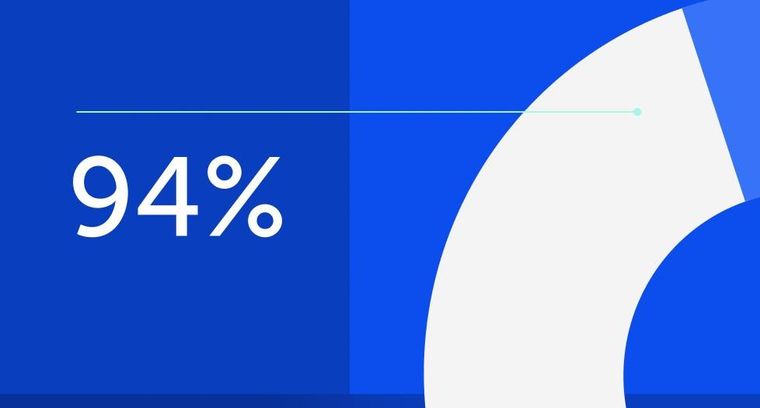
94% of researchers rate our articles as excellent or good
Learn more about the work of our research integrity team to safeguard the quality of each article we publish.
Find out more
REVIEW article
Front. Pharmacol., 25 August 2022
Sec. Experimental Pharmacology and Drug Discovery
Volume 13 - 2022 | https://doi.org/10.3389/fphar.2022.927653
This article is part of the Research TopicFibrosis: Etiology, Pathophysiology, Measurements, and TherapyView all 19 articles
Pulmonary fibrosis (PF) is a group of interstitial lung diseases that seriously endanger human life and health. Despite the current advances in research on the pathogenesis and treatment of PF, the overall quality of survival and survival rates of PF patients remain low, prompting the search for more effective therapeutic approaches. Exosomes are nanoscale vesicles with diameters ranging from approximately 30–150 nm, capable of transporting a variety of molecules in the body and mediating intercellular communication. There is an increasing number of studies focusing on the role of exosomes in PF. This review demonstrates the significance of exosomes in the pathogenesis, diagnosis, and treatment of PF. Exosomes are able to influence inflammatory, immune, and extracellular matrix deposition processes in PF and regulate the corresponding cytokines. Some exosomes detected in sputum, blood, and bronchoalveolar lavage fluid may be used as potential diagnostic and prognostic biomarkers for PF. Exosomes derived from several cells, such as mesenchymal stem cells, have demonstrated potential as PF therapeutic agents. Drug delivery systems using exosomes may also provide new insights into PF therapy.
Pulmonary fibrosis (PF) is an irreversible structural and functional change in the lung caused by excessive deposition of extra-alveolar matrix proteins resulting in fibrotic remodeling and alveolar destruction. PF is a severe risk to human life and health, with a median survival time of only 3–5 years after diagnosis of idiopathic pulmonary fibrosis (IPF). The pathogenesis of PF involves an inflammatory environment, oxidative stress, ageing, epigenetic and genetic and epithelial-mesenchymal transition (EMT) (Sundarakrishnan et al., 2018; Kinoshita and Goto, 2019; Yanagihara et al., 2020).
There are limited drugs available for the treatment of PF. Pirfenidone and nintedanib are commonly used in the treatment of PF. Although these two drugs can improve lung function and reduce clinical symptoms to some extent, they do not have significant advantages in improving survival and stopping or even reversing disease progression. And there are also uncertainties in the use of these drugs in terms of treatment duration, initial selection, etc., (Sgalla et al., 2018; Sgalla et al., 2020).
As exosomes have been refined in recent years, more and more studies have uncovered the significance of exosomes in the development of disease, and exosomes are expected to be a safe emerging method for the diagnosis and treatment of PF (Guiot et al., 2019; Xie and Zeng, 2020).
Exosomes are nanoscale vesicles ranging from approximately 30–150 nm in diameter, produced and released by most cells, and are a subtype of extracellular vesicles (EVs) (Yang D. et al., 2020). Exosomes can be found in body fluids such as blood, saliva, amniotic fluid, urine, semen, bronchial fluid, and cerebrospinal fluid (Doyle and Wang, 2019). The discovery of exosomes dates back to the 1980s when exosomes were first identified in reticulocytes (Harding et al., 1983; Pan et al., 1985; Johnstone et al., 1987), and were initially thought of simply as a means of removing unwanted cellular components (Johnstone, 1992). With the intensive study of exosomes, they are now considered to be involved in the regulation of intercellular communication, playing specific roles in biological processes such as immune response (Anel et al., 2019), antigen presentation (Arima et al., 2019), cell differentiation (Lloret-Llinares et al., 2018) and tumour invasion (Zhang and Yu, 2019; Rezaie et al., 2022).
The exosome is enclosed by a lipid bilayer and carries a variety of biologically active substances such as proteins, lipids, and nucleic acids (Wortzel et al., 2019) (Figure 1). The lipids of the exosome contain sphingolipids, phosphatidylserine, cholesterol, etc., (De Abreu et al., 2020). The nucleic acids carried by exosomes are divided into DNA and RNA, and RNA can include mRNA, microRNA (miRNA), tRNA, etc., (Keshtkar et al., 2021). Most exosomal proteins are associated with their formation and secretion, such as tetraspanins (e.g., CD9, CD63, CD81), heat shock proteins (e.g., HSP70, HSP90), TSG101, Alix, Rab GTPase, and Integrin. Furthermore, there are exosome-specific proteins secreted by specific cells, such as MHC-II from dendritic cells (Janockova et al., 2021; Liu et al., 2021).
Exosome production is associated with the endosomal system. Early-sorting endosome (ESE) formation is caused by invagination of the plasma membrane and endocytosis of the extracellular component cell surface proteins (Kalluri and LeBleu, 2020). The endoplasmic reticulum, Golgi complex, and mitochondria can participate in the maturation of the ESE (Hoyer et al., 2018; Shi et al., 2018; Amari and Germain, 2021). ESE can mature into late-sorting endosome (LSE) and eventually produce multivesicular bodies (MVBs, also known as MVEs) (Hessvik and Llorente, 2018). During this process, the endosomal membrane invaginates to produce intraluminal vesicles (ILVs), and MVBs contain a large number of ILVs (Abels and Breakefield, 2016). A portion of MVBs can be degraded by autophagosomal or lysosomal fusion, and a portion of MVBs fuse with the plasma membrane to release the contained ILVs as exosomes to be taken up and function extracellularly (Hassanpour et al., 2020) (Figure 2).
FIGURE 2. Biogenesis, secretion and uptake of exosome. Note: ESE, early-sorting endosome; LSE, late-sorting endosome; MVB, multivesicular body; ILV, intraluminal vesicle; ESCRT, endosomal sorting complex required for transport; SNARE, soluble N -ethylmaleimide-sensitive factor attachment protein receptor.
There are currently thought to be two pathways that regulate the formation of ILVs, one involving the endosomal sorting complex required for transport (ESCRT) and the other independent of ESCRT (Osaki and Okada, 2019). ESCRT is mainly composed of four complexes (ESCRT-0, ESCRT-I, ESCRT-II, and ESCRT-III) and associated proteins (e.g., VPS4, ALIX, TSG101) (Larios et al., 2020). ESCRT plays a role in ubiquitin-mediated and ubiquitin-independent cargo recognition and sorting and can induce membrane deformation, with substrates of the ESCRT pathway ultimately available in translocated to ILVs (Frankel and Audhya, 2018).
Another pathway regulating the formation of ILVs involves mainly ceramide and tetraspanins. The exosomes contain ceramides, cholesterol, sphingomyelin, and tetraspanins (Toh et al., 2018). Ceramide is essential for exosome formation and secretion, but the exact mechanism is unclear. Ceramide has been found that may promote the inward budding of MVBs to produce ILVs by affecting membrane curvature (Elsherbini and Bieberich, 2018). Ceramides, cholesterol, and sphingolipids are involved in forming lipid rafts (Wei et al., 2021). Lipid rafts play an essential function in membrane budding, may contribute to the assembly of proteins or other molecules into endosomes, and can regulate the formation of epidermal growth factor receptor-containing ILVs (Li et al., 2018; Wei et al., 2021). Tetraspanins have been shown to regulate the transport of selectively associated proteins and their function through membrane compartmentalization and play a role in membrane fusion and the production of ILVs (Böker et al., 2018; Ghossoub et al., 2020).
Exosomes contain unique contents of nucleic acids, proteins, and lipids, and the sorting mechanism of these exosomal cargoes is closely related to the ILVs formation pathway described above. Exosomal miRNAs play an essential role in intercellular communication. The type and amount of miRNAs loaded into exosomes depend on the type of parental cells, cell-specific sorting mechanisms, and stimuli such as inflammation (Wozniak et al., 2020; Xie et al., 2022). According to current studies, the potential modes of miRNA sorting into exosomes can be summarized as the following: the neural sphingomyelinase 2-dependent pathway, the sumoylated heterogeneous nuclear ribonucleoproteins-dependent pathway, the 3′miRNA sequence-dependent pathway, and the miRNA-induced silencing complex-associated pathway. In addition, other membrane proteins (e.g., Vps4A), RNA-binding proteins (e.g., Argonaute 2, YB-1), and lipid rafts have also been associated with miRNA sorting (Wei H. et al., 2021; Xie et al., 2022).
