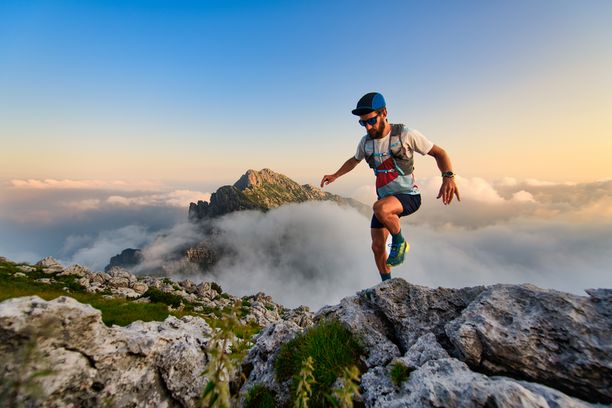
94% of researchers rate our articles as excellent or good
Learn more about the work of our research integrity team to safeguard the quality of each article we publish.
Find out more
REVIEW article
Front. Pharmacol., 15 September 2022
Sec. Pharmacology of Anti-Cancer Drugs
Volume 13 - 2022 | https://doi.org/10.3389/fphar.2022.946811
This article is part of the Research TopicNew Anti-Cancer Strategies Targeting Epigenetic Modifications and Associated Metabolism ReprogrammingView all 10 articles
Dysregulated epigenetic enzymes and resultant abnormal epigenetic modifications (EMs) have been suggested to be closely related to tumor occurrence and progression. Histone modifications (HMs) can assist in maintaining genome stability, DNA repair, transcription, and chromatin modulation within breast cancer (BC) cells. In addition, HMs are reversible, dynamic processes involving the associations of different enzymes with molecular compounds. Abnormal HMs (e.g. histone methylation and histone acetylation) have been identified to be tightly related to BC occurrence and development, even though their underlying mechanisms remain largely unclear. EMs are reversible, and as a result, epigenetic enzymes have aroused wide attention as anti-tumor therapeutic targets. At present, treatments to restore aberrant EMs within BC cells have entered preclinical or clinical trials. In addition, no existing studies have comprehensively analyzed aberrant HMs within BC cells; in addition, HM-targeting BC treatments remain to be further investigated. Histone and non-histone protein methylation is becoming an attractive anti-tumor epigenetic therapeutic target; such methylation-related enzyme inhibitors are under development at present. Consequently, the present work focuses on summarizing relevant studies on HMs related to BC and the possible mechanisms associated with abnormal HMs. Additionally, we also aim to analyze existing therapeutic agents together with those drugs approved and tested through pre-clinical and clinical trials, to assess their roles in HMs. Moreover, epi-drugs that target HMT inhibitors and HDAC inhibitors should be tested in preclinical and clinical studies for the treatment of BC. Epi-drugs that target histone methylation (HMT inhibitors) and histone acetylation (HDAC inhibitors) have now entered clinical trials or are approved by the US Food and Drug Administration (FDA). Therefore, the review covers the difficulties in applying HM-targeting treatments in clinics and proposes feasible approaches for overcoming such difficulties and promoting their use in treating BC cases.
Breast cancer (BC) accounts for a highly frequent malignancy in the female population (Winters et al., 2017; Hiatt et al., 2022). According to the statistics from the World Health Organization (WHO), BC occupies 11.7% of the overall cancer patients and takes up 6% of the overall death cases. BC displays highly variable intra-tumor and inter-tumor characteristics, cancer stages when the patient is diagnosed and morphologies; as a result, it remains a challenge to effectively treat cancer and predict patient survival. In the past 10 years, BC survival shows an increasing trend due to early screening and improvement in treatment, but its 10-year survival remains unsatisfactory (80%) (Caplan, 2014). In China, a study finds differences between high-income nations and China, which discovers that the Chinese are associated with a young age at BC onset, low BC screening rate, one-child policy, delayed BC diagnosis inducing late/advanced stage when they present with symptoms, insufficient medical resources, and the low consciousness of BC (Fan et al., 2014). Consequently, it is necessary to develop new treatments. Hormone receptors (HR), in particular, progesterone receptor (PR) and estrogen receptor (ER), have important effects on BC occurrence and development (Trabert et al., 2020). Different BC subtypes are associated with different molecular and histological features, growth rates, and endocrine therapy/chemotherapy responses. Consequently, treatments are selected based on ER/PR/human epidermal growth factor receptor 2 (HER2) expression status, tumor size and grade, lymph node metastasis (LNM), and distant metastasis (DM) (Chlebowski and Anderson, 2012).
Epigenetic and genetic alterations are suggested to have a critical effect on various cell processes such as imprinting, X chromosome inactivation, chromatin remodeling, and tumorigenesis (Han et al., 2016; Yang et al., 2021a). As for epigenetic alterations, their frequently seen subtypes are histone modifications (HMs). Epigenetic alterations can be reversible, which is different from genetic mutations; as a result, they are the safer options for anti-BC treatment (Li et al., 2021). In chromatin-associated processes such as gene modulation, histone post-translational modifications (PTMs) have an essential effect, since hub histones H2A-H2B and H3-H4 are wrapped by the 147-bp DNA fragment, forming the fundamental chromatin unit (Talbert and Henikoff, 2021). HMs have been extensively studied from diverse perspectives, but it is still necessary to understand the aforementioned processes for the sake of clarifying HMs’ functions and the related enzymatic mechanisms underlying BC. Currently, over 23 classes of HMs have been identified, but just a low portion of them are associated with BC. Therefore, the present review aims to analyze histone methylation acetylation, the most extensively investigated class. Any dysregulation in the aforementioned processes induces imbalanced gene levels within BC and results in abnormalities in cell growth, migration, invasion, and treatment resistance (Byler et al., 2014; Pasculli et al., 2018).
Multidisciplinary consultation is needed in BC treatment. The most updated treatments are surgical treatment, chemotherapy, radiotherapy, and molecularly-targeted endocrine therapy, which are selected based on the BC subtype. Recently, great efforts have been made to improve targeted therapy, especially for bevacizumab-targeting vascular endothelial growth factor (VEGF) and trastuzumab (herceptin)-targeting HER2, both of which are approved (Robert et al., 2011). Epigenetic alterations have been suggested over gene mutation because of reversibility. Epigenetic modifications (EMs) are established and maintained according to special enzyme activities, histone deacetylases together with histone methyltransferases, and they are the major targets for epigenetic treatment (Qin et al., 2019a). Epigenetic treatments that use the aforementioned enzyme inhibitors can suppress tumorigenesis (Yang et al., 2021b).
The present work aims to summarize the relevant information regarding the importance of highly abundant post-translational modifications within BC, H3Kme, H4Kme, and H3Kac for BC occurrence, migration, and prognosis. Particularly, we highlight the histone marker status within BC subtypes, and the impacts on transcriptionally regulating certain genes, erasers, and writers. We also examine the effect of histone H3K and H3K-specific methyltransferase on BC and analyze the functions of histone methyltransferases (HMTs) and histone acetyltransferases (HATs) in drug-resistant cancer, together with their relevant mechanisms. Methods to diagnose and predict prognosis based on epigenetics make great contributions to precision oncology. Some approaches to diagnose DNA methylation have been applied clinically or entered clinical trials (Cowan et al., 2010). Great efforts have been made to compensate for the abnormal epigenetic mechanisms in precision oncology, which facilitate the development of epi-drugs that target epigenetic modulators. This work collects information regarding inhibitors applied in clinical trials from the ClinicalTrials.gov database maintained by the U.S. National Library of Medicine. At present, just nine epi-drugs have been approved by the FDA, including IDH, EZH2, DNA methyltransferases (DNMTs), and histone deacetylases inhibitors (HDACis). Moreover, numerous other drugs are under clinical trials for the treatment of solid tumors (NCT01928576 and NCT03179943) or hematologic tumors (NCT02717884 and NCT03164057). It is to be noted that ER-positive (ER+) BC phase-II trials (NCT00676663, NCT00828854, and NCT04190056) are conducted to test whether epi-drugs plus conventional treatments are effective, which indicates that more is known about the epigenetic mechanisms governing the development, migration, and drug resistance of ER+ BC. This section will discuss the efficacy and mechanism of action of certain DNMT and HDAC inhibitors in treating cancers.
Histone methylation may take place in arginine and lysine residues and involves complicated modifications compared with acetylation. Lysine is mono-, di-, or trimethylated, whereas arginine is asymmetrically or symmetrically methylated (Barski et al., 2007). As a reversible process, histone methylation can be strictly modulated by different demethylases (KDMs) and methyltransferases (KMTs). A portion of such markers (H3K4, H3K36, and H3K79) is related to activation at the transcriptional level, while others (H3K9, H3K27, and H4K20) are linked to suppression at the transcriptional level (Jenuwein and Allis, 2001). Figure 1 summarizes the specific targets identified for diverse HM classes.
In eukaryotic cells, chromatin is the complex formed by DNA and histones. The basic functional unit of chromatin is the nucleosome that contains a histone octamer (H2A, H2B, H3, and H4) wrapped by DNA. Histone tails undergo numerous posttranslational modifications, which are deposited by writers, removed by erasers, and read by readers, and may either loosen or tighten DNA-histone binding with active or silent transcription.
H3K4 methylation shows high enrichment levels at transcriptional start sites (TSSs), promoter regions, and enhancer regions. In addition, H3K4me1 exhibits high enrichment levels in enhancer regions (Heintzman et al., 2007) and it can bind to H3K27me3 or H3K27ac, thus marking the suppressive or active enhancers, separately (Creyghton et al., 2010). Different from additional H3K4 methylation showing high enrichment levels in intergenic regions, H3K4me2 can mark the 5′-terminal in transcribed genes (Kim and Buratowski, 2009). H3K4me3 is canonically distributed in actively transcribed gene promoters and poised genes related to differentiation (Santos-Rosa et al., 2002). Set1 can form Complex Proteins Associated with Set1 (COMPASS) in yeast and is the unique enzyme related to every H3K4 methylation (Miller et al., 2001).
For mammals, the KMT2 (MLL) family is the main H3K4 HMT, which contains six members (KMT2A–D, KMT2F, and KMT2G). In addition, within human cells, six Set1 homologies (SET1A–SET1B and MLL1–MLL4) together with five additional H3K4 methyltransferases (SMYD1–SMYD3, SET7/9, and PRDM9) have also been identified (Wang et al., 2015). Moreover, the KMT2 family is classified into three categories according to the containing domain type, including KMT2A–KMT2B (MLL1–MLL2), KMT2C–KMT2D (MLL3–MLL4), together with KMT2F–KMT2G (SETD1A–SETD1B) (Shilatifard, 2008). As revealed by in vitro research, the core complexes of MLL1–MLL2 display mono-, di-, and low tri-methylation activities in cells (Patel et al., 2008). For instance, MLL1 is suggested to be involved in H3K4 methylation within MCF-7 cells in the estrogen-mediated transcription of ER target genes (Jeong et al., 2011). MLL1 is frequently duplicated or over-expressed within BC cells, and as a result, it may be the therapeutic target for BC treatment (Tate et al., 2019). Additionally, MLL1 can accelerate the transcription of TFF1 (the estrogen-dependent gene) by H3K4me1/2 in the enhancer region’s CpG islands and maintains the permissive chromatin architecture to bind to estrogen receptor α (ERα) and the pioneer factor (FOXA1). It results in the relaxation of chromatin for facilitating ERα binding together with its transcription within BC Jeong et al. (2014) H3K4 methyltransferase has been increasingly suggested to participate in BC occurrence. MLL2 shows a certain interaction with ERα and modulates the level of its target, thus mediating BC occurrence (Mo et al., 2006). As reported by Natarajan et al., MLL2 upregulation within BC cells was related to tissue malignancy; meanwhile, MLL2 protein upregulation was also detected in tissues from patients with breast invasive carcinomas (Natarajan et al., 2010). MLL3, a protein with high mutation frequency within BC cells, is also the main factor that regulates the ERα level (Gala et al., 2018). According to recent reports, the upregulation of SETD1A and MLL3 increases the ERα level, thus supporting the growth of tamoxifen-resistant BC. Moreover, according to genome-wide research on histone methylation, MLL3 plays an essential role in H3K4 monomethylation and H3K27 acetylation within the ERα enhancer (Kim et al., 2020). MLL4 and the H3K27 demethylase UTX (KDM6A) synergistically regulate BC growth and migration (Kim et al., 2014). Jin et al. analyzed SETD1A’s effect on tamoxifen-resistant BC. They suggested that SETD1A increased H3K4 methylation and made the chromatin region accessible to ERα targets within ER+ BC cells to activate the ER+ targets, thereby promoting the recruitment of ERα. They further discovered that SETD1A-regulated genes overlapped with specific tamoxifen-resistant genes within ER+ BC cells, which indicated the possible relation of SETD1A with tamoxifen resistance (Jin et al., 2018). SETD1A protein expression in cells increases in other BC subtypes, such as ER+, HER2+, and triple-negative breast cancer (TNBC) relative to healthy breast cells.
SMYD2 upregulation can modulate TNBC development, which predicts dismal patient survival (Li et al., 2018). SMYD3 can upregulate WNT10B (an oncogene) expression while promoting epithelial–mesenchymal transition (EMT), thus facilitating the metastasis of BC (Hamamoto et al., 2006; Fenizia et al., 2019). SET7/9 stabilizes the ER by methylating ER K302 residue, which then effectively recruits and trans-activates target genes to enhance BC occurrence (Subramanian et al., 2008). Additionally, SET7/9 deficiency promotes the cancer stem cell (CSC) features of BC while accelerating EMT, and it is associated with disease resistance, which indicates the tumor suppressor role of SET7/9 within BC (Montenegro et al., 2016).
As reported by Montenegro et al., SETD7 suppressed EMT by upregulating cadherin-1 while downregulating epidermal growth factor receptor (EGFR) and vimentin protein expression (Montenegro et al., 2016). It was evidenced by the overexpression of SETD7 within triple-negative, metastatic MDA-MB-231 cells, downregulation through siRNAs, and inhibited activity by exposing to 50 µM (R)-PFI-2 for a 3-day period within the non-metastatic estrogen receptor α (ERα/ESR1)-positive MCF-7 cells. Furthermore, SETD7 silencing within MCF-7 cells triggered the CSC phenotype (CD44+/CD24-/low) and mammosphere de-differentiation related to cadherin-1 deficiency. Such results conformed to the greater metastatic ability of MCF-7 xenografts after SETD7 silencing (Takemoto et al., 2016).
H3K9 methylation, in particular H3K9me2 and H3K9me3, is usually related to heterochromatin formation and gene suppression (Bannister et al., 2001). Apart from these, H3K9me1 can be expressed around active gene-related TSSs as well (Vavouri and Lehner, 2012). H3K9me1 and H3K9me2 show nuclear and cytoplasmic localization within mammalian cells, whereas H3K9me3 displays nuclear localization only (Towbin et al., 2012). Additionally, histones can be distributed within the cytoplasm before their chromatin assembly due to the action of histone chaperones. Actually, H3K9 shows co-translational mono- and dimethylation by SETDB1 after it is bound to ribosomes (Rivera et al., 2015). Thereafter, cytoplasmic H3 and K9me1 are assembled in the chromatin, and the product is utilized for reinforcing heterochromatin and H3K9me3 as the substrate.
Proteins belonging to the SUV39 family of human beings, including SUV39H1 (KMT1A), SUV39H2 (KMT1B), SETDB1 (KMT1E), SETDB2 (KMT1F), G9a-like protein (GLP1), and G9A (EHMT2), possess the pre-SET (N-SET) and post-SET (C-SET) domains in addition to the SET domain, which can regulate the methylation of H3K9 (Dillon et al., 2005; Wu et al., 2010). Additionally, G9a may produce homodimers or heterodimers for catalyzing H3K9me1 together with H3K9me2 within the euchromatin (Tachibana et al., 2002). In the heterochromatin, such as the pericentromeric regions, SUV39H1 can catalyze H3K9me2, while SUV39H2 catalyzes H3K9me3 (Rea et al., 2000). G9a plays a critical part in BC occurrence (Jin et al., 2022) as shown in Figure 2. Its activation can suppress anticancer genes, thereby promoting BC cell growth and migration. The overexpression of G9a can inhibit hephaestin (HEPH), thus promoting carcinogenesis of BC (Wang et al., 2017a). Additionally, G9a activation can upregulate T-Box2 (TBX2) within BC cells (Crawford et al., 2019). TBX2 overexpression promotes BC cell growth by decreasing p21WAF1 and Cdkn2a (p14Arf and p19Arf within human beings) gene expression. Suppressing G9a expression can downregulate the TBX2 level while suppressing cancer cell growth. As discovered by Zhang et al., G9a suppression induced autophagy by modulating AMPK/mTOR pathways within BC cells (Zhang et al., 2017).
Upregulation of G9a causes mono‐ or di‐methylation to lysine 9 residue of histone 3 (H3K9), resulting in an increase in the expression of TBX2, FBP1, PRC-2, and NF-κB and a decrease in the expression of DKK1, MYC, CDH10, Reptin, CASP1, ZEB2, RARRES8, and E‐cadherin in BC. G9a‐mediated up‐ and downregulation of various genes promotes cell proliferation, invasion, and metastasis and suppresses apoptosis in breast cancers.
Furthermore, SUV39H2 expression is significantly upregulated within basal-like BC, which predicts dismal BC prognostic outcomes (Liu et al., 2015a). Nonetheless, SUV39H2 mutations are detected within BC, suggesting the possibility of polymorphism within BC (Ozdag et al., 2006). TCGA-based bioinformatics analysis was carried out; as a result, SUV39H2, together with additional new genes (DNMT3B, SUV39H1, AURKB, and EZH2) was remarkably upregulated within TN disorders, which was positively related to Ki67 upregulation, tumor grade, and TN status. SUV39H2 upregulation predicted a poor survival time (Pena-Llopis et al., 2016). In ERα-positive cells, ERβ downregulates SUV39H1 and SUV39H2, and decreases the binding of ERα to p53 to abolish the suppressive heterochromatin. At last, ERβ can produce a p53-ERα transcriptional block while further suppressing proliferation and promoting apoptosis (Lu and Katzenellenbogen, 2017).
H3K27 methylation has been frequently recognized as the gene repression hallmark. H3K27me3 can generate extensive domains within the silenced gene promoters (Margueron and Reinberg, 2011). Additionally, H3K27me3 is enriched at poised enhancers along with a low level of H3K4me1 in mouse and human embryonic stem cells (ESCs) (Rada-Iglesias et al., 2011). Due to the upregulation in enhancers and promoters, H3K27me3 has a critical effect on suppressing development-related genes. Apart from upregulation in poised enhancers, H3K27me2 also shows a relation to promoters in repressive and active genes (Barski et al., 2007). Unlike H3K27me2 and H3K27me3, H3K27me1 is distributed at the actively transcribed gene promoters. The PRC2 complex, which can catalyze H3K27 methylation, has four key subunits (Ezh2, Suz12, EED, and RbAP46/48) and shows the preferential methylation of H3K27. Meanwhile, G9a represents the HMT for H3K9me1/me2, which can promote H3K27 monomethylation (Coward et al., 2018).
Some previous immunohistochemical (IHC) studies have identified the relation of H3K27me3 upregulation with luminal A-like tumors. By contrast, H3K27me3 is in a low level in highly proliferative TNBC, basal-like, ER-positive, and luminal B tumors (Holm et al., 2012; Healey et al., 2014). It is interesting that H3K27me3 is related to EZH2 upregulation in TNBC and basal-like BC, indicating the role of enhanced EZH2 activity in functions associated with non-H3K27 methylation, such as specifically regulating ubiquitination and transcription factors (TFs), and protein decomposition inducing tumor genesis and development (Park et al., 2021).
