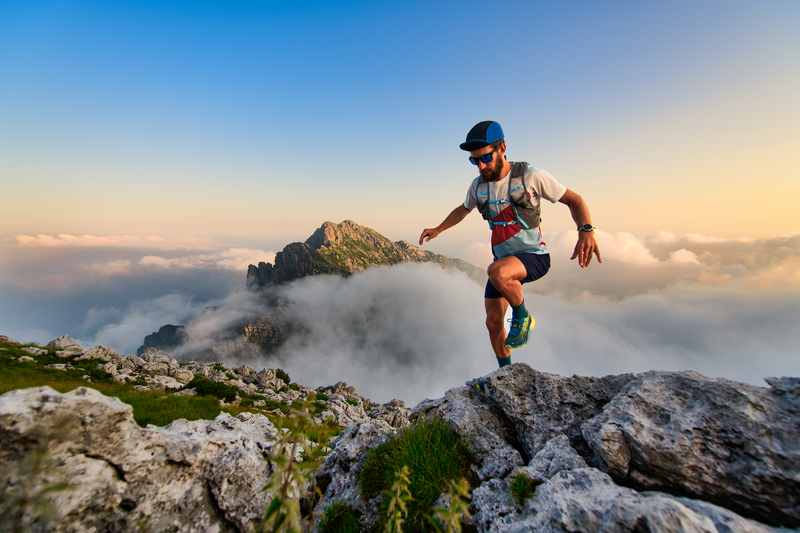
95% of researchers rate our articles as excellent or good
Learn more about the work of our research integrity team to safeguard the quality of each article we publish.
Find out more
REVIEW article
Front. Pharmacol. , 15 August 2022
Sec. Neuropharmacology
Volume 13 - 2022 | https://doi.org/10.3389/fphar.2022.971444
This article is part of the Research Topic Natural Products in the Treatment of Neurological Diseases: Identification of Novel Active Compounds and Therapeutic Targets View all 8 articles
Cryptotanshinone (CTS), a diterpenoid quinone, is found mostly in Salvia miltiorrhiza Bunge (S. miltiorrhiza) and plays a crucial role in many cellular processes, such as cell proliferation/self-renewal, differentiation and apoptosis. In particular, CTS’s profound physiological impact on various stem cell populations and their maintenance and fate determination could improve the efficiency and accuracy of stem cell therapy for high-incidence disease. However, as much promise CTS holds, these CTS-mediated processes are complex and multifactorial and many of the underlying mechanisms as well as their clinical significance for high-incidence diseases are not yet fully understood. This review aims to shed light on the impact and mechanisms of CTS on the actions of diverse stem cells and the involvement of CTS in the many processes of stem cell behavior and provide new insights for the application of CTS and stem cell therapy in treating high-incidence diseases.
GRAPHICAL ABSTRACT | This review summarizes the regulatory role of CTS in the various behaviors of different stem cell populations, and highlights the potential of CST and stem cell therapy in high-incidence diseases.
S. miltiorrhiza is a well-known traditional Chinese herb utilized as a medicine and a health-promoting food (Shi et al., 2019). Cryptotanshinone is a natural product found in S. miltiorrhiza. Many compound medicines containing CTS are currently available on the Chinese market, including Tanshinone Capsules, Danhong Injection (Wang et al., 2021c), and Compound Danshen Dropping Pills (Wang et al., 2022b). In addition, the clinical trials of chemical drugs are summarized (Table 1). CTS is being studied for a variety of pharmacological effects, including anti-inflammatory (Wu et al., 2020; Liu et al., 2021a), neuroprotective (Mao et al., 2021), cardioprotective (Wang et al., 2021e), anti-fibrosis (Lo et al., 2017; Zhang et al., 2020) and anti-tumor (Dong et al., 2018; Han et al., 2019; Vundavilli et al., 2022). The diversity of pharmacological effects of CTS demonstrates unique potential for the treatment of high-incidence diseases.
TABLE 1. Clinical trials of compound medicines containing CTS in various diseases (www.clinicaltrials.gov).
In recent years, research on some high-incidence diseases, such as neurodegenerative diseases, cancer, obesity and obesity-related complications has increased (Blüher, 2019; Siegel et al., 2022). One is that these diseases exist in all populations and age stages, the other is that these diseases have a high mortality rate (Hao et al., 2021; Gribsholt et al., 2022; Siegel et al., 2022), and because of the complexity and intractability of these diseases, the effect of treatment is limited. In contrast, the ability of CTS to modulate different signaling pathways may offer therapeutic and preventive benefits. CTS has been shown to be effective in treating these high-incidence diseases such as obesity, diabetes (Kim et al., 2007), atherosclerosis (Hao et al., 2019), neurodegenerative disease (Maione et al., 2018) and cancer (Chen et al., 2021; Shi et al., 2022). But its effects on stem cell behaviors remain unclear. CTS, as diterpenoid quinones, mostly have o-quinone or para-quinone structures with ternary or four-membered carbon rings on the skeleton (Wang et al., 2017). As lipid soluble components, CTS can more easily pass through the cell membrane (Chen et al., 2012). In addition, diterpenoid quinones can determine the fate of stem cells through a variety of different mechanisms (Li et al., 2018a; Kim et al., 2019; Liu et al., 2019). It provides a further opportunity and reference for future CTS research. Our work focuses on the diverse and critical roles of CTS in various types of stem cells, and the potential of stem cell-dependent therapy for high-incidence diseases.
Stem cells can be extracted from embryonic and postnatal animal tissues and have the dual properties of self-renewal and differentiation (De Los Angeles et al., 2015). Stem cell research has made significant progress in recent years due to its unique features that hold great promise for medicine (Madl et al., 2018). Stem cell transplantation, in which damaged tissues or organs can be healed via autologous or allogeneic stem cell transplantation, is one of the growing subjects of interest in this discipline. Based on the qualities of stem cells listed above, stem cell therapies have been widely employed in osteoporosis and obesity and intensively explored in neurodegenerative diseases and cancer (Lunn et al., 2011; Plaks et al., 2015; Pagnotti et al., 2019). Nevertheless, stem cell therapy has disadvantages, including a low transplantation rate and a low survival rate (Vissers et al., 2019; Xu et al., 2019). Combining stem cell therapy with Chinese herbs may improve stem cell therapy efficacy. CTS, for example, can enhance the development of C3H10T1/2 mesenchymal stem cells (C3H10T1/2 MSCs) into brown adipocytes (Imran et al., 2017). CTS can also enhance the development of bone marrow mesenchymal stem cells (BMSCs) into neural lineage cells (Deng et al., 2006), which may open up new avenues of investigation for obesity and spinal injuries.
To demonstrate the multifunctional potential of CTS as a treatment for high-incidence diseases, this article reviews its regulatory effects on C3H101/2 MSCs, BMSCs, cancer stem cells (CSCs), and neural stem/progenitor cells (NSCs/NPCs) (Table 2), and discusses the mechanism by which CTS promotes cell proliferation/self-renewal and differentiation. This review demonstrates the potential for CTS to improve the application of stem cell therapies and stem cell transplantation and provide new therapeutic strategies for the high-incidence diseases.
C3H10T1/2 can be used as a viable MSC model to determine the ability of potentially obese cells to differentiate into adipocytes (Wen et al., 2022). The induction of C3H10T1/2 MSCs into brown adipocytes is critical for obesity treatment. Between the mid-1970s and 2020, the World Health Organization estimates that the worldwide obesity rate has tripled and may continue to rise (Kumanyika and Dietz, 2020). Obesity is strongly associated with cardiovascular disease, type 2 diabetes, and cancer, the latter of which accounts for most human health problems (GBD 2015 Obesity Collaborators et al., 2017; Kumanyika and Dietz, 2020). Intriguingly, CTS has been revealed to induce differentiation of C3H10T1/2 MSCs into adipocyte lineages (Figure 1). Understanding the mechanism by which CTS induces the differentiation of C3H10T1/2 MSCs is critical for treating obesity.
