- State Key Laboratory of Quality Research in Chinese Medicine, Institute of Chinese Medical Sciences, University of Macau, Macao, China
- International Cooperative Laboratory of Traditional Chinese Medicine Modernization and Innovative Drug Development of Chinese Ministry of Education (MOE), College of Pharmacy, Jinan University, Guangzhou, China
- Macau Centre for Research and Development in Chinese Medicine, State Key Laboratory of Quality Research in Chinese Medicine, University of Macau, Macao, China
Parkinson’s disease (PD) is an age-related chronic neurodegenerative disease caused by the death and degeneration of dopaminergic neurons in the substantia nigra of the midbrain. The decrease of the neurotransmitter dopamine in the patient’s brain leads to various motor symptoms. PD drugs mainly enhance dopamine levels but cannot prevent or slow down the loss of dopaminergic neurons. In addition, they exhibit significant side effects and addiction issues during long-term use. Therefore, it is particularly urgent to develop novel drugs that have fewer side effects, can improve PD symptoms, and prevent the death of dopaminergic neurons. The rhizome of Gastrodia elata Blume (Tianma) is a well-known medicinal herb and has long been used as a treatment of nervous system-related diseases in China. Several clinical studies showed that formula comprising Tianma could be used as an add-on therapy for PD patients. Pharmacological studies indicated that Tianma and its bioactive components can reduce the death of dopaminergic neurons, α-synuclein accumulation, and neuroinflammation in various PD models. In this review, we briefly summarize studies regarding the effects of Tianma and its bioactive components’ effects on major PD features and explore the potential use of Tianma components for the treatment of PD.
Introduction
Parkinson’s disease (PD) is a common chronic neurodegenerative disease, which results in motor symptoms such as bradykinesia, stiffness, resting tremor, and postural instability (Kalia and Lang, 2015). Most PD symptoms are caused by the degeneration and loss of dopaminergic neurons that produce dopamine in the substantia nigra (SN) of the midbrain (Dickson et al., 2009). Reduced dopamine level results in abnormal brain transmission activity, leading to impaired movement and various motor symptoms. PD also leads to non-motor symptoms such as dizziness, cognitive changes, psychiatric and sleep problems. PD affects individuals all over the globe and is the second most prevalent neurological illness after Alzheimer’s disease. According to the statistics of PD patients worldwide from 1990 to 2015, the prevalence, disability-adjusted life-years, and deaths increased substantially, making PD the fastest-growing neurological disease (Feigin et al., 2017).
The incidence of PD increases with age, particularly in elderly individuals over 50 years of age (Bloem et al., 2021). With the increase of the aging population, the prevalence of PD will continue to grow and is expected to exceed 12 million by 2040 (Dorsey and Bloem, 2018). Meta-analysis suggests that there are significant differences in PD prevalence by geographical location. The prevalence rates in North America, Europe, and Australia are 2.4 times higher than that of Asia. In addition, the prevalence in women is three-tenths of that in men (Pringsheim et al., 2014). Environmental risk factors for PD are also identified through epidemiology. Environmental chemicals such as the pesticide paraquat are found to be a risk factor in the development of PD (Clarimon, 2020). PD is a neurological ailment that worsens with age; thus, aging is considered the major driver of PD development (Antony et al., 2013). However, since PD’s etiology and pathogenic mechanisms are still poorly understood, it is challenging to develop drugs to prevent the death of dopaminergic neurons. Currently, PD drugs including levodopa preparations, dopamine receptor agonists, and monoamine oxidase inhibitors mainly work through enhancing dopamine levels, but they exhibit a large number of side effects and addiction issues (Bastide et al., 2015). Conversely, they may generate excessive dopamine action, causing the abnormal activation of the motor system and thereby generating dyskinesias. The standard oral levodopamine (l-dopa) treatment is the most effective way to relieve PD patients’ motor symptoms. However, as the disease progresses and l-dopa continues to be taken, the movement disorder caused by the drug l-dopa, known as l-dopa-induced dyskinesia (LID), are often seen in patients who are on the treatment for over 10 years (Bastide et al., 2015). Notably, these PD drugs cannot prevent or slow down the death of dopaminergic neurons. Therefore, it is particularly urgent to find drugs that have fewer side effects, can improve the PD symptoms, and prevent the death of dopaminergic neurons.
Up to now, many medicinal herbs or natural products have been developed as PD drug candidates. Some have entered in clinical trials (Figure 1) (Prasad and Hung, 2021). For example, Ganoderma (NCT03594656) has entered phase III of a trial. And the SQJZ herbal mixture (NCT02616120), a formula consisting of Cornus officinalis, Rehmannia glutinosa, Poria cocos, Ophiopogon japonicus, Cuscuta chinensis, Trichosanthes kirilowii, Semen ziziphin spinosae, Aurantii fructus immaturus, and Schisandra chinensis (Shi et al., 2017), has entered phase II. Additionally, Hypoestoxide (NCT04858074), a natural diterpenoid found in Hypoestes rosea dry leaf powder, is in phase I. These imply that medicinal herbs may have potential in the treatment of PD without serious side effects.
Gastrodia elata blume (Tianma) is a medicinal herb first found in the Classic of the Materia Medica, also known as the Shennong Ben Cao Jing (Zhan et al., 2016; Zhu et al., 2019; Heese, 2020). According to the Global Biodiversity Information Facility database (https://www.gbif.org/), Tianma has 20 synonyms and belongs to the genus Gastrodia R. Br. Family Orchidaceae. It is mainly distributed in the mountainous regions of eastern Asia, especially in Korea, Japan, China, and India (Figure 2) (Gbif Global Biodiversity Information Facility, 2022). In ancient China, this was the preferred remedy to benefit and strengthen the qi, nourish the yin, and strengthen the body and prolong life (Zhan et al., 2016). Nowadays, it is used clinically for the treatment of dizziness, spasms, epilepsy, headache, amnesia, and hypertension (Matias et al., 2016; Zhan et al., 2016). Several clinical studies showed that the famous formula Tianma Gouteng decoction could be used as an add-on therapy for PD patients (Chen et al., 2014; Yan et al., 2014; Liu, 2015; Zhao and Hu, 2017; Zuo, 2018; Wang et al., 2019; Li et al., 2020). Furthermore, a growing number of studies have shown that Tianma and its constituents show neuroprotective effects in various PD in vitro and in vivo models. The main bioactive components of Tianma such as gastrodin, vanillyl alcohol, vanillin, vanillic acid, and anisalcohol have neuroprotective activities, which have attracted strong interest in the treatment of PD. Although the neuroprotective effects of the extracts and some compounds of Tianma have been summarized in previous reviews, new evidence from recent studies support the multiple activities of Tianma in specific PD features. Thus, in this review, we update and briefly summarize studies regarding the effects of the bioactive components of Tianma on the main features of PD and explore their potential for the treatment of PD.
The characteristics of PD brain
Dopaminergic neuronal death
The main pathological feature of PD is the decrease in dopamine caused by the death of dopaminergic neurons (Figure 3A). Although it is unknown what causes the dopaminergic neurons to die, dopaminergic neurodegeneration is accompanied by mitochondrial dysfunction and increase in reactive oxygen species (ROS) (Poewe et al., 2017). Mitochondrial dysfunction has been considered as one of the leading causes of dopaminergic neuron death. The mitochondria is an organelle found in the cytoplasmic matrix that generates energy via the mitochondrial respiratory chain (Bose and Beal, 2016), which serve an important role in metabolism, storing the intermediate products of pyruvate oxidation and Krebs cycle. Mitochondria also participates in calcium homeostasis, free radical scavenging, and programmed cell death control (Feno et al., 2019). However, other than powering and maintaining cellular function, the mitochondria is also the main source of ROS generation.
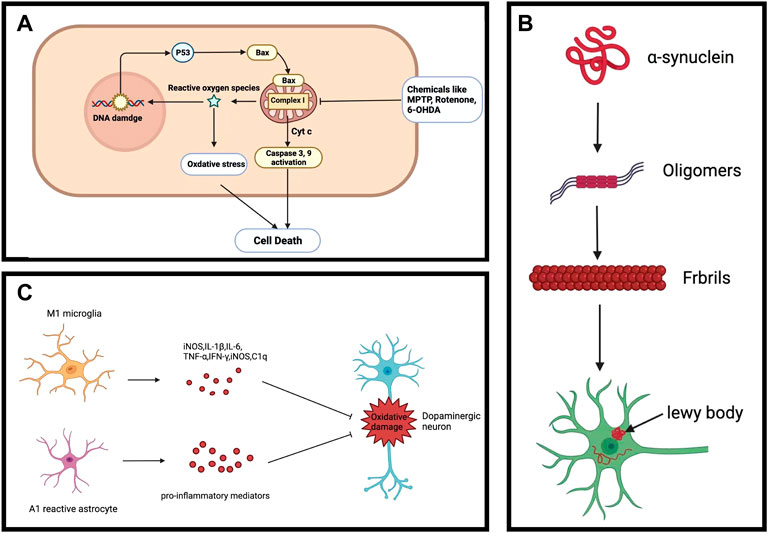
FIGURE 3. Common PD characteristics. (A) Dopaminergic neuronal death. (B) Alpha-synuclein aggregation. (C) Neuroinflammation.
