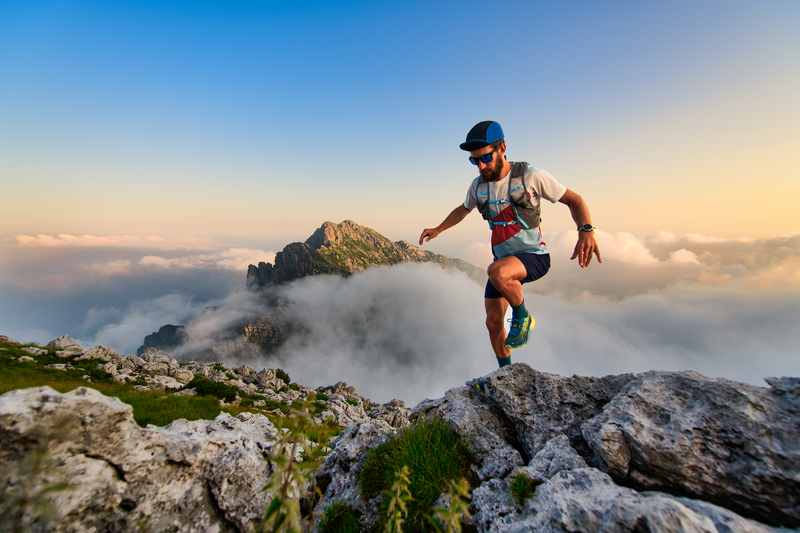
94% of researchers rate our articles as excellent or good
Learn more about the work of our research integrity team to safeguard the quality of each article we publish.
Find out more
ORIGINAL RESEARCH article
Front. Pharmacol. , 11 May 2022
Sec. Pharmacology of Infectious Diseases
Volume 13 - 2022 | https://doi.org/10.3389/fphar.2022.890955
This article is part of the Research Topic Peptides Against Infectious Diseases: From Antimicrobial Peptides to Vaccines View all 8 articles
Acinetobacter baumannii is one of the most dangerous opportunistic pathogens in the global health care setup. Its drug resistance and biofilm-forming capability are often associated with chronic infections that are difficult to treat. Therefore, the clinical treatments for highly drug-resistant A. baumannii are limited. Antimicrobial peptides are broad-spectrum antibacterial agents combined with antibiotics that minimize selective bacterial resistance and enhance antibacterial efficacy. The current study evaluated the synergistic antibacterial activities of clinically important peptide antibiotics combined with other antimicrobials against nine extensively drug-resistant A. baumannii strains in planktonic and biofilm cells in vitro. Polymyxin B and E combined with imipenem showed 100% synergy in the planktonic cell with the checkerboard. Moreover, polymyxin E with rifampicin and bacitracin with imipenem or meropenem showed 100% additive effects. In the biofilm cell, polymyxin B and E combined with azithromycin showed 100% synergy, when vancomycin with azithromycin, rifampicin, and bacitracin with azithromycin or rifampicin, and teicoplanin with tigecycline or rifampicin, all showed 100% additive effects. Therefore, peptide antibiotics combined with other antimicrobials have synergistic or additive effects on extensively drug-resistant A. baumannii in planktonic and biofilm cells. In addition, the combination of polymyxins with carbapenems or azithromycin could be an ideal therapy against extensively drug-resistant A. baumannii infections.
Acinetobacter baumannii, an opportunistic nosocomial pathogen causing severe infections, poses a significant threat to worldwide public health (Lee et al., 2017). The World Health Organization has categorized carbapenem-resistant A. baumannii, a primary member of the ESKAPE, as one of the first priority resistant bacteria globally (Mulani et al., 2019; De Oliveira et al., 2020). A. baumannii possess intrinsic antimicrobial resistance with its ability to easily adopt new resistance mechanisms that has driven the evolution of extensive-drug and even pan-drug-resistance. (Sobouti et al., 2020). A. baumannii mainly exists in two forms in the environment: planktonic and biofilm cells (Koo et al., 2017). Extensively drug-resistant A. baumannii (XDRAB) has acquired drug resistance features and strong biofilm-forming ability, a significant source of persistent nosocomial infection (Mohammed et al., 2020). Due to the lack of antibacterial drugs against XDRAB, new combinations are urgently required to replace traditional antibacterial therapy, which has become the current international research hotspot in the healthcare sector (Tacconelli et al., 2018).
