- 1Department of Anesthesiology, West China Hospital, Sichuan University, Chengdu, China
- 2Laboratory of Anesthesia and Critical Care Medicine, National-Local Joint Engineering Research Center of Translational Medicine of Anesthesiology, West China Hospital, Sichuan University, Chengdu, China
Among the advancements in drug structural modifications, the increased focus on drug metabolic and pharmacokinetic properties in the anesthetic drug design process has led to significant developments. Drug metabolism also plays a key role in optimizing the pharmacokinetics, pharmacodynamics, and safety of drug molecules. Thus, in the field of anesthesiology, the applications of pharmacokinetic strategies are discussed in the context of sedatives, analgesics, and muscle relaxants. In this review, we summarize two approaches for structural optimization to develop anesthetic drugs, by designing prodrugs and soft drugs. Drugs that both failed and succeeded during the developmental stage are highlighted to illustrate how drug metabolism and pharmacokinetic optimization strategies may help improve their physical and chemical properties.
Introduction
Drug discovery and development is an expensive process with a high failure rate commonly spanning an average of 12 years (DiMasi et al., 2010; Van Norman, 2016). Prior to the approval of compounds for human use, drug candidates are subjected to a series of in vitro and in vivo experiments examining possible efficacy and safety profiles. However, the overall failure rate in drug approval for decades has been reported to be 80–90% (Yamaguchi et al., 2021), with poor efficacy and/or unacceptable toxicity being the major causes of drug attrition at any developmental stage (Bass et al., 2009). Moreover, unforeseen toxicity accounts for 20–30% of clinical failure and remains one of the leading causes of drug recall and restriction (Berg, 2019).
During the early stages of drug discovery, screening of drug candidates is commonly undertaken to identify promising lead compounds (Roberts, 2018). After this, structural modification is carried out to improve the potency and specificity while often overlooking the pharmacokinetic (PK) parameters and toxicity at this stage (Drews, 2000). Although potency is a crucial indicator of a potential drug candidate, the PK properties are invariably affect the effectiveness of the drug. To mitigate this occurrence, understanding the interplay between PK and pharmacodynamics (PD) in therapeutic use is critical (Gabrielsson et al., 2009).
Over the past few years, the application of metabolism and PK optimization strategies in the drug design process has been gradually recognized to minimize potential safety liabilities (Buchwald, 2020; Cerny et al., 2020). The PK profile of a compound involves absorption, distribution, metabolism, and excretion (ADME), among which drug metabolism plays perhaps the most crucial role in drug development. Drug metabolism influences the pharmacological and toxicological effects and plays a key role in optimizing the PD, PK, and safety of drug molecules (Zhang and Tang, 2018). The basic principle of drug metabolism is a biotransformation process that enables efficient excretion of compounds from the body. In general, metabolic processes occur in the liver because of its high levels of metabolic enzymes (Patel et al., 2016). Although organisms have developed mechanisms for degrading and excreting foreign substances, metabolic pathways may result in reactive or toxic metabolic intermediate formation, especially through oxidative metabolism (Gillette, 1979; Attia, 2010).
Studies on drug metabolism therefore play a key role in optimizing PK/PD properties and in reducing toxicity potential associated with bioactivation. It is desirable to design a “safer” drug focused on improving the activity/toxicity ratio, such as the therapeutic index, instead of improving activity alone (Bhardwaj et al., 2014). One approach is to design metabolically stable drugs, such as pharmacologically active compounds (e.g., hard drugs) with no or very limited metabolism. Such drugs are excreted by the body after they have exerted their therapeutic effects, thereby evading the problems associated with active intermediates or metabolites. Examples of successfully designed hard drugs are bisphosphonates and certain angiotensin-converting enzyme inhibitors (Kelly and O'Malley, 1990; Lin, 1996). Another approach is to integrate the structure–activity and structure–metabolism relationship of a drug to achieve controllable metabolism while improving its biological activity and therapeutic index (Bhardwaj et al., 2014). This can be achieved by the designing of prodrugs or soft drugs (Figure 1). In this review, we provide an overview of prodrug and soft drug design for improving PK/PD and safety profiles of anesthetic drugs.
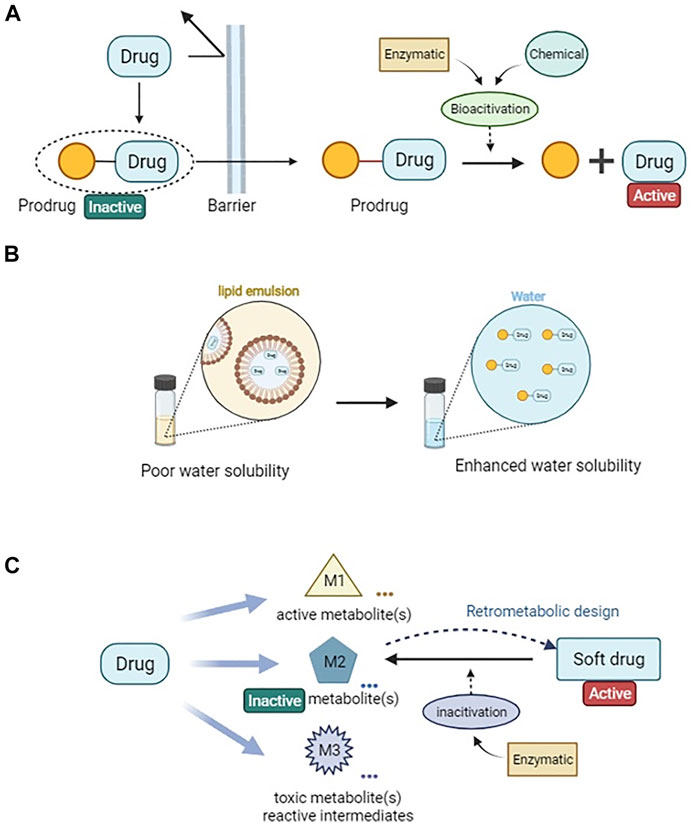
FIGURE 1. Drug metabolic and pharmacokinetic optimization strategies via prodrug and soft drug approach. (A) A simplified illustration of prodrug design for enhanced permeability. (B) Prodrug design for improving drug solubility. (C) A general sheme of soft drug design loop.
Prodrugs
Adrien Albert first introduced the term “prodrug” in 1958 (Albert, 1958). Prodrugs are pharmacologically inactive, bioreversible derivatives of active drug molecules. Prodrug designs commonly require the presence of functional groups, such as esters, amides, phosphates, carbonates, or carbamates, which are cleaved either enzymatically or chemically in the body (Rautio et al., 2008; Rautio et al., 2018).
The conversion of prodrugs to active drugs is distinct from the conversion of drugs to active metabolites. In the case of the former, the conversion of the pharmacologically inactive prodrugs to active drugs is designed with intended purposes. However, the conversion of drugs to active metabolites is an enzymatic process, and the sites of metabolism are unpredictable. While drugs and active metabolites are both pharmacologically active thus making it is an option to develop the active metabolites as new drugs, active metabolites can influence PK/PD relationships and pose considerable uncertainty in clinical trial (Anderson et al., 2009; Gabrielsson and Green, 2009).
According to the type of carrier attached, prodrugs are conventionally classified into two major types: carrier-linked prodrugs and bio-precursors. Carrier-linked prodrugs have a non-toxic carrier or pro-moiety that is covalently linked and removed enzymatically to release the active drug moiety. In contrast, the bio-precursors do not incorporate a carrier group and only yield the active compounds upon biotransformation (Jornada et al., 2015). The prodrug strategy is often implemented to modify or eliminate undesirable physicochemical properties, such as poor solubility, limited bioavailability, chemical instability, low permeability, and lack of site-specificity, of pharmacologically active drug molecules. In other words, it enables optimization of absorption, distribution, metabolism, excretion, and toxicity (ADMET) properties of pharmacologically active moieties and overcomes formulation, delivery, and toxicity hurdles and achieve optimal drug therapy and outcomes (Rautio et al., 2018; Najjar et al., 2020). From 2008 to 2017, the United States Food and Drug Administration (FDA) approved at least 30 prodrugs, accounting for nearly 10% of all novel small-molecule compounds approved (Najjar and Karaman, 2019). Some successful strategies for prodrug design are described below as examples of prodrugs used in anesthesia (Figure 2).
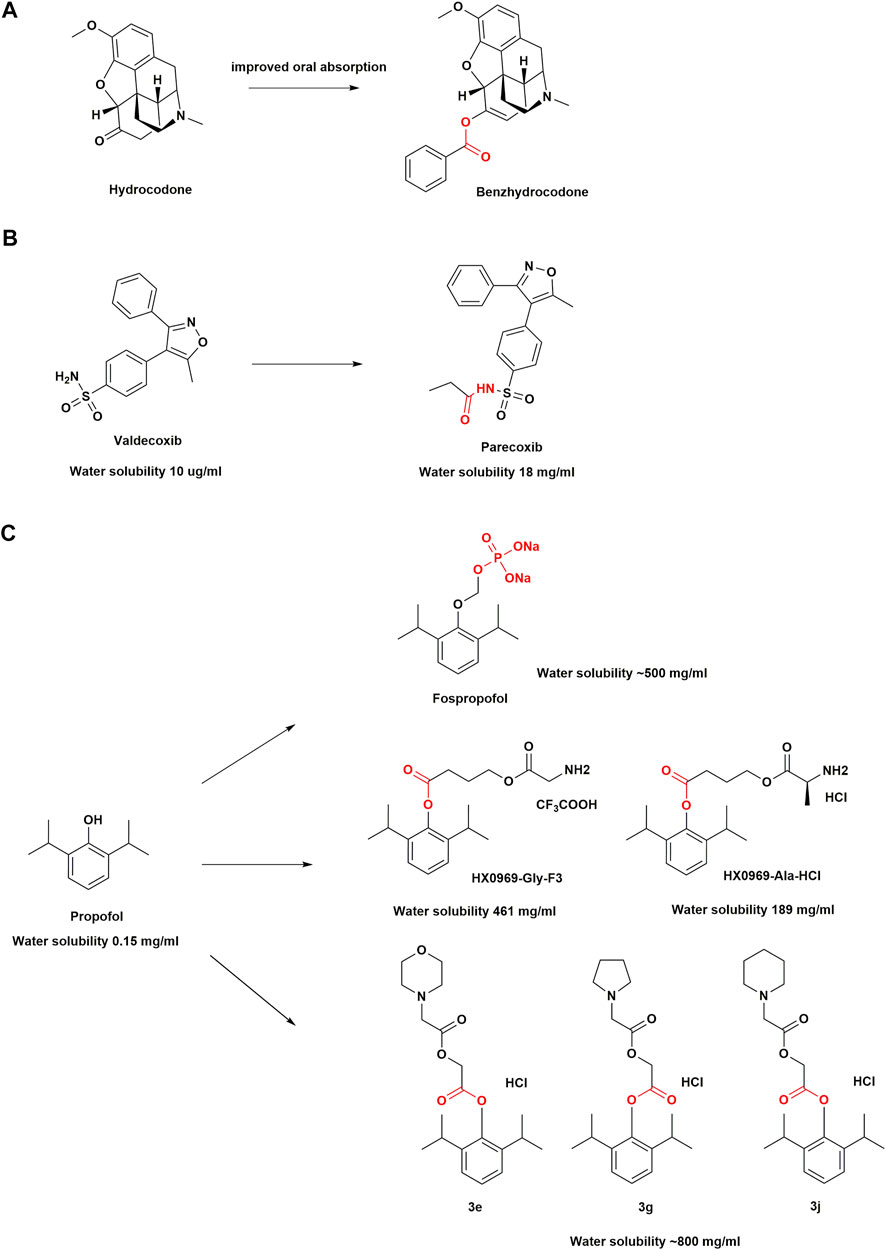
FIGURE 2. Structural optimization in anesthetic drugs with prodrug design. (A) Hydrocodone and its ester prodrug; (B) Valdecoxib and its amide prodrug; (C) Propofol prodrugs.
