- 1Key Laboratory of Environmental Pollution and Integrative Omics, Guilin Medical University, Education Department of Guangxi Zhuang Autonomous Region, Guilin, China
- 2Department of Gynecology, Guigang City People’s Hospital, The Eighth Affiliated Hospital of Guangxi Medical University, Guigang, China
- 3Key Laboratory of Tumor Immunology and Microenvironmental Regulation, Guilin Medical University, Guilin, China
Until today, the coronavirus disease 2019 (COVID-19) pandemic has caused 6,043,094 deaths worldwide, and most of the mortality cases have been related to patients with long-term diseases, especially cancer. Autophagy is a cellular process for material degradation. Recently, studies demonstrated the association of autophagy with cancer development and immune disorder, suggesting autophagy as a possible target for cancer and immune therapy. Laminarin is a polysaccharide commonly found in brown algae and has been reported to have pharmaceutic roles in treating human diseases, including cancers. In the present report, we applied network pharmacology with systematic bioinformatic analysis, including gene ontology (GO) enrichment, Kyoto Encyclopedia of Genes and Genomes (KEGG) enrichment analysis, reactome pathway analysis, and molecular docking to determine the pharmaceutic targets of laminarin against COVID-19 and cervical cancer via the autophagic process. Our results showed that the laminarin would target ten genes: CASP8, CFTR, DNMT1, HPSE, KCNH2, PIK3CA, PIK3R1, SERPINE1, TLR4, and VEGFA. The enrichment analysis suggested their involvement in cell death, immune responses, apoptosis, and viral infection. In addition, molecular docking further demonstrated the direct binding of laminarin to its target proteins, VEGFA, TLR4, CASP8, and PIK3R1. The present findings provide evidence that laminarin could be used as a combined therapy for treating patients with COVID-19 and cervical cancer.
Introduction
Since the outbreak of COVID-19 in 2019, there have been over 456,797,217 confirmed cases of COVID-19 worldwide, leading to 6,043,094 deaths, as reported to the World Health Organization (WHO) (14 March 2022). The mortality rate of the latest variant, omicron, is relatively low, but its high infection rate continues to be a pressing problem in our society (Bouzid et al., 2022). Moreover, the mortality rate is very high in patients with long-term diseases, especially cancer patients (Aghamirza Moghim Aliabadi et al., 2022). Therefore, there is an urgent need to identify alternative drug or compound to help patients with COVID-19 and cancer. Cervical carcinoma is a common malignant tumor that occurs in the female reproductive system (Cohen et al., 2019). According to the data from the WHO, cervical carcinoma is the fourth most common cancer among women, leading to 342,000 deaths in 2020 globally (https://www.who.int/news-room/fact-sheets/detail/cervical-cancer).
Autophagy is a mechanism for cellular material degradation that occurs under stressful conditions (Yun and Lee, 2018). Cumulating studies showed that autophagy disorder is closely associated with the development of cancer (Levy et al., 2017). In cervical cancer, autophagy-related genes were reported to predict the prognosis of cervical cancer (Jiang et al., 2021). In vitro and in vivo protective autophagic mechanisms resulted in antitumor activity in cervical cancer (Zhang F. et al., 2022). On the contrary, autophagy was found to interplay with the antiviral IFN-I response, suggesting the targeting of autophagy as a potential target and strategy for treating COVID-19 (Bonam et al., 2020; García-Pérez et al., 2020). Therefore, targeting autophagy could be a potential option for treating patients with COVID-19 and cervical cancer.
Laminarin is a polysaccharide commonly found in brown algae (Chen et al., 2021). Recent studies suggested the pharmaceutic roles of laminarin in human diseases, including cancers. A study of colorectal carcinoma cells demonstrated the combined anticancer effect of sulfated laminarin (Malyarenko et al., 2021). Zhang’s group also suggested that laminarin sulfate can be used as a carrier to inhibit heparinase and treat melanoma lung metastasis (Zhang et al., 2021). Besides treating cancer, laminarin was reported to regulate IL-10 production and host immunogenicity (Ferreira et al., 2007; Korotchenko et al., 2021). In this report, we applied network pharmacology with bioinformatics analysis, including gene ontology (GO) enrichment, Kyoto Encyclopedia of Genes and Genomes (KEGG) enrichment analysis, and molecular docking, to investigate the pharmaceutic targets of laminarin against COVID-19 and cervical cancer through autophagy. The findings of the present study provide evidence that laminarin could be used as a combined therapy for treating patients with COVID-19 and cervical cancer.
Materials and methods
Identification of laminarin-, cervical carcinoma-, COVID-19-, and autophagy-associated genes
The mRNA expression profile of cervical carcinoma (CC) was obtained upon searching the dataset of GSE63514 from the Gene Expression Omnibus (GEO) database, which included 24 normal and 28 cervical cancers (Barrett et al., 2013). The differentially expressed genes (DEGs) of cervical carcinoma were analyzed by the GEO2R tool (den Boon et al., 2015). The genes with |log2 fold change| > 1 and −log10 p-value > 1.3 were considered DEGs. For the identification of COVID-19- and autophagy-associated genes, the online databases including the GeneCards database (Stelzer et al., 2016), Online Mendelian Inheritance in Man (OMIM) database (Hamosh et al., 2002), and National Center for Biotechnology Information (NCBI) were accessed (National Library of Medicine National Center for Biotechnology Information (NCBI), 2022). The pharmaceutical targets of laminarin were harvested using online databases, including Comparative Toxicogenomics Database (CTD) (Davis et al., 2020), Swiss Target Prediction database (Daina et al., 2019), SuperPred database (Nickel et al., 2014), and PharmMapper (Wang X. et al., 2017). The target genes were subjected to UniProt Knowledgebase (UniProtKB) for human database correction (The UniProt Consortium, 2021). To further determine the targets of laminarin against cervical carcinoma and COVID-19 through autophagy, the laminarin-, CC-, COVID-19- and autophagy-associated genes and targets were overlapped. The common genes were considered the possible targets.
Core target screening and network analysis
The common genes were subjected to Bioconductor packages, including ClusterProfiler and ggplot2 tools, to analyze and visualize the biological processes and pathways controlled by the targets of laminarin against cervical carcinoma and COVID-19 through autophagy. ClusterProfiler provides GO enrichment, KEGG enrichment analysis, and Reactome pathway analysis (Jassal et al., 2020; Wu T. et al., 2021). Ggplot2 was used to visualize the enrichment results. The terms with adjusted p-value < 0.05 was considered statistically significant. The targets were used for protein–protein interaction (PPI) analysis using the STRING platform, and the confidence score was 0.4 (Szklarczyk et al., 2021). The NetworkAnalyzer of Cytoscape software was also used to integrate the result of targets identification, GO, and KEGG (Shannon et al., 2003).
