- Jilin Provincial Key Laboratory on Molecular and Chemical Genetic, The Second Hospital of Jilin University, Changchun, China
Paeoniflorin (PF) has been widely used for the treatment of depression in mice models, some Chinese herbal compound containing PF on treating depression, such as Xiaoyao San, Chaihu-Shugan-San, Danggui Shaoyao San etc. Many experiments are also verifying whether PF in these powders can be used as an effective component in the treatment of depression. Therefore, in this review the antidepressant effect of PF and its mechanism of action are outlined with particular focus on the following aspects: increasing the levels of monoamine neurotransmitters, inhibiting the HPA axis, promoting neuroprotection, enhancing neurogenesis in the hippocampus, and elevating levels of brain-derived neurotrophic factor (BDNF). This review may be helpful for the application of PF in the treatment of depression.
Introduction
Depression is one of the most common psychiatric sick and is characterized by significant and persistent long-term low mood with suicide being its most serious consequence. Surveys reveal that the prevalence of depression was highest among younger adults aged 18–29 years; however, the prevalence of depression in people aged over 45 is low (Wittchen et al., 1994). Depression and has become a global disease burden and its prevalence continues to increase. Studies have shown that the prevalence of depression increased significantly from 1994 to 2014, and the prevalence rate from 2004 to 2014 was higher than that from 1994 to 2003 (Lim et al., 2018). Age has also become a key factor in the incidence of depression, the prevalence of depression is constantly growing, especially during adolescence (McCarron et al., 2021). A survey in 2020 showed that college students have a high prevalence of depression (Gao et al., 2020).
Currently, there are several antidepressant drugs in clinical use, including tricyclic antidepressants (TCAs); monoamine oxidase inhibitors (MAOIs) and selective norepinephrine reuptake inhibitors (SNRIs) (Qiu et al., 2013a). However, these antidepressants have serious side-effects such as drowsiness, dryness of mouth, headaches, nausea, and sexual dysfunction. Furthermore, chronic or acute administration of antidepressants may lead to weight gain and even obesity. In general, TCAs and MAOIs may be more likely to cause weight gain than SNRIs (Fava, 2000). However, when used excessively antidepressants may cause seizures or hepatotoxicity manifesting as a transient increase in liver enzymes ultimately causing fulminant hepatic failure (FHF) (Lucena et al., 2003; Judge and Rentmeester, 2013). A previous study revealed that the onset time of hepatotoxicity caused by antidepressants ranged from 5 days to 3 years (Park and Ishino, 2013). Therefore, the clinical response and efficacy of antidepressants need to be optimized, and the development of new drugs with low toxicity is urgently needed.
Paeoniflorin (PF) is a type of monoterpene glycoside, which is derived from the root of paeonia or peony and is extracted from Radix Paeoniae Alba, which is a traditional low toxic Chinese herbal medicine widely used in the treatment of depression. Some traditional Chinese medicines containing PF have been used in the treatment of depression, such as Xiaoyao San (Chen C et al., 2020), Chaihu-Shugan-San was also proved to have a therapeutic effect on depression as early as 2004 (Kim et al., 2005). Danggui Shaoyao San is also widely used to treat depression (Xu et al., 2011; Huang et al., 2012). The pharmacological mechanisms (such as anti-neoplastic, anti-inflammatory, anti-oxidant, anti-apoptotic, or neuroprotective) of PF have been reported (Zhao et al., 2013; Tao et al., 2016; Ouyang et al., 2017; Zhang and Wei, 2020). For example, PF plays an anti-tumor role by inhibiting the expression of S-phase kinase associated protein (SKP) two in glioma cells (Ouyang et al., 2017). PF can also reduce the expression of TLR4 and MyD88, as well as the phosphorylation of IκBα and NF-κB p65, which could alleviate atherosclerosis and chronic inflammation (Li et al., 2017). However, there has been increasing emphasis on the antidepressant effects of PF in recent years. PF has been shown to elicit antidepressant activity in all of the tested mouse and rat models including chronic unpredictable mild stress (CUMS), forced swim tests (FST), tail suspension tests (TST), medicinal induction, post-stroke depression, and menopause related depression (Wang X.-L. et al., 2021).
Many other studies have proved the antidepressant effect of PF. It has been demonstrated that PF exerts neuroprotective effects via the extracellular signal-regulated kinase–cyclic adenosine monophosphate response element binding protein (ERK-CREB) signaling pathway in a CUMS-induced hippocampal damage rat model (Zhong et al., 2019). Furthermore, in a rat model of post-stroke depression (PSD), PF treatment significantly increased the levels of phosphorylated CREB (p-CREB) and brain-derived neurotrophic factor (BDNF) in the CA1 region of the hippocampal complex (Hu et al., 2019).
It has been reported that there are a number of molecular mechanisms underlying the antidepressant effects of PF, such as the effects of monoaminergic neurotransmitters on depression and the effects of the hypothalamic–pituitary–adrenal (HPA) axis on depression. In this article we will review the recent research on the antidepressant effects of PF and discuss the mechanisms underlying these effects. It is expected that PF could be exploited by studying the underlying molecular mechanisms.
Effects of monoamine neurotransmission on depression
An increasing number of studies have confirmed that monoamine neurotransmission, serotonin (5-HT), noradrenaline (NE), and dopamine (DA) are related to the neurophysiological process of depression. Physiological changes in abnormal 5-HT, NE, and DA signal transduction lead to changes in receptor regulation or function or impaired intracellular signal processing (Liu et al., 2018). Monoamine neurotransmitters have an extensive range of biological functions and are an important regulatory class for a series of physiological activities in the central nervous system (CNS, such as mental activity, behavioral states and emotion). Norepinephrine may be related to alertness, anxiety, attention, and attitude to life whereas 5-HT is involved in anxiety, and obsessive-compulsive disorders, and dopamine is involved in attention deficits, motivation, pleasure, and reward. Therefore, increasing any of these three neurotransmitters will elevate mood, but the other elements of depression may be particularly responsive to a certain neurotransmitter (Nutt, 2008). 5-HT in the brain has a significant role in mood alleviation, hunger, and sleep regulation. In addition, a healthy diet may also alleviate the symptoms of depression. One research has reported that fruits and vegetables are rich in 5-HT, which is influenced by the blood–brain barrier (BBB). Tryptophan is the precursor of 5-HT. 5-HT is synthesized through decarboxylation (Shabbir et al., 2013). The effect of NE in depression was also reported. NE is projected from the cell bodies in the locus coeruleus to the limbic system to regulate emotion, and NE transporter (NET) levels in the locus coeruleus are decreased in patients with depression who commit suicide. In addition, NET knockout mice exhibited increased extracellular NE levels and developed resistance to depression-like behaviors (Briley and Chantal, 2011). These results suggest that NE system enhancement could ameliorate depression-like behaviors in mice.
To date, the monoamine hypothesis does not address key issues, for example, drugs that enhance the delivery of 5-HT or norepinephrine are not necessarily effective in treating depression. Despite its limitations, the development of the monoamine hypothesis is of great importance for the understanding of depression and the development of novel, safe and effective drugs for its treatment (Hirschfeld, 2000).
Danzhi Xiaoyao San (DXS), a modified formula from Xiaoyao San, is a canonical Chinese medicine. This herbal formula is composed of the following herbs: Radix Bupleuri, Paeoniae Radix Alba, Angelica sinensis, bighead atractylodes rhizome, Poria cocos, bark of tree peony root, Fructus Gardeniae, Mentha haplocalyx, and licorice (Wu et al., 2016). DXS, which contains PF, has been combined with antidepressants in the clinic. It was shown that DXS exerts antidepressant-like effects in CUMS rats by inhibiting pro-inflammatory cytokine levels, inhibiting the activation of indoleamine 2,3-dioxygenase (IDO) in the hippocampus and upregulating the hippocampal contents of tryptophan and 5-HT (Zhu et al., 2015). Another study demonstrated that treatment with total glucosides of peony could inhibit monoamine oxidase activity to maintain the levels of monoamine neurotransmitters in the rat brain, while peony extract (containing 48.99% PF and 18.99% albiflorin) could increase the contents of 5-HT and NE in the brain of CUS-induced rats (Qiu et al., 2013b).
Moreover, experiments have been conducted to investigate the effects of PF in an animal model of depression in menopause. In rats which were given 10 mg/kg PF by gavage for 2 weeks, PF exerted antidepressant effects by upregulating 5-HT1A receptor (5-HT1AR) mRNA expression and downregulating 5-HT2A receptor (5-HT2AR) mRNA expression (Huang et al., 2015). PF can simultaneously exert antidepression effects via polypharmacology; for example, rats subjected to the forced swimming test (FST) exhibited decreased plasma and hippocampus 5-hydroxytryptamine, norepinephrine, and dopamine levels and reduced plasma BDNF and superoxide dismutase (SOD) levels (Mu et al., 2020).
