- 1Department of Emergency Medicine, Affiliated Hospital of Nantong University, Nantong, China
- 2Key Laboratory of Neuroregeneration of Jiangsu and Ministry of Education, Co-Innovation Center of Neuroregeneration, NMPA Key Laboratory for Research and Evaluation of Tissue Engineering Technology Products, Jiangsu Clinical Medicine Center of Tissue Engineering and Nerve Injury Repair, Nantong University, Nantong, China
- 3Department of Laboratory Medicine, Binhai County People’s Hospital Affiliated to Kangda College of Nanjing Medical University, Yancheng, China
- 4Department of Infectious Disease, Affiliated Hospital of Nantong University, Nantong, China
- 5Department of Pathology, Affiliated Hospital of Nantong University, Medical School of Nantong University, Nantong, China
Skeletal muscle is one of the largest organs in the body and the largest protein repository. Mitochondria are the main energy-producing organelles in cells and play an important role in skeletal muscle health and function. They participate in several biological processes related to skeletal muscle metabolism, growth, and regeneration. Adenosine monophosphate-activated protein kinase (AMPK) is a metabolic sensor and regulator of systemic energy balance. AMPK is involved in the control of energy metabolism by regulating many downstream targets. In this review, we propose that AMPK directly controls several facets of mitochondrial function, which in turn controls skeletal muscle metabolism and health. This review is divided into four parts. First, we summarize the properties of AMPK signal transduction and its upstream activators. Second, we discuss the role of mitochondria in myogenesis, muscle atrophy, regeneration post-injury of skeletal muscle cells. Third, we elaborate the effects of AMPK on mitochondrial biogenesis, fusion, fission and mitochondrial autophagy, and discuss how AMPK regulates the metabolism of skeletal muscle by regulating mitochondrial function. Finally, we discuss the effects of AMPK activators on muscle disease status. This review thus represents a foundation for understanding this biological process of mitochondrial dynamics regulated by AMPK in the metabolism of skeletal muscle. A better understanding of the role of AMPK on mitochondrial dynamic is essential to improve mitochondrial function, and hence promote skeletal muscle health and function.
1 Introduction
Skeletal muscle accounts for 40–50% of lean body mass, making it one of the largest organs in the body and the largest protein respository (Sartori et al., 2021). It plays an important role in posture maintenance, exercise tolerance, temperature regulation, and systemic metabolism (Leduc-Gaudet et al., 2021; Wang et al., 2022a). Reduced and discontinued use, cancer cachexia, nerve injury, diabetes, or inflammation can cause skeletal muscle atrophy (Sun et al., 2014; You and Chen, 2021). Atrophy increases the incidence of pathological fractures, deterioration of organ function, and hospitalization rate, which greatly reduces patients’ quality of life and may even be life-threatening, muscle mass is also a predictor of mortality (Andres-Mateos et al., 2013; Gu, 2021). Therefore, maintaining constant muscle mass and physiological function is important for overall health (Andres-Mateos et al., 2013; Baskin et al., 2015). Skeletal muscle consumes much energy compared to other organ systems, and are thus rich in mitochondria. Mitochondria are critical for regulating skeletal muscle metabolism due to their diverse functions such as energy production, calcium homeostasis, free radical production, triggering/regulating cell death, and the protein synthesis [Reviewed in (Hood et al., 2019)]. Therefore, maintaining the integrity of mitochondrial structure and function is important for muscle health.
Mitochondria are cellular organelles that are covered by distinct outer and inner membranes. They are the main organelles for intracellular energy production through oxidative phosphorylation (OXPHOS) (Nunnari and Suomalainen, 2012; Andrieux et al., 2021). Mitochondria are semi-autonomous organelles that have their own DNA (mtDNA), which can self-replicate under nuclear coordination and encodes a variety of subunits of electron transport chain complexes I, III, IV, and V [Reviewed in (Gustafsson et al., 2016)]. Mitochondria are highly dynamic organelles that undergo processes such as genesis, fusion, division, transportation, and autophagy with the change of cell state. These dynamic mitochondrial biological behaviors are called mitochondrial dynamics, which are essential for maintaining mitochondrial function and structure (Mishra and Chan, 2016; Heine and Hood, 2020).
Mitochondria are involved several of physiological processes including apoptosis, cell chemotaxis, autophagy, oxidative stress, signal transduction, innate immunity, calcium homeostasis, and stem cell reprogramming [Reviewed in (Deshwal et al., 2020)]. Mitochondria form a complex and interconnected cellular network structure, maintaining cell energy homeostasis through the coordination of biogenesis, dynamic fission, fusion, and autophagy (Drake et al., 2021). When cells carry out various biological activities, adenosine triphosphate (ATP) is hydrolyzed to adenosine diphosphate (ADP) or adenosine monophosphate (AMP), which liberates free energy (Ruprecht et al., 2019). When the level of intracellular ATP decreases, the cells attempt to restore the ATP level and maintain energy supply. Eukaryotes have a highly-evolved energy supply system and can regulate their metabolism according to the availability of nutrition. A key player of this system is adenosine monophosphate-activated protein kinase (AMPK) (Herzig and Shaw, 2018).
AMPK is a cellular energy sensor and one of the cellular regulatory systems to ensure that the production and consumption of ATP in the cells remain balanced (Hardie, 2018; Gonzalez et al., 2020). AMPK is activated in response to sensing increased levels of intracellular AMP and ADP, thereby promoting ATP synthesis (Carling, 2017). AMPK can also regulate mitochondrial function through multiple molecular pathways including peroxisome proliferator-activated receptor gamma coactivator-1 alpha (PGC-1a) and sirtuin 1 (SIRT1) (Chang, 2019). AMPK influences mitochondrial processes such as biogenesis, autophagy, fission, and fusion (Hardie et al., 2012; Drake et al., 2021). In view of the important role of mitochondria in skeletal muscle tissue and the regulatory role of AMPK in mitochondrial biological processes, we hypothesize that AMPK plays an important role in skeletal muscle.
There are some studies regarding AMPK’s control of mitochondrial function and the role of AMPK in skeletal muscle function (Herzig and Shaw, 2018; Kjobsted et al., 2018; Thomson, 2018; Wu and Zou, 2020; Drake et al., 2021). But no study has described in detail how AMPK affects mitochondrial dynamics, how it affects skeletal muscle growth and regeneration processes and how AMPK affects various biological processes in skeletal muscle by affecting mitochondria. In this review, we describe the effects of mitochondria on skeletal muscle metabolism. In addition, we summarize the regulatory effects of AMPK on mitochondria and how AMPK regulates skeletal muscle metabolism by regulating mitochondrial dynamics. Finally, we describe the AMPK structure and its main activators. In conclusion, current data suggest that AMPK controls skeletal muscle health and function in part through control of mitochondrial dynamics and muscle metabolism.
2 Adenosine monophosphate activated protein kinase and its activators
There are relatively few drugs based on interventions for muscle wasting (Weihrauch and Handschin, 2018). Given that AMPK is involved in multiple pathways in mitochondrial and skeletal muscle metabolism, studies are emerging on AMPK activators that may prove to help regulate mitochondrial health, thereby enhancing cellular metabolism and promoting skeletal muscle health. In this section, we describe the structure of AMPK, the major AMPK activators discovered so far, and some examples of AMPK activators can aid in improving muscle wasting.
2.1 Adenosine monophosphate activated protein kinase structure
AMPK is an αβγ heterotrimer that functions as a central regulator of energy homeostasis. It is composed of catalytic a subunit (α1 and α2), regulatory β-subunit (β1 and β2) and γ-subunit (γ1, γ2, and γ3) (Stapleton et al., 1996; Yan et al., 2018). These subunits produce 12 different complexes, all of which can be produced in mammalian tissues [Reviewed in (Ross et al., 2016)]. In muscle tissue, AMPK is the core hub of energy metabolism. All combinations of AMPK can be expressed in mammals, but their expression levels differ in different tissues, and α1β2γ1, α2β2γ1, and α2β2γ3 are mainly expressed in skeletal muscle (Birk and Wojtaszewski, 2006). Although there are different heterotrimer subtypes in tissues, their specific roles are still being studied.
2.2 Adenosine monophosphate activated protein kinase activation
AMPK signal can be activated by “physiological activators” (Table 1) and “pharmacological activators” (Table 2). The physiological activators refer to substances derived from the host’s own cells or tissues, while pharmacological activators refer to substances that do not exist in the host itself, are synthesized or exist in nature. The physiological activators include AMP/ADP, upstream kinases (liver kinase B1 (LKB1), CaMKK2, and TGF-beta-activated kinase 1 (TAK1)) and reactive oxygen species (Figure 1). Many drugs activate AMPK indirectly by mimicking physiological activators or activating physiological activators of AMPK. The pharmacological activators include antidiabetic drugs (metformin, dapagliflozin, empagliflozin), small molecules (AICAR/ZMP, A-769662 and 991, pyrrolopyridines, benzimidazoles, salsalate, PF-249, bempedoic acid, MT63-78, Compound PT1 and so on) and plant-derived extracts (Tanshinone IIA, resveratrol, berberine and quercetin).
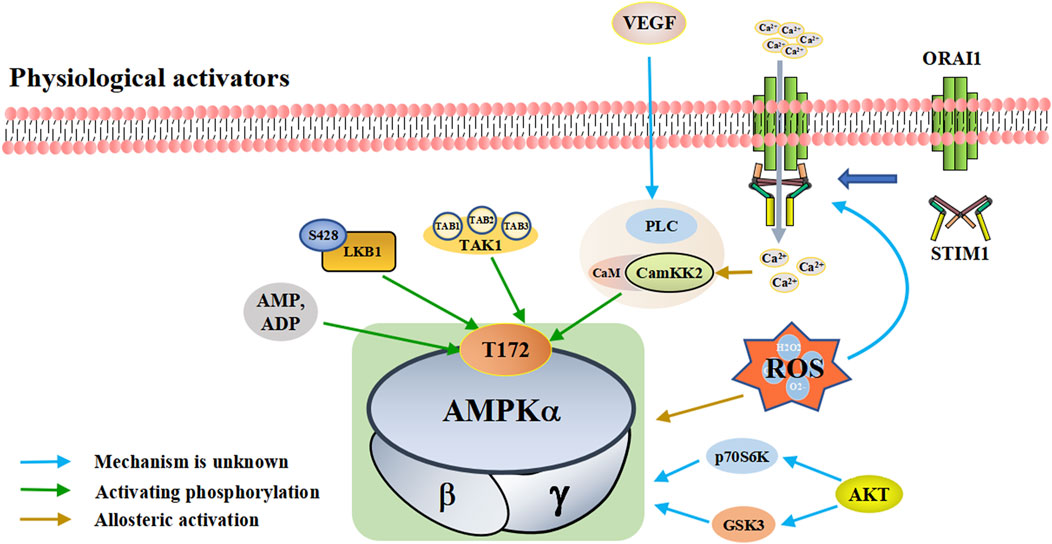
FIGURE 1. A schematic diagram of the pathways by which physiological activators activate adenosine monophosphate activated protein kinase (AMPK). The physiological activators are substances derived from the host’s own cells or tissues that activate AMPK. Physiological of AMPK include AMP/ADP, upstream kinases (LKB1, CaMKK2, TAK1, and AKT), VEGF and ROS. They are substances produced by the body that activate AMPK in different ways. AMPK is activated when the intracellular AMP/ADP ratio increases. As upstream kinases, LKB1, CaMKK2 and TAK1 can directly activate AMPK. When ROS is increased, excess ROS directly activates AMPK. He can also promote the interaction between STIM1 and Orai1 proximal to the plasma membrane, increasing calcium influx, activating CaMKK2 and subsequently AMPK. STIM1: stromal interaction molecule 1, Orai1: ORAI calcium release-activated calcium modulator 1, Akt: protein kinase B, VEGF: vascular endothelial growth factor, PLC: phospholipase C, LKB1: liver kinase B1, TAK1: TGF-beta-activated kinase 1.
2.2.1 Physiological activators
2.2.1.1 AMP/ADP
Cell metabolism and various conditions will convert ATP into AMP/ADP. The increase of intracellular AMP/ADP ratio leads to the enhanced phosphorylation of the threonine residues (Thr-172) in the AMPK a-subunit and slow down the dephosphorylation rate of Thr-172 (Hawley et al., 1996; Sanders et al., 2007). This enables AMPK activation and promotes ATP production (Gowans et al., 2013). Compared with AMP, ADP has a higher concentration and plays a major controlling role (Coccimiglio and Clarke, 2020). In addition to activating AMPK through Thr-172 phosphorylation, AMP can also bind to the regulatory γ-subunit to activate AMPK (Xiao et al., 2011; Gowans et al., 2013). AMP/ADP is the direct activator of AMPK. Some substances, such as metformin and canagliflozin, can regulate the activity of AMPK by regulating the intracellular levels of AMP/ADP.
2.2.1.2 Upstream kinases
Upstream kinases of AMPK mainly include LKB1, CaMKK2, and TAK1, all of which exert their functions by phosphorylating Thr-172 on the AMPK a-subunit (Lou et al., 2021; Zhu et al., 2022). LKB1 is a tumor suppressor gene that encodes the serine/threonine kinase of calmodulin family expressed in a variety of tissues and is highly conserved in eukaryotes [Reviewed in (Ciccarese et al., 2019)]. LKB1 plays an important role in regulating cell metabolism. LKB1 phosphorylates the AMPK a-subunit Thr-172 to activate AMPK (Sakamoto et al., 2005); LKB1 and AMPK together regulate cell growth depending on changes in environmental nutrition (Shackelford and Shaw, 2009).
CaMKK2 (also known as CaMKKβ) belongs to a serine/threonine-specific protein kinase family. When intracellular Ca2+ increases due to various reasons, Ca2+ binds to CaM to form the Ca2+/CaM complex, which activates CaMKK2 phosphorylation (Marcelo et al., 2016; Hedman et al., 2021). Activated CaMKK2 phosphorylates the AMPK a-subunit, forming a polyprotein complex composed of Ca2+/CAM, CaMKK2, and AMPK, which activates AMPK (Anderson et al., 2008; Marcelo et al., 2016; Sabbir et al., 2021). The CaMKK-AMPK pathway operates as part of signaling pathways downstream of nutrient intake, energy metabolism, adipogenesis, inflammation, and skeletal muscle metabolism (Williams and Sankar, 2019). TAK1 is a serine/threonine protein kinase of the mitogen-activated protein kinase kinase family, which functions by binding to TAB1, TAB2, and TAB3 (Mukhopadhyay and Lee, 2020; Zhu L. et al., 2021). TAK1 can be activated by lipopolysaccharide and TGF-β receptor, tumor necrosis factor-α, toll-like receptor (TLR), interleukin-1 (IL-1), and B-cell receptor (Liu et al., 2018; Jia et al., 2020). The mechanism by which TAK1 controls AMPK remains unclear. It is currently hypothesized that TAK1 regulates AMPK activity through phosphorylation (Momcilovic et al., 2006; Inokuchi-Shimizu et al., 2014).