Molecular docking
The binding affinity of laminarin with its predicted targets was assessed using molecular docking. The chemical structure of laminarin was obtained from the PubChem database (Wang Y. et al., 2017). The protein structure of VEGFA, TLR4, and CASP8 was obtained from the Protein Data Bank (PDB) (Berman et al., 2000). The ChemBio3D Draw tool of ChemBioOffice 2010 software was used to download three-dimensional structures of these proteins, followed by the MM2 energy optimization. The PDB file was converted to the pdbqt file format using AutoDock Tools 1.5.6 of the AutoDock Vina software (that can be recognized by the AutoDock Vina program for ligand docking program) (Trott and Olson, 2010). The docking parameter was determined according to the size of the root-mean-square deviation (RMSD) between the docked ligand molecule and the original ligand molecule. An RMSD of less than 4 Å was the threshold for the conformation of the ligand for matching the conformation of the original ligand in the molecular docking analysis.
Results
Identification of laminarin’s targets against cervical carcinoma and COVID-19 through autophagy
Upon analysis of the GSE63514 dataset of GEO, we identified 3,169 DEGs, including 1,093 upregulated genes and 2,076 downregulated genes in cervical carcinoma (Figure 1A). By searching the databases, we obtained 1,390 COVID-19-associated genes (Figure 1B). In addition, we found 284 pharmaceutical targets of laminarin and 1,744 autophagy-associated genes (Figure 1B). The identified gene and targets were overlapped to determine the possible targets of laminarin against cervical carcinoma and COVID-19 through autophagy. Ten common targets (CASP8, CFTR, DNMT1, HPSE, KCNH2, PIK3CA, PIK3R1, SERPINE1, TLR4, and VEGFA) were observed, and the PPI analysis showed their interaction (Figure 1B).
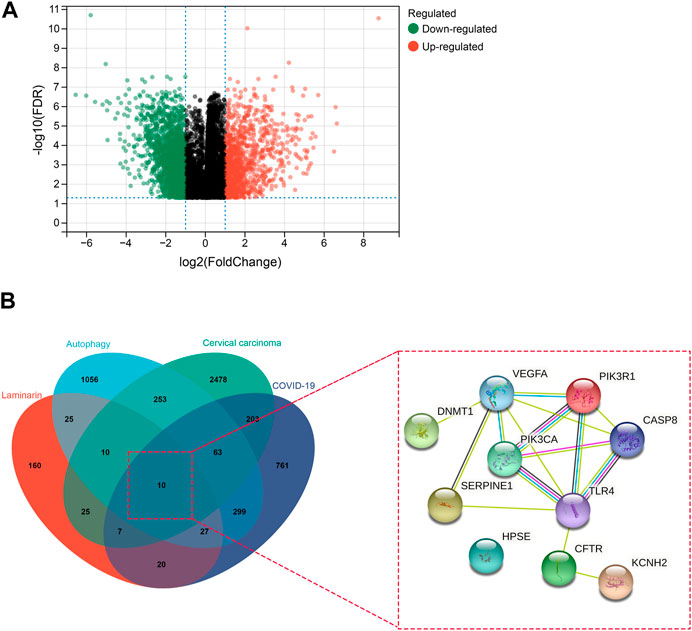
FIGURE 1. Identification of laminarin’s targets against cervical carcinoma and COVID-19 through autophagy. (A) Volcano plot showed the differential gene expression in cervical carcinoma. Genes with |log2 fold change| > 1 and −log10 p-value > 1.3 were considered differentially expressed genes. Green dots represented downregulated genes, and red dots represented upregulated genes. (B) Left panel: Venn diagram showed the number of overlapped laminarin-, COVID-19-, cervical carcinoma-, and autophagy-associated genes. Right panel: protein–protein interaction of the laminarin-, COVID-19-, cervical carcinoma-, and autophagy-associated genes.
Laminarin’s targets were involved in the cell death and immune responses
To understand the biological roles controlled by laminarin targets, GO enrichment analysis was applied. The result of GO analysis highlighted the biological processes related to cell proliferation and differentiation (Figure 2A), cell death such as extrinsic apoptotic signaling pathway, TRAIL-activated apoptotic signaling pathway, and regulation of necroptotic process (Figure 2B). In addition, laminarin’s targets played roles in immune responses such as regulation of innate immune response, regulation of leukocyte differentiation, macrophage differentiation, B-cell activation, and production of interleukin (Figure 2C). Furthermore, many kinase activities such as regulation of MAP kinase activity, regulation of I-kappaB kinase/NF-kappaB signaling, regulation of p38MAPK cascade, and protein kinase C signaling were highlighted in our result (Figure 2D). In terms of molecular function, laminarin’s targets contributed to different binding and ion channel activities, especially the functions related to immune functions such as cytokine receptor binding, tumor necrosis factor receptor binding, and chemoattractant activity (Figure 2E). These biological processes and molecular functions were suggested to occur in different cellular components, including platelet alpha granule lumen, ion channel complex, cytoplasmic vesicle lumen, perinuclear endoplasmic reticulum, pericentric heterochromatin, transmembrane transporter complex, transporter complex, early endosome, phagocytic cup, and phosphatidylinositol 3-kinase complex (Figure 2F).
