- 1Department of Rehabilitation Medicine, State Key Laboratory of Biotherapy and Cancer Center, National Clinical Research Center for Geriatrics, West China Hospital, Sichuan University, Chengdu, China
- 2West China School of Pharmacy, Sichuan University, Chengdu, China
- 3Key Laboratory of Rehabilitation Medicine in Sichuan Province/Rehabilitation Medicine Research Institute, Chengdu, China
Idiopathic pulmonary fibrosis (IPF) is a fatal interstitial lung disease. Recent studies have identified the key role of crosstalk between dysregulated epithelial cells, mesenchymal, immune, and endothelial cells in IPF. In addition, genetic mutations and environmental factors (e.g., smoking) have also been associated with the development of IPF. With the recent development of sequencing technology, epigenetics, as an intermediate link between gene expression and environmental impacts, has also been reported to be implicated in pulmonary fibrosis. Although the etiology of IPF is unknown, many novel therapeutic targets and agents have emerged from clinical trials for IPF treatment in the past years, and the successful launch of pirfenidone and nintedanib has demonstrated the promising future of anti-IPF therapy. Therefore, we aimed to gain an in-depth understanding of the underlying molecular mechanisms and pathogenic factors of IPF, which would be helpful for the diagnosis of IPF, the development of anti-fibrotic drugs, and improving the prognosis of patients with IPF. In this study, we summarized the pathogenic mechanism, therapeutic targets and clinical trials from the perspective of multiple cell types, gene mutations, epigenetic and environmental factors.
1 Introduction
Idiopathic pulmonary fibrosis (IPF) is a progressive, life-threatening, interstitial lung disease of unknown pathogenesis. IPF has a familial and sporadic onset with a poor prognosis, and death usually occurs within 2–5 years of diagnosis due to secondary respiratory failure (Noble et al., 2012). CT imaging of IPF usually shows a typical usual interstitial pneumonia (UIP) pattern, characterized by irregular reticular opacities with obligatory honeycombing, associated with traction bronchiectasis. IPF also exhibits histological features of UIP/IPF pattern characterized by architecture remodeling due to dense fibrosis with frequent honeycombing, patchy lung involvement by fibrosis, subpleural and/or paraseptal distribution, fibroblast foci at the edge of dense scars (Spagnolo et al., 2018; Baratella et al., 2021). Although the etiology of IPF is unknown, various imbalances centered on alveolar epithelial cell/fibroblast apoptosis imbalance has been shown to play an important role in the pathogenesis of IPF (Wang et al., 2021). Therefore, it is necessary to understand the respective roles and interactions of alveolar epithelial cells, fibroblasts, immune cells, and extracellular matrix (ECM) in the complex crosstalk. In addition, we discuss potential factors affecting these pro-fibrotic cells, including genetic mutations, epigenetic alterations, environmental factors and aging, with the aim of finding the underlying cause of the disease. The currently approved IPF treatment drugs are pirfenidone and nintedanib, both of which can slow the progression of IPF, but there is no evidence that they can reverse IPF-related pulmonary fibrosis (Chu et al., 2020). Lung transplantation is the only option for patients with end-stage IPF (Lederer and Martinez, 2018; Villavicencio et al., 2018). Therefore, there is a necessity to develop novel agents for the treatment of IPF. This article reviews the roles of various cells and extracellular matrix associated with pathogenic mechanisms, potential pathogenic factors, and the latest information on clinical trials of IPF.
2 The Pathological Process of Idiopathic Pulmonary Fibrosis
The current paradigm suggests that IPF occurs as a result of epithelial injury and dysregulation of the epithelial/mesenchymal crosstalk, which continuously activates multiple interconnected downstream profibrotic pathways, ultimately leading to an abnormal repair response and decreased lung function (Spagnolo et al., 2021).
In the next sections, we provide a brief overview of the pathogenic mechanisms of IPF (Figure 1). Damage to alveolar epithelial cells in response to external stimuli leads to disruption of the basement membrane and release of large amounts of cytokines. Many cytokines (interleukins, chemokines, and growth factors) are released by alveolar epithelial cells (AEC) to recruit and activate inflammatory cells and fibroblasts (Phan et al., 2021; She et al., 2021). Coagulation factors (tissue factor (TF), plasminogen activation inhibitors (PAI 1 and PAI 2), fibrinolysis inhibitors and protein C inhibitors) induce a microenvironment that promotes coagulation and inhibits fiber degradation (King et al., 2011; Selman and Pardo, 2014; Betensley et al., 2016). In addition to microenvironmental changes in the lung, cellular processes (apoptosis, senescence, epithelial-mesenchymal transition, endothelial-mesenchymal transition, and epithelial cell migration) have been shown to play a key role in IPF-associated tissue remodeling (Phan et al., 2021). In addition, fibroblasts differentiate into myofibroblasts and secrete large amounts of extracellular matrix, which eventually leads to the formation of fibroblast foci and the development of pulmonary fibrosis (Yagihashi et al., 2016). In conclusion, following injury to the AEC, the lung microenvironment and cellular processes are altered, which initiates abnormal repair and ultimately leads to the development of IPF and pulmonary function deterioration.
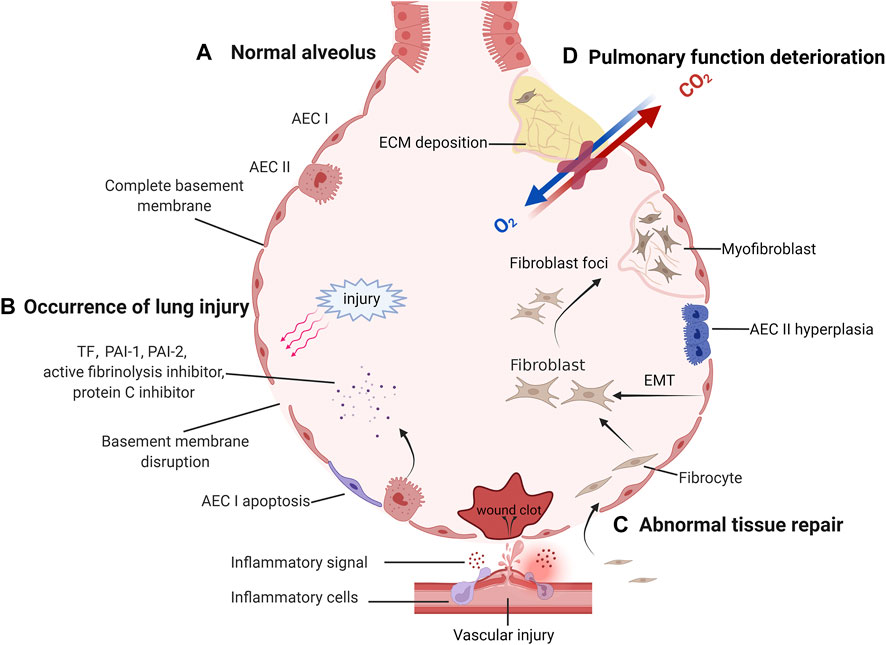
FIGURE 1. The pathological process of idiopathic pulmonary fibrosis. (A) After normal alveoli are damaged and abnormally repaired, irreversible lung function deterioration occurs. Normal alveolus has a complete basement membrane and gas exchange function. (B) When the basement membrane continuity is disrupted by external injury, the damaged capillaries and activated AECs release inflammatory signals and coagulation factors, forming a local inflammatory microenvironment. (C) If the damage persists, abnormal repair will be initiated. Lung mesenchymal progenitors, fibrocytes recruited to the lung, and endothelial cells undergoing EMT can aggregate to form fibroblasts foci and differentiate into matrix-secreting myofibroblasts. To compensate for the local blood supply to the alveoli, new blood vessels are gradually formed. (D) As fibroblast foci increased, more ECM was deposited and cross-linked together, triggering a deterioration in lung compliance and gas exchange function.
3 Important Cells and Extracellular Matrix Involved in the Pathogenesis of IPF
There are AECs, alveolar capillary endothelial cells, immune cells, fibroblasts, and mesenchymal progenitor cells near the alveoli. These cells maintain the homeostasis of the alveolar environment under normal physiological conditions. However, in the pathophysiological process of IPF, the intercellular crosstalk leads to reprogramming of cell phenotype. AECs, endothelial cells, and immune cells work together through multiple signaling pathways to regulate fibroblast phenotype. Then, fibroblasts recruitment, proliferation, differentiation, and secretion of extracellular matrix directly lead to fibrosis and exhaustion of pulmonary function. therefore, it is necessary to clarify the roles of various cells and extracellular matrix in the development of IPF.
3.1 Alveolar Epithelial Cells and Endothelial Cells
Alveolar epithelial cells/endothelial cells participate in IPF via various ways, including unfolded protein response (UPR), Epithelial-mesenchymal transition (EMT), coagulation cascade, angiogenesis, and the secretion of a variety of signaling factors (such as TGF-β) (Margaritopoulos et al., 2017; Upagupta et al., 2018; Chanda et al., 2019; Hill et al., 2019; Salton et al., 2019; Selman and Pardo, 2020). In this section, we show how AECs are involved in pulmonary fibrosis (Figure 2A).
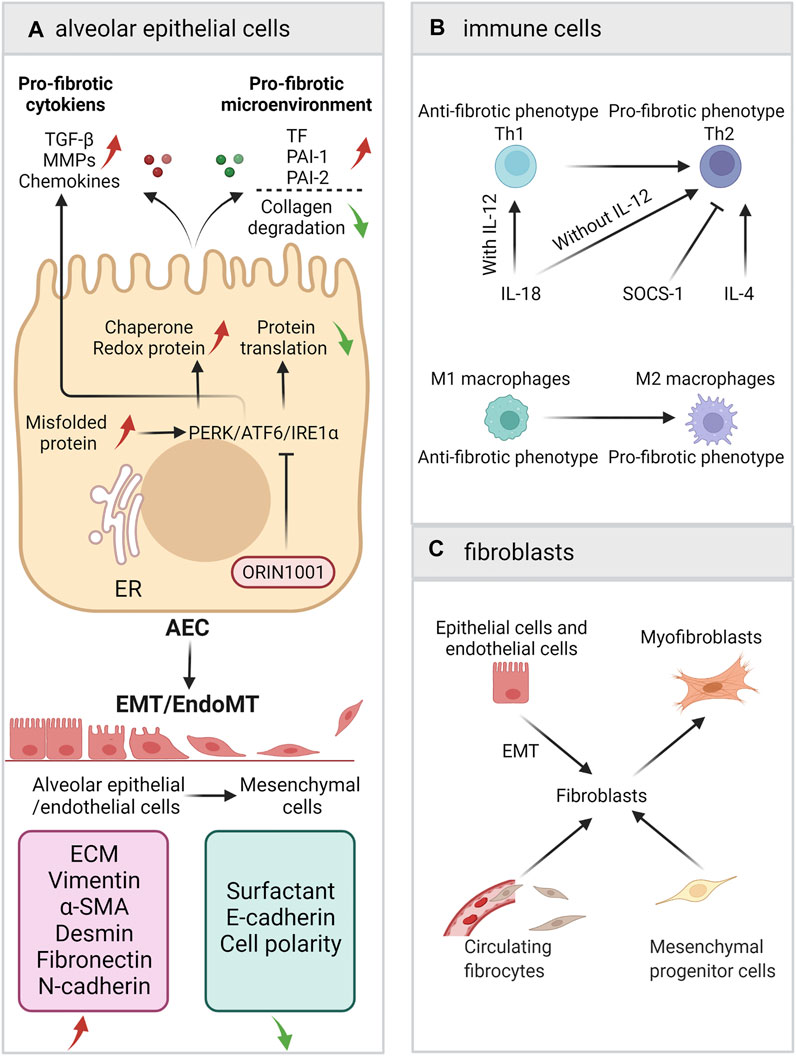
FIGURE 2. A schematic view of the roles of AECs, immune cells and fibroblasts. (A) Alveolar epithelial cells/endothelial cells participate in IPF via ER stress/UPR, EMT, coagulation cascade, and the secretion of a variety of signaling factors. (B) The balance of Th1/Th2 and M1/M2. (C) The sources of fibroblasts and the fibroblast-to-myofibroblasts differentiation.
3.1.1 Unfolded Protein Response
The UPR of AEC is one of the underlying mechanisms for the development of IPF. As an important site for cellular protein synthesis, the ER (endoplasmic reticulum) must maintain relative homeostasis. Under pathological conditions [e.g., viral infection, smoking, asbestos exposure, ROS, hypoxia, senescence, mechanical stretching, proteasome dysfunction, and autophagy disorders (Burman et al., 2018)], misfolded proteins accumulate in the ER of AECs, resulting in ER stress. To restore protein metabolism homeostasis, PERK/ATF6/IRE1α (Protein kinase R-like endoplasmic reticulum kinase/activating transcription factor 6/inositol-requiring enzyme 1α) receptors will be activated, followed by the activation of the UPR to reduce overall protein translation and increase the expression of chaperone and redox proteins. The UPR/ER stress regulates AEC apoptosis and EMT (Burman et al., 2018). However, UPR can also participate in pulmonary fibrosis by increasing the expression of profibrotic mediators, such as TGF-β1, platelet-derived growth factor (PDGF), CXCL12, and CCL2 (Zolak and de Andrade, 2012; Wolters et al., 2014). Because the upstream targets of the UPR/ER stress are of great significance for the maintenance of cell survival and organ development, pathways targeting upstream molecules may cause severe cytotoxicity. Therefore, targeting downstream molecular pathways or chaperones seems to be a better choice. (Burman et al., 2018). Currently, ORIN1001, which targets inositol-requiring transmembrane kinase endoribonuclease-1α (IRE1α), is undergoing a phase I clinical trial for the treatment of IPF(NCT04643769). The crosstalk between autophagy and endoplasmic reticulum stress is important in pulmonary fibrosis (Ghavami et al., 2018; Maciel et al., 2018). Therapeutic approaches targeting autophagy have been shown to have great potential in cancer and aging (Xia et al., 2021; Cassidy and Narita, 2022). Interestingly, studies have found that autophagy also plays an important role in IPF. TGF-β can inhibit autophagy in fibroblasts, while rapamycin and Tubastatin can promote autophagy and inhibit bleomycin-mediated pulmonary fibrosis (Patel et al., 2012; Saito et al., 2017).
3.1.2 Epithelial-Mesenchymal Transition
EMT refers to a process in which AECs gradually lose their epithelial characteristics under specific stimuli and conditions, with the subsequent appearance of interstitial cells characteristics. During this process, AECs are reprogrammed with changes in secretory phenotype, cytoskeletal proteins, intercellular junctions, and cell polarity (Salton et al., 2019). The conditions that induce EMT in AECs include alveolar epithelial cell injury and abnormal apoptosis, the UPR/ER stress, mechanical stress, smoking, and infection (Salton et al., 2019). Under the repeated stimuli, AECs are severely damaged and cannot complete repair processes and re-epithelialization normally, resulting in reprogramming and manifesting as abnormal repair processes (Salton et al., 2020). TGF-β, epidermal growth factor (EGF), fibroblast growth factor (FGF), IL-1, connective tissue growth factor factors (CTGF), insulin-like growth factor-2 (IGF-2), nuclear factor-kb (NF-kB) and Wnt can activate the transcription factors SNAIL, TWIST1 and ZEB through a variety of cytokine pathways to directly initiate EMT (Salton et al., 2019). FGFR1-3 inhibitor SKLB-YTH-60 ameliorates EMT and fibrosis in bleomycin-induced lung fibrosis mouse models (Liu et al., 2021).
However, in recent years, the central role of EMT in the pathogenesis of IPF has been questioned. The localization of type 2 epithelial cells by markers showed that the conversion of epithelial cells into myofibroblasts was incomplete (Gjorevski et al., 2012) and that the marker protein of myofibroblasts, α-SMA, and EMT epithelial cells could not be colocalized, indicating that epithelial cells may not completely convert to fibroblasts (Rock et al., 2011); additionally, mesenchymal AECs have a very limited ability to secrete ECM (Yao et al., 2019). Studies have shown that EMT indirectly promotes the formation of a profibrotic microenvironment through the dysregulation of paracrine signals between epithelial cells and mesenchymal cells (Hill et al., 2019; Yao et al., 2019). Therefore, although there is a large amount of evidence that EMT does exist in IPF, due to the latest lineage tracing results, EMT is more regarded as an indirect process. Nevertheless, the profibrotic microenvironment that regulates the occurrence of EMT is still quite promising in the inhibition of IPF (Hill et al., 2019).
3.1.3 Coagulation Cascade and Angiogenesis
Under pathological conditions, the coagulation cascade and angiogenesis are important driving forces for the promotion of pulmonary fibrosis. Because tissue factor can activate PAI 1, PAI 2, fibrinolysis inhibitors and protein C inhibitors through the coagulation cascade, resulting in a local pro-coagulation microenvironment, it inhibits the degradation of the ECM in this microenvironment and promotes the differentiation of fibrocytes (King et al., 2011; Selman and Pardo, 2014; Betensley et al., 2016). IPF patients have a relatively lower number of endothelial progenitor cells, which may potentially contribute to suppressed repair of the damaged pulmonary endothelium and thereby may drive the sequence of events in profibrogenic direction (Malli et al., 2013). Besides, it is reported that compensatory pro-angiogenic VEGF increases, which is a pro-fibrotic mediator (Malli et al., 2013).
3.1.4 Pro-Fibrotic Secretory Phenotype of AEC
In addition to the above pathways, AECs also participate in pulmonary fibrosis through the secretion of a variety of mediators, including growth factor (TGF-β, PDGF/CTGF/IGF-I/insulin-like growth factor binding proteins 3 and 5), matrix metalloproteinases (MMP1/MMP2/MMP7), chemokines (CCL17/CCL2/CXCL12), pigment epithelium-derived factor, autotaxin, sphingosine-1-phosphate, neuregulin (NRG) 1α, growth and differentiation factor 15 (GDF15), transmembrane protease serine 4 (TMPRSS4), tumor necrosis factor-alpha (TNF-α), osteopontin, and angiotensinogen. Mareike Lehmann et al. summarized the possible roles of these cytokines in detail in the occurrence and development of IPF (Selman and Pardo, 2020).
3.2 Immune Cells
A large number of studies have demonstrated that immune cells play a role in IPF. However, anti-inflammatory therapies, i.e., TNF-α monoclonal antibodies (Utz et al., 2003; Raghu et al., 2008) and glucocorticoids (Raghu et al., 2012), fail to achieve primary outcomes in clinical trials. Neither of these promising conventional treatments prevent a decline in forced vital capacity (FVC) or the progression of pulmonary fibrosis or benefit survival. In addition, a phase 3, randomized, double-blind, placebo-controlled study including 826 participants (NCT00075998) showed that the subcutaneous injection of IFNγ-1b neither improved the FVC of patients nor prolonged the survival time of patients. These clinical trials are important milestones for clinical treatment strategies for IPF, causing a shift from traditional anti-inflammatory treatment to simultaneous interventions for multiple pathogenic links. Past treatments generally treated inflammation as a whole while ignoring the dual profibrotic/antifibrotic roles of different inflammatory factors and different inflammatory cells. Perhaps by further distinguishing the roles of different inflammatory factors and pathways involved in different inflammatory cells, more precise therapeutic targets can be found. Therefore, inflammation, as one of the potential pathogeneses of IPF, remains an important focus of research.
With the deepening of research in the field of immunization, the role of immune factors in IPF has received increasing attention. Although the diagnostic criteria for IPF require the exclusion of autoimmune diseases as the underlying pathogenesis, many IPF patients still have unexplained elevated autoantibodies, and some autoantibodies are associated with acute exacerbations of IPF (AE-IPF) (Ogushi et al., 2001; Kurosu et al., 2008; Taillé et al., 2011; Kahloon et al., 2013). To inhibit the function of B cells, Ianalumab (B-cell activating factor receptor mAb) and Rituximab (CD20 mAb) have entered phase II clinical trials.