The release of exosomes involves the translocation of MVBs to the plasma membrane and the fusion of MVBs with the plasma membrane. MVBs fuse with the plasma membrane and release the contained LIVs into the extracellular space as exosomes via exocytosis. The release of exosomes is currently thought to be associated with the cytoskeleton, Rab GTPase, and the soluble N-ethylmaleimide-sensitive factor attachment protein receptor (SNARE) (Hessvik and Llorente, 2018). The cytoskeleton regulates the translocation of MVBs to the plasma membrane, depending mainly on the interaction between actin and the microtubule cytoskeleton (Villarroya-Beltri et al., 2014; Gurunathan et al., 2021). Rab GTPases play a role in vesicle transport with the main functions of regulating vesicle budding, vesicle transport along with the cytoskeleton, and membrane fusion (Jin et al., 2021). For example, Rab11 facilitates the transportation of MVBs and the fusion of MVBs with the plasma membrane (Savina et al., 2005; Messenger et al., 2018), and Rab27 can regulate the docking of MVBs with the plasma membrane (Song et al., 2019), and Rab35 is a critical factor in regulating the transport of MVBs (Yang et al., 2019). SNARE is a central mechanism that mediates membrane fusion (Wang et al., 2017). It has been found to facilitate the docking of MVBs to the plasma membrane and thus contribute to exosome release (Yu et al., 2020).
After releasing outside the cell, exosomes are taken up and acted upon by the recipient cells mainly through membrane fusion and endocytosis (Gonda et al., 2019). The exosome uptake mechanism largely depends on the recipient cell (Horibe et al., 2018).
In recent years, an increasing number of studies have focused on the role played by exosomes in PF. The initiation of PF is associated with lung injury and impaired repair, with multiple causes of lung epithelial cell injury followed by inflammatory cell recruitment and massive pro-inflammatory cytokine release, triggering an immune response. Monocytes and macrophages can drive fibrosis through an over-repair response to lung injury. The pro-fibrotic mediators act on lung fibroblasts, causing excessive proliferation and differentiation of fibroblasts, which in turn promotes the proliferation of myofibroblasts and the production of extracellular matrix (ECM), ultimately leading to PF pathological features such as excessive ECM deposition in lung tissue (Mei et al., 2021) (Figure 3). It has been reported in several publications that exosomes can influence inflammation and immunity, fibroblast proliferation and differentiation, ECM deposition, and other aspects of the PF disease process. The functions of exosomes reported so far in PF are highlighted in Figure 4 and discussed in more detail in subsequent sections.
FIGURE 3. Direct effects of regulatory immune cells, fibroblasts and extracellular matrix in pulmonary fibrosis. Note: ECM, extracellular matrix.
FIGURE 4. This figure summarizes the role of regulatory exosomes in PF. Mechanism investigation revealed that exosomes secreted by different kinds of cells, eg: human amnion epithelial cell (HAEC), Menstrual Blood-Derived Endometrial Stem Cell (MenSC), Mesenchymal stem cells (MSC), Human umbilical cord mesenchymal stem cell (HUCMSC), Bone marrow mesenchymal stem cell (BMSC), Pluripotent stem cell (iPSC), Lung spheroid cell(LSC), Vascular endothelial cell (EnC), Macrophage, M2 macrophages. The exosomes transmitted protein cargo and microRNAs regulated MAPK, NF-kb, PI3k/Akt, Wnt/β-catenin, TGFβRI/FoxM1/Smad/β-catenin, HIF-1α/Notch1/PDGFRβ/YAP1/ Twist1 signaling pathways. Finally these pathways assess their interaction with epithelial-mesenchymal transition, apoptosis and proliferation in contradicting findings.
Fibrosis is the result of excessive trauma repair and tissue remodeling, which is considered to be chronic inflammation and eventual fibrosis caused by epithelial injury (Heukels et al., 2019). Inflammatory stimulation or persistence of the inflammatory state is an essential condition for the development of PF. Following injury, damaged epithelium and recruited inflammatory cells secrete a variety of mediators, including transforming growth factor-β (TGF-β) to regulate epithelial repair and promote fibroblast recruitment and myofibroblast activation (Hewlett et al., 2018).
Macrophages can polarize to M1 and M2 phenotypes after different environmental and cytokine stimuli. Under chronic inflammatory conditions, pro-inflammatory M1 macrophages slowly convert to the more anti-inflammatory M2 phenotype, secreting mediators that promote wound healing. M2-type macrophages regulate fibrosis by secreting various growth factors (including TGF-β) and other mechanisms (Heukels et al., 2019).
Oxidative stress and inflammation are closely linked and play a pro-fibrotic role in the fibrotic process. Under sustained stimulation, abnormally functioning mitochondria overproduce reactive oxygen species (ROS) (Lv et al., 2019). ROS can be involved in inflammation by affecting NF-κB signaling, TNF signaling, and so on (Blaser et al., 2016). In addition, abnormal production and accumulation of ROS can lead to cellular damage at the level of nucleic acids, proteins, lipids, and carbohydrates, thus participating in the pathogenesis of PF (Cameli et al., 2020).
Exosomes can down-regulate inflammatory cell infiltration during PF disease and participate in the regulation of PF disease through the mediation of some classical inflammatory pathways such as NF-κB, MAPK, and other pathways. In these studies, miRNAs contained in exosomes such as miR-23a-3p and miR-182-5p could play an important role in the regulation of PF (Tan et al., 2018; Royce et al., 2019; Xiao et al., 2020; Kaur et al., 2021). Exosomes can also regulate PF by affecting ROS. For example, exosomal let-7 exerts an anti-PF effect by regulating ROS and mitochondrial DNA damage (Sun et al., 2019).
Some studies have focused on the effect of exosomes on the differentiation and proliferation of immune cells. In these studies, exosomes could alleviate PF by modulating the phenotypic switch of monocytes and macrophages, enhancing macrophage phagocytosis, and inhibiting T cell proliferation (Tan et al., 2018; Mansouri et al., 2019; Zhou et al., 2021). For example, exosomes from induced pluripotent stem cells (iPSCs) attenuate PF by suppressing M2-type macrophages during PF through the miR-302a-3p/TET1 axis (Zhou et al., 2021).
Macrophages can participate in the progression of PF through their secreted exosomes. Macrophage-derived exosomes promote differentiation, proliferation, and migration of myofibroblasts after exposure to silica (Qin et al., 2021). In bleomycin-induced PF, one study found that macrophage-derived exosomes mediated intercellular communication between macrophages and fibroblasts by transferring angiotensin II type 1 receptors to lung fibroblasts (Sun N. N. et al., 2021). Another study showed that the M2 macrophage-derived exosome miRNA-328 enhances lung interstitial fibroblast proliferation and promotes PF by regulating FAM13A (Yao et al., 2019).
Excessive ECM deposition is a characteristic feature of PF. The large number of cytokines produced after epithelial injury in PF stimulates fibroblasts to proliferate and differentiate into myofibroblasts, which leads to the production of high amounts of collagen, fibronectin, and other ECM proteins and their deposition in the interstitial lung, leading to reduced gas exchange and impaired lung function, and ultimately to respiratory failure (Chanda et al., 2019; Bueno et al., 2020; Mei et al., 2021).
A study showed that exosomes in bronchoalveolar lavage fluid (BALF) of PF rats, specifically exosome miR-204-5p, promote fibroblast proliferation and collagen deposition in lung tissue, indicating that exosomes may participate in the PF process by regulating ECM deposition (Zhu et al., 2021). Long noncoding RNA (lncRNA) HOTAIRM1 delivered by alveolar epithelial cell-derived exosomes was found to play a role in PF, and lncRNA HOTAIRM1 was able to promote lung fibroblast proliferation and differentiation and contribute to ECM remodeling by regulating the miR-30d-3p/HSF1/YY1 axis (Chen et al., 2022). Zhang et al. (2021) found that exosomes derived from bone marrow mesenchymal stem cells in a silicosis PF model reduced collagen accumulation in lung tissue and inhibited the expression of components of the Wnt/β-catenin pathway, which is closely related to EMT. Wnt5a was also found to be directly transported by EVs in PF and induced fibroblast proliferation, which incidentally consisted mainly of exosomes in that study (Martin-Medina et al., 2018). Phuong-Uyen C. Dinh et al. (2020) found that lung spheroid cell-derived exosomes (LSC-EXOs) reduced collagen deposition in PF induced by bleomycin or silica, in addition to inhibiting the proliferation of myofibroblasts. The above study illustrates the role of exosomes in regulating the proliferation of fibroblasts and myofibroblasts, which are the primary sources of ECM in PF, and this may be an entry point to investigate the mechanisms by which exosomes regulate ECM deposition (Tsukui et al., 2020).
Cytokines can be involved in multiple aspects of inflammation and ECM deposition in PF (Luzina et al., 2015). TGF-β plays a vital role in the progression of PF, mediating fibroblast recruitment and differentiation, promoting ECM deposition, and inducing EMT. TGF-β can exert pro-fibrotic effects in cooperation with platelet-derived growth factor (PDGF), which itself can be involved in lung fibroblast proliferation and collagen synthesis (Chanda et al., 2019; Wang et al., 2020). Other cytokines such as connective tissue growth factor (CTGF), insulin-like growth factor, and CCL2 also modulate PF (Luzina et al., 2015).
