- 1Pharmaceutical Sciences Research Center, Department of Pharmacy, Children’s Hospital of Nanjing Medical University, Nanjing, China
- 2School of Basic Medicine and Clinical Pharmacy, China Pharmaceutical University, Nanjing, China
- 3Visiting Graduate Student from School of Basic Medicine and Clinical Pharmacy, Pharmaceutical University, Nanjing, China
- 4Institute of Pharmaceutical Science, China Pharmaceutical University, Nanjing, China
- 5School of Institute of Pharmaceutical Science, Pharmaceutical University, Nanjing, China
- 6Hematology and Oncology Department, Children’s Hospital of Nanjing Medical University, Nanjing, China
- 7Department of Solid Oncology, Children’s Hospital of Nanjing Medical University, Nanjing, China
Thiopurines, including thioguanine (TG), 6-mercaptopurine (6-MP), and azathioprine (AZA), are extensively used in clinical practice in children with acute lymphoblastic leukemia (ALL) and inflammatory bowel diseases. However, the common adverse effects caused by myelosuppression and hepatotoxicity limit their application. Metabolizing enzymes such as thiopurine S-methyltransferase (TPMT), nudix hydrolase 15 (NUDT15), inosine triphosphate pyrophosphohydrolase (ITPA), and drug transporters like multidrug resistance-associated protein 4 (MRP4) have been reported to mediate the metabolism and transportation of thiopurine drugs. Hence, the single nucleotide polymorphisms (SNPs) in those genes could theoretically affect the pharmacokinetics and pharmacological effects of these drugs, and might also become one of the determinants of clinical efficacy and adverse effects. Moreover, long-term clinical practices have confirmed that thiopurine-related adverse reactions are associated with the systemic concentrations of their active metabolites. In this review, we mainly summarized the pharmacogenetic studies of thiopurine drugs. We also evaluated the therapeutic drug monitoring (TDM) research studies and focused on those active metabolites, hoping to continuously improve monitoring strategies for thiopurine therapy to maximize therapeutic efficacy and minimize the adverse effects or toxicity. We proposed that tailoring thiopurine dosing based on MRP4, ITPA, NUDT15, and TMPT genotypes, defined as “MINT” panel sequencing strategy, might contribute toward improving the efficacy and safety of thiopurines. Moreover, the DNA-incorporated thioguanine nucleotide (DNA-TG) metabolite level was more suitable for red cell 6-thioguanine nucleotide (6-TGNs) monitoring, which can better predict the efficacy and safety of thiopurines. Integrating the panel “MINT” sequencing strategy with therapeutic “DNA-TG” monitoring would offer a new insight into the precision thiopurine therapy for pediatric acute lymphoblastic leukemia patients.
Introduction
Thioguanine (TG), 6-mercaptopurine (6-MP), and azathioprine (AZA), collectively known as thiopurines, are broadly applied as anti-cancer and immunosuppressive agents. 6-MP is one of the backbone drugs for the maintenance therapy of acute lymphoblastic leukemia (ALL) in children and adults (Schmiegelow et al., 2014). 6-MP and AZA are also commonly prescribed in maintaining the clinical remission of patients with steroid-dependent inflammatory bowel diseases (IBD) (Andoh et al., 2021). Thiopurines are pro-drugs devoid of any intrinsic activity and need metabolic transformation to their pharmacologically active metabolites, 6-thio-guanine nucleotides (6-TGNs), which are structurally similar to the endogenous purine-base guanine, are fraudulent bases being integrated into the DNA of leucocytes, leading to the inhibition of purine de novo synthesis and cell death (Karran and Attard, 2008). It is worth noting that, while thioguanine enters the DNA to play a therapeutic role, it also creates conditions for the occurrence of common and serious adverse reactions. Hepatotoxicity, pancreatitis, gastric intolerance, and leukopenia are common adverse drug reactions associated with thiopurines (Koren et al., 1990; Lee et al., 2022). In particular, life-threatening leukopenia, caused by thiopurines, might interrupt or even discontinue the effective treatment, resulting in a high risk of subsequent disease recurrence in ALL (Fotoohi et al., 2010). Hence, the narrow therapeutic index of thiopurines indicates an urgent demand for us to utilize precision medicine strategies to make better use of these drugs.
Polymorphisms of genes encoding various drug-metabolizing enzymes and transporters could exert an effect on the efficacy and toxicity of thiopurines. In fact, the first pharmacogenetic marker by means of a pharmacology-guided approach was recognized as single nucleotide polymorphisms (SNPs) in thiopurine S-methyltransferase (TPMT) (Weinshilboum and Sladek, 1980), and its predictive role in 6-MP-related adverse effects is regarded as an important development in this field. In recent years, other related enzymes including Nudix hydrolase 15 (NUDT15) (Yang et al., 2014), inosine triphosphate pyrophosphohydrolase (ITPA) (Barba et al., 2022), and multidrug resistance-associated protein (e.g., MRP4) (Wielinga et al., 2002) have been reported to dramatically affect the pharmacokinetics and pharmacological properties of thiopurines, thereby becoming critical determinants of their therapeutic efficacy and toxicity. However, the significant differences in the distribution and frequency of these SNPs among different ethnic groups pose certain challenges for a practical clinical application of genotype-based precision therapy.
Previously, monitoring the drug concentration of thioguanine nucleotides (TGNs) in red blood cells (RBCs) has played an important role in maximizing the clinical efficacy and reducing adverse effects of these drugs, and has also been helpful for individualized dose adjustment (Nygaard et al., 2004; Hedeland et al., 2010; Nielsen et al., 2016). Unfortunately, there is a broad agreement that too high or too low TGN concentrations should be avoided, but the exact definition of both upper and lower limits, even if such rigid threshold values should be generally used at all, is still debated in different studies. Furthermore, as RBCs are not the active target of thiopurines, some researchers proposed that the cytotoxicity of thiopurines was caused by the incorporation of thioguanine nucleotides into the DNA, called DNA-TG, the true culprit in the nucleus. The concentration of DNA-TG has been quantified by using liquid chromatography–mass spectrometry (Moriyama et al., 2017; Larsen et al., 2021a). Therefore, DNA-TG monitoring is much more helpful in evaluating an inadequate dose or non-adherence, or distinguishing patients with abnormal metabolic spectrums (Nielsen et al., 2017).
In this review, we summarize the pharmacogenetic studies related to those enzymes and transporters taking responsibility for the disposition of thiopurines, focusing on the association between SNPs and the clinical efficacy and side effects of thiopurines. We also review the current status of the TDM of thiopurines in children with ALL. Combining “MINT” panel sequencing with DNA-TG monitoring is a more feasible strategy when implementing thiopurine precision medicine for those pediatric patients.
Metabolism and material action basis of thiopurines
As prodrugs that lack intrinsic activity or with low activity, thiopurines need to be transformed to 6-TGNs inside the cells through multi-enzymatic reactions and then exert pharmacological effects. The three major metabolic pathways of 6-MP are well described (Figure 1). Xanthine oxidase (XO) and thiopurine-S-methyltransferase (TPMT) are the two dominant enzymes in the metabolism of 6-MP, and produce inactive metabolites 6-thiouric acid and 6-methylmercaptopurine (6-MMP), respectively. However, the formation of 6-TGNs is a “long journey” involving multiple enzymes. 6-MP is first converted into thioIMP (6-TIMP) by hypoxanthine-guanine phosphoribosyltransferase (HGPRT), and finally, 6-TIMP metabolized to 6-TGNs, the active forms, which are successively mediated by hypoxanthine monophosphate dehydrogenase (IMPDH) and guanylate synthase (GMPS), respectively. Meanwhile, the multidrug resistance protein 4 (MRP4) can pump these active metabolites out of the cells. In this journey, TPMT can also metabolize intermediate 6-TIMP into methyl-thioIMP (6-MTIMP), an active metabolite that can suppress de novo purine synthesis, and has been reported to have an association with hepatotoxicity (Adam de Beaumais et al., 2011). 6-TIMP also can be catalyzed into 6-thioinosine diphosphate (6-TIDP) by monophosphate kinase (MPK), and into 6-thioinosine triphosphate (6-TITP) by diphosphate kinase (DPK), and finally back to 6-TIMP by ITPA forming a complete cycle.