Heukels conducted a detailed review on the role of each immune cell in IPF and the correlation of each cell type with the development of fibrosis (Heukels et al., 2019). Nevertheless, the mechanism of action of some immune cells in the pathogenesis of IPF is still not very clear. The following subsections introduce the roles of the Th1/Th2 balance and the M1/M2 balance in IPF, although these paradigms simplify the role of immune cells (Figure 2B).
3.2.1 Th1/Th2 Balance
Th1 (helper T cell type 1) cells are helper T cells produced by CD4+ cells under IFN-γ/IL-12 induction. CXC chemokine receptor 3 expressed by Th1 cells can recognize interferon-inducible T cell a chemoattractant (I-TAC), interferon g-inducible protein of 10 kD (IP-10), and monokine induced by interferon gamma (Mig) (Sumida et al., 2008). The main function of Th1 is to secrete IFN-γ. IFN-γ is considered to be antifibrotic and can reduce the production of ECM (Bouros et al., 2006; Smaldone, 2018).
Th2 (helper T cell type 2) are helper T cells produced by CD4+ cells under IL-4 induction. Th2 cells that express CC chemokine receptor 4 can recognize thymus- and activation-regulated chemokines (TARCs) and macrophage-derived chemokines (MDCs) (Sumida et al., 2008). The main function of Th2 cells is to secrete IL-4/IL-5/IL-13; these interleukins are considered to promote fibrosis (Romagnani, 2000).
Th2 polarization has been observed in IPF. In the BALF of IPF patients and in systemic circulation, the levels of Th1 cells and their secretion of IFN-γ are relatively low, and the levels of Th2 cells and their secretion of IL-4/IL-5/IL-13 are relatively high (Hams et al., 2014). In addition, in a bleomycin-induced mouse model, an increase in IFN-γ levels and a reduction in pulmonary fibrosis were observed after the administration of IL-12, an inducer of Th1 cells; in contrast, an increase in fibroblast proliferation and fibrosis was found with the use of IL-4, an inducer of Th2 cells (Hams et al., 2014; Passalacqua et al., 2017).
The inhibition of Th2 polarization is a possible direction for the treatment of IPF. Many factors can cause Th2 polarization. Galectin-1 and prostaglandin E2 promote Th2 polarization by inducing Th1 apoptosis and reducing the synthesis of Th1 inducers (Kaliński et al., 1997; Cedeno-Laurent and Dimitroff, 2012; Corapi et al., 2018), and suppressor of cytokine signaling-1 (SOCS-1) inhibits the expression of Th2 inducers to prevent excessive Th2 cell accumulation (Bao et al., 2014). Among the interleukins that regulate Th1 and Th2 differentiation, IL-18 is notable; the polarization direction mediated by IL-18 is regulated by IL-12. Under the synergistic effect of IL-12, IL-18 induces Th1 cells to produce IFN-γ, IL-12, and GM-CSF and upregulate the expression of IL-2Rα to promote an inflammatory response. In contrast, in the absence of IL-12, IL-18 induces the production of Th2-related cytokines, such as IL-13/IL-4, by T cells, NK cells, basophil cells, and mast cells and promotes the differentiation of Th2 cells (Wawrocki et al., 2016). IL-4 is another important interleukin that promotes Th2 differentiation and is an important marker of type 2 immunity. Studies have shown that significant polymorphisms are found in the IL-4 promoter of IPF patients and that these polymorphisms are strongly associated with IPF (Vasakova et al., 2006). In addition to cytokine therapy, a study used the serum IFN-γ/IL-4 ratio to represent the Th1/Th2 balance to predict the development of IPF and found that the IFN-γ/IL-4 ratio was associated with symptoms, imaging changes, FEV1 (forced expiratory volume in one second), FVC (Forced vital capacity), TLC (total lung capacity), and 6-min walking distance in IPF patients and can predict IPF progression (Peng et al., 2013).
3.2.2 M1/M2 Balance
In addition to the Th1/Th2 balance, the balance of macrophage subpopulations also plays an important role in the pathogenesis of IPF. Because cytokines secreted by Th1/Th2 cells greatly affect the differentiation of M1/M2 macrophages, Th1/Th2 cells and M1/M2 macrophages interact with each other to jointly shape the type 1 and type 2 immune microenvironments (Wang et al., 2021).
The main function of M1 (type I macrophages) is to respond to lipopolysaccharide (LPS), IL-1, and IL-6. They can secrete type 1 immune factors such as IL-12, induced nitric oxide synthase (iNOS), TNF-α, IL-1β, IL-23, IL-6, and CXCL10 (Liu G. et al., 2019), thereby playing a role in the early stage of inflammation. The main function of M2 (type II macrophages) is to respond to type 2 immune factors (IL-4, IL-10, and IL-13), glucocorticoids, and immune complexes. They can secrete cytokines that promote tissue repair, limit inflammation (Martinez et al., 2008; Zhang et al., 2018; Liu G. et al., 2019), participate in immune regulation, suppress immune responses, and remodel tissue. M2 macrophages can also be divided into M2a, M2b, and M2c macrophages based on induction conditions and functions (Martinez et al., 2008).
M2 polarization is one of the important links in the occurrence and development of pulmonary fibrosis. The M2-mediated type 2 immune response is an important component of pulmonary fibrosis. M2 macrophages provide an important microenvironment for pulmonary fibrosis by secreting profibrotic substances such as CCL18, IL-10, TIMP1 (tissue inhibitors of metalloproteinases 1), TGF-β, FGF, PDGFα, IGF1, and VEGF (Prasse et al., 2006; Heukels et al., 2019; Wang et al., 2021). Macrophages can not only secrete chemokines to recruit other cells (like CCL18), but also are attracted by chemokines themselves. CCL2 and CCL3 are important signaling molecules involved in monocyte/macrophage recruitment and help macrophages migrate to the lungs. Therefore, the antagonism of CCL2 and CCL3 may have an antifibrotic effect (Iyonaga et al., 1994; Suga et al., 1999; Deshmane et al., 2009).
3.3 Fibroblasts and Myofibroblasts
Fibrocytes are monocyte progenitor cells with differentiation potential derived from bone marrow; these cells can differentiate into adipocyte cells, chondrocytes, osteoblasts, and fibroblasts under the action of different tissue environments and humoral factors. In the in vitro environment, CD45+/CD34+ primary bone marrow fibrocytes were stimulated by the ECM of IPF patients; then the hematopoietic surface antigens CD45 and CD34 rapidly disappeared, and mesenchymal markers, i.e., α-SMA, rapidly increased. The fibrocytes transformed into fibroblasts with a contractility like that of smooth muscle cells and a strong ability to synthesize ECM (Mori et al., 2005; Lama and Phan, 2006; Kage and Borok, 2012; Chong et al., 2019). In addition to the ECM of IPF patients, TGF-β, endothelin, CTGF, interleukins (IL-3 and IL-4), serum response factors (Shi-Wen et al., 2004) and microRNA (miRNA-21, miRNA-22, miRNA-29, miRNA-125b, miRNA-126, miRNA-130a and miRNA-132, miRNA-142a) (Pandit et al., 2011; Wang et al., 2012; Cushing et al., 2015; Kuse et al., 2020) have been shown to be associated with the differentiation of fibrocytes into fibroblasts. After getting activated, fibroblasts proliferate, differentiate, resist apoptosis and can directly lead to IPF through the secretion of profibrotic factors and the remodeling of the ECM (Selman et al., 2000). Under normal circumstances, the secretion and degradation of the ECM by fibroblasts are in a dynamic balance. However, when exposed to inflammation and environmental stress, fibroblasts are reprogrammed and continue to be active and resist apoptosis (Filer and Buckley, 2013), ultimately leading to an increase in the relative rate of ECM synthesis. In patients, the number of fibrocytes and fibroblasts in the lung is positively correlated with collagen deposition and the progression of pulmonary fibrosis. When fibroblast foci start to cross-link with each other, patient lung function may decrease substantially (Snijder et al., 2019).
Although fibroblast is the chief culprit in pulmonary fibrosis, its source is still unclear. Possible sources include peripheral recruitment, mesenchymal progenitor cells in lung tissues, and EMT (Figure 2C). When local lung injury occurs, epithelial cells and endothelial cells are activated and release chemokines. Through chemokine ligand–receptor pathways (including CXCL12/SDF1-CXCR4, CCL21-CCR7 and CCL2-CCR2) (Chong et al., 2019), many circulating fibrocytes and mesenchymal progenitor cells are recruited into local tissues and undergo phenotypic transformation to become fibroblasts. In the lungs of IPF patients, there is a high level of CXCL12/CXCR4 (Mehrad et al., 2007), which is conducive to the recruitment of circulating fibrocytes to the lungs along the concentration gradient of chemokines. In addition, AECs that cannot re-epithelialize can convert into interstitial AECs through EMT, leading to a decrease in intercellular junctions and epithelial features. However, the contribution of epithelial EMT in pulmonary fibrosis is still controversial (Salton et al., 2020). In addition, local fibrocytes and fibroblasts can self-proliferate rapidly. Under the influence of cytokines, growth factors and TIMPs, local fibroblasts in IPF can resist apoptosis and continue to proliferate (Andersson-Sjöland et al., 2011).
3.4 Extracellular Matrix
In IPF, ECM remodeling and collagen deposition are the classical pathological features of the disease. ECM is mainly produced by fibroblasts/myofibroblasts, epithelial cells, inflammatory cells, and mesenchymal progenitor cells.
ECM has complex functions in IPF (Figure 3). ECM has a certain degree of mechanical stiffness, which plays an important role in the sclerosis of lung tissues (Elowsson Rendin et al., 2019). Besides, ECM also serves as a pool for variety of growth factors (bFGF, VEGF), stimulating factors (GM-CSF, M-CSF), and interleukins (IL-1, IL-8), allowing signal exchange with different cells (Andersson-Sjöland et al., 2011). The mechanical force of the ECM itself can also directly participate in pulmonary fibrosis through mechanoreceptors and some cellular pathways. Under the action of mechanical force, α6 integrin (Rahaman et al., 2014) and the transient receptor potential vanilloid 4 (TRPV4) channel (Chen et al., 2016) act as sensors to detect mechanical stimulation signals and transfer the signals into myofibroblasts. Subsequently, F-actin contracts, resulting in the phosphorylation of focal adhesion kinase (FAK). Phosphorylated FAK activates Rho kinase (ROCK) by binding Rho to ROCK. This step activates yes-associated protein (YAP)/transcriptional coactivator with PDZ-binding motif (TAZ), which ultimately activates the transcription, translation, and expression of profibrotic genes in the nucleus. In addition to the FAK-ROCK-YAP/TAZ axis, mechanical force can also release TGF-β bound by latency-associated peptide (LAP), thus activating the TGF-β-Smad dependent/independent pathway in fibroblasts and AECs and directly participating in myofibroblast differentiation (Upagupta et al., 2018).
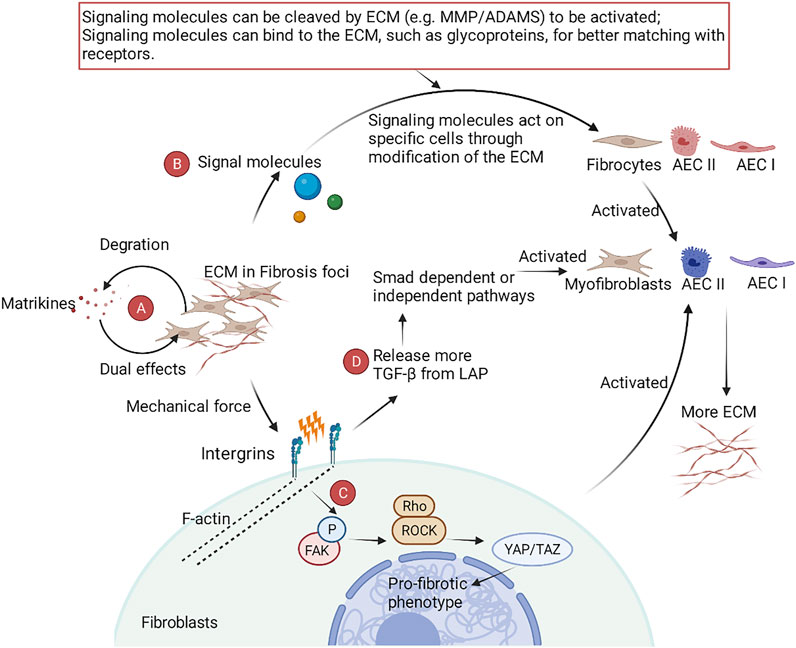
FIGURE 3. A schematic view of the role of ECM in IPF. (A) Matrkines, which contain multiple degradation products of the ECM, exert dual pro- and anti-fibrotic roles in IPF. (B) The ECM is involved in the phenotypic reprogramming of fibroblasts and AECs by modifying key signaling molecules. (C) After binding specific ECM ligands to the ectodomain of integrins, integrins bind to the cytoskeleton and various signaling proteins through their cytoplasmic tails, translating the mechanical force of cytoskeleton contraction and ECM stiffness into biochemical signals. (D) Integrins activate TGF-β-dependent fibrosis by releasing TGF-β through stretch force generated by actin-cytoskeleton interactions.
Matrikines, the degradation product of the ECM, also have special biological activities in lung diseases (Burgess and Weckmann, 2012). Matrikines are biologically active fragments derived from the degradation of ECM. These biologically active fragments exert dual biological properties by binding to integrins, heparan sulfate proteoglycans, and growth factor receptors. For example, endotrophin (the degradation product of collagen VI) promotes fibrosis, while endostatin (the degradation product of collagen XVIII) has antifibrosis effects (Ricard-Blum and Salza, 2014).
ECM includes the core matrisome and associated matrisomes. Core matrisomes include collages, ECM glycoproteins, and ECM proteoglycans. Associated matrisomes include ECM regulators, ECM-affiliated proteins, and secreted factors (Kreus et al., 2021). In the ECM of IPF patients, laminins and collagen IV associated with the basement membrane decreased, while the vast majority of core matrisomes showed an increasing trend (Elowsson Rendin et al., 2019). Although there have been numerous studies, very little is known about the role of some mediators in IPF. The various substances in the ECM are described in detail below.
3.4.1 Collagen
Collagen is the main component of ECM. Collagen is derived from activated (myo)fibroblasts, fibrocytes, epithelial cells (which can transform into mesenchymal cells through EMT), mesenchymal stromal cells, and pericytes. The vast majority of interstitial and fibrillar collagens (mainly collagen I and collagen III) are secreted by (myo)fibroblasts. These collagens constitute the main frame of the ECM and increase the mechanical stiffness of fibrotic tissue (Snijder et al., 2019). Currently, it is believed that type III collagen plays a role in the early disease stage and that type I collagen is associated with the deterioration of lung function in the late disease stage due to its involvement in collagen crosslinking mediated by lysyl oxidase (LOX) family enzymes (Rock et al., 2011). In view of the important contribution of collagen to the mechanical stiffness of pulmonary fibrosis, collagen turnover has naturally become an important means for monitoring disease progression and predicting patient prognosis. Increased concentrations of the collagen degradation markers type 1/3/6 collagen, and C-reactive protein are associated with an increased risk of overall mortality, and elevated levels of the collagen synthesis markers Pro-C3 and Pro-C6 are associated with IPF progression (Organ et al., 2019; Jessen et al., 2021).
3.4.2 Glycoproteins and Proteoglycans
There are relatively few studies on ECM glycoproteins and proteoglycans. In a radiation-induced pulmonary fibrosis model, the glycoprotein glectin-3 produced by type I AECs increased dramatically (Kasper and Hughes, 1996). In recent years, studies on proteoglycans have shown that the proteoglycan decorin reduces pulmonary fibrosis by antagonizing TGF-β and antagonizing CTGF-mediated collagen deposition (Nikaido et al., 2018). In addition, the proteoglycan lumican directly acts in the differentiate of monocytes into fibroblasts through an integrin-dependent pathway (Pilling et al., 2015). Chondroitin sulfate type E (CS-E), another proteoglycan, inhibits the expression of α-SMA, CTGF, LOXL2, and CCL2/MCP-1 by silencing the genes of CS-E and the related enzyme carbohydrate sulfotransferase 15 (CHST15) through miRNA (Kai et al., 2017). Syndecan-4 is a heparan sulfate proteoglycan. Silencing of syndecan-4 can reduce SMA-α and collagen deposition (Tanino et al., 2019). In addition, proteoglycans assist other factors in producing biological effects. For example, FXIIa must bind to the proteoglycan heparan sulfate to stimulate the migration of human lung fibroblasts (Wujak et al., 2015).
3.4.3 ECM Regulators
ECM regulators include serine protease inhibitors, cystatins, TIMPs, MMPs (Kreus et al., 2021), a disintegrin and metalloproteinases (ADAMs), and crosslinking enzymes (Ricard-Blum and Salza, 2014; Liu et al., 2016); these molecules are mainly involved in the regulation of ECM decomposition. MMPs/TIMPs play an important role in IPF and are directly involved in ECM remodeling. MMPs belong to zinc-dependent endopeptidases of the M10A subfamily, and 24 gene subtypes are expressed in the human body. As a crucial component of ECM, MMPs participate in the formation and progression of IPF via many pathways, especially TGF-β signaling pathway. Many studies have focused on MMPs and explored their potential value in resisting IPF (Table 1). Although the pathological processes of MMPs and IPF have been verified, there are no MMP inhibitors for the treatment of IPF in the clinic (Yue et al., 2021).
ECM contains a variety of signal molecules secreted by cells; therefore, the ECM is a transfer station for signal exchange. In IPF, the signaling molecules from different cells constitute a complex signaling network through the bridge function of the ECM. Upstream signals are modified and regulated by positive feedback in the ECM; this feedback, e.g., the TGF-β pathway and Rho/ROCK signaling, can affect the transcription and translation of ECM-related genes and eventually lead to pulmonary fibrosis (Snijder et al., 2019).
Although there have been many studies on active fragments, the roles of these active products in IPF are still not clear. In recent years, the ECM has shown extraordinary clinical and scientific value in the fields of anti-infection, anti-angiogenesis, and wound healing (Ricard-Blum and Salza, 2014; Ricard-Blum and Vallet, 2019). Therefore, research on active fragments and their derivatives as targets for antifibrosis therapies is innovative and has great potential.
4 Underlying Factors Associated With the Pathogenesis of IPF
4.1 Genetic Susceptibility and Epigenetic Alterations in the Development of IPF
A growing number of studies have shown that genetic mutations are associated with susceptibility, diagnosis, progression, prognosis, and adverse effects of treatment in IPF. The proportion of patients carrying genetic mutations in IPF may be underestimated. A cohort study showed that up to 36% of patients with IPF have familial genetic mutations (Barros et al., 2019). In addition, IPF mutations are present in nearly 40% of sporadic pulmonary fibrosis cases (Wolters et al., 2014). These common and rare genetic mutations mainly include mutations in telomeres, alveolar surfactant and muco-ciliary transportation system, immune and cytokine-related genes, cell adhesion and cell integrity-related genes (Table 2). MUC5B and telomere related gene mutations are more common in IPF patients, which has inspired a very large number of studies to explore the pathogenic mechanisms of gene mutations (Evans et al., 2016; Zhang et al., 2021). Besides, genetic variants have been associated with the imaging presentation of IPF. Patients with TERT mutations were more likely to exhibit the classical UIP pattern compared to patients without mutations with two-year-follow up (Baratella et al., 2021).
Notably, the majority of existing studies still remain to verify the correlation between mutations and IPF susceptibility, and there are only a few studies related to the causality and pathogenesis of IPF. Moreover, further research needs to focus on how to cross the gap between genetic testing and clinical treatment. Drugs targeting histone modifications and DNA modifications have also shown potential in preclinical studies, so we will also cover the role of epigenetics in IPF in the next sections.
