- 1Medical College, Guangxi University, Nanning, China
- 2Zhuhai Precision Medical Center, Zhuhai People’s Hospital (Zhuhai Hospital Affiliated With Jinan University), Jinan University, Zhuhai, China
Photodynamic Therapy (PDT) with the intrinsic advantages including non-invasiveness, spatiotemporal selectivity, low side-effects, and immune activation ability has been clinically approved for the treatment of head and neck cancer, esophageal cancer, pancreatic cancer, prostate cancer, and esophageal squamous cell carcinoma. Nevertheless, the PDT is only a strategy for local control of primary tumor, that it is hard to remove the residual tumor cells and inhibit the tumor metastasis. Recently, various smart nanomedicine-based strategies are developed to overcome the barriers of traditional PDT including the drawbacks of traditional photosensitizers, limited tissue penetrability of light, inefficient induction of tumor cell death and tumor resistance to the therapy. More notably, a growing number of studies have focused on improving the therapeutic efficiency by eliciting host immune system with versatile nanoplatforms, which heralds a broader clinical application prospect of PDT in the future. Herein, the pathways of PDT induced-tumor destruction, especially the host immune response is summarized, and focusing on the recent progress of nanosystems-enhanced PDT through eliciting innate immunity and adaptive immunity. We expect it will provide some insights for conquering the drawbacks current PDT and expand the range of clinical application through this review.
Introduction
Photodynamic Therapy (PDT) has been applied as adjuvant tumor therapy for more than 40 years (Li et al., 2020). It depends on a basic principles that the photosensitizer (PS) is excited by a light with an appropriate wavelength, and the excited PS directly transfers energy to the oxygen to produce reactive oxygen species (ROS), such as singlet oxygen (1O2), superoxide anions (O2−) and hydroxyl radicals (OH) in tumor cells (Dolmans et al., 2003). The highly reactive ROS will be result in the oxidation of the biomolecules in cells, including nucleic acids, lipids, and proteins, leading to severe alteration in cell signaling cascades or in gene expression regulation (Robertson et al., 2009). Consequently, the ROS directly or indirectly destroys tumor cells via apoptotic, necrotic and autophagy-associated cell death. Moreover, some photosensitizers are bound to serum protein and transfer to endothelial cells of tumor blood vessels. During laser irradiation, the tumor-associated vasculature will also be damaged, which can lead to thrombosis and hemorrhage in tumor blood vessels. Subsequently, the tumor growth can be inhibit owing to the lack of oxygen and nutrients (Janas et al., 2021; Wang et al., 2022c). In addition, PDT also induce the acute inflammation and trigger the release of cytokines and stress response proteins. Firstly, as a major characteristic of acute inflammation, the neutrophils will be activated in circulation and migrate across blood vessels to move to the infectious or injured sites (Chu et al., 2018), thus the acute inflammation induced by PDT can induce the recruitment of some neutrophils to kill cancer cells. Meanwhile, the injuries of the blood vessel and tumor cells also attract the macrophages infiltration, regulating the polarization of macrophage and improving the phagocytosis of macrophages to tumor cells (Zhou et al., 2009; Korbelik and Hamblin, 2015). Several studies have also suggested that PDT can improve the Natural Killer (NK) cells activity and initiate the immunity. Apart from the direct cytotoxicity, NK can stimulate the maturation of dendritic cells (DCs) and subsequently activate the adaptive immune cells like monocytes, cytotoxic T lymphocytes (CTLs), and B cells to enhance the whole immune response by secreting cytokines (Cantoni et al., 2016; Cerwenka and Lanier, 2016; Wang et al., 2019b). Notably, the PDT will trigger the release of adenosine triphosphate (ATP) and high-mobility group box 1 protein (HMGB1) from the dying cells, meanwhile, the calreticulin (CRT), heat shock proteins (HSPs) will be exposed on the surface of dying cells. These molecular provide “eat me” signal to initiate an immune response (Shaif-Muthana et al., 2000). It promotes the mutation of antigen presenting cells (APCs) and presentation of tumor associate antigen (TAA). Meanwhile, the secretion of cytokines (IFN-γ, IFN-α) will be boosted and further promote the APCs maturation and CTLs homing (Figure 1) (Beltran Hernandez et al., 2020). The activation of anti-tumor immunity not only contributes to the destruction of primary tumor cells, but also destroys the tumor cells even at isolated locations (Ji et al., 2022).
The mechanisms of tumor damage caused by PDT mainly include the following types: 1) PS is enriched in tumor vascular endothelial cells, causing platelet coagulation, vasoconstriction, and thrombosis. Tumor blood vessels are blocked, resulting in hypoxic infarction of tumor tissue. 2) ROS directly destroys proteins, nucleic acids, lipids, etc. of cells, causing apoptosis, necrosis and autophagy of cells. 3) PDT-triggered inflammatory responses activate innate and adaptive immune responses.
Nevertheless, the broad application of conventional PDT remains many challenges in the clinic practice. It is well-known that PS, oxygen, and light as three essential components are involved in PDT. Typically, most of the PS lacks the tumor-targeting ability and the effective dosage is insufficient in tumors (Idris et al., 2015). Furthermore, the hypoxia tumor microenvironment, limited light penetration towards tumor tissues, and insufficient T cell infiltration in tumors also greatly limited the efficacy of PDT (Zhang et al., 2018a). Recently, with the development of nanotechnology, various advanced nanoparticles were developed to overcome above drawbacks and expand the range of application, showing the great potential for improving the PDT efficacy. Numerous nanomaterials have been reported to have the ability to produce ROS under the light excitation, enabling them to serve as nano-photosensitizers in PDT (Table 1) (Wang et al., 2015; Sun et al., 2019b). Moreover, some nanoparticles are also ideal carriers for traditional photosensitizers (Lucky et al., 2015; Xie et al., 2021). The nano-photosensitizers and nano-delivery systems are able to accumulate more efficiently in tumor tissue through the enhanced permeability and retention (EPR) effect owing to the particle size and the abnormal vascular structure of tumors (Peer et al., 2007; Hao et al., 2020; Li et al., 2021b; Liang et al., 2021b). The nanomaterials can also be further modified or functionalized to improve the targeting efficacy, enhancing the dosage of in tumor and reducing toxicity to normal tissues (Zhong et al., 2015; Seidi et al., 2018; Liang et al., 2021a). Furthermore, nanomaterial-based photosensitizers have better photostability than traditional ones, which is able to produce ROS for a longer time (Wang et al., 2020b). It is worth noting that nanomaterials mediated PDT endow the excitation light source and approach more flexible and adjustable. For example, various lanthanide-doped upconversion nanoparticles (UCNPs) are developed and applied for UCNPs-based PDT. It will be excited by near infrared light (NIR), which can penetrate deep tissues (>3 cm) with lower light scattering and absorption of human tissues (Chen et al., 2017; Zhang et al., 2018a). Other excitation approach including ultrasound and X-ray gradually derived new therapeutic strategies termed sonodynamic therapy (SDT) and radiodynamic therapy (RDT), respectively, also provide novel approach for breaking through the limitation of tissue penetration depth (Chen et al., 2015; Lu et al., 2018; Pan et al., 2018; Ni et al., 2022; Nowak et al., 2022). To relieve the hypoxia in tumor microenvironment and improve the PDT efficacy, several strategies based on nanomaterials have emerged so far. In addition to the direct delivery of oxygen with nanoplatform, some nanoparticles can be served as nanoreactor to catalyze the release of oxygen in tumor microenvironment, such as MnO2, CaO2 and two-dimensional nanomaterials (Fan et al., 2016; Ji et al., 2019; Zhou et al., 2020; Wang et al., 2022a; Jin et al., 2022; Zeng et al., 2022). Besides, acceleration of intratumoral blood flow is also useful for increasing O2 concentrations in hypoxic tumors. Previous research suggests that photothermal-mediated heating will increase the blood flow and combination with photothermal therapy is regarded to enhance PDT inhibition of hypoxic tumors (Wu et al., 2019a; Chen et al., 2022). Benefiting from the unique characteristics of multifunctional nanomaterials, multiple therapeutic strategies can be simultaneously mediated by one nanoplatform to synergistically inhibit tumor, such as the combination of gas therapy (Wan et al., 2018; Wang et al., 2022b) and chemotherapy (Yang et al., 2019a; Wang et al., 2019e), etc., Recently, some emerging nanomaterials such as heterojunction-based nanoparticles have been prepared to enhance the generation efficacy of ROS through catalytic reaction, deriving a new therapeutic strategy termed catalytic therapy. The combination of PDT and catalytic therapy implied a promising strategy to strengthen the therapeutic efficiency (Pan et al., 2019; Kang et al., 2021; Kong et al., 2021; Pan et al., 2022).
Tumor recurrence and metastasis are vital causes of treatment failure and death of patients. Although PDT can induce host anti-tumor immune response, the short-acting and insufficient anti-tumor immunity is hard to control the tumor recurrence and metastasis due to the existence of immunosuppressive microenvironment. A large number of studies currently is committed to elicit robust anti-tumor immunity after PDT, and imply that activation of antitumor immune effects is a highly promising strategy to enhance PDT efficacy and expand PDT indications. Nowadays, the advanced nanomaterials have opened new promising avenues to refine and improve the anti-tumor efficacy through combining PDT with immunity activation. In addition to improve the therapeutic efficacy by excellently codelivering PS to tumors, relieve hypoxic microenvironment and combining with other treatment approaches, etc., the multifunctional nanomaterials can regulate the tumor immune microenvironment, including eliciting innate immunity and adaptive immunity (Table 2) (Xu et al., 2017; Sang et al., 2019; Chen et al., 2020; Chen et al., 2021).
Eliciting Innate Immunity
After PDT, cell debris or various cytoplasmic components resulting from tumor death and lysis can cause a strong inflammatory response in surrounding tissues. Various cells in the TME, such as surviving tumor cells, damaged endothelial cells, tumor stromal cells, etc., can release numerous pro-inflammatory mediators, including arachidonic acid, cytokines such as MIP2 (CXCL2), IL6, IL- 1β, TNFα, complement system, etc.. These components enhance chemotaxis, activation, and phagocytosis of macrophages, and aggregate neutrophils, triggering a strong innate immune response. At the same time, macrophages will present cell debris and dead tumor cells as antigens to T lymphocytes, and correspondingly activate lymphocyte-based adaptive immunity (Jin et al., 2022). Therefore, PDT-induced local tumor tissue inflammatory response activates innate and adaptive immune responses (Beltran Hernandez et al., 2020). The innate immune cells can detect tumors, induce and amplify adaptive immune responses, and exert direct effector responses. Given the crucial role of innate immune responses in antitumor immunity, harnessing manipulates innate immune responses in cancer opens up new possibilities for long-lasting, multilayered tumor control.
Potentialities of Neutrophils to Enhance Photodynamic Therapy
Neutrophils, known as polymorphonuclear leukocytes (PMN), are the major immune cell population in human blood and serve as the first line of defense against invading pathogens (Rosales, 2018). In recent years, increasing evidence has shown that neutrophils have host defense, immunomodulatory functions, and play an important role in cancer therapy (Wu et al., 2019b). The polarization of primary human neutrophils in vitro to generate N1 and N2 neutrophils was first proposed by Mareike et als. N1 polarized neutrophils have pro-inflammatory properties and promote the release of interferons, chemokines, and tumor necrosis factors (Ohms et al., 2020). Although there is an urgent need to fully understand the interaction between neutrophils and their tumor microenvironment, most of the current studies are still limited to mouse tumor models, and continued research is needed to verify whether human neutrophils have macrophage-like polarization (Eruslanov et al., 2017; Wu et al., 2020).
