- 1First Clinical Medical School, Shandong University of Traditional Chinese Medicine, Jinan, Shandong, China
- 2Affiliated Hospital of Shandong University of Traditional Chinese Medicine, Jinan, Shandong, China
- 3Department of Cardiovascular, Guang’anmen Hospital, China Academy of Chinese Medical Sciences, Beijing, China
NRG1 (Neuregulin-1) is an effective cardiomyocyte proliferator, secreted and released by endothelial vascular cells, and affects the cardiovascular system. It plays a major role in heart growth, proliferation, differentiation, apoptosis, and other cardiovascular processes. Numerous experiments have shown that NRG1 can repair the heart in the pathophysiology of atherosclerosis, myocardial infarction, ischemia reperfusion, heart failure, cardiomyopathy and other cardiovascular diseases. NRG1 can connect related signaling pathways through the NRG1/ErbB pathway, which form signal cascades to improve the myocardial microenvironment, such as regulating cardiac inflammation, oxidative stress, necrotic apoptosis. Here, we summarize recent research advances on the molecular mechanisms of NRG1, elucidate the contribution of NRG1 to cardiovascular disease, discuss therapeutic approaches targeting NRG1 associated with cardiovascular disease, and highlight areas for future research.
1 Introduction
NRG1 (Neuregulin-1) belongs to the neuregulin family of growth factors responsible for cardiac development and cardiac protection from stress (Lin et al., 2020) and is a protein that plays a central role in cell signaling in the heart, mammary gland, and central nervous system (Falls, 2003; Mei and Nave, 2014). As a cardiac regeneration growth factor, NRG1 has attracted the attention of cardiovascular medicine. NRG1 has been shown to not only stimulate cardiomyocyte proliferation (Zhao et al., 1998; Bersell et al., 2009; D'Uva et al., 2015), but also act within angiogenesis, extracellular matrix remodeling, cardiomyocyte proliferation, stem-cell recruitment, and others, that improve cardiac function (Mendes-Ferreira et al., 2016; Rebouças et al., 2016; Arora et al., 2021). Together, these mechanisms promote myocardial repair and improvement of the cardiac function, that providing a novel molecular strategy targeting at regenerating the injured myocardium.
NRG1 is a powerful cardiovascular proliferation stimulant that is essential to the differentiation, proliferation, and expression of cardiac cells (Gassmann et al., 1995; Lee et al., 1995; Meyer and Birchmeier, 1995). NRG1 administration activates multiple signaling pathways to stimulate reentry and division of the adult cardiomyocyte cycle (D'Uva et al., 2015; Lin et al., 2015) and induce myocardial regeneration. Exogenous administration of NRG1 promotes the expression of genes related to the type of work (Zhu et al., 2010) and induces cardiomyocyte differentiation into cardiac conduction system cells (Rentschler et al., 2002), promotes stem cell differentiation into working-type cardiomyocytes (Suk Kim et al., 2003), and improves cardiac tissue function after pathological injury (Mendes-Ferreira et al., 2016; Kundumani-Sridharan et al., 2021; Sun et al., 2021), and are the most promising molecules for promoting cardiac repair under experimental conditions and clinical trials (Gao et al., 2010; Jabbour et al., 2011a; Polizzotti et al., 2015). Herein, this article summarizes the molecular mechanisms of NRG1 and its recent findings in cardiovascular biology, clinical applications in cardiovascular repair, and proposed areas of future research.
2 NRG1 and its regulation
NRG1’s regulatory mechanisms are complex and include a number of signalling, molecular, and metabolic pathways. Therefore, the molecular structure of NRG1, transcription regulation modes, and the interactions of signal pathways were summarized in this part.
2.1 Basic structure of NRG1
NRG1 is the most characteristic member of the neuregulin family (Huang et al., 2015a). The NRG1 gene is found on chromosome 8 in humans and mice and on chromosome 16 in rats (Falls, 2003; Chou and Ozaki, 2010). NRG-1 encodes 21 exons (Peles et al., 1992; Steinthorsdottir et al., 2004) and generates 31 potential protein isoforms (Mei and Xiong, 2008). Depending on the structure, NRG1 isoforms are classified into six types I-VI (Meyer et al., 1997; Steinthorsdottir et al., 2004). Although different isoforms of NRG1 are expressed in cells with different efficiencies, all can complete their signaling communication with ligands (Cote et al., 2005).
NRG1 consists of NH2-terminal ECD (extracellular structural domains), transmembrane structural domains, and highly conserved COOH-terminal ICD (intracellular structural domains) (Falls et al., 1993; Buonanno and Fischbach, 2001; Bao et al., 2004). NRG1-ECD binds to ErbB to activate ErbB signaling in target cells (Marchionni et al., 1993). It can be transferred to the nucleus and inhibit the expression of several apoptotic regulators (Bao et al., 2004). The interaction between ErbB and ECD in cells can occur after the release of NRG1-ECD or directly after the synthesis of isoforms lacking isoforms lacking transmembrane structural domains or after protein hydrolysis in the form of transmembrane precursors (Stonecypher et al., 2006; Yin et al., 2015). NRG1-ErbB interactions can also occur in cell-cell contact (Leimeroth et al., 2002). NRG1-ICD is required for NRG1 function in vivo (Liu et al., 1998), interaction of CRD (cysteine-rich domain) with ErbB receptors triggers signals required for CRD-NRG1 expression cells (Bao et al., 2003), and ectopic expression of NRG1 leads to NRG1-ICD dependent apoptosis (Grimm and Leder, 1997; Grimm et al., 1998).
NRG1 is homologous to the EGF (epidemal growth factor) family (Marchionni et al., 1993; Garratt et al., 2000) and shares an EGF-like structural domain with all isoforms (Buonanno and Fischbach, 2001). The EGF-like structural domain is encoded by a common “core” exon that encodes the 5′-terminus of the EGF-like structural domain and is spliced with one of two different exons that encode the 3′-terminus of the EGF-like structural domain to produce different variants of the EGF-like structural domain (α and β) of NRG1, respectively (Buonanno and Fischbach, 2001; Falls, 2003). NRG1α is expressed at higher levels than NRG1β (Cote et al., 2005), while NRG1β having a higher affinity for ErbB4 than NRG1α (Plowman et al., 1993; Du et al., 2012). This differential may have important effects on the organism. Animal experiments have shown that NRG1β knockout mice are embryonically lethal (Meyer and Birchmeier, 1995; Kramer et al., 1996), whereas NRG1α knockout mice survive into adulthood with only significant defects in the alveolar development of the mammary lobe (Li et al., 2002). Even more important, NRG1α enhances cell adhesion molecule l1 expression in human glioma cells and promotes their migration as a function of malignancy (Zhao and Schachner, 2013). These suggest that NRG1β may play a more important role in survival than NRG1α. And another in vitro experiment on cardiac muscle cells further explored the effects of both on cardiac energy metabolism. NRG1β is a more potent activator of receptor phosphorylation and intracellular signaling than the NRG1α, and only NRG1β stimulated glucose uptake and protein synthesis (Cote et al., 2005). It means that only NRG1β are biologically active on cardiac myocytes although cardiac microvascular endothelial cells express multiple NRG1 isotypes (Cote et al., 2005) (Figure 1).
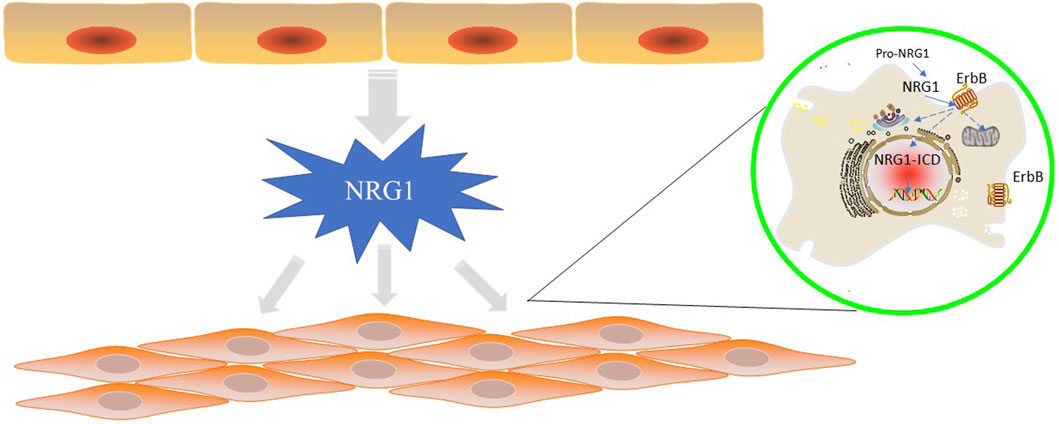
FIGURE 1. NRG1 is secreted by endothelial cells in the cardiovascular vessels and function in endothelial cells or cardiomyocytes by ErbB proteins. In “reverse signaling”, the C-terminal fragment of pro-NRG1 is generated by extracellular cleavage to release mature NRG1 and is further cleaved by γ-secretase to generate NRG1-ICD, which relocates to the nucleus to regulate gene transcription. In typical positive signaling, NRG1 binds to ErbB3 and ErbB4 ECD in differentiated cardiomyocytes and induces conformational changes, gaining higher affinity for other ErbB receptors. NRG1 ligand binding triggers homodimerization of ErbB4 and heterodimerization of ErbB2/3, ErbB2/4, ErbB3/4, followed by downstream signalling through the central pathway.
2.2 Regulation of NRG1
2.2.1 Post-translational modifications
In “reverse signaling,” the C-terminal fragment of pro-NRG1 (NRG1 precursor) is generated by extracellular cleavage to release mature NRG1 and is further cleaved by γ-secretase to generate NRG1-ICD, which relocates to the nucleus to regulate gene transcription (Loeb et al., 1998; Wang et al., 2001). NRG1 expressed in the heart is a pro-NRG1 transmembrane protein that requires protease processing for activation (Cote et al., 2005). Earlier studies showed that the ADAM (a disintegrin and metalloproteinase) family of MMP (matrix metalloproteinases), specifically ADAM17 and ADAM19, play a role in the release of pro-NRG1 from the endothelial membrane (Montero et al., 2000; Shirakabe et al., 2001; Shi et al., 2003; Kurohara et al., 2004). Follow-up studies have shown that ADAM17 is the main protease involved in pro-NRG1 release, while ADAM19 does not play a role (Horiuchi et al., 2005). This indicates that other proteins that can activate pro-NRG1 may be involved, as the ADAM17-deficient mouse was expressed differently from the NRG1-deficient mouse in terms of heart defects in the embryo (Horiuchi et al., 2005). NRG1 mRNA expression and protein synthesis are mediated by neurohormones (such as angiotensin II, phenylephrine, endothelin 1) and the mechanical pressure (Lemmens et al., 2006). These factors stimulate the expression of mRNA of NRG1 by inhibiting the expression level of NRG1 (Lemmens et al., 2006). ENOS (endothelial nitric oxide synthesis) also regulates the expression of NRG1 expression, and recent studies have found that endothelial cell increases its expression of NRG1 when NO production is eliminated (Shakeri et al., 2021), and the two complement each other through the paracrine pathway.
2.2.2 NRG1-ErbB signal pathway
NRG1 is produced mainly by endocardial vascular endothelial cells (Russell et al., 2014) and is an endogenous ligand of the ErbB family (Bouyain et al., 2005). NRG1 functioned through the receptor ErbB that all the biological effects of NRG1 were significantly reversed by the co-administration of NRG1 and ErbB inhibitor (Ding et al., 2021). The ErbB family consists of (ErbB1/EGFR), ErbB2 (HER2), ErbB3 (HER3) and ErbB4 (HER4) (Olayioye et al., 2000). All ErbB receptors are expressed on cardiac endothelial cells, fibroblasts and highly proliferative cells (Yarden and Sliwkowski, 2001), with ErbB3 and ErbB4 highly expressed on monocytes and cardiac macrophages (Carraway and Cantley, 1994; Campreciós et al., 2011), and ErbB2, ErbB4 on adult cardiomyocytes (Ma et al., 2016). The NRG1 gene encodes multiple isoforms of ErbB ligands (Pinkas-Kramarski et al., 1998; Buonanno and Fischbach, 2001). Although NRG-encoded protein isoforms by NRG differ greatly in their overall structure, the structural domain similar to EGF is sufficient to binding to receptors and trigger signals (Holmes et al., 1992).
NRG “forward” signaling, i.e., signaling from NRG-producing cells to NRG-responsive cells, leads to cellular responses, including stimulation or inhibition of proliferation, apoptosis, migration, differentiation, and adhesion (Yarden and Sliwkowski, 2001). In typical positive signaling, NRG1 binds to ErbB3 and ErbB4 ECD in differentiated cardiomyocytes and induces conformational changes (Jones et al., 1998), gaining higher affinity for other ErbB receptors (Kataria et al., 2019). NRG1 ligand binding triggers homodimerization of ErbB4 and heterodimerization of ErbB2/3, ErbB2/4, ErbB3/4 (Carraway and Cantley, 1994; Sweeney et al., 2001; Yarden and Sliwkowski, 2001), followed by downstream signaling through the central pathway (Xu et al., 2010; Fernandez-Cuesta et al., 2014). In some studies, the NRG1-ErbB pathway is believed to be a compensatory protective mechanism for cardiac injury (Bersell et al., 2009; Odiete et al., 2012; Dugaucquier et al., 2020). The NRG1-ErbB signaling axis is a critical mediator of cardiac development, and growing evidence supports a role for this system in the intricate cross-talk between the microvascular endothelium and myocytes in the adult heart.
2.2.3 Signaling cascades
1) NRG1-Hippo-YAP pathway
NRG1-ErbB4 has been shown to strongly regulate the Hippo-YAP (Yes-associated protein) pathway (Haskins et al., 2014). Haskins et al. identified the transcriptional program induced by NRG1-ErbB4 signaling as the true molecular signature of the Hippo-YAP pathway by analyzing the expression of YAP-regulated genes in several functional readouts of the NRG1 activation pathway (Haskins et al., 2014). NRG1 administration stimulates re-entry and division of the adult cardiomyocyte cycle by triggering ErbB2/ErbB4-dependent inhibition of the Hippo-YAP signaling pathway (Bersell et al., 2009; D'Uva et al., 2015; Lin et al., 2015), increasing cardiomyocyte numbers rather than hypertrophic growth (D'Uva et al., 2015; Lin et al., 2015).
The components of the Hippo pathway are divided into two main categories: core kinase modules and transcriptional modules, of which the kinase modules include MSTs (mammalian sterile 20-like kinases), LATSs (large tumor suppressor kinases), SAV1 (salvador homolog 1), MOBs (mps one binder kinase activator proteins), and RASSFs (ras associated domain family), which are responsible for the repression of the transcriptional coactivator YAP and its homologue TAZ (transcriptional coactivator with PDZ binding motif). When the Hippo signaling pathway is activated, MST1/2 forms a complex with SAV1 that phosphorylates and activates the LATS1/2-MOB1 complex, which phosphorylates YAP and TAZ, and the phosphorylated YAP and TAZ pathway complexes are moved out of the nucleus, where they remain in the cytoplasm and are degraded by the protease system to lose transcriptional activity (Zhang and Re, 2017). This prevents their nuclear localization and thus inhibits cardiomyocyte proliferation (Heallen et al., 2011a; Dey et al., 2020). When the Hippo-YAP signaling pathway is inhibited, YAP dephosphorylates and translocates to the nucleus to bind to transcription factors such as TEAD (transcriptional coactivator with PDZ binding motif) to initiate the transcriptional process to promote mitosis (Lin and Pu, 2014). Therefore, outside the regeneration window, this suggests that modulation of the NRG1-Hippo-YAP pathways could unlock adult mammals’ ability to regenerate their hearts.
There is increasing evidence that YAP signaling interacts with PI3K (phosphatidylinositol-3-kinase)-AKT (a serine/threonine kinase) signaling to positively regulate cardiomyocyte cycle progression (Sudol, 2014; Lin et al., 2015). ErbB4 activates PI3K signaling in response to IGF (insulin-like growth factor) which plays a role in promoting cardiomyocyte growth and inhibiting fibrosis (MeiKim et al., 2013). YAP activates the IGF-PI3K signaling pathway in cardiomyocytes, leading to inactivation of GSK3β (glycogen synthase kinase 3β) and an increase in its downstream β-catenin content (Xin et al., 2011a). Furthermore, YAP can directly target the expression of Pik3cb (phosphatidylinositol-4,5-bisphosphate 3-kinase catalytic subunit beta), the catalytic submitter of PI3K, to activate the PI3K-AKT pathway (Lin et al., 2015) to indirectly promote β-catenin activity.
2) NRG1-PI3K-AKT pathway
NRG1-ErbB4 can inhibit through PI3K-AKT pathway macrophages to reduce myocardial inflammation and fibrosis (Vermeulen et al., 2017), while inhibiting cardiac endoplasmic reticulum stress to reduce reperfusion injury (Fang et al., 2017). NRG1 maintains cardiac troponins by the ErbB2-PI3K pathway, which may lessen doxorubicin-induced cardiac dysfunction (Bian et al., 2009). Together, these studies confirmed that the activation of the NRG1-ErbB pathway plays a protective role in myocardial injury and may be involved in cell apoptosis and differentiation, as found in studies that NRG1/ErbB2 regulates cell differentiation and apoptosis through the PI3K/Akt pathway (Hong et al., 2021; Lu et al., 2021; Mahiny-Shahmohammady et al., 2022). In vitro, NRG1 treatment up-regulated ErbB3 phosphorylation, and increased the expression of PI3K and phosphorylation-AKT (Meng et al., 2021) to modulate the activation of PI3K-AKT pathway. In conclusion, NRG1 can act on ErbB2, ERBB3 and ERBB4, respectively activates PI3K-AKT pathway.
