- 1Resource Institute for Chinese & Ethnic Materia Medica, Guizhou University of Traditional Chinese Medicine, Guiyang, China
- 2School of Life Science and Technology, University of Electronic Science and Technology of China, Chengdu, China
Given the ability of akebia saponin D (ASD) to protect various types of stem cells, in the present study, we hypothesized that ASD could promote the proliferation, differentiation, and survival of neural stem/precursor cells (NSPCs), even in a microglia-mediated inflammatory environment, thereby mitigating inflammation-related neuropsychopathology. We established a mouse model of chronic neuroinflammation by exposing animals to low-dose lipopolysaccharide (LPS, 0.25 mg/kg/d) for 14 days. The results showed that chronic exposure to LPS strikingly reduced hippocampal levels of PI3K and pAkt and neurogenesis in mice. In the presen of a microglia-mediated inflammatory niche, the PI3K-Akt signaling in cultured NSPCs was inhibited, promoting their apoptosis and differentiation into astrocytes, while decreasing neurogenesis. Conversely, ASD strongly increased the levels of PI3K and pAkt and stimulated NSPC proliferation, survival and neuronal differentiation in the microglia-mediated inflammatory niche in vitro and in vivo. ASD also restored the synaptic function of hippocampal neurons and ameliorated depressive- and anxiety-like behaviors and cognitive impairment in mice chronically exposed to LPS. The results from network pharmacology analysis showed that the PI3K-AKT pathway is one of the targets of ASD to against major depressive disorder (MDD), anxiety and Alzheimer’s disease (AD). And the results from molecular docking based on computer modeling showed that ASD is bound to the interaction interface of the PI3K and AKT. The PI3K-Akt inhibitor LY294002 blocked the therapeutic effects of ASD in vitro and in vivo. These results suggested that ASD protects NSPCs from the microglia-mediated inflammatory niche, promoting their proliferation, survival and neuronal differentiation, as well as ameliorating depressive- and anxiety-like behaviors and cognitive impairment by activating the PI3K-AKT pathway. Our work suggests the potential of ASD for treating Alzheimer’s disease, depression and other cognitive disorders involving impaired neurogenesis by microglia-mediated inflammation.
Highlights
1) ASD protects NSPCs from the microglia-mediated inflammatory niche and stimulates NSPC proliferation and neuronal differentiation.
2) The PI3K-Akt pathway helps mediate the neuroprotective effects of ASD.
3) ASD shows preclinical potential for treatment of disorders involving impaired neurogenesis
1 Introduction
Hippocampal neurogenesis plays an important role in structural plasticity and network maintenance in adults (Cope & Gould, 2019; Gage, 2021). Perturbation of adult neurogenesis contributes to several human diseases, including depression, anxiety, cognitive impairment and neurodegenerative diseases (Snyder et al., 2011; Anacker & Hen, 2017; Zhang J. et al., 2021; Terreros-Roncal et al., 2021). During aging, hippocampal neurogenesis declines, reducing stress resistance and increasing the risk of mood disorders and progression of cognitive impairment (Deng et al., 2010; Snyder et al., 2011; Mehdipour et al., 2021). For example, as mice age, the rate at which new granular cells form in the subgranular zone (SGZ) decreases around 40-fold (Morgenstern et al., 2008).
Neurogenesis in the adult brain can mitigate the effects of aging and neurodegeneration involving amyloid-β (Cameron & Glover, 2015; Bonafina et al., 2019). This implies that promoting neurogenesis in the adult hippocampus may be an effective treatment against Alzheimer’s disease and major depressive disorder (Berger et al., 2020).
Adult hippocampal neurogenesis is supported by the proliferation and differentiation of neural stem/precursor cells (NSPCs), which can differentiate into neurons, oligodendrocytes, and astrocytes, thereby counteracting the loss of neurons during aging (Bhattarai et al., 2016; Hattiangady et al., 2020). Such neurogenesis occurs mainly in the SGZ of the dentate gyrus (DG) and in the subventricular zone (SVZ) adjacent to the lateral ventricles (Drew et al., 2013; Jurkowski et al., 2020). Therefore, targeting the proliferation, differentiation, and survival of NSPCs in these zones may alleviate Alzheimer’s disease and major depressive disorder.
A challenge to maintaining NSPC proliferation and differentiation is the microglia-mediated neuroinflammatory niche (Sato, 2015). As innate immune cells, microglia can be activated by amyloid-β, cell debris, myelin, and other harmful substances, which enhance their phagocytic activity and thereby maintain brain homeostasis (Pap et al., 2021). However, microglia appear to be hyperactivated in patients and animal models of Alzheimer’s disease and major depressive disorder (Qiao et al., 2021). Microglia-mediated inflammation inhibits neurogenesis in the hippocampus of such patients (Santos et al., 2016; Hanslik & Ulland, 2020; Perea et al., 2020) by inhibiting NSPC proliferation and differentiation (Araki et al., 2021).
Researchers have found that akebia saponin D (ASD), a triterpenoid saponin extracted from Dipsacus asper Wall, a traditional Chinese medicine, induces the proliferation and differentiation of various types of stem cells as well as angiogenesis under inflammatory conditions (Ding et al., 2019; Gu et al., 2020; Niu et al., 2021; Chen et al., 2022). Several studies have shown that ASD can cross the blood-brain barrier to improve AD pathology and Aβ-induced cognitive deficits, as well as inflammation-induced depression (Yu et al., 2012; Wang et al., 2018; Zhang et al., 2020c; Stepnik, 2021). However, the molecular mechanisms by which ASD exerts these effects remain elusive. Based on the protective and promoting effects of ASD on the function of various stem cells, we wondered whether ASD might promote NSPC proliferation and differentiation, even in a microglia-mediated inflammatory environment. This led us to wonder whether ASD might exert its neurotherapeutic effects by robustly stimulating NPSCs in the hippocampus.
Therefore we evaluated the effects of ASD on hippocampal neurogenesis and behavior in a mouse model of chronic neuroinflammation. We also evaluated the effects of ASD on NSPC proliferation, differentiation, and survival in a microglia-mediated inflammatory niche in vitro. Our studies provide a mechanistic rationale for deepening the exploration of ASD as a promising treatment against Alzheimer’s disease, depression and other cognitive disorders involving impaired neurogenesis.
2 Material and methods
2.1 Network pharmacology analysis
To screen the pathways which ASD targets for major depressive disorder (MDD), anxiety and Alzheimer’s disease (AD), the network pharmacology analysis was performed based on previous studies (Zhang et al., 2021). In brief, potential MDD-, anxiety- and AD-related targets were retrieved from the Human Gene Database (GeneCards, https://www.genecards. org/) and the DisGeNET Database (https://www.disgenet.org/home/). Targets of ASD were obtained from the TCMSP and SwissTargetPrediction databases (http://www.Swisstargetprediction.ch). Then, the targets were standardized in the UniProt (https://www.uniprot.org) database with status set as ‘reviewed’ and species set as ‘human’. After removing duplicates, a database of ASD and their targets was constructed. Screened targets of the ASD and potential MDD-, anxiety- and AD-related targets were imported into a Venn diagram webtool (http://bioinformatics.psb.ugent.be/webtools/Venn/) for analysis, and common targets were extracted for further Kyoto Encyclopedia of Genes and Genomes (KEGG) pathway enrichment analysis using R software, a free software environment for statistical computing and graphics. The KEGG pathway analyses were screened for p < 0.05. The top 20 items of KEGG analysis identified from R software were mapped as bubble plots.
2.2 Molecular docking
The Surflex-Dock module of Sybyl-X2.1 software (Tripos Associates Inc., S.H.R.; St. Louis, MO 631444, USA) was used for molecular docking to predict the binding mode of ASD with the Pi3k and AKT based on previous studies (Yu et al., 2022).
2.3 Animals
Male C57BL/6J mice (7–8 weeks old) were purchased from Changsha Tianqin Biotechnology (Changsha, China) and caged individually. The mice were habituated to their new environment for 1 week. The mice were then habituated to a 1% sucrose solution for 48 h. Sucrose preference was tested before the experiment began, defined as day 0, and this preference as well as the animal body weight served as the baseline. The mice were then divided into seven groups as described in sections 2.4. All experiments were approved by the Institutional Animal Care and Use Committee of the Guizhou University of Traditional Chinese Medicine.
2.4 Pharmacological treatments in vivo
ASD (99.92% pure) was purchased from Chengdu Alfa Biotechnology (Chengdu, China). Lipopolysaccharide (LPS; Sigma-Aldrich, MO, USA) or ASD was dissolved in 0.9% saline at a concentration of 4 mg/ml. Minocycline hydrochloride (MedChemExpress, WeVoice, USA) or LY294002 (MedChemExpress) was dissolved to a concentration of 2.5 g/ml in 0.9% saline containing 5% dimethyl sulfoxide (DMSO). Mice were habituated to 1% sucrose solution for 1 week, then allocated into seven groups such that baseline sucrose preference and body weight did not differ significantly across the groups. The seven groups were as follows: control + vehicle (Ctrl), 0.25 mg/kg/d LPS + vehicle (LPS), LPS +10 mg/kg/d ASD [LPS + ASD (10 mg)], LPS +50 mg/kg/d ASD [LPS + ASD (50 mg)], LPS +100 mg/kg/d ASD [LPS + ASD (100 mg)], LPS +50 mg/kg/d minocycline hydrochloride (LPS + Mino), and LPS +100 mg/kg/d ASD +6 μg LY294002 (LPS + ASD + LY294002).
Mice were intraperitoneally administered vehicle, ASD, minocycline hydrochloride or LY294002 once daily (at 10:00 h). The animals were also intraperitoneally administered saline or LPS once daily (at 16:00 h) for 14 days. The doses of ASD, LPS, minocycline and LY294002 were chosen based on previous studies (Guo et al., 2019; Zhang et al., 2020c; Bassett et al., 2021; Silva et al., 2021).
2.5 Behavioral testing
2.5.1 Sucrose preference test (SPT)
The SPT was performed as described (Zhang J. et al., 2021). Briefly, mice were individually housed, deprived of food and water for 12 h, and then given access to 1% sucrose solution (A) and water (B) for 2 h. The bottle positions were switched daily to avoid a side bias. The sucrose preference was calculated each week for each mouse using the formula: 100 × [VolA/(VolA + VolB)]. The sucrose consumption was normalized to body weight for each mouse.
2.5.2 Forced swimming test (FST)
The FST was performed as described (Zhang J. et al., 2021). Briefly, at 24 h before the test, each mouse was individually placed in a glass cylinder (height: 25 cm, diameter: 15 cm) filled with 26 °C water to a depth of 15 cm for 15 min. The next day, the mice were placed once again in the same situation for 6 min. The entire process was recorded with a high-definition camera. An observer blinded to animal group recorded the time spent immobile during the last 4 min.
2.5.3 Elevated plus maze test (EPMT)
The elevated plus maze contained two open arms (35 × 5 cm) and two closed arms (35 × 5 cm), connected by a central area (5 × 5 cm). The apparatus was lifted 50 cm above the floor. Tests were carried out in a quiet, dimly lit environment. The apparatus was wiped clean with 75% ethanol between tests. Mice were introduced to the maze at 1 h after the end of open field test. Mice were gently placed in the central area such that they faced one of the open arms. Spontaneous activity was monitored during 5 min. The number of entries into open arms as well as the total time spent in open arms were determined using EMP100 software (Taimeng Tech, Chengdu, China).
2.5.4 Novel object recognition test (NORT)
The novel object recognition test was performed as described (Zhang et al., 2021). Mice were individually placed for 5 min in a Plexiglass arena measuring 40 × 60 cm with walls 30 cm high, and exploration was quantified using OFT100 video tracking software (Taimeng Tech, Chengdu, China). Subsequently, mice were subjected to three habituation sessions in which two objects identical in shape, color, and odor were introduced into the arena for 3 min at a 2-min interval between trials. Before the last session, one of the objects was replaced with a novel object. The time spent exploring each object was scored during each session.
2.5.5 Morris water maze (MWM)
Spatial learning and memory were assessed in a Morris water maze. Experiments were carried out in a blue circular pool (diameter, 150 cm; height, 60 cm) filled to a depth of 30 cm with water rendered opaque by addition of 500 g milk powder and 30 ml blue food coloring. The area of the pool was divided into four virtual quadrants (NW, SW, SE, NE).
The experimental protocol consisted of four training days and an additional probe trial day. On training days, a platform was placed in the center of the SW quadrant, and the mice had to learn the location of the hidden platform with the help of visual cues placed around the maze. All animals had to perform four trials per training day. In each trial, the mice were placed into different quadrants (varied in a clockwise direction) and allowed to search for the hidden platform for 2 min. During the trials, the escape latency (time until the platform was found), the swimming path length to the platform and the swimming speed of the animals were measured and analyzed using Ethovision XT10 software (Noldus, Wageningen, Netherlands). If the platform was not found during the trial, rats were put on the platform for 10 s, and the escape latency was recorded as 2 min. The performance of the animals on the first training day was used to evaluate their short-term memory performance.
2.6 Primary culture of microglia and treatments
Primary microglia were isolated from brains of neonatal C57BL/6J mice (P0–P3) as described (Zhang et al., 2020b). After removing the meninges and blood vessels, brains were minced and centrifuged at 800 g for 5 min. The pellet was dissociated in 0.0125% trypsin (Gibco, CA, USA) for 10 min. The suspension was passed through a 70-μm cell strainer (Koch Membrane Systems, KS, USA). Cell pellets were harvested, washed, and cultured at 37°C in DMED–F12 medium containing 10% fetal bovine serum (FBS; Gibco). This procedure gave rise to mixed cultures in which microglia grew loosely atop a layer of tightly adhering astrocytes. Microglia were harvested by mechanical shaking, then transferred to new culture dishes. The purity of microglia was verified using immunofluorescence based on labeling with anti-Iba1 antibodies to identify microglia and with DAPI to identify nuclei. Cultures in which >98% of cells were Iba1+ were used in subsequent experiments.
