- 1State Key Laboratory of Southwestern Chinese Medicine Resources, School of Pharmacy, Chengdu University of Traditional Chinese Medicine, Chengdu, China
- 2Hospital of Chengdu University of Traditional Chinese Medicine, Chengdu, China
Neurodegenerative disease is a progressive neurodegeneration caused by genetic and environmental factors. Alzheimer’s disease (AD), Parkinson’s disease (PD), and Huntington’s disease (HD) are the three most common neurodegenerative diseases clinically. Unfortunately, the incidence of neurodegenerative diseases is increasing year by year. However, the current available drugs have poor efficacy and large side effects, which brings a great burden to the patients and the society. Increasing evidence suggests that occurrence and development of the neurodegenerative diseases is closely related to the mitochondrial dysfunction, which can affect mitochondrial biogenesis, mitochondrial dynamics, as well as mitochondrial mitophagy. Through the disruption of mitochondrial homeostasis, nerve cells undergo varying degrees of apoptosis. Interestingly, it has been shown in recent years that the natural agents derived from herbal medicines are beneficial for prevention/treatment of neurodegenerative diseases via regulation of mitochondrial dysfunction. Therefore, in this review, we will focus on the potential therapeutic agents from herbal medicines for treating neurodegenerative diseases via suppressing apoptosis through regulation of mitochondrial dysfunction, in order to provide a foundation for the development of more candidate drugs for neurodegenerative diseases from herbal medicine.
1 Introduction
Neurodegenerative diseases are characterized by slow and progressive dysfunction of neurons in certain areas of the brain and spinal cord (Ahat et al., 2019). Although the removal of redundant neuronal cells is essential for normal brain function, the abnormal death of different neuronal cell populations is a pathological sign of neurodegenerative diseases and will extend to other organs in the later stages of disease development (Gorman, 2008). The most common neurodegenerative diseases include a variety of complex dementia syndromes such as Alzheimer’s disease (AD), Parkinson’s disease (PD), Huntington’s disease (HD), frontotemporal dementia (FTD), mixed dementia (MD), and vascular dementia (VD). Besides, amyotrophic lateral sclerosis (ALS), and multiple sclerosis (MS) are also neurodegenerative diseases (Schrijvers et al., 2012). With the rapid growth of the elderly population worldwide, especially in developed countries, the prevalence of various age-related neurodegenerative diseases has increased sharply in recent years. It is worth noting that the incidence of AD, PD, and HD increases exponentially with age (Norton et al., 2014). According to the epidemical investigations, it is estimated that by 2050, the number of cases will increase sharply to 106.2 million. The huge medical expenses have an indelible impact on families and social economy (Tamburrino and Decressac, 2016). Therefore, exploring the pathogenesis of neurodegenerative diseases and discovering more novel effective treatments is an urgent need to alleviate the global upward trend of neurodegenerative diseases.
The researchers believe that genetic susceptibility and environmental factors, including paraquat, rotenone, metals such as iron, manganese and lead, and gaseous compounds such as carbon monoxide, contribute to the evolution of neurodegeneration (Liu et al., 2013). The etiology of neurodegenerative diseases is still in the exploratory stage. Taking AD as an example, many researchers believe that the apolipoprotein E4 gene can directly lead to excessive Aβ deposition in the brain of patients. The accumulation of aggregated protein is a common pathological feature of neurodegenerative diseases, which will lead to cell dysfunction. Besides these, the aggregated protein will cause cell death, leading to the occurrence and development of AD in turn (Baumgart et al., 2015; Livingston et al., 2017). However, after a large number of experimental verifications, it was found that the apolipoprotein E4 gene and related pathological features were not always found in AD patients, suggesting there are other causes of AD (Reitz and Mayeux, 2014). As a result, researchers put forward the mitochondrial cascade hypothesis. This hypothesis suggests that primary and secondary mitochondrial dysfunction is an important cause of AD, a driving force for Aβ plaque deposition in AD, and a typical event of neurodegeneration (Silva et al., 2011; Oliver and Reddy, 2019a).
In human body, the survival and activity of various cells are dependent on the production of energy. Mitochondria are the main production base of energy and are the key factors for generating transmembrane resting and action potentials (Johnson et al., 2021; Jessica et al., 2022). Energy can be produced through the Krebs cycle and the electron transport chain (ETC) in mitochondria (Lin and Beal, 2006). Nowadays, accumulating studies have indicated mitochondrial dysfunction and mitochondrial death may occur before the histopathological features of neurodegenerative diseases. Generally, mitochondrial DNA (mtDNA) in the human body will continue to produce new mutations as the body ages. When the mutations accumulate to a certain level, it will cause mitochondrial dysfunction, which in turn induces energy metabolism, oxidative stress and metabolic consequences (Naoi rt al., 2005; Armstrong, 2006; Schapira, 2006). The key consequences closely related to AD mainly include the destruction of intracellular calcium homeostasis and redox balance in neurons, activating the occurrence of intracellular apoptotic events, which ultimately promoting the development of neurodegenerative diseases (Swerdlow, 2018). At the same time, mitochondrial dysfunction can also interact with Aβ and p-tau to exacerbate the development of AD (Du and Yan, 2010; Spuch et al., 2012; Reddy and Oliver, 2019).
At present, the drugs used in neurodegenerative diseases cannot reverse the process of neuron loss, but only relieve the symptoms (Schneider et al., 2014; Sun et al., 2010; Solva et al., 2014). Consequently, it is of great significance to further understand the molecular mechanism behind it and discover more potential drugs for neurodegenerative diseases. For thousands of years, herbal medicine has accumulated a lot of experience in treating neurodegenerative diseases in practice. For example, in the Huangdi Neijing (Huangdi’s Internal Classic), it is recorded that single herbal medicine can be used to treat tremor, and modern pharmacological studies have further confirmed that a variety of active ingredients extracted from herbal medicine can be used to treat neurodegenerative diseases (Zhang et al., 2014; Hügel, 2015; Yang et al., 2017; Peng et al., 2022). Due to its wide curative effect and low side effects, herbal medicines have attracted increasing attention. Therefore, in this paper, we will focus on AD, PD and HD in neurodegenerative diseases, and introduce apoptosis related to mitochondrial dysfunction in neurodegenerative diseases and potential herbal medicines.
2 Mitochondrial homeostasis regulation
In the past, the classical doctrine considered mitochondria to be a static bean-like organelle. However, with the continuous development of electronic imaging technology, this doctrine has received a constant shock. In fact, as the most complex organelle in the cell, mitochondria are metabolically active and show high speed of movement in the cell. In other words, mitochondria are able to respond rapidly in various environments, which making them a dynamic organelle. Mitochondria can directly or indirectly regulate various signaling pathways in the organism, maintain calcium homeostasis, and synthesize energy for life activities mainly through the process of oxidative phosphorylation (OXPHOS) (Milane et al., 2015; Horbay and Bilyy, 2016). In neurons, mitochondria provide the energy needed to support neurite growth and neurotransmitter release, and the energy requirements are extremely high. However, the high energy production is accompanied by increased production of reactive oxygen species (ROS) in the cell (Mamelak, 2017). When intracellular mitochondrial homeostasis is dysregulated and the regulatory capacity of antioxidants is much lower than the rate of ROS production, ROS will accumulate excessively in the cell and induce a series of responses such as oxidative stress, which will eventually lead to cell death (Sarniak et al., 2016; Singh et al., 2019). Therefore, the regulation of mitochondrial homeostasis is crucial in the growth and development of neural cells.
2.1 Mitochondrial biogenesis
Mitochondrial biogenesis in neurons refers to the process of formation of new mitochondria, a process that involves the synthesis and transport of proteins and lipids, as well as the replication of mtDNA. The proteins required for mitochondrial biogenesis are mainly produced by ribosomes and later imported via outer membrane translocases. In the present study, most lipids were produced by endoplasmic reticulum and then transported to the mitochondria via vesicular transport or lipid transfer proteins, At the same time, there’s a small amount of lipid such as phosphatidylethanolamine, phosphatidylglycerol, and cardiolipin can also be generated directly in the mitochondria. Although mtDNA is the only type of DNA remaining in the cell other than nuclear DNA, it lacks a variable number of transfer RNA (tRNA) genes in its structure, so tRNA likewise needs to be transported from outside the mitochondria to inside the mitochondria (Wiedemann et al., 2004). As shown in Figure 1, mitochondrial biogenesis is mainly regulated by relevant transcriptional factors such as peroxisome proliferator-activated receptor γ (PPARγ) and coactivator 1α (PGC 1α) in the nucleus and mitochondria to increase the number of mitochondria by promoting protein synthesis and mtDNA replication, respectively (Manoli et al., 2007). In this process, nuclear respiratory factor 1 (NRF1) and nuclear respiratory factor 2 (NRF2) can be regulated by PGC 1α to bind to specific sites of mitochondrial transcription factor A (TFAM), thereby controlling the expression of multiple mtDNA-encoded genes, which contain oxidative phosphorylation system of mitochondria (OXPHOS) proteins and antioxidant-related proteins (Peng et al., 2017). In addition, AMP-activated protein kinase (AMPK) and silent mating-type information regulation 2 homolog 1 (SIRT1) regulate PGC 1α through phosphorylation and deacetylation when energy is scarce (Reznick and Shulman, 2006).
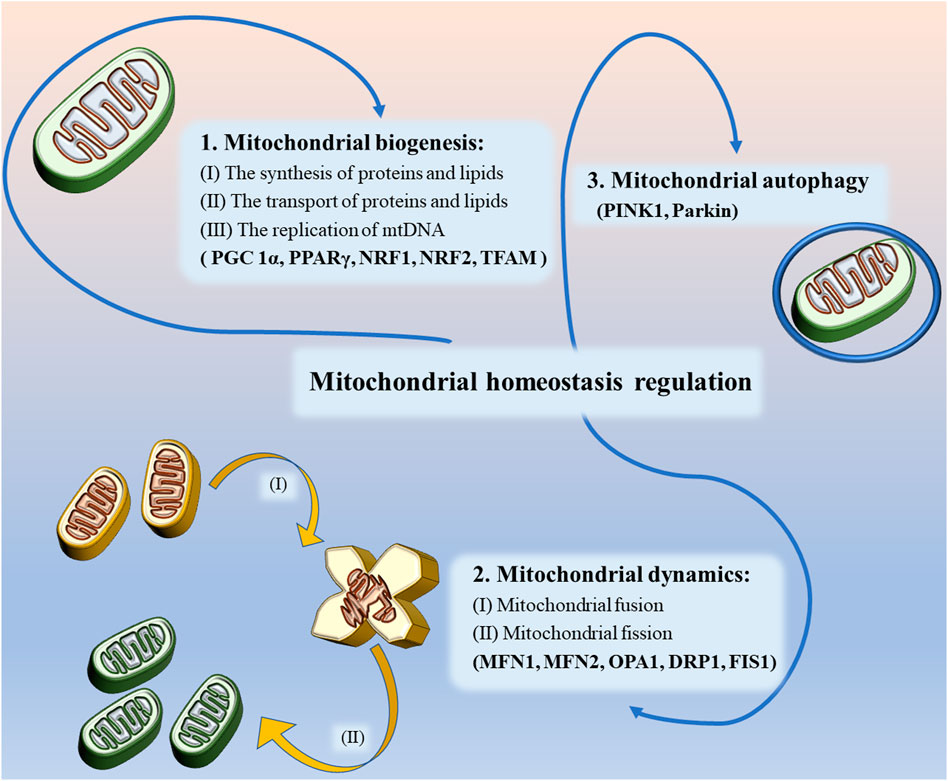
FIGURE 1. Mitochondrial homeostasis regulation includes regulation of mitochondrial biogenesis, mitochondrial dynamics and mitochondrial autophagy.
2.2 Mitochondrial dynamics
As mentioned earlier, mitochondria are dynamic organelles, and their movement is largely dependent on the strict regulation of mitochondrial dynamics to meet the energy and transport needs of the cell. Mitochondrial dynamics mainly includes fusion and fission (Valero, 2014; Peng et al., 2017). Mitochondrial fusion is a continuous process in which GTPases located on the outer mitochondrial membrane, namely outer membrane mitofusin 1 (MFN 1) and mitofusin 2 (MFN 2), tether the mitochondria to the endoplasmic reticulum and achieve mitochondrial fusion (Filadi et al., 2018). In contrast, the Optic Atrophy1 (OPA1) coordinates the mediation of mitochondrial inner membrane fusion and promotes the formation of a highly interconnected tubular network (Meyer et al., 2017). The formation of new mitochondria by fusion can repair damaged mitochondrial functions, provide conditions for efficient transfer of mtDNA during mitochondrial biogenesis and maintain cell survival, while also effectively preventing the accumulation of mtDNA mutations (Meeusen and Nunnari, 2005; Taguchi et al., 2007; Youle and van der, 2012; Burman et al., 2017).
However, in some pathological states, mitochondrial fusion is unable to repair damaged mitochondrial function, when mitochondrial fission is required (Flippo and Strack, 2017). During this process, the activity of dynamin-related protein 1 (DRP1), a key protein regulating fission and mitochondrial dynamic homeostasis, is controlled by several post-transcriptional modifications (PTM), the most important of which is the phosphorylation of residues (Ser616) and (Ser637) on DRP1 (Braschi et al., 2009; Zaja et al., 2014). Cyclin-dependent kinase 1 (CDK1) phosphorylates (Ser616) of Drp1, activating the mitochondrial fission activity of Drp1, while translocating to the outer mitochondrial membrane to interact with fission protein 1 (FIS1) and then forming a ring-like structure on the outer mitochondrial membrane to split the damaged mitochondria into two smaller spherical daughter mitochondria and eliminate the damaged portion (Smirnova et al., 2001; Zaja et al., 2014). However, in contrast, when the Ser637 site is phosphorylated by AMPK, the mitochondrial division activity will be inhibited (Li et al., 2015). During cell division, mitochondrial fission can ensure that dividing cells can obtain enough mitochondria to maintain normal cell life activities (Wai and Langer, 2015). In addition, the researchers found that downregulation of DRP1 expression could also indirectly enhance mitochondrial fusion (Wenger et al., 2013; Oliver and reddy, 2019b). Mitochondrial fusion and fission are self-balancing mechanisms necessary to maintain cellular activity, is fundamental to healthy metabolic function, and is an essential component in the regulation of mitochondrial homeostasis.
2.3 Mitochondrial autophagy
Mitochondrial autophagy refers to the removal of functionally impaired mitochondria from a cell through the mechanism of autophagy in order to maintain normal cellular activity. The process of catabolism, metabolism, and degradation that occurs in lysosomes is observed when paternal mitochondria are subject to degradation or when multiple diseases such as neurodegenerative diseases occur, and it is a form of quality control of healthy mitochondria (Lemasters, 2005). Studies have identified putative kinase 1 (PINK1) and Parkin as key molecules affecting mitochondrial autophagy. PINK1 is able to phosphorylate the Ser65 site of the ubiquitin-like structural domain, recruiting activation of the E3 ubiquitin ligase Parkin, which forms ubiquitin chains such as MFN1 and/or MFN2 with ubiquitin-binding proteins specific to mitochondrial autophagy on mitochondrial outer membrane proteins (Kondapalli et al., 2012; Lazarou et al., 2015; Wauer et al., 2015). MFN ubiquitination can promote mitochondrial autophagy and inhibit mitochondrial fusion (Ziviani et al., 2010; Scarffe et al., 2014). In addition, multiple mechanisms independent of PINK1 have been found in some studies to jointly maintain the initiation and regulation of mitochondrial autophagy in mitochondria when PINK1 is deficient (Liu et al., 2012; McWilliams et al., 2018).
3 Mitochondrial dysfunction and neuronal apoptosis
3.1 Mitochondrial dysfunction
Under physiological conditions, mitochondrial biogenesis, fusion, fission and autophagy work together to maintain mitochondrial homeostasis. Nevertheless, in some cases, mitochondrial dysfunction will lead to a series of consequences such as reduced ATP production and apoptosis (Kamil et al., 2021). In the present study, five possible mechanisms for mitochondrial dysfunction were identified, namely oxidative stress, mutation of mtDNA, opening of the mitochondrial permeability transition pore (mPTP), Ca2+ disruption, and reduced mitochondrial biosynthesis (Anna et al., 2020; Kang L. et al., 2020). Under physiological conditions, the intracellular ROS content is controlled within a certain range and the redox balance is achieved. However, when the balance between ROS and antioxidants is broken, mitochondrial dysfunction is caused by oxidative stress (Kung et al., 2021).
