- 1Guangzhou Municipal and Guangdong Provincial Key Laboratory of Molecular Target & Clinical Pharmacology, The NMPA and State Key Laboratory of Respiratory Disease, School of Pharmaceutical Sciences and the Fifth Affiliated Hospital, Guangzhou Medical University, Guangzhou, China
- 2The First Affiliated Hospital, Hainan Medical University, Haikou, China
- 3State Key Laboratory of Medical Molecular Biology, Department of Molecular Biology and Biochemistry, Institute of Basic Medical Sciences, Peking Union Medical College, Chinese Academy of Medical Sciences, Beijing, China
- 4Department of Cellular and Molecular Biology, Tuberculosis and Thoracic Tumor Research Institute, Beijing Chest Hospital, Beijing, China
- 5Department of Biochemistry and Molecular Biology, School of Integrated Chinese and Western Medicine, Anhui University of Chinese Medicine, Hefei, China
- 6State Key Laboratory of Quality Research in Chinese Medicine, Institute of Chinese Medical Sciences, University of Macau, Macao, China
- 7Department of Surgery, University Hospital of Erlangen, Friedrich-Alexander University of Erlangen-Nuremberg (FAU), Erlangen, Germany
- 8Faculty of Pharmacy, Hasanuddin University, Makassar, Indonesia
Cancer becomes one of the main causes of human deaths in the world due to the high incidence and mortality rate and produces serious economic burdens. With more and more attention is paid on cancer, its therapies are getting more of a concern. Previous research has shown that the occurrence, progression, and treatment prognosis of malignant tumors are closely related to genetic and gene mutation. CRISPR/Cas9 has emerged as a powerful method for making changes to the genome, which has extensively been applied in various cell lines. Establishing the cell and animal models by CRISPR/Cas9 laid the foundation for the clinical trials which possibly treated the tumor. CRISPR-Cas9-mediated genome editing technology brings a great promise for inhibiting migration, invasion, and even treatment of tumor. However, the potential off-target effect limits its clinical application, and the effective ethical review is necessary. The article reviews the molecular mechanisms of CRISPR/Cas9 and discusses the research and the limitation related to cancer clinical trials.
Introduction
The clustered regularly interspaced short palindromic repeats (CRISPR)/-CRISPR-associated nuclease (Cas) system is an acquired defense system to protect organisms from invading viruses and plasmids and is widespread in various bacteria and archaea (Jinek et al., 2012; Wiedenheft et al., 2012; Hsu et al., 2014). CRISPR loci are separated by a CRISPR array, comprising unique spacers consisted of short variable DNA sequences, which are flanked by diverse cas (CRISPR-associated) gene (Makarova et al., 2015; Koonin and Makarova, 2019). Among them, CRISPR is a series of short direct repeats interspaced with short sequences in E. coli that was discovered by Japanese researchers in 1987 (Ishino et al., 1987). The Cas gene encodes the Cas protein components with putative nuclease and helicase domains (Jansen et al., 2002; Haft et al., 2005). The CRISPR-Cas immune response includes three phases: adaptation; pre-CRISPR RNA (pre-crRNA) expression and processing; and interference to protect prokaryotes from succumbing to infection (Wiedenheft et al., 2012; Hille et al., 2018; and Koonin and Makarova, 2019).
The CRISPR/Cas systems are divided into two classes, which are further classified to six types and 33 subtypes that each possess signature cas gene (Makarova et al., 2020). The type II CRISPR/Cas9 system is mainly used in gene editing because of its simplicity, high efficacy, and ease to use (Liu et al., 2017). Cas9 is a large multifunctional protein that has two putative nuclease domains: HNH and RuvC to cleave DNA strands (Nierzwicki et al., 2021; Shams et al., 2021). The CRISPR/Cas9 system contains three core elements: the Cas9 protein, the CRISPR RNA (crRNA), and a trans-activating CRISPR RNA (tracrRNA), of which tracrRNA bears complementarity to the repeat regions of crRNA (Deltcheva et al., 2011). The Cas9 protein associates with a mature dual RNA (tracrRNA: crRNA), as a single-guide RNA (sgRNA) to target DNA cleavage. To interfere with the expression of target genes, Cas9 identifies the viral DNA sequence through a short protospacer adjacent motif (PAM) recognition that was able to select the target DNA among the genome (Sternberg et al., 2014). After the Cas9 protein binds to the target sequence, it results in double-stranded DNA breakings (DSBs) in specific regions of the genome (Frock et al., 2015). DSBs are repaired by different DNA damage repair mechanisms in cells: homology-directed repair (HDR), classical non-homologous end joining (cNHEJ), and microhomology-mediated end joining (MMEJ) (Rassool, 2003; Brinkman et al., 2018; Schep et al., 2021). Various DNA repair pathways might be used to repair each end at a DSB leading to the potential for asymmetric repair (Nambiar et al., 2022) (Figure 1). In clinical practice, these repair methods are used to achieve the purpose of relieving or even curing diseases.
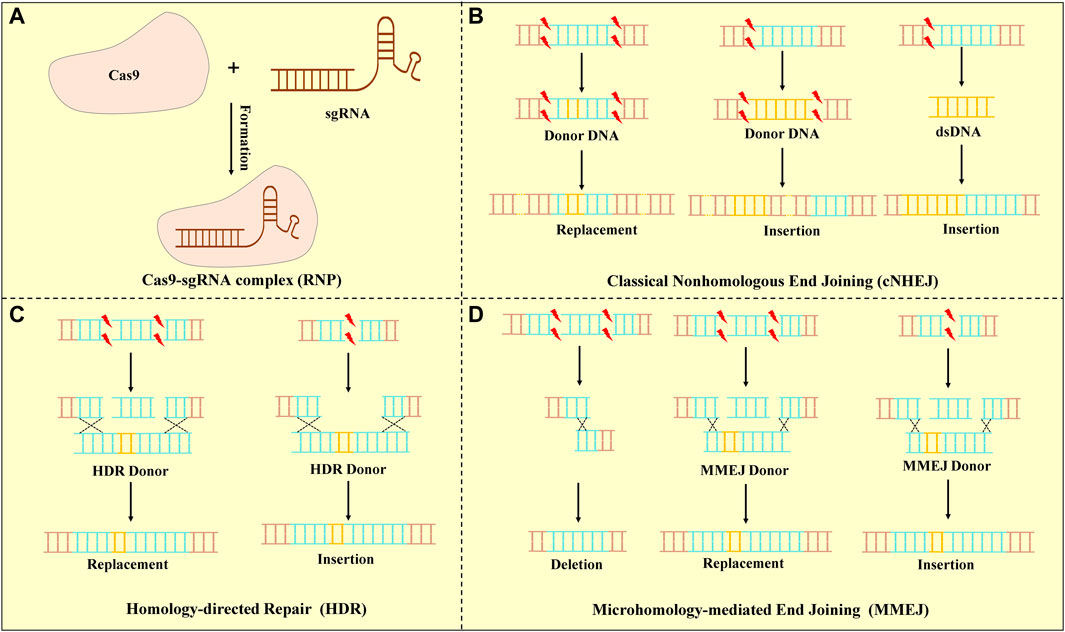
FIGURE 1. CRISPR/Cas9 system and DSB repair. (A) Mechanism of the CRISPR-Cas9 system. A short guide sgRNA associates with the Cas9 endonuclease to form the Cas9-sgRNA complex. Cas9 is a targeted DNA by PAM under the sgRNA. (B) cNHEJ : cNHEJ was the predominant pathway for repairing DSBs and was used for re-ligating broken DNA ends. Deletions or insertion mutations lead to gene frame shift mutations or premature generation of stop codes. (C) HDR: HDR was used a homologous DNA template to guide DSB repair. The DNA donor templates of HDR were used to insert or replace specific sequences into the genome. (D) MMEJ: MMEJ-mediated repair was capable of generating precise deletions between two short micro-homologous sequences (5–25 base pairs) at target loci.