2.7 Culture of adult NSPCs
Adult NSPCs were obtained from the subventricular zone of eight-week-old male C57BL/6J mice. The entire subventricular zone was dissected, and the lateral wall of the lateral ventricles was carefully removed from the surrounding brain tissue and collected in phosphate-buffered saline (PBS; BOSTER, Wuhan, China). These tissues were chopped into 1-mm cubes and digested in 0.125% trypsin (Gibco) for 5 min, then the digestion was stopped with soybean trypsin inhibitor. Cells were resuspended in complete DMEM/F12 medium (Gibco) containing 20 ng/ml recombinant murine fibroblast growth factor (FGF; PeproTech, NJ, USA), 20 ng/ml recombinant murine epidermal growth factor (EGF; PeproTech), 1% N-2 supplement (Gibco), and 2% B-27 supplement (Gibco). After culture for 7 days, neurospheres were isolated by centrifugation (600 g for 3 min), enzymatically dissociated into a single-cell suspension using 0.125% pancreatin (Sigma-Aldrich), and plated at a density of 5 × 104 cells/cm2 in proliferation medium. To permit serial cell passaging, this pancreatin dissociation process was repeated every 3–4 days.
2.8 Culture of NSPCs with conditioned medium from LPS-treated microglia
Microglia were plated at a density of 5 × 105 cells/cm2 and treated with either LPS or PBS for 24 h, washed twice with PBS, then cultured in DMEM-F12 + GlutaMax medium (Gibco) for another 24 h. The microglial medium was collected and used as conditioned medium to stimulate NSPC differentiation and proliferation. NSPCs were cultured in conditioned media from microglia treated with LPS (LPS-M-CM) or PBS (PBS-M-CM). These NSPCs were treated with 0, 10, 50 or 100 μM ASD, 50 ng/ml recombinant mouse brain-derived neurotrophic factor (BDNF; AmyJet, Wuhan, China) or 5 μM LY294002 (MedChemExpress). NSPC proliferation, differentiation, and survival were evaluated by immunofluorescence staining as described in sections 2.12.
2.9 Analysis of NSPC proliferation in vitro and in vivo
NSPCs were cultured in proliferation medium (97% DMEM/F12 + 20 ng/ml FGF +20 ng/ml EGF +1% N-2 supplement +2% B-27 supplement) and treated with different concentrations of ASD (10, 50, or 100 μM) or 50 ng/ml BDNF in the presence of PBS-M-CM or LPS-M-CM. The number and size of neurospheres were assessed after 4 days of culture.
To examine NSPC proliferation in the brain, mice received intraperitoneal injections of 5′-bromo-2′deoxyuridine (BrdU; Sigma-Aldrich) at a daily dose of 50 mg/kg for 3 days. Mice were euthanized at 24 h after the last injection, and proliferating cells in the hippocampus were labeled with anti-BrdU antibody as described below. The number of BrdU + cells was quantified.
2.10 Analysis of NSPC differentiation in vitro and in vivo
NSPCs were cultured in differentiation medium (93% DMEM/F12 + 1% N-2 supplement +2% B-27 supplement +5% FBS) and treated with different concentrations of ASD (10, 50, or 100 μM) or 50 ng/ml BDNF in the presence of PBS-M-CM or LPS-M-CM. After 5 days in culture, the rates of NSPC differentiation into neurons or astrocytes were determined by quantifying the percentages of DCX + or GFAP + or NG2+ cells as described in section 2.12.
To examine NSPC differentiation in the brain, mice received intraperitoneal injections of BrdU at a daily dose of 50 mg/kg for 3 days. Mice were euthanized at 7 days after the last injection. Using immunocytochemistry of hippocampal sections as described below, we identified neurons that differentiated from progenitor cells based on double labeling with anti-BrdU and anti-DCX antibodies, and we identified astrocytes that differentiated from progenitor cells based on double labeling with anti-BrdU and anti-GFAP antibodies. Differentiation of hippocampal NSPCs was assessed by quantifying the number and percentage of BrdU + -DCX + cells or BrdU + -GFAP + cells.
2.11 Analysis of NSPC survival and newborn neuron maturation in vitro and in vivo
NSPCs were cultured in proliferation medium for 24 h, then treated with BrdU for another 12 h. The culture medium was replaced with PBS-M-CM or LPS-M-C, to which were added different concentrations of ASD (10, 50, or 100 μM) or 50 ng/ml BDNF. After 7 days in differentiation culture, the rate of NSPC survival was determined by quantifying the percentage of BrdU + cells as described below.
To evaluate NSPC survival and newborn neuron maturation in the brain, mice received intraperitoneal injections of BrdU at a daily dose of 50 mg/kg for 3 days, after which animals were administered LPS, ASD, minocycline or LY294002 as described in sections 2.4. Mice were euthanized at 14 days after the last injection. The rate of NSPC survival was determined by quantifying the number of BrdU + cells as described below. Mature neurons derived from progenitor cells were identified based on double labeling with anti-BrdU and anti-NeuN antibodies.
2.12 Immunocytochemistry
Brain tissue was prepared and stained as described (Zhang et al., 2020b). Differentiated NSPCs were plated at a density of 105 cells/cm2 and fixed with 4% paraformaldehyde (pH 7.2) for 30 min. Hippocampal tissue or primary cells in culture were permeabilized with 0.5% Triton X-100 in PBS for 15 min, blocked in 10% donkey serum for 2 h, then incubated overnight at 4 °C with the following primary antibodies: goat anti-Iba1 (1:400, Abcam, Cambridge, United Kingdom), goat anti-Doublecortin (DCX; 1:400, Santa Cruz Biotechnology, CA, USA), mouse anti-BrdU (1:400, Cell Signaling Technology, MA, USA), and rabbit anti-GFAP (1:400, Cell Signaling Technology).
Tissue or cells were then incubated for 2 h at room temperature with DyLight 549-conjugated donkey anti-goat or DyLight 488-conjugated donkey anti-mouse secondary antibodies (both 1:300, Jackson ImmunoResearch, PA, USA). Finally, cells were incubated with DAPI (1:10,000, Roche, Basel, Switzerland) for 5 min and imaged using a fluorescence microscope (IX73, Olympus, Tokyo, Japan). Cell numbers were quantified using GraphPad Prism 5.0 (version 8.0, SPSS Inc., Chicago, USA).
2.13 Enzyme-linked immunosorbent assay (ELISA)
The dentate gyrus was dissociated from slices containing the hippocampus, flash-frozen in liquid nitrogen, and homogenized. Samples were centrifuged at 1, 000 g for 30 min. The concentration of total protein was determined using the BCA kit (BOSTER, Wuhan, China). After each sample was diluted to 1 g/ml, supernatants were assayed for interleukin (IL)-1β using an ELISA kit (BOSTER) according to the manufacturer’s protocols, and IL-1β concentration per g of total protein was calculated. The manufacturer-specified detection limit was 4 pg/ml.
2.14 Western blotting
Western blotting was used to assess levels of phosphatidylinositol 3 kinase/serine-threonine kinase (PI3K/Akt) signaling in hippocampus and in NSPCs. Mice were perfused with PBS, hippocampi were isolated and homogenized. Hippocampi or primary cultures of NSPCs were sonicated in RIPA buffer containing protease and phosphorylase kinase inhibitors (Solarbio, Beijing, China). Protein lysate was centrifuged at 1,000 g for 30 min, then the supernatant was fractionated on 12% Tris-glycine SDS-PAGE gels, transferred to PVDF membranes (0.2 or 0.45 μm), and incubated with antibodies against PI3K (1:600, Cell Signaling Technology), AKT (1:400, Abcam), or phospho-AKT (pAKT; 1:600, Abcam). Membranes were incubated with the primary antibody overnight at 4 °C, washed with PBS (BOSTER), then incubated with secondary antibodies (1:10,000, Abcam) for 2 h at room temperature. Fluorescence was developed using the ECL-Plus kit (Millipore, MA, USA) and quantified using ImageJ software (version 1.45J; National Institutes of Health, Bethesda, MD, USA).
2.15 Statistical analysis
GraphPad Prism software (version 6.0; GraphPad Software, Chicago, USA) was used for all statistical analyses. Data were presented as the mean ± standard error of the mean (SEM). Pairwise comparisons were assessed for significance using Student’s two-tailed t test, and comparisons of three or more values were assessed using one- or two-way ANOVA and Tukey’s multiple-comparisons test. Differences were considered statistically significant if p < 0.05.
3 Results
3.1 ASD protects NSPCs from the microglia-mediated inflammatory niche, promoting their proliferation, survival and neuronal differentiation in vitro by activating the PI3K-Akt pathway
To confirm that ASD directly regulates NSPCs, we examined the effects of ASD on NSPC proliferation, survival and neuronal differentiation in vitro. To simulate the microglia-mediated inflammatory niche, primary cells or neurospheres were cultured in conditioned medium from primary microglial cultures (Figure 1A). Like BDNF, 100 μM ASD increased the diameter of neurospheres in PBS-M-CM. LPS-M-CM decreased the diameter of the neurospheres, which ASD reversed at concentrations of 50 or 100 μM, but not 10 μM (Figure 1B).
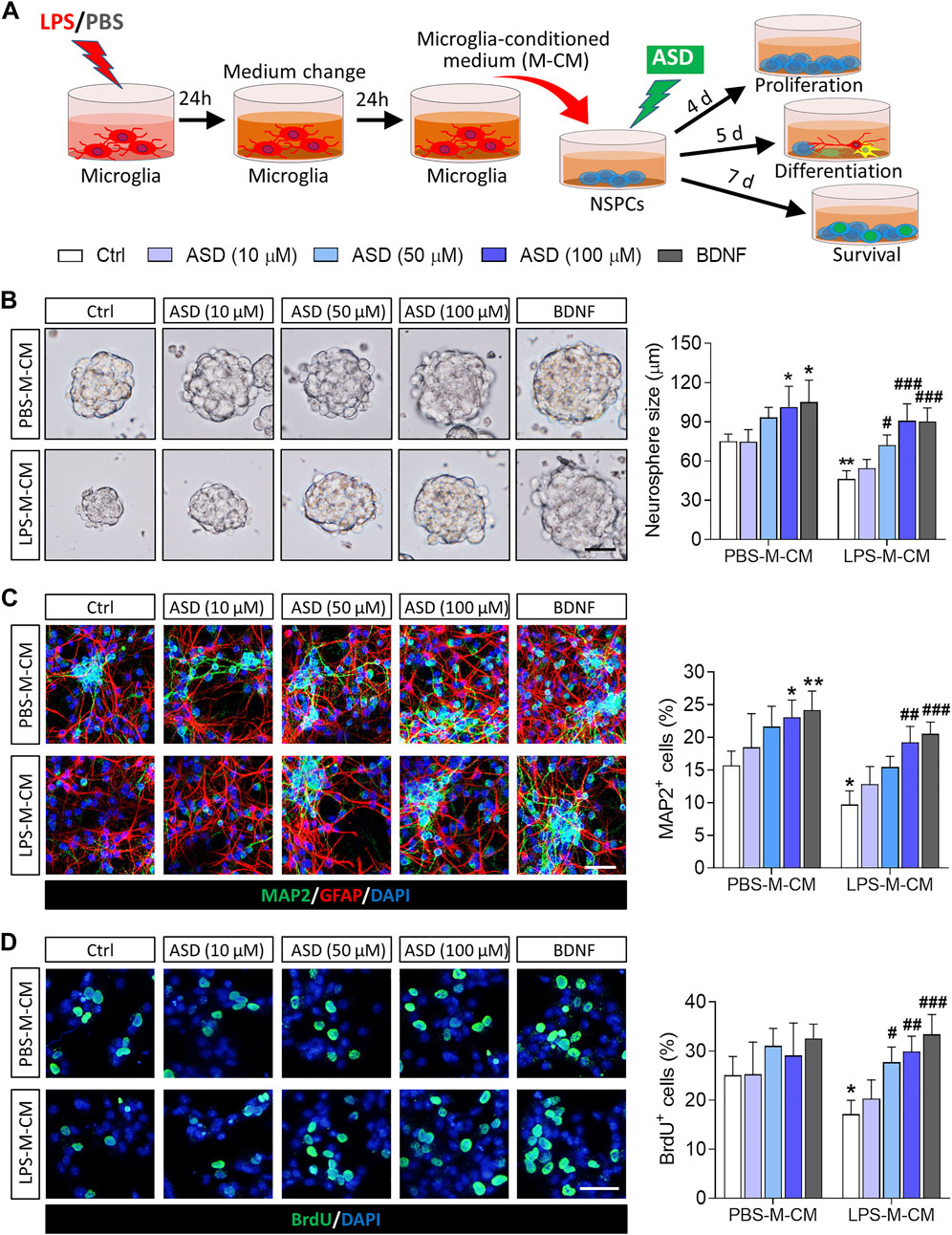
FIGURE 1. Effects of ASD on NSPC proliferation, survival and neuronal differentiation in the presence or absence of a microglia-mediated inflammatory niche. (A), Scheme describing the experimental evaluation of the effects of akebia saponin D (ASD) on survival of neurospheres in the presence or absence of an inflammatory niche. Microglia were treated with phosphate-buffered saline (PBS) or lipopolysaccharide (LPS) for 24 h, and the microglia-conditioned medium (M-CM) was collected. Neural stem/precursor cells (NSPCs) were treated with different concentrations of ASD (0, 10, 50, or 100 μM) in the presence of PBS-M-CM or LPS-M-CM. Brain-derived neurotrophic factor (BDNF) at 50 ng/ml was used as control. NSPC proliferation was measured after 4 days in culture; differentiation, after 5 days; and survival, after 7 days. (B), Micrographs and quantification of neurosphere size under different treatment conditions. Scale bar, 100 μm. (C), Micrographs and quantification of pleiotropic NSPC differentiation under different treatment conditions. Astrocytes were labeled with antibody against GFAP (green); neurons, with antibody against microtubule-associated protein 2 (MAP2) (red). Scale bar, 50 μm. (D), Micrographs and quantification of NSPC survival under different treatment conditions. Surviving NSPCs were labeled with BrdU (green). Scale bar, 20 μm. Results for each group were averaged from 5 micrographs (40×) from each of 4-6 slides. Data are mean ± standard error of the mean (SEM). *p < 0.05, **p < 0.01 vs. control microglia conditioned medium (PBS-M-CM), #p < 0.05, ##p < 0.01, ###p < 0.001 vs. LPS-treated microglia conditioned medium (LPS-M-CM) by two-way ANOVA with Tukey’s multiple-comparisons test.