H3K36 methylation within human cells can interact with transcriptional elongation and methylation of H3K9 to maintain the repressive chromatin status after gene transcription in a histone acetylation-independent manner (Fang et al., 2010). Additionally, H3K36me3 can recruit DNA methyltransferase 3A (DNMT3A) for achieving DNA methylation, which is the redundant pathway for inhibiting the false initiation of transcription (Dhayalan et al., 2010). H3K36me2, which is located in gene body regions, remains largely unclear. Nonetheless, H3K36me2 upregulation is suggested to be related to aberrant transcription (Kuo et al., 2011). H3K36 methylation is able to suppress the enzymatic activity of the PRC2 complex, thus preventing PRC2-regulated H3K27 methylation (Yuan et al., 2011). Within mammalian cells, nine H3K36 methyltransferases are discovered, among which, SMYD2, NSD1-3, SETMAR, SETD3, and ASH1L directly catalyze H3K36 mono- and dimethylation, while just testis-specific PRDM9 and SETD2 are able to catalyze H3K36me3 (Eram et al., 2014).
Jeong et al. reported that NSD3 played an important role in epigenetically regulating BC stemness, metastasis, and EMT, indicating its role as a therapeutic target for metastatic BC (Jeong et al., 2021). Other findings indicate that SETD2 alteration-mediated epigenetic modulation and downstream H3K36me3 are involved in the development of breast phyllodes tumor (PT). In PT pathogenesis, SETD2 mutations possibly take place in the early stage (Tsang et al., 2021).
H3K79 methylation shows a high enrichment level within coding regions, which is related to active transcription. H3K79 methylation occurs in the globular domains of histone H3, which is different from additional histone marks present in unstructured histone tails (Nguyen and Zhang, 2011). The aforementioned three H3K79 methylation types involve yeast Dot1 protein and the mammalian homolog DOT1L (Jones et al., 2008). It is intriguing that H3K79 methylation displays trans-tail histone modification with additional histone marks such as H4K16ac and H2B ubiquitination. In yeast, H2B ubiquitination loss reverses H3K79me2 and H3K79me3 (Ng et al., 2002). As revealed by in vitro HMT and structural assays, H2B ubiquitination plays an essential role in DOT1L’s methyltransferase activity (McGinty et al., 2008). H4’s N-terminal tail is needed for the in vitro enzymatic activity of Dot1. Furthermore, H4K16ac upregulation promotes in vivo H3K79 methylation (Altaf et al., 2007), and the latter has been suggested to disrupt transcriptional elongation, DNA damage response, and telomeric silencing (Huyen et al., 2004).
H4K20 methylation represents the suppressive hallmark for histone modification. H4K20me1 is located in the coding region of lowly transcribed genes, which can be enriched within parental nucleosomes during cell division (Sato et al., 2016). H4K20me1/me2 can recruit leucine-rich repeats and WD repeat domain containing 1 (LRWD1) and origin recognition complex subunit 1 (ORC1) in the replication origin for regulating DNA replication (Kuo et al., 2012). It is to be noted that H4K20 methylation can directly recruit L3MBTL1 (a chromatin remodeler protein) for inducing chromatin condensation (Boccuni et al., 2003). SET8 contributes to the mono-methylation of H4K20, and later H4K20me1 is methylated into H4K20me2/me3 gradually via the action of SUV4-20H1/H2 (Jorgensen et al., 2013). Additionally, H4K20 methylation has been suggested to facilitate DNA damage repair, genomic stability, nucleosome turnover, DNA replication, and chromatin compaction (Tardat et al., 2010; Yang et al., 2016). The status of histone methylation marks were mesmerized in Table 1.
Histone H2A and H2B variants are recognized as the mediators of drug resistance and also of drug sensitivity in breast cancer (Nayak et al., 2015). The histone H2A.Z depletion can also be defective in the integrity and stability of the human genome. Rangasamy et al. presented the molecular pathways linking H2A.Z to breast cancer and mechanisms were proposed to explain how the altered H2A.Z led to tumorigenesis (Rangasamy, 2010). However, monoubiquitination of histone H2B at lysine 120 (H2Bub1) has been shown to have key roles in transcription, DNA damage response, and stem cell differentiation (Cole et al., 2015). While globally depleted in breast cancer, H2Bub1 is selectively enriched in the coding region of certain highly expressed genes, including p53 target genes in response to DNA damage, functioning to exercise transcriptional control of these loci (Atanassov et al., 2016).
Dozens of lysine demethylases (KDMs) have been reported to date that are classified into two main groups (Cloos et al., 2006; Klose et al., 2006): the amine-oxidase type lysine-specific demethylases (LSDs) and the highly conserved Jumonji C (JmjC) domain-containing histone KDMs. KMTs and KDMs have both been implicated in oncogenesis. LSD1 can exhibit either pro-tumor or anti-tumor activity in breast cancer development, highlighting a context-dependent role in regulating different biological processes possibly by using different functional domains (Hu et al., 2019a; Fang et al., 2019). JMJD3 has been associated with breast cancer progression. Xun et al showed that ectopic expression of JMJD3 suppresses the stem cell-like characteristics of breast cancer cells (Xun et al., 2017).
KATs can be divided into two types according to their cellular localization, namely, cytoplasmic and nuclear KATs (Han et al., 2016; Trisciuoglio et al., 2018). Among them, nuclear KATs can be further divided according to enzyme transfer mechanisms and structural homology (Table 2). There are five different families discovered to have diverse functions and targets, namely, CREB-binding protein and its paralog p300 (p300/CBP), GCN5-related N-acetyltransferase (GNAT), nuclear receptor coactivator factor (NRCF) family, and MYST (Roth et al., 2001; Fiorentino et al., 2018). For p300/CBP, there are about 100 protein substrates detected, contributing to the acetylation of non-histone and histone proteins such as tumor suppressor protein p53 (Bowers et al., 2010; Simon et al., 2016). Among KATs, the MYST family contains the greatest gene number and shows the highest diversity, which is mainly related to gene silencing and DNA repair (Voss and Thomas, 2009), including MOZ (monocytic leukemia zinc finger protein), Tip60 (Tat-interactive protein), Sas2 (something about silencing), YBF2/Sas3, and MOF. They exhibit the features of one conserved 3-terminal histone acetyltransferase (HAT) domain (that contains a binding site for acetyl-CoA), one helix-turn-helix DNA-binding domain, and one C2HC zinc finger related to HAT catalytic performance (Roth et al., 2001; Brown et al., 2016). The KAT family is associated with great variability in structural characteristics, such as chromodomains, zinc fingers, and PHD fingers (Yang and Seto, 2007).
DNA-wrapped surrounding histones can be accessed via epigenetic mechanisms such as the acetylation of histone lysine. In each histone, KATs can acetylate 10–20 lysine residues. Histone acetylation will elevate negative charges onto DNA, thereby promoting proteins associated with DNA repair, transcription, and replication to access DNA (Vo and Goodman, 2001; Unnikrishnan et al., 2010). Histone lysine acetylation has been suggested to be related to fundamental transcriptional activation commonly seen in tumor cells, in particular for K9/K11/K18/K56 onto histone H3, and K5/K8/K13K16 onto histone H4 (Berger, 2007). Such acetylation procedure can be regulated via lysine acetyltransferases such as p300/CBP, ORC-binding HATs, monocytic leukemia zinc finger protein (MOZ), general control of amino acid synthesis 5-like 2 (GCN5), and MYST2/KAT7 (HBO1) (Kaypee et al., 2016). GCN5 silencing inhibits MDA-MB231 cell invasion, proliferation, and migration, upregulates p21, and downregulates p-AKT, p-STAT3, E2F1, and MMP9 levels within MDA-MB231 cells relative to those treated with TGF-β1. Consequently, GCN5 is the possible downstream target of the TGF-β/Smad pathway responsible for regulating EMT within BC (Zhao et al., 2018).
ERα represents the TF that binds to the growth factor (GF) and hormonal signals to be activated. Actually, ERα is extensively suggested to be acetylated post-translationally via the activation of coactivator p300. The persistently activated ERα is related to a higher risk of BC occurrence by promoting aberrant breast tissue development. ERα acetylation can be achieved within hinge/ligand domains in K229, K299, K302, and K303 (Wang et al., 2001). Also, in another research on atypical breast hyperplasia, the ERα acetylation level increases in lysines K266 and K268 via p160 and p300 coactivators (Kim et al., 2006). p300/CBP contributes to ERα acetylation and promotes cell growth within BRCA1-mutated BC cells. Cross-talk with CBP and p300 coactivators within BC cells can decrease the metastatic activity by increasing E-cadherin levels (Liu et al., 2005). H3 acetylation in the promoters of Snail, ZEB1, and ZEB2 promotes the CSC-like characteristics within BC cells (Cho et al., 2015). Metadherin (MTDH) is related to BC cell metastasis and drug resistance, which can interact with CBP, and the latter is thereby translocated into the promoter of the twist family BHLH transcription factor (TWIST) and allows for proximal H3 acetylation in the promoter (Liang et al., 2015). Certain gene mutations have been indicated to upregulate p300/CBP within BC (Tillinghast et al., 2003), which is usually related to disease relapse and chemoresistance (Xiao et al., 2011).
Human males absent on the first (hMOF) deficiency can be detected within certain cancer types, and its level is the marker for disease prognosis (Cao et al., 2014). Pfister et al. compared the non-transformed control tissues and found that the hMOF protein and mRNA levels were significantly downregulated in primary BC. In addition, the hMOF protein level was closely related to H4K16 acetylation within each tested sample. On the contrary, hMOF expression increases within certain cancer types, which is related to HBO1 acetyltransferase responsible for forming a pre-initiation complex while initiating replication (Iizuka et al., 2006). P53 shows negative regulation on HBO1 while suppressing replication in the case of cell stresses (Iizuka et al., 2008). Moreover, HBO1 expression increases within tumor cells in comparison with healthy cells (Iizuka et al., 2009); meanwhile, its phosphorylated form functions to regulate CSC genesis within BC (Duong et al., 2013). KATs are referred to as MOZ (also known as MYST3 and KAT6A), and they can form tetrameric complexes with their paralog MORF (also known as MYST4 and KAT6B). The as-formed complexes contain two small non-catalytic subunits and bromodomain- and PHD finger-containing protein 1 (BRPF1) (Kaypee et al., 2016). The aforementioned two acetyltransferases are usually mutated within BC (Lynch et al., 2013).
Numerous histone deacetyltransferases are examined in studies to achieve favorable effects (Table 3). Sirtuin (SIRT1)-mediated ERα deacetylation within BC can decrease ERα activity and suppress BC cell growth, which is the effective method for preventing BC progression. Park et al. investigated SIRT2 function using Sirt2−/− mammary tumor cell line (MMT) derived from the spontaneous mammary tumors in Sirt2−/− mice, which identified the M2 isoform of pyruvate kinase (PKM2) as a critical target of SIRT2 (Park et al., 2016c). This result was supported by Shi et al. who demonstrated that the high expression of SIRT2 by IHC (IHC score >3) was downregulated in tumor tissues compared with the normal adjacent tissues in 296 patients (Shi et al., 2020). In several cell lines and human breast cancer tissues, Nakagawa et al. analyzed the expression of class I HDACs, including HDAC1, HDAC2, HDAC 3, and HDAC 8, and investigated which subtypes of class I HDACs were overexpressed in breast cancer. They revealed the high expression levels of these class I HDACs, and IHC results for HDAC1, HDAC2, HDAC3, and HDAC8 were positive in 17 (85%), 20 (100%), 20 (100%), and 17 (85%) of 20 breast cancer cases, respectively (Nakagawa et al., 2007). HDAC6 contributes to cancer metastasis since its upregulation increases cell motility in breast cancer MCF-7 cells and its interaction with cortactin regulates motility. HDAC6 also affects transcription and translation by regulating the heat-shock protein 90 (Hsp90) and stress granules, respectively (Saji et al., 2005). HDAC11 shows different expression levels and biological functions in different systems of the human body and is among the top 1–4% of genes overexpressed in cancers, such as breast cancer (Liu et al., 2020).
At present, just a few selective small-molecular substances with the direct inhibition effect of active sites in specific KMT2 family protein enzymes are identified (Chern et al., 2020). Epi-drugs can restore the repressive TSGs or the aberrantly activated oncogenes to suppress BC development. In addition, epi-drugs can prevent drug resistance, increase anti-tumor therapeutic effects, and enhance the radiotherapeutic effect.
Chern et al. adopted the bisubstrate strategy to prepare a focused library and identified numerous strong MLL methyltransferase inhibitors. It is to be noted that compound 16 (TC-5115) shows the highest strength and displays the 16-nM IC50 value. In the complex of MLL plus another four strong inhibitors, cocrystal structures are observed, revealing the role of such inhibitors in locking the MLL SET domain within the open, inactive conformation. Further optimizing TC-5115 can assist in developing a novel anti-MLL treatment (Chern et al., 2020).
Furthermore, MLL2 expression increases in BC cells and invasive carcinomas (Natarajan et al., 2010). Similarly, MLL4 deficiency reduces H3K4me3 expression while upregulating H3K27me3 expression within SIX1, MMP9, and MMP11 genes of MDA-MD-231 cells (Rabello Ddo et al., 2013). Based on the aforementioned findings, H3K4 methyltransferase possibly connects H3K27 acetylation and H3K4 methylation within BC cells by a certain mechanism, as evidenced by research on MLL4 levels within BC cells. Afterward, the UTX-MLL4 complex significantly promoted H3K27 acetyltransferase p300 to bind to target chromatin regions, thereby additionally increasing H3K27 acetylation while enhancing the gene activation activity of the enhancer (Kim et al., 2014).
Suppressing the association of menin with HMTs is a possible new treatment. At first, macrocyclic peptidomimetic inhibitors (MCP-1) are prepared for inhibiting the interaction between menin and MLL1. Meanwhile, menin shows direct binding to MI-463 and MI-503 at the low nanomolar binding affinity, which efficiently suppresses the interaction of menin with MLL (Borkin et al., 2015). Small molecules can be used to pharmacologically inhibit the interaction between menin and MLL, which can thereby prevent in vivo MLL leukemia progression without affecting healthy hematopoiesis. MI-463 used in combination with auranofin (an inhibitor of thioredoxin reductase) shows synergistic effects on promoting BC cell apoptosis (Kato et al., 2020). Additionally, HO-1 has a strong induction effect, which facilitates synergistically promoting the efficacy of MI-463 and auranofin. Consequently, the combined application of menin-MLL inhibitors, such as MI-463, and auranofin can efficiently treat BC by inducing ferroptosis.
RANi-induced SMYD2 silencing within TNBC cells or AZ505 (an inhibitor of SMYD2)-mediated SMYD2 inhibition remarkably decreases in vivo cancer development (Li et al., 2018). SMYD2 can methylate and activate the new non-histone substrates such as the NF-κB p65 subunit and STAT3 to exert its effect, thus inducing the growth and survival of TNBC cells (Li et al., 2018). As discovered in a recent research study on BC, SMYD3 shows diverse expression levels within T47D and MCF-7 BC cell lines, which enhances cisplatin resistance of MCF-7 cells (Wang et al., 2020). In addition, SMYD3 deficiency combined with cisplatin exposure suppresses the proliferation and mitochondrial membrane potential (MMP) of cells. Based on the aforementioned findings, SMYD3 has an important effect on analyzing cancer sensitivity and resistance to cisplatin. Consequently, the SMYD3 level has an important effect on BC occurrence, while inhibiting SMYD3 is the new anti-BC treatment.
Punzi et al. found that WDR5 deficiency decreased cell metastatic ability by abolishing the mesenchymal phenotype of luminal B- and TN-derived cells, thereby promoting the epithelial phenotype. In addition, TGFβ1 regulates the aforementioned process, suggesting that WDR5 is important for inducing EMT by activating TGFβ1. Furthermore, the aforementioned EMT reversion also possibly results from the drug targeting effect of WDR5, which enhances the chemosensitivity of BC cells and the paclitaxel-mediated efficacy (Punzi et al., 2019).
As reported in a study, SETD1A activates MMP levels to modulate BC metastasis (Salz et al., 2015), and another study suggests that SETD1A amplification within mixed ductal and lobular breast cancer can upregulate the H3K4me3 marker to modulate mitosis within the mitosis and DNA damage response gene promoters. In addition, SETD1A can modulate some genes regulating DNA damage response, cell cycle, and mitosis by the promoter H3K4 methylation within LC and BC cells. SETD1A loss can trigger the efficacy of aging in suppressing tumors; as a result, SETD1A possibly has a critical effect on maintaining tumor cell growth and mitosis (Tajima et al., 2019). Furthermore, SETD1A can trigger ER+ BC cell growth and invasion by modulating genes related to cell migration and survival independent of ER. Therefore, SETD1A is essential for the development of hormone therapy resistance in BC ER independently (Jin et al., 2018). SETD1B has a similar structure to SETD1A and has a critical effect on the TNBC pathogenic mechanism and survival, no matter whether H3K4 methyltransferase is activated or not, for instance, through the formation of cytoplasmic COMPASS complexes and the regulation of ADIPOR1 via the BOD1 interaction (Wang et al., 2017b). A study shows that SET7/9’s effect can be achieved through negatively regulating stability via E2F1 and DNMT methylation. According to the aforementioned findings, SET7/9 is the biomarker utilized for predicting the invasion and treatment resistance of BC cases.
Accordingly, the G9A inhibitor treatment efficiently abolishes NDRG1-induced TBX2 suppression and decreases cell growth after functionally inhibiting TBX2. Based on the aforementioned results, TBX2 recruits the huge repressive complex into EGR1-responsive promoters to suppress physical growth control, thus inducing out-of-control BC cell growth (Crawford et al., 2019). A study reported the benzoxazole scaffold by virtual high-throughput screening (HTS), and the design and synthesis of 24 derivatives, which are later utilized to inhibit G9a. Following the repeated screening of anti-proliferative activity and kinase, this work found that GA001, the effective G9a antagonist that had a 1.32 μM IC50 value, triggered autophagy within MCF7 cells through AMPK (Zhang et al., 2017).
Using small inhibitory molecules or chromatin-modifying enzyme inhibitors to target tumor epigenome for releasing knockout genes from repressive status is the possible potent method to research cancer and develop new drugs. For erasing the H3K27me3 mark out of gene promoters, some methods have been used, such as directly or indirectly inhibiting EZH2, incorporating H3K27me3-recognizing synthetic TFs, applying natural anti-tumor agents, or combining with known anti-tumor agents. Nonetheless, targeting H3K27 methylation during anti-BC therapy possibly triggers treatment-induced side effects. In particular, apart from the effect of suppressing H3K27 methylation, EZH2 inhibitors can suppress EZH2 activity associated with additional effects. Consequently, applying drugs targeting H3K27 methylation should be properly validated, to overcome non-methylation-associated effects.
