- 1Department of Neurology, Yueyang Hospital of Integrated Traditional Chinese and Western Medicine, Shanghai University of Traditional Chinese Medicine, Shanghai, China
- 2Shanghai Frontiers Science Center of TCM Chemical Biology, Institute of Interdisciplinary Integrative Medicine Research, Shanghai University of Traditional Chinese Medicine, Shanghai, China
- 3School of Pharmacy, Zunyi Medical University, Zunyi, China
- 4Key Laboratory of Liver and Kidney Diseases (Ministry of Education), Institute of Liver Diseases, Shanghai Key Laboratory of Traditional Chinese Clinical Medicine, Shuguang Hospital Affiliated to Shanghai University of Traditional Chinese Medicine, Shanghai, China
Human cytochrome P450 3A4 (hCYP3A4) is a predominant enzyme to trigger clinically relevant drug/herb-drug interactions (DDIs or HDIs). Although a number of herbal medicines have been found with strong anti-hCYP3A4 effects in vitro, the in vivo modulatory effects of herbal medicines on hCYP3A4 and their potential risks to trigger HDIs are rarely investigated. Herein, we demonstrate a case study to efficiently find the herbal medicine(s) with potent hCYP3A4 inhibition in vitro and to accurately assess the potential HDIs risk in vivo. Following screening over 100 herbal medicines, the Chinese herb Styrax was found with the most potent hCYP3A4 inhibition in HLMs. In vitro assays demonstrated that Styrax could potently inhibit mammalian CYP3A in liver and intestinal microsomes from both humans and rats. In vivo pharmacokinetic assays showed that Styrax (i.g., 100 mg/kg) significantly elevated the plasma exposure of two CYP3A-substrate drugs (midazolam and felodipine) when midazolam or felodipine was administered orally. By contrast, the plasma exposure of either midazolam or felodipine was hardly affected by Styrax (i.g.) when the victim drug was administered intravenously. Further investigations demonstrated that seven pentacyclic triterpenoid acids (PTAs) in Styrax were key substances responsible for CYP3A inhibition, while these PTAs could be exposed to intestinal tract at relatively high exposure levels but their exposure levels in rat plasma and liver were extremely low. These findings well explained why Styrax (i.g.) could elevate the plasma exposure of victim drugs only when these agents were orally administrated. Collectively, our findings demonstrate that Styrax can modulate the pharmacokinetic behavior of CYP3A-substrate drugs via inhibiting intestinal CYP3A, which is very helpful for the clinical pharmacologists to better assess the HDIs triggered by Styrax or Styrax-related herbal products.
Introduction
The concomitant use of herbal medicines and Western medicines is widely used in clinical settings for curing various diseases including digestive diseases, cardiovascular and cerebrovascular diseases, infectious diseases, especially in China and other Asia countries (Zhang and Xu, 2012; Su and Geng, 2016; Xu et al., 2017; Qiu et al., 2020). However, the herb-drug interactions (HDIs) caused by the concomitant use of herbal medicines and Western medicines cannot be ignored (Gouws et al., 2012; Fasinu et al., 2015; Gallo et al., 2019; Parvez and Rishi, 2019). A number of studies have shown that cytochrome P450 3A4 (CYP3A4, mainly expressed in liver and small intestine, Supplementary Figure S1, https://www.proteinatlas.org/search/CYP3A4) plays a crucial role in the metabolism and detoxification of a wide range of Western medicines in vivo, thus acts as a key mediator in herb-drug interactions (HDIs) and drug-drug interactions (DDIs) (Galetin et al., 2007; Zhou, 2008; Tian and Hu, 2014). It has been reported that a wide range of herbal medicines can regulate the pharmacokinetic behavior of some important CYP3A-substrate drugs (especially for those drugs with very narrow therapeutic windows) by inhibiting or inactivating CYP3A, which may trigger clinically significant HDIs and adverse drug reactions (Zhou et al., 2019; Zhang F. et al., 2021; Zhang F. et al., 2022). Therefore, it is urgent and necessary to accurately assess the inhibitory effects of herbal medicines against human CYP3A, and to precisely evaluate the potential risks of herbal medicines to trigger clinically relevant HDIs when they are concomitantly used with CYP3A-substrate drugs.
Recently, our group and colleagues developed a novel fluorescence-based high-throughput assay for assessing the inhibitory effects of compounds/herbal extracts on human CYP3A4, which offers a powerful platform for investigating CYP3A4-related HDIs or DDIs (Ning et al., 2019; Wu et al., 2021; Jin et al., 2022; Tu et al., 2022). With the help of fluorescence-based high-throughput CYP3A4 inhibition assays, the inhibitory effects of more than 100 herbal medicines against hCYP3A4 were assessed in human liver preparations. The results clearly showed that the Chinese herb Styrax (a herbal medicine used for treating cardiovascular diseases) displayed the most potent inhibitory effect against hCYP3A4 (Figure 1). Notably, Styrax is often used as a key material for preparing some marketed herbal products (such as Suhexiang Pill, Shexiang Baoxin Pill, Subing Dropping Pill, Guanxin Suhe Pill, Shixiang fansheng Pill), owing to the high safety profiles and beneficial effects of this herb in preventing and treating cardiovascular diseases (Bian and Zhang, 2016; Guo et al., 2021). In Asia countries, Styrax and Styrax-related herbal products are frequently used in combination with a panel of marketed cardiovascular drugs (such as warfarin, digoxin and simvastatin) to treat cardiovascular diseases (Zhu, 2009; Tao et al., 2016; Yang et al., 2021). As an important ester spice, Styrax is also used for making a variety of foods (Yu, 2021). Since Styrax is widely used as a key material for making Chinese medicines or foods, the HDIs between this herb and co-administrated Western drugs should be carefully investigated.
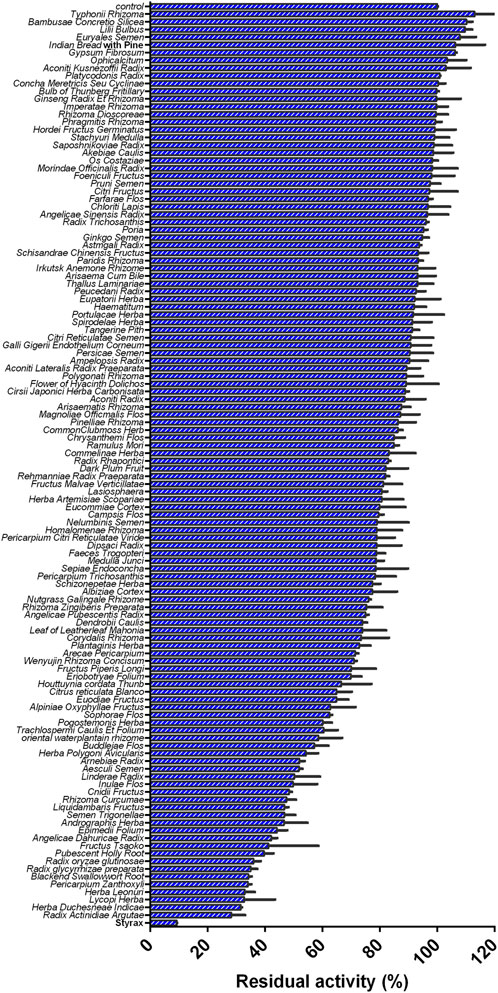
FIGURE 1. Inhibition effects of 119 Chinese medicine granules/Chinese medicines (100 μg/ml, final concentration) against CYP3A4-catalyzed NEN 4-hydroxylation in HLMs.
The major constituents in Styrax have been reported, in which cinnamic acids, phenylpropanoids and pentacyclic triterpenoid acids (PTAs) are major constituents in this herb, while the PTAs in Styrax are reported as naturally occurring CYP3A inhibitors (Zhang et al., 2020). However, the oral bioavailabilities and plasma exposure of PTAs are extremely low (Wang et al., 2020; Ban et al., 2021; Zhang Y. W. et al., 2021; Ren et al., 2021), and it is not clear whether oral administration of Styrax can inhibit intestinal CYP3A or hepatic CYP3A in living systems and then modulate the pharmacokinetic behavior of CYP3A-substrate drugs in vivo. Therefore, this study aims to solve two key questions: 1) Can Styrax change the pharmacokinetic behavior of co-administrated CYP3A-substrate drugs and trigger HDIs in vivo; 2) Styrax triggers clinically relevant HDIs via inhibiting either hepatic CYP3A4 or intestinal CYP3A4 or both.
To better solve these two questions, two CYP3A-substrate drugs (midazolam and felodipine) were selected as victim drugs in this work, while two administrated ways (oral and intravenous) were used to study the pharmacokinetic interactions between Styrax and two CYP3A-substrate drugs (midazolam and felodipine). Briefly, the inhibitory effects of Styrax against CYP3A-catalyzed midazolam 1′-hydroxylation were carefully assessed in liver and intestinal microsomes from both humans and rats. After then, the pharmacokinetic interactions between Styrax (oral) and CYP3A-substrate drugs (midazolam and felodipine) by two administrated ways (oral and intravenous) were assessed in rats. Furthermore, the inhibitory effects of the PTAs in Styrax on CYP3A were assessed, while the tissue distributions of seven major PTAs in Styrax (the key substances responsible for CYP3A inhibition) were investigated carefully.
