- 1Department of Life Sciences and Health, Faculty of Health Sciences, Oslo Metropolitan University, Oslo, Norway
- 2Department of Medical Biochemistry, Oslo University Hospital, Oslo, Norway
- 3Department of Ophthalmology, Oslo University Hospital, Oslo, Norway
Background: Although numerous nanoparticle formulations have been developed for ocular administration, concerns are being raised about a possible mismatch between potential promises made by the field of nanoparticle research and demonstration of actual therapeutic benefit. Therefore, the primary focus of this present review was to critically assess to what extent nanoencapsulation of ocular drugs improved the therapeutic outcome when treating conditions in the anterior segment of the eye.
Methods: A systematic search was conducted using Medline, PubMed, and Embase databases as well as Google Scholar for published peer-reviewed articles in English focusing on conventional nanoparticles used as drug delivery systems to the anterior segment of the eye in in vivo studies. The major therapeutic outcomes were intraocular pressure, tear secretion, number of polymorphonuclear leucocytes and pupil size. The outcome after encapsulation was compared to the non-encapsulated drug.
Results: From the search, 250 results were retrieved. Thirty-eight studies met the inclusion criteria. Rabbits were used as study subjects in all but one study, and the number of animals ranged from 3 to 10. Coated and uncoated liposomes, lipid-based and polymeric nanoparticles, as well as micelles, were studied, varying in both particle size and surface charge, and encapsulating a total of 24 different drugs, including 6 salts. The majority of the in vivo studies demonstrated some improvement after nanoencapsulation, but the duration of the benefit varied from less than 1 h to more than 20 h. The most common in vitro methods performed in the studies were drug release, transcorneal permeation, and mucin interaction.
Discussion: Nanoparticles that are small and mucoadhesive, often due to positive surface charge, appeared beneficial. Although in vitro assays can unravel more of the hidden and sophisticated interplay between the encapsulated drug and the nanoparticle structure, they suffered from a lack of in vitro—in vivo correlation. Therefore, more research should be focused towards developing predictive in vitro models, allowing rational design and systematic optimization of ocular nanoparticles with minimal animal experimentation.
1 Introduction
The burden of eye conditions is expected to increase in coming decades with a growing population and aging, behavioral and lifestyle changes (WHO, 2019). While some eye conditions, such as glaucoma, can cause vision impairment and blindness, many, such as dry eye disease (DED), ocular infection and inflammation, usually do not. Yet, these conditions are among the leading reasons for seeking medical care causing significant financial strain on the health care system (WHO, 2019) and negatively affecting quality of life (Dhouib et al., 2021; Morthen et al., 2022). Currently, there is a vast array of ocular therapeutics available, however, the main challenge has been to develop a delivery method that can overcome the anatomical and physiological barriers of the eye to improve their efficacy. Therefore, there is a growing demand for the development of safe and effective ocular drug delivery systems in the treatment of eye conditions.
The eye is a delicate organ comprised of the anterior and posterior segments (Dave et al., 2021). Conditions affecting the anterior segment of the eye such as glaucoma (Arroyo et al., 2018), DED (Chen et al., 2021), and inflammation (Katara et al., 2019) can be treated using ocular implants, intracameral or subconjunctival injections, topical and oral administration of ocular drugs. However, many of these methods are either invasive or can cause serious systemic side effects (Dave et al., 2021). Topical application remains the easiest, least invasive and safest route to deliver therapeutics (Jumelle et al., 2020; Dave et al., 2021) despite low bioavailability (Jumelle et al., 2020).
The main barrier to ocular drug delivery is the tear film. Based on the classical model, this transparent fluid is comprised of three-layers (∼10 µM thick). The outermost oily layer contains lipids; the intermediate aqueous layer contains salts, mucins, proteins, and enzymes; and the innermost mucus layer contains lysozymes and glycocalyx. Immediately upon application, ocular drugs are diluted by the tear film produced mainly by the lacrimal and Meibomian glands as a protection mechanism of the eye (Nichols et al., 1985; Yañez-Soto et al., 2014). Additionally, tear film turnover (normally ∼1–3 μl/min) that increases upon topical application can cause rapid clearance of drug molecules (within 1–2 min) via nasolacrimal drainage (Worakul and Robinson, 1997) leading to low ocular retention and absorption. The lipid and aqueous layers in the tear film impede permeation of hydrophilic and hydrophobic drugs, respectively. Lysozymes present in the tear film can also degrade the administered drugs (Worakul and Robinson, 1997; Campos et al., 2020). Another barrier is the limited precorneal surface area. It is estimated that only about 30 µl of eye drops can be applied onto the ocular surface, most of which is instantly eliminated during the first reflex blinking (Ghate and Edelhauser, 2006). Besides, tight epithelial junctions in cornea, conjunctival and scleral tissues represent a major physical barrier for drug diffusion through anterior ocular tissues, a prerequisite for pharmacological treatment of glaucoma and uveitis, for instance (Dastjerdi et al., 2011). Moreover, efflux pumps (Karla et al., 2009) and cytochrome P450 (Zhang et al., 2008) present in the corneal epithelium negatively influence drug delivery. All these barriers cause low bioavailability in which less than 5% of the drugs administered via the topical route reach the target tissues (Jumelle et al., 2020). Consequently, high drug concentrations and frequent administration are often required in traditional topical formulations to achieve the desired therapeutic effects. This is time consuming and predisposes patients to several adverse effects, such as temporary blurred vision, ocular discomfort, and damage to the ocular surface. Especially upon long-term use and in chronic eye conditions, these disadvantages lead to low patient compliance and treatment failure (Gholizadeh et al., 2021; Patel et al., 2022).
A variety of drug delivery technologies and systems, such as prodrugs, in situ gels, cul-de-sac inserts (Lacrisert®) (Abdelkader et al., 2021) and carrier systems using nanoparticles, have been investigated and developed over the past decades to address the shortcomings associated with conventional eye formulations (Jumelle et al., 2020; Gholizadeh et al., 2021). Among them, nanoparticle-based systems have gained significant attraction due to their potential to improve ocular retention (Janagam et al., 2017; Jumelle et al., 2020). In addition, encapsulation into nanoparticles may prevent biologic and enzymatic degradation of drugs and, as a result, lower concentrations may be sufficient to achieve the desired therapeutic effects (Formica et al., 2021; Patel et al., 2022). The surface properties of nanoparticles, such as hydrophobicity or hydrophilicity and charge, can be easily tuned to increase the affinity towards ocular tissues and enable closer contact with the mucin layer on the ocular surface (Janagam et al., 2017; Patel et al., 2022). Nanoparticles can also be modified to release the encapsulated drug in a sustained manner and enhance drug permeability (Jumelle et al., 2020) using excipients such as chitosan (Wadhwa et al., 2010). Owing to their small size (<1 µM), nanoparticulate formulations do not pose discomfort or irritation to the eye and they can be easily prepared as liquid dosage forms, such as eye drops, for ease of administration (Jumelle et al., 2020; Gholizadeh et al., 2021). Nanoparticle-based systems offer great potential when it comes to ocular drug delivery. However, less is known whether the recent nanomedicinal developments for ocular therapy do live up to their promises.
A few nanoparticle-based ophthalmic products are currently commercially available. These include: 1) Inveltys®, which comprises mucus-penetrating polymeric nanoparticles with loteprednol etabonate for treatment of inflammation and pain after ocular surgery; 2) the cationic nanoemulsion Cyclokat®; 3) the anionic nanoemulsion Restasis®; and 4) the nanomicellar formulation Cequa®, all containing cyclosporine A for DED treatment (Natesan et al., 2020). In the literature, a great variety of different lipid and polymeric nanoparticles, and combinations thereof, have been described and many are under development for drug delivery to the anterior segment of the eye. Most of these are based on materials that are biocompatible and generally recognized as safe both from natural sources, such as chitosan, hyaluronic acid, alginate, and gelatin, and from synthetic origin, such as polymethacrylate-based copolymers (Eudragit®), poly (lactic-co-glycolic acid) (PLGA), and polycaprolactone (Jumelle et al., 2020). Further, many clinical trials are underway for different eye diseases using mucus penetrating nanoparticles. These include: 1) KPI-121-C-001, which is under Phase III clinical trials for ocular infections, irritations, and inflammations; 2) KPI-121-C-002, which is under Phase II clinical evaluation for dry eye; 3) KPI-121-C-003, which is intended for blepharitis and is in Phase II trial; and 4) KPI-121-C-004, which is currently under investigation for retinal vein occlusion and diabetic macular edema (Campos et al., 2020).
Despite the interest and excitement in nanomedicine, critical opinion letters have recently emerged in highly ranked pharmaceutical journals raising concerns about their lack of clinical translation, further, highlighting the importance of relevant and accurate data (Allen, 2019; Park, 2019). The main objective of this systematic review was, therefore, to scrutinize the in vivo therapeutic outcomes of “common” nanoparticles (Figure 1) for treatment of conditions in the anterior segment of the eye and present our critical analyses as to whether nanoparticular encapsulation improved ocular drug treatment when compared to non-encapsulated drug administration. If granted therapeutic improvement, we also wanted to identify the most successful types of nanoparticles and search for possible patterns of physicochemical parameters, e.g., surface charge of nanoparticles and nature of encapsulated drug, that may enhance their in vivo performance. A final objective was to study the correlation between in vitro and in vivo behavior of the nanoparticles.