EGF induces YAP nuclear accumulation through PI3K-dependent blockade of YAP phosphorylation. EGF triggers the rapid translocation of YAP into the nucleus along with YAP dephosphorylation, both of which depend on LATS. EGF receptor inhibits the Hippo pathway through activation of PI3K and PDK1, but independent of AKT activity. The entire Hippo core complex dissociates in response to EGF signaling in a PI3K-PDK1-dependent manner, leading to inactivation of LATS, dephosphorylation of YAP, and YAP nuclear accumulation and transcriptional activation of its target gene. In turn, activated YAP induces transcription of amphiregulin (Zhang et al., 2009) and promotes positive feedback regulation of Hippo signaling by activation of EGF receptor.
3) other related pathways
Based on the available literature, it is known that NRG1 can regulate cardiac physiopathological processes through the interaction between Hippo pathway, Wnt pathway, ERK (extracellular signal-regulated kinase) pathway, RISK pathway and mTOR (rapamycin) pathway.
NRG1-ErbB2 is involved in the negative regulation of cardiomyocyte autophagy by activating the PI3K/PKB/mTOR and MAPK (monophosphate-activated protein kinase)/ERK/mTOR pathways (An et al., 2013; Surviladze et al., 2013). Interestingly, recent studies have found that ErbB2 signaling can lead to YAP to promote cell proliferation in an ERK-dependent manner rather than Hippo (Aharonov et al., 2020), and can also indirectly promote the onset of cardiac regeneration through interactions of the AKT and GSK3β/β-catenin pathway (D'Uva et al., 2015). For example, inactivation of cardiac SAV not only inhibits the Hippo pathway, releasing YAP into the nucleus for direct interaction with β-catenin (Heallen et al., 2013) to enhance its transcriptional activity, but also upregulates the classical Wnt pathway (Heallen et al., 2011b) to act on target genes to promote cell mitosis process.
It has been demonstrated that NRG1 induces upregulation of ligand protein expression to promote the differentiation of embryonic stem cells into working cardiomyocytes via MEK (mitogen-extracellular activated protein kinase)-ERK (Zhu et al., 2010; Wang and Huang, 2014). In cardiac transplantation, rhNRG1 (recombinant human NRG1) attenuates left ventricular remodeling and myonodal disorders by up-regulation of the RISK pathway (Liu et al., 2006; Gao et al., 2010) to create conditions to maintain donor heart function. Due to the interaction between signaling pathways and other signal crosstalk between cells, the signaling cascades of NRG1 is still unclear, and further research is still needed.
3 NRG1 with cardiovascular diseases
The downstream signaling of NRG1 acts mainly in the ventricular wall, heart valves and conduction system, and microvasculature (Rupert and Coulombe, 2015). In this section, we summarize the physiological and pathological roles of NRG1 in cellular and cardiovascular tissues to fully illustrate the important role of NRG1 in the development of cardiovascular diseases. Novel research shows that NRG1 plays an important role in the occurrence and development of cardiovascular diseases, such as atherosclerosis, MI/IR, HF, cardiotoxicity, and arrhythmia. We summarize the role of NRG1 in cardiovascular diseases (Figure 2) in conjunction with the related pathways. In addition, animal experiments and clinical studies related to the treatment of cardiovascular diseases by NRG1 are summarized in Table 1.
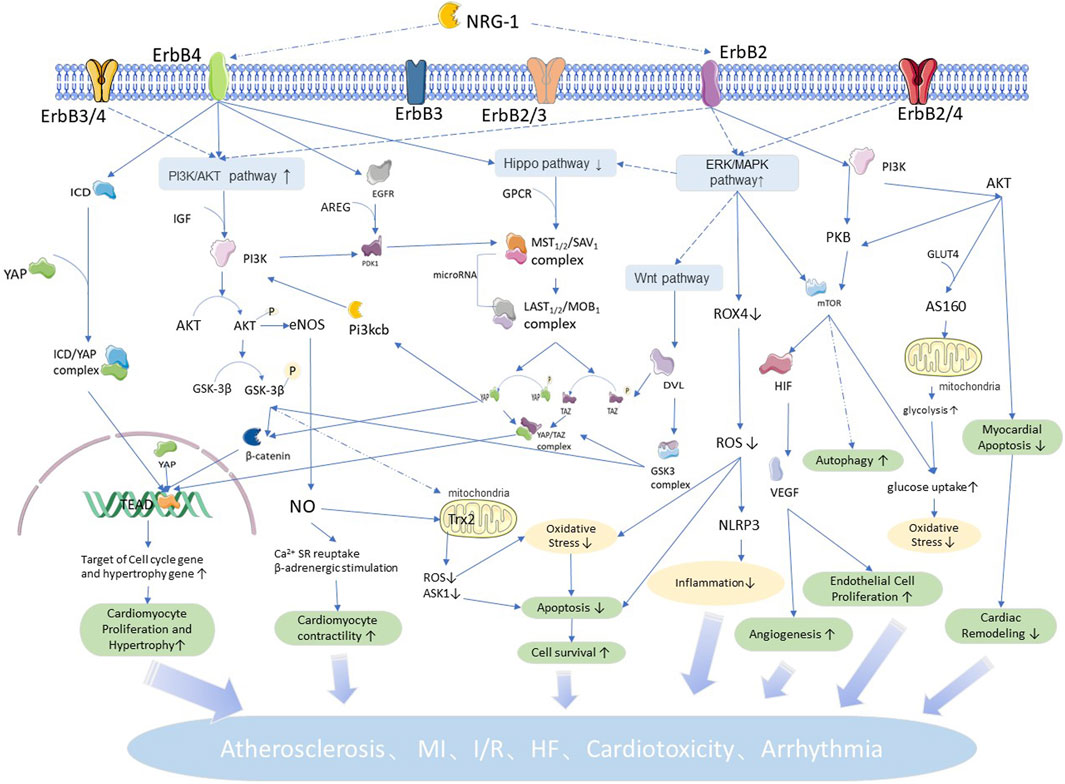
FIGURE 2. NRG1 related pathways and roles in cardiovascular: NRG1 mediated by ErbB4 regulates cell proliferation and hypertrophic growth by coordinating the Hippo pathway, and regulates apoptosis and proliferation, oxidative stress, cell autophagy and other physiopathological processes by the P13K/AKT pathway. NRG1 mediated by ErbB4 regulates cellular inflammatory response and vascular proliferation by coordinating the ERK/MAPK pathway, and the Wnt pathway is involved in regulating cardiac and vascular neogenesis. Meanwhile, the interaction between signaling pathways makes each physiopathological process intricate and complex, increasing with each other and participating in NRG1 to regulate the development of cardiovascular diseases.
3.1 NRG1 and atherosclerosis
3.1.1 NRG1 and cell aging
Cellular senescence is driven by chronic inflammation and oxidative stress. The senescence of EC (endothelial cell) and VSMC (vascular smooth muscle cell) can cause atherosclerosis (Minamino and Komuro, 2008; Nakano-Kurimoto et al., 2009; Wang and Bennett, 2012), and cellular senescence and SASP (senescence-associated secretory phenotype) play an important role in cardiovascular aging and disease (Gorenne et al., 2006; Minamino and Komuro, 2007; Sikora et al., 2011). Cells can enter a state of senescence caused by DNA damage due to intrinsic and extrinsic stressors, called “stress-induced premature senescence” (SIPS) (Correia-Melo et al., 2014). Oxidative stress and hyperglycemia are the two main factors that contribute to SIPS (Gorenne et al., 2006; Minamino and Komuro, 2007; Sikora et al., 2011).
NRG1 is known to have improved glucose metabolism (Pentassuglia et al., 2016) and antioxidant (Wang et al., 2021) effects, so it may have anti-SIPS potential. Data suggests that NRG1 significantly inhibits stress-induced premature senescence in vascular cells in vitro and in the aorta of diabetic mice in vivo (Shakeri et al., 2018), and application of rhNRG-1 for 9 weeks to type 1 diabetic mice attenuates diabetes-induced vascular senescence (Shakeri et al., 2018). Consistently, deficiency of the NRG1 receptor ErbB4 induced cellular senescence both in vitro and in vivo (Shakeri et al., 2018), and diabetes induced significantly more vascular senescence in mice lacking the ErbB4 receptor in SMC compared to wild-type littermates (Shakeri et al., 2018), suggesting the efficacy of NRG1 in anti-cellular senescence. The effects of NRG1 on cell proliferation and apoptosis have been well described (Yarden and Sliwkowski, 2001; Xu et al., 2014), but the effects of NRG1/ErbB signaling on cellular senescence remain to be elucidated.
3.1.2 NRG1 and endothelial cell damage
Previous experiments have demonstrated that NRG1 prevents foam cell formation in primary cultured human monocyte-derived macrophages (Xu et al., 2009). Also, chronic infusion of NRG1β into ApoE−/− mice inhibited macrophage infiltration in the arterial wall (Xu et al., 2009), it reduced endocytosis of acetylated LDL (low density lipoprotein) and cholesterol ACAT-1 (acyltransferase-1) activity, and it increased cholesterol efflux from human macrophages to apolipoprotein A1 (Xu et al., 2009). This again confirms the inhibitory effect of NRG1β on atherosclerotic lesions. Co-incubation of VSMCs with norepinephrine and oxidized LDL inhibited NRG1 expression and induced proliferation and phenotypic transformation of VSMCs (Jiao et al., 2008). NRG1 reduces proliferation and migration of neointima formation after vascular injury in rats by reducing VSMCs (Clement et al., 2007). These findings suggest a negative correlation between NRG1 expression and proliferation in VSMCs. Stimulation of ErbB3 by NRG1β has been reported to inhibit EC proliferation (Amin et al., 2006), and ErbB3 may be a potential therapeutic target of the NRG1-ErbB pathway against atherosclerosis.
3.1.3 NRG1 and vascular stress
In addition, the NRG1/ErbB signaling system is essential for the response to vascular stress (Parodi and Kuhn, 2014). NRG1 has anti-adrenergic effects on the cardiovascular system (Lemmens et al., 2004) leading to vasodilation. It was shown that microinjection of NRG into the ventral lateral aspect of the medulla oblongata, a major vasodilatory center, reduced blood pressure in rats (Matsukawa et al., 2013). NRG1β is an activator of nicotinic acetylcholine receptors, which may improve the atherosclerotic vascular load for cardioprotective effects by balancing the autonomic nervous system (Okoshi et al., 2004).
3.2 NRG1 and myocardial infarction
3.2.1 NRG1 and oxidative stress
Myocardial infarction is characterized by a peak in cardiomyocyte death on the first day (Whelan et al., 2010; Del Re et al., 2019). The latest research shows that no association with other variables, NRG-1 plasma levels decreased significantly following PCI/RIC and remained decreased up to 1 month following AMI (Haller et al., 2022). ROS (Reactive oxygen species) generated in cardiac ischemia-reperfusion induce NRG1β/ErbB4 activation (Kuramochi et al., 2004a), implying that NRG1β/ErbB4 signaling regulates myocardial injury in an environment of oxidative stress. NADPH oxidase 4 (NOX4) is the major ROS synthesizing enzyme in cardiac tissue (Matsushima et al., 2014). Previous experiments have demonstrated that NRG1 can inhibit NOX4 activity by activating the ERK1/2 (Wang et al., 2018) pathway in myocardial reperfusion injury, thereby downregulating ROS production (Caja et al., 2009). Specific overexpression of ErbB2 in young rat hearts induces the expression of antioxidant genes such as mitochondrial GPx1 (glutathione peroxidase 1) and catalase (Belmonte et al., 2015). NRG1 can inhibit hydrogen peroxide by acting ErbB4-Akt signaling or by direct activation of paracrine signaling by ErbB4 independent of NRG in adult rat ventricular myocytes (Kuramochi et al., 2004a), thus inhibiting hydrogen peroxide stimulated adult rat ventricular myocytes apoptosis (Kuramochi et al., 2004a) to achieve self-protection. Thus, NRG1 exerts an anti-apoptotic effect on myocardium by coordinating the oxidative stress process.
3.2.2 NRG1 and myocardial inflammatory injury
AMI (acute myocardial infarction) induces blood leukocytosis, which is negatively correlated with patient survival (Jiang et al., 2022). Excessive inflammation in endangered myocardial regions induced by MI can lead to increased cardiomyocyte death through pro-apoptotic signaling pathways and further cardiac remodeling (Nahrendorf et al., 2010; Griffiths et al., 2017). NRG1 receptor epidermal growth factor receptors ErbB2 and ErbB4 were expressed on cardiac fibroblasts in the infarct area. Systemic blockade of ErbB function in MI model mice enhanced senescence and apoptosis of cardiac fibroblasts and exacerbated inflammation (Shiraishi et al., 2022).
It has been shown that NRG1 can reduce ROS production through ERK1/2 inhibition of NOX4 and inhibit the NLRP3/caspase-1 pathway, thereby attenuating myocardial oxidative damage and inflammatory responses in MI (Wang et al., 2021). NRG1-mediated NOX4 inhibition is dependent on ERK1/2 activation (Wang et al., 2021). NOX4-mediated ROS production promotes activation of NLRP3 inflammatory vesicles (Abais et al., 2015; Moon et al., 2016), stimulates upregulation of NLRP3 inflammatory vesicles (Kawaguchi et al., 2011; Sandanger et al., 2013), and promotes activation of the inflammatory response by the organism. In a model of septic cardiomyopathy, NRG1 can inhibit NLRP3 inflammatory vesicles (Abais et al., 2015). NRG1β has also been reported to reduce the pro-inflammatory response by attenuating COX-2 (cyclooxygenase 2) expression in monocyte U937 cells (!!! INVALID CITATION) and can also exert anti-inflammatory antioxidant effects in myocardial tissues and cells by activating AKT/eNOS pathways (Kuramochi et al., 2004a; Timolati et al., 2006; Brero et al., 2010). The role of NRG1 in reducing inflammatory damage in cerebral IR has been previously reported (Wu et al., 2015). However, studies on the anti-inflammatory effects of NRG1 in myocardial tissue are limited.
3.2.3 NRG1 and energy metabolism
Under stress conditions such as insufficiency and stress overload, lipid metabolism not only cannot meet the heart’s energy needs, but also exacerbates oxidative stress (Opie, 1976), with the heart increasing glucose uptake and decreasing the consumption of fatty acids to maintain the high energy demands required for sustained contraction (Shao and Tian, 2015; Riehle and Abel, 2016; Szablewski, 2017). Under such stressful conditions, myocardial endothelial cells also release NRG1 as a cardioprotective factor (Kuramochi et al., 2004a; Hedhli et al., 2011). NRG1-ErbB2 signaling has been found to induce cardiomyocytes in the border region of the injured heart to dedifferentiate and shift their metabolism from oxidative phosphorylation to glycolysis in zebrafish, as evidenced by the decrease in mitochondrial genes and the increase in glycolytic genes (Honkoop et al., 2019). NRG1 improves the pharmacokinetic properties and produces metabolic benefits by reducing the double inhibition of hepatic gluconeogenesis and cholesterol intake in obese mice (Zhang et al., 2018). These results indicate that NRG1 promotes energy metabolism in zebra fish, which maintain regeneration, and in mammals, which significantly reduce regeneration after birth. The activation of the NRG1-ErbB2 signal helps to reduce glucose consumption after heart damage and promote the use of glucose energy. RhNRG1 increases glucose uptake by neonatal rat ventricular myocytes through ErbB2/ErbB4 and PI3K (Liu et al., 2006). In the adult heart, glucose uptake by cardiac myocytes is believed to be primarily achieved by the glucose transporter protein GLUT4 (glomerular glucose transporter 4) (Shao and Tian, 2015; Riehle and Abel, 2016). In a neonatal rat ventricular myocyte model, similar to insulin, rhNRG1 can induce glucose uptake by activating the PI3K-Akt-AS160 (Akt substrate of 160 kDa) pathway and GLUT4 translocation, and unlike insulin, the rhNRG1-induced effects are not mediated by insulin recptorsubstrate proteins (Liu et al., 2006). RhNRG-1 has been shown to enhance glucose uptake by neonatal rat ventricular myocyte through a mechanism associated with the ErbB2/ErbB4, PI3K-Akt (Pentassuglia et al., 2016).
The mammalian target of mTOR regulates human cardiac protein synthesis and energy metabolism (Laplante and Sabatini, 2012; Saxton and Sabatini, 2017). MTORC1 activity, a complex of mTOR, is associated with protein synthesis and physiological hypertrophy, and mTORC2, another complex, regulates glucose uptake (Kumar et al., 2008; Liu et al., 2014; Sato et al., 2014). NRG1β has a direct and specific stimulatory effect mediated by mTOR in the cardiac myocyte signaling cascade with direct and specific stimulatory effects (Pentassuglia et al., 2016). ErbB receptor activity is associated with mTOR signaling, and mTOR inhibitors improve the outcome of ErbB2-positive breast cancer (Vicier et al., 2014). Although mTOR inhibition appears to have therapeutic value in cancer, cardiomyocyte mTORC1 deficiency leads to cardiac dysfunction in mice (Zhang et al., 2010; Shende et al., 2011). In a rat neonatal cardiomyocyte model, NRG1/ErbB signaling enhances glucose uptake and protein synthesis, but NRG1β-induced mTORC1 plays a minor role in protein synthesis, while mTORC2 appears to be independent of glucose uptake (Pentassuglia et al., 2016).
3.2.4 NRG1 and cell multiplication
Hypertrophic growth, multinucleation, and polyploids are characteristics of cardiovascular cells in mammalians, and are the underlying cause of changes in cardiac cell growth before and after birth (Ikenishi et al., 2012; Tane et al., 2015). Cardiomyocytes undergo this process rapidly in infarcted and failing hearts in response to growth stimuli, leading to an increase in the number of multinucleated and polyploid cells in damaged myocardial tissue (Herget et al., 1997; Tevzadze et al., 2005; Stephen et al., 2009; Lázár et al., 2017; Kajstura et al., 2010), and cardiomyocytes grow hypertrophically without being able to achieve a quantitative increase. Furthermore, as the heart ages and the myocardium is released from the cell cycle, Hippo activity increases significantly, while YAP activity decreases considerably (Heallen et al., 2013; von Gise et al., 2012) and cell proliferation is endogenously inhibited. In recent years, clinical trials of the new approach to stem cell transplantation have shown limited success, but long-term effectiveness remains unknown (Wei et al., 2019; Hotham and Henson, 2020; Schwach et al., 2020). NRG1 was found to promote mitosis through ErbB (D'Uva et al., 2015) for efficient cell proliferation.