Materials and methods
Chemicals and reagents
The standard extract of Styrax was acquired by Shanghai Hutchison Pharmaceuticals (Shanghai, China). More than 100 herbal medicines granules were provided by Jiangyin Tianjiang Pharmaceutical Co., Ltd. (Wuxi, Jiangsu, China). Lansoprazole was provided by Hairong (Dujiangyan, Sichuan, China). D-glucose-6-phosphate (G-6-P), glucose-6-phosphate dehydrogenase (G-6-PDH) and β-NADP+ were purchased from Sigma-Aldrich (St. Louis, MO, United States). MgCl2, 0.9% NaCl, and sodium carboxymethyl cellulose (CMC-Na) were provided by Sinopharm Chemical Reagent (Shanghai, China). The soybean oil was supplied by COFCO Fulinmen Food Marketing Co., Ltd. (China). Midazolam was from J&K Scientific (Shanghai, China). 1′-Hydroxymidazolam was provided by Shanghai Macklin Biochemical Co., Ltd. (Shanghai, China). Felodipine was purchased from Shanghai Yuanye Biotechnology Co., Ltd. (Shanghai, China). Osalmide was ordered from Meilun Bio. Tech (Dalian, China). N-ethyl-1,8-naphthalimide (NEN) and N-ethyl-4-hydroxyl-1,8-naphthalimide (NEHN) were synthesized by using the previously reported protocol (Ning et al., 2019). Betulinic acid, epibetulinic acid, betulonic acid, oleanonic acid, oleanolic acid, maslinic acid and corosolic acid were supplied by Shanghai Standard Technology Co., Ltd. (Shanghai, China). The pooled HLMs (Lot No. H0610), pooled HIMs (Lot No. H0610.I), pooled RIMs (Lot No. R1000.I) were obtained from XenoTech (United States). The pooled RLMs (Lot No. LM-DS-02M) was ordered from Research Institute for Liver Diseases (RILD, Shanghai, China). BCA Protein Quantification Kit was acquired by Thermo Fisher Scientific Co., Ltd. Ultrapure water purified by Milli-Q® Integral Water Purification System (Millipore, United States) was used throughout. LC grade methanol, acetonitrile and formic acid were supplied by Fisher Scientific Co., Ltd. (Fair Lawn, NJ, United States). Other reagents were of the highest grade commercially available. The purities of all compounds used in this study were greater than 98%.
CYP3A inhibition assays
Inhibition of CYP3A4-catalyzed NEN 4-hydroxylation by herbal medicines or natural constituents
The inhibitory effects of more than 100 herbal medicines against CYP3A4 were assayed by using a previously reported fluorescence-based biochemical assay (Ning et al., 2019; Tu et al., 2022). In brief, PBS (pH 7.4, 100 mM), herbal medicines (100 μg/ml, final concentration)/PTAs/solvent, NEN, G-6-P, G-6-PDH, MgCl2, and HLMs were added to the 96-well plate in turn, then the samples were vortexed and pre-incubated for 3 min at 37 °C. The oxidative reactions were initiated by adding β-NADP+ to yield a final volume of 200 μl, then the formation rates of NEHN (hydroxylated metabolite of NEN) were continuously monitored for 30 min at 37 °C using a 96-well fluorescence microplate reader (SpectraMax® iD3, Molecular Devices, Austria). The percentage of organic solvent in all incubation is not over 1%. Please refer to the supplementary material (Supplementary Table S1) for detailed experimental conditions.
Inhibition of CYP3A-catalyzed midazolam 1′-hydroxylation by Styrax or its constituents
The inhibitory effects of Styrax or its constituents against CYP3A were assayed by using midazolam as the substrate (Lee et al., 2013; Dunkoksung et al., 2019; Fang et al., 2020; Tu et al., 2020). Briefly, PBS (pH 7.4, 100 mM), Styrax/PTAs/solvent, midazolam, G-6-P, G-6-PDH, MgCl2, and enzyme sources (HLMs/RLMs/HIMs/RIMs, 0.1 mg/ml, final concentrations) were added to the EP tube in turn and vortexed, then the samples were pre-incubated for 3 min at 37 °C. Next, the reactions were initiated by adding β-NADP+ to yield a final volume of 200 μl. Following incubation for 30 min at 37 °C, and 200 μl ice-cold acetonitrile containing internal standard (IS, 90 ng/ml) was subsequently added to quench the oxidative reaction. The mixture was then centrifuged at 20,000 ×g, 4 °C for 20 min, and the supernatant was quantified by LC-MS/MS analysis as described in Supplementary Table S2.
Pharmacokinetic studies
Sprague-Dawley rats (weighing 200–220 g) were purchased from Shanghai Xipuer-Bikai Experimental Animal Co., Ltd. (Shanghai, China, Permit Number: SCXK (Hu) 2018-0006). The animal experiments were carried out in Experimental Animal Center of Shanghai University of Traditional Chinese Medicine (Approval Number: PZSHUTCM210709016) and conducted in accordance with the State Committee of Science and Technology of China. In brief, forty male rats were housed under controlled environmental conditions (22 ± 2°C; 40%–80% relative humidity; 12 h light/dark cycle) for 1 week with free access to food and water. The rats were fasted overnight before dosing but were free to drink water, and provided food after finishing the study. Styrax was suspended in soybean oil. Oral midazolam/felodipine was suspended in 0.5% CMC-Na, and intravenous midazolam/felodipine was suspended in 0.9% NaCl. Styrax or soybean oil was administered orally at a dose of 100 mg/kg. The oral dose of midazolam is 20 mg/kg and the intravenous dose is 5 mg/kg. The oral dose of felodipine is 10 mg/kg and the intravenous dose is 2 mg/kg. The dose of Styrax in rats was referred to the daily recommended oral dose in humans. The dose of midazolam and felodipine in rats was referred to the doses in published literature (Lundahl et al., 1997; Kotegawa et al., 2002; Li et al., 2010; Surya Sandeep et al., 2014). Forty rats were randomly divided into eight groups.
group 1: Styrax + midazolam (oral);
group 2: soybean oil + midazolam (oral);
group 3: Styrax + midazolam (intravenous);
group 4: soybean oil + midazolam (intravenous);
group 5: Styrax + felodipine (oral);
group 6: soybean oil + felodipine (oral);
group 7: Styrax + felodipine (intravenous);
group 8: soybean oil + felodipine (intravenous);
Styrax (100 mg/kg, experiment group) or soybean oil (100 mg/kg, control group) was administered orally. After 30 min, midazolam/felodipine was administered orally and intravenously. For oral midazolam groups, blood samples were collected at 5, 10, 20, 30, 60 min, 2, 3, 4, 6, 9 h. For intravenous midazolam groups, blood samples were collected at 2, 5, 10, 20, 30, 60 min, 2, 3, 4, 6, 9 h. For oral felodipine groups, blood samples were collected at 10, 30, 60 min, 3, 4, 6, 8, 10, 24 h. For intravenous felodipine groups, blood samples were collected at 2, 5, 10, 15, 30, 45, 60 min, 4, 6, 10 h. Then blood samples were centrifuged at 8,000 rpm, 4 °C for 10 min, the supernatant was stored at −80 °C until analysis (Li et al., 2010; Xue et al., 2011; Kallem et al., 2013; Xiang et al., 2017). 50 μl plasma was mixed with 50 μl ultrapure water and 300 μl acetonitrile (containing 50 ng/ml IS). The mixture was vortexed for 1 min and then centrifuged at 10,000 × g, 4 °C for 10 min. The supernatant (320 μl) was dried by nitrogen and dissolved with 80 μl of 10% acetonitrile. The supernatant (5 μl) was injected for UHPLC-Q-Orbitrap HRMS analysis (Mass spectrometry parameters were described in Supplementary Figure S2,S3 & Supplementary Table S3,S4). Both midazolam and felodipine were quantified within the linear range of their calibration curves. The pharmacokinetic parameters of midazolam, felodipine were fitted by standard noncompartmental analyses using PKSolver.
Tissue distribution of PTAs of Styrax in rats
Animal handling and sampling
Nine Male Sprague-Dawley rats (250–300 g) were purchased from Shanghai Sippe-Bk Lab Animal Co., Ltd. (Shanghai, China) and were fed in Experimental Animal Center of SHUTCM. All animals were housed under controlled environmental conditions (22 ± 2°C; 40%–80% relative humidity; 12 h light/dark cycle) for 1 week with free access to food and water. The rats were fasted overnight before dosing but were free to drink water. Nine rats were randomly divided into three groups (1, 2, and 4 h). Styrax (100 mg/kg) was administered orally, then nine rats were anesthetized by pentobarbital after administrating 1, 2, and 4 h, respectively. Blood samples were collected from the abdominal aorta, and the tissues (including the liver, duodenum, jejunum, ileum and colon) were removed rapidly and placed on ice. After then, the blood samples were centrifuged at 8,000 rpm, 4 °C for 10 min, and the supernatant was stored at −80 °C until analysis.