Many studies have shown an antidepressant effect of PF, and its possible implication for monoamine neurotransmitters. The results show that PF can increase the levels of monoamine neurotransmitters in the mouse hippocampus (Qiu et al., 2013a). In another study, rats treated with the monoamine reuptake inhibitor reserpine received intragastric doses of peony glycosides of 40, 80, and 160 mg/kg. Total glucosides of peony (TGP) at 80 and 160 mg/kg significantly inhibited the activity of MAO-A and MAO-B (Mao et al., 2008). Monoamine oxidase inhibitors (MAOIs) can significantly alleviate depression by reducing the metabolism of monoamine neurotransmitters and also, preserve monoamine neurotransmitter concentration by inhibiting monoamine oxidase (MAO) which cause their breakdown. In addition, the activities of MAO-A and MAO-B were increased in CUS-induced rats. This phenomenon was reversed after treatment with TGP (whose major active component is PF) when TGP was given intragastrically 30 min before each stressor once every day for 24 days (Mao et al., 2009b). These findings suggest that the antidepressant effect of PF may be mediated through inhibition of MAO and therefore, has an antidepressant effect by increasing monoamine neurotransmitter levels.
As shown in Figure 1, visual representation of the pathway by which monoamine neurotransmitters are released into and removed from the synaptic space. 5-HT, NE, and DA are synthesized and released into the synaptic cleft. These neurotransmitters can interact with receptors on postsynaptic cells or bind to reuptake channels and be oxidized by MAO. Patients with depression have lower levels of monoamine neurotransmitters, and PF can increase the levels of monoamine transmitters by inhibiting the activity of MAO. The 5-HT1A and 5-HT2A receptors functionally compete with each other. The 5-HT1A receptor function decreases and the 5-HT2A receptor function increases in patients with depression. PF can increase the mRNA and protein expression levels of 5-HT1A receptors in the hypothalamus area in model rats, while the expression levels of 5-HT2A receptors are decreased.
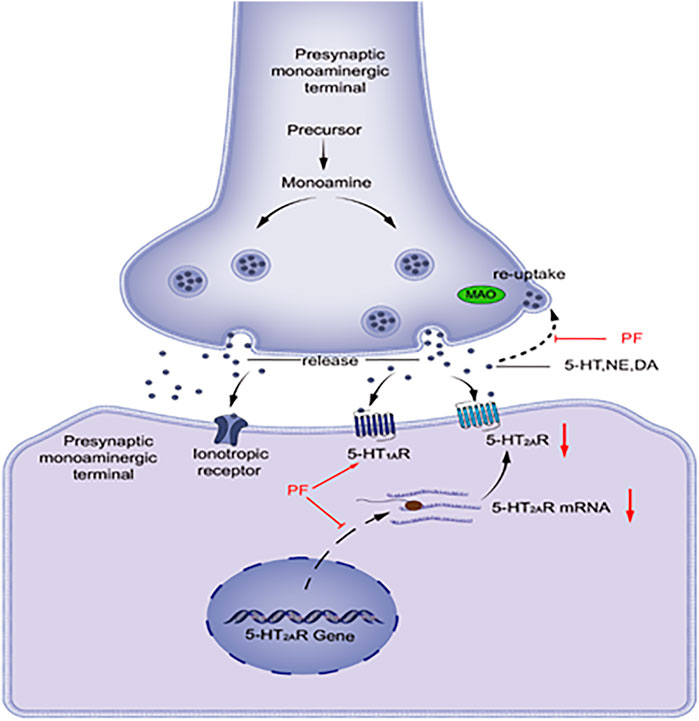
FIGURE 1. DA, dopamine; MAO, monoamine oxidase; NE, noradrenaline; PF, paeoniflorin; 5-HT, serotonin; 5-HT1AR, 5-HT1A receptor; 5-HT2AR, 5-HT2A receptor.
Effect of the hypothalamic–pituitary–adrenal axis
The HPA axis is implicated in the pathophysiology of depression, which involves the brain, the pituitary gland, and the adrenal glands, which regulate glucocorticoid (GC) production. Cortisol, a major stress hormone released by the adrenal glands, shows high affinity with mineralocorticoid receptors (MRs) and low affinity with glucocorticoid receptors (GRs). MRs primarily function in the hippocampus, while GRs play feedback roles in the brain and the pituitary gland. We speculated that the pathogenesis of depression could be caused by imbalance of GR and MR regulation in the HPA system (Keller et al., 2017). Patients with severe affective disorder of depression usually have high cortisol levels. It was found that depressive symptoms were apparently relieved when cortisol levels were reduced in either way. Therefore, it seems that steroids themselves play an important role in the occurrence and delay of depression (Pearsonmurphy, 1991).
The HPA axis is the endocrine core of the stress response in mammals, and involves corticotropin-releasing hormone (CRH) which is secreted by the hypothalamus, causing the release of adrenocorticotropic hormone (ACTH) in the anterior pituitary gland, and GC or corticosterone (CORT) in the adrenal glands. Changes in the HPA axis has been consistently found in patients with depression (Fischer et al., 2017). Misalignment of the HPA axis is believed to be mainly driven by interruption of the GR-dependent negative feedback (i.e., GR resistance) (Troubat et al., 2021). However, the change in GR levels did not directly cause depression-like behavior. Hypercortisolemia and disturbances of GR function were not always observed in the clinical manifestations of depression. Corticotropin-releasing factor (CRF) levels were increased in patients with depression, according to the autopsy reports, while the changes in CRF levels might be the basis of the influence of GR manipulation (Neigh and Nemeroff, 2006).
In rats given 80 mg/kg or 160 mg/kg CUMS through intragastric gavage once a day for 6 weeks, TGP treatment reduced the immobility time in the FST (19% and 27%, respectively), decreased serum CORT levels in a dose-dependent manner (23–30), and increased the GR mRNA level. Thus, long-term administration of TGP could effectively relieve the CUMS-induced depressive-like symptoms. TGP might exert its antidepressant effects by changing the function of the HPA axis (Mao et al., 2009a). Experiments have shown that PF can reverse the activity of the HPA axis in depressed mice, and reduce the levels of CRH, ACTH and CORT (Mu et al., 2020). In rats treated with chronic unpredictable stress (CUS), PF treatment significantly decreased the levels of ACTH and CORT. Furthermore, PF treatment attenuated the CUS-induced reduction of norepinephrine, 5-HT or its metabolite, 5-hydroxyindoleacetic acid (Qiu et al., 2013b). These results indicate that the regulation of the HPA axis and the up-regulation of the 5-HT and noradrenergic systems are important mechanisms for the antidepressant-like effect of PF in CUS-treated rats (Lichun et al., 2020).
As shown in Figure 2, depression patients with hyperactive HPA axis are mainly characterized by elevated CRH, ACTH, CORT, and metabolite levels in the central and peripheral nervous system, especially in the hypothalamus. PF can reduce the serum levels of CRH, CORT, and ACTH and restore the function of the HPA axis. This significantly improves the depression-like behavior of model rats.
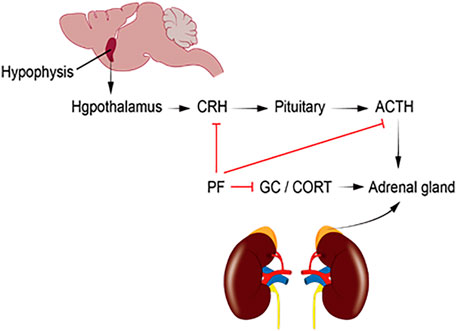
FIGURE 2. ACTH, adrenocorticotropic hormone; CORT, corticosterone; CRH, corticotropin-releasing hormone; HPA, hypothalamic–pituitary–adrenal; GC, glucocorticoid; PF, paeoniflorin.
Neuroprotection
Preclinical studies have shown that chronic stress can cause changes in the number and shape of neurons and glial cells in brain regions related to mood disorders including the hippocampus (HP), prefrontal cortex (PFC), cingulate cortex, nucleus accumbens and amygdala (AMY). Brain imaging and autopsy studies have found that the extent of branching, complexity of the dendrites and the number of neurons and glial cells in these brain regions are reduced in patients with depression (Duman, 2009).
Specific experiments have also shown that the pathogenesis of antidepressant treatment is related to HP volume reduction and PFC dysfunction. Therefore, neuronal damage is also related to depression (Schoenfeld et al., 2017; Seo et al., 2017). Currently, many in vitro and in vivo experiments have shown that PF has a neuroprotective effect on various types of neuronal damage and next we will summarize the neuroprotective mechanisms associated with its treatment (See Table 1).