DSBs are generated and repaired at specific positions in CRISPR/Cas9-mediated targeted gene segments, leading to targeted mutations because the repair process is error-prone. Though editing the targeted mutation, CRISPR/Cas9 gene editing has shown tremendous potential in oncology and has attained an encouraging achievement.
The Research of CRISPR/CAS9 for Anti-Cancer Therapy
Cancer is the first or second leading cause of mortality and ranks as an important barrier to increasing life expectancy of the world (Bray et al., 2021; Sung et al., 2021). Tumor formation involves a variety of gene mutations and epigenetic mutations (Podlaha et al., 2012; Huang et al., 2020). Cancer genome sequencing has confirmed that there was a multitude of genes and epigenetic mutations in human tumors (Gerstung et al., 2020). At present, there are various methods to treat tumor, such as conventional cancer therapy including operation, chemotherapy and radiotherapy, molecular targeted therapy, immunotherapy, and genetic therapy. Traditional methods pose a significant challenge to patients’ tolerability and adherence due to toxicity (Mun et al., 2018). Molecular targeted drugs, one of the anti-tumor drugs, have gradually replaced traditional chemotherapeutic drugs with their advantages of high targeting and efficacy, which have made revolutionary progress in the treatment of malignant tumors. However, during the clinical application, dramatic but short-lived tumor regressions and expenses limit the benefits (Vanneman and Dranoff., 2012). Tumor immunotherapy refers to the actively or passively tumor-specific responses to suppress cancer, including immune checkpoint blocks (ICBs), adoptive cell transfer (ACT), and tumor-specific vaccines (Zhang and Zhang, 2020). Despite immunotherapy marking the beginning of a new era in cancer, it only works in a subset of cancers and a fraction of patients with cancer respond to immunotherapy (Yang, 2015). Meanwhile, the existence of immune escape makes the effect less than expected.
The gene therapy contains zinc-finger nucleases (ZFNs), transcription activator-like effector nucleases (TALENs), and the CRISPR/Cas9 system, which knockout, insert, and mutate the targeted gene to treat cancer (Knott and Doudna, 2018). ZFNs and TALENs have been applied in editing targeted gene in the body; however, they are time-consuming and complicated (Gaj et al., 2013). Compared to ZFNs and TALENs, the CRISPR/Cas9 system is the method of choice for gene editing due to its simplicity, practicability, and application diversity. Hence, the CRISPR/Cas system is used widely. For tumor and immune cells, CRISPR/Cas9 gene editing is expected to provide a new strategy for tumor therapy.
The Application of CRISPR/Cas9 Technology in Tumor Cells
CRISPR-Cas9 technology is used in tumor cells to overcome cancer. The tumor cell editing mainly has the following methods:
(1) Gene knockout is the simplest and most common approach that has tremendous potential in clinical trials, mainly for pathogenic genes. In 2012, it was confirmed that Cas9 could cut programmatically various DNA sites in vitro (Jinek et al., 2012). For example, through gene editing of CCR5 in CD4+ T cells from persons who have been infected with HIV helps to combat HIV infection (Hsu et al., 2014; Tebas et al., 2014; Xu et al., 2019). Similarly, disrupting the intronic erythroid-specific enhancer for the BCL11A gene could increase HbF protein expression to possibly cure sickle-cell anemia (SCD) and β-thalassemia (Basak and Sankaran, 2016);
(2) Targeted insertion of DNA fragments helps to correct mutation to restore the normal sequence (Mali et al., 2013). Theodore et al. applied the strategy that inserting large DNA sequences (>1 kb) helps to correct IL2RA mutation in cells from patients with monogenic autoimmune disease (Roth et al., 2018);
(3) Translocation of chromosomal fragment was closely associated with tumorigenesis, for instance, modeling cancer-related chromosomal translocations was able to facilitate cancer pathogenesis research (Mani and Chinnaiyan, 2010). Irina et al. have modeled the human alveolar rhabdomyosarcoma Pax3-Foxo1 chromosome translocation in mouse myoblast by CRISPR/Cas9, which benefits researchers to explore the mechanism of the tumorigenic process (Lagutina et al., 2015);
(4) Base editing to obtain the point mutation for the purpose of various gene editing steps, such as to monitor and screen (Zhang, 2021). As we all know, the largest class of human pathogenic mutations was the point mutation (also called single nucleotide polymorphism, SNP), so that a new technology was required to change specifically an individual base pair within a vast genome (Landrum et al., 2016; Rees and Liu, 2018). Based on the CRISPR/Cas9 system, David R. Liu’s group developed a CRISPR/Cas base editor (BE) technology, also known as cytosine base editors (CBEs), to replace specific base, for instance, convert cytidine (C) to thymine (T) or guanine (G) to adenine (A) (Komor et al., 2016). Later, adenine base editors (ABEs) were developed to convert an A·T base pair to a G·C base pair (Gaudelli et al., 2017). In short, ABEs and CBEs provide four possible changes to correct point mutations (Rees and Liu, 2018).
The tumor cell models were established to test in vitro therapeutic effects, explore the mechanisms of drug actions, and clarify pathogenesis as well by the CRISPR-Cas9-mediated genome editing technology (Augert et al., 2020). Recently, the research about CRISPR/Cas9 gene editing technology in human cells was popularly increasing (Wang et al., 2014). The Human Genome Project and International HapMap Project showed more comprehensive data and have attracted considerable interest from scientists to explain the relation between gene and disease, especially tumor (Bayarsaikhan et al., 2021). With the involvement of the CRISPR/Cas system in drug discovery, the combination contains the CRISPR/Cas9 system, and stem cells would be applied to simulate at least 75,000 diseases associated with human genetic variants (Ledford, 2019). By revealing the gene–gene interactions to synthetic lethality of genes by the CRISPR system, we could obtain the candidate drug. The researchers exposed myeloid leukemia KBM7 cells to the CRISPR/Cas9 system that carrying a library of more than 70,000 sgRNAs; thus, drug-resistant genes would be identified (Wang et al., 2014). It was not only a complicated process in drug discovery but also time-consuming. Due to the high-screening knockout by the CRISPR/Cas9 system, it was increasing to elucidate the function of gene. Meanwhile, the induced pluripotent stem cells (iPSCs) with unlimited self-renewal capability were able to differentiate into cells of any lineage attract, which owned a value in cell disease modeling (Wang et al., 2017). An immunodeficiency, centromeric region instability, and facial anomalies syndrome (ICF) model was edited efficiently in human-induced pluripotent stem cells (hPSCs) by using the CRISPR system (Horii et al., 2013). Based on the CRISPR/Cas9 system, Paquet et al. introduced mono- and biallelic sequence changes to hPSCs to establish the model with Alzheimer’s disease-causing mutations in amyloid precursor protein and derived cortical neurons (Paquet et al., 2016). The CRISPR/Cas9 gene editing tool was combined with the piggyBac transposon to effectively correct the mutation of the human B hemoglobin (HBB) gene in the iPSC genome of patients with β-thalassemia (Xie et al., 2014). In a study, the Lacl gene was mutated, and several normal genes were re-expressed, which resulted in the inhibition of bladder cancer cells (Liu et al., 2014). Scientists have also cultured hPSCs in vitro to perfectly modify four colorectal cancer mutation genes including APC, P53, KRAS, and SMAD4 and then screened the mutant cells to construct the human colorectal cancer model to explore the specific mechanism of the intestinal stem cell of colon cancer (Drost et al., 2015). In summary, the CRISPR/Cas system is typically applied for the generation of cell models.
