- 1Preclinical Toxicity and Efficacy Assessment of Medicines and Chemicals Research Unit, Chulalongkorn University, Bangkok, Thailand
- 2Department of Pharmacology and Physiology, Faculty of Pharmaceutical Sciences, Chulalongkorn University, Bangkok, Thailand
The major cause of death in cancer patients is strongly associated with metastasis. While much remains to be understood, microtubule-associated proteins (MAPs) have shed light on metastatic progression’s molecular mechanisms. In this review article, we focus on the role of MAPs in cancer aggressiveness, particularly cancer metastasis activity. Increasing evidence has shown that a growing number of MAP member proteins might be fundamental regulators involved in altering microtubule dynamics, contributing to cancer migration, invasion, and epithelial-to-mesenchymal transition. MAP types have been established according to their microtubule-binding site and function in microtubule-dependent activities. We highlight that altered MAP expression was commonly found in many cancer types and related to cancer progression based on available evidence. Furthermore, we discuss and integrate the relevance of MAPs and related molecular signaling pathways in cancer metastasis. Our review provides a comprehensive understanding of MAP function on microtubules. It elucidates how MAPs regulate cancer progression, preferentially in metastasis, providing substantial scientific information on MAPs as potential therapeutic targets and prognostic markers for cancer management.
Introduction
Microtubules are important intracellular cytoskeletons that have highly dynamic structural filaments. They are hollow cylindrical structures assembled from 13 aligned protofilaments of tubulin heterodimers (Chaaban and Brouhard, 2017). The biological functions of microtubules are essential in many indispensable cellular activities, including cell division, growth, and movement (Hookway et al., 2015; Prosser and Pelletier, 2017; Singh et al., 2018). These hollow cylinder tubes are structurally rigid and, upon microtubule assembly, provide intracellular structures (Schaedel et al., 2015). The dynamic instability of the microtubule persistently alternates between polymerization and depolymerization phases (Matov et al., 2010). Mechanistically, adding GTP-tubulin dimers to the microtubule plus-end (the forming of a stabilizing cap) is the growing state. Loss of the stabilizing GTP cap, the GDP-tubulin dimers are presented, leading to the switching from a growing state to rapid shrinking (Bowne-Anderson et al., 2015). This dynamic switching activity of microtubules is associated with microtubule-associated proteins (MAPs), which bind to various microtubule sites (Akhmanova and Steinmetz, 2015). Several MAPs are coordinated with molecular trafficking along the microtubules in cells (Seiberlich et al., 2015; Bodakuntla et al., 2019).
The dynamic instability of microtubules plays a role in several cancer activities, such as cell proliferation and cell invasion, which are considered potential cancer therapy targets (Pagano et al., 2012; Li and Wang, 2020). MAPs stabilize microtubules and promote their assembly (Barbier et al., 2010; Breuzard et al., 2013). Interestingly, the dynamic properties of microtubules are indispensable for cell motility (Etienne-Manneville, 2013). Consequentially, MAPs are fundamental factors for cell motility through their regulation of microtubule dynamics (Bodakuntla et al., 2019). Alterations in MAP gene transcription and protein expression were found in many cancer types and were mostly related to cancer metastasis (Wang W. et al., 2020; Zhang et al., 2020). Nowadays, targeting MAPs has been regarded as a strategy in cancer drug research and development. In this review, we summarize currently available knowledge on MAPs, and update and discuss our information on the relationship between several types of MAPs and cancers, owing to their contribution to cancer metastasis.
Microtubule-associated protein classification
Microtubules have a critical role in cellular processes, including cell division, cell motility, and cellular trafficking of molecules and organelles (Prosser and Pelletier, 2017; Singh et al., 2018). An assembly of microtubules forms distinct arrays through dynamics and a defined architecture to accomplish the diverse roles of microtubules. Microtubule assembly requires distinctive microtubule-binding proteins, known as MAPs. Based on their localization on the microtubules, MAPs can be categorized into five groups (Figure 1): 1) microtubule lattice binding proteins are located along the microtubule length; 2) microtubule motor proteins are required for cellular transportation along the microtubule; 3) multiple site-binding proteins; 4) microtubule plus-end binding proteins, which are particularly localized to the fast-growing + TIP network of a microtubule; 5) minus-end binding proteins are associated with the slow-growing cap of microtubules (Akhmanova and Hoogenraad, 2015). Moreover, MAPs can be classified functionally into microtubule stabilizers, destabilizers, capping, and bundlers/cross-linker proteins (Goodson and Jonasson, 2018). Broadly speaking, MAPs have multiple and complex activities, making it challenging to conclude their functions because they depend on experimental aspects or assay conditions. Further details about MAPs according to their microtubule localization and activities will be specifically discussed below.
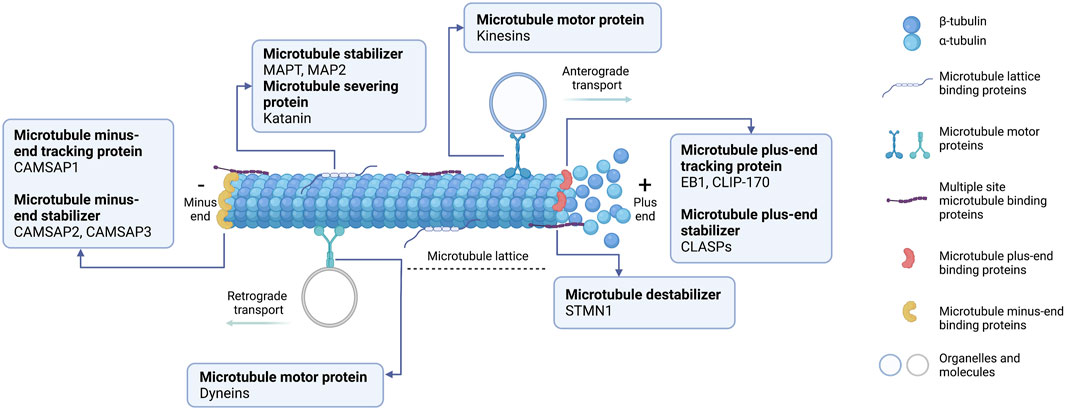
FIGURE 1. Schematic representation of microtubule-associated proteins (MAPs) binding to microtubules at different sites. (1) Microtubule lattice-binding proteins located at the middle region along the microtubule length, including MAPT and MAP2. (2) Two types of microtubule motor proteins exist: kinesins and dyneins. Kinesins carry the cellular organelle or molecules trafficking towards the microtubule plus-end (anterograde transport). Dyneins transport the cellular molecules to the microtubule minus-end (retrograde transport). (3) Multiple site microtubule-binding proteins, such as stathmin (STMN1), bind to several sites on microtubules. (4) Microtubule plus-end binding proteins (+TIPs) accumulate specifically at the growing ends of microtubules. +TIPs can affect microtubule positioning and regulate microtubules dynamically. (5) Microtubule minus-end binding proteins are preferentially anchored at the minus-end where microtubules are stabilized. This diagram was created with “Biorender.com”.
Microtubule lattice binding proteins
The microtubule lattice is typically composed of 13 protofilaments in its main body (Cross, 2019). In the middle region of the microtubule, the lattice in the GDP stage can be stabilized through classical MAPs’ activity, including microtubule-associated protein tau (MAPT). It is mainly abundant in neuronal axons, regulates microtubule assembly by inhibiting tubulin dissociation, and protects microtubules against depolymerization (Qiang et al., 2006). Similarly, microtubule-associated protein 2 (MAP2) is highly expressed in neuronal dendrites and stabilizes microtubule growth by crosslinking microtubules with intermediate filaments resulting in catastrophe rescue and microtubule stiffness activation (Teng et al., 2001). In contrast, the GDP microtubule lattice can be destabilized by the microtubule-severing protein katanin, a heterodimer protein composed of katanin P60 and katanin P80 subunits, which utilize ATP for severing existing microtubules (Ghosh et al., 2012). Mechanistically, katanin exerts its microtubule-severing function by deploying ATP hydrolysis to extract tubulin dimers at the lattice and disassemble the polymer. Consequently, new microtubule ends are created through a severe lack of GTP caps, and microtubules are rapidly depolymerized (Zehr et al., 2017). Furthermore, angiotensin II (AT2) receptor-interacting protein 3 (ATIP3) is one of the structural MAP localized along the microtubule lattice and is a potent microtubule stabilizer by associating with EB1, thereby attenuating microtubule dynamics (Nehlig et al., 2021). Comprehensively, ATIP3 interacts with EB1 in the cytosol, and the ATIP3-EB1 complex regulates the microtubule growth-shrinkage rate by preventing the accumulation of EB1 at the plus end (Velot et al., 2015).
Microtubule motor proteins
Intracellularly, molecular transport can be carried out along microtubule tracks using motor proteins, especially kinesins and dyneins (Lu and Gelfand, 2017). Kinesin motor proteins “walk” in a specific anterograde direction and intracellular transport cargoes toward microtubule plus-ends (Kevenaar et al., 2016). Kinesins are composed of different subtypes of their family proteins involved in individual cellular activities. For example, the classical kinesins, including kinesins 1 (KIF5B), 2 (KIF3A/B, 17), 3 (KIF13B), 5 (KIF11), 6 (KIF6, 9), and 8 (KIF19A, 18B), with an N-terminal motor domain and KIF14, with a middle motor domain, deploy ATP hydrolysis to generate kinesin motor movement in mechanical force toward growing microtubule plus-ends (Gigant et al., 2013; Arora et al., 2014). Furthermore, kinesin-14 or KIFC1 has a similar role as classical kinesins but has a different C-terminal motor domain, which it transports several cargos toward the microtubule minus-ends (She et al., 2017). Finally, the middle motor domain kinesin-13 or KIF2A has no motile activity, unlike other kinesin types, and has a depolymerizing microtubule activity initiated by the motor domain (Trofimova et al., 2018).
Another motor protein type, dyneins, can move toward the microtubule minus-end and transport intracellular cargoes from the cellular periphery to the center in a retrograde direction transport (Roberts et al., 2013). This motor protein guides the Golgi complex or other organelles and positions the mitotic spindle in cell division (Roberts et al., 2013). Cytoplasmic dynein is a large multi-subunit protein forming a complex called dynactin associated with the dynein motor to enhance its processivity for cargo transportation (Chowdhury et al., 2015). Because of these different functions, kinesin and dynein play important roles in distinct microtubule-dependent activities, organelle transportation, intracellular vesicle trafficking, and mitotic spindle organization (Cross and McAinsh, 2014; Kevenaar et al., 2016; Tan et al., 2018).