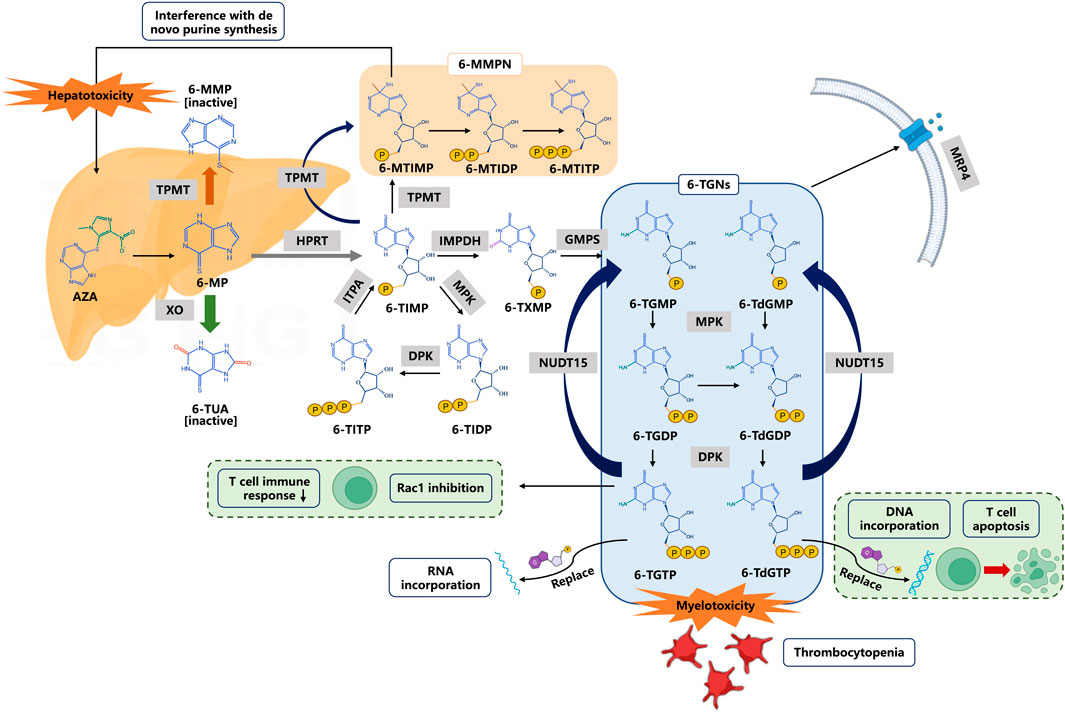
FIGURE 1. Thiopurine metabolism and transportation. 6-Thioguanine nucleotides (6-TGNs) are pharmacologic active products. 6-TGMP 6-thioguanine monophosphate, 6-TGDP 6-thioguanine diphosphate, 6-TGTP 6-thioguanine triphosphate, 6-TdGMP 6-thio-deoxyguanine monophosphate, 6-TdGDP 6-thio-deoxyguanine diphosphate, 6-TdGTP 6-thio-deoxyguanine triphosphate. Abbreviations: AZA azathioprine, 6-MP 6-mercaptopurine, 6-MMP 6-methylmercaptopurine, 6-TUA 6-thiouric acid, 6-TIMP 6-thioinosine monophosphate, 6-MMPN 6-methylmercaptopurine nucleotides, 6-MTIMP 6-methylthioinosine 5′-monophosphate, 6-MTIDP 6-methylthioinosine 5’ -diphosphate, 6-MTITP 6-methylthioinosine 5′-triphosphate, 6-TIDP 6-thioinosine diphosphate, 6-TITP 6-thioinosine triphosphate, 6-TXMP 6-thioxanthosine monophosphate. Enzymes or transporters are shown in gray boxes: TPMT thiopurine S-methyl transferase, XO xanthine oxidase, HPRT hypoxanthine phosphoribosyl transferase, MPK monophosphate kinase, DPK diphosphate kinase, ITPA inosine triphosphate pyrophosphohydrolase, IMPDH inosine monophosphate dehydrogenase, GMPS guanosine monophosphate synthetase, and MRP4 multidrug resistance-associated protein 4.
6-TGNs are active metabolites, consisting of 6-thioguanine monophosphate (6-TGMP), 6-thio-deoxyguanine monophosphate (6-TdGMP), 6-thioguanine diphosphate (6-TGDP), 6-thio-deoxyguanine diphosphate (6-TdGDP), 6-thioguanine triphosphate (6-TGTP), and 6-thio-deoxyguanine triphosphate (6-TdGTP). The aforementioned 6 metabolites are catalyzed by MPK, DPK, and NUDT15, respectively, to form another metabolic cycle. On one hand, 6-TdGTPs are integrated into DNA (6-TdGTP) and RNA (6-TGTP), leading to nucleotide suppression and protein synthesis and cause cell apoptosis (Paugh et al., 2010). On the other hand, 6-TGTPs have been reported to block the activity of Vav on RAC proteins and thus to prevent the development of an effective immune response in T cells (Poppe et al., 2006). It is generally accepted that the adverse reactions of thiopurines are closely associated with those active metabolites, especially high levels of 6-TGNs resulting in dose-dependent side effects typified by leukopenia (Relling et al., 1999). Therefore, the genetic variation of key enzymes in metabolic transformations (such as TPMT, ITPA, and NUDT15) would have an important impact on the pharmacokinetics, pharmacodynamics, and side effects of thiopurines.
Pharmacogenetics of thiopurines
Thiopurine S-methyltransferase
As one of the most critical enzymes in the biotransformation of thiopurines, TPMT is the earliest and most comprehensive pharmacogenetic predictor used in clinical practice. Loss of the TPMT function leads to excessive levels of 6-TGNs, which greatly increase the risk of leukopenia. In 1980, Weinshiboum and Sladek first reported the significant individual variations of erythrocyte TPMT activity in Caucasians, with 88.6% of high, 11.1% of intermediate, and 0.3% of undetectable activities, respectively (Weinshilboum and Sladek, 1980). Since then, this classification method has been widely used. Moreover, SNPs resulting in loss of function of the TPMT have also been identified (Ansari et al., 2002). More than 40 allelic variants (*2–*44) in the TPMT gene have been discovered (Iu et al., 2017; Zimdahl Kahlin et al., 2019), but the loss-of-function variants mainly include TPMT*2, TPMT*3A, TPMT*3B, and TPMT*3C (Azimi et al., 2014). Therefore, the TPMT phenotype is usually determined by the aforementioned mutant variants. Especially, TPMT genotypes *3A and *3C had the strongest associations with toxicity (Rudin et al., 2017). In 2011, the Clinical Pharmacogenetics Implementation Consortium (CPIC) guidelines for TPMT genotype and thiopurine dosing (Relling et al., 2011) recommend pre-emptive genotyping for TPMT before the initiation of thiopurine treatment, and that patients with heterozygous *1 allele and with *2/*3A/*3C/*4 alleles (intermediate metabolizers) should start at 30–70% of the full dose (6-MP 50 mg/m2/day or 0.75 mg/kg/day). However, for poor metabolizers, the thiopurine dose should be purposefully started with 10% of the full dose, and administered weekly thrice instead of daily. This approach has been proven to decrease the incidence of acute toxicity while having no negative effect on the relapse rates in ALL (Relling et al., 2006).
Despite the success of TPMT pharmacogenetic testing, the most important issue is that the prevalence of loss-of-function TPMT alleles varies across ethnic groups (Table 1, https://www.pharmgkb.org). In Europeans and African Americans, the common alleles that cause TPMT deficiency are TPMT*2, *3A, *3B, and *3C, while in Asians, *3C is the most common mutant allele. A recent extensive whole-genome re-sequencing study in 3,554 Japanese showed that alleles *3A and *3B were absent (not observed), and only allele *3C was confirmed to be present (0.96%) (Nagasaki et al., 2015; Yamaguchi-Kabata et al., 2015). Furthermore, in a study including 253 Chinese patients, none of the three alleles *2, *3A, and *3B were observed, and the allelic frequency of *3C was 1.6% (Zhu et al., 2016). In this study, leukopenia occurred in 25.7% of patients, and no strong association was observed between the disease and *3C genotypes. Clearly, the low-mutation frequency (<2%) of variants in the TPMT gene did not explain the high frequency of adverse reactions (>20%) occurring in East Asian populations, suggesting that pre-emptive TPMT genotyping in Asians might not be the same as that in Caucasians. On the other hand, there are a plethora of genetic variants (*1∼*44) of TPMT as mentioned previously, and it is hard to determine a patient’s exact genotype solely based on one commercial kit which usually cannot provide all target variations. Moreover, other genetic variants may contribute to inter-patient variability. Hopefully, those problems would be readily solved as testing costs are reduced and pharmacogenetic implementations become very popular.
Another concern is that results from a pharmacogenetic test are incapable of covering the other co-factors contributing to the TPMT phenotype such as age, renal insufficiency, or drug–drug interactions (Wu et al., 2019). From this perspective, measuring TPMT activity is a more accurate strategy for predicting the appropriate dose of thiopurines than genotyping test. Actually, TPMT phenotype testing is fairly common in some countries (Fargher et al., 2007; Weitzel et al., 2018). However, it is worth noting that TPMT enzyme activity is usually measured in fresh red blood cells directly (Mokhtari et al., 2020), or consumes a longer turnaround test time (Weitzel et al., 2018). Moreover, in patients who have received blood transfusions recently or in leukemia patients, because of atypical hematopoiesis, the result could not reflect the true enzymatic activity (Mokhtari et al., 2020).
Interestingly, genotype–phenotype discordance was reported at a rate of 5% in patients who underwent both tests (Weitzel et al., 2018). In patients with a discordant situation, the use of genotypes to guide thiopurine dosing is consistent with a previous study that states that optimum accuracy of the TPMT genotype test was achieved as compared with the enzyme activity assay (Donnan et al., 2011).
Nucleoside diphosphate-linked moiety X-type motif 15
In 2014, Yang and others first reported that NUDT15 (rs116855232, referred to as c.415C > T or p.R139C variant hereafter) was highly associated with thiopurine-induced leukopenia among Korean patients who suffered from IBD (Yang et al., 2014). Subsequently, loss-of-function NUDT15 diplotypes were found to be consistently related to the intolerance of thiopurine during ALL therapy (Yang et al., 2015; Moriyama et al., 2016). Based on these studies, CPIC in 2018 updated its guidelines for the dosage adjustment of 6-MP, suggesting that ALL patients should be guided by TPMT and NUDT15 genotyping for the initial dose selection of 6-MP (Relling et al., 2019).
NUDT15 is a purine-specific nudix hydrolase that controls the hydrolysis of nucleosides–diphosphates (Gad et al., 2014). This enzyme catalyzes the conversion of TGTP and deoxy-TGTP (TdGTP) metabolites to the low-toxic TGMP and deoxy-TGMP (TdGMP), respectively (Valerie et al., 2016). Defective NUDT15-mediated degradation of TdGTP results in more available TdGTP which can be used for the integration into the DNA (namely DNA-TG, the primary anti-leukemic metabolite). Simultaneously, NUDT15 deficiency also results in more TGTP, which promotes the binding of TGTP to Rac1 and also promotes the transportation of TGTP into the RNA. Therefore, it is hypothesized that NUDT15 negatively regulates thiopurine activation and its cytotoxicity (Moriyama et al., 2016). Although 6-MP-induced leukopenia is known to be related to increased 6-TGNs levels, no significant difference was found between the 6-TGN levels and NUDT15 variants (Asada et al., 2016). Interestingly, results obtained from a series of model systems in a Moriyama laboratory and ALL patients jointly indicated that NUDT15 deficiency directly led to the excessive DNA-TG levels and increased adverse effects (Moriyama et al., 2016). Therefore, the DNA-TG metabolite levels are preferred over 6-TGNs in forming NUDT15 genotype-guided dose adjustments (Moriyama et al., 2017).