Ester Prodrugs
Ester prodrugs are most commonly used and it is estimated that around half of the marketed prodrugs are activated by enzyme-mediated hydrolysis (Ettmayer et al., 2004). The prodrug approach can mask polar or charged moieties as esters to improve lipophilicity, promote membrane permeation and enhance oral absorption (Beaumont et al., 2003). Moreover, The physicochemical properties are particularly suitable for CNS analgesia drugs (Kang et al., 2021).
Benzhydrocodone, a synthetic opioid, is a prodrug of hydrocodone (Mustafa et al., 2018). The physicochemical effect after adding benzoic acid functional groups to hydrocodone results in improved oral absorption and a reduction in parenteral bioavailability of the active metabolite (Cassidy et al., 2017; Guenther et al., 2018). Benzhydrocodone is inactive and exerts its pharmacologic effects mainly through the generation of hydrocodone, which has a high affinity for µ-opioid receptors (MORs). Upon binding, hydrocodone produces profound analgesia with no ceiling (Vallejo et al., 2011). It almost completely converted into hydrocodone within 5 min of oral administration, by esterase metabolism in the gastrointestinal tract (Silver et al., 2016). In vitro data have indicated that the conversion of benzhydrocodone to hydrocodone in whole blood is a slow process that takes approximately 240 min, which may deter parenteral abuse (Silver et al., 2016). A single-center, randomized, double-blind, crossover study among 51 healthy adults reported that intranasal administration of benzhydrocodone resulted in a significantly lower hydrocodone exposure and associated decrease in Drug Liking score compared with that of hydrocodone bitartrate (Mickle et al., 2018). In the case of prodrugs where transformation is required, a lower peak plasma concentration (Cmax) and a delayed time to peak the Cmax compared with those for the parent drug may be observed (Mickle et al., 2018). In other words, a prodrug could potentially allow for better management of opioids toxicity. Benzhydrocodone in combination with acetaminophen (APAP) under the trade name Apadaz™ received FDA approval in February 2018 for the short-term management of severe acute pain (Mustafa et al., 2018). However, given that benzhydrocodone/APAP is still susceptible to oral abuse, it has not been confirmed as an abuse-deterrent opioid formulation.
Amide Prodrugs
Amide prodrug derivatives are very common among nonsteroidal anti-inflammatory drugs (NSAIDs). Studies have shown that replacing the carboxylic group of NSAIDs with an amide functional group increases cyclooxygenase-2 (COX-2) selectivity and further helps to reduce the gastrointestinal toxicity of the parent drug (Kalgutkar et al., 2000).
Few NSAIDs were previously available for the parenteral treatment of acute and chronic pain, and their use was often accompanied by serious adverse effects, such as peptic ulcers, gastrointestinal bleeding, liver and kidney dysfunction, and platelet suppression (Pirani et al., 1987; Dorais et al., 1992; Strom et al., 1996). Therefore, an amide prodrug with high water solubility and anti-inflammatory activity to develop COX-2 inhibitors was designed for parenteral delivery. Parecoxib is the first parenteral and highly selective COX-2 inhibitor (Jain, 2000; Talley et al., 2000). As an amide prodrug of a sulfonamide-based COX-2 inhibitor valdecoxib, parecoxib are inactive. An N-acylation of the prodrug moiety of valdecoxib increases the water solubility of parecoxib, which makes the sulfonamide NH group more readily ionizable. The parecoxib amide hydrolysis of the sulfonyl propionamide substituent is mainly mediated by hepatic microsomal carboxylesterases (Talley et al., 2000), with a half-life of approximately 22 min. The onset of analgesic effects occurs within 10–23 min and attains a maximum relief within 2 h (Barton et al., 2002). Early clinical studies have shown that it brings pain relief in post-surgical patients via its rapid conversion to valdecoxib in vivo (Desjardins et al., 2001). A pooled analysis of 28 randomized, placebo-controlled clinical trials with 9287 patients has shown that skin rash and cardiac complications occur infrequently with parecoxib administration, which highlight its safety in patients (Schug et al., 2017). Currently, it is approved in over 80 countries for perioperative pain control and may help reduce opioid use (Diaz-Borjon et al., 2017).
Phosphate Prodrugs
Prodrugs with ionizable functional groups (e.g., phosphate, phosphonate, and phosphinate) are designed to improve drug solubility, among which phosphate ester-based prodrugs are relatively stable and are a good substrate for alkaline phosphatases in vivo (Wiemer and Wiemer, 2015).
Propofol (2,6-diisopropylphenol) is an intravenous general anesthetic drug commonly used in clinical practice and is pharmacologically characterized by its rapid induction of anesthesia and recovery from it after discontinuation (Sahinovic et al., 2018). It is often formulated as an oil-in-water emulsion with high lipophilicity (150 μg/ml) (Rautio et al., 2008). The adverse effects associated with propofol emulsions, such as injection pain, bacterial contamination, and propofol infusion syndrome (Bennett et al., 1995; Kam and Cardone, 2007; Desousa, 2016), have led to the design of the drugs with improved water solubility, a hot spot in drug development. Therefore, phosphate prodrugs of propofol have been designed with a phosphate group attached to the hydroxyl group of propofol, such as fospropofol disodium, through an OCH2 spacer (Garnock-Jones and Scott, 2010). The substitution of the hydroxyl group by the charged phosphate group increases electronegativity, which greatly improves the water solubility of fospropofol (500 mg/ml) (Rautio et al., 2008). Once the phosphate group is hydrolyzed by alkaline phosphatase in the liver, liberating the active metabolite propofol, the resulting formaldehyde and phosphate degrade naturally (Schywalsky et al., 2003). Based on the molecular weight, 1 mg of fospropofol (332.24 g/mol) releases 0.54 mg of propofol (178.27 g/mol). Moreover, given that the “biophase” characteristics of fospropofol are different from that of propofol (Yavas et al., 2008), it results in a slower onset of the drug effect in the former group. Fospropofol has a sedation effect onset of 4–13 min and a prolonged effect duration. For prodrugs, due to the existence of enzymatic and/or chemical transformation processes, the therapeutic effects of the parent drug are usually delayed. Since the enzymatic conversion to propofol is time-dependent, fospropofol may theoretically provide a better safety profile, especially in cardiac and respiratory functions (Abdelmalak et al., 2012). For example, recent studies have shown a decreased incidence of hypotension and respiratory depression with fospropofol because of its slower onset of action (Luo et al., 2022). Moreover, contrary to what was observed in the administration of propofol, the oral and intraduodenal administration of fospropofol produced a propofol bioavailability of 30% or more in human volunteers (Wozniak et al., 2015). Therefore, the indications for propofol may be extended by nonintravenous administration of fospropofol.
Although fospropofol eliminates drawbacks associated with propofol emulsion in water-soluble formulations, common adverse events observed in patients are paresthesia (incidence 49–74%) and pruritus (incidence 16–28%), often in the perianal region (Cohen et al., 2010; Gan et al., 2010). The metabolic accumulation of phosphate components causes these adverse effects, which are transient and self-limited (Bengalorkar et al., 2011). Researchers have therefore also introduced amino acid groups into the design of propofol prodrugs. It was found that two modified amino acid prodrugs (HX0969-Ala-HCl and HX0969-Gly-F3) released propofol more rapidly than the phosphate prodrugs previously designed (fospropofol disodium and HX0969W), which was confirmed through in vitro plasma experiments in rats (Lang et al., 2014). In vivo experiments showed that the intravenous administration of amino acid prodrugs had a faster onset of action and required a lower dose than the phosphate prodrug, and prevented the generation of formaldehyde and phosphate, thereby eliminating the adverse effects associated with formaldehyde and phosphate buildup (Lang et al., 2014). This new design approach may improve the conversion efficiency of a prodrug.
Next, researchers found that the insertion of glycolic acid as a linker between propofol and cyclic amino acids could further accelerate the release of propofol into the plasma. Prodrugs (3e, 3g, 3j) have been shown to be better than fospropofol in terms of onset time, anesthesia duration time, and safety in mice (Liu et al., 2020). The molar mass, onset, and duration of action of these prodrugs were found to be comparable to those of propofol, while preserving the clinical benefits of propofol. In addition, propofol + glycolic acid + cyclic amino acids may yield a key structural feature that could contribute to the development of a safe, water-soluble, rapid-release propofol prodrug with high molecular utilization of propofol (Liu et al., 2020). However, because propofol is still to date the active ingredient in these prodrug designs, the adverse effects such as hypotension and respiratory depression remain unresolved.
Soft Drugs
The term soft drug was introduced by Bodor during the late 1970s (Bodor et al., 1980; Bodor and Kaminski, 1980). Soft drugs are pharmacologically active and undergo predictable and controllable metabolic inactivation after exhibiting their therapeutic effect (Bodor and Buchwald, 2000). In general, soft drugs are designed to control metabolism and prevent the generation of potentially reactive or toxic intermediates. If possible, the inactivation process should occur in a single, low-energy, high-volume metabolic step in which the inactive substances produced are immediately eliminated (Buchwald and Bodor, 2014).