Determination of PTAs in rat tissues
Firstly, each tissue (such as liver, duodenum, jejunum, ileum, colon) was accurately weighed after sucking the water on tissue surface. Each tissue was put into an EP tube and then cut (3-5 times) by a scissor. After then, PBS (pH 7.4) and magnetic beads were added into EP tube, grind the tissue by a tissue grinder. The tissue grinding fluid was centrifuged at 9,000 g, 4 °C for 20 min. 50 μl tissue supernatant was diluted by adding 250 μl LC grade acetonitrile. The protein was precipitated by centrifuging at 20,000 ×g, 4 °C for 30 min and the supernatant was collected to determine the protein concentration by a BCA Protein Quantification Kit. After then, the concentrations of seven PTAs were determined by using UHPLC-Q-Orbitrap HRMS analysis. Mass spectrometry parameters were described in Supplementary Figure S4 & Supplementary Table S5.
Data analysis
The dose-inhibition curves of Styrax/PTAs against CYP3A were depicted by using different dosages of Styrax/PTAs, while the half maximal inhibition concentration (IC50) was determined as the concentration of Styrax/PTAs at the catalytic activity of CYP3A was inhibited by 50% compared to the negative control (solvent only). In this study, all assays were tested in triplicate, while the data were shown as mean ± standard deviation (SD). IC50 values were fitted by GraphPad Prism 6.0 (GraphPad Software, Inc., La Jolla, United States). Meanwhile, the following formula was used to calculate the residual activities of CYP3A:
The residual activity (%) = (the Peak Area or Fluorescence value of the metabolite in the presence of inhibitor)/the Peak Area or Fluorescence value of the metabolite in negative control (solvent only) × 100%.
Results
Screening of the herbal medicines with potent CYP3A4 inhibition
Firstly, with the help of fluorescence-based high-throughput CYP3A4 inhibition assay, the inhibitory potentials of more than 100 herbal medicines against CYP3A4-catalyzed NEN 4-hydroxylation were assayed in HLMs by using a single dose (100 μg/ml, final concentration). As shown in Figure 1 and Supplementary Table S6, all tested herbal medicines showed different inhibitory effects on CYP3A4-catalyzed NEN 4-hydroxylation in HLMs. Among all the tested herbal medicines, the Chinese herb Styrax was found with the most potent hCYP3A4 inhibition in HLMs. At the dose of 100 μg/ml, Styrax inhibited CYP3A4-catalyzed NEN 4-hydroxylation near completely, with the residual activity of 9.25%. By contrast, other herbal medicines displayed relatively weak inhibitory effects on hCYP3A4 at the same dose. These findings clearly demonstrate that the Chinese herb Styrax displays strong CYP3A4 inhibition potency, which may interact with CYP3A4-substrate drugs to trigger HDIs in living systems.
Inhibitory effects of Styrax against midazolam 1′-hydroxylation
Next, the inhibitory effects of Styrax against mammalian CYP3A were validated by using a substrate-drug (midazolam) in different tissue preparations. To this end, the inhibitory effects of Styrax against CYP3A-catalyzed midazolam 1′-hydroxylation were carefully investigated in different enzyme sources including HLMs, HIMs, RLMs, and RIMs. As shown in Figure 2 and Supplementary Table S7, Styrax could potently inhibit CYP3A-catalyzed midazolam 1′-hydroxylation, with IC50 values of 3.72 μg/ml, 2.84 μg/ml, 2.90 μg/ml and 1.67 μg/ml, for HLMs, HIMs, RLMs, RIMs, respectively. These findings demonstrated that Styrax could potently inhibit mammalian CYP3A in liver and intestinal microsomes from both human and rat in vitro, with similar inhibition tendency and potency. However, it is still unclear that Styrax can inhibit hepatic CYP3A or intestinal CYP3A in vivo. Therefore, the in vivo pharmacokinetic interactions between Styrax and midazolam were carefully investigated by using two administrated ways (i.v. and i.g.).
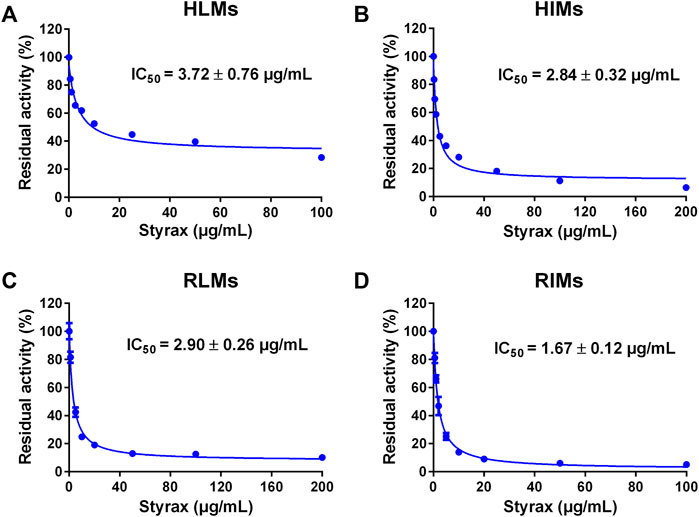
FIGURE 2. (A) Dose-inhibition curve of Styrax extract towards midazolam 1′-hydroxylation in HLMs. (B) Dose-inhibition curve of Styrax extract towards midazolam 1′-hydroxylation in HIMs. (C) Dose-inhibition curve of Styrax extract towards midazolam 1′-hydroxylation in RLMs. (D) Dose-inhibition curve of Styrax extract towards midazolam 1′-hydroxylation in RIMs. Data are expressed as mean ± SD.
Pharmacokinetic interactions between Styrax and midazolam by i.v. and i.g.
Next, the in vivo effects of Styrax on the pharmacokinetics of midazolam (i.v. and i.g.) were carefully investigated in rats, by using soybean oil (without Styrax) as control groups. The blood concentration-time profiles of midazolam with two administrated ways (oral and intravenous) were shown in Figure 3 and the pharmacokinetic parameters were detailed in Table 1. Interestingly, the plasma exposure of midazolam was hardly affected by Styrax when midazolam was administered intravenously in combination with Styrax (i.g.) to rats. By contrast, when midazolam (i.g.) was co-administered orally with Styrax (i.g.) to rats, the pharmacokinetic behavior of midazolam was significantly changed compared with the control group (soybean oil instead of Styrax). The AUC(0-inf) of midazolam (i.g.) was increased 2.04-fold (from 211.23 ng/mL·h to 431.20 ng/mL·h), while the Cmax value of midazolam (i.g.) in rats plasma was increased 2.28-fold (from 129.16 ng/ml to 294.05 ng/ml). Moreover, the metabolic half-life (t1/2) of midazolam (i.g.) was prolonged 2.11-fold (from 2.14 to 4.52 h). These findings clearly suggested that Styrax strongly elevated the plasma exposure of midazolam when midazolam was co-administered orally. Considering that both intestinal CYP3A and hepatic CYP3A participate in midazolam metabolism when this agent is administrated orally, while Styrax (i.g.) cannot influence hepatic metabolism of midazolam, suggesting that Styrax may modulate the in vivo pharmacokinetic behavior of midazolam (i.g.) via inhibiting intestinal CYP3A but not hepatic CYP3A.
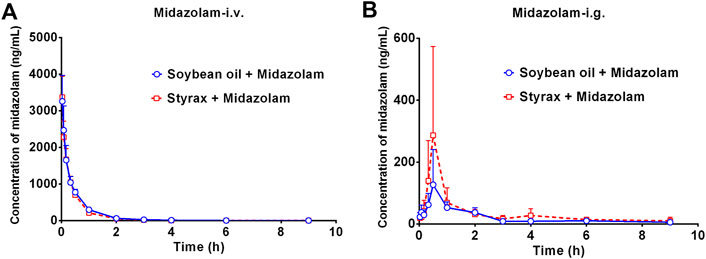
FIGURE 3. (A) The mean plasma concentration-time curves of midazolam after intravenous administration of midazolam (5 mg/kg) in rats pretreated with Soybean oil (blue) or Styrax (i.g. 100 mg/kg, red). (B) The mean plasma concentration-time curves of midazolam after intragastric administration of midazolam (20 mg/kg) in rats pretreated with (blue) or Styrax (i.g. 100 mg/kg, red). Data are expressed as the mean ± SD.