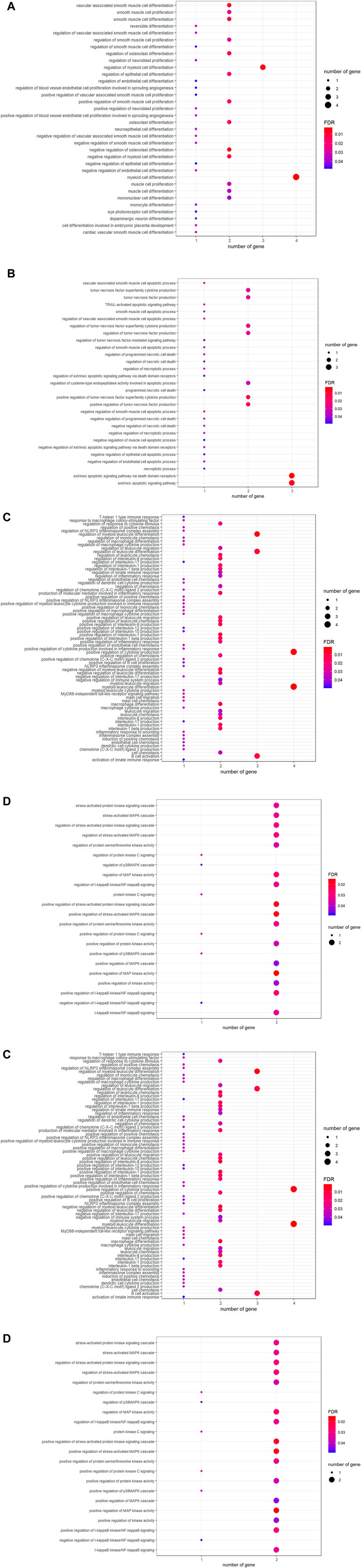
FIGURE 2. Involvement of laminarin’s targets in cell death and immune responses. Gene ontology enrichment analysis of laminarin’s targets highlighted the biological processes related to (A) cell proliferation and differentiation, (B) cell death, (C) immune responses, and (D) kinase activities. (E) Laminarin’s targets contributed to molecular functions, including binding and ion channel activities. (F) Laminarin’s targets played roles in different cellular components. The size of a bubble represents the number of genes, and the color of the bubble represents the significance of the terms.
Laminarin’s targets contributed to the pathways related to cancer and viral infection
To further investigate the signaling pathways controlled by laminarin’s targets, KEGG enrichment analysis, and Reactome pathway analysis were conducted. The result of the KEGG enrichment analysis showed the involvement of laminarin’s targets in cancer, viral infection, cell death, and immune responses (Figure 3A). More importantly, many cell signaling pathways such as Toll-like receptor signaling pathway, VEGF signaling pathway, p53 signaling pathway, PI3K-Akt signaling pathway, TNF signaling pathway, AMPK signaling pathway, NOD-like receptor signaling pathway, Rap1 signaling pathway, FoxO signaling pathway, mTOR signaling pathway, Hippo signaling pathway, JAK-STAT signaling pathway, and MAPK signaling pathway related to carcinogenicity and cell proliferation were highlighted in our result (Figure 3B). In the Reactome pathway analysis, our result highlighted the contribution of laminarin to cell apoptosis through the regulation of TRIF-mediated programmed cell death and caspase activation via death receptors and extrinsic apoptotic signaling pathway (Figure 3C). Taken together, our results suggested that the laminarin targets played important roles in carcinogenicity and cell apoptosis.
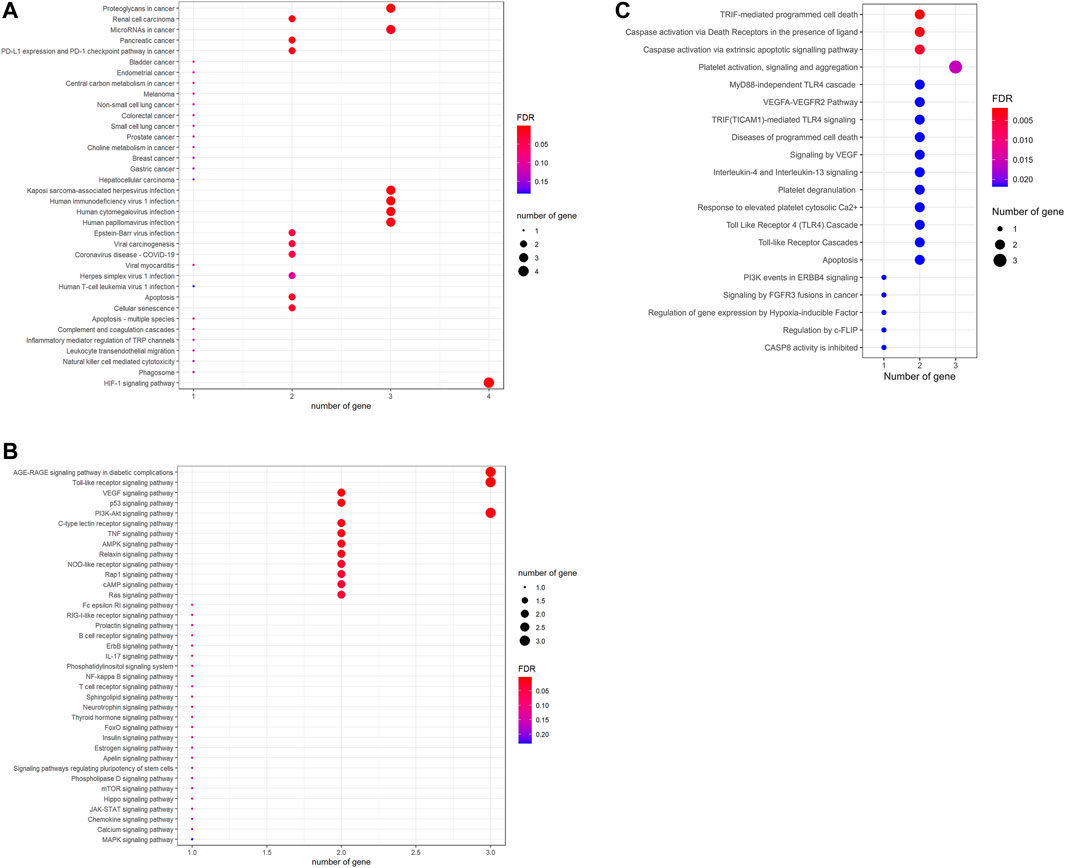
FIGURE 3. Laminarin’s targets involved in pathways related to cancer and cell apoptosis. Kyoto Encyclopedia of Genes and Genomes pathway enrichment analysis highlighted the involvement of laminarin’s targets in (A) cancer, viral infection, cell death, and immune responses; and (B) cell signaling pathways related to cancer development. (C) Reactome pathway analysis highlighted the importance of laminarin in cell apoptosis. The size of a bubble represents the number of genes, and the color of the bubble represents the significance of the pathways.