The mtDNA is a type of DNA that lacks protective histones and repair mechanisms and is highly susceptible to ROS attack. In this case, mtDNA is prone to mutation, causing damage to important functional regions within the genome such as the codon region of the oxidative phosphorylase gene and inhibit the expression and activity of OXPHOS proteins (Inna et al., 2009; Han and Chen, 2013). When mtDNA mutations accumulate to a certain threshold, mitochondrial dysfunction occurs. In addition, excessive ROS can reduce mitochondrial membrane potential and induce mPTP opening. When mPTP is irreversibly open for a long time, the mitochondrial inner membrane is fully depolarized, the inner membrane potential collapses, the OXPHOS process stops, and mitochondrial synthesis of ATP is terminated (Gjumrakch et al., 2011; Harper et al., 2004). At the same time, mitochondrial matrix efflux will also lead to massive superoxide anion production, membrane rupture, release of cytochrome C and apoptosis-inducing factors, etc. Ca2+ disruption is also an important factor contributing to mitochondrial dysfunction. It is known from previous studies that intracellular Ca2+ homeostasis can be regulated by mitochondria in conjunction with endoplasmic reticulum, etc. Ca2+ can enhance mitochondrial OXPHOS process and promote ATP synthesis, which in turn will affect calcium regulation. If the cell suffers from oxidative stress, mtDNA mutation or mTPT opening, it will likely cause the dysregulation of Ca2+ homeostasis in mitochondria, which will deepen the degree of mitochondrial dysfunction (Maassen et al., 2004; Sowers, 2004; Smith, 2007; Ito et al., 2021). From the above, it is clear that the various mechanisms complement each other and together contribute to the development of mitochondrial dysfunction. At the same time, ROS plays a central role in the pathogenesis of mitochondrial dysfunction, which runs through all the mechanisms of mitochondrial dysfunction.
3.2 Neuronal apoptosis
Apoptosis is a programmed form of cell death that is achieved by activating a specific program, the whole process is coordinated and smooth and can also be intervened, modified or stopped (Cotman and Anderson, 1995). In the initial phase of apoptosis, we can clearly observe cell shrinks, morphological rounding and loss of contact with surrounding cells. This is followed by dilation of the endoplasmic reticulum in the cytoplasm and swelling of the cisternae to form vacuoles and vesicles. In addition, nuclear modifications were taking place in parallel with it. Chromatin in the nucleus coalesces into a compact mass, which is internucleosomal disjointed by endonucleases (Tang et al., 2019; Sharma et al., 2021a). Apoptosis can affect different cell populations, which include neural precursor cells and differentiated neurons and glial cells, among others. Apoptosis ensures that only morphologically and functionally normal cells survive and are properly connected to their axons and synapses. What’s more, it is essential for nerve function. Too much or too little can lead to a variety of pathological states (Sharma et al., 2021b).
Apoptosis can be triggered by a number of pathways, which are nowadays divided into two main categories, namely the extrinsic pathway (also known as the death receptor pathway) and the intrinsic pathway. Although the two theories involve different pathways, both ultimately form apoptotic vesicles that are recognized and cleared by phagocytes (Satija et al., 2021). In the extrinsic pathway, FAS, a member of the tumor necrosis factor (TNF) superfamily, can cleave and activate caspase-8 by binding to FAS ligands to form the intracellular death-inducing signaling complex (DISC), which in turn activates the downstream caspases (caspase-3 and -7) to achieve cell death (Felderhof-Mueser et al., 2002). During this process, the extrinsic pathway of apoptosis is linked to the intrinsic pathway due to the involvement of caspase-3. The intrinsic apoptotic pathway, also known as the Bcl-2 or mitochondrial pathway, is where cytochrome C plays an extremely important role. As shown in Figure 2, when intracellular stress occurs (e.g., growth factor deprivation, endoplasmic reticulum stress, etc.), the amount of free BCL-2 decreases due to increased production of BH3-only protein, a key initiator of apoptosis, which increases the binding of the anti-apoptotic protein BCL-2 to it (Puthalakath and Strasser, 2002; Chen et al., 2005). It is reported that activation of the pro-apoptotic proteins BAX and BAK results in the formation of oligomers that induce the mitochondrial outer membrane permeablisation (MOMP) and the release of cytochrome C (Huang and Strasser, 2000; Kelly and Strasser, 2020). After release into the cytoplasm, cytochrome C activates caspase-3 and -9 via forming an apoptosome complex by binding to apoptotic protease factor 1 (Apaf 1) (Yuan et al., 2013). It has been shown experimentally that activation of caspase-3 leads to a significant release of caspase-activated deoxyribonuclease (CAD), resulting in DNA fragmentation in the cell and apoptotic phenomena such as chromatin shrinkage (Enari et al., 1998; Shakeri et al., 2017; Nijhawan et al., 1997). It is well established that when Ca2+ signaling between the endoplasmic reticulum and mitochondria is disrupted, BCL-2 family proteins will also be activated. Subsequently, the localization of Drp1 to mitochondria is further enhanced, which greatly increase mitochondrial division and the amount of mitochondrial fragmentation, resulting in mitochondrial dysfunction, which not only releases cytochrome C, but also releases apoptosis-inducing factor (AIF) to increase oxidative stress and induces apoptosis (Jeong and Seol, 2008). In addition, it is known that cardiolipin is a lipid synthesized in mitochondria and is specific to mitochondria. When oxidation of cardiolipin occurs, it can also cause MOMP and promote the release of cytochrome C (Ott et al., 2002; Gonzalvez and Gottlieb, 2007; Dingeldein et al., 2018). Therefore, mitochondrial dysfunction plays a key role in the endogenous pathway of apoptosis.
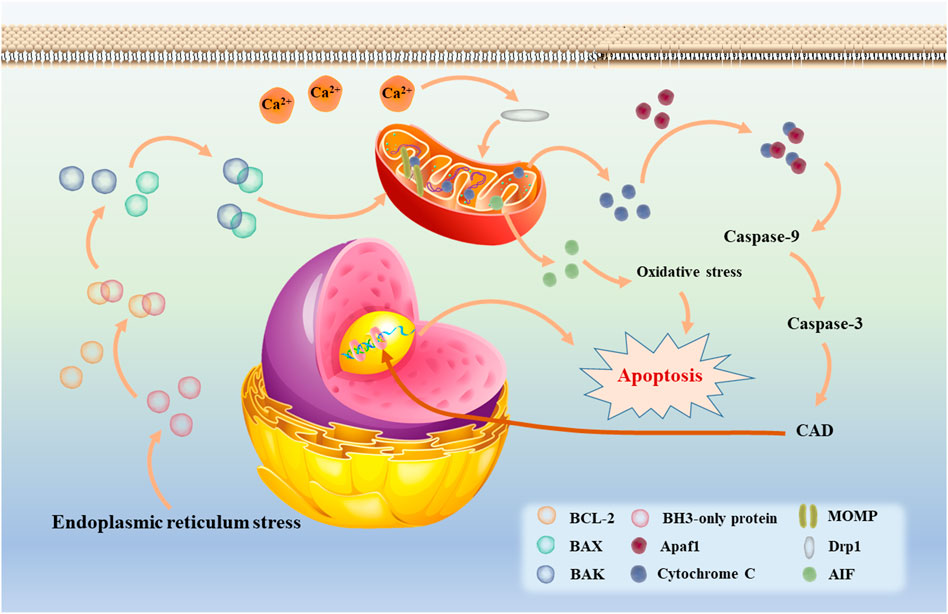
FIGURE 2. The intrinsic apoptotic mediated by mitochondria (also called Bcl-2 or mitochondrial pathway).
4 Neuronal degenerative diseases and herbal medicine
In neurodegenerative diseases, apoptosis is an important way that mediates neuronal cell death and it is essential for cell survival and homeostasis regulation. However, when apoptosis is abnormally increased, it may lead to neurodegenerative diseases. At the same time, the intrinsic apoptotic pathway is an important way of neurodegenerative diseases. Compared with other diseases, the development of therapeutic drugs for neurodegenerative diseases is relatively slow. Surprisingly, herbal medicine has many effects on endogenous apoptosis in neurodegenerative diseases, among which mitochondrial dysfunction plays an important role.
4.1 Alzheimer’s disease
4.1.1 Mitochondrial dysfunction and AD in neuronal apoptosis
Alzheimer’s disease (AD) is a typical neurodegenerative disease, accounting for 70%–80% of dementia. The incidence of AD is increasing with age. What’s more, there is an increasing prevalence of the disease among relatively young groups. Up to now, the number of AD patients in the world is up to 50 million. It is estimated that the number will double by 2050 (Bhute et al., 2020). Existing drugs have poor efficacy and cannot cure or reverse the pathogenesis of AD, which brings great economic burden to patients and the society. Existing studies have found that malnutrition, lack of physical exercise, infection and other factors may lead to AD, while depression, hyperlipidemia, cardiovascular and cerebrovascular diseases, diabetes and other complications can multiply the risk of AD (Sharma and Singh, 2020b). Currently, researchers have carried out extensive and in-depth exploration on the pathogenesis of AD, and put forward a variety of theories, including cholinergic theory, oxidative stress theory, Aβ cascade reaction and inflammation hypothesis (Su et al., 1994). But what is frustrating is that researchers still failed to accurately explain its underlying mechanism. The clinical symptoms of AD may be characterized by progressive cognitive decline, accompanied by senile plaques composed of β-amyloid peptide (Aβ) and neurofibrillary tangles composed of hyperphosphorylated tau (Anandatheerthavarada et al., 2003). Aβ has strong neurocytotoxicity and can activate intracellular apoptotic pathways through a variety of pathways, thereby inducing apoptosis and leading to pathological changes and dementia ultimately (Zhan et al., 2018; Nishizaki, 2019). It is generally believed that the deposition of Aβ and excessive ROS are involved in many pathways of endogenous apoptosis, while multiple signaling pathways related to exogenous apoptosis, such as TLR4/NF-KB and MAPKs, can also be involved in the endogenous apoptosis. For example, Aβ and ROS can initiate the phosphorylation of JNK by activating TLR4, and the phosphorylated JNK can migrate to the nucleus through c-Jun and AP-1 trans-activation to transcribed apoptosis-related genes and promote the activation of Bad and Bax. In fact, many studies have shown that amyloid precursor protein (APP) can be transported to the mitochondrial membrane and cleaved by γ secretase to form Aβ. In mitochondria, Aβ can destroy mitochondrial membrane potential by interacting with a variety of proteins, causing mitochondrial dysfunction and releasing a large amount of cytochrome C to induce apoptosis (Lustbader et al., 2004; Du et al., 2008).
As shown in Figure 3, in the current studies, Aβ was found to disrupt mitochondrial function and induce apoptosis by affecting various processes such as mitochondrial biogenesis, mitochondrial metabolism, division, fusion, and autophagy. First, Aβ can enter into the mitochondria by interaction with TOMM40 and TIM23 and then cause oxidative damage to mtDNA in the mitochondrial biogenesis phase. Transfection of AD-associated mtDNA produced hybrids with increased levels of fragmented mtDNA and a direct 50% decrease in mtDNA transcription, promoting the onset of mitochondrial dysfunction while showing the characteristics such as respiratory enzyme defects and increased ROS production (Reddy et al., 2018; Maiti et al., 2019). Subsequently, reduced expression of PGC1α, which is closely associated with biogenesis, was found in vivo and in vitro as well as brain tissue of patients. It was experimentally verified that PGC1α overexpression prevented nitrosative stress, reversed mitochondrial dysfunction and reduced neuronal apoptosis by enhancing OXPHOS levels. In neurons transfected with AD-related genes or after Aβ treatment, OXPHOS-related protein expression was reduced, with the resultant decrease in mitochondrial membrane potential, reduced mitochondrial respiration rate and reduced ATP production (Kuruva et al., 2017; Reddy et al., 2018; Maiti et al., 2019). In addition, the reduction in ATP would also decrease the expression of cyclophilin D (Cyp D), a mitochondrial matrix protein, causing a prolonged opening of the mitochondrial permeability transition pore (PTP) and ultimately apoptosis (Reddy et al., 2016). Surprisingly, PGC1α not only affects mitochondrial biogenesis, but also inhibits Aβ production by reducing the amount of β-APP cleaving enzyme (BACE1) and Aβdegrading enzymes neprilysin (Katsouri et al., 2011). In addition, its downstream target Nrf2 can also promote autophagy through the p62 pathway, leading to a decrease in Aβ (Joshi et al., 2015).
During the mitochondrial dynamics, Aβ has been shown to impair neuronal mitochondrial motility without destabilizing microtubules, causing an imbalance in mitochondrial dynamics and presenting a state of low-fusion and high-fission. In transgenic and Aβ peptide injected rat models, increased Aβ directly resulted in increased expression of mitochondrial fission proteins DRP1 and FIS1 and increased GTPase activity in neurons, whereas expression of fusion proteins MFN1, MFN2 and OPA1 was directly reduced (Wang L. et al., 2016; Kandimalla et al., 2016; Xu L. L. et al., 2017; Kandimalla et al., 2018). In contrast, when DRP1 expression is suppressed, β-secretase 1 (BACE1) expression in the brain will decrease and cognitive function is improved.
4.1.2 Potential therapeutic agents from herbal medicine for mitochondrial dysfunction in AD
Panax ginseng has been used as a tonic medicine in China for thousands of years. However, in recent studies, several active components contained in ginseng were found to have ameliorative effects on mitochondrial dysfunction in AD. Ginsenoside Rb1 inhibited mitochondrial dysfunction by reducing Bax and Cleaved Caspase-3 levels and upregulating Bcl-2 levels in the hippocampus with Aβ1-40 induced AD rats, thereby inhibiting neuronal apoptosis and improving learning and memory abilities of rats in spatial navigation (Wang et al., 2018). In 3xTg-AD mice, ginsenoside Rg1 upregulated complexxin-2 (CPLX2) and synaptosomal-associated protein 25 (SNP25), restoring mitochondria-related functions and improving behavioral deficits in mice (Nie et al., 2017). In a rat AD model induced by d-gal, ginsenoside Rg3 inhibited apoptosis by upregulating Bcl-2 expression and inhibiting caspase-3, caspase-9, Bax and AIF expression. Also, Rg3 could inhibit the expression of Cyt C in cells, suggesting that Rg3 may inhibit apoptosis through the mitochondrial pathway (Lee et al., 2013). Ginsenoside Rd, another important saponin-like component of ginseng, inhibited apoptosis induced by Aβ25-35 in hippocampal neurons by upregulating mRNA of Bcl-2 and downregulating mRNA of Bax and Cyt C (Liu et al., 2015). The same protective effect was found in the study of ginsenoside Re. In Aβ25-35-induced SH-SY5Y cells, ginsenoside Re increased the expression of Nrf2 and the ratio of Bcl-2/Bax, and inhibited the release of Cyt C and the protein levels of caspase-3 and caspase-9 in mitochondria (Liu et al., 2019). In Aβ-injected mice, ginsenoside Re also reversed learning and memory deficits in mice by restoring amino acid, lecithin and sphingolipid metabolism (Li et al., 2018). Thus, both in vitro and in vivo experiments suggest a possible protective effect of ginsenoside Re against AD. In addition to the above ginsenoside active monomer components, red ginseng oil (RGO) also exerted protective effects on Aβ25-35-induced PC12 cells. RGO could reduce BACE1 activity, inhibit apoptosis-related molecules such as Bax, caspase-3 and PARP-1, and protect neural cells from apoptotic damage. Meanwhile, it was found that RGO could inhibit the inward flow of Ca2+ in mitochondria, reverse the mitochondrial membrane potential, and reduce the content of ROS in cells, suggesting that RGO could protect neural cells by protecting the normal function of mitochondria and inhibiting apoptosis (Lee et al., 2017). Korean red ginseng extract (KRG) has been studied as a multi-component drug for AD in the clinical phase II. In vitro experiments, KRG inhibited apoptosis by activating the PI3K/Akt signaling pathway, suppressed NF-κB expression and decreased mitochondrial membrane potential, and also restored mitochondrial respiration associated with ATP production (Nguyen et al., 2015; Shin et al., 2019; Choi et al., 2020). In addition, in 5XFAD mice that expressed five familial AD mutations, it was further demonstrated that KRG could inhibit the occurrence of mitochondrial dysfunction by protecting the mitochondrial fusion and fission processes, thereby protecting AD mice (Shin et al., 2019). In conclusion, multiple active components of ginseng can play a protective role on mitochondrial dysfunction in AD. Unfortunately, it has not been specifically elucidated in some of the experiments what activities of the active ingredients influenced to restore mitochondrial function, and this needs to be further elucidated in future experiments.
In addition to ginseng, active monomeric components or extracts of many herbs can inhibit apoptosis by reversing mitochondrial dysfunction in AD. For example, puerarin from Pueraria lobata can reverse the increased expression of Bax and the decreased expression of Bcl-2 and p-BAD in Aβ25–35-induced PC12 cells through PI3K signaling pathway, and also inhibit the release of cytochrome C, which ultimately reducing cell apoptosis (Xing et al., 2011). Subsequently, researchers investigated the protective effects of puerarin on cells using mitochondrial transgenic neuronal cell cybrid models of sporadic AD. The results revealed that puerarin inhibited the generation of endogenous ROS in cells and suppressed the activation of caspase-3, p38 and JNK (Zhang et al., 2011). Urolithin A (UA), mainly produced by the metabolism of ellagitannins in pomegranate fruits and walnuts in the intestine, has the potential to penetrate BBB (Cásedas et al., 2020). Previous studies have found that UA could inhibit oxidative stress and mitochondrial autophagy in cells, thus playing a neuroprotective role (González-Sarrías et al., 2017; Pradeepkiran et al., 2022). One step further, in the latest study, it was found that UA could increase expression of genes for mitochondrial biogenesis and OXPHOS in SH-SY5Y cells transfected with the amyloid-precursor protein 695 (SY5Y-APP695) (Esselun et al., 2021). Other potential therapeutic agents from herbs are shown in Supplementary Table S1.