Antimicrobial peptides (AMPs) both natural and synthetic are one of the primary options for overcoming drug resistance (Sierra et al., 2017). Moreover, some peptides, especially those that are similar to animal defense antimicrobial peptides, have immunomodulatory activity (Jenssen et al., 2006), which have less bacterial resistance and low cytotoxicity in humans (Pletzer and Hancock, 2016). AMPs in combination with antibiotics have been shown to minimize selective bacterial resistance and enhance antibacterial efficacy (Jahangiri et al., 2021). Currently, new AMPs still require large-scale clinical trials for validation (Liu et al., 2016). Therefore, re-evaluating the existing clinical peptide antibiotics through non-traditional combinations could be a significant opportunity against XDRAB.
In this study, we investigated the antibacterial and synergistic effects of peptide antibiotics (e.g., polymyxin B, polymyxin E, vancomycin, bacitracin, and teicoplanin) in combination with other antimicrobials (e.g., imipenem, meropenem, azithromycin, and rifampicin) against XDRAB in the planktonic and biofilm cells, that could provide a new therapy for preventing and controlling XDRAB infection.
Clinically-used antibacterial agents (Magiorakos et al., 2012), such as piperacillin, ceftazidime, cefotaxime, tetracycline, minocycline, gentamicin, amikacin, ciprofloxacin, levofloxacin, chloramphenicol, imipenem, meropenem, tigecycline, polymyxin B, polymyxin E, vancomycin, bacitracin, and teicoplanin were purchased from Meilun Biological (Dalian, Liaoning, China). In addition, cation-adjusted MH broth (CAMHB), Muller-Hinton broth (MHB) and tryptic soy broth (TSB) medium were obtained from Haibo Biotechnology (Qingdao, Shandong, China).
Nine XDRAB strains were isolated from clinical specimens such as the septum, urine, or respiratory lavage fluid of patients from the respiratory intensive care unit, intensive care unit, the cardiothoracic surgery department, and the neurology department at the First Affiliated Hospital of Chengdu Medical College during the period from 2018 to 2020 (Chengdu, Sichuan, China). In addition, A. baumannii ATCC 19606, Staphylococcus aureus ATCC 29213, and Escherichia coli ATCC 25922 were used as quality control strains in antimicrobial susceptibility testing (AST) and obtained from the American Type Culture Collection (ATCC, Manassas, VA, United States).
The minimum inhibitory concentrations (MICs) were determined by the broth microdilution method for the antibacterial agents and peptide antibiotics for A. baumannii based on the guidelines provided by the Clinical and Laboratory Standards Institute (CLSI) (Humphries et al., 2021). Briefly, bacterial cells were inoculated in Luria–Bertani agar medium at 37°C for 16–20 h, and then resuspended in saline (0.9% sodium chloride) to adjust the bacterial content to reach the 0.5 McFarland turbidity standard, followed by a 20-fold dilution. Antimicrobial solutions were prepared according to the CLSI (Humphries et al., 2021). Finally, 180 μl CAMHB, 10 µl corresponding antimicrobials, and 10 µl bacterial resuspension were added into 96-well plate (NEST TC, 701001, www.cell-nest.com). The value of OD600 was measured after incubating at 37°C for 16–20 h.
The minimum biofilm inhibitory concentrations (MBICs) were detected using a previous study protocol (Kart et al., 2021) for the antibacterial agents and peptide antibiotics for A. baumannii in the biofilm cell. First, bacterial cells were inoculated in Luria–Bertani agar medium at 37°C for 16–20 h. Next, the bacterial monoclones were resuspended in saline (0.9% sodium chloride) to adjust the bacterial content to reach the 0.5 McFarland turbidity standard. Then, 190 µl TSB and 10 µl bacterial resuspension were mixed in a 96-well plate. After incubating at 37°C for 24 h, the medium was aspirated, and 200 µl PBS was added to wash three times. Finally, 190 μl MH broth medium and 10 µl corresponding antibiotics were added into 96-well plate (NEST TC, 701001, www.cell-nest.com). The optical density was measured at OD600 after incubating for 16–20 h at 37°C.
