- 1Departamento de Farmacologia, Instituto de Ciências Biológicas, Universidade Federal de Goiás, Goiânia, Brazil
- 2Campus Realengo, Instituto Federal do Rio de Janeiro, Rio de Janeiro, Brazil
- 3Laboratório de Bioquímica Celular, Universidade Federal de São João del Rei, Campus Centro-Oeste Dona Lindu, São Paulo, Brazil
- 4Laboratório de Farmacologia Bioquímica e Molecular, Instituto de Ciências Biomédicas, Universidade Federal do Rio de Janeiro, Rio de Janeiro, Brazil
- 5Departamento de Farmacologia, Instituto de Ciências Biomédicas, Universidade de São Paulo, São Paulo, Brazil
The Na+/K+-ATPase is an integral membrane ion pump, essential to maintaining osmotic balance in cells in the presence of cardiotonic steroids; more specifically, ouabain can be an endogenous modulator of the Na+/K+-ATPase. Here, we conducted a systematic review of the in vitro effects of cardiotonic steroids on Ca2+ in the brain of rats and mice. Methods: The review was carried out using the PubMed, Virtual Health Library, and EMBASE databases (between 12 June 2020 and 30 June 2020) and followed the guidelines described in the Preferred Reporting Items for Systematic Reviews and Meta-analyses (PRISMA). Results: in total, 829 references were identified in the electronic databases; however, only 20 articles were considered, on the basis of the inclusion criteria. The studies demonstrated the effects of ouabain on Ca2+ signaling in synaptosomes, brain slices, and cultures of rat and mouse cells. In addition to the well-known cytotoxic effects of high doses of ouabain, resulting from indirect stimulation of the reverse mode of the Na+/Ca2+ exchanger and increased intracellular Ca2+, other effects have been reported. Ouabain-mediated Ca2+ signaling was able to act increasing cholinergic, noradrenergic and glutamatergic neurotransmission. Furthermore, ouabain significantly increased intracellular signaling molecules such as InsPs, IP3 and cAMP. Moreover treatment with low doses of ouabain stimulated myelin basic protein synthesis. Ouabain-induced intracellular Ca2+ increase may promote the activation of important cell signaling pathways involved in cellular homeostasis and function. Thus, the study of the application of ouabain in low doses being promising for application in neurological diseases.
Systematic Review Registration: https://www.crd.york.ac.uk/prospero/display_record.php?ID=CRD42020204498, identifier CRD42020204498.
Introduction
Na+/K+-ATPase, also known as Na+-K+ pump, is a plasma membrane transporter essential for cellular homeostasis. It is responsible for the active movement of Na+ and K+ against their electrochemical gradients at the expenses of ATP hydrolysis, and supports the maintenance of the cellular osmotic balance, membrane potential and the secondary active transport of substrates and neurotransmitters (Blanco, 2005). Particularly in the brain, which is composed of highly specialized cells (i.e., neurons and glial cells), Na+/K+-ATPase is involved in neuronal excitability and astrocyte buffering of extracellular K+ ions during neuron action potential, in electrolyte balance of the cerebrospinal fluid, and in the secondary transport of molecules across the membrane, playing a fundamental role in the function of the central nervous system (Larsen et al., 2016), (Kinoshita et al., 2020). Therefore, the regulation of Na+/K+-ATPase function largely affects cell and system physiology.
This enzyme is composed of three subunits, and two of them are indispensable for enzyme activity (Figure 1). The amino acid sequence of the α subunit, also called the catalytic subunit, comprises more than 1010 residues (around 110 kDa) with 10 transmembrane domains. This subunit harbors the binding sites for ions (Na+, K+, Mg2+), ATP, and selective ligands collectively known as cardiotonic steroids, as well as for several other regulators (Blanco, 2005), (Blanco and Mercer, 1998) . All isoforms are encoded by different genes and present a high degree of homology (Clausen et al., 2017). An interesting feature is that Na+/K+-ATPase isoforms are expressed in a cell-/tissue-specific fashion (Blanco, 2005), (Cereijido et al., 2012), (Geering, 2008): the α1 isoform is ubiquitously expressed in mammalian tissues and, in rodents, it is 100–1000-fold less sensitive to cardiotonic steroids. In contrast, it has been shown not to be the case for the bufadienolide marinobufagenin (Fedorova and Bagrov, 1997), (Fedorova et al., 2001), although our group reported that it behaves similar to any known cardiotonic steroid (Godinho et al., 2017), (Carvalho et al., 2019); the α2 isoform is found in striated and smooth muscle, as well as in both astrocytes and neurons in the central nervous system, and adipose tissue; the α3 isoform is basically found in neurons but not in astrocytes and should be considered a neuronal marker (Dobretsov and Stimers, 2005); the α4 isoform is specifically found in the midpiece of sperm (Blanco, 2005). The β subunit is a type II glycoprotein of around 300 amino acids, that is, fundamental for a normal pumping activity. Enzyme kinetics modulation, Na+/K+-ATPase plasma membrane delivery and assembly, as well as cell adhesion and polarity, are characteristics of this subunit. The third subunit, γ, is a type I protein from the FXYD family (FXYD2) and it comprises around 65 amino acids. FXYD2 is not required for enzymatic activity but regulates Na+/K+-ATPase affinity for cations (Geering, 2008).
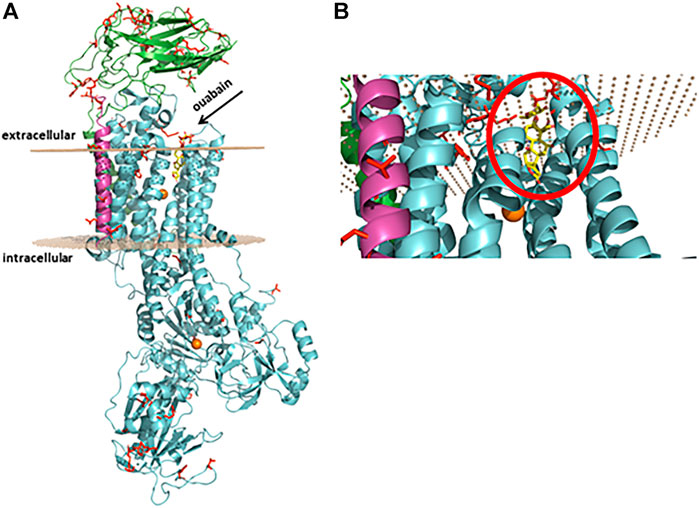
FIGURE 1. Protein representation of rat Na+/K+-ATPase α1β1γ isoform subunits. (A) In green, α1 subunit, in cyan, β1 subunit, and in pink, γ (FXYD2) subunit. The residues displaying the substitutions present in the rat compared to the human Na+/K+-ATPase protein. In orange, magnesium ions. The gray dots represent the Na+/K+-ATPase position at the plasma membrane, and the arrow points to the pocket where ouabain (in yellow) interacts. (B) Magnification of the binding pocket with ouabain (inside the red circle) seen from the bottom. The protein was constructed by homology using as reference the human crystallographic structure (pdb id:4RET) using the Swiss-model webserver, and for the representation the software PyMOL 7 was used.
Ouabain is a natural ligand of Na+/K+-ATPase and belongs to the cardiotonic steroid class of compounds, i.e., the cardenolide family, originally identified in the African plants Strophantus gratus (Ouabain, 1932) and Acokanthera ouabaio (also called wabajo or schimperi) (Kupicha, 1982). Its inhibitory capacity was reported in 1953 by Schartzmann (Schatzmamn, 1953); this discovery paved the way for research on the mechanistic pathways of other cardiotonic steroids, such as digoxin, clinically used for decades to treat heart failure (Ziff and Kotecha, 2016). Ouabain selectively binds to Na+/K+-ATPase and inhibits its pump activity, increasing intracellular Na+ and Ca2+ concentrations ([Na+]i and [Ca2+]i, respectively), the latter by inducing a lower/reverse activity of the Na+/Ca2+ exchanger that colocalizes with the Na+/K+-ATPase (Larsen et al., 2016), (Kinoshita et al., 2020). In the heart, the indirect elevation of sarcoplasmic reticulum Ca2+ levels lead to the cardiac inotropism (Akera and Brody, 1977), (Lingrel, 2009). Higher concentrations of ouabain are known to evoke cardiotoxic effects. In other organs, such as the brain, the effects are more complex. Concentrations that induce bulk inhibition of Na+/K+-ATPase generate neurotoxic effects (Lees et al., 1990), (Veldhuis et al., 2003). In contrast, ouabain at a low concentration of 10 nm increases the expression of the brain-derived neurotrophic factor (BDNF) mRNA in cerebellar cell culture (de Sá Lima et al., 2013) or after intrahippocampal injection (Tata et al., 2014) and activates the Wnt/β-catenin signaling pathway culminating with the increase of CREB/BDNF and NF-κB levels (Kawamoto et al., 2012a), indicating that the balance between ouabain-induced neuroprotective and neurotoxic effects is concentration-dependent.
Several mechanisms are involved in cellular Ca2+ homeostasis. From Ca2+-binding proteins inside cells to proteins that allow Ca2+ fluxes across biological membranes, Ca2+ concentrations are strictly controlled in cytoplasm and organelles like mitochondria, Golgi apparatus, endoplasmic reticulum and nucleus (Brini et al., 2014). Ca2+ Channels (Koh et al., 2017), the high Ca2+ affinity but low capacity plasma membrane Ca2+-ATPases and the low Ca2+ affinity but high capacity Na+/Ca2+ exchanger (Blaustein et al., 2002), sarco/endoplasmic reticulum Ca2+-ATPases (Valadares et al., 2021), and ryanodine and inositol 1,4,5-triphosphate (IP3) receptors (McGarry and Williams, 1993), (Panizza et al., 2019) are important for Ca2+ handling and secondary targets for ouabain action.