3-deazaneplanocin A (DZNep) is a known PCR2 inhibitor that can promote tumor cell apoptosis (such as MDA-MB-468, MCF7 BC cells), but it makes no difference to healthy cells (such as MCF-10A) (Tan et al., 2007). DZNep suppresses S-adenosyl-L-homocysteine (SAH) hydrolase activity and upregulates SAH to indirectly inhibit EZH2. SAH is also an antagonist of SAM, which can thereby block HMT activity (Miranda et al., 2009). Additionally, DZNep exerts the lowest effect on DNA methylation-silenced genes (Miranda et al., 2009). DZNep treatment can markedly suppress H3K27 methylation (rather than H3K9 methylation) in diverse tumor cells (such as MB-468 BC cells) by depleting PRC2 component levels in cells (EZH2, SUZ12, and EED) (Tan et al., 2007)) (Figure 3). According to the authors, DZNep treatment reactivates PRC2-suppressed genes in BC. In BRCA1-depleted BC cells with EZH2 upregulation, DZNep further induces apoptosis compared with that in BRCA1-proficient BC cells (Puppe et al., 2009). Although DZNep has aroused wide attention as a possible antitumor therapeutic agent, there is little research on its possible side reactions in vivo or in a BC model. Therefore, it is necessary to carry out in vivo experiments to examine its use in BC as an epi-drug. After DZNep, other strong and selective EZH2 inhibitors competing against SAM have also been developed (Verma et al., 2012). Typically, GSK343 and GSK926 are suggested to down-regulate histone H3K27me3 expression while suppressing EZH2 expression within BC (HCC1806 TNBC) and PCa (LNCaP) cells; nonetheless, GSK343 displays certain limitations because it is highly cleared (plasma volume where the drug is completely eliminated per unit of time) in a rat pharmacokinetic study.
Upregulation of EZH2 causes di-methylation (H3K27me2) and H3K27 tri-methylation (H3K27me3) to lysine 27 residue of histone 3 (H3K27). EZH2‐mediated up‐ and downregulation of various genes increases cell proliferation, invasion, and metastasis and decreases the apoptosis of breast cancers.
Interestingly, PARP1, one of the poly(ADP-ribose) polymerase family (PARP) members, is suggested to decrease and interact with EZH2, thus decreasing H3K27me3 expression within MDA-MB-231 cells (Yamaguchi et al., 2018). Upon alkylation and oxidative stress-induced DNA damage, PARP1 contributes to the PARylation of EZH2 while inducing PRC2 complex dissociation, decreasing EZH2 expression, and later downregulating the expression of EZH2-regulated H3K27me3 (Quintayo et al., 2012). On the contrary, PARP inhibitor (PARPi)-mediated PARP suppression can mitigate EZH2 downregulation resulting from alkylating DNA damage, thus, further increasing EZH2-induced gene knockdown and CSC characteristics in comparison with untreated cells. Consequently, the combined application of EZH2i-like GSK343 and PARPi (olaparib) is investigated within BRCA-deficient BC (Yamaguchi et al., 2018). According to results obtained from ovarian cancer (UWB1.289) and BC (HCC38 and SUM149) cells with no response to PARPi alone, adding EZH2i enhances PARPi’s efficacy (Yamaguchi et al., 2018). Based on the aforementioned findings, it is necessary to determine whether combination therapy is effective on BRCA-defective tumors in clinical trials. Actually, a phase-II clinical trial is currently recruiting HR+/HER2− advanced BC with endocrine therapy resistance to receive SHR3162 (PARPi) and SHR2554 (EZH2i) treatment (ClinicalTrials.gov Identifier: NCT04355858).
Additionally, for BC subtypes that display the lowest H3K27me3 expression and has a dismal prognostic outcome such as TNBC, the chromatin mark may be upregulated for improving patient survival. Some studies have demonstrated the crosstalk between H3K27 methylation and additional chromatin modifications, and the combined application of HDACi (MS275) or DNMTi (guadecitabine/SGI-110) has been examined within the XtMCF and LmMCF cells, and TNBC model cells exhibiting high tumorigenicity and metastasis potentials (Su et al., 2018). The monotherapies of the aforementioned two drugs can upregulate H3K27me3 expression, whereas their combination can synergistically upregulate the H3K27me3 level. Such treatment induces transcriptional reprogramming, which is evidenced by EMT suppression, protein mutant p53 (usually detected within tumor cells), ZEB1 and EZH2 promotion, and induce E-cadherin expression, H3 trimethylation, and apoptosis. Abolishing EMT induces tumor cell proliferation, clone forming, and suppression of their stemness. Additionally, MS275 alone or plus SGI suppresses XtMCF xenograft growth, whereas MS275 decreases the lung metastasis of LmMCF cells within mice (Su et al., 2018). Collectively, the aforementioned data indicate that EMT epigenetic reprogramming, such as H3K27 methylation, inhibits TNBC cells’ aggressiveness.
Epi-drug can suppress BC progression by abolishing the abnormally suppressed TSGs or abnormally activated oncogenes. Additionally, epi-drugs can prevent treatment resistance, increase antitumor drug efficacy, and enhance radiotherapeutic efficacy. Numerous epi-drugs are examined in clinical studies to achieve favorable effects (Table 3).
Some HAT-targeting inhibitors are investigated; however, none of them has been tested in clinical trials. At present, the existing HATis are library-selected inhibitors, small-molecular HATi (either synthetic or natural), and bi-substrate inhibitors. Of them, bi-substrate mimics, including Lys-CoA, exhibit potent inhibition and are rarely applied in cells due to their great molecular weight (Lau et al., 2000; Cole, 2008). It is to be noted that most potent compound, 1r, the new compound manufactured on the basis of C646, displays potent inhibition, superior drug-like properties, and low cell proliferation after removing the toxic nitro group (Liu et al., 2019). ICG-001 can suppress BC development by targeting protein–protein interactions (PPIs) between beta-catenin and CBP, but not suppressing acetyltransferase activity (Ring et al., 2018; Sulaiman et al., 2018). HATs’ acetyltransferase activity and substrate specificity can be measured through the multi-subunit protein complexes. However, the complexities have greatly hindered the translation of in vitro experiments to in vivo ones. Existing inhibitors are poorly selective and of low efficiency, which have restricted their application, even though they are possibly efficient starting points to develop novel inhibitors.
Histone lysine acetylation is related to the occurrence and development of certain disorders, thus indicating that KAT modulators may be possible therapeutic targets. Nonetheless, it remains a challenge to identify the strong and selective KATi in comparison with modulators for additional epigenetic enzymes such as KDAC inhibitors (Merarchi et al., 2019). Some methods such as computational tools and improved assay techniques are applied in identifying small-molecular KAT inhibitors; however, just a low proportion of them are verified with in vivo and in vitro activities currently (Krishna et al., 2018; Huang et al., 2019). Such KATi are divided into three categories: 1) bisubstrate inhibitors, 2) natural substances and the corresponding derivatives, and 3) synthesize small molecules.
Bisubstrate analog mimicking the ternary complex constituted by the lysine substrate and cofactor acetyl-Co is the first KAT inhibitor. Thereafter, some research groups adopted the concept for identifying specific KATi. For instance, lys-CoA is prepared by connecting coenzyme A (CoA) with the single lysine residue by means of the methylene linker (Lau et al., 2000). According to reports, lys-CoA exhibits strong activity to inhibit p300 in comparison with PCAF. Additionally, H3-CoA-20 can specifically bind to PCAF (Lau et al., 2000). Additionally, Boc-C5-CoA is also suggested to occupy two binding pockets in enzyme active sites to suppress p300 (Kwie et al., 2011). Likewise, H4K16-CoA, also a bisubstrate analog, is a strong inhibitor of MYST family enzyme Tip60 and the corresponding yeast homolog Esa1, and its IC50 values are within the micromolar range (Wu et al., 2011).
Nonetheless, bisubstrate inhibitors are poorly permeable into cells and are metabolically unstable, which is ascribed to their partial peptidic structure and polar phosphate group. The aforementioned shortcomings are managed by using cell membrane penetration technologies such as lipid permeabilization and cell micro-injection (Simon et al., 2016). Some possible CoA analog prodrugs targeting p300 are also developed (Cebrat et al., 2003). Modifications including coupling the amino acid backbone of inhibitors into the arginine-abundant peptides or TAT protein transduction domain promote p300 inhibition and transmembrane delivery (Wadia and Dowdy, 2005).
According to one study, polyamine spermidine (Spd) is connected with CoA’s S atom in a covalent manner via the thioglycolic acid linkage, which thus forms the non-toxic Spd(N1)-CoA that can be internalized in cells by the polyamine transporter (Cullis et al., 1982). Spermidinyl-CoA-based (N1) is able to change pathways related to DNA damage repair and then enhances the chemosensitivity and radiosensitivity of cells (Bandyopadhyay et al., 2009). According to the latest article, the new peptide-CoA conjugate bisubstrate inhibitor is prepared, which displays submicromolar potential to suppress HAT1 (Ngo et al., 2019).
Many articles suggest the effect of some natural substances on inhibiting KATs (Seidel et al., 2012; Kaypee et al., 2016). Curcumin, one of the natural KATi, significantly suppresses different cancers (Shanmugam et al., 2016; Mbese et al., 2019; Tajbakhsh et al., 2018). Curcumin can suppress proliferation and clone-forming abilities of MDA-MB-231 and MCF-7 cells. Based on our results, curcumin’s inhibition against BC cells is possibly associated with its resistance to EMT and CSC properties. In line with the aforementioned results, curcumin is an anti-metastatic agent for BC (Hu et al., 2019b). New curcumin preparations have also been under investigation, such as sustained-release capsules and nanoparticles (NPs) to manage inflammation and cancer (Gupta et al., 2013; Di Costanzo et al., 2014). Curcumin can suppress pure p300’s acetyltransferase activity by adopting histone H3/p53 to be the substrate. In addition, triggering receptors expressed on myeloid cells 1 (TREM-1) within tumor-associated macrophages (TAMs) cause an inflammatory response initiated by the toll-like receptor (TLR), which shows overexpression within BC cells. TREM-1 is the risk factor for BC (Pullikuth et al., 2021). Curcumin can suppress H3/H4’s p300 acetylation to regulate TREM-1 levels within the TREM-1 promoter (Yuan et al., 2012). Moreover, curcumin is also reported to suppress KAT activity within THP-1 cells (human monocytic cell line), inhibit nuclear factor-κB (NF-κB) acetylation at Lys310, and later restrain transcription activation and nuclear translocation of the corresponding downstream targets (Yun et al., 2011). Currently, only curcumin, the KAT with the lowest specificity, is under clinical trials in the treatment of different disease (Manzo et al., 2009; Gupta et al., 2013).
First separated in the shell liquid of Anacardium occidentale (cashew nut), anacardic acid (also known as 6-pentadecylsalicylic acid) (Balasubramanyam et al., 2003) is identified as a non-competitive and non-selective inhibitor of PCAF and p300/CBP. It is reported to suppress Tip60 under the same experimental conditions (Ghizzoni et al., 2012). Additionally, it can also affect RelA subunit nuclear localization and acetylation to target the NF-κB pathway, thus suppressing carcinogenesis (Hemshekhar et al., 2012). Because anacardic acid is highly lipophilic and has poor physiochemical characteristics, some new phenoxyacetic acid and 6-alkyl salicylic acid analogs are analyzed to improve KAT inhibition, cell permeability, and solubility. Phenoxyacetic acid analogs have a strong KAT inhibition effect, which is decided by their alkyl chain length and location (Eliseeva et al., 2007). Additionally, anacardic acid can alleviate Tip60-induced DNA damage to augment the radiosensitivity of cancer cells (Sun et al., 2006). Moreover, changes in salicylic acid residue or alkyl chains show specific shifts in MOF suppression of MYST family KATs (Zhang et al., 2018b). Moreover, 4-cyano-3 trifluoromethylphenylbenzamides, the other anacardic acid derivative, is able to suppress KAT3 (Souto et al., 2008). Meanwhile, pentadecylidenemalonate, anacardic acid’s simplified analog, is first identified to be a KAT activator/inhibitor, which activates PCAF and suppresses recombinant CBP and p300/CBP.
Garcinol (also known as polyisoprenylated benzophenone), a strong non-specific KATi, is extracted from Garcinia indica (Kokum fruit) (Liu et al., 2015b). Its IC50 values for PCAF and p300 are 7 and 5 μM, respectively (Balasubramanyam et al., 2004). Garcinol-induced tumor cell death is related to the inhibition of cell apoptosis and histone acetylation (Arif et al., 2009). For improving garcinol’s pharmacokinetic profiles, some derivatives that have a lower toxic effect, higher efficacy, and specificity have been prepared. Isogarcinol is prepared through intramolecular cyclization, and it is adopted to be the template for designing some new KATi. In a recent study, Milite et al. prepared the benzylidene barbituric acid derivative (EML425), which was applied as a factor to selectively block p300/CBP, and had strong inhibition within the low micromolar range (IC50 values of 1.1 and 2.9 μM for CBP and p300, respectively) (Milite et al., 2015). As discovered by Ahmad et al., garcinol exposure promoted β-catenin phosphorylation, and it decreased nuclear localization in BC (Ahmad et al., 2012). Such findings were verified in the xenograft mouse model in vivo, in which garcinol suppressed miRNAs, NF-κB, nuclear β-catenin, and vimentin. According to the aforementioned results, garcinol’s anti-BC effect is partial because of EMT phenotypic reversal, and this is related to the abnormal levels of let-7s, miR-200s, and Wnt and NF-κB pathways to some extent.
Carnosol, which takes the region binding acetyl-CoA’s pantetheine arm, is verified to be the candidate anti-BC target. In addition, it is the new natural p300 inhibitor, which can be listed in the current inhibitor panel (Alsamri et al., 2021).
In recent years, BET family-targeting small molecules (BRD2–BRD4 and testis-specific BRDT) are novel epi-drugs. The BET family functions to recognize and bind to acetylated lysine by means of bromodomain; in addition, it has critical effects on cell cycle control and transcriptional elongation. At first, BET inhibitors’ effects are examined within NUT-midline carcinoma (Filippakopoulos et al., 2010) and hepatological cancers (Dawson et al., 2011; Delmore et al., 2011; Mertz et al., 2011). Thereafter, their anticancer effects are assessed in preclinical studies on additional solid tumors such as prostatic cancer (PCa) (Asangani et al., 2014), non-small cell lung cancer (NSCLC), pancreatic cancer (Garcia et al., 2016), together with BC (Ocana et al., 2017). BET inhibitors are only effective on TNBC treated combined with PLK1 inhibitors or chemotherapy or traditional treatment-resistant TNBC (Nieto-Jimenez et al., 2017). Nonetheless, as the aforementioned studies are pre-clinical studies at present, more clinical trials are needed to verify BET inhibitors’ effect on treating BC.
HDACs can be further classified into four main types according to their sequence homology, namely, class I (HDAC1–3, and HDAC8), class II (HDAC4–7 and HDAC9–10), class III (sirtuin 1–7), and class VI (HDAC11) (Dawson and Kouzarides, 2012). The zinc metal ion is necessary for HDACs of class I/II/IV, so HDAC inhibitors can block the catalytic performance of HDACs by chelating zinc ions. Of diverse HDAC inhibitors, romidepsin and vorinostat have been approved by the FDA for the clinical treatment of cutaneous T-cell lymphoma (Olsen et al., 2007; Piekarz et al., 2009). As HDAC inhibitors display anticancer efficacy in different cancers in vitro and in vivo (Beckers et al., 2007; Kim et al., 2013b), they can be applied in clinical practice for more tumors such as BC.
According to their different structures, HDACis are classified as four types, namely, cyclic peptides, hydroxamic acids, benzamides, and aliphatic fatty acids (Table 4). At present, three HDACis of the hydroxamic acid type are approved by the FDA, which are vorinostat (SAHA), panobinostat (LBH-589), and belinostat (PXD101).
TABLE 4. Summary of HDAC inhibitors on the BC therapeutic strategy and corresponding clinical trials.
Such agents present antitumor activity in BC as well. For instance, SAHA can suppress cell growth, EMT, migration, and invasion and induce cell apoptosis, differentiation, autophagy, anoikis, and cell cycle arrest (Lee et al., 2016; Wawruszak et al., 2019; Wawruszak et al., 2021). SAHA remarkably promotes response and suppresses the resistance to tamoxifen (Lee et al., 2012), cisplatin (Wawruszak et al., 2015), olaparib (Min et al., 2015), taxol (Shi et al., 2010), epirubicin (Marchion et al., 2004), docetaxel, and trastuzumab (Bali et al., 2005). Also, SAHA efficiently promotes TNF-related apoptosis-inducing ligand (TRAIL)-mediated apoptosis, and this is achieved by triggering anoikis, increasing CD137 receptor expression, and suppressing Apo2L/TRAIL resistance (Bellarosa et al., 2012; Zhou et al., 2016). Nonetheless, SAHA can enhance TNBC cell metastasis and EMT by suppressing HDAC8, indicating that it should be cautious when treating BC with SAHA, since it might accelerate tumor metastasis (Wu et al., 2016). Meanwhile, a study showed that the combination SAHA and epigallocatechin-3-gallate (EGCG) is effective in inducing apoptosis of breast cancer cells and reducing their migratory capacity (Steed et al., 2020). In addition, Carlisi et al. showed that SAHA synergistically sensitized MDA-MB231 cells to the cytotoxic effect of parthenolide (Carlisi et al., 2015). Belinostat (PXD101) can suppress cell growth and promote cell apoptosis by PKC and Wnt/b-catenin pathways; moreover, applying belinostat in combination with the HSP90 inhibitor (17-AAG) can synergistically exert the anti-tumor effect (Lu et al., 2019; Zuo et al., 2020). Panobinostat (LBH-589) abolishes EMT in TNBC by suppressing ZEB1/2 (Rhodes et al., 2014). There are also additional HDACis of the hydroxamic acid type, including resminostat, abexinostat, and pracinostat, even though they are less tested in BC. In this regard, more research is warranted to examine whether they can be applied in BC. As discovered by Qin et al., panobinostat inhibited TNBC and non-TNBC cell growth, invasion, and migration, while promoting their apoptosis. Likewise, panobinostat suppresses BC proliferation and invasion within mouse models (Qin et al., 2019b). Romidepsin (FK2280), one of the cyclic peptide HDACi, has been approved by the FDA, and it can synergistically inhibit cell proliferation while promoting cell apoptosis when used in combination with decitabine (an inhibitor of methyltransferase) (Cooper et al., 2012). In inflammatory BC, romidepsin exposure can destroy the lymphatic vascular structure and tumor emboli by repressing HIF-1a and VEGF within inflammatory BC. Moreover, romidepsin synergistically suppresses primary tumor proliferation and multiple metastases when used in combination with paclitaxel (Robertson et al., 2013).
Valproic acid (VPA), the HDACi of the aliphatic fatty acid type, has been extensively studied, and it suppresses BC occurrence by upregulating apoptosis pathways and inducing cell cycle arrest (Fortunati et al., 2008; Travaglini et al., 2009). In addition, VPA promotes EMT by increasing ZEB1 and SNAIL levels HDAC2-dependently, but the HDAC2-related mechanism is still unknown (Zhang et al., 2019b). Additionally, VPA synergistically suppresses BC development when applied in combination with anti-tumor agents such as tamoxifen, epirubicin, cisplatin, camptothecin, and capecitabine (Marchion et al., 2005; Fortunati et al., 2008; Arakawa et al., 2009; Terranova-Barberio et al., 2016).
Entinostat (Ent, MS-275), the synthetic benzamide derivative of HDACi, exhibits potent immunomodulation on BC (McCaw et al., 2019; Connolly et al., 2021). In addition, Ent exposure can suppress EMT and the tumor-promoting cell phenotype, thereby inhibiting tumor occurrence and metastasis (Shah et al., 2014). Ent can induce the expression of retinoid acid to improve differentiation mediated by retinoic acid when it is applied together with doxorubicin and all-trans retinoic acid (ATRA); moreover, such a combination further increases doxorubicin-induced cytotoxicity. Moreover, Ent together with ATRA can manage the resistance to the aromatase inhibitor (AI) by decreasing the quantity of tumor-initiating cells (Merino et al., 2016). Additional new multifunctional inhibitors achieve favorable antitumor efficacy.