The checkerboard method, the fractional inhibitory concentration index (FICI), and the fractional biofilm inhibitory concentration index (FBIC) evaluated the synergistic effects of five peptide antibiotics combined with one of the five antimicrobials in the planktonic and biofilm cells among the nine XDRAB isolates. The planktonic cells were investigated by adding 170 µl of MHB and 10 µl of bacterial suspension (1.5 × 108 CFU/ml) into the 96 well plates. It was followed by adding 10 µl of serially diluted peptide antibiotics along the x-axis and antimicrobials along the y-axis. The biofilm cells were studied by cultivating the bacteria to form the biofilm into a 96-well plate (NEST TC, 701001, www.cell-nest.com) and washed with 200 µl of PBS three times. The following steps were consistent with the planktonic study. The value of OD600 was measured after incubating at 37°C for 20 h. The FICI and FBIC were evaluated as follows: Synergy between the two drugs was defined as either a FICI or an FBIC of ≤0.5, >0.5–1 for additivity, >1–2 for indifference, and >2 for antagonism (Kim et al., 2015; Peng et al., 2020; Lin et al., 2021).
The MICs and MBICs of 15 antibacterial agents with five peptide antibiotics are shown in Table 1, based on the interpretive standards established by CLSI (Humphries et al., 2021). These isolates from the planktonic cell were either susceptible or intermediate to polymyxin B and polymyxin E. The tigecycline sensitivity rate was 100%, but they were resistant to other antibacterial agents. The drug-resistance profiles defined the isolates as XDRAB (Magiorakos et al., 2012). Once the biofilm of XDRAB was formed, the polymyxin and tigecycline resistance in the biofilm cell was further enhanced, depicting a pan-drug resistance (PDR) trend. In addition, there was no significant difference in the antibacterial effects of the five peptide antibiotics on XDRAB under the planktonic and biofilm cells; only the drug resistance of the latter was further elevated.
TABLE 1. Minimum inhibitory concentrations (MICs) and Minimum biofilm inhibitory concentrations (MBICs) of 15 antibacterial agents with five peptide antibiotics against nine clinical isolates of A. baumannii.
Based on the FICI and FBIC values obtained from the combined drug susceptibility test (Table 2), five peptide antibiotics combined with other five antimicrobials had different degrees of synergistic or additive effects on nine XDRAB strains in the planktonic and biofilm cells. The FICI range of polymyxin B or polymyxin E combined with imipenem in the planktonic cell was 0.375–0.5 and 0.25–0.5, respectively. Synergy was noted in 100% of the isolates. When polymyxin E was combined with rifampicin and bacitracin with imipenem or meropenem, there was a 100% additive effect. In the biofilm cell, when polymyxin B and polymyxin E were combined with azithromycin, the FBIC range was 0.19–0.31 and 0.19–0.375, respectively, with a 100% synergistic effect. In addition, 100% additivity was detected when vancomycin was combined with azithromycin or rifampicin, bacitracin with azithromycin or rifampicin, and teicoplanin with tigecycline or rifampicin.
TABLE 2. Antibacterial effect of 5 peptide antibiotics in combination with 5 antimicrobials on extensively drug-resistant A. baumannii isolates (n = 9) in the planktonic cell (PC) and biofilm cell (BC).