Na+/K+-ATPase ion pumping is the classical function assigned to this transporter. Nevertheless, around 50 years after the discovery of this mechanism, a new paradigm emerged. A series of works performed by Zijian Xie and Amir Askari’s group unveiled that, besides ion transport, Na+/K+-ATPase operates as a receptor (Xie and Askari, 2002). The binding of ouabain to Na+/K+-ATPase triggers intracellular signaling networks through protein–protein interactions, generating a myriad of effects independent of the impairment of electrochemical gradients (Riganti et al., 2011). In this case, ouabain (and other cardiotonic steroids) would act as an agonist and not as a Na+/K+-ATPase inhibitor (Pierre and Xie, 2006). Actually, the assumption is that the agonist or inhibitor function of ouabain is defined depending on which Na+/K+-ATPase population ouabain binds with: in the bulk plasma membrane, Na+/K+-ATPase is an ion pump and ouabain acts as an inhibitor; in caveolae, which are small lipid raft invaginations of the plasma membrane that function as a platform for signaling cascades, Na+/K+-ATPase interacts with several molecules, such as the structural protein caveolin-1 (Quintas et al., 2010) and the nonreceptor tyrosine kinase Src (Tian et al., 2006), and ouabain induces the activation of intracellular signals. Also, Anita Aperia’s group revealed that the N-terminus of the α-subunit of the plasma membrane Na+/K+-ATPase and of the endoplasmic reticulum IP3 receptor physically interact with each other and ouabain binding to the former may stimulate repetitive cytoplasmic Ca2+ transients independent on IP3 (Zhang et al., 2006). Currently, hundreds of proteins are suggested to be involved in ouabain-induced signaling (Panizza et al., 2019). As expected, this discovery opened new horizons for research on the pharmacological effects of ouabain and other cardiotonic steroids.
Different cell functions such as growth, proliferation, differentiation and membrane excitability are regulated by Ca2+ (Clapham, 2007), (Capiod, 2016). Calcium signaling in the CNS is a finely regulated process, as alterations in Ca2+ homeostasis can alter activity and induce neuronal death seen in neurodegenerative diseases and aging (Bezprozvanny, 2009), (Kumar et al., 2009). Considering the importance of Na+/K+-ATPase in brain physiology, the fact that Ca2+ ions are involved downstream of Na+/K+-ATPase inhibition (or activation), and that cardiotonic steroids, more specifically ouabain, may be endogenous modulators of the Na+/K+-ATPase, we conducted a systematic review of the in vitro effect of cardiotonic steroids on cytoplasmic Ca2+ in rat and mouse brain, which are the most used experimental animal models. Therefore, favoring the perception of the relationship between ouabain-Na+/K+-ATPase and homeostasis Ca2+ signaling in the CNS in aging and neurodegeneration.
Materials and methods
This study followed the guidelines described in the Preferred Reporting Items for Systematic Reviews and Meta-Analyses (PRISMA), and the protocol was registered on the PROSPERO platform (Reg, No. CRD42020204498). A systematic review was performed by searching PubMed, Virtual Health Library, and EMBASE databases (between 12 June 2020 and 30 June 2020) using the following search terms: (“cardiotonic steroids” OR “cardiotonic steroid” OR “cardiac glycosides” OR “cardiac glycoside” OR digitalis OR cardenolides OR cardenolide OR bufadienolides OR bufadienolide OR ouabain OR digoxin OR digitoxin) AND (brain OR “central nervous system”) AND (calcium OR Ca2+). The following question guided the selection of articles and the development of this review: “What are the effects of cardiotonic steroids on calcium signaling in the central nervous system of rodents?” This question was structured according to the acronym PECO [P: Population; E: Exposure; C: Comparison; O: Outcome] and the eligibility criteria are described in Supplementary Table S1. First, articles were selected by title and abstract. No date restriction was used, however, only publications in Portuguese, English or Spanish were considered. The type of publication was also analyzed and review articles, case reports and papers presented in scientific events were excluded. Four reviewers, working in pairs, performed independent analysis, and included articles considering eligibility criteria. The third reviewer was consulted if there was no consensus on the decision.
We developed a data extraction sheet considering the following information about the included studies: author, date of publication, populations, species, gender, age, concentration of cardiotonic steroids, experimental conditions, calcium effect, and general conclusion of the articles with respect to cardiotonic steroids use. One reviewer performed data extraction from the articles that were included, and a second reviewer checked the information. Again, a third reviewer was consulted in cases of disagreement.
Quality analysis
Most quality features and measures of risk of bias (RoB) do not apply to or are not determined for biochemical studies of the kind here reviewed, and no standard quality assessment tool exists for in vitro studies. We based our study on quality/RoB criteria for in vitro studies presented by Prueitt et al. (Prueitt et al., 2020). Questions and criteria were modified to be relevant to the evaluation of studies of calcium homeostasis/signaling. The criteria for in vitro studies include eight domains which have been divided into three main domains, related to outcome assessment, exposure characterization and control groups. Five other quality/RoB domains that include an analysis of the number of replicates, blinding, complete data, statistical methods, and experimental conditions were also evaluated. The studies were arranged into three levels of quality based on their classification with respect to the evaluated domains. The studies were grouped into three decreasing levels of quality, according to the eight questions addressed in the RoB analysis and identified in Table 1. Tier 1 includes studies presenting a “probably low” or “definitely low” RoB for all key domains relevant to that study type AND a “probably low” or “definitely low” RoB for most (i.e., at least half) of the other domains; Tier 2 includes studies that do not meet the criteria for Tier 1 or Tier 3. Tier 3 includes studies presenting a “probably high” or “definitely high” RoB for all key domains relevant to that study type AND a “probably high " or “definitely high” RoB for most (i.e., at least half) of the other domains. Eight quality/RoB parameters were analyzed. A study may have low RoB in some parameters, but high in others, which classifies it at an intermediate level, Tiers 2. Studies classified as Tier 3 have low quality and high RoB and those classified as Tier 1 have high quality and reliability (Prueitt et al., 2020). The evaluation of the methodological quality of the studies included in this review was done by only one author.
Results
A total of 829 references were identified from electronic databases. Once duplicate entries (125) had been removed, the references were further evaluated for inclusion based on the title and/or abstract. Potentially 59 relevant articles were included in the next stage for full-text evaluation. Of these, it was not possible to gain access to 6 and 3 studies were excluded since they failed to satisfy the inclusion criteria established by the PECO. Finally, 20 studies were included in the analysis (Figure 2).
The publication period of the 20 eligible studies ranged from 1970 to 2020, and they were published in the English language. Most of the studies were conducted in the United States (n = 10; 50%), followed by Germany and Japan (n = 2; 10% each), Brazil, Canada, Denmark, and Hungary (n = 1; 5% each). Two trials were conducted in more than one country (10%).
Quality criteria for in vitro studies were applied to each article. In none of the studies experiments were blinded, and no study reported loss of data. Half of the studies did not include statistical analyses of the results. Some did not examine an adequate number of animals and poorly characterized ouabain exposure. These studies were categorized into Tier 2 or Tier 3.
Of the 20 articles, 5 (25%) were categorized into Tier 1, 13 (65%) were categorized into Tier 2, and 2 (10%) were categorized into Tier 3 based on their quality ratings across domains (Table 1). These results indicate that this systematic review presents an intermediary/low risk of bias.
Although no exclusion criteria were applied for the cardiotonic steroid studied in this systematic review, when the PECO criteria were applied, it was observed that all included studies used ouabain.
They employed different methodological strategies: six studies focused on synaptosomes, eight used brain slices, and six employed cell cultures. It was possible to group the 20 articles included in this study, on the basis of their main characteristics, which are summarized in Tables 2–4. Experimental conditions are described in Supplementary Table S2. All 20 articles are described below.
Synaptosome studies
Of six papers—from 1970 to 1982—that used synaptosomes from rodent brain, five directly evaluated Ca2+ concentrations (Table 2). One study of the effects of ouabain in Ca2+ signaling observed that Ca2+-ATPase activity in intact synaptosomes and in brain subfractions of male ICR (Institute of Cancer Research) mice, was not affected by 1 mm ouabain (Lin and Way, 1982). Except for only one study that reported the use of forebrain, the other articles indicated the nonspecific use of rat brain.
Blaustein and Wiesmann (Blaustein and Wiesmann, 1970) and Swanson et al. (Swanson et al., 1974), observed that Ca2+ uptake was enhanced when 1 M × 10−3 M or 1 M × 10−4 M ouabain, respectively, was added to presynaptic synaptosomes, and this effect was directly associated with vesicles’ Na+ content. Goddard and Robinson (Goddard and Robinson, 1976) noted that 1 M × 10−4 M ouabain augmented Na+ uptake as well. The increased uptake of Ca2+ induced by ouabain was inhibited by diphenylhydantoin (DPH, well known as phenytoin), tetrodotoxin (TTX), and ruthenium red. Despite being multitarget drugs, the first two agents inhibit voltage-gated Na+ channels, and ruthenium red inhibits Ca2+ transportation, since it competes for the Ca2+ binding site in many proteins (Sasaki et al., 1992). On the other hand, Adam-Vizi and Ligetit, (Adam-Vizi and Ligeti, 1986), when comparing membrane depolarization and Ca2+ uptake, did not observe changes in Ca2+ uptake in brain cortical synaptosomes using 5 M × 10−5 − 5 M × 10−4 M ouabain, although membrane potential was significantly affected. Ca2+ influx was only detected at higher concentrations (e.g., 1 M × 10−3 M or more).
