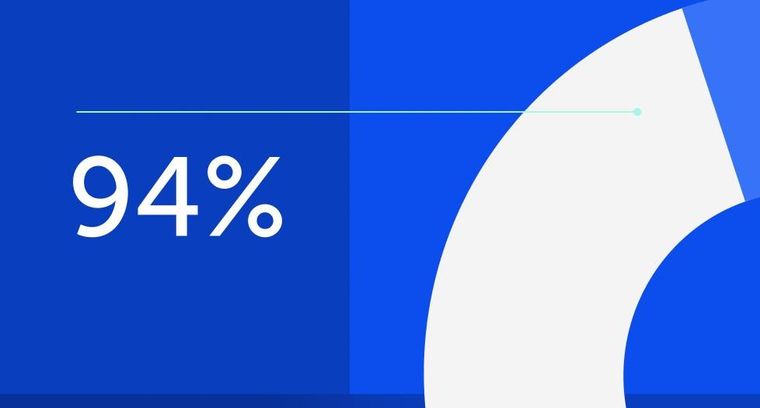
94% of researchers rate our articles as excellent or good
Learn more about the work of our research integrity team to safeguard the quality of each article we publish.
Find out more
REVIEW article
Front. Pharmacol., 16 September 2022
Sec. Experimental Pharmacology and Drug Discovery
Volume 13 - 2022 | https://doi.org/10.3389/fphar.2022.949384
This article is part of the Research TopicAdenylyl cyclase isoforms as potential drug targetsView all 10 articles
As the main secondary messengers, cyclic AMP (cAMP) and Ca2+ trigger intracellular signal transduction cascade and, in turn, regulate many aspects of cellular function in developing and mature neurons. The group I adenylyl cyclase (ADCY, also known as AC) isoforms, including ADCY1, 3, and 8 (also known as AC1, AC3, and AC8), are stimulated by Ca2+ and thus functionally positioned to integrate cAMP and Ca2+ signaling. Emerging lines of evidence have suggested the association of the Ca2+-stimulated ADCYs with bipolar disorder, schizophrenia, major depressive disorder, post-traumatic stress disorder, and autism. In this review, we discuss the molecular and cellular features as well as the physiological functions of ADCY1, 3, and 8. We further discuss the recent therapeutic development to target the Ca2+-stimulated ADCYs for potential treatments of psychiatric and neurodevelopmental disorders.
Adenylyl cyclase (ADCY) activity accounts for the basal level as well as activity-dependent production of cAMP, which is a main second messenger and regulates a wide spectrum of intracellular signaling molecules (Figure 1). In the nervous system, extracellular stimuli lead to functional responses and activity-dependent plasticity through the activation of surface neurotransmitter receptors followed by activation/inhibition of intracellular signaling molecules. With its enzymatic activity directly regulated by the G protein coupled neurotransmitter receptors (GPCR) on cell membrane, ADCY contributes to the dynamic intracellular cAMP transient following extracellular stimulation. The outcome of the cAMP-mediated signal transduction has broad impact on cellular functions including gene transcription and translation (Figure 1), the precise regulation of which is essential for neurodevelopment and activity-dependent modification of neuronal function. Thus, it is envisioned that appropriate ADCY activity is critical for neurodevelopment and neuroplasticity. Further, in addition to GPCRs, many other intracellular signaling molecules also dynamically regulate ADCY activity (Figure 1), leading to fine tuning of cAMP signaling in a task-specific manner. With regards to their physiological function, specific isoforms of ADCY have been shown to regulate distinct aspects of neuroplasticity and behavior that are essential for survival and adaptation. Emerging lines of animal study and human genome data have also suggested association of ADCY with a variety of dysfunction in the central nervous system (CNS) and the peripheral systems.
FIGURE 1. Signaling cascade triggered by the activation of Ca2+-stimulated ADCYs. Upon the activation of GPCR, Ca2+ influx through Ca2+ channels (such as VGCC, NMDAR, and Ca2+-permeable AMPAR) and/or Ca2+ release from internal store, the Ca2+-stimulated ADCYs are activated, leading to increased production of cAMP. The upregulation of cAMP activates PKA, which causes phosphorylation of CREB, ERK1/2 (indirectly), CaMKII (indirectly) and GluR1 (at S845), leading to activation of these molecules. Increase of intracellular Ca2+ leads to activation of ERK1/2 (indirectly), CaMKII, and CaMKIV, which, in turn, activate MSK1/CREB, GluR1 phosphorylation (at S831), and CBP (CREB binding protein), respectively. The activated CBP/CREB triggers CRE (cAMP responsive element)-mediated gene transcription. The ERK1/2-mediated activation of eIF4E, 4EBP1, and S6K1 may enhance ribosome activity and lead to increase of protein translation. The Ca2+-stimulated CaMKIV and CaMKII may lead to feedback inhibition of ADCY1 and ADCY3, respectively. Other main aspects of cAMP effect include the cAMP-mediated EPAC (exchange protein directly activated by cAMP) activation and cAMP-facilitated opening of the HCN (hyperpolarization-activated cyclic nucleotide-gated) channels.
To date, ten isoforms of mammalian ADCY have been identified. Nine ADCYs (i.e., ADCYs 1-9) are membrane-bound proteins and share similar structures with a short N-terminal cytoplasmic domain (the C1 domain) two transmembrane domains (i.e., TM1 and TM2) connected by the C1 cytoplasmic loop, and the C2 cytoplasmic domain at the C-terminus (Cooper, 2003) (Figure 1). Through the C1 and C2 domains, ADCYs 1-9 confer the catalytic activity and can be regulated by GPCRs and intracellular protein kinases and phosphatases (Cooper, 2003) (Figure 1). Based on structure and regulatory properties, five groups of ADCY are defined. In general, the group I ADCYs includes ADCY1, 3, and 8. They are stimulated by Gs and Ca2+ and inhibited by Gi. The group II ADCYs include ADCY2, 4, and 7. They are stimulated by Gs and not responsive to Ca2+ and Gi. The group III ADCYs include ADCY5 and 6. They are stimulated by Gs and inhibited by Ca2+ and Gi. The group IV ADCY includes ADCY9, which is only stimulated by Gs and, unlike other ADCYs, not responsive to the general ADCY activator forskolin. The group V ADCY includes ADCY10, which is a structurally distinct soluble cytoplasmic protein whose activity is regulated by Ca2+ and bicarbonate but not GPCRs (Buck et al., 1999; Litvin et al., 2003; Kleinboelting et al., 2014). ADCY10 is mainly expressed in the liver and testes but not in the central nervous system. Among these ADCYs, the group I Ca2+-stimulated ADCYs are functionally positioned to couple the two main small molecule messengers Ca2+ and cAMP in neurons (Figure 1). Human genetic studies and animal models suggest emerging roles of the Ca2+-stimulated ADCYs in regulating distinct aspects of pathology in psychiatric disorders. In this review, we focus on the Ca2+-stimulated ADCYs and discuss their potential as therapeutic targets to treat neurodevelopment and psychiatric disorders.
The existence of the calmodulin (CaM)-binding domain in the C1 or C2 region of some ADCYs suggests that certain isoforms can be regulated by Ca2+ (Wang and Storm, 2003). Affinity purification with the CaM-sepharose chromatography aided the cloning of ADCY1 followed by discovering other CaM-binding ADCYs (Krupinski et al., 1989). Among all the Ca2+-sensitive ADCYs, the group I isoforms consists of the Ca2+-stimulated ADCY1, ADCY3 and ADCY8 (Cooper, 2003). As cAMP and Ca2+ are the main second messengers in neurons, it is postulated and the Ca2+-stimulated ADCYs are at the converging hub to integrate the cAMP and Ca2+ signaling (Figure 1).
ADCY1 activity is stimulated by Ca2+ (Tang et al., 1991) with an EC50 of ∼100 nM (Fagan et al., 1996), which is slightly lower than the free Ca2+ level in resting neurons (Berridge et al., 2003). This indicates that ADCY1 is constitutively stimulated by the basal Ca2+ in resting neurons and further activated by transient increase of Ca2+ in stimulated neurons. Distinct amino acid mutations in the CaM-binding domain either reduce or abolish the Ca2+ stimulation of ADCY1 (Wu et al., 1993). Although GTPγS can directly increase ADCY1 activity (Tang et al., 1991), Gαs only stimulates ADCY1 in the presence of Ca2+ (Wayman et al., 1994); Gαi (Nielsen et al., 1996) and Gβγ (Tang et al., 1991) cause inhibition of ADCY1. Intriguingly, CaMKIV (CaM-dependent kinase IV) can directly phosphorylate ADCY1, leading to activity inhibition (Wayman et al., 1996). It is conceivable that, depending on subcellular micro-environment, Ca2+/CaM may exert dichotomy effects on ADCY1. While Ca2+ may predominantly stimulate ADCY1 in sub-cellular regions that are devoid of CaMKIV, a compromised or even inhibitory effect may be observed in the CaMKIV-enriched regions. CaMKIV may also function as a molecular break to prevent over-stimulation of ADCY1 by sustained Ca2+ elevation (Figure 1).
