- 1Department of Acupuncture and Moxibustion, Neuroscience Centre, Integrated Hospital of Traditional Chinese Medicine, Southern Medical University, Guangzhou, China
- 2Beijing Increasepharm Safety and Efficacy Co., Ltd., Beijing, China
- 3Beijing Key Laboratory of Pharmacology of Chinese Materia Region, Institute of Basic Medical Sciences, Xiyuan Hospital of China Academy of Chinese Medical Sciences, Beijing, China
- 4Department of Anesthesiology, Punan Hospital, Shanghai, China
Ischemic stroke has been considered one of the leading causes of mortality and disability worldwide, associated with a series of complex pathophysiological processes. However, effective therapeutic methods for ischemic stroke are still limited. Panax ginseng, a valuable traditional Chinese medicine, has been long used in eastern countries for various diseases. Ginsenosides, the main active ingredient of Panax ginseng, has demonstrated neuroprotective effects on ischemic stroke injury during the last decade. In this article, we summarized the pathophysiology of ischemic stroke and reviewed the literature on ginsenosides studies in preclinical and clinical ischemic stroke. Available findings showed that both major ginsenosides and minor ginsenosides (such as Rg3, Rg5, and Rh2) has a potential neuroprotective effect, mainly through attenuating the excitotoxicity, Ca2+ overload, mitochondria dysfunction, blood-brain barrier (BBB) permeability, anti-inflammation, anti-oxidative, anti-apoptosis, anti-pyroptosis, anti-autophagy, improving angiogenesis, and neurogenesis. Therefore, this review brings a current understanding of the mechanisms of ginsenosides in the treatment of ischemic stroke. Further studies, especially in clinical trials, will be important to confirm the clinical value of ginseng and ginsenosides.
Introduction
Stroke is one of the leading causes of disability and mortality worldwide, which creates a significant economic burden on the healthcare system (Johnson et al., 2019). Ischemic stroke (IS) is the primary stroke subtype, accounting for approximately 87% of stroke cases (Virani et al., 2020). The middle cerebral artery (MCA) is the most commonly affected vascular territory in cerebral ischemic stroke (Navarro-Orozco and Sánchez-Manso, 2022), and an intravascular blood clot or thrombus usually causes vascular occlusion. Clinically, thrombolytic therapy and thrombectomy are the only approved methods for treating acute ischemic stroke (Campbell and Khatri, 2020), restoring blood flow to the ischemic brain and rescuing damaged neurons in the ischemic penumbra. However, these treatments have limited time windows, intravenous alteplase (rtPA) restricted within 4.5 h, endovascular thrombectomy within 24 h (Lees et al., 2010; Powers et al., 2019), to reduce the risk of hemorrhagic transformation, and only a minority of patients benefit from the treatments timely. Therefore, exploring new drugs or therapies is necessary to prolong the therapeutic window and improve patient outcomes.
In ischemic stroke, the obstruction of brain blood vessels deprives the essential nutrients and oxygen to brain cells, causing a sudden onset of neurological deficit. The ischemic insult may lead to irreversible damage or death to neurons in the ischemic core, while the neurons in the penumbral area surrounding the ischemic core may be salvageable with effective brain-protective treatments. Ischemic stroke involves a variety of mechanisms, such as excitotoxicity, mitochondrial dysfunction, oxidative stress, inflammation, autophagy, and blood-brain barrier (BBB) damage (George and Steinberg, 2015; Chamorro et al., 2016; Wang P. et al., 2018). Mitochondrial dysfunction occurs within minutes of ischemic stroke, resulting in depletion of adenosine triphosphate (ATP) and membrane depolarization, followed by sustained glutamate release and intracellular Ca2+ overload. The increased intracellular calcium leads to the overproduction of reactive oxygen species (ROS) and activates inflammatory responses, triggering the death of damaged neurons and the leakage of BBB (Zhou et al., 2018). These pathophysiological mechanisms overlap and correlate with the development of ischemic stroke and are potential pharmacological targets for treating ischemic stroke.
As a traditional herbal medicine, Panax ginseng has been widely used in treating and preventing diseases for thousands of years in East Asian countries, especially in China, Korea, and Japan. The botanical name “Panax” implies “all-healing” in Greek, which stemmed from the traditional belief that ginseng has healing properties in all aspects of the body (Kim, 2018). Among the eleven ginseng species, Panax ginseng (Asian or Korean ginseng), Panax quinquefolius (North American ginseng), and Panax notoginseng are three particularly important for medicinal use (Wang et al., 2020). Panax ginseng contains various pharmacological components, such as ginsenosides, polysaccharides, and polyphenols (Zheng et al., 2017). Ginsenosides are considered the main active ingredients of Panax ginseng, Panax quinquefolius, and Panax notoginseng for the pharmaceutical functions, which are mainly accumulated in roots, stems, leaves, flowers buds, and berries (Kim et al., 2018). About 200 ginsenosides have been identified from ginseng, including major ginsenosides (Rd, Rb1, Rb2, Rc, Re, Rg1, etc.) and minor ginsenosides (Rh1, Rh2, Rg3, Rg5, etc.) (Hyun et al., 2022). According to the chemical structures, ginsenosides can be divided into protopanaxadiol (PPD), protopanaxatriol (PPT), and oleanolic acid. PPD mainly includes ginsenosides Rd, Rb1, Rb2, Rb3, Rg3, Rg5, Rh2, F2, and compound K. PPT includes the ginsenosides Rg1, Rg2, Re, Rf, Rh1, and F1, while the typical representative ginsenoside of the oleanolic acid is ginsenoside Ro (Lu et al., 2022). As a natural product, ginseng has a wide range of pharmacological effects, such as anti-oxidative and anti-cancer, enhancing immunity, energy, and sexuality, and combating neurological diseases, diabetes mellitus, and cardiovascular diseases (Ratan et al., 2021). Currently, growing evidence shows that ginsenosides have neuroprotective effects in vivo and in vitro and have excellent potential as novel candidate agents for ischemic stroke. It can be used to treat ischemic stroke via reducing neurotoxicity (Zhang C. et al., 2020), anti-oxidant (Chu et al., 2019), anti-inflammation (Zhu et al., 2012), anti-apoptosis (Li et al., 2010), anti-autophagy (Huang et al., 2020), regulating blood-brain barrier permeability (Zhang X. et al., 2020), promoting angiogenesis (Chen J. et al., 2019) and neurogenesis (Gao et al., 2010) to alleviate nerve damage and promote nerve repair.
This article reviews the literature on treating ischemic stroke with ginsenosides, including preclinical and clinical experimental studies. Studies of ginsenosides in treating cerebral ischemia published until March 2022 were identified from the PubMed database. We summarized the pathophysiologies of cerebral ischemia stroke and the potential mechanisms of ginsenosides in treating ischemic stroke. Our work brings a current understanding of the mechanisms of ginsenosides in the treatment of ischemic stroke.
Pathophysiologies of Ischemic Stroke
Excitotoxicity
Excitotoxicity is one of the significant events in cerebral ischemia, playing a key role in neuronal death (Rothman and Olney, 1986). After cerebral ischemia, rapid and massive release and uptake inhibition of the excitatory amino acid glutamate leads to energy failure (Chamorro et al., 2016). The function of ion pumps is required with ATP to transform the sodium (Na+), potassium (K+), and Ca2+ between intracellular and extracellular. With ATP depletion, the Ca2+ cannot be pumped out of neuron cells and causes glutamate release (Luoma et al., 2011). Postsynaptic receptors of glutamate include ionotropic receptors or metabotropic receptors (mGluRs), the ionotropic type receptor, NMDA (N-methyl-D-aspartate) receptor, which primarily regulates the excitotoxic response (Kaplan-Arabaci et al., 2022). Overactivation of glutamate receptors leads to the opening of receptor-gated calcium channels and Ca2+ influx, and the increase of intracellular Ca2+ causes a series of pathological reactions in the cytoplasm and nucleus (Lai et al., 2014). Moreover, Ca2+ overload in mitochondrial activates the downstream apoptotic pathway, inducing mitochondrial destruction and cell apoptosis (Szydlowska and Tymianski, 2010). Astrocyte glutamate transporter excitatory amino-acid transporter 2 (EAAT2 or GLT-1) is the primary glutamate transporter in the brain, playing a pivotal role in sustaining glutamate homeostasis (Tzingounis and Wadiche, 2007). Therefore, regulating the excitatory neurotransmitter glutamate and Ca2+ influx significantly reduces the excitotoxicity after cerebral ischemia.
Inflammation
Inflammatory response plays a crucial role in ischemic stroke pathogenesis, which contributes to all the stages of ischemic stroke (Drieu et al., 2018). Inflammatory response at the blood-endothelial interface, including adhesion molecules, cytokines, chemokines, and leukocytes, is an essential cerebral infarction tissue injury mechanism (Zhu et al., 2022). Astrocytes and microglia are the primary cells in the brain that mediate inflammatory responses in response to ischemic brain injury (Mo et al., 2020). Astrocyte hypertrophy and proliferation are extensive responses to neuronal injury. Stroke-induced brain injury activates microglia polarization into pro-inflammatory, classical (M1) or anti-inflammatory, alternative (M2) phenotypes (Song et al., 2019). M1 microglia produce large amounts of pro-inflammatory mediators, such as tumor necrosis factor α (TNFα), interleukin (IL)-1β, IL-6, interferon-γ (IFN-γ), inducible nitric oxide synthase (iNOS), and proteolytic enzymes (Yenari et al., 2010). While M2 microglia is characterized by the effects of pro-angiogenic and anti-inflammatory, producing IL-4, IL-10, transforming growth factor β (TGF-β), and vascular endothelial growth factor (VEGF) (Qin et al., 2019). Inflammation after cerebral ischemia with contrasting effects, as it can promote nerve repair as well as aggravate secondary brain damage. Toll-like receptors (TLRs), nuclear factor-kappa B (NF-κB), and mitogen-activated protein kinases (MAPK) signaling pathways are related to the activation of inflammation in ischemic stroke (Mo et al., 2020). TLRs are transmembrane proteins expressed in microglia, astrocytes, neurons, and cerebral endothelium (Marsh et al., 2009), which can induce inflammatory responses by regulating cytokine and chemokine production. NF-κB participates in transcriptional induction of pro-inflammatory genes, such as cell adhesion molecules, cytokines, matrix metalloproteinases (MMP), and growth factors. p38 MAPK plays a vital role in inflammation-mediated ischemic injury (Sun and Nan, 2016). In addition, the NOD-like receptor (NLR) family, pyrin domain containing 3(NLRP3) inflammasome can detect tissue damage and pathogen invasion through innate immune cell sensor components commonly known as pattern recognition receptors (PRRs). PRRs promote activation NF-κB and MAPK pathways, thus increasing the transcription of protein-coding genes associated with NLRP3 (Xu et al., 2021).
Oxidative Stress
Oxidative and nitrosative stress present a challenge to ischemic stroke, which is caused by the excessive production of reactive oxygen species (ROS) and reactive nitrogen species (RNS) (Allen and Bayraktutan, 2009). Excessive ROS can result in lipid peroxidation and damage proteins and DNA, initiating a cascade of deleterious cellular processes that promote cell death. It often results from ROS/RNS production and antioxidant systems imbalance. Under physiological conditions, ROS and RNS can be scavenged by endogenous antioxidant enzymes or non-enzyme, including superoxide dismutase (SOD), catalase (CAT), glutathione peroxidase (GPX), glutathione-S-transferase (GST), and glutathione (GSH) (Tang D. et al., 2019). After cerebral ischemia, ROS and RNS have been shown in phagocytes, vascular cells, and glial cells in the penumbra. ROS is composed of superoxide anions (O2−), hydrogen peroxide, hydroxyl radical, and hydroperoxyl radicals, a by-product of oxygen metabolism in mitochondria. RNS mainly includes nitric oxide (NO) and peroxynitrite anion (ONOO−), while ONOO− is formed by the rapid reaction of NO and O2− (He et al., 2021). Nuclear factor erythroid 2-related factor (Nrf2) is a transcription factor that regulates the expression of endogenous antioxidant enzymes, and Nrf2/ARE is an important endogenous anti-oxidative stress signaling pathway (Wu et al., 2020). Actively protecting mitochondrial function, antioxidation, free radical scavenging, and slowing down oxidative stress have become effective strategies in saving neurons from the pathological processes of cerebral ischemia-reperfusion. Anti-oxidative stress, scavenging free radicals, and protecting mitochondrial function have become effective strategies to save neurons from the pathological process of ischemic injury.
Apoptosis/Pyroptosis/Ferroptosis
Multiple cell death pathways are implicated in the pathogenesis of ischemic stroke (Tuo et al., 2022). Apoptosis is a typical form of programmed or regulated cell death. Recent studies have revealed novel programmed or regulated cell death types, including pyroptosis and ferroptosis (Galluzzi et al., 2018).