Multiple site microtubule-binding proteins
The regulation of microtubule dynamic instability is influential for cellular processes. One of the most remarkable microtubule destabilizers is stathmin (STMN1), or oncoprotein 18 (Op18) (Zeitz and Kierfeld, 2014). STMN1 efficiently promotes catastrophe through microtubule-binding activities, in which the STMN1-microtubule interaction occurs via various molecular mechanisms (Gupta et al., 2013). STMN1 is a sequestering protein that reduces microtubule polymer length by indirectly binding to tubulin subunits in a curved form, promoting depolymerization (Zeitz and Kierfeld, 2014). Moreover, STMN1 also induces microtubule destabilization by specifically interfering with the lateral binding of tubulin subunits (steric inhibition or by inducing curvature) at microtubule tips, operating both on plus- and minus-ends (Gupta et al., 2013). As a minus-end mechanism, the stathmin N-terminal peptide binds to and caps the dimer of α-tubulin that is exposed and prevents the incorporation of new tubulin dimers, resulting in catastrophe (Gupta et al., 2013). Additionally, the binding of STMN1 to the microtubule lattice can contribute to catastrophe, but is less effective than other mechanisms (Barbier et al., 2010). The STMN1-tubulin direct mechanism and STMN1-tubulin sequestering phenomenon can function together to create STMN1’s cellular activities.
Microtubule plus-end binding proteins
Microtubule dynamics are spatially mediated by MAPs, which preferentially contain polymerizing microtubule plus-ends and are known as plus-end tracking proteins (+TIPs) (Matov et al., 2010). Several + TIPs regulate microtubule dynamics, act as adaptors connecting microtubule plus-ends to other factors or cellular components, and promote microtubule assembly to membrane transportation (Galjart, 2010). The first identified + TIP protein was cytoplasmic linker protein 170 (CLIP-170). It participates in binding endocytic vesicles to microtubules (Folker et al., 2005). This + TIP protein has CAP-Gly domains that potentially bind to C-terminal EEY motifs, as present in the α-tubulin acidic tail and another + TIP protein, EB1 (Chen L. et al., 2019). Additionally, the N-terminal CAP-Gly domain of CLIP-170, in residues 1–350, tracks a polymerizing microtubule plus-ends in an EB1-dependent manner (Dixit et al., 2009). End binding proteins are another central + TIP protein type, including EB1, EB2, and EB3. They can precisely bind to the plus-end of microtubules and associate with the stabilizing GTP cap of microtubule growth (Nehlig et al., 2017). Likewise, Cytoplasmic Linker-Associated Proteins (CLASPs) are a conserved class of +TIPs proteins that contribute to microtubule stabilization (Lindeboom et al., 2019). A recent study proposed the rescue mechanism of CLASPs. They form a complex with tubulin dimers and bind along the microtubule lattice resulting in reverse microtubule depolymerization (Aher et al., 2020).
Microtubule minus-end binding proteins
Various MAPs play a crucial role in regulating microtubule dynamics at their ends, including calmodulin-regulated spectrin-associated proteins (CAMSAPs), a recently described microtubule minus-end binding proteins (Hendershott and Vale, 2014). In mammals, CAMSAP family members consist of CAMSAP1, CAMSAP2, and CAMSAP3. They potentially regulate the stability and localization of microtubule minus-ends and thus organize non-centrosomal microtubule networks, sufficient for cell division, migration, and polarity (Akhmanova and Hoogenraad, 2015; Atherton et al., 2017). In mammals, CAMSAP1 is a growing minus-end tracking protein. CAMSAP2 and CAMSAP3 promote microtubule minus-end polymerization, which initiates the stable microtubule stretches by serving as a seed for non-centrosomal microtubule outgrowth (Akhmanova and Hoogenraad, 2015). These two CAMSAPs form stretches of deposited microtubule lattice, which is highly stable, and prevent microtubule depolymerization from both ends (Atherton et al., 2017). CAMSAP2 and CAMSAP3 are mostly reported concerning microtubule dynamics and organization, providing fundamental anti-cancer drug research and development strategies.
Microtubule-associated proteins and their role in cancer metastasis
Cancer metastasis is characterized by multi-step processes in which cancer cells disseminate from their tissue of origin, leading to secondary tumor formation in other organs (Fares et al., 2020) (Figure 2). After primary tumor tissue is formed, an imbalance of nutrients supporting tumor growth mediates vascularization in terms of angiogenesis, facilitating cancer cell detachment, migration, and invasion into nearby blood vessels. In these new environments with growth factor enrichment, cancer cells extravasate and continue growing, completing secondary tumor establishment. In the metastasis process, the reorganization of microtubules through their dynamic properties occurs to support morphological changes associated with cell movement (Fife et al., 2014). Besides the direct functions of microtubules, they also serve as tracking trails for the translocation of metastasis-related signaling molecules to the leading and/or rear edges of cells (Kriebel et al., 2008; Garcin and Straube, 2019). Multiple signaling mechanisms govern these complex steps, which are potential therapeutic targets. Since several MAPs reportedly show differential expression in cancer cells compared to that in normal cells, they likely contribute to cell migration and invasion. Our recent knowledge of MAPs in cancer metastasis has been updated and discussed in (Table 1).
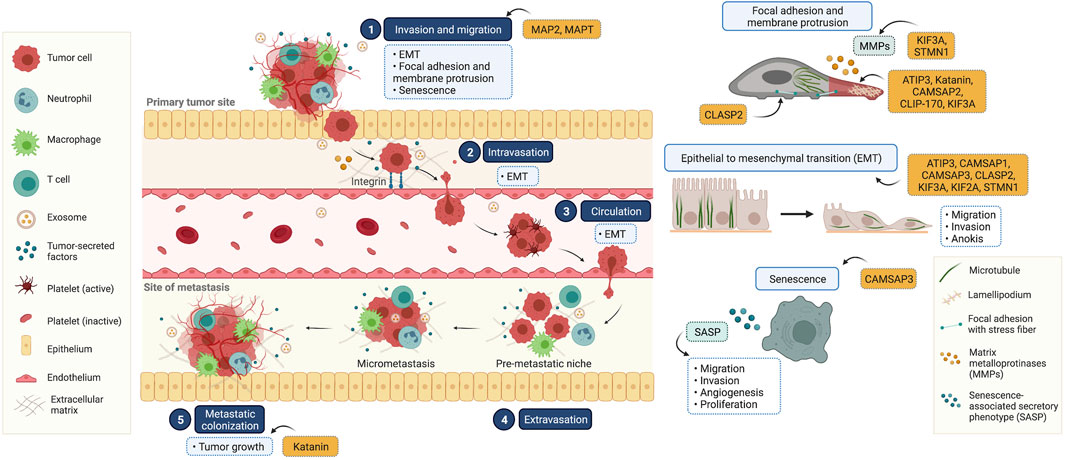
FIGURE 2. Illustrated overview of the tumor metastatic process and the participation of microtubule-associated proteins (MAPs) in this process. (1) Invasion and migration: cancer cells invade and migrate through the basement membrane and tumor stroma, respectively. This process is involved in epithelial to mesenchymal transition (EMT), focal adhesion and membrane protrusion, and senescence. (2) Intravasation: tumor cells collide with the endothelial membrane and attach firmly via integrin (cell-to-cell adhesion molecules). Subsequently, cancer cells intravasate into the surrounding vasculature or lymphatic system. (3) Circulation: circulating tumor cells survive in the circulating blood and have the ability to evade the immune clearance system. (4) Extravasation: once cancer cells reach their secondary targets, they enter through the endothelial barrier and form a premetastatic niche for tumor cell growth and survival. (5) Colonization: the premetastatic niches recruit several tumor-secreted factors, and the extracellular matrix is remodulated at these sites in a phenomenon known as micrometastasis. In the final step, micrometastasis achieves colonization at the distant organ and secondary tumors are established. This diagram was created with “Biorender.com”.
Migration and invasion
Cancer migration and invasion involve motile activity and extracellular matrix degradation. The microtubule dynamics participate in cell movement, and interference with these behaviors affects cancer migration and invasion. MAPT is abundant in neuronal axons and stabilizes microtubules by regulating microtubule polymerization and dynamic behaviors (Barbier et al., 2019). Its function has been extensively investigated in neuronal development and physiology. In neuronal-associated cancer, MAPT overexpression was tightly correlated with an increased overall survival rate in glioblastoma, being considered a tumor suppressor (Zaman et al., 2019). Consistent with renal cell carcinoma, low MAPT expression is associated with a poor overall survival rate, and MAPT RNA interference promotes cancer invasion (Han et al., 2020). Similarly, MAP2 has been identified as a microtubule stabilizer, locally restricted to neuronal dendrites (Harada et al., 2002). Due to its differential expression among neuronal epithelial tumor subtypes and limited expression in neurons, it is a powerful tumor marker (Blümcke et al., 2004; Yi et al., 2018). Among non-neuronal cancers, MAP2 upregulation, compared to adjacent normal tissue, was identified in gastric adenocarcinoma (Zheng et al., 2014). This remarkable upregulation was strongly associated with cancer invasion and lymph node metastasis. Consistently, MAP2 knockdown was shown to impair cancer cell migration (Yang et al., 2020). Contrastingly, in malignant melanoma, MAP2 was rarely expressed in metastasis tumors compared with its expression in primary melanoma (Song et al., 2010). The introduction of MAP2 into metastatic cancer cells interfered with microtubule assembly, leading to the in vitro suppression of cell migration and invasion. This suggests that MAP2 expression shows cancer-type specificity. However, the molecular mechanism behind the regulation of cancer metastasis by MAP2 is largely unknown.
As a microtubule dynamic modulator, ATIP3, a microtubule lattice binding protein, regulates breast cancer cell migration through stabilizing microtubules (Molina et al., 2013). Low expression of ATIP3 was correlated with low survival rate and lymph node metastasis in breast cancer patients (Rodrigues-Ferreira et al., 2020). Mechanically, ATIP3 deficiency enhanced microtubule dynamic at the front and rear of the cells, facilitating cell motility. Furthermore, the alteration of microtubule dynamics due to ATIP3 knockdown increased an intracellular accumulation of taxol along microtubules, thereby exerting sensitivity to taxol. This suggests that ATIP3 is a potential target for cancer therapy.
Katanin, a microtubule-severing enzyme, is a heterodimer of P60 and P80 subunits (Ghosh et al., 2012). It plays a fundamental role in regulating microtubule organization, affecting several cellular activities (Zehr et al., 2017). Recent studies have identified the role of katanin in various cancers. Clinical investigations have demonstrated that katanin expression is abundant in cancers, especially in advanced stages (Ye et al., 2012; Zuo et al., 2018). Lung cancer patients with high katanin expression show a poor survival profile, rendering katanin expression as a biomarker for predicting cancer pathogenesis (Wang L. et al., 2020). Katanin upregulation potentially enhanced breast cancer cell migration; conversely, katanin knockdown attenuated this behavior (Fu et al., 2018). The association between microtubule destabilization and cancer metastasis has not been fully elucidated. The exact mechanisms of metastasis regulation by katanin, besides its microtubule-severing properties, are unknown yet.