ADCY3 shares the most structural similarity with ADCY1 and ADCY8. However, in vitro assays with membrane preparation reveal that Ca2+ alone is not sufficient to stimulate ADCY3 activity (Choi et al., 1992; Wayman et al., 1995). When paired with the general ADCY activator forskolin or G protein activator GppNHp, high level of Ca2+ stimulates ADCY3 with an EC50 of ∼5,000 nM (Choi et al., 1992). Considering that the intracellular Ca2+ varies in the 1–10,000 nM range (Smith and Augustine, 1988), Ca2+ stimulation of ADCY3 may occur in specific subcellular domains with high Ca2+ transients in stimulated neurons. Notably, Wayman et al. found that, in the presence of GppNHp or glucagon, Ca2+ at a much lower concentration (e.g., 200 nM) can cause ∼2-fold increase of ADCY3 activity in vitro (Wayman et al., 1995). Counterintuitively, cAMP accumulation assay with live cells reveal that increase of intracellular Ca2+ (at ∼100–300 nM) significantly inhibits hormone- and Gαs-stimulated ADCY3 in vivo (Wayman et al., 1995). It is further identified that CaMKII (CaM-dependent protein kinase II) phosphorylates and inhibits ADCY3, suggesting an indirect inhibition effect of Ca2+ (Wei et al., 1996) (Figure 1). Although the physiological relevance of the Ca2+-stimulated regulation of ADCY3 remains to be elucidated, the Ca2+/CaMKII-mediated inhibition is likely to ensure a transient rather than persistent increase of cAMP increase in olfactory cilia, leading to the temporal attenuation of sensation following odor detection (Wei et al., 1998).
ADCY8 possesses a CaM-binding domain and is directly stimulated by Ca2+ with an EC50 of ∼500–800 nM (Fagan et al., 1996; Nielsen et al., 1996). Considering that ADCY8 is moderately sensitive to Ca2+, it is speculated that Ca2+ released from the internal store may not be sufficient to stimulate ADCY8. However, Martin et al. (2009) suggest that the capacitative Ca2+ entry (CCE), which is triggered by Ca2+ depletion from the internal store, may lead to more robust Ca2+ increase and stimulate ADCY8. In the non-neuronal HEK293 cells, when ADCY8 and the CCE functional molecules STIM1 and Orai1 are over-expressed, they colocalize in lipid raft domains of the cell membrane. Functionally, while Ca2+ store depletion alone fails to activate ADCY8, over-expression of STIM1 and Orai1 along with Ca2+ store depletion leads to ADCY8 activation (Martin et al., 2009). Given that there is high expression level of STIM and Orai isoforms in the central nervous system (e.g., STIM2 and Orai2 in hippocampus), it is likely that CCE activates ADCY8 as well as ADCY1 and ADCY3 in neurons (Zhang and Hu, 2020). In vitro assays with membrane preparations from ADCY8-expressing HEK293 cells detect that the Ca2+-stimulated ADCY activity can be further increased in the presence of activated Gαs (i.e., GTPγS bound Gαs) (Cali et al., 1994). However, the in vivo cAMP accumulation assay fails to detect any effect in HEK293 cells following pharmacological activation of the Gs-coupled adrenergic receptors (Cali et al., 1994; Nielsen et al., 1996). Further, the in vivo cAMP accumulation assay with the ADCY8-expressing HEK293 cells fails to detect the inhibition effects following the activation of the Gi-coupled somatostatin and dopaminergic receptors (Nielsen et al., 1996). Thus, these lines of evidence suggest that ADCY8 is exclusively regulated by Ca2+ but not by G proteins in vivo. However, overexpression of a constitutively active Gα/olf causes robust activation of both ADCY1 and ADCY8 (Regnauld et al., 2002). Regarding whether and how GPCRs can regulate ADCY8 activity, examination with neurons (rather than the heterologous HEK293 cells) and activation of full spectrum of GPCRs (in addition to adrenergic, dopaminergic and somatostatin receptors) is required.
Through the Ca2+-stimulated ADCYs, cAMP and Ca2+ signaling may converge and tune specific signaling networks and, in turn, regulate cellular functions relevant to neurotransmitter receptor activity, gene transcription, and translation (Figure 1). With regards to the function of Ca2+-stimulated ADCYs in neuroplasticity, neurodevelopment and psychiatric disorders, we focus on the Ca2+/cAMP-PKA (cAMP-dependent protein kinase)/ERK1/2 (extracellular signal-regulated kinases 1/2)-MSK1 (mitogen- and stress-activated protein kinase-1)-CREB (cAMP responsive element binding protein), the Ca2+/cAMP-ERK1/2-eIF4E (eukaryotic translation initiation factor 4E)/4EBP1 (Eukaryotic translation initiation factor 4E-binding protein 1)/S6K1 (ribosome protein S6 kinase 1), and the Ca2+/cAMP-PKA/CaMKII-GluR1 (glutamate ionotropic AMPA type subunit 1) cascades (Figure 1).
The definitive function of ADCY1 and ADCY8 in regulating the Ca2+/cAMP-mediated signaling is mainly examined with brain samples collected from mutant mice. The Ca2+-stimulated ADCY activity is reduced by ∼50% and ∼30% in the hippocampus of Adcy1 knockout (KO) and Adcy8 KO mice, respectively (Wong et al., 1999). The cAMP level is reduced by ∼25% in the Adcy1 KO hippocampus (Sethna et al., 2017). Although lack of both ADCY1 and ADCY8 surprisingly increases the basal ERK1/2 activity, the contextual fear learning- and cocaine-triggered upregulation of the ERK1/2-MSK1-CREB signaling is abolished in the Adcy1/Adcy8 double knockout (DKO) mice (Sindreu et al., 2007; DiRocco et al., 2009). The Adcy1/Adcy8 DKO mice also do not display diurnal oscillation of ERK1/2 phosphorylation in the hippocampus (Eckel-Mahan et al., 2008), suggesting lack of molecular circadian rhythm. In primary cortical neurons, ADCY1 deficiency impairs the glutamate-induced upregulation of CREB activity (Wang et al., 2007). Consistent with the function of ERK1/2-CREB in regulating the Ca2+/CRE (cAMP-responsive element)-mediated transcription of bdnf (brain-derived neurotrophic factor) (Zheng et al., 2011), mice lacking ADCY1 and ADCY8 fail to show learning- and exercise-induced up-regulation of bdnf mRNA (Zheng et al., 2012; Zheng et al., 2016). Conversely, overexpression of ADCY1 results in increase of Ca2+-stimulated ADCY activity, cAMP level, PKA activity, and basal as well as learning-induced upregulation of ERK1/2-CREB activity in the hippocampus (Wang et al., 2004). Overexpression of ADCY1 also blocks the stress-induced downregulation of bdnf transcription in hippocampus and prefrontal cortex (Yang et al., 2020).
With regards to gene expression, the cAMP-regulated ERK1/2 may impinge on ribosome activity and, in turn, regulate protein synthesis. In vitro and in vivo studies demonstrate that inhibition of ERK1/2 leads to impairments of activity-dependent upregulation of eIF4E, 4EBP1, and S6K1 phosphorylation (Kelleher et al., 2004; Zhou et al., 2010). Inhibition of ERK1/2 also suppresses various forms of protein synthesis-dependent synaptic plasticity (Gallagher et al., 2004; Kelleher et al., 2004). Whether and how the Ca2+-stimulated ADCYs regulate basal and activity-dependent changes of ribosome activity remain to be determined.
The cAMP signaling may also regulate neuronal function on cell surface. Two main phosphorylation sites (i.e., Serine 845 and 831) of GluR1 are targets of PKA and CaMKII/PKC (protein kinase C), respectively (Figure 1), and functionally involved to regulate receptor trafficking and channel conductance (Malinow, 2003). The phosphorylation of GluR1 at S845 and S831 is dynamically altered after the induction of long-term potentiation (LTP), long-term depression (LTD.), and synaptic depotentiation (Lee et al., 2000; Huang et al., 2001). Although S831 phosphorylation can be suppressed by PKC and CaMKII inhibitors, it may also be regulated indirectly through the cAMP-enhanced CaMKII activation (Makhinson et al., 1999). In mice with a natural loss-of-function mutation in Adcy1, phosphorylation of GluR1 (pGluR1) at S845, AMPAR-mediated EPSCs, and surface GluR1 are decreased at the thalamocortical synapses (Lu et al., 2003).
The mRNA transcript of Adcy1, detected by Northern blot and in situ hybridization, is predominantly expressed in the nervous system tissues including brain, adrenal gland, and retina (Figure 2A) (Xia et al., 1993; Yue et al., 2014). Within the central nervous system (CNS), Adcy1 mRNA expression is developmentally regulated (Figure 2A) and detected in distinct regions at various levels (Figures 2A, 3A) (Yue et al., 2014) (https://brainrnaseq.org). Notably, Adcy1 mRNA level is overwhelmingly higher in excitatory neurons than inhibitory neurons in the hippocampus but not in other regions (Figure 3A). Although RNA-sequencing has detected Adcy1 mRNA in neurons and glial cells (https://brainrnaseq.org), Western blot with a validated antibody (Sethna et al., 2017) detects ADCY1 protein expression only in neuron-enriched but not glial cell-enriched primary cultures (Ding et al., 2020).
FIGURE 2. Developmental and tissue-specific expression of Ca2+-stimulated Adcy mRNA transcripts in mouse. The RNAseq study by the Mouse ENCODE Consortium (Yue et al., 2014) revealed the expression of Adcy1 (A), Adcy3 (B), and Adcy8 (C) mRNA in different tissues and in CNS at different developmental stages. RPKM: Reads Per kilobase of transcript per Million mapped reads. CNS: central nervous system. E11.5, E14, and E18: embryonic day 11.5, 14, and 18.
FIGURE 3. Cell type-specific expression of Ca2+-stimulated Adcy mRNA transcripts in various regions of the mouse brain. The information of relative mRNA levels of Adcy1 (A), Adcy3 (B), and Adcy8 (C) in excitatory and inhibitory neurons of various brain regions was collected from https://mousebrain.org.
