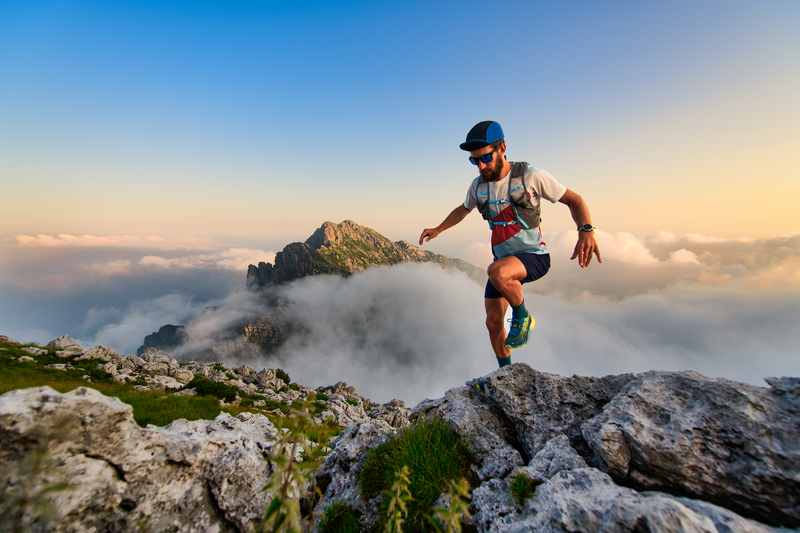
94% of researchers rate our articles as excellent or good
Learn more about the work of our research integrity team to safeguard the quality of each article we publish.
Find out more
ORIGINAL RESEARCH article
Front. Pharmacol. , 16 August 2022
Sec. Renal Pharmacology
Volume 13 - 2022 | https://doi.org/10.3389/fphar.2022.966786
This article is part of the Research Topic Diabetic Kidney Disease: Routes to drug development, pharmacology and underlying molecular mechanisms. View all 14 articles
Sirtuins, as regulators of metabolism and energy, have been found to play an important role in health and disease. Sirt1, the most widely studied member of the sirtuin family, can ameliorate oxidative stress, immune inflammation, autophagy, and mitochondrial homeostasis by deacetylating regulatory histone and nonhistone proteins. Notably, sirt1 has gradually gained attention in kidney disease research. Therefore, an evaluation of the overall distribution of publications concerning sirt1 based on bibliometric analysis methods to understand the thematic evolution and emerging research trends is necessary to discover topics with potential implications for kidney disease research. We conducted a bibliometric analysis of publications derived from the Web of Science Core Collection and found that publications concerning sirt1 have grown dramatically over the past 2 decades, especially in the past 5 years. Among these, the proportion of publications regarding kidney diseases have increased annually. China and the United States are major contributors to the study of sirt1, and Japanese researchers have made important contributions to the study of sirt1 in kidney disease. Obesity, and Alzheimer’s disease are hotspots diseases for the study of sirt1, while diabetic nephropathy is regarded as a research hotspot in the study of sirt1 in kidney disease. NAD+, oxidative stress, and p53 are the focus of the sirt1 research field. Autophagy and NLRP3 inflammasome are emerging research trends have gradually attracted the interest of scholars in sirt1, as well as in kidney disease. Notably, we also identified several potential research topics that may link sirt1 and kidney disease, which require further study, including immune function, metabolic reprogramming, and fecal microbiota.
Sirtuins belong to a highly evolutionarily conserved histone deacetylase (HDACs) family that is dependent on nicotinamide adenine dinucleotide (NAD+) (Wang and Lin, 2021). Silent information regulator 2 (Sir2) was originally identified in yeast and named in 1987 (Ivy et al., 1986). Subsequently, Sir2 was found to be involved in regulating the replicative lifespan of yeast, increasing the dose of Sir2 increased yeast lifespan by 30% (Kaeberlein et al., 1999). Sirtuins are homologous mammalian enzymes of Sir2 and consist of seven isoforms (sirt1-sirt7). The intracellular localization of Sirtuins is closely related to its function. Sirt1, sirt6, and sirt7 are mainly located in the nucleus, and primarily responsible for transcriptional regulation, repair of DNA and regulation of the cell cycle. Sirt3-sirt5 exist in mitochondria, and plays an important role in regulating cell energy metabolism. Sirt2 is the only directly available family member in the cytoplasm, and mainly functions in mitosis (Zhao et al., 2020). All members of the Sirtuins family have deacetylation properties, sirt1, sirt4, and sirt6 have been found to possess ADP-ribosyltransferase activity, sirt5 and sirt7 have also been reported to be a desuccinylase (Srivastava et al., 2020b; Gupta et al., 2022). Notably, sirt5 also has two unique properties of demalonylation and deglutarylation (Kumar and Lombard, 2018). Sirt4 has been reported to possess unique lipoamidase activity (Mathias et al., 2014) (Figure 1). Sirtuins, as a regulator of metabolism and energy, are closely associated with many aging-related diseases, including cardiovascular diseases (Soni et al., 2021), cancer (Aventaggiato et al., 2021), and neurodegenerative disorders (Leite et al., 2022). Accumulating evidence suggests that sirtuins play important roles in transcriptional regulation, cell survival, cell stress resistance, DNA damage and repair, and cell cycle regulation (Haigis and Sinclair, 2010). Interestingly, sirtuins were found to be central players in health improvement associated with calorie restriction and exercise training. In addition, some natural compounds (such as resveratrol, astragaloside IV, and honokiol) and small molecules (such as SRT1720, SRT1460, and SRT3025) have also been found to ameliorate diseases by activating sirtuins, which has made scholars realize the importance of sirtuins as potential pharmacological targets for many diseases (Dai et al., 2018).
Sirt1 is phylogenetically similar to yeast Sir2 and is the most extensively studied member of the sirtuin family. Sirt1 can regulate energy metabolism, immune inflammation, oxidative stress, mitochondrial homeostasis, autophagy, and apoptosis, and in turn ameliorates disease progression and aging by deacetylating regulatory histones and nonhistone proteins, such as peroxisome proliferator-activated receptor alpha (PPARα), PPAR gamma coactivator-1alpha (PGC-1α), and fork-head box protein (FOXO) (Zhao et al., 2020). The kidney is one of the major organs that are prone to aging-related diseases, especially in diabetes mellitus conditions. Multiple stresses lead to accelerated renal aging, including accumulation of advanced glycation end products, inflammation, autophagic damage, and oxidative stress (Guo et al., 2020). The study of sirt1 in kidney disease, especially in diabetic nephropathy (DN), has gradually gained the attention of researchers. On the one hand, sirt1 can improve renal resident cell injury and apoptosis by directly regulating oxidative stress, mitochondrial homeostasis, and autophagy. On the other hand, sirt1 can modulate ectopic lipid accumulation in the kidney, ameliorate fibrosis, and prevent the progression of renal disease. In addition, sirt1 has also been found to ameliorate vascular endothelial injury and reduce complications of kidney disease, thereby improving the vulnerability of the kidney to aging (Morigi et al., 2018). Studies have found that the knockout of renal sirt1 can lead to the aggravation of inflammation, proteinuria, and fibrosis (He et al., 2010; Liu et al., 2014), and these phenotypes of renal injury may be significantly ameliorated by using resveratrol (RSV) (Kitada et al., 2011), NMN (Yasuda et al., 2021), or SRT1720 (Funk et al., 2010) to activate sirt1. Thus, sirt1 plays an irreplaceable role in the initiation and progression of kidney diseases.
Bibliometric analysis is an effective way to assess overall trends in a field. It not only helps researchers and clinicians understand core countries, institutions, and authors of a given research area and the most influential nodal publications, but also identifies thematic changes, emerging research trends, and research gaps in this area (Sabe et al., 2022). In recent years, there has been an explosion of research on the role of sirtuins in health and disease. However, studies evaluating the overall trends of sirtuins are scarce. To our knowledge, only one bibliometric analysis has evaluated sirt6 (Lu et al., 2018). Therefore, in this study, we evaluated the overall distribution of studies on sirtuins over the past 2 decades using the bibliometric analysis method, and with a particular focus on sirt1 and kidney disease. Through an analysis of the main research areas and emerging research trends in the field of sirt1 research, we hope to shed new light and ideas on the study of sirt1 in kidney disease.
