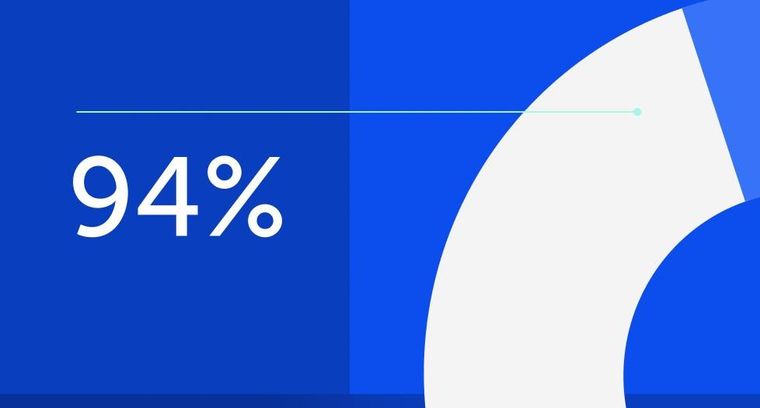
94% of researchers rate our articles as excellent or good
Learn more about the work of our research integrity team to safeguard the quality of each article we publish.
Find out more
ORIGINAL RESEARCH article
Front. Pharmacol., 16 June 2022
Sec. Experimental Pharmacology and Drug Discovery
Volume 13 - 2022 | https://doi.org/10.3389/fphar.2022.922032
This article is part of the Research TopicBone Targeting Nanoparticle Drug Delivery System in Bone Metabolism and Bone-related Tumor DiseasesView all 5 articles
In tissue engineering, hydrogel scaffolds allow various cells to be cultured and grown in vitro and then implanted to repair or replace the damaged areas. Here in this work, kartogenin (KGN), an effectively chondro-inductive non-protein bioactive drug molecule, was incorporated into a composite hydrogel comprising the positively charged chitosan (CS) and methacrylated gelatin (GelMA) polymers to fabricate appropriate microenvironments of bone marrow mesenchymal stem cells (BMSCs) for cartilage regeneration. Based on the combination of physical chain entanglements and chemical crosslinking effects, the resultant GelMA-CS@KGN composite hydrogels possessed favorable network pores and mechanical strength. In vitro cytotoxicity showed the excellent biocompatibility for facilitating the cell growth, adhesion, proliferation, and differentiation. The long-term sustainable KGN release from the hydrogel scaffolds in situ promoted the chondrogenic differentiation that can be employed as an alternative candidate for cartilage tissue regeneration.
The intrinsic properties like avascularity and low cellularity of articular cartilage have restricted its spontaneous regeneration for many years. The occurred cartilage defect always gradually gives rise to the degeneration of entire joint, thereby resulting in the severe osteoarthritis and appealing for invasive intervenes (Klein, 2009; Huey et al., 2012; Anetzberger et al., 2014; Makris et al., 2015; Li et al., 2017). To prevent the progression of aggressive lesion on the articular cartilage, surgical interventions are desperately required and updated. In this cause, several clinical managements are performed for cartilage repair in recent years, including the microfracture, mosaicplasty, and autologous chondrocyte implantation. Nevertheless, all these current clinical strategies are controversial because of the limited transplantation materials and dissatisfied long-term rehabilitation, thus necessitating the development of alternative designs and strategies (Detterline et al., 2005; Marlovits et al., 2006; Li et al., 2015; Mardones et al., 2015; Yan et al., 2020). Fortunately, the advent and development of tissue engineering and controlled drug release techniques provide the promising therapeutic for cartilage reconstruction, wherein the engineered scaffold is recognized as a crucial ingredient for cell activity, proliferation, and differentiation (Tang et al., 2020; Wang et al., 2020; Xu et al., 2020; Hua et al., 2021; Xu et al., 2021).
