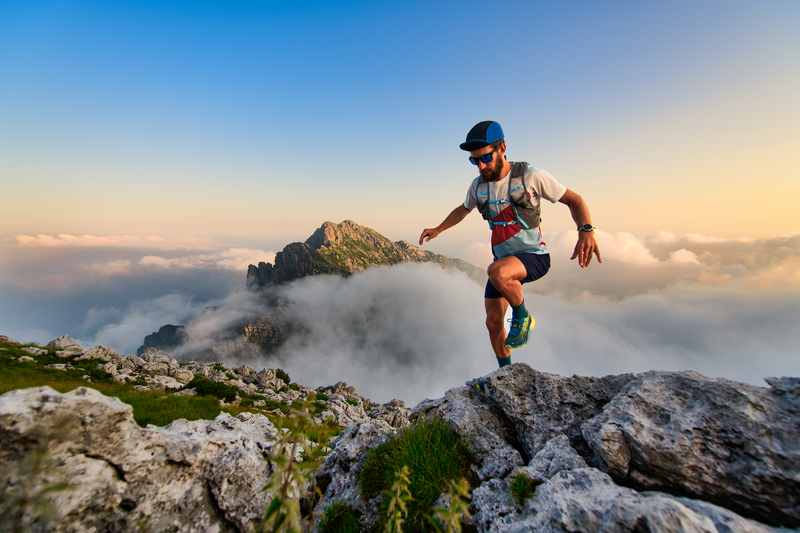
95% of researchers rate our articles as excellent or good
Learn more about the work of our research integrity team to safeguard the quality of each article we publish.
Find out more
REVIEW article
Front. Pharmacol. , 25 August 2022
Sec. Renal Pharmacology
Volume 13 - 2022 | https://doi.org/10.3389/fphar.2022.977410
Diabetic kidney disease (DKD) is one of the major public health problems in society today. It is a renal complication caused by diabetes mellitus with predominantly microangiopathy and is a major cause of end-stage renal disease (ESRD). Autophagy is a metabolic pathway for the intracellular degradation of cytoplasmic products and damaged organelles and plays a vital role in maintaining homeostasis and function of the renal cells. The dysregulation of autophagy in the hyperglycaemic state of diabetes mellitus can lead to the progression of DKD, and the activation or restoration of autophagy through drugs is beneficial to the recovery of renal function. This review summarizes the physiological process of autophagy, illustrates the close link between DKD and autophagy, and discusses the effects of drugs on autophagy and the signaling pathways involved from the perspective of podocytes, renal tubular epithelial cells, and mesangial cells, in the hope that this will be useful for clinical treatment.
Diabetes mellitus (DM) is a common and prevalent disease in recent years, characterized by chronic hyperglycaemia (Davidson et al., 2015). Diabetic kidney disease (DKD) is a serious microvascular complication of DM, which may present with a glomerular hyperfiltration state and microalbuminuria in the early stages, with disease progression to oedema and massive proteinuria and severe impairment of renal function (Reidy et al., 2014). According to reports, the number of people with DM will increase to 592 million by 2035 and about 35–40% of them will eventually progress to DKD, which is a major cause of end-stage renal disease (ESRD) and brings heavy psychological and financial burden to families and society (Cao and Cooper, 2011; Shi and Hu, 2014). Traditional treatments have focused on smoking cessation, diet control, weight loss, low protein diets, and lowering blood pressure and glucose and lipids to cope with the range of pathological changes caused by hyperglycemia (Lin et al., 2018). These methods provide some relief from DKD, but they cannot stop the progression of DKD to chronic kidney disease (McGrath and Edi, 2019). Therefore, new treatments for DKD are an urgent problem to be solved.
Autophagy is a conserved intracellular pathway that maintains cellular homeostasis by forming autolysosomes to degrade cytoplasmic components (Kitada and Koya, 2021). The term “autophagy” was first coined by Christian de Duve in 1967 as “self-eating” (Deter and De Duve, 1967). The degradation of mitochondria and other cellular components following glucagon stimulation in rats was observed and it was suggested that the autophagic process requires induction and activation of cyclic adenosine monophosphate (AMP) (Deter and De Duve, 1967). In recent years, autophagy has been intensively studied by many researchers and is considered to be a strictly cellular physiological regulatory process widely present in mammalian cells (Klionsky et al., 2021). And the molecular mechanisms associated with autophagy have been studied and discovered in yeast (Takeshige et al., 1992; Suzuki et al., 2001; Kim et al., 2002).
A growing number of studies have shown that the development of DKD is associated with a defect in autophagy in kidney (Deretic, 2021). Restoring autophagy can improve renal function, reduce the occurrence of oxidative stress, inhibit renal inflammation and fibrosis, and slow the progression of DKD, making it to be another important target for the treatment of DKD (Yang et al., 2018). But the literatures on the relationship between drugs and autophagy in DKD is currently poorly organized. Therefore, this review will discuss the important role of autophagy in DKD in terms of the two processes of autophagosome formation and autolysosome formation, in the hope of laying a theoretical foundation for the clinical treatment of DKD.
Three types of autophagy exist in mammalian cells: microautophagy, macroautophagy and chaperone-mediated autophagy (Li and Nakatogawa, 2022). Microautophagy is when proteins become trapped in vesicles formed by lysosomes and are subsequently degraded in the vacuolar lumen of lysosomes (Li and Nakatogawa, 2022). Chaperone-mediated autophagy is a highly specific form of protein catabolism mediated by the heat shock cognate 70 (Hsc70), which targets the lysosomal membrane directly into the lumen of the lysosome (Cuervo and Wong, 2014). In addition, mitophagy is a specific type of autophagy with a high degree of selectivity in its classification, which responds to organelle damage and pathogen invasion, and remove mitochondrial toxicity products (Bravo-San Pedro et al., 2017). This review focuses on the role of macroautophagy (hereafter referred to as autophagy) in DKD.
Autophagy is a highly dynamic process that includes several stages of initiation, extension, closure and degradation (Antonioli et al., 2017). Autophagy is primarily regulated by autophagy-associated proteins (ATG), which are encoded and modified by a set of highly conserved ATG genes (Levine and Kroemer, 2019). The mammalian target of rapamycin (mTOR) and AMP-activated kinase (AMPK) are two key molecules in the process of autophagy (Inoki et al., 2012). AMPK acts as an energy receptor and is activated when the AMP/ATP ratio increases in the absence of intracellular energy, while mTOR negatively regulates the process of AMPK activation and inhibits the onset of autophagy (Li and Chen, 2019). mTOR mainly works in two multi-protein complexes: mTORC1 and mTORC2 (Fantus et al., 2016). mTOR inhibits the activation of autophagy and promotes protein and nucleotide synthesis to facilitate cell growth, proliferation and differentiation, and also acts as an amino acid receptor (Kim and Guan, 2019). When cells are stimulated by amino acid signals, mTORC1 phosphorylates the downstream ribosomal protein S6 kinase (S6K) and the eukaryotic translation initiation factor 4E-binding protein 1 (4E-BP1) to promote protein synthesis (Yuan J. et al., 2020).
In the regulation of autophagy, AMPK is regulated by upstream calcium/calmodulin-dependent protein kinase 2 (CAMKK2, also called CAMKKβ) and serine/threonine-protein kinase stk11 (LKB1), while suppressing the expression of mTORC1 (Shaw et al., 2004; Hawley et al., 2005). Subsequently, AMPK phosphorylates the downstream Unc-51-like (ULK)1/2 complex (Alers et al., 2012). In mammals, ATG101 is an important component of the ULK1 complex and these components that make up ULK1 are responsible for inducing conformational changes to activate the ULK1 complex (Mercer et al., 2009; Lin and Hurley, 2016). The activation of ULK1 phosphorylates the downstream class III phosphatidylinositol three kinase (PI3K) complex (also known as VPS34 complex I), whose main target is Beclin-1. The complex is composed of the core protein PI3K, the autophagy-specific cofactor Beclin-1 and other important factors (Russell et al., 2013; Baskaran et al., 2014; Levine et al., 2015). Subsequently, the PI3K complex promotes the production of phosphatidylinositol-3-phosphate (PI3P), which recruits autophagy factors required for autophagosome formation and promotes the formation of omegasomes (omegasomes are derived from the part of the endoplasmic reticulum (ER) that is connected to other organelles) (Axe et al., 2008; Hayashi-Nishino et al., 2009; Lamb et al., 2013). In mammals, the isolation membrane is supported by ER and may be connected by membrane tubules (Hayashi-Nishino et al., 2009; Ylä-Anttila et al., 2009). The simultaneous recruitment of the effect factors double FYVE zinc finger domain containing protein 1 (DCFP1) and WD repeat domain phosphoinositide-interacting protein (WIPI) contribute to the continuous extension of the phagophore (Dooley et al., 2014; Rogov et al., 2014). ATG2 and the transmembrane protein ATG9 are thought to be autophagosomal intermembrane and interfollicular lipid transport proteins, involved in the expansion of the phagophore (Noda, 2021) (Figure 1).
Subsequently, ATG16L1 acts as an E3 ligase, promotes ATG8 lipidation and successively binds to ATG5 and ATG12, forming an ATG12-ATG5-ATG16L1 ubiquitin-like binding complex (Popelka et al., 2021). Under starvation-induced conditions, WIPI binds to ATG16L1 and recruits the ATG12-ATG5-ATG16L1 complex allowing it to aggregate to the omegasome site of the phagophore (Dooley et al., 2014). Microtubule-associated proteins 1A/1B light chain 3B (LC3), a homologue of mammalian ATG8, is hydrolytically cleaved by ATG4 protein to produce LC3I, which is then activated by ATG7 and ATG3, followed by covalent binding of LC3I by phosphatidylethanolamine, and finally conversion of LC3 to LC3II (Sou et al., 2006; Glick et al., 2010). Researchers have identified the presence of LC3II on both the internal and external surfaces of phagophore, where it plays a role in membrane fusion and sorting of degraded cargo (Glick et al., 2010; Rogov et al., 2014), as well as controlling the size of autophagosomes (Nakatogawa et al., 2007) (Figure 1).
Finally, autophagosomes transports cargo into lysosomes through fusion with lysosomes to form autolysosome (Mizushima and Komatsu, 2011). The fusion of autophagosomes with lysosomes is regulated by the VPS34 complex II, which differs from the PI3K complex in that the subunit ATG14 has been replaced with UV radiation resistance associated (UVRAG) (Lamb et al., 2013). Ras-related protein 7 (Rab7) and ADP-ribosylation factor-like GTPase Arl8 play a key role in the localization of autophagosomes and lysosomes during the fusion process (Pu et al., 2016). They bind several motor junction proteins that link autophagosomes and lysosomes to cytoskeleton-associated motor proteins that allow autophagosomes and lysosomes to move along microtubules (Garg et al., 2011; Pu et al., 2016). Homotypic fusion and vacuole protein sorting (multisubunit complex HOPS) are essential for autolysosome formation, which tether mobile vesicles along the cytoskeleton (Bröcker et al., 2012). Subsequently, the lipid component of autophagosomes regulates autophagosome-lysosome fusion, where the amount and ratio of PI(3)P and PI(3, 5)P2 regulates the formation of autolysosomes (Hasegawa et al., 2016). Finally, the lysosomes release the degraded products extracellularly in the form of cytosolic spit, facilitating the recycling of the degradation products (Buratta et al., 2020) (Figure 1).