In addition to the classic AMPK activation by phosphorylation of AMPKα-subunit Thr172, there are other kinases that control AMPK activity through other mechanisms. For example, PKD1 can inhibit AMPKα2 activity through phosphorylation at Ser491 (Coughlan et al., 2016). Protein kinase B (Akt) regulates AMPK activity by altering the activities of glycogen synthase kinase three and ribosomal protein 70 S6 kinase (Dhani et al., 2020). p70S6 kinase phosphorylates AMPKα2 Ser491 to inhibit AMPK activity (Dagon et al., 2012). Protein kinase C (PKC) results in phosphorylation at AMPKα1 Ser487, thereby inhibiting AMPK activity (Heathcote et al., 2016). Vascular endothelial growth factor (VEGF) activates AMPK through CaMKK2 in endothelial cells, but protein kinase A (PKA) inhibits AMPK activation by phosphorylation at Ser495 (Spengler et al., 2020). Further investigation will likely reveal more types of kinases and ensure better understanding of their important roles in AMPK activation and inhibition.
2.2.1.3 Reactive oxygen species
Reactive oxygen species (ROS), including hydrogen peroxide (H2O2), hydroxyl radical (OH−), single oxygen (1O2), and superoxide (O2-), are a group of molecules produced by the mitochondria, peroxisomes, endoplasmic reticulum, cytosol, plasma membrane by NADPH oxidases and so on. (Magnani and Mattevi, 2019; Perillo et al., 2020; Yang and Lian, 2020).
Reductions in nutrition, oxygen, and growth factors, can lead to excessive production of ROS (Zhao et al., 2017). Excessive ROS can result in the oxidation of cysteine residues on AMPK a- and β-subunits, which directly activate AMPK (Zmijewski et al., 2010; Cardaci et al., 2012). In addition, ROS can also affect AMPK activity by regulating Ca2+-related signaling pathways (Roca-Agujetas et al., 2019). ROS localized proximal to the plasma membrane promotes the interaction between stromal interaction molecule 1 (STIM1) and ORAI calcium release-activated calcium modulator 1 (Orai1), which stimulates Ca2+ release and activate the store-operated Ca2+ release-activated Ca2+ (CRAC), which increases calcium influx, activates CaMKK2, and subsequently activates AMPK (Mungai et al., 2011; Huang et al., 2021). The involvement of ROS in the activation of AMPK signaling pathway may also involve other mechanisms, which need to be further studied and discussed.
2.2.2 Pharmacological activators
2.2.2.1 Antidiabetic drugs
A variety of antidiabetic drugs can directly or indirectly activate AMPK (Al-Ishaq et al., 2019; LaMoia and Shulman, 2021) (Figure 2). Metformin is a first-line drug in the treatment of type II diabetes, one of its effects is to activate AMPK indirectly to affect the treatment of diabetes (Agius et al., 2020; Zhang et al., 2020; Kaneto et al., 2021). Metformin can inhibit the activity of mitochondrial complex I in vivo, thus inhibiting the oxidative phosphorylation of mitochondria, increasing ADP/ATP and AMP/ATP ratios in the cells, and activating AMPK indirectly (Rena et al., 2017; LaMoia and Shulman, 2021) (Figure 2A). Canagliflozin, Empagliflozin and Dapagliflozin are all sodium glucose cotransporter 2 (SGLT2) inhibitors, and have shown to activate AMPK in different ways. Canagliflozin inhibits respiratory chain complex I leading to an increase of intracellular AMP or ADP, so as to activate AMPK indirectly (Hawley et al., 2016; Zhou et al., 2020). Empagliflozin can activate AMPK through the LKB1/AMPK signaling pathway and Sesn2-mediated AMPK-mTOR signaling pathway and by slowing down the dephosphorylation rate of DRP1 at serine 637 (Ser-637) (Lu Q. et al., 2020; Liu et al., 2020; Sun et al., 2020). Dapagliflozin can activate AMPK by directly increasing p-AMPK/AMPK ratio (Arab et al., 2021). Although antidiabetic drugs can activate AMPK in multiple ways, their effects after AMPK activation need further investigation.
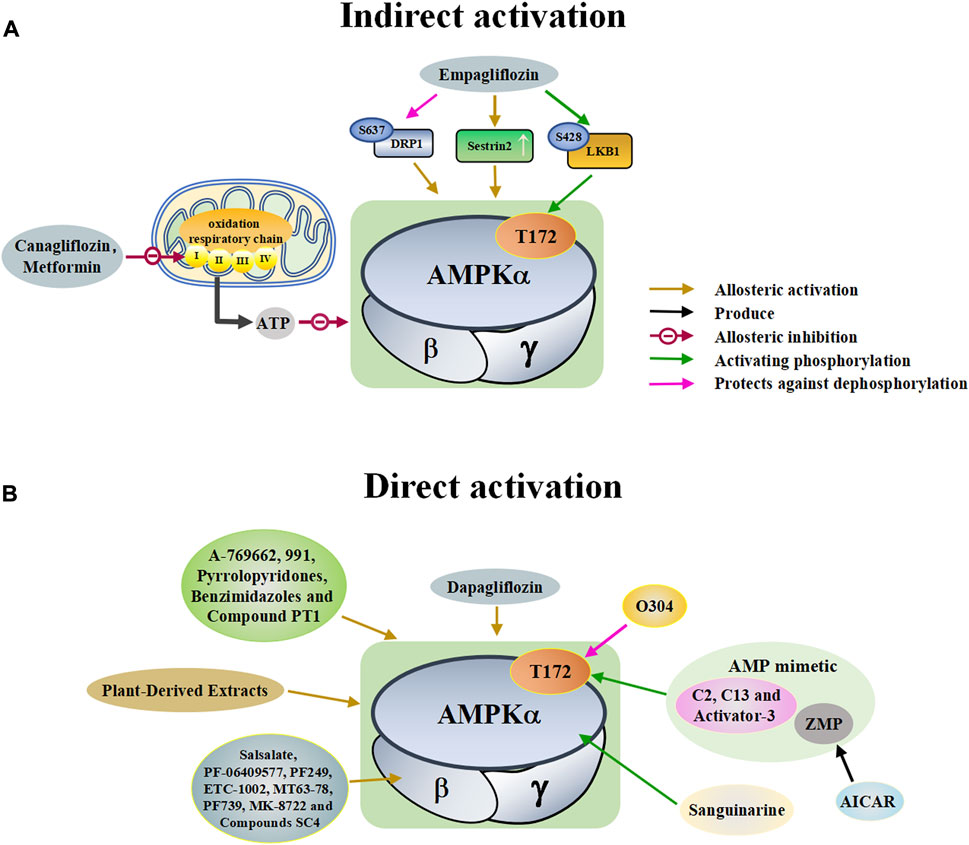
FIGURE 2. A schematic diagram of the pathways by which pharmacological activators activate adenosine monophosphate activated protein kinase (AMPK). The pharmacological activators refer to substances that do not exist in the host itself but are synthesized artificially or exist in nature that can activate AMPK. (A) Indirect activations of AMPK mainly include canagliflozin, metformin and empagliflozin. These substances enter the body and indirectly activate AMPK by controlling molecules that control AMPK. (B) Direct activations of AMPK mainly include small molecules, dapagliflozin, plant-derived extracts, O304, sanguinarine, and AICAR. These substances enter the body and directly bind to AMPK to activate AMPK. DRP1: dynamin-related protein1, LKB1: liver kinase B1, ZMP: 5-aminoimidazole-4-carboxamide ribonucleoside monophosphate, C13: Compound 13, ETC-1002: bempedoic acid.
2.2.2.2 Small molecules
Discoveries of natural compounds and druggable kinases have led to the development of small-molecule compounds that can alter AMPK activity. These small molecule compounds activate AMPK in various ways (Guigas and Viollet, 2016). AICAR is an inosine precursor and an adenosine analogue. After entering the cell, AICAR is phosphorylated by adenosine kinase to produce 5-aminoimidazole-4-carboxamide ribonucleoside monophosphate (ZMP), which is an AMP mimic that activates AMPK (Sabina et al., 1985; Ahmad et al., 2021). Similar AMP analogues include C2 and activator-3 (Langendorf et al., 2016; Bung et al., 2018; Mo et al., 2019). A variety of small molecules can activate AMPK, as shown in Table 2. All of these substances directly activate AMPK (Figure 2B).
2.2.2.3 Plant-derived extracts
Plant-derived extracts have been used in daily therapeutic activities as an effective traditional Chinese medicine, and extracts of many plants have been reported to directly activate AMPK (Francini et al., 2019; Joshi et al., 2019). Tanshinone IIA, flavonoids extracted from mulberry leaves, and resveratrol all act by activating AMPK (Zhang et al., 2019; Den Hartogh et al., 2020; Meng et al., 2020; Vlavcheski et al., 2020; Wen et al., 2020) (Table 2). In addition, many natural products such as berberine and quercetin show great potential in regulating and activating the AMPK pathway (Kjobsted et al., 2018; Wang N. et al., 2021; Xu et al., 2021). These studies suggest that plant-derived extracts can effectively activate the AMPK pathway and provide important information for the development of new drugs for many AMPK-related diseases.
2.2.3 Adenosine monophosphate activated protein kinase activators that act on skeletal muscle
Not all pharmacological activators can act on skeletal muscle due to the specific expression of three AMPK heterotrimers in skeletal muscle (Table 3). Metformin increases AMPK activity in skeletal muscle of subjects with type 2 diabetes (Musi et al., 2002). The small molecules that have been proven to activate AMPK in skeletal muscle include AICAR, 991, PF-739 and MK-8722 (Cokorinos et al., 2017; Myers et al., 2017; Olivier et al., 2018). Plant-derived extracts like flavonoids extracted from mulberry leaves and resveratrol have been shown to activate AMPK in skeletal muscle to regulate skeletal muscle state (Huang et al., 2019; Meng et al., 2020).
Although there are many activators that activate AMPK in skeletal muscle, not all of them are useful. AICAR has lost its appeal because of poor selectivity, low potency, inadequate bioavailability, and the potential “off-target” effects in cells [Reviewed in (Visnjic et al., 2021)]. Although 991, PF-739, and MK-8722 can activate AMPK in skeletal muscle and increase glucose uptake of skeletal muscle, their effects on skeletal muscle growth, atrophy, and regeneration are still unclear and need further research. Tanshinone IIA may have potential for the treatment of skeletal muscle wasting, because it can activate AMPK in various ways in different tissues (Yun et al., 2014; Zhang et al., 2014; Li et al., 2018; Zhang et al., 2019). However, whether Tanshinone IIA can activate AMPK in skeletal muscle remains to be further studied.
3 The role of mitochondria in myogenesis, regeneration, and muscle atrophy
Skeletal muscle exhibits a remearkable plasiticity, as its morphology and function can exhibit profound adaptations to the demands placed on it (Qaisar et al., 2016). Skeletal muscle tissue is the key determinant of basal metabolic rate and systemic energy metabolism, requiring a large amount of energy to maintain function. Mitochondria in the tissue maximize oxidative phosphorylation through dynamic fusion and fission to maintain cell function (Rahman and Quadrilatero, 2021a). The maintenance of normal mitochondrial function is important for the myogenesis and regeneration of skeletal muscle (Figure 3). Mitochondrial dysfunction can disorder skeletal muscle metabolism, and eventually lead to skeletal muscle atrophy.
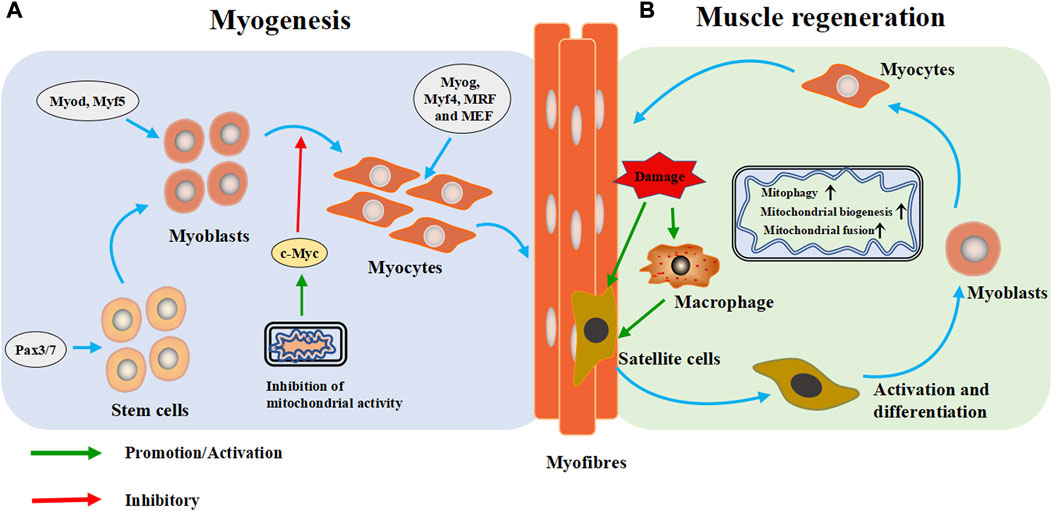
FIGURE 3. Mitochondria are extensively involved in the metabolic process of skeletal muscle cells. (A) Myogenesis: During the embryonic stage, stem cells form muscle progenitor cells under the intervention of regulatory factors and transcription factors, which are then activated and differentiate into myoblasts. Subsequently, the myoblasts exit the cell cycle and differentiate and fuse to form multinucleated myotubes. (B) Muscle regeneration: When muscle is injured, skeletal muscle heals itself through a programmed process. During degradation and inflammation, macrophages activate quiescent muscle stem cells (satellite cells) to differentiate into myoblasts, which then differentiate into muscle cells and fuse into myotubes to form muscle fibers and complete skeletal muscle repair. Pax3/Pax7: paired-box 3 and 7 transcription factors, Myod: myoblast determination protein 1, Myf5: myogenic factor 5, Myog: myogenin, Myf4: myogenin, MRF: myogenic regulatory factor, MEF2: myocyte enhancer factor 2.