Nowadays, various tissue engineered scaffolds have been fabricated for the cartilage regeneration, including natural or synthetic polymer hydrogels with three-dimensional (3D) porous architectures by the chemical or physical cross-linker (Langer and Vacanti, 1993; Das et al., 2015; You et al., 2019; Dou et al., 2020; Zhu T. et al., 2022). Naturally derived hydrogels are particularly appealing because of their inherent biocompatibility, biodegradability, and biosafety (Burdick and Prestwich, 2011; Seliktar, 2012; Li et al., 2016; Stratton et al., 2016). As the representative materials, gelatin derived from the hydrolysis of collagen possessed the extremely similar component of ECM and cell-friendly essence, while the sole alkaline polysaccharide of chitosan (CS) displayed distinct biochemical characteristics to promote the cell growth, adhesion, proliferation, and differentiation. It was found that CS-mediated expression of chondrocytes phenotype was favorable for the tissue repair in the regenerative medicine. Despite the progress achieved in the development of hydrogels over the past few decades, the poor strength and low biological activity still limited their clinical applications. Therefore, pursuit of effective physical interaction with tailored architectures and functions and the appropriate incorporation of bioactive therapeutics into hydrogels to promote cartilage regeneration are promising strategies on facilitating the therapeutic efficacy of cartilage regeneration.
Kartogenin (KGN) as a non-protein small molecule chondrogenesis-inducing agent is reported to significantly promote chondrocyte differentiation capacity in a dose-dependent manner with excellent safety. Compared to the short half-life of biological protein and/or growth factors, KGN is capable of maintaining the biological activity and stability for exerting its long-term chondrogenesis on tissue regeneration (Johnson et al., 2012; Xu et al., 2015; Yuan et al., 2021). By means of this unique advantage, development of a suitable KGN delivery system to maintain the effective drug concentration at the site of cartilage defect is essentially significant for the cartilage tissue repair. Here in this work, we designed and prepared a KGN-encapsulated GelMA-CS composite hydrogel to support BMSCs for effective cartilage regeneration. GelMA-CS hydrogel possessed suitable network pores, good mechanical strength, and excellent biocompatibility that associated with the extracellular matrix for facilitating the cell viability, adhesion, growth, and proliferation. Owing to the electrostatic interactions between the oppositely charged groups, the therapeutic drug of carboxyl modified KGN could be efficiently encapsulated and absorbed within the amine-abundant hydrogel networks, demonstrating a locally sustained delivery and elucidating therapeutic efficiency on chondrogenic differentiation and cartilage regeneration. Thereafter, these therapeutic agent-loaded GelMA-CS@KGN hydrogel scaffolds were demonstrated to exhibit great clinical potential in cartilage regeneration in the future.
Gelatin (80–100 kDa, J & K), methacrylic anhydride (94%, J & K), and CS (degree of deacetylation >90%, viscosity: 45 mPa s) were obtained from Shandong Jinhu Co., Ltd. Lithium phenyl-2,4,6-trimethylbenzoylphosphinate (LAP, J & K) and kartogenin were obtained from KGN, Selleckchem Co., Ltd. The cholecystokinin octapeptide kit was obtained from Beyotime Co., Ltd. All other reagents were purchased from Sigma-Aldrich and used as received without further purification. Cells were supplied by China Infrastructure of Cell Line Resource.
GelMA was synthesized according to the previous literature (Zhu W. et al., 2022). The GelMA-CS composite hydrogel was simply prepared by adding CS solution (3 wt%) into the GelMA solution (20 wt%), containing the photoinitiator of LAP molecule. The KGN-loaded DN hydrogel was obtained by mixing the 100.0 μg/ml of KGN into the molds under UV irradiation at room temperature.
Scanning electron microscopy (SEM) was employed to observe the microstructure of hydrogels under at acceleration voltage of 5 kV on a JSM-6700F microscope. The freeze-dried samples were sputter coated with a thin layer of Pt for 90 s to make the samples conductive before testing.
Rheology was carried out on a rheometer (Thermo Haake Rheometer, United States). The hydrogels were spread on a parallel plate (25 mm) and sealed with silicone oil to prevent the solvent evaporation. A dynamic frequency scan in the range from 0.1 to 100 rad/s was used to record the storage and loss moduli G′ and G′′. The samples were measured with the stress amplitude and temperature of 0.1% and 25°C, respectively.
The compressive measurement of hydrogels was calculated with a universal material testing machine of Instron 3365 (Instron Co., United States). The samples were cut into cylinders (diameter = 15 mm and height = 7.5 mm), and compressive measurement was carried out at a rate of 1 mm/min.