The functional changes of mitochondria are manifested in PD patients, pointing toward a critical, causal role of mitochondrial defects in the degeneration of dopaminergic neurons (Cui et al., 2012). Notably, deficiency in mitochondrial complex I was observed in the SN of PD patients (Perier et al., 2010). The Ndufs4 gene encodes the core subunit protein of reduced nicotinamide adenine dinucleotide (NADH) dehydrogenase (complex I) in the mitochondrial membrane respiratory chain. A recent study indicated that knocking out the Ndufs4 gene in mice could cause mitochondrial complex I dysfunction and was sufficient to induce human-like PD symptoms (Gonzalez-Rodriguez et al., 2021). In addition, a vicious cycle between increased cytosolic dopamine and elevated mitochondrial oxidative stress exists in PD neurons (Gegg and Schapira, 2016; Burbulla et al., 2017; Nguyen et al., 2019). A myriad of studies has shown that toxin 1-methyl-4-phenyl-1,2,3, 6-tetrahydropropyne (MPTP), rotenone, 6-hydroxydopamine (6-OHDA), pyridine toluene, trichloroethylene, and fenpyrrolazine, which are complex I inhibitors, can lead to neurodegeneration of dopaminergic neurons in animal models. These inhibitors result in increased ROS production from mitochondrial dysfunction, which contributes to the activation of the intracellular apoptotic pathway: Bax is translocated to the mitochondria and cytochrome c is released from the mitochondria into the cell matrix, triggering a caspase activation cascade, leading to cell death. On the other hand, ROS induces the activation of poly ADP-ribose polymerase (PARP), which consumes adenosine triphosphate (ATP) and nicotinamide adenine dinucleotide (NAD+), leading to programmed cell death (Bose and Beal, 2016). Enriched ROS and Ca2+ in mitochondria can cause mitochondrial swelling and dysfunction. Although it is controversial whether oxidative stress caused by mitochondrial dysfunction occurs at an early or late stage of PD (Dionisio et al., 2021), accumulated evidence supports that mitochondrial complex I defects are nevertheless involved in the loss of dopaminergic neurons.
Lewy bodies
Lewy bodies (LBs) are mainly composed of aggregated presynaptic neuron protein α-synuclein (α-Syn), whose presence is a characteristic pathologic feature of PD. In addition to the SN, LBs also occur in other areas of the midbrain, basal forebrain, and cerebral cortex as PD develops. Misfolding and aggregation of α-syn involve seeding and nucleation mechanisms and are major causes of LBs formation in PD neurons (Figure 3B). However, it is unknown what is the exact conditions that lead to aberrant misfolding and aggregation of α-Syn in PD. α-Syn aggregation was reported to be linked to proteostasis dysfunction, such as chaperone-assisted proteolysis, autophagy, and ubiquitin-proteasome system -mediated degradation (Mehra et al., 2019). Notably, loss-of-function mutations in lysosomal beta-glucocerebrosidase (GCase) result in the accumulation of α-Syn (Migdalska-Richards and Schapira, 2016). On the other hand, non-inhibitory small-molecule modulators of GCase specifically enhanced lysosomal compartment activity, leading to a reduction in GCase substrate and clearance of pathological α-Syn in human PD patient midbrain neurons (Mazzulli et al., 2016). These suggest that lysosomal degradation plays a key in the accumulation of α-Syn. Additionally, mice overexpressing α-Syn are more sensitive to MPTP toxicity (Lee et al., 2017), suggesting that α-Syn accumulation may increase the vulnerability to PD. Although the LBs do not directly lead to dopaminergic neuronal death, the accumulation of α-Syn can act as an indicator for evaluating the effects of potential drug candidates.
Neuroinflammation
Another important aspect of PD is chronic neuroinflammation. Neuroinflammation in PD is mostly linked to the reactive state of glial cells (astrocytes and microglia) in the brain. Since glial cells have bidirectional communication with neurons, loss of dopaminergic neurons is accompanied by a significant increase in the number of reactive glial cells in the SN in PD patients (Mani et al., 2021). Microglia are the primary glial cells for mediating the innate immune response against invading pathogens and scavenging damaged neurons, synapses, as well as abnormally aggregated proteins in the central nervous system through phagocytosis (Harry, 2021; Zhong et al., 2022). However, alterative immune response of microglia can be activated by secreted molecules from degenerative neurons, which leads to the secretion of pro-inflammatory cytokines such as Interleukin-1β (IL-1β), IL-6, and tumor necrosis factor-α (TNF-α) to the brain microenvironment (Figure 3C). These pro-inflammatory cytokines may accelerate neurodegeneration in PD. Reducing microglia-mediated neuroinflammation can thus decrease the degeneration of dopaminergic neurons and improve motor behavior problems (Meng et al., 2019). Although the real cause of neuroinflammation is unclear, aggregated α-synuclein, mitochondrial dysfunction, and ecological dysregulation of the gut microbiome may induce neuroinflammation (Wang et al., 2021). Given the vital role of neuroinflammation in the progression of PD, regulating it may improve the brain microenvironment and reduce neurodegeneration.
The common experimental models of PD
MPTP is a Parkinsonism-inducing neurotoxin. It is a highly lipophilic molecule that can cross the blood-brain barrier (BBB) and specifically destroy dopaminergic neurons, thus MPTP is commonly used for establishment of various animal PD models (Ke et al., 2021). In vitro PD models based on dopaminergic neuronal death are commonly used for identifying PD drug candidates. 1-Methyl-4-phenylpyridinium ion (MPP+) is a metabolite of MPTP catalyzed by monoamine oxidase-B (MAO-B) in the brain. It can be specifically taken up by dopaminergic neurons and neuron-like cells, which causes injury to the mitochondria and thereby leads to apoptosis. SHSY-5Y and the MN9D cell lines are commonly used in the MPP+ cell model since they have the ability to synthesize and release dopamine (Xie et al., 2010). They also express tyrosine hydroxylase and dopamine transporter protein (DAT) (Xie et al., 2010). In addition, these cell lines are treated with 6-OHDA and H2O2 to induce oxidative stress, which is a causative factor for dopaminergic neuronal death in PD (Gay et al., 2018). The changes in apoptosis-related markers such as Bcl-2, Bax, and caspases three are major indicators for evaluating the effects of drug candidates on cell death (Abushouk et al., 2017).
LPS-induced microglial activation is a classical model for identifying drug candidates to evaluate anti-neuroinflammation properties (Catorce and Gevorkian, 2016). BV-2 is a microglial cell line derived from C57/BL6 mice. After LPS treatment, it enters the M1 polarised state, which is the main cause of neuroinflammation in PD. The changes in proinflammatory factors such as nitric oxide (NO), TNF-α,IL-1β, and COX-2 are common indicators for evaluating the effects of drug candidates on neuroinflammation.
