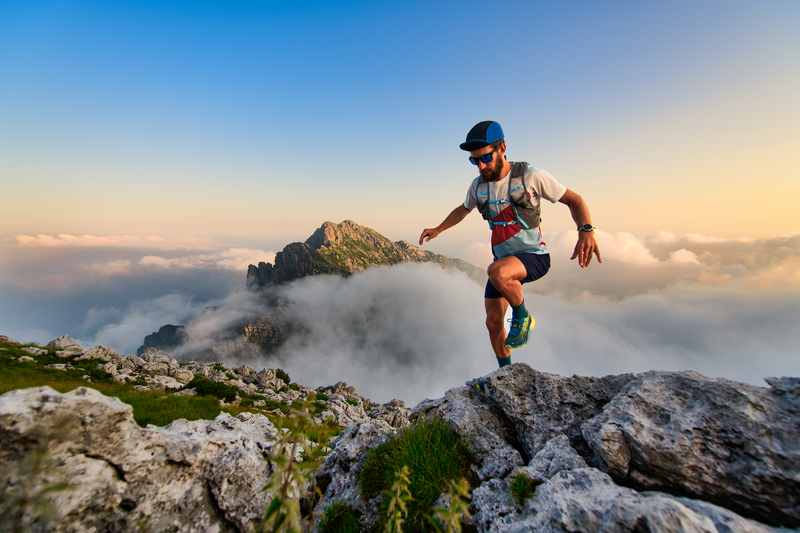
95% of researchers rate our articles as excellent or good
Learn more about the work of our research integrity team to safeguard the quality of each article we publish.
Find out more
REVIEW article
Front. Pharmacol. , 01 August 2022
Sec. Experimental Pharmacology and Drug Discovery
Volume 13 - 2022 | https://doi.org/10.3389/fphar.2022.972813
This article is part of the Research Topic Recent Advances of Minor Saponins in Panax species View all 10 articles
Panax quinquefolius L. has attracted extensive attention worldwide because of its prominent pharmacological properties on type 2 diabetes, cancers, central nervous system, and cardiovascular diseases. Ginsenosides are active phytochemicals of P. quinquefolius, which can be classified as propanaxdiol (PPD)-type, propanaxtriol (PPT)-type, oleanane-type, and ocotillol-type oligo-glycosides depending on the skeleton of aglycone. Recently, advanced analytical and isolated methods including ultra-performance liquid chromatography tandem with mass detector, preparative high-performance liquid chromatography, and high speed counter-current chromatography have been used to isolate and identify minor components in P. quinquefolius, which accelerates the clarification of the material basis. However, the poor bioavailability and undetermined bio-metabolism of most saponins have greatly hindered both the development of medicines and the identification of their real active constituents. Thus, it is essential to consider the bio-metabolism of constituents before and after absorption. In this review, we described the structures of minor ginsenosides in P. quinquefolius, including naturally occurring protype compounds and their in vivo metabolites. The preclinical and clinical pharmacological studies of the ginsenosides in the past few years were also summarized. The review will promote the reacquaint of minor saponins on the growing appreciation of their biological role in P. quinquefolius.
Ginseng root has historically been used as medicine food homology plant for thousand years in oriental countries. It occupies a prominent position in the list of best-selling natural medicines worldwide (Qi et al., 2011). Panax ginseng C.A. Meyer (known as Asian or Korean ginseng), P. quinquefolius (known as American ginseng), and P. notoginseng (Burkill) F.H. Chen (known as Sanchi ginseng) are three reputable folk medicine around the world. P. quinquefolius is one of the top 10 selling natural health products in the United States. Despite its high chemical similarity with Asian ginseng, P. quinquefolius instead exhibits heat-clearing and refreshing functions as a tonic medicinal plant (Yang et al., 2014). Modern pharmacological studies indicated P. quinquefolius exert a wide range of biological activities, such as hypoglycemic, cardiovascular protective, anti-diabetic, anti-tumor, anti-inflammatory, anti-obesity, anti-aging, and antimicrobial effects (Assinewe et al., 2003; Szczuka et al., 2019).
It is well documented that the triterpenoid saponins, called ginseng saponins or ginsenosides, are the major active compounds in P. quinquefolius (Yuan et al., 2010). The ginsenoside profile varies in this herb due to the cultivation in different areas in terms of total ginsenosides, the ratio of protopanaxadiol (PPD) to protopanaxatriol (PPT), and other marker ginsenosides. The type and contents of ginsenosides are also different in the root, stem/leaves, flower bud, and fruits. Thus, a wide spectrum of advanced analytical methods including ultra-performance liquid chromatography tandem with mass detector, preparative high-performance liquid chromatography, and high-speed counter-current chromatography have been used to isolate and identify minor components in P. quinquefolius, which accelerates the clarification of its material basis.
Rb1, Rb2, Rc, Re, and Rg1 are considered as major ginsenosides with high contents in P. quinquefolius. The multitude of sugar moieties in major ginsenosides affects their bioavailability after oral intake, as well as the biological activities. The bioactive ginsenosides in vitro do not always represent the real active form in vivo, due to the bio-metabolism of constituents by trillions of gut microbiota in the gastrointestinal tract and enzymes in blood and tissues after absorption. To link the health benefits of major ginsenosides to their effects, it is warranted to determine the profiles of P. quinquefolius and its minor metabolites.
In this review, the structural diversities of ginsenosides in different parts of P. quinquefolius are described, especially naturally occurring minor ginsenosides and those resulting from biotransformation. Preclinical and clinical studies of P. quinquefolius and ginsenosides are also delineated. Finally, special attention is paid to future research trends for P. quinquefolius, and targets identification of bioactive ginsenosides and their underlying mechanism exploration are discussed and prospected.
P. quinquefolius was first found in 1716 by father Joseph-François Lafitau, a Jesuit priest in Canada. He stumbled across P. quinquefolius growing in the woods near Montreal. It is distributed native to the temperate forest regions of North America, from 67° to 95°W longitude and 30° to 48°N latitude, including North of Quebec and Ontario and South of Mississippi, Arkansas, and Georgia. Wild ginseng is still harvested from areas in Wisconsin, Pennsylvania, and New York State. P. quinquefolius was first introduced to China in 1975, and the major producing areas are Heilongjiang, Jilin, Liaoning, Hebei, Shandong, and Shanxi Provinces (Figure 1) (Shen et al., 2019).
FIGURE 1. Geographical distribution of P. quinquefolius based on GMPGIS. The map was plotted using online ArcGIS (ESRI, Redland, CA, United States. URL: http://www.learngis2.maps.arcgis.com/). Flags showing cultivated or wild resources of P. quinquefolius.
P. quinquefolius can be cultivated in large number of countries except for the abovementioned places. Based on the environmental variables over 30 years from 1970 to 2000, and 226 global distribution areas of P. quinquefolius, the maximum entropy model (MaxEnt) was used to predict the global ecological suitable areas for P. quinquefolius. The potential ecological suitable places of P. quinquefolius were primarily in Changbai Mountain in China and Appalachian Mountain in America, in the range of 35°N–50°N, 110°E–145° and E35°N–50°N, 60°W–120°W, respectively, including Canada, the United States, China, North and South Korea, Russia and Japan. Japan and South Korea were the potential producing regions (Zhang et al., 2018).
P. quinquefolius has been used by native Americans for a long history. It was used in Cherokee medicine for coughing, shortness of breath, headaches, digestive upset, fatigue, convulsions, female reproductive problems, and general weakness. An assortment of products containing P. quinquefolius are currently available on the market, including capsule, tablet, powder, and tea. The roots are implemented in drugs, cosmetic and skin care, food and feed additives. In United States, P. quinquefolius extracts are used in candies and drinks, while in China, they are used in alcoholic beverages (Szczuka et al., 2019).