In mice, NRG1 administration has been shown to induce proliferation and cardiac regeneration in adult cardiomyocytes (Bersell et al., 2009). In fact, transient induction of ErbB2 signaling in juvenile and adult mice cardiomyocytes was sufficient to strongly induce cardiomyocyte dedifferentiation and proliferation and to trigger cardiac regeneration after myocardial infarction (D'Uva et al., 2015). In zebrafish, NRG1 is dramatically induced in perivascular cells after cardiac injury, and inhibition of its co-receptor ErbB2 disrupts the proliferative response of cardiomyocytes to injury (Gemberling et al., 2015). Following ischemic heart injury in adolescents or adults, transient expression of ErbB2 similarly triggers cardiomyocyte dedifferentiation, proliferation, neointima formation, and cardiomyocyte redifferentiation, which together lead to anatomical and functional regeneration (Lee et al., 1995; Lai et al., 2010; Liu et al., 2010; Staudt et al., 2014). This provides a definitive clinical experimental basis for the ability of NRG1-ErbB2 to effectively promote cell proliferation. Furthermore, NRG1-ErbB4 has been shown to induce mononuclear cardiomyocyte proliferation without affecting the level of apoptotic cell death (Zhu et al., 2010). These evidences suggest the feasibility of NRG1-ErbB pathway expression for myocardial regeneration as well as a clear value for clinical studies. However, as the organism matures, the cardiac level of ErbB2 decreases and its mitogenic promoting effect is significantly weaker in late postnatal development and adulthood than in the neonatal period (D'Uva et al., 2015; Polizzotti et al., 2015; Aharonov et al., 2020). Therefore, the strategy of combining NRG1 with ErbB2 overexpression or ErbB2-inducible factors should be further explored.
In the NRG1-Hippo-YAP signaling axis, deletion of YAP in the embryonic heart prevents cardiomyocyte proliferation and leads to myocardial dysplasia (von Gise et al., 2012; Xin et al., 2011b). In contrast, deletion of Sav1 or LST1/2, upstream negative regulators of YAP, and cardiomyocyte-specific hyperactivation of YAP increases embryonic cardiomyocyte proliferation (Heallen et al., 2011a; Heallen et al., 2013; von Gise et al., 2012; Xin et al., 2013). However, these experiments induce adult cardiac regeneration by inducing early cardiomyocytes with strong regenerative capacity, with limited clinical operability and application. Also, in this pathway, its downstream effector YAP/TAZ complex is overactivated in human cancers and is a hallmark of cancer. Therapeutic YAP/TAZ activation may contribute to the development of cancer (Harvey et al., 2013; Janse van Rensburg et al., 2018). The ability to harness the regenerative power of YAP/TAZ in a safe manner is critical for clinical application.
3.2.5 NRG1 and angiogenesis
NRG1 is also a proangiogenic factor that plays an important role in angiogenesis and arteriogenesis after ischemic injury (Hedhli et al., 2012). Myocardial ECs upregulate NRG1 release during ischemic injury (Fukazawa et al., 2003; Hedhli et al., 2011). The receptor for NRG1, ErbB2, was found to be expressed only in ECs, but not in cardiomyocytes from adult mouse hearts (Kundumani-Sridharan et al., 2021). NRG1-ErbB2 can regulate angiogenesis not only through the activation of HIF-1α (hypoxia-inducible factor-1α) via the PI3K/AKT/mTOR pathway (Karar and Maity, 2011), but also through paracrine up-regulation of VEGF to induce angiogenesis (Xu et al., 2010). In a study by Kundumani Sridharan (Kundumani-Sridharan et al., 2021) et al., it was shown that NRG1β-ErbB2 signaling also protects ECs through the ErbB2/4-cSrc-NO axis and the ErbB2/4-ATG5-Trx2 (thioredoxin-2) axis. Although it is difficult to perform pro-angiogenic therapy after AMI (Grines et al., 2002; Hedman et al., 2003; Henry et al., 2003; Wu et al., 2021), proangiogenic therapy still has the potential to benefit patients in the acute phase of IR (Wu et al., 2021).
NRG1 regulates endothelial progenitor cell biology by affecting their survival and differentiation capacity (Safa et al., 2011; Hedhli et al., 2012). EPDCs (epicardial-derived cells) are transformed from epicardial progenitor cells and develop into cardiac fibroblasts and VSMCs (Acharya et al., 2012; Katz et al., 2012), which are essential for myocardial and coronary vascular development (Smits et al., 2018). Consecutive genetic and pharmacological studies have also shown that the arrest of fibroblast differentiation is mediated by YAP, which controls both the composition of ECM (extracellular matrix) and vascular remodeling during cardiac development (Xiao et al., 2018). Although the role of the NRG1-Hippo-YAP pathway in noncardiomyocytes remains less clear, these findings suggest that the NRG1-Hippo-YAP pathway is required for cardiac fibroblast differentiation and coronary vascular development.
3.3 NRG1 and ischemia reperfusion
The ischemic and hypoxic myocardial microenvironment leads to myocardial injury, induces an inflammatory response (Li et al., 2021), and initiates self-repair processes such as apoptosis and autophagy (Steffens et al., 2020). In the endothelium, IR injury leads to activation of EC (i.e., expression of pro-inflammatory molecules), increased permeability and edema, and impaired vasodilation (Wan and Rodrigues, 2016; Colliva et al., 2020; Wagner and Dimmeler, 2020). In turn, endothelial injury and dysfunction promote vasoconstriction, thrombosis, and coronary occlusion, leading to cardiac inflammation and injury, adverse cardiac remodeling, and hypertrophy (Prabhu and Frangogiannis, 2016; Gibb et al., 2020; Erkens et al., 2021).
NRG1 preconditioning protects the heart against ischemia/reperfusion injury through a PI3K/Akt-dependent mechanism (Fang et al., 2010). In Langendorff analyzes, the ErbB4 inhibitor suppressed the phosphorylation of ErbB4 and the RISK pathway and aggravated myocardial edema and fiber fracture, thereby inhibited the cardioprotective effects of NRG1 (Wang et al., 2018). For assessment of downstream signals, the PI3K inhibitor and the MEK inhibitor suppressed the phosphorylation of AKT and ERK1/2 respectively and abolished the cardioprotective effects induced by NRG1 (Wang et al., 2018). Another experiment further confirmed this idea, and proposed that NRG1 might protect the heart from IR damage by inhibiting endoplasmic reticulum stress through the PI3K-Akt pathway (Fang et al., 2017). Endothelial colony-forming cells were capable of actively releasing NRG1, which, in turn, reduced apoptosis and increased the proliferation of human pluripotent stem cell-derived cardiomyocytes via the PI3K/Akt signaling pathway (Hong et al., 2021), while transcriptional silencing of NRG1 abrogated these cardioprotective effects. Moreover, endothelial colony-forming cells are uniquely suited to support human pluripotent stem cell-derived cardiomyocytes, making these progenitor cells ideal for cardiovascular regenerative medicine (Hong et al., 2021).
RIPC (remote ischemic preconditioning) is an interventional procedure in which multiple short-cycle IR cycles applied in a remote vascular bed can prevent IR injury. The endothelial NRG1β-ErbB2 signaling pathway has been shown to have a good protective effect in RIPC-induced cardiac injury (Hedhli et al., 2011; Lemmens et al., 2011; Parodi and Kuhn, 2014; Kundumani-Sridharan et al., 2021). RIPC rescues the loss of mitochondrial Trx2 mediated by IR through NRG1β-dependent protection against endothelial ErbB2 loss in mice is an important previously unrecognized mechanism of RIPC-mediated protection against MI (Kundumani-Sridharan et al., 2021). Trx2 has been shown to inhibit mitochondrial ROS generation and ASK1 (apoptosis signal-regulated kinase 1) to maintain cardiac function (Huang et al., 2015b). NO is known to alter autophagy and endothelial cell NO production may inhibit cardiomyocyte autophagy leading to loss of Trx2 (Sarkar et al., 2011). Loss of Trx2 in cardiomyocytes leads to activation of ASK1 and promotes apoptosis in cardiomyocytes (Huang et al., 2015b). Endothelial ErbB2 reduces ASK1-mediated apoptosis in cardiac tissue by mediating the phosphorylative inactivation of ATG5 (autophagy related 5), thus inhibiting Trx2 degradation in mitochondria (Kundumani-Sridharan et al., 2021). Up-regulation of the ErbB2/4-ATG5-Trx2 pathway protects against cardiomyocyte apoptosis (Nonn et al., 2003; Huang et al., 2015b), providing a new therapeutic idea against myocardial apoptosis. Although NRG1 has been shown to have a beneficial role in cardiac function, circulating NRG1 levels correlate with the severity of HF (Ky et al., 2009), which is not related to the ability to repair damaged myocardium.
In the NRG1-Hippo-YAP signaling axis, inhibition of Hippo pathway expression can somewhat reduce the level of apoptosis in peri-infarct cardiomyocytes and reduce the expansion of infarct size. YamamotoYang et al. (2003) demonstrated in myocardial infarction model mice experiments that MST1 was significantly activated upon apoptosis and that cardiac-specific overexpression of MST1 transgenes activated mouse apoptosis-associated proteins caspase3 and caspase9 in cardiac tissues, resulting in increased apoptosis in cardiomyocytes (Odashima et al., 2007). On the contrary, specific knockdown of MST1 in their cardiac tissues significantly improved apoptosis in mice (Odashima et al., 2007). Cardiac-specific overexpression of MST1 in mice leads to progressive deterioration of cardiac function (Yamamoto et al., 2003), which may be the result of increased myocardial oxygen consumption and apoptosis due to elevated wall pressure.
3.4 NRG1 and hart failure
3.4.1 NRG1 with myocardial hypertrophy and myocardial fibrosis
During the healing process of heart, damaged myocardial tissue undergoes myocardial hypertrophy, myocardial fibrosis, and ventricular remodeling, ultimately leading to myocardial hyposystolic function and heart failure (Wang et al., 2017a; Lindsey et al., 2018). In cases of heart failure, NRG1/ErbB signaling is significantly impaired (Galindo et al., 2014; Gupte et al., 2017), and administration of NRG1 attenuates the progression of HF, inhibits myocardial fibrosis and apoptosis, and reduces oxidant-producing enzymes (Gupte et al., 2017). Conditional knockout of ErbB2 in mice with accelerated heart failure after stress overload or adriamycin injury (Crone et al., 2002; Ozcelik et al., 2002) showed that the NRG/ErbB pathway acts as a stress response signal between microvascular endothelial cell and myocytes to maintain cell survival and cardiac function.
M2-like macrophage-mediated regulation of NRG1/ErbB signaling has a substantial effect on fibrotic tissue formation in the infarcted adult mouse heart and is critical for suppressing the progression of senescence and apoptosis of cardiac fibroblasts (Shiraishi et al., 2022). A recent study showed that the NRG1β-ErbB4 pathway also inhibits macrophage activation and reduces myocardial hypertrophy and fibrosis (Vermeulen et al., 2017). The molecular mechanism underlying the regulation of fibrotic tissue formation in the infarcted myocardium was shown in part to be attenuation of apoptosis and senescence of cardiac fibroblasts by the activation of NRG1/ErbB/PI3K/Akt signaling (Shiraishi et al., 2022).
However, NRG1 has also been proposed to induce cardiomyocyte hypertrophy (De Keulenaer et al., 2019). In a cardiac magnetic resonance study, it was shown that rhNRG1-treated rats had significantly increased left ventricular wall thickness after MI and increased plasma N-terminal B-type natriuretic peptide precursor levels in a rhNRG1 dose-dependent manner (Zurek et al., 2020), confirming the detrimental effects of rhNRG1-induced hypertrophy and worsened cardiac function.
3.4.2 NRG1 with left ventricular function
The NRG1/ErbB system is essential for cardiac development, is activated in the early stages of compensated HF, under conditions of myocardial stress, and decreases with disease progression and decompensation (Lemmens et al., 2007; Mendes-Ferreira et al., 2013). NRG1 administration improves cardiac dysfunction and reduces mortality in several models of LV (left ventricular) failure (Liu et al., 2006), and clinical trials have demonstrated the efficacy and safety of NRG1 in improving LV function in patients with HF (Gao et al., 2010; Jabbour et al., 2011a).
Abnormal Ca2+ handling in cardiac myocytes has been shown to affect excitation-contraction coupling and cellular function, leading to systolic dysfunction and arrhythmias (Johnson and Antoons, 2018). A recent study found that NRG1β increased Ca2+ current density in LV cardiomyocytes and promoted Ca2+ handling protein expression levels, which improved LV cardiac function in volume-overloaded HF rats (Wang et al., 2019). However, this experiment lacks direct evidence of an effect on calcium-handling function.
RhNRG1 is currently being tested in clinical trials as a new treatment for HF (Gao et al., 2010; Jabbour et al., 2011a). Early studies found that recombinant NRG1β improved myocardial contractility and diastolic capacity in rat models of chronic HF induced by ischemic, dilated, and viral cardiomyopathies (Liu et al., 2006). Similar improvements in cardiac function have been observed in dogs (Liu et al., 2006) and primates (Li et al., 2007). NRG1 attenuates the development of HF in several animal models, including diabetic cardiomyopathy (Liu et al., 2006; Odiete et al., 2013; Vandekerckhove et al., 2016). Advanced cardiomyopathy is an important cause of HF (Seidman and Seidman, 2001). Evidence suggests that weakening ErbB2 activity in the heart can directly contribute to cardiomyopathy and HF (Ozcelik et al., 2002). Therefore, ErbB2 holds promise as a potential target for clinical intervention in HF by NRG1. However, despite proposed explanations for the improvement in cardiomyocyte structure, contractility and proliferation of NRG1 (De Keulenaer et al., 2019), few studies have reported its effects on cardiac output and overall longitudinal strain, as well as LVEF and LV volume (Zurek et al., 2020), making it difficult to fully explain the benefits of NRG1 on left heart function.
3.5 NRG1 and cardiotoxicity
NRG1β is expressed in the cardiac microvascular endothelium, and promotes the growth and survival of cardiac myocytes in culture through the activation of ErbB2 and ErbB4 receptor tyrosine kinases (Fukazawa et al., 2003). On the one hand, NRG1β could reduce circulating levels of proinflammatory cytokines in rats with sepsis, adjust diaphragmatic proinflammatory cytokine level, mitigate diaphragmatic oxidative injury, and lessen diaphragm cell apoptosis, improving the function of the diaphragm and playing a role in the protection of the diaphragm by activating the signalling of PI3K/Akt (Liu et al., 2020). On the other hand, expression of NRG1 and recombinant NRG1β protects myocytes from anthracycline and β-adrenergic receptor-induced cell injury and death (Zhao et al., 1998; Sawyer et al., 2002; Fukazawa et al., 2003; Kuramochi et al., 2004b).
The pro-survival effect of NRG1 occurs through activation of ErbB2/4 in myocytes and downstream signaling in the PI3K/Akt pathway. Direct evidence for the cardiotoxic effects of NRG1 against anthracyclines is the protective activity of NRG1 against adriamycin-mediated myofibrillar disorders and prevention of troponin toxic degradation in cultured cardiomyocytes (Sawyer et al., 2002; Bian et al., 2009). NRG1 cardioprotective activity was also inferred in vivo by adriamycin-aggravated systolic dysfunction in heterozygous NRG1 mutant mice and exacerbated chamber dilation in ErbB4 knockout mice specific to the ventricles (Liu et al., 2005; Vasti et al., 2012). Therefore, expression of NRG1/ErbB not only promotes the proliferation of cardiomyocytes and reduces myocardial damage, but also reduces anthraquinone cardiotoxicity, which is undoubtedly beneficial for populations that require anthraquinone applications.
3.6 NRG1 and arrhythmia
NRG1 regulates myocardial function and sympathetic vagal homeostasis and is dynamically involved in the hemodynamic homeostasis of the cardiovascular system. NRG1 desensitizes the myocardium to the positive inotropic effects of isoprenaline through activation of eNOS, providing regulatory feedback on the autonomic imbalance present in acute cardiac stress and chronic HF (Zhao et al., 1999; Lemmens et al., 2004). Cardiomyocytes lacking NRG1 signaling are unable to adequately balance β-adrenergic activation by inhibiting parasympathetic activity (Okoshi et al., 2004; Brero et al., 2010).
Conditional ErbB2 mutant mice also exhibit prolonged ventricular repolarization time (increased QTc) and tachycardia (Ozcelik et al., 2002), which are not normally associated with dilated cardiomyopathy in humans. NRG1 has been reported to affect ventricular contractility and heart rate in perfused rat hearts as well as currents in K+-isolated sinus node myocytes (Wu et al., 2000). Changes in K+ or Na+ channel activity in humans and mice result in increased QTc and tip twisting arrhythmias (Keating and Sanguinetti, 2001). ErbB receptors classically activate various signaling cascades and affect K+ channels, non-selective cation channels and G protein-coupled receptors, all of which affect cardiac function (Wischmeyer et al., 1998; Prenzel et al., 1999; Schaefer et al., 2000).
4 NRG1 as a potential therapeutic target for cardiac repair
NRG1 treatment improves volume overload (Wang et al., 2012), adriamycin-induced LV dysfunction (Bian et al., 2009), as well as ischemia (Cohen et al., 2014) and diabetic cardiomyopathy (Li et al., 2011). Studies using NRG1 for cardiovascular regeneration and repair have focused on exogenous administration, and in vivo and in vitro experiments have confirmed its clinical effectiveness, respectively, and its greater potential and clinical value in the treatment of cardiovascular disease.