Several studies have shown that exosomes can regulate PF through cytokines such as TGF-β. Activated TGF-β binds to its receptor and regulates the expression of target genes, including pro-fibrotic genes, through Smad proteins (Massagué, 2012). Smad7 is a negative regulator of TGF-β signaling that blocks the TGF-β1/Smads signaling pathway and inhibits fibroblast differentiation. A study elucidated that in cigarette smoke-induced PF, exosomes promote abnormal epithelial-fibroblast crosstalk by inhibiting Smad7 to activate the TGF-β1/Smad3 pathway (Bai et al., 2021). TGF-β1 and its receptor can regulate the transformation of lung pericytes into myofibroblasts. Han Xie et al. found that the lung vascular endothelium-derived exosome low let-7d targets transforming growth factor β receptor 1 (TGFβR1) to regulate the TGFβRI/FoxM1/Smad/β-catenin pathway, which drives lung pericytes fibrosis (Xie et al., 2020). In addition, exosomal miR-22 was found to significantly inhibit TGF-β1-induced α-smooth muscle actin (α-SMA) expression (Kuse et al., 2020). Guiot et al. (2020) found that the macrophage-derived exosome miR-142-3p was able to reduce TGFβR1 expression and inhibit TGF-β-induced fibrotic responses in alveolar epithelial cells and lung fibroblasts by targeting TGFβR1. The finding of another study demonstrated that human umbilical cord mesenchymal stem cell-derived exosome let-7i-5p could target TGFβR1 and inhibit lung fibroblast activation via the TGFβR1/Smad3 signaling pathway in a silica-induced PF model (Xu et al., 2022).
Platelet-derived growth factor receptor β (PDGFRβ) is expressed in pericytes, and a study demonstrated that the pulmonary vascular endothelial cell-derived exosome miR-107 could target HIF-1α to regulate PDGFRβ and mitigate pericyte-promoted PF progression by inhibiting the HIF-1α/Notch1/PDGFRβ/YAP1/Twist1 signaling pathway (Wang et al., 2021). Kuse et al. (2020) found in an in vitro PF model that exosomal miR-22 could attenuate the expression of CTGF, which is an important mediator of fibrosis and stimulates lung fibroblast differentiation, thereby inhibiting CTGF-induced α-SMA expression.
The current diagnosis of PF is not straightforward and requires multidisciplinary collaboration between pulmonologists, pathologists, and radiologists, and there remains a recognized need for an accurate, early diagnosis of PF. Early diagnosis of PF is critical for prognosis with survival being poor in several fibrotic interstitial lung diseases, including IPF, and patients with PF may benefit more from early diagnosis and timely disease management (Spagnolo et al., 2012; Cottin et al., 2018). Biomarkers have objective detection and evaluation properties and can be used as indicators of physiological/pathological processes or pharmacological responses to therapeutic interventions, which are essential in PF (Guiot et al., 2017). To date, several studies have shown that exosomes detected in body fluids such as blood and sputum have the potential to be diagnostic, prognostic, and predictive biomarkers of PF (Table 1).
Hematological tests, sputum examination, and bronchoscopy are diagnostic tools for respiratory diseases, and exosomal miRNAs can be detected by these tests. Several studies have reported the potential of some exosomal miRNAs as biomarkers for the diagnosis of PF. Donato Lacedonia et al. (2021) analyzed the expression of exosomal miRNAs in the serum of IPF patients and found that let-7d and miR-16 were down-regulated compared to controls. Previous studies have shown that let-7d has a key regulatory role in the prevention of PF, while fewer works have been conducted on the association between miR-16 and PF, and further studies are necessary to determine their ability to act as biomarkers of IPF (Pandit et al., 2010; Huleihel et al., 2014; Liang et al., 2016; Min et al., 2016). Makon-Sébastien Njock and his colleagues worked on finding exosomal biomarkers of IPF in sputum. By analyzing sputum samples from IPF patients and healthy controls, they found that the significantly upregulated exosomes miR-142-3p, miR-33a-5p, and downregulated exosome let-7d-5p in sputum were promising diagnostic biomarkers, and notably, they demonstrated that exosomal miR-142-3p was negatively correlated with diffusing capacity/alveolar volume of carbon monoxide and exosomal let-7d-5p was positively correlated, which may help to evaluate the severity of IPF (Njock et al., 2019). When they further investigated the relationship between exosomal miR-142-3p and IPF, they found that in addition to its potential as a sputum biomarker for IPF, exosomal miR-142-3p was significantly upregulated in the plasma of IPF patients, making it more promising as a diagnostic modality for IPF (Guiot et al., 2020). Kaur et al. (2021) compared IPF patients with healthy nonsmokers and detected 55 differentially expressed exosomal miRNAs in IPF human lung tissue, also finding significant downregulation of exosomes miR-375-3p, miR-200a-3p, miR-200b-3p, miR-141-3p, and miR-423-5p and upregulation of exosomes miR-22-3p, miR-320a-3p, miR-320b, and miR-24-3p in BALF-derived exosomes in IPF patients. Bronchoscopic lung cryobiopsy is a promising examination technique in the diagnosis of PF, allowing safer and more effective access to lung tissue with BALF, thus providing a source of feasibility for BALF exosomal miRNAs to become a diagnostic tool for PF (Tomassetti et al., 2016). Another study showed that exosomes miR-125b, miR-128, miR-21, miR-100, miR-140-3p, and miR-374b were upregulated in BALF from IPF patients, while let-7d, miR-103, miR-26, and miR-30a-5p were downregulated (Liu et al., 2018). These findings suggest the potential of BALF-derived exosomal miRNAs as PF biomarkers.
Furthermore, Makiguchi et al. (2016) found that serum EV miR-21-5p was upregulated throughout the progression of PF compared to controls in an animal model of bleomycin-induced PF, and they observed significantly higher EV miR-21-5p in the sera of IPF patients compared to healthy controls, suggesting that EV miR-21-5p is a candidate biomarker for IPF. Tomonori Makiguchi and his colleagues also confirmed the potential of serum EV miR-21-5p as a prognostic biomarker for IPF. According to their study, serum EV miR-21-5p correlated with the rate of lung volume loss in IPF patients, and Kaplan-Meier analysis indicated that patients with higher baseline serum EV miR-21-5p levels had significantly lower survival rates. Given that the investigators obtained serum EV miR-21-5p by Total Exosome Isolation reagent, serum exosome miR-21-5p may have the same biomarker potential. Kaur et al. (2020) suggested that downregulation of an exosomal lncRNA encoding a WT1 transcription factor in the plasma of smokers may represent a possibility for them to develop PF in the future . Miriana d'Alessandro et al. examined blood exosome surface epitopes in IPF patients and found many exosome surface epitopes CD19, CD69, CD8, CD86, CD209, Cd133/1, MCSP, and ROR1 were significantly higher in IPF patients and CD42a was lower than in controls. In addition, increased expression of CD8 was associated with poor prognosis in IPF in survival analysis. These results provide the basis for the use of blood exosome surface epitopes as candidate biomarkers for the diagnosis and prognosis of IPF (D'Alessandro et al., 2021).
Lung transplantation is currently the most effective treatment for most PF. Still, only a small number of patients can benefit from it due to the limited availability of donor organs, rejection reactions, and the cost of treatment. The drug treatment options for PF are relatively limited, and taking IPF as an example, the main treatment options for IPF are nintedanib, pirfenidone, and a combination of both. Although they can slow down the disease progression of PF to some extent, these therapies have adverse drug reactions and do not have the ability to cure the disease. Many patients with PF have a low quality of life and a short survival time after diagnosis. Thus there is still an urgent need for a treatment that can stop or even reverse PF. An increasing number of studies are currently dedicated to the search for safe and effective new therapies for PF (Richeldi et al., 2017; Mei et al., 2021). As previously described, exosomes have the ability to regulate fibroblast proliferation and differentiation, ECM deposition, and EMT, and several studies have demonstrated the therapeutic potential of exosomes for PF in animal models, as shown in Table 2, suggesting that exosomes may be a valuable clue to address this issue of developing new therapies for PF.
Mesenchymal stem/stromal cell-derived exosomes (MSC-EXOs) have been proposed as promising therapeutic tools for a variety of diseases (Ahmadi and Rezaie, 2021; Janockova et al., 2021) and have shown anti-fibrotic potential such as for liver, kidney, and cardiac fibrosis (Li et al., 2013; Eirin et al., 2017; Rong et al., 2019; Chen et al., 2020). MSCs are also a preferred source of exosomes for the study of PF treatment. Mansouri et al. (2019) found that bone marrow mesenchymal stem/stromal cell-derived exosomes (BMSC-EXOs) were effective in attenuating bleomycin-induced PF and modulating lung inflammation, and interestingly, the investigators observed that BMSC-EXOs significantly restored bleomycin-induced PF in mice after intravenous injection of BMSC-EXOs on day 7 post-modeling. The ability of BMSC-EXOs to reverse PF is of interest and needs to be studied in depth. In addition to this, they found that bone marrow-derived mononuclear cell transplantation pretreated by BMSC-EXOs could exert a preventive effect on PF. Another study demonstrated the ability of BMSC-EXOs to effectively alleviate silica-induced PF in a rat model of silicosis (Zhang et al., 2021). There are no effective therapies to slow down the progression of silicosis, especially its fibrosis, and exosomes offer a therapeutic idea for PF in silicosis. Xu et al. (2020) found that human umbilical cord MSC-derived exosomes (hucMSC-EXOs) exerted anti-PF effects in a mouse silicosis model, effectively reducing collagen deposition, attenuating pulmonary fibrosis-related indices, and improving impaired lung function in silicosis mice. Chunjie Xu and colleagues found through a more in-depth study that the exosome let-7i-5p in hucMSC-EXOs effectively attenuated collagen deposition in silicosis mice and improved silica-induced PF in mice by inhibiting fibroblasts (Xu et al., 2022). Another study elucidated that hucMSC-EXOs had the effect of alleviating bleomycin-induced PF and significantly attenuated the pathological changes, and reduced collagen deposition in the lung tissue of PF mice (Yang J. et al., 2020). Acute lung injury can lead to fibrosis in lung tissue. In studying the mechanisms by which MSC regulates the progression of acute lung injury, Xiao et al. (2020) found that MSC-EXOs have the potential to attenuate lipopolysaccharide-induced PF progression, and the investigators demonstrated that miR-23a-3p and miR-182-5p transported by MSC-EXOs have the ability to reverse the EMT process that is closely associated with PF. Zhang Z. et al. (2020b) found that MSC-EXOs also had the ability to attenuate PF in a rat model of monocrotaline-induced pulmonary arterial hypertension. Another study indicated that MSC-EXOs could also attenuate PF and improve lung function in a mouse model of hyperoxia-induced bronchopulmonary dysplasia (Willis et al., 2018).