Compared with the planktonic cell, the biofilm cell of A. baumannii possesses significant biological characteristics at the transcription levels of associated virulence genes (Selasi et al., 2016). Therefore, we evaluated the antibacterial effects of nine XDRAB strains in the planktonic and biofilm cells. We also investigated the antibacterial and synergistic effects of peptide antibiotics, both alone and in combination with other antimicrobials. Our antimicrobial susceptibility results depicted that all the nine isolates in the planktonic cell were 100% sensitive to tigecycline and 33% sensitive to polymyxins. However, they were resistant to the rest of the 13 drugs. These strains all conformed to the criteria of XDRAB (Magiorakos et al., 2012). Our study indicated that the resistance rate to polymyxins was 100% after the XDRAB biofilm formation, while the intermediate tigecycline rate was 67%. The resistance rate was 22%, and the sensitivity rate was 11%, which was consistent with the results of Shenkutie et al. (2020). Various studies have demonstrated that A. baumannii drug-resistance in the biofilm cell was significantly elevated, which could be related to the extracellular polysaccharide matrix formation and efflux pump overexpression in biofilm (Singh et al., 2017). Our findings were consistent with the fact that XDRAB has posed a significant threat in global clinical settings requiring combination therapy. Indeed, the clinical options to treat XDRAB with antibacterial drugs are limited. Therefore, a non-traditional combination treatment has been pursued (Spellberg and Bonomo, 2015). New AMPs are mainly produced by living organisms and defend the host against pathogens, as a part of innate immunity (Wang et al., 2016), which could become potential antibacterial agents against XDRAB infection (Duraisamy et al., 2020). However, new AMPs are still in the process of drug discovery and development. Thus, we targeted drugs with existing clinical peptide antibiotics combined with other antimicrobials to study whether they have synergistic effects on XDRAB in planktonic and biofilm cells.
Our study depicted that the synergistic antibacterial effects of polymyxin B or E combined with imipenem were 100% against XDRAB in the planktonic cell. The optimal concentrations of polymyxin with imipenem are 1 μg/ml and 4 μg/ml, respectively. The imipenem MIC ≤4 μg/ml was thought to be susceptible in vitro. Our results were consistent with the study by Leu et al. (2014), which showed that the response of imipenem resistance reversal was dependent on polymyxin concentrations, the combination of imipenem and polymyxin at 1 μg/ml could reverse imipenem resistance in 74.6% of A. baumannii. Another study recommended polymyxin combined with imipenem as a treatment regimen for extensively drug-resistant Gram-negative bacteria (Guan et al., 2016). Moreover, our study revealed that the combination of polymyxin E with rifampicin provided a 100% additive effect, wherein polymyxin could have disrupted the integrity of the bacterial outer membrane, leading to the elevated intracellular concentration of rifampicin and enhancing the antibacterial effect (Goldstein, 2014; Khondker and Rheinstädter, 2020). Our results were consistent with the study by Park et al. (2019), which showed that the combination of polymyxin and rifampicin has been used successfully against polymyxin-resistant A. baumannii pneumonia. Bacitracin is a peptide antibiotic, it’s parenteral administration is highly restricted due to its nephrotoxicity, which prohibits its systemic therapeutic usage (Holbrook et al., 2021). Therefore, bacitracin is commonly used in combination with polymyxin and neomycin as triple antibiotic ointment for the topical treatment of wound infections (Sierra et al., 2017). When combined with imipenem or meropenem to treat XDRAB in the planktonic cell, it has 100% additive effects because bacitracin hindered bacterial cell wall synthesis by inhibiting lipid carrier dephosphorylation (Mascher et al., 2003). In contrast, carbapenems accelerated cell wall defects by restraining the secretion of mucopeptide synthase in the cell membrane. As a result, it inhibited bacterial cell wall synthesis and enhanced the antibacterial effects.