Sirtuin inhibitors suppress BC development through diverse structures, targets, and activities. In addition, they can deal with the problem of multidrug resistance through combined use with chemotherapeutics. For instance, amurensin G suppresses SIRT1 and later inhibits MDR1 and FoxO1 levels within the doxorubicin-resistant BC cells, thus potentiating doxorubicin absorption into cells and suppressing oncogenic development (Oh et al., 2010). Splitomicin can decrease cell motility while potentiating paclitaxel’s effect on resisting cell motility. Such an effect is intensified by the addition of trichostatin A (TSA), the HDAC6 inhibitor (Bonezzi et al., 2012). Some studies are conducted to evaluate SIRT1/2 inhibitors, including salermide, sirtinol, cambinol, splitomicin, nicotinamide, tenovin, suramin, indole derivatives, and analogs with similar structures. The aforementioned molecules can upregulate p53 acetylation or induce pro-apoptotic, SIRT1-epigenetically silenced gene expression to suppress BC cell growth and trigger p53-dependent apoptosis (Peck et al., 2010). Consequently, different SIRT inhibitors show synergistic effects with conventional antitumor agents in the treatment of BC. There are different pathways related to the drug resistance escape mechanism of SIRTs, indicating that more SIRT inhibitors may be prepared according to the known inhibitors for balancing efficacy and specificity. Phase-I and phase-II clinical studies have been conducted to evaluate the effects of HDAC and DNMT inhibitors on treating BC (Falahi et al., 2014). Epi-drugs exhibit poor anticancer effects on BC, and epi-drug monotherapy can just achieve an effective rate of 10% in BC cases, indicating that monotherapy may not be appropriate for treating BC. Nonetheless, according to the aforementioned clinical trials, when epi-drugs are used in combination with targeted or cytotoxic therapies, such as ER-targeted therapy, the OS and PFS are improved (Falahi et al., 2014). Consequently, the existing clinical trials mostly apply epi-drugs in combination with traditional treatments.
These encouraging preclinical findings have laid the sound basis to translate epi-drugs to clinical trials for treating BC. Table 4 summarizes epi-drug-related clinical trials for the treatment of BC (mostly from https://www.clinicaltrials.gov/). Many accomplished (NCT00262834, NCT00777049, and NCT01171924) along with ongoing (NCT00416130, NCT01638533, and NCT04676516) clinical trials have been conducted to predict the safety, pharmacodynamics, and pharmacokinetics of epi-drugs for determining the best doses and monotherapy schemes. It is to be noted that the tolerance of 300/400 mg oral SAHA (twice/day for a 14-day period, separated by a 7-day rest) has been verified (Vansteenkiste et al., 2008). However, the present work just enrolled two BC cases, making it impossible to accurately determine the response rate. More clinical trials are being conducted to predict the best SAHA dose. Apart from monotherapy, epi-drugs have been frequently utilized in combination with other drugs in clinics. For instance, one phase-II trial applied SAHA plus tamoxifen in treating BC resistant to hormone therapy, and the objective response rate (ORR) and clinical benefit rate were determined to be 19% and 40%, respectively (Munster et al., 2011). Moreover, the combined application of Ent and exemestane increased the OS from 19.8 months (as obtained after exemestane monotherapy) to 28.1 months (Yardley et al., 2013). One recent phase-II clinical trial applied SAHA in combination with tamoxifen and pembrolizumab in improving the response to immunotherapy among ER+ BC cases. The treatment strategy achieved an ORR and clinical benefit rate of 4% and 19%, respectively (Terranova-Barberio et al., 2020). Typically, as revealed by an ongoing phase-II trial (NCT04190056), the aforementioned combination strategy can trigger an immune response for treating ER+ BC, while reducing the dose and adverse reactions. BETis and SAHA synergistically treat BC with olaparib in preclinical trials (Min et al., 2015; Yang et al., 2017). Additionally, there are two ongoing trials (NCT03901469 and NCT03742245) analyzing whether epi-drugs plus PARPis are effective and safe by suppressing DNA damage repair. Taken together, these clinical trials further verify the effectiveness of epi-drugs in treating BC, which should be further investigated.
In this study, we reviewed histone modifications and their functions and potential cellular interactions, which might result in the development of potential efficient therapies with KATi and HDACi. Several synthetic compounds currently in pre-clinical studies have exhibited potent KATi activities against breast malignant cells. Moreover, they can effectively augment the anti-cancer activities of standard chemotherapeutic agents such as paclitaxel, doxorubicin, and cisplatin and sensitize drug-resistant cells to radiation therapy. Nevertheless, as shown, KATi and HDACi seem to be a promising group of anti-cancer drugs, particularly in combination with other anti-cancer drugs and/or radiotherapy. More large-scale promising evidence needs to be obtained from multi-center clinical trials. Meanwhile, their use in combination with other drugs and the schedule of such drug combinations need to be further investigated in both preclinical and clinical studies.
Currently, selectivity is one of the biggest challenges in developing drugs targeting epigenetic modifiers. Most currently developed drugs do not show selectivity to certain enzymes; instead, they target molecules that have certain common functions and structures. But epigenetic agents are most advantageous in their good tolerance and low severe adverse reaction rate, even though there are certain concerns about the safety of certain medicines (Cheng et al., 2019). Additionally, more reports indicate that the response rates are poor after short-term treatment, and resistance is developed in the end, which can be attributed to the transcriptional plasticity driven by epigenetics responding to environmental stress (Dawson, 2017). More multicenter and randomized phase-III studies should be conducted to realize the full potential and specificity of HDACis therapy in various subtypes of breast cancer. Further clinical studies should include the most promising novel HDACi and isozyme-specific inhibitors.
The present work focuses on summarizing relevant studies on HMs related to BC and the possible mechanisms associated with abnormal HMs. Additionally, we also aim to analyze existing therapeutic agents together with those drugs approved and tested through pre-clinical and clinical trials, to assess their roles in HMs. Moreover, epi-drugs that targeted HMT inhibitors and HDAC inhibitors should be tested in preclinical and clinical studies for the treatment of BC. Epi-drugs that target histone methylation (HMT inhibitors) and histone deacetylation (HDAC inhibitors) are now under clinical trials or approved by the US Food and Drug Administration (FDA). Therefore, the review covers the difficulties in applying HM-targeting treatments in clinical applications and proposes feasible approaches for overcoming such difficulties and promoting their use in treating BC cases. Indeed, the full clinical therapeutic scope and commercial value of such agents in the field of oncology are only just emerging.
JF researched data for the manuscript, wrote the manuscript, contributed to the discussion of the content, and reviewed the manuscript before the submission. XM retrieved and organized documents, modified the manuscript, and contributed to subject planning. All authors read and approved the final version of the manuscript.
The authors declare that the research was conducted in the absence of any commercial or financial relationships that could be construed as a potential conflict of interest.
All claims expressed in this article are solely those of the authors and do not necessarily represent those of their affiliated organizations, or those of the publisher, the editors, and the reviewers. Any product that may be evaluated in this article, or claim that may be made by its manufacturer, is not guaranteed or endorsed by the publisher.
BC, breast cancer; DNMTs, DNA methyltransferases; EMs, epigenetic modifications; ERα, estrogen receptor α; ESCs, embryonic stem cells; FOXA1, pioneer factor; GF, growth factor; HATs, histone acetyltransferases; HEPH, hephaestin; HER2, human epidermal growth factor receptor 2; HMs, histone modifications; HMTs, histone methyltransferases; HR, hormone receptors; KATi, KAT inhibitors; KDMs, lysine demethylases; KMTs, lysine methyltransferases; MCP-1, macrocyclic peptidomimetic inhibitors; MTDH, metadherin; NF-κB, nuclear factor-κB; NSCLC, non-small cell lung cancer; ORR, objective response rate; PCa, prostatic cancer; PPIs, protein–protein interactions; PTMs, post-translational modifications; SIRT1, sirtuin; TCGA, The Cancer Genome Atlas; Tip60, Tat-interactive protein; TSSs, transcriptional start sites; VPA, valproic acid.
Asangani, I. A., Dommeti, V. L., Wang, X., Malik, R., Cieslik, M., Yang, R., et al. (2014). Therapeutic targeting of BET bromodomain proteins in castration-resistant prostate cancer. Nature 510 (7504), 278–282. doi:10.1038/nature13229
Ahmad, A., Sarkar, S. H., Bitar, B., Ali, S., Aboukameel, A., Sethi, S., et al. (2012). Garcinol regulates EMT and Wnt signaling pathways in vitro and in vivo, leading to anticancer activity against breast cancer cells. Mol. Cancer Ther. 11 (10), 2193–2201. doi:10.1158/1535-7163.MCT-12-0232-T
Al Sarakbi, W., Sasi, W., Jiang, W. G., Roberts, T., Newbold, R. F., and Mokbel, K. (2009). The mRNA expression of SETD2 in human breast cancer: Correlation with clinico-pathological parameters. BMC Cancer 9, 290. doi:10.1186/1471-2407-9-290
Alsamri, H., Hasasna, H. E., Baby, B., Alneyadi, A., Dhaheri, Y. A., Ayoub, M. A., et al. (2021). Carnosol is a novel inhibitor of p300 acetyltransferase in breast cancer. Front. Oncol. 11, 664403. doi:10.3389/fonc.2021.664403
Altaf, M., Utley, R. T., Lacoste, N., Tan, S., Briggs, S. D., and Cote, J. (2007). Interplay of chromatin modifiers on a short basic patch of histone H4 tail defines the boundary of telomeric heterochromatin. Mol. Cell 28 (6), 1002–1014. doi:10.1016/j.molcel.2007.12.002
Arakawa, Y., Saito, S., Yamada, H., and Aiba, K. (2009). Simultaneous treatment with camptothecin and valproic acid suppresses induction of Bcl-X(L) and promotes apoptosis of MCF-7 breast cancer cells. Apoptosis 14 (9), 1076–1085. doi:10.1007/s10495-009-0384-0
Arif, M., Pradhan, S. K., Thanuja, G. R., Vedamurthy, B. M., Agrawal, S., Dasgupta, D., et al. (2009). Mechanism of p300 specific histone acetyltransferase inhibition by small molecules. J. Med. Chem. 52 (2), 267–277. doi:10.1021/jm800657z
Atanassov, B. S., Mohan, R. D., Lan, X., Kuang, X., Lu, Y., Lin, K., et al. (2016). ATXN7L3 and ENY2 Coordinate activity of multiple H2B Deubiquitinases important for cellular proliferation and tumor growth. Mol. Cell 62 (4), 558–571. doi:10.1016/j.molcel.2016.03.030
Balasubramanyam, K., Altaf, M., Varier, R. A., Swaminathan, V., Ravindran, A., Sadhale, P. P., et al. (2004). Polyisoprenylated benzophenone, garcinol, a natural histone acetyltransferase inhibitor, represses chromatin transcription and alters global gene expression. J. Biol. Chem. 279 (32), 33716–33726. doi:10.1074/jbc.M402839200
Balasubramanyam, K., Swaminathan, V., Ranganathan, A., and Kundu, T. K. (2003). Small molecule modulators of histone acetyltransferase p300. J. Biol. Chem. 278 (21), 19134–19140. doi:10.1074/jbc.M301580200
Bali, P., Pranpat, M., Swaby, R., Fiskus, W., Yamaguchi, H., Balasis, M., et al. (2005). Activity of suberoylanilide hydroxamic Acid against human breast cancer cells with amplification of her-2. Clin. Cancer Res. 11 (17), 6382–6389. doi:10.1158/1078-0432.CCR-05-0344
Bandyopadhyay, K., Baneres, J. L., Martin, A., Blonski, C., Parello, J., and Gjerset, R. A. (2009). Spermidinyl-CoA-based HAT inhibitors block DNA repair and provide cancer-specific chemo- and radiosensitization. Cell Cycle 8 (17), 2779–2788. doi:10.4161/cc.8.17.9416
Bannister, A. J., Zegerman, P., Partridge, J. F., Miska, E. A., Thomas, J. O., Allshire, R. C., et al. (2001). Selective recognition of methylated lysine 9 on histone H3 by the HP1 chromo domain. Nature 410 (6824), 120–124. doi:10.1038/35065138
Barski, A., Cuddapah, S., Cui, K., Roh, T. Y., Schones, D. E., Wang, Z., et al. (2007). High-resolution profiling of histone methylations in the human genome. Cell 129 (4), 823–837. doi:10.1016/j.cell.2007.05.009
Beckers, T., Burkhardt, C., Wieland, H., Gimmnich, P., Ciossek, T., Maier, T., et al. (2007). Distinct pharmacological properties of second generation HDAC inhibitors with the benzamide or hydroxamate head group. Int. J. Cancer 121 (5), 1138–1148. doi:10.1002/ijc.22751
Bellarosa, D., Bressan, A., Bigioni, M., Parlani, M., Maggi, C. A., and Binaschi, M. (2012). SAHA/Vorinostat induces the expression of the CD137 receptor/ligand system and enhances apoptosis mediated by soluble CD137 receptor in a human breast cancer cell line. Int. J. Oncol. 41 (4), 1486–1494. doi:10.3892/ijo.2012.1551
Berger, S. L. (2007). The complex language of chromatin regulation during transcription. Nature 447 (7143), 407–412. doi:10.1038/nature05915
Boccuni, P., MacGrogan, D., Scandura, J. M., and Nimer, S. D. (2003). The human L(3)MBT polycomb group protein is a transcriptional repressor and interacts physically and functionally with TEL (ETV6). J. Biol. Chem. 278 (17), 15412–15420. doi:10.1074/jbc.M300592200
Bonezzi, K., Belotti, D., North, B. J., Ghilardi, C., Borsotti, P., Resovi, A., et al. (2012). Inhibition of SIRT2 potentiates the anti-motility activity of taxanes: Implications for antineoplastic combination therapies. Neoplasia 14 (9), 846–854. doi:10.1593/neo.12728
Borkin, D., He, S., Miao, H., Kempinska, K., Pollock, J., Chase, J., et al. (2015). Pharmacologic inhibition of the Menin-MLL interaction blocks progression of MLL leukemia in vivo. Cancer Cell 27 (4), 589–602. doi:10.1016/j.ccell.2015.02.016
Bowers, E. M., Yan, G., Mukherjee, C., Orry, A., Wang, L., Holbert, M. A., et al. (2010). Virtual ligand screening of the p300/CBP histone acetyltransferase: Identification of a selective small molecule inhibitor. Chem. Biol. 17 (5), 471–482. doi:10.1016/j.chembiol.2010.03.006
Brown, J. A., Bourke, E., Eriksson, L. A., and Kerin, M. J. (2016). Targeting cancer using KAT inhibitors to mimic lethal knockouts. Biochem. Soc. Trans. 44 (4), 979–986. doi:10.1042/BST20160081
Byler, S., Goldgar, S., Heerboth, S., Leary, M., Housman, G., Moulton, K., et al. (2014). Genetic and epigenetic aspects of breast cancer progression and therapy. Anticancer Res. 34 (3), 1071–1077.
Cao, L., Zhu, L., Yang, J., Su, J., Ni, J., Du, Y., et al. (2014). Correlation of low expression of hMOF with clinicopathological features of colorectal carcinoma, gastric cancer and renal cell carcinoma. Int. J. Oncol. 44 (4), 1207–1214. doi:10.3892/ijo.2014.2266
Cao, Q., Yu, J., Dhanasekaran, S. M., Kim, J. H., Mani, R. S., Tomlins, S. A., et al. (2008). Repression of E-cadherin by the polycomb group protein EZH2 in cancer. Oncogene 27 (58), 7274–7284. doi:10.1038/onc.2008.333
Caplan, L. (2014). Delay in breast cancer: Implications for stage at diagnosis and survival. Front. Public Health 2, 87. doi:10.3389/fpubh.2014.00087
Carlisi, D., Lauricella, M., D'Anneo, A., Buttitta, G., Emanuele, S., di Fiore, R., et al. (2015). The synergistic effect of SAHA and parthenolide in MDA-MB231 breast cancer cells. J. Cell. Physiol. 230 (6), 1276–1289. doi:10.1002/jcp.24863
Casciello, F., Al-Ejeh, F., Kelly, G., Brennan, D. J., Ngiow, S. F., Young, A., et al. (2017). G9a drives hypoxia-mediated gene repression for breast cancer cell survival and tumorigenesis. Proc. Natl. Acad. Sci. U. S. A. 114 (27), 7077–7082. doi:10.1073/pnas.1618706114
Casciello, F., Al-Ejeh, F., Miranda, M., Kelly, G., Baxter, E., Windloch, K., et al. (2020). G9a-mediated repression of CDH10 in hypoxia enhances breast tumour cell motility and associates with poor survival outcome. Theranostics 10 (10), 4515–4529. doi:10.7150/thno.41453
Cebrat, M., Kim, C. M., Thompson, P. R., Daugherty, M., and Cole, P. A. (2003). Synthesis and analysis of potential prodrugs of coenzyme A analogues for the inhibition of the histone acetyltransferase p300. Bioorg. Med. Chem. 11 (15), 3307–3313. doi:10.1016/s0968-0896(03)00265-7
Chang, C. C., Wu, M. J., Yang, J. Y., Camarillo, I. G., and Chang, C. J. (2015). Leptin-STAT3-G9a signaling promotes Obesity-mediated breast cancer progression. Cancer Res. 75 (11), 2375–2386. doi:10.1158/0008-5472.CAN-14-3076
Chang, C. J., Yang, J. Y., Xia, W., Chen, C. T., Xie, X., Chao, C. H., et al. (2011). EZH2 promotes expansion of breast tumor initiating cells through activation of RAF1-beta-catenin signaling. Cancer Cell 19 (1), 86–100. doi:10.1016/j.ccr.2010.10.035
Cheng, Y., He, C., Wang, M., Ma, X., Mo, F., Yang, S., et al. (2019). Targeting epigenetic regulators for cancer therapy: Mechanisms and advances in clinical trials. Signal Transduct. Target. Ther. 4, 62. doi:10.1038/s41392-019-0095-0
Chern, T. R., Liu, L., Petrunak, E., Stuckey, J. A., Wang, M., Bernard, D., et al. (2020). Discovery of potent small-molecule inhibitors of MLL methyltransferase. ACS Med. Chem. Lett. 11 (6), 1348–1352. doi:10.1021/acsmedchemlett.0c00229
Chien, Y. C., Liu, L. C., Ye, H. Y., Wu, J. Y., and Yu, Y. L. (2018). EZH2 promotes migration and invasion of triple-negative breast cancer cells via regulating TIMP2-MMP-2/-9 pathway. Am. J. Cancer Res. 8 (3), 422–434.