The subcellular distribution of endogenous ADCY1, due to the lack of antibody for histochemistry, is largely unknown. With expression of the epitope-tagged recombinant protein, it is found that the HA (hemagglutinin)-ADCY1 is expressed in both dendrite and axon. The punctate and discrete HA-ADCY1 colocalizes with synaptophysin and synapsin I in cerebellar neurons, indicating enrichment in the synaptic compartment (Wang et al., 2002). Western blot analysis with synaptosome fractions detects that the endogenous ADCY1 in hippocampus is enriched in postsynaptic density and extrasynaptic fractions (Conti et al., 2007).
Consistent with the high expression level of Adcy1 mRNA in cortex, hippocampus, and cerebellum, studies with the Adcy1 KO mice have found that activity-dependent potentiation of synaptic efficacy in these brain regions requires ADCY1 (Storm et al., 1998; Liauw et al., 2005; Zheng et al., 2016). It is also evident that ADCY1 supports long-term potentiation (LTP) at both presynaptic (Villacres et al., 1998; Miao et al., 2019) and postsynaptic sites (Liauw et al., 2005; Zheng et al., 2016). It is demonstrated that ADCY1 in presynaptic neurons is required for sensory input and neurodevelopment of postsynaptic neurons. Brain region-specific knockout of Adcy1 in thalamus causes disruptive barrel patterning in layer 4 of the somatosensory cortex (Suzuki et al., 2015). Further, ADCY1 deficiency in the presynaptic RGC (retinal ganglion cell) causes map disruption of the postsynaptic tissues SC (superior colliculus) and LGN (lateral geniculate nucleus) (Dhande et al., 2012).
ADCY3 was initially identified as the major ADCY in olfactory epithelium (Bakalyar and Reed, 1990). Following the molecular cloning of Adcy3 gene, Northern blot and semiquantitative RT-PCR detected broad expression of the Adcy3 mRNA in both CNS and peripheral tissues (Xia et al., 1992; Yang et al., 1999; Yue et al., 2014) (Figure 2B). The expression level is high in brain, placenta, lung, and skeletal muscle. Intermediate expression is detected in heart, kidney, and pancreas (Yang et al., 1999; Yue et al., 2014). Within the CNS, Adcy3 mRNA and ADCY3 protein are found in many brain regions, including olfactory bulb, cortex, hippocampus, amygdala, nucleus accumbens, thalamus, hypothalamus and cerebellum (Bishop et al., 2007) (Figures 2B, 3B). It is expressed in both excitatory and inhibitory neurons (Figure 3B) as well as in glia cells (Bishop et al., 2007). Interestingly, Adcy3 mRNA level is higher in inhibitory neurons than excitatory neurons in hypothalamus (Figure 3B).
Within the olfactory epithelium, ADCY3 is predominantly localized in olfactory cilia, which is the main organelle of sensory neuron to conduct the sensation of smell. Upon activation of the Golf-coupled odorant receptors, cAMP is generated by the Golf-activated ADCY3. Binding of cAMP to the cyclic nucleotide-gated (CNG) channels causes the influx of Na+ and Ca2+, leading to sensory neuron activation and the subsequent olfactory detection process. Deficiency of ADCY3, which is the only ADCY in olfactory cilia, causes anosmia (i.e., loss of smell) (Wong et al., 2000).
In brain neurons, ADCY3 is localized in the primary cilium (Bishop et al., 2007), which is a solitary microtubule-based 2–12 μm projection from the cell surface. In contrast to typical synaptic structures, primary cilia are devoid of ionotropic neurotransmitter receptors and thought to mediate neuronal signaling via metabotropic GPCRs (Green and Mykytyn, 2014). The co-existence of ADCY3 and certain GPCRs (e.g., melanocortin 4 receptor, somatostatin receptor 3, and type 6 serotonin receptor) suggests activity-dependent cAMP signaling in primary cilia (Wang et al., 2011b; Guadiana et al., 2013; Siljee et al., 2018). Although how cilia signaling affects neuronal function is largely unknown, disruption of the cilia-enriched proteins causes alteration of neuron development and synaptic function. Notably, ADCY3 deficiency leads to reduced dendritic outgrowth and arborization, hippocampus atrophy, reduced neural transmission and impaired LTP at the schaffer collateral-CA1 synapses (Chen et al., 2016).
Adcy8 is expressed in both the CNS and the peripheral non-neuronal tissues (Muglia et al., 1999; Yue et al., 2014) (Figure 2C). Within the CNS, Adcy8 mRNA shows the highest levels in olfactory bulb, thalamus, hypothalamus and brain stem (Muglia et al., 1999; Schaefer et al., 2000) (Figure 3C). In these brain regions, ADCY8 but not ADCY1 accounts for most of the Ca2+-stimulated ADCY activity. In olfactory bulb and hypothalamus of the Adcy8 KO mice, there is no significant Ca2+-stimulated upregulation of ADCY activity (Schaefer et al., 2000). Moderately high level of Adcy8 mRNA is detected in hippocampus, in which ADCY8 deficiency causes ∼25% reduction of Ca2+-stimulated ADCY activity (Schaefer et al., 2000). In contrast to Adcy1 mRNA, robust Adcy8 mRNA is detected in both excitatory and inhibitory neurons in hippocampus (Figure 3C). This suggests that the Ca2+-stimulated cAMP signaling may be co-regulated by ADCY1 and ADCY8 in excitatory neurons, and predominantly regulated by ADCY8 in inhibitory neurons in the hippocampus.
The subcellular distribution of ADCY8 has been examined with neurons expressing the recombinant HA-ADCY8. The HA-ADCY8 displays punctate staining in both dendrites and axons of cortical and hippocampal neurons and colocalizes with both presynaptic (i.e., synaptophysin and synapsin I) and postsynaptic marker (i.e., PSD95) proteins (Wang et al., 2003). The endogenous hippocampal ADCY8 is preferentially enriched in the presynaptic active zone and also detected in extrasynaptic fractions (Conti et al., 2007). The presynaptic cellular function of ADCY8 is implicated by that the mossy fiber-CA3 LTP, which mainly relies on presynaptic Ca2+/cAMP signaling, is defective in the Adcy8 KO mice (Wang et al., 2003). The postsynaptic function of ADCY8 is implicated by that the schaffer collateral-CA1 LTD (long-term depression) is defective in the Adcy8 KO mice (Schaefer et al., 2000).
Alteration of Ca2+/cAMP-mediated signaling has been detected as molecular outcomes that are associated with various aspects of psychiatric and neurodevelopment disorders. Within the Ca2+/cAMP signaling network (Figure 1), abnormal function of GPCR (Grace, 2016), ion channel (Lee et al., 2016; Zanos and Gould, 2018; Nakazawa and Sapkota, 2020), and protein kinase (Wang et al., 2012; Robison, 2014; Gross et al., 2019) is associated with distinct malfunction and maladaptation of the brain. Here, we discuss the emerging roles of Ca2+-stimulated ADCY as risk and causal factors in regulating the cellular pathology and behavioral symptoms associated with psychiatric and neurodevelopment disorders.
Hyper-expression of ADCY1 has been identified in a mouse model of Fragile X syndrome (FXS) (Sethna et al., 2017), which is predominantly caused by mutations in the FMR1 (Fragile X messenger ribonucleoprotein 1) gene and deficient expression of its gene product FMRP (FMR1 protein). FXS is a neurodevelopment disorder and leading cause of intellectual disability and autism (Sethna et al., 2014). Among various functions of FMRP (Richter and Zhao, 2021), the RNA binding activity has been demonstrated to be directly related to the main symptoms in FXS. High-throughput screenings have revealed that FMRP binds 800–6,000 distinct mRNA targets and may suppress their translation (Brown et al., 2001; Darnell et al., 2011; Ascano et al., 2012). Along with general elevation of mRNA translation, abnormally increased expression of specific FMRP targets is linked to exaggerated signaling in FXS neurons (Wang et al., 2012; Gross et al., 2015). Mining and analysis of the multiple high-throughput screening data identified Adcy1 mRNA as a top-ranked FMRP target. Consistently, ADCY1 protein expression level is abnormally higher in the brain of Fmr1 knockout (KO) mice (Sethna et al., 2017). The enhanced ADCY1 expression is associated with the exaggerated ERK1/2/PI3K (phosphoinositide 3-kinases)-S6K1 signaling in FXS (Wang et al., 2012; Gross et al., 2015). The causal function of the elevated ADCY1 expression is supported by that genetic reduction of ADCY1 in the Fmr1 KO mice rescues the key aspects of pathology, including the exaggerated overall protein synthesis and ERK1/2/PI3K-S6K1 activity, higher dendritic spine density, audiogenic seizure, repetitive behavior and social deficits (Sethna et al., 2017). Interestingly, enhanced ADCY1 expression also results in certain behavioral abnormalities associated with FXS and autism. Forebrain overexpression of ADCY1 in transgenic mice causes hyper locomotion and social deficits (Chen et al., 2015).