3.1 The role of mitochondria in skeletal myogenesis
Skeletal myogenesis is the process of forming mature skeletal muscle tissue from precursor cells, which mainly occurs during embryonic and fetal development (Figure 3A). In the embryonic stage, stem cells form muscle progenitor cells under the influence of transcription factors such as the paired-box seven and three transcription factors (Pax7/Pax3), myoblast determination protein 1 (Myod), and myogenic factor 5 (Myf5), which then activate and differentiate into myoblasts. Subsequently, myoblasts exit the cell cycle differentiate, and fuse to form multinucleated myotubes (Bentzinger et al., 2012). As the differentiation progresses, and fuse to form multinucleated myotubes, myogenin (Myog), myogenin (Myf4), myogenic regulatory factor (MRF) and myocyte enhancer factor 2 (MEF2) catalyze subsequent gene expression (Zammit, 2017; Li et al., 2019).
The formation of skeletal muscle is accompanied by the replacement of low-function mitochondria, which eventually leads to the accumulation of high-function mitochondria (Rahman and Quadrilatero, 2021a). Mitochondria can regulate myoblast differentiation by controlling the expression of c-Myc gene. When the activity of mitochondria is inhibited, the intracellular expression of c-Myc increases, which will inhibit myogenic differentiation (Seyer et al., 2006). Mitochondrial autophagy plays a role in initiating myogenesis, at least in vitro (Rahman and Quadrilatero, 2021a). These studies suggest that normal mitochondrial function plays an important role in the genesis and formation of skeletal muscle.
3.2 Mitochondria regulate skeletal muscle regeneration
Skeletal muscle is often injured during sports, and its high regeneration efficiency is important for recovery of its function. In case of muscle injury, skeletal muscle completes self-healing through four progressive steps: degradation, inflammation, regeneration and remodeling (Huard et al., 2002). Regeneration is a programmed process. The process begins with degeneration and inflammation, and during these two steps, macrophages activate quiescent muscle stem cells (satellite cells) to differentiate into myoblasts, which then fuse into myotubes and form muscle fibers to complete skeletal muscle repair (Juban and Chazaud, 2017; Rahman and Quadrilatero, 2021b). Satellite cells are the starting point of skeletal muscle regeneration (Figure 3B).
Mitochondrial biogenesis is necessary during muscle regeneration (Wu et al., 2018; Niu et al., 2021). Under the pressure of differentiation, myoblasts require more energy to maintain cell remodeling; accordingly mitochondria are constantly splitting in cells, and mitochondrial autophagy is markedly increased (Hardy et al., 2016; Bloemberg and Quadrilatero, 2019). Mitochondrial renewal disorder has been repeatedly shown to reduce the differentiation ability of cultured myoblasts and the regeneration ability of skeletal muscle tissue (Baechler et al., 2019; Joseph and Doles, 2021; Qualls et al., 2021). Enhancing mitochondrial biogenesis can improve muscle regeneration (Niu et al., 2021). The combination of mitochondrial biogenesis and fusion promotes energy generation capacity in regenerated skeletal muscle, while inhibition of mitochondrial the protein synthesis inhibits muscle regeneration in injury models (Rahman and Quadrilatero, 2021b). Mitochondrial autophagy is necessary for skeletal muscle regeneration (Rahman and Quadrilatero, 2021a). A previous study showed that after injection of myotoxin, mitochondrial autophagy is inhibited, resulting in delayed regeneration response (Nichenko et al., 2016). Altogether, mitochondria play important roles in skeletal muscle regeneration, but the specific mechanisms remain unclear and needs further study.
3.3 The role of mitochondria in muscle atrophy
In chronic diseases, cancer and long-term infections, skeletal muscle can undergo. changes that eventually lead to atrophy (Powers et al., 2020). Muscle atrophy manifests as reductions in muscle mass, fiber cross-sectional area, strength, fatigue resistance, and exercise ability, which may lead to a decline in quality of life and increases in-hospital mortality (Boonyarom and Inui, 2006; Sartori et al., 2021). Skeletal muscle atrophy involves several signal pathways such as ubiquitin proteasome system and autophagy lysosome system (Shen et al., 2019; Wu et al., 2019; Ma et al., 2021; Wang et al., 2022b).
Skeletal muscle atrophy is also related to mitochondrial function, and regulating mitochondrial biogenesis can improve resistance to muscle atrophy (Shen et al., 2020; Jeon and Choung, 2021). When mitochondria are dysfunctional, increased intracellular ROS level activates apoptosis-related signaling pathways and the degradation of many proteins (Theilen et al., 2017).
Mitochondrial dysfunction releases mitochondrial protein apoptosis-inducing factor (AIF) and cytochrome c into the cytosol, which leads to the activation of caspase-3, promotes actin/myosin decomposition, and induces myonuclear cell apoptosis (Delavallee et al., 2020). The proteolytic system activated by AIF and cytochrome c may play an important role in the entire process of muscle atrophy in synergy with other signal transduction effectors [Reviewed in (Hyatt et al., 2019)]. Mitochondrial fission during mitochondrial dysfunction disrupts intracellular energy homeostasis, reduces ATP production, increases the relative concentration of AMP and activates AMPK. AMPK increases the expression of autophagy-specific gene proteins (ATGs) by activating the transcription factor forkhead box O 3 (FoxO3), which leads to the initiation of autophagy and ultimately to skeletal muscle atrophy (Sanchez et al., 2012; Cannavino et al., 2015). The above research results indicate that mitochondrial dysfunction can lead to muscle atrophy in various ways, and regulating mitochondrial function plays a role in resisting muscle atrophy (Figure 4).
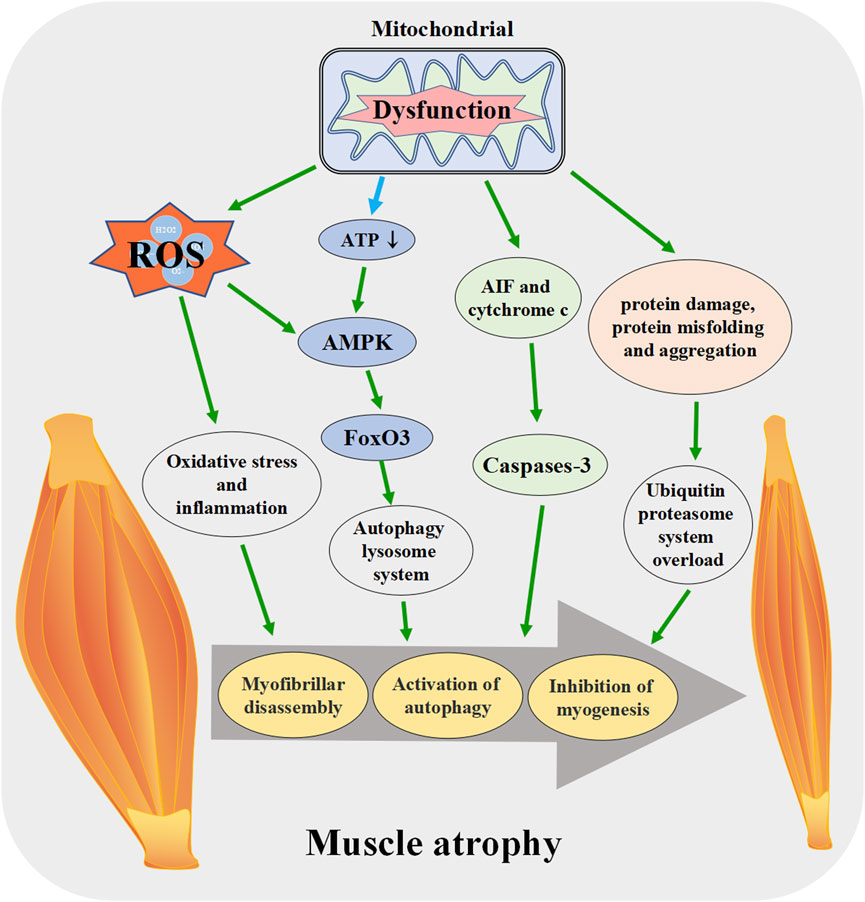
FIGURE 4. Mitochondrial dysfunction promotes the skeletal muscle atrophy. When mitochondrial function is disrupted, ROS production increases, ATP synthesis decreases and other pathways lead to the activation of apoptosis pathway in muscle tissue, enhanced protein degradation, increased autophagy, muscle fiber breakdown, and eventually induce skeletal muscle atrophy. ROS: reactive oxygen species, AIF: apoptosis-inducing factor, FoxO3: forkhead box O 3.
4 Effects of adenosine monophosphate activated protein kinase on mitochondrial dynamics and skeletal muscle
4.1 Effects of adenosine monophosphate activated protein kinase on mitochondrial biogenesis
Mitochondrial biogenesis can be considered as the growth and division of early-stage mitochondria (Jornayvaz and Shulman, 2010). It is affected by the energy demand of cells. Mitochondrial biogenesis-related pathways are activated in response to increased energy consumption conditions such as exercise, hypothermia, oxidative stress, and cell division and differentiation, resulting in changes in the number, size, and mass of mitochondria (Jornayvaz and Shulman, 2010; Popov, 2020). PGC-1α is a member of the transcriptional coactivator family. It is also considered the core molecule in mitochondrial biogenesis (Figure 5). PGC-1α interacts with transcription factors such as peroxisome proliferator-activated receptor (PPAR), estrogen-related receptor (ERR) family, and nuclear respiratory factor 1/nuclear respiratory factor 2 (NRF1/2) to activate almost all mitochondrial biogenesis pathways, including respiratory chain and fatty acid oxidation (FAO) genes, which increases the number of mitochondria and strengthens respiratory capacity (Scarpulla et al., 2012; Zhou et al., 2021).
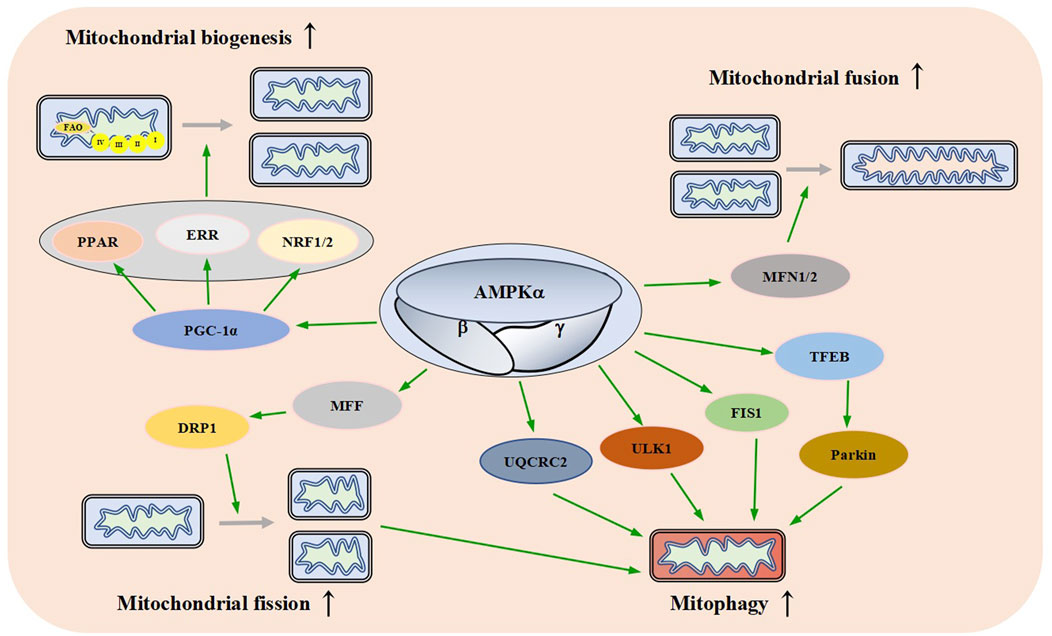
FIGURE 5. Effects of AMPK on Mitochondrial Dynamics. AMPK promotes mitochondrial biogenesis, fusion, fission, and autophagy through different signaling pathways. FAO: fatty acid oxidation, PPAR: peroxisome proliferator-activated receptor, ERR: estrogen-related receptor, NRF1/2: nuclear respiratory factor 1/nuclear respiratory factor 2, PGC-1a: peroxisome proliferator-activated receptor gamma coactivator-1 alpha, MFN1/2: mitofusin 1/2, MFF: mitochondrial fission factor, DRP1: dynamin-related protein1, UQCRC2: ubiquinol-cytochrome c reductase core protein 2, Ulk1: autophagy activating kinase 1, FIS1: mitochondrial fission one protein, TFEB: transcriptional activity of transcription factor EB.
When AMPK is activated by various stimuli, it induces the expression of PGC-1α by phosphorylation, resulting in an increased activity and thereby promoting mitochondrial biogenesis (Sun et al., 2022). These data suggest that AMPK plays an important role in mitochondrial biogenesis.
4.2 Role of adenosine monophosphate activated protein kinase in mitochondrial fusion and fission
Mitochondria are highly dynamic organelles that continuously fuse and divide in different states of cell cycle; mitochondrial fusion and division play an important role in maintaining mitochondrial homeostasis and cellular function (Lee and Yoon, 2016; Sabouny and Shutt, 2020). Fusion helps mitigate stress by mixing the contents of partially damaged mitochondria as a form of complementation. Fission is necessary for the creation of new mitochondria, it provides the raw material for new mitochondria and also contributes to quality control by the removal of damaged mitochondria and facilitates apoptosis (Adebayo et al., 2021). Mammalian mitochondrial fusion is mediated by mitofusin 1/2 (MFN1/2) and OPA1 (Mishra et al., 2014; Gao and Hu, 2021). Mitochondrial division is mainly mediated by mitochondrial fission factor (MFF), dynamin-related protein1 (DRP1), human mitochondrial dynamics proteins 49/51 (MID49/51) and mitochondrial fission one protein (FIS1) (Otera et al., 2016; Kalia et al., 2018; Hu et al., 2021; Konig et al., 2021). AMPKα1 interacts with and phosphorylates MFN2, the adenosine derivative cordycepin induces upregulation of MFN2 in cardiomyocytes in an AMPK-dependent manner to promote mitochondrial fusion (Yu et al., 2021).