FIGURE 1. Schematic diagrams of the effects of CTS on differentiation of C3H10T1/2 MSCs for the treatment of obesity.
CTS acts as an anti-diabetic and anti-obesity agent by stimulating the amp-activated protein kinase (AMPK) (Kim et al., 2007). AMPK is required for metabolic management and obesity-related disorders (Lopez, 2017; Garcia et al., 2019) since it regulates brown adipose tissue (BAT) thermogenesis and white adipose tissue browning (Lopez and Tena-Sempere, 2017). Recently, one appealing technique for combating obesity has been the use of heat generated by BAT and beige adipose tissue to treat obesity (Lu et al., 2016). CTS stimulates brown cell development in C3H10T1/2 MSCs via activating the p38 mitogen-activated protein kinase (p38-MAPK)/Adenosine 5‘-monophosphate (AMP)-activated protein kinase α (AMPKα)/drosophila mothers against decapentaplegic protein (Smad1/5) pathway (Imran et al., 2017). Among these, the expressions of Uncoupling protein 1 (Ucp1), PR domain-containing 16 (Prdm16), and peroxlsome proliferator-activated receptor-γ coactlvator-1 (Pgc-1α) specific genes are dramatically elevated in brown adipocytes. Ucp1 is found only in thermogenic adipocytes (including brown and beige adipocytes) and serves as their morphological and functional signature (Pfeifer and Hoffmann, 2015). Additionally, CTS can boost the expression of certain genes, such as TNF Receptor Superfamily Member 9 (Cd137), Heat Shock Protein Family B (Small) Member 7 (Hspb7), cyclooxygenase-2 (Cox2), and Transmembrane Protein 26 (Tmem26) in beige adipocytes induced by the adipogenic hormone 0.5 mM IBMX, 1 μM Dexamethasone, and 10 μg/ml Insulin (MDI). Surprisingly, it can also drastically decrease the mRNA levels of two specific genes in white adipocytes, Phosphoserine Aminotransferase 1 (Psat1) and Resistin (Imran et al., 2017). Notably, activation of AMPK by CTS has been verified in vitro to differentiate C3H10T1/2 MSCs into brown adipocytes. However, in vivo research will need to be conducted in the future to further elucidate the regulatory mechanism through which CTS induces MSCs.
The identification of pathways and associated variables may result in developing new CTS-based obesity treatments in the future. CTS has demonstrated therapeutic potential in treating obesity, and additional research is necessary to fully understand CTS’s role in medicine.
BMSCs are a type of pluripotent stem cell capable of self-replication and differentiation (Hao et al., 2019). BMSCs can be extracted from various tissues, including bone marrow, adipose tissue (Shanbhag et al., 2020), and umbilical cord blood (Contentin et al., 2020). BMSCs can develop into various cell types, including neurons (Hu et al., 2019), and offer distinct benefits in the treatment of spinal cord and nerve injuries. Spinal cord injury (SCI) is an incurable condition that results in the irreversible loss of motor, sensory, and sensory-motor capabilities below the level of the injury. Currently, there is no therapeutic intervention that guarantees complete recovery (Wilson et al., 2012; Chalfouh et al., 2020). CTS can induce the development of BMSCs into neural lineage cells (Figure 2), which makes them more suitable for spinal injury treatment (Deng et al., 2006). Additionally, CTS has been used to treat nervous system injuries (Hu et al., 2019; Li et al., 2019). It represents the therapeutic potential of CTS for SCI.
FIGURE 2. Schematic diagrams of the effects of CTS on differentiation of BMSCs for the treatment of spinal cord injury.
Neuron-specific enolase (NSE), glial fibrillary acidic protein (GFAP), and Neurofilament protein (NF) have been employed as markers for stem cell differentiation into neuronal lineage cells, and their expression ability can reflect stem cell development into neuronal cells (Singh et al., 2013). CTS-induced BMSCs are transplanted into spinal cord-injured monkeys. NF is discovered in 4% of Hoechst33342 stained cells. NSE and GFAP are detected in 5% of Hoechst33342 labeled cells. These findings reveal that CTS can cause BMSCs to differentiate into neuronal lineage cells. Simultaneously, the mRNA expression of GAD65 and GAD67 in the induced cells demonstrates that the differentiated cells can produce neurotransmitters. Furthermore, the monkey cortical somatosensory evoked potential (CSEP) and motor evoked potential (MEP) with SCI reverted to normal, and spinal cord tissue samples are effectively stained with hematoxylin and eosin, demonstrating spinal cord healing and regeneration (Deng et al., 2006).
Unfortunately, the specific mechanisms underlying the differentiation of BMSCs into neural lineage cells induced by CTS are still not fully illustrated yet. As a phosphoinositide 3-kinase (PI3K) inhibitor, LY294002 can stimulate the differentiation of BMSCs into neural cells (Wang et al., 2012). This study shows that inhibiting PI3K/v-akt murine thymoma viral oncogene homolog (AKT) could be a useful mechanism for stem cell research in treating neurological illnesses. A useful tool for tiny compounds that block routes (Wang et al., 2012). CTS plays a vital role by suppressing PI3K/AKT, such as reducing nerve pain after surgery and having anti-stroke properties (Zhu et al., 2017; Zhang et al., 2019). However, it is unclear whether CTS can stimulate BMSC proliferation and differentiation via modulating PI3K/AKT pathway expression.
NSCs are mostly observed in the adult central nervous system as a continuous supply of nerve cells (Draijer et al., 2019). NSCs can self-renewal and are pluripotent. As a result, NSCs can generate all neuroectodermal lineages in a manner appropriate for locations and developmental stages (Kahroba et al., 2021). Furthermore, NSCs can spontaneously develop into neurons, astrocytes, or oligodendrocytes (Wang et al., 2015b; Draijer et al., 2019). NSCs can be stimulated to proliferate, migrate, and differentiate in response to the central nervous system and pathological injury (Kernie and Parent, 2010; Sun, 2014). This, in turn, stimulates neurogenesis (Laterza et al., 2017; Wu et al., 2017). Unfortunately, due to its low cell count, endogenous neurogenesis does not fully promote repairing and regenerating nerve-damaged neurons, particularly in severe nerve injury such as stroke (Kernie and Parent, 2010). As a result, NSC transplantation for the treatment of severe neurodegenerative diseases may be a viable alternative to compensating for abnormalities in the endogenous neural repair mechanism (Boese et al., 2018).
By inhibiting Signal transducer and activator of transcription 3 (STAT3), CTS can prevent the proliferation of human embryonic stem cells-derived NSCs (Zhang et al., 2016a) (Figure 3). As a STAT3 inhibitor, CTS can reduce CSC proliferation as well (Xiong et al., 2018). It will aid future research into the interaction between CTS and stem cells and provide insight into whether the same target may be employed in diverse disorders.
FIGURE 3. Representative scheme illustrating the potential mechanisms underlying the CTS regulation in proliferation and differentiation of NSCs for the treatment of cognitive disorder.
Despite the intense interest in the impact of CTS on NSC proliferation and differentiation, the precise mechanisms behind this regulatory role remain unclear. Thus, how to control NSC and its potential mechanisms induced by CTS may provide a permissive milieu for adult neurogenesis in neurological illnesses. By inhibiting STAT3, CTS can prevent the proliferation of human NSCs. Intriguingly, blocking STAT3 may also boost NSC proliferation (Ma et al., 2018). It is worth investigating whether STAT3 inhibition has distinct effects on NSCs derived from different sources or whether varying concentrations of CST affect NSCs. Similarly, STAT3 plays a critical function in NSC differentiation (Figure 3). STAT3 inhibition can minimize the severity of spinal cord injury (Cui et al., 2017), suppress astrocyte production, and increase NSC neurogenesis (Cao et al., 2010). These findings strongly imply that CTS, via decreasing STAT3, may play a role in neurogenesis.