The Application of CRISPR/Cas9 Technology in Animals
Animal tumor models have laid a foundation for revealing the molecular mechanism of tumorigenesis and development. Animal models were ideal carriers that effectively integrate basic, clinical tumor research, which have been widely used throughout cancer research. The disease animal models played a crucial role in drug development and therapeutic approaches as an in vivo tool. Animal tumor models can be classified into four categories: carcinogen-induced models (CIMs), spontaneous and induced models, genetically engineered models, and transplant models, in which transplant models were divided into orthotopic models, heterotopic tumor models, and primary patient-derived xenografts (PDXs) (Burtin et al., 2020). Gene knockout animal tumor models, referring to genetically engineered models, use the gene knockout method to remove genes to induce tumorigenesis (Takeda et al., 2019). This is an ideal model for understanding the role of a single gene or several genes in tumorigenesis. Compared to other gene editing technologies such as ZFNs and TALENs, CRISPR/Cas9 technology has the advantages of cost, efficiency and timesaving. Scientists have creatively applied the CRISPR system for studying malignant tumors and constructed multiple animal tumor models. Precise gene editing has been successful in various animals, including rat (Li et al., 2013; Wang et al., 2013), goat (Ni et al., 2014), rabbit (Honda et al., 2015; Yuan et al., 2016), dog (Zou et al., 2015), monkey (Niu et al., 2014; Chen et al., 2015), pig (Zhou et al., 2015), C. elegans (Friedland et al., 2013), and zebrafish (Hruscha et al., 2013; Varshney et al., 2015) (Table 1).
Traditional animal models lay on the embryonic stem cells (ESCs) and HDR techniques and succeeded to establish the transgenic animal. However, it is time-consuming, and researchers spend at least 1 year to build the model. Compared with ZFNs and TALENs, CRISPR/Cas9 technology adopted three methods to build the model:
(1) Editing gene in embryos. There are 3 steps: 1) obtain zygote, 2) deliver sgRNA and Cas9 mRNA into the zygote, and 3) embryo transfer into animals to produce generation;
(2) Editing haploid embryonic stem cells (ESCs) because the haploid ESCs easily produce homozygous mutants for the generation of transgenic model;
(3) Gene editing in spermatogonia stem cells (SSCs) would be autologous transplanted to pseudo-pregnant animals (Sato et al., 2020) (Figure 2).
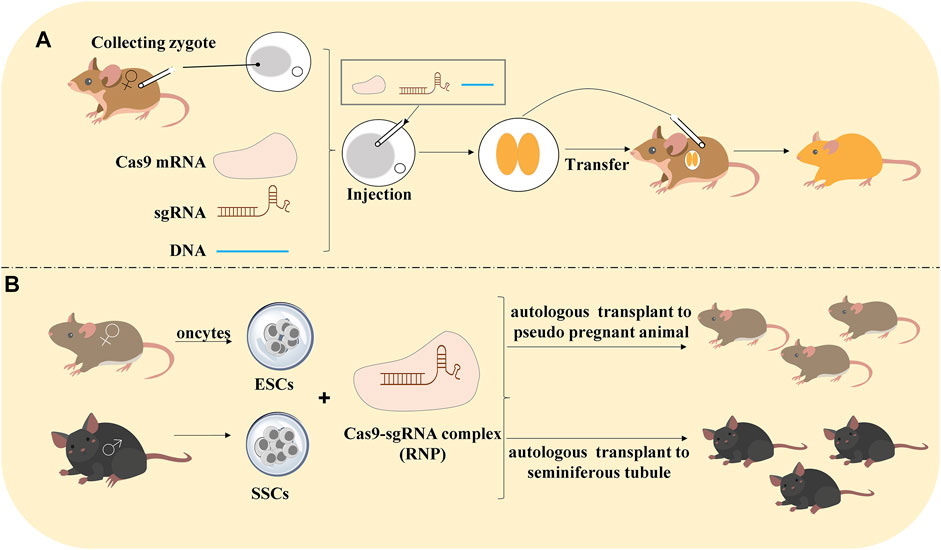
FIGURE 2. Methods of CRISPR/Cas9 technology to construct animal models. (A) Editing gene in embryos. Collecting the zygote that was injected with Cas9 mRNA, sgRNA, and DNA. After the zygote develops into an embryo, it would be transferred into the animals to produce generation. (B) Editing gene in haploid ESCs and SSCs: haploid embryonic stem cells (ESCs) are pluripotent cells generated from oocytes. CRISPR/Cas9-mediated gene editing in ESCs and autologous transplant to pseudo-pregnant animals to produce generation. Spermatogonia stem cells (SSCs) would be transfected by RNP and autologous transplant to seminiferous tubule to produce the generation.
However, editing embryos by microinjection was too time-consuming, and the microinjection required special skill, which limited the application for high-throughput genetic analysis (Hashimoto and Takemoto, 2015). On the contrary, editing in ESCs would generate multiple knockouts and large deletions at high efficiency (Safier et al., 2020). CRISPR/Cas9-mediated gene technology in SSCs did not change the paternal imprinting pattern that displayed a great promise to treat diseases (Wu et al., 2015). From the point of view of animal studies, it is more effective for the generation of transgenic animals by CRISPR/Cas9. CRISPR/Cas9 technology is integrated into tumor cell molecular biology research, allowing for accurate and quick editing of genomes, constructing animal tumor models of gene mutation and knockout to promote comprehensive research on tumor-related genes and tumor development. The CRISPR/Cas9 system was applied not only in animals but also in insects (Shirai et al., 2022). The latest research showed that “direct parental” CRISPR (DIPA-CRISPR) was defined as a method in which the RNPs were injected into the hemocoel of females to introduce the hereditary mutation in developing oocytes and successfully applied them in cockroaches and Tribolium castaneum. It is an exciting breakthrough to achieve the gene editing in cockroaches due to the unique reproduction system.
The Clinical Trial of CRISPR/Cas9 Technology
The purpose of developing new treatment methods is to achieve the purpose of preventing, alleviating, and even treating diseases. The emergence of CRISPR/Cas9 gene editing technology reflects the urgent need for treating diseases that are currently incurable, such as tumor. Applying this technology to the treatment of human diseases will bring hope to patients. In 2012, 2013, and 2015, CRISPR made the cut “breakthrough of the year,” which achieved great success such as creating a contagious gene to fight malarial infection (Hammond et al., 2016; Macias et al., 2020).