The translocation of organelles and molecules serves most cell activities, including cell migration. The trafficking of signaling molecules to membrane protrusions is necessary to generate focal adhesions (FAs) to new attachments, followed by organelle movement and cell body contraction, which are required for proper cell motility (Fourriere et al., 2019; Zinn et al., 2019). The coordination of microtubules and their binding proteins plays a crucial role in these events. CLIP-170, a +TIP MAP, is widely known to regulate cellular trafficking through microtubules (Chen Y. et al., 2019). Likewise, CLIP-170 has been reported to regulate the recycling of Met receptor tyrosine kinase (Met RTK) (Zaoui et al., 2019). An internalization of Met RTK is essential for RTK degradation and recycling to the cell surface (Barrow-McGee and Kermorgant, 2014). Disturbance to these processes affected its stability and contributed to tumorigenesis (Xiang et al., 2017). CLIP-170 is required for the endocytic trafficking of Met RTK on microtubules and relocated to the plasma membrane, where lamellipodia, flat sheet-like membrane protrusions, are formed, thereby enhancing cell motility. This study demonstrated the linkage between microtubules, MAP, and endocytic vesicles, which contributes to cancer cell aggressiveness. Nevertheless, the regulation of cellular transport by CLIP-170 was generally observed in both normal and cancer cells. Its specific functions in cancer and expression profile from clinical specimens are being further clarified. The scientific information is currently restricted to support it as a therapeutic target.
Apart from that, CLASP2, a microtubule plus-end binding protein, participates in several microtubule-associated processes (Lindeboom et al., 2019). Given its close relationship with microtubules, CLASP2 reportedly regulated FA turnover, supporting cell migration (Stehbens et al., 2014). It couples with microtubules for transportation of FA components which are required for FA formation and extracellular matrix degradation. CLASP2 expression was significantly increased in muscle-invasive bladder urothelial cancer tissues, particularly in tissues with lymph node metastasis (Chen L. et al., 2019). Its high expression was strongly associated with a poor prognosis. The introduction of exogenous CLASP2 in bladder cancer cell lines exerted in vitro cell migration and invasion (Zhu et al., 2017).
Matrix metalloproteinase (MMP) 2 and 9 play an important role in cancer invasion through their extracellular matrix enzymatic degradation. KIF3A was identified as a diagnostic parameter for breast, prostate, and bladder cancers (Liu et al., 2014; Xia et al., 2018; Zhou et al., 2021). It was overexpressed in breast cancer tissues compared to adjacent samples, being particularly associated with lymph node metastasis (Wang W. et al., 2020). RNA interference to KIF3A decreased MMP2 and MMP9 expression, suggesting that KIF3A was required for breast cancer metastasis (Wang L. et al., 2020). KIF3A knockdown in triple-negative breast cancer cell lines significantly attenuated in vitro cancer migration and invasion and in vivo metastasis (Wang W. et al., 2020). In prostate cancer, KIF3A silencing enhanced cancer migration and invasion through Wnt/β-catenin signaling (Liu et al., 2014). The upregulation of KIF3A activates this pathway by increasing DVL2 phosphorylation and β-catenin levels, promoting MMP9 and HEF1 expression and inducing cancer invasion and migration (Liu et al., 2014). Apart from direct regulation of signaling, KIF3A was shown to interact with APC and β-catenin for intracellular transport via microtubules to the membrane protrusions to exert cell migration (Jimbo et al., 2002).
Besides, proteomic analyses identified several differentially expressed proteins after STMN1 silencing. STMN1 contributes to cancer metastasis, and STMN1 RNA interference significantly inhibits lung cancer cell migration and invasion (Xun et al., 2021). STMN1 knockdown upregulated MMP1 and MMP9, responsible for cancer invasion (Shu et al., 2019). Clusterin, a glycoprotein encoded by the CLU gene, participates in EIF3I/AKT/MMP13 signaling, which facilitates cancer metastasis (Wang et al., 2015). Clusterin was downregulated in response to STMN1 suppression. Furthermore, phosphorylated AKT and STAT were gradually reduced, suggesting that the oncogenic role of STMN1 in cancer metastasis was mediated by clusterin/AKT/MMP and STAT signaling (Shu et al., 2019). Further, STMN1 negatively correlated to tumor suppressor phosphatase and tensin homolog deleted on chromosome 10 (PTEN). High STMN1 expression was detected in lung cancer with low PTEN expression (Xun et al., 2021). PTEN, classified as a tumor suppressor, is a crucial inhibitor of the phosphatidylinositol three kinase (PI3K)/AKT pathway through its phosphatase activity (Milella et al., 2015). Furthermore, PTEN loss enhanced STMN1 upregulation and ameliorated STMN-mediated cancer migration and invasion, indicating that PTEN is an upstream regulator of STMN1. These findings highlighted the interplay between STMN1 and PI3K/AKT pathways. Since cancer metastasis involves complex processes, in which STMN1 has multiple metastasis-regulating targets, STMN1 is a potential target for cancer therapy.
Interestingly, a family of non-centrosomal microtubule minus-end binding proteins, CAMSAPs, was recently identified to govern cancer metastasis. CAMSAPs are consisting of CAMSAP1, CAMSAP2, and CAMSAP3 (Hendershott and Vale, 2014). In the past decade, CAMSAPs have been found to govern neuronal development and epithelial tissue organization (Tanaka et al., 2012; Toya et al., 2016; Pongrakhananon et al., 2018a). However, their functions in cancer remain elusive. Since cancer metastasis is associated with morphological alterations to enhance cell motility, and CAMSAPs control epithelial tissue morphology, it has been hypothesized that CAMSAPs may play a significant role in cancer metastasis. Gene expression analysis obtained from tumor specimens demonstrated that CAMSAP1 mutations, mainly occurring in its CH and CKK domains, significantly correlated with better prognoses in small cell lung cancer, whereas CAMSAP1 expression was not relevant to overall survival rates (Yi et al., 2021). However, available evidence on CAMSAP1’s role in cancer metastasis remains incomplete. In contrast, according to The Cancer Genome Atlas database, significant CAMSAP2 upregulation correlated with poor overall survival rates in several malignancies, including lung squamous cell carcinoma, colon adenocarcinoma, esophageal carcinoma, and pancreatic adenocarcinoma (Chandrashekar et al., 2017). Clinical investigations reported that CAMSAP2 was abundantly expressed in hepatocellular carcinoma and associated with metastasis (Li et al., 2020). The loss of CAMSAP2, as a microtubule stabilizer, caused a transformation of microtubule patterns to radial centrosomal microtubules, whose post-translational acetylation was remarkably reduced. This phenomenon attenuated cancer cell migration and invasion, consistent with a previous study that tubulin acetylation is essential for cell motility (Boggs et al., 2015). Its underlying mechanism was further revealed, whereby CAMSAP2 suppressed histone deacetylase 6 (HDAC6), an enzyme responsible for deacetylation, through a Rac/JNK pathway. Therefore, CAMSAP2 is considered a metastasis promoter. How CAMSAP family members, which share similar conserved regions, govern cancer differently grants further studies.
Epithelial to mesenchymal transition
The transformation of epithelial to mesenchymal phenotypes plays an essential role in cancer metastasis. A tight organization of epithelial cells with high intercellular adhesion forces alters mesenchymal-like cells with low cell-cell interaction (Datta et al., 2021). The reorganization of the cytoskeleton, including microtubules, contributes to morphological changes to the spindle-like phenotype, facilitating the metastatic ability of cancer. Several MAPs were reported to govern microtubule behaviors and the transportation of signaling molecules involved in the epithelial to mesenchymal transition (EMT) process. In light of the emerging biology of microtubule motor proteins, KIFs play an essential role in the cellular trafficking of components or molecules along microtubules (Hirokawa et al., 2009). Silencing of KIF3A demonstrated that the epithelial to mesenchymal transition was strongly suppressed by the downregulation of a transcription factor, ZEB1, and vimentin, as opposed to the upregulation of the epithelial marker E-cadherin, thereby inhibiting cancer metastasis (Wang L. et al., 2020). However, the molecular mechanism for regulating EMT marker expression by KIF3A remains undiscovered.
Similarly, KIF14 was reported as a potential predictive factor for poor prognosis. It was highly expressed in several cancers including retinoblastoma, ovarian, breast, and gastric cancers (Madhavan et al., 2007; Thériault et al., 2012; Yang et al., 2019; Gerashchenko et al., 2020). Expression analyses from clinical specimens demonstrated a strong correlation between KIF14 expression levels and clinicopathological characteristics, with high expression levels found in metastasis samples (Yang et al., 2019). KIF14 silencing in gastric cancer cells inhibited cell migration and invasion by suppressing the phosphorylation of protein kinase B (AKT), a key mediator for cancer metastasis (Yang et al., 2019). Consistently, there is a molecular mechanism of KIF2A in carcinogenesis (Chen et al., 2022). However, the underlying mechanism of how KIFs control various signaling pathways requires further clarification through motor or other functions.
Stathmin (STMN1), a microtubule destabilizing protein, has gained more attention in cancer pathogenesis in the past decade. Its aberrant overexpression is extensively correlated with poor clinical outcomes in multiple cancers, being particularly associated with metastasis (Liu et al., 2015; Nie et al., 2015; Wu et al., 2018; Leiphrakpam et al., 2021). Based on the classical function, STMN1 mediated microtubule disruption and led to cellular dysfunction (Lu et al., 2014; Zhao et al., 2019). STMN1 reportedly interfered with microtubule stability and mediate EMT (Lu et al., 2014).
Microtubule instability caused by the knockdown of microtubule stabilizer ATIP3 increased an EMT feature (Zhao et al., 2015). ATIP3 restoration could downregulate vimentin and slug and upregulate epithelial E-cadherin marker. Besides affecting microtubule dynamics, ATIP3 functions as a tumor suppressor protein participating in cancer signaling. ATIP3 silencing enhanced extracellular signal-regulated kinase (ERK) phosphorylation, consequently upregulating its downstream target snail and vimentin that promoted the EMT process (Zhao et al., 2016). However, the exact mechanism of how ATIP3 regulates ERK activity was largely unidentified.