Numbers studies have shown that PDT promotes neutrophil infiltration in tumor tissues and then activates innate immunity (Cecic et al., 2006). Since neutrophils are able to migrate into inflamed tumors, the use of neutrophil-mediated drug delivery systems to enhance drug accumulation and sustained release at tumor sites opens new avenues for nanomedicine development. Therefore, Qiu et al. (2021) constructed a nanocomplex (SA-2@NCs) capable of targeting activated peripheral blood neutrophils (PBN), which was formed by encapsulating ibrutinib (IBR) drugs with targeted sialic acid (SA). First, liposomes loaded with photosensitizer DIR were injected into the tail vein, and PDT/PTT treatment was performed to induce acute inflammation and rapidly activate PBNs to infiltrate the tumor site. Then, SA-2@NCs nanocomplexes were injected into the tail vein, which accumulated at the tumor site after being internalized by activated PBN. Combining IBR-mediated immunotherapy and DIR-mediated PDT/PTT for antitumor can effectively inhibit tumor growth and metastasis (Qiu et al., 2021). However, there are some difficulties in the extraction and preservation of neutrophil membranes, and the stability of nanoparticles needs to be improved.
In summary, nanoparticles wrapped with neutrophil membranes promote the recruitment of nanoparticles in tumor tissues and improve the tumor targeting of nanoparticles. Notably, nanoparticles loaded with immune activators can be tried to activate neutrophil N1 polarization and enhance antitumor immune response.
Activation of Natural Killer Cells
NK cells are innate immune cells that are the first line of defense against infection and cancer. NK cells can spontaneously damage target cells in the absence of antigen-specific stimulation and major histocompatibility complex. Numerous studies have shown that the antitumor immune response induced by PDT enhances the activity of NK cells. Enhancing PDT-induced immune response by activating NK cells may be benefit improve the therapeutic effects (Zheng et al., 2013). Protective mechanisms such as anti-apoptotic mechanisms and therapeutic escape pathways in melanoma hinder the application of PDT in melanoma. Therefore, Wang et al. (2019b) designed a special nanoliposomes to enhance Melanoma PDT and immune activation of NK cells. The main components of nanoliposomes, including phosphatidylcholines, Ce6, and low molecular citrus pectin (LCP), are all in clinical use or biodegradable. Nanoliposomes as a trigger to enable the cytoplasmic release of LCP. LCP promotes the binding of NKG2D to MICA on tumor cell membranes and indirectly activates NK cells, giving the nanoparticles a synergistic effect of enhancing PDT and NK cell-related immunity. However, further more molecular and immune studies should be performed in the future to elucidate the role of LCP in PDT antitumor immunity. Furthermore, the natural tropism of NK cells is exploited during PDT to improve the tumor-targeting effect of nanoparticles. In antitumor immunotherapy, NK cell membrane can induce polarization of M1-macrophages, providing membrane immune-inducers for stimulating the immune response during tumor therapy. Therefore, some researchers have designed a NK cell membrane-encapsulated nanoparticle (NK-NPs), which target cancer cells and induce M1 macrophage polarization, thus induce tumor-specific immune responses (Figure 2A) (Deng et al., 2018). The in vivo results demonstrated that NK-NPs-mediated PDT could enhance NK cell membrane immunotherapy, eliminate primary tumor growth, and produce distant effects that inhibit distant untreated tumors. As shown in Figures 2B–F. In addition, blockade the immune checkpoint of NK cells may enhance the therapeutic effect of PDT. For example, some researchers have found that T-cell immunoglobulin and ITIM domain (TIGIT) is expressed on activated T cells and is also found on NK cells, memory T cells, a subset of Treg cells as well as follicular T helper cells. TIGIT directly inhibits NK and effector T cells, and it also inhibits immune responses through promoting Treg cell function (Anderson et al., 2016). In some mouse models, blocking TIGIT prevents NK cell depletion. And blocking T-cell immune receptor with TIGIT enhances NK cell-dependent tumor-specific T-cell immunity. Loading TIGIT inhibitors into nanoparticles may be a new direction for future research.
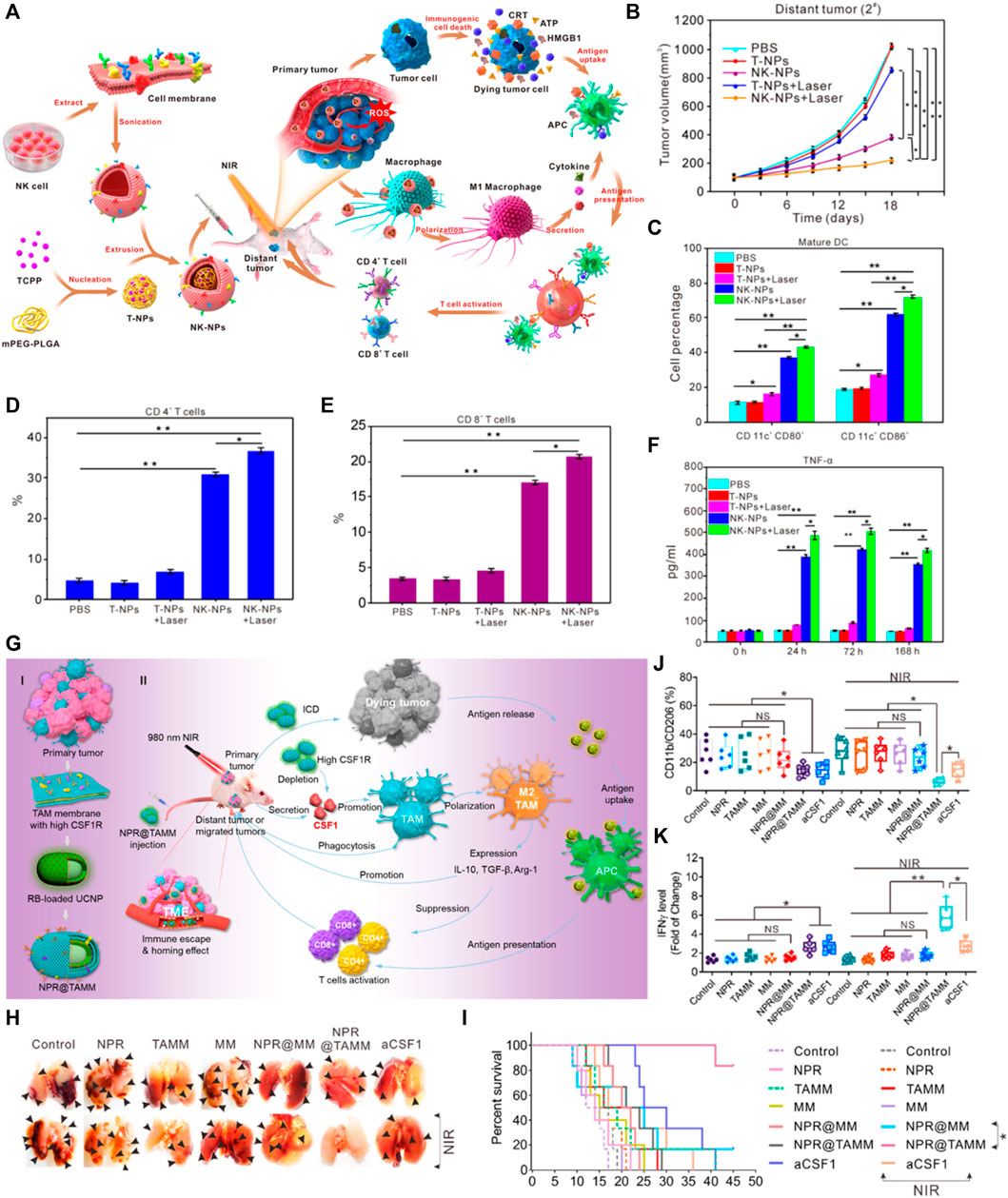
FIGURE 2. Example of nanomedicine-based PDT to activate innate immune responses. (A) Schematic Illustration of NK Cell-Membranes-Cloaked Nanoparticles for PDT-Enhanced Cell-Membrane Immunotherapy. (B) Growth curves for the distal tumors. (C) In vivo maturation of DCs (CD80+ and CD86+) from tumor-draining lymph nodes in BALB/c mice following intravenous injection of T-NPs or murine NK-NPs (n = 3 per group). (D) Proportions of tumor-infiltrating CD4+ T cells. (E) Proportions of tumor-infiltrating CD8+ T cells. (F) Pro-inflammatory cytokines (TNF-α) levels in the sera of mice treated with murine NK-NPs-mediated PDT from day 0, day 1, day 3, and day 7 (Deng et al., 2018). (G) Schematic illustration of the tumor-associated-macrophage-membrane-coated upconversion nanoparticles for improved photodynamic immunotherapy. (H) Photographs show representative external views of lung nodules. (I) The survival curve of tumor-bearing mice calculated by Kaplan−Meier estimate. (J) Quantification by flow cytometry of the ratio of CD11b + CD206 + cell populations in the different treatment groups of tumor-bearing mice. (K) ELISA assay of IFN-γ in tumor-bearing mice with different treatments (Chen et al., 2021). Data are means ± SD. *p < 0.05; **p < 0.01. NS, no significance.
In conclusion, the immune response can be improved by regulating the activation of NK cells at different targets during PDT. The natural tropism of NK cell membranes is exploited to improve the targeting of nanoparticles to tumors. The immunotherapeutic approach of the NK synergistic PDT offers a strategy for tumor immunotherapy.
Regulation of Macrophage Polarization
It is now well established from a variety of studies that macrophages can be divided into M1 macrophages, which have antitumor effects, and M2 macrophages, which promote tumor proliferation (Qian and Pollard, 2010; Lu et al., 2016). In vivo, M1 macrophages are responsible for recruiting Helper T cells, cytotoxic T lymphocyte, and NK cells, that directly target infected or tumor cells (Mantovani et al., 2004; Beatty et al., 2011; Murray and Wynn, 2011; Butcher and Galkina, 2012). Compared with M1 macrophages, M2 macrophages exhibit an anti-inflammatory phenotype and are present in infections, allergies, tissue reconstruction, and tumor development (Satoh et al., 2010). Tumor-associated macrophages (TAMs) are a prominent component of the stroma and leukocyte infiltrates in tumors (Azria et al., 2003; Balkwill, 2009). On the one hand, TAMs surround tumor cells and interact in the TME to promote tumor growth, progression, and metastasis, leading to immunosuppression. On the other hand, TAMs inhibit tumor growth after transformation to the M1 phenotype (Qiu et al., 2018). Therefore, a large number of studies support the concept of TAMs reprogramming as a sufficient and feasible approach to initiate T-cell-mediated antitumor immunity (Cassetta and Pollard, 2018). PDT induce immunogenic cell death (ICD) to release damage-associated molecular patterns (DAMPs), thus polarize macrophages from an immunosuppressive M2 phenotype to an antitumor M1 phenotype (Tan et al., 2016; Zhou et al., 2018). A large number of studies have confirmed demonstrated that the polarization of M1 macrophages could be promoted by structural modification of PDT nanoparticles and the loading of photosensitizer (Wang et al., 2019c). In addition, some nanoparticles also affect macrophage polarization by initiating ferroptosis. Ferroptosis is a new cell death format identified in recent years. Ferroptosis can effectively inhibit tumor growth and induce immune response. Considering the central role of iron in ferroptosis, many studies have focused on iron-based nanomaterials. Jiang et al. (2020) reported a PLT membrane-camouflaged Fe3O4-SAS [magnetic nanoparticle loaded with sulfasalazine (SAS)]. Fe3O4 nanoparticles are ferroptosis inducers. And the study revealed that Fe3O4-SAS@PLT-mediated ferroptosis could repolarize macrophages from immunosuppressive M2 phenotype to antitumor M1 phenotype and elicit an effective immune response. This nanoparticle-mediated ferroptosis combined with SAS immunotherapy effectively inhibited tumor growth and metastasis compared to a single treatment (Jiang et al., 2020). On the other hand, Chen et al. designed a PDT nanoparticle (NPR@TAMMs) wrapped by TAMs membrane (TAMM). Studies on the TAMM encapsulated nanoparticles showed unique antigen-homing affinity and biocompatibility. Meanwhile, TAMMs can deplete macrophage colony-stimulating factor 1 (CSF1). CSF1 is a key regulator of monocyte/macrophage differentiation and maintains the tumor-promoting function of TAMs. By displaying TAM membranes on the surface of nanoparticles, it is expected that NPR@TAMMs can mimic the source cells, thus binding to the immunomodulatory molecule CSF1, reducing serum CSF1 levels and blocking CSF1/CSF1R signaling, leading to immunosuppressive inactivation (Figures 2G–I). This PDT-immunotherapy approach based on macrophage membrane provides a new strategy for personalized cancer treatment (Chen et al., 2021). Overall, the PDT nanoparticles could improve the antitumor innate immune response through loading of agonists or modifying with TAMM and NK cell membranes.