Tumor treatment is complicated and frequently accompanied by immune escape, so that it is necessary to overcome the immune escape as a crucial treatment strategy. Chimeric antigen receptor T (CAR-T) cells have been provided with tumor cell-specific antigen chimeric domains, which can activate T cells and achieve killing effect on tumor cells (Hu et al., 2021). The signaling pathway—programmed death receptor 1 (PD-1)/programmed cell death ligand 1 (PD-L1)—led to melanoma, non-small cell lung cancer (NSCLC), colorectal cancers (CRCs), bladder cancer, and renal-cell cancer if it was activated (Ott et al., 2020; O'Neil et al., 2017; Topalian et al., 2012; Stein et al., 2021; and Yi and Li, 2016). PD-1 was encoded by the PDCD1 gene, which blocked the binding of PD-1 to its receptor PD-L1 to enhance the activation of T cells to fight cancer by improving the IFN-γ expression (Lu et al., 2020; Stadtmauer et al., 2020). Combining CRISPR/Cas9 with CAR-T cells and PD-1, the editing PDCD1 gene in T cells was an ideal method to cure cancer (Xu et al., 2022). In 2016, the first human phase I clinical trial of CRISPR was conducted in China to therapy metastatic NSCLC patients who did not respond to chemotherapy, radiotherapy, and other therapies (Lacey and Fraietta, 2020; Lu et al., 2020). Similarly, knocking TRAC region and CD52 gene in CAR-T cells by CRISPR/Cas9 avoided the host immune-mediated rejection for relapsed/refractory acute lymphoblastic leukemia (r/r ALL) (Hu et al., 2021). Su et al. have confirmed that targeting PD-1 in the T cells from patient with melanoma and gastric cancer resulted in the improvement of cytotoxicity of T cells, and the tumor cells were killed (Su et al., 2016). The successful practice of this gene therapy has laid the foundation for the clinical trial of CRISPR/Cas9 gene editing technology to inhibit tumor metastasis in human.
There are various genetic mutations in the process of tumor evolution such as proto-oncogene and tumor-suppressor gene. More and more mutated genes related to tumors have been identified by genome-sequencing technology. The efficient and specific gene editing function of the CRISPR/Cas9 system provides the possibility to directly target the mutated genes that cause cancer in vivo. As we all know, the epidermal growth factor receptor (EGFR) gene was changed in approximately 10%∼15% of NSCLC, which played an essential role in tumor progression (Koo et al., 2017). Currently, EGFR inhibitors are the first-line drugs for curing lung cancer with EGFR mutation (Janne et al., 2022). However, the development of resistance and the efficacy of drugs were limited. It was a need to develop novel tools for EGFR-mutated NSCLC, and CRISPR/Cas9 gene editing technology maybe a promising method to correct cancer-driven mutations for cancer therapy. Experiments have shown that knocking out the EGFR mutant allele (L858R) in H1975 lung cells resulted in dying of cancer cells and decreasing tumor volume . Cervical cancer was related to human papilloma virus (HPV). After targeting E6 and E7 oncogenes, tumor growth was suppressed (Khairkhah et al., 2022). However, safety and specificity of CRISPR/Cas9 need to be optimized before executing in the clinical setting. The nuclear receptor binding SET domain-containing protein 1 (NSD1) was one of the biomarkers to participate in a variety of malignancies, and human hepatocellular carcinoma (HCC) was one of them. Knocking the NSD1 gene in HCC cells led to the function of cell proliferation, migration, and invasion that were suppressed (Zhang, et al., 2019b). Targeting the reticulon 4B (Nogo-B), a negative modulator of apoptosis, was able to inhibit the ability of cell proliferation in vitro and tumor growth in vivo (Zhu et al., 2017). Based on the CRISPR/Cas9 system, it was probable to provide individualized targeted therapy, which showed potential in tumor therapy at the gene level and rises a high level. Meanwhile, the Zhang Feng’s group successfully restored vision in Leber’s congenital amaurosis type 10 (LCA10) in 2019, which showed the feasibility of CRISPR-based gene editing therapy in the treatment of genetic diseases (Maeder et al., 2019) (Table 2).
Challenges of the CRISPR/CAS9 System
As only a specific nucleic acid sequence of 20 bp was provided by using CRISPR/Cas for gene editing, its construction process was simpler and faster than ZFNs and TALENs, owing to ZFNs and TALENs must depend on the Fok I enzyme to exert. In contrast, the CRISPR technology target design was simpler and efficient, making it an ideal gene editing tool. However, it may also cause “off-target” effects, and the gene sequences should not be edited to result in unpredictable consequences. This made CRISPR more secure when used in vivo because once off-target occurred, it cannot be checked and corrected in a timely manner like in vitro experiments. The new types of Cas9, such as saCas9 and Cpf1, have been developed but cannot completely get rid of the dependence on PAM (Wang et al., 2018). This year, the newest types of Cas9-Cas9TX can inhibit the occurrence of chromosomal structural abnormalities such as chromosomal translocations and large fragment deletions in the process of gene editing and greatly improve the safety of CRISPR/Cas9 gene editing (Yin et al., 2022).
In 2018, “Gene-edited Infant” event brought a lot of controversy to the application of CRISPR at the clinical level, and direct violation of the international scientific consensus that CRISPR/Cas9 technology was not ready or appropriate for making changes to humans that could be passed on through generations until the technology matures and becomes widespread (Kofler, 2019). At this time, how to define whether accepting human beings have the right to choose their own genes in place of future generations, or whether the modified human beings can enjoy the rights of ordinary people will become a problem that needs to be pondered (Zhang et al., 2019a). The effective ethical review is the proper meaning of strengthening the ethical governance of science and technology. Therefore, it is the top priority to improve the ethical review system for human genome editing activities.
Concluding Remarks and Future Perspectives
In the past decade, CRISPR/Cas9 gene editing technology as a strategy to therapy disease successfully entered preclinical and clinical stages. With the continuous improvement of gene editing tools and the identification of new effective targets for diseases, the clinical translation and application research of gene editing technology has been expanded. Not only in insects and plants, but also in animals and even in humans, the CRISPR/Cas9 gene editing technology proves its powerful utility.
Specific gene mutation improved tumor migration, invasion, and angiogenesis, which could be reversed by targeting editing genome. At present, the in vivo gene-editing based on the CRISPR/Cas system is currently being used for diseases, such as tumor and immune diseases. At present, the clinical programs are being carrying out to verify the effects of CRISPR/Cas9 and have made outstanding achievements. However, the clinical trials have been developed only involving a small number of patients and a limited follow-up, for which the further in-depth in vivo research studies are planned (Frangoul et al., 2021). Meanwhile, long-term safety monitoring is needed to confirm the effects and unknown adverse reactions (Frangoul et al., 2021; Gillmore et al., 2021). Developing and optimizing the Cas9-based gene editing should promote the technology forward to therapeutic applications and offer a wide variety of treating strategies for human diseases, especially tumor.
In spite of the application of CRISPR/Cas9, it brings promise for tumor therapy associated with gene mutation; problems such as off-target and ethics need to resolved. Scientists must begin to observe the international consensus and strive to advance society positively by technology (Kofler, 2019). We still have a long way to go until the CRISPR/Cas9 technology is ready to treat cancer maturely.
Author Contributions
X-ZC, RG, and CZ wrote the manuscript. JX and HS designed the figures. HY, CP, and FN finished the tables. J-YZ, X-KZ, and J-QL designed and revised the manuscript. All authors have read and approved the final version of the manuscript.
Funding
This work was supported by the National Key R&D Program of China (2021YFE0202000), the Finance Science and Technology Project of Hainan Province (ZDYF2020137), the Guangdong Basic and Applied Basic Research Foundation (2020A1515010605), and the Fund of Guangdong Education Department (2021ZDZX2006).
Conflict of Interest
The authors declare that the research was conducted in the absence of any commercial or financial relationships that could be construed as a potential conflict of interest.
Publisher’s Note
All claims expressed in this article are solely those of the authors and do not necessarily represent those of their affiliated organizations, or those of the publisher, the editors and the reviewers. Any product that may be evaluated in this article, or claim that may be made by its manufacturer, is not guaranteed or endorsed by the publisher.