Despite an accumulating body of research indicates the impact of Chinese herbal monomers on NSCs (Wang et al., 2021d; Wang et al., 2021b), the precise mechanisms are still not fully understood yet. There is no doubt that STAT3 has been a hot topic in the proliferation and differentiation of NSCs (Zyuz’kov et al., 2020; Li et al., 2021). CTS can bind to the SH2 domain of STAT3 and inhibit the phosphorylation of STAT3, preventing STAT3 from forming dimer and make STAT3 unable to serve a function (Shin et al., 2009). In brief, STAT3 is a bridge between CTS and NSCs, which is conducive to the further study of NSCs by CTS.
CTS can inhibit the apoptosis of Parkinson’s disease patient-derived human-induced neuronal progenitor cells (PD-hiNPCs) by restoring the membrane potential availability in PD-hiNPCs, reducing the levels of total ROS and mitochondrial ROS, and down-regulating the expression of apoptotic protein caspase-3 (Lee et al., 2020). ROS have been recognized as an important factor in Parkinson’s disease (Hemmati-Dinarvand et al., 2019). CTS can exert antioxidant effects by activating the transcription of nuclear factor erythroid 2-related factor 2 (NRF2) signaling pathway (Figure 4). Among them, CTS can boost the expression of certain genes, such as superoxide dismutase 1 (SOD1), peroxiredoxin 1 (PRX1), peroxiredoxin 5 (PRX5), glutathione peroxidase (GPX1) and NAD(P)H quinone dehydrogenase 1 (NQO1) in PD-hiNPCs (Lee et al., 2020). These results provide evidence for CTS as a treatment for PD.
FIGURE 4. Representative scheme illustrating CTS’s inhibitory role in the apoptosis of NPCs by regulating the NRF2 signaling pathway for the treatment of parkinson’s disease.
Stem cell therapy has shown significant promise in treating neurodegenerative disorders such as Alzheimer’s and Huntington’s disease (Duncan and Valenzuela, 2017). Simultaneously, CTS has made strides in Alzheimer’s disease research (Mei et al., 2009; Maione et al., 2018). However, the therapeutic effect of transplantation of NSCs pretreated with CTS in vitro is unclear. Therefore, further studies are needed to clarify how CTS mediates the proliferation and differentiation of NSCs. These findings will provide the basis for new therapeutic strategies for the treatment of neurodegenerative diseases.
Tumor-initiating cells (TICs), also known as CSCs, are the primary causes of recurrence, metastasis, and poor prognosis in many types of cancer. As a result, it is thought to be the source of tumorigenesis (Li et al., 2018b; Atashzar et al., 2020). CSCs are more resistant to chemotherapy and radiation treatments, posing a catastrophic dilemma in cancer treatment (Hoey et al., 2009; Vermeulen et al., 2010; Takebe et al., 2015). Stem cells may be the source of all tumor cells in malignant tumors and the primary cause of drug resistance, tumor recurrence, metastasis, and poor prognosis (Maccalli et al., 2018). According to research, targeting or relieving CSCs can restrict tumor formation and progression and minimize treatment resistance, hence preventing tumor progression (Mai et al., 2017; Sun et al., 2017). At the moment, CTS is vital in limiting the proliferation of tumor stem cells (Ding et al., 2016). It has shown promise in inhibiting non-small cell lung cancer stem cells (NSCL CSCs) (Figure 5) and prostate initiating cells (Figure 6).
FIGURE 5. Representative scheme illustrating CTS’s inhibitory role in the proliferation of NSCL CSCs by regulating the Hippo signaling pathway for the treatment of non-small cell lung cancer.
FIGURE 6. Representative scheme illustrating CTS’s inhibitory role in the proliferation of LNCaP TICs by regulating the Wnt signaling pathway for the treatment of prostatic cancer.
The aberrant activation of the Hippo signaling pathway and Yes-associated protein (YAP)/transcriptional co-activator with PDZ-binding motif (TAZ)-transcriptional enhancer associate domain (TEAD) has been linked to cancer, making this pathway an appealing target for therapeutic intervention (Arthur et al., 2009). CTS controls the ectopic movement of TAZ from the nucleus to the cytoplasm, lowering the stem cell characteristics of NSCL CSCs (Jin et al., 2020). The Hippo pathway is divided into two sections. Macrophage Stimulating one and Macrophage Stimulating 2 (MST1/2), Mitogen-Activated Protein four Kinases (MAP4K), and Large Tumor Suppressor Kinase one and Large Tumor Suppressor Kinase 2 (LATS1/2) are the key inhibitory kinase modules, and YAP/TAZ and TEAD are transcription modules. When Hippo pathway is activated, LATS1/2 phosphorylates YAP/TAZ directly and inhibits nuclear YAP/TAZ through 14-three to three mediated cytoplasmic retention and ubiquitination-mediated proteasome and autolysosome degradation. Vestigial Like Family Member 4 (VGLL4) inhibits TEAD transcription activity. When Hippo pathway is closed, YAP/TAZ is dephosphorylated and delivered to the nucleus, where it combines with the transcription factor TEAD, allowing target gene transcription to contribute to cell proliferation (Park et al., 2018). CTS inhibits the expression of TAZ target genes Connective tissue growth factor (CTGF), Transcriptional Intermediary Factor 1 (TIF-1), and Mothers Against Decapentaplegic Homolog 2 (Smad2) but does not affect YAP. CTS, however, does not impact the phosphorylation of TAZ’s upstream gene LATS1/2 but instead work directly on TAZ, regulating TAZ translocation from the nucleus to the cytoplasm. Furthermore, CTS decreases the expression of NSCL CSCs specific markers octamer-binding transcription factor 4 (Oct4), Nanog, and Aldehyde Dehydrogenase one Family (ALDH1) mRNA levels while increasing the expression of Cyclin Dependent Kinase 3 (CDK3), Integrin Subunit Alpha X (CD11c), and High affinity immunoglobulin gamma Fc receptor I (CD64) mRNA levels (Jin et al., 2020).
CTS can reduce the proliferation of NSCL CSCs by reducing Hippo signaling pathway and the proliferation of LNCaP TICs by inhibiting Wnt signaling pathway (Zhang et al., 2016b). The Wingless-Int1 (Wnt)/β-catenin signaling pathway has been demonstrated to be intricately related to prostatic CSCs (Schneider and Logan, 2018). Cell fate determination has been linked to abnormalities in the regulation of Wnt/β-catenin pathway. The Wnt/β-catenin pathway could be activated in several ways, including the binding of Wnt protein to members of Frizzled family of receptors. When Wnt pathway is engaged, GSK3β may phosphorylate freshly synthesized β-catenin protein, preventing them from entering the nucleus and playing a role. When GSK3β is inactive, β-catenin cyclic protein accumulates and is transported to the nucleus, activating the downstream target genes, influencing cell self-renewal and proliferation (Valkenburg et al., 2011). The most notable property is CTS’s ability to suppress β-catenin protein expression. As a result, stem cell growth and stemness are inhibited. Additionally, CTS dose-dependently decreased the protein expression of the stem cell genes Nanog, SRY-Box Transcription Factor 2 (Sox2), and Oct4.
Intriguingly, CTS decreases C-X-C Motif Chemokine Receptor 4 (CXCR4) mRNA and protein levels. Furthermore, CTS can regulate biological activities associated with the function of stromal cell derived factor 1 (SDF1)/CXCR4 axis, such as migration and metastasis (Zhang et al., 2016b). This demonstrates that CTS may be a promising small molecule therapeutic candidate for suppressing LNCaP TIC growth.
Finally, CTS has the potential to be a good anticancer drug since it can suppress the proliferation of diverse CSCs. It is hoped that future research will delve deeper into the past association between CTS and CSCs.