The hydrogel was prepared in a container with the diameter of 15 mm and height of 7.5 mm, and 100.0 μg/ml of KGN drug was encapsulated inside the hydrogel, which was immersed into the PBS solutions, and then collected at the predetermined intervals using the UV–vis spectrophotometry with the absorption peak of 278.4 nm.
Cytotoxicity of hydrogel was measured using Cell Counting Kit-8 assay by contacting the hydrogel extracts. Bone marrow mesenchyml stem cells (MSCs) were seeded and incubated at 37°C in 5% CO2 for 12 h, and then hydrogel extracts were added into each well for further predetermined incubation time. After incubation for predetermined time, the cell culture medium was removed and 100 µl of fresh culture medium and 10 µl of CCK-8 were added to the 96 wells. Subsequently, cell viability was evaluated by comparing the absorbance of measured solutions using micro-plate reader at 450 nm. The cells cultured in DMEM medium containing 10% FBS were used as controls. The final results were assumed to be the means of triplicate. Cell viability (%) was calculated by the following equation:
Cell live and dead viability was determined by live/dead viability according to the manufacturer’s instruction. After cultivating for prescribed time intervals, BMSCs were washed by cold buffer solution for three times and immersed with 4% paraformaldehyde solution for 30 min at room temperature to fix the cell configuration. Then, fluorescent propidium iodide (PI, red) stain and fluorescent (SYTO 9, green) stain were added to the reaction mixture and incubated at room temperature for 20 min. Finally, these BMSCs were washed by buffer for three times and directly transferred to glass culture dish for further confocal laser scanning microscopy (CLSM) observation.
ELISA was used to quantitatively evaluate the extracellular protein produced by the BMSCs in hydrogels. The COL-2 content was tested by an ELISA kit following the manufacturer’s instructions. The DNA content was detected using a fluorometric assay. Aliquots of sample digestion were stained with 200 μl of Hoechst 33258 solution at 37°C for 20 min, followed by the measurement with an excitation wavelength of 360 nm and an emission wavelength of 460 nm. The DNA content was normalized with a standard curve of calf thymus DNA. In addition, the content of proteoglycan was detected from the GAG content by the 1, 9-dimethyl methylene blue dye-binding assay. Total GAG was normalized to the total DNA content by adding 20 μl of sample into 200 μl of DMMB solution with the measurement absorbance at 525 nm.
The TRIzol reagent was used to extract the total RNA when the cell-hydrogel composites were incubated for 7 and 14 days. The RNA was reversely transcribed into cDNA by using MMLV reverse kit, and RT-PCR was conducted by the real-time PCR system with SYBR Green PCR Master Mix.
All statistical results were expressed as mean ± standard deviation of more than three times for each experiment. The differences among groups were calculated by one-way analysis of variance (ANOVA) after testing for homogeneity of variances, p < 0.05 was considered statistically significant.
The preparation route of GelMA-CS@KGN composite hydrogel is observed in Figure 1. The UV irradiation could make the quick formation of the crosslinking gelatin network at the absence of the photoinitiator. However, its poor mechanical property required the incorporation of other polymers or components. Therefore, a non-covalent robust CS network was incorporated by the physically electrostatic interactions and chain entanglement effects between these two natural polymeric chains to enhance the mechanical strength. During this process, the therapeutic KGN agents could be in situ encapsulated by the electrostatic interactions among the -NH2 groups of GelMA and CS and -COOH group of KGN. Under this circumstance, the KGN molecules could be slowly released from the composite hydrogels for a long time.
FIGURE 1. Schematic illustrations of synthesis pathways of (A) GelMA macromolecule and (B) KGN-loaded GelMA-CS@KGN composite hydrogel.
As shown in Figure 2A, the occurrence of typical methacrylate peaks at 5.2–5.7 ppm testified the successful chemical modification to obtain the GelMA by the anhydride reaction. Figure 2B showed the similar morphology and the size of inner porous for these GelMA-CS and GelMA-CS@KGN composite hydrogels, which could satisfy the requirement of the pore size that allowed the cell infiltration, nutrition transfer, metabolic waste discharge, and other substance exchange. Taking consideration of the unchanged network architectures, we hypothesized the introduction of electrostatic interaction can significantly improve the drug release kinetics and encapsulated KGN drugs could also be enabled for the sustained release from the hydrogels, thereby conducting a slow release to maintain the sufficient drug concentration in situ for a long time to promote cartilage tissue regeneration.
