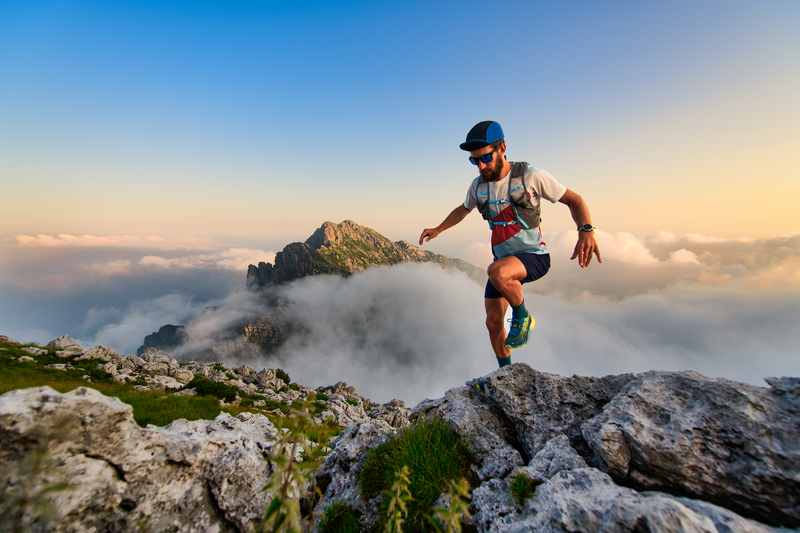
95% of researchers rate our articles as excellent or good
Learn more about the work of our research integrity team to safeguard the quality of each article we publish.
Find out more
REVIEW article
Front. Pharmacol. , 13 September 2022
Sec. Inflammation Pharmacology
Volume 13 - 2022 | https://doi.org/10.3389/fphar.2022.958022
This article is part of the Research Topic Inflammatory immune disease: Molecular Mechanisms, Translational Approaches and Therapeutics, Volume II View all 51 articles
It is worth noting that neuroinflammation is well recognized as a symptom of neurodegenerative diseases (NDs). The regulation of neuroinflammation becomes an attractive focus for innovative ND treatment technologies. There is evidence that IL-22 is associated with the development and progression of a wide assortment of NDs. For example, IL-22 can activate glial cells, causing them to generate pro-inflammatory cytokines and encourage lymphocyte infiltration in the brain. IL-22 mRNA is highly expressed in Alzheimer’s disease (AD) patients, and a high expression of IL-22 has also been detected in the brains of patients with other NDs. We examine the role of IL-22 in the development and treatment of NDs in this review, and we believe that IL-22 has therapeutic potential in these diseases.
NDs are a leading cause of death and morbidity worldwide, particularly among the elderly. The clinical manifestations and pathological changes vary among patients with NDs. However, they often share certain characteristics such as progressive malfunction and neuronal loss. These shared changes may be associated with abnormal deposition of misfolded proteins in the brains of patients with NDs. These shared changes may be related to the abnormal deposition of misfolded proteins in the brains of patients with NDs, which are inducers of neuroinflammation and glial cell activation (Cai et al., 2014). Glial cells play a key role in the occurrence and development of NDs (Heneka et al., 2015). Activated glial cells can produce and release high doses of toxic substances such as IL-1α, IL-1β, and TNF-α, as well as oxygen-free radicals and nitrous oxide, leading to an inflammatory response that affects synaptic function and kills neurons (Cao et al., 2018).
Immune cells, including a fraction of T helper (Th) cells and innate lymphocytes, generate IL-22, a unique cytokine. In diverse tissues, IL-22 exhibits opposing effects, ranging from pro-inflammatory to protective effects (Sonnenberg et al., 2011; Zenewicz and Flavell, 2011), which are largely depending on the environment. On the one hand, IL-22 can amplify inflammation alone or in combination with other cytokines and cause abnormal epithelial cell proliferation and differentiation. On the other hand, IL-22 induces the expression of pro-survival and anti-apoptotic genes like Bcl-2 and Bcl-xL and the expression of proliferation-related cytokines like c-Myc and cyclin D1. IL-22 also enhanced tissue protection by improving the barrier function. IL-22 has been implicated in the pathophysiology of a variety of organs in several studies (Table 1).
IL-22 has been linked to a number of chronic autoimmune and inflammatory illnesses, including multiple sclerosis (MS) and psoriasis (Pan et al., 2013). Significantly, higher amounts of IL-22 have also been found in the brains of patients with a range of NDs, and recent research showed that IL-22 generated by leukocytes that invade the brain leads glial cells to activate, promoting disease development. In this review, we will detail the basic properties of IL-22 and its role in the development and treatment of NDs, especially in AD and MS.
IL-22 is a cytokine belonging to the IL-10 family, which was initially discovered as an IL-10-related T-cell-derived inducer (Sabat, 2010). Like other members of the IL-10 family, the secondary structure of IL-22 is helical (Wolk and Sabat, 2006), and IL-22 in humans consists of 146 amino acids, which is 80.8% similar to IL-22 in mice. IL-22 is derived from a variety of leukocytes, and its expression is regulated by a variety of transcription factors (Pan et al., 2013) (Figure 2).
T lymphocytes were the first cell type to be recognized as an origin of IL-22. Among them, Th1 cells were the first Th cell subset found to produce IL-22 (Wolk et al., 2002). In the existence of IL-12, Th1 cells develop and release the cytokine interferon-γ (IFN-γ). On the other hand, Th17 cells that generate IL-17 and exhibit RORt are considered to be the main source of IL-22 in Th cells (Chung et al., 2006; Zheng et al., 2007). The expression of IL-22 is not linked to IL-17 or RORt expression in human CD4+ T cells (Volpe et al., 2008; Volpe et al., 2009). Only 10–18% of IL-22-producing CD4+ T cells in human peripheral blood also produce IL-17 (Duhen et al., 2009). Naive T cells develop into IL-17-producing Th17 cells when IL-6, IL-1β, and TGF-β simultaneously stimulate naive T cells, and IL-23 promotes the survival of Th17 cells. Except for TGF-β, all of these cytokines stimulate IL-22 synthesis in T cells (Zheng et al., 2007). In mouse Th17 cells, TGF-β suppresses IL-22 synthesis while increasing IL-17 synthesis (Zheng et al., 2007; Rutz et al., 2011). The foregoing clarifies why various Th17 cells are classified as IL-17-only Th17 cells or IL-17/IL-22 Th17 cells. In addition, there is a Th cell that produces only IL-22 without co-expressing IL-17 or IFN-γ. These cells are defined as Th22 cells (Duhen et al., 2009; Eyerich et al., 2009; Trifari et al., 2009). They do not express T-β and have undetectable low levels of RORt (Duhen et al., 2009). Both Th22 cells and Th17 cells require IL-6 stimulation for activation. IL-6 and TNF-β have a synergistic effect in the activation and promotion of IL-22 secretion of Th22 cells, whereas vitamin D boosts IL-22 production even more. Clones derived from Th22 cells were shown to have rather consistent cytokine profiles across a variety of polarization regimes (Eyerich et al., 2009). However, adding extra IL-1β caused the creation of IL-17 and IL-22. On the other side, TGF-β increases the synthesis of IL-17 while effectively inhibiting the production of IL-22 in Th22 cells (Duhen et al., 2009).
The IL-22 receptor (IL-22R) is a member of the cytokine receptor class II family. It is constituted by the IL-22 receptor complex, which includes IL-22R1 and IL-10R2 (also known as IL10RB). IL-22R1 expression may be seen in non-immune organs such as the skin, lungs, and kidney, as well as, greatest notably, the pancreas (Wolk et al., 2004). However, IL-10R2 is widely expressed in lymphocytes (such as T, B, and NK cells) (Wolk et al., 2004). In recent studies, researchers discovered that IL-22R is expressed in the brain tissue. The IL-22-binding protein (IL-22BP), which is produced by a separate gene and contains the ectodomain of class II cytokine receptors, is a kind of soluble IL-22 receptor. It has been demonstrated to inhibit cellular binding and signal transduction of IL-22 and modify IL-22 bioavailability in vitro (Xu et al., 2001; Nikoopour et al., 2015). IL-22 and IL-22BP have a greater affinity than IL-22 and IL-22R1 (Andoh et al., 2005). The low dissociation rate of the IL-22/IL-22BP complex indicates that it is relatively stable. The fact that IL-22 is the only IL-10 family member with additional binding proteins underscores the need to fine-tune its activity. IL-22BP may partly assist the stability and systemic spread of IL-22, in addition to limiting its local effects (Andoh et al., 2005).