Stem cell-derived exosomes other than MSCs have also demonstrated promising therapeutic effects on PF in several studies. In view of the potential therapeutic value of iPSCs and their derivatives in several diseases, Yan Zhou and colleagues, after observing the potential of iPSCs for the treatment of PF, further built on their findings that iPSC-derived exosomes (iPSC-EXOs) could alleviate bleomycin-induced PF in mice, and that miR-302a-3p carried by iPSC-EXOs was able to reduce collagen deposition and inhibit M2-type macrophage increase in mouse lungs (Zhou et al., 2016; Zhou et al., 2021). Pierre Montay-Gruel et al. administered human embryonic stem cell (hESC)-derived EVs intravenously to mice receiving chest radiation exposure, most of which were actually exosomes, and observed a significant reduction in radiation-induced PF. This study suggests that hESC-derived exosomes may have the potential to become a therapeutic pathway for radiation therapy-induced PF (Montay-Gruel et al., 2020). Menstrual blood-derived endometrial stem cells (MenSCs) have antifibrotic effects, and Lifang Sun et al. found that MenSC-derived exosomes (MenSC-EXOs) ameliorated bleomycin-induced PF and alveolar epithelial cell injury in mice, which may be closely related to exosomal let-7. The investigators observed that let-7 carried by MenSC-EXOs could inhibit the process of EMT in mice and attenuate lung histopathological changes and that MenSC-EXOs could also translocate let-7 into alveolar epithelial cells to attenuate cell damage (Sun et al., 2019).
In addition to the stem cell-derived exosomes described above, several other types of exosomes have the potential for the treatment of PF. Tan et al. (2018) endeavored to explore the feasibility of human amnion epithelial cell-derived exosomes (hAEC-EXOs) as a potential therapy for IPF. They performed early and late interventions in juvenile mice with bleomycin-induced PF via intranasal drip of hAEC-EXOs and found that hAEC-EXOs were able to exert anti-inflammatory and anti-fibrotic effects at different stages of PF, with the potential to prevent, mitigate and even reverse PF. They also showed that the aforementioned anti-PF effects of hAEC-EXOs in juvenile mice were reproduced in aged mice, which were used to simulate the clinical setting of elderly patients with IPF, demonstrating the therapeutic potential of hAEC-EXOs for various disease stages and ages of PF. Another study found that hAEC-EXOs, in combination with the anti-fibrotic drug serelaxin, exerted better anti-inflammatory, anti-fibrotic, and alleviating airway dysfunction in bleomycin-induced PF mice (Royce et al., 2019). Dinh et al. (2020) used a nebulizer to treat bleomycin-induced and silica-induced PF mice with nebulized inhaled lung spheroid cell exosomes (LSC-EXOs) and found that LSC-EXOs exerted anti-PF effects in both PF mouse models, reducing collagen accumulation and myofibroblast proliferation, and improving impaired lung function. In addition, their results suggest that LSC-EXOs may be superior to MSC-EXOs in attenuating PF. Exosomal miR-107 and let-7d produced by pulmonary vascular endothelial cells have been reported to inhibit the function of pericytes in PF, which provides a promising therapeutic target for PF (Xie et al., 2020; Wang et al., 2021). In addition, exosomal miR-22 and exosomal miR-16 demonstrated experimentally their ability to ameliorate bleomycin-induced PF in mice through their mimics (Kuse et al., 2020; Inomata et al., 2021). In most of the above studies, an intravenous injection was chosen as the mode of exosome administration. Interestingly, in exploring the therapeutic potential of hAEC-EXOs and LSC-EXOs for PF, the investigators chose intranasal drip and nebulized inhalation administration, respectively, which enriches the effective routes of administration in anti-PF exosomes.
With the development of nanomedicine, more and more literature has reported that nano-drug delivery systems can function as stable, safe, and effective drug delivery, and the progressive exploration of pulmonary drug delivery vehicles, including exosomes, has helped to advance the development of PF therapy (Skibba et al., 2020). Compared to nano-drug delivery systems such as in vitro synthesized liposomes, exosomes have unique drug delivery advantages. Exosomes are naturally secreted by organismal cells, can be widely and stably present in body fluids, and have better biocompatibility and lower immunogenicity. The lipid-rich composition of exosomes facilitates their loading of hydrophilic and lipophilic active pharmaceutical ingredients, which happens to be lipophilic for pirfenidone, a therapeutic drug for PF (Haak et al., 2017; Guiot et al., 2019). Exosomes are capable of mediating intercellular communication in the body, have the ability to target tissues and cells, and can cross biological barriers such as the blood-brain barrier (Zhang Y. et al., 2020; Saint-Pol et al., 2020). With these properties and abilities, exosomes have the potential to deliver PF therapeutic agents such as drugs and miRNAs.
There are already studies invested in the development of exosomes as drug delivery systems for anti-PF agents. Lingna Sun and coworkers designed a hybrid drug delivery system of fibroblast-derived exosomes and clodronate (CLD)-loaded liposomes (EL-CLD), which utilizes the affinity of fibroblast-derived exosomes for fibroblasts and CLD to reduce the uptake of drugs by the liver, proposing to solve the problem of poor selectivity and low delivery efficiency of PF therapeutic drugs to fibroblasts. In an animal model of PF, the investigators found that the anti-PF efficacy of Nintedanib was significantly increased when it was loaded with EL-CLD, thanks to the ability of EL-CLD to increase the delivery of nintedanib to the lung and its accumulation in fibrotic lung tissue, as well as to attenuate the macrophage-induced inflammatory response (Sun L. et al., 2021). Notably, the team has also developed another hybrid exosome-liposome drug delivery system that enhances the efficacy of nintedanib in the treatment of liver fibrosis (Ji et al., 2022). These findings highlight that the ability of exosomes to target delivery enables them to be advantageous in drug delivery, and furthermore, they demonstrate the feasibility and effectiveness of exosomes as delivery vehicles for anti-fibrotic, especially anti-PF drugs. However, the technology of exosome application for drug delivery is still immature. Problems such as how to extract exosomes efficiently and completely, how to improve the drug loading rate of exosomes, how to surface modify exosomes to improve their stability, and the types of drugs delivered are limiting the further development of this technology, and more research needs to be invested in tackling these challenges (Zhang Y. et al., 2020). Based on the barriers of exosomes as drug carriers, Yanzhen Yu and colleagues developed a functional ECM biomaterial capable of loading exosomes, which is able to capture and enrich exosomes without damaging the exosome membrane, increasing the stability of exosomes as carriers for delivery of anti-PF miR-29. The investigators observed that the application of this miR-29 exosome-enriched ECM material in bleomycin-induced PF mice resulted in good attenuation of lung tissue fibrosis in mice, which provided inspiration for optimizing the exosome delivery technology (Yu et al., 2021). Therefore, as the technology continues to improve, exosomes may hold a promising future as anti-PF agent delivery vehicles.
In recent years, research on exosomes has increased rapidly. In this context, many advances have been made in the study of the relationship between exosomes and a variety of respiratory diseases, including PF. Exosomes play an important role in communication between human cells, can participate in multiple physiopathological processes, and are closely associated with many therapeutic mechanisms (Guiot et al., 2019; Mashouri et al., 2019; Soraya et al., 2021; Yamada, 2021). According to several studies mentioned above, exosomes have the ability to participate in processes such as inflammation and immunity, ECM deposition, and EMT in PF, which is associated with the ability of exosomes to regulate inflammatory cell infiltration, immune cell proliferation, and fibroblast proliferation and differentiation in the lung, as well as to influence cytokines such as TGF-β1. Exosomes have great potential for the diagnosis and determination of prognosis in PF and show therapeutic promise for the prevention, mitigation, and even reversal of PF. In addition, the biocompatibility, low immunogenicity, and ability to target tissues of exosomes provide new insights into the development of anti-PF drug delivery systems.
However, there is still a long way to go to translate the potential of exosomes as biomarkers and therapeutics in PF into clinical applications that can benefit patients. The diagnostic and therapeutic mechanisms of exosomes in PF are currently unclear and need to be further investigated. The technical basis for the clinical application of exosomes is immature due to obstacles such as exosome extraction, preservation, and drug loading. As highlighted at the 2021 annual meeting of the International Society for Extracellular Vesicles, clinical applications of EVs such as exosomes need to explore effective, rapid, simplified, reproducible, and scalable pathways. Clinical translation of current results and further achievements require continuous efforts of multidisciplinary teams (Picciolini et al., 2021). Despite these obstacles, the prospect of clinical application of exosomes in PF currently requires more efforts to be invested in the research of exosomes as a diagnostic method and new therapies for PF to benefit PF patients.
YY, WH, and KL searched the literature and wrote the first draft of the manuscript. YL and YC drew the figures. KZ and YZ processed the tables in the manuscript. PG, QH, and CZ contributed to the revision of the manuscript. All authors read and approved the submitted version.