Apoptosis can be triggered through either the intrinsic or the extrinsic pathway. The initial morphological changes in apoptosis have been observed in post-ischemic stroke neurons, which involve cell shrinkage and cytoplasmatic condensation, nuclear membrane breakdown, and formation of apoptotic bodies (Linnik et al., 1993). The intrinsic signaling cascade of apoptosis can be mediated by calpain, ROS, and DNA damage. Excessive accumulation of Ca2+ and ROS in intracellular triggers activation of calpains and one of the substrates-B-cell leukemia/lymphoma 2 (Bcl-2). Bcl-2 is an anti-apoptotic protein that could interact with Bax on the mitochondrial membrane, causing a release of various proapoptotic factors, including cytochrome C (Cytc) and apoptosis-inducing factor (AIF) (Chao and Korsmeyer, 1998). Cystic complexes form an apoptosome with apoptotic protein-activating factor-1 and procaspase-9, activating caspase-3 and initiating cell death (Wang et al., 2019). ROS can damage the plasma membrane and DNA; DNA damage activates the nuclear pathway of cell death through the phosphorylation of p53 or translocation of nucleophosmin (Culmsee and Krieglstein, 2007). The extrinsic apoptosis pathway is triggered by the extracellular death ligands (TRAIL, FasL, TNF-α) that bind to death receptors (TRAILR, Fas, TNFR1) and Fas-associated death domain (FADD), creating a death-inducing signaling complex with procaspase-8. Activated caspase-8 activates downstream effector caspases (such as caspase-3) by direct proteolytic cleavage (Muhammad et al., 2018; Tuo et al., 2022).
Pyroptosis is an inflammatory form of programmed cell death that inflammasome activation can cause. The inflammasome is a protein complex that can be activated by infection, metabolic imbalances, and tissue injury (Broz and Dixit, 2016). Several inflammasome sensor proteins have been identified, including the NLRP1, NLRP3, NLRP4, and absent in melanoma 2 (AIM2), which trigger the downstream inflammatory response (Fann et al., 2013a). Inflammasomes, including canonical and noncanonical types, canonical inflammasomes like the NLRP3 activate caspase-1, whereas noncanonical inflammasomes activate mouse caspase-11 or human caspase-4 and caspase-5 (Hu J. J. et al., 2020). Gasdermin D (GSDMD) is the key effector of pyroptosis, downstream of inflammasome pathways, and a substrate for inflammatory caspases-1,4, 5, and 11 (Liu Z. et al., 2019). Caspase-1 or caspase-11 can cleave GSDMD into an N-terminal fragment (GSDMD-N) and C-terminal product (GSDMD-C) (Shi et al., 2015). Once caspase-1 is activated, pro-IL-1β and pro-IL18 can be divided into biologically active, mature, pro-inflammatory cytokines released into the extracellular environment, causing neuronal cell toxicity (Tuo et al., 2022). Inhibition or knockout of caspase-1 is neuroprotective in focal stroke models (Fann et al., 2013b).
Ferroptosis is an iron-dependent form of regulated cell death (Dixon et al., 2012), with iron accumulation and lipid peroxidation. Excessive intracellular iron accumulation elevates ROS by Fenton reaction, leading to ferroptosis cell death by irresistible lipid peroxidation. Studies have shown that iron deposition, lipid peroxidation, and neuronal death in the brain were significantly increased in an adult rat model of ischemic stroke (Kondo et al., 1997; Park U. J. et al., 2011). Glutathione peroxidase 4 (GPX4) plays an important role in suppressing ferroptosis, which functions to reduce lipid peroxides in cellular membranes. GPX4 uses GSH to eliminate the production of phospholipid hydroperoxides (PLOOH), the primary mediator of chain reactions in lipoxygenases (Tang D. L. et al., 2019). GSH is the most abundant antioxidant in the cell, synthesized from glutamate, cysteine, and glycine, among which cysteine is the rate-limiting precursor (Lee et al., 2020). The intracellular cysteine level mainly depends on extracellular cystine uptake by system Xc− (Koppula et al., 2018), which consists of a regulatory subunit solute carrier family 3 member 2 (SLC3A2) and a catalytic subunit solute carrier family 7 member 11 (SLC7A11). Correspondingly, the inactivation of GPX4 or SLC7A11 induces ferroptosis. The levels of GPx4 and SLC7A11 were found to be decreased in MCAO rats compared with those in the sham group (Lan et al., 2020).
Autophagy
Autophagy-dependent death, known as type 2 programmed cell death (Shen et al., 2013), plays a vital role in maintaining cellular homeostasis after cerebral ischemia. Ischemia and hypoxia cause cell dysfunction of energy metabolism, leading to the destruction of the cytoskeleton and loss of homeostasis (Mo et al., 2020). Autophagy is initiated by nucleating a double membrane, which elongates into an autophagosomal vesicle that encapsulates damaged macromolecules and organelles (Klionsky et al., 2016). A cascade of autophagy-related proteins (ATGs) plays critical roles in autophagic membrane dynamics and processes (Liu and Levine, 2015). LC3-II is a biological marker of autophagosome formation localized to the autophagosome membrane. Mammalian target of rapamycin (mTOR) is one of the critical targets for autophagy regulation, a serine/threonine-protein kinase that belongs to the phosphatidylinositol 3-kinase (PI3K) related kinase family (Glick et al., 2010). Typical autophagy is triggered through a core pre-activation complex composed of ULK1/2, ATG13, and FIP200 proteins. AMPK is a central regulator of metabolism and autophagy and can phosphorylate ULK1 to activate autophagy (Jia et al., 2020), a potential therapeutic target for ischemic stroke (Jiang et al., 2018). In moderate hypoxia, hypoxia-inducible factor-1α (HIF-1α) regulates autophagy through upregulating expression of Bcl-2 and 19-kDa interacting protein 3 (BNIP3), while BNIP3 mediates autophagy by disrupting the interaction of Beclin-1 with Bcl-2 (Matsui et al., 2008). A report showed that knockdown of Beclin-1 can prevent secondary neurodegenerative damage after focal cerebral infarction by inhibiting autophagy activation (Xing et al., 2012).
Others
Cerebral ischemia initiates a complex cascade of pathophysiological events. In addition to the pathophysiologic reviewed above, BBB permeability, angiogenesis, and neurogenesis are crucial mechanisms for cerebral ischemia and reperfusion. The BBB is a cellular barrier composed of tight junctions between vascular endothelial cells interfaced with pericytes and astrocytes (Singh et al., 2016), which protects the central nervous system (CNS) by regulating the transport of substances between the blood and brain. Inflammatory cytokines, such as TNF-α and IL-1β, can increase the permeability of BBB to entrance into the CNS (Smith et al., 2016). The increased biphasic permeability of BBB leads to cerebral angiogenic edema, hemorrhage, and mortality during ischemic stroke-reperfusion (Knowland et al., 2014). Angiogenesis involves sprouting new vessels from existing vessels, predominantly induced by vascular endothelial growth factor (VEGF) (Ferrara and Adamis, 2016). It is critical to repair tissue regeneration under wound healing, hypoxia, and chronic ischemia (Fan et al., 2018). Under hypoxic conditions, HIF-1α plays a crucial role in pathophysiological angiogenesis by directly regulating VEGF, and HIF-1α/VEGF may be an important pathway for the regulation of angiogenesis (Hu Q. et al., 2020). Neurogenesis is a complex process that generates new functional neurons and glial cells from neural stem cells (NSCs), mainly in the subventricular zone (SVZ) and the subgranular zone of the dentate gyrus (DG) of the hippocampus, involving proliferation, differentiation, migration, and maturation (Nada et al., 2014). Accumulative evidence supports that newborn neurons have critical physiological functions in neuroplasticity, learning and memory, and emotion regulation (Berg et al., 2019). After cerebral ischemia, the increased expression of brain-derived neurotrophic factor (BDNF), platelet-derived growth factor-B (PDGF-B), transforming growth factor-beta (TGF-β), fibroblast growth factor 2 (FGF2), and VEGF, may promote both angiogenesis and axonal outgrowth (Hatakeyama et al., 2020). Therefore, activation of endogenous neurogenesis plays a vital role in promoting neurological function recovery.
Neuroprotective Effects of Ginseng and Ginsenosides in Ischemic Stroke
Ginseng, the root of Panax ginseng, has been widely used to treat cerebrovascular diseases in Asian countries. Ginsenosides are the major bioactive components of ginseng, responsible for its pharmacological activities (Kim et al., 2020). Now, accumulated studies show that ginseng and ginsenosides have many positive effects on treating and preventing cerebral ischemic stroke. Ginsenosides with neuroprotective effects mainly include ginsenoside Rb1 (Liu A. et al., 2018), ginsenoside Rd (Zhang X. et al., 2020), ginsenoside Re (Chen et al., 2008), ginsenoside Rg1 (Zheng et al., 2019), ginsenoside Rg2 (Zhang G. et al., 2008), ginsenoside Rg3 (He et al., 2017), ginsenoside Rg5 (Cheng et al., 2019), ginsenoside Rh2 (Bae et al., 2006), ginsenoside F1 (Zhang et al., 2019), Compound K (Huang et al., 2020), Oleanolic acid (Lin et al., 2021) (Figure 1). Overall, the neuroprotective effects of ginseng and ginsenosides against cerebral ischemia are mediated by the regulation of excitotoxicity, Ca2+ overload, inflammation, mitochondria dysfunction, oxidative stress, apoptosis, pyroptosis, autophagy, BBB permeability, angiogenesis, and neurogenesis, as shown in Table 1 and Table 2. The content of ginsenosides in Panax ginseng is also shown in Table 1.
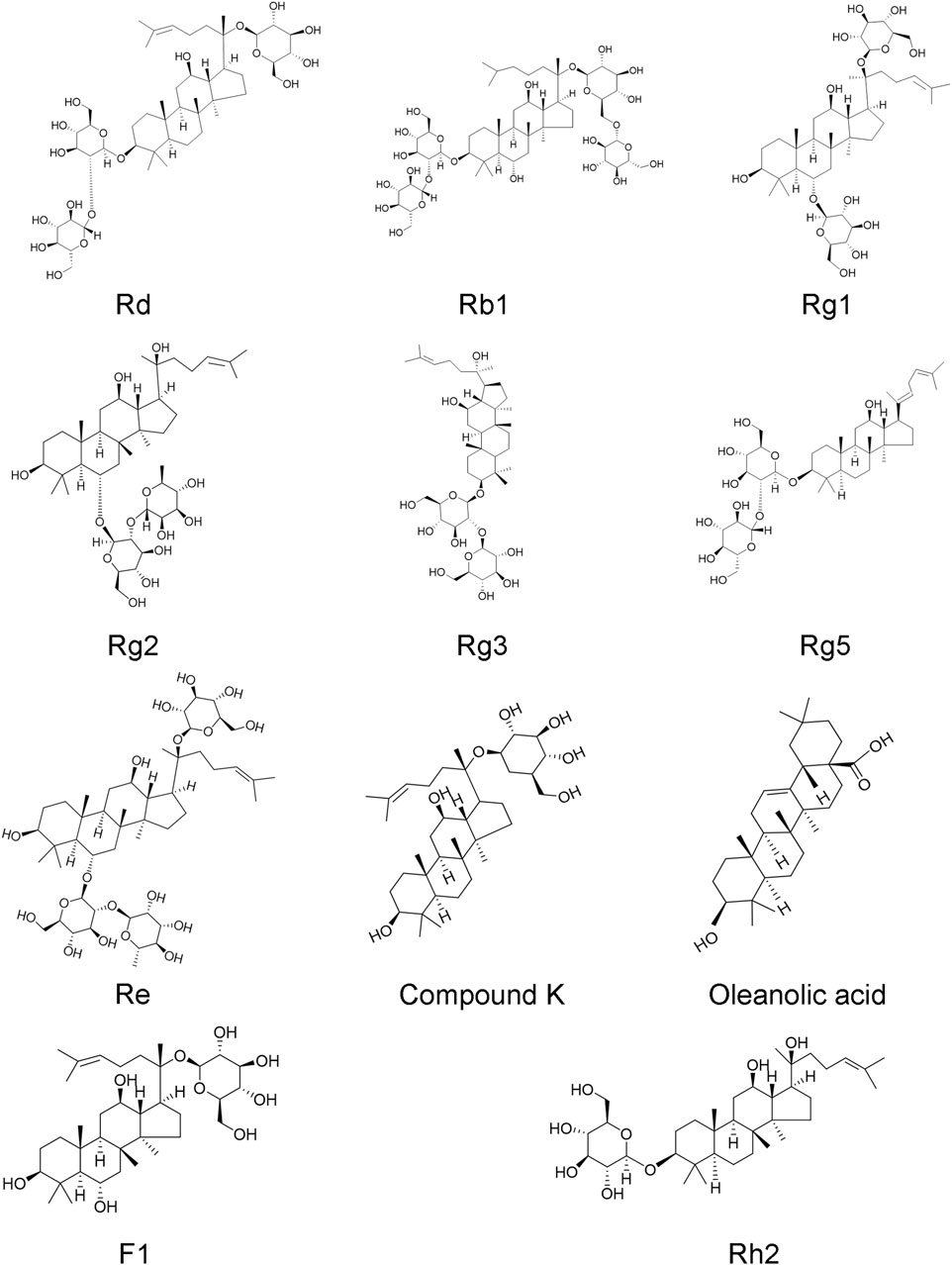
FIGURE 1. Chemical structures of ginsenoside Rd, Rb1, Rg1, Rg2, Rg3, Rg5, Re, Rh2, F1, compound K, and oleanolic acid.