As the first NUDT15 SNP linked to thiopurine toxicity, p.R139C was the most studied in patients receiving thiopurine therapy, and the results showed that NUDT15 p.R139C mutation had no influence on the enzymatic activity. Instead, it negatively affected the protein stability (Valerie et al., 2016). In children diagnosed with ALL, the tolerability of homozygotes for the p.R139C variant allele was only 8% of the standard dose of 6-MP, while the tolerable dosages were 63 and 83.5% of the standard doses of 6-MP, respectively, in those patients with heterozygous and wild-type genotypes (Yang et al., 2015). To date, a total of 20 haplotypes with star allele names of NUDT15 (*1-*20) have been identified (Moriyama et al., 2016; Moriyama et al., 2017; Schaeffeler et al., 2019) (https://www.pharmvar.org/gene/NUDT15). It was found that in Chinese IBD patients, the predictive sensitivity of NUDT15 p.R139C was 49.2 %. But, the combined analysis of Val18Ile and p.Val18_Val19insGlyVal, designed to identify diplotypes by detecting haplotypes *5 and *6, successfully increased the sensitivity to 55.4 % (Chao et al., 2017). However, compared with p.R139C, other variants are rare, and their correlations with thiopurine-induced toxicity in clinical practice remain unclear. As shown in Table 1, the frequency of the decreased function NUDT15 variants is higher in Asians and Hispanics, but lower in Europeans and Africans. Interestingly, the frequency is just reverse for TPMT variants. Therefore, as the CPIC guideline recommended, if genetic tests are available for only one gene (TPMT or NUDT15, but not both), the matched decision-making will be implemented by clinical labs. More importantly, the potential association of rare mutations with efficacy and adverse reactions of thiopurines remains to be confirmed by large cohort studies.
Inosine triphosphate pyrophosphohydrolase
ITPA can catalyze the phosphorylation of inosine triphosphate (ITP) and convert the latter to inosine monophosphate (IMP), which is a key substance in the purine metabolism. In the metabolism of 6-MP, ITPA is the catalyst that helps to complete the hydrolysis process from 6-TITP to 6-TIMP, and the ITPA deficiency leads to an abnormal accumulation of 6-TITP, which results in toxicity (Marinaki et al., 2004). There are 11 ITPA variants that have been reported (Sakamoto et al., 2020), and the two most common SNPs are 94C > A as well as IVS2 + 21A > C. Several studies have examined the role of these two variants in the ITPA gene with 6-MP metabolism, as well as adverse drug reactions including hepatotoxicity, flu-like symptoms, arthralgia, and pancreatitis (Arenas et al., 2007; Zabala-Fernandez et al., 2011; Wroblova et al., 2012) with promising results. However, consistent with TPMT polymorphism, the frequency of the ITPA (94C > A) A allele differed significantly by ethnicity and was higher in Asians (11–19%) than in Caucasian, Hispanics, and Africans (1–7%) (Okada et al., 2009). Interestingly, the reverse situation would appear for the frequency of the ITPA 94C > A allele and TPMT polymorphisms in the same populations (Marsh and Van Booven, 2009).
Naturally, research studies on the relationship between the ITPA gene polymorphism and the adverse reactions of thiopurines are inconsistent partly due to the ethnic differences in allele frequencies (Table 2). For instance, Uchiyama found that the ITPA 94C > A mutation occurred more frequently than TPMT variants in Japanese patients diagnosed with thiopurine-induced leukopenia (Uchiyama et al., 2009). Also, ALL patients with allele ITPA 94A were more likely to suffer from fever and hepatotoxicity from 6-MP, while the prevalence of TPMT variants was too low to be well applied in Malays, Chinese, and Indian populations (Wan Rosalina et al., 2012). Therefore, Asians may be more sensitive to the toxicity of AZA/6-MP based on the ITPA mutation than TPMT. However, a lack of association between the ITPA 94C > A polymorphism and AZA-related adverse effects was found in a New Zealander (Gearry et al., 2004). In addition, ITPA genotyping has no predictive significance for the clarity and development of the AZA side effects (van Dieren et al., 2005). In the Netherlands, the mean doses of 6-MP did not differ in ALL patients with or without ITPA variants (Kouwenberg et al., 2020). Another study came to a similar conclusion (Wahlund et al., 2020). The aforementioned results suggested that, before the beginning of maintenance treatment for ALL in these populations, the ITPA genotype should not to be regarded as a part of the accepted assessment.
Stocco and others analyzed a group of St. Jude patients with ALL whose 6-MP doses were not adjusted based on their TPMT genotype or TGN concentrations. Notably, the probability of grade 3–4 infections was not significantly related to the ITPA genotype in the aforementioned conditions. But if the doses were tailored for TPMT and ITPA, then they had a great effect on the likelihood of febrile neutropenia (Stocco et al., 2010). According to the authors, most studies suggested that the dose of 6-MP taken by patients was not fully adjusted for the TPMT genotype. Their results revealed that it might be the cause of why the influence of the ITPA genotype had been inconsistent in previous studies. Some researchers tend to suggest that physicians should first ponder over the genotyping for ITPA variants, together with TPMT and NUDT15, before deciding to treat a patient with 6-MP (Moradveisi et al., 2019). Of note, the association between ITPA 94 C > A and neutropenia in children with ALL was verified in recent systematic reviews and meta-analysis (Barba et al., 2022; Lee et al., 2022). A total of 1,072 and 974 ALL pediatric patients were included in the meta-analysis, respectively. Specifically, pediatric ALL patients with an ITPA 94 C > A variant had an approximately 2.5 times higher risk of suffering from neutropenia (Barba et al., 2022; Lee et al., 2022). Moreover, due to the existing ethnic differences in the ITPA 94 C > A mutation frequency, both the studies had stratified their data analysis based on the races. For neutropenia, the results did not show any different outcome between Asians and Caucasians (Barba et al., 2022), while for hepatotoxicity, the 94C > A variant was significantly associated with an increased risk in Asians and Middle Easterners (Lee et al., 2022).Moreover, IBD patients with ITPA variant alleles exhibited higher 6-TGN levels than those with the wild-type allele (Luo et al., 2022). These findings support that ITPA polymorphisms could be used as predictive biomarkers for thiopurine-related adverse effects. Nevertheless, considering the variable frequency across different ethnicities, the clinical implementation of ITPA gene tests for precision thiopurine treatment also warrants further studies.
Multi-drug resistance protein 4
MRP4 is a member of the ATP-binding cassette transporter family, encoded by the ABCC4 gene, responsible for transporting monophosphorylated nucleosides (Wielinga et al., 2002). Murine models with MRP4 deficiency confirmed that MRP4 protects against thiopurine-induced hematopoietic toxicity by reducing the accumulation of intracellular TGNs (Takenaka et al., 2007; Krishnamurthy et al., 2008). In clinics, Hiromistu Ban and others were the first to demonstrate an association of MRP4 G2269A (rs3765534) polymorphisms with thiopurine sensitivity (Ban et al., 2010). The authors found that patients with the MRP4 variant had higher 6-TGN levels in erythrocytes and a higher risk of leukopenia, compared with patients with the wild-type alleles. In addition, another study highlighted the significance of MRP4 polymorphisms related to 6-MP dose tolerance in ALL maintenance therapy (Tanaka et al., 2015). Moreover, ALL patients with intermediate active NUDT15 and ABCC4 variants experienced higher 6-MP intolerability, compared with the group with either of the variants (Tanaka et al., 2018). In a very recent study, the co-occurrence of the ABCC4 (c.912G > T, rs2274407) and ITPA (c.94C > A) variants in 145 Chinese children with ALL witnessed a significant positive association with 6-MP intolerance (Fan et al., 2022). Meanwhile, ABCC4 c.2128G > A (rs3765534) carriers experienced a significant increase in the DNA-TG to 6-MP dose ratio, which was associated with a high risk of leukopenia (Fan et al., 2022). Similarly, ABCC4 SNP rs2274407 was found to be related to the increased 6-TGN to 6-MP dose ratio (Choi et al., 2019). In addition, a study in Thai ALL pediatric patients found that the average absolute neutrophil count (ANC) at the 6th month of the maintenance phase was significantly lower in ABCC4 SNP rs3765534 carriers, compared to patients carrying wild-type alleles, and the risk of grade 4 neutropenia was higher in ABCC4 GA carriers than wild-type patients, but was not statistically significant (Khaeso et al., 2022). Of note, the allele frequency of variants in ABCC4 showed ethnic difference (Table 2), and more evidence needs to be accumulated to establish the potential association between ABCC4 variants and 6-MP-induced adverse effects in the future.
Other genetic polymorphisms
In 2012, Stocco’s team first reported that PACSIN2 (protein kinase C and casein kinase II interacting protein-2 or Syndapin 2) was the most fundamental trans-acting gene. PACSIN2 has also been shown to be a genetic variant which could affect TPMT activity (Stocco et al., 2012). Moreover, a SNP of PACSIN2 (rs2413739) was closely associated with severe gastrointestinal toxicity in children with ALL treated with 6-MP. Recently, another study confirmed these results (Franca et al., 2020). Furthermore, PACSIN2 polymorphism was shown to be linked with thiopurine-induced hematological toxicity in children receiving maintenance therapy aimed at treating ALL (Smid et al., 2016). As an earlier study had identified, PACSIN2 was a Rac1 interactor that regulated the diffusion of Rac1-mediated cells (de Kreuk et al., 2011). Therefore, the interaction of PACSIN2 with Rac1 might increase the likelihood of hematotoxicity, resulting in increased sensitivity of cells to 6-MP, thereby exposing patients to a higher risk of hematotoxicity.