Alteration of Adcy1 gene, as suggested by the human genome-wide association study (GWAS), is a potential genetic risk factor for schizophrenia (Sundararajan et al., 2018). A recent study analyzed a combined list of schizophrenia-risk genes that are collected from published GWAS data, meta-analysis data, and the OMIM and GeneCards databses (Butler et al., 2016a; Butler et al., 2016b; Wu et al., 2017). By using the GeneAnalytics program, Sundararajan et al. (2018) suggest that the schizophrenia genes have significant impact on Ca2+ signaling pathway, CREB pathway, and monoamine GPCRs. Integration analysis of the schizophrenia gene and the phenotype database (http://www.informatics.jax.org/; http://human-phenotype-ontology.github.io/) identifies an association of schizophrenia genes with reduced LTP, abnormal spatial learning, and neurodevelopment (Sundararajan et al., 2018). Based on biochemical data and functional studies with mutant mice, it is evident that ADCY1 directly regulates the CREB pathway and integrates Ca2+ and GPCR signaling. Although how ADCY1 regulates the hallmark schizophrenia symptoms remains unclear, ADCY1 deficiency leads to impaired LTP (Villacres et al., 1998; Zheng et al., 2016) and spatial memory (Wu et al., 1995) and maldevelopment of the sensory cortex (Suzuki et al., 2015). It is interesting to note that ADCY1 shows high level in cerebellum, cerebral cortex, and thalamus, which are where the schizophrenia risk genes predominantly express (Sundararajan et al., 2018). As defective cortico-cerebellar-thalamic-cortical circuit is suggested as an emerging etiological factor for schizophrenia (Andreasen and Pierson, 2008; Dorph-Petersen and Lewis, 2017), the region-specific function of ADCY1 needs to be studied.
Alterations of Adcy1 gene may affect the therapeutic efficacy of lithium in bipolar disorder (International Consortium on Lithium Genetics et al., 2018). Although bipolar disorder and schizophrenia shares significant number of genetic risk factors, patient responses to pharmacological interventions are dramatically different. While the mood stabilizer lithium is used as the first line medication in bipolar disorder, it is not effective for schizophrenia patients. Further, a significant population of bipolar disorder patients also does not show therapeutic outcome following lithium treatment. A cross-trait meta-analysis of the GWAS of schizophrenia and Consortium on Lithium Genetics has found an interesting reverse association of polygenic schizophrenia load and lithium response in bipolar disorder (International Consortium on Lithium Genetics et al., 2018). Bipolar disorder patients with low polygenic schizophrenia load show better therapeutic response to lithium. Regarding whether and how ADCY1 activity impinges on pharmacological and molecular outcome of lithium treatment, validations with in vitro cellular assays and in vivo behavioral examinations may be needed. With the available ADCY1 inhibitors and mouse models (e.g., the Adcy1 KO and overexpression mice), it is feasible to detect whether the lithium effects are attenuated or potentiated.
Although there are debates on the significance of genetic risk factors in human major depressive disorder, a GWAS study with 2,431 major depressive disorder and 3,673 control samples revealed a suggestive association of Adcy3 polymorphism with depression (Wray et al., 2012). Notably, lower PKA expression and ADCY activity (Perez et al., 2001; Hines et al., 2005) in platelets, which express only Adcy3 but not other Adcy isoforms (Katsel et al., 2003), are detected in major depressive disorder subjects and attenuated by the use of various drugs including antidepressants, analgesics, and addictive drugs (Hines et al., 2005). Consistently, Adcy3 mRNA level is reduced in the blood samples of major depressive disorder (Redei et al., 2014). Brain transcriptome analysis of human postmortem samples has also found altered level of Adcy3 transcript in autism spectrum disorder (Guan et al., 2019).
The functional relevance of Adcy3 mutations to major depressive disorder and autism spectrum disorder has been examined with the KO and knock-in mice. The conventional whole body Adcy3 KO mice display a wide spectrum of symptoms including increased REM (rapid eye movement) sleep, hypo-locomotion, neophobia, higher immobility in the tail suspension and forced swimming test, impaired nesting behavior and impaired sociability (Chen et al., 2016). The forebrain-specific pyramidal neuron conditional Adcy3 KO mice recapitulates many aspects of the major depressive disorder- and autism spectrum disorder-associated symptoms, but intriguingly display normal social interaction (Chen et al., 2016). The conventional and the conditional forebrain-specific Adcy3 KO mice show defective spatial memory and object recognition memory (Wang et al., 2011b; Chen et al., 2016). Whole body Adcy3 deficiency also causes impairments in passive avoidance memory and fear memory extinction (Wang et al., 2011b). Intriguingly, the region-specific knock-down of Adcy3 in the main olfactory epithelium not only leads to anosmia but also causes depression-like behavior (Liu et al., 2020a) and cognitive defects (Liu et al., 2020b). The results suggest a role of olfactory cAMP signaling in the association of olfaction deficiency and depression (Kohli et al., 2016); further understanding of how ADCY3 mediates the functional cross-talk among brain circuitry networks of olfaction, emotion and cognition is critical.
Further, Adcy3 loss-of-function variants are identified as a risk factor of obesity (Grarup et al., 2018), which often co-occurs with depression (Carey et al., 2014; Mannan et al., 2016). Whole body KO or knockdown of Adcy3 in ventromedial hypothalamus causes obesity (Wang et al., 2009; Yang et al., 2022). In contrast, mice overexpressing the human ADCY3 gene (Yang et al., 2022) and mice harboring a point mutation, which causes elevated enzymatic activity of ADCY3, (Pitman et al., 2014) are resistant to high fat diet-induced obesity.
Genetic variants of Adcy8 gene, as suggested by the human genetics studies, may be associated with bipolar disorder, schizophrenia, autism spectrum disorder, obsessive-compulsive disorder, and posttraumatic stress disorder. A linkage study with microsatellite markers reported an association of bipolar disorder with the genetic loci on chromosome 8q24, which covers three candidate risk genes including Adcy8 (Avramopoulos et al., 2004). Two follow-up studies using more SNP (single nucleotide polymorphism) markers revealed a finer mapping of the bipolar disorder-associated region on 8q24 and also suggest Adcy8 as a potential risk gene (Zandi et al., 2008; Zhang et al., 2010). Brain transcriptome analysis of human postmortem samples has found altered level of Adcy8 transcript in bipolar disorder and schizophrenia (Guan et al., 2019).
Clinical data have found that significant population of autism spectrum disorder and obsessive-compulsive disorder patients show overlapping pathological features (Gross-Isseroff et al., 2001; van Steensel et al., 2011). GWAS data also reveal genetic variants commonly associated with autism spectrum disorder and obsessive-compulsive disorder (Liu et al., 2019). Further, an integrative analysis of brain transcriptome in autism spectrum disorder and genomic variants in obsessive-compulsive disorder identifies Adcy8 as a common risk factor for autism spectrum disorder and obsessive-compulsive disorder (Liu et al., 2019).
Regarding functional relevance of ADCY8 alteration to symptoms associated with psychiatric and neurodevelopmental disorders, supporting data are mostly collected from mice with Adcy8 mutations. In mice with a QTL (quantitative trait loci) on a chromosome region that is homologous to human 8q24, increased Adcy8 mRNA level is detected in ventral hypothalamus and piriform cortex and associated with avoidance behavior (i.e., preference of the sheltered feeding platform over the exposed feeding platform) (de Mooij-van Malsen et al., 2009). In contrast, the Adcy8 KO mice display risk-taking traits, as indicated by more occupancy in the center area of the open field and open arm of the elevated plus maze (Schaefer et al., 2000). The Adcy8 KO mice are also hyperactive in their home cage and during the forced swimming test (Razzoli et al., 2010).
The high expression level of Adcy8 in hypothalamus suggests potential functions in regulating stress responses through the HPA (hypothalamic-pituitary-adrenal) axis. A GWAS study implicates a suggestive association of Adcy8 with posttraumatic stress disorder (Wolf et al., 2014). Adcy8 deficiency in the KO mice causes adrenal hypertrophy but normal basal plasma corticosterone level (Schaefer et al., 2000; Razzoli et al., 2010). Intriguingly, following chronic stress, the Adcy8 KO mice display higher elevation of corticosterone (than wild type mice) along with more risk-taking rather than anxiogenic behavior (Schaefer et al., 2000; Razzoli et al., 2010).
To achieve the therapeutic potential of targeting the Ca2+-stimulated ADCYs, it is necessary to develop pharmacological reagents. To date, several small molecule inhibitors against ADCY1 have been developed and tested in certain animal models. Drugs showing specific regulatory activity for ADCY3 and ADCY8 have not been reported yet.
By using a structure-based rational design compound library, Wang et al. (2011a) characterized and identified NB001 as a preferential inhibitor against ADCY1 over other ADCY isoforms. The peripherally administered NB001 can cross the blood-brain-barrier and have a half-life of about 2 h in the brain (Sethna et al., 2017). Although NB001 has a modest IC50 of 10 μM, a reasonably low dose at 1–3 mg/kg shows analgesic effect in preclinical models of neuropathic and inflammatory pain (Wang et al., 2011a). In a mouse model of FXS, NB001 at 1 mg/kg attenuates the abnormally elevated ERK1/2/Akt-S6K1 signaling and rescues repetitive behaviors and social deficits (Sethna et al., 2017).
Although NB001 is currently tested for safety in human clinical trials (Wang et al., 2022), it is not yet approved by FDA. The promising efficacy of NB001 with the FXS mouse model has motivated repurposing of the existing FDA-approved drugs. Ding et al. (2020) examined the effects of carbamazepine, which is an FDA-approved anticonvulsant and also shows pharmacological inhibition action against ADCY1 (Mann et al., 2009). It is demonstrated that carbamazepine attenuates the elevated ERK1/2/Akt activity and protein synthesis in the Fmr1 KO neurons. Peripheral administration of carbamazepine corrects hyperlocomotion and social deficits and improves learning and memory in the Fmr1 KO mice (Ding et al., 2020).
Another ADCY1 inhibitor has been identified by screening a natural product derivatives library. ST034307 shows an IC50 of 2.3 μM against ADCY1 and no detectable inhibition against other ADCYs (Brust et al., 2017). Interestingly, ST034307 at higher concentration (e.g., 30 μM) causes potentiation effect on ADCY2, 3, 5, and 6 (Brust et al., 2017). Consistent with the potential role of ADCY1 in pain (Li et al., 2020), intrathecal injection of ST034307 relieves pain in a mouse model of inflammatory pain (Brust et al., 2017).