4.1.1 Genetic Mutations in Telomeres
Telomeres are a TTAGGG repeat sequence at the end of human chromosomes, whose function is to stabilize the chromosome, prevent fusion of chromosome ends, protect structure of chromosomes, and determine cell life span, but telomerase activity in adult human cells is extremely low (Lister-Shimauchi et al., 2022).
Telomeres are protected by the shelterin complex, which consists of the protein components TRF1, TRF2, RAP1, TIN2, TPP1, and POT1. The ends of telomeres are bonded with telomerase, which is comprised of the catalytic subunit of TERT, the telomerase RNA component of TERC, and accessory proteins. The telomerase exerts its effects by adding telomeric sequences to the telomeric ends (Zhang et al., 2021). Telomere shortening is one of the important phenotypes of IPF. Telomere length of half of IPF patients rank in the lowest 1% of their age group (Stanley et al., 2016b). And telomeres are shorter in IPF patients than in other interstitial pneumonia (Snetselaar et al., 2015). However, it is questionable whether the cause of telomere shortening in IPF patients necessarily stems from telomere gene mutations. In patients with sporadic and familial pulmonary fibrosis, telomere shortening is present regardless of the presence of known telomere related gene mutations (Snetselaar et al., 2015). A possible reason for this is the presence of other unknown genetic mutations that can shorten telomere length.
The causal relationship between mutation-mediated telomere shortening and the development of IPF is inconclusive because not all individuals carrying a known telomere defective gene develop telomere shortening and IPF (Hoffman et al., 2018). Firstly, telomere gene defects in different cells may lead to different outcomes. systematically TERT knock-out does not induce spontaneous pulmonary fibrosis in mice, but can increase susceptibility to bleomycin (Degryse et al., 2012), while mesenchymal-specific TERT knock-out can alleviate bleomycin-induced pulmonary fibrosis (Liu et al., 2015); and AEC II-specific TERT defect reduces AEC II proliferation and induces AEC II cell senescence (Liu T. et al., 2019). Secondly, telomere gene defect-mediated DNA damage may be slow, which requires multiple generations of mice to become apparent (Blasco et al., 1997). Thirdly, due to the heterogeneity of the clinical manifestations, even the same genetic mutations may have different phenotypes, leading to the appearance of extra-pulmonary pathological features (rather than interstitial lung disease) (Borie et al., 2016). Fourthly, telomere length may differ in peripheral blood leukocytes and lung tissue (Snetselaar et al., 2017). Therefore, before telomere length measurements can be applied to clinical decisions, there is a need to clarify the effect of telomere length on different cells, the optimal sample type, and the corresponding intrapulmonary/extrapulmonary clinical manifestations.
The mechanism by which telomerase gene mutations lead to IPF is unclear. Available data suggest that cellular senescence or death of alveolar stem cells induced by telomere dysfunction is implicated in pulmonary fibrosis (Zhang et al., 2021). Knockdown of telomerase related genes leads to lung stem cell regenerative capacity and the senescence/death of AEC II (Jackson et al., 2011; Alder et al., 2015; Snetselaar et al., 2017; Wang et al., 2020). In addition, knockdown of telomerase related genes leads to elevated SASP (including TGF-β) levels and lung inflammation mediated by intrinsic immune cell infiltration (Chen et al., 2015; Povedano et al., 2015; Naikawadi et al., 2016; Liu et al., 2018).
Telomere length and specific telomere gene defects are instructive for susceptibility, diagnosis, progression, prognosis, and early warning of adverse effects in IPF. Shorter telomere length as an independent predictor is associated with worse survival and more pronounced imaging changes in IPF patients (Stuart et al., 2014; Newton et al., 2016; Ley et al., 2017). Pirfenidone treatment did not improve FVC and DLCO in IPF patients carrying TERT/TERC mutations (Justet et al., 2018). The relationship between TERT/TERC mutations and resistance to drug therapy needs to be further validated. Patients carrying telomere-related mutations may be at higher risk for complications after lung transplantation, such as death, chronic lung allograft dysfunction, renal disease, CMV complications (Popescu et al., 2019; Swaminathan et al., 2019), hematological complications (Silhan et al., 2014; Borie et al., 2015), anemia, leukopenia, recurrent lower respiratory tract infections (Tokman et al., 2015).
Drugs that target telomeres have also shown therapeutic potential. GRN510, a telomerase agonist, reduces inflammatory infiltration and collagen deposition in a mouse model of bleomycin-induced pulmonary fibrosis (Le Saux et al., 2013). PAP-associated domain-containing protein 5 (PAPD5) is involved in the degradation of TERC RNA. BCH001 (a PAPD5 inhibitor) and RG7834 (a PAPD5/7 inhibitor) rescue the TERC levels and telomerase activity in the cellular model of dyskeratosis congenital (Nagpal et al., 2020; Shukla et al., 2020). One study reported that hormones may regulate telomerase activity through an imperfect oestrogen response element within the TERT promoter (Bayne and Liu, 2005), which has inspired a series of subsequent clinical trials of hormones for IPF. Danazol, a synthetic androgen, was shown to increase telomere length and stabilize DLCO and FVC in a small-scale clinical trial (Townsley et al., 2016a; Townsley et al., 2016b). Danazol is currently in phase II clinical trials for plumonary fibrosis (NCT04638517). However, hepatotoxicity and worsening pulmonary fibrosis associated with long-term use of Danazol has been reported after danazol initiation and withdrawal (Chambers et al., 2020). Therefore, lower doses of danazol (200 mg) are being used in clinical trials to reduce hepatotoxicity. Nandrolone Decanoate, an anabolic androgenic steroid, is undergoing phase I/II clinical trials (NCT02055456). Telomerase reactivation therapy still has a long way to go before it becomes a therapeutic option, especially in terms of the serious adverse effects associated with hepatotoxicity. Whether peripheral blood telomere length can be used as a substitute outcome for pulmonary telomere length and how effective is telomerase reactivation therapy for IPF are still awaiting the results of current clinical trials.
In summary, details remain unclear in the complex field of the role telomeres play in the pathogenesis of ILDs and no clinical trials employing gene therapy have been initiated in patients with telomeropathies. Hopefully, adeno-associated virus-9 (AAV9)-mediated gene therapy has been reported to be successful in mouse models (Bär et al., 2016), and perhaps a telomerase-directed therapeutic strategy may be used for the treatment of IPF in the future.
4.1.2 Genetic Mutations in Alveolar Surfactant/Mucin
Phospholipids and four surfactant proteins (SFTPA, B, C, D) can be secreted by AEC II. The process of surfactant secretion is shown (Figure 4A). ATP-binding cassette subfamily A member 3 (ABCA3) is essential for the intracellular synthesis of alveolar surface-active substances (Mulugeta et al., 2015). Among four surfactant, genetic mutations of SFTPA (Wang Y. et al., 2009; Guenther et al., 2019), SFTPC (Venosa et al., 2017) and ABCA3(Zhou et al., 2017; Manali et al., 2019) have been identified in IPF patients. Although SFTPA is not directly involved in the formation of alveolar surface-active tension, it plays an important role in intrinsic lung immunity. Mouse models with SFTPA mutations exhibit intracellular retention of SFTPA and enhanced ER stress (Wang Y. et al., 2009; Maitra et al., 2010). Molecular signatures of UPR signaling and apoptotic activation associated with SFTPC have been reported in patients with IPF (Korfei et al., 2008; Lawson et al., 2008). Patients with pure mutations in ABCA3 and ABCA3-deficient mice exhibit a complete lack of alveolar surface-active substance, deformation of lamellar bodies, and death from respiratory distress shortly after birth (Shulenin et al., 2004; Cheong et al., 2007; Fitzgerald et al., 2007; Wambach et al., 2014). In lung disease, there are also reports of ABCA3 heterozygous mutations that appear to interact with SFTPC mutations (Bullard and Nogee, 2007; Crossno et al., 2010). ABCA3 mutations may induce ER stress and proteostasis failure through misfolded alveolar surface-active substances (Cheong et al., 2006; Matsumura et al., 2006; Weichert et al., 2011).
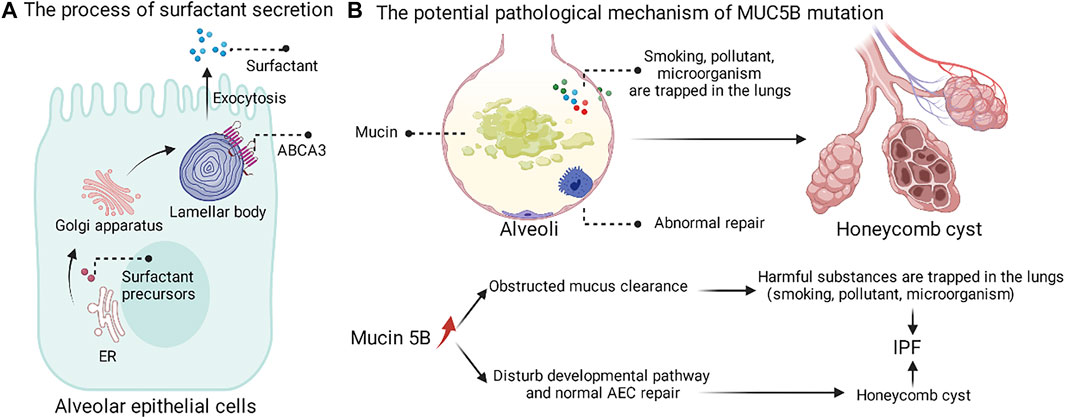
FIGURE 4. The process of surfactant secretion and the potential pathological mechanism of MUC5B mutation. (A) The surfactant precursors are synthesized on the endoplasmic reticulum, followed by Golgi modifications, and finally secreted into the periphery by cytosolic exocytosis of the lamellar body. (B) MUC5B mutation may promote the retention of harmful substances in the lung and interfere with the normal repair of AEC.
The MUC5B promoter variant, rs35705950, as the strongest genetic risk factor and common variation, was found in up to 30% of IPF (Evans et al., 2016). The rs35705950 mutation leads to increased Muc5B expression, but a causal relationship between mucus and progression of pulmonary fibrosis disease has not been established. Christopher M. Evans proposed two hypotheses for the pathogenic mechanism of MUC5B mutations (Figure 4B) (Evans et al., 2016). The first possible mechanism is that overexpression of Muc5B at the bronchoalveolar junction impairs mucociliary clearance, which in turn promotes prolonged retention of harmful particles (e.g., air pollutants, cigarette smoke, microorganisms, etc.) in the lung and induces fibrosis in the lung tissue. The second possible mechanism is that overexpression of Muc5B may lead to fibrosis and cellulitic cyst formation by interfering with the normal developmental pathway and alveolar epithelial repair. In summary, the overexpression of MUC5B at the bronchoalveolar junction in IPF patients led us to recognize that the peripheral airway also seems to influence interstitial fibrosis.
4.1.3 Genetic Mutations in Inflammatory Regulators
Genetic mutations in cytokines have also been reported in patients with IPF. IL1RN gene polymorphisms may be associated with susceptibility to fibrosing alveolitis, a disease characterized by interstitial lung fibrosis (Whyte et al., 2000). Mesenchymal stem cells (MSC) can exert anti-inflammatory and anti-fibrotic effects via IL-1RN (Ortiz et al., 2007). Association between genetic polymorphisms of IL-4 and IPF has been reported (Riha et al., 2004). IL-4 may be pro-fibrotic by promoting type 2 immunity. IL-8 gene diversity is associated with lung alveolitis and lung function decline (Ziegenhagen et al., 1998). IL-8 increases the fibrogenicity of mesenchymal progenitor cells and is involved in the proliferation, activation, and recruitment of mesenchymal cells (Yang et al., 2018). TLR3 (Toll-like receptor 3) mutation rs3775291 increase the risk for IPF patients and also reduces forced volume capacity (FVC) (Evans et al., 2016). Defectiveness of the gene TLR3 L412F causes aberrant inflammation and fibroblasts proliferation in IPF, which may be related to dysregulation of fibroblast proliferation mediated by a sluggish IFN-β response (O'Dwyer et al., 2013). The different mutations of TOOLIP (TOLL interacting protein) may be associated with different IPF susceptibility. The rs5743890 was associated with lower IPF susceptibility, while the rs5743894 was associated with higher IPF susceptibility (Oldham et al., 2015). In addition, rs5743890 was associated with increased morbidity and mortality in IPF (Noth et al., 2013). rs3750920 polymorphism is associated with the efficacy of N-Acetyl-L-cysteine (NAC) (Oldham et al., 2015). It is important to note that different studies with different populations and specimens may reach different conclusions. In a study conducted in China, except for the polymorphisms of HLA haplotype, none of the other cytokines showed an association for IPF susceptibility (Zhang et al., 2015). Therefore, the study of genetic polymorphisms of cytokines should pay more attention to the reproducibility of experimental results.
In recent years, the launch of pirfenidone and nintedanib has witnessed breakthroughs in the signaling pathways of cytokines and growth factors in the treatment of IPF, however, studies focusing on cytokine and immune-related gene polymorphisms need to be further developed.
4.1.4 Other Potential Genetic Mutations Associated With IPF
In recent years, with the availability of next-generation sequencing technologies, more and more novel mutations have been identified in IPF patients. The mutations of the gene DSP (Mathai et al., 2016; Wang et al., 2018) and DPP9 (associated with cell adhesion) (Fingerlin et al., 2013; Zhou and Wang, 2016), AKAP13 166] and FAM13A (associated with RhoA/ROCK signaling pathway) (Hirano et al., 2017; van Moorsel, 2018), and SPPL2C (associated with intramembrane proteases) (Wu et al., 2016; Lorenzo-Salazar et al., 2019) were reported to be a risk of IPF susceptivity, progression and prognosis. However, the pathogenesis of these mutations remains unclear, and current studies are limited to correlation analysis (Table 2).
4.1.5 DNA Methylation
DNA methylation is a chemical modification process in which a specific base on the DNA sequence acquires a methyl group by covalent bonding with S-adenosyl methionine (SAM) as the methyl donor. DNA methylation is catalyzed by DNA methyltransferase (DNMT). In humans, DNA methylation occurs mainly at the fifth carbon atom of cytosine in CpG dinucleotides. It is generally believed that DNA methylation can inhibit the transcription and expression of the gene.
Global methylation and methylation of specific gene have been shown to be involved in the pathogenesis of IPF. Global DNA methylation analysis based on whole lung tissue showed that DNMT expression was increased in IPF, and DNA methylation of 16 genes (including CLDN5, ZNF467, TP53INP1 and DDAH1) was associated with decreased mRNA expression (Sanders et al., 2012). In order to clarify the contribution of specific cell types to IPF, single-cell-based or cell-type-specific sequencing is necessary. The global DNA methylation analysis based on fibroblasts showed that cells derived from IPF patients differ in the methylation of multiple CpG sites (including CDKN2B, CARD10, and MGMT) (Huang et al., 2014). Hypermethylation of specific genes not only can suppress the expression of some genes [Thy-1, COX-2, PTGER2, p14ARF, IP-10 (Zhou et al., 2020), SFRP1/4, CDKN2B (Xue et al., 2021)], but also can promote the expression of some genes [α-SMA, MeCP2, KCNMB1 (Xue et al., 2021)], which eventually leads to the development of IPF (Figure 5A). Decitabine, a DNMT inhibitor, reduced fibrotic gene and DNMT-1 expression, enhanced miR-17–92 cluster expression (Dakhlallah et al., 2013). PGE2 has the capacity to limit fibrosis through its inhibition of numerous functions of these fibroblasts, and Decitabine restored PGE2 responsiveness in fibrotic fibroblasts (Huang et al., 2010).
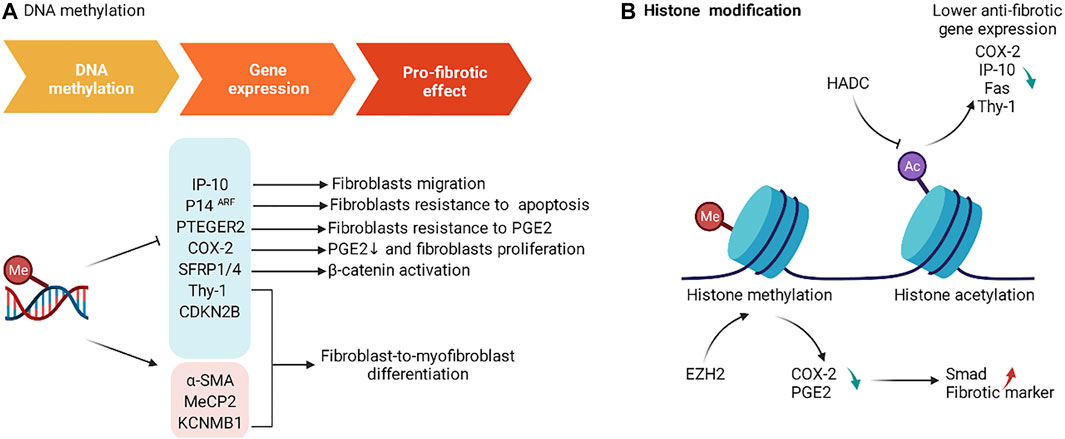
FIGURE 5. The roles of DNA methylation and histone modification in IPF. (A) DNA methylation affects the expression of gene associated with fibrosis and produces a pro-fibrotic effect. (B) EZH2 and HADC induce changes in gene expression associated with fibrosis by affecting histone modifications.
4.1.6 Histone Modification
Nucleosome, the basic unit of chromatin, is comprised of 146–147 base pairs of DNA and a histone octamer with one H2A-H2B tetramer and two H3-H4 dimers (Jenuwein and Allis, 2001).
The N- and C-terminal tails of H3 and H4 are the most frequently modified regions. The types of modifications include methylation, acetylation, phosphorylation, SUMOylation, and ubiquitination. The histone mark is named according to the histone, the modification site of these tails, and the type of modification. Histone modifications can change the electronic charge and structure of DNA-bound histone tails, thus altering chromatin state and gene expression (Kouzarides, 2007). The effect of histone acetylation and methylation in IPF has been extensively studied, and a large number of agents have been designed and validated in preclinical studies (Figure 5B).
Histone methylation is catalyzed by the histone methyltransferase (HMT), which uses S-adenosyl methionine (SAM) as the substrate to transfer methyl groups onto the lysine residues of histones. Methylation of H3K4, H3K36, and H3K79 is thought to be associated with gene activation, while H3K9, H3K27, and H4K20 are thought to be associated with gene inactivation (Black et al., 2012). Inhibition of EZH2, an H3K27me-specific methyltransferase, by 3-DZNeP and BIX01294 increased the expression of COX-2 and PGE2, and suppressed the expression of Smad signaling and fibrosis markers (Coward et al., 2014; Xiao et al., 2016).
Histone acetyltransferases (HATs) promote histone acetylation, while histone deacetylases (HDACs) reduce histone acetylation. Histone acetylation is thought to be associated with gene activation (Clayton et al., 1993). In addition, sirtuins (SIRTs) are a family of deacetylases. SIRT1 inhibits the expression of aging-related secretions by deacetylating the SASP gene (Hayakawa et al., 2015). Inhibition of SIRT3 expression promotes fibrosis in human lung fibroblasts (Sosulski et al., 2017). Histone deacetylation is associated with the repression of anti-fibrotic genes, which includes COX-2 (Coward et al., 2009), IP-10 (Coward et al., 2010), Fas (Huang et al., 2013), Thy-1 (Sanders et al., 2011).
Inhibition of histone deacetylation may have anti-pulmonary fibrosis effects. HDACi consists of a zinc-binding-group, a hydrophobic group for protein recognition and interaction, and a linker connecting both of them (Wang and Dymock, 2009). A large number of agents targeting histone modification have already demonstrated antifibrotic effects in preclinical trials or in cell levels (Table 3).