Chlebowski, R. T., and Anderson, G. L. (2012). Changing concepts: Menopausal hormone therapy and breast cancer. J. Natl. Cancer Inst. 104 (7), 517–527. doi:10.1093/jnci/djs014
Cho, M. H., Park, J. H., Choi, H. J., Park, M. K., Won, H. Y., Park, Y. J., et al. (2015). DOT1L cooperates with the c-Myc-p300 complex to epigenetically derepress CDH1 transcription factors in breast cancer progression. Nat. Commun. 6, 7821. doi:10.1038/ncomms8821
Cloos, P. A., Christensen, J., Agger, K., Maiolica, A., Rappsilber, J., Antal, T., et al. (2006). The putative oncogene GASC1 demethylates tri- and dimethylated lysine 9 on histone H3. Nature 442 (7100), 307–311. doi:10.1038/nature04837
Cole, A. J., Clifton-Bligh, R., and Marsh, D. J. (2015). Histone H2B monoubiquitination: Roles to play in human malignancy. Endocr. Relat. Cancer 22 (1), T19–T33. doi:10.1530/ERC-14-0185
Cole, P. A. (2008). Chemical probes for histone-modifying enzymes. Nat. Chem. Biol. 4 (10), 590–597. doi:10.1038/nchembio.111
Connolly, R. M., Zhao, F., Miller, K. D., Lee, M. J., Piekarz, R. L., Smith, K. L., et al. (2021). E2112: Randomized phase III trial of endocrine therapy plus entinostat or placebo in hormone receptor-positive advanced breast cancer. A trial of the ECOG-ACRIN cancer research group. J. Clin. Oncol. 39 (28), 3171–3181. doi:10.1200/JCO.21.00944
Cooper, S. J., von Roemeling, C. A., Kang, K. H., Marlow, L. A., Grebe, S. K., Menefee, M. E., et al. (2012). Reexpression of tumor suppressor, sFRP1, leads to antitumor synergy of combined HDAC and methyltransferase inhibitors in chemoresistant cancers. Mol. Cancer Ther. 11 (10), 2105–2115. doi:10.1158/1535-7163.MCT-11-0873
Cowan, L. A., Talwar, S., and Yang, A. S. (2010). Will DNA methylation inhibitors work in solid tumors? A review of the clinical experience with azacitidine and decitabine in solid tumors. Epigenomics 2 (1), 71–86. doi:10.2217/epi.09.44
Coward, W. R., Brand, O. J., Pasini, A., Jenkins, G., Knox, A. J., and Pang, L. (2018). Interplay between EZH2 and G9a regulates CXCL10 gene repression in Idiopathic Pulmonary Fibrosis. Am. J. Respir. Cell Mol. Biol. 58 (4), 449–460. doi:10.1165/rcmb.2017-0286OC
Crawford, N. T., McIntyre, A. J., McCormick, A., D'Costa, Z. C., Buckley, N. E., and Mullan, P. B. (2019). TBX2 interacts with heterochromatin protein 1 to recruit a novel repression complex to EGR1-targeted promoters to drive the proliferation of breast cancer cells. Oncogene 38 (31), 5971–5986. doi:10.1038/s41388-019-0853-z
Creyghton, M. P., Cheng, A. W., Welstead, G. G., Kooistra, T., Carey, B. W., Steine, E. J., et al. (2010). Histone H3K27ac separates active from poised enhancers and predicts developmental state. Proc. Natl. Acad. Sci. U. S. A. 107 (50), 21931–21936. doi:10.1073/pnas.1016071107
Cullis, P. M., Wolfenden, R., Cousens, L. S., and Alberts, B. M. (1982). Inhibition of histone acetylation by N-[2-(S-coenzyme A)acetyl] spermidine amide, a multisubstrate analog. J. Biol. Chem. 257 (20), 12165–12169. doi:10.1016/s0021-9258(18)33695-0
Dawson, M. A., and Kouzarides, T. (2012). Cancer epigenetics: From mechanism to therapy. Cell 150 (1), 12–27. doi:10.1016/j.cell.2012.06.013
Dawson, M. A., Prinjha, R. K., Dittmann, A., Giotopoulos, G., Bantscheff, M., Chan, W. I., et al. (2011). Inhibition of BET recruitment to chromatin as an effective treatment for MLL-fusion leukaemia. Nature 478 (7370), 529–533. doi:10.1038/nature10509
Dawson, M. A. (2017). The cancer epigenome: Concepts, challenges, and therapeutic opportunities. Science 355 (6330), 1147–1152. doi:10.1126/science.aam7304
Deb, P., Bhan, A., Hussain, I., Ansari, K. I., Bobzean, S. A., Pandita, T. K., et al. (2016). Endocrine disrupting chemical, bisphenol-A, induces breast cancer associated gene HOXB9 expression in vitro and in vivo. Gene 590 (2), 234–243. doi:10.1016/j.gene.2016.05.009
Delmore, J. E., Issa, G. C., Lemieux, M. E., Rahl, P. B., Shi, J., Jacobs, H. M., et al. (2011). BET bromodomain inhibition as a therapeutic strategy to target c-Myc. Cell 146 (6), 904–917. doi:10.1016/j.cell.2011.08.017
Dhayalan, A., Rajavelu, A., Rathert, P., Tamas, R., Jurkowska, R. Z., Ragozin, S., et al. (2010). The Dnmt3a PWWP domain reads histone 3 lysine 36 trimethylation and guides DNA methylation. J. Biol. Chem. 285 (34), 26114–26120. doi:10.1074/jbc.M109.089433
Di Costanzo, A., Del Gaudio, N., Migliaccio, A., and Altucci, L. (2014). Epigenetic drugs against cancer: An evolving landscape. Arch. Toxicol. 88 (9), 1651–1668. doi:10.1007/s00204-014-1315-6
Dillon, S. C., Zhang, X., Trievel, R. C., and Cheng, X. (2005). The SET-domain protein superfamily: Protein lysine methyltransferases. Genome Biol. 6 (8), 227. doi:10.1186/gb-2005-6-8-227
Dong, C., Wu, Y., Wang, Y., Wang, C., Kang, T., Rychahou, P. G., et al. (2013). Interaction with Suv39H1 is critical for Snail-mediated E-cadherin repression in breast cancer. Oncogene 32 (11), 1351–1362. doi:10.1038/onc.2012.169
Dong, C., Yuan, T., Wu, Y., Wang, Y., Fan, T. W., Miriyala, S., et al. (2013). Loss of FBP1 by Snail-mediated repression provides metabolic advantages in basal-like breast cancer. Cancer Cell 23 (3), 316–331. doi:10.1016/j.ccr.2013.01.022
Dong, H. T., Liu, Q., Zhao, T., Yao, F., Xu, Y., Chen, B., et al. (2020). Long non-coding RNA LOXL1-AS1 drives breast cancer invasion and metastasis by Antagonizing miR-708-5p expression and activity. Mol. Ther. Nucleic Acids 19, 696–705. doi:10.1016/j.omtn.2019.12.016
Dou, D., Ge, X., Wang, X., Xu, X., Zhang, Z., Seng, J., et al. (2019). EZH2 contributes to cisplatin resistance in breast cancer by epigenetically suppressing miR-381 expression. Onco. Targets. Ther. 12, 9627–9637. doi:10.2147/OTT.S214104
Du, D., Katsuno, Y., Meyer, D., Budi, E. H., Chen, S. H., Koeppen, H., et al. (2018). Smad3-mediated recruitment of the methyltransferase SETDB1/ESET controls Snail1 expression and epithelial-mesenchymal transition. EMBO Rep. 19 (1), 135–155. doi:10.15252/embr.201744250
Du, J., Li, L., Ou, Z., Kong, C., Zhang, Y., Dong, Z., et al. (2012). FOXC1, a target of polycomb, inhibits metastasis of breast cancer cells. Breast Cancer Res. Treat. 131 (1), 65–73. doi:10.1007/s10549-011-1396-3
Duong, M. T., Akli, S., Macalou, S., Biernacka, A., Debeb, B. G., Yi, M., et al. (2013). Hbo1 is a cyclin E/CDK2 substrate that enriches breast cancer stem-like cells. Cancer Res. 73 (17), 5556–5568. doi:10.1158/0008-5472.CAN-13-0013
Eliseeva, E. D., Valkov, V., Jung, M., and Jung, M. O. (2007). Characterization of novel inhibitors of histone acetyltransferases. Mol. Cancer Ther. 6 (9), 2391–2398. doi:10.1158/1535-7163.MCT-07-0159
Eram, M. S., Bustos, S. P., Lima-Fernandes, E., Siarheyeva, A., Senisterra, G., Hajian, T., et al. (2014). Trimethylation of histone H3 lysine 36 by human methyltransferase PRDM9 protein. J. Biol. Chem. 289 (17), 12177–12188. doi:10.1074/jbc.M113.523183
Falahi, F., van Kruchten, M., Martinet, N., Hospers, G. A., and Rots, M. G. (2014). Current and upcoming approaches to exploit the reversibility of epigenetic mutations in breast cancer. Breast Cancer Res. 16 (4), 412. doi:10.1186/s13058-014-0412-z
Fan, L., Strasser-Weippl, K., Li, J. J., St Louis, J., Finkelstein, D. M., Yu, K. D., et al. (2014). Breast cancer in China. Lancet. Oncol. 15 (7), e279–89. doi:10.1016/S1470-2045(13)70567-9
Fang, R., Barbera, A. J., Xu, Y., Rutenberg, M., Leonor, T., Bi, Q., et al. (2010). Human LSD2/KDM1b/AOF1 regulates gene transcription by modulating intragenic H3K4me2 methylation. Mol. Cell 39 (2), 222–233. doi:10.1016/j.molcel.2010.07.008
Fang, Y., Liao, G., and Yu, B. (2019). LSD1/KDM1A inhibitors in clinical trials: Advances and prospects. J. Hematol. Oncol. 12 (1), 129. doi:10.1186/s13045-019-0811-9
Fenizia, C., Bottino, C., Corbetta, S., Fittipaldi, R., Floris, P., Gaudenzi, G., et al. (2019). SMYD3 promotes the epithelial-mesenchymal transition in breast cancer. Nucleic Acids Res. 47 (3), 1278–1293. doi:10.1093/nar/gky1221
Filippakopoulos, P., Qi, J., Picaud, S., Shen, Y., Smith, W. B., Fedorov, O., et al. (2010). Selective inhibition of BET bromodomains. Nature 468 (7327), 1067–1073. doi:10.1038/nature09504
Fiorentino, F., Mai, A., and Rotili, D. (2018). Lysine acetyltransferase inhibitors: Structure-activity relationships and potential therapeutic implications. Future Med. Chem. 10 (9), 1067–1091. doi:10.4155/fmc-2017-0244
Fortunati, N., Bertino, S., Costantino, L., Bosco, O., Vercellinatto, I., Catalano, M. G., et al. (2008). Valproic acid is a selective antiproliferative agent in estrogen-sensitive breast cancer cells. Cancer Lett. 259 (2), 156–164. doi:10.1016/j.canlet.2007.10.006
Fujii, S., Ito, K., Ito, Y., and Ochiai, A. (2008). Enhancer of zeste homologue 2 (EZH2) down-regulates RUNX3 by increasing histone H3 methylation. J. Biol. Chem. 283 (25), 17324–17332. doi:10.1074/jbc.M800224200
Gala, K., Li, Q., Sinha, A., Razavi, P., Dorso, M., Sanchez-Vega, F., et al. (2018). KMT2C mediates the estrogen dependence of breast cancer through regulation of ERα enhancer function. Oncogene 37 (34), 4692–4710. doi:10.1038/s41388-018-0273-5
Garcia, P. L., Miller, A. L., Kreitzburg, K. M., Council, L. N., Gamblin, T. L., Christein, J. D., et al. (2016). The BET bromodomain inhibitor JQ1 suppresses growth of pancreatic ductal adenocarcinoma in patient-derived xenograft models. Oncogene 35 (7), 833–845. doi:10.1038/onc.2015.126
Ghizzoni, M., Wu, J., Gao, T., Haisma, H. J., Dekker, F. J., and George Zheng, Y. (2012). 6-alkylsalicylates are selective Tip60 inhibitors and target the acetyl-CoA binding site. Eur. J. Med. Chem. 47 (1), 337–344. doi:10.1016/j.ejmech.2011.11.001
Gong, C., Yao, S., Gomes, A. R., Man, E. P., Lee, H. J., Gong, G., et al. (2016). BRCA1 positively regulates FOXO3 expression by restricting FOXO3 gene methylation and epigenetic silencing through targeting EZH2 in breast cancer. Oncogenesis 5, e214. doi:10.1038/oncsis.2016.23
Gupta, S. C., Patchva, S., and Aggarwal, B. B. (2013). Therapeutic roles of curcumin: Lessons learned from clinical trials. AAPS J. 15 (1), 195–218. doi:10.1208/s12248-012-9432-8
Hamamoto, R., Silva, F. P., Tsuge, M., Nishidate, T., Katagiri, T., Nakamura, Y., et al. (2006). Enhanced SMYD3 expression is essential for the growth of breast cancer cells. Cancer Sci. 97 (2), 113–118. doi:10.1111/j.1349-7006.2006.00146.x
Han, Y., Tanios, F., Reeps, C., Zhang, J., Schwamborn, K., Eckstein, H. H., et al. (2016). Histone acetylation and histone acetyltransferases show significant alterations in human abdominal aortic aneurysm. Clin. Epigenetics 8, 3. doi:10.1186/s13148-016-0169-6
Healey, M. A., Hu, R., Beck, A. H., Collins, L. C., Schnitt, S. J., Tamimi, R. M., et al. (2014). Association of H3K9me3 and H3K27me3 repressive histone marks with breast cancer subtypes in the Nurses' Health Study. Breast Cancer Res. Treat. 147 (3), 639–651. doi:10.1007/s10549-014-3089-1
Heintzman, N. D., Stuart, R. K., Hon, G., Fu, Y., Ching, C. W., Hawkins, R. D., et al. (2007). Distinct and predictive chromatin signatures of transcriptional promoters and enhancers in the human genome. Nat. Genet. 39 (3), 311–318. doi:10.1038/ng1966
Hemshekhar, M., Sebastin Santhosh, M., Kemparaju, K., and Girish, K. S. (2012). Emerging roles of anacardic acid and its derivatives: A pharmacological overview. Basic Clin. Pharmacol. Toxicol. 110 (2), 122–132. doi:10.1111/j.1742-7843.2011.00833.x
Hiatt, R. A., Sibley, A., Venkatesh, B., Cheng, J., Dixit, N., Fox, R., et al. (2022). From cancer Epidemiology to policy and practice: The role of a comprehensive cancer center. Curr. Epidemiol. Rep. 9, 10–21. doi:10.1007/s40471-021-00280-7
Holm, K., Grabau, D., Lovgren, K., Aradottir, S., Gruvberger-Saal, S., Howlin, J., et al. (2012). Global H3K27 trimethylation and EZH2 abundance in breast tumor subtypes. Mol. Oncol. 6 (5), 494–506. doi:10.1016/j.molonc.2012.06.002
Hu, C., Li, M., Guo, T., Wang, S., Huang, W., Yang, K., et al. (2019). Anti-metastasis activity of curcumin against breast cancer via the inhibition of stem cell-like properties and EMT. Phytomedicine 58, 152740. doi:10.1016/j.phymed.2018.11.001
Hu, X., Xiang, D., Xie, Y., Tao, L., Zhang, Y., Jin, Y., et al. (2019). LSD1 suppresses invasion, migration and metastasis of luminal breast cancer cells via activation of GATA3 and repression of TRIM37 expression. Oncogene 38 (44), 7017–7034. doi:10.1038/s41388-019-0923-2
Huang, M., Huang, J., Zheng, Y., and Sun, Q. (2019). Histone acetyltransferase inhibitors: An overview in synthesis, structure-activity relationship and molecular mechanism. Eur. J. Med. Chem. 178, 259–286. doi:10.1016/j.ejmech.2019.05.078
Huyen, Y., Zgheib, O., Ditullio, R. A., Gorgoulis, V. G., Zacharatos, P., Petty, T. J., et al. (2004). Methylated lysine 79 of histone H3 targets 53BP1 to DNA double-strand breaks. Nature 432 (7015), 406–411. doi:10.1038/nature03114
Iizuka, M., Matsui, T., Takisawa, H., and Smith, M. M. (2006). Regulation of replication licensing by acetyltransferase Hbo1. Mol. Cell. Biol. 26 (3), 1098–1108. doi:10.1128/MCB.26.3.1098-1108.2006
Iizuka, M., Sarmento, O. F., Sekiya, T., Scrable, H., Allis, C. D., and Smith, M. M. (2008). Hbo1 Links p53-dependent stress signaling to DNA replication licensing. Mol. Cell. Biol. 28 (1), 140–153. doi:10.1128/MCB.00662-07
Iizuka, M., Takahashi, Y., Mizzen, C. A., Cook, R. G., Fujita, M., Allis, C. D., et al. (2009). Histone acetyltransferase Hbo1: Catalytic activity, cellular abundance, and links to primary cancers. Gene 436 (1-2), 108–114. doi:10.1016/j.gene.2009.01.020
Jenuwein, T., and Allis, C. D. (2001). Translating the histone code. Science 293 (5532), 1074–1080. doi:10.1126/science.1063127
Jeong, G. Y., Park, M. K., Choi, H. J., An, H. W., Park, Y. U., Choi, H. J., et al. (2021). NSD3-Induced methylation of H3K36 activates NOTCH signaling to drive breast tumor initiation and metastatic progression. Cancer Res. 81 (1), 77–90. doi:10.1158/0008-5472.CAN-20-0360
Jeong, K. W., Andreu-Vieyra, C., You, J. S., Jones, P. A., and Stallcup, M. R. (2014). Establishment of active chromatin structure at enhancer elements by mixed-lineage leukemia 1 to initiate estrogen-dependent gene expression. Nucleic Acids Res. 42 (4), 2245–2256. doi:10.1093/nar/gkt1236
Jeong, K. W., Kim, K., Situ, A. J., Ulmer, T. S., An, W., and Stallcup, M. R. (2011). Recognition of enhancer element-specific histone methylation by TIP60 in transcriptional activation. Nat. Struct. Mol. Biol. 18 (12), 1358–1365. doi:10.1038/nsmb.2153
Jin, M. L., Kim, Y. W., Jin, H. L., Kang, H., Lee, E. K., Stallcup, M. R., et al. (2018). Aberrant expression of SETD1A promotes survival and migration of estrogen receptor alpha-positive breast cancer cells. Int. J. Cancer 143 (11), 2871–2883. doi:10.1002/ijc.31853
Jin, Y., Park, S., Park, S. Y., Lee, C. Y., Eum, D. Y., Shim, J. W., et al. (2022). G9a knockdown suppresses cancer aggressiveness by facilitating Smad protein phosphorylation through increasing BMP5 expression in luminal A type breast cancer. Int. J. Mol. Sci. 23 (2), 589. doi:10.3390/ijms23020589
Jones, B., Su, H., Bhat, A., Lei, H., Bajko, J., Hevi, S., et al. (2008). The histone H3K79 methyltransferase Dot1L is essential for mammalian development and heterochromatin structure. PLoS Genet. 4 (9), e1000190. doi:10.1371/journal.pgen.1000190
Jorgensen, S., Schotta, G., and Sorensen, C. S. (2013). Histone H4 lysine 20 methylation: Key player in epigenetic regulation of genomic integrity. Nucleic Acids Res. 41 (5), 2797–2806. doi:10.1093/nar/gkt012
Jozwik, K. M., Chernukhin, I., Serandour, A. A., Nagarajan, S., and Carroll, J. S. (2016). FOXA1 directs H3K4 monomethylation at enhancers via recruitment of the methyltransferase MLL3. Cell Rep. 17 (10), 2715–2723. doi:10.1016/j.celrep.2016.11.028
Karthik, I. P., Desai, P., Sukumar, S., Dimitrijevic, A., Rajalingam, K., and Mahalingam, S. (2018). E4BP4/NFIL3 modulates the epigenetically repressed RAS effector RASSF8 function through histone methyltransferases. J. Biol. Chem. 293 (15), 5624–5635. doi:10.1074/jbc.RA117.000623