FIGURE 2. (A) 1H NMR spectrum of GelMA. (B) SEM images of GelMA-CS and GelMA-CS@KGN hydrogels. (C) Rheology of the GelMA-CS. (D) Compressive curves of GelMA-CS hydrogels with or without KGN laden.
Mechanical property is another important aspect for assessing the tissue engineered scaffold for cartilage repair. It is better for the mechanical parameters to be able to match the native cartilage. Therefore, we first carried out the rheological experiments in Figure 2C. The storage modulus (G′) surpassing the loss modulus (G″) within the frequency range revealed the hydrogel formation regardless of the addition of KGN molecules. The compressive strength of hydrogel scaffold is also assessed in Figure 2D. Both the GelMA-CS and GelMA-CS@KGN composite hydrogels exhibited the similar compressive strength to that of native cartilage, which was attributed to the rigid CS backbone and the dense chain entanglement network within the hydrogels. It was mentioned that the higher strength of GelMA-CS@KGN than GelMA-CS may ascribe to the ordered and evenly distributed drugs as well as the optimized electrostatic interactions among the polymeric network and small molecules, which also reflected the KGN loading did not affect the microarchitecture and mechanical performances of composite hydrogel scaffolds.
Although many traditional regulatory factors (e.g., TGF-β) can promote the cartilage repair, the short half-life and instability are the fatal defect because they always require at least 3 months achieving the reconstruction of damaged cartilage (Robson et al., 1995; Yang et al., 2001; Rey-Rico et al., 2015). In comparison, KGN is stable at room temperature and can effectively induce BMSCs into chondrocytes for a long term. The obvious superiorities in comparison to protein growth factors made KGN to be a potential chondrogenesis promoter in cartilage regeneration (Kang et al., 2017; Wang et al., 2019; Dehghan-Baniani et al., 2022). Moreover, several studies demonstrated that KGN possessed excellent biocompatibility without the toxicity on many cells even with a high concentration of 100 μM (Johnson et al., 2012). In this study, this concentration of KGN was selected and the release profile from the GelMA-CS@KGN hydrogel was detected in PBS solutions using UV−vis spectrophotometry with the absorption peak of 278.4 nm. In addition, because the absorption peak of KGN was at 278.4 nm, UV irradiation (365 nm) can not affect the KGN release for its therapeutic efficiency. A constant and sustained release of KGN is observed in Figure 3, which exhibited the cumulative release rate of KGN reached nearly 80% up to 14 days. It was mentioned that although a burst KGN release with 25% of cumulative release was displayed at the initial stage, this quick release could satisfy the high concentration demand in the early stage of defect areas for immunoregulation. This result revealed that the hydrogel scaffold got a well control release of KGN to simultaneously satisfy the initial high drug concentration demand and following long-term sustained release.
The polysaccharide CS and GelMA possessed excellent biocompatibility and favorable advantages in terms of cell attachment, growth, proliferation, and differentiation. Because KGN had no toxicity on cells even at a concentration of 100 μM, this GelMA-CS@KGN composite hydrogel was demonstrated to be an ideal engineered scaffold, which was testified using the live/dead assays. The cytotoxicity assay exhibited the gratifying result with green fluorescence after culturing GelMA-CS@KGN hydrogels into the BMSCs for 24 h in Figure 4A. Instead of cell apoptosis, extended incubation time led to the growing cell number with more than 120% of cell viability at day 2 and 3 (Figure 4B). Therefore, we further investigate if this hydrogel was able to support the cell growth and proliferation, a long-term proliferation profile of CCK-8 assay was carried out after treatment with GelMA-CS@KGN hydrogel for 6 days. Figure 4C showed that these composite hydrogels significantly promoted the cell growth and proliferation without any toxicity, and the proliferation rate slightly increased in the initial 3 days and rapidly arouse in the late 3 days, further revealing the excellent cytocompatibility of these cartilage scaffolds even if there were some KNG drugs leaking out from the GelMA-CS@KGN hydrogel. In addition, the degradation behavior of this composite hydrogel is similar to the traditional naturally derived hydrogel scaffolds, and their degradation mechanism can be attributed to the hydrolysis or enzymatic hydrolysis effects, which was affected by the degree of methyl acrylamide, the molecular weights of gelatin and CS, concentration, and the enzymatic degradation (Lin et al., 2021).