4.2 Environment Factors
Environmental factors such as asbestos, metal dust, stone dust, wood dust, chemotherapy and allergen exposure are important causes of IPF (Taskar and Coultas, 2006). Epidemiological surveys have shown that 38% of IPF patients were exposed to high-risk environments; however, it is uncertain whether these factors directly lead to the occurrence of IPF (Behr et al., 2015; Wuyts et al., 2018).
Smoking is a relatively special environmental factor. Both direct smoking and indirect smoking are independent exposure factors, and the correlation between the direct/indirect smoking and IPF increase with the increase of smoking in dose (Bellou et al., 2021). Interestingly, according to the German S2K guidelines, although 60%–70% of patients had a history of smoking, less than 7% of patients were active smokers when they developed IPF symptoms. In other words, most patients developed the disease several years after quitting smoking; the average time interval was 21 years (Behr et al., 2021). This indicates that across such a long period of time, the injury caused by smoking may continue to progress or transform into IPF.
4.3 Body Aging and Cell Senescence
According to epidemiological surveys, advanced age is one of the most relevant demographic factors for IPF (Sgalla et al., 2018). The incidence of IPF in elderly men over 50 years of age is significantly higher than that in younger men (Raghu et al., 2018). However, the mechanism of how senescence leads to IPF is still unclear. In a mouse model of fibrosis, researchers observed a series of signs of cellular senescence, including elevated senescence-associated β-galactosidase (SA-β-Gal) in lysosomes and elevated reactive oxygen species (ROS) in mitochondria, an increase in cell cycle arrest proteins such as P53/P21/P16, an increase in the apoptosis-related protein BCL-2/Bax ratio, and an increase in DNA damage in the nucleus (Blokland et al., 2020). In addition, a variety of senescence-associated secretory phenotypes (SASPs) have been observed in animal models of pulmonary fibrosis, and these SASP components are known to include powerful profibrotic molecules such as TGF-β (Cho and Stout-Delgado, 2020; Rana et al., 2020). Similarly, studies have found that the lung fibroblasts of elderly IPF patients exhibit the above characteristics (Álvarez et al., 2017). Therefore, although there is a lack of human research evidence that senescence directly causes IPF, epidemiological data from both animal experiments and cell experiments suggest that IPF is a senescence-related disease.
The main manifestations of lung senescence are decreased lung tissue elasticity, decreased effective lung volume, the thickening of intercellular substances, and decreased lung function. Cell senescence mainly manifests as cell morphology and structure disorder, cell dysfunction, and cell growth/proliferation arrest (Rana et al., 2020).
The mechanism of pulmonary fibrosis caused by aging is not very clear, but there are quite a few theories to explain the mechanism of senescence in IPF. Cell senescence can disrupt of a variety of processes and generate imbalances (for example, shortened telomeres and DNA damage, oncogene activation, redox imbalances, mitochondrial dysfunction, lysosomal and proteasome dysfunction, ER stress), resulting in increased SASP, myofibroblast resistance to apoptosis, and stem cell depletion, ultimately manifesting as abnormal repair at the lung injury site (e.g., failure of the alveolar epithelium to regenerate properly and ECM deposition) (Sgalla et al., 2018; Glass et al., 2020; Liu and Liu, 2020; Merkt et al., 2020). Aging has been reported to reprogram the hematopoietic-vascular niche to impede regeneration and promote fibrosis (Chen et al., 2021).
Because senescent cells that resist apoptosis can continuously produce profibrotic cytokines, which in turn aggravate pulmonary fibrosis, the induction of apoptosis in senescent cells (e.g., dasatinib/quercetin, ABT-263, and NOX4 inhibitors) or selective antagonism of profibrotic senescence-related factors (e.g., IL-6, TGF-β or leukotrienes) may help to alleviate IPF (Merkt et al., 2020). Many antiaging drugs have passed preclinical animal studies and entered clinical trials. For example, dasatinib (D) and quercetin (Q) can promote the apoptosis of senescent cells. The DQ combination regimen has been investigated in a phase I clinical trial (NCT02874989). A total of 14 patients were enrolled in the trial; except for one patient who had severe adverse reactions (bacterial pneumonia), the remaining patients tolerated the treatment well, and improvements in parameters such as 6-min walking distance, 4-m gait speed, and chair stand time were reported (Schafer et al., 2017; Justice et al., 2019). Interestingly, Nifurtimox (NIF), originally used to treat diarrhea, has been reported to improve pulmonary fibrosis by blocking the production of myofibroblasts (Gan et al., 2022).
5 Recent Progress in Therapeutic Targets and New Drug Development for IPF
Pirfenidone and nintedanib are two drugs approved by the FDA for the treatment of IPF. They can delay lung function deterioration and prolong patient survival in IPF patients. Although the launch of Pirfenidone and nintedanib has demonstrated the feasibility of drug treatment for IPF, they do not reverse pulmonary fibrosis and their efficacy in patients with end-stage IPF is not fully clear. Therefore, there is a need to develop additional anti-pulmonary fibrosis drugs. The development of drugs for some specific targets is currently underway, which will be described in the following sections. In addition, drug combinations for antifibrotic therapy are worth investigating. Immune checkpoint inhibitors (ICIs), either as single agents or in combination regimens, have shown great success in clinical treatment (Wu et al., 2022). A case reports indicates that the addition of nintedanib to ICIs therapy might prevent drug-induced pneumonitis or acute exacerbation of IPF (Yamakawa et al., 2019).
5.1 Summary of Emerging Therapeutic Targets for IPF in Drug Discovery
In this section we briefly describe the current therapeutic targets in clinical trials and the latest clinical trial developments. We summarize the emerging therapeutic targets and representative mechanisms in the development of IPF (Table 4).
TGF-β plays an important role in the pathogenesis of IPF by stimulating the activation and proliferation of fibroblasts (Ong et al., 2021). Both anti-TGF-β mAb and anti-TGF-β mRNA nucleic acid have entered clinical trials (NCT00125385 and NCT03727802, respectively). Monoclonal antibodies against integrins that activate latent TGF-β have also entered phase II clinical trials (NCT04072315 and NCT04396756). Tyrosine kinase inhibitors targeting the TGF-β receptor have also entered phase II clinical trials (NCT03832946). Other growth factors (PDGF, VEGF, FGF, EGF, CTGF) are also involved in the process of pulmonary fibrosis. The mAb targeting CTGF, Pamrevlumab, demonstrated clinical benefit in phase II clinical trials and is now in phase III clinical trials (NCT03955146 and NCT04419558).
Chemokines and interleukins are also involved in recruitment of pro-fibrotic cells and formation of pro-fibrotic microenvironment, as we discussed in our previous paper (Ma et al., 2022). However, CCL2, a key chemokine for monocyte/macrophage migration and infiltration, failed to demonstrate clinical benefit in a phase II clinical trial (NCT00786201). Anti-IL-13 therapy didn’t contribute to lung function in patients with IPF (NCT02345070 and NCT01872689) or was terminated due to Lack of evidence of efficacy (NCT01266135 and NCT01266135).
Leukotrienes have profibrotic effects by inducing fibroblast migration, proliferation, and matrix protein synthesis (Antoniou et al., 2007). MN-001/Tipelukast, leukotriene receptor antagonist, is undergoing phase II clinical trial (NCT02503657).
As lipid proinflammatory mediators, The ATX-LPA-LPAR axis and SPHK1-S1P-S1PR axis have also been reported as targets of IPF. LPA and S1P have been demonstrated to promote the development of fibrosis (Tager et al., 2008; Ninou et al., 2018; Huang et al., 2020; Suryadevara et al., 2020). BMS-986278 (LPA1R antagonist) has entered phase II clinical trials (NCT04308681).
PTX-2, a ligand for the Fcγ receptor, can downregulate monocyte and macrophage activity (especially M2) (Castaño et al., 2009). PRM-151, a recombinant human pentraxin-2, has entered phase II clinical trials (NCT04594707 and NCT04552899).
Multiple cellular signaling pathways play a crucial role in the development of IPF. JAK/STAT signaling (Montero et al., 2021), receptor-type tyrosine kinase/non-receptor-type tyrosine kinase signaling (e.g., Src) (Hu et al., 2014), PI3K/Akt/mTOR signaling (Hennessy et al., 2005; Mercer et al., 2016; Lawrence and Nho, 2018), and Hedgehog signaling (Effendi and Nagano, 2021) comprise complex fibrosis regulatory signaling pathway. In our previous reviews, we have discussed the aforementioned signaling pathways (Ma et al., 2022).
There are also drugs in clinical trials targeting oxidative stress (Giri, 2003), GPR40/GPR84 (Gagnon et al., 2018), and collagen cross-linking enzymes (Chen et al., 2019).
5.2 Summary of Ongoing Clinical Trials for IPF
We searched clinical trials in recent years and summarized the anti-IPF drugs undergoing clinical trials and their mechanisms of action (Table 5). We also update the latest clinical trial progress for these drugs.
6 Conclusion and Outlook
The research on the pathogenesis of IPF has made considerable progress. After years of basic and clinical studies, the pathogenesis of IPF has changed from simple inflammation to abnormal epithelial-mesenchymal crosstalk and other common pathogenic mechanisms. Based on this pathological mechanism, many studies have systematically studied the key roles of fibrocytes/(myo)fibroblasts, epithelial/endothelial cells, and ECM in the pathogenesis of IPF. With the progresses in understanding the key role of genetics and epigenetics in IPF, researchers have found that an increasing number of genetic loci and their apparent modifications are related to the maintenance of lung homeostasis although the effects of gene mutations and epigenetics on IPF still need further study.
The advent of pirfenidone and nintedanib is undoubtedly a sensational event in the field of IPF treatment, but they also have some major limitations, such as insufficient curative effect and poor pharmacokinetic properties. In addition, combination therapy based on them needs to be further studied. The number of clinical trials for therapies targeting cytokines, growth factors, and their signaling pathways is increasing rapidly. Furthermore, drugs targeting MMPs, telomerase activity, and epigenetic modifications need to be further developed.
Although IPF is idiopathic by definition, this deadly disease may become less mysterious with the progress in the understanding of the pathogenesis of IPF. The number of observed therapeutic targets is increasing, as well as the number of new drugs entering clinical trials. For patients with IPF, these advances described in this review show that slowing the IPF progression, prolonging their lives, and improving their quality of life might be possible in the future.
Author Contributions
HM contributed to the conception of this review and preparation of the manuscript, tables, and figures; XW collected information about the clinical trials; YL revised the tables and figures; YX contributed to the conception, supervision, and revision of the manuscript. All authors have approved the final article and are included in the disclosure.
Funding
This study was supported by the Department of Science and Technology of Sichuan Province (Grant No. 2021YJ0450 to YX) and the National Natural Science Foundation of China (81902287 to YL, 82173280 to YX).
Conflict of Interest
The authors declare that the research was conducted in the absence of any commercial or financial relationships that could be construed as a potential conflict of interest.
Publisher’s Note
All claims expressed in this article are solely those of the authors and do not necessarily represent those of their affiliated organizations, or those of the publisher, the editors and the reviewers. Any product that may be evaluated in this article, or claim that may be made by its manufacturer, is not guaranteed or endorsed by the publisher.
References
Álvarez, D., Cárdenes, N., Sellarés, J., Bueno, M., Corey, C., Hanumanthu, V. S., et al. (2017). IPF Lung Fibroblasts Have a Senescent Phenotype. Am. J. Physiol. Cell. Mol. Physiol. 313 (6), L1164–l1173. doi:10.1152/ajplung.00220.2017
Alder, J. K., Barkauskas, C. E., Limjunyawong, N., Stanley, S. E., Kembou, F., Tuder, R. M., et al. (2015). Telomere Dysfunction Causes Alveolar Stem Cell Failure. Proc. Natl. Acad. Sci. U. S. A. 112 (16), 5099–5104. doi:10.1073/pnas.1504780112
Allen, R. J., Porte, J., Braybrooke, R., Flores, C., Fingerlin, T. E., Oldham, J. M., et al. (2017). Genetic Variants Associated with Susceptibility to Idiopathic Pulmonary Fibrosis in People of European Ancestry: A Genome-wide Association Study. Lancet Respir. Med. 5 (11), 869–880. doi:10.1016/s2213-2600(17)30387-9
Andersson-Sjöland, A., Nihlberg, K., Eriksson, L., Bjermer, L., and Westergren-Thorsson, G. (2011). Fibrocytes and the Tissue Niche in Lung Repair. Respir. Res. 12 (1), 76. doi:10.1186/1465-9921-12-76
Antoniou, K. M., Pataka, A., Bouros, D., and Siafakas, N. M. (2007). Pathogenetic Pathways and Novel Pharmacotherapeutic Targets in Idiopathic Pulmonary Fibrosis. Pulm. Pharmacol. Ther. 20 (5), 453–461. doi:10.1016/j.pupt.2006.01.002
Aoyagi-Ikeda, K., Maeno, T., Matsui, H., Ueno, M., Hara, K., Aoki, Y., et al. (2011). Notch Induces Myofibroblast Differentiation of Alveolar Epithelial Cells via Transforming Growth Factor-{beta}-Smad3 Pathway. Am. J. Respir. Cell. Mol. Biol. 45 (1), 136–144. doi:10.1165/rcmb.2010-0140oc
Armanios, M. Y., Chen, J. J., Cogan, J. D., Alder, J. K., Ingersoll, R. G., Markin, C., et al. (2007). Telomerase mutations in families with idiopathic pulmonary fibrosis. N. Engl. J. Med. 356 (13), 1317–1326. doi:10.1056/NEJMoa066157
Bär, C., Povedano, J. M., Serrano, R., Benitez-Buelga, C., Popkes, M., Formentini, I., et al. (2016). Telomerase Gene Therapy Rescues Telomere Length, Bone Marrow Aplasia, and Survival in Mice with Aplastic Anemia. Blood 127 (14), 1770–1779. doi:10.1182/blood-2015-08-667485
Bao, Z., Zhang, Q., Wan, H., He, P., Zhou, X., and Zhou, M. (2014). Expression of Suppressor of Cytokine Signaling 1 in the Peripheral Blood of Patients with Idiopathic Pulmonary Fibrosis. Chin. Med. J. Engl. 127 (11), 2117–2120.