Direct pharmacological activation of AMPK can induce mitochondrial fission (Toyama et al., 2016; Trewin et al., 2018). Sustained energy stress activates AMPK, which binds to and phosphorylates MFF, resulting in mitochondrial translocation of DRP1 (Zhang and Lin, 2016; Zheng et al., 2018). The dynamic regulation of mitochondrial fusion and fission mediated by multiple pathways ensures the stability of mitochondrial function (Figure 5).
4.3 The role of adenosine monophosphate activated protein kinase in mitochondrial autophagy
Mitochondrial autophagy is a catabolic process that helps maintain mitochondrial quality control by transporting damaged mitochondria to the lysosome for the degradation (Pickles et al., 2018). Mitochondrial autophagy is a protective mechanism of cells, which can reduce intracellular ROS, mtDNA damage, and the accumulation of aging or damaged mitochondria (Williams and Ding, 2018; Onishi et al., 2021).
AMPK plays an important role in autophagy (Herzig and Shaw, 2018). A lot of research supports this idea. In a mouse model of leukemia, AMPK activation upregulates FIS1-mediated mitochondrial autophagy to promote the degradation of mitochondria subjected to stress and maintains the health of the mitochondrial network (Pei et al., 2018). Meanwhile, a study has found that AMPK indirectly up-regulates the expression of ubiquinol-cytochrome c reductase core protein 2 (UQCRC2) to enhance mitochondrial autophagy (Lu et al., 2021). Laker and others found that AMPK phosphorylates autophagy activating kinase 1 (Ulk1) and plays a role in mitochondrial autophagy induced by acute exercise in mouse skeletal muscle (Laker et al., 2017). A study has found that AMPK activation causes transcriptional activity of transcription factor EB (TFEB) transcription and induces Parkin-dependent mitochondrial autophagy to lessen oxidative stress, thereby enhancing mitochondrial function (Cao et al., 2020). There is also one study that has found AMPK promotes fission by phosphorylating MFF, thereby promoting autophagic clearance of damaged mitochondria (Toyama et al., 2016). These results suggest that AMPK links energy metabolism to mitochondrial autophagy through a variety of signaling pathways (Figure 5).
4.4 Adenosine monophosphate activated protein kinase influences skeletal muscle protein metabolism via mitochondrial function
The mass of adult individual skeletal muscle is mainly determined by the relative rates of the protein synthesis and degradation. When the protein synthesis efficacy is greater than protein degradation efficacy, the mass and volume of skeletal muscle increase. When protein degradation rate is greater than the protein synthesis efficacy, it causes skeletal muscle atrophy (Jaiswal et al., 2019; Romanello and Sandri, 2021).
AMPK can regulate the balance of the protein synthesis and degradation in skeletal muscle. Under healthy conditions, AMPK inhibits the protein synthesis, but under conditions of mitochondrial dysfunction, activation of AMPK might help preserve muscle the protein synthesis by promoting the synthesis of healthy mitochondria. Under physiological conditions, AMPK activation inhibits the protein synthesis and promotes protein breakdown to impair muscle hypertrophy through a variety of pathways (Thomson and Gordon, 2005; Gordon et al., 2008). AMPK inhibits the protein synthesis by inhibiting the activities of mechanistic target of rapamycin, complex 1 (mTORC1) and eukaryotic elongation factor 2 (eEF2) (Thomson, 2018). AMPK can increase FoxO activity through the NAD+/sirtuin one pathway to promote protein degradation (Canto et al., 2009). AMPK phosphorylation is negatively correlated with the growth of skeletal muscle, and overexpression of CaMKK2 inhibits the proliferation and differentiation of C2C12 myoblasts by activating AMPK (Ye et al., 2016). However, under pathological conditions, activation of AMPK promotes muscle regeneration and ameliorates muscle atrophy by promoting mitochondrial metabolic activity through different pathways. Activation of AMPK enhances PGC-1α transcription and its coactivator activity, stimulates mitochondrial biogenesis, and promotes muscle regeneration (Quattrocelli et al., 2022). Activation of AMPK enhances satellite-cell proliferation and promotes myogenic differentiation of satellite cells in regenerated muscle (Fu et al., 2016). Under normal conditions, in which sufficient energy is available to support the protein synthesis, the activation of AMPK would operate to slow this rate. In contrast, in conditions in which energy supply is insufficient to support the normal rate of the protein synthesis, such as with mitochondrial dysfunction, AMPK can help to promote the protein synthesis. In this way, AMPK can both limit and enhance muscle growth and regeneration.
In view of the positive and negative regulatory roles of AMPK in skeletal muscle metabolism, its effect on the biological process of skeletal muscle needs to be further investigated.
5 Adenosine monophosphate activated protein kinase activators can improve muscle disease status
Many studies have shown that activation of AMPK can effectively prevent or improve muscle disease status.
Qiangji Jianli decoction has been shown to improve muscle atrophy in myasthenia gravis by promoting mitochondrial biosynthesis and restoring muscle energy supply through activation of the AMPK/PGC-1α pathway (Jiao et al., 2020). Resveratrol prevents muscle atrophy caused by a high-fat diet in older adult rats by reversing mitochondrial dysfunction and oxidative stress through the PKA/LKB1/AMPK pathway (Huang et al., 2019). AMPK phosphorylation activates PGC-1α, up-regulates nuclear factor erythroid-derived 2-related factor 1 (Nrf1) expression, enhances energy metabolism, and inhibits skeletal muscle cell apoptosis (Jiang et al., 2020). AMPK can also reduce apoptosis by inhibiting mTOR signaling, increase autophagy by ULK1, and reduce fibrosis by inhibiting transforming growth factor-beta (TGF-beta) signaling (Timm and Tyler, 2020). Various other AMPK activators have shown various beneficial effects in mouse, rat, and cell studies, as shown in Table 3. AMPK activators have been noted and used in the treatment of muscle-related diseases, and as research continues, these activators may be added to the list of therapeutics for muscle-related diseases.
6 Perspectives
In recent years, several studies have confirmed that AMPK is the central hub of intracellular energy metabolism regulation. Although AMPK is not the only biological molecule regulating mitochondrial biogenesis, fusion, fission, and autophagy, it is considered to be a core molecule for the maintenance of mitochondrial homeostasis. Owing to the high energy demand of skeletal muscle tissue, mitochondria are important cellular organelles in skeletal muscle tissue. The metabolism of mitochondria affects the development, atrophy, and regeneration of skeletal muscle. Therefore, based on the relationship among AMPK, mitochondria, and skeletal muscle, it can be considered that AMPK can regulate the state of skeletal muscle by regulating mitochondria. Although many studies have shown that drugs can regulate the biological process of mitochondria by first regulating AMPK activity, followed by regulating the metabolism of skeletal muscle, the specific mechanism remains unclear, and several issues need to be addressed. Given that the subtypes of AMPK expressed in different tissues are different, it remains to be seen whether we can develop skeletal muscle-specific drugs that can regulate AMPK activity and improve skeletal muscle metabolism, thereby aiding in disease treatment.
Author contributions
Conceptualization, LQ, HS, and HJ; Methodology, YY, ML, JL, YJ, KW, DY, YS, and WW; Resources, YY, ML, JL, YJ, KW, DY, YS, and WW; Data Curation, YY, ML, JL, YJ, KW, DY, YS, and WW; Writing–Original Draft Preparation, YY, YS, HJ, HS, and LQ; Writing–Review and Editing, YY, YS, HJ, HS, and LQ; Visualization, YY and YS; Supervision, HS, ZH, and LQ; Project Administration, HS, ZH, and LQ; Funding Acquisition, ZH, HJ, HS, and LQ.
Funding
This work was supported by the National Natural Science Foundation of China (Nos. 82072160, 81901933), Jiangsu Planned Projects for Postdoctoral Research Fund (2021K031A), the Major Natural Science Research Projects in Universities of Jiangsu Province (No. 20KJA310012), the “QingLan Project” in Jiangsu Universities, the Priority Academic Program Development of Jiangsu Higher Education Institutions, Natural Science Research Project of Nantong Science and Technology Bureau (MS12021021, MS12020006, MS12020017), the Nantong Clinical Medicine Research Center (HS2019005).
Conflict of interest
The authors declare that the research was conducted in the absence of any commercial or financial relationships that could be construed as a potential conflict of interest.
Publisher’s note
All claims expressed in this article are solely those of the authors and do not necessarily represent those of their affiliated organizations, or those of the publisher, the editors and the reviewers. Any product that may be evaluated in this article, or claim that may be made by its manufacturer, is not guaranteed or endorsed by the publisher.
References
Adebayo, M., Singh, S., Singh, A. P., and Dasgupta, S. (2021). Mitochondrial fusion and fission: The fine-tune balance for cellular homeostasis. FASEB J. 35 (6), e21620. doi:10.1096/fj.202100067R
Agius, L., Ford, B. E., and Chachra, S. S. (2020). The metformin mechanism on gluconeogenesis and AMPK activation: The metabolite perspective. Int. J. Mol. Sci. 21 (9), E3240. doi:10.3390/ijms21093240
Ahmad, I., Molyvdas, A., Jian, M. Y., Zhou, T., Traylor, A. M., Cui, H., et al. (2021). AICAR decreases acute lung injury by phosphorylating AMPK and upregulating heme oxygenase-1. Eur. Respir. J. 58 (6), 2003694. doi:10.1183/13993003.03694-2020
Al-Ishaq, R. K., Abotaleb, M., Kubatka, P., Kajo, K., and Busselberg, D. (2019). Flavonoids and their anti-diabetic effects: Cellular mechanisms and effects to improve blood sugar levels. Biomolecules 9 (9), E430. doi:10.3390/biom9090430
Anderson, K. A., Ribar, T. J., Lin, F., Noeldner, P. K., Green, M. F., Muehlbauer, M. J., et al. (2008). Hypothalamic CaMKK2 contributes to the regulation of energy balance. Cell Metab. 7 (5), 377–388. doi:10.1016/j.cmet.2008.02.011
Andres-Mateos, E., Brinkmeier, H., Burks, T. N., Mejias, R., Files, D. C., Steinberger, M., et al. (2013). Activation of serum/glucocorticoid-induced kinase 1 (SGK1) is important to maintain skeletal muscle homeostasis and prevent atrophy. EMBO Mol. Med. 5 (1), 80–91. doi:10.1002/emmm.201201443
Andrieux, P., Chevillard, C., Cunha-Neto, E., and Nunes, J. P. S. (2021). Mitochondria as a cellular hub in infection and inflammation. Int. J. Mol. Sci. 22 (21), 11338. doi:10.3390/ijms222111338
Arab, H. H., Al-Shorbagy, M. Y., and Saad, M. A. (2021). Activation of autophagy and suppression of apoptosis by dapagliflozin attenuates experimental inflammatory bowel disease in rats: Targeting AMPK/mTOR, HMGB1/RAGE and Nrf2/HO-1 pathways. Chem. Biol. Interact. 335, 109368. doi:10.1016/j.cbi.2021.109368
Baechler, B. L., Bloemberg, D., and Quadrilatero, J. (2019). Mitophagy regulates mitochondrial network signaling, oxidative stress, and apoptosis during myoblast differentiation. Autophagy 15 (9), 1606–1619. doi:10.1080/15548627.2019.1591672
Baskin, K. K., Winders, B. R., and Olson, E. N. (2015). Muscle as a "mediator" of systemic metabolism. Cell Metab. 21 (2), 237–248. doi:10.1016/j.cmet.2014.12.021
Bentzinger, C. F., Wang, Y. X., and Rudnicki, M. A. (2012). Building muscle: Molecular regulation of myogenesis. Cold Spring Harb. Perspect. Biol. 4 (2), a008342. doi:10.1101/cshperspect.a008342
Bijland, S., Mancini, S. J., and Salt, I. P. (2013). Role of AMP-activated protein kinase in adipose tissue metabolism and inflammation. Clin. Sci. 124 (8), 491–507. doi:10.1042/CS20120536