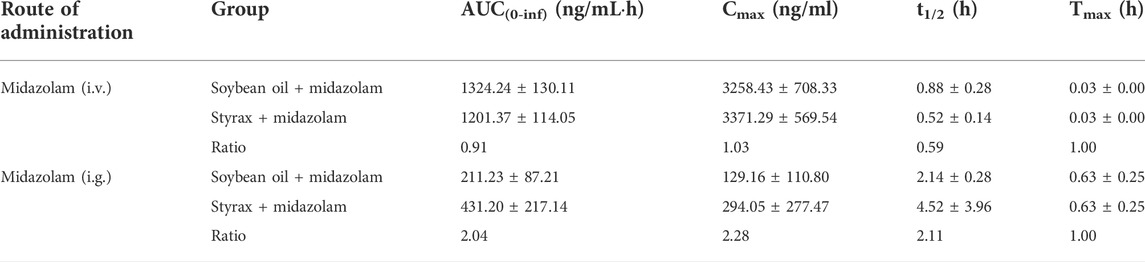
TABLE 1. Pharmacokinetic parameters of midazolam after administrating midazolam (i.v., 5 mg/kg; i.g., 20 mg/kg) in rats pretreated with Soybean oil, Styrax (100 mg/kg). Data are the mean ± SD.
Pharmacokinetic interactions between Styrax and felodipine by i.v. and i.g.
To further confirm that Styrax could influence the pharmacokinetic behavior of orally administrated agent, felodipine (an orally administrated CYP3A-substrate drug) was used as a victim drug to assess the pharmacokinetic interactions between Styrax (i.g., 100 mg/kg) and felodipine via two administrated ways (i.v. and i.g.). As shown in Figure 4A and Table 2, when felodipine was administered intravenously in rats, Styrax displayed negligible effect on the pharmacokinetic parameters of felodipine. Notably, as shown in Figure 4B and Table 2, compared with the control group (soybean oil instead of Styrax), Styrax significantly increased the values of AUC(0-inf) (1.41-fold, from 111.72 ng/mL·h to 156.98 ng/mL·h) and Cmax (2.16-fold, from 17.40 ng/ml to 37.58 ng/ml) of felodipine when felodipine was administered orally in combination with Styrax (i.g.) in rats. These findings further suggest that Styrax can modulate the pharmacokinetic behavior of orally administrated CYP3A-substrate drugs (midazolam and felodipine) by inhibiting intestinal CYP3A but not hepatic CYP3A in vivo.
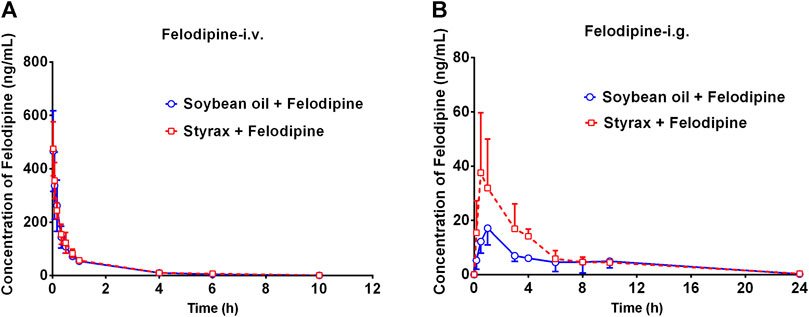
FIGURE 4. (A) The mean plasma concentration-time curves of felodipine after intravenous administration of felodipine (2 mg/kg) in rats pretreated with Soybean oil (blue) or Styrax (i.g. 100 mg/kg, red). (B) The mean plasma concentration-time curves of felodipine after intragastric administration of felodipine (10 mg/kg) in rats pretreated with (blue) or Styrax (i.g. 100 mg/kg, red). Data are expressed as the mean ± SD.
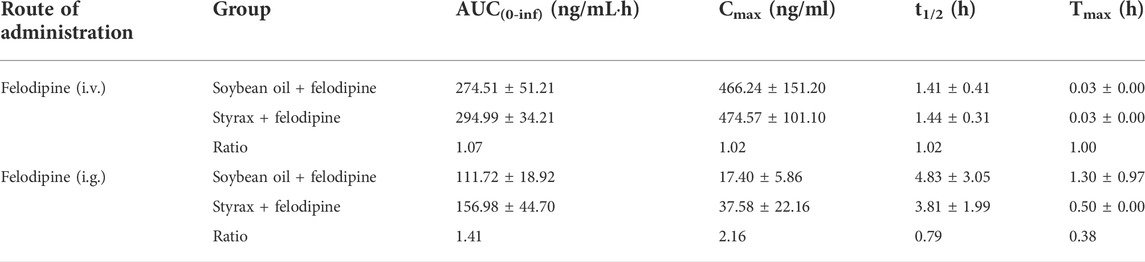
TABLE 2. Pharmacokinetic parameters of felodipine after administrating felodipine (i.v., 2 mg/kg; i.g., 10 mg/kg) in rats pretreated with Soybean oil, Styrax (100 mg/kg). Data are the mean ± SD.
Chemical profiling of Styrax
Next, the chemical constituents in Styrax were analyzed by using UHPLC-Q-Exactive Orbitrap HRMS. The total ion chromatograms (TICs) of Styrax were shown in Supplementary Figure S5. A total of 21 chemical constituents were identified in Styrax by comparing the retention times, MS fragmentation behavior, literature information and the MS/MS databases of natural products. The major constituents in Styrax are cinnamic acids, phenylpropanoids and PTAs. The mass spectrometry data of the identified constituents in Styrax were summarized in Supplementary Table S8.
The inhibitory effects of seven PTAs on CYP3A
A previous study revealed that the PTAs in Styrax displayed potent inhibition on CYP3A-catalyzed testosterone 6β-hydroxylation (Zhang et al., 2020). In this study, the inhibitory effects of these PTAs on CYP3A were assessed in HLMs by using a CYP3A-substrate drug (midazolam) and a newly reported CYP3A4 fluorescent substrate (NEN). As shown in Figures 5, 6 and Table 3, as well as Supplementary Figure S6, S7, the PTAs inhibited CYP3A-catalyzed midazolam 1′-hydroxylation in a dose-dependent manner, showing the IC50 values of 19.39, 32.20, 14.83, 7.74, 0.64, 2.51, and 0.99 μM, for maslinic acid, corosolic acid, oleanolic acid, betulinic acid, epibetulinic acid, betulonic acid and oleanonic acid, respectively. Meanwhile, all tested PTAs dose-dependently inhibited CYP3A4-catalyzed NEN 4-hydroxylation, with the IC50 values of 3.44, 41.93, 1.10, 4.11, 0.47, 0.96, and 0.80 μM, for maslinic acid, corosolic acid, oleanolic acid, betulinic acid, epibetulinic acid, betulonic acid and oleanonic acid, respectively. These results suggest that the PTAs in Styrax are key substances responsible for CYP3A inhibition, while epibetulinic acid, betulonic acid and oleanonic acid display potent CYP3A inhibitory effects.
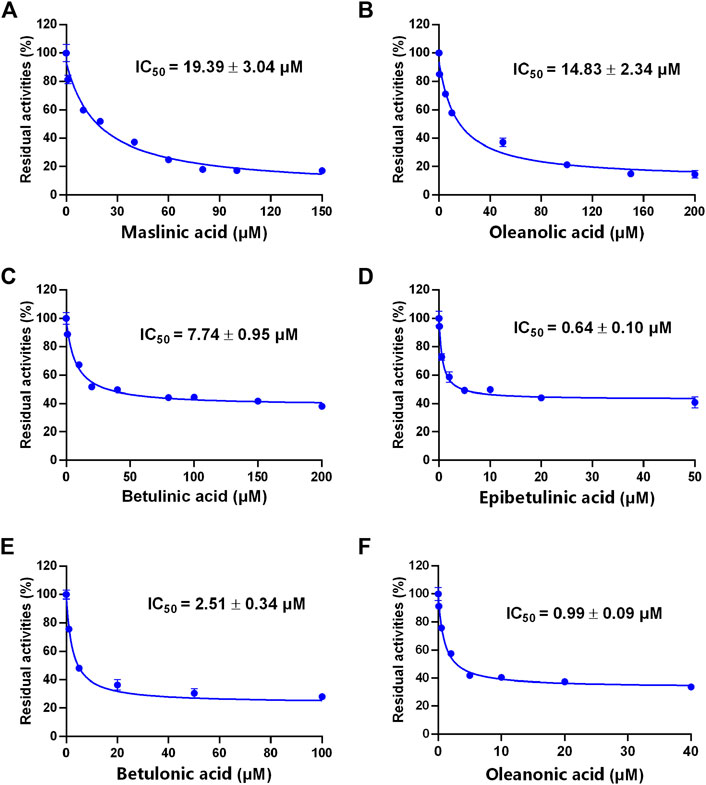
FIGURE 5. The inhibitory effects of the pentacyclic triterpenoid acids (maslinic acid (A), oleanolic acid (B), betulinic acid (C), epibetulinic acid (D), betulonic acid (E) and oleanonic acid (F)) against midazolam 1′-hydroxylation in HLMs. Data are expressed as mean ± SD.