Our study showed that polymyxin B or E combinations with azithromycin had 100% synergistic effects when XDRAB was in the biofilm cell. As a traditional biofilm inhibitor (Parnham et al., 2014), azithromycin could inhibit the synthesis of extracellular polysaccharides and alginate of Pseudomonas aeruginosa at the sub-MIC level. The synthesis indirectly affected the expression of related virulence factors, inhibiting the biofilm formation (Imperi et al., 2014). We observed that the MBIC of azithromycin was 512–1024 μg/ml and was reduced to 128 μg/ml when combined with polymyxins. This synergistic effect is due to the inhibition of biofilm-related virulent gene expression by azithromycin through biofilm destruction of XDRAB using polymyxins. On the other hand, vancomycin, teicoplanin, and bacitracin mainly treat Gram-positive bacteria. However, in our study, these three peptide antibiotics combined with rifampicin had 100% additive effects. We proposed that rifampicin inhibited the β subunit of bacterial RNA polymerase, while these three peptide antibiotics disrupted the bacterial cell wall synthesis, these combinations may disturb cell reproduction in different steps to enhance the antibacterial effects (Douthit et al., 2020; Yu et al., 2020). Therefore, rifampicin could become an ideal choice for combination therapy with peptide antibiotics. Tigecycline is used as the last line of defense against XDRAB. Our study depicted that combining five peptide antibiotics had different synergistic or additive effects on XDRAB. Notably, the synergistic and additive effects of tigecycline with polymyxins were 67% and 33%, respectively. Another study demonstrated that tigecycline at the sub-MIC level could reduce the biofilm formation of A. baumannii by controlling the expression levels of virulence factors connected with fimbriae and efflux pumps (Navidifar et al., 2019). Furthermore, the specific combination also showed a partially indifferent effect. For example, an indifferent effect occurred in 100% of XDRAB when vancomycin was combined with imipenem. However, the synergistic and additive effect of teicoplanin, a glycopeptide, in combination with imipenem, was 33% and 67%, respectively, similar to a previous study by Goldstein et al. (2004). Their study revealed that vancomycin had significant antagonism against glycopeptide intermediate Staphylococcus aureus infection (GISA) with β-lactam drugs, but teicoplanin helped enhance the antibacterial effect. The clinical implications for these findings are still unclear. Due to the limitations of the in vitro activity, further studies through in vivo models should be conducted to investigate the mechanism of action involving peptide antibiotics with other antimicrobials to combat A. baumannii associated infections.
Our study has revealed that five clinically available peptide antibiotics combined with five antimicrobials produce different antibacterial effects on nine XDRAB strains within planktonic and biofilm cells. The best combinations were polymyxins with imipenem and polymyxins with azithromycin, respectively, with 100% synergy against nine XDRAB strains in planktonic and biofilm cells. Furthermore, these combinations reduced the dose and enhanced the antibacterial effects against two forms of XDRAB, showing potential clinical significance against XDRAB infection.
The original contributions presented in the study are included in the article/Supplementary Material, further inquiries can be directed to the corresponding author.
QM carried out the experimental procedures and analyzed the data, and drafted the manuscript. FL contributed to study design and revising the manuscript. BL supervised this study and revised the manuscript. All authors reviewed and approved the final version of the manuscript.