References
Augert, A., Mathsyaraja, H., Ibrahim, A. H., Freie, B., Geuenich, M. J., Cheng, P. F., et al. (2020). MAX Functions as a Tumor Suppressor and Rewires Metabolism in Small Cell Lung Cancer. Cancer Cell 38, 97–114.e7. doi:10.1016/j.ccell.2020.04.016
Basak, A., and Sankaran, V. G. (2016). Regulation of the Fetal Hemoglobin Silencing Factor BCL11A. Ann. N. Y. Acad. Sci. 1368, 25–30. doi:10.1111/nyas.13024
Bayarsaikhan, D., Bayarsaikhan, G., and Lee, B. (2021). Recent Advances in Stem Cells and Gene Editing: Drug Discovery and Therapeutics. Prog. Mol. Biol. Transl. Sci. 181, 231–269. doi:10.1016/bs.pmbts.2021.01.019
Bray, F., Laversanne, M., Weiderpass, E., and Soerjomataram, I. (2021). The Ever-Increasing Importance of Cancer as a Leading Cause of Premature Death Worldwide. Cancer 127, 3029–3030. doi:10.1002/cncr.33587
Brinkman, E. K., Chen, T., de Haas, M., Holland, H. A., Akhtar, W., and van Steensel, B. (2018). Kinetics and Fidelity of the Repair of Cas9-Induced Double-Strand DNA Breaks. Mol. Cell 70 (5), 801–813.e6. doi:10.1016/j.molcel.2018.04.016
Bürtin, F., Mullins, C. S., and Linnebacher, M. (2020). Mouse Models of Colorectal Cancer: Past, Present and Future Perspectives. World J. Gastroenterol. 26, 1394–1426. doi:10.3748/wjg.v26.i13.1394
Chen, Y., Zheng, Y., Kang, Y., Yang, W., Niu, Y., Guo, X., et al. (2015). Functional Disruption of the Dystrophin Gene in Rhesus Monkey Using CRISPR/Cas9. Hum. Mol. Genet. 24, 3764–3774. doi:10.1093/hmg/ddv120
Deltcheva, E., Chylinski, K., Sharma, C. M., Gonzales, K., Chao, Y., Pirzada, Z. A., et al. (2011). CRISPR RNA Maturation by Trans-encoded Small RNA and Host Factor RNase III. Nature 471, 602–607. doi:10.1038/nature09886
Drost, J., van Jaarsveld, R. H., Ponsioen, B., Zimberlin, C., van Boxtel, R., Buijs, A., et al. (2015). Sequential Cancer Mutations in Cultured Human Intestinal Stem Cells. Nature 521, 43–47. doi:10.1038/nature14415
Frangoul, H., Altshuler, D., Cappellini, M. D., Chen, Y.-S., Domm, J., Eustace, B. K., et al. (2021). CRISPR-Cas9 Gene Editing for Sickle Cell Disease and β-Thalassemia. N. Engl. J. Med. 384, 252–260. doi:10.1056/NEJMoa2031054
Friedland, A. E., Tzur, Y. B., Esvelt, K. M., Colaiácovo, M. P., Church, G. M., and Calarco, J. A. (2013). Heritable Genome Editing in C. elegans via a CRISPR-Cas9 System. Nat. Methods 10, 741–743. doi:10.1038/nmeth.2532
Frock, R. L., Hu, J., Meyers, R. M., Ho, Y. J., Kii, E., and Alt, F. W. (2015). Genome-wide Detection of DNA Double-Stranded Breaks Induced by Engineered Nucleases. Nat. Biotechnol. 33, 179–186. doi:10.1038/nbt.3101
Gaj, T., Gersbach, C. A., and Barbas, C. F. (2013). ZFN, TALEN, and CRISPR/Cas-based Methods for Genome Engineering. Trends Biotechnol. 31, 397–405. doi:10.1016/j.tibtech.2013.04.004
Gaudelli, N. M., Komor, A. C., Rees, H. A., Packer, M. S., Badran, A. H., Bryson, D. I., et al. (2017). Programmable Base Editing of A•T to G•C in Genomic DNA without DNA Cleavage. Nature 551, 464–471. doi:10.1038/nature24644
Gerstung, M., Jolly, C., Leshchiner, I., Dentro, S. C., Gonzalez, S., Rosebrock, D., et al. (2020). The Evolutionary History of 2,658 Cancers. Nature 578, 122–128. doi:10.1038/s41586-019-1907-7
Gillmore, J. D., Gane, E., Taubel, J., Kao, J., Fontana, M., Maitland, M. L., et al. (2021). CRISPR-Cas9 In Vivo Gene Editing for Transthyretin Amyloidosis. N. Engl. J. Med. 385, 493–502. doi:10.1056/NEJMoa2107454
Haft, D. H., Selengut, J., Mongodin, E. F., and Nelson, K. E. (2005). A Guild of 45 CRISPR-Associated (Cas) Protein Families and Multiple CRISPR/Cas Subtypes Exist in Prokaryotic Genomes. PLoS Comput. Biol. 1 (6), e60. doi:10.1371/journal.pcbi.0010060
Hammond, A., Galizi, R., Kyrou, K., Simoni, A., Siniscalchi, C., Katsanos, D., et al. (2016). A CRISPR-Cas9 Gene Drive System Targeting Female Reproduction in the Malaria Mosquito Vector Anopheles gambiae. Nat. Biotechnol. 34, 78–83. doi:10.1038/nbt.3439
Hashimoto, M., and Takemoto, T. (2015). Electroporation Enables the Efficient mRNA Delivery into the Mouse Zygotes and Facilitates CRISPR/Cas9-based Genome Editing. Sci. Rep. 5, 11315. doi:10.1038/srep11315
Hille, F., Richter, H., Wong, S. P., Bratovič, M., Ressel, S., and Charpentier, E. (2018). The Biology of CRISPR-Cas: Backward and Forward. Cell 172, 1239–1259. doi:10.1016/j.cell.2017.11.032
Honda, A., Hirose, M., Sankai, T., Yasmin, L., Yuzawa, K., Honsho, K., et al. (2015). Single-step Generation of Rabbits Carrying a Targeted Allele of the Tyrosinase Gene Using CRISPR/Cas9. Exp. Anim. 64, 31–37. doi:10.1538/expanim.14-0034
Horii, T., Tamura, D., Morita, S., Kimura, M., and Hatada, I. (2013). Generation of an ICF Syndrome Model by Efficient Genome Editing of Human Induced Pluripotent Stem Cells Using the CRISPR System. Int. J. Mol. Sci. 14, 19774–19781. doi:10.3390/ijms141019774
Hruscha, A., Krawitz, P., Rechenberg, A., Heinrich, V., Hecht, J., Haass, C., et al. (2013). Efficient CRISPR/Cas9 Genome Editing with Low Off-Target Effects in Zebrafish. Development 140, 4982–4987. doi:10.1242/dev.099085
Hsu, P. D., Lander, E. S., and Zhang, F. (2014). Development and Applications of CRISPR-Cas9 for Genome Engineering. Cell 157, 1262–1278. doi:10.1016/j.cell.2014.05.010
Hu, Y., Zhou, Y., Zhang, M., Ge, W., Li, Y., Yang, L., et al. (2021). CRISPR/Cas9-Engineered Universal CD19/CD22 Dual-Targeted CAR-T Cell Therapy for Relapsed/Refractory B-Cell Acute Lymphoblastic Leukemia. Clin. Cancer Res. 27, 2764–2772. doi:10.1158/1078-0432.CCR-20-3863