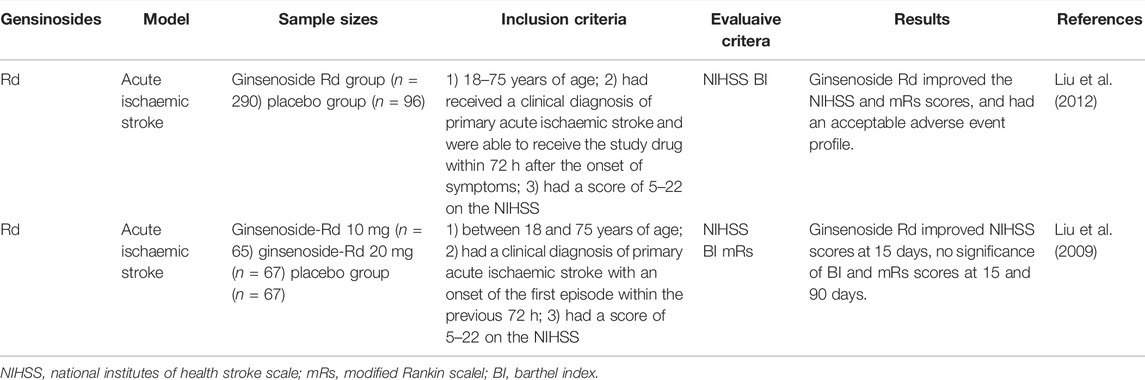
TABLE 2. Summary of clinical trials of ginsenosides interventions in cerebarl ischemic stroke patients.
Panax Ginseng and its Neuroprotective Effects
According to the manufacturing processing technique of ginseng, Panax ginseng can be divided into three types: white ginseng, red ginseng, and black ginseng (Hyun et al., 2022). White ginseng is produced by dehydration in the sun without cooking, and red ginseng is steamed at 90–100°C for 2–3 h. Until now, red ginseng is mainly processed in Korea, which is also named Korea red ginseng (KRG). While black ginseng is generated by steaming red ginseng nine times (Jo et al., 2009; Wan et al., 2021). The therapeutic effects of KRG on permanent and transient hypoxic-ischemic brain damage were studied in rats and mice at 100–360 mg/kg per day. In hypoxic-ischemic (HI) mice, 7 days before HI pretreated with KRG, reduced infarct volume, cerebral edema, and degeneration of hippocampal neurons were observed at 6 h, 24 h, 7 days, and 28 days after HI (Liu et al., 2019a; Liu et al., 2020). What’s more, red ginseng pretreatment could also suppress apoptosis in ischemic lesions (Cheon et al., 2013). Recent studies have shown that KRG pretreatment has elicited robust and prolonged anti-oxidative and anti-inflammatory effects after hypoxia-ischemia via an Nrf2-dependent manner. While Nrf2-dependent endogenous neuroprotection effects attenuate sensorimotor deficits and gliosis reactive in microglia and astrocytes, they regulate dynamic glutamine synthetase (GS) and aquaporin 4 (AQP4) expressions, thus improving long-term functional recovery (Liu L. et al., 2018; Liu et al., 2019b; Liu et al., 2020). Red ginseng could play the effect of anti-oxidant by reducing the level of lipid peroxidation (Ban et al., 2012), and increasing the expression of GSH, CAT, GST, glutathione peroxidase GPx and SOD (Kim et al., 2009) (Shah et al., 2005). Meanwhile, the neuroprotection of anti-inflammation may raise IL-10 expression and reduce the levels of TNF-α, IL-1β, and IL-6 in serum (Lee et al., 2011). In addition, black ginseng is helpful for the treatment of vascular dementia via reduced loss of cholinergic immunoreactivity and nicotinamide adenine dinucleotide phosphate-diaphorase (NADPH-d)-positive neurons in the hippocampus (Park H. J. et al., 2011). Ginseng total saponins could increase the number of BrdU+/NeuN+ cells to induce endogenous neural stem cell activation (Zheng et al., 2011), further supporting the beneficial role of ginseng in ischemic stroke.
Ginsenoside Rd and its Neuroprotective Effect
Ginsenoside Rd is one of the major ginsenosides responsible for pharmaceutical activities and has been demonstrated to exert significant neuroprotective in preclinical and clinical studies. In vitro and in vivo studies show that ginsenoside Rd could improve neuron survival and decrease neuron apoptosis. Ginsenoside Rd modulates the balance between acetylated histone H3 (Ac-H3) and histone deacetylase [histone deacetylase 2 (HDAC2)], thus upregulating BDNF in chronic cerebral hypoperfusion (CCH) mice and OGD/R neurons (Wan et al., 2017). Ginsenoside Rd significantly inhibits glutamate-induced Ca2+ entry in cortical neurons and prevents cell apoptosis (Li et al., 2010). A recent study shows that ginsenoside Rd regulates cerebral ischemia/reperfusion injury by exerting an anti-pyroptotic effect through the miR-139-5p/FoxO1/Keap1/Nrf2 axis (Yao et al., 2022). What’s more, ginsenoside Rd administration could enhance ischemic stroke-induced cognitive impairment and downregulate tau protein phosphorylation via the PI3K/AKT/GSK-3β pathway (Zhang et al., 2014). Glutamate is essential for excitatory synapse transmission; however, overstimulation of ionic glutamate receptors can trigger excessive calcium influx, leading to excitotoxicity of neurons. Ginsenoside Rd protects neurons against glutamate-induced excitotoxicity by inhibiting Ca2+ influx (Zhang C. et al., 2012), attenuating the expression of transient receptor potential melastatin 7 (TRPM7) and acid-sensing ion channels 1a (ASIC1a) (Zhang Y. et al., 2012), and mitigating DAPK1-mediated NR2b phosphorylation and attenuating calcineurin activity (Xie et al., 2016; Zhang C. et al., 2020). Ginsenoside Rd administration promotes glutamate clearance by upregulating the expression of glial glutamate transporter-1 (GLT-1) through PI3K/AKT and ERK1/2 pathways (Zhang et al., 2013).
Pretreatment of ginsenoside Rd plays antiapoptotic and anti-inflammatory effects in MCAO rats through inhibiting poly (ADP-ribose) polymerase-1, preventing the mitochondrial release of apoptosis-inducing factor (AIF), and reducing the accumulation of NF-κB p65 subunit nuclear (Hu et al., 2013). Another study showed that ginsenoside Rd could eliminate inflammatory injury by inhibiting the expression of iNOS and cyclooxygenase-2 (COX-2) (Ye et al., 2011c). Oxidative stress caused by ischemic stroke leads to DNA damage and triggers cell death. Ginsenoside Rd could upregulate the endogenous antioxidant system, preserve the mitochondrial respiratory chain complex and aconitase activities, downregulate mitochondrial hydrogen peroxide production, and stabilize mitochondrial membrane potential (Ye et al., 2009; Ye et al., 2011b; Yang et al., 2016). Another similar report showed that ginsenoside Rd minimizes mitochondria-mediated apoptosis following focal ischemia by reducing the mitochondrial release of cytochrome c (CytoC) and AIF. In vitro studies further exhibited that ginsenoside Rd could attenuate mitochondrial swelling, preserve MMP, and decrease ROS production (Ye et al., 2011d). Following ischemic stroke, impaired cell volume regulation can lead to cytotoxic cell swelling, disruption of BBB integrity, and brain edema. Ginsenoside Rd could pass through the intact BBB and exert neuroprotection effects in transient and permanent MCAO rat models (Ye et al., 2011a). In addition, ginsenoside Rd attenuates BBB by inhibiting proteasome activity and sequentially suppressing the NF-κB/MMP-9 pathway (Zhang X. et al., 2020). At the same time, ginsenoside Rd could promote neurogenesis via upregulating the expression of VEGF, BDNF, and growth-associated protein of 43 kDa (GAP-43) and activating the PI3K/Akt and ERK1/2 dependent pathways (Liu et al., 2015; Wu S. D. et al., 2016).
Two randomized, double-blind, placebo-controlled, phase II multicenter clinical trials involving 199 patients (Liu et al., 2009) and 390 patients (Liu et al., 2012) with acute ischemic stroke showed that Rd could improve patients’ neurologic deficits scores at 15 or 90 days and ameliorate disability by modified Rankin Scale (mRS) score at 90 days after stroke. The therapeutic effect of ginsenoside Rd may be related to its capability to suppress microglial proteasome and secondary inflammation (Zhang et al., 2016). The studies suggested that ginsenoside Rd is a promising neuroprotectant in acute ischemic patients.
Ginsenoside Rb1 and its Neuroprotective Effects
Ginsenoside Rb1 is one of the main bioactive saponins in ginseng, which could alleviate cerebral ischemia injury via modulating apoptosis, autophagy, oxidative, inflammation, BBB permeability, and promoting neurogenesis (Jiang et al., 2013; Luo et al., 2014; Chen et al., 2015; Cheng et al., 2019). Apoptotic caspases further classified as initiator caspases (Caspase-8, -9, -10), and effector caspases (Caspase-3, -6, -7) based on their functions (Wu Y. et al., 2016). Ginsenoside Rb1 could inhibit apoptosis and attenuate damaged neurons by downregulation of the expression of caspase-3, caspase-9 (Liu A. et al., 2018), nitric oxide, and superoxide (Ke et al., 2014), and up-regulating the expression of the mitochondrion associated antiapoptotic factor Bcl-xL (Zhang et al., 2006). Ginsenoside Rb1 could inhibit the expression of Beclin-1 and LC3-II via activation of PI3K/Akt pathway (Lu et al., 2011; Luo et al., 2014). The neuroprotective effect of ginsenoside Rb1 is also related to the activation of Akt/mTOR signaling pathway and inhibition of P-PTEN protein (Guo et al., 2018).
Free radicals can be excessively produced following cerebral ischemia. Ginsenoside Rbl protects the cerebral cortex and hippocampal CA1 neurons against ischemic damage by scavenging free radicals (Lim et al., 1997; Zhang et al., 1998). Administration of Rb1 or Rg1 could improve the mitochondrial and reduce ROS production in OGD/R cultured astrocytes, with increased activity of CAT, complexes I, II, III, and V, elevated level of mtDNA and ATP, and attenuated the MMP depolarization (Xu et al., 2019). Furthermore, ginsenoside Rb1 also showed an antioxidative effect in aged mice (Dong et al., 2017). Inflammation plays an important role in the pathophysiological process after ischemic stroke, which could induce secondary brain damage (Rajkovic et al., 2018). Ginsenoside Rbl could exert anti-inflammatory effects by downregulating the expression of IL-6, and TNF-α (Zhu et al., 2012), which is associated with TLR4/MyD88 and SIRT1 signaling pathways (Cheng et al., 2019). High mobility group box1 (HMGB1) is a highly abundant non-histone DNA-binding nuclear protein and is a crucial pro-inflammatory factor in ischemic stroke. Administration of Ginsenoside Rb1 could also attenuate cerebral ischemic reperfusion-induced apoptosis and inflammation via inhibiting HMGB1inflammatory signals (Liu A. et al., 2018). In addition, ginsenoside Rb1 protects BBB integrity following cerebral ischemia and reduces brain edema by suppressing neuroinflammation induction of MMP-9 and NOX4-derived free radicals (Chen et al., 2015). Ginsenoside Rb1 has a positive effect on neurogenesis, probably via improving the expression of NeuN, BDNF, glial-derived neurotrophic factor (GDNF), and growth-associated protein 43 (GAP43), while decreasing the expression of TUNEL, caspase-3, and GFAP (Yuan et al., 2007; Yoshikawa et al., 2008) (Jiang et al., 2013) (Gao et al., 2010). Intravenous infusion of ginsenoside Rb1 prevents ischemic brain damage through upregulation of VEGF and Bcl-xL (Sakanaka et al., 2007). In addition, ginsenoside Rb1could promote functional motor recovery in post-stroke mice by stimulating axonal regeneration and brain repair by regulating the cAMP/PKA/CREB pathway (Gao X. et al., 2020).
Ginsenosides Rg1, Rg2, Rg3, Rg5 and Their Neuroprotective Effects
Ginsenoside Rg, including Rg1, Rg2, Rg3, and Rg5, has been widely used in cerebral ischemic stroke with therapeutic effects of anti-apoptosis (Zhang G. et al., 2008), antioxidant (Li et al., 2017a), anti-inflammation (Zheng et al., 2019), regulating energy metabolism (Gao J. et al., 2020), and promoting angiogenesis (Chen J. et al., 2019) and neurogenesis (Shen and Zhang, 2003).