The therapeutic effects of chinese medicine formula containing tianma
According to the ancient record of Chinese medicine, Tianma Gouteng decoction is a well-known formula containing Tianma, Uncaria Ramulus Cum Uncis, Haliotidis Concha, Gardeniae Fructus, Scutellariae Radix, Cyathulae Radix, Leonur Iherba, Eucommiae Cortex, Taxilli Herba, Caulis Polygoni Multiflori, and Zhu Fushen, originally used in the treatment of various disorders such as hypertension, migraine (Chang et al., 2014; Chong et al., 2021), and dementia (Lin et al., 2015). In preclinical studies, this formula inhibited lipid peroxidation by attenuating the lipid peroxidation-related protein ALOX15, thereby improving behavioral deficits and reducing the loss of dopaminergic neurons in MPTP-induced mice, 6-OHDA-induced rat, and α-Syn A53T overexpressed mice (Jiang Y.N et al., 2020). In clinical studies, Tianma Gouteng decoction was found to be a potential candidate for the treatment of PD. Its therapeutic effects are based on usage in combination with clinical drugs such as selegiline, levodopa, medopar, or pramipexole (Chen et al., 2014; Yan et al., 2014; Liu, 2015; Zhao and Hu, 2017; Zuo, 2018; Wang et al., 2019; Li et al., 2020). Compared to single treatment with these drugs, the combined treatment with Tianma Gouteng decoction leads to significantly lower scores in Parts I, II, III, and IV of the Unified PD Rating Scale (UPDRS). The treatment of Tianma Gouteng Decoction combined with selegiline decreased the serum levels of TNF-α, IL-6, malondialdehyde (MDA), and Cys-C when compared to the control group (Zuo, 2018). In the treatment of Tianma Gouteng decoction combined with Levodopa, it was found that the levels of IL-2, IL-6, TNF-α, MDA, and Cys-C in the treatment group decreased significantly and the SOD level increased significantly after 3 months (Gu et al., 2018). In the treatment of Tianma Gouteng decoction combined with Madopar, the levels of Glu and GABA in the treatment group were significantly increased compared to the control group (Liu et al., 2020). Serum homocysteine (Hcy) decreased, serum uric acid (UA) and adiponectin (APN) levels increased in Tianma Gouteng decoction combined with pramipexole treatment (Liu, 2020; Gui, 2021). These clinical evidences support that Tianma Gouteng decoction can be used as an add-on therapy for improving PD motor symptoms and blood biochemical parameters in PD.
The effects of tianma extract on various PD models
Tianma is a major medicinal herb in Tianma Gouteng decoction, hinting that it may have some potential therapeutic effects for PD. Evidence suggests that Tianma display strong antioxidant properties and neuroprotection in vitro and in vivo PD models. In MPP+-treated SH-SY5Y cells, the ethanol extract of Tianma conferred protective effects against MPP+-induced cell death (An et al., 2010). Researchers also demonstrated that the water extract of Tianma is an effective treatment for alleviating the motor symptoms of parkinsonian and loss of dopaminergic neurons in the fly model with LRRK2-G2019S mutation, which is the most common familial PD mutation (Lin et al., 2021). They found that the extract of Tianma activated the Akt-Nrf2 pathway in glia but not in neurons, contributing to its neuroprotective effects. Simultaneously, the extract of Tianma also worked as a neuroprotectant to improve locomotor performance, reduce dopaminergic neuron loss, and microglial activation in mice with LRRK2-G2019S mutation (Lin et al., 2021). Tianma extract could reduce lipopolysaccharides (LPS)-stimulated neuroinflammation BV-2 microglia model by down-regulating JNK/nuclear factor-κB (NF-κB) pathway (Kim et al., 2012), supporting the anti-neuroinflammation activity of Tianma. In 6-OHDA mice, l-dopa’s adverse effect LID was alleviated by Tianma extract via normalizing FosB and ERK activation (Doo et al., 2014). On the other hand, GABA levels in the SN pars reticulata can be increased by high-frequency stimulation of the subthalamic nucleus which is a therapy for patients with severe PD (Windels et al., 2005). Tianma extract could improve cognitive impairment and recover normal GABA levels in aluminum-treated rats (Shuchang et al., 2008). Tianma also provides anxiolytic-like effects via regulating the GABAergic nervous system (Ha et al., 2000). Taken together, due to the multiple activities of Tianma, we believe the bioactive components from Tianma may have the potential to alleviate various PD characteristics and symptoms.
The effects of bioactive compounds of tianma on various PD models
To present, at least 81 compounds have been isolated from Tianma, with five major groups including phenols and their glycosides, polysaccharides, sterols, organic acids, and other compounds (Zhu et al., 2019). The contents of these compounds are comprised of total phenolics (0.0485%), total polysaccharides (21.6%), total flavonoids (0.235%), total amino acids (1.92%), β sitosterol (0.113%), and other undetermined compounds (77.8%) (Zhan et al., 2016). Amongst them, the bioactive components of Tianma include gastrodin, vanillyl alcohol, vanillin, vanillic acid, and anisalcohol, which exhibit neuroprotective activities in various PD models. Gastrodin is considered as the major reference standard and the main bioactive component of Tianma. The maximum content of gastrodin reaches 0.24%, while other active components such as vanillin, vanillyl alcohol, vanillic acid, and anisalcohol belong to phenolic compounds that in total reach 0.0485% detected by the Folin-Ciocaileu colorimetric method (Zhan et al., 2016). The EtOEt extract of Tianma was subjected to the phenolic separation of compounds and showed to comprise Vanillyl Alcohol (0.00195%), Vanillin (0.00282%), and Vanillic acid (0.00195%) (Jang et al., 2010). Another report found that Tianma extracted with ethyl acetate to obtain the ethyl acetate fraction of Tianma after the crude extract of ethanol gives rise to anisyl alcohol (0.009445%) (Duan et al., 2013).
Gastrodin
Gastrodin (4-hydroxybenzyl alcohol 4-O-bata-d-glucoside) is a major active compound found in Tianma. The potential of gastrodin in various PD models attracts scientists’ strong attention. Pretreatment of gastrodin inhibited apoptosis in MPP+/SH-SY5Y cells model through modulating oxidative stress, cleaved PARP, Bax/Bcl ratio, and caspase-3 (Kumar et al., 2013). Some reports showed that gastrodin reduced MPP+-induced death of human dopaminergic SH-SY5Y cells through a reduction in Bcl-2/Bax ratio and ROS production (Jiang et al., 2014). Gastrodin increased the Nrf2 nuclear translocation and further enhanced heme oxygenase-1 (HO-1) expression via the p38 mitogen-activated protein kinase (MAPK) pathway. Pretreatment of rat glioma cell line C6 with gastrodin could reduce hydrogen peroxide-induced ferroptosis, which is a type of necrosis caused by iron-induced accumulation of lipid hydroperoxide (Jiang T et al., 2020). In this model, gastrodin decreased released lactate dehydrogenase (LDH) and intracellular MDA level. It up-regulated the expression of nuclear Nrf2, glutathione peroxidase 4 (GPX4), ferroportin-1 (FPN1), and HO-1, contributing to its cytoprotective effects against hydrogen peroxide-induced oxidative injury. Gastrodin can penetrate the BBB (Lin et al., 2007); thus it is well studied in various PD animal models. Others reported that gastrodin could ameliorate MPTP-induced motor deficits of mice in the open-field test and the rotating test (Wang et al., 2014). Oxidative stress in MPTP-induced PD mice was reduced by the antioxidant effects of gastrodin. In the striatum of MPTP mice, gastrodin not only robustly enhanced the expression of HO-1 and superoxide dismutase (SOD), but also promoted Nrf2 nuclear translocation (Wang et al., 2014). They further found that ERK1/2 phosphorylation contributes to the Nrf2 antioxidant system activated by gastrodin. Nrf2-mediated the expression of antioxidants and detoxification proteins, which are major antioxidant responses to defend against ROS, linking neuroprotective effects of gastrodin to the ability to enhance intracellular antioxidant responses. In 6-OHDA rat model of PD, pretreatment with gastrodin not only significantly improved motor incoordination and rigidity but also reduced lipid peroxidation levels, NO production, and increased total antioxidant capacity in the SN. In a rotenone-induced PD rat model, gastrodin could improve motor deficits and reduced the death of dopaminergic neurons in the SN (Li et al., 2012). Others found that gastrodin enhanced the food clearance as well as decreased pumping rate and the loss of dopaminergic neurons in the 6-OHDA Caenorhabditis elegans (C. elegans) model of PD via the DAF-2/DAF-16 insulin-like pathway (Yan et al., 2019). Pink1B9 mutant Drosophila can generate various PD-like phenotypes. Gastrodin significantly prolonged lifespan and enhanced the climbing ability in this PD fly model (He et al., 2021). In addition, gastrodin treatment increased the dopamine level and rescued the progressive death of dopaminergic neurons in the protocerebral posterial lateral one region of the PD fly brain (He et al., 2021). Notably, gastrodin also extended the lifespan, physical activity, and antioxidant ability in wild-type Drosophila, revealing that gastrodin may display anti-aging activity.