Cytosolic 5′-nucleotidase II (NT5C2) is an allosteric catabolic enzyme that hydrolyzes IMP, GMP, and AMP. The thiopurine nucleotides thio-GMP, thio-IMP, and methylthioIMP are all converted by NT5C2 (Brouwer et al., 2005). Increased in vitro nucleotidase activity has been identified in NT5C2 mutant proteins. Also, when expressed in ALL lymphoblasts, these proteins were resistant to the chemotherapy with 6-MP/6-TG (Tzoneva et al., 2013). Tulstrup and others found that the thiopurine metabolism can be altered by NT5C2-germline variants associated with acquired recurrent NT5C2 mutations in childhood acute lymphoblastic leukemia (Tulstrup et al., 2018). Moreover, sub-clonal NT5C2 mutations determine relapses related to the high risks in treatment failure in patients, while emphasizing their complicated role in the outcome over mutant NT5C2, which acts as a targetable driver during relapse progression. Therefore, a thorough, rigorous, and retrospective study is warranted so that we could identify NT5C2 mutations, further deepening our understanding and better treating the relapse subtype of aggressive ALL (Barz et al., 2020).
Recently, folate metabolic variants including thymidylate synthetase (TYMS) and dihydrofolate reductase (DHFR) were shown to be potential biomarkers of 6-MP-induced myelotoxicity, which could be employed for the individualization of 6-MP therapy when childhood ALL treatment reaches its maintenance phase (Milosevic et al., 2019). In Chinese pediatric patients, the MTHFR rs1801133 variant was found to have a 4.46-fold higher risk of hepatotoxicity than the wild-type genotype (Zhou et al., 2020). In this study, the authors also found that IMPDH1 (rs2278293) was associated with a high risk of leukopenia (Zhou et al., 2020), which is consistent with Rihwa Choi’s earlier study in Korean ALL patients (Choi et al., 2019). Moreover, Rihwa Choi and others analyzed 103 SNPs and found that, in addition to the TPMT genotype, thiopurine metabolism and any adverse effects were linked with a total of 32 SNPs in 24 genes (Choi et al., 2019). A series of genetic polymorphisms such as ADK, ATIC, GART, GMPS, GSTP1, SLC29A1, KCNMA1, SLC19A1, MOCOS, MTRR, SLC28A3, SLCO1B1, and XDH have been linked with thiopurine-related adverse effects that have not been previously assessed.
Generally, the association between genotype and phenotype in pharmacogenetic studies is complex. As shown in Supplementary Table S1, we summarize the effects of TMPT, NUDT15, ITPA, and MRP4 polymorphisms on the clinical outcomes of thiopurines in ALL pediatric patients. We propose that the ongoing research will surely manifest the value of pharmacogenetics in predicting the optimal dose and reducing adverse events, thereby contributing to better treatment for ALL patients.
Therapeutic drug monitoring of thiopurines
The pharmacodynamics and pharmacogenetics of thiopurines have evolved considerably over the past 30 years. In addition to genotype–phenotype related research studies, long-term clinical practice has confirmed that thiopurine-associated adverse events and ALL relapses are related to metabolite levels, including 6-TGNs, 6-methylmercaptopurine nucleotides (6-MMPN), or the ratio of 6-MMPN/6-TGNs, and DNA-TG levels (Table 3). Therefore, integrating TDM of these active metabolites with pharmacogenetics may facilitate the development of a more personalized dosing method than the traditional weight-based one.
Concentrations of 6-TGNs in RBCs is related to neutropenia and relapsed risk
In ALL patients, the 6-TGN concentrations in RBCs have been recognized to correlate with neutropenia and tolerable 6-MP doses in the 1980s (Lennard et al., 1983; Lilleyman et al., 1984; Lennard and Lilleyman, 1989), whereas the well-established reference range of 6-TGN therapeutic levels has not yet been agreed (Schmiegelow and Bruunshuus, 1990; Lancaster et al., 1998; Innocenti et al., 2000; Stoneham et al., 2003; Halonen et al., 2006; Lennard et al., 2006). A study by Chrzanowska et al. showed the 6-TGN concentrations that ranged from <60 to 833 pmol/8 × 108 RBC in patients receiving 6-MP dosed at 50 mg/m2/day (Chrzanowska et al., 1999). In the study by Bhatia et al., patients received a higher dose of 6-MP, 75 mg/m2/day and the 6-TGN levels remained 0.3–714.1 pmol/8 × 108 RBC (Bhatia et al., 2015). Nevertheless, the levels obtained in the study by Rosdiana et al. were 6–234.04 pmol/8 × 108 RBC with a 6-MP dose of 50 mg/m2/day (Rosdiana et al., 2021). Interestingly, Zhou Y and others proposed the existence of a target threshold of 197.50 pmol/8 × 108 RBCs to predict the risk of leukopenia in Chinese pediatric patients tormented by ALL (Zhou et al., 2020). Notably, not all studies found a correlation between metabolite concentrations and adverse effects (Halonen et al., 2006), which may due to the different therapy regimens (Lancaster et al., 1998; Lennard et al., 2006). More importantly, 6-TGN concentrations were associated with relapsed risk (Lennard et al., 1990; Wojtuszkiewicz et al., 2014). Therefore, there is an urgent to conduct a multicenter study involving more patients to find a suitable target range of therapeutic targets.
Ratio of 6-MMPN/6-TGN is associated with the efficacy and tolerance of thiopurines
As previously described, a clear correlation between 6-MMPN concentrations and the development of hepatotoxicity was found in ALL patients treated with 6-MP (Nygaard et al., 2004; Adam de Beaumais et al., 2011). The findings of Nygaard et al. (Nygaard et al., 2004) indicated that the 6-MMPN contents were the most important pharmacological determinants of elevated aminotransferase levels during the 6-MP maintenance therapy in childhood ALL. A later study of Beaumais et al. showed that the threshold concentration of 6-MMPN, 4,884 pmol/8 × 108 RBC could predict the risk of hepatotoxicity with a positive predictive value of 95.7% (Adam de Beaumais et al., 2011). As well known, patients carrying TPMT mutations had higher TGN levels than their wild-type counterparts, but this genetic variation only interprets the intolerance in 30–60% patients who received full doses of 6-MP or AZA (Relling et al., 2011). Some heterozygotes might be sufficiently thiopurine-tolerant because they have lower 6-MMPN concentrations than those with homozygous wild-type carriers (and thus fewer toxic effects), and therefore tolerated higher 6-TGNs. For example, in Dervieux’s study, wild-type patients experienced higher 6-MMPN concentrations (median: 6,137 pmol/8 × 108 cells) than those carrying TPMT mutations (median: 307 pmol/8 × 108 cells) (Dervieux et al., 2001). In this study, the 6-MMPN concentrations in RBCs were determined in the last patients enrolled in the trail, with a median concentration of 5,749 pmol/8 × 108 cells (range 20–19682). The authors confirmed that higher 6-MP dosage and infectious events were associated with higher 6-MMPN concentrations. More recently, studies have shown a link between the ratio of 6-MMPN/6-TGN and the incidence of grade 3–4 neutropenia (Rosdiana et al., 2021). The researchers therefore proposed that the balance between RBC 6-MMPN and 6-TGN levels was important for predicting efficacy and improving the tolerance of thiopurines. Unfortunately, the therapeutic ratio of 6-MMPN/6-TGN is also unclear up to now.
DNA-incorporated thioguanine nucleotides and relapse-free survival during ALL maintenance therapy
Pharmacologically, leukocyte DNA-TG, a cytotoxic agent and the end-point metabolite of 6-MP (Karran and Attard, 2008), appears to be more appropriately and accurately reflecting therapy intensity in the nucleated target cells in ALL, and it has recently been associated with 6-TGNs and 6-MMPN in RBCs (Hedeland et al., 2010; Ebbesen et al., 2013; Vang et al., 2015). Moreover, using an on-therapy blood count model, Nielsen and others attempted to estimate the degree of myelosuppression using the DNA-TG levels, a parameter available on-therapy that could be indicative of the treatment intensity. However, the results were contradictory. On-therapy ANC decreased with increasing DNA-TGN level (p < 0.001, model adjusted for off-therapy ANC), whereas on-therapy absolute lymphocyte counts (ALC) could not be modeled reliably (Nielsen et al., 2016). The authors claimed that measurements of the DNA-TG levels could provide blood counts when evaluating therapy intensity, but required prospective validation. Subsequently, a prospective sub-study of a phase 3 trial (NOPHO ALL 2008) has confirmed that the DNA-TG concentration (adjusted hazard ratio 0·81 per 100 fmol/μg DNA increase, 95%CI 0.67–0.98; p = 0.029) was closely related to relapse-free survival (Nielsen et al., 2017). Although higher DNA-TG was associated with a decreased relapse rate, it was worth noting that the cytotoxicity of maintenance therapy in ALL was also dependent on DNA-TG formation. Larsen and others added a low-dose 6-TG to MTX/6 MP maintenance therapy, termed as “TEAM” strategy, which proved to be a novel and practicable method to enhance the maintenance therapy, resulting in higher DNA-TGs without causing additional toxicity (Larsen et al., 2021b). They recommended that a reliable profile of DNA-TG levels could be provided by measuring DNA-TGs in leucocytes every 2–4 weeks (Larsen et al., 2021a). On the other hand, as pharmacogenomics significantly influence the metabolism of 6-MP, numerous studies have investigated the influence of genotypes on the DNA-TG levels. In Gerbek’s study, Gerbek found that TPMT heterozygous patients held notably higher DNA-TG levels than TPMT wild-type carriers (Gerbek et al., 2018). Another study of Nielsen was consistent with those results (Nielsen et al., 2021). Gerbek and others also found that the DNA-TG levels were significantly elevated in ITPA heterozygotes compared with ITPA wild-type carriers (Gerbek et al., 2018). In addition, DNA-TG accumulated with higher efficiency in vivo as the amount of risk alleles increased in the NUDT15 gene (Moriyama et al., 2017).
We speculate that those large inter-individual variations in previous studies might be caused by variations in 6-MP absorption and disposition, drug–drug interactions, different TPMT enzyme activities, ethnic differences, and by patients’ compliance. In addition, the stratification according to those risk factors could be performed, but many chemotherapies are still used in the same way when treating all types of ALL. Hence, as optimal metabolite levels may vary by indications, it is important for physicians to adapt posology so that toxicity can be reduced while efficacy is not affected.