4.2 Parkinson’s disease
4.2.1 Mitochondrial dysfunction and AD in neuronal apoptosis in PD
Due to the global demographic transition, Parkinson’s disease (PD) has become the second most common neurodegenerative disease after AD (de Lau and Breteler, 2006). Clinical symptoms of PD can manifest as non-motor symptoms such as insomnia and dementia, as well as motor dysfunction, including bradykinesia, resting tremor and muscle tonus (Tysnes and Storstein, 2017). In China, PD affect about 3.9% of older people aging 50 years old, and this number will grow to 4.94 million by 2030, narrating half of the people with PD worldwide (Dorsey et al., 2007). PD can be induced by a combination of genetic factors (familial PD) and environmental factors (Sporadic PD) (Koros et al., 2017). The pathological features of PD are mainly manifest as retarded dopaminergic neurons in region of substantia nigra pars compacta and the aggregation of Lewy vesicles containing misfolded α-synuclein (α-syn). (Dickson, 2018). Although the genetic and cellular processes of PD have been extensively studied in recent decades, the exact pathogenesis of the disease has not been well explained. There is growing evidence that mitochondrial dysfunction-induced apoptosis is closely related to neurological damage in PD.
Genetic and environmental factors can affect mitochondrial function through a variety of pathways, including those affecting mitochondrial biogenesis, mitochondrial dynamics, mitochondrial autophagy, aberrant electron transport chain complexes, Ca2+ homeostasis, axonal transport, and reactive oxygen species production (Georgiou et al., 2017; Billingsley et al., 2019; Sun et al., 2019). All these functional changes will cause mitochondrial dysfunction and neuronal apoptosis, which in turn induce PD. α-synuclein plays a critical role in dopamine synthesis, storage, and release in dopaminergic neurons, while it also can be imported into mitochondria membrane (Burré, 2015). Under physiological conditions, misfolded α-syn can be cleared by the ubiquitin-proteasome and autophagy-lysosomal systems. However, when the generation of misfolded α-syn exceeds the cellular clearance capacity, α-syn aggregates to form oligomers and generates insoluble protofibrils which are the core of Lewy vesicles, and thus lead to the development of neurotoxicity (Parnetti et al., 2019). In new studies it has been shown that α-syn interacts with outer mitochondrial membrane proteins such as translocase of the outer membrane 20 (TOM20) and voltage-dependent anion-selective channel 1 (VDAC1), cutting off transport channels for proteins required for mitochondrial biosynthesis and inhibiting mitochondrial-endoplasmic reticulum interactions to dysregulate intracellular Ca2+ homeostasis, which are shown in Figure 4 (Guardia-Laguarta et al., 2014; Di Maio et al., 2016; Paillusson et al., 2017). In addition, aggregated or mutated α-syn can disrupt mitochondrial kinetic homeostasis and autophagic processes by regulating the actin cytoskeleton and inducing the opening of the mitochondrial permeability transition pore (PTP) (Ludtmann et al., 2018). After mitochondrial dysfunction, mitochondrial complex I and IV activities are inhibited, oxidative stress is enhanced, cytochrome C is released, and apoptosis occurs in neuronal cells (Paillusson et al., 2017). At the same time, a mitochondrial matrix protease, ATP-dependent Clp protease (ClpP), was discovered in recent studies, which can reduce the formation of α-synuclein fibrillary by inhibiting the phosphorylation of α-synuclein Ser129. This finding suggests that ClpP may be an effective therapeutic target for α-synuclein induced PD (Hu and Wang, 2019).
In addition to α-syn, genes such as Parkin, PTEN-inducible putative kinase 1 (PINK1), leucine-rich repeat kinase 2 (LRRK2) and DJ-1 have also shown a high correlation with mitochondria in PD. The role of the PINK1/Parkin pathway in the maintenance of mitochondrial quality control has been thoroughly investigated, while its role in PD pathology have also been progressively identified. PINK1 can recruit Parkin into dysfunctional mitochondria and regulate mitochondrial dynamics by activating mitochondrial fission or mitochondrial autophagy (Gegg et al., 2009; Pickles et al., 2018). Also, PINK1 can inhibit apoptosis by suppressing cytochrome C release and caspase activation (Petit et al., 2005). However, when the mitochondrial membrane potential is impaired on a large scale, the stability of PINK1 at the outer mitochondrial membrane is affected by the ripple effect. PINK1 deficiency leads to altered mitochondrial morphology and impaired autophagy, while evidence suggests that this defect also impairs mtDNA and ATP levels during mitochondrial biogenesis (Tufi et al., 2014). In addition, PINK1, if overexpressed in PD models, can protect neuronal cells by inducing α-syn autophagy (Liu J. et al., 2017). Mutations in LRRK2 are the most common cause of autosomal dominant familial PD and some sporadic PD. LRRK2 can mediate mitochondria by co-localizing with the kinetic superfamily of GTPases (Drp1, Mfn1, Mfn2 and OPA1) Kinetics (Stafa et al., 2014). Oral administration of low doses of rotenone results in mice with LRRK2 mutations exhibiting significant motor defects. Mutations in LRRK2 will result in increased DRP1 Ser616 phosphorylation and mitochondrial fission activity, and mitochondrial dysfunction will occur (Liu H. F. et al., 2017). Mutations in the DJ1 gene are associated with autosomal recessive juvenile PD. When cells undergo oxidative stress, DJ1 cysteine residues (C106) acidify and localize to mitochondria, interacting with monomeric or multimeric α-syn to inhibit oligomer formation thereby exerting a protective effect (Canet-Avilés et al., 2004). In addition, when mitochondria are stimulated by apoptosis, high-temperature requirement protein A2 (HTRA2) can be released from the mitochondrial membrane gap and bind to apoptosis-inhibiting proteins to further activate caspase activity and caspase-independent death (Martins et al., 2004). In contrast, under normal physiological state, HTRA2 can be responsible for the degradation of denatured proteins in mitochondria and can exert neuroprotective effects by targeting DJ1 mutations (Dagda and Chu, 2009).
4.2.2 Potential therapeutic agents from herbal medicine for mitochondrial dysfunction in PD
As mentioned above, PD is closely related to mitochondrial dysfunction, so mitochondrial dysfunction can be found in many studies on herbal therapy for PD. Theacrine is one of the main purine alkaloids in tea. It is easily absorbed by the gastrointestinal tract and can penetrate the central nervous system. Therefore, in recent years, attempts have been made to apply it to neurodegenerative diseases such as PD, with surprising results. In 6-hydroxydopamine (6-OHDA)-treated rats and MPTP-treated mice/zebrafish, theacrine prevented the loss of dopaminergic neurons and impaired behavioral performance. What’s more, 1-methyl-4-phenylpyridinium (MPP+)-treated SH-SY5Y cells showed that theacrine could prevent cell apoptosis through SIRT3-mediated SOD2 deacetylation, reduce ROS accumulation and restore mitochondrial function, thus alleviating cell apoptosis caused by oxidative damage and mitochondrial dysfunction (Duan et al., 2020). Unfortunately, it has not been clarified in this study what biological functions of mitochondria are mediated by theacrine.
Quercetin is a common flavonoid with antioxidant activity, which plays a neuroprotective role in a variety of neurodegenerative diseases. Notably, in (MPP+) -induced SH-SY5Y cells, quercetin preconditioning effectively protected cells from mitochondrial damage in a dose-dependent manner. For example, quercetin could protect mtDNA replication and transcription in mitochondria by upregulating TFAM expression, and could also up-regulate the expression of H2AX and dopaminergic neuron marker tyrosine hydroxylase (TH). However, it has no significant effect on the expression of ND9, a nuclear coding subunit of complex I in plasmid electron transport chain, and PGC-1α. Through above measures, quercetin can effectively reverse mitochondrial dysfunction induced by MPP+, and ensure the normal production of ATP and the balance of ROS in cells (Kang S. et al., 2020).
Baicalein is one of the main flavonoids in herbal medicine Scutellaria baicalensis, which can be used to treat inflammation, hypertension and cardiovascular diseases in modern pharmacological studies. After years of research, it has been found that baicalein also has significant efficacy on PD, and the clinical trial phase I has been completed in China. Has neuroprotective effect in various PD models, and its protective effect is directly related to mitochondria mediated apoptosis. For example, in 6-OHDA-induced SH-SY5Y cells, baicalin could reverse MMP, inhibit the activation of mitochondrial downstream caspase-9 and caspase-3, and reduce the protein level of phosphorylated JNK (Lee et al., 2005). In rotenone-induced PC12 cells, baicalein could inhibit ROS production, MMP dissipation and ATP deficiency. Meanwhile, the activation of caspase-3 and caspase-7 was down-regulated by baicalein (Li et al., 2012). The similar results were found in isolated rat brain mitochondria, while the protection effect of baicalein was performed by promoting active mitochondrial respiration and inhibiting mitochondrial swelling (Li et al., 2012). In another study, N2A cells were co-transfected with E46K α-synuclein to establish PD models, and it was found that baicalein could reduce mitochondrial depolarization (Jiang et al., 2010). In the in vivo model, intraperitoneal injection of baicalein for MPP + induced rats could reduce α-synuclein aggregates and inflammation-related factors in rats. In addition, baicalein could also inhibit the activation of caspase-9 and caspase-12 in the dopaminergic system of rat substantia nigra striatum (Hung et al., 2016). Other potential therapeutic agents from herbal medicine for mitochondrial dysfunction in PD are shown in Table 1.
4.3 Huntington’s disease
4.3.1 Cell apoptosis mediated by mitochondrial dysfunction in HD
Huntington’s chorea is an autosomal dominant neurodegenerative genetic disorder with a lower global prevalence of approximately 0.005%–0.01% than AD and PD (Bates et al., 2015). However, there are significant regional differences in the prevalence of HD. In the UK, approximately 12–13 out of 100,000 people have HD, in contrast to only 1 in every million people of Asian and African descent (Pringsheim et al., 2012). The clinical symptoms of HD can be manifest as chorea, dystonia and other motor disorders, as well as cognitive decline (Walker, 2007). Prior to the onset of HD, CAG trinucleotide repeat expansion in exon 1 of the Huntington (HTT) gene and leads to polyglutamine repeats in the HTT protein. Subsequently, it leads to the occurrence of mitochondrial dysfunction and preferentially leading to the loss of striatal medium spiny neurons (MSNs), and ultimately to the onset and progression of HD (Ross and Tabrizi, 2011). In HD, altered mitochondrial morphology can be considered as a hallmark event of the disease and its morphological changes are variable in different cell types. For example, in peripheral tissue cells such as lymphoblasts and fibroblasts, increased mitochondrial morphology often occurs, whereas neuronal cells exhibit increased mitochondrial fragmentation (Kim et al., 2010; Jin et al., 2013). Excessive morphological alterations will certainly lead to mitochondrial dysfunction, which in turn induces neuronal cell apoptosis. It has been shown that mitochondria in the striatum of adult rats are more sensitive to Ca2+-induced membrane permeability than those in the cerebral cortex and are highly susceptible to various stresses (Brustovetsky et al., 2003). In addition, the high energy demand of MSNs, the specific localization of mutant HTT (mHTT) and its aberrant interactions with brain region-specific protein partners also make MSNs more susceptible to mitochondrial dysfunction (Li et al., 2000; Goula et al., 2012).
As shown in Figure 5, HTT can affect normal mitochondrial function through multiple pathways in HD. PGC-1α, as a key transcriptional co-activator, is involved in the regulation of mtDNA production during the mitochondrial biogenesis (Puigserver and Spiegelman, 2003). However, mHTT can directly inhibit PGC-1α transcription by interfering with the CREB/TAF4 signaling pathway (Cui et al., 2006). Meanwhile, typical HD pathological features such as striatal neuron deficiency were exhibited in PGC-1α-free mice (Lucas et al., 2012). Notably, the CREB/TAF4 content did not differ significantly in striatal-like cells of wild mice and HD mice (Cui et al., 2006). This result suggests that although the interaction between mHTT and PGC-1α may result in reduced PGC-1α content, it is not the only cause of PGC-1α impairment. P53 is a tumor suppressor protein that exerts effects by regulating various target genes, including cell cycle arrest, apoptosis, and metabolism (Bae et al., 2005). In HD, mHTT binds to p53 and inhibits the recruitment of the E3 ligase Mdm2, resulting in increased p53 transcriptional activity (Bae et al., 2005). P53 has an extremely strong and sustained localization effect on mitochondria and can activate apoptosis not only by directly interacting with anti-apoptotic Bcl-2 and BAX proteins to promote BAX expression and inhibit Bcl-2 expression, but also by directly promoting the release of cytochrome C to induce apoptosis (Mahyar-Roemer et al., 2004). In previous studies, it was also found that in addition to p53 inducing mitochondrial necrosis by binding Drp1, mHTT can also promote mitochondrial fragmentation by enhancing Drp1 and FIS1 expression and inhibiting MFN1/2 expression (Shirendeb et al., 2012; Guo et al., 2013; Guo et al., 2014). Also, mHTT can interact with p62 or the autophagy protein BNIP3 to disrupt mitochondrial autophagy, further exacerbating mitochondrial dysfunction and contributing to the onset of apoptosis (Ochaba et al., 2014; Rui et al., 2015).
4.3.2 Potential therapeutic agents from herbal medicine for mitochondrial dysfunction in HD
After previous studies, it has been clear that HD is closely related to mitochondrial dysfunction. However, up to now, there have been just a bit of therapeutic herbal medicine for HD, and little of herbal medicines have entered clinical studies. Among the existing research, epigallocatechin gallate and L-theanine, which are isolated from green tea, can alleviate HD symptoms of the rats induced by 3-nitro propionic acid (NP). Epigallocatechin gallate and L-theanine restored mitochondrial respiratory enzyme I, II, and IV respectively, improved REDOX enzymes such as superoxide dismutase (SOD) and catalase enzyme (CAT) (Kumar and Kumar, 2009a; Thangarajan et al., 2014).
As shown in Table 2, praeruptorin C is an active ingredient derived from herbal medicine Peucedanum praeruptorum dunn, which effectively alleviates motor deficits and depression-like behavior in HD mice induced by 3-NP. The underlying mechanism was found to be related to the upregulation of BDNF, DARPP and Huntingtin proteins in the striatum of 3-NP mice by Praeruptorin C (Wang L. et al., 2017). Puerarin, another flavonoid extracted from Pueraria lobata, was applied to 3-NP induced HD rat model. The results showed that puerarin could reduce apoptosis by decreasing caspase-3 activity, inhibiting mitochondrial cytochrome C release and Bax/Bcl-2 level, which was an effective method to prevent HD (Mahdy et al., 2014).
In addition to the active monomer components, herbal extracts are also a potentially effective way to treat HD. Gastrodia elata is a common Chinese herbal medicine used to treat dizziness, headaches and epilepsy. In recent studies, it was found that extract of Gastrodia elata (EG) might have a potential therapeutic effect on HD. In PC12 cells overexpressing mutant Huntingtin protein (mTT), EG could reverse mitochondrial dynamic dysregulation by up-regulating MFN1, MFN2 and OPA1 and down-regulating FIS1. At the same time, EG could promote mitochondrial biogenesis by enhancing PGC-1 α expression and CREB phosphorylation, reducing mHTT aggregation and alleviating HD symptoms (Huang et al., 2019).
5 Discussion
AD, PD and HD are the main types of neurodegenerative diseases. Many studies have been carried out on the pathogenesis of AD, PD and HD in order to find effective treatment strategies. In current studies, neuroinflammation, apoptosis, autophagy and oxidative stress are all involved in the pathogenesis of neurodegenerative diseases, among which apoptosis mediated by mitochondrial dysfunction is indispensable. Mitochondria are the key centers coordinating antioxidant, energy production and apoptosis in cells. As a cell with high demand for energy, neurons have a strong dependence on mitochondria. Therefore, proper maintenance of mitochondrial function is critical for neuronal cells and is a potential therapeutic target for neurodegenerative diseases such as AD, PD and HD. Herbal medicine is widely used in modern drug development because of its multi-function and multi-target function. In this review, we focus on apoptosis-related mitochondrial dysfunction and related potential therapeutic herbs. The results show that herbal medicines can be effective against mitochondrial dysfunction in a variety of models of AD, PD and HD, and are a class of potential drug sources with outstanding effects. However, in the current study, there are still many herbal medicines that have not fully elucidated the specific relationship between them and mitochondrial dysfunction, and further research is needed. All in all, herbal medicine can be used as an important class of drugs for the treatment of neurodegenerative diseases and needs further development.