Direct binding of laminarin to its target proteins, VEGFA, TLR4, CASP8, and PIK3R1
Before conducting the molecular docking analysis, laminarin’s targets were prioritized using the Cytoscape_v3.8.2 tool. Four core targets of laminarin (VEGFA, TLR4, CASP8, and PIK3R1) were obtained under the setting with a medium of freedom of 2.5 and the highest freedom of 5 (Figure 4A). The protein structures of VEGFA (ID: 4KZN) (Shaik et al., 2020), TLR4 (ID: 5V3Q) (Menon et al., 2017), CASP8 (ID: 2VUW) (Eswaran et al., 2009), and PIK3R1 (ID: 4JPS) (Furet et al., 2013) were obtained from the PDB database. Then, these protein structures were subjected to molecular docking analysis with laminarin using the AutoDock Vina program, and the results were displayed using PyMOL (version 2.3). Our results showed the possible direct binding of laminarin to these four target proteins, which was reflected by the negative value of the binding affinity (Figure 4). We found the formation of hydrogen bonds between laminarin with LYS-48 (3.2Å), HIS-86 (3.2 Å), GLN-87 (3.3Å), GLY-88 (2.2Å), and GLN-89 (3.4 Å) of VEGFA (ID: 4KZN) (Figure 4A). The binding affinity was −4.2 kcal/mol. For the TLR4 (ID: 5V3Q), its amino acid residues LYS-59 (3.1 Å), LYS-57 (2.8 Å), TYR-229 (3.0Å), PHE-31 (2.3 Å), ARG-183 (3.3 Å), and TYP-222 (3.0Å) formed hydrogen bonds with laminarin, and the binding affinity was −6.2 kcal/mol (Figure 4B). Similar bindings were observed between laminarin and CASP8 (ID: 2VUW) through the amino acid residues of ASP-687 (2.7 Å), GLU-492 (2.2 Å), GLN-614 (2.7 Å), LYS-511 (2.5Å), ILE-490 (2.4 Å), and ASP-611 (2.2 Å) with −8.4 kcal/mol (Figure 4C). In addition, laminarin formed hydrogen bonds with amino acid residues, ASP-933 (2.3Å), SER-774 (2.9 Å), ARG-770 (3.3Å), SER-854 (3.0Å), and VAL-851 (2.8 Å) of PIK3R1 (ID: 4JPS) (Figure 4D). The binding affinity was −6.9 kcal/mol. Taken together, our data suggested that laminarin could directly bind to its target proteins.
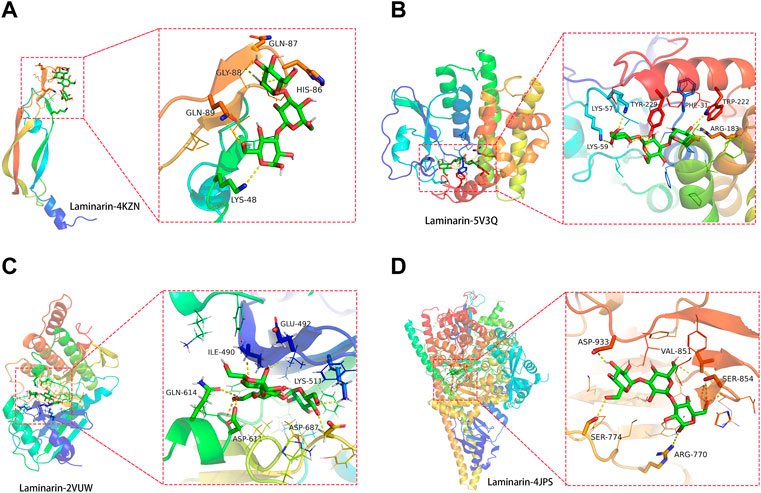
FIGURE 4. Direct binding of laminarin with its target proteins. Molecular docking analysis showed the hydrogen bond formation between laminarin with the amino residues of (A) VEGFA (ID: 4KZN), (B) TLR4 (ID: 5V3Q), (C) CASP8 (ID: 2VUW), and (D) PIK3R1 (ID: 4JPS).
Discussion
This study aimed to investigate the possible use of laminarin for treating cervical carcinoma and COVID-19 by targeting autophagy (Figure 5). By searching different databases, we identified a cluster of common associated genes of laminarin, cervical carcinoma, COVID-19, and autophagy. The following genes were considered the possible targets of laminarin against cervical carcinoma and COVID-19 via autophagy: CASP8, CFTR, DNMT1, HPSE, KCNH2, PIK3CA, PIK3R1, SERPINE1, TLR4, and VEGFA. Bioinformatic analysis, including network pharmacology, GO enrichment analysis, and molecular docking, was further used to characterize the biological functions and the underlying mechanisms controlled by these genes. The result of GO highlighted the involvement of these genes in cell death and immune responses, suggesting that laminarin could mediate autophagic cell death. Autophagy plays a dual role as a proviral and an antiviral factor during virus replication and is considered a target for developing an effective anti-SARS-CoV-2 treatment (Liang et al., 2021). Some reports demonstrated the close association between autophagy and SARS-CoV-2. A kinase phosphorylation study showed that SARS-CoV-2 regulated various signaling pathways, including autophagy (Chatterjee and Thakur, 2022). A genome-wide CRISPR/Cas-9 knockout (KO) screening suggested that autophagy-related genes were required for SARS-CoV-2 replication (Kratzel et al., 2021). More importantly, studies found that induction of autophagy is essential for the infection and replication of SARS-CoV-2 through the regulation of acute inflammatory responses (Zhang X. et al., 2022). On the contrary, autophagy also played an important role in cervical cancer. It was reported that autophagy controlled the epithelial-to-mesenchymal transition and metastasis of cervical cancer through the regulation of the NOTCH1 intracellular domain (Zada et al., 2022). In addition, autophagy was crucial for the sensitivity of cervical cancer cells to cisplatin chemotherapy (Huang et al., 2021). The application of laminarin to therefore target autophagy could be an alternative strategy for patients with COVID-19 and cervical carcinoma.