Huang, W., Yan, Y., Liu, Y., Lin, M., Ma, J., Zhang, W., et al. (2020). Exosomes with Low miR-34c-3p Expression Promote Invasion and Migration of Non-small Cell Lung Cancer by Upregulating Integrin α2β1. Signal Transduct. Target Ther. 5, 39. doi:10.1038/s41392-020-0133-y
Ishino, Y., Shinagawa, H., Makino, K., Amemura, M., and Nakata, A. (1987). Nucleotide Sequence of the Iap Gene, Responsible for Alkaline Phosphatase Isozyme Conversion in Escherichia coli, and Identification of the Gene Product. J. Bacteriol. 169, 5429–5433. doi:10.1128/jb.169.12.5429-5433.1987
Jänne, P. A., Baik, C., Su, W. C., Johnson, M. L., Hayashi, H., Nishio, M., et al. (2022). Efficacy and Safety of Patritumab Deruxtecan (HER3-DXd) in EGFR Inhibitor-Resistant, EGFR-Mutated Non-small Cell Lung Cancer. Cancer Discov. 12, 74–89. doi:10.1158/2159-8290.CD-21-0715
Jansen, R., Embden, J. D., Gaastra, W., and Schouls, L. M. (2002). Identification of Genes that Are Associated with DNA Repeats in Prokaryotes. Mol. Microbiol. 43, 1565–1575. doi:10.1046/j.1365-2958.2002.02839.x
Jinek, M., Chylinski, K., Fonfara, I., Hauer, M., Doudna, J. A., and Charpentier, E. (2012). A Programmable Dual-RNA-Guided DNA Endonuclease in Adaptive Bacterial Immunity. Science 337, 816–821. doi:10.1126/science.1225829
Khairkhah, N., Bolhassani, A., and Najafipour, R. (2022). Current and Future Direction in Treatment of HPV-Related Cervical Disease. J. Mol. Med. 100, 829–845. doi:10.1007/s00109-022-02199-y
Knott, G. J., and Doudna, J. A. (2018). CRISPR-cas Guides the Future of Genetic Engineering. Science 361, 866–869. doi:10.1126/science.aat5011
Kofler, N. (2019). Why Were Scientists Silent over Gene-Edited Babies? Nature 566, 427. doi:10.1038/d41586-019-00662-4
Komor, A. C., Kim, Y. B., Packer, M. S., Zuris, J. A., and Liu, D. R. (2016). Programmable Editing of a Target Base in Genomic DNA without Double-Stranded DNA Cleavage. Nature 533, 420–424. doi:10.1038/nature17946
Koo, T., Yoon, A. R., Cho, H. Y., Bae, S., Yun, C. O., and Kim, J. S. (2017). Selective Disruption of an Oncogenic Mutant Allele by CRISPR/Cas9 Induces Efficient Tumor Regression. Nucleic Acids Res. 45, 7897–7908. doi:10.1093/nar/gkx490
Koonin, E. V., and Makarova, K. S. (2019). Origins and Evolution of CRISPR-Cas Systems. Philos. Trans. R. Soc. Lond B Biol. Sci. 374, 20180087. doi:10.1098/rstb.2018.0087
Lacey, S. F., and Fraietta, J. A. (2020). First Trial of CRISPR-Edited T Cells in Lung Cancer. Trends Mol. Med. 26, 713–715. doi:10.1016/j.molmed.2020.06.001
Lagutina, I. V., Valentine, V., Picchione, F., Harwood, F., Valentine, M. B., Villarejo-Balcells, B., et al. (2015). Modeling of the Human Alveolar Rhabdomyosarcoma Pax3-Foxo1 Chromosome Translocation in Mouse Myoblasts Using CRISPR-Cas9 Nuclease. PLoS Genet. 11, e1004951. doi:10.1371/journal.pgen.1004951
Landrum, M. J., Lee, J. M., Benson, M., Brown, G., Chao, C., Chitipiralla, S., et al. (2016). ClinVar: Public Archive of Interpretations of Clinically Relevant Variants. Nucleic Acids Res. 44, D862–D868. doi:10.1093/nar/gkv1222
Ledford, H. (2019). Super-precise New CRISPR Tool Could Tackle a Plethora of Genetic Diseases. Nature 574, 464–465. doi:10.1038/d41586-019-03164-5
Li, W., Teng, F., Li, T., and Zhou, Q. (2013). Simultaneous Generation and Germline Transmission of Multiple Gene Mutations in Rat Using CRISPR-Cas Systems. Nat. Biotechnol. 31, 684–686. doi:10.1038/nbt.2652
Liu, C., Zhang, L., Liu, H., and Cheng, K. (2017). Delivery Strategies of the CRISPR-Cas9 Gene-Editing System for Therapeutic Applications. J. Control Release 266, 17–26. doi:10.1016/j.jconrel.2017.09.012
Liu, Y., Zeng, Y., Liu, L., Zhuang, C., Fu, X., Huang, W., et al. (2014). Synthesizing and Gate Genetic Circuits Based on CRISPR-Cas9 for Identification of Bladder Cancer Cells. Nat. Commun. 5, 5393. doi:10.1038/ncomms6393
Lu, Y., Xue, J., Deng, T., Zhou, X., Yu, K., Deng, L., et al. (2020). Safety and Feasibility of CRISPR-Edited T Cells in Patients with Refractory Non-small-cell Lung Cancer. Nat. Med. 26, 732–740. doi:10.1038/s41591-020-0840-5
Macias, V. M., McKeand, S., Chaverra-Rodriguez, D., Hughes, G. L., Fazekas, A., Pujhari, S., et al. (2020). Cas9-Mediated Gene-Editing in the Malaria Mosquito Anopheles stephensi by ReMOT Control. G3 (Bethesda) 10, 1353–1360. doi:10.1534/g3.120.401133
Maeder, M. L., Stefanidakis, M., Wilson, C. J., Baral, R., Barrera, L. A., Bounoutas, G. S., et al. (2019). Development of a Gene-Editing Approach to Restore Vision Loss in Leber Congenital Amaurosis Type 10. Nat. Med. 25, 229–233. doi:10.1038/s41591-018-0327-9
Makarova, K. S., Wolf, Y. I., Alkhnbashi, O. S., Costa, F., Shah, S. A., Saunders, S. J., et al. (2015). An Updated Evolutionary Classification of CRISPR-Cas Systems. Nat. Rev. Microbiol. 13, 722–736. doi:10.1038/nrmicro3569
Makarova, K. S., Wolf, Y. I., Iranzo, J., Shmakov, S. A., Alkhnbashi, O. S., Brouns, S. J. J., et al. (2020). Evolutionary Classification of CRISPR-Cas Systems: a Burst of Class 2 and Derived Variants. Nat. Rev. Microbiol. 18, 67–83. doi:10.1038/s41579-019-0299-x
Mali, P., Yang, L., Esvelt, K. M., Aach, J., Guell, M., DiCarlo, J. E., et al. (2013). RNA-guided Human Genome Engineering via Cas9. Science 339, 823–826. doi:10.1126/science.1232033
Mani, R. S., and Chinnaiyan, A. M. (2010). Triggers for Genomic Rearrangements: Insights into Genomic, Cellular and Environmental Influences. Nat. Rev. Genet. 11, 819–829. doi:10.1038/nrg2883
Mun, E. J., Babiker, H. M., Weinberg, U., Kirson, E. D., and Von Hoff, D. D. (2018). Tumor-Treating Fields: A Fourth Modality in Cancer Treatment. Clin. Cancer Res. 24, 266–275. doi:10.1158/1078-0432.CCR-17-1117