Oxidative stress and depression
Oxidative stress is defined as an imbalance between reactive oxygen species (ROS) production and the antioxidant capacity of the cell. Oxidative stress is a major cause of depressive disorders (Bhatt et al., 2020). Excess production of ROS by the cells can cause damage to DNA, proteins, and lipids, and eventually lead to cell death (Black et al., 2015). Oxidative stress plays an important role in the pathophysiology of major depression through the actions of free radicals, non-free radical molecules, ROS and nitrogen-based molecules (Vaváková et al., 2015). As the brain is the major consumer of oxygen, oxidative stress can easily damage the CNS and accelerate the occurrence of mental illnesses, such as depression. Therefore, abnormal levels of oxidative stress markers are often seen in many depressive patients. DNA oxidation in Brodmann area 10 of the brain is significantly increased in depression (Szebeni et al., 2016) and it has been shown that 8-hydroxy-2′-deoxyguanosine (8-OHdG) and F2-isoprostanes (markers of DNA oxidation and lipid damage) act as reliable marker for increased oxidative stress (Black et al., 2015). A study found that 8-OHdG and F2-isoprostane levels were increased in patients with depression and suggested that oxidative stress increased and reduced the levels of antioxidants, indicating that depression is accompanied by increased oxidative damage (Black et al., 2015). High concentrations of glutamate can also induce neurotoxicity in rat pheochromocytoma cells (PC12) and oxidative stress may induce glutamate-induced neuronal damage. Whereas PF has a neuroprotective effect on PC12 cell neurotoxicity induced by glutamate, as it can inhibiting oxidative stress (Mao et al., 2010b).
Nerve growth factor (NGF) has powerful neuroprotective and nerve reparatory functions. One study reported that antidepressants could increase mRNA levels of NGF in CORT-induced PC12 cells, activate adenylate cyclase (AC) in the cell membrane, increase intracellular cAMP levels, and upregulate the cAMP-response element binding protein (cAMP-CREB) cascade and NGF expression (Yun-Feng et al., 2003). In CORT-treated PC12 cells (cell viability 41%), PF (1, 10, and 50 μM) can increase cell viability (to 49, 55, and 61%, respectively) and reduce intracellular ROS levels (to 169%, 149%, and 132% of the control value, respectively) and malondialdehyde (MDA) levels (to 180%, 161%, and 146% of the control value, respectively). PF can also reverse the decrease in NGF mRNA levels caused by CORT (51% of the control value) in PC12 cells, indicating that PF exerts its neuroprotective effect by inhibiting oxidative stress and up-regulating NGF expression (72%, 82%, and 87% of the control value, respectively) (Mao et al., 2012). PF can also inhibit mitochondrial 6-hydroxydopamine (6-OHDA)-induced apoptosis in PC12 cells by increasing the antioxidant capacity of glutathione (GSH), and can significantly attenuate 6-OHDA-induced nuclear factor κB (NF-κB) translocation and block the 6-OHDA-induced upregulation of protein kinase Cδ (PKCδ). These results indicate that the inhibition of apoptosis in PC12 cells caused by PF may be mediated through the inhibition of the ROS/PKCδ/NF-κB signaling pathway (Dong et al., 2015). The survival rate of CORT-induced PC12 cells decreased to 30%, and it significantly increased after TGP (1, 5, or 10 mg/L) treatment (to 38%, 45%, and 49%, respectively). Similarly, TGP (1, 5, or 10 mg/L) treatment decreased the intracellular ROS (160%, 148%, and 129% of the control value, respectively; CORT-treated 195%) and MDA (183%, 160%, and 145% of the control value, respectively; CORT-treated 214%) levels and increased the GSH (55%, 63%, and 71% of the control value, respectively; CORT-treated 42%) and SOD (52%, 57%, and 60% of the control value, respectively; CORT-treated 40%) levels. The level of catalase (CAT) (59% and 60% of the control value, respectively; CORT-treated 43%) also increased after TGP (5 and 10 mg/L) treatment. This suggested that TGP partially reversed the CORT-induced neurotoxicity (Mao et al., 2011a). These data strongly suggest that one of the mechanisms of neuronal protection produced by PF is the inhibition of oxidative stress.
Balance between ion concentration and ion channel activity
Glial calcium (Ca2+) is involved in various forms of pathological processes in the nervous system (Nedergaard et al., 2010). Calcium pumps have high affinity for intracellular calcium ions and can stabilize the intracellular Ca2+ concentration. The calcium pump is the main factor reducing intracellular Ca2+ concentrations in hippocampal neurons (Mata and Sepúlveda, 2005). Disruption of mitochondrial dynamics can lead to calcium overload in cells and cause nerve cell damage or death (DeCoster, 1995). Ca2+ participates in the transmission of the depolarizing signal and contributes to synaptic activity. Ca2+ controls specific neuronal processes, such as long-term potentiation (LTP) or depression of synaptic transmission (Brini et al., 2014). Homeostatic control of intracellular Ca2+ levels plays an important role in the regulation of neuronal activity, including neurotransmitter release, synaptic plasticity, memory storage, and neuronal cell death (Calvo-Rodriguez et al., 2020). Glutamate activates postsynaptic receptors in excitotoxicity, including the ionotropic N-methyl-d-aspartate (NMDA) and 2-amino-3-(3-hydroxy-5-methylisoxazol-4-yl) proprionate (AMPA). Therefore, the excessive synaptic release of glutamate will lead to the imbalance of Ca2+ homeostasis, and eventually lead to apoptosis (Arundine and Tymianski, 2003). Elevated intracellular Ca2+ levels can activate a range of calcium-dependent signaling pathways (Takemoto-Kimura et al., 2017). Calmodulin (CaM) is expressed ubiquitously in eukaryotic cells and is involved in many key signaling pathways such as the control of growth, proliferation and motility, and changes in intracellular Ca2+ concentrations can regulate CaM (Chin and Means, 2000). Ca2+/calmodulin-dependent protein kinase II type (CaMK-II) is present in the brain and can increase Ca2+ levels through voltage-dependent channels and interact with CaM (Rothschild and Tombes, 2020) demonstrating that Ca2+, CaM, CaMK-II all play a key role in the nervous system.
Research has shown that abnormal serum calcium levels cause cognitive impairment in patients with depression and in the nervous system, NMDA receptors allow the influx of extracellular Ca2+ causing the activation of signaling pathways: calcineurin induces long-term depression (Grützner et al., 2018). Therefore, maintaining the correct regulation of calcium channels is one of the mechanisms that protect the nervous system. Calbindin-D28 K is one of the primary calcium-binding proteins in the brain and maintains the intracellular calcium balance by binding excessive Ca2+, which can protect normal activation and function of neurons and inhibit neuronal apoptosis induced by intracellular Ca2+ overload. Experiments showed that PF could reverse the mRNA level of Calbindin-D28 K in PC12 cells treated with glutamate and decrease the intracellular free calcium concentration (Shifman et al., 2006; Mao et al., 2010b).
PF exerts neuroprotective effects by maintaining Ca2+ homeostasis and regulating Ca2+ signaling pathways
NMDA receptors are prevalent in the cerebral cortex, hippocampus, striatum, and amygdala. Importantly, damage to these regions can cause emotional and cognitive deficits. Activated NMDA receptors cause Ca2+ influx, and calcium overload could activate nitric oxide synthase (NOS), producing nitric oxide (NO) (Li et al., 2006). It has been found that PF has a protective effect on NMDA-induced neurotoxicity in rat PC12 cells as it can improve cell viability, reduce the release of lactate dehydrogenase (LDH), reverse the increase in intracellular Ca2+ concentrations and the decrease the mRNA levels of D28 K (calcium-binding protein) (Mao et al., 2011b). These results indicate that PF exerts a neuroprotective effect on NMDA-induced PC12 cell neurotoxicity at least partially through Ca2+ antagonism. Lots of experiments demonstrated that PF suppressed intracellular Ca2+ overload and the expression of CaMKII (Zhong et al., 2009; Wang et al., 2013a; Zhang et al., 2017). In a premenstrual syndrome (PMS) depression model, PF could restore the phosphorylation level of CaMKII in Cav1.2-induced CaM/CaMKII signaling pathway. In addition, experiments have shown that PF can significantly inhibit the increase in intracellular Ca2+ concentration and Cav1.2 current density induced by KCl, may regulate the CaM/CaMKII pathway through the regulation of Cav1.2 (Song C et al., 2017).