This work was supported by the National Natural Science Foundation of China (grants no. 81373454).
The authors declare that the research was conducted in the absence of any commercial or financial relationships that could be construed as a potential conflict of interest.
All claims expressed in this article are solely those of the authors and do not necessarily represent those of their affiliated organizations, or those of the publisher, the editors and the reviewers. Any product that may be evaluated in this article, or claim that may be made by its manufacturer, is not guaranteed or endorsed by the publisher.
De Oliveira, D. M. P., Forde, B. M., Kidd, T. J., Harris, P. N. A., Schembri, M. A., Beatson, S. A., et al. (2020). Antimicrobial Resistance in ESKAPE Pathogens. Clin. Microbiol. Rev. 33 (3). doi:10.1128/cmr.00181-19
Douthit, C., Gudenkauf, B., Hamood, A., Mudaliar, N., Caroom, C., and Jenkins, M. (2020). Effects of Powdered Rifampin and Vancomycin Solutions on Biofilm Production of staphylococcus Aureus on Orthopedic Implants. J. Clin. Orthop. Trauma 11 (Suppl. 1), S113–s117. doi:10.1016/j.jcot.2019.10.002
Duraisamy, S., Balakrishnan, S., Ranjith, S., Husain, F., Sathyan, A., Peter, A. S., et al. (2020). Bacteriocin-a Potential Antimicrobial Peptide towards Disrupting and Preventing Biofilm Formation in the Clinical and Environmental Locales. Environ. Sci. Pollut. Res. Int. 27 (36), 44922–44936. doi:10.1007/s11356-020-10989-5
Goldstein, B. P. (2014). Resistance to Rifampicin: a Review. J. Antibiot. (Tokyo) 67 (9), 625–630. doi:10.1038/ja.2014.107
Goldstein, F. W., Atoui, R., Ben Ali, A., Nguyen, J. C., Ly, A., and Kitzis, M. D. (2004). False Synergy between Vancomycin and Beta-Lactams against Glycopeptide-Intermediate Staphylococcus aureus (GISA) Caused by Inappropriate Testing Methods. Clin. Microbiol. Infect. 10 (4), 342–345. doi:10.1111/j.1198-743X.2004.00856.x
Guan, X., Guan, X., He, L., Hu, B., Hu, J., Huang, X., et al. (2016). Laboratory Diagnosis, Clinical Management and Infection Control of the Infections Caused by Extensively Drug-Resistant Gram-Negative Bacilli: a Chinese Consensus Statement. Clin. Microbiol. Infect. 22 (Suppl. 1), S15–S25. doi:10.1016/j.cmi.2015.11.004
Holbrook, L. M., Keeton, S. J., Sasikumar, P., Nock, S., Gelzinis, J., Brunt, E., et al. (2021). Zafirlukast Is a Broad-Spectrum Thiol Isomerase Inhibitor that Inhibits Thrombosis without Altering Bleeding Times. Br. J. Pharmacol. 178 (3), 550–563. doi:10.1111/bph.15291
Humphries, R., Bobenchik, A. M., Hindler, J. A., and Schuetz, A. N. (2021). Overview of Changes to the Clinical and Laboratory Standards Institute Performance Standards for Antimicrobial Susceptibility Testing, M100, 31st Edition. J. Clin. Microbiol. 59, e002132. doi:10.1128/jcm.00213-21
Imperi, F., Leoni, L., and Visca, P. (2014). Antivirulence Activity of Azithromycin in Pseudomonas aeruginosa. Front. Microbiol. 5, 178. doi:10.3389/fmicb.2014.00178
Jahangiri, A., Neshani, A., Mirhosseini, S. A., Ghazvini, K., Zare, H., and Sedighian, H. (2021). Synergistic Effect of Two Antimicrobial Peptides, Nisin and P10 with Conventional Antibiotics against Extensively Drug-Resistant Acinetobacter Baumannii and Colistin-Resistant Pseudomonas aeruginosa Isolates. Microb. Pathog. 150, 104700. doi:10.1016/j.micpath.2020.104700
Jenssen, H., Hamill, P., and Hancock, R. E. (2006). Peptide Antimicrobial Agents. Clin. Microbiol. Rev. 19 (3), 491–511. doi:10.1128/cmr.00056-05
Kart, D., Reçber, T., Nemutlu, E., and Sagiroglu, M. (2021). Sub-Inhibitory Concentrations of Ciprofloxacin Alone and Combinations with Plant-Derived Compounds against P. aeruginosa Biofilms and Their Effects on the Metabolomic Profile of P. aeruginosa Biofilms. Antibiot. (Basel) 10 (4). doi:10.3390/antibiotics10040414
Khondker, A., and Rheinstädter, M. C. (2020). How Do Bacterial Membranes Resist Polymyxin Antibiotics? Commun. Biol. 3 (1), 77. doi:10.1038/s42003-020-0803-x