4.1 RhNRG1 and engineered bivalent NRG1β
The previously research shown that treatment with rhNRG1 improves pulmonary arterial hypertension by decreasing pulmonary arterial remodelling and endothelial dysfunction, as well as by restoring right ventricular function (Mendes-Ferreira et al., 2016). And recent study demonstrated that rhNRG1 treatment can decrease right ventricular intrinsic diastolic stiffness, through the improvement of calcium handling and cardiac remodelling signalling (Adão et al., 2019). The conclusion in Australia trial showed that short-term administration of rhNRG-1 results in acute and sustained improvement in cardiac function but it is an open-label study without a placebo group. In phase II clinical trials of rhNRG1 in the treatment of patients with chronic HF (Gao et al., 2010; Jabbour et al., 2011a) of China, Short-term administration of rhNRG-1 (0.6 μg/kg) in chronic HF patients could result in sustained improvement of cardiac pumping and inhibition or reversal of ventricular remodeling even 3 months after treatment, which is safe and well tolerated. RhNRG1 is emerging as a promising therapeutic option for cardiovascular disease and cardiac dysfunction.
RhNRG1 stimulates the proliferation of embryonic/fetal/neonatal cardiomyocytes, hypertrophic growth, sarcoma formation, and survival in isolation (Zhao et al., 1998; Lai et al., 2010). Although ErbB2 and ErbB4 play an important role in NRG1-promoted cardiac repair, overexpression of ErbB2 receptor subunits can promote uncontrolled cancer growth (Wadugu and Kühn, 2012), which would be the greatest limitation of clinical interventions to achieve myocardial regeneration with ErbB2. Previous studies have shown that ErbB3 play an adaptive role in overcoming the heart pressure overload (Yin et al., 2021) and the human protein database shows that the expression of ErbB3 is similar between immune cells and heart fibroblasts. Therefore, its therapeutic effect on heart disease cannot be ignored and is expected to be a potential target for overcoming rhNRG1 defects. Based on this theory, Lee et al. (Jay et al., 2013) designed engineered bivalent NRG1β, an effect mediated by receptor biasing toward ErbB3 homotypic interactions uncommonly formed by native NRG1β, resulting in some cases in decreased migration, inhibited proliferation, and increased apoptosis (Jay et al., 2011). Engineered bivalent NRG1β exhibits reduced tumor potential compared to NRG1 and still retains its cardioprotective properties (Jay et al., 2011; Jay et al., 2013).
4.2 NRG1 gene transduction
Gene fusions are heterozygous genes generated by structural DNA rearrangements, including translocations and insertions, transcriptional passages or splicing (Latysheva and Babu, 2016), resulting in dysregulated activity. The mechanism involving NRG1 gene fusions that induce cancer is due to ErbB-mediated pathway activation (Laskin et al., 2020) leading to aberrant cell proliferation. NRG1 fusions are enriched in aggressive mucinous adenocarcinoma of the lung, but have a low incidence in multiple tumor types (Nakaoku et al., 2014; Jones et al., 2017; Heining et al., 2018; Trombetta et al., 2018). Gene based therapies using gene delivery systems (eg., viral and nonviral vectors) to regulate gene expression at the cellular level can treat post-infarction pathological changes (Cao et al., 2019). In an experimental study in which a lentivirus carrying the human NRG1 gene was injected into the infarcted myocardium of rats, NRG1 gene transduction of the established a stable expression system in the infarcted heart and further activated the PI3K/Akt/eNOS pathway to promote neovascularization and prevent apoptosis (Xiao et al., 2012). In general, gene-based NRG1 therapy helps to reduce post-infarction cardiomyocyte loss, promote neoangiogenesis, and improve cardiac function. Furthermore, two studies have confirmed that endogenous up-regulation of NRG1/ErbB2 signaling and the promotion of NRG1 expression can also be achieved through exercise training (Cai et al., 2016; Cai et al., 2018), suggesting that there is great room for the development of methods to up-regulate NRG1 expression.
4.3 NRG1 and other growth factors
VEGF (vascular endothelial growth factor) and angiopoietin (Ang)-1 may regulate myocardial angiogenesis and survival via the NRG1/ErbB signaling pathway. VEGF or Ang-1 can significantly promote NRG1 expression and secretion in human cardiac microvascular endothelial cells (Wu et al., 2018). In turn, NRG1 treatment also increased significantly the expression of VEGF and Ang-1 in human coronary artery smooth muscle cells (Gui et al., 2018). Qiliqiangxin could attenuate anoxia-induced injuries in cardiac microvascular endothelial cells via NRG1/ErbB signalling which involves repairing damaged myocardial endothelial cells by affecting the expression and secretion levels of NRG1 and VEGF (Wang et al., 2017b).
These findings indicated NRG1 could increase the myocardial angiogenesis, probably via the direct effects of NRG1 and via the increasing expression of VEGF and Ang1 which provide a reliable basis for NRG combined with VEGF and other growth factors for cardiac repair. Furthermore, Lemmens et al. reported that mechanical strain increases endothelial NRG1 synthesis and release, but ang-II and adrenergic agonists decrease endothelial NRG1 synthesis and release (Lemmens et al., 2006; Lemmens et al., 2007). The mechanisms of the inverse relationship of Ang-2 on NRG1 are not clear and further research is still needed.
5 Concluding remarks and future directions
Successful cardiac repair requires three key phenomena: myocardial cell replenishment, removal of interstitial fibrosis, and hematologic reconstitution of the regenerating myocardium. Current research has focused on the direction of post-infarction myocardial proliferation, with less research in the cardiac microenvironment. Promotion of cardiac repair through the intervention of cardiac growth factor NRG1 has become a hot topic of current research. NRG1 has therapeutic effects on many forms of heart disease by directly acting on cardiomyocytes, endothelial cells, macrophages, and fibroblasts to promote cell proliferation, anti-apoptosis, anti-inflammatory and antioxidant effects, and regulate myocardial energy metabolism.
Biomaterials have emerged as innovative scaffolds for the delivery of both cells and proteins in tissue engineering applications. The combination of NRG-encapsulating scaffolds with cells capable of inducing cardiac regeneration could represent an ambitious and promising therapeutic strategy for the repair of diseased or damaged myocardial tissue (Simón-Yarza et al., 2015). Because nanoscale phenomena play an important role in cell signal transduction, enzyme action and cell cycle (Li et al., 2018), and shows excellent performance in the field of targeted drug therapy and the development of biomaterials, nanotechnology will hopefully be used in conjunction with NRG1 to repair the heart. Other emerging technology, such as DNA nano-threads (Baig et al., 2020), it can achieve deliver targeted drug through circular DNA scaffolding for the potential applications. These emerging technologies can accelerate the development of NRG1 therapy for cardiovascular diseases.
The mechanisms by which NRG/ErbB signaling is cardioprotective have been elucidated. The results of promising clinical trials of two different forms of recombinant NRG1 in systolic heart failure support further investigation of this biologic therapy. However, NRG1/ErbB still needs a lot of experiments to explore safe and feasible methods because it is hampered by the low efficiency of improving cardiac regeneration and side effects. And current experimental models are mainly limited to nonhuman animal models and to in vitro cellular models, it is difficult to completely replicate the real myocardial microenvironment of human. But it provides a direction for future research on cardiac repair in cardiovascular diseases.
Author contributions
YW wrote the main text. JW contributed equally to this work. PZ, XZ, YW retrieve and organize the documents. YZ and XC had great contribution in second time revision, polishing manuscript and helping in revising figures.
Funding
This work was funded by grants from the National Natural Science Foundation of China (No.82004369).
Acknowledgments
We thank YZ for the guideness and revision of this manuscript.
Conflict of interest
The authors declare that the research was conducted in the absence of any commercial or financial relationships that could be construed as a potential conflict of interest.
Publisher’s note
All claims expressed in this article are solely those of the authors and do not necessarily represent those of their affiliated organizations, or those of the publisher, the editors and the reviewers. Any product that may be evaluated in this article, or claim that may be made by its manufacturer, is not guaranteed or endorsed by the publisher.
References
Abais, J. M., Xia, M., Zhang, Y., Boini, K. M., and Li, P. L. (2015). Redox regulation of NLRP3 inflammasomes: ROS as trigger or effector? Antioxid. Redox Signal. 22 (13), 1111–1129. doi:10.1089/ars.2014.5994
Acharya, A., Baek, S. T., Huang, G., Eskiocak, B., Goetsch, S., Sung, C. Y., et al. (2012). The bHLH transcription factor Tcf21 is required for lineage-specific EMT of cardiac fibroblast progenitors. Development 139 (12), 2139–2149. doi:10.1242/dev.079970
Adão, R., Mendes-Ferreira, P., Maia-Rocha, C., Santos-Ribeiro, D., Rodrigues, P. G., Vidal-Meireles, A., et al. (2019). Neuregulin-1 attenuates right ventricular diastolic stiffness in experimental pulmonary hypertension. Clin. Exp. Pharmacol. Physiol. 46 (3), 255–265. doi:10.1111/1440-1681.13043
Aharonov, A., Shakked, A., Umansky, K. B., Savidor, A., Genzelinakh, A., Kain, D., et al. (2020). ERBB2 drives YAP activation and EMT-like processes during cardiac regeneration. Nat. Cell Biol. 22 (11), 1346–1356. doi:10.1038/s41556-020-00588-4
Amin, D. N., Hida, K., Bielenberg, D. R., and Klagsbrun, M. (2006). Tumor endothelial cells express epidermal growth factor receptor (EGFR) but not ErbB3 and are responsive to EGF and to EGFR kinase inhibitors. Cancer Res. 66 (4), 2173–2180. doi:10.1158/0008-5472.CAN-05-3387
An, T., Huang, Y., Zhou, Q., Wei, B. Q., Zhang, R. C., Yin, S. J., et al. (2013). Neuregulin-1 attenuates doxorubicin-induced autophagy in neonatal rat cardiomyocytes. J. Cardiovasc. Pharmacol. 62 (2), 130–137. doi:10.1097/FJC.0b013e318291c094
Arora, H., Lavin, A. C., Balkan, W., Hare, J. M., and White, I. A. (2021). Neuregulin-1, in a conducive milieu with Wnt/BMP/retinoic acid, prolongs the epicardial-mediated cardiac regeneration capacity of neonatal heart explants. J. Stem Cells Regen. Med. 17 (1), 18–27. doi:10.46582/jsrm.1701003
Kajstura, J., Gurusamy, N., Ogórek, B., Goichberg, P., Clavo-Rondon, C., Hosoda, T., et al. (2010). Myocyte turnover in the aging human heart [retracted in: Circ Res. 2019 Feb 15;124(4): e23]. Circ. Res. 107 (11), 1374–1386. doi:10.1161/CIRCRESAHA.110.231498
Baig, M., Lai, W. F., Ahsan, A., Jabeen, M., Farooq, M. A., Mikrani, R., et al. (2020). Synthesis of ligand functionalized ErbB-3 targeted novel DNA nano-threads loaded with the low dose of doxorubicin for efficient in vitro evaluation of the resistant anti-cancer activity. Pharm. Res. 37 (4), 75. doi:10.1007/s11095-020-02803-1
Bao, J., Lin, H., Ouyang, Y., Lei, D., Osman, A., Kim, T. W., et al. (2004). Activity-dependent transcription regulation of PSD-95 by neuregulin-1 and Eos. Nat. Neurosci. 7 (11), 1250–1258. doi:10.1038/nn1342
Bao, J., Wolpowitz, D., Role, L. W., and Talmage, D. A. (2003). Back signaling by the Nrg-1 intracellular domain. J. Cell Biol. 161 (6), 1133–1141. doi:10.1083/jcb.200212085
Belmonte, F., Das, S., Sysa-Shah, P., Sivakumaran, V., Stanley, B., Guo, X., et al. (2015). ErbB2 overexpression upregulates antioxidant enzymes, reduces basal levels of reactive oxygen species, and protects against doxorubicin cardiotoxicity. Am. J. Physiol. Heart Circ. Physiol. 309 (8), H1271–H1280. doi:10.1152/ajpheart.00517.2014
Bersell, K., Arab, S., Haring, B., and Kuhn, B. (2009). Neuregulin1/ErbB4 signaling induces cardiomyocyte proliferation and repair of heart injury. Cell 138 (2), 257–270. doi:10.1016/j.cell.2009.04.060
Bian, Y., Sun, M., Silver, M., Ho, K. K. L., Marchionni, M. A., Caggiano, A. O., et al. (2009). Neuregulin-1 attenuated doxorubicin-induced decrease in cardiac troponins. Am. J. Physiol. Heart Circ. Physiol. 297 (6), H1974–H1983. doi:10.1152/ajpheart.01010.2008
Bouyain, S., Longo, P. A., Li, S., Ferguson, K. M., and Leahy, D. J. (2005). The extracellular region of ErbB4 adopts a tethered conformation in the absence of ligand. Proc. Natl. Acad. Sci. U. S. A. 102 (42), 15024–15029. doi:10.1073/pnas.0507591102
Brero, A., Ramella, R., Fitou, A., Dati, C., Alloatti, G., Gallo, M. P., et al. (2010). Neuregulin-1beta1 rapidly modulates nitric oxide synthesis and calcium handling in rat cardiomyocytes. Cardiovasc. Res. 88 (3), 443–452. doi:10.1093/cvr/cvq238
Buonanno, A., and Fischbach, G. D. (2001). Neuregulin and ErbB receptor signaling pathways in the nervous system. Curr. Opin. Neurobiol. 11 (3), 287–296. doi:10.1016/s0959-4388(00)00210-5
Cai, M., Wang, Q., Liu, Z., Jia, D., Feng, R., and Tian, Z. (2018). Effects of different types of exercise on skeletal muscle atrophy, antioxidant capacity and growth factors expression following myocardial infarction. Life Sci. 213, 40–49. doi:10.1016/j.lfs.2018.10.015
Cai, M. X., Shi, X. C., Chen, T., Tan, Z. N., Lin, Q. Q., Du, S. J., et al. (2016). Exercise training activates neuregulin 1/ErbB signaling and promotes cardiac repair in a rat myocardial infarction model. Life Sci. 149, 1–9. doi:10.1016/j.lfs.2016.02.055
Caja, L., Sancho, P., Bertran, E., Iglesias-Serret, D., Gil, J., and Fabregat, I. (2009). Overactivation of the MEK/ERK pathway in liver tumor cells confers resistance to TGF-{beta}-induced cell death through impairing up-regulation of the NADPH oxidase NOX4. Cancer Res. 69 (19), 7595–7602. doi:10.1158/0008-5472.CAN-09-1482
Campreciós, G., Lorita, J., Pardina, E., Peinado-Onsurbe, J., Soley, M., and Ramirez, I. (2011). Expression, localization, and regulation of the neuregulin receptor ErbB3 in mouse heart. J. Cell. Physiol. 226 (2), 450–455. doi:10.1002/jcp.22354
Cao, Y., Tan, Y. F., Wong, Y. S., Liew, M. W. J., and Venkatraman, S. (2019). Recent advances in chitosan-based carriers for gene delivery. Mar. Drugs 17 (6), E381. doi:10.3390/md17060381
Carraway, K. L., and Cantley, L. C. (1994). A neu acquaintance for erbB3 and erbB4: A role for receptor heterodimerization in growth signaling. Cell 78 (1), 5–8. doi:10.1016/0092-8674(94)90564-9
Chou, C. F., and Ozaki, M. (2010). In silico analysis of neuregulin 1 evolution in vertebrates. Biosci. Rep. 30 (4), 267–275. doi:10.1042/BSR20090097
Clement, C. M., Thomas, L. K., Mou, Y., Croslan, D. R., Gibbons, G. H., and Ford, B. D. (2007). Neuregulin-1 attenuates neointimal formation following vascular injury and inhibits the proliferation of vascular smooth muscle cells. J. Vasc. Res. 44 (4), 303–312. doi:10.1159/000101776
Cohen, J. E., Purcell, B. P., MacArthur, J. W., Mu, A., Shudo, Y., Patel, J. B., et al. (2014). A bioengineered hydrogel system enables targeted and sustained intramyocardial delivery of neuregulin, activating the cardiomyocyte cell cycle and enhancing ventricular function in a murine model of ischemic cardiomyopathy. Circ. Heart Fail. 7 (4), 619–626. doi:10.1161/CIRCHEARTFAILURE.113.001273
Colliva, A., Braga, L., Giacca, M., and Zacchigna, S. (2020). Endothelial cell-cardiomyocyte crosstalk in heart development and disease. J. Physiol. 598 (14), 2923–2939. doi:10.1113/JP276758
Correia-Melo, C., Hewitt, G., and Passos, J. F. (2014). Telomeres, oxidative stress and inflammatory factors: Partners in cellular senescence? Longev. Heal. 3 (1), 1. doi:10.1186/2046-2395-3-1
Cote, G. M., Miller, T. A., Lebrasseur, N. K., Kuramochi, Y., and Sawyer, D. B. (2005). Neuregulin-1alpha and beta isoform expression in cardiac microvascular endothelial cells and function in cardiac myocytes in vitro. Exp. Cell Res. 311 (1), 135–146. doi:10.1016/j.yexcr.2005.08.017
Crone, S. A., Zhao, Y. Y., Fan, L., Gu, Y., Minamisawa, S., Liu, Y., et al. (2002). ErbB2 is essential in the prevention of dilated cardiomyopathy. Nat. Med. 8 (5), 459–465. doi:10.1038/nm0502-459
D'Uva, G., Aharonov, A., Lauriola, M., Kain, D., Yahalom-Ronen, Y., Carvalho, S., et al. (2015). ERBB2 triggers mammalian heart regeneration by promoting cardiomyocyte dedifferentiation and proliferation. Nat. Cell Biol. 17 (5), 627–638. doi:10.1038/ncb3149
De Keulenaer, G. W., Feyen, E., Dugaucquier, L., Shakeri, H., Shchendrygina, A., Belenkov, Y. N., et al. (2019). Mechanisms of the multitasking endothelial protein NRG-1 as a compensatory factor during chronic heart failure. Circ. Heart Fail. 12 (10), e006288. doi:10.1161/CIRCHEARTFAILURE.119.006288
Del Re, D. P., Amgalan, D., Linkermann, A., Liu, Q., and Kitsis, R. N. (2019). Fundamental mechanisms of regulated cell death and implications for heart disease. Physiol. Rev. 99 (4), 1765–1817. doi:10.1152/physrev.00022.2018
Dey, A., Varelas, X., and Guan, K. L. (2020). Targeting the Hippo pathway in cancer, fibrosis, wound healing and regenerative medicine. Nat. Rev. Drug Discov. 19 (7), 480–494. doi:10.1038/s41573-020-0070-z
Ding, Z., Dai, C., Zhong, L., Liu, R., Gao, W., Zhang, H., et al. (2021). Neuregulin-1 converts reactive astrocytes toward oligodendrocyte lineage cells via upregulating the PI3K-AKT-mTOR pathway to repair spinal cord injury. Biomed. Pharmacother. 134, 111168. doi:10.1016/j.biopha.2020.111168
Du, Y., Yang, H., Xu, Y., Cang, X., Luo, C., Mao, Y., et al. (2012). Conformational transition and energy landscape of ErbB4 activated by neuregulin1β: One microsecond molecular dynamics simulations. J. Am. Chem. Soc. 134 (15), 6720–6731. doi:10.1021/ja211941d
Dugaucquier, L., Feyen, E., Mateiu, L., Bruyns, T. A. M., De Keulenaer, G. W., and Segers, V. F. M. (2020). The role of endothelial autocrine NRG1/ERBB4 signaling in cardiac remodeling. Am. J. Physiol. Heart Circ. Physiol. 319 (2), H443–H455. doi:10.1152/ajpheart.00176.2020
Erkens, R., Totzeck, M., Brum, A., Duse, D., Botker, H. E., Rassaf, T., et al. (2021). Endothelium-dependent remote signaling in ischemia and reperfusion: Alterations in the cardiometabolic continuum. Free Radic. Biol. Med. 165, 265–281. doi:10.1016/j.freeradbiomed.2021.01.040
Falls, D. L. (2003). Neuregulins: Functions, forms, and signaling strategies. Exp. Cell Res. 284 (1), 14–30. doi:10.1016/s0014-4827(02)00102-7
Falls, D. L., Rosen, K. M., Corfas, G., Lane, W. S., and Fischbach, G. D. (1993). ARIA, a protein that stimulates acetylcholine receptor synthesis, is a member of the neu ligand family. Cell 72 (5), 801–815. doi:10.1016/0092-8674(93)90407-h
Fang, S. J., Li, P. Y., Wang, C. M., Xin, Y., Lu, W. W., Zhang, X. X., et al. (2017). Inhibition of endoplasmic reticulum stress by neuregulin-1 protects against myocardial ischemia/reperfusion injury. Peptides 88, 196–207. doi:10.1016/j.peptides.2016.12.009
Fang, S. J., Wu, X. S., Han, Z. H., Zhang, X. X., Wang, C. M., Li, X. Y., et al. (2010). Neuregulin-1 preconditioning protects the heart against ischemia/reperfusion injury through a PI3K/Akt-dependent mechanism. Chin. Med. J. 123 (24), 3597–3604.