Therefore, it is desirable that soft drugs are metabolized by a broad class of hydrolytic enzymes rather than undergoing oxidative metabolism. Mammalian carboxylesterases (EC 3.1.1.1) play an important role as enzymes for drug biotransformation and constitute a polygenic family with low substrate specificity (Laizure et al., 2013). Together with other carboxylate hydrolases, such as butyrylcholinesterase (BChE, EC 3.1.1.8) and arylesterase (ArE, EC 3.1.1.1.2), they effectively catalyze the hydrolysis of various chemicals containing functional groups, such as carboxylic acid esters, amides, and thioesters, to their respective free acids (Laizure et al., 2013; Di, 2019). As esterases are ubiquitous in mammals and widely expressed in various tissues, they provide a more reliable source of inactivation relative to metabolic enzymes that are expressed primarily in organs such as the liver and kidney, especially in critically ill patients with severely impaired liver and kidney function (Laizure et al., 2013). Therefore, many soft drug strategies are focused on hydrolysis by esterases. However, this does not imply that molecular structures containing ester bonds have the PK characteristics of rapid metabolism because steric hindrance around ester bonds can have a great impact on enzymatic reactions where the larger the steric hindrance, the longer the half-life (Buchwald and Bodor, 1999). Moreover, excessively rapid metabolism should be avoided to ensure the prolonged activity of the parent compound at the desired site (Bodor and Buchwald, 2000). Pharmaceutical development in anesthesiology has gravitated toward soft drugs because they cater to the high degree of control required over the rapidly changing clinical process allowing for a state of anesthesia that can be turned on immediately when desired and can be turned off in a controlled manner (Egan, 2009). It is desirable that not only should anesthetic drugs provide the advantage of predictable control, but also that the rapid metabolism of soft drugs should be independent of liver and kidney function and thus should not be altered by continuous infusion and multiple intermittent repeated doses. The drug development in anesthesia has therefore gradually focused on designing soft drugs for sedation, analgesia, and muscle relaxation in recent years (Figure 3, Figure 4) (Egan, 2009; Birgenheier et al., 2020). Since esmolol was first marketed as an ultra short-acting ß-blocker in the early 1980s (Erhardt et al., 1982), this aforementioned successful drug design approach has been rapidly applied to opioid analgesics, benzodiazepine scaffolds, and other ester derivatives (Feldman et al., 1991; James et al., 1991; Stafford et al., 2002). In most cases, such drugs are rapidly metabolized, and the pharmacological activity of the corresponding metabolites is one or more orders of magnitude lower than that of the parent drug (Shaffer et al., 1988; Westmoreland et al., 1993; Kilpatrick et al., 2007). Some well-designed soft drugs have been approved for clinical use; however, many novel soft drug development programs have discontinued such as those of novel etomidate and propanidid soft drug analogs (Egan et al., 2012; Husain et al., 2012). Therefore, ongoing attempts to develop soft drugs in the field of anesthesia will need to see some type of success in order to provide options for subsequent drug development.
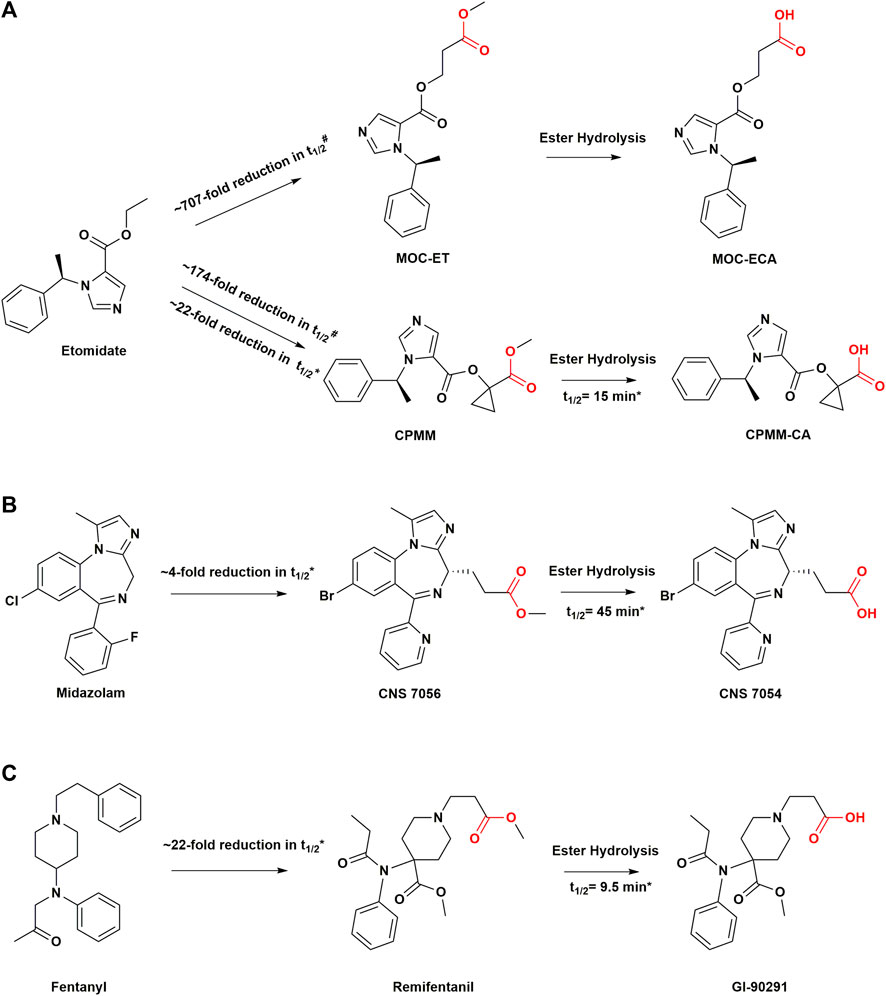
FIGURE 3. Structural modifications in sedatives and analgesics with soft drug design. (A) Soft analogs of etomidate and their metabolites. (B) Soft analog of midazolam and its metabolite. (C) soft analog of fentanyl and its metabolite. #Values are from measurements of in vitro metabolic half-lives in rat blood. *Values are from measurements of in vivo metabolic half-lives in human body. t1/2, half-life; MOC-ET, methoxycarbonyl etomidate; CPMM, cyclopropyl-methoxycarbonyl metomidate.
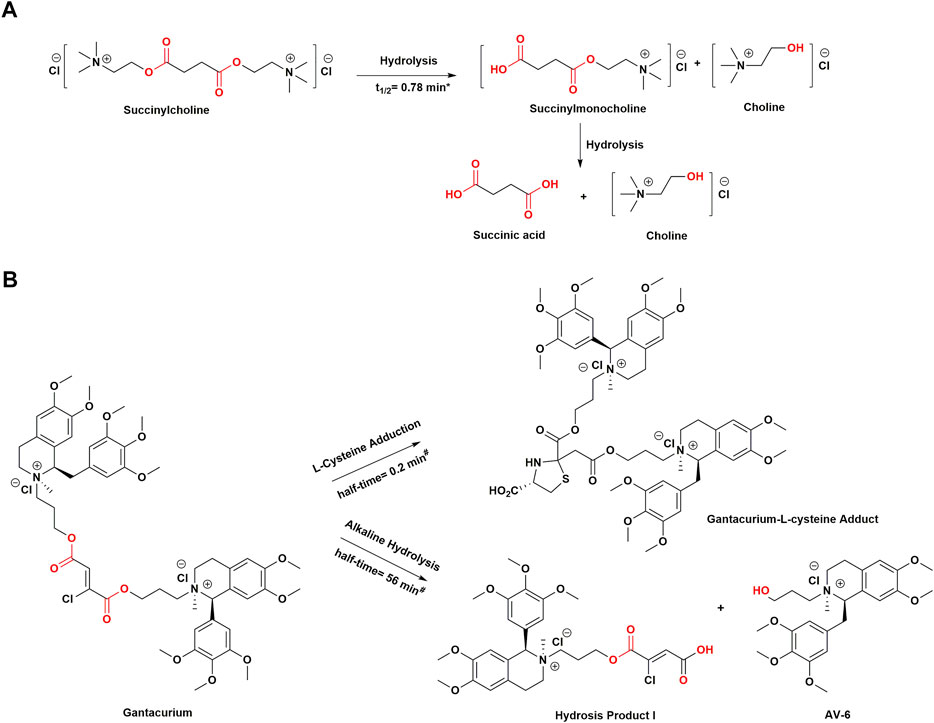
FIGURE 4. Structural modifications in muscle relaxants drugs with soft drug design. (A) Chemical structure of succinylcholine and its hydrolysis process. (B) Chemical structure of gantacurium and its breakdown products. *Values are from measurements of in vivo metabolic half-lives in human body. #Values are from measurements of in vitro reaction half-times in phosphate buffer at pH 7.4 and 37°C. t1/2, half-life.
Sedatives
Etomidate and Analogues
Etomidate is an ester-containing, short-acting imidazole-type derivative discovered in 1964 (Godefroi et al., 1965). As a nonbarbiturate intravenous general anesthetic, it has outstanding pharmacological characteristics and causes rapid induction of the state of anesthesia. Etomidate is hemodynamically stable during anesthesia and has little effect on respiratory effects (Morgan et al., 1975). Etomidate is inactivated by hepatic esterases, which leads to the formation of corresponding carboxylic acid (ET-acid) (Van Hamme et al., 1978). Although etomidate also contains a carboxylic ester structure that can be hydrolyzed to carboxylic acids by hepatic esterases, it is a poor substrate for these esterases (Van Hamme et al., 1978). Owing to the structural proximity of the ester bond and the imidazole ring, the steric hindrance of hydrolysis increases, prolonging the duration of action with a terminal metabolic half-life of approximately 2–5 h (Van Hamme et al., 1978; Forman, 2011). In critically ill patients, induction with etomidate could increase mortality by inhibiting adrenocortical steroid synthesis (Ledingham and Watt, 1983; Wagner and White, 1984). Studies have shown that etomidate could cause adrenocortical function of up to 6-8 h from a single administration, and more than 24 h from continuous infusion (Allolio et al., 1984; Wanscher et al., 1985), severely limiting its clinical application. A valid explanation for this could be its high affinity for 11β-hydroxylase and the cholesterol side-chain cleavage enzyme, which are the key enzymes involved in steroidogenesis (de Jong et al., 1984; Pejo et al., 2016). Therefore, it has been hypothesized that “soft” etomidate derivatives would ameliorate this side effect since the drugs would be rapidly metabolized (McGrath and Raines, 2018).
Methoxycarbonyl etomidate (MOC-etomidate) is the first soft analog of etomidate, which can be rapidly hydrolyzed by nonspecific esterase activity because of its metabolically labile ester (Cotten et al., 2009). The main metabolite is methoxycarbonyl etomidate carboxylic acid (MOC-ECA). Owing to the two-carbon spacer, which increases the length between the labile ester moiety and the imidazole ring in MOC-etomidate, the steric hindrance of ester hydrolysis decreases significantly (Cotten et al., 2009). Therefore, compared with etomidate, MOC-etomidate can be deactivated by rapid hydrolysis in vivo, with a half-life of only a few minutes (Husain et al., 2012). As a result, it maintains the hypnotic effect with a shorter duration of action and is devoid of the suppression of adrenocortical function after a single bolus (Cotten et al., 2009). It is also demonstrated that PK modifications can reduce the inhibitory effect of etomidate analogs on adrenocortical function (Cotten et al., 2009). However, studies have shown that MOC-ECA accumulates gradually over time during continuous infusion, leading to the suppression of electroencephalographic activity (Ge et al., 2012). The related hypothesis could be that ultrafast metabolism results in marked context sensitivity with a slow clearance of metabolites in the brain in which case even minimal active metabolites may accumulate to concentrations sufficient to produce significant pharmacological effects despite the metabolite potency of MOC-ECA being 350-fold lower than that of MOC-etomidate (Ge et al., 2012; Pejo et al., 2012).