Birk, J. B., and Wojtaszewski, J. F. (2006). Predominant alpha2/beta2/gamma3 AMPK activation during exercise in human skeletal muscle. J. Physiol. 577 (3), 1021–1032. doi:10.1113/jphysiol.2006.120972
Bloemberg, D., and Quadrilatero, J. (2019). Autophagy, apoptosis, and mitochondria: Molecular integration and physiological relevance in skeletal muscle. Am. J. Physiol. Cell Physiol. 317 (1), C111–C130. doi:10.1152/ajpcell.00261.2018
Boonyarom, O., and Inui, K. (2006). Atrophy and hypertrophy of skeletal muscles: Structural and functional aspects. Acta Physiol. 188 (2), 77–89. doi:10.1111/j.1748-1716.2006.01613.x
Bultot, L., Jensen, T. E., Lai, Y. C., Madsen, A. L., Collodet, C., Kviklyte, S., et al. (2016). Benzimidazole derivative small-molecule 991 enhances AMPK activity and glucose uptake induced by AICAR or contraction in skeletal muscle. Am. J. Physiol. Endocrinol. Metab. 311 (4), E706–E719. doi:10.1152/ajpendo.00237.2016
Bung, N., Surepalli, S., Seshadri, S., Patel, S., Peddasomayajula, S., Kummari, L. K., et al. (2018). 2-[2-(4-(trifluoromethyl)phenylamino)thiazol-4-yl]acetic acid (Activator-3) is a potent activator of AMPK. Sci. Rep. 8 (1), 9599. doi:10.1038/s41598-018-27974-1
Cameron, K. O., Kung, D. W., Kalgutkar, A. S., Kurumbail, R. G., Miller, R., Salatto, C. T., et al. (2016). Discovery and preclinical characterization of 6-Chloro-5-[4-(1-hydroxycyclobutyl)phenyl]-1H-indole-3-carboxylic acid (PF-06409577), a direct activator of adenosine monophosphate-activated protein kinase (AMPK), for the potential treatment of diabetic nephropathy. J. Med. Chem. 59 (17), 8068–8081. doi:10.1021/acs.jmedchem.6b00866
Cannavino, J., Brocca, L., Sandri, M., Grassi, B., Bottinelli, R., and Pellegrino, M. A. (2015). The role of alterations in mitochondrial dynamics and PGC-1α over-expression in fast muscle atrophy following hindlimb unloading. J. Physiol. 593 (8), 1981–1995. doi:10.1113/jphysiol.2014.286740
Canto, C., Gerhart-Hines, Z., Feige, J. N., Lagouge, M., Noriega, L., Milne, J. C., et al. (2009). AMPK regulates energy expenditure by modulating NAD+ metabolism and SIRT1 activity. Nature 458 (7241), 1056–1060. doi:10.1038/nature07813
Cao, S., Wang, C., Yan, J., Li, X., Wen, J., and Hu, C. (2020). Curcumin ameliorates oxidative stress-induced intestinal barrier injury and mitochondrial damage by promoting Parkin dependent mitophagy through AMPK-TFEB signal pathway. Free Radic. Biol. Med. 147, 8–22. doi:10.1016/j.freeradbiomed.2019.12.004
Cardaci, S., Filomeni, G., and Ciriolo, M. R. (2012). Redox implications of AMPK-mediated signal transduction beyond energetic clues. J. Cell Sci. 125 (9), 2115–2125. doi:10.1242/jcs.095216
Carling, D. (2017). AMPK signalling in health and disease. Curr. Opin. Cell Biol. 45, 31–37. doi:10.1016/j.ceb.2017.01.005
Chang, E. (2019). 1, 25-dihydroxyvitamin D decreases tertiary butyl-hydrogen peroxide-induced oxidative stress and increases AMPK/SIRT1 activation in C2C12 muscle cells. Molecules 24 (21), E3903. doi:10.3390/molecules24213903
Ciccarese, F., Zulato, E., and Indraccolo, S. (2019). LKB1/AMPK pathway and drug response in cancer: A therapeutic perspective. Oxid. Med. Cell. Longev. 2019, 8730816. doi:10.1155/2019/8730816
Coccimiglio, I. F., and Clarke, D. C. (2020). ADP is the dominant controller of AMP-activated protein kinase activity dynamics in skeletal muscle during exercise. PLoS Comput. Biol. 16 (7), e1008079. doi:10.1371/journal.pcbi.1008079
Cokorinos, E. C., Delmore, J., Reyes, A. R., Albuquerque, B., Kjobsted, R., Jorgensen, N. O., et al. (2017). Activation of skeletal muscle AMPK promotes glucose disposal and glucose lowering in non-human primates and mice. Cell Metab. 25 (5), 1147–1159. doi:10.1016/j.cmet.2017.04.010
Coughlan, K. A., Valentine, R. J., Sudit, B. S., Allen, K., Dagon, Y., Kahn, B. B., et al. (2016). PKD1 inhibits AMPKα2 through phosphorylation of serine 491 and impairs insulin signaling in skeletal muscle cells. J. Biol. Chem. 291 (11), 5664–5675. doi:10.1074/jbc.M115.696849
Dagon, Y., Hur, E., Zheng, B., Wellenstein, K., Cantley, L. C., and Kahn, B. B. (2012). p70S6 kinase phosphorylates AMPK on serine 491 to mediate leptin's effect on food intake. Cell Metab. 16 (1), 104–112. doi:10.1016/j.cmet.2012.05.010
Day, E. A., Ford, R. J., Smith, B. K., Houde, V. P., Stypa, S., Rehal, S., et al. (2021). Salsalate reduces atherosclerosis through AMPKβ1 in mice. Mol. Metab. 53, 101321. doi:10.1016/j.molmet.2021.101321
Delavallee, L., Mathiah, N., Cabon, L., Mazeraud, A., Brunelle-Navas, M. N., Lerner, L. K., et al. (2020). Mitochondrial AIF loss causes metabolic reprogramming, caspase-independent cell death blockade, embryonic lethality, and perinatal hydrocephalus. Mol. Metab. 40, 101027. doi:10.1016/j.molmet.2020.101027
Den Hartogh, D. J., Vlavcheski, F., Giacca, A., and Tsiani, E. (2020). Attenuation of free fatty acid (FFA)-Induced skeletal muscle cell insulin resistance by resveratrol is linked to activation of AMPK and inhibition of mTOR and p70 S6K. Int. J. Mol. Sci. 21 (14), E4900. doi:10.3390/ijms21144900
Deshwal, S., Fiedler, K. U., and Langer, T. (2020). Mitochondrial proteases: Multifaceted regulators of mitochondrial plasticity. Annu. Rev. Biochem. 89, 501–528. doi:10.1146/annurev-biochem-062917-012739
Dhani, S., Ghazi, T., Nagiah, S., Baijnath, S., Singh, S. D., and Chuturgoon, A. A. (2020). Fusaric acid alters Akt and ampk signalling in c57bl/6 mice brain tissue. Food Chem. Toxicol. 138, 111252. doi:10.1016/j.fct.2020.111252
DiTacchio, K. A., Heinemann, S. F., and Dziewczapolski, G. (2015). Metformin treatment alters memory function in a mouse model of Alzheimer's disease. J. Alzheimers Dis. 44 (1), 43–48. doi:10.3233/JAD-141332
Drake, J. C., Wilson, R. J., Laker, R. C., Guan, Y., Spaulding, H. R., Nichenko, A. S., et al. (2021). Mitochondria-localized AMPK responds to local energetics and contributes to exercise and energetic stress-induced mitophagy. Proc. Natl. Acad. Sci. U. S. A. 118 (37), e2025932118. doi:10.1073/pnas.2025932118
Duca, F. A., Cote, C. D., Rasmussen, B. A., Zadeh-Tahmasebi, M., Rutter, G. A., Filippi, B. M., et al. (2015). Metformin activates a duodenal Ampk-dependent pathway to lower hepatic glucose production in rats. Nat. Med. 21 (5), 506–511. doi:10.1038/nm.3787
Ericsson, M., Steneberg, P., Nyren, R., and Edlund, H. (2021). AMPK activator O304 improves metabolic and cardiac function, and exercise capacity in aged mice. Commun. Biol. 4 (1), 1306. doi:10.1038/s42003-021-02837-0
Filippov, S., Pinkosky, S. L., Lister, R. J., Pawloski, C., Hanselman, J. C., Cramer, C. T., et al. (2013). ETC-1002 regulates immune response, leukocyte homing, and adipose tissue inflammation via LKB1-dependent activation of macrophage AMPK. J. Lipid Res. 54 (8), 2095–2108. doi:10.1194/jlr.M035212
Francini, F., Schinella, G. R., and Rios, J. L. (2019). Activation of AMPK by medicinal plants and natural products: Its role in type 2 diabetes mellitus. Mini Rev. Med. Chem. 19 (11), 880–901. doi:10.2174/1389557519666181128120726
Fu, X., Zhu, M., Zhang, S., Foretz, M., Viollet, B., and Du, M. (2016). Obesity impairs skeletal muscle regeneration through inhibition of AMPK. Diabetes 65 (1), 188–200. doi:10.2337/db15-0647
Gao, S., and Hu, J. (2021). Mitochondrial fusion: The machineries in and out. Trends Cell Biol. 31 (1), 62–74. doi:10.1016/j.tcb.2020.09.008
Gonzalez, A., Hall, M. N., Lin, S. C., and Hardie, D. G. (2020). AMPK and TOR: The yin and Yang of cellular nutrient sensing and growth control. Cell Metab. 31 (3), 472–492. doi:10.1016/j.cmet.2020.01.015
Gordon, S. E., Lake, J. A., Westerkamp, C. M., and Thomson, D. M. (2008). Does AMP-activated protein kinase negatively mediate aged fast-twitch skeletal muscle mass? Exerc. Sport Sci. Rev. 36 (4), 179–186. doi:10.1097/JES.0b013e3181877e13
Gowans, G. J., Hawley, S. A., Ross, F. A., and Hardie, D. G. (2013). AMP is a true physiological regulator of AMP-activated protein kinase by both allosteric activation and enhancing net phosphorylation. Cell Metab. 18 (4), 556–566. doi:10.1016/j.cmet.2013.08.019
Gu, X. (2021). Biodegradable materials and the tissue engineering of nerves. Engineering 7 (12), 1700–1703. doi:10.1016/j.eng.2021.10.011
Guigas, B., and Viollet, B. (2016). Targeting AMPK: From ancient drugs to new small-molecule activators. Exp. Suppl. 107, 327–350. doi:10.1007/978-3-319-43589-3_13
Gustafsson, C. M., Falkenberg, M., and Larsson, N. G. (2016). Maintenance and expression of mammalian mitochondrial DNA. Annu. Rev. Biochem. 85, 133–160. doi:10.1146/annurev-biochem-060815-014402
Hardie, D. G. (2018). Keeping the home fires burning: AMP-activated protein kinase. J. R. Soc. Interface 15 (138), 20170774. doi:10.1098/rsif.2017.0774
Hardie, D. G., Ross, F. A., and Hawley, S. A. (2012). Ampk: A nutrient and energy sensor that maintains energy homeostasis. Nat. Rev. Mol. Cell Biol. 13 (4), 251–262. doi:10.1038/nrm3311
Hardy, D., Besnard, A., Latil, M., Jouvion, G., Briand, D., Thepenier, C., et al. (2016). Comparative study of injury models for studying muscle regeneration in mice. PLoS One 11 (1), e0147198. doi:10.1371/journal.pone.0147198
Hasan, R., Lasker, S., Hasan, A., Zerin, F., Zamila, M., Parvez, F., et al. (2020). Canagliflozin ameliorates renal oxidative stress and inflammation by stimulating AMPK-Akt-eNOS pathway in the isoprenaline-induced oxidative stress model. Sci. Rep. 10 (1), 14659. doi:10.1038/s41598-020-71599-2
Hawley, S. A., Davison, M., Woods, A., Davies, S. P., Beri, R. K., Carling, D., et al. (1996). Characterization of the AMP-activated protein kinase kinase from rat liver and identification of threonine 172 as the major site at which it phosphorylates AMP-activated protein kinase. J. Biol. Chem. 271 (44), 27879–27887. doi:10.1074/jbc.271.44.27879
Hawley, S. A., Ford, R. J., Smith, B. K., Gowans, G. J., Mancini, S. J., Pitt, R. D., et al. (2016). The Na+/Glucose cotransporter inhibitor canagliflozin activates AMPK by inhibiting mitochondrial function and increasing cellular AMP levels. Diabetes 65 (9), 2784–2794. doi:10.2337/db16-0058
Heathcote, H. R., Mancini, S. J., Strembitska, A., Jamal, K., Reihill, J. A., Palmer, T. M., et al. (2016). Protein kinase C phosphorylates AMP-activated protein kinase α1 Ser48. Biochem. J. 473 (24), 4681–4697. doi:10.1042/BCJ20160211
Hedman, A. C., Li, Z., Gorisse, L., Parvathaneni, S., Morgan, C. J., and Sacks, D. B. (2021). IQGAP1 binds AMPK and is required for maximum AMPK activation. J. Biol. Chem. 296, 100075. doi:10.1074/jbc.RA120.016193
Heine, K. B., and Hood, W. R. (2020). Mitochondrial behaviour, morphology, and animal performance. Biol. Rev. Camb. Philos. Soc. 95 (3), 730–737. doi:10.1111/brv.12584
Herzig, S., and Shaw, R. J. (2018). Ampk: Guardian of metabolism and mitochondrial homeostasis. Nat. Rev. Mol. Cell Biol. 19 (2), 121–135. doi:10.1038/nrm.2017.95
Hood, D. A., Memme, J. M., Oliveira, A. N., and Triolo, M. (2019). Maintenance of skeletal muscle mitochondria in health, exercise, and aging. Annu. Rev. Physiol. 81, 19–41. doi:10.1146/annurev-physiol-020518-114310
Hu, Y., Chen, H., Zhang, L., Lin, X., Li, X., Zhuang, H., et al. (2021). The AMPK-MFN2 axis regulates MAM dynamics and autophagy induced by energy stresses. Autophagy 17 (5), 1142–1156. doi:10.1080/15548627.2020.1749490
Huang, L., Dai, K., Chen, M., Zhou, W., Wang, X., Chen, J., et al. (2016). The AMPK agonist PT1 and mTOR inhibitor 3HOI-BA-01 protect cardiomyocytes after ischemia through induction of autophagy. J. Cardiovasc. Pharmacol. Ther. 21 (1), 70–81. doi:10.1177/1074248415581177
Huang, W., Liu, Y., Luz, A., Berrong, M., Meyer, J. N., Zou, Y., et al. (2021). Calcium/calmodulin dependent protein kinase kinase 2 regulates the expansion of tumor-induced myeloid-derived suppressor cells. Front. Immunol. 12, 754083. doi:10.3389/fimmu.2021.754083