FIGURE 4. Cytotoxicity of GelMA-CS@KGN hydrogel in vitro. (A) Live/dead staining of BMSCs. Cells in green manifest living ones while cells in red manifest dead ones. (B) Cell viability. (C) Cell proliferation of GelMA-CS@KGN hydrogel after cultivating for various time (n = 3, **p < 0.01).
Ideal cartilage repair materials should enhance the chondrogenic differentiation of BMSCs. To reveal the effect of hydrogel scaffolds on the chondrogenic differentiation in vitro, the expression of cartilage-specific genes like ACAN, COL-2, PRG4, and SOX-9 are investigated in Figure 5. Compared with the traditional GelMA hydrogel, real-time PCR showed that GelMA-CS and GelMA-CS@KGN (100 μg/ml) hydrogel scaffolds exhibited higher expression of cartilage-specific genes. It was mentioned that the improved mechanical property of GelMA-CS may contribute to the favorable cartilage differentiation ability in vitro. In addition, on account of the sustainable release of KGN drug, the mRNA expression levels of cartilaginous markers of ACAN, COL-2, PRG4, and SOX-9 were significantly upregulated in the GelMA-CS@KGN (100 μg/ml) group after incubation for 7 and 14 days (n = 3, p < 0.05), suggesting that the KGN agents could effectively promote chondrogenic differentiation of the BMSCs in vitro for a long term.
FIGURE 5. RT-PCR analysis. The mRNA expression of cartilage-specific marker genes of (A) ACAN, (B) COL-2, (C) PRG4, and (D) SOX-9 for (A) GelMA, (B) GelMA-CS, and (C) GelMA-CS@KGN (100 μg/ml) scaffolds determined by RT-PCR tests on days 7 and 14 (n = 3, *p < 0.05, and **p < 0.01).
ELISA analysis was used to quantitatively evaluate the extracellular protein produced by the MSCs in hydrogels in Figure 6. Along with the extension of time, DNA contents were continuously increased for these three groups. Wherein, the average DNA content in GelMA-CS@KGN was higher than the other groups within 2 weeks. Similarly, GAG and COL-2 contents in GelMA-CS@KGN hydrogels also increased over time, and reached their highest levels after 14 days that was more than other groups at any time points. To the best of our knowledge, GAG was an important ECM component in articular cartilage, and its production was directly related to the level of chondrogenesis. Thereafter, these results suggested that the KGN release from the hydrogel scaffold could significantly promote the chondrogenic differentiation of BMSCs.
FIGURE 6. GAG production, COL-2 proteins and DNA contents in hydrogels after culturing for 7 and 14 days (A) GAG production, (B) COL-2 deposition, and (C) DNA contents in various hydrogels scaffolds of (A) GelMA, (B) GelMA-CS, and (C) GelMA-CS@KGN by the BMSCs on 7 and 14 days (n = 3, **p < 0.01).
In summary, we developed a KGN-loaded GelMA-CS composite hydrogel with the long-term drug release. On account of the good compatibility, suitable porous architectures and favorable mechanical strength, this GelMA-CS@KGN hydrogel scaffold could promote the cell activity, growth, proliferation, and differentiation to favor the cartilage regeneration. Cytotoxicity assay indicated the good cell proliferation, while the sustained KGN release could significantly promote the cartilaginous differentiation in situ. The RT-PCR test also verified that the hydrogel scaffolds could enhance the expression of cartilage-specific genes as well as matrix deposition of the increased level of GAG, capable of facilitating cartilage tissue regeneration. Importantly, this composite hydrogel system could overcome the limitations of protein growth factor-based scaffolds and reach a stable chondrogenesis-inducing status for a long repair time. We believe this study demonstrated it may serve as an appropriate strategy for cartilage defect repair by locally delivering the stable chondrogenesis KGN promoter, which will exhibit great potential for clinical cartilage regeneration.
The raw data supporting the conclusion of this article will be made available by the authors, without undue reservation.
WZ, RC, and XX contributed equally to this article. GT and XY initiated the project. WZ, RC, XX, LZ, and YL searched the data base, wrote, and finalized the manuscript. LZ and YL made important suggestions and helped revising the article. All authors reviewed and commented on the manuscript.