Eliciting Adaptive Immunity
A growing number of studies have shown that acute inflammation triggered by PDT recruits leukocytes to the tumor area and secretes chemokines, then promote the maturation of dendritic cells (DCs) and activating T and B cells, leading to adaptive immune responses (Brackett and Gollnick, 2011; Yang et al., 2016). Although some studies have suggested that B cells play a role in PDT-induced antitumor immunity and mechanism of B cell-mediated antitumor immunity has not been elucidated (Preise et al., 2009; Brackett and Gollnick, 2011; Rocha et al., 2015). Therefore, in this section, we focus on PDT-induced T-cell-mediated tumor-specific immune responses.
Promotion of Dendritic Cells Maturation
Currently, the mechanism of antitumor immune response by PDT has been described mainly by the immunogenic cell death (ICD), which can transform a “cold” tumor into a “hot” (Kumar et al., 2021). ICD induces DAMP such as calreticulin (CRT), high mobility group protein 1 (HMGB1), and heat shock protein (HSP-70/90) (Adkins et al., 2014; Radogna and Diederich, 2018; Li et al., 2019). These danger signals cause APC to present antigens (Turubanova et al., 2019). Subsequently, T lymphocyte infiltration increases and its mediated adaptive immune response is activated (Kabingu et al., 2007; Duan et al., 2016). Consequently, activation of DC maturation and promotion of antigen presentation may contribute to the improvement of the antitumor efficacy of PDT.
Numerous studies have reported enhanced DC maturation and activation and increased secretion of pro-inflammatory cytokines after PDT (Gollnick and Brackett, 2010; Kushibiki et al., 2010; Brackett and Gollnick, 2011; Zheng et al., 2016). The infiltration of DCs in tumor tissues is essential for antigen presentation in the immune response. On the one hand, adoptive DCs can be attempted to promote the immune response. Sur et al. (2008) appropriately transferred DCs of homozygous bone marrow origin into PDT-treated tumors and found that this treatment improved the survival rate of rats. On the other hand, designing DCs vaccines for tumor-targeted immunotherapy. Studies have shown that a DC-based tumor vaccine would be a safe and promising tool for cancer therapy. Yang et al. (2019b) reported a multimeric nanoformulation capable of producing tumor-associated antigens (TAAs) and acting as DC immune adjuvants. The nanoformulation was based on a chimeric cross-linked polymersome (CCP) as a carrier to encapsulate doxorubicin hydrochloride (DOX) and a photosensitizer 2-(1-hexyloxyethyl)-2-devinyl pyropheophorbide-a (HPPH). The chemotherapeutic drug DOX can induce immunogenic cell death (ICD), the cell debris generated by PDT acts as TAAs, and in addition, CCPS with amine groups acts as an adjuvant for DCs. Combined PDT and immune adjuvants can enhance the recruitment of TAAs and DCs, promote the maturation of DC cells, and trigger a cascade of immune responses (Figure 3A) (Yang et al., 2019b). About 3.02% of the DCs were activated in the tumor tissue of the CCPS/HPPH/DOX treated group and about 1.7% of the cytotoxic T lymphocyte population infiltrated into the mouse tumor tissue, which was significantly higher than that of the control group (Figures 3B,C). Garg et al. (2016) developed a DC-based vaccine using chrysin-PDT that induced a strong immune response and improved overall survival in a mouse glioma model. Numerous trials have shown that dendritic cell-based vaccination can induce tumor-specific T-cell responses. However, the therapeutic effect of activating DCs to promote antigen presentation is limited due to the lack of prevalent antigens in the immune system. There are several other studies that focus on immune regulation during PDT. A novel therapeutic strategy is to construct the nanoparticles loaded with photosensitizers and DCs agonists. Im et al. (2019) reported a low oxygen response mesoporous silica nanocapsule (CAGE) for PDT. The nanocapsules were loaded with Ce6 and their surfaces were modified with glycol chitosan (GC) and PEG. The negatively charged CpG is loaded onto the CAGE surface by electrostatic interaction with GC. CpG oligonucleotides (CpG) bind to TLR9 and activate dendritic cells, upregulating costimulatory markers for antigen presentation. In this study, modification of nanoparticles activated DCs and increased DCs antigen presentation, which subsequently enhanced antigen presentation (Im et al., 2019). The developed immune adjuvant delivery system reflects its function in the tumor-associated immune microenvironment, demonstrating the potential of adjuvant immunotherapy combined with PDT in antitumor therapy.
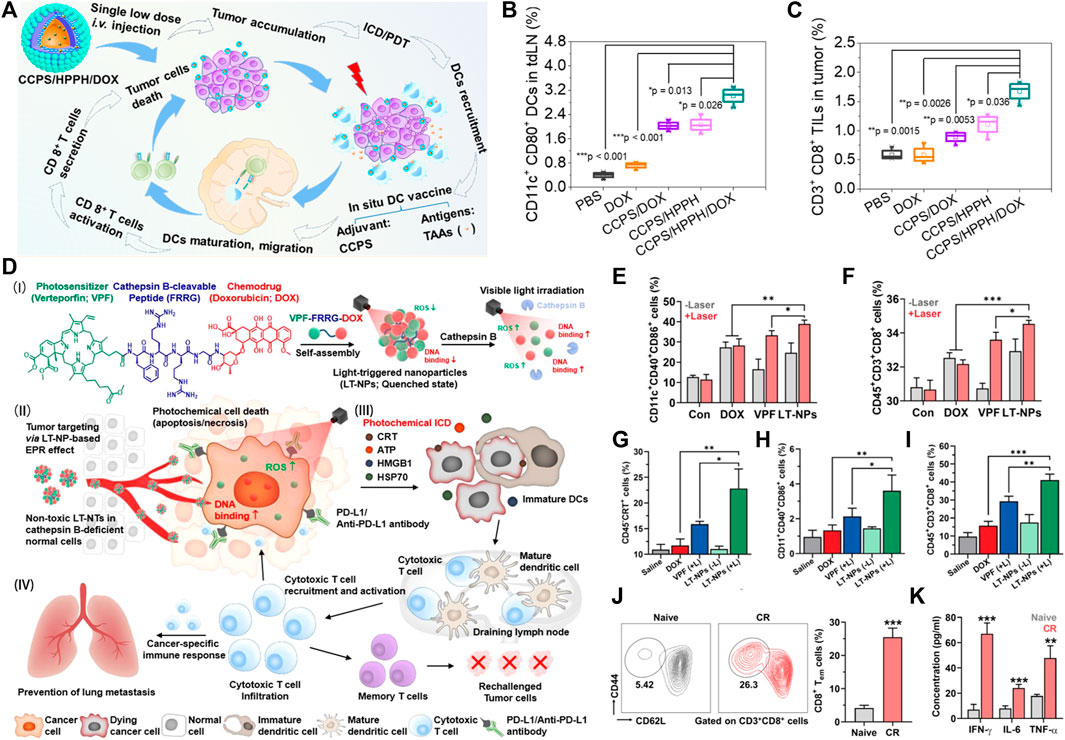
FIGURE 3. Example of nanomedicine-based PDT to activate adaptive immune responses. (A) Schematic illustration of an in situ DC vaccine exploiting chimeric cross-linked polymersomes (CCPS) as adjuvant combined with tumor-associated antigens (TAAs) induced by PDT and ICD for MC38 colorectal cancer immunotherapy. (B) Activated DC ratio in tumor-draining lymph nodes (tdLNs) for mice treated with different nanoformulations (n = 3). (C) Tumor infiltrating lymphocytes in tumor sites after treatment (n = 3) (Yang et al., 2019b). (D) Schematic illustration of visible-light-triggered prodrug nanoparticles (LT-NPs) combined chemotherapy and PDT to potentiate checkpoint blockade cancer immunotherapy. (E,F) Percentage of (E) matured DCs (CD11c + CD40 + CD86+) and (F) cytotoxic T cells (CD45 + CD3+ CD8+) in lymphocytes after coculture with culture medium containing CT26 cells treated with DOX, VPF, or LT-NPs in the presence or absence of light irradiation (n = 5). (G–I) Percentage of (G) CRT-positive cancer cells (CD45−CRT+), (H) tumor-infiltrating matured dendritic cells (CD11c + CD40 + CD86+), and (I) tumor-infiltrating cytotoxic T cells (CD45 + CD3+ CD8+) on day 7 after treatments (n = 5). (J) Percentage of splenic effector/memory T cells among the CD8+ T cells (CD3+CD8+CD44 + CD62Llow) in mice that experienced CR by LT-NPs (+L) with anti-PD-L1 antibody on day 100 after treatment and naive mice (n = 5). (K) Cytokine levels in serum isolated 20 days after CR mice were rechallenged with secondary tumors, compared to naive mice (n = 5) (Choi et al., 2021). Data are means ± SD. *p < 0.05, **p < 0.01, ***p < 0.001.
In sum up, activating DC to enhance the antitumor immune efficacy of PDT is a promising approach. Adoptive DC, DC vaccine and the DCs agonists could promote DC maturation, thus facilitate antigen presentation and subsequent immune response.
Depletion of Tumor-Associated T Regulatory Cells
Previous studies have shown that PDT induces adaptive immune responses in a CTL-dependent manner (Zheng et al., 2013; Kleinovink et al., 2017). An important aspect of PDT-mediated antitumor immunity is the effective infiltration of T cells into tumors (Ino et al., 2013). Clinical studies also have shown that PDT activates CD4+ helper T cells (Hou et al., 2020). And a number of experiments have demonstrated that patients with vulvar intraepithelial neoplasia who responded to PDT had increased levels of infiltrating CD8+ T cells after treatment. However, the tumor tissue makes use of all kinds of immunosuppressive mechanisms to establish an immunosuppressive microenvironment to resist and suppress antitumor immune response. The presence of immune checkpoint molecules and tumor-infiltrating immunosuppressive cells in tumor tissue limits the activation and effective functioning of antigen-reactive T cells and enables tumors to escape from immune elimination.
Numerous tumor-associated T regulatory cells (Tregs) inhibit the activation and expansion of tumor antigen-specific effector T cells, which provides a favorable environment for tumor growth (Nishikawa and Sakaguchi, 2014). Depletion of tumor-infiltrating Tregs would be beneficial to trigger antitumor immune response. Remarkably, systemic depletion of Tregs may induce severe autoimmune diseases and hyperimmune responses (Wing and Sakaguchi, 2010). CD25-targeted PDT as reported by Oh et al. (2017) can induce Tregs apoptosis in tumors and inhibit tumor growth. It is well known that immunosuppressive cells, including myeloid-derived suppressive cells (MDSCs), M2-like tumor-associated macrophages (TAMs), and regulatory T cells (Tregs), are responsible for tumor immune escape. Loading drugs against immunosuppressive cells into nanoparticles can down-regulate the activity of immunosuppressive cells, providing a new path for promoting PDT antitumor immunotherapy. Ding et al. (2021) prepared a liposome (LIC) composed of phosphoinositol 3-kinase (PI3Kγ) inhibitor IPI-549 and photosensitizer Chlore6 (Ce6). IPI-549 is a selective PI3Kγ inhibitor that inhibits PI3Kγ in MDSCs, leading to downregulation of arginase 1 (Arg-1) and ROS, promoting apoptosis of MDSCs and reducing their immunosuppressive activity against CD8+T cells. It promotes the proliferation and activation of cytotoxic T lymphocytes (CTL), reduces Tregs, and repolarizes TAMs to an M1-like phenotype (Ding et al., 2021).