Reactive microglia-mediated neuroinflammation plays a role in the pathological process of PD (Li et al., 2012). In LPS-induced bv-2 microglia, gastrodin can be found to reduce the expression of pro-inflammatory cytokines such as COX-2 and NO by inhibiting MAPK phosphorylation and the NF-κB signaling pathway (Dai et al., 2011). Another report also showed that gastrodin decreased the expression and production of pro-inflammatory cytokines in LPS-induced bv-2 microglia by inhibiting the Wnt/β-catenin signaling pathway (Yao et al., 2019). Neuroinflammation parameters such as increased pro-inflammatory cytokines IL-1β, IL-6, and TNF-α as well as decreased resting microglia are observed in the SN of rotenone-induced PD rat, whereas gastrodin could decrease them. Myeloperoxidase (MPO) is a critical peroxidase enzyme increased in reactive microglial and contributes to the degeneration of dopaminergic neurons (Chen et al., 2020). In 6-OHDA-induced PD rat model, MPO activity in the SN was reduced by gastrodin (Haddadi et al., 2018), revealing that gastrodin has the effects in reducing microglial activation in various PD models. Additionally, gastrodin was found to attenuate α-Syn accumulation in α-Syn overexpressing transgenic worms (Yan et al., 2019). These pieces of evidence support that gastrodin can enhance the intracellular antioxidant system, reduce neuroinflammation and α-Syn accumulation, and contribute to reducing the death of dopaminergic neurons and PD-like motor problems.
Vanillin
Vanillin (4-hydroxy-3-methoxybenzaldehyde), a fragrant organic phenolic molecule, is widely used as a flavoring agent in food, beverage, cosmetics, and pharmaceutical industries. Vanillin is also identified in Tianma and can cross BBB (Salau et al., 2020). It has been reported to provide neuroprotection in different in vivo PD neurotoxin models. In a rotenone-induced PD rat model, improvements in both motor and depressive like behavior were observed after vanillin treatment. Vanillin could reduce rotenone-induced striatal dopamine depletion and oxidative stress. It also reduced rotenone-induced changes of apoptotic protein markers such as increased cytochrome C, decreased Bax, and increased Bcl-2 (Dhanalakshmi et al., 2016). In 6-OHDA PD rats, vanillin treatment could significantly decrease apomorphine-induced tight contralateral rotation and maintain dopamine levels (Abuthawabeh et al., 2020). In addition, pretreatment of vanillin could attenuate rotenone-induced mitochondrial dysfunction, oxidative stress, and apoptotic cascade in human SH-SY5Y cells (Dhanalakshmi et al., 2015).
Moreover, anti-neuroinflammatory effect of vanillin is mainly mediated via microglial activation, which has been demonstrated both in vivo and in vitro. LPS leads to motor dysfunction and dopaminergic neuron death in the SN of mice, whereas vanillin decreases neuroinflammation. Vanilin reduces LPS-induced expression of cyclooxygenase-2 (COX-2), inducible NO synthase (iNOS), and pro-inflammatory factors such as IL-1β and IL-6 through modulation of p38, ERK1/2, and NF-κB signaling (Yan et al., 2017). An in vitro study showed that vanillin inhibits the phosphorylation of MAPKs and NF-κB in LPS-treated microglia, and also reduces the protein levels of COX-2 and iNOS, as well as the mRNA levels of IL-1β, TNF-α and IL-6 (Kim et al., 2019). These reports suggest that vanillin is a potential drug candidate for the prevention of PD-related neuroinflammation through the inhibition of microglial activation. Thus, the neuroprotective effects of vanillin may be mediated through both anti-neuroinflammatory and anti-apoptotic activities.
Vanillic acid
Vanillic acid (4-hydroxy-3-methoxybenzoic acid) is an oxidized form of vanillin and can be isolated from Tianma. Vanillic acid has antioxidant activity. Vanillic acid was found to reduce hydrogen peroxide-induced death and ROS in human SH-SY5Y cells (Gay et al., 2018). It can maintain the levels of FoxO3a, manganese superoxide dismutase (MnSOD), and catalase, contributing to its anti-oxidative stress activity. It also has the potential to be used in combination with clinical medications to provide better neuroprotective effects for PD. According to one study in a rotenone-induced PD rat model (Sharma et al., 2021), vanillin combined with levodopa-carbidopa co-treatment was found to significantly improve rotenone-induced motor deficits and reduce various oxidative stress indicators such as increased lipid peroxidation and decreased GSH and catalase in the brain. This combined treatment also increased dopamine level in rotenone-treated rats. However, vanillic acid treatment alone did not provide any effects. In silico prediction showed that vanillic acid could not cross the BBB (Salau et al., 2020), hinting that vanillic acid may have unknow mechanism to enhance the effects of levodopa-carbidopa.
Vanillyl alcohol
Vanillyl alcohol (4-Hydroxy-3-methoxybenzyl alcohol) is a bioactive compound of Tianma that has antioxidant properties (Bezerra-Filho et al., 2019). It has been reported to have neuroprotection in a neurotoxin-induced PD cell model. Vanillyl alcohol exhibited anti-apoptotic effects in MPP+-induced dopaminergic MN9D cells by significantly reducing the ratio of Bax/Bcl-2 and the activation of PARP (Kim et al., 2011). It also reduced ROS level in MPP+-treated MN9D cells. The results of electron spin resonance spectrometry further supported that vanillyl alcohol is a strong antioxidant with scavenging activity for DPPH and alkyl radicals. It illustrates that the protective activity of vanillyl alcohol against MPP+-induced cell injury is dependent on its antioxidant ability; however, the activity of vanillyl alcohol lacks evidence in PD animal models.
Anisalcohol
Anisalcohol (p-methoxybenzyl alcohol or 4-methoxybenzyl alcohol) is a phenolic compound that can be isolated from Tianma and is widely used in food flavoring and fragrances (Duan et al., 2013). One report indicated that anisalcohol was found to have anti-inflammatory effects in LPS-activated BV-2 microglia. LPS-stimulated microglia become M1 type and release pro-inflammatory factors such as TNF-α, IL-6, and PGE2, leading to neurotoxicity. Treatment with anisalcohol selectively modulates microglia polarization and also reduces LPS-induced pro-inflammatory factor production (Xiang et al., 2018). Notably, the generation of anti-inflammatory cytokine IL-10 and transforming growth factor -β (TGF-β) was enhanced by anisalcohol treatment in LPS-activated BV-2 cells (Xiang et al., 2018). They also performed flow cytometry using antibodies against CD16/32 and CD206 to confirm the polarization states of microglia and found that anisalcohol treatment could promote microglia to become M2 type microglia, which serve a protective role in the brain. In a mechanistic study, anisalcohol was found to display anti-inflammatory activity by inhibiting JNK/NF-κB activation, supporting the anti-neuroinflammation activity of anisalcohol.
Discussion
Dopaminergic neuron death, α-Syn aggregation, and neuroinflammation are major characteristics of PD and can be used as key indicators for evaluating the efficacy of promising PD drugs. In this review, we assessed the effects of five constituents of Tianma on these major features of PD (Table 1). Although all these components are phenolic compounds with similar structures, their bioactivities are different in PD models. Gastrodin and vanillyl alcohol are reported to have protective effects against MPP+-induced cytotoxicity by upregulating Bcl-2 protein and thereby inhibiting the apoptotic pathway in PD cell models (Kumar et al., 2013; Jiang et al., 2014; Gay et al., 2018). Comparing their working concentrations (Table 2), gastrodin (1,5 and 25 μM) works at lower concentrations than vanillyl alcohol (10 and 20 μM). In the H2O2 cell model, vanillic acid treatment maintains the levels of SOD2, catalase, and Bcl-2 protein expression (Kim et al., 2011), suggesting that vanillic acid works through mediating oxidative stress-induced cell death. However, evidence to support the protective effect of vanillic acid against MPP+-induced cytotoxicity is still lacking. In PD animal models, only gastrodin and vanillin exert neuroprotective effects, probably due to their ability to cross BBB. According to mechanistic studies, gastrodin can enhance Nrf2-mediated intracellular antioxidant system, which is critical in maintaining mitochondrial functions under oxidative damage (Jiang T et al., 2020). Therefore, current evidences do support that gastrodin is able to reduce PD neurotoxin-induced mitochondrial dysfunction and oxidative stress as well as enhance the survival of neurons in different PD models. In contrast, there is no report regarding the action mechanism of vanillin and further study is needed to identify what contributes to the neuoprotective effects of vanillin.