Many clinical therapeutic agents for DKD have been shown to activate autophagy and influence the progression of DKD through different signaling pathways, including the classical drug for lowering sugar - metformin, the classic mTOR target inhibitor - rapamycin, and emerging therapeutic agents such as sodium-glucose cotransporter (SGLT)2 inhibitors and glucagon-like peptide 1 (GLP-1) activators (Gonzalez et al., 2021). In addition, in the ongoing research on Chinese herbal extracts, active ingredients such as resveratrol, hispidulin and berberine have shown renoprotective effects based on the induction of autophagy in kidney (which we will discuss in more detail in the following subsections) (Wang et al., 2019c).
The traditional pathogenesis of DKD is mainly associated with hyperglycemia: elevated blood glucose promotes metabolic disturbances in DKD, like activating oxidative stress in the kidney, contributing to excessive production of reactive oxygen species (ROS) and reducing activity of antioxidant substances (Fang et al., 2012; Tuttle, 2017). The protein kinase C-α and polyol and hexosamine pathways are activated, upregulating ROS production and increasing the expression of the associated inflammatory factors interleukin (IL) 6, monocyte chemoattractant protein-1, tumour necrosis factor alpha and intercellular adhesion molecule-1 in DKD, accelerating the progress of DKD (Wu X. Q. et al., 2021; Dusabimana et al., 2021; Jha et al., 2022). In addition, imbalances in lipid metabolism, including increased lipid uptake or synthesis, are also important triggers for the development of DKD (Mitrofanova et al., 2021). In a hyperglycaemic environment, small arteries have abnormal systolic-diastolic function, endothelin-1secretion is increased, promoting vascular dysfunction (Potenza et al., 2009; Tuttle, 2017). At the same time, genetic factors and activation of the renin-angiotensin-aldosterone system also contribute to the development of DKD (Vegter et al., 2012; Kato and Natarajan, 2019).
Controlling blood glucose, lipids and blood pressure are common methods in the treatment of DKD (Doshi and Friedman, 2017). Moreover, lifestyle changes such as: limiting protein and sodium intake, exercise, weight loss and smoking cessation, which can slow the progression of DKD and reduce the risk of cardiovascular events (Lin et al., 2018).
Proteinuria is a key indicator in the diagnosis of DKD and an important factor in the development of DKD (Colhoun and Marcovecchio, 2018). Coresh J et al. showed that changes in proteinuria were consistently associated with subsequent risk of ESRD through a cohort study (Coresh et al., 2019). Results from an 11-years observational cohort study from Japan showed that non-albuminuric DKD had a lower risk of mortality, cardiovascular events or abnormal kidney function compared with other DKD phenotypes (Yokoyama et al., 2020). Blood passes through the glomerular capillary network, passing through the glomerular filtration barrier to allow primary urine to enter the Bowman’s space, which is then reabsorbed by the renal tubules and finally excreted as a virtually protein-free urine (Mora-Fernández et al., 2014). Here, researchers have introduced an important concept: the glomerular filtration barrier (GFB), the disruption of which is an important cause of DKD proteinuria (Pavenstädt et al., 2003). It is a physiological barrier structure composed of glomerular endothelium, glomerular basement membrane (GBM) and podocytes and slit-diaphragm (SD) from the inside out, allowing a highly selective ultrafiltration of plasma components (Mora-Fernández et al., 2014; Lin and Susztak, 2016). The glycocalyx of the glomerular endothelium has specific molecular and charge properties and therefore constitutes a charge barrier for the glomerulus (Weinbaum et al., 2007). Thus, when endothelial cell dysfunction, GBM and podocyte damage occur, these lead to the production of proteinuria followed by glomerulosclerosis and a decrease in glomerular filtration (Jefferson et al., 2008).
The foot-process is an essential component of the podocyte, with interlocking foot-process wrapping around the capillary bulb to form filtering slits that bridge with the electron-dense membrane-like structure SD (Huber and Benzing, 2005). High glucose-induced ROS production during DKD promotes podocyte detachment and apoptosis (Susztak et al., 2006). The non-renewable nature of the podocyte leads to a reduction in the number of podocytes, with subsequent disruption of the normal structure of the GFB and podocytes, weakened the connections between podocytes and finally the leakage of non-selective proteins (Susztak et al., 2006; Jefferson et al., 2008). Downregulation of nephrin expression, a key component of SD in the DKD environment, results in abnormal podocyte function (Doublier et al., 2003). The imbalance in activation of the small GTP-binding proteins Rac1 and Cdc42 (Cdc42 regulates the podocyte actin cytoskeleton), validates the link between podocyte injury and proteinuria as well as glomerulosclerosis at another level (Blattner et al., 2013). Autophagy deficiency is also an important participant in podocyte damage and will be discussed in the next section of the article.
In the course of DKD, damage to renal tubular epithelial cells has a greater correlation with kidney dysfunction (Carney, 2016). A series of inflammatory and oxidative stress responses generated by the massive protein leakage in DKD patients leads to morphological and functional dysfunction of the renal tubules, with epithelial mesenchymal transformation and cell detachment as well as apoptosis, ultimately leading to fibrosis (Chen et al., 2020). In addition to this, pathological changes in DKD glomeruli are often accompanied by proliferation and expansion of the mesangial matrix, and activation of mesangial cells driven by factors such as hyperglycaemia and dyslipidaemia, accelerating glomerulosclerosis (Ebefors et al., 2021).
By reviewing the literatures, We have learned that advanced glycation end products (AGEs) are irreversible metabolites produced by hyperglycaemia and AGEs contribute to the production of ROS and mediate inflammatory processes (Matoba et al., 2020; Wu X. Q. et al., 2021; Jha et al., 2022). However, AGEs can be degraded by autophagy and inhibit the inflammatory response (Takahashi et al., 2017). A study of autophagy knockout followed by streptozotocin (STZ) induced DM showed severe microalbuminuria, podocyte and endothelial cell damage in the model mice compared to the control mice (Lenoir et al., 2015). And the renal pathological findings also showed extensive mesangium expansion and glomerulosclerosis, thus demonstrating the close relationship between DKD and autophagy (Lenoir et al., 2015).
As mentioned previously, we have discussed the biological process of autophagy and its function in cellular metabolism (Kitada and Koya, 2021); and have argued for a close link between autophagy and DKD by reviewing the literatures (Lenoir et al., 2015). Therefore, we will explore how do drugs target autophagy for the treatment of DKD based on two processes, one is autophagosome formation and another is autolysosome formation, in the hope of enhancing the understanding of the relationship between autophagy and DKD.
In diabetic mice and murine podocyte cell (MPC-5) models, ruxolitinib or wogonin affected autophagy and delayed the progression of DKD through JAK/STAT and Bcl-2-mediated pathways (Chen D. et al., 2021; Liu et al., 2022). In another experimental study, overexpression of KLF4 (a zinc finger-containing transcription factor) showed that KLF4 could play a nephroprotective role by regulating the mTOR/S6K pathway to activate autophagy (Gong et al., 2019). Paecilomyces cicadae-fermented radix astragali played a role in activating autophagy by inhibiting the PI3K/AKT/mTOR signaling pathway, thereby alleviating the progression of DKD (Yang F. et al., 2020). In two experiments using db/db mice and MPC-5 cells as the primary targets, the calcium-sensitive receptor type II agonist cinacalcet worked by activating the CaMKKβ-LKB1-AMPK pathway, while the exosome generated by adipose-derived stem cells achieved therapeutic effects by inhibiting the Smad1/mTOR pathway (Lim et al., 2018; Jin et al., 2019). Similarly, sarsaapogenin (the main active ingredient in Anemarrhena asphodeloides Bunge) and spermine, both of which promoted autophagy by targeting GSK3β and AMPK/mTOR, respectively (Li et al., 2021; Zhang et al., 2021). In a podocyte model induced by high glucose, celastrol restored autophagy through an HO-1-mediated pathway while alleviating the inflammation and damage induced by high glucose in podocytes (Zhan et al., 2018). At the same time, in two additional experiments with high glucose induced podocytes, the expression of G-protein-coupled receptor 43 (GPR43) or sperm-associated antigen 5 (SPAG5), both of which promoted autophagy via the ERK/EGR1 and SPAG5/AKT/mTOR signaling pathways, were inhibited by GPR43 gene knockout or SPAG5 gene silencing, it could be used as potential therapeutic targets for DKD (Xu et al., 2020; Lu et al., 2022). In animal and cellular models of DKD, Astragaloside IV (AS-IV) induced autophagy by activating the AMPK pathway (Guo et al., 2017). In KK-Ay mice and podocyte models, treatment with apelin or AS-IV was administered and both were shown to play an important role in alleviating DKD by regulating the ERK/AKT/mTOR and SIRT1-NF-κB P65 signaling pathways (Liu et al., 2017; Wang X. et al., 2019). Interestingly, the use of hispidulin or berberine in DKD cell models, both of which promoted autophagy by inhibiting mTOR-related pathways (Wu et al., 2018; Li et al., 2020). Inhibition of mTOR and TFEB affected the formation and conversion of autophagy in DKD mice and cellular models, respectively (Zhao X. et al., 2018). Moreover, many researches have also revealed the effects of protein tyrosine phosphatase 1B, resveratrol, ursodeoxycholic acid and 4-phenylbutyrate as well as histone deacetylase 4 on the autophagic process of podocytes in DKD models (Wang et al., 2014; Cao et al., 2016; Huang et al., 2017; Ito et al., 2017). Therefore, autophagy affects cell metabolism in podocytes through mTOR, AMPK, PI3K and other related pathways, inhibits inflammation and kidney damage, and delays the progress of DKD (Figure 2; Tables 1-3, Table.5).
In DKD mice and renal tubular epithelial cell models, treatment with LNA-anti-miR-214 or overexpression of Klotho (an anti-aging factor expressed in the kidney) modulated the P53/miR-214/ULK1 and AMPK/ERK signaling pathways to exert nephroprotective effects (Ma et al., 2020; Xue et al., 2021). In a different study model, Korean red ginseng was shown to promote autophagy and thus reduced hyperglycaemia-induced renal inflammation and fibrosis, exerting a nephroprotective effect (Karunasagara et al., 2020). In a study using high glucose induced human proximal tubular cells (HK-2 cells) as a model, the investigators found that renal injury could be ameliorated by altering the expression of miR-155 and SIRT1, or by administering dapagliflozin and astilbin, respectively (Chen et al., 2018; Wang Y. et al., 2018; Xu et al., 2021). However, the difference between these two drugs was that dapagliflozin acted to suppress inflammation by regulating the AMPK/mTOR/NF-κB pathway, whereas astilbin worked on the PI3K/AKT signaling pathway to attenuate high glucose-induced autophagy and apoptosis (Chen et al., 2018; Xu et al., 2021). Carbon monoxide positively regulated autophagy via the Beclin-1-Bcl-2 pathway in the DKD model to protect the kidney from injury and aging; meanwhile, wogonin attenuated renal fibrosis by targeting PI3K/AKT/NF-κB to regulate renal inflammation and autophagic activity (Chen L. et al., 2021; Lei et al., 2021). In palmitic acid-induced HK-2 cells, alprostadil ameliorated renal tubular insulin resistance and restored autophagy via an autophagy-dependent fibroblast growth factor 21-associated pathway (Wei et al., 2018). Cyclocarya paliurus triterpenic acids or reduced expression of thioredoxin interacting protein (TXNIP) acted through mTOR or in combination with the AMPK pathway to induce autophagy (Huang et al., 2016; Zhang et al., 2019). In contrast, the Chinese herbal extract icariin from Epimedium restored autophagy and attenuated renal tubulointerstitial fibrosis by targeting miR-192-5p/GLP-1R (GLP-1 receptor) in a DKD model (Jia et al., 2021). At the same time, the overexpression of TXNIP (one of the factors of autophagy) induced by high glucose led to autophagy dysfunction and participated in the occurrence of DKD (Huang et al., 2014). Studies had shown that the drug empagliflozin or paricalcitol (an activated vitamin D analog) restored autophagy via AMPK-related pathways in DKD models (Lee et al., 2019; Li et al., 2022). Treatment with dencichine or Cathelicidin-BF peptide in DKD rats and cellular models showed that both positively regulated autophagy through an AMPK-mediated pathway and improved renal function by reducing oxidative stress and fibrosis (Huang et al., 2020; Liu et al., 2020). However, experiments in which metformin treated DKD rats and renal tubular epithelial cells models, metformin exerted therapeutic effects through an AMPK-mediated pathway (Wang F. et al., 2021). To investigate the specific mechanism of fenofibrate on the DKD model, researchers found that fenofibrate induced autophagy and acted as an antioxidant via the AMPK pathway (Sohn et al., 2017). In addition, researchers have experimentally demonstrated that the use of therapeutic agents and approaches such as mitoQ (mitochondria-targeted antioxidant) and NSC697923 inhibitor and t-AUCB (an inhibitor of soluble epoxide hydrolase) can slow down the progression of DKD by improving autophagy (Xiao et al., 2017; Pontrelli et al., 2018; Jiang et al., 2020; Han et al., 2021; Liang et al., 2021; Zheng et al., 2021). In summary, autophagy plays an important role in maintaining the homeostasis and function of renal tubular epithelial cells. If autophagy is imbalanced, it leads to intracellular metabolic changes and accelerates the onset and progression of disease, but these could be restored with drugs such as metformin and empagliflozin (Figure 2; Table.1-3, Table.5).