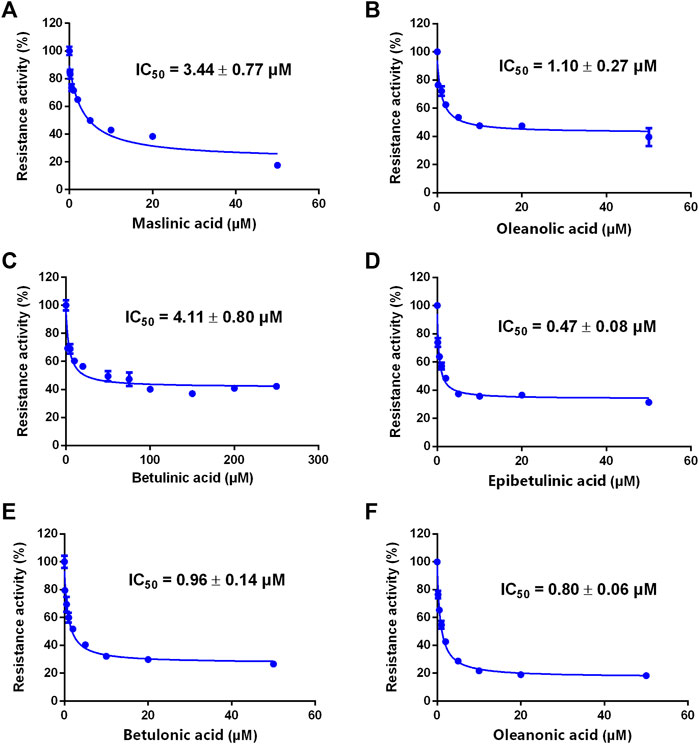
FIGURE 6. The inhibitory effects of the pentacyclic triterpenoid acids (maslinic acid (A), oleanolic acid (B), betulinic acid (C), epibetulinic acid (D), betulonic acid (E) and oleanonic acid (F)) against NEN-hydroxylation in HLMs. Data are expressed as mean ± SD.
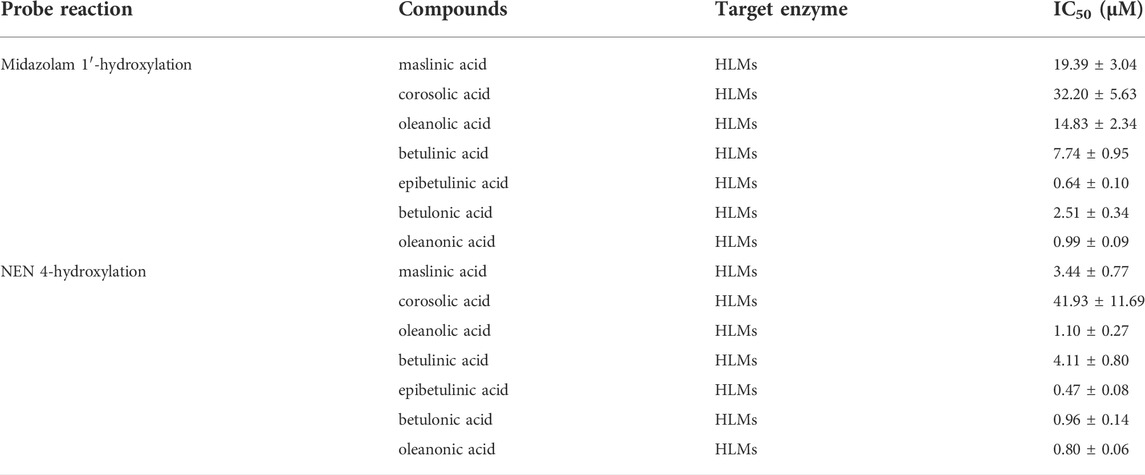
TABLE 3. Inhibitory effects of pentacyclic triterpenoid acids on CYP3A in HLMs. Data are the mean ± SD.
Tissue distribution of seven PTAs from Styrax in rats
Next, the tissue distribution (in plasma, liver, duodenum, jejunum, ileum and colon) of seven PTAs from Styrax was determined in rats after single oral dose of Styrax (100 mg/kg). As shown in Figure 7, Supplementary Figure S8, and Supplementary Tables S9–S12, maslinic acid, corosolic acid and betulinic acid could not be detected (lower the detection limit) in rat plasma and rat liver, while very low exposure levels of epibetulinic acid, betulonic acid, oleanonic acid, oleanolic acid were detected in rat plasma and rat liver. By contrast, these PTAs could be easily detected from the intestinal tract (especially in jejunum, ileum and colon) in rats. Notably, the local exposure of oleanonic acid to rat jejunum could reach 745.69 nM/mg tissue protein (1 h), which was near to the IC50 value of oleanonic acid against CYP3A4. These results suggested that the major PTAs in Styrax could be distributed in intestinal tract at relatively high exposure levels but their plasma and liver exposure in rats was extremely low. These findings well explain why Styrax (i.g.) significantly elevates the plasma exposure of CYP3A-substrate drugs only when these agents are orally administrated, and why Styrax cannot modulate the pharmacokinetic behavior of CYP3A-substrate drugs when these agents administered intravenously.
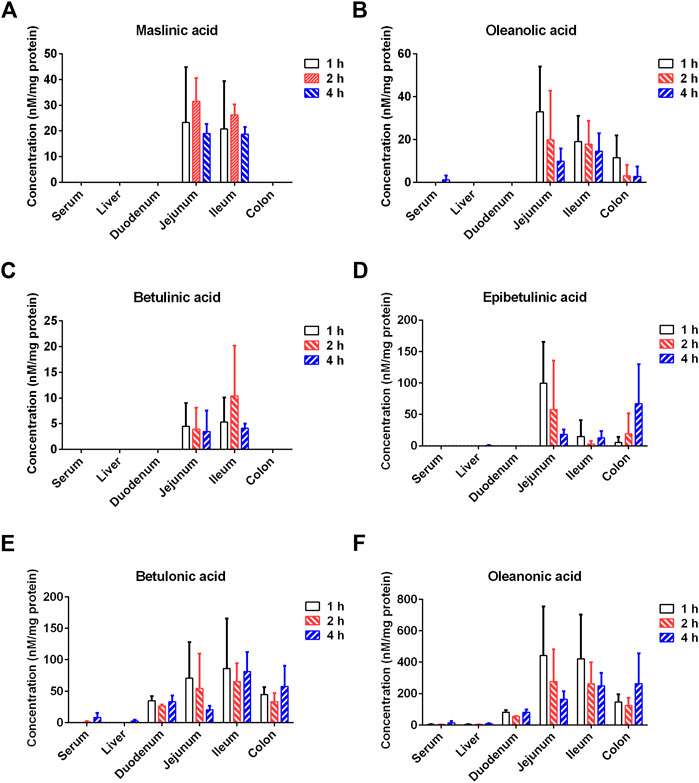
FIGURE 7. Tissue distribution of the major constituents of Styrax (maslinic acid (A), oleanolic acid (B), betulinic acid (C), epibetulinic acid (D), betulonic acid (E), and oleanonic acid (F)) after a single oral dose of Styrax (100 mg/kg) to rats.
Discussion
Over the past few decades, the concomitant use of Chinese medicines and Western medicines has widely been used to cure a range of human diseases, such as digestive diseases, cardiovascular diseases, infectious diseases and various types of cancer (Klotz, 2009; Cong et al., 2015; Mair et al., 2020). In most cases, the concomitant use of Chinese medicines and Western medicines can bring better therapeutic effects via acting on various therapeutic targets or prolonging the half-lives of active compounds in either Chinese medicines or Western medicines (Wu et al., 2011; Shao et al., 2021; Zhang T. et al., 2022). However, in some cases, the concomitant use of Chinese medicines and Western medicines (especially for those agents with very narrow therapeutic windows) has a high risk of triggering potential HDIs (Meijerman et al., 2006; Han et al., 2020). It is well-known that most herbal medicines are administered orally, while Western medicines are administered in various ways including oral administration and injection. Oral drugs are generally absorbed through the mesentery, through the portal vein to the liver, and then enter into the blood circulation system. Thus, it should be clarified whether herbal medicines can modulate the pharmacokinetic behavior of Western medicine(s) by inhibiting/inactivating intestinal or hepatic drug-metabolizing enzymes in vivo.
As one of the most abundant drug-metabolizing enzymes (DMEs) in human liver and intestine, CYP3A4 plays a crucial role in the metabolic clearance and detoxification of a wide range of Western medicines in vivo, thus acts as a key mediator in HDIs and DDIs (Galetin et al., 2007; Zhou, 2008; Tian and Hu, 2014). In this study, following screening more than 100 kinds of clinical commonly used Chinese medicines, Styrax is found with the most potent CYP3A4 inhibitory effect (almost completely inhibit CYP3A4 at 100 μg/ml). Considering that Styrax and Styrax-related herbal products are frequently used in combination with a panel of marketed cardiovascular drugs (such as warfarin, digoxin and simvastatin) to treat cardiovascular diseases (Zhu, 2009; Tao et al., 2016; Yang et al., 2021). It is well-known that Styrax/Styrax-related herbal products are often taken orally for the treatment of human diseases, while a number of studies have reported that the local distribution of herbal constituents in the intestine is much higher than that in the liver (Lai et al., 2009; Wang et al., 2018). In these cases, it is necessary to clarify whether Styrax can trigger HDIs in vivo and to reveal the key organ(s) and molecular mechanisms involved in the pharmacokinetic HDIs triggered by Styrax.