Ginsenosides, which share a unique dammarane type triterpenoid saponin structure (Fuzzati, 2004), are the major characteristic constituents of P. quinquefolius. More than 100 ginsenosides have been identified in P. quinquefolius, including naturally occurring compounds and those resulting from steaming and biotransformation (Yuan et al., 2010). The contents and types of ginsenosides vary from the roots, leaves, stems, flower buds and fruits of P. quinquefolius (Table 1). A comprehensive study was conducted to compare the components among different parts of P. quinquefolius and found that the root contains much more abundant Rb1, Ro, and mRb1 isomer, compared with the other parts. The stem leaf and flower bud show similar saponin composition, with richer m-Rb2, Rb3, and p-F11, than the root (Wang et al., 2019). Differences were found in sugar moieties, numbers, and sugar attachment at positions C-3, C-6, or C-20 and they provided diversity in ginsenoside structures (Qi et al., 2011). The carbonylation at C-3, dehydrogenation at C-5, 6 and changeable C-20 side-chain, and stereoisomerism further enrich the structural diversity of ginsenosides.
As summarized in Figure 2, ginsenosides in P. quinquefolius are generally classified into four groups, consisting of protopanaxadiol-type (PPD), protopanaxatriol-type (PPT), ocotillol-type, and oleanolic acid-type. PPD and PPT are the major groups of ginsenosides and are usually found in neutral forms. In the PPD-type, sugar residues are attached to β-OH at C-3 and/or C-20. Natural occurring PPD compounds include compounds 1–28. Compounds 29–30 with modified PPD structure were characterized by a double bond between C-5 and C-6 and a hydroxyl group in C-7 was isolated from the roots of P. quinquefolius (Yoshikawa et al., 1998). Compounds 31–39 were clarified as modified PPD structures with variable C-20 side-chains. In the PPT group, sugar moieties are attached to the α-OH at C-6 and/or β-OH at C-20. PPT constituents include compounds 40–60. PPD and PPT type ginsenosides constitute the main saponins in P. quinquefolius, and reports have shown that Rb1, Rb2, Rc, Rg1, Re, and Rd account for 90% of the total saponins (Wang et al., 2015b). Minor ginsenosides isolated from P. quinquefolius include ocotillol-type (compounds 61–69), oleanane-type (compounds 70–71), and dammarane saponins with a modified aglycone skeleton (compounds 29–39 and 54–60). A variety of minor ginsenosides have been isolated and the structures were elucidated via MS/MS, and NMR analysis. For example, in 1998, Yoshikawa et al. identified 5 dammarane-type triterpene oligoglycosides named quinquenosides I–V from the root of P. quinquefolius, along with notoginsenoside A, C, G, K, malonyl G-Rb1, pseudo-G-Rc1, gypenoside XVII, and chikusetsusaponin Iva (Yoshikawa et al., 1998). Three new dammarane-type saponins named quinquenosides L1–3 were isolated from the leaves and stems of P. quinquefolius collected in Canada (Wang et al., 1998; Wang et al., 2001). By using LC/MS/MS, the ginsenosides malonyl G-Rb2 and malonyl G-Rc were characterized in the root of P. quinquefolius (Wang et al., 1999). In 2004, a new dammarane-type triterpenoid saponin, ginsenoside Rg8, was isolated from the roots of P. quinquefolius, along with (20E)-ginsenoside F4, Rh1, and F1 (Dou et al., 2006). In 2007, from the flower buds of P. quinquefolius, 5 new dammarane-type triterpene glycosides, floralquinquenosides A, B, C, D, and E, along with 18 known ginsenosides were isolated and identified by NMR analysis (Nakamura et al., 2007). Four new triterpenoid saponin quinquenoside L10, 14, 16, and 17 were isolated from the leaves and stems of P. quinquefolius in 2009 (Chen et al., 2009; Li et al., 2009). Quinquenoside F6 was isolated from the fruits of P. quinquefolius (Lu et al., 2012). Two new dammarane-type saponins quinquefoloside-Ld and Le with a novel heptatomic ring between C-12 and C-17 from leaves of P. quinquefolius were elucidated (Xiang et al., 2013). Two new ocotillol-type compounds were isolated from the leaves and stems of P. quinquefolium L. and identified as pseudo-ginsenoside RT6 and pseudoginsengenin R1 (Liu et al., 2013). A new ocotillol-type ginsenoside, namely 12-one-pseudoginsenoside F11 (12-one-Pseudo-G-F11), was isolated from stems and leaves of P. quinquefolium (Qi et al., 2020).
FIGURE 2. Ginsenosides characterized from P. quinquefolius. PPD, Protopanaxadiol; PPT, protopanaxatriol; G, ginsenoside; Q, quinquenoside.
The ginsenosides Rb1, Rb2, Rc, Re, and Rg1 are usually characterized as major ginsenosides, and account for more than 80% of total ginsenosides (Yu et al., 2017). The bioactive ginsenosides have been widely utilized in medical and chemical fields, which created a demand for their availability. However, the large size and poor cell membrane permeability of major ginsenosides restricted their absorption and bioavailability in human body after oral administration (Liu et al., 2010a). Therefore, the production of rare or minor smaller ginsenosides by transformation is urgently requisite. On the one hand, in vitro or in vivo biotransformation of major ginsenosides can generate an assortment of novel structural ginsenosides, resulted from the reduction of sugar moieties, substituent groups alteration and aglycone backbone changes. The small molecular ginsenosides are easily absorbed in the gastrointestinal tract after oral administration due to the deglucosylation (Ryu et al., 2017). In addition, a multitude of evidence showed that biotransformation of major ginsenosides to minor ginsenosides result in improved pharmacological activities (Quan et al., 2015; Ryu et al., 2017).
The deglycosylation of sugar moieties is mainly occurred in the transformation of major ginsenosides. Thermal and mild acid hydrolysis treatments show inefficient and low selective decomposition, while biotransformation including microbial enzymatic transformation, microbial transformation, and in vivo transformation, exerts high selectivity, lower by-products, and high targets yields. Thus, research on the biotransformation of major ginsenosides for increasing the bioavailability and pharmacological activities by structural modification of ginsenosides attracts more attention. The types, pathways, conditions, and yields of biotransformation were shown in Table 2.
Ginsenosides Rb1, Rb2, and Rc belong to protopanaxadiol (PPD) triterpenoid saponins, which are further modified by the glycosidation at the positions of C-3 and C-20 with different sugar moieties. The variable origin of microbial β-glucosidase determines the position and efficiency of deglycosylation. Microbacterium esteraromaticum derived β-glucosidase bgp1 catalyses ginsenoside Rb1 into 20(S)-Rg3 via intermediate product Rd, while β-glucosidase bgp3 transforms Rb1 into Compound K (C-K) via Rd (Quan et al., 2012b; Quan et al., 2012d). Intriguingly, crude glycosidase obtained from Leuconostoc mesenteroides DC102 transforms Rb1 into compound K with a yield of 99% after 3 days cultivation (Quan et al., 2011). In addition, an enzyme immobilization method was developed for the effective biotransformation of Rb1to Rd, and the catalytic efficiency of the immobilized β-glucosidase from Aspergillus niger was 3.30-fold higher than that of the free enzyme (Wu et al., 2021). Ginsenoside Rb2 can be transformed to Rd in the treatment of α-L-Arabinopyranosidase (Kim et al., 2020). While, after coculture Rb2 with β-glucosidase from M. esteraromaticum or Armillaria mellea mycelium, the product compound K was obtained via intermediate compounds Y, Rd, and C-O (Quan et al., 2012a; Kim et al., 2018). The a-L-arabinofuranosidase purified from thermarum DSM5069 catalyses ginsenoside Rc to Rd with a high yield of 99.4% (Xie et al., 2016). The biotransformation pathways were shown in Figure 3.