Treatment of induced rat mesangial cells and animals with metformin and paeoniflorin showed that metformin alleviated oxidative stress and enhanced autophagy via the AMPK/SIRT1-FOXO1 pathway (Ren et al., 2020). In contrast, paeoniflorin reduced LC3II/LC3I expression through the RAGE/mTOR pathway, decreased the number of autophagosomes and inhibited AGEs-induced autophagy (Chen et al., 2017). To investigate the role of lncRNA SOX2OT and tissue inhibitor of metalloproteinase 3 (TIMP3) in the progression of DKD, overexpression or gene deletion was used in treated mice and mouse mesangial cells, suggesting that the overexpression of lncRNA SOX2OT (SOX2 overlapping transcript) restored autophagy via AKT/mTOR, while TIMP3 deletion led to reduced FOXO1 expression of the autophagy key factor and increased expression of the FOXO1 transcriptional repressor STAT1, driving renal function to deteriorate in DKD patients and mice (Fiorentino et al., 2013; Chen K. et al., 2021). In addition to this, AS-IV induced autophagy and inhibited activation of mesangial cells in the SIRT1-NF-κB pathway, alleviating mesangial matrix expansion and proliferation in the KK-Ay mouse model (Wang et al., 2018b). In DKD rat and HMC models, icariin promoted G-protein-coupled estrogen receptor-mediated P62-dependent Keap1 degradation as well as Nrf2 activation, and played a therapeutic role in the progression of type 1 DKD in rats (Wang et al., 2020). Similarly, triptolide targeted the miR-141-3p/PTEN/AKT/mTOR pathway to induce autophagy and protect renal function (Li et al., 2017). By summarizing the above literature, we found that autophagy exists in glomerular mesangial cells. Oxidative stress and inflammation inhibit autophagy in mesangial cells, but the application of drugs such as AS-IV and Icariin can upregulate the occurrence of autophagy by regulating autophagy-related pathways (Figure 2; Tables.1-3, Table.5).
The use of GLP-1 in Zucker diabetic fatty rats and AGEs induced Ins 1 cells model showed that GLP-1 showed renoprotective effects independent of hypoglycaemia (Yang S. et al., 2020). Using liraglutide in an animal model of spontaneously diabetic tori fatty rats, it was concluded that liraglutide protected the kidney and activated autophagy in early DKD via the AMPK/eNOS pathway independent of reductions in blood glucose and blood pressure (Yamada et al., 2021). Caffeic acid, ethyl acetate or Huang-Gui Solid Dispersion (HGSD) was used in a model of Wistar rats with established DM, and it was ultimately concluded that all three drugs could activate autophagy through AMPK-mediated pathways, switching on of key downstream genes (Matboli et al., 2017; Zhang et al., 2020; Ezzat et al., 2021). Purinergic receptors P2Y2R knockout impaired autophagy via the AKT/SIRT-1/FOXO3a signaling pathway in an experiment using unilateral nephrectomy combined with high fat diet and STZ (Dusabimana et al., 2020). However, in two other studies using the same animal model, the use of the drug geniposide or Abelmoschus manihot activated autophagy, reduced fibrosis and inflammation, as a result slowed the progression of DKD (Kim et al., 2018; Dusabimana et al., 2021). Meanwhile, erlotinib, Cordyceps militaris polysaccharides, and calcium dobesilate were used in STZ-induced mice as a model to demonstrate that erlotinib activated AMPK, inhibited mTOR activity and thus epidermal growth factor receptor, and played a role in the renal protective mechanism (Zhang et al., 2014). And another drug calcium dobesilate restored autophagy by blocking the VEGF/PI3K/AKT/mTOR cascade reaction and exerted nephroprotective effects together with Cordyceps militaris polysaccharides (Wang et al., 2019b; Chen et al., 2019). In a DKD rat model, mangiferin increased the number of autophagosomes in DKD rat podocytes and reduce proteinuria in diabetic rats, and this result was achieved by upregulating phosphorylated AMPK and ULK1 as well as downregulating mTOR phosphorylation (Wang et al., 2018a). Similarly, in a study on jujuboside A (JuA), it was demonstrated that JuA also promoted autophagy and mitophagy by activating CaMKK2, an upstream regulator of AMPK, alleviating the hyperglycaemic state and reducing various indicators of renal function (Zhong et al., 2022). The Chinese herbal formula Jiedu Tongluo Baoshen formula and valproate could counteract renal injury by modulating PI3K/AKT/mTOR or NF-κB/iNOS pathways, respectively (Khan et al., 2015; Jin et al., 2022). The use of paricalcitol (vitamin D receptor agonist) improved autophagic fluxes and improved slit diaphragm-tight junction conversion in DKD rats and db/db mice (Wang B. et al., 2021). A study on the protective effects of isorhamnetin in DKD rats had also demonstrated that isorhamnetin had good hypoglycaemic and hypolipidaemic properties, increased the number of autophagosomes and had a nephroprotective effect (Matboli et al., 2021). Finally, very low protein diets restored autophagy by inhibiting mTOR-related pathways (Kitada et al., 2016). In addition, by consulting the literatures, we also know that IL-17A and SCO-792 (an enteropeptidase inhibitor) improved DKD by regulating autophagy (Kim et al., 2021; Sugama et al., 2021). In a conclusion, in experiments using only animals as research models, researchers have found that drugs can activate autophagy, regulate autophagy-related pathways and key autophagy factors, and exert nephroprotective effects (Figure 2; Table.1-3, Table.5).
In experiments with DKD patients and AGEs-induced podocytes, researchers found that AGEs did not induce autophagy in podocytes, but increased LC3-II and P62-positive vacuoles, demonstrating that the autophagic degradation pathway was disrupted. This was caused by increased lysosomal membrane permeability inducing lysosomal dysfunction; however, therapeutic measures of resveratrol plus vitamin E could improve this situation (Liu et al., 2019). In db/db mice and AGE-BSA-induced HK-2 cells, restoration of Smad3 expression promoted TFEB-dependent lysosomogenesis (Yang C. et al., 2021). AGEs was able to induce an increase in the number of autophagosomes, the accumulation of sequestosome 1 protein and a decrease in the number of autolysosomes in AGEs-induced HK-2 cells. Therefore, this experiment concluded that AGEs was able to cause inactivation of autophagy, accelerate increased lysosomal permeability and dysfunction in HK-2 cells (Liu et al., 2015). Hepatocyte growth factor was used on high glucose-induced MPC-5 and STZ-induced mice, which regulated the PI3K/AKT-GSK3β-TFEB signaling pathway, improved lysosomal function and increased autophagic activity (Hou et al., 2020). In the ATG5 knockout mouse model and proximal tubular epithelial cells model, defective autophagy downregulated lysosomal biogenesis and promote the accumulation of AGEs, leading to DKD progression (Takahashi et al., 2017). In a study using STZ-induced Sprague-Dawley rats after high-glucose and high-fat model, the drug Keluoxin improved biochemical indicators, reduce podocyte damage and modulate lysosomal function in DKD rats, mitigating the progression of DKD (Yang X. et al., 2021). In a separate study, a group of STZ-induced male BALB/c mice were injected with mesenchymal stem cells (MSCs) via tail vein while primary MSCs were extracted from BALB/c mice for culture and then transfected with TFEB RNA. The results demonstrated that MSCs shifted the M2 phenotype from M1/M2 to macrophages and produce a nephroprotective effect, which was mediated by TFEB (Yuan Y. et al., 2020). Meanwhile, a study on the mechanism of high doses of vitamin E treatment with DKD had shown that high doses of vitamin E could promote lysosomal function and reduce autophagic stress to delay the progression of DKD (Zhao Y. et al., 2018). In addition to this, in order to investigate the role of the vaspin-HSPA1L (heat shock protein family A member 1 like) complex in the development of DKD, the present study made use of patients with obesity-related nephropathy and DKD as well as high-fat and high-glucose-induced mice as models, and then concluded that the vapsin/HSPA1L-mediated pathway played a role in ER stress, impaire autophagy and lysosomal function (Nakatsuka et al., 2021). In summary, the formation of the autolysosome is another key pathway in the autophagic process and is related to the degradation of intracellular metabolites (Wu M. et al., 2021). Excessive production of AGEs or knockdown of key autophagy factors leads to increased lysosomal membrane permeability or downregulated lysosomal biogenesis and impaired lysosomal function, resulting in disruption of the autophagic degradation pathway (Levy et al., 2017; Liu et al., 2019). However, therapeutic measures such as resveratrol plus vitamin E can promote autophagy and slow down the progression of DKD in terms of restoring lysosomal function (Figure 2; Table.4).
Defective autophagy is present in the pathophysiology of many diseases and has been studied in the fields of oncology, neurodegenerative diseases, metabolic diseases and renaldiseases, where it is closely related to the pathological process of DKD (Mizushima and Levine, 2020).
AMPK activation by upstream kinases rapidly phosphorylates the ULK-1 complex while inhibiting the activation of mTOR (Alers et al., 2012). Subsequent phosphorylation of downstream Beclin-1 activates the PI3K complex, and promotes the synthesis of PI3P (Axe et al., 2008; Russell et al., 2013; Baskaran et al., 2014). Then PI3P recruits autophagy factors, inducing the formation of omegasomes which work as the initial part of the phagophore (Lamb et al., 2013). Subsequently, omegasomes are continuously extended by the action of DCFP1 and WIPI (Lamb et al., 2013). In parallel, two downstream ubiquitin complexes (ATG12-ATG5-ATG16L1 and LC3-II) are formed and then recruited to the phagophore upon induction of WIPI and ATG16L1 (Sou et al., 2006; Dooley et al., 2014; Popelka et al., 2021). Subsequently, the autophagosome wrapped around the cargo fuses with the lysosome and is regulated by the VPS34 complex II and the HOPS complex as well as Rab7 (Bröcker et al., 2012; Lamb et al., 2013; Pu et al., 2016). It is eventually degraded by the lysosome and excreted from the cell in the form of exocytosis for subsequent recycling and use (Buratta et al., 2020).