Huang, Y., Zhu, X., Chen, K., Lang, H., Zhang, Y., Hou, P., et al. (2019). Resveratrol prevents sarcopenic obesity by reversing mitochondrial dysfunction and oxidative stress via the PKA/LKB1/AMPK pathway. Aging (Albany NY) 11 (8), 2217–2240. doi:10.18632/aging.101910
Huard, J., Li, Y., and Fu, F. H. (2002). Muscle injuries and repair: Current trends in research. J. Bone Jt. Surgery-American Volume 84 (5), 822–832. doi:10.2106/00004623-200205000-00022
Hunter, R. W., Foretz, M., Bultot, L., Fullerton, M. D., Deak, M., Ross, F. A., et al. (2014). Mechanism of action of compound-13: An α1-selective small molecule activator of AMPK. Chem. Biol. 21 (7), 866–879. doi:10.1016/j.chembiol.2014.05.014
Hyatt, H., Deminice, R., Yoshihara, T., and Powers, S. K. (2019). Mitochondrial dysfunction induces muscle atrophy during prolonged inactivity: A review of the causes and effects. Arch. Biochem. Biophys. 662, 49–60. doi:10.1016/j.abb.2018.11.005
Inokuchi-Shimizu, S., Park, E. J., Roh, Y. S., Yang, L., Zhang, B., Song, J., et al. (2014). TAK1-mediated autophagy and fatty acid oxidation prevent hepatosteatosis and tumorigenesis. J. Clin. Invest. 124 (8), 3566–3578. doi:10.1172/JCI74068
Jaikumkao, K., Promsan, S., Thongnak, L., Swe, M. T., Tapanya, M., Htun, K. T., et al. (2021). Dapagliflozin ameliorates pancreatic injury and activates kidney autophagy by modulating the AMPK/mTOR signaling pathway in obese rats. J. Cell. Physiol. 236 (9), 6424–6440. doi:10.1002/jcp.30316
Jaiswal, N., Gavin, M. G., Quinn, W. J., Luongo, T. S., Gelfer, R. G., Baur, J. A., et al. (2019). The role of skeletal muscle Akt in the regulation of muscle mass and glucose homeostasis. Mol. Metab. 28, 1–13. doi:10.1016/j.molmet.2019.08.001
Jeon, S. H., and Choung, S. Y. (2021). Oyster hydrolysates attenuate muscle atrophy via regulating protein turnover and mitochondria biogenesis in C2C12 cell and immobilized mice. Nutrients 13 (12), 4385. doi:10.3390/nu13124385
Jia, J., Bissa, B., Brecht, L., Allers, L., Choi, S. W., Gu, Y., et al. (2020). AMPK is activated during lysosomal damage via a galectin-ubiquitin signal transduction system. Autophagy 16 (8), 1550–1552. doi:10.1080/15548627.2020.1788890
Jiang, B., Yang, Y. J., Dang, W. Z., Li, H., Feng, G. Z., Yu, X. C., et al. (2020). Astragaloside IV reverses simvastatin-induced skeletal muscle injury by activating the AMPK-PGC-1α signalling pathway. Phytother. Res. 34 (5), 1175–1184. doi:10.1002/ptr.6593
Jiang, L., Liu, T., Xie, L., Ouyang, C., Ji, J., and Huang, T. (2019). AICAR prolongs corneal allograft survival via the AMPK-mTOR signaling pathway in mice. Biomed. Pharmacother. 113, 108558. doi:10.1016/j.biopha.2019.01.019
Jiao, W., Hu, F., Li, J., Song, J., Liang, J., Li, L., et al. (2020). Qiangji Jianli Decoction promotes mitochondrial biogenesis in skeletal muscle of myasthenia gravis rats via AMPK/PGC-1α signaling pathway. Biomed. Pharmacother. 129, 110482. doi:10.1016/j.biopha.2020.110482
Jimenez de Oya, N., Blazquez, A. B., Casas, J., Saiz, J. C., and Martin-Acebes, M. A. (2018). Direct activation of adenosine monophosphate-activated protein kinase (AMPK) by PF-06409577 inhibits flavivirus infection through modification of host cell lipid metabolism. Antimicrob. Agents Chemother. 62 (7), 003600–18. doi:10.1128/AAC.00360-18
Jorgensen, N. O., Kjobsted, R., Larsen, M. R., Birk, J. B., Andersen, N. R., Albuquerque, B., et al. (2021). Direct small molecule ADaM-site AMPK activators reveal an AMPKγ3-independent mechanism for blood glucose lowering. Mol. Metab. 51, 101259. doi:10.1016/j.molmet.2021.101259
Jornayvaz, F. R., and Shulman, G. I. (2010). Regulation of mitochondrial biogenesis. Essays Biochem. 47, 69–84. doi:10.1042/bse0470069
Joseph, J., and Doles, J. D. (2021). Disease-associated metabolic alterations that impact satellite cells and muscle regeneration: Perspectives and therapeutic outlook. Nutr. Metab. 18 (1), 33. doi:10.1186/s12986-021-00565-0
Joshi, T., Singh, A. K., Haratipour, P., Sah, A. N., Pandey, A. K., Naseri, R., et al. (2019). Targeting AMPK signaling pathway by natural products for treatment of diabetes mellitus and its complications. J. Cell. Physiol. 234 (10), 17212–17231. doi:10.1002/jcp.28528
Juban, G., and Chazaud, B. (2017). Metabolic regulation of macrophages during tissue repair: Insights from skeletal muscle regeneration. FEBS Lett. 591 (19), 3007–3021. doi:10.1002/1873-3468.12703
Jung, T. W., Youn, B. S., Choi, H. Y., Lee, S. Y., Hong, H. C., Yang, S. J., et al. (2013). Salsalate and adiponectin ameliorate hepatic steatosis by inhibition of the hepatokine fetuin-A. Biochem. Pharmacol. 86 (7), 960–969. doi:10.1016/j.bcp.2013.07.034
Kalia, R., Wang, R. Y., Yusuf, A., Thomas, P. V., Agard, D. A., Shaw, J. M., et al. (2018). Structural basis of mitochondrial receptor binding and constriction by DRP1. Nature 558 (7710), 401–405. doi:10.1038/s41586-018-0211-2
Kaneto, H., Kimura, T., Obata, A., Shimoda, M., and Kaku, K. (2021). Multifaceted mechanisms of action of metformin which have been unraveled one after another in the long history. Int. J. Mol. Sci. 22 (5), 2596. doi:10.3390/ijms22052596
Kjobsted, R., Hingst, J. R., Fentz, J., Foretz, M., Sanz, M. N., Pehmoller, C., et al. (2018). AMPK in skeletal muscle function and metabolism. FASEB J. 32 (4), 1741–1777. doi:10.1096/fj.201700442R
Konig, T., Nolte, H., Aaltonen, M. J., Tatsuta, T., Krols, M., Stroh, T., et al. (2021). MIROs and DRP1 drive mitochondrial-derived vesicle biogenesis and promote quality control. Nat. Cell Biol. 23 (12), 1271–1286. doi:10.1038/s41556-021-00798-4
Kopietz, F., Berggreen, C., Larsson, S., Sall, J., Ekelund, M., Sakamoto, K., et al. (2018). AMPK activation by A-769662 and 991 does not affect catecholamine-induced lipolysis in human adipocytes. Am. J. Physiol. Endocrinol. Metab. 315 (5), E1075–E1085. doi:10.1152/ajpendo.00110.2018
Laker, R. C., Drake, J. C., Wilson, R. J., Lira, V. A., Lewellen, B. M., Ryall, K. A., et al. (2017). Ampk phosphorylation of Ulk1 is required for targeting of mitochondria to lysosomes in exercise-induced mitophagy. Nat. Commun. 8 (1), 548. doi:10.1038/s41467-017-00520-9
LaMoia, T. E., and Shulman, G. I. (2021). Cellular and molecular mechanisms of metformin action. Endocr. Rev. 42 (1), 77–96. doi:10.1210/endrev/bnaa023
Langendorf, C. G., Ngoei, K. R. W., Scott, J. W., Ling, N. X. Y., Issa, S. M. A., Gorman, M. A., et al. (2016). Structural basis of allosteric and synergistic activation of AMPK by furan-2-phosphonic derivative C2 binding. Nat. Commun. 7, 10912. doi:10.1038/ncomms10912
Leduc-Gaudet, J. P., Hussain, S. N. A., Barreiro, E., and Gouspillou, G. (2021). Mitochondrial dynamics and mitophagy in skeletal muscle health and aging. Int. J. Mol. Sci. 22 (15), 8179. doi:10.3390/ijms22158179
Lee, H., and Yoon, Y. (2016). Mitochondrial fission and fusion. Biochem. Soc. Trans. 44 (6), 1725–1735. doi:10.1042/BST20160129
Li, H., Zhang, P., Lin, H., Gao, H., and Yin, J. (2022). ETC-1002 attenuates Porphyromonas gingivalis lipopolysaccharide-induced inflammation in RAW264.7 cells via the AMPK/NF-κB pathway and exerts ameliorative effects in experimental periodontitis in mice. Dis. Markers 2022, 8583674. doi:10.1155/2022/8583674
Li, J., Aponte Paris, S., Thakur, H., Kapiloff, M. S., and Dodge-Kafka, K. L. (2019). Muscle A-kinase-anchoring protein-beta-bound calcineurin toggles active and repressive transcriptional complexes of myocyte enhancer factor 2D. J. Biol. Chem. 294 (7), 2543–2554. doi:10.1074/jbc.RA118.005465
Li, J., Chen, C., Zhang, W., Bi, J., Yang, G., and Li, E. (2021a). Salsalate reverses metabolic disorders in a mouse model of non-alcoholic fatty liver disease through AMPK activation and caspase-6 activity inhibition. Basic Clin. Pharmacol. Toxicol. 128 (3), 394–409. doi:10.1111/bcpt.13535
Li, L., Li, Q., Huang, W., Han, Y., Tan, H., An, M., et al. (2021b). Dapagliflozin alleviates hepatic steatosis by restoring autophagy via the AMPK-mTOR pathway. Front. Pharmacol. 12, 589273. doi:10.3389/fphar.2021.589273
Li, T., Fang, T., Xu, L., Liu, X., Li, X., Xue, M., et al. (2020). Empagliflozin alleviates hepatic steatosis by activating the AMPK-TET2-autophagy pathway in vivo and in vitro. Front. Pharmacol. 11, 622153. doi:10.3389/fphar.2020.622153
Li, X., Park, S. J., Jin, F., Deng, Y., Yang, J. H., Chang, J. H., et al. (2018). Tanshinone IIA suppresses FcεRI-mediated mast cell signaling and anaphylaxis by activation of the Sirt1/LKB1/AMPK pathway. Biochem. Pharmacol. 152, 362–372. doi:10.1016/j.bcp.2018.04.015
Liu, W., Jiang, Y., Sun, J., Geng, S., Pan, Z., Prinz, R. A., et al. (2018). Activation of TGF-beta-activated kinase 1 (TAK1) restricts Salmonella Typhimurium growth by inducing AMPK activation and autophagy. Cell Death Dis. 9 (5), 570. doi:10.1038/s41419-018-0612-z
Liu, X., Xu, C., Xu, L., Li, X., Sun, H., Xue, M., et al. (2020). Empagliflozin improves diabetic renal tubular injury by alleviating mitochondrial fission via AMPK/SP1/PGAM5 pathway. Metabolism. 111, 154334. doi:10.1016/j.metabol.2020.154334
Logie, L., Lees, Z., Allwood, J. W., McDougall, G., Beall, C., and Rena, G. (2018). Regulation of hepatic glucose production and AMPK by AICAR but not by metformin depends on drug uptake through the equilibrative nucleoside transporter 1 (ENT1). Diabetes Obes. Metab. 20 (12), 2748–2758. doi:10.1111/dom.13455
Lou, Z. L., Zhang, C. X., Li, J. F., Chen, R. H., Wu, W. J., Hu, X. F., et al. (2021). Apelin/APJ-manipulated CaMKK/AMPK/GSK3β signaling works as an endogenous counterinjury mechanism in promoting the vitality of random-pattern skin flaps. Oxid. Med. Cell. Longev. 2021, 8836058. doi:10.1155/2021/8836058
Lu, Q., Liu, J., Li, X., Sun, X., Zhang, J., Ren, D., et al. (2020a). Empagliflozin attenuates ischemia and reperfusion injury through LKB1/AMPK signaling pathway. Mol. Cell. Endocrinol. 501, 110642. doi:10.1016/j.mce.2019.110642
Lu, X., Xuan, W., Li, J., Yao, H., Huang, C., and Li, J. (2021). AMPK protects against alcohol-induced liver injury through UQCRC2 to up-regulate mitophagy. Autophagy 17 (11), 3622–3643. doi:10.1080/15548627.2021.1886829
Lu, Y. H., Chang, Y. P., Li, T., Han, F., Li, C. J., Li, X. Y., et al. (2020b). Empagliflozin attenuates hyperuricemia by upregulation of ABCG2 via AMPK/AKT/CREB signaling pathway in type 2 diabetic mice. Int. J. Biol. Sci. 16 (3), 529–542. doi:10.7150/ijbs.33007
Ma, W., Cai, Y., Shen, Y., Chen, X., Zhang, L., Ji, Y., et al. (2021). HDAC4 knockdown alleviates denervation-induced muscle atrophy by inhibiting myogenin-dependent atrogene activation. Front. Cell. Neurosci. 15, 663384. doi:10.3389/fncel.2021.663384
Madhavi, Y. V., Gaikwad, N., Yerra, V. G., Kalvala, A. K., Nanduri, S., and Kumar, A. (2019). Targeting AMPK in diabetes and diabetic complications: Energy homeostasis, autophagy and mitochondrial health. Curr. Med. Chem. 26 (27), 5207–5229. doi:10.2174/0929867325666180406120051
Magnani, F., and Mattevi, A. (2019). Structure and mechanisms of ROS generation by NADPH oxidases. Curr. Opin. Struct. Biol. 59, 91–97. doi:10.1016/j.sbi.2019.03.001
Marcelo, K. L., Means, A. R., and York, B. (2016). The Ca(2+)/calmodulin/CaMKK2 Axis: Nature's metabolic CaMshaft. Trends Endocrinol. Metab. 27 (10), 706–718. doi:10.1016/j.tem.2016.06.001
Meng, Q., Qi, X., Fu, Y., Chen, Q., Cheng, P., Yu, X., et al. (2020). Flavonoids extracted from mulberry (Morus alba L.) leaf improve skeletal muscle mitochondrial function by activating AMPK in type 2 diabetes. J. Ethnopharmacol. 248, 112326. doi:10.1016/j.jep.2019.112326
Mishra, P., Carelli, V., Manfredi, G., and Chan, D. C. (2014). Proteolytic cleavage of Opa1 stimulates mitochondrial inner membrane fusion and couples fusion to oxidative phosphorylation. Cell Metab. 19 (4), 630–641. doi:10.1016/j.cmet.2014.03.011
Mishra, P., and Chan, D. C. (2016). Metabolic regulation of mitochondrial dynamics. J. Cell Biol. 212 (4), 379–387. doi:10.1083/jcb.201511036
Mo, Y., Zhu, J. L., Jiang, A., Zhao, J., Ye, L., and Han, B. (2019). Compound 13 activates AMPK-Nrf2 signaling to protect neuronal cells from oxygen glucose deprivation-reoxygenation. Aging (Albany NY) 11 (24), 12032–12042. doi:10.18632/aging.102534