Cyclopropyl-methoxycarbonyl-metomidate (CPMM, currently known as ABP-700) is a second-generation soft analog of etomidate, a member of the MOC-etomidate analogs family, with an optimal onset and offset profile (Husain et al., 2012). The researchers concluded that incorporating different aliphatic groups on the two-carbon intervals of MOC-etomidate and the introduction of steric hindrance could help reduce the rate of ester bond hydrolysis and prolong the duration of anesthesia. This strategy was based on previous studies which found that the insertion of large chemical groups near metabolically unstable ester bonds slowed down the rate of ester bond hydrolysis (Buchwald, 2001; Buchwald and Bodor, 2002). Therefore, although ABP-700 contains an ester bond which can be metabolized to a carboxylic acid metabolite by nonspecific esterases, it is metabolized as a soft drug more slowly than MOC-etomidate (Pejo et al., 2016). Compared to MOC-etomidate, ABP-700 has an additional aliphatic group, namely, cyclopropyl, between the etomidate backbone and the labile ester, which slows down the metabolic rate (Husain et al., 2012). At the same time, such a structure leads to unexpected potency, which is approximately an order of magnitude higher than that of MOC-etomidate (Husain et al., 2012). These chemical modifications combine optimized PK and PD properties while improving the overall therapeutic index.
Preclinical studies in rodent models have shown that the ABP-700 group recovered faster than the etomidate group and that the recovery time was independent of the infusion time. Furthermore, the electroencephalographic burst suppression ratio (BSR) was reversed within minutes after discontinuation of the closed-loop infusion that lasted 2 h (Pejo et al., 2012). Cortisol concentrations in beagles did not differ from those of beagles in the propofol group after 2 h of continuous infusion of ABP-700 in response to extraneous corticotropic hormone stimulation (Campagna et al., 2014). Clinical studies on the safety and efficacy of ABP-700 have shown that ABP-700 retains the desirable properties of etomidate with hemodynamic stability and no respiratory depression (Struys et al., 2017; Valk et al., 2018). However, patients in the ABP-700 group often experienced excitatory phenomena and abnormal involuntary muscle movement excitation at clinical doses (Valk et al., 2018), which led to discontinued development by The Medicines Company in 2017. Recent studies have shown no correlation between involuntary muscle movement and epilepsy (Valk et al., 2019), although, disinhibitory effects of the Bispectral Index (BIS) are associated with involuntary muscle movements (Valk et al., 2021). The mechanisms underlying clinical excitation are still unclear, and ABP-700 was restarted in 2020 with funding by Mass General Brigham (Boston, MA, United States), which still needs further investigation (Valk and Struys, 2021).
Benzodiazepines
Benzodiazepines constitute a class of psychotropic drugs with hypnotic, sedative, anti-anxiety, and anterograde amnesia properties that have been widely used in clinical practice. Midazolam remains one of the most commonly used sedatives. However, its elimination half-life is approximately 1.5–3 h and it has a prolonged sedation time due to its production of active metabolites and dependence on liver metabolism (Allonen et al., 1981; Smith et al., 1981). Moreover, the cytochrome P450 enzyme has individual differences, making the sedative duration of midazolam unpredictable (Kanto, 1985; Wandel et al., 1994). Remimazolam, formerly known as CNS 7056, is an ester-modified benzodiazepine derivative with a rapid offset of drug effect. It has a typical pharmacological profile of benzodiazepines while exhibiting the soft PK properties of remifentanil (Goudra and Singh, 2014). Pharmacodynamically, remimazolam is similar to midazolam and acts on gamma-aminobutyric acid (GABAA) receptors which can be antagonized by the benzodiazepine antagonist flumazenil (Kilpatrick et al., 2007). Similar to remifentanil, its metabolism is organ-independent, and it can be administered to patients with renal or hepatic impairment (Stöhr et al., 2021). The carboxylic acid ester bond in the molecular structure of remimazolam is readily inactivated in vivo by nonspecific esterases in the blood and tissues. Its carboxylic acid metabolite, CNS 7054, has 1/320 to 1/410 times the affinity for the benzodiazepine receptor than that of remimazolam (Kilpatrick et al., 2007). These characteristics lend remimazolam a shorter action time (<10 min) than midazolam, with an elimination half-life of only 0.75 h (Antonik et al., 2012). Clinical trials have shown that remimazolam has a rapid onset of action and rapid recovery after sedation. In phase II and III clinical trials, remimazolam was safely used in outpatient gastrocolonoscopy, and patients recovered more quickly than those treated with midazolam (Borkett et al., 2015; Pambianco et al., 2016; Rex et al., 2018). Remimazolam demonstrates deeper sedation and quicker recovery than midazolam during continuous infusion in healthy Chinese participants (Sheng et al., 2020). It demonstrated a controllable pharmacological effect profile even after long-lasting continuous infusion, and a high clearance (Lohmer et al., 2020; Schüttler et al., 2020). The sedative effect of this drug is comparable to that of propofol, with rapid peak sedation within 1–2 min and only moderate hemodynamic side effects (Goudra and Singh, 2014; Birgenheier et al., 2020). Currently, remimazolam is seen as the future of sedatives, which has already received regulatory approval in Japan for general anesthesia in adults. It has been approved by the United States Food and Drug Administration (US FDA) for the induction and maintenance of procedural sedation (Keam, 2020). A more complete picture of the clinical potential of remimazolam will be available in the coming years as the drug moves from late-stage development to more widespread postmarketing use.
Analgesics
As an ultra short-acting MOR agonist, remifentanil synthesized by Feldman et al., has a strong analgesic effect (Feldman et al., 1991; Bürkle et al., 1996). The introduction of a methyl ester group on the N-acyl side chain of the piperidine ring improves sensitivity to esterase hydrolytic metabolism (Feldman et al., 1991). Studies have shown that the metabolic clearance rate of remifentanil is faster than that of liver blood flow, indicating that the metabolism of remifentanil is independent of the liver (Glass et al., 1999). It can be easily metabolized by nonspecific plasma and tissue esterases into the carboxylic acid metabolite GI-90291 (Glass et al., 1999). GI-90291 has a potency of 1/300 to 1/1000 times that of remifentanil and is inactive at clinical doses. The renal excretion rate of this metabolite has been estimated to be over 80% (Glass et al., 1993). Remifentanil has a short onset of action of 1–2 min and a terminal half-life of 10–20 min (Westmoreland et al., 1993). After continuous infusion for 3 h, the time-dose-related half-life of remifentanil was only approximately 3 min, preventing undesirable accumulation (Kapila et al., 1995). This also explains why hydrolases are popular for soft drug inactivation. In the design of etomidate analogs, the idea of inserting two CH2 groups between the labile ester group and the imidazole ring was also derived from the structure of remifentanil (Cotten et al., 2009). Remifentanil had the same potency as fentanyl in a rat model of tail removal response, and the duration of action was four times shorter (Feldman et al., 1991). The potency of remifentanil was 20–30 times higher than that of alfentanil in both healthy adult volunteers and those who underwent surgery (Egan et al., 1996; Scott and Perry, 2005). Remifentanil, as an effective opioid analgesic, has been widely used for perioperative pain management (Wilhelm and Kreuer, 2008).
Muscle Relaxants
Succinylcholine, an accidental soft drug introduced in the 1950s, is the only currently available depolarizing muscle relaxant (DMR) with favorable PK properties (Thesleff et al., 1952). It contains two acetylcholine molecules linked together by methyl acetate (Jonas and Hunter, 2004). Succinylcholine is normally rapidly degraded in plasma by pseudocholinesterase (PChE) to succinylmonocholine, succinic acid, and choline (Viby-Mogensen, 1980; Curran et al., 1987). However, given that PChE is synthesized in the liver and is present in the plasma, liver-related diseases may decrease enzyme activity and prolong the duration of associated neuromuscular block. In addition, patients with PChE deficiency are incapable of metabolizing suxamethonium, resulting in prolonged apnea (Al-Emam, 2021). In general, the onset time of succinylcholine is within 60 s and lasts for 4–6 min when administered to patients with normal plasma PChE activity (Alvarellos et al., 2015). Thus, because of its short half-life, it is often used in clinical procedures requiring short periods of muscle relaxation, such as endotracheal intubation, fiberoptic bronchoscopy, and electroconvulsive therapy (Sluga et al., 2005; Li et al., 2016). However, it suffers some serious side effects, such arrhythmia, hyperkalemia, increased intraocular or gastric pressure, and sometimes even malignant hyperthermia, precluding it from being the “ideal” ultra short-acting DMR (Galindo and Davis, 1962; Walton and Farman, 1973; Meyers et al., 1978).
In the past few decades, there have been some nondepolarizing muscle relaxants (NDMRs) that may be used instead of succinylcholine, such as short-acting gantacurium. Gantacurium chloride, formerly recognized as GW280430A, is a rapid onset NDMR (Belmont et al., 2004; de Boer and Carlos, 2018). As a bis-tetrahydroisoquinolinium chlorofumarate, it is a single isomer such as cisatracurium, whereas atracurium and mivacurium consist of a mixture of isomers (Boros et al., 1999). In preclinical and clinical trials, gantacurium was regarded as a promising candidate because it seemed to have a nearly identical kinetic profile to succinylcholine. In human volunteers, its effect onset time was less than 3 min, which was capable of being shortened to approximately 1.5 min by increasing the dose to four times the effective dose of 95% (ED95) with a duration of action of 15 min (Belmont et al., 2004). There are two routes of inactivation that are unrelated to PChE activity. One is a slow process, in which it is metabolized by alkaline ester hydrolysis in plasma, and the other is a fast process that involves adduction of the amino acid cysteine (L-cysteine) to saturate the fumarate double bond (Savarese et al., 2004). The latter method of chemical degradation most likely accounts for its ultrashort duration of effect. Moreover, the unique means of elimination involve neither the kidney nor the liver, and the metabolites of gantacurium are pharmacologically inactive (Belmont et al., 2004). Numerous studies have indicated that L-cysteine adduction can reverse neuromuscular blockade of gantacurium and its analogs (CW 002 and CW 011), which is the same as sugammadex reversal of rocuronium (Savarese et al., 2010; Sunaga et al., 2010).
Conclusion
From pharmacological and toxicological perspectives, metabolic considerations in the drug design process help improve PK/PD and safety profiles. For both prodrugs and soft drugs, the applied strategies are driven by unmet medical needs and are used to overcome undesirable drug properties to achieve optimal clinical use. In fact, from a broad perspective, prodrugs and soft drugs seem to be two extremes of a continuum of possibilities (Stańczak and Ferra, 2006). Prodrugs design is a very useful approach for improving the drug-like properties of a molecule to circumvent formulation and delivery difficulties. In the case of the soft drug approach, the retrometabolic drug design strategy allows a predictable metabolic route via a single inactivation. Prodrug design strategies have a wide range of applications, and soft drug design represents an approach that meets the unique needs of modern anesthesia practice.
In recent years, new drug development programs for the analogs of anesthetics have resulted in only a handful of compounds with market approval (Liu et al., 2016; Keam, 2020). For soft sedative-hypnotics, abnormal excitatory activity has been the main reason for discontinuing the development programs. This is the case for the etomidate and propanidid soft drug analogs. To compete with existing drugs, novel anesthetic drugs should possess a high therapeutic index and minimal side effects to optimize the benefit/risk ratios in patients.