Fernandez-Cuesta, L., Plenker, D., Osada, H., Sun, R., Menon, R., Leenders, F., et al. (2014). CD74-NRG1 fusions in lung adenocarcinoma. Cancer Discov. 4 (4), 415–422. doi:10.1158/2159-8290.CD-13-0633
Fukazawa, R., Miller, T. A., Kuramochi, Y., Frantz, S., Kim, Y. D., Marchionni, M. A., et al. (2003). Neuregulin-1 protects ventricular myocytes from anthracycline-induced apoptosis via erbB4-dependent activation of PI3-kinase/Akt. J. Mol. Cell. Cardiol. 35 (12), 1473–1479. doi:10.1016/j.yjmcc.2003.09.012
Galindo, C. L., Ryzhov, S., and Sawyer, D. B. (2014). Neuregulin as a heart failure therapy and mediator of reverse remodeling. Curr. Heart Fail. Rep. 11 (1), 40–49. doi:10.1007/s11897-013-0176-2
Gao, R., Zhang, J., Cheng, L., Wu, X., Dong, W., Yang, X., et al. (2010). A Phase II, randomized, double-blind, multicenter, based on standard therapy, placebo-controlled study of the efficacy and safety of recombinant human neuregulin-1 in patients with chronic heart failure. J. Am. Coll. Cardiol. 55 (18), 1907–1914. doi:10.1016/j.jacc.2009.12.044
Garratt, A. N., Britsch, S., and Birchmeier, C. (2000). Neuregulin, a factor with many functions in the life of a schwann cell. Bioessays 22 (11), 987–996. doi:10.1002/1521-1878(200011)22:11<987::AID-BIES5>3.0.CO;2-5
Gassmann, M., CasagrandaF., , Orioli, D., Simon, H., Lai, C., Klein, R., et al. (1995). Aberrant neural and cardiac development in mice lacking the ErbB4 neuregulin receptor. Nature 378 (6555), 390–394. doi:10.1038/378390a0
Gemberling, M., Karra, R., Dickson, A. L., and Poss, K. D. (2015). Nrg1 is an injury-induced cardiomyocyte mitogen for the endogenous heart regeneration program in zebrafish. Elife 4, e05871. doi:10.7554/eLife.05871
Gibb, A. A., Lazaropoulos, M. P., and Elrod, J. W. (2020). Myofibroblasts and fibrosis: Mitochondrial and metabolic control of cellular differentiation. Circ. Res. 127 (3), 427–447. doi:10.1161/CIRCRESAHA.120.316958
Gorenne, I., Kavurma, M., Scott, S., and Bennett, M. (2006). Vascular smooth muscle cell senescence in atherosclerosis. Cardiovasc. Res. 72 (1), 9–17. doi:10.1016/j.cardiores.2006.06.004
Griffiths, H. R., Gao, D., and Pararasa, C. (2017). Redox regulation in metabolic programming and inflammation. Redox Biol. 12 (C), 50–57. doi:10.1016/j.redox.2017.01.023
Grimm, S., and Leder, P. (1997). An apoptosis-inducing isoform of neu differentiation factor (NDF) identified using a novel screen for dominant, apoptosis-inducing genes. J. Exp. Med. 185 (6), 1137–1142. doi:10.1084/jem.185.6.1137
Grimm, S., Weinstein, E. J., Krane, I. M., and Leder, P. (1998). Neu differentiation factor (NDF), a dominant oncogene, causes apoptosis in vitro and in vivo. J. Exp. Med. 188 (8), 1535–1539. doi:10.1084/jem.188.8.1535
Grines, C. L., Watkins, M. W., Helmer, G., Penny, W., Brinker, J., Marmur, J. D., et al. (2002). Angiogenic Gene Therapy (AGENT) trial in patients with stable angina pectoris. Circulation 105 (11), 1291–1297. doi:10.1161/hc1102.105595
Gu, X., Liu, X., Xu, D., Li, X., Yan, M., Qi, Y., et al. (2010). Cardiac functional improvement in rats with myocardial infarction by up-regulating cardiac myosin light chain kinase with neuregulin. Cardiovasc. Res. 88 (2), 334–343. doi:10.1093/cvr/cvq223
Gui, C., Zeng, Z. Y., Chen, Q., Luo, Y. W., Li, L., and Chen, L. L. (2018). Neuregulin-1 promotes myocardial angiogenesis in the rat model of diabetic cardiomyopathy. Cell. Physiol. biochem. 46 (6), 2325–2334. doi:10.1159/000489622
Guo, Y. F., Zhang, X. x., Liu, Y., Duan, H. y., Jie, B. z., and Wu, X. s. (2012). Neuregulin-1 attenuates mitochondrial dysfunction in a rat model of heart failure. Chin. Med. J. 125 (5), 807–814.
Gupte, M., Lal, H., Ahmad, F., Sawyer, D. B., and Hill, M. F. (2017). Chronic neuregulin-1β treatment mitigates the progression of postmyocardial infarction heart failure in the setting of type 1 diabetes mellitus by suppressing myocardial apoptosis, fibrosis, and key oxidant-producing enzymes. J. Card. Fail. 23 (12), 887–899. doi:10.1016/j.cardfail.2017.08.456
Haller, P. M., Goncalves, I. F., Acar, E., Jager, B., Pilz, P. M., Wojta, J., et al. (2022). Relationship between plasma Neuregulin-1 and cardiac function in patients with ST-elevation myocardial infarction. Rev. Cardiovasc. Med. 23 (2), 63. doi:10.31083/j.rcm2302063
Harvey, K. F., Zhang, X., and Thomas, D. M. (2013). The Hippo pathway and human cancer. Nat. Rev. Cancer 13 (4), 246–257. doi:10.1038/nrc3458
Haskins, J. W., Nguyen, D. X., and Stern, D. F. (2014). Neuregulin 1-activated ERBB4 interacts with YAP to induce Hippo pathway target genes and promote cell migration. Sci. Signal. 7 (355), ra116. doi:10.1126/scisignal.2005770
Heallen, T., Morikawa, Y., Leach, J., Tao, G., Willerson, J. T., Johnson, R. L., et al. (2013). Hippo signaling impedes adult heart regeneration. Development 140 (23), 4683–4690. doi:10.1242/dev.102798
Heallen, T., Zhang, M., Wang, J., Bonilla-Claudio, M., Klysik, E., Johnson, R. L., et al. (2011). Hippo pathway inhibits Wnt signaling to restrain cardiomyocyte proliferation and heart size. Science 332 (6028), 458–461. doi:10.1126/science.1199010
Heallen, T., Zhang, M., Wang, J., Bonilla-Claudio, M., Klysik, E., Johnson, R. L., et al. (2011). Hippo pathway inhibits Wnt signaling to restrain cardiomyocyte proliferation and heart size. Science 332 (6028), 458–461. doi:10.1126/science.1199010
Hedhli, N., Dobrucki, L. W., Kalinowski, A., Zhuang, Z. W., Wu, X., Russell, R. R., et al. (2012). Endothelial-derived neuregulin is an important mediator of ischaemia-induced angiogenesis and arteriogenesis. Cardiovasc. Res. 93 (3), 516–524. doi:10.1093/cvr/cvr352
Hedhli, N., Huang, Q., Kalinowski, A., Palmeri, M., Hu, X., Russell, R. R., et al. (2011). Endothelium-derived neuregulin protects the heart against ischemic injury. Circulation 123 (20), 2254–2262. doi:10.1161/CIRCULATIONAHA.110.991125
Hedman, M., Hartikainen, J., Syvanne, M., Stjernvall, J., Hedman, A., Kivela, A., et al. (2003). Safety and feasibility of catheter-based local intracoronary vascular endothelial growth factor gene transfer in the prevention of postangioplasty and in-stent restenosis and in the treatment of chronic myocardial ischemia: Phase II results of the kuopio angiogenesis trial (KAT). Circulation 107 (21), 2677–2683. doi:10.1161/01.CIR.0000070540.80780.92
Heining, C., Horak, P., Uhrig, S., Codo, P. L., Klink, B., Hutter, B., et al. (2018). NRG1 fusions in KRAS wild-type pancreatic cancer. Cancer Discov. 8 (9), 1087–1095. doi:10.1158/2159-8290.CD-18-0036
Henry, T. D., Annex, B. H., McKendall, G. R., Azrin, M. A., Lopez, J. J., Giordano, F. J., et al. (2003). The VIVA trial: Vascular endothelial growth factor in Ischemia for Vascular Angiogenesis. Circulation 107 (10), 1359–1365. doi:10.1161/01.cir.0000061911.47710.8a
Herget, G. W., NeuburgerM., , Plagwitz, R., and Adler, C. P. (1997). DNA content, ploidy level and number of nuclei in the human heart after myocardial infarction. Cardiovasc. Res. 36 (1), 45–51. doi:10.1016/s0008-6363(97)00140-5
Hintsanen, M., Elovainio, M., Puttonen, S., Kivimaki, M., Raitakari, O. T., Lehtimaki, T., et al. (2007). Neuregulin-1 genotype moderates the association between job strain and early atherosclerosis in young men. Ann. Behav. Med. 33 (2), 148–155. doi:10.1007/BF02879896
Holmes, W. E., Sliwkowski, M. X., Akita, R. W., Henzel, W. J., Lee, J., Park, J. W., et al. (1992). Identification of heregulin, a specific activator of p185erbB2. Science 256 (5060), 1205–1210. doi:10.1126/science.256.5060.1205
Hong, X., Oh, N., Wang, K., Neumeyer, J., Lee, C. N., Lin, R. Z., et al. (2021). Human endothelial colony-forming cells provide trophic support for pluripotent stem cell-derived cardiomyocytes via distinctively high expression of neuregulin-1. Angiogenesis 24 (2), 327–344. doi:10.1007/s10456-020-09765-3
Honkoop, H., de Bakker, D. E., Aharonov, A., Kruse, F., Shakked, A., Nguyen, P. D., et al. (2019). Single-cell analysis uncovers that metabolic reprogramming by ErbB2 signaling is essential for cardiomyocyte proliferation in the regenerating heart. Elife 8, e50163. doi:10.7554/eLife.50163
Horiuchi, K., Zhou, H. M., Kelly, K., Manova, K., and Blobel, C. P. (2005). Evaluation of the contributions of ADAMs 9, 12, 15, 17, and 19 to heart development and ectodomain shedding of neuregulins beta1 and beta2. Dev. Biol. 283 (2), 459–471. doi:10.1016/j.ydbio.2005.05.004
Hotham, W. E., and Henson, F. M. D. (2020). The use of large animals to facilitate the process of MSC going from laboratory to patient-'bench to bedside. Cell Biol. Toxicol. 36 (2), 103–114. doi:10.1007/s10565-020-09521-9
Huang, Q., Zhang, J., Liang, L., Lan, Z., Huo, T., and Li, S. (2015). The significance of neuregulin-1/ErbB expression in autogenous vein grafts in a diabetic rat model. J. Cardiovasc. Pharmacol. 66 (3), 300–306. doi:10.1097/FJC.0000000000000279
Huang, Q., Zhou, H. J., Zhang, H., Huang, Y., Hinojosa-Kirschenbaum, F., Fan, P., et al. (2015). Thioredoxin-2 inhibits mitochondrial reactive oxygen species generation and apoptosis stress kinase-1 activity to maintain cardiac function. Circulation 131 (12), 1082–1097. doi:10.1161/CIRCULATIONAHA.114.012725
Ikenishi, A., Okayama, H., Iwamoto, N., Yoshitome, S., Tane, S., Nakamura, K., et al. (2012). Cell cycle regulation in mouse heart during embryonic and postnatal stages. Dev. Growth Differ. 54 (8), 731–738. doi:10.1111/j.1440-169X.2012.01373.x
Jabbour, A., Gao, L., Kwan, J., Watson, A., Sun, L., Qiu, M. R., et al. (2011). A recombinant human neuregulin-1 peptide improves preservation of the rodent heart after prolonged hypothermic storage. Transplantation 91 (9), 961–967. doi:10.1097/TP.0b013e3182115b4b
Jabbour, A., Hayward, C. S., Keogh, A. M., Kotlyar, E., McCrohon, J. A., England, J. F., et al. (2011). Parenteral administration of recombinant human neuregulin-1 to patients with stable chronic heart failure produces favourable acute and chronic haemodynamic responses. Eur. J. Heart Fail. 13 (1), 83–92. doi:10.1093/eurjhf/hfq152
Janse van Rensburg, H. J., Azad, T., Ling, M., Hao, Y., Snetsinger, B., Khanal, P., et al. (2018). The Hippo pathway component TAZ promotes immune evasion in human cancer through PD-L1. Cancer Res. 78 (6), 1457–1470. doi:10.1158/0008-5472.CAN-17-3139
Jay, S. M., Kurtagic, E., Alvarez, L. M., de Picciotto, S., Sanchez, E., Hawkins, J. F., et al. (2011). Engineered bivalent ligands to bias ErbB receptor-mediated signaling and phenotypes. J. Biol. Chem. 286 (31), 27729–27740. doi:10.1074/jbc.M111.221093
Jay, S. M., Murthy, A. C., Hawkins, J. F., Wortzel, J. R., Steinhauser, M. L., Alvarez, L. M., et al. (2013). An engineered bivalent neuregulin protects against doxorubicin-induced cardiotoxicity with reduced proneoplastic potential. Circulation 128 (2), 152–161. doi:10.1161/CIRCULATIONAHA.113.002203
Jiang, K., Tu, Z., Chen, K., Xu, Y., Chen, F., Xu, S., et al. (2022). Gasdermin D inhibition confers antineutrophil-mediated cardioprotection in acute myocardial infarction. J. Clin. Invest. 132 (1), e151268. doi:10.1172/JCI151268
Jiao, L., Wang, M. C., Yang, Y. A., Chen, E. Q., Xu, H. T., Wu, K. Y., et al. (2008). Norepinephrine reversibly regulates the proliferation and phenotypic transformation of vascular smooth muscle cells. Exp. Mol. Pathol. 85 (3), 196–200. doi:10.1016/j.yexmp.2008.09.007
Johnson, D. M., and Antoons, G. (2018). Arrhythmogenic mechanisms in heart failure: Linking β-adrenergic stimulation, stretch, and calcium. Front. Physiol. 9, 1453. doi:10.3389/fphys.2018.01453
Jones, J. T., Ballinger, M. D., Pisacane, P. I., Lofgren, J. A., Fitzpatrick, V. D., Fairbrother, W. J., et al. (1998). Binding interaction of the heregulinbeta egf domain with ErbB3 and ErbB4 receptors assessed by alanine scanning mutagenesis. J. Biol. Chem. 273 (19), 11667–11674. doi:10.1074/jbc.273.19.11667
Jones, M. R., Lim, H., Shen, Y., PlEasancE, E., Ch'ng, C., Reisle, C., et al. (2017). Successful targeting of the NRG1 pathway indicates novel treatment strategy for metastatic cancer. Ann. Oncol. 28 (12), 3092–3097. doi:10.1093/annonc/mdx523
Karar, J., and Maity, A. (2011). PI3K/AKT/mTOR pathway in angiogenesis. Front. Mol. Neurosci. 4 (51), 51. doi:10.3389/fnmol.2011.00051
Kataria, H., Alizadeh, A., and Karimi-Abdolrezaee, S. (2019). Neuregulin-1/ErbB network: An emerging modulator of nervous system injury and repair. Prog. Neurobiol. 180, 101643. doi:10.1016/j.pneurobio.2019.101643
Katz, T. C., Singh, M. K., Degenhardt, K., Rivera-Feliciano, J., Johnson, R. L., Epstein, J. A., et al. (2012). Distinct compartments of the proepicardial organ give rise to coronary vascular endothelial cells. Dev. Cell 22 (3), 639–650. doi:10.1016/j.devcel.2012.01.012
Kawaguchi, M., Takahashi, M., Hata, T., Kashima, Y., Usui, F., Morimoto, H., et al. (2011). Inflammasome activation of cardiac fibroblasts is essential for myocardial ischemia/reperfusion injury. Circulation 123 (6), 594–604. doi:10.1161/CIRCULATIONAHA.110.982777
Keating, M. T., and Sanguinetti, M. C. (2001). Molecular and cellular mechanisms of cardiac arrhythmias. Cell 104 (4), 569–580. doi:10.1016/s0092-8674(01)00243-4
Kramer, R., BucayN., , Kane, D. J., Martin, L. E., Tarpley, J. E., and Theill, L. E. (1996). Neuregulins with an Ig-like domain are essential for mouse myocardial and neuronal development. Proc. Natl. Acad. Sci. U. S. A. 93 (10), 4833–4838. doi:10.1073/pnas.93.10.4833
Kumar, A., Harris, T. E., Keller, S. R., Choi, K. M., Magnuson, M. A., and Lawrence, J. C. (2008). Muscle-specific deletion of rictor impairs insulin-stimulated glucose transport and enhances Basal glycogen synthase activity. Mol. Cell. Biol. 28 (1), 61–70. doi:10.1128/MCB.01405-07
Kundumani-Sridharan, V., Subramani, J., Owens, C., and Das, K. C. (2021). Nrg1β released in remote ischemic preconditioning improves myocardial perfusion and decreases ischemia/reperfusion injury via ErbB2-mediated rescue of endothelial nitric oxide synthase and abrogation of Trx2 autophagy. Arterioscler. Thromb. Vasc. Biol. 41 (8), 2293–2314. doi:10.1161/ATVBAHA.121.315957