This review summarizes current evidences on the role and impact of CTS in various stem cells and provides a new perspective on the prevention and treatment of high-incidence diseases. More importantly, CTS exhibits great potential in various high-incidence diseases and influences the cell proliferation/self-renewal and differentiation of stem cells. It is critical to investigate the potential mechanism of CTS in the proliferation and differentiation of enormous stem cells, as this may provide further opportunities and references for future CTS research. So far, research on the impact of CTS on stem cells remains in its early phases, and it is unclear how CTS affects stem cell destiny regulation and integration. The effect of CTS on stem cells progresses to in vivo research, which will eventually alter disease treatment. Although significant progress has been made in understanding the role of CTS in stem cell proliferation and destiny regulation, we are only now beginning to understand its significance in stem cell development. Stem cells provide a wonderful platform for understanding CTS function and a unique possibility for developing new therapeutics and treatments for high-incidence diseases. It is critical to focus on stem cell biology and molecular mechanisms in future studies. As a result, additional research into the effects of CTS on stem cells can assist the medical community in realizing its therapeutic potential through stem cell treatment.
The introduction of several compound drugs, including CTS, gives us complete confidence in the clinical prospects of CTS. The ability of CTS to cross the blood-brain barrier fulfills the FDA’s quantitative drug analysis standard (Wang et al., 2022a). However, it should be recognized that CTS carries potential hazards. CTS has been demonstrated to have pharmacological toxicity in both in vivo zebrafish research (Wang et al., 2021a) and in vitro cell experiments (Wu et al., 2016; Kim et al., 2017), which is the most important problem for CTS to be solved. Furthermore, CTS has poor water solubility, poor oral absorption, low bioavailability, and high photosensitivity, which are some of the factors limiting its development (Wang et al., 2022c; Zhang et al., 2022), but there have been many studies to improve CTS bioavailability through spray formulations (Wang et al., 2022c), nanoparticles (Zhang et al., 2022), and nano-emulsions (Chengxi et al., 2019). CTS requires large-scale, randomized, double-blind, and controlled clinical trials to validate the safety and increase clinical efficacy. Although progress has been made in understanding the role of CTS in high-incidence diseases, what is urgently needed is to explore its impact on different stem cells and future therapeutic potential for various diseases.
YL and XC contributed to the conception, designed the paper, supervised the work, administered the project and its final editing. XG drafted the work and revised it critically for important intellectual content. RM performed figure illustration. All authors participated in the revision of the paper and approved the final manuscript.
This work was supported by the National Natural Science Foundation of China (Grant No. 82073832 to YL; 81903915 to XC); Tianjin Natural Science Fund for Distinguished Young Scholars (Grant No. 20JCJQJC00070 to YL); Tianjin Municipal Education Commission Scientific Research Project (Natural Science, Grant No. 2019ZD11 to YL). Hong Kong General Research Fund (No. 15105621 to WM-L).
The authors declare that the research was conducted in the absence of any commercial or financial relationships that could be construed as a potential conflict of interest.
All claims expressed in this article are solely those of the authors and do not necessarily represent those of their affiliated organizations, or those of the publisher, the editors and the reviewers. Any product that may be evaluated in this article, or claim that may be made by its manufacturer, is not guaranteed or endorsed by the publisher.
The Supplementary Material for this article can be found online at: https://www.frontiersin.org/articles/10.3389/fphar.2022.971444/full#supplementary-material
Arthur, A., Zannettino, A., and Gronthos, S. (2009). The therapeutic applications of multipotential mesenchymal/stromal stem cells in skeletal tissue repair. J. Cell. Physiol. 218 (2), 237–245. doi:10.1002/jcp.21592
Atashzar, M. R., Baharlou, R., Karami, J., Abdollahi, H., Rezaei, R., Pourramezan, F., et al. (2020). Cancer stem cells: a review from origin to therapeutic implications. J. Cell. Physiol. 235 (2), 790–803. doi:10.1002/jcp.29044
Blüher, M. (2019). Obesity: global epidemiology and pathogenesis. Nat. Rev. Endocrinol. 15 (5), 288–298. doi:10.1038/s41574-019-0176-8
Boese, A. C., Le, Q. E., Pham, D., Hamblin, M. H., and Lee, J. P. (2018). Neural stem cell therapy for subacute and chronic ischemic stroke. Stem Cell Res. Ther. 9 (1), 154. doi:10.1186/s13287-018-0913-2
Cao, F., Hata, R., Zhu, P., Nakashiro, K., and Sakanaka, M. (2010). Conditional deletion of Stat3 promotes neurogenesis and inhibits astrogliogenesis in neural stem cells. Biochem. Biophys. Res. Commun. 394 (3), 843–847. doi:10.1016/j.bbrc.2010.03.092
Chalfouh, C., Guillou, C., Hardouin, J., Delarue, Q., Li, X., Duclos, C., et al. (2020). The regenerative effect of trans-spinal magnetic stimulation after spinal cord injury: Mechanisms and pathways underlying the effect. Neurotherapeutics 17 (4), 2069–2088. doi:10.1007/s13311-020-00915-5
Chen, L., Fu, G., Hua, Q., Zhu, H. Y., Deng, Y., Wu, W., et al. (2022). Efficacy of add-on Danhong injection in patients with unstable angina pectoris: a double-blind, randomized, placebo-controlled, multicenter clinical trial. J. Ethnopharmacol. 284, 114794. doi:10.1016/j.jep.2021.114794
Chen, X., Deng, Y., Xue, Y., and Liang, J. (2012). Screening of bioactive compounds in Radix Salviae Miltiorrhizae with liposomes and cell membranes using HPLC. J. Pharm. Biomed. Anal. 70, 194–201. doi:10.1016/j.jpba.2012.06.030
Chen, Z. M., Hu, J., Xu, Y. M., He, W., Meng, L., Huang, T., et al. (2021). Cryptotanshinone inhibits cytotoxin-associated gene A-associated development of gastric cancer and mucosal erosions. World J. Gastrointest. Oncol. 13 (7), 693–705. doi:10.4251/wjgo.v13.i7.693
Chengxi, W., Rishuo, G., QuLiang, G., Laiyou, W., Lianbing, H., and Linghao, Q. (2019). The prevention effects of cryptotanshinone nanoemulsion on postoperative peritoneal adhesions. Drug Dev. Ind. Pharm. 45 (5), 695–702. doi:10.1080/03639045.2018.1529788
Contentin, R., Demoor, M., Concari, M., Desance, M., Audigie, F., Branly, T., et al. (2020). Comparison of the chondrogenic potential of mesenchymal stem cells derived from bone marrow and umbilical cord blood intended for cartilage tissue engineering. Stem Cell Rev. Rep. 16 (1), 126–143. doi:10.1007/s12015-019-09914-2
Cui, M., Ma, X., Sun, J., He, J., Shen, L., and Li, F. (2017). Effects of STAT3 inhibitors on neural functional recovery after spinal cord injury in rats. Biosci. Trends 10 (6), 460–466. doi:10.5582/bst.2016.01160
De Los Angeles, A., Ferrari, F., Xi, R., Fujiwara, Y., Benvenisty, N., Deng, H., et al. (2015). Hallmarks of pluripotency. Nature 525 (7570), 469–478. doi:10.1038/nature15515
Deng, Y. B., Liu, X. G., Liu, Z. G., Liu, X. L., Liu, Y., and Zhou, G. Q. (2006). Implantation of BM mesenchymal stem cells into injured spinal cord elicits de novo neurogenesis and functional recovery: evidence from a study in rhesus monkeys. Cytotherapy 8 (3), 210–214. doi:10.1080/14653240600760808