AD is an ND and the most prevalent cause of dementia. Up to now, about 50 million people worldwide suffer from dementia due to aging populations, and the number of people with dementia is projected to reach 152 million by mid-century, with the largest increase in low- and middle-income countries (Qiu et al., 2009). Over the past few decades, community surveys in Japan and China have found a marked increase in the prevalence of AD (Chan et al., 2013; Ohara et al., 2017). In particular, women have higher morbidity and mortality than men (Collaborators G. B. D. D., 2019). Additionally, from 2000 to 2018, the number of patients dying from AD in the United States increased by 146.2%, making AD the fifth leading cause of death among older adults in the United States. The pathological hallmarks of AD are neurofibrillary tangles and amyloid-β peptide (Aβ) deposition in the brain. Aβ is a major cause of neuronal death in vulnerable areas including the neocortex and hippocampus, which may contribute to behavioral and functional abnormalities in AD (Gandy and Greengard, 1994). The buildup of Aβ in the brain, according to the amyloid hypothesis, is the key factor underlying the pathogenesis of AD. Tau-containing neurofibrillary tangles arise when extracellular Aβ production and clearance are out of equilibrium. However, the amyloid cascade theory cannot completely explain the pathophysiology of AD (Hardy and Selkoe, 2002). Later studies on AD pathogenesis have extensively recognized the close functional relationship between the immune system and the central nervous system (CNS). Microglia are the most significant immune cells in the neural milieu in the CNS, and they may be stimulated to phagocytose and remove Aβ, as well as enhance the production of inflammatory cytokines to speed up a neuronal injury (Hong et al., 2016). These findings suggest that immune system dysfunction is linked to the onset of AD. Additionally, in AD, peripheral immune processes interact with the CNS. Monocytes, macrophages, neutrophils, and T cells from the peripheral blood may infiltrate the brain and play a major role in the genesis of AD (Polfliet et al., 2001; Ziegler-Heitbrock, 2007; Baik et al., 2014; Gate et al., 2020). Recent research found that high levels of activated CD4+ and CD8+ T lymphocytes in the peripheral blood were highly linked to cognitive impairment and magnetic resonance imaging (MRI) abnormalities in particular brain areas in AD patients (Lueg et al., 2015). The etiology of AD is unknown, but it may be linked to the activation of cytokine production in the brain by Aβ since research found that peripheral T cells activated with Aβ in vitro secreted more pro-inflammatory cytokines (Monsonego et al., 2006).
Prior research found that AD patients had no change in the total number of CD4+ and CD8+ cells but an increased percentage of circulating immune cells, particularly lymphocytes that generate IL-17, IL-6, IL-22, and IFN-γ (Gouya et al., 2014). In AD patients, interleukins (IL-21 and IL-22, etc.) generated by Th17 cells, as well as transcription factors (RORγ, etc.) involved in Th17 cell growth, are significantly increased (Saresella et al., 2011). Although further research is needed on the role and regulatory mechanism of Th17 cells in AD occurrence and development, current research practices reveal that their functions are related to released cytokines, such as Th17 cell infiltration in AD patients’ brains induced by β-42 injection, and in an AD rat model, levels of IL-17 and IL-22 were found to be higher in the brain parenchyma, cerebrospinal fluid, and serum (Zhang et al., 2013). According to Kebir et al. (2007), IL-22 seems to be involved in the breakdown of the blood–brain barrier (BBB). IL-17R and IL-22R were increased in AD patients’ BBB endothelial cells, according to Vitaliti et al. (2019). When IL-22 and IL-17 were co-cultured with human brain endothelial cells, occludin and zonula occludens (ZO)-1 levels were found to be dramatically decreased; this result was also confirmed in experimental autoimmune encephalomyelitis (EAE) mice. Nevertheless, the specific mechanism through which IL-22 causes BBB permeability is unknown. In addition, immune cells that penetrate the brain, such as Th17 cells, may create cytokines that bind to receptors on neurons, resulting in neuronal death and NDs (Tzartos et al., 2011).
MS is by far the most prevalent chronic inflammatory disease of the CNS in the world, impacting over 2.5 million individuals globally. According to a systematic analysis of MS (Collaborators G. B. D. M. S., 2019), in 2016, an estimated 2,221,188 people worldwide were living with MS, equivalent to a prevalence of 30·1 cases per 100,000 people. From 1990 to 2016, the age-standardized prevalence rate was estimated to have increased by 22·47 cases per 100,000 people. Age-standardized prevalence rates are higher than 120 per 100,000 in North America and some Nordic countries, moderate (60–120 per 100,000) in some countries in Europe and Oceania, and lowest (<60 per 100,000) in northern Africa and the Middle East, Latin America, Asia, Oceania, the Caribbean, and Sub-Saharan Africa. Notably, the global prevalence of MS varies by gender. Among prepubertal children, the prevalence of MS is similar in boys and girls. During adolescence, the curve begins to diverge, with the prevalence increasing more in girls than in boys. This pattern continued until the end of the sixth decade of life when the sex ratio was 2:1 in favor of women. Among older adults, prevalence rates generally continue to climb in women but decline slowly in men. Although the exact etiology of MS is uncertain, it is assumed to be an autoimmune disease caused by autoreactive lymphocytes reacting to CNS autoantigens (Dendrou et al., 2015). MS has a wide range of clinical symptoms, including sensory and visual difficulties, tiredness, cognitive abnormalities, and motor dysfunction (Compston and Coles, 2008). BBB damage, infiltration of macrophages, T cells, and B cells into the CNS, and local activation of microglia and astrocytes all contribute to inflammation, demyelination, gliosis, neurodegeneration, and primary axonal damage in MS (Frischer et al., 2009; Dendrou et al., 2015). This process can be explained by the “molecular mimicry” theory (Figure 1).
FIGURE 1. Overview of molecular mimicry. Mononuclear macrophages in the body phagocytose and digest antigenic substances (such as erroneous protein deposits in neurodegenerative diseases) and transmit antigenic signals that have common antigenicity with myelin basic protein to helper T cells. After activation, it enters the CNS, activates effector T cells, releases a large number of cytokines, and activates complement and B cells, resulting in the activation of microglia and astrocytes and the release of neurotoxic substances, oligodendrocyte apoptosis, and myeloid cells. The sheath is damaged, eventually leading to the onset of neurodegenerative diseases.
T cells, B cells, and activated macrophages/microglia are the main immune cells in the immune infiltrating brain of MS patients (Pittock and Lucchinetti, 2007). Different CD4+ T cell subsets all play essential roles in MS immunopathogenesis. Th1 and Th17 cells play an important role in the initiation and progression of MS. IL-17, IL-6, IL-21, IL-22, IL-23, and TNF-α are inflammatory cytokines generated by Th17 cells, of which IL-17A is the most important cytokine in Th17-mediated encephalopathy. However, new research has shown that IL-17A has only a minor impact on the onset and course of MS. In contrast, the importance of IL-22 in MS advancement has increased significantly. IL-23 is crucial for the development of autoimmune diseases. IL-23 can encourage Th cells to generate IL-17. However, a new study has shown that the IL-22 gene is the most highly expressed gene in Th cells after IL-23 stimulation. IL-22 receptor (IL-22R) is a heterodimer composed of IL-10 receptor-2 (IL-10R2) and IL-22R1, and its expression site is similar to that of the IL-17A receptor (IL-17RA), which mainly exists on the stromal cells of the CNS, such as endothelial cells, epithelial cells, and IL-17RA (Weiss et al., 2004; Boniface et al., 2005; Bettelli et al., 2007). Because of the apparent close relationship between IL-22 and IL-17, including that their receptors are expressed by similar cell types, IL-22 may have a synergistic role with IL-17 in the development of CNS disease. IL-22 also affects barrier surface integrity in a variety of clinical diseases, and when combined with IL-17, IL-22 appears to disrupt the integrity of the BBB (Sonnenberg et al., 2010). This procedure has been demonstrated in the brains of AD patients. The mechanism by which they alter BBB permeability in MS patients may be similar to that in AD patients’ brains. Lymphocytes penetrate the CNS via a breach in the BBB, and the infiltrating lymphocytes secrete numerous cytokines that exacerbate disease progression (Kebir et al., 2007). To further explore the relationship between IL-22 and MS, Katharina K et al. (Kreymborg et al., 2007) induced EAE using IL22−/− mice, which were surprisingly fully sensitive to EAE compared to controls. Further study found that these EAE mice had significantly increased the expression levels of IL-22 in the brain and spinal cord. The finding in human patients is also consistent with the results of animal experiments, with MS patients having elevated IL-22 levels in the plasma, white matter, and MS plaques compared with normal controls (Perriard et al., 2015). During EAE onset (Vellios and van der Zee, 2020), IL-22 levels increased markedly at the peak of the disease and dropped sharply during recovery, while IL-10 and IL-17 levels remained unchanged (Almolda et al., 2011). These findings suggest that IL-22 may have a more important role in the development of MS than IL-10 and IL-17.