Momcilovic, M., Hong, S. P., and Carlson, M. (2006). Mammalian TAK1 activates Snf1 protein kinase in yeast and phosphorylates AMP-activated protein kinase in vitro. J. Biol. Chem. 281 (35), 25336–25343. doi:10.1074/jbc.M604399200
Mukhopadhyay, H., and Lee, N. Y. (2020). Multifaceted roles of TAK1 signaling in cancer. Oncogene 39 (7), 1402–1413. doi:10.1038/s41388-019-1088-8
Mungai, P. T., Waypa, G. B., Jairaman, A., Prakriya, M., Dokic, D., Ball, M. K., et al. (2011). Hypoxia triggers AMPK activation through reactive oxygen species-mediated activation of calcium release-activated calcium channels. Mol. Cell. Biol. 31 (17), 3531–3545. doi:10.1128/MCB.05124-11
Musi, N., Hirshman, M. F., Nygren, J., Svanfeldt, M., Bavenholm, P., Rooyackers, O., et al. (2002). Metformin increases AMP-activated protein kinase activity in skeletal muscle of subjects with type 2 diabetes. Diabetes 51 (7), 2074–2081. doi:10.2337/diabetes.51.7.2074
Myers, R. W., Guan, H. P., Ehrhart, J., Petrov, A., Prahalada, S., Tozzo, E., et al. (2017). Systemic pan-AMPK activator MK-8722 improves glucose homeostasis but induces cardiac hypertrophy. Science 357 (6350), 507–511. doi:10.1126/science.aah5582
Ngoei, K. R. W., Langendorf, C. G., Ling, N. X. Y., Hoque, A., Varghese, S., Camerino, M. A., et al. (2018). Structural determinants for small-molecule activation of skeletal muscle AMPK α2β2γ1 by the glucose importagog SC4. Cell Chem. Biol. 25 (6), 728–737. doi:10.1016/j.chembiol.2018.03.008
Nichenko, A. S., Southern, W. M., Atuan, M., Luan, J., Peissig, K. B., Foltz, S. J., et al. (2016). Mitochondrial maintenance via autophagy contributes to functional skeletal muscle regeneration and remodeling. Am. J. Physiol. Cell Physiol. 311 (2), C190–C200. doi:10.1152/ajpcell.00066.2016
Niu, W., Wang, H., Wang, B., Mao, X., and Du, M. (2021). Resveratrol improves muscle regeneration in obese mice through enhancing mitochondrial biogenesis. J. Nutr. Biochem. 98, 108804. doi:10.1016/j.jnutbio.2021.108804
Nunnari, J., and Suomalainen, A. (2012). Mitochondria: In sickness and in health. Cell 148 (6), 1145–1159. doi:10.1016/j.cell.2012.02.035
Olivier, S., Foretz, M., and Viollet, B. (2018). Promise and challenges for direct small molecule AMPK activators. Biochem. Pharmacol. 153, 147–158. doi:10.1016/j.bcp.2018.01.049
Onishi, M., Yamano, K., Sato, M., Matsuda, N., and Okamoto, K. (2021). Molecular mechanisms and physiological functions of mitophagy. EMBO J. 40 (3), e104705. doi:10.15252/embj.2020104705
Otera, H., Miyata, N., Kuge, O., and Mihara, K. (2016). Drp1-dependent mitochondrial fission via MiD49/51 is essential for apoptotic cristae remodeling. J. Cell Biol. 212 (5), 531–544. doi:10.1083/jcb.201508099
Pang, T., Zhang, Z. S., Gu, M., Qiu, B. Y., Yu, L. F., Cao, P. R., et al. (2008). Small molecule antagonizes autoinhibition and activates AMP-activated protein kinase in cells. J. Biol. Chem. 283 (23), 16051–16060. doi:10.1074/jbc.M710114200
Pei, S., Minhajuddin, M., Adane, B., Khan, N., Stevens, B. M., Mack, S. C., et al. (2018). AMPK/FIS1-Mediated mitophagy is required for self-renewal of human AML stem cells. Cell Stem Cell 23 (1), 86–100. doi:10.1016/j.stem.2018.05.021
Perillo, B., Di Donato, M., Pezone, A., Di Zazzo, E., Giovannelli, P., Galasso, G., et al. (2020). ROS in cancer therapy: The bright side of the moon. Exp. Mol. Med. 52 (2), 192–203. doi:10.1038/s12276-020-0384-2
Pickles, S., Vigie, P., and Youle, R. J. (2018). Mitophagy and quality control mechanisms in mitochondrial maintenance. Curr. Biol. 28 (4), R170–R185. doi:10.1016/j.cub.2018.01.004
Pinkosky, S. L., Newton, R. S., Day, E. A., Ford, R. J., Lhotak, S., Austin, R. C., et al. (2016). Liver-specific ATP-citrate lyase inhibition by bempedoic acid decreases LDL-C and attenuates atherosclerosis. Nat. Commun. 7, 13457. doi:10.1038/ncomms13457
Popov, L. D. (2020). Mitochondrial biogenesis: An update. J. Cell. Mol. Med. 24 (9), 4892–4899. doi:10.1111/jcmm.15194
Powers, S. K., Ozdemir, M., and Hyatt, H. (2020). Redox control of proteolysis during inactivity-induced skeletal muscle atrophy. Antioxid. Redox Signal. 33 (8), 559–569. doi:10.1089/ars.2019.8000
Qaisar, R., Bhaskaran, S., and Van Remmen, H. (2016). Muscle fiber type diversification during exercise and regeneration. Free Radic. Biol. Med. 98, 56–67. doi:10.1016/j.freeradbiomed.2016.03.025
Qualls, A. E., Southern, W. M., and Call, J. A. (2021). Mitochondria-cytokine crosstalk following skeletal muscle injury and disuse: A mini-review. Am. J. Physiol. Cell Physiol. 320 (5), C681–C688. doi:10.1152/ajpcell.00462.2020
Quattrocelli, M., Wintzinger, M., Miz, K., Levine, D. C., Peek, C. B., Bass, J., et al. (2022). Muscle mitochondrial remodeling by intermittent glucocorticoid drugs requires an intact circadian clock and muscle PGC1α. Sci. Adv. 8 (7), eabm1189. doi:10.1126/sciadv.abm1189
Rahman, F. A., and Quadrilatero, J. (2021a). Emerging role of mitophagy in myoblast differentiation and skeletal muscle remodeling. Semin. Cell Dev. Biol. doi:10.1016/j.semcdb.2021.11.026
Rahman, F. A., and Quadrilatero, J. (2021b). Mitochondrial network remodeling: An important feature of myogenesis and skeletal muscle regeneration. Cell. Mol. Life Sci. 78 (10), 4653–4675. doi:10.1007/s00018-021-03807-9
Rameshrad, M., Soraya, H., Maleki-Dizaji, N., Vaez, H., and Garjani, A. (2016). A-769662, a direct AMPK activator, attenuates lipopolysaccharide-induced acute heart and lung inflammation in rats. Mol. Med. Rep. 13 (3), 2843–2849. doi:10.3892/mmr.2016.4821
Rena, G., Hardie, D. G., and Pearson, E. R. (2017). The mechanisms of action of metformin. Diabetologia 60 (9), 1577–1585. doi:10.1007/s00125-017-4342-z
Roca-Agujetas, V., de Dios, C., Leston, L., Mari, M., Morales, A., and Colell, A. (2019). Recent insights into the mitochondrial role in autophagy and its regulation by oxidative stress. Oxid. Med. Cell. Longev. 2019, 3809308. doi:10.1155/2019/3809308
Romanello, V., and Sandri, M. (2021). The connection between the dynamic remodeling of the mitochondrial network and the regulation of muscle mass. Cell. Mol. Life Sci. 78 (4), 1305–1328. doi:10.1007/s00018-020-03662-0
Ross, F. A., MacKintosh, C., and Hardie, D. G. (2016). AMP-Activated protein kinase: A cellular energy sensor that comes in 12 flavours. FEBS J. 283 (16), 2987–3001. doi:10.1111/febs.13698
Ruprecht, J. J., King, M. S., Zogg, T., Aleksandrova, A. A., Pardon, E., Crichton, P. G., et al. (2019). The molecular mechanism of transport by the mitochondrial ADP/ATP carrier. Cell 176 (3), 435–447. doi:10.1016/j.cell.2018.11.025
Sabbir, M. G., Taylor, C. G., and Zahradka, P. (2021). CAMKK2 regulates mitochondrial function by controlling succinate dehydrogenase expression, post-translational modification, megacomplex assembly, and activity in a cell-type-specific manner. Cell Commun. Signal. 19 (1), 98. doi:10.1186/s12964-021-00778-z
Sabina, R. L., Patterson, D., and Holmes, E. W. (1985). 5-Amino-4-imidazolecarboxamide riboside (Z-riboside) metabolism in eukaryotic cells. J. Biol. Chem. 260 (10), 6107–6114. doi:10.1016/s0021-9258(18)88943-8
Sabouny, R., and Shutt, T. E. (2020). Reciprocal regulation of mitochondrial fission and fusion. Trends biochem. Sci. 45 (7), 564–577. doi:10.1016/j.tibs.2020.03.009
Sakamoto, K., McCarthy, A., Smith, D., Green, K. A., Grahame Hardie, D., Ashworth, A., et al. (2005). Deficiency of LKB1 in skeletal muscle prevents AMPK activation and glucose uptake during contraction. EMBO J. 24 (10), 1810–1820. doi:10.1038/sj.emboj.7600667
Sanchez, A. M., Csibi, A., Raibon, A., Cornille, K., Gay, S., Bernardi, H., et al. (2012). AMPK promotes skeletal muscle autophagy through activation of forkhead FoxO3a and interaction with Ulk1. J. Cell. Biochem. 113 (2), 695–710. doi:10.1002/jcb.23399
Sanders, M. J., Grondin, P. O., Hegarty, B. D., Snowden, M. A., and Carling, D. (2007). Investigating the mechanism for AMP activation of the AMP-activated protein kinase cascade. Biochem. J. 403 (1), 139–148. doi:10.1042/BJ20061520
Sartori, R., Romanello, V., and Sandri, M. (2021). Mechanisms of muscle atrophy and hypertrophy: Implications in health and disease. Nat. Commun. 12 (1), 330. doi:10.1038/s41467-020-20123-1
Scarpulla, R. C., Vega, R. B., and Kelly, D. P. (2012). Transcriptional integration of mitochondrial biogenesis. Trends Endocrinol. Metab. 23 (9), 459–466. doi:10.1016/j.tem.2012.06.006
Seabright, A. P., Fine, N. H. F., Barlow, J. P., Lord, S. O., Musa, I., Gray, A., et al. (2020). AMPK activation induces mitophagy and promotes mitochondrial fission while activating TBK1 in a PINK1-Parkin independent manner. FASEB J. 34 (5), 6284–6301. doi:10.1096/fj.201903051R
Seyer, P., Grandemange, S., Busson, M., Carazo, A., Gamaleri, F., Pessemesse, L., et al. (2006). Mitochondrial activity regulates myoblast differentiation by control of c-Myc expression. J. Cell. Physiol. 207 (1), 75–86. doi:10.1002/jcp.20539
Shackelford, D. B., and Shaw, R. J. (2009). The LKB1-AMPK pathway: Metabolism and growth control in tumour suppression. Nat. Rev. Cancer 9 (8), 563–575. doi:10.1038/nrc2676
Shen, Y., Zhang, Q., Huang, Z., Zhu, J., Qiu, J., Ma, W., et al. (2020). Isoquercitrin delays denervated soleus muscle atrophy by inhibiting oxidative stress and inflammation. Front. Physiol. 11, 988. doi:10.3389/fphys.2020.00988
Shen, Y., Zhang, R., Xu, L., Wan, Q., Zhu, J., Gu, J., et al. (2019). Microarray analysis of gene expression provides new insights into denervation-induced skeletal muscle atrophy. Front. Physiol. 10 (1298). doi:10.3389/fphys.2019.01298
Spengler, K., Zibrova, D., Woods, A., Langendorf, C. G., Scott, J. W., Carling, D., et al. (2020). Protein kinase A negatively regulates VEGF-induced AMPK activation by phosphorylating CaMKK2 at serine 495. Biochem. J. 477 (17), 3453–3469. doi:10.1042/BCJ20200555
Stapleton, D., Mitchelhill, K. I., Gao, G., Widmer, J., Michell, B. J., Teh, T., et al. (1996). Mammalian AMP-activated protein kinase subfamily. J. Biol. Chem. 271 (2), 611–614. doi:10.1074/jbc.271.2.611
Sun, H., Qiu, J., Chen, Y., Yu, M., Ding, F., and Gu, X. (2014). Proteomic and bioinformatic analysis of differentially expressed proteins in denervated skeletal muscle. Int. J. Mol. Med. 33 (6), 1586–1596. doi:10.3892/ijmm.2014.1737
Sun, J., Song, F. H., Wu, J. Y., Zhang, L. Q., Li, D. Y., Gao, S. J., et al. (2022). Sestrin2 overexpression attenuates osteoarthritis pain via induction of AMPK/PGC-1α-mediated mitochondrial biogenesis and suppression of neuroinflammation. Brain Behav. Immun. 102, 53–70. doi:10.1016/j.bbi.2022.02.015
Sun, X., Han, F., Lu, Q., Li, X., Ren, D., Zhang, J., et al. (2020). Empagliflozin ameliorates obesity-related cardiac dysfunction by regulating sestrin2-mediated AMPK-mTOR signaling and redox homeostasis in high-fat diet-induced obese mice. Diabetes 69 (6), 1292–1305. doi:10.2337/db19-0991
Theilen, N. T., Kunkel, G. H., and Tyagi, S. C. (2017). The role of exercise and TFAM in preventing skeletal muscle atrophy. J. Cell. Physiol. 232 (9), 2348–2358. doi:10.1002/jcp.25737
Thomson, D. M., and Gordon, S. E. (2005). Diminished overload-induced hypertrophy in aged fast-twitch skeletal muscle is associated with AMPK hyperphosphorylation. J. Appl. Physiol. 98 (2), 557–564. doi:10.1152/japplphysiol.00811.2004
Thomson, D. M. (2018). The role of AMPK in the regulation of skeletal muscle size, hypertrophy, and regeneration. Int. J. Mol. Sci. 19 (10), E3125. doi:10.3390/ijms19103125
Tian, J., Zhang, M., Suo, M., Liu, D., Wang, X., Liu, M., et al. (2021). Dapagliflozin alleviates cardiac fibrosis through suppressing EndMT and fibroblast activation via AMPKα/TGF-β/Smad signalling in type 2 diabetic rats. J. Cell. Mol. Med. 25 (16), 7642–7659. doi:10.1111/jcmm.16601
Timm, K. N., and Tyler, D. J. (2020). The role of AMPK activation for cardioprotection in doxorubicin-induced cardiotoxicity. Cardiovasc. Drugs Ther. 34 (2), 255–269. doi:10.1007/s10557-020-06941-x