To confirm that ASD promotes NSPC differentiation, NSPCs were allowed to differentiate for 7 days in differentiation medium in the presence or absence of ASD. Then, we analyzed the percentages of cells that were developmentally committed to becoming neurons (DCX + cells) or astrocytes (GFAP + cells). LPS-M-CM reduced the neuronal differentiation of NSPCs, while either BDNF or ASD (50 or 100 μM) promoted it in the presence or absence of LPS-M-CM (Figure 1C).
To confirm that ASD promotes NSPC survival, we used BrdU to label proliferating NSPCs, then these cells were allowed to differentiate for 7 days in differentiation medium. Exposing NSPCs to LPS-M-CM decreased the percentage of BrdU + cells, which 50 or 100 μM ASD partially reversed (Figure 1D).
Next, we confirmed in this in vitro system that the PI3K-Akt pathway mediates the effects of ASD on NSPC proliferation, survival and neuronal differentiation. Conditioned medium from microglia treated only with LPS reduced levels of PI3K, Akt, and pAkt in primary cultures of NSPCs, while ASD (100 μM) strongly increased those levels in the presence or absence of LPS-M-CM (Figure 2A). In addition, the PI3K-Akt pathway inhibitor LY294002 blocked the effects of ASD on NSPC proliferation and neuronal differentiation in the presence or absence of LPS-M-CM (Figures 2B–D).
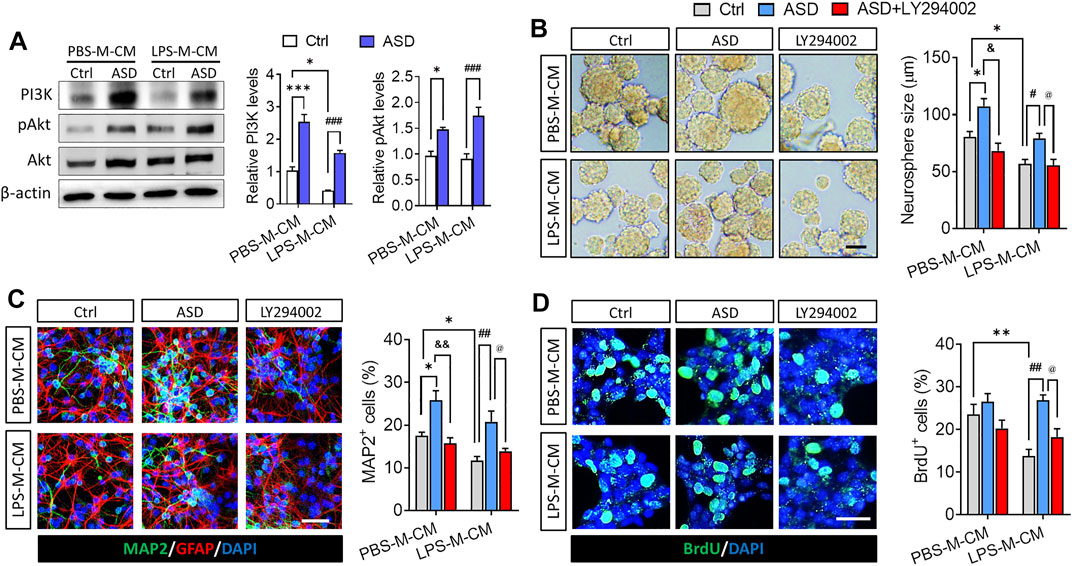
FIGURE 2. ASD protects NSPCs from the microglia-mediated inflammatory niche by activating the PI3K-AKT pathway. (A), Western blotting showing activation of the PI3K-AKT pathway in NSPCs after ASD treatment (100 μM) in the presence of PBS-M-CM or LPS-M-CM. Levels of PI3K and AKT were normalized to those of β-actin, and levels of phospho-AKT (p-AKT) were normalized to those of AKT. Ctrl, control without ASD. (B), Micrographs and quantification of neurosphere size, showing that the PI3K-AKT pathway inhibitor LY294002 blocked the effects of ASD on neurosphere proliferation in the presence of PBS-M-CM or LPS-M-CM. Scale bar, 100 μm. (C), Micrographs and quantification of the percentage of MAP2+ cells, showing that LY294002 also blocked the effects of ASD on neurogenesis in the presence of PBS-M-CM or LPS-M-CM. Astrocytes were labeled with antibody against GFAP (green); neurons, with antibody against MAP2 (red). Scale bar, 50 μm. (D), Micrographs and quantification of the percentage of BrdU + cells, showing that LY294002 blocked the effects of ASD on NSPC survival in the presence of LPS-M-CM. Surviving NSPCs were labeled with BrdU (green). Scale bar, 20 μm. Results for each group were averaged from 5 micrographs (40×) from each of 4-6 slides. Data are mean ± standard error of the mean (SEM), *p < 0.05, **p < 0.01, ***p < 0.001 vs. control microglia conditioned medium (PBS-M-CM), #p < 0.05, ##p < 0.01, ###p < 0.001 vs. LPS-treated microglia conditioned medium (LPS-M-CM), &p < 0.05, &&p < 0.01 vs. ASD + PBS-treated microglia conditioned medium (ASD + PBS-M-CM), @p < 0.05 vs. ASD + LPS-treated microglia conditioned medium (ASD + LPS-M-CM) by two-way ANOVA with Tukey’s multiple-comparisons test.
These results suggest that ASD protects NSPCs from the microglia-mediated inflammatory niche and promotes their proliferation and neurogenesis by activating the PI3K-Akt pathway.
3.2 ASD protects hippocampal neurogenesis from the chronic LPS-induced inflammatory niche in vivo by activating the PI3K-Akt pathway
Given the neuroprotective effects of ASD, we evaluated its effects on hippocampal neurogenesis and behavior in a mouse model of chronic neuroinflammation, induced by chronic exposure to LPS (Figure 3A). Given the role of PI3K-Akt signaling in regulation of stem cell function and improvement of cognitive deficits (Yu et al., 2012; Ke et al., 2016), we first examined the effects of ASD on PI3K-Akt signaling in the hippocampus. We found that chronic LPS exposure caused a striking reduction in the levels of PI3K and pAkt in hippocampus, which ASD reversed in a dose-dependent manner (Figures 3B,C).
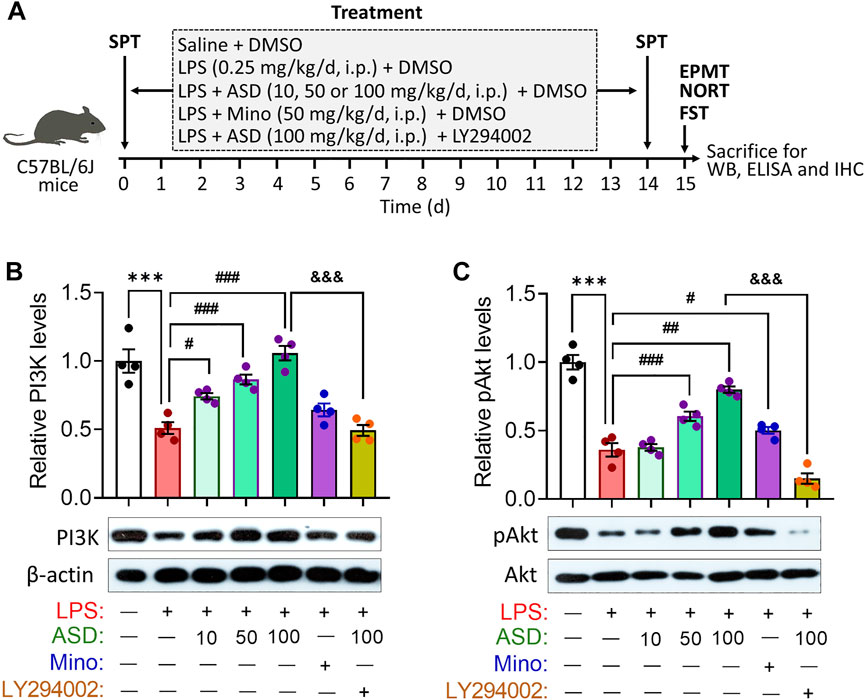
FIGURE 3. ASD activates the PI3K-Akt pathway in hippocampus of mice chronically exposed to LPS. (A), Scheme of the experimental procedure. ASD, akebia saponin D; DMSO, dimethyl sulphoxide; ELISA, enzyme-linked immunosorbent assay; EPMT, elevated plus maze test; FST, forced swimming test; IHC, immunocytochemistry; LPS, lipopolysaccharide; Mino, minocycline; NORT, novel object recognition test; SPT, sucrose preference test; WB, western blotting. (B,C), Western blotting shows the levels of PI3K and pAkt in the hippocampus of mice treated with saline (Ctrl) or lipopolysaccharide (LPS), then with akebia saponin D (ASD), minocycline (Mino) or PI3K-Akt inhibitor (LY294002). Levels of PI3K were normalized to those of β-actin, and levels of pAkt were normalized to those of Akt. Data are mean ± standard error of the mean (SEM) (n = 4), ***p < 0.001 vs. Ctrl group, #p < 0.05, ##p < 0.01, ###p < 0.001 vs. LPS group, &&&p < 0.001 vs. ASD (100 mg/kg) + LPS group based on one-way ANOVA with Tukey’s multiple-comparisons test.
LPS-induced microglial hyperactivation should supress neurogenesis (Littlefield et al., 2015; Bassett et al., 2021), which we confirmed by showing that LPS strongly reduced the numbers of BrdU + cells (proliferating cells) (Figures 4A,B), BrdU + -DCX + cells (newborn neurons) (Figures 4C–E) and BrdU + -NeuN + cells (mature neurons) (Figures 5A–D) in hippocampus, while increasing the number of GFAP + -BrdU + cells in the SGZ of the hippocampus (Figures 4F–H). Like minocycline, ASD at 100 mg/kg/d for 14 days strongly reversed these LPS effects, while the effects of ASD were blocked by the PI3K-Akt signaling inhibitor LY294002 (Figures 4A–H, 5A–D). We also found that ASD at 100 mg/kg/d for 14 days promoted the proliferation of neural stem cells in normal mice, but did not affect hippocampal neurogenesis and the maturation of newborn neurons (Supplementary Figure S1).
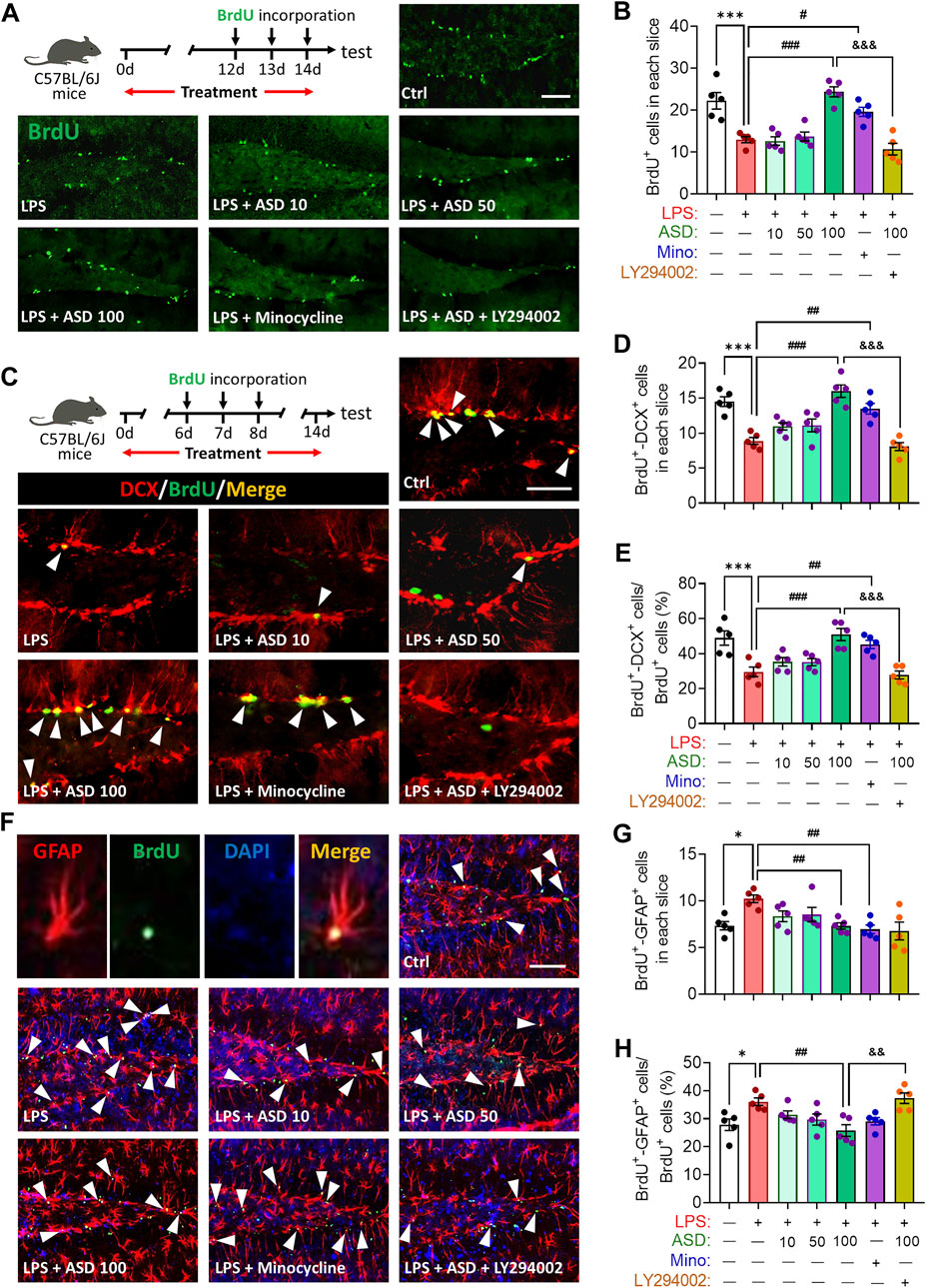
FIGURE 4. Effects of ASD on NSPC proliferation and differentiation in dentate gyrus of mice chronically exposed to LPS. (A), Timeline for detecting proliferation of neural stem/progenitor cells (NSPCs) based on immunofluorescence micrographs of BrdU + cells in dentate gyrus (DG) of mice. Proliferating NSPCs were labeled using 5′-bromo-2′deoxyuridine (BrdU) (green). Scale bar, 100 μm. (B), Quantification of hippocampal BrdU + cells. Five mice from each group were examined, and five hippocampal micrographs (40×) from each animal were quantified. (C), Timeline for evaluating NSPC differentiation based on immunofluorescence micrographs of BrdU + -DCX + cells in dentate gyrus (DG) of mice. Proliferating NSPCs were labelled with BrdU (green); immature neurons, with antibody against doublecortin (DCX); and newborn neurons differentiated from NSPCs, with both BrdU and anti-DCX antibody (white arrowheads). Scale bar, 100 μm. (D), Quantification of the hippocampal BrdU + -DCX + cells in each slice. (E), Quantification of the percentage of total BrdU + cells in the DG that were BrdU + -DCX+. (F), Immunofluorescence micrographs of BrdU + -GFAP + cells in the DG. Proliferating NSPCs were labelled with BrdU (green); astrocytes, with antibody against GFAP; and newborn astrocytes differentiated from NSPCs, with BrdU and anti-GFAP antibody (white arrowheads). Scale bar, 100 μm. (G), Quantification of hippocampal BrdU + -GFAP + cells in each slice. (H), Quantification of the percentage of total BrdU + cells in the DG that were BrdU + -GFAP+. Five mice from each group were examined, and five hippocampal micrographs (40×) from each animal were quantified. Each dot in the bar graph represents the average of all micrographs for each mouse. Data are mean ± standard error of the mean (SEM) (n = 5), *p < 0.05, ***p < 0.001 vs. Ctrl group, #p < 0.05, ##p < 0.01, ###p < 0.001 vs. LPS group, &&p < 0.01, &&&p < 0.001 vs. ASD (100 mg/kg) + LPS group by one-way ANOVA with Tukey’s multiple-comparisons test. Each dot in the bar graph represents the average of all micrographs for each mouse.