Another key subset of CD4+ T cells, Tregs, is impaired in MS patients. Tregs are one of the most critical cells in autoimmune illnesses, and they may play a role in both controlling and preventing disease progression. Despite the fact that the amount of Tregs in MS is unaffected, their function is diminished, which may contribute to disease development and progression (Huan et al., 2005). CD4+CD25+FOXP3+ Tregs have been reported to inhibit effector T-cell activation and proliferation. The expression of the cellular marker CD25 and the transcription factor forkhead box P3 (FOXP3) characterizes them. Compared with the healthy control group, the FOXP3 mRNA level in MS patients was decreased, and further detection showed that the proportion of CD4+CD25+FOXP3+ Tregs in the total peripheral blood mononuclear cells (PBMC) of the patients was decreased. Jin Z et al. (Zhen et al., 2017) suggested that IL-22 is involved in inhibiting the function of Tregs cells in MS patients, thereby indirectly promoting the activation and proliferation of effector T cells and promoting the development of the disease. When Jin Z et al. stimulated CD4+ T cells from MS patients with IL-22, the expression of FOXP3 was significantly downregulated.
However, the mechanism of action of IL-22 in the pathogenesis of MS is not fully understood. Existing studies suggest that IL-22 may play a pathogenic role by regulating the activity of glial cells. In EAE-induced animal brain tissue, IL-22R expression increased with disease progression, and high expression levels of IL-22R were found in microglia, astrocytes, and oligodendrocytes.
In active MS lesions, “classically activated” microglia are assumed to be important for myelin phagocytosis, antigen presentation to T cells, and the production of pro-inflammatory cytokines (Lassmann et al., 2012). In a mouse model of EAE, microglial paralysis had been found to postpone the development of EAE and lower clinical severity (Heppner et al., 2005). However, whereas IL-22 activation of microglia in the brain has been extensively described in other ND models, the effects of IL-22 on microglia in MS have not been explored. Microglia as a therapeutic target for MS has been a hot topic in recent years, and thoroughly understanding the control of IL-22 on the activity of microglial cells in MS patients might aid with targeted treatment.
Traditionally, astrocytes are thought to respond only when neurons are demyelinated and formed glial scars (Brosnan and Raine, 2013). However, recent research has shown that astrocytes are important players in the early stages of MS (Farina et al., 2007; Sofroniew, 2015; Ponath et al., 2017). Astrocytes perform several functions in the progression of MS, including recruiting lymphocytes (Farina et al., 2007; Ransohoff and Brown, 2012; Liddelow and Hoyer, 2016), encouraging tissue damage (Bleasel and Frost, 1986; Warren et al., 1992; Pitt et al., 2000; Smith et al., 2000; Nourbakhsh et al., 2021), limiting inflammation, and promoting lesion healing (Sofroniew, 2015; Tschoe et al., 2020). In recent years, it has also been found that astrocytes in the brain also support B-cell survival and have been implicated in CNS-compartmentalized inflammation and progressive CNS damage (Touil et al., 2018). IL-22R is strongly expressed on astrocytes in the brains of MS patients, particularly in MS plaques or surrounding blood vessels. IL-22 has been found to protect astrocytes in an inflammatory environment in a study as by Perriard et al. (2015). They found that primary human astrocytes treated with IL-22 had a better survival rate. It is possible that anti-apoptotic pathways are involved (Ponath et al., 2018).
The development of MS is accompanied by oligodendrocyte (OL) dysfunction and death, which leads to demyelination and neurodegeneration that exacerbate MS. Furthermore, increased expression of Fas on the glial membrane surface in MS lesions has been observed (Dowling et al., 1996; Hoftberger et al., 2004). FasL on CD8+ T cells increased Fas on glial cell interaction, leading to the apoptosis of glial cells (Hovelmeyer et al., 2005).
Most NDs, including AD, Parkinson’s disease (PD), MS, and amyotrophic lateral sclerosis (ALS), are related to neuroinflammatory processes (Lucin and Wyss-Coray, 2009), for example, highly insoluble Aβ peptide deposits and neurofibrillary tangles constitute a unique trigger for inflammation in the brains of AD patients (Akiyama et al., 2000; Gonzalez et al., 2014). Inflammatory mediators also increase the processing of amyloid precursor protein (APP) in multiple ways, generating a vicious cycle of AD development (Heneka et al., 2010). This adds to the evidence that IL-22 has a function in NDs.
IL-22 is a powerful pro-inflammatory cytokine. Inflammatory cytokines including COX-2, PGE2, IL-6, and TNF-α have a role in various degenerative brain illnesses, according to pathological research (Ennerfelt and Lukens, 2020; Shahdadi Sardou et al., 2020; Daily et al., 2021). The expression levels of inflammatory cytokines were upregulated in IL-22-treated neuronal cells and glial cells. IL-22R is constitutively expressed in BV2 mouse microglia and HT22 hippocampal neurons, and the same results were obtained in mouse brain tissue, particularly its expression in the hippocampus and cerebellum with increased inflammation. Although microglia have protective activity, hyperactivated microglia release high levels of inflammatory cytokines such as IL-6, IL-1β, and TNF-α, leading to neuronal death and chronic inflammation, which is the degenerative main cause of encephalopathy (Cervellati et al., 2020; Wu et al., 2021). In addition, the expression level of COX-2 is quickly upregulated in inflammatory circumstances, and IL-22-treated BV2 and HT22 cells revealed a considerable rise in COX-2 mRNA expression (Lee et al., 2022), and PGE2 synthesis in both cells enhanced (Nikoopour et al., 2015). This shows that by activating COX-2, IL-22 may boost PGE2 synthesis. Using the Affymetrix GeneChip Mouse Gene 2.0 ST array, Dahae Lee et al. (2022) evaluated the expression levels of inflammatory cytokine genes in HT22 cells, following IL-22 administration and discovered that roughly one-third of inflammatory cytokines were upregulated.
A lot of research has been conducted on NDs in recent years. However, in-depth research into its molecular processes is still lacking. It is worth mentioning that the involvement of the JAK/STAT signaling pathway in NDs has been steadily uncovered, and it is safe to say that the JAK/STAT signaling pathway plays a key role in ND pathogenesis (Trager et al., 2013; Yan et al., 2018; Jain et al., 2021).
The JAK/STAT signaling pathway is a signal transduction pathway stimulated by cytokines involving the activation of two families of proteins discovered and cloned in the early 1990s: the Janus kinase (JAK), which comprises four tyrosine kinases (JAK1, JAK2, JAK3, and TYK2), and the signal transducer and activator of transcription (STAT) contains seven structurally and functionally related proteins (STAT1, STAT2, STAT3, STAT4, STAT5A, STAT5B, and STAT6). In the CNS, the aberrant JAK/STAT signaling pathway is associated with hormone secretion, neuroinflammation, and tumorigenesis, which will lead to CNS dysregulation. Dysregulation of the CNS mainly affects the state of neurons/glial cells. Nevado-Holgado et al. (2019) studied AD patients, demonstrated that the JAK/STAT signaling pathway has a key role in the development and progression of AD, and pointed out some potential AD therapeutic targets in this signaling pathway. Reichenbach et al. (2019) studied STAT3-deficient APP/PS1 mice and found that STAT3-mediated astrogliosis is an important therapeutic target for AD. In addition, in the EAE animal model, the role of the JAK/STAT signaling pathway was also confirmed. In the EAE animal model, STAT4 regulates IFN-γ production by autoreactive Th1 cells. IFN-γ can promote macrophage STAT1 expression and stimulate macrophage M1 differentiation. Th17 cells also generate GM-CSF in the CNS, which promotes macrophage pro-inflammatory polarization through the JAK2/STAT5 signaling pathway (Andersson et al., 2015).
Although the JAK/STAT signaling pathway has been shown to be activated in NDs, the underlying mechanisms remain unknown. The relationship between IL-22 and the JAK/STAT signaling pathway is well known (Figure 2). JAKs (JAK1 and Tyk2) are used in IL-22 signaling to transmit downstream phosphorylation signals such as mitogen-activated protein kinase (MAPK) signaling (ERK1/2, MEK1/2, JNK, and p38 kinase), signal transducers, and transcription activators (STAT1, STAT3, and STAT5) (Lejeune et al., 2002). When IL-22 binds to its receptor complex, receptor-associated JAK1/Tyk2 kinases are activated, causing phosphorylation of downstream proteins. Meng et al. described the function of Src homologue-2, consisting of protein tyrosine phosphatase 2 (Shp2), in the IL-22 signaling pathway. Studies had shown that IL-22 induced IL-22R1 phosphorylation, and Shp2 bound to tyrosine-phosphorylated IL-22R1 upon IL-22 stimulation. Furthermore, Tyr251 and Tyr301 of IL-22R1 were required for Shp2 binding to IL-22R1. The binding of Shp2 to IL-22R1 and activation of Shp2 protein tyrosine phosphatase were critical for MAP kinase activity, signal transduction, and IL-22-activated STAT3 transcriptional phosphorylation. These research studies showed that Shp2 plays a crucial role in the IL-22-mediated signal transduction pathway (Meng et al., 2010).