Moreover, acetylcholine release was not detected upon ouabain treatment, despite the degree of depolarization being comparable to those of other depolarizing agents that induce neurotransmitter exocytosis. Satoh and Nakazato (Satoh and Nakazato, 1989), showed that ouabain elicited a concentration-dependent (5 M × 10−8 −5 M × 10−4 M) release of acetylcholine from synaptosomes regardless of the presence or absence of extracellular Ca2+, but such effect was impaired when the protein kinase C (PKC) and ryanodine receptor blocker TMB‐8 was coincubated with ouabain, suggesting the importance of intracellular Ca2+.
Brain slices studies
Of eight papers—from 1973 to 2017—that used slices from rodent brain, five directly evaluated Ca2+ concentrations, and all observed an increase of it (Table 3). Furthermore, three studies evaluated proteins that contribute to the control of Ca2+ signaling and homeostasis. These studies, using 1 M × 10−4 M ouabain and rat brain cortical slices, demonstrated that ouabain increased IP3 levels but had minimal effects on inositol 1,3,4,5-tetrakisphosphate (IP4) accumulation (Myles and Fain, 1994). Ouabain induced the formation of cAMP, dependently on extracellular Ca2+, and this process was blocked by verapamil, an inhibitor of L-type voltage-gated Ca2+-channels, also known as dihydropyridine receptors, that are responsible for Ca2+ entry during the potential action (Mørk et al., 1993). In addition, ouabain induced a concentration-dependent accumulation of inositol phosphates (InsPs), which was much higher in neonatal rat brain than in adult brain. Furthermore, the accumulation of InsPs induced by ouabain was dependent on extracellular Ca2+ and was blocked by EGTA (Balduini and Costa, 1990).
A large range of ouabain concentrations, from 1 × 10−5 to 1 × 10−3 M, was demonstrated to increase the [Ca2+]i in different rodent brain slices. Using 1 M × 10−5 M ouabain, Okamoto et al. (Okamoto et al., 1995) showed a progressive elevation of [Ca2+]i in hippocampal slices, and this was significantly suppressed with the coadministration of Li+, since lithium appears to antagonize the ouabain Na+/K+-ATPase inhibition, enhancing the extrusion of intracellular Ca2+ by Na+/Ca2+ exchanger as a consequence. Also, enhanced Ca2+ uptake was demonstrated using 1 M × 10−4 M ouabain, with a subsequent release of norepinephrine (Pincus and Lee, 1973). Ouabain at 1 M × 10−3 M increased the [Ca2+]i, reduced transmembrane water flux, and raised the mean neuron and glial cell volume (Bai et al., 2018).
In addition, concentrations of ouabain, 1 M × 10−4 M and 3 M × 10−5 M, generated in vitro spreading depression (SD) in freshly prepared hippocampal and neocortical slices. Interestingly, although ouabain produced a significant elevation of in [Ca2+ ]i, Ca2+ by itself was shown not to be responsible for SD (Basarsky et al., 1998), (Dietz et al., 2008).
Cell culture studies
Of six papers—from 1995 to 2020—that used cell culture from rodent brain, all directly evaluated the Ca2+ concentration, and observed an increase of it (Table 4). Of the six articles, five carried out studies focused on primary cell culture. Two studies used cultures of primary brain oligodendrocyte precursor cells (OPCs), one used cortical culture, one cerebellar culture, and one hippocampal cell cultures. Only one article investigated the effects of ouabain on immortalized cells (SN56 cells). The largest increase in the Ca2+ level was observed at the highest ouabain concentration (1 M × 10−3 M) (Stelmashook et al., 1999), and the smallest increase at the lowest ouabain concentration (1 M × 10−4 M) (Bassetti et al., 2020). It is interesting to note that studies from the 1990s used higher concentrations of ouabain in their investigations, whereas more recent studies utilized lower concentrations. In addition to the concentration-dependent increase of the [Ca2+]i induced by ouabain, some articles also observed a time-dependent variation in [Ca2+]i (Stelmashook et al., 1999)- (Xiao et al., 2002).
In primary cerebellar culture, Stelmashook et al. (1999) showed that addition of 1 M × 10−3 M ouabain had a toxic effect leading to death 62 ± 3% of the total amount of granule cells against 3 % in control. This effect was abolished when the antagonist of NMDA receptors APH (0.1 mm) was added to the incubation medium together with ouabain. APH also prevented cells from swelling, mitochondrial deenergization, neuronal death. Furthermore, in primary cortical cultures, the neuronal apoptotic and necrotic death associated with Na+/K+-ATPase inhibition, caused by the application of 1 M × 10−4 M ouabain, was consistent with the intracellular depletion of K+ and the accumulation of Ca2+ and Na+, In addition, exposure for 20 h to ouabain induced DNA fragmentation (Xiao et al., 2002). In the same way, Mark et al. (1995) using primary hippocampal cell culture using calcium indicator dye Fura-2 showed that 30 min incubation with 2 M × 10−3 M ouabain leads to an increase in [Ca2+]i levels and neuronal degeneration. They also demonstrated that the addition of ouabain promotes a decrease in neuron survival in a concentration dependent manner. Moreover, the use of Hoescht dye and ethidium bromide homodimer also revealed nuclear condensation and DNA fragmentation induced by ouabain (Mark et al., 1995).
Using SN56 cells Lomeo at al (2003) showed that ouabain (2 M × 10−4 M) had a calcium dependent effect on [Ca2+]i levels, leading to enhanced acetylcholine release. This effect of ouabain on acetylcholine release was dose and time dependent, achieving the maximum value after 30 min and was not inhibited by the addition of 1 µM tetrodotoxin (TTX). However, the effect of ouabain was suppressed with the addition of BAPTA-AM (Lomeo et al., 2003).
Interestingly, studies using cultures of oligodendrocyte precursor cells have shown that a long incubation of oligodendrocyte precursor cells (OPC) cultures with ouabain (5 M × 10−7 M, 24 h) failed to significantly change [Na+]i levels, but ouabain treatment significantly increased [Ca2+]i and stimulated myelin basic protein synthesis (Friess et al., 2016).
Figure 3 is a schematic summary of the finding of this study.
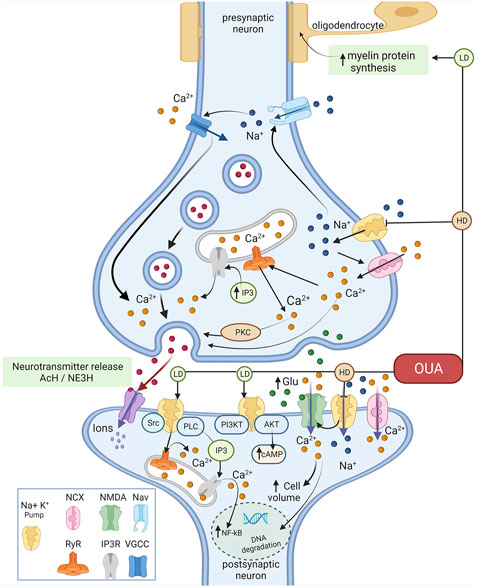
FIGURE 3. Representative schedule of the effects of ouabain on Ca2+ signaling. Ouabain in high concentrations selectively binds to Na+/K+-ATPase, inhibiting its pump activity, leading to increased intracellular Na+ and Ca2+ concentrations. The latter by inducing a lower/reverse action of the Na+–Ca2+ exchanger (NCX) colocalizes with the Na+/K+-ATPase. Ouabain effects on intracellular Ca2+ influence the release of acetylcholine (Ach) and norepinephrine (NE3H) and glutamate (Glu). Binding of ouabain to Na+/K+-ATPase triggers intracellular signaling networks in the glutamate signaling cascade through protein-protein interactions, generating many effects independent of the impairment of electrochemical gradients. In this case, ouabain (and other cardiotonic steroids) would act as an agonist, stimulating these pathways, acting in a different way observed as Na+/K+-ATPase inhibitor. In low concentrations ouabain can stimulate changes in Na+/K+-ATPase that triggers signaling complexes such PI3K-AKT pathways, increasing cAMP. Also, ouabain modulates Ca2+ intracellular oscillation through activation of ryanodine receptor (RyR) and IP3 receptor (IP3R) that provoke the increasing of NF-κB and activation of PKC. LD- Low Dose and HD- High dose (Figure were created with BioRender.com).
Discussion
The most studied effects of cardiotonic steroids refer to their action on the cardiovascular system. Here in this systematic review, we demonstrated the effects of ouabain on Ca2+ oscillation and signaling in the nervous system of rodents, indicating that the balance between ouabain-induced neuroprotective and neurotoxic effects is concentration-dependent. Furthermore, the action of ouabain is broad, acting not only on neurons but also on glial cells.
Ca2+ homeostasis plays a crucial role in the maintenance of different cellular functions. Ca2+ has been described as an important second messenger, regulating many different cellular processes, including cell division, proliferation differentiation, apoptosis, necrosis, neurotransmission and synaptic plasticity (Arundine and Tymianski, 2003), (Berridge et al., 2003). The concentration of this free ion in the cytosol is kept about 10,000 times below the extracellular concentration (Nicotera and Orrenius, 1998). This high electrochemical Ca2+ gradient between the intra and extracellular compartments enables the transduction of biochemical signals into cells (Berridge et al., 2003).