Hyperglycaemia stimulates the production of metabolites such as ROS and AGEs, which promote oxidative stress, inflammation and impair autophagy, while drugs can slow the progression of DKD by inducing or restoring autophagy (Zheng et al., 2020; Winiarska et al., 2021). In recent years relevant reports have discussed drugs that target autophagy in the treatment of DKD, such as the herbal extracts, the emerging GLP-1 activators and SGLT2 inhibitors and so on, all of which have been shown to modulate the autophagic process and improve renal function (Huang et al., 2017; Wang et al., 2018b; Winiarska et al., 2021; Yamada et al., 2021).
Autophagy acts as a reactive protective mechanism in the kidney in order to protect cells from stressors, and mTOR is generally inhibited in this situation (Kim et al., 2011). However, the inhibition of autophagy that results from mTOR activation does not imply cell damage, but rather promotes cell proliferation and contributes to kidney repair (Xie et al., 2015; Viana et al., 2018). Similarly, in a separate study, autophagy was found to have a possible role in promoting renal fibrosis: in severe acute kidney injury, tubular cells undergo arrest in the G2/M phase, leading to the secretion of pro-fibrotic factors that promotes the progression of renal fibrosis, accompanied by the emergence of TOR-autophagic spatially coupled compartments structures (distinct cytoplasmic compartments rich in mTORC1) (Yang et al., 2010; Canaud et al., 2019; Tang et al., 2020). In addition, side effects associated with the mTOR inhibitor rapamycin for DKD, such as hyperglycaemia, insulin resistance and dyslipidaemia, have also been reported, which may be related to the metabolic profile of the pancreas and other insulin-resistant tissues, including the liver and muscle (Viana et al., 2018). Therefore, the existence of autophagy is therefore like a double-edged sword.
However, there are many questions about autophagy that have not been clearly described. Three types of autophagy are known, namely microautophagy, macroautophagy and chaperone-mediated autophagy, and have been described and studied in details (Li et al., 2012; Feng et al., 2014; Dash et al., 2019). However, it is not clear whether there is an interconnection among the three of them, and the specific triggering mechanism of autophagy in them is also lacking. Further discoveries are needed about the specific mechanisms by which autophagy produces stress responding to human metabolites. On the other hand, there are no extensive experimental studies on the exact mechanism of autophagosome-lysosome fusion regulation and degradation and a large body of evidence is lacking to support this view. In addition, the exact mechanism of action of drugs targeting autophagy is still unclear, which has prevented the translation of some drugs from animal studies to human clinical studies for the time being, and therefore lacks theoretical support for human clinical studies. We have now affirmed the positive role of autophagy for the treatment of DKD and it can be one of the key directions for the future treatment of DKD (Ding and Choi, 2015). However, autophagy also has side effects, so how to manipulate autophagy is an important issue for researchers today. The above issues will need to be refined over time with further research.
Activation of autophagy protects renal function, while impaired autophagy is involved in DKD progression. Although drugs targeting autophagy are still being investigated and some have already been used clinically, the incidence of DKD is still on the rise, so maintaining autophagy in the kidney’s intrinsic cells is now an urgent issue in the medical field. By reviewing the physiological process of autophagy and the roles of DKD therapeutic agents as well as related pathways, we hope to provide new perspectives and inspiration for the clinical treatment of DKD.
ZZ, YS, JX, DJ, XL, DZ designed the idea, checked the literature, made tables and figures. ZZ and YS wrote the manuscript. FL, WQ and XT modified the manuscript. All authors have read and approved the final manuscript.
Authors’ work has been supported by Innovation Team and Talents Cultivation Program of National Administration of Traditional Chinese Medicine (No: ZYYCXTD-D-202001), and State Administration of Traditional Chinese Medicine Special Project for Scientific and Technological Research in Chinese Medicine (No: 2016ZX03), and Science and Technology Innovation Project of the Chinese Academy of Traditional Chinese Medicine (No: C12021A02610).
The authors declare that the research was conducted in the absence of any commercial or financial relationships that could be construed as a potential conflict of interest.
All claims expressed in this article are solely those of the authors and do not necessarily represent those of their affiliated organizations, or those of the publisher, the editors and the reviewers. Any product that may be evaluated in this article, or claim that may be made by its manufacturer, is not guaranteed or endorsed by the publisher.
Alers, S., Löffler, A. S., Wesselborg, S., and Stork, B. (2012). Role of AMPK-mTOR-Ulk1/2 in the regulation of autophagy: Cross talk, shortcuts, and feedbacks.Mol. Cell Biol. 32 (1), 2–11. doi:10.1128/mcb.06159-11
Antonioli, M., Di Rienzo, M., Piacentini, M., and Fimia, G. M. (2017). Emerging mechanisms in initiating and terminating autophagy. Trends Biochem. Sci. 42 (1), 28–41. doi:10.1016/j.tibs.2016.09.008
Axe, E. L., Walker, S. A., Manifava, M., Chandra, P., Roderick, H. L., Habermann, A., et al. (2008). Autophagosome formation from membrane compartments enriched in phosphatidylinositol 3-phosphate and dynamically connected to the endoplasmic reticulum. J. Cell Biol. 182 (4), 685–701. doi:10.1083/jcb.200803137
Baskaran, S., Carlson, L. A., Stjepanovic, G., Young, L. N., Kim, D. J., Grob, P., et al. (2014). Architecture and dynamics of the autophagic phosphatidylinositol 3-kinase complex. Elife 3, e05115. doi:10.7554/eLife.05115
Blattner, S. M., Hodgin, J. B., Nishio, M., Wylie, S. A., Saha, J., Soofi, A. A., et al. (2013). Divergent functions of the rho GTPases Rac1 and Cdc42 in podocyte injury. Kidney Int. 84 (5), 920–930. doi:10.1038/ki.2013.175
Bravo-San Pedro, J. M., Kroemer, G., and Galluzzi, L. (2017). Autophagy and mitophagy in cardiovascular disease. Circ. Res. 120 (11), 1812–1824. doi:10.1161/circresaha.117.311082
Bröcker, C., Kuhlee, A., Gatsogiannis, C., Balderhaar, H. J., Hönscher, C., Engelbrecht-Vandré, S., et al. (2012). Molecular architecture of the multisubunit homotypic fusion and vacuole protein sorting (HOPS) tethering complex. Proc. Natl. Acad. Sci. U. S. A. 109 (6), 1991–1996. doi:10.1073/pnas.1117797109
Buratta, S., Tancini, B., Sagini, K., Delo, F., Chiaradia, E., Urbanelli, L., et al. (2020). Lysosomal exocytosis, exosome release and secretory autophagy: The autophagic- and endo-lysosomal systems go extracellular. Ijms 21 (7), 2576. doi:10.3390/ijms21072576
Canaud, G., Brooks, C., Kishi, S., Taguchi, K., Nishimura, K., Magassa, S., et al. (2019). Cyclin G1 and TASCC regulate kidney epithelial cell G 2 -M arrest and fibrotic maladaptive repair. Sci. Transl. Med. 11 (476), eaav4754. doi:10.1126/scitranslmed.aav4754
Cao, A. L., Wang, L., Chen, X., Wang, Y. M., Guo, H. J., Chu, S., et al. (2016). Ursodeoxycholic acid and 4-phenylbutyrate prevent endoplasmic reticulum stress-induced podocyte apoptosis in diabetic nephropathy. Lab. Invest. 96 (6), 610–622. doi:10.1038/labinvest.2016.44
Cao, Z., and Cooper, M. E. (2011). Pathogenesis of diabetic nephropathy. J. Diabetes Investig. 2 (4), 243–247. doi:10.1111/j.2040-1124.2011.00131.x
Carney, E. F. (2016). Diabetic nephropathy: The genetic architecture of DKD in T1DM. Nat. Rev. Nephrol. 12 (12), 714. doi:10.1038/nrneph.2016.149
Chen, D. D., Xu, R., Zhou, J. Y., Chen, J. Q., Wang, L., Liu, X. S., et al. (2019). Cordyceps militaris polysaccharides exerted protective effects on diabetic nephropathy in mice via regulation of autophagy. Food Funct. 10 (8), 5102–5114. doi:10.1039/c9fo00957d
Chen, D., Liu, Y., Chen, J., Lin, H., Guo, H., Wu, Y., et al. (2021a). JAK/STAT pathway promotes the progression of diabetic kidney disease via autophagy in podocytes. Eur. J. Pharmacol. 902, 174121. doi:10.1016/j.ejphar.2021.174121
Chen, F., Sun, Z., Zhu, X., and Ma, Y. (2018). Astilbin inhibits high glucose-induced autophagy and apoptosis through the PI3K/Akt pathway in human proximal tubular epithelial cells. Biomed. Pharmacother. 106, 1175–1181. doi:10.1016/j.biopha.2018.07.072
Chen, J., Zhao, D., Zhu, M., Zhang, M., Hou, X., Ding, W., et al. (2017). Paeoniflorin ameliorates AGEs-induced mesangial cell injury through inhibiting RAGE/mTOR/autophagy pathway. Biomed. Pharmacother. 89, 1362–1369. doi:10.1016/j.biopha.2017.03.016
Chen, K., Yu, B., and Liao, J. (2021b). LncRNA SOX2OT alleviates mesangial cell proliferation and fibrosis in diabetic nephropathy via Akt/mTOR-mediated autophagy. Mol. Med. 27 (1), 71. doi:10.1186/s10020-021-00310-6
Chen, L., Mei, G., Jiang, C., Cheng, X., Li, D., Zhao, Y., et al. (2021c). Carbon monoxide alleviates senescence in diabetic nephropathy by improving autophagy. Cell Prolif. 54 (6), e13052. doi:10.1111/cpr.13052
Chen, S. J., Lv, L. L., Liu, B. C., and Tang, R. N. (2020). Crosstalk between tubular epithelial cells and glomerular endothelial cells in diabetic kidney disease. Cell Prolif. 53 (3), e12763. doi:10.1111/cpr.12763
