and gut health are central to the development of Parkinson's disease. Biomolecules 11 (1), E30. doi:10.3390/biom11010030
Wang, L., Xu, S., Xu, X., and Chan, P. (2009). (-)-Epigallocatechin-3-Gallate protects SH-SY5Y cells against 6-OHDA-induced cell death through STAT3 activation. J. Alzheimers Dis. 17 (2), 295–304. doi:10.3233/JAD-2009-1048
Wang, Q., Liu, Y., and Zhou, J. (2015). Neuroinflammation in Parkinson's disease and its potential as therapeutic target. Transl. Neurodegener. 4, 19. doi:10.1186/s40035-015-0042-0
Wang, Y., Gao, L., Chen, J., Li, Q., Huo, L., Wang, Y., et al. (2021). Pharmacological modulation of Nrf2/HO-1 signaling pathway as a therapeutic target of Parkinson's disease. Front. Pharmacol. 12, 757161. doi:10.3389/fphar.2021.757161
Wu, L., Liu, M., Liang, J., Li, N., Yang, D., Cai, J., et al. (2021). Ferroptosis as a new mechanism in Parkinson's disease therapy using traditional Chinese medicine. Front. Pharmacol. 12, 659584. doi:10.3389/fphar.2021.659584
Xu, Q., Langley, M., Kanthasamy, A. G., and Reddy, M. B. (2017). Epigallocatechin gallate has a neurorescue effect in a mouse model of Parkinson disease. J. Nutr. 147 (10), 1926–1931. doi:10.3945/jn.117.255034
Xu, Y., Xie, M., Xue, J., Xiang, L., Li, Y., Xiao, J., et al. (2020). EGCG ameliorates neuronal and behavioral defects by remodeling gut microbiota and TotM expression in Drosophila models of Parkinson's disease. FASEB J. 34 (4), 5931–5950. doi:10.1096/fj.201903125RR
Xu, Y., Zhang, Y., Quan, Z., Wong, W., Guo, J., Zhang, R., et al. (2016). Epigallocatechin gallate (EGCG) inhibits alpha-synuclein aggregation: A potential agent for Parkinson's disease. Neurochem. Res. 41 (10), 2788–2796. doi:10.1007/s11064-016-1995-9
Yang, J. E., Rhoo, K. Y., Lee, S., Lee, J. T., Park, J. H., Bhak, G., et al. (2017). EGCG-Mediated protection of the membrane disruption and cytotoxicity caused by the 'active oligomer' of α-synuclein. Sci. Rep. 7 (1), 17945. doi:10.1038/s41598-017-18349-z
Yang, L., Mao, K., Yu, H., and Chen, J. (2020). Neuroinflammatory responses and Parkinson' disease: Pathogenic mechanisms and therapeutic targets. J. Neuroimmune Pharmacol. 15 (4), 830–837. doi:10.1007/s11481-020-09926-7
Yao, Y., Tang, Y., and Wei, G. (2020). Epigallocatechin gallate destabilizes α-synuclein fibril by disrupting the E46-K80 salt-bridge and inter-protofibril interface. ACS Chem. Neurosci. 11 (24), 4351–4361. doi:10.1021/acschemneuro.0c00598
Ye, Q., Ye, L., Xu, X., Huang, B., Zhang, X., Zhu, Y., et al. (2012). Epigallocatechin-3-gallate suppresses 1-methyl-4-phenyl-pyridine-induced oxidative stress in PC12 cells via the SIRT1/PGC-1α signaling pathway. BMC Complement. Altern. Med. 12, 82. doi:10.1186/1472-6882-12-82
Zhang, J., Nie, S., and Wang, S. (2013). Nanoencapsulation enhances epigallocatechin-3-gallate stability and its antiatherogenic bioactivities in macrophages. J. Agric. Food Chem. 61 (38), 9200–9209. doi:10.1021/jf4023004
Zhang, S., Zhu, Q., Chen, J. Y., OuYang, D., and Lu, J. H. (2020). The pharmacological activity of epigallocatechin-3-gallate (EGCG) on alzheimer's disease animal model: A systematic review. Phytomedicine 79, 153316. doi:10.1016/j.phymed.2020.153316
Zhao, J., Xu, L., Liang, Q., Sun, Q., Chen, C., Zhang, Y., et al. (2017). Metal chelator EGCG attenuates Fe(III)-induced conformational transition of α-synuclein and protects AS-PC12 cells against Fe(III)-induced death. J. Neurochem. 143 (1), 136–146. doi:10.1111/jnc.14142
Zheng, J., and Conrad, M. (2020). The metabolic underpinnings of ferroptosis. Cell Metab. 32 (6), 920–937. doi:10.1016/j.cmet.2020.10.011
Zhou, T., Zhu, M., and Liang, Z. (2018). (-)-Epigallocatechin-3-gallate modulates peripheral immunity in the MPTP-induced mouse model of Parkinson's disease. Mol. Med. Rep. 17 (4), 4883–4888. doi:10.3892/mmr.2018.8470
Zoey, F. L., Palanivel, M., Padmanabhan, P., and Gulyás, B. (2021). Parkinson's disease: A nanotheranostic approach targeting alpha-synuclein aggregation. Front. Cell Dev. Biol. 9, 707441. doi:10.3389/fcell.2021.707441
Keywords: Parkinson’s disease, epigallocatechin 3-gallate, oxidative stress, apoptosis, neuroinflammation, α-synuclein
Citation: Wang Y, Wu S, Li Q, Lang W, Li W, Jiang X, Wan Z, Chen J and Wang H (2022) Epigallocatechin-3-gallate: A phytochemical as a promising drug candidate for the treatment of Parkinson’s disease. Front. Pharmacol. 13:977521. doi: 10.3389/fphar.2022.977521
Received: 24 June 2022; Accepted: 01 August 2022;
Published: 12 September 2022.
Edited by:
Matilde Otero-Losada, Consejo Nacional de Investigaciones Científicas y Técnicas. CAECIHS.UAI-CONICET, ArgentinaReviewed by:
Maria Raposo, New University of Lisbon, PortugalNeha Atulkumar Singh, Mayo Clinic, United States
Copyright © 2022 Wang, Wu, Li, Lang, Li, Jiang, Wan, Chen and Wang. This is an open-access article distributed under the terms of the Creative Commons Attribution License (CC BY). The use, distribution or reproduction in other forums is permitted, provided the original author(s) and the copyright owner(s) are credited and that the original publication in this journal is cited, in accordance with accepted academic practice. No use, distribution or reproduction is permitted which does not comply with these terms.
*Correspondence: Jichao Chen, Y2hlbl9odHp4eXlAc2luYS5jb20=; Hongquan Wang, d2hvbmdxdWFuQGFsaXl1bi5jb20=
†ORCID: Hongquan Wang, orcid.org/0000-0002-5447-2017
‡These authors have contributed equally to this work