Author contributions
All authors made a significant contribution to the work reported, whether that is in the conception, execution, acquisition of data, analysis and interpretation. WP and CW conceived this paper; R-LL, WP, and L-YW took part in drafting, revising and critically reviewing the article; H-XD and QZ gave final approval of the version to be published; XG and CW have agreed on the journal to which the article has been submitted and agree to be accountable for all aspects of the work.
Funding
This work was supported by Natural Science Foundation of Sichuan Province (No. 2022NSFSC0720, 2020YFS0523), State Administration of Traditional Chinese Medicine of Sichuan Province of China (No. 2021MS460, No. 2020HJZX001), and the Xinglin scholar discipline promotion talent program of Chengdu University of traditional Chinese medicine (No. BSH2018006).
Conflict of interest
The authors declare that the research was conducted in the absence of any commercial or financial relationships that could be construed as a potential conflict of interest.
Publisher’s note
All claims expressed in this article are solely those of the authors and do not necessarily represent those of their affiliated organizations, or those of the publisher, the editors and the reviewers. Any product that may be evaluated in this article, or claim that may be made by its manufacturer, is not guaranteed or endorsed by the publisher.
Supplementary Material
The Supplementary Material for this article can be found online at: https://www.frontiersin.org/articles/10.3389/fphar.2022.937289/full#supplementary-material
References
Ahat, E., Li, J., and Wang, Y. (2019). New insights into the golgi stacking proteins. Front. Cell. Dev. Biol. 7, 131. doi:10.3389/fcell.2019.00131
Ahmad Rather, M., Justin-Thenmozhi, A., Manivasagam, T., Saravanababu, C., Guillemin, G. J., and Essa, M. M. (2019). Asiatic acid attenuated aluminum chloride-induced tau pathology, oxidative stress and apoptosis via AKT/GSK-3β signaling pathway in wistar rats. Neurotox. Res. 35 (4), 955–968. doi:10.1007/s12640-019-9999-2
Ahmed, H., Abushouk, A. I., Gabr, M., Negida, A., and Abdel-Daim, M. M. (2017). Parkinson's disease and pesticides: A meta-analysis of disease connection and genetic alterations. Biomed. Pharmacother. = Biomedecine Pharmacother. 90, 638–649. doi:10.1016/j.biopha.2017.03.100
Aliev, G., Li, Y., Palacios, H. H., and Obrenovich, M. E. (2011). Oxidative stress induced mitochondrial DNA deletion as a hallmark for the drug development in the context of the cerebrovascular diseases. Recent Pat. cardiovasc. Drug Discov. 6 (3), 222–241. doi:10.2174/157489011797376942
Anandatheerthavarada, H. K., Biswas, G., Robin, M. A., and Avadhani, N. G. (2003). Mitochondrial targeting and a novel transmembrane arrest of Alzheimer's amyloid precursor protein impairs mitochondrial function in neuronal cells. J. Cell. Biol. 161 (1), 41–54. doi:10.1083/jcb.200207030
Armstrong, J. S. (2006). The role of the mitochondrial permeability transition in cell death. Mitochondrion 6 (5), 225–234. doi:10.1016/j.mito.2006.07.006
Bae, B. I., Xu, H., Igarashi, S., Fujimuro, M., Agrawal, N., Taya, Y., et al. (2005). p53 mediates cellular dysfunction and behavioral abnormalities in Huntington's disease. Neuron 47 (1), 29–41. doi:10.1016/j.neuron.2005.06.005
Bai, D., Jin, G., Yin, S., Zou, D., Zhu, Q., Yang, Z., et al. (2017). Antioxidative and anti-apoptotic roles of Silibinin in reversing learning and memory deficits in APP/PS1 mice. Neurochem. Res. 42 (12), 3439–3445. doi:10.1007/s11064-017-2389-3
Baluchnejadmojarad, T., Mohamadi-Zarch, S. M., and Roghani, M. (2019). Safranal, an active ingredient of saffron, attenuates cognitive deficits in amyloid β-induced rat model of alzheimer's disease: Underlying mechanisms. Metab. Brain Dis. 34 (6), 1747–1759. doi:10.1007/s11011-019-00481-6
Baumgart, M., Snyder, H. M., Carrillo, M. C., Fazio, S., Kim, H., and Johns, H. (2015). Summary of the evidence on modifiable risk factors for cognitive decline and dementia: A population-based perspective. Alzheimers Dement. 11 (6), 718–726. doi:10.1016/j.jalz.2015.05.016
Bhute, S., Sarmah, D., Datta, A., Rane, P., Shard, A., Goswami, A., et al. (2020). Molecular pathogenesis and interventional strategies for alzheimer's disease: Promises and pitfalls. ACS Pharmacol. Transl. Sci. 3 (3), 472–488. doi:10.1021/acsptsci.9b00104
Billingsley, K. J., Barbosa, I. A., Bandrés-Ciga, S., Quinn, J. P., Bubb, V. J., Deshpande, C., et al. (2019). International Parkinson’s Disease Genomics Consortium (IPDGC), 5, 8. doi:10.1038/s41531-019-0080-xMitochondria function associated genes contribute to Parkinson's Disease risk and later age at onsetNPJ Parkinson's Dis.
Binawade, Y., and Jagtap, A. (2013). Neuroprotective effect of lutein against 3-nitropropionic acid-induced Huntington's disease-like symptoms: Possible behavioral, biochemical, and cellular alterations. J. Med. Food 16 (10), 934–943. doi:10.1089/jmf.2012.2698
Braschi, E., Zunino, R., and McBride, H. M. (2009). MAPL is a new mitochondrial SUMO E3 ligase that regulates mitochondrial fission. EMBO Rep. 10 (7), 748–754. doi:10.1038/embor.2009.86
Brustovetsky, N., Brustovetsky, T., Purl, K. J., Capano, M., Crompton, M., and Dubinsky, J. M. (2003). Increased susceptibility of striatal mitochondria to calcium-induced permeability transition. J. Neurosci. 23 (12), 4858–4867. doi:10.1523/jneurosci.23-12-04858.2003
Burman, J. L., Pickles, S., Wang, C., Sekine, S., Vargas, J., Zhang, Z., et al. (2017). Mitochondrial fission facilitates the selective mitophagy of protein aggregates. J. Cell. Biol. 216 (10), 3231–3247. doi:10.1083/jcb.201612106
Burré, J. (2015). The synaptic function of α-synuclein. J. Park. Dis. 5 (4), 699–713. doi:10.3233/JPD-150642
Canet-Avilés, R. M., Wilson, M. A., Miller, D. W., Ahmad, R., McLendon, C., Bandyopadhyay, S., et al. (2004). The Parkinson's disease protein DJ-1 is neuroprotective due to cysteine-sulfinic acid-driven mitochondrial localization. Proc. Natl. Acad. Sci. U. S. A. 101 (24), 9103–9108. doi:10.1073/pnas.0402959101
Cao, Q., Qin, L., Huang, F., Wang, X., Yang, L., Shi, H., et al. (2017). Amentoflavone protects dopaminergic neurons in MPTP-induced Parkinson's disease model mice through PI3K/Akt and ERK signaling pathways. Toxicol. Appl. Pharmacol. 319, 80–90. doi:10.1016/j.taap.2017.01.019
Carmona, V., Martín-Aragón, S., Goldberg, J., Schubert, D., and Bermejo-Bescós, P. (2020). Several targets involved in Alzheimer's disease amyloidogenesis are affected by morin and isoquercitrin. Nutr. Neurosci. 23 (8), 575–590. doi:10.1080/1028415X.2018.1534793
Cásedas, G., Les, F., Choya-Foces, C., Hugo, M., and López, V. (2020). The metabolite urolithin-A ameliorates oxidative stress in neuro-2a cells, becoming a potential neuroprotective agent. Antioxidants 9 (2), 177. doi:10.3390/antiox9020177
Chen, L., Feng, P., Peng, A., Qiu, X., Lai, W., Zhang, L., et al. (2020). Protective effects of isoquercitrin on streptozotocin-induced neurotoxicity. J. Cell.. Mol. Med. 24 (18), 10458–10467. doi:10.1111/jcmm.15658
Chen, L., Willis, S. N., Wei, A., Smith, B. J., Fletcher, J. I., Hinds, M. G., et al. (2005). Differential targeting of prosurvival Bcl-2 proteins by their BH3-only ligands allows complementary apoptotic function. Mol. Cell 17 (3), 393–403. doi:10.1016/j.molcel.2004.12.030
Chen, S., Gu, C., Xu, C., Zhang, J., Xu, Y., Ren, Q., et al. (2014). Celastrol prevents cadmium-induced neuronal cell death via targeting JNK and PTEN-Akt/mTOR network. J. Neurochem. 128 (2), 256–266. doi:10.1111/jnc.12474
Cheng, W., Chen, W., Wang, P., and Chu, J. (2018). Asiatic acid protects differentiated PC12 cells from Aβ25-35-induced apoptosis and tau hyperphosphorylation via regulating PI3K/Akt/GSK-3β signaling. Life Sci. 208, 96–101. doi:10.1016/j.lfs.2018.07.016
Chi, T. Y., Wang, L. H., Ji, X. F., Shen, L., and Zou, L. B. (2013). Protective effect of xanthoceraside against β-amyloid-induced neurotoxicity in neuroblastoma SH-SY5Y cells. J. Asian Nat. Prod. Res. 15 (9), 1013–1022. doi:10.1080/10286020.2013.821982
Choi, I. S., Lee, Y. J., Choi, D. Y., Lee, Y. K., Lee, Y. H., Kim, K. H., et al. (2011). 4-O-methylhonokiol attenuated memory impairment through modulation of oxidative damage of enzymes involving amyloid-β generation and accumulation in a mouse model of Alzheimer's disease. J. Alzheimers Dis. 27 (1), 127–141. doi:10.3233/JAD-2011-110545
Choi, S., Lim, J. W., and Kim, H. (2020). Korean red ginseng inhibits amyloid-β-induced apoptosis and nucling expression in human neuronal cells. Pharmacology 105 (9-10), 586–597. doi:10.1159/000505592
Cotman, C. W., and Anderson, A. J. (1995). A potential role for apoptosis in neurodegeneration and Alzheimer's disease. Mol. Neurobiol. 10 (1), 19–45. doi:10.1007/BF02740836
Cui, L., Jeong, H., Borovecki, F., Parkhurst, C. N., Tanese, N., and Krainc, D. (2006). Transcriptional repression of PGC-1alpha by mutant huntingtin leads to mitochondrial dysfunction and neurodegeneration. Cell. 127 (1), 59–69. doi:10.1016/j.cell.2006.09.015
Dagda, R. K., and Chu, C. T. (2009). Mitochondrial quality control: Insights on how Parkinson's disease related genes PINK1, parkin, and omi/HtrA2 interact to maintain mitochondrial homeostasis. J. Bioenerg. Biomembr. 41 (6), 473–479. doi:10.1007/s10863-009-9255-1
de Lau, L. M., and Breteler, M. M. (2006). Epidemiology of Parkinson's disease. Lancet. Neurol. 5 (6), 525–535. doi:10.1016/S1474-4422(06)70471-9
Deng, Q., and Yang, X. (2014). Protective effects of Gynostemma pentaphyllum polysaccharides on PC12 cells impaired by MPP(+). Int. J. Biol. Macromol. 69, 171–175. doi:10.1016/j.ijbiomac.2014.05.049
Di Maio, R., Barrett, P. J., Hoffman, E. K., Barrett, C. W., Zharikov, A., Borah, A., et al. (2016). α-Synuclein binds to TOM20 and inhibits mitochondrial protein import in Parkinson's disease. Sci. Transl. Med. 8 (342), 342ra78. doi:10.1126/scitranslmed.aaf3634
Dickson, D. W. (2018). Neuropathology of Parkinson disease. Park. Relat. Disord. 46 (1), S30–S33. doi:10.1016/j.parkreldis.2017.07.033
Dikalova, A., Mayorov, V., Xiao, L., Panov, A., Amarnath, V., Zagol-Ikapitte, I., et al. (2020). Mitochondrial isolevuglandins contribute to vascular oxidative stress and mitochondria-targeted scavenger of isolevuglandins reduces mitochondrial dysfunction and hypertension. Hypertension 76 (6), 1980–1991. doi:10.1161/HYPERTENSIONAHA.120.15236
Dingeldein, A., Sparrman, T., and Gröbner, G. (2018). Oxidatively stressed mitochondria-mimicking membranes: A molecular insight into their organization during apoptosis. Biochim. Biophys. Acta. Biomembr. 1860 (12), 2644–2654. doi:10.1016/j.bbamem.2018.10.007
Dong, H., Li, R., Yu, C., Xu, T., Zhang, X., and Dong, M. (2015). Paeoniflorin inhibition of 6-hydroxydopamine-induced apoptosis in PC12 cells via suppressing reactive oxygen species-mediated PKCδ/NF-κB pathway. Neuroscience 285, 70–80. doi:10.1016/j.neuroscience.2014.11.008
Dorsey, E. R., Constantinescu, R., Thompson, J. P., Biglan, K. M., Holloway, R. G., Kieburtz, K., et al. (2007). Projected number of people with Parkinson disease in the most populous nations, 2005 through 2030. Neurology 68 (5), 384–386. doi:10.1212/01.wnl.0000247740.47667.03
Dragicevic, N., Smith, A., Lin, X., Yuan, F., Copes, N., Delic, V., et al. (2011). Green tea epigallocatechin-3-gallate (EGCG) and other flavonoids reduce Alzheimer's amyloid-induced mitochondrial dysfunction. J. Alzheimers Dis. 26 (3), 507–521. doi:10.3233/JAD-2011-101629
Drever, B. D., Anderson, W. G., Riedel, G., Kim, D. H., Ryu, J. H., Choi, D. Y., et al. (2008). The seed extract of Cassia obtusifolia offers neuroprotection to mouse hippocampal cultures. J. Pharmacol. Sci. 107 (4), 380–392. doi:10.1254/jphs.08034fp
Du, H., Guo, L., Fang, F., Chen, D., Sosunov, A. A., McKhann, G. M., et al. (2008). Cyclophilin D deficiency attenuates mitochondrial and neuronal perturbation and ameliorates learning and memory in Alzheimer's disease. Nat. Med. 14 (10), 1097–1105. doi:10.1038/nm.1868
Du, H., and Yan, S. S. (2010). Mitochondrial permeability transition pore in Alzheimer's disease: cyclophilin D and amyloid beta. Biochim. Biophys. Acta 1802 (1), 198–204. doi:10.1016/j.bbadis.2009.07.005
Duan, W. J., Liang, L., Pan, M. H., Lu, D. H., Wang, T. M., Li, S. B., et al. (2020). Theacrine, a purine alkaloid from kucha, protects against Parkinson's disease through SIRT3 activation. Phytomedicine. 77, 153281. doi:10.1016/j.phymed.2020.153281
Enari, M., Sakahira, H., Yokoyama, H., Okawa, K., Iwamatsu, A., and Nagata, S. (1998). A caspase-activated DNase that degrades DNA during apoptosis, and its inhibitor ICAD. Nature 391 (6662), 43–50. doi:10.1038/34112
Esselun, C., Theyssen, E., and Eckert, G. P. (2021). Effects of urolithin A on mitochondrial parameters in a cellular model of early alzheimer disease. Int. J. Mol. Sci. 22 (15), 8333. doi:10.3390/ijms22158333
Felderhoff-Mueser, U., Sifringer, M., Pesditschek, S., Kuckuck, H., Moysich, A., Bittigau, P., et al. (2002). Pathways leading to apoptotic neurodegeneration following trauma to the developing rat brain. Neurobiol. Dis. 11 (2), 231–245. doi:10.1006/nbdi.2002.0521
Filadi, R., Pendin, D., and Pizzo, P. (2018). Mitofusin 2: From functions to disease. Cell. Death Dis. 9 (3), 330. doi:10.1038/s41419-017-0023-6
Flippo, K. H., and Strack, S. (2017). Mitochondrial dynamics in neuronal injury, development and plasticity. J. Cell. Sci. 130 (4), 671–681. doi:10.1242/jcs.171017
Gao, J., He, H., Jiang, W., Chang, X., Zhu, L., Luo, F., et al. (2015). Salidroside ameliorates cognitive impairment in a d-galactose-induced rat model of Alzheimer's disease. Behav. Brain Res. 293, 27–33. doi:10.1016/j.bbr.2015.06.045
Gao, X., and Tang, X. C. (2006). Huperzine A attenuates mitochondrial dysfunction in beta-amyloid-treated PC12 cells by reducing oxygen free radicals accumulation and improving mitochondrial energy metabolism. J. Neurosci. Res. 83 (6), 1048–1057. doi:10.1002/jnr.20791
Gegg, M. E., Cooper, J. M., Schapira, A. H., and Taanman, J. W. (2009). Silencing of PINK1 expression affects mitochondrial DNA and oxidative phosphorylation in dopaminergic cells. PloS one 4 (3), e4756. doi:10.1371/journal.pone.0004756
Georgiou, A., Demetriou, C. A., Heraclides, A., Christou, Y. P., Leonidou, E., Loukaides, P., et al. (2017). Mitochondrial superclusters influence age of onset of Parkinson's disease in a gender specific manner in the Cypriot population: A case-control study. PloS one 12 (9), e0183444. doi:10.1371/journal.pone.0183444