The regulation of EMT marker by a microtubule plus-end binding protein was also reported. CLASP2 overexpression mediated the upregulation of vimentin, a mesenchymal marker (Zhu et al., 2017). In contrast, its knockdown resulted in the upregulation of E-cadherin, an epithelial marker. However, the mechanism whereby CLASP2 promotes EMT remained unknown. CLASP2 may govern the nuclear translocation of EMT regulatory transcription factors, which requires further studies. Additionally, the information on CLASP2 was limited only to bladder cancer, and investigations in other malignancies could provide benefits for clinical applications.
Besides, a non-centrosomal microtubule minus-end binding protein CAMSAP3 plays a distinct suppressive role on cancer metastasis, unlike other CAMSAPs. Our group demonstrated that CAMSAP3 knockout promoted lung cancer cell migration in an AKT-dependent mechanism (Pongrakhananon et al., 2018b). As a fundamental role, CAMSAP3 maintains microtubule stability, and CAMSAP3 depletion contributes to microtubule hyperacetylation, facilitating cancer migration. The association between microtubule acetylation and oncogenic signaling was further identified. In the absence of CAMSAP3, AKT phosphorylation became more stabilized on microtubules, especially acetylated ones. Since AKT signaling is a key driver for EMT, increases in AKT phosphorylation in response to CAMSAP3 knockout mediated cell migration. However, the mechanism of CAMSAP3’s effect on tubulin acetylation requires further verification.
Senescence
Generally, cellular senescence is a process involving potentially irreversible growth arrest (Muñoz-Espín and Serrano, 2014). It has been reported that senescence is a therapeutic target for anti-cancer drugs to suppress tumor growth and enhance immune filtration, suggesting a tumor suppressive activity (Bian et al., 2020; Fitsiou et al., 2022). However, senescence plays a dual role in cancers that also acts as an intermediate stage, and secreted senescence-associated secretory phenotypes (SASPs) that were required for cancer metastasis (Capparelli et al., 2012; Guccini et al., 2021). During senescence, microtubule stability appeared to increase since senescence affects both structural and functional changes (Moujaber et al., 2019). The impact of MAPs on senescence was recently reported. CAMSAP3 played a significant role in cellular senescence (Wattanathamsan et al., 2021). CAMSAP3 loss mediated in vitro and in vivo senescence-like phenotypes and increased SASP expression by suppressing ERK (Wattanathamsan et al., 2021). Besides the involvement of microtubule stabilization, proteomic analysis revealed that vimentin is essential for CAMSAP3-regulated ERK signaling. These findings have shed light on the molecular mechanism of MAPs in cancer senescence. However, an interplay of MAPs in the regulation of microtubule dynamic during senescence was not fully identified.
Tumor growth and tissue colonization
To complete metastasis, the tumor colonization was established at a distant site in which several growth activators were enriched, as opposed to growth suppressors being attenuated. A tumor suppressor p53 is a key DNA damage sensor preventing uncontrolled cell proliferation. The association of p53 and MAPs in the regulation of cancer growth was reported. p53 binds to the KATNA1 promoter and positively regulates katanin transcription in human colorectal carcinoma cells. Furthermore, this activity is more pronounced under hypoxic conditions (Kirimtay et al., 2020). In the absence of p53, katanin expression is strongly reduced and microtubule organization is disturbed, suggesting a linkage between hypoxia and microtubule stability. Katanin p60 was shown to regulate the spindle pole during cytokinesis and its inhibition caused an incomplete cell division (Matsuo et al., 2013). Nevertheless, it is controversial that clinical observations indicated a function of katanin as a tumor promoter, but the regulation of p53 on katanin transcription provided an opposite activity. It has been reported that katanin overexpression inhibited cell growth but promoted metastasis (Fu et al., 2018). It possibly depended on the dominant regulators facilitating transcription or post-translation of katanin under specific circumstances and the cellular responsiveness to the tumor microenvironment.
Conclusion and perspectives
Cancer is a life-threatening disease, mainly caused by its ability to metastasize. The eradication of metastatic cancer cells remains a challenge in anti-cancer drug research and discovery. Due to its complex process, incorporating various signaling pathways, which are largely unidentified, several attempts have been made to identify key players as potential therapeutic targets. Microtubules are fundamental cytoskeleton components, participating in various cellular activities, including supporting cell morphology, chromosome segregation, cell motility, and trafficking. Although microtubules are found in both normal and malignant cells, some differences have been identified in microtubule behavior, dynamics, and post-translational modifications (Wattanathamsan and Pongrakhananon, 2021), which MAPs mediate. The biological role of MAPs has gained increasing interest. Importantly, several MAPs have expression and/or mutation profiles distinctive from normal tissue, rendering them value as potential predictive markers.
Based on the classical function of microtubules, some MAPs regulate cancer metastasis through microtubule reorganization and/or microtubule dynamic alterations. For example, microtubule disruption by microtubule-severing proteins can disturb cell migratory activity. The enhancement of microtubule stability or post-translational modifications can alter the trafficking of signals related to metastasis. Besides, emerging evidence has shed light on the direct mechanism whereby MAPs are key drivers regulating metastasis. Many MAPs have direct effects on signaling pathways independently from their microtubule association, likely by altering the expression of signaling proteins. At present, little is known about the molecular mechanism of MAPs, and their further identification might provide us with predictive/prognostic markers and/or therapeutic targets, thereby improving clinical outcomes.
Recently, MAPs have been discovered as biomarkers for drug sensitivity of metastasis tumors, particularly taxane-based chemotherapy. Transcriptomic analysis from breast cancer patients treated with taxanes showed that some of the genes encoding MAPs were differently expressed, and one of the interesting genes was MTSU1, which encodes ATIP3. The expression of ATIP3 was decreased in taxane-sensitive breast tumors (Rodrigues-Ferreira et al., 2019), and downregulation of MTSU1 was associated with low lymph node metastasis after obtaining taxane-based chemotherapy (Rodrigues-Ferreira et al., 2020). MAPT was also downregulated in ovarian cancer patients who had well responsive to paclitaxel (Smoter et al., 2013). Besides, breast cancer patients with a lower level of STMN1 displayed a higher sensitivity to docetaxel-containing chemotherapy (Meng et al., 2012). These studies provide intriguing biomarkers for predictive chemotherapy responsiveness, and substantial experiments for validation may contribute to a therapeutic approach for restoring the resistance to taxane-based chemotherapy, facilitating the translation of research to clinical application.
Currently, drugs targeting MAPs are under the drug design process, while some are in clinical trials. One upcoming strategy is to interact with and suppress MAP’s function that regulates microtubules participating in cancer cell division. The quinazolinone-based kinesin inhibitors were in clinical phases 1-2. Mode of action is involved with the inhibition of kinesin binding to microtubules, thereby suppressing mitotic spindle assembly and mediating cell cycle arrest (Matsuno et al., 2008). Ispinesib mesylate selectively inhibits kinesin spindle protein (KSP) which is under phase II clinical trial for breast cancer treatment (Khwaja et al., 2021). The other KSP inhibitors, such as MK-0731, SB743921, and ARRY-520, are under clinical trial in solid tumors (Holen et al., 2012; Khoury et al., 2012; Khwaja et al., 2021). The second strategy is to target MAPs associated with microtubule stability. Since taxane- and vinca alkaloid-based drugs that bind to and interfere with microtubule stabilization have low clinical outcomes due to drug resistance after prolonged regimens, MAPs regulating microtubule stability become attractive targets in drug discovery. GRC0321, a purine-based compound, was sought to be a katanin-targeting agent, which showed a potent apoptosis-inducing effect in lung cancer cells (Kuo et al., 2016). These purine-type agents directly target katanin via stabilizing the katanin p60 hexamer and inducing its microtubule-severing activity. Recently, compound 20b was identified targeting katanin. Compound 20b prevented cancer cell proliferation by interacting with the katanin in non-small cell lung cancer (Gao et al., 2019). As an aberrant expression in cancers, the target at katanin may provide an advantage in the aspect of selectivity to cancer with a lower effect on normal cells. However, this concept requires verification.
Another drug design and development strategy is hybrid drugs that have multiple modes of action such as disrupting microtubule dynamics in combination with another anti-cancer mechanism. This strategy is a rationally attractive approach to enhance efficacy and reduce severe toxicity, in which hybrid drugs are a combination of at least two pharmacophores with covalent linkage in a single molecule to cooperate with multiple targets. Hybridized microtubule targeting drugs were developed including combretastatin hybrids, vinca alkaloid hybrids, and a-noscapine hybrids (Gherbovet et al., 2014; Vilanova et al., 2014; Mahaddalkar et al., 2016; Devi Tangutur et al., 2017). Although this strategy first came up for interrupting microtubule dynamics, this concept provides a more expandable approach for drug design targeting MAPs. Recently, arylaldoxime/5-nitroimidazole hybrids were synthesized to inhibit microtubule affinity-regulatory kinase 4 (MARK4), along with exerting anti-oxidant activity (Peerzada et al., 2020). MARK4 belongs to the serine/threonine kinases family that is required for phosphorylating MAPs, including MAPT and MAP2, thereby affecting the microtubule dynamics (Trinczek et al., 2004). As MARK4 promotes cancer progression (Heidary Arash et al., 2017) and oxidative stress associated with cancer pathogenesis (Zahra et al., 2021), the molecular hybridization of this molecule provided a significant anti-cancer activity. However, the anti-metastatic activity of these agents requires further investigation. Hence, we can at least infer that MAPs are promising therapeutic targets for anti-cancer drug discovery and development.
Microtubule post-translational modifications (PTMs) are implicated as a mechanism underlying the MAP-mediated regulation of cancer behaviors. Generally, microtubule PTMs are maintained in a balanced state and play a role in cellular activity, in which they are regulated by several enzymes. One such enzyme is histone deacetylase 6 (HDAC6), which is localized predominantly in the cytoplasm and controls nonhistone acetylation, such as that of α-tubulin (Li C. et al., 2018). High expression levels of HDAC6 have been detected in numerous types of cancer and such expression is strongly associated with a poor overall survival rate (Zhang et al., 2017; Li T. et al., 2018). HDAC6 regulates essential biological processes in cancer through α-tubulin deacetylation (Li C. et al., 2018). Additionally, HDAC6 was reported to participate with MAPs, including CLIP-170 and CAMSAP2, in promoting cancer metastasis (Li et al., 2014; Li et al., 2020). HDAC6 inhibition is, therefore, one possible approach in cancer therapy. Several selective HDAC6 inhibitors are now the subject of clinical trials for cancer treatment (Pulya et al., 2021). Ricolinostat (ACY-1215) is well-recognized as monotherapy or can be used in combination with dexamethasone, bortezomib, or lenalidomide in refractory or relapsed multiple myeloma (Vogl et al., 2017). Citarinostat (ACY-241), the second-generation HDAC6 inhibitor, is an orally active HDAC6 inhibitor with improved potency (Niesvizky et al., 2015). Given that its pharmacophores have been identified, the selectivity and efficacy of HDAC6 inhibitors are of interest concerning further drug design and development.