Conclusion and outlook
Thiopurines have been broadly used for over 5 decades in the treatment of a wide range of diseases. However, side effects for thiopurines ranging from mild rashes, flu-like symptoms to severe life-threatening myelosuppression, and hepatotoxicity have hindered their clinical application. Although pharmacogenetics and TDM for thiopurines may contribute to and influence clinical practice, there are still several problems that need to be addressed urgently.
Although the pharmacogenetics of thiopurines is one of the most successful clinical applications, the large intra- and inter-individual variations of thiopurines, especially the high incidence of side effects in Asian populations, remain difficult to explain well. One possible explanation is that, thiopurine-related toxicity phenotype could not be determined only by one or two genotyping analyses (e.g. TPMT and NUDT15). Conversely, while high-throughput techniques allow researchers to map thousands of genetic polymorphisms in a single test, it still remains an enormous challenge to identify and figure out which one exerts the most considerable impact on both efficacy and toxicity. We now propose in this review, that a comprehensive consideration of the “MINT” sequencing strategy including MRP4, ITPA, NUDT15, and TPMT genes, combined with patients’ clinical characteristics, will provide more accurate information for the precise medication of thiopurines. Furthermore, if rare SNPs could dramatically alter the properties of transporter proteins or enzymes in victims with thiopurine-related side effects, it would be beneficial to continue further research. Unfortunately, we still have a long way to go before incorporating pharmacogenetic tests into routine clinical practice, which can help predict the outcomes and effects of thiopurine therapy.
For TDM of active metabolites, the therapeutic levels of 6-TGNs, 6-MMPN, or their cut-offs remain inconsistent. Therefore, in clinical practice, physicians should be cautious about the calculated optimal threshold for adverse events. On the other hand, most studies measure the level of metabolites in RBCs to evaluate the effect of drug treatment, which are not representative of the drugs in lymphocytes. This may be the reason why various studies have come to different conclusions. Recent studies have found that the levels of DNA-TG may be more related to the clinical efficacy and adverse reactions of thiopurines, which is the development direction of TDM monitoring in the future. The standardization of procedures for the evaluation of metabolites should be attached great importance in the near future.
In conclusion, we propose that integrating the “MINT” sequencing strategy with routine DNA-TG- and 6-MMPN-monitoring might be more feasible toward improving the efficacy and tolerability of thiopurines. Nevertheless, multicenter studies with large samples in different ethnic populations need to be performed in the future.
Author contributions
H-LG, FC, LZ and TL: Concept and design. H-LG: Principal investigators for the review, literature summary, and primary author of the manuscript. Y-TZ, W-JW, ND, Y-HH, and Y-YZ: Performed the original articles’ collection and analysis, made the figures. H-LG and FC: Drafted of the manuscript. LZ, and TL: Assisted in the design and performance of the study and the writing of the manuscript. FC: Critical revision of the manuscript.
Funding
This research was supported by the Specially Appointed Medical Expert Project of the Jiangsu Commission of Health (2019), Jiangsu Research Hospital Association for Precision Medication (JY202108). This study was also sponsored by the Scientific Research Support Foundation for Top Young Scholars at the Children’s Hospital of Nanjing Medical University (2020).
Conflict of interest
The authors declare that the research was conducted in the absence of any commercial or financial relationships that could be construed as a potential conflict of interest.
Publisher’s note
All claims expressed in this article are solely those of the authors and do not necessarily represent those of their affiliated organizations, or those of the publisher, the editors, and the reviewers. Any product that may be evaluated in this article, or claim that may be made by its manufacturer, is not guaranteed or endorsed by the publisher.
Supplementary material
The Supplementary Material for this article can be found online at: https://www.frontiersin.org/articles/10.3389/fphar.2022.941182/full#supplementary-material
References
Adam de Beaumais, T., Fakhoury, M., Medard, Y., Azougagh, S., Zhang, D., Yakouben, K., et al. (2011). Determinants of mercaptopurine toxicity in paediatric acute lymphoblastic leukemia maintenance therapy. Br. J. Clin. Pharmacol. 71, 575–584. doi:10.1111/j.1365-2125.2010.03867.x
Andoh, A., Kawahara, M., Imai, T., Tatsumi, G., Inatomi, O., and Kakuta, Y. (2021). Thiopurine pharmacogenomics and pregnancy in inflammatory bowel disease. J. Gastroenterol. 56, 881–890. doi:10.1007/s00535-021-01805-z
Ansari, A., Hassan, C., Duley, J., Marinaki, A., Shobowale-Bakre, E. M., Seed, P., et al. (2002). Thiopurine methyltransferase activity and the use of azathioprine in inflammatory bowel disease. Aliment. Pharmacol. Ther. 16, 1743–1750. doi:10.1046/j.1365-2036.2002.01353.x
Arenas, M., Duley, J., Sumi, S., Sanderson, J., and Marinaki, A. (2007). The ITPA c.94C>A and g.IVS2+21A>C sequence variants contribute to missplicing of the ITPA gene. Biochim. Biophys. Acta 1772, 96–102. doi:10.1016/j.bbadis.2006.10.006
Asada, A., Nishida, A., Shioya, M., Imaeda, H., Inatomi, O., Bamba, S., et al. (2016). NUDT15 R139C-related thiopurine leukocytopenia is mediated by 6-thioguanine nucleotide-independent mechanism in Japanese patients with inflammatory bowel disease. J. Gastroenterol. 51, 22–29. doi:10.1007/s00535-015-1142-4
Azimi, F., Jafariyan, M., Khatami, S., Mortazavi, Y., and Azad, M. (2014). Assessment of thiopurine-based drugs according to thiopurine S-methyltransferase genotype in patients with acute lymphoblastic leukemia. Iran. J. Ped. Hematol. Oncol. 4, 32–38.
Ban, H., Andoh, A., Imaeda, H., Kobori, A., Bamba, S., Tsujikawa, T., et al. (2010). The multidrug-resistance protein 4 polymorphism is a new factor accounting for thiopurine sensitivity in Japanese patients with inflammatory bowel disease. J. Gastroenterol. 45, 1014–1021. doi:10.1007/s00535-010-0248-y
Banerjee, R., Ravikanth, V. V., Pal, P., Bale, G., Avanthi, U. S., Goren, I., et al. (2020). NUDT15 C415T variant compared with TPMT genotyping in predicting azathioprine-induced leucopenia: Prospective analysis of 1014 inflammatory bowel disease patients in India. Aliment. Pharmacol. Ther. 52, 1683–1694. doi:10.1111/apt.16137
Barba, E., Kontou, P. I., Michalopoulos, I., Bagos, P. G., and Braliou, G. G. (2022). Association of ITPA gene polymorphisms with adverse effects of AZA/6-MP administration: A systematic review and meta-analysis. Pharmacogenomics J. 22, 39–54. doi:10.1038/s41397-021-00255-3
Barz, M. J., Hof, J., Groeneveld-Krentz, S., Loh, J. W., Szymansky, A., Astrahantseff, K., et al. (2020). Subclonal NT5C2 mutations are associated with poor outcomes after relapse of pediatric acute lymphoblastic leukemia. Blood 135, 921–933. doi:10.1182/blood.2019002499
Berkovitch, M., Matsui, D., Zipursky, A., Blanchette, V. S., Verjee, Z., Giesbrecht, E., et al. (1996). Hepatotoxicity of 6-mercaptopurine in childhood acute lymphocytic leukemia: Pharmacokinetic characteristics. Med. Pediatr. Oncol. 26, 85–89. doi:10.1002/(SICI)1096-911X(199602)26:2<85::AID-MPO3>3.0.CO;2-Q
Bhatia, S., Landier, W., Hageman, L., Chen, Y., Kim, H., Sun, C. L., et al. (2015). Systemic exposure to thiopurines and risk of relapse in children with acute lymphoblastic leukemia: A Children's oncology group study. JAMA Oncol. 1, 287–295. doi:10.1001/jamaoncol.2015.0245
Brouwer, C., Vogels-Mentink, T. M., Keizer-Garritsen, J. J., Trijbels, F. J., Bokkerink, J. P., Hoogerbrugge, P. M., et al. (2005). Role of 5'-nucleotidase in thiopurine metabolism: Enzyme kinetic profile and association with thio-GMP levels in patients with acute lymphoblastic leukemia during 6-mercaptopurine treatment. Clin. Chim. Acta. 361, 95–103. doi:10.1016/j.cccn.2005.05.006
Chao, K., Wang, X., Cao, Q., Qian, J., Wu, K., Zhu, X., et al. (2017). Combined detection of NUDT15 variants could highly predict thiopurine-induced leukopenia in Chinese patients with inflammatory bowel disease: A multicenter analysis. Inflamm. Bowel Dis. 23, 1592–1599. doi:10.1097/MIB.0000000000001148
Choi, R., Sohn, I., Kim, M. J., Woo, H. I., Lee, J. W., Ma, Y., et al. (2019). Pathway genes and metabolites in thiopurine therapy in Korean children with acute lymphoblastic leukaemia. Br. J. Clin. Pharmacol. 85, 1585–1597. doi:10.1111/bcp.13943
Chrzanowska, M., Kolecki, P., Duczmal-Cichocka, B., and Fiet, J. (1999). Metabolites of mercaptopurine in red blood cells: A relationship between 6-thioguanine nucleotides and 6-methylmercaptopurine metabolite concentrations in children with lymphoblastic leukemia. Eur. J. Pharm. Sci. 8, 329–334. doi:10.1016/s0928-0987(99)00027-5
de Kreuk, B. J., Nethe, M., Fernandez-Borja, M., Anthony, E. C., Hensbergen, P. J., Deelder, A. M., et al. (2011). The F-BAR domain protein PACSIN2 associates with Rac1 and regulates cell spreading and migration. J. Cell Sci. 124, 2375–2388. doi:10.1242/jcs.080630