Kato, I., Kasukabe, T., and Kumakura, S. (2020). MeninMLL inhibitors induce ferroptosis and enhance the antiproliferative activity of auranofin in several types of cancer cells. Int. J. Oncol. 57 (4), 1057–1071. doi:10.3892/ijo.2020.5116
Kaypee, S., Sudarshan, D., Shanmugam, M. K., Mukherjee, D., Sethi, G., and Kundu, T. K. (2016). Aberrant lysine acetylation in tumorigenesis: Implications in the development of therapeutics. Pharmacol. Ther. 162, 98–119. doi:10.1016/j.pharmthera.2016.01.011
Kim, J., Shin, Y., Lee, S., Kim, M., Punj, V., Lu, J. F., et al. (2018). Regulation of breast cancer-induced Osteoclastogenesis by MacroH2A1.2 involving EZH2-mediated H3K27me3. Cell Rep. 24 (1), 224–237. doi:10.1016/j.celrep.2018.06.020
Kim, J. H., Sharma, A., Dhar, S. S., Lee, S. H., Gu, B., Chan, C. H., et al. (2014). UTX and MLL4 coordinately regulate transcriptional programs for cell proliferation and invasiveness in breast cancer cells. Cancer Res. 74 (6), 1705–1717. doi:10.1158/0008-5472.CAN-13-1896
Kim, M. Y., Woo, E. M., Chong, Y. T., Homenko, D. R., and Kraus, W. L. (2006). Acetylation of estrogen receptor alpha by p300 at lysines 266 and 268 enhances the deoxyribonucleic acid binding and transactivation activities of the receptor. Mol. Endocrinol. 20 (7), 1479–1493. doi:10.1210/me.2005-0531
Kim, S. S., Lee, M. H., and Lee, M. O. (2020). Histone methyltransferases regulate the transcriptional expression of ERα and the proliferation of tamoxifen-resistant breast cancer cells. Breast Cancer Res. Treat. 180 (1), 45–54. doi:10.1007/s10549-019-05517-0
Kim, T., and Buratowski, S. (2009). Dimethylation of H3K4 by Set1 recruits the Set3 histone deacetylase complex to 5' transcribed regions. Cell 137 (2), 259–272. doi:10.1016/j.cell.2009.02.045
Kim, Y., Kim, Y. S., Kim, D. E., Lee, J. S., Song, J. H., Kim, H. G., et al. (2013). BIX-01294 induces autophagy-associated cell death via EHMT2/G9a dysfunction and intracellular reactive oxygen species production. Autophagy 9 (12), 2126–2139. doi:10.4161/auto.26308
Kim, Y. J., Greer, C. B., Cecchini, K. R., Harris, L. N., Tuck, D. P., and Kim, T. H. (2013). HDAC inhibitors induce transcriptional repression of high copy number genes in breast cancer through elongation blockade. Oncogene 32 (23), 2828–2835. doi:10.1038/onc.2013.32
Klose, R. J., Kallin, E. M., and Zhang, Y. (2006). JmjC-domain-containing proteins and histone demethylation. Nat. Rev. Genet. 7 (9), 715–727. doi:10.1038/nrg1945
Krishna, S., Kumar, S., Singh, D. K., Lakra, A. D., Banerjee, D., and Siddiqi, M. I. (2018). Multiple Machine Learning based-Chemoinformatics models for identification of histone acetyl Transferase inhibitors. Mol. Inf. 37 (8), e1700150. doi:10.1002/minf.201700150
Kuo, A. J., Cheung, P., Chen, K., Zee, B. M., Kioi, M., Lauring, J., et al. (2011). NSD2 links dimethylation of histone H3 at lysine 36 to oncogenic programming. Mol. Cell 44 (4), 609–620. doi:10.1016/j.molcel.2011.08.042
Kuo, A. J., Song, J., Cheung, P., Ishibe-Murakami, S., Yamazoe, S., Chen, J. K., et al. (2012). The BAH domain of ORC1 links H4K20me2 to DNA replication licensing and Meier-Gorlin syndrome. Nature 484 (7392), 115–119. doi:10.1038/nature10956
Kwie, F. H., Briet, M., Soupaya, D., Hoffmann, P., Maturano, M., Rodriguez, F., et al. (2011). New potent bisubstrate inhibitors of histone acetyltransferase p300: Design, synthesis and biological evaluation. Chem. Biol. Drug Des. 77 (1), 86–92. doi:10.1111/j.1747-0285.2010.01056.x
Lau, O. D., Kundu, T. K., Soccio, R. E., Ait-Si-Ali, S., Khalil, E. M., Vassilev, A., et al. (2000). HATs off: Selective synthetic inhibitors of the histone acetyltransferases p300 and PCAF. Mol. Cell 5 (3), 589–595. doi:10.1016/s1097-2765(00)80452-9
Lee, J. Y., Kuo, C. W., Tsai, S. L., Cheng, S. M., Chen, S. H., Chan, H. H., et al. (2016). Inhibition of HDAC3- and HDAC6-promoted Survivin expression plays an important role in SAHA-induced autophagy and Viability reduction in breast cancer cells. Front. Pharmacol. 7, 81. doi:10.3389/fphar.2016.00081
Lee, Y. J., Won, A. J., Lee, J., Jung, J. H., Yoon, S., Lee, B. M., et al. (2012). Molecular mechanism of SAHA on regulation of autophagic cell death in tamoxifen-resistant MCF-7 breast cancer cells. Int. J. Med. Sci. 9 (10), 881–893. doi:10.7150/ijms.5011
Li, L. X., Zhou, J. X., Calvet, J. P., Godwin, A. K., Jensen, R. A., and Li, X. (2018). Lysine methyltransferase SMYD2 promotes triple negative breast cancer progression. Cell Death Dis. 9 (3), 326. doi:10.1038/s41419-018-0347-x
Li, W., Wu, H., Sui, S., Wang, Q., Xu, S., and Pang, D. (2021). Targeting histone modifications in breast cancer: A Precise Weapon on the Way. Front. Cell Dev. Biol. 9, 736935. doi:10.3389/fcell.2021.736935
Li, Z., Yu, D., Li, H., Lv, Y., and Li, S. (2019). Long noncoding RNA UCA1 confers tamoxifen resistance in breast cancer endocrinotherapy through regulation of the EZH2/p21 axis and the PI3K/AKT signaling pathway. Int. J. Oncol. 54 (3), 1033–1042. doi:10.3892/ijo.2019.4679
Liang, Y., Hu, J., Li, J., Liu, Y., Yu, J., Zhuang, X., et al. (2015). Epigenetic activation of TWIST1 by MTDH promotes cancer stem-like cell Traits in breast cancer. Cancer Res. 75 (17), 3672–3680. doi:10.1158/0008-5472.CAN-15-0930
Liu, C., Ho, P. C., Wong, F. C., Sethi, G., Wang, L. Z., and Goh, B. C. (2015). Garcinol: Current status of its anti-oxidative, anti-inflammatory and anti-cancer effects. Cancer Lett. 362 (1), 8–14. doi:10.1016/j.canlet.2015.03.019
Liu, L., Kimball, S., Liu, H., Holowatyj, A., and Yang, Z. Q. (2015). Genetic alterations of histone lysine methyltransferases and their significance in breast cancer. Oncotarget 6 (4), 2466–2482. doi:10.18632/oncotarget.2967
Liu, R., Zhang, Z., Yang, H., Zhou, K., Geng, M., Zhou, W., et al. (2019). Design, synthesis, and biological evaluation of a new class of histone acetyltransferase p300 inhibitors. Eur. J. Med. Chem. 180, 171–190. doi:10.1016/j.ejmech.2019.07.026
Liu, S. S., Wu, F., Jin, Y. M., Chang, W. Q., and Xu, T. M. (2020). HDAC11: A rising star in epigenetics. Biomed. Pharmacother. 131, 110607. doi:10.1016/j.biopha.2020.110607
Liu, X., Li, C., Zhang, R., Xiao, W., Niu, X., Ye, X., et al. (2018). The EZH2- H3K27me3-DNMT1 complex orchestrates epigenetic silencing of the wwc1 gene, a Hippo/YAP pathway upstream effector, in breast cancer epithelial cells. Cell. Signal. 51, 243–256. doi:10.1016/j.cellsig.2018.08.011
Liu, Y. N., Lee, W. W., Wang, C. Y., Chao, T. H., Chen, Y., and Chen, J. H. (2005). Regulatory mechanisms controlling human E-cadherin gene expression. Oncogene 24 (56), 8277–8290. doi:10.1038/sj.onc.1208991
Liu, Z., Chen, P., Gao, H., Gu, Y., Yang, J., Peng, H., et al. (2014). Ubiquitylation of autophagy receptor Optineurin by HACE1 activates selective autophagy for tumor suppression. Cancer Cell 26 (1), 106–120. doi:10.1016/j.ccr.2014.05.015
Lu, P., Gu, Y., Li, L., Wang, F., Yang, X., and Yang, Y. (2019). Belinostat suppresses cell proliferation by inactivating Wnt/β-catenin pathway and promotes apoptosis through regulating PKC pathway in breast cancer. Artif. Cells Nanomed. Biotechnol. 47 (1), 3955–3960. doi:10.1080/21691401.2019.1671855
Lu, W., and Katzenellenbogen, B. S. (2017). Estrogen receptor-β modulation of the ERα-p53 loop regulating gene expression, proliferation, and apoptosis in breast cancer. Horm. Cancer 8 (4), 230–242. doi:10.1007/s12672-017-0298-1
Luan, Q. X., Zhang, B. G., Li, X. J., and Guo, M. Y. (2016). MiR-129-5p is downregulated in breast cancer cells partly due to promoter H3K27m3 modification and regulates epithelial-mesenchymal transition and multi-drug resistance. Eur. Rev. Med. Pharmacol. Sci. 20 (20), 4257–4265.
Luo, X. G., Zhang, C. L., Zhao, W. W., Liu, Z. P., Liu, L., Mu, A., et al. (2014). Histone methyltransferase SMYD3 promotes MRTF-A-mediated transactivation of MYL9 and migration of MCF-7 breast cancer cells. Cancer Lett. 344 (1), 129–137. doi:10.1016/j.canlet.2013.10.026
Lynch, H., Wen, H., Kim, Y. C., Snyder, C., Kinarsky, Y., Chen, P. X., et al. (2013). Can unknown predisposition in familial breast cancer be family-specific? Breast J. 19 (5), 520–528. doi:10.1111/tbj.12145
Mu, X., Chen, M., Xiao, B., Yang, B., Singh, S., and Zhang, B. (2019). EZH2 confers sensitivity of breast cancer cells to taxol by Attenuating p21 expression epigenetically. DNA Cell Biol. 38 (7), 651–659. doi:10.1089/dna.2019.4699
Manzo, F., Tambaro, F. P., Mai, A., and Altucci, L. (2009). Histone acetyltransferase inhibitors and preclinical studies. Expert Opin. Ther. Pat. 19 (6), 761–774. doi:10.1517/13543770902895727
Marchion, D. C., Bicaku, E., Daud, A. I., Richon, V., Sullivan, D. M., and Munster, P. N. (2004). Sequence-specific potentiation of topoisomerase II inhibitors by the histone deacetylase inhibitor suberoylanilide hydroxamic acid. J. Cell. Biochem. 92 (2), 223–237. doi:10.1002/jcb.20045
Marchion, D. C., Bicaku, E., Daud, A. I., Sullivan, D. M., and Munster, P. N. (2005). In vivo synergy between topoisomerase II and histone deacetylase inhibitors: Predictive correlates. Mol. Cancer Ther. 4 (12), 1993–2000. doi:10.1158/1535-7163.MCT-05-0194
Margueron, R., and Reinberg, D. (2011). The Polycomb complex PRC2 and its mark in life. Nature 469 (7330), 343–349. doi:10.1038/nature09784
Matkar, S., Sharma, P., Gao, S., Gurung, B., Katona, B. W., Liao, J., et al. (2015). An epigenetic pathway regulates sensitivity of breast cancer cells to HER2 inhibition via FOXO/c-Myc Axis. Cancer Cell 28 (4), 472–485. doi:10.1016/j.ccell.2015.09.005
Mbese, Z., Khwaza, V., and Aderibigbe, B. A. (2019). Curcumin and its derivatives as potential therapeutic agents in prostate, colon and breast cancers. Molecules 24 (23), E4386. doi:10.3390/molecules24234386
McCaw, T. R., Li, M., Starenki, D., Liu, M., Cooper, S. J., Arend, R. C., et al. (2019). Histone deacetylase inhibition promotes intratumoral CD8(+) T-cell responses, sensitizing murine breast tumors to anti-PD1. Cancer Immunol. Immunother. 68 (12), 2081–2094. doi:10.1007/s00262-019-02430-9
McGinty, R. K., Kim, J., Chatterjee, C., Roeder, R. G., and Muir, T. W. (2008). Chemically ubiquitylated histone H2B stimulates hDot1L-mediated intranucleosomal methylation. Nature 453 (7196), 812–816. doi:10.1038/nature06906
Merarchi, M., Sethi, G., Shanmugam, M. K., Fan, L., Arfuso, F., and Ahn, K. S. (2019). Role of natural products in modulating histone deacetylases in cancer. Molecules 24 (6), E1047. doi:10.3390/molecules24061047
Merino, V. F., Nguyen, N., Jin, K., Sadik, H., Cho, S., Korangath, P., et al. (2016). Combined treatment with epigenetic, differentiating, and chemotherapeutic agents Cooperatively targets tumor-initiating cells in triple-negative breast cancer. Cancer Res. 76 (7), 2013–2024. doi:10.1158/0008-5472.CAN-15-1619
Mertz, J. A., Conery, A. R., Bryant, B. M., Sandy, P., Balasubramanian, S., Mele, D. A., et al. (2011). Targeting MYC dependence in cancer by inhibiting BET bromodomains. Proc. Natl. Acad. Sci. U. S. A. 108 (40), 16669–16674. doi:10.1073/pnas.1108190108
Milite, C., Feoli, A., Sasaki, K., La Pietra, V., Balzano, A. L., Marinelli, L., et al. (2015). A novel cell-permeable, selective, and noncompetitive inhibitor of KAT3 histone acetyltransferases from a combined molecular pruning/classical isosterism approach. J. Med. Chem. 58 (6), 2779–2798. doi:10.1021/jm5019687
Miller, T., Krogan, N. J., Dover, J., Erdjument-Bromage, H., Tempst, P., Johnston, M., et al. (2001). Compass: A complex of proteins associated with a trithorax-related SET domain protein. Proc. Natl. Acad. Sci. U. S. A. 98 (23), 12902–12907. doi:10.1073/pnas.231473398
Min, A., Im, S. A., Kim, D. K., Song, S. H., Kim, H. J., Lee, K. H., et al. (2015). Histone deacetylase inhibitor, suberoylanilide hydroxamic acid (SAHA), enhances anti-tumor effects of the poly (ADP-ribose) polymerase (PARP) inhibitor olaparib in triple-negative breast cancer cells. Breast Cancer Res. 17, 33. doi:10.1186/s13058-015-0534-y
Miranda, T. B., Cortez, C. C., Yoo, C. B., Liang, G., Abe, M., Kelly, T. K., et al. (2009). DZNep is a global histone methylation inhibitor that reactivates developmental genes not silenced by DNA methylation. Mol. Cancer Ther. 8 (6), 1579–1588. doi:10.1158/1535-7163.MCT-09-0013
Mo, R., Rao, S. M., and Zhu, Y. J. (2006). Identification of the MLL2 complex as a coactivator for estrogen receptor alpha. J. Biol. Chem. 281 (23), 15714–15720. doi:10.1074/jbc.M513245200
Montenegro, M. F., Sanchez-Del-Campo, L., Gonzalez-Guerrero, R., Martinez-Barba, E., Pinero-Madrona, A., Cabezas-Herrera, J., et al. (2016). Tumor suppressor SET9 guides the epigenetic plasticity of breast cancer cells and serves as an early-stage biomarker for predicting metastasis. Oncogene 35 (47), 6143–6152. doi:10.1038/onc.2016.154
Munster, P. N., Thurn, K. T., Thomas, S., Raha, P., Lacevic, M., Miller, A., et al. (2011). A phase II study of the histone deacetylase inhibitor vorinostat combined with tamoxifen for the treatment of patients with hormone therapy-resistant breast cancer. Br. J. Cancer 104 (12), 1828–1835. doi:10.1038/bjc.2011.156
Nakagawa, M., Oda, Y., Eguchi, T., Aishima, S., Yao, T., Hosoi, F., et al. (2007). Expression profile of class I histone deacetylases in human cancer tissues. Oncol. Rep. 18 (4), 769–774. doi:10.3892/or.18.4.769
Natarajan, T. G., Kallakury, B. V., Sheehan, C. E., Bartlett, M. B., Ganesan, N., Preet, A., et al. (2010). Epigenetic regulator MLL2 shows altered expression in cancer cell lines and tumors from human breast and colon. Cancer Cell Int. 10, 13. doi:10.1186/1475-2867-10-13
Nayak, S. R., Harrington, E., Boone, D., Hartmaier, R., Chen, J., Pathiraja, T. N., et al. (2015). A role for histone H2B variants in endocrine-resistant breast cancer. Horm. Cancer 6 (5-6), 214–224. doi:10.1007/s12672-015-0230-5
Ng, H. H., Xu, R. M., Zhang, Y., and Struhl, K. (2002). Ubiquitination of histone H2B by Rad6 is required for efficient Dot1-mediated methylation of histone H3 lysine 79. J. Biol. Chem. 277 (38), 34655–34657. doi:10.1074/jbc.C200433200
Ngo, L., Brown, T., and Zheng, Y. G. (2019). Bisubstrate inhibitors to target histone acetyltransferase 1. Chem. Biol. Drug Des. 93 (5), 865–873. doi:10.1111/cbdd.13476
Nguyen, A. T., and Zhang, Y. (2011). The diverse functions of Dot1 and H3K79 methylation. Genes Dev. 25 (13), 1345–1358. doi:10.1101/gad.2057811
Nie, L., Wei, Y., Zhang, F., Hsu, Y. H., Chan, L. C., Xia, W., et al. (2019). CDK2-mediated site-specific phosphorylation of EZH2 drives and maintains triple-negative breast cancer. Nat. Commun. 10 (1), 5114. doi:10.1038/s41467-019-13105-5
Nieto-Jimenez, C., Alcaraz-Sanabria, A., Perez-Pena, J., Corrales-Sanchez, V., Serrano-Heras, G., Galan-Moya, E. M., et al. (2017). Targeting basal-like breast tumors with bromodomain and extraterminal domain (BET) and polo-like kinase inhibitors. Oncotarget 8 (12), 19478–19490. doi:10.18632/oncotarget.14465
Ocana, A., Nieto-Jimenez, C., and Pandiella, A. (2017). BET inhibitors as novel therapeutic agents in breast cancer. Oncotarget 8 (41), 71285–71291. doi:10.18632/oncotarget.19744
Oh, W. K., Cho, K. B., Hien, T. T., Kim, T. H., Kim, H. S., Dao, T. T., et al. (2010). Amurensin G, a potent natural SIRT1 inhibitor, rescues doxorubicin responsiveness via down-regulation of multidrug resistance 1. Mol. Pharmacol. 78 (5), 855–864. doi:10.1124/mol.110.065961
Oktyabri, D., Ishimura, A., Tange, S., Terashima, M., and Suzuki, T. (2016). DOT1L histone methyltransferase regulates the expression of BCAT1 and is involved in sphere formation and cell migration of breast cancer cell lines. Biochimie 123, 20–31. doi:10.1016/j.biochi.2016.01.005
Olsen, E. A., Kim, Y. H., Kuzel, T. M., Pacheco, T. R., Foss, F. M., Parker, S., et al. (2007). Phase IIb multicenter trial of vorinostat in patients with persistent, progressive, or treatment refractory cutaneous T-cell lymphoma. J. Clin. Oncol. 25 (21), 3109–3115. doi:10.1200/JCO.2006.10.2434