Apoptosis and depression
Dysregulation of apoptosis can damage neuroblasts, and ultimately lead to neurodegenerative diseases (Ghavami et al., 2014), suggesting a very important role for neuronal apoptosis in many CNS disorders. Diabetes-related depression rats exhibit hippocampal neuronal apoptosis by the aberrant Glu–GluR2–Parkin pathway, which is also responsible for depressive-like behaviors and monoamine neurotransmitter deficiency in rats (Liu et al., 2021). Stress also results in apoptosis (Lucassen et al., 2006). As is well known, apoptosis is controlled by pro-apoptotic family members (such as Bax) and anti-apoptotic family members (such as Bcl-2). A study has shown that a combination of isolation and CUMS stimulation after cerebral ischemia significantly upregulated neuronal apoptosis and the expression levels of pro-apoptotic proteins in the hippocampus (Wang A. et al., 2021). MicroRNAs (miRNAs) and connective tissue growth factor (CTGF) are also involved in hippocampal neuronal apoptosis in CUMS-induced depression-like mice (Pei et al., 2020). Prolactin receptor (PRLR), an antidepressant factor, participates in depression by the JAK2–STAT5 signaling pathway. Additionally, increased numbers of apoptotic cells and necrotic cells were observed in chronic mild stress (CMS)-induced depression mice, and the expression of caspase-3, Bax, and proteins involved in the JAK2–STAT5 signaling pathway was decreased after PRLR silencing, along with increased expression of BDNF and Bcl-2 (Tian et al., 2019).
Many studies have shown that the protective effect of PF is related to the anti-apoptotic pathway. As a potent neurotoxin, methyl-4-phenylpyridine ions (MPP+) can induce neuronal apoptosis and neurodegeneration. For example, PF (the most significant effect was observed at a dose of 200 μM) improves the viability of PC12 cells differentiated in MPP+ and also inhibits excessive release of LDH. It can also protect cells from apoptosis induced by DNA damage and reverse the concentration of MPP+ on B cell lymphoma and inhibits the increase of ADP-ribose polymerase which is involved in apoptosis (Wang et al., 2013b). Moreover, studies have shown that PF could protect neurons against oxidative damage and the role of mitophagy in apoptosis (Mayer and Oberbauer, 2003; Haeberlein, 2004; Cao et al., 2010). In addition, PF can inhibit the mitogen-activated protein kinase 4- c-Jun N-terminal kinase (MKK4-JNK) signaling pathway, down-regulate the level of cleaved-caspase-3 and up-regulate the ratio of Bcl-2/Bax, and significantly protect hypothalamic neurons from TBTC-induced cytotoxicity, apoptosis and the reduction in MMP (Cong et al., 2019). Further, PF also inhibits neuronal apoptosis by many pathways, such as by activating the Nrf2/ARE pathway [73] [74]. The survival rate of PC12 cells was 37% when the CORT concentration was 200 μM, and the survival rate of PC12 cells increased gradually (45–55%) after treatment with TGP. TGP could also reduce caspase-3 activity induced by CORT and upregulate the Bcl-2/Bax ratio. All of these results indicate that TGP inhibits apoptosis by suppressing the mitochondrial apoptosis pathway (Mao et al., 2009c). These data suggest that the regulation of apoptosis-related proteins and their signaling pathways is the key to the anti-apoptotic effect of PF.
Neuroinflammation and depression
An increasing number of studies have shown that immune cells are involved in the neurodegenerative response of the CNS and that neuroinflammation has been associated with many neurodegenerative diseases. Furthermore, Microglia and astrocytes have been found to trigger inflammatory processes in neurodegenerative diseases (Ising and Heneka, 2018). Neuroinflammation caused by stress could impair adult hippocampal neurogenesis and result in cognitive deficits. Stress could also activate microglia (Wu et al., 2021). Depression can lead to characteristic glial cell changes and increase the levels of pro-inflammatory cytokines. These are crucial factors in the event of neuroinflammation in the brain (Benatti et al., 2016). Induction of inflammation was also accompanied by changes in behavior and emotional changes, including depressive-like behaviors. These are closely related to the brain immune system and neuroinflammation (Nettis and Pariante, 2020). A study revealed that the levels of interleukin-6 (IL-6), tumor necrosis factor-alpha (TNF-α), and interleukin-8 (IL-8) were increased in cerebrospinal fluid (CSF) of patients with depression (Enache et al., 2019). An increase of pro-inflammatory cytokines levels can activate the NF-κB signaling pathway, which could upregulate expression of inflammatory genes, such as cyclooxygenase-2 (COX-2). COX-2 produces prostaglandin, especially prostaglandin E2 (PGE2), which can enhance complex inflammatory cascades (Pitsillou et al., 2020). Increasing evidence demonstrates that neuroinflammation is inextricably linked with depression; thus, inhibiting neuroinflammation is also becoming a critical therapeutic strategy for depression treatment.
Fibroblast growth factor 2 (FGF-2) can regulate neuronal proliferation and differentiation and is involved in treatment outcomes of the antidepressant fluoxetine (Simard et al., 2018). A study revealed that lipopolysaccharide (LPS)-induced neuroinflammation decreases the level of FGF-2 (Chen M et al., 2020). PF (20, 40, or 80 mg/kg) given daily by gavage for a week prevented the decrease in FGF-2 levels in LPS-injected mice. PF also reversed the increased levels of TLR4, NF-κB phosphorylation, and NLRP3 in the hippocampus and the increased levels of the pro-inflammatory cytokines IL-6, IL-8, and TNF-α. PF also reversed the increased Iba1-labeled microglia and COX-2 levels. PF reduced the release of pro-inflammatory cytokines through binding with TLR4/NF-κB/NLRP3 and alleviated neuronal injury by cytokines (Cheng et al., 2021). In some neuroinflammatory models, the anti-inflammatory effect of PF on the nervous system has been confirmed where it has been shown to inhibit the excessive activation of astrocytes and microglia induced by arterial occlusion (MCAO) and prevent the production of pro-inflammatory mediators. PF treatment can prevent TNFα-induced apoptosis (Guo et al., 2012). Apoptosis signal-regulated kinase 1 (ASK1) can activate p38, JNK and MKK, and induce apoptosis and inflammation (Liles et al., 2018). PF has also been found to mimic the ASK1 inhibitor NQDI1 and inhibit ASK1 phosphorylation thereby reducing astrocyte and microglia responses and lowering the expression of inflammatory factors such as IL-1β and TNF-α. In LPS-treated hippocampal slices, PF reversed hippocampal cell death and reduced NO and interleukin (IL)-1β production, while also reducing the release of pro-inflammatory factors such as tumor necrosis factor-α and IL-1β, thereby preventing nerve damage caused by inflammation (Zhou et al., 2019).
Hippocampal neurogenesis and brain-derived neurotrophic factor
Brain-derived neurotrophic factor (BDNF) is derived from neuronal cells in the brain. BDNF synthesis takes place in the areas involved in emotional and cognitive functions, such as the hippocampus and frontal areas (Phillips, 2017). BDNF reduction was also found in hippocampus of patients with depression (Kim et al., 2007; Birkenhäger et al., 2012). CUS and depressive-like symptoms are associated with a reduction in BDNF levels in the hippocampus and frontal cortex and decreased tropomycin receptor kinase B (TrkB) activity (Russo-Neustadt et al., 2001; Zhang et al., 2014). It was found that antidepressants enhanced BDNF levels in the hippocampus (Duman and Monteggia, 2006), neurogenesis (Caviedes et al., 2017), and the viability of hippocampal cells (Bergami et al., 2008). Antidepressants increase hippocampal neurogenesis (Hill et al., 2015) and studies have shown that serum- and glucocorticoid-inducible kinase 1 (SGK1) was related to depression, which is a mediator of the influence of cortisol on neurogenesis and glucocorticoid receptor (GR) function. It has also been shown that the level of SGK1 in the peripheral blood of patients with depression increases, and CORT reduces neuronal differentiation through SGK1-dependent mechanisms (Anacker et al., 2013). BDNF is a growth factor that affects the proliferation, differentiation, survival, and death of both neuronal and non-neuronal cells and also can control emotional and cognitive function. The level of BDNF in the neurons of patients with depression is very low. However, the expression of BDNF increases after taking antidepressants (Song M et al., 2017) suggesting that the deficiency of BDNF has an important impact on the physiology and pathology of patients with depression.
PF exerts a significant antidepressant effect by regulating hippocampal neurogenesis and BDNF levels
Long-term treatment with TGP (80 and 160 mg/kg, intragastrically) can reverse CUMS-induced depressive-like behavior in rats. PF increased the BDNF protein and mRNA levels in the hippocampus and frontal cortex of rats exposed to CUMS (Mao et al., 2010a). PF can reverse depression-like behaviors induced by CUMS and PF treatment prevents the reduction in dendritic spine density the expression of BDNF and postsynaptic density protein 95 (PSD95) in the hippocampus of these mice (Liu et al., 2019). In the acute depression animal model caused by forced swimming in rats, PF significantly increased rat serum and hippocampal levels of BDNF, and has a protective effect on hippocampal pathomorphology (Mu et al., 2020). PF can significantly increase the expression of hippocampal complex CA1 and phosphorylated CREB (p-CREB) in post-stroke depression (Hu et al., 2019) and significantly increases the rate of sucrose consumption and 5-bromo-2-deoxyuridine-positive cells in the dentate gyrus of rats induced by CUMS. In addition to the enhanced protein expression and gene transcription of BDNF, PF can also activate the expression of tropomyosin receptor kinase B (high-affinity receptor for BDNF) (Chen et al., 2019) and importantly can promote hippocampal neurogenesis and up-regulate BDNF levels and thus help to relieve the symptoms of depression (see Table 2).