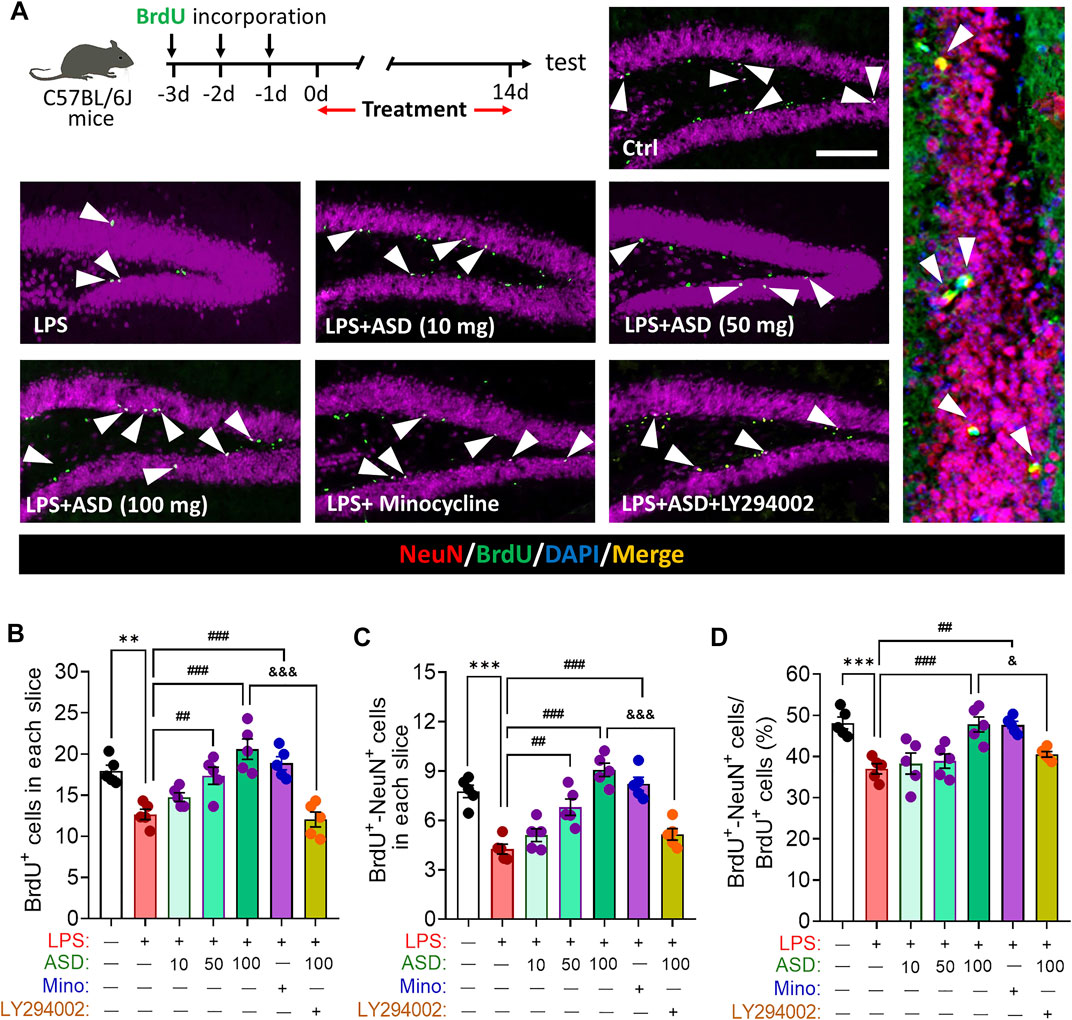
FIGURE 5. Effects of ASD on survival and maturation of newborn neurons in dentate gyrus of mice chronically exposed to LPS. (A), Timeline for evaluating newborn neuron survival and maturation based on immunofluorescence micrographs of BrdU + -NeuN + cells in the dentate gyrus of mice. Surviving cells were labelled with BrdU (green); mature neurons, with antibody against neuron-specific nucleoprotein (NeuN); and mature neurons differentiated from NSPCs, with both BrdU and anti-NeuN antibody (white arrowheads). Scale bar, 100 μm. (B), Quantification of hippocampal BrdU + cells in each slice. (C), Quantification of hippocampal BrdU + -NeuN + cells in each slice. (D), Quantification of the percentage of total BrdU + cells in DG that were BrdU + -NeuN+. Five mice from each group were examined, and five hippocampal micrographs (40×) from each animal were quantified. Each dot in the bar graph represents the average of all micrographs for each mouse. Data are mean ± standard error of the mean (SEM) (n = 5), **p < 0.01, ***p < 0.001 vs. Ctrl group, ##p < 0.01, ###p < 0.001 vs. LPS group, &p < 0.05, &&&p < 0.001 vs. ASD (100 mg/kg) + LPS group by one-way ANOVA with Tukey’s multiple-comparisons test.
Abnormal neurogenesis in hippocampus will lead to synaptic dysfunction which link to depression- and anxiety-related symptoms aand cognitive function. Indeed, our results showed that chronic LPS exposure decreased the hippocampal levels of glutamate receptor 1 (GluA1) and 2 (GluA2) subunits of the a-amino-3-hydroxy-5-methyl-4-isoxazole-propionicacid (AMPA), which mediated rapid excitatory synaptic transmission in the central nervous system. These changes in synaptic function-related markers were partially reversed by ASD, while the effects of ASD were blocked by the PI3K-Akt signaling inhibitor LY294002 (Figures 6A,B).
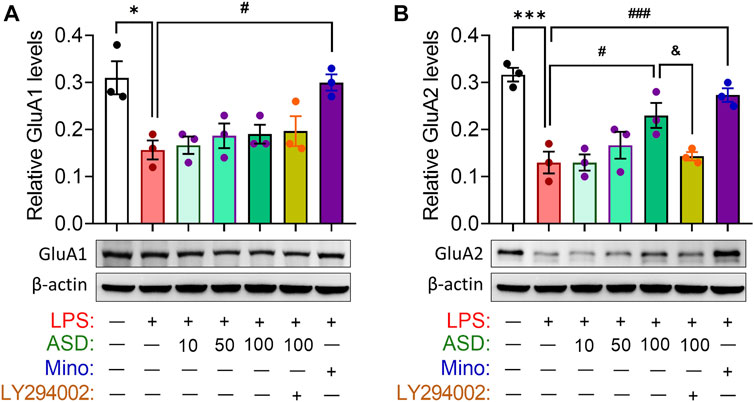
FIGURE 6. Effects of ASD on synaptic function in hippocampus of mice chronically exposed to LPS. (A,B), Western blotting shows the levels of GluA1 and GluA2 in the hippocampus of mice treated with saline (Ctrl) or lipopolysaccharide (LPS), then with akebia saponin D (ASD), minocycline (Mino) or PI3K-Akt inhibitor (LY294002). Levels of GluA1 and GluA2 were normalized to those of β-actin. Figures 6A,B share the same β-actin. Data are mean ± standard error of the mean (SEM) (n = 4), *p < 0.05, ***p < 0.001 vs. Ctrl group, #p < 0.05, ###p < 0.001 vs. LPS group, &p < 0.05 vs. ASD (100 mg/kg) + LPS group based on one-way ANOVA with Tukey’s multiple-comparisons test.
Given the observed improvement in hippocampal neurogenesis of LPS-administrated mice that were treated with ASD, we determined whether ASD inhibits microglial activation or acts directly on neural stem cells in neurogenic niche of mice exposed LPS stimulation. We found that LPS induced increases in the area of Iba1+ cells in hippocampus and in the level of IL-1β in hippocampus or hippocampal CA1, CA3 and dentate gyrus (Figures 7A–G), reflecting proliferation of microglia and their activation to secrete pro-inflammatory cytokines. Minocycline and ASD at 100 mg, but not 10 mg or 50 mg, reversed these effects of LPS in hippocampal CA1, CA3 (Figures 7A–G). However, even the 100 mg of ASD did not completely reverse the LPS-induce changes in morphology and number of microglia and the increase in IL-1β in hippocampal dentate gyrus (Figures 7A–G). These results suggest that ASD maybe directly activate PI3K-Akt signaling of hippocampal NSPC to promote neurognegesis rather than inhibition of microglial activation in dentate gyrus of LPS-exposed mice.
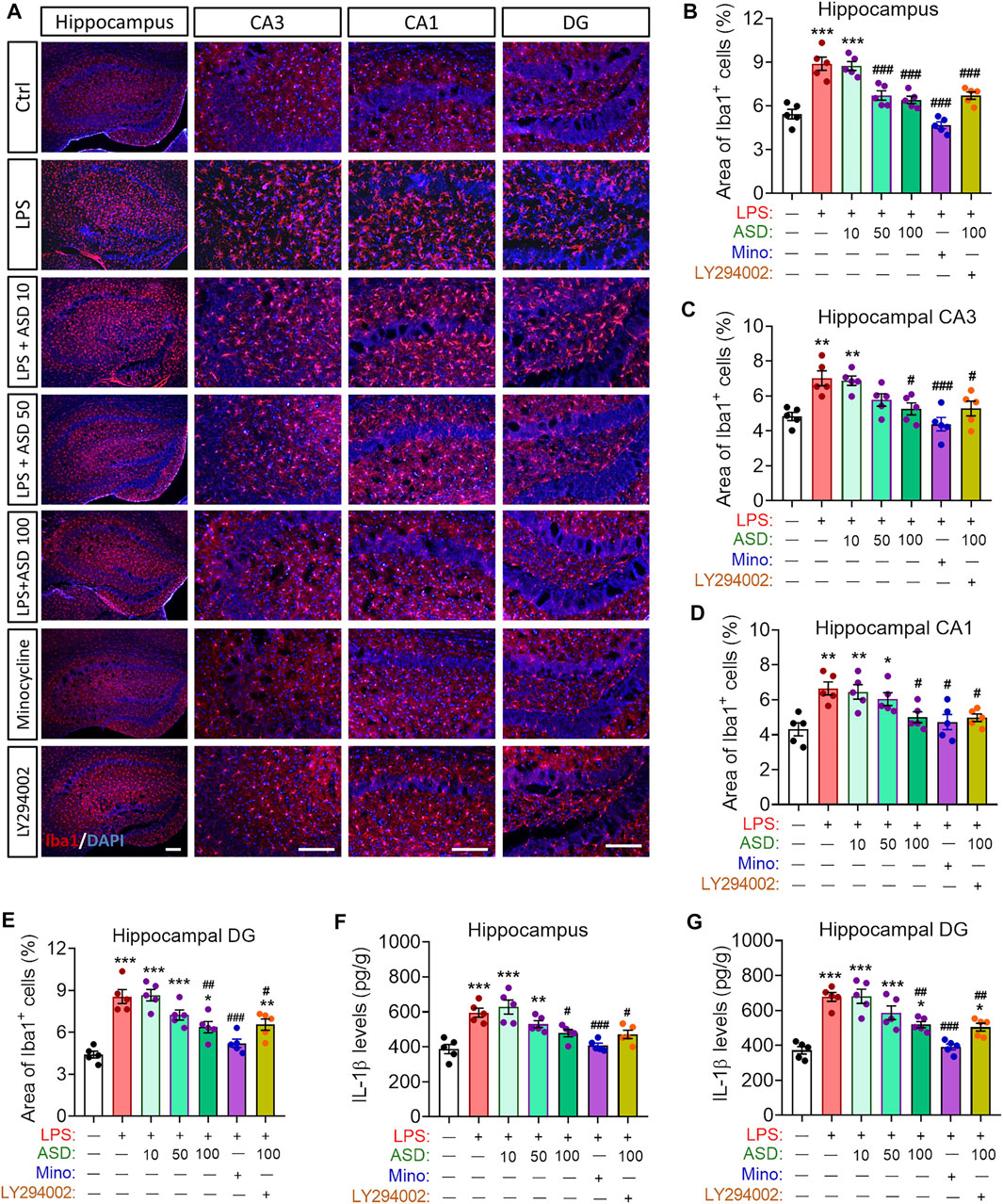
FIGURE 7. Effects of ASD on microglia, astrocyte numbers and IL-1β levels in neurogenic niche of mice chronically exposed to LPS. (A), Representative fluorescence micrographs showing the morphology and density of microglia in the hippocampus of mice treated with saline (Ctrl) or lipopolysaccharide (LPS), followed by akebia saponin D (ASD) or minocycline. Microglia were labeled with antibody against ionized calcium binding adapter molecule 1 (Iba1) (red) and nuclei, with 4’,6-diamidino-2-phenylindole (DAPI) (blue). Scale bar, 100 μm. (B–E), Quantification of the percentages of total area containing Iba1+ cells in hippocampal CA3 (C), CA1 (D) and dentate gyrus (DG) (E) for evaluating changes in the morphology and density of microglia. Five mice from each group were examined, and five hippocampal micrographs (40×) from each animal were quantified. Each dot in the bar graph represents the average of all micrographs for each mouse. (F,G), Quantification of the concentration of IL-1β in hippocampus and hippocampal DG as an index of neuroinflammation. Data are mean ± standard error of the mean (SEM) (n = 5), *p < 0.05, **p < 0.01, ***p < 0.001 vs. Ctrl group, #p < 0.05, ##p < 0.01, ###p < 0.001 vs. LPS group by one-way ANOVA with Tukey’s multiple-comparisons test.