Regarding neurotransmission modulated by ouabain, Ca2+ triggers synaptic vesicle exocytosis, thereby releasing the neurotransmitters contained in the vesicles and initiating synaptic transmission (Katz and Miledi, 1967). Whereas ouabain selectively binds to Na+/K+-ATPase and inhibits its ion pump activity, increasing intracellular Na+ and Ca2+ concentrations, the latter by inducing a lower/reverse activity of the Na+/Ca2+ exchanger that colocalizes with the Na+/K+-ATPase (Larsen et al., 2016), (Kinoshita et al., 2020), it is expected that it can modulate neurotransmission. Inhibition of the Na+/K+-ATPase could lead to depolarization of the neuron, followed by Ca2+ influx and transmitter release by exocytosis (Banks, 1967). It could also lead indirectly to a rise in intracellular Ca2+, through mobilization of intracellular Ca2+ stores (Baker and Crawford, 1975). The results collected in this study represents the literature. In fact, ouabain is capable of interfering with different neurotransmission systems, such as cholinergic (Satoh and Nakazato, 1989), (v Blasi et al., 1988), noradrenergic (Pincus and Lee, 1973), (Yamazaki et al., 2007) and glutamatergic (Jacobson et al., 1986), (Stelmashook et al., 1999). Moreover, other studies have demonstrated that ouabain also interferes with the release of dopamine in animal models (Sui et al., 1199).
The toxic effect of high concentrations of ouabain has been widely described in the literature, as well as the involvement of high levels of Ca2+ influx, promoting neuronal excitotoxicity (Veldhuis et al., 2003). This effect was observed in synaptosomes as well as in brain slices.
Excitotoxicity is a term used to indicate the death of nerve cells by glutamate (Glu) as well as other amino acids, resulting in neurodegenerative diseases (Lewerenz and Maher, 2015), (Olney, 1986), increased release of Glu that occurs under neurological disorders may be a result of metabolic changes and reduced Na+,K+-ATPase activity (Shi et al., 2019), (Beal et al., 1993). The findings indicate that NMDA receptors are involved in ouabain effects on [Ca2+]i and cell toxicity. It is known that Na+/K+-ATPase inhibition leads to a decrease in Glu uptake in cortical astrocytes cell cultures (Volterra et al., 1994), interfering with GluT transport in astrocytes (Nguyen et al., 2010), favoring the neurotoxic effects of high doses of ouabain. This suggesting that Na+/K+-ATPase inhibition by ouabain led to Glu accumulation of extracellular Glu, hyperstimulation of glutamate receptors, and higher Ca2+ and Na+ influxes into the cells through N-methyl-d-aspartate (NMDA) receptors in neuro–glial cell cultures of the cerebellum (Stelmashook et al., 1999). These authors associated the exposure of high ouabain concentrations with a toxic effect on cerebellar and hippocampal cells.
Interestingly, ouabain in nanomolar concentration consistently reduces the Ca2+ response to NMDA. Downregulation of the NMDA response is not associated with internalization of the receptor or with alterations in its state of Src phosphorylation (Akkuratov et al., 2020). It has been observed that ouabain activates NF-κB by an NMDA–Src–Ras-like protein through MAPK pathways in cultured cerebellar cells (de Sá Lima et al., 2013). In addition, the intra-hippocampal administration of ouabain in a low concentration that did not alter the activity of Na+/K+-ATPase promoted the activation of NF-κB, leading to increased brain-derived neurotrophic factor (BDNF) levels, similar to NMDA treatment, which was reversed by the NMDA antagonist MK-801 (Kawamoto et al., 2012b). Moreover, intrahippocampal injection of ouabain 10 nM activated the Wnt/β-catenin signaling pathway and to increase CREB/BDNF and NFκB levels. These effects contribute to important changes in the cellular microenvironment, resulting in enhanced levels of dendritic branching in hippocampal neurons, in association with an improvement in spatial reference memory and the inhibition of long-term memory extinction (Orellana et al., 2018).
Altered levels of acetylcholine, as well as its receptors, have been observed in neurodegenerative and neuropsychiatric diseases (Tata et al., 2014). In order to obtain a concentration-dependent curve of [3H] acetylcholine release, Satoh and Nakazato (Satoh and Nakazato, 1989) utilized 5 × 10−8-5x10−4 M ouabain, possibly inhibiting initially the α2/α3 isoforms—which are, in rodents, much more sensitive to cardiotonic steroids—and subsequently the ouabain-resistant α1 (O’brien et al., 1994), (Lopez et al., 2002). Interestingly Lomeo at al (2003) using SN56 cells showed that ouabain had a Ca2+ dependent effect on [Ca2+]i levels, leading to enhanced acetylcholine release. This effect of ouabain on acetylcholine release was dose and time dependent, achieving the maximum value after 30 min. This effect was not inhibited by the addition of 1 µM tetrodotoxin (TTX), discarding the involvement of TTX-sensitive Na+ channels. However, the effect of ouabain was suppressed with the addition of BAPTA-AM, suggesting the involvement of intracellular calcium stores. The authors suggested that in cholinergic neurons the ouabain induced increase in [Na+]i results in intracellular calcium alterations inducing an increase in [Ca2+]i, causing a release of acetylcholine independent of Ca2+ (Lomeo et al., 2003). Despite the effect of high doses of ouabain on cholinergic neurotransmission, no studies were found on the role of lower doses of this cardiotonic on the cholinergic system.
Another phenomenon observed on the effect of the micromolar application of ouabain was the Spreading depression (SD), which is a wave of profound depolarization that propagates throughout the brain tissue after traumatic or vascular brain insults (Somjen, 2001). Interestingly, it was observed that the spreading depression is not only caused by the increase of in [Ca2+ ]i, but also the partition of Zn2+ and mitochondrial stress, since it was observed that selective chelation of Zn2+ with N,N,N,N-tetrakis (2-pyridylmethyl) ethylenediamine (TPEN) eliminated ouabain-SD, implying that Zn2+ entry and mitochondrial dysfunction may play a critical role in the Ouabain-induced SD mechanism (Dietz et al., 2008). Thus, this is a useful model for studying the pathways involved in this phenomenon and that can lead to the search for more efficient treatments.
Different studies have demonstrated that neuronal activity promotes an elevation in extracellular [K+], stimulating an increase in intracellular Ca2+transient in oligodendrocytes, due to the reversion of the Na+/Ca2+ exchanger, which led to increased synthesis of myelin basic protein (MBP) (Belachew et al., 2000), (Chen et al., 2007). Interestingly, using primary brain OPCs cultures, Friess et al. (Friess et al., 2016) showed that incubation with 5 M × 10−7 M ouabain increased the intracellular Ca2+ levels and stimulated MBP synthesis, and this effect of ouabain on Ca2+ transients was eliminated by the addition to the incubation medium of 1 µMKB-R734 9, a Na+/Ca2+ exchanger inhibitor. In addition, it was observed that the activity of the Na+/K+-ATPase α2 isoform, present in oligodendrocyte lineage cell (OLCs), changed the Na+/Ca2+ exchanger-mediated [Ca2+]i, modulating MBP synthesis in OLCs (Hammann et al., 2018). Using the same experimental model of primary OPCs cultures, Basseti et al. (Bassetti et al., 2020) demonstrated that lower concentrations of ouabain (1 M × 10−7 M) also increased Ca2+ transient frequency. Additionally, this work showed that the inhibition of ryanodine receptor type 3 with 10 µM ryanodine also blocked Ca2+ transient.
The process of myelination of neuronal axons through oligodendrocyte activity is a process controlled by the release of neurotransmitters and changes in ionic concentrations (Butt and Bay, 2011). Among the important proteins in the myelination process, there is myelin basic protein (MBP), and failures in its production result in CNS hypomyelination processes (Readhead and Hood, 1990), (Wiecien et al., 1998). Interestingly, studies using cultures of oligodendrocyte precursor cells (OPC) have shown that a long incubation (24 h) of these cells with nanomolar concentrations of ouabain failed to significantly change [Na+]i levels, but ouabain treatment significantly increased [Ca2+]i and stimulated MPB synthesis (Friess et al., 2016). The same group further suggested that the crosstalk among ryanodine receptors, Na+/–Ca2+ exchangers, and possibly Na+/K+-ATPase may evoke Ca2+ transients for the development of isolated oligodendrocytes (Bassetti et al., 2020), thus demonstrating the importance of Na+,K+-ATPase in the synthesis of axonal myelin by oligodendrocytes.
Interestingly, in addition to the existence of a Na+/Ca2+ exchanger colocalized with Na+/K+-ATPase, the discovery of ancillary signaling pathways, involving protein–protein interactions, revealed that the physical association between Na+/K+-ATPase and IP3 receptors allows intracellular Ca2+ oscillations evoked by ouabain (Fontana et al., 2013), (Miyakawa-Naito et al., 2003). Moreover, Src kinase activation would promote the stimulation of phospholipase C and PKC independently of ion modulation (Mohammadi et al., 2001), (Yuan et al., 2005).