Dervieux, T., Medard, Y., Verpillat, P., Guigonis, V., Duval, M., Lescoeur, B., et al. (2001). Possible implication of thiopurine S-methyltransferase in occurrence of infectious episodes during maintenance therapy for childhood lymphoblastic leukemia with mercaptopurine. Leukemia 15, 1706–1712. doi:10.1038/sj.leu.2402259
Donnan, J. R., Ungar, W. J., Mathews, M., and Rahman, P. (2011). Systematic review of thiopurine methyltransferase genotype and enzymatic testing strategies. Ther. Drug Monit. 33, 192–199. doi:10.1097/FTD.0b013e31820810cd
Ebbesen, M. S., Nersting, J., Jacobsen, J. H., Frandsen, T. L., Vettenranta, K., Abramsson, J., et al. (2013). Incorporation of 6-thioguanine nucleotides into DNA during maintenance therapy of childhood acute lymphoblastic leukemia-the influence of thiopurine methyltransferase genotypes. J. Clin. Pharmacol. 53, 670–674. doi:10.1002/jcph.81
Fan, P. O. L., Leung, K. T., Chan, K. Y. Y., Leung, A. W. K., Lam, G. K. S., Chow, T. T. W., et al. (2022). ABCC4, ITPA, NUDT15, TPMT and their interaction as genetic predictors of 6-mercaptopurine intolerance in Chinese patients with acute lymphoblastic leukemia. Pediatr. Hematol. Oncol. 39, 254–266. doi:10.1080/08880018.2021.1973628
Fargher, E. A., Tricker, K., Newman, W., Elliott, R., Roberts, S. A., Shaffer, J. L., et al. (2007). Current use of pharmacogenetic testing: A national survey of thiopurine methyltransferase testing prior to azathioprine prescription. J. Clin. Pharm. Ther. 32, 187–195. doi:10.1111/j.1365-2710.2007.00805.x
Fotoohi, A. K., Coulthard, S. A., and Albertioni, F. (2010). Thiopurines: Factors influencing toxicity and response. Biochem. Pharmacol. 79, 1211–1220. doi:10.1016/j.bcp.2010.01.006
Franca, R., Stocco, G., Favretto, D., Giurici, N., Del Rizzo, I., Locatelli, F., et al. (2020). PACSIN2 rs2413739 influence on thiopurine pharmacokinetics: Validation studies in pediatric patients. Pharmacogenomics J. 20, 415–425. doi:10.1038/s41397-019-0130-0
Gad, H., Koolmeister, T., Jemth, A. S., Eshtad, S., Jacques, S. A., Strom, C. E., et al. (2014). MTH1 inhibition eradicates cancer by preventing sanitation of the dNTP pool. Nature 508, 215–221. doi:10.1038/nature13181
Ganping Zhou, Z. C., Zhou, S., Feng, Z., Xu, F., and Xu, F. (2008). A simplistic individualization method for 6-mercaptopurine in acute lymphoblastic leukemia children. Int. J. Pharmacol. 4, 64–66. doi:10.3923/ijp.2008.64.66
Gearry, R. B., Roberts, R. L., Barclay, M. L., and Kennedy, M. A. (2004). Lack of association between the ITPA 94C>A polymorphism and adverse effects from azathioprine. Pharmacogenetics 14, 779–781. doi:10.1097/00008571-200411000-00010
Gerbek, T., Ebbesen, M., Nersting, J., Frandsen, T. L., Appell, M. L., and Schmiegelow, K. (2018). Role of TPMT and ITPA variants in mercaptopurine disposition. Cancer Chemother. Pharmacol. 81, 579–586. doi:10.1007/s00280-018-3525-8
Halonen, P., Mattila, J., Mäkipernaa, A., Ruuska, T., and Schmiegelow, K. (2006). Erythrocyte concentrations of metabolites or cumulative doses of 6-mercaptopurine and methotrexate do not predict liver changes in children treated for acute lymphoblastic leukemia. Pediatr. Blood Cancer 46, 762–766. doi:10.1002/pbc.20442
Hedeland, R. L., Hvidt, K., Nersting, J., Rosthoj, S., Dalhoff, K., Lausen, B., et al. (2010). DNA incorporation of 6-thioguanine nucleotides during maintenance therapy of childhood acute lymphoblastic leukaemia and non-Hodgkin lymphoma. Cancer Chemother. Pharmacol. 66, 485–491. doi:10.1007/s00280-009-1184-5
Innocenti, F., Danesi, R., Favre, C., Nardi, M., Menconi, M. C., Di Paolo, A., et al. (2000). Variable correlation between 6-mercaptopurine metabolites in erythrocytes and hematologic toxicity: Implications for drug monitoring in children with acute lymphoblastic leukemia. Ther. Drug Monit. 22, 375–382. doi:10.1097/00007691-200008000-00002
Iu, Y. P. H., Helander, S., Kahlin, A. Z., Cheng, C. W., Shek, C. C., Leung, M. H., et al. (2017). One amino acid makes a difference-Characterization of a new TPMT allele and the influence of SAM on TPMT stability. Sci. Rep. 7, 46428. doi:10.1038/srep46428
Ju, H. Y., Lee, J. W., Cho, H. W., Hyun, J. K., Ma, Y., Yi, E. S., et al. (2021). DNA-thioguanine nucleotide as a treatment marker in acute lymphoblastic leukemia patients with NUDT15 variant genotypes. PLoS One 16, e0245667. doi:10.1371/journal.pone.0245667
Karran, P., and Attard, N. (2008). Thiopurines in current medical practice: Molecular mechanisms and contributions to therapy-related cancer. Nat. Rev. Cancer 8, 24–36. doi:10.1038/nrc2292
Khaeso, K., Komvilaisak, P., Chainansamit, S. O., Nakkam, N., Suwannaying, K., Kuwatjanakul, P., et al. (2022). NUDT15 is a key genetic factor for prediction of hematotoxicity in pediatric patients who received a standard low dosage regimen of 6-mercaptopurine. Drug Metab. Pharmacokinet. 43, 100436. doi:10.1016/j.dmpk.2021.100436
Koren, G., Ferrazini, G., Sulh, H., Langevin, A. M., Kapelushnik, J., Klein, J., et al. (1990). Systemic exposure to mercaptopurine as a prognostic factor in acute lymphocytic leukemia in children. N. Engl. J. Med. 323, 17–21. doi:10.1056/NEJM199007053230104
Kouwenberg, T. W., van den Bosch, B. J. C., Bierau, J., Te Loo, D., Coenen, M. J. H., and Hagleitner, M. M. (2020). Dosage of 6-mercaptopurine in relation to genetic TPMT and ITPA variants: Toward individualized pediatric acute lymphoblastic leukemia maintenance treatment. J. Pediatr. Hematol. Oncol. 42, e94–e97. doi:10.1097/MPH.0000000000001707
Krishnamurthy, P., Schwab, M., Takenaka, K., Nachagari, D., Morgan, J., Leslie, M., et al. (2008). Transporter-mediated protection against thiopurine-induced hematopoietic toxicity. Cancer Res. 68, 4983–4989. doi:10.1158/0008-5472.CAN-07-6790
Lancaster, D. L., Lennard, L., Rowland, K., Vora, A. J., and Lilleyman, J. S. (1998). Thioguanine versus mercaptopurine for therapy of childhood lymphoblastic leukaemia: A comparison of haematological toxicity and drug metabolite concentrations. Br. J. Haematol. 102, 439–443. doi:10.1046/j.1365-2141.1998.00812.x
Larsen, R. H., Hjalgrim, L. L., Degn, M., Nersting, J., Als-Nielsen, B., Grell, K., et al. (2021). Dynamics of leucocyte DNA thioguanine nucleotide levels during maintenance therapy of childhood acute lymphoblastic leukemia. Cancer Chemother. Pharmacol. 88, 53–60. doi:10.1007/s00280-020-04219-5
Larsen, R. H., Utke Rank, C., Grell, K., Norgaard Moller, L., Malthe Overgaard, U., Kampmann, P., et al. (2021). Increments in DNA-thioguanine level during thiopurine enhanced maintenance therapy of acute lymphoblastic leukemia. Haematologica 106 (11), 2824–2833. doi:10.3324/haematol.2020.278166
Lee, Y., Jang, E. J., Yoon, H. Y., Yee, J., and Gwak, H. S. (2022). Effect of ITPA polymorphism on adverse drug reactions of 6-mercaptopurine in pediatric patients with acute lymphoblastic leukemia: A systematic review and meta-analysis. Pharm. (Basel) 15, 416. doi:10.3390/ph15040416
Lennard, L., Lilleyman, J. S., Van Loon, J., and Weinshilboum, R. M. (1990). Genetic variation in response to 6-mercaptopurine for childhood acute lymphoblastic leukaemia. Lancet 336, 225–229. doi:10.1016/0140-6736(90)91745-v
Lennard, L., and Lilleyman, J. S. (1989). Variable mercaptopurine metabolism and treatment outcome in childhood lymphoblastic leukemia. J. Clin. Oncol. 7, 1816–1823. doi:10.1200/JCO.1989.7.12.1816
Lennard, L., Rees, C. A., Lilleyman, J. S., and Maddocks, J. L. (1983). Childhood leukaemia: A relationship between intracellular 6-mercaptopurine metabolites and neutropenia. Br. J. Clin. Pharmacol. 16, 359–363. doi:10.1111/j.1365-2125.1983.tb02178.x
Lennard, L., Richards, S., Cartwright, C. S., Mitchell, C., Lilleyman, J. S., Vora, A., et al. (2006). The thiopurine methyltransferase genetic polymorphism is associated with thioguanine-related veno-occlusive disease of the liver in children with acute lymphoblastic leukemia. Clin. Pharmacol. Ther. 80, 375–383. doi:10.1016/j.clpt.2006.07.002
Lilleyman, J. S., Lennard, L., Rees, C. A., Morgan, G., and Maddocks, J. L. (1984). Childhood lymphoblastic leukaemia: Sex difference in 6-mercaptopurine utilization. Br. J. Cancer 49, 703–707. doi:10.1038/bjc.1984.111
Luo, X., Yan, S., Jin, L., Zhu, H., Zhang, X., and Ge, W. (2022). Inosine triphosphate pyrophosphatase and NUDT15 are good predictors of clinical outcomes in thiopurine-treated Chinese patients with inflammatory bowel disease. Ther. Drug Monit. 44, 391–395. doi:10.1097/FTD.0000000000000965
Marinaki, A. M., Ansari, A., Duley, J. A., Arenas, M., Sumi, S., Lewis, C. M., et al. (2004). Adverse drug reactions to azathioprine therapy are associated with polymorphism in the gene encoding inosine triphosphate pyrophosphatase (ITPase). Pharmacogenetics 14, 181–187. doi:10.1097/00008571-200403000-00006
Marsh, S., and Van Booven, D. J. (2009). The increasing complexity of mercaptopurine pharmacogenomics. Clin. Pharmacol. Ther. 85, 139–141. doi:10.1038/clpt.2008.219
Milosevic, G., Kotur, N., Lazic, J., Krstovski, N., Stankovic, B., Zukic, B., et al. (2019). Influence of variants in folate metabolism genes on 6-mercaptopurine induced toxicity during treatment for childhood acute lymphocytic leukemia. J. BUON 24, 2075–2083.