FIGURE 2. Overview of the production and action signaling pathways of IL-22. IL-22 is often, but not always, correlated with IL-17 expression. Subpopulations of CD4+ T cells, CD8+ T cells, innate T cells, and innate lymphoid cells are sources of IL-22, IL-22, and IL-17 or IL-17. The main cytokines driving IL-22 expression are IL-23, IL-6, and IL-1β. Certain cell types require IL-12, IL-18, IL-21, and TNF-α to produce IL-22. TGF-β inhibits IL-22 production in all T-cell subsets but induces IL-17 in these cells; IL-22 signals through its cognate receptor/JAK complex, resulting in downstream phosphorylation of STAT (homo- or heterodimer). Translocation of these STAT complexes to the nucleus drives transcription of genes involved in processes ranging from inflammation to angiogenesis and survival. The control of activity of this pathway involves different mechanisms including regulation of the phosphorylation state of JAK and STAT by phosphatases, or of the JAK kinase activity by SOCS (suppressor of cytokine signaling), for example.
In addition to the JAK/STAT signaling pathway, the binding of IL-22 to cellular receptors also promotes the activation of MAPK and the p38 signaling pathway (Andoh et al., 2005; Ikeuchi et al., 2005; Wolk et al., 2006; Liu et al., 2012; Mitra et al., 2012). However, these IL-22-activated MAPK and p38 signaling pathways are both cross-linked with the JAK/STAT signaling pathway. The NF-κB signaling pathway is also involved in the development of NDs, with increased cell surface FAS expression in IL-22-treated CG4 cells and mouse oligodendrocytes. Compared with the controls, IL-22 greatly enhanced the phosphorylation of MSK-1 and then activated p65, a key protein in the NF-κB signaling pathway, demonstrating that IL-22 activated the MSK-1/NF-κB signaling pathway and enhanced the Fas expression on the surface of glial cells (Zhen et al., 2017). In NDs, all of the aforementioned signaling pathways influence disease progression (Jiang et al., 2009; Jia et al., 2011; Noubade et al., 2011; Arumugam et al., 2012; Tagoe and Putterman, 2012). Therefore, manipulating IL-22 and its signaling pathway might be a viable treatment method for NDs (Pan et al., 2013).
Disease-modifying therapy (DMT) has been widely used in recent decades as an effective disease-modifying therapy to treat NDs (Gross and Corboy, 2019; Cole et al., 2021), and there are now two approved drugs used to treat AD, namely, cholinesterase inhibitors and N-methyl-D-aspartate (NMDA) antagonists. However, cholinesterase inhibitors and NMDA antagonists are only effective for treating AD symptoms but do not completely cure or prevent AD. In addition, the enormous limitations to the vast majority of drugs (98% of small-molecule drugs and 100% of large-molecule drugs) in crossing the BBB further increase the therapeutic difficulty of treating NDs. Stem cell therapy, also known as regenerative therapy, uses stem cells or their derivatives to improve the repair response of dysfunctional and damaged tissue. The goal of stem cell therapy is often focused on cell replacement or providing environmental enrichment. Stem cell therapy has revolutionized medicine over the years, and its therapeutic applications provide a valuable and attractive option for the treatment of a variety of diseases, including NDs (Sakthiswary and Raymond, 2012). However, there are some inevitable adverse consequences to this strategy. As a result, developing better therapies is critical. Immunotherapy has received increased attention in recent years, and excellent therapeutic benefits have been established in the clinical treatment of a variety of disorders. IL-22 has promise as a possible target for treating AD, MS, and other NDs due to its crucial involvement in the inflammatory and proliferative cascades. As previously observed, IL-22BP has an inhibitory influence on the function of IL-22 and has been identified as a risk gene for MS (Beyeen et al., 2010). We think that IL-22 neutralizers are prospective medications not only just for MS but also for other NDs since soluble cytokine receptors have been demonstrated to be useful as a treatment for inflammatory diseases (Moreland, 1999). Wang et al. (2012) found that the expression levels of inflammatory cytokines (TNF-α and IL-6) were considerably lowered in the mouse brain using the IL-22−/− mouse model. TNF-α and IL-6, as previously indicated, aid in the recruitment and maintenance of inflammatory Th17 cells in the tissue (Kebir et al., 2007) and may contribute to disease development and progression (Paganelli et al., 2002; Ng et al., 2018). Wang et al. (2017) injected Aβ1-42 into the mouse hippocampus to create an Alzheimer’s animal model, and they found that the model mice had a higher level of IL-22 in the hippocampus and cortex. After IL-22 was highly expressed in the mouse brains, the mice’s disease improved significantly. According to the findings, IL-22 may be a possible therapeutic target for NDs, and this is a promising therapeutic strategy.
Hu et al. (2019)analyzed cerebrospinal fluid samples from AD, MS, PD, and DLB patients and found that four cytokines (IL-10, IP-10, IL-8, and TNF-α) related to innate immunity and subtypes of helper T cells (Th1, Th2, and Th17) have much greater levels of expression in the cerebral fluid of patients with degenerative disorders than healthy people. Rodrguez-Sáinz Model C et al. performed a cross-sectional investigation of 17 randomly chosen patients during an MS episode, measuring TNF-α and IL-10 expression levels in the blood and cerebrospinal fluid (CSF) of the patients. The results showed that TNF-α and IL-10 expression levels in the blood and CSF of MS patients were greater than in healthy controls. The high levels of TNF-α and IL-10 (in the serum and cerebrospinal fluid) that accompany the onset of NDs indicate simultaneous expression of cytokines Th1, Th2, and Th17, and given the relationship of IL-22 to these three cells, we suspect IL-22 also has untapped clinical potential in patients with NDs.
Nanotherapy is a new treatment method with lower toxicity and fewer side effects that has become more popular in recent years. Therapeutics and theranostics based on nanoparticles (NPs) have been developed for a variety of ailments, particularly for the treatment of different kinds of malignancies (Muntimadugu et al., 2017; Gong et al., 2019). However, crossing the BBB with a carrier for small-molecule therapies is particularly challenging because the BBB significantly restricts the use of nanotherapeutics in CNS illnesses like NDs. Finding effective delivery systems to help drugs cross the BBB and successfully localize and bind to targets is a challenge in the field of treatment of NDs.
Lohan et al. (2017) employed carbon nanotubes as a carrier for the medication BRB, effectively delivering neuro drugs to brain microglia and slowing the course of AD. BRB has several limitations, including low bioavailability, poor intestinal absorption, and limited CNS penetration, despite its great therapeutic benefits. Conti et al. (2017) developed a functional liposome that removed Aβ42 and delayed disease progression. NLCs offer excellent biodegradability, physical durability, low toxicity, and superior degradability, but they have drawbacks such as gelation, lipid compartment development, and fundamentally poor integration rates, among others. These issues obstruct their use in clinical practice (Naseri et al., 2015). Li et al. (2021) recently developed poly (lactic-co-glycolic acid) (PLGA)-based NPs. The researchers discovered that the nanoparticles’ surface coating interacted with the BBB and nerve cells in many ways to improve drug absorption and that PS 80-coated NPs (PS 80-NPs) loaded with tau siRNA effectively suppressed tau expression in mouse nerve cells. In addition, inorganic nanoparticles, such as magnetic nanoparticles (Cui et al., 2018), metal-rich nanoparticles (Naziroglu et al., 2017), and quantum nanoparticles (Gupta et al., 2010), are also promising options for treating NDs.
Despite the fact that there have been several studies published on the use of nanocarriers to cure and regulate NDs, no relevant clinical trials have been reported. The use of simple, repeatable, and low-cost nanomaterial preparation methods is a key barrier to scaling up these materials. The protein corona effect, the impact of cell sex, type, and passage number on cell therapy, and the need for patient-specific cells are all difficulties that must be addressed in nanocarrier clinical trials. The majority of the scholarly literature on nanomedicine ignores these difficulties (Mahmoudi, 2018). Overall, there are still numerous issues in the clinical translation of nanocarriers to be resolved. The creation of vectors that target IL-22 silencing has considerable advantages for ND remission, and it may be a major approach for ND therapy.
A variety of cytokines are related to the occurrence and development of NDs, among which IL-22 is a key factor in the process of NDs. Although the mechanism of IL-22 in NDs is yet unknown, a growing body of evidence from in vivo and in vitro models suggests that IL-22 might be a feasible therapeutic target for NDs. However, it should be emphasized that the majority of the data on IL-22 came from animal models, which may or may not be relevant to people (or other experimental ones). Furthermore, the function of IL-22 in prevalent NDs such as PD and ALS has not been investigated. As a result, further research is required, particularly in human systems, to fully comprehend the function of IL-22 in NDs.
WJ and JP collected the related literature and drafted the manuscript. HZ and YK participated in the design of the review and drafted the manuscript. WJ, JP, and BJ revised and edited the manuscript. M and JF supervised the review process. YC and YX participated in the revision work of the manuscript.
The authors declare that the research was conducted in the absence of any commercial or financial relationships that could be construed as a potential conflict of interest.
All claims expressed in this article are solely those of the authors and do not necessarily represent those of their affiliated organizations, or those of the publisher, the editors, and the reviewers. Any product that may be evaluated in this article, or claim that may be made by its manufacturer, is not guaranteed or endorsed by the publisher.