Toyama, E. Q., Herzig, S., Courchet, J., Lewis, T. L., Loson, O. C., Hellberg, K., et al. (2016). Metabolism. AMP-activated protein kinase mediates mitochondrial fission in response to energy stress. Science 351 (6270), 275–281. doi:10.1126/science.aab4138
Trewin, A. J., Berry, B. J., and Wojtovich, A. P. (2018). Exercise and mitochondrial dynamics: Keeping in shape with ROS and AMPK. Antioxidants (Basel) 7 (1). doi:10.3390/antiox7010007
Tsogbadrakh, B., Ryu, H., Ju, K. D., Lee, J., Yun, S., Yu, K. S., et al. (2019). AICAR, an AMPK activator, protects against cisplatin-induced acute kidney injury through the JAK/STAT/SOCS pathway. Biochem. Biophys. Res. Commun. 509 (3), 680–686. doi:10.1016/j.bbrc.2018.12.159
Visnjic, D., Lalic, H., Dembitz, V., Tomic, B., and Smoljo, T. (2021). AICAr, a widely used AMPK activator with important AMPK-independent effects: A systematic review. Cells 10 (5), 1095. doi:10.3390/cells10051095
Vlavcheski, F., Den Hartogh, D. J., Giacca, A., and Tsiani, E. (2020). Amelioration of high-insulin-induced skeletal muscle cell insulin resistance by resveratrol is linked to activation of AMPK and restoration of GLUT4 translocation. Nutrients 12 (4), E914. doi:10.3390/nu12040914
Wang, C., Huang, B., Sun, L., Wang, X., Zhou, B., Tang, H., et al. (2021a). MK8722, an AMPK activator, inhibiting carcinoma proliferation, invasion and migration in human pancreatic cancer cells. Biomed. Pharmacother. 144, 112325. doi:10.1016/j.biopha.2021.112325
Wang, N., Wang, L., Yang, J., Wang, Z., and Cheng, L. (2021b). Quercetin promotes osteogenic differentiation and antioxidant responses of mouse bone mesenchymal stem cells through activation of the AMPK/SIRT1 signaling pathway. Phytotherapy Res. 35, 2639–2650. doi:10.1002/ptr.7010
Wang, W., Li, M., Chen, Z., Xu, L., Chang, M., Wang, K., et al. (2022a). Biogenesis and function of extracellular vesicles in pathophysiological processes of skeletal muscle atrophy. Biochem. Pharmacol. 198, 114954. doi:10.1016/j.bcp.2022.114954
Wang, W., Shen, D., Zhang, L., Ji, Y., Xu, L., Chen, Z., et al. (2022b). SKP-SC-EVs mitigate denervated muscle atrophy by inhibiting oxidative stress and inflammation and improving microcirculation. Antioxidants (Basel) 11 (1), 66. doi:10.3390/antiox11010066
Wang, Y., An, H., Liu, T., Qin, C., Sesaki, H., Guo, S., et al. (2019). Metformin improves mitochondrial respiratory activity through activation of AMPK. Cell Rep. 29 (6), 1511–1523. e1515. doi:10.1016/j.celrep.2019.09.070
Wang, Y., Ding, Y., Sun, P., Zhang, W., Xin, Q., Wang, N., et al. (2022c). Empagliflozin-enhanced antioxidant defense attenuates lipotoxicity and protects hepatocytes by promoting FoxO3a- and nrf2-mediated nuclear translocation via the CAMKK2/AMPK pathway. Antioxidants (Basel) 11 (5), 799. doi:10.3390/antiox11050799
Weihrauch, M., and Handschin, C. (2018). Pharmacological targeting of exercise adaptations in skeletal muscle: Benefits and pitfalls. Biochem. Pharmacol. 147, 211–220. doi:10.1016/j.bcp.2017.10.006
Wen, W., Chen, X., Huang, Z., Chen, D., Chen, H., Luo, Y., et al. (2020). Resveratrol regulates muscle fiber type conversion via miR-22-3p and AMPK/SIRT1/PGC-1α pathway. J. Nutr. Biochem. 77, 108297. doi:10.1016/j.jnutbio.2019.108297
Williams, J. A., and Ding, W. X. (2018). Mechanisms, pathophysiological roles and methods for analyzing mitophagy - recent insights. Biol. Chem. 399 (2), 147–178. doi:10.1515/hsz-2017-0228
Williams, J. N., and Sankar, U. (2019). CaMKK2 signaling in metabolism and skeletal disease: A new Axis with therapeutic potential. Curr. Osteoporos. Rep. 17 (4), 169–177. doi:10.1007/s11914-019-00518-w
Wu, C., Tang, L., Ni, X., Xu, T., Fang, Q., Xu, L., et al. (2019). Salidroside attenuates denervation-induced skeletal muscle atrophy through negative regulation of pro-inflammatory cytokine. Front. Physiol. 10 (665). doi:10.3389/fphys.2019.00665
Wu, Q., Zhu, J., Liu, F., Liu, J., and Li, M. (2018). Downregulation of 14-3-3β inhibits proliferation and migration in osteosarcoma cells. Mol. Med. Rep. 17 (2), 2493–2500. doi:10.3892/mmr.2017.8144
Wu, S., and Zou, M. H. (2020). AMPK, mitochondrial function, and cardiovascular disease. Int. J. Mol. Sci. 21 (14), E4987. doi:10.3390/ijms21144987
Xiao, B., Sanders, M. J., Underwood, E., Heath, R., Mayer, F. V., Carmena, D., et al. (2011). Structure of mammalian AMPK and its regulation by ADP. Nature 472 (7342), 230–233. doi:10.1038/nature09932
Xu, Y., Yu, T., Ma, G., Zheng, L., Jiang, X., Yang, F., et al. (2021). Berberine modulates deacetylation of PPARγ to promote adipose tissue remodeling and thermogenesis via AMPK/SIRT1 pathway. Int. J. Biol. Sci. 17 (12), 3173–3187. doi:10.7150/ijbs.62556
Yan, Y., Zhou, X. E., Xu, H. E., and Melcher, K. (2018). Structure and physiological regulation of AMPK. Int. J. Mol. Sci. 19 (11), E3534. doi:10.3390/ijms19113534
Yang, F., Qin, Y., Wang, Y., Meng, S., Xian, H., Che, H., et al. (2019). Metformin inhibits the NLRP3 inflammasome via AMPK/mTOR-dependent effects in diabetic cardiomyopathy. Int. J. Biol. Sci. 15 (5), 1010–1019. doi:10.7150/ijbs.29680
Yang, S., and Lian, G. (2020). ROS and diseases: Role in metabolism and energy supply. Mol. Cell. Biochem. 467 (1-2), 1–12. doi:10.1007/s11010-019-03667-9
Yang, X., Liu, Q., Li, Y., Tang, Q., Wu, T., Chen, L., et al. (2020). The diabetes medication canagliflozin promotes mitochondrial remodelling of adipocyte via the AMPK-Sirt1-Pgc-1α signalling pathway. Adipocyte 9 (1), 484–494. doi:10.1080/21623945.2020.1807850
Yang, Z., Wang, X., He, Y., Qi, L., Yu, L., Xue, B., et al. (2012). The full capacity of AICAR to reduce obesity-induced inflammation and insulin resistance requires myeloid SIRT1. PLoS One 7 (11), e49935. doi:10.1371/journal.pone.0049935
Ye, C., Zhang, D., Zhao, L., Li, Y., Yao, X., Wang, H., et al. (2016). CaMKK2 suppresses muscle regeneration through the inhibition of myoblast proliferation and differentiation. Int. J. Mol. Sci. 17 (10), E1695. doi:10.3390/ijms17101695
You, J. S., and Chen, J. (2021). Aging does not exacerbate muscle loss during denervation and lends unique muscle-specific atrophy resistance with Akt activation. Front. Physiol. 12, 779547. doi:10.3389/fphys.2021.779547
Yu, H., Hong, X., Liu, L., Wu, Y., Xie, X., Fang, G., et al. (2021). Cordycepin decreases ischemia/reperfusion injury in diabetic hearts via upregulating AMPK/Mfn2-dependent mitochondrial fusion. Front. Pharmacol. 12, 754005. doi:10.3389/fphar.2021.754005
Yu, X., Meng, Z., Fang, T., Liu, X., Cheng, Y., Xu, L., et al. (2022). Empagliflozin inhibits hepatic gluconeogenesis and increases glycogen synthesis by AMPK/CREB/GSK3β signalling pathway. Front. Physiol. 13, 817542. doi:10.3389/fphys.2022.817542
Yun, S. M., Jung, J. H., Jeong, S. J., Sohn, E. J., Kim, B., and Kim, S. H. (2014). Tanshinone IIA induces autophagic cell death via activation of AMPK and ERK and inhibition of mTOR and p70 S6K in KBM-5 leukemia cells. Phytother. Res. 28 (3), 458–464. doi:10.1002/ptr.5015
Zadra, G., Photopoulos, C., Tyekucheva, S., Heidari, P., Weng, Q. P., Fedele, G., et al. (2014). A novel direct activator of AMPK inhibits prostate cancer growth by blocking lipogenesis. EMBO Mol. Med. 6 (4), 519–538. doi:10.1002/emmm.201302734
Zammit, P. S. (2017). Function of the myogenic regulatory factors Myf5, MyoD, Myogenin and MRF4 in skeletal muscle, satellite cells and regenerative myogenesis. Semin. Cell Dev. Biol. 72, 19–32. doi:10.1016/j.semcdb.2017.11.011
Zhang, C. S., and Lin, S. C. (2016). AMPK promotes autophagy by facilitating mitochondrial fission. Cell Metab. 23 (3), 399–401. doi:10.1016/j.cmet.2016.02.017
Zhang, D. H., Yin, H. D., Li, J. J., Wang, Y., Yang, C. W., Jiang, X. S., et al. (2020). KLF5 regulates chicken skeletal muscle atrophy via the canonical Wnt/β-catenin signaling pathway. Exp. Anim. 69 (4), 430–440. doi:10.1538/expanim.20-0046
Zhang, F., Xie, J., Wang, G., Zhang, G., and Yang, H. (2018). Anti-osteoporosis activity of Sanguinarine in preosteoblast MC3T3-E1 cells and an ovariectomized rat model. J. Cell. Physiol. 233 (6), 4626–4633. doi:10.1002/jcp.26187
Zhang, H. S., Chen, X. Y., Wu, T. C., and Zhang, F. J. (2014). Tanshinone II A inhibits tat-induced HIV-1 transactivation through redox-regulated AMPK/Nampt pathway. J. Cell. Physiol. 229 (9), 1193–1201. doi:10.1002/jcp.24552
Zhang, X., Wang, Q., Wang, X., Chen, X., Shao, M., Zhang, Q., et al. (2019). Tanshinone IIA protects against heart failure post-myocardial infarction via AMPKs/mTOR-dependent autophagy pathway. Biomed. Pharmacother. 112, 108599. doi:10.1016/j.biopha.2019.108599
Zhao, H., Zhu, H., Lin, Z., Lin, G., and Lv, G. (2015). Compound 13, an α1-selective small molecule activator of AMPK, inhibits Helicobacter pylori-induced oxidative stresses and gastric epithelial cell apoptosis. Biochem. Biophys. Res. Commun. 463 (4), 510–517. doi:10.1016/j.bbrc.2015.05.059
Zhao, Y., Hu, X., Liu, Y., Dong, S., Wen, Z., He, W., et al. (2017). ROS signaling under metabolic stress: Cross-talk between AMPK and AKT pathway. Mol. Cancer 16 (1), 79. doi:10.1186/s12943-017-0648-1
Zheng, Q., Zhao, Y., Guo, J., Zhao, S., Fei, C., Xiao, C., et al. (2018). Iron overload promotes mitochondrial fragmentation in mesenchymal stromal cells from myelodysplastic syndrome patients through activation of the AMPK/MFF/Drp1 pathway. Cell Death Dis. 9 (5), 515. doi:10.1038/s41419-018-0552-7
Zhou, J., Zhu, J., Yu, S. J., Ma, H. L., Chen, J., Ding, X. F., et al. (2020). Sodium-glucose co-transporter-2 (SGLT-2) inhibition reduces glucose uptake to induce breast cancer cell growth arrest through AMPK/mTOR pathway. Biomed. Pharmacother. 132, 110821. doi:10.1016/j.biopha.2020.110821
Zhou, Q., Xu, H., Yan, L., Ye, L., Zhang, X., Tan, B., et al. (2021). PGC-1α promotes mitochondrial respiration and biogenesis during the differentiation of hiPSCs into cardiomyocytes.. Genes Dis. 8 (6), 891–906. doi:10.1016/j.gendis.2020.12.006
Zhu, L., Lama, S., Tu, L., Dusting, G. J., Wang, J. H., and Liu, G. S. (2021a). TAK1 signaling is a potential therapeutic target for pathological angiogenesis. Angiogenesis 24 (3), 453–470. doi:10.1007/s10456-021-09787-5
Zhu, M., Shen, W., Li, J., Jia, N., Xiong, Y., Miao, J., et al. (2022). AMPK activator O304 protects against kidney aging through promoting energy metabolism and autophagy. Front. Pharmacol. 13, 836496. doi:10.3389/fphar.2022.836496
Zhu, Y. R., Zhang, X. Y., Wu, Q. P., Yu, C. J., Liu, Y. Y., and Zhang, Y. Q. (2021b). PF-06409577 activates AMPK signaling and inhibits osteosarcoma cell growth. Front. Oncol. 11, 659181. doi:10.3389/fonc.2021.659181
Keywords: AMPK, mitochondria, skeletal muscle, muscle atrophy, muscle regeneration
Citation: Yan Y, Li M, Lin J, Ji Y, Wang K, Yan D, Shen Y, Wang W, Huang Z, Jiang H, Sun H and Qi L (2022) Adenosine monophosphate activated protein kinase contributes to skeletal muscle health through the control of mitochondrial function. Front. Pharmacol. 13:947387. doi: 10.3389/fphar.2022.947387
Received: 18 May 2022; Accepted: 06 October 2022;
Published: 20 October 2022.
Edited by:
Arild C. Rustan, University of Oslo, NorwayReviewed by:
David C. Clarke, Simon Fraser University, CanadaChristine Skagen, Oslo University Hospital, Norway
Copyright © 2022 Yan, Li, Lin, Ji, Wang, Yan, Shen, Wang, Huang, Jiang, Sun and Qi. This is an open-access article distributed under the terms of the Creative Commons Attribution License (CC BY). The use, distribution or reproduction in other forums is permitted, provided the original author(s) and the copyright owner(s) are credited and that the original publication in this journal is cited, in accordance with accepted academic practice. No use, distribution or reproduction is permitted which does not comply with these terms.
*Correspondence: Haiyan Jiang, amh5QG50dS5lZHUuY24=; Hualin Sun, c3VuaGxAbnR1LmVkdS5jbg==; Lei Qi, cWlsZWk3MjNAbnR1LmVkdS5jbg==
†These authors have contributed equally to this work