Although the roles of MAPs have been explored in some respects, our scientific understanding of MAPs can still be improved and validated. Nevertheless, it is now clear that some MAPs, such as MAPT, ATIP3, and STMN1, as well as enzymes that regulate microtubule behaviors, such as HDAC6 and MARK4, are potentially important cancer therapeutic targets and/or predictive/prognostic biomarkers. Furthermore, the development of molecular hybrids as microtubule- or MAP-targeting agents is in progress, and such agents will improve drug efficacy and selectivity while reducing side effects. Overall, this review collects the recent important evidence that will help guide researchers conducting further research on MAPs in cancer.
Author contributions
OW and VP searched the literature; created the figures and tables; and drafted, edited, and revised the manuscript. All authors read and approved the final manuscript.
Funding
The Ratchadapiseksompotch Fund at Chulalongkorn University (Rein_65_03_33_17) supported this work.
Conflict of interest
The authors declare that the research was conducted in the absence of any commercial or financial relationships that could be construed as a potential conflict of interest.
Publisher’s note
All claims expressed in this article are solely those of the authors and do not necessarily represent those of their affiliated organizations, or those of the publisher, the editors and the reviewers. Any product that may be evaluated in this article, or claim that may be made by its manufacturer, is not guaranteed or endorsed by the publisher.
References
Aher, A., Rai, D., Schaedel, L., Gaillard, J., John, K., Liu, Q., et al. (2020). CLASP mediates microtubule repair by restricting lattice damage and regulating tubulin incorporation. Curr. Biol. 30, 2175–2183. doi:10.1016/j.cub.2020.03.070
Akhmanova, A., and Hoogenraad, C. C. (2015). Microtubule minus-end-targeting proteins. Curr. Biol. 25, R162–R171. doi:10.1016/j.cub.2014.12.027
Akhmanova, A., and Steinmetz, M. O. (2015). Control of microtubule organization and dynamics: Two ends in the limelight. Nat. Rev. Mol. Cell. Biol. 16, 711–726. doi:10.1038/nrm4084
Arora, K., Talje, L., Asenjo, A. B., Andersen, P., Atchia, K., Joshi, M., et al. (2014). KIF14 binds tightly to microtubules and adopts a rigor-like conformation. J. Mol. Biol. 426, 2997–3015. doi:10.1016/j.jmb.2014.05.030
Atherton, J., Jiang, K., Stangier, M. M., Luo, Y., Hua, S., Houben, K., et al. (2017). A structural model for microtubule minus-end recognition and protection by CAMSAP proteins. Nat. Struct. Mol. Biol. 24, 931–943. doi:10.1038/nsmb.3483
Barbier, P., Dorléans, A., Devred, F., Sanz, L., Allegro, D., Alfonso, C., et al. (2010). Stathmin and interfacial microtubule inhibitors recognize a naturally curved conformation of tubulin dimers. J. Biol. Chem. 285, 31672–31681. doi:10.1074/jbc.M110.141929
Barrow-McGee, R., and Kermorgant, S. (2014). Met endosomal signalling: In the right place, at the right time. Int. J. Biochem. Cell. Biol. 49, 69–74. doi:10.1016/j.biocel.2014.01.009
Bian, Y., Wei, J., Zhao, C., and Li, G. (2020). Natural polyphenols targeting senescence: A novel prevention and therapy strategy for cancer. Int. J. Mol. Sci. 21, 684. doi:10.3390/ijms21020684
Blümcke, I., Müller, S., Buslei, R., Riederer, B. M., and Wiestler, O. D. (2004). Microtubule-associated protein-2 immunoreactivity: A useful tool in the differential diagnosis of low-grade neuroepithelial tumors. Acta Neuropathol. 108, 89–96. doi:10.1007/s00401-004-0873-8
Bodakuntla, S., Jijumon, A. S., Villablanca, C., Gonzalez-Billault, C., and Janke, C. (2019). Microtubule-associated proteins: Structuring the cytoskeleton. Trends Cell. Biol. 29, 804–819. doi:10.1016/j.tcb.2019.07.004
Boggs, A. E., Vitolo, M. I., Whipple, R. A., Charpentier, M. S., Goloubeva, O. G., Ioffe, O. B., et al. (2015). α-Tubulin acetylation elevated in metastatic and basal-like breast cancer cells promotes microtentacle formation, adhesion, and invasive migration. Cancer Res. 75, 203–215. doi:10.1158/0008-5472.CAN-13-3563
Bowne-Anderson, H., Hibbel, A., and Howard, J. (2015). Regulation of microtubule growth and catastrophe: Unifying theory and experiment. Trends Cell. Biol. 25, 769–779. doi:10.1016/j.tcb.2015.08.009
Breuzard, G., Hubert, P., Nouar, R., De Bessa, T., Devred, F., Barbier, P., et al. (2013). Molecular mechanisms of Tau binding to microtubules and its role in microtubule dynamics in live cells. J. Cell. Sci. 126, 2810–2819. doi:10.1242/jcs.120832
Capparelli, C., Whitaker-Menezes, D., Guido, C., Balliet, R., Pestell, T. G., Howell, A., et al. (2012). CTGF drives autophagy, glycolysis and senescence in cancer-associated fibroblasts via HIF1 activation, metabolically promoting tumor growth. Cell. Cycle 11, 2272–2284. doi:10.4161/cc.20717
Chaaban, S., and Brouhard, G. J. (2017). A microtubule bestiary: Structural diversity in tubulin polymers. Mol. Biol. Cell. 28, 2924–2931. doi:10.1091/mbc.E16-05-0271
Chandrashekar, D. S., Bashel, B., Balasubramanya, S. A. H., Creighton, C. J., Ponce-Rodriguez, I., Chakravarthi, B. V., et al. (2017). Ualcan: A portal for facilitating tumor subgroup gene expression and survival analyses. Neoplasia 19, 649–658. doi:10.1016/j.neo.2017.05.002
Chen, J., Wen, J., Di Liu, X. X., Fan, M., and Zhang, Z. (2022). The molecular mechanism of kinesin family member 2A (KIF2A) underlying non-small cell lung cancer: The effect of its knockdown on malignant behaviors, stemness, chemosensitivity, and potential regulated signaling pathways. Am. J. Transl. Res. 14, 68–85. 1943-8141/AJTR0124034.
Chen, L., Xiong, W., Guo, W., Su, S., Qi, L., Zhu, B., et al. (2019). Significance of CLASP2 expression in prognosis for muscle-invasive bladder cancer patients: A propensity score-based analysis. Urol. Oncol. 37, 800–807. doi:10.1016/j.urolonc.2019.05.003
Chen, Y., Wang, P., and Slep, K. C. (2019). Mapping multivalency in the CLIP-170-EB1 microtubule plus-end complex. J. Biol. Chem. 294, 918–931. doi:10.1074/jbc.RA118.006125
Chowdhury, S., Ketcham, S. A., Schroer, T. A., and Lander, G. C. (2015). Structural organization of the dynein-dynactin complex bound to microtubules. Nat. Struct. Mol. Biol. 22, 345–347. doi:10.1038/nsmb.2996
Cross, R. A., and McAinsh, A. (2014). Prime movers: The mechanochemistry of mitotic kinesins. Nat. Rev. Mol. Cell. Biol. 15, 257–271. doi:10.1038/nrm3768
Cross, R. A. (2019). Microtubule lattice plasticity. Curr. Opin. Cell. Biol. 56, 88–93. doi:10.1016/j.ceb.2018.10.004
Datta, A., Deng, S., Gopal, V., Yap, K. C. H., Halim, C. E., Lye, M. L., et al. (2021). Cytoskeletal dynamics in epithelial-mesenchymal transition: Insights into therapeutic targets for cancer metastasis. Cancers 13, 1882. doi:10.3390/cancers13081882
Devi Tangutur, A., Kumar, D., Vamsi Krishna, K., and Kantevari, S. (2017). Microtubule targeting agents as cancer chemotherapeutics: An overview of molecular hybrids as stabilizing and destabilizing agents. Curr. Top. Med. Chem. 17, 2523–2537. doi:10.2174/1568026617666170104145640
Dixit, R., Barnett, B., Lazarus, J. E., Tokito, M., Goldman, Y. E., and Holzbaur, E. L. (2009). Microtubule plus-end tracking by CLIP-170 requires EB1. Proc. Natl. Acad. Sci. U. S. A. 106, 492–497. doi:10.1073/pnas.0807614106
Etienne-Manneville, S. (2013). Microtubules in cell migration. Annu. Rev. Cell. Dev. Biol. 29, 471–499. doi:10.1146/annurev-cellbio-101011-155711
Fares, J., Fares, M. Y., Khachfe, H. H., Salhab, H. A., and Fares, Y. (2020). Molecular principles of metastasis: A hallmark of cancer revisited. Signal Transduct. Target. Ther. 5, 28–17. doi:10.1038/s41392-020-0134-x
Fife, C. M., McCarroll, J. A., and Kavallaris, M. (2014). Movers and shakers: Cell cytoskeleton in cancer metastasis. Br. J. Pharmacol. 171, 5507–5523. doi:10.1111/bph.12704
Fitsiou, E., Soto-Gamez, A., and Demaria, M. (2022). Biological functions of therapy-induced senescence in cancer. Semin. Cancer Biol. 81, 5–13. doi:10.1016/j.semcancer.2021.03.021