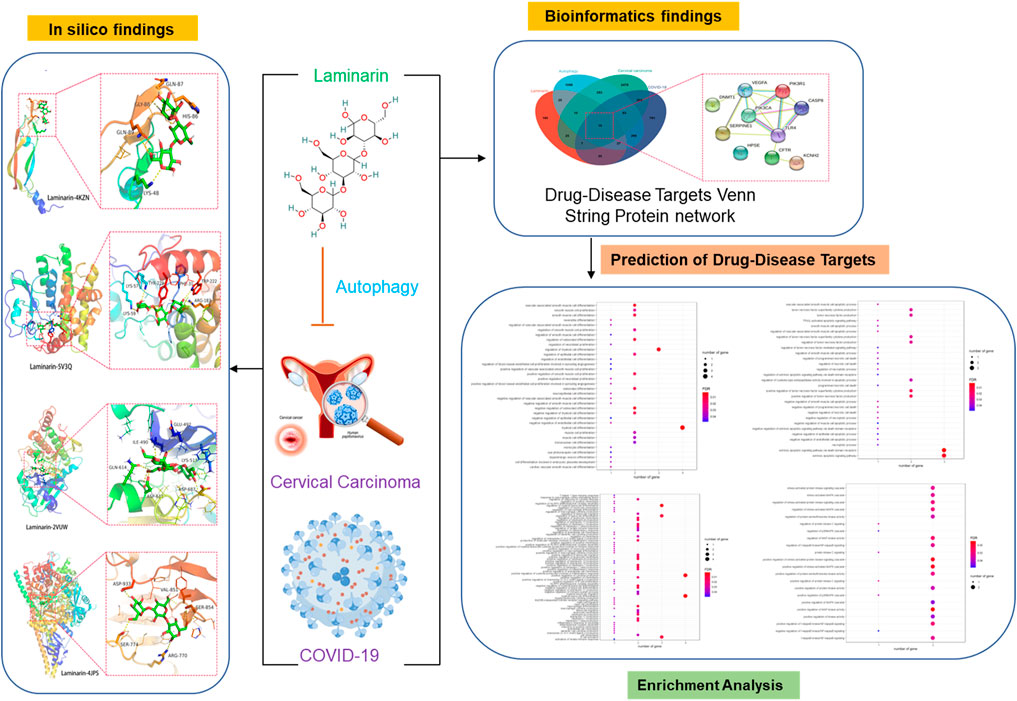
FIGURE 5. Workflow of the study. The pharmaceutic targets and their function roles were identified using network pharmacology, gene ontology enrichment analysis, and molecular docking.
In the pathway analysis, we found that laminarin’s targets played a role in many cell signaling pathways related to COVID-19 and cervical carcinoma. The VEGF signaling pathway plays a vital role in the pathologies of COVID-19-associated pulmonary edema, sepsis, and acute lung injury (Wang et al., 2021). A clinical comparison study among the COVID-19 patient categories found a significantly lower level of VEGF in the mildly symptomatic patients (Tripathy et al., 2021). In addition, VEGF blockade and antagonist were reported to be helpful in the treatment of COVID-19 (Sahebnasagh et al., 2021; Lampropoulou et al., 2022). In cervical cancer, VEGF signaling regulated several oncogenic signaling pathways and cancer-regulated angiogenesis (Prasad et al., 2022). Functionally, VEGF controlled proliferation and apoptosis in cervical cancer. (Wu X. et al., 2021). Hence, VEGF was considered a therapeutic target for recurrent and metastatic cervical cancer (Chuai et al., 2021). The VEGF inhibitor bevacizumab was reportedly used in advanced metastatic cervical cancer (Singh et al., 2022). Besides VEGF signaling, we also found the regulation of the p53 signaling pathway by laminarin’s targets. This pathway was reported to be associated with COVID-19 infection. A clinical study demonstrated that peripheral blood mononuclear cells of COVID-19 patients had a higher p53 expression, which positively correlated with the level of inflammatory cytokines (Bordoni et al., 2021). In addition, p53 signaling is related to immune and apoptosis-related functions of SARS-CoV-2 infected cells (Rahaman et al., 2021) because SARS-CoV-2 could favor tumor growth by inducing MDM2-mediated p53 downregulation (Tanda et al., 2022) and target mTOR and RPS6KB1 to inhibit viral replication in the human respiratory tract and lung cells (Ramaiah, 2020). Furthermore, single-nucleotide polymorphism of p53 played a role in the innate immune response and restricted the SARS-CoV-2-mediated mortality rate (Lodhi et al., 2021). By targeting these signaling pathways, laminarin could be a potential compound to treat patients with cervical cancer and COVID-19.
For the latter part, we conducted a molecular docking study to investigate the possible direct binding of laminarin to its core target proteins. Molecular docking is a key tool commonly used to predict the predominant binding of a ligand with a protein of known three-dimensional structure (Morris and Lim-Wilby, 2008). Our result showed that laminarin had a good binding affinity to VEGFA, TLR4, CASP8, and PIK3R1. Vascular endothelial growth factor A (VEGFA) is involved in the VEGF signaling pathway, and we have discussed its roles in COVID-19 and cervical cancer above. Toll-like receptor 4 (TLR4) played an important role in the generation of an antiviral state after being infected with pathogenic viruses (Boodhoo et al., 2022). A study of the human pluripotent stem cell–based model of SARS-CoV-2 infection demonstrated the involvement of TLR4 in the inflammatory activation of vascular endothelial cells (Ma et al., 2022), and TLR4 was reported to contribute to anti-inflammatory effects and SARS-CoV-2 infection in vitro (Numata and Voelker, 2022). Therefore, targeting the TLR4 inflammatory pathway could serve as a potential strategy in reducing inflammatory lung injury (Bermudez et al., 2022). Caspase 8 (CASP8) is a key regulator of extrinsic apoptosis and necroptosis pathways (Tummers and Green, 2017), which is associated with COVID-19 infection. A study of 101 plasma proteins of hospitalized COVID-19 patients demonstrated a positive correlation between inflammatory markers and CASP8 (Haljasmägi et al., 2020). More importantly, SARS-CoV-2 infection activated CASP8 to trigger cell apoptosis and inflammatory cytokine processing in the lung epithelial cells (Li et al., 2020), suggesting CASP8 to be a target to relieve the severity of SARS-CoV-2 infection.
In conclusion, our data, for the first time, provide evidence that laminarin could be an alternative treatment for patients with cervical cancer and COVID-19 by targeting autophagy. However, as our findings were drawn from network pharmacology, further clinical verification and molecular functional characterization are needed to warrant the present findings from bioinformatic analysis before clinical use of laminarin.
Data availability statement
The original contributions presented in the study are included in the article/Supplementary Material. Further inquiries can be directed to the corresponding authors.
Author contributions
WH and RL conceived and designed the study. JL, YC, LN, and XL performed the data analysis and data interpretation. JL, YC, LN, and XL conducted bioinformatics and statistical analyses. WH and RL prepared the manuscript.
Conflict of interest
The authors declare that the research was conducted in the absence of any commercial or financial relationships that could be construed as a potential conflict of interest.
Publisher’s note
All claims expressed in this article are solely those of the authors and do not necessarily represent those of their affiliated organizations or those of the publisher, the editors, and the reviewers. Any product that may be evaluated in this article, or claim that may be made by its manufacturer, is not guaranteed or endorsed by the publisher.