One study in brain slices demonstrated that ouabain caused the stimulation of PtdIns hydrolysis, causing an accumulation of InsPs in the cytoplasm, which may be related to cell signaling modulated by Na+/K+-ATPase inhibition, activation of Na+/Ca2+ exchanger, mobilization of Ca2+ and PKC (Balduini and Costa, 1990). Interestingly, there was no direct correlation between the stimulus leading to PtdIns hydrolysis and the binding of radioactive ouabain (number of Na+/K+-pumps) or strophantidin effect (an equipotent inhibitor), and this may suggest that this outcome is modulated by cell signaling mechanisms besides the classic inhibitory effect. A similar effect was found in the study by Myles et al. (Myles and Fain, 1994), where 1 M × 10−4 M ouabain stimulated an 82% increase in intracellular IP3 levels but had a minimal effect on IP4 accumulation. Moreover, in addition to InsPs, treatment with 1 M × 10−4 M ouabain in brain slices also increased intracellular cAMP (Mørk et al., 1993). Since only few studies utilized slices, it is not possible to say whether accumulation of cytosolic Ca2+ found in these studies is an effect modulated only by the regulation of the Na+/Ca2+ exchanger, which is the classic effect of cardiotonic steroids. It is well known that Ca2+ mobilization and influx is linked to the activation of cellular signaling pathways, especially those associated with G proteins, with the involvement of cAMP and IP3 (Satoh and Nakazato, 1989), (Liu et al., 2007).
In addition, studies that include the nervous system and others demonstrate that Na+/K+-ATPase acts as a receptor and a signal transducer, involving many pathways such as that dependent on the membrane-associated nonreceptor tyrosine kinase Src (Tian et al., 2006) the Ras/Raf/ERK1/2 pathway (Eckert et al., 2008), the phosphate inositol 3-kinase (PI3K) pathway, the PI3K-dependent protein kinase B pathway, phospholipase C signaling, [Ca2+]i oscillations (Liu et al., 2007), (Schoner and Scheiner-Bobis, 2007), (Aperia et al., 2015), and gene transcription (Li et al., 2006). These pathways are also triggered by the interaction of ouabain with Na+/K+-ATPase. Na+/K+- ATPase inhibition or activation is dependent on ouabain concentration. It has been shown that inhibition of pump activity requires micromolar concentrations of ouabain (Fontana et al., 2013), (Blaustein and Hamlyn, 2020), while activation of Na+/K+-ATPase signaling pathways occurs in the presence of nanomolar concentrations of ouabain (Fontana et al., 2013).
As discussed above, several studies using different models have shown that the effects of ouabain at low concentrations are due to the activation of cell signaling pathways. Studies have shown that in renal epithelial cells, as well as in astrocytes, ouabain, at low concentrations, binds with Na+/K+-ATPase directly activating the IP3 receptors (physical interaction), and triggering slow Ca2+ oscillations and the activation of NF-kB; this ultimately leads to the proliferation of these cells (Liu et al., 2007), (Li et al., 2006), (Aizman et al., 2001). Furthermore, studies in cardiomyocytes have observed that low ouabain concentrations promote [Ca2+]i oscillations due to the activation of Src kinase followed by the stimulation of the Ras/Raf/MEK/MAPK cascade regulating cell hypertrophic growth (Yuan et al., 2005), (Zhu et al., 1996). It is important to note that in such signaling conditions, Ca2+ is a key factor, and positive feedback may occur.
The therapeutic range for the use of ouabain and other cardiac steroids is very narrow. Added to this problem of narrow therapeutic index, we have the majority use of this drug by elderly patients, contributing to toxicity problems (Whayne, 12018). This reality caused mistrust and disuse of cardiac steroids in clinical practice. Thus, in addition to expanding knowledge about signaling pathways and neuroprotective effects of low doses of ouabain and other cardiotonic, a strategy for the safe therapeutic use of cardiotonic steroids for neuroprotection would be the chemical modification of their structure to increase the therapeutic index of this class. Gamma-benzylidene digoxin derivatives are digoxin-derived molecules that have demonstrated low toxicity to cells (de Oliveira et al., 2021) and have already demonstrated neuroprotection for chemical ischemia (de Souza Gonçalves et al., 2019) and increased α3 activity and increased antioxidant defenses such as GSH, desired drug characteristics of neuroprotection (Parreira et al., 2021).
Conclusion
Ca2+ mobilization is a canonical effect of cardiotonic steroids such as ouabain. In all models studied - synaptosomes, brain slices or cell cultures—an increase in [Ca2+]i was observed. In addition to the well known cytotoxic effects of ouabain, resulting from stimulation of the Na+/Ca2+ exchanger reverse mode and increased Ca2+, other effects have been reported, since Ca2+ may play a role in major cellular effects, mainly by activating signaling pathways. Ouabain-induced Ca2+ signaling was able to increment cholinergic, noradrenergic and glutamatergic neurotransmission. Treatment with ouabain stimulated MBP synthesis and significantly increased biological second messengers such as InsPs, IP3 and cAMP. This review deepens the knowledge about the effects and signaling mediated by cardiotonic steroids (ouabain) in the nervous system, which has been shown to be concentration dependent. Structural modifications of cardiotonic steroids may be useful for the generation of new agents that are less toxic and with neuroprotective action.
Data availability statement
The raw data supporting the conclusions of this article will be made available by the authors, without undue reservation.
Author contributions
AL, EP, GSM, LB, LEMQ, MLCS, CS, and LEDC. Performed the selection of the studies and the extraction of the data JAL, EP, GSM, MLCS, and LEDC. Performed the quality analysis of the studies LEDC. Write and elaborate the figures and tables of the manuscript: JAL, EP, GSM, LB, LEMQ, MLCS, and LEDC. Reviewed topics and discussed concepts in the manuscript: JAL, EP, GSM, LB, LEMQ, EMK, MLCS, CS, and LEDC.
Funding
ML is supported by a master fellowship from National Council for Scientific and Technological Development (CNPq). CS, EK, LB, and LQ are research fellows of the National Council for Scientific and Technological Development (CNPq). This publication was made possible by grants from São Paulo Research Foundation (FAPESP) to CS (2016/07427-8); CNPq 405089/2018-0; CAPES—STINT program 88887.125409/2016-00 (Joint Brazilian-Swedish Research Collaboration) and USP Neuroscience Research Support Centres (NAPNA) to CS; CNPq Universal #409436/2016-0 and Fundação de Amparo à Pesquisa do Rio de Janeiro (FAPERJ APQ1 # 210.379/2019) to LEMQ. Fundação de Amparo à Pesquisa do Estado de Minas Gerais (FAPEMIG APQ#01176-16) to LEDC and UFSJ support.
Acknowledgments
We thank BioRender.com and Larissa de Sá Lima for technical support. The authors also thank MSc. Pedro Azalim Neto for building the rat Na+/K+-ATPase protein structure.
Conflict of interest
The authors declare that the research was conducted in the absence of any commercial or financial relationships that could be construed as a potential conflict of interest.
Publisher’s note
All claims expressed in this article are solely those of the authors and do not necessarily represent those of their affiliated organizations, or those of the publisher, the editors and the reviewers. Any product that may be evaluated in this article, or claim that may be made by its manufacturer, is not guaranteed or endorsed by the publisher.
Supplementary material
The Supplementary Material for this article can be found online at: https://www.frontiersin.org/articles/10.3389/fphar.2022.916312/full#supplementary-material
References
Adam-Vizi, V., and Ligeti, E. (1986). Calcium uptake of rat brain synaptosomes as a function of membrane potential under different depolarizing conditions. J. Physiol. 372 (1), 363–377. doi:10.1113/jphysiol.1986.sp016013
Aizman, O., Uhlén, P., Lal, M., Brismar, H., and Aperia, A. (2001). Ouabain, a steroid hormone that signals with slow calcium oscillations. Proc. Natl. Acad. Sci. U. S. A. 98 (23), 13420–13424. doi:10.1073/pnas.221315298
Akera, T., and Brody, T. M. (1977). The role of Na+, K+-ATPase in the inotropic action of digitalis. Pharmacol. Rev. 29 (3), 187–220.