Gastrodin, vanillin, and anisalcohol are reported to reduce microglial activation and pro-inflammatory cytokine generation (Table 3). In the LPS/BV-2 cells, the working concentrations of anisalcohol (0.1, 1, 10 μM) were found to be much lower than those of vanillin (100, 200, 300, 400 μM) and gastrodin (20, 30, 40, 60 μM). Vanillin and gastrodin mediate anti-inflammatory activity via inhibiting LPS-induced phosphorylation of MAPK and NF-κB. Anisalcohol also inhibits p65 phosphorylation of NF-κB and JNK phosphorylation, while anisalcohol can significantly inhibit the M1 microglia marker CD16/32 and promote M2 microglia CD206 expression. These evidences support that anisalcohol has anti-inflammatory effects at the cellular level. However, there is no report regarding anti-neuroinflammation of anisalcohol in animal models. Notably, anisalcohol can promote microglia to become M2 type microglia. It is possible that gastrodin and vanillin also have similar effects on the changes of microglia polarisations. Both gastrodin and vanillin display in vivo anti-neuroinflammation activity, but the working concentration of gastrodin (200 mg/kg) is far higher than vanillin (20 mg/kg). The possible reason is that in vivo rapid metabolism of gastrodin into 4-hydroxybenzyl alcohol (4-HBA) may reduce its application in vivo because 4-HBA exhibits limited biological activity (Liu et al., 2018). Therefore, maintaining the in vivo stability of gastrodin may be a critical issue to solve for future applications.
Gastrodin is the only candidate that may clear the accumulation of α-Syn. Strikingly, gastrodin is reported to exhibit anti-aging activity in Drosophila (He et al., 2021). Since aging is the major driver of PD development, it would be interesting examine whether this activity can be observed in other species. Compared with other compounds, gastrodin not only decreases three major PD features but also slows aging, providing vital support for its potential for PD.
These studies still have limitations because none of the current experimental models can mimic all clinical features of PD (Ke et al., 2021). The classical neurotoxin (MPTP, 6-OHDA, and rotenone)-induced PD animal models can rapidly generate the loss of dopaminergic neurons and neuroinflammation without disease progression. These in vivo models help define the benefits of drug candidates to reduce PD symptoms. Cell models are treated with neurotoxins to induce oxidative stress, mitochondria dysfunction, and cell death. LPS-induced BV-2 microglia activation is commonly used for investigating neuroinflammation. Undoubtedly, these in vitro models can mimic some PD features for drug screening and mechanistic study. However, a poor understanding of PD etiology and pathogenesis still limits us from identifying the suitability of drug candidates. In addition, these models fail to reflect the impact of aging. Thus, it may be necessary to evaluate the effects of drug candidates via using more diverse models such as transgenic animal models and neurons derived from induced pluripotent stem cells of human PD patients; alternatively, establishing more precise models to mimic PD development may help assess the effects of potential drug candidates.
There are many similarities between Tianma and Ganoderma. Both have complicated components, but have been used for centuries in Chinese medicine, which may support their safety as therapeutics. In vitro and in vivo studies, they can improve motor symptoms and various major PD features (Zhang et al., 2011; Ren et al., 2019). In toxicology experiments, it was found that a 28-day oral intake of Tianma water extract (8,065 mg/kg body weight) did not have adverse effects on mortality, body weight, organ weight, behavior, hematology, and clinical biochemistry (Lu et al., 2020). Notably, gastrodin was found in a clinical study (NCT00297245) to prevent cognitive decline after cardiac surgery with cardiopulmonary bypass. Results indicated that gastrodin is an effective and safe drug for preventing the neurocognitive decline in patients (Zhang et al., 2011). These studies support that Tianma and its active compounds are safe and have the potential to relieve neurological symptoms.
Conclusion
So far, the exact pathogenic mechanisms of PD are not well understood, thereby resulting a lack of effective drugs for preventing PD development. The bioactive components of Tianma have various positive effects on dopaminergic neuron death, α-synuclein aggregation, and neuroinflammation, further supporting the therapeutic potential of Tianma for PD. Of these, gastrodin shows greatest potential as it reduces the characteristics of PD and displays anti-aging activity in preclinical studies. Further studies on Tianma and its major compounds are warranted to fully understand the toxicological profiles, pharmacokinetics, and the underlying molecular mechanisms of these naturally occurring compounds and their potential for the prevention and treatment of PD.
Author contributions
CL and C-MC designed and wrote the manuscript. SQ, ZZ, HL, SL, H-JZ, HS, and YW reviewed and critically read the manuscript. All authors read and approved the submitted manuscript.
Funding
This work was supported by the grants from the Science and Technology Development Fund, Macau S. A. R (FDCT) (File no. 0029/2021/ITP, 0071/2021/A, and 007/2020/ALC), Shenzhen-Hong Kong-Macau S&T Program (Category C) (File no. SGDX2020110309420200), and the Research Fund of University of Macau (File no. SRG2021-00013-ICMS).
Conflict of interest
The authors declare that the research was conducted in the absence of any commercial or financial relationships that could be construed as a potential conflict of interest.
Publisher’s note
All claims expressed in this article are solely those of the authors and do not necessarily represent those of their affiliated organizations, or those of the publisher, the editors and the reviewers. Any product that may be evaluated in this article, or claim that may be made by its manufacturer, is not guaranteed or endorsed by the publisher.
References
Abushouk, A. I., Negida, A., Ahmed, H., and Abdel-Daim, M. M. (2017). Neuroprotective mechanisms of plant extracts against MPTP induced neurotoxicity: Future applications in Parkinson's disease. Biomed. Pharmacother. 85, 635–645. doi:10.1016/j.biopha.2016.11.074
Abuthawabeh, R., Abuirmeileh, A. N., and Alzoubi, K. H. (2020). The beneficial effect of vanillin on 6-hydroxydopamine rat model of Parkinson's disease. Restor. Neurol. Neurosci. 38 (5), 369–373. doi:10.3233/rnn-201028
An, H., Kim, I. S., Koppula, S., Kim, B. W., Park, P. J., Lim, B. O., et al. (2010). Protective effects of Gastrodia elata Blume on MPP+-induced cytotoxicity in human dopaminergic SH-SY5Y cells. J. Ethnopharmacol. 130 (2), 290–298. doi:10.1016/j.jep.2010.05.006
Antony, P. M. A., Diederich, N. J., Kruger, R., and Balling, R. (2013). The hallmarks of Parkinson's disease. Febs J. 280 (23), 5981–5993. doi:10.1111/febs.12335