This work was supported by the National Science Foundation for Postdoctoral Scientists of China (2020M683733) and Shanghai Changning Committee of Science and Technology of China (CNKW2020Y01).
The authors declare that the research was conducted in the absence of any commercial or financial relationships that could be construed as a potential conflict of interest.
All claims expressed in this article are solely those of the authors and do not necessarily represent those of their affiliated organizations, or those of the publisher, the editors, and the reviewers. Any product that may be evaluated in this article, or claim that may be made by its manufacturer, is not guaranteed or endorsed by the publisher.
Anetzberger, H., Mayer, A., Glaser, C., Lorenz, S., Birkenmaier, C., and Müller-Gerbl, M. (2014). Meniscectomy Leads to Early Changes in the Mineralization Distribution of Subchondral Bone Plate. Knee Surg. Sports Traumatol. Arthrosc. 22, 112–119. doi:10.1007/s00167-012-2297-7
Burdick, J. A., and Prestwich, G. D. (2011). Hyaluronic Acid Hydrogels for Biomedical Applications. Adv. Mat. 23, H41–H56. doi:10.1002/adma.201003963
Das, S., Pati, F., Choi, Y. J., Rijal, G., Shim, J. H., Kim, S. W., et al. (2015). Bioprintable, Cell-Laden Silk Fibroin-Gelatin Hydrogel Supporting Multilineage Differentiation of Stem Cells for Fabrication of Three-Dimensional Tissue Constructs. Acta Biomater. 11, 233–246. doi:10.1016/j.actbio.2014.09.023
Dehghan-Baniani, D., Mehrjou, B., Wang, D., Bagheri, R., Solouk, A., Chu, P. K., et al. (2022). A Dual Functional Chondro-Inductive Chitosan Thermogel with High Shear Modulus and Sustained Drug Release for Cartilage Tissue Engineering. Int. J. Biol. Macromol. 205, 638–650. doi:10.1016/j.ijbiomac.2022.02.115
Detterline, A. J., Goldberg, S., Bach, B. R., and Cole, B. J. (2005). Treatment Options for Articular Cartilage Defects of the Knee. Orthop. Nurs. 24, 361–368. doi:10.1097/00006416-200509000-00012
Dou, X., Cao, Q., Sun, F., Wang, Y., Wang, H., Shen, H., et al. (2020). Synergistic Control of Dual Cross-Linking Strategy toward Tailor-Made Hydrogels. Sci. China Chem. 63, 1793–1798. doi:10.1007/s11426-020-9821-2
Hua, Y. J., Xia, H. T., Jia, L. T., Zhao, J. Z., Zhao, D. D., Yan, X. Y., et al. (2021). Ultrafast, Tough, and Adhesive Hydrogel Based on Hybrid Photocrosslinking for Articular Cartilage Repair in Water-Filled Arthroscopy. Sci. Adv. 7, eabg0628. doi:10.1126/sciadv.abg0628
Huey, D. J., Hu, J. C., and Athanasiou, K. A. (2012). Unlike Bone, Cartilage Regeneration Remains Elusive. Science 338, 917–921. doi:10.1126/science.1222454
Johnson, K., Zhu, S., Tremblay, M. S., Payette, J. N., Wang, J., Bouchez, L. C., et al. (2012). A Stem Cell-Based Approach to Cartilage Repair. Science 336, 717–721. doi:10.1126/science.1215157
Kang, M.-L., Jeong, S.-Y., and Im, G.-I. (2017). Hyaluronic Acid Hydrogel Functionalized with Self-Assembled Micelles of Amphiphilic Pegylated Kartogenin for the Treatment of Osteoarthritis. Tissue Eng. Part A 23, 630–639. doi:10.1089/ten.tea.2016.0524
Klein, J. (2009). Chemistry. Repair or Replacement-Aa Joint Perspective. Science 323, 47–48. doi:10.1126/science.1166753
Langer, R., and Vacanti, J. P. (1993). Tissue Engineering. Science 260, 920–926. doi:10.1126/science.8493529
Li, X., Ding, J., Wang, J., Zhuang, X., and Chen, X. (2015). Biomimetic Biphasic Scaffolds for Osteochondral Defect Repair. Regen. Biomater. 2, 221–228. doi:10.1093/rb/rbv015