Ding, X., Cao, Y., Yuan, Y., Gong, Z., Liu, Y., Zhao, L., et al. (2016). Development of APTES-decorated HepG2 cancer stem cell membrane chromatography for screening active components from Salvia miltiorrhiza. Anal. Chem. 88 (24), 12081–12089. doi:10.1021/acs.analchem.6b02709
Dong, B., Liang, Z., Chen, Z., Li, B., Zheng, L., Yang, J., et al. (2018). Cryptotanshinone suppresses key onco-proliferative and drug-resistant pathways of chronic myeloid leukemia by targeting STAT5 and STAT3 phosphorylation. Sci. China. Life Sci. 61 (9), 999–1009. doi:10.1007/s11427-018-9324-y
Draijer, S., Chaves, I., and Hoekman, M. F. M. (2019). The circadian clock in adult neural stem cell maintenance. Prog. Neurobiol. 173, 41–53. doi:10.1016/j.pneurobio.2018.05.007
Duncan, T., and Valenzuela, M. (2017). Alzheimer's disease, dementia, and stem cell therapy. Stem Cell Res. Ther. 8 (1), 111. doi:10.1186/s13287-017-0567-5
Garcia, D., Hellberg, K., Chaix, A., Wallace, M., Herzig, S., Badur, M. G., et al. (2019). Genetic liver-specific AMPK activation protects against diet-induced obesity and NAFLD. Cell Rep. 26 (1), 192–208. e6. doi:10.1016/j.celrep.2018.12.036
GBD 2015 Obesity Collaborators, Afshin, A., Forouzanfar, M. H., Reitsma, M. B., Sur, P., Estep, K., et al. (2017). Health effects of overweight and obesity in 195 countries over 25 years. N. Engl. J. Med. 377 (1), 13–27. doi:10.1056/NEJMoa1614362
Gribsholt, S. B., Farkas, D. K., Thomsen, R. W., Richelsen, B., and Sørensen, H. T. (2022). Mortality among Danish patients with a hospital diagnosis of overweight or obesity over a 40-year period. Clin. Epidemiol. 14, 309–325. doi:10.2147/clep.S350459
Han, Z., Liu, S., Lin, H., Trivett, A. L., Hannifin, S., Yang, D., et al. (2019). Inhibition of murine hepatoma tumor growth by cryptotanshinone involves TLR7-dependent activation of macrophages and induction of adaptive antitumor immune defenses. Cancer Immunol. Immunother. 68 (7), 1073–1085. doi:10.1007/s00262-019-02338-4
Hao, D., Danbin, W., Maojuan, G., Chun, S., Bin, L., Lin, Y., et al. (2019). Ethanol extracts of Danlou tablet attenuate atherosclerosis via inhibiting inflammation and promoting lipid effluent. Pharmacol. Res. 146, 104306. doi:10.1016/j.phrs.2019.104306
Hao, Z., Ruggiano, N., Li, Q., Guo, Y., and Pan, X. (2021). Disparities in depression among Chinese older adults with neurodegenerative diseases. Aging Ment. Health 26 (3), 632–638. doi:10.1080/13607863.2021.1871879
Hemmati-Dinarvand, M., Saedi, S., Valilo, M., Kalantary-Charvadeh, A., Alizadeh Sani, M., Kargar, R., et al. (2019). Oxidative stress and Parkinson's disease: conflict of oxidant-antioxidant systems. Neurosci. Lett. 709, 134296. doi:10.1016/j.neulet.2019.134296
Hoey, T., Yen, W. C., Axelrod, F., Basi, J., Donigian, L., Dylla, S., et al. (2009). DLL4 blockade inhibits tumor growth and reduces tumor-initiating cell frequency. Cell Stem Cell 5 (2), 168–177. doi:10.1016/j.stem.2009.05.019
Hu, Y., Li, X., Huang, G., Wang, J., and Lu, W. (2019). Fasudil may induce the differentiation of bone marrow mesenchymal stem cells into neuronlike cells via the Wnt/betacatenin pathway. Mol. Med. Rep. 19 (4), 3095–3104. doi:10.3892/mmr.2019.9978
Imran, K. M., Rahman, N., Yoon, D., Jeon, M., Lee, B. T., and Kim, Y. S. (2017). Cryptotanshinone promotes commitment to the brown adipocyte lineage and mitochondrial biogenesis in C3H10T1/2 mesenchymal stem cells via AMPK and p38-MAPK signaling. Biochim. Biophys. Acta. Mol. Cell Biol. Lipids 1862 (10), 1110–1120. doi:10.1016/j.bbalip.2017.08.001
Jin, L., Wu, Z., Wang, Y., and Zhao, X. (2020). Cryptotanshinone attenuates the stemness of non-small cell lung cancer cells via promoting TAZ translocation from nuclear to cytoplasm. Chin. Med. 15, 66. doi:10.1186/s13020-020-00348-4
Kahroba, H., Ramezani, B., Maadi, H., Sadeghi, M. R., Jaberie, H., and Ramezani, F. (2021). The role of Nrf2 in neural stem/progenitors cells: from maintaining stemness and self-renewal to promoting differentiation capability and facilitating therapeutic application in neurodegenerative disease. Ageing Res. Rev. 65, 101211. doi:10.1016/j.arr.2020.101211
Kernie, S. G., and Parent, J. M. (2010). Forebrain neurogenesis after focal ischemic and traumatic brain injury. Neurobiol. Dis. 37 (2), 267–274. doi:10.1016/j.nbd.2009.11.002
Kim, E. J., Jung, S. N., Son, K. H., Kim, S. R., Ha, T. Y., Park, M. G., et al. (2007). Antidiabetes and antiobesity effect of cryptotanshinone via activation of AMP-activated protein kinase. Mol. Pharmacol. 72 (1), 62–72. doi:10.1124/mol.107.034447
Kim, E. J., Kim, S. Y., Kim, S. M., and Lee, M. (2017). A novel topoisomerase 2a inhibitor, cryptotanshinone, suppresses the growth of PC3 cells without apparent cytotoxicity. Toxicol. Appl. Pharmacol. 330, 84–92. doi:10.1016/j.taap.2017.07.007
Kim, S. L., Choi, H. S., Kim, J. H., Jeong, D. K., Kim, K. S., and Lee, D. S. (2019). Dihydrotanshinone-induced NOX5 activation inhibits breast cancer stem cell through the ROS/Stat3 signaling pathway. Oxid. Med. Cell. Longev. 2019, 9296439. doi:10.1155/2019/9296439
Kumanyika, S., and Dietz, W. H. (2020). Solving population-wide obesity - progress and future prospects. N. Engl. J. Med. 383 (23), 2197–2200. doi:10.1056/NEJMp2029646
Laterza, C., Wattananit, S., Uoshima, N., Ge, R., Pekny, R., Tornero, D., et al. (2017). Monocyte depletion early after stroke promotes neurogenesis from endogenous neural stem cells in adult brain. Exp. Neurol. 297, 129–137. doi:10.1016/j.expneurol.2017.07.012
Lee, J. E., Sim, H., Yoo, H. M., Lee, M., Baek, A., Jeon, Y. J., et al. (2020). Neuroprotective effects of cryptotanshinone in a direct reprogramming model of Parkinson's disease. Molecules 25 (16), 3602. doi:10.3390/molecules25163602
Li, B., Wang, Y., Lu, J., Liu, J., Yuan, Y., Yu, Y., et al. (2015). Evaluating the effects of Danhong injection in treatment of acute ischemic stroke: study protocol for a multicenter randomized controlled trial. Trials 16, 561. doi:10.1186/s13063-015-1076-4
Li, H., Wang, C., He, T., Zhao, T., Chen, Y. Y., Shen, Y. L., et al. (2019). Mitochondrial transfer from bone marrow mesenchymal stem cells to motor neurons in spinal cord injury rats via gap junction. Theranostics 9 (7), 2017–2035. doi:10.7150/thno.29400
Li, K., Song, J., Zhao, Q., Wang, B., Zhang, Y., Wang, X., et al. (2018a). Effective component of Salvia miltiorrhiza in promoting cardiomyogenic differentiation of human placentaderived mesenchymal stem cells. Int. J. Mol. Med. 41 (2), 962–968. doi:10.3892/ijmm.2017.3293
Li, K., Zhang, T. T., Hua, F., and Hu, Z. W. (2018b). Metformin reduces TRIB3 expression and restores autophagy flux: an alternative antitumor action. Autophagy 14 (7), 1278–1279. doi:10.1080/15548627.2018.1460022
Li, T., Zhao, X., Duan, J., Cui, S., Zhu, K., Wan, Y., et al. (2021). Targeted inhibition of STAT3 in neural stem cells promotes neuronal differentiation and functional recovery in rats with spinal cord injury. Exp. Ther. Med. 22 (1), 711. doi:10.3892/etm.2021.10143
Liu, H., Zhan, X., Xu, G., Wang, Z., Li, R., Wang, Y., et al. (2021a). Cryptotanshinone specifically suppresses NLRP3 inflammasome activation and protects against inflammasome-mediated diseases. Pharmacol. Res. 164, 105384. doi:10.1016/j.phrs.2020.105384
Liu, J., Li, D. D., Dong, W., Liu, Y. Q., Wu, Y., Tang, D. X., et al. (2021b). Detection of an anti-angina therapeutic module in the effective population treated by a multi-target drug Danhong injection: a randomized trial. Signal Transduct. Target. Ther. 6 (1), 329. doi:10.1038/s41392-021-00741-x
Liu, X., Niu, Y., Xie, W., Wei, D., and Du, Q. (2019). Tanshinone IIA promotes osteogenic differentiation of human periodontal ligament stem cells via ERK1/2-dependent Runx2 induction. Am. J. Transl. Res. 11 (1), 340–350.