Akkuratov, E. E., Westin, L., Vazquez-Juarez, E., de Marothy, M., Melnikova, A. K., Blom, H., et al. (40302020). Ouabain modulates the functional interaction between Na, K-ATPase and NMDA receptor. Mol. Neurobiol. 57 (10), 4018–4030. doi:10.1007/s12035-020-01984-5
Aperia, A., Akkuratov, E. E., Fontana, J. M., and Brismar, H. (2015). Hugh davson distinguished lectureship of the cell and molecular physiology sectionNa+-k+-ATPase, a new class of plasma membrane receptors. Am. J. Physiol. Cell Physiol. 310 (7), C491–C495. doi:10.1152/ajpcell.00359.2015
Arundine, M., and Tymianski, M. (2003). Molecular mechanisms of calcium-dependent neurodegeneration in excitotoxicity. Cell Calcium 34 (4–5), 325–337. doi:10.1016/S0143-4160(03)00141-6
Bai, R., Springer, C. S., Plenz, D., and Basser, P. J. (2018). Fast, Na+/K+ pump driven, steady-state transcytolemmal water exchange in neuronal tissue: A study of rat brain cortical cultures. Magn. Reson. Med. 79 (6), 3207–3217. doi:10.1002/mrm.26980
Baker, P. F., and Crawford, A. C. (1975). A note of the mechanism by which inhibitors of the sodium pump accelerate spontaneous release of transmitter from motor nerve terminals. J. Physiol. 247 (1), 209–226. doi:10.1113/jphysiol.1975.sp010928
Balduini, W., and Costa, L. G. (1990). Characterization of ouabain-induced phosphoinositide hydrolysis in brain slices of the neonatal rat. Neurochem. Res. 15 (10), 1023–1029. doi:10.1007/BF00965749
Banks, P. (1967). The effect of ouabain on the secretion of catecholamines and on the intracellular concentration of potassium. J. Physiol. 193 (3), 631–637. doi:10.1113/jphysiol.1967.sp008383
Basarsky, T. A., Duffy, S. N., Andrew, R. D., and MacVicar, B. A. (1998). Imaging spreading depression and associated intracellular calcium waves in brain slices. J. Neurosci. 18 (18), 7189–7199. doi:10.1523/jneurosci.18-18-07189.1998
Bassetti, D., Hammann, J., Luhmann, H. J., White, R., and Kirischuk, S. (2020). Ryanodine receptor- and sodium-calcium exchanger-mediated spontaneous calcium activity in immature oligodendrocytes in cultures. Neurosci. Lett. 732. doi:10.1016/j.neulet.2020.134913
Beal, M. F., Hyman, B. T., and Koroshetz, W. (1993). Do defects in mitochondrial energy metabolism underlie the pathology of neurodegenerative diseases? Trends Neurosci. 16 (4), 125–131. doi:10.1016/0166-2236(93)90117-5
Belachew, S., Malgrange, B., Rigo, J. M., Rogister, B., LePrince, P., Hans, G., et al. (2000). Glycine triggers an intracellular calcium influx in oligodendrocyte progenitor cells which is mediated by the activation of both the ionotropic glycine receptor and Na+-dependent transporters. Eur. J. Neurosci. 12 (6), 1924–1930. doi:10.1046/j.1460-9568.2000.00085.x
Berridge, M. J., Bootman, M. D., and Roderick, H. L. (2003). Calcium signalling: Dynamics, homeostasis and remodelling. Nat. Rev. Mol. Cell Biol. 4 (7), 517–529. doi:10.1038/nrm1155
Bezprozvanny, I. (2009). Calcium signaling and neurodegenerative diseases. Trends Mol. Med. 15 (3), 89–100. doi:10.1016/j.molmed.2009.01.001
Blanco, G., and Mercer, R. W. (1998). Isozymes of the Na-K-ATPase: Heterogeneity in structure, diversity in function. The American Physiological Society, 245–247. doi:10.1177/153857449803200307
Blanco, G. (2005). Na, K-ATPase subunit heterogeneity as a mechanism for tissue-specific ion regulation. Semin. Nephrol. 25 (5), 292–303. doi:10.1016/j.semnephrol.2005.03.004
Blaustein, M. P., and Hamlyn, J. M. (2020). Ouabain, endogenous ouabain and ouabain-like factors: The Na+ pump/ouabain receptor, its linkage to NCX, and its myriad functions. Cell Calcium 86, 102159. doi:10.1016/j.ceca.2020.102159
Blaustein, M. P., Juhaszova, M., Golovina, V. A., Church, P. J., and Stanley, E. F. (2002). Na/Ca exchanger and PMCA localization in neurons and astrocytes: Functional implications. Ann. N. Y. Acad. Sci. 976, 356–366. doi:10.1111/j.1749-6632.2002.tb04762.x
Blaustein, M. P., and Wiesmann, W. P. (1970). Effect of sodium ions on calcium movements in isolated synaptic terminals. Proc. Natl. Acad. Sci. U. S. A. 66 (3), 664–671. doi:10.1073/pnas.66.3.664
Brini, M., Calì, T., Ottolini, D., and Carafoli, E. (2014). Neuronal calcium signaling: Function and dysfunction. Cell. Mol. Life Sci. 71 (15), 2787–2814. doi:10.1007/s00018-013-1550-7
Butt, A. M., and Bay, V. (2011). Axon-glial interactions in the central nervous system. J. Anat. 219 (1), 1. doi:10.1111/j.1469-7580.2011.01401.x
Capiod, T. (2016). Extracellular calcium has multiple targets to control cell proliferation. Adv. Exp. Med. Biol. 898, 133–156. doi:10.1007/978-3-319-26974-0_7
Carvalho, D. C. M., Cavalcante-Silva, L. H. A., Lima, E. d. A., Galvao, J. G. F. M., Alves, A. K. d. A., Feijo, P. R. O., et al. (2019). Marinobufagenin inhibits neutrophil migration and proinflammatory cytokines. J. Immunol. Res. 2019, 1094520. doi:10.1155/2019/1094520
Cereijido, M., Contreras, R. G., Shoshani, L., and Larre, I. (2012). The Na +-K +-ATPase as self-adhesion molecule and hormone receptor. Am. J. Physiol. Cell Physiol. 302 (3), C473–C481. doi:10.1152/ajpcell.00083.2011
Chen, H., Kintner, D. B., Jones, M., Matsuda, T., Baba, A., Kiedrowski, L., et al. (2007). AMPA-mediated excitotoxicity in oligodendrocytes: Role for Na +-K+-Cl- co-transport and reversal of Na +/Ca2+ exchanger. J. Neurochem. 102 (6), 1783–1795. doi:10.1111/j.1471-4159.2007.04638.x
Clausen, M. v., Hilbers, F., and Poulsen, H. (2017). The structure and function of the Na, K-ATPase isoforms in health and disease. Front. Physiol. 8 (JUN), 371–416. doi:10.3389/fphys.2017.00371
de Oliveira, G. C., Rocha, S. C., da Silva Lopes, M. A., Paixão, N., Alves, S. L. G., Pessoa, M. T. C., et al. (2021). Implications of synthetic modifications of the cardiotonic steroid lactone ring on cytotoxicity. J. Membr. Biol. 254 (5–6), 487–497. doi:10.1007/s00232-021-00186-x
de Sá Lima, L., Kawamoto, E. M., Munhoz, C. D., Kinoshita, P. F., Orellana, A. M., Curi, R., et al. (2013). Ouabain activates NFκB through an NMDA signaling pathway in cultured cerebellar cells. Neuropharmacology 73, 327–336. doi:10.1016/j.neuropharm.2013.06.006
de Souza Gonçalves, B., de Moura Valadares, J. M., Alves, S. L. G., Silva, S. C., Rangel, L. P., Cortes, V. F., et al. (2019). Evaluation of neuroprotective activity of digoxin and semisynthetic derivatives against partial chemical ischemia. J. Cell. Biochem. 120 (10), 17108–17122. doi:10.1002/jcb.28971
Dietz, R. M., Weiss, J. H., and Shuttleworth, C. W. (2008). Zn2+ influx is critical for some forms of spreading depression in brain slices. J. Neurosci. 28 (32), 8014–8024. doi:10.1523/JNEUROSCI.0765-08.2008
Dobretsov, M., and Stimers, J. R. (2005). Neuronal function and alpha3 isoform of the Na/K-ATPase. Front. Biosci. 10 (1–3), 2373–2396. doi:10.2741/1704
Eckert, A., Tagscherer, K. E., Haas, T. L., Grund, K., Sykora, J., et al. (2008). The PEA-15/PED protein protects glioblastoma cells from glucose deprivation-induced apoptosis via the ERK/MAP kinase pathway. Oncogene 27 (8), 1155–1166. doi:10.1038/sj.onc.1210732
Fedorova, O. v., and Bagrov, A. Y. (1997). Inhibition of Na/K ATPase from rat aorta by two Na/K pump inhibitors, ouabain and marinobufagenin: Evidence of interaction with different α- subunit isoforms. Am. J. Hypertens. 10 (8), 929–935. doi:10.1016/S0895-7061(97)00096-4
Fedorova, O. v., Kolodkin, N. I., Agalakova, N. I., Lakatta, E. G., and Bagrov, A. Y. (2001). Marinobufagenin, an endogenous alpha-1 sodium pump ligand, in hypertensive Dahl salt-sensitive rats. Hypertension 37 (2 II), 462–466. doi:10.1161/01.hyp.37.2.462
Fontana, J. M., Burlaka, I., Khodus, G., Brismar, H., and Aperia, A. (2013). Calcium oscillations triggered by cardiotonic steroids. FEBS J. 280 (21), 5450–5455. doi:10.1111/febs.12448
Friess, M., Hammann, J., Unichenko, P., Luhmann, H. J., White, R., and Kirischuk, S. (2016). Intracellular ion signaling influences myelin basic protein synthesis in oligodendrocyte precursor cells. Cell Calcium 60 (5), 322–330. doi:10.1016/j.ceca.2016.06.009