FIGURE 3. Schematic illustration of biotransformation of major PPD-type ginsenosides Rb1, Rb2, and Rc into minor ginsenosides.
Ginsenosides Re, Rf, and Rg1 are another type of major ginsenosides belong to protopanaxatriol (PPT) triterpenoid saponins, and the positions of C-6 and C-20 are glycosidased with different sugar moieties. The β-glucosidase bgp1 gene consists of 2,496 bp encoding 831 amino acids which have homology to the glycosyl hydrolase families 3 protein domain. Recombinant β-glucosidase bgp1 transformed ginsenosides Re and Rg1 to ginsenosides Rg2 and Rh1, respectively (Quan et al., 2012c). A β-glucosidase gene isolated from A. niger, bgl1, was able to transform ginsenoside Rf into Rh1 (Ruan et al., 2009). The β-glucosidase finally transform Rh1 into PPT with a yield of 90.4% (Liu et al., 2010). Another β-glucosidase gene bglSk, isolated from Sanguibacter keddieii, consists of 1,857 bp and revealed significant homology to that of glycoside hydrolase family 3, which could convert major ginsenosides Rb1, Rb2, Rc, Rd, Re, and Rg1 into rare ginsenosides such as Compound Y, C-Mc, Compound K, Rg2(S), and F1. Kim et al. (2012) found bglSk could completely convert the Rg1 into F1. The biotransformation pathways were shown in Figure 4.
FIGURE 4. Schematic illustration of biotransformation of major PPT-type ginsenosides Re, Rf, and Rg1 into minor ginsenosides.
Collectively, the different β-glucosidase showed specialized catalysed position, and β-glucosidase bgp1 prefers to hydrolyse the glucosides at C-20 position, while β-glucosidase bglSk recognizes C-3 and C-6 position. However, β-glucosidase bgp3 and β-glucosidase isolated from A. niger do not show selectivity at C-6 and C-20.
Microbial transformation is effective in modifying ginsenosides to obtain new chemical derivatives and is also a major production method of minor ginsenosides. The enzymatic transformation showed advantages of a short reaction time, superior environmental protection, and high product yield and purity. However, the separation and purification processes of enzymes are high-cost and complicated, and the reaction conditions are strictly controlled due to the susceptible enzyme activity. In contrast, microbial transformation is characterized by wide applications and low costs, but a dearth of high selectivity and a long conversion time. Thus, the combination of enzymatic and microbial transformation of ginsenosides could warrant the actual production process.
Burkholderia pyrrocinia GP16, Bacillus megaterium GP27, and Sphingomonas echinoides GP50 were screened from 70 strains of aerobic bacteria with β-glucosidase activity, and they almost completely transformed Rb1 to Rd (Kim et al., 2005). With the aid of bacteria L. mesenteroides KFRI 690 or Fungi Arthrinium sp. GE 17–18, ginsenoside Rb1 can be converted to Compound K efficiently with yields of 97.8% and 100%, respectively (Park et al., 2012; Fu et al., 2016). In addition, gypenoside LXXV and F2 were finally obtained via intermediate product Ginsenoside XVII without further conversion by Fungus Esteya vermicola CNU 120806 and bacteria Intrasporangium sp. GS603 transformation, respectively (Cheng et al., 2007; Hou et al., 2012). The scale-up fermentation was carried out using Paecilomyces bainier sp. 229, and ginsenoside Rb1 was converted to a known 3-keto C-K and two new dehydrogenated C-K metabolites (Figure 3), which were isolated through repeated silica gel column chromatography and high-pressure liquid chromatography (Zhou et al., 2018). Furthermore, several kinds of bacteria, such as Microbacterium sp. GS514, Burkholderia sp. GE 17–7, and Flavobacterium sp. GE 32, can transform Rb1 to Rg3 via the intermediate product Rd (Cheng et al., 2008; Fu et al., 2017; Fu, 2019). Rc was converted into minor ginsenosides Rg3 and C-MC1 with bacteria Leuconostoc sp. BG78 and Sphingopyxis sp. BG97, respectively (Ten et al., 2014a and Ten et al., 2014b). Sui et al. (2020) demonstrated that ginsenoside Rg1 could be thoroughly converted into 20(S/R)-Rh1 and 25-OH-20(S/R)-Rh1 by Cordyceps Sinensis, with a biocatalytic pathway established as Rg1→20(S/R)-Rh1→25-OH-20(S/R)-Rh1, and the molar bioconversion rate for total 25-OH-20(S/R)-Rh1 was 82.5%. Aside from bacteria and fungi, human fecal, and intestinal microflora could also transform ginsenosides. While human fecal microflora was prepared from a healthy Chinese man and subsequently incubated with P. quinquefolius saponins at 37°C for 24 h, three most abundant metabolites are identified with liquid chromatography/quadrupole time-of-flight mass spectrometry (LC–Q-TOF-MS) as 20(S)-ginsenoside Rg3, ginsenoside F2, and Compound K (Wan et al., 2013). Additionally, human intestinal bacteria were incubated with ginsenosides Rb1, Rb2, Rb3 and Rc at 37°C under anaerobic conditions, and ginsenoside Compound K was identified as the transformed product after 48 h with transformation rates of 83.5%, 88.7%, 85.6%, and 84.2%, respectively (Zheng et al., 2021).
Gut microbiota mainly transform prototype ginsenosides into rare bioactive metabolites. Unlike in vitro enzyme and microbial transformation, the ginsenosides underlying anaerobically with pooled gut bacteria resulted in some novel metabolites in the plasma, bile, urine, and feces. After Rb1, Rg3, and Rh2 were administered to male Sprague Dawley rats at a dose of 100 mg/kg body weight, Rb1 and Rg3 could be metabolized to Rh2, while Rb1 could be metabolized to Rg3. The final products of Rb1, Rg3, and Rh2 were protopanaxadiol and monooxygenated protopanaxadiol (Qian and Cai, 2010). To further clarify the role of microbiota on metabolism of Rb1, ginsenoside Rb1 was administered to normal and antimicrobials treated rats, and the metabolites of Rb1, such as Rd, F2, and Compound K were detected in normal rat plasma but not in antimicrobials treated rats (Kang et al., 2016). Oxygenated metabolites have been considered as the major circulating metabolites of ginsenosides. After ginsenosides Rb1 and Rg1 were oral administered to rats for 24 h, totally 10 and 9 oxygenated metabolites were characterized by UHPLC-QTOF MS analysis, respectively (Figure 5) (Wang et al., 2015a; Wang et al., 2016). The degradation of ginsenosides has been thoroughly investigated in animals and in vitro using enzymes and microbiota, thus the elucidation of metabolites reaching the systemic circulation in human is of great importance. Six healthy male volunteers ingested 1 g of P. quinquefolius twice a day for 7 days. Totally, 5, 10, and 20 metabolites were detected in plasma, urine, and feces, respectively. And Compound K is found to be the major metabolite in all three samples (Wan et al., 2016).
FIGURE 5. The oxygenated metabolites involved in in vivo biotransformation pathways of ginsenosides Rb1 and Rg1.