Baratella, E., Ruaro, B., Giudici, F., Wade, B., Santagiuliana, M., Salton, F., et al. (2021). Evaluation of correlations between genetic variants and high-resolution computed tomography patterns in idiopathic pulmonary fibrosis. Diagnostics 11 (5), 762. doi:10.3390/diagnostics11050762
Barros, A., Oldham, J., and Noth, I. (2019). Genetics of idiopathic pulmonary fibrosis. Am. J. Med. Sci. 357 (5), 379–383. doi:10.1016/j.amjms.2019.02.009
Bayne, S., and Liu, J. P. (2005). Hormones and Growth Factors Regulate Telomerase Activity in Ageing and Cancer. Mol Cell Endocrinol. 240 (1–2), 11–22. doi:10.1016/j.mce.2005.05.009
Behr, J., Günther, A., Bonella, F., Dinkel, J., Fink, L., Geiser, T., et al. (2021). S2K guideline for diagnosis of idiopathic pulmonary fibrosis. Respiration 100 (3), 238–271. doi:10.1159/000512315
Behr, J., Kreuter, M., Hoeper, M. M., Wirtz, H., Klotsche, J., Koschel, D., et al. (2015). Management of Patients with Idiopathic Pulmonary Fibrosis in Clinical Practice: The INSIGHTS-IPF Registry. Eur. Respir. J. 46 (1), 186–196. doi:10.1183/09031936.00217614
Bellou, V., Belbasis, L., and Evangelou, E. (2021). Tobacco smoking and risk for pulmonary fibrosis: A prospective cohort study from the UK biobank. Chest 160 (3), 983–993. doi:10.1016/j.chest.2021.04.035
Betensley, A., Sharif, R., and Karamichos, D. (2016). A systematic review of the role of dysfunctional wound healing in the pathogenesis and treatment of idiopathic pulmonary fibrosis. J. Clin. Med. 6 (1), 2. doi:10.3390/jcm6010002
Black, J. C., Van Rechem, C., and Whetstine, J. R. (2012). Histone Lysine Methylation Dynamics: Establishment, Regulation, and Biological Impact. Mol. Cell. 48 (4), 491–507. doi:10.1016/j.molcel.2012.11.006
Blasco, M. A., Lee, H. W., Hande, M. P., Samper, E., Lansdorp, P. M., DePinho, R. A., et al. (1997). Telomere Shortening and Tumor Formation by Mouse Cells Lacking Telomerase RNA. Cell. 91 (1), 25–34. doi:10.1016/s0092-8674(01)80006-4
Blokland, K. E. C., Pouwels, S. D., Schuliga, M., Knight, D. A., and Burgess, J. K. (2020). Regulation of Cellular Senescence by Extracellular Matrix during Chronic Fibrotic Diseases. Clin. Sci. (Lond) 134 (20), 2681–2706. doi:10.1042/cs20190893
Borie, R., Kannengiesser, C., Hirschi, S., Le Pavec, J., Mal, H., Bergot, E., et al. (2015). Severe Hematologic Complications after Lung Transplantation in Patients with Telomerase Complex Mutations. J. Heart Transpl. 34 (4), 538–546. doi:10.1016/j.healun.2014.11.010
Borie, R., Tabèze, L., Thabut, G., Nunes, H., Cottin, V., Marchand-Adam, S., et al. (2016). Prevalence and Characteristics of TERT and TERC Mutations in Suspected Genetic Pulmonary Fibrosis. Eur. Respir. J. 48 (6), 1721–1731. doi:10.1183/13993003.02115-2015
Bouros, D., Antoniou, K. M., Tzouvelekis, A., and Siafakas, N. M. (2006). Interferon-gamma 1b for the Treatment of Idiopathic Pulmonary Fibrosis. Expert Opin. Biol. Ther. 6 (10), 1051–1060. doi:10.1517/14712598.6.10.1051
Bratcher, P. E., Weathington, N. M., Nick, H. J., Jackson, P. L., Snelgrove, R. J., and Gaggar, A. (2012). MMP-9 Cleaves SP-D and Abrogates its Innate Immune Functions In Vitro. PLoS One 7 (7), e41881. doi:10.1371/journal.pone.0041881
Bullard, J. E., and Nogee, L. M. (2007). Heterozygosity for ABCA3 Mutations Modifies the Severity of Lung Disease Associated with a Surfactant Protein C Gene (SFTPC) Mutation. Pediatr. Res. 62 (2), 176–179. doi:10.1203/PDR.0b013e3180a72588
Burgess, J. K., and Weckmann, M. (2012). Matrikines and the Lungs. Pharmacol. Ther. 134 (3), 317–337. doi:10.1016/j.pharmthera.2012.02.002
Burman, A., Tanjore, H., and Blackwell, T. S. (2018). Endoplasmic Reticulum Stress in Pulmonary Fibrosis. Matrix Biol. 68-69, 355–365. doi:10.1016/j.matbio.2018.03.015
Cassidy, L. D., and Narita, M. (2022). Autophagy at the Intersection of Aging, Senescence, and Cancer. Mol. Oncol. doi:10.1002/1878-0261.13269
Castaño, A. P., Lin, S. L., Surowy, T., Nowlin, B. T., Turlapati, S. A., Patel, T., et al. (2009). Serum Amyloid P Inhibits Fibrosis through Fc Gamma R-dependent Monocyte-Macrophage Regulation In Vivo. Sci. Transl. Med. 1 (5), 5ra13. doi:10.1126/scitranslmed.3000111
Cedeno-Laurent, F., and Dimitroff, C. J. (2012). Galectin-1 Research in T Cell Immunity: Past, Present and Future. Clin. Immunol. 142 (2), 107–116. doi:10.1016/j.clim.2011.09.011
Chambers, D. C., Lutzky, V. P., Apte, S. H., Godbolt, D., Feenstra, J., and Mackintosh, J. (2020). Successful Treatment of Telomeropathy-Related Interstitial Lung Disease with Immunosuppression and Danazol. Respirol. Case Rep. 8 (6), e00607. doi:10.1002/rcr2.607
Chanda, D., Otoupalova, E., Smith, S. R., Volckaert, T., De Langhe, S. P., and Thannickal, V. J. (2019). Developmental Pathways in the Pathogenesis of Lung Fibrosis. Mol. Asp. Med. 65, 56–69. doi:10.1016/j.mam.2018.08.004
Chen, H., Qu, J., Huang, X., Kurundkar, A., Zhu, L., Yang, N., et al. (2016). Mechanosensing by the α6-integrin Confers an Invasive Fibroblast Phenotype and Mediates Lung Fibrosis. Nat. Commun. 7, 12564. doi:10.1038/ncomms12564
Chen, L., Li, S., and Li, W. (2019). LOX/LOXL in Pulmonary Fibrosis: Potential Therapeutic Targets. J. Drug Target 27 (7), 790–796. doi:10.1080/1061186x.2018.1550649
Chen, R., Zhang, K., Chen, H., Zhao, X., Wang, J., Li, L., et al. (2015). Telomerase deficiency causes alveolar stem cell senescence-Associated low-Grade inflammation in lungs. J. Biol. Chem. 290 (52), 30813–30829. doi:10.1074/jbc.M115.681619
Chen, Y., Pu, Q., Ma, Y., Zhang, H., Ye, T., Zhao, C., et al. (2021). Aging reprograms the hematopoietic-vascular niche to impede regeneration and promote fibrosis. Cell. Metab. 33 (2), 395–e4. e394. doi:10.1016/j.cmet.2020.11.019
Cheong, N., Madesh, M., Gonzales, L. W., Zhao, M., Yu, K., Ballard, P. L., et al. (2006). Functional and Trafficking Defects in ATP Binding Cassette A3 Mutants Associated with Respiratory Distress Syndrome. J. Biol. Chem. 281 (14), 9791–9800. doi:10.1074/jbc.M507515200
Cheong, N., Zhang, H., Madesh, M., Zhao, M., Yu, K., Dodia, C., et al. (2007). ABCA3 Is Critical for Lamellar Body Biogenesis In Vivo. J. Biol. Chem. 282 (33), 23811–23817. doi:10.1074/jbc.M703927200
Cho, S. J., and Stout-Delgado, H. W. (2020). Aging and lung disease. Annu. Rev. Physiol. 82, 433–459. doi:10.1146/annurev-physiol-021119-034610
Chong, S. G., Sato, S., Kolb, M., and Gauldie, J. (2019). Fibrocytes and Fibroblasts-Where Are We Now. Int. J. Biochem. Cell. Biol. 116, 105595. doi:10.1016/j.biocel.2019.105595
Chu, K. A., Yeh, C. C., Kuo, F. H., Lin, W. R., Hsu, C. W., Chen, T. H., et al. (2020). Comparison of Reversal of Rat Pulmonary Fibrosis of Nintedanib, Pirfenidone, and Human Umbilical Mesenchymal Stem Cells from Wharton's Jelly. Stem Cell. Res. Ther. 11 (1), 513. doi:10.1186/s13287-020-02012-y
Clayton, A. L., Hebbes, T. R., Thorne, A. W., and Crane-Robinson, C. (1993). Histone Acetylation and Gene Induction in Human Cells. FEBS Lett. 336 (1), 23–26. doi:10.1016/0014-5793(93)81601-u
Cogan, J. D., Kropski, J. A., Zhao, M., Mitchell, D. B., Rives, L., Markin, C., et al. (2015). Rare Variants in RTEL1 Are Associated with Familial Interstitial Pneumonia. Am. J. Respir. Crit. Care Med. 191 (6), 646–655. doi:10.1164/rccm.201408-1510OC
Conforti, F., Davies, E. R., Calderwood, C. J., Thatcher, T. H., Jones, M. G., Smart, D. E., et al. (2017). The Histone Deacetylase Inhibitor, Romidepsin, as a Potential Treatment for Pulmonary Fibrosis. Oncotarget 8 (30), 48737–48754. doi:10.18632/oncotarget.17114
Conti, C., Montero-Fernandez, A., Borg, E., Osadolor, T., Viola, P., De Lauretis, A., et al. (2016). Mucins MUC5B and MUC5AC in distal airways and honeycomb spaces: Comparison Among idiopathic pulmonary fibrosis/usual interstitial pneumonia, fibrotic nonspecific interstitial pneumonitis, and control lungs. Am. J. Respir. Crit. Care Med. 193 (4), 462–464. doi:10.1164/rccm.201507-1322LE
Corapi, E., Carrizo, G., Compagno, D., and Laderach, D. (2018). Endogenous galectin-1 in T lymphocytes regulates anti-prostate cancer immunity. Front. Immunol. 9, 2190. doi:10.3389/fimmu.2018.02190
Coward, W. R., Feghali-Bostwick, C. A., Jenkins, G., Knox, A. J., and Pang, L. (2014). A Central Role for G9a and EZH2 in the Epigenetic Silencing of Cyclooxygenase-2 in Idiopathic Pulmonary Fibrosis. Faseb J. 28 (7), 3183–3196. doi:10.1096/fj.13-241760
Coward, W. R., Watts, K., Feghali-Bostwick, C. A., Jenkins, G., and Pang, L. (2010). Repression of IP-10 by Interactions between Histone Deacetylation and Hypermethylation in Idiopathic Pulmonary Fibrosis. Mol. Cell. Biol. 30 (12), 2874–2886. doi:10.1128/mcb.01527-09
Coward, W. R., Watts, K., Feghali-Bostwick, C. A., Knox, A., and Pang, L. (2009). Defective Histone Acetylation Is Responsible for the Diminished Expression of Cyclooxygenase 2 in Idiopathic Pulmonary Fibrosis. Mol. Cell. Biol. 29 (15), 4325–4339. doi:10.1128/mcb.01776-08
Craig, V. J., Quintero, P. A., Fyfe, S. E., Patel, A. S., Knolle, M. D., Kobzik, L., et al. (2013). Profibrotic Activities for Matrix Metalloproteinase-8 during Bleomycin-Mediated Lung Injury. J. Immunol. 190 (8), 4283–4296. doi:10.4049/jimmunol.1201043
Crossno, P. F., Polosukhin, V. V., Blackwell, T. S., Johnson, J. E., Markin, C., Moore, P. E., et al. (2010). Identification of Early Interstitial Lung Disease in an Individual with Genetic Variations in ABCA3 and SFTPC. Chest 137 (4), 969–973. doi:10.1378/chest.09-0790
Cushing, L., Kuang, P., and Lü, J. (2015). The Role of miR-29 in Pulmonary Fibrosis. Biochem. Cell. Biol. 93 (2), 109–118. doi:10.1139/bcb-2014-0095
Dakhlallah, D., Batte, K., Wang, Y., Cantemir-Stone, C. Z., Yan, P., Nuovo, G., et al. (2013). Epigenetic Regulation of miR-17∼92 Contributes to the Pathogenesis of Pulmonary Fibrosis. Am. J. Respir. Crit. Care Med. 187 (4), 397–405. doi:10.1164/rccm.201205-0888OC
Davies, E. R., Haitchi, H. M., Thatcher, T. H., Sime, P. J., Kottmann, R. M., Ganesan, A., et al. (2012). Spiruchostatin A Inhibits Proliferation and Differentiation of Fibroblasts from Patients with Pulmonary Fibrosis. Am. J. Respir. Cell. Mol. Biol. 46 (5), 687–694. doi:10.1165/rcmb.2011-0040OC
Degryse, A. L., Xu, X. C., Newman, J. L., Mitchell, D. B., Tanjore, H., Polosukhin, V. V., et al. (2012). Telomerase Deficiency Does Not Alter Bleomycin-Induced Fibrosis in Mice. Exp. Lung Res. 38 (3), 124–134. doi:10.3109/01902148.2012.658148
Deshmane, S. L., Kremlev, S., Amini, S., and Sawaya, B. E. (2009). Monocyte Chemoattractant Protein-1 (MCP-1): An Overview. J. Interferon Cytokine Res. 29 (6), 313–326. doi:10.1089/jir.2008.0027
Effendi, W. I., and Nagano, T. (2021). The Hedgehog signaling pathway in idiopathic pulmonary fibrosis: Resurrection time. Int. J. Mol. Sci. 23 (1), 171. doi:10.3390/ijms23010171
Elowsson Rendin, L., Löfdahl, A., Åhrman, E., Müller, C., Notermans, T., Michaliková, B., et al. (2019). Matrisome properties of scaffolds direct fibroblasts in idiopathic pulmonary fibrosis. Int. J. Mol. Sci. 20 (16), 4013. doi:10.3390/ijms20164013
Evans, C. M., Fingerlin, T. E., Schwarz, M. I., Lynch, D., Kurche, J., Warg, L., et al. (2016). Idiopathic pulmonary fibrosis: A genetic disease that involves mucociliary dysfunction of the peripheral airways. Physiol. Rev. 96 (4), 1567–1591. doi:10.1152/physrev.00004.2016
Filer, A., and Buckley, C. D. (2013). Fibroblasts and fibroblast-like synoviocytes, 215–231. doi:10.1016/b978-1-4377-1738-9.00015-3
Filippakopoulos, P., Qi, J., Picaud, S., Shen, Y., Smith, W. B., Fedorov, O., et al. (2010). Selective Inhibition of BET Bromodomains. Nature 468 (7327), 1067–1073. doi:10.1038/nature09504
Fingerlin, T. E., Murphy, E., Zhang, W., Peljto, A. L., Brown, K. K., Steele, M. P., et al. (2013). Genome-wide Association Study Identifies Multiple Susceptibility Loci for Pulmonary Fibrosis. Nat. Genet. 45 (6), 613–620. doi:10.1038/ng.2609
Fitzgerald, M. L., Xavier, R., Haley, K. J., Welti, R., Goss, J. L., Brown, C. E., et al. (2007). ABCA3 Inactivation in Mice Causes Respiratory Failure, Loss of Pulmonary Surfactant, and Depletion of Lung Phosphatidylglycerol. J. Lipid Res. 48 (3), 621–632. doi:10.1194/jlr.M600449-JLR200
Fukuhara, A., Tanino, Y., Ishii, T., Inokoshi, Y., Saito, K., Fukuhara, N., et al. (2013). Pulmonary Fibrosis in Dyskeratosis Congenita with TINF2 Gene Mutation. Eur. Respir. J. 42 (6), 1757–1759. doi:10.1183/09031936.00149113
Gagnon, L., Leduc, M., Thibodeau, J. F., Zhang, M. Z., Grouix, B., Sarra-Bournet, F., et al. (2018). A newly discovered antifibrotic pathway regulated by two fatty acid receptors: GPR40 and GPR84. Am. J. Pathol. 188 (5), 1132–1148. doi:10.1016/j.ajpath.2018.01.009
Gan, C., Zhang, Q., Liu, H., Wang, G., Wang, L., Li, Y., et al. (2022). Nifuroxazide Ameliorates Pulmonary Fibrosis by Blocking Myofibroblast Genesis: A Drug Repurposing Study. Respir. Res. 23 (1), 32. doi:10.1186/s12931-022-01946-6
García-Prieto, E., González-López, A., Cabrera, S., Astudillo, A., Gutiérrez-Fernández, A., Fanjul-Fernandez, M., et al. (2010). Resistance to Bleomycin-Induced Lung Fibrosis in MMP-8 Deficient Mice Is Mediated by Interleukin-10. PLoS One 5 (10), e13242. doi:10.1371/journal.pone.0013242
Gaysinskaya, V., Stanley, S. E., Adam, S., and Armanios, M. (2020). Synonymous mutation in DKC1 causes telomerase RNA insufficiency manifesting as familial pulmonary fibrosis. Chest 158 (6), 2449–2457. doi:10.1016/j.chest.2020.07.025
Ghavami, S., Yeganeh, B., Zeki, A. A., Shojaei, S., Kenyon, N. J., Ott, S., et al. (2018). Autophagy and the Unfolded Protein Response Promote Profibrotic Effects of TGF-Β1 in Human Lung Fibroblasts. Am. J. Physiol. Cell. Mol. Physiol. 314 (3), L493–l504. doi:10.1152/ajplung.00372.2017
Giri, S. N. (2003). Novel Pharmacological Approaches to Manage Interstitial Lung Fibrosis in the Twenty-First Century. Annu. Rev. Pharmacol. Toxicol. 43, 73–95. doi:10.1146/annurev.pharmtox.43.100901.135740
Gjorevski, N., Boghaert, E., and Nelson, C. M. (2012). Regulation of epithelial-mesenchymal transition by transmission of mechanical stress through epithelial tissues. Cancer Microenviron. 5 (1), 29–38. doi:10.1007/s12307-011-0076-5
Glass, D. S., Grossfeld, D., Renna, H. A., Agarwala, P., Spiegler, P., Kasselman, L. J., et al. (2020). Idiopathic Pulmonary Fibrosis: Molecular Mechanisms and Potential Treatment Approaches. Respir. Investig. 58 (5), 320–335. doi:10.1016/j.resinv.2020.04.002
Guenther, K., Seeger, W., Guenther, A., and Korfei, M. (2019). Cellular Consequences of Uncharacterized Surfactant Protein-A2 (SFTPA2)-Gene Mutations Associated with Familial IPF and Lung Cancer. J Eur. Respir. J. 54 (Suppl. 63), OA1608. doi:10.1183/13993003.congress-2019.OA1608
Guo, W., Shan, B., Klingsberg, R. C., Qin, X., and Lasky, J. A. (2009). Abrogation of TGF-Beta1-Induced Fibroblast-Myofibroblast Differentiation by Histone Deacetylase Inhibition. Am. J. Physiol. Cell. Mol. Physiol. 297 (5), L864–L870. doi:10.1152/ajplung.00128.2009
Hams, E., Armstrong, M. E., Barlow, J. L., Saunders, S. P., Schwartz, C., Cooke, G., et al. (2014). IL-25 and Type 2 Innate Lymphoid Cells Induce Pulmonary Fibrosis. Proc. Natl. Acad. Sci. U. S. A. 111 (1), 367–372. doi:10.1073/pnas.1315854111
Hayakawa, T., Iwai, M., Aoki, S., Takimoto, K., Maruyama, M., Maruyama, W., et al. (2015). SIRT1 Suppresses the Senescence-Associated Secretory Phenotype through Epigenetic Gene Regulation. PLoS One 10 (1), e0116480. doi:10.1371/journal.pone.0116480
Hennessy, B. T., Smith, D. L., Ram, P. T., Lu, Y., and Mills, G. B. (2005). Exploiting the PI3K/AKT Pathway for Cancer Drug Discovery. Nat. Rev. Drug Discov. 4 (12), 988–1004. doi:10.1038/nrd1902
Herrera, I., Cisneros, J., Maldonado, M., Ramírez, R., Ortiz-Quintero, B., Anso, E., et al. (2013). Matrix Metalloproteinase (MMP)-1 Induces Lung Alveolar Epithelial Cell Migration and Proliferation, Protects from Apoptosis, and Represses Mitochondrial Oxygen Consumption. J. Biol. Chem. 288 (36), 25964–25975. doi:10.1074/jbc.M113.459784
Heukels, P., Moor, C. C., von der Thüsen, J. H., Wijsenbeek, M. S., and Kool, M. (2019). Inflammation and Immunity in IPF Pathogenesis and Treatment. Respir. Med. 147, 79–91. doi:10.1016/j.rmed.2018.12.015
Hill, C., Jones, M. G., Davies, D. E., and Wang, Y. (2019). Epithelial-mesenchymal Transition Contributes to Pulmonary Fibrosis via Aberrant Epithelial/fibroblastic Cross-Talk. J. Health Dis. 3 (2), 31–35. doi:10.29245/2689-999x/2019/2.1149
Hirano, C., Ohshimo, S., Horimasu, Y., Iwamoto, H., Fujitaka, K., Hamada, H., et al. (2017). FAM13A Polymorphism as a Prognostic Factor in Patients with Idiopathic Pulmonary Fibrosis. Respir. Med. 123, 105–109. doi:10.1016/j.rmed.2016.12.007
Hoffman, T. W., van Moorsel, C. H. M., Borie, R., and Crestani, B. (2018). Pulmonary Phenotypes Associated with Genetic Variation in Telomere-Related Genes. Curr. Opin. Pulm. Med. 24 (3), 269–280. doi:10.1097/mcp.0000000000000475
Hu, M., Che, P., Han, X., Cai, G. Q., Liu, G., Antony, V., et al. (2014). Therapeutic Targeting of SRC Kinase in Myofibroblast Differentiation and Pulmonary Fibrosis. J. Pharmacol. Exp. Ther. 351 (1), 87–95. doi:10.1124/jpet.114.216044
Huang, L. S., Sudhadevi, T., Fu, P., Punathil-Kannan, P. K., Ebenezer, D. L., Ramchandran, R., et al. (2020). Sphingosine kinase 1/S1P signaling contributes to pulmonary fibrosis by activating hippo/YAP pathway and mitochondrial reactive oxygen species in lung fibroblasts. Int. J. Mol. Sci. 21 (6), 2064. doi:10.3390/ijms21062064
Huang, S. K., Fisher, A. S., Scruggs, A. M., White, E. S., Hogaboam, C. M., Richardson, B. C., et al. (2010). Hypermethylation of PTGER2 Confers Prostaglandin E2 Resistance in Fibrotic Fibroblasts from Humans and Mice. Am. J. Pathol. 177 (5), 2245–2255. doi:10.2353/ajpath.2010.100446
Huang, S. K., Scruggs, A. M., Donaghy, J., Horowitz, J. C., Zaslona, Z., Przybranowski, S., et al. (2013). Histone Modifications Are Responsible for Decreased Fas Expression and Apoptosis Resistance in Fibrotic Lung Fibroblasts. Cell. Death Dis. 4 (5), e621. doi:10.1038/cddis.2013.146
Huang, S. K., Scruggs, A. M., McEachin, R. C., White, E. S., and Peters-Golden, M. (2014). Lung Fibroblasts from Patients with Idiopathic Pulmonary Fibrosis Exhibit Genome-wide Differences in DNA Methylation Compared to Fibroblasts from Nonfibrotic Lung. PLoS One 9 (9), e107055. doi:10.1371/journal.pone.0107055
Humphries, D. C., Mills, R., Dobie, R., Henderson, N. C., Sethi, T., and Mackinnon, A. C. (2021). Selective myeloid depletion of galectin-3 offers protection against acute and chronic lung injury. Front. Pharmacol. 12, 715986. doi:10.3389/fphar.2021.715986
Hwang, S. Y., Park, S. Y., Hong, J. Y., Lee, S. Y., Shin, J. H., Na, Y., et al. (2020). Field-based Rational Design of P300 Histone Acetyltransferase Inhibitor and Systematic Evaluation as an Anti-fibrotic Agent. Chem. Commun. (Camb) 56 (68), 9795–9798. doi:10.1039/d0cc03553j
Iyonaga, K., Takeya, M., Saita, N., Sakamoto, O., Yoshimura, T., Ando, M., et al. (1994). Monocyte Chemoattractant Protein-1 in Idiopathic Pulmonary Fibrosis and Other Interstitial Lung Diseases. Hum. Pathol. 25 (5), 455–463. doi:10.1016/0046-8177(94)90117-1
Jackson, S. R., Lee, J., Reddy, R., Williams, G. N., Kikuchi, A., Freiberg, Y., et al. (2011). Partial Pneumonectomy of Telomerase Null Mice Carrying Shortened Telomeres Initiates Cell Growth Arrest Resulting in a Limited Compensatory Growth Response. Am. J. Physiol. Lung Cell. Mol. Physiol. 300 (6), L898–L909. doi:10.1152/ajplung.00409.2010
Jara, P., Calyeca, J., Romero, Y., Plácido, L., Yu, G., Kaminski, N., et al. (2015). Matrix Metalloproteinase (MMP)-19-deficient Fibroblasts Display a Profibrotic Phenotype. Am. J. Physiol. Lung Cell. Mol. Physiol. 308 (6), L511–L522. doi:10.1152/ajplung.00043.2014
Jenuwein, T., and Allis, C. D. (2001). Translating the Histone Code. Science 293 (5532), 1074–1080. doi:10.1126/science.1063127
Jessen, H., Hoyer, N., Prior, T. S., Frederiksen, P., Karsdal, M. A., Leeming, D. J., et al. (2021). Turnover of Type I and III Collagen Predicts Progression of Idiopathic Pulmonary Fibrosis. Respir. Res. 22 (1), 205. doi:10.1186/s12931-021-01801-0
Justet, A., Thabut, G., Manali, E., Molina Molina, M., Kannengiesser, C., Cadranel, J., et al. (2018). Safety and Efficacy of Pirfenidone in Patients Carrying Telomerase Complex Mutation. Eur. Respir. J. 51 (3), 1701875. doi:10.1183/13993003.01875-2017
Justice, J. N., Nambiar, A. M., Tchkonia, T., LeBrasseur, N. K., Pascual, R., Hashmi, S. K., et al. (2019). Senolytics in Idiopathic Pulmonary Fibrosis: Results from a First-In-Human, Open-Label, Pilot Study. EBioMedicine 40, 554–563. doi:10.1016/j.ebiom.2018.12.052
Kage, H., and Borok, Z. (2012). EMT and Interstitial Lung Disease: A Mysterious Relationship. Curr. Opin. Pulm. Med. 18 (5), 517–523. doi:10.1097/MCP.0b013e3283566721
Kahloon, R. A., Xue, J., Bhargava, A., Csizmadia, E., Otterbein, L., Kass, D. J., et al. (2013). Patients with Idiopathic Pulmonary Fibrosis with Antibodies to Heat Shock Protein 70 Have Poor Prognoses. Am. J. Respir. Crit. Care Med. 187 (7), 768–775. doi:10.1164/rccm.201203-0506OC
Kai, Y., Tomoda, K., Yoneyama, H., Kitabatake, M., Nakamura, A., Ito, T., et al. (2017). Silencing of carbohydrate sulfotransferase 15 hinders murine pulmonary fibrosis development. Mol. Ther. Nucleic Acids 6, 163–172. doi:10.1016/j.omtn.2016.12.008
Kaliński, P., Hilkens, C. M., Snijders, A., Snijdewint, F. G., and Kapsenberg, M. L. (1997). IL-12-deficient Dendritic Cells, Generated in the Presence of Prostaglandin E2, Promote Type 2 Cytokine Production in Maturing Human Naive T Helper Cells. J. Immunol. 159 (1), 28–35.