In addition to Tregs, the other natural immunosuppressive cells including MDSCs and M2 macrophages are also attracted to the tumor microenvironment. Thus, it would be highly desirable to develop anti-immunosuppressive cells-based therapies to achieve therapeutic feasibility.
The Combination of Photodynamic Therapy With Immune Checkpoint Blockade
Immune checkpoints are a plethora of inhibitory pathways present in the immune system initiated by ligand-receptor interactions that regulate immune responses in surrounding tissues (Pardoll, 2012). Immunosuppression is mediated by cytotoxic T lymphocyte-associated antigen-4 (CTLA-4) and programmed death-1 (PD-1). These two immunomodulatory receptors are expressed on T cells (Quezada et al., 2011; Pardoll, 2012). T-cell receptors (TCR) initiate an immune response by recognizing antigens. This is regulated by the balance between co-stimulatory and inhibitory signals (i.e., immune checkpoints) (Zou and Chen, 2008). Immune checkpoints are easily blocked by antibodies or regulated by recombinant forms of ligands or receptors, preventing antitumor immunity. Combining immune checkpoint inhibitors with other therapies including PDT may improve the effectiveness of antitumor treatment (He et al., 2016; Wang et al., 2016; Yang et al., 2017; Yuan et al., 2021).
CTLA-4 is the first clinically targeted immune checkpoint receptor that is expressed exclusively on T cells, where it primarily regulates the early stages of T-cell activation. CTLA-4 neutralizes the activity of the T-cell co-stimulatory receptor CD28 (Lenschow et al., 1996). Once antigen recognition occurs, CD28 signaling amplifies TCR signaling to activate T cells (Schneider et al., 2006). CTLA-4 blockade modulates immunosuppression within tumors and has been approved by the US Food and Drug Administration for the treatment of several types of cancer therapies (Wang et al., 2016; Xu et al., 2017). Wang et al. (2019b) reported a nanoparticle UCNP-CE6-R837, which promoted CTLA-4 checkpoint blockade by loading immune adjuvant, effectively inhibited the immunosuppressive activity of Treg cells, increased the ratio of CTL to Treg cells, and promoted antitumor cell immunity. Surprisingly, PDT based on UCNP-CE6-R837 combined with CTLA-4 blockade can effectively induce the generation of immune memory response to prevent tumor recurrence, similar to the function of cancer vaccine. This study proved that PDT combined with cancer immunotherapy can achieve significant synergistic therapeutic effects (Wang et al., 2019b).
In contrast to CTLA-4, PD-1 has a remarkably promising immune checkpoint receptor, and has the primary role of regulating effector T-cell activity within tissues and tumors and limiting autoimmunity (Keir et al., 2006; Okazaki and Honjo, 2007; Keir et al., 2008). PD1 is highly expressed on Treg, and promotes Tregs proliferation in the presence of its ligands. Since many tumors have highly infiltrating Treg, blocking the PD-1 pathway may enhance the antitumor immune response by reducing the number or inhibiting the activity of Treg (Francisco et al., 2009). Two ligands of PD-1 are PD-L1 and PD-L2 (Tseng et al., 2001). On solid tumor cells, the main PD1 ligand expressed is PD-L1. Clinical evidence has been shown that PD-L1 expression by tumor cells suppresses local T-cell-mediated antitumor responses (Konishi et al., 2004). PD-1 monoclonal antibodies are currently commercialized and used clinically to treat several types of tumors (Pardoll, 2012; Wolchok et al., 2013; Larkin et al., 2015). The combination therapy strategy based on PD-1 inhibitors is expected to enhance the effect of PDT. Nanoparticles simultaneously encapsulated or loaded with photosensitizers and immune checkpoint inhibitors systematically exert antitumor immune efficacy. Choi et al. (2021) reported a nanoparticle (LT-NPs) that directly binds chemical drugs, photosensitizers and lysable peptide precursors. LT-NPs highly accumulate within tumor tissues via the EPR effect, and they are specifically cleaved to VPF and cathepsin B overexpression occurs in tumor cells, resulting in minimized side effects. At the same time, Because of LT-NPs containing PD-L1 blockers, the combinatorial treatment with anti-PD-L1 antibody not only leads to a powerful antitumor immune response but also efficiently inhibits the progression of distant pulmonary metastatic tumors (Figures 3D–K). However, it should be noted that due to the complex chemical synthesis process, it is difficult to control the quality of the functional nanoparticles in mass production. At the same time, the structure and function of nanoparticles need to be adjusted according to the specific clinical needs in the future study of PDT.
Indoleamine-2, 3-dioxygenase (IDO) is an immunomodulatory enzyme that is highly expressed in many types of solid tumors and catalyzes the oxidative metabolism of tryptophan to kynurenine (Feng et al., 2018). Deficiency of tryptophan or accumulation of kynurenine in the tumor microenvironment can inhibit T cell proliferation, promote Tregs production and activation (Awuah et al., 2015). It has been reported that some nanoparticles amplify PDT-induced immune responses by inhibiting IDO activity. Gao et al. (2020) investigated exfoliable prodrug vesicles in the tumor microenvironment in combination with a photosensitizer and IDO-1 inhibitor. Prodrug vesicles remain stable in the blood, avoiding drug leakage, and specifically accumulate at the tumor site. Meanwhile, the NLG919 prodrug was reactivated in the tumor microenvironment to inhibit IDO-1. Notably, the antitumor efficacy of the nanoparticles in the CT26 subcutaneous tumor model was relatively superior to that in the 4T1 tumor model, which may be due to more pronounced IDO-mediated immune evasion in CT26 tumors. It can be proved that in different tumor models, the inhibition of IDO shows different therapeutic effects, which has certain reference significance for the personalized adjustment of drug clinical application in the future (Gao et al., 2020).
Conclusion
Numerous preclinical studies have shown that PDT is considered a promising strategy for the treatment of several types of tumors. PDT damages tumor cells and induces ICD to activates cellular subsequent immune response. As more has been learned about the immune regulatory mechanisms of PDT, PDT based on nano delivery systems are becoming the focus of clinical trials for cancer. According to the purpose and use of the treatment, nanoparticles are synthesized from different types of materials, such as lipids, polymers and inorganic compounds. Overcoming tumor-mediated immunosuppression by these nanocarriers via a combination or conjugation with immune checkpoint inhibitors would offer the possibility of amplifying antitumor immune responses and improving therapeutic efficacy. Other studies focused on induction of ICD, reprogramming of TAMs and regulating the tumor immune microenvironment by nanomedicine. Nevertheless, challenges and opportunities also remain for nanosystems driven photodynamic immunotherapy. Firstly, some nanopolymers and inorganic nanomaterials with poor biodegradability and biocompatibility significantly limits their clinical transformation. Optimized liposomes, extracellular vehicles, and biodegradable organic polymers etc. may be facilitate to clinical application (Cabeza et al., 2020; Pinto et al., 2021). Secondly, PDT initiated immune response and duration are relatively weak and short. There are still needed to optimize advanced biomaterials for eliciting effective immune responses and simultaneous activation of multiple immune signaling pathways. In addition to the combination of chemical drug and immune adjuvant to strengthen the host anti-tumor effect, the development of autologous tumor cell–based vaccines are emerging as a transformable and promising approach for personalized tumor therapy (Doix et al., 2019; Fang et al., 2020). Last but not least, more effective photosensitizers or nanocarriers is urgent to develop based on the further exploration of anti-tumor mechanism. Recently, PDT acts as a source of ROS is regarded to closely associated with a new form of regulated cell death, ferroptosis, which is characterized by the accumulation of iron-dependent ROS and lipid peroxides (LPO) to lethal levels. PDT can work in synergism with ferroptosis inducers and achieve a synergistic effect (Mishchenko et al., 2021). The combination action may lead to uncontrolled lipid ROS accumulation and cancer cell death by ferroptosis (Xu et al., 2020; Shui et al., 2021). More interestingly, PDT activates T lymphocytes to release IFN-γ which has been proved to downregulates both system xc- subunits (SLC3A2 and SLC7A11) at the transcriptional level, thereby causing depletion of the intracellular GSH pool and triggering ferroptosis in cancer cells (Wang et al., 2019d; Zitvogel and Kroemer, 2019). The ferroptosis also induce tumor cells death through ICD, which is expected to continuously elicit and maintain the host’s anti-tumor immune effect after PDT. Therefore, ferroptosis induction in PDT is regarded as a powerful alternative strategy for reinforcing tumor therapeutic efficiency. It is clear that more work is required to develop novel integrated platform of PDT that induce prominent anti-tumor immunity effect in tumor microenvironment and limited toxic side effects in normal cells.
Author Contributions
YM and FX write the manuscript, LW and CL revised manuscript and supervised this work. All authors have read and agreed to the published version of the manuscript.
Funding
This research was funded by the Guangdong Basic and Applied Basic Research Foundation (2020A1515011101, 2021A1515011703), the Guangxi Natural Science Foundation (2020GXNSFBA297123) and the National Natural Science Foundation of China (61805049).
Conflict of Interest
The authors declare that the research was conducted in the absence of any commercial or financial relationships that could be construed as a potential conflict of interest.
Publisher’s Note
All claims expressed in this article are solely those of the authors and do not necessarily represent those of their affiliated organizations, or those of the publisher, the editors and the reviewers. Any product that may be evaluated in this article, or claim that may be made by its manufacturer, is not guaranteed or endorsed by the publisher.
Acknowledgments
We would like to acknowledge the Open Fund of MOE Key Laboratory of Laser Life Science and Institute of Laser Life Science.