Kim, H. A., Ryu, S. Y., Seo, I., Suh, S. I., Suh, M. H., and Baek, W. K. (2015). Biofilm Formation and Colistin Susceptibility of Acinetobacter Baumannii Isolated from Korean Nosocomial Samples. Microb. Drug Resist 21 (4), 452–457. doi:10.1089/mdr.2014.0236
Koo, H., Allan, R. N., Howlin, R. P., Stoodley, P., and Hall-Stoodley, L. (2017). Targeting Microbial Biofilms: Current and Prospective Therapeutic Strategies. Nat. Rev. Microbiol. 15 (12), 740–755. doi:10.1038/nrmicro.2017.99
Lee, C. R., Lee, J. H., Park, M., Park, K. S., Bae, I. K., Kim, Y. B., et al. (2017). Biology of Acinetobacter Baumannii: Pathogenesis, Antibiotic Resistance Mechanisms, and Prospective Treatment Options. Front. Cell. Infect. Microbiol. 7, 55. doi:10.3389/fcimb.2017.00055
Leu, H. S., Ye, J. J., Lee, M. H., Su, L. H., Huang, P. Y., Wu, T. L., et al. (2014). Synergy of Imipenem/colistin Methanesulfonate Combinations against Imipenem-Nonsusceptible Multidrug-Resistant Acinetobacter Baumannii. J. Microbiol. Immunol. Infect. 47 (5), 406–411. doi:10.1016/j.jmii.2013.05.007
Lin, F., Yu, B., Wang, Q., Yuan, M., and Ling, B. (2021). Combination Inhibition Activity of Chlorhexidine and Antibiotics on Multidrug-Resistant Acinetobacter Baumannii In Vitro. BMC Infect. Dis. 21 (1), 266. doi:10.1186/s12879-021-05963-6
Liu, X., Yin, H., Weng, C. X., and Cai, Y. (2016). Low-Frequency Ultrasound Enhances Antimicrobial Activity of Colistin-Vancomycin Combination against Pan-Resistant Biofilm of Acinetobacter Baumannii. Ultrasound Med. Biol. 42 (8), 1968–1975. doi:10.1016/j.ultrasmedbio.2016.03.016
Magiorakos, A. P., Srinivasan, A., Carey, R. B., Carmeli, Y., Falagas, M. E., Giske, C. G., et al. (2012). Multidrug-resistant, Extensively Drug-Resistant and Pandrug-Resistant Bacteria: an International Expert Proposal for Interim Standard Definitions for Acquired Resistance. Clin. Microbiol. Infect. 18 (3), 268–281. doi:10.1111/j.1469-0691.2011.03570.x
Mascher, T., Margulis, N. G., Wang, T., Ye, R. W., and Helmann, J. D. (2003). Cell Wall Stress Responses in Bacillus Subtilis: the Regulatory Network of the Bacitracin Stimulon. Mol. Microbiol. 50 (5), 1591–1604. doi:10.1046/j.1365-2958.2003.03786.x
Mohammed, M. A., Ahmed, M. T., Anwer, B. E., Aboshanab, K. M., and Aboulwafa, M. M. (2020). Propranolol, Chlorpromazine and Diclofenac Restore Susceptibility of Extensively Drug-Resistant (XDR)-Acinetobacter Baumannii to Fluoroquinolones. PLoS One 15 (8), e0238195. doi:10.1371/journal.pone.0238195
Mulani, M. S., Kamble, E. E., Kumkar, S. N., Tawre, M. S., and Pardesi, K. R. (2019). Emerging Strategies to Combat ESKAPE Pathogens in the Era of Antimicrobial Resistance: A Review. Front. Microbiol. 10, 539. doi:10.3389/fmicb.2019.00539
Navidifar, T., Amin, M., and Rashno, M. (2019). Effects of Sub-inhibitory Concentrations of Meropenem and Tigecycline on the Expression of Genes Regulating Pili, Efflux Pumps and Virulence Factors Involved in Biofilm Formation by Acinetobacter Baumannii. Infect. Drug Resist 12, 1099–1111. doi:10.2147/idr.S199993
Park, H. J., Cho, J. H., Kim, H. J., Han, S. H., Jeong, S. H., and Byun, M. K. (2019). Colistin Monotherapy versus Colistin/rifampicin Combination Therapy in Pneumonia Caused by Colistin-Resistant Acinetobacter Baumannii: A Randomised Controlled Trial. J. Glob. Antimicrob. Resist 17, 66–71. doi:10.1016/j.jgar.2018.11.016
Parnham, M. J., Erakovic Haber, V., Giamarellos-Bourboulis, E. J., Perletti, G., Verleden, G. M., and Vos, R. (2014). Azithromycin: Mechanisms of Action and Their Relevance for Clinical Applications. Pharmacol. Ther. 143 (2), 225–245. doi:10.1016/j.pharmthera.2014.03.003
Peng, Q., Lin, F., and Ling, B. (2020). In Vitro activity of Biofilm Inhibitors in Combination with Antibacterial Drugs against Extensively Drug-Resistant Acinetobacter Baumannii. Sci. Rep. 10 (1), 18097. doi:10.1038/s41598-020-75218-y