PPD and PPT types of ginsenosides were purified from the leaves of P. quinquefolius, and the porcine pancreatic lipase activity was determined in vitro. PDG inhibited the pancreatic lipase activity in a dose-dependent manner at the concentrations of 0.25–1 mg/ml, while PPT showed no inhibitory activity. Moreover, PPD was effective in preventing and healing obesity, fatty liver and hypertriglyceridemia in mice fed with a high-fat diet (Liu et al., 2010b). Another clinical study indicated that the oral intake of P. quinquefolius extract with 1 g/meal (3 g/day) significantly reduced HbA1c and fasting blood glucose, and systolic blood pressure was also lowered (Vuksan et al., 2019). A dammarane from acid hydrolysates of P. quinquefolius total saponins, named 20(R)-dammarane-3β,12β,20,25-tetrahydroxy-3β-O-β-D-glucopyranoside, exhibited significantly inhibitory activity against α-glucosidase, and the IC50 value [(0.22 ± 0.21) μmol/L] was about 43-fold lower than the positive control acarbose, indicating the potential effects of saponins on diabetes (Han et al., 2020). After a 5-weeks treatment of malonyl ginsenosides from P. quinquefolius, the fasting blood glucose (FBG), triglyceride (TG), total cholesterol (TC), low-density lipoprotein cholesterol (LDL-C), nonesterified fatty acid (NEFA), alanine transaminase (ALT), and aspartate transaminase (AST) levels were significantly reduced and glucose tolerance and insulin resistance were improved (Liu et al., 2021). IRS1/PI3K/Akt and IRS1/PI3K/Akt pathways are involved in the anti-T2DM effects of malonyl ginsenosides.
20(S)-PPD is a metabolite of ginseng saponin of P. quinquefolius, which significantly inhibited the growth and induced cell cycle arrest in HCT116 cells. An in vivo study showed that when i.p. administered (30 mg/kg) PPD once every 2 days for 3 weeks, xenograft tumor growth in athymic nude mice bearing HCT116 cells were inhibited (Gao et al., 2013). A structure-function relationship study indicated that sugar numbers within a ginsenoside exerted an inverse impact on tumor cells, and the sugar moiety at C-6 possess higher anti-cancer activity than that with linkages at C-3 or C-20, due to the increased steric hindrance to target proteins after C-6 was sugar substituted (Qi et al., 2010).
The number and position of hydroxyl groups in ginsenosides also affect their pharmacological activities. The substitution of hydroxyl or methoxyl groups at C-25 increases the anti-tumor effects of ginsenosides. Compared with 20(S)-Rh2, 20(S)-PPD and 20(S)-Rg3, 20(S)-25-OH-PPD showed the most apoptotic, antiproliferative, cell cycle arrest, and tumor growth inhibition effects in vivo (Wang et al., 2008b). In addition, usually 20(S) stereoisomers of ginsenosides show stronger chemopreventive effects than 20(R) stereoisomers (Qi et al., 2010).
When fifty-two healthy volunteers (40–60 years old, mean age 51.63) received 200 mg of P. quinquefolius or a matching placebo for 1, 3, and 6 h according to a double-blind, placebo-controlled, balanced, crossover design, the result showed that cognitive performance on “Working Memory” was significantly improved after treatment for 3 h (Ossoukhova et al., 2015). In addition, Cereboost™, an extract of P. quinquefolius extract, restored Aβ1-42 which insulted downregulation of brain microtubule-associated protein 2 and synaptophysin as well as acetylcholine concentration, thus recovered the cognitive function (Shin et al., 2016). When APP/PS1 AD mice was administered by pseudoginsenoside-F11 at 8 mg/kg for 4 weeks, the expressions of β-amyloid precursor protein (APP) and Aβ1-40 in the cortex and hippocampus were significantly inhibited, and the activities of superoxide dismutase (SOD) and glutathione peroxidase (GSH-Px) were restored (Wang et al., 2013a). Additionally, pseudoginsenoside-F11 exerts anti-Parkinson effects through inhibiting free radical formation and stimulating endogenous antioxidant release in a 6-hydroxydopamine-lesioned rat model (Wang et al., 2013b).
Experimental autoimmune encephalomyelitis (EAE) is a commonly used experimental model for the demyelinating disease, multiple sclerosis (MS). An aqueous extract of ginseng (150 mg/kg body mass) was oral administered to MOG (35–55) peptide induced EAE mice, and the clinical signs of EAE, TNF-α expression, and iNOS and demyelination scores were significantly improved compared with model mice (Bowie et al., 2012). Pseudoginsenoside-F11 (4 and 8 mg/kg bw twice at a 4 h interval) significantly mitigated anxiety-like behavior in methamphetamine-induced rats, shortened the time of immobility in forced swimming test, and significantly decreased the number of errors in the T-maze test (Wu et al., 2003).
The saponins from the leaves of P. quinquefolius showed a renoprotective effect in a mouse model of cisplatin-induced acute kidney injury. The further mechanism study clarified that saponins administration significantly suppressed the protein expression levels of Nox4, cleaved-Caspase-3, cleaved-Caspase-9, Bax, NF-κB, COX-2, and iNOS (Ma et al., 2017).
A MI/R model was constructed to investigate whether P. quinquefolius saponins decrease no-reflow phenomenon via suppression of inflammation, and the results showed that the inhibition of NLRP3 inflammasome via TLR4/MyD88/NF-κB signaling pathway is involved in P. quinquefolius saponins effects on cardiac functional improvement and pathological morphology changes of myocardium (Yu et al., 2021). Mice pretreated with saponins from the leaves of P. quinquefolius (150 or 300 mg/kg) by oral gavage for 7 days significantly reversed acetaminophen induced liver injury. Further study indicated that anti-oxidant, anti-apoptotic and anti-inflammatory activities were involved in its mechanism (Xu et al., 2017).
The heated P. quinquefolius could protect cell viability against H2O2-induced oxidative damage, and enhance the activities of superoxide dismutase and catalase dose dependently in V79-4 cells (Kim et al., 2007). Heat-processing reduced the content of ginsenosides Rb1, Re, Rc, and Rd, and increased the content of Rg2 and Rg3 in P. quinquefolius. After 2 h steaming, the percent content of ginsenoside Rg3 increased from 0.06% to 5.9%, and Rg3 showed the best antiproliferative effects in human breast cancer cell line MCF-7 via arresting cancer cells in G1-phase (Wang et al., 2008a).
Ginsenoside C-Y can be used as a potential botanical agent to protect premature skin from UVB-induced photodamage and prevent skin hyperpigmentation (Liu et al., 2019). Taken together, P. quinquefolius and its derived ginsenosides possess a variety of pharmacological activities (Figure 6), which is a promising medicinal plant for human health.
FIGURE 6. Biological and pharmacological activities of P. quinquefolius and its derived ginsenosides.
Collectively, recent advances on the cultivation, chemical diversity, biotransformation, pharmacological, and clinical studies of P. quinquefolius were summarized in this review. A total of 75 naturally occurring ginsenosides have been identified from the roots, leaves and stems, flower buds, and fruits of wild or cultivated P. quinquefolius. With the aid of advanced chemical and analytical techniques and the characterization of novel compounds, the diversity of ginsenosides is constantly revealed.
Major ginsenosides, the main components in P. quinquefolius, are usually difficult to be absorbed and exhibit low bioavailability. However, minor ginsenosides with relatively high bioavailability and pharmacological activities can be obtained by biotransformation. Some of P. quinquefolius associated bacteria, fungus or their enzymes were purified, with highly selectivity to the substituted sugar moieties in C-3, C-6 and C-20. The in vitro and in vivo metabolic pathways of major ginsenosides are also discussed. Moreover, the pharmacological activities of P. quinquefolius or its derived ginsenosides, including anti-tumor, anti-diabetes and obesity, anti-colitis, anti-hepatotoxicity, anti-neurodegenerative disease, myocardial, and renoprotection were exhibited and summarized.
In conclusion, P. quinquefolius is a very promising medicinal plant for the treatment of diverse diseases, while the greater attention of the following issues should be focused in the future: 1) Due to the low yields of naturally occurring minor ginsenosides, most of the novel compounds are not screened for their biological activities, and total or semi-synthesis and directional biotransformation may be efficient ways. 2) Although the pharmacological effects of some ginsenosides were investigated, the direct targets and mechanism are rarely discovered, which need to be further elucidated.
ZY generated the main idea, prepared the figures and tables, and wrote the manuscript. JD and CH performed literature search on pharmacological effects and biotransformation of ginsenosides. XF and SL performed literature search on ginsenoside structures. ZY performed the experiments and analysed the data. ML and SW performed a critical review of data and literature, edited the paper content and its final content.