Ozdag, H., Teschendorff, A. E., Ahmed, A. A., Hyland, S. J., Blenkiron, C., Bobrow, L., et al. (2006). Differential expression of selected histone modifier genes in human solid cancers. BMC Genomics 7, 90. doi:10.1186/1471-2164-7-90
Park, S. E., Yi, H. J., Suh, N., Park, Y. Y., Koh, J. Y., Jeong, S. Y., et al. (2016). Inhibition of EHMT2/G9a epigenetically increases the transcription of Beclin-1 via an increase in ROS and activation of NF-κB. Oncotarget 7 (26), 39796–39808. doi:10.18632/oncotarget.9290
Park, S. H., Fong, K. W., Mong, E., Martin, M. C., Schiltz, G. E., and Yu, J. (2021). Going beyond polycomb: EZH2 functions in prostate cancer. Oncogene 40 (39), 5788–5798. doi:10.1038/s41388-021-01982-4
Park, S. H., Ozden, O., Liu, G., Song, H. Y., Zhu, Y., Yan, Y., et al. (2016). SIRT2-Mediated deacetylation and Tetramerization of pyruvate kinase directs Glycolysis and tumor growth. Cancer Res. 76 (13), 3802–3812. doi:10.1158/0008-5472.CAN-15-2498
Park, U. H., Kang, M. R., Kim, E. J., Kwon, Y. S., Hur, W., Yoon, S. K., et al. (2016). ASXL2 promotes proliferation of breast cancer cells by linking ERα to histone methylation. Oncogene 35 (28), 3742–3752. doi:10.1038/onc.2015.443
Pasculli, B., Barbano, R., and Parrella, P. (2018). Epigenetics of breast cancer: Biology and clinical implication in the era of precision medicine. Semin. Cancer Biol. 51, 22–35. doi:10.1016/j.semcancer.2018.01.007
Patel, A., Vought, V. E., Dharmarajan, V., and Cosgrove, M. S. (2008). A conserved arginine-containing motif crucial for the assembly and enzymatic activity of the mixed lineage leukemia protein-1 core complex. J. Biol. Chem. 283 (47), 32162–32175. doi:10.1074/jbc.M806317200
Peck, B., Chen, C. Y., Ho, K. K., Di Fruscia, P., Myatt, S. S., Coombes, R. C., et al. (2010). SIRT inhibitors induce cell death and p53 acetylation through targeting both SIRT1 and SIRT2. Mol. Cancer Ther. 9 (4), 844–855. doi:10.1158/1535-7163.MCT-09-0971
Pena-Llopis, S., Wan, Y., and Martinez, E. D. (2016). Unique epigenetic gene profiles define human breast cancers with poor prognosis. Oncotarget 7 (52), 85819–85831. doi:10.18632/oncotarget.13334
Piao, L., Suzuki, T., Dohmae, N., Nakamura, Y., and Hamamoto, R. (2015). SUV39H2 methylates and stabilizes LSD1 by inhibiting polyubiquitination in human cancer cells. Oncotarget 6 (19), 16939–16950. doi:10.18632/oncotarget.4760
Piekarz, R. L., Frye, R., Turner, M., Wright, J. J., Allen, S. L., Kirschbaum, M. H., et al. (2009). Phase II multi-institutional trial of the histone deacetylase inhibitor romidepsin as monotherapy for patients with cutaneous T-cell lymphoma. J. Clin. Oncol. 27 (32), 5410–5417. doi:10.1200/JCO.2008.21.6150
Pullikuth, A. K., Routh, E. D., Zimmerman, K. D., Chifman, J., Chou, J. W., Soike, M. H., et al. (2021). Bulk and single-cell profiling of breast tumors Identifies TREM-1 as a dominant immune suppressive marker associated with poor outcomes. Front. Oncol. 11, 734959. doi:10.3389/fonc.2021.734959
Punzi, S., Balestrieri, C., D'Alesio, C., Bossi, D., Dellino, G. I., Gatti, E., et al. (2019). WDR5 inhibition halts metastasis dissemination by repressing the mesenchymal phenotype of breast cancer cells. Breast Cancer Res. 21 (1), 123. doi:10.1186/s13058-019-1216-y
Puppe, J., Drost, R., Liu, X., Joosse, S. A., Evers, B., Cornelissen-Steijger, P., et al. (2009). BRCA1-deficient mammary tumor cells are dependent on EZH2 expression and sensitive to Polycomb Repressive Complex 2-inhibitor 3-deazaneplanocin A. Breast Cancer Res. 11 (4), R63. doi:10.1186/bcr2354
Qin, G., Li, Y., Xu, X., Wang, X., Zhang, K., Tang, Y., et al. (2019). Panobinostat (LBH589) inhibits Wnt/β-catenin signaling pathway via upregulating APCL expression in breast cancer. Cell. Signal. 59, 62–75. doi:10.1016/j.cellsig.2019.03.014
Qin, Y., Vasilatos, S. N., Chen, L., Wu, H., Cao, Z., Fu, Y., et al. (2019). Inhibition of histone lysine-specific demethylase 1 elicits breast tumor immunity and enhances antitumor efficacy of immune checkpoint blockade. Oncogene 38 (3), 390–405. doi:10.1038/s41388-018-0451-5
Quintayo, M. A., Munro, A. F., Thomas, J., Kunkler, I. H., Jack, W., Kerr, G. R., et al. (2012). GSK3β and cyclin D1 expression predicts outcome in early breast cancer patients. Breast Cancer Res. Treat. 136 (1), 161–168. doi:10.1007/s10549-012-2229-8
Rabello Ddo, A., de Moura, C. A., de Andrade, R. V., Motoyama, A. B., and Silva, F. P. (2013). Altered expression of MLL methyltransferase family genes in breast cancer. Int. J. Oncol. 43 (2), 653–660. doi:10.3892/ijo.2013.1981
Rada-Iglesias, A., Bajpai, R., Swigut, T., Brugmann, S. A., Flynn, R. A., and Wysocka, J. (2011). A unique chromatin signature uncovers early developmental enhancers in humans. Nature 470 (7333), 279–283. doi:10.1038/nature09692
Rangasamy, D. (2010). Histone variant H2A.Z can serve as a new target for breast cancer therapy. Curr. Med. Chem. 17 (28), 3155–3161. doi:10.2174/092986710792231941
Rea, S., Eisenhaber, F., O'Carroll, D., Strahl, B. D., Sun, Z. W., Schmid, M., et al. (2000). Regulation of chromatin structure by site-specific histone H3 methyltransferases. Nature 406 (6796), 593–599. doi:10.1038/35020506
Ren, G., Baritaki, S., Marathe, H., Feng, J., Park, S., Beach, S., et al. (2012). Polycomb protein EZH2 regulates tumor invasion via the transcriptional repression of the metastasis suppressor RKIP in breast and prostate cancer. Cancer Res. 72 (12), 3091–3104. doi:10.1158/0008-5472.CAN-11-3546
Rhodes, L. V., Tate, C. R., Segar, H. C., Burks, H. E., Phamduy, T. B., Hoang, V., et al. (2014). Suppression of triple-negative breast cancer metastasis by pan-DAC inhibitor panobinostat via inhibition of ZEB family of EMT master regulators. Breast Cancer Res. Treat. 145 (3), 593–604. doi:10.1007/s10549-014-2979-6
Ring, A., Nguyen, C., Smbatyan, G., Tripathy, D., Yu, M., Press, M., et al. (2018). CBP/β-Catenin/FOXM1 is a novel therapeutic target in triple negative breast cancer. Cancers (Basel) 10 (12), E525. doi:10.3390/cancers10120525
Rivera, C., Saavedra, F., Alvarez, F., Diaz-Celis, C., Ugalde, V., Li, J., et al. (2015). Methylation of histone H3 lysine 9 occurs during translation. Nucleic Acids Res. 43 (19), 9097–9106. doi:10.1093/nar/gkv929
Robert, N. J., Dieras, V., Glaspy, J., Brufsky, A. M., Bondarenko, I., Lipatov, O. N., et al. (2011). RIBBON-1: Randomized, double-blind, placebo-controlled, phase III trial of chemotherapy with or without bevacizumab for first-line treatment of human epidermal growth factor receptor 2-negative, locally recurrent or metastatic breast cancer. J. Clin. Oncol. 29 (10), 1252–1260. doi:10.1200/JCO.2010.28.0982
Robertson, F. M., Chu, K., Boley, K. M., Ye, Z., Liu, H., Wright, M. C., et al. (2013). The class I HDAC inhibitor Romidepsin targets inflammatory breast cancer tumor emboli and synergizes with paclitaxel to inhibit metastasis. J. Exp. Ther. Oncol. 10 (3), 219–233.
Roth, S. Y., Denu, J. M., and Allis, C. D. (2001). Histone acetyltransferases. Annu. Rev. Biochem. 70, 81–120. doi:10.1146/annurev.biochem.70.1.81
Saji, S., Kawakami, M., Hayashi, S., Yoshida, N., Hirose, M., Horiguchi, S., et al. (2005). Significance of HDAC6 regulation via estrogen signaling for cell motility and prognosis in estrogen receptor-positive breast cancer. Oncogene 24 (28), 4531–4539. doi:10.1038/sj.onc.1208646
Salz, T., Deng, C., Pampo, C., Siemann, D., Qiu, Y., Brown, K., et al. (2015). Histone methyltransferase hSETD1A is a novel regulator of metastasis in breast cancer. Mol. Cancer Res. 13 (3), 461–469. doi:10.1158/1541-7786.MCR-14-0389
Santos-Rosa, H., Schneider, R., Bannister, A. J., Sherriff, J., Bernstein, B. E., Emre, N. C., et al. (2002). Active genes are tri-methylated at K4 of histone H3. Nature 419 (6905), 407–411. doi:10.1038/nature01080
Sato, Y., Kujirai, T., Arai, R., Asakawa, H., Ohtsuki, C., Horikoshi, N., et al. (2016). A Genetically Encoded Probe for Live-cell Imaging of H4K20 monomethylation. J. Mol. Biol. 428 (20), 3885–3902. doi:10.1016/j.jmb.2016.08.010
Seidel, C., Florean, C., Schnekenburger, M., Dicato, M., and Diederich, M. (2012). Chromatin-modifying agents in anti-cancer therapy. Biochimie 94 (11), 2264–2279. doi:10.1016/j.biochi.2012.05.012
Shah, P., Gau, Y., and Sabnis, G. (2014). Histone deacetylase inhibitor entinostat reverses epithelial to mesenchymal transition of breast cancer cells by reversing the repression of E-cadherin. Breast Cancer Res. Treat. 143 (1), 99–111. doi:10.1007/s10549-013-2784-7
Shanmugam, M. K., Lee, J. H., Chai, E. Z., Kanchi, M. M., Kar, S., Arfuso, F., et al. (2016). Cancer prevention and therapy through the modulation of transcription factors by bioactive natural compounds. Semin. Cancer Biol. 40-41, 35–47. doi:10.1016/j.semcancer.2016.03.005
Shi, P., Zhou, M., and Yang, Y. (2020). Upregulated tumor sirtuin 2 expression correlates with reduced TNM stage and better overall survival in surgical breast cancer patients. Ir. J. Med. Sci. 189 (1), 83–89. doi:10.1007/s11845-019-02071-y
Shi, Y. K., Li, Z. H., Han, X. Q., Yi, J. H., Wang, Z. H., Hou, J. L., et al. (2010). The histone deacetylase inhibitor suberoylanilide hydroxamic acid induces growth inhibition and enhances taxol-induced cell death in breast cancer. Cancer Chemother. Pharmacol. 66 (6), 1131–1140. doi:10.1007/s00280-010-1455-1
Shilatifard, A. (2008). Molecular implementation and physiological roles for histone H3 lysine 4 (H3K4) methylation. Curr. Opin. Cell Biol. 20 (3), 341–348. doi:10.1016/j.ceb.2008.03.019
Si, X., Zang, R., Zhang, E., Liu, Y., Shi, X., Zhang, E., et al. (2016). LncRNA H19 confers chemoresistance in ERα-positive breast cancer through epigenetic silencing of the pro-apoptotic gene BIK. Oncotarget 7 (49), 81452–81462. doi:10.18632/oncotarget.13263
Simon, R. P., Robaa, D., Alhalabi, Z., Sippl, W., and Jung, M. (2016). KATching-up on small molecule modulators of lysine acetyltransferases. J. Med. Chem. 59 (4), 1249–1270. doi:10.1021/acs.jmedchem.5b01502
Siouda, M., Dujardin, A. D., Barbollat-Boutrand, L., Mendoza-Parra, M. A., Gibert, B., Ouzounova, M., et al. (2020). CDYL2 epigenetically regulates MIR124 to control NF-κB/STAT3-Dependent breast cancer cell plasticity. iScience 23 (6), 101141. doi:10.1016/j.isci.2020.101141
Song, Z., Zhang, X., Lin, Y., Wei, Y., Liang, S., and Dong, C. (2019). LINC01133 inhibits breast cancer invasion and metastasis by negatively regulating SOX4 expression through EZH2. J. Cell. Mol. Med. 23 (11), 7554–7565. doi:10.1111/jcmm.14625
Souto, J. A., Conte, M., Alvarez, R., Nebbioso, A., Carafa, V., Altucci, L., et al. (2008). Synthesis of benzamides related to anacardic acid and their histone acetyltransferase (HAT) inhibitory activities. ChemMedChem 3 (9), 1435–1442. doi:10.1002/cmdc.200800096
Steed, K. L., Jordan, H. R., and Tollefsbol, T. O. (2020). SAHA and EGCG promote apoptosis in triple-negative breast cancer cells, possibly through the modulation of cIAP2. Anticancer Res. 40 (1), 9–26. doi:10.21873/anticanres.13922
Su, C. H., Lin, I. H., Tzeng, T. Y., Hsieh, W. T., and Hsu, M. T. (2016). Regulation of IL-20 expression by Estradiol through KMT2B-mediated epigenetic modification. PLoS One 11 (11), e0166090. doi:10.1371/journal.pone.0166090
Su, Y., Hopfinger, N. R., Nguyen, T. D., Pogash, T. J., Santucci-Pereira, J., and Russo, J. (2018). Epigenetic reprogramming of epithelial mesenchymal transition in triple negative breast cancer cells with DNA methyltransferase and histone deacetylase inhibitors. J. Exp. Clin. Cancer Res. 37 (1), 314. doi:10.1186/s13046-018-0988-8
Subramanian, K., Jia, D., Kapoor-Vazirani, P., Powell, D. R., Collins, R. E., Sharma, D., et al. (2008). Regulation of estrogen receptor alpha by the SET7 lysine methyltransferase. Mol. Cell 30 (3), 336–347. doi:10.1016/j.molcel.2008.03.022
Sulaiman, A., McGarry, S., Li, L., Jia, D., Ooi, S., Addison, C., et al. (2018). Dual inhibition of Wnt and Yes-associated protein signaling retards the growth of triple-negative breast cancer in both mesenchymal and epithelial states. Mol. Oncol. 12 (4), 423–440. doi:10.1002/1878-0261.12167
Sun, Y., Jiang, X., Chen, S., and Price, B. D. (2006). Inhibition of histone acetyltransferase activity by anacardic acid sensitizes tumor cells to ionizing radiation. FEBS Lett. 580 (18), 4353–4356. doi:10.1016/j.febslet.2006.06.092
Tachibana, M., Sugimoto, K., Nozaki, M., Ueda, J., Ohta, T., Ohki, M., et al. (2002). G9a histone methyltransferase plays a dominant role in euchromatic histone H3 lysine 9 methylation and is essential for early embryogenesis. Genes Dev. 16 (14), 1779–1791. doi:10.1101/gad.989402
Tajbakhsh, A., Hasanzadeh, M., Rezaee, M., Khedri, M., Khazaei, M., ShahidSales, S., et al. (2018). Therapeutic potential of novel formulated forms of curcumin in the treatment of breast cancer by the targeting of cellular and physiological dysregulated pathways. J. Cell. Physiol. 233 (3), 2183–2192. doi:10.1002/jcp.25961
Tajima, K., Matsuda, S., Yae, T., Drapkin, B. J., Morris, R., Boukhali, M., et al. (2019). SETD1A protects from senescence through regulation of the mitotic gene expression program. Nat. Commun. 10 (1), 2854. doi:10.1038/s41467-019-10786-w
Takemoto, Y., Ito, A., Niwa, H., Okamura, M., Fujiwara, T., Hirano, T., et al. (2016). Identification of Cyproheptadine as an inhibitor of SET domain containing lysine methyltransferase 7/9 (Set7/9) that regulates estrogen-dependent transcription. J. Med. Chem. 59 (8), 3650–3660. doi:10.1021/acs.jmedchem.5b01732
Talbert, P. B., and Henikoff, S. (2021). Histone variants at a glance. J. Cell Sci. 134 (6), jcs244749. doi:10.1242/jcs.244749
Tan, J., Yang, X., Zhuang, L., Jiang, X., Chen, W., Lee, P. L., et al. (2007). Pharmacologic disruption of Polycomb-repressive complex 2-mediated gene repression selectively induces apoptosis in cancer cells. Genes Dev. 21 (9), 1050–1063. doi:10.1101/gad.1524107
Taniguchi, H., Jacinto, F. V., Villanueva, A., Fernandez, A. F., Yamamoto, H., Carmona, F. J., et al. (2012). Silencing of Kruppel-like factor 2 by the histone methyltransferase EZH2 in human cancer. Oncogene 31 (15), 1988–1994. doi:10.1038/onc.2011.387
Tardat, M., Brustel, J., Kirsh, O., Lefevbre, C., Callanan, M., Sardet, C., et al. (2010). The histone H4 Lys 20 methyltransferase PR-Set7 regulates replication origins in mammalian cells. Nat. Cell Biol. 12 (11), 1086–1093. doi:10.1038/ncb2113
Tate, J. G., Bamford, S., Jubb, H. C., Sondka, Z., Beare, D. M., Bindal, N., et al. (2019). Cosmic: The Catalogue of Somatic mutations in cancer. Nucleic Acids Res. 47 (D1), D941–D947. doi:10.1093/nar/gky1015
Terranova-Barberio, M., Pawlowska, N., Dhawan, M., Moasser, M., Chien, A. J., Melisko, M. E., et al. (2020). Exhausted T cell signature predicts immunotherapy response in ER-positive breast cancer. Nat. Commun. 11 (1), 3584. doi:10.1038/s41467-020-17414-y
Terranova-Barberio, M., Roca, M. S., Zotti, A. I., Leone, A., Bruzzese, F., Vitagliano, C., et al. (2016). Valproic acid potentiates the anticancer activity of capecitabine in vitro and in vivo in breast cancer models via induction of thymidine phosphorylase expression. Oncotarget 7 (7), 7715–7731. doi:10.18632/oncotarget.6802
Tillinghast, G. W., Partee, J., Albert, P., Kelley, J. M., Burtow, K. H., and Kelly, K. (2003). Analysis of genetic stability at the EP300 and CREBBP loci in a panel of cancer cell lines. Genes Chromosom. Cancer 37 (2), 121–131. doi:10.1002/gcc.10195
Towbin, B. D., Gonzalez-Aguilera, C., Sack, R., Gaidatzis, D., Kalck, V., Meister, P., et al. (2012). Step-wise methylation of histone H3K9 positions heterochromatin at the nuclear periphery. Cell 150 (5), 934–947. doi:10.1016/j.cell.2012.06.051
Trabert, B., Sherman, M. E., Kannan, N., and Stanczyk, F. Z. (2020). Progesterone and breast cancer. Endocr. Rev. 41 (2), bnz001. doi:10.1210/endrev/bnz001