NDs, neurodegenerative diseases; TNF-α, tumor necrosis factor-α; AD, Alzheimer’s disease; MS, multiple sclerosis; IFN-γ, interferon-γ; TGF-β, transforming growth factor-β; Aβ, amyloid-β peptides; CNS, central nervous system; EAE, experimental autoimmune encephalomyelitis; Tregs, regulatory T cells; OL, oligodendrocyte; PD, Parkinson’s disease; ALS, amyotrophic lateral sclerosis; APP, amyloid precursor protein; STAT, signal transducer and activator of transcription; JAK, Janus kinase; MAPK, mitogen-activated protein kinase; DMT, disease-modifying treatment; CSF, cerebrospinal fluid; NPs, nanoparticles; BRB, berberine; NLCs, nanostructured lipid carriers; MRI, magnetic resonance imaging.
Akiyama, H., Barger, S., Barnum, S., Bradt, B., Bauer, J., Cole, G. M., et al. (2000). Inflammation and Alzheimer's disease. Neurobiol. Aging 21, 383–421. doi:10.1016/s0197-4580(00)00124-x
Almolda, B., Costa, M., Montoya, M., Gonzalez, B., and Castellano, B. (2011). Increase in Th17 and T-reg lymphocytes and decrease of IL22 correlate with the recovery phase of acute EAE in rat. PLoS One 6, e27473. doi:10.1371/journal.pone.0027473
Andersson, K. M., Cavallini, N. F., Hu, D., Brisslert, M., Cialic, R., Valadi, H., et al. (2015). Pathogenic transdifferentiation of Th17 cells contribute to perpetuation of rheumatoid arthritis during anti-TNF treatment. Mol. Med. 21, 536–543. doi:10.2119/molmed.2015.00057
Andoh, A., Zhang, Z., Inatomi, O., Fujino, S., Deguchi, Y., Araki, Y., et al. (2005). Interleukin-22, a member of the IL-10 subfamily, induces inflammatory responses in colonic subepithelial myofibroblasts. Gastroenterology 129, 969–984. doi:10.1053/j.gastro.2005.06.071
Arumugam, S., Thandavarayan, R. A., Veeraveedu, P. T., Giridharan, V. V., Soetikno, V., Harima, M., et al. (2012). Involvement of AMPK and MAPK signaling during the progression of experimental autoimmune myocarditis in rats and its blockade using a novel antioxidant. Exp. Mol. Pathol. 93, 183–189. doi:10.1016/j.yexmp.2012.04.012
Baik, S. H., Cha, M. Y., Hyun, Y. M., Cho, H., Hamza, B., Kim, D. K., et al. (2014). Migration of neutrophils targeting amyloid plaques in Alzheimer's disease mouse model. Neurobiol. Aging 35, 1286–1292. doi:10.1016/j.neurobiolaging.2014.01.003
Bettelli, E., Oukka, M., and Kuchroo, V. K. (2007). T(H)-17 cells in the circle of immunity and autoimmunity. Nat. Immunol. 8, 345–350. doi:10.1038/ni0407-345
Beyeen, A. D., Adzemovic, M. Z., Ockinger, J., Stridh, P., Becanovic, K., Laaksonen, H., et al. (2010). IL-22RA2 associates with multiple sclerosis and macrophage effector mechanisms in experimental neuroinflammation. J. Immunol. 185, 6883–6890. doi:10.4049/jimmunol.1001392
Bleasel, K. F., and Frost, R. B. (1986). A new neurosurgical irrigating sucking cutter. Technical note. J. Neurosurg. 65, 120–121. doi:10.3171/jns.1986.65.1.0120
Boniface, K., Bernard, F. X., Garcia, M., Gurney, A. L., Lecron, J. C., and Morel, F. (2005). IL-22 inhibits epidermal differentiation and induces proinflammatory gene expression and migration of human keratinocytes. J. Immunol. 174, 3695–3702. doi:10.4049/jimmunol.174.6.3695
Brosnan, C. F., and Raine, C. S. (2013). The astrocyte in multiple sclerosis revisited. Glia 61, 453–465. doi:10.1002/glia.22443
Cai, Z., Hussain, M. D., and Yan, L. J. (2014). Microglia, neuroinflammation, and beta-amyloid protein in Alzheimer's disease. Int. J. Neurosci. 124, 307–321. doi:10.3109/00207454.2013.833510
Cao, J., Hou, J., Ping, J., and Cai, D. (2018). Advances in developing novel therapeutic strategies for Alzheimer's disease. Mol. Neurodegener. 13, 64. doi:10.1186/s13024-018-0299-8
Cervellati, C., Trentini, A., Pecorelli, A., and Valacchi, G. (2020). Inflammation in neurological disorders: The thin boundary between brain and periphery. Antioxid. Redox Signal. 33, 191–210. doi:10.1089/ars.2020.8076
Chan, K. Y., Wang, W., Wu, J. J., Liu, L., Theodoratou, E., Car, J., et al. (2013). Epidemiology of Alzheimer's disease and other forms of dementia in China, 1990-2010: A systematic review and analysis. Lancet 381, 2016–2023. doi:10.1016/S0140-6736(13)60221-4
Chung, Y., Yang, X., Chang, S. H., Ma, L., Tian, Q., and Dong, C. (2006). Expression and regulation of IL-22 in the IL-17-producing CD4+ T lymphocytes. Cell Res. 16, 902–907. doi:10.1038/sj.cr.7310106
Cole, T. A., Zhao, H., Collier, T. J., Sandoval, I., Sortwell, C. E., Steece-Collier, K., et al. (2021). α-Synuclein antisense oligonucleotides as a disease-modifying therapy for Parkinson's disease. JCI Insight 6, 135633. doi:10.1172/jci.insight.135633
Collaborators, G. B. D. D. (2019a). Global, regional, and national burden of Alzheimer's disease and other dementias, 1990-2016: A systematic analysis for the global burden of disease study 2016. Lancet. Neurol. 18, 88–106. doi:10.1016/S1474-4422(18)30403-4
Collaborators, G. B. D. M. S. (2019b). Global, regional, and national burden of multiple sclerosis 1990-2016: A systematic analysis for the global burden of disease study 2016. Lancet. Neurol. 18, 269–285. doi:10.1016/S1474-4422(18)30443-5
Compston, A., and Coles, A. (2008). Multiple sclerosis. Lancet 372, 1502–1517. doi:10.1016/S0140-6736(08)61620-7
Conti, E., Gregori, M., Radice, I., Da Re, F., Grana, D., Re, F., et al. (2017). Multifunctional liposomes interact with Abeta in human biological fluids: Therapeutic implications for Alzheimer's disease. Neurochem. Int. 108, 60–65. doi:10.1016/j.neuint.2017.02.012
Cui, N., Lu, H., and Li, M. (2018). Magnetic nanoparticles associated PEG/PLGA block copolymer targeted with anti-transferrin receptor antibodies for Alzheimer's disease. J. Biomed. Nanotechnol. 14, 1017–1024. doi:10.1166/jbn.2018.2512
Daily, J. W., Kang, S., and Park, S. (2021). Protection against Alzheimer's disease by luteolin: Role of brain glucose regulation, anti-inflammatory activity, and the gut microbiota-liver-brain axis. Biofactors 47, 218–231. doi:10.1002/biof.1703
Dendrou, C. A., Fugger, L., and Friese, M. A. (2015). Immunopathology of multiple sclerosis. Nat. Rev. Immunol. 15, 545–558. doi:10.1038/nri3871
Dowling, P., Shang, G., Raval, S., Menonna, J., Cook, S., and Husar, W. (1996). Involvement of the CD95 (APO-1/Fas) receptor/ligand system in multiple sclerosis brain. J. Exp. Med. 184, 1513–1518. doi:10.1084/jem.184.4.1513
Duhen, T., Geiger, R., Jarrossay, D., Lanzavecchia, A., and Sallusto, F. (2009). Production of interleukin 22 but not interleukin 17 by a subset of human skin-homing memory T cells. Nat. Immunol. 10, 857–863. doi:10.1038/ni.1767
Ennerfelt, H. E., and Lukens, J. R. (2020). The role of innate immunity in Alzheimer's disease. Immunol. Rev. 297, 225–246. doi:10.1111/imr.12896
Eyerich, S., Eyerich, K., Pennino, D., Carbone, T., Nasorri, F., Pallotta, S., et al. (2009). Th22 cells represent a distinct human T cell subset involved in epidermal immunity and remodeling. J. Clin. Invest. 119, 3573–3585. doi:10.1172/JCI40202
Farina, C., Aloisi, F., and Meinl, E. (2007). Astrocytes are active players in cerebral innate immunity. Trends Immunol. 28, 138–145. doi:10.1016/j.it.2007.01.005
Frischer, J. M., Bramow, S., Dal-Bianco, A., Lucchinetti, C. F., Rauschka, H., Schmidbauer, M., et al. (2009). The relation between inflammation and neurodegeneration in multiple sclerosis brains. Brain. 132, 1175–1189. doi:10.1093/brain/awp070