References
Adkins, I., Fucikova, J., Garg, A. D., Agostinis, P., and Špíšek, R. (2014). Physical Modalities Inducing Immunogenic Tumor Cell Death for Cancer Immunotherapy. Oncoimmunology 3 (12), e968434. doi:10.4161/21624011.2014.968434
Anderson, A. C., Joller, N., and Kuchroo, V. K. (2016). Lag-3, Tim-3, and TIGIT: Co-inhibitory Receptors with Specialized Functions in Immune Regulation. Immunity 44 (5), 989–1004. doi:10.1016/j.immuni.2016.05.001
Awuah, S. G., Zheng, Y. R., Bruno, P. M., Hemann, M. T., and Lippard, S. J. (2015). A Pt(IV) Pro-drug Preferentially Targets Indoleamine-2,3-Dioxygenase, Providing Enhanced Ovarian Cancer Immuno-Chemotherapy. J. Am. Chem. Soc. 137 (47), 14854–14857. doi:10.1021/jacs.5b10182
Azria, D., Larbouret, C., Garambois, V., Kramar, A., Martineau, P., Robert, B., et al. (2003). Potentiation of Ionising Radiation by Targeting Tumour Necrosis Factor Alpha Using a Bispecific Antibody in Human Pancreatic Cancer. Br. J. Cancer 89 (10), 1987–1994. doi:10.1038/sj.bjc.6601362
Babič, A., Herceg, V., Bastien, E., Lassalle, H. P., Bezdetnaya, L., and Lange, N. (2018). 5-Aminolevulinic Acid-Squalene Nanoassemblies for Tumor Photodetection and Therapy: In Vitro Studies. Nanoscale Res. Lett. 13 (1), 10. doi:10.1186/s11671-017-2408-y
Balkwill, F. (2009). Tumour Necrosis Factor and Cancer. Nat. Rev. Cancer 9 (5), 361–371. doi:10.1038/nrc2628
Beatty, G. L., Chiorean, E. G., Fishman, M. P., Saboury, B., Teitelbaum, U. R., Sun, W., et al. (2011). CD40 Agonists Alter Tumor Stroma and Show Efficacy against Pancreatic Carcinoma in Mice and Humans. Science 331 (6024), 1612–1616. doi:10.1126/science.1198443
Beltrán Hernández, I., Yu, Y., Ossendorp, F., Korbelik, M., and Oliveira, S. (2020). Preclinical and Clinical Evidence of Immune Responses Triggered in Oncologic Photodynamic Therapy: Clinical Recommendations. J. Clin. Med. 9 (2). doi:10.3390/jcm9020333
Brackett, C. M., and Gollnick, S. O. (2011). Photodynamic Therapy Enhancement of Anti-tumor Immunity. Photochem Photobiol. Sci. 10 (5), 649–652. doi:10.1039/c0pp00354a
Butcher, M. J., and Galkina, E. V. (2012). Phenotypic and Functional Heterogeneity of Macrophages and Dendritic Cell Subsets in the Healthy and Atherosclerosis-Prone Aorta. Front. Physiol. 3, 44. doi:10.3389/fphys.2012.00044
Cabeza, L., Perazzoli, G., Peña, M., Cepero, A., Luque, C., Melguizo, C., et al. (2020). Cancer Therapy Based on Extracellular Vesicles as Drug Delivery Vehicles. J. Control Release 327, 296–315. doi:10.1016/j.jconrel.2020.08.018
Cantoni, C., Huergo-Zapico, L., Parodi, M., Pedrazzi, M., Mingari, M. C., Moretta, A., et al. (2016). NK Cells, Tumor Cell Transition, and Tumor Progression in Solid Malignancies: New Hints for NK-Based Immunotherapy? J. Immunol. Res. 2016, 4684268. doi:10.1155/2016/4684268
Cassetta, L., and Pollard, J. W. (2018). Targeting Macrophages: Therapeutic Approaches in Cancer. Nat. Rev. Drug Discov. 17 (12), 887–904. doi:10.1038/nrd.2018.169
Cecic, I., Sun, J., and Korbelik, M. (2006). Role of Complement Anaphylatoxin C3a in Photodynamic Therapy-Elicited Engagement of Host Neutrophils and Other Immune Cells. Photochem Photobiol. 82 (2), 558–562. doi:10.1562/2005-09-09-RA-681
Cerwenka, A., and Lanier, L. L. (2016). Natural Killer Cell Memory in Infection, Inflammation and Cancer. Nat. Rev. Immunol. 16 (2), 112–123. doi:10.1038/nri.2015.9
Chen, C., Song, M., Du, Y., Yu, Y., Li, C., Han, Y., et al. (2021). Tumor-Associated-Macrophage-Membrane-Coated Nanoparticles for Improved Photodynamic Immunotherapy. Nano Lett. 21 (13), 5522–5531. doi:10.1021/acs.nanolett.1c00818
Chen, G., Jaskula-Sztul, R., Esquibel, C. R., Lou, I., Zheng, Q., Dammalapati, A., et al. (2017). Neuroendocrine Tumor-Targeted Upconversion Nanoparticle-Based Micelles for Simultaneous NIR-Controlled Combination Chemotherapy and Photodynamic Therapy, and Fluorescence Imaging. Adv. Funct. Mater 27 (8). doi:10.1002/adfm.201604671
Chen, H., Wang, G. D., Chuang, Y. J., Zhen, Z., Chen, X., Biddinger, P., et al. (2015). Nanoscintillator-mediated X-Ray Inducible Photodynamic Therapy for In Vivo Cancer Treatment. Nano Lett. 15 (4), 2249–2256. doi:10.1021/nl504044p
Chen, H. H., Lu, I. L., Liu, T. I., Tsai, Y. C., Chiang, W. H., Lin, S. C., et al. (2019). Indocyanine Green/doxorubicin-Encapsulated Functionalized Nanoparticles for Effective Combination Therapy against Human MDR Breast Cancer. Colloids Surf. B Biointerfaces 177, 294–305. doi:10.1016/j.colsurfb.2019.02.001
Chen, J., Fan, T., Xie, Z., Zeng, Q., Xue, P., Zheng, T., et al. (2020). Advances in Nanomaterials for Photodynamic Therapy Applications: Status and Challenges. Biomaterials 237, 119827. doi:10.1016/j.biomaterials.2020.119827
Chen, Y., Huang, W., Dong, Y., Yu, X., Mo, A., and Peng, Q. (2022). Enhanced Antibacterial Activity of Indocyanine Green-Loaded Graphene Oxide via Synergistic Contact Killing, Photothermal and Photodynamic Therapy. J. Biomed. Nanotechnol. 18 (1), 185–192. doi:10.1166/jbn.2022.3236
Cheng, H. B., Qiao, B., Li, H., Cao, J., Luo, Y., Kotraiah Swamy, K. M., et al. (2021). Protein-Activatable Diarylethene Monomer as a Smart Trigger of Noninvasive Control over Reversible Generation of Singlet Oxygen: A Facile, Switchable, Theranostic Strategy for Photodynamic-Immunotherapy. J. Am. Chem. Soc. 143 (5), 2413–2422. doi:10.1021/jacs.0c12851
Choi, J., Shim, M. K., Yang, S., Hwang, H. S., Cho, H., Kim, J., et al. (2021). Visible-Light-Triggered Prodrug Nanoparticles Combine Chemotherapy and Photodynamic Therapy to Potentiate Checkpoint Blockade Cancer Immunotherapy. ACS Nano 15, 12086–12098. doi:10.1021/acsnano.1c03416
Choi, Y., Chang, J. E., Jheon, S., Han, S. J., and Kim, J. K. (2018). Enhanced Production of Reactive Oxygen Species in HeLa Cells under Concurrent Low-dose C-arboplatin and Photofrin® P-hotodynamic T-herapy. Oncol. Rep. 40 (1), 339–345. doi:10.3892/or.2018.6415
Chu, D., Dong, X., Shi, X., Zhang, C., and Wang, Z. (2018). Neutrophil-Based Drug Delivery Systems. Adv. Mater 30 (22), e1706245. doi:10.1002/adma.201706245
Deng, G., Sun, Z., Li, S., Peng, X., Li, W., Zhou, L., et al. (2018). Cell-Membrane Immunotherapy Based on Natural Killer Cell Membrane Coated Nanoparticles for the Effective Inhibition of Primary and Abscopal Tumor Growth. ACS Nano 12 (12), 12096–12108. doi:10.1021/acsnano.8b05292
Deng, K., Chen, Y., Li, C., Deng, X., Hou, Z., Cheng, Z., et al. (2017). 808 Nm Light Responsive Nanotheranostic Agents Based on Near-Infrared Dye Functionalized Manganese Ferrite for Magnetic-Targeted and Imaging-Guided Photodynamic/photothermal Therapy. J. Mater Chem. B 5 (9), 1803–1814. doi:10.1039/c6tb03233h
Ding, D., Zhong, H., Liang, R., Lan, T., Zhu, X., Huang, S., et al. (2021). Multifunctional Nanodrug Mediates Synergistic Photodynamic Therapy and MDSCsγTargeting Immunotherapy of Colon Cancer. Adv. Sci. 8 (14), 2100712. doi:10.1002/advs.202100712
Doix, B., Trempolec, N., Riant, O., and Feron, O. (2019). Low Photosensitizer Dose and Early Radiotherapy Enhance Antitumor Immune Response of Photodynamic Therapy-Based Dendritic Cell Vaccination. Front. Oncol. 9, 811. doi:10.3389/fonc.2019.00811
Dolmans, D. E., Fukumura, D., and Jain, R. K. (2003). Photodynamic Therapy for Cancer. Nat. Rev. Cancer 3 (5), 380–387. doi:10.1038/nrc1071
Duan, X., Chan, C., Guo, N., Han, W., Weichselbaum, R. R., and Lin, W. (2016). Photodynamic Therapy Mediated by Nontoxic Core-Shell Nanoparticles Synergizes with Immune Checkpoint Blockade to Elicit Antitumor Immunity and Antimetastatic Effect on Breast Cancer. J. Am. Chem. Soc. 138 (51), 16686–16695. doi:10.1021/jacs.6b09538
Eruslanov, E. B., Singhal, S., and Albelda, S. M. (2017). Mouse versus Human Neutrophils in Cancer: A Major Knowledge Gap. Trends Cancer 3 (2), 149–160. doi:10.1016/j.trecan.2016.12.006
Fan, W., Huang, P., and Chen, X. (2016). Overcoming the Achilles' Heel of Photodynamic Therapy. Chem. Soc. Rev. 45 (23), 6488–6519. doi:10.1039/c6cs00616g
Fang, L., Zhao, Z., Wang, J., Zhang, P., Ding, Y., Jiang, Y., et al. (2020). Engineering Autologous Tumor Cell Vaccine to Locally Mobilize Antitumor Immunity in Tumor Surgical Bed. Sci. Adv. 6 (25), eaba4024. doi:10.1126/sciadv.aba4024
Feng, B., Zhou, F., Hou, B., Wang, D., Wang, T., Fu, Y., et al. (2018). Binary Cooperative Prodrug Nanoparticles Improve Immunotherapy by Synergistically Modulating Immune Tumor Microenvironment. Adv. Mater 30 (38), e1803001. doi:10.1002/adma.201803001
Francisco, L. M., Salinas, V. H., Brown, K. E., Vanguri, V. K., Freeman, G. J., Kuchroo, V. K., et al. (2009). PD-L1 Regulates the Development, Maintenance, and Function of Induced Regulatory T Cells. J. Exp. Med. 206 (13), 3015–3029. doi:10.1084/jem.20090847
Gao, A., Chen, B., Gao, J., Zhou, F., Saeed, M., Hou, B., et al. (2020). Sheddable Prodrug Vesicles Combating Adaptive Immune Resistance for Improved Photodynamic Immunotherapy of Cancer. Nano Lett. 20 (1), 353–362. doi:10.1021/acs.nanolett.9b04012
Garg, A. D., Vandenberk, L., Koks, C., Verschuere, T., Boon, L., Van Gool, S. W., et al. (2016). Dendritic Cell Vaccines Based on Immunogenic Cell Death Elicit Danger Signals and T Cell-Driven Rejection of High-Grade Glioma. Sci. Transl. Med. 8 (328), 328ra27. doi:10.1126/scitranslmed.aae0105
Gollnick, S. O., and Brackett, C. M. (2010). Enhancement of Anti-tumor Immunity by Photodynamic Therapy. Immunol. Res. 46 (1-3), 216–226. doi:10.1007/s12026-009-8119-4
Hao, Q., Wang, Z., Zhao, W., Wen, L., Wang, W., Lu, S., et al. (2020). Dual-Responsive Polyprodrug Nanoparticles with Cascade-Enhanced Magnetic Resonance Signals for Deep-Penetration Drug Release in Tumor Therapy. ACS Appl. Mater Interfaces 12 (44), 49489–49501. doi:10.1021/acsami.0c16110