Kuramochi, Y., Cote, G. M., Guo, X., Lebrasseur, N. K., Cui, L., Liao, R., et al. (2004). Cardiac endothelial cells regulate reactive oxygen species-induced cardiomyocyte apoptosis through neuregulin-1beta/erbB4 signaling. J. Biol. Chem. 279 (49), 51141–51147. doi:10.1074/jbc.M408662200
Kuramochi, Y., Lim, C. C., Guo, X., Colucci, W. S., Liao, R., and Sawyer, D. B. (2004). Myocyte contractile activity modulates norepinephrine cytotoxicity and survival effects of neuregulin-1beta. Am. J. Physiol. Cell Physiol. 286 (2), C222–C229. doi:10.1152/ajpcell.00312.2003
Kurohara, K., Komatsu, K., Kurisaki, T., Masuda, A., Irie, N., Asano, M., et al. (2004). Essential roles of Meltrin beta (ADAM19) in heart development. Dev. Biol. 267 (1), 14–28. doi:10.1016/j.ydbio.2003.10.021
Ky, B., Kimmel, S. E., Safa, R. N., Putt, M. E., Sweitzer, N. K., Fang, J. C., et al. (2009). Neuregulin-1 beta is associated with disease severity and adverse outcomes in chronic heart failure. Circulation 120 (4), 310–317. doi:10.1161/CIRCULATIONAHA.109.856310
Lai, D., Liu, X., Forrai, A., Wolstein, O., Michalicek, J., Ahmed, I., et al. (2010). Neuregulin 1 sustains the gene regulatory network in both trabecular and nontrabecular myocardium. Circ. Res. 107 (6), 715–727. doi:10.1161/CIRCRESAHA.110.218693
Laplante, M., and Sabatini, D. M. (2012). mTOR signaling in growth control and disease. Cell 149 (2), 274–293. doi:10.1016/j.cell.2012.03.017
Laskin, J., Liu, S. V., Tolba, K., Heining, C., Schlenk, R. F., Cheema, P., et al. (2020). NRG1 fusion-driven tumors: Biology, detection, and the therapeutic role of afatinib and other ErbB-targeting agents. Ann. Oncol. 31 (12), 1693–1703. doi:10.1016/j.annonc.2020.08.2335
Latysheva, N. S., and Babu, M. M. (2016). Discovering and understanding oncogenic gene fusions through data intensive computational approaches. Nucleic Acids Res. 44 (10), 4487–4503. doi:10.1093/nar/gkw282
Lázár, E., Sadek, H. A., and Bergmann, O. (2017). Cardiomyocyte renewal in the human heart: Insights from the fall-out. Eur. Heart J. 38 (30), 2333–2342. doi:10.1093/eurheartj/ehx343
Lee, K. F., Simon, H., CHen, H., Bates, B., Hung, M. C., and Hauser, C. (1995). Requirement for neuregulin receptor erbB2 in neural and cardiac development. Nature 378 (6555), 394–398. doi:10.1038/378394a0
Leimeroth, R., Lobsiger, C., Lussi, A., Taylor, V., Suter, U., and Sommer, L. (2002). Membrane-bound neuregulin1 type III actively promotes Schwann cell differentiation of multipotent Progenitor cells. Dev. Biol. 246 (2), 245–258. doi:10.1006/dbio.2002.0670
Lemmens, K., Doggen, K., and De Keulenaer, G. W. (2011). Activation of the neuregulin/ErbB system during physiological ventricular remodeling in pregnancy. Am. J. Physiol. Heart Circ. Physiol. 300 (3), H931–H942. doi:10.1152/ajpheart.00385.2010
Lemmens, K., Doggen, K., and De Keulenaer, G. W. (2007). Role of neuregulin-1/ErbB signaling in cardiovascular physiology and disease: Implications for therapy of heart failure. Circulation 116 (8), 954–960. doi:10.1161/CIRCULATIONAHA.107.690487
Lemmens, K., Fransen, P., Sys, S. U., Brutsaert, D. L., and De Keulenaer, G. W. (2004). Neuregulin-1 induces a negative inotropic effect in cardiac muscle: Role of nitric oxide synthase. Circulation 109 (3), 324–326. doi:10.1161/01.CIR.0000114521.88547.5E
Lemmens, K., Segers, V. F. M., Demolder, M., and De Keulenaer, G. W. (2006). Role of neuregulin-1/ErbB2 signaling in endothelium-cardiomyocyte cross-talk. J. Biol. Chem. 281 (28), 19469–19477. doi:10.1074/jbc.M600399200
Li, B., Zheng, Z., Wei, Y., Wang, M., Peng, J., Kang, T., et al. (2011). Therapeutic effects of neuregulin-1 in diabetic cardiomyopathy rats. Cardiovasc. Diabetol. 10, 69. doi:10.1186/1475-2840-10-69
Li, J., Gu, X. h., Duan, J. c., Zeng, L., Li, Y., and Wang, L. (2007). [Effects of recombined human neuregulin on the contractibility of cardiac muscles of rhesus monkeys with pacing-induced heart failure]. Sichuan Da Xue Xue Bao Yi Xue Ban. 38 (1), 105–108.
Li, L., Cleary, S., Mandarano, M. A., Long, W., Birchmeier, C., and Jones, F. E. (2002). The breast proto-oncogene, HRGalpha regulates epithelial proliferation and lobuloalveolar development in the mouse mammary gland. Oncogene 21 (32), 4900–4907. doi:10.1038/sj.onc.1205634
Li, T., Liang, W., Xiao, X., and Qian, Y. (2018). Nanotechnology, an alternative with promising prospects and advantages for the treatment of cardiovascular diseases. Int. J. Nanomedicine 13, 7349–7362. doi:10.2147/IJN.S179678
Li, X., Zhang, Y., Ren, X., Wang, Y., Chen, D., Li, Q., et al. (2021). Ischemic microenvironment-responsive therapeutics for cardiovascular diseases. Adv. Mat. 33 (52), e2105348. doi:10.1002/adma.202105348
Liang, X., Ding, Y., Lin, F., Zhang, Y., Zhou, X., Meng, Q., et al. (2019). Overexpression of ERBB4 rejuvenates aged mesenchymal stem cells and enhances angiogenesis via PI3K/AKT and MAPK/ERK pathways. Faseb J. 33 (3), 4559–4570. doi:10.1096/fj.201801690R
Lin, Y., Liu, H., and Wang, X. (2020). Neuregulin-1, a microvascular endothelial-derived protein, protects against myocardial ischemia-reperfusion injury (Review). Int. J. Mol. Med. 46 (3), 925–935. doi:10.3892/ijmm.2020.4662
Lin, Z., and Pu, W. T. (2014). Harnessing Hippo in the heart: Hippo/Yap signaling and applications to heart regeneration and rejuvenation. Stem Cell Res. 13 (3), 571–581. doi:10.1016/j.scr.2014.04.010
Lin, Z., Zhou, P., von Gise, A., Gu, F., Ma, Q., Chen, J., et al. (2015). Pi3kcb links Hippo-YAP and PI3K-AKT signaling pathways to promote cardiomyocyte proliferation and survival. Circ. Res. 116 (1), 35–45. doi:10.1161/CIRCRESAHA.115.304457
Lindsey, M. L., Bolli, R., Canty, J. M., Du, X. J., Frangogiannis, N. G., Frantz, S., et al. (2018). Guidelines for experimental models of myocardial ischemia and infarction. Am. J. Physiol. Heart Circ. Physiol. 314 (4), H812–H838. doi:10.1152/ajpheart.00335.2017
Liu, F. F., Stone, J. R., Schuldt, A. J. T., Okoshi, K., Okoshi, M. P., Nakayama, M., et al. (2005). Heterozygous knockout of neuregulin-1 gene in mice exacerbates doxorubicin-induced heart failure. Am. J. Physiol. Heart Circ. Physiol. 289 (2), H660–H666. doi:10.1152/ajpheart.00268.2005
Liu, H., Liu, R., Xiong, Y., Li, X., Wang, X., Ma, Y., et al. (2014). Leucine facilitates the insulin-stimulated glucose uptake and insulin signaling in skeletal muscle cells: Involving mTORC1 and mTORC2. Amino Acids 46 (8), 1971–1979. doi:10.1007/s00726-014-1752-9
Liu, H., Weng, X. J., Yao, J. Y., Zheng, J., Lv, X., Zhou, X. H., et al. (2020). Neuregulin-1β protects the rat diaphragm during sepsis against oxidative stress and inflammation by activating the PI3K/Akt pathway. Oxid. Med. Cell. Longev. 2020, 1720961. doi:10.1155/2020/1720961
Liu, J., Bressan, M., Hassel, D., Huisken, J., Staudt, D., Kikuchi, K., et al. (2010). A dual role for ErbB2 signaling in cardiac trabeculation. Development 137 (22), 3867–3875. doi:10.1242/dev.053736
Liu, X., Gu, X., Li, Z., Li, X., Li, H., Chang, J., et al. (2006). Neuregulin-1/erbB-activation improves cardiac function and survival in models of ischemic, dilated, and viral cardiomyopathy. J. Am. Coll. Cardiol. 48 (7), 1438–1447. doi:10.1016/j.jacc.2006.05.057
Liu, X., Hwang, H., Cao, L., BucklandM., , CunninghAm, A., Chen, J., et al. (1998). Domain-specific gene disruption reveals critical regulation of neuregulin signaling by its cytoplasmic tail. Proc. Natl. Acad. Sci. U. S. A. 95 (22), 13024–13029. doi:10.1073/pnas.95.22.13024
Loeb, J. A., Susanto, E. T., and Fischbach, G. D. (1998). The neuregulin precursor proARIA is processed to ARIA after expression on the cell surface by a protein kinase C-enhanced mechanism. Mol. Cell. Neurosci. 11 (1-2), 77–91. doi:10.1006/mcne.1998.0676
Lu, F., Wei, L., Yang, C., Qiao, Y., Liu, Y. S., Chen, X. D., et al. (2021). Nrg1/ErbB2 regulates differentiation and apoptosis of neural stem cells in the cochlear nucleus through PI3K/Akt pathway. Neurosci. Lett. 751, 135803. doi:10.1016/j.neulet.2021.135803
Ma, H., Yin, C., Zhang, Y., Qian, L., and Liu, J. (2016). ErbB2 is required for cardiomyocyte proliferation in murine neonatal hearts. Gene 592 (2), 325–330. doi:10.1016/j.gene.2016.07.006
Mahiny-Shahmohammady, D., Hauck, L., and Billia, F. (2022). Defining the molecular underpinnings controlling cardiomyocyte proliferation. Clin. Sci. 136 (12), 911–934. doi:10.1042/CS20211180
Marchionni, M. A., Goodearl, A. D., Chen, M. S., Bermingham-McDOnOgh, O., Kirk, C., HendricksM., , et al. (1993). Glial growth factors are alternatively spliced erbB2 ligands expressed in the nervous system. Nature 362 (6418), 312–318. doi:10.1038/362312a0
Matsukawa, R., Hirooka, Y., Ito, K., and Sunagawa, K. (2013). Inhibition of neuregulin-1/ErbB signaling in the rostral ventrolateral medulla leads to hypertension through reduced nitric oxide synthesis. Am. J. Hypertens. 26 (1), 51–57. doi:10.1093/ajh/hps005
Matsushima, S., Tsutsui, H., and Sadoshima, J. (2014). Physiological and pathological functions of NADPH oxidases during myocardial ischemia-reperfusion. Trends cardiovasc. Med. 24 (5), 202–205. doi:10.1016/j.tcm.2014.03.003
Mei, M., Kim, Y., Sutherland, L. B., Murakami, M., Qi, X., McAnally, J., et al. (2013). Hippo pathway effector Yap promotes cardiac regeneration. Proc. Natl. Acad. Sci. U. S. A. 110 (34), 13839–13844. doi:10.1073/pnas.1313192110
Mei, L., and Nave, K. A. (2014). Neuregulin-ERBB signaling in the nervous system and neuropsychiatric diseases. Neuron 83 (1), 27–49. doi:10.1016/j.neuron.2014.06.007
Mei, L., and Xiong, W. C. (2008). Neuregulin 1 in neural development, synaptic plasticity and schizophrenia. Nat. Rev. Neurosci. 9 (6), 437–452. doi:10.1038/nrn2392
Mendes-Ferreira, P., De Keulenaer, G. W., Leite-Moreira, A. F., and Bras-Silva, C. (2013). Therapeutic potential of neuregulin-1 in cardiovascular disease. Drug Discov. Today 18 (17-18), 836–842. doi:10.1016/j.drudis.2013.01.010
Mendes-Ferreira, P., Maia-Rocha, C., Adao, R., Mendes, M. J., Santos-Ribeiro, D., Alves, B. S., et al. (2016). Neuregulin-1 improves right ventricular function and attenuates experimental pulmonary arterial hypertension. Cardiovasc. Res. 109 (1), 44–54. doi:10.1093/cvr/cvv244
Meng, D., Pan, H., Chen, Y., Ding, J., and Dai, Y. (2021). Roles and mechanisms of NRG1 in modulating the pathogenesis of NAFLD through ErbB3 signaling in hepatocytes (NRG1 modulates NAFLD through ErbB3 signaling). Obes. Res. Clin. Pract. 15 (2), 145–151. doi:10.1016/j.orcp.2021.01.003
Meyer, D., and Birchmeier, C. (1995). Multiple essential functions of neuregulin in development. Nature 378 (6555), 386–390. doi:10.1038/378386a0
Meyer, D., Yamaai, T., GArrAtt, A., RiEthmachEr-SonnEnbErg, E., Kane, D., Theill, L. E., et al. (1997). Isoform-specific expression and function of neuregulin. Development 124 (18), 3575–3586. doi:10.1242/dev.124.18.3575
Minamino, T., and Komuro, I. (2008). Vascular aging: Insights from studies on cellular senescence, stem cell aging, and progeroid syndromes. Nat. Clin. Pract. Cardiovasc. Med. 5 (10), 637–648. doi:10.1038/ncpcardio1324
Minamino, T., and Komuro, I. (2007). Vascular cell senescence: Contribution to atherosclerosis. Circ. Res. 100 (1), 15–26. doi:10.1161/01.RES.0000256837.40544.4a
Montero, J. C., Yuste, L., Diaz-RodriguEz, E., EspAris-OgAndo, A., and PAndiellA, A. (2000). Differential shedding of transmembrane neuregulin isoforms by the tumor necrosis factor-alpha-converting enzyme. Mol. Cell. Neurosci. 16 (5), 631–648. doi:10.1006/mcne.2000.0896
Moon, J. S., Nakahira, K., Chung, K. P., DeNicola, G. M., Koo, M. J., Pabon, M. A., et al. (2016). NOX4-dependent fatty acid oxidation promotes NLRP3 inflammasome activation in macrophages. Nat. Med. 22 (9), 1002–1012. doi:10.1038/nm.4153
Nahrendorf, M., Pittet, M. J., and Swirski, F. K. (2010). Monocytes: Protagonists of infarct inflammation and repair after myocardial infarction. Circulation 121 (22), 2437–2445. doi:10.1161/CIRCULATIONAHA.109.916346
Nakano-Kurimoto, R., Ikeda, K., Uraoka, M., Nakagawa, Y., Yutaka, K., Koide, M., et al. (2009). Replicative senescence of vascular smooth muscle cells enhances the calcification through initiating the osteoblastic transition. Am. J. Physiol. Heart Circ. Physiol. 297 (5), H1673–H1684. doi:10.1152/ajpheart.00455.2009
Nakaoku, T., Tsuta, K., Ichikawa, H., Shiraishi, K., Sakamoto, H., Enari, M., et al. (2014). Druggable oncogene fusions in invasive mucinous lung adenocarcinoma. Clin. Cancer Res. 20 (12), 3087–3093. doi:10.1158/1078-0432.CCR-14-0107
Nonn, L., Williams, R. R., Erickson, R. P., and Powis, G. (2003). The absence of mitochondrial thioredoxin 2 causes massive apoptosis, exencephaly, and early embryonic lethality in homozygous mice. Mol. Cell. Biol. 23 (3), 916–922. doi:10.1128/mcb.23.3.916-922.2003
Odashima, M., Usui, S., Takagi, H., Hong, C., Liu, J., Yokota, M., et al. (2007). Inhibition of endogenous Mst1 prevents apoptosis and cardiac dysfunction without affecting cardiac hypertrophy after myocardial infarction. Circ. Res. 100 (9), 1344–1352. doi:10.1161/01.RES.0000265846.23485.7a
Odiete, O., Hill, M. F., and Sawyer, D. B. (2012). Neuregulin in cardiovascular development and disease. Circ. Res. 111 (10), 1376–1385. doi:10.1161/CIRCRESAHA.112.267286
Odiete, O., Konik, E. A., Sawyer, D. B., and Hill, M. F. (2013). Type 1 diabetes mellitus abrogates compensatory augmentation of myocardial neuregulin-1β/ErbB in response to myocardial infarction resulting in worsening heart failure. Cardiovasc. Diabetol. 12, 52. doi:10.1186/1475-2840-12-52
Okoshi, K., Nakayama, M., Yan, X., Okoshi, M. P., Schuldt, A. J. T., Marchionni, M. A., et al. (2004). Neuregulins regulate cardiac parasympathetic activity: Muscarinic modulation of beta-adrenergic activity in myocytes from mice with neuregulin-1 gene deletion. Circulation 110 (6), 713–717. doi:10.1161/01.CIR.0000138109.32748.80
Olayioye, M. A., Neve, R. M., Lane, H. A., and Hynes, N. E. (2000). The ErbB signaling network: Receptor heterodimerization in development and cancer. Embo J. 19 (13), 3159–3167. doi:10.1093/emboj/19.13.3159
Opie, L. H. (1976). Effects of regional ischemia on metabolism of glucose and fatty acids. Relative rates of aerobic and anaerobic energy production during myocardial infarction and comparison with effects of anoxia. Circ. Res. 38 (5), I52–I74.