Folker, E. S., Baker, B. M., and Goodson, H. V. (2005). Interactions between CLIP-170, tubulin, and microtubules: Implications for the mechanism of clip-170 plus-end tracking behavior. Mol. Biol. Cell. 16, 5373–5384. doi:10.1091/mbc.e04-12-1106
Fourriere, L., Kasri, A., Gareil, N., Bardin, S., Bousquet, H., Pereira, D., et al. (2019). RAB6 and microtubules restrict protein secretion to focal adhesions. J. Cell. Biol. 218, 2215–2231. doi:10.1083/jcb.201805002
Fu, W., Wu, H., Cheng, Z., Huang, S., and Rao, H. (2018). The role of katanin p60 in breast cancer bone metastasis. Oncol. Lett. 15, 4963–4969. doi:10.3892/ol.2018.7942
Galjart, N. (2010). Plus-end-tracking proteins and their interactions at microtubule ends. Curr. Biol. 20, R528–R537. doi:10.1016/j.cub.2010.05.022
Gao, F., Liang, Y., Zhou, P., Cheng, J., Ding, K., and Wang, Y. (2019). Design, synthesis, antitumor activities and biological studies of novel diaryl substituted fused heterocycles as dual ligands targeting tubulin and katanin. Eur. J. Med. Chem. 178, 177–194. doi:10.1016/j.ejmech.2019.05.072
Garcin, C., and Straube, A. (2019). Microtubules in cell migration. Essays Biochem. 63, 509–520. doi:10.1042/EBC20190016
Gerashchenko, T. S., Zolotaryova, S. Y., Kiselev, A. M., Tashireva, L. A., Novikov, N. M., Krakhmal, N. V., et al. (2020). The activity of KIF14, Mieap, and EZR in a new type of the invasive component, torpedo-like structures, predetermines the metastatic potential of breast cancer. Cancers 12, 1909. doi:10.3390/cancers12071909
Gherbovet, O., Coderch, C., Alvarez, M. C. G., Bignon, J., Thoret, S., Guéritte, F., et al. (2014). One-pot synthesis of Vinca alkaloids-phomopsin hybrids. J. Med. Chem. 57, 5470–5476. doi:10.1021/jm500530v
Ghosh, D. K., Dasgupta, D., and Guha, A. (2012). Models, regulations, and functions of microtubule severing by Katanin. ISRN Mol. Biol. 2012, 596289. doi:10.5402/2012/596289
Gigant, B., Wang, W., Dreier, B., Jiang, Q., Pecqueur, L., Plückthun, A., et al. (2013). Structure of a kinesin-tubulin complex and implications for kinesin motility. Nat. Struct. Mol. Biol. 20, 1001–1007. doi:10.1038/nsmb.2624
Goodson, H. V., and Jonasson, E. M. (2018). Microtubules and microtubule-associated proteins. Cold Spring Harb. Perspect. Biol. 10, a022608. doi:10.1101/cshperspect.a022608
Guccini, I., Revandkar, A., D'Ambrosio, M., Colucci, M., Pasquini, E., Mosole, S., et al. (2021). Senescence reprogramming by TIMP1 deficiency promotes prostate cancer metastasis. Cancer Cell. 39, 68–82.e9. doi:10.1016/j.ccell.2020.10.012
Gupta, K. K., Li, C., Duan, A., Alberico, E. O., Kim, O. V., Alber, M. S., et al. (2013). Mechanism for the catastrophe-promoting activity of the microtubule destabilizer Op18/stathmin. Proc. Natl. Acad. Sci. U. S. A. 110, 20449–20454. doi:10.1073/pnas.1309958110
Han, X., Sekino, Y., Babasaki, T., Goto, K., Inoue, S., Hayashi, T., et al. (2020). Microtubule-associated protein tau (MAPT) is a promising independent prognostic marker and tumor suppressive protein in clear cell renal cell carcinoma. Urol. Oncol. 38, 9–605. doi:10.1016/j.urolonc.2020.02.010
Harada, A., Teng, J., Takei, Y., Oguchi, K., and Hirokawa, N. (2002). MAP2 is required for dendrite elongation, PKA anchoring in dendrites, and proper PKA signal transduction. J. Cell. Biol. 158, 541–549. doi:10.1083/jcb.200110134
Heidary Arash, E., Shiban, A., Song, S., and Attisano, L. (2017). MARK4 inhibits Hippo signaling to promote proliferation and migration of breast cancer cells. EMBO Rep. 18, 420–436. doi:10.15252/embr.201642455
Hendershott, M. C., and Vale, R. D. (2014). Regulation of microtubule minus-end dynamics by CAMSAPs and Patronin. Proc. Natl. Acad. Sci. U. S. A. 111, 5860–5865. doi:10.1073/pnas.1404133111
Hirokawa, N., Noda, Y., Tanaka, Y., and Niwa, S. (2009). Kinesin superfamily motor proteins and intracellular transport. Nat. Rev. Mol. Cell. Biol. 10, 682–696. doi:10.1038/nrm2774
Holen, K., DiPaola, R., Liu, G., Tan, A. R., Wilding, G., Hsu, K., et al. (2012). A phase I trial of MK-0731, a kinesin spindle protein (KSP) inhibitor, in patients with solid tumors. Invest. New Drugs 30, 1088–1095. doi:10.1007/s10637-011-9653-1
Hookway, C., Ding, L., Davidson, M. W., Rappoport, J. Z., Danuser, G., and Gelfand, V. I. (2015). Microtubule-dependent transport and dynamics of vimentin intermediate filaments. Mol. Biol. Cell. 26, 1675–1686. doi:10.1091/mbc.E14-09-1398
Jimbo, T., Kawasaki, Y., Koyama, R., Sato, R., Takada, S., Haraguchi, K., et al. (2002). Identification of a link between the tumour suppressor APC and the kinesin superfamily. Nat. Cell. Biol. 4, 323–327. doi:10.1038/ncb779
Kevenaar, J. T., Bianchi, S., Van Spronsen, M., Olieric, N., Lipka, J., Frias, C. P., et al. (2016). Kinesin-binding protein controls microtubule dynamics and cargo trafficking by regulating kinesin motor activity. Curr. Biol. 26, 849–861. doi:10.1016/j.cub.2016.01.048
Khoury, H. J., Garcia‐Manero, G., Borthakur, G., Kadia, T., Foudray, M. C., Arellano, M., et al. (2012). A phase 1 dose‐escalation study of ARRY‐520, a kinesin spindle protein inhibitor, in patients with advanced myeloid leukemias. Cancer 118, 3556–3564. doi:10.1002/cncr.26664
Khwaja, S., Kumar, K., Das, R., and Negi, A. S. (2021). Microtubule associated proteins as targets for anticancer drug development. Bioorg. Chem. 116, 105320. doi:10.1016/j.bioorg.2021.105320
Kirimtay, K., Selçuk, E., Kelle, D., Erman, B., and Karabay, A. (2020). p53 regulates katanin-p60 promoter in HCT 116 cells. Gene 727, 144241. doi:10.1016/j.gene.2019.144241
Kriebel, P. W., Barr, V. A., Rericha, E. C., Zhang, G., and Parent, C. A. (2008). Collective cell migration requires vesicular trafficking for chemoattractant delivery at the trailing edge. J. Cell. Biol. 183, 949–961. doi:10.1083/jcb.200808105
Kuo, T. C., Li, L. W., Pan, S. H., Fang, J. M., Liu, J. H., Cheng, T. J., et al. (2016). Purine-type compounds induce microtubule fragmentation and lung cancer cell death through interaction with katanin. J. Med. Chem. 59, 8521–8534. doi:10.1021/acs.jmedchem.6b00797
Leiphrakpam, P. D., Lazenby, A. J., Smith, L. M., Brattain, M. G., and Are, C. (2021). Stathmin expression in metastatic colorectal cancer. J. Surg. Oncol. 123, 1764–1772. doi:10.1002/jso.26464
Li, C., Cao, L., Xu, C., Liu, F., Xiang, G., Liu, X., et al. (2018). The immunohistochemical expression and potential prognostic value of HDAC6 and AR in invasive breast cancer. Hum. Pathol. 75, 16–25. doi:10.1016/j.humpath.2017.11.010
Li, D., Ding, X., Xie, M., Huang, Z., Han, P., Tian, D., et al. (2020). CAMSAP2-mediated noncentrosomal microtubule acetylation drives hepatocellular carcinoma metastasis. Theranostics 10, 3749–3766. doi:10.7150/thno.42596
Li, D., Sun, X., Zhang, L., Yan, B., Xie, S., Liu, R., et al. (2014). Histone deacetylase 6 and cytoplasmic linker protein 170 function together to regulate the motility of pancreatic cancer cells. Protein Cell. 5, 214–223. doi:10.1007/s13238-013-0010-3
Li, T., Zhang, C., Hassan, S., Liu, X., Song, F., Chen, K., et al. (2018). Histone deacetylase 6 in cancer. J. Hematol. Oncol. 11, 111. doi:10.1186/s13045-018-0654-9
Li, X., and Wang, J. (2020). Mechanical tumor microenvironment and transduction: Cytoskeleton mediates cancer cell invasion and metastasis. Int. J. Biol. Sci. 16, 2014–2028. doi:10.7150/ijbs.44943
Lindeboom, J. J., Nakamura, M., Saltini, M., Hibbel, A., Walia, A., Ketelaar, T., et al. (2019). CLASP stabilization of plus ends created by severing promotes microtubule creation and reorientation. J. Cell. Biol. 218, 190–205. doi:10.1083/jcb.201805047
Liu, X., Liu, H., Liang, J., Yin, B., Xiao, J., Li, J., et al. (2015). Stathmin is a potential molecular marker and target for the treatment of gastric cancer. Int. J. Clin. Exp. Med. 8, 6502–6509. 1940-5901/IJCEM0006281.