The small intestine is the key organ for absorption, metabolism, and excretion of orally administered drugs in mammals (Yamashita et al., 2021). Many oral drugs can be metabolized by mammalian CYPs, while intestinal CYP3A4 makes a significant contribution to the metabolism of drugs (Galetin et al., 2007). In this study, in vitro assays demonstrated that Styrax and its major constituents (seven PTAs) potently inhibited CYP3A-catalyzed oxidative metabolism of various substrates. In vivo assays showed that Styrax only affected the pharmacokinetic behavior of oral victim CYP3A-substrate drugs (midazolam and felodipine), which prompted us to carefully evaluate the tissue distribution of Styrax/its active constituents (seven PTAs) after oral administration of this herbal extract in rats. These results clearly showed that seven PTAs from Styrax could be exposed to the intestinal tract (especially in jejunum, ileum and colon) at relatively high levels in rats after single oral dose of Styrax (100 mg/kg), but these PTAs were hardly exposed to the liver. The extremely low exposure of these PTAs from Styrax to mammal liver could be attributed to the poor oral bioavailability and the first-pass metabolism of PTAs (Zhang et al., 2019; Yin et al., 2022). The high local exposure of PTAs in the intestinal tract indicated that intestinal CYP3A could be significantly inhibited by these natural compounds in Styrax, while the extremely low exposure of PTAs to mammal liver well-explained why Styrax hardly modulated the in vivo pharmacokinetic behaviors of the CYP3A-substrate drugs administrated by intravenous injection.
It should be noted that some CYP3A4 potent inhibitors (such as ritonavir) have been approved by FDA in combination with CYP3A4-substrate drugs to slow down the metabolic clearance of some important CYP3A4-substrate drugs in vivo, such as Aluvia/Kaletra (lopinavir and ritonavir) and paxlovid (nirmatrelvir and ritonavir) (Hurst and Faulds, 2000; Agarwal and Agarwal, 2021; Eng et al., 2022; Lamb, 2022). Thus, the potent CYP3A4 inhibitors with improved safety profiles could be developed as oral agents for improving the systemic exposure of CYP3A4-substrate drugs in vivo. Considering that hepatic CYP3A plays an important role in endogenous metabolism (such as bile acids and hormones) and the metabolic clearance of toxins, potent inhibition of hepatic CYP3A may lead to serious consequences (such as drug-induced liver injury and imbalance of hormone metabolism) (Li et al., 1995; Burk and Wojnowski, 2004; Banankhah et al., 2016; Qin et al., 2021). In this study, our findings show that the PTAs in Styrax are difficult to enter into the circulation system after oral administration of Styrax, Styrax or its main constituents (PTAs) can be intentionally used in combination with some CYP3A-substrate drugs with low oral bioavailability, aiming to improve its oral bioavailability without affecting the function of hepatic CYP3A. In future, the naturally occurring PTAs in Styrax could be used as promising lead compounds to develop more efficacious CYP3A inhibitors with strong anti-CYP3A effects, improved cell membrane permeability and high safety profiles.
Conclusion
In summary, we demonstrate a case study to efficiently find the herbal medicine(s) with potent hCYP3A4 inhibitory effect in vitro and to accurately assess the potential HDIs risk in vivo. With the help of fluorescence-based high-throughput hCYP3A4 inhibition assay, the Chinese herb Styrax was found with the most potent hCYP3A4 inhibition in HLMs. In vivo pharmacokinetic assays demonstrated that Styrax (i.g., 100 mg/kg) only affected the pharmacokinetic behavior of orally administrated CYP3A-substrate drugs (midazolam and felodipine). Further investigations demonstrated that seven PTAs in Styrax were key substances responsible for CYP3A inhibition, while these PTAs could be exposed to intestinal tract at relatively high exposure levels but the exposure levels of these PTAs in rat plasma and liver were extremely low. Collectively, our findings showed that the Chinese herb Styrax (i.g.) could significantly elevate the plasma exposure of orally administrated CYP3A-substrate drugs but hardly modulated the pharmacokinetic profiles of intravenously administrated CYP3A-substrate drugs, which could be attributed to the high exposure levels of the PTAs in Styrax in intestinal tract and the extremely low exposure levels in rat liver. All these findings clearly suggest that the tissue exposure of some key constituents in herbal medicines strongly affect their in vivo inhibitory effects on CYP3A4, which are very helpful for the clinical pharmacologists to better understand the pharmacokinetic HDIs triggered by herbal products that contains naturally occurring CYP3A4 inhibitors.
Data availability statement
The raw data supporting the conclusions of this article will be made available by the authors, without undue reservation.
Ethics statement
The animal study was reviewed and approved by Animal experiment was carried out in Experimental Animal Center of SHUTCM (Approval Number: PZSHUTCM210709016) and conducted in accordance with the State Committee of Science and Technology of China.
Author contributions
GG, YH, and WL designed the study. FZ, TZ, and JG performed the experiments. GG, YH, ML, QF, and SQ analyzed the data. GG, WL, and FZ wrote the paper. YH critically revised the manuscript.
Funding
This work was supported by the National Key Research and Development Program of China (2017YFC1700200, 2017YFC1702000), the NSF of China (81922070, 81973286, 82141203), Shanghai Science and Technology Innovation Action Plans (20S21901500, 20S21900900) supported by Shanghai Science and Technology Committee, Innovation Team and Talents Cultivation Program of National Administration of Traditional Chinese Medicine (ZYYCXTDD-202004), Three-year Action Plan for Shanghai TCM Development and Inheritance Program [ZY(2021-2023)-0401], and Clinical Research Plan of SHDC (SHDC2020CR 2046B).
Conflict of interest
The authors declare that the research was conducted in the absence of any commercial or financial relationships that could be construed as a potential conflict of interest.
Publisher’s note
All claims expressed in this article are solely those of the authors and do not necessarily represent those of their affiliated organizations, or those of the publisher, the editors and the reviewers. Any product that may be evaluated in this article, or claim that may be made by its manufacturer, is not guaranteed or endorsed by the publisher.
Supplementary material
The Supplementary Material for this article can be found online at: https://www.frontiersin.org/articles/10.3389/fphar.2022.974578/full#supplementary-material
Abbreviations
AUC, area under the plasma concentration; CMC-Na, sodium carboxymethyl cellulose; CYPs/P450s, cytochrome P450 enzymes; DDIs, drug-drug interactions; DMEs, drug-metabolizing enzymes; ESI, electrospray ionization; G-6-P, D-Glucose-6-phosphate; G-6-PDH, glucose-6-phosphate dehydrogenase; HDIs, herb-drug interactions; hCYP3A4, human cytochrome P450 3A4; HIMs, human intestine microsomes; HLMs, human liver microsomes; IC50, half maximal inhibition concentration; IS, internal standard; i.v., intravenous; i.g., intragastrical; MRM, multiple reaction monitoring; NEN, N-ethyl-1,8-naphthalimide; NEHN, N-ethyl-4-hydroxyl-1,8-naphthalimide; PBS, potassium phosphate buffer; PTAs, pentacyclic triterpenoid acids; RIMs, rat intestine microsomes; RLMs, rat liver microsomes; SHUTCM, Shanghai University of Traditional Chinese Medicine; t1/2, half-life; TICs, total ion chromatograms.