The Web of Science Core Collection (WoSCC), the most comprehensive database for bibliometric analysis, was used to conduct a comprehensive search of publications related to sirtuins. We used topic subject (TS) as our search strategy, and the retrieval formula was set as TS = (“sirt” OR “sirtuin” OR “sirtuins” OR “SIRT1” OR “sirtuin1” OR “SIRT2” OR “sirtuin2” OR “SIRT3” OR “sirtuin3” OR “SIRT4” OR “sirtuin4” OR “SIRT5” OR “sirtuin5” OR “SIRT6” OR “sirtuin6” OR “SIRT7” OR “sirtuin7”). Publication type was restricted to “article” and “review”. In addition, we performed additional analyses of sirtuin family members (sirt1-sirt7) separately, with a focus on the analysis of sirt1 and sirt1 in kidney disease. The retrieval formula is shown in Supplementary Table S1. The above process was completed in 1 day on 25 April 2022, to avoid bias from data updates.
Citespace (version 6.1.R1), VOSviewer (version 1.6.16), Bibliometrix 4.1.0 package (https://www.bibliometrix.org), and the Arrowsmith project (http://arrowsmith.psych.uic.edu) were used to analyze the collected data, including the overall distribution of publications, leading countries and institutions, core journals, active authors, co-citation references, and keyword analysis. The Journal Citation Reports (JCR) and Hirsch index (H-index) have also been used to assist in assessing the academic impact of journals and authors.
Citespace (Chen, 2004) was used to perform cluster analysis and burst analysis of co-references and keywords to understand the main research areas and emerging research trends of sirt1, and automated labelling for cluster interpretation. We defined modularity Q > 0.3 and mean silhouette >0.5 as indicators that the clustering results were sufficiently stable and reliable. Bibliometrix, based on the R project (Aria and Cuccurullo, 2017), was used to construct the thematic evolution of keywords, and the time cutting points were set as 2018 and 2020, respectively (recent five and 3 years). Next, VOSviewer (van Eck and Waltman, 2010) was used to extract and visualize the keywords for sirt1 in kidney disease research. Finally, the Arrowsmith project (Smalheiser et al., 2009) was used to construct an association between sirt1 and kidney disease. The retrieval formula of A-query (sirt1) was set as [sirt1 (MeSH Terms)] OR [sirtuin1 (MeSH Terms]), and the retrieval formula of C-query (kidney disease) was set as [kidney disease (MeSH Terms)] OR [renal disease (MeSH Terms)] OR [nephropathy (MeSH Terms)]. Using the keywords obtained from the Arrowsmith Project as the prediction group and the keywords extracted from VOSviewer was used as the confirmation group, and a Venn diagram of the two groups was drawn to obtain the potential links between sirt1 research and kidney disease. String (https://cn.string-db.org) was used to construct protein-protein interaction (PPI) networks for the target proteins.
A total of 18225 publications from 1994 to 2022 were retrieved from the WoSCC. Through curve fitting analysis, we found that the publications about sirtuins showed a sharp growth trend with an annual growth rate of 13.10% in the last 5 years (R2 = 0.8386, Figure 2A). From 1994 to 2004, studies on sirtuins were in their infancy, with no more than 50 articles published annually. Since 2005, the number of publications on sirtuins has increased dramatically at an average rate of 100 per year. Even from 2013, the growth rate of publications on sirtuins reached an average of 200 publications per year, illustrating that research on sirtuins has gradually emerged in numerous fields.
FIGURE 2. Overall distribution of sirtuins study. (A). Global annual production trends in studies on sirtuins. (B). Key diseases in sirtuins related research. (C). Stream plots of annual production trends for the sirtuins family. (D). The annual proportion of kidney disease in studies on sirt1.
Through co-occurrence analysis of the keywords of the retrieved publications, it was found that the diseases related to sirtuins research are mainly concentrated in aging, obesity, neurodegenerative diseases, cancer, and cardiovascular diseases (Figure 2B; Table 1). Publications about sirt1, growing rapidly (R2 = 0.9733), were the most studied (n = 11265, 61.81%) and had the highest h-index (250) (Figure 2C; Table 2). There were 744 articles focusing on kidney disease, and the proportion of research investigating kidney disease and sirt1 increased annually (Figure 2D), suggesting that an increasing number of scholars have focused on the potential role of sirt1 in kidney disease. Next, we present an in-depth analysis of publications on sirt1, with a particular focus on the field of kidney disease.
In total, 6941 institutions from 92 countries contributed to publications related to sirt1. The United States is a central player in international collaboration and has partnered with many countries, most notably with China (Figure 3A).75.68% of publications were represented by the top ten countries with the largest number of publications (Table 3). China and the United States are far ahead of other countries in terms of the number of publications and total citations. In terms of average citation frequency, the United States (average cited 83.60 times) continues to rank first, followed by Canada (average cited 67.18 times) and the United Kingdom (average cited 53.94 times) (Figure 3B). Furthermore, seven of the ten most cited institutions worldwide are from the United States, which explains why the United States is far ahead of the world in terms of the total and average number of citations. In the field of kidney disease, China and the United States are still far ahead in terms of the number of publications and citations. However, Japan ranked first in terms of average citation frequency, followed by Italy and the United States (Supplemental Table S2). Kanazawa Medical University (cited 1799 times) and Shiga University of Medical Science (cited 1495 times) from Japan and Fudan University (cited 958 times) from China are the most influential institutions in the field of sirt1 in kidney diseases.
FIGURE 3. National contributions of the sirt1 study. (A) Global geographic distribution and collaboration regarding the study of sirt1. (B) The top ten highest productive countries for the study of sirt1.
A total of 39208 authors contributed to the study of sirt1. Frye RA from the University of Pittsburgh was the first scholar to publish publications on sirt1 in 1999 (Frye, 1999). Sinclair DA (cited 20460 times) from Harvard Medical School and Guarente L (cited 18758 times) from Massachusetts Institute of Technology (MIT), with the highest number of citations, were the most influential authors in terms of sirt1 research (Table 4). In the field of kidney disease, 3789 authors have been involved in the study of sirt1. Koya D from Kanazawa Medical University (cited 1799 times) and Kume S from Shiga University of Medical Science (cited 1446 times), as the most cited authors, were the most influential scholars (Supplemental Table S3). Koya D and Kume S were also the first to publish that sirt1 plays a key role in kidney disease.
A total of 1665 journals published publications on Sirt1. According to the JCR, 582 (34.96%) journals appeared in JCR quartile 1 (Q1) and 444 (26.67%) journals were ranked in Q2 (Figure 4A), revealing the enormous academic impact of sirt1 research. Furthermore, a total of 5153 publications were published in these Q1 journals and 3690 in Q2 journals (Figure 4B), with mean impact factors of 8.55 and 4.18, respectively (Figure 4C). The Journal of Biological Chemistry, Cell, and Nature, as the journals with the highest academic impact in this field, are the three most cited journals, and eight of the ten most cited journals belong to the Q1, where Science, Nature, and Cell were the most frequently cited journals on average (Table 5). However, only three of the ten highest-volume journals appeared among the highly cited journals (Table 5). The dual-map overlay shows a total of three main citation paths in publications related to sirt1, where the publications performing citations were focused on the molecular, biology, immunology and medicine, medical, clinical field and the cited references were focused on the molecular, biology, genencs and health, nursing, medicine field (Figure 4D). In the field of kidney disease, 321 journals published relevant publications, 130 (40.5%) of which belonged to Q1, and 90 (28.04%) appeared in Q2 (Figure 4E), with mean impact factors of 18.91 and 10.18, respectively, which were better than all the publications on sirt1 (Figures 4F,G), illustrating the potential promise of sirt1 in kidney disease research. Journal of Clinical Investigation, Journal of the American Society of Nephrology (JASN), Aging Cell, and Kidney International (KI), as the most cited journals, were identified as the most influential journals (Supplemental Table S4).
FIGURE 4. Characteristics of the core journals involved in the study of sirt1. (A) The quartile ranking of journals concerned with sirt1. (B) Radar plot of publication volume of sirt1 for journals in different quartile rankings. (C) Impact factor distribution of journals in different quartile rankings. (D) The dual-map overlay of the publications concerning sirt1. (E) The quartile ranking of journals concerned with sirt1 in kidney disease. (F) Radar plot of the proportion of different quartile rankings with respect to the number of publications of sirt1 and sirt1 in kidney disease. (G) Radar plot of Impact factor distribution of journals in different quartile rankings of sirt1 and sirt1 in kidney disease.
TABLE 5. Top ten journals with the largest number of publications and the most cited related to sirt1.