Financial support was provided by Sichuan Science and technology program, Grant/Award Numbers: 2020JDRC0114, 2020YFH0164; Special subject of scientific research on traditional Chinese Medicine of Sichuan Administration of Traditional Chinese Medicine, Grant/Award Numbers: 2021MS093, 2021MS539; “100 Talent Plan” Project of Hospital of Chengdu University of Traditional Chinese Medicine, Grant/Award Number: Hospital office [2021] 42; The fifth batch of training objects of the National TCM Clinical Excellent Talents Training Program, Grant/Award Number: National TCM Renjiao Letter [2022] No. 1; Training objects of the National Traditional Chinese Medicine Innovation Backbone Talents Training Program, Grant/Award Number: National TCM Renjiao Letter [2019] No. 128.
The authors declare that the research was conducted in the absence of any commercial or financial relationships that could be construed as a potential conflict of interest.
All claims expressed in this article are solely those of the authors and do not necessarily represent those of their affiliated organizations, or those of the publisher, the editors and the reviewers. Any product that may be evaluated in this article, or claim that may be made by its manufacturer, is not guaranteed or endorsed by the publisher.
Abels, E. R., and Breakefield, X. O. (2016). Introduction to extracellular vesicles: Biogenesis, RNA cargo selection, content, release, and uptake. Cell. Mol. Neurobiol. 36, 301–312. doi:10.1007/s10571-016-0366-z
Ahmadi, M., and Rezaie, J. (2021). Ageing and mesenchymal stem cells derived exosomes: Molecular insight and challenges. Cell biochem. Funct. 39, 60–66. doi:10.1002/cbf.3602
Amari, L., and Germain, M. (2021). Mitochondrial extracellular vesicles - origins and roles. Front. Mol. Neurosci. 14, 767219. doi:10.3389/fnmol.2021.767219
Anel, A., Gallego-Lleyda, A., De Miguel, D., Naval, J., and MARTíNEZ-Lostao, L. (2019). Role of exosomes in the regulation of T-cell mediated immune responses and in autoimmune disease. Cell 8, E154. doi:10.3390/cells8020154
Arima, Y., Liu, W., Takahashi, Y., Nishikawa, M., and Takakura, Y. (2019). Effects of localization of antigen proteins in antigen-loaded exosomes on efficiency of antigen presentation. Mol. Pharm. 16, 2309–2314. doi:10.1021/acs.molpharmaceut.8b01093
Bai, J., Deng, J., Han, Z., Cui, Y., He, R., Gu, Y., et al. (2021). CircRNA_0026344 via exosomal miR-21 regulation of Smad7 is involved in aberrant cross-talk of epithelium-fibroblasts during cigarette smoke-induced pulmonary fibrosis. Toxicol. Lett. 347, 58–66. doi:10.1016/j.toxlet.2021.04.017
Blaser, H., Dostert, C., Mak, T. W., and Brenner, D. (2016). TNF and ROS crosstalk in inflammation. Trends Cell Biol. 26, 249–261. doi:10.1016/j.tcb.2015.12.002
Böker, K., Lemus-Diaz, N., Rinaldi Ferreira, R., Schiller, L., Schneider, S., and Gruber, J. (2018). The impact of the CD9 tetraspanin on lentivirus infectivity and exosome secretion. Mol. Ther. 26, 634–647. doi:10.1016/j.ymthe.2017.11.008
Bueno, M., Calyeca, J., Rojas, M., and Mora, A. L. (2020). Mitochondria dysfunction and metabolic reprogramming as drivers of idiopathic pulmonary fibrosis. Redox Biol. 33, 101509. doi:10.1016/j.redox.2020.101509
Cameli, P., Carleo, A., Bergantini, L., Landi, C., Prasse, A., and Bargagli, E. (2020). Oxidant/antioxidant disequilibrium in idiopathic pulmonary fibrosis pathogenesis. Inflammation 43, 1–7. doi:10.1007/s10753-019-01059-1
Chanda, D., Otoupalova, E., , Smith, S. R., Volckaert, T., De Langhe, S. P., and Thannickal, V. J. (2019). Developmental pathways in the pathogenesis of lung fibrosis. Mol. Asp. Med. 65, 56–69. doi:10.1016/j.mam.2018.08.004,
Chen, F., Li, X., Zhao, J., Geng, J., Xie, J., and Xu, B. (2020). Bone marrow mesenchymal stem cell-derived exosomes attenuate cardiac hypertrophy and fibrosis in pressure overload induced remodeling. Vitro Cell. Dev. Biol. Anim. 56, 567–576. doi:10.1007/s11626-020-00481-2
Chen, L., Yang, Y., Yue, R., Peng, X., Yu, H., and Huang, X. (2022). Exosomes derived from hypoxia-induced alveolar epithelial cells stimulate interstitial pulmonary fibrosis through a HOTAIRM1-dependent mechanism. Lab. Invest.. doi:10.1038/s41374-022-00782-y
Cottin, V., Hirani, N., Hotchkin, D., Nambiar, A., Ogura, T., Otaola, M., et al. (2018). Presentation, diagnosis and clinical course of the spectrum of progressive-fibrosing interstitial lung diseases. Eur. Respir. Rev. 27, 180076. doi:10.1183/16000617.0076-2018
D'alessandro, M., Soccio, P., Bergantini, L., Cameli, P., Scioscia, G., Foschino Barbaro, M. P., et al. (2021). Extracellular vesicle surface signatures in IPF patients: A multiplex bead-based flow cytometry approach. Cell, 10. 1045. doi:10.3390/cells10051045
De Abreu, R. C., Fernandes, H., Da Costa Martins, P. A., Sahoo, S., Emanueli, C., and Ferreira, L. (2020). Native and bioengineered extracellular vesicles for cardiovascular therapeutics. Nat. Rev. Cardiol. 17, 685–697. doi:10.1038/s41569-020-0389-5
Dinh, P. C., Paudel, D., Brochu, H., Popowski, K. D., Gracieux, M. C., Cores, J., et al. (2020). Inhalation of lung spheroid cell secretome and exosomes promotes lung repair in pulmonary fibrosis. Nat. Commun. 11, 1064. doi:10.1038/s41467-020-14344-7
Doyle, L. M., and Wang, M. Z. (2019). Overview of extracellular vesicles, their origin, composition, purpose, and methods for exosome isolation and analysis. Cells 8, E727. doi:10.3390/cells8070727
Eirin, A., Zhu, X., Puranik, A., Tang, H., Mcgurren, K., van Wijnen, A., et al. (2017). Mesenchymal stem cell-derived extracellular vesicles attenuate kidney inflammation. Kidney Int. 92, 114–124. doi:10.1016/j.kint.2016.12.023
Elsherbini, A., and Bieberich, E. (2018). Ceramide and exosomes: A novel target in cancer biology and therapy. Adv. Cancer Res. 140, 121–154. doi:10.1016/bs.acr.2018.05.004
Frankel, E., and Audhya, A. (2018). ESCRT-dependent cargo sorting at multivesicular endosomes. Semin. Cell Dev. Biol. 74, 4–10. doi:10.1016/j.semcdb.2017.08.020
Ghossoub, R., CHéRY, M., Audebert, S., Leblanc, R., Egea-Jimenez, A., Lembo, F., et al. (2020). Tetraspanin-6 negatively regulates exosome production. Proc. Natl. Acad. Sci. U. S. A. 117, 5913–5922. doi:10.1073/pnas.1922447117
Gonda, A., Kabagwira, J., Senthil, G., and Wall, N. (2019). Internalization of exosomes through receptor-mediated endocytosis. Mol. Cancer Res. 17, 337–347. doi:10.1158/1541-7786.MCR-18-0891
Guiot, J., Cambier, M., Boeckx, A., Henket, M., Nivelles, O., Gester, F., et al. (2020). Macrophage-derived exosomes attenuate fibrosis in airway epithelial cells through delivery of antifibrotic miR-142-3p. Thorax 75, 870–881. doi:10.1136/thoraxjnl-2019-214077
Guiot, J., Moermans, C., Henket, M., Corhay, J., and Louis, R. (2017). Blood biomarkers in idiopathic pulmonary fibrosis. Lung 195, 273–280. doi:10.1007/s00408-017-9993-5
Guiot, J., Struman, I., Louis, E., Louis, R., Malaise, M., and Njock, M. S. (2019). Exosomal miRNAs in lung diseases: From biologic function to therapeutic targets. J. Clin. Med. 8, E1345. doi:10.3390/jcm8091345
Gurunathan, S., Kang, M., Qasim, M., Khan, K., and Kim, J. (2021). Biogenesis, membrane trafficking, functions, and next generation nanotherapeutics medicine of extracellular vesicles. Int. J. Nanomedicine 16, 3357–3383. doi:10.2147/IJN.S310357
Haak, A. J., Girtman, M. A., Ali, M. F., Carmona, E. M., Limper, A. H., and Tschumperlin, D. J. (2017). Phenylpyrrolidine structural mimics of pirfenidone lacking antifibrotic activity: A new tool for mechanism of action studies. Eur. J. Pharmacol. 811, 87–92. doi:10.1016/j.ejphar.2017.05.050
Harding, C., Heuser, J., and Stahl, P. (1983). Receptor-mediated endocytosis of transferrin and recycling of the transferrin receptor in rat reticulocytes. J. Cell Biol. 97, 329–339. doi:10.1083/jcb.97.2.329