Li, X., Ding, J., Zhang, Z., Yang, M., Yu, J., Wang, J., et al. (2016). Kartogenin-incorporated Thermogel Supports Stem Cells for Significant Cartilage Regeneration. ACS Appl. Mat. Interfaces 8, 5148–5159. doi:10.1021/acsami.5b12212
Li, Y., Xiao, Y., and Liu, C. (2017). The Horizon of Materiobiology: A Perspective on Material-Guided Cell Behaviors and Tissue Engineering. Chem. Rev. 117, 4376–4421. doi:10.1021/acs.chemrev.6b00654
Lin, Y. T., Hsu, T. T., Liu, Y. W., Kao, C. T., and Huang, T. H. (2021). Bidirectional Differentiation of Human-Derived Stem Cells Induced by Biomimetic Calcium Silicate-Reinforced Gelatin Methacrylate Bioink for Odontogenic Regeneration. Biomedicines 9, 929. doi:10.3390/biomedicines9080929
Makris, E. A., Gomoll, A. H., Malizos, K. N., Hu, J. C., and Athanasiou, K. A. (2015). Repair and Tissue Engineering Techniques for Articular Cartilage. Nat. Rev. Rheumatol. 11, 21–34. doi:10.1038/nrrheum.2014.157
Mardones, R., Jofré, C. M., and Minguell, J. J. (2015). Cell Therapy and Tissue Engineering Approaches for Cartilage Repair And/or Regeneration. Int. J. Stem Cells 8, 48–53. doi:10.15283/ijsc.2015.8.1.48
Marlovits, S., Zeller, P., Singer, P., Resinger, C., and Vécsei, V. (2006). Cartilage Repair: Generations of Autologous Chondrocyte Transplantation. Eur. J. Radiol. 57, 24–31. doi:10.1016/j.ejrad.2005.08.009
Rey-Rico, A., Venkatesan, J. K., Sohier, J., Moroni, L., Cucchiarini, M., and Madry, H. (2015). Adapted Chondrogenic Differentiation of Human Mesenchymal Stem Cells via Controlled Release of TGF-Β1 from Poly(ethylene Oxide)-Terephtalate/poly(butylene Terepthalate) Multiblock Scaffolds. J. Biomed. Mater Res. A 103, 371–383. doi:10.1002/jbm.a.35181
Robson, M. C., Phillip, L. G., Cooper, D. M., Lyle, W. G., Robson, L. E., Odom, L., et al. (1995). Safety and Effect of Transforming Growth Factor-Beta(2) for Treatment of Venous Stasis Ulcers. Wound Repair Regen. 3, 157–167. doi:10.1046/j.1524-475X.1995.30207.x
Seliktar, D. (2012). Designing Cell-Compatible Hydrogels for Biomedical Applications. Science 336, 1124–1128. doi:10.1126/science.1214804
Stratton, S., Shelke, N. B., Hoshino, K., Rudraiah, S., and Kumbar, S. G. (2016). Bioactive Polymeric Scaffolds for Tissue Engineering. Bioact. Mat. 1, 93–108. doi:10.1016/j.bioactmat.2016.11.001
Tang, G., Zhou, B., Li, F., Wang, W., Liu, Y., Wang, X., et al. (2020). Advances of Naturally Derived and Synthetic Hydrogels for Intervertebral Disk Regeneration. Front. Bioeng. Biotechnol. 8, 745. doi:10.3389/fbioe.2020.00745
Wang, S. J., Qin, J. Z., Zhang, T. E., and Xia, C. (2019). Intra-articular Injection of Kartogenin-Incorporated Thermogel Enhancing Osteoarthritis Treatment. Front. Chem. 7, 677. doi:10.3389/fchem.2019.00677
Wang, X., Yang, Y., Shi, Y., and Jia, F. (2020). Editorial: Smart Hydrogels in Tissue Engineering and Regenerative Medicine. Front. Chem. 8, 245. doi:10.3389/fchem.2020.00245
Xu, B., Ye, J., Yuan, F. Z., Zhang, J. Y., Chen, Y. R., Fan, B. S., et al. (2020). Advances of Stem Cell-Laden Hydrogels with Biomimetic Microenvironment for Osteochondral Repair. Front. Bioeng. Biotechnol. 8, 247. doi:10.3389/fbioe.2020.00247
Xu, X., Shi, D., Shen, Y., Xu, Z., Dai, J., Chen, D., et al. (2015). Full-thickness Cartilage Defects Are Repaired via a Microfracture Technique and Intraarticular Injection of the Small-Molecule Compound Kartogenin. Arthritis Res. Ther. 17, 20. doi:10.1186/s13075-015-0537-1