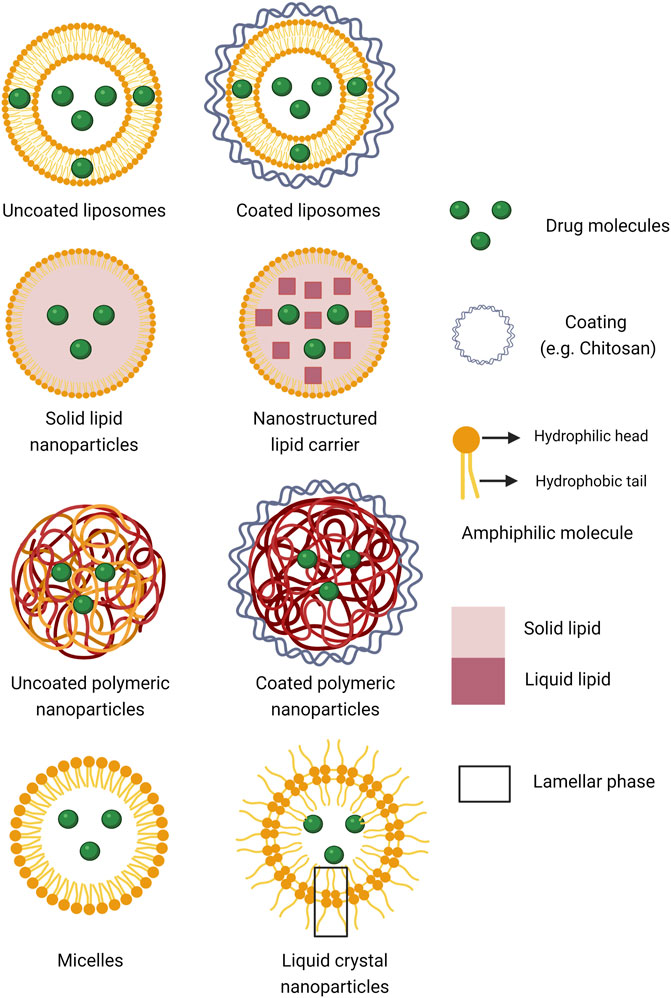
FIGURE 1. Schematic presentation of the different types of nanoparticulate systems for drug encapsulation scrutinized in the present analysis (Created with BioRender.com).
2 Methods
A systematic search for published peer-reviewed articles focusing on drug delivery to the anterior segment of the eye was performed in Medline and Embase databases using the Ovid interface in October 2021. The search was limited to articles written in English, but not by publication date. The systematic search was thematically structured into four categories: 1) nanoparticles, 2) used as a drug delivery system, 3) installed on the ocular surface, 4) in in vivo systems. Both “free text words” and “medical subject headings (MeSH) terms”—used by the databases for indexing articles—were used for the search. Overlapping search words were used in each category, which were combined with “OR” to broaden the search within the category. The search results from all four categories were then combined with “AND” to narrow the search (Table 1). Hits of the type “original article” were screened based on inclusion and exclusion criteria (Table 2). Two authors reviewed each article independently, and a third one was involved in case of disagreement. Hits of the type “review article” were first screened for relevance, based on title and abstract, by two authors. Then the bibliographies of the most relevant review articles were scrutinized for relevant original articles, using the procedure already described for original articles. Four authors screened at least 10 bibliographies each, covering 48 bibliographies in total. Finally, a non-systematic search was conducted in Google Scholar to look for studies that were missing from the systematic search.

TABLE 1. Systematic search thematically structured into four categories of “MeSH terms” and “free text words” used for literature search in Medline and Embase databases using the Ovid interface. The “MeSH terms” and “free text words” in each category were combined with “OR” to broaden the search within the category. The search results from all four categories were then combined with “AND” to narrow the search.
Our research question, search strategy and inclusion/exclusion criteria can be framed to the PICO process as described in the following. The population (P) was human and animal subjects, both healthy and with conditions in the anterior segment of the eye. The intervention (I) was drug encapsulated in conventional nanoformulations installed on the ocular surface. The comparison (C) was the non-encapsulated (“free”) therapeutic agent. The outcome (O) was initially not limited to any specific clinical parameter, as we wanted the search to include a variety of ocular conditions. However, the outcome was limited to a quantifiable therapeutic outcome of a condition occurring on the ocular surface or after the drug has been released on the ocular surface.
Two authors retrieved and synthesized the results together. The therapeutic outcome of encapsulation within all reviewed ocular conditions was compared to the non-encapsulated drug including in some cases the commercial reference. In DED, ocular inflammation, and endotoxin-induced uveitis, this comparison was expressed as a ratio further used to rank the nanoparticles based on their performances. For DED, we focused on tear (secretion) volume and higher ratios were interpreted as improved therapeutic effect of nanoparticles. In ocular inflammation and endotoxin-induced uveitis, we focused on the number of polymorphonuclear cells, and lower ratios suggested better treatment outcome with the nanoparticles. An emphasis was given to the polymorphonuclear leucocytes values at specific time points that reflect a high degree of inflammation, as the effect of treatment should be greatest when the inflammation is at a maximum. For pupillary constriction/dilation evaluation, the ability of nanoparticles to extend the amount or duration of pupil diameter was considered following topical application to rabbit eyes. In case of glaucoma, the results were presented in various ways either as intraocular pressure (IOP) reduction (or its percentage) or IOP values. Due to such varied ways of data presentation in glaucoma studies, we, for ease of evaluation, ranked the therapeutic outcome within each data set by four categories of in vivo efficacy. These categories were based on the superior (1), good (2), moderate (3), and marginal (4) performance of the nanoparticles. The ranking criteria are summarized in Table 3.
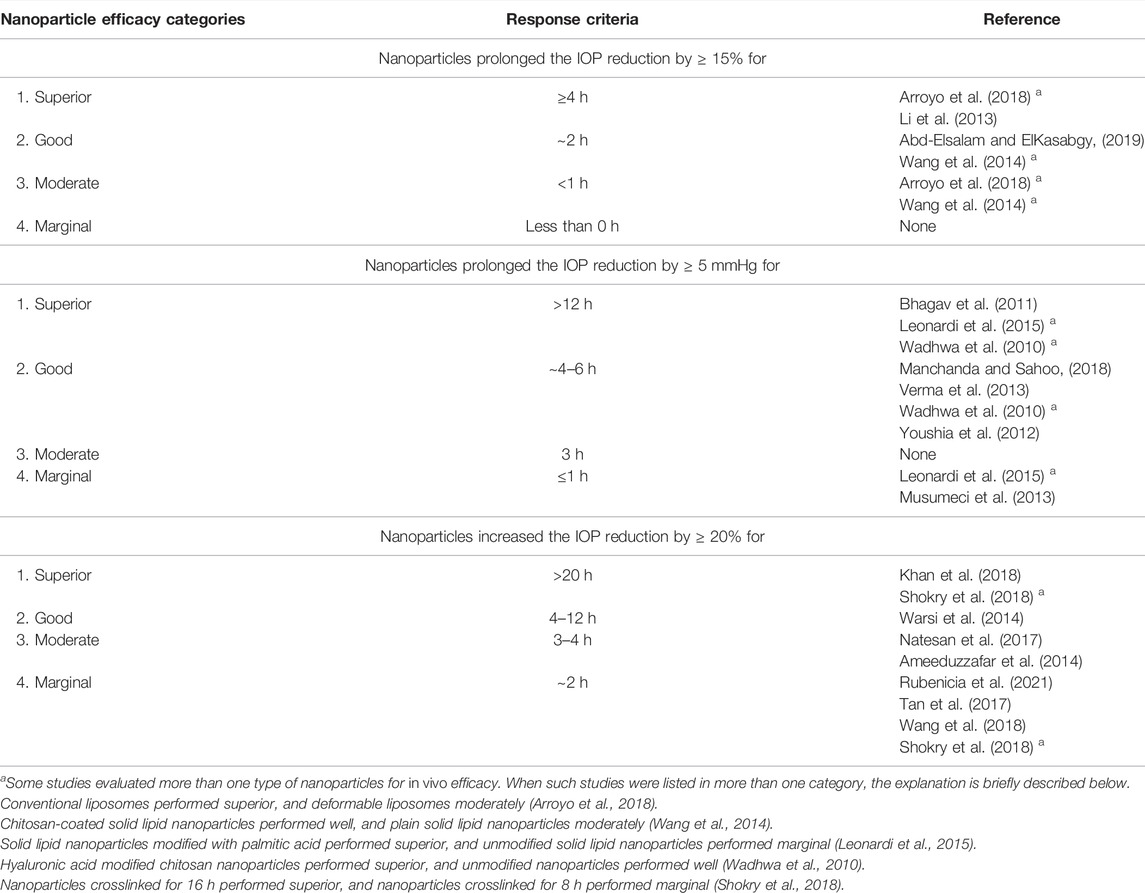
TABLE 3. Categorization of nanoparticle efficacy based on response criteria when evaluating in vivo efficacy of nanoencapsulation of anti-glaucoma drugs when compared to non-encapsulated drug, in the reviewed studies.