Liu, Z., Rebowe, R. E., Wang, Z., Li, Y., Wang, Z., DePaolo, J. S., et al. (2014). KIF3a promotes proliferation and invasion via Wnt signaling in advanced prostate cancer. Mol. Cancer Res. 12, 491–503. doi:10.1158/1541-7786.MCR-13-0418
Lu, W., and Gelfand, V. I. (2017). Moonlighting motors: Kinesin, dynein, and cell polarity. Trends Cell. Biol. 27, 505–514. doi:10.1016/j.tcb.2017.02.005
Lu, Y., Liu, C., Xu, Y. F., Cheng, H., Shi, S., Wu, C. T., et al. (2014). Stathmin destabilizing microtubule dynamics promotes malignant potential in cancer cells by epithelial-mesenchymal transition. Hepatobiliary Pancreat. Dis. Int. 13, 386–394. doi:10.1016/S1499-3872(14)60038-2
Madhavan, J., Coral, K., Mallikarjuna, K., Corson, T. W., Amit, N., Khetan, V., et al. (2007). High expression of KIF14 in retinoblastoma: Association with older age at diagnosis. Invest. Ophthalmol. Vis. Sci. 48, 4901–4906. doi:10.1167/iovs.07-0063
Mahaddalkar, T., Naik, P. K., Choudhary, S., Manchukonda, N., Kantevari, S., and Lopus, M. (2016). Structural investigations into the binding mode of a novel noscapine analogue, 9-(4-vinylphenyl) noscapine, with tubulin by biochemical analyses and molecular dynamic simulations. J. Biomol. Struct. Dyn. 31, 2475–2484. doi:10.1080/07391102.2016.1222969
Matov, A., Applegate, K., Kumar, P., Thoma, C., Krek, W., Danuser, G., et al. (2010). Analysis of microtubule dynamic instability using a plus-end growth marker. Nat. Methods 7, 761–768. doi:10.1038/nmeth.1493
Matsuno, K., Sawada, J. I., and Asai, A. (2008). Therapeutic potential of mitotic kinesin inhibitors in cancer. Expert Opin. Ther. Pat. 18, 253–274. doi:10.1517/13543776.18.3.253
Matsuo, M., Shimodaira, T., Kasama, T., Hata, Y., Echigo, A., Okabe, M., et al. (2013). Katanin p60 contributes to microtubule instability around the midbody and facilitates cytokinesis in rat cells. PLoS One 8, e80392. doi:10.1371/journal.pone.0080392
Meng, X. L., Su, D., Wang, L., Gao, Y., Hu, Y. J., Yang, H. J., et al. (2012). Low expression of stathmin in tumor predicts high response to neoadjuvant chemotherapy with docetaxel-containing regimens in locally advanced breast cancer. Genet. Test. Mol. Biomarkers 16, 689–694. doi:10.1089/gtmb.2011.0298
Milella, M., Falcone, I., Conciatori, F., Cesta Incani, U., Del Curatolo, A., Inzerilli, N., et al. (2015). Pten: Multiple functions in human malignant tumors. Front. Oncol. 5, 24. doi:10.3389/fonc.2015.00024
Molina, A., Velot, L., Ghouinem, L., Abdelkarim, M., Bouchet, B. P., Luissint, A. C., et al. (2013). ATIP3, a novel prognostic marker of breast cancer patient survival, limits cancer cell migration and slows metastatic progression by regulating microtubule dynamics. Cancer Res. 73, 2905–2915. doi:10.1158/0008-5472.CAN-12-3565
Moujaber, O., Fishbein, F., Omran, N., Liang, Y., Colmegna, I., Presley, J. F., et al. (2019). Cellular senescence is associated with reorganization of the microtubule cytoskeleton. Cell. Mol. Life Sci. 76, 1169–1183. doi:10.1007/s00018-018-2999-1
Muñoz-Espín, D., and Serrano, M. (2014). Cellular senescence: From physiology to pathology. Nat. Rev. Mol. Cell. Biol. 15, 482–496. doi:10.1038/nrm3823
Nehlig, A., Molina, A., Rodrigues-Ferreira, S., Honoré, S., and Nahmias, C. (2017). Regulation of end-binding protein EB1 in the control of microtubule dynamics. Cell. Mol. Life Sci. 74, 2381–2393. doi:10.1007/s00018-017-2476-2
Nehlig, A., Seiler, C., Steblyanko, Y., Dingli, F., Arras, G., Loew, D., et al. (2021). Reciprocal regulation of Aurora kinase A and ATIP3 in the control of metaphase spindle length. Cell. Mol. Life Sci. 78, 1765–1779. doi:10.1007/s00018-020-03614-8
Nie, W., Xu, M. D., Gan, L., Huang, H., Xiu, Q., and Li, B. (2015). Overexpression of stathmin 1 is a poor prognostic biomarker in non-small cell lung cancer. Lab. Invest. 95, 56–64. doi:10.1038/labinvest.2014.124
Niesvizky, R., Richardson, P. G., Gabrail, N. Y., Madan, S., Yee, A. J., Quayle, S. N., et al. (2015). ACY-241, a novel, HDAC6 selective inhibitor: Synergy with immunomodulatory (IMiD®) drugs in multiple myeloma (MM) cells and early clinical results (ACE-MM-200 study). Blood 126, 3040. doi:10.1182/blood.V126.23.3040.3040
Pagano, A., Honoré, S., Mohan, R., Berges, R., Akhmanova, A., and Braguer, D. (2012). Epothilone B inhibits migration of glioblastoma cells by inducing microtubule catastrophes and affecting EB1 accumulation at microtubule plus ends. Biochem. Pharmacol. 84, 432–443. doi:10.1016/j.bcp.2012.05.010
Peerzada, M. N., Khan, P., Khan, N. S., Avecilla, F., Siddiqui, S. M., Hassan, M. I., et al. (2020). Design and development of small-molecule arylaldoxime/5-nitroimidazole hybrids as potent inhibitors of MARK4: A promising approach for target-based cancer therapy. ACS omega 5, 22759–22771. doi:10.1021/acsomega.0c01703
Pongrakhananon, V., Saito, H., Hiver, S., Abe, T., Shioi, G., Meng, W., et al. (2018a). CAMSAP3 maintains neuronal polarity through regulation of microtubule stability. Proc. Natl. Acad. Sci. U. S. A. 115, 9750–9755. doi:10.1073/pnas.1803875115
Pongrakhananon, V., Wattanathamsan, O., Takeichi, M., Chetprayoon, P., and Chanvorachote, P. (2018b). Loss of CAMSAP3 promotes EMT via the modification of microtubule-Akt machinery. J. Cell. Sci. 131, jcs216168. doi:10.1242/jcs.216168
Prosser, S. L., and Pelletier, L. (2017). Mitotic spindle assembly in animal cells: A fine balancing act. Nat. Rev. Mol. Cell. Biol. 18, 187–201. doi:10.1038/nrm.2016.162
Pulya, S., Amin, S. A., Adhikari, N., Biswas, S., Jha, T., and Ghosh, B. (2021). HDAC6 as privileged target in drug discovery: A perspective. Pharmacol. Res. 163, 105274. doi:10.1016/j.phrs.2020.105274
Qiang, L., Yu, W., Andreadis, A., Luo, M., and Baas, P. W. (2006). Tau protects microtubules in the axon from severing by katanin. J. Neurosci. 26, 3120–3129. doi:10.1523/JNEUROSCI.5392-05.2006
Roberts, A. J., Kon, T., Knight, P. J., Sutoh, K., and Burgess, S. A. (2013). Functions and mechanics of dynein motor proteins. Nat. Rev. Mol. Cell. Biol. 14, 713–726. doi:10.1038/nrm3667
Rodrigues-Ferreira, S., Nehlig, A., Kacem, M., and Nahmias, C. (2020). ATIP3 deficiency facilitates intracellular accumulation of paclitaxel to reduce cancer cell migration and lymph node metastasis in breast cancer patients. Sci. Rep. 10, 13217. doi:10.1038/s41598-020-70142-7
Rodrigues-Ferreira, S., Nehlig, A., Moindjie, H., Monchecourt, C., Seiler, C., Marangoni, E., et al. (2019). Improving breast cancer sensitivity to paclitaxel by increasing aneuploidy. Proc. Natl. Acad. Sci. U. S. A. 116, 23691–23697. doi:10.1073/pnas.1910824116
Schaedel, L., John, K., Gaillard, J., Nachury, M. V., Blanchoin, L., and Théry, M. (2015). Microtubules self-repair in response to mechanical stress. Nat. Mat. 14, 1156–1163. doi:10.1038/nmat4396
Seiberlich, V., Bauer, N. G., Schwarz, L., Ffrench‐Constant, C., Goldbaum, O., and Richter‐Landsberg, C. (2015). Downregulation of the microtubule associated protein Tau impairs process outgrowth and myelin basic protein m RNA transport in oligodendrocytes. Glia 63, 1621–1635. doi:10.1002/glia.22832
She, Z. Y., Pan, M. Y., Tan, F. Q., and Yang, W. X. (2017). Minus end-directed kinesin-14 KIFC1 regulates the positioning and architecture of the Golgi apparatus. Oncotarget 8, 36469–36483. doi:10.18632/oncotarget.16863
Shu, F., Zou, X., Tuo, H., She, S., Huang, J., Ren, H., et al. (2019). Stathmin gene silencing suppresses proliferation, migration and invasion of gastric cancer cells via AKT/sCLU and STAT3 signaling. Int. J. Oncol. 54, 1086–1098. doi:10.3892/ijo.2019.4674
Singh, A., Saha, T., Begemann, I., Ricker, A., Nüsse, H., Thorn-Seshold, O., et al. (2018). Polarized microtubule dynamics directs cell mechanics and coordinates forces during epithelial morphogenesis. Nat. Cell. Biol. 20, 1126–1133. doi:10.1038/s41556-018-0193-1
Smoter, M., Bodnar, L., Grala, B., Stec, R., Zieniuk, K., Kozlowski, W., et al. (2013). Tau protein as a potential predictive marker in epithelial ovarian cancer patients treated with paclitaxel/platinum first-line chemotherapy. J. Exp. Clin. Cancer Res. 32, 25–28. doi:10.1186/1756-9966-32-25
Song, Z., He, C. D., Sun, C., Xu, Y., Jin, X., Zhang, Y., et al. (2010). Increased expression of MAP2 inhibits melanoma cell proliferation, invasion and tumor growth in vitro and in vivo. Exp. Dermatol. 19, 958–964. doi:10.1111/j.1600-0625.2009.01020.x
Stehbens, S. J., Paszek, M., Pemble, H., Ettinger, A., Gierke, S., and Wittmann, T. (2014). CLASPs link focal-adhesion-associated microtubule capture to localized exocytosis and adhesion site turnover. Nat. Cell. Biol. 16, 561–573. doi:10.1038/ncb2975
Tan, R., Foster, P. J., Needleman, D. J., and McKenney, R. J. (2018). Cooperative accumulation of dynein-dynactin at microtubule minus-ends drives microtubule network reorganization. Dev. Cell. 44, 233–247. doi:10.1016/j.devcel.2017.12.023
Tanaka, N., Meng, W., Nagae, S., and Takeichi, M. (2012). Nezha/CAMSAP3 and CAMSAP2 cooperate in epithelial-specific organization of noncentrosomal microtubules. Proc. Natl. Acad. Sci. U. S. A. 109, 20029–20034. doi:10.1073/pnas.1218017109
Teng, J., Takei, Y., Harada, A., Nakata, T., Chen, J., and Hirokawa, N. (2001). Synergistic effects of MAP2 and MAP1B knockout in neuronal migration, dendritic outgrowth, and microtubule organization. J. Cell. Biol. 155, 65–76. doi:10.1083/jcb.200106025
Thériault, B. L., Pajovic, S., Bernardini, M. Q., Shaw, P. A., and Gallie, B. L. (2012). Kinesin family member 14: An independent prognostic marker and potential therapeutic target for ovarian cancer. Int. J. Cancer 130, 1844–1854. doi:10.1002/ijc.26189