Bastide, M. F., Meissner, W. G., Picconi, B., Fasano, S., Fernagut, P. O., Feyder, M., et al. (2015). Pathophysiology of L-dopa-induced motor and non-motor complications in Parkinson's disease. Prog. Neurobiol. 132, 96–168. doi:10.1016/j.pneurobio.2015.07.002
Bezerra-Filho, C. S. M., Barboza, J. N., Souza, M. T. S., Sabry, P., Ismail, N. S. M., and de Sousa, D. P. (2019). Therapeutic potential of vanillin and its main metabolites to regulate the inflammatory response and oxidative stress. Mini Rev. Med. Chem. 19 (20), 1681–1693. doi:10.2174/1389557519666190312164355
Bloem, B. R., Okun, M. S., and Klein, C. (2021). Parkinson's disease. Lancet 397 (10291), 2284–2303. doi:10.1016/s0140-6736(21)00218-x
Bose, A., and Beal, M. F. (2016). Mitochondrial dysfunction in Parkinson's disease. J. Neurochem. 139, 216–231. doi:10.1111/jnc.13731
Burbulla, L. F., Song, P., Mazzulli, J. R., Zampese, E., Wong, Y. C., Jeon, S., et al. (2017). Dopamine oxidation mediates mitochondrial and lysosomal dysfunction in Parkinson’s disease. Science 357 (6357), 1255–1261. doi:10.1126/science.aam9080
Catorce, M. N., and Gevorkian, G. (2016). LPS-Induced murine neuroinflammation model: Main features and suitability for pre-clinical assessment of nutraceuticals. Curr. Neuropharmacol. 14 (2), 155–164. doi:10.2174/1570159x14666151204122017
Chang, Y. Y., Tsai, Y. T., Lai, J. N., Yeh, C. H., and Lin, S. K. (2014). The traditional Chinese medicine prescription patterns for migraine patients in taiwan: a population-based study. J. Ethnopharmacol. 151 (3), 1209–1217. doi:10.1016/j.jep.2013.12.040
Chen, S., Chen, H., Du, Q., and Shen, J. (2020). Targeting myeloperoxidase (MPO) mediated oxidative stress and inflammation for reducing brain ischemia injury: potential application of natural compounds. Front. Physiol. 11, 433. doi:10.3389/fphys.2020.00433
Chen, Y., He, F. L., and Yang, H. (2014). Tianma Gouteng yin zhiliao pajinsenbing linchuang liaoxiao guancha clinical observation on the treatment of Parkinson's disease with Tianma Gouteng decoction. Prev. Treat. Cardio-Cerebral-Vascular Dis. 14 (3). doi:10.3969/j.issn.1009-816x.2014.03.31
Chong, C. M., Zhong, Z., Vong, C. T., Wang, S., Lu, J. J., Zhong, H. J., et al. (2021). The potentials of uncariae Ramulus Cum Uncis for the treatment of migraine: targeting CGRP in the trigeminovascular system. Curr. Neuropharmacol. 19 (7), 1090–1100. doi:10.2174/1570159X18666201029150937
Clarimon, J. (2020). Genetic-environmental factors finally assessed together in Parkinson's disease. J. Neurol. Neurosurg. Psychiatry 91 (10), 1030. doi:10.1136/jnnp-2020-324472
Cui, H., Kong, Y., and Zhang, H. (2012). Oxidative stress, mitochondrial dysfunction, and aging. J. Signal Transduct. 2012, 646354. doi:10.1155/2012/646354
Dai, J.-N., Zong, Y., Zhong, L.-M., Li, Y.-M., Zhang, W., Bian, L.-G., et al. (2011). Gastrodin inhibits expression of inducible NO synthase, cyclooxygenase-2 and proinflammatory cytokines in cultured LPS-stimulated microglia via MAPK pathways. PLoS ONE 6 (7), e21891. doi:10.1371/journal.pone.0021891
Dhanalakshmi, C., Janakiraman, U., Manivasagam, T., Justin Thenmozhi, A., Essa, M. M., Kalandar, A., et al. (2016). Vanillin attenuated behavioural impairments, neurochemical deficts, oxidative stress and apoptosis against rotenone induced rat model of Parkinson's disease. Neurochem. Res. 41 (8), 1899–1910. doi:10.1007/s11064-016-1901-5
Dhanalakshmi, C., Manivasagam, T., Nataraj, J., Justin Thenmozhi, A., and Essa, M. M. (2015). Neurosupportive role of vanillin, a natural phenolic compound, on rotenone induced neurotoxicity in SH-SY5Y neuroblastoma cells. Evid. Based. Complement. Altern. Med. 2015, 626028. doi:10.1155/2015/626028
Dickson, D. W., Braak, H., Duda, J. E., Duyckaerts, C., Gasser, T., Halliday, G. M., et al. (2009). Neuropathological assessment of Parkinson's disease: refining the diagnostic criteria. Lancet. Neurol. 8 (12), 1150–1157. doi:10.1016/s1474-4422(09)70238-8
Dionisio, P. A., Amaral, J. D., and Rodrigues, C. M. P. (2021). Oxidative stress and regulated cell death in Parkinson's disease. Ageing Res. Rev. 67, 101263. doi:10.1016/j.arr.2021.101263
Doo, A. R., Kim, S. N., Hahm, D. H., Yoo, H. H., Park, J. Y., Lee, H., et al. (2014). Gastrodia elata Blume alleviates L-DOPA-induced dyskinesia by normalizing FosB and ERK activation in a 6-OHDA-lesioned Parkinson's disease mouse model. BMC Complement. Altern. Med. 14, 107. doi:10.1186/1472-6882-14-107
Dorsey, E. R., and Bloem, B. R. (2018). The Parkinson pandemic-A call to action. JAMA Neurol. 75 (1), 9–10. doi:10.1001/jamaneurol.2017.3299
Duan, X. H., Li, Z. L., Yang, D. S., Zhang, F. L., Lin, Q., and Dai, R. (2013). Study on the chemical constituents of Gastrodia elata. Zhong Yao Cai 36 (10), 1608–1611.
Feigin, V. L., Abajobir, A. A., Abate, K. H., Abd-Allah, F., Abdulle, A. M., Abera, S. F., et al. (2017). Global, regional, and national burden of neurological disorders during 1990–2015: a systematic analysis for the global burden of disease study 2015. Lancet Neurology 16 (11), 877–897. doi:10.1016/s1474-4422(17)30299-5
Feno, S., Butera, G., Vecellio Reane, D., Rizzuto, R., and Raffaello, A. (2019). Crosstalk between calcium and ROS in pathophysiological conditions. Oxid. Med. Cell. Longev. 2019, 9324018. doi:10.1155/2019/9324018
Gay, N. H., Phopin, K., Suwanjang, W., Songtawee, N., Ruankham, W., Wongchitrat, P., et al. (2018). Neuroprotective effects of phenolic and carboxylic acids on oxidative stress-induced toxicity in human neuroblastoma SH-SY5Y cells. Neurochem. Res. 43 (3), 619–636. doi:10.1007/s11064-017-2463-x
Gegg, M. E., and Schapira, A. H. (2016). Mitochondrial dysfunction associated with glucocerebrosidase deficiency. Neurobiol. Dis. 90, 43–50. doi:10.1016/j.nbd.2015.09.006
Global Biodiversity Information Facility (2022). Species in the GBIF taxonomy. [Online]. Available: https://www.gbif.org/[Accessed (Accessed July 07, 2022)]].
Gonzalez-Rodriguez, P., Zampese, E., Stout, K. A., Guzman, J. N., Ilijic, E., Yang, B., et al. (2021). Disruption of mitochondrial complex I induces progressive parkinsonism. Nature 599 (7886), 650–656. doi:10.1038/s41586-021-04059-0
Gu, L. L., Fu, G. H., and Zhang, B. C. (2018). Tianma Gouteng Keli lianhe zuoxuanduoba zhiliao Pajinshenbing de liaoxiao ji dui xueqingyanxingyinzi he SOD, MDA, Cys-C de yingxiang[The efficacy of Tianma Gouteng granules combined with levodopa in the treatment of Parkinson's disease and the effect on serum inflammatory factors and SOD, MDA and Cys-C]. Chin. J. Integr. Med. Cardio-Cerebrovascular Dis. 16 (01), 95–98. doi:10.3969/i.sn.1672-1349.2018.01.026
Gui, Y. S. (2021). Tianma Gouteng yin lianhe pulakesuo zhiliao pajinsen de Liaoxiao guancha[observation on the curative effect of Tianma Gouteng decoction combined with pramipexole in the treatment of Parkinson's disease]. Mod. Med. Health Res. 5 (21), 56–59.