Ginsenoside Rg1 could reduce the neurological deficit scores, brain edema, and infarct volume in MCAO mice and inhibit intracellular Ca2+ overload and ROS production in astrocytes (Sun et al., 2014). Neuron apoptosis, inflammation, and oxidative stress are the main pathological characteristics of cerebral ischemia stroke. Ginsenoside Rg1 protects NSCs from OGD-induced cell apoptosis and oxidative stress via inhibiting the phosphorylation of p38/JNK2 (Li et al., 2017b), while Rg1 combined with mannitol protects neurons against apoptosis through the PERK-eIF2-α-ATF4 signaling pathway (Gu et al., 2020). PPARγ/Heme oxygenase-1 (HO-1) signaling was critical in mediating apoptosis and inflammation, while ginsenoside Rg1 could activate PPARγ/HO-1 and provide neuroprotective effects via modulating the expression of levels of PPARγ, Bcl-2, cleaved caspase-3, cleaved caspase-9, IL-1β, TNF-α, HMGB1 (Yang et al., 2015). Similarly, ginsenoside Rg2 and Rg3 exert a neuroprotective effect against apoptosis by decreasing the levels of Bax, and increasing the levels of Bcl-2 (He et al., 2012; He et al., 2017) [86]. Ginsenoside Rg1, Rg3, Rg5, Rb1, Rh2, and Re could reduce cerebral ischemic damage by inhibiting NF-κB transcriptional activity and the expression of pro-inflammatory cytokines (Cheng et al., 2019; Zheng et al., 2019). Administration of ginsenoside Rg1 in combination with geniposide protected against focal cerebral ischemia injury via microglial microRNA-155-5p inhibition (Wang J. et al., 2018). Moreover, the neuroprotection of ginsenoside Rg3 against ischemic injury is associated with multiple lncRNAs, miRNAs sand mRNAs, which mainly related to the tumor necrosis factor (TNF), NF-κB, cytokine, and cGMP-PKG, cAMP and MAPK signaling pathways (Yang et al., 2022; Zhang et al., 2022).
A previous study confirmed that ginsenoside Rg1 exerts the neuroprotective effect of antioxidant via downregulation of the NF-kB signaling pathway, and activation of Akt and ERK1/2 in H2O2-induced cell injury (Liu et al., 2011). In vitro and in vivo studies showed that Ginsenoside Rg1 significantly increased PPARγ expression and regulated the oxidative stress and inflammation after ischemic injury (Li et al., 2017a). Additionally, ginsenoside Rg1 could alleviate oxidative stress via inhibiting miR-144 and promoting the Nrf2/ARE pathway after ischemic/reperfusion injury (Chu et al., 2019). What’s more, ginsenoside Rg1 exerts neuroprotective effects by blocking the intracellular calcium overload and decreasing the concentration of free calcium and iNOS activity after OGD exposure (He et al., 2014), the inhibition of calcium influx via NMDA receptors and L-type voltage-dependent Ca2+ channels (Zhang Y. F. et al., 2008). Metabolic changes play an important role in cerebral ischemic damage. The potential therapeutic effect of ginsenoside Rg1 is possible via suppressing the systemic metabolic changes in cerebral injury rats (Lin et al., 2015). NSCs transplantation combined with ginsenoside Rg1 could significantly improve the cerebral infarct and neurological deficits via intervening energy metabolism, amino acids metabolism, and lipids metabolism (Gao J. et al., 2020). Besides, ginsenoside Rg3 could decrease the activities of SOD and GSH-Px, and enhance MDA and ATP levels after cerebral ischemia, which provide neuroprotection via reducing lipid peroxides, scavenging free radicals, and improving mitochondrial energy metabolism (Tian et al., 2005; Tian et al., 2009).
Angiogenesis plays a crucial role in reconstructing brain tissue and recovering neurological function after an ischemic stroke. Ginsenoside Rg1 could promote cerebral angiogenesis through the PI3K/Akt/mTOR signaling pathway, via upregulating the expressions of VEGF, HIF-1α, PI3K, p-Akt, and p-mTOR, and significantly increase the proliferation, migration and tube formation of endothelial cells (Chen J. et al., 2019). Besides, ginsenoside Rg1 exerts neuroprotection in cerebral ischemic injury via increasing the expression of BDNF in the hippocampal CA1 region and decreasing the expression of IL-1β, IL-6, and TNF-α in serum (Wang L. et al., 2018), as well as promoting the neurogenesis in the dentate gyrus of gerbils after global ischemia (Shen and Zhang, 2003). Ginsenoside Rg1 could also ameliorate neurological injury by attenuating BBB permeability, which is related to the downregulation of PAR-1 and aquaporin 4 expressions (Xie et al., 2015) (Zhou et al., 2014).
Other Ginsenosides and Their Neuroprotective Effects
In addition to ginsenosides summarized above, ginsenoside Re, Rh2, F1, and Compound K, Oleanolic acid may also play a neuroprotective role in treating cerebral ischemic stroke. Ginsenoside Re significantly improved mitochondrial membrane fluidity and decreased mitochondrial swelling, which ameliorated lipid peroxidation and protected neurons via improving the activities of SOD and GSH-Px, and reducing the content of MDA in the rat brain (Zhou et al., 2006; Chen et al., 2008). Oleanolic acid (OA) exerts neuroprotective effects via reducing ROS production and suppressing the activation of GSK-3β, and upregulating the expression of HO-1throgh GSK-3β/HO-1 signaling pathway in OGD/R induced SH-SY5Y cells and MCAO rats (Lin et al., 2021). Compound K (CK), a ginseng saponin metabolite, showed the neuroprotective effect of anti-inflammatory via suppressing microglial activation through inhibiting ROS, MAPK, and NF-κB/activator protein-1 (AP-1) and enhancement of HO-1 signaling (Park et al., 2012). Pretreatment of CK protects against neuron damage by increasing cell viability and decreasing ROS generation, mitochondrial damage, and Ca2+ overload. What’s more, OGD/R-induced autophagy and apoptosis in neurons could be regulated by modulating the AMP-activated protein kinase (AMPK) and mTOR pathway (Huang et al., 2020). Ginsenoside Rh2 inhibited the expression of COX-2, TNF-α, and IL-1β, and promoted the anti-inflammatory cytokine IL-10, depending on the AP-1 and protein kinase A (PKA) pathway, which is more potent than the anti-inflammatory effect of ginsenoside Rg3 (Bae et al., 2006). Ginsenoside F1 could promote angiogenesis through the insulin-like growth factor 1 (IGF-1)/insulin-like growth factor 1 receptor (IGF1R) pathway and might also enhance focal cerebral blood perfusion and increase cerebral microvessel density in MCAO rats (Zhang et al., 2019).
Conclusion and Perspectives
Currently, effective therapies for preventing and treating patients with ischemic stroke remain a challenge. Panax ginseng has been widely used in eastern countries for various diseases. The neuroprotective effects of ginseng or ginsenosides on preclinical and clinical ischemic stroke injury have been demonstrated during the last decade. This review concludes our recent findings related to the effects of ginseng and ginsenosides against ischemic stroke. As shown in Figure 2, ginsenoside Rd, Rb1 and Rg1 are the most commonly used in treating ischemic stroke. Mechanisms underlying the neuroprotective effects of ginseng or ginsenosides include regulation of excitotoxicity, Ca2+ overload, inflammation, mitochondria dysfunction, oxidative stress, apoptosis, pyroptosis, autophagy, BBB permeability, improving angiogenesis and neurogenesis. These effects can potentially improve abnormal neurobehaviors, such as sensorimotor or cognitive deficits. Ginseng and ginsenosides exert neuroprotective effects via modulating multiple signaling pathways, such as MAPK/ERK, PI3K/AKT, cAMP/PKA, AMPK/mTOR, NF-κB, Nrf2, GSK-3β/HO-1, IGF-1/IGF1R pathways, to block the pathological damage of neurons and promote the neural remodeling of stroke (Figure 3).
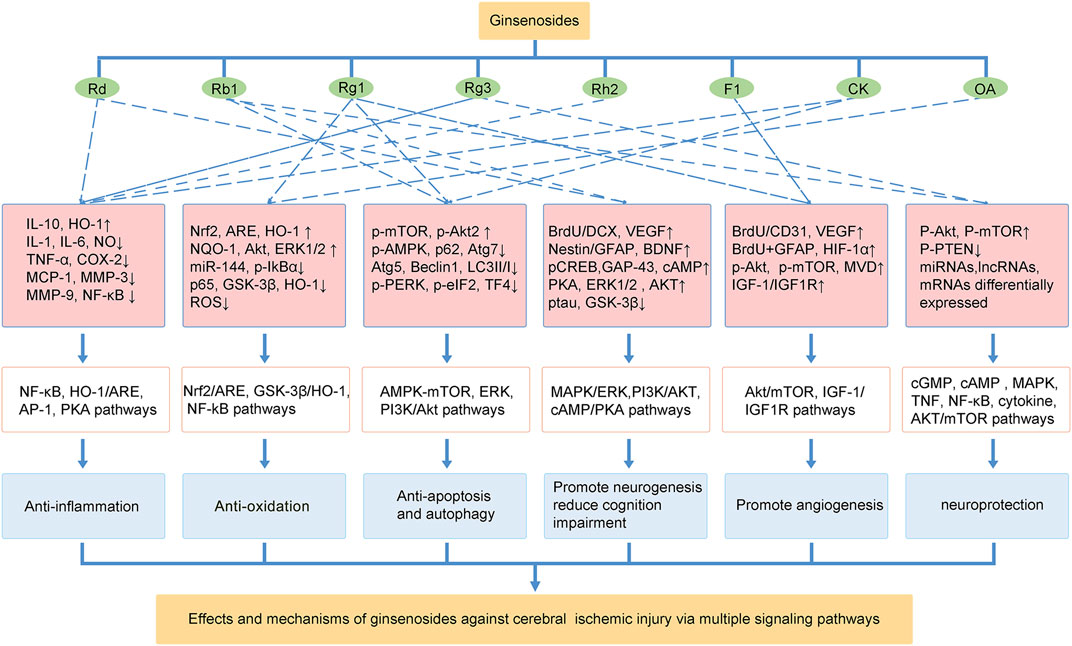
FIGURE 3. The effects and mechanisms of ginsenosides against cerebral ischemic injury via multiple signaling pathways.
Although numerous preclinical studies have been conducted on the neuroprotective effects of ginseng and ginsenosides in the treatment of ischemic stroke, there are few clinical trials of ginsenosides in treating ischemic stroke. Thus, further high-quality studies are needed to establish the clinical efficacy of ginsenosides. In addition, most experimental stroke models were induced by MCAO in young rats or mice, and only a few aged animals or models with diabetes were used, while the clinical patients are more likely to be associated with hypertension, hyperlipidemia, hyperglycemia, or other diseases. Therefore, it is necessary to study the neuroprotective effects of Panax ginseng or ginsenosides against ischemic stroke with pseudo-clinical models, which will provide a reliable basis for the clinical application of ginseng. Overall, this review describes the recent progress of pharmacological research on ginseng and ginsenosides in ischemic stroke and points out the issues that future research should focus on, which is of great importance for understanding the use of ginseng in the prevention and treatment of ischemic stroke.
Author Contributions
JL and GZ designed and supervised the review, AZ and NL collected, analyzed, and drafted the main manuscript; YZ, ZY, and YF checked references, figures and tables; MY revised the manuscript. All authors have read and approved the final version.
Funding
This study was supported by the National Natural Science Foundation of China (NSFC 82030124, NSFC 81873041).
Conflict of Interest
NL was employed by the company Beijing Increasepharm Safety and Efficacy Co., Ltd.
The remaining authors declare that the research was conducted in the absence of any commercial or financial relationships that could be construed as a potential conflict of interest.
Publisher’s Note
All claims expressed in this article are solely those of the authors and do not necessarily represent those of their affiliated organizations, or those of the publisher, the editors and the reviewers. Any product that may be evaluated in this article, or claim that may be made by its manufacturer, is not guaranteed or endorsed by the publisher.