These experiments indicate that 100 mg/kg/d ASD activated the PI3K-Akt pathway to restore hippocampal NSPC proliferation, survival and neuronal differentiation, as well as the synaptic function of hippocampal neurons in a microenvironment of chronic neuroinflammation.
3.3 ASD ameliorates depressive- and anxiety-like behaviors and cognitive impairment in a mouse model of chronic neuroinflammation
In healthy individuals, a steady stream of new neurons in the DG can project into the cerebral cortex to regulate emotional and cognitive functions (Zhang et al., 2021), but this steady stream is likely compromised in patients with Alzheimer’s disease or depression, who suffer hippocampal atrophy. The impaired neurogenesis likely contributes to depression, anxiety and cognitive impairments. Given our observations above that ASD can promote NSPC proliferation and neuronal differentiation in vitro and in vivo, even in a chronically inflammatory microenvironment, we wanted to examine whether these effects of ASD could mitigate depression, anxiety and cognitive impairment due to chronic inflammation.
Depressive-like behaviors of mice were evaluated using the SPT, which evaluates anhedonia, and the FST, which assesses behavioral despair. Chronic exposure to LPS decreased the sucrose preference of mice, which ASD (at a daily dose of at least 100 mg/kg) or minocyline reversed (Figures 8A,B). Chronic exposure to LPS shortened the latency time and prolonged immobility time in the FST, which ASD reversed (Figures 8C–E).
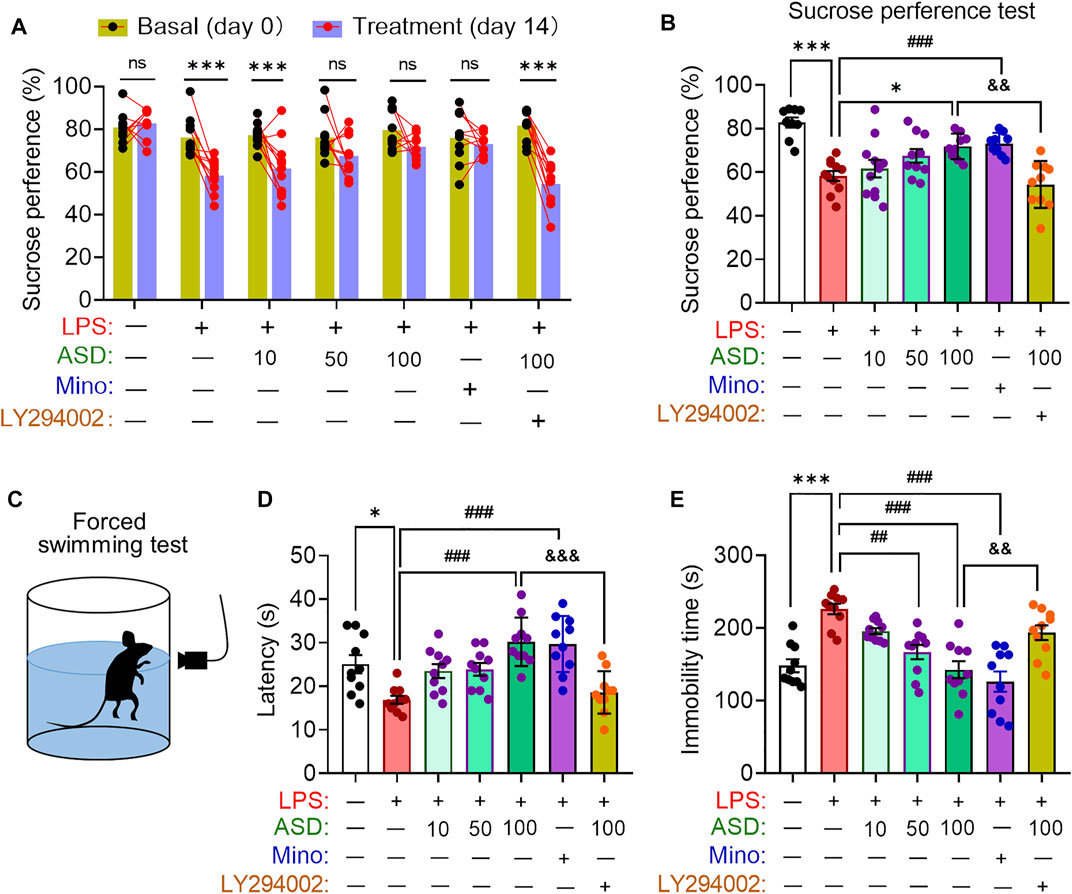
FIGURE 8. Effects of ASD on depressive-like behaviors of mice chronically exposed to LPS in the presence or absence of PI3K-Akt inhibitor. (A), Changes in sucrose preference of individual saline-treated (Ctrl) or lipopolysaccharide (LPS)-treated mice, before treatment (day 0) and afterward (day 14). (B), Changes in sucrose preference following treatment with akebia saponin D (ASD), minocycline (Mino) or PI3K-Akt inhibitor (LY294002) for 14 days. (C–E), Effects of ASD on immobility time and latency in the forced swimming test. Data are mean ± standard error of the mean (SEM) (n = 8-11). Panel (A): ***p < 0.001 vs. basal (0-days) by a paired Student’s t test for. Panels (B), (D) and (E): *p < 0.05, ***p < 0.001 vs. Ctrl group, ##p < 0.01, ###p < 0.001 vs. LPS group, &&p < 0.01, &&&p < 0.001 vs. ASD (100 mg/kg) + LPS group by one-way ANOVA with Tukey’s multiple-comparisons test.
Anxiety-like behaviors of mice were evaluated using the elevated plus maze test (Figure 9A). Chronic exposure to LPS reduced the number of open-arm entries and time spent in open arms, which ASD (at a daily dose of at least 100 mg/kg) or minocyline reversed (Figures 9B,C).
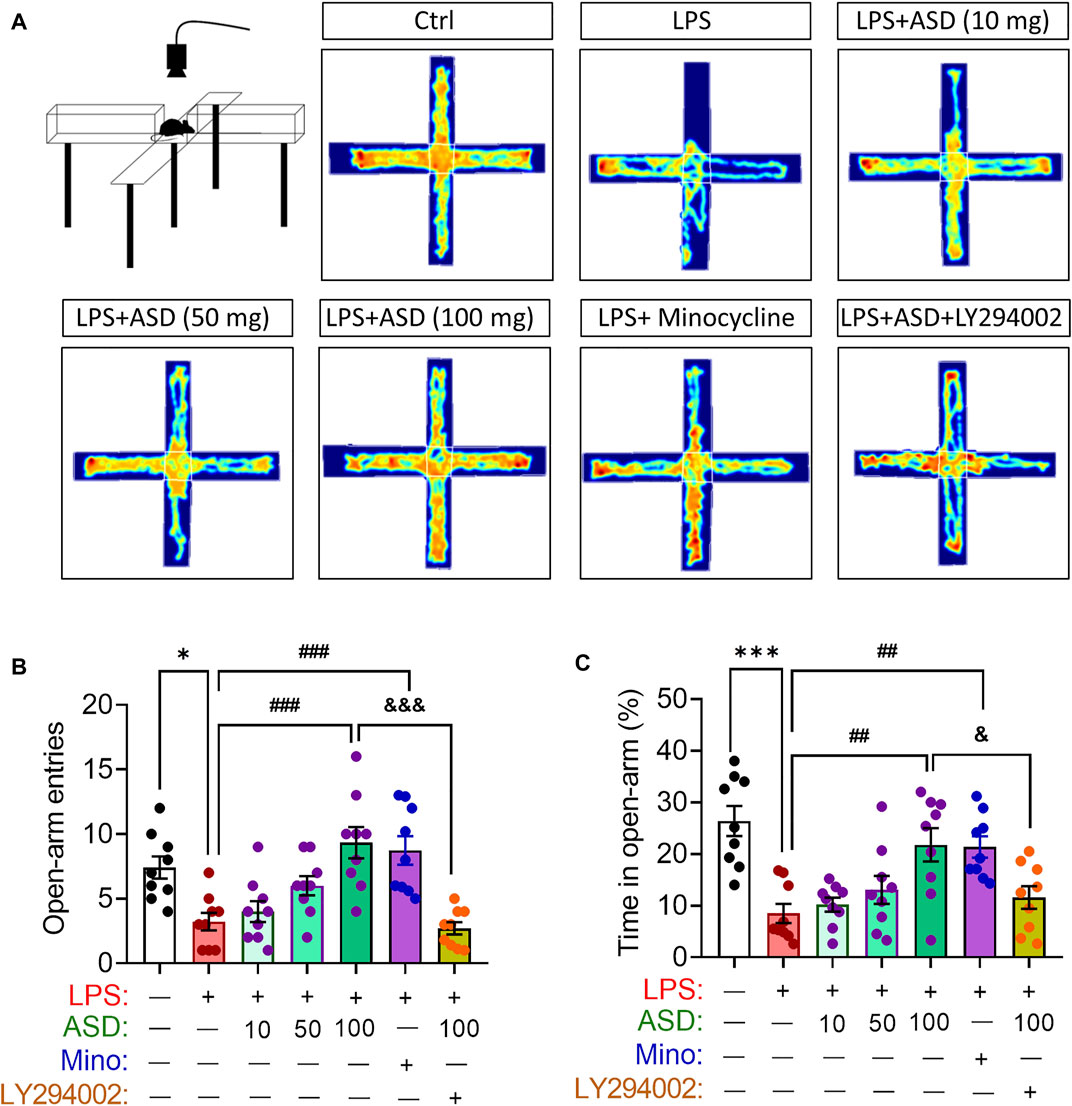
FIGURE 9. Effects of ASD on anxiety-like behaviors of mice chronically exposed to LPS in the presence or absence of PI3K-Akt inhibitor. (A), Scheme describing the experimental evaluation of anxiety-like behaviors of mice using the elevated plus maze test, and the heatmap of mouse behavior in this test. (B,C), Changes in open-arm entries and time in open-arms in mice treated with saline (Ctrl) or lipopolysaccharide (LPS), followed by akebia saponin D (ASD), minocycline (Mino) or PI3K-Akt inhibitor (LY294002) for 14 days. Data are mean ± standard error of the mean (SEM) (n = 8-11). *p < 0.05, ***p < 0.001 vs. Ctrl group, ##p < 0.01, ###p < 0.001 vs. LPS group, &p < 0.05, &&&p < 0.001 vs. ASD (100 mg/kg) + LPS group by one-way ANOVA with Tukey’s multiple-comparisons test.
Cognition was tested through the novel object recognition test (Figure 10A). No preference was shown for the novel item, regardless of whether it replaced the familiar object on the right or left (Figure 10B). Chronic exposure to LPS induced a significant cognitive defect (Figure 10C), which ASD (at 10–100 mg/kg/d) or minocyline ameliorated (Figures 10A–C).