Lo, S. H., Hsu, C. T., Niu, H. S., Niu, C. S., Cheng, J. T., and Chen, Z. C. (2017). Cryptotanshinone inhibits STAT3 signaling to alleviate cardiac fibrosis in type 1-like diabetic rats. Phytother. Res. 31 (4), 638–646. doi:10.1002/ptr.5777
Lopez, M. (2017). EJE PRIZE 2017: Hypothalamic AMPK: a golden target against obesity? Eur. J. Endocrinol. 176 (5), R235–R246. doi:10.1530/EJE-16-0927
Lopez, M., and Tena-Sempere, M. (2017). Estradiol effects on hypothalamic AMPK and BAT thermogenesis: a gateway for obesity treatment? Pharmacol. Ther. 178, 109–122. doi:10.1016/j.pharmthera.2017.03.014
Lu, P., Zhang, F. C., Qian, S. W., Li, X., Cui, Z. M., Dang, Y. J., et al. (2016). Artemisinin derivatives prevent obesity by inducing browning of WAT and enhancing BAT function. Cell Res. 26 (10), 1169–1172. doi:10.1038/cr.2016.108
Lunn, J. S., Sakowski, S. A., Hur, J., and Feldman, E. L. (2011). Stem cell technology for neurodegenerative diseases. Ann. Neurol. 70 (3), 353–361. doi:10.1002/ana.22487
Ma, X. X., Liu, J., Wang, C. M., Zhou, J. P., He, Z. Z., and Lin, H. (2018). Low-dose curcumin stimulates proliferation of rat embryonic neural stem cells through glucocorticoid receptor and STAT3. CNS Neurosci. Ther. 24 (10), 940–946. doi:10.1111/cns.12843
Maccalli, C., Rasul, K. I., Elawad, M., and Ferrone, S. (2018). The role of cancer stem cells in the modulation of anti-tumor immune responses. Semin. Cancer Biol. 53, 189–200. doi:10.1016/j.semcancer.2018.09.006
Madl, C. M., Heilshorn, S. C., and Blau, H. M. (2018). Bioengineering strategies to accelerate stem cell therapeutics. Nature 557 (7705), 335–342. doi:10.1038/s41586-018-0089-z
Mai, T. T., Hamai, A., Hienzsch, A., Caneque, T., Muller, S., Wicinski, J., et al. (2017). Salinomycin kills cancer stem cells by sequestering iron in lysosomes. Nat. Chem. 9 (10), 1025–1033. doi:10.1038/nchem.2778
Maione, F., Piccolo, M., De Vita, S., Chini, M. G., Cristiano, C., De Caro, C., et al. (2018). Down regulation of pro-inflammatory pathways by tanshinone IIA and cryptotanshinone in a non-genetic mouse model of Alzheimer's disease. Pharmacol. Res. 129, 482–490. doi:10.1016/j.phrs.2017.11.018
Mao, Y., Qu, Y., and Wang, Q. (2021). Cryptotanshinone reduces neurotoxicity induced by cerebral ischemia-reperfusion injury involving modulation of microglial polarization. Restor. Neurol. Neurosci. 39 (3), 209–220. doi:10.3233/RNN-201070
Mei, Z., Zhang, F., Tao, L., Zheng, W., Cao, Y., Wang, Z., et al. (2009). Cryptotanshinone, a compound from Salvia miltiorrhiza modulates amyloid precursor protein metabolism and attenuates beta-amyloid deposition through upregulating alpha-secretase in vivo and in vitro. Neurosci. Lett. 452 (2), 90–95. doi:10.1016/j.neulet.2009.01.013
Pagnotti, G. M., Styner, M., Uzer, G., Patel, V. S., Wright, L. E., Ness, K. K., et al. (2019). Combating osteoporosis and obesity with exercise: leveraging cell mechanosensitivity. Nat. Rev. Endocrinol. 15 (6), 339–355. doi:10.1038/s41574-019-0170-1
Park, J. H., Shin, J. E., and Park, H. W. (2018). The role of hippo pathway in cancer stem cell biology. Mol. Cells 41 (2), 83–92. doi:10.14348/molcells.2018.2242
Pfeifer, A., and Hoffmann, L. S. (2015). Brown, beige, and white: the new color code of fat and its pharmacological implications. Annu. Rev. Pharmacol. Toxicol. 55, 207–227. doi:10.1146/annurev-pharmtox-010814-124346
Plaks, V., Kong, N., and Werb, Z. (2015). The cancer stem cell niche: how essential is the niche in regulating stemness of tumor cells? Cell Stem Cell 16 (3), 225–238. doi:10.1016/j.stem.2015.02.015
Schneider, J. A., and Logan, S. K. (2018). Revisiting the role of Wnt/β-catenin signaling in prostate cancer. Mol. Cell. Endocrinol. 462, 3–8. doi:10.1016/j.mce.2017.02.008
Shanbhag, S., Mohamed-Ahmed, S., Lunde, T. H. F., Suliman, S., Bolstad, A. I., Hervig, T., et al. (2020). Influence of platelet storage time on human platelet lysates and platelet lysate-expanded mesenchymal stromal cells for bone tissue engineering. Stem Cell Res. Ther. 11 (1), 351. doi:10.1186/s13287-020-01863-9
Shi, D., Li, H., Zhang, Z., He, Y., Chen, M., Sun, L., et al. (2022). Cryptotanshinone inhibits proliferation and induces apoptosis of breast cancer MCF-7 cells via GPER mediated PI3K/AKT signaling pathway. PLoS One 17 (1), e0262389. doi:10.1371/journal.pone.0262389
Shi, M., Huang, F., Deng, C., Wang, Y., and Kai, G. (2019). Bioactivities, biosynthesis and biotechnological production of phenolic acids in Salvia miltiorrhiza. Crit. Rev. Food Sci. Nutr. 59 (6), 953–964. doi:10.1080/10408398.2018.1474170
Shi, Y., Liu, D., Yuan, J., Yan, L., Zhan, Z., Pan, D., et al. (2020). Compound danshen dripping Pills prevented leptin deficiency-induced hepatic ER stress, stimulated autophagy, and improved Insulin resistance of ob/ob mice. Evid. Based. Complement. Altern. Med. 2020, 5368657. doi:10.1155/2020/5368657
Shin, D. S., Kim, H. N., Shin, K. D., Yoon, Y. J., Kim, S. J., Han, D. C., et al. (2009). Cryptotanshinone inhibits constitutive signal transducer and activator of transcription 3 function through blocking the dimerization in DU145 prostate cancer cells. Cancer Res. 69 (1), 193–202. doi:10.1158/0008-5472.CAN-08-2575
Siegel, R. L., Miller, K. D., Fuchs, H. E., and Jemal, A. (2022). Cancer statistics, 2022. CA. Cancer J. Clin. 72 (1), 7–33. doi:10.3322/caac.21708
Singh, S. P., Tripathy, N. K., and Nityanand, S. (2013). Comparison of phenotypic markers and neural differentiation potential of multipotent adult progenitor cells and mesenchymal stem cells. World J. Stem Cells 5 (2), 53–60. doi:10.4252/wjsc.v5.i2.53
Sun, D. (2014). The potential of endogenous neurogenesis for brain repair and regeneration following traumatic brain injury. Neural Regen. Res. 9 (7), 688–692. doi:10.4103/1673-5374.131567
Sun, J., Luo, Q., Liu, L., Yang, X., Zhu, S., and Song, G. (2017). Salinomycin attenuates liver cancer stem cell motility by enhancing cell stiffness and increasing F-actin formation via the FAK-ERK1/2 signalling pathway. Toxicology 384, 1–10. doi:10.1016/j.tox.2017.04.006