Geering, K. (2008). Functional roles of Na, K-ATPase subunits. Curr. Opin. Nephrol. Hypertens. 17 (5), 526–532. doi:10.1097/MNH.0b013e3283036cbf
Goddard, G. A., and Robinson, J. D. (1976). Uptake and release of calcium by rat brain synaptosomes. Brain Res. 110 (2), 331–350. doi:10.1016/0006-8993(76)90406-6
Godinho, A. N., Costa, G. T., Oliveira, N. O., Cardi, B. A., Uchoa, D. E. A., Silveira, E. R., et al. (2017). Effects of cardiotonic steroids on isolated perfused kidney and NHE3 activity in renal proximal tubules. Biochim. Biophys. Acta. Gen. Subj. 1861 (8), 1943–1950. doi:10.1016/j.bbagen.2017.05.012
Hammann, J., Bassetti, D., White, R., Luhmann, H. J., and Kirischuk, S. (2018). α2 isoform of Na + , K + -ATPase via Na + , Ca 2+ exchanger modulates myelin basic protein synthesis in oligodendrocyte lineage cells in vitro. Cell Calcium 73, 1–10. doi:10.1016/j.ceca.2018.03.003
Jacobson, I., Hagberg, H., Sandberg, M., and Hamberger, A. (1986). Ouabain-induced changes in extracellular aspartate, glutamate and GABA levels in the rabbit olfactory bulb in vivo. Neurosci. Lett. 64 (2), 211–215. doi:10.1016/0304-3940(86)90102-3
Katz, B., and Miledi, R. (1967). Ionic requirements of synaptic transmitter release. Nature 215 (5101), 651. doi:10.1038/215651a0
Kawamoto, E. M., Lima, L. S., Munhoz, C. D., Yshii, L. M., Kinoshita, P. F., Amara, F. G., et al. (2012). Influence of N-methyl-D-aspartate receptors on ouabain activation of nuclear factor-κB in the rat hippocampus. J. Neurosci. Res. 90 (1), 213–228. doi:10.1002/jnr.22745
Kawamoto, S., Tran, T. H., Maruya, M., Suzuki, K., Doi, Y., Tsutsui, Y., et al. (2012). The inhibitory receptor PD-1 regulates IgA selection and bacterial composition in the gut. Science 821, 485–489. doi:10.1126/science.1217718
Kinoshita, P. F., Orellana, A. M. M., Nakao, V. W., de Souza Port's, N. M., Quintas, L. E. M., Kawamoto, E. M., et al. (2020). The Janus face of ouabain in Na+/K+-ATPase and calcium signalling in neurons. Br. J. Pharmacol. 179, 1512–1524. doi:10.1111/bph.15419
Koh, C. H., Wu, J., Chung, Y. Y., Liu, Z., Zhang, R. R., Chong, K., et al. (2017). Identification of Na+/K+-ATPase inhibition-independent proarrhythmic ionic mechanisms of cardiac glycosides. Sci. Rep. 7 (1), 2465. doi:10.1038/s41598-017-02496-4
Kumar, A., Bodhinathan, K., and Foster, T. C. (2009). Susceptibility to calcium dysregulation during brain aging. Front. Aging Neurosci. 1 (NOV), 2–13. doi:10.3389/neuro.24.002.2009
Kupicha, F. K. (1982). Studies on african apocynaceae: The genus Acokanthera. Kew Bull. 37 (1), 41. doi:10.2307/4114719
Larsen, B. R., Stoica, A., and MacAulay, N. (2016). Managing brain extracellular K+ during neuronal activity: The physiological role of the Na+/K+-ATPase subunit isoforms. Front. Physiol. 7 (APR), 141–210. doi:10.3389/fphys.2016.00141
Lees, G. J., Lehmann, A., Sandberg, M., and Hamberger, A. (1990). The neurotoxicity of ouabain, a sodium-potassium ATPase inhibitor, in the rat hippocampus. Neurosci. Lett. 120 (2), 159–162. doi:10.1016/0304-3940(90)90027-7
Lewerenz, J., and Maher, P. (2015). Chronic glutamate toxicity in neurodegenerative diseases-What is the evidence? Front. Neurosci. 9 (DEC), 469–520. doi:10.3389/fnins.2015.00469
Li, J., Zelenin, S., Aperia, A., and Aizman, O. (2006). Low doses of ouabain protect from serum deprivation-triggered apoptosis and stimulate kidney cell proliferation via activation of NF-kappaB. J. Am. Soc. Nephrol. 17 (7), 1848–1857. doi:10.1681/ASN.2005080894
Lin, S. C., and Way, E. L. (1982). Calcium‐activated ATPases in presynaptic nerve endings. J. Neurochem. 39 (6), 1641–1651. doi:10.1111/j.1471-4159.1982.tb07998.x
Lingrel, J. B. (2009). The physiological significance of the cardiotonic steroid/ouabain-binding site of the Na, K-ATPase. Annu. Rev. Physiol. 72, 395–412. doi:10.1146/annurev-physiol-021909-135725
Liu, X. L., Miyakawa, A., Aperia, A., and Krieger, P. (2007). Na, K-ATPase generates calcium oscillations in hippocampal astrocytes. NeuroReport 18 (6), 597–600. doi:10.1097/WNR.0b013e3280b07bc9
Lomeo, R. S., Gomez, R. S., Prado, M. A. M., Romano-Silva, M. A., Massensini, A. R., and Gomez, M. v. (2003). Exocytotic release of [3H]-Acetylcholine by ouabain involves intracellular Ca2+ stores in rat brain cortical slices. Cell. Mol. Neurobiol. 23 (6), 917–927. doi:10.1023/B:CEMN.0000005320.06215.80
Lopez, L. B., Eduardo Quintas, L. M., and Noel, F. U. (2002). Influence of development on Na(+)/K(+)-ATPase expression: Isoform- and tissue-dependency. Comp. Biochem. Physiol. A Mol. Integr. Physiol. 131 (2), 323–333. doi:10.1016/s1095-6433(01)00482-2
Mark, R. J., Hensley, K., Butterfield, D. A., and Mattson, M. P. (1995). Amyloid β-peptide impairs ion-motive ATPase activities: Evidence for a role in loss of neuronal Ca2+ homeostasis and cell death. J. Neurosci. 15 (9), 6239–6249. doi:10.1523/jneurosci.15-09-06239.1995
McGarry, S. J., and Williams, A. J. (1993). Digoxin activates sarcoplasmic reticulum Ca2+‐release channels: A possible role in cardiac inotropy. Br. J. Pharmacol. 108 (4), 1043–1050. doi:10.1111/j.1476-5381.1993.tb13503.x
Miyakawa-Naito, A., Uhlen, P., Lal, M., Aizman, O., Mikoshiba, K., Brismar, H., et al. (2003). Cell signaling microdomain with Na, K-ATPase and inositol 1, 4, 5-trisphosphate receptor generates calcium oscillations. J. Biol. Chem. 278 (50), 50355–50361. doi:10.1074/jbc.M305378200
Mohammadi, K., Kometiani, P., Xie, Z., and Askari, A. (2001). Role of protein kinase C in the signal pathways that link Na +/K+-ATPase to ERK1/2. J. Biol. Chem. 276 (45), 42050–42056. doi:10.1074/jbc.M107892200
Mørk, A., Geisler, A., and Mork, A. (1993). Effects of minocycline on accumulation of cyclic AMP in cerebral cortex of rat. A comparison with lithium. Neuropharmacology 32 (8), 793–798. doi:10.1016/0028-3908(93)90188-9
Myles, M. E., and Fain, J. N. (1994). Carbachol, but not norepinephrine, NMDA, ionomycin, ouabain, or phorbol myristate acetate, increases inositol 1, 3, 4, 5-tetrakisphosphate accumulation in rat brain cortical slices. J. Neurochem. 62 (6), 2333–2339. doi:10.1046/j.1471-4159.1994.62062333.x
Nguyen, K. T., Buljan, V., Else, P. L., Pow, D. V., and Balcar, V. J. (2010). Cardiac glycosides ouabain and digoxin interfere with the regulation of glutamate transporter GLAST in astrocytes cultured from neonatal rat brain. Neurochem. Res. 35 (12), 2062–2069. doi:10.1007/s11064-010-0274-4
Nicotera, P., and Orrenius, S. (1998). The role of calcium in apoptosis. Biometals. 11 (4), 375–382. doi:10.1023/A:1009226316146
O’brien, W. J., Lingrel, J. B., and Wallick, E. T. (1994). Ouabain binding kinetics of the rat alpha two and alpha three isoforms of the sodium-potassium adenosine triphosphate. Arch. Biochem. Biophys. 310 (1), 32–39. doi:10.1006/abbi.1994.1136
Okamoto, Y., Kagaya, A., Motohashi, N., and Yamawaki, S. (1995). Inhibitory effects of lithium ion on intracellular Ca2+ mobilization in the rat hippocampal slices. Neurochem. Int. 26 (3), 233–238. doi:10.1016/0197-0186(94)00130-M
Olney, J. W. (1986). Inciting excitotoxic cytocide among central neurons. Adv. Exp. Med. Biol. 203, 631–645. doi:10.1007/978-1-4684-7971-3_48
Orellana, A. M., Leite, J. A., Kinoshita, P. F., Vasconcelos, A. R., Andreotti, D. Z., de Sa Lima, L., et al. (2018). Ouabain increases neuronal branching in hippocampus and improves spatial memory. Neuropharmacology 140, 260–274. doi:10.1016/j.neuropharm.2018.08.008
Panizza, E., Zhang, L., Fontana, J. M., Hamada, K., Svensson, D., Akkuratov, E. E., et al. (2019). Ouabain-regulated phosphoproteome reveals molecular mechanisms for Na+, K+-ATPase control of cell adhesion, proliferation, and survival. FASEB J. 33 (9), 10193–10206. doi:10.1096/fj.201900445R
Parreira, G. M., Faria, J. A., Marques, S. M. S., Garcia, I. J. P., Silva, I. F., De Carvalho, L. E. D., et al. (2021). The γ-benzylidene digoxin derivative BD-15 increases the α3-Na, K-ATPase activity in rat Hippocampus and prefrontal cortex and no change on heart. J. Membr. Biol. 254 (2), 189–199. doi:10.1007/s00232-021-00173-2