He, C., Duan, X., Guo, N., Chan, C., Poon, C., Weichselbaum, R. R., et al. (2016). Core-shell Nanoscale Coordination Polymers Combine Chemotherapy and Photodynamic Therapy to Potentiate Checkpoint Blockade Cancer Immunotherapy. Nat. Commun. 7, 12499. doi:10.1038/ncomms12499
Hou, Y. J., Yang, X. X., Liu, R. Q., Zhao, D., Guo, C. X., Zhu, A. C., et al. (2020). Pathological Mechanism of Photodynamic Therapy and Photothermal Therapy Based on Nanoparticles. Int. J. Nanomedicine 15, 6827–6838. doi:10.2147/IJN.S269321
Hu, X., Tian, H., Jiang, W., Song, A., Li, Z., and Luan, Y. (2018). Rational Design of IR820- and Ce6-Based Versatile Micelle for Single NIR Laser-Induced Imaging and Dual-Modal Phototherapy. Small 14 (52), e1802994. doi:10.1002/smll.201802994
Idris, N. M., Jayakumar, M. K., Bansal, A., and Zhang, Y. (2015). Upconversion Nanoparticles as Versatile Light Nanotransducers for Photoactivation Applications. Chem. Soc. Rev. 44 (6), 1449–1478. doi:10.1039/c4cs00158c
Im, S., Lee, J., Park, D., Park, A., Kim, Y. M., and Kim, W. J. (2019). Hypoxia-Triggered Transforming Immunomodulator for Cancer Immunotherapy via Photodynamically Enhanced Antigen Presentation of Dendritic Cell. ACS Nano 13 (1), 476–488. doi:10.1021/acsnano.8b07045
Ino, Y., Yamazaki-Itoh, R., Shimada, K., Iwasaki, M., Kosuge, T., Kanai, Y., et al. (2013). Immune Cell Infiltration as an Indicator of the Immune Microenvironment of Pancreatic Cancer. Br. J. Cancer 108 (4), 914–923. doi:10.1038/bjc.2013.32
Janas, K., Boniewska-Bernacka, E., Dyrda, G., and Słota, R. (2021). Porphyrin and Phthalocyanine Photosensitizers Designed for Targeted Photodynamic Therapy of Colorectal Cancer. Bioorg Med. Chem. 30, 115926. doi:10.1016/j.bmc.2020.115926
Ji, B., Wei, M., and Yang, B. (2022). Recent Advances in Nanomedicines for Photodynamic Therapy (PDT)-driven Cancer Immunotherapy. Theranostics 12 (1), 434–458. doi:10.7150/thno.67300
Ji, X., Kang, Y., Ouyang, J., Chen, Y., Artzi, D., Zeng, X., et al. (2019). Synthesis of Ultrathin Biotite Nanosheets as an Intelligent Theranostic Platform for Combination Cancer Therapy. Adv. Sci. (Weinh) 6 (19), 1901211. doi:10.1002/advs.201901211
Jiang, Q., Wang, K., Zhang, X., Ouyang, B., Liu, H., Pang, Z., et al. (2020). Platelet Membrane-Camouflaged Magnetic Nanoparticles for Ferroptosis-Enhanced Cancer Immunotherapy. Small 16 (22), e2001704. doi:10.1002/smll.202001704
Jin, Z. Y., Fatima, H., Zhang, Y., Shao, Z., and Chen, X. J. (2022). Recent Advances in BioγCompatible Oxygen Singlet Generation and its Tumor Treatment. Adv. Ther. 5 (1), 2100176. doi:10.1002/adtp.202100176
Jogdand, A., Alvi, S. B., Rajalakshmi, P. S., and Rengan, A. K. (2020). NIR-dye Based Mucoadhesive Nanosystem for Photothermal Therapy in Breast Cancer Cells. J. Photochem Photobiol. B 208, 111901. doi:10.1016/j.jphotobiol.2020.111901
Kabingu, E., Vaughan, L., Owczarczak, B., Ramsey, K. D., and Gollnick, S. O. (2007). CD8+ T Cell-Mediated Control of Distant Tumours Following Local Photodynamic Therapy Is Independent of CD4+ T Cells and Dependent on Natural Killer Cells. Br. J. Cancer 96 (12), 1839–1848. doi:10.1038/sj.bjc.6603792
Kang, Y., Lei, L., Zhu, C., Zhang, H., Mei, L., and Ji, X. (2021). Piezo-photocatalytic Effect Mediating Reactive Oxygen Species Burst for Cancer Catalytic Therapy. Mater Horiz. 8 (8), 2273–2285. doi:10.1039/d1mh00492a
Keir, M. E., Butte, M. J., Freeman, G. J., and Sharpe, A. H. (2008). PD-1 and its Ligands in Tolerance and Immunity. Annu. Rev. Immunol. 26, 677–704. doi:10.1146/annurev.immunol.26.021607.090331
Keir, M. E., Liang, S. C., Guleria, I., Latchman, Y. E., Qipo, A., Albacker, L. A., et al. (2006). Tissue Expression of PD-L1 Mediates Peripheral T Cell Tolerance. J. Exp. Med. 203 (4), 883–895. doi:10.1084/jem.20051776
Kleinovink, J. W., Fransen, M. F., Löwik, C. W., and Ossendorp, F. (2017). Photodynamic-Immune Checkpoint Therapy Eradicates Local and Distant Tumors by CD8+ T Cells. Cancer Immunol. Res. 5 (10), 832–838. doi:10.1158/2326-6066.CIR-17-0055
Kong, N., Zhang, H., Feng, C., Liu, C., Xiao, Y., Zhang, X., et al. (2021). Arsenene-mediated Multiple Independently Targeted Reactive Oxygen Species Burst for Cancer Therapy. Nat. Commun. 12 (1), 4777. doi:10.1038/s41467-021-24961-5
Konishi, J., Yamazaki, K., Azuma, M., Kinoshita, I., Dosaka-Akita, H., and Nishimura, M. (2004). B7-H1 Expression on Non-small Cell Lung Cancer Cells and its Relationship with Tumor-Infiltrating Lymphocytes and Their PD-1 Expression. Clin. Cancer Res. 10 (15), 5094–5100. doi:10.1158/1078-0432.CCR-04-0428
Korbelik, M., and Hamblin, M. R. (2015). The Impact of Macrophage-Cancer Cell Interaction on the Efficacy of Photodynamic Therapy. Photochem Photobiol. Sci. 14 (8), 1403–1409. doi:10.1039/c4pp00451e
Kumar, A., Moralès, O., Mordon, S., Delhem, N., and Boleslawski, E. (2021). Could Photodynamic Therapy Be a Promising Therapeutic Modality in Hepatocellular Carcinoma Patients? A Critical Review of Experimental and Clinical Studies. Cancers 13 (20), 5176. doi:10.3390/cancers13205176
Kushibiki, T., Tajiri, T., Tomioka, Y., and Awazu, K. (2010). Photodynamic Therapy Induces Interleukin Secretion from Dendritic Cells. Int. J. Clin. Exp. Med. 3 (2), 110–114.
Larkin, J., Chiarion-Sileni, V., Gonzalez, R., Grob, J. J., Cowey, C. L., Lao, C. D., et al. (2015). Combined Nivolumab and Ipilimumab or Monotherapy in Untreated Melanoma. N. Engl. J. Med. 373 (1), 23–34. doi:10.1056/NEJMoa1504030
Lee, S. Y., Lee, R., Kim, E., Lee, S., and Park, Y. I. (2020). Near-Infrared Light-Triggered Photodynamic Therapy and Apoptosis Using Upconversion Nanoparticles with Dual Photosensitizers. Front. Bioeng. Biotechnol. 8, 275. doi:10.3389/fbioe.2020.00275
Lenschow, D. J., Walunas, T. L., and Bluestone, J. A. (1996). CD28/B7 System of T Cell Costimulation. Annu. Rev. Immunol. 14, 233–258. doi:10.1146/annurev.immunol.14.1.233
Li, K., Dong, W., Miao, Y., Liu, Q., Qiu, L., and Lin, J. (2021a). Dual-targeted 5-aminolevulinic Acid Derivatives with Glutathione Depletion Function for Enhanced Photodynamic Therapy. J. Photochem Photobiol. B 215, 112107. doi:10.1016/j.jphotobiol.2020.112107
Li, W., Yang, J., Luo, L., Jiang, M., Qin, B., Yin, H., et al. (2019). Targeting Photodynamic and Photothermal Therapy to the Endoplasmic Reticulum Enhances Immunogenic Cancer Cell Death. Nat. Commun. 10 (1), 3349. doi:10.1038/s41467-019-11269-8
Li, X., Lovell, J. F., Yoon, J., and Chen, X. (2020). Clinical Development and Potential of Photothermal and Photodynamic Therapies for Cancer. Nat. Rev. Clin. Oncol. 17 (11), 657–674. doi:10.1038/s41571-020-0410-2
Li, Y., Ye, F., Zhang, S., Ni, W., Wen, L., and Qin, H. (2021b). Carbon-Coated Magnetic Nanoparticle Dedicated to MRI/Photoacoustic Imaging of Tumor in Living Mice. Front. Bioeng. Biotechnol. 9, 800744. doi:10.3389/fbioe.2021.800744
Liang, L., Wen, L., Weng, Y., Song, J., Li, H., Zhang, Y., et al. (2021a). Homologous-targeted and Tumor Microenvironment-Activated Hydroxyl Radical Nanogenerator for Enhanced Chemoimmunotherapy of Non-small Cell Lung Cancer. Chem. Eng. J. 425, 131451. doi:10.1016/j.cej.2021.131451
Liang, X., Chen, M., Bhattarai, P., Hameed, S., Tang, Y., and Dai, Z. (2021b). Complementing Cancer Photodynamic Therapy with Ferroptosis through Iron Oxide Loaded Porphyrin-Grafted Lipid Nanoparticles. ACS Nano 15 (12), 20164–20180. doi:10.1021/acsnano.1c08108
Lin, S. L., Chen, Z. R., and Chang, C. A. (2018). Nd3+ Sensitized Core-Shell-Shell Nanocomposites Loaded with IR806 Dye for Photothermal Therapy and Up-Conversion Luminescence Imaging by a Single Wavelength NIR Light Irradiation. Nanotheranostics 2 (3), 243–257. doi:10.7150/ntno.25901
Lu, C., Zhou, F., Wu, S., Liu, L., and Xing, D. (2016). Phototherapy-Induced Antitumor Immunity: Long-Term Tumor Suppression Effects via Photoinactivation of Respiratory Chain Oxidase-Triggered Superoxide Anion Burst. Antioxid. Redox Signal 24 (5), 249–262. doi:10.1089/ars.2015.6334
Lu, K., He, C., Guo, N., Chan, C., Ni, K., Lan, G., et al. (2018). Low-dose X-Ray Radiotherapy-Radiodynamic Therapy via Nanoscale Metal-Organic Frameworks Enhances Checkpoint Blockade Immunotherapy. Nat. Biomed. Eng. 2 (8), 600–610. doi:10.1038/s41551-018-0203-4
Lucky, S. S., Soo, K. C., and Zhang, Y. (2015). Nanoparticles in Photodynamic Therapy. Chem. Rev. 115 (4), 1990–2042. doi:10.1021/cr5004198
Mantovani, A., Sica, A., Sozzani, S., Allavena, P., Vecchi, A., and Locati, M. (2004). The Chemokine System in Diverse Forms of Macrophage Activation and Polarization. Trends Immunol. 25 (12), 677–686. doi:10.1016/j.it.2004.09.015
Mao, M., Liu, S., Zhou, Y., Wang, G., Deng, J., and Tian, L. (2020). Nanostructured lipid carrier delivering chlorins e6 as In Situ dendritic cell vaccine for immunotherapy of gastric cancer. J. Mater Res. 35 (23), 3257–3264. doi:10.1557/jmr.2020.227
Marydasan, B., Nair, R. R., Babu, P. S. S., Ramaiah, D., and Nair, S. A. (2019). Picolyl Porphyrin Nanostructures as a Functional Drug Entrant for Photodynamic Therapy in Human Breast Cancers. ACS Omega 4 (7), 12808–12816. doi:10.1021/acsomega.9b01380
Mishchenko, T. A., Balalaeva, I. V., Vedunova, M. V., and Krysko, D. V. (2021). Ferroptosis and Photodynamic Therapy Synergism: Enhancing Anticancer Treatment. Trends Cancer 7 (6), 484–487. doi:10.1016/j.trecan.2021.01.013
Murray, P. J., and Wynn, T. A. (2011). Protective and Pathogenic Functions of Macrophage Subsets. Nat. Rev. Immunol. 11 (11), 723–737. doi:10.1038/nri3073
Ni, K., Xu, Z., Culbert, A., Luo, T., Guo, N., Yang, K., et al. (2022). Synergistic Checkpoint-Blockade and Radiotherapy-Radiodynamic Therapy via an Immunomodulatory Nanoscale Metal-Organic Framework. Nat. Biomed. Eng. 6 (2), 144–156. doi:10.1038/s41551-022-00846-w
Nishikawa, H., and Sakaguchi, S. (2014). Regulatory T Cells in Cancer Immunotherapy. Curr. Opin. Immunol. 27, 1–7. doi:10.1016/j.coi.2013.12.005