Colhoun, H. M., and Marcovecchio, M. L. (2018). Biomarkers of diabetic kidney disease. Diabetologia 61 (5), 996–1011. doi:10.1007/s00125-018-4567-5
Coresh, J., Heerspink, H. J. L., Sang, Y., Matsushita, K., Arnlov, J., Astor, B. C., et al. (2019). Change in albuminuria and subsequent risk of end-stage kidney disease: An individual participant-level consortium meta-analysis of observational studies. Lancet Diabetes Endocrinol. 7 (2), 115–127. doi:10.1016/s2213-8587(18)30313-9
Cuervo, A. M., and Wong, E. (2014). Chaperone-mediated autophagy: Roles in disease and aging. Cell Res. 24 (1), 92–104. doi:10.1038/cr.2013.153
Dash, S., Aydin, Y., and Moroz, K. (2019). Chaperone-mediated autophagy in the liver: Good or bad? Cells 8 (11), 1308. doi:10.3390/cells8111308
Davidson, P., Kwiatkowski, C. A., and Wien, M. (2015). Management of hyperglycemia and enteral nutrition in the hospitalized patient. Nutr. Clin. Pract. 30 (5), 652–659. doi:10.1177/0884533615591057
Deretic, V. (2021). Autophagy in inflammation, infection, and immunometabolism. Immunity 54 (3), 437–453. doi:10.1016/j.immuni.2021.01.018
Deter, R. L., and De Duve, C. (1967). Influence of glucagon, an inducer of cellular autophagy, on some physical properties of rat liver lysosomes. J. Cell Biol. 33 (2), 437–449. doi:10.1083/jcb.33.2.437
Ding, Y., and Choi, M. E. (2015). Autophagy in diabetic nephropathy. J. Endocrinol. 224 (1), R15–R30. doi:10.1530/joe-14-0437
Dooley, H. C., Razi, M., Polson, H. E., Girardin, S. E., Wilson, M. I., and Tooze, S. A. (2014). WIPI2 links LC3 conjugation with PI3P, autophagosome formation, and pathogen clearance by recruiting Atg12-5-16L1. Mol. Cell 55 (2), 238–252. doi:10.1016/j.molcel.2014.05.021
Doshi, S. M., and Friedman, A. N. (2017). Diagnosis and management of type 2 diabetic kidney disease. Clin. J. Am. Soc. Nephrol. 12 (8), 1366–1373. doi:10.2215/cjn.11111016
Doublier, S., Salvidio, G., Lupia, E., Ruotsalainen, V., Verzola, D., Deferrari, G., et al. (2003). Nephrin expression is reduced in human diabetic nephropathy: Evidence for a distinct role for glycated albumin and angiotensin II. Diabetes 52 (4), 1023–1030. doi:10.2337/diabetes.52.4.1023
Dusabimana, T., Kim, S. R., Park, E. J., Je, J., Jeong, K., Yun, S. P., et al. (2020). P2Y2R contributes to the development of diabetic nephropathy by inhibiting autophagy response. Mol. Metab. 42, 101089. doi:10.1016/j.molmet.2020.101089
Dusabimana, T., Park, E. J., Je, J., Jeong, K., Yun, S. P., Kim, H. J., et al. (2021). Geniposide improves diabetic nephropathy by enhancing ULK1-mediated autophagy and reducing oxidative stress through AMPK activation. Ijms 22 (4), 1651. doi:10.3390/ijms22041651
Ebefors, K., Bergwall, L., and Nyström, J. (2021). The glomerulus according to the mesangium. Front. Med. (Lausanne) 8, 740527. doi:10.3389/fmed.2021.740527
Ezzat, S. M., Abdallah, H. M. I., Yassen, N. N., Radwan, R. A., Mostafa, E. S., Salama, M. M., et al. (2021). Phenolics from Physalis peruviana fruits ameliorate streptozotocin-induced diabetes and diabetic nephropathy in rats via induction of autophagy and apoptosis regression. Biomed. Pharmacother. 142, 111948. doi:10.1016/j.biopha.2021.111948
Fang, D., Wan, X., Deng, W., Guan, H., Ke, W., Xiao, H., et al. (2012). Fufang Xue Shuan Tong capsules inhibit renal oxidative stress markers and indices of nephropathy in diabetic rats. Exp. Ther. Med. 4 (5), 871–876. doi:10.3892/etm.2012.680
Fantus, D., Rogers, N. M., Grahammer, F., Huber, T. B., and Thomson, A. W. (2016). Roles of mTOR complexes in the kidney: Implications for renal disease and transplantation. Nat. Rev. Nephrol. 12 (10), 587–609. doi:10.1038/nrneph.2016.108
Feng, Y., He, D., Yao, Z., and Klionsky, D. J. (2014). The machinery of macroautophagy. Cell Res. 24 (1), 24–41. doi:10.1038/cr.2013.168
Fiorentino, L., Cavalera, M., Menini, S., Marchetti, V., Mavilio, M., Fabrizi, M., et al. (2013). Loss of TIMP3 underlies diabetic nephropathy via FoxO1/STAT1 interplay. EMBO Mol. Med. 5 (3), 441–455. doi:10.1002/emmm.201201475
Garg, S., Sharma, M., Ung, C., Tuli, A., Barral, D. C., Hava, D. L., et al. (2011). Lysosomal trafficking, antigen presentation, and microbial killing are controlled by the Arf-like GTPase Arl8b. Immunity 35 (2), 182–193. doi:10.1016/j.immuni.2011.06.009
Glick, D., Barth, S., and Macleod, K. F. (2010). Autophagy: Cellular and molecular mechanisms. J. Pathol. 221 (1), 3–12. doi:10.1002/path.2697
Gong, J., Zhan, H., Li, Y., Zhang, W., Jin, J., and He, Q. (2019). Krüppel‑like factor 4 ameliorates diabetic kidney disease by activating autophagy via the mTOR pathway. Mol. Med. Rep. 20 (4), 3240–3248. doi:10.3892/mmr.2019.10585
Gonzalez, C., Carro Negueruela, M., Nicora Santamarina, C., Resnik, R., and Vaccaro, M. J. C. (2021). Autophagy dysregulation in diabetic kidney disease: From pathophysiology to pharmacological interventions. Cells 10 (9), 2497. doi:10.3390/cells10092497
Guo, H., Wang, Y., Zhang, X., Zang, Y., Zhang, Y., Wang, L., et al. (2017). Astragaloside IV protects against podocyte injury via SERCA2-dependent ER stress reduction and AMPKα-regulated autophagy induction in streptozotocin-induced diabetic nephropathy. Sci. Rep. 7 (1), 6852. doi:10.1038/s41598-017-07061-7
Han, Y., Xiong, S., Zhao, H., Yang, S., Yang, M., Zhu, X., et al. (2021). Lipophagy deficiency exacerbates ectopic lipid accumulation and tubular cells injury in diabetic nephropathy. Cell Death Dis. 12 (11), 1031. doi:10.1038/s41419-021-04326-y
Hasegawa, J., Iwamoto, R., Otomo, T., Nezu, A., Hamasaki, M., and Yoshimori, T. (2016). Autophagosome-lysosome fusion in neurons requires INPP5E, a protein associated with Joubert syndrome. Embo J. 35 (17), 1853–1867. doi:10.15252/embj.201593148
Hawley, S. A., Pan, D. A., Mustard, K. J., Ross, L., Bain, J., Edelman, A. M., et al. (2005). Calmodulin-dependent protein kinase kinase-beta is an alternative upstream kinase for AMP-activated protein kinase. Cell Metab. 2 (1), 9–19. doi:10.1016/j.cmet.2005.05.009
Hayashi-Nishino, M., Fujita, N., Noda, T., Yamaguchi, A., Yoshimori, T., and Yamamoto, A. (2009). A subdomain of the endoplasmic reticulum forms a cradle for autophagosome formation. Nat. Cell Biol. 11 (12), 1433–1437. doi:10.1038/ncb1991
Hou, B., Li, Y., Li, X., Zhang, C., Zhao, Z., Chen, Q., et al. (2020). HGF protected against diabetic nephropathy via autophagy-lysosome pathway in podocyte by modulating PI3K/Akt-GSK3β-TFEB axis. Cell Signal 75, 109744. doi:10.1016/j.cellsig.2020.109744
Huang, C., Cheng, L., Feng, X., Li, X., and Wang, L. (2020). Dencichine ameliorates renal injury by improving oxidative stress, apoptosis and fibrosis in diabetic rats. Life Sci. 258, 118146. doi:10.1016/j.lfs.2020.118146
Huang, C., Lin, M. Z., Cheng, D., Braet, F., Pollock, C. A., and Chen, X. M. (2014). Thioredoxin-interacting protein mediates dysfunction of tubular autophagy in diabetic kidneys through inhibiting autophagic flux. Lab. Invest. 94 (3), 309–320. doi:10.1038/labinvest.2014.2
Huang, C., Zhang, Y., Kelly, D. J., Tan, C. Y., Gill, A., Cheng, D., et al. (2016). Thioredoxin interacting protein (TXNIP) regulates tubular autophagy and mitophagy in diabetic nephropathy through the mTOR signaling pathway. Sci. Rep. 6, 29196. doi:10.1038/srep29196
Huang, S. S., Ding, D. F., Chen, S., Dong, C. L., Ye, X. L., Yuan, Y. G., et al. (2017). Resveratrol protects podocytes against apoptosis via stimulation of autophagy in a mouse model of diabetic nephropathy. Sci. Rep. 7, 45692. doi:10.1038/srep45692
Huber, T. B., and Benzing, T. (2005). The slit diaphragm: A signaling platform to regulate podocyte function. Curr. Opin. Nephrol. Hypertens. 14 (3), 211–216. doi:10.1097/01.mnh.0000165885.85803.a8
Inoki, K., Kim, J., and Guan, K. L. (2012). AMPK and mTOR in cellular energy homeostasis and drug targets. Annu. Rev. Pharmacol. Toxicol. 52, 381–400. doi:10.1146/annurev-pharmtox-010611-134537
Ito, Y., Hsu, M. F., Bettaieb, A., Koike, S., Mello, A., Calvo-Rubio, M., et al. (2017). Protein tyrosine phosphatase 1B deficiency in podocytes mitigates hyperglycemia-induced renal injury. Metabolism 76, 56–69. doi:10.1016/j.metabol.2017.07.009
Jefferson, J. A., Shankland, S. J., and Pichler, R. H. (2008). Proteinuria in diabetic kidney disease: A mechanistic viewpoint. Kidney Int. 74 (1), 22–36. doi:10.1038/ki.2008.128
Jha, J. C., Dai, A., Garzarella, J., Charlton, A., Urner, S., Østergaard, J. A., et al. (2022). Independent of renox, NOX5 promotes renal inflammation and fibrosis in diabetes by activating ROS-sensitive pathways. Diabetes 71 (6), 1282–1298. doi:10.2337/db21-1079
Jia, Z., Wang, K., Zhang, Y., Duan, Y., Xiao, K., Liu, S., et al. (2021). Icariin ameliorates diabetic renal tubulointerstitial fibrosis by restoring autophagy via regulation of the miR-192-5p/GLP-1R pathway. Front. Pharmacol. 12, 720387. doi:10.3389/fphar.2021.720387
Jiang, X. S., Xiang, X. Y., Chen, X. M., He, J. L., Liu, T., Gan, H., et al. (2020). Inhibition of soluble epoxide hydrolase attenuates renal tubular mitochondrial dysfunction and ER stress by restoring autophagic flux in diabetic nephropathy. Cell Death Dis. 11 (5), 385. doi:10.1038/s41419-020-2594-x
Jin, D., Liu, F., Yu, M., Zhao, Y., Yan, G., Xue, J., et al. (2022). Jiedu Tongluo Baoshen formula enhances podocyte autophagy and reduces proteinuria in diabetic kidney disease by inhibiting PI3K/Akt/mTOR signaling pathway. J. Ethnopharmacol. 293, 115246. doi:10.1016/j.jep.2022.115246