Ha, J. H., Lee, D. U., Lee, J. T., Kim, J. S., Yong, C. S., Kim, J. A., et al. (2000). 4-Hydroxybenzaldehyde from Gastrodia elata B1. is active in the antioxidation and GABAergic neuromodulation of the rat brain. J. Ethnopharmacol. 73 (1-2), 329–333. doi:10.1016/s0378-8741(00)00313-5
Haddadi, R., Poursina, M., Zeraati, F., and Nadi, F. (2018). Gastrodin microinjection suppresses 6-OHDA-induced motor impairments in parkinsonian rats: Insights into oxidative balance and microglial activation in SNc. Inflammopharmacology 26 (5), 1305–1316. doi:10.1007/s10787-018-0470-4
Harry, G. J. (2021). Microglia in neurodegenerative events-an initiator or a significant other? Int. J. Mol. Sci. 22 (11), 5818. doi:10.3390/ijms22115818
He, J., Li, X., Yang, S., Li, Y., Lin, X., Xiu, M., et al. (2021). Gastrodin extends the lifespan and protects against neurodegeneration in the Drosophila PINK1 model of Parkinson's disease. Food Funct. 12 (17), 7816–7824. doi:10.1039/d1fo00847a
Heese, K. (2020). Gastrodia elata blume (Tianma): hope for brain aging and dementia. Evid. Based. Complement. Altern. Med. 2020, 8870148. doi:10.1155/2020/8870148
Jang, Y. W., Lee, J. Y., and Kim, C. J. (2010). Anti-asthmatic activity of phenolic compounds from the roots of Gastrodia elata Bl. Int. Immunopharmacol. 10 (2), 147–154. doi:10.1016/j.intimp.2009.10.009
Jiang, G., Hu, Y., Liu, L., Cai, J., Peng, C., and Li, Q. (2014). Gastrodin protects against MPP(+)-induced oxidative stress by up regulates heme oxygenase-1 expression through p38 MAPK/Nrf2 pathway in human dopaminergic cells. Neurochem. Int. 75, 79–88. doi:10.1016/j.neuint.2014.06.003
Jiang, T., Chu, J., Chen, H. J. T., Cheng, H., Su, J. J., Wang, X. C., et al. (2020). Gastrodin inhibits H2O2-induced ferroptosis through its antioxidative effect in rat glioma cell line C6. Biol. Pharm. Bull. 43 (3), 480–487. doi:10.1248/bpb.b19-00824
Jiang, Y. N., Guo, Y. Z., Lu, D. H., Pan, M. H., Liu, H. Z., Jiao, G. L., et al. (2020). Tianma Gouteng granules decreases the susceptibility of Parkinson's disease by inhibiting ALOX15-mediated lipid peroxidation. J. Ethnopharmacol. 256, 112824. doi:10.1016/j.jep.2020.112824
Kalia, L. V., and Lang, A. E. (2015). Parkinson's disease. Lancet 386 (9996), 896–912. doi:10.1016/s0140-6736(14)61393-3
Ke, M., Chong, C. M., Zhu, Q., Zhang, K., Cai, C. Z., Lu, J. H., et al. (2021). Comprehensive perspectives on experimental models for Parkinson's disease. Aging Dis. 12 (1), 223–246. doi:10.14336/AD.2020.0331
Kim, B. W., Koppula, S., Kim, J. W., Lim, H. W., Hwang, J. W., Kim, I. S., et al. (2012). Modulation of LPS-stimulated neuroinflammation in BV-2 microglia by gastrodia elata: 4-hydroxybenzyl alcohol is the bioactive candidate. J. Ethnopharmacol. 139 (2), 549–557. doi:10.1016/j.jep.2011.11.048
Kim, I. S., Choi, D. K., and Jung, H. J. (2011). Neuroprotective effects of vanillyl alcohol in gastrodia elata blume through suppression of oxidative stress and anti-apoptotic activity in toxin-induced dopaminergic MN9D cells. Molecules 16 (7), 5349–5361. doi:10.3390/molecules16075349
Kim, M. E., Na, J. Y., Park, Y.-D., and Lee, J. S. (2019). Anti-neuroinflammatory effects of vanillin through the regulation of inflammatory factors and NF-κB signaling in LPS-stimulated microglia. Appl. Biochem. Biotechnol. 187 (3), 884–893. doi:10.1007/s12010-018-2857-5
Kumar, H., Kim, I. S., More, S. V., Kim, B. W., Bahk, Y. Y., and Choi, D. K. (2013). Gastrodin protects apoptotic dopaminergic neurons in a toxin-induced Parkinson's disease model. Evid. Based. Complement. Altern. Med. 2013, 514095. doi:10.1155/2013/514095
Lee, S., Oh, S. T., Jeong, H. J., Pak, S. C., Park, H. J., Kim, J., et al. (2017). MPTP-induced vulnerability of dopamine neurons in A53T alpha-synuclein overexpressed mice with the potential involvement of DJ-1 downregulation. Korean J. Physiol. Pharmacol. 21 (6), 625–632. doi:10.4196/kjpp.2017.21.6.625
Li, C., Chen, X., Zhang, N., Song, Y., and Mu, Y. (2012). Gastrodin inhibits neuroinflammation in rotenone-induced Parkinson's disease model rats. Neural Regen. Res. 7 (5), 325–331. doi:10.3969/j.issn.1673-5374.2012.05.001
Li, Y., Wang, Z. F., and Liu, W. (2020). Meiduoba lianhe Tianma Gouteng yin jianjian zhiliao laonian pajinsenbing de Linchuang guancha[clinical observation of madopa combined with Tianma Gouteng decoction in the treatment of senile Parkinson's disease]. Electron. J. Clin. Med. Literature 7 (14), 146. doi:10.16281/j.cnki.jocml.2020.14.132
Lin, L. C., Chen, Y. F., Tsai, T. R., and Tsai, T. H. (2007). Analysis of brain distribution and biliary excretion of a nutrient supplement, gastrodin, in rat. Anal. Chim. Acta 590 (2), 173–179. doi:10.1016/j.aca.2007.03.035
Lin, S. K., Tsai, Y. T., Lai, J. N., and Wu, C. T. (2015). Demographic and medication characteristics of traditional Chinese medicine users among dementia patients in taiwan: A nationwide database study. J. Ethnopharmacol. 161, 108–115. doi:10.1016/j.jep.2014.12.015
Lin, Y. E., Lin, C. H., Ho, E. P., Ke, Y. C., Petridi, S., Elliott, C. J., et al. (2021). Glial Nrf2 signaling mediates the neuroprotection exerted by Gastrodia elata Blume in Lrrk2-G2019S Parkinson's disease. Elife 10, e73753. doi:10.7554/eLife.73753
Liu, R. N. (2020). Effect of pramipexole combined with modified Tianma Gouteng decoction on serum hcy and blood uric acid levels for patients with early Parkinson's disease. J. Sichuan Traditional Chin. Med. 38 (05), 145–148.
Liu, R. N., Wang, Z., and Chen, Q. Q. (2020). Tianma Gouteng yin zhiliao pajinsenbing dui xuejiang Glu, GABA de Yingxiang[effect of Tianma Gouteng decoction on plasma Glu and GABA in the treatment of Parkinson's disease]. Chin. J. Mod. Drug Appl. 14 (14), 220–222. doi:10.14164/j.cnki.cn11-5581/r.2020.14.101
Liu, Y., Gao, J., Peng, M., Meng, H., Ma, H., Cai, P., et al. (2018). A review on central nervous system effects of gastrodin. Front. Pharmacol. 9, 24. doi:10.3389/fphar.2018.00024
Liu, Z. B. (2015). Tianma Gouteng yin jiajian lianhe meiduoba zhiliao 67 li pajinsen liaoxiao guancha[observation of curative effect of modified Tianma Gouteng decoction combined with madopar in the treatment of 67 cases of Parkinson's disease]. China's Naturop. 23 (7), 69–70. doi:10.19621/j.cnki.11-3555/r.2015.07.056
Lu, K. H., Ou, G. L., Chang, H. P., Chen, W. C., Liu, S. H., and Sheen, L. Y. (2020). Safety evaluation of water extract of Gastrodia elata Blume: Genotoxicity and 28-day oral toxicity studies. Regul. Toxicol. Pharmacol. 114, 104657. doi:10.1016/j.yrtph.2020.104657
Mani, S., Sevanan, M., Krishnamoorthy, A., and Sekar, S. (2021). A systematic review of molecular approaches that link mitochondrial dysfunction and neuroinflammation in Parkinson’s disease. Neurol. Sci. 42 (11), 4459–4469. doi:10.1007/s10072-021-05551-1
Matias, M., Silvestre, S., Falcao, A., and Alves, G. (2016). Gastrodia elata and epilepsy: Rationale and therapeutic potential. Phytomedicine 23 (12), 1511–1526. doi:10.1016/j.phymed.2016.09.001
Mazzulli, J. R., Zunke, F., Tsunemi, T., Toker, N. J., Jeon, S., Burbulla, L. F., et al. (2016). Activation of -glucocerebrosidase reduces pathological -synuclein and restores lysosomal function in Parkinson's patient midbrain neurons. J. Neurosci. 36 (29), 7693–7706. doi:10.1523/jneurosci.0628-16.2016
Mehra, S., Sahay, S., and Maji, S. K. (2019). α-Synuclein misfolding and aggregation: Implications in Parkinson's disease pathogenesis. Biochim. Biophys. Acta. Proteins Proteom. 1867 (10), 890–908. doi:10.1016/j.bbapap.2019.03.001
Meng, F., Guo, Z. G., Hu, Y. L., Mai, W. H., Zhang, Z. J., Zhang, B., et al. (2019). CD73-derived adenosine controls inflammation and neurodegeneration by modulating dopamine signalling. Brain 142, 700–718. doi:10.1093/brain/awy351
Migdalska-Richards, A., and Schapira, A. H. V. (2016). The relationship between glucocerebrosidase mutations and Parkinson disease. J. Neurochem. 139, 77–90. doi:10.1111/jnc.13385
Nguyen, M., Wong, Y. C., Ysselstein, D., Severino, A., and Krainc, D. (2019). Synaptic, mitochondrial, and lysosomal dysfunction in Parkinson’s disease. Trends Neurosci. 42 (2), 140–149. doi:10.1016/j.tins.2018.11.001
Perier, C., Bové, J., Dehay, B., Jackson-Lewis, V., Rabinovitch, P. S., Przedborski, S., et al. (2010). Apoptosis-inducing factor deficiency sensitizes dopaminergic neurons to parkinsonian neurotoxins. Ann. Neurol. 68, 184–192. doi:10.1002/ana.22034
Poewe, W., Seppi, K., Tanner, C. M., Halliday, G. M., Brundin, P., Volkmann, J., et al. (2017). Parkinson disease. Nat. Rev. Dis. Prim. 3, 17013. doi:10.1038/nrdp.2017.13
Prasad, E. M., and Hung, S. Y. (2021). Current therapies in clinical trials of Parkinson's disease: A 2021 update. Pharm. (Basel) 14 (8), 717. doi:10.3390/ph14080717
Pringsheim, T., Jette, N., Frolkis, A., and Steeves, T. D. L. (2014). The prevalence of Parkinson's disease: a systematic review and meta-analysis. Mov. Disord. 29 (13), 1583–1590. doi:10.1002/mds.25945
Ren, Z. L., Wang, C. D., Wang, T., Ding, H., Zhou, M., Yang, N., et al. (2019). Ganoderma lucidum extract ameliorates MPTP-induced parkinsonism and protects dopaminergic neurons from oxidative stress via regulating mitochondrial function, autophagy, and apoptosis. Acta Pharmacol. Sin. 40 (4), 441–450. doi:10.1038/s41401-018-0077-8
Salau, V. F., Erukainure, O. L., Ibeji, C. U., Olasehinde, T. A., Koorbanally, N. A., and Islam, M. S. (2020). Vanillin and vanillic acid modulate antioxidant defense system via amelioration of metabolic complications linked to Fe(2+)-induced brain tissues damage. Metab. Brain Dis. 35 (5), 727–738. doi:10.1007/s11011-020-00545-y
Sharma, N., Khurana, N., Muthuraman, A., and Utreja, P. (2021). Pharmacological evaluation of vanillic acid in rotenone-induced Parkinson's disease rat model. Eur. J. Pharmacol. 903, 174112. doi:10.1016/j.ejphar.2021.174112
Shi, J., Tian, J. Z., Li, T., Qin, B., Fan, D. S., Ni, J. N. A., et al. (2017). Efficacy and safety of SQJZ herbal mixtures on nonmotor symptoms in Parkinson disease patients Protocol for a randomized, double-blind, placebo-controlled trial. Medicine 96 (50), e8824. doi:10.1097/md.0000000000008824
Shuchang, H., Qiao, N., Piye, N., Mingwei, H., Xiaoshu, S., Feng, S., et al. (2008). Protective effects of gastrodia elata on aluminium-chloride-induced learning impairments and alterations of amino acid neurotransmitter release in adult rats. Restor. Neurol. Neurosci. 26 (6), 467–473.