In the top 10 most cited publications, a paper published by Lagouge et al. (2006) in Cell in 2006 was the most co-cited, with 3029 citations. This research reported that RSV, as an activator of sirt1, can promote energy and metabolic homeostasis and improve aerobic capacity. The article published in Nature by Howitz et al. (2003) was cited 2833 times and ranked second. They similarly reported that small molecules, such as RSV, can activate sirt1 and extend the lifespan of Saccharomyces cerevisiae. This underscores the importance of investigating sirt1 activating compounds (STACs). In addition, we analyzed the top ten most-cited publications in the last 5 years. The most cited article was written by Das et al. (2018) in 2018 and published in Cell. They reported that endothelial sirt1 deficiency is a reversible cause of vascular aging, and the NAD precursor NMN can reverse this change through sirt1. The second ranked article by citation number was published in the Journal of Neuroinflammation by Chen et al. (2018) in 2018, which showed that ω-3 polyunsaturated fatty acid supplementation can regulate HMGB1/NF-κB pathways through sirt1-mediated deacetylation to attenuate inflammatory responses. In terms of kidney disease, the most cited articles reported that RSV attenuates proteinuria, renal immunoglobulin deposition, and glomerulonephritis in lupus nephritis mice (Wang et al., 2014). In the last 5 years, the most cited articles were published by Ren et al. (2020), who reported that metformin, a classic hypoglycemic agent, produced renoprotective effects against diabetes via the AMPK/SIRT1-FoxO1 pathway. The second most focused article was a review published by Ralto et al.(2020), who reported that NAD+ homeostasis plays an important role in kidney disease and health.
Next, we performed a cluster analysis of the co-cited references, resulting in seven clusters with a modularity Q of 0.646 and a mean silhouette value of 0.8156 (Figure 5A). The top five clusters were enriched for diseases associated with sirt1, including diabetes, skeletal muscle, cancer, and cognitive deficit. It is important to note that nicotinamide mononucleotides (NAD) appear to be a hotspot that gains considerable interest. When focusing on kidney disease, five clusters were extracted, with a modularity Q of 0.4116 and a mean silhouette value of 0.6787 (Figure 5B). We found that NAD+ has also been the focus of research regarding sirt1 in kidney disease.
FIGURE 5. Co-cited references analysis. (A) Timeline plot of clustering concerning sirt1. (B) Timeline plot of clustering concerning sirt1 in kidney disease. (C) Burst analysis concerning sirt1. (D) Burst analysis concerning sirt1 in kidney disease.
In addition, we performed an analysis of burst and obtained 778 burst references (Figure 5C). The reference with the strongest beginning of citation burst was published in Cell by Vaziri et al. (2001) in 2001. They described that sirt1 can deacetylate regulated p53, thus improving growth arrest or apoptosis. Similarly, the references with the second strongest beginning of citation burst were published by Luo et al. (2001) in Cell in 2001 and reported similar results. This illustrates that the regulation of deacetylation of p53 by sirt1 is a major discovery in sirt1 research. When focusing on the last 3 years, the reference with the strongest beginning of citation burst was published by Alves-fernandes D et al. and Zhang W et al., and they jointly focused on the important crosstalk role of sirt1 and oxidative stress in different diseases (Zhang et al., 2017; Alves-Fernandes and Jasiulionis, 2019). In terms of kidney disease, 49 burst references were found (Figure 5D), the publication by Brunet A et al. in Science in 2004 had the strongest beginning of citation burst, followed by Rodgers J et al., published in Nature in 2005, indicating that the regulation of FOXO and PGC-1α by sirt1 has received much attention from scholars in the field of kidney disease (Brunet et al., 2004; Rodgers et al., 2005). In the past 5 years, the publication by Liu R et al. in Diabetes in 2014 showed the strongest beginning of citation burst and found that transcription factors, such as p65 and STAT3, acetylation plays an important role in DN (Liu et al., 2014), followed by an article in JASN published by Morigi M et al. in 2018, who reviewed the central role of sirt1 in kidney health and disease (Morigi et al., 2018).
We performed clustering analysis on the extracted 24819 keywords and obtained eight clusters (Figure 6A). Obesity and Alzheimer’s disease appear to be continuing hotspots for comparative attention among scholars investigating sirt1. In addition, regulation of oxidative stress and p53 by sirt1 appears to be a hotspot of ongoing interest in mechanistic studies. In terms of kidney disease, DN is a disease of considerable interest, and oxidative stress and mitochondrial biogenesis are mechanistic studies that have received continued attention (Figure 6B).
FIGURE 6. Keywords timeline of clustering. (A) Keywords timeline of clustering of sirt1. (B) Keywords timeline of clustering of sirt1 in kidney disease.
A total of 331 keywords were retrieved from the burst analysis (Figure 7A). “Calorie restriction,” “life span,” and “saccharomyces cerevisiae,” as the keywords with the strongest citation bursts, indicate major areas in the study of sirt1. In the past 5 years, “autophagy,” “injury,” and “NLRP3 inflammasome” represent emerging areas in the study of sirt1. In addition, we found that the research theme of sirt1 changed over time (Figure 7B), in the last 3 years, “AMPK,” “diabetic nephropathy” and “autophagy” have been the themes of greatest interest. In terms of kidney disease (Figure 7C), 13 keywords were retrieved from burst analysis. “Calorie restriction,” “autophagy,” and “acetylation,” as the keywords with the strongest citation bursts, indicate major areas in the study of sirt1 in kidney disease, and “autophagy” and “NLRP3 inflammasome” also represent emerging areas in the past 5 years in the study of sirt1 in kidney disease.
FIGURE 7. Keyword analysis. (A) Burst analysis concerning sirt1. (B) The thematic evolution of keywords. (C) Burst analysis concerning sirt1 in kidney disease. (D) Clustering of keywords related to sirt1 in kidney disease. (E) Venn diagram for the keywords linking sirt1 and kidney diseases. (F) Potential research topics linking sirt1 and kidney diseases. (G) PPI network for the targets related to sirt1 in kidney diseases.
In addition, we extracted and clustered the keywords of publications based on sirt1 studies involving kidney disease using VOSviewer. A total of 3004 keywords appeared more than five times, and these keywords formed eight clusters (Figure 7D), including sirt1-associated kidney disease (green clusters), mechanisms (purple, blue, yellow, and aquamarine clusters), and treatment options (red clusters).
Next, we explored possible connections between sirt1 and kidney disease based on the Arrowsmith project. After removing duplicates and synonyms, a total of 2309 keywords with correlations greater than 0.5 were obtained. We defined the keywords obtained from the Arrowsmith project as the “prediction” group and the keywords extracted from VOSviewer as the “confirmation” group. We constructed a Venn diagram for these two groups of keywords in the hope of discovering the keywords revealing kidney disease (Figure 7E). A total of 1834 keywords were found to serve as potential research topics linking sirt1 and kidney diseases, and the keywords with a correlation greater than 0.95 are shown in Table 6 and Figure 7F. We further constructed a PPI network for these targets and found that IL6, SP1, CREB1, VEGFA, and PTGS2 were the targets most closely related to sirt1 in kidney diseases (Figure 7G).
In this study, we conducted a holistic evaluation of sirtuin-related research over nearly 2 decades. We found that since 2013, research on sirtuins has exploded in various fields, likely because the crucial role of sirtuins in health and disease has gradually gained the attention of researchers and clinicians. We reviewed the retrieved publications by timeline and identified a few landmark publications, such as Frye, RA, in 1999, which reported five sirtuin (SIRT1-SIRT5) homologs to yeast sir2 and found that these proteins can metabolize NAD (Frye, 1999). NAD+ has also received widespread attention as a fuel for sirtuins. In the last 3 years, two of the most cited articles have reported the important role of NAD+ homeostasis in disease and health, and elevated NAD+ levels have shown beneficial effects in various diseases (Katsyuba et al., 2020; Covarrubias et al., 2021). Cardiovascular diseases and neurodegenerative disorders are the main forces of sirtuin research. A recent highly cited article reported that NAD+ supplementation can reverse vascular aging by activating sirt1, and this effect could be further enhanced by hydrogen sulfide supplementation (another dietary restriction mimetic) (Das et al., 2018). Similarly, in another highly cited paper, NAD+ supplementation was found to ameliorate neuroinflammation, DNA damage, and synaptic dysfunction in Alzheimer’s disease (Hou et al., 2018). These results illustrate that NAD+, an important branch in the study of sirtuins, has received extensive attention, which also provides inspiration for the study of sirtuins in kidney diseases. Notably, in this study, we found that sirt3 is the most widely studied member of sirtuins family after sirt1. Deficiency of sirt3 was shown to result in HIF1α accumulation and PKM2 dimer formation, and subsequently leads to aberrant glycolysis and mesenchymal transformations, ultimately promoting fibrosis in DN (Srivastava et al., 2018). Recently, sirt3 was reported to contribute to the improvement of metabolic reprogramming and endothelial-to-mesenchymal transition (EndMT) in endothelial cells of DN, leading to the amelioration of fibrosis (Srivastava et al., 2021a). Interestingly, deficiency of Fibroblast Growth Factor Receptor 1 (FGFR1) has been reported to mediate the EndMT in DN and aggravate fibrosis (Li et al., 2020). N-acetyl-seryl-aspartyl-lysyl-proline (AcSDKP) restores the expression of sirt3 and ameliorates fibrosis in DN (Srivastava et al., 2020a), an effect that was found to be partially dependent on FGFR1 (Li et al., 2020).