Jin, J., Shi, Y., Gong, J., Zhao, L., Li, Y., He, Q., et al. (2019). Exosome secreted from adipose-derived stem cells attenuates diabetic nephropathy by promoting autophagy flux and inhibiting apoptosis in podocyte. Stem Cell Res. Ther. 10 (1), 95. doi:10.1186/s13287-019-1177-1
Karunasagara, S., Hong, G. L., Park, S. R., Lee, N. H., Jung, D. Y., Kim, T. W., et al. (2020). Korean red ginseng attenuates hyperglycemia-induced renal inflammation and fibrosis via accelerated autophagy and protects against diabetic kidney disease. J. Ethnopharmacol. 254, 112693. doi:10.1016/j.jep.2020.112693
Kato, M., and Natarajan, R. (2019). Epigenetics and epigenomics in diabetic kidney disease and metabolic memory. Nat. Rev. Nephrol. 15 (6), 327–345. doi:10.1038/s41581-019-0135-6
Khan, S., Jena, G., Tikoo, K., and Kumar, V. (2015). Valproate attenuates the proteinuria, podocyte and renal injury by facilitating autophagy and inactivation of NF-κB/iNOS signaling in diabetic rat. Biochimie 110, 1–16. doi:10.1016/j.biochi.2014.12.015
Kim, H., Dusabimana, T., Kim, S. R., Je, J., Jeong, K., Kang, M. C., et al. (2018). Supplementation of Abelmoschus manihot ameliorates diabetic nephropathy and hepatic steatosis by activating autophagy in mice. Nutrients 10 (11), 1703. doi:10.3390/nu10111703
Kim, J., and Guan, K. L. (2019). mTOR as a central hub of nutrient signalling and cell growth. Nat. Cell Biol. 21 (1), 63–71. doi:10.1038/s41556-018-0205-1
Kim, J., Huang, W. P., Stromhaug, P. E., and Klionsky, D. J. (2002). Convergence of multiple autophagy and cytoplasm to vacuole targeting components to a perivacuolar membrane compartment prior to de novo vesicle formation. J. Biol. Chem. 277 (1), 763–773. doi:10.1074/jbc.M109134200
Kim, J., Kundu, M., Viollet, B., and Guan, K. L. (2011). AMPK and mTOR regulate autophagy through direct phosphorylation of Ulk1. Nat. Cell Biol. 13 (2), 132–141. doi:10.1038/ncb2152
Kim, K. H., Hong, G. L., Jung, D. Y., Karunasagara, S., Jeong, W. I., and Jung, J. Y. (2021). IL-17 deficiency aggravates the streptozotocin-induced diabetic nephropathy through the reduction of autophagosome formation in mice. Mol. Med. 27 (1), 25. doi:10.1186/s10020-021-00285-4
Kitada, M., and Koya, D. (2021). Autophagy in metabolic disease and ageing. Nat. Rev. Endocrinol. 17 (11), 647–661. doi:10.1038/s41574-021-00551-9
Kitada, M., Ogura, Y., Suzuki, T., Sen, S., Lee, S. M., Kanasaki, K., et al. (2016). A very-low-protein diet ameliorates advanced diabetic nephropathy through autophagy induction by suppression of the mTORC1 pathway in Wistar fatty rats, an animal model of type 2 diabetes and obesity. Diabetologia 59 (6), 1307–1317. doi:10.1007/s00125-016-3925-4
Klionsky, D. J., Petroni, G., Amaravadi, R. K., Baehrecke, E. H., Ballabio, A., Boya, P., et al. (2021). Autophagy in major human diseases. Embo J. 40 (19), e108863. doi:10.15252/embj.2021108863
Lamb, C. A., Yoshimori, T., and Tooze, S. A. (2013). The autophagosome: Origins unknown, biogenesis complex. Nat. Rev. Mol. Cell Biol. 14 (12), 759–774. doi:10.1038/nrm3696
Lee, Y. H., Kim, S. H., Kang, J. M., Heo, J. H., Kim, D. J., Park, S. H., et al. (2019). Empagliflozin attenuates diabetic tubulopathy by improving mitochondrial fragmentation and autophagy. Am. J. Physiol. Ren. Physiol. 317 (4), F767–f780. doi:10.1152/ajprenal.00565.2018
Lei, L., Zhao, J., Liu, X. Q., Chen, J., Qi, X. M., Xia, L. L., et al. (2021). Wogonin alleviates kidney tubular epithelial injury in diabetic nephropathy by inhibiting PI3K/akt/NF-κB signaling pathways. Drug Des. Devel Ther. 15, 3131–3150. doi:10.2147/dddt.S310882
Lenoir, O., Jasiek, M., Hénique, C., Guyonnet, L., Hartleben, B., Bork, T., et al. (2015). Endothelial cell and podocyte autophagy synergistically protect from diabetes-induced glomerulosclerosis. Autophagy 11 (7), 1130–1145. doi:10.1080/15548627.2015.1049799
Levine, B., and Kroemer, G. (2019). Biological functions of autophagy genes: A disease perspective. Cell 176 (1-2), 11–42. doi:10.1016/j.cell.2018.09.048
Levine, B., Liu, R., Dong, X., and Zhong, Q. (2015). Beclin orthologs: Integrative hubs of cell signaling, membrane trafficking, and physiology. Trends Cell Biol. 25 (9), 533–544. doi:10.1016/j.tcb.2015.05.004
Levy, J. M. M., Towers, C. G., and Thorburn, A. (2017). Targeting autophagy in cancer. Nat. Rev. Cancer 17 (9), 528–542. doi:10.1038/nrc.2017.53
Li, A., Yi, B., Han, H., Yang, S., Hu, Z., Zheng, L., et al. (2022). Vitamin D-VDR (vitamin D receptor) regulates defective autophagy in renal tubular epithelial cell in streptozotocin-induced diabetic mice via the AMPK pathway. Autophagy 18 (4), 877–890. doi:10.1080/15548627.2021.1962681
Li, C., Guan, X. M., Wang, R. Y., Xie, Y. S., Zhou, H., Ni, W. J., et al. (2020). Berberine mitigates high glucose-induced podocyte apoptosis by modulating autophagy via the mTOR/P70S6K/4EBP1 pathway. Life Sci. 243, 117277. doi:10.1016/j.lfs.2020.117277
Li, W. W., Li, J., and Bao, J. K. (2012). Microautophagy: Lesser-known self-eating. Cell Mol. Life Sci. 69 (7), 1125–1136. doi:10.1007/s00018-011-0865-5
Li, X. Y., Wang, S. S., Han, Z., Han, F., Chang, Y. P., Yang, Y., et al. (2017). Triptolide restores autophagy to alleviate diabetic renal fibrosis through the miR-141-3p/PTEN/Akt/mTOR pathway. Mol. Ther. Nucleic Acids 9, 48–56. doi:10.1016/j.omtn.2017.08.011
Li, X. Z., Jiang, H., Xu, L., Liu, Y. Q., Tang, J. W., Shi, J. S., et al. (2021). Sarsasapogenin restores podocyte autophagy in diabetic nephropathy by targeting GSK3β signaling pathway. Biochem. Pharmacol. 192, 114675. doi:10.1016/j.bcp.2021.114675
Li, Y., and Chen, Y. (2019). AMPK and autophagy. Adv. Exp. Med. Biol. 1206, 85–108. doi:10.1007/978-981-15-0602-4_4
Li, Z., and Nakatogawa, H. (2022). Degradation of nuclear components via different autophagy pathways. Trends Cell Biol. 32, 574–584. doi:10.1016/j.tcb.2021.12.008
Liang, Q., Liu, T., Guo, T., Tao, W., Chen, X., Chen, W., et al. (2021). ATF4 promotes renal tubulointerstitial fibrosis by suppressing autophagy in diabetic nephropathy. Life Sci. 264, 118686. doi:10.1016/j.lfs.2020.118686
Lim, J. H., Kim, H. W., Kim, M. Y., Kim, T. W., Kim, E. N., Kim, Y., et al. (2018). Cinacalcet-mediated activation of the CaMKKβ-LKB1-AMPK pathway attenuates diabetic nephropathy in db/db mice by modulation of apoptosis and autophagy. Cell Death Dis. 9 (3), 270. doi:10.1038/s41419-018-0324-4
Lin, J. S., and Susztak, K. (2016). Podocytes: The weakest link in diabetic kidney disease? Curr. Diab Rep. 16 (5), 45. doi:10.1007/s11892-016-0735-5
Lin, M. G., and Hurley, J. H. (2016). Structure and function of the ULK1 complex in autophagy. Curr. Opin. Cell Biol. 39, 61–68. doi:10.1016/j.ceb.2016.02.010
Lin, Y. C., Chang, Y. H., Yang, S. Y., Wu, K. D., and Chu, T. S. (2018). Update of pathophysiology and management of diabetic kidney disease. J. Formos. Med. Assoc. 117 (8), 662–675. doi:10.1016/j.jfma.2018.02.007
Liu, W. J., Gan, Y., Huang, W. F., Wu, H. L., Zhang, X. Q., Zheng, H. J., et al. (2019). Lysosome restoration to activate podocyte autophagy: A new therapeutic strategy for diabetic kidney disease. Cell Death Dis. 10 (11), 806. doi:10.1038/s41419-019-2002-6
Liu, W. J., Shen, T. T., Chen, R. H., Wu, H. L., Wang, Y. J., Deng, J. K., et al. (2015). Autophagy-lysosome pathway in renal tubular epithelial cells is disrupted by advanced glycation end products in diabetic nephropathy. J. Biol. Chem. 290 (33), 20499–20510. doi:10.1074/jbc.M115.666354
Liu, X. Q., Jiang, L., Li, Y. Y., Huang, Y. B., Hu, X. R., Zhu, W., et al. (2022). Wogonin protects glomerular podocytes by targeting Bcl-2-mediated autophagy and apoptosis in diabetic kidney disease. Acta Pharmacol. Sin. 43 (1), 96–110. doi:10.1038/s41401-021-00721-5
Liu, Y., Kou, D., Chu, N., and Ding, G. (2020). Cathelicidin-BF attenuate kidney injury through inhibiting oxidative stress, inflammation and fibrosis in streptozotocin-induced diabetic rats. Life Sci. 257, 117918. doi:10.1016/j.lfs.2020.117918
Liu, Y., Zhang, J., Wang, Y., and Zeng, X. (2017). Apelin involved in progression of diabetic nephropathy by inhibiting autophagy in podocytes. Cell Death Dis. 8 (8), e3006. doi:10.1038/cddis.2017.414
Lu, J., Chen, P. P., Zhang, J. X., Li, X. Q., Wang, G. H., Yuan, B. Y., et al. (2022). GPR43 activation-mediated lipotoxicity contributes to podocyte injury in diabetic nephropathy by modulating the ERK/EGR1 pathway. Int. J. Biol. Sci. 18 (1), 96–111. doi:10.7150/ijbs.64665
Ma, Z., Li, L., Livingston, M. J., Zhang, D., Mi, Q., Zhang, M., et al. (2020). p53/microRNA-214/ULK1 axis impairs renal tubular autophagy in diabetic kidney disease. J. Clin. Invest. 130 (9), 5011–5026. doi:10.1172/jci135536
Matboli, M., Eissa, S., Ibrahim, D., Hegazy, M. G. A., Imam, S. S., and Habib, E. K. (2017). Caffeic acid attenuates diabetic kidney disease via modulation of autophagy in a high-fat diet/streptozotocin- induced diabetic rat. Sci. Rep. 7 (1), 2263. doi:10.1038/s41598-017-02320-z
Matboli, M., Ibrahim, D., Hasanin, A. H., Hassan, M. K., Habib, E. K., Bekhet, M. M., et al. (2021). Epigenetic modulation of autophagy genes linked to diabetic nephropathy by administration of isorhamnetin in Type 2 diabetes mellitus rats. Epigenomics 13 (3), 187–202. doi:10.2217/epi-2020-0353
Matoba, K., Takeda, Y., Nagai, Y., Yokota, T., Utsunomiya, K., and Nishimura, R. (2020). Targeting redox imbalance as an approach for diabetic kidney disease. Biomedicines 8 (2), 40. doi:10.3390/biomedicines8020040
McGrath, K., and Edi, R. (2019). Diabetic kidney disease: Diagnosis, treatment, and prevention. Am. Fam. Physician 99 (12), 751–759.