Takebe, N., Miele, L., Harris, P. J., Jeong, W., Bando, H., Kahn, M., et al. (2015). Targeting notch, hedgehog, and Wnt pathways in cancer stem cells: clinical update. Nat. Rev. Clin. Oncol. 12 (8), 445–464. doi:10.1038/nrclinonc.2015.61
Valkenburg, K. C., Graveel, C. R., Zylstra-Diegel, C. R., Zhong, Z., and Williams, B. O. (2011). Wnt/β-catenin signaling in normal and cancer stem cells. Cancers (Basel) 3 (2), 2050–2079. doi:10.3390/cancers3022050
Vermeulen, L., De Sousa, E. M. F., van der Heijden, M., Cameron, K., de Jong, J. H., Borovski, T., et al. (2010). Wnt activity defines colon cancer stem cells and is regulated by the microenvironment. Nat. Cell Biol. 12 (5), 468–476. doi:10.1038/ncb2048
Vissers, C., Ming, G. L., and Song, H. (2019). Nanoparticle technology and stem cell therapy team up against neurodegenerative disorders. Adv. Drug Deliv. Rev. 148, 239–251. doi:10.1016/j.addr.2019.02.007
Vundavilli, H., Datta, A., Sima, C., Hua, J., Lopes, R., Bittner, M., et al. (2022). Anti-tumor effects of cryptotanshinone (C19H20O3) in human osteosarcoma cell lines. Biomed. Pharmacother. 150, 112993. doi:10.1016/j.biopha.2022.112993
Wang, C., Wang, T., Lian, B. W., Lai, S., Li, S., Li, Y. M., et al. (2021a). Developmental toxicity of cryptotanshinone on the early-life stage of zebrafish development. Hum. Exp. Toxicol. 40 (12), S278–S289. doi:10.1177/09603271211009954
Wang, H. D., Wang, H. M., Wang, X. Y., Xu, X. Y., Hu, Y., Li, X., et al. (2022b). A novel hybrid scan approach enabling the ion-mobility separation and the alternate data-dependent and data-independent acquisitions (HDDIDDA): its combination with off-line two-dimensional liquid chromatography for comprehensively characterizing the multicomponents from compound danshen dripping pill. Anal. Chim. Acta 1193, 339320. doi:10.1016/j.aca.2021.339320
Wang, H., Hou, X., Li, B., Yang, Y., Li, Q., and Si, Y. (2021d). Study on active components of cuscuta chinensis promoting neural stem cells proliferation: bioassay-guided fractionation. Molecules 26 (21), 6634. doi:10.3390/molecules26216634
Wang, H., Zhang, M., Fang, J., He, Y., Liu, M., Hong, Z., et al. (2022a). Simultaneous determination of seven lipophilic and hydrophilic components in Salvia miltiorrhiza Bunge by LC-MS/MS method and its application to a transport study in a blood-brain-barrier cell model. Molecules 27 (3), 657. doi:10.3390/molecules27030657
Wang, J., Hu, J., Chen, X., Lei, X., Feng, H., Wan, F., et al. (2021b). Traditional Chinese medicine monomers: novel strategy for endogenous neural stem cells activation after stroke. Front. Cell. Neurosci. 15, 628115. doi:10.3389/fncel.2021.628115
Wang, P. Q., Li, D. D., Dong, W., Liu, J., Yu, Y. N., Shen, C. T., et al. (2015a). Danhong injection in the treatment of chronic stable angina: study protocol for a randomized controlled trial. Trials 16, 474. doi:10.1186/s13063-015-0998-1
Wang, Q., Chuikov, S., Taitano, S., Wu, Q., Rastogi, A., Tuck, S. J., et al. (2015b). Dimethyl fumarate protects neural stem/progenitor cells and neurons from oxidative damage through nrf2-ERK1/2 MAPK pathway. Int. J. Mol. Sci. 16 (6), 13885–13907. doi:10.3390/ijms160613885
Wang, X., Sun, Q., Jiang, Q., Jiang, Y., Zhang, Y., Cao, J., et al. (2021e). Cryptotanshinone ameliorates doxorubicin-induced cardiotoxicity by targeting akt-GSK-3β-mPTP pathway in vitro. Molecules 26 (5), 1460. doi:10.3390/molecules26051460
Wang, X., Wan, W., Lu, J., Zhang, Y., Quan, G., Pan, X., et al. (2022c). Inhalable cryptotanshinone spray-dried swellable microparticles for pulmonary fibrosis therapy by regulating TGF-β1/Smad3, STAT3 and SIRT3 pathways. Eur. J. Pharm. Biopharm. 172, 177–192. doi:10.1016/j.ejpb.2022.02.012
Wang, Y., Bryant, S. H., Cheng, T., Wang, J., Gindulyte, A., Shoemaker, B. A., et al. (2017). PubChem BioAssay: 2017 update. Nucleic Acids Res. 45 (D1), D955–D963. doi:10.1093/nar/gkw1118
Wang, Y., He, W., Bian, H., Liu, C., and Li, S. (2012). Small molecule induction of neural-like cells from bone marrow-mesenchymal stem cells. J. Cell. Biochem. 113 (5), 1527–1536. doi:10.1002/jcb.24021
Wang, Z., Wan, H., Tong, X., He, Y., Yang, J., Zhang, L., et al. (2021c). An integrative strategy for discovery of functional compound combination from traditional Chinese medicine: danhong Injection as a model. Biomed. Pharmacother. 138, 111451. doi:10.1016/j.biopha.2021.111451
Wen, Q., Xie, X., Ren, Q., and Du, Y. (2022). Polybrominated diphenyl ether congener 99 (PBDE 99) promotes adipocyte lineage commitment of C3H10T1/2 mesenchymal stem cells. Chemosphere 290, 133312. doi:10.1016/j.chemosphere.2021.133312
Wilson, J. R., Cadotte, D. W., and Fehlings, M. G. (2012). Clinical predictors of neurological outcome, functional status, and survival after traumatic spinal cord injury: a systematic review. J. Neurosurg. Spine. 17 (1), 11–26. doi:10.3171/2012.4.AOSPINE1245
Wu, C. F., Bohnert, S., Thines, E., and Efferth, T. (2016). Cytotoxicity of Salvia miltiorrhiza against multidrug-resistant cancer cells. Am. J. Chin. Med. 44 (4), 871–894. doi:10.1142/S0192415X16500488
Wu, J. S., Meng, Q. Y., Shi, X. H., Liu, L. X., Zhang, Z. K., Guan, H. S., et al. (2020). The oxygenated products of cryptotanshinone by biotransformation with Cunninghamella elegans exerting anti-neuroinflammatory effects by inhibiting TLR 4-mediated MAPK signaling pathway. Bioorg. Chem. 104, 104246. doi:10.1016/j.bioorg.2020.104246
Wu, K.-J., Yu, S., Lee, J.-Y., Hoffer, B., and Wang, Y. (2017). Improving neurorepair in stroke brain through endogenous neurogenesis-enhancing drugs. Cell Transpl. 26 (9), 1596–1600. doi:10.1177/0963689717721230
Xiong, S., Wang, R., Chen, Q., Luo, J., Wang, J., Zhao, Z., et al. (2018). Cancer-associated fibroblasts promote stem cell-like properties of hepatocellular carcinoma cells through IL-6/STAT3/Notch signaling. Am. J. Cancer Res. 8 (2), 302–316.