As the backbone structures of the adenosine-based NB001 and chromone-based ST034307 predict limitation and drawback of these compounds as practical therapeutic reagents, recent effort aims to identify ADCY1 inhibitors with different structures. The oxadiazole-based AC10065 at micromolar concentrations can suppress both ADCY1 and ADCY8 (Kaur et al., 2019). New drug screening followed by structure optimization has revealed several pyrimidinone-based compounds that show selectivity of ADCY1 over other ADCYs with an IC50 at the sub-micromolar level (Scott et al., 2022). As these newly identified ADCY1 inhibitors show moderate therapeutic efficacy in an inflammatory pain model (Scott et al., 2022), further optimization may be needed.
In summary, previous studies have demonstrated the function of Ca2+-stimulated ADCYs in regulating various aspects of neuronal property and behavior. It is evident that a distinct ADCY isoform, rather than general and overall cAMP level, may specifically control an isoform-specific cellular and physiological function. Elucidation of Ca2+-stimulated ADCY function in distinct brain regions and distinct cell types may help to develop precise intervention of the disorder-specific pathology. Development of high affinity isoform-specific drugs with favorable pharmacokinetics and toxicity profile will lead to practical intervention to promote mental health and alleviate symptoms associated with certain psychiatric and neurodevelopment disorders. Further, interpretation with the current animal models should consider non-specific effects of global gene deficiency, complication of different genetic backgrounds and validity of the behavior outcome. Precision preclinical models with more direct face and construct validity need to be developed.
JC, QD, and LA wrote the first draft of the manuscript. HW wrote the manuscript and obtained funding.
This research was supported by NIH grants R01MH124992 and R01MH119149 (to HW).
The authors declare that the research was conducted in the absence of any commercial or financial relationships that could be construed as a potential conflict of interest.
All claims expressed in this article are solely those of the authors and do not necessarily represent those of their affiliated organizations, or those of the publisher, the editors and the reviewers. Any product that may be evaluated in this article, or claim that may be made by its manufacturer, is not guaranteed or endorsed by the publisher.
Andreasen, N. C., and Pierson, R. (2008). The role of the cerebellum in schizophrenia. Biol. Psychiatry 64 (2), 81–88. doi:10.1016/j.biopsych.2008.01.003
Ascano, M., Mukherjee, N., Bandaru, P., Miller, J. B., Nusbaum, J. D., Corcoran, D. L., et al. (2012). FMRP targets distinct mRNA sequence elements to regulate protein expression. Nature 492 (7429), 382–386. doi:10.1038/nature11737
Avramopoulos, D., Willour, V. L., Zandi, P. P., Huo, Y., MacKinnon, D. F., Potash, J. B., et al. (2004). Linkage of bipolar affective disorder on chromosome 8q24: Follow-up and parametric analysis. Mol. Psychiatry 9 (2), 191–196. doi:10.1038/sj.mp.4001388
Bakalyar, H. A., and Reed, R. R. (1990). Identification of a specialized adenylyl cyclase that may mediate odorant detection. Science 250 (4986), 1403–1406. doi:10.1126/science.2255909
Berridge, M. J., Bootman, M. D., and Roderick, H. L. (2003). Calcium signalling: Dynamics, homeostasis and remodelling. Nat. Rev. Mol. Cell Biol. 4 (7), 517–529. doi:10.1038/nrm1155
Bishop, G. A., Berbari, N. F., Lewis, J., and Mykytyn, K. (2007). Type III adenylyl cyclase localizes to primary cilia throughout the adult mouse brain. J. Comp. Neurol. 505 (5), 562–571. doi:10.1002/cne.21510
Brown, V., Jin, P., Ceman, S., Darnell, J. C., O'Donnell, W. T., Tenenbaum, S. A., et al. (2001). Microarray identification of FMRP-associated brain mRNAs and altered mRNA translational profiles in fragile X syndrome. Cell 107 (4), 477–487. doi:10.1016/s0092-8674(01)00568-2
Brust, T. F., Alongkronrusmee, D., Soto-Velasquez, M., Baldwin, T. A., Ye, Z., Dai, M., et al. (2017). Identification of a selective small-molecule inhibitor of type 1 adenylyl cyclase activity with analgesic properties. Sci. Signal. 10 (467), eaah5381. doi:10.1126/scisignal.aah5381
Buck, J., Sinclair, M. L., Schapal, L., Cann, M. J., and Levin, L. R. (1999). Cytosolic adenylyl cyclase defines a unique signaling molecule in mammals. Proc. Natl. Acad. Sci. U. S. A. 96 (1), 79–84. doi:10.1073/pnas.96.1.79
Butler, M. G., McGuire, A. B., Masoud, H., and Manzardo, A. M. (2016a). Currently recognized genes for schizophrenia: High-resolution chromosome ideogram representation. Am. J. Med. Genet. B Neuropsychiatr. Genet. 171B (2), 181–202. doi:10.1002/ajmg.b.32391
Butler, M. G., Rafi, S. K., McGuire, A., and Manzardo, A. M. (2016b). Currently recognized clinically relevant and known genes for human reproduction and related infertility with representation on high-resolution chromosome ideograms. Gene 575 (1), 149–159. doi:10.1016/j.gene.2015.08.057
Cali, J. J., Zwaagstra, J. C., Mons, N., Cooper, D. M., and Krupinski, J. (1994). Type VIII adenylyl cyclase. A Ca2+/calmodulin-stimulated enzyme expressed in discrete regions of rat brain. J. Biol. Chem. 269 (16), 12190–12195. doi:10.1016/s0021-9258(17)32700-x
Carey, M., Small, H., Yoong, S. L., Boyes, A., Bisquera, A., and Sanson-Fisher, R. (2014). Prevalence of comorbid depression and obesity in general practice: A cross-sectional survey. Br. J. Gen. Pract. 64 (620), e122–127. doi:10.3399/bjgp14X677482
Chen, X., Cao, H., Saraf, A., Zweifel, L. S., and Storm, D. R. (2015). Overexpression of the type 1 adenylyl cyclase in the forebrain leads to deficits of behavioral inhibition. J. Neurosci. 35 (1), 339–351. doi:10.1523/JNEUROSCI.2478-14.2015
Chen, X., Luo, J., Leng, Y., Yang, Y., Zweifel, L. S., Palmiter, R. D., et al. (2016). Ablation of type III adenylyl cyclase in mice causes reduced neuronal activity, altered sleep pattern, and depression-like phenotypes. Biol. Psychiatry 80 (11), 836–848. doi:10.1016/j.biopsych.2015.12.012
Choi, E. J., Xia, Z., and Storm, D. R. (1992). Stimulation of the type III olfactory adenylyl cyclase by calcium and calmodulin. Biochemistry 31 (28), 6492–6498. doi:10.1021/bi00143a019
Conti, A. C., Maas, J. W., Muglia, L. M., Dave, B. A., Vogt, S. K., Tran, T. T., et al. (2007). Distinct regional and subcellular localization of adenylyl cyclases type 1 and 8 in mouse brain. Neuroscience 146 (2), 713–729. doi:10.1016/j.neuroscience.2007.01.045
Cooper, D. M. (2003). Regulation and organization of adenylyl cyclases and cAMP. Biochem. J. 375 (3), 517–529. doi:10.1042/BJ20031061
Darnell, J. C., Van Driesche, S. J., Zhang, C., Hung, K. Y., Mele, A., Fraser, C. E., et al. (2011). FMRP stalls ribosomal translocation on mRNAs linked to synaptic function and autism. Cell 146 (2), 247–261. doi:10.1016/j.cell.2011.06.013
de Mooij-van Malsen, A. J., van Lith, H. A., Oppelaar, H., Hendriks, J., de Wit, M., Kostrzewa, E., et al. (2009). Interspecies trait genetics reveals association of Adcy8 with mouse avoidance behavior and a human mood disorder. Biol. Psychiatry 66 (12), 1123–1130. doi:10.1016/j.biopsych.2009.06.016
Dhande, O. S., Bhatt, S., Anishchenko, A., Elstrott, J., Iwasato, T., Swindell, E. C., et al. (2012). Role of adenylate cyclase 1 in retinofugal map development. J. Comp. Neurol. 520 (7), 1562–1583. doi:10.1002/cne.23000
Ding, Q., Zhang, F., Feng, Y., and Wang, H. (2020). Carbamazepine restores neuronal signaling, protein synthesis, and cognitive function in a mouse model of fragile X syndrome. Int. J. Mol. Sci. 21 (23), E9327. doi:10.3390/ijms21239327
DiRocco, D. P., Scheiner, Z. S., Sindreu, C. B., Chan, G. C., and Storm, D. R. (2009). A role for calmodulin-stimulated adenylyl cyclases in cocaine sensitization. J. Neurosci. 29 (8), 2393–2403. doi:10.1523/JNEUROSCI.4356-08.2009
Dorph-Petersen, K. A., and Lewis, D. A. (2017). Postmortem structural studies of the thalamus in schizophrenia. Schizophr. Res. 180, 28–35. doi:10.1016/j.schres.2016.08.007