References
Aghamirza Moghim Aliabadi, H., Eivazzadeh-Keihan, R., Beig Parikhani, A., Fattahi Mehraban, S., Maleki, A., Fereshteh, S., et al. (2022). COVID-19: a systematic review and update on prevention, diagnosis, and treatment. MedComm 3 (1), e115. doi:10.1002/mco2.115
Barrett, T., Wilhite, S. E., Ledoux, P., Evangelista, C., Kim, I. F., Tomashevsky, M., et al. (2013). NCBI GEO: archive for functional genomics data sets--update. Nucleic Acids Res. 41 (Database issue), D991–D995. doi:10.1093/nar/gks1193
Berman, H. M., Westbrook, J., Feng, Z., Gilliland, G., Bhat, T. N., Weissig, H., et al. (2000). The protein Data Bank. Nucleic Acids Res. 28, 235–242. doi:10.1093/nar/28.1.235
Bermudez, T., Sammani, S., Song, J. H., Hernon, V. R., Kempf, C. L., Garcia, A. N., et al. (2022). eNAMPT neutralization reduces preclinical ARDS severity via rectified NFkB and Akt/mTORC2 signaling. Sci. Rep. 12 (1), 696. doi:10.1038/s41598-021-04444-9
Bonam, S. R., Muller, S., Bayry, J., and Klionsky, D. J. (2020). Autophagy as an emerging target for COVID-19: lessons from an old friend, chloroquine. Autophagy 16 (12), 2260–2266. doi:10.1080/15548627.2020.1779467
Boodhoo, N., Matsuyama-Kato, A., Shojadoost, B., Behboudi, S., and Sharif, S. (2022). The severe acute respiratory syndrome coronavirus 2 non-structural proteins 1 and 15 proteins mediate antiviral immune evasion. Curr. Res. Virol. Sci. 3, 100021. doi:10.1016/j.crviro.2022.100021
Bordoni, V., Tartaglia, E., Sacchi, A., Fimia, G. M., Cimini, E., Casetti, R., et al. (2021). The unbalanced p53/SIRT1 axis may impact lymphocyte homeostasis in COVID-19 patients. Int. J. Infect. Dis. 105, 49–53. doi:10.1016/j.ijid.2021.02.019
Bouzid, D., Visseaux, B., Kassasseya, C., Daoud, A., Fémy, F., Hermand, C., et al. (2022). Comparison of patients infected with delta versus omicron COVID-19 variants presenting to paris emergency departments : a retrospective cohort study. Ann. Intern Med. 175 (6), 831–837. doi:10.7326/M22-0308
Chatterjee, B., and Thakur, S. S. (2022). SARS-CoV-2 infection triggers phosphorylation: Potential target for anti-COVID-19 therapeutics. Front. Immunol. 13, 829474. doi:10.3389/fimmu.2022.829474
Chen, J., Yang, J., Du, H., Aslam, M., Wang, W., Chen, W., et al. (2021). Laminarin, a major polysaccharide in stramenopiles. Mar. Drugs 19 (10), 576. doi:10.3390/md19100576
Chuai, Y., Rizzuto, I., Zhang, X., Li, Y., Dai, G., Otter, S. J., et al. (2021). Vascular endothelial growth factor (VEGF) targeting therapy for persistent, recurrent, or metastatic cervical cancer. Cochrane Database Syst. Rev. 3 (3), CD013348. doi:10.1002/14651858.CD013348.pub2
Cohen, P. A., Jhingran, A., Oaknin, A., and Denny, L. (2019). Cervical cancer. Lancet 393 (10167), 169–182. doi:10.1016/S0140-6736(18)32470-X
Daina, A., Michielin, O., and Zoete, V. (2019). Swiss target prediction: Updated data and new features for efficient prediction of protein targets of small molecules. Nucleic Acids Res. 47, W357–W364. doi:10.1093/nar/gkz382
Davis, A. P., Grondin, C. J., Johnson, R. J., Sciaky, D., Wiegers, J., Wiegers, T. C., et al. (2020). The comparative Toxicogenomics database: update 2021. Nucleic Acids Res. 49, D1138–D1143. doi:10.1093/nar/gkaa891
den Boon, J. A., Pyeon, D., Wang, S. S., Horswill, M., Schiffman, M., Sherman, M., et al. (2015). Molecular transitions from papillomavirus infection to cervical precancer and cancer: Role of stromal estrogen receptor signaling. Proc. Natl. Acad. Sci. U. S. A. 112 (25), E3255–E3264. doi:10.1073/pnas.1509322112
Eswaran, J., Patnaik, D., Filippakopoulos, P., Wang, F., Stein, R. L., Murray, J. W., et al. (2009). Structure and functional characterization of the atypical human kinase haspin. Proc. Natl. Acad. Sci. U. S. A. 106, 20198–20203. doi:10.1073/pnas.0901989106
Ferreira, K. S., Bastos, K. R., Russo, M., and Almeida, S. R. (2007). Interaction between paracoccidioides brasiliensis and pulmonary dendritic cells induces interleukin-10 production and toll-like receptor-2 expression: Possible mechanisms of susceptibility. J. Infect. Dis. 196 (7), 1108–1115. doi:10.1086/521369
Furet, P., Guagnano, V., Fairhurst, R. A., Imbach-Weese, P., Bruce, I., Knapp, M., et al. (2013). Discovery of NVP-BYL719 a potent and selective phosphatidylinositol-3 kinase alpha inhibitor selected for clinical evaluation. Bioorg. Med. Chem. Lett. 23, 3741–3748. doi:10.1016/j.bmcl.2013.05.007
García-Pérez, B. E., González-Rojas, J. A., Salazar, M. I., Torres-Torres, C., and Castrejón-Jiménez, N. S. (2020). Taming the autophagy as a strategy for treating COVID-19. Cells 9 (12), 2679. doi:10.3390/cells9122679
Haljasmägi, L., Salumets, A., Rumm, A. P., Jürgenson, M., Krassohhina, E., Remm, A., et al. (2020). Longitudinal proteomic profiling reveals increased early inflammation and sustained apoptosis proteins in severe COVID-19. Sci. Rep. 10 (1), 20533. doi:10.1038/s41598-020-77525-w
Hamosh, A., Scott, A. F., Amberger, J. S., Bocchini, C., Valle, D., and McKusick, V. A. (2002). Online Mendelian Inheritance in Man (OMIM), a knowledgebase of human genes and genetic disorders. Nucleic Acids Res. 33 (1), 52–55. doi:10.1093/nar/30.1.52
Huang, H., Han, Q., Zheng, H., Liu, M., Shi, S., Zhang, T., et al. (2021). MAP4K4 mediates the SOX6-induced autophagy and reduces the chemosensitivity of cervical cancer. Cell Death Dis. 13 (1), 13. doi:10.1038/s41419-021-04474-1