Mokhtari, M., Mostanbet, F., Nekooee Fard, S., Shekarkhar, G., Sepaskhah, M., and Sadati, M. S. (2020). Thiopurine S-methyltransferase and pemphigus vulgaris: A phenotype-genotype study. Iran. J. Pathol. 15, 299–305. doi:10.30699/ijp.2020.121365.2320
Moradveisi, B., Muwakkit, S., Zamani, F., Ghaderi, E., Mohammadi, E., and Zgheib, N. K. (2019). ITPA, TPMT, and NUDT15 genetic polymorphisms predict 6-mercaptopurine toxicity in Middle eastern children with acute lymphoblastic leukemia. Front. Pharmacol. 10, 916. doi:10.3389/fphar.2019.00916
Moriyama, T., Nishii, R., Lin, T. N., Kihira, K., Toyoda, H., Jacob, N., et al. (2017). The effects of inherited NUDT15 polymorphisms on thiopurine active metabolites in Japanese children with acute lymphoblastic leukemia. Pharmacogenet. Genomics 27, 236–239. doi:10.1097/FPC.0000000000000282
Moriyama, T., Nishii, R., Perez-Andreu, V., Yang, W., Klussmann, F. A., Zhao, X., et al. (2016). NUDT15 polymorphisms alter thiopurine metabolism and hematopoietic toxicity. Nat. Genet. 48, 367–373. doi:10.1038/ng.3508
Nagasaki, M., Yasuda, J., Katsuoka, F., Nariai, N., Kojima, K., Kawai, Y., et al. (2015). Rare variant discovery by deep whole-genome sequencing of 1, 070 Japanese individuals. Nat. Commun. 6, 8018. doi:10.1038/ncomms9018
Nielsen, S. N., Grell, K., Nersting, J., Abrahamsson, J., Lund, B., Kanerva, J., et al. (2017). DNA-Thioguanine nucleotide concentration and relapse-free survival during maintenance therapy of childhood acute lymphoblastic leukaemia (NOPHO ALL2008): A prospective substudy of a phase 3 trial. Lancet. Oncol. 18, 515–524. doi:10.1016/S1470-2045(17)30154-7
Nielsen, S. N., Grell, K., Nersting, J., Frandsen, T. L., Hjalgrim, L. L., and Schmiegelow, K. (2016). Measures of 6-mercaptopurine and methotrexate maintenance therapy intensity in childhood acute lymphoblastic leukemia. Cancer Chemother. Pharmacol. 78, 983–994. doi:10.1007/s00280-016-3151-2
Nielsen, S. N., Toksvang, L. N., Grell, K., Nersting, J., Abrahamsson, J., Lund, B., et al. (2021). No association between relapse hazard and thiopurine methyltransferase geno- or phenotypes in non-high risk acute lymphoblastic leukemia: A NOPHO ALL2008 sub-study. Cancer Chemother. Pharmacol. 88, 271–279. doi:10.1007/s00280-021-04281-7
Nygaard, U., Toft, N., and Schmiegelow, K. (2004). Methylated metabolites of 6-mercaptopurine are associated with hepatotoxicity. Clin. Pharmacol. Ther. 75, 274–281. doi:10.1016/j.clpt.2003.12.001
Okada, Y., Nakamura, K., Hiromura, K., Nojima, Y., Horiuchi, R., and Yamamoto, K. (2009). Pro32Thr polymorphism of inosine triphosphate pyrophosphatase gene predicts efficacy of low-dose azathioprine for patients with systemic lupus erythematosus. Clin. Pharmacol. Ther. 85, 527–530. doi:10.1038/clpt.2008.261
Paugh, S. W., Stocco, G., and Evans, W. E. (2010). Pharmacogenomics in pediatric leukemia. Curr. Opin. Pediatr. 22, 703–710. doi:10.1097/MOP.0b013e32833fde85
Poppe, D., Tiede, I., Fritz, G., Becker, C., Bartsch, B., Wirtz, S., et al. (2006). Azathioprine suppresses ezrin-radixin-moesin-dependent T cell-APC conjugation through inhibition of Vav guanosine exchange activity on Rac proteins. J. Immunol. 176, 640–651. doi:10.4049/jimmunol.176.1.640
Relling, M. V., Gardner, E. E., Sandborn, W. J., Schmiegelow, K., Pui, C. H., Yee, S. W., et al. (2011). Clinical Pharmacogenetics Implementation Consortium guidelines for thiopurine methyltransferase genotype and thiopurine dosing. Clin. Pharmacol. Ther. 89, 387–391. doi:10.1038/clpt.2010.320
Relling, M. V., Hancock, M. L., Rivera, G. K., Sandlund, J. T., Ribeiro, R. C., Krynetski, E. Y., et al. (1999). Mercaptopurine therapy intolerance and heterozygosity at the thiopurine S-methyltransferase gene locus. J. Natl. Cancer Inst. 91, 2001–2008. doi:10.1093/jnci/91.23.2001
Relling, M. V., Pui, C. H., Cheng, C., and Evans, W. E. (2006). Thiopurine methyltransferase in acute lymphoblastic leukemia. Blood 107, 843–844. doi:10.1182/blood-2005-08-3379
Relling, M. V., Schwab, M., Whirl-Carrillo, M., Suarez-Kurtz, G., Pui, C. H., Stein, C. M., et al. (2019). Clinical pharmacogenetics implementation Consortium guideline for thiopurine dosing based on TPMT and NUDT15 genotypes: 2018 update. Clin. Pharmacol. Ther. 105, 1095–1105. doi:10.1002/cpt.1304
Rosdiana, D. S., Setiabudy, R., Andalusia, R., Gatot, D., Louisa, M., Bardosono, S., et al. (2021). TPMT genetic variability and its association with hematotoxicity in Indonesian children with acute lymphoblastic leukemia in maintenance therapy. Pharmgenomics. Pers. Med. 14, 199–210. doi:10.2147/PGPM.S288988
Rudin, S., Marable, M., and Huang, R. S. (2017). The promise of pharmacogenomics in reducing toxicity during acute lymphoblastic leukemia maintenance treatment. Genomics Proteomics Bioinforma. 15, 82–93. doi:10.1016/j.gpb.2016.11.003
Sakamoto, M., Kouhei, D., Haniffa, M., Silva, S., Troncoso, M., Santander, P., et al. (2020). A novel ITPA variant causes epileptic encephalopathy with multiple-organ dysfunction. J. Hum. Genet. 65, 751–757. doi:10.1038/s10038-020-0765-3
Schaeffeler, E., Jaeger, S. U., Klumpp, V., Yang, J. J., Igel, S., Hinze, L., et al. (2019). Impact of NUDT15 genetics on severe thiopurine-related hematotoxicity in patients with European ancestry. Genet. Med. 21, 2145–2150. doi:10.1038/s41436-019-0448-7
Schmiegelow, K., and Bruunshuus, I. (1990). 6-Thioguanine nucleotide accumulation in red blood cells during maintenance chemotherapy for childhood acute lymphoblastic leukemia, and its relation to leukopenia. Cancer Chemother. Pharmacol. 26, 288–292. doi:10.1007/BF02897232
Schmiegelow, K., Nielsen, S. N., Frandsen, T. L., and Nersting, J. (2014). Mercaptopurine/methotrexate maintenance therapy of childhood acute lymphoblastic leukemia: Clinical facts and fiction. J. Pediatr. Hematol. Oncol. 36, 503–517. doi:10.1097/MPH.0000000000000206
Smid, A., Karas-Kuzelicki, N., Jazbec, J., and Mlinaric-Rascan, I. (2016). PACSIN2 polymorphism is associated with thiopurine-induced hematological toxicity in children with acute lymphoblastic leukaemia undergoing maintenance therapy. Sci. Rep. 6, 30244. doi:10.1038/srep30244
Stocco, G., Crews, K. R., and Evans, W. E. (2010). Genetic polymorphism of inosine-triphosphate-pyrophosphatase influences mercaptopurine metabolism and toxicity during treatment of acute lymphoblastic leukemia individualized for thiopurine-S-methyl-transferase status. Expert Opin. Drug Saf. 9, 23–37. doi:10.1517/14740330903426151
Stocco, G., Yang, W., Crews, K. R., Thierfelder, W. E., Decorti, G., Londero, M., et al. (2012). PACSIN2 polymorphism influences TPMT activity and mercaptopurine-related gastrointestinal toxicity. Hum. Mol. Genet. 21, 4793–4804. doi:10.1093/hmg/dds302
Stoneham, S., Lennard, L., Coen, P., Lilleyman, J., and Saha, V. (2003). Veno-occlusive disease in patients receiving thiopurines during maintenance therapy for childhood acute lymphoblastic leukaemia. Br. J. Haematol. 123, 100–102. doi:10.1046/j.1365-2141.2003.04578.x
Takenaka, K., Morgan, J. A., Scheffer, G. L., Adachi, M., Stewart, C. F., Sun, D., et al. (2007). Substrate overlap between Mrp4 and Abcg2/Bcrp affects purine analogue drug cytotoxicity and tissue distribution. Cancer Res. 67, 6965–6972. doi:10.1158/0008-5472.CAN-06-4720
Tanaka, Y., Manabe, A., Fukushima, H., Suzuki, R., Nakadate, H., Kondoh, K., et al. (2015). Multidrug resistance protein 4 (MRP4) polymorphisms impact the 6-mercaptopurine dose tolerance during maintenance therapy in Japanese childhood acute lymphoblastic leukemia. Pharmacogenomics J. 15, 380–384. doi:10.1038/tpj.2014.74