Eckel-Mahan, K. L., Phan, T., Han, S., Wang, H., Chan, G. C., Scheiner, Z. S., et al. (2008). Circadian oscillation of hippocampal MAPK activity and cAmp: Implications for memory persistence. Nat. Neurosci. 11 (9), 1074–1082. doi:10.1038/nn.2174
Fagan, K. A., Mahey, R., and Cooper, D. M. (1996). Functional co-localization of transfected Ca(2+)-stimulable adenylyl cyclases with capacitative Ca2+ entry sites. J. Biol. Chem. 271 (21), 12438–12444. doi:10.1074/jbc.271.21.12438
Gallagher, S. M., Daly, C. A., Bear, M. F., and Huber, K. M. (2004). Extracellular signal-regulated protein kinase activation is required for metabotropic glutamate receptor-dependent long-term depression in hippocampal area CA1. J. Neurosci. 24 (20), 4859–4864. doi:10.1523/JNEUROSCI.5407-03.2004
Grace, A. A. (2016). Dysregulation of the dopamine system in the pathophysiology of schizophrenia and depression. Nat. Rev. Neurosci. 17 (8), 524–532. doi:10.1038/nrn.2016.57
Grarup, N., Moltke, I., Andersen, M. K., Dalby, M., Vitting-Seerup, K., Kern, T., et al. (2018). Loss-of-function variants in ADCY3 increase risk of obesity and type 2 diabetes. Nat. Genet. 50 (2), 172–174. doi:10.1038/s41588-017-0022-7
Green, J. A., and Mykytyn, K. (2014). Neuronal primary cilia: An underappreciated signaling and sensory organelle in the brain. Neuropsychopharmacology 39 (1), 244–245. doi:10.1038/npp.2013.203
Gross, C., Banerjee, A., Tiwari, D., Longo, F., White, A. R., Allen, A. G., et al. (2019). Isoform-selective phosphoinositide 3-kinase inhibition ameliorates a broad range of fragile X syndrome-associated deficits in a mouse model. Neuropsychopharmacology 44 (2), 324–333. doi:10.1038/s41386-018-0150-5
Gross, C., Chang, C. W., Kelly, S. M., Bhattacharya, A., McBride, S. M., Danielson, S. W., et al. (2015). Increased expression of the PI3K enhancer PIKE mediates deficits in synaptic plasticity and behavior in fragile X syndrome. Cell Rep. 11 (5), 727–736. doi:10.1016/j.celrep.2015.03.060
Gross-Isseroff, R., Hermesh, H., and Weizman, A. (2001). Obsessive compulsive behaviour in autism--towards an autistic-obsessive compulsive syndrome? World J. Biol. Psychiatry 2 (4), 193–197. doi:10.3109/15622970109026809
Guadiana, S. M., Semple-Rowland, S., Daroszewski, D., Madorsky, I., Breunig, J. J., Mykytyn, K., et al. (2013). Arborization of dendrites by developing neocortical neurons is dependent on primary cilia and type 3 adenylyl cyclase. J. Neurosci. 33 (6), 2626–2638. doi:10.1523/JNEUROSCI.2906-12.2013
Guan, J., Cai, J. J., Ji, G., and Sham, P. C. (2019). Commonality in dysregulated expression of gene sets in cortical brains of individuals with autism, schizophrenia, and bipolar disorder. Transl. Psychiatry 9 (1), 152. doi:10.1038/s41398-019-0488-4
Hines, L. M., Tabakoff, B., and State, W. I. S. (2005). Trait markers of alcohol, U., and dependence, IPlatelet adenylyl cyclase activity: A biological marker for major depression and recent drug use. Biol. Psychiatry 58 (12), 955–962. doi:10.1016/j.biopsych.2005.05.040
Huang, C. C., Liang, Y. C., and Hsu, K. S. (2001). Characterization of the mechanism underlying the reversal of long term potentiation by low frequency stimulation at hippocampal CA1 synapses. J. Biol. Chem. 276 (51), 48108–48117. doi:10.1074/jbc.M106388200
International Consortium on Lithium Genetics., , Amare, A. T., Schubert, K. O., Hou, L., Clark, S. R., Papiol, S., et al. (2018). Association of polygenic score for schizophrenia and hla antigen and inflammation genes with response to lithium in bipolar affective disorder: A genome-wide association study. JAMA Psychiatry 75 (1), 65–74. doi:10.1001/jamapsychiatry.2017.3433
Katsel, P. L., Tagliente, T. M., Schwarz, T. E., Craddock-Royal, B. D., Patel, N. D., and Maayani, S. (2003). Molecular and biochemical evidence for the presence of type III adenylyl cyclase in human platelets. Platelets 14 (1), 21–33. doi:10.1080/0953710021000062905
Kaur, J., Soto-Velasquez, M., Ding, Z., Ghanbarpour, A., Lill, M. A., van Rijn, R. M., et al. (2019). Optimization of a 1, 3, 4-oxadiazole series for inhibition of Ca(2+)/calmodulin-stimulated activity of adenylyl cyclases 1 and 8 for the treatment of chronic pain. Eur. J. Med. Chem. 162, 568–585. doi:10.1016/j.ejmech.2018.11.036
Kelleher, R. J., Govindarajan, A., Jung, H. Y., Kang, H., and Tonegawa, S. (2004). Translational control by MAPK signaling in long-term synaptic plasticity and memory. Cell 116 (3), 467–479. doi:10.1016/s0092-8674(04)00115-1
Kleinboelting, S., Diaz, A., Moniot, S., van den Heuvel, J., Weyand, M., Levin, L. R., et al. (2014). Crystal structures of human soluble adenylyl cyclase reveal mechanisms of catalysis and of its activation through bicarbonate. Proc. Natl. Acad. Sci. U. S. A. 111 (10), 3727–3732. doi:10.1073/pnas.1322778111
Kohli, P., Soler, Z. M., Nguyen, S. A., Muus, J. S., and Schlosser, R. J. (2016). The association between olfaction and depression: A systematic review. Chem. Senses 41 (6), 479–486. doi:10.1093/chemse/bjw061
Krupinski, J., Coussen, F., Bakalyar, H. A., Tang, W. J., Feinstein, P. G., Orth, K., et al. (1989). Adenylyl cyclase amino acid sequence: Possible channel- or transporter-like structure. Science 244 (4912), 1558–1564. doi:10.1126/science.2472670
Lee, H. K., Barbarosie, M., Kameyama, K., Bear, M. F., and Huganir, R. L. (2000). Regulation of distinct AMPA receptor phosphorylation sites during bidirectional synaptic plasticity. Nature 405 (6789), 955–959. doi:10.1038/35016089
Lee, K., Goodman, L., Fourie, C., Schenk, S., Leitch, B., and Montgomery, J. M. (2016). AMPA receptors as therapeutic targets for neurological disorders. Adv. Protein Chem. Struct. Biol. 103, 203–261. doi:10.1016/bs.apcsb.2015.10.004
Li, X. H., Chen, Q. Y., and Zhuo, M. (2020). Neuronal adenylyl cyclase targeting central plasticity for the treatment of chronic pain. Neurotherapeutics 17 (3), 861–873. doi:10.1007/s13311-020-00927-1
Liauw, J., Wu, L. J., and Zhuo, M. (2005). Calcium-stimulated adenylyl cyclases required for long-term potentiation in the anterior cingulate cortex. J. Neurophysiol. 94 (1), 878–882. doi:10.1152/jn.01205.2004
Litvin, T. N., Kamenetsky, M., Zarifyan, A., Buck, J., and Levin, L. R. (2003). Kinetic properties of "soluble" adenylyl cyclase. Synergism between calcium and bicarbonate. J. Biol. Chem. 278 (18), 15922–15926. doi:10.1074/jbc.M212475200
Liu, D., Cao, H., Kural, K. C., Fang, Q., and Zhang, F. (2019). Integrative analysis of shared genetic pathogenesis by autism spectrum disorder and obsessive-compulsive disorder. Biosci. Rep. 39 (12), BSR20191942. doi:10.1042/BSR20191942
Liu, X., Zhou, Y., Li, S., Yang, D., Jiao, M., Liu, X., et al. (2020a). Type 3 adenylyl cyclase in the main olfactory epithelium participates in depression-like and anxiety-like behaviours. J. Affect. Disord. 268, 28–38. doi:10.1016/j.jad.2020.02.041
Liu, X., Zhou, Y., Yang, D., Li, S., Liu, X., and Wang, Z. (2020b). Type 3 adenylyl cyclase in the MOE is involved in learning and memory in mice. Behav. Brain Res. 383, 112533. doi:10.1016/j.bbr.2020.112533
Lu, H. C., She, W. C., Plas, D. T., Neumann, P. E., Janz, R., and Crair, M. C. (2003). Adenylyl cyclase I regulates AMPA receptor trafficking during mouse cortical 'barrel' map development. Nat. Neurosci. 6 (9), 939–947. doi:10.1038/nn1106
Makhinson, M., Chotiner, J. K., Watson, J. B., and O'Dell, T. J. (1999). Adenylyl cyclase activation modulates activity-dependent changes in synaptic strength and Ca2+/calmodulin-dependent kinase II autophosphorylation. J. Neurosci. 19 (7), 2500–2510. doi:10.1523/jneurosci.19-07-02500.1999
Malinow, R. (2003). AMPA receptor trafficking and long-term potentiation. Philos. Trans. R. Soc. Lond. B Biol. Sci. 358 (1432), 707–714. doi:10.1098/rstb.2002.1233
Mann, L., Heldman, E., Bersudsky, Y., Vatner, S. F., Ishikawa, Y., Almog, O., et al. (2009). Inhibition of specific adenylyl cyclase isoforms by lithium and carbamazepine, but not valproate, may be related to their antidepressant effect. Bipolar Disord. 11 (8), 885–896. doi:10.1111/j.1399-5618.2009.00762.x