For reporting the studies, we consulted and followed the PRISMA guidelines where relevant. PRISMA checklist primarily focuses on the reporting of studies assessing the effects of intervention, often in a clinical setting aimed at a particular condition. The studies we found evaluated a technological intervention applied in several different conditions and studied using a small number of animal subjects.
3 Results
We retrieved 250 hits from our systematic search in Medline and Embase databases: 123 original research articles and 105 review articles, of which 48 reviews were regarded relevant. From the 123 original research articles, only three met all inclusion criteria as shown in the flowchart in Figure 2. After screening the bibliography of the 48 review articles, 35 more original research articles meeting all inclusion criteria were identified. Finally, 38 articles in total met all the inclusion criteria (Table 4). The search from Google Scholar did not yield additional articles.
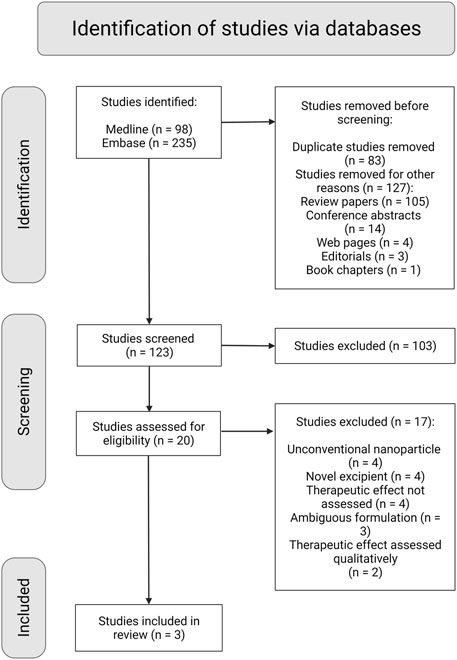
FIGURE 2. PRISMA flow diagram with schematic representation of the screening and selection process of studies retrieved from the systematic search (Created with BioRender.com).

TABLE 4. Summary of in vivo studies on nanoparticle-based systems used for drug delivery to anterior segment of the eye.
Table 4 shows articles on conventional nanoparticles developed for glaucoma, DED, ocular inflammation, endotoxin-induced uveitis, pupillary constriction/dilation, and corneal wound healing. Within each ocular condition, the articles were sorted based on the ranking criteria previously described. Based on 21 articles, glaucoma was the most common condition in the anterior segment of the eye for the development of nanoparticular drug delivery systems. Antiglaucoma drugs entrapped in liposomes (Arroyo et al., 2018), liquid crystal (Li et al., 2013), polymeric (Wadhwa et al., 2010; Bhagav et al., 2011; Khan et al., 2018; Shokry et al., 2018), and solid lipid nanoparticles (Leonardi et al., 2015) showed superior in vivo outcomes when compared to the non-encapsulated drugs (Table 3). When timolol maleate was encapsulated into polymeric nanoparticles, the IOP reduction (by ≥ 20%) was prolonged for over 20 h (Shokry et al., 2018). Similarly, encapsulation prolonged the IOP reduction (by ≥ 5 mmHg) for more than 12 h in several other studies (Wadhwa et al., 2010; Bhagav et al., 2011; Leonardi et al., 2015).
Five articles on DED were reviewed. Among these, polymeric gelatin (Huang et al., 2018) and coated PLGA (Chen et al., 2021) nanoparticles enhanced tear production by ∼1.8- and 1.5-fold, respectively. Liposomes (Shafaa et al., 2011; Karn et al., 2014) and micelles (Kang et al., 2016) increased the tear volume by only ∼1.2-fold. The actual tear secretion (in millimeters) after treatment with both encapsulated and non-encapsulated drugs are presented in Table 4. For ocular inflammation and endotoxin-induced uveitis, eight articles were assessed. A noticeable reduction in the number of polymorphonuclear leucocytes in aqueous humor was observed with drug-incorporated nanoparticles compared to the drug in solution or suspension. After a single instillation, the lowest ratio (0.64) was obtained with Eudragit® nanoparticles (Katara and Majumdar, 2013), while the highest ratio (0.86) was obtained with solid lipid nanoparticles (Sharma AK. et al., 2016b). After multiple instillations, Eudragit® nanoparticles resulted in even lower ratios, ranging from 0.17 to 0.60 (Table 4) (Adibkia et al., 2007a; Adibkia et al., 2007b).
Three articles studied pupil constriction or dilation in healthy rabbits using different types of nanoparticles (Table 4). Pepić et al. (2004) showed that micelles only achieved a ∼10% increase in pupillary constriction and a 30 min increase in duration, while Pignatello et al. (2002a) reported that Eudragit® nanoparticles minimally increased the pupil diameter. We found one report of corneal wound healing where liquid crystal nanoparticles were used. The nanoformulation decreased the injured corneal area by ∼50% and reduced inflammation after 82 h of administration compared to non-encapsulated drug (Silva et al., 2019).
In all studied eye conditions, rabbit models were commonly used for investigating the in vivo performance of the nanoparticles. The number of animals used per experimental group ranged from 3 to 10 (Table 4). For glaucoma studies, rabbits with both normal as well as elevated IOP were used. For DED, rabbits used were pretreated topically with eye drops containing either 0.1% benzalkonium chloride (Huang et al., 2018; Chen et al., 2021) or 1% atropine sulfate in order to induce disease symptoms (Shafaa et al., 2011; Karn et al., 2014; Kang et al., 2016). Ocular inflammation in rabbits was induced with topical application of arachidonic acid (Bucolo et al., 2002; Katara and Majumdar, 2013; Sharma A. K. et al., 2016a; Sharma AK. et al., 2016b; Weng et al., 2018; Katara et al., 2019) or endotoxin (Adibkia et al., 2007a; Adibkia et al., 2007b). Pupillary constriction was studied in initially healthy animals (Pignatello et al., 2002a; Pignatello et al., 2002b; Pepić et al., 2004) whereas ocular wound was created by topically exposing rabbit eyes to ethanol causing corneal chemical burn (Silva et al., 2019).
As with all research, a risk of bias might be involved when assessing only published studies, as most likely studies with positive therapeutic outcomes are published more often compared to those where no improvements are found. Due to such a bias trend in publication, we might have encountered mostly those studies where nanoparticles showed enhanced therapeutic effect in vivo when compared to the non-encapsulated drug.
4 Discussion
4.1 Nanoencapsulation in Different Ocular Conditions
In the following sections, we present a summary of our main findings. Additionally, characteristics of nanoparticles and encapsulated drug are discussed in relation to their performance in the various conditions of the anterior segment of the eye.
4.1.1 Glaucoma
Glaucoma is an ocular disorder characterized by optic nerve damage in a specific pattern, which if left untreated can potentially result in permanent vision loss (Morrison et al., 2005; Osborne, 2010). Neuroprotection in glaucoma is, thus, a sought-after approach to reduce the disease progression (Vishwaraj et al., 2022). An increase in IOP, usually above 21 mmHg (Heijl et al., 2002), is another critical risk factor of glaucoma. Therefore, effective clinical management of glaucoma can also be achieved by a mean IOP reduction of ∼ ≥ 15% or ≥ 5 mmHg (Akman et al., 2005). Current anti-glaucoma therapies act by either reducing aqueous humor formation or increasing the outflow of the fluid (Aggarwal and Kaur, 2005). Although conventional formulations can reduce the IOP to the normal level (16–18 mmHg), a persistent challenge has been to achieve a prolonged hypotensive effect due to short ocular residence time of the formulations. Pharmacological therapy for glaucoma often implies lifelong treatment. Reducing the frequency of drug administration is crucial to increase patient adherence to therapy and prevent disease progression. The potential of nanoparticles to increase ocular retention of anti-glaucomatous drugs has, therefore, lead to their great interest.
Four studies presented their results as percentage IOP reduction. Among them, the highest improvement of in vivo efficacy was obtained upon encapsulation of hydrophilic drugs into liquid crystalline nanoparticles (Li et al., 2013) and conventional liposomes (Arroyo et al., 2018) compared to non-encapsulated drugs. Looking at their physicochemical properties, it seems that small particle size may be beneficial as sizes of ∼15–200 nm (Li et al., 2013; Arroyo et al., 2018) performed better than particle sizes of ∼350 nm (Arroyo et al., 2018). Moreover, surface modification of the nanoparticles seems also to positively influence the therapeutic outcome. For instance, chitosan coated liposomes (Abd-Elsalam and ElKasabgy, 2019) and solid lipid nanoparticles fell into efficacy category 2 (Wang et al., 2014), while plain solid lipid nanoparticles fell into category 3 (Table 3) (Wang et al., 2014).