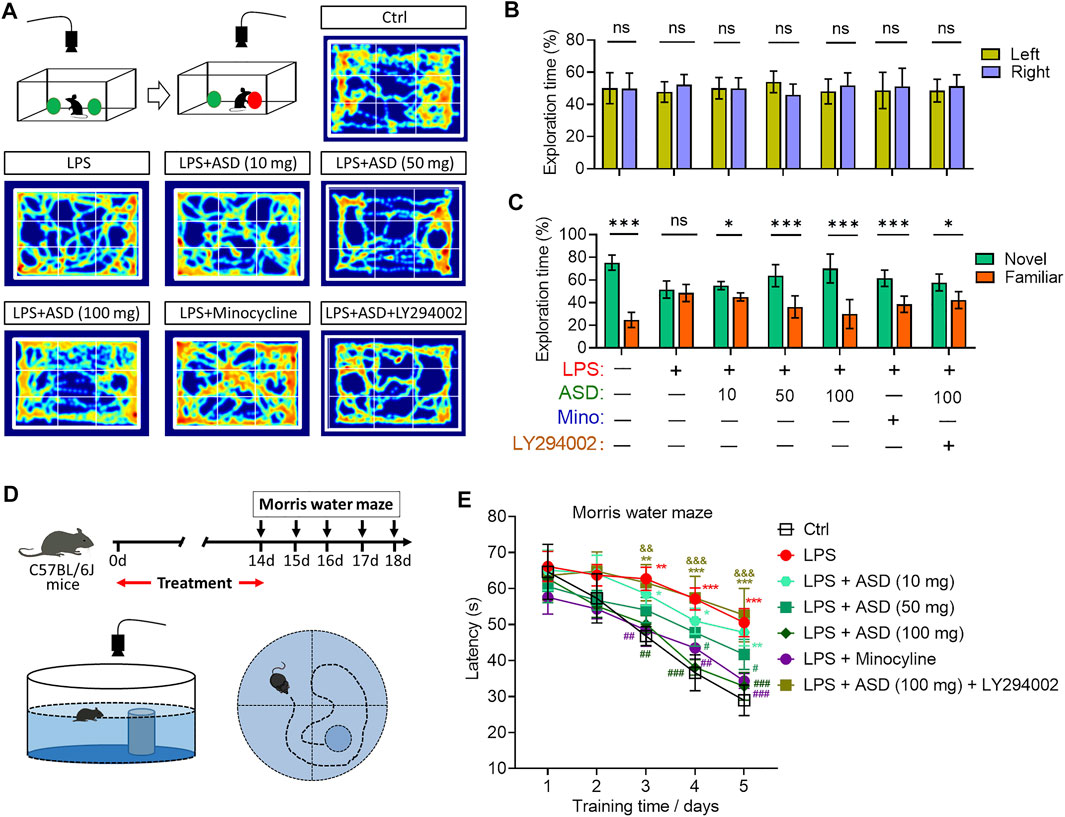
FIGURE 10. Effects of ASD on cognitive impairment of mice chronically exposed to LPS in the presence or absence of PI3K-Akt inhibitor. (A), Scheme describing the experimental evaluation of mice using the novel object recognition test, and heatmap of mouse behavior in this test. (B), Mice showed a preference for the novel item, regardless of whether it replaced the familiar object on the right or left. (C), Comparison of preference for the novel or familiar object. (D), Scheme describing the experimental evaluation of learning and memory using the Morris water maze test. (E), Morris water maze latency of mice treated with saline (Ctrl) or lipopolysaccharide (LPS), followed by akebia saponin D (ASD), minocycline or PI3K-Akt inhibitor (LY294002). Data are mean ± standard error of the mean (SEM) (n = 8-11). Panels (B) and (C): *p < 0.05, ***p < 0.001 vs. left (B) or novel (C) by a paired Student’s t-test. Panel (E): *p < 0.05, **p < 0.01, ***p < 0.001 vs. Ctrl group, #p < 0.05, ##p < 0.01, ###p < 0.001 vs. LPS group, &&p < 0.01, &&&p < 0.001 vs. ASD (100 mg/kg) + LPS group by one-way ANOVA with Tukey’s multiple-comparisons test.
Learning and memory were assessed using a Morris water maze (Figure 10D). Chronic exposure to LPS prolonged latency time in the maze, which ASD (at a dose of at least 50 mg/kg/d) or minocyline reversed (Figure 10E).
To further expound the pharmacological mechanisms of ASD against disorders involving impaired neurogenesis, we performed network pharmacology-based analysis of ASD for the treatment of major depressive disorder (MDD), anxiety and Alzheimer’s disease (AD). We identified 4795 MDD-related targets, 2663 AD-related targets, 4097 AD-related targets, 2,663 anxiety-related targets (Supplementary Figure S2). A total of 1,386 targets involving MDD, AD and anxiety were identified, of which 84 were ASD targets (Figure 11A). These common targets were extracted for further Kyoto Encyclopedia of Genes and Genomes (KEGG) pathway enrichment analysis using R software. The results showed that the PI3K-Akt pathway ranks fourth in KEGG pathway enrichment (Figure 11B).
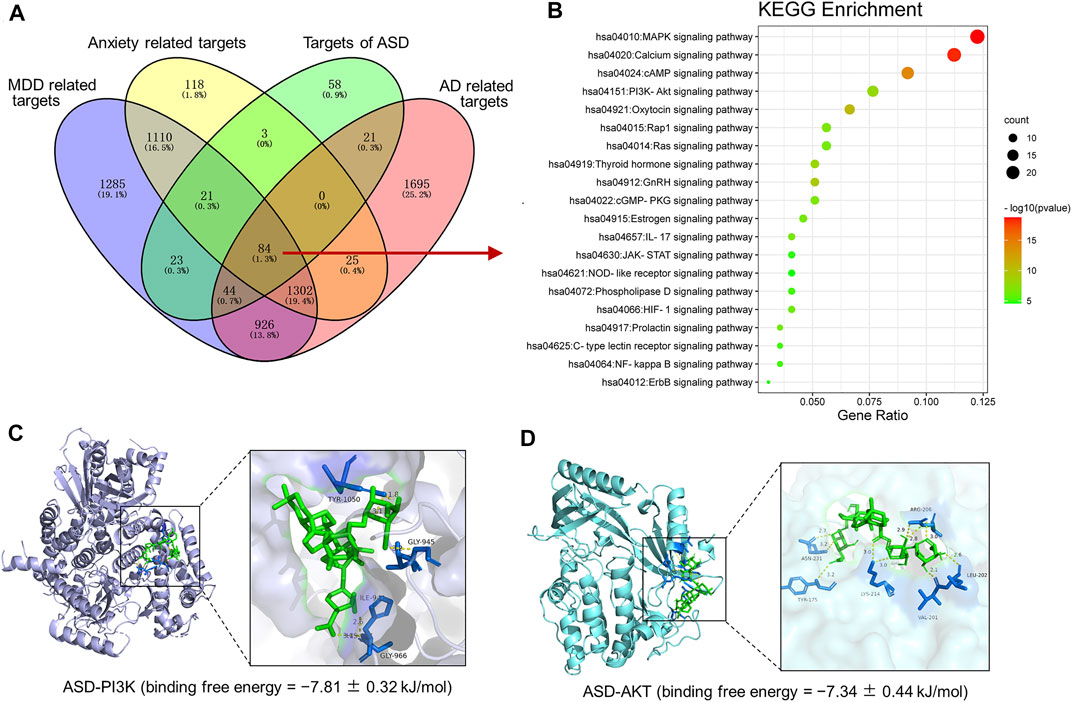
FIGURE 11. Pharmacological mechanisms of ASD against disorders involving impaired neurogenesis. (A), Venn diagram summarizing the intersection targets of the akebia saponin D (ASD), major depressive disorder (MDD), anxiety and Alzheimer’s disease (AD). (B), KEGG analysis of key targets of ASD in treatment of MDD, anxiety and AD. Bubble plot of top 20 KEGG pathways. (C,D), Molecular docking diagram of ASD (green) to target PI3K (C) and Akt (D). The yellow lines represent the hydrogen bond interaction force, which is the main force promoting molecule binding with the active site. The blue positions indicate the amino acid residues of the receptors (PI3K/AKT).
To probe the possible binding mode of ASD with the PI3K/Akt, molecular docking was performed. ASD is bound to the interaction interface of the PI3K and AKT (Figures 11C,D). Upon calculation of the MM/GBSA binding energy, the ligand ASD bound to the PI3K with a stability of −7.81 ± 0.32 kJ/mol (Figure 11C); and the ligand ASD bound to the AKT with a stability of −7.34 ± 0.44 kJ/mol (Figure 11D).
In support of PI3K-Akt signaling as mediator of the neuroprotective effects of ASD, PI3K-Akt inhibitor LY294002 blocked the compound’s ability to reverse the LPS-induced defects on all these behavioral tests (Figures 8A,10E). These results suggested that PI3K-Akt pathway is one of the targets of ASD to against the disorders involving impaired neurogenesis.
4 Discussion
Neurogenesis plays a fundamental role in the postnatal brain, where it is required for neuronal plasticity (Puderbaugh & Emmady, 2022). Promoting neurogenesis in the adult brain may be an effective strategy for treating major depressive disorder and Alzheimer’s disease, and the compound ASD from traditional Chinese medicine has shown promise in this regard. In the present work, we found that ASD can promote NSPC proliferation, survival and neuronal differentiation in vitro and in vivo, even in a microglia-mediated inflammatory niche. These effects correlate with milder behavioral symptoms of depression, anxiety and cognitive impairment due to chronic neuroinflammation. Finally, we provide evidence that the therapeutic effects of ASD involve activation of the PI3K-AKT pathway. To our knowledge, this study is the first to report the direct regulation of ASD on adult hippocampal NSPCs.
The hippocampus, a brain area critical for learning, memory and emotion, is especially vulnerable to damage in early stages of AD, chronic stress or inflammation, when neurogenesis in the adult hippocampus is altered (Zonis et al., 2015; Chesnokova et al., 2016; Babcock et al., 2021; Du Preez et al., 2021). Various key molecules involved in the pathogenesis of major depression disorder or AD have been linked to the inhibition of neurogenesis (Berger et al., 2020; Babcock et al., 2021; Walgrave et al., 2021). Brain imaging and postmortem studies of patients with either of these disorders indicate reduction in hippocampal volume perhaps due to reduced neurogenesis and loss of mature neurons (Frimodt-Møller et al., 2019; Zarate-Garza et al., 2021).
This reduced neurogenesis seems to be at least partly the fault of microglia. These immune cells create an inflammatory microenvironment that inhibits NSPC proliferation and differentiation (Xiong et al., 2016; Zhang et al., 2020b). In a variety of neurodegenerative settings, microglia alter their transcriptional profile, morphology, and function to exert negative effects in disease models (Mattei et al., 2017; Wolf et al., 2017). Activation of microglia results in phagocytosis and production of pro-inflammatory cytokines, reactive oxygen species, and inducible NO synthase (iNOS), which can alter the hippocampal neurogenic niche, reducing NSPC proliferation, survival and neuronal differentiation. These injuries can contribute to cognitive dysfunction and depression (Snyder et al., 2011; Berger et al., 2020; Zhang et al., 2020b; Zhang J. et al., 2021; Mizuno et al., 2021). In the present study, we found that mice chronically exposed to LPS exhibited obvious depressive- and anxiety-like behaviors and cognitive impairment, which were accompanied by microglial overactivation and inhibition of hippocampal neurogenesis. These results support the idea that microglia-mediated neuroinflammation inhibits hippocampal neurogenesis, with diverse behavioral consequences related to depression, anxiety and cognitive impairment.
Adult hippocampal neurogenesis is supported by NSPC proliferation and neuronal differentiation, as well as maturation and survival of the resulting newborn neurons (Deierborg et al., 2010; Pilz et al., 2018). These processes are strongly dependent on a supportive hippocampal neurogenic niche, which activated microglia antagonize by creating an inflammatory microenvironment (Toda et al., 2019; Zhang et al., 2020b). Consistent with this, we found that chronic LPS exposure reduced the numbers of BrdU + cells, BrdU + -DCX + cells and BrdU + -NeuN + cells in mouse hippocampus. Indeed, conditioned medium from microglia treated with LPS was sufficient to induce NSPC apoptosis and suppress their proliferation and neuronal differentiation.
Several studies have shown that inhibitors of microglial activation such as minocycline can suppress pro-inflammatory cytokine expression and restore hippocampal neurogenesis, as well as ameliorate depressive-like behaviors and cognitive impairment (Zhang et al., 2020b; Alavi et al., 2021; Bassett et al., 2021). Our results are consistent with the observation that variations in the relatively small number of new neurons in adult brains of humans and rodents is strongly linked to the pathogenesis and remission of neuropsychiatric disorders (Grace et al., 2021). We show here that ASD exerted therapeutic effects similar to those of minocycline, yet it did not reverse the LPS-induced increase in the area of Iba1+ cells or IL-1β level. Thus, it appears that ASD restores hippocampal NSPC proliferation, survival and neuronal differentiation, as well as the synaptic function of hippocampal neurons in a microenvironment of chronic neuroinflammation. However, different from what we found in chronic mild stress model mice (Jiang et al., 2022), ASD does not appear to completely inhibit microglia-mediated inflammation in hippocampus of chronic LPS-treated mice as minocycline does. Instead, ASD acts directly on NSPCs to promote their proliferation, survival and neuronal differentiation. It is interesting that ASD at 100 mg/kg/d for 14 days promoted the proliferation of NSPCs in normal mice, but did not affect hippocampal neurogenesis and the maturation of newborn neurons. It may be that the healthy body itself monitors neurogenesis and limits the number of newborn neurons by inducing apoptosis of excess immature neurons.
Inhibited neurogenesis may exacerbate neuronal vulnerability to Alzheimer’s disease, stress or immune challenge, leading to memory impairment, anxiety or depression; under these conditions, enhanced neurogenesis may be a compensatory response to repair and protect the brain (Bassani et al., 2018; Moreno-Jiménez et al., 2019; Tobin et al., 2019). Adult neurogenesis in the hippocampus subgranular zone (SGZ) is associated with the etiology and efficacy of treatments against Alzheimer’s disease and major depressive disorder (Anacker & Hen, 2017; Babcock et al., 2021; Zhang J. et al., 2021). Adult hippocampal neurogenesis appears to occur in humans as well as in rodents, although this idea is still controversial (Spalding et al., 2013; Kempermann et al., 2018; Moreno-Jiménez et al., 2021). In this study, we isolated NSPCs from the hippocampal subgranular zone of adult mice and induced their proliferation and differentiation, suggesting that NSPCs do indeed exist in the adult hippocampus of rodents.