Mannan, M., Mamun, A., Doi, S., and Clavarino, A. (2016). Prospective associations between depression and obesity for adolescent males and females- A systematic review and meta-analysis of longitudinal studies. PLoS One 11 (6), e0157240. doi:10.1371/journal.pone.0157240
Martin, A. C., Willoughby, D., Ciruela, A., Ayling, L. J., Pagano, M., Wachten, S., et al. (2009). Capacitative Ca2+ entry via Orai1 and stromal interacting molecule 1 (STIM1) regulates adenylyl cyclase type 8. Mol. Pharmacol. 75 (4), 830–842. doi:10.1124/mol.108.051748
Miao, H. H., Li, X. H., Chen, Q. Y., and Zhuo, M. (2019). Calcium-stimulated adenylyl cyclase subtype 1 is required for presynaptic long-term potentiation in the insular cortex of adult mice. Mol. Pain 15, 1744806919842961. doi:10.1177/1744806919842961
Muglia, L. M., Schaefer, M. L., Vogt, S. K., Gurtner, G., Imamura, A., and Muglia, L. J. (1999). The 5'-flanking region of the mouse adenylyl cyclase type VIII gene imparts tissue-specific expression in transgenic mice. J. Neurosci. 19 (6), 2051–2058. doi:10.1523/jneurosci.19-06-02051.1999
Nakazawa, K., and Sapkota, K. (2020). The origin of NMDA receptor hypofunction in schizophrenia. Pharmacol. Ther. 205, 107426. doi:10.1016/j.pharmthera.2019.107426
Nielsen, M. D., Chan, G. C., Poser, S. W., and Storm, D. R. (1996). Differential regulation of type I and type VIII Ca2+-stimulated adenylyl cyclases by Gi-coupled receptors in vivo. J. Biol. Chem. 271 (52), 33308–33316. doi:10.1074/jbc.271.52.33308
Perez, J., Tardito, D., Racagni, G., Smeraldi, E., and Zanardi, R. (2001). Protein kinase A and Rap1 levels in platelets of untreated patients with major depression. Mol. Psychiatry 6 (1), 44–49. doi:10.1038/sj.mp.4000795
Pitman, J. L., Wheeler, M. C., Lloyd, D. J., Walker, J. R., Glynne, R. J., and Gekakis, N. (2014). A gain-of-function mutation in adenylate cyclase 3 protects mice from diet-induced obesity. PLoS One 9 (10), e110226. doi:10.1371/journal.pone.0110226
Razzoli, M., Andreoli, M., Maraia, G., Di Francesco, C., and Arban, R. (2010). Functional role of calcium-stimulated adenylyl cyclase 8 in adaptations to psychological stressors in the mouse: Implications for mood disorders. Neuroscience 170 (2), 429–440. doi:10.1016/j.neuroscience.2010.07.022
Redei, E. E., Andrus, B. M., Kwasny, M. J., Seok, J., Cai, X., Ho, J., et al. (2014). Blood transcriptomic biomarkers in adult primary care patients with major depressive disorder undergoing cognitive behavioral therapy. Transl. Psychiatry 4, e442. doi:10.1038/tp.2014.66
Regnauld, K. L., Leteurtre, E., Gutkind, S. J., Gespach, C. P., and Emami, S. (2002). Activation of adenylyl cyclases, regulation of insulin status, and cell survival by G(alpha)olf in pancreatic beta-cells. Am. J. Physiol. Regul. Integr. Comp. Physiol. 282 (3), R870–R880. doi:10.1152/ajpregu.00374.2001
Richter, J. D., and Zhao, X. (2021). The molecular biology of FMRP: New insights into fragile X syndrome. Nat. Rev. Neurosci. 22 (4), 209–222. doi:10.1038/s41583-021-00432-0
Robison, A. J. (2014). Emerging role of CaMKII in neuropsychiatric disease. Trends Neurosci. 37 (11), 653–662. doi:10.1016/j.tins.2014.07.001
Schaefer, M. L., Wong, S. T., Wozniak, D. F., Muglia, L. M., Liauw, J. A., Zhuo, M., et al. (2000). Altered stress-induced anxiety in adenylyl cyclase type VIII-deficient mice. J. Neurosci. 20 (13), 4809–4820. doi:10.1523/jneurosci.20-13-04809.2000
Scott, J. A., Soto-Velasquez, M., Hayes, M. P., LaVigne, J. E., Miller, H. R., Kaur, J., et al. (2022). Optimization of a pyrimidinone series for selective inhibition of Ca(2+)/calmodulin-stimulated adenylyl cyclase 1 activity for the treatment of chronic pain. J. Med. Chem. 65 (6), 4667–4686. doi:10.1021/acs.jmedchem.1c01759
Sethna, F., Feng, W., Ding, Q., Robison, A. J., Feng, Y., and Wang, H. (2017). Enhanced expression of ADCY1 underlies aberrant neuronal signalling and behaviour in a syndromic autism model. Nat. Commun. 8, 14359. doi:10.1038/ncomms14359
Sethna, F., Moon, C., and Wang, H. (2014). From FMRP function to potential therapies for fragile X syndrome. Neurochem. Res. 39 (6), 1016–1031. doi:10.1007/s11064-013-1229-3
Siljee, J. E., Wang, Y., Bernard, A. A., Ersoy, B. A., Zhang, S., Marley, A., et al. (2018). Subcellular localization of MC4R with ADCY3 at neuronal primary cilia underlies a common pathway for genetic predisposition to obesity. Nat. Genet. 50 (2), 180–185. doi:10.1038/s41588-017-0020-9
Sindreu, C. B., Scheiner, Z. S., and Storm, D. R. (2007). Ca2+ -stimulated adenylyl cyclases regulate ERK-dependent activation of MSK1 during fear conditioning. Neuron 53 (1), 79–89. doi:10.1016/j.neuron.2006.11.024
Smith, S. J., and Augustine, G. J. (1988). Calcium ions, active zones and synaptic transmitter release. Trends Neurosci. 11 (10), 458–464. doi:10.1016/0166-2236(88)90199-3
Storm, D. R., Hansel, C., Hacker, B., Parent, A., and Linden, D. J. (1998). Impaired cerebellar long-term potentiation in type I adenylyl cyclase mutant mice. Neuron 20 (6), 1199–1210. doi:10.1016/s0896-6273(00)80500-0
Sundararajan, T., Manzardo, A. M., and Butler, M. G. (2018). Functional analysis of schizophrenia genes using GeneAnalytics program and integrated databases. Gene 641, 25–34. doi:10.1016/j.gene.2017.10.035
Suzuki, A., Lee, L. J., Hayashi, Y., Muglia, L., Itohara, S., Erzurumlu, R. S., et al. (2015). Thalamic adenylyl cyclase 1 is required for barrel formation in the somatosensory cortex. Neuroscience 290, 518–529. doi:10.1016/j.neuroscience.2015.01.043
Tang, W. J., Krupinski, J., and Gilman, A. G. (1991). Expression and characterization of calmodulin-activated (type I) adenylylcyclase. J. Biol. Chem. 266 (13), 8595–8603. doi:10.1016/s0021-9258(18)93016-4
van Steensel, F. J., Bogels, S. M., and Perrin, S. (2011). Anxiety disorders in children and adolescents with autistic spectrum disorders: A meta-analysis. Clin. Child. Fam. Psychol. Rev. 14 (3), 302–317. doi:10.1007/s10567-011-0097-0
Villacres, E. C., Wong, S. T., Chavkin, C., and Storm, D. R. (1998). Type I adenylyl cyclase mutant mice have impaired mossy fiber long-term potentiation. J. Neurosci. 18 (9), 3186–3194. doi:10.1523/jneurosci.18-09-03186.1998
Wang, H., Chan, G. C., Athos, J., and Storm, D. R. (2002). Synaptic concentration of type-I adenylyl cyclase in cerebellar neurons. J. Neurochem. 83 (4), 946–954. doi:10.1046/j.1471-4159.2002.01206.x
Wang, H., Ferguson, G. D., Pineda, V. V., Cundiff, P. E., and Storm, D. R. (2004). Overexpression of type-1 adenylyl cyclase in mouse forebrain enhances recognition memory and LTP. Nat. Neurosci. 7 (6), 635–642. doi:10.1038/nn1248
Wang, H., Gong, B., Vadakkan, K. I., Toyoda, H., Kaang, B. K., and Zhuo, M. (2007). Genetic evidence for adenylyl cyclase 1 as a target for preventing neuronal excitotoxicity mediated by N-methyl-D-aspartate receptors. J. Biol. Chem. 282 (2), 1507–1517. doi:10.1074/jbc.M607291200
Wang, H., Pineda, V. V., Chan, G. C., Wong, S. T., Muglia, L. J., and Storm, D. R. (2003). Type 8 adenylyl cyclase is targeted to excitatory synapses and required for mossy fiber long-term potentiation. J. Neurosci. 23 (30), 9710–9718. doi:10.1523/jneurosci.23-30-09710.2003
Wang, H., and Storm, D. R. (2003). Calmodulin-regulated adenylyl cyclases: Cross-talk and plasticity in the central nervous system. Mol. Pharmacol. 63 (3), 463–468. doi:10.1124/mol.63.3.463
Wang, H., Xu, H., Wu, L. J., Kim, S. S., Chen, T., Koga, K., et al. (2011a). Identification of an adenylyl cyclase inhibitor for treating neuropathic and inflammatory pain. Sci. Transl. Med. 3 (65), 65ra3. doi:10.1126/scitranslmed.3001269
Wang, W., Chen, Q. Y., Zhao, P., Zhong, J., Wang, Y., Li, X., et al. (2022). Human safety study of a selective neuronal adenylate cyclase 1 inhibitor NB001 which relieves the neuropathic pain and blocks ACC in adult mice. Mol. Pain 2022, 17448069221089596. doi:10.1177/17448069221089596
Wang, X., Snape, M., Klann, E., Stone, J. G., Singh, A., Petersen, R. B., et al. (2012). Activation of the extracellular signal-regulated kinase pathway contributes to the behavioral deficit of fragile x-syndrome. J. Neurochem. 121 (4), 672–679. doi:10.1111/j.1471-4159.2012.07722.x