This research was funded by the Natural Science Foundation of Guangdong Province of China (Nos. 2020A1515010779 and 2022A1515011419), National Natural Science Foundation of China (No. 62171143), and Special Program for Key Field of Guangdong Colleges (No. 2021ZDZX1060).
The authors declare that the research was conducted in the absence of any commercial or financial relationships that could be construed as a potential conflict of interest.
All claims expressed in this article are solely those of the authors and do not necessarily represent those of their affiliated organizations, or those of the publisher, the editors and the reviewers. Any product that may be evaluated in this article, or claim that may be made by its manufacturer, is not guaranteed or endorsed by the publisher.
Assinewe, V. A., Baum, B. R., Gagnon, D., and Arnason, J. T. (2003). Phytochemistry of wild populations of Panax quinquefolius L. (North American ginseng). J. Agric. Food Chem. 51, 4549–4553. doi:10.1021/jf030042h
Bowie, L. E., Roscoe, W. A., Lui, E. M., Smith, R., and Karlik, S. J. (2012). Effects of an aqueous extract of North American ginseng on MOG(35-55)-induced EAE in mice. Can. J. Physiol. Pharmacol. 90, 933–939. doi:10.1139/y2012-092
Chen, S. E., Staba, E. J., Taniyasu, S., Kasai, R., and Tanaka, O. (1981). Further study on dammarane-saponins of leaves and stems of American Ginseng, Panax quinquefolium. Planta Med. 42, 406–409. doi:10.1055/s-2007-971664
Chen, J., Zhao, R., Zeng, Y. M., Meng, H., Zuo, W. J., Li, X., et al. (2009). Three new triterpenoid saponins from the leaves and stems of Panax quinquefolium. J. Asian Nat. Prod. Res. 11, 195–201. doi:10.1080/10286020802682734
Cheng, L. Q., Na, J. R., Kim, M. K., Bang, M. H., and Yang, D. C. (2007). Microbial conversion of ginsenoside Rb1 to minor ginsenoside F2 and gypenoside XVII by Intrasporangium sp. GS603 isolated from soil. J. Microbiol. Biotechnol. 17, 1937–1943.
Cheng, L. Q., Na, J. R., Bang, M. H., Kim, M. K., and Yang, D. C. (2008). Conversion of major ginsenoside Rb1 to 20(S)-ginsenoside Rg3 by Microbacterium sp. GS514. Phytochemistry 69, 218–224. doi:10.1016/j.phytochem.2007.06.035
Dong, W. W., Han, X. Z., Zhao, J., Zhong, F. L., Ma, R., Wu, S., et al. (2018). Metabolite profiling of ginsenosides in rat plasma, urine and feces by LC-MS/MS and its application to a pharmacokinetic study after oral administration of Panax ginseng extract. Biomed. Chromatogr. 32, e4105. doi:10.1002/bmc.4105
Dou, D., Li, W., Guo, N., Fu, R., Pei, Y., Koike, K., et al. (2006). Ginsenoside Rg8, a new dammarane-type triterpenoid saponin from roots of Panax quinquefolium. Chem. Pharm. Bull. 54, 751–753. doi:10.1248/cpb.54.751
Fu, Y., Yin, Z. H., Wu, L. P., and Yin, C. R. (2016). Biotransformation of ginsenoside Rb1 to ginsenoside C-K by endophytic fungus Arthrinium sp. GE 17-18 isolated from Panax ginseng. Lett. Appl. Microbiol. 63, 196–201. doi:10.1111/lam.12606
Fu, Y., Yin, Z. H., and Yin, C. Y. (2017). Biotransformation of ginsenoside Rb1 to ginsenoside Rg3 by endophytic bacterium Burkholderia sp. GE 17-7 isolated from Panax ginseng. J. Appl. Microbiol. 122, 1579–1585. doi:10.1111/jam.13435
Fu, Y. (2019). Biotransformation of ginsenoside Rb1 to Gyp-XVII and minor ginsenoside Rg3 by endophytic bacterium Flavobacterium sp. GE 32 isolated from Panax ginseng. Lett. Appl. Microbiol. 68, 134–141. doi:10.1111/lam.13090
Fuzzati, N. (2004). Analysis methods of ginsenosides. J. Chromatogr. B Anal. Technol. Biomed. Life Sci. 812, 119–133. doi:10.1016/j.jchromb.2004.07.039
Gao, J. L., Lv, G. Y., He, B. C., Zhang, B. Q., Zhang, H., Wang, N., et al. (2013). Ginseng saponin metabolite 20(S)-protopanaxadiol inhibits tumor growth by targeting multiple cancer signaling pathways. Oncol. Rep. 30, 292–298. doi:10.3892/or.2013.2438
Han, L., Lin, M. Y., Zheng, Q., Liu, H. Y., Liu, H. Y., Dong, G., et al. (2014). A new epimer of ocotillol from stems and leaves of American ginseng. Nat. Prod. Res. 28, 935–939. doi:10.1080/14786419.2014.896008
Han, L., Li, Z., Zheng, Q., Liu, J. P., and Li, P. Y. (2016). A new triterpenoid compound from stems and leaves of American ginseng. Nat. Prod. Res. 30, 13–19. doi:10.1080/14786419.2015.1030403
Han, S., Shi, S., Zou, Y., Wang, Z., Wang, Y., Shi, L., et al. (2020). Chemical constituents from acid hydrolyzates of Panax quinquefolius total saponins and their inhibition activity to α-glycosidase and protein tyrosine phosphatase 1B. Chin. Herb. Med. 12, 195–199. doi:10.1016/j.chmed.2020.03.003
Hou, J. G., Xue, J. J., Sun, M. Q., Wang, C. Y., Liu, L., Zhang, D. L., et al. (2012). Highly selective microbial transformation of major ginsenoside Rb1 to gypenoside LXXV by Esteya vermicola CNU120806. J. Appl. Microbiol. 113, 807–814. doi:10.1111/j.1365-2672.2012.05400.x
Kang, A., Zhang, S., Zhu, D., Dong, Y., Shan, J., Xie, T., et al. (2016). Gut microbiota in the pharmacokinetics and colonic deglycosylation metabolism of ginsenoside Rb1 in rats: Contrary effects of antimicrobials treatment and restraint stress. Chem. Biol. Interact. 258, 187–196. doi:10.1016/j.cbi.2016.09.005
Kim, K. K., Lee, J. W., Lee, K. Y., and Yang, D. C. (2005). Microbial conversion of major ginsenoside rb(1) to pharmaceutically active minor ginsenoside rd. J. Microbiol. 43, 456–462. doi:10.1016/j.jgr.2015.11.004
Kim, K. T., Yoo, K. M., Lee, J. W., Eom, S. H., Hwang, I. K., Lee, C. Y., et al. (2007). Protective effect of steamed American ginseng (Panax quinquefolius L.) on V79-4 cells induced by oxidative stress. J. Ethnopharmacol. 111, 443–450. doi:10.1016/j.jep.2007.01.004