Sirt1 is the most extensively studied member of the sirtuin family, with more than half of the sirtuin studies focused on sirt1. The United States and China were central participants in sirt1 studies. Harvard Medical School in the United States was a major contributor to sirt1 studies, and Sinclair DA’s team was the representative. They previously reported that RSV acts as a sirt1 activator to mimic calorie restriction, improve the DNA stability of yeast, and prolong its lifespan by 70% (Howitz et al., 2003). Recently, Sinclair DA et al. focused on the significance of NAD+ metabolism in disease and health. They found that activating sirt1 or increasing NAD+ levels improved the health of patients with cardiovascular and metabolic diseases (Das et al., 2018; Kane and Sinclair, 2018). In addition, they also found that NAD+ supplementation maintains telomere length and inhibits DNA damage, thus improving fibrotic disorders and premature aging (Amano et al., 2019). MIT in the United States is another important contributor to sirt1 research, and Guarente L’s team is the leader. They found early that sirt1 can deacetylate and regulate p53 to improve cell aging and apoptosis (Vaziri et al., 2001), and a recent study found that NAD+ supplementation rejuvenates stem cells in the aging intestine (Igarashi et al., 2019).
More than one-third of the publications were published in Q1 journals, showing the high academic influence of the research on sirt1, and with Cell, Nature, and Science being the most representative journals. Recently, an article published in Cell reported that the deacetylation of sirt1 produces an important protective effect against non-aging-related brain injury (Shin et al., 2021). Nature has recently reported dynamic changes in miR-34a targeting sirt1 (Baronti et al., 2020). In addition, based on co-cited references and keyword clusters and burst analysis, we found that diabetes, skeletal muscle, cancer, and cognitive deficit are hotspots diseases for the study of sirt1, and NAD+, oxidative stress, and deacetylation regulation of p53 are hotspots in the research field of sirt1. In addition, we also found that autophagy, NLRP3 inflammasome, AMPK pathway have received much attention from researchers as emerging research trends in recent years. Both autophagy and sirt1 have been recognized as important players in the aging process, and the connection between them has attracted much interest. Cui et al. (2006) suggested that blockade of autophagy is accompanied by a decrease in sirt1 expression. The most cited articles reported that sirt1, as an important regulator of autophagy, can regulate protein expression via deacetylation of autophagy-related genes (Atg), such as Atg5, Atg7, and Atg8, while sirt1 deficiency resulted in impaired autophagy activation (Lee et al., 2008). A recent study identified sirt1 as a novel and selective substrate of nuclear autophagy and an important regulator of sirt1 protein homeostasis (Xu et al., 2020), while inhibition of autophagy could promote sirt1-mediated health benefits (Wang et al., 2021). NLRP3 inflammasome are reportedly involved in sirt1. Fu et al. (2013) showed that RSV, as a sirt1 activator, inhibits NLRP3 inflammasome activation, while sirt1 inhibition can significantly enhance the expression of inflammatory factors. The latest findings that the sirt1 agonist SRT1720 reduces NLRP3 inflammasome activation and pyroptosis in an Akt signaling-dependent manner (Han et al., 2020) and that NLRP3 knockout leads to increased NAD+ levels and increased sirt1 expression, illustrate that the NLRP3 inflammasome appears to be involved in sirt1-associated-aging.
We found that kidney disease has gained increasing attention in recent years in the study of sirt1. China maintained its leading position both in terms of total publications and total citations, followed by researchers at the United States. Fudan University from China have been working on sirt1, and Hao CM’s team is the main representative among these researchers. They found that sirt1 may serve as a potential pharmacological target for kidney injury (Guan et al., 2017), and that sirt1 activation effectively improves renal fibrosis (Huang et al., 2014), which may be related to the improvement of renal oxidative stress (He et al., 2010). In addition, it is worth mentioning that Japan has made an important contribution to research investigating sirt1 in kidney disease due to the high citation frequency. Koya D from Kanazawa Medical University and Kume S from Shiga University of Medical Science are the main representatives of this group of researchers. Koya D and Kume S were early scholars in the field of kidney disease who focused on sirt1. As early as 2006, they reported that sirt1 deacetylates and regulates p53, and reduces mesangial cell apoptosis (Kume et al., 2006). The most influential article reported that calorie restriction enhances the adaptability of cells in aging kidneys to hypoxia through sirt1-dependent mitochondrial autophagy (Kume et al., 2010). Recently, a review by Koya D et al. discussed the relationship between sirt1 and oxidative stress in nephropathy, emphasizing that sirt1 activation in the kidney may represent a novel therapeutic strategy (Ogura et al., 2021). Furthermore, JASN, PLOS One, and KI are the most influential journals for sirt1 research in kidney disease. A recent publication in JASN reported that short-term treatment with NMN ameliorated the renal injury phenotype and survival of diabetic nephropathy by upregulating SIRT1 expression (Yasuda et al., 2021). Similarly, a recent article published in KI also reported that BF175 may reduce podocyte loss and renal dysfunction in diabetic nephropathy by activating sirt1 (Feng et al., 2021).
Oxidative stress and mitochondrial biogenesis were the main mechanisms involved in kidney disease. “Autophagy,” “acetylation,” and “NLRP3 inflammasome” have received much attention in the field of kidney disease as emerging research trends. It is widely accepted that sirt1 acts as a major driver of autophagy in kidney disease; however, the exact mechanism remains to be explored. As sirt1 is a novel substrate of autophagy, crosstalk between autophagy and sirt1 deserves significant attention in kidney disease (Xu et al., 2020; Wang et al., 2021). In addition, a recent study reported that the AMPK pathway maintains a high basal level of autophagy in podocytes independent of the mTOR pathway (Bork et al., 2020), this appears to provide important evidence for a link between autophagy and sirt1 in kidney disease. Notably, recent studies have shown that acetylation has emerged as an important regulatory mechanism of autophagy, and that acetylation not only regulates autophagy-related proteins but also affects autophagic function through the regulation of histones and transcription factors, indicating that deacetylation of sirt1 plays an important role in kidney disease, especially in autophagy (Xu and Wan, 2022). Crosstalk between sirt1 and the NLRP3 inflammasome plays an important role in kidney disease. Jiang et al. (2021) found that sirt1 knockout aggravated NLRP3 inflammasome activation in a mouse model of aldosterone infusion, and a recent report also found that sirt1 blunted NLRP3 inflammasome activation via autophagy to ameliorate IgA nephropathy (Wu et al., 2020). However, the complex connection between sirt1 and the NLRP3 inflammasome in kidney disease is poorly understood.
In addition, some neglected areas of kidney disease deserve attention as potential emerging hotspots in the study of Sirt1, such as immune, metabolic reprogramming and fecal microbiota. Immunity is a major cause of kidney diseases, including membranous nephropathy, lupus nephritis, and IgA nephropathy (Liu et al., 2020). Innate and adaptive immunity are involved in the initiation and maintenance of kidney injury (Dellepiane et al., 2020). Sirt1 has recently been found to act as an important regulator of immune cells and immune responses (Shen et al., 2021), which deserves the attention of researchers studying kidney diseases. In addition, metabolic reprogramming has been an emerging hotspot of kidney disease research in recent years, and changes in fatty acid metabolism and glucose metabolism have been found to be closely related to renal fibrosis (Zhu et al., 2021). Sirt1 plays an important role in energy and metabolic regulation (Ong and Ramasamy, 2018), and therefore, sirt1 may mediate metabolic reprogramming and play an important role in renal diseases. In addition, gut microbes and their derived metabolites were found to promote the progression of kidney disease and the development of severe complications, especially cardiovascular diseases (Mahmoodpoor et al., 2017; Feng et al., 2019). Recent studies have reported that deletion of sirt1 causes alterations in the gut microbiota, and sirt1 may be an important mediator of the interaction between the host and gut microbes (Wellman et al., 2017), which may provide important inspiration for the study of the gut-kidney axis. Notably, some natural ingredients, such as barbarum polysaccharides, Panax notoginseng, oridonin, triptolide, and hesperetin, deserve attention as potential therapeutic agents influencing sirt1 in kidney diseases.