Toya, M., Kobayashi, S., Kawasaki, M., Shioi, G., Kaneko, M., Ishiuchi, T., et al. (2016). CAMSAP3 orients the apical-to-basal polarity of microtubule arrays in epithelial cells. Proc. Natl. Acad. Sci. U. S. A. 113, 332–337. doi:10.1073/pnas.1520638113
Trinczek, B., Brajenovic, M., Ebneth, A., and Drewes, G. (2004). MARK4 is a novel microtubule-associated proteins/microtubule affinity-regulating kinase that binds to the cellular microtubule network and to centrosomes. J. Biol. Chem. 279, 5915–5923. doi:10.1074/jbc.M304528200
Trofimova, D., Paydar, M., Zara, A., Talje, L., Kwok, B. H., and Allingham, J. S. (2018). Ternary complex of Kif2A-bound tandem tubulin heterodimers represents a kinesin-13-mediated microtubule depolymerization reaction intermediate. Nat. Commun. 9, 2628. doi:10.1038/s41467-018-05025-7
Velot, L., Molina, A., Rodrigues-Ferreira, S., Nehlig, A., Bouchet, B. P., Morel, M., et al. (2015). Negative regulation of EB1 turnover at microtubule plus ends by interaction with microtubule-associated protein ATIP3. Oncotarget 6, 43557–43570. doi:10.18632/oncotarget.6196
Vilanova, C., Torijano-Gutiérrez, S., Díaz-Oltra, S., Murga, J., Falomir, E., Carda, M., et al. (2014). Design and synthesis of pironetin analogue/combretastatin A-4 hybrids containing a 1, 2, 3- triazole ring and evaluation of their cytotoxic activity. Eur. J. Med. Chem. 87, 125–130. doi:10.1016/j.ejmech.2014.09.053
Vogl, D. T., Raje, N., Jagannath, S., Richardson, P., Hari, P., Orlowski, R., et al. (2017). Ricolinostat, the first selective histone deacetylase 6 inhibitor, in combination with bortezomib and dexamethasone for relapsed or refractory multiple myeloma. Clin. Cancer Res. 23, 3307–3315. doi:10.1158/1078-0432.CCR-16-2526
Wang, C., Jin, G., Jin, H., Wang, N., Luo, Q., Zhang, Y., et al. (2015). Clusterin facilitates metastasis by EIF3I/Akt/MMP13 signaling in hepatocellular carcinoma. Oncotarget 6, 2903–2916. doi:10.18632/oncotarget.3093
Wang, L., Tantai, J., and Zhu, X. (2020). Katanin P60: A potential biomarker for lymph node metastasis and prognosis for non-small cell lung cancer. World J. Surg. Oncol. 18, 157–158. doi:10.1186/s12957-020-01939-z
Wang, W., Zhang, R., Wang, X., Wang, N., Zhao, J., Wei, Z., et al. (2020). Suppression of KIF3A inhibits triple negative breast cancer growth and metastasis by repressing Rb‐E2F signaling and epithelial‐mesenchymal transition. Cancer Sci. 111, 1422–1434. doi:10.1111/cas.14324
Wattanathamsan, O., Chetprayoon, P., Chantaravisoot, N., Wongkongkathep, P., Chanvorachote, P., and Pongrakhananon, V. (2021). CAMSAP3 depletion induces lung cancer cell senescence‐associated phenotypes through extracellular signal‐regulated kinase inactivation. Cancer Med. 10, 8961–8975. doi:10.1002/cam4.4380
Wattanathamsan, O., and Pongrakhananon, V. (2021). Post-translational modifications of tubulin: Their role in cancers and the regulation of signaling molecules. Cancer Gene Ther., 1–8. doi:10.1038/s41417-021-00396-4
Wu, H., Deng, W. W., Yang, L. L., Zhang, W. F., and Sun, Z. J. (2018). Expression and phosphorylation of Stathmin 1 indicate poor survival in head and neck squamous cell carcinoma and associate with immune suppression. Biomark. Med. 12, 759–769. doi:10.2217/bmm-2017-0443
Xia, P., Chu, S., Liu, G., Chen, G., Yi, T., Feng, S., et al. (2018). High expression of KIF3A is a potential new parameter for the diagnosis and prognosis of breast cancer. Biomed. Rep. 8, 343–349. doi:10.3892/br.2018.1061
Xiang, C., Chen, J., and Fu, P. (2017). HGF/Met signaling in cancer invasion: The impact on cytoskeleton remodeling. Cancers 9, 44. doi:10.3390/cancers9050044
Xun, G., Hu, W., and Li, B. (2021). PTEN loss promotes oncogenic function of STMN1 via PI3K/AKT pathway in lung cancer. Sci. Rep. 11, 14318–8. doi:10.1038/s41598-021-93815-3
Yang, L., Xu, X., and Zheng, J. (2020). Microtubule-associated protein 2 knockdown sensitizes glioma cells to vincristine treatment. NeuroReport 31, 197–204. doi:10.1097/WNR.0000000000001378
Yang, Z., Li, C., Yan, C., Li, J., Yan, M., Liu, B., et al. (2019). KIF14 promotes tumor progression and metastasis and is an independent predictor of poor prognosis in human gastric cancer. Biochim. Biophys. Acta. Mol. Basis Dis. 1865, 181–192. doi:10.1016/j.bbadis.2018.10.039
Ye, X., Lee, Y. C., Choueiri, M., Chu, K., Huang, C. F., Tsai, W. W., et al. (2012). Aberrant expression of katanin p60 in prostate cancer bone metastasis. Prostate 72, 291–300. doi:10.1002/pros.21431
Yi, R., Feng, J., Yang, S., Huang, X., Liao, Y., Hu, Z., et al. (2018). miR-484/MAP2/c-Myc-positive regulatory loop in glioma promotes tumor-initiating properties through ERK1/2 signaling. J. Mol. Histol. 49, 209–218. doi:10.1007/s10735-018-9760-9
Yi, Y., Qiu, Z., Yao, Z., Lin, A., Qin, Y., Sha, R., et al. (2021). CAMSAP1 mutation correlates with improved prognosis in small cell lung cancer patients treated with platinum-based chemotherapy. Front. Cell. Dev. Biol. 9, 770811. doi:10.3389/fcell.2021.770811
Zahra, K. F., Lefter, R., Ali, A., Abdellah, E. C., Trus, C., Ciobica, A., et al. (2021). The involvement of the oxidative stress status in cancer pathology: A double view on the role of the antioxidants. Oxid. Med. Cell. Longev. 2021, 9965916. doi:10.1155/2021/9965916
Zaman, S., Chobrutskiy, B. I., Sikaria, D., and Blanck, G. (2019). MAPT (Tau) expression is a biomarker for an increased rate of survival for low-grade glioma. Oncol. Rep. 41, 1359–1366. doi:10.3892/or.2018.6896
Zaoui, K., Duhamel, S., Parachoniak, C. A., and Park, M. (2019). CLIP‐170 spatially modulates receptor tyrosine kinase recycling to coordinate cell migration. Traffic 20, 187–201. doi:10.1111/tra.12629
Zehr, E., Szyk, A., Piszczek, G., Szczesna, E., Zuo, X., and Roll-Mecak, A. (2017). Katanin spiral and ring structures shed light on power stroke for microtubule severing. Nat. Struct. Mol. Biol. 24, 717–725. doi:10.1038/nsmb.3448
Zeitz, M., and Kierfeld, J. (2014). Feedback mechanism for microtubule length regulation by stathmin gradients. Biophys. J. 107, 2860–2871. doi:10.1016/j.bpj.2014.10.056
Zhang, L., Liu, X., Song, L., Zhai, H., and Chang, C. (2020). MAP7 promotes migration and invasion and progression of human cervical cancer through modulating the autophagy. Cancer Cell. Int. 20, 17–18. doi:10.1186/s12935-020-1095-4
Zhang, Z., Cao, Y., Zhao, W., Guo, L., and Liu, W. (2017). HDAC6 serves as a biomarker for the prognosis of patients with renal cell carcinoma. Cancer Biomark. 19, 169–175. doi:10.3233/CBM-160298
Zhao, L., Zhang, D., Shen, Q., Jin, M., Lin, Z., Ma, H., et al. (2019). KIAA1199 promotes metastasis of colorectal cancer cells via microtubule destabilization regulated by a PP2A/stathmin pathway. Oncogene 38, 935–949. doi:10.1038/s41388-018-0493-8
Zhao, T., Ding, X., Chang, B., Zhou, X., and Wang, A. (2015). MTUS1/ATIP3a down-regulation is associated with enhanced migration, invasion and poor prognosis in salivary adenoid cystic carcinoma. BMC Cancer 15, 203. doi:10.1186/s12885-015-1209-x
Zhao, T., He, Q., Liu, Z., Ding, X., Zhou, X., and Wang, A. (2016). Angiotensin II type 2 receptor-interacting protein 3a suppresses proliferation, migration and invasion in tongue squamous cell carcinoma via the extracellular signal-regulated kinase-Snai2 pathway. Oncol. Lett. 11, 340–344. doi:10.3892/ol.2015.3898
Zheng, S., Shi, L., Zhang, Y., and He, T. (2014). Expression of SNCG, MAP2, SDF-1 and CXCR4 in gastric adenocarcinoma and their clinical significance. Int. J. Clin. Exp. Pathol. 7, 6606–6615.
Zhou, Q., Yu, J., Zheng, Q., Wu, T., Ji, Z., and Zhuo, Y. (2021). Kinesin family member 3A stimulates cell proliferation, migration, and invasion of bladder cancer cells in vitro and in vivo. FEBS open bio 11, 1487–1496. doi:10.1002/2211-5463.12768
Zhu, B., Qi, L., Liu, S., Liu, W., Ou, Z., Chen, M., et al. (2017). CLASP2 is involved in the EMT and early progression after transurethral resection of the bladder tumor. BMC cancer 17, 105–110. doi:10.1186/s12885-017-3101-3
Zinn, A., Goicoechea, S. M., Kreider-Letterman, G., Maity, D., Awadia, S., Cedeno-Rosario, L., et al. (2019). The small GTPase RhoG regulates microtubule-mediated focal adhesion disassembly. Sci. Rep. 9, 5163. doi:10.1038/s41598-019-41558-7
Keywords: cancer, epithelial to mesenchymal transition, metastasis, microtubules, microtubule-associated protein, migration and invasion
Citation: Wattanathamsan O and Pongrakhananon V (2022) Emerging role of microtubule-associated proteins on cancer metastasis. Front. Pharmacol. 13:935493. doi: 10.3389/fphar.2022.935493
Received: 04 May 2022; Accepted: 29 August 2022;
Published: 14 September 2022.
Edited by:
Ivan Vorobjev, Lomonosov Moscow State University, RussiaReviewed by:
Songbo Xie, Shandong Normal University, ChinaAlexander Lang, University Hospital of Düsseldorf, Germany
Satish Bodakuntla, National Institutes of Health (NIH), United States
Copyright © 2022 Wattanathamsan and Pongrakhananon. This is an open-access article distributed under the terms of the Creative Commons Attribution License (CC BY). The use, distribution or reproduction in other forums is permitted, provided the original author(s) and the copyright owner(s) are credited and that the original publication in this journal is cited, in accordance with accepted academic practice. No use, distribution or reproduction is permitted which does not comply with these terms.
*Correspondence: Varisa Pongrakhananon, dmFyaXNhLnBAcGhhcm0uY2h1bGEuYWMudGg=