Mercer, C. A., Kaliappan, A., and Dennis, P. B. (2009). A novel, human Atg13 binding protein, Atg101, interacts with ULK1 and is essential for macroautophagy. Autophagy 5 (5), 649–662. doi:10.4161/auto.5.5.8249
Mitrofanova, A., Burke, G., Merscher, S., and Fornoni, A. (2021). New insights into renal lipid dysmetabolism in diabetic kidney disease. World J. Diabetes 12 (5), 524–540. doi:10.4239/wjd.v12.i5.524
Mizushima, N., and Komatsu, M. (2011). Autophagy: Renovation of cells and tissues. Cell 147 (4), 728–741. doi:10.1016/j.cell.2011.10.026
Mizushima, N., and Levine, B. (2020). Autophagy in human diseases. N. Engl. J. Med. 383 (16), 1564–1576. doi:10.1056/NEJMra2022774
Mora-Fernández, C., Domínguez-Pimentel, V., de Fuentes, M. M., Górriz, J. L., Martínez-Castelao, A., and Navarro-González, J. F. (2014). Diabetic kidney disease: From physiology to therapeutics. J. Physiol. 592 (18), 3997–4012. doi:10.1113/jphysiol.2014.272328
Nakatogawa, H., Ichimura, Y., and Ohsumi, Y. (2007). Atg8, a ubiquitin-like protein required for autophagosome formation, mediates membrane tethering and hemifusion. Cell 130 (1), 165–178. doi:10.1016/j.cell.2007.05.021
Nakatsuka, A., Yamaguchi, S., Eguchi, J., Kakuta, S., Iwakura, Y., Sugiyama, H., et al. (2021). A Vaspin-HSPA1L complex protects proximal tubular cells from organelle stress in diabetic kidney disease. Commun. Biol. 4 (1), 373. doi:10.1038/s42003-021-01902-y
Noda, N. N. (2021). Atg2 and Atg9: Intermembrane and interleaflet lipid transporters driving autophagy. Biochim. Biophys. Acta Mol. Cell Biol. Lipids 1866 (8), 158956. doi:10.1016/j.bbalip.2021.158956
Pavenstädt, H., Kriz, W., and Kretzler, M. (2003). Cell biology of the glomerular podocyte. Physiol. Rev. 83 (1), 253–307. doi:10.1152/physrev.00020.2002
Pontrelli, P., Oranger, A., Barozzino, M., Divella, C., Conserva, F., Fiore, M. G., et al. (2018). Deregulation of autophagy under hyperglycemic conditions is dependent on increased lysine 63 ubiquitination: A candidate mechanism in the progression of diabetic nephropathy. J. Mol. Med. Berl. 96 (7), 645–659. doi:10.1007/s00109-018-1656-3
Popelka, H., Reinhart, E. F., Metur, S. P., Leary, K. A., Ragusa, M. J., and Klionsky, D. J. (2021). Membrane binding and homodimerization of Atg16 via two distinct protein regions is essential for autophagy in yeast. J. Mol. Biol. 433 (5), 166809. doi:10.1016/j.jmb.2021.166809
Potenza, M. A., Gagliardi, S., Nacci, C., Carratu', M. R., and Montagnani, M. (2009). Endothelial dysfunction in diabetes: From mechanisms to therapeutic targets. Curr. Med. Chem. 16 (1), 94–112. doi:10.2174/092986709787002853
Pu, J., Guardia, C. M., Keren-Kaplan, T., and Bonifacino, J. S. (2016). Mechanisms and functions of lysosome positioning. J. Cell Sci. 129 (23), 4329–4339. doi:10.1242/jcs.196287
Reidy, K., Kang, H. M., Hostetter, T., and Susztak, K. (2014). Molecular mechanisms of diabetic kidney disease. J. Clin. Invest. 124 (6), 2333–2340. doi:10.1172/jci72271
Ren, H., Shao, Y., Wu, C., Ma, X., Lv, C., and Wang, Q. (2020). Metformin alleviates oxidative stress and enhances autophagy in diabetic kidney disease via AMPK/SIRT1-FoxO1 pathway. Mol. Cell Endocrinol. 500, 110628. doi:10.1016/j.mce.2019.110628
Rogov, V., Dötsch, V., Johansen, T., and Kirkin, V. (2014). Interactions between autophagy receptors and ubiquitin-like proteins form the molecular basis for selective autophagy. Mol. Cell 53 (2), 167–178. doi:10.1016/j.molcel.2013.12.014
Russell, R. C., Tian, Y., Yuan, H., Park, H. W., Chang, Y. Y., Kim, J., et al. (2013). ULK1 induces autophagy by phosphorylating Beclin-1 and activating VPS34 lipid kinase. Nat. Cell Biol. 15 (7), 741–750. doi:10.1038/ncb2757
Shaw, R. J., Kosmatka, M., Bardeesy, N., Hurley, R. L., Witters, L. A., DePinho, R. A., et al. (2004). The tumor suppressor LKB1 kinase directly activates AMP-activated kinase and regulates apoptosis in response to energy stress. Proc. Natl. Acad. Sci. U. S. A. 101 (10), 3329–3335. doi:10.1073/pnas.0308061100
Shi, Y., and Hu, F. B. (2014). The global implications of diabetes and cancer. Lancet 383 (9933), 1947–1948. doi:10.1016/s0140-6736(14)60886-2
Sohn, M., Kim, K., Uddin, M. J., Lee, G., Hwang, I., Kang, H., et al. (2017). Delayed treatment with fenofibrate protects against high-fat diet-induced kidney injury in mice: The possible role of AMPK autophagy. Am. J. Physiol. Ren. Physiol. 312 (2), F323–f334. doi:10.1152/ajprenal.00596.2015
Sou, Y. S., Tanida, I., Komatsu, M., Ueno, T., and Kominami, E. (2006). Phosphatidylserine in addition to phosphatidylethanolamine is an in vitro target of the mammalian Atg8 modifiers, LC3, GABARAP, and GATE-16. J. Biol. Chem. 281 (6), 3017–3024. doi:10.1074/jbc.M505888200
Sugama, J., Katayama, Y., Moritoh, Y., and Watanabe, M. (2021). Enteropeptidase inhibition improves kidney function in a rat model of diabetic kidney disease. Diabetes Obes. Metab. 23 (1), 86–96. doi:10.1111/dom.14190
Susztak, K., Raff, A. C., Schiffer, M., and Böttinger, E. P. (2006). Glucose-induced reactive oxygen species cause apoptosis of podocytes and podocyte depletion at the onset of diabetic nephropathy. Diabetes 55 (1), 225–233. doi:10.2337/diabetes.55.01.06.db05-0894
Suzuki, K., Kirisako, T., Kamada, Y., Mizushima, N., Noda, T., and Ohsumi, Y. (2001). The pre-autophagosomal structure organized by concerted functions of APG genes is essential for autophagosome formation. Embo J. 20 (21), 5971–5981. doi:10.1093/emboj/20.21.5971
Takahashi, A., Takabatake, Y., Kimura, T., Maejima, I., Namba, T., Yamamoto, T., et al. (2017). Autophagy inhibits the accumulation of advanced glycation end products by promoting lysosomal biogenesis and function in the kidney proximal tubules. Diabetes 66 (5), 1359–1372. doi:10.2337/db16-0397
Takeshige, K., Baba, M., Tsuboi, S., Noda, T., and Ohsumi, Y. (1992). Autophagy in yeast demonstrated with proteinase-deficient mutants and conditions for its induction. J. Cell Biol. 119 (2), 301–311. doi:10.1083/jcb.119.2.301
Tang, C., Livingston, M. J., Liu, Z., and Dong, Z. (2020). Autophagy in kidney homeostasis and disease. Nat. Rev. Nephrol. 16 (9), 489–508. doi:10.1038/s41581-020-0309-2
Tuttle, K. R. (2017). Back to the future: Glomerular hyperfiltration and the diabetic kidney. Diabetes 66 (1), 14–16. doi:10.2337/dbi16-0056
Vegter, S., Perna, A., Postma, M. J., Navis, G., Remuzzi, G., and Ruggenenti, P. (2012). Sodium intake, ACE inhibition, and progression to ESRD. J. Am. Soc. Nephrol. 23 (1), 165–173. doi:10.1681/asn.2011040430
Viana, S. D., Reis, F., and Alves, R. (2018). Therapeutic Use of mTOR inhibitors in renal diseases: Advances, drawbacks, and challenges. Oxid. Med. Cell Longev. 2018, 3693625. doi:10.1155/2018/3693625
Wang, B., Qian, J. Y., Tang, T. T., Lin, L. L., Yu, N., Guo, H. L., et al. (2021a). VDR/Atg3 Axis regulates slit diaphragm to tight junction transition via p62-mediated autophagy pathway in diabetic nephropathy. Diabetes 70 (11), 2639–2651. doi:10.2337/db21-0205
Wang, F., Sun, H., Zuo, B., Shi, K., Zhang, X., Zhang, C., et al. (2021b). Metformin attenuates renal tubulointerstitial fibrosis via upgrading autophagy in the early stage of diabetic nephropathy. Sci. Rep. 11 (1), 16362. doi:10.1038/s41598-021-95827-5
Wang, K., Zheng, X., Pan, Z., Yao, W., Gao, X., Wang, X., et al. (2020). Icariin prevents extracellular matrix accumulation and ameliorates experimental diabetic kidney disease by inhibiting oxidative stress via GPER mediated p62-dependent Keap1 degradation and Nrf2 activation. Front. Cell Dev. Biol. 8, 559. doi:10.3389/fcell.2020.00559
Wang, X., Gao, L., Lin, H., Song, J., Wang, J., Yin, Y., et al. (2018a). Mangiferin prevents diabetic nephropathy progression and protects podocyte function via autophagy in diabetic rat glomeruli. Eur. J. Pharmacol. 824, 170–178. doi:10.1016/j.ejphar.2018.02.009
Wang, X., Gao, Y., Tian, N., Wang, T., Shi, Y., Xu, J., et al. (2019a). Astragaloside IV inhibits glucose-induced epithelial-mesenchymal transition of podocytes through autophagy enhancement via the SIRT-NF-κB p65 axis. Sci. Rep. 9 (1), 323. doi:10.1038/s41598-018-36911-1
Wang, X., Gao, Y., Tian, N., Zhu, Z., Wang, T., Xu, J., et al. (2018b). Astragaloside IV represses high glucose-induced mesangial cells activation by enhancing autophagy via SIRT1 deacetylation of NF-κB p65 subunit. Drug Des. Devel Ther. 12, 2971–2980. doi:10.2147/dddt.S174058
Wang, X., Liu, J., Zhen, J., Zhang, C., Wan, Q., Liu, G., et al. (2014). Histone deacetylase 4 selectively contributes to podocyte injury in diabetic nephropathy. Kidney Int. 86 (4), 712–725. doi:10.1038/ki.2014.111
Wang, Y., Lu, Y. H., Tang, C., Xue, M., Li, X. Y., Chang, Y. P., et al. (2019b). Calcium dobesilate restores autophagy by inhibiting the VEGF/PI3K/AKT/mTOR signaling pathway. Front. Pharmacol. 10, 886. doi:10.3389/fphar.2019.00886
Wang, Y., Zhao, H., Wang, Q., Zhou, X., Lu, X., Liu, T., et al. (2019c). Chinese herbal medicine in ameliorating diabetic kidney disease via activating autophagy. J. Diabetes Res. 2019, 9030893. doi:10.1155/2019/9030893
Wang, Y., Zheng, Z. J., Jia, Y. J., Yang, Y. L., and Xue, Y. M. (2018c). Role of p53/miR-155-5p/sirt1 loop in renal tubular injury of diabetic kidney disease. J. Transl. Med. 16 (1), 146. doi:10.1186/s12967-018-1486-7
Wei, W., An, X. R., Jin, S. J., Li, X. X., and Xu, M. (2018). Inhibition of insulin resistance by PGE1 via autophagy-dependent FGF21 pathway in diabetic nephropathy. Sci. Rep. 8 (1), 9. doi:10.1038/s41598-017-18427-2