Noh, J., Jung, E., Yoo, D., Kang, C., Kim, C., Park, S., et al. (2018). Dual Imaging-Guided Oxidative-Photothermal Combination Anticancer Therapeutics. ACS Appl. Mater Interfaces 10 (47), 40424–40433. doi:10.1021/acsami.8b14968
Nowak, K. M., Schwartz, M. R., Breza, V. R., and Price, R. J. (2022). Sonodynamic Therapy: Rapid Progress and New Opportunities for Non-invasive Tumor Cell Killing with Sound. Cancer Lett. 532, 215592. doi:10.1016/j.canlet.2022.215592
Oh, D. S., Kim, H., Oh, J. E., Jung, H. E., Lee, Y. S., Park, J. H., et al. (2017). Intratumoral Depletion of Regulatory T Cells Using CD25-Targeted Photodynamic Therapy in a Mouse Melanoma Model Induces Antitumoral Immune Responses. Oncotarget 8 (29), 47440–47453. doi:10.18632/oncotarget.17663
Ohms, M., Möller, S., and Laskay, T. (2020). An Attempt to Polarize Human Neutrophils toward N1 and N2 Phenotypes In Vitro. Front. Immunol. 11, 532. doi:10.3389/fimmu.2020.00532
Okazaki, T., and Honjo, T. (2007). PD-1 and PD-1 Ligands: from Discovery to Clinical Application. Int. Immunol. 19 (7), 813–824. doi:10.1093/intimm/dxm057
Pan, C., Mao, Z., Yuan, X., Zhang, H., Mei, L., and Ji, X. (2022). Heterojunction Nanomedicine. Adv. Sci. (Weinh) 9 (11), e2105747. doi:10.1002/advs.202105747
Pan, C., Ou, M., Cheng, Q., Zhou, Y., Yu, Y., Li, Z., et al. (2019). ZγScheme Heterojunction Functionalized Pyrite Nanosheets for Modulating Tumor Microenvironment and Strengthening Photo/Chemodynamic Therapeutic Effects. Adv. Funct. Mat. 30 (3), 1906466. doi:10.1002/adfm.201906466
Pan, X., Wang, H., Wang, S., Sun, X., Wang, L., Wang, W., et al. (2018). Sonodynamic Therapy (SDT): a Novel Strategy for Cancer Nanotheranostics. Sci. China Life Sci. 61 (4), 415–426. doi:10.1007/s11427-017-9262-x
Pardoll, D. M. (2012). The Blockade of Immune Checkpoints in Cancer Immunotherapy. Nat. Rev. Cancer 12 (4), 252–264. doi:10.1038/nrc3239
Peer, D., Karp, J. M., Hong, S., Farokhzad, O. C., Margalit, R., and Langer, R. (2007). Nanocarriers as an Emerging Platform for Cancer Therapy. Nat. Nanotechnol. 2 (12), 751–760. doi:10.1038/nnano.2007.387
Pinto, A., Marangon, I., Méreaux, J., Nicolás-Boluda, A., Lavieu, G., Wilhelm, C., et al. (2021). Immune Reprogramming Precision Photodynamic Therapy of Peritoneal Metastasis by Scalable Stem-Cell-Derived Extracellular Vesicles. ACS Nano 15 (2), 3251–3263. doi:10.1021/acsnano.0c09938
Preise, D., Oren, R., Glinert, I., Kalchenko, V., Jung, S., Scherz, A., et al. (2009). Systemic Antitumor Protection by Vascular-Targeted Photodynamic Therapy Involves Cellular and Humoral Immunity. Cancer Immunol. Immunother. 58 (1), 71–84. doi:10.1007/s00262-008-0527-0
Qian, B. Z., and Pollard, J. W. (2010). Macrophage Diversity Enhances Tumor Progression and Metastasis. Cell 141 (1), 39–51. doi:10.1016/j.cell.2010.03.014
Qiu, Q., Li, C., Yan, X., Zhang, H., Luo, X., Gao, X., et al. (2021). Photodynamic/ Photothermal Therapy Enhances Neutrophil-Mediated Ibrutinib Tumor Delivery for Potent Tumor Immunotherapy: More Than One Plus One? Biomaterials 269, 120652. doi:10.1016/j.biomaterials.2021.120652
Qiu, S. Q., Waaijer, S. J. H., Zwager, M. C., de Vries, E. G. E., van der Vegt, B., and Schröder, C. P. (2018). Tumor-associated Macrophages in Breast Cancer: Innocent Bystander or Important Player? Cancer Treat. Rev. 70, 178–189. doi:10.1016/j.ctrv.2018.08.010
Quezada, S. A., Peggs, K. S., Simpson, T. R., and Allison, J. P. (2011). Shifting the Equilibrium in Cancer Immunoediting: from Tumor Tolerance to Eradication. Immunol. Rev. 241 (1), 104–118. doi:10.1111/j.1600-065X.2011.01007.x
Radogna, F., and Diederich, M. (2018). Stress-induced Cellular Responses in Immunogenic Cell Death: Implications for Cancer Immunotherapy. Biochem. Pharmacol. 153, 12–23. doi:10.1016/j.bcp.2018.02.006
Robertson, C. A., Evans, D. H., and Abrahamse, H. (2009). Photodynamic Therapy (PDT): a Short Review on Cellular Mechanisms and Cancer Research Applications for PDT. J. Photochem Photobiol. B 96 (1), 1–8. doi:10.1016/j.jphotobiol.2009.04.001
Rocha, L. B., Gomes-da-Silva, L. C., Dąbrowski, J. M., and Arnaut, L. G. (2015). Elimination of Primary Tumours and Control of Metastasis with Rationally Designed Bacteriochlorin Photodynamic Therapy Regimens. Eur. J. Cancer 51 (13), 1822–1830. doi:10.1016/j.ejca.2015.06.002
Rosales, C. (2018). Neutrophil: A Cell with Many Roles in Inflammation or Several Cell Types? Front. Physiol. 9, 113. doi:10.3389/fphys.2018.00113
Sang, W., Zhang, Z., Dai, Y., and Chen, X. (2019). Recent Advances in Nanomaterial-Based Synergistic Combination Cancer Immunotherapy. Chem. Soc. Rev. 48 (14), 3771–3810. doi:10.1039/c8cs00896e
Satoh, T., Takeuchi, O., Vandenbon, A., Yasuda, K., Tanaka, Y., Kumagai, Y., et al. (2010). The Jmjd3-Irf4 axis Regulates M2 Macrophage Polarization and Host Responses against Helminth Infection. Nat. Immunol. 11 (10), 936–944. doi:10.1038/ni.1920
Schneider, H., Downey, J., Smith, A., Zinselmeyer, B. H., Rush, C., Brewer, J. M., et al. (2006). Reversal of the TCR Stop Signal by CTLA-4. Science 313 (5795), 1972–1975. doi:10.1126/science.1131078
Seidi, K., Neubauer, H. A., Moriggl, R., Jahanban-Esfahlan, R., and Javaheri, T. (2018). Tumor Target Amplification: Implications for Nano Drug Delivery Systems. J. Control Release 275, 142–161. doi:10.1016/j.jconrel.2018.02.020
Shaif-Muthana, M., McIntyre, C., Sisley, K., Rennie, I., and Murray, A. (2000). Dead or Alive: Immunogenicity of Human Melanoma Cells when Presented by Dendritic Cells. Cancer Res. 60 (22), 6441–6447.
Shui, S., Zhao, Z., Wang, H., Conrad, M., and Liu, G. (2021). Non-enzymatic Lipid Peroxidation Initiated by Photodynamic Therapy Drives a Distinct Ferroptosis-like Cell Death Pathway. Redox Biol. 45, 102056. doi:10.1016/j.redox.2021.102056
Sun, W., Shi, T., Luo, L., Chen, X., Lv, P., Lv, Y., et al. (2019a). Monodisperse and Uniform Mesoporous Silicate Nanosensitizers Achieve Low-Dose X-Ray-Induced Deep-Penetrating Photodynamic Therapy. Adv. Mater 31 (16), e1808024. doi:10.1002/adma.201808024
Sun, W., Zhao, X., Fan, J., Du, J., and Peng, X. (2019b). Boron Dipyrromethene Nano-Photosensitizers for Anticancer Phototherapies. Small 15 (32), e1804927. doi:10.1002/smll.201804927
Sur, B. W., Nguyen, P., Sun, C. H., Tromberg, B. J., and Nelson, E. L. (2008). Immunophototherapy Using PDT Combined with Rapid Intratumoral Dendritic Cell Injection. Photochem Photobiol. 84 (5), 1257–1264. doi:10.1111/j.1751-1097.2008.00356.x
Tan, H. Y., Wang, N., Li, S., Hong, M., Wang, X., and Feng, Y. (2016). The Reactive Oxygen Species in Macrophage Polarization: Reflecting its Dual Role in Progression and Treatment of Human Diseases. Oxid. Med. Cell Longev. 2016, 2795090. doi:10.1155/2016/2795090
Tseng, S. Y., Otsuji, M., Gorski, K., Huang, X., Slansky, J. E., Pai, S. I., et al. (2001). B7-DC, a New Dendritic Cell Molecule with Potent Costimulatory Properties for T Cells. J. Exp. Med. 193 (7), 839–846. doi:10.1084/jem.193.7.839
Turubanova, V. D., Balalaeva, I. V., Mishchenko, T. A., Catanzaro, E., Alzeibak, R., Peskova, N. N., et al. (2019). Immunogenic Cell Death Induced by a New Photodynamic Therapy Based on Photosens and Photodithazine. J. Immunother. Cancer 7 (1), 350. doi:10.1186/s40425-019-0826-3
Wan, S. S., Zeng, J. Y., Cheng, H., and Zhang, X. Z. (2018). ROS-induced NO Generation for Gas Therapy and Sensitizing Photodynamic Therapy of Tumor. Biomaterials 185, 51–62. doi:10.1016/j.biomaterials.2018.09.004
Wang, C., Du, W., Wu, C., Dan, S., Sun, M., Zhang, T., et al. (2022a). Cathespin BγInitiated Cypate Nanoparticle Formation for Tumor Photoacoustic Imaging. Angew. Chem. Int. Ed. 61 (5), e202114766. doi:10.1002/anie.202114766
Wang, D., Wang, T., Liu, J., Yu, H., Jiao, S., Feng, B., et al. (2016). Acid-Activatable Versatile Micelleplexes for PD-L1 Blockade-Enhanced Cancer Photodynamic Immunotherapy. Nano Lett. 16 (9), 5503–5513. doi:10.1021/acs.nanolett.6b01994
Wang, H., Yang, X., Shao, W., Chen, S., Xie, J., Zhang, X., et al. (2015). Ultrathin Black Phosphorus Nanosheets for Efficient Singlet Oxygen Generation. J. Am. Chem. Soc. 137 (35), 11376–11382. doi:10.1021/jacs.5b06025
Wang, M., Song, J., Zhou, F., Hoover, A. R., Murray, C., Zhou, B., et al. (2019a). NIR-triggered Phototherapy and Immunotherapy via an Antigen-Capturing Nanoplatform for Metastatic Cancer Treatment. Adv. Sci. (Weinh) 6 (10), 1802157. doi:10.1002/advs.201802157
Wang, R., Xia, X., Yang, Y., Rong, X., Liu, T., Su, Z., et al. (2022b). A Glutathione Activatable Photosensitizer for Combined Photodynamic and Gas Therapy under Red Light Irradiation. Adv. Healthc. Mater 11 (4), e2102017. doi:10.1002/adhm.202102017
Wang, S., Liu, H., Xin, J., Rahmanzadeh, R., Wang, J., Yao, C., et al. (2019b). Chlorin-Based Photoactivable Galectin-3-Inhibitor Nanoliposome for Enhanced Photodynamic Therapy and NK Cell-Related Immunity in Melanoma. ACS Appl. Mater Interfaces 11 (45), 41829–41841. doi:10.1021/acsami.9b09560
Wang, T., Zhang, H., Han, Y., Liu, H., Ren, F., Zeng, J., et al. (2019c). Light-Enhanced O2-Evolving Nanoparticles Boost Photodynamic Therapy to Elicit Antitumor Immunity. ACS Appl. Mater Interfaces 11 (18), 16367–16379. doi:10.1021/acsami.9b03541
Wang, W., Green, M., Choi, J. E., Gijón, M., Kennedy, P. D., Johnson, J. K., et al. (2019d). CD8+ T Cells Regulate Tumour Ferroptosis during Cancer Immunotherapy. Nature 569 (7755), 270–274. doi:10.1038/s41586-019-1170-y
Wang, X., Tian, Y., Liao, X., Tang, Y., Ni, Q., Sun, J., et al. (2020a). Enhancing Selective Photosensitizer Accumulation and Oxygen Supply for High-Efficacy Photodynamic Therapy toward Glioma by 5-aminolevulinic Acid Loaded Nanoplatform. J. Colloid Interface Sci. 565, 483–493. doi:10.1016/j.jcis.2020.01.020
Wang, X., Zhong, X., and Cheng, L. (2020b). Titanium-based Nanomaterials for Cancer Theranostics. Coord. Chem. Rev. 430, 213662.