References
Agarwal, S., and Agarwal, S. K. (2021). Lopinavir-ritonavir in SARS-CoV-2 infection and drug-drug interactions with cardioactive medications. Cardiovasc. Drugs Ther. 35 (3), 427–440. doi:10.1007/s10557-020-07070-1
Ban, Y. J., Chen, R., Zhang, Y., Zhang, L., Liu, W. X., Zhu, G. F., et al. (2021). Research progress on oral absorption and metabolism of pentacyclic triterpenoids. Chem. Reagents 43 (07), 906–916. doi:10.13822/j.cnki.hxsj.2021008033
Banankhah, P. S., Garnick, K. A., and Greenblatt, D. J. (2016). Ketoconazole-associated liver injury in drug-drug interaction studies in healthy volunteers. J. Clin. Pharmacol. 56 (10), 1196–1202. doi:10.1002/jcph.711
Bian, J., and Zhang, H. Y. (2016). A preliminary study on the ancient and modern application of Suhexiang pills. Clin. J. Traditional Chin. Med. 28 (06), 875–878. doi:10.16448/j.cjtcm.2016.0310
Burk, O., and Wojnowski, L. (2004). Cytochrome P450 3A and their regulation. Naunyn. Schmiedeb. Arch. Pharmacol. 369 (1), 105–124. doi:10.1007/s00210-003-0815-3
Cong, Y., Sun, K., He, X., Li, J., Dong, Y., Zheng, B., et al. (2015). A traditional Chinese medicine xiao-ai-tong suppresses pain through modulation of cytokines and prevents adverse reactions of morphine treatment in bone cancer pain patients. Mediat. Inflamm. 2015, 961635. doi:10.1155/2015/961635
Dunkoksung, W., Vardhanabhuti, N., Siripong, P., and Jianmongkol, S. (2019). Rhinacanthin-C mediated herb-drug interactions with drug transporters and phase I drug-metabolizing enzymes. Drug Metab. Dispos. 47 (10), 1040–1049. doi:10.1124/dmd.118.085647
Eng, H., Dantonio, A. L., Kadar, E. P., Obach, R. S., Di, L., Lin, J., et al. (2022). Disposition of nirmatrelvir, an orally bioavailable inhibitor of SARS-CoV-2 3C-like protease, across animals and humans. Drug Metab. Dispos. 50 (5), 576–590. doi:10.1124/dmd.121.000801
Fang, S. Q., Huang, J., Zhang, F., Ni, H. M., Chen, Q. L., Zhu, J. R., et al. (2020). Pharmacokinetic interaction between a Chinese herbal formula Huosu Yangwei oral liquid and apatinib in vitro and in vivo. J. Pharm. Pharmacol. 72 (7), 979–989. doi:10.1111/jphp.13268
Fasinu, P. S., Gurley, B. J., and Walker, L. A. (2015). Clinically relevant pharmacokinetic herb-drug interactions in antiretroviral therapy. Curr. Drug Metab. 17 (1), 52–64. doi:10.2174/1389200216666151103115053
Galetin, A., Hinton, L. K., Burt, H., Obach, R. S., and Houston, J. B. (2007). Maximal inhibition of intestinal first-pass metabolism as a pragmatic indicator of intestinal contribution to the drug-drug interactions for CYP3A4 cleared drugs. Curr. Drug Metab. 8 (7), 685–693. doi:10.2174/138920007782109805
Gallo, P., De Vincentis, A., Pedone, C., Nobili, A., Tettamanti, M., Gentilucci, U. V., et al. (2019). Drug-drug interactions involving CYP3A4 and p-glycoprotein in hospitalized elderly patients. Eur. J. Intern. Med. 65, 51–57. doi:10.1016/j.ejim.2019.05.002
Gouws, C., Steyn, D., Du Plessis, L., Steenekamp, J., and Hamman, J. H. (2012). Combination therapy of western drugs and herbal medicines: Recent advances in understanding interactions involving metabolism and efflux. Expert Opin. Drug Metab. Toxicol. 8 (8), 973–984. doi:10.1517/17425255.2012.691966
Guo, J., Qin, Z., He, Q., Fong, T. L., Lau, N. C., Cho, W. C. S., et al. (2021). Shexiang Baoxin Pill for acute myocardial infarction: Clinical evidence and molecular mechanism of antioxidative stress. Oxid. Med. Cell. Longev. 2021, 7644648. doi:10.1155/2021/7644648
Han, M. Z., Li, S. S., Li, J., Li, X. C., Gao, L. L., Lu, Y., et al. (2020). Qingfei Paidu Decoction causes hyperkalemia in patients with novel coronavirus pneumonia. J. Adverse Drug React. 22 (06), 375–376.
Hurst, M., and Faulds, D. (2000). Lopinavir. Drugs 60 (6), 1371–1379. discussion 1380-1. doi:10.2165/00003495-200060060-00009
Jin, Q., Wu, J., Wu, Y., Li, H., Finel, M., Wang, D., et al. (2022). Optical substrates for drug-metabolizing enzymes: Recent advances and future perspectives. Acta Pharm. Sin. B 12 (3), 1068–1099. doi:10.1016/j.apsb.2022.01.009
Kallem, R. R., Ramesh, M., and Seshagirirao, J. V. (2013). Validated LC-ESI-MS/MS method for simultaneous quantitation of felodipine and metoprolol in rat plasma: Application to a pharmacokinetic study in rats. Biomed. Chromatogr. 27 (6), 784–791. doi:10.1002/bmc.2861
Klotz, U. (2009). Pharmacokinetics and drug metabolism in the elderly. Drug Metab. Rev. 41 (2), 67–76. doi:10.1080/03602530902722679
Kotegawa, T., Laurijssens, B. E., Von Moltke, L. L., Cotreau, M. M., Perloff, M. D., Venkatakrishnan, K., et al. (2002). In vitro, pharmacokinetic, and pharmacodynamic interactions of ketoconazole and midazolam in the rat. J. Pharmacol. Exp. Ther. 302 (3), 1228–1237. doi:10.1124/jpet.102.035972
Lai, L., Hao, H., Wang, Q., Zheng, C., Zhou, F., Liu, Y., et al. (2009). Effects of short-term and long-term pretreatment of Schisandra lignans on regulating hepatic and intestinal CYP3A in rats. Drug Metab. Dispos. 37 (12), 2399–2407. doi:10.1124/dmd.109.027433
Lamb, Y. N. (2022). Nirmatrelvir plus ritonavir: First approval. Drugs 82 (5), 585–591. doi:10.1007/s40265-022-01692-5
Lee, S. Y., Lee, J. Y., Kang, W., Kwon, K. I., Park, S. K., Oh, S. J., et al. (2013). Cytochrome P450-mediated herb-drug interaction potential of Galgeun-tang. Food Chem. Toxicol. 51, 343–349. doi:10.1016/j.fct.2012.10.012
Li, A. P., Kaminski, D. L., and Rasmussen, A. (1995). Substrates of human hepatic cytochrome P450 3A4. Toxicology 104 (1-3), 1–8. doi:10.1016/0300-483x(95)03155-9
Li, H. Y., Xu, W., Su, J., Zhang, X., Hu, L. W., and Zhang, W. D. (2010). In vitro and in vivo inhibitory effects of glycyrrhetinic acid on cytochrome P450 3A activity. Pharmacology 86 (5-6), 287–292. doi:10.1159/000320956
Lundahl, J., Regårdh, C. G., Edgar, B., and Johnsson, G. (1997). Effects of grapefruit juice ingestion-pharmacokinetics and haemodynamics of intravenously and orally administered felodipine in healthy men. Eur. J. Clin. Pharmacol. 52 (2), 139–145. doi:10.1007/s002280050263
Mair, A., Wilson, M., and Dreischulte, T. (2020). Addressing the challenge of polypharmacy. Annu. Rev. Pharmacol. Toxicol. 60, 661–681. doi:10.1146/annurev-pharmtox-010919-023508
Meijerman, I., Beijnen, J. H., and Schellens, J. H. (2006). Herb-drug interactions in oncology: Focus on mechanisms of induction. Oncologist 11 (7), 742–752. doi:10.1634/theoncologist.11-7-742
Ning, J., Wang, W., Ge, G., Chu, P., Long, F., Yang, Y., et al. (2019). Target enzyme-activated two-photon fluorescent probes: A case study of CYP3A4 using a two-dimensional design strategy. Angew. Chem. Int. Ed. Engl. 58 (29), 9959–9963. doi:10.1002/anie.201903683
Parvez, M. K., and Rishi, V. (2019). Herb-drug interactions and hepatotoxicity. Curr. Drug Metab. 20 (4), 275–282. doi:10.2174/1389200220666190325141422
Qin, X., Zhang, Y., Lu, J., Huang, S., Liu, Z., and Wang, X. (2021). CYP3A deficiency alters bile acid homeostasis and leads to changes in hepatic susceptibility in rats. Toxicol. Appl. Pharmacol. 429, 115703. doi:10.1016/j.taap.2021.115703
Qiu, R., Zhao, C., Liang, T., Hao, X., Huang, Y., Zhang, X., et al. (2020). Core outcome set for clinical trials of COVID-19 based on traditional Chinese and western medicine. Front. Pharmacol. 11, 781. doi:10.3389/fphar.2020.00781
Ren, C., Kong, D., Ning, C., Xing, H., Cheng, Y., Zhang, Y., et al. (2021). Improved pharmacokinetic characteristics of ursolic acid in rats following intratracheal instillation and nose-only inhalation exposure. J. Pharm. Sci. 110 (2), 905–913. doi:10.1016/j.xphs.2020.10.006
Shao, H., He, X., Zhang, L., Du, S., Yi, X., Cui, X., et al. (2021). Efficacy of ligustrazine injection as adjunctive therapy in treating acute cerebral infarction: A systematic review and meta-analysis. Front. Pharmacol. 12, 761722. doi:10.3389/fphar.2021.761722
Su, J. L., and Geng, N. Z. (2016). Observation on the efficacy of traditional Chinese medicine Yiqi Tongmai Decoction combined with conventional Western medicine in the treatment of angina pectoris after myocardial infarction in the elderly. Clin. Res. Traditional Chin. Med. 8 (27), 11–12.