Researchers have gradually applied artificial intelligence-assisted drug design strategies to drug metabolism studies in recent years (Wang et al., 2019; Smith, 2022). As enzymes (usually cytochrome P450) are essential for drug metabolism, the three-dimensional crystal structures of various enzymes and carrier proteins have been analyzed, the results of which have provided the basis for structural information (Pochapsky and Pochapsky, 2019). This would render the prediction of interactions achievable at the beginning of drug design (Smith, 2022). Although there are several challenges and failures in drug development, these experiences have driven the development of new compounds. In this review, the important roles of drug metabolism and pharmacokinetics strategies in drug design are emphasized and expounded through examples of various prodrugs and soft drugs in anesthesia. The rational use of these strategies will help develop more effective and safer drugs in the future.
Author Contributions
CD collected the documentations and wrote the original manuscript; WZ and JL proposed amendments and modified the paper. All authors contributed to the article and approved the submitted version.
Funding
This work was supported by the Post-Doctor Research Project, West China Hospital, Sichuan University (2021HXBH077).
Conflict of Interest
The authors declare that the research was conducted in the absence of any commercial or financial relationships that could be construed as a potential conflict of interest.
Publisher’s Note
All claims expressed in this article are solely those of the authors and do not necessarily represent those of their affiliated organizations, or those of the publisher, the editors and the reviewers. Any product that may be evaluated in this article, or claim that may be made by its manufacturer, is not guaranteed or endorsed by the publisher.
Acknowledgments
We would like to thank Editage (www.editage.cn) for English language editing.
References
Abdelmalak, B., Khanna, A., and Tetzlaff, J. (2012). Fospropofol, a New Sedative Anesthetic, and its Utility in the Perioperative Period. Curr. Pharm. Des. 18 (38), 6241–6252. doi:10.2174/138161212803832308
Al-Emam, A. (2021). Butyryl-Cholinesterase Deficiency: A Case Report of Delayed Recovery After General Anaesthesia. Toxicol Rep. 108, 1226–1228. doi:10.1016/j.toxrep.2021.06.016
Albert, A. (1958). Chemical Aspects of Selective Toxicity. Nature 182 (4633), 421–422. doi:10.1038/182421a0
Allolio, B., Stuttmann, R., Leonhard, U., Fischer, H., and Winkelmann, W. (1984). Adrenocortical Suppression by a Single Induction Dose of Etomidate. Klin. Wochenschr 62 (21), 1014–1017. doi:10.1007/bf01711723
Allonen, H., Ziegler, G., and Klotz, U. (1981). Midazolam Kinetics. Clin. Pharmacol. Ther. 30 (5), 653–661. doi:10.1038/clpt.1981.217
Alvarellos, M. L., McDonagh, E. M., Patel, S., McLeod, H. L., Altman, R. B., and Klein, T. E. (2015). PharmGKB Summary: Succinylcholine Pathway, Pharmacokinetics/pharmacodynamics. Pharmacogenet Genomics 25 (12), 622–630. doi:10.1097/fpc.0000000000000170
Anderson, S., Luffer-Atlas, D., and Knadler, M. P. (2009). Predicting Circulating Human Metabolites: How Good Are We?. Chem. Res. Toxicol. 22 (2), 243–256. doi:10.1021/tx8004086
Antonik, L. J., Goldwater, D. R., Kilpatrick, G. J., Tilbrook, G. S., and Borkett, K. M. (2012). A Placebo- and Midazolam-Controlled Phase I Single Ascending-Dose Study Evaluating the Safety, Pharmacokinetics, and Pharmacodynamics of Remimazolam (CNS 7056): Part I. Safety, Efficacy, and Basic Pharmacokinetics. Anesth. Analg. 115 (2), 274–283. doi:10.1213/ANE.0b013e31823f0c28
Attia, S. M. (2010). Deleterious Effects of Reactive Metabolites. Oxid. Med. Cell. Longev. 3 (4), 238–253. doi:10.4161/oxim.3.4.13246
Barton, S. F., Langeland, F. F., Snabes, M. C., LeComte, D., Kuss, M. E., Dhadda, S. S., et al. (2002). Efficacy and Safety of Intravenous Parecoxib Sodium in Relieving Acute Postoperative Pain Following Gynecologic Laparotomy Surgery. Anesthesiology 97 (2), 306–314. doi:10.1097/00000542-200208000-00004
Bass, A. S., Cartwright, M. E., Mahon, C., Morrison, R., Snyder, R., McNamara, P., et al. (2009). Exploratory Drug Safety: a Discovery Strategy to Reduce Attrition in Development. J. Pharmacol. Toxicol. Methods 60 (1), 69–78. doi:10.1016/j.vascn.2009.04.194
Belmont, M. R., Lien, C. A., Tjan, J., Bradley, E., Stein, B., Patel, S. S., et al. (2004). Clinical Pharmacology of GW280430A in Humans. Anesthesiology 100 (4), 768–773. doi:10.1097/00000542-200404000-00004
Bengalorkar, G. M., Bhuvana, K., Sarala, N., and Kumar, T. (2011). Fospropofol: Clinical Pharmacology. J. Anaesthesiol. Clin. Pharmacol. 27 (1), 79–83. doi:10.4103/0970-9185.76656
Bennett, S. N., McNeil, M. M., Bland, L. A., Arduino, M. J., Villarino, M. E., Perrotta, D. M., et al. (1995). Postoperative Infections Traced to Contamination of an Intravenous Anesthetic, Propofol. N. Engl. J. Med. 333 (3), 147–154. doi:10.1056/nejm199507203330303
Berg, E. L. (2019). Human Cell-Based In Vitro Phenotypic Profiling for Drug Safety-Related Attrition. Front. Big Data 2, 47. doi:10.3389/fdata.2019.00047
Bhardwaj, Y. R., Pareek, A., Jain, V., and Kishore, D. (2014). Chemical Delivery Systems and Soft Drugs: Retrometabolic Approaches of Drug Design. Saudi Pharm. J. 22 (4), 290–302. doi:10.1016/j.jsps.2013.04.004
Birgenheier, N. M., Stuart, A. R., and Egan, T. D. (2020). Soft Drugs in Anesthesia: Remifentanil as Prototype to Modern Anesthetic Drug Development. Curr. Opin. Anaesthesiol. 33 (4), 499–505. doi:10.1097/aco.0000000000000879
Bodor, N., and Buchwald, P. (2000). Soft Drug Design: General Principles and Recent Applications. Med. Res. Rev. 20 (1), 58–101. doi:10.1002/(sici)1098-1128(200001)20
Bodor, N., Kaminski, J. J., and Selk, S. (1980). Soft Drugs. 1. Labile Quaternary Ammonium Salts as Soft Antimicrobials. J. Med. Chem. 23 (5), 469–474. doi:10.1021/jm00179a001
Bodor, N., and Kaminski, J. J. (1980). Soft Drugs. 2. Soft Alkylating Compounds as Potential Antitumor Agents. J. Med. Chem. 23 (5), 566–569. doi:10.1021/jm00179a018
Borkett, K. M., Riff, D. S., Schwartz, H. I., Winkle, P. J., Pambianco, D. J., Lees, J. P., et al. (2015). A Phase IIa, Randomized, Double-Blind Study of Remimazolam (CNS 7056) versus Midazolam for Sedation in Upper Gastrointestinal Endoscopy. Anesth. Analg. 120 (4), 771–780. doi:10.1213/ane.0000000000000548
Boros, E. E., Bigham, E. C., Boswell, G. E., Mook, R. A., Patel, S. S., Savarese, J. J., et al. (1999). Bis- and Mixed-Tetrahydroisoquinolinium Chlorofumarates: New Ultra-short-acting Nondepolarizing Neuromuscular Blockers. J. Med. Chem. 42 (2), 206–209. doi:10.1021/jm980597h
Buchwald, P., and Bodor, N. (2002). Physicochemical Aspects of the Enzymatic Hydrolysis of Carboxylic Esters. Pharmazie 57 (2), 87–93. doi:10.1080/1057563029001/4881
Buchwald, P., and Bodor, N. (1999). Quantitative Structure-Metabolism Relationships: Steric and Nonsteric Effects in the Enzymatic Hydrolysis of Noncongener Carboxylic Esters. J. Med. Chem. 42 (25), 5160–5168. doi:10.1021/jm990145k
Buchwald, P., and Bodor, N. (2014). Recent Advances in the Design and Development of Soft Drugs. Pharmazie 69 (6), 403–413. doi:10.1691/ph.2014.3911R
Buchwald, P. (2020). Soft Drugs: Design Principles, Success Stories, and Future Perspectives. Expert Opin. Drug Metab. Toxicol. 16 (8), 645–650. doi:10.1080/17425255.2020.1777280
Buchwald, P. (2001). Structure-metabolism Relationships: Steric Effects and the Enzymatic Hydrolysis of Carboxylic Esters. Mini Rev. Med. Chem. 1 (1), 101–111. doi:10.2174/1389557013407403
Burkle, H., Dunbar, S., and Van Aken, H. (1996). Remifentanil: A Novel, Short-Acting, mu-Opioid. Anesth. Analgesia 83 (3), 646–651. doi:10.1097/00000539-199609000-00038
Campagna, J. A., Pojasek, K., Grayzel, D., Randle, J., and Raines, D. E. (2014). Advancing Novel Anesthetics: Pharmacodynamic and Pharmacokinetic Studies of Cyclopropyl-Methoxycarbonyl Metomidate in Dogs. Anesthesiology 121 (6), 1203–1216. doi:10.1097/aln.0000000000000416