Seven studies used IOP reduction to report their findings. A distinctive property that appeared from the nanoparticles categorized as “superior” and “good” in terms of efficacy from these studies (category 1 and 2 of Table 3) was positive surface charge. These formulations were either based on the positively charged polymers Eudragit® (Bhagav et al., 2011; Verma et al., 2013) and chitosan (Wadhwa et al., 2010; Manchanda and Sahoo, 2018), or positively charged lipids (Youshia et al., 2012; Leonardi et al., 2015). Similarly, the nanoparticles were small, ranging from ∼118 nm to ∼320 nm (Wadhwa et al., 2010; Bhagav et al., 2011; Youshia et al., 2012; Verma et al., 2013; Leonardi et al., 2015; Manchanda and Sahoo, 2018). However, positive surface charge alone did not guarantee a successful outcome. Positively charged solid lipid nanoparticles have been categorized both as “superior” and “marginal”, depending on the composition of the lipid core (Leonardi et al., 2015). This indicates that other factors than surface charge may also play a role. Especially when palmitic acid was included in the formulation, solid lipid nanoparticles demonstrated “superior” therapeutic outcome (category 1) (Leonardi et al., 2015). Moreover, when positively charged chitosan was modified with hyaluronic acid, the performance was improved; changing from “good” to “superior” (Wadhwa et al., 2010; Manchanda and Sahoo, 2018). According to the authors, this observation could be explained by a synergistic effect for mucoadhesion provided by hyaluronic acid in the formulation (Wadhwa et al., 2010). However, depending on the composition and the overall nanoparticle property, changes in the formulation did not always improve nanoparticle efficacy. Plain PLGA nanoparticles were not more effective than the aqueous drug solution, and modification with polyethylene glycol only marginally improved the therapeutic outcome (Musumeci et al., 2013). When looking at the properties of the encapsulated substance, there were some indications that charged drugs benefitted the most from being encapsulated (Wadhwa et al., 2010; Bhagav et al., 2011; Manchanda and Sahoo, 2018). However, there were some ambiguity as, for example, solid lipid nanoparticles encapsulating melatonin performed “superior” (Leonardi et al., 2015) while incorporation of melatonin into PLGA enhanced efficacy only marginally. The “marginal” performance of PLGA might be explained by its slow melatonin release (Musumeci et al., 2013), as discussed further in section 4.2.1.
Eight studies reported the findings as IOP values in mmHg at different time points (Table 3). In contrast to previous observation (Musumeci et al., 2013), when forskolin (Khan et al., 2018) and dorzolamide hydrochloride were encapsulated into PLGA nanoparticles (Warsi et al., 2014), the performance of the drugs improved substantially (category 1 and 2, respectively). The “superior” PLGA nanoparticles had mucoadhesive chitosan-coating (Khan et al., 2018). The importance of mucoadhesion was also confirmed by Shokry et al. (2018) using gelatin nanoparticles. Smaller gelatin particles (∼206 nm vs. ∼782 nm) performed better in vivo (Shokry et al., 2018) following the pattern on particle size. Again, nanoparticles belonging to the “superior” category had positive surface charge (Khan et al., 2018; Shokry et al., 2018). Chitosan-based nanoparticles, however, only performed moderately (category 3) (Ameeduzzafar et al., 2014; Natesan et al., 2017), and after coating with hyaluronic acid, the performance further decreased to “marginal” (Rubenicia et al., 2021). This was surprising as these results contradicted with those from Wadhwa et al. (2010) and Manchanda and Sahoo (2018) discussed earlier. Such inconsistency may be explained by the nature of the encapsulated drugs, where chitosan encapsulation potentially increased the therapeutic effect of a charged/hydrophilic molecule (Wadhwa et al., 2010; Manchanda and Sahoo, 2018) more than an uncharged/lipophilic one (Ameeduzzafar et al., 2014; Natesan et al., 2017; Rubenicia et al., 2021). Liposomes were the least effective delivery system, both plain (Wang et al., 2018) as well as chitosan-coated (Tan et al., 2017), which was contradictory to Arroyo et al. (2018) and Abd-Elsalam and ElKasabgy (2019). Again, drug release could be a possible explanation for such discrepancy further elaborated for Tan et al. (2017) in section 4.2.3.
4.1.2 Dry Eye Disease
DED causes changes in the quality and quantity of the tear film leading to dryness and irritation of the ocular mucosa (Yazdani et al., 2019). Multiple new approaches have been developed in the recent years for effective clinical assessment and treatment of DED (Heidari et al., 2019; Aragona et al., 2021). Here, we have evaluated the therapeutic outcome of nanoparticles in the treatment of DED by using tear secretion as the major parameter.
Compared to non-encapsulated drug, gelatin nanoparticles showed the highest increase in tear production. These nanoparticles were additionally coated with the mucoadhesive polymer hyaluronic acid (Huang et al., 2018). The positive effect of ocular adhesion was also seen in Chen et al. (2021), where both plain and coated PLGA nanoparticles were investigated. Sebocyte membranes engineered to overexpress integrin-β1 that promoted binding to the ocular epithelium was used to coat the PLGA nanoparticles. While coated PLGA nanoparticles increased tear production compared to non-encapsulated drug, plain PLGA nanoparticles decreased the production. However, in this study, dexamethasone suspension as the control was applied more frequently than the PLGA nanoparticles. Therefore, the results might have been underestimated for the latter (Chen et al., 2021). Nanoparticles without obvious adhesive properties, such as liposomes (Shafaa et al., 2011; Karn et al., 2014) and micelles (Kang et al., 2016), only marginally enhanced tear secretion.
4.1.3 Inflammation and Endotoxin-Induced Uveitis
Eye injuries, infection, irritation, and ocular surgery are some of the common causes of ocular inflammation, which can lead to vision-threatening outcomes (Katara and Majumdar, 2013). Ocular inflammation is characterized by infiltration of macrophages and neutrophils of the eye (Katara et al., 2019). Thus, measurement of the number of polymorphonuclear leucocytes in the aqueous humor of the eye can help assess the anti-inflammatory activity of nanoparticles upon their instillation.
Polymeric nanoparticles comprising of Eudragit® most effectively reduced the number of polymorphonuclear leucocytes compared to the non-encapsulated drug (Bucolo et al., 2002; Katara and Majumdar, 2013; Katara et al., 2019). These nanoparticles were positively charged, smaller than 250 nm, and encapsulated both the charged sodium ibuprofen (Bucolo et al., 2002) and uncharged aceclofenac (Katara and Majumdar, 2013; Katara et al., 2019). Poly-ε-caprolactone (Sharma A. K. et al., 2016a) and solid lipid nanoparticles were less effective systems (Sharma AK. et al., 2016b), probably due to a lack of sufficient release of the drug celecoxib from the nanoparticles, as discussed further in section 4.2.1.
In two other studies, Eudragit® nanoparticles were installed multiple times over a course of 36 h (Adibkia et al., 2007a; Adibkia et al., 2007b). Here, encapsulation did not improve the therapeutic outcome of the drugs methylprednisolone acetate and piroxicam for the first 6–12 h, but an increased effect was shown in the 24–36 h time span. Therefore, improved in vivo performance of nanoparticles may be revealed after multiple administrations.
4.1.4 Constriction of the Pupil
Surgical or mechanical traumas of the anterior segment of the eye can induce excessive constriction of the pupil (Kulkarni, 1991). The pupil diameter can be increased by pharmacological intervention, which requires a certain availability of the drug at the intraocular level (Pignatello et al., 2002a).
In contrast to earlier promising observations involving Eudragit® nanoparticles (Bucolo et al., 2002; Adibkia et al., 2007a; Adibkia et al., 2007b; Katara and Majumdar, 2013; Katara et al., 2019), Pignatello et al. (2002a) did not manage to further inhibit the pupillary response to surgical trauma with this delivery system. Micelles only achieved a marginal increase of pupillary response reflected in a very low degree of drug encapsulation (Pepić et al., 2004).
4.1.5 Nanoparticle and Drug Characteristics Potentially Influencing the Therapeutic Outcome
Based on our previous discussions, small nanoparticular size and adhesive properties, often related to a positive surface charge, were key factors benefitting the effect of encapsulation. Adhesive properties of nanoparticles could increase their ocular residence time, thereby prolonging the presence of encapsulated drug at the target tissues and, thus, prolonging their action. Larger particle size, on the other hand, may hinder the admittance to the target site (Shokry et al., 2018). The potential benefit of encapsulation might not be the same for charged/hydrophilic drug and an uncharged/lipophilic drug (Pepić et al., 2004). Therefore, it was difficult to independently evaluate the effect of encapsulation when the control and encapsulated drugs were not in the same chemical form (Pignatello et al., 2002b; Ibrahim et al., 2015; Weng et al., 2018). Non-irritant and non-toxic nanoformulations avoid tear stimulation, leading to longer retention times on the corneal surface. Several of the reported studies have shown absence of signs of irritation, inflammation, and histopathological changes in the ocular tissues associated with the nanoformulations. This was shown for different nanoparticular systems as well as excipients; plain (Shafaa et al., 2011; Karn et al., 2014) as well as chitosan-coated (Tan et al., 2017; Abd-Elsalam and ElKasabgy, 2019) liposomes, plain (Ameeduzzafar et al., 2014; Warsi et al., 2014; Huang et al., 2018; Khan et al., 2018) as well as coated (Wadhwa et al., 2010; Ibrahim et al., 2015) polymeric nanoparticles, solid lipid nanoparticles (Wang et al., 2014), nanostructured lipid matrix (Youshia et al., 2012), and liquid crystal nanoparticles (Li et al., 2013; Huang et al., 2017) were well tolerated upon topical instillation.