Cluster and burst analyses showed that DN was a primary focus in sirt1 research. Sirt1 plays an important role in the epigenetic regulations of renal tubules and podocytes in DN. Sirt1 can deacetylate regulated STAT3, p53, FOXO4 and PGC1-α to maintain podocyte function (Nakatani and Inagi, 2016), and activation of sirt1 promotes the expression of PGC1-α in podocytes, thereby ameliorating podocyte injury and proteinuria in DN (Hong et al., 2018). Similarly, sirt1 could also deacetylate regulated Beclin1 and p53 to improve tubular autophagy (Deng et al., 2021; Sun et al., 2021) and attenuate high glucose induced tubular injury and apoptosis (Wang et al., 2016). Interestingly, renal tubular sirt1 was found to regulate the expression of Claudin-1 to mediate crosstalk with podocytes to ameliorate proteinuria in DN (Hasegawa et al., 2013). In addition, recent studies have found that glucocorticoid receptor (GR) plays an important role in DN. Deficiency of GR of endothelial accelerates renal fibrosis in DN mice (Srivastava et al., 2021c), and the same results were found in DN mice with podocyte GR loss (Srivastava et al., 2021b). Importantly, sirt1 was found to be a transcriptional enhancer of GR and is independent of its deacetylase activity role (Suzuki et al., 2018). Interestingly, some emerging drugs also hold promise for improving DN through sirt1, such as glycolysis inhibitors (Lv et al., 2018), DPP-4 inhibitors (linagliptin) (Elbaz et al., 2018), JAK/STA3 inhibitors (Wang et al., 2018), mineralocorticoid receptor agonists (Moore et al., 2012) and N-acetyl-seryl-aspartyl-lysyl-proline (Srivastava et al., 2020a).
In summary, sirt1 plays an important role in the regulation of inflammation, autophagy, oxidative stress, and mitochondrial homeostasis in kidney disease, especially in DN. In vivo and in vitro evidence shows that Sirt1 can serve as an important potential pharmacological target in kidney disease (Figure 8). Several drugs available in kidney disease have also been shown to be partially contribute to the activation of the sirt1, such as sodium glucose co-transporter two inhibitors (Packer, 2020), angiotensin-converting enzyme inhibitor (enalapril) (Veitch et al., 2021), angiotensin Ⅱ receptor blocker (olmesartan) (Gu et al., 2016), and statins (Khayatan et al., 2022). In addition, some small molecule compounds, especially some natural compounds, targeting sirt1 may also serve as potentially promising candidates for the treatment of kidney diseases, like RSV, Catalpol and Astragaloside IV.
FIGURE 8. Implication of sirt1 in diabetic kidney disease. Sirt1 attenuated podocyte and tubule injury and apoptosis, improve endothelial-to-mesenchymal transition (EndMT), and regulated autophagy and NLRP3 inflammasome activation in DKD by deacetylating regulatory ATG, Beclin1, STAT3, p53, FOXOs and PGC1-α. Several drugs available in kidney disease have been shown to be partially contribute to the activation of the sirt1, including SGLT2i, ACEI, ARB, and statins. Some natural compounds have also been found to have the same effect, such as resveratrol, catalpol and astragaloside IV.
Compared with the traditional review, bibliometrics is more tend to objective sort out a research field as a whole, which is suitable for the beginning of a research, to find the mainstream direction and emerging hot spots, so as to make more meaningful research. This study suggests our sirt1 as highly promising in the study of kidney diseases, especially in DN, and the relationship between Sirt1 and immune, metabolic reprogramming and fecal microbiota in kidney diseases deserves significant attention. However, this study has some limitations that need to be considered. First, we developed a search formula in as much detail as possible; however, that is difficult to avoid some studies were not included in our analysis. Second, we selected as many landmark articles as possible; however, it is possible that some important publications and research directions were missed due to differences in the evaluation indicators on which we focused. Finally, we combined various bibliometric analysis methods in order to obtain more useful and instructive results, but due to the limitations of the algorithm, some newer views may not be presented.
Based on bibliometric analysis, we found that publications regarding sirt1 have increased dramatically in the last 2 decades, especially in the last 5 years, in which studies on kidney diseases have gained increasing attention. China and the United States are major contributors to sirt1 studies, and Japanese scholars have also made important contributions to studies about sirt1 in kidney disease. NAD+, oxidative stress, and p53 are the main focus of scholars studying sirt1. Autophagy and NLRP3 inflammasome are emerging research trends that have gradually attracted the interest of researchers, especially regarding kidney diseases. In addition, some neglected potential research topics deserve further attention and in-depth studies.
The original contributions presented in the study are included in the article/Supplementary Material, further inquiries can be directed to the corresponding author.
TL, SM, and YZ designed the study. FM and YW collected the data. TL, LY, and HM analyzed the data and drafted the manuscript. All authors contributed to the article and approved the final version of the manuscript.
This work was supported by grants from National Nature Science Foundation of China (82074393).
The authors declare that the research was conducted in the absence of any commercial or financial relationships that could be construed as a potential conflict of interest.
All claims expressed in this article are solely those of the authors and do not necessarily represent those of their affiliated organizations, or those of the publisher, the editors and the reviewers. Any product that may be evaluated in this article, or claim that may be made by its manufacturer, is not guaranteed or endorsed by the publisher.
The Supplementary Material for this article can be found online at: https://www.frontiersin.org/articles/10.3389/fphar.2022.966786/full#supplementary-material
Alves-Fernandes, D. K., and Jasiulionis, M. G. (2019). The role of SIRT1 on DNA damage response and epigenetic alterations in cancer. Int. J. Mol. Sci. 20 (13), E3153. doi:10.3390/ijms20133153
Amano, H., Chaudhury, A., Rodriguez-Aguayo, C., Lu, L., Akhanov, V., Catic, A., et al. (2019). Telomere dysfunction induces sirtuin repression that drives telomere-dependent disease. Cell Metab. 29 (6), 1274–1290. e1279. doi:10.1016/j.cmet.2019.03.001
Aria, M., and Cuccurullo, C. (2017). Bibliometrix : an R-tool for comprehensive science mapping analysis. J. Inf. 11 (4), 959–975. doi:10.1016/j.joi.2017.08.007
Aventaggiato, M., Vernucci, E., Barreca, F., Russo, M. A., and Tafani, M. (2021). Sirtuins' control of autophagy and mitophagy in cancer. Pharmacol. Ther. 221, 107748. doi:10.1016/j.pharmthera.2020.107748
Baronti, L., Guzzetti, I., Ebrahimi, P., Friebe Sandoz, S., Steiner, E., Schlagnitweit, J., et al. (2020). Base-pair conformational switch modulates miR-34a targeting of Sirt1 mRNA. Nature 583 (7814), 139–144. doi:10.1038/s41586-020-2336-3