Conclusion
To sum up, we mainly discussed two commonly used models of PF antidepressant. In the depression model, PF significantly improved the depression like behavior of mice, such as reducing the immobility time of FST and TST. In the neuron injury model, PF protected neuron damage caused by different toxins by inhibiting oxidative stress, neuronal apoptosis and modulation of ion channel etc.
Although many experiments have confirmed an antidepressant effect of PF, there are also a few questions. For example, PF is a hydrophilic compound with low fat solubility, and it has difficulty crossing the blood–brain barrier (Yang et al., 2018). Therefore, more experiments are needed in the future to find new neuroprotective effects of PF, explore new ways for PF to smoothly pass the blood–brain barrier, to improve the antidepressant effect of PF, and to optimize treatment regimens. It is also necessary to seek the optimal concentration and dose of PF for depression. Most importantly, PF can be used as a very effective treatment target for depression. We hope to make up for the deficiencies through experiments and apply PF to the clinical treatment of depression as soon as possible.
Author contributions
LW and WG wrote the first draft. LW and XY provided the organization and framework of the article. WY and RC provided critical revisions. All authors approved the final version of the manuscript for submission.
Funding
This work was supported by the Jilin Science and Technology Agency (20180520124JH, 20180519003JH, 20190701078GH, and 20180414050 GH) and a Jilin Province Medical and Health Talents grant (2019SCZT007).
Conflict of interest
The authors declare that the research was conducted in the absence of any commercial or financial relationships that could be construed as a potential conflict of interest.
Publisher’s note
All claims expressed in this article are solely those of the authors and do not necessarily represent those of their affiliated organizations, or those of the publisher, the editors and the reviewers. Any product that may be evaluated in this article, or claim that may be made by its manufacturer, is not guaranteed or endorsed by the publisher.
References
Anacker, C., Cattaneo, A., Musaelyan, K., Zunszain, P. A., Horowitz, M., Molteni, R., et al. (2013). Role for the kinase SGK1 in stress, depression, and glucocorticoid effects on hippocampal neurogenesis. Proc. Natl. Acad. Sci. U. S. A. 110, 8708–8713. doi:10.1073/pnas.1300886110
Arundine, M., and Tymianski, M. (2003). Molecular mechanisms of calcium-dependent neurodegeneration in excitotoxicity. Cell Calcium 34, 325–337. doi:10.1016/S0143-4160(03)00141-6
Benatti, C., Blom, M. C., Torta, R., Rigillo, G., Alboni, S., Zizzi, F., et al. (2016). Disease-induced neuroinflammation and depression. CNS Neurol. Disord. Drug Targets 15, 414–433. doi:10.2174/1871527315666160321104749
Bergami, M., Rimondini, R., Santi, S., and Blum, R. (2008). Deletion of TrkB in adult progenitors alters newborn neuron integration into hippocampal circuits and increases anxiety-like behavior. Biol. Sci. 105, 15570. doi:10.1073/pnas.0803702105
Bhatt, S., Nagappa, A. N., and Patil, C. R. (2020). Role of oxidative stress in depression. Drug Discov. Today 25, 1270–1276. doi:10.1016/j.drudis.2020.05.001
Birkenhäger, T. K., Geldermans, S., Van den Broek, W. W., van Beveren, N., and Fekkes, D. (2012). Serum brain-derived neurotrophic factor level in relation to illness severity and episode duration in patients with major depression. J. Psychiatr. Res. 46, 285–289. doi:10.1016/j.jpsychires.2011.12.006
Black, C. N., Bot, M., Scheffer, P. G., Cuijpers, P., and Penninx, B. W. J. H. (2015). Is depression associated with increased oxidative stress? A systematic review and meta-analysis. Psychoneuroendocrinology 51, 164–175. doi:10.1016/j.psyneuen.2014.09.025
Briley, M., and Chantal, M. (2011). The importance of norepinephrine in depression. Neuropsychiatr. Dis. Treat. 9–13. doi:10.2147/NDT.S19619
Brini, M., Calì, T., Ottolini, D., and Carafoli, E. (2014). Neuronal calcium signaling: function and dysfunction. Cell. Mol. Life Sci. 71, 2787–2814. doi:10.1007/s00018-013-1550-7
Calvo-Rodriguez, M., Hernando-Pérez, E., López-Vázquez, S., Núñez, J., Villalobos, C., and Núñez, L. (2020). Remodeling of intracellular Ca2+ homeostasis in rat hippocampal neurons aged in vitro. Int. J. Mol. Sci. 21, 1549. doi:10.3390/ijms21041549
Cao, B.-Y., Yang, Y.-P., Luo, W.-F., Mao, C.-J., Han, R., Sun, X., et al. (2010). Paeoniflorin, a potent natural compound, protects PC12 cells from MPP+ and acidic damage via autophagic pathway. J. Ethnopharmacol. 131, 122–129. doi:10.1016/j.jep.2010.06.009
Caviedes, A., Lafourcade, C., Soto, C., and Wyneken, U. (2017). BDNF/NF-κB signaling in the neurobiology of depression. Curr. Pharm. Des. 23, 3154–3163. doi:10.2174/1381612823666170111141915
Chen, C., Yin, Q., Tian, J., Gao, X., Qin, X., Du, G., et al. (2020). Studies on the potential link between antidepressant effect of Xiaoyao San and its pharmacological activity of hepatoprotection based on multi-platform metabolomics. J. Ethnopharmacol. 249, 112432. doi:10.1016/j.jep.2019.112432
Chen, L.-B., Qiu, F.-M., Zhong, X.-M., Hong, C., and Huang, Z. (2019). Promoting neurogenesis in hippocampal dentate gyrus of chronic unpredictable stress-induced depressive-like rats with paeoniflorin. J. Integr. Neurosci. 18, 43–49. doi:10.31083/j.jin.2019.01.116
Chen, M., Zhang, Q.-P., Zhu, J.-X., Cheng, J., Liu, Q., Xu, G.-H., et al. (2020). Involvement of FGF-2 modulation in the antidepressant-like effects of liquiritin in mice. Eur. J. Pharmacol. 881, 173297. doi:10.1016/j.ejphar.2020.173297
Cheng, J., Chen, M., Wan, H.-Q., Chen, X.-Q., Li, C.-F., Zhu, J.-X., et al. (2021). Paeoniflorin exerts antidepressant-like effects through enhancing neuronal FGF-2 by microglial inactivation. J. Ethnopharmacol. 274, 114046. doi:10.1016/j.jep.2021.114046
Chin, D., and Means, A. R. (2000). Calmodulin: a prototypical calcium sensor. Trends Cell Biol. 10, 322–328. doi:10.1016/S0962-8924(00)01800-6
Cong, C., Kluwe, L., Li, S., Liu, X., Liu, Y., Liu, H., et al. (2019). Paeoniflorin inhibits tributyltin chloride-induced apoptosis in hypothalamic neurons via inhibition of MKK4-JNK signaling pathway. J. Ethnopharmacol. 237, 1–8. doi:10.1016/j.jep.2019.03.030
DeCoster, M. A. (1995). Calcium dynamics in the central nervous system. Adv. Neuroimmunol. 5, 233–239. doi:10.1016/0960-5428(95)00015-T
Dong, H., Li, R., Yu, C., Xu, T., Zhang, X., and Dong, M. (2015). Paeoniflorin inhibition of 6-hydroxydopamine-induced apoptosis in PC12 cells via suppressing reactive oxygen species-mediated PKCδ/NF-κB pathway. Neuroscience 285, 70–80. doi:10.1016/j.neuroscience.2014.11.008
Duman, R. S., and Monteggia, L. M. (2006). A neurotrophic model for stress-related mood disorders. Biol. Psychiatry 59, 1116–1127. doi:10.1016/j.biopsych.2006.02.013
Duman, R. S. (2009). Neuronal damage and protection in the pathophysiology and treatment of psychiatric illness: stress and depression. Dialogues Clin. Neurosci. 11, 239–255. doi:10.31887/DCNS.2009.11.3/rsduman
Enache, D., Pariante, C. M., and Mondelli, V. (2019). Markers of central inflammation in major depressive disorder: A systematic review and meta-analysis of studies examining cerebrospinal fluid, positron emission tomography and post-mortem brain tissue. Brain Behav. Immun. 81, 24–40. doi:10.1016/j.bbi.2019.06.015
Fischer, S., Macare, C., and Cleare, A. J. (2017). Hypothalamic-pituitary-adrenal (HPA) axis functioning as predictor of antidepressant response–Meta-analysis. Neurosci. Biobehav. Rev. 83, 200–211. doi:10.1016/j.neubiorev.2017.10.012
Gao, L., Xie, Y., Jia, C., and Wang, W. (2020). Prevalence of depression among Chinese university students: a systematic review and meta-analysis. Sci. Rep. 10, 15897. doi:10.1038/s41598-020-72998-1
Ghavami, S., Shojaei, S., Yeganeh, B., Ande, S. R., Jangamreddy, J. R., Mehrpour, M., et al. (2014). Autophagy and apoptosis dysfunction in neurodegenerative disorders. Prog. Neurobiol. 112, 24–49. doi:10.1016/j.pneurobio.2013.10.004
Grützner, T. M., Listunova, L., Fabian, G. A., Kramer, B. A., Flach, D., Weisbrod, M., et al. (2018). Serum calcium levels and neuropsychological performance in depression and matched healthy controls: Reversal of correlation a marker of the aging cognitive clock? Psychoneuroendocrinology 91, 198–205. doi:10.1016/j.psyneuen.2018.03.012
Guo, R.-B., Wang, G.-F., Zhao, A.-P., Gu, J., Sun, X.-L., and Hu, G. (2012). Paeoniflorin protects against ischemia-induced brain damages in rats via inhibiting MAPKs/NF-κB-Mediated inflammatory responses. PLoS ONE 7, e49701. doi:10.1371/journal.pone.0049701
Haeberlein, S. L. B. (2004). Mitochondrial function in apoptotic neuronal cell death. Neurochem. Res. 29, 521–530. doi:10.1023/B:NERE.0000014823.74782.b7
Hill, A. S., Sahay, A., and Hen, R. (2015). Increasing adult hippocampal neurogenesis is sufficient to reduce anxiety and depression-like behaviors. Neuropsychopharmacology 40, 2368–2378. doi:10.1038/npp.2015.85
Hirschfeld, R. M. A. (2000). History and evolution of the monoamine hypothesis of depression. J. Clin. Psychiatry 3, 4–6.