4.2 Associations Between Therapeutic Outcome and in vitro Studies
Neither the physicochemical properties of the nanoparticles, such as their size, surface charge, type of core and surface coating, nor the type of encapsulated drug, could solely determine nanoparticles’ behavior in vivo. For instance, liposomes showed both “superior” (Arroyo et al., 2018) and “marginal” therapeutic efficacy in glaucomatous rabbits. Similarly, PLGA formulations were the best (Warsi et al., 2014; Khan et al., 2018) as well as poor (Musumeci et al., 2013) performing systems in vivo. Eudragit® nanoparticles displayed “good” therapeutic efficacy in rabbits with glaucoma (Bhagav et al., 2011; Verma et al., 2013) and inflammation (Bucolo et al., 2002; Katara and Majumdar, 2013; Katara et al., 2019), but the in vivo effect was poor in pupillary constriction/dilation (Pignatello et al., 2002a). We, therefore, searched for a more complex interplay between the properties of nanoparticles and encapsulated drug, and their therapeutic efficacy, which might be revealed in their drug release, mucoadhesion or transcorneal permeation properties, often measured in vitro/ex vivo as part of the development work.
4.2.1 Drug Release
To achieve prolonged therapeutic effect, as desired for ocular drug delivery, the drug should be released from the nanoparticles in a sustained manner. Several studies have shown correlation of improved therapeutic outcome of nanoencapsulated drug with sustained drug release in vitro (Li et al., 2013; Ameeduzzafar et al., 2014; Tan et al., 2017; Khan et al., 2018; Wang et al., 2018). Such correlation was also demonstrated among different nanoparticular compositions, made with varying proportions of secondary emulsifier and polymer. The Eudragit® formulation with higher burst release followed by a fast drug release in vitro had a shorter duration of drug action compared to the one that displayed a sustained release profile (IOP reduction for ∼36 h vs. 72 h) (Bhagav et al., 2011). Nevertheless, converse correlation has also been demonstrated. Solid lipid nanoparticles based on heterolipids, also termed nanostructured lipid matrices (Youshia et al., 2012), and chitosan nanoparticles (Manchanda and Sahoo, 2018) with fast drug release displayed a more pronounced and longer lasting IOP reduction. The reason for this discrepancy might be that drug release first and foremost must align with the residence time of the respective nanoparticle. In other words, time and place for drug release should coincide for an optimal therapeutic effect. A consequence of non-alignment was encountered in Abd-Elsalam and ElKasabgy (2019). Here, an in vivo therapeutic effect was achieved for 8 h indicating an even shorter residence time. However, the slowest releasing nanoparticle only released ∼50% of the drug in vitro within these 8 h. An increased pharmacological effect was observed when the drug release was increased to ∼70%. The therapeutic effect of this formulation could have increased had a higher amount of drug been released within the ocular residence time. In another study, there was only marginal improvement in IOP reduction with polyethylene glycol modified PLGA nanoparticles compared to the aqueous melatonin solution. Such poor performance was evident in the non-alignment of drug release from nanoparticles in vitro and the time of effect in vivo. The results showed that only 20% of the drug was released after 24 h from the nanoparticles (Musumeci et al., 2013). A similar situation was encountered in Sharma A. K. et al. (2016a) and Sharma AK. et al. (2016b) where the number of polymorphonuclear leucocytes were measured after 4 h, however, only ∼20% of the drug was released by that time.
Drug release is an important factor for influencing therapeutic outcome. This was also demonstrated in Warsi et al. (2014), where various PLGA nanoparticles had similar drug release rates that were reflected by their therapeutic effects. However, the case is not always that simple. Gelatin nanoparticles crosslinked for 16 or 8 h had almost superimposable release profiles, despite different therapeutic outcomes (Shokry et al., 2018) attributed to the positive effect of small particle size (∼206 nm vs. ∼782 nm). And in Leonardi et al. (2015), the release of melatonin from various solid lipid nanoparticles at different time points could not be used to explain the in vivo outcome. The findings from Shokry et al. (2018) and Leonardi et al. (2015) indicated that additional parameters besides drug release might also be important, such as residence time and transcorneal drug permeability.
4.2.2 Ocular Surface Residence Time
Increased ocular surface residence time of nanoparticles will counteract the highly efficient ocular lacrimal drainage system. As an estimation of the in vivo ocular residence time, the interaction between mucus and the nanoparticles has been tested in vitro (Wadhwa et al., 2010; Ameeduzzafar et al., 2014; Tan et al., 2017; Manchanda and Sahoo, 2018; Abd-Elsalam and ElKasabgy, 2019). The mucin-particle method is commonly used, where changes in zeta potential and the mean particle size of nanoparticles are measured following mixing with negatively charged mucin (de Sá et al., 2015; Tan et al., 2017; Abd-Elsalam and ElKasabgy, 2019).
Manchanda and Sahoo (2018) examined the mucus-interaction of various chitosan nanoparticles and found associations with their in vivo therapeutic performance. When chitosan nanoparticles were coated with hyaluronic acid, the therapeutic effect increased (Wadhwa et al., 2010). The modification did not affect the in vitro drug release, which remained almost unchanged. The mucus-interaction increased slightly, although this increase (91% vs. 87%) was not significant enough to truly reflect the pronounced therapeutic benefit of this modification. This shows that in vitro mucus interaction assays alone do not sufficiently predict the in vivo ocular retention and, thus, the therapeutic effect of the nanoparticles. Some studies have used gamma scintigraphy for better prediction of nanoparticle behavior (Ameeduzzafar et al., 2014; Warsi et al., 2014; Tan et al., 2017; Khan et al., 2018). This technique, if available, gives sufficient information to design an effective delivery system where the drug release rate is targeted at the actual retention time of the nanoparticle. In the above-mentioned studies, however, in vivo retention was only traced for 10 min (Tan et al., 2017), 30 min (Ameeduzzafar et al., 2014; Warsi et al., 2014) or up to 6 h (Khan et al., 2018). Although the latter is a long time, it did not match the drug release, which was monitored for at least 12 h.
4.2.3 Transcorneal Drug Permeability
Nanoencapsulation can also facilitate co-delivery of drug and permeation enhancers. The multifunctional polymer chitosan has permeation enhancing effect in addition to being mucoadhesive (Wadhwa et al., 2010; Ameeduzzafar et al., 2014; Tan et al., 2017; Khan et al., 2018). Other examples include surfactants, such as glyceryl monoolein (Li et al., 2013) or vitamin E tocopheryl polyethylene glycol 1,000 succinate (TPGS) (Warsi et al., 2014). Indeed, a correlation between the therapeutic outcome of nanoparticles and permeation through excised goat or rabbit cornea has been demonstrated in several studies. The ex vivo transcorneal transport of drug from nanoparticles was higher than from drug solution or suspension (Li et al., 2013; Ameeduzzafar et al., 2014; Tan et al., 2017; Khan et al., 2018).
On the contrary, the ex vivo enhanced permeability of vitamin E TPGS over polyvinyl alcohol-incorporated PLGA nanoparticles failed to corroborate in vivo results (Warsi et al., 2014). Their performance in vivo was comparable as were their drug release rates as discussed earlier. This may indicate an overestimation of permeation in ex vivo experiments. Similar observations were made in Manchanda and Sahoo (2018), where the difference in ex vivo transcorneal transport after 2 h was much larger than the difference in therapeutic outcome, which was better reflected in in vitro drug release and mucus-interaction. Thus, the ex vivo permeation assay is probably limited to comparing and ranking various nanoparticles and has less merit for predicting their in vivo performance. Advantages and limitations of in vitro and ex vivo corneal permeation assays have been reviewed previously (Agarwal and Rupenthal, 2016). A lack of alignment between drug release and permeation was apparent in Tan et al. (2017). There, close to 100% of the drug permeated within 6 h, while only ∼60% was released in the same period in the in vitro drug release experiment.
There were also examples where the relationship between ex vivo permeation and therapeutic outcome was ambiguous. In one instance, two different types of nanoparticles (chitosan and hyaluronic acid modified chitosan) with similar drug release rates were investigated. An improved therapeutic effect was observed with hyaluronic acid modified chitosan nanoparticles. The mucus-interaction predicted to some degree this improvement. The permeability assay was also capable of predicting this improvement, but only after 4 h and not for the first 2 h of the ex vivo study (Wadhwa et al., 2010). Additionally, the benefit of nanoencapsulation was overestimated at 4 h. In another study, permeation was lower for the encapsulated drug although the therapeutic outcome improved (Wang et al., 2014).