Tanaka, Y., Nakadate, H., Kondoh, K., Nakamura, K., Koh, K., and Manabe, A. (2018). Interaction between NUDT15 and ABCC4 variants enhances intolerability of 6-mercaptopurine in Japanese patients with childhood acute lymphoblastic leukemia. Pharmacogenomics J. 18, 275–280. doi:10.1038/tpj.2017.12
Toksvang, L. N., Andrés-Jensen, L., Rank, C. U., Niinimäki, R., Nersting, J., Nielsen, S. N., et al. (2021). Maintenance therapy and risk of osteonecrosis in children and young adults with acute lymphoblastic leukemia: A NOPHO ALL2008 sub-study. Cancer Chemother. Pharmacol. 88, 911–917. doi:10.1007/s00280-021-04316-z
Tulstrup, M., Grosjean, M., Nielsen, S. N., Grell, K., Wolthers, B. O., Wegener, P. S., et al. (2018). NT5C2 germline variants alter thiopurine metabolism and are associated with acquired NT5C2 relapse mutations in childhood acute lymphoblastic leukaemia. Leukemia 32, 2527–2535. doi:10.1038/s41375-018-0245-3
Tzoneva, G., Perez-Garcia, A., Carpenter, Z., Khiabanian, H., Tosello, V., Allegretta, M., et al. (2013). Activating mutations in the NT5C2 nucleotidase gene drive chemotherapy resistance in relapsed ALL. Nat. Med. 19, 368–371. doi:10.1038/nm.3078
Uchiyama, K., Nakamura, M., Kubota, T., Yamane, T., Fujise, K., and Tajiri, H. (2009). Thiopurine S-methyltransferase and inosine triphosphate pyrophosphohydrolase genes in Japanese patients with inflammatory bowel disease in whom adverse drug reactions were induced by azathioprine/6-mercaptopurine treatment. J. Gastroenterol. 44, 197–203. doi:10.1007/s00535-008-2307-1
Valerie, N. C., Hagenkort, A., Page, B. D., Masuyer, G., Rehling, D., Carter, M., et al. (2016). NUDT15 hydrolyzes 6-thio-DeoxyGTP to mediate the anticancer efficacy of 6-thioguanine. Cancer Res. 76, 5501–5511. doi:10.1158/0008-5472.CAN-16-0584
van Dieren, J. M., van Vuuren, A. J., Kusters, J. G., Nieuwenhuis, E. E., Kuipers, E. J., and van der Woude, C. J. (2005). ITPA genotyping is not predictive for the development of side effects in AZA treated inflammatory bowel disease patients. Gut 54, 1664.
Vang, S. I., Schmiegelow, K., Frandsen, T., Rosthoj, S., and Nersting, J. (2015). Mercaptopurine metabolite levels are predictors of bone marrow toxicity following high-dose methotrexate therapy of childhood acute lymphoblastic leukaemia. Cancer Chemother. Pharmacol. 75, 1089–1093. doi:10.1007/s00280-015-2717-8
Wahlund, M., Nilsson, A., Kahlin, A. Z., Broliden, K., Myrberg, I. H., Appell, M. L., et al. (2020). The role of TPMT, ITPA, and NUDT15 variants during mercaptopurine treatment of Swedish pediatric patients with acute lymphoblastic leukemia. J. Pediatr. 216, 150–157. doi:10.1016/j.jpeds.2019.09.024
Wan Rosalina, W. R., Teh, L. K., Mohamad, N., Nasir, A., Yusoff, R., Baba, A. A., et al. (2012). Polymorphism of ITPA 94C>A and risk of adverse effects among patients with acute lymphoblastic leukaemia treated with 6-mercaptopurine. J. Clin. Pharm. Ther. 37, 237–241. doi:10.1111/j.1365-2710.2011.01272.x
Weinshilboum, R. M., and Sladek, S. L. (1980). Mercaptopurine pharmacogenetics: Monogenic inheritance of erythrocyte thiopurine methyltransferase activity. Am. J. Hum. Genet. 32, 651–662.
Weitzel, K. W., Smith, D. M., Elsey, A. R., Duong, B. Q., Burkley, B., Clare-Salzler, M., et al. (2018). Implementation of standardized clinical processes for TPMT testing in a diverse multidisciplinary population: Challenges and lessons learned. Clin. Transl. Sci. 11, 175–181. doi:10.1111/cts.12533
Wielinga, P. R., Reid, G., Challa, E. E., van der Heijden, I., van Deemter, L., de Haas, M., et al. (2002). Thiopurine metabolism and identification of the thiopurine metabolites transported by MRP4 and MRP5 overexpressed in human embryonic kidney cells. Mol. Pharmacol. 62, 1321–1331. doi:10.1124/mol.62.6.1321
Wojtuszkiewicz, A., Barcelos, A., Dubbelman, B., De Abreu, R., Brouwer, C., Bökkerink, J. P., et al. (2014). Assessment of mercaptopurine (6MP) metabolites and 6MP metabolic key-enzymes in childhood acute lymphoblastic leukemia. Nucleosides Nucleotides Nucleic Acids 33, 422–433. doi:10.1080/15257770.2014.904519
Wroblova, K., Kolorz, M., Batovsky, M., Zboril, V., Suchankova, J., Bartos, M., et al. (2012). Gene polymorphisms involved in manifestation of leucopenia, digestive intolerance, and pancreatitis in azathioprine-treated patients. Dig. Dis. Sci. 57, 2394–2401. doi:10.1007/s10620-012-2163-y
Wu, F., Melis, R., McMillin, G. A., and Johnson-Davis, K. L. (2019). Retrospective data analysis of the influence of age and sex on TPMT activity and its phenotype-genotype correlation. J. Appl. Lab. Med. 3, 827–838. doi:10.1373/jalm.2018.027276
Yamaguchi-Kabata, Y., Nariai, N., Kawai, Y., Sato, Y., Kojima, K., Tateno, M., et al. (2015). iJGVD: an integrative Japanese genome variation database based on whole-genome sequencing. Hum. Genome Var. 2, 15050. doi:10.1038/hgv.2015.50
Yang, J. J., Landier, W., Yang, W., Liu, C., Hageman, L., Cheng, C., et al. (2015). Inherited NUDT15 variant is a genetic determinant of mercaptopurine intolerance in children with acute lymphoblastic leukemia. J. Clin. Oncol. 33, 1235–1242. doi:10.1200/JCO.2014.59.4671
Yang, S. K., Hong, M., Baek, J., Choi, H., Zhao, W., Jung, Y., et al. (2014). A common missense variant in NUDT15 confers susceptibility to thiopurine-induced leukopenia. Nat. Genet. 46, 1017–1020. doi:10.1038/ng.3060
Zabala-Fernandez, W., Barreiro-de Acosta, M., Echarri, A., Carpio, D., Lorenzo, A., Castro, J., et al. (2011). A pharmacogenetics study of TPMT and ITPA genes detects a relationship with side effects and clinical response in patients with inflammatory bowel disease receiving Azathioprine. J. Gastrointestin. Liver Dis. 20, 247–253.
Zhou, Y., Wang, L., Zhai, X. Y., Wen, L., Tang, F., Yang, F., et al. (2020). Precision therapy of 6-mercaptopurine in Chinese children with acute lymphoblastic leukaemia. Br. J. Clin. Pharmacol. 86, 1519–1527. doi:10.1111/bcp.14258
Zhu, X., Wang, X. D., Chao, K., Zhi, M., Zheng, H., Ruan, H. L., et al. (2016). NUDT15 polymorphisms are better than thiopurine S-methyltransferase as predictor of risk for thiopurine-induced leukopenia in Chinese patients with Crohn's disease. Aliment. Pharmacol. Ther. 44, 967–975. doi:10.1111/apt.13796
Keywords: thiopurines, myelosuppression, pharmacogenetics, “MINT” sequencing strategy, therapeutic “DNA-TG” monitoring
Citation: Guo H-L, Zhao Y-T, Wang W-J, Dong N, Hu Y-H, Zhang Y-Y, Chen F, Zhou L and Li T (2022) Optimizing thiopurine therapy in children with acute lymphoblastic leukemia: A promising “MINT” sequencing strategy and therapeutic “DNA-TG” monitoring. Front. Pharmacol. 13:941182. doi: 10.3389/fphar.2022.941182
Received: 11 May 2022; Accepted: 02 August 2022;
Published: 27 September 2022.
Edited by:
Xiaoling Wang, Capital Medical University, ChinaReviewed by:
Nikola Kotur, University of Belgrade, SerbiaSarah McGarrity, Harvard Medical School, Boston, United States
Copyright © 2022 Guo, Zhao, Wang, Dong, Hu, Zhang, Chen, Zhou and Li. This is an open-access article distributed under the terms of the Creative Commons Attribution License (CC BY). The use, distribution or reproduction in other forums is permitted, provided the original author(s) and the copyright owner(s) are credited and that the original publication in this journal is cited, in accordance with accepted academic practice. No use, distribution or reproduction is permitted which does not comply with these terms.
*Correspondence: Feng Chen, Y3kuY2hlbjUwOEBnbWFpbC5jb20=; Li Zhou, emhvdWxpbmpleUAxNjMuY29t; Tao Li, SXRjcmxjcUB5ZWFoLm5ldA==