Hu, M., Wang, A., Zhao, Z., Chen, X., Li, Y., and Liu, B. (2019). Antidepressant-like effects of paeoniflorin on post-stroke depression in a rat model. Neurol. Res. 41, 446–455. doi:10.1080/01616412.2019.1576361
Huang, H., Zhao, J., Jiang, L., Xie, Y., Xia, Y., Lv, R., et al. (2015). Paeoniflorin improves menopause depression in ovariectomized rats under chronic unpredictable mild stress. Int. J. Clin. Exp. Med. 8, 5103.
Huang, Z., Mao, Q.-Q., Zhong, X.-M., Li, Z.-Y., Qiu, F.-M., and Ip, S.-P. (2012). Mechanistic study on the antidepressant-like effect of danggui-shaoyao-san, a Chinese herbal formula. Evid. Based. Complement. Altern. Med. 2012, 173565–173567. doi:10.1155/2012/173565
Ising, C., and Heneka, M. T. (2018). Functional and structural damage of neurons by innate immune mechanisms during neurodegeneration. Cell Death Dis. 9, 120. doi:10.1038/s41419-017-0153-x
Judge, B. S., and Rentmeester, L. L. (2013). Antidepressant overdose–induced seizures. Psychiatr. Clin. North Am. 36, 245–260. doi:10.1016/j.psc.2013.02.004
Keller, J., Gomez, R., Williams, G., Lembke, A., Lazzeroni, L., Murphy, G. M., et al. (2017). HPA axis in major depression: cortisol, clinical symptomatology and genetic variation predict cognition. Mol. Psychiatry 22, 527–536. doi:10.1038/mp.2016.120
Kim, S.-H., Han, J., Seog, D.-H., Chung, J.-Y., Kim, N., Hong Park, Y., et al. (2005). Antidepressant effect of Chaihu-Shugan-San extract and its constituents in rat models of depression. Life Sci. 76, 1297–1306. doi:10.1016/j.lfs.2004.10.022
Kim, Y.-K., Lee, H.-P., Won, S.-D., Park, E.-Y., Lee, H.-Y., Lee, B.-H., et al. (2007). Low plasma BDNF is associated with suicidal behavior in major depression. Prog. Neuropsychopharmacol. Biol. Psychiatry 31, 78–85. doi:10.1016/j.pnpbp.2006.06.024
Li, H., Jiao, Y., and Xie, M. (2017). Paeoniflorin ameliorates atherosclerosis by suppressing TLR4-mediated NF-κB activation. Inflammation 40, 2042–2051. doi:10.1007/s10753-017-0644-z
Li, Y.-F., Zhang, Y.-Z., Liu, Y.-Q., Wang, H.-L., Cao, J.-B., Guan, T.-T., et al. (2006). Inhibition of N-methyl-D-aspartate receptor function appears to be one of the common actions for antidepressants. J. Psychopharmacol. 20, 629–635. doi:10.1177/0269881106059692
Li, Y., Zheng, X., Xia, S., Li, Y., Deng, H., Wang, X., et al. (2020). Paeoniflorin ameliorates depressive-like behavior in prenatally stressed offspring by restoring the HPA axis- and glucocorticoid receptor- associated dysfunction. J. Affect. Disord. 274, 471–481. doi:10.1016/j.jad.2020.05.078
Liles, J. T., Corkey, B. K., Notte, G. T., Budas, G. R., Lansdon, E. B., Hinojosa-Kirschenbaum, F., et al. (2018). ASK1 contributes to fibrosis and dysfunction in models of kidney disease. J. Clin. Invest. 128, 4485–4500. doi:10.1172/JCI99768
Lim, G. Y., Tam, W. W., Lu, Y., Ho, C. S., Zhang, M. W., and Ho, R. C. (2018). Prevalence of depression in the community from 30 countries between 1994 and 2014. Sci. Rep. 8, 2861. doi:10.1038/s41598-018-21243-x
Liu, J., Liu, L., Han, Y., Yi, J., Guo, C., Zhao, H., et al. (2021). The molecular mechanism underlying mitophagy-mediated hippocampal neuron apoptosis in diabetes-related depression. J. Cell. Mol. Med. 25, 7342–7353. doi:10.1111/jcmm.16763
Liu, S.-C., Hu, W.-Y., Zhang, W.-Y., Yang, L., Li, Y., Xiao, Z.-C., et al. (2019). Paeoniflorin attenuates impairment of spatial learning and hippocampal long-term potentiation in mice subjected to chronic unpredictable mild stress. Psychopharmacology 236, 2823–2834. doi:10.1007/s00213-019-05257-5
Liu, Y., Zhao, J., and Guo, W. (2018). Emotional roles of mono-aminergic neurotransmitters in major depressive disorder and anxiety disorders. Front. Psychol. 9, 2201. doi:10.3389/fpsyg.2018.02201
Lucassen, P., Heine, V., Muller, M., van der Beek, E., Wiegant, V., Ron De Kloet, E., et al. (2006). Stress, depression and hippocampal apoptosis. CNS Neurol. Disord. Drug Targets 5, 531–546. doi:10.2174/187152706778559273
Lucena, M. I., Carvajal, A., Andrade, R. J., and Velasco, A. (2003). Antidepressant-induced hepatotoxicity. Expert Opin. Drug Saf. 14, 249–262. doi:10.1517/14740338.2.3.249
Mao, Q.-Q., Ip, S.-P., Ko, K.-M., Tsai, S.-H., and Che, C.-T. (2009a). Peony glycosides produce antidepressant-like action in mice exposed to chronic unpredictable mild stress: Effects on hypothalamic-pituitary-adrenal function and brain-derived neurotrophic factor. Prog. Neuropsychopharmacol. Biol. Psychiatry 33, 1211–1216. doi:10.1016/j.pnpbp.2009.07.002
Mao, Q.-Q., Ip, S.-P., Ko, K.-M., Tsai, S.-H., Xian, Y.-F., and Che, C.-T. (2009b). Effects of peony glycosides on mice exposed to chronic unpredictable stress: Further evidence for antidepressant-like activity. J. Ethnopharmacol. 124, 316–320. doi:10.1016/j.jep.2009.04.019
Mao, Q.-Q., Ip, S.-P., Ko, K.-M., Tsai, S.-H., Zhao, M., and Che, C.-T. (2009c). Peony glycosides protect against corticosterone-induced neurotoxicity in PC12 cells. Cell. Mol. Neurobiol. 29, 643–647. doi:10.1007/s10571-009-9357-7
Mao, Q.-Q., Ip, S.-P., Tsai, S.-H., and Che, C.-T. (2008). Antidepressant-like effect of peony glycosides in mice. J. Ethnopharmacol. 119, 272–275. doi:10.1016/j.jep.2008.07.008
Mao, Q.-Q., Xian, Y.-F., Ip, S.-P., Tsai, S.-H., and Che, C.-T. (2010a). Long-term treatment with peony glycosides reverses chronic unpredictable mild stress-induced depressive-like behavior via increasing expression of neurotrophins in rat brain. Behav. Brain Res. 7, 171–177. doi:10.1016/j.bbr.2010.02.026
Mao, Q.-Q., Xian, Y.-F., Ip, S.-P., Tsai, S.-H., and Che, C.-T. (2011a). Protective effects of peony glycosides against corticosterone-induced cell death in PC12 cells through antioxidant action. J. Ethnopharmacol. 133, 1121–1125. doi:10.1016/j.jep.2010.11.043
Mao, Q.-Q., Zhong, X.-M., Feng, C.-R., Pan, A.-J., Li, Z.-Y., and Huang, Z. (2010b). Protective effects of paeoniflorin against glutamate-induced neurotoxicity in PC12 cells via antioxidant mechanisms and Ca2+ antagonism. Cell. Mol. Neurobiol. 30, 1059–1066. doi:10.1007/s10571-010-9537-5
Mao, Q.-Q., Zhong, X.-M., Li, Z.-Y., and Huang, Z. (2011b). Paeoniflorin protects against NMDA-induced neurotoxicity in PC12 cells via Ca 2+ antagonism: NEUROPROTECTIVE EFFECT OF PAEONIFLORIN. Phytother. Res. 25, 681–685. doi:10.1002/ptr.3321