4.3 Challenges With Clinical Translation
Our investigation revealed that the in vivo correlation of commonly used in vitro tests during development and characterization of nanoparticles was limited. At present, these methodologies are limited in their usefulness unless accompanied by an in vivo proof of concept. Although each individual test can be used for comparative evaluation between different types of nanoparticles, we observed that the test results of various in vitro and ex vivo studies could not always be successfully combined. Therefore, designing a nanoparticle whose in vivo drug release aligns with the residence time in vivo has posed a challenge. Even after optimization of the formulation, the clinical translation of nanoparticles is faced with several technological, development and production issues (Weng et al., 2017; Jumelle et al., 2020). In addition, not every nanoparticle with promising therapeutic outcome in animal models performs well in clinical trials. One problem is that there are anatomical and physiological differences between humans and commonly used animal models, such as mice, rats and rabbits (Morrison et al., 2005). For instance, rabbit ocular anatomy, although being similar and comparable to human, does not completely mimic the latter. Rabbit eyes have higher mucus production, higher surface sensitivity and lower rate of blinking, which can result in better drug retention and drug penetration compared to human eyes, thus, resulting in overestimation of therapeutic outcome that cannot be extrapolated to humans (Destruel et al., 2017; Weng et al., 2017).
5 Conclusion
In the present study, we have evaluated and ranked various types of nanoparticles based on their therapeutic merits compared to non-encapsulated drug for ocular delivery. The majority of the studies demonstrated some improved efficacy of drugs after encapsulation, however, to variable degrees. The greatest achievement was quite substantial. For instance, the successful nanoparticles prolonged IOP reduction for over 20 h. Other promising nanoformulations increased tear production by ∼80%. Similarly, nanoparticles reduced polymorphonuclear leucocytes by ∼36% on single administration, which increased to an impressive ∼83% after multiple instillations. We have noticed trends that small and mucoadhesive nanoparticles, often caused by a positive surface charge, might be beneficial. However, this picture is ambiguous, possibly due to the complex interplay between the physicochemical properties of the drug along with the core and surface properties of the nanoparticles. This interplay was sometimes revealed in in vitro drug release, in vitro mucus interaction and/or ex vivo permeation tests.
Future work should be directed towards designing nanoparticles by systematic evaluation of one formulation parameter at a time. It is also crucial that the drug release aligns with formulation residence time in vivo. Additionally, development of more in vivo relevant in vitro assays, particularly for adhesion and permeability, may improve the characterization of nanoparticles for a particular drug and for a specific condition. Rational extrapolation of data from relevant in vitro experiments would then be used to predict in vivo behavior, thereby minimizing the need for animal testing, which is already limited due to ethical, economic, and technical reasons.
Data Availability Statement
The original contributions presented in the study are included in the article/Supplementary Material, further inquiries can be directed to the corresponding author.
Author Contributions
EH and SN conceived the idea. MB led the literature search, screened hits, and reviewed papers for results, and wrote the first draft. EH, SN, and MY contributed to the literature search process, and screened hits and reviewed papers for results. MB and EH extracted, interpreted, and discussed the results. EH, SN, MY, and TU revised the manuscript before submission.
Conflict of Interest
The authors declare that the research was conducted in the absence of any commercial or financial relationships that could be construed as a potential conflict of interest.
Publisher’s Note
All claims expressed in this article are solely those of the authors and do not necessarily represent those of their affiliated organizations, or those of the publisher, the editors and the reviewers. Any product that may be evaluated in this article, or claim that may be made by its manufacturer, is not guaranteed or endorsed by the publisher.
Acknowledgments
We acknowledge the generous support of Niclas Karlsson with the format of the figures.
References
Abd-Elsalam, W. H., and ElKasabgy, N. A. (2019). Mucoadhesive Olaminosomes: A Novel Prolonged Release Nanocarrier of Agomelatine for the Treatment of Ocular Hypertension. Int. J. Pharm. 560, 235–245. doi:10.1016/j.ijpharm.2019.01.070
Abdelkader, H., Fathalla, Z., Seyfoddin, A., Farahani, M., Thrimawithana, T., Allahham, A., et al. (2021). Polymeric Long-Acting Drug Delivery Systems (LADDS) for Treatment of Chronic Diseases: Inserts, Patches, Wafers, and Implants. Adv. Drug Deliv. Rev. 177, 113957. doi:10.1016/j.addr.2021.113957
Adibkia, K., Omidi, Y., Siahi, M. R., Javadzadeh, A. R., Barzegar-Jalali, M., Barar, J., et al. (2007a). Inhibition of Endotoxin-Induced Uveitis by Methylprednisolone Acetate Nanosuspension in Rabbits. J. Ocul. Pharmacol. Ther. 23 (5), 421–432. doi:10.1089/jop.2007.0039
Adibkia, K., Siahi Shadbad, M. R., Nokhodchi, A., Javadzedeh, A., Barzegar-Jalali, M., Barar, J., et al. (2007b). Piroxicam Nanoparticles for Ocular Delivery: Physicochemical Characterization and Implementation in Endotoxin-Induced Uveitis. J. Drug Target 15 (6), 407–416. doi:10.1080/10611860701453125
Agarwal, P., and Rupenthal, I. D. (2016). In Vitro and Ex Vivo Corneal Penetration and Absorption Models. Drug Deliv. Transl. Res. 6 (6), 634–647. doi:10.1007/s13346-015-0275-6
Aggarwal, D., and Kaur, I. P. (2005). Improved Pharmacodynamics of Timolol Maleate from a Mucoadhesive Niosomal Ophthalmic Drug Delivery System. Int. J. Pharm. 290 (1), 155–159. doi:10.1016/j.ijpharm.2004.10.026
Akman, A., Cetinkaya, A., Akova, Y. A., and Ertan, A. (2005). Comparison of Additional Intraocular Pressure-Lowering Effects of Latanoprost vs Brimonidine in Primary Open-Angle Glaucoma Patients with Intraocular Pressure Uncontrolled by Timolol-Dorzolamide Combination. Eye (Lond) 19 (2), 145–151. doi:10.1038/sj.eye.6701428
Allen, C. (2019). Nanomedicine Researchers: Slow Down to Speed up. J. Control Release 315, 214–215. doi:10.1016/j.jconrel.2019.11.012
Ameeduzzafar, Z. A., Ali, J., Bhatnagar, A., Kumar, N., and Ali, A. (2014). Chitosan Nanoparticles Amplify the Ocular Hypotensive Effect of Cateolol in Rabbits. Int. J. Biol. Macromol. 65, 479–491. doi:10.1016/j.ijbiomac.2014.02.002
Aragona, P., Giannaccare, G., Mencucci, R., Rubino, P., Cantera, E., and Rolando, M. (2021). Modern Approach to the Treatment of Dry Eye, a Complex Multifactorial Disease: a P.I.C.A.S.S.O. Board Review. Br. J. Ophthalmol. 105 (4), 446–453. doi:10.1136/bjophthalmol-2019-315747
Arroyo, C. M., Quinteros, D., Cózar-Bernal, M. J., Palma, S. D., Rabasco, A. M., and González-Rodríguez, M. L. (2018). Ophthalmic Administration of a 10-Fold-Lower Dose of Conventional Nanoliposome Formulations Caused Levels of Intraocular Pressure Similar to Those Induced by Marketed Eye Drops. Eur. J. Pharm. Sci. 111, 186–194. doi:10.1016/j.ejps.2017.09.024
Bhagav, P., Upadhyay, H., and Chandran, S. (2011). Brimonidine Tartrate-Eudragit Long-Acting Nanoparticles: Formulation, Optimization, In Vitro and In Vivo Evaluation. AAPS PharmSciTech 12 (4), 1087–1101. doi:10.1208/s12249-011-9675-1