References
Allen, C. L., and Bayraktutan, U. (2009). Oxidative Stress and its Role in the Pathogenesis of Ischaemic Stroke. Int. J. Stroke 4, 461–470. doi:10.1111/j.1747-4949.2009.00387.x
Bae, E. A., Kim, E. J., Park, J. S., Kim, H. S., Ryu, J. H., and Kim, D. H. (2006). Ginsenosides Rg3 and Rh2 Inhibit the Activation of AP-1 and Protein Kinase A Pathway in Lipopolysaccharide/interferon-Gamma-Stimulated BV-2 Microglial Cells. Planta Med. 72, 627–633. doi:10.1055/s-2006-931563
Ban, J. Y., Kang, S. W., Lee, J. S., Chung, J. H., Ko, Y. G., and Choi, H. S. (2012). Korean Red Ginseng Protects against Neuronal Damage Induced by Transient Focal Ischemia in Rats. Exp. Ther. Med. 3, 693–698. doi:10.3892/etm.2012.449
Berg, D. A., Su, Y., Jimenez-Cyrus, D., Patel, A., Huang, N., Morizet, D., et al. (2019). A Common Embryonic Origin of Stem Cells Drives Developmental and Adult Neurogenesis. Cell. 177, 654–e15. doi:10.1016/j.cell.2019.02.010
Broz, P., and Dixit, V. M. (2016). Inflammasomes: Mechanism of Assembly, Regulation and Signalling. Nat. Rev. Immunol. 16, 407–420. doi:10.1038/nri.2016.58
Campbell, B. C. V., and Khatri, P. (2020). Stroke. Lancet 396, 129–142. doi:10.1016/s0140-6736(20)31179-x
Chamorro, Á., Dirnagl, U., Urra, X., and Planas, A. M. (2016). Neuroprotection in Acute Stroke: Targeting Excitotoxicity, Oxidative and Nitrosative Stress, and Inflammation. Lancet Neurol. 15, 869–881. doi:10.1016/S1474-4422(16)00114-9
Chao, D. T., and Korsmeyer, S. J. (1998). BCL-2 Family: Regulators of Cell Death. Annu. Rev. Immunol. 16, 395–419. doi:10.1146/annurev.immunol.16.1.395
Chen, J., Zhang, X., Liu, X., Zhang, C., Shang, W., Xue, J., et al. (2019a). Ginsenoside Rg1 Promotes Cerebral Angiogenesis via the PI3K/Akt/mTOR Signaling Pathway in Ischemic Mice. Eur. J. Pharmacol. 856, 172418. doi:10.1016/j.ejphar.2019.172418
Chen, L. M., Zhou, X. M., Cao, Y. L., and Hu, W. X. (2008). Neuroprotection of Ginsenoside Re in Cerebral Ischemia-Reperfusion Injury in Rats. J. Asian Nat. Prod. Res. 10, 439–445. doi:10.1080/10286020801892292
Chen, W., Balan, P., and Popovich, D. G. (2019b). Analysis of Ginsenoside Content (Panax Ginseng) from Different Regions. Molecules 24. doi:10.3390/molecules24193491
Chen, W., Guo, Y., Yang, W., Zheng, P., Zeng, J., and Tong, W. (2015). Protective Effect of Ginsenoside Rb1 on Integrity of Blood-Brain Barrier Following Cerebral Ischemia. Exp. Brain Res. 233, 2823–2831. doi:10.1007/s00221-015-4352-3
Cheng, Z., Zhang, M., Ling, C., Zhu, Y., Ren, H., Hong, C., et al. (2019). Neuroprotective Effects of Ginsenosides against Cerebral Ischemia. Molecules 24. doi:10.3390/molecules24061102
Cheon, S. Y., Cho, K. J., Lee, J. E., Kim, H. W., Lee, S. K., Kim, H. J., et al. (2013). Cerebroprotective Effects of Red Ginseng Extract Pretreatment against Ischemia-Induced Oxidative Stress and Apoptosis. Int. J. Neurosci. 123, 269–277. doi:10.3109/00207454.2012.758120
Chu, S. F., Zhang, Z., Zhou, X., He, W. B., Chen, C., Luo, P., et al. (2019). Ginsenoside Rg1 Protects against Ischemic/reperfusion-Induced Neuronal Injury through miR-144/Nrf2/ARE Pathway. Acta Pharmacol. Sin. 40, 13–25. doi:10.1038/s41401-018-0154-z
Culmsee, C., and Krieglstein, J. (2007). Ischaemic Brain Damage after Stroke: New Insights into Efficient Therapeutic Strategies. International Symposium on Neurodegeneration and Neuroprotection. EMBO Rep. 8, 129–133. doi:10.1038/sj.embor.7400892
Dixon, S. J., Lemberg, K. M., Lamprecht, M. R., Skouta, R., Zaitsev, E. M., Gleason, C. E., et al. (2012). Ferroptosis: an Iron-dependent Form of Nonapoptotic Cell Death. Cell. 149, 1060–1072. doi:10.1016/j.cell.2012.03.042
Dong, X., Zheng, L., Lu, S., and Yang, Y. (2017). Neuroprotective Effects of Pretreatment of Ginsenoside Rb1 on Severe Cerebral Ischemia-Induced Injuries in Aged Mice: Involvement of Anti-oxidant Signaling. Geriatr. Gerontol. Int. 17, 338–345. doi:10.1111/ggi.12699
Drieu, A., Levard, D., Vivien, D., and Rubio, M. (2018). Anti-inflammatory Treatments for Stroke: from Bench to Bedside. Ther. Adv. Neurol. Disord. 11, 1756286418789854. doi:10.1177/1756286418789854
Fan, Y., Lu, H., Liang, W., Garcia-Barrio, M. T., Guo, Y., Zhang, J., et al. (2018). Endothelial TFEB (Transcription Factor EB) Positively Regulates Postischemic Angiogenesis. Circ. Res. 122, 945–957. doi:10.1161/CIRCRESAHA.118.312672
Fann, D. Y., Lee, S. Y., Manzanero, S., Chunduri, P., Sobey, C. G., and Arumugam, T. V. (2013a). Pathogenesis of Acute Stroke and the Role of Inflammasomes. Ageing Res. Rev. 12, 941–966. doi:10.1016/j.arr.2013.09.004
Fann, D. Y., Lee, S. Y., Manzanero, S., Tang, S. C., Gelderblom, M., Chunduri, P., et al. (2013b). Intravenous Immunoglobulin Suppresses NLRP1 and NLRP3 Inflammasome-Mediated Neuronal Death in Ischemic Stroke. Cell. Death Dis. 4, e790. doi:10.1038/cddis.2013.326
Ferrara, N., and Adamis, A. P. (2016). Ten Years of Anti-vascular Endothelial Growth Factor Therapy. Nat. Rev. Drug Discov. 15, 385–403. doi:10.1038/nrd.2015.17
Galluzzi, L., Vitale, I., Aaronson, S. A., Abrams, J. M., Adam, D., Agostinis, P., et al. (2018). Molecular Mechanisms of Cell Death: Recommendations of the Nomenclature Committee on Cell Death 2018. Cell. Death Differ. 25, 486–541. doi:10.1038/s41418-017-0012-4
Gao, J., Bai, P., Li, Y., Li, J., Jia, C., Wang, T., et al. (2020a). Metabolomic Profiling of the Synergistic Effects of Ginsenoside Rg1 in Combination with Neural Stem Cell Transplantation in Ischemic Stroke Rats. J. Proteome Res. 19, 2676–2688. doi:10.1021/acs.jproteome.9b00639
Gao, X. Q., Yang, C. X., Chen, G. J., Wang, G. Y., Chen, B., Tan, S. K., et al. (2010). Ginsenoside Rb1 Regulates the Expressions of Brain-Derived Neurotrophic Factor and Caspase-3 and Induces Neurogenesis in Rats with Experimental Cerebral Ischemia. J. Ethnopharmacol. 132, 393–399. doi:10.1016/j.jep.2010.07.033
Gao, X., Zhang, X., Cui, L., Chen, R., Zhang, C., Xue, J., et al. (2020b). Ginsenoside Rb1 Promotes Motor Functional Recovery and Axonal Regeneration in Post-stroke Mice through cAMP/PKA/CREB Signaling Pathway. Brain Res. Bull. 154, 51–60. doi:10.1016/j.brainresbull.2019.10.006
George, P. M., and Steinberg, G. K. (2015). Novel Stroke Therapeutics: Unraveling Stroke Pathophysiology and its Impact on Clinical Treatments. Neuron 87, 297–309. doi:10.1016/j.neuron.2015.05.041
Glick, D., Barth, S., and Macleod, K. F. (2010). Autophagy: Cellular and Molecular Mechanisms. J. Pathol. 221, 3–12. doi:10.1002/path.2697
Gu, Y., Ren, K., Wang, L., Jiang, C., and Yao, Q. (2020). Rg1 in Combination with Mannitol Protects Neurons against Glutamate-Induced ER Stress via the PERK-eIF2 α-ATF4 Signaling Pathway. Life Sci. 263, 118559. doi:10.1016/j.lfs.2020.118559
Guo, Y., Wang, L. P., Li, C., Xiong, Y. X., Yan, Y. T., Zhao, L. Q., et al. (2018). Effects of Ginsenoside Rb1 on Expressions of Phosphorylation Akt/Phosphorylation mTOR/Phosphorylation PTEN in Artificial Abnormal Hippocampal Microenvironment in Rats. Neurochem. Res. 43, 1927–1937. doi:10.1007/s11064-018-2612-x
Hatakeyama, M., Ninomiya, I., and Kanazawa, M. (2020). Angiogenesis and Neuronal Remodeling after Ischemic Stroke. Neural Regen. Res. 15, 16–19. doi:10.4103/1673-5374.264442
He, B., Chen, P., Xie, Y., Li, S., Zhang, X., Yang, R., et al. (2017). 20(R)-Ginsenoside Rg3 Protects SH-Sy5y Cells against Apoptosis Induced by Oxygen and Glucose Deprivation/reperfusion. Bioorg Med. Chem. Lett. 27, 3867–3871. doi:10.1016/j.bmcl.2017.06.045
He, B., Chen, P., Yang, J., Yun, Y., Zhang, X., Yang, R., et al. (2012). Neuroprotective Effect of 20(R)-ginsenoside Rg(3) against Transient Focal Cerebral Ischemia in Rats. Neurosci. Lett. 526, 106–111. doi:10.1016/j.neulet.2012.08.022
He, J., Liu, J., Huang, Y., Tang, X., Xiao, H., and Hu, Z. (2021). Oxidative Stress, Inflammation, and Autophagy: Potential Targets of Mesenchymal Stem Cells-Based Therapies in Ischemic Stroke. Front. Neurosci. 15. doi:10.3389/fnins.2021.641157
He, Q., Sun, J., Wang, Q., Wang, W., and He, B. (2014). Neuroprotective Effects of Ginsenoside Rg1 against Oxygen-Glucose Deprivation in Cultured Hippocampal Neurons. J. Chin. Med. Assoc. 77, 142–149. doi:10.1016/j.jcma.2014.01.001
Hu, G., Wu, Z., Yang, F., Zhao, H., Liu, X., Deng, Y., et al. (2013). Ginsenoside Rd Blocks AIF Mitochondrio-Nuclear Translocation and NF-Κb Nuclear Accumulation by Inhibiting poly(ADP-Ribose) Polymerase-1 after Focal Cerebral Ischemia in Rats. Neurol. Sci. 34, 2101–2106. doi:10.1007/s10072-013-1344-6
Hu, J. J., Liu, X., Xia, S., Zhang, Z., Zhang, Y., Zhao, J., et al. (2020a). FDA-Approved Disulfiram Inhibits Pyroptosis by Blocking Gasdermin D Pore Formation. Nat. Immunol. 21, 736–745. doi:10.1038/s41590-020-0669-6
Hu, Q., Liu, L., Zhou, L., Lu, H., Wang, J., Chen, X., et al. (2020b). Effect of Fluoxetine on HIF-1α- Netrin/VEGF Cascade, Angiogenesis and Neuroprotection in a Rat Model of Transient Middle Cerebral Artery Occlusion. Exp. Neurol. 329, 113312. doi:10.1016/j.expneurol.2020.113312
Huang, Q., Lou, T., Wang, M., Xue, L., Lu, J., Zhang, H., et al. (2020). Compound K Inhibits Autophagy-Mediated Apoptosis Induced by Oxygen and Glucose Deprivation/reperfusion via Regulating AMPK-mTOR Pathway in Neurons. Life Sci. 254, 117793. doi:10.1016/j.lfs.2020.117793
Hyun, S. H., Bhilare, K. D., In, G., Park, C.-K., and Kim, J.-H. (2022). Effects of Panax Ginseng and Ginsenosides on Oxidative Stress and Cardiovascular Diseases: Pharmacological and Therapeutic Roles. J. Ginseng Res. 46, 33–38. doi:10.1016/j.jgr.2021.07.007
Jia, J., Bissa, B., Brecht, L., Allers, L., Choi, S. W., Gu, Y., et al. (2020). AMPK, a Regulator of Metabolism and Autophagy, Is Activated by Lysosomal Damage via a Novel Galectin-Directed Ubiquitin Signal Transduction System. Mol. Cell. 77, 951–969. doi:10.1016/j.molcel.2019.12.028
Jiang, S., Li, T., Ji, T., Yi, W., Yang, Z., Wang, S., et al. (2018). AMPK: Potential Therapeutic Target for Ischemic Stroke. Theranostics 8, 4535–4551. doi:10.7150/thno.25674
Jiang, Z., Wang, Y., Zhang, X., Peng, T., Lu, Y., Leng, J., et al. (2013). Preventive and Therapeutic Effects of Ginsenoside Rb1 for Neural Injury during Cerebral Infarction in Rats. Am. J. Chin. Med. 41, 341–352. doi:10.1142/S0192415X13500250
Jo, E.-J., Kang, S. J., and Kim, A.-J. (2009). Effects of Steam- and Dry-Processing Temperatures on the Benzo(a)pyrene Content of Black and Red Ginseng. Korean J. Food Nutr. 22, 199–204.