Kim, J. K., Cui, C. H., Yoon, M. H., Kim, S. C., and Im, W. T. (2012). Bioconversion of major ginsenosides Rg1 to minor ginsenoside F1 using novel recombinant ginsenoside hydrolyzing glycosidase cloned from Sanguibacter keddieii and enzyme characterization. J. Biotechnol. 161, 294–301. doi:10.1016/j.jbiotec.2012.06.021
Kim, M. J., Upadhyaya, J., Yoon, M. S., Ryu, N. S., Song, Y. E., Park, H. W., et al. (2018). Highly regioselective biotransformation of ginsenoside Rb2 into compound Y and compound K by β-glycosidase purified from Armillaria mellea mycelia. J. Ginseng Res. 42, 504–511. doi:10.1016/j.jgr.2017.07.001
Kim, J. H., Oh, J. M., Chun, S., Park, H. Y., and Im, W. T. (2020). Enzymatic biotransformation of ginsenoside Rb2 into rd by recombinant α-L-arabinopyranosidase from Blastococcus saxobsidens. J. Microbiol. Biotechnol. 30, 391–397. doi:10.4014/jmb.1910.10065
Li, T. S. C., Mazza, G., Cottrell, A. C., and Gao, L. (1996). Ginsenosides in roots and leaves of American ginseng. J. Agric. Food Chem. 44, 717–720. doi:10.1021/jf950309f
Li, G. Y., Zeng, Y. M., Meng, H., Li, X., and Wang, J. H. (2009). A new triterpenoid saponin from the leaves and stems of Panax quinquefolium L. Chin. Chem. Lett. 20, 1207–1210. doi:10.1016/j.cclet.2009.05.017
Liu, J. P., Tian, X., Liu, H. Y., Zhang, Q. H., Lu, D., Li, P. Y., et al. (2013). Two novel dammarane-type compounds from the leaves and stems of Panax quinquefolium L. J. Asian Nat. Prod. Res. 15, 974–978. doi:10.1080/10286020.2013.794416
Liu, X. Y., Xiao, Y. K., Hwang, E., Haeng, J. J., and Yi, T. H. (2019). Antiphotoaging and antimelanogenesis properties of ginsenoside C-Y, a ginsenoside Rb2 metabolite from American ginseng PDD-ginsenoside. Photochem. Photobiol. 95, 1412–1423. doi:10.1111/php.13116
Liu, Z., Qu, C. Y., Li, J. X., Wang, Y. F., Li, W., Wang, C. Z., et al. (2021). Hypoglycemic and hypolipidemic effects of malonyl ginsenosides from American ginseng (Panax quinquefolius L.) on type 2 diabetic mice. ACS Omega 6, 33652–33664. doi:10.1021/acsomega.1c04656
Liu, L., Gu, L. J., Zhang, D. L., Wang, Z., Wang, C. Y., Li, Z., et al. (2010a). Microbial conversion of rare ginsenoside Rf to 20(S)-protopanaxatriol by Aspergillus niger. Biosci. Biotechnol. Biochem. 74, 96–100. doi:10.1271/bbb.90596
Liu, R., Zhang, J., Liu, W., Kimura, Y., and Zheng, Y. (2010b). Anti-Obesity effects of protopanaxdiol types of Ginsenosides isolated from the leaves of American ginseng (Panax quinquefolius L.) in mice fed with a high-fat diet. Fitoterapia 81, 1079–1087. doi:10.1016/j.fitote.2010.07.002
Lu, D., Li, P., and Liu, J. (2012). Quinquenoside F₆, a new triterpenoid saponin from the fruits of Panax quinquefolium L. Nat. Prod. Res. 26, 1395–1401. doi:10.1080/14786419.2011.592833
Ma, Z. N., Li, Y. Z., Li, W., Yan, X. T., Yang, G., Zhang, J., et al. (2017). Nephroprotective effects of saponins from leaves of Panax quinquefolius against cisplatin-induced acute kidney injury. Int. J. Mol. Sci. 18, 1407. doi:10.3390/ijms18071407
Nakamura, S., Sugimoto, S., Matsuda, H., and Yoshikawa, M. (2007). Medicinal flowers. XVII. New dammarane-type triterpene glycosides from flower buds of American ginseng, Panax quinquefolium L. Chem. Pharm. Bull. 55, 1342–1348. doi:10.1248/cpb.55.1342
Ossoukhova, A., Owen, L., Savage, K., Meyer, M., Ibarra, A., Roller, M., et al. (2015). Improved working memory performance following administration of a single dose of American ginseng (Panax quinquefolius L.) to healthy middle-age adults. Hum. Psychopharmacol. 30, 108–122. doi:10.1002/hup.2463
Park, S. J., Youn, S. Y., Ji, G. E., and Park, M. S. (2012). Whole cell biotransformation of major ginsenosides using leuconostocs and lactobacilli. Food Sci. Biotechnol. 21, 839–844. doi:10.1007/s10068-012-0108-z
Popovich, D. G., and Kitts, D. D. (2004). Mechanistic studies on protopanaxadiol, Rh2, and ginseng (Panax quinquefolius) extract induced cytotoxicity in intestinal Caco-2 cells. J. Biochem. Mol. Toxicol. 18, 143–149. doi:10.1002/jbt.20019
Qi, L. W., Wang, C. Z., and Yuan, C. S. (2010). American ginseng: potential structure-function relationship in cancer chemoprevention. Biochem. Pharmacol. 80, 947–954. doi:10.1016/j.bcp.2010.06.023
Qi, L. W., Wang, C. Z., and Yuan, C. S. (2011). Ginsenosides from American ginseng: chemical and pharmacological diversity. Phytochemistry 72, 689–699. doi:10.1016/j.phytochem.2011.02.012
Qi, Z., Wang, Z., Zhou, B., Fu, S., Hong, T., Li, P., et al. (2020). A new ocotillol-type ginsenoside from stems and leaves of Panax quinquefolium L. and its anti-oxidative effect on hydrogen peroxide exposed A549 cells. Nat. Prod. Res. 34, 2474–2481. doi:10.1080/14786419.2018.1543677
Qian, T., and Cai, Z. (2010). Biotransformation of ginsenosides Rb1, Rg3 and Rh2 in rat gastrointestinal tracts. Chin. Med. 5, 19. doi:10.1186/1749-8546-5-19
Quan, L. H., Cheng, L. Q., Kim, H. B., Kim, J. H., Son, N. R., Kim, S. Y., et al. (2010). Bioconversion of ginsenoside rd into compound k by lactobacillus pentosus dc101 isolated from kimchi. J. Ginseng Res. 34, 288–295. doi:10.5142/jgr.2010.34.4.288
Quan, L. H., Piao, J. Y., Min, J. W., Kim, H. B., Kim, S. R., Yang, D. U., et al. (2011). Biotransformation of ginsenoside Rb1 to prosapogenins, gypenoside XVII, ginsenoside rd, ginsenoside F2, and compound K by Leuconostoc mesenteroides DC102. J. Ginseng Res. 35, 344–351. doi:10.5142/jgr.2011.35.3.344