Cassidy, T. A., Oyedele, N., Mickle, T. C., Guenther, S., and Budman, S. H. (2017). Patterns of Abuse and Routes of Administration for Immediate-Release Hydrocodone Combination Products. Pharmacoepidemiol Drug Saf. 26 (9), 1071–1082. doi:10.1002/pds.4249
Cerny, M. A., Kalgutkar, A. S., Obach, R. S., Sharma, R., Spracklin, D. K., and Walker, G. S. (2020). Effective Application of Metabolite Profiling in Drug Design and Discovery. J. Med. Chem. 63 (12), 6387–6406. doi:10.1021/acs.jmedchem.9b01840
Cohen, L. B., Cattau, E., Goetsch, A., Shah, A., Weber, J. R., Rex, D. K., et al. (2010). A Randomized, Double-Blind, Phase 3 Study of Fospropofol Disodium for Sedation during Colonoscopy. J. Clin. Gastroenterol. 44 (5), 345–353. doi:10.1097/MCG.0b013e3181c2987e
Cotten, J. F., Husain, S. S., Forman, S. A., Miller, K. W., Kelly, E. W., Nguyen, H. H., et al. (2009). Methoxycarbonyl-etomidate: a Novel Rapidly Metabolized and Ultra-short-acting Etomidate Analogue that Does Not Produce Prolonged Adrenocortical Suppression. Anesthesiology 111 (2), 240–249. doi:10.1097/ALN.0b013e3181ae63d1
Curran, M. J., Donati, F., and Bevan, D. R. (1987). Onset and Recovery of Atracurium and Suxamethonium-Induced Neuromuscular Blockade with Simultaneous Train-Of-Four and Single Twitch Stimulation. Br. J. Anaesth. 59 (8), 989–994. doi:10.1093/bja/59.8.989
de Boer, H. D., and Carlos, R. V. (2018). New Drug Developments for Neuromuscular Blockade and Reversal: Gantacurium, CW002, CW011, and Calabadion. Curr. Anesthesiol. Rep. 8 (2), 119–124. doi:10.1007/s40140-018-0262-9
de Jong, F. H., Mallios, C., Jansen, C., Scheck, P. A., and Lamberts, S. W. (1984). Etomidate Suppresses Adrenocortical Function by Inhibition of 11 Beta-Hydroxylation. J. Clin. Endocrinol. Metab. 59 (6), 1143–1147. doi:10.1210/jcem-59-6-1143
Desjardins, P. J., Grossman, E. H., Kuss, M. E., Talwalker, S., Dhadda, S., Baum, D., et al. (2001). The Injectable Cyclooxygenase-2-Specific Inhibitor Parecoxib Sodium has Analgesic Efficacy When Administered Preoperatively. Anesth. Analg. 93 (3), 721–727. doi:10.1097/00000539-200109000-00036
Desousa, K. A. (2016). Pain on Propofol Injection: Causes and Remedies. Indian J. Pharmacol. 48 (6), 617–623. doi:10.4103/0253-7613.194845
Di, L. (2019). The Impact of Carboxylesterases in Drug Metabolism and Pharmacokinetics. Curr. Drug Metab. 20 (2), 91–102. doi:10.2174/1389200219666180821094502
Diaz-Borjon, E., Torres-Gomez, A., Essex, M. N., Salomon, P., Li, C., Cheung, R., et al. (2017). Parecoxib Provides Analgesic and Opioid-Sparing Effects Following Major Orthopedic Surgery: A Subset Analysis of a Randomized, Placebo-Controlled Clinical Trial. Pain Ther. 6 (1), 61–72. doi:10.1007/s40122-017-0066-5
DiMasi, J. A., Feldman, L., Seckler, A., and Wilson, A. (2010). Trends in Risks Associated with New Drug Development: Success Rates for Investigational Drugs. Clin. Pharmacol. Ther. 87 (3), 272–277. doi:10.1038/clpt.2009.295
Dorais, J., Grégoire, G., and LeLorier, J. (1992). Gastrointestinal Damage Associated with Nonsteroidal Antiinflammatory Drugs. N. Engl. J. Med. 327 (26), 1882–1883. doi:10.1056/nejm199212243272612
Drews, J. (2000). Drug Discovery: a Historical Perspective. Science 287 (5460), 1960–1964. doi:10.1126/science.287.5460.1960
Egan, T. D. (2009). Is Anesthesiology Going Soft?: Trends in Fragile Pharmacology. Anesthesiology 111 (2), 229–230. doi:10.1097/ALN.0b013e3181ae8460
Egan, T. D., Minto, C. F., Hermann, D. J., Barr, J., Muir, K. T., and Shafer, S. L. (1996). Remifentanil versus Alfentanil: Comparative Pharmacokinetics and Pharmacodynamics in Healthy Adult Male Volunteers. Anesthesiology 84 (4), 821–833. doi:10.1097/00000542-199604000-00009
Egan, T. D., Obara, S., Jenkins, T. E., Jaw-Tsai, S. S., Amagasu, S., Cook, D. R., et al. (2012). AZD-3043: a Novel, Metabolically Labile Sedative-Hypnotic Agent with Rapid and Predictable Emergence from Hypnosis. Anesthesiology 116 (6), 1267–1277. doi:10.1097/ALN.0b013e31825685a6
Erhardt, P. W., Woo, C. M., Anderson, W. G., and Gorczynski, R. J. (1982). Ultra-short-acting Beta-Adrenergic Receptor Blocking Agents. 2. (Aryloxy)propanolamines Containing Esters on the Aryl Function. J. Med. Chem. 25 (12), 1408–1412. doi:10.1021/jm00354a003
Feldman, P. L., James, M. K., Brackeen, M. F., Bilotta, J. M., Schuster, S. V., Lahey, A. P., et al. (1991). Design, Synthesis, and Pharmacological Evaluation of Ultrashort- to Long-Acting Opioid Analgetics. J. Med. Chem. 34 (7), 2202–2208. doi:10.1021/jm00111a041
Forman, S. A. (2011). Clinical and Molecular Pharmacology of Etomidate. Anesthesiology 114 (3), 695–707. doi:10.1097/ALN.0b013e3181ff72b5
Gabrielsson, J., Dolgos, H., Gillberg, P. G., Bredberg, U., Benthem, B., and Duker, G. (2009). Early Integration of Pharmacokinetic and Dynamic Reasoning Is Essential for Optimal Development of Lead Compounds: Strategic Considerations. Drug Discov. Today 14 (7-8), 358–372. doi:10.1016/j.drudis.2008.12.011
Gabrielsson, J., and Green, A. R. (2009). Quantitative Pharmacology or Pharmacokinetic Pharmacodynamic Integration Should be a Vital Component in Integrative Pharmacology. J. Pharmacol. Exp. Ther. 331 (3), 767–774. doi:10.1124/jpet.109.157172
Galindo, A. H., and Davis, T. B. (1962). Succinylcholine and Cardiac Excitability. Anesthesiology 23, 32–40. doi:10.1097/00000542-196201000-00006
Gan, T. J., Berry, B. D., Ekman, E. F., Muckerman, R. C., Shore, N., and Hardi, R. (2010). Safety Evaluation of Fospropofol for Sedation during Minor Surgical Procedures. J. Clin. Anesth. 22 (4), 260–267. doi:10.1016/j.jclinane.2009.08.007
Garnock-Jones, K. P., and Scott, L. J. (2010). Fospropofol. Drugs 70 (4), 469–477. doi:10.2165/11204450-000000000-00000
Ge, R. L., Pejo, E., Haburcak, M., Husain, S. S., Forman, S. A., and Raines, D. E. (2012). Pharmacological Studies of Methoxycarbonyl Etomidate's Carboxylic Acid Metabolite. Anesth. Analg. 115 (2), 305–308. doi:10.1213/ANE.0b013e318239c6ca
Gillette, J. R. (1979). Effects of Induction of Cytochrome P-450 Enzymes on the Concentration of Foreign Compounds and Their Metabolites and on the Toxicological Effects of These Compounds. Drug Metab. Rev. 10 (1), 59–87. doi:10.3109/03602537908993901
Glass, P. S., Gan, T. J., and Howell, S. (1999). A Review of the Pharmacokinetics and Pharmacodynamics of Remifentanil. Anesth. Analg. 89 (4 Suppl. l), S7–S14. doi:10.1097/00000539-199910001-00003
Glass, P. S., Hardman, D., Kamiyama, Y., Quill, T. J., Marton, G., Donn, K. H., et al. (1993). Preliminary Pharmacokinetics and Pharmacodynamics of an Ultra-short-acting Opioid: Remifentanil (GI87084B). Anesth. Analg. 77 (5), 1031–1040. doi:10.1213/00000539-199311000-00028
Godefroi, E. F., Janssen, P. A., Vandereycken, C. A., Vanheertum, A. H., and Niemegeers, C. J. (1965). Dl-1-(1-Arylalkyl)Imidazole-5-Carboxylate Esters. A Novel Type of Hypnotic Agents. J. Med. Chem. 8, 220–223. doi:10.1021/jm00326a017
Goudra, B. G., and Singh, P. M. (2014). Remimazolam: The Future of its Sedative Potential. Saudi J. Anaesth. 8 (3), 388–391. doi:10.4103/1658-354x.136627
Guenther, S. M., Mickle, T. C., Barrett, A. C., Roupe, K. A., Zhou, J., and Lam, V. (2018). Relative Bioavailability, Intranasal Abuse Potential, and Safety of Benzhydrocodone/Acetaminophen Compared with Hydrocodone Bitartrate/Acetaminophen in Recreational Drug Abusers. Pain Med. 19 (5), 955–966. doi:10.1093/pm/pnx195
Husain, S. S., Pejo, E., Ge, R., and Raines, D. E. (2012). Modifying Methoxycarbonyl Etomidate Inter-ester Spacer Optimizes In Vitro Metabolic Stability and In Vivo Hypnotic Potency and Duration of Action. Anesthesiology 117 (5), 1027–1036. doi:10.1097/ALN.0b013e31826d3bef
Jain, K. K. (2000). Evaluation of Intravenous Parecoxib for the Relief of Acute Post-surgical Pain. Expert Opin. Investig. Drugs 9 (11), 2717–2723. doi:10.1517/13543784.9.11.2717
James, M. K., Feldman, P. L., Schuster, S. V., Bilotta, J. M., Brackeen, M. F., and Leighton, H. J. (1991). Opioid Receptor Activity of GI 87084B, a Novel Ultra-short Acting Analgesic, in Isolated Tissues. J. Pharmacol. Exp. Ther. 259 (2), 712–718.
Kanto, J. H. (1985). Midazolam: the First Water-Soluble Benzodiazepine. Pharmacology, Pharmacokinetics and Efficacy in Insomnia and Anesthesia. Pharmacotherapy 5 (3), 138–155.