Hassanpour, M., Rezabakhsh, A., Rezaie, J., Nouri, M., and Rahbarghazi, R. (2020). Exosomal cargos modulate autophagy in recipient cells via different signaling pathways. Cell Biosci. 10, 92. doi:10.1186/s13578-020-00455-7
Hessvik, N., and Llorente, A. (2018). Current knowledge on exosome biogenesis and release. Cell. Mol. Life Sci. 75, 193–208. doi:10.1007/s00018-017-2595-9
Heukels, P., Moor, C. C., von der THüSEN, J. H., Wijsenbeek, M. S., and Kool, M. (2019). Inflammation and immunity in IPF pathogenesis and treatment. Respir. Med. 147, 79–91. doi:10.1016/j.rmed.2018.12.015
Hewlett, J. C., Kropski, J. A., and Blackwell, T. S. (2018). Idiopathic pulmonary fibrosis: Epithelial-mesenchymal interactions and emerging therapeutic targets. Matrix Biol. 71-72, 112–127. doi:10.1016/j.matbio.2018.03.021
Horibe, S., Tanahashi, T., Kawauchi, S., Murakami, Y., and Rikitake, Y. (2018). Mechanism of recipient cell-dependent differences in exosome uptake. BMC Cancer 18, 47. doi:10.1186/s12885-017-3958-1
Hoyer, M. J., Chitwood, P. J., Ebmeier, C. C., Striepen, J. F., Qi, R. Z., Old, W. M., et al. (2018). A novel class of ER membrane proteins regulates ER-associated endosome fission. Cell 175, 254–265. e14. doi:10.1016/j.cell.2018.08.030
Huleihel, L., Ben-Yehudah, A., Milosevic, J., Yu, G., Pandit, K., Sakamoto, K., et al. (2014). Let-7d microRNA affects mesenchymal phenotypic properties of lung fibroblasts. Am. J. Physiol. Lung Cell. Mol. Physiol. 306, L534–L542. doi:10.1152/ajplung.00149.2013
Inomata, M., Kamio, K., Azuma, A., Matsuda, K., Usuki, J., Morinaga, A., et al. (2021). Rictor-targeting exosomal microRNA-16 ameliorates lung fibrosis by inhibiting the mTORC2-SPARC axis. Exp. Cell Res. 398, 112416. doi:10.1016/j.yexcr.2020.112416
Janockova, J., Slovinska, L., Harvanova, D., Spakova, T., and Rosocha, J. (2021). New therapeutic approaches of mesenchymal stem cells-derived exosomes. J. Biomed. Sci. 28, 39. doi:10.1186/s12929-021-00736-4
Ji, K., Fan, M., Huang, D., Sun, L., Li, B., Xu, R., et al. (2022). Clodronate-nintedanib-loaded exosome-liposome hybridization enhances the liver fibrosis therapy by inhibiting Kupffer cell activity. Biomater. Sci. 10, 702–713. doi:10.1039/d1bm01663f
Jin, H., Tang, Y., Yang, L., Peng, X., Li, B., Fan, Q., et al. (2021). Rab GTPases: Central coordinators of membrane trafficking in cancer. Front. Cell Dev. Biol. 9, 648384. doi:10.3389/fcell.2021.648384
Johnstone, R., Adam, M., Hammond, J., Orr, L., and Turbide, C. (1987). Vesicle formation during reticulocyte maturation. Association of plasma membrane activities with released vesicles (exosomes). J. Biol. Chem. 262, 9412–9420. doi:10.1016/s0021-9258(18)48095-7
Johnstone, R. (1992). The jeanne manery-Fisher memorial lecture 1991. Maturation of reticulocytes: formation of exosomes as a mechanism for shedding membrane proteins. Biochem. Cell Biol. 70, 179–190. doi:10.1139/o92-028
Kalluri, R., and Lebleu, V. (2020). The biology function and biomedical applications of exosomes. Science 367, eaau6977. doi:10.1126/science.aau6977
Kaur, G., Maremanda, K. P., Campos, M., Chand, H. S., Li, F., Hirani, N., et al. (2021). Distinct exosomal miRNA profiles from BALF and lung tissue of COPD and IPF patients. Int. J. Mol. Sci. 22, 11830. doi:10.3390/ijms222111830
Kaur, G., Singh, K., Maremanda, K. P., Li, D., Chand, H. S., and Rahman, I. (2020). Differential plasma exosomal long non-coding RNAs expression profiles and their emerging role in E-cigarette users, cigarette, waterpipe, and dual smokers. PloS One 15, e0243065. doi:10.1371/journal.pone.0243065
Keshtkar, S., Kaviani, M., Soleimanian, S., Azarpira, N., Asvar, Z., and Pakbaz, S. (2021). Stem cell-derived exosome as potential therapeutics for microbial diseases. Front. Microbiol. 12, 786111. doi:10.3389/fmicb.2021.786111
Kinoshita, T., and Goto, T. (2019). Molecular mechanisms of pulmonary fibrogenesis and its progression to lung cancer: A review. Int. J. Mol. Sci. 20, E1461. doi:10.3390/ijms20061461
Kuse, N., Kamio, K., Azuma, A., Matsuda, K., Inomata, M., Usuki, J., et al. (2020). Exosome-derived microRNA-22 ameliorates pulmonary fibrosis by regulating fibroblast-to-myofibroblast differentiation in vitro and in vivo. J. Nippon. Med. Sch. 87, 118–128. doi:10.1272/jnms.JNMS.2020_87-302
Lacedonia, D., Scioscia, G., Soccio, P., Conese, M., Catucci, L., Palladino, G. P., et al. (2021). Downregulation of exosomal let-7d and miR-16 in idiopathic pulmonary fibrosis. BMC Pulm. Med. 21, 188. doi:10.1186/s12890-021-01550-2
Larios, J., Mercier, V., Roux, A., and Gruenberg, J. (2020). ALIX- and ESCRT-III-dependent sorting of tetraspanins to exosomes. J. Cell Biol. 219. doi:10.1083/jcb.201904113
Li, S., Lin, Z., Jiang, X., and Yu, X. (2018). Exosomal cargo-loading and synthetic exosome-mimics as potential therapeutic tools. Acta Pharmacol. Sin. 39, 542–551. doi:10.1038/aps.2017.178
Li, T., Yan, Y., Wang, B., Qian, H., Zhang, X., Shen, L., et al. (2013). Exosomes derived from human umbilical cord mesenchymal stem cells alleviate liver fibrosis. Stem Cells Dev. 22, 845–854. doi:10.1089/scd.2012.0395
Liang, H., Liu, S., Chen, Y., Bai, X., Liu, L., Dong, Y., et al. (2016). miR-26a suppresses EMT by disrupting the Lin28B/let-7d axis: potential cross-talks among miRNAs in IPF. J. Mol. Med. 94, 655–665. doi:10.1007/s00109-016-1381-8
Liu, B., Jiang, T., Hu, X., Liu, Z., Zhao, L., Liu, H., et al. (2018). Downregulation of microRNA-30a in bronchoalveolar lavage fluid from idiopathic pulmonary fibrosis patients. Mol. Med. Rep. 18, 5799–5806. doi:10.3892/mmr.2018.9565
Liu, J., Ren, L., Li, S., Li, W., Zheng, X., Yang, Y., et al. (2021). The biology, function, and applications of exosomes in cancer. Acta Pharm. Sin. B 11, 2783–2797. doi:10.1016/j.apsb.2021.01.001
Lloret-Llinares, M., Karadoulama, E., Chen, Y., Wojenski, L., Villafano, G., Bornholdt, J., et al. (2018). The RNA exosome contributes to gene expression regulation during stem cell differentiation. Nucleic Acids Res. 46, 11502–11513. doi:10.1093/nar/gky817
Luzina, I. G., Todd, N. W., Sundararajan, S., and Atamas, S. P. (2015). The cytokines of pulmonary fibrosis: Much learned, much more to learn. Cytokine 74, 88–100. doi:10.1016/j.cyto.2014.11.008
Lv, M., Liu, Y., Ma, S., and Yu, Z. (2019). Current advances in idiopathic pulmonary fibrosis: the pathogenesis, therapeutic strategies and candidate molecules. Future Med. Chem. 11, 2595–2620. doi:10.4155/fmc-2019-0111
Makiguchi, T., Yamada, M., Yoshioka, Y., Sugiura, H., Koarai, A., Chiba, S., et al. (2016). Serum extracellular vesicular miR-21-5p is a predictor of the prognosis in idiopathic pulmonary fibrosis. Respir. Res. 17, 110. doi:10.1186/s12931-016-0427-3
Mansouri, N., Willis, G. R., Fernandez-Gonzalez, A., Reis, M., Nassiri, S., Mitsialis, S. A., et al. (2019). Mesenchymal stromal cell exosomes prevent and revert experimental pulmonary fibrosis through modulation of monocyte phenotypes. JCI Insight 4, 128060. doi:10.1172/jci.insight.128060
Martin-Medina, A., Lehmann, M., Burgy, O., Hermann, S., Baarsma, H. A., Wagner, D. E., et al. (2018). Increased extracellular vesicles mediate WNT5A signaling in idiopathic pulmonary fibrosis. Am. J. Respir. Crit. Care Med. 198, 1527–1538. doi:10.1164/rccm.201708-1580OC
Mashouri, L., Yousefi, H., Aref, A. R., Ahadi, A. M., Molaei, F., and Alahari, S. K. (2019). Exosomes: composition, biogenesis, and mechanisms in cancer metastasis and drug resistance. Mol. Cancer 18, 75. doi:10.1186/s12943-019-0991-5
Massagué, J. (2012). TGFβ signalling in context. Nat. Rev. Mol. Cell Biol. 13, 616–630. doi:10.1038/nrm3434
Mei, Q., Liu, Z., Zuo, H., Yang, Z., and Qu, J. (2021). Idiopathic pulmonary fibrosis: An update on pathogenesis. Front. Pharmacol. 12, 797292. doi:10.3389/fphar.2021.797292