Kamio, K., Azuma, A., Usuki, J., Matsuda, K., Inomata, M., Nishijima, N., et al. (2017). XPLN Is Modulated by HDAC Inhibitors and Negatively Regulates SPARC Expression by Targeting mTORC2 in Human Lung Fibroblasts. Pulm. Pharmacol. Ther. 44, 61–69. doi:10.1016/j.pupt.2017.03.003
Kasper, M., and Hughes, R. C. (1996). Immunocytochemical Evidence for a Modulation of Galectin 3 (Mac-2), a Carbohydrate Binding Protein, in Pulmonary Fibrosis. J. Pathol. 179 (3), 309–316. doi:10.1002/(SICI)1096-9896(199607)179:3<309:AID-PATH572>3.0.CO;2-D
Kim, Y. S., Cha, H., Kim, H. J., Cho, J. M., and Kim, H. R. (2019). The anti-fibrotic effects of CG-745, an HDAC inhibitor, in bleomycin and PHMG-induced mouse models. Molecules 24 (15), 2792. doi:10.3390/molecules24152792
King, T. E., Pardo, A., and Selman, M. (2011). Idiopathic Pulmonary Fibrosis. Lancet 378 (9807), 1949–1961. doi:10.1016/s0140-6736(11)60052-4
Korfei, M., Ruppert, C., Mahavadi, P., Henneke, I., Markart, P., Koch, M., et al. (2008). Epithelial Endoplasmic Reticulum Stress and Apoptosis in Sporadic Idiopathic Pulmonary Fibrosis. Am. J. Respir. Crit. Care Med. 178 (8), 838–846. doi:10.1164/rccm.200802-313OC
Korfei, M., Skwarna, S., Henneke, I., MacKenzie, B., Klymenko, O., Saito, S., et al. (2015). Aberrant Expression and Activity of Histone Deacetylases in Sporadic Idiopathic Pulmonary Fibrosis. Thorax 70 (11), 1022–1032. doi:10.1136/thoraxjnl-2014-206411
Kouzarides, T. (2007). Chromatin Modifications and Their Function. Cell. 128 (4), 693–705. doi:10.1016/j.cell.2007.02.005
Kreus, M., Lehtonen, S., Skarp, S., and Kaarteenaho, R. (2021). Extracellular Matrix Proteins Produced by Stromal Cells in Idiopathic Pulmonary Fibrosis and Lung Adenocarcinoma. PLoS One 16 (4), e0250109. doi:10.1371/journal.pone.0250109
Kropski, J. A., Young, L. R., Cogan, J. D., Mitchell, D. B., Lancaster, L. H., Worrell, J. A., et al. (2017). Genetic evaluation and testing of patients and families with idiopathic pulmonary fibrosis. Am. J. Respir. Crit. Care Med. 195 (11), 1423–1428. doi:10.1164/rccm.201609-1820PP
Kurosu, K., Takiguchi, Y., Okada, O., Yumoto, N., Sakao, S., Tada, Y., et al. (2008). Identification of Annexin 1 as a Novel Autoantigen in Acute Exacerbation of Idiopathic Pulmonary Fibrosis. J. Immunol. 181 (1), 756–767. doi:10.4049/jimmunol.181.1.756
Kuse, N., Kamio, K., Azuma, A., Matsuda, K., Inomata, M., Usuki, J., et al. (2020). Exosome-derived microRNA-22 ameliorates pulmonary fibrosis by regulating fibroblast-To-myofibroblast differentiation In vitro and In vivo. J. Nippon. Med. Sch. 87 (3), 118–128. doi:10.1272/jnms.JNMS.2020_87-302
Lam, A. P., Herazo-Maya, J. D., Sennello, J. A., Flozak, A. S., Russell, S., Mutlu, G. M., et al. (2014). Wnt Coreceptor Lrp5 Is a Driver of Idiopathic Pulmonary Fibrosis. Am. J. Respir. Crit. Care Med. 190 (2), 185–195. doi:10.1164/rccm.201401-0079OC
Lama, V. N., and Phan, S. H. (2006). The Extrapulmonary Origin of Fibroblasts: Stem/progenitor Cells and beyond. Proc. Am. Thorac. Soc. 3 (4), 373–376. doi:10.1513/pats.200512-133TK
Lawrence, J., and Nho, R. (2018). The role of the mammalian target of rapamycin (mTOR) in pulmonary fibrosis. Int. J. Mol. Sci. 19 (3), 778. doi:10.3390/ijms19030778
Lawson, W. E., Crossno, P. F., Polosukhin, V. V., Roldan, J., Cheng, D. S., Lane, K. B., et al. (2008). Endoplasmic Reticulum Stress in Alveolar Epithelial Cells Is Prominent in IPF: Association with Altered Surfactant Protein Processing and Herpesvirus Infection. Am. J. Physiol. Lung Cell. Mol. Physiol. 294 (6), L1119–L1126. doi:10.1152/ajplung.00382.2007
Le Saux, C. J., Davy, P., Brampton, C., Ahuja, S. S., Fauce, S., Shivshankar, P., et al. (2013). A Novel Telomerase Activator Suppresses Lung Damage in a Murine Model of Idiopathic Pulmonary Fibrosis. PLoS One. 8 (3), e58423. doi:10.1371/journal.pone.0058423
Lederer, D. J., and Martinez, F. J. (2018). Idiopathic pulmonary fibrosis. N. Engl. J. Med. 378 (19), 1811–1823. doi:10.1056/NEJMra1705751
Ley, B., Newton, C. A., Arnould, I., Elicker, B. M., Henry, T. S., Vittinghoff, E., et al. (2017). The MUC5B Promoter Polymorphism and Telomere Length in Patients with Chronic Hypersensitivity Pneumonitis: An Observational Cohort-Control Study. Lancet Respir. Med. 5 (8), 639–647. doi:10.1016/s2213-2600(17)30216-3
Li, G., Jin, F., Du, J., He, Q., Yang, B., and Luo, P. (2019). Macrophage-secreted TSLP and MMP9 Promote Bleomycin-Induced Pulmonary Fibrosis. Toxicol. Appl. Pharmacol. 366, 10–16. doi:10.1016/j.taap.2019.01.011
Lipson, K. E., Wong, C., Teng, Y., and Spong, S. (2012). CTGF Is a Central Mediator of Tissue Remodeling and Fibrosis and its Inhibition Can Reverse the Process of Fibrosis. Fibrogenes. Tissue Repair 5 (Suppl. 1), S24. doi:10.1186/1755-1536-5-s1-s24
Lister-Shimauchi, E. H., McCarthy, B., Lippincott, M., and Ahmed, S. (2022). Genetic and epigenetic inheritance at telomeres. Epigenomes 6 (1), 9. doi:10.3390/epigenomes6010009
Liu, G., Zhai, H., Zhang, T., Li, S., Li, N., Chen, J., et al. (2019a). New Therapeutic Strategies for IPF: Based on the "Phagocytosis-Secretion-Immunization" Network Regulation Mechanism of Pulmonary Macrophages. Biomed. Pharmacother. 118, 109230. doi:10.1016/j.biopha.2019.109230
Liu, H., Wu, X., Gan, C., Wang, L., Wang, G., Yue, L., et al. (2021). A Novel Multikinase Inhibitor SKLB-YTH-60 Ameliorates Inflammation and Fibrosis in Bleomycin-Induced Lung Fibrosis Mouse Models. Cell. Prolif. 54 (7), e13081. doi:10.1111/cpr.13081
Liu, L., Yin, H., Huang, M., He, J., Yi, G., Wang, Z., et al. (2016). miR-21 Promotes Pulmonary Fibrosis in Rats via Down-Regulating the Expression of ADAMTS-1. Xi Bao Yu Fen Zi Mian Yi Xue Za Zhi 32 (12), 1636–1640.
Liu, R. M., and Liu, G. (2020). Cell Senescence and Fibrotic Lung Diseases. Exp. Gerontol. 132, 110836. doi:10.1016/j.exger.2020.110836
Liu, T., Gonzalez De Los Santos, F., Zhao, Y., Wu, Z., Rinke, A. E., Kim, K. K., et al. (2019b). Telomerase Reverse Transcriptase Ameliorates Lung Fibrosis by Protecting Alveolar Epithelial Cells against Senescence. J. Biol. Chem. 294 (22), 8861–8871. doi:10.1074/jbc.RA118.006615
Liu, T., Yu, H., Ding, L., Wu, Z., Gonzalez De Los Santos, F., Liu, J., et al. (2015). Conditional knockout of telomerase reverse transcriptase in mesenchymal cells impairs mouse pulmonary fibrosis. PLoS One 10 (11), e0142547. doi:10.1371/journal.pone.0142547
Liu, Y. Y., Shi, Y., Liu, Y., Pan, X. H., and Zhang, K. X. (2018). Telomere Shortening Activates TGF-β/Smads Signaling in Lungs and Enhances Both Lipopolysaccharide and Bleomycin-Induced Pulmonary Fibrosis. Acta Pharmacol. Sin. 39 (11), 1735–1745. doi:10.1038/s41401-018-0007-9
Lorenzo-Salazar, J. M., Ma, S. F., Jou, J., Hou, P. C., Guillen-Guio, B., Allen, R. J., et al. (2019). Novel Idiopathic Pulmonary Fibrosis Susceptibility Variants Revealed by Deep Sequencing. ERJ Open Res. 5 (2), 00071–02019. doi:10.1183/23120541.00071-2019
Ma, H., Liu, S., Li, S., and Xia, Y. (2022). Targeting growth factor and cytokine pathways to treat idiopathic pulmonary fibrosis. Front. Pharmacol. 13. doi:10.3389/fphar.2022.918771
Maciel, M., Hernández-Barrientos, D., Herrera, I., Selman, M., Pardo, A., and Cabrera, S. (2018). Impaired Autophagic Activity and ATG4B Deficiency Are Associated with Increased Endoplasmic Reticulum Stress-Induced Lung Injury. Aging (Albany NY) 10 (8), 2098–2112. doi:10.18632/aging.101532
Maitra, M., Wang, Y., Gerard, R. D., Mendelson, C. R., and Garcia, C. K. (2010). Surfactant Protein A2 Mutations Associated with Pulmonary Fibrosis Lead to Protein Instability and Endoplasmic Reticulum Stress. J. Biol. Chem. 285 (29), 22103–22113. doi:10.1074/jbc.M110.121467
Malli, F., Koutsokera, A., Paraskeva, E., Zakynthinos, E., Papagianni, M., Makris, D., et al. (2013). Endothelial Progenitor Cells in the Pathogenesis of Idiopathic Pulmonary Fibrosis: An Evolving Concept. PLoS One 8 (1), e53658. doi:10.1371/journal.pone.0053658
Manali, E. D., Legendre, M., Nathan, N., Kannengiesser, C., Coulomb-L'Hermine, A., Tsiligiannis, T., et al. (2019). Bi-allelic Missense ABCA3 Mutations in a Patient with Childhood ILD Who Reached Adulthood. ERJ Open Res. 5 (3), 00066–02019. doi:10.1183/23120541.00066-2019
Manicone, A. M., Huizar, I., and McGuire, J. K. (2009). Matrilysin (Matrix Metalloproteinase-7) Regulates Anti-inflammatory and Antifibrotic Pulmonary Dendritic Cells that Express CD103 (alpha(E)beta(7)-integrin). Am. J. Pathol. 175 (6), 2319–2331. doi:10.2353/ajpath.2009.090101
Margaritopoulos, G. A., Lasithiotaki, I., and Antoniou, K. M. (2017). Toll-like Receptors and Autophagy in Interstitial Lung Diseases. Eur. J. Pharmacol. 808, 28–34. doi:10.1016/j.ejphar.2016.09.032
Martinez, F. O., Sica, A., Mantovani, A., and Locati, M. (2008). Macrophage Activation and Polarization. Front. Biosci. 13, 453–461. doi:10.2741/2692
Mathai, S. K., Pedersen, B. S., Smith, K., Russell, P., Schwarz, M. I., Brown, K. K., et al. (2016). Desmoplakin variants are associated with idiopathic pulmonary fibrosis. Am. J. Respir. Crit. Care Med. 193 (10), 1151–1160. doi:10.1164/rccm.201509-1863OC
Matsumura, Y., Ban, N., Ueda, K., and Inagaki, N. (2006). Characterization and Classification of ATP-Binding Cassette Transporter ABCA3 Mutants in Fatal Surfactant Deficiency. J. Biol. Chem. 281 (45), 34503–34514. doi:10.1074/jbc.M600071200
McGuire, J. K., Li, Q., and Parks, W. C. (2003). Matrilysin (Matrix Metalloproteinase-7) Mediates E-Cadherin Ectodomain Shedding in Injured Lung Epithelium. Am. J. Pathol. 162 (6), 1831–1843. doi:10.1016/s0002-9440(10)64318-0
Mehrad, B., Burdick, M. D., Zisman, D. A., Keane, M. P., Belperio, J. A., and Strieter, R. M. (2007). Circulating Peripheral Blood Fibrocytes in Human Fibrotic Interstitial Lung Disease. Biochem. Biophys. Res. Commun. 353 (1), 104–108. doi:10.1016/j.bbrc.2006.11.149
Mercer, P. F., Woodcock, H. V., Eley, J. D., Platé, M., Sulikowski, M. G., Durrenberger, P. F., et al. (2016). Exploration of a Potent PI3 kinase/mTOR Inhibitor as a Novel Anti-fibrotic Agent in IPF. Thorax 71 (8), 701–711. doi:10.1136/thoraxjnl-2015-207429
Merkt, W., Bueno, M., Mora, A. L., and Lagares, D. (2020). Senotherapeutics: Targeting Senescence in Idiopathic Pulmonary Fibrosis. Semin. Cell. Dev. Biol. 101, 104–110. doi:10.1016/j.semcdb.2019.12.008
Montero, P., Milara, J., Roger, I., and Cortijo, J. (2021). Role of JAK/STAT in interstitial lung diseases; molecular and cellular mechanisms. Int. J. Mol. Sci. 22 (12), 6211. doi:10.3390/ijms22126211
Mori, L., Bellini, A., Stacey, M. A., Schmidt, M., and Mattoli, S. (2005). Fibrocytes Contribute to the Myofibroblast Population in Wounded Skin and Originate from the Bone Marrow. Exp. Cell. Res. 304 (1), 81–90. doi:10.1016/j.yexcr.2004.11.011
Mulugeta, S., Nureki, S., and Beers, M. F. (2015). Lost after Translation: Insights from Pulmonary Surfactant for Understanding the Role of Alveolar Epithelial Dysfunction and Cellular Quality Control in Fibrotic Lung Disease. Am. J. Physiol. Lung Cell. Mol. Physiol. 309 (6), L507–L525. doi:10.1152/ajplung.00139.2015
Munger, J. S., Huang, X., Kawakatsu, H., Griffiths, M. J., Dalton, S. L., Wu, J., et al. (1999). The Integrin Alpha V Beta 6 Binds and Activates Latent TGF Beta 1: A Mechanism for Regulating Pulmonary Inflammation and Fibrosis. Cell. 96 (3), 319–328. doi:10.1016/s0092-8674(00)80545-0
Murray, M. Y., Birkland, T. P., Howe, J. D., Rowan, A. D., Fidock, M., Parks, W. C., et al. (2013). Macrophage Migration and Invasion Is Regulated by MMP10 Expression. PLoS One 8 (5), e63555. doi:10.1371/journal.pone.0063555
Murthy, S., Ryan, A., He, C., Mallampalli, R. K., and Carter, A. B. (2010). Rac1-mediated Mitochondrial H2O2 Generation Regulates MMP-9 Gene Expression in Macrophages via Inhibition of SP-1 and AP-1. J. Biol. Chem. 285 (32), 25062–25073. doi:10.1074/jbc.M109.099655
Nagpal, N., Wang, J., Zeng, J., Lo, E., Moon, D. H., Luk, K., et al. (2020). Small-Molecule PAPD5 Inhibitors Restore Telomerase Activity in Patient Stem Cells. Cell Stem Cell 26 (6), 896–909.e898. doi:10.1016/j.stem.2020.03.016
Naikawadi, R. P., Disayabutr, S., Mallavia, B., Donne, M. L., Green, G., La, J. L., et al. (2016). Telomere Dysfunction in Alveolar Epithelial Cells Causes Lung Remodeling and Fibrosis. JCI Insight 1 (14), e86704. doi:10.1172/jci.insight.86704
Newton, C. A., Batra, K., Torrealba, J., Kozlitina, J., Glazer, C. S., Aravena, C., et al. (2016). Telomere-related Lung Fibrosis Is Diagnostically Heterogeneous but Uniformly Progressive. Eur. Respir. J. 48 (6), 1710–1720. doi:10.1183/13993003.00308-2016
Nguyen, M., Arkell, J., and Jackson, C. J. (2001). Human Endothelial Gelatinases and Angiogenesis. Int. J. Biochem. Cell. Biol. 33 (10), 960–970. doi:10.1016/s1357-2725(01)00007-3
Nikaido, T., Tanino, Y., Wang, X., Sato, Y., Togawa, R., Kikuchi, M., et al. (2018). Serum Decorin Is a Potential Prognostic Biomarker in Patients with Acute Exacerbation of Idiopathic Pulmonary Fibrosis. J. Thorac. Dis. 10 (9), 5346–5358. doi:10.21037/jtd.2018.08.60
Ninou, I., Magkrioti, C., and Aidinis, V. (2018). Autotaxin in pathophysiology and pulmonary fibrosis. Front. Med. (Lausanne) 5, 180. doi:10.3389/fmed.2018.00180
Noble, P. W., Barkauskas, C. E., and Jiang, D. (2012). Pulmonary Fibrosis: Patterns and Perpetrators. J. Clin. Investig. 122 (8), 2756–2762. doi:10.1172/jci60323
Noth, I., Zhang, Y., Ma, S. F., Flores, C., Barber, M., Huang, Y., et al. (2013). Genetic Variants Associated with Idiopathic Pulmonary Fibrosis Susceptibility and Mortality: A Genome-wide Association Study. Lancet Respir. Med. 1 (4), 309–317. doi:10.1016/s2213-2600(13)70045-6
O'Dwyer, D. N., Armstrong, M. E., Kooblall, M., and Donnelly, S. C. (2015). Targeting Defective Toll-like Receptor-3 Function and Idiopathic Pulmonary Fibrosis. Expert Opin. Ther. Targets 19 (4), 507–514. doi:10.1517/14728222.2014.988706
O'Dwyer, D. N., Armstrong, M. E., Trujillo, G., Cooke, G., Keane, M. P., Fallon, P. G., et al. (2013). The Toll-like Receptor 3 L412F Polymorphism and Disease Progression in Idiopathic Pulmonary Fibrosis. Am. J. Respir. Crit. Care Med. 188 (12), 1442–1450. doi:10.1164/rccm.201304-0760OC
Ogushi, F., Tani, K., Endo, T., Tada, H., Kawano, T., Asano, T., et al. (2001). Autoantibodies to IL-1 Alpha in Sera from Rapidly Progressive Idiopathic Pulmonary Fibrosis. J. Med. Investig. 48 (3-4), 181–189.