Nambiar, T. S., Baudrier, L., Billon, P., and Ciccia, A. (2022). CRISPR-based Genome Editing through the Lens of DNA Repair. Mol. Cell 82, 348–388. doi:10.1016/j.molcel.2021.12.026
Ni, W., Qiao, J., Hu, S., Zhao, X., Regouski, M., Yang, M., et al. (2014). Efficient Gene Knockout in Goats Using CRISPR/Cas9 System. PLoS One 9, e106718. doi:10.1371/journal.pone.0106718
Nierzwicki, Ł., Arantes, P. R., Saha, A., and Palermo, G. (2021). Establishing the Allosteric Mechanism in CRISPR‐Cas9. WIREs Comput. Mol. SciMol Sci. 11 (3), e1503. doi:10.1002/wcms.1503
Niu, Y., Shen, B., Cui, Y., Chen, Y., Wang, J., Wang, L., et al. (2014). Generation of Gene-Modified Cynomolgus Monkey via Cas9/RNA-Mediated Gene Targeting in One-Cell Embryos. Cell 156, 836–843. doi:10.1016/j.cell.2014.01.027
O'Neil, B. H., Wallmark, J. M., Lorente, D., Elez, E., Raimbourg, J., Gomez-Roca, C., et al. (2017). Safety and Antitumor Activity of the Anti-PD-1 Antibody Pembrolizumab in Patients with Advanced Colorectal Carcinoma. PLoS One 12, e0189848. doi:10.1371/journal.pone.0189848
Ott, P. A., Hu-Lieskovan, S., Chmielowski, B., Govindan, R., Naing, A., Bhardwaj, N., et al. (2020). A Phase Ib Trial of Personalized Neoantigen Therapy Plus Anti-PD-1 in Patients with Advanced Melanoma, Non-small Cell Lung Cancer, or Bladder Cancer. Cell 183, 347–e24. doi:10.1016/j.cell.2020.08.053
Paquet, D., Kwart, D., Chen, A., Sproul, A., Jacob, S., Teo, S., et al. (2016). Efficient Introduction of Specific Homozygous and Heterozygous Mutations Using CRISPR/Cas9. Nature 533, 125–129. doi:10.1038/nature17664
Podlaha, O., Riester, M., De, S., and Michor, F. (2012). Evolution of the Cancer Genome. Trends Genet. 28, 155–163. doi:10.1016/j.tig.2012.01.003
Rassool, F. V. (2003). DNA Double Strand Breaks (DSB) and Non-homologous End Joining (NHEJ) Pathways in Human Leukemia. Cancer Lett. 193, 1–9. doi:10.1016/s0304-3835(02)00692-4
Rees, H. A., and Liu, D. R. (2018). Base Editing: Precision Chemistry on the Genome and Transcriptome of Living Cells. Nat. Rev. Genet. 19, 770–788. doi:10.1038/s41576-018-0059-1
Roth, T. L., Puig-Saus, C., Yu, R., Shifrut, E., Carnevale, J., Li, P. J., et al. (2018). Reprogramming Human T Cell Function and Specificity with Non-viral Genome Targeting. Nature 559, 405–409. doi:10.1038/s41586-018-0326-5
Safier, L. Z., Zuccaro, M. V., and Egli, D. (2020). Efficient SNP Editing in Haploid Human Pluripotent Stem Cells. J. Assist. Reprod. Genet. 37, 735–745. doi:10.1007/s10815-020-01723-8
Sato, M., Takabayashi, S., Akasaka, E., and Nakamura, S. (2020). Recent Advances and Future Perspectives of In Vivo Targeted Delivery of Genome-Editing Reagents to Germ Cells, Embryos, and Fetuses in Mice. Cells 9, 799. doi:10.3390/cells9040799
Schep, R., Brinkman, E. K., Leemans, C., Vergara, X., van der Weide, R. H., Morris, B., et al. (2021). Impact of Chromatin Context on Cas9-Induced DNA Double-Strand Break Repair Pathway Balance. Mol. Cell 81, 2216–e10. doi:10.1016/j.molcel.2021.03.032
Shams, A., Higgins, S. A., Fellmann, C., Laughlin, T. G., Oakes, B. L., Lew, R., et al. (2021). Comprehensive Deletion Landscape of CRISPR-Cas9 Identifies Minimal RNA-Guided DNA-Binding Modules. Nat. Commun. 12, 5664. doi:10.1038/s41467-021-25992-8
Shirai, Y., Piulachs, M. D., Belles, X., and Daimon, T. (2022). DIPA-CRISPR Is a Simple and Accessible Method for Insect Gene Editing. Cell Rep. Methods 2, 100215. doi:10.1016/j.crmeth.2022.100215
Stadtmauer, E. A., Fraietta, J. A., Davis, M. M., Cohen, A. D., Weber, K. L., Lancaster, E., et al. (2020). CRISPR-engineered T Cells in Patients with Refractory Cancer. Science 367 (6481), eaba7365. doi:10.1126/science.aba7365
Stein, A., Simnica, D., Schultheiss, C., Scholz, R., Tintelnot, J., Gökkurt, E., et al. (2021). PD-L1 Targeting and Subclonal Immune Escape Mediated by PD-L1 Mutations in Metastatic Colorectal Cancer. J. Immunother. Cancer 9 (7), e002844. doi:10.1136/jitc-2021-002844
Sternberg, S. H., Redding, S., Jinek, M., Greene, E. C., and Doudna, J. A. (2014). DNA Interrogation by the CRISPR RNA-Guided Endonuclease Cas9. Nature 507, 62–67. doi:10.1038/nature13011
Su, S., Hu, B., Shao, J., Shen, B., Du, J., Du, Y., et al. (2016). CRISPR-Cas9 Mediated Efficient PD-1 Disruption on Human Primary T Cells from Cancer Patients. Sci. Rep. 6, 20070. doi:10.1038/srep20070
Sung, H., Ferlay, J., Siegel, R. L., Laversanne, M., Soerjomataram, I., Jemal, A., et al. (2021). Global Cancer Statistics 2020: GLOBOCAN Estimates of Incidence and Mortality Worldwide for 36 Cancers in 185 Countries. CA Cancer J. Clin. 71, 209–249. doi:10.3322/caac.21660
Takeda, H., Kataoka, S., Nakayama, M., Ali, M. A. E., Oshima, H., Yamamoto, D., et al. (2019). CRISPR-Cas9-mediated Gene Knockout in Intestinal Tumor Organoids Provides Functional Validation for Colorectal Cancer Driver Genes. Proc. Natl. Acad. Sci. U. S. A. 116, 15635–15644. doi:10.1073/pnas.1904714116
Tebas, P., Stein, D., Tang, W. W., Frank, I., Wang, S. Q., Lee, G., et al. (2014). Gene Editing of CCR5 in Autologous CD4 T Cells of Persons Infected with HIV. N. Engl. J. Med. 370, 901–910. doi:10.1056/NEJMoa1300662
Topalian, S. L., Hodi, F. S., Brahmer, J. R., Gettinger, S. N., Smith, D. C., McDermott, D. F., et al. (2012). Safety, Activity, and Immune Correlates of Anti-PD-1 Antibody in Cancer. N. Engl. J. Med. 366, 2443–2454. doi:10.1056/NEJMoa1200690
Vanneman, M., and Dranoff, G. (2012). Combining Immunotherapy and Targeted Therapies in Cancer Treatment. Nat. Rev. Cancer 12, 237–251. doi:10.1038/nrc3237