Jassal, B., Matthews, L., Viteri, G., Gong, C., Lorente, P., Fabregat, A., et al. (2020). The reactome pathway knowledgebase. Nucleic Acids Res. 48 (D1), D498–D503. doi:10.1093/nar/gkz1031
Jiang, J., Xu, H., Wang, Y., and Lu, H. (2021). Identification and validation of autophagy-related gene nomograms to predict the prognostic value of patients with cervical cancer. J. Oncol. 25, 5583400. doi:10.1155/2021/5583400
Korotchenko, E., Schießl, V., Scheiblhofer, S., Schubert, M., Dall, E., Joubert, I. A., et al. (2021). Laser-facilitated epicutaneous immunotherapy with hypoallergenic beta-glucan neoglycoconjugates suppresses lung inflammation and avoids local side effects in a mouse model of allergic asthma. Allergy 76 (1), 210–222. doi:10.1111/all.14481
Kratzel, A., Kelly, J. N., V'kovski, P., Portmann, J., Brüggemann, Y., Todt, D., et al. (2021). A genome-wide CRISPR screen identifies interactors of the autophagy pathway as conserved coronavirus targets. PLoS Biol. 19 (12), e3001490. doi:10.1371/journal.pbio.3001490
Lampropoulou, D. I., Bala, V. M., Zerva, E., Pliakou, E., Filippou, D., Gazouli, M., et al. (2022). The potential role of the combined PARP-1 and VEGF inhibition in severe SARS-CoV-2 (COVID-19) infection. J. Infect. Dev. Ctries. 16 (1), 101–111. doi:10.3855/jidc.15386
Levy, J. M. M., Towers, C. G., and Thorburn, A. (2017). Targeting autophagy in cancer. Nat. Rev. Cancer 17 (9), 528–542. doi:10.1038/nrc.2017.53
Li, S., Zhang, Y., Guan, Z., Li, H., Ye, M., Chen, X., et al. (2020). SARS-CoV-2 triggers inflammatory responses and cell death through caspase-8 activation. Signal Transduct. Target. Ther. 5 (1), 235. doi:10.1038/s41392-020-00334-0
Liang, W., Liu, H., He, J., Ai, L., Meng, Q., Zhang, W., et al. (2021). Studies progression on the function of autophagy in viral infection. Front. Cell Dev. Biol. 9, 772965. doi:10.3389/fcell.2021.772965
Lodhi, N., Singh, R., Rajput, S. P., and Saquib, Q. (2021). SARS-CoV-2: Understanding the transcriptional regulation of ACE2 and TMPRSS2 and the role of single nucleotide polymorphism (SNP) at codon 72 of p53 in the innate immune response against virus infection. Int. J. Mol. Sci. 22 (16), 8660. doi:10.3390/ijms22168660
Ma, Z., Li, X., Fan, R. L. Y., Yang, K. Y., Ng, C. S. H., Lau, R. W. H., et al. (2022). A human pluripotent stem cell-based model of SARS-CoV-2 infection reveals an ACE2-independent inflammatory activation of vascular endothelial cells through TLR4. Stem Cell Rep. 17 (3), 538–555. doi:10.1016/j.stemcr.2022.01.015
Malyarenko, O. S., Malyarenko, T. V., Usoltseva, R. V., Surits, V. V., Kicha, A. A., Ivanchina, N. V., et al. (2021). Combined anticancer effect of sulfated laminaran from the Brown alga Alaria angusta and polyhydroxysteroid glycosides from the starfish Protoreaster lincki on 3D colorectal carcinoma HCT 116 cell line. Mar. Drugs 19 (10), 540. doi:10.3390/md19100540
Menon, D., Innes, A., Oakley, A. J., Dahlstrom, J. E., Jensen, L. M., Brustle, A., et al. (2017). GSTO1-1 plays a pro-inflammatory role in models of inflammation, colitis and obesity. Sci. Rep. 7, 17832. doi:10.1038/s41598-017-17861-6
Morris, G. M., and Lim-Wilby, M. (2008). Molecular docking. Methods Mol. Biol. 443, 365–382. doi:10.1007/978-1-59745-177-2_19
National Library of Medicine National Center for Biotechnology Information (NCBI) (2022). Gene. Bethesda MD, 20894 USA: U.S. National Library of Medicine 8600 Rockville Pike. Available at: https://www.ncbi.nlm.nih.gov/gene.
Nickel, J., Gohlke, B. O., Erehman, J., Banerjee, P., Rong, W. W., Goede, A., et al. (2014). SuperPred: update on drug classification and target prediction. Nucleic Acids Res. 42 (Web Server issue), W26–W31. doi:10.1093/nar/gku477
Numata, M., and Voelker, D. R. (2022). Anti-inflammatory and anti-viral actions of anionic pulmonary surfactant phospholipids. Biochim. Biophys. Acta. Mol. Cell Biol. Lipids 1867 (6), 159139. doi:10.1016/j.bbalip.2022.159139
Prasad, C. B., Singh, D., Pandey, L. K., Pradhan, S., Singh, S., Narayan, G., et al. (2022). VEGFa/VEGFR2 autocrine and paracrine signaling promotes cervical carcinogenesis via β-catenin and snail. Int. J. Biochem. Cell Biol. 142, 106122. doi:10.1016/j.biocel.2021.106122
Rahaman, M., Komanapalli, J., Mukherjee, M., Byram, P. K., Sahoo, S., Chakravorty, N., et al. (2021). Decrypting the role of predicted SARS-CoV-2 miRNAs in COVID-19 pathogenesis: a bioinformatics approach. Comput. Biol. Med. 136, 104669. doi:10.1016/j.compbiomed.2021.104669
Ramaiah, M. J. (2020). mTOR inhibition and p53 activation, microRNAs: The possible therapy against pandemic COVID-19. Gene Rep. 20, 100765. doi:10.1016/j.genrep.2020.100765
Sahebnasagh, A., Nabavi, S. M., Kashani, H. R. K., Abdollahian, S., Habtemariam, S., Rezabakhsh, A., et al. (2021). Anti-VEGF agents: As appealing targets in the setting of COVID-19 treatment in critically ill patients. Int. Immunopharmacol. 101 (Pt B), 108257. doi:10.1016/j.intimp.2021.108257
Shannon, P., Markiel, A., Ozier, O., Baliga, N. S., Wang, J. T., Ramage, D., et al. (2003). Cytoscape: a software environment for integrated models of biomolecular interaction networks. Genome Res. 13 (11), 2498–2504. doi:10.1101/gr.1239303
Shaik, F., Cuthbert, G. A., Homer-Vanniasinkam, S., Muench, S. P., Ponnambalam, S., and Harrison, M. A. (2020). Structural basis for vascular endothelial growth factor receptor activation and implications for disease therapy. Biomolecules 10 (12), 1673.