The PI3K/Akt signaling pathway is the classical anti-apoptotic and pro-survival signal transduction pathway (Gabbouj et al., 2019; Xie et al., 2019). When PI3K binds to growth factor receptors such as the epidermal growth factor receptor, Akt becomes activated, leading to the activation or inhibition of downstream substrates such as the apoptotic proteins Bad and Caspase-9 (Jeong et al., 2008). These substrates go on to regulate cell proliferation, differentiation, apoptosis, migration, and other processes (Darici et al., 2020). Base on the network pharmacology analysis and molecular docking, we found PI3K-Akt pathway is one of the targets of ASD to against the disorders involving impaired neurogenesis, such as Alzheimer’s disease, major depressive disorder and anxiety disorder. In our mouse model of chronic neuroinflammation, levels of PI3K and pAkt were strikingly reduced in hippocampus, and this was associated with reduced NSPC proliferation and differentiation. The PI3K-Akt signaling pathway in adult NSPCs is strongly inhibited in an environment of neuroinflammation mediated by microglia, leading to increased apoptosis and differentiation into astrocytes. Conversely, ASD strongly increased levels of PI3K, Akt, and pAkt proteins, even in the presence of LPS-M-CM. These results suggest that the PI3K-Akt pathway mediates the effects of ASD on NSPC proliferation, survival and neuronal differentiation. Consistent with this, we found that LY294002, an inhibitor of the PI3K/Akt pathway, blocked the effects of ASD on NSPC apoptosis, proliferation and neuronal differentiation in the presence or absence of LPS-M-CM. LY294002 treatment also blocked the effects of ASD on hippocampal neurogenesis and depressive- and anxiety-like behaviors and cognitive impairment. Our results add to the number of cellular contexts in which ASD activates PI3K/Akt signaling with therapeutic effects, including bone regeneration (Ke et al., 2016) as well as Alzheimer’s disease (Zheng et al., 2017; Razani et al., 2021), anxiety (Qiao et al., 2018) and depression (Guo et al., 2019; Shan et al., 2020). This growing literature argues for activating PI3K/Akt signaling as a strategy against neurological disorders involving impaired neurogenesis.
Our results provide strong evidence that ASD protects NSPCs from the microglia-mediated inflammatory niche and promotes their proliferation, survival and neuronal differentiation by activating the PI3K-Akt pathway (Figure 12). Our results support further evaluation of ASD for the treatment of Alzheimer’s disease, major depressive disorder and other conditions associated with impaired neurogenesis.
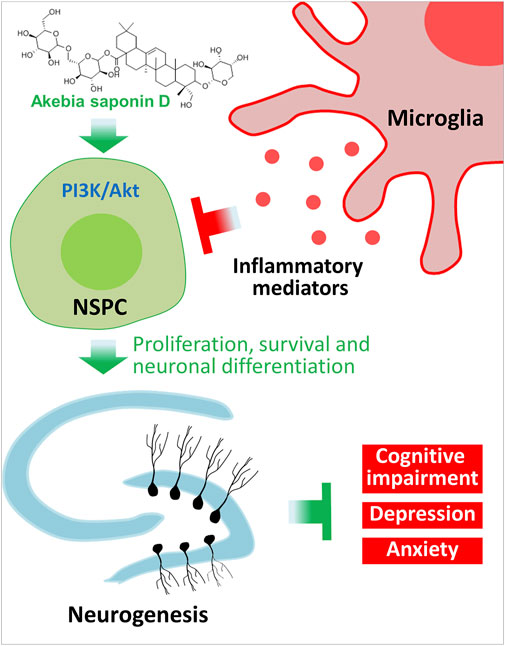
FIGURE 12. Schematic diagram of how akebia saponin D may protect hippocampal neurogenesis. Akebia saponin D activates the PI3K-Akt pathway to protect NSPCs from the microglia-mediated inflammatory niche, promoting their proliferation, survival and neuronal differentiation, as well as ameliorating depressive- and anxiety-like behaviors and cognitive impairment.
Data availability statement
The original contributions presented in the study are included in the article/Supplementary Material, further inquiries can be directed to the corresponding authors.
Ethics statement
The animal study was reviewed and approved by Institutional Animal Care and Use Committee of the Guizhou University of Traditional Chinese Medicine.
Author contributions
JZ and TZ conceived and designed the study. JZ and QL wrote the manuscript, which was reviewed by TZ and ZY, and approved by all the authors. CX and CY performed behavioral and immunofluorescence studies. WJ and LL cultured NSPCs and microglia. DS and ZZ performed statistical analysis of the data.
Funding
This work was supported by the National Natural Science Foundation of China (82060726, 81860675), the Department of Science and Technology of Guizhou High-level Innovative Talents (20185638-2), Sichuan Science and Technology Program (2020YJ0225) and Guizhou Science and Technology Plan Project (20195611).
Acknowledgments
We are grateful to Creaducate Consulting GmbH for help in editing the manuscript.
Conflict of interest
The authors declare that the research was conducted in the absence of any commercial or financial relationships that could be construed as a potential conflict of interest.
Publisher’s note
All claims expressed in this article are solely those of the authors and do not necessarily represent those of their affiliated organizations, or those of the publisher, the editors and the reviewers. Any product that may be evaluated in this article, or claim that may be made by its manufacturer, is not guaranteed or endorsed by the publisher.
Supplementary material
The Supplementary Material for this article can be found online at: https://www.frontiersin.org/articles/10.3389/fphar.2022.927419/full#supplementary-material
Abbreviations
AD, Alzheimer’s disease; ASD, Akebia saponin D; BDNF, brain-derived neurotrophic factor; BrdU, 5′-bromo-2′deoxyuridine; DAPI, 4′,6-diamidino-2-phenylindole; DCX, doublecortin; DG, dentate gyrus; GCL, granular cell layer; GFAP, glial fibrillary acidic protein; Iba1, ionized calcium binding adapter molecule 1; MAP2, Microtubule-associated protein 2; MDD, major depressive disorder; NeuN, neuron-specific nucleoprotein; NSPC, neural stem/progenitor cell; PBS, phosphate-buffered saline; PI3K/AKT, phosphatidylinositol 3 kinase/serine-threonine kinase; LPS, lipopolysaccharide; SGZ, subgranular zone.
References
Alavi, S., Darharaj, M., Bilehsavar, S. H., Amini, M., Zafarghandi, M. B. S., Berenji, V., et al. (2021). Successful use of minocycline for the treatment of methamphetamine-induced psychosis and cognitive impairments: An open-label case series. Clin. Neuropharmacol. 44, 126–131. doi:10.1097/WNF.0000000000000460
Anacker, C., and Hen, R. (2017). Adult hippocampal neurogenesis and cognitive flexibility - linking memory and mood. Nat. Rev. Neurosci. 18, 335–346. doi:10.1038/nrn.2017.45
Araki, T., Ikegaya, Y., and Koyama, R. (2021). The effects of microglia- and astrocyte-derived factors on neurogenesis in health and disease. Eur. J. Neurosci. 54, 5880–5901. doi:10.1111/ejn.14969
Babcock, K. R., Page, J. S., Fallon, J. R., and Webb, A. E. (2021). Adult hippocampal neurogenesis in aging and Alzheimer's disease. Stem Cell. Rep. 16, 681–693. doi:10.1016/j.stemcr.2021.01.019
Bassani, T. B., Bonato, J. M., Machado, M. M. F., Cóppola-Segovia, V., Moura, E. L. R., Zanata, S. M., et al. (2018). Decrease in adult neurogenesis and neuroinflammation are involved in spatial memory impairment in the streptozotocin-induced model of sporadic Alzheimer's disease in rats. Mol. Neurobiol. 55, 4280–4296. doi:10.1007/s12035-017-0645-9
Bassett, B., Subramaniyam, S., Fan, Y., Varney, S., Pan, H., Carneiro, A. M. D., et al. (2021). Minocycline alleviates depression-like symptoms by rescuing decrease in neurogenesis in dorsal hippocampus via blocking microglia activation/phagocytosis. Brain Behav. Immun. 91, 519–530. doi:10.1016/j.bbi.2020.11.009
Berger, T., Lee, H., Young, A. H., Aarsland, D., and Thuret, S. (2020). Adult hippocampal neurogenesis in major depressive disorder and Alzheimer's disease. Trends Mol. Med. 26, 803–818. doi:10.1016/j.molmed.2020.03.010
Bhattarai, P., Thomas, A. K., Cosacak, M. I., Papadimitriou, C., Mashkaryan, V., Froc, C., et al. (2016). IL4/STAT6 signaling activates neural stem cell proliferation and neurogenesis upon amyloid-β42 aggregation in adult zebrafish brain. Cell. Rep. 17, 941–948. doi:10.1016/j.celrep.2016.09.075
Bonafina, A., Trinchero, M. F., Ríos, A. S., Bekinschtein, P., Schinder, A. F., Paratcha, G., et al. (2019). GDNF and GFRα1 are required for proper integration of adult-born hippocampal neurons. Cell. Rep. 29, 4308–4319.e4. e4. doi:10.1016/j.celrep.2019.11.100
Cameron, H. A., and Glover, L. R. (2015). Adult neurogenesis: Beyond learning and memory. Annu. Rev. Psychol. 66, 53–81. doi:10.1146/annurev-psych-010814-015006
Chen, F., Liang, Q., Mao, L., Yin, Y., Zhang, L., Li, C., et al. (2022). Synergy effects of Asperosaponin VI and bioactive factor BMP-2 on osteogenesis and anti-osteoclastogenesis. Bioact. Mat. 10, 335–344. doi:10.1016/j.bioactmat.2021.09.001
Chesnokova, V., Pechnick, R. N., and Wawrowsky, K. (2016). Chronic peripheral inflammation, hippocampal neurogenesis, and behavior. Brain Behav. Immun. 58, 1–8. doi:10.1016/j.bbi.2016.01.017
Cope, E. C., and Gould, E. (2019). Adult neurogenesis, glia, and the extracellular matrix. Cell. stem Cell. 24, 690–705. doi:10.1016/j.stem.2019.03.023
Darici, S., Alkhaldi, H., Horne, G., Jørgensen, H. G., Marmiroli, S., and Huang, X. (2020). Targeting PI3K/Akt/mTOR in AML: Rationale and clinical evidence. J. Clin. Med. 9, E2934. doi:10.3390/jcm9092934
Deierborg, T., Roybon, L., Inacio, A. R., Pesic, J., and Brundin, P. (2010). Brain injury activates microglia that induce neural stem cell proliferation ex vivo and promote differentiation of neurosphere-derived cells into neurons and oligodendrocytes. Neuroscience 171, 1386–1396. doi:10.1016/j.neuroscience.2010.09.045
Deng, W., Aimone, J. B., and Gage, F. H. (2010). New neurons and new memories: How does adult hippocampal neurogenesis affect learning and memory? Nat. Rev. Neurosci. 11, 339–350. doi:10.1038/nrn2822
Ding, X., Li, W., Chen, D., Zhang, C., Wang, L., Zhang, H., et al. (2019). Asperosaponin VI stimulates osteogenic differentiation of rat adipose-derived stem cells. Regen. Ther. 11, 17–24. doi:10.1016/j.reth.2019.03.007
Drew, L. J., Fusi, S., and Hen, R. (2013). Adult neurogenesis in the mammalian hippocampus: Why the dentate gyrus? Learn. Mem. 20, 710–729. doi:10.1101/lm.026542.112
Du Preez, A., Onorato, D., Eiben, I., Musaelyan, K., Egeland, M., Zunszain, P. A., et al. (2021). Chronic stress followed by social isolation promotes depressive-like behaviour, alters microglial and astrocyte biology and reduces hippocampal neurogenesis in male mice. Brain Behav. Immun. 91, 24–47. doi:10.1016/j.bbi.2020.07.015
Frimodt-Møller, K. E., Møllegaard Jepsen, J. R., Feldt-Rasmussen, U., and Krogh, J. (2019). Hippocampal volume, cognitive functions, depression, anxiety, and quality of life in patients with cushing syndrome. J. Clin. Endocrinol. Metab. 104, 4563–4577. doi:10.1210/jc.2019-00749
Gabbouj, S., Ryhänen, S., Marttinen, M., Wittrahm, R., Takalo, M., Kemppainen, S., et al. (2019). Altered insulin signaling in Alzheimer's disease brain - special emphasis on PI3K-Akt pathway. Front. Neurosci. 13, 629. doi:10.3389/fnins.2019.00629
Gage, F. H. (2021). Adult neurogenesis in neurological diseases. Science 374, 1049–1050. doi:10.1126/science.abm7468
Grace, P. M., Tawfik, V. L., Svensson, C. I., Burton, M. D., Loggia, M. L., and Hutchinson, M. R. (2021). The neuroimmunology of chronic pain: From rodents to humans. J. Neurosci. 41, 855–865. doi:10.1523/JNEUROSCI.1650-20.2020
Gu, M., Jin, J., Ren, C., Chen, X., Gao, W., Wang, X., et al. (2020). Akebia Saponin D suppresses inflammation in chondrocytes via the NRF2/HO-1/NF-κB axis and ameliorates osteoarthritis in mice. Food Funct. 11, 10852–10863. doi:10.1039/d0fo01909g
Guo, L. T., Wang, S. Q., Su, J., Xu, L. X., Ji, Z. Y., Zhang, R. Y., et al. (2019). Baicalin ameliorates neuroinflammation-induced depressive-like behavior through inhibition of toll-like receptor 4 expression via the PI3K/AKT/FoxO1 pathway. J. Neuroinflammation 16, 95. doi:10.1186/s12974-019-1474-8
Hanslik, K. L., and Ulland, T. K. (2020). The role of microglia and the Nlrp3 inflammasome in alzheimer's disease. Front. Neurol. 11, 570711. doi:10.3389/fneur.2020.570711
Hattiangady, B., Kuruba, R., Shuai, B., Grier, R., and Shetty, A. K. (2020). Hippocampal neural stem cell grafting after status epilepticus alleviates chronic epilepsy and abnormal plasticity, and maintains better memory and mood function. Aging Dis. 11, 1374–1394. doi:10.14336/AD.2020.1020