Jonas, A. A., and Hunter, J. M. (2004). Pharmacology of Neuromuscular Blocking Drugs. Continuing Educ. Anaesth. Crit. Care & Pain 4 (1), 2–0037. doi:10.1093/bjaceaccp/mkh002
Jornada, D. H., dos Santos Fernandes, G. F., Chiba, D. E., de Melo, T. R., dos Santos, J. L., and Chung, M. C. (2015). The Prodrug Approach: A Successful Tool for Improving Drug Solubility. Molecules 21 (1), 42. doi:10.3390/molecules21010042
Kalgutkar, A. S., Marnett, A. B., Crews, B. C., Remmel, R. P., and Marnett, L. J. (2000). Ester and Amide Derivatives of the Nonsteroidal Antiinflammatory Drug, Indomethacin, as Selective Cyclooxygenase-2 Inhibitors. J. Med. Chem. 43 (15), 2860–2870. doi:10.1021/jm000004e
Kam, P. C., and Cardone, D. (2007). Propofol Infusion Syndrome. Anaesthesia 62 (7), 690–701. doi:10.1111/j.1365-2044.2007.05055.x
Kang, T., Miao, Z., Liu, S., and Ke, B. (2021). Prodrug Strategies in the CNS Drugs: Small Modification Makes Big Improvements. Ctmc 21, 2157–2169. doi:10.2174/1568026621666210727163827
Kapila, A., Glass, P. S., Jacobs, J. R., Muir, K. T., Hermann, D. J., Shiraishi, M., et al. (1995). Measured Context-Sensitive Half-Times of Remifentanil and Alfentanil. Anesthesiology 83 (5), 968–975. doi:10.1097/00000542-199511000-00009
Keam, S. J. (2020). Remimazolam: First Approval. Drugs 80 (6), 625–633. doi:10.1007/s40265-020-01299-8
Kelly, J. G., and O'Malley, K. (1990). Clinical Pharmacokinetics of the Newer ACE Inhibitors. A Review. Clin. Pharmacokinet. 19 (3), 177–196. doi:10.2165/00003088-199019030-00003
Kilpatrick, G. J., McIntyre, M. S., Cox, R. F., Stafford, J. A., Pacofsky, G. J., Lovell, G. G., et al. (2007). CNS 7056: a Novel Ultra-short-acting Benzodiazepine. Anesthesiology 107 (1), 60–66. doi:10.1097/01.anes.0000267503.85085.c0
Laizure, S. C., Herring, V., Hu, Z., Witbrodt, K., and Parker, R. B. (2013). The Role of Human Carboxylesterases in Drug Metabolism: Have We Overlooked Their Importance? Pharmacotherapy 33 (2), 210–222. doi:10.1002/phar.1194
Lang, B. C., Yang, J., Wang, Y., Luo, Y., Kang, Y., Liu, J., et al. (2014). An Improved Design of Water-Soluble Propofol Prodrugs Characterized by Rapid Onset of Action. Anesth. Analg. 118 (4), 745–754. doi:10.1213/ane.0000000000000124
Ledingham, I. M., and Watt, I. (1983). Influence of Sedation on Mortality in Critically Ill Multiple Trauma Patients. Lancet 1 (8336), 1270. doi:10.1016/s0140-6736(83)92712-5
Li, E. H., Bryson, E. O., and Kellner, C. H. (2016). Muscle Relaxation With Succinylcholine in Electroconvulsive Therapy. Anesth. Analg. 123 (5), 1329. doi:10.1213/ane.0000000000001475
Lin, J. H. (1996). Bisphosphonates: a Review of Their Pharmacokinetic Properties. Bone 18 (2), 75–85. doi:10.1016/8756-3282(95)00445-9
Liu, L. Q., Hong, P. X., Song, X. H., Zhou, C. C., Ling, R., Kang, Y., et al. (2020). Design, Synthesis, and Activity Study of Water-Soluble, Rapid-Release Propofol Prodrugs. J. Med. Chem. 63 (14), 7857–7866. doi:10.1021/acs.jmedchem.0c00698
Liu, R., Luo, C., Liu, J., Zhang, W., Li, Y., and Xu, J. (2016). Efficacy and Safety of FospropofolFD Compared to Propofol When Given During the Induction of General Anaesthesia: A Phase II, Multi-Centre, Randomized, Parallel-Group, Active-Controlled, Double-Blind, Double-Dummy Study. Basic Clin. Pharmacol. Toxicol. 119 (1), 93–100. doi:10.1111/bcpt.12552
Lohmer, L. L., Schippers, F., Petersen, K. U., Stoehr, T., and Schmith, V. D. (2020). Time-to-Event Modeling for Remimazolam for the Indication of Induction and Maintenance of General Anesthesia. J. Clin. Pharmacol. 60 (4), 505–514. doi:10.1002/jcph.1552
Luo, Z., Tu, H., Zhang, X., Wang, X., Ouyang, W., Wei, X., et al. (2022). Efficacy and Safety of HSK3486 for Anesthesia/Sedation in Patients Undergoing Fiberoptic Bronchoscopy: A Multicenter, Double-Blind, Propofol-Controlled, Randomized, Phase 3 Study. CNS Drugs 36 (3), 301–313. doi:10.1007/s40263-021-00890-1
McGrath, M., and Raines, D. E. (2018). Anesthetic Drug Discovery and Development: A Case Study of Novel Etomidate Analogs. Methods Enzymol. 603, 153–169. doi:10.1016/bs.mie.2018.01.026
Meyers, E. F., Krupin, T., Johnson, M., and Zink, H. (1978). Failure of Nondepolarizing Neuromuscular Blockers to Inhibit Succinylcholine-Induced Increased Intraocular Pressure, a Controlled Study. Anesthesiology 48 (2), 149–151. doi:10.1097/00000542-197802000-00013
Mickle, T. C., Guenther, S. M., Barrett, A. C., Roupe, K. A., Zhou, J., Dickerson, D., et al. (2018). Pharmacokinetics and Abuse Potential of Benzhydrocodone, a Novel Prodrug of Hydrocodone, After Intranasal Administration in Recreational Drug Users. Pain Med. 19 (12), 2438–2449. doi:10.1093/pm/pnx247
Morgan, M., Lumley, J., and Whitwam, J. G. (1975). Etomidate, a New Water-Soluble Non-barbiturate Intravenous Induction Agent. Lancet 1 (7913), 955–956. doi:10.1016/s0140-6736(75)92011-5
Mustafa, A. A., Rajan, R., Suarez, J. D., and Alzghari, S. K. (2018). A Review of the Opioid Analgesic Benzhydrocodone-Acetaminophen. Cureus 10 (6), e2844. doi:10.7759/cureus.2844
Najjar, A., and Karaman, R. (2019). The Prodrug Approach in the Era of Drug Design. Expert Opin. Drug Deliv. 16 (1), 1–5. doi:10.1080/17425247.2019.1553954
Najjar, A., Najjar, A., and Karaman, R. (2020). Newly Developed Prodrugs and Prodrugs in Development; an Insight of the Recent Years. Molecules 25 (4), 884. doi:10.3390/molecules25040884
Pambianco, D. J., Borkett, K. M., Riff, D. S., Winkle, P. J., Schwartz, H. I., Melson, T. I., et al. (2016). A Phase IIb Study Comparing the Safety and Efficacy of Remimazolam and Midazolam in Patients Undergoing Colonoscopy. Gastrointest. Endosc. 83 (5), 984–992. doi:10.1016/j.gie.2015.08.062
Patel, M., Taskar, K. S., and Zamek-Gliszczynski, M. J. (2016). Importance of Hepatic Transporters in Clinical Disposition of Drugs and Their Metabolites. J. Clin. Pharmacol. 56, S23–S39. doi:10.1002/jcph.671
Pejo, E., Ge, R., Banacos, N., Cotten, J. F., Husain, S. S., and Raines, D. E. (2012). Electroencephalographic Recovery, Hypnotic Emergence, and the Effects of Metabolite after Continuous Infusions of a Rapidly Metabolized Etomidate Analog in Rats. Anesthesiology 116 (5), 1057–1065. doi:10.1097/ALN.0b013e3182515403
Pejo, E., Liu, J., Lin, X., and Raines, D. E. (2016). Distinct Hypnotic Recoveries After Infusions of Methoxycarbonyl Etomidate and Cyclopropyl Methoxycarbonyl Metomidate: The Role of the Metabolite. Anesth. Analg. 122 (4), 1008–1014. doi:10.1213/ane.0000000000001146
Pirani, C. L., Valeri, A., D'Agati, V., and Appel, G. B. (1987). Renal Toxicity of Nonsteroidal Anti-inflammatory Drugs. Contrib. Nephrol. 55, 159–175. doi:10.1159/000413416
Pochapsky, T. C., and Pochapsky, S. S. (2019). What Your Crystal Structure Will Not Tell You about Enzyme Function. Acc. Chem. Res. 52 (5), 1409–1418. doi:10.1021/acs.accounts.9b00066
Rautio, J., Kumpulainen, H., Heimbach, T., Oliyai, R., Oh, D., Järvinen, T., et al. (2008). Prodrugs: Design and Clinical Applications. Nat. Rev. Drug Discov. 7 (3), 255–270. doi:10.1038/nrd2468
Rautio, J., Meanwell, N. A., Di, L., and Hageman, M. J. (2018). The Expanding Role of Prodrugs in Contemporary Drug Design and Development. Nat. Rev. Drug Discov. 17 (8), 559–587. doi:10.1038/nrd.2018.46
Rex, D. K., Bhandari, R., Desta, T., DeMicco, M. P., Schaeffer, C., Etzkorn, K., et al. (2018). A Phase III Study Evaluating the Efficacy and Safety of Remimazolam (CNS 7056) Compared with Placebo and Midazolam in Patients Undergoing Colonoscopy. Gastrointest. Endosc. 88 (3), 427. doi:10.1016/j.gie.2018.04.2351
Roberts, R. A. (2018). Understanding Drug Targets: No Such Thing as Bad News. Drug Discov. Today 23 (12), 1925–1928. doi:10.1016/j.drudis.2018.05.028
Sahinovic, M. M., Struys, M. M. R. F., and Absalom, A. R. (2018). Clinical Pharmacokinetics and Pharmacodynamics of Propofol. Clin. Pharmacokinet. 57 (12), 1539–1558. doi:10.1007/s40262-018-0672-3
Savarese, J. J., Belmont, M. R., Hashim, M. A., Mook, R. A., Boros, E. E., Samano, V., et al. (2004). Preclinical Pharmacology of GW280430A (AV430A) in the Rhesus Monkey and in the Cat: a Comparison with Mivacurium. Anesthesiology 100 (4), 835–845. doi:10.1097/00000542-200404000-00013
Savarese, J. J., McGilvra, J. D., Sunaga, H., Belmont, M. R., Van Ornum, S. G., Savard, P. M., et al. (2010). Rapid Chemical Antagonism of Neuromuscular Blockade by L-Cysteine Adduction to and Inactivation of the Olefinic (Double-bonded) Isoquinolinium Diester Compounds Gantacurium (AV430A), CW 002, and CW 011. Anesthesiology 113 (1), 58–73. doi:10.1097/ALN.0b013e3181dc1b5b
Schug, S. A., Parsons, B., Li, C., and Xia, F. (2017). The Safety Profile of Parecoxib for the Treatment of Postoperative Pain: a Pooled Analysis of 28 Randomized, Double-Blind, Placebo-Controlled Clinical Trials and a Review of over 10 Years of Postauthorization Data. J. Pain Res. 10, 2451–2459. doi:10.2147/jpr.S136052
Schüttler, J., Eisenried, A., Lerch, M., Fechner, J., Jeleazcov, C., and Ihmsen, H. (2020). Pharmacokinetics and Pharmacodynamics of Remimazolam (CNS 7056) after Continuous Infusion in Healthy Male Volunteers: Part I. Pharmacokinetics and Clinical Pharmacodynamics. Anesthesiology 132 (4), 636–651. doi:10.1097/aln.0000000000003103
Schywalsky, M., Ihmsen, H., Tzabazis, A., Fechner, J., Burak, E., Vornov, J., et al. (2003). Pharmacokinetics and Pharmacodynamics of the New Propofol Prodrug GPI 15715 in Rats. Eur. J. Anaesthesiol. 20 (3), 182–190. doi:10.1017/s0265021503000322
Scott, L. J., and Perry, C. M. (2005). Remifentanil: a Review of its Use during the Induction and Maintenance of General Anaesthesia. Drugs 65 (13), 1793–1823. doi:10.2165/00003495-200565130-00007
Shaffer, J. E., Quon, C. Y., and Gorczynski, R. J. (1988). Beta-adrenoreceptor Antagonist Potency and Pharmacodynamics of ASL-8123, the Primary Acid Metabolite of Esmolol. J. Cardiovasc Pharmacol. 11 (2), 187–192. doi:10.1097/00005344-198811020-00010
Sheng, X. Y., Liang, Y., Yang, X. Y., Li, L. E., Ye, X., Zhao, X., et al. (2020). Safety, Pharmacokinetic and Pharmacodynamic Properties of Single Ascending Dose and Continuous Infusion of Remimazolam Besylate in Healthy Chinese Volunteers. Eur. J. Clin. Pharmacol. 76 (3), 383–391. doi:10.1007/s00228-019-02800-3
Silver, S. (2016). Joint Meeting of Anesthetic and Analgesic Drug Products Advisory Committee and Drug Safety and Risk Management Advisory Committee. FDA Briefing Materials for Apadaz. US Food and Drug Administration. Available at: http://www.fda.gov/NewsEvents/Newsroom/PressAnnouncements/ucm491739.htm (Accessed June 15, 2016).