Gandy, S., and Greengard, P. (1994). Processing of alzheimer A beta-amyloid precursor protein: Cell biology, regulation, and role in alzheimer disease. Int. Rev. Neurobiol. 36, 29–50. doi:10.1016/s0074-7742(08)60302-5
Gate, D., Saligrama, N., Leventhal, O., Yang, A. C., Unger, M. S., Middeldorp, J., et al. (2020). Clonally expanded CD8 T cells patrol the cerebrospinal fluid in Alzheimer's disease. Nature 577, 399–404. doi:10.1038/s41586-019-1895-7
Gong, B. S., Wang, R., Xu, H. X., Miao, M. Y., and Yao, Z. Z. (2019). Nanotherapy targeting the tumor microenvironment. Curr. Cancer Drug Targets 19, 525–533. doi:10.2174/1568009619666181220103714
Gonzalez, H., Elgueta, D., Montoya, A., and Pacheco, R. (2014). Neuroimmune regulation of microglial activity involved in neuroinflammation and neurodegenerative diseases. J. Neuroimmunol. 274, 1–13. doi:10.1016/j.jneuroim.2014.07.012
Gouya, G., Arrich, J., Wolzt, M., Huber, K., Verheugt, F. W., Gurbel, P. A., et al. (2014). Antiplatelet treatment for prevention of cerebrovascular events in patients with vascular diseases: A systematic review and meta-analysis. Stroke 45, 492–503. doi:10.1161/STROKEAHA.113.002590
Gross, R. H., and Corboy, J. R. (2019). Monitoring, switching, and stopping multiple sclerosis disease-modifying therapies. Contin. (Minneap Minn) 25, 715–735. doi:10.1212/CON.0000000000000738
Gupta, S., Babu, P., and Surolia, A. (2010). Biphenyl ethers conjugated CdSe/ZnS core/shell quantum dots and interpretation of the mechanism of amyloid fibril disruption. Biomaterials 31, 6809–6822. doi:10.1016/j.biomaterials.2010.05.031
Hardy, J., and Selkoe, D. J. (2002). The amyloid hypothesis of Alzheimer's disease: Progress and problems on the road to therapeutics. Science 297, 353–356. doi:10.1126/science.1072994
Heneka, M. T., Carson, M. J., El Khoury, J., Landreth, G. E., Brosseron, F., Feinstein, D. L., et al. (2015). Neuroinflammation in Alzheimer's disease. Lancet. Neurol. 14, 388–405. doi:10.1016/S1474-4422(15)70016-5
Heneka, M. T., O'Banion, M. K., Terwel, D., and Kummer, M. P. (2010). Neuroinflammatory processes in Alzheimer's disease. J. Neural Transm. 117, 919–947. doi:10.1007/s00702-010-0438-z
Heppner, F. L., Greter, M., Marino, D., Falsig, J., Raivich, G., Hovelmeyer, N., et al. (2005). Experimental autoimmune encephalomyelitis repressed by microglial paralysis. Nat. Med. 11, 146–152. doi:10.1038/nm1177
Hoftberger, R., Aboul-Enein, F., Brueck, W., Lucchinetti, C., Rodriguez, M., Schmidbauer, M., et al. (2004). Expression of major histocompatibility complex class I molecules on the different cell types in multiple sclerosis lesions. Brain Pathol. 14, 43–50. doi:10.1111/j.1750-3639.2004.tb00496.x
Hong, S., Beja-Glasser, V. F., Nfonoyim, B. M., Frouin, A., Li, S., Ramakrishnan, S., et al. (2016). Complement and microglia mediate early synapse loss in Alzheimer mouse models. Science 352, 712–716. doi:10.1126/science.aad8373
Hovelmeyer, N., Hao, Z., Kranidioti, K., Kassiotis, G., Buch, T., Frommer, F., et al. (2005). Apoptosis of oligodendrocytes via Fas and TNF-R1 is a key event in the induction of experimental autoimmune encephalomyelitis. J. Immunol. 175, 5875–5884. doi:10.4049/jimmunol.175.9.5875
Hu, W. T., Howell, J. C., Ozturk, T., Gangishetti, U., Kollhoff, A. L., Hatcher-Martin, J. M., et al. (2019). CSF cytokines in aging, multiple sclerosis, and dementia. Front. Immunol. 10, 480. doi:10.3389/fimmu.2019.00480
Huan, J., Culbertson, N., Spencer, L., Bartholomew, R., Burrows, G. G., Chou, Y. K., et al. (2005). Decreased FOXP3 levels in multiple sclerosis patients. J. Neurosci. Res. 81, 45–52. doi:10.1002/jnr.20522
Ikeuchi, H., Kuroiwa, T., Hiramatsu, N., Kaneko, Y., Hiromura, K., Ueki, K., et al. (2005). Expression of interleukin-22 in rheumatoid arthritis: Potential role as a proinflammatory cytokine. Arthritis Rheum. 52, 1037–1046. doi:10.1002/art.20965
Jain, M., Singh, M. K., Shyam, H., Mishra, A., Kumar, S., Kumar, A., et al. (2021). Role of JAK/STAT in the neuroinflammation and its association with neurological disorders. Ann. Neurosci. 28, 191–200. doi:10.1177/09727531211070532
Jia, Y., Jing, J., Bai, Y., Li, Z., Liu, L., Luo, J., et al. (2011). Amelioration of experimental autoimmune encephalomyelitis by plumbagin through down-regulation of JAK-STAT and NF-κB signaling pathways. PLoS One 6, e27006. doi:10.1371/journal.pone.0027006
Jiang, Z., Li, H., Fitzgerald, D. C., Zhang, G. X., and Rostami, A. (2009). MOG(35-55) i.v suppresses experimental autoimmune encephalomyelitis partially through modulation of Th17 and JAK/STAT pathways. Eur. J. Immunol. 39, 789–799. doi:10.1002/eji.200838427
Kebir, H., Kreymborg, K., Ifergan, I., Dodelet-Devillers, A., Cayrol, R., Bernard, M., et al. (2007). Human TH17 lymphocytes promote blood-brain barrier disruption and central nervous system inflammation. Nat. Med. 13, 1173–1175. doi:10.1038/nm1651
Kreymborg, K., Etzensperger, R., Dumoutier, L., Haak, S., Rebollo, A., Buch, T., et al. (2007). IL-22 is expressed by Th17 cells in an IL-23-dependent fashion, but not required for the development of autoimmune encephalomyelitis. J. Immunol. 179, 8098–8104. doi:10.4049/jimmunol.179.12.8098
Lassmann, H., Van Horssen, J., and Mahad, D. (2012). Progressive multiple sclerosis: Pathology and pathogenesis. Nat. Rev. Neurol. 8, 647–656. doi:10.1038/nrneurol.2012.168
Lee, D., Jo, H., Go, C., Jang, Y., Chu, N., Bae, S., et al. (2022). The roles of IL-22 and its receptor in the regulation of inflammatory responses in the brain. Int. J. Mol. Sci. 23, 757. doi:10.3390/ijms23020757
Lejeune, D., Dumoutier, L., Constantinescu, S., Kruijer, W., Schuringa, J. J., and Renauld, J. C. (2002). Interleukin-22 (IL-22) activates the JAK/STAT, ERK, JNK, and p38 MAP kinase pathways in a rat hepatoma cell line. Pathways that are shared with and distinct from IL-10. J. Biol. Chem. 277, 33676–33682. doi:10.1074/jbc.M204204200
Li, W., Qiu, J., Li, X. L., Aday, S., Zhang, J., Conley, G., et al. (2021). BBB pathophysiology-independent delivery of siRNA in traumatic brain injury. Sci. Adv. 7, eabd6889. doi:10.1126/sciadv.abd6889
Liddelow, S., and Hoyer, D. (2016). Astrocytes: Adhesion molecules and immunomodulation. Curr. Drug Targets 17, 1871–1881. doi:10.2174/1389450117666160101120703
Liu, Y., Pan, W., Yang, S., Wu, X., Wu, J., Ma, J., et al. (2012). Interleukin-22 protects rat PC12 pheochromocytoma cells from serum deprivation-induced cell death. Mol. Cell. Biochem. 371, 137–146. doi:10.1007/s11010-012-1430-8
Lohan, S., Raza, K., Mehta, S. K., Bhatti, G. K., Saini, S., and Singh, B. (2017). Anti-Alzheimer's potential of berberine using surface decorated multi-walled carbon nanotubes: A preclinical evidence. Int. J. Pharm. 530, 263–278. doi:10.1016/j.ijpharm.2017.07.080
Lucin, K. M., and Wyss-Coray, T. (2009). Immune activation in brain aging and neurodegeneration: Too much or too little? Neuron 64, 110–122. doi:10.1016/j.neuron.2009.08.039
Lueg, G., Gross, C. C., Lohmann, H., Johnen, A., Kemmling, A., Deppe, M., et al. (2015). Clinical relevance of specific T-cell activation in the blood and cerebrospinal fluid of patients with mild Alzheimer's disease. Neurobiol. Aging 36, 81–89. doi:10.1016/j.neurobiolaging.2014.08.008
Mahmoudi, M. (2018). Debugging nano-bio interfaces: Systematic strategies to accelerate clinical translation of nanotechnologies. Trends Biotechnol. 36, 755–769. doi:10.1016/j.tibtech.2018.02.014