Singh, V., Gor, D., Azad, S., Ricca, A., Xu, Y., Meghal, T., et al. (2022). Dysphagia as a rare presentation of cervical cancer with mediastinal metastasis. Eur. J. Case Rep. Intern. Med. 9 (1), 003136. doi:10.12890/2022_003136
Stelzer, G., Rosen, R., Plaschkes, I., Zimmerman, S., Twik, M., Fishilevich, S., et al. (2016). GeneCards suite: from gene data mining to disease genome sequence analysis. Curr. Protoc. Bioinforma. 54, 1.30.1–1.30.33. doi:10.1002/cpbi.5
Szklarczyk, D., Gable, A. L., Nastou, K. C., Lyon, D., Kirsch, R., Pyysalo, S., et al. (2021). The STRING database in 2021: customizable protein–protein networks, and functional characterization of user-uploaded gene/measurement sets. Nucleic Acids Res. 49 (D1), D605–D612. doi:10.1093/nar/gkaa1074
Tanda, M. L., Ippolito, S., Gallo, D., Baj, A., Novazzi, F., Genoni, A., et al. (2022). SARS-CoV-2 detection in primary thyroid sarcoma: coincidence or interaction? J. Endocrinol. Invest. 5, 1059–1063. doi:10.1007/s40618-021-01722-1
The UniProt Consortium (2021). UniProt: the universal protein knowledgebase in 2021. Nucleic Acids Res. 49, D480–D489. doi:10.1093/nar/gkaa1100
Tripathy, A. S., Vishwakarma, S., Trimbake, D., Gurav, Y. K., Potdar, V. A., Mokashi, N. D., et al. (2021). Pro-inflammatory CXCL-10, TNF-α, IL-1β, and IL-6: biomarkers of SARS-CoV-2 infection. Arch. Virol. 166 (12), 3301–3310. doi:10.1007/s00705-021-05247-z
Trott, O., and Olson, A. J. (2010). AutoDock Vina: improving the speed and accuracy of docking with a new scoring function, efficient optimization, and multithreading. J. Comput. Chem. 31, 455–461. doi:10.1002/jcc.21334
Tummers, B., and Green, D. R. (2017). Caspase-8: regulating life and death. Immunol. Rev. 277 (1), 76–89. doi:10.1111/imr.12541
Wang, L., Astone, M., Alam, S. K., Zhu, Z., Pei, W., Frank, D. A., et al. (2021). Suppressing STAT3 activity protects the endothelial barrier from VEGF-mediated vascular permeability. Dis. Model. Mech. 14 (11), dmm049029. doi:10.1242/dmm.049029
Wang, X., Shen, Y., Wang, S., Li, S., Zhang, W., Liu, X., et al. (2017). PharmMapper 2017 update: A web server for potential drug target identification with a comprehensive target pharmacophore database. Nucleic Acids Res. 45, W356–W360. doi:10.1093/nar/gkx374
Wang, Y., Bryant, S. H., Cheng, T., Wang, J., Gindulyte, A., Shoemaker, B. A., et al. (2017). PubChem BioAssay: 2017 update. Nucleic Acids Res. 45 (D1), D955–D963. doi:10.1093/nar/gkw1118
Wu, T., Hu, E., Xu, S., Chen, M., Guo, P., Dai, Z., et al. (2021). clusterProfiler 4.0: A universal enrichment tool for interpreting omics data. Innov. (Camb). 2 (3), 100141. doi:10.1016/j.xinn.2021.100141
Wu, X., Lei, J., Zhou, B., Sun, Q., Gao, Y., Shi, F., et al. (2021). MiR-628-5p inhibits cervical carcinoma proliferation and promotes apoptosis by targeting VEGF. Am. J. Med. Sci. 361 (4), 499–508. doi:10.1016/j.amjms.2020.11.031
Yun, C. W., and Lee, S. H. (2018). The roles of autophagy in cancer. Int. J. Mol. Sci. 19 (11), 3466. doi:10.3390/ijms19113466
Zada, S., Hwang, J. S., Lai, T. H., Pham, T. M., Ahmed, M., Elashkar, O., et al. (2022). Autophagy-mediated degradation of NOTCH1 intracellular domain controls the epithelial to mesenchymal transition and cancer metastasis. Cell Biosci. 12 (1), 17. doi:10.1186/s13578-022-00752-3
Zhang, F., Zhang, H., Qian, W., Xi, Y., Chang, L., Wu, X., et al. (2022). Matrine exerts antitumor activity in cervical cancer by protective autophagy via the Akt/mTOR pathway in vitro and in vivo. Oncol. Lett. 23 (4), 110. doi:10.3892/ol.2022.13230
Zhang, X., Yang, Z., Pan, T., Long, X., Sun, Q., Wang, P. H., et al. (2022). SARS-CoV-2 ORF3a induces RETREG1/FAM134B-dependent reticulophagy and triggers sequential ER stress and inflammatory responses during SARS-CoV-2 infection. Autophagy 3, 1–17. doi:10.1080/15548627.2022.2039992
Keywords: functional foods, laminarin, cervical carcinoma, COVID-19, biotargets
Citation: Liu J, Chen Y, Nie L, Liang X, Huang W and Li R (2022) In silico analysis and preclinical findings uncover potential targets of anti-cervical carcinoma and COVID-19 in laminarin, a promising nutraceutical. Front. Pharmacol. 13:955482. doi: 10.3389/fphar.2022.955482
Received: 30 May 2022; Accepted: 04 July 2022;
Published: 09 August 2022.
Edited by:
Kawsar Ahmed, Mawlana Bhashani Science and Technology University, BangladeshReviewed by:
Md. Tanvir Hasan, Daffodil International University, BangladeshTasnimul Alam Taz, Wayne State University, United States
Md Mamun Ali, Daffodil International University, Bangladesh
Copyright © 2022 Liu, Chen, Nie, Liang, Huang and Li. This is an open-access article distributed under the terms of the Creative Commons Attribution License (CC BY). The use, distribution or reproduction in other forums is permitted, provided the original author(s) and the copyright owner(s) are credited and that the original publication in this journal is cited, in accordance with accepted academic practice. No use, distribution or reproduction is permitted which does not comply with these terms.
*Correspondence: Wenjun Huang, dXB0b3duNzhAeWVhaC5uZXQ=; Rong Li, bGlyb25nMTI3OEAxNjMuY29t
†These authors have contributed equally to this work