Quan, L. H., Jin, Y., Wang, C., Min, J. W., Kim, Y. J., Yang, D. C., et al. (2012a). Enzymatic transformation of the major ginsenoside Rb2 to minor compound Y and compound K by a ginsenoside-hydrolyzing β-glycosidase from Microbacterium esteraromaticum. J. Ind. Microbiol. Biotechnol. 39, 1557–1562. doi:10.1007/s10295-012-1158-1
Quan, L. H., Min, J. W., Jin, Y., Wang, C., Kim, Y. J., Yang, D. C., et al. (2012b). Enzymatic biotransformation of ginsenoside Rb1 to compound K by recombinant β-glucosidase from Microbacterium esteraromaticum. J. Agric. Food Chem. 60, 3776–3781. doi:10.1021/jf300186a
Quan, L. H., Min, J. W., Sathiyamoorthy, S., Yang, D. U., Kim, Y. J., and Yang, D. C. (2012c). Biotransformation of ginsenosides Re and Rg1 into ginsenosides Rg2 and Rh1 by recombinant β-glucosidase. Biotechnol. Lett. 34, 913–917. doi:10.1007/s10529-012-0849-z
Quan, L. H., Min, J. W., Yang, D. U., Kim, Y. J., and Yang, D. C. (2012d). Enzymatic biotransformation of ginsenoside Rb1 to 20(S)-Rg3 by recombinant β-glucosidase from Microbacterium esteraromaticum. Appl. Microbiol. Biotechnol. 94, 377–384. doi:10.1007/s00253-011-3861-7
Quan, K., Liu, Q., Wan, J. Y., Zhao, Y. J., Guo, R. Z., Alolga, R. N., et al. (2015). Rapid preparation of rare ginsenosides by acid transformation and their structure-activity relationships against cancer cells. Sci. Rep. 5, 8598. doi:10.1038/srep08598
Ruan, C. C., Zhang, H., Zhang, L. X., Liu, Z., Sun, G. Z., Lei, J., et al. (2009). Biotransformation of ginsenoside Rf to Rh1 by recombinant beta-glucosidase. Molecules 14, 2043–2048. doi:10.3390/molecules14062043
Ryu, J., Lee, H. W., Yoon, J., Seo, B., Kwon, D. E., Shin, U. M., et al. (2017). Effect of hydrothermal processing on ginseng extract. J. Ginseng Res. 41, 572–577. doi:10.1016/j.jgr.2016.12.002
Shen, L., Li, X. W., Meng, X. X., Wu, J., Tang, H., Huang, L. F., et al. (2019). Prediction of the globally ecological suitability of Panax quinquefolius by the geographic information system for global medicinal plants (GMPGIS). Chin. J. Nat. Med. 17, 481–489. doi:10.1016/S1875-5364(19)30069-X
Shin, K., Guo, H., Cha, Y., Ban, Y. H., Seo, da. W., Choi, Y., et al. (2016). Cereboost™, an American ginseng extract, improves cognitive function via up-regulation of choline acetyltransferase expression and neuroprotection. Regul. Toxicol. Pharmacol. 78, 53–58. doi:10.1016/j.yrtph.2016.04.006
Sui, X., Liu, J., Xin, Y., Qu, M., Qiu, Y., He, T., et al. (2020). Highly regioselective biotransformation of ginsenoside Rg1 to 25-OH derivatives of 20(S/R)-Rh1 by Cordyceps Sinensis. Bioorg. Med. Chem. Lett. 30, 127504. doi:10.1016/j.bmcl.2020.127504
Szczuka, D., Nowak, A., Zakłos-Szyda, M., Kochan, E., Szymańska, G., Motyl, I., et al. (2019). American ginseng (Panax quinquefolium L.) as a source of bioactive phytochemicals with pro-health properties. Nutrients 11, 1041. doi:10.3390/nu11051041
Tawab, M. A., Bahr, U., Karas, M., Wurglics, M., and Schubert-Zsilavecz, M. (2003). Degradation of ginsenosides in humans after oral administration. Drug Metab. Dispos. 31, 1065–1071. doi:10.1124/dmd.31.8.1065
Ten, L. N., Chae, S. M., and Yoo, S. A. (2014a). Transformation of ginsenoside rc into (20S)-Rg3 by the bacterium Leuconostoc sp. BG78. Chem. Nat. Compd. 50, 562–564. doi:10.1007/s10600-014-1018-5
Ten, L. N., Chae, S. M., and Yoo, S. A. (2014b). Biotransformation of ginsenoside rc into c-mc1 by the bacterium sphingopyxis sp. bg97. Chem. Nat. Compd. 50, 565–567. doi:10.1007/s10600-014-1019-4
Vuksan, V., Xu, Z. Z., Jovanovski, E., Jenkins, A. L., Beljan-Zdravkovic, U., Sievenpiper, J. L., et al. (2019). Efficacy and safety of American ginseng (Panax quinquefolius L.) extract on glycemic control and cardiovascular risk factors in individuals with type 2 diabetes: a double-blind, randomized, cross-over clinical trial. Eur. J. Nutr. 58, 1237–1245. doi:10.1007/s00394-018-1642-0
Wan, J. Y., Liu, P., Wang, H. Y., Qi, L. W., Wang, C. Z., Li, P., et al. (2013). Biotransformation and metabolic profile of American ginseng saponins with human intestinal microflora by liquid chromatography quadrupole time-of-flight mass spectrometry. J. Chromatogr. A 1286, 83–92. doi:10.1016/j.chroma.2013.02.053
Wan, J. Y., Wang, C. Z., Liu, Z., Zhang, Q. H., Musch, M. W., Bissonnette, M., et al. (2016). Determination of American ginseng saponins and their metabolites in human plasma, urine and feces samples by liquid chromatography coupled with quadrupole time-of-flight mass spectrometry. J. Chromatogr. B Anal. Technol. Biomed. Life Sci. 1015-1016, 62–73. doi:10.1016/j.jchromb.2016.02.008
Wang, J., Li, W., and Li, X. (1998). A new saponin from the leaves and stems of Panax quinquefolium L. collected in Canada. J. Asian Nat. Prod. Res. 1, 93–97. doi:10.1080/10286029808039849
Wang, X., Sakuma, T., Asafu-Adjaye, E., and Shiu, G. K. (1999). Determination of ginsenosides in plant extracts from Panax ginseng and Panax quinquefolius L. by LC/MS/MS. Anal. Chem. 71, 1579–1584. doi:10.1021/ac980890p
Wang, J. H., Li, W., Sha, Y., Tezuka, Y., Kadota, S., Li, X., et al. (2001). Triterpenoid saponins from leaves and stems of Panax quinquefolium L. J. Asian Nat. Prod. Res. 3, 123–130. doi:10.1080/10286020108041379
Wang, C. Z., Aung, H. H., Zhang, B., Sun, S., Li, X. L., He, H., et al. (2008a). Chemopreventive effects of heat-processed Panax quinquefolius root on human breast cancer cells. Anticancer Res. 28, 2545–2551.