González-Sarrías, A., Núñez-Sánchez, M. Á., Tomás-Barberán, F. A., and Espín, J. C. (2017). Neuroprotective effects of bioavailable polyphenol-derived metabolites against oxidative stress-induced cytotoxicity in human neuroblastoma SH-SY5Y cells. J. Agric. Food Chem. 65 (4), 752–758. doi:10.1021/acs.jafc.6b04538
Gonzalvez, F., and Gottlieb, E. (2007). Cardiolipin: Setting the beat of apoptosis. Apoptosis. 12 (5), 877–885. doi:10.1007/s10495-007-0718-8
Gorman, A. M. (2008). Neuronal cell death in neurodegenerative diseases: Recurring themes around protein handling. J. Cell. Mol. Med. 12 (6A), 2263–2280. doi:10.1111/j.1582-4934.2008.00402.x
Goula, A. V., Stys, A., Chan, J. P., Trottier, Y., Festenstein, R., and Merienne, K. (2012). Transcription elongation and tissue-specific somatic CAG instability. PLoS Genet. 8 (11), e1003051. doi:10.1371/journal.pgen.1003051
Gu, L., Yu, Q., Li, Q., Zhang, L., Lu, H., and Zhang, X. (2018). Andrographolide protects PC12 cells against β-amyloid-induced autophagy-associated cell death through activation of the nrf2-mediated p62 signaling pathway. Int. J. Mol. Sci. 19 (9), 2844. doi:10.3390/ijms19092844
Guardia-Laguarta, C., Area-Gomez, E., Rüb, C., Liu, Y., Magrané, J., Becker, D., et al. (2014). α-Synuclein is localized to mitochondria-associated ER membranes. J. Neurosci. 34 (1), 249–259. doi:10.1523/JNEUROSCI.2507-13.2014
Gucev, Z. (2017). Comments on: Kocova M, anastasovska V. Comments on 'newborn screening in southeastern europe. Publ. Mol. Genet. Metabolism 113 (1-2), 42–45. doi:10.1016/j.neures.2014.12.003
Guo, X., Disatnik, M. H., Monbureau, M., Shamloo, M., Mochly-Rosen, D., and Qi, X. (2013). Inhibition of mitochondrial fragmentation diminishes Huntington's disease-associated neurodegeneration. J. Clin. Investig. 123 (12), 5371–5388. doi:10.1172/jci70911
Guo, X., Sesaki, H., and Qi, X. (2014). Drp1 stabilizes p53 on the mitochondria to trigger necrosis under oxidative stress conditions in vitro and in vivo. Biochem. J. 461 (1), 137–146. doi:10.1042/bj20131438
Han, Y., and Chen, J. Z. (2013). Oxidative stress induces mitochondrial DNA damage and cytotoxicity through independent mechanisms in human cancer cells. Biomed. Res. Int. 2013, 825065. doi:10.1155/2013/825065
Han, Y., Yang, H., Li, L., Du, X., and Sun, C. (2019). Schisanhenol improves learning and memory in scopolamine-treated mice by reducing acetylcholinesterase activity and attenuating oxidative damage through SIRT1-PGC-1α-Tau signaling pathway. Int. J. Neurosci. 129 (2), 110–118. doi:10.1080/00207454.2018.1503183
Harper, M. E., Bevilacqua, L., Hagopian, K., Weindruch, R., and Ramsey, J. J. (2004). Ageing, oxidative stress, and mitochondrial uncoupling. Acta Physiol. Scand. 182 (4), 321–331. doi:10.1111/j.1365-201X.2004.01370.x
He, H., Wang, S., Tian, J., Chen, L., Zhang, W., Zhao, J., et al. (2015). Protective effects of 2, 3, 5, 4'-tetrahydroxystilbene-2-O-β-D-glucoside in the MPTP-induced mouse model of Parkinson's disease: Involvement of reactive oxygen species-mediated JNK, P38 and mitochondrial pathways. Eur. J. Pharmacol. 767, 175–182. doi:10.1016/j.ejphar.2015.10.023
Horbay, R., and Bilyy, R. (2016). Mitochondrial dynamics during cell cycling. Apoptosis. 21 (12), 1327–1335. doi:10.1007/s10495-016-1295-5
Hu, S., Han, R., Mak, S., and Han, Y. (2011). Protection against 1-methyl-4-phenylpyridinium ion (MPP+)-induced apoptosis by water extract of ginseng (Panax ginseng C.A. Meyer) in SH-SY5Y cells. J. Ethnopharmacol. 135 (1), 34–42. doi:10.1016/j.jep.2011.02.017
Hu, Y., and Wang, J. (2019). Interactions between clopidogrel and traditional Chinese medicine. J. Thromb. Thrombolysis 48 (3), 491–499. doi:10.1007/s11239-019-01945-3
Huang, C. L., Wang, K. C., Yang, Y. C., Chiou, C. T., Tan, C. H., Lin, Y. L., et al. (2018). Gastrodia elata alleviates mutant huntingtin aggregation through mitochondrial function and biogenesis mediation. Phytomedicine. 39, 75–84. doi:10.1016/j.phymed.2017.12.017
Huang, D. C., and Strasser, A. (2000). BH3-Only proteins-essential initiators of apoptotic cell death. Cell. 103 (6), 839–842. doi:10.1016/s0092-8674(00)00187-2
Huang, J. Y., Yuan, Y. H., Yan, J. Q., Wang, Y. N., Chu, S. F., Zhu, C. G., et al. (2016). 20C, a bibenzyl compound isolated from Gastrodia elata, protects PC12 cells against rotenone-induced apoptosis via activation of the Nrf2/ARE/HO-1 signaling pathway. Acta Pharmacol. Sin. 37 (6), 731–740. doi:10.1038/aps.2015.154
Huang, N. K., Lin, C. C., Lin, Y. L., Huang, C. L., Chiou, C. T., Lee, Y. C., et al. (2019). Morphological control of mitochondria as the novel mechanism of Gastrodia elata in attenuating mutant huntingtin-induced protein aggregations. Phytomedicine. 59, 152756. doi:10.1016/j.phymed.2018.11.016
Hügel, H. M. (2015). Brain food for alzheimer-free ageing: Focus on herbal medicines. Adv. Exp. Med. Biol. 863, 95–116. doi:10.1007/978-3-319-18365-7_5
Hung, K. C., Huang, H. J., Wang, Y. T., and Lin, A. M. (2016). Baicalein attenuates α-synuclein aggregation, inflammasome activation and autophagy in the MPP+-treated nigrostriatal dopaminergic system in vivo. J. Ethnopharmacol. 194, 522–529. doi:10.1016/j.jep.2016.10.040
Im, A. R., Chae, S. W., Zhang, G. J., and Lee, M. Y. (2014). Neuroprotective effects of Psoralea corylifolia Linn seed extracts on mitochondrial dysfunction induced by 3-nitropropionic acid. BMC Complement. Altern. Med. 14, 370. doi:10.1186/1472-6882-14-370
Ito, H., Kurokawa, H., and Matsui, H. (2021). Mitochondrial reactive oxygen species and heme, non-heme iron metabolism. Arch. Biochem. Biophys. 700, 108695. doi:10.1016/j.abb.2020.108695
Jeong, S. Y., and Seol, D. W. (2008). The role of mitochondria in apoptosis. BMB Rep. 41 (1), 11–22. doi:10.5483/bmbrep.2008.41.1.011
Jiang, G., Hu, Y., Liu, L., Cai, J., Peng, C., and Li, Q. (2014). Gastrodin protects against MPP(+)-induced oxidative stress by up regulates heme oxygenase-1 expression through p38 MAPK/Nrf2 pathway in human dopaminergic cells. Neurochem. Int. 75, 79–88. doi:10.1016/j.neuint.2014.06.003
Jiang, M., Porat-Shliom, Y., Pei, Z., Cheng, Y., Xiang, L., Sommers, K., et al. (2010). Baicalein reduces E46K alpha-synuclein aggregation in vitro and protects cells against E46K alpha-synuclein toxicity in cell models of familiar Parkinsonism. J. Neurochem. 114 (2), 419–429. doi:10.1111/j.1471-4159.2010.06752.x
Jin, Y. N., Yu, Y. V., Gundemir, S., Jo, C., Cui, M., Tieu, K., et al. (2013). Impaired mitochondrial dynamics and Nrf2 signaling contribute to compromised responses to oxidative stress in striatal cells expressing full-length mutant huntingtin. PloS one 8 (3), e57932. doi:10.1371/journal.pone.00.57932
Johnson, J., Mercado-Ayon, E., Mercado-Ayon, Y., Dong, Y. N., Halawani, S., Ngaba, L., et al. (2021). Mitochondrial dysfunction in the development and progression of neurodegenerative diseases. Arch. Biochem. Biophys. 702, 108698. doi:10.1016/j.abb.2020.108698
Joshi, G., Gan, K. A., Johnson, D. A., and Johnson, J. A. (2015). Increased Alzheimer's disease-like pathology in the APP/PS1ΔE9 mouse model lacking Nrf2 through modulation of autophagy. Neurobiol. Aging 36 (2), 664–679. doi:10.1016/j.neurobiolaging.2014.09.004
Kandimalla, R., Manczak, M., Fry, D., Suneetha, Y., Sesaki, H., and Reddy, P. H. (2016). Reduced dynamin-related protein 1 protects against phosphorylated Tau-induced mitochondrial dysfunction and synaptic damage in Alzheimer's disease. Hum. Mol. Genet. 25 (22), 4881–4897. doi:10.1093/hmg/ddw312
Kandimalla, R., Manczak, M., Yin, X., Wang, R., and Reddy, P. H. (2018). Hippocampal phosphorylated tau induced cognitive decline, dendritic spine loss and mitochondrial abnormalities in a mouse model of Alzheimer's disease. Hum. Mol. Genet. 27 (1), 30–40. doi:10.1093/hmg/ddx381
Kang, L., Liu, S., Li, J., Tian, Y., Xue, Y., and Liu, X. (2020a). The mitochondria-targeted anti-oxidant MitoQ protects against intervertebral disc degeneration by ameliorating mitochondrial dysfunction and redox imbalance. Cell. Prolif. 53 (3), e12779. doi:10.1111/cpr.12779
Kang, S., Piao, Y., Kang, Y. C., Lim, S., and Pak, Y. K. (2020b). Qi-activating quercetin alleviates mitochondrial dysfunction and neuroinflammation in vivo and in vitro. Arch. Pharm. Res. 43 (5), 553–566. doi:10.1007/s12272-020-01238-x
Katsouri, L., Parr, C., Bogdanovic, N., Willem, M., and Sastre, M. (2011). PPARγ co-activator-1α (PGC-1α) reduces amyloid-β generation through a PPARγ-dependent mechanism. J. Alzheimers Dis. 25 (1), 151–162. doi:10.3233/JAD-2011-101356
Kelly, G. L., and Strasser, A. (2020). Toward targeting antiapoptotic MCL-1 for cancer therapy. Annu. Rev. Cancer Biol. 4, 299–313. doi:10.1146/annurev-cancerbio-030419-033510
Kim, H. G., Park, G., Piao, Y., Kang, M. S., Pak, Y. K., Hong, S. P., et al. (2014). Effects of the root bark of Paeonia suffruticosa on mitochondria-mediated neuroprotection in an MPTP-induced model of Parkinson's disease. Food Chem. Toxicol. 65, 293–300. doi:10.1016/j.fct.2013.12.037
Kim, I. S., Choi, D. K., and Jung, H. J. (2011). Neuroprotective effects of vanillyl alcohol in Gastrodia elata Blume through suppression of oxidative stress and anti-apoptotic activity in toxin-induced dopaminergic MN9D cells. Mol. (Basel, Switz. 16 (7), 5349–5361. doi:10.3390/molecules16075349
Kim, I. S., Koppula, S., Park, P. J., Kim, E. H., Kim, C. G., Choi, W. S., et al. (2009). Chrysanthemum morifolium Ramat (CM) extract protects human neuroblastoma SH-SY5Y cells against MPP+-induced cytotoxicity. J. Ethnopharmacol. 126 (3), 447–454. doi:10.1016/j.jep.2009.09.017
Kim, J., Moody, J. P., Edgerly, C. K., Bordiuk, O. L., Cormier, K., Smith, K., et al. (2010). Mitochondrial loss, dysfunction and altered dynamics in Huntington's disease. Hum. Mol. Genet. 19 (20), 3919–3935. doi:10.1093/hmg/ddq306
Kim, S. M., Park, Y. J., Shin, M. S., Kim, H. R., Kim, M. J., Lee, S. H., et al. (2017). Acacetin inhibits neuronal cell death induced by 6-hydroxydopamine in cellular Parkinson's disease model. Bioorg. Med. Chem. Lett. 27 (23), 5207–5212. doi:10.1016/j.bmcl.2017.10.048
Kondapalli, C., Kazlauskaite, A., Zhang, N., Woodroof, H. I., Campbell, D. G., Gourlay, R., et al. (2012). PINK1 is activated by mitochondrial membrane potential depolarization and stimulates Parkin E3 ligase activity by phosphorylating Serine 65. Open Biol. 2 (5), 120080. doi:10.1098/rsob.120080
Koros, C., Simitsi, A., and Stefanis, L. (2017). Genetics of Parkinson's disease: Genotype-phenotype correlations. Int. Rev. Neurobiol. 132, 197–231. doi:10.1016/bs.irn.2017.01.009
Kou, X., Liu, X., Chen, X., Li, J., Yang, X., Fan, J., et al. (2016). Ampelopsin attenuates brain aging of D-gal-induced rats through miR-34a-mediated SIRT1/mTOR signal pathway. Oncotarget 7 (46), 74484–74495. doi:10.18632/oncotarget.12811
Kumar, P., and Kumar, A. (2009b). Possible neuroprotective effect of Withania somnifera root extract against 3-nitropropionic acid-induced behavioral, biochemical, and mitochondrial dysfunction in an animal model of Huntington's disease. J. Med. Food 12 (3), 591–600. doi:10.1089/jmf.2008.0028
Kumar, P., and Kumar, A. (2009a). Protective effects of epigallocatechin gallate following 3-nitropropionic acid-induced brain damage: Possible nitric oxide mechanisms. Psychopharmacology 207 (2), 257–270. doi:10.1007/s00213-009-1652-y
Kung, H. C., Lin, K. J., Kung, C. T., and Lin, T. K. (2021). Oxidative stress, mitochondrial dysfunction, and neuroprotection of polyphenols with respect to resveratrol in Parkinson's disease. Biomedicines 9 (8), 918. doi:10.3390/biomedicines9080918
Kuruva, C. S., Manczak, M., Yin, X., Ogunmokun, G., Reddy, A. P., and Reddy, P. H. (2017). Aqua-soluble DDQ reduces the levels of Drp1 and Aβ and inhibits abnormal interactions between Aβ and Drp1 and protects Alzheimer's disease neurons from Aβ- and Drp1-induced mitochondrial and synaptic toxicities. Hum. Mol. Genet. 26 (17), 3375–3395. doi:10.1093/hmg/ddx226
Lazarou, M., Sliter, D. A., Kane, L. A., Sarraf, S. A., Wang, C., Burman, J. L., et al. (2015). The ubiquitin kinase PINK1 recruits autophagy receptors to induce mitophagy. Nature 524 (7565), 309–314. doi:10.1038/nature14893
Lee, B., Sur, B., Park, J., Kim, S. H., Kwon, S., Yeom, M., et al. (2013). Ginsenoside rg3 alleviates lipopolysaccharide-induced learning and memory impairments by anti-inflammatory activity in rats. Biomol. Ther. 21 (5), 381–390. doi:10.4062/biomolther.2013.053
Lee, C. H., Hwang, D. S., Kim, H. G., Oh, H., Park, H., Cho, J. H., et al. (2010). Protective effect of Cyperi rhizoma against 6-hydroxydopamine-induced neuronal damage. J. Med. Food 13 (3), 564–571. doi:10.1089/jmf.2009.1252
Lee, H. J., Noh, Y. H., Lee, D. Y., Kim, Y. S., Kim, K. Y., Chung, Y. H., et al. (2005). Baicalein attenuates 6-hydroxydopamine-induced neurotoxicity in SH-SY5Y cells. Eur. J. Cell. Biol. 84 (11), 897–905. doi:10.1016/j.ejcb.2005.07.003
Lee, S., Youn, K., Jeong, W. S., Ho, C. T., and Jun, M. (2017). Protective effects of red ginseng oil against aβ25-35-induced neuronal apoptosis and inflammation in PC12 cells. Int. J. Mol. Sci. 18 (10), 2218. doi:10.3390/ijms18102218
Lei, H., Zhao, C. Y., Liu, D. M., Zhang, Y., Li, L., Wang, X. L., et al. (2014). l-3-n-Butylphthalide attenuates β-amyloid-induced toxicity in neuroblastoma SH-SY5Y cells through regulating mitochondrion-mediated apoptosis and MAPK signaling. J. Asian Nat. Prod. Res. 16 (8), 854–864. doi:10.1080/10286020.2014.939586
Lei, Y., Yang, L., Ye, C. Y., Qin, M. Y., Yang, H. Y., Jiang, H. L., et al. (2015). Involvement of intracellular and mitochondrial Aβ in the ameliorative effects of huperzine A against oligomeric aβ42-induced injury in primary rat neurons. PloS one 10 (5), e0128366. doi:10.1371/journal.pone.0128366
Lemasters, J. J. (2005). Selective mitochondrial autophagy, or mitophagy, as a targeted defense against oxidative stress, mitochondrial dysfunction, and aging. Rejuvenation Res. 8 (1), 3–5. doi:10.1089/rej.2005.8.3
Leung, K. W., Yung, K. K., Mak, N. K., Chan, Y. S., Fan, T. P., and Wong, R. N. (2007). Neuroprotective effects of ginsenoside-Rg1 in primary nigral neurons against rotenone toxicity. Neuropharmacology 52 (3), 827–835. doi:10.1016/j.neuropharm.2006.10.001
Li, C., Xing, G., Dong, M., Zhou, L., Li, J., Wang, G., et al. (2010). Beta-asarone protection against beta-amyloid-induced neurotoxicity in PC12 cells via JNK signaling and modulation of Bcl-2 family proteins. Eur. J. Pharmacol. 635 (1-3), 96–102. doi:10.1016/j.ejphar.2010.03.013
Li, H., Li, S. H., Johnston, H., Shelbourne, P. F., and Li, X. J. (2000). Amino-terminal fragments of mutant huntingtin show selective accumulation in striatal neurons and synaptic toxicity. Nat. Genet. 25 (4), 385–389. doi:10.1038/78054