Wang, T., Shi, C., Luo, H., Zheng, H., Fan, L., Tang, M., et al. (2021). Neuroinflammation in Parkinson's disease: Triggers, mechanisms, and immunotherapies. Neuroscientist 28 (4), 364–381. doi:10.1177/1073858421991066
Wang, X. L., Xing, G. H., Hong, B., Li, X. M., Zou, Y., Zhang, X. J., et al. (2014). Gastrodin prevents motor deficits and oxidative stress in the MPTP mouse model of Parkinson's disease: Involvement of ERK1/2-nrf2 signaling pathway. Life Sci. 114 (2), 77–85. doi:10.1016/j.lfs.2014.08.004
Wang, Z., Lai, T. B., Wang, X. X., and Li, X. F. (2019). Clinical study of Tianma Gouteng granules combined with madopar in the treatment of Parkinson disease. China Med. Her. 16 (2), 6371–6466.
Windels, F., Carcenac, C., Poupard, A., and Savasta, M. (2005). Pallidal origin of GABA release within the substantia nigra pars reticulata during high-frequency stimulation of the subthalamic nucleus. J. Neurosci. 25 (20), 5079–5086. doi:10.1523/JNEUROSCI.0360-05.2005
Xiang, B., Xiao, C., Shen, T., and Li, X. (2018). Anti-inflammatory effects of anisalcohol on lipopolysaccharide-stimulated BV2 microglia via selective modulation of microglia polarization and down-regulation of NF-κB p65 and JNK activation. Mol. Immunol. 95, 39–46. doi:10.1016/j.molimm.2018.01.011
Xie, H. R., Hu, L. S., and Li, G. Y. (2010). SH-SY5Y human neuroblastoma cell line: in vitro cell model of dopaminergic neurons in Parkinson's disease. Chin. Med. J. 123 (8), 1086–1092.
Yan, J. H., Liang, Y., Liu, C., Ouyang, S., and Tang, H. Y. (2014). Clinical observation of decoction of gastrodia and uncariacombined with madopar in the treatment of Parkinson. Asia-Pacific Tradit. Med. 10 (4), 111–113.
Yan, J., Yang, Z., Zhao, N., Li, Z., and Cao, X. (2019). Gastrodin protects dopaminergic neurons via insulin-like pathway in a Parkinson's disease model. BMC Neurosci. 20 (1), 31. doi:10.1186/s12868-019-0512-x
Yan, X., Liu, D. F., Zhang, X. Y., Liu, D., Xu, S. Y., Chen, G. X., et al. (2017). Vanillin protects dopaminergic neurons against inflammation-mediated cell death by inhibiting ERK1/2, P38 and the NF-κB signaling pathway. Int. J. Mol. Sci. 18 (2), 389. doi:10.3390/ijms18020389
Yao, Y. Y., Bian, L. G., Yang, P., Sui, Y., Li, R., Chen, Y. L., et al. (2019). Gastrodin attenuates proliferation and inflammatory responses in activated microglia through Wnt/β-catenin signaling pathway. Brain Res. 1717, 190–203. doi:10.1016/j.brainres.2019.04.025
Zhan, H. D., Zhou, H. Y., Sui, Y. P., Du, X. L., Wang, W. H., Dai, L., et al. (2016). The rhizome of Gastrodia elata Blume - an ethnopharmacological review. J. Ethnopharmacol. 189, 361–385. doi:10.1016/j.jep.2016.06.057
Zhang, R. P., Xu, S. L., Cai, Y. N., Zhou, M., Zuo, X. H., and Chan, P. (2011). Ganoderma lucidum protects dopaminergic neuron degeneration through inhibition of microglial activation. Evid. Based. Complement. Altern. Med. 2011, 156810. doi:10.1093/ecam/nep075
Zhang, Z., Ma, P., Xu, Y., Zhan, M., Zhang, Y., Yao, S., et al. (2011). Preventive effect of gastrodin on cognitive decline after cardiac surgery with cardiopulmonary bypass: a double-blind, randomized controlled study. J. Huazhong Univ. Sci. Technol. Med. Sci. 31 (1), 120–127. doi:10.1007/s11596-011-0162-4
Zhao, Y. M., and Hu, Q. (2017). Clinical observation of Tianma Gouteng Granules combined with levodopa in treatment of Parkinson’s disease. Drugs & Clin. 32 (3), 403–406.
Zhong, Z., Vong, C. T., Chen, F., Tan, H., Zhang, C., Wang, N., et al. (2022). Immunomodulatory potential of natural products from herbal medicines as immune checkpoints inhibitors: Helping to fight against cancer via multiple targets. Med. Res. Rev. 42 (3), 1246–1279. doi:10.1002/med.21876
Zhu, H., Liu, C., Hou, J., Long, H., Wang, B., Guo, D., et al. (2019). Gastrodia elata blume polysaccharides: A review of their acquisition, analysis, modification, and pharmacological activities. Molecules 24 (13), 2436. doi:10.3390/molecules24132436
Keywords: Parkisnon’s disease, tianma (Gastrodia elata blume), dopaminergic neurons, neuroinflmamation, synuclein
Citation: Lu C, Qu S, Zhong Z, Luo H, Lei SS, Zhong H-J, Su H, Wang Y and Chong C-M (2022) The effects of bioactive components from the rhizome of gastrodia elata blume (Tianma) on the characteristics of Parkinson’s disease. Front. Pharmacol. 13:963327. doi: 10.3389/fphar.2022.963327
Received: 07 June 2022; Accepted: 07 November 2022;
Published: 30 November 2022.
Edited by:
Kah Hui Wong, University of Malaya, MalaysiaReviewed by:
Rohit Sharma, Banaras Hindu University, IndiaSaeideh Momtaz, Academic Center for Education, Iran
Copyright © 2022 Lu, Qu, Zhong, Luo, Lei, Zhong, Su, Wang and Chong. This is an open-access article distributed under the terms of the Creative Commons Attribution License (CC BY). The use, distribution or reproduction in other forums is permitted, provided the original author(s) and the copyright owner(s) are credited and that the original publication in this journal is cited, in accordance with accepted academic practice. No use, distribution or reproduction is permitted which does not comply with these terms.
*Correspondence: Cheong-Meng Chong, Y21jaG9uZ0B1bS5lZHUubW8=; Yitao Wang, eXR3YW5nQHVtLmVkdS5tbw==