Bork, T., Liang, W., Yamahara, K., Lee, P., Tian, Z., Liu, S., et al. (2020). Podocytes maintain high basal levels of autophagy independent of mtor signaling. Autophagy 16 (11), 1932–1948. doi:10.1080/15548627.2019.1705007
Brunet, A., Sweeney, L. B., Sturgill, J. F., Chua, K. F., Greer, P. L., Lin, Y., et al. (2004). Stress-dependent regulation of FOXO transcription factors by the SIRT1 deacetylase. Science 303 (5666), 2011–2015. doi:10.1126/science.1094637
Chen, C. (2004). Searching for intellectual turning points: progressive knowledge domain visualization. Proc. Natl. Acad. Sci. U. S. A. 101 (1), 5303–5310. doi:10.1073/pnas.0307513100
Chen, X., Chen, C., Fan, S., Wu, S., Yang, F., Fang, Z., et al. (2018). Omega-3 polyunsaturated fatty acid attenuates the inflammatory response by modulating microglia polarization through SIRT1-mediated deacetylation of the HMGB1/NF-κB pathway following experimental traumatic brain injury. J. Neuroinflammation 15 (1), 116. doi:10.1186/s12974-018-1151-3
Covarrubias, A. J., Perrone, R., Grozio, A., and Verdin, E. (2021). NAD(+) metabolism and its roles in cellular processes during ageing. Nat. Rev. Mol. Cell Biol. 22 (2), 119–141. doi:10.1038/s41580-020-00313-x
Cui, Q., Tashiro, S., Onodera, S., and Ikejima, T. (2006). Augmentation of oridonin-induced apoptosis observed with reduced autophagy. J. Pharmacol. Sci. 101 (3), 230–239. doi:10.1254/jphs.fpj06003x
Dai, H., Sinclair, D. A., Ellis, J. L., and Steegborn, C. (2018). Sirtuin activators and inhibitors: promises, achievements, and challenges. Pharmacol. Ther. 188, 140–154. doi:10.1016/j.pharmthera.2018.03.004
Das, A., Huang, G. X., Bonkowski, M. S., Longchamp, A., Li, C., Schultz, M. B., et al. (2018). Impairment of an endothelial NAD(+)-H(2)S signaling network is a reversible cause of vascular aging. Cell 173 (1), 74–89. e20. doi:10.1016/j.cell.2018.02.008
Dellepiane, S., Leventhal, J. S., and Cravedi, P. (2020). T cells and acute kidney injury: a two-way relationship. Front. Immunol. 11, 1546. doi:10.3389/fimmu.2020.01546
Deng, Z., Sun, M., Wu, J., Fang, H., Cai, S., An, S., et al. (2021). SIRT1 attenuates sepsis-induced acute kidney injury via Beclin1 deacetylation-mediated autophagy activation. Cell Death Dis. 12 (2), 217. doi:10.1038/s41419-021-03508-y
Elbaz, E. M., Senousy, M. A., El-Tanbouly, D. M., and Sayed, R. H. (2018). Neuroprotective effect of linagliptin against cuprizone-induced demyelination and behavioural dysfunction in mice: a pivotal role of AMPK/SIRT1 and JAK2/STAT3/NF-κB signalling pathway modulation. Toxicol. Appl. Pharmacol. 352, 153–161. doi:10.1016/j.taap.2018.05.035
Feng, J., Bao, L., Wang, X., Li, H., Chen, Y., Xiao, W., et al. (2021). Low expression of HIV genes in podocytes accelerates the progression of diabetic kidney disease in mice. Kidney Int. 99 (4), 914–925. doi:10.1016/j.kint.2020.12.012
Feng, Y. L., Cao, G., Chen, D. Q., Vaziri, N. D., Chen, L., Zhang, J., et al. (2019). Microbiome-metabolomics reveals gut microbiota associated with glycine-conjugated metabolites and polyamine metabolism in chronic kidney disease. Cell. Mol. Life Sci. 76 (24), 4961–4978. doi:10.1007/s00018-019-03155-9
Frye, R. A. (1999). Characterization of five human cDNAs with homology to the yeast SIR2 gene: Sir2-like proteins (sirtuins) metabolize NAD and may have protein ADP-ribosyltransferase activity. Biochem. Biophys. Res. Commun. 260 (1), 273–279. doi:10.1006/bbrc.1999.0897
Fu, Y., Wang, Y., Du, L., Xu, C., Cao, J., Fan, T., et al. (2013). Resveratrol inhibits ionising irradiation-induced inflammation in MSCs by activating SIRT1 and limiting NLRP-3 inflammasome activation. Int. J. Mol. Sci. 14 (7), 14105–14118. doi:10.3390/ijms140714105
Funk, J. A., Odejinmi, S., and Schnellmann, R. G. (2010). SRT1720 induces mitochondrial biogenesis and rescues mitochondrial function after oxidant injury in renal proximal tubule cells. J. Pharmacol. Exp. Ther. 333 (2), 593–601. doi:10.1124/jpet.109.161992
Gu, J., Yang, M., Qi, N., Mei, S., Chen, J., Song, S., et al. (2016). Olmesartan prevents microalbuminuria in db/db diabetic mice through inhibition of angiotensin II/p38/SIRT1-Induced podocyte apoptosis. Kidney Blood Press. Res. 41 (6), 848–864. doi:10.1159/000452588
Guan, Y., Wang, S. R., Huang, X. Z., Xie, Q. H., Xu, Y. Y., Shang, D., et al. (2017). Nicotinamide mononucleotide, an NAD(+) precursor, rescues age-associated susceptibility to AKI in a sirtuin 1-dependent manner. J. Am. Soc. Nephrol. 28 (8), 2337–2352. doi:10.1681/asn.2016040385
Guo, J., Zheng, H. J., Zhang, W., Lou, W., Xia, C., Han, X. T., et al. (2020). Accelerated kidney aging in diabetes mellitus. Oxid. Med. Cell. Longev. 2020, 1234059. doi:10.1155/2020/1234059
Gupta, R., Ambasta, R. K., and Kumar, P. (2022). Multifaced role of protein deacetylase sirtuins in neurodegenerative disease. Neurosci. Biobehav. Rev. 132, 976–997. doi:10.1016/j.neubiorev.2021.10.047
Haigis, M. C., and Sinclair, D. A. (2010). Mammalian sirtuins: biological insights and disease relevance. Annu. Rev. Pathol. 5, 253–295. doi:10.1146/annurev.pathol.4.110807.092250
Han, Y., Sun, W., Ren, D., Zhang, J., He, Z., Fedorova, J., et al. (2020). SIRT1 agonism modulates cardiac NLRP3 inflammasome through pyruvate dehydrogenase during ischemia and reperfusion. Redox Biol. 34, 101538. doi:10.1016/j.redox.2020.101538
Hasegawa, K., Wakino, S., Simic, P., Sakamaki, Y., Minakuchi, H., Fujimura, K., et al. (2013). Renal tubular Sirt1 attenuates diabetic albuminuria by epigenetically suppressing Claudin-1 overexpression in podocytes. Nat. Med. 19 (11), 1496–1504. doi:10.1038/nm.3363
He, W., Wang, Y., Zhang, M. Z., You, L., Davis, L. S., Fan, H., et al. (2010). Sirt1 activation protects the mouse renal medulla from oxidative injury. J. Clin. Invest. 120 (4), 1056–1068. doi:10.1172/jci41563
Hong, Q., Zhang, L., Das, B., Li, Z., Liu, B., Cai, G., et al. (2018). Increased podocyte Sirtuin-1 function attenuates diabetic kidney injury. Kidney Int. 93 (6), 1330–1343. doi:10.1016/j.kint.2017.12.008
Hou, Y., Lautrup, S., Cordonnier, S., Wang, Y., Croteau, D. L., Zavala, E., et al. (2018). NAD(+) supplementation normalizes key Alzheimer's features and DNA damage responses in a new AD mouse model with introduced DNA repair deficiency. Proc. Natl. Acad. Sci. U. S. A. 115 (8), E1876–e1885. doi:10.1073/pnas.1718819115
Howitz, K. T., Bitterman, K. J., Cohen, H. Y., Lamming, D. W., Lavu, S., Wood, J. G., et al. (2003). Small molecule activators of sirtuins extend Saccharomyces cerevisiae lifespan. Nature 425 (6954), 191–196. doi:10.1038/nature01960
Huang, X. Z., Wen, D., Zhang, M., Xie, Q., Ma, L., Guan, Y., et al. (2014). Sirt1 activation ameliorates renal fibrosis by inhibiting the TGF-β/Smad3 pathway. J. Cell. Biochem. 115 (5), 996–1005. doi:10.1002/jcb.24748
Igarashi, M., Miura, M., Williams, E., Jaksch, F., Kadowaki, T., Yamauchi, T., et al. (2019). NAD(+) supplementation rejuvenates aged gut adult stem cells. Aging Cell 18 (3), e12935. doi:10.1111/acel.12935
Ivy, J. M., Klar, A. J., and Hicks, J. B. (1986). Cloning and characterization of four SIR genes of Saccharomyces cerevisiae. Mol. Cell. Biol. 6 (2), 688–702. doi:10.1128/mcb.6.2.688