Jeong, S. J., Dasgupta, A., Jung, K. J., Um, J. H., Burke, A., Park, H. U., et al. (2008). PI3K/AKT inhibition induces caspase-dependent apoptosis in HTLV-1-transformed cells. Virology 370, 264–272. doi:10.1016/j.virol.2007.09.003
Jiang, X., Yi, S., Liu, Q., Su, D., Li, L., Xiao, C., et al. (2022). Asperosaponin VI ameliorates the CMS-induced depressive-like behaviors by inducing a neuroprotective microglial phenotype in hippocampus via PPAR-gamma pathway. J. Neuroinflammation 19, 115. doi:10.1186/s12974-022-02478-y
Jurkowski, M. P., Bettio, L., Woo, K. E, Patten, A., Yau, S. Y., and Gil-Mohapel, J. (2020). Beyond the Hippocampus and the SVZ: Adult neurogenesis throughout the brain. Front. Cell. Neurosci. 14, 576444. doi:10.3389/fncel.2020.576444
Ke, K., Li, Q., Yang, X., Xie, Z., Wang, Y., Shi, J., et al. (2016). Asperosaponin VI promotes bone marrow stromal cell osteogenic differentiation through the PI3K/AKT signaling pathway in an osteoporosis model. Sci. Rep. 6, 35233. doi:10.1038/srep35233
Kempermann, G., Gage, F. H., Aigner, L., Song, H., Curtis, M. A., Thuret, S., et al. (2018). Human adult neurogenesis: Evidence and remaining questions. Cell. stem Cell. 23, 25–30. doi:10.1016/j.stem.2018.04.004
Littlefield, A. M., Setti, S. E., Priester, C., and Kohman, R. A. (2015). Voluntary exercise attenuates LPS-induced reductions in neurogenesis and increases microglia expression of a proneurogenic phenotype in aged mice. J. Neuroinflammation 12, 138. doi:10.1186/s12974-015-0362-0
Mattei, D., Ivanov, A., Ferrai, C., Jordan, P., Guneykaya, D., Buonfiglioli, A., et al. (2017). Maternal immune activation results in complex microglial transcriptome signature in the adult offspring that is reversed by minocycline treatment. Transl. Psychiatry 7, e1120. doi:10.1038/tp.2017.80
Mehdipour, M., Mehdipour, T., Skinner, C. M., Wong, N., Liu, C., Chen, C. C., et al. (2021). Plasma dilution improves cognition and attenuates neuroinflammation in old mice. GeroScience 43, 1–18. doi:10.1007/s11357-020-00297-8
Mizuno, Y., Abolhassani, N., Mazzei, G., Sakumi, K., Saito, T., Saido, T. C., et al. (2021). MUTYH actively contributes to microglial activation and impaired neurogenesis in the pathogenesis of Alzheimer's disease. Oxid. Med. Cell. Longev. 2021, 8635088. doi:10.1155/2021/8635088
Moreno-Jiménez, E. P., Flor-García, M., Terreros-Roncal, J., Rábano, A., Cafini, F., Pallas-Bazarra, N., et al. (2019). Adult hippocampal neurogenesis is abundant in neurologically healthy subjects and drops sharply in patients with Alzheimer's disease. Nat. Med. 25, 554–560. doi:10.1038/s41591-019-0375-9
Moreno-Jiménez, E. P., Terreros-Roncal, J., Flor-García, M., Rábano, A., and Llorens-Martín, M. (2021). Evidences for adult hippocampal neurogenesis in humans. J. Neurosci. 41, 2541–2553. doi:10.1523/JNEUROSCI.0675-20.2020
Morgenstern, N. A., Lombardi, G., and Schinder, A. F. (2008). Newborn granule cells in the ageing dentate gyrus. J. physiology 586, 3751–3757. doi:10.1113/jphysiol.2008.154807
Niu, Y. T., Xie, L., Deng, R. R., and Zhang, X. Y. (2021). In the presence of TGF-β1, Asperosaponin VI promotes human mesenchymal stem cell differentiation into nucleus pulposus like- cells. BMC Complement. Med. Ther. 21, 32. doi:10.1186/s12906-020-03169-y
Pap, R., Pandur, E., Jánosa, G., Sipos, K., Agócs, A., and Deli, J. (2021). Lutein exerts antioxidant and anti-Inflammatory effects and influences iron utilization of BV-2 microglia, 10.Antioxidants (Basel)
Perea, J. R., Bolós, M., and Avila, J. (2020). Microglia in alzheimer's disease in the context of tau pathology. Biomolecules 10, 1439. doi:10.3390/biom10101439
Pilz, G. A., Bottes, S., Betizeau, M., Jörg, D. J., Carta, S., Simons, B. D., et al. (2018). Live imaging of neurogenesis in the adult mouse hippocampus. Science 359, 658–662. doi:10.1126/science.aao5056
Puderbaugh, M., and Emmady, P. D. (2022). “Neuroplasticity,” in StatPearls, (FL): StatPearls publishing copyright © 2022 (Treasure Island: StatPearls Publishing LLC.).
Qiao, O., Ji, H., Zhang, Y., Zhang, X., Zhang, X., Liu, N., et al. (2021). New insights in drug development for Alzheimer's disease based on microglia function. Biomed. Pharmacother. 140, 111703. doi:10.1016/j.biopha.2021.111703
Qiao, X., Gai, H., Su, R., Deji, C., Cui, J., Lai, J., et al. (2018). PI3K-AKT-GSK3β-CREB signaling pathway regulates anxiety-like behavior in rats following alcohol withdrawal. J. Affect. Disord. 235, 96–104. doi:10.1016/j.jad.2018.04.039
Razani, E., Pourbagheri-Sigaroodi, A., Safaroghli-Azar, A., Zoghi, A., Shanaki-Bavarsad, M., and Bashash, D. (2021). The PI3K/Akt signaling axis in alzheimer's disease: A valuable target to stimulate or suppress? Cell. Stress Chaperones 26, 871–887. doi:10.1007/s12192-021-01231-3
Santos, L. E., Beckman, D., and Ferreira, S. T. (2016). Microglial dysfunction connects depression and Alzheimer's disease. Brain Behav. Immun. 55, 151–165. doi:10.1016/j.bbi.2015.11.011
Shan, X., Chen, J., Dai, S., Wang, J., Huang, Z., Lv, Z., et al. (2020). Cyanidin-related antidepressant-like efficacy requires PI3K/AKT/FoxG1/FGF-2 pathway modulated enhancement of neuronal differentiation and dendritic maturation. Phytomedicine. 76, 153269. doi:10.1016/j.phymed.2020.153269
Silva, S. P., Zago, A. M., Carvalho, F. B., Germann, L., Colombo, G. M., Rahmeier, F. L., et al. (2021). Neuroprotective effect of taurine against cell death, glial changes, and neuronal loss in the cerebellum of rats exposed to chronic-recurrent neuroinflammation induced by LPS. J. Immunol. Res. 2021, 7497185. doi:10.1155/2021/7497185
Snyder, J. S., Soumier, A., Brewer, M., Pickel, J., and Cameron, H. A. (2011). Adult hippocampal neurogenesis buffers stress responses and depressive behaviour. Nature 476, 458–461. doi:10.1038/nature10287
Spalding, K. L., Bergmann, O., Alkass, K., Bernard, S., Salehpour, M., Huttner, H. B., et al. (2013). Dynamics of hippocampal neurogenesis in adult humans. Cell. 153, 1219–1227. doi:10.1016/j.cell.2013.05.002
Stepnik, K. (2021). Biomimetic chromatographic studies combined with the computational approach to investigate the ability of triterpenoid saponins of plant origin to cross the blood-brain barrier. Int. J. Mol. Sci. 22, 3573. doi:10.3390/ijms22073573
Terreros-Roncal, J., Moreno-Jiménez, E. P., Flor-García, M., Rodríguez-Moreno, C. B., Trinchero, M. F., Cafini, F., et al. (2021). Impact of neurodegenerative diseases on human adult hippocampal neurogenesis. Science 374, 1106–1113. doi:10.1126/science.abl5163
Tobin, M. K., Musaraca, K., Disouky, A., Shetti, A., Bheri, A., Honer, W. G., et al. (2019). Human hippocampal neurogenesis persists in aged adults and Alzheimer's disease patients. Cell. stem Cell. 24, 974–982.e3. e3. doi:10.1016/j.stem.2019.05.003
Toda, T., Parylak, S. L., Linker, S. B., and Gage, F. H. (2019). The role of adult hippocampal neurogenesis in brain health and disease. Mol. Psychiatry 24, 67–87. doi:10.1038/s41380-018-0036-2
Walgrave, H., Balusu, S., Snoeck, S., Vanden Eynden, E., Craessaerts, K., Thrupp, N., et al. (2021). Restoring miR-132 expression rescues adult hippocampal neurogenesis and memory deficits in Alzheimer's disease. Cell. stem Cell. 28, 1805–1821.e8. e8. doi:10.1016/j.stem.2021.05.001
Wang, Y., Shen, J., Yang, X., Jin, Y., Yang, Z., Wang, R., et al. (2018). Akebia saponin D reverses corticosterone hypersecretion in an Alzheimer's disease rat model. Biomed. Pharmacother. 107, 219–225. doi:10.1016/j.biopha.2018.07.149
Wolf, S. A., Boddeke, H. W., and Kettenmann, H. (2017). Microglia in physiology and disease. Annu. Rev. Physiol. 79, 619–643. doi:10.1146/annurev-physiol-022516-034406
Xie, Y., Shi, X., Sheng, K., Han, G., Li, W., Zhao, Q., et al. (2019). PI3K/Akt signaling transduction pathway, erythropoiesis and glycolysis in hypoxia (Review). Mol. Med. Rep. 19, 783–791. doi:10.3892/mmr.2018.9713
Xiong, X. Y., Liu, L., and Yang, Q. W. (2016). Functions and mechanisms of microglia/macrophages in neuroinflammation and neurogenesis after stroke. Prog. Neurobiol. 142, 23–44. doi:10.1016/j.pneurobio.2016.05.001
Yu, X., Wang, L. N., Du, Q. M., Ma, L., Chen, L., You, R., et al. (2012). Akebia saponin D attenuates amyloid β-induced cognitive deficits and inflammatory response in rats: Involvement of Akt/NF-κB pathway. Behav. Brain Res. 235, 200–209. doi:10.1016/j.bbr.2012.07.045
Yu, Z., Gao, J., Zhang, X., Peng, Y., Wei, W., Xu, J., et al. (2022). Characterization of a small-molecule inhibitor targeting NEMO/IKKβ to suppress colorectal cancer growth. Signal Transduct. Target. Ther. 7, 71. doi:10.1038/s41392-022-00888-1
Zarate-Garza, P. P., Ortega-Balderas, J. A., Ontiveros-Sanchez de la Barquera, J. A., Lugo-Guillen, R. A., Marfil-Rivera, A., Quiroga-Garza, A., et al. (2021). Hippocampal volume as treatment predictor in antidepressant naïve patients with major depressive disorder. J. Psychiatr. Res. 140, 323–328. doi:10.1016/j.jpsychires.2021.06.008
Zhang, H., Yao, S., Zhang, Z., Zhou, C., Fu, F., Bian, Y., et al. (2021). Network pharmacology and experimental validation to reveal the pharmacological mechanisms of liuwei dihuang decoction against intervertebral disc degeneration. Drug Des. devel. Ther. 15, 4911–4924. doi:10.2147/DDDT.S338439
Zhang, J., He, H., Qiao, Y., Zhou, T., He, H., Yi, S., et al. (2020b). Priming of microglia with IFN-gamma impairs adult hippocampal neurogenesis and leads to depression-like behaviors and cognitive defects. Glia 68, 2674–2692. doi:10.1002/glia.23878
Zhang, J., Rong, P., Zhang, L., He, H., Zhou, T., Fan, Y., et al. (2021b). IL4-driven microglia modulate stress resilience through BDNF-dependent neurogenesis. Sci. Adv. 7, eabb9888. doi:10.1126/sciadv.abb9888
Zhang, J., Yi, S., Li, Y., Xiao, C., Liu, C., Jiang, W., et al. (2020c). The antidepressant effects of asperosaponin VI are mediated by the suppression of microglial activation and reduction of TLR4/NF-κB-induced Ido expression. Psychopharmacology 237, 2531–2545. doi:10.1007/s00213-020-05553-5
Zheng, R., Zhang, Z. H., Chen, C., Chen, Y., Jia, S. Z., Liu, Q., et al. (2017). Selenomethionine promoted hippocampal neurogenesis via the PI3K-Akt-GSK3β-Wnt pathway in a mouse model of Alzheimer's disease. Biochem. Biophys. Res. Commun. 485, 6–15. doi:10.1016/j.bbrc.2017.01.069
Keywords: neuroinflammation, akebia saponin D, cognitive impairment, depression, neurogenesis, microglia, neural stem/precursor cell, PI3K-Akt signaling pathway
Citation: Liu Q, Zhang J, Xiao C, Su D, Li L, Yang C, Zhao Z, Jiang W, You Z and Zhou T (2022) Akebia saponin D protects hippocampal neurogenesis from microglia-mediated inflammation and ameliorates depressive-like behaviors and cognitive impairment in mice through the PI3K-Akt pathway. Front. Pharmacol. 13:927419. doi: 10.3389/fphar.2022.927419
Received: 24 April 2022; Accepted: 09 August 2022;
Published: 30 August 2022.
Edited by:
Riham Salah El Dine, Cairo University, EgyptReviewed by:
Fabiola Paciello, Catholic University of the Sacred Heart, ItalyMing Fang, Guangdong Academy of Medical Sciences, China
Copyright © 2022 Liu, Zhang, Xiao, Su, Li, Yang, Zhao, Jiang, You and Zhou. This is an open-access article distributed under the terms of the Creative Commons Attribution License (CC BY). The use, distribution or reproduction in other forums is permitted, provided the original author(s) and the copyright owner(s) are credited and that the original publication in this journal is cited, in accordance with accepted academic practice. No use, distribution or reproduction is permitted which does not comply with these terms.
*Correspondence: Jinqiang Zhang, NTUyNDUwMzc0QHFxLmNvbQ== Tao Zhou, dGFvemhvdTg4QDE2My5jb20=