Johnson, C. O., Minh, N., Roth, G. A., Nichols, E., Alam, T., Abate, D., et al. (2019). Global, Regional, and National Burden of Stroke, 1990-2016: a Systematic Analysis for the Global Burden of Disease Study 2016. Lancet Neurol. 18, 439–458. doi:10.1016/S1474-4422(19)30034-1
Kaplan-Arabaci, O., Acari, A., Ciftci, P., and Gozuacik, D. (2022). Glutamate Scavenging as a Neuroreparative Strategy in Ischemic Stroke. Front. Pharmacol. 13, 866738. doi:10.3389/fphar.2022.866738
Ke, L., Guo, W., Xu, J., Zhang, G., Wang, W., and Huang, W. (2014). Ginsenoside Rb1 Attenuates Activated Microglia-Induced Neuronal Damage. Neural Regen. Res. 9, 252–259. doi:10.4103/1673-5374.128217
Kim, D., Park, M., Haleem, I., Lee, Y., Koo, J., Na, Y. C., et al. (2020). Natural Product Ginsenoside 20(S)-25-Methoxyl-Dammarane-3β, 12β, 20-Triol in Cancer Treatment: A Review of the Pharmacological Mechanisms and Pharmacokinetics. Front. Pharmacol. 11, 521. doi:10.3389/fphar.2020.00521
Kim, J. H. (2018). Pharmacological and Medical Applications of Panax Ginseng and Ginsenosides: a Review for Use in Cardiovascular Diseases. J. Ginseng Res. 42, 264–269. doi:10.1016/j.jgr.2017.10.004
Kim, N. H., Jayakodi, M., Lee, S. C., Choi, B. S., Jang, W., Lee, J., et al. (2018). Genome and Evolution of the Shade-Requiring Medicinal Herb Panax Ginseng. Plant Biotechnol. J. 16, 1904–1917. doi:10.1111/pbi.12926
Kim, Y. O., Kim, H. J., Kim, G. S., Park, H. G., Lim, S. J., Seong, N. S., et al. (2009). Panax Ginseng Protects against Global Ischemia Injury in Rat hippocampus. J. Med. Food 12, 71–76. doi:10.1089/jmf.2007.0614
Klionsky, D. J., Abdelmohsen, K., Abe, A., Abedin, M. J., Abeliovich, H., Acevedo Arozena, A., et al. (2016). Guidelines for the Use and Interpretation of Assays for Monitoring Autophagy. Autophagy 12, 1–222. doi:10.1080/15548627.2015.1100356. 3rd edition
Knowland, D., Arac, A., Sekiguchi, K. J., Hsu, M., Lutz, S. E., Perrino, J., et al. (2014). Stepwise Recruitment of Transcellular and Paracellular Pathways Underlies Blood-Brain Barrier Breakdown in Stroke. Neuron 82, 603–617. doi:10.1016/j.neuron.2014.03.003
Kondo, Y., Asanuma, M., Nishibayashi, S., Iwata, E., and Ogawa, N. (1997). Late-onset Lipid Peroxidation and Neuronal Cell Death Following Transient Forebrain Ischemia in Rat Brain. Brain Res. 772, 37–44. doi:10.1016/s0006-8993(97)00836-6
Koppula, P., Zhang, Y., Zhuang, L., and Gan, B. (2018). Amino Acid Transporter SLC7A11/xCT at the Crossroads of Regulating Redox Homeostasis and Nutrient Dependency of Cancer. Cancer Commun. 38. doi:10.1186/s40880-018-0288-x
Lai, T. W., Zhang, S., and Wang, Y. T. (2014). Excitotoxicity and Stroke: Identifying Novel Targets for Neuroprotection. Prog. Neurobiol. 115, 157–188. doi:10.1016/j.pneurobio.2013.11.006
Lan, B., Ge, J. W., Cheng, S. W., Zheng, X. L., Liao, J., He, C., et al. (2020). Extract of Naotaifang, a Compound Chinese Herbal Medicine, Protects Neuron Ferroptosis Induced by Acute Cerebral Ischemia in Rats. J. Integr. Medicine-Jim 18, 344–350. doi:10.1016/j.joim.2020.01.008
Lee, H., Zandkarimi, F., Zhang, Y., Meena, J. K., Kim, J., Zhuang, L., et al. (2020). Energy-stress-mediated AMPK Activation Inhibits Ferroptosis. Nat. Cell. Biol. 22, 225–234. doi:10.1038/s41556-020-0461-8
Lee, J. S., Choi, H. S., Kang, S. W., Chung, J. H., Park, H. K., Ban, J. Y., et al. (2011). Therapeutic Effect of Korean Red Ginseng on Inflammatory Cytokines in Rats with Focal Cerebral Ischemia/reperfusion Injury. Am. J. Chin. Med. 39, 83–94. doi:10.1142/S0192415X1100866X
Lees, K. R., Bluhmki, E., Von Kummer, R., Brott, T. G., Toni, D., Grotta, J. C., et al. (2010). Time to Treatment with Intravenous Alteplase and Outcome in Stroke: an Updated Pooled Analysis of ECASS, ATLANTIS, NINDS, and EPITHET Trials. Lancet 375, 1695–1703. doi:10.1016/S0140-6736(10)60491-6
Li, X. Y., Liang, J., Tang, Y. B., Zhou, J. G., and Guan, Y. Y. (2010). Ginsenoside Rd Prevents Glutamate-Induced Apoptosis in Rat Cortical Neurons. Clin. Exp. Pharmacol. Physiol. 37, 199–204. doi:10.1111/j.1440-1681.2009.05286.x
Li, Y., Guan, Y., Wang, Y., Yu, C. L., Zhai, F. G., and Guan, L. X. (2017a). Neuroprotective Effect of the Ginsenoside Rg1 on Cerebral Ischemic Injury In Vivo and In Vitro Is Mediated by PPARγ-Regulated Antioxidative and Anti-inflammatory Pathways. Evid. Based Complement. Altern. Med. 2017, 7842082. doi:10.1155/2017/7842082
Li, Y., Suo, L., Liu, Y., Li, H., and Xue, W. (2017b). Protective Effects of Ginsenoside Rg1 against Oxygen-Glucose-Deprivation-Induced Apoptosis in Neural Stem Cells. J. Neurol. Sci. 373, 107–112. doi:10.1016/j.jns.2016.12.036
Lim, J. H., Wen, T. C., Matsuda, S., Tanaka, J., Maeda, N., Peng, H., et al. (1997). Protection of Ischemic Hippocampal Neurons by Ginsenoside Rb1, a Main Ingredient of Ginseng Root. Neurosci. Res. 28, 191–200. doi:10.1016/s0168-0102(97)00041-2
Lin, K., Zhang, Z., Zhang, Z., Zhu, P., Jiang, X., Wang, Y., et al. (2021). Oleanolic Acid Alleviates Cerebral Ischemia/Reperfusion Injury via Regulation of the GSK-3β/HO-1 Signaling Pathway. Pharm. (Basel) 15. doi:10.3390/ph15010001
Lin, M., Sun, W., Gong, W., Ding, Y., Zhuang, Y., and Hou, Q. (2015). Ginsenoside Rg1 Protects against Transient Focal Cerebral Ischemic Injury and Suppresses its Systemic Metabolic Changes in Cerabral Injury Rats. Acta Pharm. Sin. B 5, 277–284. doi:10.1016/j.apsb.2015.02.001
Linnik, M. D., Zobrist, R. H., and Hatfield, M. D. (1993). Evidence Supporting a Role for Programmed Cell Death in Focal Cerebral Ischemia in Rats. Stroke 24, 2002–2008. discussion 2008-2009. doi:10.1161/01.str.24.12.2002
Liu, A., Zhu, W., Sun, L., Han, G., Liu, H., Chen, Z., et al. (2018a). Ginsenoside Rb1 Administration Attenuates Focal Cerebral Ischemic Reperfusion Injury through Inhibition of HMGB1 and Inflammation Signals. Exp. Ther. Med. 16, 3020–3026. doi:10.3892/etm.2018.6523
Liu, L., Kelly, M. G., Wierzbicki, E. L., Escober-Nario, I. C., Vollmer, M. K., and Doré, S. (2019a). Nrf2 Plays an Essential Role in Long-Term Brain Damage and Neuroprotection of Korean Red Ginseng in a Permanent Cerebral Ischemia Model. Antioxidants (Basel) 8, 273. doi:10.3390/antiox8080273
Liu, L., Vollmer, M. K., Ahmad, A. S., Fernandez, V. M., Kim, H., and Doré, S. (2019b). Pretreatment with Korean Red Ginseng or Dimethyl Fumarate Attenuates Reactive Gliosis and Confers Sustained Neuroprotection against Cerebral Hypoxic-Ischemic Damage by an Nrf2-dependent Mechanism. Free Radic. Biol. Med. 131, 98–114. doi:10.1016/j.freeradbiomed.2018.11.017
Liu, L., Vollmer, M. K., Fernandez, V. M., Dweik, Y., Kim, H., and Doré, S. (2018b). Korean Red Ginseng Pretreatment Protects against Long-Term Sensorimotor Deficits after Ischemic Stroke Likely through Nrf2. Front. Cell. Neurosci. 12, 74. doi:10.3389/fncel.2018.00074
Liu, L., Vollmer, M. K., Kelly, M. G., Fernandez, V. M., Fernandez, T. G., Kim, H., et al. (2020). Reactive Gliosis Contributes to Nrf2-dependent Neuroprotection by Pretreatment with Dimethyl Fumarate or Korean Red Ginseng against Hypoxic-Ischemia: Focus on Hippocampal Injury. Mol. Neurobiol. 57, 105–117. doi:10.1007/s12035-019-01760-0
Liu, Q., Kou, J. P., and Yu, B. Y. (2011). Ginsenoside Rg1 Protects against Hydrogen Peroxide-Induced Cell Death in PC12 Cells via Inhibiting NF-Κb Activation. Neurochem. Int. 58, 119–125. doi:10.1016/j.neuint.2010.11.004
Liu, X., Wang, L., Wen, A., Yang, J., Yan, Y., Song, Y., et al. (2012). Ginsenoside-Rd Improves Outcome of Acute Ischaemic Stroke - a Randomized, Double-Blind, Placebo-Controlled, Multicenter Trial. Eur. J. Neurol. 19, 855–863. doi:10.1111/j.1468-1331.2011.03634.x
Liu, X., Xia, J., Wang, L., Song, Y., Yang, J., Yan, Y., et al. (2009). Efficacy and Safety of Ginsenoside-Rd for Acute Ischaemic Stroke: a Randomized, Double-Blind, Placebo-Controlled, Phase II Multicenter Trial. Eur. J. Neurol. 16, 569–575. doi:10.1111/j.1468-1331.2009.02534.x
Liu, X. Y., Zhou, X. Y., Hou, J. C., Zhu, H., Wang, Z., Liu, J. X., et al. (2015). Ginsenoside Rd Promotes Neurogenesis in Rat Brain after Transient Focal Cerebral Ischemia via Activation of PI3K/Akt Pathway. Acta Pharmacol. Sin. 36, 421–428. doi:10.1038/aps.2014.156
Liu, Y., and Levine, B. (2015). Autosis and Autophagic Cell Death: the Dark Side of Autophagy. Cell. Death Differ. 22, 367–376. doi:10.1038/cdd.2014.143
Liu, Z., Wang, C., Yang, J., Zhou, B., Yang, R., Ramachandran, R., et al. (2019c). Crystal Structures of the Full-Length Murine and Human Gasdermin D Reveal Mechanisms of Autoinhibition, Lipid Binding, and Oligomerization. Immunity 51, 43–49. doi:10.1016/j.immuni.2019.04.017
Lu, J., Wang, X., Wu, A., Cao, Y., Dai, X., Liang, Y., et al. (2022). Ginsenosides in Central Nervous System Diseases: Pharmacological Actions, Mechanisms, and Therapeutics. Phytother. Res. doi:10.1002/ptr.7395
Lu, T., Jiang, Y., Zhou, Z., Yue, X., Wei, N., Chen, Z., et al. (2011). Intranasal Ginsenoside Rb1 Targets the Brain and Ameliorates Cerebral Ischemia/reperfusion Injury in Rats. Biol. Pharm. Bull. 34, 1319–1324. doi:10.1248/bpb.34.1319
Luo, T., Liu, G., Ma, H., Lu, B., Xu, H., Wang, Y., et al. (2014). Inhibition of Autophagy via Activation of PI3K/Akt Pathway Contributes to the Protection of Ginsenoside Rb1 against Neuronal Death Caused by Ischemic Insults. Int. J. Mol. Sci. 15, 15426–15442. doi:10.3390/ijms150915426
Luoma, J. I., Kelley, B. G., and Mermelstein, P. G. (2011). Progesterone Inhibition of Voltage-Gated Calcium Channels Is a Potential Neuroprotective Mechanism against Excitotoxicity. Steroids 76, 845–855. doi:10.1016/j.steroids.2011.02.013
Marsh, B., Stevens, S. L., Packard, A. E. B., Gopalan, B., Hunter, B., Leung, P. Y., et al. (2009). Systemic Lipopolysaccharide Protects the Brain from Ischemic Injury by Reprogramming the Response of the Brain to Stroke: A Critical Role for IRF3. J. Neurosci. 29, 9839–9849. doi:10.1523/JNEUROSCI.2496-09.2009
Matsui, Y., Kyoi, S., Takagi, H., Hsu, C. P., Hariharan, N., Ago, T., et al. (2008). Molecular Mechanisms and Physiological Significance of Autophagy during Myocardial Ischemia and Reperfusion. Autophagy 4, 409–415. doi:10.4161/auto.5638
Mo, Y., Sun, Y.-Y., and Liu, K.-Y. (2020). Autophagy and Inflammation in Ischemic Stroke. Neural Regen. Res. 15, 1388–1396. doi:10.4103/1673-5374.274331
Muhammad, I. F., Borné, Y., Melander, O., Orho-Melander, M., Nilsson, J., Söderholm, M., et al. (2018). FADD (Fas-Associated Protein with Death Domain), Caspase-3, and Caspase-8 and Incidence of Ischemic Stroke. Stroke 49, 2224–2226. doi:10.1161/STROKEAHA.118.022063
Nada, S. E., Tulsulkar, J., and Shah, Z. A. (2014). Heme Oxygenase 1-mediated Neurogenesis Is Enhanced by Ginkgo Biloba (EGb 761®) after Permanent Ischemic Stroke in Mice. Mol. Neurobiol. 49, 945–956. doi:10.1007/s12035-013-8572-x
Navarro-Orozco, D., and Sánchez-Manso, J. C. (2022). “Neuroanatomy, Middle Cerebral Artery,” in StatPearls. (Treasure Island (FL) (Florida: StatPearls Publishing LLC.). StatPearls Publishing Copyright © 2022.