Bucolo, C., Maltese, A., Puglisi, G., and Pignatello, R. (2002). Enhanced Ocular Anti-inflammatory Activity of Ibuprofen Carried by an Eudragit RS100 Nanoparticle Suspension. Ophthalmic Res. 34 (5), 319–323. doi:10.1159/000065608
Campos, P. M., Petrilli, R., and Lopez, R. F. V. (2020). The Prominence of the Dosage Form Design to Treat Ocular Diseases. Int. J. Pharm. 586, 119577. doi:10.1016/j.ijpharm.2020.119577
Chen, L., Wu, F., Pang, Y., Yan, D., Zhang, S., Chen, F., et al. (2021). Therapeutic Nanocoating of Ocular Surface. Nano Today 41, 101309. doi:10.1016/j.nantod.2021.101309
Dastjerdi, M. H., Sadrai, Z., Saban, D. R., Zhang, Q., and Dana, R. (2011). Corneal Penetration of Topical and Subconjunctival Bevacizumab. Invest. Ophthalmol. Vis. Sci. 52 (12), 8718–8723. doi:10.1167/iovs.11-7871
Dave, R. S., Goostrey, T. C., Ziolkowska, M., Czerny-Holownia, S., Hoare, T., and Sheardown, H. (2021). Ocular Drug Delivery to the Anterior Segment Using Nanocarriers: A Mucoadhesive/mucopenetrative Perspective. J. Control Release 336, 71–88. doi:10.1016/j.jconrel.2021.06.011
de Sá, F. A., Taveira, S. F., Gelfuso, G. M., Lima, E. M., and Gratieri, T. (2015). Liposomal Voriconazole (VOR) Formulation for Improved Ocular Delivery. Colloids Surf. B Biointerfaces 133, 331–338. doi:10.1016/j.colsurfb.2015.06.036
Destruel, P. L., Zeng, N., Maury, M., Mignet, N., and Boudy, V. (2017). In Vitro and In Vivo Evaluation of In Situ Gelling Systems for Sustained Topical Ophthalmic Delivery: State of the Art and beyond. Drug Discov. Today 22 (4), 638–651. doi:10.1016/j.drudis.2016.12.008
Dhouib, W., Abdallah, S., Bannour, R., Ben Cheikh, A., Bhiri, S., Ghali, H., et al. (2021). Quality of Life in Adult Patients with Open-Angle Glaucoma: a Systematic Review. Eur. J. Public Health 31 (Suppl. 3), ckab165. 180. doi:10.1093/eurpub/ckab165.180
Formica, M. L., Awde Alfonso, H. G., and Palma, S. D. (2021). Biological Drug Therapy for Ocular Angiogenesis: Anti-VEGF Agents and Novel Strategies Based on Nanotechnology. Pharmacol. Res. Perspect. 9 (2), e00723. doi:10.1002/prp2.723
Ghate, D., and Edelhauser, H. F. (2006). Ocular Drug Delivery. Expert Opin. Drug Deliv. 3 (2), 275–287. doi:10.1517/17425247.3.2.275
Gholizadeh, S., Wang, Z., Chen, X., Dana, R., and Annabi, N. (2021). Advanced Nanodelivery Platforms for Topical Ophthalmic Drug Delivery. Drug Discov. Today 26 (6), 1437–1449. doi:10.1016/j.drudis.2021.02.027
Heidari, M., Noorizadeh, F., Wu, K., Inomata, T., and Mashaghi, A. (2019). Dry Eye Disease: Emerging Approaches to Disease Analysis and Therapy. J. Clin. Med. 8 (9), 1439. doi:10.3390/jcm8091439
Heijl, A., Leske, M. C., Bengtsson, B., Hyman, L., Bengtsson, B., and Hussein, M. (2002). Reduction of Intraocular Pressure and Glaucoma Progression: Results from the Early Manifest Glaucoma Trial. Arch. Ophthalmol. 120 (10), 1268–1279. doi:10.1001/archopht.120.10.1268
Huang, H. Y., Wang, M. C., Chen, Z. Y., Chiu, W. Y., Chen, K. H., Lin, I. C., et al. (2018). Gelatin-epigallocatechin Gallate Nanoparticles with Hyaluronic Acid Decoration as Eye Drops Can Treat Rabbit Dry-Eye Syndrome Effectively via Inflammatory Relief. Int. J. Nanomedicine 13, 7251–7273. doi:10.2147/IJN.S173198
Huang, J., Peng, T., Li, Y., Zhan, Z., Zeng, Y., Huang, Y., et al. (2017). Ocular Cubosome Drug Delivery System for Timolol Maleate: Preparation, Characterization, Cytotoxicity, Ex Vivo, and In Vivo Evaluation. AAPS PharmSciTech 18 (8), 2919–2926. doi:10.1208/s12249-017-0763-8
Ibrahim, M. M., Abd-Elgawad, A. H., Soliman, O. A., and Jablonski, M. M. (2015). Natural Bioadhesive Biodegradable Nanoparticle-Based Topical Ophthalmic Formulations for Management of Glaucoma. Transl. Vis. Sci. Technol. 4 (3), 12. doi:10.1167/tvst.4.3.12
Janagam, D. R., Wu, L., and Lowe, T. L. (2017). Nanoparticles for Drug Delivery to the Anterior Segment of the Eye. Adv. Drug Deliv. Rev. 122, 31–64. doi:10.1016/j.addr.2017.04.001
Jumelle, C., Gholizadeh, S., Annabi, N., and Dana, R. (2020). Advances and Limitations of Drug Delivery Systems Formulated as Eye Drops. J. Control Release 321, 1–22. doi:10.1016/j.jconrel.2020.01.057
Kang, H., Cha, K. H., Cho, W., Park, J., Park, H. J., Sun, B. K., et al. (2016). Cyclosporine Amicellar Delivery System for Dry Eyes. Int. J. Nanomedicine 11, 2921–2933. doi:10.2147/IJN.S107569
Karla, P. K., Earla, R., Boddu, S. H., Johnston, T. P., Pal, D., and Mitra, A. (2009). Molecular Expression and Functional Evidence of a Drug Efflux Pump (BCRP) in Human Corneal Epithelial Cells. Curr. Eye Res. 34 (1), 1–9. doi:10.1080/02713680802518251
Karn, P. R., Kim, H. D., Kang, H., Sun, B. K., Jin, S. E., and Hwang, S. J. (2014). Supercritical Fluid-Mediated Liposomes Containing Cyclosporin A for the Treatment of Dry Eye Syndrome in a Rabbit Model: Comparative Study with the Conventional Cyclosporin A Emulsion. Int. J. Nanomedicine 9, 3791–3800. doi:10.2147/IJN.S65601
Katara, R., and Majumdar, D. K. (2013). Eudragit RL 100-based Nanoparticulate System of Aceclofenac for Ocular Delivery. Colloids Surf. B Biointerfaces 103, 455–462. doi:10.1016/j.colsurfb.2012.10.056
Katara, R., Sachdeva, S., and Majumdar, D. K. (2019). Design, Characterization, and Evaluation of Aceclofenac-Loaded Eudragit RS 100 Nanoparticulate System for Ocular Delivery. Pharm. Dev. Technol. 24 (3), 368–379. doi:10.1080/10837450.2018.1486424
Khan, N., AmeeduzzafarKhanna, K., Khanna, K., Bhatnagar, A., Ahmad, F. J., and Ali, A. (2018). Chitosan Coated PLGA Nanoparticles Amplify the Ocular Hypotensive Effect of Forskolin: Statistical Design, Characterization and In Vivo Studies. Int. J. Biol. Macromol. 116, 648–663. doi:10.1016/j.ijbiomac.2018.04.122
Kulkarni, P. S. (1991). The Role of Endogenous Eicosanoids in Rabbit-Intraocular Inflammation. J. Ocul. Pharmacol. 7 (3), 227–241. doi:10.1089/jop.1991.7.227
Leonardi, A., Bucolo, C., Drago, F., Salomone, S., and Pignatello, R. (2015). Cationic Solid Lipid Nanoparticles Enhance Ocular Hypotensive Effect of Melatonin in Rabbit. Int. J. Pharm. 478 (1), 180–186. doi:10.1016/j.ijpharm.2014.11.032
Li, J., Wu, L., Wu, W., Wang, B., Wang, Z., Xin, H., et al. (2013). A Potential Carrier Based on Liquid Crystal Nanoparticles for Ophthalmic Delivery of Pilocarpine Nitrate. Int. J. Pharm. 455 (1), 75–84. doi:10.1016/j.ijpharm.2013.07.057
Manchanda, S., and Sahoo, P. K. (2018). Fabrication and Characterization of Mucoadhesive Topical Nanoformulations of Dorzolamide HCl for Ocular Hypertension. J. Pharm. Investig. 48 (3), 323–332. doi:10.1007/s40005-017-0324-x
Morrison, J. C., Johnson, E. C., Cepurna, W., and Jia, L. (2005). Understanding Mechanisms of Pressure-Induced Optic Nerve Damage. Prog. Retin Eye Res. 24 (2), 217–240. doi:10.1016/j.preteyeres.2004.08.003
Morthen, M. K., Magno, M. S., Utheim, T. P., Snieder, H., Jansonius, N., Hammond, C. J., et al. (2022). The Vision-Related Burden of Dry Eye. Ocul. Surf. 23, 207–215. doi:10.1016/j.jtos.2021.10.007
Musumeci, T., Bucolo, C., Carbone, C., Pignatello, R., Drago, F., and Puglisi, G. (2013). Polymeric Nanoparticles Augment the Ocular Hypotensive Effect of Melatonin in Rabbits. Int. J. Pharm. 440 (2), 135–140. doi:10.1016/j.ijpharm.2012.10.014
Natesan, S., Boddu, S. H. S., Krishnaswami, V., and Shahwan, M. (2020). The Role of Nano-Ophthalmology in Treating Dry Eye Disease. Pharm. Nanotechnol. 8 (4), 258–289. doi:10.2174/2211738508666200628034227
Natesan, S., Pandian, S., Ponnusamy, C., Palanichamy, R., Muthusamy, S., and Kandasamy, R. (2017). Co-encapsulated Resveratrol and Quercetin in Chitosan and Peg Modified Chitosan Nanoparticles: For Efficient Intra Ocular Pressure Reduction. Int. J. Biol. Macromol. 104, 1837–1845. doi:10.1016/j.ijbiomac.2017.04.117
Nichols, B. A., Chiappino, M. L., and Dawson, C. R. (1985). Demonstration of the Mucous Layer of the Tear Film by Electron Microscopy. Invest. Ophthalmol. Vis. Sci. 26 (4), 464–473.