Jiang, M., Zhao, M., Bai, M., Lei, J., Yuan, Y., Huang, S., et al. (2021). SIRT1 alleviates aldosterone-induced podocyte injury by suppressing mitochondrial dysfunction and NLRP3 inflammasome activation. Kidney Dis. 7 (4), 293–305. doi:10.1159/000513884
Kaeberlein, M., McVey, M., and Guarente, L. (1999). The SIR2/3/4 complex and SIR2 alone promote longevity in Saccharomyces cerevisiae by two different mechanisms. Genes Dev. 13 (19), 2570–2580. doi:10.1101/gad.13.19.2570
Kane, A. E., and Sinclair, D. A. (2018). Sirtuins and NAD(+) in the development and treatment of metabolic and cardiovascular diseases. Circ. Res. 123 (7), 868–885. doi:10.1161/circresaha.118.312498
Katsyuba, E., Romani, M., Hofer, D., and Auwerx, J. (2020). NAD(+) homeostasis in health and disease. Nat. Metab. 2 (1), 9–31. doi:10.1038/s42255-019-0161-5
Khayatan, D., Razavi, S. M., Arab, Z. N., Khanahmadi, M., Momtaz, S., Butler, A. E., et al. (2022). Regulatory effects of statins on SIRT1 and other sirtuins in cardiovascular diseases. Life (Basel) 12 (5), 760. doi:10.3390/life12050760
Kitada, M., Kume, S., Imaizumi, N., and Koya, D. (2011). Resveratrol improves oxidative stress and protects against diabetic nephropathy through normalization of Mn-SOD dysfunction in AMPK/SIRT1-independent pathway. Diabetes 60 (2), 634–643. doi:10.2337/db10-0386
Kumar, S., and Lombard, D. B. (2018). Functions of the sirtuin deacylase SIRT5 in normal physiology and pathobiology. Crit. Rev. Biochem. Mol. Biol. 53 (3), 311–334. doi:10.1080/10409238.2018.1458071
Kume, S., Haneda, M., Kanasaki, K., Sugimoto, T., Araki, S., Isono, M., et al. (2006). Silent information regulator 2 (SIRT1) attenuates oxidative stress-induced mesangial cell apoptosis via p53 deacetylation. Free Radic. Biol. Med. 40 (12), 2175–2182. doi:10.1016/j.freeradbiomed.2006.02.014
Kume, S., Uzu, T., Horiike, K., Chin-Kanasaki, M., Isshiki, K., Araki, S., et al. (2010). Calorie restriction enhances cell adaptation to hypoxia through Sirt1-dependent mitochondrial autophagy in mouse aged kidney. J. Clin. Invest. 120 (4), 1043–1055. doi:10.1172/jci41376
Lagouge, M., Argmann, C., Gerhart-Hines, Z., Meziane, H., Lerin, C., Daussin, F., et al. (2006). Resveratrol improves mitochondrial function and protects against metabolic disease by activating SIRT1 and PGC-1alpha. Cell 127 (6), 1109–1122. doi:10.1016/j.cell.2006.11.013
Lee, I. H., Cao, L., Mostoslavsky, R., Lombard, D. B., Liu, J., Bruns, N. E., et al. (2008). A role for the NAD-dependent deacetylase Sirt1 in the regulation of autophagy. Proc. Natl. Acad. Sci. U. S. A. 105 (9), 3374–3379. doi:10.1073/pnas.0712145105
Leite, J. A., Ghirotto, B., Targhetta, V. P., de Lima, J., and Câmara, N. O. S. (2022). Sirtuins as pharmacological targets in neurodegenerative and neuropsychiatric disorders. Br. J. Pharmacol. 179 (8), 1496–1511. doi:10.1111/bph.15570
Li, J., Liu, H., Srivastava, S. P., Hu, Q., Gao, R., Li, S., et al. (2020). Endothelial FGFR1 (Fibroblast growth factor receptor 1) deficiency contributes differential fibrogenic effects in kidney and heart of diabetic mice. Hypertension 76 (6), 1935–1944. doi:10.1161/hypertensionaha.120.15587
Liu, R., Zhong, Y., Li, X., Chen, H., Jim, B., Zhou, M. M., et al. (2014). Role of transcription factor acetylation in diabetic kidney disease. Diabetes 63 (7), 2440–2453. doi:10.2337/db13-1810
Liu, W., Gao, C., Liu, Z., Dai, H., Feng, Z., Dong, Z., et al. (2020). Idiopathic membranous nephropathy: Glomerular pathological pattern caused by extrarenal immunity activity. Front. Immunol. 11, 1846. doi:10.3389/fimmu.2020.01846
Lu, K., Yu, S., Sun, D., Xing, H., An, J., Kong, C., et al. (2018). Scientometric analysis of SIRT6 studies. Med. Sci. Monit. 24, 8357–8371. doi:10.12659/msm.913644
Luo, J., Nikolaev, A. Y., Imai, S., Chen, D., Su, F., Shiloh, A., et al. (2001). Negative control of p53 by Sir2alpha promotes cell survival under stress. Cell 107 (2), 137–148. doi:10.1016/s0092-8674(01)00524-4
Lv, Q., Wang, K., Qiao, S., Yang, L., Xin, Y., Dai, Y., et al. (2018). Norisoboldine, a natural AhR agonist, promotes Treg differentiation and attenuates colitis via targeting glycolysis and subsequent NAD(+)/SIRT1/SUV39H1/H3K9me3 signaling pathway. Cell Death Dis. 9 (3), 258. doi:10.1038/s41419-018-0297-3
Mahmoodpoor, F., Rahbar Saadat, Y., Barzegari, A., Ardalan, M., and Zununi Vahed, S. (2017). The impact of gut microbiota on kidney function and pathogenesis. Biomed. Pharmacother. 93, 412–419. doi:10.1016/j.biopha.2017.06.066
Mathias, R. A., Greco, T. M., Oberstein, A., Budayeva, H. G., Chakrabarti, R., Rowland, E. A., et al. (2014). Sirtuin 4 is a lipoamidase regulating pyruvate dehydrogenase complex activity. Cell 159 (7), 1615–1625. doi:10.1016/j.cell.2014.11.046
Moore, R. L., Dai, Y., and Faller, D. V. (2012). Sirtuin 1 (SIRT1) and steroid hormone receptor activity in cancer. J. Endocrinol. 213 (1), 37–48. doi:10.1530/joe-11-0217
Morigi, M., Perico, L., and Benigni, A. (2018). Sirtuins in renal health and disease. J. Am. Soc. Nephrol. 29 (7), 1799–1809. doi:10.1681/asn.2017111218
Nakatani, Y., and Inagi, R. (2016). Epigenetic regulation through SIRT1 in podocytes. Curr. Hypertens. Rev. 12 (2), 89–94. doi:10.2174/1573402112666160302102515
Ogura, Y., Kitada, M., and Koya, D. (2021). Sirtuins and renal oxidative stress. Antioxidants (Basel) 10 (8), 1198. doi:10.3390/antiox10081198
Ong, A. L. C., and Ramasamy, T. S. (2018). Role of Sirtuin1-p53 regulatory axis in aging, cancer and cellular reprogramming. Ageing Res. Rev. 43, 64–80. doi:10.1016/j.arr.2018.02.004
Packer, M. (2020). Interplay of adenosine monophosphate-activated protein kinase/sirtuin-1 activation and sodium influx inhibition mediates the renal benefits of sodium-glucose co-transporter-2 inhibitors in type 2 diabetes: a novel conceptual framework. Diabetes Obes. Metab. 22 (5), 734–742. doi:10.1111/dom.13961
Ralto, K. M., Rhee, E. P., and Parikh, S. M. (2020). NAD(+) homeostasis in renal health and disease. Nat. Rev. Nephrol. 16 (2), 99–111. doi:10.1038/s41581-019-0216-6
Ren, H., Shao, Y., Wu, C., Ma, X., Lv, C., and Wang, Q. (2020). Metformin alleviates oxidative stress and enhances autophagy in diabetic kidney disease via AMPK/SIRT1-FoxO1 pathway. Mol. Cell. Endocrinol. 500, 110628. doi:10.1016/j.mce.2019.110628
Rodgers, J. T., Lerin, C., Haas, W., Gygi, S. P., Spiegelman, B. M., and Puigserver, P. (2005). Nutrient control of glucose homeostasis through a complex of PGC-1alpha and SIRT1. Nature 434 (7029), 113–118. doi:10.1038/nature03354
Sabe, M., Pillinger, T., Kaiser, S., Chen, C., Taipale, H., Tanskanen, A., et al. (2022). Half a century of research on antipsychotics and schizophrenia: a scientometric study of hotspots, nodes, bursts, and trends. Neurosci. Biobehav. Rev. 136, 104608. doi:10.1016/j.neubiorev.2022.104608
Shen, P., Deng, X., Chen, Z., Ba, X., Qin, K., Huang, Y., et al. (2021). SIRT1: a potential therapeutic target in autoimmune diseases. Front. Immunol. 12, 779177. doi:10.3389/fimmu.2021.779177
Shin, M. K., Vázquez-Rosa, E., Koh, Y., Dhar, M., Chaubey, K., Cintrón-Pérez, C. J., et al. (2021). Reducing acetylated tau is neuroprotective in brain injury. Cell 184 (10), 2715–2732. e2723. doi:10.1016/j.cell.2021.03.032