Li, J., Liu, Y., Li, W., Wang, Z., Guo, P., Li, L., et al. (2018). Metabolic profiling of the effects of ginsenoside Re in an Alzheimer's disease mouse model. Behav. Brain Res. 337, 160–172. doi:10.1016/j.bbr.2017.09.027
Li, J., Wang, Y., Wang, Y., Wen, X., Ma, X. N., Chen, W., et al. (2015). Pharmacological activation of AMPK prevents Drp1-mediated mitochondrial fission and alleviates endoplasmic reticulum stress-associated endothelial dysfunction. J. Mol. Cell. Cardiol. 86, 62–74. doi:10.1016/j.yjmcc.2015.07.010
Li, P., Nijhawan, D., Budihardjo, I., Srinivasula, S. M., Ahmad, M., Alnemri, E. S., et al. (1997). Cytochrome c and dATP-dependent formation of Apaf-1/caspase-9 complex initiates an apoptotic protease cascade. Cell. 91 (4), 479–489. doi:10.1016/s0092-8674(00)80434-1
Li, R., Lu, F., Sun, X., He, L., Duan, H., Peng, W., et al. (2022). Development and in vivo evaluation of hydroxy-α-sanshool intranasal liposomes as a potential remedial treatment for alzheimer's disease. Int. J. Nanomedicine 17, 185–201. doi:10.2147/IJN.S339979
Li, R. L., Zhang, Q., Liu, J., Sun, J. Y., He, L. Y., Duan, H. X., et al. (2020). Oxidative medicine and cellular longevity. doi: 10.1155/2020/3481758.Hydroxy-α-sanshool possesses protective potentials on H2O2-stimulated PC12 cells by suppression of oxidative stress-induced apoptosis through regulation of PI3K/akt signal pathway3481758
Li, X. X., He, G. R., Mu, X., Xu, B., Tian, S., Yu, X., et al. (2012). Protective effects of baicalein against rotenone-induced neurotoxicity in PC12 cells and isolated rat brain mitochondria. Eur. J. Pharmacol. 674 (2-3), 227–233. doi:10.1016/j.ejphar.2011.09.181
Liang, Z., Shi, F., Wang, Y., Lu, L., Zhang, Z., Wang, X., et al. (2011). Neuroprotective effects of tenuigenin in a SH-SY5Y cell model with 6-OHDA-induced injury. Neurosci. Lett. 497 (2), 104–109. doi:10.1016/j.neulet.2011.04.041
Liao, Z. L., Su, H., Tan, Y. F., Qiu, Y. J., Zhu, J. P., Chen, Y., et al. (2019). Salidroside protects PC-12 cells against amyloid β-induced apoptosis by activation of the ERK1/2 and AKT signaling pathways. Int. J. Mol. Med. 43 (4), 1769–1777. doi:10.3892/ijmm.2019.4088
Lin, C. M., Lin, R. D., Chen, S. T., Lin, Y. P., Chiu, W. T., Lin, J. W., et al. (2010). Neurocytoprotective effects of the bioactive constituents of Pueraria thomsonii in 6-hydroxydopamine (6-OHDA)-treated nerve growth factor (NGF)-differentiated PC12 cells. Phytochemistry 71 (17-18), 2147–2156. doi:10.1016/j.phytochem.2010.08.015
Lin, M. T., and Beal, M. F. (2006). Mitochondrial dysfunction and oxidative stress in neurodegenerative diseases. Nature 443 (7113), 787–795. doi:10.1038/nature05292
Liu, H. F., Ho, P. W., Leung, G. C., Lam, C. S., Pang, S. Y., Li, L., et al. (2017a). Combined LRRK2 mutation, aging and chronic low dose oral rotenone as a model of Parkinson's disease. Sci. Rep. 7, 40887. doi:10.1038/srep40887
Liu, J. F., Yan, X. D., Qi, L. S., Li, L., Hu, G. Y., Li, P., et al. (2015). Ginsenoside Rd attenuates Aβ25-35-induced oxidative stress and apoptosis in primary cultured hippocampal neurons. Chem. Biol. Interact. 239, 12–18. doi:10.1016/j.cbi.2015.06.030
Liu, J., Wang, X., Lu, Y., Duan, C., Gao, G., Lu, L., et al. (2017b). Pink1 interacts with α-synuclein and abrogates α-synuclein-induced neurotoxicity by activating autophagy. Cell. Death Dis. 8 (9), e3056. doi:10.1038/cddis.2017.427
Liu, L., Feng, D., Chen, G., Chen, M., Zheng, Q., Song, P., et al. (2012). Mitochondrial outer-membrane protein FUNDC1 mediates hypoxia-induced mitophagy in mammalian cells. Nat. Cell. Biol. 14 (2), 177–185. doi:10.1038/ncb2422
Liu, M., Bai, X., Yu, S., Zhao, W., Qiao, J., Liu, Y., et al. (2019). Ginsenoside Re inhibits ROS/ASK-1 dependent mitochondrial apoptosis pathway and activation of nrf2-antioxidant response in beta-amyloid-challenged SH-SY5Y cells. Mol. (Basel, Switz. 24 (15), 2687. doi:10.3390/molecules24152687
Liu, T., Jin, H., Sun, Q. R., Xu, J. H., and Hu, H. T. (2010). The neuroprotective effects of tanshinone IIA on β-amyloid-induced toxicity in rat cortical neurons. Neuropharmacology 59 (7-8), 595–604. doi:10.1016/j.neuropharm.2010.08.013
Liu, X., Ma, T., Qu, B., Ji, Y., and Liu, Z. (2013). Pesticide-induced gene mutations and Parkinson disease risk: A meta-analysis. Genet. Test. Mol. Biomarkers 17 (11), 826–832. doi:10.1089/gtmb.2013.0313
Livingston, G., Sommerlad, A., Orgeta, V., Costafreda, S. G., Huntley, J., Ames, D., et al. (2017). Dementia prevention, intervention, and care. Lancet (London, Engl. 390 (10113), 2673–2734. doi:10.1016/S0140-6736(17)31363-6
Lu, Y. H., Su, M. Y., Huang, H. Y., Lin-Li, , and Yuan, C. G. (2010). Protective effects of the citrus flavanones to PC12 cells against cytotoxicity induced by hydrogen peroxide. Neurosci. Lett. 484 (1), 6–11. doi:10.1016/j.neulet.2010.07.078
Lucas, E. K., Dougherty, S. E., McMeekin, L. J., Trinh, A. T., Reid, C. S., and Cowell, R. M. (2012). Developmental alterations in motor coordination and medium spiny neuron markers in mice lacking pgc-1α. PloS one 7 (8), e42878. doi:10.1371/journal.pone.0042878
Ludtmann, M., Angelova, P. R., Horrocks, M. H., Choi, M. L., Rodrigues, M., Baev, A. Y., et al. (2018). α-synuclein oligomers interact with ATP synthase and open the permeability transition pore in Parkinson's disease. Nat. Commun. 9 (1), 2293. doi:10.1038/s41467-018-04422-2
Luo, F. C., Wang, S. D., Qi, L., Song, J. Y., Lv, T., and Bai, J. (2011). Protective effect of panaxatriol saponins extracted from Panax notoginseng against MPTP-induced neurotoxicity in vivo. J. Ethnopharmacol. 133 (2), 448–453. doi:10.1016/j.jep.2010.10.017
Lustbader, J. W., Cirilli, M., Lin, C., Xu, H. W., Takuma, K., Wang, N., et al. (2004). ABAD directly links Abeta to mitochondrial toxicity in Alzheimer's disease. Sci. (New York, N.Y.) 304 (5669), 448–452. doi:10.1126/science.1091230
Ma, X. W., and Guo, R. Y. (2017). Dose-dependent effect of Curcuma longa for the treatment of Parkinson's disease. Exp. Ther. Med. 13 (5), 1799–1805. doi:10.3892/etm.2017.4225
Maassen, J. A., 'T Hart, L. M., Van Essen, E., Heine, R. J., Nijpels, G., Jahangir Tafrechi, R. S., et al. (2004). Mitochondrial diabetes: Molecular mechanisms and clinical presentation. Diabetes 53 (1), S103–S109. S103–S109. doi:10.2337/diabetes.53.2007.s103
Mahdy, H. M., Mohamed, M. R., Emam, M. A., Karim, A. M., Abdel-Naim, A. B., Khalifa, A. E., et al. (2014). The anti-apoptotic and anti-inflammatory properties of puerarin attenuate 3-nitropropionic-acid induced neurotoxicity in rats. Can. J. Physiol. Pharmacol. 92 (3), 252–258. doi:10.1139/cjpp-2013-0398
Mahyar-Roemer, M., Fritzsche, C., Wagner, S., Laue, M., and Roemer, K. (2004). Mitochondrial p53 levels parallel total p53 levels independent of stress response in human colorectal carcinoma and glioblastoma cells. Oncogene 23 (37), 6226–6236. doi:10.1038/sj.onc.1207637
Maiti, P., Plemmons, A., Bowers, Z., Weaver, C., and Dunbar, G. (2019). Labeling and imaging of amyloid plaques in brain tissue using the natural polyphenol curcumin. J. Vis. Exp. 153. doi:10.3791/60377
Mamelak, M. (2017). Energy and the alzheimer brain. Neurosci. Biobehav. Rev. 75, 297–313. doi:10.1016/j.neubiorev.2017.02.001
Manoli, I., Alesci, S., Blackman, M. R., Su, Y. A., Rennert, O. M., and Chrousos, G. P. (2007). Mitochondria as key components of the stress response. Trends Endocrinol. Metab. 18 (5), 190–198. doi:10.1016/j.tem.2007.04.004
Martins, L. M., Morrison, A., Klupsch, K., Fedele, V., Moisoi, N., Teismann, P., et al. (2004). Neuroprotective role of the Reaper-related serine protease HtrA2/Omi revealed by targeted deletion in mice. Mol. Cell. Biol. 24 (22), 9848–9862. doi:10.1128/MCB.24.22.9848-9862.2004
Matsuzaki, K., Yamakuni, T., Hashimoto, M., Haque, A. M., Shido, O., Mimaki, Y., et al. (2006). Nobiletin restoring beta-amyloid-impaired CREB phosphorylation rescues memory deterioration in Alzheimer's disease model rats. Neurosci. Lett. 400 (3), 230–234. doi:10.1016/j.neulet.2006.02.077
McWilliams, T. G., Prescott, A. R., Montava-Garriga, L., Ball, G., Singh, F., Barini, E., et al. (2018). Basal mitophagy occurs independently of PINK1 in mouse tissues of high metabolic demand. Cell. Metab. 27 (2), 439–449.e5. e5. doi:10.1016/j.cmet.2017.12.008
Meeusen, S. L., and Nunnari, J. (2005). How mitochondria fuse. Curr. Opin. Cell. Biol. 17 (4), 389–394. doi:10.1016/j.ceb.2005.06.014
Meng, X. B., Sun, G. B., Wang, M., Sun, J., Qin, M., and Sun, X. B. (2013). Evidence-based complementary and alternative medicine. doi:10.1155/2013/971712P90RSK and Nrf2 activation via MEK1/2-ERK1/2 pathways mediated by Notoginsenoside R2 to prevent 6-hydroxydopamine-induced apoptotic death in SH-SY5Y cellseCAM971712
Meyer, J. N., Leuthner, T. C., and Luz, A. L. (2017). Mitochondrial fusion, fission, and mitochondrial toxicity. Toxicology 391, 42–53. doi:10.1016/j.tox.2017.07.019
Milane, L., Trivedi, M., Singh, A., Talekar, M., and Amiji, M. (2015). Mitochondrial biology, targets, and drug delivery. J. Control. Release 207, 40–58. doi:10.1016/j.jconrel.2015.03.036
Naoi, M., Maruyama, W., Shamoto-Nagai, M., Yi, H., Akao, Y., and Tanaka, M. (2005). Oxidative stress in mitochondria: Decision to survival and death of neurons in neurodegenerative disorders. Mol. Neurobiol. 31 (1-3), 81–93. doi:10.1385/MN:31:1-3:081
Nguyen, C. T., Luong, T. T., Kim, G. L., Pyo, S., and Rhee, D. K. (2015). Korean Red Ginseng inhibits apoptosis in neuroblastoma cells via estrogen receptor β-mediated phosphatidylinositol-3 kinase/Akt signaling. J. Ginseng Res. 39 (1), 69–75. doi:10.1016/j.jgr.2014.06.005
Nie, L., Xia, J., Li, H., Zhang, Z., Yang, Y., Huang, X., et al. (2017). Ginsenoside Rg1 ameliorates behavioral abnormalities and modulates the hippocampal proteomic change in triple transgenic mice of alzheimer's disease. Oxid. Med. Cell. Longev., 6473506. doi:10.1155/2017/6473506
Nishizaki, T. (2019). Fe3+ facilitates endocytic internalization of extracellular aβ1-42 and enhances aβ1-42-induced caspase-3/caspase-4 activation and neuronal cell death. Mol. Neurobiol. 56 (7), 4812–4819. doi:10.1007/s12035-018-1408-y
Norton, S., Matthews, F. E., Barnes, D. E., Yaffe, K., and Brayne, C. (2014). Potential for primary prevention of alzheimer's disease: An analysis of population-based data. Lancet. Neurol. 13 (8), 788–794. doi:10.1016/S1474-4422(14)70136-X
Ochaba, J., Lukacsovich, T., Csikos, G., Zheng, S., Margulis, J., Salazar, L., et al. (2014). Potential function for the Huntingtin protein as a scaffold for selective autophagy. Proc. Natl. Acad. Sci. U. S. A. 111 (47), 16889–16894. doi:10.1073/pnas.1420103111
Oliver, D., and Reddy, P. H. (2019b). Dynamics of dynamin-related protein 1 in alzheimer's disease and other neurodegenerative diseases. Cells 8 (9), 961. doi:10.3390/cells8090961
Oliver, D., and Reddy, P. H. (2019a). Molecular basis of alzheimer's disease: Focus on mitochondria. J. Alzheimers Dis. 72 (1), S95–S116. doi:10.3233/JAD-190048
Ott, M., Robertson, J. D., Gogvadze, V., Zhivotovsky, B., and Orrenius, S. (2002). Cytochrome c release from mitochondria proceeds by a two-step process. Proc. Natl. Acad. Sci. U. S. A. 99 (3), 1259–1263. doi:10.1073/pnas.241655498
Paillusson, S., Gomez-Suaga, P., Stoica, R., Little, D., Gissen, P., Devine, M. J., et al. (2017). α-Synuclein binds to the ER-mitochondria tethering protein VAPB to disrupt Ca2+ homeostasis and mitochondrial ATP production. Acta Neuropathol. 134 (1), 129–149. doi:10.1007/s00401-017-1704-z
Panes, J. D., Wendt, A., Ramirez-Molina, O., Castro, P. A., and Fuentealba, J. (2022). Deciphering the role of PGC-1α in neurological disorders: From mitochondrial dysfunction to synaptic failure. Neural Regen. Res. 17 (2), 237–245. doi:10.4103/1673-5374.317957
Parnetti, L., Gaetani, L., Eusebi, P., Paciotti, S., Hansson, O., El-Agnaf, O., et al. (2019). CSF and blood biomarkers for Parkinson's disease. Lancet. Neurol. 18 (6), 573–586. doi:10.1016/S1474-4422(19)30024-9
Peng, K., Yang, L., Wang, J., Ye, F., Dan, G., Zhao, Y., et al. (2017). The interaction of mitochondrial biogenesis and fission/fusion mediated by PGC-1α regulates rotenone-induced dopaminergic neurotoxicity. Mol. Neurobiol. 54 (5), 3783–3797. doi:10.1007/s12035-016-9944-9
Peng, W., Chen, Y., Tumilty, S., Liu, L., Luo, L., Yin, H., et al. (2022). Paeoniflorin is a promising natural monomer for neurodegenerative diseases via modulation of Ca2+ and ROS homeostasis. Curr. Opin. Pharmacol. 62, 97–102. doi:10.1016/j.coph.2021.11.009
Peng, Y., Hou, C., Yang, Z., Li, C., Jia, L., Liu, J., et al. (2016). Hydroxytyrosol mildly improve cognitive function independent of APP processing in APP/PS1 mice. Mol. Nutr. Food Res. 60 (11), 2331–2342. doi:10.1002/mnfr.201600332
Peng, Y., Xing, C., Xu, S., Lemere, C. A., Chen, G., Liu, B., et al. (2009). L-3-n-butylphthalide improves cognitive impairment induced by intracerebroventricular infusion of amyloid-beta peptide in rats. Eur. J. Pharmacol. 621 (1-3), 38–45. doi:10.1016/j.ejphar.2009.08.036
Petit, A., Kawarai, T., Paitel, E., Sanjo, N., Maj, M., Scheid, M., et al. (2005). Wild-type PINK1 prevents basal and induced neuronal apoptosis, a protective effect abrogated by Parkinson disease-related mutations. J. Biol. Chem. 280 (40), 34025–34032. doi:10.1074/jbc.M505143200
Pickles, S., Vigié, P., and Youle, R. J. (2018). Mitophagy and quality control mechanisms in mitochondrial maintenance. Curr. Biol. 28 (4), R170–R185. doi:10.1016/j.cub.2018.01.004
Pradeepkiran, J. A., Hindle, A., Kshirsagar, S., and Reddy, P. H. (2022). Are mitophagy enhancers therapeutic targets for Alzheimer's disease? Biomed. Pharmacother. 149, 112918. doi:10.1016/j.biopha.2022.112918
Prakash, A., and Kumar, A. (2014). Implicating the role of lycopene in restoration of mitochondrial enzymes and BDNF levels in β-amyloid induced Alzheimer׳s disease. Eur. J. Pharmacol. 741, 104–111. doi:10.1016/j.ejphar.2014.07.036
Pringsheim, T., Wiltshire, K., Day, L., Dykeman, J., Steeves, T., and Jette, N. (2012). The incidence and prevalence of Huntington's disease: A systematic review and meta-analysis. Mov. Disord. 27 (9), 1083–1091. doi:10.1002/mds.25075
Puigserver, P., and Spiegelman, B. M. (2003). Peroxisome proliferator-activated receptor-gamma coactivator 1 alpha (PGC-1 alpha): Transcriptional coactivator and metabolic regulator. Endocr. Rev. 24 (1), 78–90. doi:10.1210/er.2002-0012