Mao, Q.-Q., Zhong, X.-M., Qiu, F.-M., Li, Z.-Y., and Huang, Z. (2012). Protective effects of paeoniflorin against corticosterone-induced neurotoxicity in PC12 cells: Neuroprotective effect of paeoniflorin. Phytother. Res. 26, 969–973. doi:10.1002/ptr.3673
Mata, A. M., and Sepúlveda, M. R. (2005). Calcium pumps in the central nervous system. Brain Res. Brain Res. Rev. 49, 398–405. doi:10.1016/j.brainresrev.2004.11.004
Mayer, B., and Oberbauer, R. (2003). Mitochondrial regulation of apoptosis. News Physiol. Sci. 18, 89–94. doi:10.1152/nips.01433.2002
McCarron, R. M., Shapiro, B., Rawles, J., and Luo, J. (2021). Depression. Ann. Intern. Med. 174, ITC65–ITC80. doi:10.7326/AITC202105180
Mu, D., Xue, M., Xu, J., Hu, Y., Chen, Y., Ren, P., et al. (2020). Antidepression and prokinetic effects of paeoniflorin on rats in the forced swimming test via polypharmacology. Evid. Based. Complement. Altern. Med. 2020, 2153571–2153610. doi:10.1155/2020/2153571
Nedergaard, M., Rodríguez, J. J., and Verkhratsky, A. (2010). Glial calcium and diseases of the nervous system. Cell Calcium 47, 140–149. doi:10.1016/j.ceca.2009.11.010
Neigh, G. N., and Nemeroff, C. B. (2006). Reduced glucocorticoid receptors: consequence or cause of depression? Trends Endocrinol. Metab. 17, 124–125. doi:10.1016/j.tem.2006.03.002
Nettis, M. A., and Pariante, C. M. (2020). “Is there neuroinflammation in depression? Understanding the link between the brain and the peripheral immune system in depression,” in International review of neurobiology (Netherland: Elsevier), 23–40. doi:10.1016/bs.irn.2019.12.004
Nutt, D. J. (2008). Relationship of neurotransmitters to the symptoms of major depressive disorder. J. Clin. Psychiatry 4.
Ouyang, J., Xu, H., Li, M., Dai, X., Fu, F., Zhang, X., et al. (2017). Paeoniflorin exerts antitumor effects by inactivating S phase kinase-associated protein 2 in glioma cells. Oncol. Rep. 39, 1052–1062. doi:10.3892/or.2017.6175
Park, S., and Ishino, R. (2013). Liver injury associated with antidepressants. Curr. Drug Saf. 8, 207–223. doi:10.2174/1574886311308030011
Pearsonmurphy, B. (1991). General review steroids and depression. J Steroid Biochem Molecular Bio 38.537 doi:10.1016/0960-0760(91)90312-S
Pei, G., Xu, L., Huang, W., and Yin, J. (2020). The protective role of microRNA-133b in restricting hippocampal neurons apoptosis and inflammatory injury in rats with depression by suppressing CTGF. Int. Immunopharmacol. 78, 106076. doi:10.1016/j.intimp.2019.106076
Phillips, C. (2017). Brain-derived neurotrophic factor, depression, and physical activity: Making the neuroplastic connection. Neural Plast. 17, 7260130. doi:10.1155/2017/7260130
Pitsillou, E., Bresnehan, S. M., Kagarakis, E. A., Wijoyo, S. J., Liang, J., Hung, A., et al. (2020). The cellular and molecular basis of major depressive disorder: towards a unified model for understanding clinical depression. Mol. Biol. Rep. 47, 753–770. doi:10.1007/s11033-019-05129-3
Qiu, F., Zhong, X.-M., Mao, Q.-Q., and Huang, Z. (2013b). Antidepressant-like effects of paeoniflorin on the behavioural, biochemical, and neurochemical patterns of rats exposed to chronic unpredictable stress. Neurosci. Lett. 541, 209–213. doi:10.1016/j.neulet.2013.02.029
Qiu, F., Zhong, X., Mao, Q., and Huang, Z. (2013a). The antidepressant-like effects of paeoniflorin in mouse models. Exp. Ther. Med. 5, 1113–1116. doi:10.3892/etm.2013.925
Rothschild, S. C., and Tombes, R. M. (2020). “Widespread roles of CaMK-II in developmental pathways,” in Calcium signaling advances in experimental medicine and biology. Editor Md. S. Islam (Cham: Springer International Publishing), 519–535. doi:10.1007/978-3-030-12457-1_21
Russo-Neustadt, A., Ha, T., Ramirez, R., and Kesslak, J. P. (2001). Physical activity–antidepressant treatment combination: impact on brain-derived neurotrophic factor and behavior in an animal model. Behav. Brain Res. 120, 87–95. doi:10.1016/S0166-4328(00)00364-8
Schoenfeld, T. J., McCausland, H. C., Morris, H. D., Padmanaban, V., and Cameron, H. A. (2017). Stress and loss of adult neurogenesis differentially reduce hippocampal volume. Biol. Psychiatry 82, 914–923. doi:10.1016/j.biopsych.2017.05.013
Seo, J.-S., Wei, J., Qin, L., Kim, Y., Yan, Z., and Greengard, P. (2017). Cellular and molecular basis for stress-induced depression. Mol. Psychiatry 22, 1440–1447. doi:10.1038/mp.2016.118
Shabbir, F., Patel, A., Mattison, C., Bose, S., Krishnamohan, R., Sweeney, E., et al. (2013). Effect of diet on serotonergic neurotransmission in depression. Neurochem. Int. 62, 324–329. doi:10.1016/j.neuint.2012.12.014
Shifman, J. M., Choi, M. H., Mihalas, S., Mayo, S. L., and Kennedy, M. B. (2006). Ca 2+/calmodulin-dependent protein kinase II (CaMKII) is activated by calmodulin with two bound calciums. Proc. Natl. Acad. Sci. U. S. A. 103, 13968–13973. doi:10.1073/pnas.0606433103
Simard, S., Shail, P., MacGregor, J., El Sayed, M., Duman, R. S., Vaccarino, F. M., et al. (2018). Fibroblast growth factor 2 is necessary for the antidepressant effects of fluoxetine. PLoS ONE 13, e0204980. doi:10.1371/journal.pone.0204980
Song, C., Wang, J., Gao, D., Yu, Y., Li, F., Wei, S., et al. (2017). Paeoniflorin, the main active ingredient of shuyu capsule, inhibits Ca v 1.2 and regulates calmodulin/calmodulin-dependent protein kinase II signalling. Biomed. Res. Int. 20171, 8459287. doi:10.1155/2017/8459287
Song, M., Martinowich, K., and Lee, F. S. (2017). BDNF at the synapse: why location matters. Mol. Psychiatry 22, 1370–1375. doi:10.1038/mp.2017.144
Szebeni, A., Szebeni, K., DiPeri, T. P., Johnson, L. A., Stockmeier, C. A., Crawford, J. D., et al. (2016). Elevated DNA oxidation and DNA repair enzyme expression in brain white matter in major depressive disorder. Int. J. Neuropsychopharmacol. 20, 363–373. doi:10.1093/ijnp/pyw114
Takemoto-Kimura, S., Suzuki, K., Horigane, S., Kamijo, S., Inoue, M., Sakamoto, M., et al. (2017). Calmodulin kinases: essential regulators in health and disease. J. Neurochem. 141, 808–818. doi:10.1111/jnc.14020
Tao, Y., Wen, Z., Song, Y., and Wang, H. (2016). Paeoniflorin attenuates hepatic ischemia/reperfusion injury via anti-oxidative, anti-inflammatory and anti-apoptotic pathways. Exp. Ther. Med. 11, 263–268. doi:10.3892/etm.2015.2902
Tian, R.-H., Bai, Y., Li, J.-Y., and Guo, K.-M. (2019). Reducing PRLR expression and JAK2 activity results in an increase in BDNF expression and inhibits the apoptosis of CA3 hippocampal neurons in a chronic mild stress model of depression. Brain Res. 1725, 146472. doi:10.1016/j.brainres.2019.146472