Wang, W., Wang, H., Rayburn, E. R., Zhao, Y., Hill, D. L., Zhang, R., et al. (2008b). 20(S)-25-methoxyl-dammarane-3beta, 12beta, 20-triol, a novel natural product for prostate cancer therapy: activity in vitro and in vivo and mechanisms of action. Br. J. Cancer 98, 792–802. doi:10.1038/sj.bjc.6604227
Wang, L., Liu, Q. M., Sung, B. H., An, D. S., Lee, H. G., Kim, S. G., et al. (2011). Bioconversion of ginsenosides Rb(1), Rb(2), rc and rd by novel β-glucosidase hydrolyzing outer 3-O glycoside from Sphingomonas sp. 2F2: cloning, expression, and enzyme characterization. J. Biotechnol. 156, 125–133. doi:10.1016/j.jbiotec.2011.07.024
Wang, C. M., Liu, M. Y., Wang, F., Wei, M. J., Wang, S., Wu, C. F., et al. (2013a). Anti-amnesic effect of pseudoginsenoside-F11 in two mouse models of Alzheimer's disease. Pharmacol. Biochem. Behav. 106, 57–67. doi:10.1016/j.pbb.2013.03.010
Wang, J. Y., Yang, J. Y., Wang, F., Fu, S. Y., Hou, Y., Jiang, B., et al. (2013b). Neuroprotective effect of pseudoginsenoside-f11 on a rat model of Parkinson's disease induced by 6-hydroxydopamine. Evid. Based. Complement. Altern. Med. 2013, 152798. doi:10.1155/2013/152798
Wang, J. R., Yau, L. F., Tong, T. T., Feng, Q. T., Bai, L. P., Ma, J., et al. (2015a). Characterization of oxygenated metabolites of ginsenoside Rb1 in plasma and urine of rat. J. Agric. Food Chem. 63, 2689–2700. doi:10.1021/acs.jafc.5b00710
Wang, Y., Choi, H. K., Brinckmann, J. A., Jiang, X., and Huang, L. (2015b). Chemical analysis of Panax quinquefolius (North American ginseng): A review. J. Chromatogr. A 1426, 1–15. doi:10.1016/j.chroma.2015.11.012
Wang, Y. S., Jin, Y. P., Gao, W., Xiao, S. Y., Zhang, Y. W., Zheng, P. H., et al. (2015c). Complete (1)H-NMR and (13)C-NMR spectral assignment of five malonyl ginsenosides from the fresh flower buds of Panax ginseng. J. Ginseng Res. 40, 245–250. doi:10.1016/j.jgr.2015.08.003
Wang, J. R., Tong, T. T., Yau, L. F., Chen, C. Y., Bai, L. P., Ma, J., et al. (2016). Characterization of oxygenated metabolites of ginsenoside Rg1 in plasma and urine of rat. J. Chromatogr. B Anal. Technol. Biomed. Life Sci. 1026, 75–86. doi:10.1016/j.jchromb.2015.12.028
Wang, H., Zhang, C., Zuo, T., Li, W., Jia, L., Wang, X., et al. (2019). In-depth profiling, characterization, and comparison of the ginsenosides among three different parts (the root, stem leaf, and flower bud) of Panax quinquefolius L. by ultra-high performance liquid chromatography/quadrupole-Orbitrap mass spectrometry. Anal. Bioanal. Chem. 411, 7817–7829. doi:10.1007/s00216-019-02180-8
Wu, C. F., Liu, Y. L., Song, M., Liu, W., Wang, J. H., Li, X., et al. (2003). Protective effects of pseudoginsenoside-F11 on methamphetamine-induced neurotoxicity in mice. Pharmacol. Biochem. Behav. 76, 103–109. doi:10.1016/s0091-3057(03)00215-6
Wu, L., Jin, Y., Yin, C., and Bai, L. (2012). Co-transformation of Panax major ginsenosides Rb₁ and Rg₁ to minor ginsenosides C-K and F₁ by Cladosporium cladosporioides. J. Ind. Microbiol. Biotechnol. 39, 521–527. doi:10.1007/s10295-011-1058-9
Wu, X., Qu, B., Liu, Y., Ren, X., Wang, S., Quan, Y., et al. (2021). Highly enhanced activity and stability via affinity induced immobilization β-glucosidase from Aspergillus niger onto amino-based silica for the biotransformation of ginsenoside Rb1. J. Chromatogr. A 1653, 462388. doi:10.1016/j.chroma.2021.462388
Xiang, Z., Lv, J., Zhou, Z., Li, Y., Dou, D., Zhao, J., et al. (2013). Two new dammarane-type saponins from leaves of Panax quinquefolium. Nat. Prod. Res. 27, 1271–1276. doi:10.1080/14786419.2012.730045
Xie, J., Zhao, D., Zhao, L., Pei, J., Xiao, W., Ding, G., et al. (2016). Characterization of a novel arabinose-tolerant α-L-arabinofuranosidase with high ginsenoside Rc to ginsenoside Rd bioconversion productivity. J. Appl. Microbiol. 120, 647–660. doi:10.1111/jam.13040
Xu, X. Y., Hu, J. N., Liu, Z., Zhang, R., He, Y. F., Hou, W., et al. (2017). Saponins (ginsenosides) from the leaves of Panax quinquefolius ameliorated acetaminophen-induced hepatotoxicity in mice. J. Agric. Food Chem. 65, 3684–3692. doi:10.1021/acs.jafc.7b00610
Yan, C., Hao, C., Jin, W., Dong, W., and Quan, L. (2021). Biotransformation of ginsenoside Rb1 to ginsenoside F2 by recombinant β-glucosidase from rat intestinal Enterococcus gallinarum. Biotechnol. Bioproc. E. 26, 968–975. doi:10.1007/s12257-021-0008-2
Yang, W. Z., Hu, Y., Wu, W. Y., Ye, M., and Guo, D. A. (2014). Saponins in the genus panax L. (Araliaceae): a systematic review of their chemical diversity. Phytochemistry 106, 7–24. doi:10.1016/j.phytochem.2014.07.012
Yoshikawa, M., Murakami, T., Yashiro, K., Yamahara, J., Matsuda, H., Saijoh, R., et al. (1998). Bioactive saponins and glycosides. XI. Structures of new dammarane-type triterpene oligoglycosides, quinquenosides I, II, III, IV, and V, from American ginseng, the roots of Panax quinquefolium L. Chem. Pharm. Bull. 46, 647–654. doi:10.1248/cpb.46.647
Yu, S., Zhou, X., Li, F., Xu, C., Zheng, F., Li, J., et al. (2017). Microbial transformation of ginsenoside Rb1, Re and Rg1 and its contribution to the improved anti-inflammatory activity of ginseng. Sci. Rep. 7, 138. doi:10.1038/s41598-017-00262-0
Yu, P., Li, Y., Fu, W., Li, X., Liu, Y., Wang, Y., et al. (2021). Panax quinquefolius L. Saponins protect myocardial ischemia reperfusion No-reflow through inhibiting the activation of NLRP3 inflammasome via TLR4/MyD88/NF-κB signaling pathway. Front. Pharmacol. 11, 607813. doi:10.3389/fphar.2020.607813
Yuan, C. S., Wang, C. Z., Wicks, S. M., and Qi, L. W. (2010). Chemical and pharmacological studies of saponins with a focus on American ginseng. J. Ginseng Res. 34, 160–167. doi:10.5142/jgr.2010.34.3.160
Zhang, Q., Wen, J., Chang, Z. Q., Xie, C. X., and Song, J. Y. (2018). Evaluation and prediction of ecological suitability of medicinal plant American ginseng (panax quinquefolius). Chin. Herb. Med. 1, 80–85. doi:10.1016/j.chmed.2018.01.003
Zheng, F., Zhang, M. Y., Wu, Y. X., Wang, Y. Z., Li, F. T., Han, M. X., et al. (2021). Biotransformation of ginsenosides (Rb1, Rb2, Rb3, rc) in human intestinal bacteria and its effect on intestinal flora. Chem. Biodivers. 18, e2100296. doi:10.1002/cbdv.202100296
Zhou, W., Huang, H., Zhu, H., Zhou, P., and Shi, X. (2018). New metabolites from the biotransformation of ginsenoside Rb1 by Paecilomyces bainier sp.229 and activities in inducing osteogenic differentiation by Wnt/β-catenin signaling activation. J. Ginseng Res. 42, 199–207. doi:10.1016/j.jgr.2017.03.004
Keywords: Panax quinquefolius, minor ginsenosides, metabolites, structural diversity, pharmacological effects
Citation: Yang Z, Deng J, Liu M, He C, Feng X, Liu S and Wei S (2022) A review for discovering bioactive minor saponins and biotransformative metabolites in Panax quinquefolius L.. Front. Pharmacol. 13:972813. doi: 10.3389/fphar.2022.972813
Received: 19 June 2022; Accepted: 04 July 2022;
Published: 01 August 2022.
Edited by:
Weicheng Hu, Huaiyin Normal University, ChinaReviewed by:
Lun Wang, Chengdu Institute of Biology (CAS), ChinaCopyright © 2022 Yang, Deng, Liu, He, Feng, Liu and Wei. This is an open-access article distributed under the terms of the Creative Commons Attribution License (CC BY). The use, distribution or reproduction in other forums is permitted, provided the original author(s) and the copyright owner(s) are credited and that the original publication in this journal is cited, in accordance with accepted academic practice. No use, distribution or reproduction is permitted which does not comply with these terms.
*Correspondence: Mingxin Liu, bGl1bXhAZ2RvdS5lZHUuY24=; Shuai Wei, d2Vpc2h1YWl3c0AxMjYuY29t
Disclaimer: All claims expressed in this article are solely those of the authors and do not necessarily represent those of their affiliated organizations, or those of the publisher, the editors and the reviewers. Any product that may be evaluated in this article or claim that may be made by its manufacturer is not guaranteed or endorsed by the publisher.
Research integrity at Frontiers
Learn more about the work of our research integrity team to safeguard the quality of each article we publish.