Xu, J., Xiong, Y. Y., Li, Q., Hu, M. J., Huang, P. S., Xu, J. Y., et al. (2019). Optimization of timing and times for administration of atorvastatin-pretreated mesenchymal stem cells in a preclinical model of acute myocardial infarction. Stem Cells Transl. Med. 8 (10), 1068–1083. doi:10.1002/sctm.19-0013
Zhang, L., Yu, L., and Wei, Y. (2022). Oral administration of cryptotanshinone-encapsulated nanoparticles for the amelioration of ulcerative colitis. Cell. Mol. Bioeng. 15 (1), 129–136. doi:10.1007/s12195-021-00711-x
Zhang, Q., Gan, C., Liu, H., Wang, L., Li, Y., Tan, Z., et al. (2020). Cryptotanshinone reverses the epithelial-mesenchymal transformation process and attenuates bleomycin-induced pulmonary fibrosis. Phytother. Res. 34 (10), 2685–2696. doi:10.1002/ptr.6699
Zhang, W., Suo, M., Yu, G., and Zhang, M. (2019). Antinociceptive and anti-inflammatory effects of cryptotanshinone through PI3K/Akt signaling pathway in a rat model of neuropathic pain. Chem. Biol. Interact. 305, 127–133. doi:10.1016/j.cbi.2019.03.016
Zhang, X. Z., Qian, S. S., Zhang, Y. J., and Wang, R. Q. (2016a). Salvia miltiorrhiza: a source for anti-Alzheimer's disease drugs. Pharm. Biol. 54 (1), 18–24. doi:10.3109/13880209.2015.1027408
Zhang, Y., Cabarcas, S. M., Zheng, J. I., Sun, L., Mathews, L. A., Zhang, X., et al. (2016b). Cryptotanshinone targets tumor-initiating cells through down-regulation of stemness genes expression. Oncol. Lett. 11 (6), 3803–3812. doi:10.3892/ol.2016.4444
Zhu, W., Qiu, W., and Lu, A. (2017). Cryptotanshinone exhibits therapeutical effects on cerebral stroke through the PI3K/AKTeNOS signaling pathway. Mol. Med. Rep. 16 (6), 9361–9366. doi:10.3892/mmr.2017.7824
Zyuz'kov, G. N., Miroshnichenko, L. A., Polyakova, T. Y., Stavrova, L. A., Simanina, E. V., and Zhdanov, V. V. (2020). Specific roles of JAKs and STAT3 in functions of neural stem cells and committed neuronal progenitors during ethanol-induced neurodegeneration. Bull. Exp. Biol. Med. 168 (3), 356–360. doi:10.1007/s10517-020-04708-w
CTS Cryptotanshinone
S. miltiorrhiza Salvia miltiorrhiza Bunge
C3H10T1/2 MSCs C3H10T1/2 mesenchymal stem cells
BMSCs bone marrow mesenchymal stem cells
NSCs/NPCs neural stem/progenitor cells
CSCs cancer stem cells
AMPK amp-activated protein kinase
p38-AMPK p38 mitogen-activated protein kinase
AMPKα Adenosine 5‘-monophosphate (AMP)-activated protein kinase α
Smad1/5 drosophila mothers against decapentaplegic protein
BAT brown adipose tissue
Ucp1 Uncoupling protein 1
Prdm16 PR domain-containing 16
Pgc-1α peroxlsome proliferator-activated receptor-γ coactlvator-1
Cd137 TNF Receptor Superfamily Member 9
Hspb7 Heat Shock Protein Family B (Small) Member 7
Cox2 cyclooxygenase-2
Tmem26 Transmembrane Protein 26
MDI 0.5 mM IBMX, 1 μM Dexamethasone and 10 μg/ml Insulin
Psat1 Phosphoserine Aminotransferase 1
SCI Spinal cord injury
NSE Neuron specific enolase
GFAP glial fibrillary acidic protein
NF Neurofilament protein
BMSCs Bone marrow mesenchymal stem cells
CSEP cortex somatosensory evoked potential
MEP motor evoked potential
PI3K/AKT phosphoinositide 3-kinase/v-akt murine thymoma viral oncogene homolog
STAT3 Signal transducer and activator of transcription 3
PD-hiNPCs Parkinson’s disease patient-derived human-induced neuronal progenitor cells
NRF2 nuclear factor erythroid 2-related factor 2
SOD1 superoxide dismutase 1
PRX1 peroxiredoxin 1
PRX5 peroxiredoxin 5
GPX1 glutathione peroxidase
NQO1 NAD(P)H quinone dehydrogenase 1
TICs tumor-initiating cells
NSCL non-small cell lung
CSCs cancer stem cells
YAP Yes-associated protein
TAZ transcriptional co-activator with PDZ-binding motif
TEAD transcriptional enhancer associate domain
MST1/2 Macrophage Stimulating 1 and Macrophage Stimulating 2
MAP4K Mitogen-Activated Protein Kinase Kinase Kinase Kinase
LATS1/2 Large Tumor Suppressor Kinase 1 and Large Tumor Suppressor Kinase 2
VGLL4 Vestigial Like Family Member 4
CTGF Connective tissue growth factor
TIF-1 Transcriptional Intermediary Factor 1
Smad2 Mothers Against Decapentaplegic Homolog 2
Oct4 octamer-binding transcription factor 4
ALDH1 Aldehyde Dehydrogenase 1 Family
CDK3 Cyclin Dependent Kinase 3
CD11c Integrin Subunit Alpha X
CD64 High affinity immunoglobulin gamma Fc receptor I
Wnt/β-Catenin Wingless-Int1/β-Catenin
Sox2 SRY-Box Transcription Factor 2
CXCR4 C-X-C Motif Chemokine Receptor 4
SDF1 stromal cell derived factor 1
Keywords: cryptotanshinone, high-incidence diseases, proliferation, differentiation, apoptosis, stem cell, self-renewal
Citation: Guo X, Ma R, Wang M, Wui-Man Lau B, Chen X and Li Y (2022) Novel perspectives on the therapeutic role of cryptotanshinone in the management of stem cell behaviors for high-incidence diseases. Front. Pharmacol. 13:971444. doi: 10.3389/fphar.2022.971444
Received: 17 June 2022; Accepted: 18 July 2022;
Published: 15 August 2022.
Edited by:
Wei Cui, Ningbo University, ChinaReviewed by:
Xuefeng Jiang, East China Normal University, ChinaCopyright © 2022 Guo, Ma, Wang, Wui-Man Lau, Chen and Li. This is an open-access article distributed under the terms of the Creative Commons Attribution License (CC BY). The use, distribution or reproduction in other forums is permitted, provided the original author(s) and the copyright owner(s) are credited and that the original publication in this journal is cited, in accordance with accepted academic practice. No use, distribution or reproduction is permitted which does not comply with these terms.
*Correspondence: Xiaopeng Chen, eHBjaGVuQHRqdXRjbS5lZHUuY24=; Yue Li, bGl5dWUyMDE4QHRqdXRjbS5lZHUuY24=
Disclaimer: All claims expressed in this article are solely those of the authors and do not necessarily represent those of their affiliated organizations, or those of the publisher, the editors and the reviewers. Any product that may be evaluated in this article or claim that may be made by its manufacturer is not guaranteed or endorsed by the publisher.
Research integrity at Frontiers
Learn more about the work of our research integrity team to safeguard the quality of each article we publish.