Messenger, S., Woo, S., Sun, Z., and Martin, T. (2018). A Ca 2+-stimulated exosome release pathway in cancer cells is regulated by Munc13-4. J. Cell Biol. 217, 2877–2890. doi:10.1083/jcb.201710132
Min, H., Fan, S., Song, S., Zhuang, Y., Li, H., Wu, Y., et al. (2016). Plasma microRNAs are associated with acute exacerbation in idiopathic pulmonary fibrosis. Diagn. Pathol. 11, 135. doi:10.1186/s13000-016-0583-2
Montay-Gruel, P., Zhu, Y., Petit, B., Leavitt, R., Warn, M., Giedzinski, E., et al. (2020). Extracellular vesicles for the treatment of radiation-induced normal tissue toxicity in the lung. Front. Oncol. 10, 602763. doi:10.3389/fonc.2020.602763
Njock, M. S., Guiot, J., Henket, M. A., Nivelles, O., Thiry, M., Dequiedt, F., et al. (2019). Sputum exosomes: promising biomarkers for idiopathic pulmonary fibrosis. Thorax 74, 309–312. doi:10.1136/thoraxjnl-2018-211897
Osaki, M., and Okada, F. (2019). Exosomes and their role in cancer progression. Yonago Acta Med. 62, 182–190. doi:10.33160/yam.2019.06.002
Pan, B., Teng, K., Wu, C., Adam, M., and Johnstone, R. (1985). Electron microscopic evidence for externalization of the transferrin receptor in vesicular form in sheep reticulocytes. J. Cell Biol. 101, 942–948. doi:10.1083/jcb.101.3.942
Pandit, K., Corcoran, D., Yousef, H., Yarlagadda, M., Tzouvelekis, A., Gibson, K., et al. (2010). Inhibition and role of let-7d in idiopathic pulmonary fibrosis. Am. J. Respir. Crit. Care Med. 182, 220–229. doi:10.1164/rccm.200911-1698OC
Picciolini, S., RODà, F., Bedoni, M., and Gualerzi, A. (2021). Advances in the field of micro- and nanotechnologies applied to extracellular vesicle research: Take-home message from ISEV2021. Micromachines 12, 1563. doi:10.3390/mi12121563
Qin, X., Lin, X., Liu, L., Li, Y., Li, X., Deng, Z., et al. (2021). Macrophage-derived exosomes mediate silica-induced pulmonary fibrosis by activating fibroblast in an endoplasmic reticulum stress-dependent manner. J. Cell. Mol. Med. 25, 4466–4477. doi:10.1111/jcmm.16524
Rezaie, J., Ahmadi, M., Ravanbakhsh, R., Mojarad, B., Mahbubfam, S., Shaban, S. A., et al. (2022). Tumor-derived extracellular vesicles: The metastatic organotropism drivers. Life Sci. 289, 120216. doi:10.1016/j.lfs.2021.120216
Richeldi, L., Collard, H. R., and Jones, M. G. (2017). Idiopathic pulmonary fibrosis. Lancet (London, Engl. 389, 1941–1952. doi:10.1016/S0140-6736(17)30866-8
Rong, X., Liu, J., Yao, X., Jiang, T., Wang, Y., and Xie, F. (2019). Human bone marrow mesenchymal stem cells-derived exosomes alleviate liver fibrosis through the Wnt/β-catenin pathway. Stem Cell Res. Ther. 10, 98. doi:10.1186/s13287-019-1204-2
Royce, S. G., Patel, K. P., Mao, W., Zhu, D., Lim, R., and Samuel, C. S. (2019). Serelaxin enhances the therapeutic effects of human amnion epithelial cell-derived exosomes in experimental models of lung disease. Br. J. Pharmacol. 176, 2195–2208. doi:10.1111/bph.14666
Saint-Pol, J., Gosselet, F., Duban-Deweer, S., Pottiez, G., and Karamanos, Y. (2020). Targeting and crossing the blood-brain barrier with extracellular vesicles. Cells 9, E851. doi:10.3390/cells9040851
Savina, A., Fader, C., Damiani, M., and Colombo, M. (2005). Rab11 promotes docking and fusion of multivesicular bodies in a calcium-dependent manner. Traffic 6, 131–143. doi:10.1111/j.1600-0854.2004.00257.x
Sgalla, G., Franciosa, C., Simonetti, J., and Richeldi, L. (2020). Pamrevlumab for the treatment of idiopathic pulmonary fibrosis. Expert Opin. Investig. Drugs 29, 771–777. doi:10.1080/13543784.2020.1773790
Sgalla, G., Iovene, B., Calvello, M., Ori, M., Varone, F., and Richeldi, L. (2018). Idiopathic pulmonary fibrosis: pathogenesis and management. Respir. Res. 19, 32. doi:10.1186/s12931-018-0730-2
Shi, M., Chen, B., Mahajan, D., Boh, B. K., Zhou, Y., Dutta, B., et al. (2018). Amino acids stimulate the endosome-to-Golgi trafficking through Ragulator and small GTPase Arl5. Nat. Commun. 9, 4987. doi:10.1038/s41467-018-07444-y
Skibba, M., Drelich, A., Poellmann, M., Hong, S., and Brasier, A. R. (2020). Nanoapproaches to modifying epigenetics of epithelial mesenchymal transition for treatment of pulmonary fibrosis. Front. Pharmacol. 11, 607689. doi:10.3389/fphar.2020.607689
Song, L., Tang, S., Han, X., Jiang, Z., Dong, L., Liu, C., et al. (2019). KIBRA controls exosome secretion via inhibiting the proteasomal degradation of Rab27a. Nat. Commun. 10, 1639. doi:10.1038/s41467-019-09720-x
Soraya, H., Sani, N. A., Jabbari, N., and Rezaie, J. (2021). Metformin increases exosome biogenesis and secretion in U87 MG human glioblastoma cells: A possible mechanism of therapeutic resistance. Arch. Med. Res. 52, 151–162. doi:10.1016/j.arcmed.2020.10.007
Spagnolo, P., Tonelli, R., Cocconcelli, E., Stefani, A., and Richeldi, L. (2012). Idiopathic pulmonary fibrosis: diagnostic pitfalls and therapeutic challenges. Multidiscip. Respir. Med. 7, 42. doi:10.1186/2049-6958-7-42
Sun, L., Fan, M., Huang, D., Li, B., Xu, R., Gao, F., et al. (2021a). Clodronate-loaded liposomal and fibroblast-derived exosomal hybrid system for enhanced drug delivery to pulmonary fibrosis. Biomaterials 271, 120761. doi:10.1016/j.biomaterials.2021.120761
Sun, L., Zhu, M., Feng, W., Lin, Y., Yin, J., Jin, J., et al. (2019). Exosomal miRNA let-7 from menstrual blood-derived endometrial stem cells alleviates pulmonary fibrosis through regulating mitochondrial DNA damage. Oxid. Med. Cell. Longev. 2019, 4506303. doi:10.1155/2019/4506303
Sun, N. N., Zhang, Y., Huang, W. H., Zheng, B. J., Jin, S. Y., Li, X., et al. (2021b). Macrophage exosomes transfer angiotensin II type 1 receptor to lung fibroblasts mediating bleomycin-induced pulmonary fibrosis. Chin. Med. J. 134, 2175–2185. doi:10.1097/CM9.0000000000001605
Sundarakrishnan, A., Chen, Y., Black, L. D., Aldridge, B. B., and Kaplan, D. L. (2018). Engineered cell and tissue models of pulmonary fibrosis. Adv. Drug Deliv. Rev. 129, 78–94. doi:10.1016/j.addr.2017.12.013
Tan, J. L., Lau, S. N., Leaw, B., Nguyen, H. P. T., Salamonsen, L. A., Saad, M. I., et al. (2018). Amnion epithelial cell-derived exosomes restrict lung injury and enhance endogenous lung repair. Stem Cells Transl. Med. 7, 180–196. doi:10.1002/sctm.17-0185
Toh, W., Lai, R., Zhang, B., and Lim, S. (2018). MSC exosome works through a protein-based mechanism of action. Biochem. Soc. Trans. 46, 843–853. doi:10.1042/BST20180079
Tomassetti, S., Wells, A. U., Costabel, U., Cavazza, A., Colby, T. V., Rossi, G., et al. (2016). Bronchoscopic lung cryobiopsy increases diagnostic confidence in the multidisciplinary diagnosis of idiopathic pulmonary fibrosis. Am. J. Respir. Crit. Care Med. 193, 745–752. doi:10.1164/rccm.201504-0711OC
Tsukui, T., Sun, K.-H., Wetter, J. B., Wilson-Kanamori, J. R., Hazelwood, L. A., Henderson, N. C., et al. (2020). Collagen-producing lung cell atlas identifies multiple subsets with distinct localization and relevance to fibrosis. Nat. Commun. 11, 1920. doi:10.1038/s41467-020-15647-5
Villarroya-Beltri, C., Baixauli, F., GUTIéRREZ-VáZQUEZ, C., SáNCHEZ-Madrid, F., and Mittelbrunn, M. (2014). Sorting it out: regulation of exosome loading. Semin. Cancer Biol. 28, 3–13. doi:10.1016/j.semcancer.2014.04.009
Wang, F., Xia, H., and Yao, S. (2020). Regulatory T cells are a double-edged sword in pulmonary fibrosis. Int. Immunopharmacol. 84, 106443. doi:10.1016/j.intimp.2020.106443
Wang, T., Li, L., and Hong, W. (2017). SNARE proteins in membrane trafficking. Traffic 18, 767–775. doi:10.1111/tra.12524
Wang, Y. C., Xie, H., Zhang, Y. C., Meng, Q. H., Xiong, M. M., Jia, M. W., et al. (2021). Exosomal miR-107 antagonizes profibrotic phenotypes of pericytes by targeting a pathway involving HIF-1α/Notch1/PDGFRβ/YAP1/Twist1 axis in vitro. Am. J. Physiol. Heart Circ. Physiol. 320, H520–h534. doi:10.1152/ajpheart.00373.2020