Park, H. J., Shim, H. S., Kim, K. S., and Shim, I. (2011a). The Protective Effect of Black Ginseng against Transient Focal Ischemia-Induced Neuronal Damage in Rats. Korean J. Physiol. Pharmacol. 15, 333–338. doi:10.4196/kjpp.2011.15.6.333
Park, H. W., In, G., Han, S. T., Lee, M. W., Kim, S. Y., Kim, K. T., et al. (2013). Simultaneous Determination of 30 Ginsenosides in Panax Ginseng Preparations Using Ultra Performance Liquid Chromatography. J. Ginseng Res. 37, 457–467. doi:10.5142/jgr.2013.37.457
Park, J. S., Shin, J. A., Jung, J. S., Hyun, J. W., Van Le, T. K., Kim, D. H., et al. (2012). Anti-inflammatory Mechanism of Compound K in Activated Microglia and its Neuroprotective Effect on Experimental Stroke in Mice. J. Pharmacol. Exp. Ther. 341, 59–67. doi:10.1124/jpet.111.189035
Park, U. J., Lee, Y. A., Won, S. M., Lee, J. H., Kang, S. H., Springer, J. E., et al. (2011b). Blood-derived Iron Mediates Free Radical Production and Neuronal Death in the Hippocampal CA1 Area Following Transient Forebrain Ischemia in Rat. Acta Neuropathol. 121, 459–473. doi:10.1007/s00401-010-0785-8
Powers, W. J., Rabinstein, A. A., Ackerson, T., Adeoye, O. M., Bambakidis, N. C., Becker, K., et al. (2019). Amer Heart Assoc StrokeGuidelines for the Early Management of Patients with Acute Ischemic Stroke: 2019 Update to the 2018 Guidelines for the Early Management of Acute Ischemic Stroke: A Guideline for Healthcare Professionals from the American Heart Association/American Stroke Association. Stroke 50, E344–E418. doi:10.1161/str.0000000000000211
Qin, C., Zhou, L. Q., Ma, X. T., Hu, Z. W., Yang, S., Chen, M., et al. (2019). Dual Functions of Microglia in Ischemic Stroke. Neurosci. Bull. 35, 921–933. doi:10.1007/s12264-019-00388-3
Rajkovic, O., Potjewyd, G., and Pinteaux, E. (2018). Regenerative Medicine Therapies for Targeting Neuroinflammation after Stroke. Front. Neurol. 9, 734. doi:10.3389/fneur.2018.00734
Ratan, Z. A., Haidere, M. F., Hong, Y. H., Park, S. H., Lee, J.-O., Lee, J., et al. (2021). Pharmacological Potential of Ginseng and its Major Component Ginsenosides. J. Ginseng Res. 45, 199–210. doi:10.1016/j.jgr.2020.02.004
Rothman, S. M., and Olney, J. W. (1986). Glutamate and the Pathophysiology of Hypoxic-Iischemic Brain Damage. Ann. Neurol. 19, 105–111. doi:10.1002/ana.410190202
Sakanaka, M., Zhu, P., Zhang, B., Wen, T. C., Cao, F., Ma, Y. J., et al. (2007). Intravenous Infusion of Dihydroginsenoside Rb1 Prevents Compressive Spinal Cord Injury and Ischemic Brain Damage through Upregulation of VEGF and Bcl-XL. J. Neurotrauma 24, 1037–1054. doi:10.1089/neu.2006.0182
Shah, Z. A., Gilani, R. A., Sharma, P., and Vohora, S. B. (2005). Cerebroprotective Effect of Korean Ginseng Tea against Global and Focal Models of Ischemia in Rats. J. Ethnopharmacol. 101, 299–307. doi:10.1016/j.jep.2005.05.002
Shen, J., Zheng, H., Ruan, J., Fang, W., Li, A., Tian, G., et al. (2013). Autophagy Inhibition Induces Enhanced Proapoptotic Effects of ZD6474 in Glioblastoma. Br. J. Cancer 109, 164–171. doi:10.1038/bjc.2013.306
Shen, L., and Zhang, J. (2003). Ginsenoside Rg1 Increases Ischemia-Induced Cell Proliferation and Survival in the Dentate Gyrus of Adult Gerbils. Neurosci. Lett. 344, 1–4. doi:10.1016/s0304-3940(03)00318-5
Shi, J., Zhao, Y., Wang, K., Shi, X., Wang, Y., Huang, H., et al. (2015). Cleavage of GSDMD by Inflammatory Caspases Determines Pyroptotic Cell Death. Nature 526, 660–665. doi:10.1038/nature15514
Singh, A., Kim, W., Kim, Y., Jeong, K., Kang, C. S., Kim, Y., et al. (2016). Multifunctional Photonics Nanoparticles for Crossing the Blood-Brain Barrier and Effecting Optically Trackable Brain Theranostics. Adv. Funct. Mater 26, 7057–7066. doi:10.1002/adfm.201602808
Smith, J. R., Galie, P. A., Slochower, D. R., Weisshaar, C. L., Janmey, P. A., and Winkelstein, B. A. (2016). Salmon-Derived Thrombin Inhibits Development of Chronic Pain through an Endothelial Barrier Protective Mechanism Dependent on APC. Biomaterials 80, 96–105. doi:10.1016/j.biomaterials.2015.11.062
Song, Y., Li, Z., He, T., Qu, M., Jiang, L., Li, W., et al. (2019). M2 Microglia-Derived Exosomes Protect the Mouse Brain from Ischemia-Reperfusion Injury via Exosomal miR-124. Theranostics 9, 2910–2923. doi:10.7150/thno.30879
Sun, C., Lai, X., Huang, X., and Zeng, Y. (2014). Protective Effects of Ginsenoside Rg1 on Astrocytes and Cerebral Ischemic-Reperfusion Mice. Biol. Pharm. Bull. 37, 1891–1898. doi:10.1248/bpb.b14-00394
Sun, J., and Nan, G. (2016). The Mitogen-Activated Protein Kinase (MAPK) Signaling Pathway as a Discovery Target in Stroke. J. Mol. Neurosci. 59, 90–98. doi:10.1007/s12031-016-0717-8
Szydlowska, K., and Tymianski, M. (2010). Calcium, Ischemia and Excitotoxicity. Cell. Calcium 47, 122–129. doi:10.1016/j.ceca.2010.01.003
Tang, D., Kang, R., Berghe, T. V., Vandenabeele, P., and Kroemer, G. (2019a). The Molecular Machinery of Regulated Cell Death. Cell. Res. 29, 347–364. doi:10.1038/s41422-019-0164-5
Tang, D. L., Kang, R., Vanden Berghe, T., Vandenabeele, P., and Kroemer, G. (2019b). The Molecular Machinery of Regulated Cell Death. Cell. Res. 29, 347–364. doi:10.1038/s41422-019-0164-5
Tian, J., Fu, F., Geng, M., Jiang, Y., Yang, J., Jiang, W., et al. (2005). Neuroprotective Effect of 20(S)-ginsenoside Rg3 on Cerebral Ischemia in Rats. Neurosci. Lett. 374, 92–97. doi:10.1016/j.neulet.2004.10.030
Tian, J., Zhang, S., Li, G., Liu, Z., and Xu, B. (2009). 20(S)-ginsenoside Rg3, a Neuroprotective Agent, Inhibits Mitochondrial Permeability Transition Pores in Rat Brain. Phytother. Res. 23, 486–491. doi:10.1002/ptr.2653
Tuo, Q. Z., Zhang, S. T., and Lei, P. (2022). Mechanisms of Neuronal Cell Death in Ischemic Stroke and Their Therapeutic Implications. Med. Res. Rev. 42, 259–305. doi:10.1002/med.21817
Tzingounis, A. V., and Wadiche, J. I. (2007). Glutamate Transporters: Confining Runaway Excitation by Shaping Synaptic Transmission. Nat. Rev. Neurosci. 8, 935–947. doi:10.1038/nrn2274
Virani, S. S., Alonso, A., Benjamin, E. J., Bittencourt, M. S., Callaway, C. W., Carson, A. P., et al. (2020). Heart Disease and Stroke Statistics-2020 Update: A Report from the American Heart Association. Circulation 141, e139–e596. doi:10.1161/CIR.0000000000000757
Wan, Q., Ma, X., Zhang, Z. J., Sun, T., Xia, F., Zhao, G., et al. (2017). Ginsenoside Reduces Cognitive Impairment during Chronic Cerebral Hypoperfusion through Brain-Derived Neurotrophic Factor Regulated by Epigenetic Modulation. Mol. Neurobiol. 54, 2889–2900. doi:10.1007/s12035-016-9868-4
Wan, Y., Wang, J., Xu, J.-F., Tang, F., Chen, L., Tan, Y.-Z., et al. (2021). Panax Ginseng and its Ginsenosides: Potential Candidates for the Prevention and Treatment of Chemotherapy-Induced Side Effects. J. Ginseng Res. 45, 617–630. doi:10.1016/j.jgr.2021.03.001
Wang, J., Li, D., Hou, J., and Lei, H. (2018a). Protective Effects of Geniposide and Ginsenoside Rg1 Combination Treatment on Rats Following Cerebral Ischemia Are Mediated via Microglial microRNA-155-5p Inhibition. Mol. Med. Rep. 17, 3186–3193. doi:10.3892/mmr.2017.8221
Wang, L. J., Huang, Y., Yin, G., Wang, J., Wang, P., Chen, Z. Y., et al. (2020). Antimicrobial Activities of Asian Ginseng, American Ginseng, and Notoginseng. Phytotherapy Res. 34, 1226–1236. doi:10.1002/ptr.6605
Wang, L., Zhao, H., Zhai, Z. Z., and Qu, L. X. (2018b). Protective Effect and Mechanism of Ginsenoside Rg1 in Cerebral Ischaemia-Reperfusion Injury in Mice. Biomed. Pharmacother. 99, 876–882. doi:10.1016/j.biopha.2018.01.136
Wang, P., Shao, B. Z., Deng, Z., Chen, S., Yue, Z., and Miao, C. Y. (2018c). Autophagy in Ischemic Stroke. Prog. Neurobiol. 163-164, 98–117. doi:10.1016/j.pneurobio.2018.01.001
Wang, R., Dong, Y., Lu, Y., Zhang, W., Brann, D. W., and Zhang, Q. (2019). Photobiomodulation for Global Cerebral Ischemia: Targeting Mitochondrial Dynamics and Functions. Mol. Neurobiol. 56, 1852–1869. doi:10.1007/s12035-018-1191-9
Wu, L., Xiong, X., Wu, X., Ye, Y., Jian, Z., Zhi, Z., et al. (2020). Targeting Oxidative Stress and Inflammation to Prevent Ischemia-Reperfusion Injury. Front. Mol. Neurosci. 13, 28. doi:10.3389/fnmol.2020.00028
Wu, S. D., Xia, F., Lin, X. M., Duan, K. L., Wang, F., Lu, Q. L., et al. (2016a). Ginsenoside-Rd Promotes Neurite Outgrowth of PC12 Cells through MAPK/ERK- and PI3K/AKT-dependent Pathways. Int. J. Mol. Sci. 17. doi:10.3390/ijms17020177
Wu, Y., Lindblad, J. L., Garnett, J., Kamber Kaya, H. E., Xu, D., Zhao, Y., et al. (2016b). Genetic Characterization of Two Gain-Of-Function Alleles of the Effector Caspase DrICE in Drosophila. Cell. Death Differ. 23, 723–732. doi:10.1038/cdd.2015.144
Xie, C. L., Li, J. H., Wang, W. W., Zheng, G. Q., and Wang, L. X. (2015). Neuroprotective Effect of Ginsenoside-Rg1 on Cerebral Ischemia/reperfusion Injury in Rats by Downregulating Protease-Activated Receptor-1 Expression. Life Sci. 121, 145–151. doi:10.1016/j.lfs.2014.12.002
Xie, Z., Shi, M., Zhang, C., Zhao, H., Hui, H., and Zhao, G. (2016). Ginsenoside Rd Protects against Cerebral Ischemia-Reperfusion Injury via Decreasing the Expression of the NMDA Receptor 2B Subunit and its Phosphorylated Product. Neurochem. Res. 41, 2149–2159. doi:10.1007/s11064-016-1930-0
Xing, S., Zhang, Y., Li, J., Zhang, J., Li, Y., Dang, C., et al. (2012). Beclin 1 Knockdown Inhibits Autophagic Activation and Prevents the Secondary Neurodegenerative Damage in the Ipsilateral Thalamus Following Focal Cerebral Infarction. Autophagy 8, 63–76. doi:10.4161/auto.8.1.18217
Xu, M., Ma, Q., Fan, C., Chen, X., Zhang, H., and Tang, M. (2019). Ginsenosides Rb1 and Rg1 Protect Primary Cultured Astrocytes against Oxygen-Glucose Deprivation/Reoxygenation-Induced Injury via Improving Mitochondrial Function. Int. J. Mol. Sci. 20. doi:10.3390/ijms20236086
Xu, Q. X., Zhao, B., Ye, Y. Z., Li, Y. N., Zhang, Y. G., Xiong, X. X., et al. (2021). Relevant Mediators Involved in and Therapies Targeting the Inflammatory Response Induced by Activation of the NLRP3 Inflammasome in Ischemic Stroke. J. Neuroinflammation 18. doi:10.1186/s12974-021-02137-8
Yang, L. X., Zhang, X., and Zhao, G. (2016). Ginsenoside Rd Attenuates DNA Damage by Increasing Expression of DNA Glycosylase Endonuclease VIII-like Proteins after Focal Cerebral Ischemia. Chin. Med. J. Engl. 129, 1955–1962. doi:10.4103/0366-6999.187851
Yang, Y., He, B., Yang, R., Chen, D., Zhang, X., Li, F., et al. (2022). Comprehensive Analysis of lncRNA Expression Profiles in Rats with Cerebral Ischemia-Reperfusion Injury after Treatment with 20(R)-ginsenoside Rg3. J. Integr. Neurosci. 21, 16. doi:10.31083/j.jin2101016
Yang, Y., Li, X., Zhang, L., Liu, L., Jing, G., and Cai, H. (2015). Ginsenoside Rg1 Suppressed Inflammation and Neuron Apoptosis by Activating PPARγ/HO-1 in hippocampus in Rat Model of Cerebral Ischemia-Reperfusion Injury. Int. J. Clin. Exp. Pathol. 8, 2484–2494.