Smalheiser, N. R., Torvik, V. I., and Zhou, W. (2009). Arrowsmith two-node search interface: a tutorial on finding meaningful links between two disparate sets of articles in MEDLINE. Comput. Methods Programs Biomed. 94 (2), 190–197. doi:10.1016/j.cmpb.2008.12.006
Soni, S. K., Basu, P., Singaravel, M., Sharma, R., Pandi-Perumal, S. R., Cardinali, D. P., et al. (2021). Sirtuins and the circadian clock interplay in cardioprotection: focus on sirtuin 1. Cell. Mol. Life Sci. 78 (6), 2503–2515. doi:10.1007/s00018-020-03713-6
Srivastava, S. P., Goodwin, J. E., Kanasaki, K., and Koya, D. (2020a). Metabolic reprogramming by N-acetyl-seryl-aspartyl-lysyl-proline protects against diabetic kidney disease. Br. J. Pharmacol. 177 (16), 3691–3711. doi:10.1111/bph.15087
Srivastava, S. P., Kanasaki, K., and Goodwin, J. E. (2020b). Loss of mitochondrial control impacts renal health. Front. Pharmacol. 11, 543973. doi:10.3389/fphar.2020.543973
Srivastava, S. P., Li, J., Kitada, M., Fujita, H., Yamada, Y., Goodwin, J. E., et al. (2018). SIRT3 deficiency leads to induction of abnormal glycolysis in diabetic kidney with fibrosis. Cell Death Dis. 9 (10), 997. doi:10.1038/s41419-018-1057-0
Srivastava, S. P., Li, J., Takagaki, Y., Kitada, M., Goodwin, J. E., Kanasaki, K., et al. (2021a). Endothelial SIRT3 regulates myofibroblast metabolic shifts in diabetic kidneys. iScience 24 (5), 102390. doi:10.1016/j.isci.2021.102390
Srivastava, S. P., Zhou, H., Setia, O., Dardik, A., Fernandez-Hernando, C., and Goodwin, J. (2021b). Podocyte glucocorticoid receptors are essential for glomerular endothelial cell homeostasis in diabetes mellitus. J. Am. Heart Assoc. 10 (15), e019437. doi:10.1161/jaha.120.019437
Srivastava, S. P., Zhou, H., Setia, O., Liu, B., Kanasaki, K., Koya, D., et al. (2021c). Loss of endothelial glucocorticoid receptor accelerates diabetic nephropathy. Nat. Commun. 12 (1), 2368. doi:10.1038/s41467-021-22617-y
Sun, M., Li, J., Mao, L., Wu, J., Deng, Z., He, M., et al. (2021). p53 deacetylation alleviates sepsis-induced acute kidney injury by promoting autophagy. Front. Immunol. 12, 685523. doi:10.3389/fimmu.2021.685523
Suzuki, S., Iben, J. R., Coon, S. L., and Kino, T. (2018). SIRT1 is a transcriptional enhancer of the glucocorticoid receptor acting independently to its deacetylase activity. Mol. Cell. Endocrinol. 461, 178–187. doi:10.1016/j.mce.2017.09.012
van Eck, N. J., and Waltman, L. (2010). Software survey: VOSviewer, a computer program for bibliometric mapping. Scientometrics 84 (2), 523–538. doi:10.1007/s11192-009-0146-3
Vaziri, H., Dessain, S. K., Ng Eaton, E., Imai, S. I., Frye, R. A., Pandita, T. K., et al. (2001). hSIR2(SIRT1) functions as an NAD-dependent p53 deacetylase. Cell 107 (2), 149–159. doi:10.1016/s0092-8674(01)00527-x
Veitch, M. R., Thai, K., Zhang, Y., Desjardins, J. F., Kabir, G., Connelly, K. A., et al. (2021). Late intervention in the remnant kidney model attenuates proteinuria but not glomerular filtration rate decline. Nephrol. Carlt. 26 (3), 270–279. doi:10.1111/nep.13828
Wang, L., Xu, C., Johansen, T., Berger, S. L., and Dou, Z. (2021). SIRT1 - a new mammalian substrate of nuclear autophagy. Autophagy 17 (2), 593–595. doi:10.1080/15548627.2020.1860541
Wang, M., and Lin, H. (2021). Understanding the function of mammalian sirtuins and protein lysine acylation. Annu. Rev. Biochem. 90, 245–285. doi:10.1146/annurev-biochem-082520-125411
Wang, W., Li, F., Xu, Y., Wei, J., Zhang, Y., Yang, H., et al. (2018). JAK1-mediated Sirt1 phosphorylation functions as a negative feedback of the JAK1-STAT3 pathway. J. Biol. Chem. 293 (28), 11067–11075. doi:10.1074/jbc.RA117.001387
Wang, X. L., Wu, L. Y., Zhao, L., Sun, L. N., Liu, H. Y., Liu, G., et al. (2016). SIRT1 activator ameliorates the renal tubular injury induced by hyperglycemia in vivo and in vitro via inhibiting apoptosis. Biomed. Pharmacother. 83, 41–50. doi:10.1016/j.biopha.2016.06.009
Wang, Z. L., Luo, X. F., Li, M. T., Xu, D., Zhou, S., Chen, H. Z., et al. (2014). Resveratrol possesses protective effects in a pristane-induced lupus mouse model. PLoS One 9 (12), e114792. doi:10.1371/journal.pone.0114792
Wellman, A. S., Metukuri, M. R., Kazgan, N., Xu, X., Xu, Q., Ren, N. S. X., et al. (2017). Intestinal epithelial sirtuin 1 regulates intestinal inflammation during aging in mice by altering the intestinal microbiota. Gastroenterology 153 (3), 772–786. doi:10.1053/j.gastro.2017.05.022
Wu, C. Y., Hua, K. F., Yang, S. R., Tsai, Y. S., Yang, S. M., Hsieh, C. Y., et al. (2020). Tris DBA ameliorates IgA nephropathy by blunting the activating signal of NLRP3 inflammasome through SIRT1- and SIRT3-mediated autophagy induction. J. Cell. Mol. Med. 24 (23), 13609–13622. doi:10.1111/jcmm.15663
Xu, C., Wang, L., Fozouni, P., Evjen, G., Chandra, V., Jiang, J., et al. (2020). SIRT1 is downregulated by autophagy in senescence and ageing. Nat. Cell Biol. 22 (10), 1170–1179. doi:10.1038/s41556-020-00579-5
Xu, Y., and Wan, W. (2022). Acetylation in the regulation of autophagy. Autophagy, 1–9. doi:10.1080/15548627.2022.2062112
Yasuda, I., Hasegawa, K., Sakamaki, Y., Muraoka, H., Kawaguchi, T., Kusahana, E., et al. (2021). Pre-emptive short-term nicotinamide mononucleotide treatment in a mouse model of diabetic nephropathy. J. Am. Soc. Nephrol. 32 (6), 1355–1370. doi:10.1681/asn.2020081188
Zhang, W., Huang, Q., Zeng, Z., Wu, J., Zhang, Y., and Chen, Z. (2017). Sirt1 inhibits oxidative stress in vascular endothelial cells. Oxid. Med. Cell. Longev. 2017, 7543973. doi:10.1155/2017/7543973
Zhao, L., Cao, J., Hu, K., He, X., Yun, D., Tong, T., et al. (2020). Sirtuins and their biological relevance in aging and age-related diseases. Aging Dis. 11 (4), 927–945. doi:10.14336/ad.2019.0820
Keywords: bibliometric analysis, SIRT1, kidney disease, NAD, oxidative stress, autophagy
Citation: Liu T, Mu S, Yang L, Mao H, Ma F, Wang Y and Zhan Y (2022) Comprehensive bibliometric analysis of sirtuins: Focus on sirt1 and kidney disease. Front. Pharmacol. 13:966786. doi: 10.3389/fphar.2022.966786
Received: 11 June 2022; Accepted: 25 July 2022;
Published: 16 August 2022.
Edited by:
Swayam Prakash Srivastava, Yale University, United StatesReviewed by:
Lining Miao, Second Hospital of Jilin University, ChinaCopyright © 2022 Liu, Mu, Yang, Mao, Ma, Wang and Zhan. This is an open-access article distributed under the terms of the Creative Commons Attribution License (CC BY). The use, distribution or reproduction in other forums is permitted, provided the original author(s) and the copyright owner(s) are credited and that the original publication in this journal is cited, in accordance with accepted academic practice. No use, distribution or reproduction is permitted which does not comply with these terms.
*Correspondence: Yongli Zhan, emhhbnlvbmdsaTg4QHNpbmEuY29t
†These authors have contributed equally to this work
Disclaimer: All claims expressed in this article are solely those of the authors and do not necessarily represent those of their affiliated organizations, or those of the publisher, the editors and the reviewers. Any product that may be evaluated in this article or claim that may be made by its manufacturer is not guaranteed or endorsed by the publisher.
Research integrity at Frontiers
Learn more about the work of our research integrity team to safeguard the quality of each article we publish.