Oldham, J. M., Ma, S. F., Martinez, F. J., Anstrom, K. J., Raghu, G., Schwartz, D. A., et al. (2015). TOLLIP, MUC5B, and the response to N-acetylcysteine Among individuals with idiopathic pulmonary fibrosis. Am. J. Respir. Crit. Care Med. 192 (12), 1475–1482. doi:10.1164/rccm.201505-1010OC
Ong, C. H., Tham, C. L., Harith, H. H., Firdaus, N., and Israf, D. A. (2021). TGF-β-induced Fibrosis: A Review on the Underlying Mechanism and Potential Therapeutic Strategies. Eur. J. Pharmacol. 911, 174510. doi:10.1016/j.ejphar.2021.174510
Ono, S., Tanaka, T., Ishida, M., Kinoshita, A., Fukuoka, J., Takaki, M., et al. (2011). Surfactant Protein C G100S Mutation Causes Familial Pulmonary Fibrosis in Japanese Kindred. Eur. Respir. J. 38 (4), 861–869. doi:10.1183/09031936.00143610
Organ, L. A., Duggan, A. R., Oballa, E., Taggart, S. C., Simpson, J. K., Kang'ombe, A. R., et al. (2019). Biomarkers of Collagen Synthesis Predict Progression in the PROFILE Idiopathic Pulmonary Fibrosis Cohort. Respir. Res. 20 (1), 148. doi:10.1186/s12931-019-1118-7
Ortiz, L. A., Dutreil, M., Fattman, C., Pandey, A. C., Torres, G., Go, K., et al. (2007). Interleukin 1 Receptor Antagonist Mediates the Antiinflammatory and Antifibrotic Effect of Mesenchymal Stem Cells during Lung Injury. Proc. Natl. Acad. Sci. U. S. A. 104 (26), 11002–11007. doi:10.1073/pnas.0704421104
Pandit, K. V., Milosevic, J., and Kaminski, N. (2011). MicroRNAs in Idiopathic Pulmonary Fibrosis. Transl. Res. 157 (4), 191–199. doi:10.1016/j.trsl.2011.01.012
Pardo, A., Gibson, K., Cisneros, J., Richards, T. J., Yang, Y., Becerril, C., et al. (2005). Up-regulation and Profibrotic Role of Osteopontin in Human Idiopathic Pulmonary Fibrosis. PLoS Med. 2 (9), e251. doi:10.1371/journal.pmed.0020251
Passalacqua, G., Mincarini, M., Colombo, D., Troisi, G., Ferrari, M., Bagnasco, D., et al. (2017). IL-13 and Idiopathic Pulmonary Fibrosis: Possible Links and New Therapeutic Strategies. Pulm. Pharmacol. Ther. 45, 95–100. doi:10.1016/j.pupt.2017.05.007
Patel, A. S., Lin, L., Geyer, A., Haspel, J. A., An, C. H., Cao, J., et al. (2012). Autophagy in Idiopathic Pulmonary Fibrosis. PLoS One 7 (7), e41394. doi:10.1371/journal.pone.0041394
Peljto, A. L., Zhang, Y., Fingerlin, T. E., Ma, S. F., Garcia, J. G., Richards, T. J., et al. (2013). Association between the MUC5B Promoter Polymorphism and Survival in Patients with Idiopathic Pulmonary Fibrosis. Jama 309 (21), 2232–2239. doi:10.1001/jama.2013.5827
Peng, S. C., Hu, X., Wei, L. Q., and Li, Z. H. (2013). The Correlation of Helper T Lymphocyte 1/helper T Lymphocyte 2 with Clinical and Image Features in Patients with Idiopathic Pulmonary Fibrosis. Zhonghua Nei Ke Za Zhi 52 (6), 489–493.
Phan, T. H. G., Paliogiannis, P., Nasrallah, G. K., Giordo, R., Eid, A. H., Fois, A. G., et al. (2021). Emerging Cellular and Molecular Determinants of Idiopathic Pulmonary Fibrosis. Cell. Mol. Life Sci. 78 (5), 2031–2057. doi:10.1007/s00018-020-03693-7
Pilling, D., Vakil, V., Cox, N., and Gomer, R. H. (2015). TNF-α-stimulated Fibroblasts Secrete Lumican to Promote Fibrocyte Differentiation. Proc. Natl. Acad. Sci. U. S. A. 112 (38), 11929–11934. doi:10.1073/pnas.1507387112
Popescu, I., Mannem, H., Winters, S. A., Hoji, A., Silveira, F., McNally, E., et al. (2019). Impaired cytomegalovirus immunity in idiopathic pulmonary fibrosis lung transplant recipients with short telomeres. Am. J. Respir. Crit. Care Med. 199 (3), 362–376. doi:10.1164/rccm.201805-0825OC
Povedano, J. M., Martinez, P., Flores, J. M., Mulero, F., and Blasco, M. A. (2015). Mice with pulmonary fibrosis driven by telomere dysfunction. Cell. Rep. 12 (2), 286–299. doi:10.1016/j.celrep.2015.06.028
Prasse, A., Pechkovsky, D. V., Toews, G. B., Jungraithmayr, W., Kollert, F., Goldmann, T., et al. (2006). A Vicious Circle of Alveolar Macrophages and Fibroblasts Perpetuates Pulmonary Fibrosis via CCL18. Am. J. Respir. Crit. Care Med. 173 (7), 781–792. doi:10.1164/rccm.200509-1518OC
Raghu, G., Brown, K. K., Costabel, U., Cottin, V., du Bois, R. M., Lasky, J. A., et al. (2008). Treatment of Idiopathic Pulmonary Fibrosis with Etanercept: An Exploratory, Placebo-Controlled Trial. Am. J. Respir. Crit. Care Med. 178 (9), 948–955. doi:10.1164/rccm.200709-1446OC
Raghu, G., Remy-Jardin, M., Myers, J. L., Richeldi, L., Ryerson, C. J., Lederer, D. J., et al. (2018). Diagnosis of idiopathic pulmonary fibrosis. An official ATS/ERS/JRS/ALAT clinical practice guideline. Am. J. Respir. Crit. Care Med. 198 (5), e44–e68. doi:10.1164/rccm.201807-1255ST
Raghu, G., Raghu, G., Anstrom, K. J., King, T. E., Lasky, J. A., and Martinez, F. J. (2012). Prednisone, Azathioprine, and N-Acetylcysteine for Pulmonary Fibrosis. N. Engl. J. Med. 366 (21), 1968–1977. doi:10.1056/NEJMoa1113354
Rahaman, S. O., Grove, L. M., Paruchuri, S., Southern, B. D., Abraham, S., Niese, K. A., et al. (2014). TRPV4 Mediates Myofibroblast Differentiation and Pulmonary Fibrosis in Mice. J. Clin. Investig. 124 (12), 5225–5238. doi:10.1172/jci75331
Rana, T., Jiang, C., Liu, G., Miyata, T., Antony, V., Thannickal, V. J., et al. (2020). PAI-1 regulation of TGF-Β1-Induced alveolar type II cell senescence, SASP secretion, and SASP-Mediated activation of alveolar macrophages. Am. J. Respir. Cell. Mol. Biol. 62 (3), 319–330. doi:10.1165/rcmb.2019-0071OC
Ricard-Blum, S., and Salza, R. (2014). Matricryptins and Matrikines: Biologically Active Fragments of the Extracellular Matrix. Exp. Dermatol 23 (7), 457–463. doi:10.1111/exd.12435
Ricard-Blum, S., and Vallet, S. D. (2019). Fragments Generated upon Extracellular Matrix Remodeling: Biological Regulators and Potential Drugs. Matrix Biol. 75-76, 170–189. doi:10.1016/j.matbio.2017.11.005
Richter, A. G., McKeown, S., Rathinam, S., Harper, L., Rajesh, P., McAuley, D. F., et al. (2009). Soluble Endostatin Is a Novel Inhibitor of Epithelial Repair in Idiopathic Pulmonary Fibrosis. Thorax 64 (2), 156–161. doi:10.1136/thx.2008.102814
Riha, R. L., Yang, I. A., Rabnott, G. C., Tunnicliffe, A. M., Fong, K. M., and Zimmerman, P. V. (2004). Cytokine Gene Polymorphisms in Idiopathic Pulmonary Fibrosis. Intern Med. J. 34 (3), 126–129. doi:10.1111/j.1444-0903.2004.00503.x
Rock, J. R., Barkauskas, C. E., Cronce, M. J., Xue, Y., Harris, J. R., Liang, J., et al. (2011). Multiple Stromal Populations Contribute to Pulmonary Fibrosis without Evidence for Epithelial to Mesenchymal Transition. Proc. Natl. Acad. Sci. U. S. A. 108 (52), E1475–E1483. doi:10.1073/pnas.1117988108
Rohani, M. G., McMahan, R. S., Razumova, M. V., Hertz, A. L., Cieslewicz, M., Pun, S. H., et al. (2015). MMP-10 regulates collagenolytic activity of alternatively activated resident macrophages. J. Investig. Dermatol 135 (10), 2377–2384. doi:10.1038/jid.2015.167
Romagnani, S. (2000). T-Cell Subsets (Th1 versus Th2). Ann. Allergy Asthma Immunol. 85 (1), 9–21. quiz 18, 21. doi:10.1016/s1081-1206(10)62426-x
Ruffin, M., Thompson, K. E., Corvol, H., and Guillot, L. (2020). Two-hybrid Screening of FAM13A Protein Partners in Lung Epithelial Cells. BMC Res. Notes 12 (1), 804. doi:10.1186/s13104-019-4840-9
Sabeh, F., Li, X. Y., Saunders, T. L., Rowe, R. G., and Weiss, S. J. (2009). Secreted versus Membrane-Anchored Collagenases: Relative Roles in Fibroblast-dependent Collagenolysis and Invasion. J. Biol. Chem. 284 (34), 23001–23011. doi:10.1074/jbc.M109.002808
Saito, S., Zhuang, Y., Shan, B., Danchuk, S., Luo, F., Korfei, M., et al. (2017). Tubastatin Ameliorates Pulmonary Fibrosis by Targeting the TGFβ-Pi3k-Akt Pathway. PLoS One 12 (10), e0186615. doi:10.1371/journal.pone.0186615
Salton, F., Ruaro, B., Confalonieri, P., and Confalonieri, M. (2020). Epithelial-mesenchymal transition: A major pathogenic driver in idiopathic pulmonary fibrosis? Med. Kaunas. 56 (11), 608. doi:10.3390/medicina56110608
Salton, F., Volpe, M. C., and Confalonieri, M. (2019). Epithelial⁻Mesenchymal transition in the pathogenesis of idiopathic pulmonary fibrosis. Med. Kaunas. 55 (4), 83. doi:10.3390/medicina55040083
Sanders, Y. Y., Ambalavanan, N., Halloran, B., Zhang, X., Liu, H., Crossman, D. K., et al. (2012). Altered DNA Methylation Profile in Idiopathic Pulmonary Fibrosis. Am. J. Respir. Crit. Care Med. 186 (6), 525–535. doi:10.1164/rccm.201201-0077OC
Sanders, Y. Y., Tollefsbol, T. O., Varisco, B. M., and Hagood, J. S. (2011). Epigenetic Regulation of Thy-1 by Histone Deacetylase Inhibitor in Rat Lung Fibroblasts. Am. J. Respir. Cell. Mol. Biol. 45 (1), 16–23. doi:10.1165/rcmb.2010-0154OC
Schafer, M. J., White, T. A., Iijima, K., Haak, A. J., Ligresti, G., Atkinson, E. J., et al. (2017). Cellular Senescence Mediates Fibrotic Pulmonary Disease. Nat. Commun. 8, 14532. doi:10.1038/ncomms14532
Selman, M., and Pardo, A. (2014). Revealing the Pathogenic and Aging-Related Mechanisms of the Enigmatic Idiopathic Pulmonary Fibrosis. An Integral Model. Am. J. Respir. Crit. Care Med. 189 (10), 1161–1172. doi:10.1164/rccm.201312-2221PP
Selman, M., and Pardo, A. (2020). The Leading Role of Epithelial Cells in the Pathogenesis of Idiopathic Pulmonary Fibrosis. Cell. Signal 66, 109482. doi:10.1016/j.cellsig.2019.109482
Selman, M., Ruiz, V., Cabrera, S., Segura, L., Ramírez, R., Barrios, R., et al. (2000). TIMP-1, -2, -3, and -4 in Idiopathic Pulmonary Fibrosis. A Prevailing Nondegradative Lung Microenvironment? Am. J. Physiol. Lung Cell. Mol. Physiol. 279 (3), L562–L574. doi:10.1152/ajplung.2000.279.3.L562
Sgalla, G., Iovene, B., Calvello, M., Ori, M., Varone, F., and Richeldi, L. (2018). Idiopathic Pulmonary Fibrosis: Pathogenesis and Management. Respir. Res. 19 (1), 32. doi:10.1186/s12931-018-0730-2
She, Y. X., Yu, Q. Y., and Tang, X. X. (2021). Role of Interleukins in the Pathogenesis of Pulmonary Fibrosis. Cell. Death Discov. 7 (1), 52. doi:10.1038/s41420-021-00437-9
Shi-Wen, X., Chen, Y., Denton, C. P., Eastwood, M., Renzoni, E. A., Bou-Gharios, G., et al. (2004). Endothelin-1 Promotes Myofibroblast Induction through the ETA Receptor via a Rac/phosphoinositide 3-kinase/Akt-dependent Pathway and Is Essential for the Enhanced Contractile Phenotype of Fibrotic Fibroblasts. Mol. Biol. Cell. 15 (6), 2707–2719. doi:10.1091/mbc.e03-12-0902
Shifeng, L., Hong, X., Xue, Y., Siyu, N., Qiaodan, Z., Dingjie, X., et al. (2019). Ac-SDKP Increases α-TAT 1 and Promotes the Apoptosis in Lung Fibroblasts and Epithelial Cells Double-Stimulated with TGF-Β1 and Silica. Toxicol. Appl. Pharmacol. 369, 17–29. doi:10.1016/j.taap.2019.02.015
Shukla, S., Jeong, H. C., Sturgeon, C. M., Parker, R., and Batista, L. F. Z. (2020). Chemical Inhibition of PAPD5/7 Rescues Telomerase Function and Hematopoiesis in Dyskeratosis Congenita. Blood Adv. 4 (12), 2717–2722. doi:10.1182/bloodadvances.2020001848
Shulenin, S., Nogee, L. M., Annilo, T., Wert, S. E., Whitsett, J. A., and Dean, M. (2004). ABCA3 Gene Mutations in Newborns with Fatal Surfactant Deficiency. N. Engl. J. Med. 350 (13), 1296–1303. doi:10.1056/NEJMoa032178
Silhan, L. L., Shah, P. D., Chambers, D. C., Snyder, L. D., Riise, G. C., Wagner, C. L., et al. (2014). Lung Transplantation in Telomerase Mutation Carriers with Pulmonary Fibrosis. Eur. Respir. J. 44 (1), 178–187. doi:10.1183/09031936.00060014
Smaldone, G. C. (2018). Repurposing of Gamma Interferon via Inhalation Delivery. Adv. Drug Deliv. Rev. 133, 87–92. doi:10.1016/j.addr.2018.06.004
Snetselaar, R., van Batenburg, A. A., van Oosterhout, M. F. M., Kazemier, K. M., Roothaan, S. M., Peeters, T., et al. (2017). Short Telomere Length in IPF Lung Associates with Fibrotic Lesions and Predicts Survival. PLoS One 12 (12), e0189467. doi:10.1371/journal.pone.0189467
Snetselaar, R., van Moorsel, C. H. M., Kazemier, K. M., van der Vis, J. J., Zanen, P., van Oosterhout, M. F. M., et al. (2015). Telomere Length in Interstitial Lung Diseases. Chest 148 (4), 1011–1018. doi:10.1378/chest.14-3078
Snijder, J., Peraza, J., Padilla, M., Capaccione, K., and Salvatore, M. M. (2019). Pulmonary Fibrosis: A Disease of Alveolar Collapse and Collagen Deposition. Expert Rev. Respir. Med. 13 (7), 615–619. doi:10.1080/17476348.2019.1623028
Sosulski, M. L., Gongora, R., Feghali-Bostwick, C., Lasky, J. A., and Sanchez, C. G. (2017). Sirtuin 3 deregulation promotes pulmonary fibrosis. J. Gerontol. A Biol. Sci. Med. Sci. 72 (5), 595–602. doi:10.1093/gerona/glw151
Spagnolo, P., Kropski, J. A., Jones, M. G., Lee, J. S., Rossi, G., Karampitsakos, T., et al. (2021). Idiopathic Pulmonary Fibrosis: Disease Mechanisms and Drug Development. Pharmacol. Ther. 222, 107798. doi:10.1016/j.pharmthera.2020.107798
Spagnolo, P., Tzouvelekis, A., and Bonella, F. (2018). The management of patients with idiopathic pulmonary fibrosis. Front. Med. (Lausanne) 5, 148. doi:10.3389/fmed.2018.00148
Stanley, S. E., Gable, D. L., Wagner, C. L., Carlile, T. M., Hanumanthu, V. S., Podlevsky, J. D., et al. (2016a). Loss-of-function Mutations in the RNA Biogenesis Factor NAF1 Predispose to Pulmonary Fibrosis-Emphysema. Sci. Transl. Med. 8 (351), 351ra107. doi:10.1126/scitranslmed.aaf7837
Stanley, S. E., Merck, S. J., and Armanios, M. (2016b). Telomerase and the genetics of emphysema susceptibility. Implications for pathogenesis paradigms and patient care. Ann. Am. Thorac. Soc. 13 Suppl 5 (Suppl. 5), S447–s451. doi:10.1513/AnnalsATS.201609-718AW
Stuart, B. D., Choi, J., Zaidi, S., Xing, C., Holohan, B., Chen, R., et al. (2015). Exome Sequencing Links Mutations in PARN and RTEL1 with Familial Pulmonary Fibrosis and Telomere Shortening. Nat. Genet. 47 (5), 512–517. doi:10.1038/ng.3278
Stuart, B. D., Lee, J. S., Kozlitina, J., Noth, I., Devine, M. S., Glazer, C. S., et al. (2014). Effect of Telomere Length on Survival in Patients with Idiopathic Pulmonary Fibrosis: An Observational Cohort Study with Independent Validation. Lancet Respir. Med. 2 (7), 557–565. doi:10.1016/s2213-2600(14)70124-9
Suga, M., Iyonaga, K., Ichiyasu, H., Saita, N., Yamasaki, H., and Ando, M. (1999). Clinical Significance of MCP-1 Levels in BALF and Serum in Patients with Interstitial Lung Diseases. Eur. Respir. J. 14 (2), 376–382. doi:10.1034/j.1399-3003.1999.14b23.x