Meng, S., Gui, Q., Xu, Q., Lu, K., Jiao, X., Fan, J., et al. (2010). Association of Shp2 with phosphorylated IL-22R1 is required for interleukin-22-induced MAP kinase activation. J. Mol. Cell Biol. 2, 223–230. doi:10.1093/jmcb/mjq017
Mitra, A., Raychaudhuri, S. K., and Raychaudhuri, S. P. (2012). IL-22 induced cell proliferation is regulated by PI3K/Akt/mTOR signaling cascade. Cytokine 60, 38–42. doi:10.1016/j.cyto.2012.06.316
Monsonego, A., Imitola, J., Petrovic, S., Zota, V., Nemirovsky, A., Baron, R., et al. (2006). Abeta-induced meningoencephalitis is IFN-gamma-dependent and is associated with T cell-dependent clearance of Abeta in a mouse model of Alzheimer's disease. Proc. Natl. Acad. Sci. U. S. A. 103, 5048–5053. doi:10.1073/pnas.0506209103
Moreland, L. W. (1999). Clinical and pharmacological experience with etanercept. Expert Opin. Investig. Drugs 8, 1443–1451. doi:10.1517/13543784.8.9.1443
Muntimadugu, E., Kommineni, N., and Khan, W. (2017). Exploring the potential of nanotherapeutics in targeting tumor microenvironment for cancer therapy. Pharmacol. Res. 126, 109–122. doi:10.1016/j.phrs.2017.05.010
Naseri, N., Valizadeh, H., and Zakeri-Milani, P. (2015). Solid lipid nanoparticles and nanostructured lipid carriers: Structure, preparation and application. Adv. Pharm. Bull. 5, 305–313. doi:10.15171/apb.2015.043
Naziroglu, M., Muhamad, S., and Pecze, L. (2017). Nanoparticles as potential clinical therapeutic agents in Alzheimer's disease: Focus on selenium nanoparticles. Expert Rev. Clin. Pharmacol. 10, 773–782. doi:10.1080/17512433.2017.1324781
Nevado-Holgado, A. J., Ribe, E., Thei, L., Furlong, L., Mayer, M. A., Quan, J., et al. (2019). Cells, 8.Genetic and real-world clinical data, combined with empirical validation, nominate jak-stat signaling as a target for Alzheimer's disease therapeutic development
Ng, A., Tam, W. W., Zhang, M. W., Ho, C. S., Husain, S. F., Mcintyre, R. S., et al. (2018). IL-1β, IL-6, TNF- α and CRP in elderly patients with depression or Alzheimer's disease: Systematic review and meta-analysis. Sci. Rep. 8, 12050. doi:10.1038/s41598-018-30487-6
Nikoopour, E., Bellemore, S. M., and Singh, B. (2015). IL-22, cell regeneration and autoimmunity. Cytokine 74, 35–42. doi:10.1016/j.cyto.2014.09.007
Noubade, R., Krementsov, D. N., Del Rio, R., Thornton, T., Nagaleekar, V., Saligrama, N., et al. (2011). Activation of p38 MAPK in CD4 T cells controls IL-17 production and autoimmune encephalomyelitis. Blood 118, 3290–3300. doi:10.1182/blood-2011-02-336552
Nourbakhsh, F., Read, M. I., Barreto, G. E., and Sahebkar, A. (2021). Astrocytes and inflammasome: A possible crosstalk in neurological diseases. Curr. Med. Chem. 28, 4972–4994. doi:10.2174/0929867328666210301105422
Ohara, T., Hata, J., Yoshida, D., Mukai, N., Nagata, M., Iwaki, T., et al. (2017). Trends in dementia prevalence, incidence, and survival rate in a Japanese community. Neurology 88, 1925–1932. doi:10.1212/WNL.0000000000003932
Paganelli, R., Di Iorio, A., Patricelli, L., Ripani, F., Sparvieri, E., Faricelli, R., et al. (2002). Proinflammatory cytokines in sera of elderly patients with dementia: Levels in vascular injury are higher than those of mild-moderate Alzheimer's disease patients. Exp. Gerontol. 37, 257–263. doi:10.1016/s0531-5565(01)00191-7
Pan, H. F., Li, X. P., Zheng, S. G., and Ye, D. Q. (2013). Emerging role of interleukin-22 in autoimmune diseases. Cytokine Growth Factor Rev. 24, 51–57. doi:10.1016/j.cytogfr.2012.07.002
Perriard, G., Mathias, A., Enz, L., Canales, M., Schluep, M., Gentner, M., et al. (2015). Interleukin-22 is increased in multiple sclerosis patients and targets astrocytes. J. Neuroinflammation 12, 119. doi:10.1186/s12974-015-0335-3
Pitt, D., Werner, P., and Raine, C. S. (2000). Glutamate excitotoxicity in a model of multiple sclerosis. Nat. Med. 6, 67–70. doi:10.1038/71555
Pittock, S. J., and Lucchinetti, C. F. (2007). The pathology of MS: New insights and potential clinical applications. Neurologist 13, 45–56. doi:10.1097/01.nrl.0000253065.31662.37
Polfliet, M. M., Goede, P. H., Van Kesteren-Hendrikx, E. M., Van Rooijen, N., Dijkstra, C. D., and Van Den Berg, T. K. (2001). A method for the selective depletion of perivascular and meningeal macrophages in the central nervous system. J. Neuroimmunol. 116, 188–195. doi:10.1016/s0165-5728(01)00282-x
Ponath, G., Park, C., and Pitt, D. (2018). The role of astrocytes in multiple sclerosis. Front. Immunol. 9, 217. doi:10.3389/fimmu.2018.00217
Ponath, G., Ramanan, S., Mubarak, M., Housley, W., Lee, S., Sahinkaya, F. R., et al. (2017). Myelin phagocytosis by astrocytes after myelin damage promotes lesion pathology. Brain. 140, 399–413. doi:10.1093/brain/aww298
Qiu, C., Kivipelto, M., and Von Strauss, E. (2009). Epidemiology of Alzheimer's disease: Occurrence, determinants, and strategies toward intervention. Dialogues Clin. Neurosci. 11, 111–128. doi:10.31887/dcns.2009.11.2/cqiu
Ransohoff, R. M., and Brown, M. A. (2012). Innate immunity in the central nervous system. J. Clin. Invest. 122, 1164–1171. doi:10.1172/JCI58644
Reichenbach, N., Delekate, A., Plescher, M., Schmitt, F., Krauss, S., Blank, N., et al. (2019). Inhibition of Stat3-mediated astrogliosis ameliorates pathology in an Alzheimer's disease model. EMBO Mol. Med. 11, e9665. doi:10.15252/emmm.201809665
Rutz, S., Noubade, R., Eidenschenk, C., Ota, N., Zeng, W., Zheng, Y., et al. (2011). Transcription factor c-Maf mediates the TGF-beta-dependent suppression of IL-22 production in T(H)17 cells. Nat. Immunol. 12, 1238–1245. doi:10.1038/ni.2134
Sabat, R. (2010). IL-10 family of cytokines. Cytokine Growth Factor Rev. 21, 315–324. doi:10.1016/j.cytogfr.2010.11.001
Sakthiswary, R., and Raymond, A. A. (2012). Stem cell therapy in neurodegenerative diseases: From principles to practice. Neural Regen. Res. 7, 1822–1831. doi:10.3969/j.issn.1673-5374.2012.23.009
Saresella, M., Calabrese, E., Marventano, I., Piancone, F., Gatti, A., Alberoni, M., et al. (2011). Increased activity of Th-17 and Th-9 lymphocytes and a skewing of the post-thymic differentiation pathway are seen in Alzheimer's disease. Brain Behav. Immun. 25, 539–547. doi:10.1016/j.bbi.2010.12.004
Shahdadi Sardou, H., Jebali, A., and Iman, M. (2020). Dual function of interleukin-23 Aptamer to suppress brain inflammation via attachment to macrophage stimulating 1 kinase and interleukin-23. Colloids Surf. B Biointerfaces 185, 110619. doi:10.1016/j.colsurfb.2019.110619
Smith, T., Groom, A., Zhu, B., and Turski, L. (2000). Autoimmune encephalomyelitis ameliorated by AMPA antagonists. Nat. Med. 6, 62–66. doi:10.1038/71548
Sofroniew, M. V. (2015). Astrocyte barriers to neurotoxic inflammation. Nat. Rev. Neurosci. 16, 249–263. doi:10.1038/nrn3898
Sonnenberg, G. F., Fouser, L. A., and Artis, D. (2011). Border patrol: Regulation of immunity, inflammation and tissue homeostasis at barrier surfaces by IL-22. Nat. Immunol. 12, 383–390. doi:10.1038/ni.2025
Sonnenberg, G. F., Fouser, L. A., and Artis, D. (2010). Functional biology of the IL-22-IL-22R pathway in regulating immunity and inflammation at barrier surfaces. Adv. Immunol. 107, 1–29. doi:10.1016/B978-0-12-381300-8.00001-0
Tagoe, C., and Putterman, C. (2012). JAK2 inhibition in murine systemic lupus erythematosus. Immunotherapy 4, 369–372. doi:10.2217/imt.12.20