Wang, Y., Zhao, J., Chen, Z., Zhang, F., Wang, Q., Guo, W., et al. (2019e). Construct of MoSe2/Bi2Se3 Nanoheterostructure: Multimodal CT/PT Imaging-Guided PTT/PDT/chemotherapy for Cancer Treating. Biomaterials 217, 119282. doi:10.1016/j.biomaterials.2019.119282
Wang, Z., Zhan, M., and Hu, X. (2022c). Pulsed Laser Excited Photoacoustic Effect for Disease Diagnosis and Therapy. Chem. A Eur. J. doi:10.1002/chem.202200042
Wing, K., and Sakaguchi, S. (2010). Regulatory T Cells Exert Checks and Balances on Self Tolerance and Autoimmunity. Nat. Immunol. 11 (1), 7–13. doi:10.1038/ni.1818
Wolchok, J. D., Kluger, H., Callahan, M. K., Postow, M. A., Rizvi, N. A., Lesokhin, A. M., et al. (2013). Nivolumab Plus Ipilimumab in Advanced Melanoma. N. Engl. J. Med. 369 (2), 122–133. doi:10.1056/NEJMoa1302369
Wu, K., Zhao, H., Sun, Z., Wang, B., Tang, X., Dai, Y., et al. (2019a). Endogenous Oxygen Generating Multifunctional Theranostic Nanoplatform for Enhanced Photodynamic-Photothermal Therapy and Multimodal Imaging. Theranostics 9 (25), 7697–7713. doi:10.7150/thno.38565
Wu, L., Saxena, S., Awaji, M., and Singh, R. K. (2019b). Tumor-Associated Neutrophils in Cancer: Going Pro. Cancers (Basel) 11 (4). doi:10.3390/cancers11040564
Wu, L., Saxena, S., and Singh, R. K. (2020). Neutrophils in the Tumor Microenvironment. Adv. Exp. Med. Biol. 1224, 1–20. doi:10.1007/978-3-030-35723-8_1
Xie, J., Wang, Y., Choi, W., Jangili, P., Ge, Y., Xu, Y., et al. (2021). Overcoming Barriers in Photodynamic Therapy Harnessing Nano-Formulation Strategies. Chem. Soc. Rev. 50 (16), 9152–9201. doi:10.1039/d0cs01370f
Xing, Y., Ding, T., Wang, Z., Wang, L., Guan, H., Tang, J., et al. (2019). Temporally Controlled Photothermal/Photodynamic and Combined Therapy for Overcoming Multidrug Resistance of Cancer by Polydopamine Nanoclustered Micelles. ACS Appl. Mater Interfaces 11 (15), 13945–13953. doi:10.1021/acsami.9b00472
Xu, D., Baidya, A., Deng, K., Li, Y. S., Wu, B., and Xu, H. B. (2021). Multifunctional Nanoparticle PEG-Ce6-Gd for MRI-guided P-hotodynamic T-herapy. Oncol. Rep. 45 (2), 547–556. doi:10.3892/or.2020.7871
Xu, J., Xu, L., Wang, C., Yang, R., Zhuang, Q., Han, X., et al. (2017). Near-Infrared-Triggered Photodynamic Therapy with Multitasking Upconversion Nanoparticles in Combination with Checkpoint Blockade for Immunotherapy of Colorectal Cancer. ACS Nano 11 (5), 4463–4474. doi:10.1021/acsnano.7b00715
Xu, T., Ma, Y., Yuan, Q., Hu, H., Hu, X., Qian, Z., et al. (2020). Enhanced Ferroptosis by Oxygen-Boosted Phototherapy Based on a 2-in-1 Nanoplatform of Ferrous Hemoglobin for Tumor Synergistic Therapy. ACS Nano 14 (3), 3414–3425. doi:10.1021/acsnano.9b09426
Yang, G., Tian, J., Chen, C., Jiang, D., Xue, Y., Wang, C., et al. (2019a). An Oxygen Self-Sufficient NIR-Responsive Nanosystem for Enhanced PDT and Chemotherapy against Hypoxic Tumors. Chem. Sci. 10 (22), 5766–5772. doi:10.1039/c9sc00985j
Yang, G., Xu, L., Chao, Y., Xu, J., Sun, X., Wu, Y., et al. (2017). Hollow MnO2 as a Tumor-Microenvironment-Responsive Biodegradable Nano-Platform for Combination Therapy Favoring Antitumor Immune Responses. Nat. Commun. 8 (1), 902. doi:10.1038/s41467-017-01050-0
Yang, W., Zhu, G., Wang, S., Yu, G., Yang, Z., Lin, L., et al. (2019b). In Situ Dendritic Cell Vaccine for Effective Cancer Immunotherapy. ACS Nano 13 (3), 3083–3094. doi:10.1021/acsnano.8b08346
Yang, Y., Hu, Y., and Wang, H. (2016). Targeting Antitumor Immune Response for Enhancing the Efficacy of Photodynamic Therapy of Cancer: Recent Advances and Future Perspectives. Oxid. Med. Cell Longev. 2016, 5274084. doi:10.1155/2016/5274084
Yao, X., Yang, B., Wang, S., Dai, Z., Zhang, D., Zheng, X., et al. (2020). A Novel Multifunctional FePt/BP Nanoplatform for Synergistic Photothermal/photodynamic/chemodynamic Cancer Therapies and Photothermally-Enhanced Immunotherapy. J. Mater Chem. B 8 (35), 8010–8021. doi:10.1039/d0tb00411a
Yuan, Z., Fan, G., Wu, H., Liu, C., Zhan, Y., Qiu, Y., et al. (2021). Photodynamic Therapy Synergizes with PD-L1 Checkpoint Blockade for Immunotherapy of CRC by Multifunctional Nanoparticles. Mol. Ther. 29 (10), 2931–2948. doi:10.1016/j.ymthe.2021.05.017
Zaharie-Butucel, D., Potara, M., Suarasan, S., Licarete, E., and Astilean, S. (2019). Efficient Combined Near-Infrared-Triggered Therapy: Phototherapy over Chemotherapy in Chitosan-Reduced Graphene Oxide-Ir820 Dye-Doxorubicin Nanoplatforms. J. Colloid Interface Sci. 552, 218–229. doi:10.1016/j.jcis.2019.05.050
Zeng, W., Zhang, H., Yuan, X., Chen, T., Pei, Z., and Ji, X. (2022). Two-Dimensional Nanomaterial-Based Catalytic Medicine: Theories, Advanced Catalyst and System Design. Adv. Drug Deliv. Rev. 184, 114241. doi:10.1016/j.addr.2022.114241
Zhang, D., Wen, L., Huang, R., Wang, H., Hu, X., and Xing, D. (2018a). Mitochondrial Specific Photodynamic Therapy by Rare-Earth Nanoparticles Mediated Near-Infrared Graphene Quantum Dots. Biomaterials 153, 14–26. doi:10.1016/j.biomaterials.2017.10.034
Zhang, L., Qin, Y., Zhang, Z., Fan, F., Huang, C., Lu, L., et al. (2018b). Dual pH/reduction-Responsive Hybrid Polymeric Micelles for Targeted Chemo-Photothermal Combination Therapy. Acta Biomater. 75, 371–385. doi:10.1016/j.actbio.2018.05.026
Zhang, X., Li, L., Liu, Q., Wang, Y., Yang, J., Qiu, T., et al. (2019). Co-Delivery of Rose Bengal and Doxorubicin Nanoparticles for Combination Photodynamic and Chemo-Therapy. J. Biomed. Nanotechnol. 15 (1), 184–195. doi:10.1166/jbn.2019.2674
Zheng, L., Li, Y., Cui, Y., Yin, H., Liu, T., Yu, G., et al. (2013). Generation of an Effective Anti-lung Cancer Vaccine by DTPP-Mediated Photodynamic Therapy and Mechanistic Studies. Lasers Med. Sci. 28 (5), 1383–1392. doi:10.1007/s10103-013-1270-0
Zheng, Y., Yin, G., Le, V., Zhang, A., Chen, S., Liang, X., et al. (2016). Photodynamic-therapy Activates Immune Response by Disrupting Immunity Homeostasis of Tumor Cells, Which Generates Vaccine for Cancer Therapy. Int. J. Biol. Sci. 12 (1), 120–132. doi:10.7150/ijbs.12852
Zhong, J., Wen, L., Yang, S., Xiang, L., Chen, Q., and Xing, D. (2015). Imaging-guided High-Efficient Photoacoustic Tumor Therapy with Targeting Gold Nanorods. Nanomedicine 11 (6), 1499–1509. doi:10.1016/j.nano.2015.04.002
Zhou, F., Xing, D., and Chen, W. R. (2009). Regulation of HSP70 on Activating Macrophages Using PDT-Induced Apoptotic Cells. Int. J. Cancer 125 (6), 1380–1389. doi:10.1002/ijc.24520
Zhou, L., Chen, L., Hu, X., Lu, Y., Liu, W., Sun, Y., et al. (2020). A Cu9S5 Nanoparticle-Based CpG Delivery System for Synergistic Photothermal-, Photodynamic- and Immunotherapy. Commun. Biol. 3 (1), 343. doi:10.1038/s42003-020-1070-6
Zhou, Y., Que, K. T., Zhang, Z., Yi, Z. J., Zhao, P. X., You, Y., et al. (2018). Iron Overloaded Polarizes Macrophage to Proinflammation Phenotype through ROS/acetyl-p53 Pathway. Cancer Med. 7 (8), 4012–4022. doi:10.1002/cam4.1670
Zhou, Y., Ren, X., Hou, Z., Wang, N., Jiang, Y., and Luan, Y. (2021). Engineering a Photosensitizer Nanoplatform for Amplified Photodynamic Immunotherapy via Tumor Microenvironment Modulation. Nanoscale Horiz. 6 (2), 120–131. doi:10.1039/d0nh00480d
Zhu, M., Sheng, Z., Jia, Y., Hu, D., Liu, X., Xia, X., et al. (2017). Indocyanine Green-holo-Transferrin Nanoassemblies for Tumor-Targeted Dual-Modal Imaging and Photothermal Therapy of Glioma. ACS Appl. Mater Interfaces 9 (45), 39249–39258. doi:10.1021/acsami.7b14076
Zitvogel, L., and Kroemer, G. (2019). Interferon-γ Induces Cancer Cell Ferroptosis. Cell Res. 29 (9), 692–693. doi:10.1038/s41422-019-0186-z
Keywords: nanosystems, photosensitizers, photodynamic therapy, innate immunity, adaptive immunity
Citation: Ma Y, Xiao F, Lu C and Wen L (2022) Multifunctional Nanosystems Powered Photodynamic Immunotherapy. Front. Pharmacol. 13:905078. doi: 10.3389/fphar.2022.905078
Received: 26 March 2022; Accepted: 25 April 2022;
Published: 11 May 2022.
Edited by:
Renu John, Indian Institute of Technology Hyderabad, IndiaCopyright © 2022 Ma, Xiao, Lu and Wen. This is an open-access article distributed under the terms of the Creative Commons Attribution License (CC BY). The use, distribution or reproduction in other forums is permitted, provided the original author(s) and the copyright owner(s) are credited and that the original publication in this journal is cited, in accordance with accepted academic practice. No use, distribution or reproduction is permitted which does not comply with these terms.
*Correspondence: Cuixia Lu, bHVjdWl4aWFAZ3h1LmVkdS5jbg==; Liewei Wen, d2VubmVyMTk4OUAxNjMuY29t
†These authors have contributed equally to this work