Puthalakath, H., and Strasser, A. (2002). Keeping killers on a tight leash: Transcriptional and post-translational control of the pro-apoptotic activity of BH3-only proteins. Cell. Death Differ. 9 (5), 505–512. doi:10.1038/sj.cdd.4400998
Radad, K., Gille, G., Moldzio, R., Saito, H., Ishige, K., and Rausch, W. D. (2004a1996)., 111. Vienna, Austria, 37–45. doi:10.1007/s00702-003-0063-1Ginsenosides Rb1 and Rg1 effects on survival and neurite growth of MPP+-affected mesencephalic dopaminergic cellsJ. Neural Transm.1
Radad, K., Gille, G., Moldzio, R., Saito, H., and Rausch, W. D. (2004b). Ginsenosides Rb1 and Rg1 effects on mesencephalic dopaminergic cells stressed with glutamate. Brain Res. 1021 (1), 41–53. doi:10.1016/j.brainres.2004.06.030
Ramazani, E., YazdFazeli, M., Emami, S. A., Mohtashami, L., Javadi, B., Asili, J., et al. (2020). Protective effects of Cinnamomum verum, Cinnamomum cassia and cinnamaldehyde against 6-OHDA-induced apoptosis in PC12 cells. Mol. Biol. Rep. 47 (4), 2437–2445. doi:10.1007/s11033-020-05284-y
Reddy, P. H., Manczak, M., Yin, X., Grady, M. C., Mitchell, A., Kandimalla, R., et al. (2016). Protective effects of a natural product, curcumin, against amyloid β induced mitochondrial and synaptic toxicities in Alzheimer's disease. J. Investig. Med. 64 (8), 1220–1234. doi:10.1136/jim-2016-000240
Reddy, P. H., Manczak, M., Yin, X., and Reddy, A. P. (2018). Synergistic protective effects of mitochondrial division inhibitor 1 and mitochondria-targeted small peptide SS31 in alzheimer's disease. J. Alzheimers Dis. 62 (4), 1549–1565. doi:10.3233/JAD-170988
Reddy, P. H., and Oliver, D. M. (2019). Amyloid beta and phosphorylated tau-induced defective autophagy and mitophagy in alzheimer's disease. Cells 8 (5), 488. doi:10.3390/cells8050488
Reitz, C., and Mayeux, R. (2014). Alzheimer disease: Epidemiology, diagnostic criteria, risk factors and biomarkers. Biochem. Pharmacol. 88 (4), 640–651. doi:10.1016/j.bcp.2013.12.024
Reznick, R. M., and Shulman, G. I. (2006). The role of AMP-activated protein kinase in mitochondrial biogenesis. J. Physiol. 574 (1), 33–39. doi:10.1113/jphysiol.2006.109512
Ross, C. A., and Tabrizi, S. J. (2011). Huntington's disease: From molecular pathogenesis to clinical treatment. Lancet. Neurol. 10 (1), 83–98. doi:10.1016/S1474-4422(10)70245-3
Rui, Y. N., Xu, Z., Patel, B., Chen, Z., Chen, D., Tito, A., et al. (2015). Huntingtin functions as a scaffold for selective macroautophagy. Nat. Cell. Biol. 17 (3), 262–275. doi:10.1038/ncb3101
Ryu, Y. K., Kang, Y., Go, J., Park, H. Y., Noh, J. R., Kim, Y. H., et al. (2017). Humulus japonicus prevents dopaminergic neuron death in 6-hydroxydopamine-induced models of Parkinson's disease. J. Med. Food 20 (2), 116–123. doi:10.1089/jmf.2016.3851
Sabogal-Guáqueta, A. M., Hobbie, F., Keerthi, A., Oun, A., Kortholt, A., Boddeke, E., et al. (2019). Linalool attenuates oxidative stress and mitochondrial dysfunction mediated by glutamate and NMDA toxicity. Biomed. Pharmacother. = Biomedecine Pharmacother. 118, 109295. doi:10.1016/j.biopha.2019.109295
Sapkota, K., Kim, S., Park, S. E., and Kim, S. J. (2011). Detoxified extract of Rhus verniciflua Stokes inhibits rotenone-induced apoptosis in human dopaminergic cells, SH-SY5Y.. Cell. Mol. Neurobiol. 31 (2), 213–223. doi:10.1007/s10571-010-9609-6
Sarniak, A., Lipińska, J., Tytman, K., and Lipińska, S. (2016). Endogenous mechanisms of reactive oxygen species (ROS) generation. Postepy Hig. Med. Dosw. 70 (0), 1150–1165. doi:10.5604/17322693.1224259
Satija, S., Kaur, H., Tambuwala, M. M., Sharma, P., Vyas, M., Khurana, N., et al. (2021). Hypoxia-inducible factor (HIF): Fuel for cancer progression. Curr. Mol. Pharmacol. 14 (3), 321–332. doi:10.2174/1874467214666210120154929
Scarffe, L. A., Stevens, D. A., Dawson, V. L., and Dawson, T. M. (2014). Parkin and PINK1: Much more than mitophagy. Trends Neurosci. 37 (6), 315–324. doi:10.1016/j.tins.2014.03.004
Schapira, A. H. (2006). Mitochondrial disease. Lancet (London, Engl. 368 (9529), 70–82. doi:10.1016/S0140-6736(06)68970-8
Schneider, L. S., Mangialasche, F., Andreasen, N., Feldman, H., Giacobini, E., Jones, R., et al. (2014). Clinical trials and late-stage drug development for alzheimer's disease: An appraisal from 1984 to 2014. J. Intern. Med. 275 (3), 251–283. doi:10.1111/joim.12191
Schrijvers, E. M., Verhaaren, B. F., Koudstaal, P. J., Hofman, A., Ikram, M. A., and Breteler, M. M. (2012). Is dementia incidence declining? Trends in dementia incidence since 1990 in the Rotterdam Study. Neurology 78 (19), 1456–1463. doi:10.1212/WNL.0b013e3182553be6
Shakeri, R., Kheirollahi, A., and Davoodi, J. (2017). Apaf-1: Regulation and function in cell death. Biochimie 135, 111–125. doi:10.1016/j.biochi.2017.02.001
Sharma, R., and Padwad, Y. (2020a). Nutraceuticals-Based immunotherapeutic concepts and opportunities for the mitigation of cellular senescence and aging: A narrative review. Ageing Res. Rev. 63, 101141. doi:10.1016/j.arr.2020.101141
Sharma, V. K., Singh, T. G., and Mehta, V. (2021b). Stressed mitochondria: A target to intrude alzheimer's disease. Mitochondrion 59, 48–57. doi:10.1016/j.mito.2021.04.004
Sharma, V. K., and Singh, T. G. (2020b). Navigating alzheimer's disease via chronic stress: The role of glucocorticoids. Curr. Drug Targets 21 (5), 433–444. doi:10.2174/1389450120666191017114735
Sharma, V. K., Singh, T. G., Singh, S., Garg, N., and Dhiman, S. (2021a). Apoptotic pathways and alzheimer's disease: Probing therapeutic potential. Neurochem. Res. 46 (12), 3103–3122. doi:10.1007/s11064-021-03418-7
Sheng, G. Q., Zhang, J. R., Pu, X. P., Ma, J., and Li, C. L. (2002). Protective effect of verbascoside on 1-methyl-4-phenylpyridinium ion-induced neurotoxicity in PC12 cells. Eur. J. Pharmacol. 451 (2), 119–124. doi:10.1016/s0014-2999(02)02240-9
Shin, S. J., Jeon, S. G., Kim, J. I., Jeong, Y. O., Kim, S., Park, Y. H., et al. (2019). Red ginseng attenuates aβ-induced mitochondrial dysfunction and aβ-mediated pathology in an animal model of alzheimer's disease. Int. J. Mol. Sci. 20 (12), 3030. doi:10.3390/ijms20123030
Shinomol, G. K., and Muralidhara, (2008). Prophylactic neuroprotective property of Centella asiatica against 3-nitropropionic acid induced oxidative stress and mitochondrial dysfunctions in brain regions of prepubertal mice. Neurotoxicology 29 (6), 948–957. doi:10.1016/j.neuro.2008.09.009
Shirendeb, U. P., Calkins, M. J., Manczak, M., Anekonda, V., Dufour, B., McBride, J. L., et al. (2012). Mutant huntingtin's interaction with mitochondrial protein Drp1 impairs mitochondrial biogenesis and causes defective axonal transport and synaptic degeneration in Huntington's disease. Hum. Mol. Genet. 21 (2), 406–420. doi:10.1093/hmg/ddr475
Shokolenko, I., Venediktova, N., Bochkareva, A., Wilson, G. L., and Alexeyev, M. F. (2009). Oxidative stress induces degradation of mitochondrial DNA. Nucleic Acids Res. 37 (8), 2539–2548. doi:10.1093/nar/gkp100
Siekacz, K., Piotrowski, W. J., Iwański, M. A., Górski, P., and Białas, A. J. (2021). The role of interaction between mitochondria and the extracellular matrix in the development of idiopathic pulmonary fibrosis. Oxid. Med. Cell. Longev. 2021, 9932442. doi:10.1155/2021/9932442
Silva, D. F., Esteves, A. R., Oliveira, C. R., and Cardoso, S. M. (2011). Mitochondria: The common upstream driver of amyloid-β and tau pathology in alzheimer's disease. Curr. Alzheimer Res. 8 (5), 563–572. doi:10.2174/156720511796391872
Silva, T., Reis, J., Teixeira, J., and Borges, F. (2014). Alzheimer's disease, enzyme targets and drug discovery struggles: From natural products to drug prototypes. Ageing Res. Rev. 15, 116–145. doi:10.1016/j.arr.2014.03.008
Singh, A., Kukreti, R., Saso, L., and Kukreti, S. (2019). Oxidative stress: A key modulator in neurodegenerative diseases. Mol. (Basel, Switz. 24 (8), 1583. doi:10.3390/molecules24081583
Smirnova, E., Griparic, L., Shurland, D. L., and van der Bliek, A. M. (2001). Dynamin-related protein Drp1 is required for mitochondrial division in mammalian cells. Mol. Biol. Cell. 12 (8), 2245–2256. doi:10.1091/mbc.12.8.2245
Smith, S. C. (2007). Multiple risk factors for cardiovascular disease and diabetes mellitus. Am. J. Med. 120 (3)–S11. S3–S11. doi:10.1016/j.amjmed.2007.01.002
Sowers, J. R. (2004). Insulin resistance and hypertension. Am. J. Physiol. Heart Circ. Physiol. 286 (5), H1597–H1602. doi:10.1152/ajpheart.00026.2004
Spuch, C., Ortolano, S., and Navarro, C. (2012). New insights in the amyloid-Beta interaction with mitochondria. J. Aging Res., 324968. doi:10.1155/2012/324968
Stafa, K., Tsika, E., Moser, R., Musso, A., Glauser, L., Jones, A., et al. (2014). Functional interaction of Parkinson's disease-associated LRRK2 with members of the dynamin GTPase superfamily. Hum. Mol. Genet. 23 (8), 2055–2077. doi:10.1093/hmg/ddt600
Su, J. H., Anderson, A. J., Cummings, B. J., and Cotman, C. W. (1994). Immunohistochemical evidence for apoptosis in Alzheimer's disease. Neuroreport 5 (18), 2529–2533. doi:10.1097/00001756-199412000-00031
Sun, F., Deng, Y., Han, X., Liu, Q., Zhang, P., Manzoor, R., et al. (2019). A secret that underlies Parkinson's disease: The damaging cycle. Neurochem. Int. 129, 104484. doi:10.1016/j.neuint.2019.104484
Sun, F. L., Zhang, L., Zhang, R. Y., and Li, L. (2011). Tetrahydroxystilbene glucoside protects human neuroblastoma SH-SY5Y cells against MPP+-induced cytotoxicity. Eur. J. Pharmacol. 660 (2-3), 283–290. doi:10.1016/j.ejphar.2011.03.046
Sun, X., Wei, X., Qu, S., Zhao, Y., and Zhang, X. (2010). Hydroxysafflor Yellow A suppresses thrombin generation and inflammatory responses following focal cerebral ischemia-reperfusion in rats. Bioorg. Med. Chem. Lett. 20 (14), 4120–4124. doi:10.1016/j.bmcl.2010.05.076
Swerdlow, R. H. (2018). Mitochondria and mitochondrial cascades in alzheimer's disease. J. Alzheimers Dis. 62 (3), 1403–1416. doi:10.3233/JAD-170585
Taguchi, N., Ishihara, N., Jofuku, A., Oka, T., and Mihara, K. (2007). Mitotic phosphorylation of dynamin-related GTPase Drp1 participates in mitochondrial fission. J. Biol. Chem. 282 (15), 11521–11529. doi:10.1074/jbc.M607279200
Tamburrino, A., and Decressac, M. (2016). Aged and diseased neurons get lost in transport. Trends Neurosci. 39 (4), 199–201. doi:10.1016/j.tins.2016.02.007
Tang, D., Kang, R., Berghe, T. V., Vandenabeele, P., and Kroemer, G. (2019). The molecular machinery of regulated cell death. Cell. Res. 29 (5), 347–364. doi:10.1038/s41422-019-0164-5
Thangarajan, S., Deivasigamani, A., Natarajan, S. S., Krishnan, P., and Mohanan, S. K. (2014). Neuroprotective activity of L-theanine on 3-nitropropionic acid-induced neurotoxicity in rat striatum. Int. J. Neurosci. 124 (9), 673–684. doi:10.3109/00207454.2013.872642
Tian, X., Zhang, L., Wang, J., Dai, J., Shen, S., Yang, L., et al. (2013). The protective effect of hyperbaric oxygen and Ginkgo biloba extract on Aβ25-35-induced oxidative stress and neuronal apoptosis in rats. Behav. Brain Res. 242, 1–8. doi:10.1016/j.bbr.2012.12.026
Tong, Y., Bai, L., Gong, R., Chuan, J., Duan, X., and Zhu, Y. (2018). Shikonin protects PC12 cells against β-amyloid peptide-induced cell injury through antioxidant and antiapoptotic activities. Sci. Rep. 8 (1), 26. doi:10.1038/s41598-017-18058-7
Tufi, R., Gandhi, S., de Castro, I. P., Lehmann, S., Angelova, P. R., Dinsdale, D., et al. (2014). Enhancing nucleotide metabolism protects against mitochondrial dysfunction and neurodegeneration in a PINK1 model of Parkinson's disease. Nat. Cell. Biol. 16 (2), 157–166. doi:10.1038/ncb2901
Tysnes, O. B., and Storstein, A. (2017)., 124. Vienna, Austria, 901–905. doi:10.1007/s00702-017-1686-yEpidemiology of Parkinson's diseaseJ. Neural Transm.8
Valero, T. (2014). Mitochondrial biogenesis: Pharmacological approaches. Curr. Pharm. Des. 20 (35), 5507–5509. doi:10.2174/138161282035140911142118
Wai, T., and Langer, T. (2016). Mitochondrial dynamics and metabolic regulation. Trends Endocrinol. Metab. 27 (2), 105–117. doi:10.1016/j.tem.2015.12.001
Walker, F. O. (2007). Huntington's disease. Lancet (London, Engl. 369 (9557), 218–228. doi:10.1016/S0140-6736(07)60111-1
Wang, C., Cai, X., Hu, W., Li, Z., Kong, F., Chen, X., et al. (2019a). Investigation of the neuroprotective effects of crocin via antioxidant activities in HT22 cells and in mice with Alzheimer's disease. Int. J. Mol. Med. 43 (2), 956–966. doi:10.3892/ijmm.2018.4032
Wang, D. M., Li, S. Q., Wu, W. L., Zhu, X. Y., Wang, Y., and Yuan, H. Y. (2014a). Effects of long-term treatment with quercetin on cognition and mitochondrial function in a mouse model of Alzheimer's disease. Neurochem. Res. 39 (8), 1533–1543. doi:10.1007/s11064-014-1343-x
Wang, K., Zhu, L., Zhu, X., Zhang, K., Huang, B., Zhang, J., et al. (2014b). Protective effect of paeoniflorin on Aβ25-35-induced SH-SY5Y cell injury by preventing mitochondrial dysfunction. Cell. Mol. Neurobiol. 34 (2), 227–234. doi:10.1007/s10571-013-0006-9
Wang, L., Guo, L., Lu, L., Sun, H., Shao, M., Beck, S. J., et al. (2016a). Synaptosomal mitochondrial dysfunction in 5xFAD mouse model of alzheimer's disease. PloS one 11 (3), e0150441. doi:10.1371/journal.pone.0150441
Wang, L., Jin, G. F., Yu, H. H., Lu, X. H., Zou, Z. H., Liang, J. Q., et al. (2019b). Protective effects of tenuifolin isolated from Polygala tenuifolia Willd roots on neuronal apoptosis and learning and memory deficits in mice with Alzheimer's disease. Food Funct. 10 (11), 7453–7460. doi:10.1039/c9fo00994a
Wang, L., Wang, J., Yang, L., Zhou, S. M., Guan, S. Y., Yang, L. K., et al. (2017a). Effect of Praeruptorin C on 3-nitropropionic acid induced Huntington's disease-like symptoms in mice. Biomed. Pharmacother. = Biomedecine Pharmacother. 86, 81–87. doi:10.1016/j.biopha.2016.11.111
Wang, M., Li, Y., Ni, C., and Song, G. (2017b). Honokiol attenuates oligomeric amyloid β1-42-induced alzheimer's disease in mice through attenuating mitochondrial apoptosis and inhibiting the nuclear factor kappa-B signaling pathway. Cell. Physiol. biochem. 43 (1), 69–81. doi:10.1159/000480320
Wang, X. J., and Xu, J. X. (2005). Salvianic acid A protects human neuroblastoma SH-SY5Y cells against MPP+-induced cytotoxicity. Neurosci. Res. 51 (2), 129–138. doi:10.1016/j.neures.2004.10.001
Wang, Y. H., Xuan, Z. H., Tian, S., and Du, G. H. (2015a). Echinacoside protects against 6-hydroxydopamine-induced mitochondrial dysfunction and inflammatory responses in PC12 cells via reducing ROS production. Evid. Based Complement Alternat. Med. 2015, 189239. doi:10.1155/2015/189239
Wang, Y., Li, Y., Yang, W., Gao, S., Lin, J., Wang, T., et al. (2018). Ginsenoside Rb1 inhibit apoptosis in rat model of Alzheimer's disease induced by Aβ1-40. Am. J. Transl. Res. 10 (3), 796–805.