Touil, H., Kobert, A., Lebeurrier, N., Rieger, A., Saikali, P., Lambert, C., et al. (2018). Human central nervous system astrocytes support survival and activation of B cells: Implications for MS pathogenesis. J. Neuroinflammation 15, 114. doi:10.1186/s12974-018-1136-2
Trager, U., Magnusson, A., Lahiri Swales, N., Wild, E., North, J., Lowdell, M., et al. (2013). JAK/STAT signalling in huntington's disease immune cells. PLoS Curr. 5. doi:10.1371/currents.hd.5791c897b5c3bebeed93b1d1da0c0648
Trifari, S., Kaplan, C. D., Tran, E. H., Crellin, N. K., and Spits, H. (2009). Identification of a human helper T cell population that has abundant production of interleukin 22 and is distinct from T(H)-17, T(H)1 and T(H)2 cells. Nat. Immunol. 10, 864–871. doi:10.1038/ni.1770
Tschoe, C., Bushnell, C. D., Duncan, P. W., Alexander-Miller, M. A., and Wolfe, S. Q. (2020). Neuroinflammation after intracerebral hemorrhage and potential therapeutic targets. J. Stroke 22, 29–46. doi:10.5853/jos.2019.02236
Tzartos, J. S., Craner, M. J., Friese, M. A., Jakobsen, K. B., Newcombe, J., Esiri, M. M., et al. (2011). IL-21 and IL-21 receptor expression in lymphocytes and neurons in multiple sclerosis brain. Am. J. Pathol. 178, 794–802. doi:10.1016/j.ajpath.2010.10.043
Vellios, N., and van der Zee, K. (2020). Dataset on cigarette smokers in six South African townships. Data Brief. 32, 106260. doi:10.1016/j.dib.2020.106260
Vitaliti, G., Pavone, P., Marino, S., Saporito, M. A. N., Corsello, G., and Falsaperla, R. (2019). Molecular mechanism involved in the pathogenesis of early-onset epileptic encephalopathy. Front. Mol. Neurosci. 12, 118. doi:10.3389/fnmol.2019.00118
Volpe, E., Servant, N., Zollinger, R., Bogiatzi, S. I., Hupe, P., Barillot, E., et al. (2008). A critical function for transforming growth factor-beta, interleukin 23 and proinflammatory cytokines in driving and modulating human T(H)-17 responses. Nat. Immunol. 9, 650–657. doi:10.1038/ni.1613
Volpe, E., Touzot, M., Servant, N., Marloie-Provost, M. A., Hupe, P., Barillot, E., et al. (2009). Multiparametric analysis of cytokine-driven human Th17 differentiation reveals a differential regulation of IL-17 and IL-22 production. Blood 114, 3610–3614. doi:10.1182/blood-2009-05-223768
Wang, L., Xiaokaiti, Y., Wang, G., Xu, X., Chen, L., Huang, X., et al. (2017). Inhibition of PDE2 reverses beta amyloid induced memory impairment through regulation of PKA/PKG-dependent neuro-inflammatory and apoptotic pathways. Sci. Rep. 7, 12044. doi:10.1038/s41598-017-08070-2
Wang, P., Bai, F., Zenewicz, L. A., Dai, J., Gate, D., Cheng, G., et al. (2012). IL-22 signaling contributes to West Nile encephalitis pathogenesis. PLoS One 7, e44153. doi:10.1371/journal.pone.0044153
Warren, E. T., Heath, B. J., and Brand, W. W. (1992). A staged expanding pulmonary artery band. Ann. Thorac. Surg. 54, 240–242. discussion 243. doi:10.1016/0003-4975(92)91376-k
Weiss, B., Wolk, K., Grunberg, B. H., Volk, H. D., Sterry, W., Asadullah, K., et al. (2004). Cloning of murine IL-22 receptor alpha 2 and comparison with its human counterpart. Genes Immun. 5, 330–336. doi:10.1038/sj.gene.6364104
Wolk, K., Kunz, S., Asadullah, K., and Sabat, R. (2002). Cutting edge: Immune cells as sources and targets of the IL-10 family members? J. Immunol. 168, 5397–5402. doi:10.4049/jimmunol.168.11.5397
Wolk, K., Kunz, S., Witte, E., Friedrich, M., Asadullah, K., and Sabat, R. (2004). IL-22 increases the innate immunity of tissues. Immunity 21, 241–254. doi:10.1016/j.immuni.2004.07.007
Wolk, K., and Sabat, R. (2006). Interleukin-22: A novel T- and NK-cell derived cytokine that regulates the biology of tissue cells. Cytokine Growth Factor Rev. 17, 367–380. doi:10.1016/j.cytogfr.2006.09.001
Wolk, K., Witte, E., Wallace, E., Docke, W. D., Kunz, S., Asadullah, K., et al. (2006). IL-22 regulates the expression of genes responsible for antimicrobial defense, cellular differentiation, and mobility in keratinocytes: A potential role in psoriasis. Eur. J. Immunol. 36, 1309–1323. doi:10.1002/eji.200535503
Wu, Y., Zhang, H., Wang, C., Broekman, B. F. P., Chong, Y. S., Shek, L. P., et al. (2021). Inflammatory modulation of the associations between prenatal maternal depression and neonatal brain. Neuropsychopharmacology 46, 470–477. doi:10.1038/s41386-020-0774-0
Xu, W., Presnell, S. R., Parrish-Novak, J., Kindsvogel, W., Jaspers, S., Chen, Z., et al. (2001). A soluble class II cytokine receptor, IL-22RA2, is a naturally occurring IL-22 antagonist. Proc. Natl. Acad. Sci. U. S. A. 98, 9511–9516. doi:10.1073/pnas.171303198
Yan, Z., Gibson, S. A., Buckley, J. A., Qin, H., and Benveniste, E. N. (2018). Role of the JAK/STAT signaling pathway in regulation of innate immunity in neuroinflammatory diseases. Clin. Immunol. 189, 4–13. doi:10.1016/j.clim.2016.09.014
Zenewicz, L. A., and Flavell, R. A. (2011). Recent advances in IL-22 biology. Int. Immunol. 23, 159–163. doi:10.1093/intimm/dxr001
Zhang, J., Ke, K. F., Liu, Z., Qiu, Y. H., and Peng, Y. P. (2013). Th17 cell-mediated neuroinflammation is involved in neurodegeneration of aβ1-42-induced Alzheimer's disease model rats. PLoS One 8, e75786. doi:10.1371/journal.pone.0075786
Zhen, J., Yuan, J., Fu, Y., Zhu, R., Wang, M., Chang, H., et al. (2017). IL-22 promotes Fas expression in oligodendrocytes and inhibits FOXP3 expression in T cells by activating the NF-κB pathway in multiple sclerosis. Mol. Immunol. 82, 84–93. doi:10.1016/j.molimm.2016.12.020
Zheng, Y., Danilenko, D. M., Valdez, P., Kasman, I., Eastham-Anderson, J., Wu, J., et al. (2007). Interleukin-22, a T(H)17 cytokine, mediates IL-23-induced dermal inflammation and acanthosis. Nature 445, 648–651. doi:10.1038/nature05505
Keywords: neurodegenerative disease, IL-22, central nervous system, neuroinflammatory, immune infiltration, nanotherapy
Citation: Chen W, Wang J, Yang H, Sun Y, Chen B, Liu Y, Han Y, Shan M and Zhan J (2022) Interleukin 22 and its association with neurodegenerative disease activity. Front. Pharmacol. 13:958022. doi: 10.3389/fphar.2022.958022
Received: 31 May 2022; Accepted: 26 July 2022;
Published: 13 September 2022.
Edited by:
Cheng Chen, Hefei Institutes of Physical Science (CAS), ChinaReviewed by:
Yang Yang, University of Texas Health Science Center at Houston, United StatesCopyright © 2022 Chen, Wang, Yang, Sun, Chen, Liu, Han, Shan and Zhan. This is an open-access article distributed under the terms of the Creative Commons Attribution License (CC BY). The use, distribution or reproduction in other forums is permitted, provided the original author(s) and the copyright owner(s) are credited and that the original publication in this journal is cited, in accordance with accepted academic practice. No use, distribution or reproduction is permitted which does not comply with these terms.
*Correspondence: Ming Shan, c2hhbm1pbmcwMDAwQDE2My5jb20=; Junfeng Zhan, empmbG92ZTIwMDJAMTYzLmNvbQ==
†These authors have contributed equally to this work
Disclaimer: All claims expressed in this article are solely those of the authors and do not necessarily represent those of their affiliated organizations, or those of the publisher, the editors and the reviewers. Any product that may be evaluated in this article or claim that may be made by its manufacturer is not guaranteed or endorsed by the publisher.
Research integrity at Frontiers
Learn more about the work of our research integrity team to safeguard the quality of each article we publish.