Sluga, M., Ummenhofer, W., Studer, W., Siegemund, M., and Marsch, S. C. (2005). Rocuronium versus Succinylcholine for Rapid Sequence Induction of Anesthesia and Endotracheal Intubation: a Prospective, Randomized Trial in Emergent Cases. Anesth. Analg. 101 (5), 1356–1361. doi:10.1213/01.Ane.0000180196.58567.Fe
Smith, G. F. (2022). Artificial Intelligence in Drug Safety and Metabolism. Methods Mol. Biol. 2390, 483–501. doi:10.1007/978-1-0716-1787-8_22
Smith, M. T., Eadie, M. J., and Brophy, T. O. (1981). The Pharmacokinetics of Midazolam in Man. Eur. J. Clin. Pharmacol. 19 (4), 271–278. doi:10.1007/bf00562804
Stafford, J. A., Pacofsky, G. J., Cox, R. F., Cowan, J. R., Dorsey, G. F., Gonzales, S. S., et al. (2002). Identification and Structure-Activity Studies of Novel Ultrashort-Acting Benzodiazepine Receptor Agonists. Bioorg Med. Chem. Lett. 12 (21), 3215–3218. doi:10.1016/s0960-894x(02)00512-7
Stöhr, T., Colin, P. J., Ossig, J., Pesic, M., Borkett, K., Winkle, P., et al. (2021). Pharmacokinetic Properties of Remimazolam in Subjects with Hepatic or Renal Impairment. Br. J. Anaesth. 127 (3), 415–423. doi:10.1016/j.bja.2021.05.027
Strom, B. L., Berlin, J. A., Kinman, J. L., Spitz, P. W., Hennessy, S., Feldman, H., et al. (1996). Parenteral Ketorolac and Risk of Gastrointestinal and Operative Site Bleeding. A Postmarketing Surveillance Study. Jama 275 (5), 376–382. doi:10.1001/jama.1996.03530290046036
Struys, M. M. R. F., Valk, B. I., Eleveld, D. J., Absalom, A. R., Meyer, P., Meier, S., et al. (2017). A Phase 1, Single-Center, Double-Blind, Placebo-Controlled Study in Healthy Subjects to Assess the Safety, Tolerability, Clinical Effects, and Pharmacokinetics-Pharmacodynamics of Intravenous Cyclopropyl-Methoxycarbonylmetomidate (ABP-700) after a Single Ascending Bolus Dose. Anesthesiology 127 (1), 20–35. doi:10.1097/aln.0000000000001662
Sunaga, H., Zhang, Y., Savarese, J. J., and Emala, C. W. (2010). Gantacurium and CW002 Do Not Potentiate Muscarinic Receptor-Mediated Airway Smooth Muscle Constriction in guinea Pigs. Anesthesiology 112 (4), 892–899. doi:10.1097/ALN.0b013e3181d32016
Talley, J. J., Bertenshaw, S. R., Brown, D. L., Carter, J. S., Graneto, M. J., Kellogg, M. S., et al. (2000). N-[[(5-methyl-3-phenylisoxazol-4-yl)-phenyl]sulfonyl]propanamide, Sodium Salt, Parecoxib Sodium: A Potent and Selective Inhibitor of COX-2 for Parenteral Administration. J. Med. Chem. 43 (9), 1661–1663. doi:10.1021/jm000069h
Thesleff, S., Dardel, O. V., and Holmberg, G. (1952). Succinylcholine Iodide; a New Muscular Relaxant. Br. J. Anaesth. 24 (4), 238–244. doi:10.1093/bja/24.4.238
Valk, B. I., Absalom, A. R., Meyer, P., Meier, S., den Daas, I., van Amsterdam, K., et al. (2018). Safety and Clinical Effect of i.V. Infusion of Cyclopropyl-Methoxycarbonyl Etomidate (ABP-700), a Soft Analogue of Etomidate, in Healthy Subjects. Br. J. Anaesth. 120 (6), 1401–1411. doi:10.1016/j.bja.2018.01.038
Valk, B. I., Eleveld, D. J., Meyer, P., Meier, S., den Daas, I., van Amsterdam, K., et al. (2021). Modeling the Effect of Excitation on Depth of Anesthesia Monitoring in γ-Aminobutyric Acid Type A Receptor Agonist ABP-700. Anesthesiology 134 (1), 35–51. doi:10.1097/aln.0000000000003590
Valk, B. I., McGrath, M., Lehoux, D., Zerler, B., Marota, J. J. A., and Raines, D. E. (2019). Toxicologic and Inhibitory Receptor Actions of the Etomidate Analog ABP-700 and its Metabolite CPM-Acid. Anesthesiology 131 (2), 287–304. doi:10.1097/aln.0000000000002758
Valk, B. I., and Struys, M. M. R. F. (2021). Etomidate and its Analogs: A Review of Pharmacokinetics and Pharmacodynamics. Clin. Pharmacokinet. 60 (10), 1253–1269. doi:10.1007/s40262-021-01038-6
Vallejo, R., Barkin, R. L., and Wang, V. C. (2011). Pharmacology of Opioids in the Treatment of Chronic Pain Syndromes. Pain Physician 14 (4), E343–E360. doi:10.36076/ppj.2011/14/e343
Van Hamme, M. J., Ghoneim, M. M., and Ambre, J. J. (1978). Pharmacokinetics of Etomidate, a New Intravenous Anesthetic. Anesthesiology 49 (4), 274–277. doi:10.1097/00000542-197810000-00010
Van Norman, G. A. (2016). Drugs, Devices, and the FDA: Part 1: An Overview of Approval Processes for Drugs. JACC Basic Transl. Sci. 1 (3), 170–179. doi:10.1016/j.jacbts.2016.03.002
Viby-Mogensen, J. (1980). Correlation of Succinylcholine Duration of Action with Plasma Cholinesterase Activity in Subjects with the Genotypically Normal Enzyme. Anesthesiology 53 (6), 517–520. doi:10.1097/00000542-198012000-00017
Wagner, R. L., and White, P. F. (1984). Etomidate Inhibits Adrenocortical Function in Surgical Patients. Anesthesiology 61 (6), 647–651. doi:10.1097/00000542-198412000-00003
Walton, J. D., and Farman, J. V. (1973). Suxamethonium Hyperkalaemia in Uraemic Neuropathy. Anaesthesia 28 (6), 666–668. doi:10.1111/j.1365-2044.1973.tb00552.x
Wandel, C., Böcker, R., Böhrer, H., Browne, A., Rügheimer, E., and Martin, E. (1994). Midazolam Is Metabolized by at Least Three Different Cytochrome P450 Enzymes. Br. J. Anaesth. 73 (5), 658–661. doi:10.1093/bja/73.5.658
Wang, D., Liu, W., Shen, Z., Jiang, L., Wang, J., Li, S., et al. (2019). Deep Learning Based Drug Metabolites Prediction. Front. Pharmacol. 10, 1586. doi:10.3389/fphar.2019.01586
Wanscher, M., Tønnesen, E., Hüttel, M., and Larsen, K. (1985). Etomidate Infusion and Adrenocortical Function. A Study in Elective Surgery. Acta Anaesthesiol. Scand. 29 (5), 483–485. doi:10.1111/j.1399-6576.1985.tb02238.x
Westmoreland, C. L., Hoke, J. F., Sebel, P. S., Hug, C. C., and Muir, K. T. (1993). Pharmacokinetics of Remifentanil (GI87084B) and its Major Metabolite (GI90291) in Patients Undergoing Elective Inpatient Surgery. Anesthesiology 79 (5), 893–903. doi:10.1097/00000542-199311000-00005
Wiemer, A. J., and Wiemer, D. F. (2015). Prodrugs of Phosphonates and Phosphates: Crossing the Membrane Barrier. Top. Curr. Chem. 360, 115–160. doi:10.1007/128_2014_561
Wilhelm, W., and Kreuer, S. (2008). The Place for Short-Acting Opioids: Special Emphasis on Remifentanil. Crit. Care 12, S5. doi:10.1186/cc6152
Wozniak, K. M., Vornov, J. J., Mistry, B. M., Wu, Y., Rais, R., and Slusher, B. S. (2015). Gastrointestinal Delivery of Propofol from Fospropofol: its Bioavailability and Activity in Rodents and Human Volunteers. J. Transl. Med. 13, 170. doi:10.1186/s12967-015-0526-9
Yamaguchi, S., Kaneko, M., and Narukawa, M. (2021). Approval Success Rates of Drug Candidates Based on Target, Action, Modality, Application, and Their Combinations. Clin. Transl. Sci. 14 (3), 1113–1122. doi:10.1111/cts.12980
Yavas, S., Lizdas, D., Gravenstein, N., and Lampotang, S. (2008). Interactive Web Simulation for Propofol and Fospropofol, a New Propofol Prodrug. Anesth. Analg. 106 (3), 880–883. doi:10.1213/ane.0b013e3181614fae
Keywords: anesthetics, prodrugs, soft drugs, pharmacokinetic strategies, drug metabolism
Citation: Deng C, Liu J and Zhang W (2022) Structural Modification in Anesthetic Drug Development for Prodrugs and Soft Drugs. Front. Pharmacol. 13:923353. doi: 10.3389/fphar.2022.923353
Received: 19 April 2022; Accepted: 01 June 2022;
Published: 01 July 2022.
Edited by:
Takeo Nakanishi, Takasaki University of Health and Welfare, JapanReviewed by:
Qidong You, China Pharmaceutical University, ChinaFlorence Williams, The University of Iowa, United States
Skylar J. Ferrara, Oregon Health and Science University, United States
Beatrijs Valk, University Medical Center Groningen, Netherlands
Copyright © 2022 Deng, Liu and Zhang. This is an open-access article distributed under the terms of the Creative Commons Attribution License (CC BY). The use, distribution or reproduction in other forums is permitted, provided the original author(s) and the copyright owner(s) are credited and that the original publication in this journal is cited, in accordance with accepted academic practice. No use, distribution or reproduction is permitted which does not comply with these terms.
*Correspondence: Wensheng Zhang, emhhbmdfd3NAc2N1LmVkdS5jbg==