Sumida, A., Hasegawa, Y., Okamoto, M., Hashimoto, N., Imaizumi, K., Yatsuya, H., et al. (2008). TH1/TH2 Immune Response in Lung Fibroblasts in Interstitial Lung Disease. Arch. Med. Res. 39 (5), 503–510. doi:10.1016/j.arcmed.2008.02.005
Suryadevara, V., Ramchandran, R., Kamp, D. W., and Natarajan, V. (2020). Lipid mediators regulate pulmonary fibrosis: Potential mechanisms and signaling pathways. Int. J. Mol. Sci. 21 (12), 4257. doi:10.3390/ijms21124257
Swaminathan, A. C., Neely, M. L., Frankel, C. W., Kelly, F. L., Petrovski, S., Durheim, M. T., et al. (2019). Lung transplant outcomes in patients with pulmonary fibrosis with telomere-related gene variants. Chest 156 (3), 477–485. doi:10.1016/j.chest.2019.03.030
Tager, A. M., LaCamera, P., Shea, B. S., Campanella, G. S., Selman, M., Zhao, Z., et al. (2008). The Lysophosphatidic Acid Receptor LPA1 Links Pulmonary Fibrosis to Lung Injury by Mediating Fibroblast Recruitment and Vascular Leak. Nat. Med. 14 (1), 45–54. doi:10.1038/nm1685
Taillé, C., Grootenboer-Mignot, S., Boursier, C., Michel, L., Debray, M. P., Fagart, J., et al. (2011). Identification of Periplakin as a New Target for Autoreactivity in Idiopathic Pulmonary Fibrosis. Am. J. Respir. Crit. Care Med. 183 (6), 759–766. doi:10.1164/rccm.201001-0076OC
Tanino, Y., Wang, X., Nikaido, T., Misa, K., Sato, Y., Togawa, R., et al. (2019). Syndecan-4 inhibits the development of pulmonary fibrosis by attenuating TGF-β signaling. Int. J. Mol. Sci. 20 (20), 4989. doi:10.3390/ijms20204989
Taskar, V. S., and Coultas, D. B. (2006). Is Idiopathic Pulmonary Fibrosis an Environmental Disease? Proc. Am. Thorac. Soc. 3 (4), 293–298. doi:10.1513/pats.200512-131TK
Tokman, S., Singer, J. P., Devine, M. S., Westall, G. P., Aubert, J. D., Tamm, M., et al. (2015). Clinical Outcomes of Lung Transplant Recipients with Telomerase Mutations. J. Heart Lung Transpl. 34 (10), 1318–1324. doi:10.1016/j.healun.2015.05.002
Townsley, D. M., Dumitriu, B., Liu, D., Biancotto, A., Weinstein, B., Chen, C., et al. (2016a). Danazol Treatment for Telomere Diseases. N. Engl. J. Med. 374 (20), 1922–1931. doi:10.1056/NEJMoa1515319
Townsley, D. M., Dumitriu, B., and Young, N. S. (2016b). Danazol Treatment for Telomere Diseases. N. Engl. J. Med. 375 (11), 1095–1096. doi:10.1056/NEJMc1607752
Upagupta, C., Shimbori, C., Alsilmi, R., and Kolb, M. (2018). Matrix Abnormalities in Pulmonary Fibrosis. Eur. Respir. Rev. 27 (148), 180033. doi:10.1183/16000617.0033-2018
Utz, J. P., Limper, A. H., Kalra, S., Specks, U., Scott, J. P., Vuk-Pavlovic, Z., et al. (2003). Etanercept for the Treatment of Stage II and III Progressive Pulmonary Sarcoidosis. Chest 124 (1), 177–185. doi:10.1378/chest.124.1.177
van Moorsel, C. H. M. (2018). Trade-offs in Aging Lung Diseases: A Review on Shared but Opposite Genetic Risk Variants in Idiopathic Pulmonary Fibrosis, Lung Cancer and Chronic Obstructive Pulmonary Disease. Curr. Opin. Pulm. Med. 24 (3), 309–317. doi:10.1097/mcp.0000000000000476
Vasakova, M., Sterclova, M., Matej, R., Olejar, T., Kolesar, L., Skibova, J., et al. (2013). IL-4 Polymorphisms, HRCT Score and Lung Tissue Markers in Idiopathic Pulmonary Fibrosis. Hum. Immunol. 74 (1), 1346–51. doi:10.1016/j.humimm.2013.07.011
Vasakova, M., Striz, I., Slavcev, A., Jandova, S., Kolesar, L., and Sulc, J. (2006). Th1/Th2 Cytokine Gene Polymorphisms in Patients with Idiopathic Pulmonary Fibrosis. Tissue Antigens 67 (3), 229–232. doi:10.1111/j.1399-0039.2006.00560.x
Venosa, A., Tomer, Y., Jamil, S., and Beers, M. (2017). Expression of A pulmonary fibrosis associated surfactant protein C mutant, SP-Ci73t, in alveolar type 2 cells induces lung inflammation and aberrant parenchymal remodeling. Physiology 31, 694.5–694.5. doi:10.1096/fasebj.31.1_supplement.694.5
Villavicencio, M. A., Axtell, A. L., Osho, A., Astor, T., Roy, N., Melnitchouk, S., et al. (2018). Single- versus double-lung transplantation in pulmonary fibrosis: Impact of age and pulmonary hypertension. Ann. Thorac. Surg. 106 (3), 856–863. doi:10.1016/j.athoracsur.2018.04.060
Wambach, J. A., Casey, A. M., Fishman, M. P., Wegner, D. J., Wert, S. E., Cole, F. S., et al. (2014). Genotype-phenotype Correlations for Infants and Children with ABCA3 Deficiency. Am. J. Respir. Crit. Care Med. 189 (12), 1538–1543. doi:10.1164/rccm.201402-0342OC
Wang, H., and Dymock, B. W. (2009). New Patented Histone Deacetylase Inhibitors. Expert Opin. Ther. Pat. 19 (12), 1727–1757. doi:10.1517/13543770903393789
Wang, L., Chen, R., Li, G., Wang, Z., Liu, J., Liang, Y., et al. (2020). FBW7 mediates senescence and pulmonary fibrosis through telomere uncapping. Cell. Metab. 32 (5), 860–e9. e869. doi:10.1016/j.cmet.2020.10.004
Wang, Q., Xie, Z. L., Wu, Q., Jin, Z. X., Yang, C., and Feng, J. (2021). Role of Various Imbalances Centered on Alveolar Epithelial Cell/fibroblast Apoptosis Imbalance in the Pathogenesis of Idiopathic Pulmonary Fibrosis. Chin. Med. J. Engl. 134 (3), 261–274. doi:10.1097/cm9.0000000000001288
Wang, T., Feng, Y., Sun, H., Zhang, L., Hao, L., Shi, C., et al. (2012). miR-21 Regulates Skin Wound Healing by Targeting Multiple Aspects of the Healing Process. Am. J. Pathol. 181 (6), 1911–1920. doi:10.1016/j.ajpath.2012.08.022
Wang, W., Yu, Y., Xiao, J., Gao, Y., Sang, L., Lian, Y., et al. (2018). A novel variant of desmoplakin is potentially associated with silicosis risk. DNA Cell. Biol. 37 (11), 925–931. doi:10.1089/dna.2018.4370
Wang, Y., Kuan, P. J., Xing, C., Cronkhite, J. T., Torres, F., Rosenblatt, R. L., et al. (2009a). Genetic Defects in Surfactant Protein A2 Are Associated with Pulmonary Fibrosis and Lung Cancer. Am. J. Hum. Genet. 84 (1), 52–59. doi:10.1016/j.ajhg.2008.11.010
Wang, Z., Chen, C., Finger, S. N., Kwajah, S., Jung, M., Schwarz, H., et al. (2009b). Suberoylanilide Hydroxamic Acid: A Potential Epigenetic Therapeutic Agent for Lung Fibrosis? Eur. Respir. J. 34 (1), 145–155. doi:10.1183/09031936.00084808
Wawrocki, S., Druszczynska, M., Kowalewicz-Kulbat, M., and Rudnicka, W. (2016). Interleukin 18 (IL-18) as a Target for Immune Intervention. Acta Biochim. Pol. 63 (1), 59–63. doi:10.18388/abp.2015_1153
Weichert, N., Kaltenborn, E., Hector, A., Woischnik, M., Schams, A., Holzinger, A., et al. (2011). Some ABCA3 Mutations Elevate ER Stress and Initiate Apoptosis of Lung Epithelial Cells. Respir. Res. 12 (1), 4. doi:10.1186/1465-9921-12-4
Whyte, M., Hubbard, R., Meliconi, R., Whidborne, M., Eaton, V., Bingle, C., et al. (2000). Increased Risk of Fibrosing Alveolitis Associated with Interleukin-1 Receptor Antagonist and Tumor Necrosis Factor-Alpha Gene Polymorphisms. Am. J. Respir. Crit. Care Med. 162 (2 Pt 1), 755–758. doi:10.1164/ajrccm.162.2.9909053
Wolters, P. J., Collard, H. R., and Jones, K. D. (2014). Pathogenesis of Idiopathic Pulmonary Fibrosis. Annu. Rev. Pathol. 9, 157–179. doi:10.1146/annurev-pathol-012513-104706
Wu, B., Crampton, S. P., and Hughes, C. C. (2007). Wnt Signaling Induces Matrix Metalloproteinase Expression and Regulates T Cell Transmigration. Immunity 26 (2), 227–239. doi:10.1016/j.immuni.2006.12.007
Wu, M., Assassi, S., Salazar, G. A., Pedroza, C., Gorlova, O. Y., Chen, W. V., et al. (2016). Genetic Susceptibility Loci of Idiopathic Interstitial Pneumonia Do Not Represent Risk for Systemic Sclerosis: A Case Control Study in caucasian Patients. Arthritis Res. Ther. 18, 20. doi:10.1186/s13075-016-0923-3
Wu, M., Huang, Q., Xie, Y., Wu, X., Ma, H., Zhang, Y., et al. (2022). Improvement of the Anticancer Efficacy of PD-1/pd-L1 Blockade via Combination Therapy and PD-L1 Regulation. J. Hematol. Oncol. 15 (1), 24. doi:10.1186/s13045-022-01242-2
Wujak, L., Didiasova, M., Zakrzewicz, D., Frey, H., Schaefer, L., and Wygrecka, M. (2015). Heparan Sulfate Proteoglycans Mediate Factor XIIa Binding to the Cell Surface. J. Biol. Chem. 290 (11), 7027–7039. doi:10.1074/jbc.M114.606343
Wuyts, W. A., Dahlqvist, C., Slabbynck, H., Schlesser, M., Gusbin, N., Compere, C., et al. (2018). Baseline Clinical Characteristics, Comorbidities and Prescribed Medication in a Real-World Population of Patients with Idiopathic Pulmonary Fibrosis: The PROOF Registry. BMJ Open Respir. Res. 5 (1), e000331. doi:10.1136/bmjresp-2018-000331
Xia, Y., Xu, F., Xiong, M., Yang, H., Lin, W., Xie, Y., et al. (2021). Repurposing of Antipsychotic Trifluoperazine for Treating Brain Metastasis, Lung Metastasis and Bone Metastasis of Melanoma by Disrupting Autophagy Flux. Pharmacol. Res. 163, 105295. doi:10.1016/j.phrs.2020.105295
Xiao, X., Senavirathna, L. K., Gou, X., Huang, C., Liang, Y., and Liu, L. (2016). EZH2 Enhances the Differentiation of Fibroblasts into Myofibroblasts in Idiopathic Pulmonary Fibrosis. Physiol. Rep. 4 (17), e12915. doi:10.14814/phy2.12915
Xiong, Y., Zhang, J., Shi, L., Ning, Y., Zhu, Y., Chen, S., et al. (2017). NOGO-B Promotes EMT in Lung Fibrosis via MMP14 Mediates Free TGF-Beta1 Formation. Oncotarget 8 (41), 71024–71037. doi:10.18632/oncotarget.20297
Xue, J., Gochuico, B. R., Alawad, A. S., Feghali-Bostwick, C. A., Noth, I., Nathan, S. D., et al. (2011). The HLA Class II Allele DRB1*1501 Is Over-represented in Patients with Idiopathic Pulmonary Fibrosis. PLoS One 6 (2), e14715. doi:10.1371/journal.pone.0014715
Xue, T., Qiu, X., Liu, H., Gan, C., Tan, Z., Xie, Y., et al. (2021). Epigenetic Regulation in Fibrosis Progress. Pharmacol. Res. 173, 105910. doi:10.1016/j.phrs.2021.105910
Yagihashi, K., Huckleberry, J., Colby, T. V., Tazelaar, H. D., Zach, J., Sundaram, B., et al. (2016). Radiologic-pathologic Discordance in Biopsy-Proven Usual Interstitial Pneumonia. Eur. Respir. J. 47 (4), 1189–1197. doi:10.1183/13993003.01680-2015
Yamakawa, H., Oba, T., Ohta, H., Tsukahara, Y., Kida, G., Tsumiyama, E., et al. (2019). Nintedanib Allows Retreatment with Atezolizumab of Combined Non-small Cell Lung Cancer/idiopathic Pulmonary Fibrosis after Atezolizumab-Induced Pneumonitis: A Case Report. BMC Pulm. Med. 19 (1), 156. doi:10.1186/s12890-019-0920-9
Yamashita, C. M., Dolgonos, L., Zemans, R. L., Young, S. K., Robertson, J., Briones, N., et al. (2011). Matrix Metalloproteinase 3 Is a Mediator of Pulmonary Fibrosis. Am. J. Pathol. 179 (4), 1733–1745. doi:10.1016/j.ajpath.2011.06.041
Yang, L., Herrera, J., Gilbertsen, A., Xia, H., Smith, K., Benyumov, A., et al. (2018). IL-8 Mediates Idiopathic Pulmonary Fibrosis Mesenchymal Progenitor Cell Fibrogenicity. Am. J. Physiol. Lung Cell. Mol. Physiol. 314 (1), L127–l136. doi:10.1152/ajplung.00200.2017
Yao, L., Conforti, F., Hill, C., Bell, J., Drawater, L., Li, J., et al. (2019). Paracrine Signalling during ZEB1-Mediated Epithelial-Mesenchymal Transition Augments Local Myofibroblast Differentiation in Lung Fibrosis. Cell. Death Differ. 26 (5), 943–957. doi:10.1038/s41418-018-0175-7
Yu, G., Kovkarova-Naumovski, E., Jara, P., Parwani, A., Kass, D., Ruiz, V., et al. (2012). Matrix Metalloproteinase-19 Is a Key Regulator of Lung Fibrosis in Mice and Humans. Am. J. Respir. Crit. Care Med. 186 (8), 752–762. doi:10.1164/rccm.201202-0302OC
Yue, L., Shi, Y., Su, X., Ouyang, L., Wang, G., and Ye, T. (2021). Matrix Metalloproteinases Inhibitors in Idiopathic Pulmonary Fibrosis: Medicinal Chemistry Perspectives. Eur. J. Med. Chem. 224, 113714. doi:10.1016/j.ejmech.2021.113714
Zhang, H. P., Zou, J., Xie, P., Gao, F., and Mu, H. J. (2015). Association of HLA and Cytokine Gene Polymorphisms with Idiopathic Pulmonary Fibrosis. Kaohsiung J. Med. Sci. 31 (12), 613–620. doi:10.1016/j.kjms.2015.10.007
Zhang, K., Xu, L., and Cong, Y.-S. (2021). Telomere dysfunction in idiopathic pulmonary fibrosis. Front. Med. 8, 739810. doi:10.3389/fmed.2021.739810
Zhang, L., Wang, Y., Wu, G., Xiong, W., Gu, W., and Wang, C. Y. (2018). Macrophages: Friend or Foe in Idiopathic Pulmonary Fibrosis? Respir. Res. 19 (1), 170. doi:10.1186/s12931-018-0864-2
Zhang, Q., Tu, W., Tian, K., Han, L., Wang, Q., Chen, P., et al. (2019). Sirtuin 6 Inhibits Myofibroblast Differentiation via Inactivating Transforming Growth Factor-β1/Smad2 and Nuclear Factor-Κb Signaling Pathways in Human Fetal Lung Fibroblasts. J. Cell. Biochem. 120 (1), 93–104. doi:10.1002/jcb.27128
Zhou, S., Wang, X., Gao, H., and Zeng, Y. (2020). DNA methylation in pulmonary fibrosis. Adv. Exp. Med. Biol. 1255, 51–62. doi:10.1007/978-981-15-4494-1_4
Zhou, W., and Wang, Y. (2016). Candidate Genes of Idiopathic Pulmonary Fibrosis: Current Evidence and Research. Appl. Clin. Genet. 9, 5–13. doi:10.2147/tacg.S61999
Zhou, W., Zhuang, Y., Sun, J., Wang, X., Zhao, Q., Xu, L., et al. (2017). Variants of the ABCA3 Gene Might Contribute to Susceptibility to Interstitial Lung Diseases in the Chinese Population. Sci. Rep. 7 (1), 4097. doi:10.1038/s41598-017-04486-y
Ziegenhagen, M. W., Zabel, P., Zissel, G., Schlaak, M., and Müller-Quernheim, J. (1998). Serum Level of Interleukin 8 Is Elevated in Idiopathic Pulmonary Fibrosis and Indicates Disease Activity. Am. J. Respir. Crit. Care Med. 157 (3 Pt 1), 762–768. doi:10.1164/ajrccm.157.3.9705014
Keywords: idiopathic pulmonary fibrosis, cells crosstalk, gene mutations, epigenetics, emerging drugs
Citation: Ma H, Wu X, Li Y and Xia Y (2022) Research Progress in the Molecular Mechanisms, Therapeutic Targets, and Drug Development of Idiopathic Pulmonary Fibrosis. Front. Pharmacol. 13:963054. doi: 10.3389/fphar.2022.963054
Received: 07 June 2022; Accepted: 24 June 2022;
Published: 21 July 2022.
Edited by:
Barbara Ruaro, University of Trieste, ItalyReviewed by:
Riccardo Pozzan, University of Trieste, ItalyChiara Bozzi, University of Trieste, Italy
Copyright © 2022 Ma, Wu, Li and Xia. This is an open-access article distributed under the terms of the Creative Commons Attribution License (CC BY). The use, distribution or reproduction in other forums is permitted, provided the original author(s) and the copyright owner(s) are credited and that the original publication in this journal is cited, in accordance with accepted academic practice. No use, distribution or reproduction is permitted which does not comply with these terms.
*Correspondence: Yong Xia, eXhpYTRAc2N1LmVkdS5jbg==
†These authors have contributed equally to this work and share first authorship