Surya Sandeep, M., Sridhar, V., Puneeth, Y., Ravindra Babu, P., and Naveen Babu, K. (2014). Enhanced oral bioavailability of felodipine by naringenin in Wistar rats and inhibition of P-glycoprotein in everted rat gut sacs in vitro. Drug Dev. Ind. Pharm. 40 (10), 1371–1377. doi:10.3109/03639045.2013.819885
Tao, J., Jiang, P., Peng, C., Li, M., Liu, R., and Zhang, W. (2016). The pharmacokinetic characters of simvastatin after co-administration with Shexiang Baoxin Pill in healthy volunteers' plasma. J. Chromatogr. B Anal. Technol. Biomed. Life Sci. 1026, 162–167. doi:10.1016/j.jchromb.2016.01.018
Tian, D., and Hu, Z. (2014). CYP3A4-mediated pharmacokinetic interactions in cancer therapy. Curr. Drug Metab. 15 (8), 808–817. doi:10.2174/1389200216666150223152627
Tu, D., Ning, J., Zou, L., Wang, P., Zhang, Y., Tian, X., et al. (2022). Unique oxidative metabolism of bufalin generates two reactive metabolites that strongly inactivate human cytochrome P450 3A. J. Med. Chem. 65 (5), 4018–4029. doi:10.1021/acs.jmedchem.1c01875
Tu, D. Z., Mao, X., Zhang, F., He, R. J., Wu, J. J., Wu, Y., et al. (2020). Reversible and irreversible inhibition of cytochrome P450 enzymes by methylophiopogonanone A. Drug Metab. Dispos. 49 (6), 459–469. doi:10.1124/dmd.120.000325
Wang, M., Xu, Z. Q., Li, Z. X., and Liu, Y. L. (2020). Effects of intestinal network barrier on the absorption mechanism of pentacyclic triterpenoid saponins from Pulsatilla chinensis. Jiangxi Tradit. Chin. Med. 51 (01), 69–71.
Wang, Z., You, L., Cheng, Y., Hu, K., Wang, Z., Cheng, Y., et al. (2018). Investigation of pharmacokinetics, tissue distribution and excretion of schisandrin B in rats by HPLC-MS/MS. Biomed. Chromatogr. 32 (2), e4069. doi:10.1002/bmc.4069
Wu, B., Zhao, S., Wang, X., Dong, Q., and Zheng, G. (2011). Mechanism of traditional Chinese medicine on animal model of Parkinson's disease. Zhongguo Zhong Yao Za Zhi 36 (18), 2588–2591.
Wu, J. J., Guan, X. Q., Dai, Z. R., He, R. J., Ding, X. X., Yang, L., et al. (2021). Molecular probes for human cytochrome P450 enzymes: Recent progress and future perspectives. Coord. Chem. Rev. 427, 213600. doi:10.1016/j.ccr.2020.213600
Xiang, Q., Li, C., Zhao, X., and Cui, Y. M. (2017). The influence of CYP3A5*3 and BCRPC421A genetic polymorphisms on the pharmacokinetics of felodipine in healthy Chinese volunteers. J. Clin. Pharm. Ther. 42 (3), 345–349. doi:10.1111/jcpt.12505
Xu, Z. L., Zhang, Y., Tao, X. J., Zhang, M. B., Dou, D. Q., and Kang, T. G. (2017). Interaction of synergism and detoxification in the combined use of traditional Chinese medicine and anti-infective Western medicine. Shizhen Tradit. Chin. Med. 28 (01), 202–204.
Xue, X., Huang, M., Xiao, H., Qin, X., Huang, L., Zhong, G., et al. (2011). Rapid and simultaneous measurement of midazolam, 1'-hydroxymidazolam and digoxin by liquid chromatography/tandem mass spectrometry: Application to an in vivo study to simultaneously measure P-glycoprotein and cytochrome P450 3A activity. J. Pharm. Biomed. Anal. 28 (551), 187–193. doi:10.1016/j.jpba.2011.01.018
Yamashita, T., Inui, T., Yokota, J., Kawakami, K., Morinaga, G., Takatani, M., et al. (2021). Monolayer platform using human biopsy-derived duodenal organoids for pharmaceutical research. Mol. Ther. Methods Clin. Dev. 22, 263–278. doi:10.1016/j.omtm.2021.05.005
Yang, Y., Gao, S., Fang, Q., and Zhu, M. (2021). Efficacy and safety of Shexiang Baoxin Pill combined with western medicine in the treatment of acute myocardial infarction: A single-center, double-blind, randomized controlled trial. Med. Baltim. 100 (3), e24246. doi:10.1097/MD.0000000000024246
Yin, Y., Sheng, L., Zhang, J., Zhang, L., Liu, J., Wen, X., et al. (2022). Synthesis and in vitro/in vivo anticancer evaluation of pentacyclic triterpenoid derivatives linked with l-phenylalanine or l-proline. Bioorg. Chem. 126, 105865. doi:10.1016/j.bioorg.2022.105865
Yu, Q. (2021). A brief analysis of the application of styraxine series spices in the food field. Food Saf. Guide (12), 181–182. doi:10.16043/j.cnki.cfs.2021.12.101
Zhang, B., Lu, Y., Li, P., Wen, X., and Yang, J. (2019). Study on the absorption of corosolic acid in the gastrointestinal tract and its metabolites in rats. Toxicol. Appl. Pharmacol. 378, 114600. doi:10.1016/j.taap.2019.114600
Zhang, F., Huang, J., He, R. J., Wang, L., Huo, P. C., Guan, X. Q., et al. (2020). Herb-drug interaction between Styrax and warfarin: Molecular basis and mechanism. Phytomedicine. 77, 153287. doi:10.1016/j.phymed.2020.153287
Zhang, F., Huang, J., Liu, W., Wang, C. R., Liu, Y. F., Tu, D. Z., et al. (2021). Inhibition of drug-metabolizing enzymes by Qingfei Paidu decoction: Implication of herb-drug interactions in COVID-19 pharmacotherapy. Food Chem. Toxicol. 149, 111998. doi:10.1016/j.fct.2021.111998
Zhang, F., Liu, W., Huang, J., Chen, Q. L., Wang, D. D., Zou, L. W., et al. (2022). Inhibition of drug-metabolizing enzymes by jingyin granules: Implications of herb-drug interactions in antiviral therapy. Acta Pharmacol. Sin. 43 (4), 1072–1081. doi:10.1038/s41401-021-00697-2
Zhang, L. G., and Xu, X. S. (2012). 30 cases of peptic ulcer treated with Western medicine combined with traditional Chinese medicine Xiaokuiping decoction. Chin. Ethn. Folk. Med. 21 (23), 101.
Zhang, T., Li, X., Chen, Y., Zhao, L., Tian, J., and Zhang, J. (2022). Evidence mapping of 23 systematic reviews of traditional Chinese medicine combined with western medicine approaches for COVID-19. Front. Pharmacol. 12, 807491. doi:10.3389/fphar.2021.807491
Zhang, Y. W., Tu, L. L., Zhang, Y., Pan, J. C., Zheng, G. L., and Yin, L. N. (2021). Liver-targeted delivery of asiatic acid nanostructured lipid carrier for the treatment of liver fibrosis. Drug Deliv. 28 (1), 2534–2547. doi:10.1080/10717544.2021.2008054
Zhou, Q. H., Zhu, Y. D., Zhang, F., Song, Y. Q., Jia, S. N., Zhu, L., et al. (2019). Interactions of drug-metabolizing enzymes with the Chinese herb Psoraleae Fructus. Chin. J. Nat. Med. 17 (11), 858–870. doi:10.1016/S1875-5364(19)30103-7
Zhou, S. F. (2008). Drugs behave as substrates, inhibitors and inducers of human cytochrome P450 3A4. Curr. Drug Metab. 9 (4), 310–322. doi:10.2174/138920008784220664
Keywords: herb-drug interactions (HDIs), styrax, pentacyclic triterpenoid acids (PTAs), intestinal CYP3A, CYP3A-substrate drugs
Citation: Zhang F, Zhang T, Gong J, Fang Q, Qi S, Li M, Han Y, Liu W and Ge G (2022) The Chinese herb Styrax triggers pharmacokinetic herb-drug interactions via inhibiting intestinal CYP3A. Front. Pharmacol. 13:974578. doi: 10.3389/fphar.2022.974578
Received: 21 June 2022; Accepted: 28 July 2022;
Published: 29 August 2022.
Edited by:
Michael Heinrich, University College London, United KingdomCopyright © 2022 Zhang, Zhang, Gong, Fang, Qi, Li, Han, Liu and Ge. This is an open-access article distributed under the terms of the Creative Commons Attribution License (CC BY). The use, distribution or reproduction in other forums is permitted, provided the original author(s) and the copyright owner(s) are credited and that the original publication in this journal is cited, in accordance with accepted academic practice. No use, distribution or reproduction is permitted which does not comply with these terms.
*Correspondence: Guangbo Ge, Z2VndWFuZ2JvQHNodXRjbS5lZHUuY24=; Wei Liu, bHdoemF5bEBzaHV0Y20uZWR1LmNu; Yan Han, aGFueWFuQHNodXRjbS5lZHUuY24=