Wang, Z., Li, V., Chan, G. C., Phan, T., Nudelman, A. S., Xia, Z., et al. (2009). Adult type 3 adenylyl cyclase-deficient mice are obese. PLoS One 4 (9), e6979. doi:10.1371/journal.pone.0006979
Wang, Z., Phan, T., and Storm, D. R. (2011b). The type 3 adenylyl cyclase is required for novel object learning and extinction of contextual memory: Role of cAMP signaling in primary cilia. J. Neurosci. 31 (15), 5557–5561. doi:10.1523/JNEUROSCI.6561-10.2011
Wayman, G. A., Impey, S., and Storm, D. R. (1995). Ca2+ inhibition of type III adenylyl cyclase in vivo. J. Biol. Chem. 270 (37), 21480–21486. doi:10.1074/jbc.270.37.21480
Wayman, G. A., Impey, S., Wu, Z., Kindsvogel, W., Prichard, L., and Storm, D. R. (1994). Synergistic activation of the type I adenylyl cyclase by Ca2+ and Gs-coupled receptors in vivo. J. Biol. Chem. 269 (41), 25400–25405. doi:10.1016/s0021-9258(18)47263-8
Wayman, G. A., Wei, J., Wong, S., and Storm, D. R. (1996). Regulation of type I adenylyl cyclase by calmodulin kinase IV in vivo. Mol. Cell. Biol. 16 (11), 6075–6082. doi:10.1128/MCB.16.11.6075
Wei, J., Wayman, G., and Storm, D. R. (1996). Phosphorylation and inhibition of type III adenylyl cyclase by calmodulin-dependent protein kinase II in vivo. J. Biol. Chem. 271 (39), 24231–24235. doi:10.1074/jbc.271.39.24231
Wei, J., Zhao, A. Z., Chan, G. C., Baker, L. P., Impey, S., Beavo, J. A., et al. (1998). Phosphorylation and inhibition of olfactory adenylyl cyclase by CaM kinase II in neurons: A mechanism for attenuation of olfactory signals. Neuron 21 (3), 495–504. doi:10.1016/s0896-6273(00)80561-9
Wolf, E. J., Rasmusson, A. M., Mitchell, K. S., Logue, M. W., Baldwin, C. T., and Miller, M. W. (2014). A genome-wide association study of clinical symptoms of dissociation in a trauma-exposed sample. Depress. Anxiety 31 (4), 352–360. doi:10.1002/da.22260
Wong, S. T., Athos, J., Figueroa, X. A., Pineda, V. V., Schaefer, M. L., Chavkin, C. C., et al. (1999). Calcium-stimulated adenylyl cyclase activity is critical for hippocampus-dependent long-term memory and late phase LTP. Neuron 23 (4), 787–798. doi:10.1016/s0896-6273(01)80036-2
Wong, S. T., Trinh, K., Hacker, B., Chan, G. C., Lowe, G., Gaggar, A., et al. (2000). Disruption of the type III adenylyl cyclase gene leads to peripheral and behavioral anosmia in transgenic mice. Neuron 27 (3), 487–497. doi:10.1016/s0896-6273(00)00060-x
Wray, N. R., Pergadia, M. L., Blackwood, D. H., Penninx, B. W., Gordon, S. D., Nyholt, D. R., et al. (2012). Genome-wide association study of major depressive disorder: New results, meta-analysis, and lessons learned. Mol. Psychiatry 17 (1), 36–48. doi:10.1038/mp.2010.109
Wu, Y., Yao, Y. G., and Luo, X. J. (2017). Szdb: A database for schizophrenia genetic research. Schizophr. Bull. 43 (2), 459–471. doi:10.1093/schbul/sbw102
Wu, Z. L., Thomas, S. A., Villacres, E. C., Xia, Z., Simmons, M. L., Chavkin, C., et al. (1995). Altered behavior and long-term potentiation in type I adenylyl cyclase mutant mice. Proc. Natl. Acad. Sci. U. S. A. 92 (1), 220–224. doi:10.1073/pnas.92.1.220
Wu, Z., Wong, S. T., and Storms, D. R. (1993). Modification of the calcium and calmodulin sensitivity of the type I adenylyl cyclase by mutagenesis of its calmodulin binding domain. J. Biol. Chem. 268 (32), 23766–23768. doi:10.1016/s0021-9258(20)80447-5
Xia, Z., Choi, E. J., Wang, F., Blazynski, C., and Storm, D. R. (1993). Type I calmodulin-sensitive adenylyl cyclase is neural specific. J. Neurochem. 60 (1), 305–311. doi:10.1111/j.1471-4159.1993.tb05852.x
Xia, Z., Choi, E. J., Wang, F., and Storm, D. R. (1992). The type III calcium/calmodulin-sensitive adenylyl cyclase is not specific to olfactory sensory neurons. Neurosci. Lett. 144 (1-2), 169–173. doi:10.1016/0304-3940(92)90742-p
Yang, B., He, B., Abdel-Halim, S. M., Tibell, A., Brendel, M. D., Bretzel, R. G., et al. (1999). Molecular cloning of a full-length cDNA for human type 3 adenylyl cyclase and its expression in human islets. Biochem. Biophys. Res. Commun. 254 (3), 548–551. doi:10.1006/bbrc.1998.9983
Yang, D., Wu, X., Wang, W., Zhou, Y., and Wang, Z. (2022). Ciliary type III adenylyl cyclase in the VMH is crucial for high-fat diet-induced obesity mediated by autophagy. Adv. Sci. 9 (3), e2102568. doi:10.1002/advs.202102568
Yang, M., Ding, Q., Zhang, M., Moon, C., and Wang, H. (2020). Forebrain overexpression of type 1 adenylyl cyclase promotes molecular stability and behavioral resilience to physical stress. Neurobiol. Stress 13, 100237. doi:10.1016/j.ynstr.2020.100237
Yue, F., Cheng, Y., Breschi, A., Vierstra, J., Wu, W., Ryba, T., et al. (2014). A comparative encyclopedia of DNA elements in the mouse genome. Nature 515 (7527), 355–364. doi:10.1038/nature13992
Zandi, P. P., Zollner, S., Avramopoulos, D., Willour, V. L., Chen, Y., Qin, Z. S., et al. (2008). Family-based SNP association study on 8q24 in bipolar disorder. Am. J. Med. Genet. B Neuropsychiatr. Genet. 147B (5), 612–618. doi:10.1002/ajmg.b.30651
Zanos, P., and Gould, T. D. (2018). Mechanisms of ketamine action as an antidepressant. Mol. Psychiatry 23 (4), 801–811. doi:10.1038/mp.2017.255
Zhang, I., and Hu, H. (2020). Store-operated calcium channels in physiological and pathological States of the nervous system. Front. Cell. Neurosci. 14, 600758. doi:10.3389/fncel.2020.600758
Zhang, P., Xiang, N., Chen, Y., Sliwerska, E., McInnis, M. G., Burmeister, M., et al. (2010). Family-based association analysis to finemap bipolar linkage peak on chromosome 8q24 using 2, 500 genotyped SNPs and 15, 000 imputed SNPs. Bipolar Disord. 12 (8), 786–792. doi:10.1111/j.1399-5618.2010.00883.x
Zheng, F., Zhang, M., Ding, Q., Sethna, F., Yan, L., Moon, C., et al. (2016). Voluntary running depreciates the requirement of Ca2+-stimulated cAMP signaling in synaptic potentiation and memory formation. Learn. Mem. 23 (8), 442–449. doi:10.1101/lm.040642.115
Zheng, F., Zhou, X., Luo, Y., Xiao, H., Wayman, G., and Wang, H. (2011). Regulation of brain-derived neurotrophic factor exon IV transcription through calcium responsive elements in cortical neurons. PLoS One 6 (12), e28441. doi:10.1371/journal.pone.0028441
Zheng, F., Zhou, X., Moon, C., and Wang, H. (2012). Regulation of brain-derived neurotrophic factor expression in neurons. Int. J. Physiol. Pathophysiol. Pharmacol. 4 (4), 188–200.
Keywords: autism, Ca2+-stimulated adenylyl cyclase, bipolar disorder, schizophrenia, major depressive disorder, post-traumatic stress disorder, therapeutics
Citation: Chen J, Ding Q, An L and Wang H (2022) Ca2+-stimulated adenylyl cyclases as therapeutic targets for psychiatric and neurodevelopmental disorders. Front. Pharmacol. 13:949384. doi: 10.3389/fphar.2022.949384
Received: 20 May 2022; Accepted: 05 September 2022;
Published: 16 September 2022.
Edited by:
Rennolds S. Ostrom, Chapman University, United StatesReviewed by:
Muhammad Aslam, University of Giessen, GermanyCopyright © 2022 Chen, Ding, An and Wang. This is an open-access article distributed under the terms of the Creative Commons Attribution License (CC BY). The use, distribution or reproduction in other forums is permitted, provided the original author(s) and the copyright owner(s) are credited and that the original publication in this journal is cited, in accordance with accepted academic practice. No use, distribution or reproduction is permitted which does not comply with these terms.
*Correspondence: Hongbing Wang, d2FuZ2hvQG1zdS5lZHU=
Disclaimer: All claims expressed in this article are solely those of the authors and do not necessarily represent those of their affiliated organizations, or those of the publisher, the editors and the reviewers. Any product that may be evaluated in this article or claim that may be made by its manufacturer is not guaranteed or endorsed by the publisher.
Research integrity at Frontiers
Learn more about the work of our research integrity team to safeguard the quality of each article we publish.