Wang, Y., Miao, Y., Mir, A. Z., Cheng, L., Wang, L., Zhao, L., et al. (2016b). Inhibition of beta-amyloid-induced neurotoxicity by pinocembrin through Nrf2/HO-1 pathway in SH-SY5Y cells. J. Neurol. Sci. 368, 223–230. doi:10.1016/j.jns.2016.07.010
Wang, Y. Q., Wang, M. Y., Fu, X. R., Peng-Yu, , Gao, G. F., Fan, Y. M., et al. (2015b). Neuroprotective effects of ginkgetin against neuroinjury in Parkinson's disease model induced by MPTP via chelating iron. Free Radic. Res. 49 (9), 1069–1080. doi:10.3109/10715762.2015.1032958
Wauer, T., Swatek, K. N., Wagstaff, J. L., Gladkova, C., Pruneda, J. N., Michel, M. A., et al. (2015). Ubiquitin Ser65 phosphorylation affects ubiquitin structure, chain assembly and hydrolysis. EMBO J. 34 (3), 307–325. doi:10.15252/embj.201489847
Wenger, J., Klinglmayr, E., Fröhlich, C., Eibl, C., Gimeno, A., Hessenberger, M., et al. (2013). Functional mapping of human dynamin-1-like GTPase domain based on x-ray structure analyses. PloS one 8 (8), e71835. doi:10.1371/journal.pone.0071835
Wiedemann, N., Frazier, A. E., and Pfanner, N. (2004). The protein import machinery of mitochondria. J. Biol. Chem. 279 (15), 14473–14476. doi:10.1074/jbc.R400003200
Wong, L. R., Tan, E. A., Lim, M., Shen, W., Lian, X. L., Wang, Y., et al. (2021). Functional effects of berberine in modulating mitochondrial dysfunction and inflammatory response in the respective amyloidogenic cells and activated microglial cells - in vitro models simulating Alzheimer's disease pathology. Life Sci. 282, 119824. doi:10.1016/j.lfs.2021.119824
Wong, V. K., Wu, A. G., Wang, J. R., Liu, L., and Law, B. Y. (2015). Neferine attenuates the protein level and toxicity of mutant huntingtin in PC-12 cells via induction of autophagy. Mol. (Basel, Switz. 20 (3), 3496–3514. doi:10.3390/molecules20033496
Xia, M. L., Xie, X. H., Ding, J. H., Du, R. H., and Hu, G. (2020). Astragaloside IV inhibits astrocyte senescence: Implication in Parkinson's disease. J. Neuroinflammation 17 (1), 105. doi:10.1186/s12974-020-01791-8
Xian, Y. F., Ip, S. P., Mao, Q. Q., and Lin, Z. X. (2016). Neuroprotective effects of honokiol against beta-amyloid-induced neurotoxicity via GSK-3β and β-catenin signaling pathway in PC12 cells. Neurochem. Int. 97, 8–14. doi:10.1016/j.neuint.2016.04.014
Xian, Y. F., Lin, Z. X., Mao, Q. Q., Chen, J. N., Su, Z. R., Lai, X. P., et al. (2013). Evidence-based complementary and alternative medicine. doi:10.1155/2013/163057Isorhynchophylline protects PC12 cells against beta-amyloid-induced apoptosis via PI3K/akt signaling pathwayeCAM163057
Xian, Y. F., Mao, Q. Q., Wu, J. C., Su, Z. R., Chen, J. N., Lai, X. P., et al. (2014). Isorhynchophylline treatment improves the amyloid-β-induced cognitive impairment in rats via inhibition of neuronal apoptosis and tau protein hyperphosphorylation. J. Alzheimers Dis. 39 (2), 331–346. doi:10.3233/JAD-131457
Xiao, X., Chen, Q., Zhu, X., and Wang, Y. (2019). ABAD/17β-HSD10 reduction contributes to the protective mechanism of huperzine a on the cerebral mitochondrial function in APP/PS1 mice. Neurobiol. Aging 81, 77–87. doi:10.1016/j.neurobiolaging.2019.05.016
Xing, G., Dong, M., Li, X., Zou, Y., Fan, L., Wang, X., et al. (2011). Neuroprotective effects of puerarin against beta-amyloid-induced neurotoxicity in PC12 cells via a PI3K-dependent signaling pathway. Brain Res. Bull. 85 (3-4), 212–218. doi:10.1016/j.brainresbull.2011.03.024
Xu, L. L., Shen, Y., Wang, X., Wei, L. F., Wang, P., Yang, H., et al. (2017a). Mitochondrial dynamics changes with age in an APPsw/PS1dE9 mouse model of Alzheimer's disease. Neuroreport 28 (4), 222–228. doi:10.1097/WNR.0000000000000739
Xu, P., Wang, K., Lu, C., Dong, L., Gao, L., Yan, M., et al. (2017b). Protective effects of linalool against amyloid beta-induced cognitive deficits and damages in mice. Life Sci. 174, 21–27. doi:10.1016/j.lfs.2017.02.010
Yang, A., Bagit, A., and MacPherson, R. (2021). Resveratrol, metabolic dysregulation, and alzheimer's disease: Considerations for neurogenerative disease. Int. J. Mol. Sci. 22 (9), 4628. doi:10.3390/ijms22094628
Yang, L., Ye, C. Y., Huang, X. T., Tang, X. C., and Zhang, H. Y. (2012). Decreased accumulation of subcellular amyloid-β with improved mitochondrial function mediates the neuroprotective effect of huperzine A. J. Alzheimers Dis. 31 (1), 131–142. doi:10.3233/JAD-2012-120274
Yang, T., Zhao, X., Zhang, Y., Xie, J., and Zhou, A. (2020). 6‴-Feruloylspinosin alleviated beta-amyloid induced toxicity by promoting mitophagy in Caenorhabditis elegans (GMC101) and PC12 cells. Sci. Total Environ. 715, 136953. doi:10.1016/j.scitotenv.2020.136953
Yang, W. T., Zheng, X. W., Chen, S., Shan, C. S., Xu, Q. Q., Zhu, J. Z., et al. (2017). Chinese herbal medicine for Alzheimer's disease: Clinical evidence and possible mechanism of neurogenesis. Biochem. Pharmacol. 141, 143–155. doi:10.1016/j.bcp.2017.07.002
Ye, M., Lee, S. G., Chung, E. S., Lim, S. J., Kim, W. S., Yoon, H., et al. (2014). Neuroprotective effects of cuscutae semen in a mouse model of Parkinson's diseaseeCAM. In Evidence-based complementary and alternative medicine, 150153. doi:10.1155/2014/150153
Youle, R. J., and van der Bliek, A. M. (2012). Mitochondrial fission, fusion, and stress. Sci. (New York, N.Y.) 337 (6098), 1062–1065. doi:10.1126/science.1219855
Yu, X., Li, Y., and Mu, X. (2020). Effect of quercetin on PC12 alzheimer's disease cell model induced by aβ25-35 and its mechanism based on sirtuin1/nrf2/HO-1 pathway. Biomed. Res. Int. 2020, 8210578. doi:10.1155/2020/8210578
Yuan, S., Topf, M., Reubold, T. F., Eschenburg, S., and Akey, C. W. (2013). Changes in Apaf-1 conformation that drive apoptosome assembly. Biochemistry 52 (13), 2319–2327. doi:10.1021/bi301721g
Zaja, I., Bai, X., Liu, Y., Kikuchi, C., Dosenovic, S., Yan, Y., et al. (2014). Cdk1, PKCδ and calcineurin-mediated Drp1 pathway contributes to mitochondrial fission-induced cardiomyocyte death. Biochem. Biophys. Res. Commun. 453 (4), 710–721. doi:10.1016/j.bbrc.2014.09.144
Zhan, X., Stamova, B., and Sharp, F. R. (2018). Lipopolysaccharide associates with amyloid plaques, neurons and oligodendrocytes in alzheimer's disease brain: A review. Front. Aging Neurosci. 10, 42. doi:10.3389/fnagi.2018.00042
Zhang, C., Li, C., Chen, S., Li, Z., Ma, L., Jia, X., et al. (2017a). Hormetic effect of panaxatriol saponins confers neuroprotection in PC12 cells and zebrafish through PI3K/AKT/mTOR and AMPK/SIRT1/FOXO3 pathways. Sci. Rep. 7, 41082. doi:10.1038/srep41082
Zhang, H., Liu, Y., Lao, M., Ma, Z., and Yi, X. (2011). Puerarin protects Alzheimer's disease neuronal cybrids from oxidant-stress induced apoptosis by inhibiting pro-death signaling pathways. Exp. Gerontol. 46 (1), 30–37. doi:10.1016/j.exger.2010.09.013
Zhang, L., Yu, H., Zhao, X., Lin, X., Tan, C., Cao, G., et al. (2010). Neuroprotective effects of salidroside against beta-amyloid-induced oxidative stress in SH-SY5Y human neuroblastoma cells. Neurochem. Int. 57 (5), 547–555. doi:10.1016/j.neuint.2010.06.021
Zhang, R., Zhang, N., Zhang, H., Liu, C., Dong, X., Wang, X., et al. (2017b). Celastrol prevents cadmium-induced neuronal cell death by blocking reactive oxygen species-mediated mammalian target of rapamycin pathway. Br. J. Pharmacol. 174 (1), 82–100. doi:10.1111/bph.13655
Zhang, X., Wu, J., Dou, Y., Xia, B., Rong, W., Rimbach, G., et al. (2012). Asiatic acid protects primary neurons against C2-ceramide-induced apoptosis. Eur. J. Pharmacol. 679 (1-3), 51–59. doi:10.1016/j.ejphar.2012.01.006
Zhang, Y., Wang, J., Wang, C., Li, Z., Liu, X., Zhang, J., et al. (2018). Pharmacological basis for the use of evodiamine in alzheimer's disease: Antioxidation and antiapoptosis. Int. J. Mol. Sci. 19 (5), 1527. doi:10.3390/ijms19051527
Zhang, Y., Wang, Z. Z., Sun, H. M., Li, P., Li, Y. F., and Chen, N. H. (2014). Systematic review of traditional Chinese medicine for depression in Parkinson's disease. Am. J. Chin. Med. 42 (5), 1035–1051. doi:10.1142/S0192415X14500657
Zhang, Z., Li, X., Li, D., Luo, M., Li, Y., Song, L., et al. (2017c). Asiaticoside ameliorates β-amyloid-induced learning and memory deficits in rats by inhibiting mitochondrial apoptosis and reducing inflammatory factors. Exp. Ther. Med. 13 (2), 413–420. doi:10.3892/etm.2016.4004
Zhang, Z. X., Li, Y. B., and Zhao, R. P. (2017d). Epigallocatechin gallate attenuates β-amyloid generation and oxidative stress involvement of PPARγ in N2a/app695 cells. Neurochem. Res. 42 (2), 468–480. doi:10.1007/s11064-016-2093-8
Zhong, S. Z., Ge, Q. H., Li, Q., Qu, R., and Ma, S. P. (2009). Peoniflorin attentuates Abeta((1-42))-mediated neurotoxicity by regulating calcium homeostasis and ameliorating oxidative stress in hippocampus of rats. J. Neurol. Sci. 280 (1-2), 71–78. doi:10.1016/j.jns.2009.01.027
Keywords: neurodegenerative disease, apoptosis, herbal medicine, mitochondrial dysfunction, therapeutic strategy
Citation: Li R-L, Wang L-Y, Duan H-X, Zhang Q, Guo X, Wu C and Peng W (2022) Regulation of mitochondrial dysfunction induced cell apoptosis is a potential therapeutic strategy for herbal medicine to treat neurodegenerative diseases. Front. Pharmacol. 13:937289. doi: 10.3389/fphar.2022.937289
Received: 06 May 2022; Accepted: 11 August 2022;
Published: 22 September 2022.
Edited by:
Md. Ataur Rahman, Kyung Hee University, South KoreaReviewed by:
Jangampalli Adi Pradeepkiran, Texas Tech University, United StatesMd. Saidur Rahman, Chung-Ang University, South Korea
Copyright © 2022 Li, Wang, Duan, Zhang, Guo, Wu and Peng. This is an open-access article distributed under the terms of the Creative Commons Attribution License (CC BY). The use, distribution or reproduction in other forums is permitted, provided the original author(s) and the copyright owner(s) are credited and that the original publication in this journal is cited, in accordance with accepted academic practice. No use, distribution or reproduction is permitted which does not comply with these terms.
*Correspondence: Xiaohui Guo, Z3VveGlhb2h1aUBjZHV0Y20uZWR1LmNu; Chunjie Wu, d3VjamNkdGNtQDE2My5jb20=; Wei Peng, cGVuZ3dlaUBjZHV0Y20uZWR1LmNu