Travaglini, L., Vian, L., Billi, M., Grignani, F., and Nervi, C. (2009). Epigenetic reprogramming of breast cancer cells by valproic acid occurs regardless of estrogen receptor status. Int. J. Biochem. Cell Biol. 41 (1), 225–234. doi:10.1016/j.biocel.2008.08.019
Trisciuoglio, D., Di Martile, M., and Del Bufalo, D. (2018). Emerging role of histone acetyltransferase in stem cells and cancer. Stem Cells Int. 2018, 8908751. doi:10.1155/2018/8908751
Truax, A. D., Thakkar, M., and Greer, S. F. (2012). Dysregulated recruitment of the histone methyltransferase EZH2 to the class II transactivator (CIITA) promoter IV in breast cancer cells. PLoS One 7 (4), e36013. doi:10.1371/journal.pone.0036013
Tsang, J. Y., Lai, S. T., Ni, Y. B., Shao, Y., Poon, I. K., Kwan, J. S., et al. (2021). SETD2 alterations and histone H3K36 trimethylation in phyllodes tumor of breast. Breast Cancer Res. Treat. 187 (2), 339–347. doi:10.1007/s10549-021-06181-z
Tu, W. B., Shiah, Y. J., Lourenco, C., Mullen, P. J., Dingar, D., Redel, C., et al. (2018). MYC interacts with the G9a histone methyltransferase to drive transcriptional repression and tumorigenesis. Cancer Cell 34 (4), 579–595. doi:10.1016/j.ccell.2018.09.001
Unnikrishnan, A., Gafken, P. R., and Tsukiyama, T. (2010). Dynamic changes in histone acetylation regulate origins of DNA replication. Nat. Struct. Mol. Biol. 17 (4), 430–437. doi:10.1038/nsmb.1780
Vansteenkiste, J., Van Cutsem, E., Dumez, H., Chen, C., Ricker, J. L., Randolph, S. S., et al. (2008). Early phase II trial of oral vorinostat in relapsed or refractory breast, colorectal, or non-small cell lung cancer. Invest. New Drugs 26 (5), 483–488. doi:10.1007/s10637-008-9131-6
Vavouri, T., and Lehner, B. (2012). Human genes with CpG island promoters have a distinct transcription-associated chromatin organization. Genome Biol. 13 (11), R110. doi:10.1186/gb-2012-13-11-r110
Verma, S. K., Tian, X., LaFrance, L. V., Duquenne, C., Suarez, D. P., Newlander, K. A., et al. (2012). Identification of potent, selective, cell-active inhibitors of the histone lysine methyltransferase EZH2. ACS Med. Chem. Lett. 3 (12), 1091–1096. doi:10.1021/ml3003346
Vo, N., and Goodman, R. H. (2001). CREB-binding protein and p300 in transcriptional regulation. J. Biol. Chem. 276 (17), 13505–13508. doi:10.1074/jbc.R000025200
Voss, A. K., and Thomas, T. (2009). MYST family histone acetyltransferases take center stage in stem cells and development. Bioessays 31 (10), 1050–1061. doi:10.1002/bies.200900051
Vougiouklakis, T., Saloura, V., Park, J. H., Takamatsu, N., Miyamoto, T., Nakamura, Y., et al. (2018). Development of novel SUV39H2 inhibitors that exhibit growth suppressive effects in mouse xenograft models and regulate the phosphorylation of H2AX. Oncotarget 9 (61), 31820–31831. doi:10.18632/oncotarget.25806
Wadia, J. S., and Dowdy, S. F. (2005). Transmembrane delivery of protein and peptide drugs by TAT-mediated transduction in the treatment of cancer. Adv. Drug Deliv. Rev. 57 (4), 579–596. doi:10.1016/j.addr.2004.10.005
Wang, C., Fu, M., Angeletti, R. H., Siconolfi-Baez, L., Reutens, A. T., Albanese, C., et al. (2001). Direct acetylation of the estrogen receptor alpha hinge region by p300 regulates transactivation and hormone sensitivity. J. Biol. Chem. 276 (21), 18375–18383. doi:10.1074/jbc.M100800200
Wang, J., Duan, Z., Nugent, Z., Zou, J. X., Borowsky, A. D., Zhang, Y., et al. (2016). Reprogramming metabolism by histone methyltransferase NSD2 drives endocrine resistance via coordinated activation of pentose phosphate pathway enzymes. Cancer Lett. 378 (2), 69–79. doi:10.1016/j.canlet.2016.05.004
Wang, J. J., Zou, J. X., Wang, H., Duan, Z. J., Wang, H. B., Chen, P., et al. (2019). Histone methyltransferase NSD2 mediates the survival and invasion of triple-negative breast cancer cells via stimulating ADAM9-EGFR-AKT signaling. Acta Pharmacol. Sin. 40 (8), 1067–1075. doi:10.1038/s41401-018-0199-z
Wang, L., Collings, C. K., Zhao, Z., Cozzolino, K. A., Ma, Q., Liang, K., et al. (2017). A cytoplasmic COMPASS is necessary for cell survival and triple-negative breast cancer pathogenesis by regulating metabolism. Genes Dev. 31 (20), 2056–2066. doi:10.1101/gad.306092.117
Wang, L., Xu, M. L., Wang, C., Dong, Q. Q., Miao, Z., Chen, X. Y., et al. (2020). SET and MYND domain-containing protein 3 inhibits tumor cell sensitivity to cisplatin. Oncol. Lett. 19 (5), 3469–3476. doi:10.3892/ol.2020.11465
Wang, T., Mao, B., Cheng, C., Zou, Z., Gao, J., Yang, Y., et al. (2018). YAP promotes breast cancer metastasis by repressing growth differentiation factor-15. Biochim. Biophys. Acta. Mol. Basis Dis. 1864 (5), 1744–1753. doi:10.1016/j.bbadis.2018.02.020
Wang, Y., Han, Y., Fan, E., and Zhang, K. (2015). Analytical strategies used to identify the readers of histone modifications: A review. Anal. Chim. Acta 891, 32–42. doi:10.1016/j.aca.2015.06.049
Wang, Y. F., Zhang, J., Su, Y., Shen, Y. Y., Jiang, D. X., Hou, Y. Y., et al. (2017). G9a regulates breast cancer growth by modulating iron homeostasis through the repression of ferroxidase hephaestin. Nat. Commun. 8 (1), 274. doi:10.1038/s41467-017-00350-9
Wawruszak, A., Borkiewicz, L., Okon, E., Kukula-Koch, W., Afshan, S., and Halasa, M. (2021). Vorinostat (SAHA) and breast cancer: An overview. Cancers (Basel) 13 (18), 4700. doi:10.3390/cancers13184700
Wawruszak, A., Gumbarewicz, E., Okon, E., Jeleniewicz, W., Czapinski, J., Halasa, M., et al. (2019). Histone deacetylase inhibitors reinforce the phenotypical markers of breast epithelial or mesenchymal cancer cells but inhibit their migratory properties. Cancer Manag. Res. 11, 8345–8358. doi:10.2147/CMAR.S210029
Wawruszak, A., Luszczki, J. J., Grabarska, A., Gumbarewicz, E., Dmoszynska-Graniczka, M., Polberg, K., et al. (2015). Assessment of interactions between cisplatin and two histone deacetylase inhibitors in MCF7, T47D and MDA-MB-231 human breast cancer cell lines - an Isobolographic analysis. PLoS One 10 (11), e0143013. doi:10.1371/journal.pone.0143013
Winters, S., Martin, C., Murphy, D., and Shokar, N. K. (2017). Breast cancer Epidemiology, prevention, and screening. Prog. Mol. Biol. Transl. Sci. 151, 1–32. doi:10.1016/bs.pmbts.2017.07.002
Wu, H., Min, J., Lunin, V. V., Antoshenko, T., Dombrovski, L., Zeng, H., et al. (2010). Structural biology of human H3K9 methyltransferases. PLoS One 5 (1), e8570. doi:10.1371/journal.pone.0008570
Wu, H. T., Liu, Y. E., Hsu, K. W., Wang, Y. F., Chan, Y. C., Chen, Y., et al. (2020). MLL3 induced by Luteolin causes apoptosis in tamoxifen-resistant breast cancer cells through H3K4 monomethylation and suppression of the PI3K/AKT/mTOR pathway. Am. J. Chin. Med. 48 (5), 1221–1241. doi:10.1142/S0192415X20500603
Wu, J., Wang, J., Li, M., Yang, Y., Wang, B., and Zheng, Y. G. (2011). Small molecule inhibitors of histone acetyltransferase Tip60. Bioorg. Chem. 39 (1), 53–58. doi:10.1016/j.bioorg.2010.11.003
Wu, S., Luo, Z., Yu, P. J., Xie, H., and He, Y. W. (2016). Suberoylanilide hydroxamic acid (SAHA) promotes the epithelial mesenchymal transition of triple negative breast cancer cells via HDAC8/FOXA1 signals. Biol. Chem. 397 (1), 75–83. doi:10.1515/hsz-2015-0215
Xiao, X. S., Cai, M. Y., Chen, J. W., Guan, X. Y., Kung, H. F., Zeng, Y. X., et al. (2011). High expression of p300 in human breast cancer correlates with tumor Recurrence and predicts adverse prognosis. Chin. J. Cancer Res. 23 (3), 201–207. doi:10.1007/s11670-011-0201-5
Xun, J., Wang, D., Shen, L., Gong, J., Gao, R., Du, L., et al. (2017). JMJD3 suppresses stem cell-like characteristics in breast cancer cells by downregulation of Oct4 independently of its demethylase activity. Oncotarget 8 (13), 21918–21929. doi:10.18632/oncotarget.15747
Yi, C., Li, G., Wang, W., Sun, Y., Zhang, Y., Zhong, C., et al. (2021). Disruption of YY1-EZH2 interaction using synthetic peptides inhibits breast cancer development. Cancers 13 (10), 2402. doi:10.3390/cancers13102402
Yamaguchi, H., Du, Y., Nakai, K., Ding, M., Chang, S. S., Hsu, J. L., et al. (2018). EZH2 contributes to the response to PARP inhibitors through its PARP-mediated poly-ADP ribosylation in breast cancer. Oncogene 37 (2), 208–217. doi:10.1038/onc.2017.311
Yang, F., Sun, L., Li, Q., Han, X., Lei, L., Zhang, H., et al. (2012). SET8 promotes epithelial-mesenchymal transition and confers TWIST dual transcriptional activities. EMBO J. 31 (1), 110–123. doi:10.1038/emboj.2011.364
Yang, G., Yuan, Y., Yuan, H., Wang, J., Yun, H., Geng, Y., et al. (2021). Histone acetyltransferase 1 is a succinyltransferase for histones and non-histones and promotes tumorigenesis. EMBO Rep. 22 (2), e50967. doi:10.15252/embr.202050967
Yang, H., Kwon, C. S., Choi, Y., and Lee, D. (2016). Both H4K20 mono-methylation and H3K56 acetylation mark transcription-dependent histone turnover in fission yeast. Biochem. Biophys. Res. Commun. 476 (4), 515–521. doi:10.1016/j.bbrc.2016.05.155
Yang, L., Zhang, Y., Shan, W., Hu, Z., Yuan, J., Pi, J., et al. (2017). Repression of BET activity sensitizes homologous recombination-proficient cancers to PARP inhibition. Sci. Transl. Med. 9 (400), eaal1645. doi:10.1126/scitranslmed.aal1645
Yang, T., Wang, P., Yin, X., Zhang, J., Huo, M., Gao, J., et al. (2021). The histone deacetylase inhibitor PCI-24781 impairs calcium influx and inhibits proliferation and metastasis in breast cancer. Theranostics 11 (5), 2058–2076. doi:10.7150/thno.48314
Yang, X. J., and Seto, E. (2007). HATs and HDACs: From structure, function and regulation to novel strategies for therapy and prevention. Oncogene 26 (37), 5310–5318. doi:10.1038/sj.onc.1210599
Yardley, D. A., Ismail-Khan, R. R., Melichar, B., Lichinitser, M., Munster, P. N., Klein, P. M., et al. (2013). Randomized phase II, double-blind, placebo-controlled study of exemestane with or without entinostat in postmenopausal women with locally recurrent or metastatic estrogen receptor-positive breast cancer progressing on treatment with a nonsteroidal aromatase inhibitor. J. Clin. Oncol. 31 (17), 2128–2135. doi:10.1200/JCO.2012.43.7251
Yomtoubian, S., Lee, S. B., Verma, A., Izzo, F., Markowitz, G., Choi, H., et al. (2020). Inhibition of EZH2 catalytic activity selectively targets a metastatic Subpopulation in triple-negative breast cancer. Cell Rep. 30 (3), 755–770. doi:10.1016/j.celrep.2019.12.056
Yu, C. W., Cheng, K. C., Chen, L. C., Lin, M. X., Chang, Y. C., and Hwang-Verslues, W. W. (2018). Pro-inflammatory cytokines IL-6 and CCL2 suppress expression of circadian gene Period2 in mammary epithelial cells. Biochim. Biophys. Acta. Gene Regul. Mech. 1861 (11), 1007–1017. doi:10.1016/j.bbagrm.2018.09.003
Yu, Y., Qi, J., Xiong, J., Jiang, L., Cui, D., He, J., et al. (2019). Epigenetic Co-Deregulation of EZH2/TET1 is a senescence-Countering, actionable Vulnerability in triple-negative breast cancer. Theranostics 9 (3), 761–777. doi:10.7150/thno.29520
Yuan, W., Xu, M., Huang, C., Liu, N., Chen, S., and Zhu, B. (2011). H3K36 methylation antagonizes PRC2-mediated H3K27 methylation. J. Biol. Chem. 286 (10), 7983–7989. doi:10.1074/jbc.M110.194027
Yuan, Z., Syed, M. A., Panchal, D., Rogers, D., Joo, M., and Sadikot, R. T. (2012). Curcumin mediated epigenetic modulation inhibits TREM-1 expression in response to lipopolysaccharide. Int. J. Biochem. Cell Biol. 44 (11), 2032–2043. doi:10.1016/j.biocel.2012.08.001
Yun, J. M., Jialal, I., and Devaraj, S. (2011). Epigenetic regulation of high glucose-induced proinflammatory cytokine production in monocytes by curcumin. J. Nutr. Biochem. 22 (5), 450–458. doi:10.1016/j.jnutbio.2010.03.014
Zeng, Y., Qiu, R., Yang, Y., Gao, T., Zheng, Y., Huang, W., et al. (2019). Regulation of EZH2 by SMYD2-mediated lysine methylation is implicated in tumorigenesis. Cell Rep. 29 (6), 1482–1498. doi:10.1016/j.celrep.2019.10.004
Zhao, L., Pang, A., and Li, Y. (2018). Function of GCN5 in the TGF-β1-induced epithelial-to-mesenchymal transition in breast cancer. Oncol. Lett. 16 (3), 3955–3963. doi:10.3892/ol.2018.9134
Zhang, J., Sui, S., Wu, H., Zhang, J., Zhang, X., Xu, S., et al. (2019). The transcriptional landscape of lncRNAs reveals the oncogenic function of LINC00511 in ER-negative breast cancer. Cell Death Dis. 10 (8), 599. doi:10.1038/s41419-019-1835-3
Zhang, H., Zhang, Y., Chen, C., Zhu, X., Zhang, C., Xia, Y., et al. (2018). A double-negative feedback loop between DEAD-box protein DDX21 and Snail regulates epithelial-mesenchymal transition and metastasis in breast cancer. Cancer Lett. 437, 67–78. doi:10.1016/j.canlet.2018.08.021
Zhang, J., Yao, D., Jiang, Y., Huang, J., Yang, S., and Wang, J. (2017). Synthesis and biological evaluation of benzimidazole derivatives as the G9a Histone Methyltransferase inhibitors that induce autophagy and apoptosis of breast cancer cells. Bioorg. Chem. 72, 168–181. doi:10.1016/j.bioorg.2017.04.005
Zhang, K. J., Tan, X. L., and Guo, L. (2020). The long non-coding RNA DANCR regulates the inflammatory phenotype of breast cancer cells and promotes breast cancer progression via EZH2-dependent suppression of SOCS3 transcription. Mol. Oncol. 14 (2), 309–328. doi:10.1002/1878-0261.12622
Zhang, R., Li, X., Liu, Z., Wang, Y., Zhang, H., and Xu, H. (2020). EZH2 inhibitors-mediated epigenetic reactivation of FOSB inhibits triple-negative breast cancer progress. Cancer Cell Int. 20, 175. doi:10.1186/s12935-020-01260-5
Zhang, R., Wang, J., Zhao, L., Liu, S., Du, D., Ding, H., et al. (2018). Identification of novel inhibitors of histone acetyltransferase hMOF through high throughput screening. Eur. J. Med. Chem. 157, 867–876. doi:10.1016/j.ejmech.2018.08.026
Zhang, S., Tang, Z., Qing, B., Tang, R., Duan, Q., Ding, S., et al. (2019). Valproic acid promotes the epithelial-to-mesenchymal transition of breast cancer cells through stabilization of Snail and transcriptional upregulation of Zeb1. Eur. J. Pharmacol. 865, 172745. doi:10.1016/j.ejphar.2019.172745
Zhang, Y., Liu, J., Lin, J., Zhou, L., Song, Y., Wei, B., et al. (2016). The transcription factor GATA1 and the histone methyltransferase SET7 interact to promote VEGF-mediated angiogenesis and tumor growth and predict clinical outcome of breast cancer. Oncotarget 7 (9), 9859–9875. doi:10.18632/oncotarget.7126
Zhou, W., Feng, X., Han, H., Guo, S., and Wang, G. (2016). Synergistic effects of combined treatment with histone deacetylase inhibitor suberoylanilide hydroxamic acid and TRAIL on human breast cancer cells. Sci. Rep. 6, 28004. doi:10.1038/srep28004
Keywords: epi-drugs, histone modification, tumor suppressor gene, breast cancer, epigenetics
Citation: Feng J and Meng X (2022) Histone modification and histone modification-targeted anti-cancer drugs in breast cancer: Fundamentals and beyond. Front. Pharmacol. 13:946811. doi: 10.3389/fphar.2022.946811
Received: 18 May 2022; Accepted: 15 August 2022;
Published: 15 September 2022.
Edited by:
Lixiang Xue, Peking University Third Hospital, ChinaReviewed by:
Sonia Emanuele, University of Palermo, ItalyCopyright © 2022 Feng and Meng. This is an open-access article distributed under the terms of the Creative Commons Attribution License (CC BY). The use, distribution or reproduction in other forums is permitted, provided the original author(s) and the copyright owner(s) are credited and that the original publication in this journal is cited, in accordance with accepted academic practice. No use, distribution or reproduction is permitted which does not comply with these terms.
*Correspondence: Xinyue Meng, bWVuZ3hpbnl1ZTg4QDE2My5jb20=
Disclaimer: All claims expressed in this article are solely those of the authors and do not necessarily represent those of their affiliated organizations, or those of the publisher, the editors and the reviewers. Any product that may be evaluated in this article or claim that may be made by its manufacturer is not guaranteed or endorsed by the publisher.
Research integrity at Frontiers
Learn more about the work of our research integrity team to safeguard the quality of each article we publish.