Troubat, R., Barone, P., Leman, S., Desmidt, T., Cressant, A., Atanasova, B., et al. (2021). Neuroinflammation and depression: A review. Eur. J. Neurosci. 53, 151–171. doi:10.1111/ejn.14720
Vaváková, M., Ďuračková, Z., and Trebatická, J. (2015). Markers of oxidative stress and neuroprogression in depression disorder. Oxid. Med. Cell. Longev. 2015, 898393–898412. doi:10.1155/2015/898393
Wang, A., Mi, L., Zhang, Z., Hu, M., Zhao, Z., Liu, B., et al. (2021a). Saikosaponin A improved depression-like behavior and inhibited hippocampal neuronal apoptosis after cerebral ischemia through p-CREB/BDNF pathway. Behav. Brain Res. 403, 113138. doi:10.1016/j.bbr.2021.113138
Wang, D., Tan, Q.-R., and Zhang, Z.-J. (2013a). Neuroprotective effects of paeoniflorin, but not the isomer albiflorin, are associated with the suppression of intracellular calcium and calcium/calmodulin protein kinase II in PC12 cells. J. Mol. Neurosci. 51, 581–590. doi:10.1007/s12031-013-0031-7
Wang, D., Wong, H. K., Feng, Y.-B., and Zhang, Z.-J. (2013b). Paeoniflorin, a natural neuroprotective agent, modulates multiple anti-apoptotic and pro-apoptotic pathways in differentiated PC12 cells. Cell. Mol. Neurobiol. 33, 521–529. doi:10.1007/s10571-013-9914-y
Wang, X.-L., Feng, S.-T., Wang, Y.-T., Chen, N.-H., Wang, Z.-Z., and Zhang, Y. (2021b). Paeoniflorin: A neuroprotective monoterpenoid glycoside with promising anti-depressive properties. Phytomedicine. 90, 153669. doi:10.1016/j.phymed.2021.153669
Willaime-Morawek, S., Brami-Cherrier, K., Mariani, J., Caboche, J., and Brugg, B. (2003). C-jun N-terminal kinases/c-Jun and p38 pathways cooperate in ceramide-induced neuronal apoptosis. Neuroscience 119, 387–397. doi:10.1016/S0306-4522(02)00996-X
Wittchen, H.-U., Knäuper, B., and Kessler, R. C. (1994). Lifetime risk of depression. Br. J. Psychiatry 165, 16–22. doi:10.1192/S0007125000293240
Wu, L.-L., Liu, Y., Yan, C., Pan, Y., Su, J.-F., and Wu, W.-K. (2016). Antidepressant-like effects of fractions prepared from danzhi-xiaoyao-san decoction in rats with chronic unpredictable mild stress: Effects on hypothalamic-pituitary-adrenal Axis, arginine vasopressin, and neurotransmitters. Evid. Based. Complement. Altern. Med. 2016, 6784689–6784711. doi:10.1155/2016/6784689
Wu, Z., Xiao, L., Wang, H., and Wang, G. (2021). Neurogenic hypothesis of positive psychology in stress-induced depression: Adult hippocampal neurogenesis, neuroinflammation, and stress resilience. Int. Immunopharmacol. 97, 107653. doi:10.1016/j.intimp.2021.107653
Xu, F., Peng, D., Tao, C., Yin, D., Kou, J., Zhu, D., et al. (2011). Anti-depression effects of Danggui-Shaoyao-San, a fixed combination of Traditional Chinese Medicine, on depression model in mice and rats. Phytomedicine 18, 1130–1136. doi:10.1016/j.phymed.2011.05.002
Yang, B., Du, S., Lu, Y., Jia, S., Zhao, M., Bai, J., et al. (2018). Influence of paeoniflorin and menthol on puerarin transport across MDCK and MDCK-MDR1 cells as blood–brain barrier in vitro model. J. Pharm. Pharmacol. 70, 349–360. doi:10.1111/jphp.12853
Yang, X., Yao, W., Shi, H., Liu, H., Li, Y., Gao, Y., et al. (2016). Paeoniflorin protects Schwann cells against high glucose induced oxidative injury by activating Nrf2/ARE pathway and inhibiting apoptosis. J. Ethnopharmacol. 185, 361–369. doi:10.1016/j.jep.2016.03.031
Yun-Feng, L., Yan-Qin, L., Wen-Chao, H., and Zhi-Pu, L. (2003). Cytoprotective effect is one of common action pathways for antidepressants. Acta Pharmacol. Sin. 5.
Zhang, L., and Wei, W. (2020). Anti-inflammatory and immunoregulatory effects of paeoniflorin and total glucosides of paeony. Pharmacol. Ther. 207, 107452. doi:10.1016/j.pharmthera.2019.107452
Zhang, R., Peng, Z., Wang, H., Xue, F., Chen, Y., Wang, Y., et al. (2014). Gastrodin ameliorates depressive-like behaviors and up-regulates the expression of BDNF in the Hippocampus and hippocampal-derived astrocyte of rats. Neurochem. Res. 8, 172–179. doi:10.1007/s11064-013-1203-0
Zhang, Y., Qiao, L., Xu, W., Wang, X., Li, H., Xu, W., et al. (2017). Paeoniflorin attenuates cerebral ischemia-induced injury by regulating Ca2+/CaMKII/CREB signaling pathway. Molecules 22, 359. doi:10.3390/molecules22030359
Zhao, Y., Zhou, G., Wang, J., Jia, L., Zhang, P., Li, R., et al. (2013). Paeoniflorin protects against ANIT-induced cholestasis by ameliorating oxidative stress in rats. Food Chem. Toxicol. 58, 242–248. doi:10.1016/j.fct.2013.04.030
Zhong, S.-Z., Ge, Q.-H., Li, Q., Qu, R., and Ma, S.-P. (2009). Peoniflorin attentuates Abeta((1-42))-mediated neurotoxicity by regulating calcium homeostasis and ameliorating oxidative stress in hippocampus of rats. J. Neurol. Sci. 280, 71–78. doi:10.1016/j.jns.2009.01.027
Zhong, X., Li, G., Qiu, F., and Huang, Z. (2019). Paeoniflorin ameliorates chronic stress-induced depression-like behaviors and neuronal damages in rats via activation of the ERK-CREB pathway. Front. Psychiatry 9, 772. doi:10.3389/fpsyt.2018.00772
Zhou, D., Zhang, S., Hu, L., Gu, Y.-F., Cai, Y., Wu, D., et al. (2019). Inhibition of apoptosis signal-regulating kinase by paeoniflorin attenuates neuroinflammation and ameliorates neuropathic pain. J. Neuroinflammation 16, 83. doi:10.1186/s12974-019-1476-6
Keywords: depression, paeoniflorin, antidepressant, neuroprotection, apoptosis, neuroinflammation
Citation: Guo W, Yao X, Cui R, Yang W and Wang L (2023) Mechanisms of paeoniaceae action as an antidepressant. Front. Pharmacol. 13:934199. doi: 10.3389/fphar.2022.934199
Received: 02 May 2022; Accepted: 05 August 2022;
Published: 08 February 2023.
Edited by:
Fushun Wang, Nanjing University of Chinese Medicine, ChinaReviewed by:
Razia Khanam, Gulf Medical University, United Arab EmiratesZhe Shi, Hunan University of Chinese Medicine, China
Copyright © 2023 Guo, Yao, Cui, Yang and Wang. This is an open-access article distributed under the terms of the Creative Commons Attribution License (CC BY). The use, distribution or reproduction in other forums is permitted, provided the original author(s) and the copyright owner(s) are credited and that the original publication in this journal is cited, in accordance with accepted academic practice. No use, distribution or reproduction is permitted which does not comply with these terms.
*Correspondence: Wei Yang, d3lhbmcyMDAyQGpsdS5lZHUuY24=; Lei Wang, OTk5NjE4NjRAcXEuY29t