Ozcelik, C., Erdmann, B., Pilz, B., Wettschureck, N., Britsch, S., Hubner, N., et al. (2002). Conditional mutation of the ErbB2 (HER2) receptor in cardiomyocytes leads to dilated cardiomyopathy. Proc. Natl. Acad. Sci. U. S. A. 99 (13), 8880–8885. doi:10.1073/pnas.122249299
Parodi, E. M., and Kuhn, B. (2014). Signalling between microvascular endothelium and cardiomyocytes through neuregulin. Cardiovasc. Res. 102 (2), 194–204. doi:10.1093/cvr/cvu021
Peles, E., Bacus, S. S., Koski, R. A., Lu, H. S., Wen, D., Ogden, S. G., et al. (1992). Isolation of the neu/HER-2 stimulatory ligand: A 44 kd glycoprotein that induces differentiation of mammary tumor cells. Cell 69 (1), 205–216. doi:10.1016/0092-8674(92)90131-u
Pentassuglia, L., Heim, P., Lebboukh, S., Morandi, C., Xu, L., and Brink, M. (2016). Neuregulin-1β promotes glucose uptake via PI3K/Akt in neonatal rat cardiomyocytes. Am. J. Physiol. Endocrinol. Metab. 310 (9), E782–E794. doi:10.1152/ajpendo.00259.2015
Pilz, P. M., Hamza, O., Gidlof, O., Goncalves, I. F., Tretter, E. V., Trojanek, S., et al. (2019). Remote ischemic perconditioning attenuates adverse cardiac remodeling and preserves left ventricular function in a rat model of reperfused myocardial infarction. Int. J. Cardiol. 285, 72–79. doi:10.1016/j.ijcard.2019.03.003
Pinkas-Kramarski, R., ShellyM., , Guarino, B. C., Wang, L. M., Lyass, L., Alroy, I., et al. (1998). ErbB tyrosine kinases and the two neuregulin families constitute a ligand-receptor network. Mol. Cell. Biol. 18 (10), 6090–6101. doi:10.1128/mcb.18.10.6090
Plowman, G. D., Green, J. M., Culouscou, J. M., Carlton, G. W., Rothwell, V. M., and Buckley, S. (1993). Heregulin induces tyrosine phosphorylation of HER4/p180erbB4. Nature 366 (6454), 473–475. doi:10.1038/366473a0
Polizzotti, B. D., Ganapathy, B., Walsh, S., Choudhury, S., Ammanamanchi, N., Bennett, D. G., et al. (2015). Neuregulin stimulation of cardiomyocyte regeneration in mice and human myocardium reveals a therapeutic window. Sci. Transl. Med. 7 (281), 281ra45. doi:10.1126/scitranslmed.aaa5171
Prabhu, S. D., and Frangogiannis, N. G. (2016). The biological basis for cardiac repair after myocardial infarction: From inflammation to fibrosis. Circ. Res. 119 (1), 91–112. doi:10.1161/CIRCRESAHA.116.303577
Prenzel, N., Zwick, E., Daub, H., LesererM., , AbRaham, R., WallasCh, C., et al. (1999). EGF receptor transactivation by G-protein-coupled receptors requires metalloproteinase cleavage of proHB-EGF. Nature 402 (6764), 884–888. doi:10.1038/47260
Rebouças, J. S., Santos-Magalhães, N. S., and Formiga, F. R. (2016). Cardiac regeneration using growth factors: Advances and challenges. Arq. Bras. Cardiol. 107 (3), 271–275. doi:10.5935/abc.20160097
Rentschler, S., Zander, J., Meyers, K., France, D., Levine, R., Porter, G., et al. (2002). Neuregulin-1 promotes formation of the murine cardiac conduction system. Proc. Natl. Acad. Sci. U. S. A. 99 (16), 10464–10469. doi:10.1073/pnas.162301699
Riehle, C., and Abel, E. D. (2016). Insulin signaling and heart failure. Circ. Res. 118 (7), 1151–1169. doi:10.1161/CIRCRESAHA.116.306206
Rupert, C. E., and Coulombe, K. L. (2015). The roles of neuregulin-1 in cardiac development, homeostasis, and disease. Biomark. Insights 10 (1), 1–9. doi:10.4137/BMI.S20061
Russell, K. S., Kalinowski, A., and Hedhli, N. (2014). Cardiovascular effects of neuregulin-1/ErbB signaling: Role in vascular signaling and angiogenesis. Curr. Pharm. Des. 20 (30), 4899–4905. doi:10.2174/1381612819666131125151058
Safa, R. N., Peng, X. Y., Pentassuglia, L., Lim, C. C., Lamparter, M., Silverstein, C., et al. (2011). Neuregulin-1β regulation of embryonic endothelial progenitor cell survival. Am. J. Physiol. Heart Circ. Physiol. 300 (4), H1311–H1319. doi:10.1152/ajpheart.01104.2009
Sandanger, Ø., Ranheim, T., Vinge, L. E., Bliksoen, M., Alfsnes, K., Finsen, A. V., et al. (2013). The NLRP3 inflammasome is up-regulated in cardiac fibroblasts and mediates myocardial ischaemia-reperfusion injury. Cardiovasc. Res. 99 (1), 164–174. doi:10.1093/cvr/cvt091
Sarkar, S., Korolchuk, V. I., Renna, M., Imarisio, S., Fleming, A., Williams, A., et al. (2011). Complex inhibitory effects of nitric oxide on autophagy. Mol. Cell 43 (1), 19–32. doi:10.1016/j.molcel.2011.04.029
Sato, M., Dehvari, N., Oberg, A. I., Dallner, O. S., Sandstrom, A. L., Olsen, J. M., et al. (2014). Improving type 2 diabetes through a distinct adrenergic signaling pathway involving mTORC2 that mediates glucose uptake in skeletal muscle. Diabetes 63 (12), 4115–4129. doi:10.2337/db13-1860
Sawyer, D. B., Zuppinger, C., Miller, T. A., Eppenberger, H. M., and Suter, T. M. (2002). Modulation of anthracycline-induced myofibrillar disarray in rat ventricular myocytes by neuregulin-1beta and anti-erbB2: Potential mechanism for trastuzumab-induced cardiotoxicity. Circulation 105 (13), 1551–1554. doi:10.1161/01.cir.0000013839.41224.1c
Saxton, R. A., and Sabatini, D. M. (2017). mTOR signaling in growth, metabolism, and disease. Cell 168 (6), 960–976. doi:10.1016/j.cell.2017.02.004
Schaefer, M., Plant, T. D., Obukhov, A. G., Hofmann, T., Gudermann, T., and Schultz, G. (2000). Receptor-mediated regulation of the nonselective cation channels TRPC4 and TRPC5. J. Biol. Chem. 275 (23), 17517–17526. doi:10.1074/jbc.275.23.17517
Schwach, V., Gomes Fernandes, M., Maas, S., Gerhardt, S., Tsonaka, R., van der Weerd, L., et al. (2020). Expandable human cardiovascular progenitors from stem cells for regenerating mouse heart after myocardial infarction. Cardiovasc. Res. 116 (3), 545–553. doi:10.1093/cvr/cvz181
Seidman, J. G., and Seidman, C. (2001). The genetic basis for cardiomyopathy: From mutation identification to mechanistic paradigms. Cell 104 (4), 557–567. doi:10.1016/s0092-8674(01)00242-2
Shakeri, H., Boen, J. R. A., De Moudt, S., Hendrickx, J. O., Leloup, A. J. A., Jacobs, G., et al. (2021). Neuregulin-1 compensates for endothelial nitric oxide synthase deficiency. Am. J. Physiol. Heart Circ. Physiol. 320 (6), H2416–h2428. doi:10.1152/ajpheart.00914.2020
Shakeri, H., Gevaert, A. B., Schrijvers, D. M., De Meyer, G. R. Y., De Keulenaer, G. W., Guns, P. J. D. F., et al. (2018). Neuregulin-1 attenuates stress-induced vascular senescence. Cardiovasc. Res. 114 (7), 1041–1051. doi:10.1093/cvr/cvy059
Shao, D., and Tian, R. (2015). Glucose transporters in cardiac metabolism and hypertrophy. Compr. Physiol. 6 (1), 331–351. doi:10.1002/cphy.c150016
Shende, P., Plaisance, I., Morandi, C., Pellieux, C., Berthonneche, C., Zorzato, F., et al. (2011). Cardiac raptor ablation impairs adaptive hypertrophy, alters metabolic gene expression, and causes heart failure in mice. Circulation 123 (10), 1073–1082. doi:10.1161/CIRCULATIONAHA.110.977066
Shi, W., Chen, H., Sun, J., Buckley, S., Zhao, J., Anderson, K. D., et al. (2003). TACE is required for fetal murine cardiac development and modeling. Dev. Biol. 261 (2), 371–380. doi:10.1016/s0012-1606(03)00315-4
Shiraishi, M., Yamaguchi, A., and Suzuki, K. (2022). Nrg1/ErbB signaling-mediated regulation of fibrosis after myocardial infarction. Faseb J. 36 (2), e22150. doi:10.1096/fj.202101428RR
Shirakabe, K., WakatSuki, S., Kurisaki, T., and FujisAwA-SehArA, A. (2001). Roles of Meltrin beta/ADAM19 in the processing of neuregulin. J. Biol. Chem. 276 (12), 9352–9358. doi:10.1074/jbc.M007913200
Sikora, E., Arendt, T., Bennett, M., and Narita, M. (2011). Impact of cellular senescence signature on ageing research. Ageing Res. Rev. 10 (1), 146–152. doi:10.1016/j.arr.2010.10.002
Simón-Yarza, T., Rossi, A., Heffels, K. H., Prosper, F., Groll, J., and Blanco-Prieto, M. J. (2015). Polymeric electrospun scaffolds: Neuregulin encapsulation and biocompatibility studies in a model of myocardial ischemia. Tissue Eng. Part A 21 (9-10), 1654–1661. doi:10.1089/ten.TEA.2014.0523
Smits, A. M., Dronkers, E., and Goumans, M. J. (2018). The epicardium as a source of multipotent adult cardiac progenitor cells: Their origin, role and fate. Pharmacol. Res. 127, 129–140. doi:10.1016/j.phrs.2017.07.020
Staudt, D. W., Liu, J., Thorn, K. S., Stuurman, N., Liebling, M., and Stainier, D. Y. R. (2014). High-resolution imaging of cardiomyocyte behavior reveals two distinct steps in ventricular trabeculation. Development 141 (3), 585–593. doi:10.1242/dev.098632
Steffens, S., Van Linthout, S., Sluijter, J. P. G., Tocchetti, C. G., Thum, T., and Madonna, R. (2020). Stimulating pro-reparative immune responses to prevent adverse cardiac remodelling: Consensus document from the joint 2019 meeting of the ESC working groups of cellular biology of the heart and myocardial function. Cardiovasc. Res. 116 (11), 1850–1862. doi:10.1093/cvr/cvaa137
Steinthorsdottir, V., Stefansson, H., Ghosh, S., Birgisdottir, B., Bjornsdottir, S., Fasquel, A. C., et al. (2004). Multiple novel transcription initiation sites for NRG1. Gene 342 (1), 97–105. doi:10.1016/j.gene.2004.07.029
Stephen, M. J., Poindexter, B. J., Moolman, J. A., Sheikh-Hamad, D., and Bick, R. J. (2009). Do binucleate cardiomyocytes have a role in myocardial repair? Insights using isolated rodent myocytes and cell culture. Open cardiovasc. Med. J. 3, 1–7. doi:10.2174/1874192400903010001
Stonecypher, M. S., Chaudhury, A. R., Byer, S. J., and Carroll, S. L. (2006). Neuregulin growth factors and their ErbB receptors form a potential signaling network for schwannoma tumorigenesis. J. Neuropathol. Exp. Neurol. 65 (2), 162–175. doi:10.1097/01.jnen.0000199575.93794.2f
Sudol, M. (2014). Neuregulin 1-activated ERBB4 as a "dedicated" receptor for the Hippo-YAP pathway. Sci. Signal. 7 (355), pe29. doi:10.1126/scisignal.aaa2710
Suk Kim, H., Hidaka, K., and Morisaki, T. (2003). Expression of ErbB receptors in ES cell-derived cardiomyocytes. Biochem. Biophys. Res. Commun. 309 (1), 241–246. doi:10.1016/s0006-291x(03)01521-3
Sun, K., Li, Y. Y., and Jin, J. (2021). A double-edged sword of immuno-microenvironment in cardiac homeostasis and injury repair. Signal Transduct. Target. Ther. 6 (1), 79. doi:10.1038/s41392-020-00455-6
Surviladze, Z., Sterk, R. T., DeHaro, S. A., and Ozbun, M. A. (2013). Cellular entry of human papillomavirus type 16 involves activation of the phosphatidylinositol 3-kinase/Akt/mTOR pathway and inhibition of autophagy. J. Virol. 87 (5), 2508–2517. doi:10.1128/JVI.02319-12
Sweeney, C., Fambrough, D., Huard, C., Diamonti, A. J., Lander, E. S., Cantley, L. C., et al. (2001). Growth factor-specific signaling pathway stimulation and gene expression mediated by ErbB receptors. J. Biol. Chem. 276 (25), 22685–22698. doi:10.1074/jbc.M100602200
Szablewski, L. (2017). Glucose transporters in healthy heart and in cardiac disease. Int. J. Cardiol. 230, 70–75. doi:10.1016/j.ijcard.2016.12.083
Tane, S., Okayama, H., Ikenishi, A., Amemiya, Y., Nakayama, K. I., and Takeuchi, T. (2015). Two inhibitory systems and CKIs regulate cell cycle exit of mammalian cardiomyocytes after birth. Biochem. Biophys. Res. Commun. 466 (2), 147–154. doi:10.1016/j.bbrc.2015.08.102
Tevzadze, N., Rukhadze, R., and Dzidziguri, D. (2005). The age related changes in cell cycle of mice cardiomyocytes. Georgian Med. News 1 (128), 87–90.