Weinbaum, S., Tarbell, J. M., and Damiano, E. R. (2007). The structure and function of the endothelial glycocalyx layer. Annu. Rev. Biomed. Eng. 9, 121–167. doi:10.1146/annurev.bioeng.9.060906.151959
Winiarska, A., Knysak, M., Nabrdalik, K., Gumprecht, J., and Stompór, T. (2021). Inflammation and oxidative stress in diabetic kidney disease: The targets for SGLT2 inhibitors and GLP-1 receptor agonists. Int. J. Mol. Sci. 22 (19). doi:10.3390/ijms221910822
Wu, F., Li, S., Zhang, N., Huang, W., Li, X., Wang, M., et al. (2018). Hispidulin alleviates high-glucose-induced podocyte injury by regulating protective autophagy. Biomed. Pharmacother. 104, 307–314. doi:10.1016/j.biopha.2018.05.017
Wu, M., Zhang, M., Zhang, Y., Li, Z., Li, X., Liu, Z., et al. (2021a). Relationship between lysosomal dyshomeostasis and progression of diabetic kidney disease. Cell Death Dis. 12 (11), 958. doi:10.1038/s41419-021-04271-w
Wu, X. Q., Zhang, D. D., Wang, Y. N., Tan, Y. Q., Yu, X. Y., and Zhao, Y. Y. (2021b). AGE/RAGE in diabetic kidney disease and ageing kidney. Free Radic. Biol. Med. 171, 260–271. doi:10.1016/j.freeradbiomed.2021.05.025
Xiao, L., Xu, X., Zhang, F., Wang, M., Xu, Y., Tang, D., et al. (2017). The mitochondria-targeted antioxidant MitoQ ameliorated tubular injury mediated by mitophagy in diabetic kidney disease via Nrf2/PINK1. Redox Biol. 11, 297–311. doi:10.1016/j.redox.2016.12.022
Xie, Y., Kang, R., Sun, X., Zhong, M., Huang, J., Klionsky, D. J., et al. (2015). Posttranslational modification of autophagy-related proteins in macroautophagy. Autophagy 11 (1), 28–45. doi:10.4161/15548627.2014.984267
Xu, J., Deng, Y., Wang, Y., Sun, X., Chen, S., and Fu, G. (2020). SPAG5‐AS1 inhibited autophagy and aggravated apoptosis of podocytes via SPAG5/AKT/mTOR pathway. Cell Prolif. 53 (2), e12738. doi:10.1111/cpr.12738
Xu, J., Kitada, M., Ogura, Y., Liu, H., and Koya, D. (2021). Dapagliflozin restores impaired autophagy and suppresses inflammation in high glucose-treated HK-2 cells. Cells 10 (6), 1457. doi:10.3390/cells10061457
Xue, M., Yang, F., Le, Y., Yang, Y., Wang, B., Jia, Y., et al. (2021). Klotho protects against diabetic kidney disease via AMPK- and ERK-mediated autophagy. Acta Diabetol. 58 (10), 1413–1423. doi:10.1007/s00592-021-01736-4
Yamada, S., Tanabe, J., Ogura, Y., Nagai, Y., Sugaya, T., Ohata, K., et al. (2021). Renoprotective effect of GLP-1 receptor agonist, liraglutide, in early-phase diabetic kidney disease in spontaneously diabetic Torii fatty rats. Clin. Exp. Nephrol. 25 (4), 365–375. doi:10.1007/s10157-020-02007-2
Yang, C., Chen, X. C., Li, Z. H., Wu, H. L., Jing, K. P., Huang, X. R., et al. (2021a). SMAD3 promotes autophagy dysregulation by triggering lysosome depletion in tubular epithelial cells in diabetic nephropathy. Autophagy 17 (9), 2325–2344. doi:10.1080/15548627.2020.1824694
Yang, D., Livingston, M. J., Liu, Z., Dong, G., Zhang, M., Chen, J. K., et al. (2018). Autophagy in diabetic kidney disease: Regulation, pathological role and therapeutic potential. Cell Mol. Life Sci. 75 (4), 669–688. doi:10.1007/s00018-017-2639-1
Yang, F., Qu, Q., Zhao, C., Liu, X., Yang, P., Li, Z., et al. (2020a). Paecilomyces cicadae-fermented Radix astragali activates podocyte autophagy by attenuating PI3K/AKT/mTOR pathways to protect against diabetic nephropathy in mice. Biomed. Pharmacother. 129, 110479. doi:10.1016/j.biopha.2020.110479
Yang, L., Besschetnova, T. Y., Brooks, C. R., Shah, J. V., and Bonventre, J. V. (2010). Epithelial cell cycle arrest in G2/M mediates kidney fibrosis after injury. Nat. Med. 16 (5), 535–543. doi:10.1038/nm.2144
Yang, S., Lin, C., Zhuo, X., Wang, J., Rao, S., Xu, W., et al. (2020b). Glucagon-like peptide-1 alleviates diabetic kidney disease through activation of autophagy by regulating AMP-activated protein kinase-mammalian target of rapamycin pathway. Am. J. Physiol. Endocrinol. Metab. 319 (6), E1019–e1030. doi:10.1152/ajpendo.00195.2019
Yang, X., Han, X., Wen, Q., Qiu, X., Deng, H., and Chen, Q. (2021b). Protective effect of Keluoxin against diabetic nephropathy in type 2 diabetic mellitus models. Evidence-Based Complementary Altern. Med. 2021, 8455709. doi:10.1155/2021/8455709
Ylä-Anttila, P., Vihinen, H., Jokitalo, E., and Eskelinen, E. L. (2009). 3D tomography reveals connections between the phagophore and endoplasmic reticulum. Autophagy 5 (8), 1180–1185. doi:10.4161/auto.5.8.10274
Yokoyama, H., Araki, S. I., Kawai, K., Yamazaki, K., Shirabe, S. I., Sugimoto, H., et al. (2020). The prognosis of patients with type 2 diabetes and nonalbuminuric diabetic kidney disease is not always poor: Implication of the effects of coexisting macrovascular complications (JDDM 54). Diabetes Care 43 (5), 1102–1110. doi:10.2337/dc19-2049
Yuan, J., Dong, X., Yap, J., and Hu, J. (2020a). The MAPK and AMPK signalings: Interplay and implication in targeted cancer therapy. J. Hematol. Oncol. 13 (1), 113. doi:10.1186/s13045-020-00949-4
Yuan, Y., Li, L., Zhu, L., Liu, F., Tang, X., Liao, G., et al. (2020b). Mesenchymal stem cells elicit macrophages into M2 phenotype via improving transcription factor EB-mediated autophagy to alleviate diabetic nephropathy. Stem Cells 38 (5), 639–652. doi:10.1002/stem.3144
Zhan, X., Yan, C., Chen, Y., Wei, X., Xiao, J., Deng, L., et al. (2018). Celastrol antagonizes high glucose-evoked podocyte injury, inflammation and insulin resistance by restoring the HO-1-mediated autophagy pathway. Mol. Immunol. 104, 61–68. doi:10.1016/j.molimm.2018.10.021
Zhang, M., Zhang, Y., Xiao, D., Zhang, J., Wang, X., Guan, F., et al. (2020). Highly bioavailable berberine formulation ameliorates diabetic nephropathy through the inhibition of glomerular mesangial matrix expansion and the activation of autophagy. Eur. J. Pharmacol. 873, 172955. doi:10.1016/j.ejphar.2020.172955
Zhang, M. Z., Wang, Y., Paueksakon, P., and Harris, R. C. (2014). Epidermal growth factor receptor inhibition slows progression of diabetic nephropathy in association with a decrease in endoplasmic reticulum stress and an increase in autophagy. Diabetes 63 (6), 2063–2072. doi:10.2337/db13-1279
Zhang, X. X., Jiang, C. H., Liu, Y., Lou, D. X., Huang, Y. P., Gao, M., et al. (2019). Cyclocarya paliurus triterpenic acids fraction attenuates kidney injury via AMPK-mTOR-regulated autophagy pathway in diabetic rats. Phytomedicine 64, 153060. doi:10.1016/j.phymed.2019.153060
Zhang, X., Zhang, L., Chen, Z., Li, S., Che, B., Wang, N., et al. (2021). Exogenous spermine attenuates diabetic kidney injury in rats by inhibiting AMPK/mTOR signaling pathway. Int. J. Mol. Med. 47 (3). doi:10.3892/ijmm.2021.4860
Zhao, X., Chen, Y., Tan, X., Zhang, L., Zhang, H., Li, Z., et al. (2018a). Advanced glycation end-products suppress autophagic flux in podocytes by activating mammalian target of rapamycin and inhibiting nuclear translocation of transcription factor EB. J. Pathol. 245 (2), 235–248. doi:10.1002/path.5077
Zhao, Y., Zhang, W., Jia, Q., Feng, Z., Guo, J., Han, X., et al. (2018b). High dose vitamin E attenuates diabetic nephropathy via alleviation of autophagic stress. Front. Physiol. 9, 1939. doi:10.3389/fphys.2018.01939
Zheng, D., Tao, M., Liang, X., Li, Y., Jin, J., and He, Q. (2020). p66Shc regulates podocyte autophagy in high glucose environment through the Notch-PTEN-PI3K/Akt/mTOR pathway. Histol. Histopathol. 35 (4), 405–415. doi:10.14670/hh-18-178
Zheng, G. S., Tan, Y. M., Shang, Y. Y., Liu, Y. P., Hu, B. A., Wang, D., et al. (2021). CIDEC silencing attenuates diabetic nephropathy via inhibiting apoptosis and promoting autophagy. J. Diabetes Investig. 12 (8), 1336–1345. doi:10.1111/jdi.13534
Keywords: diabetic kidney disease, autophagy, autophagosome, lysosome, podocytes, renal tubular epithelial cells
Citation: Zhang Z, Sun Y, Xue J, Jin D, Li X, Zhao D, Lian F, Qi W and Tong X (2022) The critical role of dysregulated autophagy in the progression of diabetic kidney disease. Front. Pharmacol. 13:977410. doi: 10.3389/fphar.2022.977410
Received: 24 June 2022; Accepted: 04 August 2022;
Published: 25 August 2022.
Edited by:
Jun-Yan Liu, Chongqing Medical University, ChinaReviewed by:
Yuan Xinxu, Virginia Commonwealth University, United StatesCopyright © 2022 Zhang, Sun, Xue, Jin, Li, Zhao, Lian, Qi and Tong. This is an open-access article distributed under the terms of the Creative Commons Attribution License (CC BY). The use, distribution or reproduction in other forums is permitted, provided the original author(s) and the copyright owner(s) are credited and that the original publication in this journal is cited, in accordance with accepted academic practice. No use, distribution or reproduction is permitted which does not comply with these terms.
*Correspondence: Fengmei Lian, bGZtNTY1QHNvaHUuY29t; Wenxiu Qi, cWl3ZW54aXUwNTE3QDE2My5jb20=; Xiaolin Tong, dG9uZ3hpYW9saW5AdmlwLjE2My5jb20=
†These authors have contributed equally to this work
Disclaimer: All claims expressed in this article are solely those of the authors and do not necessarily represent those of their affiliated organizations, or those of the publisher, the editors and the reviewers. Any product that may be evaluated in this article or claim that may be made by its manufacturer is not guaranteed or endorsed by the publisher.
Research integrity at Frontiers
Learn more about the work of our research integrity team to safeguard the quality of each article we publish.