Xu, Z., Fan, C., Zhang, Q., Liu, Y., Cui, C., Liu, B., et al. (2021). A Self‐Thickening and Self‐Strengthening Strategy for 3D Printing High‐Strength and Antiswelling Supramolecular Polymer Hydrogels as Meniscus Substitutes. Adv. Funct. Mat. 31, 2100462. doi:10.1002/adfm.202100462
Yan, X., Chen, Y. R., Song, Y. F., Ye, J., Yang, M., Xu, B. B., et al. (2020). Advances in the Application of Supramolecular Hydrogels for Stem Cell Delivery and Cartilage Tissue Engineering. Front. Bioeng. Biotechnol. 8, 847. doi:10.3389/fbioe.2020.00847
Yang, X., Chen, L., Xu, X., Li, C., Huang, C., and Deng, C. X. (2001). TGF-beta/Smad3 Signals Repress Chondrocyte Hypertrophic Differentiation and Are Required for Maintaining Articular Cartilage. J. Cell Biol. 153, 35–46. doi:10.1083/jcb.153.1.35
You, F., Chen, X., Cooper, D. M. L., Chang, T., and Eames, B. F. (2019). Homogeneous Hydroxyapatite/alginate Composite Hydrogel Promotes Calcified Cartilage Matrix Deposition with Potential for Three-Dimensional Bioprinting. Biofabrication 11, 015015. doi:10.1088/1758-5090/aaf44a
Yuan, F. Z., Wang, H. F., Guan, J., Fu, J. N., Yang, M., Zhang, J. Y., et al. (2021). Fabrication of Injectable Chitosan-Chondroitin Sulfate Hydrogel Embedding Kartogenin-Loaded Microspheres as an Ultrasound-Triggered Drug Delivery System for Cartilage Tissue Engineering. Pharmaceutics 13, 1487. doi:10.3390/pharmaceutics13091487
Zhu, T., Wang, H., Jing, Z., Fan, D., Liu, Z., Wang, X., et al. (2022). High Efficacy of Tetra-PEG Hydrogel Sealants for Sutureless Dural Closure. Bioact. Mater. 8, 12–19. doi:10.1016/j.bioactmat.2021.06.022
Keywords: BMSCs, cartilage regeneration, hydrogel scaffold, KGN, long-term release
Citation: Zhang W, Chen R, Xu X, Zhu L, Liu Y, Yu X and Tang G (2022) Construction of Biocompatible Hydrogel Scaffolds With a Long-Term Drug Release for Facilitating Cartilage Repair. Front. Pharmacol. 13:922032. doi: 10.3389/fphar.2022.922032
Received: 17 April 2022; Accepted: 06 May 2022;
Published: 16 June 2022.
Edited by:
Xing Wang, Institute of Chemistry (CAS), ChinaReviewed by:
Daoyang Fan, Peking University Third Hospital, ChinaCopyright © 2022 Zhang, Chen, Xu, Zhu, Liu, Yu and Tang. This is an open-access article distributed under the terms of the Creative Commons Attribution License (CC BY). The use, distribution or reproduction in other forums is permitted, provided the original author(s) and the copyright owner(s) are credited and that the original publication in this journal is cited, in accordance with accepted academic practice. No use, distribution or reproduction is permitted which does not comply with these terms.
*Correspondence: GuoKe Tang, R0tUYW5nQHNoc211LmVkdS5jbg==; XiaoJie Yu, eXUzNzAyODJAc2luYS5jb20=
†These authors have contributed equally to this work
Disclaimer: All claims expressed in this article are solely those of the authors and do not necessarily represent those of their affiliated organizations, or those of the publisher, the editors and the reviewers. Any product that may be evaluated in this article or claim that may be made by its manufacturer is not guaranteed or endorsed by the publisher.
Research integrity at Frontiers
Learn more about the work of our research integrity team to safeguard the quality of each article we publish.