Timolati, F., Ott, D., Pentassuglia, L., Giraud, M. N., Perriard, J. C., Suter, T. M., et al. (2006). Neuregulin-1 beta attenuates doxorubicin-induced alterations of excitation-contraction coupling and reduces oxidative stress in adult rat cardiomyocytes. J. Mol. Cell. Cardiol. 41 (5), 845–854. doi:10.1016/j.yjmcc.2006.08.002
Trombetta, D., Graziano, P., Scarpa, A., Sparaneo, A., Rossi, G., Rossi, A., et al. (2018). Frequent NRG1 fusions in Caucasian pulmonary mucinous adenocarcinoma predicted by Phospho-ErbB3 expression. Oncotarget 9 (11), 9661–9671. doi:10.18632/oncotarget.23800
Vandekerckhove, L., Vermeulen, Z., Liu, Z. Z., Boimvaser, S., Patzak, A., Segers, V. F. M., et al. (2016). Neuregulin-1 attenuates development of nephropathy in a type 1 diabetes mouse model with high cardiovascular risk. Am. J. Physiol. Endocrinol. Metab. 310 (7), E495–E504. doi:10.1152/ajpendo.00432.2015
Vasti, C., Witt, H., Said, M., Sorroche, P., Garcia-Rivello, H., Ruiz-Noppinger, P., et al. (2012). Doxorubicin and NRG-1/erbB4-deficiency affect gene expression profile: Involving protein homeostasis in mouse. ISRN Cardiol. 2012, 745185. doi:10.5402/2012/745185
Vermeulen, Z., Hervent, A. S., Dugaucquier, L., Vandekerckhove, L., Rombouts, M., Beyens, M., et al. (2017). Inhibitory actions of the NRG-1/ErbB4 pathway in macrophages during tissue fibrosis in the heart, skin, and lung. Am. J. Physiol. Heart Circ. Physiol. 313 (5), H934–H945. doi:10.1152/ajpheart.00206.2017
Vicier, C., Dieci, M. V., Arnedos, M., Delaloge, S., Viens, P., and Andre, F. (2014). Clinical development of mTOR inhibitors in breast cancer. Breast Cancer Res. 16 (1), 203. doi:10.1186/bcr3618
von Gise, A., Lin, Z., Schlegelmilch, K., Honor, L. B., Pan, G. M., Buck, J. N., et al. (2012). YAP1, the nuclear target of Hippo signaling, stimulates heart growth through cardiomyocyte proliferation but not hypertrophy. Proc. Natl. Acad. Sci. U. S. A. 109 (7), 2394–2399. doi:10.1073/pnas.1116136109
Wadugu, B., and Kühn, B. (2012). The role of neuregulin/ErbB2/ErbB4 signaling in the heart with special focus on effects on cardiomyocyte proliferation. Am. J. Physiol. Heart Circ. Physiol. 302 (11), H2139–H2147. doi:10.1152/ajpheart.00063.2012
Wagner, J. U. G., and Dimmeler, S. (2020). Cellular cross-talks in the diseased and aging heart. J. Mol. Cell. Cardiol. 138, 136–146. doi:10.1016/j.yjmcc.2019.11.152
Wan, A., and Rodrigues, B. (2016). Endothelial cell-cardiomyocyte crosstalk in diabetic cardiomyopathy. Cardiovasc. Res. 111 (3), 172–183. doi:10.1093/cvr/cvw159
Wang, F., Wang, H., Liu, X., Yu, H., Huang, X., Huang, W., et al. (2021). Neuregulin-1 alleviate oxidative stress and mitigate inflammation by suppressing NOX4 and NLRP3/caspase-1 in myocardial ischaemia-reperfusion injury. J. Cell. Mol. Med. 25 (3), 1783–1795. doi:10.1111/jcmm.16287
Wang, F., Wang, H., Liu, X., Yu, H., Zuo, B., Song, Z., et al. (2018). Pharmacological postconditioning with Neuregulin-1 mimics the cardioprotective effects of ischaemic postconditioning via ErbB4-dependent activation of reperfusion injury salvage kinase pathway. Mol. Med. 24 (1), 39. doi:10.1186/s10020-018-0040-7
Wang, J. C., and Bennett, M. (2012). Aging and atherosclerosis: Mechanisms, functional consequences, and potential therapeutics for cellular senescence. Circ. Res. 111 (2), 245–259. doi:10.1161/CIRCRESAHA.111.261388
Wang, J. Y., Miller, S. J., and Falls, D. L. (2001). The N-terminal region of neuregulin isoforms determines the accumulation of cell surface and released neuregulin ectodomain. J. Biol. Chem. 276 (4), 2841–2851. doi:10.1074/jbc.M005700200
Wang, J., Zhou, J., Wang, Y., Yang, C., Fu, M., Zhang, J., et al. (2017). Qiliqiangxin protects against anoxic injury in cardiac microvascular endothelial cells via NRG-1/ErbB-PI3K/Akt/mTOR pathway. J. Cell. Mol. Med. 21 (9), 1905–1914. doi:10.1111/jcmm.13111
Wang, X. H., Zhuo, X. Z., Ni, Y. J., Gong, M., Wang, T. Z., Lu, Q., et al. (2012). Improvement of cardiac function and reversal of gap junction remodeling by Neuregulin-1β in volume-overloaded rats with heart failure. J. Geriatr. Cardiol. 9 (2), 172–179. doi:10.3724/SP.J.1263.2012.03271
Wang, X., Zhuo, X., Gao, J., Liu, H., Lin, F., and Ma, A. (2019). Neuregulin-1β partially improves cardiac function in volume-overload heart failure through regulation of abnormal calcium handling. Front. Pharmacol. 10, 616. doi:10.3389/fphar.2019.00616
Wang, Y. Y., Li, T., Liu, Y. W., Liu, B. J., Hu, X. M., et al. (2017). [Effect of the ischemic post-conditioning on the prevention of the cardio-renal damage in patients with acute ST-segment elevation myocardial infarction after primary percutaneous coronary intervention]. Zhonghua Xin Xue Guan Bing Za Zhi 45 (4), 277–282. doi:10.3760/cma.j.issn.0253-3758.2017.04.005
Wang, Z., and Huang, J. (2014). Neuregulin-1 increases connexin-40 and connexin-45 expression in embryonic stem cell-derived cardiomyocytes. Appl. Biochem. Biotechnol. 174 (2), 483–493. doi:10.1007/s12010-014-1089-6
Wei, H., Wang, C., Guo, R., Takahashi, K., and Naruse, K. (2019). Development of a model of ischemic heart disease using cardiomyocytes differentiated from human induced pluripotent stem cells. Biochem. Biophys. Res. Commun. 520 (3), 600–605. doi:10.1016/j.bbrc.2019.09.119
Whelan, R. S., Kaplinskiy, V., and Kitsis, R. N. (2010). Cell death in the pathogenesis of heart disease: Mechanisms and significance. Annu. Rev. Physiol. 72, 19–44. doi:10.1146/annurev.physiol.010908.163111
Wischmeyer, E., Döring, F., and Karschin, A. (1998). Acute suppression of inwardly rectifying Kir2.1 channels by direct tyrosine kinase phosphorylation. J. Biol. Chem. 273 (51), 34063–34068. doi:10.1074/jbc.273.51.34063
Wu, C., Gui, C., Li, L., Pang, Y., Tang, Z., and Wei, J. (2018). Expression and secretion of neuregulin-1 in cardiac microvascular endothelial cells treated with angiogenic factors. Exp. Ther. Med. 15 (4), 3577–3581. doi:10.3892/etm.2018.5811
Wu, J. Y., Yu, H., and Cohen, I. S. (2000). Epidermal growth factor increases i(f) in rabbit SA node cells by activating a tyrosine kinase. Biochim. Biophys. Acta 1463 (1), 15–19. doi:10.1016/s0005-2736(99)00233-3
Wu, L., Walas, S., Leung, W., Sykes, D. B., Wu, J., Lo, E. H., et al. (2015). Neuregulin1-β decreases IL-1β-induced neutrophil adhesion to human brain microvascular endothelial cells. Transl. Stroke Res. 6 (2), 116–124. doi:10.1007/s12975-014-0347-9
Wu, X., Reboll, M. R., Korf-Klingebiel, M., and Wollert, K. C. (2021). Angiogenesis after acute myocardial infarction. Cardiovasc. Res. 117 (5), 1257–1273. doi:10.1093/cvr/cvaa287
Xiao, J., Li, B., Zheng, Z., Wang, M., Peng, J., Li, Y., et al. (2012). Therapeutic effects of neuregulin-1 gene transduction in rats with myocardial infarction. Coron. Artery Dis. 23 (7), 460–468. doi:10.1097/MCA.0b013e32835877da
Xiao, Y., Hill, M. C., Zhang, M., Martin, T. J., Morikawa, Y., Wang, S., et al. (2018). Hippo signaling plays an essential role in cell state transitions during cardiac fibroblast development. Dev. Cell 45 (2), 153–169. doi:10.1016/j.devcel.2018.03.019
Xin, M., Kim, Y., Sutherland, L. B., Murakami, M., Qi, X., McAnally, J., et al. (2013). Hippo pathway effector Yap promotes cardiac regeneration. Proc. Natl. Acad. Sci. U. S. A. 110 (34), 13839–13844. doi:10.1073/pnas.1313192110
Xin, M., Kim, Y., Sutherland, L. B., Qi, X., McAnally, J., Schwartz, R. J., et al. (2011). Regulation of insulin-like growth factor signaling by yap governs cardiomyocyte proliferation and embryonic heart size. Sci. Signal. 4 (196), ra70. doi:10.1126/scisignal.2002278
Xin, M., Kim, Y., Sutherland, L. B., Qi, X., McAnally, J., Schwartz, R. J., et al. (2011). Regulation of insulin-like growth factor signaling by Yap governs cardiomyocyte proliferation and embryonic heart size. Sci. Signal. 4 (196), ra70. doi:10.1126/scisignal.2002278
Xu, G., Watanabe, T., Iso, Y., Koba, S., Sakai, T., Nagashima, M., et al. (2009). Preventive effects of heregulin-beta1 on macrophage foam cell formation and atherosclerosis. Circ. Res. 105 (5), 500–510. doi:10.1161/CIRCRESAHA.109.193870
Xu, M., Wu, X., Jie, B., Zhang, X., Zhang, J., Xin, Y., et al. (2014). Neuregulin-1 protects myocardial cells against H2 O2 -induced apoptosis by regulating endoplasmic reticulum stress. Cell biochem. Funct. 32 (5), 464–469. doi:10.1002/cbf.3038
Xu, Y., Li, X., Liu, X., and Zhou, M. (2010). Neuregulin-1/ErbB signaling and chronic heart failure. Adv. Pharmacol. 59, 31–51. doi:10.1016/S1054-3589(10)59002-1
Yamamoto, S., Yang, G., Zablocki, D., Liu, J., Hong, C., Kim, S. J., et al. (2003). Activation of Mst1 causes dilated cardiomyopathy by stimulating apoptosis without compensatory ventricular myocyte hypertrophy. J. Clin. Invest. 111 (10), 1463–1474. doi:10.1172/JCI17459
Yamamoto, , Yang, G., Zablocki, D., Liu, J., Hong, C., Kim, S. J., et al. (2003). Activation of Mst1 causes dilated cardiomyopathy by stimulating apoptosis without compensatory ventricular myocyte hypertrophy. J. Clin. Invest. 111 (10), 1463–1474. doi:10.1172/JCI17459
Yarden, Y., and Sliwkowski, M. X. (2001). Untangling the ErbB signalling network. Nat. Rev. Mol. Cell Biol. 2 (2), 127–137. doi:10.1038/35052073
Yiadom, M. Y., Greenberg, J., Smith, H. M., Sawyer, D. B., Liu, D., Carlise, J., et al. (2016). Diagnostic utility of neuregulin for acute coronary syndrome. Dis. Markers 2016, 8025271. doi:10.1155/2016/8025271
Yin, D. M., Chen, Y. J., Liu, S., Jiao, H., Shen, C., SAthyAmurthy, A., et al. (2015). Calcyon stimulates neuregulin 1 maturation and signaling. Mol. Psychiatry 20 (10), 1251–1260. doi:10.1038/mp.2014.131
Yin, H., Favreau-Lessard, A. J., deKay, J. T., Herrmann, Y. R., Robich, M. P., Koza, R. A., et al. (2021). Protective role of ErbB3 signaling in myeloid cells during adaptation to cardiac pressure overload. J. Mol. Cell. Cardiol. 152, 1–16. doi:10.1016/j.yjmcc.2020.11.009
Zhang, D., Contu, R., Latronico, M. V. G., Zhang, J., Zhang, J. L., Rizzi, R., et al. (2010). MTORC1 regulates cardiac function and myocyte survival through 4E-BP1 inhibition in mice. J. Clin. Invest. 120 (8), 2805–2816. doi:10.1172/JCI43008
Zhang, J., Ji, J. Y., Yu, M., Overholtzer, M., Smolen, G. A., Wang, R., et al. (2009). YAP-dependent induction of amphiregulin identifies a non-cell-autonomous component of the Hippo pathway. Nat. Cell Biol. 11 (12), 1444–1450. doi:10.1038/ncb1993
Zhang, P., Kuang, H., He, Y., Idiga, S. O., Li, S., Chen, Z., et al. (2018). NRG1-Fc improves metabolic health via dual hepatic and central action. JCI Insight 3 (5), 98522. doi:10.1172/jci.insight.98522
Zhang, Y., and Re, D. D. (2017). A growing role for the Hippo signaling pathway in the heart. J. Mol. Med. 95 (5), 465–472. doi:10.1007/s00109-017-1525-5
Zhao, W. J., and Schachner, M. (2013). Neuregulin 1 enhances cell adhesion molecule l1 expression in human glioma cells and promotes their migration as a function of malignancy. J. Neuropathol. Exp. Neurol. 72 (3), 244–255. doi:10.1097/NEN.0b013e3182863dc5
Zhao, Y. Y., FerOn, O., Dessy, C., Han, X., Marchionni, M. A., and Kelly, R. A. (1999). Neuregulin signaling in the heart. Dynamic targeting of erbB4 to caveolar microdomains in cardiac myocytes. Circ. Res. 84 (12), 1380–1387. doi:10.1161/01.res.84.12.1380
Zhao, Y. Y., Sawyer, D. R., Baliga, R. R., Opel, D. J., Han, X., Marchionni, M. A., et al. (1998). Neuregulins promote survival and growth of cardiac myocytes. Persistence of ErbB2 and ErbB4 expression in neonatal and adult ventricular myocytes. J. Biol. Chem. 273 (17), 10261–10269. doi:10.1074/jbc.273.17.10261
Zhu, W. Z., Xie, Y., Moyes, K. W., Gold, J. D., Askari, B., and Laflamme, M. A. (2010). Neuregulin/ErbB signaling regulates cardiac subtype specification in differentiating human embryonic stem cells. Circ. Res. 107 (6), 776–786. doi:10.1161/CIRCRESAHA.110.223917
Zurek, M., Johansson, E., Palmer, M., Albery, T., Johansson, K., Ryden-Markinhutha, K., et al. (2020). Neuregulin-1 induces cardiac hypertrophy and impairs cardiac performance in post-myocardial infarction rats. Circulation 142 (13), 1308–1311. doi:10.1161/CIRCULATIONAHA.119.044313
Glossary
ADAM a disintegrin and metalloproteinase
AREG amphiregulin
ATG5 autophagy related 5
AMI acute myocardial infarction
ACAT-1 acyltransferase-1
COX-2 cyclooxygenase 2
CRD cystein-rich domain
cMLCK cardiac myosin light chain kinase
ECD extracellular structural domain
EGF epidermal growth factor
eNOS Endothelial nitric oxide synthase
ERK extracellular signal-regulated kinase
EPDCs epicardial derived cells
ECM extracellular matrix
FGF2 fibroblast growth factor 2
GSK3β glycogen synthase kinase 3β
GLUT4 glomerular glucose transporter 4
GPx1 glutathione peroxidase 1
HF heart failure
HIF-1α hypoxia-inducible factor-1α
IR ischemia/reperfusion
ICD intracellular structural domain
IGF insulin-like growth factor
LATSs large tumor suppressor kinases
LVEF left ventricular ejection fraction
LV left ventricular
LDL low-density lipoprotein
MI myocardial infarction
MMP matrix metalloproteinases
MSTs Mammalian sterile 20-like kinases
MOBs mps one binder kinase activator proteins
mTOR rapamycin
MEK mitogen-extracellular activated protein kinase
MAPK adenosine monophosphate-activated protein kinase
NRG-1 Neuregulin-1
PI3K phosphatidylinositol-3-kinase
Pik3cb phosphatidylinositol-4,5-bisphosphate 3-kinase catalytic subunit beta
Akt-AS160 Akt substrate of 160 kDa (AS160)
RASSFs ras associated domain family
PDK1 phosphatidylinositol-dependent kinase 1
rhNRG1 recombinant human NRG1
ROS reactive oxygen species
RIPC remote ischemic preconditioning
SAV1 salvador homolog 1
SASPs senescence-associated secretory phenotypes
SIPS stress-induced premature senescence
TAZ transcriptional coactivator with PDZ-binding motif
TEAD transcriptional enhanced associate domain
Trx2 thioredoxin-2
VEGFA vascular endothelial growth factor A
VSMCs vascular smooth muscle cells
YAP yes-associated protein
VEGF vascular endothelial growth factor
Keywords: cardiomyocyte proliferation, cardiac repair, cardiovascular disease, neuregulin-1 (Nrg1), therapeutic target
Citation: Wang Y, Wei J, Zhang P, Zhang X, Wang Y, Chen W, Zhao Y and Cui X (2022) Neuregulin-1, a potential therapeutic target for cardiac repair. Front. Pharmacol. 13:945206. doi: 10.3389/fphar.2022.945206
Received: 18 May 2022; Accepted: 01 August 2022;
Published: 31 August 2022.
Edited by:
Prasanth Puthanveetil, Midwestern University, United StatesReviewed by:
Attila Kiss, Medical University of Vienna, AustriaFabio Rocha Formiga, University of Pernambuco (UPE), Brazil
Copyright © 2022 Wang, Wei, Zhang, Zhang, Wang, Chen, Zhao and Cui. This is an open-access article distributed under the terms of the Creative Commons Attribution License (CC BY). The use, distribution or reproduction in other forums is permitted, provided the original author(s) and the copyright owner(s) are credited and that the original publication in this journal is cited, in accordance with accepted academic practice. No use, distribution or reproduction is permitted which does not comply with these terms.
*Correspondence: Yanan Zhao, 71001818@sdutcm.edu.cn; Xiangning Cui, cuixiangning@126.com
†These authors share first authorship