Yao, Y., Hu, S., Zhang, C., Zhou, Q., Wang, H., Yang, Y., et al. (2022). Ginsenoside Rd Attenuates Cerebral Ischemia/reperfusion Injury by Exerting an Anti-pyroptotic Effect via the miR-139-5p/FoxO1/Keap1/Nrf2 axis. Int. Immunopharmacol. 105, 108582. doi:10.1016/j.intimp.2022.108582
Ye, R., Kong, X., Yang, Q., Zhang, Y., Han, J., Li, P., et al. (2011a). Ginsenoside Rd in Experimental Stroke: Superior Neuroprotective Efficacy with a Wide Therapeutic Window. Neurotherapeutics 8, 515–525. doi:10.1007/s13311-011-0051-3
Ye, R., Kong, X., Yang, Q., Zhang, Y., Han, J., and Zhao, G. (2011b). Ginsenoside Rd Attenuates Redox Imbalance and Improves Stroke Outcome after Focal Cerebral Ischemia in Aged Mice. Neuropharmacology 61, 815–824. doi:10.1016/j.neuropharm.2011.05.029
Ye, R., Li, N., Han, J., Kong, X., Cao, R., Rao, Z., et al. (2009). Neuroprotective Effects of Ginsenoside Rd against Oxygen-Glucose Deprivation in Cultured Hippocampal Neurons. Neurosci. Res. 64, 306–310. doi:10.1016/j.neures.2009.03.016
Ye, R., Yang, Q., Kong, X., Han, J., Zhang, X., Zhang, Y., et al. (2011c). Ginsenoside Rd Attenuates Early Oxidative Damage and Sequential Inflammatory Response after Transient Focal Ischemia in Rats. Neurochem. Int. 58, 391–398. doi:10.1016/j.neuint.2010.12.015
Ye, R., Zhang, X., Kong, X., Han, J., Yang, Q., Zhang, Y., et al. (2011d). Ginsenoside Rd Attenuates Mitochondrial Dysfunction and Sequential Apoptosis after Transient Focal Ischemia. Neuroscience 178, 169–180. doi:10.1016/j.neuroscience.2011.01.007
Yenari, M. A., Kauppinen, T. M., and Swanson, R. A. (2010). Microglial Activation in Stroke: Therapeutic Targets. Neurotherapeutics 7, 378–391. doi:10.1016/j.nurt.2010.07.005
Yoshikawa, T., Akiyoshi, Y., Susumu, T., Tokado, H., Fukuzaki, K., Nagata, R., et al. (2008). Ginsenoside Rb1 Reduces Neurodegeneration in the Peri-Infarct Area of a Thromboembolic Stroke Model in Non-human Primates. J. Pharmacol. Sci. 107, 32–40. doi:10.1254/jphs.fp0071297
Yuan, Q. L., Yang, C. X., Xu, P., Gao, X. Q., Deng, L., Chen, P., et al. (2007). Neuroprotective Effects of Ginsenoside Rb1 on Transient Cerebral Ischemia in Rats. Brain Res. 1167, 1–12. doi:10.1016/j.brainres.2007.06.024
Zhang, B., Hata, R., Zhu, P., Sato, K., Wen, T. C., Yang, L., et al. (2006). Prevention of Ischemic Neuronal Death by Intravenous Infusion of a Ginseng Saponin, Ginsenoside Rb(1), that Upregulates Bcl-X(L) Expression. J. Cereb. Blood Flow. Metab. 26, 708–721. doi:10.1038/sj.jcbfm.9600225
Zhang, B., Matsuda, S., Tanaka, J., Tateishi, N., Maeda, N., Wen, T. C., et al. (1998). Ginsenoside Rb(1) Prevents Image Navigation Disability, Cortical Infarction, and Thalamic Degeneration in Rats with Focal Cerebral Ischemia. J. Stroke Cerebrovasc. Dis. 7, 1–9. doi:10.1016/s1052-3057(98)80015-3
Zhang, C., Du, F., Shi, M., Ye, R., Cheng, H., Han, J., et al. (2012a). Ginsenoside Rd Protects Neurons against Glutamate-Induced Excitotoxicity by Inhibiting Ca(2+) Influx. Cell. Mol. Neurobiol. 32, 121–128. doi:10.1007/s10571-011-9742-x
Zhang, C., Liu, X., Xu, H., Hu, G., Zhang, X., Xie, Z., et al. (2020a). Protopanaxadiol Ginsenoside Rd Protects against NMDA Receptor-Mediated Excitotoxicity by Attenuating Calcineurin-Regulated DAPK1 Activity. Sci. Rep. 10, 8078. doi:10.1038/s41598-020-64738-2
Zhang, G., Liu, A., Zhou, Y., San, X., Jin, T., and Jin, Y. (2008a). Panax Ginseng Ginsenoside-Rg2 Protects Memory Impairment via Anti-apoptosis in a Rat Model with Vascular Dementia. J. Ethnopharmacol. 115, 441–448. doi:10.1016/j.jep.2007.10.026
Zhang, G., Xia, F., Zhang, Y., Zhang, X., Cao, Y., Wang, L., et al. (2016). Ginsenoside Rd Is Efficacious against Acute Ischemic Stroke by Suppressing Microglial Proteasome-Mediated Inflammation. Mol. Neurobiol. 53, 2529–2540. doi:10.1007/s12035-015-9261-8
Zhang, J., Liu, M., Huang, M., Chen, M., Zhang, D., Luo, L., et al. (2019). Ginsenoside F1 Promotes Angiogenesis by Activating the IGF-1/IGF1R Pathway. Pharmacol. Res. 144, 292–305. doi:10.1016/j.phrs.2019.04.021
Zhang, R., Chen, D. Y., Luo, X. W., Yang, Y., Zhang, X. C., Yang, R. H., et al. (2022). Comprehensive Analysis of the Effect of 20(R)-Ginsenoside Rg3 on Stroke Recovery in Rats via the Integrative miRNA-mRNA Regulatory Network. Molecules 27. doi:10.3390/molecules27051573
Zhang, X., Liu, X., Hu, G., Zhang, G., Zhao, G., and Shi, M. (2020b). Ginsenoside Rd Attenuates Blood-Brain Barrier Damage by Suppressing Proteasome-Mediated Signaling after Transient Forebrain Ischemia. Neuroreport 31, 466–472. doi:10.1097/WNR.0000000000001426
Zhang, X., Shi, M., Bjørås, M., Wang, W., Zhang, G., Han, J., et al. (2013). Ginsenoside Rd Promotes Glutamate Clearance by Up-Regulating Glial Glutamate Transporter GLT-1 via PI3K/AKT and ERK1/2 Pathways. Front. Pharmacol. 4, 152. doi:10.3389/fphar.2013.00152
Zhang, X., Shi, M., Ye, R., Wang, W., Liu, X., Zhang, G., et al. (2014). Ginsenoside Rd Attenuates Tau Protein Phosphorylation via the PI3K/AKT/GSK-3β Pathway after Transient Forebrain Ischemia. Neurochem. Res. 39, 1363–1373. doi:10.1007/s11064-014-1321-3
Zhang, Y. F., Fan, X. J., Li, X., Peng, L. L., Wang, G. H., Ke, K. F., et al. (2008b). Ginsenoside Rg1 Protects Neurons from Hypoxic-Ischemic Injury Possibly by Inhibiting Ca2+ Influx through NMDA Receptors and L-type Voltage-dependent Ca2+ Channels. Eur. J. Pharmacol. 586, 90–99. doi:10.1016/j.ejphar.2007.12.037
Zhang, Y., Zhou, L., Zhang, X., Bai, J., Shi, M., and Zhao, G. (2012b). Ginsenoside-Rd Attenuates TRPM7 and ASIC1a but Promotes ASIC2a Expression in Rats after Focal Cerebral Ischemia. Neurol. Sci. 33, 1125–1131. doi:10.1007/s10072-011-0916-6
Zheng, G. Q., Cheng, W., Wang, Y., Wang, X. M., Zhao, S. Z., Zhou, Y., et al. (2011). Ginseng Total Saponins Enhance Neurogenesis after Focal Cerebral Ischemia. J. Ethnopharmacol. 133, 724–728. doi:10.1016/j.jep.2010.01.064
Zheng, Q., Bao, X. Y., Zhu, P. C., Tong, Q., Zheng, G. Q., and Wang, Y. (2017). Ginsenoside Rb1 for Myocardial Ischemia/Reperfusion Injury: Preclinical Evidence and Possible Mechanisms. Oxid. Med. Cell. Longev. 2017, 6313625. doi:10.1155/2017/6313625
Zheng, T., Jiang, H., Jin, R., Zhao, Y., Bai, Y., Xu, H., et al. (2019). Ginsenoside Rg1 Attenuates Protein Aggregation and Inflammatory Response Following Cerebral Ischemia and Reperfusion Injury. Eur. J. Pharmacol. 853, 65–73. doi:10.1016/j.ejphar.2019.02.018
Zhou, X. M., Cao, Y. L., and Dou, D. Q. (2006). Protective Effect of Ginsenoside-Re against Cerebral Ischemia/reperfusion Damage in Rats. Biol. Pharm. Bull. 29, 2502–2505. doi:10.1248/bpb.29.2502
Zhou, Y., Li, H. Q., Lu, L., Fu, D. L., Liu, A. J., Li, J. H., et al. (2014). Ginsenoside Rg1 Provides Neuroprotection against Blood Brain Barrier Disruption and Neurological Injury in a Rat Model of Cerebral Ischemia/reperfusion through Downregulation of Aquaporin 4 Expression. Phytomedicine 21, 998–1003. doi:10.1016/j.phymed.2013.12.005
Zhou, Z., Lu, J., Liu, W. W., Manaenko, A., Hou, X., Mei, Q., et al. (2018). Advances in Stroke Pharmacology. Pharmacol. Ther. 191, 23–42. doi:10.1016/j.pharmthera.2018.05.012
Zhu, H., Hu, S. P., Li, Y. T., Sun, Y., Xiong, X. X., Hu, X. Y., et al. (2022). Interleukins and Ischemic Stroke. Front. Immunol. 13. doi:10.3389/fimmu.2022.828447
Keywords: panax ginseng, ginsenosides, traditional Chinese medicine, cerebral ischemic stroke, neuroprotection mechanisms
Citation: Zhao A, Liu N, Yao M, Zhang Y, Yao Z, Feng Y, Liu J and Zhou G (2022) A Review of Neuroprotective Effects and Mechanisms of Ginsenosides From Panax Ginseng in Treating Ischemic Stroke. Front. Pharmacol. 13:946752. doi: 10.3389/fphar.2022.946752
Received: 18 May 2022; Accepted: 14 June 2022;
Published: 07 July 2022.
Edited by:
Guangbo Fu, Huaian No. 1 People’s Hospital Nanjing Medical University, ChinaReviewed by:
Hongliang Li, Yangzhou University, ChinaJian Huang, Princeton University, United States
Copyright © 2022 Zhao, Liu, Yao, Zhang, Yao, Feng, Liu and Zhou. This is an open-access article distributed under the terms of the Creative Commons Attribution License (CC BY). The use, distribution or reproduction in other forums is permitted, provided the original author(s) and the copyright owner(s) are credited and that the original publication in this journal is cited, in accordance with accepted academic practice. No use, distribution or reproduction is permitted which does not comply with these terms.
*Correspondence: Jianxun Liu, bGl1angwMzI0QHNpbmEuY29t; Guoping Zhou, ZG9jdG9yemdwQHNpbmEuY29t
†These authors have contributed equally to this work and share first authorship