Osborne, N. N. (2010). Mitochondria: Their Role in Ganglion Cell Death and Survival in Primary Open Angle Glaucoma. Exp. Eye Res. 90 (6), 750–757. doi:10.1016/j.exer.2010.03.008
Park, K. (2019). The Beginning of the End of the Nanomedicine Hype. J. Control Release 305, 221–222. doi:10.1016/j.jconrel.2019.05.044
Patel, K. D., Barrios Silva, L., Park, Y., Shakouri, T., Keskin-Erdogan, Z., Sawadkar, P., et al. (2022). Recent Advances in Drug Delivery Systems for Glaucoma Treatment. Mater. Today Nano 18, 100178. doi:10.1016/j.mtnano.2022.100178
Pepić, I., Jalsenjak, N., and Jalsenjak, I. (2004). Micellar Solutions of Triblock Copolymer Surfactants with Pilocarpine. Int. J. Pharm. 272 (1-2), 57–64. doi:10.1016/j.ijpharm.2003.11.032
Pignatello, R., Bucolo, C., Ferrara, P., Maltese, A., Puleo, A., and Puglisi, G. (2002a). Eudragit RS100 Nanosuspensions for the Ophthalmic Controlled Delivery of Ibuprofen. Eur. J. Pharm. Sci. 16 (1-2), 53–61. doi:10.1016/s0928-0987(02)00057-x
Pignatello, R., Bucolo, C., Spedalieri, G., Maltese, A., and Puglisi, G. (2002b). Flurbiprofen-loaded Acrylate Polymer Nanosuspensions for Ophthalmic Application. Biomaterials 23 (15), 3247–3255. doi:10.1016/s0142-9612(02)00080-7
Rubenicia, A. M. L., Cubillan, L. D. P., Sicam, V. A. D. P., Macabeo, A. P. G., Villaflores, O. B., and Castillo, A. L. (2021). Intraocular Pressure Reduction Effect of 0.005% Latanoprost Eye Drops in a Hyaluronic Acid-Chitosan Nanoparticle Drug Delivery System in Albino Rabbits. Transl. Vis. Sci. Technol. 10 (4), 2. doi:10.1167/tvst.10.4.2
Shafaa, M. W., El Shazly, L. H., El Shazly, A. H., El Gohary, A. A., and El Hossary, G. G. (2011). Efficacy of Topically Applied Liposome-Bound Tetracycline in the Treatment of Dry Eye Model. Vet. Ophthalmol. 14 (1), 18–25. doi:10.1111/j.1463-5224.2010.00834.x
Sharma, A. K., Sahoo, P. K., Majumdar, D. K., Sharma, N., Sharma, R. K., and Kumar, A. (2016b). Fabrication and Evaluation of Lipid Nanoparticulates for Ocular Delivery of a COX-2 Inhibitor. Drug Deliv. 23 (9), 3364–3373. doi:10.1080/10717544.2016.1183720
Sharma, A. K., Sahoo, P. K., Majumdar, D. K., and Panda, A. K. (2016a). Topical Ocular Delivery of a COX-II Inhibitor via Biodegradable Nanoparticles. Nanotechnol. Rev. 5 (5), 435–444. doi:10.1515/ntrev-2016-0004
Shokry, M., Hathout, R. M., and Mansour, S. (2018). Exploring Gelatin Nanoparticles as Novel Nanocarriers for Timolol Maleate: Augmented In-Vivo Efficacy and Safe Histological Profile. Int. J. Pharm. 545 (1), 229–239. doi:10.1016/j.ijpharm.2018.04.059
Silva, R. O., Costa, B. L. D., Silva, F. R. D., Silva, C. N. D., Paiva, M. B., Dourado, L. F. N., et al. (2019). Treatment for Chemical Burning Using Liquid Crystalline Nanoparticles as an Ophthalmic Delivery System for Pirfenidone. Int. J. Pharm. 568, 118466. doi:10.1016/j.ijpharm.2019.118466
Tan, G., Yu, S., Pan, H., Li, J., Liu, D., Yuan, K., et al. (2017). Bioadhesive Chitosan-Loaded Liposomes: A More Efficient and Higher Permeable Ocular Delivery Platform for Timolol Maleate. Int. J. Biol. Macromol. 94, 355–363. doi:10.1016/j.ijbiomac.2016.10.035
Verma, P., Gupta, R. N., Jha, A. K., and Pandey, R. (2013). Development, In Vitro and In Vivo Characterization of Eudragit RL 100 Nanoparticles for Improved Ocular Bioavailability of Acetazolamide. Drug Deliv. 20 (7), 269–276. doi:10.3109/10717544.2013.834417
Vishwaraj, C. R., Kavitha, S., Venkatesh, R., Shukla, A. G., Chandran, P., and Tripathi, S. (2022). Neuroprotection in Glaucoma. Indian J. Ophthalmol. 70 (2), 380–385. doi:10.4103/ijo.IJO_1158_21
Wadhwa, S., Paliwal, R., Paliwal, S. R., and Vyas, S. P. (2010). Hyaluronic Acid Modified Chitosan Nanoparticles for Effective Management of Glaucoma: Development, Characterization, and Evaluation. J. Drug Target 18 (4), 292–302. doi:10.3109/10611860903450023
Wang, F., Bao, X., Fang, A., Li, H., Zhou, Y., Liu, Y., et al. (2018). Nanoliposome-Encapsulated Brinzolamide-Hydropropyl-β-Cyclodextrin Inclusion Complex: A Potential Therapeutic Ocular Drug-Delivery System. Front. Pharmacol. 9 (91), 91. doi:10.3389/fphar.2018.00091
Wang, F., Chen, L., Zhang, D., Jiang, S., Shi, K., Huang, Y., et al. (2014). Methazolamide-loaded Solid Lipid Nanoparticles Modified with Low-Molecular Weight Chitosan for the Treatment of Glaucoma: Vitro and Vivo Study. J. Drug Target 22 (9), 849–858. doi:10.3109/1061186X.2014.939983
Warsi, M. H., Anwar, M., Garg, V., Jain, G. K., Talegaonkar, S., Ahmad, F. J., et al. (2014). Dorzolamide-loaded PLGA/vitamin E TPGS Nanoparticles for Glaucoma Therapy: Pharmacoscintigraphy Study and Evaluation of Extended Ocular Hypotensive Effect in Rabbits. Colloids Surf. B Biointerfaces 122, 423–431. doi:10.1016/j.colsurfb.2014.07.004
Weng, Y., Liu, J., Jin, S., Guo, W., Liang, X., and Hu, Z. (2017). Nanotechnology-based Strategies for Treatment of Ocular Disease. Acta Pharm. Sin. B 7 (3), 281–291. doi:10.1016/j.apsb.2016.09.001
Weng, Y. H., Ma, X. W., Che, J., Li, C., Liu, J., Chen, S. Z., et al. (2018). Nanomicelle-Assisted Targeted Ocular Delivery with Enhanced Antiinflammatory Efficacy In Vivo. Adv. Sci. (Weinh) 5 (1), 1700455. doi:10.1002/advs.201700455
Worakul, N., and Robinson, J. R. (1997). Ocular Pharmacokinetics/pharmacodynamics. Eur. J. Pharm. Biopharm. 44 (1), 71–83. doi:10.1016/S0939-6411(97)00064-7
Yañez-Soto, B., Mannis, M. J., Schwab, I. R., Li, J. Y., Leonard, B. C., Abbott, N. L., et al. (2014). Interfacial Phenomena and the Ocular Surface. Ocul. Surf. 12 (3), 178–201. doi:10.1016/j.jtos.2014.01.004
Yazdani, M., Elgstøen, K. B. P., Rootwelt, H., Shahdadfar, A., Utheim, Ø. A., and Utheim, T. P. (2019). Tear Metabolomics in Dry Eye Disease: A Review. Int. J. Mol. Sci. 20 (15), 3755. doi:10.3390/ijms20153755
Youshia, J., Kamel, A. O., El Shamy, A., and Mansour, S. (2012). Design of Cationic Nanostructured Heterolipid Matrices for Ocular Delivery of Methazolamide. Int. J. Nanomedicine 7, 2483–2496. doi:10.2147/IJN.S28307
Keywords: ocular drug delivery, topical administration, nanoparticle, drug delivery, encapsulation, animal studies, in vivo efficacy
Citation: Bhandari M, Nguyen S, Yazdani M, Utheim TP and Hagesaether E (2022) The Therapeutic Benefits of Nanoencapsulation in Drug Delivery to the Anterior Segment of the Eye: A Systematic Review. Front. Pharmacol. 13:903519. doi: 10.3389/fphar.2022.903519
Received: 24 March 2022; Accepted: 25 April 2022;
Published: 13 May 2022.
Edited by:
Paolo Giunchedi, University of Sassari, ItalyReviewed by:
Kaihui Nan, Wenzhou Medical University, ChinaNarendar Dudhipala, University of Mississippi, United States
Carla Marcella Caramella, University of Pavia, Italy
Copyright © 2022 Bhandari, Nguyen, Yazdani, Utheim and Hagesaether. This is an open-access article distributed under the terms of the Creative Commons Attribution License (CC BY). The use, distribution or reproduction in other forums is permitted, provided the original author(s) and the copyright owner(s) are credited and that the original publication in this journal is cited, in accordance with accepted academic practice. No use, distribution or reproduction is permitted which does not comply with these terms.
*Correspondence: Madhavi Bhandari, bWFkaGF2aWJAb3Nsb21ldC5ubw==