Pierre, S. v., and Xie, Z. (2006). The Na, K-ATPase receptor complex: Its organization and membership. Cell biochem. Biophys. 46 (3), 303–316. doi:10.1385/CBB:46:3:303
Pincus, J. H., and Lee, S. (1973). Diphenylhydantoin and calcium. Relation to norepinephrine release from brain slices. Arch. Neurol. 29 (4), 239–244. doi:10.1001/archneur.1973.00490280051007
Prueitt, R. L., Li, W., Chang, Y. C., Boffetta, P., and Goodman, J. E. (2020). Systematic review of the potential respiratory carcinogenicity of metallic nickel in humans. Crit. Rev. Toxicol. 50 (7), 605–639. doi:10.1080/10408444.2020.1803792
Quintas, L. E. M., Pierre, S. v., Liu, L., Bai, Y., Liu, X., and Xie, Z. J. (2010). Alterations of Na+/K+-ATPase function in caveolin-1 knockout cardiac fibroblasts. J. Mol. Cell. Cardiol. 49 (3), 525–531. doi:10.1016/j.yjmcc.2010.04.015
Readhead, C., and Hood, L. (1990). The dysmyelinating mouse mutations shiverer (shi) and myelin deficient (shimld). Behav. Genet. 20 (2), 213–234. doi:10.1007/BF01067791
Riganti, C., Campia, I., Kopecka, J., Gazzano, E., Doublier, S., Aldieri, E., et al. (2011). Pleiotropic effects of cardioactive glycosides. Curr. Med. Chem. 18 (6), 872–885. doi:10.2174/092986711794927685
Sasaki, T., Naka, M., Nakamura, F., and Tanaka, T. (1992). Ruthenium red inhibits the binding of calcium to calmodulin required for enzyme activation. J. Biol. Chem. 267 (30), 21518–21523. doi:10.1016/s0021-9258(19)36640-2
Satoh, E., and Nakazato, Y. (1989). [3H]acetylcholine release and the change in cytosolic free calcium level induced by high K+ and ouabain in rat brain synaptosomes. Neurosci. Lett. 107 (1–3), 284–288. doi:10.1016/0304-3940(89)90832-X
Schatzmamn, H. J. (1953). Cardiac glycosides as inhibitors of active potassium and sodium transport by erythrocyte membrane. Helv. Physiol. Pharmacol. Acta 11 (4), 346–354. doi:10.1038/cddis.2013.140
Schoner, W., and Scheiner-Bobis, G. (2007). Endogenous and exogenous cardiac glycosides: Their roles in hypertension, salt metabolism, and cell growth. Am. J. Physiol. Cell Physiol. 293 (2), C509–C536. doi:10.1152/ajpcell.00098.2007
Shi, M., Cao, L., Cao, X., Zhu, M., Zhang, X., Wu, Z., et al. (2019). DR-region of Na +/K + ATPase is a target to treat excitotoxicity and stroke. Cell Death Dis. 10 (1), 6. doi:10.1038/s41419-018-1230-5
Somjen, G. G. (2001). Mechanisms of spreading depression and hypoxic spreading depression-like depolarization. Physiol. Rev. 81 (3), 1065–1096. doi:10.1152/physrev.2001.81.3.1065
Stelmashook, E. v., Weih, M., Zorov, D., Victorov, I., Dirnagl, U., and Isaev, N. (1999). Short-term block of Na+/K+-ATPase in neuro-glial cell cultures of cerebellum induces glutamate dependent damage of granule cells. FEBS Lett. 456 (1), 41–44. doi:10.1016/S0014-5793(99)00922-9
Sui, L., Song, X. J., Ren, J., Ju, L. H., and Wang, Y. (11992013). Intracerebroventricular administration of ouabain alters synaptic plasticity and dopamine release in rat medial prefrontal cortex. J. Neural Transm. 120 (8), 1191–1199. doi:10.1007/s00702-013-0973-5
Swanson, P. D., Anderson, L., and Stahl, W. L. (1974). Uptake of calcium ions by synaptosomes from rat brain. Biochim. Biophys. Acta 356 (2), 174–183. doi:10.1016/0005-2736(74)90281-8
Tata, A., Velluto, L., D’Angelo, C., and Reale, M. (2014). Cholinergic system dysfunction and neurodegenerative diseases: Cause or effect? CNS Neurol. Disord. Drug Targets 13 (7), 1294–1303. doi:10.2174/1871527313666140917121132
Tian, J., Cai, T., Yuan, Z., Wang, H., Liu, L., Haas, M., et al. (2006). Binding of Src to Na +/K + -ATPase forms a functional signaling complex. Mol. Biol. Cell 17 (1), 317–326. doi:10.1091/mbc.e05-08-0735
v Blasi, J. M., Cefia, V., Gonzfilez-Garcia, C., Marsal, J., and Solsona, C. (1988). Ouabain induces acetylcholine release from pure cholinergic synaptosomes independently of extracellular calcium concentration. Neurochem. Res. 13 (11), 1035–1041. doi:10.1007/BF00973147
Valadares, J. M. de M., Bajaj, S. O., Li, H., Wang, H. L., Silva, S. C., Garcia, I. J. P., et al. (2021). Cytotoxic effect of carbohydrate derivatives of digitoxigenin involves modulation of plasma membrane Ca2+-ATPase. J. Cell. Biochem. 122 (12), 1903–1914. doi:10.1002/jcb.30150
Veldhuis, W. B., van der Stelt, M., Delmas, F., Gillet, B., Veldink, G. A., Vliegenthart, J. F., et al. (2003). In vivo excitotoxicity induced by ouabain, a Na+/K+-ATPase inhibitor. J. Cereb. Blood Flow. Metab. 23 (1), 62–74. doi:10.1097/01.WCB.0000039287.37737.50
Volterra, A., Trotti, D., Tromba, C., Floridi, S., and Racagni, G. (1994). Glutamate uptake inhibition by oxygen free radicals in rat cortical astrocytes. J. Neurosci. 14 (1), 2924–2932. doi:10.1523/JNEUROSCI.14-05-02924.1994
Whayne, T. F. (0120). “Clinical use of digitalis: A state of the art review,” in American journal of cardiovascular drugs (Springer International Publishing), 18, 427–440. doi:10.1007/s40256-018-0292-1
Wiecien, D. K. J. M., O’connor, , , O’connor, L. T., Goetz, B. D., Delaney, K. H., and Duncan, I. D. (1998). Morphological and morphometric studies of the dysmyelinating mutant, the Long Evans shaker rat. J. Neurocytol. 27 (8), 581–591. doi:10.1023/a:1006922227791
Xiao, A. Y., Wei, L., Xia, S., Rothman, S., and Yu, S. P. (2002). Ionic mechanism of ouabain-induced concurrent apoptosis and necrosis in individual cultured cortical neurons. J. Neurosci. 22 (4), 1350–1362. doi:10.1523/jneurosci.22-04-01350.2002
Xie, Z., and Askari, A. (2002). Na+/K+-ATPase as a signal transducer. Eur. J. Biochem. 269 (10), 2434–2439. doi:10.1046/j.1432-1033.2002.02910.x
Yamazaki, T., Akiyama, T., Kitagawa, H., KomakiF., , , Mori, H., Kawada, T., et al. (2007). Characterization of ouabain-induced noradrenaline and acetylcholine release from in situ cardiac autonomic nerve endings. Acta Physiol. 191 (4), 275–284. doi:10.1111/j.1748-1716.2007.01749.x
Yuan, Z., Cai, T., Tian, J., v Ivanov, A., Giovannucci, D. R., and Xie, Z. (2005). Na/K-ATPase tethers phospholipase C and IP3 receptor into a calcium-regulatory complex. Mol. Biol. Cell 16, 4034–4045. doi:10.1091/mbc.e05-04-0295
Zhang, S., Malmersjö, S., Li, J., Ando, H., Aizman, O., Uhlén, P., et al. (2006). Distinct role of the N-terminal tail of the Na, K-ATPase catalytic subunit as a signal transducer. J. Biol. Chem. 281 (31), 21954–21962. doi:10.1074/jbc.M601578200
Zhu, Z., Tepel, M., Neusser, M., and Zidek, W. (1996). Low concentrations of ouabain increase cytosolic free calcium concentration in rat vascular smooth muscle cells. Clin. Sci. 90 (1), 9–12. doi:10.1042/cs0900009
Keywords: ouabain, Na+/K+-ATPase, calcium, signaling, nervous system
Citation: Leite JA, Pôças E, Maia GS, Barbosa L, Quintas LEM, Kawamoto EM, Silva MLCd, Scavone C and de Carvalho LED (2022) Effect of ouabain on calcium signaling in rodent brain: A systematic review of in vitro studies. Front. Pharmacol. 13:916312. doi: 10.3389/fphar.2022.916312
Received: 09 April 2022; Accepted: 20 July 2022;
Published: 29 August 2022.
Edited by:
Anna Boguszewska-Czubara, Medical University of Lublin, PolandReviewed by:
Elena Bossi, University of Insubria, ItalyClemens Möller, Hochschule Albstadt-Sigmaringen, Germany
Copyright © 2022 Leite, Pôças, Maia, Barbosa, Quintas, Kawamoto, Silva, Scavone and de Carvalho. This is an open-access article distributed under the terms of the Creative Commons Attribution License (CC BY). The use, distribution or reproduction in other forums is permitted, provided the original author(s) and the copyright owner(s) are credited and that the original publication in this journal is cited, in accordance with accepted academic practice. No use, distribution or reproduction is permitted which does not comply with these terms.
*Correspondence: Luciana E. Drumond de Carvalho, bHVpYW5hZHJ1bW9uZEB1ZnNqLmVkdS5icg==