Pletzer, D., and Hancock, R. E. (2016). Antibiofilm Peptides: Potential as Broad-Spectrum Agents. J. Bacteriol. 198 (19), 2572–2578. doi:10.1128/jb.00017-16
Selasi, G. N., Nicholas, A., Jeon, H., Na, S. H., Kwon, H. I., Kim, Y. J., et al. (2016). Differences in Biofilm Mass, Expression of Biofilm-Associated Genes, and Resistance to Desiccation between Epidemic and Sporadic Clones of Carbapenem-Resistant Acinetobacter Baumannii Sequence Type 191. PLoS One 11 (9), e0162576. doi:10.1371/journal.pone.0162576
Shenkutie, A. M., Yao, M. Z., Siu, G. K., Wong, B. K. C., and Leung, P. H. (2020). Biofilm-Induced Antibiotic Resistance in Clinical Acinetobacter Baumannii Isolates. Antibiot. (Basel) 9 (11). doi:10.3390/antibiotics9110817
Sierra, J. M., Fusté, E., Rabanal, F., Vinuesa, T., and Viñas, M. (2017). An Overview of Antimicrobial Peptides and the Latest Advances in Their Development. Expert Opin. Biol. Ther. 17 (6), 663–676. doi:10.1080/14712598.2017.1315402
Singh, S., Singh, S. K., Chowdhury, I., and Singh, R. (2017). Understanding the Mechanism of Bacterial Biofilms Resistance to Antimicrobial Agents. Open Microbiol. J. 11, 53–62. doi:10.2174/1874285801711010053
Sobouti, B., Mirshekar, M., Fallah, S., Tabaei, A., Fallah Mehrabadi, J., and Darbandi, A. (2020). Pan Drug-Resistant Acinetobacter Baumannii Causing Nosocomial Infections Among Burnt Children. Med. J. Islam Repub. Iran. 34, 24. doi:10.34171/mjiri.34.24
Spellberg, B., and Bonomo, R. A. (2015). Combination Therapy for Extreme Drug-Resistant Acinetobacter Baumannii: Ready for Prime Time? Crit. Care Med. 43 (6), 1332–1334. doi:10.1097/ccm.0000000000001029
Tacconelli, E., Carrara, E., Savoldi, A., Harbarth, S., Mendelson, M., Monnet, D. L., et al. (2018). Discovery, Research, and Development of New Antibiotics: the WHO Priority List of Antibiotic-Resistant Bacteria and Tuberculosis. Lancet Infect. Dis. 18 (3), 318–327. doi:10.1016/s1473-3099(17)30753-3
Wang, G., Li, X., and Wang, Z. (2016). APD3: the Antimicrobial Peptide Database as a Tool for Research and Education. Nucleic Acids Res. 44 (D1), D1087–D1093. doi:10.1093/nar/gkv1278
Keywords: peptide antibiotics, antimicrobials, Acinetobacter baumannii, synergy, antibacterial
Citation: Meng Q, Lin F and Ling B (2022) In Vitro Activity of Peptide Antibiotics in Combination With Other Antimicrobials on Extensively Drug-Resistant Acinetobacter baumannii in the Planktonic and Biofilm Cell. Front. Pharmacol. 13:890955. doi: 10.3389/fphar.2022.890955
Received: 07 March 2022; Accepted: 28 April 2022;
Published: 11 May 2022.
Edited by:
Karl Hassan, The University of Newcastle, AustraliaReviewed by:
Hasan Ejaz, Al Jouf University, Saudi ArabiaCopyright © 2022 Meng, Lin and Ling. This is an open-access article distributed under the terms of the Creative Commons Attribution License (CC BY). The use, distribution or reproduction in other forums is permitted, provided the original author(s) and the copyright owner(s) are credited and that the original publication in this journal is cited, in accordance with accepted academic practice. No use, distribution or reproduction is permitted which does not comply with these terms.
*Correspondence: Baodong Ling, bGluZ2Jhb2RvbmdAY21jLmVkdS5jbg==
Disclaimer: All claims expressed in this article are solely those of the authors and do not necessarily represent those of their affiliated organizations, or those of the publisher, the editors and the reviewers. Any product that may be evaluated in this article or claim that may be made by its manufacturer is not guaranteed or endorsed by the publisher.
Research integrity at Frontiers
Learn more about the work of our research integrity team to safeguard the quality of each article we publish.