Wei, D., Zhan, W., Gao, Y., Huang, L., Gong, R., Wang, W., et al. (2021). RAB31 marks and controls an ESCRT-independent exosome pathway. Cell Res. 31, 157–177. doi:10.1038/s41422-020-00409-1
Wei, H., Chen, Q., Lin, L., Sha, C., Li, T., Liu, Y., et al. (2021b). Regulation of exosome production and cargo sorting. Int. J. Biol. Sci. 17, 163–177. doi:10.7150/ijbs.53671
Willis, G. R., Fernandez-Gonzalez, A., Anastas, J., Vitali, S. H., Liu, X., Ericsson, M., et al. (2018). Mesenchymal stromal cell exosomes ameliorate experimental bronchopulmonary dysplasia and restore lung function through macrophage immunomodulation. Am. J. Respir. Crit. Care Med. 197, 104–116. doi:10.1164/rccm.201705-0925OC
Wortzel, I., Dror, S., Kenific, C. M., and Lyden, D. (2019). Exosome-mediated metastasis: Communication from a distance. Dev. Cell 49, 347–360. doi:10.1016/j.devcel.2019.04.011
Wozniak, A. L., Adams, A., King, K. E., Dunn, W., Christenson, L. K., Hung, W. T., et al. (2020). The RNA binding protein FMR1 controls selective exosomal miRNA cargo loading during inflammation. J. Cell Biol. 219, e201912074. doi:10.1083/jcb.201912074
Xiao, K., He, W., Guan, W., Hou, F., Yan, P., Xu, J., et al. (2020). Mesenchymal stem cells reverse EMT process through blocking the activation of NF-κB and Hedgehog pathways in LPS-induced acute lung injury. Cell Death Dis. 11, 863. doi:10.1038/s41419-020-03034-3
Xie, H., Gao, Y. M., Zhang, Y. C., Jia, M. W., Peng, F., Meng, Q. H., et al. (2020). Low let-7d exosomes from pulmonary vascular endothelial cells drive lung pericyte fibrosis through the TGFβRI/FoxM1/Smad/β-catenin pathway. J. Cell. Mol. Med. 24, 13913–13926. doi:10.1111/jcmm.15989
Xie, L., and Zeng, Y. (2020). Therapeutic potential of exosomes in pulmonary fibrosis. Front. Pharmacol. 11, 590972. doi:10.3389/fphar.2020.590972
Xie, S., Zhang, Q., and Jiang, L. (2022). Current Knowledge on exosome biogenesis, cargo-sorting mechanism and therapeutic implications. Membranes 12, 498. doi:10.3390/membranes12050498
Xu, C., Hou, L., Zhao, J., Wang, Y., Jiang, F., Jiang, Q., et al. (2022). Exosomal let-7i-5p from three-dimensional cultured human umbilical cord mesenchymal stem cells inhibits fibroblast activation in silicosis through targeting TGFBR1. Ecotoxicol. Environ. Saf. 233, 113302. doi:10.1016/j.ecoenv.2022.113302
Xu, C., Zhao, J., Li, Q., Hou, L., Wang, Y., Li, S., et al. (2020). Exosomes derived from three-dimensional cultured human umbilical cord mesenchymal stem cells ameliorate pulmonary fibrosis in a mouse silicosis model. Stem Cell Res. Ther. 11, 503. doi:10.1186/s13287-020-02023-9
Yamada, M. (2021). Extracellular vesicles: Their emerging roles in the pathogenesis of respiratory diseases. Respir. Investig. 59, 302–311. doi:10.1016/j.resinv.2021.02.006
Yanagihara, T., Chong, S. G., Vierhout, M., Hirota, J. A., Ask, K., and Kolb, M. (2020). Current models of pulmonary fibrosis for future drug discovery efforts. Expert Opin. Drug Discov. 15, 931–941. doi:10.1080/17460441.2020.1755252
Yang, D., Zhang, W., Zhang, H., Zhang, F., Chen, L., Ma, L., et al. (2020a). Progress, opportunity, and perspective on exosome isolation - efforts for efficient exosome-based theranostics. Theranostics 10, 3684–3707. doi:10.7150/thno.41580
Yang, J., Hu, H., Zhang, S., Jiang, L., Cheng, Y., Xie, H., et al. (2020b). Human umbilical cord mesenchymal stem cell-derived exosomes alleviate pulmonary fibrosis in mice by inhibiting epithelial-mesenchymal transition. Nan Fang. Yi Ke Da Xue Xue Bao 40, 988–994. doi:10.12122/j.issn.1673-4254.2020.07.11
Yang, L., Peng, X., Li, Y., Zhang, X., Ma, Y., Wu, C., et al. (2019). Long non-coding RNA HOTAIR promotes exosome secretion by regulating RAB35 and SNAP23 in hepatocellular carcinoma. Mol. Cancer 18, 78. doi:10.1186/s12943-019-0990-6
Yao, M. Y., Zhang, W. H., Ma, W. T., Liu, Q. H., Xing, L. H., and Zhao, G. F. (2019). microRNA-328 in exosomes derived from M2 macrophages exerts a promotive effect on the progression of pulmonary fibrosis via FAM13A in a rat model. Exp. Mol. Med. 51, 1–16. doi:10.1038/s12276-019-0255-x
Yu, Y., Liu, X., Zhao, Z., Xu, Z., Qiao, Y., Zhou, Y., et al. (2021). The extracellular matrix enriched with exosomes for the treatment on pulmonary fibrosis in mice. Front. Pharmacol. 12, 747223. doi:10.3389/fphar.2021.747223
Yu, Z., Shi, M., Stewart, T., Fernagut, P., Huang, Y., Tian, C., et al. (2020). Reduced oligodendrocyte exosome secretion in multiple system atrophy involves SNARE dysfunction. Brain. 143, 1780–1797. doi:10.1093/brain/awaa110
Zhang, E., Geng, X., Shan, S., Li, P., Li, S., Li, W., et al. (2021). Exosomes derived from bone marrow mesenchymal stem cells reverse epithelial-mesenchymal transition potentially via attenuating Wnt/β-catenin signaling to alleviate silica-induced pulmonary fibrosis. Toxicol. Mech. Methods 31, 655–666. doi:10.1080/15376516.2021.1950250
Zhang, L., and Yu, D. (2019). Exosomes in cancer development, metastasis, and immunity. Biochim. Biophys. Acta. Rev. Cancer 1871, 455–468. doi:10.1016/j.bbcan.2019.04.004
Zhang, Y., Bi, J., Huang, J., Tang, Y., du, S., and Li, P. (2020a). Exosome: A review of its classification, isolation techniques, storage, diagnostic and targeted therapy applications. Int. J. Nanomedicine 15, 6917–6934. doi:10.2147/IJN.S264498
Zhang, Z., Ge, L., Zhang, S., Wang, J., Jiang, W., Xin, Q., et al. (2020b). The protective effects of MSC-EXO against pulmonary hypertension through regulating Wnt5a/BMP signalling pathway. J. Cell. Mol. Med. 24, 13938–13948. doi:10.1111/jcmm.16002
Zhou, Y., Gao, Y., Zhang, W., Chen, Y., Jin, M., and Yang, Z. (2021). Exosomes derived from induced pluripotent stem cells suppresses M2-type macrophages during pulmonary fibrosis via miR-302a-3p/TET1 axis. Int. Immunopharmacol. 99, 108075. doi:10.1016/j.intimp.2021.108075
Zhou, Y., He, Z., Gao, Y., Zheng, R., Zhang, X., Zhao, L., et al. (2016). Induced pluripotent stem cells inhibit bleomycin-induced pulmonary fibrosis in mice through suppressing TGF-β1/smad-mediated epithelial to mesenchymal transition. Front. Pharmacol. 7, 430. doi:10.3389/fphar.2016.00430
Keywords: exosomes, pulmonary fibrosis, pathogenesis, therapeutics, biomarkers
Citation: Yang Y, Liu Y, Chai Y, Liu K, Hu W, Zhao K, Zhu Y, Gao P, Huang Q and Zhang C (2022) Exosomes in pathogenesis, diagnosis, and treatment of pulmonary fibrosis. Front. Pharmacol. 13:927653. doi: 10.3389/fphar.2022.927653
Received: 24 April 2022; Accepted: 26 July 2022;
Published: 25 August 2022.
Edited by:
Yanping Liu, Shandong University, ChinaReviewed by:
Anshul S. Jadli, University of Calgary, CanadaCopyright © 2022 Yang, Liu, Chai, Liu, Hu, Zhao, Zhu, Gao, Huang and Zhang. This is an open-access article distributed under the terms of the Creative Commons Attribution License (CC BY). The use, distribution or reproduction in other forums is permitted, provided the original author(s) and the copyright owner(s) are credited and that the original publication in this journal is cited, in accordance with accepted academic practice. No use, distribution or reproduction is permitted which does not comply with these terms.
*Correspondence: Peiyang Gao, Z2FvcHk5MzBAMTI2LmNvbQ==; Qingsong Huang, aHVhbmdxaW5nc29uZzU4MDJAMTYzLmNvbQ==; Chuantao Zhang, emhhbmdjaHVhbnRhb0BjZHV0Y20uZWR1LmNu
†These authors have contributed equally to this work and share first authorship
Disclaimer: All claims expressed in this article are solely those of the authors and do not necessarily represent those of their affiliated organizations, or those of the publisher, the editors and the reviewers. Any product that may be evaluated in this article or claim that may be made by its manufacturer is not guaranteed or endorsed by the publisher.
Research integrity at Frontiers
Learn more about the work of our research integrity team to safeguard the quality of each article we publish.