Varshney, G. K., Pei, W., LaFave, M. C., Idol, J., Xu, L., Gallardo, V., et al. (2015). High-throughput Gene Targeting and Phenotyping in Zebrafish Using CRISPR/Cas9. Genome Res. 25, 1030–1042. doi:10.1101/gr.186379.114
Wang, H., Yang, H., Shivalila, C. S., Dawlaty, M. M., Cheng, A. W., Zhang, F., et al. (2013). One-step Generation of Mice Carrying Mutations in Multiple Genes by CRISPR/Cas-mediated Genome Engineering. Cell 153, 910–918. doi:10.1016/j.cell.2013.04.025
Wang, H. X., Li, M., Lee, C. M., Chakraborty, S., Kim, H. W., Bao, G., et al. (2017). CRISPR/Cas9-Based Genome Editing for Disease Modeling and Therapy: Challenges and Opportunities for Nonviral Delivery. Chem. Rev. 117, 9874–9906. doi:10.1021/acs.chemrev.6b00799
Wang, T., Wei, J. J., Sabatini, D. M., and Lander, E. S. (2014). Genetic Screens in Human Cells Using the CRISPR-Cas9 System. Science 343, 80–84. doi:10.1126/science.1246981
Wang, Y., Liu, K. I., Sutrisnoh, N. B., Srinivasan, H., Zhang, J., Li, J., et al. (2018). Systematic Evaluation of CRISPR-Cas Systems Reveals Design Principles for Genome Editing in Human Cells. Genome Biol. 19, 62. doi:10.1186/s13059-018-1445-x
Wang, Z., Li, N., Feng, K., Chen, M., Zhang, Y., Liu, Y., et al. (2021). Phase I Study of CAR-T Cells with PD-1 and TCR Disruption in Mesothelin-Positive Solid Tumors. Cell Mol. Immunol. 18, 2188–2198. doi:10.1038/s41423-021-00749-x
Wiedenheft, B., Sternberg, S. H., and Doudna, J. A. (2012). RNA-guided Genetic Silencing Systems in Bacteria and Archaea. Nature 482, 331–338. doi:10.1038/nature10886
Wu, Y., Zhou, H., Fan, X., Zhang, Y., Zhang, M., Wang, Y., et al. (2015). Correction of a Genetic Disease by CRISPR-Cas9-Mediated Gene Editing in Mouse Spermatogonial Stem Cells. Cell Res. 25, 67–79. doi:10.1038/cr.2014.160
Xie, F., Ye, L., Chang, J. C., Beyer, A. I., Wang, J., Muench, M. O., et al. (2014). Seamless Gene Correction of β-thalassemia Mutations in Patient-specific iPSCs Using CRISPR/Cas9 and piggyBac. Genome Res. 24, 1526–1533. doi:10.1101/gr.173427.114
Xu, L., Wang, J., Liu, Y., Xie, L., Su, B., Mou, D., et al. (2019). CRISPR-edited Stem Cells in a Patient with HIV and Acute Lymphocytic Leukemia. N. Engl. J. Med. 381, 1240–1247. doi:10.1056/NEJMoa1817426
Xu, Y., Chen, C., Guo, Y., Hu, S., and Sun, Z. (2022). Effect of CRISPR/Cas9-Edited PD-1/pd-L1 on Tumor Immunity and Immunotherapy. Front. Immunol. 13, 848327. doi:10.3389/fimmu.2022.848327
Yang, Y. (2015). Cancer Immunotherapy: Harnessing the Immune System to Battle Cancer. J. Clin. Invest. 125, 3335–3337. doi:10.1172/JCI83871
Yi, L., and Li, J. (2016). CRISPR-Cas9 Therapeutics in Cancer: Promising Strategies and Present Challenges. Biochim. Biophys. Acta 1866, 197–207. doi:10.1016/j.bbcan.2016.09.002
Yin, J., Lu, R., Xin, C., Wang, Y., Ling, X., Li, D., et al. (2022). Cas9 Exo-Endonuclease Eliminates Chromosomal Translocations during Genome Editing. Nat. Commun. 13, 1204. doi:10.1038/s41467-022-28900-w
Yuan, L., Sui, T., Chen, M., Deng, J., Huang, Y., Zeng, J., et al. (2016). CRISPR/Cas9-mediated GJA8 Knockout in Rabbits Recapitulates Human Congenital Cataracts. Sci. Rep. 6, 22024. doi:10.1038/srep22024
Zhang, B., Chen, Z., Yi, J., Tang, H., Wang, C., and Chinese Academy of, E. (2019a). Chinese Academy of Engineering Calls for Actions on the Birth of Gene-Edited Infants. Lancet 393, 25. doi:10.1016/S0140-6736(18)33081-2
Zhang, S., Zhang, F., Chen, Q., Wan, C., Xiong, J., and Xu, J. (2019b). CRISPR/Cas9-mediated Knockout of NSD1 Suppresses the Hepatocellular Carcinoma Development via the NSD1/H3/Wnt10b Signaling Pathway. J. Exp. Clin. Cancer Res. 38, 467. doi:10.1186/s13046-019-1462-y
Zhang, Y., and Zhang, Z. (2020). The History and Advances in Cancer Immunotherapy: Understanding the Characteristics of Tumor-Infiltrating Immune Cells and Their Therapeutic Implications. Cell Mol. Immunol. 17, 807–821. doi:10.1038/s41423-020-0488-6
Zhou, X., Xin, J., Fan, N., Zou, Q., Huang, J., Ouyang, Z., et al. (2015). Generation of CRISPR/Cas9-mediated Gene-Targeted Pigs via Somatic Cell Nuclear Transfer. Cell Mol. Life Sci. 72, 1175–1184. doi:10.1007/s00018-014-1744-7
Zhu, B., Chen, S., Hu, X., Jin, X., Le, Y., Cao, L., et al. (2017). Knockout of the Nogo-B Gene Attenuates Tumor Growth and Metastasis in Hepatocellular Carcinoma. Neoplasia 19, 583–593. doi:10.1016/j.neo.2017.02.007
Keywords: CRISPR/Cas9, gene editing technology, anti-cancer therapy, off-target effect, ethics
Citation: Chen X-Z, Guo R, Zhao C, Xu J, Song H, Yu H, Pilarsky C, Nainu F, Li J-Q, Zhou X-K and Zhang J-Y (2022) A Novel Anti-Cancer Therapy: CRISPR/Cas9 Gene Editing. Front. Pharmacol. 13:939090. doi: 10.3389/fphar.2022.939090
Received: 08 May 2022; Accepted: 14 June 2022;
Published: 22 July 2022.
Edited by:
Liang Ouyang, Sichuan University, ChinaReviewed by:
Bing Liu, Guangdong Pharmaceutical University, ChinaXiaokun Wang, Wayne State University, United States
Copyright © 2022 Chen, Guo, Zhao, Xu, Song, Yu, Pilarsky, Nainu, Li, Zhou and Zhang. This is an open-access article distributed under the terms of the Creative Commons Attribution License (CC BY). The use, distribution or reproduction in other forums is permitted, provided the original author(s) and the copyright owner(s) are credited and that the original publication in this journal is cited, in accordance with accepted academic practice. No use, distribution or reproduction is permitted which does not comply with these terms.
*Correspondence: Jing-Quan Li, bGlqaW5ncXVhbjIwMDhAMTYzLmNvbQ==; Xin-Ke Zhou, enhrc3RhckAxMjYuY29t; Jian-Ye Zhang, amlhbnllekAxNjMuY29t
†These authors have contributed equally to this work